- 1Max-Planck-Institute of Immunobiology and Epigenetics, Freiburg, Germany
- 2Faculty of Biology, Albert Ludwig University, Freiburg, Germany
- 3International Max Planck Research School for Immunobiology, Epigenetics, and Metabolism (IMPRS-IEM), Freiburg, Germany
In the nervous system, alternative RNA processing is particularly prevalent, which results in the expression of thousands of transcript variants found in no other tissue. Neuron-specific RNA-binding proteins co-transcriptionally regulate alternative splicing, alternative polyadenylation, and RNA editing, thereby shaping the RNA identity of nervous system cells. Recent evidence suggests that interactions between RNA-binding proteins and cis-regulatory elements such as promoters and enhancers play a role in the determination of neuron-specific expression profiles. Here, we discuss possible mechanisms through which transcription and RNA processing cross-talk to generate the uniquely complex neuronal transcriptome, with a focus on alternative 3′-end formation.
Introduction
Neurons are structurally and functionally complex cells that constantly adapt to their environment and to external stimuli. This necessitates a rapid, dynamic yet robust coordination of gene expression, a task that neurons achieve by specifically modulating transcription and RNA processing. Alternative splicing (AS) and alternative polyadenylation (APA) of mRNA precursors can generate multiple mRNA isoforms from the same transcription unit. In APA, the use of several functional polyadenylation [poly(A)] sites results in mRNA isoforms with different 3′-ends. When alternative [poly(A)] sites are located upstream of the stop codon, transcripts differ in their protein coding potential. More commonly, mRNAs with different 3′ UTRs are generated (Mitschka and Mayr, 2022). Notably, in animals from flies to humans, hundreds of genes undergo a shift toward more distal [poly(A)] sites exclusively in neurons, thus producing longer, often ultra-long, 3′ UTRs, termed “neuronal 3′ UTRs” (nUTRs) (Hilgers et al., 2011; Smibert et al., 2012; Miura et al., 2013; Carrasco et al., 2020; Wei et al., 2020). The alternative use of splice sites through AS is also particularly prevalent in neurons; the selective inclusion or exclusion of exons results in thousands of neuron-specific transcript variants (Carrasco et al., 2020; Lee et al., 2021). One particularly striking example of neural-regulated AS is the systematic inclusion of <30 nucleotide “microexons” (Irimia et al., 2014) that is mediated by the eMIC protein domain across Bilateria (Torres-Méndez et al., 2019). Interestingly, the splicing programs independently evolved in nonvertebrate and vertebrate bilaterians, but ultimately regulate neuronal excitability: in mammals, neuronal microexons encode amino acids on the surface of interaction domains of proteins involved in neurogenesis, whereas in flies, top splicing targets are enriched in ion channels (Torres-Méndez et al., 2022).
An integral and conserved feature of neurogenesis, neuronal RNA processing generates mRNA isoforms that differ in their coding or UTR sequence, thereby increasing proteome diversity and fine-tuning gene expression [reviewed in (Bhat et al., 2022, Hilgers, 2022, Wei and Lai, 2022, Lee et al., 2023)]. Neuron-specific RNA isoforms play an important role in neurogenesis (Zhang et al., 2019; Bae et al., 2020; Bae and Miura, 2020; Carrasco et al., 2020) and contribute to the versatility of neuronal cells by helping coordinate specialized processes. Although the importance of RNA-based regulation in human neurological disease has been known for decades, the underlying pathogenic mechanisms are still not well understood.
RNA-binding proteins regulate alternative RNA processing in neurons
RNA processing is regulated by a myriad of RNA-binding proteins (RBPs) that usually act in a cell-, gene-, and context-specific manner. Many RBPs are enriched or exclusively expressed in neural tissues, and consequently mediate RNA processing in a nervous-system-specific manner. Such RBPs and their molecular roles are typically well-conserved across metazoans; they include members of the protein families ELAV (Embryonic Lethal Abnormal Vision)/Hu (Human antigen) PTBP (Polypyrimidine Tract-Binding Protein), NOVA antigen (Neuro-oncological ventral), RBFOX (RNA-binding Fox-1 homolog), and CELF (CUGBP Elav-like family). The role of these protein families in neuronal RNA processing have been recently described in an excellent review (Lee et al., 2023). In this perspective article, we will maintain a focus on the well-studied protein ELAV as a representative model for neuron-specific RBPs and their interactions with transcriptional processing.
ELAV/Hu proteins highly conserved RBPs critical for neuronal differentiation, maturation and function (Mirisis and Carew, 2019; Hilgers, 2022; Wei and Lai, 2022; Mulligan and Bicknell, 2023). Typically, at least one member of the ELAV/Hu protein family is expressed specifically in neurons, and ELAV/Hu proteins serve as markers for neuronal cell types throughout the animal kingdom (Pascale et al., 2008). In Drosophila, where it was first described, ELAV regulates AS as well as APA (Koushika et al., 1996, 2000; Soller and White, 2003). Genome-wide studies in Drosophila revealed that ELAV/Hu operate on the transcriptome scale, with hundreds of genes undergoing ELAV-dependent alternative processing (Hilgers et al., 2012; Carrasco et al., 2020; Wei et al., 2020; Lee et al., 2021). Strikingly, all neuron-specific APA events were shown to depend on ELAV; the RNA signatures mediated by ELAV/Hu proteins are so manifold and distinct that the RBP is considered a “master regulator” of neuronal RNA processing in Drosophila. It remains to be seen whether ELAV/Hu proteins possess a similar monopoly in other systems; evidence from individual genes in human and mouse systems suggests that they do (Zhu et al., 2007; Dai et al., 2012; Mansfield and Keene, 2012; Dorrity et al., 2023), although the molecular intricacies remain to be solved. In Drosophila, ELAV binds nascent transcripts in the vicinity of proximal poly(A) and splice sites to inhibit their usage and foster APA and AS, respectively. Nearly all mRNAs found to be deregulated in elav mutants were direct binding targets of ELAV as seen by iCLIP in fly brains (Carrasco et al., 2020), suggesting that ELAV regulates its functional APA targets through direct physical interaction. In contrast, while ELAV binds to many AS targets at relevant splice sites (Carrasco et al., 2020; Lee et al., 2021), indicative of a direct effect, an indirect role was also described: ELAV mediates neuronal APA of Srrm234, and the resulting eMIC-containing isoform of Srrm234 in turn globally promotes the inclusion of neural microexons (Torres-Méndez et al., 2022).
