- 1Centre for Discovery Brain Sciences, University of Edinburgh, Edinburgh, United Kingdom
- 2Simons Initiative for the Developing Brain, Patrick Wild Centre, University of Edinburgh, Edinburgh, United Kingdom
Ubiquitination is one of the most conserved post-translational modifications and together with mRNA translation contributes to cellular protein homeostasis (proteostasis). Temporal and spatial regulation of proteostasis is particularly important during synaptic plasticity, when translation of specific mRNAs requires tight regulation. Mutations in genes encoding regulators of mRNA translation and in ubiquitin ligases have been associated with several neurodevelopmental disorders. RNA metabolism and translation are regulated by RNA-binding proteins, critical for the spatial and temporal control of translation in neurons. Several ubiquitin ligases also regulate RNA-dependent mechanisms in neurons, with numerous ubiquitination events described in splicing factors and ribosomal proteins. Here we will explore how ubiquitination regulates translation in neurons, from RNA biogenesis to alternative splicing and how dysregulation of ubiquitin signaling can be the underlying cause of pathology in neurodevelopmental disorders, such as Fragile X syndrome. Finally we propose that targeting ubiquitin signaling is an attractive novel therapeutic strategy for neurodevelopmental disorders where mRNA translation and ubiquitin signaling are disrupted.
Introduction
Protein abundance is regulated by the coordination of synthesis and degradation, and these two processes sculpt the molecular architecture of a neuron during development and plasticity. The fate of proteins within cells is directly regulated by ubiquitination. This post-translational modification involves the covalent attachment of a small protein, ubiquitin, to target proteins. Ubiquitination is a cascade of events that start with the activation of ubiquitin by the E1 activating enzyme. Active ubiquitin is then transferred to the E2 conjugating enzyme, which is in charge of interacting with the E3 ligase to ultimately transfer ubiquitin into E3 ligase substrates. Mono-ubiquitination of proteins can regulate cellular processes such as gene transcription, signal transduction and DNA damage response by altering protein localization, protein-protein interactions or endocytosis (Greer et al., 2003; Pelzer et al., 2013; Fukushima et al., 2015; Zhou et al., 2017; Wang et al., 2019). In addition, ubiquitin can generate different types of chains via its seven lysine residues, and each type of poly-ubiquitination (N1, K6, K11, K27, K29, K33, K48, and K63) will have a distinct intracellular role. For instance, the K48 chain-type has been widely described to target proteins into degradation by the Ubiquitin Proteasome System (UPS; Thrower et al., 2000), while K63 ubiquitin chains can drive changes in protein localization or regulate endocytosis and innate immune response (Richard et al., 2020; Madiraju et al., 2022; Saeed et al., 2023).
Ubiquitin signaling has been widely described to be involved in receptor trafficking, synapse formation (Haas and Broadie, 2008; Iwai, 2021; Lei et al., 2021; Pérez-Villegas et al., 2022; Dikic and Schulman, 2023) and remodeling (Mei et al., 2020) by regulating the turnover of synaptic proteins at synapses (Tai and Schuman, 2008; Bingol and Sheng, 2011). Disruption of any ubiquitin-mediated pathways leads to aberrant neuronal morphology, connectivity or synapse formation, which are hallmark features of neurodevelopmental disorders (NDDs; Louros and Osterweil, 2016; Batool et al., 2019).
Neurodevelopmental disorders affect more than 15% of children worldwide (Romero-Ayuso, 2021), and include intellectual disability (ID) and autism spectrum disorder (ASD; Ismail and Shapiro, 2019). Large-scale sequencing studies contributed to the understanding of NDDs pathophysiology by unveiling their genetic etiology. More precisely, these studies report mutations in genes involved in synaptic function and structure, transcriptional and translational regulators (De Rubeis et al., 2014; Krumm et al., 2014; de la Torre-Ubieta et al., 2016), as well as mutations in genes involved in ubiquitin-dependent protein degradation (Louros and Osterweil, 2016; Trost et al., 2022). Several components of the UPS implicated in NDDs, also play crucial roles in RNA synthesis and splicing (Cho et al., 2014; Jewell et al., 2015; Saez et al., 2020; Pitts et al., 2022). Therefore, there is a growing interest in exploring the crosstalk between protein synthesis and protein degradation in the context of NDDs. This mini-review aims to compile all pertinent information on the regulatory role of ubiquitination in RNA biogenesis in the context of NDDs.
Ubiquitin ligases as new players in translation control
Neurons maintain efficient crosstalk between protein translation and degradation to adjust to their physiological needs. Among the intermediaries between protein synthesis and degradation pathways, ubiquitin ligases emerge as central regulators. Ubiquitination of ribosomal proteins has been described over three decades in a seminal paper showing that ubiquitination regulates ribosomal proteins abundance and that the assembly into the ribosome is facilitated by ubiquitin (Finley et al., 1989). More recent studies showed that K63-linked ubiquitination of ribosomal proteins and translation elongation factors promote translation in yeast (Silva et al., 2015). Similarly, in human cells ribosomal proteins were found to be ubiquitinated after the inhibition of translation (Higgins et al., 2015). Moreover, Culin-3, an E3 ubiquitin ligase previously implicated in NDDs (De Rubeis et al., 2014), has been involved in the formation of a ribosome modification platform that alters the translation of specific mRNAs (Werner et al., 2015). Ubiquitination can also regulate translation is by modulating translational surveillance pathways. When aberrant nascent polypeptides are stalled in ribosomes during translation and ribosomes collide, the ribosome quality control (RQC) surveillance pathway is activated, in which ubiquitinated ribosomal subunits are recognized to assist into the ribosome-splitting event (Matsuo et al., 2023). Although dysfunction of RQC is suggested to elicit neurological disorders, the molecular mechanisms involved remain poorly understood. Makorin ring finger protein (MRKN1), a ubiquitin ligase previously shown to control local translation in neurons during synaptic plasticity (Miroci et al., 2012), was recently implicated in the RQC pathway, promoting ribosome stalling at poly(A) sequences and starting RQC by ubiquitinating RPS10 and other RQC factors (Hildebrandt et al., 2019). Interestingly, MRKN1 is member of a family of ubiquitin ligases that also binds RNA, known as the RNA-binding ubiquitin ligases (RBULs). So far, over 30 RBULs have been identified (Thapa et al., 2020) but their function in the brain remains elusive.
