- 1Department of Surgery, University of Arizona, Tucson, AZ, United States
- 2Department of Neurobiology, University of Texas Medical Branch (UTMB) at Galvestion, Galvestion, TX, United States
- 3Sealy Institute for Drug Discovery (SIDD), University of Texas Medical Branch (UTMB) at Galvestion, Galvestion, TX, United States
Uncontrolled and chronic inflammatory states in the Central Nervous System (CNS) are the hallmark of neurodegenerative pathology and every injury or stroke-related insult. The key mediators of these neuroinflammatory states are glial cells known as microglia, the resident immune cell at the core of the inflammatory event, and astroglia, which encapsulate inflammatory insults in proteoglycan-rich scar tissue. Since the majority of neuroinflammation is exclusively based on the responses of said glia, their phenotypes have been identified to be on an inflammatory spectrum encompassing developmental, homeostatic, and reparative behaviors as opposed to their ability to affect devastating cell death cascades and scar tissue formation. Recently, research groups have focused on peptide discovery to identify these phenotypes, find novel mechanisms, and mediate or re-engineer their actions. Peptides retain the diverse function of proteins but significantly reduce the activity dependence on delicate 3D structures. Several peptides targeting unique phenotypes of microglia and astroglia have been identified, along with several capable of mediating deleterious behaviors or promoting beneficial outcomes in the context of neuroinflammation. A comprehensive review of the peptides unique to microglia and astroglia will be provided along with their primary discovery methodologies, including top-down approaches using known biomolecules and naïve strategies using peptide and phage libraries.
1 Introduction
Neuroinflammation, inflammation centering in the central nervous system (CNS), is the core biological feature of every neural pathology be it insult or injury, including spinal cord or traumatic brain injury (SCI/TBI), and neurodegenerative disorder, including but not limited to Alzheimer's disease (AD), Parkinson's disease (PD), Huntington's disease (HD), amyotrophic lateral sclerosis (ALS), and multiple sclerosis (MS). The key arbitrators in these inflammatory events are neuroglia, known as microglia, the singular immune cells, and astrocytes, the support cell that builds the scar tissue known as gliosis (Figure 1). Microglia are highly mobile surveyors of CNS tissues with archetypical ramified morphologies that are involved in developmental (Mehl et al., 2022; Hattori, 2023), homeostatic (Li and Barres, 2018; Mordelt and de Witte, 2023), and reparative roles (Lloyd et al., 2019). This is constrasted with their roles in cell death cascades and immunity, where they behave similarly to macrophages with M1 and M2 phenotypes, representing polar ends of the pro and anti-inflammatory spetrum (Yunna et al., 2020). Astrocytes have comparable phenotypical roles in support and maintenance (Liddelow and Barres, 2017; Liddelow et al., 2020; Garland et al., 2022), including synaptic plasticity and gliotransmission (Koyama, 2015), as well as neuro and myelin protection (Burda et al., 2016; Zhou et al., 2020). As microglia react to a potent pro-inflammatory injury/neurodegenerative event, they initiate an intense necrotic cascade and recruit astrocytes to generate a proteoglycan-rich network, or gliotic scar, effectively quarantining the cascade and blocking meaningful axonal regrowth (Gao et al., 2013). Newer phenotypes are emerging, indicative of priming due to chronic inflammation, which is considered to be associated with neuropsychiatric and neurodegenerative disorders (Perry and Holmes, 2014). This allows for the identification of microglia unique to a broad spectrum of clinical issues, such as the gut-brain axis (Huang et al., 2023), neurodegenerative reactivities (Kang et al., 2018; Prater et al., 2023), and substance abuse-related cell behavior (Lacagnina et al., 2017; Melbourne et al., 2021). Astrocytes often work in subsequent tandem with microglia being first responders, often generating shared phenotypes under inflammatory and support conditions, and as such, likely have complimentary roles in these novel phenotypes. It has become impactful to understand the unique molecular patterns of these cells under their various states toward developing disease-specific biomarkers and custom therapeutics.
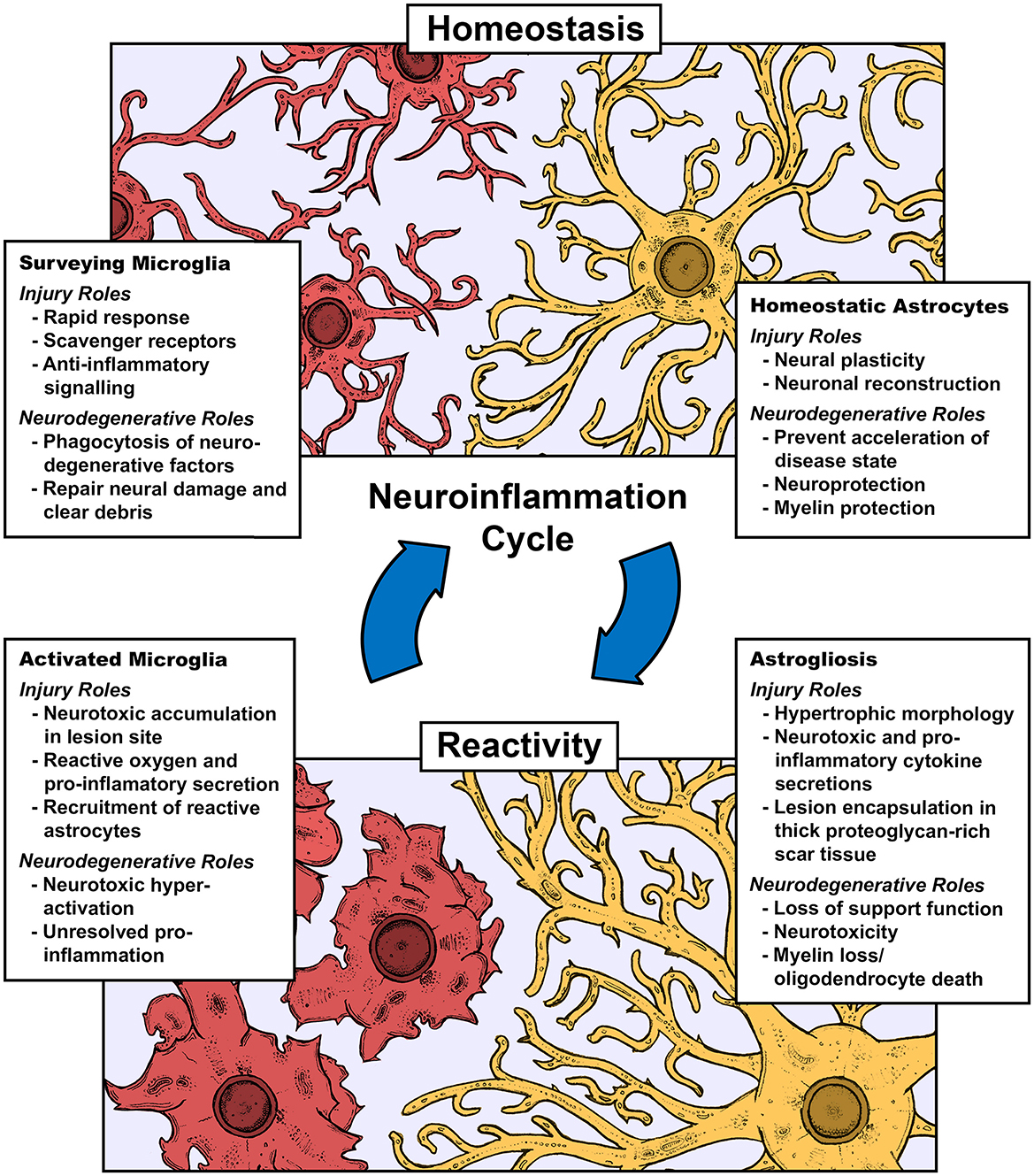
Figure 1. Role of microglia and astrocytes in the cycle of neuroinflammation defining key aspects of their roles under homeostasis, injury, and neurodegenerative prgression.
Many new tools and methods are emerging to characterize and facilitate the unique changes in cellular paradigms, of which peptides are rapidly becoming popular (Wang et al., 2022). With respect to targeting and mediating microglia and astroglia in phenotypically complex neuropathologies and pathophysiologies, peptide-based approaches are valid strategies. Retaining the diverse function of their protein counterparts, small protein fragments, or peptides does not require delicate 3D structures to function accordingly. As such, they have several advantages. They are robust in production methods, yields, and storage methods. Countless chemistries are available for unique modifications technically challenging or impossible with cellular translation and post-translation, which could include lipid/glycolic additions (Kowalczyk et al., 2017; Crijns et al., 2021), branching (Tam and Zavala, 1989; Elter et al., 2024), cyclization (Chung et al., 2017), organometallic additions (Reginato and Taddei, 2002), photo-labile/sensitive groups (Glatthar and Giese, 2000; Mikkelsen et al., 2018), isotopes (Zheng et al., 2016), and non-natural amino acids (Castro et al., 2023). Several biopanning and discovery tools make use of their small sequence lengths, such as display techniques and libraries (Wu et al., 2016). Peptides typically have lower computational requirements for molecular and drug discovery simulations (Rodrigues et al., 2022). Solid phase chemistry allows precise planning and the incorporation into higher order bioengineering strategies (bioconjugates, scaffolds, and drug delivery) through “lock and key” chemistries, such as click (Li et al., 2013). Although small and short in sequence, peptides can still confer secondary structures, high specificity, and potent cellular responses.
Herein, we summarize and report these strategies and functional studies on peptides effective at interacting with neuroglia. Families of neuropeptides or hormonal peptides are known to have systemic and cell-specific responses across the entire spectrum of mammalian biology (Hook et al., 2008; Burbach, 2011). Endogenously secreted in the CNS, a large family of neuropeptides have been characterized to affect a significant functional response from microglia and astrocytes, including neuroinflammatory mediation and gliotransmission (Carniglia et al., 2016). Although a few peptides have been discovered in studies for glial biology, many glial-related peptides have been discovered in extracellular matrices (ECMs) (de Castro Brás and Frangogiannis, 2020) and growth factor sources (Sporn and Roberts, 1988), such as cell adhesion molecules and transforming growth factors. More recently, system-wide immune biology and bioengineering have become clinically meaningful, which has led to a focus on inflammatory mediating peptides (La Manna et al., 2018a). Peptides are being engineered and designed to regulate inflammatory cells, including but not limited to monocytes, T cells, and organ-specific macrophages. As microglia emulate many of the pathways of immune cells, these peptides could be an excellent source for the glial researcher's toolbox.
Given that many of these peptides have been discovered indirectly, they are expected to confer limited specificity to glia subtypes and phenotypes. Using naïve blindfolded biopanning techniques, such as phage display and peptide libraries, a few studies have yielded microglia or astrocyte-specific peptides. Bacteriophages can display randomized peptide sequences on their coat at a specified length, which generates libraries with millions in sequence diversity. Further, polar M1/M2 or activated/ramified specific peptides have been discovered for the first time (Terashima et al., 2018; Koss et al., 2023). Although these peptides may have produced little to no activity beyond binding, the potential for bio-imaging and cytometric assessment is excellent. This review discusses these strategies and systematically lists the peptide studies.
2 Neuropeptides
One of the most intuitive sources of glial-interacial peptides are those native to the CNS. Neuropeptides are the most extensive family of molecules involved in signaling within the CNS. The hallmark characteristics of neuropeptides are their involvement in the biosynthesis and expression of genes within neurons and their role in chemical communication via regulated storage and release from secretory pathways (Hook et al., 2008). Additionally, neuropeptides are directly involved in the modulation and mediation of various neural functions through their interactions with neural receptors (Burbach, 2011). In their review, Carniglia et al. (2016) comprehensively characterized a variety of neuropeptides and their functional relation to microglia. Below is a summary of these peptides, with an added emphasis on their function in astrocytes and additional novel peptides. All neuropeptides with known interactions with microglia, astrocytes, and their receptors are fully summarized in Table 1.
2.1 Pro-opiomelanocortin peptides
Pro-opiomelanocortin (POMC) peptides are a family of precursor proteins with various functions derived from anterior and intermediate lobes of the pituitary and some neurons in the arcuate nucleus within the hypothalamus (Harno et al., 2018). After post-translational cleavage, its derivatives include peptide hormones known as melanocortins, such as Adrenocorticotropin or adrenocorticotropic hormone (ACTH), α, β, γ-Melanocyte-Stimulating Hormones (MSH), β & γ Lipotropin, and β-Endorphins (Cawley et al., 2016). These peptides have diverse functions, including involvement in caloric intake and energy expenditure, learning and memory, and sexual behaviors (Wikberg et al., 2000). Additionally, melanocortins are tightly associated with inflammatory processes on immune cells such as macrophages, monocytes, and lymphocytes in both the central and peripheral nervous systems (Catania et al., 2004). Although the involvement of melanocortins with immune cells has been characterized, their effects on microglia are not widely understood, especially in the context of β and γ-MSH and β-Endorphins (Carniglia et al., 2017).
Bettenworth et al. used an α-MSH C-terminus fragment tripeptide K(d)PT in their in vitro/in vivo interleukin 10 (IL10) deficient colitis mice models to note a reduced severity in inflammation and improved transepithelial electrical resistance after interferon-gamma (IFNγ) and tumor necrosis factor-alpha (TNFα) activation (Bettenworth et al., 2011). α-MSH peptides also have a profound effect on microglia through the inhibition of toll-like receptors 2 and 4 (TLR2 and TLR4) (Carniglia et al., 2016) and the attenuation of amyloid beta (Aβ) activation (nitric oxides, TNFα, and IL6) (Galimberti et al., 1999; Lindberg et al., 2005). α-MSH has been shown to inhibit the CNS production of TNFα in a mouse-based model of neuroinflammation after lipopolysaccharide (LPS) was injected (Rajora et al., 1997). Setmelanotide, a novel melanocortin-4 receptor (MC4R) agonist, was shown to attenuate a reactive astrocyte phenotype in an in-vitro study on active MS lesions through the induction of IL6 and IL11. MC4R is abundant throughout the CNS, especially within astrocytes (Kamermans et al., 2019). Hippocampal rat astrocytes were found to have doubled vascular endothelial growth factor (VEGF) expression 1 h after exposure to α-MSH, which is suggestive of neuroprotective qualities of the peptide related to the subsequent release of neurotrophic growth factors (Dubynina et al., 2009). α-MSH has also been shown to be neuroprotective of neurons and glial cells through a PPAR-γ and brain-derived neurotrophic factor (BDNF) mediated mechanism in the striatum of rats exposed to 3-nitropropionic acid (3-NP), which induces the degeneration of the striatum (Saba et al., 2019). In murine microglia, αMSH reduced TNFα, nitric oxide, and IL6 production and reduced TNFα in human astrocytes (Lipton et al., 1998).
ACTH is a pituitary hormone stimulated by neuropeptides, which bind to specific G-protein coupled receptors (GPCRs), or corticotropin-releasing hormone receptors, and are crucial in regulating cortisol and stress (Lim and Khoo, 2000). Neuropeptides in this class include corticotrophin-releasing factor (CRF) and Urocortin I (UROC), which have a more complex effect on glial-mediated inflammation. CRF can activate both microglia and astrocytes related to neuropsychiatric depression (Jiang et al., 2019), while UROC has an inflammatory-mediated neuroprotective effect on hippocampal neurons (Pedersen et al., 2002). Thymopentin (TP-5) is a small peptide secreted by the thymic system known for its immunomodulatory effects on T cells (Fan et al., 2006). It can also enhance levels of ACTHs and endorphins (Malaise et al., 1987). TP-5 is capable of inhibiting LPS reactive microglia in the nod-like receptor protein 3 (NLRP3) and nuclear factor kappa B (NFκB) pathways, facilitating a neuroprotective outcome (Peng et al., 2023).
2.2 Opioid peptides
Some of the most potent neuropeptides are opioids that target respective opioid receptors (OPs), which include the μ (MOP), δ (DOP), κ (KOP), and nociceptin/orphanin FQ (NOP) classes (Henry et al., 2017). These GPCRs are expressed amongst a wide distribution throughout the central and peripheral nervous systems and multiple organ systems. The enkephalins play a role in analgesia, the stress response, peristalsis, the cardiovascular system, and neuroprotection, amongst many other functions (Hughes et al., 1975). In the CNS, these receptors are responsible for pain mediation via neuronal neurotransmitter release and postsynaptic hyperpolarization and are also expressed by both microglia and astrocytes (Eriksson et al., 1993; Liang et al., 2014; Gavioli et al., 2015; Corkrum et al., 2019; Maduna et al., 2019; Machelska and Celik, 2020; Reiss et al., 2021; Xu et al., 2023).
2.2.1 Endogenous opioid enkephalins
Endogenous opioid enkephalins (ENK) are peptide derivatives of the endorphin family that signal through various OPs, including the MOP peptide receptor and the DOP opioid peptide receptor (Gupta et al., 2021). Enkephalins are pentapeptides derived from the precursor proenkephalin (PENK) and are subdivided into two groups based on the amino acids present on their carboxy-terminal, either leucine or methionine (Cullen and Cascella, 2024). These are the leu-enkephalins (LENK) and the met-enkephalins (MENK) (Reiner, 1987). Qin et al. demonstrated that pre-treatment of neuron-glia cultures with LENK had a neuroprotective effect on dopaminergic neurons against LPS-induced neurotoxicity via attenuation of the reactive oxygen species (ROS) mediated amplification of TNFα in microglia as well as the inhibition of microglial NADPH oxidase mediated damage (Qin et al., 2005). LENK also boosted both cell viability and induction of autophagy in astrocytes exposed to oxygen-glucose deprivation, exhibiting a cryoprotective effect in the central nervous system (Wang et al., 2019). Stiene-Martin and Hauser (1990) demonstrated a total decrease in cell numbers of astrocytes of mixed glial cultures treated with MENK as compared to basal cell treatments and those treated with naloxone, an opioid-receptor antagonist, indicating MENK-mediated suppression of astrocyte growth in culture. MENK treatment not only induced microglia to the M1 phenotype via significantly increased levels of M1-associated genes including TNFα, IL12, and cluster differentiation 40 and 86 (CD40 and CD86) but also boosted microglial cytotoxicity toward glioblastoma cells, indicating the peptide's functional relevance regarding the polarization of microglial phenotypes as well as its tumoricidal properties (Xu et al., 2016).
Although LENK can have a degree of specificity to DOP, these peptides are small and may suffer from poor bioavailability (Mosberg et al., 2014). The desire to generate longer-lasting PENK peptides with unique specificities led to the generation of DADLE, capable of binding to DOP and the one subset of MOP, as well as DAMGO and DPDPE, which are specific to MOP and DOP, respectively. Like other DOP agonists, DADLE and DPDPE produce an anti-inflammatory response in microglia and generate a cyto/neuroprotective response from astrocytes (Liang et al., 2014; Shrivastava et al., 2017; Wang et al., 2019; Mali and Novotny, 2022). DAMGO, like other MOPs, stimulates a pro-inflammatory outcome with higher rates of glutamate-mediated gliotransmission (Corkrum et al., 2019; Cuitavi et al., 2023). The amphibian-derived-PENK dermorphin and its derivative DALDA are also potent targets for the MOPs. However, they have demonstrated potent inhibition of neuroinflammatory cascades and oxide-nitrosative stress through down-regulation of Trp ion channels in a chemotherapy-induced neuropathic pain rodent model (Gadepalli et al., 2023). Other noteworthy PENKs have been discovered from amphibians, including dermenkephalin or deltorphins, which include deltorphin A, I, and II. As their name suggests, their excellent affinity to DOPs has had noteworthy effects on the glial mitigating of neuropathic cold allodynia (Mika et al., 2014; Reiss et al., 2021). Astrocytes were the most affected by the deltorphins as Mika et al. showed that DOP expression in primary microglia was lacking, suggesting that DOP neuroinflammatory pain mediation might require the cooperative effort of mixed glial interactions. These peptides have rapidly become stand-ins for OP specificity studies. How these peptides interact with OPs may necessitate further study, as the expected mediation of inflammation is not always clear.
2.2.2 Prodynorphins
The prodynorphins (PDYN) are also a class of PENK-proenkaphalin B opioid polypeptides. Of these, endomorphin 1 and 2 are tetrapeptides capable of having an inhibitory function on MOPs, which leads to the regulation of neuroinflammatory functions in microglia, including phagocytosis, chemotaxis, and the secretion of superoxide anions (Azuma et al., 2001; Lu et al., 2022). Dynorphins A and B are PDYNs uniquely specific to KOPs and capable of mediating neuroinflammation and pain through microglia and astrocytes (Liu et al., 2020; Lee et al., 2022). PDYNs also include endorphins, of which the MOP specific β-Endorphin inhibits microglial neuroinflammation comparable to IL10 (Mao et al., 2019; Ma et al., 2021; Belo et al., 2023), which is considered one of the more potent inflammatory regulating cytokines (Carlini et al., 2023). The dynorphin peptide α-neoendorphin can bind to MOP, DOP, and KOP but has only been observed to regulate pain when secreted by spinal astrocytes (Wahlert et al., 2013).
2.2.3 Prepronociceptins
The nociceptin receptors (NOPs) are involved in emotional and pain regulation and have also been discovered in microglia and astrocytes (Brown and Cox, 2006; Meyer et al., 2017). The opioids specific to MOP, DOP, and KOP are usually not effective on NOPs. The primary agonists of NOPs are prepronociceptin (PNOC) peptides, with Nociceptin/ Orphanin (N/OFQ) being the natural peptide used in neuropeptide signaling. In astrocytes, N/OFQ regulates glutamate/aspartate homeostasis in maturation and development (Meyer et al., 2017). N/OFQ has also been shown to attenuate interleukin one beta (IL1β) in astrocytes and microglia due to either LPS agonism of TLR4 or traumatic injury (Mallimo and Kusnecov, 2013). The NOP does see an increase in LPS and PD models of microglia (Brown and Cox, 2006), suggesting there may be a natural inflammatory mediation due to this pathway. Although these receptors are prevalent in microglia, which are known to be involved in pain regulation, the role of NOPs in microglial pain mediation is still unclear (Machelska and Celik, 2020).
2.3 Bombesins
The bombesins (BB) are a class of neuropeptides that target the GPCRs called bombesin receptors (BBR1, 2, and 3), which owe their name to the first bombesin peptide discovered from the skin of the European fire-bellied toad Bombinabombina (LaPelusa and Jan, 2024). BB is known chiefly for its exogenous effects on appetite suppression in the CNS. However, gastrin release peptide (GRP) and neuromedins (NMB, NMC, and NMU) were later discovered to be the mammalian peptide of the bombesin family (Majumdar and Weber, 2012). GRP has a role in pain modulation and signaling for astrocytes related to chronic itch (Ji et al., 2019), which microglia can exacerbate (Liu et al., 2023). NMB has a role in astrocyte-mediated depolarization of CA1 pyramidal neurons by increasing the number/amplitude of small inhibitory postsynaptic currents (Lee et al., 1999). Mason et al. (2002) compared NMB to NMC, which was able to cause astrocytes to hyperpolarize (higher amplitude and frequency) with Ca2+ 32-fold higher in NMC. NMU, although abundantly secreted in the hippocampus, functionality might be linked to protection against neuroinflammation. Iwai et al. (2008) demonstrated the promotion of neuroprotection against mixed glial (astrocytes and microglia) LPS conditioning with increased BDNF secretions and sparing rodent memory loss.
2.4 Pancreatic peptides
Neuropeptide Y (NPY) belongs to the family of pancreatic peptides, which includes both peptide YY and pancreatic polypeptide (PP) (Holzer et al., 2012). PP and Peptide YY have been studied concerning the gut-brain axis and the regulation of appetite and obesity (Karra et al., 2009; Holzer et al., 2012). NPY has a wide distribution in both the PNS and CNS and has diverse functional involvement in feeding behaviors, blood pressure control, memory, anxiety, and circadian rhythms (Thorsell and Heilig, 2002). Ferreira et al. (2010) demonstrated NPY-mediated inhibition of NO, IL1β, and subsequent NFκB activation in N9 murine microglia exposed to LPS. NPY also exhibited protective effects toward N9 microglia and a reduction of CD11b immunoreactivity in hippocampal microglial cultures exposed to methamphetamines, suggesting a neuroprotective role of NPY in meth-induced microglial injury (Gonçalves et al., 2012). Li et al. (2014) found that in rat microglia exposed to LPS and co-treated with NPY, there was a reduction in LPS-induced increases in TNFα, IL1β, and N-methyl-D-aspartate (NMDA) current excitotoxicity via inhibition of microglial reactivity. NPY is also expressed in astrocytes, and Barnea et al. demonstrated an increase in both proNPY-mRNA and production of NPY in cultures exposed to IL1β, suggesting immune involvement of this peptide in astrocytic inflammatory pathways (Barnea et al., 2001).
Amylin is a peptide hormone that has co-secretion with insulin from the beta-cells of the pancreas (Ludvik et al., 1997). Functionally, its primary role is a glucoregulatory hormone, with significant importance in energy/glucose metabolism, inhibition of glucagon secretion, satiety, and delayed gastric emptying (Hay et al., 2015). Amylin easily traverses the blood-brain barrier (BBB) and plays a part in a variety of functions in the CNS, including a role in appetite inhibition, the relaxation of cerebrovascular structures, and its potential for neural regenerative capabilities (Westfall and Curfman-Falvey, 1995; Trevaskis et al., 2010; Roth, 2013). In a transgenic model of AD, administration of an amylin antagonist reduced inflammatory microglial markers, including ionized calcium binding adaptor molecule 1 (Iba1) and CD68, caspase-1, TNFα, and IL1β, and a concordant reduction in Aβ plaque burden and size compared to controls, implicating amylin in the pathogenesis of AD (Fu et al., 2017). Wang et al. demonstrated that peripheral amylin treatment reduced levels of the inflammatory markers CD68 and Iba1—two markers directly correlated with the levels of neurofibrillary tangles in AD's models—via amylin's effect on CD68 in microglial cells (Wang E. et al., 2017).
