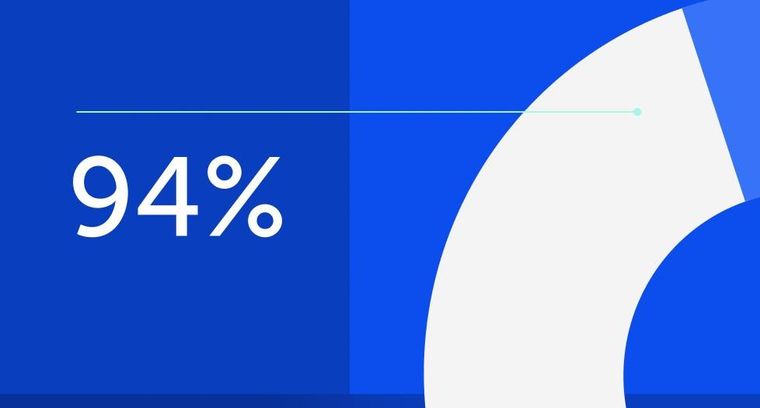
94% of researchers rate our articles as excellent or good
Learn more about the work of our research integrity team to safeguard the quality of each article we publish.
Find out more
REVIEW article
Front. Mol. Neurosci., 07 June 2024
Sec. Molecular Signalling and Pathways
Volume 17 - 2024 | https://doi.org/10.3389/fnmol.2024.1371738
This article is part of the Research TopicPerspectives in Neuroscience: Mechanical Forces for the Modulation of Axonal Mechanics and Nerve RegenerationView all 5 articles
Biological structures have evolved to very efficiently generate, transmit, and withstand mechanical forces. These biological examples have inspired mechanical engineers for centuries and led to the development of critical insights and concepts. However, progress in mechanical engineering also raises new questions about biological structures. The past decades have seen the increasing study of failure of engineered structures due to repetitive loading, and its origin in processes such as materials fatigue. Repetitive loading is also experienced by some neurons, for example in the peripheral nervous system. This perspective, after briefly introducing the engineering concept of mechanical fatigue, aims to discuss the potential effects based on our knowledge of cellular responses to mechanical stresses. A particular focus of our discussion are the effects of mechanical stress on axons and their cytoskeletal structures. Furthermore, we highlight the difficulty of imaging these structures and the promise of new microscopy techniques. The identification of repair mechanisms and paradigms underlying long-term stability is an exciting and emerging topic in biology as well as a potential source of inspiration for engineers.
Biological structures have evolved to generate, transmit, and withstand mechanical forces in a highly sophisticated manner. Biological examples have inspired mechanical engineers for centuries and led to the development of critical insights and concepts. For example, “tensegrity” refers to the emergence of stability as a result of a balance of tensile and compressive elements in a mechanical structure. It was inspired by the human musculoskeletal system, applied to architectural designs, and returned to biology as a framework to understand cellular mechanics (Wang N. et al., 2001; Ingber et al., 2014).
However, progress in the engineering discipline of mechanics also raises new questions about biological structures. The past decades have seen the increasing study of failure of engineered structures, and its origin in processes such as materials fatigue. Now engineers ask not only “Which mechanical design elements enable a human to walk upright?” but also “What enables a human to walk upright for 100 years, given what we know about mechanical degradation”? At the tissue level, we now understand that some tissues, such as bone, are in a dynamic equilibrium between assembly and disassembly (Florencio-Silva et al., 2015) which extends their lifetime dramatically by enabling constant repair (Einhorn, 1998; Bates et al., 2018). Other tissues, such as the spinal cord, are formed from long-lived cells which maintain their function over the entire lifetime of the organism (Liddell, 1960). How do these cells maintain their operation despite being constantly subjected to mechanical stresses? How do the mechanical stresses degrade intracellular structures, and what mechanisms are activated to effect repair? These are the fundamental biological questions currently under investigation.
Of particular interest is the axon, because – in addition to transmitting information – it is a unique mechanical structure. Mechanical structures such as rods are the default structures in mechanical engineering used to illustrate how applied forces generate locally varying stresses. These stresses are translated into locally varying strains based on the local materials properties, which are then integrated into deformations. Repeated deformations can lead to failure at subcritical stresses due to materials fatigue (Suresh, 1998), as was discovered by Wöhler in the study of axles of rail cars (again slender rods) in the 19th century (Spangenberg, 1875). While the neuron is shaped like a long slender rod, it differs from rods by its ability to heal which makes it the perfect candidate to examine dynamic materials.
The effect of mechanical forces on neurons has of course been extensively studied in biophysics and cell biology, but primarily when a constant or transient load is applied (Karafyllidis and Lagoudas, 2007; Hamant et al., 2019; Thompson et al., 2019). These studies yielded, e.g., insights into the response of neurons to stretching in the course of traumatic brain injury (Farkas et al., 2006), or the effect of applied mechanical forces on axonal regeneration (Shibuya et al., 2009). The mechanical integrity of axons is not only challenged by external mechanical forces but also internal mechanical forces, including forces generated by motor protein and forces generated by microtubule polymerization (Padmanabhan and Goodhill, 2018; Hahn et al., 2019; Franze, 2020; Raffa, 2023). Microtubules not only play a role in stabilizing axons mechanically but also in axon functionality (Kapitein and Hoogenraad, 2015; Che et al., 2016; Kaplan et al., 2018; Kelliher et al., 2019). In vivo, they are essential for axon elongation, guidance and connectivity (Miller and Suter, 2018). They provide the cytoskeletal “tracks” for transportation of proteins, vesicles and granules (Namba et al., 2011; Maday et al., 2014). However, the functional response of microtubules in homeostasis and under repetitive mechanical stimulation remain largely unknown.
The increased stability of neuronal microtubules compared to other microtubules and the effect of drugs on it has been highlighted in the recent literature (Baas et al., 2016; Hamant et al., 2019). The effects of mechanical stress have been studied in the context of traumatic brain injury. Short-term large (>30%) stretching of axons has been found to lead to breaking of microtubules and subsequent axon degeneration (Tang-Schomer et al., 2010). Local complete photo-damage to microtubules has highlighted molecular mechanisms of repair (Aumeier et al., 2016). The mechanisms of repair and adaptation have not yet been elucidated. Recent efforts to model the damage and failure of microtubules using molecular dynamics simulations apply unrealistic strain rates due to computational time limitations (Manuchehrfar and Shamloo, 2018; Wu and Adnan, 2018). Hahn et al. have synthesized the existing knowledge about axon homeostasis and highlighted the complexity of axonal structure and regulation as well as the prominent role of microtubule bundles (Hahn et al., 2019).
Understanding the fundamental mechanobiology of axonal homeostasis is of potentially significant biomedical value, due to the proposed role of axon decay as key trigger for neuronal decay observed in aging and neurodegenerative disorders (Adalbert and Coleman, 2013; Salvadores et al., 2017; Jakobs and Franze, 2020). However, our primary interest is fundamental biology and its intersection with biomechanics at the molecular and nanoscale.
Here, we would like to aggregate the accumulating information about the mechanisms responsible for axonal homeostasis and develop a better appreciation of the cell as a “self-repairing machine.” The novelty of our discussion is rooted in that an engineering perspective is taken and applied to cell biology by asking not “How does it work?” or “How does it break?” but “How does it keep working for so long”? For example, the functioning of healthy axons and their response to acute damage (e.g., as a result of traumatic brain injury) has been extensively studied, but the response to everyday stresses is a frontier. Biological strategies to maintain homeostasis of mechanically stressed cellular structures must exist but are largely undiscovered.
Mechanical structures can be maintained in a functional state by a design which maximizes lifetime through the use of hard materials (e.g., steel or the enamel of teeth) or through frequent repair (e.g., tires or bone). Recent advances in nanotechnology have enabled the study of the mechanical properties of biological nanostructures such as cytoskeletal filaments (Duan et al., 2015) and have even employed these biological nanostructures in hybrid nanodevices (Hess and Ross, 2017; Saper and Hess, 2019). In these devices, the disruption of the biological nanostructures by mechanical stresses often limits the device lifetime (Hess and Dumont, 2011; Dumont et al., 2015; Keya et al., 2017; Saper and Hess, 2019). It has been a recent discovery that these biological nanostructures are self-healing and can reverse mechanical damage if supplied with new building blocks in a microfluidics device (Schaedel et al., 2015, 2019; Aumeier et al., 2016). Long-lasting nanodevices enabled by the incorporation of self-repair mechanisms represent a frontier in nanotechnology.
Cells are faced with a similar need to maintain their subcellular structures in a working state, sometimes for over a century (Boateng and Goldspink, 2008). Generally speaking, the attention of science is currently focused more on the mechanisms of cellular functions than the long-term maintenance of the structures involved in these mechanisms or on acute injuries. However, aging and disease are often associated first with a breakdown of the repair mechanisms before functional decay becomes observable. It is our goal to advance our understanding of the cellular repair mechanisms counteracting damage resulting from repeatedly applied mechanical stresses.
Exerting defined mechanical stresses on cells and their subcellular mechanical structure is generally challenging, but easier for the axons of neurons. The axon is a cellular structure to which we can adapt our existing tools developed for the investigation of the nanomechanics of microtubules (Kabir et al., 2012, 2015). Its growth pattern is affected by mechanical constraints (Shefi et al., 2004) and physical mechanical interactions (Baranes et al., 2012). The axon is also of particular relevance since its functioning is impacted by neurodegenerative diseases (Gunawardena et al., 2000), and lends itself to the controlled application of mechanical stress cycles and the observation of the effects, as well as to the modeling of the nanoscale mechanics of deformation.
Here, we aim to outline the mechanical stresses applied to axons, summarize how engineered structures respond to repeated mechanical stresses, discuss how controlled mechanical stresses can be applied repeatedly to biological structures, discuss how cytoskeletal structures respond to repeated stresses, and how failure events resulting from mechanical stresses may be imaged with modern microscopy techniques.
The impact of daily movement on the internal structure of nerves is unclear and few studies have been done to date. While some studies have investigated the effects of traumatic brain injury, others have analyzed cultured neurons in the absence of mechanical motion (Bray and Bunge, 1981; Jafari et al., 1997; Antman-Passig et al., 2017; Ju et al., 2017). However, the peripheral nervous system – unlike the central nervous system – is subjected to constant movements within the joints and could be affected by mechanical fatigue, which is damage caused by repeated loading with fluctuating stresses and strains. The nerves, and therefore the axon bundles that are part of them, are regularly exposed to three types of mechanical stress: tension, compression and bending (Topp and Boyd, 2006). For example, in the human arm, the median and ulnar nerves are subjected to one of the most common movements in daily life, cycles of flexion and extension at different arm joints. Bending an elbow by 90° results in a radius of curvature of approximately 4 cm for the median nerve and 3 cm for the ulnar nerve. In the human body, nerves in the resting position are stretched by 10% (Topp and Boyd, 2006), while daily movements can increase the strain up to 14% (Wright et al., 2001). Force measurements of flexion and extension movements of the elbow or wrist performed on embalmed bodies (allowing for direct measurements) using a buckle force transducer indicated a 2 to 4% stretching (Byl et al., 2002). Furthermore, while the nerve is elongated, there is a reduction in the cross-section, which leads to lateral compression. Forces on the nerves are reduced by excursion, in which the peripheral nerves slide or glide relative to the surrounding tissue mainly in the joint area (Topp and Boyd, 2012). Tension and compression stresses have not shown any effect on the axons over a low number of cycles. Studies agree that the viscoelastic properties of nerves allow them to cope with the mechanical stresses they experience on a daily basis (Phillips et al., 2004). The strain-limit value, the value below which there is no damage on the internal structures, is not reached during daily stretching and compressing movement (Dennerll et al., 1989). However, these results were obtained from studies on cadavers or static cultured neurons. The number of flexion and extension cycles sustained by the axons of the median and ulnar nerves, assuming a human lifetime of 80 years, movement for 16 h a day, and 5 flexion and extension movements per minute, is estimated to be approximately 100 million. Human median and ulnar nerves, therefore, sustain over their lifetime approximately 100 million extension and flexion cycles with a strain of 2–4% and a radius of curvature of 3–4 cm. The peripheral nerves of smaller animals experience a roughly similar number of cycles since a shorter lifespan is balanced by more frequent movement, similar strain levels due to geometric similarity and smaller radii of curvature (in proportion to linear size). Many engineered structures, such as electric cables, would not perform reliably when subjected to mechanical stresses of this magnitude, as we will discuss in the next section.
