- 1Laboratory Neurobiology of the Retina, Department of Neurobiology and Program of Biomedical Sciences, Biology Institute, Fluminense Federal University Niterói, Rio de Janeiro, Brazil
- 2Laboratory Neurobiology of the Retina, Department of Neurobiology and Program of Neurosciences, Biology Institute, Fluminense Federal University, Rio de Janeiro, Brazil
- 3Department of Health Sciences, Clinical Pharmacology and Oncology Section, University of Florence, Florence, Italy
- 4Laboratory of Neuroplasticity, Program of Neurosciences, Department of Neurobiology, Biology Institute, Niteroi, Brazil
- 5Laboratory of Neurobiology of Development, Program of Neurosciences, Department of Neurobiology, Biology Institute, Niteroi, Brazil
The Transient Receptor Potential (TRP) constitutes a family of channels subdivided into seven subfamilies: Ankyrin (TRPA), Canonical (TRPC), Melastatin (TRPM), Mucolipin (TRPML), no-mechano-potential C (TRPN), Polycystic (TRPP), and Vanilloid (TRPV). Although they are structurally similar to one another, the peculiarities of each subfamily are key to the response to stimuli and the signaling pathway that each one triggers. TRPs are non-selective cation channels, most of which are permeable to Ca2+, which is a well-established second messenger that modulates several intracellular signaling pathways and is involved in physiological and pathological conditions in various cell types. TRPs depolarize excitable cells by increasing the influx of Ca2+, Na+, and other cations. Most TRP families are activated by temperature variations, membrane stretching, or chemical agents and, therefore, are defined as polymodal channels. All TPRs are expressed, at some level, in the central nervous system (CNS) and ocular-related structures, such as the retina and optic nerve (ON), except the TRPP in the ON. TRPC, TRPM, TRPV, and TRPML are found in the retinal pigmented cells, whereas only TRPA1 and TRPM are detected in the uvea. Accordingly, several studies have focused on the search to unravel the role of TRPs in physiological and pathological conditions related to the eyes. Thus, this review aims to shed light on endogenous and exogenous modulators, triggered cell signaling pathways, and localization and roles of each subfamily of TRP channels in physiological and pathological conditions in the retina, optic nerve, and retinal pigmented epithelium of vertebrates.
1 Introduction
The visual system is one of the most important senses, contributing to the defense and survival of animals, especially humans, and playing a crucial role in daily activities and complex behaviors (Potier et al., 2018). Visual loss tremendously impacts quality of life, affecting work and leisure activities, such as reading, driving, and watching television. Further, visual deficits/blindness are associated with psychological and social well-being, increasing the probability of developing anxiety and depression (Bradley and Delaffon, 2020).
The external part of the eyeball is composed of the cornea and sclera, followed by the iris, pupil, crystalline, and ciliary body; the posterior part comprises the retina and the choroid (Irsch and Guyton, 2009; Kels et al., 2015). Although all these components are essential to vision, the majority of non-prevented, untreatable visual deficits or blindness results from retinal pathologies (GBD 2019 Blindness and Vision Impairment Collaborators, 2021).
The Transient Receptor Potential (TRP) is an evolutionarily conserved group of channels found in invertebrates, such as the phylum nematodes and arthropods, and in vertebrates, such as fish and mammals (Vriens et al., 2004). They form ion-permeable transmembrane polymodal receptors, classically described in somatosensory neurons but also found in ocular structures, and they are sensitive to different stimulus types (Ma et al., 2022; Samanta et al., 2018). Accordingly, they can be modulated by thermal stimuli, plasma membrane stretching, endogenous/exogenous chemical molecules, fragments of pathogens, and inflammatory signals (Gilliam and Wensel, 2011). The majority of TRPs is permeable to Ca2+, a well-known second messenger, which induces several cellular responses (Reinach et al., 2015; Vangeel and Voets, 2019). Currently, seven subfamilies of TRPs are known: Ankyrin (TRPA), Canonical (TRPC), Melastatin (TRPM), Mucolipin (TRPML), NO-mechano-potential C (TRPN), Polycystic (TRPP) and Vanilloid (TRPV) (Samanta et al., 2018). TRPN was not included in this review as it has not been described in the retina.
Since the discovery of these channels, experimental studies seek the structural detail of each subfamily to reach a greater understanding of the modulatory mechanisms, as well as the action of TRP antagonists and agonists in the respective binding sites or through indirect stimulation, to broaden the understanding of the roles of this superfamily in a physiological and pathological context. In the present review, we focus on shedding light on information about the expression, modulation, and physiological function of TRPs and their putative involvement in the main human retinal pathologies.
2 Search method
The present review searched for published studies on PubMed, Mendeley, and Scopus platforms, using keywords related to the subject topics. In the introductory topic, the combination of terms used was: “TRP” (AND) “brain” (129 articles), OR “vision” (102 articles), OR “structure” (171 articles). For all other topics, the terms “TRPC OR TRPV OR TRPM OR TRPA1 OR TRPML OR TRPP” were combined (AND) with a specific keyword related to the topic, as follows:
• Structure, functioning, exogenous/endogenous modulators, and signaling pathways: “structure OR modulation OR signaling.”
• Expression and functions in ocular structures: “Retina and retinal OR optic nerve OR pigment epithelium.”
• Ocular diseases: “Macular degeneration.” “Diabetic retinopathy,” “retina,” “methylglyoxal,” “glaucoma,” “intraocular pressure,” “retina” “retinitis pigmentosa,” “rd10,” “rd1.”
For this review, we highlighted studies published in English in the last 5 years that brought new information to the subject up to publication. However, some reviews and data published 10 years ago or more were also included. This was necessary to contextualize information about TRP structure and signal/function in retinal physiology or retinopathies. Supplementary material consists of a diagram of search strategies and the total number of articles for each one.
3 TRPs: from modulators to functioning
The first description of the TRP channels was in the Drosophila melanogaster, where abnormal retinal responses to high luminosity were documented (Reinach et al., 2015; Samanta et al., 2018). Since then, there has been an increase in research demonstrating the involvement of TRPs in retinal physiology and pathologies that lead to loss or partial impairment of vision in models of glaucoma (de Araújo et al., 2020; Choi et al., 2015; Irnaten et al., 2020; Oda et al., 2020), diabetic retinopathy (Sachdeva et al., 2018), retinitis pigmentosa (Nelidova et al., 2020), and retinal dysfunction promoted in non-ocular diseases such as mucolipidosis type IV (ML-IV) (Grishchuk et al., 2016; Venugopal et al., 2007).
All TRP subfamilies show some structural similarities. They are tetramers, where each monomer consists of six transmembrane domains (TM) (S1-S6) (Bae et al., 2018; Huang et al., 2020; Huffer et al., 2020). S5 and S6 (Huffer et al., 2020) are connected to the p-loop (Bae et al., 2018; Huang et al., 2020), and the three structures comprise the channel selectivity filter (Bae et al., 2018; de Baaij et al., 2014; Huang et al., 2020).
TRPs are a remarkably diverse group of proteins with several characteristics, which will be highlighted in the present review. Most TRP channels are permeable to monovalent and divalent ions, except for TRPV5 and TRPV6, which are highly ion-selective and have the response amplitude dependent on the extracellular Ca2+ concentration (10 and 30 mM, respectively) (Hoenderop et al., 2003; Nilius et al., 2001; Montell, 2005; Voets et al., 2001; Figure 1A). There is also an extension originating right after TM at the end of the N-terminal, called the TRP box, whose activity is related to channel activation and inactivation (Feng, 2017; Huffer et al., 2020).
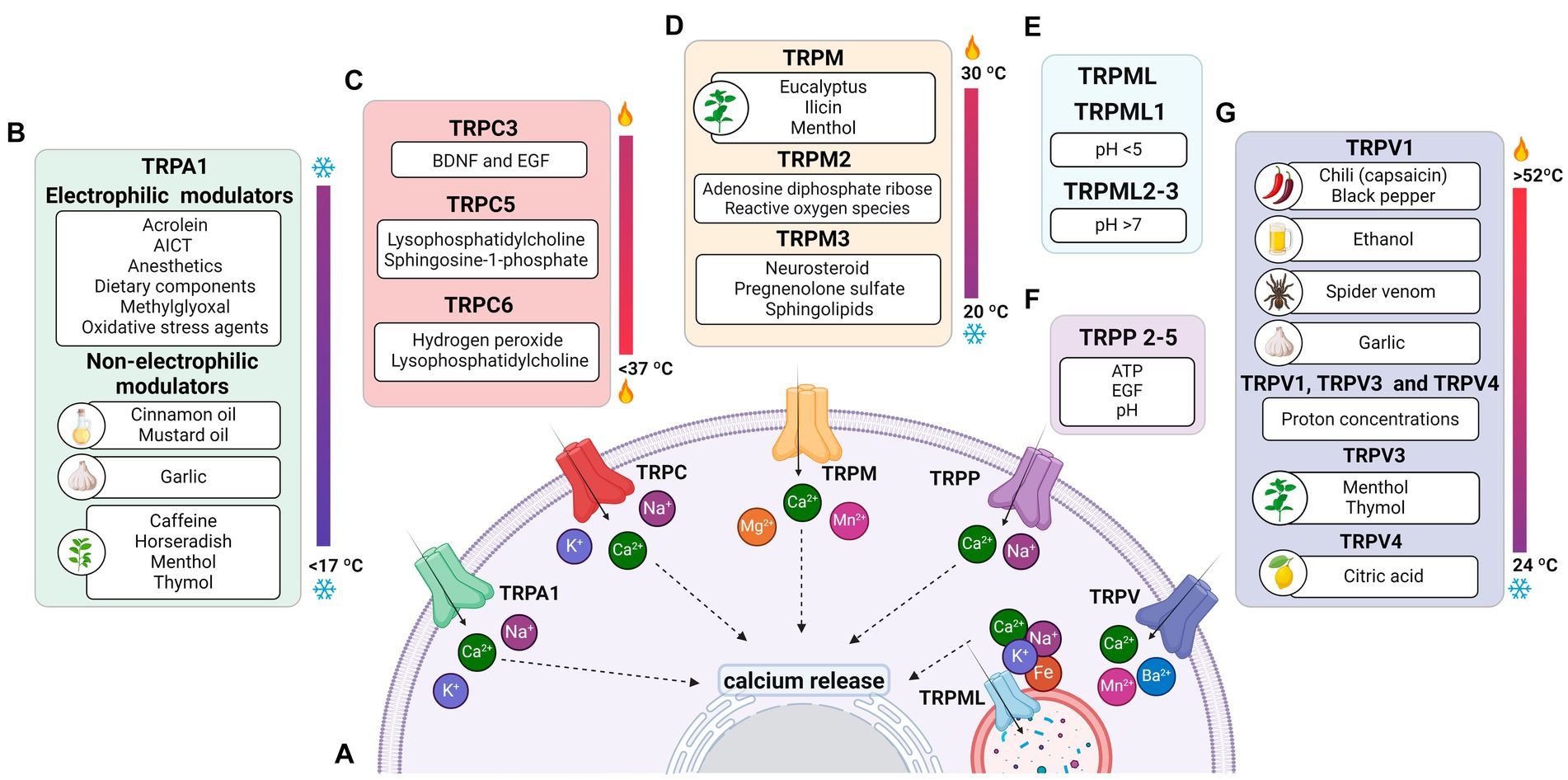
Figure 1. Permeability of TRP receptors and exogenous modulators. (A) TRPA1 receptors and TRPCs are permeable to potassium (K+), sodium (Na+), and calcium (Ca2+) ions; TRPM, TRPP and TRPVs receptors are permeable to calcium; the TRPML receptor is permeable to calcium, sodium (Na+), iron (Fe), and potassium (K+) ions. Since all receptors are permeable to calcium, their activation contributes to an increase in intracellular calcium levels and the release of ions stored in the endoplasmic reticulum. (B) Modulators of TRPA1 receptors, including acrylamide-induced cytotoxicity (AICT), dietary components, and oxidative stress agents. (C) Regulators of TRPC receptors, including brain-derived neurotrophic factor (BDNF) and epidermal growth factor (EGF). (D) Extrinsic modulators of TRPM receptors. (E) Extrinsic modulators of TRPML receptors, including adenosine triphosphate (ATP) and changes in acidity/alkalinity (pH). (F) Regulators of TRPP receptors. (G) Modulators of TRPV receptors.
The C- and N-termini constitute the cytoplasmic region of TRPs (Huffer et al., 2020). At the C-terminus of the TRPC, TRPM, and TRPA1 subfamilies, the coiled-coil (CC) domain appears to play a role in ligand anchoring and assembly of these channels in the lipid bilayer (Feng, 2017; Hilton et al., 2019; Nooren et al., 1999). Furthermore, the N-terminus of TRPA1, TRPC, TRPV, and TRPM exhibits repeats of the ankyrin protein, whose function is related to conformational changes of the ionic pore and target of specific molecules in some TRPs (Feng, 2017; Hilton et al., 2019; Li et al., 2019).
The central pore and the TRP box are highly conserved in the different TRP subfamilies, while S1-S4 show structural modifications, and the C-terminus and N-terminus are quite variable between subfamilies. These differences contribute to establishing specificities and modulatory mechanisms in TRP channels (Huffer et al., 2020; Li et al., 2011). Similarities and differences in the structural components between TRP subfamily members, modulating molecules, as well as the response after activation of these channels, are summarized in Table 1.
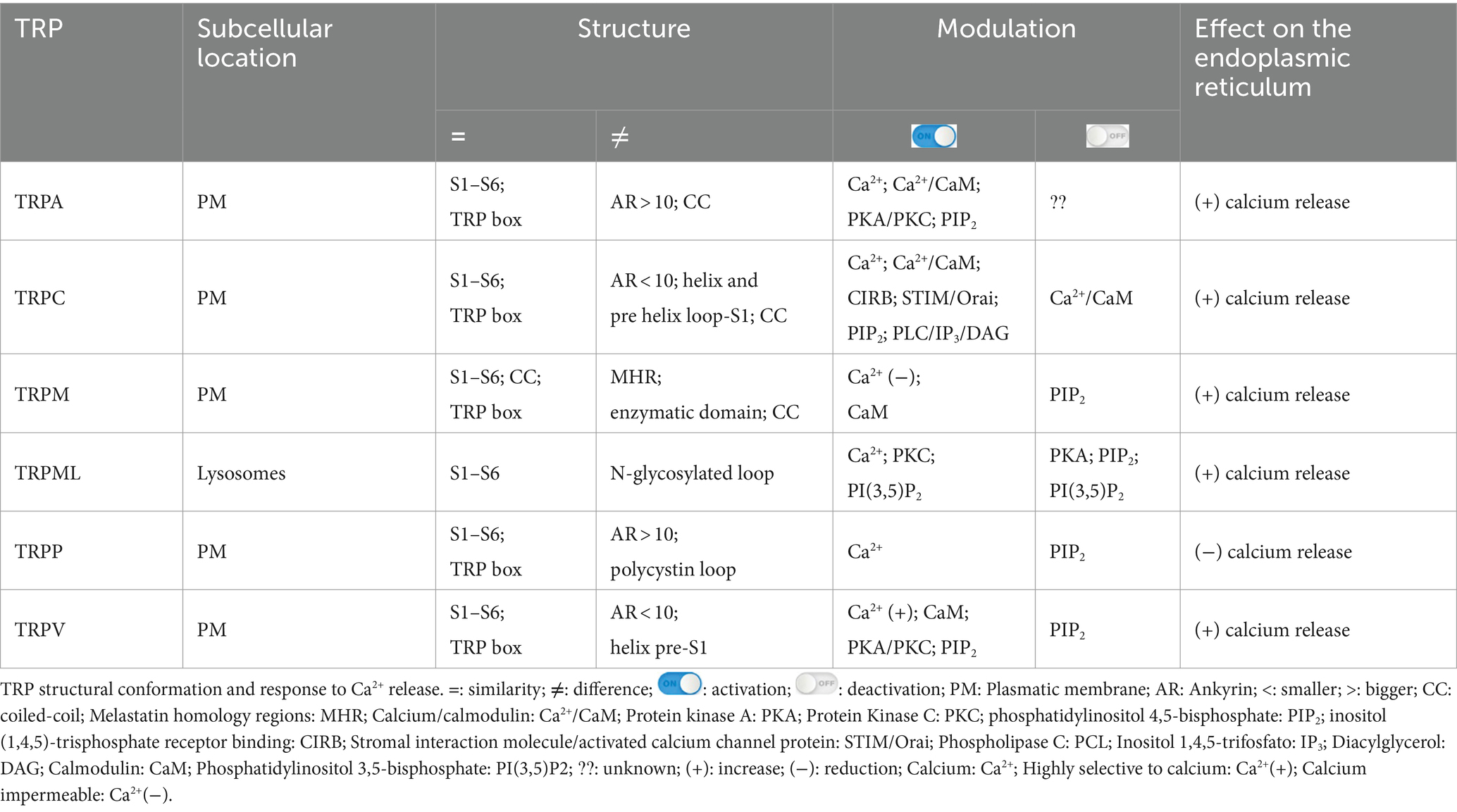
Table 1. Structural components, modulatory and response mechanism peculiarities among all TRP subfamilies.
3.1 TRPA1
TRPA1 is a cation-permeable channel, especially to Ca2+ (Talavera et al., 2020; Figure 1A). This receptor is mechanical-, thermo-, and chemo-sensitive and can be activated by exogenous and endogenous agents (Kádková et al., 2017). There are binding sites for channel-modulating substances in TRPA1 transmembrane domains, which also appear important for voltage-sensitivity and -dependence. Marsakova and collaborators suggest a binding site for Ca2+ in the external region of the TRPA1 protein and that the S1-S2 domains are physically coupled to the gating regions of the channel (Marsakova et al., 2017). On the other hand, recent experiments by Zhao et al. (2020) suggest that Ca2+-dependent modulations appear to be concentrated in S2–S3 (Zhao et al., 2020). The size of the NH2-terminal is equivalent to all the rest of the protein, consisting of 14–18 repetitions of ankyrin, a characteristic that gave TRPA1 its name (Meents et al., 2017; Nilius et al., 2011). In addition to being a binding site for several molecules, such as cytosolic Ca2+, it also appears to participate in thermal and chemical responsiveness (Cordero-Morales et al., 2011; Jabba et al., 2014).
The N-terminal region can regulate and integrate stimuli (Zayats et al., 2013; Hynkova et al., 2016). Many possible forms of channel modulation involving the N-terminal region have been described: (1) irritating agonists and electrophiles (isocyanates, cinnamaldehyde, garlic diallyl disulfide, acrolein) can chemically react with primary amines and cysteines (Bahia et al., 2016; Suo et al., 2020) inducing channel opening (Hinman et al., 2006; Macpherson et al., 2007); (2) direct calcium binding participating in desensitization (Cordero-Morales et al., 2011); (3) direct interaction with proteins such as FGFR2, which inhibit TRPA1 (Berrout et al., 2017); (4) phosphorylation, for example by protein kinase A (PKA) or protein kinase C (PKC), which can increase levels of the channel on the plasma membrane. The C-terminal termination also has Ca2+ binding sites but seems more associated with voltage-dependent responses (Sura et al., 2012). A summary of the stimuli of TRP is shown in Table 2.
It is known that the activation of all TRPs promotes the entry of Ca2+ into the cell, which, in turn, triggers the activation of biochemical pathways, such as the calcium-dependent-phospholipase C (PLC) pathway (Kouchi et al., 2004), which hydrolyzes phosphatidylinositol 4,5-bisphosphate (PIP2) into inositol 1,4,5-trifosfato (IP3) and diacylglycerol (DAG) (Wang et al., 2020) and then IP3 binds to IP3 receptors located in the endoplasmic reticulum (ER), promoting a transient increase in cytosolic Ca2+ (Curcic et al., 2019; Mori et al., 2015; Figure 1A). PIP2 is a major modulator of TRP channels, and it can sometimes inhibit or activate, as will be discussed later (Pumroy et al., 2020). Usually, one of the interaction sites with PIP2 is located at the N-terminus of TRPs, and in the case of TRPA1, it is also located at the C-terminus (Macikova et al., 2019).
TRPA1 can be modulated by a huge variety of chemical compounds, divided into electrophilic and non-electrophilic modulators, according to the ability of each group to interact covalently or noncovalently, respectively (Talavera et al., 2020; Figure 1B). Among the former group, there are dietary components such as cinnamaldehyde, allicin (Bandell et al., 2004), isothiocyanates (Bandell et al., 2004), thymol, methyl salicylate; anesthetics as isoflurane, lidocaine, and propofol (Matta et al., 2008); and oxidative stress agents such as nitric oxide (NO), hydrogen peroxide (H2O2), and methylglyoxal (MG) (Cao et al., 2012; Meents et al., 2017), although reactive oxygen (ROS), nitrogen (RNS), and carbonyl (RCS) species are all, in general, potential activators (Bessac and Jordt, 2008; Nilius et al., 2011). In the context of oxidative stress, the product of lipid peroxidation, 4-hydroxynonenal (4-HNE), is also a potent activator of TRPA1 (Trevisani et al., 2007). The non-electrophilic group includes some commonly consumed components, such as menthol (Karashima et al., 2009), thymol (Lee et al., 2008), carvacrol (Xu et al., 2006), nicotine, caffeine, and Δ9–tetrahydrocannabinol; medication like nifedipine and nonsteroidal anti-inflammatory drugs (Jordt et al., 2004; Nassini et al., 2015; Talavera et al., 2009); and protons (Nilius et al., 2011). Interestingly, some ligands have a bimodal effect, activating the channel in low concentrations while inhibiting it at higher doses, as it is the case of nicotine and menthol (Karashima et al., 2009; Talavera et al., 2009). Furthermore, there are also components with species-specific effects when it comes to modulation of the channel; for example, caffeine can activate TRPA1 in mice but suppresses it in humans (Nagatomo and Kubo, 2008).