Additionally, administration of an amylin antagonist improved learning and spatial memory deficits in AD mice, with associated suppression in microglial activation and neuroinflammation (Soudy et al., 2017). Amylin was also shown to produce effects in astrocytes that mimic those of Aβ proteins, such as the inhibition of astrocytic redox activity and the induction of reactive gliosis (Abe et al., 1997). Collectively, these studies indicate amylin and its resultant receptor cascades as another promising therapeutic target for future AD treatments.
2.5 Intestinal peptides
Vasoactive intestinal peptide (VIP) is a 28 amino acid peptide from the secretin and glucagon family. Initially, it was found to have vasodilatory effects, and it has a wide distribution in the CNS and peripheral nervous system (PNS), cardiopulmonary, reproductive, and digestive systems (Iwasaki et al., 2019). VIP also shares 68% sequence similarity with neuropeptide pituitary adenylate cyclase-activating polypeptide, also known as PACAP (Moody et al., 2011). PACAP is a 38-amino acid peptide mainly found in the hypothalamus and has been used as a biomarker for TBI (Toth et al., 2020). These peptides act as ligands for many of the same GPCRs, including PAC1, VPAC1, and VPAC2 (Carniglia et al., 2017). Both PAC1 and VPAC1 have been detected in rat microglia, and VIP and PACAP reduced TNFα mRNA production in cultured microglia exposed to LPS in a cyclic adenosine monophosphate (cAMP) dependent fashion (Kim et al., 2000). Delgado et al. have examined these peptides in a variety of studies. They found that VIP and PACAP reduced pro-inflammatory cytokine production of nitric oxide (NO), TNFα, IL1β, and IL6 in LPS-exposed microglia (Delgado et al., 2003). In a PD study with mice exposed to neurotoxic 1-methyl-4-phenyl-1,2,3,6-tetrahydropyridine (MPTP), VIP treatment produced a significant reduction in the loss of dopaminergic neurons in the substantia nigra. It reduced MPTP-induced microglial activation and release of inflammatory cytokines. VIP also reduced Aβ plaque-induced neurodegeneration in activated microglia by p38 mitogen-activated protein kinase (MAPK), p42/p44 MAPK, and NFkB signal blockade (Delgado et al., 2008).
PACAP-27, a 27-amino acid-based derivative, reduced neurodegenerative in CA3 pyramidal neurons via reduced microglial activation and boosted learning/memory performance in aged mice treated with neurotoxic prostaglandin (Avila et al., 2019). PACAP-38 was found to modulate the inflammatory response by reprogramming microglia into the M2 phenotype in a degenerative retinal animal model (Denes et al., 2023). Additionally, PACAP-38 reduced neuronal damage via attenuation of IL6 and reduction of mitochondrial cytochrome c release in an ischemic mouse model (Ohtaki et al., 2006). VIP and PACAP induced increased activity-dependent neuroprotective protein (ADNP) in cortical astrocytes from newborn rats in a VPAC2-dependent mechanism (Zusev and Gozes, 2004). In astrocytes exposed to PACAP, the maximal velocity of glutamate uptake was increased, with a similar effect also produced on exposure to higher concentrations of VIP, suggesting these two peptides are implicated in glutamate-mediated neuropsychological disorders. A comprehensive review of the role of both PACAP and VIP regarding the functions of astrocytes can be found in the work of Masmoudi-Kouki et al. with implications evidence in glial cell activity and proliferation, glycogen metabolism, cell plasticity and release of neuroprotective factors (Masmoudi-Kouki et al., 2007).
Glucagon-like peptide 2 (GLP-2) is another peptide derivative of the proglucagon gene. It is a member of the glucagon-like peptide family of intestinal hormones known for their diverse roles in intestinal function and growth, gastric motility, and acid secretion, and regulation of appetite and energy homeostasis, amongst other functions (Burrin et al., 2001). These actions are mediated via the GLP-2 receptor (GLP-2R) in a GPCR-mediated cascade (Drucker, 2001, p. 2). The GLP-2 receptor has been localized within several regions of the mouse and rat CNS, including but not limited to the cerebellum, amygdala, cerebral cortex, and hippocampus (Lovshin et al., 2001). Velázquez et al. (2009) demonstrated a synergistic effect of GLP-2 on the proliferation of rat astrocytes, DNA synthesis, and astrocyte density, suggesting a regenerative role for GLP-2 in glial cells of the CNS. In a PD mouse model, a GLP-2 analog reduced the inflammatory response by modulating microglial activation and reducing levels of inflammatory cytokines. It improved the mitochondrial dysfunction induced by MPTP within the substantia nigra (Zhang et al., 2021).
Exendin-4 (Ex-4) is a GLP-1R agonist that is found within the saliva of the Gila monster lizard. GLP-1 affects insulin secretion, the inhibition of food intake, gastric emptying, and glucagon secretion (Ding et al., 2006). The effect of GLP-1 is limited due to rapid breakdown by peptidases, limiting its clinical applications. Ex-4 is a more stable GLP-1 analog used for treating type II diabetes and has also been found to cross the blood-brain barrier and exhibit neuroprotective effects within the CNS. In an MPTP mouse model of PD, treatment of Ex-4 systemically prevented microglial activation induced by MPTP and release of pro-inflammatory mediators such as TNFα and IL-1β, suggesting Ex-4's role as a potential therapeutic in neurodegenerative diseases (Kim et al., 2009). In astrocyte cultures exposed to oxygen-glucose deprivation, treatment with Ex-4 reduced levels of inflammatory mediators derived from astrocytes in ischemic brain tissue after middle cerebral artery occlusion and mitigated the resultant breakdown of the BBB (Shan et al., 2019).
Uroguanylin—a member of the natriuretic family of guanylin peptides—is an intestinal peptide hormone most prominently found in the epithelium of the gastrointestinal tract with a diverse impact on physiological processes, including its role in digestive fluid secretions and renal salt balance (Forte, 2004a). Uroguanylin activates guanylate cyclase C (GC-C), particularly in the intestine, where GC-C is an enterotoxin receptor. GC-C has also been characterized in other non-intestinal tissues such as the kidneys, lungs, reproductive system, and the brain (Forte, 2004b). In the CNS, GC-C is localized within the dopaminergic neurons of the midbrain and ventral tegmental area. GC-C activation heightens glutamate and acetylcholine receptor-mediated excitatory responses, and GC-C knockout mice develop attention deficits and hyperactive behavior (Gong et al., 2011). Uroguanylin also has a GC-C-independent mechanism via modulation of Ca2+ in astrocytes by influencing their intracellular pH (Habek et al., 2021). This suggests that uroguanylin plays a role in Ca2+-mediated signaling pathways in astrocytes, such as regulating neuronal circuits (Guerra-Gomes et al., 2017).
2.6 Statins
Somatostatin (SST) is a neuropeptide of the statin family that exists in a cyclic form due to its disulfide bonds between cysteine residues and acts as an endocrine and exocrine inhibitor in systems, including the liver, lungs, pancreas, gastrointestinal tract, adrenals, and thyroid (O'Toole and Sharma, 2024). SST has two molecular formulations known as somatostatin 14 (SST-14) and somatostatin 28 (SST-28), based on the presence of 14 or 28 amino acids that are derivatives from proteolysis from a precursor molecule, pre-pro-SST (Barbieri et al., 2013). These molecules interact with five subtypes of GPCRs known as sst1-sst5. Not only is the presence of mRNA for several of these receptors—sst2, sst3, and sst4— characterized in primary cultured rat microglia, but SST is functionally active and inhibits microglial activation via reduced IL-3 and granulocyte-macrophage colony-stimulating factor (GM-CSF) signaling (Feindt et al., 1998). Bai et al. examined the effects of SST in a PD in which LPS was injected into the substantia nigra of rats. SST treatment reduced the LPS-induced loss of dopaminergic neurons in the substantia nigra due to suppressed activation of microglia and the NFkB pathway. Additionally, there was decreased production of TNFα, IL1β, ROS, and prostaglandin E2, known to be produced by active microglia in PD (Bai et al., 2015). SST was also found to boost the production of insulin-degrading-enzyme (IDE) in rat microglia and mouse BV2 cells. IDE is the main extracellular protease secreted by microglial cells to degrade Aβ plaques, suggesting that SST levels in microglia may be crucial to the pathogenesis of AD (Tundo et al., 2012). SST is commonly co-expressed with Aβ, although SST does not typically co-express with astrocytes despite sharing a similar spatial distribution in the CNS (Gonzalez-Rodriguez et al., 2021). In 2020, Hernandez et al. examined the effect of administering topical SST in a mouse model of diabetic retinal neurodegeneration. They found SST exhibited an anti-inflammatory effect by inhibiting the inflammatory M1 microglial response to an LPS trigger (Hernández et al., 2020). Likewise, Mazzeo et al. demonstrated a reduction of pro-inflammatory and pro-apoptotic mediators in human retinal pericytes exposed to microglial cells treated with LPS and SST vs. LPS alone (Mazzeo et al., 2017).
Despite its vast array of functions, somatostatin's clinical efficacy was reduced by shortcomings related to its short half-life, necessity for intravenous administration, and hypersecretion of hormones such as insulin, glucagon, and growth hormone after SST administration (Yuen and Samson, 2022). Due to this, SST analogs such as octreotide were developed. Octreotide is a cyclic SST analog with different receptor-binding characteristics. It has an improved half-life of 2-h compared to SST's half-life of 3 min and does not cause rebound hypersecretions of hormones. Feindt et al. demonstrated that octreotide inhibits microglial activation in their study alongside SST via reduced IL3 and GM-CSF signaling (Feindt et al., 1998).
Cortistatin is another cyclic neuropeptide belonging to the somatostatin family expressed in 14 and 17 amino-acid isomers known as cortistatin 14 (CST-14) and cortistatin 17 (CST-17). These two peptides share 11 amino acids with SST-14 and can bind the same SST receptor motifs with an affinity similar to that of SST (de Lecea, 2008). Despite shared homology with SST receptors, cortistatin has unique effects in the CNS that are different from SST, including its role in locomotor activity and slow-wave sleep induction, suggesting alternative signaling pathways distinct to CST (Carniglia et al., 2017). In addition, evidence suggests that CST plays a role in neuroinflammatory pathways. In the CNS, cortistatin-deficient mice were predisposed to weakening of the endothelium, disruption of tight junctions, BBB leakage, and dysregulation of immune activity that was reversible upon treatment with cortistatin in an in-vitro barrier model simulating an ischemic environment (Castillo-González et al., 2023). In a well-established model of PD based on exposure to the neurotoxic compound MPTP, cortistatin treatment improved locomotor activity and reduced glial activation in affected brain regions, reduced the production of inflammatory mediators, and boosted the production of neurotrophic factors in the striatum (Serrano-Martínez et al., 2024). These anti-inflammatory and neuroprotective properties of cortistatin indicate the peptide's potential as a novel therapeutic agent in treating PD.
2.7 Kinins
Tachykinins are a group of peptide hormones that have diverse expression throughout both the nervous and immune systems. The main mammalian tachykinins include substance P (SP), neurokinin A (NKA), neurokinin B (NKB), neuropeptide K (NPK), and hemokinin-1 (HK-1). The tachykinins signal utilizes three neurokinin receptor subtypes: NK-1R, NK-2R, and NK3-R (Steinhoff et al., 2014). Of the tachykinins, SP has been widely examined with many different pathophysiological effects, including roles in nociception, regulation of bone metabolism, and inflammatory bowel disease. Human microglial and astrocytic cells both express robust amounts of the NK-1R isoform, which is functionally augmented by SP in inflammatory and neurotoxic glial responses (Burmeister et al., 2017). SP has been shown to induce pro-inflammatory cytokines and stimulate immune cells in an NFkB-mediated manner (Johnson et al., 2017). In neurogenic inflammation, the SP receptor NK-1R has been found in human fetal microglia (Lai et al., 2000). Martin et al. (1993) examined that SP alone did not enhance IL1 or TNFα production in rat microglia but found that SP and LPS synergistically quadrupled the release of IL1β compared to LPS by itself, suggesting an implication for SP in inflammatory pathology within the CNS. More recently, Zhu et al. demonstrated that SP can induce the activation of microglia and the subsequent release of pro-inflammatory factors such as Il1 and TNFα in primary cultured microglia (Zhu et al., 2014). SP also has a role in microglial density in chemotaxis of the substantia nigra (Wang Q. et al., 2015). Additionally, SP potentiated increased class II major histocompatibility protein expression in the microglia of rats treated with interferon-gamma (McCluskey and Lampson, 2001). SP is found at a high concentration within the substantia nigra, suggesting that this peptide might be implicated in the pathology of PD, especially considering that SP potentiates the release of dopamine in the striatum. Block et al. examined that SP-activated nicotinamide adenine dinucleotide phosphate (NADPH) oxidase in microglial cells, subsequently producing excess intracellular ROS and extracellular superoxide that was neurotoxic to dopaminergic neurons in a microglia-dependent manner (Block et al., 2006). Inhibition of the NK-1R receptor attenuated both the microglial inflammatory response process and dopaminergic neurotoxicity induced by LPS-activated BV2 microglia, further suggesting the role of SP in PD (Jiang et al., 2020).
Hemokinin-1 (HK-1) is another member of the tachykinin family, named for its unique role in the hematopoiesis of B-lymphocytes (Zhang et al., 2000). Encoded by the Tac4 gene, HK-1 is the only tachykinin peptide primarily produced outside neural tissues. Sakai et al. discovered that the Tac4 tachykinin gene is predominantly expressed in primary cultures of microglia in comparison to the Tac1 gene that encodes SP. Tac4 mRNA expression in the microglial cells was also upregulated in response to LPS compared to the Tac1 gene, suggesting HK-1 may play a more prominent role in microglial activation in the CNS with SP in neuroinflammatory disorders relating to pathological microglial activation (Sakai et al., 2012). In a model of inflammatory orofacial pain within the trigeminal ganglia, Tac4 was upregulated in neurons and satellite glial cells, suggesting a role for HK-1 release in neuroglial interactions under inflammatory conditions within the CNS (Aczél et al., 2020).
Cholecystokinin (CCK) is a 33-amino acid long peptide hormone found abundantly within the gastrointestinal tract and the CNS. CCK is implicated in regulating nociception, feeding, and learning/memory by interacting with two G-protein coupled receptors known as CCK1 and CCK2 (Okonkwo et al., 2024). CCK has a variety of isoforms, including cholecystokinin octapeptide (CCK-8), which is abundantly expressed within the CNS (Rehfeld, 2021). CCK has been studied in the context of neurodegenerative diseases such as AD, with a relationship found between higher levels of CCK and reduced probability of mild cognitive impairment and AD (Plagman et al., 2019). Intestinal protein level expression of CCK was reduced significantly in a PD model, indicating a possible role of CCK in the gut-brain axis of PD pathology (Choi et al., 2021). In an aged mice model of delayed neurocognitive recovery, CCK-8 treatment reduced the activation of microglia and A1 reactive astrocytes and suppressed the expression of inflammatory mediators in the hippocampus (Chen et al., 2021). Müller et al. (1997) demonstrated that CCK-8 induced repetitively increased calcium signaling in rat and mouse hippocampal astrocytes, signifying astrocytes as a significant target for CCK in the CNS. CCK-8 also suppressed methamphetamine-induced microglial activation and production of both IL6 and TNFα both in vitro and in vivo (Gou et al., 2020).
Another peptide involved in the kinin system is known as bradykinin, which is a vasoactive peptide that is of particular importance in relation to inflammatory reactions and the regulation of blood pressure (Pirahanchi and Sharma, 2024). Functionally, bradykinin can increase vascular permeability and vasodilation of the gastrointestinal system, along with the urethra, uterus, and aorta. Bradykinin is also implicated in various pathophysiological conditions such as hereditary acquired angioedema, hypertension, allergic respiratory reactions, and AD, among others (Golias et al., 2007). These biological effects are mediated by specific GPCRs known as B1 and B2. In the CNS, bradykinin is released endogenously after injury or stroke. It can interact with bradykinin receptors on microglia to induce an anti-inflammatory cascade and subsequently produce a neuroprotective effect. Bradykinin has also been demonstrated to attract microglia to the site of a CNS lesion and boost microglial motility in vitro within mixed cultures of cerebrocortical cells of rats. This effect was reversed with a B1 receptor antagonist (Huisman et al., 2008). Liu et al. demonstrated that the bradykinin-mediated stimulus of B2 receptors induced increased Ca2+ signaling and generation of ROS in astrocytes. The increased ROS boosted the downstream release of glutamate from these astrocytes, which subsequently interacted with NMDA receptors and increased Ca2+ in the cytosol of neurons, indicating bradykinin's role in the crosstalk between astrocytes and neurons within the CNS (Liu et al., 2009). In BV2 microglial cells, a B1 receptor antagonist caused a significant increase in LPS-induced nitric oxide release nitric oxide synthase production, and TNFα release. Additionally, intranasal administration of the B1 receptor antagonist increased the amyloid plaque burden and accumulation of microglia in the cortex of mice in an AD model, indicating that bradykinin has a role in neuroinflammatory diseases (Asraf et al., 2017).
2.8 Galaninergic peptides
Galanin (GALA-2-11) is a 30-amino acid-long regulatory peptide with a diverse distribution within the central and peripheral nervous system that functions in a variety of physiological states, including feeding and gastrointestinal motility, nociception, learning/memory, and epileptic activities within the brain (Vrontakis, 2002). These functions are mediated by interactions with three GPCRs known as GAL1 − 3-R. GALA-2-11 was demonstrated to boost the induction of c-Fos, JunB, and Tis11 mRNA production within cultured astrocytes, indicating that there are functional GALA-2-11 receptors on neuroglial cells in the CNS (Priller et al., 1998). Koller et al. (2019) demonstrated that exposure to exogenous GALA-2-11 influenced the differentiation/polarization of macrophages by modulating the expression of chemokines and inflammatory cytokines such as TGF-β, IL-10, and IL-1Ra, most prominently in Type-1 macrophages. Additionally, the reduced mRNA of the Gal-1-receptor (GALR1) via null mutation or administration of galantine—a GALR1 antagonist—in a mouse model of status epilepticus was found to increase damage within the hippocampus, suggesting a role for the GAL1R as a potential therapeutic target in modulating cell death during epileptic insults within the CNS (Schauwecker, 2010).
2.9 Adrenal peptides
Adrenomedullin (AM) and calcitonin gene-related peptide (CGRP) are neuropeptides of the CGRP/calcitonin family. AM was initially isolated from the adrenal gland, has a significant vasodilatory effect, and plays vital regulatory roles in the cardiovascular, renal, and lymphatic systems (Schönauer et al., 2017). Over-expression of AM promotes astrocyte mediated neuroprotection in a cerebral ischemic stroke (Xia et al., 2004). CGRP and AM were demonstrated to reduce LPS-induced microglial activation in vitro and reduce pro-inflammatory mediators such as IL6, TNFα, and NO (Consonni et al., 2011). In a model of experimental autoimmune encephalomyelitis (EAE), ADM reduced the clinical severity of EAE, decreased the production of inflammatory mediators in microglia and astrocytes such as IL6, IL12, and TNFα, and boosted the expression of neuroprotective factors such as BDNF and activity-dependent neuroprotector protein (ADNP) (Pedreño et al., 2014). In a different model of chronic murine EAE, CGRP also reduced the clinical severity of disease and attenuated microglial activation (Sardi et al., 2014). Together, this evidence suggests the role of both AM and CGRP in the modulation of microglial activation in neuroinflammatory diseases. CGRP induces the production of both microglia and astrocytes at the transcription level, and increases the release of tissue plasminogen activator, implicated in the tissue remodeling process. This suggests a role for CGRP in glial activation during motor neuron injury (Reddington et al., 1995). Intrathecal administration of CGRP was also demonstrated to increase the number of astrocytes that act on receptors within these astrocytes and lead to H3K9 acetylation—associated with inflammatory gene expression, proliferation, and autophagy. Increased amounts of astrocytes with higher amounts of H3K9 acetylation are seen after nerve injuries, suggesting a role for CGRP in attenuating neuropathic pain in an astrocyte-mediated mechanism (Sun C. et al., 2021).
Intermedin—also known as adrenomedullin-2 (AM2)—is a peptide related to CGRP and adrenomedullin, with shared overlap and homology in various receptor activities (Roh et al., 2004). AM2 is primarily expressed in the gastrointestinal system, the pituitary gland, and the renal system. AM2 plays a significant role in water and electrolyte balance, systemic and pulmonary vasodilation, and cardiac contractility (Bell and McDermott, 2008; Cui et al., 2008). AM2 inhibited inflammation induced by LPS in BV2 microglial cells via the reduction of inflammatory mediators, including TNFα, IL1β, cyclooxygenase 2 (COX2), and inducible nitric oxide synthase (iNOS) (Sun et al., 2021). AM2 also exhibited an antioxidant effect within the hippocampus and modulated inflammation in BV2 microglial cells. It inhibited the nuclear translocation of inflammatory mediators, including NFκB p-65 and nuclear factor of kappa light polypeptide gene enhancer in B-cells inhibitor alpha (IκBα). Additionally, AM2 reduced the generation of ROS, indicating its neuroprotective properties (Lee et al., 2023). This collectively suggests the role of AM2 in neuroinflammatory diseases and indicates this peptide's potential as a future therapeutic target.
2.10 Adipose regulators
Leptin (LEP) is a peptide hormone mainly known for its involvement in energy and appetite homeostasis. It is produced predominantly in adipose tissues, with LEP expression varying with changes in nutritional states (Ramos-Lobo and Donato, 2017). LEP is too large of a molecule to cross the BBB and is transported centrally via a regulated transport system. However, some studies suggest that LEP can be produced in the CNS in areas such as the hypothalamus, cortex, and cerebellum, indicating there may be local, specific functions of this hormone (Morash et al., 1999). Both the short (LEPRa) and long (LEPRb) isoforms of the LEP receptor were detected in primary cultures of mouse microglia, and LEP treatment resulted in a dose-dependent increase in IL1β via a STAT3-dependent mechanism (Pinteaux et al., 2007). Leptin knockouts in mouse astrocytes resulted in mediated hypothalamic pSTAT3-related hypothatlamic gliosis and excacerbated diet-induce obesity (Wang Y. et al., 2015). Previously, pharmacologic blockage of microglial phagocytosis in obese mice reduced obesity-associated cognitive decline and dendritic spine loss (Cope et al., 2018). Ma et al. demonstrated that LEP treatment reduced Aβ plaque burden, increased microglial immunoreactivity, and increased both IL1β and IL6 levels in the hippocampus of adult mice compared to the saline control group, implicating LEP signaling in microglial activation and the release of central inflammatory mediators (Ma et al., 2023). In a rat model of spinal cord injury (SCI), LEP administration reduced microglial activation and boosted functional recovery (Fernández-Martos et al., 2012). In a mouse model of adult-onset obesity, obesity receptor (OBR)—the primary LEP transport receptor at the BBB—exhibited significantly increased immunofluorescent staining within astrocytes of the hypothalamus of the obese mice as compared to the control, suggesting astrocyte's role in the pathogenesis of obesity within the CNS (Pan et al., 2008).
Obestatin is a 23-amino acid long gastrointestinal peptide hormone with a diverse range of physiological functions, mainly known for its role in the reduction of both food intake and weight gain (Cowan et al., 2016). It operates along the gut-brain axis and is also involved in regulating sleep, memory improvement, secretion of pancreatic enzymes, and blood pressure regulation (Lacquaniti et al., 2011). Obestatin also exhibits a cardioprotective effect via inhibition of apoptosis in reperfusion injuries within models of cardiac ischemia (Alloatti et al., 2010). In the CNS, obestatin exerted a neuroprotective effect and antioxidant properties against ischemic injury via attenuation of astrocyte activation and reduction of neuronal cell apoptosis (Mirarab et al., 2019).
2.11 Growth hormone secratogues
Growth hormone secretogues (GHS) are neuropeptides that induce the secretion of growth hormones. They were discovered in studies attempting to understand the underlying causes of endocrine-related aging and are crucial in maintaining metabolic radicals (Poudel et al., 2020; Cai, 2021; Tresguerres et al., 2022). Ghrelin or acylated Acyl-Ghrelin (AG) is a GHS that is involved in hunger regulation but also has an -inflammatory effect on microglia involved in NLRP3 inflammasomes, pyroptosis, and the NFκB and TNFα pathways (Liu et al., 2019; Maldonado-Ruiz et al., 2019). In astrocytes, AG is involved in glucose and glutamate homeostasis and metabolism (Fuente-Martín et al., 2016; Frago and Chowen, 2017). The endocrine-secreted growth hormone-releasing factor's (GRF) primary function is to bind to receptors and induce the production of growth hormone; however, this peptide is produced across many other systems, including the reproductive and immune systems. In the ocular system, GRF has been noted as an inflammatory mediator lending to the protection of ocular neurons (Cen et al., 2022). Other GHSs exist, such as insulin-like growth factor; however, there is limited understanding of their interaction with neuroglia, to date.
2.12 Other hormone peptides
Some neuropeptides significantly affect glial and overall endocrine signaling related to social bonding and stress. Oxytocin (OXT) is a hormonal peptide that affects maternal bonding and reproduction (Lee et al., 2009). Although the OXT receptor is not entirely understood in glia, OXT does have a role in neuroinflammation and glial signaling. When treated with microglia, it inhibits inflammation in vitro and in vivo with AD Aβ plaque models (Selles et al., 2023). With astrocytes, OXT is crucial in regulating neuromodulation, gliotransmission, and neural plasticity (Wang P. et al., 2017; Baudon et al., 2022). Vasopressin/arginine vasopressin (VPN/AVP) is a neuropeptide with a significant role in social bonding and human reproduction and has a complementary function to OXT. Like OXT, VPN has a role in neuroinflammation, being secreted by reactive microglia (Szmydynger-Chodobska et al., 2011) or exacerbating microglial into pro-inflammatory cytokine production in a TBI rodent model (Szmydynger-Chodobska et al., 2010). Fundamentally, VPN is responsible for osmotic regulation in a variety of cells. In astrocytes, this is characterized by swelling and uptake, which remains functionally unclear (Sarfaraz and Fraser, 1999). Apelin (APJ) is a peptide that targets the apelin GPCR, is related to body fluid uptake and appetite, and reduces the secretion of VPN. In rats with SCI, an APJ's attenuation on microglial and astrocyte-mediated neuroinflammation promoted the survival of endogenous neural stem cells (Liu et al., 2022). Apelin-13 (APEL) was able to limit a neuroinflammatory response in lower NF-κB and IκB kinase β (IKKβ) levels in depressive rodents (Zhang et al., 2019), LPS stimulated N9 microglia, and astrocytes, and microglia in LPS injected mice (Zhou et al., 2019).