The application of mechanical stress to materials can lead to deformation and, ultimately, fracture or failure. The progression from elastic deformation over yielding to eventual fracture under uniaxial load conditions is depicted in the stress–strain curve (Figure 1A) (Courtney, 2005). Repeated application of stress over thousands or millions of cycles can lead to failure at loads significantly below the yield stress due to the formation and growth of cracks. This phenomenon is termed mechanical fatigue (Suresh, 1998). Mechanical fatigue assumes a pivotal role in evaluating the endurance and dependability of structural components subjected to the cyclic loading conditions often present in aerospace, automotive, and civil engineering. The fatigue behavior of a material is often captured in a SN plot, where the number of cycles to failure N at a given stress level is shown on the abscissa and the corresponding stress level on the ordinate. (Figure 1B). For some engineering materials cyclic stresses below a stress level termed “endurance limit” do not ever lead to fracture and permit an infinite lifetime.
Figure 1. (A) Stress strain curve: the plot represents an example of a stress strain curve typical for steel where different properties of the material can be assessed (Yun and Gardner, 2017). (B) S-N Diagrams and Healing Capability: This figure presents S-N diagrams, where S represents fatigue strength and N the number of cycles. The diagrams are constructed through subjecting components to cycles of predetermined load until failure. The failure line (solid black) on the graph indicates the cycle count at which 50% of the tested samples fail under a specific load. Notably, some materials exhibit an endurance limit (Se), denoting a threshold below which fatigue failure does not occur. Conversely, other materials eventually experience fatigue failure regardless of the applied load. The dashed blue line illustrates how the curve may be altered due to healing, which is activated during normal usage.
For example, the Ti Al6 V4 titanium alloy stands out for its durability, tolerating 104 cycles at 700 MPa and a practically infinite number of cycles below its endurance limit of 600 MPa (Fleck and Eifler, 2010). For comparison, cold-rolled mild steel can endure 300 MPa over 106 cycles and exhibits an endurance limit of 270 MPa (Forrest, 2013). In biomechanics, fatigue testing has been conducted for bones which are cyclically loaded during walking and running (Taylor, 1998; Acevedo et al., 2018; Edwards, 2018). Bone fatigue arises from microcracks within the bone matrix that may undergo partial repair before the repair cycle is disrupted, leading to crack propagation. This phenomenon is accentuated in older individuals due to a decline in bone density.
Mechanical fatigue is also experienced by biological materials (Arola et al., 2010; Edwards, 2018; Qiang et al., 2019). For example, Nasrin et al. conducted fatigue measurements on microtubules and extrapolated that microtubules can withstand 103 cycles of 20% compression and 5 × 106 cycles of 12.5% compression. However, further experiments are required to ascertain the existence of an endurance limit for microtubules (Nasrin et al., 2023). Unlike inanimate materials, which often incur irreparable damage from fractures or fatigue, living organisms possess an exceptional capability to self-heal. When a biological material begins to accumulate damage, it can engage in a regenerative process whereby the compromised region is renewed and fortified with fresh components. This process can occur at multiple scales, from the molecular to the tissue level. At the molecular scale for example, microtubules can undergo replenishment through the replacement or addition of new tubulin building blocks (Ganser and Uchihashi, 2019), and biomolecular motors in muscles are systematically replaced (Boateng and Goldspink, 2008). In instances where the healing process is impaired or absent, the biological material becomes more susceptible to fracture (Schaedel et al., 2015). An illustration of this is that bones experiencing a physiological stress level are estimated to fracture during their lifetime, thus necessitating repair to avert failure (Taylor, 1998). This inherent mechanism serves as a safeguard, shielding the material from the accumulation of damage, as it perpetually renews and rehabilitates itself. This cycle of rejuvenation ensures the resilience and functionality of biological structures over time, presenting a potent countermeasure against the mechanical fatigue associated with non-living materials.
Neurons, heart, lungs, and muscles of a mammal are constantly under tensile-compressive stress cycles throughout development, growth, and adult life. Mechanical stress can trigger biological responses and promote biological processes like embryogenesis (Fruleux et al., 2019), development (Lecuit and Lenne, 2007; Le et al., 2016), and tissue homeostasis (Matthews et al., 2006; Barnes et al., 2017). The response of cells has been investigated by applying mechanical stresses in different modes, constant, transient, and repetitive.
Constant applied stress exerts a continuous force on cells modulating cellular structure and function. For instance, continuous tensile stress on endothelial cells alters cytoskeletal organization and focal adhesion dynamics (Bao and Suresh, 2003).
Mechanical stress suppresses the growth and proliferation of cardiac stem cells (Kurazumi et al., 2011) and human parametrial ligament fibroblasts (Hu et al., 2017) led by cytoskeletal depolymerization and rearrangement, indicating damage to F-actin in cells. Additionally, research demonstrated the impact of constant stress on neuronal cells, showing alterations in synaptic plasticity and neurotransmitter release dynamics (Uchida et al., 2014). Constant stress can lead to cell stiffening through tensegrity mechanisms, with structural remodeling occurring over time to solidify the cytoskeleton. Steady loading generally results in stiffening rather than softening of cells (Walker et al., 2020; Putra et al., 2023).
Transient stresses introduce temporary variations in mechanical load on cells. Over longer timescales, transient stretching of cells induces structural disorder within the cytoskeleton, causing a loss of stability and softening (Stamenović and Wang, 2011). A quantitative cell-based model suggested that cells adapt their volume with a certain delay in response to pressure changes due to internal friction and cytoskeletal remodeling (Van Liedekerke et al., 2019). Stretching under mechanical stress generates internal pressure to deform the cell membrane and contribute to volume changes in cells. This membrane stress can partially compensate for osmotic gradients and help maintain cell volume (Khmelinskii and Makarov, 2020). Cancer cells showed increased plasticity in behavior due to increasing stress (Onal et al., 2023).
Cyclic stress is the most pronounced mode of stress in living beings that imposes rhythmic mechanical cues on cells, triggering adaptive responses and physiological changes. The effects of cyclic stress on cellular behavior have provided insights into mechanotransduction mechanisms and biomechanical regulation by modulating cell adhesion and migration processes through alterations in cellular orientation and alignment. When cyclic stress is exerted in a single direction (uniaxially), causing deformation or strain along that specific axis, cells align perpendicular to the stress. In contrast, under equibiaxial stress, where stress is simultaneously applied along two balanced axes with equal intensity, resulting in uniform deformation or strain across both axes, cells show no clear orientation (Wang J. H. C. et al., 2001; Pennisi et al., 2011; Tondon and Kaunas, 2014). Mechanical stress applied to human fibroblasts leads to changes in the number, length, and area of vinculin-positive focal adhesion contacts. This is related to the roles of Akt and RhoA in focal adhesion assembly and maturation (Boccafoschi et al., 2010). The cardiac fibroblasts respond to mechanical stress by revealing changes in gene expression profiles associated with extracellular matrix remodeling and tissue homeostasis (Jungbauer et al., 2008; Greiner et al., 2013). A similar effect of cyclic stress is observed on vascular smooth muscle cells (Liu et al., 2008) and osteosarcoma cells (Alloisio et al., 2023), where the cell morphology was changed to a larger cell area and more elongated shape and expression of genes related to proliferation was increased two to three fold under stress. The frequency of cyclic stress and cell proliferation are negatively correlated (Duveau et al., 2024).
In summary, mechanical stress has diverse effects on different cell types, including reduced proliferation, cytoskeletal changes, altered adhesion and signaling, and changes in membrane mechanics and cell volume regulation. The specific responses depend on the mode of the mechanical stress being applied. The cytoskeleton is the primary means of sensing, integrating, and coordinating cellular responses to mechanical stimuli and structural cues (McCain and Parker, 2011; Table 1).
Biological processes indispensably require responses to external mechanical stress (Hamant and Haswell, 2017; Sadhanasatish et al., 2023). Stress applied to a cell is distributed broadly by its cytoskeleton, but the magnitude of transmitted stress to a particular location depends on network mechanics and architecture and can have marked effects on cellular processes, from individual filament polymerization up to entire network reorganization (Fletcher and Mullins, 2010). When repetitive stretching is applied, cellular reorientation occurs in an alignment perpendicular to the direction of the stretching, as shown in Figure 2 (Liu et al., 2008; Faust et al., 2011; Sakamoto et al., 2017; Lin et al., 2020). Such cellular reorientation is assisted by the reorganization of the contacts of the cells to the extracellular matrix (ECM). Cells maintain a set point in the ECM and reorganize their stress fibers, adhesions, and traction forces (Saez et al., 2005). The cytoskeletal components contribute to the direct communication between cells and their ECMs by mediating integrins, associated with actin through formation of regulatory molecules (Verma et al., 2012). The repetitive application of stress causes progressive adaptation in cytoskeletal structures, leading to reversible compression in proteins like α-actinin (Verma et al., 2012). This adaptation occurs over multiple time scales, involving rapid and reversible changes in cytoskeletal stresses followed by chronic rearrangements. Myotubes, when subjected to repetitive stretching, exhibited increased synthesis of myosin compared to unstimulated conditions (Vandenburgh and Kaufman, 1979).
Figure 2. (A) A Scheme of an experimental setup for applying cyclic stretch on neurons. One end of an elastic polydimethylsiloxane (PDMS) film is attached to a fixed clamp and the other end is attached to a movable clamp. The PDMS is cyclically elongated. The direction of the elongation is indicated by the red arrow. (B) Snapshot of a PC12 cell subjected to cyclic stretch, where εapp is the cyclic stretch, L is the length of the axon, and θ is the angle between axon alignment and stretch direction. (C) Morphological images of PC12 cells after 24, 72, and 120 h with and without applying cyclic stretching. Images in the upper row are obtained under no stretch and in the lower row are obtained under cyclic stretch (10% amplitude and 0.25 Hz frequency). Scale bar: 100 μm. Figure adapted from Lin et al. (2020).
In response to repetitive mechanical stress, the cytoskeleton exhibits dynamic responses characterized by changes in stiffness, self-repair mechanisms and enhanced stabilization. Microtubules soften under mechanical stress, with repeated bending cycles causing incremental softening and material fatigue. The maximal deflection of microtubules increases with each mechanical cycle, indicating a progressive softening effect under repeated stress (Schaedel et al., 2015). As response to cycles of compressive forces in living cells, microtubules become distorted, less dynamic and more stable (Li et al., 2023). Actin filaments responds to cyclic stretch through reversible stress softening (Chaudhuri et al., 2007). Actin depolymerization and repolymerization play a significant role in strain softening and recovery responses under mechanical stress (Walker et al., 2020).