Although previous studies demonstrate a direct link between ribosomal protein ubiquitination and changes in translation, the role of ribosomal protein ubiquitination in neurons hasn't been explored. The majority of ribosomal proteins is produced in the nucleus where ribosomes are assembled, but the enrichment of mRNAs of ribosomal proteins in dendrites and axons is a long-standing observation (Moccia et al., 2003). Proteomic studies show that over 80% of ribosomal proteins are ubiquitinated in neurons (Schreiber et al., 2015; Sun et al., 2023), 20 of those putatively ubiquitinated in synaptic fractions (Na et al., 2012; Table 1). Recent studies confirmed that ribosomal proteins are locally synthesized and incorporated into existing ribosomes in axons (Shigeoka et al., 2019) as well as in dendrites (Fusco et al., 2021). Both studies show that a subset of ribosomal proteins is more frequently incorporated or exchanged into mature ribosomes. Interestingly, a fraction of the exchanging ribosomal proteins is also ubiquitinated in neurons (Table 1; Na et al., 2012; Schreiber et al., 2015), suggesting an additional layer of regulation of ribosomal protein exchange in neurons that may be essential to regulate local protein synthesis in response to synaptic plasticity. Whether these processes are affected in NDDs is an open question, but since changes in ribosome abundance have been reported in several NDDs (Griesi-Oliveira et al., 2020; Seo et al., 2022), it would be interesting to investigate if their ubiquitination is aberrant in NDDs, and if that can be targeted to normalize ribosome levels and translation rates.
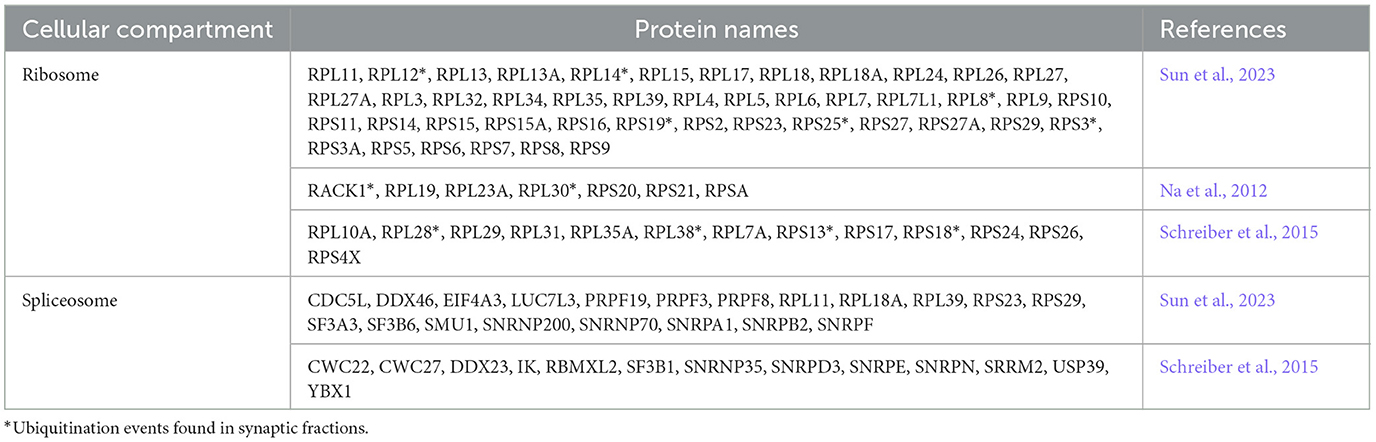
Table 1. Several components of the splicing machinery as well as ribosomal proteins are ubiquitinated in the brain.
Alternative splicing regulation by ubiquitin and its dysfunction in NDDs
Most protein-coding genes in humans are transcribed as pre-mRNAs that contain a series of exons and introns. Following transcription, the removal of introns during the process of pre-mRNA splicing is required before the nascent transcript is translated into a protein. Alternative splicing generates multiple proteins from a single pre-mRNA by including and/or excluding alternative exons, thereby diversifying cellular proteomes (Han et al., 2011; Wang et al., 2015). This process is particularly important in neurons that rely on the function of heavily spliced genes such as Neurexins, n-Cadherins, and calcium-activated potassium channels that can produce hundreds of mRNA isoforms through alternative splicing. Indeed, some NDDs occur when alternative splicing goes awry. For example, extensive transcriptomics studies using post-mortem brain tissue from ASD patients have shown pervasive mis-regulation of microexon splicing (Irimia et al., 2014; Chanarat and Mishra, 2018; Su et al., 2018).
The molecular machinery responsible for pre-mRNA splicing is called the spliceosome complex. It is composed of five small nuclear RNAs (U1, U2, U4, U5, and U6) pre-assembled with proteins into small ribonucleoproteins (snRNPs), together with hundreds of auxiliary proteins that help the spliceosome recognize splice sites (Wassarman and Steitz, 1992; Zhou et al., 2002; Matlin and Moore, 2007). High-throughput genetic studies showed a possible link between ubiquitin ligases and the process of pre-mRNA splicing. For instance, ubiquitin binds to the highly conserved spliceosomal core protein PRPF8 via its C-terminal domain (Grainger and Beggs, 2005; Bellare et al., 2006, 2008). Additionally, the literature also suggests that ubiquitination of other splicing factors may modulate spliceosomal activity through reversible protein-protein interactions (Bellare et al., 2008). For example, PRPF3 and PRPF31 undergo K63-linked ubiquitination by an RBUL, PRPF19 (Chanarat and Mishra, 2018), an essential step for spliceosomal activation (Hogg et al., 2010). Ubiquitinated PRPF3 and PRPF31 then bind PRPF8 and stabilize the tri-snRNP complex (Park et al., 2016). As the splicing cycle progresses, PRPF3 and PRPF31 are deubiquitinated by USP4 and USP15, respectively (Song et al., 2010; Das et al., 2017). Altogether, this shows that the ubiquitination state of several components of the spliceosome tightly regulate its assembly and activation, therefore affecting splicing.
The regulation of the spliceosome by ubiquitination in neurons is less elucidated, but proteomic studies identify several ubiquitinated splicing factors such as PRPF3, PRP9, as well as the RBUL, PRPF19 (Table 1). Considering that neurons express highly spliced genes, dysregulated ubiquitination of the spliceosome could have major consequences in neuronal development and function and contribute to NDDs. Importantly, a recent study identified mutations in three spliceosome factors in NDDs, including six individuals who harbored mostly de novo heterozygous variants in PRPF19. This study demonstrated that these pathogenic variants lead to converging neurodevelopmental phenotypes, including, but not limited to developmental delay, ID and autism (Li et al., 2024).
Ubiquitination of RNA-binding proteins: contribution to NDDs
RNA metabolism is regulated at different stages by specific RNA-binding proteins (RBPs). RBPs are responsible for mRNA transport and translation regulation within dendrites and are required for long-lasting forms of synaptic plasticity (Glock et al., 2017). The loss of RBP function leads to numerous disorders, including ASD, Fragile X Syndrome (FXS; Bhakar et al., 2012; Zoghbi and Bear, 2012; Darnell and Klann, 2013; Lee et al., 2016; Popovitchenko et al., 2016) and epilepsy (Lee et al., 2016).