Ca2+ is another important modulator of TRPA1 activity. It interacts intracellularly first to activate and then increases the response to other activators, followed by an inactivation process (Sura et al., 2012). Both potentiation and inactivation appear to be mediated by independent processes (Wang et al., 2008). In addition, there is evidence that modulation by Ca2+ is dependent on the direct binding of calmodulin to the channel, which affects both the activation and inactivation steps (Hasan et al., 2017), although the direct action of Ca2+ on human TRPA1 has also been demonstrated by calmodulin-independent mechanisms (Moparthi et al., 2020).
Protons can also activate TRPA1 in the context of acidosis, a common event in ischemia, for example. Intracellular protons are more commonly accepted as modulators, while extracellular activation is species specific (de la Roche et al., 2013). Additionally, phosphorylation sites for kinases, such as PKA and cyclin-dependent kinase 5 (Cdk5), have been identified and correlated with the capacity to promote sensitization of the channel, enabling increased responsiveness to agonist stimulation (Meents et al., 2017; Hall et al., 2018). As demonstrated recently, PKC can also sensitize the channel (McMillan et al., 2021), but data concerning more direct interaction needs further analysis.
3.2 TRPC
TRPC 1–7 are classified as non-selective channels, permeable to Na+, K+, and Ca2+ (DeHaven et al., 2009; Figure 1A). TRPCs are abundantly expressed in immune cells, endothelium, heart, striated muscle, pancreas, kidneys, placenta, retina, pituitary gland, cerebellum, limbic system, and substantia nigra (Wang et al., 2020; Warren et al., 2006). Only TRPC2 is not expressed in human cells (de Souza and Ambudkar, 2014).
The carboxy-terminal region commonly comprises domains that bind Ca2+/CaM complex and inositol (1,4,5)-trisphosphate receptor binding (CIRB), and other molecules, such as trimeric G proteins (Friedlova et al., 2010; Wang et al., 2010; Wang et al., 2020). Although the mechanisms of TRPC activation are not yet well described (Wang et al., 2020), it is known that they are channels dependent on PLC, either activated by G protein-coupled receptors or tyrosine kinase receptors (Feng, 2017; Li et al., 2019; Wang et al., 2020; Zhu, 2005).
In response to PLC signaling, there is an elevation in calcium levels in the cytoplasm, with a consequent depletion of Ca2+ stores in the ER, resulting in activation of TRPC, which promotes robust and sustained Ca2+ entry by the channel (Curcic et al., 2019; Hofmann et al., 1999; Mori et al., 2015; Zhu, 2005).
Therefore, it is proposed that TRPC represent store-operated Ca2+ entry channels (SOCE), as they play a key role in increasing cytoplasmic Ca2+ (DeHaven et al., 2009; Feng, 2017; Li et al., 2019). Furthermore, intracellular Ca2+ moderately potentiates TRPC4 responses and considerably TRPC5, possibly due to a longer opening time of both ionic pores (Blair et al., 2009; Mori et al., 2015). Although TRPC1 alone functions as a SOCE in human adult retinal pigment epithelial-19 (ARPE-19) cells (Bollimuntha et al., 2005), TRPC3 and 4 only function as SOCE when co-expressed with TRPC1 (de Souza and Ambudkar, 2014). It has already been demonstrated that these TRPCs function as a SOCE channel in cultured Müller glia and 129/SvImJ mice (Molnár et al., 2016; Da Silva et al., 2008).
Physical stimuli have been linked to the activation of TRPCs. TRPC1, C5, and C6 are characterized as mechanosensitive receptors (Choi et al., 2015; Irnaten et al., 2020), while TRPC5 is also stimulated by temperatures below 37°C (Oda et al., 2020; Figure 1C).
TRPC1, 4, and 5 are activated directly by phospholipase C (Duan et al., 2018; Chen et al., 2020). Both TRPC3 and C5 can be stimulated by IP3, while C3, 6, and 7 exhibit varying sensitivity in the presence of PIP2 (Wang et al., 2020). TRPC2, 3, 6, and 7 are directly stimulated by DAG (Fan et al., 2018; Li et al., 2019; Wang et al., 2020), while TRPC may not be stimulated by DAG in ipRGCs culture (Graham et al., 2008). Although TRPC4 and C5 are not activated by DAG, they may become sensitive to the molecule and its derivatives (Curcic et al., 2019; Storch et al., 2017). This sensitivity change in TRPC4-5 occurs while PKC activity is inhibited since the kinase deactivates these TRPCs as well as PIP2 (Storch et al., 2017).
3.3 TRPM
The TRPM family consists of eight members (TRPM1–TRPM8), expressed in cells distributed in different tissues, such as the retina, kidney, pancreas, prostate, heart, intestine, and immune system (Huang et al., 2020; Sawamura et al., 2017). In the nervous system, TRPM is detected in neurons and glial cells (astrocytes, oligodendrocytes, and microglia) (Sawamura et al., 2017).
TRPM1, TRPM2, TRPM3, TRPM6, TRPM7, and TRPM8 share similarities in generating responses through Ca2+ influx (Bae et al., 2018), while TRPM4 and TRPM5 are voltage-dependent and Ca2+ impermeable (Huang et al., 2020; Samanta et al., 2018). TRPMs are also permeable to Mg2+ and Mn2+; Mg2+ negatively modulates the TRPM1 channel expressed in the photoreceptor (Jimenez et al., 2020; Figure 1A). In addition, TRPM5 is activated by intracellular Ca2+ with greater sensitivity than TRPM4 (Ruan et al., 2021; Zierler et al., 2017).
The TRPM has particular structural characteristics that are distinct from other TRPs. They are the receptors with the highest amount of amino acid residues in the cytosolic region and do not have the N-terminal ankyrin repeats (Huang et al., 2020; Kraft and Harteneck, 2005; Samanta et al., 2018). The N-terminus comprises four melastatin homology regions (MHRs), while the C-terminus exposes two domains (Chen et al., 2019; Guo et al., 2017).
Furthermore, the cytoplasmic domain may show differences between members of the TRPM family (Huang et al., 2020; Jirku et al., 2015; Zierler et al., 2017). For example, most members of the TRPM family, including TRPM1, exhibit binding sites in both the transmembrane domain and the N-terminus for interaction with PIP2 (Huang et al., 2020; Jirku et al., 2015; Zierler et al., 2017). The C-terminus of TRPM2, 6, and 7 have enzymatic domains (Guo et al., 2017; Jirku et al., 2015; Samanta et al., 2018). The enzymatic domains reported in experimental data at the C-terminus of TRPM6 and 7 are comprised of alpha kinases, a class of kinases that preferentially phosphorylate on the alpha helix of the target molecule. These kinases can induce positive modulatory functions related to Mg2+ (Cabezas-Bratesco et al., 2015; Cai et al., 2017); the ion also plays a negative role in the activation of TRPM1 in retinal cells (Rampino and Nawy, 2011). Conditions of increased ROS production and oxidative stress induce ADPR release and TRPM2 activation (Tóth et al., 2014; Huang et al., 2019; Huang et al., 2018; Figure 1D).
It has been described that at the N-terminus of TRPM2, there is an isoleucine glutamine (IQ) domain, reported as the main binding site for the Ca2+/CaM complex (Du et al., 2009; Kim et al., 2008; Syed Mortadza et al., 2015). Experiments involving mutations in amino acids of the IQ motif resulted in inactivity of TRPM2 when the channel was stimulated by increased intracellular Ca2+ or ADPR, suggesting that the Ca2+/CaM complex plays a crucial role in the activation of TRPM2 (Du et al., 2009).
TRPMs play a variety of physiological and biochemical roles in health and disease (Jimenez et al., 2020). For example, TRPM1 plays a key role in the response of retinal ON-bipolar cells (Rampino and Nawy, 2011; Wong et al., 2019); TRPM2 is an oxidative stress sensor in human monocytic cells (Belrose et al., 2012; Sawamura et al., 2017; Wehrhahn et al., 2010); TRPM3, TRPM4, and TRPM5 are involved in the recognition of high temperatures, and TRPM8 in cold (Talavera et al., 2005; Vriens et al., 2011; Figure 1D). In addition, this channel has also been classically described to be activated by organic compounds such as menthol, eucalyptus, and ilicin (Jimenez et al., 2020; Kraft and Harteneck, 2005; Venkatachalam and Montell, 2007; Figure 1D). TRPM6 and TRPM7 play a role in Mg2+ homeostasis (Chen et al., 2019; Jin et al., 2008; Wong et al., 2019).
TRPMs are also stimulated by a variety of endogenous agents: TRPM2 activation occurs by ADPR and its analogs, in addition to reactive oxygen species (ROS) (Jimenez et al., 2020; Kraft and Harteneck, 2005; Montell, 2005); TRPM3 is activated by sphingolipids, a class of membrane lipids (Jimenez et al., 2020; Kraft and Harteneck, 2005), and neurosteroid pregnenolone sulfate (PS) during the development of mice retinal cells (Samanta et al., 2018; Shiels, 2020); both TRPM4 and TRPM5 are activated by increased intracellular Ca2+ and the co-expression of TRPM6 and TRPM7 turn them responsive to intracellular Mg2+ concentrations ranging from 0.3 to 1 mM (Ferioli et al., 2017; Montell, 2005; Figure 1A). TRPM5 is positively stimulated by extracellular adenosine triphosphate (ATP) (Hofmann et al., 2003; Jimenez et al., 2020), and there is evidence that TRPM7 shows the same modulation (Jimenez et al., 2020; Kraft and Harteneck, 2005; Montell, 2005).
The TRPM family is blocked by a variety of molecules and microenvironmental conditions (Jimenez et al., 2020). TRPM1 is down-regulated by Zn2+ (Lambert et al., 2011); TRPM2 is inactivated by AMP and pH < 7 (Du et al., 2009; Lange et al., 2008); TRPM3 is blocked by Mg2+ and cholesterol (Lambert et al., 2011); TRPM4 is inactivated by cytosolic ATP (Guo et al., 2017); TRPM5 is blocked by acidic pH (D. Liu et al., 2005); and TRPM6 and TRPM7 are blocked by Mg2+ (Ferioli et al., 2017). TRPM6 dependent-P2X4 inhibition has also been demonstrated (de Baaij et al., 2014), while the inactivation of TRPM8 occurs by the activity of bradykinin and histamine signaling during inflammatory processes (Zhang et al., 2012a,b).
3.4 TRPML/TRPP
TRPML (1–3) are expressed in kidneys, lungs, endocrine tissues, heart, and brain (Samanta et al., 2018; Wang et al., 2014). TRPML1 is the best known and studied within the subfamily, while the other two channels have not yet been reported to impact the health or pathologies of the organism. Therefore, in relation to permeability, it is only known that TRPML1 is permeable to Ca2+, Na+, K+, Fe2+, and Zn2+ (LaPlante et al., 2002; LaPlante et al., 2004; Zhang et al., 2018; Pedersen et al., 2005; Figure 1A).
TRPML channel proteins have fewer amino acids than other TRPs, making them smaller, with no ankyrin repeats, with an incomplete TRP box, and. Binding sites for PKC between S1 and S2 (Samanta et al., 2018; Spix et al., 2020; Wang et al., 2014).
Moreover, TRPML1 participates in phagocytosis and protein trafficking (LaPlante et al., 2002; Spix et al., 2020). Importantly, TRPML1 mutations, or deletions in the channel, cause ML-IV with serious deleterious ophthalmologic symptoms, including strabismus, myopia, light sensitivity, and retinal degeneration (Smith et al., 2002; Grishchuk et al., 2016).
As illustrated in Figure 1E, variations of pH activate these channels: TRPML1 by pH <5 and TRPML2 and 3 by pH >7 (Spix et al., 2020). Similar to most TRPs, TRPML is mainly up-regulated, but in some cases down-regulated, by phosphatidylinositol 3,5-bisphosphate [PI(3,5)P2], found in lysosomes (Dayam et al., 2015; Zhang et al., 2012a,b). The engulfment of potentially harmful particles by phagocytic cells, for example, induces the formation of PI(3,5)P2, which acts directly by stimulating TRPML1, expressed in the lumen of organelle, to evoke Ca2+ release (Dayam et al., 2015; Zhang et al., 2012a,b; Wang et al., 2014).
PKA can inhibit TRPML1, and when the channel is expressed in the plasma membrane, it is inactivated by low extracellular pH and PIP2. PIP2 inactivates TRPML1 even in the presence of the agonist, while phospholipid hydrolysis has been shown to activate the channel in HEK293 (Venkatachalam et al., 2015; Wang et al., 2014; Zhang et al., 2012a,b).
Similar to TRPML channels, there is still little information about the permeability of the TRPP subfamily, with only Ca2+permeability known for TRPP2-5 and, specifically, for P2 being permeable to Na+ as well (Pedersen et al., 2005).
Due to structural differences from TRP and other TRPPs, TRPP1 was considered to form another family known as polycystin1 (PC1), which is a non-functional channel. TRPPs are channels found in various tissues, such as the nervous system, heart, placenta, intestine, and pancreas (Hofherr and Köttgen, 2011; Samanta et al., 2018).
TRPPs have only one specific structural feature, an extensive loop called polycystin, which projects to the cytoplasm and is found in the middle of S1-S2. This is similar to TRPML, whereas in TRPP this structure is related to the stabilization of the channels in the plasma membrane (Hofherr and Köttgen, 2011; Samanta et al., 2018).
Wegierski’s group proposes that Ca2+ release in ER is reduced in the presence of TRPP2, and the absence of the channel promotes a huge outflow of Ca2+ from the organelle (Wegierski et al., 2009). Similar to TRPC, TRPP2 plays a role in balancing ER Ca2+ concentrations (Hofherr and Köttgen, 2011). On the other hand, Zheng and colleagues suggest that PIP2 has an inhibitory role in TRPP2-3 (Zheng et al., 2018). Further studies are needed for TRPP to elucidate the role of modulators. It has been mentioned that pH (Huang et al., 2006; Ng et al., 2019), EGF (Zhang et al., 2013) and ATP (Cantero Mdel et al., 2015) are activators and that natural exogenous ligands are unknown (Figure 1F).
3.5 TRPV
The TRPV subfamily comprises six channels (1–6), permeable to Ca2+, Ba2+, Mn2+ (Owsianik et al., 2006; Figure 1A). These channels are commonly expressed in the dorsal root, trigeminal, vagus nerves (Samanta et al., 2018; Seebohm and Schreiber, 2021), retina, and optic nerve (Choi et al., 2015; Leonelli et al., 2010; Leonelli et al., 2009), but also in other tissues, smooth muscle, digestive system, kidneys, and pancreas (Samanta et al., 2018; Seebohm and Schreiber, 2021).
TRPV has a smaller helix that precedes S1 and six ankyrin repeats that recognize modulatory molecules (Garcia-Elias et al., 2015; Pumroy et al., 2020). In the amino and carboxy terminal regions, there are binding sites to CaM, ATP (Phelps et al., 2010; Seebohm and Schreiber, 2021), PKA and PKC (Mamenko et al., 2013; Seebohm and Schreiber, 2021) that can regulate the activity of these channels (Mamenko et al., 2013; Niemeyer, 2005; Phelps et al., 2010). In particular, PIP2 has a controversial function in TRPs (Pumroy et al., 2020). Low levels of the phospholipid inhibit TRPV1, as well as partially inactivate V2, and completely V6, under the same conditions (Cao et al., 2013; Lukacs et al., 2007; Mercado et al., 2010). PIP2 can stimulate V1 in high concentrations of TRPV1 agonists (Lukacs et al., 2007); the reduction of PIP2 activates V3, and in V4, the phospholipid stimulates the channel during response to heat and decreases resistance to muscle stretch; V5 is activated by elevated PIP2 levels (Doerner et al., 2011; Garcia-Elias et al., 2013; Lee et al., 2005), PIP2 also has the potential to enhance TRPV3 functionality and regulate TRPV5 inhibitory responses to Mg2+, highlighting the complexity and controversial activity of inositol phospholipid (Lee et al., 2005; Pumroy et al., 2020; Seebohm and Schreiber, 2021).
Originally, most TRPV channels respond to thermal stimuli: TRPV1 (> 42°C), TRPV2 (> 52°C), TRPV3 (31–39°C), and TRPV4 (24–33°C). TRPV5 and TRPV6 are not heat sensors but playa relevant role in Ca2+ regulation, given their high ionic selectivity. The TRPV subfamily responds to elevated osmotic pressure, as seen in a glaucoma model, by activating TRPV1 (Sappington and Calkins, 2008). Additionally, it activates TRPV2 and TRPV4 (Muraki et al., 2003; Vriens et al., 2004) while inactivating TRPV5 and TRPV6 (Gangwar et al., 2017; Lewis et al., 2011; Figure 1E).
Capsaicin was the first molecule described to stimulate TRPV1 (Caterina et al., 1997; Figure 1E). Furthermore, V1 was reported to be activated by ethanol in sensory nerves, while garlic extract activated V1 channels expressed in Xenopus oocytes. The amplitude of the TRPV3 response depends on the increase in menthol concentration; the channel is also activated by thymol. TRPV4 has been reported to be stimulated by citric acid solution (Buday et al., 2017; Macpherson et al., 2005; Nicoletti et al., 2008; Vogt-Eisele et al., 2007). TRPV1 and TRPV4 detect high concentrations of protons, while TRPV3 responds to low concentrations (Seebohm and Schreiber, 2021). Furthermore, cholesterol acts on TRPV1 and TRPV3 (Klein et al., 2014; Picazo-Juárez et al., 2011).
It is possible that activation of V1-V2 promotes Ca2+ entry into the cell, which, in turn, triggers activation of a type of PLC sensitive to calcium (Lukacs et al., 2007; Mercado et al., 2010). This hydrolyzes PIP2 into IP3 and DAG (Wang et al., 2020). Then, IP3 binds to IP3 receptors located in the ER, promoting a transient increase of cytosolic Ca2+ (Curcic et al., 2019; Mori et al., 2015).
Mercado and collaborators suggest that PIP2 binds directly to active TRPV2, and the hydrolysis of the phospholipid in the plasma membrane is dependent on the entry of Ca2+ into the channel; this negative modulation of PIP2 levels attenuates the responses triggered by TRPV2 (Mercado et al., 2010). Interestingly, the Ca2+/CaM complex did not bind to the channel’s ankyrin repeats, contrary to what was observed in the other TRPVs. Mercado et al. (2010) have described that this complex might not be functional and therefore would have no role in TRPV2 desensitization (Mercado et al., 2010; Rosenbaum et al., 2004; Strotmann et al., 2003). It was later reported that deletion of the Ca2+/CaM complex binding site from the N-terminus prevented interaction with IP3 receptors, and although TRPV4 still triggered Ca2+ influx, no current amplification was observed in the presence of ATP. These results indicate that the channel activity does not depend on the Ca2+/CaM complex, but the responses are potentiated by communication with the protein binding site (Garcia-Elias et al., 2008; Niemeyer, 2005).
TRPV4 current can be significantly enhanced by cytoplasmic ATP. Interestingly, this modulation only occurs when the channel is activated by osmotic changes, but not by temperature (Fernandes et al., 2008).
4 TRPs expression and functions in the healthy retina and disease conditions
The retina is organized into three cellular layers interspersed with two plexiform layers (Wässle, 2004). The cell layers include the ganglion cell layer (GCL), composed by cell bodies of ganglion cells and displaced amacrine cells; the inner nuclear layer (INL), constituted of bipolar, amacrine, horizontal cells and Müller cells; and the outer nuclear layer (ONL), composed by photoreceptor cells (rods and cones) (Masland, 2001). Synaptic contacts in the retina occur in the inner plexiform layer (IPL), located between the GCL and INL, and the outer plexiform layer (OPL) between INL and ONL. Therefore, the IPL contains synapses of ganglion cells, amacrine, and bipolar cells, while the OPL presents synapses between photoreceptors and bipolar and horizontal cells (Wässle, 2004). Ganglion cell axons form the optic nerve, which connects the retina to brain visual areas. The retinal pigmented epithelium (RPE) is the outermost layer of the retina, adjacent to the choroid, and is important for the physiology of the healthy retina (Wimmers and Strauss, 2007). The RPE controls the trafficking of substances by removing debris from degenerated photoreceptors, ion flux, and releases protective signals, among other functions. Pigmentation of epithelial cells plays a crucial role in preventing light scattering, important to a better resolution of image formation (Wimmers and Strauss, 2007). TRPs family members are widely distributed in the retina (Figure 2).