A role for cis-regulatory elements in alternative RNA processing
In addition to trans-acting factors such as RBPs, recent findings point to a role for cis-regulatory sequences —promoters, enhancers— and their associated effectors —transcription factors— in the regulation of RNA processing. Early studies showed a physical association of RNA processing factors with the transcription machinery, as well as a positive influence of transcriptional activation on 3′-end processing (Dantonel et al., 1997; Calvo and Manley, 2003; Glover-Cutter et al., 2008; Wang et al., 2010; Nagaike et al., 2011; Yang et al., 2016; Carminati et al., 2023). Conversely, effective co-transcriptional processing is necessary for RNA Polymerase II (Pol II) processivity (Tellier et al., 2020). The mechanistic underpinnings of the regulatory coupling between transcription initiation, processing and transcription termination are not well understood. Accumulating evidence has shown that these couplings are important for context-, tissue-, and gene-specific APA and AS. Correlations between the use of distinct transcription start sites (TSSs) and 3′-end processing at different poly(A) sites have been observed, for example in different cell types (Anvar et al., 2018; Hardwick et al., 2022) and in the disease context (Demircioğlu et al., 2019). Recent studies have now established a causal link between sites of transcription initiation and sites of RNA processing: in Drosophila brains and human cerebral organoids, specific TSSs —so-called “dominant promoters”— foster the selection of distinct splice and 3′-end processing sites. Promoter dominance is highly prevalent in the nervous system, occurring in about 40–60% of genes, and broadly regulates mRNA isoform selection (Alfonso-Gonzalez et al., 2023). A role for distal gene enhancers and the relative position of the TSS relative to 3′-end sites on the DNA template have also been shown to influence 3′-end processing activity, and consequently, the expression of alternative 3′ UTR isoforms (Kwon et al., 2022; Calvo-Roitberg et al., 2024). These couplings between transcription initiation and RNA processing choices suggest a widespread coordination of events that occur during transcription; they also imply that many RNA processing events are regulated as soon as transcription initiates, many kilobases upstream.
Regulation of RNA processing by transcription factors
Transcription factors (TFs), the key effectors and regulators of transcription, likely play an important role in coordinating transcription initiation and RNA processing. While it is commonly understood that they primarily function at the chromatin level by binding directly to DNA, it is less recognized that a subset of TFs, termed DRBPs (DNA- and RNA-binding proteins), also have the capability to bind RNA.
For example, the Hox transcription factor Ultrabithorax (Ubx) binds to nascent pre-mRNAs at alternative cassette exons through its homeodomain, thereby promoting exon inclusion in the Drosophila mesoderm (Carnesecchi et al., 2022). Interestingly, Ubx interacts with chromatin in a dynamic, transcription elongation-dependent manner, indicating that Ubx may accompany Pol II from initiation to processing using different nucleic acid binding modules or assembling distinct functional complexes “on the go.”
A potentially widespread function for TFs in AS arose from studies in which knockdown of TFs with C2H2-type zinc finger (ZnF) DNA-binding domains had pronounced effects on splicing events in K562 and mouse neural cells (Han et al., 2017; Ullah et al., 2023). For at least a subset of ZnF TFs, the modulation of exon inclusion/exclusion and intron retention seemed to occur through direct binding of nascent RNA at intronic regions. One such ZnF, Zfp871, regulates hundreds of neural-differential exons in genes typically associated with neuronal morphology and function, hinting at a broad role for ZnF TFs in the regulation of neuron-specific RNA processing (Han et al., 2017).
Recent findings also reveal an involvement of the transcriptional co-activators CREB-binding protein (CBP)/p300 in alternative 3′-end site selection. In Drosophila brains, CBP was found enriched at dominant promoters as well as at their associated, usually distal, 3′-end site. Strikingly, genetic deletion of CBP resulted in a broad disruption of the 3′-end expression landscape in developing embryos (Alfonso-Gonzalez et al., 2023). How CBP connects sites of transcription initiation and alternative processing, remains unknown; given the essential role of CBP in neuronal differentiation (Lipinski et al., 2019), understanding this interaction could provide clues into the promoter-mediated establishment and maintenance of the neuron-specific 3′-end landscape.
A recent, genome-wide study found that nearly half of all TFs can bind RNA in human cells. Interactions occur through a novel, highly conserved arginine-rich motif (ARM) and were shown to enhance the TF’s association with chromatin, thereby promoting target gene expression (Oksuz et al., 2023). Missense mutations in ARM motifs were associated with human diseases, including cancer and developmental syndromes; perturbations of key TF’s ARMs without affecting DNA binding resulted in developmental defects in zebrafish, which suggests that RNA binding constitutes a widespread property of TFs that contribute to their function in vivo.
In contrast, several recent studies report that multiple chromatin proteins previously described as DRBPs, including PRC2, JARID2, and YY1, do not appear to bind RNA in vivo. PRC2 core subunits did not associate with RNA under stringent experimental conditions (Guo et al., 2024); the loss of PRC2 enrichment at chromatin upon RNase treatment can be explained, at least in part, by a concomitant, unspecific enrichment of non-target regions (Hall Hickman and Jenner, 2024; Healy et al., 2024). It will be important to verify the actual binding of TFs to RNA on a case-by-case basis in order to distinguish direct and indirect effects on the regulation of RNA metabolism (Nielsen and Ulitksy, 2024). Although many protein-RNA interactions remain to be functionally validated and mechanisms to be elucidated, chromatin proteins have emerged as key players in the regulation of RNA processing.