3 Extracellular matrix glial peptides
Several peptides and peptide derivatives from ECM have been discovered to have some degree of effect on glia (Table 2). In neurons and glial cells, a membrane-linked glycoprotein known as the neural cell adhesion molecule (NCAM) is involved in cell-cell interactions and plays an important role in the development of the nervous system along with synaptic plasticity, learning, and memory (Weledji and Assob, 2014). NCAM modulates an intracellular signaling cascade with its interaction between the fibroblast growth factor receptor (FGFR) and downstream activation of kinases, including protein kinase C, phosphatidylinositol-3 kinase (PI-3), and extracellular signal-regulated kinase (ERK) (Niethammer et al., 2002). Fibroblast growth loop —or FGL—is an NCAM-derived peptide mimetic that has been shown to emulate the interaction between FGFR and influence cytokine levels in glial cells (Cox et al., 2013). Specifically, FGL promotes the production of IL4. This anti-inflammatory cytokine acts on neurons to induce the expression of a ligand at the neural cell membrane known as CD200, which provides a central inhibitory signal to the microglial response (Casali and Reed-Geaghan, 2021). In typical aging brains, there is a decline in IGF-1, a polypeptide hormone that inhibits the activation of microglia. This activation occurs through an IFNγ induced mechanism (Ivan et al., 2023). Downer et al. examined the interaction of FGL concerning imbalances among pro-inflammatory IFNγ and inhibitory IGF-1 levels in aging rat hippocampi with associated increased glial reactivity. FGL was shown to reverse the decline of IGF-1 in the aging neurons and promote CD200 and reduced antigen presentation of MHCII and CD40 expression in a robust manner, reducing IFNγ induced activation of glial cells that is more prominent during aging (Downer et al., 2009, 2010). Ojo et al. examined the effect of systemic FGL treatments on microglia and astrocyte population densities and activation in aging rat hippocampi (Ojo et al., 2011). In this study, FGL reduced the density of CD11b and MHCII-positive microglia and decreased glial fibrillary acidic protein (GFAP)-associated immunoreactivity in the subfields of all the aged hippocampi. Zellinger et al. (2014) showed new astrocyte growth and secretion of IL4 in their epileptic kindling electrode mouse model, which might be associated with promoting the development of a hyperexcitable network.
NCAM and putative homophilic binding domain regions of NCAM (KHIFSDDSSE) have been shown to have an anti-proliferative effect on astrocyte cell division and growth (Sporns et al., 1995), which was later assessed on silica surfaces (Kam et al., 2002). Sridar et al. (2017) also measured astrogliosic attenuation when this peptide was coated on inert platinum-iridium electrode surfaces in a mixed glial 3D culture model. A fibroblast growth factor (FGF) receptor activation motif (FRM) peptide (DRVEPYSSTA) was modified into a multimeric form. It was shown to promote survival and outgrowth in myelinated oligodendrocytes (Palser et al., 2009). These studies demonstrate the novelty of NCAM peptide derivatives to promote myelin viability and attenuate the glial response in the context of reducing inflammatory neurodegenerative changes.
The basement membrane is an ECM that holds cells and tissues together, mainly due to the activity and function of laminins. These large glycoproteins (~400 kDa) are a central component of the basal lamina and have robust functions, including their involvement in the migration, adhesion, and attachment of cells (Aumailley, 2013). Laminins consist of three polypeptides linked by disulfide bonds that were initially known as A, B1, and B2 (Tashiro et al., 1989). The self-assembly of these proteins is essential in promoting basement membrane development, and their involvement in ECM affects the function of different neural cell types. For this reason, the sequences of their primary amino acids are being heavily studied. We will review the application and data on one such sequence known as IKVAV, a pentapeptide on the A chain of the laminin protein. It was shown that the adhesion of astrocytes on silica surfaces modified with IKVAV was not improved (Kam et al., 2002). The adhesion of astrocytes is critical in the early cell-to-substrate response, revealing that IKVAV plays a role in the attenuation of astrocytes and their subsequent functionality within the nervous system. In 2016, Koss et al. examined this sequence in the context of RADA4-IKVAV-derived nano scaffolds and their effect on primary microglia and astrocyte activity. It was found that IKVAV did not affect astrogliosis and microglial activation (Koss et al., 2016). YIGSR is another laminin-based peptide that was studied alongside IKVAV in the 2002 Kam et al. study, also shown to attenuate the adhesion of astrocytes on silica substrates. These results reveal that these laminin-based peptide motifs are involved in the bioactivity of microglia and astrocytes, especially concerning the adhesion of these cells in the early cell-substrate response.
Along with laminin, fibronectin is another large glycoprotein within the ECM. In contrast to laminin—which exists exclusively in the basement membranes—fibronectin is found in highly abundant organized structures within the membranes of both basement and interstitial ECMs. Fibronectin ranges in size from 230 to 270 kDa and is typically secreted by cells in a dimer form covalently linked by disulfide bonds (Dalton and Lemmon, 2021). It plays a significant role in cellular growth, differentiation, migration, and adhesion (Pankov and Yamada, 2002). Fibronectin self-assembles into a scaffold that initiates cell adhesion in a cell-mediated process in which this dimer is converted into a fibrillar network (Wierzbicka-Patynowski and Schwarzbauer, 2003). Due to its involvement in the ECM and cellular processes, amino acid sequences are being identified for their application in tissue engineering. One such sequence, RGD, has been studied regarding its application to neuronal nano scaffolds and adhesive biomaterials (Ruoslahti, 1996). Fibronectin binds to integrin receptors, which, aside from cellular adhesion, also mediate intracellular responses that support cellular survival and differentiation. This is a feature of embryonic radial glial cells, with large amounts of cellular surfaces interacting with fibronectin as the predominant ECM molecule (Campos, 2005). Markó et al. (2011) demonstrated that radial-glia-like neural progenitor cells had rapid adhesion to surfaces coated with an RGD motif peptide. In the CNS, molecules with RGD are induced following damage. In 2007, Peluffo et al. examined if these RGD motifs mediated neuroprotective effects after synthetic peptides containing RGD in a genetic vector were injected into rat brains within NMDA excitotoxicity models. They evaluated the effect of RGD on glial damage and inflammatory response. They found that the rats treated with the RGD peptide had significant increases in both microglia cell number and reactivity, although there was no difference in the response of astroglia (Peluffo et al., 2007).
Vitronectin is another glycoprotein in ECM-cell adhesion comparable to fibronectin but complexes with alternate specificity to laminin anchoring integrins (Schvartz et al., 1999). The RGD peptide is also present in vitronectin, yet other glial-responsive peptides are found in vitronectin (Raman et al., 2020). Specifically, Raman et al. used a vitronectin-derived peptide (VDP) to differentiate neural precursors into highly pure astrocyte populations effectively. The astrocytes were an effective model for inflammation with respect to Aβ uptake and apolipoprotein E (ApoE) production, which are hallmarks of AD.
4 Growth factor glial peptides
Both astrocytes and microglia are primary producers of growth factors for the development, maintenance, and repair of the CNS; however, a different panel of factors plays multiple critical roles in glial function. Transforming growth factor alpha (TGF-α) is crucial in maintaining glutamate transporter function in astrocytes, which provides neuroprotection associated with most neurodegenerative diseases (Karki et al., 2014). Several peptides associated with glial biology have been noted (Table 3). TGF-α is a 50 amino acid peptide with several cyclic loops (Tam et al., 1991), where the B loop beta sheet had the highest activity (Bettenworth et al., 2011). Other peptides based on the cyclic form of TGF-α were optimized with binding to monoclonal antibodies (Hahn et al., 2001). Transforming growth factor beta (TGF-β) variants 1, 2, and colony-stimulating factor 1 (CSF1) promotes proliferation and viability in microglia, and inhibitors have been designed with the intent of mediating inflammation associated with neuropathic and cancer pain (Bureta et al., 2019). The TGFβ1 binding domain mimicking peptide pm26TGF-β1 was used by Vaz et al. as a potent anti-inflammatory agent to favor IL10 over TNFα secretion and promoted differentiation in T cells while noting leukocyte rolling and neutrophil migration in their inflammatory mouse models (Vaz et al., 2015). The TGFβ1 inhibitor peptides P17 and P144 have been used with great effect to reduce SMAD phosphorylation and over-expression of collagens (Dotor et al., 2007; Hanafy et al., 2020), ameliorated inflammation and redox balance in a mouse ear injury (Murillo-Cuesta et al., 2015), and a fibrotic inhibitor across liver, skin scleroderma, and myocardial rodent models (Ezquerro et al., 2003; Santiago et al., 2005; Hermida et al., 2009). A capped CSF1 peptide was discovered alongside a tachykinin-like peptide in a systematic bioinformatic analysis. However, the in vitro potential of these peptides is yet unknown (Wiggenhorn et al., 2023).
Epidermal growth factor (EGF) receptor pathways are highly upregulated after neuronal injury, activating astrocytes (Liu and Neufeld, 2007) and chemotactically mobilizing microglia into lesion sites (Nolte et al., 1997). Cardó-Vila et al. (2010) designed a decoy replicating binding features of the EGF receptor as an EGF pathway inhibitor, while Tombling et al. used the EGF-like domain of the low-density lipoprotein receptor to engineered a peptide therapeutic inhibitor of proprotein convertase subtilisin/kexin type 9 (PCSK9) (Tombling et al., 2021), which could mediate levels of phosphorylated NFκB in reactive astrocytes and microglia (O'Connell and Lohoff, 2020).
IFG-1 has a complex pleiotropic role in neuroinflammation, and is secreted by microglia for various neurotropic and immunomodulatory roles (Myhre et al., 2019). Microglia and astrocytes exposed to high levels of IFG-1 have neuroprotective, inhibit BBB permeability, phagocytic reactivity, and aging-related neurodegeneration (Labandeira-Garcia et al., 2017; Pinto-Benito et al., 2022). Several groups have developed peptides to emulate the IFG-1 binding function (Cascieri et al., 1989; Chrudinová et al., 2018). One cyclic peptide (CYAAPLKPAKSC) has been used successfully to suppress proliferation in prostate carcinoma lines and as a standard agonist for IFG-1 (Bonnefont et al., 2007). Camarero et al. used this peptide to promote neurogenesis and neuritogenesis in the cochleovestibular ganglion, while Croci et al. were able to prolong the survival of Purkinje neurons in the developing cerebellum (Camarero et al., 2003; Croci et al., 2011). Considering this peptide was treated with complex mixtures of CNS cells, it is possible that a pleiotropic response was initiated by neuroglia and could be a potent tool in unlocking their beneficial roles.
5 Peptides in glial biology
As microglia are unique phenotypes of neural and immune cells, a handful of peptides are known to be characterized as direct ligands for microglia and astrocytes (Table 4). The ocellatin peptides [K1(1–16) and K1(1–21)] were derived from the amphibian Leptodactylus vastus for their antioxidant properties (Sousa et al., 2020). When tested with primary microglia, these helical peptides attenuated ROS, nitrites, and neuroinflammation related to TLR4 and the production of NFκB. One of the more specific microglial-focused peptides was discovered by Kurinami et al. (2016), who developed microglial healing peptides (MHP) based on receptor activator NFκB ligand (RANKL) and its target receptor (RANK). The RANK/RANKL system is a known microglial mediator for ischemic brain injury by inhibiting TLR4 (Iadecola and Anrather, 2011; Shimamura et al., 2014). One major drawback of using RANKL as a direct therapeutic is the induction of osteoclast differentiation (Ferrari-Lacraz and Ferrari, 2011) and eventual bone loss (Tomimori et al., 2009), therefore Kurinami sought to design as a peptide analog that could inhibit TLR4 without the potential osteoporotic complication. To this end, MHP1 inhibited RANKL osteoclast differentiation while attenuating neuroinflammatory behavior and ischemic injury in primary mixed glia-neuronal culture with LPS-induced neuronal death and with an intracerebroventricular peptide injection after a transient middle cerebral artery occlusion.
Although it may be an unexpected strategy to prime microglia to particular pathogens, it is essential in developing immunotherapeutics. Apolipoproteins are involved in Aβ fibrils and AD, but several peptides have been noteworthy in antimicrobial anti-infective studies (Kelly et al., 2010). Comparable ApoE suppression of TNFα and nitrite in primary murine microglia activation has also been noted with peptides (Laskowitz et al., 2001). Pocivavsek et al. (2009) used the ApoE141 − 149 peptide EP to attenuate TLR4 activation, inhibiting the c-Jun N-terminal kinase (JNK) pathway while activating ERK. Dimer form ApoE peptide was the most significant in stimulating secreted amyloid precursor protein alpha (sAPPα) and the microglia-attenuation of Aβ plaques, in vivo (Minami et al., 2010).
6 Inflammatory peptide candidates in glial mediation of neural pathologies
6.1 Cytokine analog peptides
Several peptides were developed with immune targets in mind (Table 5), although their effect on microglia and astrocytes has witnessed minimal evaluation. As microglia bear macrophage/monocyte phenotypes and express most of the same receptors and pathways as many other immune cells, inflammatory-mediating peptides could make excellent candidates to explore in neuroinflammatory systems. Growth factors, neuropeptides, and the cytokine families of interleukins and TNFα are some of the most potent inflammatory mediators that have inspired many peptides and peptide inhibitors. TNFα is one of the most potent pro-inflammatory cytokines in apoptosis and necrosis and has been a primary target for inflammatory mediation in cancer and rheumatic systemic diseases (Idriss and Naismith, 2000). TNFα receptors are prevalent in both microglia and astrocytes. There has been a focus on generating monoclonal antibody inhibitors (Chang et al., 2007; Qin et al., 2007), and several have been clinically evaluated (Benucci et al., 2012), but a demand for more cost-effective peptide variants led to several unique peptides. Chirinos-Rojas et al. first observed an anti-TNFα peptide SEP-7, whose activity could be mediated by retaining 1-3 arginine-based protection groups 4-methoxy-2,3,6-trimethylbenzenesulphonyl (Mtr). They later used an alternative peptide to attenuate cytotoxicity in fibroblasts (Chirinos-Rojas et al., 1997, 1998). Guo et al. (2002) generated a family of cyclic TNFα-targeting peptides, of which binding can be tuned by amino acid amphilicity proximal to the C-terminus. Qin et al. (2006) generated a TNFα antagonist peptide that could competitively inhibit antibody binding and prevent cytotoxicity in L929 cells. Brunetti et al. (2014) synthesized a tri-lysine tetramer peptide and assessed significant binding with Surface Plasmon Resonance and were able to target TNFα receptors in melanoma cells. Sclavons et al. (2013) generated cyclic peptide variants to target TNFα, one of which had specificity to liver tissue in their murine hepatitis model.
IL1β is one of the more potent pro-inflammatory cytokines. It has a trophic, proliferative, and reactive effect on microglia (Monif et al., 2016). It has been shown to improve neuronal survival in the retinal system (Todd et al., 2019), but it can compromise and result in cell death for astrocytes (Kralingen et al., 2013). A tripeptide IL1β analog DKPT was used by Ferreira et al. (1988) to antagonize IL1β-invoked hyperalgesia in their rat model. Several specialized peptides were designed by Geranurimi et al. (2020) with α-lactam substitutions for D-threonine. Diverse amine and triazole substituents were fabricated to refine the allosteric modulation of the IL1 receptor and tested with in vitro assays (retinal microglia) and preterm birth/oxygen-induced retinopathy models. Peptides were found to inhibit Rho/Rho-associated coiled-coil-containing protein kinase 2 (ROCK2) and JNK pathways while ineffective at activating the expected NFκB system.
IL2 is often the focus of T-regulatory cell mediation in neuroinflammatory disease, where the BBB is breached to allow the general immune system to become involved (Yshii et al., 2022). IL2 does share the identical receptor subunits as IL15 and has a pro-inflammatory effect on microglia (nitrites), as opposed to IL15's attenuating effect (Hanisch, 2002). Gründemann et al. (2013) found that the Kalata B1-based cyclotide, a unique peptide that forms intramolecular cyclic bridges across three locations, was capable of inhibiting T-lymphocyte proliferation and down-regulating both the expression of IL2, its receptor, and the subsequent maintenance of baseline TNFα and IL1β. Wang et al. (2014) modified this cyclotide with myelin oligodendrocyte glycoprotein (MOG) epitopes (MOG3) and were able to observe significant myelin remediation in their MS mouse model. The Kalata B1 cyclotide was evaluated to have poor BBB permeability (Melander et al., 2023), yet the BBB is damaged in the late stages of MS to allow encephalitogenic T-cell infiltration. Microglia are known to collaborate with these T-cells (Dong and Yong, 2019) and could have a singular response to cyclotides worth utilizing in earlier disease-stage therapeutics. Although these IL2-mediating peptides might require T-cell intervention to have a more significant effect, IL2 treatments are beneficial in targeting activated astrocytes and Aβ fibrils (Alves et al., 2017), which could be beneficial in treatments for AD.
IL8, also known as the C-X-C chemokine (CXC), has a core role in neutrophil recruitment/degranulation and chemotaxis at core inflammatory insults and infections but can be secreted by many cells with TLRs (Harada et al., 2014), which include microglia and astrocytes (Ehrlich et al., 1998; Robinson et al., 2020). CXC and their receptors have a core role in microglial activation in the NLRP3 inflammasome pathway, as noted in stroke (Werner et al., 2020) and perinatal brain injury (Serdar et al., 2020). One IL8 inhibitor, hexapeptide Antileukinate, has shown promising results in mediating inflammation in lung injury (Hayashi et al., 2002) and acute pancreatitis (Bhatia and Hegde, 2007). Hayashi et al. reduced neutrophil mobilization, significantly reducing interstitial lung fluid buildup in their rodent model. Fukuno et al. (2003) used this peptide and noted a reduction in eosinophil chemotactically-mediated thickening of submucosal tissue in a chronic antigen-exposed rodent model. Bhatia and Hegde noted a decrease in pancreatic myeloperoxidase (MPO) and macrophage inflammatory protein-2 (MIP-2) associated with acute pancreatic inflammation. Antileukinate may have some benefit in treating acute brain insults such as stroke and traumatic brain injury, especially when BBB breach and immune cell infiltration may be a concern.
IL10 is a pleiotropic cytokine that has a primary role in regulating inflammation and is considered one of the most potent 'shut off' mechanisms for restoring microglia homeostasis after a variety of pro-inflammatory insults (Lobo-Silva et al., 2016; Shemer et al., 2020). Unsurprisingly, peptide analogs have been in high demand, and several sequences have emerged recently. Ni et al. generated IL10 inhibitor peptides (P1 and P2) to promote chronic inflammatory resolution using helical repeating amphiphilic residues (Ni et al., 2016). These were tested with T cells and monocytes (U-937 and primary human) and found to enhance IL12 and papillomavirus 16 CD8+ mediated immunity. Chang et al. used in silico modeling to generate one candidate IL10 analog (IL10NM25) (Chang et al., 2020), which selectively bound to IL10Ra and reduced the proliferation of monocyte and lymphoblastoid cells. Computational/machine learning approaches by Nagpal et al. (2017) and Singh et al. (2021) have led to hundreds of peptides emulating IL10, which, to date, are untested in cells and tissues.
IL15 is an essential component of the inflammatory signaling cascade, being a pro-inflammatory cytokine regulating homeostasis following insult to the CNS. It is critical in scar tissue formation with microglial accumulation and secretion of IL1β and TNFα, as well as astrocyte production of GFAP (Gomez-Nicola et al., 2010; Shi et al., 2020). As these microglia-astrocyte crosstalks are core to gliosis in every neuroinflammatory pathology, an IL15 peptide analog could be a potent therapeutic tool. The IL15 sequence was derived (Santos et al., 2008) using peptide spot synthesis to target the IL15 receptor alpha (IL15Rα). This peptide was evaluated for IL15 activity in two cell lines and was able to attenuate IL15Rα in a human prostate carcinoma cell line. The free cysteine was used to promote a dimer structure, which significantly improved the bioactivity of the peptide. It should be noted that IL15 binding epitopes, as part of antibody structures, have shown promising results in rheumatoid arthritic (Baslund et al., 2005) and psoriatic therapeutic development (Villadsen et al., 2003); therefore, specific molecular machinery may need to be considered in the peptide design for interleukin-based peptides.
6.2 Suppressor of cytokine singnalling 2/Janus kinas 2 pathway
Flowers et al. (2004) generated a peptide Tkip to target the autophosphorylation site on Janus kinase 2 (JAK2), which retained some homology to the suppressor of cytokine signaling 2 (SOCS2). The JAK family is a known entity with a crucial role in signaling IFNγ receptors, and JAK2 is specific to IFNγ (Kotenko and Pestka, 2000), which is a potent driver in TLR activation and switch for the adaptive immune response over the innate microglial response (Kann et al., 2022). Comparable to JAK2, Flowers et al. found that Tkip inhibits IFNγ's antiviral behavior and promotes an adaptive presentation response associated with major histocompatible complex class I (MHCI) (Flowers et al., 2004). Another peptide associated with the kinase inhibitory region (KIR) for binding to JAK2, named SOCS1-KIR, was evaluated by Waiboci et al. (2007). After confirming binding to pJAK2 (1001–1013), the SOCS1-KIR peptide functioned as an agonist to IFNγ and blocked SOCS1-induced inhibition of signal transducer and activator of transcription 3 (STAT3) phosphorylation, suggesting its use as a SOCS-1 antagonist. Such potent microglial neuro-inflammatory mediating peptides could lead to treatments for oncological and immunological disorders, which are often associated with uncontrollable tyrosine kinase activity (Blume-Jensen and Hunter, 2001; Tsygankov, 2003). Specific to the CNS, the JAK2/SOCS could be a core target in mediating cancer and neurodegenerative disorder (Nicolas et al., 2013) and has been associated with microglia and astrocytes in neurological disorders comparably to other components of the immune system (Jain et al., 2021).
One of the more prevalent families of JAK2-targeting peptides was discovered and characterized in D. Marasco's group. Initially discovered in a peptide library engineered to screen comparable KIR peptides by Doti et al. (2012), a new combinatorial peptide PS-5 was found with binding constant values in the nanomolar range able to reduce the phosphorylation of STAT1 and expression of IRF-1 in keratinocytes. Madonna et al. (2013) corroborated PS-5's JAK2 impairment in keratinocytes and T lymphocytes in vitro and with an IFNγ treatment of human skin explants. La Manna et al. evaluated PS-5's ability to mimic SOCS-1 in vascular smooth muscle cells (VSMCs). They could derive cyclic and non-natural naphthyl (Nal1) containing variants with better stability (La Manna et al., 2017). They employed a surface plasmon resonance technique to characterize their peptide binding with a more robust quality. La Manna et al. (2021) used the cyclic forms PS-5 to inhibit the oxidative stress associated with JAK-mediated tyrosine phosphorylation and pro-inflammatory cytokine expression in VSCMs. La Manna's team was also able to generate SOCS3 mimetic peptides (KIRESS) to inhibit the cytokine production of triple-negative breast cancer subtypes and their subsequent tumor growth and pulmonary metastasis in murine xenografts (La Manna et al., 2018b). La Manna et al. (2022b) performed a systematic ad-hoc assessment of several cyclic lactam bridge KIR-SOCS1 peptide modifications to refine micromolar affinity toward JAK2. Modifications included subtle alterations in ring stability/flexibility with citrulline (Cit), ornithine (Orn), and diaminopimelic acid (Dap) substitutions to generate several inhibitor variants. La Manna et al. have compiled more comprehensive reviews on anti-inflammatory peptides involved in the JAK2/SOCS system (La Manna et al., 2018a, 2022a). Despite their wide success, these KIR peptides have yet to be applied to CNS-related neuroinflammatory mediation or tumor suppression.
Considering its potent pro-inflammatory effect on microglia and astrocytes, there has been interest in generating IFNγ antagonist peptide analogs (Seelig et al., 1995). In microglia, IFNγ antagonist has been linked to inhibiting neuroinflammation associated with AD and PD, as well as being clinically relevant to the pathophysiology of schizophrenia (Kato et al., 2007). IFNγ antagonism in astrocytes has a protective mediation of autoimmune-related neurological disability (Hindinger et al., 2012). It should be noted that autoimmune antagonism has been shown to have opposing effects in microglia and astrocytes signaling (Ding et al., 2015), so some degree of specificity in targeting glia might be required to produce the most beneficial response, which complex peptide ligands could produce.
6.3 Aminopetidase N/cluster differentiation 13 pathway
Metalloproteinase peptide cleavage is critical in activating peptide fragments in various inflammatory and wound-healing events. The M1 aminopeptidase N (APN) or CD13 cleavage event promotes viral activation (Chen et al., 2012) cancer angiogenesis and metastasis (Zhang and Xu, 2008; Wickström et al., 2011) making it a noteworthy target for the mediation of corona viral inflammation (Ruan et al., 2023) and anti-cancer, some of which reached clinical evaluation (Xu et al., 2011; Jiang et al., 2016). Microglia produce higher levels of APN than peripheral monocytes and macrophages when assessing enkephalin inactivation (Lucius et al., 1995), associated with pain sensation and mood disorders (Henry et al., 2017). Furthermore, APN is a known regulator for astrocyte-microglial crosstalk in the brain renin-angiotensin pathway during neuroinflammation, which is crucial in regulating CNS blood pressure (Kim et al., 2022). Joshi et al. (2017) generated a selective APN-inhibitor peptide (cyclic HSPW) through structural profiling and X-ray crystallographic assessment. They demonstrated the peptide's inhibitory and anti-tumorgenic activity in PC3/DU145 cells and DU145 grafted mice. Although one application was tested, the peptide inhibition of this system could lead to analgesics, mood stabilizers, and neuroinflammation/blood pressure attenuating peptides if focused toward CNS glia.