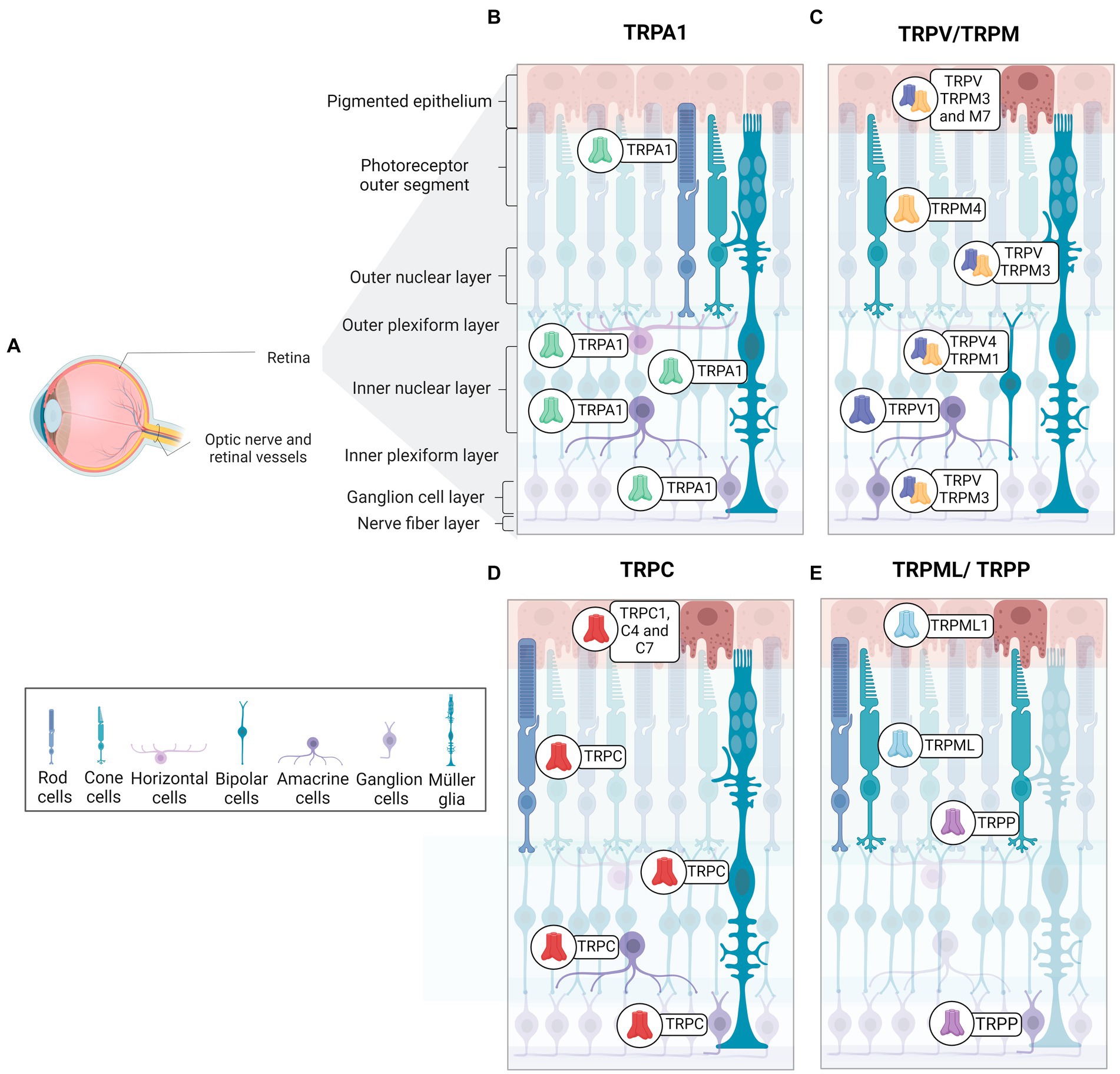
Figure 2. Distribution and expression of TRP receptors in retinal cells. (A) Schematic representation of a radial section of the eye indicating the location of the retina and optic nerve. (B) TRPA1 receptors are present in photoreceptors, horizontal cells, Müller glia, amacrine cells, and ganglion cells. (C) TRPM receptors are identified as follow in epithelial cells (TRPM2, TRPM3 and TRPM7), Müller glia and ganglion cells (TRPM3), cone-type photoreceptors (TRPM4), bipolar cells (TRPM1). (D) TRPC receptors are distributed in retinal pigmented epithelium cells (TRPC1, TRPC4, TRPC7), rod-type photoreceptors, Müller glia (TRPC1, TRPC5, TRPC6), bipolar cells (TRPC6), amacrine cells (TRPC5, TRPC6), and ganglion cells (TRPC5, TRPC6). (E)TRPML1 receptors are expressed in retinal pigment cells and photoreceptors. TRPP2 receptors are found in cone photoreceptors and ganglion cells. TRPV receptors are observed in pigment epithelium (TRPV1-TRPV4), Müller glia (TRPV1, TRPV4), amacrine cells (TRPV1), bipolar cells (TRPV4) and ganglion cells (TRPV1).
Araújo and collaborators detected TRPA1 in amacrine, horizontal, ganglion cells, Müller glia, and photoreceptors in mouse and human retinas (de Araújo et al., 2020; Figure 2B). Although TRPA1 seems to play a crucial role in the cell death promoted by ischemia (de Araújo et al., 2020; Araújo et al., 2017), the function in the healthy retina is still undescribed. The same group also demonstrated the presence of TRPA1 in the chick retina, and its role in cell death induced by acute ischemia (Araújo et al., 2017). Table 3 summarizes the expression of TRPs in regions of retina.
TRPC5 channels are expressed in Müller glial cells, amacrine cells, displaced amacrine, and ganglion cells of C57BL/6 mice retina (Oda et al., 2020; Wang et al., 2010; Figure 2C). Müller glia responds to various stimuli, such as mechanical stimuli, and indirectly to light, with an intense increase in cytoplasmic Ca2+ (Da Silva et al., 2008). Müller glia in vitro express TRPC1 and C6 mRNA and responds to DAG and its analog with potentiated Ca2+ current, suggesting a pivotal role for TRPC as SOCE channels in this cell (Molnár et al., 2016; Da Silva et al., 2008). In agreement, TRPC1/3 knockouts (Molnár et al., 2016) and specific pharmacological blockers of TRPC promote a marked decrease in intracellular Ca2+ currents in Müller cells, supporting the hypothesis that SOCE is a TRPC channel (Molnár et al., 2016; Da Silva et al., 2008).
TRPC6 actively participates in phototransduction in intrinsically photosensitive RGCs (ipRGCs), but C3 and C7 may have a role in the response mechanism of ipRGCs, a small group of ganglion cells that contain melanopsin and respond to light with depolarization and Ca2+ influx (Berson, 2007; Perez-Leighton et al., 2011). ipRGCs are involved with contrast control, day-night cycle, pupil diameter modulation, and humor (Berson, 2007; Contreras et al., 2021; Sonoda et al., 2018).
TRPC6 and C7 are expressed in ganglion cells, INL, and OPL (Perez-Leighton et al., 2011; Warren et al., 2006), and TRPC6 also occurs in ipRGCs (Perez-Leighton et al., 2011; Figure 2C). TRPC3, 6, and 7 channels are activated by high light intensity (Sonoda et al., 2018).
Many studies have examined phototransduction in ipRGCs. It is suggested that Gq protein activation induces PLC/PIP2 hydrolysis and generates IP3 and DAG, which stimulate TRPC and increase intracellular Ca2+ in response to light stimulation (Contreras et al., 2021; Bailes and Lucas, 2010; Graham et al., 2008). However, despite data proposing that neither DAG nor its derivatives were able to trigger action potentials in ipRGCs in an attempt to activate TRPC channels (Graham et al., 2008), other results have shown that phototransduction was blocked pharmacologically through the use of inhibitors for TRPC, which also act on IP3 (Bailes and Lucas, 2010; Sekaran et al., 2007).
TRPC4 occurs in human retinal microvascular endothelial cells (HRMECs) and it seems to be related to angiogenesis. The vascular endothelial growth factor (VEGF), the main modulator of the growth of new blood vessels, lost its angiogenic property in TRPC4 knockdown HRMECs cell culture, suggesting a role for TRPC4 as a modulator in retinal neovascularization (Song et al., 2015).
Furthermore, it was recently demonstrated that TRPC5 inhibition induces axon elongation through the optic nerve and, analogously, increases TRPC5 expression and decreases axonal extension, suggesting that TRPC5 modulates the projection of cell axonal processes of ganglion cells during and after retinal development (Oda et al., 2020).
TRPC1, C4, and C7 are expressed in the RPE, as represented in Figure 2D. Intracellular Ca2+ currents decreased considerably with specific TRPC subfamily blockers, suggesting that the basal Ca2+ influx, essential for RPE functions, is mainly due to the activity of TRPC (Wimmers and Strauss, 2007; Cordeiro and Strauss, 2011).
TRPM1 has been described in the INL, specifically in ON bipolar cells, as well as in the OPL of mouse, macaque, and human retina (Choi et al., 2015; Klooster et al., 2011; Križaj et al., 2010; Morgans et al., 2009; Figure 2D). It is well documented that ON bipolar cells hyperpolarize in response to glutamate, released in the dark, due the activation of mGluR6. The molecular mechanism involves the closure of TRPM1 through alpha and the beta-gamma complex of the Go-protein stimulated by mGluR6 (Koike et al., 2010; Xu et al., 2016). Thus, ON-bipolar cells show a depolarizing response to light, with the decrease of glutamate release. In agreement, TRPM1 knockout in mice results in a clinical pattern similar to the night blindness pathology (Morgans et al., 2009; Irie and Furukawa, 2014).
Previous studies have described how Mg2+ modulates some members of the TRP family (Huang et al., 2020; Sawamura et al., 2017; Zierler et al., 2017). Intracellular Mg2+ (1.7–2 mM) decreases the TRPM1 current in rod and ON-cone bipolar cells, and stimulation of the PLC-PKCα pathway alleviates this inhibition only in rod bipolar cells (Rampino and Nawy, 2011).
Recently, studies addressed the effects of TRPM2-mediated calcium influx when the channel was stimulated by oxidative stress, as well as the neuroprotective effect of antioxidants for TRP modulation in ARPE-19 cells and a role on sirtuin 2-dependent cell death (Figure 2D; Meléndez García et al., 2015; Daldal and Nazıroğlu, 2022a, 2022b).
In mice, TRPM3 has been found in the iris, ciliary body, retinal pigment epithelium, GCL, INL, IPL, and OPL (Brown et al., 2015; Hughes et al., 2012; Webster et al., 2020; Figure 2D). Expression of TRPM3 has also been described in Müller glial cells during mouse retinal development (Webster et al., 2020). A recent study showed an increase in Ca2+ influx induced by TRPM3 agonist into TRPM3-positive ganglion cells in developing mice, whereas TRPM3 knockouts presented a reduction in these currents (Webster et al., 2020). Knockouts for TRPM3, but not for TRPM1, have shown a lower percentage of pupil contraction in response to low light intensity stimuli when compared to control mice (Hughes et al., 2012).
TRPM4 is detected in the Müller glial, GCL, ONL, and, specifically, in cone photoreceptors of the human retina (Berdugo et al., 2021; Figure 2D). In addition, TRPM4 in the outer limiting membrane of the human retina partially colocalizes with internal rectifier potassium channel 6.2 (Kir6.2) and sulfonylurea receptor (SUR1), both subunits being constituents of ATP-dependent potassium channels (Berdugo et al., 2021; Omri et al., 2010). SUR1 or Kir6.2 also co-localizes with Müller glial cells and cone photoreceptors in monkey retinas (Berdugo et al., 2021).
RPE undergoes blue wavelength-induced apoptotic cell death. Blue light is emitted by sunlight and light-emitting diode (LED) lamps (Hu and Xu, 2021; Tao et al., 2019). TRPM7 overexpression protects RPE cells from cell death induced by blue light, while TRPM7 ablation results in a low proliferation rate and lower cell viability, a decrease in the levels of PKC, ERK, and Bcl-2 proteins, and an increase in Bax (Hu and Xu, 2021). These data suggest a key role for TRPM7 in the survival of RPE cells exposed to blue light via PKC/ERK signaling (Hu and Xu, 2021).
TRPP2 has been detected in all layers of the mouse retina, with strong intensity in the outer segments of cones and INL (Gilliam and Wensel, 2011; Figure 2E). Patients with ML-IV have severe deleterious neurological, motor, and ocular consequences (Smith et al., 2002; Grishchuk et al., 2016). In the genetic ablation of TRPML1, it was seen that there was a thinning of the ONL and shortening of the outer segments of the rods in an age-dependent manner. Furthermore, except for INL, accumulation of lysosomal aggregates was found in almost all retina layers in Trpml1−/− (Grishchuk et al., 2016).
In animals with retinal detachment, agonist stimulation of TRPML1 decreases rod and cone apoptosis, preserving the photoreceptor layer. In addition, the activity of the channel changes in these retinas and therefore, ROS concentrations are markedly smaller. In this way, there is an improvement in the visual capacity in the injured animals treated with an agonist. These results make the channel an excellent therapeutic target (Yan et al., 2021).
Except for TRPML, TRPP1-3 mRNA expression has been demonstrated in the optic nerve. Furthermore, TRPP1-2 is one of the most abundant in astrocytes around the ocular structure (Choi et al., 2015). Mucolipidosis IV can also induce optic nerve thinning and neuronal demyelination at the level of the brain, which results in severe motor problems (Smith et al., 2002; Grishchuk et al., 2016). As expected, TRPML1 knockout mice show a decrease in the thickness of myelinated axonal projections and optic nerve thickness, possibly caused by a decrease in blood supply (Grishchuk et al., 2016).
Toll-like receptor 3 (TLR3) has recently been linked to ATP production from lysosomes in RPE cells and astrocytes expressed in the murine optic nerve head (ONH). The activation of TLR3 in these cells induces the stimulation of TRPML1, which, in turn, by inducing the release of lysosomal Ca2+ into the extracellular environment, can promote the fusion of the plasma membrane with the lysosomal membrane and increase the release of ATP from the organelle (Beckel et al., 2018). Gomez and colleagues (2018) observed, in Trpml1−/− mice, low extracellular Ca2+ concentrations even with pharmacological stimulation and confirmed that ion efflux occurred through channel activity (Gómez et al., 2018).
It is known that the inadequate functioning of the lysosome can lead to the accumulation of metabolites, including lysosomal lipids, which generate oxidative stress in the tissue. Experimental data indicate that TRPML1 gene expression remained at normal levels in RPE, but extracellular Ca2+ elevation was impaired under conditions of lipid accumulation, suggesting that TRPML1 responses are essential for lysosomal ATP release (Gómez et al., 2018).
Finally, TRPVs are expressed in astrocytes, microglia, and endothelial cells (Ho et al., 2014; Leonelli et al., 2010). TRPV1 is located in all retina layers during development and in adult mice. V1 shows higher immunofluorescence intensity in INL, GCL, and IPL in the mature mouse retina, while V2 is weakly restricted to INL, OPL, IPL, and GCL (Choi et al., 2015; Leonelli et al., 2010; Leonelli et al., 2009). As illustrated in Figure 2C, TRPV1 is expressed in amacrine cells, and TRPV4 was recently found in bipolar cells, while the V4 homodimer was found in Müller glia and RCGs. However, there are also reports of TRPV1/TRPV4 co-localization in these regions of the retina of mice (Gao et al., 2019; Leonelli et al., 2011; Sappington et al., 2015).
In the retina, TRPVs play a role in mechanical damage (Ho et al., 2014; Leonelli et al., 2010). As reported by Ho et al. (2014), in astrocytes that express TRPV1 and when scratching retinal tissue, there is an increase in the shortening and migration of these cells, through an increase of intracellular Ca2+ mediated by the channel in response to mechanical stress (Ho et al., 2014).
Interestingly, TRPV1 levels increase in the GCL and optic nerve axons after optic nerve injury in mice, whereas 21 days after injury, there is a decrease in TRPV1 staining in microglia and astrocytes. On the other hand, capsaicin, a TRPV1 agonist, accelerated cellular degeneration, induced retinal thinning, and intensified glial fibrillary acidic protein (GFAP) immunofluorescence in Müller’s glia. Capsaicin increases the generation of lipid peroxidation products in vitro but not in vivo, and this response is blocked by receptor antagonist capsazepine (Leonelli et al., 2010).
TRPV1-4 is expressed in the RPE of mice and is a target of type 1 angiotensin II receptor (AT1R) signaling triggered by its activation by angiotensin II (AngII) (Barro-Soria et al., 2012). Indirect activation of V2 contributes to Ca2+ influx to the cytoplasm and release of ions stored in the endoplasmic reticulum. This role, attributed to TRPV2 in RPE was observed through the inhibition of AngII, caused by the application of the PLC blocker, abolishing the influx of Ca2+ and demonstrating the involvement of the pathway. Corroborating with the data reported above, the researchers also used SKF96365, a TRPV2 antagonist, and AngII simultaneously to evaluate the channel activation responses by AngII. As expected, the Ca2+ currents were suppressed in RPE cultures (Barro-Soria et al., 2012).
Similarly, VEGF production is also mediated by the interaction of the insulin-like growth factor (IGF)-1 receptor with AngII, which stimulates phosphoinositide 3-kinase (PI3K) activity to induce Ca2+ influx into TRPV1 and V2. Interestingly, IGF-1 increases the expression of TRPV2 channels, and inhibition of IGF-1 and its specific receptor leads to a drastic reduction in the effects of the Ang II pathway and in the formation of new blood vessels in the human RPE in a pathological context, thus suggesting a relationship between TRPVs and neovascularization (Cordeiro et al., 2010; Reichhart et al., 2015; Smith et al., 1999).
The optic nerves of mice and rats have been found to express TRPA1, TRPM1-M4, M6-M7, TRPC1-C6, TRPV1-V2, and V4 (Choi et al., 2015; Leonelli et al., 2009; Papanikolaou et al., 2017).
5 Retinal pathologies
5.1 TRPs, angiogenesis, and age-related macular degeneration
Age-related macular degeneration is a leading cause of blindness, affecting 200 million people around the globe. With expectations to increase in the next few years, AMD represents a major public health problem (Wong et al., 2014). It is a multifactorial disorder that is driven by genetic and environmental influences. The disease affects the macular region of the retina, a cone-enriched region of high-acuity vision, particularly in the primates. Pathogenesis includes characteristic lesions through the formation of drusen or basal laminar deposits at initial stages, while neovascular abnormalities account for late-stage phenomena in the wet or exudative AMD, but not for dry AMD (Mitchell et al., 2018).
Genome-wide association studies have been performed throughout the years to identify candidate genes associated with the progression of AMD (Fritsche et al., 2014). Recently, two studies identified the TRPM1 gene as one of the candidates correlated to AMD incidence (Persad et al., 2017; Orozco et al., 2023). Although there is little evidence of a direct correlation between TRP channels and the progression of AMD, there are associations that could be made to provide new insight into this area (Wong and Yao, 2011).
Wet AMD is a treatable form of the disease, and anti-VEGF compounds represent the current treatment, since VEGF is one of the most studied angiogenic factors leading to neovascularization. Endothelial cells (EC) express many forms of TRP family members (Wong and Yao, 2011) and are key cell types to promote angiogenesis. Angiogenic factors like VEGF can activate TRP channels, increasing intracellular Ca2+ and modulating angiogenic pathways in a diversity of situations (Kwan et al., 2006; Smani et al., 2018; Negri et al., 2019).
Several studies demonstrate the coupling of TRP channels to angiogenesis in the retina, which is essential to understanding the potential of these proteins to modulate neovascular disease progression, like AMD. It has been shown that the silencing of TRPC4 with siRNA inhibits neovascularization in an oxygen-induced retinopathy (OIR) model performed from P7 to P12 mice, and evaluated at P17 when neovascularization peaks (Song et al., 2015). This model is important to simulate and understand conditions like retinopathy of prematurity, a leading cause of blindness among children. These results were replicated in HRMECs in which silencing TRPC4 inhibited VEGF-induced migration and tube formation capacity, also inhibiting signaling pathways triggered by the vascular growth factor, indicating that this channel is crucial for VEGF effects (Song et al., 2015).
Using the same parameter of OIR, Zhu et al. (2019) found that TRPC5 is also a regulator of angiogenesis in mouse retina, seeing that TRPC5 knockout reduces sprouting and tube formation and increases avascular areas and neovascular tufts. The mechanism of TRPC5 signaling seems to involve the activation of nuclear factors activating T cell isoform c3 (NFATc3) and angiopoietin-1 (Zhu et al., 2019). The study also suggests that the FDA approved drug, riluzole, used for amyotrophic lateral sclerosis (ALS) and activator of TRPC5, could be an option to treat ischemic diseases, as it increased perfusion in the hindlimb ischemic model.
The role of TRPV1 and 4 was also investigated in HRMECs. Pharmacological inhibitors of TRPV1 or 4 channels reduce sprouting angiogenesis, although they are ineffective in reducing VEGF-induced sprouting, suggesting that these channels are not required for the vascular factor’s effect (O'Leary et al., 2019). Interestingly, inhibition of the channels reduces neovascularization while increasing normal vascular area in the OIR model. Both channels have been linked to involvement in corneal injury (Okada et al., 2021); thus, in vivo and in vitro, genetic or pharmacological ablation reduced fibrotic proliferation and inflammatory cytokines in the case of V4 (Okada et al., 2016), in addition to the absence of V1 impairing wound healing and cell migration (Sumioka et al., 2014). Therefore, modulation of TRP channels could arise as alternative treatments for patients not responding to regular therapeutic methods in ischemic and neovascular retinal diseases. Figure 3A illustrates the putative relationship between the activity of TRP channels and AMD.
