- 1Laboratory for Neurochemistry and Molecular Neurobiology, Croatian Institute for Brain Research, School of Medicine, University of Zagreb, Zagreb, Croatia
- 2Laboratory for Cell Biology and Signalling, Department for Molecular Biology, Institute Ruđer Bošković, Zagreb, Croatia
- 3Department for Chemistry and Biochemistry, School of Medicine, University of Zagreb, Zagreb, Croatia
Neurodevelopment encompasses a complex series of molecular events occuring at defined time points distinguishable by the specific genetic readout and active protein machinery. Due to immense intricacy of intertwined molecular pathways, extracting and describing all the components of a single pathway is a demanding task. In other words, there is always a risk of leaving potential transient molecular partners unnoticed while investigating signaling cascades with core functions—and the very neglected ones could be the turning point in understanding the context and regulation of the signaling events. For example, signaling pathways of Notch and Toll-like receptors (TLRs) have been so far unrelated in the vast body of knowledge about neurodevelopment, however evidence from available literature points to their remarkable overlap in influence on identical molecular processes and reveals their potential functional links. Based on data demonstrating Notch and TLR structural engagement and functions during neurodevelopment, along with our description of novel molecular binding models, here we hypothesize that TLR proteins act as likely crucial components in the Notch signaling cascade. We advocate for the hypothesized role of TLRs in Notch signaling by: elaborating components and features of their pathways; reviewing their effects on fates of neural progenitor cells during neurodevelopment; proposing molecular and functional aspects of the hypothesis, along with venues for testing it. Finally, we discuss substantial indications of environmental influence on the proposed Notch-TLR system and its impact on neurodevelopmental outcomes.
1 Notch and Toll—structural and signaling features
During neurodevelopment, neural progenitor cells (NPCs) settled in the neuroectodermal zone act as a self-sustainable pool of cells that subsequently differentiate into neurons, oligodendrocytes, and astrocytes. In the decision-making time points, NPCs mobilize crucial developmental pathways, creating the molecular crossroads for diverse cellular fates. Across metazoan clades, the Notch signaling cascade propels developmental mechanisms and represents a fundamental pathway for development of the central nervous system (CNS) (Gaiano and Fishell, 2002; Stasiulewicz et al., 2015; Gozlan and Sprinzak, 2023). Architecture and molecular organization of the developing brain is under the influence of Notch in synergy with multiple pathways, such as Sonic Hedgehog (Shh) and Wnt signaling pathways (Akai et al., 2005; Keilani and Sugaya, 2008; Jacobs and Huang, 2019, 2021; Marczenke et al., 2021). Being a cell contact-dependent pathway, the Notch signaling hub events occur on the membranes of signal-sending and signal-receiving cells (Fiúza and Arias, 2007; D’Souza et al., 2010; Lovendahl et al., 2018). The principle of the signaling cascade comprises binding of Notch receptors on the signal-receiving cell with the Delta/Serrate/LAG-1(DSL) family of ligands, mostly Jagged and Delta-like (J/D), on the signal-sending cell. The juxtacrine J/D complex association and endocytosis-induced tension generation are vital for exposure of S2 and S3 cleavage sites (Sprinzak and Blacklow, 2021). A series of ADAM and γ-secretase proteolytic cleavages release the membrane-bound Notch intracellular domain (NICD)(Brou et al., 2000; Mumm et al., 2000; Sanchez-Irizarry et al., 2004; Fiúza and Arias, 2007; Lovendahl et al., 2018; Sprinzak and Blacklow, 2021; Wolfe and Miao, 2022), and its nuclear translocation activates Notch-targeted HES and HEY genes (Figure 1) (Kageyama and Ohtsuka, 1999). The selection of receptor and ligand isoforms sets up a large number of binding combinations related to unique (Six et al., 2003; Gordon et al., 2007; D’Souza et al., 2010; Lovendahl et al., 2018; Zeronian et al., 2021; Gozlan and Sprinzak, 2023) molecular and cellular outcomes. Let us first describe Notch and its J/D ligand extracellular engagement mechanism in order to explain in more depth the hypothesis about Toll-like receptors (TLRs) as novel and essential partners in Notch signaling during neurodevelopment.
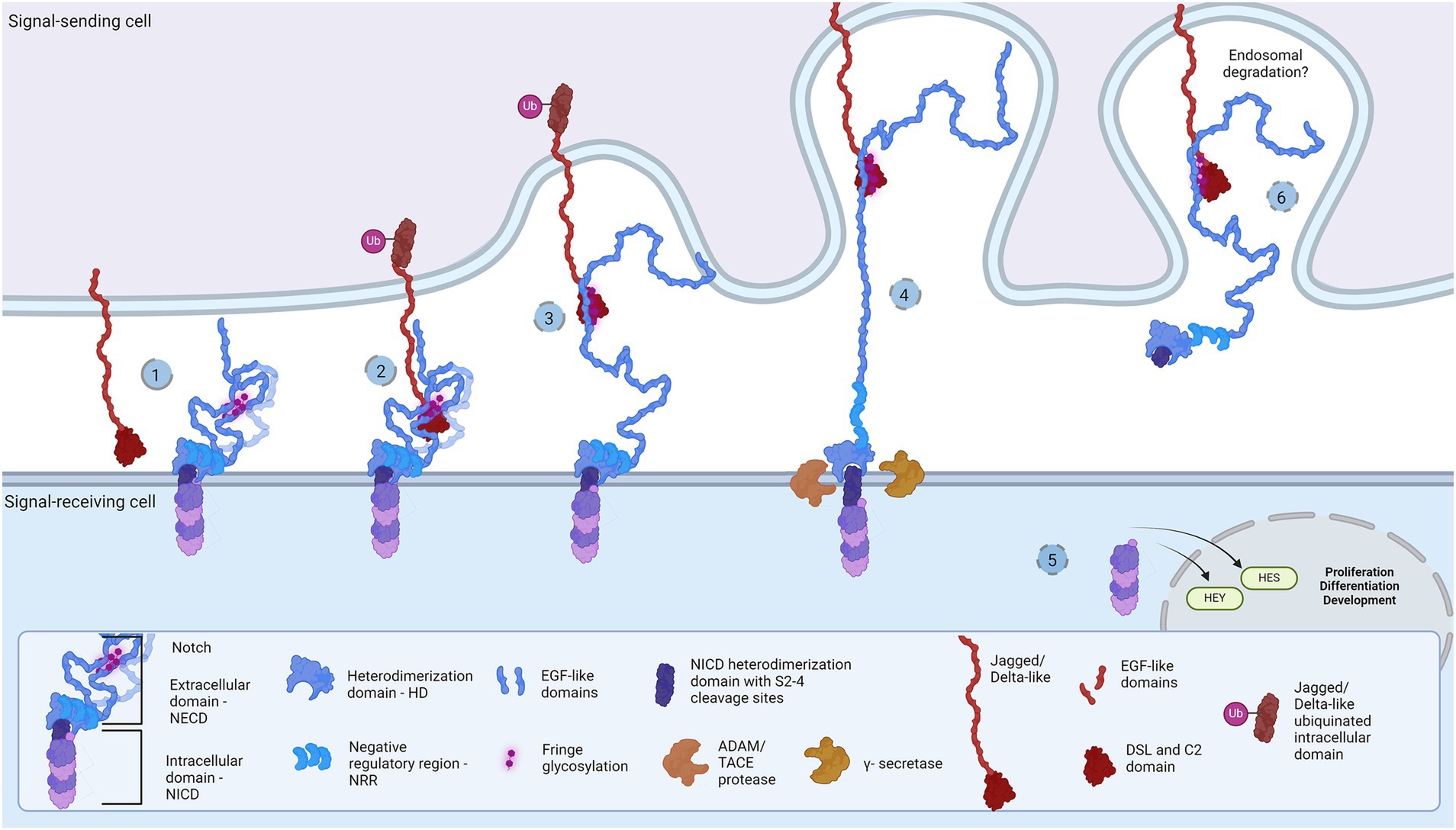
Figure 1. Representation of the Notch signaling pathway: The core proteins Notch and DSL ligands, Jagged and Delta-like, bind in cis (inhibition) and trans (activation) modes. Notch is a dimer of intracellular (Notch intracellular domain, NICD) and extracellular domain (Notch extracellular domain, NECD). (1) The protective dome-like NECD is glycosylated by Fringe glycotransferases. DSL ligand is composed of EGF-like domains with a Notch-interacting DSL/C2 domain. Posttranslational modifications regulate ligand affinity. Upon binding (2), the interaction groove firm binding and tension induction triggers endocytosis of DSL ligand (3) and NECD dissociation from NICD. Exposed NCID is cleaved by ADAM and γ-secretase (4) to release transcription activator NICD domain that regulates HES and HEY gene expression (5). The NECD/DSL complex undergoes endocytosis and is proposed to be degraded in the endosome (6). Created in BioRender. BioRender.com/f38j163.
Notch receptors (Notch1-4) are transmembrane proteins comprised of two non-covalently bound heterodimers (Sanchez-Irizarry et al., 2004; Lovendahl et al., 2018). The extracellular monomer (Notch extracellular domain, NECD) consists of multiple epidermal growth factor (EGF) domains with DSL-interacting EGF domains wrapping the mechanosensory Negative Regulatory Region (NRR) domain (Sanchez-Irizarry et al., 2004; Lovendahl et al., 2018; Sprinzak and Blacklow, 2021). The dimerization complex resembles a mushroom: buried under folds of the LIN-12/Notch repeat (LNR) domain, the heterodimerization (HD) domain protects the intracellular monomer protruding stem with S2 and S3 cleavage sites (Lovendahl et al., 2018; Sprinzak and Blacklow, 2021). In mammals, two Jagged (Jag1-2) and two out of three Delta-like (Dll1, Dll4) ligands bind Notch and pull off the protecting NECD to reveal the cleavage sites, while Dll3 does not bind Notch and is localized in endosome (Nichols et al., 2007; Musse et al., 2012; Lovendahl et al., 2018; Sprinzak and Blacklow, 2021). The JD’s extracellular portion also has multiple EGF repeats between the N-terminal C2-like immunoglobulin domain connected to the DSL domain and the cysteine-rich region proximally to the membrane (Lovendahl et al., 2018). Both proteins form rod-like open solenoids as a result of variable EGF repeats (Bork et al., 1996; Wouters et al., 2005; Kelly et al., 2010) and are glycosylated which is a standard structural feature of adhesion molecules (Moloney et al., 2000; Chen et al., 2001; Wouters et al., 2005; Yu et al., 2021). The extracellular portion of the Notch receptor responsible for J/D engagement is between EGF8 and EGF13, while the adhering part of the J/D are C2-like and DSL domains (Cordle et al., 2008a; Musse et al., 2012; Luca et al., 2015; Handford et al., 2018). C2-like domain has lipid-binding properties probably acting as a membrane proximity sensor: the lipids in question are glycosphingolipids (GSLs) in Drosophila (Hamel et al., 2010) and phospholipids (PL) in mammals (Ca2+-dependent in Jag) (Chillakuri et al., 2013; Suckling et al., 2017; Handford et al., 2018). Notch EGF10-12 and Jag/Dll C2-DSL interaction groove covers an area of approximately 1,100 Å2 and provides variety and strength of interactions (Cordle et al., 2008a, 2008b; Luca et al., 2015; Handford et al., 2018), i.e., stabilization of glycosylated residues, hydrophobic and H-bond establishment, salt-bridge formation, and Ca2+-dependent structural rigidity induction, enable the conformational change, generate tension which activates endocytosis and NECD dissociation (Nichols et al., 2007; D’Souza et al., 2010; Musse et al., 2012). This action reveals NICD cleavage stem while following proteolytic action leads to subsequent intracellular signaling events (Nichols et al., 2007; Musse et al., 2012; Luca et al., 2017; Sprinzak and Blacklow, 2021). The destiny of the Notch/J/D ligand complex after endocytosis is elusive but is believed to undergo endosomal degradation. In order to bind the Notch receptor, J/D has to be in ‘flexed’ conformation which exposes the binding groove, and it is supposed that J/D spontaneously transitions between ‘flexed’ and ‘stiff’ conformation (Luca et al., 2015, 2017; Handford et al., 2018). The dynamics and steps of conformational transition, how exactly the Jag and Dll achieve their flexed conformation, find and bind Notch, and what happens with the pulled NECD/J/D ligand complex in the signal-sending cell remains unclear. This leaves space for speculations: is there an additional molecule which delivers and presents ligands to Notch receptors; does the signal-sending cell recognizes the final response and if so is there a response-reading machinery in the signal-sending cell? Here we aim to clear up these questions and offer a novel perspective on the Notch signaling cascade, by introducing the TLR family as potential member of the Notch cascade.
The Toll-like receptor (TLR) family of proteins first emerged in Cnidaria (Beutler and Rehli, 2002; Brennan and Gilmore, 2018; Liu et al., 2019) and has since evolved as a patterning tool in development (Barak et al., 2014; Noordstra and Yap, 2021; Umetsu, 2022; Weiss and O’Neill, 2022; Sommariva et al., 2023) and a pattern-recognition tool in immunity (Crack and Bray, 2007; Ma et al., 2007; Park et al., 2009; Scheffel et al., 2012; Anthoney et al., 2018; Chen et al., 2019). The first mention of TLR proteins was the Toll receptor in Drosophila melanogaster embryology (Parthier et al., 2014; Al Asafen et al., 2020; Umetsu, 2022), where it serves as a molecular agent for cellular organization in dorsoventral body symmetry formation. Namely, the dosage of ligand Spätzle is measured with the Toll receptor and translated into Dorsal, Snail, and Twist protein gradients, resulting in a cellular patterning of ectoderm, neurogenic ectoderm, and mesoderm (Umetsu, 2022). Later, the same receptor was revealed as a signaling hub in innate immunity upon external pathogen stimuli (Takeda and Akira, 2004; Botos et al., 2011). These two functions are preserved with evolutionary modifications in mammals. For instance, mammalian TLR actions associated with innate immunity differ from Drosophila’s due to an additional direct ligand-binding capacity; TLR expression in NPCs and involvement in developmental processes, without apparent endogenous ligand, confirms its role in neurodevelopment. With that said, let us explore the structural and functional features of TLRs that will speak in favor of the hypothesis.
Structurally, the Toll-like receptors form an open solenoid by packing leucine-rich repeats (LRRs) in a horseshoe-shaped extracellular domain with adhesive and binding capacity (Jin et al., 2007; Kang et al., 2009; Park et al., 2009; Botos et al., 2011; Salunke et al., 2012). The function of LRR proteins depends on their structure (specifically, radii and number of LRR modules) (Kobe and Deisenhofer, 1994; Kobe and Kajava, 2001; Enkhbayar et al., 2004) and regarding the LRR composition, TLRs are divided into long and short structures (Figure 2). The intracellular domain of TLRs is a Toll-interleukine-1 receptor (TIR) homology domain that targets TIR-containing proteins, e.g., MyD88 and TRIF, activating TAB2/3/TAK1 and TBK1 relay, TRAF3/6-operated molecular switch. In brief, TLR1,2,4–9, dimers bind TIRAP and MyD88 that scaffold IRAK4/IRAK1/2 and activated TRAF3/6 proteins. Described down-stream effects of signalization upon TLR3 and TLR4 dimerization (Beutler and Rehli, 2002; Ge et al., 2002; Matsumura et al., 2003; Verstak et al., 2009; Smoak et al., 2010; Tukhvatulin et al., 2010) which inlude TRAM and TRIF adaptors, activation of TRAF3 and TRAF6, formation of TAB2/3/TAK1 signalosome and regulation of IKK and MAPK signaling events are presented in Figure 3. TRAF3 activates additional families of IKK-like kinases (TBK1, IKKi). Activated genetic readouts and protein machinery are described in immune answer (Vabulas et al., 2001; Jack et al., 2019; Chen et al., 2021), proliferation and differentiation (Lathia et al., 2008; Ulrich et al., 2015; Song et al., 2019; Mann et al., 2023), mature and apoptotic phenotypes (Cameron et al., 2007; Ma et al., 2007; Stridh et al., 2011; Ishizuka et al., 2013; Li et al., 2013, 2020; Shi et al., 2013; Church et al., 2016; Abdul et al., 2019; Werkman et al., 2020). The library of TLR-activating ligands induces TLR homodimerization in antiparallel orientation, except for versatile TLR2, which builds heterodimers with TLR1, TLR4, or TLR6 in a ligand-dependent fashion (Kang et al., 2009; Schenk et al., 2009; Fernandez-Lizarbe et al., 2013; van Bergenhenegouwen et al., 2013; Koymans et al., 2015; Liu et al., 2019; Ved et al., 2021; Galleguillos et al., 2022). TLR structural features, LRR concave β-sheet, and outer α-helices surface delineate their specificity to ligands, creating a multifaceted recognition hub (Figures 2, 4A) (Werling et al., 2009). Membrane localization of TLR2, TLR4, and TLR5 enables them to recognize and bind di-and triacylated lipoproteins (TLR2/1, TLR2/6) (Jin et al., 2007; Kang et al., 2009), LPS presented by coreceptors (TLR4) (Kim et al., 2007; Park et al., 2009) and flagellin (TLR5) (Smith and Ozinsky, 2002; Yoon et al., 2012). Endosomal TLRs bind viral dsRNA (TLR3), ssRNA (TLR7, TLR8) and ssDNA (TLR9) (Choe et al., 2005; Wang et al., 2006; Botos et al., 2009; Gosu et al., 2019). Mammalian TLRs are designed to fit and bind pathogen-associated molecular pattern molecules (PAMPs), damage-associated molecular pattern molecules (DAMPs), and protein coreceptors (Akashi-Takamura and Miyake, 2008; Park and Lee, 2013; van Bergenhenegouwen et al., 2013). Interestingly, the evolutionary older ligands, particularly in Drosophila development, are protein ones, and we could consider that TLRs’ protein-binding mode of action makes a conserved modus operandi in developmental processes. TLRs, specifically TLR4, recognize and interact with distinct structural features of protein ligands (Vabulas et al., 2001; Kim et al., 2005; Liu et al., 2012; van Bergenhenegouwen et al., 2013; Mehmeti et al., 2019; Ved et al., 2021), particularly protein folds and carbohydrate moieties, opening the possibility that a (glyco)protein could be a potential TLR4 neurodevelopmental ligand. The protein coreceptors that bind to mammalian TLR4 have a distinct C2 globulin fold with a lipid binding pocket; e.g., globulin MD-2 in a complex with LPS is TLR4 binding partner (Gioannini et al., 2004; Kim et al., 2007; Schumann, 2011). TLR4/MD-2 quaternary complex consists of two MD-2 molecules engaged with both TLR4 monomers. Unique structural features of TLR4 allow MD-2 binding at the concave dimerization face of the first and the back of the second TLR4 monomer (Figure 4A) (Kim et al., 2007). Protein MD-2 and Jag/Dll C2-like domains have evolutionarily conserved folds, showing overall similarity between overlapped models of MD-2 and Jag/Dll C2-like domains (Figure 4A2). This structural similarity between the Notch ligands and the TLR4 coreceptor is the first indication that TLRs possibly act as a binding partners of Notch ligands.