Interaction of RBPs with cis-regulatory elements
The connection between RNA processing and transcription regulation in cis is supported by the widespread occurrence of physical and genetic interactions between splicing/ polyadenylation factors and the transcription machinery [reviewed in Bentley (2014), Shenasa and Bentley (2023), and Shine et al. (2024)]. In addition to an effect of RBPs on Pol II processivity through their interaction with transcribing RNA, multiple lines of evidence also indicate that RBPs interact with chromatin to regulate promoter activity in a promoter- and gene-specific manner. Several genome-wide studies suggest that many nuclear RBPs exert their function at the chromatin level. By ChIP-seq, RBPs were found to pervasively, extensively, and specifically associate with DNA at gene promoters (Xiao et al., 2019); in another study, RBPs even constituted nearly half of all proteins obtained from the chromatome (Rafiee et al., 2021). One RBP with a demonstrated role at gene promoters is the splicing factor Rbm25, which co-associates with the TF YY1 at numerous genetic loci; the physical interaction between the two proteins is necessary for YY1 recruitment to chromatin and transcriptional output (Xiao et al., 2019), suggesting a role of RBPs in the regulation of promoter activity. Whether RBPs are recruited to the DNA template via RNA binding, through the RBP’s intrinsically disordered regions (IDRs), or through chromatin-associated proteins, likely differs on a gene- and RBP-dependent basis.
The first evidence of promoter sequences in the regulation of APA was shown in the context of neuron-specific RNA processing. In the Drosophila nervous system, the RBP ELAV physically associates with the promoters of genes that undergo ELAV-dependent APA and 3′ UTR lengthening. Selection of the distal, neuron-specific 3′-end site was abrogated upon replacing the native promoter of an ELAV target gene by a generic one; moreover, ectopic ELAV expression in muscle cells induced neuronal 3′ UTRs from transgenes carrying the native, but not the generic promoter. The ELAV binding pattern coincided with the signature of Pol II promoter-proximal pausing, indicating that ELAV may be loaded onto the transcription machinery during transcription initiation (Oktaba et al., 2015). It remains unclear how ELAV then finds its way to its functional sites on the nascent RNA —proximal poly(A) sites potentially located kilobases further downstream (Hilgers, 2015; Slobodin and Agami, 2015).
The RNA and DNA-binding protein Fused in Sarcoma (FUS), linked to amyotrophic lateral sclerosis (ALS) (Kwiatkowski et al., 2009; Vance et al., 2009), functions in multiple RNA processes in neuronal cell nuclei. FUS was shown to co-transcriptionally binds to pre-mRNAs to regulate AS (Ishigaki et al., 2012; Lagier-Tourenne et al., 2012); FUS iCLIP clusters on nascent RNA positionally coincide with RNA Pol II pausing sites (Masuda et al., 2015). Moreover, in vitro experiments indicate that FUS mediates the physical and functional interactions between the transcription and splicing machineries (Yu and Reed, 2015). Interestingly, the histone mark H3K36me3 in actively elongating genes was recently shown to recruit FUS to chromatin and away from nascent RNA, thereby ensuring proper poly(A) site selection (Jia et al., 2024).
As more examples arise of transcription factors and cis-regulatory elements that control RBP-mediated AS and APA, it will be interesting to determine whether common mechanisms govern these interactions, or if they differ based on the gene and cellular context.
Possible mechanisms linking transcription to co-transcriptional processing
Several scenarios can be envisaged to explain how RBPs interact with gene activation and transcription processes, regulating AS and APA in a tissue-specific manner. Although our hypotheses are formulated with neuronal RBPs in mind, the proposed mechanisms are not mutually exclusive, and each of them may operate in different contexts or tissues.
Interestingly, enhancer regions help modulate 3′-end processing choices (Kwon et al., 2022; Calvo-Roitberg et al., 2024); it is conceivable that RNA processing factors are recruited to specific genes through enhancer-promoter interactions and the TFs that mediate them. One possible mechanism is exemplified by the histone acetyltransferase and chromatin remodeler CBP, which binds RNAs transcribed from enhancer regions (eRNAs) and stimulates transcription at target promoters (Bose et al., 2017). CBP binds both to neuron-specific TSSs and associated, often neuron-specific 3′-end sites (Alfonso-Gonzalez et al., 2023), thereby creating a link between the spatiotemporal regulation of gene activation and RNA processing. TFs like CBP may guide RBPs to gene promoters by first promoting recruitment to enhancer regions through direct (TF-RBP) or indirect (TF-eRNA-RBP) interactions (Figure 1A).