Neurons, as all cells, interact physically with the surrounding environment and through a process known as mechanotransduction perceive the mechanical stimuli and convert them into a biochemical response (Watson, 1991; French, 1992). Through this process they adapt to and survive in a changing environment. Neurons experience mechanical stimuli during neurodevelopment, aging, pathologic conditions and in everyday function, homeostatic process, and movement (Butler, 1989; Sarkis et al., 2017; Chighizola et al., 2019; Javier-Torrent et al., 2021). Neuronal mechanical interactions affect their expression regulation (Baranes et al., 2019). However, the signaling cascade of mechanotransduction in neurons is still only partially discovered. The repeated observations that mechanical strains can induce a cytoskeletal remodeling inside cells suggest that the cytoskeleton could be strongly involved in the transduction process. Exogenous mechanical stimuli are perceived by neurons and propagated within the cell through a complex network that spans from the cell membrane to the nuclear membrane (Figure 3). The interaction with matrix is mediated by cell adhesion molecules of the immunoglobulin superfamily (IgSF), including NCAM and L1 family members (Leshchyns'ka and Sytnyk, 2016). Their extracellular domain, containing one or several immunoglobulin-like (Ig) repeats typically mediate interactions with proteins of the extracellular matrix (ECM) (Grumet and Sakurai, 1996). The intracellular domains of IgSF cell adhesion molecules (CAMs) interact with the components of the cytoskeleton including the submembrane actin-spectrin meshwork, actin microfilaments, and microtubules. Similarly to non-neuronal adhesion points, IgSF CAMs can trigger the recruitment of multiple scaffold proteins thereby amplifying the interactions with the cytoskeleton, leading to the maturation the point contact (PC) adhesion and promotion of intracellular force generation by actomyosin contraction. Forces generated intracellularly by the actin cytoskeleton can be transmitted to the cell cytoskeleton via physical coupling between the F-actin filaments and the microtubules through a number of common binding proteins and regulators (Dogterom and Koenderink, 2019). Interestingly, the cell cytoskeleton is also coupled to the nucleoskeleton through the LINC (LInkers of the Nucleoskeleton to the Cytoskeleton) complex formed by the SUN and KASH protein domains. SUN proteins cross the inner nuclear membrane (INM), enabling their N-termini to bind to the nuclear lamina, while KASH domain proteins cross the outer nuclear membrane (ONM), enabling their N-termini to bind to the cytoskeleton. Similarly to the actin stress fibers anchored by the PC adhesions that are involved in ECM / cytockeleton transmission of contractile forces through actin-myosin dynamics, it has been reported that an actin cap, composed by bundles of highly contractile acto-myosin filaments anchored to the apical surface of the interphase nucleus, is involved in force transmission from cytoskeleton to nucleoskeleton (Kim et al., 2013). Through this network, mechanical forces can also propagate from cell periphery to the nucleus (Li et al., 2014). Ingber et al. proposed a “tensegrity” model in which the cytoskeleton supports the tension inside cells by maintaining a balance between the forces at play. Dynamic cytoskeletal elements, like the contractile actomyosin and the compression-bearing microtubules, would participate in the propagation and diffusion of the mechanical stresses across the cytoskeleton of the cell (Ingber et al., 1981; Ingber, 1993; Wang et al., 1993; Ingber, 1997; Brangwynne et al., 2006; Figure 4). Even if myosin and actin have been first proposed to be involved in mechanosensing, now many articles are identifying microtubules as important players in force transduction and sensing events (Falconieri et al., 2023a).
Figure 3. Scheme of the neural cytoskeleton: actin fibers (red), microtubules (green), myosin (purple), spectrin (blue). Top insert highlights vesicles transport in the axon. Central insert illustrates actin-spectrin rings. Bottom inset shows dendritic spines.
Figure 4. Scheme of mechanical strain consequences on neurons. (A) Strains with high magnitude and high rate can cause morphological alteration of neurons, degeneration and disconnection, membrane poration, GC collapse, Tau detachment from bundles, loss and break of MTs causing transport interruption and formation of swelling. (B) Possible mechanisms involved in neuron resilience to mechanical strain comprehend activation of mechano-sensible channels, GC re-orientation, tension buffering mediated by actin-spectrin rings re-organization, MTs assembly and polymerization that lead to an accumulation of vesicles and organelles with local translation events, interactions between MAPs and MTs and post-translational modification of tubulin such as acetylation.
The forces that are experienced by neurons can be divided into internal forces, determined mainly by re-adjustments of the cytoskeleton in response to external stimuli, and exogenous forces, which are the focus of this review. The exogenous forces can be divided into forces of extension or stretching, compression, and bending.
The effects of stretching are the most investigated; they have been studied since the 1940s in relation to body growth, neurodevelopment, and axon elongation (Weiss, 1941; Bray, 1984; Van Essen, 1997). Nowadays, it is known that low stretching forces (from very low pN to a few nN) can modulate a plethora of events like axon elongation, branching, axon excitability, transport alteration, orientation, and cytoskeletal dynamics (De Vincentiis et al., 2020b). Several thresholds for tensile forces have been identified that discriminate between elongation or axon breakage / growth cone (GC) collapse. For example, a threshold of sensitivity to mechanical strain required to promote neurite initiation was identified, and it was observed that the sensitivity changed with the neuronal type: a force of 0.5–1.5 nN was required in chick sensory neurons, 3–10 nN in PC12 neurons, and 0.31 ± 0.06 nN in chick forebrain neurons (Zheng et al., 1991; Chada et al., 1997; Lamoureux et al., 1997). Furthermore, axons cannot tolerate forces that exceed a maximum limit: Smith and colleagues found that axons of neurons could tolerate a 1 mm/day stretch rate, but when the stretch rate was doubled axons disconnected and were unable to sustain the elongation (Smith et al., 2001); Franze and colleagues determined that strains above 274 pN/mm2 caused GC collapse, neurite retraction and growth in another direction (Franze et al., 2009). Interestingly, Pfister and colleagues showed that the use of an acclimatation time could increase the threshold of tolerance for DRG neurons to stretching above which axons underwent disconnection (Pfister et al., 2004).
The effect of mechanical strain is not only related to the magnitude but also to the strain direction and the rate of application. Uniaxial strain on axons seem to be most damaging when the applied strain is parallel to the axons rather than inclined (Nakadate et al., 2014, 2017). Moreover, axons can tolerate high extension (until twice their original length) if the applied strain rate is low, but if the strain is applied quickly (< 50 ms) consequences are more drastic (Tang-Schomer et al., 2010); for instance, uniaxial strain above 65% applied with a 26–35 1/s strain rate has been found to cause primary axotomy (Smith et al., 1999). When the insult is above the threshold, cells cannot put in place counteracting mechanisms due to a failure of their structures. Tang-Shomer and colleagues have shown that application of high dynamic strains on cortical neurons causes distortions and axon swelling along axons after they relax back within the first hours after stimulation, and degeneration in most axons within 24 h. They hypothesize that the appearance of varicosities along axons is due to transport interruption, as they observed that breakage, buckling and reduction of microtubules take place immediately after the injury (Tang-Schomer et al., 2010, 2012). The sensibility of microtubules to high strain rates has been also highlighted by in vitro studies showing how the high rate can cause microtubules breaking and detachment from bundles due to the breaking of tau-tau bonds (Ahmadzadeh et al., 2014, 2015).
Interestingly, a comprehensive analysis of the works published in the last four decades shows that the elongation rate per applied force calculated in previous studies is surprisingly similar (0.1–1 μm/h/pN) (De Vincentiis et al., 2020a), irrespective of the force magnitude (from 1 pN to 100 nN) and the model. The interpretation of this fact is that when axons are chronically stimulated with low forces, similarly to those generated physiologically (generally below 500 pN) (De Vincentiis et al., 2020b), they can sustain the stress over days to weeks, promoting axon elongation. When axons are stimulated acutely with high intensity forces, e.g., those generated in traumatic events (>1 nN), the axons thin and break in minutes if a resting time is not allowed to transport or neo-synthesize the mass necessary to counteract the applied strain.
Neurite elongation induced by mechanical stimulation can be productive, and it has been highlighted that MTs assembly and polymerization may be involved (Zheng et al., 1993; Putnam et al., 2001; Lee and Suter, 2008; Miller and Suter, 2018; Raffa et al., 2018; De Vincentiis et al., 2020a; Falconieri et al., 2022, 2023b). It has been proposed that mechanical signals could influence MTs stability by acting on the MT lattice structure (Gudimchuk et al., 2020), by a modulation of MAP function (Franck et al., 2007; Trushko et al., 2013), or by post-translational modification of tubulin for review (see Kerr et al., 2015; Robison et al., 2016; Swiatlowska et al., 2020; Coleman et al., 2021; Torrino et al., 2021; Seetharaman et al., 2022; Falconieri et al., 2023a). Recently, our group demonstrated a connection between axonal elongation and the modulation of MT dynamics in hippocampal neurons. Specifically, we found that forces generated by magnetic nanoparticles promote axonal elongation which was accompanied by an increase in microtubule stability and density (De Vincentiis et al., 2020a; Falconieri et al., 2022, 2023b). Stable MTs present a different surface that is recognized by motor proteins or MAPs, remaining stably bound to long-lived MTs (Jansen et al., 2021). We proposed that the addition of mass induced by force application is caused by this increase in microtubule stabilization/density that results in accumulation of organelles and vesicles in the axon, which, in turn, increases the probability of local translational events. This increase in axonal transport and the activation of local translation can provide the necessary mass to sustain axon elongation and neuronal maturation.
In contrast to stretching forces, the mechanotransduction of compressive strain is investigated less in neurons. Interesting studies in this field have been conducted on plant cells. These works have shown how microtubules can undergo a re-orientation during anisotropic wall stress or constrain and microindentation of apical meristems (Williamson, 1990; Hejnowicz et al., 2000; Hamant et al., 2008; Louveaux et al., 2016). In neural cells, many studies have been done in relation to traumatic brain injuries and spinal cord injuries, where the compressive force has been applied in-plane or perpendicular to the plane of the tissue. Similarly to the threshold behavior observed for tensile strain, a threshold has to be exceeded for compression to generate a response by cells, while overcoming a maximum limit induces cell damage and death. The application of a low compression force (<55 kPa), perpendicular to the axon plane, showed in hippocampal neurons that most axons are still able to grow after the injury, but when the applied stress rises to 55 kPa the percentage of growing axons decreased to 8% with swelling phenomena and thinning of the axonal membrane, while severe stress (>95 kPa) caused an immediate transection of the axon (Hosmane et al., 2011). By using a similar approach on axons of hippocampal neurons, Fournier and colleagues showed that a vertical focal compression prompted axon swelling and changes in cytoskeletal distribution. More in detail, they observed a decrease in neurofilament and MT density, a decrease in their number and MT break point and disorganization (Fournier et al., 2015). Compressive stresses higher than 0.5 MPa have been seen to strongly impact neuron viability. Most of these studies rely on instruments based on compressed gas to apply a vertical pressure, in the range of 0.5 to 1 MPa, to neurons. In these cases, data show a decrease of cell viability and an increase of apoptotic processes (magnitude and time dependent), oxidative stress, an increase of intracellular calcium, mitochondrial dysfunction, and ER stress (Ye et al., 2012; Quan et al., 2014; Chen et al., 2019). Similarly, to high stretching strain, these high compressive strains have been found to impact the cytoskeleton leading to its remodeling and events like microtubules disruption, disorganization and degeneration (Ye et al., 2012; Quan et al., 2014).