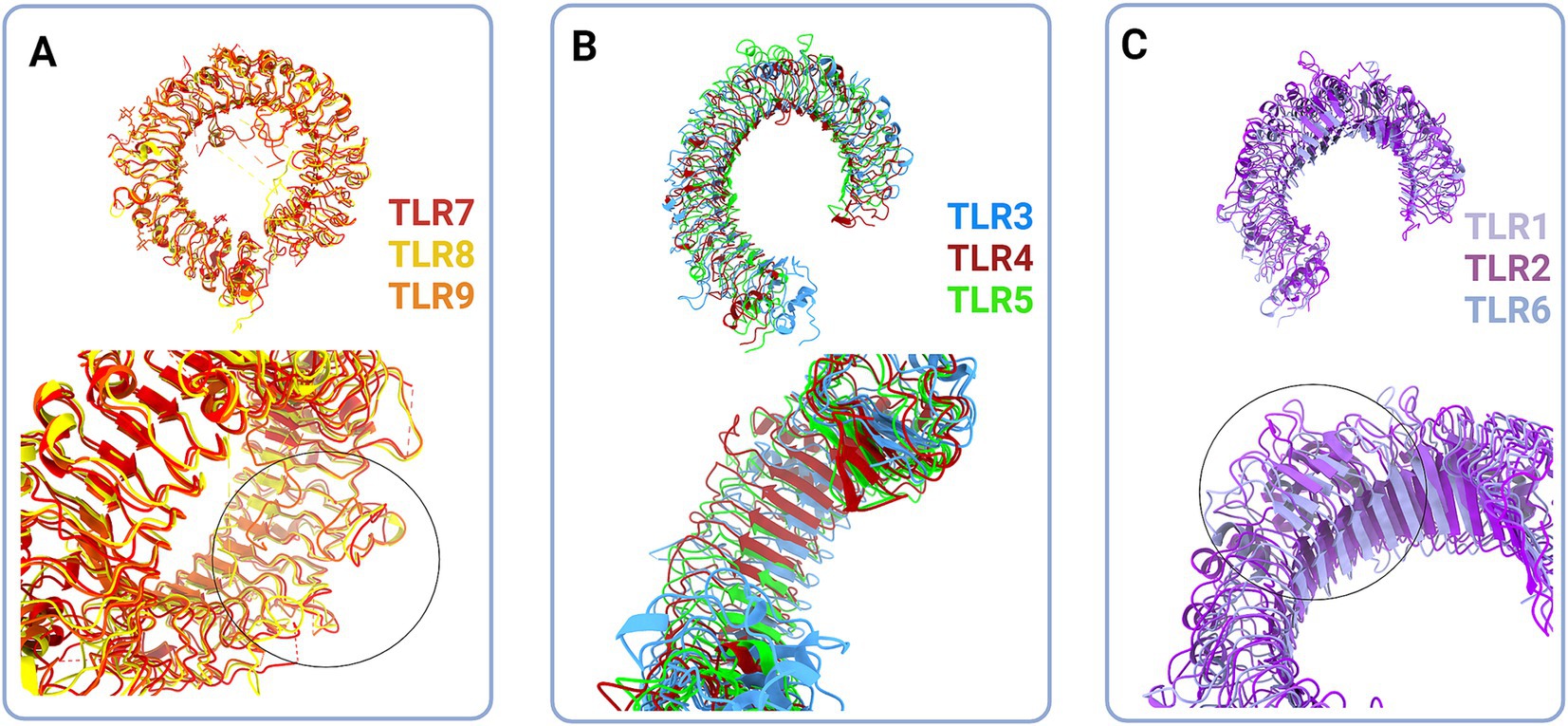
Figure 2. Structural classes of Toll-like receptors (TLRs) are long (A and B) and short (C) TLRs. (A) Superimposed structural models of TLR7 (red, hTLR7 PDB:7CYN), TLR8 (yellow, hTLR8 PDB:7R54) and TLR9 (tangerine, mTLR PDB:3WPG) (upper panel). Zooming in on the loops on dimerization side of long TLRs reveals that loops enclose cavity between two dimers (bottom panel, circle). (B) Superimposed structural models of TLR3 (blue, hTLR3 PDB:7C76), TLR 4 (deep red, hTLR4 PBD:3FXI) and TLR5 (green, hTLR5 PDB:3J0A) (upper panel). Zoom in on the LRR types in TLR3, TLR4 and TLR5 (bottom panel). (C) Superimposed structural models of TLR1 (lilac, hTLR1 PDB:6NIH), TLR2 (purple, hTLR2 PDB:6NIG), and TLR6 (light blue, mTLR6 PDB: 3479) (upper panel). Closing up of TLR2, TLR1 and TLR6 β sheets on dimerisation side dictate the ligand cavity depth on top of TLR monomer (bottom panel). Created in BioRender. BioRender.com/o67p300.
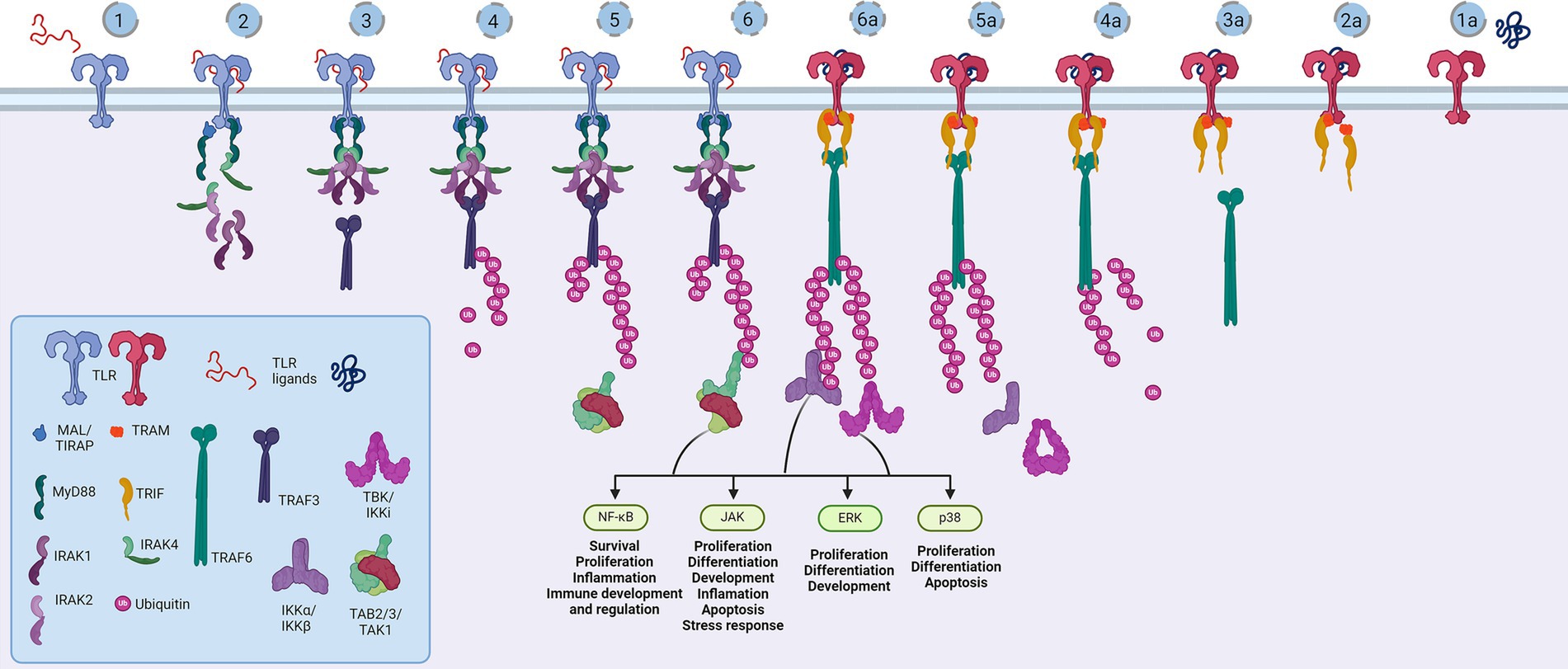
Figure 3. Representation of the Toll-like receptor pathway. Toll receptors scaffold signalosomes in membrane proximity, e.g., TLR dimers scaffold Mydosome (TLR2/1, TLR2/6, TLR4, TLR5, TLR7, TLR8 and TLR9) (1–6) and TRIFosome (TLR3 and TLR4) (1a-6a). Ligand binding to TLR (1) primes Mydosome. TLR recruits MAL/TIRAP,MyD88, IRAK1/2 and IRAK4 scaffold in hexameric structures (2) activating TRAF3 and TRAF6 (3) which are able to auto-ubiquinate (4). Poly-ubiquitin chains associate (5) and activate TAB2/3/TAK1 kinases (6). Trifosome is primed with TLR ligand binding (1a). Associated TRAM and TRIF (2a) activate TRAF3 directly (3a) and TRAF6 in a RIP1-dependant fashion. TRAF3 polyubiquitination (4a) recruits (5a) and activates TBK/IKKi kinases (6a). These kinases regulate IKKα/IKKβ kinases and input of NF-κB, JAK, ERK and p38 regulated genes and proceses. Created in BioRender. BioRender.com/a29t370.
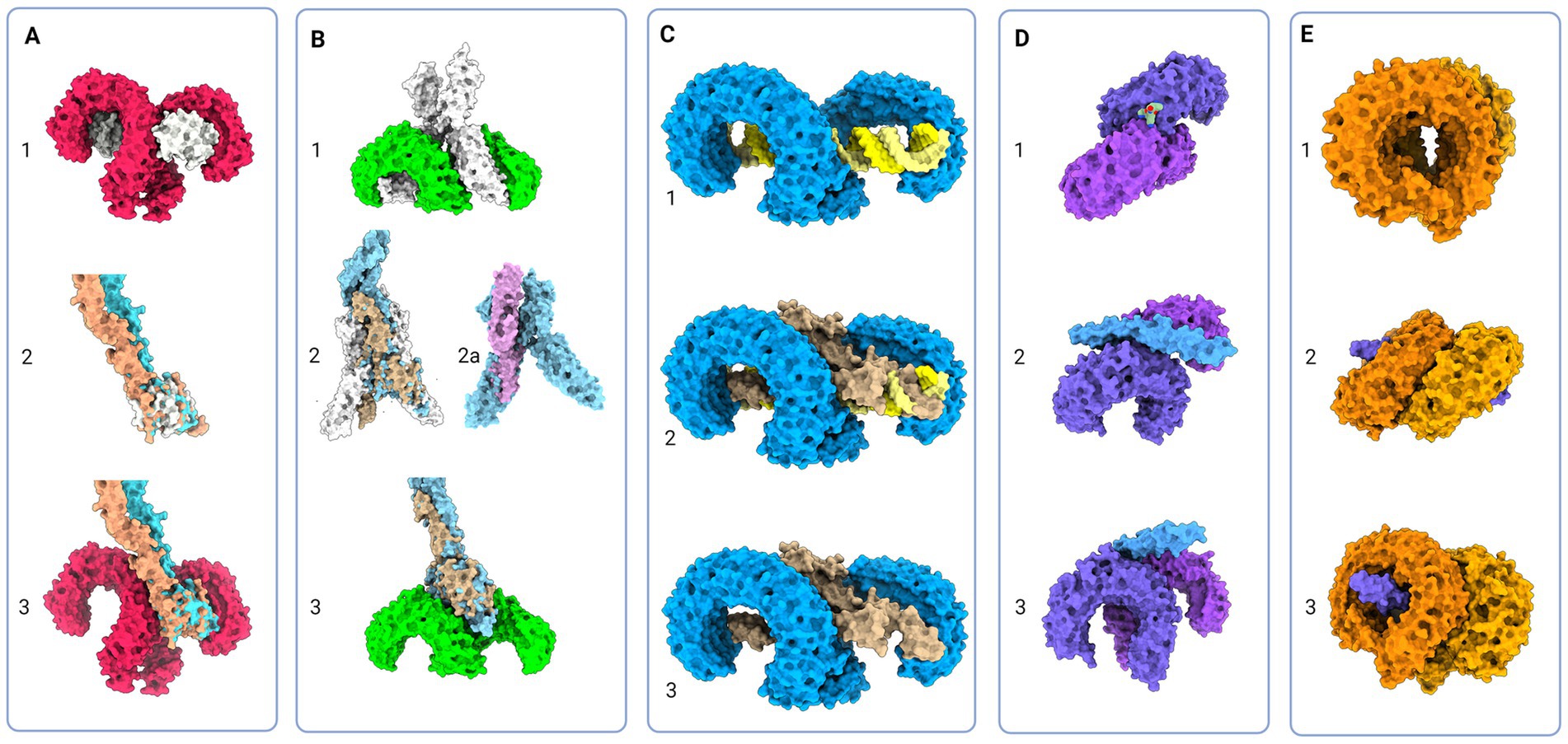
Figure 4. Protein models of TLRs with natural ligands and superimposition of TLR and Notch signaling components; (A) 1 - Molecular visualization of TLR4 dimer with coreceptor MD-2 (PDB:3FXI), 2 – Aligned models of MD-2, Jagged (apricot, PDB:5UK5) and Delta-like (tan, PDB:4XL1), 3 – Proposed model of Jagged and Delta-like in complex with TLR4; (B) 1 – TLR5 model in complex with flagellin (PDB:3 V47), 2 – Flagellin model aligned with NECD/Delta-like complex (tan, PDB:4XLW, light blue, PDB:4XL1), 2a – Flagellin model (PDB:3 V47) aligned with APP core domain (lilac, PDB:3NYL), 3 – Proposed model of NECD/Delta-like in complex with TLR5; (C): 1 - TLR3 in complex with dsDNA (PDB:7DAS), 2 – Superimposed NECD/Delta-like complex over dsRNA in complex with TLR3, and 3 – Theoretical binding model of TLR3 and NECD/Delta-like complex; (D) 1 - TLR1/2 in complex with LPS (PDB:2Z7X), 2 and 3 - Theoretical model of TLR2/1 interaction with Notch EGF-like domains (PDB:4D0E) (E) 1 - Model of TLR7 dimers (PDB:5GMF), 2 and 3 – Theoretical model of EGF-like domains (PDB:4D0E) in complex with TLR7. Created in BioRender. BioRender.com/t26j594.
2 Notch and Tolls in neurodevelopment
To investigate the hypothesis on shared Notch and Toll signaling mechanisms with implications for developing nervous system, we demonstrate systematically the striking functional overlaps of Notch and TLR signaling during neurodevelopment documented in the literature (Table 1).
