- 1Department of Neurobiology, University of Massachusetts Chan Medical School, Worcester, MA, United States
- 2Department of Veterans Affairs, San Francisco Veterans Affairs Medical Center, San Francisco, CA, United States
- 3Department of Orthopaedic Surgery, University of California, San Francisco, San Francisco, CA, United States
- 4Department of Neurological Surgery, University of California, San Francisco, San Francisco, CA, United States
- 5Brain and Spinal Injury Center, Zuckerberg San Francisco General Hospital and Trauma Center, San Francisco, CA, United States
- 6San Francisco Veterans Affairs Healthcare System, San Francisco, CA, United States
- 7Zuckerberg San Francisco General Hospital and Trauma CenterOrthopaedic Trauma Institute,, San Francisco, CA, United States
Mechanical stimuli, such as stretch, shear stress, or compression, activate a range of biomolecular responses through cellular mechanotransduction. In the nervous system, studies on mechanical stress have highlighted key pathophysiological mechanisms underlying traumatic injury and neurodegenerative diseases. However, the biomolecular pathways triggered by mechanical stimuli in the nervous system has not been fully explored, especially compared to other body systems. This gap in knowledge may be due to the wide variety of methods and definitions used in research. Additionally, as mechanical stimulation techniques such as ultrasound and electromagnetic stimulation are increasingly utilized in psychological and neurorehabilitation treatments, it is vital to understand the underlying biological mechanisms in order to develop accurate pathophysiological models and enhance therapeutic interventions. This review aims to summarize the cellular signaling pathways activated by various mechanical and electromagnetic stimuli with a particular focus on the mammalian nervous system. Furthermore, we briefly discuss potential cellular mechanosensors involved in these processes.
Introduction
The mammalian brain and spinal cord are viscoelastic tissues composed of heterogeneous cell populations, including neurons, astrocytes, oligodendrocytes, vascular endothelial, and microglia (Goriely et al., 2015). The stiffness of human and rodent brain tissue, with a shear modulus of approximately 1 kPa, is notably softer than that of other organ tissues (Elkin et al., 2010; Budday et al., 2017). Thus, nervous tissue is particularly sensitive to mechanical stimulation and easily susceptible to stress-induced cellular and axonal deformation (Reiter et al., 2023). However, it is well-established that mechanical stimuli influence cellular development, proliferation, and function, depending on the type and intensity of the stimuli. Therefore, the impact of mechanical stimuli on the brain and spinal cord must be considered in different contexts and applications.
Studies on traumatic injury and neurodegenerative disorders provide great insight into how mechanobiological forces influence the central nervous system (CNS). Focal traumatic injuries often result from the tension, compression and tearing of nervous tissue due to external stress (Blennow et al., 2012). Additionally, shear stress can occur when the brain experiences longitudinal tension and compression when acceleration is misaligned with the brain’s axis (Proces et al., 2022). These types of injuries serve as prime examples of how high levels of external stress impact the CNS. In neurodegenerative cases of such as Alzheimer disease or gliosis, an overall softening of nervous tissue is commonly observed, indicating a decrease in tissue stiffness (Hall et al., 2021). Beyond pathological conditions, mechanical forces generated during activities like sports or running can influence the brain and spinal cord tissues through flexion, extension, compression and shearing.
Numerous studies have investigated the effect of mechanical stress on the CNS through various methods of mechanical stimulation. However, these experiments have mainly focused on tissue-level effects and their macroscopic negative outcomes, such as diffuse axonal injury. Interestingly, mechanical stimulation can also have positive effects on the CNS. For instance, mechanical stretching promotes neurite outgrowth (Higgins et al., 2013) and induces changes in extracellular matrix stiffness, both known to regulate adult neurogenesis in the brain (Kjell et al., 2020). Therefore, the potential of mechanical stimulation to promote neural regeneration warrants consideration. To establish a standard for mechanical stimulation experiments and gain a more comprehensive understanding of its impact, it is essential to first evaluate the existing knowledge on the cellular and molecular outcomes of such stimuli.
Mechanical stimuli generated by dynamic movements, physical forces, and surrounding tissue are likely to influence the functions, maintenance and fates of neuronal and glial cells (Pillai and Franze, 2024). Additionally, these stimuli may share critical features in mechanotransduction. To fully understand the effects of mechanical stimuli on the CNS, it is necessary to investigate the key cellular signaling pathways involved. Several insightful reviews have examined the relationship between mechanical stimuli and the nervous system (Goriely et al., 2015; Proces et al., 2022; Motz et al., 2021; Rocha et al., 2022; Keating and Cullen, 2021). These reviews have explored mechanosensation, potential sensors, relevant protein channels (Proces et al., 2022; Hall et al., 2021; Higgins et al., 2013; Kjell et al., 2020), and the impact of various subtypes of mechanical stimuli in the context of CNS injuries and pathological conditions (Goriely et al., 2015; Rocha et al., 2022; Keating and Cullen, 2021). However, unlike other organs and cell types, few reviews address the causal effects of mechanical stimuli on neurons and glial cells, and the subsequent activation of cellular signaling pathways.
To summarize cellular pathways triggered by mechanical stimuli in the CNS, we first selected types of stimulation commonly studied in neurotraumatic research, such as stretching, compression and shear stress. We have also included fluid shear stress, which is known to be generated when external forces are applied to the brain or spinal cord (Keating and Cullen, 2021). Although secondary, mixed cascades, such as inflammation, inevitably occur after in vivo application of stress, leading to what is often termed ‘mechanical injury’, these studies still provided valuable insight. We prioritized studies that explicitly targeted mechanobiogical approaches or applied in vitro techniques specific to certain cell types.
In addition to mechanical stimulation, this review also encompasses cellular signaling pathways in the CNS driven by ultrasound and electromagnetic stimulation. Ultrasound is a mechanical pressure wave, while electromagnetic stimulation, such as transcranial magnetic stimulation (TMS), mediates biomolecular transduction through electromagnetic forces (Adey, 1993). Emerging studies suggest that non-invasive ultrasound and electromagnetic stimulation to the brain can induce therapeutic effects on psychological and neurodegenerative disorders (Nardone et al., 2014; George et al., 1999; Meng et al., 2021; Qiu et al., 2021). Therefore, it is valuable to consider how these external stimuli activate biomolecular pathways compared to classical mechanical stimuli.
In this review, we examine prominent mechanotransduction cellular signaling pathways, including MAPK, PKC, Akt, YAP/TAZ, and Rho-ROCK, which are activated by various types of mechanical and electromagnetic stimuli within the mammalian nervous system. These types of mechanical stimulation were selected for their practical interpretations and potential clinical implications. Additionally, we briefly discuss the mechanosensors and channels expressed on neurons and glial cells that are associated with the mechanotransduction of these stimuli.
Mechanical and electromagnetic stimuli induced intracellular signaling pathways in the nervous system
MAPK signaling pathway
The mitogen-activated protein kinase (MAPK) pathway is one of the most well-known signal transduction cascades activated by various mechanical stimuli. MAPKs are categorized into three distinct types: extracellular signal-regulated kinases (ERKs), c-Jun N-terminal kinases (JNKs), and p38 MAPKs (Zhang and Liu, 2002). While the upstream signals within the MAPK network are interconnected, the activation of downstream MAPK family members is tightly regulated. These pathways have been studied in depth due to their significance in cell processes including, proliferation, differentiation, maintenance, and development in mammalian cells. In the nervous system, MAPK signaling has been implicated in neuronal development, axonal outgrowth, differentiation, maturation, and synaptic plasticity (Thomas and Huganir, 2004). In particular, JNKs, which are known to be activated by cellular stress, play key roles in regulating apoptosis and neuronal development (Coffey, 2014). Additionally, various cellular stresses such as oxidative stress, inflammatory cytokines or UV radiation, can trigger p38 MAPK activation, which regulates neuroinflammatory responses, survival and synaptic plasticity (Asih et al., 2020) (Figure 1).
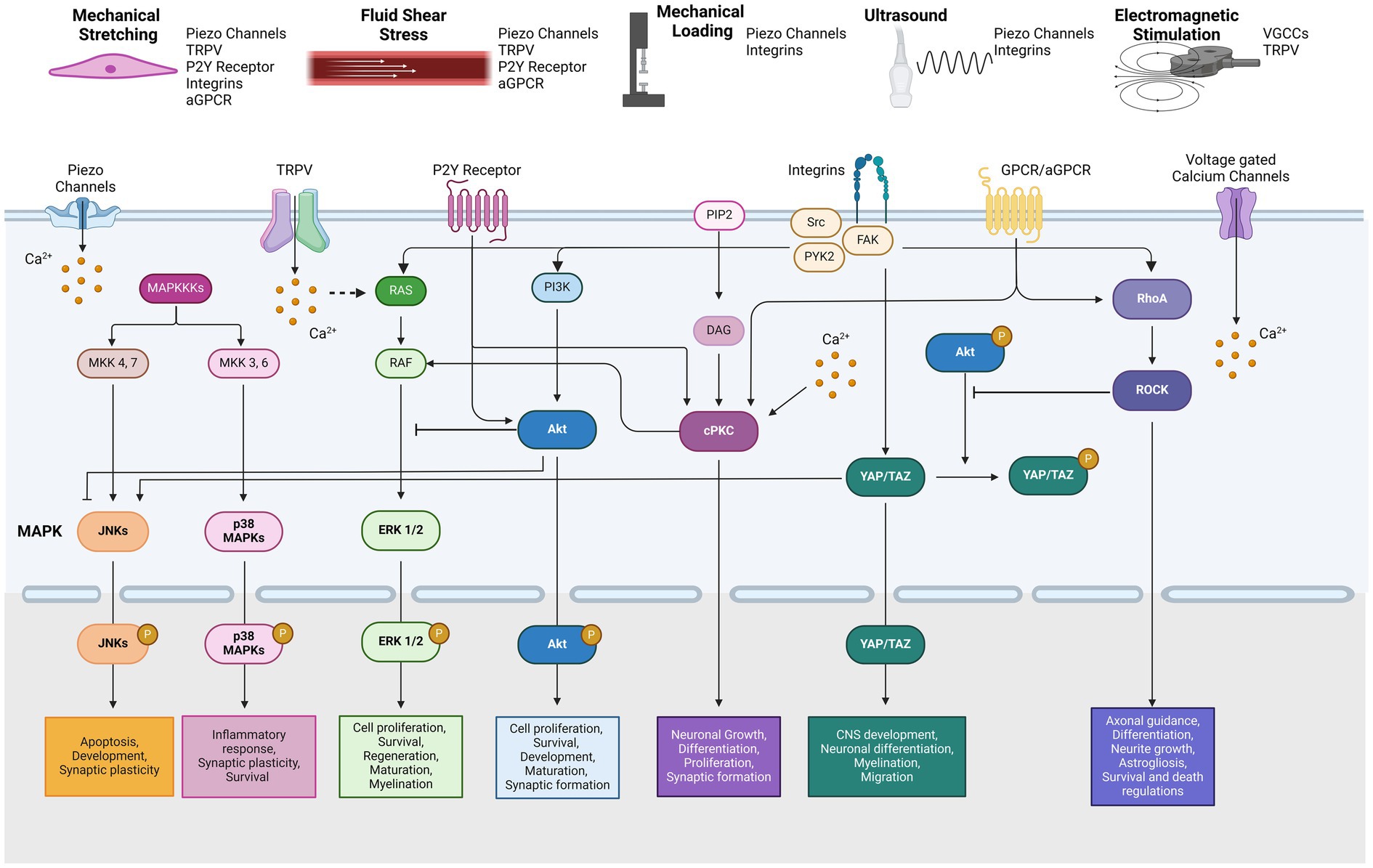
Figure 1. Types of mechanical stimulation and related mechanotransduction pathways by reported mechanosensors.
Mechanical stretching has been shown to induce of phosphorylation of ERK1/2, JNKs, and p38 MAPKs across various cell types including cardiomyocytes, skeletal muscle cells, osteoblasts, and vascular endothelial cells (Hsu et al., 2010; Suzuma et al., 2002; Zou et al., 2004; Boppart et al., 2001; Kanno et al., 2007). The degree of MAPK activation correlates positively with both the percentage (Aikawa et al., 2002) and frequency (Hz) of stretch (Hsu et al., 2010). Studies indicate that mechanical stretching activates MAPK signaling in astrocytes, cortical neurons, and oligodendroglial cell lines (Neary et al., 2003; Chierto et al., 2019; Ralay et al., 2011; Bhowmick et al., 2019). For example, in primary cultured rat astrocytes, 30% elongation under mechanical stretch initially elevates phospho-ERK levels, while prolonged stretching up 24 h is needed to increase activation of JNKs (Ralay et al., 2011). However, levels of activated p38 MAPKs were not significantly impacted by stretch. The stretch-induced ERK activation in primary rat astrocytes is hypothesized to be calcium-dependent and mediated by ATP-release in response to strain (Neary et al., 2003). These studies suggest that MAPK activation is related to the cellular mechanism of astrogliosis formation and inflammatory responses after mechanical stress.
In biaxially stretched cortical neurons, 30% stretch resulted in increased phosphorylation of ERK 1/2 and JNKs, but not p38 MAPKs (Bhowmick et al., 2019). These findings imply that ERK and JNK activations significantly regulate apoptotic neuronal cell death. In contrast, a study using the oligodendroglial cell line 158 N found that a 30% stretch stress did not significantly alter phosphorylated ERK 1/2 and JNKs levels, but reduced the levels of phosphorylated p38 MAPKs (Chierto et al., 2019). This tensile strain led to increased reactive oxygen species and changes in myelination protein expression. However, an ex vivo stretch test on mouse cerebellar tissue showed ERK 1/2 activation, while activation of p38 MAPKs was not observed. Additionally, in the retinal tissue layer, positive ERK 1/2 immunosignals increased following 20% stretch stress (Lindqvist et al., 2010).
Fluid shear stress has also been shown to induce phosphorylation of JNKs, ERKs, and p38 MAPKs in in vitro systems using vascular endothelial cells, osteosarcoma cell lines, and osteoblasts (Kadohama et al., 2006; Mengistu et al., 2011; Kapur et al., 2003). The extent of phosphorylation is influenced by both the duration (minutes) and the magnitude of stress (dyn/cm2 or Pa) applied. However, it has also been reported that laminar fluid shear stress inhibits TNF-α and IL-1-mediated activation of JNKs in human umbilical vein endothelial cells (Surapisitchat et al., 2001). Although fluid shear stress influences N-methyl-D-aspartic acid (NMDA) receptor-mediated calcium influx in primary cultured rat astrocytes (Maneshi et al., 2017), there have been no reports on the direct application of fluid shear stress to other glial or neuronal cells in vitro in relation to MAPK signaling.