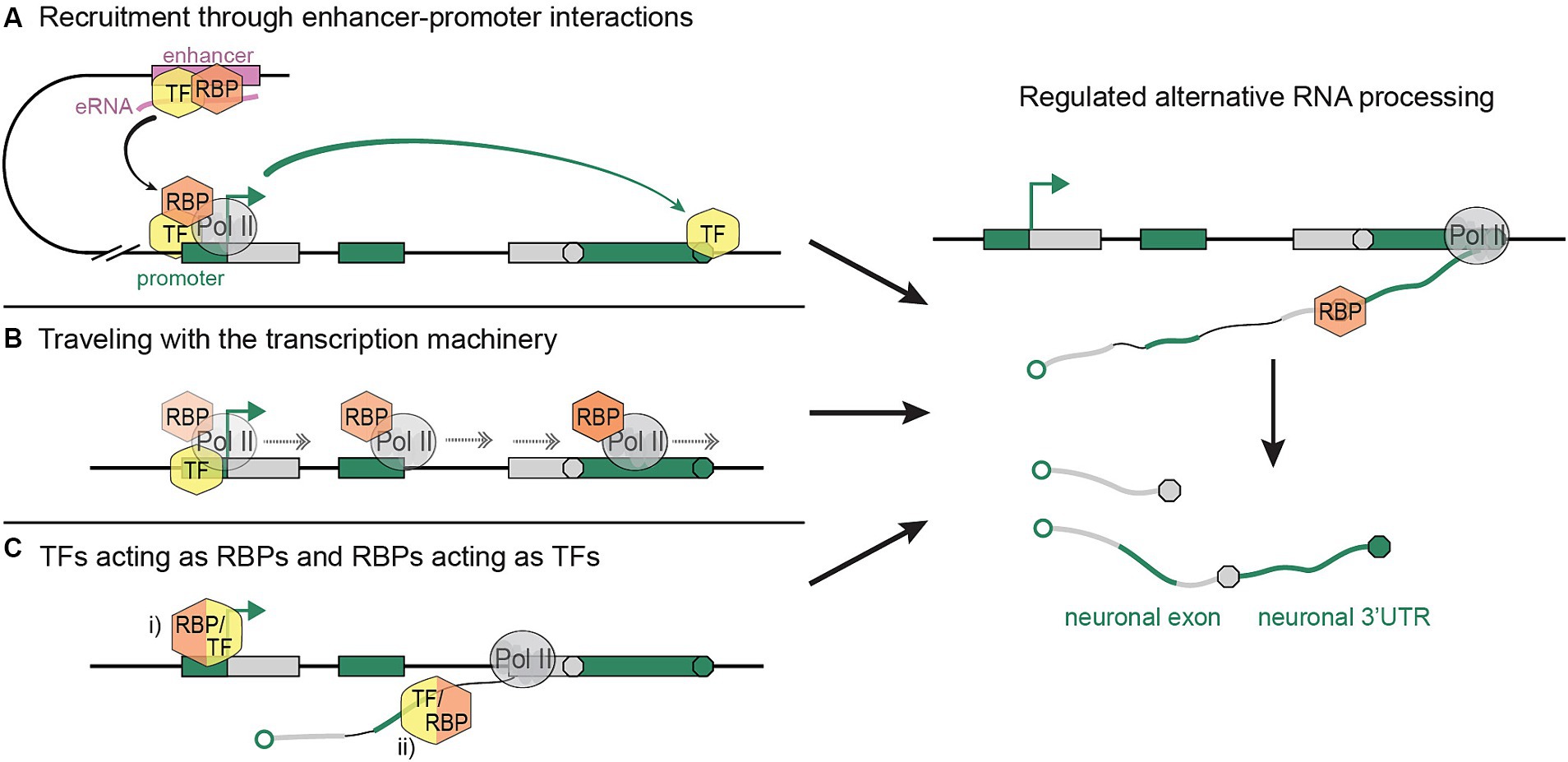
Figure 1. Possible mechanisms linking cis- and trans-regulation of tissue-specific RNA processing. (A) RBPs are recruited to tissue-specific enhancers and their target promoter through binding to TFs and/or enhancer RNAs. The activation of a dominant promoter fosters the expression of the linked, tissue-specific 3′-end. (B) RBPs associate with the transcription machinery at gene promoters by binding to TFs or the Pol II CTD, and accompany the elongating transcription complex to downstream sites of alternative RNA processing. (C) Intertwined regulation of transcription and RNA processing through (i) RBPs acting as TFs at the gene promoter, and/or (ii) TFs acting as RBPs on nascent pre-mRNAs. Resulting tissue-specific (neuronal) RNA processing events are represented on the right. Sequences that are expressed in a tissue-specific (neuronal) fashion are shown as green boxes (DNA) and lines (RNA).
Transcriptional processivity and elongation speed are essential for proper RNA processing, especially in long genes; Pol II slowing, pausing, and fastening can disrupt exon selection and 3′-end patterns (Fong et al., 2014, 2015; Liu et al., 2017; Aslanzadeh et al., 2018; Muniz et al., 2021; Debès et al., 2023; Welsh and Gardini, 2023; Zukher et al., 2023). We propose that in specific contexts, RNA processing choices are modulated through recruitment of RBPs to sites of transcription initiation, allowing them to subsequently hitchhike on the elongating transcription machinery, and to be released onto nascent RNA during Pol II pausing or other changes in elongation dynamics (Figure 1B). In this process, the Pol II C-Terminal Domain (CTD) or tissue-specific TFs may provide a scaffold for interactions with RBPs.
Finally, the increasingly appreciated ability of RBPs to associate with DNA, and of TFs to bind RNA, raises the question whether the two processes —transcription initiation and RNA processing— are as separately controlled by each of the two protein groups as previously thought. Individual examples of RBPs activating transcription have been reported (Zeng et al., 2016; Bi et al., 2019; Ren et al., 2021; Xu et al., 2023), indicating that tissue-specific regulators of RNA processing may also be involved in the activation of distinct promoters. In light of promoter dominance, the RBP-mediated activation of neuron-specific promoters may constitute one mechanism by which neuronal 3′-ends and, more generally, tissue-specific mRNA isoforms are selected. In this context, it is important that nascent pre-mRNAs represent not only mere products, but also important regulators of transcription and RNA processing (Skalska et al., 2017). RBPs may bind nascent RNAs as early as transcription initiation and influence the transcription process (Figure 1C). Similarly, TFs may be recruited to sites of APA and AS by initially binding nascent RNAs in the vicinity of promoter regions.
Conclusion
Neuron-specific RNA processing is pervasive and occurs in all animals that have been studied, including humans. Variations in 3′ UTR length and sequence contribute to neurological disorders, emphasizing the importance of alternative mRNA processing in nervous system development and physiology (Mohanan et al., 2021; LaForce et al., 2022; Wilson et al., 2023). It has become more and more evident that cis-regulatory elements and their associated biomolecules —transcription factors, coding and non-coding RNAs— contribute to the generation of neuron-specific exons and 3′ UTRs. Recent advances in long-read RNA sequencing, chromatin capture studies, protein-nucleic acid interaction analyses, and imaging of nascent mRNAs, have already provided glimpses into the coordination of co-and post-transcriptional processes. Systematically applying these approaches to nervous system tissues, in combination, will shed light on the mechanisms that link transcription and RNA processing, and help identifying and possibly targeting disease-causing mutations.
Author contributions
HO: Conceptualization, Visualization, Writing – original draft, Writing – review & editing. VH: Conceptualization, Visualization, Writing – original draft, Writing – review & editing.
Funding
The author(s) declare that financial support was received for the research, authorship, and/or publication of this article. This work was funded by the Max Planck Society, the Deutsche Forschungsgemeinschaft (DFG, German Research Foundation) SFB 1381 (Project-ID 403222702) and under Germany’s Excellence Strategy (CIBSS – EXC-2189 – Project-ID 390939984), and the European Research Council (ERC) under the European Union’s Horizon 2020 research and innovation program (grant agreement ERC-2018-STG-803258).