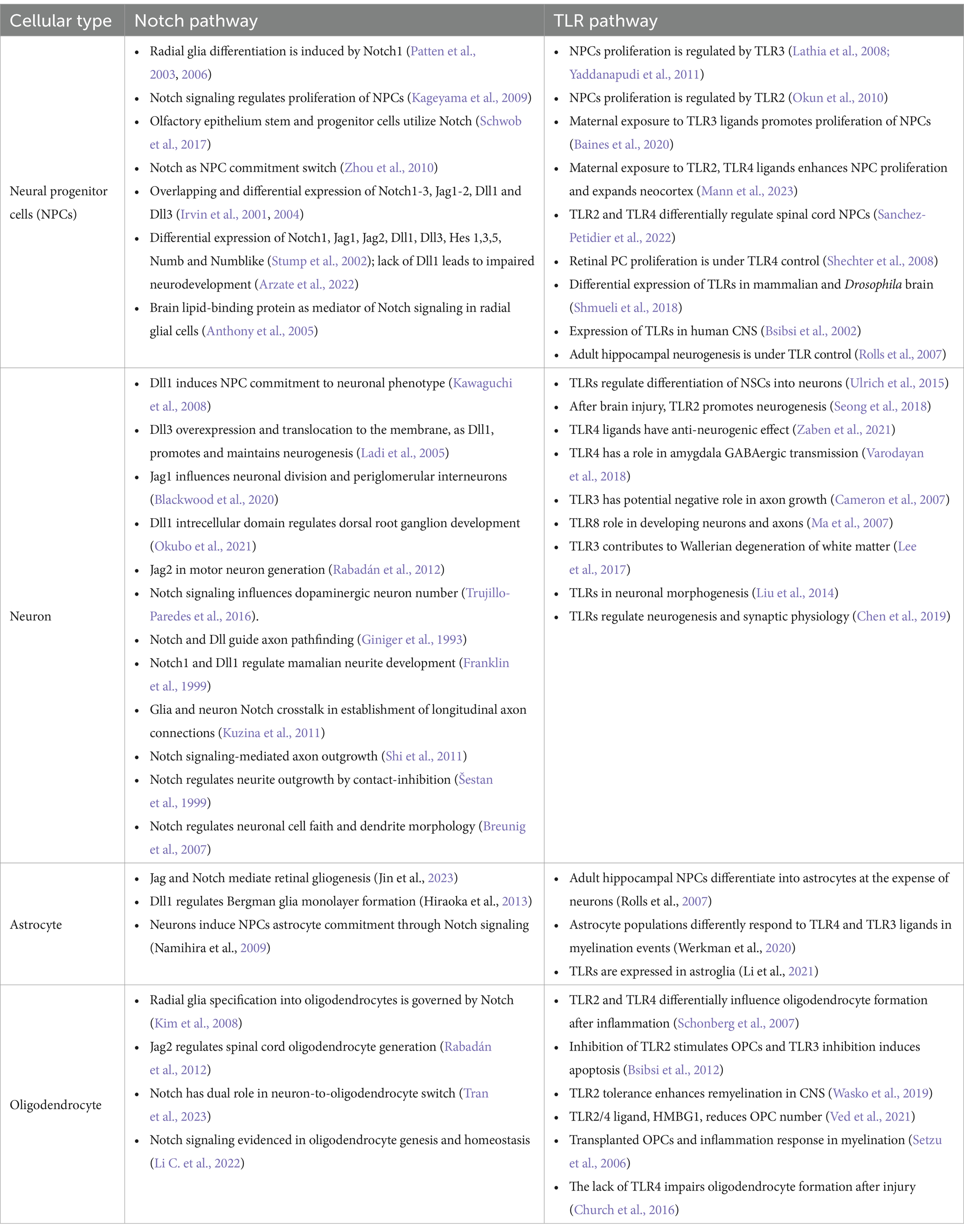
Table 1. Cell-specific expression and functions of Notch and TLR signaling pathways during neurodevelopment.
Described functions of Notch signaling during neurodevelopment encompass NPCs number, identity, spatial organization (Irvin et al., 2001, 2004; Stump et al., 2002; Patten et al., 2006; Keilani and Sugaya, 2008; Kim et al., 2008; Zhou et al., 2010; Rabadán et al., 2012; Blackwood et al., 2020; Marczenke et al., 2021; Mase et al., 2021; Okubo et al., 2021; Li C. et al., 2022; Tran et al., 2023) and connectome formation events (Franklin et al., 1999; Šestan et al., 1999; Breunig et al., 2007; Kuzina et al., 2011; Shi et al., 2011). Notch signaling seems to be crucial for asymmetric NPCs division (Casas Gimeno and Paridaen, 2022) and may be viewed as a switch between NPC differentiation, neurogenesis, and gliogenesis. Also, Notch1 is implicated in radial glia (RG) identity establishment and differentiation to ventral RG (Patten et al., 2003, 2006; Mase et al., 2021). These findings confirm that Notch orchestrates morphology, outgrowth and density ratio of neurons, oligodendrocytes, and astrocytes which eventually has implications on the axonal, dendritic, and synaptic network formation (Šestan et al., 1999; Shi et al., 2011; Kuzina et al., 2011).
Evidenced extensive impact of Notch signaling on critical neurodevelopmental events overlaps with so far known neurodevelopmental functions of TLRs. Firstly, TLR activation affects the immune response during and after (neuro)development, and its effect was observed in cooperation with the Notch pathway and as a Hh and Wnt signaling regulator, respectively (Opitz et al., 2001; Re and Strominger, 2001; Vabulas et al., 2001; Frantz et al., 2001; Matsumura et al., 2003; Leemans et al., 2005; Crack and Bray, 2007; Ziegler et al., 2007; Palaga et al., 2008; Park et al., 2008; Hu et al., 2008; Nichols et al., 2009; Lalancette-Hbert et al., 2009; Cameron and Landreth, 2010; Foldi et al., 2010; Tsao et al., 2011; Liu et al., 2012; Winters et al., 2013; Kim et al., 2013; Okun et al., 2014; Hamidi et al., 2014; Rangasamy et al., 2018; Mehmeti et al., 2019; Martínez-García et al., 2020). Secondly, the expression of TLRs on all cell types across the developing and adult brain unquestionably affirms their impact on neurodevelopment (Barak et al., 2014; Okun et al., 2019).
Clearly, a large body of evidence confirms that certain TLR functions match the scope of Notch functions during embryonic and postnatal brain development (Table 1). Observed functional overlaps indicate that different TLR and Notch proteins govern switches between cellular commitments. For example, the actions of Dll1, TLR2, and TLR4 overlap to some extent: Dll1 induces neurogenesis; loss of Dll1 and TLR4 induces proliferation and gliogenesis; the loss of TLR2 induces proliferation and neurogenesis. From that perspective, TLR2 and Dll are required for proliferation cessation, while TLR4 and Dll1 combinations become inductors of the same cellular fate, suggesting they are a part of the same signaling machinery. It has to be noted that interplay of Notch and TLR signaling has been described in parts, however majority of studies focuses on coordinated responses of the immune system components, based on widely recognized functions of TLRs in innate immunity (Hu et al., 2008; Palaga et al., 2008; Tsao et al., 2011; Shang et al., 2016). The most striking interplay between TLR and Notch in immunity was documented in the study by Hu et al. which confirmed synergistic cooperation between the Notch and TLR pathways mediated by RBP-J. Specifically, the acute TLR induction has been shown to activate HES1 and HEY1 genes, while TLR interleukin genes expression were dependant on NICD nuclear translocation and RBP-J binding. This finding fully supports here presented theory and it would be certainly interesting to confirm the same interplay during neurodevelopment. So far, direct involvement of TLRs in neurodevelopment has been underappreciated, but the herein reviewed findings indicate that TLRs stand at the core program of the nervous system development, and deciphering the role of TLR signaling, particularly as the part of the proposed TLR-Notch complex could be valuable for understanding the spatiotemporal cellular organization of CNS.
3 Hypothesis
We suggest that TLRs are an integral part of the Notch signaling cascade acting as a Notch signaling transductor scaffold and deciphering machinery in both signal-sending and signal-receiving cells. Events that presumably lead to Notch binding and signaling on the signal-receiving cell membrane are organized with TLR1, TLR2, TLR4, and TLR6, while TLR5, TLR3, TLR7, TLR8, and TLR9 are an answer interpreting machinery for the signal-sending cell. The proposed functions for each TLR would occur sequentially as follows: TLR4—ligand bait; TLR1,2,6 heterodimers—Notch sorter and selector; TLR5—answer catcher; TLR3—answer reader; TLR7,8 and 9—answer reader and tuner.
4 Proposed experimental paradigms
To prove the hypothesis, one would need to check for biochemical and physiological evidence of TLR involvement in the Notch pathway and correlate them to the functional and eventually behavior patterns. The biochemistry of the proposed system should first describe chemico-physical interactions, followed by observations using models in vitro and in vivo, and it could be investigated in several ways:
1. Interactive visualization and analysis of molecular structures utilizing available models from PDB library,1 bioinformatics tools such as PDBeFold2 and Chimera program3;
2. Molecular simulation, the fast-science approach using already existing structural data collected on Notch, Jag, Dll, and TLR which could be uploaded into programs providing us with information on the proposed interaction parameters (orientation, energies, contacts, electrostatic, polar, and non-polar interactions) and enabling understanding and locating target protein interactions;
3. Cross-linking mass spectrometry (XL-MS) analysis of NPCs, in vitro and in vivo, would provide a series of data regarding the molecular system of interest. Data collected in controlled conditions, the basis for the interaction matrix, would be challenged with the data collected on TLR knock-out (KO) systems and TLR-challenged systems to untangle the communication code of Notch/TLR signaling. The TLR-challenged system could also be a simulation model to investigate the dose-dependent changes introduced by the environmental setup;
4. State-of-the-art cryo-electron microscopy (EM) technology and its further advancements could be implemented for structural and topographical research of the Notch/TLR pathway. In vitro models of NPCs, organoids, and ex vivo brain imaging would provide starting material for investigation and help describe the exact 3D model and mechanics of Notch/TLR initial signaling events.
The proposed solutions for checking the hypothesis are methodologically feasible and could provide the scientific community with valuable answers. Another way to test the hypothesis would be to use data collected on individuals exposed to TLR agonists in utero during critical neurodevelopment stages. Such data may reveal whether TLR challenge acts on neurodevelopment and whether discrete changes in neuronal number, branching and networks strength establishes neurophenotype basis for different neurobehavioral patterns.
However, the limitations of proposed structural analysis approaches should not be overlooked and require to take into account that any posttranslational modification could be responsible for maximal efficiency in binding between Notch/Jag/TLR, thus not fully resolved from available data. Other challenges might arise from the following facts: extracellular proteins are the most lavishly modified proteins; native conformations and molecular dynamics in the cellular environment are entropy-rich and challenging to simulate; glycosylated residues are intrinsic to cellular communication and serve as recognition residues; sugar code enables additional structural recognition means and induces differential affinity towards interaction partners.
5 Supportive evidence for novel interactions within proposed TLR-Notch assembly
Whilst there are currently no in vivo supportive data, the interactive visualization and analysis of molecular structures utilizing informatics tools (see footnote 2) and Chimera program (see footnote 3) yields several exciting findings. More specifically, the analyses of molecular structure and potential binding models of TLRs and Notch suggest their novel roles and interactions (also see Figures 4, 5).
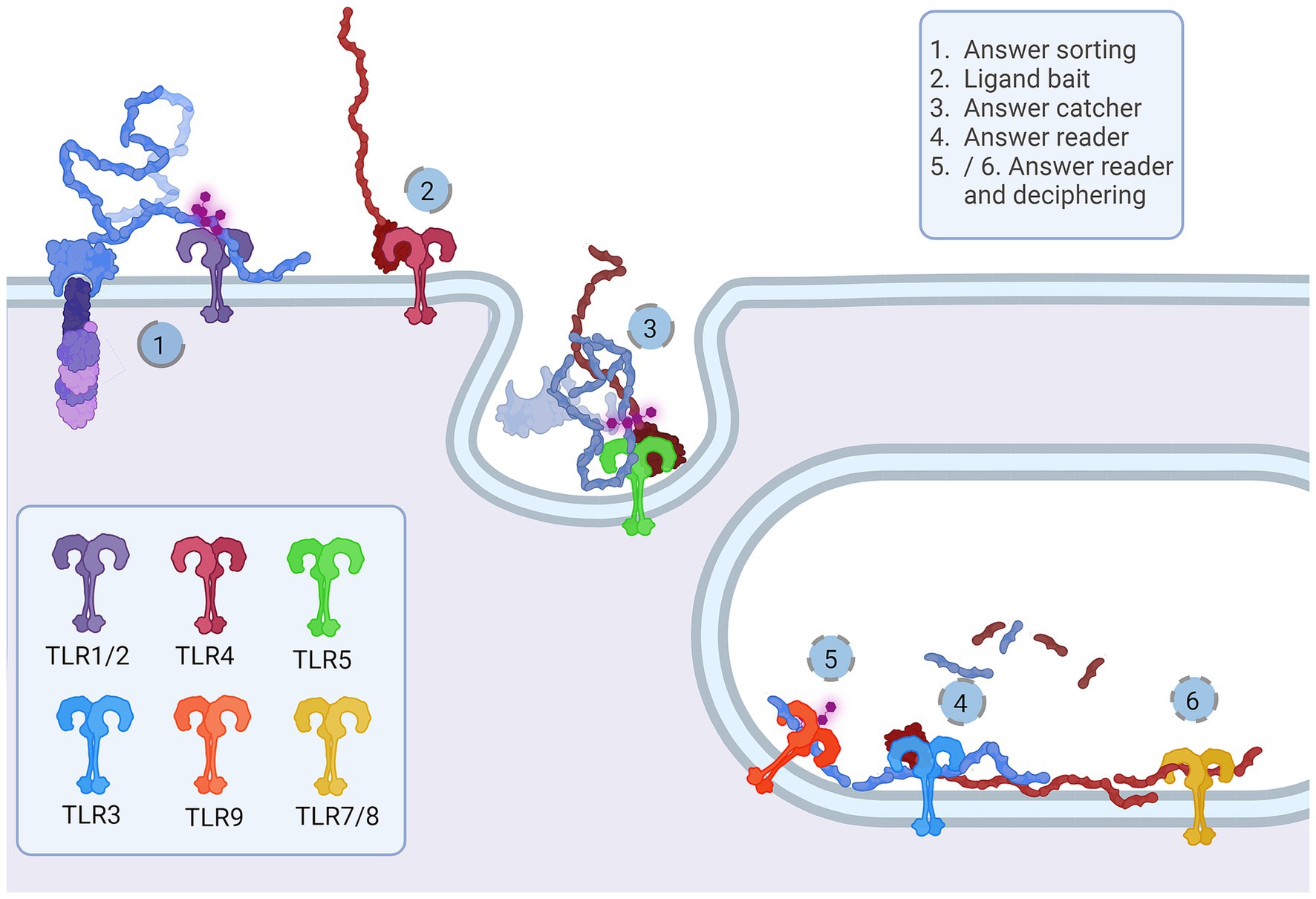
Figure 5. Representation of the proposed Notch-TLR pathway. Each step of the Notch cascade is accompanied by TLR dimerization and downstream signal amplification. TLR1/2/6 Notch sorting and choosing (1) binds DSL ligand fetched by TLR4 (2). The other cell senses the tension and pulls of NECD/DSL complex where TLR5 confirmes catch (5). In endosome, TLR3 signals if NECD/DSL complex is present (4) while TLR7/8/9 fine tunes the answer by examination of EGF PTMs (5 and 6). The accumulation of Notch stimulation of individual TLR proteins regulates NF-κB, JNK, ERK and p38 programes input driving cellular behavior. Created in BioRender. BioRender.com/a30x851.
The majority representation of the Notch/J/D binding model assumes Notch, Jag, and Dll as stiff sticks protruding perpendicularly to the cellular membrane and interacting similarly, deep within the extracellular matrix and away from a cell membrane. From the above-given information, Notch is a tangled dome-like protein complex that needs ligands to remove protection mechanically and enable message transduction. For this to happen, J/D must be in the precise structural orientation for groove exposure and proximity of potential binding partners (Luca et al., 2015, 2017; Handford et al., 2018). The lipophilic property of J/D C2 domain (Chillakuri et al., 2013; Suckling et al., 2017) could prime the interaction with a signal-receiving cell. It is reasonable to suggest that Jag and Dll span the distance and are loosely tensioned between two cells. Lipid-C2 interactions are an open invitation for anchoring protein that will maximize the conformational flex of the J/D structure and be a docking tool for signal-receiving cells. The cross-talk between the C2 domain of J/D and lipids on signal-receiving cells is the point where TLR4 enters the story. By interactive visualization of molecular structures we were able to observe that TLR4 recognizes J/D, binds and locks it in an active conformation, and delivers it to selected Notch (Figure 4A). The described interaction indicates a potential role of TLR4 as a molecular anchorage in signal-receiving cells. We assume that the J/D tethered with a TLR4 would provide an energetically favorable environment and support for flex conformation to ensure its availability for interaction with Notch. The TLR2 with interacting partners TLR1 and TLR6 could be a Notch sorting and ‘decision-making’ tool. Distinct TLR2 heterodimer complexes would recognize the ligand fetched by TLR4 and choose the appropriately accessorized Notch receptor. The glycosylation status of Notch receptors and J/D ligands may be a motif that allows TLR2 to sort and choose binding combinations (Figure 4D). This mode of action indicates that establishing interaction between TLR4/J/D leads to a Notch-binding tension-generating step in the cascade. We propose that TLR4 and TLR2 act as a fly ‘buttoning’ Notch and J/D. The J/D/TLR4-tether generates tension between two membranes, and the tension’s release might be a cue for the signal-sending cell membrane invagination resulting in Notch dissociation and proteolysis on the signal-receiving cell. We hypothesize that TLR4 and TLR2 support Notch and J/D ligand binding, resulting with promotion of NICD downstream cascade in signal-receiving cells.