Mechanical pressure can generate compression and shear strain in the tissue. It has been reported that in vitro compression and shear strain can activate ERKs, JNKs, and p38 MAPKs in chondrocytes as well as osteoblasts (Song et al., 2016; Fitzgerald et al., 2008). However, there is a paucity of similar studies using neural cells. In vivo models mild traumatic brain injury can be viewed as mechanical stimuli models that incorporate both compression and shear stress (Keating and Cullen, 2021). In these models, significant phosphorylation of ERKs and p38 MAPKs have been observed in mouse brain tissue (Bachstetter et al., 2013; Zhao et al., 2012), while JNK activation was not detected (Mori et al., 2002). Additionally, chronic compression of the dorsal root ganglion (DRG) in vivo resulted in activation of ERKs and p38 MAPKs in neurons and microglia (Han et al., 2012; Qu et al., 2016). These findings suggest that mechanical compression stimuli generally facilitate the activation of ERKs and p38 MAPKs, which regulate neuronal apoptosis and inflammatory responses, such as cytokines release. Although secondary inflammatory responses following in vivo mechanical stress may contribute to these effects, the involvement of MAPK cascades in neuroprotection is suggested.
Ultrasound can generate mechanical pressure waves that are typically applied both in vitro and in vivo at frequencies in the MHz range. In the medical setting, ultrasound is conducted at a frequency range between 1 to 15 MHz, while therapeutic applications typically utilize frequencies of around 1 MHz or lower (Tufail et al., 2011). Ultrasound can be delivered through various tissues as pulsed or continuous waves. Its physiological effects on cells and tissues have been attributed to a combination of heat generation, micro-vibrations, microstreaming, and shear stress (VanBavel, 2007; Xin et al., 2016). However, low-intensity pulsed ultrasound (LIPUS), which operates at intensities below 3 W/cm2, has minimal thermal effects and is primarily used as a therapeutic intervention (Jiang et al., 2019).
In mammalian osteoblast and synovial cells, LIPUS at frequencies between 1.5 and 3 MHz has been shown to upregulate phosphorylation levels of ERKs, JNKs, and p38 MAPKs (Chiu et al., 2008; Sato et al., 2014). In contrast, LIPUS stimulation in dental pulp stem cells led to increased activation of ERKs and JNKs, but not p38 MAPKs (Gao et al., 2017). In rodent 3 T3-L1, MC3T3-E1, and ST2 cells, LIPUS application at 1.5 MHz induced phosphorylation of ERK 1/2 only, without affecting JNKs and p38 MAPKs (Kusuyama et al., 2014). In addition, Louw et al. found that phosphorylated ERK levels did not change with ultrasound frequencies above 2 MHz in chondrocytes (Louw et al., 2013). However, the phosphorylation of ERKs was did not significantly change in rat astrocytes treated with LIPUS at 1 MHz frequency (Liu et al., 2017). Similarly, in vivo ultrasound application at 0.5 MHz did not promote the phosphorylation of JNKs or p44/42 MAPK in rat brain tissue (Jalali et al., 2010) although the applied frequency ranges in these reports are generally lower than those used in other system.
Electromagnetic stimulation also alters the cellular activation and function in the nervous system (Siebner et al., 2022). Theoretically, an electromagnetic field induces the vibration of free ions on the cellular membrane (Panagopoulos et al., 2002), modulating ion influx and membrane potential due to microelectronic activities. It has been suggested that electromagnetic stimuli, such as weak electromagnetic fields (EMF), can activate the MAPK pathway (Blank and Goodman, 2009). Extremely low-frequency EMF in the range of 0–100 Hz are commonly used and are considered to have negligible thermal effects at the cellular level (Adey, 1993). A study using low frequency electromagnetic stimulation of 50 Hz on various cell lines has shown an increase in the phosphorylation of ERK 1/2 and p38 MAPKs, but did not promote the phosphorylation of JNKs (Kapri-Pardes et al., 2017).
Focusing on the nervous system’s response to EMF, in vitro use of a repetitive transcranial magnetic stimulation (TMS) system at 10 Hz on rat neural stem cell cultures has demonstrated activation of ERK 1/2 and p38 MAPKs, while JNKs were not significantly affected (Cui et al., 2019). This suggests that the TMS-activated MAPK pathway may enhance the proliferation and differentiation of neural stem cells. Similarly, magnetic stimulation applied to mouse neuronal cells and oligodendrocyte precursor cell line CG4 resulted in a significant increase in the ERK 1/2 phosphorylation (Ma et al., 2013; Dolgova et al., 2021). In the mouse hippocampal neuronal cell line HT22, EMS at 10 Hz and 1.75 T intensity increased the levels of phosphorylated ERK 1/2 and JNKs, but not p38 MAPKs (Kim et al., 2023). Also, in the rat Schwann cells, 50 Hz magnetic stimulation at 0.1 T intensity transiently increased phospho-ERK 1/2 levels (Colciago et al., 2015). Overall, these studies indicate that activation of MAPKs by electromagnetic stimulation enhances synaptic plasticity, attenuates of cell death and promotes remyelination in vitro. Furthermore, in vitro exposure of electromagnetic pulses to rat microglia resulted in p38 MAPKs phosphorylation, but did not affect ERKs and JNKs (Yang et al., 2016). In vivo, repetitive TMS at 15 Hz frequency and 1.26 T intensity led to elevated ERK 1/2 phosphorylation and increased neuronal proliferation in hippocampal tissue (Chen et al., 2015). Additionally, TMS at 15 Hz induced robust axonal elongation and MAP2K phosphorylation in cortical neurons (Boato et al., 2023). Collectively, both mechanical and electromagnetic stimuli typically enhance ERK phosphorylation, whereas the activation of JNK or p38 MAPK is inconsistent and varies depending on the type of stimulus and cell type (Table 1).
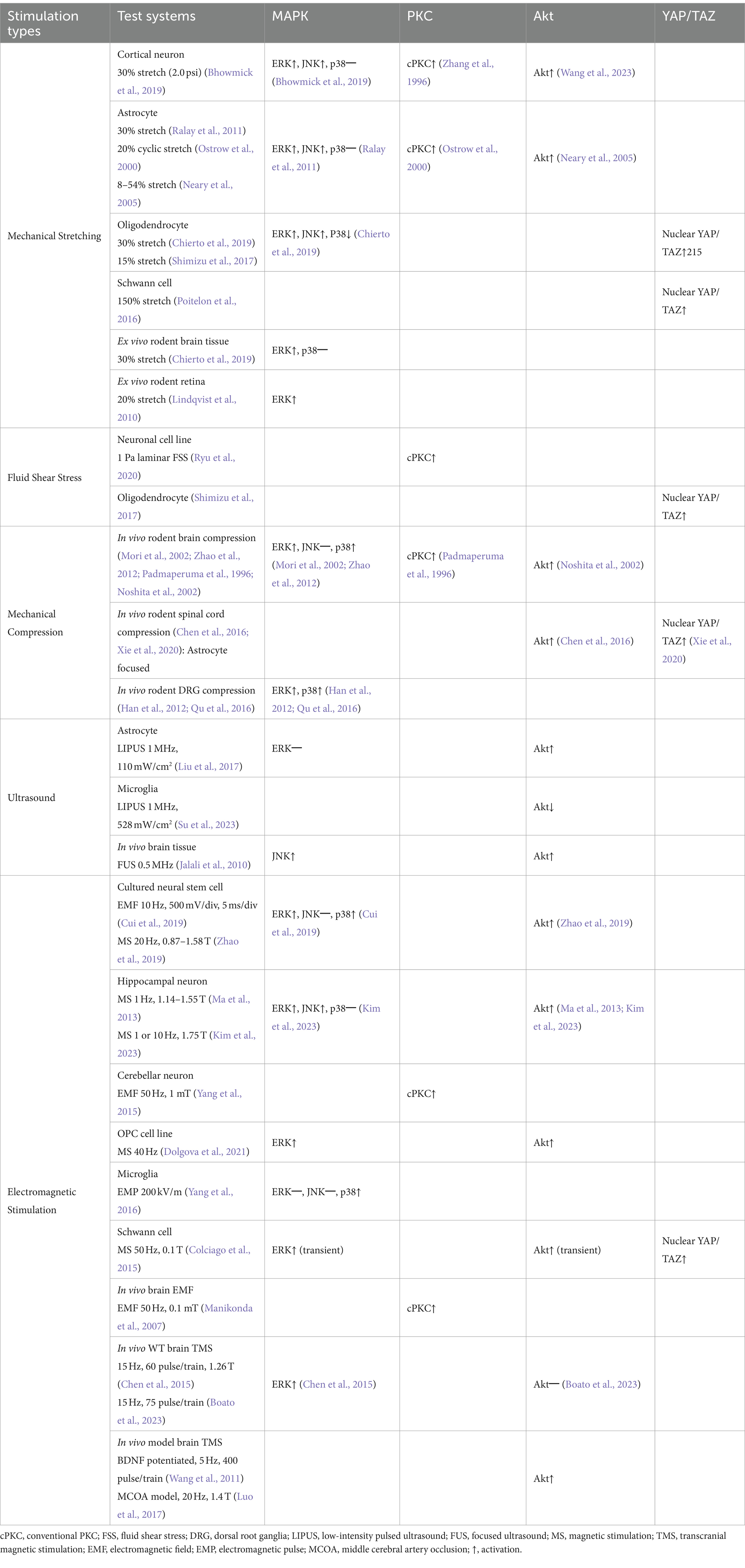
Table 1. Summarized results of mechanically or electromagnetically activated intracellular pathways from studies using the mammalian nervous system.
PKC signaling pathway
Protein kinase C (PKC) is activated by mechanical stress via gated channels and receptors including the NMDA receptor, TRPV4, and the purinergic P2Y2 receptor (Lea et al., 2002; Wang et al., 2005; Adapala et al., 2011). Activation of PKC typically involves the participation of phospholipase and diacylglycerol leading to a cascade of downstream cellular signaling, including involvement of the MAPK pathway. PKC is classified into several subgroups: conventional (calcium-dependent;α, β, and γ), novel (calcium-independent; δ, ε, η and θ), and atypical types (Parekh et al., 2000). The expression of PKC isozymes varies depending on the cell type and tissue, influencing their specific signaling pathways. For example, PKCγ is highly expressed in the nervous tissue, PKCδ is predominantly found in the soma of neurons, and PKCε is concentrated in the presynaptic terminals (Liu and Heckman, 1998). PKC activation regulates many functions in the CNS, including neurotransmitter release, neuronal growth, proliferation and differentiation, and synaptic plasticity (Huang, 1989) (Figure 1).
Mechanical stretch activates various PKC isoforms across different cell types, including conventional PKC in fibroblast and smooth muscle cells, PKCζ in vascular pericytes and PKCα/ε in endothelial cells (Suzuma et al., 2002; Cheng et al., 2001; Husse et al., 2007; Persson et al., 1995). In cortical neurons and astrocytes, mechanical stretch-induced PKC activation is linked to calcium influx via the NMDA receptor (Lea et al., 2002; Zhang et al., 1996; Ostrow and Sachs, 2005). In vitro studies suggest that mechanical stretching not only activates the NMDA receptor, but also modifies the α-amino-3-hydroxyl-5-methyl-4-isoxazolepropionate (AMPA) receptor leading to potentiated calcium influx in neurons (Spaethling et al., 2012; Goforth et al., 2004). This stretch-induced calcium influx is thought to directly trigger PKC activation. A study investigating the activation of PKCα through mechanical stretching found that this process led to the internalization of the GluR2 subunit of the AMPA receptor in rat cortical neurons (Bell et al., 2009). This suggests a connection between traumatic CNS injury and neuronal toxicity due to the loss of GluA2-lacking AMPA receptor (Ferguson et al., 2008).
In vitro studies on endothelial cells have shown that both conventional and novel types of PKC isoforms are activated by fluid shear stress (Ishida et al., 1997; Berk et al., 1995). Conventional PKC activity is trigged through direct calcium influx, while the calcium-independent PKCε isoform is also activated by fluid shear stress, both mediate ERK 1/2 phosphorylation (Traub et al., 1997). In vitro studies utilizing neuronal cell lines have also shown PKC activation and elevated intracellular calcium levels in response to fluid shear stress (Ryu et al., 2020). However, research on PKC activation in other neural cell types in response to shear stress remains limited.
Compressive stimulation has been shown to activate the PKCα pathway in chondrocytes and keratinocytes through integrin-associated mechanotransduction (Takei et al., 1997; Lee et al., 2002). In human nucleus pulposus cells, mechanical compression specifically activates the PKCε isoform (Sun et al., 2013). In the nervous system, in vivo studies using compressive methods have reported upregulation of conventional PKC isoforms in brain and spinal cord neurons, particularly those associated pain sensitivity (Padmaperuma et al., 1996; Mao et al., 1992). Although these studies closely resemble ‘mechanical injury’, and should be interpreted as reflecting a combination of various effects on the tissue, the majority of PKC activation is still primarily attributed to mechanical stimulation. These studies observed significant membrane translocation of PKC in ipsilateral compressed nervous tissue, linking PKC activation with mechanical stress. This activation could result from either mechanically induced calcium influx or as part of cellular injury response, such as apoptosis. Direct evidence from in vitro studies or specific effects of PKC activation in neurons or glial cells induced solely by compression has yet to be reported.
Studies exploring the relationship between LIPUS and PKC activation are sparse. However, a study by Lee et al. demonstrated that exposure to 1 MHz ultrasound significantly elevated intracellular calcium levels and enhanced calcium-independent PKCδ activation in a murine skeletal muscle cell line (Lee et al., 2014). While there are currently no reports specifically addressing ultrasound-induced PKC activation in the CNS, these findings suggest the potential of a similar PKC activation mechanism in neural tissues.