Due to its significant role in translation regulation and its impact on neuronal homeostasis, Fragile X messenger ribonucleoprotein (FMRP) stands out as one of the most extensively studied RBPs. Evidence suggests that FMRP is transported into dendrites and synapses where it acts as a central regulator of local translation (Darnell and Klann, 2013; Schieweck et al., 2021). Additionally, FMRP has a dual role in both RNA localization and translation; localizes to polyribosome complexes and is well-documented for its role as a translational repressor (Laggerbauer et al., 2001; Mazroui et al., 2002). Studies of Fmr1 mutant models have revealed alterations in plasticity and excitability in several brain circuits, as a consequence of the excessive protein synthesis (Osterweil et al., 2013; Louros et al., 2023). Deficiency of FMRP, the underlying cause of Fragile X Syndrome, causes dysregulation of the translation of mRNAs that bind to FMRP. Interestingly, the majority of FMRP target mRNAs are less translated in the hippocampus (Ceolin et al., 2017; Thomson et al., 2017; Sawicka et al., 2019; Sharma et al., 2019; Seo et al., 2022) and this is reflected in the synapse-enriched proteome of Fmr1 KO mouse (Louros et al., 2023).
FMRP undergoes degradation primarily through the ubiquitin-proteasome system (UPS), which is a major pathway for targeted protein degradation in cells (Chanarat and Mishra, 2018; Ebstein et al., 2021; Winden et al., 2023). Consistent with this, FMRP undergoes regulation by ubiquitination, a tightly controlled process that can be triggered by specific events such as dephosphorylation at key sites such as S499 (Wilkerson et al., 2023). Various factors contribute to this dephosphorylation, including activation of PP2A by the activation of metabotropic glutamate receptors (Nalavadi et al., 2012). Additionally, developmental cues play a crucial role during specific stages of development by regulating dephosphorylation and subsequent ubiquitination of FMRP (Schieweck et al., 2021). Once dephosphorylated, FMRP becomes a target for specific E3 ubiquitin ligases such as APC/Cdh1, and once ubiquitinated, it is targeted for degradation (Nalavadi et al., 2012; Valdez-Sinon et al., 2020). FMRP is also known for its role in mRNA-protein interactions within ribonucleoprotein (RNP) granules, which are crucial for mRNA transport and localization (Valdez-Sinon et al., 2020). Ubiquitination-induced degradation of FMRP may disrupt these interactions, impairing the transport and proper localization of mRNAs, thereby affecting gene expression programs that are essential for normal cellular function (Valdez-Sinon et al., 2020; Wilkerson et al., 2023).
In addition to FMRP other RBPs related to NDDs are regulated by ubiquitination. One example is the ELAVL family, which undertakes essential functions across spatiotemporal windows in brain development to help regulate and specify transcriptomic programs for cell specialization (Mulligan and Bicknell, 2023). Different components of this family have been related to ASDs, behavioral abnormalities or seizures (Mulligan and Bicknell, 2023), with ELAV2 showing a clear role in neurodevelopment and listed by SFARI as a candidate gene for ASD. ELAV2 targets are also involved in synaptic function and neurodevelopmental disorders (Berto et al., 2016). Even though in the context of cancer, ELAV1 has been described to be ubiquitinated, facilitating its proteasome mediated degradation and leading to an increase in the survival rate of cells under heat-shock response (Daks et al., 2021), the ubiquitination of this RBP has not been demonstrated in neurons. RBFOX1 is another RBP strongly implicated in ASD. This protein regulates both splicing and transcriptional networks in human neuronal development (Fogel et al., 2012) and it has been found to be ubiquitinated in Alzheimer's disease post mortem human brain tissue, particularly in axons, tangles and neuropil threads, suggesting a role in axonal proteostasis (Fernandez et al., 2021).
Altogether, these evidences show that RNA binding proteins are regulated by ubiquitination in the brain and despite lacking detailed mechanisms it is possible that aberrant ubiquitination of RNA binding proteins contributes to the pathophysiology of NDDs.
Dysregulated proteostasis in NDDs: new therapeutic opportunities
Dysregulation of translational represents a common endpoint of familial and sporadic ASD-associated signaling pathways (De Rubeis et al., 2014; Krumm et al., 2014). The identification of this dysregulated pathway has been used to develop several therapeutic strategies for FXS and other NDDs, however, due to the limited success in clinical trials there is an urgent need for identification of new pathways amenable for therapeutic development.
A recent development was the discovery of upregulated protein degradation machinery in FXS, downstream of the increased protein translation rates that characterize this disorder (Louros et al., 2023). This study shows that the increase in protein degradation is primarily a consequence of excessive translation of proteasomal subunits and ubiquitin ligases in excitatory neurons from Fmr1 mutant mice. Importantly, pharmacological reduction of proteasome activity and ubiquitin ligases was sufficient to normalize protein synthesis rates, demonstrating the intricate relationship between translation and degradation in FXS. This could be a consequence of modulating ribosomal subunits turnover since the authors found ribosomal subunits excessively targeted for degradation in synaptic enriched fractions, possibly through increased ubiquitination rates. Finally, this study found that increased proteasome activity contributes to hyperexcitability and audiogenic seizures in Fmr1 KO mice, and that this phenotype was corrected by pharmacological and genetic manipulation of the proteasome (Louros et al., 2023). This study opens the door to more investigations into the dysfunction of ubiquitin signaling and proteasomal degradation in other NDDs, and it demonstrates that targeting ubiquitin signaling could be a new pathway for therapeutic development.
One of the most studied ubiquitin ligases linked to neurodevelopmental disorders is UBE3A, with loss of function mutations causing Angelman syndrome (AS; Kalsner and Chamberlain, 2015). AS is characterized by intellectual disability, developmental delay, seizures, motor disruptions, and an unusually positive demeanor (LaSalle et al., 2015). Many studies have identified targets of Ube3a in mouse, rats and human AS samples (Pandya et al., 2022) including some regulators of protein synthesis. One interesting target of Ube3a is the mTOR suppressor protein TSC2 (Zheng et al., 2008), directly involved in the regulation of protein synthesis. Recent work suggests that degradation of TSC2 following ubiquitination by Ube3a may contribute to pathology, as treatment with the mTOR inhibitor rapamycin rescued motor deficits and abnormal dendritic branching in AS mutant mice (Sun et al., 2015). Furthermore, lovastatin, previously shown to correct excessive protein synthesis rates and seizures in FXS (Osterweil et al., 2013; Asiminas et al., 2019), was also shown to correct seizures in the AS mouse model (Chung et al., 2018), suggesting that protein synthesis rates could be increased in the AS mutant mouse. This was indeed confirmed in a recent study that found increased de novo protein synthesis in the hippocampus of the AS mutant mouse (Aria et al., 2023), as well as impaired autophagy that when enhanced was able to ameliorate cognitive impairments in AS mice.