Conflict of interest
The authors declare that the research was conducted in the absence of any commercial or financial relationships that could be construed as a potential conflict of interest.
Publisher’s note
All claims expressed in this article are solely those of the authors and do not necessarily represent those of their affiliated organizations, or those of the publisher, the editors and the reviewers. Any product that may be evaluated in this article, or claim that may be made by its manufacturer, is not guaranteed or endorsed by the publisher.
References
Alfonso-Gonzalez, C., Legnini, I., Holec, S., Arrigoni, L., Ozbulut, H. C., Mateos, F., et al. (2023). Sites of transcription initiation drive mRNA isoform selection. Cell 186, 2438–2455.e22. doi: 10.1016/j.cell.2023.04.012
Anvar, S. Y., Allard, G., Tseng, E., Sheynkman, G. M., De Klerk, E., Vermaat, M., et al. (2018). Full-length mRNA sequencing uncovers a widespread coupling between transcription initiation and mRNA processing. Genome Biol. 19:46. doi: 10.1186/s13059-018-1418-0
Aslanzadeh, V., Huang, Y., Sanguinetti, G., and Beggs, J. D. (2018). Transcription rate strongly affects splicing fidelity and cotranscriptionality in budding yeast. Genome Res. 28, 203–213. doi: 10.1101/gr.225615.117
Bae, B., Gruner, H. N., Lynch, M., Feng, T., So, K., Oliver, D., et al. (2020). Elimination of Calm1 long 3'-UTR mRNA isoform by CRISPR-Cas9 gene editing impairs dorsal root ganglion development and hippocampal neuron activation in mice. RNA 26, 1414–1430. doi: 10.1261/rna.076430.120
Bae, B., and Miura, P. (2020). Emerging roles for 3' UTRs in neurons. Int. J. Mol. Sci. 21:3413. doi: 10.3390/ijms21103413
Bentley, D. L. (2014). Coupling mRNA processing with transcription in time and space. Nat. Rev. Genet. 15, 163–175. doi: 10.1038/nrg3662
Bhat, V. D., Jayaraj, J., and Babu, K. (2022). RNA and neuronal function: the importance of post-transcriptional regulation. Oxford Open Neuroscience 1. doi: 10.1093/oons/kvac011
Bi, X., Xu, Y., Li, T., Li, X., Li, W., Shao, W., et al. (2019). RNA targets Ribogenesis factor WDR43 to chromatin for transcription and pluripotency control. Mol. Cell 75:e9. doi: 10.1016/j.molcel.2019.05.007
Bose, D. A., Donahue, G., Reinberg, D., Shiekhattar, R., Bonasio, R., and Berger, S. L. (2017). RNA binding to CBP stimulates histone acetylation and transcription. Cell 168, 135–149.e22. doi: 10.1016/j.cell.2016.12.020
Calvo, O., and Manley, J. L. (2003). Strange bedfellows: polyadenylation factors at the promoter. Genes Dev. 17, 1321–1327. doi: 10.1101/gad.1093603
Calvo-Roitberg, E., Carroll, C. L., Venev, S. V., Kim, G., Mick, S. T., Dekker, J., et al. (2024). mRNA initiation and termination are spatially coordinated. bioRxiv. [Preprint]. doi: 10.1101/2024.01.05.574404
Carminati, M., Rodriguez-Molina, J. B., Manav, M. C., Bellini, D., and Passmore, L. A. (2023). A direct interaction between CPF and RNA pol II links RNA 3′ end processing to transcription. Mol. Cell 83, 4461–4478.e13. doi: 10.1016/j.molcel.2023.11.004
Carnesecchi, J., Boumpas, P., Van Nierop, Y. S. P., Domsch, K., Pinto, H. D., Borges Pinto, P., et al. (2022). The Hox transcription factor Ultrabithorax binds RNA and regulates co-transcriptional splicing through an interplay with RNA polymerase II. Nucleic Acids Res. 50, 763–783. doi: 10.1093/nar/gkab1250
Carrasco, J., Rauer, M., Hummel, B., Grzejda, D., Alfonso-Gonzalez, C., Lee, Y., et al. (2020). ELAV and FNE determine neuronal transcript signatures through EXon-activated rescue. Mol. Cell 80, 156–163.e6. doi: 10.1016/j.molcel.2020.09.011
Dai, W., Zhang, G., and Makeyev, E. V. (2012). RNA-binding protein HuR autoregulates its expression by promoting alternative polyadenylation site usage. Nucleic Acids Res. 40, 787–800. doi: 10.1093/nar/gkr783
Dantonel, J.-C., Murthy, K. G. K., Manley, J. L., and Tora, L. (1997). Transcription factor TFIID recruits factor CPSF for formation of 3′ end of mRNA. Nature 389, 399–402. doi: 10.1038/38763
Debès, C., Papadakis, A., Grönke, S., Karalay, Ö., Tain, L. S., Mizi, A., et al. (2023). Ageing-associated changes in transcriptional elongation influence longevity. Nature 616, 814–821. doi: 10.1038/s41586-023-05922-y
Demircioğlu, D., Cukuroglu, E., Kindermans, M., Nandi, T., Calabrese, C., Fonseca, N. A., et al. (2019). A Pan-cancer transcriptome analysis reveals pervasive regulation through alternative promoters. Cell 178, 1465–1477.e17. doi: 10.1016/j.cell.2019.08.018
Dorrity, T. J., Shin, H., Wiegand, K. A., Aruda, J., Closser, M., Jung, E., et al. (2023). Long 3'UTRs predispose neurons to inflammation by promoting immunostimulatory double-stranded RNA formation. Sci. Immunol. 8:eadg2979. doi: 10.1126/sciimmunol.adg2979