The effects of EMF on PKC activation have been explored in lymphocytic cell lines such as HL60 and NALM6 (Uckun et al., 1995; Nie and Henderson, 2003), indicating that PKCα is particularly activated among the isozymes of PKC. In rat cerebellar neurons exposed to 50 Hz EMF, increased phosphorylation of PKC was observed with enhanced neuronal GABAA receptor-mediated currents (Yang et al., 2015). Similarly, in vivo exposure of rats to 50 Hz EMF resulted in increased PKC activity and intracellular calcium levels, likely due to NMDA receptor activation (Manikonda et al., 2007). Even direct mild electrical stimulation at 10 Hz activated PKC in the astroglia cells, leading to the release of nerve growth factor (Koyama et al., 1997). However, these studies did not differentiate between PKC subgroups. In contrast, high-frequency 2–10 GHz microwave exposure in rats demonstrated reduced PKC activation in the cerebral cortex with accompanied by aggregation of glial cell populations and morphological changes (Paulraj and Behari, 2006). Overall, PKC activation has been commonly linked with an increase in intracellular calcium levels, regardless of the type of stimulation, highlighting its potential role in cellular responses to electromagnetic fields (Table 1).
Akt signaling pathway
The Akt signaling pathway, also known as Protein Kinase B, plays a crucial role in cellular proliferation, maintenance, and survival, similar to the well-studied MAPK pathway. Akt activation is primarily mediated by the downstream effects of phosphoinositide 3-kinases (PI3K), which are transduced by various biological factors, including brain-derived neurotrophic factor (BDNF) and insulin-like growth factor 1 (IGF-1) (Brunet et al., 2001). The cascade of Akt phosphorylation leads to the activation of NF-κB and mTOR, key regulators of cellular processes. In the nervous system, Akt signaling is implicated in neuronal survival, development, maturation, axonal regeneration, and synapse formation (Sanchez-Alegria et al., 2018) (Figure 1).
Cyclic stretching has been shown to increase PI3K activity and Akt phosphorylation in osteoblasts, epidermal cells, endothelial cells, and vascular pericytes (Suzuma et al., 2002; Danciu et al., 2003; Kippenberger et al., 2005; Hu et al., 2013). Additionally, in vivo stretching has been found to enhance Akt activity in rat muscle tissue (Sakamoto et al., 2003). In primary cultured rat astrocytes, mechanical strain ranging from 8 to 54% significantly increased the levels of phosphorylated Akt (Neary et al., 2005). Neary et al. highlighted that activation of Akt in astrocytes is dependent on calcium influx triggered by mechanical stretching which is crucial for their survival and growth (Neary et al., 2005). Similarly, mechanical stretching of primary cultured mouse neurons also resulted in upregulation of Akt activity (Wang et al., 2023). Although detailed analyses on the mechanism behind Akt in response to mechanical stretching are limited, it is generally attributed to calcium influx and PI3K activation.
Akt activation has also been observed in endothelial cells and osteocytes subjected to fluid shear stress (Batra et al., 2014; Haga et al., 2003). However, there is no direct or indirect evidence of fluid shear stress affecting neurons or glial cells in relation to the Akt pathway. In regard to mechanical compressive stimulation, Akt activation has been observed in numerous cell types including osteoblasts, fibroblasts, and chondrocytes in rodent models (Song et al., 2016; Niehoff et al., 2008; Tanaka et al., 2018). Although in vitro studies on neuronal or glial cells are limited, in vivo studies have shown increased Akt phosphorylation in neurons and astrocytes within mouse brain and rat spinal cord tissues under compression (Chen et al., 2016; Noshita et al., 2002). These studies employed classical ‘mechanical injury’ techniques to induce stress, suggesting that the Akt signaling is activated in the ipsilateral tissues to prevent neuronal death. However, it remains uncertain whether Akt signaling would be activated under precise compression methods at the cellular level. Further studies are needed to explore this question.
LIPUS at 1.5 MHz has been shown to activate the PI3K-Akt signaling pathway in osteoblast (Tang et al., 2006).Similarly, LIPUS application at 110 mW/cm2 and 1 MHz significantly increased phosphorylated Akt in rat astrocytes and elevated BDNF levels (Liu et al., 2017). In vivo studies on rat brains exposed to LIPUS also demonstrated enhanced Akt activation which correlated with increased VEGF expression and paracellular permeability (Jalali et al., 2010). However, in rat microglia, PI3K-Akt signaling was reduced after LIPUS application at 528 mW/cm2 and 1 MHz, accompanied by decreased microglial activities and cytokine releases (Su et al., 2023). These conflicting findings suggest that activation of Akt by ultrasound varies depending on the specific cell type within the CNS.
Studies on electromagnetic stimulation have shown that exposure to 50 Hz EMF does not significantly alter Akt phosphorylation in several non-neural cell lines (Kapri-Pardes et al., 2017). However, rodent-cultured neuronal cells exposed to magnetic stimulation experienced increased phosphorylated Akt in neural stem/precursor cells, oligodendrocyte precursor cell lines, and hippocampal cell lines (Ma et al., 2013; Dolgova et al., 2021; Kim et al., 2023; Zhao et al., 2019). Furthermore, in rat Schwann cells, 50 Hz of electromagnetic exposure resulted in a transient increase in phosphorylated Akt levels (Colciago et al., 2015). In specific conditions, such as the rat brain ischemic model and BDNF-induction pathway, TMS has been shown to significantly increase the levels of phosphorylated Akt in brain tissue, along with BDNF and tyrosine kinase B (Luo et al., 2017; Wang et al., 2011). Conversely, in vivo TMS at 15 Hz in wild-type adult mice did not activate Akt signaling in cortical tissue (Boato et al., 2023). Similarly, in vivo exposure of rats to high-frequency microwaves (1–2 GHz) did not result in significant changes of Akt phosphorylation levels in brain tissue (Tan et al., 2021). These findings suggest that the effects of electromagnetic stimulation on Akt activation vary depending on cell type and experimental conditions, including whether the study is conducted in vitro, in vivo, or pre-conditioned models (Table 1).
YAP/TAZ signaling pathway
Yes-associated protein (YAP) and its coactivator PDZ-binding motif (TAZ) are critical downstream effectors of the Hippo signaling pathway (Piccolo et al., 2014). YAP/TAZ acts as a transcriptional regulator with essential roles in developing the CNS, including neuronal differentiation, cellular migration, and apoptosis. Moreover, YAP/TAZ dysregulation has been implicated in neurodegenerative disorders such as Huntington’s or Alzheimer’s diseases (Jin et al., 2020). Phosphorylation of YAP is mediated by the Hippo pathway, particularly through the action of MOB1 and LATS1/2. Once phosphorylated, YAP/TAZ is sequestered in the cytoplasm, blocking its translocation to the nucleus and inhibiting transcriptional activity. It should be noted that YAP is not detectable in mature neurons (Huang et al., 2016). The nuclear activity of YAP/TAZ is regulated by integrin activation, which is triggered by a range of mechanical stimuli, including matrix stiffness, stretching, and cell density (Piccolo et al., 2014) (Figure 1).
The nuclear translocation of YAP/TAZ induced by mechanical stretching has been extensively studied in in vitro systems using epithelial cells, various stem cell lineages, and endothelial cells (Aragona et al., 2013; Elbediwy et al., 2016; Panciera et al., 2017). In rat oligodendrocyte progenitor cells, a 15% stretch was sufficient to promote nuclear localization of YAP (Shimizu et al., 2017). Similarly, Schwann cells exhibited increased YAP/TAZ nuclear activity under 150% mechanical stretch (Poitelon et al., 2016). While the role of matrix stiffness-related in regulating YAP/TAZ activity has been highlighted in glial cells and the differentiation of neural stem cells (Rammensee et al., 2017; Marinval and Chew, 2021), studies specifically employing direct stretching systems have not been reported. Nonetheless, it can be hypothesized that mechanical stretching could trigger YAP/TAZ nuclear activity, potentially influencing processes like myelination and cell morphology (Hong et al., 2023). Similarly, fluid flow-mediated nuclear translocation of YAP has been documented in endothelial cells and various tumor cell types (Yu et al., 2021; Qin et al., 2019; Nakajima et al., 2017). In oligodendrocytes, YAP activity was regulated by shear stress generated by rotating culture flasks (Shibasaki et al., 2010). However, studies explicitly investigating YAP/TAZ translocation in glial cells beyond this finding are scare.
Mechanical compression applied to rodent osteocytes and chondrocytes has been shown to promote the nuclear translocation of YAP/TAZ (Zarka et al., 2021; Jing et al., 2020). In the CNS, rodent models have demonstrated that compressive stimulation increases YAP/TAZ nuclear translocation in astrocytes (Xie et al., 2020; Pu et al., 2023). This YAP activation was found to enhance astrocyte proliferation and is associated with the formation of astrogliosis. This process may play a role in regulating glial scar and promoting functional recovery following mechanical compression. Regarding ultrasound stimulation, LIPUS at 1.5 MHz for 20 min has been shown to increase YAP phosphorylation in human umbilical vein endothelial cells (Xu et al., 2018). However, in mouse C2C12 myoblast cell line, LIPUS at 3.6 MHz for 5 min reduced YAP phosphorylation and induced its nuclear translocation (Puts et al., 2018). These conflicting results, coupled with limited research on LIPUS effects on the nervous system, necessitate further investigation into the influence of ultrasound stimulation on YAP/TAZ activity.
There are several reports examining the effects of electromagnetic stimulation on YAP/TAZ activity. In human tendon cells, exposure to a 2 Hz EMF did not affect YAP/TAZ nuclear translocation (Pesqueira et al., 2017). Conversely, a static EMF of 1 mT applied to human dental pulp stem cells resulted in slight activation of YAP/TAZ nuclear activity (Zheng et al., 2018). Interestingly, when rat Schwann cells were exposed to a 50 Hz EMF, there was upregulation of cytoplasmic localization of YAP (Colciago et al., 2015). This shift was accompanied by enhanced proliferation and migration in EMF-exposed Schwann cells compared to the control group. However, studies specifically investigating the effects of EMF on YAP/TAZ in glial cells are lacking. From the current literature it can be reasonably assumed that the impact of electromagnetic stimulation on YAP/TAZ in the nervous system may be rather limited (Table 1).
Rho-ROCK signaling pathway
The Rho GTPase family, which includes Rho, Rac, and Cdc42, plays an essential role in common cellular processes including growth, migration, morphology, cell cycle regulation, and cytoskeletal structure remodeling (Etienne-Manneville and Hall, 2002). Among these, RhoA and its downstream effector, Rho-associated coiled-coil containing protein kinase 1 (ROCK), mediates mechanical signals through interactions between GPCRs and integrins (Buchsbaum, 2007). In the nervous system, the Rho-ROCK signaling pathway has been extensively studied for its role in promoting axonal guidance, neurite outgrowth, neuronal differentiation, and the regulation of astrocyte morphology astrogliosis (John et al., 2004; Stankiewicz and Linseman, 2014). Numerous studies have demonstrated that changes in the stiffness of the extracellular matrix (ECM) can activate the Rho-ROCK signaling pathway, which in turn regulates a range of cellular responses to these external mechanical cues (Zhang et al., 2017; Huang et al., 2012; Huang and Ingber, 2005).
Research using rodent cardiomyocytes and pluripotent stem cells has demonstrated that mechanical stretch activates the Rho-ROCK signaling pathway (Torsoni et al., 2005; Teramura et al., 2012). Similarly, fluid shear stress in mammalian endothelial cells induces Rho translocation to the cytoplasmic membrane, activating the Rho-ROCK pathway and its downstream effectors (Lin et al., 2003). In fibroblast, this pathway also mediates cellular stiffening in response to shear stress (Lee et al., 2006). Additionally, LIPUS has been shown to trigger the Rho-MAPK pathway in mouse phagocytes and mesenchymal stem/progenitor cells (Kusuyama et al., 2014; Zhou et al., 2008), while 50 Hz EMF promotes Rho-ROCK activation in human mesenchymal stem cells (Zhang et al., 2018). However, studies have yet to explore the effects of direct mechanical stress on Rho-ROCK signaling in the nervous system both in vitro or in vivo, leaving the impact of mechanical or electromagnetic intervention on this pathway in the nervous system unclear.
In summary, we have consolidated the findings on intracellular pathways modulated by various mechanical and electromagnetic stimulation (Table 1). These cellular pathways are highly interconnected, though the extent of crosstalk between pathways is context-dependent (Figure 1). Generally, PKC activates Raf and MEK, which are upstream of the MAPK signaling pathway (Aksamitiene et al., 2012). Conversely, activation of Akt signaling provides negative feedback to the Raf–ERK pathway (Mendoza et al., 2011), while inhibition of Akt in neurons enhances and promotes JNK activity (Hollville et al., 2019). Akt activation also phosphorylates YAP, leading to its sequestration in the cytoplasm (Basu et al., 2003). Meanwhile, Rho signaling is implicated in the mechanically induced activation of YAP/TAZ (Dupont, 2016). It also inhibits Akt while promoting p38 MAPKs and JNK in neuronal cells (Stankiewicz and Linseman, 2014; Moya and Halder, 2019). Consistent with these interactions, YAP/TAZ activation further enhances JNK cascades (Wang et al., 2016). Moreover, studies have shown that mechanical force-induced integrin-Src-FAK activation triggers multiple related pathways, including MAPK, PI3K-Akt, YAP/TAZ, and Rho-ROCK signaling (Moya and Halder, 2019; Schwartz, 2010).
Mechanoreceptors in neuronal and glial cells
Cytoskeletal components and adhesion molecules
Currently, it is understood that cells sense mechanical axial and shear stress through integrin-actomyosin dynamics (Schwartz, 2010). Integrins, which bind to ECM proteins like fibronectin or laminin, connect to the cytoskeleton-like actin when forces are applied. These mechanical cues trigger changes in integrins, initiating the formation of linkages with actin and other focal adhesion complex molecules, including Src, focal adhesion kinase (FAK), and proline-rich tyrosine kinase 2 (PYK2). Subsequent mechanical stretching of cells or use of rigid extracellular materials activates of various signaling cascades, including MAPK, Rho GTPases, PI3K, and increased cytoplasmic calcium levels (Schwartz, 2010). These cascades, in turn, further regulate cytoskeletal dynamics. In particular, YAP/TAZ signaling is influenced by ECM-integrin interactions with cytoskeletal F-actin, which promotes TEAD transcription factor activation (Panciera et al., 2017). This integrin-FAK-mediated response has also been implicated in ultrasound-mediated cellular responses (Tang et al., 2006).