Altogether, these findings show the intricate crosstalk between ubiquitin signaling and translation in NDDs. Targeted protein degradation technologies have emerged over 20 years ago with potential for targeting undruggable protein targets. PROTAC (proteolysis-targeting chimera) or molecular glue (MG)-driven ternary complex formation with an ubiquitin E3 ligase utilizes cells' UPS to degrade target proteins. Several such molecules have entered clinical development (Kong and Jones, 2023). Recent clinical proof-of-concept for PROTAC molecules against two cancer targets confirmed the successful clinical targeting of proteins previously considered “undruggable.” There are currently over 20 new PROTACs under clinical development (Békés et al., 2022). The application of these strategies to brain disorders offers several advantages and challenges but recent studies have shown promise in the context of neurodegenerative disorders [recently reviewed by Farrell and Jarome (2021)] suggesting that this new therapeutic avenue for NDDs could offer increased specificity and lower off-target effects.
Conclusions and perspectives
Molecular analysis of patient-derived tissues and mouse models of the monogenic ID has shown widespread changes at the epigenetic, transcriptional, and translational gene expression levels. The interplay between changes at multiple levels is essential to the pathophysiology of NDDs. Importantly, coordination between the translational machinery, RBPs and the ubiquitin proteasome system regulates dendritic proteostasis in response to neuronal activity (Hanus and Schuman, 2013). Indeed, mutations in components of these systems are associated with altered plasticity and may underlie the pathogenesis of NDDs. Considering that in several models of NDDs protein synthesis rates are affected (Auerbach et al., 2011; Barnes et al., 2015; Aria et al., 2023), ribosome abundance is increased and the ubiquitin proteasome system is overexpressed (Seo et al., 2022; Louros et al., 2023), it is pertinent to investigate the contributions of ubiquitin signaling dysfunction to ribosome quality control and alternative splicing. However, the isolation and identification of ubiquitinated proteins under physiological conditions from in vivo tissues is a challenging task, particularly in the brain, as the ubiquitinated proteins are generally found at very low levels within the cells. Besides, the fast kinetics at which some of the proteins conjugated with ubiquitin are degraded (Ronchi and Haas, 2012), the action of the deubiquitinating enzymes (Stegmeier et al., 2007) or the fact that proteins might be modified with ubiquitin only in well-defined temporal windows (Clute and Pines, 1999), make their analysis even more challenging. Considering that ubiquitin signaling modulates so many aspects of RNA biogenesis that are affected in NDDs (Figure 1), we believe it is vital to develop methods to improve the identification of dysregulated ubiquitination in the brain to accelerate the development of novel therapeutic options for NDDs.
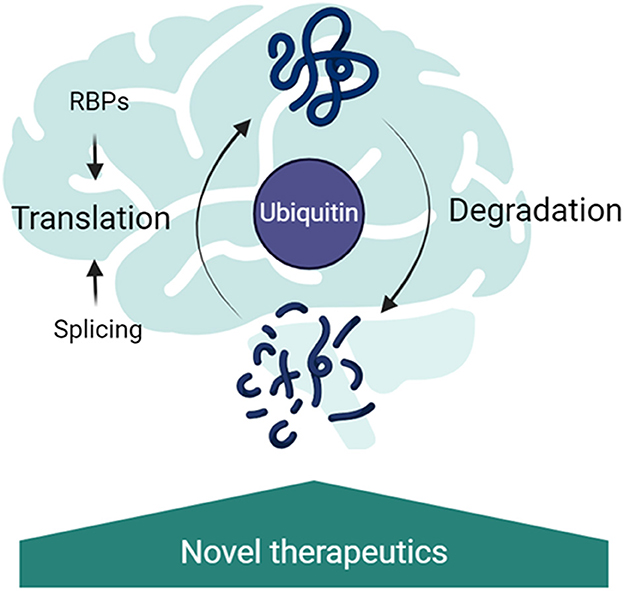
Figure 1. Ubiquitin signaling as a central regulator of RNA metabolism in the brain. Ubiquitination regulates splicing, through regulation of the spliceosome remodeling; RNA-binding protein abundance and binding partners; translation rates through ribosomal protein ubiquitination and the turnover rates of proteins in the brain. Disruption of these pathways has been implicated in neurodevelopmental disorders, therefore targeting the ubiquitin signaling is a promising new therapeutic strategy.
Author contributions
NE: Writing – original draft, Writing – review & editing. SS: Writing – original draft. SRL: Conceptualization, Funding acquisition, Visualization, Writing – original draft, Writing – review & editing.
Funding
The author(s) declare financial support was received for the research, authorship, and/or publication of this article. This work was supported by the Simons Initiative for the Developing Brain and by the College of Medicine and Veterinary Medicine, University of Edinburgh.
Acknowledgments
The authors would like to acknowledge all the colleagues that discussed the manuscript prior to submission.
Conflict of interest
The authors declare that the research was conducted in the absence of any commercial or financial relationships that could be construed as a potential conflict of interest.
Publisher's note
All claims expressed in this article are solely those of the authors and do not necessarily represent those of their affiliated organizations, or those of the publisher, the editors and the reviewers. Any product that may be evaluated in this article, or claim that may be made by its manufacturer, is not guaranteed or endorsed by the publisher.