Studies conducted by application of in-plane compressions have instead alighted other interesting consequences on the cytoskeleton. For instance, by cell membrane compression, microtubule buckling has been observed in beating cardiac myocytes, cells compressed with a glass microneedle, and in cells in the proximal region of the cell membrane (Wang N. et al., 2001; Brangwynne et al., 2006). A recent study on retinal pigmental epithelial cells has shown that a compressive strain of 40% induces cell detachment, but when the strain is decreased to 10% there is no impact on cell shape; however, with the same strain, 1 Hz rate causes cell detachment while 0.1 Hz is well tolerated. In the same work, authors report a reduction of microtubule growth and an increase of microtubule stability through the relocation of plus-tip proteins (EB1 and CLASP2) from the microtubule end to the microtubule shaft, suggesting their central role in mechano-response (Li et al., 2023). Although these studies focused on non-neuronal cells, they offer insights into how compression frequency and force impacts cells, potentially affecting neurons in a similar way.
Neurons can be subjected to a bending load through body movements, or the shear stress generated by body fluids. One of the models used to study the effect of axon bending on signal transduction pathways is C. elegans. The motion of C. elegans consists in a dorso-ventral bending by which the animal can move through a process modulated by proprioception (Goulding, 2012; Yeon et al., 2018). DVA, PMD, SMD proprioceptive neurons and the motor neurons can be activated by body bending and mechanosensitive channels could be involved in their activation and in the signal transduction (Li et al., 2006; Wen et al., 2012; Schafer, 2015; Yeon et al., 2018; Das et al., 2019, 2021; Tao et al., 2019; Liu et al., 2020). In DVA neurons, for instance, an increase of calcium level takes place following body bending, and the neuron activation seems to be dependent on TRP4 channels (Li et al., 2006). In SMDD neurons, in contrast, trp1 and trp2 channels have been found activated during body movement (Yeon et al., 2018). Similarly, in Drosophila nociceptors have been found activated by shear stress through TrpA1 (Gong et al., 2022).
Studies on plants models suggest that microtubules also may have a key role in signal mechanotransduction induced by a bending load (Nick, 2008). For instance, when the epidermis of maize coleoptiles is subjected to bending, MTs re-orient acquiring a longitudinal and transversal orientation at the inner (compressed) and outer (extended) side (Zandomeni and Schopfer, 1994; Fischer and Schopfer, 1997). Another study, on Azuki epicotyl, showed that microtubule orientation was altered by an inhibitor of stretch activated channels suggesting that mechanosensitive channels and microtubules could be strongly involved in this process (Ikushima and Shimmen, 2005).
The direction of axonal growth was found to change in response to the application of a pico-Newton shear stress. Growth cones of individual axons turn in response to a shear force of 0.17 pN generated by the controlled rotation of an optically driven particle (Wu et al., 2012). Similarly, neurites were found to preferentially align to the direction of the applied force, when a tangential force of a few pN was generated on MNP-loaded neurites through magnetic fields (Riggio et al., 2014). However, studies about shear stress in neurons have highlighted the presence of a threshold of tolerability. Kilinc and coworkers designed a microfluidic device to generate hydrodynamic shear stress (Blackman et al., 2000), observing that a fluid shear stress above 6 μPa caused the detachment of primary chick forebrain neurons, whereas at 4.5 μPa shear stress beading events took place but cells survived (Kilinc et al., 2008). Shear stress caused mechanical damage to the axolemma (axolemmal pores), calcium influx, Calpain activity, disruption of the cytoskeleton, and accumulation of mitochondria in the points of axonal bending, suggesting a causal relationship between membrane damage, Ca2+ influx, calpain dynamics, microtubule breaking and the formation of axonal beads (Kilinc et al., 2008, 2009). A microfluidic device was used to generate a cyclic shear stress to investigate how the stress repetition could impact on cells. In presence of high bending load, polymerizing tubulin was reduced together with an increase of cell elasticity; this observation suggests that the loss of microtubules could be related to a reduced resistance to deformation. From these findings, authors speculated that the cytoskeleton and the time for it to reassemble could play a crucial role in the protection from injury (Edwards et al., 2001). Taken together these studies suggest that shear stress can influence axonal growth at low levels, but high or repetitive stress can damage neurons through membrane disruption and cytoskeletal impairment.
All these insights show that neural mechanotransduction is a complex mechanism in which many factors are involved. A high strain magnitude and fast rate of application can severely affect neurons, even if neurons tolerate repeated strain with a resting time in between so that counteracting mechanisms can be put in place (Pfister et al., 2004). Cells cannot repair the damage if the applied strains are above a threshold, because of failure in cellular structure and function, as microtubules break and axonal transport interruption. Which homeostatic mechanisms help cell resilience at lower strain is still unclear, however, here we discussed some examples in which microtubules, their posttranslational modifications and associated proteins seem to be involved. Long-lived microtubules are acetylated on lysine 40 of α-tubulin (αK40) inside their lumen. Microtubules of neurons that are submitted to repetitive mechanical stress are highly acetylated (Janke and Montagnac, 2017). It was reported that αK40 acetylation modifies the microtubule lattice to better adapt to mechanical stress, facilitating microtubule self-repair mechanisms (Janke and Montagnac, 2017). It reduces inter-protofilament interactions and confers resilience against repeated mechanical stresses (Portran et al., 2017). Consistently, the depletion of the αK40 writer (the tubulin acetyltransferase TAT1) led to a significant increase in the frequency of microtubule breakage (Xu et al., 2017). Loss of this modification is associated with neuronal degeneration (Neumann and Hilliard, 2014). In the absence of K40 acetylation, microtubules in c3da neurons can be mechanically damaged (Yan et al., 2018).
Actin/spectrin rings also appear to be involved in the tolerance to mechanical stress. They act as load-bearing elements able to discharge the mechanical stress by the unfolding and re-folding of spectrin tetramers, working as “shock absorber” (Dubey et al., 2020). Krieg and colleagues showed in touch receptor neurons of C. elegans that more players could contribute to mechanical neuroprotection, with the actin-spectrin networks providing tension, MT bundles conferring stiffness, and microtubule-associate proteins like tau acting as dissipator of torsional forces (Krieg et al., 2017).
In summary, many elements could work in combination to confer resilience against mechanical stress to neurons for all our long life; the emerging understanding will yield important insights on aging and neurodegeneration studies.
Uncovering the effects of repetitive stress on neurons necessitates high-resolution imaging techniques to visualize subcellular changes within the cytoskeleton. The cytoskeleton of neurons is tightly packed composed of nanometric elements, including microtubule individual fibers spaced as close as 50 nm apart (Yamada et al., 1971). The microtubule density can reach up to 150 fibers within just one μm square (Wortman et al., 2014). While it is clear that mechanical load affects the microtubules within a living cell as discussed in the previous section, the diffraction limit which is typically over 200 nm (Born and Wolf, 1986) prevents us from getting to the single microtubule fiber or even to a bundle resolution in vitro (Kabir et al., 2020). Therefore, imaging the details of the neuronal cytoskeleton requires advanced microscopy techniques that can bypass this limit (Leterrier et al., 2017; Werner et al., 2021).
While electron microscopy (EM) with its resolution of a few nanometers provides the capability to resolve individual microtubules as seen in Figure 5 (Lunn et al., 1997; Yang et al., 1999; Ventura Santos et al., 2023), it faces significant challenges when it comes to simultaneously imaging multiple targets as it involves heavy metal staining, which can affect sample integrity and hinder antibody binding to its target resulting in a poor contrast and inability to differentiate between different targets (Kim et al., 2020; Lelek et al., 2021). Moreover, it requires complicated sample preparation with harsh conditions which can introduce artifacts and are not suitable for live cells (Inkson, 2016). Therefore, super-resolution (SR) optical techniques that have been developed over the past two decades offer valuable complementary approaches, as they allow to overcome those challenges and open new possibilities for more comprehensive analysis.
Figure 5. (A,B) Transmission electron microscopy (TEM) images of hippocampal rat neurons cell’s axon with microtubules indicated by black arrows (B), Scale bar: 100 nm. Figure was adapted from Fournier et al. (2015).
Among the common SR techniques, single-molecule localization microscopy (SMLM) stands out due to its ability to achieve resolutions as fine as 10 nm (Rust et al., 2006), compared to 100 nm and 30 nm of Structured Illumination Microscopy (SIM) and Stimulated Emission Depletion microscopy STED, respectively, (Gustafsson, 2000; Willig et al., 2006), and were able to resolve the complex structure of the cytoskeleton, revealing actin rings (Xu et al., 2013; Papandréou and Leterrier, 2018) and single microtubule bundles and even fibers within the neurite, as can be seen in Figure 6 (Mikhaylova et al., 2015; Tas et al., 2017). Furthermore, some SMLM based techniques are compatible with live cells (Shroff et al., 2013; Zhang et al., 2013). These characteristics are fundamental when examining the characterization and dynamic behavior of a minute structure such as the neuronal cytoskeleton (Wang and Brown, 2002; Sakakibara et al., 2013). However, even though SMLM holds the theoretical capability to achieve the desired resolution, it is challenged by a densely packed structure such as the neuronal cytoskeleton. Images of these structures are often characterized by increased noise levels, resulting in diminished SNR and consequently inferior resolution. To overcome this challenge, certain modifications to the SMLM approach are necessary.
Figure 6. (A) SMLM reconstruction of microtubule bundles labeled with nanobodies in the dendrites of a hippocampal primary neuron. Yellow arrows indicate microtubule ends and yellow lines were used to create the profiles (B,C). Inset shows the diffraction-limited fluorescence image. Scale bar, 2 μm. Figure was adapted from Mikhaylova et al. (2015).
One approach that was explored in the last decade, is to combine SMLM with other advanced techniques, such as expansion microscopy (ExM) resulting in Ex-SMLM (Tong et al., 2016; Zwettler et al., 2020). This method increases the physical size of the sample and thereby mitigates its dense structure, enabling us to achieve a resolution like electron microscopy (Wang et al., 2014; Alon et al., 2021). ExM operates by introducing a hydrogel matrix to the sample and anchoring the target of interest, such as proteins, within the sample to the hydrogel. Subsequently, a chemical reaction, often as simple as water absorption, is employed to expand the hydrogel, leading to a uniform expansion of the entire sample (Chen et al., 2015; Alon et al., 2019; Gambarotto et al., 2021). Unfortunately, ExM must use fixed cells, and as a result does not allow the observation of dynamic events, such as damage processes resulting from mechanical stimulation and the ensuing repair mechanisms.
Measuring intracellular dynamics and integrity are crucial to comprehend cellular responses to mechanical stress that may serve as indicators of potential self-repair mechanisms (Schaedel et al., 2015; Théry and Blanchoin, 2021). One central factor in the characterization of these dynamics is the diffusion coefficient, a metric that quantifies the movement of particles across a predefined area. As mechanical stress progresses, any change in dynamics (e.g., diffusion coefficient) will indicate on the possible existence of self-repair mechanisms (Robert et al., 1990). Measuring these changes requires the determination of the diffusion coefficients of ensembles and individual particles and various microscopy techniques are employed to measure them. Fluorescent Recovery After Photobleaching (FRAP) enables us to access the diffusion coefficient for entire populations of molecules (Edson et al., 1993; Ishikawa-Ankerhold et al., 2012; Blumenthal et al., 2015). Single Particle Tracking (SPT) based techniques provide the means to measure several parameters on the single molecule level such as diffusion coefficient and directionality over time (Tinevez et al., 2017) even in densely packed areas by using photoactivatable dyes (Subach et al., 2010). Both approaches provide complementary information and improve our comprehension of cellular responses to mechanical stress.