The signal-sending cell supposedly integrates the molecular events by gathering stimuli from the pulled NECD/J/D complex. Here, based on analyzed molecular structure, we propose that TLR5 acts as an answer-receiving tool. TLR5 may sense the tension and recognize non-interacting J/D and NECDs EGF domains (Figure 4C). The NECD/J/D complex interface facing the signal-sending cell is bifurcated and shaped like ligands recognized by TLR5. With the help of TLR5, the complex is taken inside the cell, where it would be contextualized. In the endosome, degradation releases strands of Notch EGF repeats, NECD/J/D binding complex, unbound J/D ligands, and J/D ligand intracellular domain. Each degradation product is a piece of valuable information for signaling cells. We propose TLR3 recognizes and gives context to cleaved NECD/J/D binding complex (Figure 4C) while TLR7, TLR8, and TLR9 bind EGF repeats (Figure 4E) giving necessary information about cell-receiving cells responses. One single strand-recognizing TLR could also be sensing the degradation product of the cell’s unused ligands and help the signal-sending cell react appropriately to the neighboring population dynamics.
During differentiation, asymmetric inheritance is induced by cellular touch (Casas Gimeno and Paridaen, 2022). TLR links Notch inductors’ direct genetic input to TAB/TAK adductors’ rearrangements in cytoplasmic and membrane phenotypes to accommodate genetic identity-specific protein output. Notch signaling cascades may form an activated membrane patch that integrates changes in signal-receiving cell. Sequestering and ratio of specific IKK(α/β/γ) and IKK-like (TBK1 and IKKi) pathways are most likely needed for modulation of NF-κB-dependent and independent pathways, creating identity commitment signaling and metabolic landscapes. It is likely that TLR signaling modulates NF-κB signals induced by growth factors and synergistically affects PI3K signaling. TLR also activates IKK complex and its downstream mTORC1 and mTORC2 metabolic programmes that are highly active in neurodevelopment (Switon et al., 2017). The mTORC1 conducts neuronal cytoskeletal organization and mTORC2, which is located in endosomes and orchestrates lipid metabolism, crucial for genesis of membranes—cellular ‘skin’ and communication platform of all neural cells (Switon et al., 2017). TLRs also induce MAPK cascades, like p38, JNK and ERK which are known to participate in proliferative and differentiative cellular programs in stem cells (Johnson and Lapadat, 2002). MAPK/ERK pathway is IKK-dependant - these pathways could change and dictate daughter cell fate, behavior and metabolism and could be crucial for the timeline of neurodevelopment (Figure 6).
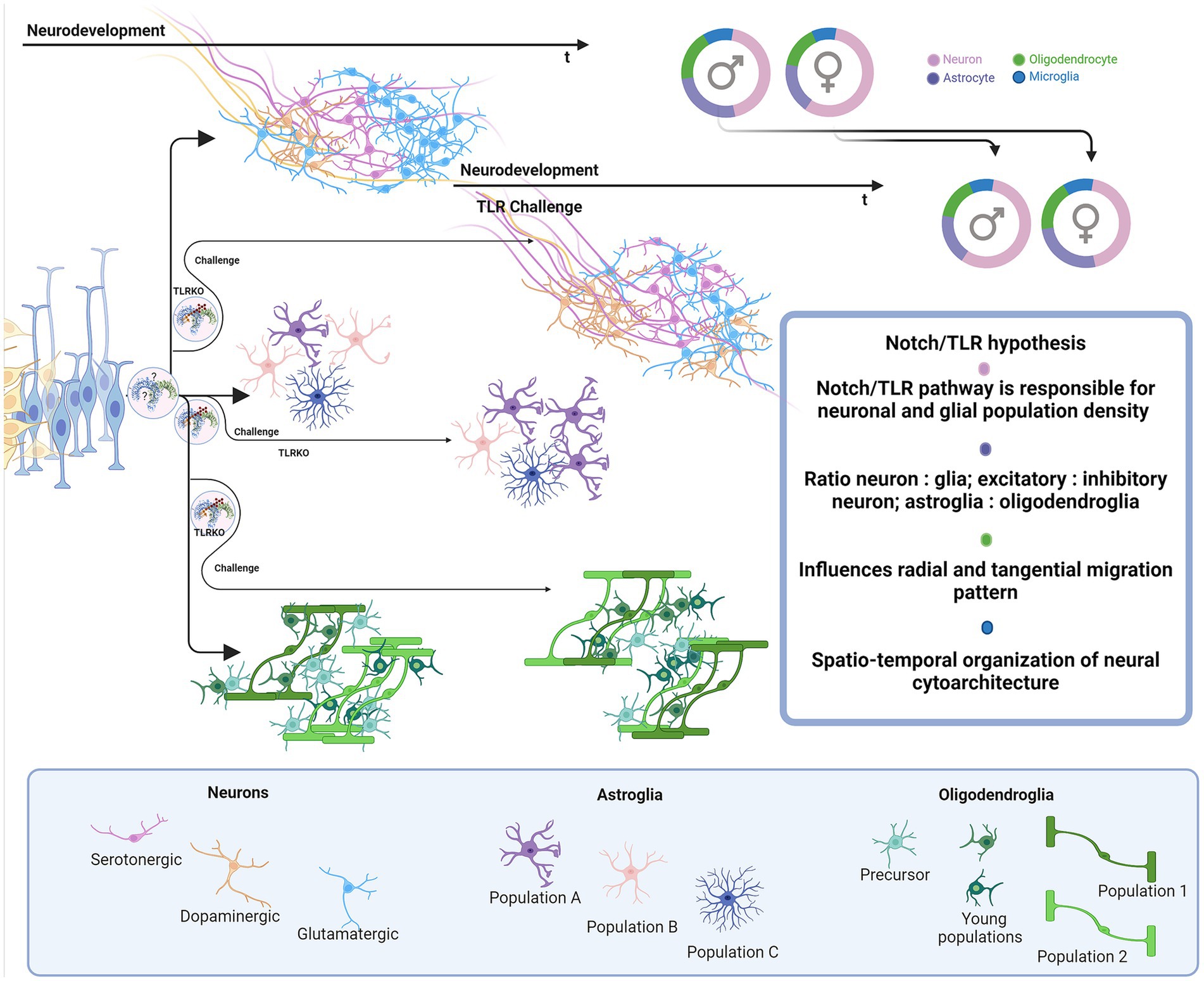
Figure 6. Proposed impact of Notch/TLR system on neurodevelopment. Presuming that Notch/TLR system is responsible for cellular adaptation to various stimuli and subsequent formation of cellular identity, challenged TLR system could evolve different environmentally dictated phenotypes in a sex-dependent manner. Male and female neurophenotypes could be challenged to generate variations in density and identity of cellular populations which eventually influences on neural cytoarchitecture, circuitres and behavioral phenotypes. Created in BioRender. BioRender.com/d80v300.
We believe that the hypothesized model introduces the needed complexity and integrative perspective of the mammalian Notch signaling in brain development. Namely, the current model focuses mostly on the signal-receiving cell as the active player, while the role of the signal-sending cell in Notch signaling is not fully understood and is thus overlooked. The current model resembles instruction-giving rather than communication-based decision-making between the cells. We advocate that TLRs untangle the information given by Notch and J/D ligand structure features and provide necessary information and instruction both to signal-sending and signal-receiving cells. Here hypothesized model involving the TLR family in the Notch signaling pathway takes into account two-way intercellular communication in which both cells actively interpret responses in order to efficiently coordinate developmental and homeostatic processes.
6 Relevance of the hypothesized Notch/Toll system for the molecular basis of neurodevelopment
Notch signaling is one of the central cellular homeostatic mechanisms able to exert adaptive responses both during development and in mature cells. In this respect, incorporating TLRs and their putative role as fundamental parts of Notch signaling provides a new perspective for neurodevelopment- and neuroimmune-related studies.
It is reasonable to assume that TLRs play a role in two overlapping pathways in the brain—the TLR Notch-dependent (ND) cellular development/homeostasis pathway and the environment-dependent (ED) cellular (immune) response. Differentiation between the two pathways would add to the understanding of molecular aspects of neurodevelopment at the phylogenetic and ontogenic levels. The TLR-ND pathway would be best studied in the course of development to confirm the exact steps of how specific TLR, Notch, and J/D ligands achieve formation of cellular identity and communication during maturation and adulthood when established identity becomes a matter of innate immunity. The TLR-ED pathway is intertwined with ND-mediated cellular crosstalk, homeostatic neural cell-to-cell communication and its environmental influence surveillance by immune cells. In this context, innate immunity proves that TLR proteins are of utter importance for the brain and its homeostasis. In order to prevent cellular miscommunication, microglia, like all innate immunity cells, express the entire TLR repertoire, functioning as an affinity bead that siphons environmental molecular patterns, PAMPs and DAMPs. The immune component of TLR-ED has been widely studied, and some of the phenomena described involve developmental/homeostatic ND pathway. The way the environmental stimuli influence individual cellular TLR-ND and ED pathways will be further discussed.
First, we briefly recapitulate the roles of TLRs and their cellular and time-dependent expression patterns during brain development (Table 1) and propose the following sequence of neurodevelopmental events accompanied by TLRs actions. Let us imagine the developing nervous system and the emerging complexity course depending on the cellular ability to communicate with the environment, more precisely, neighboring cells. TLR scaffold, Jag and Dll ligand type on the signal-sending cell, and available Notch receptor on the signal-receiving cell drive the course of proliferation, differentiation, and specialization, e.g., axon, dendrite, and synapse formation.We suggest that NPC TLR-ND system starts as a simple TLR5/TLR3 answer-reading machine for low-specificity Notch binding events. With increasing number of NPCs, the growing density of TLR3-sensing Notch events may allow the emergence of TLR4, TLR7, TLR8, and TLR9. Hipothetically, this quartet slowly silences proliferation and guides NPC asymmetric division and gradual specialization in progenitor subtypes (radial glia, ventral and basal radial glia, retinal progenitor cells, immediate progenitors, oligodendrocyte progenitor cells). The colonization of the brain is now set off.
Waves of climbing young neurons create layers upon layers, as they travel from densely populated, supposedly high-Notch regions, to unpopulated, presumed low-Notch regions.We anticipate that the juxtacrine input of “older” neurons maintains progenitor cell identity on the way to its destination within growing layers. As development proceeds, documented specific changes of TLRs expression paterns (Bsibsi et al., 2002; Shmueli et al., 2018) suggest that TLR3 could be potentially a progenitor and immature phenotype program switch, while TLR4 and TLR9 a differentiative and mature program switches. When situated, TLR7-driven axonal outgrowth (Ma et al., 2007) creates a foundation for establishing the regional-specific neuronal circuitries and networks. The upcoming gliogenesis builds the cell-type diversity; thus, new TLRs emerge (TLR1 and TLR6) which most likely support the novel identity choice. Once positioned glial cells could shed J/D ligands and convey signal to incoming glial progenitors about area population density, creating regions of cell-diverse clusters. When colonization is complete, maturation can take place. Young neurons start to branch, forming inter-regional connections, and oligodendrocytes start myelination in the presumed TLR-ND fashion, where TLR2 and partners support the ongoing Notch events. Astrocytes and already infiltrated microglia mature and continue supporting decades-long network formation and calibration (Schafer et al., 2012, 2013; Miyamoto et al., 2013; Ikegami et al., 2019). Microglial action acquires the brain’s environmental experience and plastic potential, e.g., network malleability, progenitor number, and potency, providing necessary adaptation mechanisms for survival and growth. TLR proteins react to the differences in environmental stimuli, creating unique cellular makeup, connection variety, cellular mosaicism, and organism-specific circuitry, resulting in various neurophenotypes, behaviors, conditions, and pathologies. To address these varieties, one would need to explore the immune response landscape by tracking TLRs involved in ED and address the influence on the ND counterpart pathway.
Multiple neurodivergent phenotypes and conditions are indicated to stem from environmental challenges during pregnancy and neonatal period of neurodevelopment and involve TLRs. For example, autism spectrum disorder (ASD) (Chung et al., 2021; Yu et al., 2023; Vacharasin et al., 2024), attention-deficit/hyperactivity disorder (ADHD) (Ganna et al., 2018; Kim et al., 2020), schizophrenia and neurodevelopmental disorders (NDDs) are associated with maternal immune activation (MIA)(Baghel et al., 2018; Baines et al., 2020; Talukdar et al., 2021; Anderson et al., 2022). In all mentioned NDDs, specific differences in total brain volume or volume of particular regions compared to controls have been documented by neuroimaging studies (Courchesne et al., 2001; Hyakkoku et al., 2010; Lukito et al., 2020; Li T. et al., 2022). Is it possible that these NDDs are associated with MIA disturbance of TLR-ND pathway? If the TLR challenge coincides with neurogenesis, depending on infectious agent and the spatio-temporal neurodevelopmental stage, there is a firm possibility that diversity in neuronal type and number is introduced. The number of neurons residing in specific brain regions (amygdala, hippocampus, cortex, cerebellum) or even sub-structural clusters, can vary, induce mosaicism, and build the potential for alterations of neuronal networks and connections. As described earlier, bacterial LPS induces neuronal overgrowth (Humann et al., 2016; Mann et al., 2023) and dsRNA silences proliferation (Yaddanapudi et al., 2011). Importantly, the essential role of TLR signaling in neurodevelopment is corroborated by a recent finding of the 16p11.2 deletion syndrome which has phenotypic features of ASD (Chung et al., 2021). One of the affected genes in the 16p11.2 deletion syndrome is coding for ERK1 protein (Pucilowska et al., 2015), a downstream element of the TLR cascade. In other words, ASD neurophenotypes may be exacerbated through inhibition of TLR cascade. Viral and bacterial coinfection effects on the TLR2/4 and TLR3 systems probably participate in molecular events underlying an ASD-described increase and decrease in the volume of particular regions. Persistence and duration of infection could be attributed to the range of ASD phenotypic diversity in individuals.
On the other hand, TLR3 inhibition by viral dsRNA could lower proliferation and neurogenesis capacity which may be associated with decreased number of neurons, atypical cellular density and changed brain volumes in ADHD and schizophrenia. Further, in challenging periods that coincide with neuronal outgrowth and branching involving the TLR7/8/9 system, neurons may shape unusual connections resulting in differential synaptic landscape formation and neural circuitries. The same applies to gliogenesis - if progenitor pool potential is disturbed, the fate of oligodendrocytes and astrocytes can change, and individuals become susceptible to various pathological processes.
When elaborating neuroimmunity in the context of TLRs actions, we should also consider infections caused by the herpes virus family, Epstein–Barr (EBV) and herpes simplex (HSV) virus, and related pathologies, multiple sclerosis (MS) (Hernández-Pedro et al., 2013; Baecher-Allan et al., 2018) and Alzheimer’s disease (AD) (Landreth and Reed-Geaghan, 2009; Cameron and Landreth, 2010). Pathogenesis of MS involves innate and adaptive immunity responses related to demyelination, neuronal loss, and lesion formation (Hernández-Pedro et al., 2013). Potential role of TLRs in MS pathogenesis may be related to viral microRNA. Namely, it has been shown that HSV and EBV regulate immune answer and viral life cycle via viral microRNA-filled extracellular vesicles (Forte and Luftig, 2011; Kim et al., 2017; Wang et al., 2018; Cone et al., 2019). EBV infection in adolescence and later life could affect the pool of brain-residing progenitor cells involving the EBV infected cells release of microRNA, exhibiting immune-and viral cycle-regulating properties (Forte and Luftig, 2011; Wang et al., 2018). It is possible that distinct microRNA, circulating via extracellular vesicles, is taken by OPC and NPC. Once in cells, it could be that microRNAs doublestranded harpins bind TLR3 and dysregulate its activity causing the loss of TLR3 information. This drives progenitor cells to premature differentiation (Yaddanapudi et al., 2011; Bsibsi et al., 2012), apoptosis, and lower plastic ability. The myelinating oligodendrocyte has to be in contact with the neuron, astrocyte, progenitor, and immune cell to successfully fullfill its duty to become a new oligodendrocyte and if not, it is non-invasively removed by microglia (Berghoff et al., 2021). If there is a lack of Notch input due to TLR3 inhibition following a viral infection, the dying OPC/oligodendrocyte identity may be obscured and astrocytes and microglia could mistake it for an invading agent. The resulting innate immunity response might instruct adaptive immunity to attack specific subgroups of oligodendrocytes. We suggest that as adaptive immunity attacks myelin, dying cells produce other TLR ligands (particularly ligands of the TLR2 and TLR4, previously shown to affect regulation of oligodendrocyte life-cycle)(Church et al., 2016; Sanchez-Petidier et al., 2022; Brandt et al., 2023) which continue to disrupt homeostasis, leading to loss of neuronal networks integrity and emergence of MS symptoms as an autoimmune condition. Similarly, sporadic AD is frequent in individuals infected with HSV. The reoccurring infection could be detrimental to the hippocampal progenitor cell pool (Walker et al., 2018) and compromise astrocyte-dependent neuron identity and homeostasis by releasing TLR2 and TLR9 ligands (Lima et al., 2010; Zolini et al., 2014). Interestingly, utilizing a molecular visualization tool, we have observed probable processing of amyloid-precursor protein (APP) similarly to Notch: α-secretase cleavage releases the extracellular domain with two distinct structural motifs. APP E2 domain resembles TLR5 ligand flagellin and functions in cis-and trans-ligation (Rossjohn et al., 1999; Barnham et al., 2003; Wang and Ha, 2004). The extracellular part of APP has many similarities with the Notch/J/D complex, and APP presence is confirmed in dimers with Notch (Di Chen et al., 2006) (Figure 4B2a). Is it possible that APP is one of the natural Notch and TLR ligands, and its ligation and shedding give information about cellular environment and condition of neurons, resulting with growth and repair? Astrocytes seem to be most affected by miscommunication and may play an essential role in bridging the connection between neurons, oligodendrocytes, and microglia (Krasowska-Zoladek et al., 2007; Guerrero-García, 2020; Werkman et al., 2020; Wang et al., 2021). MS and AD-like pathologies have been previously considered in the context of viral infections, however, taking into account TLR signaling may shed light on events which include cellular response to environmental cues leading to disruption in developing and homeostatic pathways.