References
Aria, F., Pandey, K., and Alberini, C. M. (2023). Excessive protein accumulation and impaired autophagy in the hippocampus of angelman syndrome modeled in mice. Biol. Psychiat. 94, 68–83. doi: 10.1016/j.biopsych.2022.11.016
Asiminas, A., Jackson, A. D., Louros, S. R., Till, S. M., Spano, T., Dando, O., et al. (2019). Sustained correction of associative learning deficits after brief, early treatment in a rat model of Fragile X Syndrome. Sci. Transl. Med. 11:eaao0498. doi: 10.1126/scitranslmed.aao0498
Auerbach, B. D., Osterweil, E. K., and Bear, M. F. (2011). Mutations causing syndromic autism define an axis of synaptic pathophysiology. Nature 480, 63–68. doi: 10.1038/nature10658
Barnes, S. A., Wijetunge, L. S., Jackson, A. D., Katsanevaki, D., Osterweil, E. K., Komiyama, N. H., et al. (2015). Convergence of hippocampal pathophysiology in Syngap+/- and Fmr1-/y mice. J. Neurosci. 35, 15073–15081. doi: 10.1523/JNEUROSCI.1087-15.2015
Batool, S., Raza, H., Zaidi, J., Riaz, S., Hasan, S., and Syed, N. I. (2019). Synapse formation: from cellular and molecular mechanisms to neurodevelopmental and neurodegenerative disorders. J. Neurophysiol. 121, 1381–1397. doi: 10.1152/jn.00833.2018
Békés, M., Langley, D. R., and Crews, C. M. (2022). PROTAC targeted protein degraders: the past is prologue. Nat. Rev. Drug Discov. 21, 181–200. doi: 10.1038/s41573-021-00371-6
Bellare, P., Kutach, A. K., Rines, A. K., Guthrie, C., and Sontheimer, E. J. (2006). Ubiquitin binding by a variant Jab1/MPN domain in the essential pre-mRNA splicing factor Prp8p. RNA 12, 292–302. doi: 10.1261/rna.2152306
Bellare, P., Small, E. C., Huang, X., Wohlschlegel, J. A., Staley, J. P., and Sontheimer, E. J. (2008). A role for ubiquitin in the spliceosome assembly pathway. Nat. Struct. Mol. Biol. 15, 444–451. doi: 10.1038/nsmb.1401
Berto, S., Usui, N., Konopka, G., and Fogel, B. L. (2016). ELAVL2-regulated transcriptional and splicing networks in human neurons link neurodevelopment and autism. Hum. Mol. Genet. 2016:ddw110. doi: 10.1093/hmg/ddw110
Bhakar, A. L., Dölen, G., and Bear, M. F. (2012). The pathophysiology of fragile X (and what it teaches us about synapses). Annu. Rev. Neurosci. 35, 417–443. doi: 10.1146/annurev-neuro-060909-153138
Bingol, B., and Sheng, M. (2011). Deconstruction for reconstruction: the role of proteolysis in neural plasticity and disease. Neuron 69, 22–32. doi: 10.1016/j.neuron.2010.11.006
Ceolin, L., Bouquier, N., Vitre-Boubaker, J., Rialle, S., Severac, D., Valjent, E., et al. (2017). Cell type-specific mRNA dysregulation in hippocampal CA1 pyramidal neurons of the fragile X syndrome mouse model. Front. Mol. Neurosci. 10:340. doi: 10.3389/fnmol.2017.00340
Chanarat, S., and Mishra, S. K. (2018). Emerging roles of ubiquitin-like proteins in pre-mRNA splicing. Trends Biochem. Sci. 43, 896–907. doi: 10.1016/j.tibs.2018.09.001
Cho, S. K., Chaabane, S. B., Shah, P., Poulsen, C. P., and Yang, S. W. (2014). COP1 E3 ligase protects HYL1 to retain microRNA biogenesis. Nat. Commun. 5:5867. doi: 10.1038/ncomms6867
Chung, L., Bey, A. L., Towers, A. J., Cao, X., Kim, I. H., and Jiang, Y. (2018). Lovastatin suppresses hyperexcitability and seizure in Angelman syndrome model. Neurobiol. Dis. 110, 12–19. doi: 10.1016/j.nbd.2017.10.016
Clute, P., and Pines, J. (1999). Temporal and spatial control of cyclin B1 destruction in metaphase. Nat. Cell Biol. 1, 82–87. doi,: 10.1038/10049
Daks, A., Petukhov, A., Fedorova, O., Shuvalov, O., Kizenko, A., Tananykina, E., et al. (2021). The RNA-binding protein HuR is a novel target of Pirh2 E3 ubiquitin ligase. Cell Death Dis. 12:581. doi: 10.1038/s41419-021-03871-w
Darnell, J. C., and Klann, E. (2013). The translation of translational control by FMRP: therapeutic targets for fragile X syndrome. Nat. Neurosci. 16:1530. doi: 10.1038/nn.3379
Das, T., Park, J. K., Park, J., Kim, E., Rape, M., Kim, E. E. K., et al. (2017). USP15 regulates dynamic protein-protein interactions of the spliceosome through deubiquitination of PRP31. Nucl. Acids Res. 45, 4866–4880. doi: 10.1093/nar/gkw1365
de la Torre-Ubieta, L., Won, H., Stein, J. L., and Geschwind, D. H. (2016). Advancing the understanding of autism disease mechanisms through genetics. Nat. Med. 22, 345–361. doi: 10.1038/nm.4071
De Rubeis, S., He, X., Goldberg, A. P., Poultney, C. S., Samocha, K., Cicek, A. E., et al. (2014). Synaptic, transcriptional and chromatin genes disrupted in autism. Nature 515, 209–215. doi: 10.1038/nature13772
Dikic, I., and Schulman, B. A. (2023). An expanded lexicon for the ubiquitin code. Nat. Rev. Mol. Cell Biol. 24, 273–287. doi: 10.1038/s41580-022-00543-1
Ebstein, F., Küry, S., Papendorf, J. J., and Krüger, E. (2021). Neurodevelopmental disorders (NDD) caused by genomic alterations of the ubiquitin-proteasome system (UPS): the possible contribution of immune dysregulation to disease pathogenesis. Front. Mol. Neurosci. 14:733012. doi: 10.3389/fnmol.2021.733012
Farrell, K., and Jarome, T. J. (2021). Is PROTAC technology really a game changer for central nervous system drug discovery? Expert Opin. Drug Discov. 16, 833–840. doi: 10.1080/17460441.2021.1915979
Fernandez, W. R., Solopova, E., Shostak, A., Balotin, K. M., Mobley, B., Harmsen, H., et al. (2021). RBFOX1 is regulated by the adenosine 2a receptor and is ubiquitinated in tau tangles in Alzheimer's disease. Alzheimer's Dement. 17:e056367. doi: 10.1002/alz.056367
Finley, D., Bartel, B., and Varshavsky, A. (1989). The tails of ubiquitin precursors are ribosomal proteins whose fusion to ubiquitin facilitates ribosome biogenesis. Nature 338, 394–401. doi: 10.1038/338394a0
Fogel, B. L., Wexler, E., Wahnich, A., Friedrich, T., Vijayendran, C., Gao, F., et al. (2012). RBFOX1 regulates both splicing and transcriptional networks in human neuronal development. Hum. Mol. Genet. 21, 4171–4186. doi: 10.1093/hmg/dds240