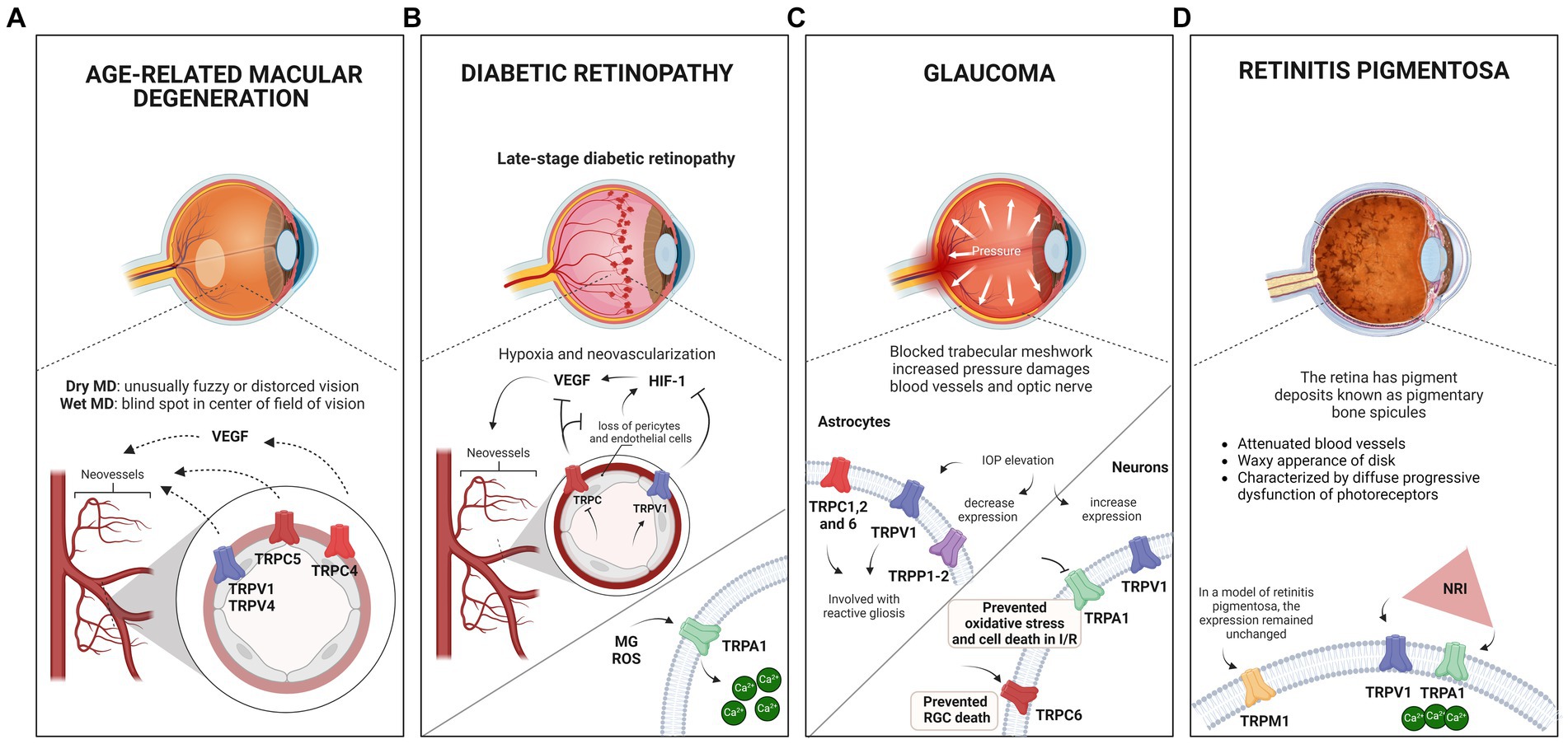
Figure 3. Eye pathologies and the involvement of TRP channels. (A) Schematic illustration of macular degeneration. In the wet form of the disease, abnormal blood vessel growth in the retina can lead to fluid and blood leakage, damaging the macula and causing sudden or rapid loss of central vision. Research has suggested that when activated, TRPV1, TRPV4, TRPC5, and TRPC4 receptors may contribute to the neovascularization process associated with the disease. Notably, TRPC4 activation occurs via VEGF stimulation. (B) Schematic showing the late stage of diabetic retinopathy (DR). Disruption of the blood-retinal barrier results in microaneurysms, increased vascular permeability, and capillary obstruction, leading to inadequate retinal oxygen distribution. Increased VEGF expression and hypoxia-inducible factor 1 (HIF-1) activity have been observed, suggesting the concept of DR to neurovascular diseases. Studies have indicated that TRPV1 activation suppresses the PPARγ-poldip2-Nox4 pathway, thereby inhibiting DR-associated increases in HIF-1, VEGF, ROS, and H₂O₂, while reducing retinal microvessel hyperpermeability. In addition, TRPC inhibition decreased VEGF expression and inhibited neovascularization. TRPA1 activation by methylglyoxal (MG) and reactive oxygen species (ROS) increases Ca2+ levels, that could lead to tissue damage and exacerbation of oxidative stress. (C) Schematic illustrating glaucoma and its association with elevated intraocular pressure (IOP). Glaucoma complex mechanisms, including genetic mutations, reduced ocular blood supply, neurotoxicity due to increased ROS production, cell death, and phagocytosis. In astrocytes, TRPCs, mainly TRPC1, TRPC2, and C6, appear to contribute to the disease, particularly to reactive gliosis. This can also be induced by elevated IOP, which activates TRPV1 receptors. Studies have revealed a significant decrease in TRPP1-2 expression 24 h after IOP elevation. In neuronal cells, elevated IOP increases TRPV1 expression in the inner plexiform layer (IPL) and retinal ganglion cells (RGCs), leading to an elevated rate of spontaneous action potential. The protective role of TRPV1 in ganglion cells promotes the stress response, and the absence of the channel appears to accelerate degeneration. Inhibition of TRPA1 during ischemia protects against retinal cell death in chick and mouse models, preventing oxidative stress and cell death induced by IOP reperfusion, highlighting its role in triggering the degeneration and elevation of IOP in glaucoma. Studies have also suggested that TRPC6 may mediate a neuroprotective response in a model of ischemia and reperfusion by increasing the number of ganglion cells. (D) Schematic illustrating retinitis pigmentosa, characterized by pigment deposits known as pigmentary bone spicules. TRPM1 expression in Rd1 mice (a model of retinitis pigmentosa) remained similar to that in wild-type mice at P21, despite retinal remodeling due to degeneration. Near-infrared (NIR) light therapy has been shown to protect the retina against neurodegeneration and inflammatory processes in a retinitis pigmentosa model. This treatment stimulates retinal cell signaling without causing tissue damage, increases Ca2+ influx, and triggers ganglion cell responses. The thermal sensitivity of TRPV1 and TRPA1 channels to the treatment was evaluated based on their sensitivity to temperature variations.
5.2 Diabetic retinopathy
Diabetic retinopathy (DR) is among the leading causes of blindness in working-age adults. Classically and clinically, DR has been considered a vascular disease (Wang and Lo, 2018; Stitt et al., 2016). A key event is the blood-retinal barrier disruption, with loss of pericytes and endothelial cells, leading to microaneurysms, increased vascular permeability, and capillary obstruction, resulting in poor oxygen distribution in the retina (Wang and Lo, 2018; Sachdeva et al., 2018; Stitt et al., 2016). Consequently, VEGF has increased expression through the hypoxia-inducible factor 1 (HIF-1) (Wang and Lo, 2018). However, a great amount of data has demonstrated many early modifications in neural aspects of retinal physiology, extending the current concept of DR to a neurovascular disease (Carpi-Santos et al., 2022). Blindness arising from this pathology can result from different mechanisms. The most frequent is due to diabetic maculopathy, characterized by an increased concentration of macular fluid (Wang and Lo, 2018; Stitt et al., 2016).
The role of oxidative stress in the retina of hyperglycemic patients is well documented, being marked by accentuated production of ROS, as well as RCS and RNS (Wang and Lo, 2018; Sachdeva et al., 2018). Additionally, a sustained generation of MG promotes a series of structural changes in macromolecules and transcriptional modifications (Sachdeva et al., 2018; Schlotterer et al., 2019), and other end products of advanced glycation (AGEs) are generated from MG (Sachdeva et al., 2018; Schlotterer et al., 2019; Stitt et al., 2016).
TRPA1 is activated by MG (Andersson et al., 2013; Eberhardt et al., 2012) and ROS (Andersson et al., 2008; Mori et al., 2016), through covalent modifications of N terminal cysteine residues (Hinman et al., 2006), increasing Ca2+ levels, leading to tissue damage and exacerbation of oxidative stress, as shown in Figure 3B. There is evidence that hypoxia can lead to TRPA1 activation through produced-lipid peroxidation metabolites (Pires and Earley, 2018). Conversely, TRPA1 knockout or pharmacological inhibition plays a pivotal role in reducing ischemia/reperfusion oxidative damage and apoptosis in the retina (de Araújo et al., 2020), in traumatic brain injury (Yang et al., 2022), and preventing itching and hyperalgesia in mice with methylglyoxal and streptozotocin-induced diabetes, with similar effects to methylglyoxal scavengers, such as D-arginine and aminoguanidine (Cheng et al., 2019). TRPA1 deficiency and antagonism are also able to reduce hypoxia-induced painful dysesthesia, similarly to ROS scavengers (So et al., 2016), and attenuate cold hypersensitivity caused by diabetic peripheral neuropathy (Hiyama et al., 2018). Since DR progression depends on oxidative stress (Carpi-Santos et al., 2022), these results raise an interesting possibility of searching for the role of TRPA1 in DR models. An exciting target to study TRPA1 is Müller glia, which express TRPA1 (de Araújo et al., 2020), and show oxidative stress as an early response to hyperglycemia conditions, upregulating VEGF (Rossino et al., 2020), which contributes to the injurious events in DR, such as increased endothelial permeability, angiogenesis, and apoptosis (Wang et al., 2015). As oxidative stress is a key stimulus to several biochemical and neurochemical alterations in DR (Fanaro et al., 2023), and VEGF is a crucial signal to induce angiogenesis, the study of TRPA1 in Müller glial cells appears to be a promising target to develop future therapeutic agents.
Several studies also point to a strong impact of TRPV channels in DR pathogenesis. For instance, TRPV1 activation by capsaicin suppresses the PPARγ-poldip2-Nox4 pathway, inhibiting DR increases in HIF-1, VEGF, ROS, and H₂O2 and decreased hyperpermeability of retinal microvessels (Liu et al., 2022). Moreover, TRPV4 is downregulated by hyperglycemia in diabetic rats, which suggests a possible contribution to protection from endothelial dysfunction in diabetes (Monaghan et al., 2015). In one study, this channel’s inhibition by vasoinhibins prevented excessive blood retinal barrier (BRB) permeability in diabetes (Arredondo Zamarripa et al., 2017). Another study found that TRPV4 gene deletion abolished retinal edema, which is highly important to BRB disruption, corroborating the possible role of BRB dysfunction in DR (Orduña Ríos et al., 2019). Regarding neuronal dysfunction, it has already been described that TRPV4 contributes to ganglion cell stretch and to the activation of proapoptotic signaling pathways in the mouse retina (Ryskamp et al., 2011), which could, therefore, contribute to an increase of damage in DR. Therefore, modulation of TRPV4 in early periods of DR, which shows harmful effects on neural retina, with no angiogenesis effect, could be harmful to the progression of DR. The data suggest that TRPV4 could be an attractive target to study in the context of later stages of DR with neovascularization.
TRPC channels are widely present in the retina and have been studied as possible targets for their contribution to exacerbating DR harm. Although their expression in hyperglycemic contexts needs to be better established, there has been evidence of an increase in a diabetic model compared to control (Sachdeva et al., 2018). STZ-treated TRPC1/4/5/6−/− mice presented decreased endothelial and pericyte cell death that led to retinal thickness preservation, as well as lower concentrations of MG in plasma and red blood cells, and enhanced expression and activity of glyoxalase 1 (GLO1), the major MG detoxification enzyme, compared to control (Sachdeva et al., 2018). TRPC channels have also been strongly associated with angiogenesis in several models, mediating VEGF-induced Ca2+ influx (Smani et al., 2018). Moreover, TRPC inhibition by SKF96365 decreased VEGF expression and downregulated proliferation, migration, and lumen formation in HRECs exposed to high glucose, inhibiting neovascularization (Lang et al., 2020). Another study documented aggravation in the pathogenesis of DR by activating TRPC6 by Hyp9, a highly selective TRPC6 agonist, increasing ROS accumulation and enhancing the augmented expression of IL-6 and VEGF in cells exposed to high glucose. Adversely, its silencing and knockdown inhibited the decrease in Müller cell viability and gliosis in rMC-1 cells while decreasing IL-6, VEGF, ROS, and intracellular Ca2+ levels.
TRPM2 was shown to be activated by hypoxia-induced ADP-ribose and oxidative stress, common events in DR pathogenesis, and its blockage restored pro-inflammatory cytokines (TNF-α and IL-1β) and ROS levels (Özkaya et al., 2021). In contrast, TRPM8 activation by linalool produces anti-angiogenic effects through inhibition of beta 1 integrin/focal adhesion kinase (FAK) signaling (Becker et al., 2021), which could be of great relevance to attenuating DR implications.
Together, these findings portray the relevance of TRP channels in diabetic retinopathy pathogenesis, each type presenting its characteristics in the signaling pathways. It is important to highlight, however, that information about TRP channels in DR is still very scarce, and further research is needed. Considering the effects of some TRPs in the retinal function, together with the scarce data available in the DR context, it would be interesting to invest in the better characterization of the potential protective effect of the inhibition of TRPV1, TRPV4, TRPC1/4/5/6 in later stages of DR, due to their regulation of angiogenesis. On the other hand, the inhibition of TRPA1 and TRPM2 could be interesting in the context of early alterations that occur in the neural retina of diabetic animals. Finally, the activation of TRPM8 could also be a promising target to evaluate a protective effect in the DR context with neovascularization.
5.3 Glaucoma
Glaucoma is a group of ophthalmological conditions that leads to retinal/optic nerve degeneration, initially affecting the periphery of the retina (Fan Gaskin et al., 2021; Guedes, 2021; Jayaram et al., 2015). It is estimated that about 76 million individuals are affected by glaucoma, representing the main cause of irreversible vision loss.
Depending on the causes that initiate and develop the disease, it is possible to divide glaucoma into primary and secondary (Moazzeni et al., 2020). Although open-angle glaucoma is the most common type of neuropathy, representing around 80% of cases (Guedes, 2021; Moazzeni et al., 2020; Okumus et al., 2013), both primary open and closed-angle glaucoma involve a complex and heterogeneous set of mechanisms, such as increased intraocular pressure (IOP), genetic mutation, decreased blood supply to the eye, neurotoxicity from increased ROS production, cell death, and phagocytosis (Fan Gaskin et al., 2021; Moazzeni et al., 2020; Okumus et al., 2013). Thus, IOP regulation is currently the main approach for clinical treatment, intervention, and subject of study aiming for a better understanding of glaucoma (Jayaram et al., 2015). Despite data implicating TRP family channels in ocular pathologies (Choi et al., 2015; Irnaten et al., 2020; Oda et al., 2020), research on the role of TRP in glaucoma is still scarce.
The role of TRPA1 in retinal ischemic conditions has been evaluated, and the inhibition results to be a protective effect. Treatment with a first generation TRPA1 antagonist (HC-030031) during acute ischemia promotes a protection of cell death in the chick retina (Araújo et al., 2017). In a murine model of glaucoma, pharmacological inhibition (A-967079 or HC-030031) or genetic ablation of TRPA1 also prevents oxidative stress and cell death promoted by IOP-reperfusion (2 and 7 days) in mouse retinas (de Araújo et al., 2020). These findings point to an active role of TRPA1 in modulating degeneration in ischemic conditions and IOP present in glaucoma regulating oxidative stress (de Araújo et al., 2020; Araújo et al., 2017; Ling et al., 2016). These data reveal a promising possibility for developing new treatments and encourage research to describe better the protective mechanisms involved. In addition to the retina, TRPA1 is also present in intraocular trigeminal nerves (TG) (Ling et al., 2016). Since previous data state that the channel responds to membrane stretching and is expressed in TG, it could be involved with regulating intraocular pressure. Accordingly, Bimatoprost, a prostanoid analog used to control IOP in glaucomatous patients, can activate TRPA1 in TG neurons (Ling et al., 2016). Thus, it would be interesting to test the effect of Bimatoprost on retinal cell death detected in different glaucoma models.
TRPCs, mainly TRPC1 and TRPC2 and C6, have been detected in the optic nerve and adjacent astrocytes and appear to play a role in this eye disease, as shown in Figure 3C (Choi et al., 2015; Molnár et al., 2016; Irnaten et al., 2020). In mice with glaucoma, Trpc1−/− exhibited marked and rapid reactive gliosis when compared to the wild type; the authors suggest that this is due to the mechanosensitive properties of TRPC1 in response to ocular pressure, which would be involved in the suppression of gliosis (Molnár et al., 2016).
The increase in IOP also causes significant changes in the lamina cribrosa (LC), which is responsible for involving the optic nerve. This region is obstructed, making it difficult for axons to exit the retina to the brain nuclei. In addition, LC is rich in astrocytes that organize the exit of axons from the eye, and these astrocytic cells become reactive in the face of some damage, such as optic nerve crush. Another important finding in LC in glaucomatous is that there is an increase in matrix proteins that induce the development of fibrous tissue in response to oxidative stress in the tissue. Thus, it has been reported that both gene silencing and pharmacological inhibition of TRPC1 and C6 reduce matrix protein gene expression, suggesting that TRPC channels play a role in the oxidative context involving LC with glaucoma (Irnaten et al., 2020). On the other hand, an increase in intracellular Ca2+ in LC has been reported, possibly due to TRPC activity (Irnaten et al., 2020). It has been suggested that the influx of extracellular Ca2+ also acts on the regulation of the contractile properties of trabecular meshwork (TM) via TRPC1 and TRPC4 (Abad et al., 2008), possibly via depletion of intracellular Ca2+ stock concentrations in the endoplasmic reticulum, a mechanism similar to that described for the activation of SOCE channels (Abad et al., 2008; Yuan et al., 2009). In addition, it has been shown that TRPC5 modulation regulates axon growth in the optic nerve, with TRPC5 inhibition promoting the elongation of axons while its activation reduces axon extension (Oda et al., 2020). Interestingly, in ganglion cells, there is a temporary increase in TRPC6 mRNA in response to ischemia and reperfusion in mice. In addition, the stimulation of TRPC6 with OAG in injured retinas increases the number of RGCs, whereas its inhibition triggers a decrease in ganglion cells. These data suggest that TRPC6 may mediate a neuroprotective response in an ischemia and reperfusion model (Wang et al., 2010). Therefore, in the context of glaucoma, which shows ganglion cell axon degeneration in the optic nerve, this seems to be a good opportunity to study the protective effect of the inhibition of TRPC5 together with the activation of TRPC6. It would also be interesting to evaluate the possible facilitation by the combination with inhibitors of TRPC1 to assess the elongation of the ganglion cell axons due to the reduction of matrix proteins and the development of fibrous tissue in LC.
In wild-type mice, expression of TRPP1-3, TRPP1 is much lower than TRPP2, while TRPP3 is weakly marked in ONH. In addition, elevated levels of TRPP1-2 have also been found in ONH-associated astrocytes. However, after 24 h of IOP elevation, there was a marked decrease in TRPP1-2 expression (Choi et al., 2015). Although there are very few studies evaluating TRPPs in glaucoma, the presence and the alteration observed in IOP indicate that they could represent an interesting target for the study of the axon degeneration present in this retinopathy.
IOP elevation increases TRPV1 expression in IPL and RGCs, accompanied by an increase in spontaneous action potential rate, and in Trpv1−/− ganglion cells, the high firing rate was not observed (Weitlauf et al., 2014). This suggests a protective role of TRPV1 in ganglion cells by promoting a stress response and, furthermore, the absence of the channel appears to accelerate degeneration (Weitlauf et al., 2014).
On the other hand, Sappington et al. (2015) reported that both TRPV1 knockout and pharmacological inhibition of the channel in an ex vivo model of increased IOP, resulted in fewer RGCs labeled for an apoptotic marker, suggesting that the entry pathway of TRPV1 Ca2+ could be related to cell death (Sappington et al., 2015). Corroborating these data, McGrady and colleagues observed no changes in pressure in the presence or absence of TRPV1 (McGrady et al., 2020; Risner et al., 2020), and TRPV1 knockouts did not show any protection against IOP-induced cell death after 2 or 7 days of reperfusion (de Araújo et al., 2020).
Intraocular injection of capsaicin promotes microglial gliosis (Leonelli et al., 2010). Interestingly, in an animal model, increased IOP leads to Ca2+ influx via TRPV1, which, in turn, stimulates interleukin-6 (IL-6) production and secretion by retinal microglia close to ganglion cells. However, in cell culture, pharmacological inhibition of TRPV1 associated with increased hydrostatic pressure resulted in decreased cytokine levels (Sappington and Calkins, 2008).
TRPV1 retinal content is inversely regulated by IOP in young and old mice, with lower pressure increasing TRPV1 expression (and vice-versa) (Sappington et al., 2015), as shown in Figure 3C. It appears that RGC also responds to an increase in salinity with Ca2+ influx through TRPV4, and prolonged periods in this condition decrease the viability of these cells (Ryskamp et al., 2011).
Other studies have demonstrated the presence of TRPs in different structures involved in the pathophysiology of glaucoma and raised the possibility of their involvement. In the case of TRPM3, it is possible that the increase in Ca2+ influx, mediated by the channel, participates in trabecular contraction and relaxation to promote contraction of the smooth muscle associated with these regions (Bennett et al., 2014; Hughes et al., 2012; Wiederholt et al., 2000).
It is well documented that these contractions of the trabecular meshwork coordinate the inflow and outflow of aqueous humor (Wiederholt et al., 2000), and that the ciliary body also plays a role in fluid production (Bennett et al., 2014). Thus, the balance between the production and drainage of aqueous humor is directly associated with IOP stability. Furthermore, as TRPM3 is abundantly expressed in both the trabecular meshwork and ciliary body, it is possible that the channel has a role in maintaining IOP by promoting a sustained Ca2+ current (Bennett et al., 2014; Shiels, 2020).