Fong, N., Brannan, K., Erickson, B., Kim, H., Cortazar, M. A., Sheridan, R. M., et al. (2015). Effects of transcription elongation rate and Xrn2 exonuclease activity on RNA polymerase II termination suggest widespread kinetic competition. Mol. Cell 60, 256–267. doi: 10.1016/j.molcel.2015.09.026
Fong, N., Kim, H., Zhou, Y., Ji, X., Qiu, J., Saldi, T., et al. (2014). Pre-mRNA splicing is facilitated by an optimal RNA polymerase II elongation rate. Genes Dev. 28, 2663–2676. doi: 10.1101/gad.252106.114
Glover-Cutter, K., Kim, S., Espinosa, J., and Bentley, D. L. (2008). RNA polymerase II pauses and associates with pre-mRNA processing factors at both ends of genes. Nat. Struct. Mol. Biol. 15, 71–78. doi: 10.1038/nsmb1352
Guo, J. K., Blanco, M. R., Walkup, W. G. T., Bonesteele, G., Urbinati, C. R., Banerjee, A. K., et al. (2024). Denaturing purifications demonstrate that PRC2 and other widely reported chromatin proteins do not appear to bind directly to RNA in vivo. Mol. Cell 84, 1271–1289.e12. doi: 10.1016/j.molcel.2024.01.026
Hall Hickman, A., and Jenner, R. G. (2024). Apparent RNA bridging between PRC2 and chromatin is an artifact of non-specific chromatin precipitation upon RNA degradation. Cell Rep. 43:113856. doi: 10.1016/j.celrep.2024.113856
Han, H., Braunschweig, U., Gonatopoulos-Pournatzis, T., Weatheritt, R. J., Hirsch, C. L., Ha, K. C. H., et al. (2017). Multilayered control of alternative splicing regulatory networks by transcription factors. Mol. Cell 65:e7. doi: 10.1016/j.molcel.2017.01.011
Hardwick, S. A., Hu, W., Joglekar, A., Fan, L., Collier, P. G., Foord, C., et al. (2022). Single-nuclei isoform RNA sequencing unlocks barcoded exon connectivity in frozen brain tissue. Nat. Biotechnol. 40, 1082–1092. doi: 10.1038/s41587-022-01231-3
Healy, E., Zhang, Q., Gail, E. H., Agius, S. C., Sun, G., Bullen, M., et al. (2024). The apparent loss of PRC2 chromatin occupancy as an artifact of RNA depletion. Cell Rep. 43:113858. doi: 10.1016/j.celrep.2024.113858
Hilgers, V. (2015). Alternative polyadenylation coupled to transcription initiation: insights from ELAV-mediated 3 ' UTR extension. RNA Biol. 12, 918–921. doi: 10.1080/15476286.2015.1060393
Hilgers, V. (2022). Regulation of neuronal RNA signatures by ELAV/Hu proteins. Wiley Interdiscip rev. RNA. 14:e1733. doi: 10.1002/wrna.1733
Hilgers, V., Lemke, S. B., and Levine, M. (2012). ELAV mediates 3 ' UTR extension in the Drosophila nervous system. Genes Dev. 26, 2259–2264. doi: 10.1101/gad.199653.112
Hilgers, V., Perry, M. W., Hendrix, D., Stark, A., Levine, M., and Haley, B. (2011). Neural-specific elongation of 3 ' UTRs during Drosophila development. Proc. Natl. Acad. Sci. USA 108, 15864–15869. doi: 10.1073/pnas.1112672108
Irimia, M., Weatheritt, R. J., Ellis, J. D., Parikshak, N. N., Gonatopoulos-Pournatzis, T., Babor, M., et al. (2014). A highly conserved program of neuronal microexons is misregulated in autistic brains. Cell 159, 1511–1523. doi: 10.1016/j.cell.2014.11.035
Ishigaki, S., Masuda, A., Fujioka, Y., Iguchi, Y., Katsuno, M., Shibata, A., et al. (2012). Position-dependent FUS-RNA interactions regulate alternative splicing events and transcriptions. Sci. Rep. 2:529. doi: 10.1038/srep00529
Jia, J., Fan, H., Wan, X., Fang, Y., Li, Z., Tang, Y., et al. (2024). FUS reads histone H3K36me3 to regulate alternative polyadenylation. Nucleic Acids Res. 52, 5549–5571. doi: 10.1093/nar/gkae184
Koushika, S. P., Lisbin, M. J., and White, K. (1996). ELAV, a Drosophila neuron-specific protein, mediates the generation of an alternatively spliced neural protein isoform. Curr. Biol. 6, 1634–1641. doi: 10.1016/S0960-9822(02)70787-2
Koushika, S. P., Soller, M., and White, K. (2000). The neuron-enriched splicing pattern of Drosophila erect wing is dependent on the presence of ELAV protein. Mol. Cell. Biol. 20, 1836–1845. doi: 10.1128/MCB.20.5.1836-1845.2000
Kwiatkowski, T. J. Jr., Bosco, D. A., Leclerc, A. L., Tamrazian, E., Vanderburg, C. R., Russ, C., et al. (2009). Mutations in the FUS/TLS gene on chromosome 16 cause familial amyotrophic lateral sclerosis. Science 323, 1205–1208. doi: 10.1126/science.1166066
Kwon, B., Fansler, M. M., Patel, N. D., Lee, J., Ma, W., and Mayr, C. (2022). Enhancers regulate 3′ end processing activity to control expression of alternative 3′UTR isoforms. Nat. Commun. 13:2709. doi: 10.1038/s41467-022-30525-y
Laforce, G. R., Philippidou, P., and Schaffer, A. E. (2022). mRNA isoform balance in neuronal development and disease. Wiley Interdiscip. Rev. RNA. 14:e1762. doi: 10.1002/wrna.1762
Lagier-Tourenne, C., Polymenidou, M., Hutt, K. R., Vu, A. Q., Baughn, M., Huelga, S. C., et al. (2012). Divergent roles of ALS-linked proteins FUS/TLS and TDP-43 intersect in processing long pre-mRNAs. Nat. Neurosci. 15, 1488–1497. doi: 10.1038/nn.3230
Lee, S., Aubee, J. I., and Lai, E. C. (2023). Regulation of alternative splicing and polyadenylation in neurons. Life Sci. Alliance 6:e202302000. doi: 10.26508/lsa.202302000