The axons of peripheral nerves undoubtedly experience repetitive mechanical motion causing significant deformations. The effects of these deformations on the internal structure of an axon are still unknown, due to the difficulty of replicating the motion in cell culture and observing the dynamic changes with an imaging technique of sufficient resolution. While electron microscopy yielded detailed static images of the axon ultrastructure, we anticipate that the imaging of dynamic changes will require advances in optical imaging techniques. Continuous progress is being made in creating devices which allow the application of repetitive stresses to cells and cellular substructures, such as microtubules and microtubule bundles (Kabir et al., 2015, 2020; Abraham et al., 2018). However, the number of cycles is typically limited to hundreds rather than the 100 million deformation cycles experienced by a peripheral neuron in a human, which requires the extrapolation of the responses observed in such experiments (Abraham et al., 2018; Qiang et al., 2019). In the testing of engineered systems, “accelerated aging” where the stress level (e.g., by elevating temperature) is increased above the typically experienced values during testing has been proven to be of value (Hukins et al., 2008), and may be replicated here. Previous work on the response of cells to single and repeated mechanical stresses has highlighted to complexity of the cellular response, which depends on the specific nature of the deformation (e.g., tensile or compressive) and the precise time course of stress application. Often a threshold behavior is observed, where damage is detectable above a certain level of stress, and cell death occurs at an even higher level. An intriguing question is of course if mechanical stress can have a hormetic effect (Calabrese and Mattson, 2017). Despite the theoretical and experimental challenges associated with this research topic, new information about the mechanical response of cells and subcellular components is constantly being generated and contributes to the elucidation of the key mechanisms. Progress in our understanding of this topic promises clinical benefits related to diseases of aging as well as lessons for the bioinspired design of engineered structures.
AC: Writing – original draft. AF: Writing – original draft. OM: Writing – original draft. MR: Writing – original draft. GS: Writing – original draft, Writing – review & editing. HH: Writing – original draft, Writing – review & editing. AK: Writing – original draft, Writing – review & editing. VR: Writing – original draft, Writing – review & editing. OS: Writing – original draft, Writing – review & editing. SRN: Writing – original draft.
The author(s) declare that financial support was received for the research, authorship, and/or publication of this article. The authors gratefully acknowledge support from the Human Frontiers Science Program grant RGP0026/2021, the JSPS Postdoctoral Fellowship Standard Program (SN), the Future AI and Robot Technology Research and Development by New Energy and Industrial Technology Development Organization (NEDO) JPNP20006, the Grant-in-Aid for Scientific Research on Innovative Areas “Molecular Engine” JP18H05423, the Grant-in-Aid for Scientific Research (AK) JP21H04434, and the Grant-in-Aid for Challenging Research (Pioneering) JP17K19211 (AK).
OS and OM would like to express their gratitude to Zehavit Shapira for a fruitful discussions and to Eli Varon for helpful comments and discussions. Figures 3 and 4 were created with BioRender (Biorender.com).
The authors declare that the research was conducted in the absence of any commercial or financial relationships that could be construed as a potential conflict of interest.
All claims expressed in this article are solely those of the authors and do not necessarily represent those of their affiliated organizations, or those of the publisher, the editors and the reviewers. Any product that may be evaluated in this article, or claim that may be made by its manufacturer, is not guaranteed or endorsed by the publisher.
Abraham, J.-A., Linnartz, C., Dreissen, G., Springer, R., Blaschke, S., Rueger, M. A., et al. (2018). Directing neuronal outgrowth and network formation of rat cortical neurons by cyclic substrate stretch. Langmuir 35, 7423–7431. doi: 10.1021/acs.langmuir.8b02003
Acevedo, C., Stadelmann, V. A., Pioletti, D. P., Alliston, T., and Ritchie, R. O. (2018). Fatigue as the missing link between bone fragility and fracture. Nat. Biomed. Eng. 2, 62–71. doi: 10.1038/s41551-017-0183-9
Adalbert, R., and Coleman, M. P. (2013). Axon pathology in age-related neurodegenerative disorders. Neuropathol. Appl. Neurobiol. 39, 90–108. doi: 10.1111/j.1365-2990.2012.01308.x
Ahmadzadeh, H., Smith, D. H., and Shenoy, V. B. (2014). Viscoelasticity of tau proteins leads to strain rate-dependent breaking of microtubules during axonal stretch injury: predictions from a mathematical model. Biophys. J. 106, 1123–1133. doi: 10.1016/j.bpj.2014.01.024
Ahmadzadeh, H., Smith, D. H., and Shenoy, V. B. (2015). Mechanical effects of dynamic binding between tau proteins on microtubules during axonal injury. Biophys. J. 109, 2328–2337. doi: 10.1016/j.bpj.2015.09.010
Alloisio, G., Rodriguez, D. B., Luce, M., Ciaccio, C., Marini, S., Cricenti, A., et al. (2023). Cyclic stretch-induced mechanical stress applied at 1 Hz frequency can Alter the metastatic potential properties of SAOS-2 osteosarcoma cells. Int. J. Mol. Sci. 24:7686. doi: 10.3390/ijms24097686
Alon, S., Goodwin, D. R., Sinha, A., Wassie, A. T., Chen, F., Daugharthy, E. R., et al. (2021). Expansion sequencing: spatially precise in situ transcriptomics in intact biological systems. Science 371:eaax2656. doi: 10.1126/science.aax2656
Alon, S., Huynh, G. H., and Boyden, E. S. (2019). Expansion microscopy: enabling single cell analysis in intact biological systems. FEBS J. 286, 1482–1494. doi: 10.1111/febs.14597
Antman-Passig, M., Levy, S., Gartenberg, C., Schori, H., and Shefi, O. (2017). Mechanically oriented 3D collagen hydrogel for directing neurite growth. Tissue Eng. Part A 23, 403–414. doi: 10.1089/ten.tea.2016.0185
Arola, D., Bajaj, D., Ivancik, J., Majd, H., and Zhang, D. (2010). Fatigue of biomaterials: hard tissues. Int. J. Fatigue 32, 1400–1412. doi: 10.1016/j.ijfatigue.2009.08.007
Aumeier, C., Schaedel, L., Gaillard, J., John, K., Blanchoin, L., and Théry, M. (2016). Self-repair promotes microtubule rescue. Nat. Cell Biol. 18, 1054–1064. doi: 10.1038/ncb3406
Baas, P. W., Rao, A. N., Matamoros, A. J., and Leo, L. (2016). Stability properties of neuronal microtubules. Cytoskeleton 73, 442–460. doi: 10.1002/cm.21286
Bao, G., and Suresh, S. (2003). Cell and molecular mechanics of biological materials. Nat. Mater. 2, 715–725. doi: 10.1038/nmat1001
Baranes, K., Chejanovsky, N., Alon, N., Sharoni, A., and Shefi, O. (2012). Topographic cues of nano-scale height direct neuronal growth pattern. Biotechnol. Bioeng. 109, 1791–1797. doi: 10.1002/bit.24444
Baranes, K., Hibsh, D., Cohen, S., Yamin, T., Efroni, S., Sharoni, A., et al. (2019). Comparing transcriptome profiles of neurons interfacing adjacent cells and Nanopatterned substrates reveals fundamental neuronal interactions. Nano Lett. 19, 1451–1459. doi: 10.1021/acs.nanolett.8b03879
Barnes, J. M., Przybyla, L., and Weaver, V. M. (2017). Tissue mechanics regulate brain development, homeostasis and disease. J. Cell Sci. 130, 71–82. doi: 10.1242/jcs.191742
Bates, P., Yeo, A., and Ramachandran, M. (2018). Bone injury, healing and grafting. In: M. Ramachandran Basic Orthopaedic Sciences. London: CRC Press, pp. 205–222.
Blackman, B. R., Barbee, K. A., and Thibault, L. E. (2000). In vitro cell shearing device to investigate the dynamic response of cells in a controlled hydrodynamic environment. Ann. Biomed. Eng. 28, 363–372. doi: 10.1114/1.286
Blumenthal, D., Goldstien, L., Edidin, M., and Gheber, L. A. (2015). Universal approach to FRAP analysis of arbitrary bleaching patterns. Sci. Rep. 5:11655. doi: 10.1038/srep11655
Boateng, S. Y., and Goldspink, P. H. (2008). Assembly and maintenance of the sarcomere night and day. Cardiovasc. Res. 77, 667–675. doi: 10.1093/cvr/cvm048
Boccafoschi, F., Bosetti, M., Sandra, P. M., Leigheb, M., and Cannas, M. (2010). Effects of mechanical stress on cell adhesion: a possible mechanism for morphological changes. Cell Adhes. Migr. 4, 19–25. doi: 10.4161/cam.4.1.9569
Born, M., and Wolf, E. (1986). Principles of optics. 6th Edn. Cambridge: Cambridge University Press, 199.