The TRPM3 gene is composed of an intron that harbors the non-coding micro-RNA (miR204) (Jayaram et al., 2015; Shiels, 2020). miR204 is one of the most abundant micro-RNAs in most layers of the human retina, excluding only IPL and OPL. In addition, the micro-RNA is also one of the most expressed in retinal regions that suffer ocular damage, but in a murine model of glaucoma, miR204 is downregulated, possibly via TGF-β modulation (Jayaram et al., 2015; Bennett et al., 2014). In glaucoma, several studies have reported that increased TGF-β concentration is associated with the emergence of dense fibers in the TM, contributing to increased IOP (Jayaram et al., 2015; Robertson et al., 2010; Shiels, 2020).
5.4 Retinitis pigmentosa
Retinitis pigmentosa (RP) comprises a group of neurodegenerative and genetic diseases, the heredity causes for which may be autosomal recessive, dominant, X-linked, or mitochondrial (Niwa et al., 2016). Furthermore, the pathology may result in visual loss alone and be classified as “non-syndromic” RP or, if associated with other syndromes such as Usher and Bardet-Biedl syndrome, be categorized as “syndromic” RP (Daiger et al., 2014; O'Neal and Luther, 2024). The visual deficit arises from the degeneration of photoreceptors, first rods, followed by cones (Guadagni et al., 2015).
In ophthalmological examinations of the fundus of the eye, the depression of blood vessels can be observed; bone spicules between photoreceptors that morphologically alter the environment and facilitate the diffusion of non-resident cells (Daiger et al., 2014).
There is still no cure for the disease, given the variety of genetic mutations responsible for the appearance of retinitis pigmentosa; however, one alternative is the development of palliative treatments aimed at prolonging the useful life of photoreceptors, delaying degeneration (Hartong et al., 2006; Massengill et al., 2020). Recently, Nelidova and colleagues studied near-infrared (NIR) light therapy in the Rd1 model involving TRPV1 and TRPA1 channels (Nelidova et al., 2020). Since both thermal activity and the presence of these channels are well documented in the vertebrate retina, the group used a gold nanomaterial viral system. Thus, by exciting the nanorods with NIR, heat is generated; this thermal energy activates TRPV1 and TRPA1 expressed in the surviving functional cones, and the response extends to the GCL of the Rd1 retina, a result similar to that obtained in the wild-type (Nelidova et al., 2020). This type of treatment is based on the concept that light absorption in the low radiation range could stimulate and modulate retinal cell signaling without causing tissue damage. When cones are stimulated with NIR, Ca2+ influx increases and triggers ganglion cell responses (Zhu et al., 2021). NIR also increases the activity of neurons in the visual cortex in animal models of retinitis pigmentosa when compared to control (Nelidova et al., 2020). Figure 3D summarizes the present data of TRAP1 and TRPV1 in RP.
Finally, the expression of TRPM1 in Rd1 mice, one of the animal models for retinitis pigmentosa, was similar to the wild type at P21 despite retinal remodeling occurring due to degeneration (Križaj et al., 2010).
The few data available on TRPs in retinitis pigmentosa highlight the need to explore this field for an innovative and effective treatment. In addition, we currently do not find any data to address the signaling mechanisms that trigger the symptoms described involving any TRP (Maneu et al., 2022; Nelidova et al., 2020; Zhu et al., 2021).
6 Concluding remarks
TRPs are a heterogeneous family of ion channels with some structural similarities, highly modulated by endogenous and exogenous stimuli. It has been demonstrated that potentially harmful sensory and mechanical stimuli can modulate TRPs in physiological and pathological contexts. Indeed, TRPs are important for development and maintenance health and retinal pathologies.
The present review discusses the potential role of TRP channels in the pathophysiology of, and possible therapeutic targets for, the most prevalent retinal diseases. Several studies have revealed that the TRP channel family is ubiquitously present in the retina; these studies shed light on findings demonstrating their involvement in the physiology of virtually all retinal cells. Using different disease models, much evidence shows their clear involvement in distinct retinopathies. Here, we highlighted the studies that analyze strategies to delay or prevent the progression of these diseases using TRP modulation, with some encouraging results, as well as highlighting unanswered questions yet to be studied.
It is extremely important to understand the factors, agents, and biochemical and/or genetic contexts that induce the appearance of the pathologies addressed in this review. It is also necessary to have therapeutic approaches that promote neuroprotection, alleviating degeneration with no or few side effects, through, for example, drugs that modulate TRP channels.
Author contributions
TN: Investigation, Methodology, Writing – original draft, Writing – review & editing. DP-F: Investigation, Methodology, Writing – original draft. LV: Investigation, Methodology, Writing – original draft. AN: Investigation, Writing – review & editing. FL: Data curation, Writing – review & editing. RN: Data curation, Writing – review & editing. PC-C: Data curation, Writing – review & editing. AF-M: Data curation, Writing – review & editing, Writing – original draft. DS: Data curation, Methodology, Writing – original draft, Writing – review & editing. KC: Conceptualization, Data curation, Methodology, Project administration, Writing – original draft, Writing – review & editing.
Funding
The author(s) declare that financial support was received for the research, authorship, and/or publication of this article. Work in the laboratories of the authors were supported by grants from the Fundação Carlos Chagas Filho de Amparo à Pesquisa do Estado do Rio de Janeiro (FAPERJ): AF-M and PC-C : E-26/010.002428/2019, PC-C : E-26/200.341/2023, KC: E-26/211.289/2021, E-26/201.025/2021; Conselho Nacional de Desenvolvimento Científico e Tecnológico (CNPq): KC: Instituto Nacional de Ciência e Tecnologia/National Institute for Translational Neuroscience (INCT-INNT) 465346/2014–6, KC and PC-C are supported by a special scholarship for senior scientists of the Rio de Janeiro State (CNE/FAPERJ, grant no. E-26/202.977/2017 and PC-C : E-26/200.341/2023) and CNPq (KC: 316553/2023–9 and PC-C : 316573/2021–3).
Acknowledgments
DP-F thanks Fundação Carlos Chagas Filho de Amparo à Pesquisa do Estado do Rio de Janeiro (FAPERJ), TN, and AN thanks CAPES, and KC and PC-C thank Conselho Nacional de Desenvolvimento Científico e Tecnológico (CNPq) for individual research fellowship.
Conflict of interest
The authors declare that the research was conducted in the absence of any commercial or financial relationships that could be construed as a potential conflict of interest.
The author(s) declared that they were an editorial board member of Frontiers, at the time of submission. This had no impact on the peer review process and the final decision.
Publisher’s note
All claims expressed in this article are solely those of the authors and do not necessarily represent those of their affiliated organizations, or those of the publisher, the editors and the reviewers. Any product that may be evaluated in this article, or claim that may be made by its manufacturer, is not guaranteed or endorsed by the publisher.
Supplementary material
The Supplementary material for this article can be found online at: https://www.frontiersin.org/articles/10.3389/fnmol.2024.1459083/full#supplementary-material
Abbreviations
ADPKD, Autosomal dominant polycystic kidney disease; ADPR, ADP-ribose; AGEs, Advanced glycation; ALS, amyotrophic lateral sclerosis; AngII, Angiotensin II; AMD, Macular degeneration; AMP, adenosine monophosphate; AP1, activator protein-1; ATP, Adenosine triphosphate; AT1R, Angiotensin II type 1 receptor; BDNF, Brain-derived neurotrophic factor; BRB, Blood retinal barrier; CaM, Calmodulin; CC, Coiled-coil domain; Cdk5, Cyclin-dependent kinase 5; CIRB, inositol (1,4,5)-trisphosphate receptor binding; CNS, Central nervous system; DR, Diabetic retinopathy; DAG, Diacylglycerol; DkTx, Double knot toxin; EC, Endothelial cells; EGF, Epidermal growth factor; ER, Endoplasmic reticulum; FAK, Beta 1 integrin/focal adhesion kinase; GCL, ganglion cell layer; GFAP, Glial fibrillary acidic protein; 4-HNE, 4-hydroxynonenal; H2O2, hydrogen peroxide; HEK293, Immortalized human embryonic kidney cells 293; HIF-1, Hypoxia-inducible factor 1; HRMECs, Human retinal microvascular endothelial cells; CIRB, Inositol (1,4,5)-triphosphate receptor binding; IGF-1, Insulin-like growth factor; IQ, Isoleucine–glutamine; IL-6, Interleukin-6; INL, inner nuclear layer; IOP, Intraocular pressure; IP3, inositol 1,4,5-trifosfato; IPL, inner plexiform layer; ipRGCs, Intrinsically photosensitive RGCs; LC, Lamina cribrosa; LED, Light-emitting diode; MHRs, Melastatin homology regions; mGluR6, Metabotropic glutamate receptors 6; MG, Methylglyoxal; ML-IV, Mucolipidosis type IV; NIR, Near-infrared light; NO, nitric oxide; miR204, Non-coding micro-RNA; NFATc3, Nuclear factor activating T cell isoform c3; NUDT9-H, NUDT9-homology; OIR, Oxygen induced retinopathy; PC1, Polycystin1; PI(3,5)P2, Phosphatidylinositol 3,5-bisphosphate; PIP2, Phosphatidylinositol 4, 5-biphosphate; PI3K, Phosphoinositide 3-kinase; PLC, Phospholipase C; ProTx-1, Protoxin 1; OAG, One-oleoy1–1 acetyl-sn glycerol; ON, optic nerve; ONH, optic nerve head; ONL, outer nuclear layer; OPL, outer plexiform layer; PAX6, combiner box 6; PKA, Protein kinases A; PKC, Protein kinase C; PS, Pregnenolone sulfate; RCS, Reactive carbonyl species; RNS, Nitrogen species; ROS, Reactive oxygen species; Kir6.2, Rectifier potassium channel 6.2; RP, Retinitis pigmentosa; RPE, Retinal pigment epithelium; SERCA, Sarco/endoplasmic reticulum Ca2+ -ATPase inhibitor; SUR1, Sulfonylurea receptor; SOCE, Store-operated Ca2+ entry channels; STIM1, Stromal interacting molecule 1; TG, Trigeminal nerves; TM, Transmembrane Domains; TRP, Transient Receptor Potential; TRPA1, The Transient Receptor Potential 1; TRPA1−/−, TRPA1 knockouts; TRPC, The Transient Receptor Potential Canonical; TRPC1−/−, TRPC1 knockouts; TRPC4−/−, TRPC4 knockouts; TRPC5−/−, TRPC5 knockouts; TRPC6−/−, TRPC6 knockouts; TRPM, Transient Receptor Potential Melastatin; TRPML, The Transient Receptor Potential Mucolipin; TRPML1−/−, TRPML1 knockouts; TRPN, NO-mechano-potential; TRPV, Transient Receptor Potential Vanilloid; TLR3, Toll-like receptor 3; VEGF, Vascular endothelial growth factor.
References
Abad, E., Lorente, G., Gavara, N., Morales, M., Gual, A., and Gasull, X. (2008). Activation of store-operated Ca(2+) channels in trabecular meshwork cells. Invest. Ophthalmol. Vis. Sci. 49, 677–686. doi: 10.1167/iovs.07-1080
Andersson, D. A., Gentry, C., Light, E., Vastani, N., Vallortigara, J., Bierhaus, A., et al. (2013). Methylglyoxal evokes pain by stimulating TRPA1. PLoS One 8:e77986. doi: 10.1371/journal.pone.0077986
Andersson, D. A., Gentry, C., Moss, S., and Bevan, S. (2008). Transient receptor potential A1 is a sensory receptor for multiple products of oxidative stress. J. Neurosci. 28, 2485–2494. doi: 10.1523/jneurosci.5369-07.2008
Araújo, D. S. M., Miya-Coreixas, V. S., Pandolfo, P., and Calaza, K. C. (2017). Cannabinoid receptors and TRPA1 on neuroprotection in a model of retinal ischemia. Exp. Eye Res. 154, 116–125. doi: 10.1016/j.exer.2016.11.015
Arredondo Zamarripa, D., Noguez Imm, R., Bautista Cortés, A. M., Vázquez Ruíz, O., Bernardini, M., Fiorio Pla, A., et al. (2017). Dual contribution of TRPV4 antagonism in the regulatory effect of vasoinhibins on blood-retinal barrier permeability: diabetic milieu makes a difference. Sci. Rep. 7:13094. doi: 10.1038/s41598-017-13621-8
Bae, C., Jara-Oseguera, A., and Swartz, K. J. (2018). TRPM channels come into focus. Science 359, 160–161. doi: 10.1126/science.aar6205
Bahia, P. K., Parks, T. A., Stanford, K. R., Mitchell, D. A., Varma, S., Stevens, S. M. Jr., et al. (2016). The exceptionally high reactivity of Cys 621 is critical for electrophilic activation of the sensory nerve ion channel TRPA1. J. Gen. Physiol. 147, 451–465. doi: 10.1085/jgp.201611581
Bailes, H. J., and Lucas, R. J. (2010). Melanopsin and inner retinal photoreception. Cell. Mol. Life Sci. 67, 99–111. doi: 10.1007/s00018-009-0155-7
Bandell, M., Story, G. M., Hwang, S. W., Viswanath, V., Eid, S. R., Petrus, M. J., et al. (2004). Noxious cold ion channel TRPA1 is activated by pungent compounds and bradykinin. Neuron 41, 849–857. doi: 10.1016/s0896-6273(04)00150-3
Barro-Soria, R., Stindl, J., Müller, C., Foeckler, R., Todorov, V., Castrop, H., et al. (2012). Angiotensin-2-mediated Ca2+ signaling in the retinal pigment epithelium: role of angiotensin-receptor-associated-protein and TRPV2 channel. PLoS One 7:e49624. doi: 10.1371/journal.pone.0049624
Beckel, J. M., Gómez, N. M., Lu, W., Campagno, K. E., Nabet, B., Albalawi, F., et al. (2018). Stimulation of TLR3 triggers release of lysosomal ATP in astrocytes and epithelial cells that requires TRPML1 channels. Sci. Rep. 8:5726. doi: 10.1038/s41598-018-23877-3
Becker, V., Hui, X., Nalbach, L., Ampofo, E., Lipp, P., Menger, M. D., et al. (2021). Linalool inhibits the angiogenic activity of endothelial cells by downregulating intracellular ATP levels and activating TRPM8. Angiogenesis 24, 613–630. doi: 10.1007/s10456-021-09772-y
Belrose, J. C., Xie, Y. F., Gierszewski, L. J., MacDonald, J. F., and Jackson, M. F. (2012). Loss of glutathione homeostasis associated with neuronal senescence facilitates TRPM2 channel activation in cultured hippocampal pyramidal neurons. Mol. Brain 5:11. doi: 10.1186/1756-6606-5-11
Bennett, T. M., Mackay, D. S., Siegfried, C. J., and Shiels, A. (2014). Mutation of the melastatin-related cation channel, TRPM3, underlies inherited cataract and glaucoma. PLoS One 9:e104000. doi: 10.1371/journal.pone.0104000
Berdugo, M., Delaunay, K., Naud, M. C., Guegan, J., Moulin, A., Savoldelli, M., et al. (2021). The antidiabetic drug glibenclamide exerts direct retinal neuroprotection. Transl. Res. 229, 83–99. doi: 10.1016/j.trsl.2020.10.003
Berrout, J., Kyriakopoulou, E., Moparthi, L., Hogea, A. S., Berrout, L., Ivan, C., et al. (2017). TRPA1-FGFR2 binding event is a regulatory oncogenic driver modulated by miRNA-142-3p. Nat. Commun. 8:947. doi: 10.1038/s41467-017-00983-w
Berson, D. M. (2007). Phototransduction in ganglion-cell photoreceptors. Pflugers Arch. 454, 849–855. doi: 10.1007/s00424-007-0242-2
Bessac, B. F., and Jordt, S. E. (2008). Breathtaking TRP channels: TRPA1 and TRPV1 in airway chemosensation and reflex control. Physiology 23, 360–370. doi: 10.1152/physiol.00026.2008
Blair, N. T., Kaczmarek, J. S., and Clapham, D. E. (2009). Intracellular calcium strongly potentiates agonist-activated TRPC5 channels. J. Gen. Physiol. 133, 525–546. doi: 10.1085/jgp.200810153
Bollimuntha, S., Cornatzer, E., and Singh, B. B. (2005). Plasma membrane localization and function of TRPC1 is dependent on its interaction with beta-tubulin in retinal epithelium cells. Vis. Neurosci. 22, 163–170. doi: 10.1017/s0952523805222058
Bradley, E. R., and Delaffon, V. (2020). Diabetic retinopathy screening in persons with mental illness: a literature review. BMJ Open Ophthalmol. 5:e000437. doi: 10.1136/bmjophth-2020-000437
Brown, R. L., Xiong, W. H., Peters, J. H., Tekmen-Clark, M., Strycharska-Orczyk, I., Reed, B. T., et al. (2015). TRPM3 expression in mouse retina. PLoS One 10:e0117615. doi: 10.1371/journal.pone.0117615
Buday, T., Kovacikova, L., Ruzinak, R., and Plevkova, J. (2017). TRPV4 antagonist GSK2193874 does not modulate cough response to osmotic stimuli. Respir. Physiol. Neurobiol. 236, 1–4. doi: 10.1016/j.resp.2016.10.010
Cabezas-Bratesco, D., Brauchi, S., González-Teuber, V., Steinberg, X., Valencia, I., and Colenso, C. (2015). The different roles of the channel-kinases TRPM6 and TRPM7. Curr. Med. Chem. 22, 2943–2953. doi: 10.2174/0929867322666150716115644
Cai, N., Bai, Z., Nanda, V., and Runnels, L. W. (2017). Mass spectrometric analysis of TRPM6 and TRPM7 phosphorylation reveals regulatory mechanisms of the channel-kinases. Sci. Rep. 7:42739. doi: 10.1038/srep42739
Cantero Mdel, R., Velázquez, I. F., Streets, A. J., Ong, A. C., and Cantiello, H. F. (2015). The cAMP signaling pathway and direct protein kinase a phosphorylation regulate Polycystin-2 (TRPP2) channel function. J. Biol. Chem. 290, 23888–23896. doi: 10.1074/jbc.M115.661082
Cao, C., Zakharian, E., Borbiro, I., and Rohacs, T. (2013). Interplay between calmodulin and phosphatidylinositol 4,5-bisphosphate in Ca2+−induced inactivation of transient receptor potential vanilloid 6 channels. J. Biol. Chem. 288, 5278–5290. doi: 10.1074/jbc.M112.409482
Cao, D. S., Zhong, L., Hsieh, T. H., Abooj, M., Bishnoi, M., Hughes, L., et al. (2012). Expression of transient receptor potential ankyrin 1 (TRPA1) and its role in insulin release from rat pancreatic beta cells. PLoS One 7:e38005. doi: 10.1371/journal.pone.0038005
Carpi-Santos, R., de Melo Reis, R. A., Gomes, F. C. A., and Calaza, K. C. (2022). Contribution of Müller cells in the diabetic retinopathy development: focus on oxidative stress and inflammation. Antioxidants 11:617. doi: 10.3390/antiox11040617
Caterina, M. J., Schumacher, M. A., Tominaga, M., Rosen, T. A., Levine, J. D., and Julius, D. (1997). The capsaicin receptor: a heat-activated ion channel in the pain pathway. Nature 389, 816–824. doi: 10.1038/39807
Chen, X., Sooch, G., Demaree, I. S., White, F. A., and Obukhov, A. G. (2020). Transient receptor potential canonical (TRPC) channels: then and now. Cells 9:1983. doi: 10.3390/cells9091983
Chen, Y., Zhang, X., Yang, T., Bi, R., Huang, Z., Ding, H., et al. (2019). Emerging structural biology of TRPM subfamily channels. Cell Calcium 79, 75–79. doi: 10.1016/j.ceca.2019.02.011
Cheng, R. X., Feng, Y., Liu, D., Wang, Z. H., Zhang, J. T., Chen, L. H., et al. (2019). The role of Na(v)1.7 and methylglyoxal-mediated activation of TRPA1 in itch and hypoalgesia in a murine model of type 1 diabetes. Theranostics 9, 4287–4307. doi: 10.7150/thno.36077
Choi, H. J., Sun, D., and Jakobs, T. C. (2015). Astrocytes in the optic nerve head express putative mechanosensitive channels. Mol. Vis. 21, 749–766.