Lee, S., Wei, L., Zhang, B., Goering, R., Majumdar, S., Wen, J., et al. (2021). ELAV/Hu RNA binding proteins determine multiple programs of neural alternative splicing. PLoS Genet. 17:e1009439. doi: 10.1371/journal.pgen.1009439
Lipinski, M., Del Blanco, B., and Barco, A. (2019). CBP/p300 in brain development and plasticity: disentangling the KAT's cradle. Curr. Opin. Neurobiol. 59, 1–8. doi: 10.1016/j.conb.2019.01.023
Liu, X., Freitas, J., Zheng, D., Oliveira, M. S., Hoque, M., Martins, T., et al. (2017). Transcription elongation rate has a tissue-specific impact on alternative cleavage and polyadenylation in Drosophila melanogaster. RNA 23, 1807–1816. doi: 10.1261/rna.062661.117
Mansfield, K. D., and Keene, J. D. (2012). Neuron-specific ELAV/Hu proteins suppress HuR mRNA during neuronal differentiation by alternative polyadenylation. Nucleic Acids Res. 40, 2734–2746. doi: 10.1093/nar/gkr1114
Masuda, A., Takeda, J., Okuno, T., Okamoto, T., Ohkawara, B., Ito, M., et al. (2015). Position-specific binding of FUS to nascent RNA regulates mRNA length. Genes Dev. 29, 1045–1057. doi: 10.1101/gad.255737.114
Mirisis, A. A., and Carew, T. J. (2019). The ELAV family of RNA-binding proteins in synaptic plasticity and long-term memory. Neurobiol. Learn. Mem. 161, 143–148. doi: 10.1016/j.nlm.2019.04.007
Mitschka, S., and Mayr, C. (2022). Context-specific regulation and function of mRNA alternative polyadenylation. Nat. Rev. Mol. Cell Biol. 23, 779–796. doi: 10.1038/s41580-022-00507-5
Miura, P., Shenker, S., Andreu-Agullo, C., Westholm, J. O., and Lai, E. C. (2013). Widespread and extensive lengthening of 3' UTRs in the mammalian brain. Genome Res. 23, 812–825. doi: 10.1101/gr.146886.112
Mohanan, N. K., Shaji, F., Koshre, G. R., and Laishram, R. S. (2021). Alternative polyadenylation: an enigma of transcript length variation in health and disease. Wiley Interdiscip. Rev. RNA. 13:e1692. doi: 10.1002/wrna.1692
Mulligan, M. R., and Bicknell, L. S. (2023). The molecular genetics of nELAVL in brain development and disease. Eur. J. Hum. Genet. 31, 1209–1217. doi: 10.1038/s41431-023-01456-z
Muniz, L., Nicolas, E., and Trouche, D. (2021). RNA polymerase II speed: a key player in controlling and adapting transcriptome composition. EMBO J. 40:e105740. doi: 10.15252/embj.2020105740
Nagaike, T., Logan, C., Hotta, I., Rozenblatt-Rosen, O., Meyerson, M., and Manley, J. L. (2011). Transcriptional activators enhance polyadenylation of mRNA precursors. Mol. Cell 41, 409–418. doi: 10.1016/j.molcel.2011.01.022
Nielsen, M., and Ulitksy, I. (2024). The links are still missing: revisiting the role of RNA as a guide for chromatin-associated proteins. Mol. Cell 84, 1178–1179. doi: 10.1016/j.molcel.2024.03.005
Oksuz, O., Henninger, J. E., Warneford-Thomson, R., Zheng, M. M., Erb, H., Vancura, A., et al. (2023). Transcription factors interact with RNA to regulate genes. Mol. Cell 83, 2449–2463.e13. doi: 10.1016/j.molcel.2023.06.012
Oktaba, K., Zhang, W., Lotz, T. S., Jun, D. J., Lemke, S. B., Ng, S. P., et al. (2015). ELAV links paused pol II to alternative polyadenylation in the Drosophila nervous system. Mol. Cell 57, 341–348. doi: 10.1016/j.molcel.2014.11.024
Pascale, A., Amadio, M., and Quattrone, A. (2008). Defining a neuron: neuronal ELAV proteins. Cell. Mol. Life Sci. 65, 128–140. doi: 10.1007/s00018-007-7017-y
Rafiee, M. R., Zagalak, J. A., Sidorov, S., Steinhauser, S., Davey, K., Ule, J., et al. (2021). Chromatin-contact atlas reveals disorder-mediated protein interactions and moonlighting chromatin-associated RBPs. Nucleic Acids Res. 49, 13092–13107. doi: 10.1093/nar/gkab1180
Ren, Y., Huo, Y., Li, W., He, M., Liu, S., Yang, J., et al. (2021). A global screening identifies chromatin-enriched RNA-binding proteins and the transcriptional regulatory activity of QKI5 during monocytic differentiation. Genome Biol. 22:290. doi: 10.1186/s13059-021-02508-7
Shenasa, H., and Bentley, D. L. (2023). Pre-mRNA splicing and its cotranscriptional connections. Trends Genet. 39, 672–685. doi: 10.1016/j.tig.2023.04.008
Shine, M., Gordon, J., Scharfen, L., Zigackova, D., Herzel, L., and Neugebauer, K. M. (2024). Co-transcriptional gene regulation in eukaryotes and prokaryotes. Nat. Rev. Mol. Cell Biol. 25, 534–554. doi: 10.1038/s41580-024-00706-2
Skalska, L., Beltran-Nebot, M., Ule, J., and Jenner, R. G. (2017). Regulatory feedback from nascent RNA to chromatin and transcription. Nat. Rev. Mol. Cell Biol. 18, 331–337. doi: 10.1038/nrm.2017.12
Slobodin, B., and Agami, R. (2015). Transcription initiation determines its end. Mol. Cell 57, 205–206. doi: 10.1016/j.molcel.2015.01.006
Smibert, P., Miura, P., Westholm, J. O., Shenker, S., May, G., Duff, M. O., et al. (2012). Global patterns of tissue-specific alternative polyadenylation in Drosophila. Cell Rep. 1, 277–289. doi: 10.1016/j.celrep.2012.01.001