Brangwynne, C. P., MacKintosh, F. C., Kumar, S., Geisse, N. A., Talbot, J., Mahadevan, L., et al. (2006). Microtubules can bear enhanced compressive loads in living cells because of lateral reinforcement. J. Cell Biol. 173, 733–741. doi: 10.1083/jcb.200601060
Bray, D. (1984). Axonal growth in response to experimentally applied mechanical tension. Dev. Biol. 102, 379–389. doi: 10.1016/0012-1606(84)90202-1
Bray, D., and Bunge, M. B. (1981). Serial analysis of microtubules in cultured rat sensory axons. J. Neurocytol. 10, 589–605. doi: 10.1007/BF01262592
Butler, D. S. (1989). Adverse mechanical tension in the nervous system: a model for assessment and treatment. Aust. J. Physiother. 35, 227–238. doi: 10.1016/S0004-9514(14)60511-0
Byl, C., Puttlitz, C., Byl, N., Lotz, J., and Topp, K. (2002). Strain in the median and ulnar nerves during upper-extremity positioning. J. Hand Surg. Am. 27, 1032–1040. doi: 10.1053/jhsu.2002.35886
Calabrese, E. J., and Mattson, M. P. (2017). How does hormesis impact biology, toxicology, and medicine? NPJ Aging Mech. Dis. 3:13. doi: 10.1038/s41514-017-0013-z
Chada, S., Lamoureux, P., Buxbaum, R. E., and Heidemann, S. R. (1997). Cytomechanics of neurite outgrowth from chick brain neurons. J. Cell Sci. 110, 1179–1186. doi: 10.1242/jcs.110.10.1179
Chaudhuri, O., Parekh, S. H., and Fletcher, D. A. (2007). Reversible stress softening of actin networks. Nature 445, 295–298. doi: 10.1038/nature05459
Che, D. L., Chowdary, P. D., and Cui, B. (2016). A close look at axonal transport: cargos slow down when crossing stationary organelles. Neurosci. Lett. 610, 110–116. doi: 10.1016/j.neulet.2015.10.066
Chen, F., Tillberg, P. W., and Boyden, E. S. (2015). Expansion microscopy. Science 347, 543–548. doi: 10.1126/science.1260088
Chen, T., Zhu, J., Wang, Y.-H., and Hang, C.-H. (2019). ROS-mediated mitochondrial dysfunction and ER stress contribute to compression-induced neuronal injury. Neuroscience 416, 268–280. doi: 10.1016/j.neuroscience.2019.08.007
Chighizola, M., Dini, T., Lenardi, C., Milani, P., Podestà, A., and Schulte, C. (2019). Mechanotransduction in neuronal cell development and functioning. Biophys. Rev. 11, 701–720. doi: 10.1007/s12551-019-00587-2
Coleman, A. K., Joca, H. C., Shi, G., Lederer, W. J., and Ward, C. W. (2021). Tubulin acetylation increases cytoskeletal stiffness to regulate mechanotransduction in striated muscle. J. Gen. Physiol. 153:e202012743. doi: 10.1085/jgp.202012743
Das, R., Lin, L.-C., Català-Castro, F., Malaiwong, N., Sanfeliu-Cerdán, N., Porta-de-la-Riva, M., et al. (2021). An asymmetric mechanical code ciphers curvature-dependent proprioceptor activity. Sci. Adv. 7:eabg4617. doi: 10.1126/sciadv.abg4617
Das, R., Wieser, S., and Krieg, M. (2019). Neuronal stretch reception–making sense of the mechanosense. Exp. Cell Res. 378, 104–112. doi: 10.1016/j.yexcr.2019.01.028
De Vincentiis, S., Falconieri, A., Mainardi, M., Cappello, V., Scribano, V., Bizzarri, R., et al. (2020a). Extremely low forces induce extreme axon growth. J. Neurosci. 40, 4997–5007. doi: 10.1523/JNEUROSCI.3075-19.2020
De Vincentiis, S., Falconieri, A., Scribano, V., Ghignoli, S., and Raffa, V. (2020b). Manipulation of axonal outgrowth via exogenous low forces. Int. J. Mol. Sci. 21:8009. doi: 10.3390/ijms21218009
Dennerll, T. J., Lamoureux, P., Buxbaum, R. E., and Heidemann, S. R. (1989). The cytomechanics of axonal elongation and retraction. J. Cell Biol. 109, 3073–3083. doi: 10.1083/jcb.109.6.3073
Dogterom, M., and Koenderink, G. H. (2019). Actin–microtubule crosstalk in cell biology. Nat. Rev. Mol. Cell Biol. 20, 38–54. doi: 10.1038/s41580-018-0067-1
Duan, L., Che, D., Zhang, K., Ong, Q., Guo, S., and Cui, B. (2015). Optogenetic control of molecular motors and organelle distributions in cells. Chem. Biol. 22, 671–682. doi: 10.1016/j.chembiol.2015.04.014
Dubey, S., Bhembre, N., Bodas, S., Veer, S., Ghose, A., Callan-Jones, A., et al. (2020). The axonal actin-spectrin lattice acts as a tension buffering shock absorber. eLife 9:e51772. doi: 10.7554/eLife.51772
Dumont, E. L. P., Do, C., and Hess, H. (2015). Molecular wear of microtubules propelled by surface-adhered kinesins. Nat. Nanotechnol. 10, 166–169. doi: 10.1038/nnano.2014.334
Duveau, F., Cordier, C., Chiron, L., Le Bec, M., Pouzet, S., Séguin, J., et al. (2024). Yeast cell responses and survival during periodic osmotic stress are controlled by glucose availability. eLife 12:RP88750. doi: 10.7554/eLife.88750
Edson, K. J., Lim, S., Borisy, G. G., and Letourneau, P. C. (1993). FRAP analysis of the stability of the microtubule population along the neurites of chick sensory neurons. Cell Motil. Cytoskeleton 25, 59–72. doi: 10.1002/cm.970250108
Edwards, W. B. (2018). Modeling overuse injuries in sport as a mechanical fatigue phenomenon. Exerc. Sport Sci. Rev. 46, 224–231. doi: 10.1249/JES.0000000000000163
Edwards, M. E., Wang, S. S., and Good, T. A. (2001). Role of viscoelastic properties of differentiated SH-SY5Y human neuroblastoma cells in cyclic shear stress injury. Biotechnol. Prog. 17, 760–767. doi: 10.1021/bp010040m
Einhorn, T. A. (1998). The cell and molecular biology of fracture healing. Clin. Orthop. Relat. Res. 355S, S7–S21. doi: 10.1097/00003086-199810001-00003
Falconieri, A., Coppini, A., and Raffa, V. (2023a). Microtubules as a signal hub for axon growth in response to mechanical force. Biol. Chem. 405, 67–77. doi: 10.1515/hsz-2023-0173
Falconieri, A., De Vincentiis, S., Cappello, V., Convertino, D., Das, R., Ghignoli, S., et al. (2023b). Axonal plasticity in response to active forces generated through magnetic nano-pulling. Cell Rep. 42:111912. doi: 10.1016/j.celrep.2022.111912
Falconieri, A., Taparia, N., De Vincentiis, S., Cappello, V., Sniadecki, N. J., and Raffa, V. (2022). Magnetically-actuated microposts stimulate axon growth. Biophys. J. 121, 374–382. doi: 10.1016/j.bpj.2021.12.041
Farkas, O., Lifshitz, J., and Povlishock, J. T. (2006). Mechanoporation induced by diffuse traumatic brain injury: an irreversible or reversible response to injury? J. Neurosci. 26, 3130–3140. doi: 10.1523/JNEUROSCI.5119-05.2006
Faust, U., Hampe, N., Rubner, W., Kirchgessner, N., Safran, S., Hoffmann, B., et al. (2011). Cyclic stress at mHz frequencies aligns fibroblasts in direction of zero strain. PLoS One 6:e28963. doi: 10.1371/journal.pone.0028963
Fischer, K., and Schopfer, P. (1997). Interaction of auxin, light, and mechanical stress in orienting microtubules in relation to tropic curvature in the epidermis of maize coleoptiles. Protoplasma 196, 108–116. doi: 10.1007/BF01281064
Fleck, C., and Eifler, D. (2010). Corrosion, fatigue and corrosion fatigue behaviour of metal implant materials, especially titanium alloys. Int. J. Fatigue 32, 929–935. doi: 10.1016/j.ijfatigue.2009.09.009
Fletcher, D. A., and Mullins, R. D. (2010). Cell mechanics and the cytoskeleton. Nature 463, 485–492. doi: 10.1038/nature08908
Florencio-Silva, R., Da Sasso, G. R., Sasso-Cerri, E., Simões, M. J., and Cerri, P. S. (2015). Biology of bone tissue: structure, function, and factors that influence bone cells. Biomed. Res. Int. 2015, 1–17. doi: 10.1155/2015/421746
Fournier, A. J., Hogan, J. D., Rajbhandari, L., Shrestha, S., Venkatesan, A., and Ramesh, K. T. (2015). Changes in neurofilament and microtubule distribution following focal axon compression. PLoS One 10:e0131617. doi: 10.1371/journal.pone.0131617
Franck, A. D., Powers, A. F., Gestaut, D. R., Gonen, T., Davis, T. N., and Asbury, C. L. (2007). Tension applied through the Dam1 complex promotes microtubule elongation providing a direct mechanism for length control in mitosis. Nat. Cell Biol. 9, 832–837. doi: 10.1038/ncb1609
Franze, K. (2020). Integrating chemistry and mechanics: the forces driving axon growth. Annu. Rev. Cell Dev. Biol. 36, 61–83. doi: 10.1146/annurev-cellbio-100818-125157
Franze, K., Gerdelmann, J., Weick, M., Betz, T., Pawlizak, S., Lakadamyali, M., et al. (2009). Neurite branch retraction is caused by a threshold-dependent mechanical impact. Biophys. J. 97, 1883–1890. doi: 10.1016/j.bpj.2009.07.033
French, A. S. (1992). Mechanotransduction. Annu. Rev. Physiol. 54, 135–152. doi: 10.1146/annurev.ph.54.030192.001031
Fruleux, A., Verger, S., and Boudaoud, A. (2019). Feeling stressed or strained? A biophysical model for cell wall mechanosensing in plants. Front. Plant Sci. 10:757. doi: 10.3389/fpls.2019.00757
Gambarotto, D., Hamel, V., and Guichard, P. (2021, 2021). Ultrastructure expansion microscopy (U-ExM). Methods Cell Biol. 161, 57–81. doi: 10.1016/bs.mcb.2020.05.006
Ganser, C., and Uchihashi, T. (2019). Microtubule self-healing and defect creation investigated by in-line force measurements during high-speed atomic force microscopy imaging. Nanoscale 11, 125–135. doi: 10.1039/C8NR07392A
Gong, J., Chen, J., Gu, P., Shang, Y., Ruppell, K. T., Yang, Y., et al. (2022). Shear stress activates nociceptors to drive Drosophila mechanical nociception. Neuron 110, 3727–3742.e8. doi: 10.1016/j.neuron.2022.08.015
Goulding, M. (2012). Motor neurons that multitask. Neuron 76, 669–670. doi: 10.1016/j.neuron.2012.11.011
Greiner, A. M., Chen, H., Spatz, J. P., and Kemkemer, R. (2013). Cyclic tensile strain controls cell shape and directs actin stress fiber formation and focal adhesion alignment in spreading cells. PLoS One 8:e77328. doi: 10.1371/journal.pone.0077328
Grumet, M., and Sakurai, T. (1996). Heterophilic interactions of the neural cell adhesion molecules ng-CAM and Nr-CAM with neural receptors and extracellular matrix proteins. Semin. Neurosci. 8, 379–389. doi: 10.1006/smns.1996.0046
Gudimchuk, N. B., Ulyanov, E. V., O’Toole, E., Page, C. L., Vinogradov, D. S., Morgan, G., et al. (2020). Mechanisms of microtubule dynamics and force generation examined with computational modeling and electron cryotomography. Nat. Commun. 11:3765. doi: 10.1038/s41467-020-17553-2
Gunawardena, S. D. S., Kamal, A., and Goldstein, L. S. B. (2000). Axonal and vesicular transport in the pathology of Alzheimer’s disease (AD): genetic evidence for a motor-receptor function. Mol. Biol. Cell 11:553A.
Gustafsson, M. G. L. (2000). Surpassing the lateral resolution limit by a factor of two using structured illumination microscopy. J. Microsc. 198, 82–87. doi: 10.1046/j.1365-2818.2000.00710.x
Hahn, I., Voelzmann, A., Liew, Y.-T., Costa-Gomes, B., and Prokop, A. (2019). The model of local axon homeostasis-explaining the role and regulation of microtubule bundles in axon maintenance and pathology. Neural Dev. 14:11. doi: 10.1186/s13064-019-0134-0
Hamant, O., and Haswell, E. S. (2017). Life behind the wall: sensing mechanical cues in plants. BMC Biol. 15, 1–9. doi: 10.1186/s12915-017-0403-5
Hamant, O., Heisler, M. G., Jonsson, H., Krupinski, P., Uyttewaal, M., Bokov, P., et al. (2008). Developmental patterning by mechanical signals in Arabidopsis. Science 322, 1650–1655. doi: 10.1126/science.1165594
Hamant, O., Inoue, D., Bouchez, D., Dumais, J., and Mjolsness, E. (2019). Are microtubules tension sensors? Nat. Commun. 10:2360. doi: 10.1038/s41467-019-10207-y
Hejnowicz, Z., Rusin, A., and Rusin, T. (2000). Tensile tissue stress affects the orientation of cortical microtubules in the epidermis of sunflower hypocotyl. J. Plant Growth Regul. 19, 31–44. doi: 10.1007/s003440000005
Hess, H., and Dumont, E. L. P. (2011). Fatigue failure and molecular machine design. Small 7, 1619–1623. doi: 10.1002/smll.201100240
Hess, H., and Ross, J. L. (2017). Non-equilibrium assembly of microtubules: from molecules to autonomous chemical robots. Chem. Soc. Rev. 46, 5570–5587. doi: 10.1039/C7CS00030H
Hosmane, S., Fournier, A., Wright, R., Rajbhandari, L., Siddique, R., Yang, I. H., et al. (2011). Valve-based microfluidic compression platform: single axon injury and regrowth. Lab Chip 11, 3888–3895. doi: 10.1039/c1lc20549h
Hu, M., Hong, L., Hong, S., Min, J., Zhao, Y., Yang, Q., et al. (2017). Mechanical stress influences the viability and morphology of human parametrial ligament fibroblasts. Mol. Med. Rep. 15, 853–858. doi: 10.3892/mmr.2016.6052
Hukins, D. W. L., Mahomed, A., and Kukureka, S. N. (2008). Accelerated aging for testing polymeric biomaterials and medical devices. Med. Eng. Phys. 30, 1270–1274. doi: 10.1016/j.medengphy.2008.06.001
Ikushima, T., and Shimmen, T. (2005). Mechano-sensitive orientation of cortical microtubules during gravitropism in azuki bean epicotyls. J. Plant Res. 118, 19–26. doi: 10.1007/s10265-004-0189-8
Ingber, D. E. (1993). Cellular tensegrity: defining new rules of biological design that govern the cytoskeleton. J. Cell Sci. 104, 613–627. doi: 10.1242/jcs.104.3.613
Ingber, D. E. (1997). Tensegrity: the architectural basis of cellular mechanotransduction. Annu. Rev. Physiol. 59, 575–599. doi: 10.1146/annurev.physiol.59.1.575
Ingber, D. E., Madri, J. A., and Jamieson, J. D. (1981). Role of basal lamina in neoplastic disorganization of tissue architecture. Proc. Natl. Acad. Sci. 78, 3901–3905. doi: 10.1073/pnas.78.6.3901
Ingber, D. E., Wang, N., and Stamenović, D. (2014). Tensegrity, cellular biophysics, and the mechanics of living systems. Rep. Prog. Phys. 77:046603. doi: 10.1088/0034-4885/77/4/046603
Inkson, B. J. (2016). Scanning electron microscopy (SEM) and transmission electron microscopy (TEM) for materials characterization. In: G. Huebschen, I. Altpeter, R. Tschuncky, and H. G. Herrmann Materials characterization using nondestructive evaluation (NDE) methods. Amsterdam, Netherlands: Elsevier, pp. 17–43.