Neurons and glial cells express distinct integrin subtypes depending on the brain regions and cell type (Pinkstaff et al., 1999). Notably, integrin α3β1 plays a crucial role in synaptic plasticity, while integrin αv regulates the migratory functions of glial cells (Wang et al., 2005; Lilja and Ivaska, 2018; Milner et al., 1996). In hippocampal neurons, the activation of integrin-dependent receptor-like protein tyrosine phosphatase α (RPTPα) has been implicated in mechanical sensory signaling (Kostic et al., 2007). In addition, PYK2 expression, along with FAK, are highly enriched in CNS neurons compared to other organ tissues and is involved in synapse and neurite formation (Menegon et al., 1999; de Pins et al., 2021). Although mature neurons typically do not express YAP and show low levels of integrin expression post-development, mechanical stretching might have a more limited impact on neurons than on other glial cells. Nonetheless, promoting stretch signaling appropriately could support axonal regeneration and plasticity, as overexpression or the activation of integrins has been shown to enhance neurite outgrowth in adult neurons (Condic, 2001; Ivins et al., 2000).
Activation of cellular membrane mechanosensory receptors and mechanical stress-gated channels
Members of the transient receptor potential (TRP) ion channel family serve as key sensors of mechanical stimulation in the nervous system. These channels are broadly expressed in the brain, spinal cord, and DRG neurons (Pedersen et al., 2005) and play a crucial role in mechanosensation, responding to a wide range of mechanical stimuli (Pedersen et al., 2005). Among them, the TRP Vanilloid receptor family (TRPV), which consists of Ca2+-permeable ion channels, is particularly significant for its activation by mechanical stress in the mammalian nervous system. For instance, TRPV2 is activated by cell stretching in neurons (Shibasaki et al., 2010), while TRPV4 in astrocytes responds to various mechanical stresses, including osmotic pressure (Kanju and Liedtke, 2016; Benfenati et al., 2007). Other members of the TRP family, such as TRPC, TRPM, and TRPA1, are also highly expressed in the mammalian CNS (Sawamura et al., 2017; Kheradpezhouh et al., 2017). Although the expression of these channels is abundant in neurons and glial cells, their activation in response to mechanical stress has not been well-characterized. Recently, TRPA1 has been highlighted for the role it serves in sensing fluid shear stress and regulating calcium influx in Drosophila enteroendocrine cells (Gong et al., 2023).
Purinergic P2 receptors also play a role in mechanosensation. The P2X and P2Y receptor subtypes are widely expressed in the brain and spinal cord, including in neurons and glial cells (Franke et al., 2006). Among them, the G protein-coupled P2Y2 receptor contains an integrin-binding site that mediates cytoskeletal signaling and stimulates PKC activation (Peterson et al., 2010). Furthermore, the P2Y2 receptor is particularly implicated in nerve injury and the pathology of neurodegenerative diseases such as Alzheimer’s disease (Peterson et al., 2010). In terms of mechanical stress, the P2Y2 receptor is activated by fluid shear stress, initiating intracellular cascades in endothelial cells (Sathanoori et al., 2017; Wang et al., 2015). In rat astrocytes, research indicates that stretch-induced Akt phosphorylation is mediated by P2 receptors (Neary et al., 2005). While P2 receptors appear to function as mechanosensors for various cell types, their specific roles in neurons, particularly in the context of mechanical or electromagnetic stimulation, remain unclear.
Piezo 1 and 2 are among of the most studied mechanically activated ion channels over the past decade. Initially discovered in the neuronal cell line Neuro2A and DRG cells, these channels play crucial roles in mechanotransduction (Coste et al., 2010). In the nervous system of mammals, Piezo1 is broadly expressed, whereas the expression of Piezo2 is more selectively found in sensory neurons, cortical and hippocampal pyramidal neurons, cerebellar Purkinje cells, and olfactory bulb neurons (Zong et al., 2023; Wang and Hamill, 2021). Piezo channels are now recognized as key mechanosensors that transduce signals by opening cation channels in response to mechanical stimuli including stretch, fluid shear stress, and ultrasound stimulation.
Recently, the role of Piezo channels in neurophysiology and related pathologies has garnered increasing attention, leading to several comprehensive reviews on their significance in the nervous system (Zong et al., 2023; Zheng et al., 2023; Fang et al., 2021). While Piezo1 primarily mediates cation influx in response to mechanical stimuli, its activation through classical cellular pathways, rather than mechanical sensation, yields varying outcomes. For example, activation of Piezo1 has been shown to reduce axonal outgrowth in mammalian neurons (Song et al., 2019), whereas, pharmacological activation of Piezo1 has been linked to demyelination in the mouse brain (Velasco-Estevez et al., 2020). These findings underscore the need for further research to elucidate the specific conditions required to trigger classical intracellular pathways and how these pathways may differ compared to other mechanosensors.
Several types of G protein-coupled receptors (GPCRs), including angiotensin II type 1 receptors and adhesion GPCRs (aGPCRs), have also been recognized for their mechanosensitive properties (Wilde et al., 2022). In particular, aGPCRs are known to be responsive to various mechanical stimuli including, shear stress, pressure, and stretching. These are hypothesized to regulate processes in the nervous system involved in development, synapse formation, and myelination (Langenhan et al., 2016). Various subfamilies of aGPCRs are widely expressed in the mammalian CNS, with their expression patterns varying by region and developmental stage (Ehrlich et al., 2018). Among them, GPR 56 and 68, which are expressed in both mouse and human brains, have been shown to be activated in response to mechanical stretching and fluid shear stress in vitro (Wei et al., 2018; Yeung et al., 2020; Ozkan et al., 2021). Activation of GPR56 is known to promote PKC activation and the Rho-ROCK signaling pathway (Ganesh et al., 2020). These findings suggest that other aGPCRs expressed in neurons and glial cells may also respond to mechanical forces, highlighting their potential mechanosensitive role and opening a new avenue for further investigation.
Studies suggest cells can sense electromagnetic stimulation through direct activation of voltage-gated calcium channels, resulting in an influx of calcium ions (Pall, 2013; Grassi et al., 2004). It is hypothesized that ion oscillations induced by electromagnetic stimulation disrupt the electrochemical balance of cell membranes (Panagopoulos et al., 2002). However, the specific types of calcium channels involved in this response have not been clearly elucidated. Another possibility is that thermosensitive TRPV channels are activated in response to the heat generated during electromagnetic stimulation (Stanley and Friedman, 2019). Additionally, given the abundant expression of TRPV channels in the brain and spinal cord, TRPV-mediated calcium influx may also serve as another plausible mechanism for cellular signaling in response to electromagnetic stimuli in the nervous system (Gunthorpe et al., 2002). In summary, the key mechanosensors discussed are succinctly illustrated in Figure 1.
Discussion
This review explores the key features of mechanically activated cellular pathways and related mechanosensors, with a particular focus on the mammalian nervous system. The studies discussed here primarily focused on two types of stimulation: mechanical stretching and electromagnetic stimulation. Electromagnetic stimulation has recently gained attention, particularly with the U.S. FDA’s approval of repetitive TMS in 2008 for treating major depressive disorder, and the approval of pulsed EMF for musculoskeletal disorders (George et al., 2013; Waldorff et al., 2017). Beyond TMS, there has been a growing interest in the use of ultrasound stimulation to modulate the nervous system, particularly in the context of mental health (Hameroff et al., 2013). The immense potential for practical applications of these techniques continues to attract significant interest in the field. While these methods can activate substantial cellular signaling pathways, the specific mechanosensitive channels or receptors remain largely undefined. As electromagnetic and ultrasound stimulation continues to expand in therapies and rehabilitations for neurodegenerative disorders and injuries, further elucidation of related mechanisms on neural cells is highly anticipated.
Among the cellular pathways responsive to mechanical and electromagnetic stimulation, the MAPK and Akt pathways have been extensively studied. Akt activation is commonly reported across various types of stimuli with the exception of high-frequency electromagnetic stimulation. However, the activation of specific subgroups of MAPKs may depend on the type of stimuli and cells involved. In neurons, phosphorylation of ERK and JNK is commonly triggered by various stimuli in vitro, while p38 MAPKs were not. In contrast, in vivo studies using compression models have shown upregulated ERK and p38 MAPK. However, these results should be cautiously interpreted as in vivo application using compression may cause cellular damage and inflammatory responses that confound the effects of mechanical stimulation.
Microglia exhibited different responses to ultrasound and electromagnetic stimuli compared to other cell types. Reports on the YAP/TAZ pathway have primarily focused on oligodendrocytes and Schwann cells, particularly in relation to changes in their myelination functions. In contrast, reports on the Rho-ROCK pathway have largely focused on matrix stiffness-mediated cellular differentiation in neural stem cells (Kang et al., 2020; Oyama et al., 2021).
A significant limitation of the current methodology lies in the difficulty of determining the appropriate range of force or intensity thresholds required for different types of mechanical stressors. While this is relatively straightforward in the case of using low-frequency electromagnetic stimulation (e.g., ~50 Hz) or high GHz microwave exposure, the precise magnitude of stimulation needed to achieve beneficial effects or avoid pathophysiological consequences remains an area requiring careful consideration. This issue necessitates thorough investigation across various contexts to accurately assess the cellular-level impacts that could lead to either positive outcomes or adverse effects. Additionally, distinguishing between calcium influx due to inflammatory reactions (e.g., injury) and that induced by mechanical stimuli in vivo is challenging. For example, Akt and p38 MAPK activation observed in in vivo models may be associated with subsequent neuroinflammation (Asih et al., 2020; Pu et al., 2023). Thus, we have prioritized in vitro studies to interpret results, though it is crucial to acknowledge in vivo results. Translating promising in vitro findings into in vivo contexts is necessary for identifying precise mechanisms. Micro-level techniques, such as AFM-based compression or microfluidic chips, offer the potential for more precise in vitro experimentation on mammalian neurons and glial cells (Onal et al., 2022). These methods could enable more detailed investigations into the activation and deactivation of specific cell signaling pathways.
Tissue stiffness-mediated mechanical stress was not explicitly addressed in this review because stretching and mechanical compression methods offer similar forms of mechanical stress. Comprehensive reviews done on tissue stiffness sensing, and related cellular pathways are available for further reading (Pillai and Franze, 2024; Motz et al., 2021; Schwartz, 2010; Janmey et al., 2020).While we did not discuss all types of mechanical stimuli and their molecular, it is important to emphasize that understanding stress type-specific molecular pathways is critical for comprehending clinically relevant biological responses in the CNS. This consideration is essential even for models not directly related to the traumatic CNS injury field.
In conclusion, various mechanical and electromagnetic stimuli can activate significant cellular signaling pathways in different ways, leading to diverse outcomes in the nervous system. Further research is needed to deepen our understanding of the precise biomolecular mechanisms underlying the therapeutic effects of mechanical stimulation on neuroregeneration and neurorehabilitation (Huie et al., 2017).
Author contributions
YR: Conceptualization, Investigation, Project administration, Resources, Supervision, Visualization, Writing – original draft, Writing – review & editing. AW: Writing – review & editing. XL: Supervision, Writing – review & editing. BF: Supervision, Writing – review & editing. AF: Supervision, Writing – review & editing. KM: Conceptualization, Funding acquisition, Project administration, Resources, Supervision, Writing – original draft, Writing – review & editing.
Funding
The author(s) declare that financial support was received for the research, authorship, and/or publication of this article. Research reported in this publication was supported by the National Institute of Arthritis and Musculoskeletal and Skin Diseases of the National Institutes of Health under Award Number P30AR075055. The content is solely the responsibility of the authors and does not necessarily represent the official views of the National Institutes of Health.
Acknowledgments
The authors would like to thank Dr. Futai Kensuke for his critical comments and valuable support.
Conflict of interest
The authors declare that the research was conducted in the absence of any commercial or financial relationships that could be construed as a potential conflict of interest.
Publisher’s note
All claims expressed in this article are solely those of the authors and do not necessarily represent those of their affiliated organizations, or those of the publisher, the editors and the reviewers. Any product that may be evaluated in this article, or claim that may be made by its manufacturer, is not guaranteed or endorsed by the publisher.