The APN-targeting NGR peptide sequence has limited investigation as an immune mediating peptide but has led to several advances in binding affinity refinement (Xu and Li, 2005). Cyanine 5.5 (Cy5.5) imaging in cancer-based cell lines HT-1080 and MCF-7 was performed by von Wallbrunn et al. (2008), while Hahnenkamp et al. substituted poly ethylene glycol (PEG) spacers on benzoyl moieties of NGR derivatives to improve fluorescent targeting (Hahnenkamp et al., 2013). Li et al. (2016) added an anti-tumor pro-drug property with a cytosine deaminase chimeric peptide capable of converting 5-fluorocytosine (5-CF) to a cytotoxic 5-fluorouracil (5-FU) at the CD13 of cells receptor modeled across a broad spectrum of cell lines (A375, A431, MCF-7, HT-1080, MDA-MB231, MDA-MB468). Uddin et al. (2019) introduced a Knob-Socket protein packing modeling system to generate two 5-mer and one 8-mer peptide (PEP20, 24, and 173) with an order of magnitude higher binding affinity over the cyclic NGR control. Although the NGR peptide may have little effect on APN inhibition compared to other targets, it certainly provides a strong candidate for shuttling other effective ligands to APN-expressing glia.
6.4 Intestinal marcrophage-mediating peptides
Several small inflammatory regulating peptides, some as small as three amino acids, have been discovered to mitigate bowel inflammation through intestinal macrophages. Wada et al. (2013) used a gluten hydrolysate-derived pyroEL peptide in an acute DSS-based colitis model as an anti-inflammatory/antimicrobial agent. Kovacs-Nolan et al. (2012) treated their DSS-colitis model with the soy-derived VPY in DSS-induced rodent colitis. They noted a significant reduction in pro-inflammatory cytokines: TNFα, IFNγ, IL1β, IL6, and IL17. The tripeptide KVP was used by Dalmasso et al. (2008) to inhibit pro-inflammatory cytokine secretion through the NFκB and mitogen-activated protein (MAP) kinase pathway in intestinal epithelial and T cells and eventually attenuate dextran sulfate sodium (DSS)/2,4,6-trinitrobenzene sulfonic acid (TNBS)-induced colitis in mice.
Several groups derived small peptides from Annexing A1 (ANXA1), a potent mediator of glucocorticoid anti-inflammation through NFκB. The NFκB/MAP pathways are crucial for induction and maintenance in the CNS (Mendonca et al., 2018). They are hallmarks of microglial activation in AD-related Aβ plaque-induced inflammation (Tilstra et al., 2011). Zhang et al. (2010) used the ANXA1 tripeptide QAW in their intestinal cancer xenograft model and reduced tumor tissue growth by 58%. The ANXA1-derived peptide MC-12 was used by Ouyang et al. (2012) as a NFκB inhibitor in mice with DSS-induced colitis and were able to restore cytokines TNFα, IFNγ, and IL1β, IL6, and IL10 to a baseline. Cobos Caceres et al. (2017) grafted a cyclic form of MC-12 into a sunflower trypsin inhibitor scaffold. The cyclic MC-12 peptide significantly improved over the linear MC-12 in reducing the inflammation in their acute colitis model in mice while maintaining stability in plasma.
Neuropeptide derivatives have been used in a handful of studies. Bettenworth et al. (2011) used an α-MSH C-terminus fragment tripeptide K(d)PT in their in vitro/in vivo IL10 deficient colitis mice models to note a reduced severity in inflammation and improved transepithelial electrical resistance after IFNγ and TNFα activation. As noted previously, α-MSH peptides also have a profound effect on microglia through the inhibition of TLR2 and TLR4 (Carniglia et al., 2016) and the attenuation of Aβ activation (nitric oxides, TNFα, and IL6) (Galimberti et al., 1999; Lindberg et al., 2005). Akgül et al. (2006) noted a decrease in rodent NFκB and pancreatic inflammation when they treated their rat model with their TNBS acid-induced colitis with octreotide, a somatostatin derivative. Also mentioned previously, somatostatins have a potent effect on microglia, including the inhibition of IL3-stimulated proliferation, tyrosine phosphorylation, and anti-amyloidosis in AD (Feindt et al., 1998; Tundo et al., 2012).
Chromogranin-A (CHGA) is a vesicle-based acidic protein and peptide precursor in various immune regulating functions (Chanat and Huttner, 1991; D'amico et al., 2014). One CHGA-derived peptide, Chromofungin (CHR: CHGA47 − 66), is a promising candidate that is involved in antimicrobial (Yoo, 1992; Maget-Dana, 1999; Lugardon et al., 2001) and inflammatory regulation (Ghia et al., 2004; Metz-Boutigue et al., 2010). Eissa et al. (2017) used the CHR peptide to activate gut macrophages into an alternative phenotype capable of attenuating oxidative stress and pro-inflammatory cytokine release, reducing colitis in a mouse model. Microglia bear many phenotypical similarities and have a fundamental crosstalk role with intestinal macrophages (Verheijden et al., 2015). CHGA is considered to promote a neurotoxic phenotype in brain microglia (Ciesielski-Treska et al., 1998), but this has also been shown to promote a phenotypical switch (Taupenot et al., 1996), leading to a neuronal-apoptotic response (Ulrich et al., 2002). CHGA is also a hallmark of neurodegenerative cerebral spinal fluid (Kaiserova et al., 2021) and tissue lesions (van Luijn et al., 2016). It may be the apoptotic outcome over a necrotic cascade that allows the favorable colitis remediation seen by Eissa et al., but this has yet to be verified with CHR-treated microglia.
7 Innovative tools and techniques in the discovery of neuroglial specific peptides
With a few exceptions, most of the peptides discussed were found to be targets for many other cell types or known receptors. Using naïve approaches from library techniques, few efforts have been made to discover peptides specific to glia or mechanisms of glia with a focus on microglia and/or astrocytes. The major benefit to employing such tools allows for the discovery of new mechanisms focused on cellular or bio molecular targets. In particular, phage display has yielded the most significant results, which utilizes a peptide-coat expressing bacterial virus with extensive sequence diversity (<billion sequences). The M13 filamentous phage has been a popular choice due to its non-essential regions for modification. Despite their prevalence use across many types of cells and tissue, only a few studies have utilized M13 or other filamentous phage for microglial and astrocyte panning (Table 6). Examples include the identification of a select peptide (SFTYWTN) from a family S/TFT/XYW consensus site from E20 microglia (Samoylova et al., 2002) and a small number of binders to primary murine microglia using fd phage (Lundin et al., 2003). Terashima et al. (2018) employed a combination of M13 phage display for isolating peptides specific to M1 and M2 microglia phenotypes. Koss et al. (2019, 2023) observed changes in binding patterns of 58 candidate peptides to primary rat microglia across various phenotypes using M13 phage display. Functional analysis suggested roles in synaptic pruning, neuroprotection, and neurodegenerative diseases. Additionally, Zhou et al. used a C7C phage system to discover a heptapeptide against Aβ42 that reduced reactive oxygen species and microgliosis in vivo.
The bone marrow homing peptide 1 (BMHP1) was discovered against hematopoietic stem cells (Nowakowski et al., 2004). Bjornson et al. (1999) demonstrated shared differentiation and adhesion pathways between stem cells of neural and bone marrow origins, making these motifs attractive in the context of neural tissue engineering. SKPPGTSS is also involved in the family of neuronal apoptosis inhibitor proteins (Deveraux and Reed, 1999). BMHP1 was also eventually used to differentiate neural precursors into astrocytes in a 3D culture environment (Gelain et al., 2006) and investigated the viability of neural and glial cells (radial glia/astrocytes) within peptide hydrogel scaffolds. The highest percentage of living cells in the hydrogels containing the SKPPGTSS peptide remained after 3 months, indicating that this motif is essential in supporting neuronal cell viability (Koutsopoulos and Zhang, 2013).
The T7 phage system is emerging as the preferred method due to several advantages over filamentous phage. These include faster bacterial growth, direct insertion of large cDNA libraries into the T7 genome for capsid fusion, broader compatibility with various libraries, simpler and more efficient affinity elution, and enhanced viability during recombination (Deng et al., 2018). To date, Caberoy et al. (2009, 2010a, 2012) have conducted a series of T7 studies focused on microglial phagocytosis, identifying peptides that mediate the engulfment of debris through the c-mer proto-oncogene tyrosine kinase (MerTK) receptor and “eat-me” signals. Notably, only one of these studies directly employed primary microglia and BV-2 cells for panning (Caberoy et al., 2009), while the rest used full-length protein display to facilitate a phagocytic response.
Despite the limited peptide discovery studies available, the peptides have yielded a treasure of new information. With clever experimental design, these display tools can be expanded into other pathologies and classes of neuroglia. Further, glial are anatomically disctinct across regions of the CNS (Tan et al., 2020) and mammalian physiologies (Toledano Furman et al., 2020), which could also be explored. New molecular features are being added to the phage themselves to tailor phage toward unique targets or applications; cyclic and glycopeptides (Ng et al., 2012; Ng and Derda, 2016), chelates (Wright and Deonarain, 2007), and BBB traffickers (Majerova et al., 2014; Urich et al., 2015), which could be pivotal in challenging in vivo phage display techniques. New mRNA display technique are immerging with sequence diversities reaching the trillions (Newton et al., 2020), yet these require transfection, which is more successful with astroglia than microglia. Recently, functionally microglia have been discovered in cerebral organoids (Ormel et al., 2018), which are excellent for modeling genetic disorders from patient-derived induced pluripotent stem cells. Considering all of the tools emerging in the peptide-display fields, many exciting projects and discoveries await the glial researcher.
8 Conclusions and future directions
At a cursory glance, there may be limited options for choosing methods for the immune and bioengineering of immune cells, microglia, and support cells astrocytes, which are crucial in the homeostasis and maintenance of neural health and neuroinflammatory mediation. However, many peptides have been characterized in the distant and recent literature. Neuropeptides and hormonal peptides are powerful tools for the control of inflammation, neuroprotection, and gliotransmission. However, they are also potent ligands, albeit highly specific, for receptors in many cells across several mammalian systems, making them useful in specific cases (isolated cell culture studies, ex vivo treatments, and drug delivery systems). Despite neuroglia's role in ECM reconstruction and growth factor secretion in wound healing and pathophysiology, a handful of peptides have been discovered to replicate these features effectively. Peptides are known to be excellent candidates in replicating cytokine analogs, targeting cytokine receptors, and regulating immune and inflammatory responses from macrophages and monocytes in other systems. With further exploration, these could lead to many potent therapeutics, as these pathways are also known in microglia and astrocytes. Specificity to neuroglia remains elusive for many of these peptides. However, recent biopanning techniques, such as phage display, have uncovered many peptides capable of distinguishing glia from other cell types. Further, these peptides can identify sub-phenotypes of glia, including M1 and M2, or activated and ramified, which is of great interest in screening and immunotechnologies (blotting, microscopy, and cytometry). The specificity of such peptides may not confer a biological response, and their target and origin proteins are often difficult to elucidate. Nevertheless, these options allow for the exciting and pleiotropic design of chimeric peptides and bioconjugates that could unlock meaningful tools and therapeutics for the glial researcher.
Author contributions
BB: Conceptualization, Visualization, Writing – original draft, Writing – review & editing. KK: Conceptualization, Visualization, Writing – original draft, Writing – review & editing, Funding acquisition, Supervision.
Funding
The author(s) declare that financial support was received for the research, authorship, and/or publication of this article. This work was supported by the Canadian Institutes of Health Research (CIHR) (RN409371 – 430628).
Conflict of interest
The authors declare that the research was conducted in the absence of any commercial or financial relationships that could be construed as a potential conflict of interest.
Publisher's note
All claims expressed in this article are solely those of the authors and do not necessarily represent those of their affiliated organizations, or those of the publisher, the editors and the reviewers. Any product that may be evaluated in this article, or claim that may be made by its manufacturer, is not guaranteed or endorsed by the publisher.
References
Abe, K., Kato, M., and Saito, H. (1997). Human amylin mimicks amyloid β protein-induced reactive gliosis and inhibition of cellular redox activity in cultured astrocytes. Brain Res. 762, 285–288. doi: 10.1016/S0006-8993(97)00595-7
Aczél, T., Kecskés, A., Kun, J., Szenthe, K., Bánáti, F., Szathmary, S., et al. (2020). Hemokinin-1 gene expression is upregulated in trigeminal ganglia in an inflammatory orofacial pain model: potential role in peripheral sensitization. Int. J. Mol. Sci. 21:2938. doi: 10.3390/ijms21082938
Akgül, S., Erbil, Y., Giris, M., Alis, H., Yanik, B. T., Olgaç, V., et al. (2006). The effect of octreotide on pancreatic damage in TNBS-induced colitis. Surg. Innov. 13, 102–108. doi: 10.1177/1553350606291337
Alloatti, G., Arnoletti, E., Bassino, E., Penna, C., Perrelli, M. G., Ghé, C., et al. (2010). Obestatin affords cardioprotection to the ischemic-reperfused isolated rat heart and inhibits apoptosis in cultures of similarly stressed cardiomyocytes. Am. J. Physiol. Heart Circ. Physiol. 299, H470–H481. doi: 10.1152/ajpheart.00800.2009
Alves, S., Churlaud, G., Audrain, M., Michaelsen-Preusse, K., Fol, R., Souchet, B., et al. (2017). Interleukin-2 improves amyloid pathology, synaptic failure and memory in Alzheimer's disease mice. Brain 140, 826–842. doi: 10.1093/brain/aww330
Asraf, K., Torika, N., Danon, A., and Fleisher-Berkovich, S. (2017). Involvement of the bradykinin B1 receptor in microglial activation: in vitro and in vivo studies. Front. Endocrinol. 8:82. doi: 10.3389/fendo.2017.00082
Avila, J. A., Kiprowska, M., Jean-Louis, T., Rockwell, P., Figueiredo-Pereira, M. E., and Serrano, P. A. (2019). PACAP27 mitigates an age-dependent hippocampal vulnerability to PGJ2-induced spatial learning deficits and neuroinflammation in mice. Brain Behav. 10:e01465. doi: 10.1002/brb3.1465
Azuma, Y., Ohura, K., Wang, P. L., and Shinohara, M. (2001). Endomorphins 1 and 2 modulate chemotaxis, phagocytosis and superoxide anion production by microglia. J. Neuroimmunol. 119, 51–56. doi: 10.1016/S0165-5728(01)00363-0
Bai, L., Zhang, X., Li, X., Liu, N., Lou, F., Ma, H., et al. (2015). Somatostatin prevents lipopolysaccharide-induced neurodegeneration in the rat substantia nigra by inhibiting the activation of microglia. Mol. Med. Rep. 12, 1002–1008. doi: 10.3892/mmr.2015.3494
Barbieri, F., Bajetto, A., Pattarozzi, A., Gatti, M., Würth, R., Thellung, S., et al. (2013). Peptide receptor targeting in cancer: the somatostatin paradigm. Int. J. Pept. 2013:926295. doi: 10.1155/2013/926295
Barnea, A., Roberts, J., Keller, P., and Word, R. A. (2001). Interleukin-1β induces expression of neuropeptide Y in primary astrocyte cultures in a cytokine-specific manner: induction in human but not rat astrocytes. Brain Res. 896, 137–145. doi: 10.1016/S0006-8993(01)02141-2
Baslund, B., Tvede, N., Danneskiold-Samsoe, B., Larsson, P., Panayi, G., Petersen, J., et al. (2005). Targeting interleukin-15 in patients with rheumatoid arthritis: a proof-of-concept study. Arthrit. Rheum. 52, 2686–2692. doi: 10.1002/art.21249
Baudon, A., Clauss Creusot, E., Althammer, F., Schaaf, C. P., and Charlet, A. (2022). Emerging role of astrocytes in oxytocin-mediated control of neural circuits and brain functions. Prog. Neurobiol. 217:102328. doi: 10.1016/j.pneurobio.2022.102328
Bell, D., and McDermott, B. J. (2008). Intermedin (adrenomedullin-2): a novel counter-regulatory peptide in the cardiovascular and renal systems. Br. J. Pharmacol. 153(Suppl. 1), S247–S262. doi: 10.1038/sj.bjp.0707494
Belo, T. C. A., Santos, G. X., da Silva, B. E. G., Rocha, B. L. G., Abdala, D. W., Freire, L. A. M., et al. (2023). IL-10/β-endorphin-mediated neuroimmune modulation on microglia during antinociception. Brain Sci. 13:789. doi: 10.3390/brainsci13050789
Benucci, M., Saviola, G., Manfredi, M., Sarzi-Puttini, P., and Atzeni, F. (2012). Tumor necrosis factors blocking agents: analogies and differences. Acta Biomed. 83, 72–80.
Bettenworth, D., Buyse, M., Böhm, M., Mennigen, R., Czorniak, I., Kannengiesser, K., et al. (2011). The tripeptide KdPT protects from intestinal inflammation and maintains intestinal barrier function. Am. J. Pathol. 179, 1230–1242. doi: 10.1016/j.ajpath.2011.05.013
Bhatia, M., and Hegde, A. (2007). Treatment with antileukinate, a CXCR2 chemokine receptor antagonist, protects mice against acute pancreatitis and associated lung injury. Regul. Pept. 138, 40–48. doi: 10.1016/j.regpep.2006.08.006
Bjornson, C. R., Rietze, R. L., Reynolds, B. A., Magli, M. C., and Vescovi, A. L. (1999). Turning brain into blood: a hematopoietic fate adopted by adult neural stem cells in vivo. Science 283, 534–537. doi: 10.1126/science.283.5401.534
Block, M. L., Li, G., Qin, L., Wu, X., Pei, Z., Wang, T., et al. (2006). Potent regulation of microglia-derived oxidative stress and dopaminergic neuron survival: substance P vs. dynorphin. FASEB J. 20, 251–258. doi: 10.1096/fj.05-4553com
Blume-Jensen, P., and Hunter, T. (2001). Oncogenic kinase signalling. Nature 411, 355–365. doi: 10.1038/35077225
Bonnefont, J., Daulhac, L., Etienne, M., Chapuy, E., Mallet, C., Ouchchane, L., et al. (2007). Acetaminophen recruits spinal p42/p44 MAPKs and GH/IGF-1 receptors to produce analgesia via the serotonergic system. Mol. Pharmacol. 71, 407–415. doi: 10.1124/mol.106.025775
Brown, J. M., and Cox, B. M. (2006). Microglia expression of nociceptin receptor variants; implications for Mptp-induced dopaminergic deficits. FASEB J. 20, A1132–A1133. doi: 10.1096/fasebj.20.5.A1132-d
Brunetti, J., Lelli, B., Scali, S., Falciani, C., Bracci, L., and Pini, A. (2014). A novel phage-library-selected peptide inhibits human TNF-α binding to its receptors. Molecules 19, 7255–7268. doi: 10.3390/molecules19067255
Burbach, J. P. H. (2011). “What are neuropeptides?,” in Neuropeptides: Methods and Protocols, ed A. Merighi (Totowa, NJ: Humana Press), 1–36.
Burda, J. E., Bernstein, A. M., and Sofroniew, M. V. (2016). Astrocyte roles in traumatic brain injury. Exp. Neurol. 275 (Pt 3), 305–315. doi: 10.1016/j.expneurol.2015.03.020
Bureta, C., Setoguchi, T., Saitoh, Y., Tominaga, H., Maeda, S., Nagano, S., et al. (2019). TGF-β promotes the proliferation of microglia in vitro. Brain Sci. 10:20. doi: 10.3390/brainsci10010020
Burmeister, A. R., Johnson, M. B., Chauhan, V. S., Moerdyk-Schauwecker, M. J., Young, A. D., Cooley, I. D., et al. (2017). Human microglia and astrocytes constitutively express the neurokinin-1 receptor and functionally respond to substance P. J. Neuroinflamm. 14:245. doi: 10.1186/s12974-017-1012-5
Burrin, D. G., Stoll, B., Petersen, Y., and Sangild, P. (2001). Glucagon-like peptide 2: a nutrient-responsive gut growth factor. J. Nutr. 131, 709–712. doi: 10.1093/jn/131.3.709
Caberoy, N. B., Alvarado, G., and Li, W. (2012). Tubby regulates microglial phagocytosis through MerTK. J. Neuroimmunol. 252, 40–48. doi: 10.1016/j.jneuroim.2012.07.009
Caberoy, N. B., Maiguel, D., Kim, Y., and Li, W. (2010a). Identification of tubby and tubby-like protein 1 as eat-me signals by phage display. Exp. Cell Res. 316, 245–257. doi: 10.1016/j.yexcr.2009.10.008
Caberoy, N. B., Zhou, Y., Jiang, X., Alvarado, G., and Li, W. (2010b). Efficient identification of tubby-binding proteins by an improved system of T7 phage display. J. Mol. Recognit. 23, 74–83. doi: 10.1002/jmr.983
Caberoy, N. B., Zhou, Y., and Li, W. (2009). Can phage display be used as a tool to functionally identify endogenous eat-me signals in phagocytosis? J. Biomol. Screen. 14, 653–661. doi: 10.1177/1087057109335679
Cai, Z.-J. (2021). “Chapter two - hypothalamic aging and hormones,” in Vitamins and Hormones, ed. G. Litwack (Cambridge, MA; San Diego, CA: Elsevier), 15–37.