Fukushima, T., Yoshihara, H., Furuta, H., Kamei, H., Hakuno, F., Luan, J., et al. (2015). Nedd4-induced monoubiquitination of IRS-2 enhances IGF signalling and mitogenic activity. Nat. Commun. 6:6780. doi: 10.1038/ncomms7780
Fusco, C. M., Desch, K., Dörrbaum, A. R., Wang, M., Staab, A., Chan, I. C. W., et al. (2021). Neuronal ribosomes exhibit dynamic and context-dependent exchange of ribosomal proteins. Nat. Commun. 12:6127. doi: 10.1038/s41467-021-26365-x
Glock, C., Heumüller, M., and Schuman, E. M. (2017). mRNA transport and local translation in neurons. Curr. Opin. Neurobiol. Mol. Neurosci. 45, 169–177. doi: 10.1016/j.conb.2017.05.005
Grainger, R. J., and Beggs, J. D. (2005). Prp8 protein: at the heart of the spliceosome. RNA 11, 533–557. doi: 10.1261/rna.2220705
Greer, S. F., Zika, E., Conti, B., Zhu, X.-S., and Ting, J. P.-Y. (2003). Enhancement of CIITA transcriptional function by ubiquitin. Nat. Immunol. 4, 1074–1082. doi: 10.1038/ni985
Griesi-Oliveira, K., Fogo, M. S., Pinto, B. G. G., Alves, A. Y., Suzuki, A. M., Morales, A. G., et al. (2020). Transcriptome of iPSC-derived neuronal cells reveals a module of co-expressed genes consistently associated with autism spectrum disorder. Mol. Psychiat. 26, 1589–1605. doi: 10.1038/s41380-020-0669-9
Haas, K. F., and Broadie, K. (2008). Roles of ubiquitination at the synapse. Biochim. Biophys. Acta 1779, 495–506. doi: 10.1016/j.bbagrm.2007.12.010
Han, J., Xiong, J., Wang, D., and Fu, X. D. (2011). Pre-mRNA splicing: where and when in the nucleus. Trends Cell Biol. 3:3. doi: 10.1016/j.tcb.2011.03.003
Hanus, C., and Schuman, E. M. (2013). Proteostasis in complex dendrites. Nat. Rev. Neurosci. 14, 638–648. doi: 10.1038/nrn3546
Higgins, R., Gendron, J. M., Rising, L., Mak, R., Webb, K., Kaiser, S. E., et al. (2015). The unfolded protein response triggers site-specific regulatory ubiquitylation of 40S ribosomal proteins. Mol. Cell 59, 35–49. doi: 10.1016/j.molcel.2015.04.026
Hildebrandt, A., Brüggemann, M., Rücklé, C., Boerner, S., Heidelberger, J. B., Busch, A., et al. (2019). The RNA-binding ubiquitin ligase MKRN1 functions in ribosome-associated quality control of poly(A) translation. Genome Biol. 20:216. doi: 10.1186/s13059-019-1814-0
Hogg, R., McGrail, J. C., and O'Keefe, R. T. (2010). The function of the NineTeen Complex (NTC) in regulating spliceosome conformations and fidelity during pre-mRNA splicing. Biochem. Soc. Transact. 38, 1110–1115. doi: 10.1042/BST0381110
Irimia, M., Weatheritt, R. J., Ellis, J. D., Parikshak, N. N., Gonatopoulos-Pournatzis, T., Babor, M., et al. (2014). A highly conserved program of neuronal microexons is misregulated in autistic brains. Cell 159, 1511–1523. doi: 10.1016/j.cell.2014.11.035
Ismail, F. Y., and Shapiro, B. K. (2019). What are neurodevelopmental disorders? Curr. Opin. Neurol. 32, 611–616. doi: 10.1097/WCO.0000000000000710
Iwai, K. (2021). Discovery of linear ubiquitination, a crucial regulator for immune signaling and cell death. FEBS J. 288, 1060–1069. doi: 10.1111/febs.15471
Jewell, J. L., Flores, F., and Guan, K.-L. (2015). Micro(RNA) managing by mTORC1. Mol. Cell 57, 575–576. doi: 10.1016/j.molcel.2015.02.006
Kalsner, L., and Chamberlain, S. J. (2015). Prader-Willi, Angelman, and 15q11-q13 duplication syndromes. Pediatr. Clin. North Am. 62, 587–606. doi: 10.1016/j.pcl.2015.03.004
Kong, N. R., and Jones, L. H. (2023). Clinical translation of targeted protein degraders. Clin. Pharmacol. Therapeut. 114, 558–568. doi: 10.1002/cpt.2985
Krumm, N., O'Roak, B. J., Shendure, J., and Eichler, E. E. (2014). A de novo convergence of autism genetics and molecular neuroscience. Trends Neurosci. 37, 95–105. doi: 10.1016/j.tins.2013.11.005
Laggerbauer, B., Ostareck, D., Keidel, E. M., Ostareck-Lederer, A., and Fischer, U. (2001). Evidence that fragile X mental retardation protein is a negative regulator of translation. Hum. Mol. Genet. 10, 329–338. doi: 10.1093/hmg/10.4.329
LaSalle, J. M., Reiter, L. T., and Chamberlain, S. J. (2015). Epigenetic regulation of UBE3A and roles in human neurodevelopmental disorders. Epigenomics 7, 1213–1228. doi: 10.2217/epi.15.70
Lee, J. A., Damianov, A., Lin, C. H., Fontes, M., Parikshak, N. N., Anderson, E. S., et al. (2016). Cytoplasmic Rbfox1 regulates the expression of synaptic and autism-related genes. Neuron. 89, 113–28. doi: 10.1016/j.neuron.2015.11.025
Lei, Z., Wang, J., Zhang, L., and Liu, C. H. (2021). Ubiquitination-dependent regulation of small GTPases in membrane trafficking: from cell biology to human diseases. Front. Cell Dev. Biol. 9:688352. doi: 10.3389/fcell.2021.688352
Li, D., Wang, Q., Bayat, A., Battig, M. R., Zhou, Y., Bosch, D. G., et al. (2024). Spliceosome malfunction causes neurodevelopmental disorders with overlapping features. J. Clin. Invest. 134:e171235. doi: 10.1172/JCI171235
Louros, S. R., and Osterweil, E. K. (2016). Perturbed proteostasis in autism spectrum disorders. J. Neurochem. 139, 1081–1092. doi: 10.1111/jnc.13723
Louros, S. R., Seo, S. S., Maio, B., Martinez-Gonzalez, C., Gonzalez-Lozano, M. A., Muscas, M., et al. (2023). Excessive proteostasis contributes to pathology in fragile X syndrome. Neuron 111, 508–525.e7. doi: 10.1016/j.neuron.2022.11.012
Madiraju, C., Novack, J. P., Reed, J. C., and Matsuzawa, S. (2022). K63 ubiquitination in immune signaling. Trends Immunol. 43, 148–162. doi: 10.1016/j.it.2021.12.005
Matlin, A. J., and Moore, M. J. (2007). Spliceosome assembly and composition. Adv. Exp. Med. Biol. 623, 14–35. doi: 10.1007/978-0-387-77374-2_2
Matsuo, Y., Uchihashi, T., and Inada, T. (2023). Decoding of the ubiquitin code for clearance of colliding ribosomes by the RQT complex. Nat. Commun. 14:79. doi: 10.1038/s41467-022-35608-4