References
Adapala, R. K., Talasila, P. K., Bratz, I. N., Zhang, D. X., Suzuki, M., Meszaros, J. G., et al. (2011). PKCalpha mediates acetylcholine-induced activation of TRPV4-dependent calcium influx in endothelial cells. Am. J. Physiol. Heart Circ. Physiol. 301, H757–H765. doi: 10.1152/ajpheart.00142.2011
Adey, W. R. (1993). Biological effects of electromagnetic fields. J. Cell. Biochem. 51, 410–416. doi: 10.1002/jcb.2400510405
Aikawa, R., Nagai, T., Kudoh, S., Zou, Y., Tanaka, M., Tamura, M., et al. (2002). Integrins play a critical role in mechanical stress-induced p38 MAPK activation. Hypertension 39, 233–238. doi: 10.1161/hy0202.102699
Aksamitiene, E., Kiyatkin, A., and Kholodenko, B. N. (2012). Cross-talk between mitogenic Ras/MAPK and survival PI3K/Akt pathways: a fine balance. Biochem. Soc. Trans. 40, 139–146. doi: 10.1042/BST20110609
Aragona, M., Panciera, T., Manfrin, A., Giulitti, S., Michielin, F., Elvassore, N., et al. (2013). A mechanical checkpoint controls multicellular growth through YAP/TAZ regulation by actin-processing factors. Cell 154, 1047–1059. doi: 10.1016/j.cell.2013.07.042
Asih, P. R., Prikas, E., Stefanoska, K., Tan, A. R. P., Ahel, H. I., and Ittner, A. (2020). Functions of p38 MAP kinases in the central nervous system. Front. Mol. Neurosci. 13:570586. doi: 10.3389/fnmol.2020.570586
Bachstetter, A. D., Rowe, R. K., Kaneko, M., Goulding, D., Lifshitz, J., and Van Eldik, L. J. (2013). The p38alpha MAPK regulates microglial responsiveness to diffuse traumatic brain injury. J. Neurosci. 33, 6143–6153. doi: 10.1523/JNEUROSCI.5399-12.2013
Basu, S., Totty, N. F., Irwin, M. S., Sudol, M., and Downward, J. (2003). Akt phosphorylates the yes-associated protein, YAP, to induce interaction with 14-3-3 and attenuation of p73-mediated apoptosis. Mol. Cell 11, 11–23. doi: 10.1016/S1097-2765(02)00776-1
Batra, N., Riquelme, M. A., Burra, S., Kar, R., Gu, S., and Jiang, J. X. (2014). Direct regulation of osteocytic connexin 43 hemichannels through AKT kinase activated by mechanical stimulation. J. Biol. Chem. 289, 10582–10591. doi: 10.1074/jbc.M114.550608
Bell, J. D., Park, E., Ai, J., and Baker, A. J. (2009). PICK1-mediated GluR2 endocytosis contributes to cellular injury after neuronal trauma. Cell Death Differ. 16, 1665–1680. doi: 10.1038/cdd.2009.106
Benfenati, V., Amiry-Moghaddam, M., Caprini, M., Mylonakou, M. N., Rapisarda, C., Ottersen, O. P., et al. (2007). Expression and functional characterization of transient receptor potential vanilloid-related channel 4 (TRPV4) in rat cortical astrocytes. Neuroscience 148, 876–892. doi: 10.1016/j.neuroscience.2007.06.039
Berk, B. C., Corson, M. A., Peterson, T. E., and Tseng, H. (1995). Protein kinases as mediators of fluid shear stress stimulated signal transduction in endothelial cells: a hypothesis for calcium-dependent and calcium-independent events activated by flow. J. Biomech. 28, 1439–1450. doi: 10.1016/0021-9290(95)00092-5
Bhowmick, S., D'Mello, V., and Abdul-Muneer, P. M. (2019). Synergistic inhibition of ERK1/2 and JNK, not p38, phosphorylation ameliorates neuronal damages after traumatic brain injury. Mol. Neurobiol. 56, 1124–1136. doi: 10.1007/s12035-018-1132-7
Blank, M., and Goodman, R. (2009). Electromagnetic fields stress living cells. Pathophysiology 16, 71–78. doi: 10.1016/j.pathophys.2009.01.006
Blennow, K., Hardy, J., and Zetterberg, H. (2012). The neuropathology and neurobiology of traumatic brain injury. Neuron 76, 886–899. doi: 10.1016/j.neuron.2012.11.021
Boato, F., Guan, X., Zhu, Y., Ryu, Y., Voutounou, M., Rynne, C., et al. (2023). Activation of MAP2K signaling by genetic engineering or HF-rTMS promotes corticospinal axon sprouting and functional regeneration. Sci. Transl. Med. 15:eabq6885. doi: 10.1126/scitranslmed.abq6885
Boppart, M. D., Hirshman, M. F., Sakamoto, K., Fielding, R. A., and Goodyear, L. J. (2001). Static stretch increases c-Jun NH2-terminal kinase activity and p38 phosphorylation in rat skeletal muscle. Am. J. Physiol. Cell Physiol. 280, C352–C358. doi: 10.1152/ajpcell.2001.280.2.C352
Brunet, A., Datta, S. R., and Greenberg, M. E. (2001). Transcription-dependent and -independent control of neuronal survival by the PI3K-Akt signaling pathway. Curr. Opin. Neurobiol. 11, 297–305. doi: 10.1016/S0959-4388(00)00211-7
Buchsbaum, R. J. (2007). Rho activation at a glance. J. Cell Sci. 120, 1149–1152. doi: 10.1242/jcs.03428
Budday, S., Sommer, G., Birkl, C., Langkammer, C., Haybaeck, J., Kohnert, J., et al. (2017). Mechanical characterization of human brain tissue. Acta Biomater. 48, 319–340. doi: 10.1016/j.actbio.2016.10.036
Chen, C. H., Sung, C. S., Huang, S. Y., Feng, C. W., Hung, H. C., Yang, S. N., et al. (2016). The role of the PI3K/Akt/mTOR pathway in glial scar formation following spinal cord injury. Exp. Neurol. 278, 27–41. doi: 10.1016/j.expneurol.2016.01.023
Chen, Y. H., Zhang, R. G., Xue, F., Wang, H. N., Chen, Y. C., Hu, G. T., et al. (2015). Quetiapine and repetitive transcranial magnetic stimulation ameliorate depression-like behaviors and up-regulate the proliferation of hippocampal-derived neural stem cells in a rat model of depression: the involvement of the BDNF/ERK signal pathway. Pharmacol. Biochem. Behav. 136, 39–46. doi: 10.1016/j.pbb.2015.07.005
Cheng, J. J., Wung, B. S., Chao, Y. J., and Wang, D. L. (2001). Sequential activation of protein kinase C (PKC)-alpha and PKC-epsilon contributes to sustained Raf/ERK1/2 activation in endothelial cells under mechanical strain. J. Biol. Chem. 276, 31368–31375. doi: 10.1074/jbc.M011317200
Chierto, E., Simon, A., Castoldi, F., Meffre, D., Cristinziano, G., Sapone, F., et al. (2019). Mechanical stretch of high magnitude provokes axonal injury, elongation of Paranodal junctions, and signaling alterations in oligodendrocytes. Mol. Neurobiol. 56, 4231–4248. doi: 10.1007/s12035-018-1372-6
Chiu, Y. C., Huang, T. H., Fu, W. M., Yang, R. S., and Tang, C. H. (2008). Ultrasound stimulates MMP-13 expression through p38 and JNK pathway in osteoblasts. J. Cell. Physiol. 215, 356–365. doi: 10.1002/jcp.21322
Coffey, E. T. (2014). Nuclear and cytosolic JNK signalling in neurons. Nat. Rev. Neurosci. 15, 285–299. doi: 10.1038/nrn3729
Colciago, A., Melfi, S., Giannotti, G., Bonalume, V., Ballabio, M., Caffino, L., et al. (2015). Tumor suppressor Nf2/merlin drives Schwann cell changes following electromagnetic field exposure through hippo-dependent mechanisms. Cell Death Discov. 1:15021. doi: 10.1038/cddiscovery.2015.21
Condic, M. L. (2001). Adult neuronal regeneration induced by transgenic integrin expression. J. Neurosci. 21, 4782–4788. doi: 10.1523/JNEUROSCI.21-13-04782.2001
Coste, B., Mathur, J., Schmidt, M., Earley, T. J., Ranade, S., Petrus, M. J., et al. (2010). Piezo1 and Piezo2 are essential components of distinct mechanically activated cation channels. Science 330, 55–60. doi: 10.1126/science.1193270
Cui, M., Ge, H., Zeng, H., Yan, H., Zhang, L., Feng, H., et al. (2019). Repetitive transcranial magnetic stimulation promotes neural stem cell proliferation and differentiation after intracerebral hemorrhage in mice. Cell Transplant. 28, 568–584. doi: 10.1177/0963689719834870
Danciu, T. E., Adam, R. M., Naruse, K., Freeman, M. R., and Hauschka, P. V. (2003). Calcium regulates the PI3K-Akt pathway in stretched osteoblasts. FEBS Lett. 536, 193–197. doi: 10.1016/S0014-5793(03)00055-3
de Pins, B., Mendes, T., Giralt, A., and Girault, J. A. (2021). The non-receptor tyrosine kinase Pyk2 in brain function and neurological and psychiatric diseases. Front Synaptic Neurosci. 13:749001. doi: 10.3389/fnsyn.2021.749001
Dolgova, N., Wei, Z., Spink, B., Gui, L., Hua, Q., Truong, D., et al. (2021). Low-field magnetic stimulation accelerates the differentiation of oligodendrocyte precursor cells via non-canonical TGF-beta signaling pathways. Mol. Neurobiol. 58, 855–866. doi: 10.1007/s12035-020-02157-0
Dupont, S. (2016). Role of YAP/TAZ in cell-matrix adhesion-mediated signalling and mechanotransduction. Exp. Cell Res. 343, 42–53. doi: 10.1016/j.yexcr.2015.10.034
Ehrlich, A. T., Maroteaux, G., Robe, A., Venteo, L., Nasseef, M. T., van Kempen, L. C., et al. (2018). Expression map of 78 brain-expressed mouse orphan GPCRs provides a translational resource for neuropsychiatric research. Commun. Biol. 1:102. doi: 10.1038/s42003-018-0106-7
Elbediwy, A., Vincent-Mistiaen, Z. I., and Thompson, B. J. (2016). YAP and TAZ in epithelial stem cells: a sensor for cell polarity, mechanical forces and tissue damage. BioEssays 38, 644–653. doi: 10.1002/bies.201600037
Elkin, B. S., Ilankovan, A., and Morrison, B. 3rd. (2010). Age-dependent regional mechanical properties of the rat hippocampus and cortex. J. Biomech. Eng. 132:011010. doi: 10.1115/1.4000164
Etienne-Manneville, S., and Hall, A. (2002). Rho GTPases in cell biology. Nature 420, 629–635. doi: 10.1038/nature01148
Fang, X. Z., Zhou, T., Xu, J. Q., Wang, Y. X., Sun, M. M., He, Y. J., et al. (2021). Structure, kinetic properties and biological function of mechanosensitive piezo channels. Cell Biosci. 11:13. doi: 10.1186/s13578-020-00522-z
Ferguson, A. R., Christensen, R. N., Gensel, J. C., Miller, B. A., Sun, F., Beattie, E. C., et al. (2008). Cell death after spinal cord injury is exacerbated by rapid TNF alpha-induced trafficking of GluR2-lacking AMPARs to the plasma membrane. J. Neurosci. 28, 11391–11400. doi: 10.1523/JNEUROSCI.3708-08.2008
Fitzgerald, J. B., Jin, M., Chai, D. H., Siparsky, P., Fanning, P., and Grodzinsky, A. J. (2008). Shear- and compression-induced chondrocyte transcription requires MAPK activation in cartilage explants. J. Biol. Chem. 283, 6735–6743. doi: 10.1074/jbc.M708670200
Franke, H., Krugel, U., and Illes, P. (2006). P2 receptors and neuronal injury. Pflugers Arch. 452, 622–644. doi: 10.1007/s00424-006-0071-8
Ganesh, R. A., Venkataraman, K., and Sirdeshmukh, R. (2020). GPR56: an adhesion GPCR involved in brain development, neurological disorders and cancer. Brain Res. 1747:147055. doi: 10.1016/j.brainres.2020.147055
Gao, Q., Cooper, P. R., Walmsley, A. D., and Scheven, B. A. (2017). Role of piezo channels in ultrasound-stimulated dental stem cells. J. Endod. 43, 1130–1136. doi: 10.1016/j.joen.2017.02.022
George, M. S., Lisanby, S. H., and Sackeim, H. A. (1999). Transcranial magnetic stimulation: applications in neuropsychiatry. Arch. Gen. Psychiatry 56, 300–311. doi: 10.1001/archpsyc.56.4.300
George, M. S., Taylor, J. J., and Short, E. B. (2013). The expanding evidence base for rTMS treatment of depression. Curr. Opin. Psychiatry 26, 13–18. doi: 10.1097/YCO.0b013e32835ab46d
Goforth, P. B., Ellis, E. F., and Satin, L. S. (2004). Mechanical injury modulates AMPA receptor kinetics via an NMDA receptor-dependent pathway. J. Neurotrauma 21, 719–732. doi: 10.1089/0897715041269704
Gong, J., Nirala, N. K., Chen, J., Wang, F., Gu, P., Wen, Q., et al. (2023). TrpA1 is a shear stress mechanosensing channel regulating intestinal stem cell proliferation in Drosophila. Sci. Adv. 9:eadc9660. doi: 10.1126/sciadv.adc9660
Goriely, A., Geers, M. G., Holzapfel, G. A., Jayamohan, J., Jerusalem, A., Sivaloganathan, S., et al. (2015). Mechanics of the brain: perspectives, challenges, and opportunities. Biomech. Model. Mechanobiol. 14, 931–965. doi: 10.1007/s10237-015-0662-4
Grassi, C., D'Ascenzo, M., Torsello, A., Martinotti, G., Wolf, F., Cittadini, A., et al. (2004). Effects of 50 Hz electromagnetic fields on voltage-gated Ca2+ channels and their role in modulation of neuroendocrine cell proliferation and death. Cell Calcium 35, 307–315. doi: 10.1016/j.ceca.2003.09.001
Gunthorpe, M. J., Benham, C. D., Randall, A., and Davis, J. B. (2002). The diversity in the vanilloid (TRPV) receptor family of ion channels. Trends Pharmacol. Sci. 23, 183–191. doi: 10.1016/S0165-6147(02)01999-5