Brain tissue damage and injuries are cellular environment-changing events that also affect the microglial TLR system and influence the presumed TLR-ND cellular response. TLR is implicated as an essential player in traumatic injuries, tissue damage, and repair, and its many immunity-related roles in responding to tissue damage have been resolved by studies in genetically modified mouse models with TLR deficiency (Zhang et al., 2011; Ishizuka et al., 2013; Winters et al., 2013; Church et al., 2016; Gorup et al., 2019; Wasko et al., 2019; Polsek et al., 2020). The cellular environment in brain injury becomes saturated with DAMPs, and binding events affect the microglial TLR system, influencing TLR-ND cellular communication (Shi et al., 2013). TLR2 and TLR4 influence post-injury cellular recruitment and axonal tissue regeneration potency (Winters et al., 2013; Gorup et al., 2019). Lack of TLR2 is shown to protect the brain from immune invasion and has a poor prognosis in injury repair and tissue regeneration (Gorup et al., 2019). TLR4 activation/inhibition, on the other hand, has regenerative quality, and aids OPC recruitment and axon remyelination (Schonberg et al., 2007). The basic cellular load of mRNA coding for TLR2-engaged downstream scaffold proteins is differential in TLR2KO mice; an increase in load of IRAK1, IRAK4, IKKβ, and IL-6 and a decrease in IRAK3 has been observed (Winters et al., 2013). Since increased neuronal numbers are a phenotypical trait, we can assume that IRAK1, IRAK4, and IKKβ echo neuronal identity metabolism. At the same time, the observed IL-6 increase could be a constant signal for OPC premature maturation and apoptosis, lowering OPC regenerative capacity.
Finally, TLR and Notch signaling pathways may interfere with modeling and functional effects of hormones during sex-dependent differentiation of the brain. Since the presented data suggests that the Notch-TLR pathway is involved in the formation of cellular identity during neurodevelopment, we could make another intriguing assumption as to whether the building up of individual sex-related behavioral (psychological) patterns depends on the same signaling system, activated in response to myriads of environmental stimuli (Figure 6). Certainly, the resolving in more details the interplay of the environment with central cellular homeostatic and adaptive signaling pathways during neurodevelopment and brain maturation may substantially broaden our understanding of sex-related differences of clinical phenotypes reported for several neurodevelopmental, neurological and psychiatric disorders (ASD, ADHD, schizophrenia, MS, AD) (Krementsov et al., 2014; Carney, 2019; Lins et al., 2019; Hui et al., 2020; Merikangas and Almasy, 2020; Dunstan et al., 2024).
7 Conclusion
We proposed a theoretical framework backed up by preliminary modelling of structural and functional assembly of TLRs and Notch which enables highly coordinated actions of both signaling pathways crucial for intercellular communication and cellular adaptation to environment. We point out so far underappreciated roles of TLRs in neurodevelopment and describe striking functional similarities of TLRs and Notch signaling during embryonic and postnatal brain development. Several possible interactions of TLRs and Notch are introduced and evidenced by preliminary analysis of visualized molecular structures which speaks in favor of the hypothesized intriguing cooperation of Notch and specific TLR isoforms in signal-sending and signal-receiving cellular milieu. We expect that a pilot supportive evidence for here presented hypothesis may provide sound basis for revisiting current viewpoints about interplay of TLR and Notch signaling. Future experimental studies, by including some of our proposed approaches, should better delineate the likely fundamental molecular Notch-TLR interactions which in the nervous system may underlie formation of cellular identity, cytoarchitecture, neural circuitries and diverse neurobehavioral phenotypes.
Data availability statement
The original contributions presented in the study are included in the article/supplementary material, further inquiries can be directed to the corresponding authors.
Author contributions
MS: Conceptualization, Data curation, Investigation, Visualization, Writing – original draft, Writing – review & editing, Methodology, Validation. SK-B: Conceptualization, Funding acquisition, Supervision, Validation, Writing – original draft, Writing – review & editing.
Funding
The author(s) declare that financial support was received for the research, authorship, and/or publication of this article. This work was supported by research project funded by the Croatian Science Foundation grant NeuroReact, IP-2016-06-8636 (to SKB), University of Zagreb support grant, 1123025 (to SKB) and European Union through the European Regional Development Fund, Operational Programme “Competitiveness and Cohesion 2014–2020”, grant agreement no. KK.01.1.1.02.0015.
Acknowledgments
Authors express their gratitude to Kristina Mlinac-Jerković and Katarina Ilić, for continuous inspiring discussions, brain-stormings, detail-oriented and playfull scientific imagination which greatly contributed to integration of ideas resulting with this works. Authors also deeply appreciate encouragement, the critical reading and insightful comments by Ivana Rosenzweig and Dinko Mitrečić who made crucial influence on the final version of the manuscript. Figures were created with Biorender.com (CC-BY license issued by BioRender).
Conflict of interest
The authors declare that the research was conducted in the absence of any commercial or financial relationships that could be construed as a potential conflict of interest.
Publisher’s note
All claims expressed in this article are solely those of the authors and do not necessarily represent those of their affiliated organizations, or those of the publisher, the editors and the reviewers. Any product that may be evaluated in this article, or claim that may be made by its manufacturer, is not guaranteed or endorsed by the publisher.
Footnotes
References
Abdul, Y., Abdelsaid, M., Li, W., Webb, R. C., Sullivan, J. C., Dong, G., et al. (2019). Inhibition of toll-like Receptor-4 (TLR-4) improves neurobehavioral outcomes after acute ischemic stroke in diabetic rats: possible role of vascular endothelial TLR-4. Mol. Neurobiol. 56, 1607–1617. doi: 10.1007/s12035-018-1184-8
Akai, J., Halley, P. A., and Storey, K. G. (2005). FGF-dependent notch signaling maintains the spinal cord stem zone. Genes Dev. 19, 2877–2887. doi: 10.1101/gad.357705
Akashi-Takamura, S., and Miyake, K. (2008). TLR accessory molecules. Curr. Opin. Immunol. 20, 420–425. doi: 10.1016/j.coi.2008.07.001
Asafen, H.Al, Bandodkar, P. U., Carrell-Noel, S., Schloop, A. E., Friedman, J., and Reeves, G. T. (2020). Robustness of the dorsal morphogen gradient with respect to morphogen dosage. PLoS Comput. Biol. 16, e1007750–e1007721. doi: 10.1371/journal.pcbi.1007750
Anderson, R. C., O’Keeffe, G. W., and McDermott, K. W. (2022). Characterisation of the consequences of maternal immune activation on distinct cell populations in the developing rat spinal cord. J. Anat. 241, 938–950. doi: 10.1111/joa.13726
Anthoney, N., Foldi, I., and Hidalgo, A. (2018). Toll and toll-like receptor signalling in development. Devleopment 145, 1–6. doi: 10.1242/dev.156018
Anthony, T. E., Mason, H. A., Gridley, T., Fishell, G., and Heintz, N. (2005). Brain lipid-binding protein is a direct target of notch signaling in radial glial cells. Genes Dev. 19, 1028–1033. doi: 10.1101/gad.1302105
Arzate, D. M., Valencia, C., Dimas, M. A., Antonio-Cabrera, E., Domínguez-Salazar, E., Guerrero-Flores, G., et al. (2022). Dll1 haploinsufficiency causes brain abnormalities with functional relevance. Front. Neurosci. 16, 1–19. doi: 10.3389/fnins.2022.951418
Baecher-Allan, C., Kaskow, B. J., and Weiner, H. L. (2018). Multiple sclerosis: mechanisms and immunotherapy. Neuron 97, 742–768. doi: 10.1016/j.neuron.2018.01.021
Baghel, M. S., Singh, B., Dhuriya, Y. K., Shukla, R. K., Patro, N., Khanna, V. K., et al. (2018). Postnatal exposure to poly (I:C) impairs learning and memory through changes in synaptic plasticity gene expression in developing rat brain. Neurobiol. Learn. Mem. 155, 379–389. doi: 10.1016/j.nlm.2018.09.005
Baines, K. J., Hillier, D. M., Haddad, F. L., Rajakumar, N., Schmid, S., and Renaud, S. J. (2020). Maternal immune activation alters fetal brain development and enhances proliferation of neural precursor cells in rats. Front. Immunol. 11:1145. doi: 10.3389/fimmu.2020.01145
Barak, B., Feldman, N., and Okun, E. (2014). Toll-like receptors as developmental tools that regulate neurogenesis during development: an update. Front. Neurosci. 8, 1–6. doi: 10.3389/fnins.2014.00272
Barnham, K. J., McKinstry, W. J., Multhaup, G., Galatis, D., Morton, C. J., Curtain, C. C., et al. (2003). Structure of the Alzheimer’s disease amyloid precursor protein copper binding domain. A regulator of neuronal copper homeostasis. J. Biol. Chem. 278, 17401–17407. doi: 10.1074/jbc.M300629200
Berghoff, S. A., Spieth, L., Sun, T., Hosang, L., Schlaphoff, L., Depp, C., et al. (2021). Microglia facilitate repair of demyelinated lesions via post-squalene sterol synthesis. Nat. Neurosci. 24, 47–60. doi: 10.1038/s41593-020-00757-6
Beutler, B., and Rehli, M. (2002). Evolution of the TIR, tolls and TLRs: functional inferences from computational biology. Curr. Top. Microbiol. Immunol. 270, 1–21. doi: 10.1007/978-3-642-59430-4_1
Blackwood, C. A., Bailetti, A., Nandi, S., Gridley, T., and Hébert, J. M. (2020). Notch dosage: Jagged1 Haploinsufficiency is associated with reduced neuronal division and disruption of Periglomerular interneurons in mice. Front. Cell Dev. Biol. 8, 1–14. doi: 10.3389/fcell.2020.00113
Bork, P., Downing, A. K., Kieffer, B., and Campbell, I. D. (1996). Structure and distribution of modules in extracellular proteins. Q. Rev. Biophys. 29, 119–167. doi: 10.1017/s0033583500005783
Botos, I., Liu, L., Wang, Y., Segal, D. M., and Davies, D. R. (2009). The toll-like receptor 3:dsRNA signaling complex. Biochim. Biophys. Acta Gene Regul. Mech. 1789, 667–674. doi: 10.1016/j.bbagrm.2009.06.005
Botos, I., Segal, D. M., and Davies, D. R. (2011). The structural biology of toll-like receptors. Structure 19, 447–459. doi: 10.1016/j.str.2011.02.004
Brandt, A., Kromm, F., Hernández-Arriaga, A., Martínez Sánchez, I., Bozkir, H. Ö., Staltner, R., et al. (2023). Cognitive alterations in old mice are associated with intestinal barrier dysfunction and induced toll-like receptor 2 and 4 signaling in different brain regions. Cells 12:2153. doi: 10.3390/cells12172153
Brennan, J. J., and Gilmore, T. D. (2018). Evolutionary origins of toll-like receptor signaling. Mol. Biol. Evol. 35, 1576–1587. doi: 10.1093/molbev/msy050
Breunig, J. J., Silbereis, J., Vaccarino, F. M., Šestan, N., and Rakic, P. (2007). Notch regulates cell fate and dendrite morphology of newborn neurons in the postnatal dentate gyrus. Proc. Natl. Acad. Sci. USA 104, 20558–20563. doi: 10.1073/pnas.0710156104
Brou, C., Logeat, F., Gupta, N., Bessia, C., LeBail, O., Doedens, J. R., et al. (2000). A novel proteolytic cleavage involved in notch signaling: the role of the disintegrin-metalloprotease TACE. Mol. Cell 5, 207–216. doi: 10.1016/S1097-2765(00)80417-7
Bsibsi, M., Nomden, A., van Noort, J. M., and Baron, W. (2012). Toll-like receptors 2 and 3 agonists differentially affect oligodendrocyte survival, differentiation, and myelin membrane formation. J. Neurosci. Res. 90, 388–398. doi: 10.1002/jnr.22767
Bsibsi, M., Ravid, R., Gveric, D., and Van Noort, J. M. (2002). Broad expression of toll-like receptors in the human central nervous system. J. Neuropathol. Exp. Neurol. 61, 1013–1021. doi: 10.1093/jnen/61.11.1013
Cameron, J. S., Alexopoulou, L., Sloane, J. A., DiBernardo, A. B., Ma, Y., Kosaras, B., et al. (2007). Toll-like receptor 3 is a potent negative regulator of axonal growth in mammals. J. Neurosci. 27, 13033–13041. doi: 10.1523/JNEUROSCI.4290-06.2007
Cameron, B., and Landreth, G. E. (2010). Inflammation, microglia, and alzheimer’s disease. Neurobiol. Dis. 37, 503–509. doi: 10.1016/j.nbd.2009.10.006
Carney, R. S. E. (2019). Does prenatal exposure to maternal inflammation causes sex differences in schizophrenia-related behavioral outcomes in adult rats? eNeuro 6:ENEURO.0393–19.2019. doi: 10.1523/ENEURO.0393-19.2019
Casas Gimeno, G., and Paridaen, J. T. M. L. (2022). The symmetry of neural stem cell and progenitor divisions in the vertebrate brain. Front. Cell Dev. Biol. 10:885269. doi: 10.3389/fcell.2022.885269
Chen, Y., Lin, J., Zhao, Y., Ma, X., and Yi, H. (2021). Toll-like receptor 3 (TLR3) regulation mechanisms and roles in antiviral innate immune responses. J. Zhejiang Univ. B 22, 609–632. doi: 10.1631/jzus.B2000808
Chen, J., Moloney, D. J., and Stanley, P. (2001). Fringe modulation of Jagged1-induced notch signaling requires the action of β4galactosyltransferase-1. Proc. Natl. Acad. Sci. USA 98, 13716–13721. doi: 10.1073/pnas.241398098
Chen, C. Y., Shih, Y. C., Hung, Y. F., and Hsueh, Y. P. (2019). Beyond defense: regulation of neuronal morphogenesis and brain functions via toll-like receptors. J. Biomed. Sci. 26, 90–13. doi: 10.1186/s12929-019-0584-z
Chillakuri, C. R., Sheppard, D., Ilagan, M. X. G., Holt, L. R., Abbott, F., Liang, S., et al. (2013). Structural analysis uncovers lipid-binding properties of notch ligands. Cell Rep. 5, 861–867. doi: 10.1016/j.celrep.2013.10.029
Choe, J., Kelker, M. S., and Wilson, I. A. (2005). Structural biology: crystal structure of human toll-like receptor 3 (TLR3) ectodomain. Science 309, 581–585. doi: 10.1126/science.1115253
Chung, W. K., Roberts, T. P., Sherr, E. H., Snyder, L. A. G., and Spiro, J. E. (2021). 16P11.2 Deletion Syndrome. Curr. Opin. Genet. Dev. 68, 49–56. doi: 10.1016/j.gde.2021.01.011
Church, J. S., Kigerl, K. A., Lerch, J. K., Popovich, P. G., and McTigue, D. M. (2016). TLR4 deficiency impairs oligodendrocyte formation in the injured spinal cord. J. Neurosci. 36, 6352–6364. doi: 10.1523/JNEUROSCI.0353-16.2016
Cone, A. S., York, S. B., and Meckes, D. G. (2019). Extracellular Vesicles in Epstein-Barr Virus Pathogenesis. Curr. Clin. Microbiol. Rep. 6, 121–131. doi: 10.1007/s40588-019-00123-6