Camarero, G., Leon, Y., Gorospe, I., De Pablo, F., Alsina, B., Giraldez, F., et al. (2003). Insulin-like growth factor 1 is required for survival of transit-amplifying neuroblasts and differentiation of otic neurons. Dev. Biol. 262, 242–253. doi: 10.1016/S0012-1606(03)00387-7
Campos, L. S. (2005). β1 integrins and neural stem cells: making sense of the extracellular environment. Bioessays 27, 698–707. doi: 10.1002/bies.20256
Cardó-Vila, M., Giordano, R. J., Sidman, R. L., Bronk, L. F., Fan, Z., Mendelsohn, J., et al. (2010). From combinatorial peptide selection to drug prototype (II): targeting the epidermal growth factor receptor pathway. Proc. Natl. Acad. Sci. U. S. A. 107, 5118–5123. doi: 10.1073/pnas.0915146107
Carlini, V., Noonan, D. M., Abdalalem, E., Goletti, D., Sansone, C., Calabrone, L., et al. (2023). The multifaceted nature of IL-10: regulation, role in immunological homeostasis and its relevance to cancer, COVID-19 and post-COVID conditions. Front. Immunol. 14:1161067. doi: 10.3389/fimmu.2023.1161067
Carniglia, L., Ramírez, D., Durand, D., Saba, J., Caruso, C., and Lasaga, M. (2016). [Nle4, D-Phe7]-α-MSH inhibits toll-like receptor (TLR)2- and TLR4-induced microglial activation and promotes a M2-like phenotype. PLoS ONE 11:e0158564. doi: 10.1371/journal.pone.0158564
Carniglia, L., Ramírez, D., Durand, D., Saba, J., Turati, J., Caruso, C., et al. (2017). Neuropeptides and microglial activation in inflammation, pain, and neurodegenerative diseases. Mediat. Inflamm. 2017:5048616. doi: 10.1155/2017/5048616
Casali, B. T., and Reed-Geaghan, E. G. (2021). Microglial function and regulation during development, homeostasis and Alzheimer's disease. Cells 10:957. doi: 10.3390/cells10040957
Cascieri, M. A., Chicchi, G. G., Applebaum, J., Green, B. G., Hayes, N. S., and Bayne, M. L. (1989). Structural analogs of human insulin-like growth factor (IGF) I with altered affinity for type 2 IGF receptors. J. Biol. Chem. 264, 2199–2202. doi: 10.1016/S0021-9258(18)94162-1
Castillo-González, J., Ruiz, J. L., Serrano-Martínez, I., Forte-Lago, I., Ubago-Rodriguez, A., Caro, M., et al. (2023). Cortistatin deficiency reveals a dysfunctional brain endothelium with impaired gene pathways, exacerbated immune activation, and disrupted barrier integrity. J. Neuroinflammation 20:226. doi: 10.1186/s12974-023-02908-5
Castro, T. G., Melle-Franco, M., Sousa, C. E. A., Cavaco-Paulo, A., and Marcos, J. C. (2023). Non-canonical amino acids as building blocks for peptidomimetics: structure, function, and applications. Biomolecules 13:981. doi: 10.3390/biom13060981
Catania, A., Gatti, S., Colombo, G., and Lipton, J. M. (2004). Targeting melanocortin receptors as a novel strategy to control inflammation. Pharmacol. Rev. 56, 1–29. doi: 10.1124/pr.56.1.1
Cawley, N. X., Li, Z., and Loh, Y. P. (2016). Biosynthesis, trafficking and secretion of pro-opiomelanocortin-derived peptides. J. Mol. Endocrinol. 56, T77–T97. doi: 10.1530/JME-15-0323
Cen, L.-P., Ng, T. K., Chu, W. K., and Pang, C. P. (2022). Growth hormone-releasing hormone receptor signaling in experimental ocular inflammation and neuroprotection. Neural Regen. Res. 17, 2643–2648. doi: 10.4103/1673-5374.336135
Chamberlin, S. G., Sargood, K. J., Richter, A., Mellor, J. M., Anderson, D. W., Richards, N. G. J., et al. (1995). Constrained peptide analogues of transforming growth factor-α residues cysteine 21-32 are mitogenically active: use of proline mimetics to enhance biological potency (*). J. Biol. Chem. 270, 21062–21067. doi: 10.1074/jbc.270.36.21062
Chanat, E., and Huttner, W. B. (1991). Milieu-induced, selective aggregation of regulated secretory proteins in the trans-Golgi network. J. Cell Biol. 115, 1505–1519. doi: 10.1083/jcb.115.6.1505
Chang, C.-C., Liu, C.-D., Pan, S.-F., Huang, W.-H., Peng, C.-W., and Hsu, H.-J. (2020). Targeting of interleukin-10 receptor by a potential human interleukin-10 peptide efficiently blocks interleukin-10 pathway-dependent cell proliferation. Tzu Chin. Med. J. 32, 245–253. doi: 10.4103/tcmj.tcmj_237_19
Chang, H., Qin, W., Li, Y., Zhang, J., Lin, Z., Lv, M., et al. (2007). A novel human scFv fragment against TNF-alpha from de novo design method. Mol. Immunol. 44, 3789–3796. doi: 10.1016/j.molimm.2007.03.020
Chen, L., Lin, Y.-L., Peng, G., and Li, F. (2012). Structural basis for multifunctional roles of mammalian aminopeptidase N. Proc. Natl. Acad. Sci. U. S. A. 109, 17966–17971. doi: 10.1073/pnas.1210123109
Chen, L., Yang, N., Li, Y., Li, Y., Hong, J., Wang, Q., et al. (2021). Cholecystokinin octapeptide improves hippocampal glutamatergic synaptogenesis and postoperative cognition by inhibiting induction of A1 reactive astrocytes in aged mice. CNS Neurosci. Ther. 27, 1374–1384. doi: 10.1111/cns.13718
Chirinos-Rojas, C. L., Steward, M. W., and Partidos, C. D. (1997). Use of a solid-phase random peptide library to identify inhibitors of TNF-alpha mediated cytotoxicity in vitro. Cytokine 9, 226–232. doi: 10.1006/cyto.1996.0158
Chirinos-Rojas, C. L., Steward, M. W., and Partidos, C. D. (1998). A peptidomimetic antagonist of TNF-alpha-mediated cytotoxicity identified from a phage-displayed random peptide library. J. Immunol. 161, 5621–5626. doi: 10.4049/jimmunol.161.10.5621
Choi, J. G., Jeong, M., Joo, B. R., Ahn, J.-H., Woo, J.-H., Kim, D.-H., et al. (2021). Reduced levels of intestinal neuropeptides and neurotrophins in neurotoxin-induced parkinson disease mouse models. J. Neuropathol. Exp. Neurol. 80, 15–20. doi: 10.1093/jnen/nlaa113
Chrudinová, M., Žáková, L., Marek, A., Socha, O., Buděšínský, M., Hubálek, M., et al. (2018). A versatile insulin analog with high potency for both insulin and insulin-like growth factor 1 receptors: structural implications for receptor binding. J. Biol. Chem. 293, 16818–16829. doi: 10.1074/jbc.RA118.004852
Chung, B. K. W., White, C. J., and Yudin, A. K. (2017). Solid-phase synthesis, cyclization, and site-specific functionalization of aziridine-containing tetrapeptides. Nat. Protoc. 12, 1277–1287. doi: 10.1038/nprot.2017.035
Ciesielski-Treska, J., Ulrich, G., Taupenot, L., Chasserot-Golaz, S., Corti, A., Aunis, D., et al. (1998). Chromogranin A induces a neurotoxic phenotype in brain microglial cells*. J. Biol. Chem. 273, 14339–14346. doi: 10.1074/jbc.273.23.14339
Cobos Caceres, C., Bansal, P. S., Navarro, S., Wilson, D., Don, L., Giacomin, P., et al. (2017). An engineered cyclic peptide alleviates symptoms of inflammation in a murine model of inflammatory bowel disease. J. Biol. Chem. 292, 10288–10294. doi: 10.1074/jbc.M117.779215
Consonni, A., Morara, S., Codazzi, F., Grohovaz, F., and Zacchetti, D. (2011). Inhibition of lipopolysaccharide-induced microglia activation by calcitonin gene related peptide and adrenomedullin. Mol. Cell. Neurosci. 48, 151–160. doi: 10.1016/j.mcn.2011.07.006
Cope, E. C., LaMarca, E. A., Monari, P. K., Olson, L. B., Martinez, S., Zych, A. D., et al. (2018). Microglia play an active role in obesity-associated cognitive decline. J. Neurosci. 38, 8889–8904. doi: 10.1523/JNEUROSCI.0789-18.2018
Corkrum, M., Rothwell, P. E., Thomas, M. J., Kofuji, P., and Araque, A. (2019). Opioid-mediated astrocyte–neuron signaling in the nucleus accumbens. Cells 8:586. doi: 10.3390/cells8060586
Cowan, E., Burch, K. J., Green, B. D., and Grieve, D. J. (2016). Obestatin as a key regulator of metabolism and cardiovascular function with emerging therapeutic potential for diabetes. Br. J. Pharmacol. 173, 2165–2181. doi: 10.1111/bph.13502
Cox, F. F., Berezin, V., Bock, E., and Lynch, M. A. (2013). The neural cell adhesion molecule-derived peptide, FGL, attenuates lipopolysaccharide-induced changes in glia in a CD200-dependent manner. Neuroscience 235, 141–148. doi: 10.1016/j.neuroscience.2012.12.030
Crijns, H., Adyns, L., Ganseman, E., Cambier, S., Vandekerckhove, E., Pörtner, N., et al. (2021). Affinity and specificity for binding to glycosaminoglycans can be tuned by adapting peptide length and sequence. Int. J. Mol. Sci. 23, 447. doi: 10.3390/ijms23010447
Croci, L., Barili, V., Chia, D., Massimino, L., van Vugt, R., Masserdotti, G., et al. (2011). Local insulin-like growth factor I expression is essential for Purkinje neuron survival at birth. Cell Death Differ. 18, 48–59. doi: 10.1038/cdd.2010.78
Cui, H., Kohsaka, A., Waki, H., Gouraud, S., Nakamura, T., Yukawa, K., et al. (2008). Adrenomedullin 2 microinjection into the nucleus tractus solitarius elevates arterial pressure and heart rate in rats. Auton. Neurosci. 142, 45–50. doi: 10.1016/j.autneu.2008.07.009
Cuitavi, J., Andrés-Herrera, P., Meseguer, D., Campos-Jurado, Y., Lorente, J. D., Caruana, H., et al. (2023). Focal mu-opioid receptor activation promotes neuroinflammation and microglial activation in the mesocorticolimbic system: alterations induced by inflammatory pain. Glia 71, 1906–1920. doi: 10.1002/glia.24374
Cullen, J. M., and Cascella, M. (2024). “Physiology, Enkephalin,” in StatPearls (Treasure Island, FL: StatPearls Publishing). Available online at: http://www.ncbi.nlm.nih.gov/books/NBK557764/ (accessed June 27, 2024).
Dalmasso, G., Charrier-Hisamuddin, L., Nguyen, H. T. T., Yan, Y., Sitaraman, S., and Merlin, D. (2008). PepT1-mediated tripeptide KPV uptake reduces intestinal inflammation. Gastroenterology 134, 166–178. doi: 10.1053/j.gastro.2007.10.026
Dalton, C. J., and Lemmon, C. A. (2021). Fibronectin: molecular structure, fibrillar structure and mechanochemical signaling. Cells 10:2443. doi: 10.3390/cells10092443
D'amico, M. A., Ghinassi, B., Izzicupo, P., Manzoli, L., and Di Baldassarre, A. (2014). Biological function and clinical relevance of chromogranin A and derived peptides. Endocr. Connect. 3, R45–54. doi: 10.1530/EC-14-0027
de Castro Brás, L. E., and Frangogiannis, N. G. (2020). Extracellular matrix-derived peptides in tissue remodeling and fibrosis. Matrix Biol. 91–92, 176–187. doi: 10.1016/j.matbio.2020.04.006
de Lecea, L. (2008). Cortistatin–functions in the central nervous system. Mol. Cell. Endocrinol. 286, 88–95. doi: 10.1016/j.mce.2007.12.014
Delgado, M., and Ganea, D. (2003). Neuroprotective effect of vasoactive intestinal peptide (VIP) in a mouse model of Parkinson's disease by blocking microglial activation. FASEB J. 17, 944–946. doi: 10.1096/fj.02-0799fje
Delgado, M., Leceta, J., and Ganea, D. (2003). Vasoactive intestinal peptide and pituitary adenylate cyclase-activating polypeptide inhibit the production of inflammatory mediators by activated microglia. J. Leukoc. Biol. 73, 155–164. doi: 10.1189/jlb.0702372
Delgado, M., Varela, N., and Gonzalez-Rey, E. (2008). Vasoactive intestinal peptide protects against beta-amyloid-induced neurodegeneration by inhibiting microglia activation at multiple levels. Glia 56, 1091–1103. doi: 10.1002/glia.20681
Denes, V., Lukats, A., Szarka, G., Subicz, R., Mester, A., Kovacs-Valasek, A., et al. (2023). Long-term Effects of the pituitary-adenylate cyclase-activating Polypeptide (PACAP38) in the adult mouse retina: microglial activation and induction of neural proliferation. Neurochem. Res. 48, 3430–3446. doi: 10.1007/s11064-023-03989-7
Deng, X., Wang, L., You, X., Dai, P., and Zeng, Y. (2018). Advances in the T7 phage display system (review). Mol. Med. Rep. 17, 714–720. doi: 10.3892/mmr.2017.7994
Deveraux, Q. L., and Reed, J. C. (1999). IAP family proteins—suppressors of apoptosis. Genes Dev. 13, 239–252. doi: 10.1101/gad.13.3.239
Ding, X., Saxena, N. K., Lin, S., Gupta, N., and Anania, F. A. (2006). Exendin-4, a glucagon-like protein-1 (GLP-1) receptor agonist, reverses hepatic steatosis in ob/ob mice. Hepatology 43, 173–181. doi: 10.1002/hep.21006
Ding, X., Yan, Y., Li, X., Li, K., Ciric, B., Yang, J., et al. (2015). Silencing IFN-γ binding/signaling in astrocytes vs. microglia leads to opposite effects on CNS autoimmunity. J. Immunol. 194, 4251–4264. doi: 10.4049/jimmunol.1303321
Dong, Y., and Yong, V. W. (2019). When encephalitogenic T cells collaborate with microglia in multiple sclerosis. Nat. Rev. Neurol. 15, 704–717. doi: 10.1038/s41582-019-0253-6
Doti, N., Scognamiglio, P. L., Madonna, S., Scarponi, C., Ruvo, M., Perretta, G., et al. (2012). New mimetic peptides of the kinase-inhibitory region (KIR) of SOCS1 through focused peptide libraries. Biochem. J. 443, 231–240. doi: 10.1042/BJ20111647
Dotor, J., López-Vázquez, A. B., Lasarte, J. J., Sarobe, P., García-Granero, M., Riezu-Boj, J. I., et al. (2007). Identification of peptide inhibitors of transforming growth factor beta 1 using a phage-displayed peptide library. Cytokine 39, 106–115. doi: 10.1016/j.cyto.2007.06.004
Downer, E. J., Cowley, T. R., Cox, F., Maher, F. O., Berezin, V., Bock, E., et al. (2009). A synthetic NCAM-derived mimetic peptide, FGL, exerts anti-inflammatory properties via IGF-1 and interferon-gamma modulation. J. Neurochem. 109, 1516–1525. doi: 10.1111/j.1471-4159.2009.06076.x
Downer, E. J., Cowley, T. R., Lyons, A., Mills, K. H. G., Berezin, V., Bock, E., et al. (2010). A novel anti-inflammatory role of NCAM-derived mimetic peptide, FGL. Neurobiol. Aging 31, 118–128. doi: 10.1016/j.neurobiolaging.2008.03.017
Drucker, D. J. (2001). Glucagon-like peptide 21. J. Clin. Endocrinol. Metab. 86, 1759–1764. doi: 10.1210/jcem.86.4.7386
Dubynina, E. V., Inozemtseva, L. S., Markov, D. D., Yatsenko, K. A., Dolotov, O. V., and Grivennikov, I. A. (2009). Alpha-melanocyte-stimulating hormone increases the expression of vascular endothelial growth factor in rat hippocampal astrocytes in vitro. Neurochem. J. 3, 267–271. doi: 10.1134/S1819712409040059
Ehrlich, L. C., Hu, S., Sheng, W. S., Sutton, R. L., Rockswold, G. L., Peterson, P. K., et al. (1998). Cytokine regulation of human microglial cell IL-8 production. J. Immunol. 160, 1944–1948. doi: 10.4049/jimmunol.160.4.1944
Eissa, N., Hussein, H., Kermarrec, L., Grover, J., Metz-Boutigue, M.-H. E., Bernstein, C. N., et al. (2017). Chromofungin ameliorates the progression of colitis by regulating alternatively activated macrophages. Front. Immunol. 8:1131. doi: 10.3389/fimmu.2017.01131
Elter, J. K., Liščáková, V., Moravec, O., Vragović, M., Filipová, M., Štěpánek, P., et al. (2024). Solid-phase synthesis as a tool to create exactly defined, branched polymer vectors for cell membrane targeting. Macromolecules 57, 1050–1071. doi: 10.1021/acs.macromol.3c02600
Eriksson, P. S., Nilsson, M., Wågberg, M., Hansson, E., and Rönnbäck, L. (1993). Kappa-opioid receptors on astrocytes stimulate L-type Ca2+ channels. Neuroscience 54, 401–407. doi: 10.1016/0306-4522(93)90261-D
Ezquerro, I.-J., Lasarte, J.-J., Dotor, J., Castilla-Cortázar, I., Bustos, M., Peñuelas, I., et al. (2003). A synthetic peptide from transforming growth factor beta type III receptor inhibits liver fibrogenesis in rats with carbon tetrachloride liver injury. Cytokine 22, 12–20. doi: 10.1016/S1043-4666(03)00101-7
Fan, Y., Chang, H., Yu, Y., Liu, J., Zhao, L., Yang, D., et al. (2006). Thymopentin (TP5), an immunomodulatory peptide, suppresses proliferation and induces differentiation in HL-60 cells. Biochim. Biophys. Acta 1763, 1059–1066. doi: 10.1016/j.bbamcr.2006.07.004
Feindt, J., Schmidt, A., and Mentlein, R. (1998). Receptors and effects of the inhibitory neuropeptide somatostatin in microglial cells. Brain Res. Mol. Brain Res. 60, 228–233. doi: 10.1016/S0169-328X(98)00184-3
Fernández-Martos, C. M., González, P., and Rodriguez, F. J. (2012). Acute leptin treatment enhances functional recovery after spinal cord injury. PLoS ONE 7:e35594. doi: 10.1371/journal.pone.0035594
Ferrari-Lacraz, S., and Ferrari, S. (2011). Do RANKL inhibitors (denosumab) affect inflammation and immunity? Osteoporos. Int. 22, 435–446. doi: 10.1007/s00198-010-1326-y
Ferreira, R., Xapelli, S., Santos, T., Silva, A. P., Cristóvão, A., Cortes, L., et al. (2010). Neuropeptide Y modulation of interleukin-1β (IL-1β)-induced nitric oxide production in microglia. J. Biol. Chem. 285, 41921–41934. doi: 10.1074/jbc.M110.164020
Ferreira, S. H., Lorenzetti, B. B., Bristow, A. F., and Poole, S. (1988). Interleukin-1 beta as a potent hyperalgesic agent antagonized by a tripeptide analogue. Nature 334, 698–700. doi: 10.1038/334698a0
Flowers, L. O., Johnson, H. M., Mujtaba, M. G., Ellis, M. R., Haider, S. M. I., and Subramaniam, P. S. (2004). Characterization of a peptide inhibitor of Janus kinase 2 that mimics suppressor of cytokine signaling 1 function. J. Immunol. 172, 7510–7518. doi: 10.4049/jimmunol.172.12.7510
Forte, L. R. (2004a). “Guanylin,” in Encyclopedia of Gastroenterology, ed L. R. Johnson (New York, NY: Elsevier), 262–264.
Forte, L. R. (2004b). Uroguanylin and guanylin peptides: pharmacology and experimental therapeutics. Pharmacol. Ther. 104, 137–162. doi: 10.1016/j.pharmthera.2004.08.007
Frago, L. M., and Chowen, J. A. (2017). Involvement of astrocytes in mediating the central effects of ghrelin. Int. J. Mol. Sci. 18, 536. doi: 10.3390/ijms18030536
Fu, W., Shi, D., Westaway, D., and Jhamandas, J. H. (2015). Bioenergetic mechanisms in astrocytes may contribute to amyloid plaque deposition and toxicity. J. Biol. Chem. 290, 12504–12513. doi: 10.1074/jbc.M114.618157
Fu, W., Vukojevic, V., Patel, A., Soudy, R., MacTavish, D., Westaway, D., et al. (2017). Role of microglial amylin receptors in mediating beta amyloid (Aβ)-induced inflammation. J. Neuroinflamm. 14:199. doi: 10.1186/s12974-017-0972-9
Fuente-Martín, E., García-Cáceres, C., Argente-Arizón, P., Díaz, F., Granado, M., Freire-Regatillo, A., et al. (2016). Ghrelin regulates glucose and glutamate transporters in hypothalamic astrocytes. Sci. Rep. 6:23673. doi: 10.1038/srep23673
Fukuno, Y., Hayashi, S., Kohsa, K., Fujisawa, N., Tominaga, M., Miller, E. J., et al. (2003). Chemokine receptor inhibitor, Antileukinate, suppressed ovalbumin-induced eosinophilic inflammation in the airway. Cytokine 22, 116–125. doi: 10.1016/S1043-4666(03)00121-2
Gadepalli, A., Ummadisetty, O., Akhilesh Chouhan, D., Yadav, K. E., and Tiwari, V. (2023). Peripheral mu-opioid receptor activation by dermorphin [D-Arg2, Lys4] (1–4) amide alleviates behavioral and neurobiological aberrations in rat model of chemotherapy-induced neuropathic pain. Neurotherapeutics 21:e00302. doi: 10.1016/j.neurot.2023.10.012
Galimberti, D., Baron, P., Meda, L., Prat, E., Scarpini, E., Delgado, R., et al. (1999). α-MSH peptides inhibit production of nitric oxide and tumor necrosis factor-α by microglial cells activated with β-amyloid and interferon γ. Biochem. Biophys. Res. Commun. 263, 251–256. doi: 10.1006/bbrc.1999.1276
Gao, Z., Zhu, Q., Zhang, Y., Zhao, Y., Cai, L., Shields, C. B., et al. (2013). Reciprocal modulation between microglia and astrocyte in reactive gliosis following the CNS injury. Mol. Neurobiol. 48, 690–701. doi: 10.1007/s12035-013-8460-4
Garland, E. F., Hartnell, I. J., and Boche, D. (2022). Microglia and astrocyte function and communication: what do we know in humans? Front. Neurosci. 16:824888. doi: 10.3389/fnins.2022.824888
Gavioli, E. C., de Medeiros, I. U., Monteiro, M. C., Calo, G., and Romão, P. R. T. (2015). “Chapter nine - nociceptin/orphanin FQ-NOP receptor system in inflammatory and immune-mediated diseases,” in Vitamins & Hormones, ed. G. Litwack (Cambridge, MA; San Diego, CA: Elsevier), 241–266.
Gelain, F., Bottai, D., Vescovi, A., and Zhang, S. (2006). Designer self-assembling peptide nanofiber scaffolds for adult mouse neural stem cell 3-dimensional cultures. PLoS ONE 1:e119. doi: 10.1371/journal.pone.0000119
Geranurimi, A., Cheng, C. W. H., Quiniou, C., Côté, F., Hou, X., Lahaie, I., et al. (2020). Interleukin-1 receptor modulation using β-substituted α-amino-γ-lactam peptides from solid-phase synthesis and diversification. Front. Chem. 8:610431. doi: 10.3389/fchem.2020.610431
Ghia, J.-E., Crenner, F., Metz-Boutigue, M.-H., Aunis, D., and Angel, F. (2004). Effects of a chromogranin-derived peptide (CgA 47-66) in the writhing nociceptive response induced by acetic acid in rats. Regul. Pept. 119, 199–207. doi: 10.1016/j.regpep.2004.02.014
Glatthar, R., and Giese, B. (2000). A new photocleavable linker in solid-phase chemistry for ether cleavage. Org. Lett. 2, 2315–2317. doi: 10.1021/ol006076h
Golias, C., Charalabopoulos, A., Stagikas, D., Charalabopoulos, K., and Batistatou, A. (2007). The kinin system - bradykinin: biological effects and clinical implications. Multiple role of the kinin system - bradykinin. Hippokratia 11, 124–128.
Gomez-Nicola, D., Valle-Argos, B., and Nieto-Sampedro, M. (2010). Blockade of IL-15 activity inhibits microglial activation through the NFkappaB, p38, and ERK1/2 pathways, reducing cytokine and chemokine release. Glia 58, 264–276. doi: 10.1002/glia.20920
Gonçalves, J., Ribeiro, C. F., Malva, J. O., and Silva, A. P. (2012). Protective role of neuropeptide Y Y2 receptors in cell death and microglial response following methamphetamine injury. Eur. J. Neurosci. 36, 3173–3183. doi: 10.1111/j.1460-9568.2012.08232.x
Gong, R., Ding, C., Hu, J., Lu, Y., Liu, F., Mann, E., et al. (2011). Role for the membrane receptor guanylyl cyclase-C in attention deficiency and hyperactive behavior. Science 333, 1642–1646. doi: 10.1126/science.1207675
Gonzalez-Rodriguez, M., Astillero-Lopez, V., Villanueva-Anguita, P., Paya-Rodriguez, M. E., Flores-Cuadrado, A., Villar-Conde, S., et al. (2021). Somatostatin and astroglial involvement in the human limbic system in Alzheimer's disease. Int. J. Mol. Sci. 22:8434. doi: 10.3390/ijms22168434
Gou, H., Sun, D., Hao, L., An, M., Xie, B., Cong, B., et al. (2020). Cholecystokinin-8 attenuates methamphetamine-induced inflammatory activation of microglial cells through CCK2 receptor. Neurotoxicology 81, 70–79. doi: 10.1016/j.neuro.2020.09.001
Gründemann, C., Thell, K., Lengen, K., Garcia-Käufer, M., Huang, Y.-H., Huber, R., et al. (2013). Cyclotides suppress human T-lymphocyte proliferation by an interleukin 2-dependent mechanism. PLoS ONE 8:e68016. doi: 10.1371/journal.pone.0068016
Guerra-Gomes, S., Sousa, N., Pinto, L., and Oliveira, J. F. (2017). Functional roles of astrocyte calcium elevations: from synapses to behavior. Front. Cell. Neurosci. 11:427. doi: 10.3389/fncel.2017.00427
Guo, H.-P., Luo, H.-B., Liu, Y.-J., Zhu, P., and Fu, N. (2002). Screening of tumor necrosis factor-alpha-binding peptides by phage display peptide library. Di Yi Jun. Yi Da Xue Xue Bao 22, 597–599.