Haga, M., Chen, A., Gortler, D., Dardik, A., and Sumpio, B. E. (2003). Shear stress and cyclic strain may suppress apoptosis in endothelial cells by different pathways. Endothelium 10, 149–157. doi: 10.1080/10623320390233463
Hall, C. M., Moeendarbary, E., and Sheridan, G. K. (2021). Mechanobiology of the brain in ageing and Alzheimer's disease. Eur. J. Neurosci. 53, 3851–3878. doi: 10.1111/ejn.14766
Hameroff, S., Trakas, M., Duffield, C., Annabi, E., Gerace, M. B., Boyle, P., et al. (2013). Transcranial ultrasound (TUS) effects on mental states: a pilot study. Brain Stimul. 6, 409–415. doi: 10.1016/j.brs.2012.05.002
Han, S. R., Yang, G. Y., Ahn, M. H., Kim, M. J., Ju, J. S., Bae, Y. C., et al. (2012). Blockade of microglial activation reduces mechanical allodynia in rats with compression of the trigeminal ganglion. Prog. Neuro-Psychopharmacol. Biol. Psychiatry 36, 52–59. doi: 10.1016/j.pnpbp.2011.10.007
Higgins, S., Lee, J. S., Ha, L., and Lim, J. Y. (2013). Inducing neurite outgrowth by mechanical cell stretch. Biores Open Access 2, 212–216. doi: 10.1089/biores.2013.0008
Hollville, E., Romero, S. E., and Deshmukh, M. (2019). Apoptotic cell death regulation in neurons. FEBS J. 286, 3276–3298. doi: 10.1111/febs.14970
Hong, J., Kirkland, J. M., Acheta, J., Marziali, L. N., Beck, B., Jeanette, H., et al. (2023). YAP and TAZ regulate remyelination in the central nervous system. Glia 72, 156–166. doi: 10.1002/glia.24467
Hsu, H. J., Lee, C. F., Locke, A., Vanderzyl, S. Q., and Kaunas, R. (2010). Stretch-induced stress fiber remodeling and the activations of JNK and ERK depend on mechanical strain rate, but not FAK. PLoS One 5:e12470. doi: 10.1371/journal.pone.0012470
Hu, Z., Xiong, Y., Han, X., Geng, C., Jiang, B., Huo, Y., et al. (2013). Acute mechanical stretch promotes eNOS activation in venous endothelial cells mainly via PKA and Akt pathways. PLoS One 8:e71359. doi: 10.1371/journal.pone.0071359
Huang, K. P. (1989). The mechanism of protein kinase C activation. Trends Neurosci. 12, 425–432. doi: 10.1016/0166-2236(89)90091-X
Huang, Z., Hu, J., Pan, J., Wang, Y., Hu, G., Zhou, J., et al. (2016). YAP stabilizes SMAD1 and promotes BMP2-induced neocortical astrocytic differentiation. Development 143, 2398–2409. doi: 10.1242/dev.130658
Huang, S., and Ingber, D. E. (2005). Cell tension, matrix mechanics, and cancer development. Cancer Cell 8, 175–176. doi: 10.1016/j.ccr.2005.08.009
Huang, X., Yang, N., Fiore, V. F., Barker, T. H., Sun, Y., Morris, S. W., et al. (2012). Matrix stiffness-induced myofibroblast differentiation is mediated by intrinsic mechanotransduction. Am. J. Respir. Cell Mol. Biol. 47, 340–348. doi: 10.1165/rcmb.2012-0050OC
Huie, J. R., Morioka, K., Haefeli, J., and Ferguson, A. R. (2017). What is being trained? How divergent forms of plasticity compete to shape locomotor recovery after spinal cord injury. J. Neurotrauma 34, 1831–1840. doi: 10.1089/neu.2016.4562
Husse, B., Briest, W., Homagk, L., Isenberg, G., and Gekle, M. (2007). Cyclical mechanical stretch modulates expression of collagen I and collagen III by PKC and tyrosine kinase in cardiac fibroblasts. Am. J. Physiol. Regul. Integr. Comp. Physiol. 293, R1898–R1907. doi: 10.1152/ajpregu.00804.2006
Ishida, T., Takahashi, M., Corson, M. A., and Berk, B. C. (1997). Fluid shear stress-mediated signal transduction: how do endothelial cells transduce mechanical force into biological responses? Ann. NY. Acad. Sci. 811, 12–24. doi: 10.1111/j.1749-6632.1997.tb51984.x
Ivins, J. K., Yurchenco, P. D., and Lander, A. D. (2000). Regulation of neurite outgrowth by integrin activation. J. Neurosci. 20, 6551–6560. doi: 10.1523/JNEUROSCI.20-17-06551.2000
Jalali, S., Huang, Y., Dumont, D. J., and Hynynen, K. (2010). Focused ultrasound-mediated bbb disruption is associated with an increase in activation of AKT: experimental study in rats. BMC Neurol. 10:114. doi: 10.1186/1471-2377-10-114
Janmey, P. A., Fletcher, D. A., and Reinhart-King, C. A. (2020). Stiffness sensing by cells. Physiol. Rev. 100, 695–724. doi: 10.1152/physrev.00013.2019
Jiang, X., Savchenko, O., Li, Y., Qi, S., Yang, T., Zhang, W., et al. (2019). A review of low-intensity pulsed ultrasound for therapeutic applications. I.E.E.E. Trans. Biomed. Eng. 66, 2704–2718. doi: 10.1109/TBME.2018.2889669
Jin, J., Zhao, X., Fu, H., and Gao, Y. (2020). The effects of YAP and its related mechanisms in central nervous system diseases. Front. Neurosci. 14:595. doi: 10.3389/fnins.2020.00595
Jing, X., Yang, X., Zhang, W., Wang, S., Cui, X., Du, T., et al. (2020). Mechanical loading induces HIF-1alpha expression in chondrocytes via YAP. Biotechnol. Lett. 42, 1645–1654. doi: 10.1007/s10529-020-02910-4
John, G. R., Chen, L., Rivieccio, M. A., Melendez-Vasquez, C. V., Hartley, A., and Brosnan, C. F. (2004). Interleukin-1beta induces a reactive astroglial phenotype via deactivation of the rho GTPase-Rock axis. J. Neurosci. 24, 2837–2845. doi: 10.1523/JNEUROSCI.4789-03.2004
Kadohama, T., Akasaka, N., Nishimura, K., Hoshino, Y., Sasajima, T., and Sumpio, B. E. (2006). p38 mitogen-activated protein kinase activation in endothelial cell is implicated in cell alignment and elongation induced by fluid shear stress. Endothelium 13, 43–50. doi: 10.1080/10623320600660219
Kang, P. H., Schaffer, D. V., and Kumar, S. (2020). Angiomotin links ROCK and YAP signaling in mechanosensitive differentiation of neural stem cells. Mol. Biol. Cell 31, 386–396. doi: 10.1091/mbc.E19-11-0602
Kanju, P., and Liedtke, W. (2016). Pleiotropic function of TRPV4 ion channels in the central nervous system. Exp. Physiol. 101, 1472–1476. doi: 10.1113/EP085790
Kanno, T., Takahashi, T., Tsujisawa, T., Ariyoshi, W., and Nishihara, T. (2007). Mechanical stress-mediated Runx2 activation is dependent on Ras/ERK1/2 MAPK signaling in osteoblasts. J. Cell. Biochem. 101, 1266–1277. doi: 10.1002/jcb.21249
Kapri-Pardes, E., Hanoch, T., Maik-Rachline, G., Murbach, M., Bounds, P. L., Kuster, N., et al. (2017). Activation of signaling cascades by weak extremely low frequency electromagnetic fields. Cell. Physiol. Biochem. 43, 1533–1546. doi: 10.1159/000481977
Kapur, S., Baylink, D. J., and Lau, K. H. (2003). Fluid flow shear stress stimulates human osteoblast proliferation and differentiation through multiple interacting and competing signal transduction pathways. Bone 32, 241–251. doi: 10.1016/S8756-3282(02)00979-1
Keating, C. E., and Cullen, D. K. (2021). Mechanosensation in traumatic brain injury. Neurobiol. Dis. 148:105210. doi: 10.1016/j.nbd.2020.105210
Kheradpezhouh, E., Choy, J. M. C., Daria, V. R., and Arabzadeh, E. (2017). TRPA1 expression and its functional activation in rodent cortex. Open Biol. 7:160314. doi: 10.1098/rsob.160314
Kim, S. K., Lee, G. Y., Kim, S. K., Kwon, Y. J., Seo, E. B., Lee, H., et al. (2023). Protective effects of repetitive transcranial magnetic stimulation against Streptozotocin-induced Alzheimer's disease. Mol. Neurobiol. 61, 1687–1703. doi: 10.1007/s12035-023-03573-8
Kippenberger, S., Loitsch, S., Guschel, M., Muller, J., Knies, Y., Kaufmann, R., et al. (2005). Mechanical stretch stimulates protein kinase B/Akt phosphorylation in epidermal cells via angiotensin II type 1 receptor and epidermal growth factor receptor. J. Biol. Chem. 280, 3060–3067. doi: 10.1074/jbc.M409590200
Kjell, J., Fischer-Sternjak, J., Thompson, A. J., Friess, C., Sticco, M. J., Salinas, F., et al. (2020). Defining the adult neural stem cell niche proteome identifies key regulators of adult neurogenesis. Cell Stem Cell 26, 277–293.e8. doi: 10.1016/j.stem.2020.01.002
Kostic, A., Sap, J., and Sheetz, M. P. (2007). RPTPalpha is required for rigidity-dependent inhibition of extension and differentiation of hippocampal neurons. J. Cell Sci. 120, 3895–3904. doi: 10.1242/jcs.009852
Koyama, S., Haruyama, T., Kobatake, E., and Aizawa, M. (1997). Electrically induced NGF production by astroglial cells. Nat. Biotechnol. 15, 164–166. doi: 10.1038/nbt0297-164
Kusuyama, J., Bandow, K., Shamoto, M., Kakimoto, K., Ohnishi, T., and Matsuguchi, T. (2014). Low intensity pulsed ultrasound (LIPUS) influences the multilineage differentiation of mesenchymal stem and progenitor cell lines through ROCK-cot/Tpl2-MEK-ERK signaling pathway. J. Biol. Chem. 289, 10330–10344. doi: 10.1074/jbc.M113.546382
Langenhan, T., Piao, X., and Monk, K. R. (2016). Adhesion G protein-coupled receptors in nervous system development and disease. Nat. Rev. Neurosci. 17, 550–561. doi: 10.1038/nrn.2016.86
Lea, P. M., Custer, S. J., Vicini, S., and Faden, A. I. (2002). Neuronal and glial mGluR5 modulation prevents stretch-induced enhancement of NMDA receptor current. Pharmacol. Biochem. Behav. 73, 287–298. doi: 10.1016/S0091-3057(02)00825-0
Lee, J. L., Lo, C. W., Inserra, C., Bera, J. C., and Chen, W. S. (2014). Ultrasound enhanced PEI-mediated gene delivery through increasing the intracellular calcium level and PKC-delta protein expression. Pharm. Res. 31, 2354–2366. doi: 10.1007/s11095-014-1332-4
Lee, H. S., Millward-Sadler, S. J., Wright, M. O., Nuki, G., Al-Jamal, R., and Salter, D. M. (2002). Activation of integrin-RACK1/PKCalpha signalling in human articular chondrocyte mechanotransduction. Osteoarthr. Cartil. 10, 890–897. doi: 10.1053/joca.2002.0842
Lee, J. S., Panorchan, P., Hale, C. M., Khatau, S. B., Kole, T. P., Tseng, Y., et al. (2006). Ballistic intracellular nanorheology reveals ROCK-hard cytoplasmic stiffening response to fluid flow. J. Cell Sci. 119, 1760–1768. doi: 10.1242/jcs.02899
Lilja, J., and Ivaska, J. (2018). Integrin activity in neuronal connectivity. J. Cell Sci. 131:jcs212803. doi: 10.1242/jcs.212803
Lin, T., Zeng, L., Liu, Y., DeFea, K., Schwartz, M. A., Chien, S., et al. (2003). Rho-ROCK-LIMK-cofilin pathway regulates shear stress activation of sterol regulatory element binding proteins. Circ. Res. 92, 1296–1304. doi: 10.1161/01.RES.0000078780.65824.8B
Lindqvist, N., Liu, Q., Zajadacz, J., Franze, K., and Reichenbach, A. (2010). Retinal glial (Muller) cells: sensing and responding to tissue stretch. Invest. Ophthalmol. Vis. Sci. 51, 1683–1690. doi: 10.1167/iovs.09-4159
Liu, W. S., and Heckman, C. A. (1998). The sevenfold way of PKC regulation. Cell. Signal. 10, 529–542. doi: 10.1016/S0898-6568(98)00012-6
Liu, S. H., Lai, Y. L., Chen, B. L., and Yang, F. Y. (2017). Ultrasound enhances the expression of brain-derived neurotrophic factor in astrocyte through activation of TrkB-Akt and calcium-CaMK signaling pathways. Cereb. Cortex 27, 3152–3160. doi: 10.1093/cercor/bhw169
Louw, T. M., Budhiraja, G., Viljoen, H. J., and Subramanian, A. (2013). Mechanotransduction of ultrasound is frequency dependent below the cavitation threshold. Ultrasound Med. Biol. 39, 1303–1319. doi: 10.1016/j.ultrasmedbio.2013.01.015
Luo, J., Zheng, H., Zhang, L., Zhang, Q., Li, L., Pei, Z., et al. (2017). High-frequency repetitive transcranial magnetic stimulation (rTMS) improves functional recovery by enhancing neurogenesis and activating BDNF/TrkB signaling in ischemic rats. Int. J. Mol. Sci. 18:455. doi: 10.3390/ijms18020455
Ma, J., Zhang, Z., Su, Y., Kang, L., Geng, D., Wang, Y., et al. (2013). Magnetic stimulation modulates structural synaptic plasticity and regulates BDNF-TrkB signal pathway in cultured hippocampal neurons. Neurochem. Int. 62, 84–91. doi: 10.1016/j.neuint.2012.11.010
Maneshi, M. M., Maki, B., Gnanasambandam, R., Belin, S., Popescu, G. K., Sachs, F., et al. (2017). Mechanical stress activates NMDA receptors in the absence of agonists. Sci. Rep. 7:39610. doi: 10.1038/srep39610
Manikonda, P. K., Rajendra, P., Devendranath, D., Gunasekaran, B., Channakeshava, R. S. S., et al. (2007). Influence of extremely low frequency magnetic fields on Ca2+ signaling and NMDA receptor functions in rat hippocampus. Neurosci. Lett. 413, 145–149. doi: 10.1016/j.neulet.2006.11.048