Cordle, J., Johnson, S., Zi Yan Tay, J., Roversi, P., Wilkin, M. B., de Madrid, B. H., et al. (2008a). A conserved face of the jagged/serrate DSL domain is involved in notch trans-activation and cis-inhibition. Nat. Struct. Mol. Biol. 15, 849–857. doi: 10.1038/nsmb.1457
Cordle, J., Redfield, C., Stacey, M., Van Der Merwe, P. A., Willis, A. C., Champion, B. R., et al. (2008b). Localization of the delta-like-1-binding site in human Notch-1 and its modulation by calcium affinity. J. Biol. Chem. 283, 11785–11793. doi: 10.1074/jbc.M708424200
Courchesne, E., Karns, C. M., Davis, H. R., Ziccardi, R., Carper, R. A., Tigue, Z. D., et al. (2001). Unusual brain growth patterns in early life in patients with autistic disorder: an MRI study. Neurology 57, 245–254. doi: 10.1212/WNL.57.2.245
Crack, P. J., and Bray, P. J. (2007). Toll-like receptors in the brain and their potential roles in neuropathology. Immunol. Cell Biol. 85, 476–480. doi: 10.1038/sj.icb.7100103
D’Souza, B., Meloty-Kapella, L., and Weinmaster, G. (2010). Canonical and non-canonical notch ligands. Curr. Top. Dev. Biol. 92, 73–129. doi: 10.1016/S0070-2153(10)92003-6
Chen, C.Di, Oh, S. Y., Hinman, J. D., and Abraham, C. R. (2006). Visualization of APP dimerization and APP-Notch2 heterodimerization in living cells using bimolecular fluorescence complementation. J. Neurochem. 97, 30–43. doi: 10.1111/j.1471-4159.2006.03705.x
Dunstan, I. K., McLeod, R., Radford-Smith, D. E., Xiong, W., Pate, T., Probert, F., et al. (2024). Unique pathways downstream of TLR-4 and TLR-7 activation: sex-dependent behavioural, cytokine, and metabolic consequences. Front. Cell. Neurosci. 18:1345441. doi: 10.3389/fncel.2024.1345441
Enkhbayar, P., Kamiya, M., Osaki, M., Matsumoto, T., and Matsushima, N. (2004). Structural principles of leucine-rich repeat (LRR) proteins. Proteins Struct. Funct. Genet. 54, 394–403. doi: 10.1002/prot.10605
Fernandez-Lizarbe, S., Montesinos, J., and Guerri, C. (2013). Ethanol induces TLR4/TLR2 association, triggering an inflammatory response in microglial cells. J. Neurochem. 126, 261–273. doi: 10.1111/jnc.12276
Fiúza, U. M., and Arias, A. M. (2007). Cell and molecular biology of notch. J. Endocrinol. 194, 459–474. doi: 10.1677/JOE-07-0242
Foldi, J., Chung, A. Y., Xu, H., Zhu, J., Outtz, H. H., Kitajewski, J., et al. (2010). Autoamplification of notch signaling in macrophages by TLR-induced and RBP-J–dependent induction of Jagged1. J. Immunol. 185, 5023–5031. doi: 10.4049/jimmunol.1001544
Forte, E., and Luftig, M. A. (2011). The role of microRNAs in Epstein-Barr virus latency and lytic reactivation. Microbes Infect. 13, 1156–1167. doi: 10.1016/j.micinf.2011.07.007
Franklin, J. L., Berechid, B. E., Cutting, F. B., Presente, A., Chambers, C. B., Foltz, D. R., et al. (1999). Autonomous and non-autonomous regulation of mammalian neurite development by Notch1 and Delta1. Curr. Biol. 9, 1448–1457. doi: 10.1016/S0960-9822(00)80114-1
Frantz, S., Kelly, R. A., and Bourcier, T. (2001). Role of TLR-2 in the activation of nuclear factor κB by oxidative stress in cardiac myocytes. J. Biol. Chem. 276, 5197–5203. doi: 10.1074/jbc.M009160200
Gaiano, N., and Fishell, G. (2002). The role of notch in promoting glial and neural stem cell fates. Annu. Rev. Neurosci. 25, 471–490. doi: 10.1146/annurev.neuro.25.030702.130823
Galleguillos, D., Wang, Q., Steinberg, N., Zaidi, A., Shrivastava, G., Dhami, K., et al. (2022). Anti-inflammatory role of GM1 and other gangliosides on microglia. J. Neuroinflammation 19:9. doi: 10.1186/s12974-021-02374-x
Ganna, A., Satterstrom, F. K., Zekavat, S. M., Das, I., Kurki, M. I., Churchhouse, C., et al. (2018). Quantifying the impact of rare and ultra-rare coding variation across the phenotypic Spectrum. Am. J. Hum. Genet. 102, 1204–1211. doi: 10.1016/j.ajhg.2018.05.002
Ge, B., Gram, H., Di Padova, F., Huang, B., New, L., Ulevitch, R. J., et al. (2002). MAPKK-independent activation of p38α mediated by TAB1-dependent autophosphorylation of p38α. Science 295, 1291–1294. doi: 10.1126/science.1067289
Giniger, E., Jan, L. Y., and Jan, Y. N. (1993). Specifying the path of the intersegmental nerve of the Drosophila embryo: a role for Delta and notch. Development 117, 431–440. doi: 10.1242/dev.117.2.431
Gioannini, T. L., Teghanemt, A., Zhang, D., Coussens, N. P., Dockstader, W., Ramaswamy, S., et al. (2004). Isolation of an endotoxin–MD-2 complex that produces toll-like receptor 4-dependent cell activation at picomolar concentrations. Proc. Natl. Acad. Sci. 101, 4186–4191. doi: 10.1073/pnas.0306906101
Gordon, W. R., Vardar-Ulu, D., Histen, G., Sanchez-Irizarry, C., Aster, J. C., and Blacklow, S. C. (2007). Structural basis for autoinhibition of notch. Nat. Struct. Mol. Biol. 14, 295–300. doi: 10.1038/nsmb1227
Gorup, D., Škokić, S., Kriz, J., and Gajović, S. (2019). Tlr2 deficiency is associated with enhanced elements of neuronal repair and caspase 3 activation following brain ischemia. Sci. Rep. 9, 2821–2810. doi: 10.1038/s41598-019-39541-3
Gosu, V., Son, S., Shin, D., and Song, K. D. (2019). Insights into the dynamic nature of the dsRNA-bound TLR3 complex. Sci. Rep. 9, 3652–3614. doi: 10.1038/s41598-019-39984-8
Gozlan, O., and Sprinzak, D. (2023). Notch signaling in development and homeostasis. Development 150, 1–4. doi: 10.1242/dev.201138
Guerrero-García, J. J. (2020). The role of astrocytes in multiple sclerosis pathogenesis. Neurologia 35, 400–408. doi: 10.1016/j.nrl.2017.07.021
Hamel, S., Fantini, J., and Schweisguth, F. (2010). Notch ligand activity is modulated by glycosphingolipid membrane composition in Drosophila melanogaster. J. Cell Biol. 188, 581–594. doi: 10.1083/jcb.200907116
Hamidi, S., Schäfer-Korting, M., and Weindl, G. (2014). TLR2/1 and sphingosine 1-phosphate modulate inflammation, myofibroblast differentiation and cell migration in fibroblasts. Biochim. Biophys. Acta Mol. Cell Biol. Lipids 1841, 484–494. doi: 10.1016/j.bbalip.2014.01.008
Handford, P. A., Korona, B., Suckling, R., Redfield, C., and Lea, S. M. (2018). Structural insights into notch receptor-ligand interactions. Adv. Exp. Med. Biol. 1066, 33–46. doi: 10.1007/978-3-319-89512-3_2
Hernández-Pedro, N. Y., Espinosa-Ramirez, G., De La Cruz, V. P., Pineda, B., and Sotelo, J. (2013). Initial immunopathogenesis of multiple sclerosis: innate immune response. Clin. Dev. Immunol. 2013, 1–15. doi: 10.1155/2013/413465
Hiraoka, Y., Komine, O., Nagaoka, M., Bai, N., Hozumi, K., and Tanaka, K. (2013). Delta-like 1 regulates Bergmann glial monolayer formation during cerebellar development. Mol. Brain 6:1. doi: 10.1186/1756-6606-6-25
Hu, X., Chung, A. Y., Wu, I., Foldi, J., Chen, J., Ji, J. D., et al. (2008). Integrated regulation of toll-like receptor responses by notch and interferon-γ pathways. Immunity 29, 691–703. doi: 10.1016/j.immuni.2008.08.016
Hui, C. W., Vecchiarelli, H. A., Gervais, É., Luo, X., Michaud, F., Scheefhals, L., et al. (2020). Sex differences of microglia and synapses in the hippocampal dentate gyrus of adult mouse offspring exposed to maternal immune activation. Front. Cell. Neurosci. 14, 1–12. doi: 10.3389/fncel.2020.558181
Humann, J., Mann, B., Gao, G., Moresco, P., Ramahi, J., Loh, L. N., et al. (2016). Bacterial peptidoglycan traverses the placenta to induce fetal Neuroproliferation and aberrant postnatal behavior. Cell Host Microbe 19, 388–399. doi: 10.1016/j.chom.2016.02.009
Hyakkoku, K., Hamanaka, J., Tsuruma, K., Shimazawa, M., Tanaka, H., Uematsu, S., et al. (2010). Toll-like receptor 4 (TLR4), but not TLR3 or TLR9, knock-out mice have neuroprotective effects against focal cerebral ischemia. Neuroscience 171, 258–267. doi: 10.1016/j.neuroscience.2010.08.054
Ikegami, A., Haruwaka, K., and Wake, H. (2019). Microglia: lifelong modulator of neural circuits. Neuropathology 39, 173–180. doi: 10.1111/neup.12560
Irvin, D. K., Nakano, I., Paucar, A., and Kornblum, H. I. (2004). Patterns of Jagged1, Jagged2, Delta-like 1 and Delta-like 3 expression during late embryonic and postnatal brain development suggest multiple functional roles in progenitors and differentiated cells. J. Neurosci. Res. 75, 330–343. doi: 10.1002/jnr.10843
Irvin, D. K., Zurcher, S. D., Nguyen, T., Weinmaster, G., and Kornblum, H. I. (2001). Expression patterns of Notch1, Notch2, and Notch3 suggest multiple functional roles for the notch-DSL signaling system during brain development. J. Comp. Neurol. 436, 167–181. doi: 10.1002/cne.1059
Ishizuka, F., Shimazawa, M., Inoue, Y., Nakano, Y., Ogishima, H., Nakamura, S., et al. (2013). Toll-like receptor 4 mediates retinal ischemia/reperfusion injury through nuclear factor-κB and spleen tyrosine kinase activation. Investig. Ophthalmol. Vis. Sci. 54, 5807–5816. doi: 10.1167/iovs.13-11932
Jack, C. S., Arbour, N., Manusow, J., Montgrain, V., Blain, M., Mccrea, E., et al. (2019). TLR signaling tailors innate immune responses in human microglia and astrocytes. J. Immunol. 175, 4320–4330. doi: 10.4049/jimmunol.175.7.4320
Jacobs, C. T., and Huang, P. (2019). Notch signalling maintains hedgehog responsiveness via a gli-dependent mechanism during spinal cord patterning in zebrafish. eLife 8, 1–24. doi: 10.7554/eLife.49252
Jacobs, C. T., and Huang, P. (2021). Complex crosstalk of notch and hedgehog signalling during the development of the central nervous system. Cell. Mol. Life Sci. 78, 635–644. doi: 10.1007/s00018-020-03627-3
Jin, M. S., Kim, S. E., Heo, J. Y., Lee, M. E., Kim, H. M., Paik, S. G., et al. (2007). Crystal structure of the TLR1-TLR2 heterodimer induced by binding of a tri-Acylated Lipopeptide. Cell 130, 1071–1082. doi: 10.1016/j.cell.2007.09.008
Jin, M., Zhang, H., Xu, B., Li, Y., Qin, H., Yu, S., et al. (2023). Jag2b-Notch3 /1b-mediated neuron-to-glia crosstalk controls retinal gliogenesis. EMBO Rep. 24:e57072. doi: 10.15252/embr.202357072
Johnson, G. L., and Lapadat, R. (2002). Mitogen-activated protein kinase pathways mediated by ERK, JNK, and p38 protein kinases. Science 298, 1911–1912. doi: 10.1126/science.1072682
Kageyama, R., and Ohtsuka, T. (1999). The notch-Hes pathway in mammalian neural development. Cell Res. 9, 179–188. doi: 10.1038/sj.cr.7290016
Kageyama, R., Ohtsuka, T., Shimojo, H., and Imayoshi, I. (2009). Dynamic regulation of notch signaling in neural progenitor cells. Curr. Opin. Cell Biol. 21, 733–740. doi: 10.1016/j.ceb.2009.08.009
Kang, J. Y., Nan, X., Jin, M. S., Youn, S. J., Ryu, Y. H., Mah, S., et al. (2009). Recognition of Lipopeptide patterns by toll-like receptor 2-toll-like receptor 6 heterodimer. Immunity 31, 873–884. doi: 10.1016/j.immuni.2009.09.018
Kawaguchi, D., Yoshimatsu, T., Hozumi, K., and Gotoh, Y. (2008). Selection of differentiating cells by different levels of delta-like 1 among neural precursor cells in the developing mouse telencephalon. Development 135, 3849–3858. doi: 10.1242/dev.024570
Keilani, S., and Sugaya, K. (2008). Reelin induces a radial glial phenotype in human neural progenitor cells by activation of Notch-1. BMC Dev. Biol. 8, 1–9. doi: 10.1186/1471-213X-8-69
Kelly, D. F., Lake, R. J., Middelkoop, T. C., Fan, H. Y., Artavanis-Tsakonas, S., and Walz, T. (2010). Molecular structure and dimeric organization of the notch extracellular domain as revealed by electron microscopy. PLoS One 5, 1–6. doi: 10.1371/journal.pone.0010532
Kim, J. I., Chang, J. L., Mi, S. J., Lee, C. H., Paik, S. G., Lee, H., et al. (2005). Crystal structure of CD14 and its implications for lipopolysaccharide signaling. J. Biol. Chem. 280, 11347–11351. doi: 10.1074/jbc.M414607200
Kim, Y. S., Choi, J., and Yoon, B. E. (2020). Neuron-glia interactions in neurodevelopmental disorders. Cells 9:176. doi: 10.3390/cells9102176
Kim, C., Ho, D. H., Suk, J. E., You, S., Michael, S., Kang, J., et al. (2013). Neuron-released oligomeric α-synuclein is an endogenous agonist of TLR2 for paracrine activation of microglia. Nat. Commun. 4:1562. doi: 10.1038/ncomms2534
Kim, H., Iizasa, H., Kanehiro, Y., Fekadu, S., and Yoshiyama, H. (2017). Herpesviral microRNAs in cellular metabolism and immune responses. Front. Microbiol. 8:1318. doi: 10.3389/fmicb.2017.01318
Kim, H. M., Park, B. S., Kim, J. I., Kim, S. E., Lee, J., Oh, S. C., et al. (2007). Crystal structure of the TLR4-MD-2 complex with bound endotoxin antagonist Eritoran. Cell 130, 906–917. doi: 10.1016/j.cell.2007.08.002
Kim, H., Shin, J., Kim, S., Poling, J., Park, H. C., and Appel, B. (2008). Notch-regulated oligodendrocyte specification from radial glia in the spinal cord of zebrafish embryos. Dev. Dyn. 237, 2081–2089. doi: 10.1002/dvdy.21620
Kobe, B., and Deisenhofer, J. (1994). The leucine-rich repeat: a versatile binding motif. Trends Biochem. Sci. 19, 415–421. doi: 10.1016/0968-0004(94)90090-6
Kobe, B., and Kajava, A. V. (2001). The leucine-rich repeat as a protein recognition motif. Curr. Opin. Struct. Biol. 11, 725–732. doi: 10.1016/S0959-440X(01)00266-4
Koymans, K. J., Feitsma, L. J., Brondijk, T. H. C., Aerts, P. C., Lukkien, E., Lössl, P., et al. (2015). Structural basis for inhibition of TLR2 by staphylococcal superantigen-like protein 3 (SSL3). Proc. Natl. Acad. Sci. 112, 11018–11023. doi: 10.1073/pnas.1502026112
Krasowska-Zoladek, A., Banaszewska, M., Kraszpulski, M., and Konat, G. W. (2007). Kinetics of inflammatory response of astrocytes induced by TLR 3 and TLR4 ligation. J. Neurosci. Res. 85, 205–212. doi: 10.1002/jnr.21088
Krementsov, D. N., Noubade, R., Dragon, J. A., Otsu, K., Rincon, M., and Teuscher, C. (2014). Sex-specific control of central nervous system autoimmunity by p38 mitogen-activated protein kinase signaling in myeloid cells. Ann. Neurol. 75, 50–66. doi: 10.1002/ana.24020
Kuzina, I., Song, J. K., and Giniger, E. (2011). How notch establishes longitudinal axon connections between successive segments of the Drosophila CNS. Development 138, 1839–1849. doi: 10.1242/dev.062471
Ladi, E., Nichols, J. T., Ge, W., Miyamoto, A., Yao, C., Yang, L. T., et al. (2005). The divergent DSL ligand Dll3 does not activate notch signaling but cell autonomously attenuates signaling induced by other DSL ligands. J. Cell Biol. 170, 983–992. doi: 10.1083/jcb.200503113
Lalancette-Hbert, M., Phaneuf, D., Soucy, G., Weng, Y. C., and Kriz, J. (2009). Live imaging of toll-like receptor 2 response in cerebral ischaemia reveals a role of olfactory bulb microglia as modulators of inflammation. Brain 132, 940–954. doi: 10.1093/brain/awn345