Gupta, A., Gullapalli, S., Pan, H., Ramos-Ortolaza, D. L., Hayward, M. D., Low, M. J., et al. (2021). Regulation of opioid receptors by their endogenous opioid peptides. Cell. Mol. Neurobiol. 41, 1103–1118. doi: 10.1007/s10571-020-01015-w
Habek, N., Ratko, M., and DugandŽić, A. (2021). Uroguanylin increases Ca2+ concentration in astrocytes via guanylate cyclase C-independent signaling pathway. Croat. Med. J. 62, 250–263. doi: 10.3325/cmj.2021.62.250
Hahn, M., Winkler, D., Welfle, K., Misselwitz, R., Welfle, H., Wessner, H., et al. (2001). Cross-reactive binding of cyclic peptides to an anti-TGFalpha antibody Fab fragment: an X-ray structural and thermodynamic analysis. J. Mol. Biol. 314, 293–309. doi: 10.1006/jmbi.2001.5135
Hahnenkamp, A., Schäfers, M., Bremer, C., and Höltke, C. (2013). Design and synthesis of small-molecule fluorescent photoprobes targeted to aminopeptdase N (APN/CD13) for optical imaging of angiogenesis. Bioconjug. Chem. 24, 1027–1038. doi: 10.1021/bc400074w
Hanafy, N. A. N., Fabregat, I., Leporatti, S., and El Kemary, M. (2020). Encapsulating TGF-β1 inhibitory peptides P17 and P144 as a promising strategy to facilitate their dissolution and to improve their functionalization. Pharmaceutics 12:421. doi: 10.3390/pharmaceutics12050421
Hanisch, U.-K. (2002). Microglia as a source and target of cytokines. Glia 40, 140–155. doi: 10.1002/glia.10161
Harada, R., Okamura, N., Furumoto, S., Yoshikawa, T., Arai, H., Yanai, K., et al. (2014). Use of a benzimidazole derivative BF-188 in fluorescence multispectral imaging for selective visualization of tau protein fibrils in the Alzheimer's disease brain. Mol. Imaging Biol. 16, 19–27. doi: 10.1007/s11307-013-0667-2
Harno, E., Gali Ramamoorthy, T., Coll, A. P., and White, A. (2018). POMC: the physiological power of hormone processing. Physiol. Rev. 98, 2381–2430. doi: 10.1152/physrev.00024.2017
Hattori, Y. (2023). The multifaceted roles of embryonic microglia in the developing brain. Front. Cell. Neurosci. 17:988952. doi: 10.3389/fncel.2023.988952
Hay, D. L., Chen, S., Lutz, T. A., Parkes, D. G., and Roth, J. D. (2015). Amylin: pharmacology, physiology, and clinical potential. Pharmacol. Rev. 67, 564–600. doi: 10.1124/pr.115.010629
Hayashi, S., Yatsunami, J., Fukuno, Y., Kawashima, M., and Miller, E. J. (2002). Antileukinate, a hexapeptide inhibitor of CXC-chemokine receptor, suppresses bleomycin-induced acute lung injury in mice. Lung 180, 339–348. doi: 10.1007/s00408-002-0106-7
Henry, M. S., Gendron, L., Tremblay, M.-E., and Drolet, G. (2017). Enkephalins: endogenous analgesics with an emerging role in stress resilience. Neural Plast. 2017:1546125. doi: 10.1155/2017/1546125
Hermida, N., López, B., González, A., Dotor, J., Lasarte, J. J., Sarobe, P., et al. (2009). A synthetic peptide from transforming growth factor-beta1 type III receptor prevents myocardial fibrosis in spontaneously hypertensive rats. Cardiovasc. Res. 81, 601–609. doi: 10.1093/cvr/cvn315
Hernández, C., Arroba, A. I., Bogdanov, P., Ramos, H., Simó-Servat, O., Simó, R., et al. (2020). Effect of topical administration of somatostatin on retinal inflammation and neurodegeneration in an experimental model of diabetes. J. Clin. Med. 9:2579. doi: 10.3390/jcm9082579
Hindinger, C., Bergmann, C. C., Hinton, D. R., Phares, T. W., Parra, G. I., Hussain, S., et al. (2012). IFN-γ signaling to astrocytes protects from autoimmune mediated neurological disability. PLoS ONE 7:e42088. doi: 10.1371/journal.pone.0042088
Holzer, P., Reichmann, F., and Farzi, A. (2012). Neuropeptide Y, peptide YY and pancreatic polypeptide in the gut–brain axis. Neuropeptides 46, 261–274. doi: 10.1016/j.npep.2012.08.005
Hook, V., Funkelstein, L., Lu, D., Bark, S., Wegrzyn, J., and Hwang, S.-R. (2008). Proteases for processing proneuropeptides into peptide neurotransmitters and hormones. Annu. Rev. Pharmacol. Toxicol. 48, 393–423. doi: 10.1146/annurev.pharmtox.48.113006.094812
Huang, Y., Wu, J., Zhang, H., Li, Y., Wen, L., Tan, X., et al. (2023). The gut microbiome modulates the transformation of microglial subtypes. Mol. Psychiatry 28, 1611–1621. doi: 10.1038/s41380-023-02017-y
Hughes, J., Smith, T. W., Kosterlitz, H. W., Fothergill, L. A., Morgan, B. A., and Morris, H. R. (1975). Identification of two related pentapeptides from the brain with potent opiate agonist activity. Nature 258, 577–579. doi: 10.1038/258577a0
Huisman, C., Kok, P., Schmaal, L., and Verhoog, P. (2008). Bradykinin: a microglia attractant in vivo? J. Neurosci. 28, 3531–3532. doi: 10.1523/JNEUROSCI.0374-08.2008
Iadecola, C., and Anrather, J. (2011). The immunology of stroke: from mechanisms to translation. Nat. Med. 17, 796–808. doi: 10.1038/nm.2399
Idriss, H. T., and Naismith, J. H. (2000). TNF alpha and the TNF receptor superfamily: structure-function relationship(s). Microsc. Res. Tech. 50, 184–195. doi: 10.1002/1097-0029(20000801)50:3<184::AID-JEMT2>3.0.CO;2-H
Ivan, D. C., Berve, K. C., Walthert, S., Monaco, G., Borst, K., Bouillet, E., et al. (2023). Insulin-like growth factor-1 receptor controls the function of CNS-resident macrophages and their contribution to neuroinflammation. Acta Neuropathol. Commun. 11:35. doi: 10.1186/s40478-023-01535-8
Iwai, T., Iinuma, Y., Kodani, R., and Oka, J.-I. (2008). Neuromedin U inhibits inflammation-mediated memory impairment and neuronal cell-death in rodents. Neurosci. Res. 61, 113–119. doi: 10.1016/j.neures.2008.01.018
Iwasaki, M., Akiba, Y., and Kaunitz, J. D. (2019). Recent advances in vasoactive intestinal peptide physiology and pathophysiology: focus on the gastrointestinal system. F1000Res 8:F1000 Faculty Rev-1629. doi: 10.12688/f1000research.18039.1
Jain, M., Singh, M. K., Shyam, H., Mishra, A., Kumar, S., Kumar, A., et al. (2021). Role of JAK/STAT in the neuroinflammation and its association with neurological disorders. Ann. Neurosci. 28:191. doi: 10.1177/09727531211070532
Ji, R.-R., Donnelly, C. R., and Nedergaard, M. (2019). Astrocytes in chronic pain and itch. Nat. Rev. Neurosci. 20, 667–685. doi: 10.1038/s41583-019-0218-1
Jiang, W., Wang, X., Wang, W., Hua, F., Zhang, Z., Zhang, Z., et al. (2020). Inhibition of NK1R attenuates LPS-induced microglial inflammation and consequent death of PC12 cells. Brain Res. Bull. 162, 115–124. doi: 10.1016/j.brainresbull.2020.05.015
Jiang, Y., Li, X., Hou, J., Huang, Y., Jia, Y., Zou, M., et al. (2016). Discovery of BC-01, a novel mutual prodrug (hybrid drug) of ubenimex and fluorouracil as anticancer agent. Eur. J. Med. Chem. 121, 649–657. doi: 10.1016/j.ejmech.2016.05.068
Jiang, Y., Peng, T., Gaur, U., Silva, M., Little, P., Chen, Z., et al. (2019). Role of corticotropin releasing factor in the neuroimmune mechanisms of depression: examination of current pharmaceutical and herbal therapies. Front. Cell. Neurosci. 13:290. doi: 10.3389/fncel.2019.00290
Johnson, M. B., Young, A. D., and Marriott, I. (2017). The therapeutic potential of targeting substance P/NK-1R interactions in inflammatory CNS disorders. Front. Cell. Neurosci. 10:296. doi: 10.3389/fncel.2016.00296
Joshi, S., Chen, L., Winter, M. B., Lin, Y.-L., Yang, Y., Shapovalova, M., et al. (2017). The rational design of therapeutic peptides for aminopeptidase N using a substrate-based approach. Sci. Rep. 7:1424. doi: 10.1038/s41598-017-01542-5
Kaiserova, M., Chudackova, M., Mensikova, K., Vastik, M., Kurcova, S., Prikrylova Vranova, H., et al. (2021). Cerebrospinal fluid levels of chromogranin a in Parkinson's disease and multiple system atrophy. Brain Sci. 11, 141. doi: 10.3390/brainsci11020141
Kam, L., Shain, W., Turner, J. N., and Bizios, R. (2002). Selective adhesion of astrocytes to surfaces modified with immobilized peptides. Biomaterials 23, 511–515. doi: 10.1016/S0142-9612(01)00133-8
Kamermans, A., Verhoeven, T., van het Hof, B., Koning, J. J., Borghuis, L., Witte, M., et al. (2019). Setmelanotide, a novel, selective melanocortin receptor-4 agonist exerts anti-inflammatory actions in astrocytes and promotes an anti-inflammatory macrophage phenotype. Front. Immunol. 10:2312. doi: 10.3389/fimmu.2019.02312
Kang, S. S., Ebbert, M. T. W., Baker, K. E., Cook, C., Wang, X., Sens, J. P., et al. (2018). Microglial translational profiling reveals a convergent APOE pathway from aging, amyloid, and tau. J. Exp. Med. 215, 2235–2245. doi: 10.1084/jem.20180653
Kann, O., Almouhanna, F., and Chausse, B. (2022). Interferon γ: a master cytokine in microglia-mediated neural network dysfunction and neurodegeneration. Trends Neurosci. 45, 913–927. doi: 10.1016/j.tins.2022.10.007
Karki, P., Smith, K., Johnson, J., and Lee, E. (2014). Astrocyte-derived growth factors and estrogen neuroprotection: role of transforming growth factor-α in estrogen-induced upregulation of glutamate transporters in astrocytes. Mol. Cell. Endocrinol. 389, 58–64. doi: 10.1016/j.mce.2014.01.010
Karra, E., Chandarana, K., and Batterham, R. L. (2009). The role of peptide YY in appetite regulation and obesity. J. Physiol. 587, 19–25. doi: 10.1113/jphysiol.2008.164269
Kato, T., Monji, A., Hashioka, S., and Kanba, S. (2007). Risperidone significantly inhibits interferon-gamma-induced microglial activation in vitro. Schizophr. Res. 92, 108–115. doi: 10.1016/j.schres.2007.01.019
Kelly, B. A., Harrison, I., McKnight, Á., and Dobson, C. B. (2010). Anti-infective activity of apolipoprotein domain derived peptides in vitro: identification of novel antimicrobial peptides related to apolipoprotein B with anti-HIV activity. BMC Immunol. 11:13. doi: 10.1186/1471-2172-11-13
Kim, J.-H., Afridi, R., Cho, E., Yoon, J. H., Lim, Y.-H., Lee, H.-W., et al. (2022). Soluble ANPEP released from human astrocytes as a positive regulator of microglial activation and neuroinflammation: brain renin–angiotensin system in astrocyte–microglia crosstalk. Mol. Cell. Proteom. 21:100424. doi: 10.1016/j.mcpro.2022.100424
Kim, S., Moon, M., and Park, S. (2009). Exendin-4 protects dopaminergic neurons by inhibition of microglial activation and matrix metalloproteinase-3 expression in an animal model of Parkinson's disease. J. Endocrinol. 202, 431–439. doi: 10.1677/JOE-09-0132
Kim, W. K., Kan, Y., Ganea, D., Hart, R. P., Gozes, I., and Jonakait, G. M. (2000). Vasoactive intestinal peptide and pituitary adenylyl cyclase-activating polypeptide inhibit tumor necrosis factor-alpha production in injured spinal cord and in activated microglia via a cAMP-dependent pathway. J. Neurosci. 20, 3622–3630. doi: 10.1523/JNEUROSCI.20-10-03622.2000
Koller, A., Brunner, S. M., Bianchini, R., Ramspacher, A., Emberger, M., Sternberg, F., et al. (2019). Galanin is a potent modulator of cytokine and chemokine expression in human macrophages. Sci. Rep. 9:7237. doi: 10.1038/s41598-019-43704-7
Koss, K., Churchward, M. A., Tsui, C., and Todd, K. G. (2019). In vitro priming and hyper-activation of brain microglia: an assessment of phenotypes. Mol. Neurobiol. 56, 6409–6425. doi: 10.1007/s12035-019-1529-y
Koss, K. M., Churchward, M. A., Nguyen, A. T., Yager, J. Y., Todd, K. G., and Unsworth, L. D. (2016). Brain biocompatibility and microglia response towards engineered self-assembling (RADA)4 nanoscaffolds. Acta Biomater. 35, 127–137. doi: 10.1016/j.actbio.2016.02.001
Koss, K. M., Son, T., Li, C., Hao, Y., Cao, J., Churchward, M. A., et al. (2023). Toward discovering a novel family of peptides targeting neuroinflammatory states of brain microglia and astrocytes. J. Neurochem. doi: 10.1111/jnc.15840
Kotenko, S. V., and Pestka, S. (2000). Jak-Stat signal transduction pathway through the eyes of cytokine class II receptor complexes. Oncogene 19, 2557–2565. doi: 10.1038/sj.onc.1203524
Koutsopoulos, S., and Zhang, S. (2013). Long-term three-dimensional neural tissue cultures in functionalized self-assembling peptide hydrogels, matrigel and collagen I. Acta Biomater. 9, 5162–5169. doi: 10.1016/j.actbio.2012.09.010
Kovacs-Nolan, J., Zhang, H., Ibuki, M., Nakamori, T., Yoshiura, K., Turner, P. V., et al. (2012). The PepT1-transportable soy tripeptide VPY reduces intestinal inflammation. Biochim. Biophys. Acta 1820, 1753–1763. doi: 10.1016/j.bbagen.2012.07.007
Kowalczyk, R., Harris, P. W. R., Williams, G. M., Yang, S.-H., and Brimble, M. A. (2017). Peptide lipidation – a synthetic strategy to afford peptide based therapeutics. Peptides Peptide Based Biomater. Biomed. Appl. 1030, 185–227. doi: 10.1007/978-3-319-66095-0_9
Koyama, Y. (2015). Functional alterations of astrocytes in mental disorders: pharmacological significance as a drug target. Front. Cell. Neurosci. 9:261. doi: 10.3389/fncel.2015.00261
Kralingen, C., van Kho, D. T., Costa, J., Angel, C. E., and Graham, E. S. (2013). Exposure to inflammatory cytokines IL-1β and tnfα induces compromise and death of astrocytes; implications for chronic neuroinflammation. PLoS ONE 8:e84269. doi: 10.1371/journal.pone.0084269
Kurinami, H., Shimamura, M., Nakagami, H., Shimizu, H., Koriyama, H., Kawano, T., et al. (2016). A novel therapeutic peptide as a partial agonist of RANKL in ischemic stroke. Sci. Rep. 6:38062. doi: 10.1038/srep38062
La Manna, S., De Benedictis, I., and Marasco, D. (2022a). Proteomimetics of natural regulators of JAK–STAT pathway: novel therapeutic perspectives. Front. Mol. Biosci. 8:792546. doi: 10.3389/fmolb.2021.792546
La Manna, S., Di Natale, C., Florio, D., and Marasco, D. (2018a). Peptides as therapeutic agents for inflammatory-related diseases. Int. J. Mol. Sci. 19:2714. doi: 10.3390/ijms19092714
La Manna, S., Fortuna, S., Leone, M., Mercurio, F. A., Di Donato, I., Bellavita, R., et al. (2022b). Ad-hoc modifications of cyclic mimetics of SOCS1 protein: structural and functional insights. Eur. J. Med. Chem. 243:114781. doi: 10.1016/j.ejmech.2022.114781
La Manna, S., Lee, E., Ouzounova, M., Di Natale, C., Novellino, E., Merlino, A., et al. (2018b). Mimetics of suppressor of cytokine signaling 3: novel potential therapeutics in triple breast cancer. Int. J. Cancer 143, 2177–2186. doi: 10.1002/ijc.31594
La Manna, S., Lopez-Sanz, L., Bernal, S., Fortuna, S., Mercurio, F. A., Leone, M., et al. (2021). Cyclic mimetics of kinase-inhibitory region of suppressors of cytokine signaling 1: progress toward novel anti-inflammatory therapeutics. Eur. J. Med. Chem. 221, 113547. doi: 10.1016/j.ejmech.2021.113547
La Manna, S., Lopez-Sanz, L., Leone, M., Brandi, P., Scognamiglio, P. L., Morelli, G., et al. (2017). Structure-activity studies of peptidomimetics based on kinase-inhibitory region of suppressors of cytokine signaling 1. Biopolymers. doi: 10.1002/bip.23082
Labandeira-Garcia, J. L., Costa-Besada, M. A., Labandeira, C. M., Villar-Cheda, B., and Rodríguez-Perez, A. I. (2017). Insulin-like growth factor-1 and neuroinflammation. Front. Aging Neurosci. 9:365. doi: 10.3389/fnagi.2017.00365
Lacagnina, M. J., Rivera, P. D., and Bilbo, S. D. (2017). Glial and neuroimmune mechanisms as critical modulators of drug use and abuse. Neuropsychopharmacol 42, 156–177. doi: 10.1038/npp.2016.121
Lacquaniti, A., Donato, V., Chirico, V., Buemi, A., and Buemi, M. (2011). Obestatin: an interesting but controversial gut hormone. Ann. Nutr. Metab. 59, 193–199. doi: 10.1159/000334106
Lai, J. P., Zhan, G. X., Campbell, D. E., Douglas, S. D., and Ho, W. Z. (2000). Detection of substance P and its receptor in human fetal microglia. Neuroscience 101, 1137–1144. doi: 10.1016/S0306-4522(00)00398-5
LaPelusa, A., and Jan, A. (2024). “Biochemistry, bombesin,” in StatPearls (Treasure Island, FL: StatPearls Publishing). Available online at: http://www.ncbi.nlm.nih.gov/books/NBK541129/ (accessed June 27, 2024).
Laskowitz, D. T., Thekdi, A. D., Thekdi, S. D., Han, S. K., Myers, J. K., Pizzo, S. V., et al. (2001). Downregulation of microglial activation by apolipoprotein E and apoE-mimetic peptides. Exp. Neurol. 167, 74–85. doi: 10.1006/exnr.2001.7541
Lee, H., Liu, Z., Dong, L., Lee, D. Y., Yoon, D., Oh, H., et al. (2023). Anti-neuroinflammatory and neuroprotective effect of intermedin B isolated from the Curcuma longa L. via NF-κB and ROS inhibition in BV2 microglia and HT22 hippocampal cells. Int. J. Mol. Sci. 24:7390. doi: 10.3390/ijms24087390
Lee, H.-J., Macbeth, A. H., Pagani, J., and Young, W. S. (2009). Oxytocin: the great facilitator of life. Prog. Neurobiol. 88, 127–151. doi: 10.1016/j.pneurobio.2009.04.001
Lee, K., Dixon, A. K., Gonzalez, I., Stevens, E. B., McNulty, S., Oles, R., et al. (1999). Bombesin-like peptides depolarize rat hippocampal interneurones through interaction with subtype 2 bombesin receptors. J. Physiol. 518 (Pt 3), 791–802. doi: 10.1111/j.1469-7793.1999.0791p.x
Lee, S. J., Logsdon, A. F., Yagi, M., Baskin, B. M., Peskind, Elaine, R., et al. (2022). The dynorphin/kappa opioid receptor mediates adverse immunological and behavioral outcomes induced by repetitive blast trauma. J. Neuroinflamm. 19:288. doi: 10.1186/s12974-022-02643-3
Li, H., Aneja, R., and Chaiken, I. (2013). Click chemistry in peptide-based drug design. Molecules 18, 9797–9817. doi: 10.3390/molecules18089797
Li, J.-J., Chang, S.-F., Liau, I.-I., Chan, P.-C., Liu, R.-S., Yen, S.-H., et al. (2016). Targeted antitumor prodrug therapy using CNGRC-yCD fusion protein in combination with 5-fluorocytosine. J. Biomed. Sci. 23:15. doi: 10.1186/s12929-016-0227-6
Li, Q., and Barres, B. A. (2018). Microglia and macrophages in brain homeostasis and disease. Nat. Rev. Immunol. 18, 225–242. doi: 10.1038/nri.2017.125
Li, Q., Dong, C., Li, W., Bu, W., Wu, J., and Zhao, W. (2014). Neuropeptide Y protects cerebral cortical neurons by regulating microglial immune function. Neural Regen. Res. 9, 959–967. doi: 10.4103/1673-5374.133140
Liang, J., Chao, D., Sandhu, H. K., Yu, Y., Zhang, L., Balboni, G., et al. (2014). δ-Opioid receptors up-regulate excitatory amino acid transporters in mouse astrocytes. Br. J. Pharmacol. 171, 5417–5430. doi: 10.1111/bph.12857
Liddelow, S. A., and Barres, B. A. (2017). Reactive astrocytes: production, function, and therapeutic potential. Immunity 46, 957–967. doi: 10.1016/j.immuni.2017.06.006
Liddelow, S. A., Marsh, S. E., and Stevens, B. (2020). Microglia and astrocytes in disease: dynamic duo or partners in crime? Trends Immunol. 41, 820–835. doi: 10.1016/j.it.2020.07.006
Lim, C. T., and Khoo, B. (2000). “Normal physiology of ACTH and GH release in the hypothalamus and anterior pituitary in man,” in Endotext, eds. K. R. Feingold, B. Anawalt, M. R. Blackman, A. Boyce, G. Chrousos, E. Corpas, et al. (South Dartmouth, MA: MDText.com, Inc.). Available online at: http://www.ncbi.nlm.nih.gov/books/NBK279116/ (accessed June 27, 2024).