Contreras, E., Nobleman, A. P., Robinson, P. R., and Schmidt, T. M. (2021). Melanopsin phototransduction: beyond canonical cascades. J. Exp. Biol. 224:jeb226522. doi: 10.1242/jeb.226522
Cordeiro, S., Seyler, S., Stindl, J., Milenkovic, V. M., and Strauss, O. (2010). Heat-sensitive TRPV channels in retinal pigment epithelial cells: regulation of VEGF-A secretion. Invest. Ophthalmol. Vis. Sci. 51, 6001–6008. doi: 10.1167/iovs.09-4720
Cordeiro, S., and Strauss, O. (2011). Expression of Orai genes and I(CRAC) activation in the human retinal pigment epithelium. Graefes Arch. Clin. Exp. Ophthalmol. 249, 47–54. doi: 10.1007/s00417-010-1445-3
Cordero-Morales, J. F., Gracheva, E. O., and Julius, D. (2011). Cytoplasmic ankyrin repeats of transient receptor potential A1 (TRPA1) dictate sensitivity to thermal and chemical stimuli. Proc. Natl. Acad. Sci. USA 108, E1184–E1191. doi: 10.1073/pnas.1114124108
Curcic, S., Schober, R., Schindl, R., and Groschner, K. (2019). TRPC-mediated Ca(2+) signaling and control of cellular functions. Semin. Cell Dev. Biol. 94, 28–39. doi: 10.1016/j.semcdb.2019.02.001
Da Silva, N., Herron, C. E., Stevens, K., Jollimore, C. A., Barnes, S., and Kelly, M. E. (2008). Metabotropic receptor-activated calcium increases and store-operated calcium influx in mouse Müller cells. Invest. Ophthalmol. Vis. Sci. 49, 3065–3073. doi: 10.1167/iovs.07-1118
Daiger, S. P., Bowne, S. J., and Sullivan, L. S. (2014). Genes and mutations causing autosomal dominant retinitis Pigmentosa. Cold Spring Harb. Perspect. Med. 5:a017129. doi: 10.1101/cshperspect.a017129
Daldal, H., and Nazıroğlu, M. (2022a). Carvacrol protects the ARPE19 retinal pigment epithelial cells against high glucose-induced oxidative stress, apoptosis, and inflammation by suppressing the TRPM2 channel signaling pathways. Graefes Arch. Clin. Exp. Ophthalmol. 260, 2567–2583. doi: 10.1007/s00417-022-05731-5
Daldal, H., and Nazıroğlu, M. (2022b). Rituximab attenuated lipopolysaccharide-induced oxidative cytotoxicity, apoptosis, and inflammation in the human retina cells via modulating the TRPM2 signaling pathways. Ocul. Immunol. Inflamm. 30, 1315–1328. doi: 10.1080/09273948.2022.2075400
Dayam, R. M., Saric, A., Shilliday, R. E., and Botelho, R. J. (2015). The phosphoinositide-gated lysosomal Ca(2+) channel, TRPML1, is required for phagosome maturation. Traffic 16, 1010–1026. doi: 10.1111/tra.12303
de Araújo, D. S. M., De Logu, F., Adembri, C., Rizzo, S., Janal, M. N., Landini, L., et al. (2020). TRPA1 mediates damage of the retina induced by ischemia and reperfusion in mice. Cell Death Dis. 11:633. doi: 10.1038/s41419-020-02863-6
de Baaij, J. H., Blanchard, M. G., Lavrijsen, M., Leipziger, J., Bindels, R. J., and Hoenderop, J. G. (2014). P2X4 receptor regulation of transient receptor potential melastatin type 6 (TRPM6) Mg2+ channels. Pflugers Arch. 466, 1941–1952. doi: 10.1007/s00424-014-1440-3
de la Roche, J., Eberhardt, M. J., Klinger, A. B., Stanslowsky, N., Wegner, F., Koppert, W., et al. (2013). The molecular basis for species-specific activation of human TRPA1 protein by protons involves poorly conserved residues within transmembrane domains 5 and 6. J. Biol. Chem. 288, 20280–20292. doi: 10.1074/jbc.M113.479337
de Souza, L. B., and Ambudkar, I. S. (2014). Trafficking mechanisms and regulation of TRPC channels. Cell Calcium 56, 43–50. doi: 10.1016/j.ceca.2014.05.001
DeHaven, W. I., Jones, B. F., Petranka, J. G., Smyth, J. T., Tomita, T., Bird, G. S., et al. (2009). TRPC channels function independently of STIM1 and Orai1. J. Physiol. 587, 2275–2298. doi: 10.1113/jphysiol.2009.170431
Doerner, J. F., Hatt, H., and Ramsey, I. S. (2011). Voltage- and temperature-dependent activation of TRPV3 channels is potentiated by receptor-mediated PI(4,5)P2 hydrolysis. J. Gen. Physiol. 137, 271–288. doi: 10.1085/jgp.200910388
Du, J., Xie, J., and Yue, L. (2009). Modulation of TRPM2 by acidic pH and the underlying mechanisms for pH sensitivity. J. Gen. Physiol. 134, 471–488. doi: 10.1085/jgp.200910254
Duan, J., Li, J., Zeng, B., Chen, G. L., Peng, X., Zhang, Y., et al. (2018). Structure of the mouse TRPC4 ion channel. Nat. Commun. 9:3102. doi: 10.1038/s41467-018-05247-9
Eberhardt, C., Amann, B., Stangassinger, M., Hauck, S. M., and Deeg, C. A. (2012). Isolation, characterization and establishment of an equine retinal glial cell line: a prerequisite to investigate the physiological function of Müller cells in the retina. J Anim Physiol Anim Nutr 96, 260–269. doi: 10.1111/j.1439-0396.2011.01147.x
Fan, C., Choi, W., Sun, W., Du, J., and Lü, W. (2018). Structure of the human lipid-gated cation channel TRPC3. eLife 7:e36852. doi: 10.7554/eLife.36852
Fan Gaskin, J. C., Shah, M. H., and Chan, E. C. (2021). Oxidative stress and the role of NADPH oxidase in Glaucoma. Antioxidants 10:238. doi: 10.3390/antiox10020238
Fanaro, G. B., Marques, M. R., Calaza, K. D. C., Brito, R., Pessoni, A. M., Mendonça, H. R., et al. (2023). New insights on dietary polyphenols for the Management of Oxidative Stress and Neuroinflammation in diabetic retinopathy. Antioxidants 12:1237. doi: 10.3390/antiox12061237
Feng, S. (2017). TRPC Channel structure and properties. Adv. Exp. Med. Biol. 976, 9–23. doi: 10.1007/978-94-024-1088-4_2
Ferioli, S., Zierler, S., Zaißerer, J., Schredelseker, J., Gudermann, T., and Chubanov, V. (2017). TRPM6 and TRPM7 differentially contribute to the relief of heteromeric TRPM6/7 channels from inhibition by cytosolic Mg(2+) and mg·ATP. Sci. Rep. 7:8806. doi: 10.1038/s41598-017-08144-1
Fernandes, J., Lorenzo, I. M., Andrade, Y. N., Garcia-Elias, A., Serra, S. A., Fernández-Fernández, J. M., et al. (2008). IP3 sensitizes TRPV4 channel to the mechano- and osmotransducing messenger 5′-6′-epoxyeicosatrienoic acid. J. Cell Biol. 181, 143–155. doi: 10.1083/jcb.200712058
Friedlova, E., Grycova, L., Holakovska, B., Silhan, J., Janouskova, H., Sulc, M., et al. (2010). The interactions of the C-terminal region of the TRPC6 channel with calmodulin. Neurochem. Int. 56, 363–366. doi: 10.1016/j.neuint.2009.11.009
Fritsche, L. G., Fariss, R. N., Stambolian, D., Abecasis, G. R., Curcio, C. A., and Swaroop, A. (2014). Age-related macular degeneration: genetics and biology coming together. Annu. Rev. Genomics Hum. Genet. 15, 151–171. doi: 10.1146/annurev-genom-090413-025610
Gangwar, R., Meena, A. S., Shukla, P. K., Nagaraja, A. S., Dorniak, P. L., Pallikuth, S., et al. (2017). Calcium-mediated oxidative stress: a common mechanism in tight junction disruption by different types of cellular stress. Biochem. J. 474, 731–749. doi: 10.1042/bcj20160679
Gao, F., Yang, Z., Jacoby, R. A., Wu, S. M., and Pang, J. J. (2019). The expression and function of TRPV4 channels in primate retinal ganglion cells and bipolar cells. Cell Death Dis. 10:364. doi: 10.1038/s41419-019-1576-3
Garcia-Elias, A., Berna-Erro, A., Rubio-Moscardo, F., Pardo-Pastor, C., Mrkonjić, S., Sepúlveda, R. V., et al. (2015). Interaction between the linker, pre-S1, and TRP domains determines folding, assembly, and trafficking of TRPV channels. Structure 23, 1404–1413. doi: 10.1016/j.str.2015.05.018
Garcia-Elias, A., Lorenzo, I. M., Vicente, R., and Valverde, M. A. (2008). IP3 receptor binds to and sensitizes TRPV4 channel to osmotic stimuli via a calmodulin-binding site. J. Biol. Chem. 283, 31284–31288. doi: 10.1074/jbc.C800184200
Garcia-Elias, A., Mrkonjic, S., Pardo-Pastor, C., Inada, H., Hellmich, U. A., Rubio-Moscardó, F., et al. (2013). Phosphatidylinositol-4,5-biphosphate-dependent rearrangement of TRPV4 cytosolic tails enables channel activation by physiological stimuli. Proc. Natl. Acad. Sci. USA 110, 9553–9558. doi: 10.1073/pnas.1220231110
GBD 2019 Blindness and Vision Impairment Collaborators (2021). Causes of blindness and vision impairment in 2020 and trends over 30 years, and prevalence of avoidable blindness in relation to VISION 2020: the right to sight: an analysis for the global burden of disease study. Lancet Glob. Health 9, e144–e160. doi: 10.1016/s2214-109x(20)30489-7
Gilliam, J. C., and Wensel, T. G. (2011). TRP channel gene expression in the mouse retina. Vis. Res. 51, 2440–2452. doi: 10.1016/j.visres.2011.10.009
Gómez, N. M., Lu, W., Lim, J. C., Kiselyov, K., Campagno, K. E., Grishchuk, Y., et al. (2018). Robust lysosomal calcium signaling through channel TRPML1 is impaired by lysosomal lipid accumulation. FASEB J. 32, 782–794. doi: 10.1096/fj.201700220RR
Graham, D. M., Wong, K. Y., Shapiro, P., Frederick, C., Pattabiraman, K., and Berson, D. M. (2008). Melanopsin ganglion cells use a membrane-associated rhabdomeric phototransduction cascade. J. Neurophysiol. 99, 2522–2532. doi: 10.1152/jn.01066.2007
Grishchuk, Y., Stember, K. G., Matsunaga, A., Olivares, A. M., Cruz, N. M., King, V. E., et al. (2016). Retinal dystrophy and optic nerve pathology in the mouse model of Mucolipidosis IV. Am. J. Pathol. 186, 199–209. doi: 10.1016/j.ajpath.2015.09.017
Guadagni, V., Novelli, E., Piano, I., Gargini, C., and Strettoi, E. (2015). Pharmacological approaches to retinitis pigmentosa: a laboratory perspective. Prog. Retin. Eye Res. 48, 62–81. doi: 10.1016/j.preteyeres.2015.06.005
Guo, J., She, J., Zeng, W., Chen, Q., Bai, X. C., and Jiang, Y. (2017). Structures of the calcium-activated, non-selective cation channel TRPM4. Nature 552, 205–209. doi: 10.1038/nature24997
Hall, B. E., Prochazkova, M., Sapio, M. R., Minetos, P., Kurochkina, N., Binukumar, B. K., et al. (2018). Phosphorylation of the transient receptor potential Ankyrin 1 by cyclin-dependent kinase 5 affects chemo-nociception. Sci. Rep. 8:1177. doi: 10.1038/s41598-018-19532-6
Hartong, D. T., Berson, E. L., and Dryja, T. P. (2006). Retinitis pigmentosa. Lancet 368, 1795–1809. doi: 10.1016/s0140-6736(06)69740-7
Hasan, R., Leeson-Payne, A. T., Jaggar, J. H., and Zhang, X. (2017). Calmodulin is responsible for Ca(2+)-dependent regulation of TRPA1 channels. Sci. Rep. 7:45098. doi: 10.1038/srep45098
Hilton, J. K., Kim, M., and Van Horn, W. D. (2019). Structural and evolutionary insights point to allosteric regulation of TRP ion channels. Acc. Chem. Res. 52, 1643–1652. doi: 10.1021/acs.accounts.9b00075
Hinman, A., Chuang, H. H., Bautista, D. M., and Julius, D. (2006). TRP channel activation by reversible covalent modification. Proc. Natl. Acad. Sci. USA 103, 19564–19568. doi: 10.1073/pnas.0609598103
Hiyama, H., Yano, Y., So, K., Imai, S., Nagayasu, K., Shirakawa, H., et al. (2018). TRPA1 sensitization during diabetic vascular impairment contributes to cold hypersensitivity in a mouse model of painful diabetic peripheral neuropathy. Mol. Pain 14:1744806918789812. doi: 10.1177/1744806918789812
Ho, K. W., Lambert, W. S., and Calkins, D. J. (2014). Activation of the TRPV1 cation channel contributes to stress-induced astrocyte migration. Glia 62, 1435–1451. doi: 10.1002/glia.22691
Hoenderop, J. G., van Leeuwen, J. P., van der Eerden, B. C., Kersten, F. F., van der Kemp, A. W., Mérillat, A. M., et al. (2003). Renal Ca2+ wasting, hyperabsorption, and reduced bone thickness in mice lacking TRPV5. J. Clin. Invest. 112, 1906–1914. doi: 10.1172/jci19826
Hofherr, A., and Köttgen, M. (2011). TRPP channels and polycystins. Adv. Exp. Med. Biol. 704, 287–313. doi: 10.1007/978-94-007-0265-3_16
Hofmann, T., Chubanov, V., Gudermann, T., and Montell, C. (2003). TRPM5 is a voltage-modulated and ca(2+)-activated monovalent selective cation channel. Curr. Biol. 13, 1153–1158. doi: 10.1016/s0960-9822(03)00431-7
Hofmann, T., Obukhov, A. G., Schaefer, M., Harteneck, C., Gudermann, T., and Schultz, G. (1999). Direct activation of human TRPC6 and TRPC3 channels by diacylglycerol. Nature 397, 259–263. doi: 10.1038/16711
Hu, L., and Xu, G. (2021). Potential protective role of TRPM7 and involvement of PKC/ERK pathway in blue Light-induced apoptosis in retinal pigment epithelium cells in vitro. Asia Pac. J. Ophthalmol. 10, 572–578. doi: 10.1097/apo.0000000000000447
Huang, A. L., Chen, X., Hoon, M. A., Chandrashekar, J., Guo, W., Tränkner, D., et al. (2006). The cells and logic for mammalian sour taste detection. Nature 442, 934–938. doi: 10.1038/nature05084
Huang, Y., Fliegert, R., Guse, A. H., Lü, W., and Du, J. (2020). A structural overview of the ion channels of the TRPM family. Cell Calcium 85:102111. doi: 10.1016/j.ceca.2019.102111
Huang, Y., Roth, B., Lü, W., and Du, J. (2019). Ligand recognition and gating mechanism through three ligand-binding sites of human TRPM2 channel. eLife 8:e50175. doi: 10.7554/eLife.50175
Huang, Y., Winkler, P. A., Sun, W., Lü, W., and Du, J. (2018). Architecture of the TRPM2 channel and its activation mechanism by ADP-ribose and calcium. Nature 562, 145–149. doi: 10.1038/s41586-018-0558-4
Huffer, K. E., Aleksandrova, A. A., Jara-Oseguera, A., Forrest, L. R., and Swartz, K. J. (2020). Global alignment and assessment of TRP channel transmembrane domain structures to explore functional mechanisms. eLife 9:e58660. doi: 10.7554/eLife.58660
Hughes, S., Pothecary, C. A., Jagannath, A., Foster, R. G., Hankins, M. W., and Peirson, S. N. (2012). Profound defects in pupillary responses to light in TRPM-channel null mice: a role for TRPM channels in non-image-forming photoreception. Eur. J. Neurosci. 35, 34–43. doi: 10.1111/j.1460-9568.2011.07944.x
Hynkova, A., Marsakova, L., Vaskova, J., and Vlachova, V. (2016). N-terminal tetrapeptide T/SPLH motifs contribute to multimodal activation of human TRPA1 channel. Sci. Rep. 6:28700. doi: 10.1038/srep28700
Irie, S., and Furukawa, T. (2014). TRPM1. Handb. Exp. Pharmacol. 222, 387–402. doi: 10.1007/978-3-642-54215-2_15
Irnaten, M., O'Malley, G., Clark, A. F., and O'Brien, C. J. (2020). Transient receptor potential channels TRPC1/TRPC6 regulate lamina cribrosa cell extracellular matrix gene transcription and proliferation. Exp. Eye Res. 193:107980. doi: 10.1016/j.exer.2020.107980
Irsch, K., and Guyton, D. L. (2009). “Anatomy of eyes” in Encyclopedia of biometrics. eds. S. Z. Li and A. Jain (Boston, MA: Springer US), 11–16.