Ishikawa-Ankerhold, H. C., Ankerhold, R., and Drummen, G. P. C. (2012). Advanced fluorescence microscopy techniques—Frap, Flip, flap, fret and flim. Molecules 17, 4047–4132. doi: 10.3390/molecules17044047
Jafari, S. S., Maxwell, W. L., Neilson, M., and Graham, D. I. (1997). Axonal cytoskeletal changes after non-disruptive axonal injury. J. Neurocytol. 26, 201–221. doi: 10.1023/A:1018588114648
Jakobs, M. A., and Franze, K. (2020). Microtubule polarity in axons is sorted by a molecular gradient of dynactin. Biophys. J. 118:598a. doi: 10.1016/j.bpj.2019.11.3233
Janke, C., and Montagnac, G. (2017). Causes and consequences of microtubule acetylation. Curr. Biol. 27, R1287–R1292. doi: 10.1016/j.cub.2017.10.044
Jansen, K. I., Burute, M., and Kapitein, L. C. (2021, 2023). A live-cell marker to visualize the dynamics of stable microtubules. J Cell Biol 222:e202106105. doi: 10.1083/jcb.202106105
Javier-Torrent, M., Zimmer-Bensch, G., and Nguyen, L. (2021). Mechanical forces orchestrate brain development. Trends Neurosci. 44, 110–121. doi: 10.1016/j.tins.2020.10.012
Ju, M.-S., Lin, C.-C. K., and Chang, C.-T. (2017). Researches on biomechanical properties and models of peripheral nerves-a review. J. Biomech. Sci. Eng. 12, 16–678. doi: 10.1299/jbse.16-00678
Jungbauer, S., Gao, H., Spatz, J. P., and Kemkemer, R. (2008). Two characteristic regimes in frequency-dependent dynamic reorientation of fibroblasts on cyclically stretched substrates. Biophys. J. 95, 3470–3478. doi: 10.1529/biophysj.107.128611
Kabir, A. M. R., Inoue, D., Afrin, T., Mayama, H., Sada, K., and Kakugo, A. (2015). Buckling of microtubules on a 2D elastic medium. Sci. Rep. 5:17222. doi: 10.1038/srep17222
Kabir, A. M. R., Inoue, D., Kakugo, A., Sada, K., and Gong, J. P. (2012). Active self-organization of microtubules in an inert chamber system. Polym. J. 44, 607–611. doi: 10.1038/pj.2012.26
Kabir, A. M. R., Sada, K., and Kakugo, A. (2020). Breaking of buckled microtubules is mediated by kinesins. Biochem. Biophys. Res. Commun. 524, 249–254. doi: 10.1016/j.bbrc.2020.01.082
Kapitein, L. C., and Hoogenraad, C. C. (2015). Building the neuronal microtubule cytoskeleton. Neuron 87, 492–506. doi: 10.1016/j.neuron.2015.05.046
Kaplan, L., Ierokomos, A., Chowdary, P., Bryant, Z., and Cui, B. (2018). Rotation of endosomes demonstrates coordination of molecular motors during axonal transport. Sci. Adv. 4:e1602170. doi: 10.1126/sciadv.1602170
Karafyllidis, I. G., and Lagoudas, D. C. (2007). Microtubules as mechanical force sensors. Biosystems 88, 137–146. doi: 10.1016/j.biosystems.2006.05.003
Kelliher, M. T., Saunders, H. A. J., and Wildonger, J. (2019). Microtubule control of functional architecture in neurons. Curr. Opin. Neurobiol. 57, 39–45. doi: 10.1016/j.conb.2019.01.003
Kerr, J. P., Robison, P., Shi, G., Bogush, A. I., Kempema, A. M., Hexum, J. K., et al. (2015). Detyrosinated microtubules modulate mechanotransduction in heart and skeletal muscle. Nat. Commun. 6:8526. doi: 10.1038/ncomms9526
Keya, J. J., Inoue, D., Suzuki, Y., Kozai, T., Ishikuro, D., Kodera, N., et al. (2017). High-resolution imaging of a single gliding protofilament of tubulins by HS-AFM. Sci. Rep. 7:6166. doi: 10.1038/s41598-017-06249-1
Khmelinskii, I., and Makarov, V. I. (2020). On the effects of mechanical stress of biological membranes in modeling of swelling dynamics of biological systems. Sci. Rep. 10:8395. doi: 10.1038/s41598-020-65217-4
Kilinc, D., Gallo, G., and Barbee, K. A. (2008). Mechanically-induced membrane poration causes axonal beading and localized cytoskeletal damage. Exp. Neurol. 212, 422–430. doi: 10.1016/j.expneurol.2008.04.025
Kilinc, D., Gallo, G., and Barbee, K. A. (2009). Mechanical membrane injury induces axonal beading through localized activation of calpain. Exp. Neurol. 219, 553–561. doi: 10.1016/j.expneurol.2009.07.014
Kim, D.-H., Chambliss, A. B., and Wirtz, D. (2013). The multi-faceted role of the actin cap in cellular mechanosensation and mechanotransduction. Soft Matter 9, 5516–5523. doi: 10.1039/c3sm50798j
Kim, E., Lee, J., Noh, S., Kwon, O., and Mun, J. Y. (2020). Double staining method for array tomography using scanning electron microscopy. Appl Microsc 50:14. doi: 10.1186/s42649-020-00033-8
Krieg, M., Stühmer, J., Cueva, J. G., Fetter, R., Spilker, K., Cremers, D., et al. (2017). Genetic defects in β-spectrin and tau sensitize C. elegans axons to movement-induced damage via torque-tension coupling. eLife 6:e20172. doi: 10.7554/eLife.20172
Kurazumi, H., Kubo, M., Ohshima, M., Yamamoto, Y., Takemoto, Y., Suzuki, R., et al. (2011). The effects of mechanical stress on the growth, differentiation, and paracrine factor production of cardiac stem cells. PLoS One 6:e28890. doi: 10.1371/journal.pone.0028890
Lamoureux, P., Altun-Gultekin, Z. F., Lin, C., Wagner, J. A., and Heidemann, S. R. (1997). Rac is required for growth cone function but not neurite assembly. J. Cell Sci. 110, 635–641. doi: 10.1242/jcs.110.5.635
Le, H. Q., Ghatak, S., Yeung, C.-Y. C., Tellkamp, F., Günschmann, C., Dieterich, C., et al. (2016). Mechanical regulation of transcription controls Polycomb-mediated gene silencing during lineage commitment. Nat. Cell Biol. 18, 864–875. doi: 10.1038/ncb3387
Lecuit, T., and Lenne, P.-F. (2007). Cell surface mechanics and the control of cell shape, tissue patterns and morphogenesis. Nat. Rev. Mol. Cell Biol. 8, 633–644. doi: 10.1038/nrm2222
Lee, A. C., and Suter, D. M. (2008). Quantitative analysis of microtubule dynamics during adhesion-mediated growth cone guidance. Dev. Neurobiol. 68, 1363–1377. doi: 10.1002/dneu.20662
Lelek, M., Gyparaki, M. T., Beliu, G., Schueder, F., Griffié, J., Manley, S., et al. (2021). Single-molecule localization microscopy. Nat. Rev. Methods Prim. 1:39. doi: 10.1038/s43586-021-00038-x
Leshchyns'ka, I., and Sytnyk, V. (2016). Reciprocal interactions between cell adhesion molecules of the immunoglobulin superfamily and the cytoskeleton in neurons. Front. Cell Dev. Biol. 4:9. doi: 10.3389/fcell.2016.00009
Leterrier, C., Dubey, P., and Roy, S. (2017). The nano-architecture of the axonal cytoskeleton. Nat. Rev. Neurosci. 18, 713–726. doi: 10.1038/nrn.2017.129
Li, W., Feng, Z., Sternberg, P. W., and Shawn Xu, X. Z. (2006). A C. elegans stretch receptor neuron revealed by a mechanosensitive TRP channel homologue. Nature 440, 684–687. doi: 10.1038/nature04538
Li, Y., Kučera, O., Cuvelier, D., Rutkowski, D. M., Deygas, M., Rai, D., et al. (2023). Compressive forces stabilize microtubules in living cells. Nat. Mater. 22, 913–924. doi: 10.1038/s41563-023-01578-1
Li, Q., Kumar, A., Makhija, E., and Shivashankar, G. V. (2014). The regulation of dynamic mechanical coupling between actin cytoskeleton and nucleus by matrix geometry. Biomaterials 35, 961–969. doi: 10.1016/j.biomaterials.2013.10.037
Lin, J., Li, X., Yin, J., and Qian, J. (2020). Effect of cyclic stretch on neuron reorientation and axon outgrowth. Front. Bioeng. Biotechnol. 8:597867. doi: 10.3389/fbioe.2020.597867
Liu, P., Chen, B., and Wang, Z.-W. (2020). GABAergic motor neurons bias locomotor decision-making in C. elegans. Nat. Commun. 11:5076. doi: 10.1038/s41467-020-18893-9
Liu, B., Qu, M.-J., Qin, K.-R., Li, H., Li, Z.-K., Shen, B.-R., et al. (2008). Role of cyclic strain frequency in regulating the alignment of vascular smooth muscle cells in vitro. Biophys. J. 94, 1497–1507. doi: 10.1529/biophysj.106.098574
Louveaux, M., Rochette, S., Beauzamy, L., Boudaoud, A., and Hamant, O. (2016). The impact of mechanical compression on cortical microtubules in Arabidopsis: a quantitative pipeline. Plant J. 88, 328–342. doi: 10.1111/tpj.13290
Lunn, K. F., Baas, P. W., and Duncan, I. D. (1997). Microtubule organization and stability in the oligodendrocyte. J. Neurosci. 17, 4921–4932. doi: 10.1523/JNEUROSCI.17-13-04921.1997
Maday, S., Twelvetrees, A. E., Moughamian, A. J., and Holzbaur, E. L. F. (2014). Axonal transport: cargo-specific mechanisms of motility and regulation. Neuron 84, 292–309. doi: 10.1016/j.neuron.2014.10.019
Manuchehrfar, F., and Shamloo, A. (2018). Mechanical behavior of axonal microtubules; the effect of fluid on the rupture of axonal microtubules. BioRxiv 2018:378455. doi: 10.1101/378455
Matthews, B. D., Overby, D. R., Mannix, R., and Ingber, D. E. (2006). Cellular adaptation to mechanical stress: role of integrins, rho, cytoskeletal tension and mechanosensitive ion channels. J. Cell Sci. 119, 508–518. doi: 10.1242/jcs.02760
McCain, M. L., and Parker, K. K. (2011). Mechanotransduction: the role of mechanical stress, myocyte shape, and cytoskeletal architecture on cardiac function. Pflügers Arch. J. Physiol. 462, 89–104. doi: 10.1007/s00424-011-0951-4
Mikhaylova, M., Cloin, B. M. C., Finan, K., Van Den Berg, R., Teeuw, J., Kijanka, M. M., et al. (2015). Resolving bundled microtubules using anti-tubulin nanobodies. Nat. Commun. 6:7933. doi: 10.1038/ncomms8933
Miller, K. E., and Suter, D. M. (2018). An integrated cytoskeletal model of neurite outgrowth. Front. Cell. Neurosci. 12:447. doi: 10.3389/fncel.2018.00447
Nakadate, H., Fukumura, Y., Kaneko, Y., Kakuta, A., Furukawa, H., and Aomura, S. (2014). In vitro uniaxial stretch model for evaluating the effect of strain along axon on damage to neurons. J. Biomech. Sci. Eng. 9, 14–136. doi: 10.1299/jbse.14-00136
Nakadate, H., Kurtoglu, E., Furukawa, H., Oikawa, S., Aomura, S., Kakuta, A., et al. (2017). Strain-rate dependency of axonal tolerance for uniaxial stretching. United States: SAE Technical Paper.