Mao, J., Price, D. D., Mayer, D. J., and Hayes, R. L. (1992). Pain-related increases in spinal cord membrane-bound protein kinase C following peripheral nerve injury. Brain Res. 588, 144–149. doi: 10.1016/0006-8993(92)91354-H
Marinval, N., and Chew, S. Y. (2021). Mechanotransduction assays for neural regeneration strategies: a focus on glial cells. APL Bioeng. 5:021505. doi: 10.1063/5.0037814
Mendoza, M. C., Er, E. E., and Blenis, J. (2011). The Ras-ERK and PI3K-mTOR pathways: cross-talk and compensation. Trends Biochem. Sci. 36, 320–328. doi: 10.1016/j.tibs.2011.03.006
Menegon, A., Burgaya, F., Baudot, P., Dunlap, D. D., Girault, J. A., and Valtorta, F. (1999). FAK+ and PYK2/CAKbeta, two related tyrosine kinases highly expressed in the central nervous system: similarities and differences in the expression pattern. Eur. J. Neurosci. 11, 3777–3788. doi: 10.1046/j.1460-9568.1999.00798.x
Meng, Y., Hynynen, K., and Lipsman, N. (2021). Applications of focused ultrasound in the brain: from thermoablation to drug delivery. Nat. Rev. Neurol. 17, 7–22. doi: 10.1038/s41582-020-00418-z
Mengistu, M., Brotzman, H., Ghadiali, S., and Lowe-Krentz, L. (2011). Fluid shear stress-induced JNK activity leads to actin remodeling for cell alignment. J. Cell. Physiol. 226, 110–121. doi: 10.1002/jcp.22311
Milner, R., Edwards, G., Streuli, C., and Ffrench-Constant, C. (1996). A role in migration for the alpha V beta 1 integrin expressed on oligodendrocyte precursors. J. Neurosci. 16, 7240–7252. doi: 10.1523/JNEUROSCI.16-22-07240.1996
Mori, T., Wang, X., Jung, J. C., Sumii, T., Singhal, A. B., Fini, M. E., et al. (2002). Mitogen-activated protein kinase inhibition in traumatic brain injury: in vitro and in vivo effects. J. Cereb. Blood Flow Metab. 22, 444–452. doi: 10.1097/00004647-200204000-00008
Motz, C. T., Kabat, V., Saxena, T., Bellamkonda, R. V., and Zhu, C. (2021). Neuromechanobiology: an expanding field driven by the force of greater focus. Adv. Healthc. Mater. 10:e2100102. doi: 10.1002/adhm.202100102
Moya, I. M., and Halder, G. (2019). Hippo-YAP/TAZ signalling in organ regeneration and regenerative medicine. Nat. Rev. Mol. Cell Biol. 20, 211–226. doi: 10.1038/s41580-018-0086-y
Nakajima, H., Yamamoto, K., Agarwala, S., Terai, K., Fukui, H., Fukuhara, S., et al. (2017). Flow-dependent endothelial YAP regulation contributes to vessel maintenance. Dev. Cell 40, 523–536.e6. doi: 10.1016/j.devcel.2017.02.019
Nardone, R., Tezzon, F., Holler, Y., Golaszewski, S., Trinka, E., and Brigo, F. (2014). Transcranial magnetic stimulation (TMS)/repetitive TMS in mild cognitive impairment and Alzheimer's disease. Acta Neurol. Scand. 129, 351–366. doi: 10.1111/ane.12223
Neary, J. T., Kang, Y., Tran, M., and Feld, J. (2005). Traumatic injury activates protein kinase B/Akt in cultured astrocytes: role of extracellular ATP and P2 purinergic receptors. J. Neurotrauma 22, 491–500. doi: 10.1089/neu.2005.22.491
Neary, J. T., Kang, Y., Willoughby, K. A., and Ellis, E. F. (2003). Activation of extracellular signal-regulated kinase by stretch-induced injury in astrocytes involves extracellular ATP and P2 purinergic receptors. J. Neurosci. 23, 2348–2356. doi: 10.1523/JNEUROSCI.23-06-02348.2003
Nie, K., and Henderson, A. (2003). MAP kinase activation in cells exposed to a 60 Hz electromagnetic field. J. Cell. Biochem. 90, 1197–1206. doi: 10.1002/jcb.10704
Niehoff, A., Offermann, M., Dargel, J., Schmidt, A., Bruggemann, G. P., and Bloch, W. (2008). Dynamic and static mechanical compression affects Akt phosphorylation in porcine patellofemoral joint cartilage. J. Orthop. Res. 26, 616–623. doi: 10.1002/jor.20542
Noshita, N., Lewen, A., Sugawara, T., and Chan, P. H. (2002). Akt phosphorylation and neuronal survival after traumatic brain injury in mice. Neurobiol. Dis. 9, 294–304. doi: 10.1006/nbdi.2002.0482
Onal, S., Alkaisi, M. M., and Nock, V. (2022). Microdevice-based mechanical compression on living cells. iScience 25:105518. doi: 10.1016/j.isci.2022.105518
Ostrow, L. W., Langan, T. J., and Sachs, F. (2000). Stretch-induced endothelin-1 production by astrocytes. J. Cardiovasc. Pharmacol. 36, S274–S277. doi: 10.1097/00005344-200036051-00081
Ostrow, L. W., and Sachs, F. (2005). Mechanosensation and endothelin in astrocytes—hypothetical roles in CNS pathophysiology. Brain Res. Brain Res. Rev. 48, 488–508. doi: 10.1016/j.brainresrev.2004.09.005
Oyama, H., Nukuda, A., Ishihara, S., and Haga, H. (2021). Soft surfaces promote astrocytic differentiation of mouse embryonic neural stem cells via dephosphorylation of MRLC in the absence of serum. Sci. Rep. 11:19574. doi: 10.1038/s41598-021-99059-5
Ozkan, A. D., Gettas, T., Sogata, A., Phaychanpheng, W., Zhou, M., and Lacroix, J. J. (2021). Mechanical and chemical activation of GPR68 probed with a genetically encoded fluorescent reporter. J. Cell Sci. 134:jcs255455. doi: 10.1242/jcs.255455
Padmaperuma, B., Mark, R., Dhillon, H. S., Mattson, M. P., and Prasad, M. R. (1996). Alterations in brain protein kinase C after experimental brain injury. Brain Res. 714, 19–26. doi: 10.1016/0006-8993(95)01579-5
Pall, M. L. (2013). Electromagnetic fields act via activation of voltage-gated calcium channels to produce beneficial or adverse effects. J. Cell. Mol. Med. 17, 958–965. doi: 10.1111/jcmm.12088
Panagopoulos, D. J., Karabarbounis, A., and Margaritis, L. H. (2002). Mechanism for action of electromagnetic fields on cells. Biochem. Biophys. Res. Commun. 298, 95–102. doi: 10.1016/S0006-291X(02)02393-8
Panciera, T., Azzolin, L., Cordenonsi, M., and Piccolo, S. (2017). Mechanobiology of YAP and TAZ in physiology and disease. Nat. Rev. Mol. Cell Biol. 18, 758–770. doi: 10.1038/nrm.2017.87
Parekh, D. B., Ziegler, W., and Parker, P. J. (2000). Multiple pathways control protein kinase C phosphorylation. EMBO J. 19, 496–503. doi: 10.1093/emboj/19.4.496
Paulraj, R., and Behari, J. (2006). Protein kinase C activity in developing rat brain cells exposed to 2.45 GHz radiation. Electromagn. Biol. Med. 25, 61–70. doi: 10.1080/15368370600581939
Pedersen, S. F., Owsianik, G., and Nilius, B. (2005). TRP channels: an overview. Cell Calcium 38, 233–252. doi: 10.1016/j.ceca.2005.06.028
Persson, K., Sando, J. J., Tuttle, J. B., and Steers, W. D. (1995). Protein kinase C in cyclic stretch-induced nerve growth factor production by urinary tract smooth muscle cells. Am. J. Phys. 269, C1018–C1024. doi: 10.1152/ajpcell.1995.269.4.C1018
Pesqueira, T., Costa-Almeida, R., and Gomes, M. E. (2017). Uncovering the effect of low-frequency static magnetic field on tendon-derived cells: from mechanosensing to tenogenesis. Sci. Rep. 7:10948. doi: 10.1038/s41598-017-11253-6
Peterson, T. S., Camden, J. M., Wang, Y., Seye, C. I., Wood, W. G., Sun, G. Y., et al. (2010). P2Y2 nucleotide receptor-mediated responses in brain cells. Mol. Neurobiol. 41, 356–366. doi: 10.1007/s12035-010-8115-7
Piccolo, S., Dupont, S., and Cordenonsi, M. (2014). The biology of YAP/TAZ: hippo signaling and beyond. Physiol. Rev. 94, 1287–1312. doi: 10.1152/physrev.00005.2014
Pillai, E. K., and Franze, K. (2024). Mechanics in the nervous system: from development to disease. Neuron 112, 342–361. doi: 10.1016/j.neuron.2023.10.005
Pinkstaff, J. K., Detterich, J., Lynch, G., and Gall, C. (1999). Integrin subunit gene expression is regionally differentiated in adult brain. J. Neurosci. 19, 1541–1556. doi: 10.1523/JNEUROSCI.19-05-01541.1999
Poitelon, Y., Lopez-Anido, C., Catignas, K., Berti, C., Palmisano, M., Williamson, C., et al. (2016). YAP and TAZ control peripheral myelination and the expression of laminin receptors in Schwann cells. Nat. Neurosci. 19, 879–887. doi: 10.1038/nn.4316
Proces, A., Luciano, M., Kalukula, Y., Ris, L., and Gabriele, S. (2022). Multiscale Mechanobiology in brain physiology and diseases. Front. Cell Dev. Biol. 10:823857. doi: 10.3389/fcell.2022.823857
Pu, P. M., Li, Z. Y., Dai, Y. X., Sun, Y. L., Wang, Y. J., Cui, X. J., et al. (2023). Analysis of gene expression profiles and experimental validations of a rat chronic cervical cord compression model. Neurochem. Int. 168:105564. doi: 10.1016/j.neuint.2023.105564
Puts, R., Rikeit, P., Ruschke, K., Knaus, P., Schreivogel, S., and Raum, K. (2018). Functional regulation of YAP mechanosensitive transcriptional coactivator by focused low-intensity pulsed ultrasound (FLIPUS) enhances proliferation of murine mesenchymal precursors. PLoS One 13:e0206041. doi: 10.1371/journal.pone.0206041
Qin, X., Li, J., Sun, J., Liu, L., Chen, D., and Liu, Y. (2019). Low shear stress induces ERK nuclear localization and YAP activation to control the proliferation of breast cancer cells. Biochem. Biophys. Res. Commun. 510, 219–223. doi: 10.1016/j.bbrc.2019.01.065
Qiu, W., Bouakaz, A., Konofagou, E. E., and Zheng, H. (2021). Ultrasound for the brain: a review of physical and engineering principles, and clinical applications. IEEE Trans. Ultrason. Ferroelectr. Freq. Control 68, 6–20. doi: 10.1109/TUFFC.2020.3019932
Qu, Y. J., Jia, L., Zhang, X., Wei, H., and Yue, S. W. (2016). MAPK pathways are involved in neuropathic pain in rats with chronic compression of the dorsal root ganglion. Evid. Based Complement. Alternat. Med. 2016:6153215. doi: 10.1155/2016/6153215
Ralay, H. R., Zunich, S. M., Choi, N., Hodge, J. N., and Wainwright, M. S. (2011). Mild stretch-induced injury increases susceptibility to interleukin-1beta-induced release of matrix metalloproteinase-9 from astrocytes. J. Neurotrauma 28, 1757–1766. doi: 10.1089/neu.2011.1799
Rammensee, S., Kang, M. S., Georgiou, K., Kumar, S., and Schaffer, D. V. (2017). Dynamics of mechanosensitive neural stem cell differentiation. Stem Cells 35, 497–506. doi: 10.1002/stem.2489
Reiter, N., Paulsen, F., and Budday, S. (2023). Mechanisms of mechanical load transfer through brain tissue. Sci. Rep. 13:8703. doi: 10.1038/s41598-023-35768-3
Rocha, D. N., Carvalho, E. D., Relvas, J. B., Oliveira, M. J., and Pego, A. P. (2022). Mechanotransduction: exploring new therapeutic avenues in central nervous system pathology. Front. Neurosci. 16:861613. doi: 10.3389/fnins.2022.861613
Ryu, Y., Maekawa, T., Yoshino, D., Sakitani, N., Takashima, A., Inoue, T., et al. (2020). Mechanical regulation underlies effects of exercise on serotonin-induced signaling in the prefrontal cortex neurons. iScience. 23:100874. doi: 10.1016/j.isci.2020.100874
Sakamoto, K., Aschenbach, W. G., Hirshman, M. F., and Goodyear, L. J. (2003). Akt signaling in skeletal muscle: regulation by exercise and passive stretch. Am. J. Physiol. Endocrinol. Metab. 285, E1081–E1088. doi: 10.1152/ajpendo.00228.2003
Sanchez-Alegria, K., Flores-Leon, M., Avila-Munoz, E., Rodriguez-Corona, N., and Arias, C. (2018). PI3K signaling in neurons: a central node for the control of multiple functions. Int. J. Mol. Sci. 19:3725. doi: 10.3390/ijms19123725
Sathanoori, R., Bryl-Gorecka, P., Muller, C. E., Erb, L., Weisman, G. A., Olde, B., et al. (2017). P2Y (2) receptor modulates shear stress-induced cell alignment and actin stress fibers in human umbilical vein endothelial cells. Cell. Mol. Life Sci. 74, 731–746. doi: 10.1007/s00018-016-2365-0
Sato, M., Nagata, K., Kuroda, S., Horiuchi, S., Nakamura, T., Karima, M., et al. (2014). Low-intensity pulsed ultrasound activates integrin-mediated mechanotransduction pathway in synovial cells. Ann. Biomed. Eng. 42, 2156–2163. doi: 10.1007/s10439-014-1081-x
Sawamura, S., Shirakawa, H., Nakagawa, T., Mori, Y., and Kaneko, S. (2017). “TRP channels in the brain: what are they there for?” in Neurobiology of TRP channels. Frontiers in neuroscience. ed. T. L. R. Emir (Boca Raton, FL: CRC Press/Taylor & Francis), 295–322.