Lindberg, C., Hjorth, E., Post, C., Winblad, B., and Schultzberg, M. (2005). Cytokine production by a human microglial cell line: effects of beta-amyloid and alpha-melanocyte-stimulating hormone. Neurotox. Res. 8, 267–276. doi: 10.1007/BF03033980
Lipton, J. M., Catania, A., and Delgado, R. (1998). Peptide modulation of inflammatory processes within the brain. Neuroimmunomodulation 5, 178–183. doi: 10.1159/000026335
Liu, B., and Neufeld, A. H. (2007). Activation of epidermal growth factor receptors in astrocytes: from development to neural injury. J. Neurosci. Res. 85, 3523–3529. doi: 10.1002/jnr.21364
Liu, F., Li, Z., He, X., Yu, H., and Feng, J. (2019). Ghrelin attenuates neuroinflammation and demyelination in experimental autoimmune encephalomyelitis involving NLRP3 inflammasome signaling pathway and pyroptosis. Front. Pharmacol. 10:1320. doi: 10.3389/fphar.2019.01320
Liu, H.-T., Akita, T., Shimizu, T., Sabirov, R. Z., and Okada, Y. (2009). Bradykinin-induced astrocyte–neuron signalling: glutamate release is mediated by ROS-activated volume-sensitive outwardly rectifying anion channels. J. Physiol. 587, 2197–2209. doi: 10.1113/jphysiol.2008.165084
Liu, L., Xu, Y., Dai, H., Tan, S., Mao, X., and Chen, Z. (2020). Dynorphin activation of kappa opioid receptor promotes microglial polarization toward M2 phenotype via TLR4/NF-κB pathway. Cell Biosci. 10, 42. doi: 10.1186/s13578-020-00387-2
Liu, Q., Zhou, S., Wang, X., Gu, C., Guo, Q., Li, X., et al. (2022). Apelin alleviated neuroinflammation and promoted endogenous neural stem cell proliferation and differentiation after spinal cord injury in rats. J. Neuroinflamm. 19:160. doi: 10.1186/s12974-022-02518-7
Liu, X., Wang, Y., Zeng, Y., Wang, D., Wen, Y., Fan, L., et al. (2023). Microglia-neuron interactions promote chronic itch via the NLRP3-IL-1β-GRPR axis. Allergy 78, 1570–1584. doi: 10.1111/all.15699
Lloyd, A. F., Davies, C. L., Holloway, R. K., Labrak, Y., Ireland, G., Carradori, D., et al. (2019). Central nervous system regeneration is driven by microglia necroptosis and repopulation. Nat. Neurosci. 22, 1046–1052. doi: 10.1038/s41593-019-0418-z
Lobo-Silva, D., Carriche, G. M., Castro, A. G., Roque, S., and Saraiva, M. (2016). Balancing the immune response in the brain: IL-10 and its regulation. J. Neuroinflamm. 13:297. doi: 10.1186/s12974-016-0763-8
Lovshin, J., Estall, J., Yusta, B., Brown, T. J., and Drucker, D. J. (2001). Glucagon-like peptide (GLP)-2 action in the murine central nervous system is enhanced by elimination of GLP-1 receptor signaling. J. Biol. Chem. 276, 21489–21499. doi: 10.1074/jbc.M009382200
Lu, Y.-C., Yin, J.-B., Bai, Y., Li, X., Zhang, T., Yang, J., et al. (2022). Morphological features of endomorphin-2-immunoreactive ultrastructures in the dorsal root ganglion and spinal dorsal horn of the rat. J. Chem. Neuroanat. 125:102142. doi: 10.1016/j.jchemneu.2022.102142
Lucius, R., Sievers, J., and Mentlein, R. (1995). Enkephalin metabolism by microglial aminopeptidase N (CD13). J. Neurochem. 64, 1841–1847. doi: 10.1046/j.1471-4159.1995.64041841.x
Ludvik, B., Kautzky-Willer, A., Prager, R., Thomaseth, K., and Pacini, G. (1997). Amylin: history and overview. Diabet. Med. 14(Suppl. 2), S9–13. doi: 10.1002/(SICI)1096-9136(199706)14:2+<S9::AID-DIA397>3.0.CO;2-D
Lugardon, K., Chasserot-Golaz, S., Kieffer, A. E., Maget-Dana, R., Nullans, G., Kieffer, B., et al. (2001). Structural and biological characterization of chromofungin, the antifungal chromogranin A-(47-66)-derived peptide. J. Biol. Chem. 276, 35875–35882. doi: 10.1074/jbc.M104670200
Lundin, K., Aarum, J., Appelsved, L., Johansson-Borg, A., von Garrelts, E., Dypbukt, J., et al. (2003). Generation of microglia specific reagents from phage displayed peptide libraries. J. Immunol. Methods 278, 235–247. doi: 10.1016/S0022-1759(03)00241-2
Ma, J., Hou, Y.-H., Liao, Z.-Y., Ma, Z., Zhang, X.-X., Wang, J.-L., et al. (2023). Neuroprotective effects of leptin on the APP/PS1 Alzheimer's disease mouse model: role of microglial and neuroinflammation. Degener. Neurol. Neuromuscul. Dis. 13, 69–79. doi: 10.2147/DNND.S427781
Ma, L., Peng, S., Wei, J., Zhao, M., Ahmad, K. A., Chen, J., et al. (2021). Spinal microglial β-endorphin signaling mediates IL-10 and exenatide-induced inhibition of synaptic plasticity in neuropathic pain. CNS Neurosci. Ther. 27, 1157–1172. doi: 10.1111/cns.13694
Machelska, H., and Celik, M. Ö. (2020). Opioid receptors in immune and glial cells—implications for pain control. Front. Immunol. 11:300. doi: 10.3389/fimmu.2020.00300
Madonna, S., Scarponi, C., Doti, N., Carbone, T., Cavani, A., Scognamiglio, P. L., et al. (2013). Therapeutical potential of a peptide mimicking the SOCS1 kinase inhibitory region in skin immune responses. Eur. J. Immunol. 43, 1883–1895. doi: 10.1002/eji.201343370
Maduna, T., Audouard, E., Dembélé, D., Mouzaoui, N., Reiss, D., Massotte, D., et al. (2019). Microglia express mu opioid receptor: insights from transcriptomics and fluorescent reporter mice. Front. Psychiatry 9:726. doi: 10.3389/fpsyt.2018.00726
Maget-Dana, R. (1999). The monolayer technique: a potent tool for studying the interfacial properties of antimicrobial and membrane-lytic peptides and their interactions with lipid membranes. Biochim. Biophys. Acta 1462, 109–140. doi: 10.1016/S0005-2736(99)00203-5
Majerova, P., Zilkova, M., Kazmerova, Z., Kovac, A., Paholikova, K., Kovacech, B., et al. (2014). Microglia display modest phagocytic capacity for extracellular tau oligomers. J. Neuroinflamm. 11:161. doi: 10.1186/s12974-014-0161-z
Majumdar, I. D., and Weber, H. C. (2012). Appetite-modifying effects of bombesin receptor subtype-3 agonists. Handb. Exp. Pharmacol. 405–432. doi: 10.1007/978-3-642-24716-3_19
Malaise, M. G., Hazee-Hagelstein, M. T., Reuter, A. M., Vrinds-Gevaert, Y., Goldstein, G., and Franchimont, P. (1987). Thymopoietin and thymopentin enhance the levels of ACTH, beta-endorphin and beta-lipotropin from rat pituitary cells in vitro. Acta Endocrinol. 115, 455–460. doi: 10.1530/acta.0.1150455
Maldonado-Ruiz, R., Cárdenas-Tueme, M., Montalvo-Martínez, L., Vidaltamayo, R., Garza-Ocañas, L., Reséndez-Perez, D., et al. (2019). Priming of hypothalamic ghrelin signaling and microglia activation exacerbate feeding in rats' offspring following maternal overnutrition. Nutrients 11:1241. doi: 10.3390/nu11061241
Mali, A. S., and Novotny, J. (2022). Opioid receptor activation suppresses the neuroinflammatory response by promoting microglial M2 polarization. Mol. Cell. Neurosci. 121:103744. doi: 10.1016/j.mcn.2022.103744
Mallimo, E. M., and Kusnecov, A. W. (2013). The role of orphanin FQ/nociceptin in neuroplasticity: relationship to stress, anxiety and neuroinflammation. Front. Cell. Neurosci. 7:173. doi: 10.3389/fncel.2013.00173
Mao, X.-F., Wu, H.-Y., Tang, X.-Q., Ali, U., Liu, H., and Wang, Y.-X. (2019). Activation of GPR40 produces mechanical antiallodynia via the spinal glial interleukin-10/β-endorphin pathway. J. Neuroinflamm. 16:84. doi: 10.1186/s12974-019-1457-9
Markó, K., Kohidi, T., Hádinger, N., Jelitai, M., Mezo, G., and Madarász, E. (2011). Isolation of radial glia-like neural stem cells from fetal and adult mouse forebrain via selective adhesion to a novel adhesive peptide-conjugate. PLoS ONE 6:e28538. doi: 10.1371/journal.pone.0028538
Martin, F. C., Anton, P. A., Gornbein, J. A., Shanahan, F., and Merrill, J. E. (1993). Production of interleukin-1 by microglia in response to substance P: role for a non-classical NK-1 receptor. J. Neuroimmunol. 42, 53–60. doi: 10.1016/0165-5728(93)90212-H
Masmoudi-Kouki, O., Gandolfo, P., Castel, H., Leprince, J., Fournier, A., Dejda, A., et al. (2007). Role of PACAP and VIP in astroglial functions. Peptides 28, 1753–1760. doi: 10.1016/j.peptides.2007.05.015
Mason, S., Smart, D., Marshall, I. C. B., McKnight, A., Skepper, J. N., and McNulty, S. (2002). Identification and characterisation of functional bombesin receptors in human astrocytes. Eur. J. Pharmacol. 438, 25–34. doi: 10.1016/S0014-2999(02)01268-2
Mazzeo, A., Arroba, A. I., Beltramo, E., Valverde, A. M., and Porta, M. (2017). Somatostatin protects human retinal pericytes from inflammation mediated by microglia. Exp. Eye Res. 164, 46–54. doi: 10.1016/j.exer.2017.07.011
McCluskey, L. P., and Lampson, L. A. (2001). Local immune regulation in the central nervous system by substance P vs. glutamate. J Neuroimmunol 116, 136–146. doi: 10.1016/S0165-5728(01)00295-8
Mehl, L. C., Manjally, A. V., Bouadi, O., Gibson, E. M., and Leng Tay, T. (2022). Microglia in brain development and regeneration. Development 149:dev200425. doi: 10.1242/dev.200425
Melander, E., Eriksson, C., Wellens, S., Hosseini, K., Fredriksson, R., Gosselet, F., et al. (2023). Differential blood–brain barrier transport and cell uptake of cyclic peptides in vivo and in vitro. Pharmaceutics 15:1507. doi: 10.3390/pharmaceutics15051507
Melbourne, J. K., Chandler, C. M., Van Doorn, C. E., Bardo, M. T., Pauly, J. R., Peng, H., et al. (2021). Primed for addiction: a critical review of the role of microglia in the neurodevelopmental consequences of adolescent alcohol drinking. Alcohol. Clin. Exp. Res. 45, 1908–1926. doi: 10.1111/acer.14694
Mendonca, P., Taka, E., Bauer, D., Reams, R. R., and Soliman, K. F. A. (2018). The attenuating effects of 1,2,3,4,6 penta-O-galloyl-β-d-glucose on pro-inflammatory responses of LPS/IFNγ-activated BV-2 microglial cells through NF?B and MAPK signaling pathways. J. Neuroimmunol. 324, 43–53. doi: 10.1016/j.jneuroim.2018.09.004
Metz-Boutigue, M., Zhang, D., Lavaux, T., Schneider, F., and Aunis, D. (2010). Two chromogranin A-derived peptides, chromofungin and catestatin, induce neutrophil activation via a store-operated channel-dependent mechanism. Crit. Care 14:P32. doi: 10.1186/cc9135
Meyer, L. C., Paisley, C. E., Mohamed, E., Bigbee, J. W., Kordula, T., Richard, H., et al. (2017). Novel role of the nociceptin system as a regulator of glutamate transporter expression in developing astrocytes. Glia 65, 2003–2023. doi: 10.1002/glia.23210
Mika, J., Popiolek-Barczyk, K., Rojewska, E., Makuch, W., Starowicz, K., and Przewlocka, B. (2014). Delta-opioid receptor analgesia is independent of microglial activation in a rat model of neuropathic pain. PLoS ONE 9:e104420. doi: 10.1371/journal.pone.0104420
Mikkelsen, R. J. T., Grier, K. E., Mortensen, K. T., Nielsen, T. E., and Qvortrup, K. (2018). Photolabile linkers for solid-phase synthesis. ACS Comb. Sci. 20, 377–399. doi: 10.1021/acscombsci.8b00028
Minami, S. S., Cordova, A., Cirrito, J. R., Tesoriero, J. A., Babus, L. W., Davis, G. C., et al. (2010). ApoE mimetic peptide decreases Aβ production in vitro and in vivo. Mol. Neurodegener. 5:16. doi: 10.1186/1750-1326-5-16
Mirarab, E., Hojati, V., Vaezi, G., Shiravi, A., and Khaksari, M. (2019). Obestatin inhibits apoptosis and astrogliosis of hippocampal neurons following global cerebral ischemia reperfusion via antioxidant and anti-inflammatory mechanisms. Iran. J. Basic Med. Sci. 22, 617–622. doi: 10.22038/ijbms.2019.34118.8110
Monif, M., Reid, C. A., Powell, K. L., Drummond, K. J., O'Brien, T. J., and Williams, D. A. (2016). Interleukin-1β has trophic effects in microglia and its release is mediated by P2X7R pore. J. Neuroinflamm. 13:173. doi: 10.1186/s12974-016-0621-8
Moody, T. W., Ito, T., Osefo, N., and Jensen, R. T. (2011). VIP and PACAP. Recent insights into their functions/roles in physiology and disease from molecular and genetic studies. Curr. Opin. Endocrinol. Diabetes Obes. 18, 61–67. doi: 10.1097/MED.0b013e328342568a
Morash, B., Li, A., Murphy, P. R., Wilkinson, M., and Ur, E. (1999). Leptin gene expression in the brain and pituitary gland. Endocrinology 140, 5995–5998. doi: 10.1210/endo.140.12.7288
Mordelt, A., and de Witte, L. D. (2023). Microglia-mediated synaptic pruning as a key deficit in neurodevelopmental disorders: hype or hope? Curr. Opin. Neurobiol. 79:102674. doi: 10.1016/j.conb.2022.102674
Mosberg, H. I., Yeomans, L., Anand, J. P., Porter, V., Sobczyk-Kojiro, K., Traynor, J. R., et al. (2014). Development of a bioavailable μ opioid receptor (MOPr) agonist, δ opioid receptor (DOPr) antagonist peptide that evokes antinociception without development of acute tolerance. J. Med. Chem. 57, 3148–3153. doi: 10.1021/jm5002088
Müller, W., Heinemann, U., and Berlin, K. (1997). Cholecystokinin activates CCKB-receptor–mediated Ca-signaling in hippocampal astrocytes. J. Neurophysiol. 78, 1997–2001. doi: 10.1152/jn.1997.78.4.1997
Murillo-Cuesta, S., Rodríguez-de la Rosa, L., Contreras, J., Celaya, A. M., Camarero, G., Rivera, T., et al. (2015). Transforming growth factor β1 inhibition protects from noise-induced hearing loss. Front. Aging Neurosci. 7:32. doi: 10.3389/fnagi.2015.00032
Myhre, C. L., Thygesen, C., Villadsen, B., Vollerup, J., Ilkjær, L., Krohn, K. T., et al. (2019). Microglia express insulin-like growth factor-1 in the hippocampus of aged APPswe/PS1ΔE9 transgenic mice. Front. Cell. Neurosci. 13:308. doi: 10.3389/fncel.2019.00308
Nagpal, G., Usmani, S. S., Dhanda, S. K., Kaur, H., Singh, S., Sharma, M., et al. (2017). Computer-aided designing of immunosuppressive peptides based on IL-10 inducing potential. Sci. Rep. 7:42851. doi: 10.1038/srep42851
Newton, M. S., Cabezas, Y., and Seelig, B. (2020). Advantages of mRNA display. ACS Synth. Biol. 9, 181–190. doi: 10.1021/acssynbio.9b00419
Ng, S., and Derda, R. (2016). Phage-displayed macrocyclic glycopeptide libraries. Org. Biomol. Chem. 14, 5539–5545. doi: 10.1039/C5OB02646F
Ng, S., Jafari, M. R., Matochko, W. L., and Derda, R. (2012). Quantitative synthesis of genetically encoded glycopeptide libraries displayed on M13 phage. ACS Chem. Biol. 7, 1482–1487. doi: 10.1021/cb300187t
Ni, G., Chen, S., Yang, Y., Cummins, S. F., Zhan, J., Li, Z., et al. (2016). Investigation the possibility of using peptides with a helical repeating pattern of hydro-phobic and hydrophilic residues to inhibit IL-10. PLoS ONE 11:e0153939. doi: 10.1371/journal.pone.0153939
Nicolas, C. S., Amici, M., Bortolotto, Z. A., Doherty, A., Csaba, Z., Fafouri, A., et al. (2013). The role of JAK-STAT signaling within the CNS. JAKSTAT 2:e22925. doi: 10.4161/jkst.22925
Niethammer, P., Delling, M., Sytnyk, V., Dityatev, A., Fukami, K., and Schachner, M. (2002). Cosignaling of NCAM via lipid rafts and the FGF receptor is required for neuritogenesis. J. Cell Biol. 157, 521–532. doi: 10.1083/jcb.200109059
Nolte, C., Kirchhoff, F., and Kettenmann, H. (1997). Epidermal growth factor is a motility factor for microglial cells in vitro: evidence for EGF receptor expression. Eur. J. Neurosci. 9, 1690–1698. doi: 10.1111/j.1460-9568.1997.tb01526.x
Nowakowski, G. S., Dooner, M. S., Valinski, H. M., Mihaliak, A. M., Quesenberry, P. J., and Becker, P. S. (2004). A specific heptapeptide from a phage display peptide library homes to bone marrow and binds to primitive hematopoietic stem cells. Stem Cells 22, 1030–1038. doi: 10.1634/stemcells.22-6-1030
O'Connell, E. M., and Lohoff, F. W. (2020). Proprotein convertase subtilisin/Kexin type 9 (PCSK9) in the brain and relevance for neuropsychiatric disorders. Front. Neurosci. 14:609. doi: 10.3389/fnins.2020.00609
Ohtaki, H., Nakamachi, T., Dohi, K., Aizawa, Y., Takaki, A., Hodoyama, K., et al. (2006). Pituitary adenylate cyclase-activating polypeptide (PACAP) decreases ischemic neuronal cell death in association with IL-6. Proc. Natl. Acad. Sci. U.S.A. 103, 7488–7493. doi: 10.1073/pnas.0600375103
Ojo, B., Rezaie, P., Gabbott, P. L., Cowely, T. R., Medvedev, N. I., Lynch, M. A., et al. (2011). A neural cell adhesion molecule-derived peptide, FGL, attenuates glial cell activation in the aged hippocampus. Exp. Neurol. 232, 318–328. doi: 10.1016/j.expneurol.2011.09.025
Okonkwo, O., Zezoff, D., and Adeyinka, A. (2024). “Biochemistry, cholecystokinin,” in StatPearls (Treasure Island, FL: StatPearls Publishing). Available online at: http://www.ncbi.nlm.nih.gov/books/NBK534204/ (accessed June 27, 2024).
Ormel, P. R., Vieira de Sá, R., van Bodegraven, E. J., Karst, H., Harschnitz, O., Sneeboer, M. A. M., et al. (2018). Microglia innately develop within cerebral organoids. Nat. Commun. 9:4167. doi: 10.1038/s41467-018-06684-2
O'Toole, T. J., and Sharma, S. (2024). “Physiology, somatostatin,” in StatPearls (Treasure Island, FL: StatPearls Publishing). Available online at: http://www.ncbi.nlm.nih.gov/books/NBK538327/ (accessed June 27, 2024).
Ouyang, N., Zhu, C., Zhou, D., Nie, T., Go, M. F., Richards, R. J., et al. (2012). MC-12, an Annexin A1-based peptide, is effective in the treatment of experimental colitis. PLoS ONE 7:e41585. doi: 10.1371/journal.pone.0041585
Palser, A. L., Norman, A. L., Saffell, J. L., and Reynolds, R. (2009). Neural cell adhesion molecule stimulates survival of premyelinating oligodendrocytes via the fibroblast growth factor receptor. J. Neurosci. Res. 87, 3356–3368. doi: 10.1002/jnr.22248
Pan, W., Hsuchou, H., He, Y., Sakharkar, A., Cain, C., Yu, C., et al. (2008). Astrocyte leptin receptor (ObR) and leptin transport in adult-onset obese mice. Endocrinology 149, 2798–2806. doi: 10.1210/en.2007-1673
Pankov, R., and Yamada, K. M. (2002). Fibronectin at a glance. J. Cell Sci. 115, 3861–3863. doi: 10.1242/jcs.00059
Pedersen, W. A., Wan, R., Zhang, P., and Mattson, M. P. (2002). Urocortin, but not urocortin II, protects cultured hippocampal neurons from oxidative and excitotoxic cell death via corticotropin-releasing hormone receptor type I. J. Neurosci. 22, 404–412. doi: 10.1523/JNEUROSCI.22-02-00404.2002
Pedreño, M., Morell, M., Robledo, G., Souza-Moreira, L., Forte-Lago, I., Caro, M., et al. (2014). Adrenomedullin protects from experimental autoimmune encephalomyelitis at multiple levels. Brain Behav. Immun. 37:152. doi: 10.1016/j.bbi.2013.11.021
Peluffo, H., González, P., Arís, A., Acarin, L., Saura, J., Villaverde, A., et al. (2007). RGD domains neuroprotect the immature brain by a glial-dependent mechanism. Ann. Neurol. 62, 251–261. doi: 10.1002/ana.21170
Peng, S.-J., Feng, Y., Li, X., Wang, X.-X., Wang, Y., Zhou, B.-T., et al. (2023). Thymopentin (TP-5) prevents lipopolysaccharide-induced neuroinflammation and dopaminergic neuron injury by inhibiting the NF-κB/NLRP3 signaling pathway. Int. Immunopharmacol. 119:110109. doi: 10.1016/j.intimp.2023.110109
Perry, V. H., and Holmes, C. (2014). Microglial priming in neurodegenerative disease. Nat. Rev. Neurol. 10, 217–224. doi: 10.1038/nrneurol.2014.38
Pinteaux, E., Inoue, W., Schmidt, L., Molina-Holgado, F., Rothwell, N. J., and Luheshi, G. N. (2007). Leptin induces interleukin-1beta release from rat microglial cells through a caspase 1 independent mechanism. J. Neurochem. 102, 826–833. doi: 10.1111/j.1471-4159.2007.04559.x
Pinto-Benito, D., Paradela-Leal, C., Ganchala, D., de Castro-Molina, P., and Arevalo, M.-A. (2022). IGF-1 regulates astrocytic phagocytosis and inflammation through the p110α isoform of PI3K in a sex-specific manner. Glia 70, 1153–1169. doi: 10.1002/glia.24163
Pirahanchi, Y., and Sharma, S. (2024). “Physiology, bradykinin,” in StatPearls (Treasure Island, FL: StatPearls Publishing). Available online at: http://www.ncbi.nlm.nih.gov/books/NBK537187/ (accessed May 27, 2024).
Plagman, A., Hoscheidt, S., McLimans, K. E., Klinedinst, B., Pappas, C., Anantharam, V., et al. (2019). Cholecystokinin and Alzheimer's disease: a biomarker of metabolic function, neural integrity, and cognitive performance. Neurobiol. Aging 76, 201–207. doi: 10.1016/j.neurobiolaging.2019.01.002
Pocivavsek, A., Burns, M. P., and Rebeck, G. W. (2009). Low density lipoprotein receptors regulate microglial inflammation through C-Jun N-terminal kinase. Glia 57, 444–453. doi: 10.1002/glia.20772
Poudel, S. B., Dixit, M., Neginskaya, M., Nagaraj, K., Pavlov, E., Werner, H., et al. (2020). Effects of GH/IGF on the aging mitochondria. Cells 9:1384. doi: 10.3390/cells9061384
Prater, K. E., Green, K. J., Mamde, S., Sun, W., Cochoit, A., Smith, C. L., et al. (2023). Human microglia show unique transcriptional changes in Alzheimer's disease. Nat Aging 3, 894–907. doi: 10.1038/s43587-023-00424-y
Priller, J., Haas, C. A., Reddington, M., and Kreutzberg, G. W. (1998). Cultured astrocytes express functional receptors for galanin. Glia 24, 323–328. doi: 10.1002/(SICI)1098-1136(199811)24:3<323::AID-GLIA6>3.0.CO;2-2
Qin, L., Liu, Y., Qian, X., Hong, J.-S., and Block, M. L. (2005). Microglial NADPH oxidase mediates leucine enkephalin dopaminergic neuroprotection. Ann. N. Y. Acad. Sci. 1053, 107–120. doi: 10.1111/j.1749-6632.2005.tb00016.x
Qin, W., Feng, J., Li, Y., Lin, Z., and Shen, B. (2006). De novo design TNF-alpha antagonistic peptide based on the complex structure of TNF-alpha with its neutralizing monoclonal antibody Z12. J. Biotechnol. 125, 57–63. doi: 10.1016/j.jbiotec.2006.01.036
Qin, W., Feng, J., Li, Y., Lin, Z., and Shen, B. (2007). A novel domain antibody rationally designed against TNF-alpha using variable region of human heavy chain antibody as scaffolds to display antagonistic peptides. Mol. Immunol. 44, 2355–2361. doi: 10.1016/j.molimm.2006.10.016
Rajora, N., Boccoli, G., Burns, D., Sharma, S., Catania, A. P., and Lipton, J. M. (1997). alpha-MSH modulates local and circulating tumor necrosis factor-alpha in experimental brain inflammation. J. Neurosci. 17, 2181–2186. doi: 10.1523/JNEUROSCI.17-06-02181.1997
Raman, S., Srinivasan, G., Brookhouser, N., Nguyen, T., Henson, T., Morgan, D., et al. (2020). A defined and scalable peptide-based platform for the generation of human pluripotent stem cell-derived astrocytes. ACS Biomater. Sci. Eng. 6, 3477–3490. doi: 10.1021/acsbiomaterials.0c00067
Ramos-Lobo, A. M., and Donato, J. (2017). The role of leptin in health and disease. Temperature 4, 258–291. doi: 10.1080/23328940.2017.1327003
Reddington, M., Priller, J., Treichel, J., Haas, C., and Kreutzberg, G. W. (1995). Astrocytes and microglia as potential targets for calcitonin gene related peptide in the central nervous system. Can. J. Physiol. Pharmacol. 73, 1047–1049. doi: 10.1139/y95-148
Reginato, G., and Taddei, M. (2002). Organometallic chemistry on solid phase. An overview. Farmaco 57, 373–384. doi: 10.1002/chin.200247246
Rehfeld, J. F. (2021). Cholecystokinin and the hormone concept. Endocr. Connect. 10, R139–R150. doi: 10.1530/EC-21-0025
Reiner, A. (1987). The distribution of proenkephalin-derived peptides in the central nervous system of turtles. J. Comp. Neurol. 259, 65–91. doi: 10.1002/cne.902590106
Reiss, D., Maurin, H., Audouard, E., Martínez-Navarro, M., Xue, Y., Herault, Y., et al. (2021). Delta opioid receptor in astrocytes contributes to neuropathic cold pain and analgesic tolerance in female mice. Front. Cell. Neurosci. 15:745178. doi: 10.3389/fncel.2021.745178
Robinson, K. F., Narasipura, S. D., Wallace, J., Ritz, E. M., and Al-Harthi, L. (2020). Negative regulation of IL-8 in human astrocytes depends on β-catenin while positive regulation is mediated by TCFs/LEF/ATF2 interaction. Cytokine 136:155252. doi: 10.1016/j.cyto.2020.155252
Rodrigues, C. H. M., Garg, A., Keizer, D., Pires, D. E. V., and Ascher, D. B. (2022). CSM-peptides: a computational approach to rapid identification of therapeutic peptides. Prot. Sci. 31:e4442. doi: 10.1002/pro.4442
Roh, J., Chang, C. L., Bhalla, A., Klein, C., and Hsu, S. Y. T. (2004). Intermedin Is a calcitonin/calcitonin gene-related peptide family peptide acting through the calcitonin receptor-like receptor/receptor activity-modifying protein receptor complexes *. J. Biol. Chem. 279, 7264–7274. doi: 10.1074/jbc.M305332200
Roth, J. D. (2013). Amylin and the regulation of appetite and adiposity: recent advances in receptor signaling, neurobiology and pharmacology. Curr. Opin. Endocrinol. Diabetes Obes. 20, 8–13. doi: 10.1097/MED.0b013e32835b896f
Ruan, Z., Tang, J., Zeng, M., and Fan, P. (2023). Virtual high-throughput screening: Potential inhibitors targeting aminopeptidase N (CD13) and PIKfyve for SARS-CoV-2. Open Life Sci. 18:20220637. doi: 10.1515/biol-2022-0637
Ruoslahti, E. (1996). Rgd and other recognition sequences for integrins. Annu. Rev. Cell Dev. Biol. 12, 697–715. doi: 10.1146/annurev.cellbio.12.1.697
Saba, J., Carniglia, L., Ramírez, D., Turati, J., Imsen, M., Durand, D., et al. (2019). Melanocortin 4 receptor activation protects striatal neurons and glial cells from 3-nitropropionic acid toxicity. Mol. Cell. Neurosci. 94, 41–51. doi: 10.1016/j.mcn.2018.12.002
Sakai, A., Takasu, K., Sawada, M., and Suzuki, H. (2012). Hemokinin-1 gene expression is upregulated in microglia activated by lipopolysaccharide through NF-κB and p38 MAPK signaling pathways. PLoS ONE 7:e32268. doi: 10.1371/journal.pone.0032268
Samoylova, T. I., Ahmed, B. Y., Vodyanoy, V., Morrison, N. E., Samoylov, A. M., Globa, L. P., et al. (2002). Targeting peptides for microglia identified via phage display. J. Neuroimmunol. 127, 13–21. doi: 10.1016/S0165-5728(02)00071-1
Santiago, B., Gutierrez-Cañas, I., Dotor, J., Palao, G., Lasarte, J. J., Ruiz, J., et al. (2005). Topical application of a peptide inhibitor of transforming growth factor-beta1 ameliorates bleomycin-induced skin fibrosis. J. Invest. Dermatol. 125, 450–455. doi: 10.1111/j.0022-202X.2005.23859.x
Santos, A., Cabrales, A., Reyes, O., Gerónimo, H., Rodríguez, Y., Hilda, G., et al. (2008). Identification of an interleukin-15 antagonist peptide that binds to IL-15Rα. Biotecnol. Aplicada 25, 320–324.