Soller, M., and White, K. (2003). ELAV inhibits 3′-end processing to promote neural splicing of ewg pre-mRNA. Genes Dev. 17, 2526–2538. doi: 10.1101/gad.1106703
Tellier, M., Maudlin, I., and Murphy, S. (2020). Transcription and splicing: A two-way street. Wiley Interdiscip. Rev. 11, –e1593. doi: 10.1002/wrna.1593
Torres-Méndez, A., Bonnal, S., Marquez, Y., Roth, J., Iglesias, M., Permanyer, J., et al. (2019). A novel protein domain in an ancestral splicing factor drove the evolution of neural microexons. Nat. Ecol. Evol. 3, 691–701. doi: 10.1038/s41559-019-0813-6
Torres-Méndez, A., Pop, S., Bonnal, S., Almudi, I., Avola, A., Roberts, R. J. V., et al. (2022). Parallel evolution of a splicing program controlling neuronal excitability in flies and mammals. Sci. Adv. 8:eabk0445. doi: 10.1126/sciadv.abk0445
Ullah, F., Jabeen, S., Salton, M., Reddy, A. S. N., and Ben-Hur, A. (2023). Evidence for the role of transcription factors in the co-transcriptional regulation of intron retention. Genome Biol. 24:53. doi: 10.1186/s13059-023-02885-1
Vance, C., Rogelj, B., Hortobágyi, T., De Vos, K. J., Nishimura, A. L., Sreedharan, J., et al. (2009). Mutations in FUS, an RNA processing protein, cause familial amyotrophic lateral sclerosis type 6. Science 323, 1208–1211. doi: 10.1126/science.1165942
Wang, Y., Fairley, J. A., and Roberts, S. G. (2010). Phosphorylation of TFIIB links transcription initiation and termination. Curr. Biol. 20, 548–553. doi: 10.1016/j.cub.2010.01.052
Wei, L., and Lai, E. C. (2022). Regulation of the alternative neural transcriptome by ELAV/Hu RNA binding proteins. Front. Genet. 13:848626. doi: 10.3389/fgene.2022.848626
Wei, L., Lee, S., Majumdar, S., Zhang, B., Sanfilippo, P., Joseph, B., et al. (2020). Overlapping activities of ELAV/Hu family RNA binding proteins specify the extended neuronal 3' UTR landscape in Drosophila. Mol. Cell 80, 140–155.e6. doi: 10.1016/j.molcel.2020.09.007
Welsh, S. A., and Gardini, A. (2023). Genomic regulation of transcription and RNA processing by the multitasking integrator complex. Nat. Rev. Mol. Cell Biol. 24, 204–220. doi: 10.1038/s41580-022-00534-2
Wilson, D. M. III, Cookson, M. R., Van Den Bosch, L., Zetterberg, H., Holtzman, D. M., and Dewachter, I. (2023). Hallmarks of neurodegenerative diseases. Cell 186, 693–714. doi: 10.1016/j.cell.2022.12.032
Xiao, R., Chen, J.-Y., Liang, Z., Luo, D., Chen, G., Lu, Z. J., et al. (2019). Pervasive chromatin-RNA binding protein interactions enable RNA-based regulation of transcription. Cell 178, 107–121.e18. doi: 10.1016/j.cell.2019.06.001
Xu, F., Li, R., Von Gromoff, E. D., Drepper, F., Knapp, B., Warscheid, B., et al. (2023). Reprogramming of the transcriptome after heat stress mediates heat hormesis in Caenorhabditis elegans. Nat. Commun. 14:4176. doi: 10.1038/s41467-023-39882-8
Yang, Y., Li, W., Hoque, M., Hou, L., Shen, S., Tian, B., et al. (2016). PAF complex plays novel subunit-specific roles in alternative cleavage and polyadenylation. PLoS Genet. 12:e1005794. doi: 10.1371/journal.pgen.1005794
Yu, Y., and Reed, R. (2015). FUS functions in coupling transcription to splicing by mediating an interaction between RNAP II and U1 snRNP. Proc. Natl. Acad. Sci. USA 112, 8608–8613. doi: 10.1073/pnas.1506282112
Zeng, Y., Yao, B., Shin, J., Lin, L., Kim, N., Song, Q., et al. (2016). Lin28A binds active promoters and recruits Tet1 to regulate gene expression. Mol. Cell 61, 153–160. doi: 10.1016/j.molcel.2015.11.020
Zhang, Z., So, K., Peterson, R., Bauer, M., Ng, H., Zhang, Y., et al. (2019). Elav-mediated exon skipping and alternative polyadenylation of the Dscam1 gene are required for axon outgrowth. Cell Rep. 27, 3808–3817.e7. doi: 10.1016/j.celrep.2019.05.083
Zhu, H., Zhou, H. L., Hasman, R. A., and Lou, H. (2007). Hu proteins regulate polyadenylation by blocking sites containing U-rich sequences. J. Biol. Chem. 282, 2203–2210. doi: 10.1074/jbc.M609349200
Keywords: neuronal RNA processing, transcription factors, RNA-binding proteins, alternative polyadenylation, RNA, nervous system
Citation: Ozbulut HC and Hilgers V (2024) Neuronal RNA processing: cross-talk between transcriptional regulation and RNA-binding proteins. Front. Mol. Neurosci. 17:1426410. doi: 10.3389/fnmol.2024.1426410
Edited by:
Oriane Mauger, Max Planck Institute of Psychiatry, GermanyCopyright © 2024 Ozbulut and Hilgers. This is an open-access article distributed under the terms of the Creative Commons Attribution License (CC BY). The use, distribution or reproduction in other forums is permitted, provided the original author(s) and the copyright owner(s) are credited and that the original publication in this journal is cited, in accordance with accepted academic practice. No use, distribution or reproduction is permitted which does not comply with these terms.
*Correspondence: Valérie Hilgers, aGlsZ2Vyc0BpZS1mcmVpYnVyZy5tcGcuZGU=