Landreth, G. E., and Reed-Geaghan, E. G. (2009). Toll-like receptors in Alzheimer’s disease. Curr. Top. Microbiol. Immunol. 336, 137–153. doi: 10.1007/978-3-642-00549-7_8
Lathia, J. D., Okun, E., Tang, S. C., Griffioen, K., Cheng, A., Mughal, M. R., et al. (2008). Toll-like receptor 3 is a negative regulator of embryonic neural progenitor cell proliferation. J. Neurosci. 28, 13978–13984. doi: 10.1523/JNEUROSCI.2140-08.2008
Lee, H., Baek, J., Min, H., Cho, I. H., Yu, S. W., and Lee, S. J. (2017). Toll-like receptor 3 contributes to wallerian degeneration after peripheral nerve injury. Neuroimmunomodulation 23, 209–216. doi: 10.1159/000449134
Leemans, J. C., Weening, J. J., Florquin, S., Leemans, J. C., Stokman, G., Claessen, N., et al. (2005). Ischemia / reperfusion injury in the kidney find the latest version: renal-associated TLR2 mediates ischemia / reperfusion injury in the kidney. J. Clin. Invest. 115, 2894–2903. doi: 10.1172/JCI22832
Li, L., Acioglu, C., Heary, R. F., and Elkabes, S. (2021). Role of astroglial toll-like receptors (TLRs) in central nervous system infections, injury and neurodegenerative diseases. Brain Behav. Immun. 91, 740–755. doi: 10.1016/j.bbi.2020.10.007
Li, G., Forero, M. G., Wentzell, J., Durmus, I., Wolf, R., Anthoney, N., et al. (2020). A toll-receptor map underlies structural brain plasticity. eLife 9, 1–32. doi: 10.7554/eLife.52743
Li, T., Hoogman, M., Roth Mota, N., Buitelaar, J. K., Vasquez, A. A., Franke, B., et al. (2022). Dissecting the heterogeneous subcortical brain volume of autism spectrum disorder using community detection. Autism Res. 15, 42–55. doi: 10.1002/aur.2627
Li, C., Xie, Z., Xing, Z., Zhu, H., Zhou, W., Xie, S., et al. (2022). The notch signaling pathway regulates differentiation of NG2 cells into oligodendrocytes in demyelinating diseases. Cell. Mol. Neurobiol. 42, 1–11. doi: 10.1007/s10571-021-01089-0
Li, X., Yun, Z., Tan, Z., Li, S., Wang, D., Ma, K., et al. (2013). The role of toll-like receptor (TLR) 2 and 9 in renal ischemia and reperfusion injury. Urology 81, 1379.e15–1379.e20. doi: 10.1016/j.urology.2013.02.016
Lima, G. K., Zolini, G. P., Mansur, D. S., Lima, B. H. F., Wischhoff, U., Astigarraga, R. G., et al. (2010). Toll-like receptor (TLR) 2 and TLR9 expressed in trigeminal ganglia are critical to viral control during herpes simplex virus 1 infection. Am. J. Pathol. 177, 2433–2445. doi: 10.2353/ajpath.2010.100121
Lins, B. R., Marks, W. N., Zabder, N. K., Greba, Q., and Howland, J. G. (2019). Maternal immune activation during pregnancy alters the behavior profile of female offspring of Sprague dawley rats. eNeuro 6, ENEURO.0437–ENEU18.2019. doi: 10.1523/ENEURO.0437-18.2019
Liu, H. Y., Chen, C. Y., and Hsueh, Y. P. (2014). Innate immune responses regulate morphogenesis and degeneration: roles of toll-like receptors and Sarm1 in neurons. Neurosci. Bull. 30, 645–654. doi: 10.1007/s12264-014-1445-5
Liu, S., Liu, Y., Hao, W., Wolf, L., Kiliaan, A. J., Penke, B., et al. (2012). TLR2 is a primary receptor for Alzheimer’s amyloid peptide to trigger Neuroinflammatory activation. J. Immunol. 188, 1098–1107. doi: 10.4049/jimmunol.1101121
Liu, G., Zhang, H., Zhao, C., and Zhang, H. (2019). Evolutionary history of the toll-like receptor gene family across vertebrates. Genome Biol. Evol. 12, 3615–3634. doi: 10.1093/gbe/evz266
Lovendahl, K. N., Blacklow, S. C., and Gordon, W. R. (2018). The molecular mechanism of notch activation. Adv. Exp. Med. Biol. 1066, 47–58. doi: 10.1007/978-3-319-89512-3_3
Luca, V. C., Jude, K. M., Pierce, N. W., Nachury, M. V., Fischer, S., and Garcia, K. C. (2015). Structural basis for Notch1 engagement of Delta-like 4. Science 347, 847–853. doi: 10.1126/science.1261093
Luca, V. C., Kim, B. C., Ge, C., Kakuda, S., Wu, D., Roein-Peikar, M., et al. (2017). Notch-jagged complex structure implicates a catch bond in tuning ligand sensitivity. Science 355, 1320–1324. doi: 10.1126/science.aaf9739
Lukito, S., Norman, L., Carlisi, C., Radua, J., Hart, H., Simonoff, E., et al. (2020). Comparative meta-analyses of brain structural and functional abnormalities during cognitive control in attention-deficit/hyperactivity disorder and autism spectrum disorder. Psychol. Med. 50, 894–919. doi: 10.1017/S0033291720000574
Ma, Y., Haynes, R. L., Sidman, R. L., and Vartanian, T. (2007). An innate immune receptor in brain, neurons and axons. Cell Cycle 6, 2859–2868. doi: 10.4161/cc.6.23.5018
Mann, B., Crawford, J. C., Reddy, K., Lott, J., Youn, Y. H., Gao, G., et al. (2023). Bacterial TLR2/6 ligands block Ciliogenesis, Derepress hedgehog signaling, and expand the neocortex. MBio 14, e0051023–e0051019. doi: 10.1128/mbio.00510-23
Marczenke, M., Sunaga-Franze, D. Y., Popp, O., Althaus, I. W., Sauer, S., Mertins, P., et al. (2021). GAS1 is required for NOTCH-dependent facilitation of SHH signaling in the ventral forebrain neuroepithelium. Development 148, 1–14. doi: 10.1242/dev.200080
Martínez-García, M. Á., Ojeda-Ojeda, M., Rodríguez-Martín, E., Insenser, M., Moncayo, S., Álvarez-Blasco, F., et al. (2020). TLR2 and TLR4 surface and gene expression in White blood cells after fasting and Oral glucose, lipid and protein challenges: influence of obesity and sex hormones. Biomol. Ther. 10:111. doi: 10.3390/biom10010111
Mase, S., Shitamukai, A., Wu, Q., Morimoto, M., Gridley, T., and Matsuzaki, F. (2021). Notch1 and Notch2 collaboratively maintain radial glial cells in mouse neurogenesis. Neurosci. Res. 170, 122–132. doi: 10.1016/j.neures.2020.11.007
Matsumura, T., Degawa, T., Takii, T., Hayashi, H., Okamoto, T., Inoue, J. I., et al. (2003). TRAF6-NF-κB pathway is essential for interleukin-1-induced TLR2 expression and its functional response to TLR2 ligand in murine hepatocytes. Immunology 109, 127–136. doi: 10.1046/j.1365-2567.2003.01627.x
Mehmeti, M., Bergenfelz, C., Källberg, E., Millrud, C. R., Björk, P., Ivars, F., et al. (2019). Wnt5a is a TLR2/4-ligand that induces tolerance in human myeloid cells. Commun. Biol. 2, 176–113. doi: 10.1038/s42003-019-0432-4
Merikangas, A. K., and Almasy, L. (2020). Using the tools of genetic epidemiology to understand sex differences in neuropsychiatric disorders. Genes Brain Behav. 19, e12660–e12616. doi: 10.1111/gbb.12660
Miyamoto, A., Wake, H., Moorhouse, A. J., and Nabekura, J. (2013). Microglia and synapse interactions: fine tuning neural circuits and candidate molecules. Front. Cell. Neurosci. 7, 1–6. doi: 10.3389/fncel.2013.00070
Moloney, D. J., Panin, V. M., Johnston, S. H., Chen, J., Shao, L., Wilson, R., et al. (2000). Fringe is a glycosyltransferase that modifies notch. Nature 406, 369–375. doi: 10.1038/35019000
Mumm, J. S., Schroeter, E. H., Saxena, M. T., Griesemer, A., Tian, X., Pan, D. J., et al. (2000). A ligand-induced extracellular cleavage regulates γ-secretase-like proteolytic activation of Notch1. Mol. Cell 5, 197–206. doi: 10.1016/S1097-2765(00)80416-5
Musse, A. A., Meloty-Kapella, L., and Weinmaster, G. (2012). Notch ligand endocytosis: mechanistic basis of signaling activity. Semin. Cell Dev. Biol. 23, 429–436. doi: 10.1016/j.semcdb.2012.01.011
Namihira, M., Kohyama, J., Semi, K., Sanosaka, T., Deneen, B., Taga, T., et al. (2009). Committed neuronal precursors confer astrocytic potential on residual neural precursor cells. Dev. Cell 16, 245–255. doi: 10.1016/j.devcel.2008.12.014
Nichols, J. R., Aldrich, A. L., Mariani, M. M., Vidlak, D., Esen, N., and Kielian, T. (2009). TLR2 deficiency leads to increased Th17 infiltrates in experimental brain abscesses. J. Immunol. 182, 7119–7130. doi: 10.4049/jimmunol.0802656
Nichols, J. T., Miyamoto, A., Olsen, S. L., D’Souza, B., Yao, C., and Weinmaster, G. (2007). DSL ligand endocytosis physically dissociates Notch1 heterodimers before activating proteolysis can occur. J. Cell Biol. 176, 445–458. doi: 10.1083/jcb.200609014
Noordstra, I., and Yap, A. S. (2021). For whom the cell tolls. Dev. Cell 56, 1555–1557. doi: 10.1016/j.devcel.2021.05.013
Okubo, Y., Ohtake, F., Igarashi, K., Yasuhiko, Y., Hirabayashi, Y., Saga, Y., et al. (2021). Cleaved Delta like 1 intracellular domain regulates neural development via notch signal-dependent and-independent pathways. Development 148:664. doi: 10.1242/dev.193664
Okun, E., Griffioen, K. J., Gen Son, T., Lee, J. H., Roberts, N. J., Mughal, M. R., et al. (2010). TLR2 activation inhibits embryonic neural progenitor cell proliferation. J. Neurochem. 114, 462–474. doi: 10.1111/j.1471-4159.2010.06778.x
Okun, E., Griffioen, K. J., and Mattson, M. P. (2019). Toll-like receptor signaling in neural plasticity and disease. Trends Neurosci. 34, 269–281. doi: 10.1016/j.tins.2011.02.005
Okun, E., Griffioen, K. J., Rothman, S., Wan, R., Cong, W. N., De Cabo, R., et al. (2014). Toll-like receptors 2 and 4 modulate autonomic control of heart rate and energy metabolism. Brain Behav. Immun. 36, 90–100. doi: 10.1016/j.bbi.2013.10.013
Opitz, B., Schröder, N. W. J., Spreitzer, I., Michelsen, K. S., Kirschning, C. J., Hallatschek, W., et al. (2001). Toll-like Receptor-2 mediates Treponema glycolipid and Lipoteichoic acid-induced NF-κB translocation. J. Biol. Chem. 276, 22041–22047. doi: 10.1074/jbc.M010481200
Palaga, T., Buranaruk, C., Rengpipat, S., Fauq, A. H., Golde, T. E., Kaufmann, S. H. E., et al. (2008). Notch signaling is activated by TLR stimulation and regulates macrophage functions. Eur. J. Immunol. 38, 174–183. doi: 10.1002/eji.200636999
Park, C., Cho, I., Kim, D., Jo, E., Choi, S., Bae, S., et al. (2008). Toll-like receptor 2 contributes to glial cell activation and heme oxygenase-1 expression in traumatic brain injury. Neurosci. Lett. 431, 123–128. doi: 10.1016/j.neulet.2007.11.057
Park, B. S., and Lee, J. O. (2013). Recognition of lipopolysaccharide pattern by TLR4 complexes. Exp. Mol. Med. 45:e66. doi: 10.1038/emm.2013.97
Park, B. S., Song, D. H., Kim, H. M., Choi, B. S., Lee, H., and Lee, J. O. (2009). The structural basis of lipopolysaccharide recognition by the TLR4-MD-2 complex. Nature 458, 1191–1195. doi: 10.1038/nature07830
Parthier, C., Stelter, M., Ursel, C., Fandrich, U., Lilie, H., Breithaupt, C., et al. (2014). Structure of the toll-Spätzle complex, a molecular hub in Drosophila development and innate immunity. Proc. Natl. Acad. Sci. USA 111, 6281–6286. doi: 10.1073/pnas.1320678111
Patten, B. A., Peyrin, J. M., Weinmaster, G., and Corfas, G. (2003). Sequential signaling through Notch1 and erbB receptors mediates radial glia differentiation. J. Neurosci. 23, 6132–6140. doi: 10.1523/jneurosci.23-14-06132.2003
Patten, B. A., Sardi, S. P., Koirala, S., Nakafuku, M., and Corfas, G. (2006). Notch1 signaling regulates radial glia differentiation through multiple transcriptional mechanisms. J. Neurosci. 26, 3102–3108. doi: 10.1523/JNEUROSCI.4829-05.2006
Polsek, D., Cash, D., Veronese, M., Ilic, K., Wood, T. C., Milosevic, M., et al. (2020). The innate immune toll-like-receptor-2 modulates the depressogenic and anorexiolytic neuroinflammatory response in obstructive sleep apnoea. Sci. Rep. 10, 11475–11413. doi: 10.1038/s41598-020-68299-2
Pucilowska, J., Vithayathil, J., Tavares, E. J., Kelly, C., Colleen Karlo, J., and Landreth, G. E. (2015). The 16p11.2 deletion mouse model of autism exhibits altered cortical progenitor proliferation and brain cytoarchitecture linked to the ERK MAPK pathway. J. Neurosci. 35, 3190–3200. doi: 10.1523/JNEUROSCI.4864-13.2015
Rabadán, M. A., Cayuso, J., Le Dréau, G., Cruz, C., Barzi, M., Pons, S., et al. (2012). Jagged2 controls the generation of motor neuron and oligodendrocyte progenitors in the ventral spinal cord. Cell Death Differ. 19, 209–219. doi: 10.1038/cdd.2011.84
Rangasamy, S. B., Jana, M., Roy, A., Corbett, G. T., Kundu, M., Chandra, S., et al. (2018). Selective disruption of TLR2-MyD88 interaction inhibits inflammation and attenuates Alzheimer’s pathology. J. Clin. Invest. 128, 4297–4312. doi: 10.1172/JCI96209
Re, F., and Strominger, J. L. (2001). Toll-like receptor 2 (TLR2) and TLR4 differentially activate human dendritic cells. J. Biol. Chem. 276, 37692–37699. doi: 10.1074/jbc.M105927200
Rolls, A., Shechter, R., London, A., Ziv, Y., Ronen, A., Levy, R., et al. (2007). Toll-like receptors modulate adult hippocampal neurogenesis. Nat. Cell Biol. 9, 1081–1088. doi: 10.1038/ncb1629
Rossjohn, J., Cappai, R., Feil, S. C., Henry, A., McKinstry, W. J., Galatis, D., et al. (1999). Crystal structure of the Nterminal, growth factor-like domain of Alzheimer amyloid precursor protein. Nat. Struct. Biol. 6, 327–331. doi: 10.1038/7562
Salunke, D. B., Shukla, N. M., Yoo, E., Crall, B. M., Balakrishna, R., Malladi, S. S., et al. (2012). Structure-activity relationships in human toll-like receptor 2-specific monoacyl lipopeptides. J. Med. Chem. 55, 3353–3363. doi: 10.1021/jm3000533
Sanchez-Irizarry, C., Carpenter, A. C., Weng, A. P., Pear, W. S., Aster, J. C., and Blacklow, S. C. (2004). Notch subunit Heterodimerization and prevention of ligand-independent proteolytic activation depend, respectively, on a novel domain and the LNR repeats. Mol. Cell. Biol. 24, 9265–9273. doi: 10.1128/mcb.24.21.9265-9273.2004
Sanchez-Petidier, M., Guerri, C., and Moreno-Manzano, V. (2022). Toll-like receptors 2 and 4 differentially regulate the self-renewal and differentiation of spinal cord neural precursor cells. Stem Cell Res Ther 13, 1–16. doi: 10.1186/s13287-022-02798-z
Schafer, D. P., Lehrman, E. K., Kautzman, A. G., Koyama, R., Mardinly, A. R., Yamasaki, R., et al. (2012). Microglia sculpt postnatal neural circuits in an activity and complement-dependent manner. Neuron 74, 691–705. doi: 10.1016/j.neuron.2012.03.026
Schafer, D. P., Lehrman, E. K., and Stevens, B. (2013). The “quad-partite” synapse: microglia-synapse interactions in the developing and mature CNS. Glia 61, 24–36. doi: 10.1002/glia.22389
Scheffel, J., Regen, T., Van Rossum, D., Seifert, S., Ribes, S., Nau, R., et al. (2012). Toll-like receptor activation reveals developmental reorganization and unmasks responder subsets of microglia. Glia 60, 1930–1943. doi: 10.1002/glia.22409
Schenk, M., Belisle, J. T., and Modlin, R. L. (2009). TLR2 looks at lipoproteins. Immunity 31, 847–849. doi: 10.1016/j.immuni.2009.11.008
Schonberg, D. L., Popovich, P. G., and McTigue, D. M. (2007). Oligodendrocyte generation is differentially influenced by toll-like receptor (TLR) 2 and TLR4-mediated intraspinal macrophage activation. J. Neuropathol. Exp. Neurol. 66, 1124–1135. doi: 10.1097/nen.0b013e31815c2530