Jabba, S., Goyal, R., Sosa-Pagán, J. O., Moldenhauer, H., Wu, J., Kalmeta, B., et al. (2014). Directionality of temperature activation in mouse TRPA1 ion channel can be inverted by single-point mutations in ankyrin repeat six. Neuron 82, 1017–1031. doi: 10.1016/j.neuron.2014.04.016
Jayaram, H., Cepurna, W. O., Johnson, E. C., and Morrison, J. C. (2015). MicroRNA expression in the glaucomatous retina. Invest. Ophthalmol. Vis. Sci. 56, 7971–7982. doi: 10.1167/iovs.15-18088
Jimenez, I., Prado, Y., Marchant, F., Otero, C., Eltit, F., Cabello-Verrugio, C., et al. (2020). TRPM channels in human diseases. Cells 9:2604. doi: 10.3390/cells9122604
Jin, J., Desai, B. N., Navarro, B., Donovan, A., Andrews, N. C., and Clapham, D. E. (2008). Deletion of Trpm7 disrupts embryonic development and thymopoiesis without altering Mg2+ homeostasis. Science 322, 756–760. doi: 10.1126/science.1163493
Jirku, M., Bumba, L., Bednarova, L., Kubala, M., Sulc, M., Franek, M., et al. (2015). Characterization of the part of N-terminal PIP2 binding site of the TRPM1 channel. Biophys. Chem. 207, 135–142. doi: 10.1016/j.bpc.2015.10.005
Jordt, S. E., Bautista, D. M., Chuang, H. H., McKemy, D. D., Zygmunt, P. M., Högestätt, E. D., et al. (2004). Mustard oils and cannabinoids excite sensory nerve fibres through the TRP channel ANKTM1. Nature 427, 260–265. doi: 10.1038/nature02282
Kádková, A., Synytsya, V., Krusek, J., Zímová, L., and Vlachová, V. (2017). Molecular basis of TRPA1 regulation in nociceptive neurons. A review. Physiol. Res. 66, 425–439. doi: 10.33549/physiolres.933553
Karashima, Y., Talavera, K., Everaerts, W., Janssens, A., Kwan, K. Y., Vennekens, R., et al. (2009). TRPA1 acts as a cold sensor in vitro and in vivo. Proc. Natl. Acad. Sci. USA 106, 1273–1278. doi: 10.1073/pnas.0808487106
Kels, B. D., Grzybowski, A., and Grant-Kels, J. M. (2015). Human ocular anatomy. Clin. Dermatol. 33, 140–146. doi: 10.1016/j.clindermatol.2014.10.006
Kim, E. Y., Rumpf, C. H., Fujiwara, Y., Cooley, E. S., Van Petegem, F., and Minor, D. L. Jr. (2008). Structures of CaV2 Ca2+/CaM-IQ domain complexes reveal binding modes that underlie calcium-dependent inactivation and facilitation. Structure 16, 1455–1467. doi: 10.1016/j.str.2008.07.010
Klein, A. S., Tannert, A., and Schaefer, M. (2014). Cholesterol sensitises the transient receptor potential channel TRPV3 to lower temperatures and activator concentrations. Cell Calcium 55, 59–68. doi: 10.1016/j.ceca.2013.12.001
Klooster, J., Blokker, J., Ten Brink, J. B., Unmehopa, U., Fluiter, K., Bergen, A. A., et al. (2011). Ultrastructural localization and expression of TRPM1 in the human retina. Invest. Ophthalmol. Vis. Sci. 52, 8356–8362. doi: 10.1167/iovs.11-7575
Koike, C., Obara, T., Uriu, Y., Numata, T., Sanuki, R., Miyata, K., et al. (2010). TRPM1 is a component of the retinal ON bipolar cell transduction channel in the mGluR6 cascade. Proc. Natl. Acad. Sci. USA 107, 332–337. doi: 10.1073/pnas.0912730107
Kouchi, Z., Fukami, K., Shikano, T., Oda, S., Nakamura, Y., Takenawa, T., et al. (2004). Recombinant phospholipase Czeta has high Ca2+ sensitivity and induces Ca2+ oscillations in mouse eggs. J. Biol. Chem. 279, 10408–10412. doi: 10.1074/jbc.M313801200
Kraft, R., and Harteneck, C. (2005). The mammalian melastatin-related transient receptor potential cation channels: an overview. Pflugers Arch. 451, 204–211. doi: 10.1007/s00424-005-1428-0
Križaj, D., Huang, W., Furukawa, T., Punzo, C., and Xing, W. (2010). Plasticity of TRPM1 expression and localization in the wild type and degenerating mouse retina. Vis. Res. 50, 2460–2465. doi: 10.1016/j.visres.2010.08.034
Kwan, K. Y., Allchorne, A. J., Vollrath, M. A., Christensen, A. P., Zhang, D. S., Woolf, C. J., et al. (2006). TRPA1 contributes to cold, mechanical, and chemical nociception but is not essential for hair-cell transduction. Neuron 50, 277–289. doi: 10.1016/j.neuron.2006.03.042
Lambert, S., Drews, A., Rizun, O., Wagner, T. F., Lis, A., Mannebach, S., et al. (2011). Transient receptor potential melastatin 1 (TRPM1) is an ion-conducting plasma membrane channel inhibited by zinc ions. J. Biol. Chem. 286, 12221–12233. doi: 10.1074/jbc.M110.202945
Lang, H. B., Xie, R. X., Huang, M. L., Fang, L. Y., Tang, Y. B., and Zhang, F. (2020). The effect and mechanism of TRPC1, 3, and 6 on the proliferation, migration, and lumen formation of retinal vascular endothelial cells induced by high glucose. Ophthalmic Res. 63, 284–294. doi: 10.1159/000503724
Lange, I., Penner, R., Fleig, A., and Beck, A. (2008). Synergistic regulation of endogenous TRPM2 channels by adenine dinucleotides in primary human neutrophils. Cell Calcium 44, 604–615. doi: 10.1016/j.ceca.2008.05.001
LaPlante, J. M., Falardeau, J., Sun, M., Kanazirska, M., Brown, E. M., Slaugenhaupt, S. A., et al. (2002). Identification and characterization of the single channel function of human mucolipin-1 implicated in mucolipidosis type IV, a disorder affecting the lysosomal pathway. FEBS Lett. 532, 183–187. doi: 10.1016/s0014-5793(02)03670-0
LaPlante, J. M., Ye, C. P., Quinn, S. J., Goldin, E., Brown, E. M., Slaugenhaupt, S. A., et al. (2004). Functional links between mucolipin-1 and Ca2+−dependent membrane trafficking in mucolipidosis IV. Biochem. Biophys. Res. Commun. 322, 1384–1391. doi: 10.1016/j.bbrc.2004.08.045
Lee, S. P., Buber, M. T., Yang, Q., Cerne, R., Cortés, R. Y., Sprous, D. G., et al. (2008). Thymol and related alkyl phenols activate the hTRPA1 channel. Br. J. Pharmacol. 153, 1739–1749. doi: 10.1038/bjp.2008.85
Lee, J., Cha, S. K., Sun, T. J., and Huang, C. L. (2005). PIP2 activates TRPV5 and releases its inhibition by intracellular Mg2+. J. Gen. Physiol. 126, 439–451. doi: 10.1085/jgp.200509314
Leonelli, M., Martins, D. O., and Britto, L. R. (2010). TRPV1 receptors are involved in protein nitration and Müller cell reaction in the acutely axotomized rat retina. Exp. Eye Res. 91, 755–768. doi: 10.1016/j.exer.2010.08.026
Leonelli, M., Martins, D. O., and Britto, L. R. (2011). TRPV1 receptors modulate retinal development. Int. J. Dev. Neurosci. 29, 405–413. doi: 10.1016/j.ijdevneu.2011.03.002
Leonelli, M., Martins, D. O., Kihara, A. H., and Britto, L. R. (2009). Ontogenetic expression of the vanilloid receptors TRPV1 and TRPV2 in the rat retina. Int. J. Dev. Neurosci. 27, 709–718. doi: 10.1016/j.ijdevneu.2009.07.003
Lewis, R., Asplin, K. E., Bruce, G., Dart, C., Mobasheri, A., and Barrett-Jolley, R. (2011). The role of the membrane potential in chondrocyte volume regulation. J. Cell. Physiol. 226, 2979–2986. doi: 10.1002/jcp.22646
Li, M., Yu, Y., and Yang, J. (2011). Structural biology of TRP channels. Adv. Exp. Med. Biol. 704, 1–23. doi: 10.1007/978-94-007-0265-3_1
Li, J., Zhang, X., Song, X., Liu, R., Zhang, J., and Li, Z. (2019). The structure of TRPC ion channels. Cell Calcium 80, 25–28. doi: 10.1016/j.ceca.2019.03.005
Ling, Y., Hu, Z., Meng, Q., Fang, P., and Liu, H. (2016). Bimatoprost increases Mechanosensitivity of trigeminal ganglion neurons innervating the inner walls of rat anterior chambers via activation of TRPA1. Invest. Ophthalmol. Vis. Sci. 57, 567–576. doi: 10.1167/iovs.15-18108
Liu, K., Gao, X., Hu, C., Gui, Y., Gui, S., Ni, Q., et al. (2022). Capsaicin ameliorates diabetic retinopathy by inhibiting poldip2-induced oxidative stress. Redox Biol. 56:102460. doi: 10.1016/j.redox.2022.102460
Liu, D., Zhang, Z., and Liman, E. R. (2005). Extracellular acid block and acid-enhanced inactivation of the Ca2+−activated cation channel TRPM5 involve residues in the S3-S4 and S5-S6 extracellular domains. J. Biol. Chem. 280, 20691–20699. doi: 10.1074/jbc.M414072200
Lukacs, V., Thyagarajan, B., Varnai, P., Balla, A., Balla, T., and Rohacs, T. (2007). Dual regulation of TRPV1 by phosphoinositides. J. Neurosci. 27, 7070–7080. doi: 10.1523/jneurosci.1866-07.2007
Ma, L., Liu, X., Liu, Q., Jin, S., Chang, H., and Liu, H. (2022). The roles of transient receptor potential ion channels in pathologies of Glaucoma. Front. Physiol. 13:806786. doi: 10.3389/fphys.2022.806786
Macikova, L., Sinica, V., Kadkova, A., Villette, S., Ciaccafava, A., Faherty, J., et al. (2019). Putative interaction site for membrane phospholipids controls activation of TRPA1 channel at physiological membrane potentials. FEBS J. 286, 3664–3683. doi: 10.1111/febs.14931
Macpherson, L. J., Dubin, A. E., Evans, M. J., Marr, F., Schultz, P. G., Cravatt, B. F., et al. (2007). Noxious compounds activate TRPA1 ion channels through covalent modification of cysteines. Nature 445, 541–545. doi: 10.1038/nature05544
Macpherson, L. J., Geierstanger, B. H., Viswanath, V., Bandell, M., Eid, S. R., Hwang, S., et al. (2005). The pungency of garlic: activation of TRPA1 and TRPV1 in response to allicin. Curr. Biol. 15, 929–934. doi: 10.1016/j.cub.2005.04.018
Mamenko, M., Zaika, O. L., Boukelmoune, N., Berrout, J., O'Neil, R. G., and Pochynyuk, O. (2013). Discrete control of TRPV4 channel function in the distal nephron by protein kinases a and C. J. Biol. Chem. 288, 20306–20314. doi: 10.1074/jbc.M113.466797
Maneu, V., Lax, P., De Diego, A. M. G., Cuenca, N., and García, A. G. (2022). Combined drug triads for synergic neuroprotection in retinal degeneration. Biomed. Pharmacother. 149:112911. doi: 10.1016/j.biopha.2022.112911
Marsakova, L., Barvik, I., Zima, V., Zimova, L., and Vlachova, V. (2017). The first extracellular linker is important for several aspects of the gating mechanism of human TRPA1 channel. Front. Mol. Neurosci. 10:16. doi: 10.3389/fnmol.2017.00016
Masland, R. H. (2001). The fundamental plan of the retina. Nat. Neurosci. 4, 877–886. doi: 10.1038/nn0901-877
Massengill, M. T., Ash, N. F., Young, B. M., Ildefonso, C. J., and Lewin, A. S. (2020). Sectoral activation of glia in an inducible mouse model of autosomal dominant retinitis pigmentosa. Sci. Rep. 10:16967. doi: 10.1038/s41598-020-73749-y
Matta, J. A., Cornett, P. M., Miyares, R. L., Abe, K., Sahibzada, N., and Ahern, G. P. (2008). General anesthetics activate a nociceptive ion channel to enhance pain and inflammation. Proc. Natl. Acad. Sci. USA 105, 8784–8789. doi: 10.1073/pnas.0711038105
McGrady, N. R., Risner, M. L., Vest, V., and Calkins, D. J. (2020). TRPV1 Tunes optic nerve axon excitability in Glaucoma. Front. Physiol. 11:249. doi: 10.3389/fphys.2020.00249
McMillan, H., Lundy, F. T., Dunne, O. M., Al-Natour, B., Jeanneau, C., About, I., et al. (2021). Endogenous mas-related G-protein-coupled receptor X1 activates and sensitizes TRPA1 in a human model of peripheral nerves. FASEB J. 35:e21492. doi: 10.1096/fj.202001667RR
Meents, J. E., Fischer, M. J., and McNaughton, P. A. (2017). Sensitization of TRPA1 by protein kinase a. PLoS One 12:e0170097. doi: 10.1371/journal.pone.0170097
Meléndez García, R., Arredondo Zamarripa, D., Arnold, E., Ruiz-Herrera, X., Noguez Imm, R., Baeza Cruz, G., et al. (2015). Prolactin protects retinal pigment epithelium by inhibiting sirtuin 2-dependent cell death. EBioMedicine 7, 35–49. doi: 10.1016/j.ebiom.2016.03.048
Mercado, J., Gordon-Shaag, A., Zagotta, W. N., and Gordon, S. E. (2010). Ca2+−dependent desensitization of TRPV2 channels is mediated by hydrolysis of phosphatidylinositol 4,5-bisphosphate. J. Neurosci. 30, 13338–13347. doi: 10.1523/jneurosci.2108-10.2010
Mitchell, P., Liew, G., Gopinath, B., and Wong, T. Y. (2018). Age-related macular degeneration. Lancet 392, 1147–1159. doi: 10.1016/s0140-6736(18)31550-2
Moazzeni, H., Khani, M., and Elahi, E. (2020). Insights into the regulatory molecules involved in glaucoma pathogenesis. Am. J. Med. Genet. C Semin. Med. Genet. 184, 782–827. doi: 10.1002/ajmg.c.31833
Molnár, T., Yarishkin, O., Iuso, A., Barabas, P., Jones, B., Marc, R. E., et al. (2016). Store-operated calcium entry in Müller glia is controlled by synergistic activation of TRPC and Orai channels. J. Neurosci. 36, 3184–3198. doi: 10.1523/jneurosci.4069-15.2016
Monaghan, K., McNaughten, J., McGahon, M. K., Kelly, C., Kyle, D., Yong, P. H., et al. (2015). Hyperglycemia and diabetes downregulate the functional expression of TRPV4 channels in retinal microvascular endothelium. PLoS One 10:e0128359. doi: 10.1371/journal.pone.0128359
Montell, C. (2005). The TRP superfamily of cation channels. Sci STKE 2005:re3. doi: 10.1126/stke.2722005re3
Moparthi, L., Moparthi, S. B., Wenger, J., and Zygmunt, P. M. (2020). Calcium activates purified human TRPA1 with and without its N-terminal ankyrin repeat domain in the absence of calmodulin. Cell Calcium 90:102228. doi: 10.1016/j.ceca.2020.102228
Morgans, C. W., Zhang, J., Jeffrey, B. G., Nelson, S. M., Burke, N. S., Duvoisin, R. M., et al. (2009). TRPM1 is required for the depolarizing light response in retinal ON-bipolar cells. Proc. Natl. Acad. Sci. USA 106, 19174–19178. doi: 10.1073/pnas.0908711106
Mori, M. X., Itsuki, K., Hase, H., Sawamura, S., Kurokawa, T., Mori, Y., et al. (2015). Dynamics of receptor-operated ca(2+) currents through TRPC channels controlled via the PI(4,5)P2-PLC signaling pathway. Front. Pharmacol. 6:22. doi: 10.3389/fphar.2015.00022
Mori, Y., Takahashi, N., Polat, O. K., Kurokawa, T., Takeda, N., and Inoue, M. (2016). Redox-sensitive transient receptor potential channels in oxygen sensing and adaptation. Pflugers Arch. 468, 85–97. doi: 10.1007/s00424-015-1716-2
Muraki, K., Iwata, Y., Katanosaka, Y., Ito, T., Ohya, S., Shigekawa, M., et al. (2003). TRPV2 is a component of osmotically sensitive cation channels in murine aortic myocytes. Circ. Res. 93, 829–838. doi: 10.1161/01.Res.0000097263.10220.0c
Nagatomo, K., and Kubo, Y. (2008). Caffeine activates mouse TRPA1 channels but suppresses human TRPA1 channels. Proc. Natl. Acad. Sci. USA 105, 17373–17378. doi: 10.1073/pnas.0809769105
Nassini, R., Fusi, C., Materazzi, S., Coppi, E., Tuccinardi, T., Marone, I. M., et al. (2015). The TRPA1 channel mediates the analgesic action of dipyrone and pyrazolone derivatives. Br. J. Pharmacol. 172, 3397–3411. doi: 10.1111/bph.13129
Negri, S., Faris, P., Berra-Romani, R., Guerra, G., and Moccia, F. (2019). Endothelial transient receptor potential channels and vascular remodeling: extracellular ca(2 +) entry for angiogenesis, Arteriogenesis and Vasculogenesis. Front. Physiol. 10:1618. doi: 10.3389/fphys.2019.01618
Nelidova, D., Morikawa, R. K., Cowan, C. S., Raics, Z., Goldblum, D., Scholl, H. P. N., et al. (2020). Restoring light sensitivity using tunable near-infrared sensors. Science 368, 1108–1113. doi: 10.1126/science.aaz5887
Ng, L. C. T., Vien, T. N., Yarov-Yarovoy, V., and DeCaen, P. G. (2019). Opening TRPP2 (PKD2L1) requires the transfer of gating charges. Proc. Natl. Acad. Sci. USA 116, 15540–15549. doi: 10.1073/pnas.1902917116
Nicoletti, P., Trevisani, M., Manconi, M., Gatti, R., De Siena, G., Zagli, G., et al. (2008). Ethanol causes neurogenic vasodilation by TRPV1 activation and CGRP release in the trigeminovascular system of the guinea pig. Cephalalgia 28, 9–17. doi: 10.1111/j.1468-2982.2007.01448.x
Niemeyer, B. A. (2005). Structure-function analysis of TRPV channels. Naunyn Schmiedeberg's Arch. Pharmacol. 371, 285–294. doi: 10.1007/s00210-005-1053-7
Nilius, B., Prenen, J., and Owsianik, G. (2011). Irritating channels: the case of TRPA1. J. Physiol. 589, 1543–1549. doi: 10.1113/jphysiol.2010.200717
Nilius, B., Prenen, J., Vennekens, R., Hoenderop, J. G., Bindels, R. J., and Droogmans, G. (2001). Modulation of the epithelial calcium channel, ECaC, by intracellular Ca2+. Cell Calcium 29, 417–428. doi: 10.1054/ceca.2001.0201
Niwa, M., Aoki, H., Hirata, A., Tomita, H., Green, P. G., and Hara, A. (2016). Retinal cell degeneration in animal models. Int. J. Mol. Sci. 17:110. doi: 10.3390/ijms17010110
Nooren, I. M., Kaptein, R., Sauer, R. T., and Boelens, R. (1999). The tetramerization domain of the Mnt repressor consists of two right-handed coiled coils. Nat. Struct. Biol. 6, 755–759. doi: 10.1038/11531
Oda, M., Yamamoto, H., Matsumoto, H., Ishizaki, Y., and Shibasaki, K. (2020). TRPC5 regulates axonal outgrowth in developing retinal ganglion cells. Lab. Investig. 100, 297–310. doi: 10.1038/s41374-019-0347-1
Okada, Y., Shirai, K., Miyajima, M., Reinach, P. S., Yamanaka, O., Sumioka, T., et al. (2016). Loss of TRPV4 function suppresses inflammatory fibrosis induced by alkali-burning mouse corneas. PLoS One 11:e0167200. doi: 10.1371/journal.pone.0167200
Okada, Y., Sumioka, T., Reinach, P. S., Miyajima, M., and Saika, S. (2021). Roles of epithelial and mesenchymal TRP channels in mediating inflammatory fibrosis. Front. Immunol. 12:731674. doi: 10.3389/fimmu.2021.731674
Okumus, S., Demiryürek, S., Gürler, B., Coskun, E., Bozgeyik, I., Oztuzcu, S., et al. (2013). Association transient receptor potential melastatin channel gene polymorphism with primary open angle glaucoma. Mol. Vis. 19, 1852–1858
O'Leary, C., McGahon, M. K., Ashraf, S., McNaughten, J., Friedel, T., Cincolà, P., et al. (2019). Involvement of TRPV1 and TRPV4 channels in retinal angiogenesis. Invest. Ophthalmol. Vis. Sci. 60, 3297–3309. doi: 10.1167/iovs.18-26344
Omri, S., Omri, B., Savoldelli, M., Jonet, L., Thillaye-Goldenberg, B., Thuret, G., et al. (2010). The outer limiting membrane (OLM) revisited: clinical implications. Clin. Ophthalmol. 4, 183–195. doi: 10.2147/opth.s5901
O'Neal, T. B., and Luther, E. E. (2024). “Retinitis Pigmentosa” in StatPearls (Treasure Island, FL: StatPearls Publishing LLC).
Orduña Ríos, M., Noguez Imm, R., Hernández Godínez, N. M., Bautista Cortes, A. M., López Escalante, D. D., Liedtke, W., et al. (2019). TRPV4 inhibition prevents increased water diffusion and blood-retina barrier breakdown in the retina of streptozotocin-induced diabetic mice. PLoS One 14:e0212158. doi: 10.1371/journal.pone.0212158
Orozco, L. D., Chen, H. H., Cox, C., Katschke, K. J., Arceo Rommel, C., Espiritu, P., et al. (2023). Integration of eQTL and a single-cell atlas in the human eye identifies causal genes for age-related macular degeneration. Cell Rep. 42:112298. doi: 10.1016/j.celrep.2023.112298
Owsianik, G., Talavera, K., Voets, T., and Nilius, B. (2006). Permeation and selectivity of TRP channels. Annu. Rev. Physiol. 68, 685–717. doi: 10.1146/annurev.physiol.68.040204.101406
Özkaya, D., Nazıroğlu, M., Vanyorek, L., and Muhamad, S. (2021). Involvement of TRPM2 channel on hypoxia-induced oxidative injury, inflammation, and cell death in retinal pigment epithelial cells: modulator action of selenium nanoparticles. Biol. Trace Elem. Res. 199, 1356–1369. doi: 10.1007/s12011-020-02556-3
Papanikolaou, M., Lewis, A., and Butt, A. M. (2017). Store-operated calcium entry is essential for glial calcium signalling in CNS white matter. Brain Struct. Funct. 222, 2993–3005. doi: 10.1007/s00429-017-1380-8
Pedersen, S. F., Owsianik, G., and Nilius, B. (2005). TRP channels: an overview. Cell Calcium 38, 233–252. doi: 10.1016/j.ceca.2005.06.028
Perez-Leighton, C. E., Schmidt, T. M., Abramowitz, J., Birnbaumer, L., and Kofuji, P. (2011). Intrinsic phototransduction persists in melanopsin-expressing ganglion cells lacking diacylglycerol-sensitive TRPC subunits. Eur. J. Neurosci. 33, 856–867. doi: 10.1111/j.1460-9568.2010.07583.x
Persad, P. J., Heid, I. M., Weeks, D. E., Baird, P. N., de Jong, E. K., Haines, J. L., et al. (2017). Joint analysis of nuclear and mitochondrial variants in age-related macular degeneration identifies novel loci TRPM1 and ABHD2/RLBP1. Invest. Ophthalmol. Vis. Sci. 58, 4027–4038. doi: 10.1167/iovs.17-21734
Phelps, C. B., Wang, R. R., Choo, S. S., and Gaudet, R. (2010). Differential regulation of TRPV1, TRPV3, and TRPV4 sensitivity through a conserved binding site on the ankyrin repeat domain. J. Biol. Chem. 285, 731–740. doi: 10.1074/jbc.M109.052548
Picazo-Juárez, G., Romero-Suárez, S., Nieto-Posadas, A., Llorente, I., Jara-Oseguera, A., Briggs, M., et al. (2011). Identification of a binding motif in the S5 helix that confers cholesterol sensitivity to the TRPV1 ion channel. J. Biol. Chem. 286, 24966–24976. doi: 10.1074/jbc.M111.237537
Pires, P. W., and Earley, S. (2018). Neuroprotective effects of TRPA1 channels in the cerebral endothelium following ischemic stroke. eLife 7:e35316. doi: 10.7554/eLife.35316
Potier, S., Mitkus, M., and Kelber, A. (2018). High resolution of colour vision, but low contrast sensitivity in a diurnal raptor. Proc. Biol. Sci. 285:20181036. doi: 10.1098/rspb.2018.1036
Pumroy, R. A., Fluck, E. C. 3rd, Ahmed, T., and Moiseenkova-Bell, V. Y. (2020). Structural insights into the gating mechanisms of TRPV channels. Cell Calcium 87:102168. doi: 10.1016/j.ceca.2020.102168
Rampino, M. A., and Nawy, S. A. (2011). Relief of Mg2+-dependent inhibition of TRPM1 by PKCα at the rod bipolar cell synapse. J. Neurosci. 31, 13596–13603. doi: 10.1523/jneurosci.2655-11.2011
Reichhart, N., Keckeis, S., Fried, F., Fels, G., and Strauss, O. (2015). Regulation of surface expression of TRPV2 channels in the retinal pigment epithelium. Graefes Arch. Clin. Exp. Ophthalmol. 253, 865–874. doi: 10.1007/s00417-014-2917-7
Reinach, P. S., Chen, W., and Mergler, S. (2015). Polymodal roles of transient receptor potential channels in the control of ocular function. Eye Vis. 2:5. doi: 10.1186/s40662-015-0016-4
Risner, M. L., McGrady, N. R., Boal, A. M., Pasini, S., and Calkins, D. J. (2020). TRPV1 supports Axogenic enhanced excitability in response to neurodegenerative stress. Front. Cell. Neurosci. 14:603419. doi: 10.3389/fncel.2020.603419
Robertson, J. V., Golesic, E., Gauldie, J., and West-Mays, J. A. (2010). Ocular gene transfer of active TGF-beta induces changes in anterior segment morphology and elevated IOP in rats. Invest. Ophthalmol. Vis. Sci. 51, 308–318. doi: 10.1167/iovs.09-3380
Rosenbaum, T., Gordon-Shaag, A., Munari, M., and Gordon, S. E. (2004). Ca2+/calmodulin modulates TRPV1 activation by capsaicin. J. Gen. Physiol. 123, 53–62. doi: 10.1085/jgp.200308906
Rossino, M. G., Lulli, M., Amato, R., Cammalleri, M., Monte, M. D., and Casini, G. (2020). Oxidative stress induces a VEGF autocrine loop in the retina: relevance for diabetic retinopathy. Cells 9:1452. doi: 10.3390/cells9061452
Ruan, Y., Jiang, S., Musayeva, A., Pfeiffer, N., and Gericke, A. (2021). Corneal epithelial stem cells-physiology, pathophysiology and therapeutic options. Cells 10:2302. doi: 10.3390/cells10092302
Ryskamp, D. A., Witkovsky, P., Barabas, P., Huang, W., Koehler, C., Akimov, N. P., et al. (2011). The polymodal ion channel transient receptor potential vanilloid 4 modulates calcium flux, spiking rate, and apoptosis of mouse retinal ganglion cells. J. Neurosci. 31, 7089–7101. doi: 10.1523/jneurosci.0359-11.2011
Sachdeva, R., Schlotterer, A., Schumacher, D., Matka, C., Mathar, I., Dietrich, N., et al. (2018). TRPC proteins contribute to development of diabetic retinopathy and regulate glyoxalase 1 activity and methylglyoxal accumulation. Mol. Metab. 9, 156–167. doi: 10.1016/j.molmet.2018.01.003
Samanta, A., Hughes, T. E. T., and Moiseenkova-Bell, V. Y. (2018). Transient receptor potential (TRP) channels. Subcell. Biochem. 87, 141–165. doi: 10.1007/978-981-10-7757-9_6
Sappington, R. M., and Calkins, D. J. (2008). Contribution of TRPV1 to microglia-derived IL-6 and NFkappaB translocation with elevated hydrostatic pressure. Invest. Ophthalmol. Vis. Sci. 49, 3004–3017. doi: 10.1167/iovs.07-1355
Sappington, R. M., Sidorova, T., Ward, N. J., Chakravarthy, R., Ho, K. W., and Calkins, D. J. (2015). Activation of transient receptor potential vanilloid-1 (TRPV1) influences how retinal ganglion cell neurons respond to pressure-related stress. Channels 9, 102–113. doi: 10.1080/19336950.2015.1009272
Sawamura, S., Shirakawa, H., Nakagawa, T., Mori, Y., and Kaneko, S. (2017). “TRP channels in the brain: what are they there for?” in Neurobiology of TRP channels. ed. T. L. R. Emir (Boca Raton, FL: CRC Press), 295–322.