Mazroui, R., Huot, M.-E., Tremblay, S., Filion, C., Labelle, Y., and Khandjian, E. W. (2002). Trapping of messenger RNA by Fragile X Mental Retardation protein into cytoplasmic granules induces translation repression. Hum. Mol. Genet. 11, 3007–3017. doi: 10.1093/hmg/11.24.3007
Mei, S., Ruan, H., Ma, Q., and Yao, W.-D. (2020). The ubiquitin-editing enzyme A20 regulates synapse remodeling and efficacy. Brain Res. 1727:146569. doi: 10.1016/j.brainres.2019.146569
Miroci, H., Schob, C., Kindler, S., Ölschläger-Schütt, J., Fehr, S., Jungenitz, T., et al. (2012). Makorin ring zinc finger protein 1 (MKRN1), a novel poly(A)-binding protein-interacting protein, stimulates translation in nerve cells. J. Biol. Chem. 287, 1322–1334. doi: 10.1074/jbc.M111.315291
Moccia, R., Chen, D., Lyles, V., Kapuya, E. E. Y., Kalachikov, S., Spahn, C. M. T., et al. (2003). An unbiased cDNA library prepared from isolated aplysia sensory neuron processes is enriched for cytoskeletal and translational mRNAs. J. Neurosci. 23, 9409–9417. doi: 10.1523/JNEUROSCI.23-28-09409.2003
Mulligan, M. R., and Bicknell, L. S. (2023). The molecular genetics of nELAVL in brain development and disease. Eur. J. Hum. Genet. 31, 1209–1217. doi: 10.1038/s41431-023-01456-z
Na, C.-H., Jones, D. R., Yang, Y., Wang, X., Xu, Y., and Peng, J. (2012). Synaptic protein ubiquitination in rat brain revealed by antibody-based ubiquitome analysis. J. Proteome Res. 11, 4722–4732. doi: 10.1021/pr300536k
Nalavadi, V. C., Muddashetty, R. S., Gross, C., and Bassell, G. J. (2012). Dephosphorylation-induced ubiquitination and degradation of FMRP in dendrites: a role in immediate early mGluR-stimulated translation. J. Neurosci. 32, 2582–2587. doi: 10.1523/JNEUROSCI.5057-11.2012
Osterweil, E. K., Chuang, S. C., Chubykin, A. A., Sidorov, M., Bianchi, R., Wong, R. K. S., et al. (2013). Lovastatin corrects excess protein synthesis and prevents epileptogenesis in a mouse model of fragile X syndrome. Neuron 77:243. doi: 10.1016/j.neuron.2012.01.034
Pandya, N. J., Meier, S., Tyanova, S., Terrigno, M., Wang, C., Punt, A. M., et al. (2022). A cross-species spatiotemporal proteomic analysis identifies UBE3A-dependent signaling pathways and targets. Mol. Psychiat. 27, 2590–2601. doi: 10.1038/s41380-022-01484-z
Park, J. K., Das, T., Song, E. J., and Kim, E. E. K. (2016). Structural basis for recruiting and shuttling of the spliceosomal deubiquitinase USP4 by SART3. Nucl. Acids Res. 44, 5424–5437. doi: 10.1093/nar/gkw218
Pelzer, C., Cabalzar, K., Wolf, A., Gonzalez, M., Lenz, G., and Thome, M. (2013). The protease activity of the paracaspase MALT1 is controlled by monoubiquitination. Nat. Immunol. 14, 337–345. doi: 10.1038/ni.2540
Pérez-Villegas, E. M., Ruiz, R., Bachiller, S., Ventura, F., Armengol, J. A., and Rosa, J. L. (2022). The HERC proteins and the nervous system. Semin. Cell Dev. Biol. 132, 5–15. doi: 10.1016/j.semcdb.2021.11.017
Pitts, S., Liu, H., Ibrahim, A., Garg, A., Felgueira, C. M., Begum, A., et al. (2022). Identification of an E3 ligase that targets the catalytic subunit of RNA Polymerase I upon transcription stress. J. Biol. Chem. 298:102690. doi: 10.1016/j.jbc.2022.102690
Popovitchenko, T., Thompson, K., Viljetic, B., Jiao, X., Kontonyiannis, D. L., Kiledjian, M., et al. (2016). The RNA binding protein HuR determines the differential translation of autism-associated FoxP subfamily members in the developing neocortex. Sci. Rep. 6:28998. doi: 10.1038/srep28998
Richard, T. J. C., Herzog, L. K., Vornberger, J., Rahmanto, A. S., Sangfelt, O., Salomons, F. A., et al. (2020). K63-linked ubiquitylation induces global sequestration of mitochondria. Sci. Rep. 10:22334. doi: 10.1038/s41598-020-78845-7
Romero-Ayuso, D. (2021). Future challenges in research in children with neurodevelopmental disorders. Children 8:328. doi: 10.3390/children8050328
Ronchi, V. P., and Haas, A. L. (2012). Measuring rates of ubiquitin chain formation as a functional readout of ligase activity. Methods Mol. Biol. 832, 197–218. doi: 10.1007/978-1-61779-474-2_14
Saeed, B., Deligne, F., Brillada, C., Dünser, K., Ditengou, F. A., Turek, I., et al. (2023). K63-linked ubiquitin chains are a global signal for endocytosis and contribute to selective autophagy in plants. Curr. Biol. 33, 1337–1345.e5. doi: 10.1016/j.cub.2023.02.024
Saez, I., Gerbracht, J. V., Koyuncu, S., Lee, H. J., Horn, M., Kroef, V., et al. (2020). The E3 ubiquitin ligase UBR5 interacts with the H/ACA ribonucleoprotein complex and regulates ribosomal RNA biogenesis in embryonic stem cells. FEBS Lett. 594, 175–188. doi: 10.1002/1873-3468.13559
Sawicka, K., Hale, C. R., Park, C. Y., Fak, J. J., Gresack, J. E., Driesche, S. J. V., et al. (2019). FMRP has a cell-type-specific role in CA1 pyramidal neurons to regulate autism-related transcripts and circadian memory. eLife 8:46919. doi: 10.7554/eLife.46919.sa2
Schieweck, R., Ninkovic, J., and Kiebler, M. A. (2021). RNA-binding proteins balance brain function in health and disease. Physiol. Rev. 47:2019. doi: 10.1152/physrev.00047.2019
Schreiber, J., Végh, M. J., Dawitz, J., Kroon, T., Loos, M., Labonté, D., et al. (2015). Ubiquitin ligase TRIM3 controls hippocampal plasticity and learning by regulating synaptic γ-actin levels. J. Cell Biol. 211, 569–586. doi: 10.1083/jcb.201506048
Seo, S. S., Louros, S. R., Anstey, N., Gonzalez-Lozano, M. A., Harper, C. B., Verity, N. C., et al. (2022). Excess ribosomal protein production unbalances translation in a model of Fragile X Syndrome. Nat. Commun. 13:30979. doi: 10.1038/s41467-022-30979-0
Sharma, S. D., Metz, J. B., Li, H., Hobson, B. D., Hornstein, N., Sulzer, D., et al. (2019). Widespread alterations in translation elongation in the brain of juvenile Fmr1 knockout mice. Cell Rep. 26, 3313–3322.e5. doi: 10.1016/j.celrep.2019.02.086