Schumann, R. R. (2011). Old and new findings on lipopolysaccharide-binding protein: a soluble pattern-recognition molecule. Biochem. Soc. Trans. 39, 989–993. doi: 10.1042/BST0390989
Schwob, J. E., Jang, W., Holbrook, E. H., Lin, B., Herrick, D. B., Peterson, J. N., et al. (2017). Stem and progenitor cells of the mammalian olfactory epithelium: taking poietic license. J. Comp. Neurol. 525, 1034–1054. doi: 10.1002/cne.24105
Seong, K. J., Kim, H. J., Cai, B., Kook, M. S., Jung, J. Y., and Kim, W. J. (2018). Toll-like receptor 2 promotes neurogenesis from the dentate gyrus after photothrombotic cerebral ischemia in mice. Korean J. Physiol. Pharmacol. 22, 145–153. doi: 10.4196/kjpp.2018.22.2.145
Šestan, N., Artavanis-Tsakonas, S., and Rakic, P. (1999). Contact-dependent inhibition of cortical neurite growth mediated by notch signaling. Science 286, 741–746. doi: 10.1126/science.286.5440.741
Setzu, A., Lathia, J. D., Zhao, C., Wells, K., Rao, M. S., Ffrench-Constant, C., et al. (2006). Inflammation stimulates myelination by transplanted oligodendrocyte precursor cells. Glia 54, 297–303. doi: 10.1002/glia.20371
Shang, Y., Smith, S., and Hu, X. (2016). Role of notch signaling in regulating innate immunity and inflammation in health and disease. Protein Cell 7, 159–174. doi: 10.1007/s13238-016-0250-0
Shechter, R., Ronen, A., Rolls, A., London, A., Bakalash, S., Young, M. J., et al. (2008). Toll-like receptor 4 restricts retinal progenitor cell proliferation. J. Cell Biol. 183, 393–400. doi: 10.1083/jcb.200804010
Shi, H., Gabarin, N., Hickey, E., and Askalan, R. (2013). TLR-3 receptor activation protects the very immature brain from ischemic injury. J. Neuroinflammation 10:1. doi: 10.1186/1742-2094-10-104
Shi, M., Liu, Z., Lv, Y., Zheng, M., Du, F., Zhao, G., et al. (2011). Forced notch signaling inhibits commissural axon outgrowth in the developing chick central nerve system. PLoS One 6:e14570. doi: 10.1371/journal.pone.0014570
Shmueli, A., Shalit, T., Okun, E., and Shohat-Ophir, G. (2018). The toll pathway in the central nervous system of flies and mammals. Neuro Mol. Med. 20, 419–436. doi: 10.1007/s12017-018-8515-9
Six, E., Ndiaye, D., Laâbi, Y., Brou, C., Gupta-Rossi, N., Israël, A., et al. (2003). The notch ligand Delta1 is sequentially cleaved by an ADAM protease and γ-secretase. Proc. Natl. Acad. Sci. USA 100, 7638–7643. doi: 10.1073/pnas.1230693100
Smith, K. D., and Ozinsky, A. (2002). Toll-like receptor-5 and the innate immune response to bacterial flagellin. Curr. Top. Microbiol. Immunol. 270, 93–108. doi: 10.1007/978-3-642-59430-4_6
Smoak, K. A., Aloor, J. J., Madenspacher, J., Merrick, B. A., Collins, J. B., Zhu, X., et al. (2010). Myeloid differentiation primary response protein 88 couples reverse cholesterol transport to inflammation. Cell Metab. 11, 493–502. doi: 10.1016/j.cmet.2010.04.006
Sommariva, M., Busnelli, M., Menegola, E., Di Renzo, F., Indino, S., Menon, A., et al. (2023). Immunostaining patterns reveal potential morphogenetic role of toll-like receptors 4 and 7 in the development of mouse respiratory system, liver and pancreas. Anat. Cell Biol. 56, 228–235. doi: 10.5115/acb.22.221
Song, Y., Shou, L. M., Ai, L. Y., Bei, Y., and Chen, M. T. (2019). Mini-review: the non-immune functions of toll-like receptors. Crit. Rev. Eukaryot. Gene Expr. 29, 37–45. doi: 10.1615/CritRevEukaryotGeneExpr.2018027399
Sprinzak, D., and Blacklow, S. C. (2021). Biophysics of notch signaling. Annu. Rev. Biophys. 50, 157–189. doi: 10.1146/annurev-biophys-101920-082204
Stasiulewicz, M., Gray, S. D., Mastromina, I., Silva, J. C., Björklund, M., Seymour, P. A., et al. (2015). A conserved role for notch signaling in priming the cellular response to Shh through ciliary localisation of the key Shh transducer Smo. Development 142, 2291–2303. doi: 10.1242/dev.125237
Stridh, L., Smith, P. L. P., Naylor, A. S., Wang, X., and Mallard, C. (2011). Regulation of toll-like receptor 1 and-2 in neonatal mice brains after hypoxia-ischemia. J. Neuroinflammation 8:45. doi: 10.1186/1742-2094-8-45
Stump, G., Durrer, A., Klein, A. L., Lütolf, S., Suter, U., and Taylor, V. (2002). Notch1 and its ligands Delta-like and jagged are expressed and active in distinct cell populations in the postnatal mouse brain. Mech. Dev. 114, 153–159. doi: 10.1016/S0925-4773(02)00043-6
Suckling, R. J., Korona, B., Whiteman, P., Chillakuri, C., Holt, L., Handford, P. A., et al. (2017). Structural and functional dissection of the interplay between lipid and notch binding by human notch ligands. EMBO J. 36, 2204–2215. doi: 10.15252/embj.201796632
Switon, K., Kotulska, K., Janusz-Kaminska, A., Zmorzynska, J., and Jaworski, J. (2017). Molecular neurobiology of mTOR. Neuroscience 341, 112–153. doi: 10.1016/j.neuroscience.2016.11.017
Takeda, K., and Akira, S. (2004). TLR signaling pathways. Semin. Immunol. 16, 3–9. doi: 10.1016/j.smim.2003.10.003
Talukdar, P. M., Abdul, F., Maes, M., Berk, M., Venkatasubramanian, G., Kutty, B. M., et al. (2021). A proof-of-concept study of maternal immune activation mediated induction of toll-like receptor (TLR) and inflammasome pathways leading to neuroprogressive changes and schizophrenia-like behaviours in offspring. Eur. Neuropsychopharmacol. 52, 48–61. doi: 10.1016/j.euroneuro.2021.06.009
Tran, L. N., Loew, S. K., and Franco, S. J. (2023). Notch signaling plays a dual role in regulating the neuron-to-oligodendrocyte switch in the developing dorsal forebrain. J. Neurosci. 43, 6854–6871. doi: 10.1523/JNEUROSCI.0144-23.2023
Trujillo-Paredes, N., Valencia, C., Guerrero-Flores, G., Arzate, D. M., Baizabal, J. M., Guerra-Crespo, M., et al. (2016). Regulation of differentiation flux by notch signalling influences the number of dopaminergic neurons in the adult brain. Biol. Open 5, 336–347. doi: 10.1242/bio.013383
Tsao, P. N., Wei, S. C., Huang, M. T., Lee, M. C., Chou, H. C., Chen, C. Y., et al. (2011). Lipopolysaccharide-induced notch signaling activation through JNK-dependent pathway regulates inflammatory response. J. Biomed. Sci. 18, 56–59. doi: 10.1186/1423-0127-18-56
Tukhvatulin, A. I., Logunov, D. Y., Shcherbinin, D. N., Shmarov, M. M., Naroditsky, B. S., Gudkov, A. V., et al. (2010). Toll-like receptors and their adapter molecules. Biochemist 75, 1098–1114. doi: 10.1134/S0006297910090038
Ulrich, H., Do Nascimento, I. C., Bocsi, J., and Tárnok, A. (2015). Immunomodulation in stem cell differentiation into neurons and brain repair. Stem Cell Rev. Rep. 11, 474–486. doi: 10.1007/s12015-014-9556-6
Umetsu, D. (2022). Cell mechanics and cell-cell recognition controls by toll-like receptors in tissue morphogenesis and homeostasis. Fly 16, 233–247. doi: 10.1080/19336934.2022.2074783
Vabulas, R. M., Ahmad-Nejad, P., da Costa, C., Miethke, T., Kirschning, C. J., Häcker, H., et al. (2001). Endocytosed HSP60s use TLR2 and TLR4 to activate the TIR signaling pathway in innate immune cells. J. Biol. Chem. 276, 31332–31339. doi: 10.1074/jbc.M103217200
Vacharasin, J. M., Ward, J. A., McCord, M. M., Cox, K., Imitola, J., and Lizarraga, S. B. (2024). Neuroimmune mechanisms in autism etiology - untangling a complex problem using human cellular models. Oxford Open Neurosci. 3, 1–17. doi: 10.1093/oons/kvae003
van Bergenhenegouwen, J., Plantinga, T. S., Joosten, L. A. B., Netea, M. G., Folkerts, G., Kraneveld, A. D., et al. (2013). TLR2 & co: a critical analysis of the complex interactions between TLR2 and coreceptors. J. Leukoc. Biol. 94, 885–902. doi: 10.1189/jlb.0113003
Varodayan, F. P., Khom, S., Patel, R. R., Steinman, M. Q., Hedges, D. M., Oleata, C. S., et al. (2018). Role of TLR4 in the modulation of central amygdala GABA transmission by CRF following restraint stress. Alcohol Alcohol. 53, 642–649. doi: 10.1093/alcalc/agx114
Ved, R., Sharouf, F., Harari, B., Muzaffar, M., Manivannan, S., Ormonde, C., et al. (2021). Disulfide HMGB1 acts via TLR2/4 receptors to reduce the numbers of oligodendrocyte progenitor cells after traumatic injury in vitro. Sci. Rep. 11, 6181–6114. doi: 10.1038/s41598-021-84932-0
Verstak, B., Nagpal, K., Bottomley, S. P., Golenbock, D. T., Hertzog, P. J., and Mansell, A. (2009). MyD88 adapter-like (mal)/TIRAP interaction with TRAF6 is critical for TLR2-and TLR4-mediated NF-κB proinflammatory responses. J. Biol. Chem. 284, 24192–24203. doi: 10.1074/jbc.M109.023044
Walker, D. G., Tang, T. M., and Lue, L.-F. (2018). Increased expression of toll-like receptor 3, an anti-viral signaling molecule, and related genes in Alzheimer’s disease brains. Exp. Neurol. 309, 91–106. doi: 10.1016/j.expneurol.2018.07.016
Wang, Y., and Ha, Y. (2004). The X-ray structure of an antiparallel dimer of the human amyloid precursor protein E2 domain. Mol. Cell 15, 343–353. doi: 10.1016/j.molcel.2004.06.037
Wang, H., Kulas, J. A., Wang, C., Holtzman, D. M., Ferris, H. A., and Hansen, S. B. (2021). Regulation of beta-amyloid production in neurons by astrocyte-derived cholesterol. Proc. Natl. Acad. Sci. USA 118:e2102191118. doi: 10.1073/pnas.2102191118
Wang, J., Shao, Y., Bennett, T. A., Shankar, R. A., Wightman, P. D., and Reddy, L. G. (2006). The functional effects of physical interactions among toll-like receptors 7, 8, and 9. J. Biol. Chem. 281, 37427–37434. doi: 10.1074/jbc.M605311200
Wang, M., Yu, F., Wu, W., Wang, Y., Ding, H., and Qian, L. (2018). Epstein-Barr virus-encoded microRNAs as regulators in host immune responses. Int. J. Biol. Sci. 14, 565–576. doi: 10.7150/ijbs.24562
Wasko, N. J., Kulak, M. H., Paul, D., Nicaise, A. M., Yeung, S. T., Nichols, F. C., et al. (2019). Systemic TLR2 tolerance enhances central nervous system remyelination. J. Neuroinflammation 16, 158–115. doi: 10.1186/s12974-019-1540-2
Weiss, H. J., and O’Neill, L. A. J. (2022). Of flies and men—the discovery of TLRs. Cells 11:127. doi: 10.3390/cells11193127
Werkman, I. L., Dubbelaar, M. L., van der Vlies, P., de Boer-Bergsma, J. J., Eggen, B. J. L., and Baron, W. (2020). Transcriptional heterogeneity between primary adult grey and white matter astrocytes underlie differences in modulation of in vitro myelination. J. Neuroinflammation 17, 373–318. doi: 10.1186/s12974-020-02045-3
Werling, D., Jann, O. C., Offord, V., Glass, E. J., and Coffey, T. J. (2009). Variation matters: TLR structure and species-specific pathogen recognition. Trends Immunol. 30, 124–130. doi: 10.1016/j.it.2008.12.001
Winters, L., Winters, T., Gorup, D., Mitrečić, D., Ćurlin, M., Križ, J., et al. (2013). Expression analysis of genes involved in TLR2-related signaling pathway: inflammation and apoptosis after ischemic brain injury. Neuroscience 238, 87–96. doi: 10.1016/j.neuroscience.2013.02.001
Wolfe, M. S., and Miao, Y. (2022). Structure and mechanism of the γ-secretase intramembrane protease complex. Curr. Opin. Struct. Biol. 74:102373. doi: 10.1016/j.sbi.2022.102373
Wouters, M. A., Rigoutsos, I., Chu, C. K., Feng, L. L., Sparrow, D. B., and Dunwoodie, S. L. (2005). Evolution of distinct EGF domains with specific functions. Protein Sci. 14, 1091–1103. doi: 10.1110/ps.041207005
Yaddanapudi, K., de Miranda, J., Hornig, M., and Lipkin, W. I. (2011). Toll-like receptor 3 regulates neural stem cell proliferation by modulating the sonic hedgehog pathway. PLoS One 6:e26766. doi: 10.1371/journal.pone.0026766
Yoon, S., Kurnasov, O., Natarajan, V., Hong, M., Gudkov, A. V., Osterman, A. L., et al. (2012). Structural basis of TLR5-Flagellin recognition and signaling. Science 335, 859–864. doi: 10.1126/science.1215584
Yu, X., Mostafijur Rahman, M., Carter, S. A., Lin, J. C., Zhuang, Z., Chow, T., et al. (2023). Prenatal air pollution, maternal immune activation, and autism spectrum disorder. Environ. Int. 179:108148. doi: 10.1016/j.envint.2023.108148
Yu, S., Wang, W., Albakri, M., Yu, X., Majihail, G., Lim, S., et al. (2021). O-Fucose and fringe-modified NOTCH1 extracellular domain fragments as decoys to release niche-lodged hematopoietic progenitor cells. Glycobiology 31, 582–592. doi: 10.1093/glycob/cwaa113
Zaben, M., Haan, N., Sharouf, F., Ahmed, A., Sundstrom, L. E., and Gray, W. P. (2021). IL-1β and HMGB1 are anti-neurogenic to endogenous neural stem cells in the sclerotic epileptic human hippocampus. J. Neuroinflammation 18, 218–215. doi: 10.1186/s12974-021-02265-1
Zeronian, M. R., Klykov, O., Portell i de Montserrat, J., Konijnenberg, M. J., Gaur, A., Scheltema, R. A., et al. (2021). Notch–jagged signaling complex defined by an interaction mosaic. Proc. Natl. Acad. Sci. USA 118, 1–12. doi: 10.1073/pnas.2102502118
Zhang, Z., Zhang, Z. Y., Wu, Y., and Schluesener, H. J. (2011). Immunolocalization of toll-like receptors 2 and 4 as well as their endogenous ligand, heat shock protein 70, in rat traumatic brain injury. Neuroimmunomodulation 19, 10–19. doi: 10.1159/000326771
Zhou, Z. D., Kumari, U., Xiao, Z. C., and Tan, E. K. (2010). Notch as a molecular switch in neural stem cells. IUBMB Life 62, 618–623. doi: 10.1002/iub.362
Ziegler, G., Harhausen, D., Schepers, C., Hoffmann, O., Röhr, C., Prinz, V., et al. (2007). TLR2 has a detrimental role in mouse transient focal cerebral ischemia. Biochem. Biophys. Res. Commun. 359, 574–579. doi: 10.1016/j.bbrc.2007.05.157
Keywords: neurodevelopment, neural progenitor cells, signaling pathways, cell–cell communication, behavior pattern
Citation: Stojanovic M and Kalanj-Bognar S (2024) Toll-like receptors as a missing link in Notch signaling cascade during neurodevelopment. Front. Mol. Neurosci. 17:1465023. doi: 10.3389/fnmol.2024.1465023
Edited by:
Oliver von Bohlen und Halbach, Universitätsmedizin Greifswald, GermanyReviewed by:
Stephan Maxeiner, Saarland University, GermanyAlberto Pascual, Spanish National Research Council (CSIC), Spain
Copyright © 2024 Stojanovic and Kalanj-Bognar. This is an open-access article distributed under the terms of the Creative Commons Attribution License (CC BY). The use, distribution or reproduction in other forums is permitted, provided the original author(s) and the copyright owner(s) are credited and that the original publication in this journal is cited, in accordance with accepted academic practice. No use, distribution or reproduction is permitted which does not comply with these terms.
*Correspondence:Mario Stojanovic, bWFyaW8uc3RvamFub3ZpY0BpcmIuaHI=; Svjetlana Kalanj-Bognar, c3ZqZXRsYW5hLmthbGFuai5ib2duYXJAbWVmLmhy