Sardi, C., Zambusi, L., Finardi, A., Ruffini, F., Tolun, A. A., Dickerson, I. M., et al. (2014). Involvement of calcitonin gene-related peptide and receptor component protein in experimental autoimmune encephalomyelitis. J. Neuroimmunol. 271, 18–29. doi: 10.1016/j.jneuroim.2014.03.008
Sarfaraz, D., and Fraser, C. L. (1999). Effects of arginine vasopressin on cell volume regulation in brain astrocyte in culture. Am. J. Physiol. 276, E596–601. doi: 10.1152/ajpendo.1999.276.3.E596
Schauwecker, P. E. (2010). Galanin receptor 1 deletion exacerbates hippocampal neuronal loss after systemic kainate administration in mice. PLoS ONE 5:e15657. doi: 10.1371/journal.pone.0015657
Schönauer, R., Els-Heindl, S., and Beck-Sickinger, A. G. (2017). Adrenomedullin – new perspectives of a potent peptide hormone. J. Pept. Sci. 23, 472–485. doi: 10.1002/psc.2953
Schvartz, I., Seger, D., and Shaltiel, S. (1999). Vitronectin. Int. J. Biochem. Cell Biol. 31, 539–544. doi: 10.1016/S1357-2725(99)00005-9
Sclavons, C., Burtea, C., Boutry, S., Laurent, S., Vander Elst, L., and Muller, R. N. (2013). Phage display screening for tumor necrosis factor-α-binding peptides: detection of inflammation in a mouse model of hepatitis. Int. J. Pept. 2013:348409. doi: 10.1155/2013/348409
Seelig, G. F., Prosise, W. W., Hawkins, J. C., and Senior, M. M. (1995). Development of a receptor peptide antagonist to human gamma-interferon and characterization of its ligand-bound conformation using transferred nuclear Overhauser effect spectroscopy. J. Biol. Chem. 270, 9241–9249. doi: 10.1074/jbc.270.16.9241
Selles, M. C., Fortuna, J. T. S., de Faria, Y. P. R., Siqueira, L. D., Lima-Filho, R., Longo, B. M., et al. (2023). Oxytocin attenuates microglial activation and restores social and non-social memory in APP/PS1 Alzheimer model mice. iScience 26:106545. doi: 10.1016/j.isci.2023.106545
Serdar, M., Kempe, K., Herrmann, R., Picard, D., Remke, M., Herz, J., et al. (2020). Involvement of CXCL1/CXCR2 during microglia activation following inflammation-sensitized hypoxic-ischemic brain injury in neonatal rats. Front. Neurol. 11:540878. doi: 10.3389/fneur.2020.540878
Serrano-Martínez, I., Pedreño, M., Castillo-González, J., Ferraz-de-Paula, V., Vargas-Rodríguez, P., Forte-Lago, I., et al. (2024). Cortistatin as a novel multimodal therapy for the treatment of Parkinson's disease. Int. J. Mol. Sci. 25, 694. doi: 10.3390/ijms25020694
Shan, Y., Tan, S., Lin, Y., Liao, S., Zhang, B., Chen, X., et al. (2019). The glucagon-like peptide-1 receptor agonist reduces inflammation and blood-brain barrier breakdown in an astrocyte-dependent manner in experimental stroke. J. Neuroinflamm. 16:242. doi: 10.1186/s12974-019-1638-6
Shemer, A., Scheyltjens, I., Frumer, G. R., Kim, J.-S., Grozovski, J., Ayanaw, S., et al. (2020). Interleukin-10 prevents pathological microglia hyperactivation following peripheral endotoxin challenge. Immunity 53, 1033–1049.e7. doi: 10.1016/j.immuni.2020.09.018
Shi, S. X., Li, Y.-J., Shi, K., Wood, K., Ducruet, A. F., and Liu, Q. (2020). IL (interleukin)-15 bridges astrocyte-microglia crosstalk and exacerbates brain injury following intracerebral hemorrhage. Stroke 51, 967–974. doi: 10.1161/STROKEAHA.119.028638
Shimamura, M., Nakagami, H., Osako, M. K., Kurinami, H., Koriyama, H., Zhengda, P., et al. (2014). OPG/RANKL/RANK axis is a critical inflammatory signaling system in ischemic brain in mice. Proc. Natl. Acad. Sci. U. S. A. 111, 8191–8196. doi: 10.1073/pnas.1400544111
Shrivastava, P., Cabrera, M. A., Chastain, L. G., Boyadjieva, N. I., Jabbar, S., Franklin, T., et al. (2017). Mu-opioid receptor and delta-opioid receptor differentially regulate microglial inflammatory response to control proopiomelanocortin neuronal apoptosis in the hypothalamus: effects of neonatal alcohol. J. Neuroinflamm. 14:83. doi: 10.1186/s12974-017-0844-3
Singh, O., Hsu, W.-L., and Su, E. C.-Y. (2021). ILeukin10Pred: a computational approach for predicting IL-10-inducing immunosuppressive peptides using combinations of amino acid global features. Biology 11:5. doi: 10.3390/biology11010005
Soudy, R., Kimura, R., Patel, A., Fu, W., Kaur, K., Westaway, D., et al. (2019). Short amylin receptor antagonist peptides improve memory deficits in Alzheimer's disease mouse model. Sci. Rep. 9:10942. doi: 10.1038/s41598-019-47255-9
Soudy, R., Patel, A., Fu, W., Kaur, K., MacTavish, D., Westaway, D., et al. (2017). Cyclic AC253, a novel amylin receptor antagonist, improves cognitive deficits in a mouse model of Alzheimer's disease. Alzheimers Dementia 3, 44–56. doi: 10.1016/j.trci.2016.11.005
Sousa, N. A., Oliveira, G. A. L., de Oliveira, A. P., Lopes, A. L. F., Iles, B., Nogueira, K. M., et al. (2020). Novel ocellatin peptides mitigate LPS-induced ROS formation and NF-kB activation in microglia and hippocampal neurons. Sci. Rep. 10:2696. doi: 10.1038/s41598-020-59665-1
Sporn, M. B., and Roberts, A. B. (1988). Peptide growth factors are multifunctional. Nature 332, 217–219. doi: 10.1038/332217a0
Sporns, O., Edelman, G. M., and Crossin, K. L. (1995). The neural cell adhesion molecule (N-CAM) inhibits proliferation in primary cultures of rat astrocytes. Proc. Natl. Acad. Sci. U. S. A. 92, 542–546. doi: 10.1073/pnas.92.2.542
Sridar, S., Churchward, M. A., Mushahwar, V. K., Todd, K. G., and Elias, A. L. (2017). Peptide modification of polyimide-insulated microwires: towards improved biocompatibility through reduced glial scarring. Acta Biomater. 60, 154–166. doi: 10.1016/j.actbio.2017.07.026
Steinhoff, M. S., von Mentzer, B., Geppetti, P., Pothoulakis, C., and Bunnett, N. W. (2014). Tachykinins and their receptors: contributions to physiological control and the mechanisms of disease. Physiol. Rev. 94, 265–301. doi: 10.1152/physrev.00031.2013
Stiene-Martin, A., and Hauser, K. F. (1990). Opioid-dependent growth of glial cultures: suppression of astrocyte DNA synthesis by met-enkephalin. Life Sci. 46, 91–98. doi: 10.1016/0024-3205(90)90041-O
Sun, C., An, Q., Li, R., Chen, S., Gu, X., An, S., et al. (2021). Calcitonin gene-related peptide induces the histone H3 lysine 9 acetylation in astrocytes associated with neuroinflammation in rats with neuropathic pain. CNS Neurosci. Ther. 27, 1409–1424. doi: 10.1111/cns.13720
Sun, J., Qian, P., Kang, Y., Dai, H.-B., Wang, F.-Z., Wang, H.-Y., et al. (2021). Adrenomedullin 2 attenuates LPS-induced inflammation in microglia cells by receptor-mediated cAMP-PKA pathway. Neuropeptides 85:102109. doi: 10.1016/j.npep.2020.102109
Sun, L., Jiang, W.-W., Wang, Y., Yuan, Y.-S., Rong, Z., Wu, J., et al. (2021). Phosphorylated α-synuclein aggregated in Schwann cells exacerbates peripheral neuroinflammation and nerve dysfunction in Parkinson's disease through TLR2/NF-κB pathway. Cell Death Discov. 7, 1–10. doi: 10.1038/s41420-021-00676-w
Szmydynger-Chodobska, J., Fox, L. M., Lynch, K. M., Zink, B. J., and Chodobski, A. (2010). Vasopressin amplifies the production of proinflammatory mediators in traumatic brain injury. J. Neurotrauma 27, 1449–1461. doi: 10.1089/neu.2010.1331
Szmydynger-Chodobska, J., Zink, B. J., and Chodobski, A. (2011). Multiple sites of vasopressin synthesis in the injured brain. J. Cereb. Blood Flow Metab. 31, 47–51. doi: 10.1038/jcbfm.2010.188
Tam, J. P., Lin, Y.-Z., Liu, W., Wang, D.-X., Ke, X.-H., and Zhang, J.-W. (1991). Mapping the receptor-recognition site of human transforming growth factor-α. Int. J. Pept. Protein Res. 38, 204–211. doi: 10.1111/j.1399-3011.1991.tb01430.x
Tam, J. P., and Zavala, F. (1989). Multiple antigen peptide. A novel approach to increase detection sensitivity of synthetic peptides in solid-phase immunoassays. J. Immunol. Methods 124, 53–61. doi: 10.1016/0022-1759(89)90185-3
Tan, Y.-L., Yuan, Y., and Tian, L. (2020). Microglial regional heterogeneity and its role in the brain. Mol. Psychiatry 25, 351–367. doi: 10.1038/s41380-019-0609-8
Tashiro, K., Sephel, G. C., Weeks, B., Sasaki, M., Martin, G. R., Kleinman, H. K., et al. (1989). A synthetic peptide containing the IKVAV sequence from the A chain of laminin mediates cell attachment, migration, and neurite outgrowth. J. Biol. Chem. 264, 16174–16182. doi: 10.1016/S0021-9258(18)71604-9
Taupenot, L., Ciesielski-Treska, J., Ulrich, G., Chasserot-Golaz, S., Aunis, D., and Bader, M.-F. (1996). Chromogranin a triggers a phenotypic transformation and the generation of nitric oxide in brain microglial cells. Neuroscience 72, 377–389. doi: 10.1016/0306-4522(96)83172-1
Terashima, T., Ogawa, N., Nakae, Y., Sato, T., Katagi, M., Okano, J., et al. (2018). Gene therapy for neuropathic pain through siRNA-IRF5 gene delivery with homing peptides to microglia. Mol. Ther. Nucl. Acids 11, 203–215. doi: 10.1016/j.omtn.2018.02.007
Thorsell, A., and Heilig, M. (2002). Diverse functions of neuropeptide Y revealed using genetically modified animals. Neuropeptides 36, 182–193. doi: 10.1054/npep.2002.0897
Tilstra, J. S., Clauson, C. L., Niedernhofer, L. J., and Robbins, P. D. (2011). NF-κB in aging and disease. Aging Dis. 2, 449–465.
Todd, L., Palazzo, I., Suarez, L., Liu, X., Volkov, L., Hoang, T. V., et al. (2019). Reactive microglia and IL1β/IL-1R1-signaling mediate neuroprotection in excitotoxin-damaged mouse retina. J. Neuroinflamm. 16:118. doi: 10.1186/s12974-019-1505-5
Toledano Furman, N., Gottlieb, A., Prabhakara, K. S., Bedi, S., Caplan, H. W., Ruppert, K. A., et al. (2020). High-resolution and differential analysis of rat microglial markers in traumatic brain injury: conventional flow cytometric and bioinformatics analysis. Sci. Rep. 10:11991. doi: 10.1038/s41598-020-68770-0
Tombling, B. J., Lammi, C., Lawrence, N., Li, J., Arnoldi, A., Craik, D. J., et al. (2021). Engineered EGF-A peptides with improved affinity for proprotein convertase subtilisin/kexin type 9 (PCSK9). ACS Chem. Biol. 16, 429–439. doi: 10.1021/acschembio.0c00991
Tomimori, Y., Mori, K., Koide, M., Nakamichi, Y., Ninomiya, T., Udagawa, N., et al. (2009). Evaluation of pharmaceuticals with a novel 50-hour animal model of bone loss. J. Bone Miner. Res. 24, 1194–1205. doi: 10.1359/jbmr.090217
Toth, D., Tamas, A., and Reglodi, D. (2020). The neuroprotective and biomarker potential of PACAP in human traumatic brain injury. Int. J. Mol. Sci. 21:827. doi: 10.3390/ijms21030827
Tresguerres, J. Á. F., Fernández-Tresguerres, I., Viña, J., Rancan, L., Paredes, S. D., Linillos-Pradillo, B., et al. (2022). Effects of GH on the aging process in several organs: mechanisms of action. Int. J. Mol. Sci. 23, 7848. doi: 10.3390/ijms23147848
Trevaskis, J. L., Turek, V. F., Wittmer, C., Griffin, P. S., Wilson, J. K., Reynolds, J. M., et al. (2010). Enhanced amylin-mediated body weight loss in estradiol-deficient diet-induced obese rats. Endocrinology 151, 5657–5668. doi: 10.1210/en.2010-0590
Tsygankov, A. Y. (2003). Non-receptor protein tyrosine kinases. Front. Biosci. 8, s595–635. doi: 10.2741/1106
Tundo, G., Ciaccio, C., Sbardella, D., Boraso, M., Viviani, B., Coletta, M., et al. (2012). Somatostatin modulates insulin-degrading-enzyme metabolism: implications for the regulation of microglia activity in AD. PLoS ONE 7:e34376. doi: 10.1371/journal.pone.0034376
Uddin, M. Z., Li, X., Joo, H., Tsai, J., Wrischnik, L., and Jasti, B. (2019). Rational design of peptide ligands based on knob–socket protein packing model using CD13 as a prototype receptor. ACS Omega 4, 5126–5136. doi: 10.1021/acsomega.8b03421
Ulrich, G., Ciesielski-Treska, J., Taupenot, L., and Bader, M.-F. (2002). Chromogranin A-activated microglial cells induce neuronal apoptosis. Ann. N. Y. Acad. Sci. 971, 560–562. doi: 10.1111/j.1749-6632.2002.tb04527.x
Urich, E., Schmucki, R., Ruderisch, N., Kitas, E., Certa, U., Jacobsen, H., et al. (2015). Cargo delivery into the brain by in vivo identified transport peptides. Sci. Rep. 5:14104. doi: 10.1038/srep14104
van Luijn, M. M., van Meurs, M., Stoop, M. P., Verbraak, E., Wierenga-Wolf, A. F., Melief, M.-J., et al. (2016). Elevated expression of the cerebrospinal fluid disease markers chromogranin a and clusterin in astrocytes of multiple sclerosis white matter lesions. J. Neuropathol. Exp. Neurol. 75, 86–98. doi: 10.1093/jnen/nlv004
Vaz, E. R., Fujimura, P. T., Araujo, G. R., da Silva, C. A. T., Silva, R. L., Cunha, T. M., et al. (2015). A short peptide that mimics the binding domain of TGF-β1 presents potent anti-inflammatory activity. PLoS ONE 10:e0136116. doi: 10.1371/journal.pone.0136116
Velázquez, E., Blázquez, E., and Ruiz-Albusac, J. M. (2009). Synergistic effect of glucagon-like peptide 2 (GLP-2) and of key growth factors on the proliferation of cultured rat astrocytes. evidence for reciprocal upregulation of the mRNAs for GLP-2 and IGF-I receptors. Mol. Neurobiol. 40, 183–193. doi: 10.1007/s12035-009-8080-1
Verheijden, S., Schepper, S. D., and Boeckxstaens, G. E. (2015). Neuron-macrophage crosstalk in the intestine: a “microglia” perspective. Front. Cell. Neurosci. 9:403. doi: 10.3389/fncel.2015.00403
Villadsen, L. S., Schuurman, J., Beurskens, F., Dam, T. N., Dagnaes-Hansen, F., Skov, L., et al. (2003). Resolution of psoriasis upon blockade of IL-15 biological activity in a xenograft mouse model. J. Clin. Invest. 112, 1571–1580. doi: 10.1172/JCI200318986
von Wallbrunn, A., Waldeck, J., Höltke, C., Zühlsdorf, M., Mesters, R., Heindel, W., et al. (2008). In vivo optical imaging of CD13/APN-expression in tumor xenografts. J. Biomed. Opt. 13:011007. doi: 10.1117/1.2839046
Vrontakis, M. E. (2002). Galanin: a biologically active peptide. Curr. Drug Targets CNS Neurol. Disord. 1, 531–541. doi: 10.2174/1568007023338914
Wada, S., Sato, K., Ohta, R., Wada, E., Bou, Y., Fujiwara, M., et al. (2013). Ingestion of low dose pyroglutamyl leucine improves dextran sulfate sodium-induced colitis and intestinal microbiota in mice. J. Agric. Food Chem. 61, 8807–8813. doi: 10.1021/jf402515a
Wahlert, A., Funkelstein, L., Fitzsimmons, B., Yaksh, T., and Hook, V. (2013). Spinal astrocytes produce and secrete dynorphin neuropeptides. Neuropeptides 47, 109–115. doi: 10.1016/j.npep.2012.10.006
Waiboci, L. W., Ahmed, C. M., Mujtaba, M. G., Flowers, L. O., Martin, J. P., Haider, M. I., et al. (2007). Both the suppressor of cytokine signaling 1 (SOCS-1) kinase inhibitory region and SOCS-1 mimetic bind to JAK2 autophosphorylation site: implications for the development of a SOCS-1 antagonist. J. Immunol. 178, 5058–5068. doi: 10.4049/jimmunol.178.8.5058
Wang, C. K., Gruber, C. W., Cemazar, M., Siatskas, C., Tagore, P., Payne, N., et al. (2014). Molecular grafting onto a stable framework yields novel cyclic peptides for the treatment of multiple sclerosis. ACS Chem. Biol. 9, 156–163. doi: 10.1021/cb400548s
Wang, E., Zhu, H., Wang, X., Gower, A. C., Wallack, M., Blusztajn, J. K., et al. (2017). Amylin treatment reduces neuroinflammation and ameliorates abnormal patterns of gene expression in the cerebral cortex of an Alzheimer's disease mouse model. J. Alzheimers. Dis. 56, 47–61. doi: 10.3233/JAD-160677
Wang, L., Wang, N., Zhang, W., Cheng, X., Yan, Z., Shao, G., et al. (2022). Therapeutic peptides: current applications and future directions. Sig. Transduct. Target Ther. 7, 1–27. doi: 10.1038/s41392-022-00904-4
Wang, P., Qin, D., and Wang, Y.-F. (2017). Oxytocin rapidly changes astrocytic GFAP plasticity by differentially modulating the expressions of pERK 1/2 and protein kinase A. Front. Mol. Neurosci. 10:262. doi: 10.3389/fnmol.2017.00262
Wang, Q., Oyarzabal, E., Wilson, B., Qian, L., and Hong, J.-S. (2015). Substance P enhances microglial density in the substantia nigra through neurokinin-1 receptor/NADPH oxidase-mediated chemotaxis in mice. Clin. Sci. 129, 757–767. doi: 10.1042/CS20150008
Wang, S., Cao, X., Duan, Y., and Zhang, G. (2019). Delta opioid peptide [d-Ala2, d-Leu5] enkephalin (DADLE) exerts a cytoprotective effect in astrocytes exposed to oxygen-glucose deprivation by inducing autophagy. Cell Transplant. 28:775. doi: 10.1177/0963689719825619
Wang, Y., Hsuchou, H., He, Y., Kastin, A. J., and Pan, W. (2015). Role of astrocytes in leptin signaling. J. Mol. Neurosci. 56, 829–839. doi: 10.1007/s12031-015-0518-5
Weledji, E. P., and Assob, J. C. (2014). The ubiquitous neural cell adhesion molecule (N-CAM). Ann. Med. Surg. 3, 77–81. doi: 10.1016/j.amsu.2014.06.014
Werner, Y., Mass, E., Ashok Kumar, P., Ulas, T., Händler, K., Horne, A., et al. (2020). Cxcr4 distinguishes HSC-derived monocytes from microglia and reveals monocyte immune responses to experimental stroke. Nat. Neurosci. 23, 351–362. doi: 10.1038/s41593-020-0585-y
Westfall, T. C., and Curfman-Falvey, M. (1995). Amylin-induced relaxation of the perfused mesenteric arterial bed: meditation by calcitonin gene-related peptide receptors. J. Cardiovasc. Pharmacol. 26, 932–936. doi: 10.1097/00005344-199512000-00012
Wickström, M., Larsson, R., Nygren, P., and Gullbo, J. (2011). Aminopeptidase N (CD13) as a target for cancer chemotherapy. Cancer Sci. 102, 501–508. doi: 10.1111/j.1349-7006.2010.01826.x
Wierzbicka-Patynowski, I., and Schwarzbauer, J. E. (2003). The ins and outs of fibronectin matrix assembly. J. Cell Sci. 116, 3269–3276. doi: 10.1242/jcs.00670
Wiggenhorn, A. L., Abuzaid, H. Z., Coassolo, L., Li, V. L., Tanzo, J. T., Wei, W., et al. (2023). A class of secreted mammalian peptides with potential to expand cell-cell communication. bioRxiv [preprint]. doi: 10.1038/s41467-023-43857-0
Wikberg, J. E., Muceniece, R., Mandrika, I., Prusis, P., Lindblom, J., Post, C., et al. (2000). New aspects on the melanocortins and their receptors. Pharmacol. Res. 42, 393–420. doi: 10.1006/phrs.2000.0725
Wright, M. J., and Deonarain, M. P. (2007). Phage display of chelating recombinant antibody libraries. Mol. Immunol. 44, 2860–2869. doi: 10.1016/j.molimm.2007.01.026
Wu, C.-H., Liu, I.-J., Lu, R.-M., and Wu, H.-C. (2016). Advancement and applications of peptide phage display technology in biomedical science. J. Biomed. Sci. 23:8. doi: 10.1186/s12929-016-0223-x
Xia, C.-F., Yin, H., Borlongan, C. V., Chao, J., and Chao, L. (2004). Adrenomedullin gene delivery protects against cerebral ischemic injury by promoting astrocyte migration and survival. Hum. Gene Ther. 15, 1243–1254. doi: 10.1089/hum.2004.15.1243
Xu, J.-W., Li, C.-G., Huang, X.-E., Li, Y., and Huo, J.-G. (2011). Ubenimex capsule improves general performance and chemotherapy related toxicity in advanced gastric cancer cases. Asian Pac. J. Cancer Prev. 12, 985–987.
Xu, W., and Li, Q. (2005). Progress in the development of aminopeptidase N (APN/CD13) inhibitors. Curr. Med. Chem. Anticancer. Agents 5, 281–301. doi: 10.2174/1568011053765949
Xu, X., Gao, Y., Wen, L., Zhai, Z., Zhang, S., Shan, F., et al. (2016). Methionine enkephalin regulates microglia polarization and function. Int. Immunopharmacol. 40, 90–97. doi: 10.1016/j.intimp.2016.08.037
Xu, Y., Chen, R., Zhi, F., Sheng, S., Khiati, L., Yang, Y., et al. (2023). δ-opioid receptor, microglia and neuroinflammation. Aging Dis. 14, 778–793. doi: 10.14336/AD.2022.0912
Yoo, S. H. (1992). Identification of the Ca(2+)-dependent calmodulin-binding region of chromogranin A. Biochemistry 31, 6134–6140. doi: 10.1021/bi00141a025
Yshii, L., Pasciuto, E., Bielefeld, P., Mascali, L., Lemaitre, P., Marino, M., et al. (2022). Astrocyte-targeted gene delivery of interleukin 2 specifically increases brain-resident regulatory T cell numbers and protects against pathological neuroinflammation. Nat. Immunol. 23, 878–891. doi: 10.1038/s41590-022-01208-z
Yuen, K. C. J., and Samson, S. L. (2022). Oral octreotide: a review of recent clinical trials and practical recommendations for its use in the treatment of patients with acromegaly. Endocr. Pract. 28, 637–645. doi: 10.1016/j.eprac.2022.04.009
Yunna, C., Mengru, H., Lei, W., and Weidong, C. (2020). Macrophage M1/M2 polarization. Eur. J. Pharmacol. 877:173090. doi: 10.1016/j.ejphar.2020.173090
Zellinger, C., Salvamoser, J. D., Seeger, N., Russmann, V., and Potschka, H. (2014). Impact of the neural cell adhesion molecule-derived peptide FGL on seizure progression and cellular alterations in the mouse kindling model. ACS Chem. Neurosci. 5, 185–193. doi: 10.1021/cn400153g
Zhang, X., and Xu, W. (2008). Aminopeptidase N (APN/CD13) as a target for anti-cancer agent design. Curr. Med. Chem. 15, 2850–2865. doi: 10.2174/092986708786242840
Zhang, Y., Lu, L., Furlonger, C., Wu, G. E., and Paige, C. J. (2000). Hemokinin is a hematopoietic-specific tachykinin that regulates B lymphopoiesis. Nat. Immunol. 1, 392–397. doi: 10.1038/80826
Zhang, Z., Hao, L., Shi, M., Yu, Z., Shao, S., Yuan, Y., et al. (2021). Neuroprotective effects of a GLP-2 analogue in the MPTP Parkinson's disease mouse model. J. Parkinsons Dis. 11, 529–543. doi: 10.3233/JPD-202318
Zhang, Z., Huang, L., Zhao, W., and Rigas, B. (2010). Annexin 1 induced by anti-inflammatory drugs binds to NF-kappaB and inhibits its activation: anticancer effects in vitro and in vivo. Cancer Res. 70, 2379–2388. doi: 10.1158/0008-5472.CAN-09-4204
Zhang, Z.-X., Li, E., Yan, J.-P., Fu, W., Shen, P., Tian, S.-W., et al. (2019). Apelin attenuates depressive-like behavior and neuroinflammation in rats co-treated with chronic stress and lipopolysaccharide. Neuropeptides 77:101959. doi: 10.1016/j.npep.2019.101959
Zheng, J.-S., He, Y., Zuo, C., Cai, X.-Y., Tang, S., Wang, Z. A., et al. (2016). Robust chemical synthesis of membrane proteins through a general method of removable backbone modification. J. Am. Chem. Soc. 138, 3553–3561. doi: 10.1021/jacs.6b00515
Zhou, S., Guo, X., Chen, S., Xu, Z., Duan, W., and Zeng, B. (2019). Apelin-13 regulates LPS-induced N9 microglia polarization involving STAT3 signaling pathway. Neuropeptides 76:101938. doi: 10.1016/j.npep.2019.101938
Zhou, W., Lu, S., Su, Y., Xue, D., Yu, X., Wang, S., et al. (2014). Decreasing oxidative stress and neuroinflammation with a multifunctional peptide rescues memory deficits in mice with Alzheimer disease. Free Radic. Biol. Med. 74, 50–63. doi: 10.1016/j.freeradbiomed.2014.06.013
Zhou, Y., Shao, A., Yao, Y., Tu, S., Deng, Y., and Zhang, J. (2020). Dual roles of astrocytes in plasticity and reconstruction after traumatic brain injury. Cell Commun. Signal. 18:62. doi: 10.1186/s12964-020-00549-2
Zhu, J., Qu, C., Lu, X., and Zhang, S. (2014). Activation of microglia by histamine and substance P. Cell. Physiol. Biochem. 34, 768–780. doi: 10.1159/000363041
Zusev, M., and Gozes, I. (2004). Differential regulation of activity-dependent neuroprotective protein in rat astrocytes by VIP and PACAP. Regul. Pept. 123, 33–41. doi: 10.1016/j.regpep.2004.05.021
Glossary
Keywords: peptide, glia, microglia, astrocytes, neuroinflammation
Citation: Benita BA and Koss KM (2024) Peptide discovery across the spectrum of neuroinflammation; microglia and astrocyte phenotypical targeting, mediation, and mechanistic understanding. Front. Mol. Neurosci. 17:1443985. doi: 10.3389/fnmol.2024.1443985
Received: 04 June 2024; Accepted: 24 July 2024;
Published: 20 November 2024.
Edited by:
Tiziana Bachetti, Ospedale Policlinico San Martino, ItalyReviewed by:
Juan Carlos Martínez-Lazcano, Manuel Velasco Suárez National Institute of Neurology and Neurosurgery, MexicoSimona Dedoni, University of Cagliari, Italy
Copyright © 2024 Benita and Koss. This is an open-access article distributed under the terms of the Creative Commons Attribution License (CC BY). The use, distribution or reproduction in other forums is permitted, provided the original author(s) and the copyright owner(s) are credited and that the original publication in this journal is cited, in accordance with accepted academic practice. No use, distribution or reproduction is permitted which does not comply with these terms.
*Correspondence: Kyle M. Koss, a2tvc3NAdWFsYmVydGEuY2E=