Shigeoka, T., Koppers, M., Wong, H. H. W., Lin, J. Q., Cagnetta, R., Dwivedy, A., et al. (2019). On-site ribosome remodeling by locally synthesized ribosomal proteins in axons. Cell Rep. 29, 3605–3619.e10. doi: 10.1016/j.celrep.2019.11.025
Silva, G. M., Finley, D., and Vogel, C. (2015). K63 polyubiquitination is a new modulator of the oxidative stress response. Nat. Struct. Mol. Biol. 22, 116–123. doi: 10.1038/nsmb.2955
Song, E. J., Werner, S. L., Neubauer, J., Stegmeier, F., Aspden, J., Rio, D., et al. (2010). The Prp19 complex and the Usp4Sart3 deubiquitinating enzyme control reversible ubiquitination at the spliceosome. Genes Dev. 24, 1434–1447. doi: 10.1101/gad.1925010
Stegmeier, F., Rape, M., Draviam, V. M., Nalepa, G., Sowa, M. E., Ang, X. L., et al. (2007). Anaphase initiation is regulated by antagonistic ubiquitination and deubiquitination activities. Nature 446, 876–881. doi: 10.1038/nature05694
Su, C. H., Dhananjaya, D., and Tarn, W. Y. (2018). Alternative splicing in neurogenesis and brain development. Front. Mol. Biosci. 2018:12. doi: 10.3389/fmolb.2018.00012
Sun, C., Desch, K., Nassim-Assir, B., Giandomenico, S. L., Nemcova, P., Langer, J. D., et al. (2023). An abundance of free regulatory (19S) proteasome particles regulates neuronal synapses. Science 380:eadf2018. doi: 10.1126/science.adf2018
Sun, J., Liu, Y., Moreno, S., Baudry, M., and Bi, X. (2015). Imbalanced mechanistic target of rapamycin C1 and C2 activity in the cerebellum of Angelman syndrome mice impairs motor function. J. Neurosci. 35, 4706–4718. doi: 10.1523/JNEUROSCI.4276-14.2015
Tai, H. C., and Schuman, E. M. (2008). Ubiquitin, the proteasome and protein degradation in neuronal function and dysfunction. Nat. Rev. Neurosci. 9, 826–838. doi: 10.1038/nrn2499
Thapa, P., Shanmugam, N., and Pokrzywa, W. (2020). Ubiquitin signaling regulates RNA biogenesis, processing, and metabolism. BioEssays 2020:171. doi: 10.1002/bies.201900171
Thomson, S. R., Seo, S. S., Barnes, S. A., Louros, S. R., Muscas, M., Dando, O., et al. (2017). Cell-type-specific translation profiling reveals a novel strategy for treating fragile X syndrome. Neuron 95, 550–563.e5. doi: 10.1016/j.neuron.2017.07.013
Thrower, J. S., Hoffman, L., Rechsteiner, M., and Pickart, C. M. (2000). Recognition of the polyubiquitin proteolytic signal. EMBO J. 19, 94–102. doi: 10.1093/emboj/19.1.94
Trost, B., Thiruvahindrapuram, B., Chan, A. J. S., Engchuan, W., Higginbotham, E. J., Howe, J. L., et al. (2022). Genomic architecture of autism from comprehensive whole-genome sequence annotation. Cell 185, 4409–4427.e18. doi: 10.1016/j.cell.2022.10.009
Valdez-Sinon, A. N., Lai, A., Shi, L., Lancaster, C. L., Gokhale, A., Faundez, V., et al. (2020). Cdh1-APC regulates protein synthesis and stress granules in neurons through an FMRP-dependent mechanism. iScience 23:101132. doi: 10.1016/j.isci.2020.101132
Wang, Y., Liu, J., Huang, B., Xu, Y. M., Li, J., Huang, L. F., et al. (2015). Mechanism of alternative splicing and its regulation. Biomed. Rep. 3:152. doi: 10.3892/br.2014.407
Wang, Y., Yang, L., Zhang, X., Cui, W., Liu, Y., Sun, Q., et al. (2019). Epigenetic regulation of ferroptosis by H2B monoubiquitination and p53. EMBO Rep. 20, e47563. doi: 10.15252/embr.201847563
Wassarman, D. A., and Steitz, J. A. (1992). Interactions of small nuclear RNA's with precursor messenger RNA during in vitro splicing. Science 257, 1918–1925. doi: 10.1126/science.1411506
Werner, A., Iwasaki, S., McGourty, C., Medina-Ruiz, S., Teerikorpi, N., Fedrigo, I., et al. (2015). Cell fate determination by ubiquitin-dependent regulation of translation. Nature 525, 523–527. doi: 10.1038/nature14978
Wilkerson, J. R., Ifrim, M. F., Valdez-Sinon, A. N., Hahn, P., Bowles, J. E., Molinaro, G., et al. (2023). FMRP phosphorylation and interactions with Cdh1 regulate association with dendritic RNA granules and MEF2-triggered synapse elimination. Neurobiol. Dis. 182:106136. doi: 10.1016/j.nbd.2023.106136
Winden, K. D., Pham, T. T., Teaney, N. A., Ruiz, J., Chen, R., Chen, C., et al. (2023). Increased degradation of FMRP contributes to neuronal hyperexcitability in tuberous sclerosis complex. Cell Rep. 42:112838. doi: 10.1016/j.celrep.2023.112838
Zheng, L., Ding, H., Lu, Z., Li, Y., Pan, Y., Ning, T., et al. (2008). E3 ubiquitin ligase E6AP-mediated TSC2 turnover in the presence and absence of HPV16 E6. Genes Cells 13, 285–294. doi: 10.1111/j.1365-2443.2008.01162.x
Zhou, F., Xie, F., Jin, K., Zhang, Z., Clerici, M., Gao, R., et al. (2017). USP4 inhibits SMAD4 monoubiquitination and promotes activin and BMP signaling. EMBO J. 36, 1623–1639. doi: 10.15252/embj.201695372
Zhou, Z., Licklider, L. J., Gygi, S. P., and Reed, R. (2002). Comprehensive proteomic analysis of the human spliceosome. Nature 419, 182–185. doi: 10.1038/nature01031
Keywords: ubiquitin, translation, splicing, ribosome, neurodevelopmental disorders, FMRP, UBE3A
Citation: Elu N, Subash S and R. Louros S (2024) Crosstalk between ubiquitination and translation in neurodevelopmental disorders. Front. Mol. Neurosci. 17:1398048. doi: 10.3389/fnmol.2024.1398048
Received: 08 March 2024; Accepted: 12 August 2024;
Published: 02 September 2024.
Edited by:
Clémence Bernard, University of Exeter, United KingdomReviewed by:
Beatriz Alvarez, Complutense University of Madrid, SpainHanna Hörnberg, Helmholtz Association of German Research Centers (HZ), Germany
Copyright © 2024 Elu, Subash and R. Louros. This is an open-access article distributed under the terms of the Creative Commons Attribution License (CC BY). The use, distribution or reproduction in other forums is permitted, provided the original author(s) and the copyright owner(s) are credited and that the original publication in this journal is cited, in accordance with accepted academic practice. No use, distribution or reproduction is permitted which does not comply with these terms.
*Correspondence: Susana R. Louros, c2xvdXJvc0BlZC5hYy51aw==