Schwartz, M. A. (2010). Integrins and extracellular matrix in mechanotransduction. Cold Spring Harb. Perspect. Biol. 2:a005066. doi: 10.1101/cshperspect.a005066
Shibasaki, K., Murayama, N., Ono, K., Ishizaki, Y., and Tominaga, M. (2010). TRPV2 enhances axon outgrowth through its activation by membrane stretch in developing sensory and motor neurons. J. Neurosci. 30, 4601–4612. doi: 10.1523/JNEUROSCI.5830-09.2010
Shimizu, T., Osanai, Y., Tanaka, K. F., Abe, M., Natsume, R., Sakimura, K., et al. (2017). YAP functions as a mechanotransducer in oligodendrocyte morphogenesis and maturation. Glia 65, 360–374. doi: 10.1002/glia.23096
Siebner, H. R., Funke, K., Aberra, A. S., Antal, A., Bestmann, S., Chen, R., et al. (2022). Transcranial magnetic stimulation of the brain: what is stimulated? - a consensus and critical position paper. Clin. Neurophysiol. 140, 59–97. doi: 10.1016/j.clinph.2022.04.022
Song, Y., Li, D., Farrelly, O., Miles, L., Li, F., Kim, S. E., et al. (2019). The mechanosensitive Ion Channel piezo inhibits axon regeneration. Neuron 102, 373–389.e6. doi: 10.1016/j.neuron.2019.01.050
Song, F., Wang, Y., Jiang, D., Wang, T., Zhang, Y., Ma, H., et al. (2016). Cyclic compressive stress regulates apoptosis in rat osteoblasts: involvement of PI3K/Akt and JNK MAPK signaling pathways. PLoS One 11:e0165845. doi: 10.1371/journal.pone.0165845
Spaethling, J., Le, L., and Meaney, D. F. (2012). NMDA receptor mediated phosphorylation of GluR1 subunits contributes to the appearance of calcium-permeable AMPA receptors after mechanical stretch injury. Neurobiol. Dis. 46, 646–654. doi: 10.1016/j.nbd.2012.03.003
Stankiewicz, T. R., and Linseman, D. A. (2014). Rho family GTPases: key players in neuronal development, neuronal survival, and neurodegeneration. Front. Cell. Neurosci. 8:314. doi: 10.3389/fncel.2014.00314
Stanley, S. A., and Friedman, J. M. (2019). Electromagnetic regulation of cell activity. Cold Spring Harb. Perspect. Med. 9:p.a034322. doi: 10.1101/cshperspect.a034322
Su, W. S., Wu, C. H., Song, W. S., Chen, S. F., and Yang, F. Y. (2023). Low-intensity pulsed ultrasound ameliorates glia-mediated inflammation and neuronal damage in experimental intracerebral hemorrhage conditions. J. Transl. Med. 21:565. doi: 10.1186/s12967-023-04377-z
Sun, Z., Guo, Y. S., Yan, S. J., Wan, Z. Y., Gao, B., Wang, L., et al. (2013). CK8 phosphorylation induced by compressive loads underlies the downregulation of CK8 in human disc degeneration by activating protein kinase C. Lab. Investig. 93, 1323–1330. doi: 10.1038/labinvest.2013.122
Surapisitchat, J., Hoefen, R. J., Pi, X., Yoshizumi, M., Yan, C., and Berk, B. C. (2001). Fluid shear stress inhibits TNF-alpha activation of JNK but not ERK1/2 or p38 in human umbilical vein endothelial cells: inhibitory crosstalk among MAPK family members. Proc. Natl. Acad. Sci. USA 98, 6476–6481. doi: 10.1073/pnas.101134098
Suzuma, I., Suzuma, K., Ueki, K., Hata, Y., Feener, E. P., King, G. L., et al. (2002). Stretch-induced retinal vascular endothelial growth factor expression is mediated by phosphatidylinositol 3-kinase and protein kinase C (PKC)-zeta but not by stretch-induced ERK1/2, Akt, Ras, or classical/novel PKC pathways. J. Biol. Chem. 277, 1047–1057. doi: 10.1074/jbc.M105336200
Takei, T., Han, O., Ikeda, M., Male, P., Mills, I., and Sumpio, B. E. (1997). Cyclic strain stimulates isoform-specific PKC activation and translocation in cultured human keratinocytes. J. Cell. Biochem. 67, 327–337. doi: 10.1002/(SICI)1097-4644(19971201)67:3<327::AID-JCB5>3.0.CO;2-Y
Tan, S., Wang, H., Xu, X., Zhao, L., Zhang, J., Dong, J., et al. (2021). Acute effects of 2.856 GHz and 1.5 GHz microwaves on spatial memory abilities and CREB-related pathways. Sci. Rep. 11:12348. doi: 10.1038/s41598-021-91622-4
Tanaka, R., Umemura, M., Narikawa, M., Fujita, T., Yokoyama, U., Ishigami, T., et al. (2018). Hydrostatic pressure suppresses fibrotic changes via Akt/GSK-3 signaling in human cardiac fibroblasts. Physiol. Rep. 6:e13687. doi: 10.14814/phy2.13687
Tang, C. H., Yang, R. S., Huang, T. H., Lu, D. Y., Chuang, W. J., Huang, T. F., et al. (2006). Ultrasound stimulates cyclooxygenase-2 expression and increases bone formation through integrin, focal adhesion kinase, phosphatidylinositol 3-kinase, and Akt pathway in osteoblasts. Mol. Pharmacol. 69, 2047–2057. doi: 10.1124/mol.105.022160
Teramura, T., Takehara, T., Onodera, Y., Nakagawa, K., Hamanishi, C., and Fukuda, K. (2012). Mechanical stimulation of cyclic tensile strain induces reduction of pluripotent related gene expressions via activation of rho/ROCK and subsequent decreasing of AKT phosphorylation in human induced pluripotent stem cells. Biochem. Biophys. Res. Commun. 417, 836–841. doi: 10.1016/j.bbrc.2011.12.052
Thomas, G. M., and Huganir, R. L. (2004). MAPK cascade signalling and synaptic plasticity. Nat. Rev. Neurosci. 5, 173–183. doi: 10.1038/nrn1346
Torsoni, A. S., Marin, T. M., Velloso, L. A., and Franchini, K. G. (2005). RhoA/ROCK signaling is critical to FAK activation by cyclic stretch in cardiac myocytes. Am. J. Physiol. Heart Circ. Physiol. 289, H1488–H1496. doi: 10.1152/ajpheart.00692.2004
Traub, O., Monia, B. P., Dean, N. M., and Berk, B. C. (1997). PKC-epsilon is required for mechano-sensitive activation of ERK1/2 in endothelial cells. J. Biol. Chem. 272, 31251–31257. doi: 10.1074/jbc.272.50.31251
Tufail, Y., Yoshihiro, A., Pati, S., Li, M. M., and Tyler, W. J. (2011). Ultrasonic neuromodulation by brain stimulation with transcranial ultrasound. Nat. Protoc. 6, 1453–1470. doi: 10.1038/nprot.2011.371
Uckun, F. M., Kurosaki, T., Jin, J., Jun, X., Morgan, A., Takata, M., et al. (1995). Exposure of B-lineage lymphoid cells to low energy electromagnetic fields stimulates Lyn kinase. J. Biol. Chem. 270, 27666–27670. doi: 10.1074/jbc.270.46.27666
VanBavel, E. (2007). Effects of shear stress on endothelial cells: possible relevance for ultrasound applications. Prog. Biophys. Mol. Biol. 93, 374–383. doi: 10.1016/j.pbiomolbio.2006.07.017
Velasco-Estevez, M., Gadalla, K. K. E., Linan-Barba, N., Cobb, S., Dev, K. K., and Sheridan, G. K. (2020). Inhibition of Piezo1 attenuates demyelination in the central nervous system. Glia 68, 356–375. doi: 10.1002/glia.23722
Waldorff, E. I., Zhang, N., and Ryaby, J. T. (2017). Pulsed electromagnetic field applications: a corporate perspective. J. Orthop. Transl. 9, 60–68. doi: 10.1016/j.jot.2017.02.006
Wang, H. Y., Crupi, D., Liu, J., Stucky, A., Cruciata, G., Di Rocco, A., et al. (2011). Repetitive transcranial magnetic stimulation enhances BDNF-TrkB signaling in both brain and lymphocyte. J. Neurosci. 31, 11044–11054. doi: 10.1523/JNEUROSCI.2125-11.2011
Wang, J., and Hamill, O. P. (2021). Piezo2-peripheral baroreceptor channel expressed in select neurons of the mouse brain: a putative mechanism for synchronizing neural networks by transducing intracranial pressure pulses. J. Integr. Neurosci. 20, 825–837. doi: 10.31083/j.jin2004085
Wang, S., Iring, A., Strilic, B., Albarran Juarez, J., Kaur, H., Troidl, K., et al. (2015). P2Y (2) and Gq/G (1) (1) control blood pressure by mediating endothelial mechanotransduction. J. Clin. Invest. 125, 3077–3086. doi: 10.1172/JCI81067
Wang, C., Ji, Y., Zhang, H., Ye, Y., Zhang, G., Zhang, S., et al. (2023). Increased level of exosomal miR-20b-5p derived from hypothermia-treated microglia promotes neurite outgrowth and synapse recovery after traumatic brain injury. Neurobiol. Dis. 179:106042. doi: 10.1016/j.nbd.2023.106042
Wang, M., Kong, Q., Gonzalez, F. A., Sun, G., Erb, L., Seye, C., et al. (2005). P2Y nucleotide receptor interaction with alpha integrin mediates astrocyte migration. J. Neurochem. 95, 630–640. doi: 10.1111/j.1471-4159.2005.03408.x
Wang, L., Luo, J. Y., Li, B., Tian, X. Y., Chen, L. J., Huang, Y., et al. (2016). Integrin-YAP/TAZ-JNK cascade mediates atheroprotective effect of unidirectional shear flow. Nature 540, 579–582. doi: 10.1038/nature20602
Wei, W. C., Bianchi, F., Wang, Y. K., Tang, M. J., Ye, H., and Glitsch, M. D. (2018). Coincidence detection of membrane stretch and extracellular pH by the proton-sensing receptor OGR1 (GPR68). Curr. Biol. 28, 3815–3823.e4. doi: 10.1016/j.cub.2018.10.046
Wilde, C., Mitgau, J., Suchy, T., Schoneberg, T., and Liebscher, I. (2022). Translating the force-mechano-sensing GPCRs. Am. J. Physiol. Cell Physiol. 322, C1047–C1060. doi: 10.1152/ajpcell.00465.2021
Xie, C., Shen, X., Xu, X., Liu, H., Li, F., Lu, S., et al. (2020). Astrocytic YAP promotes the formation of glia scars and neural regeneration after spinal cord injury. J. Neurosci. 40, 2644–2662. doi: 10.1523/JNEUROSCI.2229-19.2020
Xin, Z., Lin, G., Lei, H., Lue, T. F., and Guo, Y. (2016). Clinical applications of low-intensity pulsed ultrasound and its potential role in urology. Transl. Androl. Urol. 5, 255–266. doi: 10.21037/tau.2016.02.04
Xu, X. M., Xu, T. M., Wei, Y. B., Gao, X. X., Sun, J. C., Wang, Y., et al. (2018). Low-intensity pulsed ultrasound treatment accelerates angiogenesis by activating YAP/TAZ in human umbilical vein endothelial cells. Ultrasound Med. Biol. 44, 2655–2661. doi: 10.1016/j.ultrasmedbio.2018.07.007
Yang, G., Ren, Z., and Mei, Y. A. (2015). Exposure to 50 Hz magnetic field modulates GABAA currents in cerebellar granule neurons through an EP receptor-mediated PKC pathway. J. Cell. Mol. Med. 19, 2413–2422. doi: 10.1111/jcmm.12626
Yang, L. L., Zhou, Y., Tian, W. D., Li, H. J., Kang Chu, L., Miao, X., et al. (2016). Electromagnetic pulse activated brain microglia via the p38 MAPK pathway. Neurotoxicology 52, 144–149. doi: 10.1016/j.neuro.2015.12.008
Yeung, J., Adili, R., Stringham, E. N., Luo, R., Vizurraga, A., Rosselli-Murai, L. K., et al. (2020). GPR56/ADGRG1 is a platelet collagen-responsive GPCR and hemostatic sensor of shear force. Proc. Natl. Acad. Sci. USA 117, 28275–28286. doi: 10.1073/pnas.2008921117
Yu, H., He, J., Su, G., Wang, Y., Fang, F., Yang, W., et al. (2021). Fluid shear stress activates YAP to promote epithelial-mesenchymal transition in hepatocellular carcinoma. Mol. Oncol. 15, 3164–3183. doi: 10.1002/1878-0261.13061
Zarka, M., Etienne, F., Bourmaud, M., Szondi, D., Schwartz, J. M., Kampmann, K., et al. (2021). Mechanical loading activates the YAP/TAZ pathway and chemokine expression in the MLO-Y4 osteocyte-like cell line. Lab. Investig. 101, 1597–1604. doi: 10.1038/s41374-021-00668-5
Zhang, T., Lin, S., Shao, X., Zhang, Q., Xue, C., Zhang, S., et al. (2017). Effect of matrix stiffness on osteoblast functionalization. Cell Prolif. 50:e12338. doi: 10.1111/cpr.12338
Zhang, W., and Liu, H. T. (2002). MAPK signal pathways in the regulation of cell proliferation in mammalian cells. Cell Res. 12, 9–18. doi: 10.1038/sj.cr.7290105
Zhang, L., Rzigalinski, B. A., Ellis, E. F., and Satin, L. S. (1996). Reduction of voltage-dependent Mg2+ blockade of NMDA current in mechanically injured neurons. Science 274, 1921–1923. doi: 10.1126/science.274.5294.1921
Zhang, Y., Yan, J., Xu, H., Yang, Y., Li, W., Wu, H., et al. (2018). Extremely low frequency electromagnetic fields promote mesenchymal stem cell migration by increasing intracellular ca (2+) and activating the FAK/rho GTPases signaling pathways in vitro. Stem Cell Res Ther 9:143. doi: 10.1186/s13287-018-0883-4
Zhao, Y., Luo, P., Guo, Q., Li, S., Zhang, L., Zhao, M., et al. (2012). Interactions between SIRT1 and MAPK/ERK regulate neuronal apoptosis induced by traumatic brain injury in vitro and in vivo. Exp. Neurol. 237, 489–498. doi: 10.1016/j.expneurol.2012.07.004
Zhao, C. G., Qin, J., Sun, W., Ju, F., Zhao, Y. L., Wang, R., et al. (2019). rTMS regulates the balance between proliferation and apoptosis of spinal cord derived neural stem/progenitor cells. Front. Cell. Neurosci. 13:584. doi: 10.3389/fncel.2019.00584
Zheng, Q., Liu, H., Yu, W., Dong, Y., Zhou, L., Deng, W., et al. (2023). Mechanical properties of the brain: focus on the essential role of Piezo1-mediated mechanotransduction in the CNS. Brain Behav. 13:e3136. doi: 10.1002/brb3.3136
Zheng, L., Zhang, L., Chen, L., Jiang, J., Zhou, X., Wang, M., et al. (2018). Static magnetic field regulates proliferation, migration, differentiation, and YAP/TAZ activation of human dental pulp stem cells. J. Tissue Eng. Regen. Med. 12, 2029–2040. doi: 10.1002/term.2737
Zhou, S., Bachem, M. G., Seufferlein, T., Li, Y., Gross, H. J., and Schmelz, A. (2008). Low intensity pulsed ultrasound accelerates macrophage phagocytosis by a pathway that requires actin polymerization, rho, and Src/MAPKs activity. Cell. Signal. 20, 695–704. doi: 10.1016/j.cellsig.2007.12.005
Zong, B., Yu, F., Zhang, X., Pang, Y., Zhao, W., Sun, P., et al. (2023). Mechanosensitive Piezo1 channel in physiology and pathophysiology of the central nervous system. Ageing Res. Rev. 90:102026. doi: 10.1016/j.arr.2023.102026
Keywords: mechanotransduction, nervous system, mechanical stress, cellular signaling, mechanosensor
Citation: Ryu Y, Wague A, Liu X, Feeley BT, Ferguson AR and Morioka K (2024) Cellular signaling pathways in the nervous system activated by various mechanical and electromagnetic stimuli. Front. Mol. Neurosci. 17:1427070. doi: 10.3389/fnmol.2024.1427070
Edited by:
Ryo Hotta, Harvard Medical School, United StatesReviewed by:
Daniel Marcel Suter, Purdue University, United StatesEmily Welby, Medical College of Wisconsin, United States
Copyright © 2024 Ryu, Wague, Liu, Feeley, Ferguson and Morioka. This is an open-access article distributed under the terms of the Creative Commons Attribution License (CC BY). The use, distribution or reproduction in other forums is permitted, provided the original author(s) and the copyright owner(s) are credited and that the original publication in this journal is cited, in accordance with accepted academic practice. No use, distribution or reproduction is permitted which does not comply with these terms.
*Correspondence: Kazuhito Morioka, a2F6dWhpdG8ubW9yaW9rYUB1Y3NmLmVkdQ==; Youngjae Ryu, eW91bmdqYWUucnl1QHVtYXNzbWVkLmVkdQ==