Schlotterer, A., Kolibabka, M., Lin, J., Acunman, K., Dietrich, N., Sticht, C., et al. (2019). Methylglyoxal induces retinopathy-type lesions in the absence of hyperglycemia: studies in a rat model. FASEB J. 33, 4141–4153. doi: 10.1096/fj.201801146RR
Seebohm, G., and Schreiber, J. A. (2021). Beyond hot and spicy: TRPV channels and their pharmacological modulation. Cell. Physiol. Biochem. 55, 108–130. doi: 10.33594/000000358
Sekaran, S., Lall, G. S., Ralphs, K. L., Wolstenholme, A. J., Lucas, R. J., Foster, R. G., et al. (2007). 2-Aminoethoxydiphenylborane is an acute inhibitor of directly photosensitive retinal ganglion cell activity in vitro and in vivo. J. Neurosci. 27, 3981–3986. doi: 10.1523/jneurosci.4716-06.2007
Shiels, A. (2020). TRPM3_miR-204: a complex locus for eye development and disease. Hum. Genomics 14:7. doi: 10.1186/s40246-020-00258-4
Smani, T., Gómez, L. J., Regodon, S., Woodard, G. E., Siegfried, G., Khatib, A. M., et al. (2018). TRP channels in angiogenesis and other endothelial functions. Front. Physiol. 9:1731. doi: 10.3389/fphys.2018.01731
Smith, J. A., Chan, C. C., Goldin, E., and Schiffmann, R. (2002). Noninvasive diagnosis and ophthalmic features of mucolipidosis type IV. Ophthalmology 109, 588–594. doi: 10.1016/s0161-6420(01)00968-x
Smith, L. E., Shen, W., Perruzzi, C., Soker, S., Kinose, F., Xu, X., et al. (1999). Regulation of vascular endothelial growth factor-dependent retinal neovascularization by insulin-like growth factor-1 receptor. Nat. Med. 5, 1390–1395. doi: 10.1038/70963
So, K., Tei, Y., Zhao, M., Miyake, T., Hiyama, H., Shirakawa, H., et al. (2016). Hypoxia-induced sensitisation of TRPA1 in painful dysesthesia evoked by transient hindlimb ischemia/reperfusion in mice. Sci. Rep. 6:23261. doi: 10.1038/srep23261
Song, H. B., Jun, H. O., Kim, J. H., Fruttiger, M., and Kim, J. H. (2015). Suppression of transient receptor potential canonical channel 4 inhibits vascular endothelial growth factor-induced retinal neovascularization. Cell Calcium 57, 101–108. doi: 10.1016/j.ceca.2015.01.002
Sonoda, T., Lee, S. K., Birnbaumer, L., and Schmidt, T. M. (2018). Melanopsin Phototransduction is repurposed by ipRGC subtypes to shape the function of distinct visual circuits. Neuron 99, 754–767.e4. doi: 10.1016/j.neuron.2018.06.032
Spix, B., Chao, Y. K., Abrahamian, C., Chen, C. C., and Grimm, C. (2020). TRPML cation channels in inflammation and immunity. Front. Immunol. 11:225. doi: 10.3389/fimmu.2020.00225
Stitt, A. W., Curtis, T. M., Chen, M., Medina, R. J., McKay, G. J., Jenkins, A., et al. (2016). The progress in understanding and treatment of diabetic retinopathy. Prog. Retin. Eye Res. 51, 156–186. doi: 10.1016/j.preteyeres.2015.08.001
Storch, U., Forst, A. L., Pardatscher, F., Erdogmus, S., Philipp, M., Gregoritza, M., et al. (2017). Dynamic NHERF interaction with TRPC4/5 proteins is required for channel gating by diacylglycerol. Proc. Natl. Acad. Sci. USA 114, E37–e46. doi: 10.1073/pnas.1612263114
Strotmann, R., Schultz, G., and Plant, T. D. (2003). Ca2+−dependent potentiation of the nonselective cation channel TRPV4 is mediated by a C-terminal calmodulin binding site. J. Biol. Chem. 278, 26541–26549. doi: 10.1074/jbc.M302590200
Sumioka, T., Okada, Y., Reinach, P. S., Shirai, K., Miyajima, M., Yamanaka, O., et al. (2014). Impairment of corneal epithelial wound healing in a TRPV1-deficient mouse. Invest. Ophthalmol. Vis. Sci. 55, 3295–3302. doi: 10.1167/iovs.13-13077
Suo, Y., Wang, Z., Zubcevic, L., Hsu, A. L., He, Q., Borgnia, M. J., et al. (2020). Structural insights into electrophile irritant sensing by the human TRPA1 channel. Neuron 105, 882–894.e5. doi: 10.1016/j.neuron.2019.11.023
Sura, L., Zíma, V., Marsakova, L., Hynkova, A., Barvík, I., and Vlachova, V. (2012). C-terminal acidic cluster is involved in Ca2+−induced regulation of human transient receptor potential ankyrin 1 channel. J. Biol. Chem. 287, 18067–18077. doi: 10.1074/jbc.M112.341859
Syed Mortadza, S. A., Wang, L., Li, D., and Jiang, L. H. (2015). TRPM2 channel-mediated ROS-sensitive ca(2+) signaling mechanisms in immune cells. Front. Immunol. 6:407. doi: 10.3389/fimmu.2015.00407
Talavera, K., Gees, M., Karashima, Y., Meseguer, V. M., Vanoirbeek, J. A., Damann, N., et al. (2009). Nicotine activates the chemosensory cation channel TRPA1. Nat. Neurosci. 12, 1293–1299. doi: 10.1038/nn.2379
Talavera, K., Startek, J. B., Alvarez-Collazo, J., Boonen, B., Alpizar, Y. A., Sanchez, A., et al. (2020). Mammalian transient receptor potential TRPA1 channels: from structure to disease. Physiol. Rev. 100, 725–803. doi: 10.1152/physrev.00005.2019
Talavera, K., Yasumatsu, K., Voets, T., Droogmans, G., Shigemura, N., Ninomiya, Y., et al. (2005). Heat activation of TRPM5 underlies thermal sensitivity of sweet taste. Nature 438, 1022–1025. doi: 10.1038/nature04248
Tao, J. X., Zhou, W. C., and Zhu, X. G. (2019). Mitochondria as potential targets and initiators of the blue Light Hazard to the retina. Oxidative Med. Cell. Longev. 2019, 6435364–6435320. doi: 10.1155/2019/6435364
Tóth, B., Iordanov, I., and Csanády, L. (2014). Putative chanzyme activity of TRPM2 cation channel is unrelated to pore gating. Proc. Natl. Acad. Sci. USA 111, 16949–16954. doi: 10.1073/pnas.1412449111
Trevisani, M., Siemens, J., Materazzi, S., Bautista, D. M., Nassini, R., Campi, B., et al. (2007). 4-Hydroxynonenal, an endogenous aldehyde, causes pain and neurogenic inflammation through activation of the irritant receptor TRPA1. Proc. Natl. Acad. Sci. USA 104, 13519–13524. doi: 10.1073/pnas.0705923104
Vangeel, L., and Voets, T. (2019). Transient receptor potential channels and calcium signaling. Cold Spring Harb. Perspect. Biol. 11:a035048. doi: 10.1101/cshperspect.a035048
Venkatachalam, K., and Montell, C. (2007). TRP channels. Annu. Rev. Biochem. 76, 387–417. doi: 10.1146/annurev.biochem.75.103004.142819
Venkatachalam, K., Wong, C. O., and Zhu, M. X. (2015). The role of TRPMLs in endolysosomal trafficking and function. Cell Calcium 58, 48–56. doi: 10.1016/j.ceca.2014.10.008
Venugopal, B., Browning, M. F., Curcio-Morelli, C., Varro, A., Michaud, N., Nanthakumar, N., et al. (2007). Neurologic, gastric, and opthalmologic pathologies in a murine model of mucolipidosis type IV. Am. J. Hum. Genet. 81, 1070–1083. doi: 10.1086/521954
Voets, T., Prenen, J., Fleig, A., Vennekens, R., Watanabe, H., Hoenderop, J. G., et al. (2001). CaT1 and the calcium release-activated calcium channel manifest distinct pore properties. J. Biol. Chem. 276, 47767–47770. doi: 10.1074/jbc.C100607200
Vogt-Eisele, A. K., Weber, K., Sherkheli, M. A., Vielhaber, G., Panten, J., Gisselmann, G., et al. (2007). Monoterpenoid agonists of TRPV3. Br. J. Pharmacol. 151, 530–540. doi: 10.1038/sj.bjp.0707245
Vriens, J., Owsianik, G., Hofmann, T., Philipp, S. E., Stab, J., Chen, X., et al. (2011). TRPM3 is a nociceptor channel involved in the detection of noxious heat. Neuron 70, 482–494. doi: 10.1016/j.neuron.2011.02.051
Vriens, J., Watanabe, H., Janssens, A., Droogmans, G., Voets, T., and Nilius, B. (2004). Cell swelling, heat, and chemical agonists use distinct pathways for the activation of the cation channel TRPV4. Proc. Natl. Acad. Sci. USA 101, 396–401. doi: 10.1073/pnas.0303329101
Wang, Y. Y., Chang, R. B., Waters, H. N., McKemy, D. D., and Liman, E. R. (2008). The nociceptor ion channel TRPA1 is potentiated and inactivated by permeating calcium ions. J. Biol. Chem. 283, 32691–32703. doi: 10.1074/jbc.M803568200
Wang, H., Cheng, X., Tian, J., Xiao, Y., Tian, T., Xu, F., et al. (2020). TRPC channels: structure, function, regulation and recent advances in small molecular probes. Pharmacol. Ther. 209:107497. doi: 10.1016/j.pharmthera.2020.107497
Wang, W., and Lo, A. C. Y. (2018). Diabetic retinopathy: pathophysiology and treatments. Int. J. Mol. Sci. 19:1816. doi: 10.3390/ijms19061816
Wang, J., Shanmugam, A., Markand, S., Zorrilla, E., Ganapathy, V., and Smith, S. B. (2015). Sigma 1 receptor regulates the oxidative stress response in primary retinal Müller glial cells via NRF2 signaling and system xc(−), the Na(+)-independent glutamate-cystine exchanger. Free Radic. Biol. Med. 86, 25–36. doi: 10.1016/j.freeradbiomed.2015.04.009
Wang, X., Teng, L., Li, A., Ge, J., Laties, A. M., and Zhang, X. (2010). TRPC6 channel protects retinal ganglion cells in a rat model of retinal ischemia/reperfusion-induced cell death. Invest. Ophthalmol. Vis. Sci. 51, 5751–5758. doi: 10.1167/iovs.10-5451
Wang, W., Zhang, X., Gao, Q., and Xu, H. (2014). TRPML1: an ion channel in the lysosome. Handb. Exp. Pharmacol. 222, 631–645. doi: 10.1007/978-3-642-54215-2_24
Warren, E. J., Allen, C. N., Brown, R. L., and Robinson, D. W. (2006). The light-activated signaling pathway in SCN-projecting rat retinal ganglion cells. Eur. J. Neurosci. 23, 2477–2487. doi: 10.1111/j.1460-9568.2006.04777.x
Wässle, H. (2004). Parallel processing in the mammalian retina. Nat. Rev. Neurosci. 5, 747–757. doi: 10.1038/nrn1497
Webster, C. M., Tworig, J., Caval-Holme, F., Morgans, C. W., and Feller, M. B. (2020). The impact of steroid activation of TRPM3 on spontaneous activity in the developing retina. eNeuro 7, ENEURO.0175–ENEU19.2020. doi: 10.1523/eneuro.0175-19.2020
Wegierski, T., Steffl, D., Kopp, C., Tauber, R., Buchholz, B., Nitschke, R., et al. (2009). TRPP2 channels regulate apoptosis through the Ca2+ concentration in the endoplasmic reticulum. EMBO J. 28, 490–499. doi: 10.1038/emboj.2008.307
Wehrhahn, J., Kraft, R., Harteneck, C., and Hauschildt, S. (2010). Transient receptor potential melastatin 2 is required for lipopolysaccharide-induced cytokine production in human monocytes. J. Immunol. 184, 2386–2393. doi: 10.4049/jimmunol.0902474
Weitlauf, C., Ward, N. J., Lambert, W. S., Sidorova, T. N., Ho, K. W., Sappington, R. M., et al. (2014). Short-term increases in transient receptor potential vanilloid-1 mediate stress-induced enhancement of neuronal excitation. J. Neurosci. 34, 15369–15381. doi: 10.1523/jneurosci.3424-14.2014
Wiederholt, M., Thieme, H., and Stumpff, F. (2000). The regulation of trabecular meshwork and ciliary muscle contractility. Prog. Retin. Eye Res. 19, 271–295. doi: 10.1016/s1350-9462(99)00015-4
Wimmers, S., and Strauss, O. (2007). Basal calcium entry in retinal pigment epithelial cells is mediated by TRPC channels. Invest. Ophthalmol. Vis. Sci. 48, 5767–5772. doi: 10.1167/iovs.07-0412
Wong, K. K., Banham, A. H., Yaacob, N. S., and Nur Husna, S. M. (2019). The oncogenic roles of TRPM ion channels in cancer. J. Cell. Physiol. 234, 14556–14573. doi: 10.1002/jcp.28168
Wong, W. L., Su, X., Li, X., Cheung, C. M., Klein, R., Cheng, C. Y., et al. (2014). Global prevalence of age-related macular degeneration and disease burden projection for 2020 and 2040: a systematic review and meta-analysis. Lancet Glob. Health 2, e106–e116. doi: 10.1016/s2214-109x(13)70145-1
Wong, C. O., and Yao, X. (2011). TRP channels in vascular endothelial cells. Adv. Exp. Med. Biol. 704, 759–780. doi: 10.1007/978-94-007-0265-3_40
Xu, H., Delling, M., Jun, J. C., and Clapham, D. E. (2006). Oregano, thyme and clove-derived flavors and skin sensitizers activate specific TRP channels. Nat. Neurosci. 9, 628–635. doi: 10.1038/nn1692
Xu, Y., Orlandi, C., Cao, Y., Yang, S., Choi, C. I., Pagadala, V., et al. (2016). The TRPM1 channel in ON-bipolar cells is gated by both the α and the βγ subunits of the G-protein go. Sci. Rep. 6:20940. doi: 10.1038/srep20940
Yan, Y., Wang, Y., Ding, J., Lu, L., Ke, G. J., and Dong, K. (2021). TRPML1 inhibited photoreceptor apoptosis and protected the retina by activation of autophagy in experimental retinal detachment. Ophthalmic Res. 64, 587–594. doi: 10.1159/000512104
Yang, X. J., Ling, S., Zhou, M. L., Deng, H. J., Qi, M., Liu, X. L., et al. (2022). Inhibition of TRPA1 attenuates oxidative stress-induced damage after traumatic brain injury via the ERK/AKT signaling pathway. Neuroscience 494, 51–68. doi: 10.1016/j.neuroscience.2022.02.003
Yuan, J. P., Kim, M. S., Zeng, W., Shin, D. M., Huang, G., Worley, P. F., et al. (2009). TRPC channels as STIM1-regulated SOCs. Channels 3, 221–225. doi: 10.4161/chan.3.4.9198
Zayats, V., Samad, A., Minofar, B., Roelofs, K. E., Stockner, T., and Ettrich, R. (2013). Regulation of the transient receptor potential channel TRPA1 by its N-terminal ankyrin repeat domain. J. Mol. Model. 19, 4689–4700. doi: 10.1007/s00894-012-1505-1
Zhang, Z. R., Chu, W. F., Song, B., Gooz, M., Zhang, J. N., Yu, C. J., et al. (2013). TRPP2 and TRPV4 form an EGF-activated calcium permeable channel at the apical membrane of renal collecting duct cells. PLoS One 8:e73424. doi: 10.1371/journal.pone.0073424
Zhang, X., Hu, M., Yang, Y., and Xu, H. (2018). Organellar TRP channels. Nat. Struct. Mol. Biol. 25, 1009–1018. doi: 10.1038/s41594-018-0148-z
Zhang, X., Li, X., and Xu, H. (2012). Phosphoinositide isoforms determine compartment-specific ion channel activity. Proc. Natl. Acad. Sci. USA 109, 11384–11389. doi: 10.1073/pnas.1202194109
Zhang, X., Mak, S., Li, L., Parra, A., Denlinger, B., Belmonte, C., et al. (2012). Direct inhibition of the cold-activated TRPM8 ion channel by Gαq. Nat. Cell Biol. 14, 851–858. doi: 10.1038/ncb2529
Zhao, J., Lin King, J. V., Paulsen, C. E., Cheng, Y., and Julius, D. (2020). Irritant-evoked activation and calcium modulation of the TRPA1 receptor. Nature 585, 141–145. doi: 10.1038/s41586-020-2480-9
Zheng, W., Cai, R., Hofmann, L., Nesin, V., Hu, Q., Long, W., et al. (2018). Direct binding between pre-S1 and TRP-like domains in TRPP channels mediates gating and functional regulation by PIP2. Cell Rep. 22, 1560–1573. doi: 10.1016/j.celrep.2018.01.042
Zhu, M. X. (2005). Multiple roles of calmodulin and other ca(2+)-binding proteins in the functional regulation of TRP channels. Pflugers Arch. 451, 105–115. doi: 10.1007/s00424-005-1427-1
Zhu, Y., Gao, M., Zhou, T., Xie, M., Mao, A., Feng, L., et al. (2019). The TRPC5 channel regulates angiogenesis and promotes recovery from ischemic injury in mice. J. Biol. Chem. 294, 28–37. doi: 10.1074/jbc.RA118.005392
Zhu, Q., Xiao, S., Hua, Z., Yang, D., Hu, M., Zhu, Y. T., et al. (2021). Near infrared (NIR) Light therapy of eye diseases: a review. Int. J. Med. Sci. 18, 109–119. doi: 10.7150/ijms.52980
Keywords: age-related macular degeneration, glaucoma, retinitis pigmentosa, diabetic retinopathy, TRPs channels
Citation: Nascimento THOd, Pereira-Figueiredo D, Veroneze L, Nascimento AA, De Logu F, Nassini R, Campello-Costa P, Faria-Melibeu AdC, Souza Monteiro de Araújo D and Calaza KC (2024) Functions of TRPs in retinal tissue in physiological and pathological conditions. Front. Mol. Neurosci. 17:1459083. doi: 10.3389/fnmol.2024.1459083
Edited by:
Hongzhen Hu, Icahn School of Medicine at Mount Sinai, United StatesReviewed by:
Stéphanie C. Thébault, National Autonomous University of Mexico, MexicoMasayuki X. Mori, University of Occupational and Environmental Health Japan, Japan
Copyright © 2024 Nascimento, Pereira-Figueiredo, Veroneze, Nascimento, De Logu, Nassini, Campello-Costa, Faria-Melibeu, Souza Monteiro de Araújo and Calaza. This is an open-access article distributed under the terms of the Creative Commons Attribution License (CC BY). The use, distribution or reproduction in other forums is permitted, provided the original author(s) and the copyright owner(s) are credited and that the original publication in this journal is cited, in accordance with accepted academic practice. No use, distribution or reproduction is permitted which does not comply with these terms.
*Correspondence: Daniel Souza Monteiro de Araújo, ZGFuaWVsLnNvdXphbW9udGVpcm9kZWFyYXVqb0B1bmlmaS5pdA==; ZGFuaWVsLnNvdXphbW9udGVpcm9kZWFyYXVqb0BnbWFpbC5jb20=