Namba, T., Nakamuta, S., Funahashi, Y., and Kaibuchi, K. (2011). The role of selective transport in neuronal polarization. Dev. Neurobiol. 71, 445–457. doi: 10.1002/dneu.20876
Nasrin, S. R., Kazeruni, N. M. B., Stanislav Tsitkov, J. B. R., Kakugo, A., and Hess, H. (2023). Mechanical fatigue in microtubules.
Neumann, B., and Hilliard, M. A. (2014). Loss of MEC-17 leads to microtubule instability and axonal degeneration. Cell Rep. 6, 93–103. doi: 10.1016/j.celrep.2013.12.004
Nick, P. (2008). Microtubules as sensors for abiotic stimuli. In: P. Nick Plant Microtubules: Development and Flexibility. Berlin: Springer Science & Business Media, pp. 175–203.
Onal, S., Alkaisi, M. M., and Nock, V. (2023). Application of sequential cyclic compression on cancer cells in a flexible microdevice. PLoS One 18:e0279896. doi: 10.1371/journal.pone.0279896
Padmanabhan, P., and Goodhill, G. J. (2018). Axon growth regulation by a bistable molecular switch. Proc. R. Soc. B Biol. Sci. 285:20172618. doi: 10.1098/rspb.2017.2618
Papandréou, M.-J., and Leterrier, C. (2018). The functional architecture of axonal actin. Mol. Cell. Neurosci. 91, 151–159. doi: 10.1016/j.mcn.2018.05.003
Pennisi, C. P., Olesen, C. G., de Zee, M., Rasmussen, J., and Zachar, V. (2011). Uniaxial cyclic strain drives assembly and differentiation of skeletal myocytes. Tissue Eng. Part A 17, 2543–2550. doi: 10.1089/ten.tea.2011.0089
Pfister, B. J., Iwata, A., Meaney, D. F., and Smith, D. H. (2004). Extreme stretch growth of integrated axons. J. Neurosci. 24, 7978–7983. doi: 10.1523/JNEUROSCI.1974-04.2004
Phillips, J. B., Smit, X., De Zoysa, N., Afoke, A., and Brown, R. A. (2004). Peripheral nerves in the rat exhibit localized heterogeneity of tensile properties during limb movement. J. Physiol. 557, 879–887. doi: 10.1113/jphysiol.2004.061804
Portran, D., Schaedel, L., Xu, Z., Théry, M., and Nachury, M. V. (2017). Tubulin acetylation protects long-lived microtubules against mechanical ageing. Nat. Cell Biol. 19, 391–398. doi: 10.1038/ncb3481
Putnam, A. J., Schultz, K., and Mooney, D. J. (2001). Control of microtubule assembly by extracellular matrix and externally applied strain. Am. J. Physiol. Physiol. 280, C556–C564. doi: 10.1152/ajpcell.2001.280.3.C556
Putra, V. D. L., Kilian, K. A., and Knothe Tate, M. L. (2023). Biomechanical, biophysical and biochemical modulators of cytoskeletal remodelling and emergent stem cell lineage commitment. Commun. Biol. 6:75. doi: 10.1038/s42003-022-04320-w
Qiang, Y., Liu, J., Dao, M., Suresh, S., and Du, E. (2019). Mechanical fatigue of human red blood cells. Proc. Natl. Acad. Sci. 116, 19828–19834. doi: 10.1073/pnas.1910336116
Quan, X., Guo, K., Wang, Y., Huang, L., Chen, B., Ye, Z., et al. (2014). Mechanical compression insults induce nanoscale changes of membrane-skeleton arrangement which could cause apoptosis and necrosis in dorsal root ganglion neurons. Biosci. Biotechnol. Biochem. 78, 1631–1639. doi: 10.1080/09168451.2014.932664
Raffa, V. (2023). Force: a messenger of axon outgrowth. Semin. Cell Dev. Biol. 140, 3–12. doi: 10.1016/j.semcdb.2022.07.004
Raffa, V., Falcone, F., De Vincentiis, S., Falconieri, A., Calatayud, M. P., Goya, G. F., et al. (2018). Piconewton mechanical forces promote neurite growth. Biophys. J. 115, 2026–2033. doi: 10.1016/j.bpj.2018.10.009
Riggio, C., Calatayud, M. P., Giannaccini, M., Sanz, B., Torres, T. E., Fernández-Pacheco, R., et al. (2014). The orientation of the neuronal growth process can be directed via magnetic nanoparticles under an applied magnetic field. Nanomedicine nanotechnology. Biol. Med. 10, 1549–1558. doi: 10.1016/j.nano.2013.12.008
Robert, C., Bouchiba, M., Robert, R., Margolis, R. L., and Job, D. (1990). Self organization of the microtubule network. A diffusion based model. Biol. Cell 68, 177–181. doi: 10.1016/0248-4900(90)90306-N
Robison, P., Caporizzo, M. A., Ahmadzadeh, H., Bogush, A. I., Chen, C. Y. X., Margulies, K. B., et al. (2016). Detyrosinated microtubules buckle and bear load in contracting cardiomyocytes. Science 352:428. doi: 10.1126/science.aaf0659
Rust, M. J., Bates, M., and Zhuang, X. (2006). Stochastic optical reconstruction microscopy (STORM) provides sub-diffraction-limit image resolution. Nat. Methods 3, 793–796. doi: 10.1038/nmeth929
Sadhanasatish, T., Augustin, K., Windgasse, L., Chrostek-Grashoff, A., Rief, M., and Grashoff, C. (2023). A molecular optomechanics approach reveals functional relevance of force transduction across Talin and desmoplakin. Sci. Adv. 9:eadg3347. doi: 10.1126/sciadv.adg3347
Saez, A., Buguin, A., Silberzan, P., and Ladoux, B. (2005). Is the mechanical activity of epithelial cells controlled by deformations or forces? Biophys. J. 89, L52–L54. doi: 10.1529/biophysj.105.071217
Sakakibara, A., Ando, R., Sapir, T., and Tanaka, T. (2013). Microtubule dynamics in neuronal morphogenesis. Open Biol. 3:130061. doi: 10.1098/rsob.130061
Sakamoto, N., Ogawa, M., Sadamoto, K., Takeuchi, M., and Kataoka, N. (2017). Mechanical role of Nesprin-1-mediated nucleus–actin filament binding in cyclic stretch-induced fibroblast elongation. Cell. Mol. Bioeng. 10, 327–338. doi: 10.1007/s12195-017-0487-6
Salvadores, N., Sanhueza, M., Manque, P., and Court, F. A. (2017). Axonal degeneration during aging and its functional role in neurodegenerative disorders. Front. Neurosci. 11:451. doi: 10.3389/fnins.2017.00451
Saper, G., and Hess, H. (2019). Synthetic systems powered by biological molecular motors. Chem. Rev. 120, 288–309. doi: 10.1021/acs.chemrev.9b00249
Sarkis, G. A., Mangaonkar, M. D., Moghieb, A., Lelling, B., Guertin, M., Yadikar, H., et al. (2017). The application of proteomics to traumatic brain and spinal cord injuries. Curr. Neurol. Neurosci. Rep. 17, 1–11. doi: 10.1007/s11910-017-0736-z
Schaedel, L., John, K., Gaillard, J., Nachury, M. V., Blanchoin, L., and Thery, M. (2015). Microtubules self-repair in response to mechanical stress. Nat. Mater. 14, 1156–1163. doi: 10.1038/nmat4396
Schaedel, L., Triclin, S., Chrétien, D., Abrieu, A., Aumeier, C., Gaillard, J., et al. (2019). Lattice defects induce microtubule self-renewal. Nat. Phys. 15, 830–838. doi: 10.1038/s41567-019-0542-4
Schafer, W. R. (2015). Mechanosensory molecules and circuits in C. elegans. Pflügers Arch. J. Physiol. 467, 39–48. doi: 10.1007/s00424-014-1574-3
Seetharaman, S., Vianay, B., Roca, V., Farrugia, A. J., De Pascalis, C., Boëda, B., et al. (2022). Microtubules tune mechanosensitive cell responses. Nat. Mater. 21, 366–377. doi: 10.1038/s41563-021-01108-x
Shefi, O., Harel, A., Chklovskii, D. B., Ben-Jacob, E., and Ayali, A. (2004). Biophysical constraints on neuronal branching. Neurocomputing 58-60, 487–495. doi: 10.1016/j.neucom.2004.01.085
Shibuya, S., Yamamoto, T., and Itano, T. (2009). Glial and axonal regeneration following spinal cord injury. Cell Adhes. Migr. 3, 99–106. doi: 10.4161/cam.3.1.7372
Shroff, H., White, H., and Betzig, E. (2013). Photoactivated localization microscopy (PALM) of adhesion complexes. Curr. Protoc. Cell Biol. 58, 4–21. doi: 10.1002/0471143030.cb0421s58
Smith, D. H., Wolf, J. A., Lusardi, T. A., Lee, V. M.-Y., and Meaney, D. F. (1999). High tolerance and delayed elastic response of cultured axons to dynamic stretch injury. J. Neurosci. 19, 4263–4269. doi: 10.1523/JNEUROSCI.19-11-04263.1999
Smith, D. H., Wolf, J. A., and Meaney, D. F. (2001). A new strategy to produce sustained growth of central nervous system axons: continuous mechanical tension. Tissue Eng. 7, 131–139. doi: 10.1089/107632701300062714
Spangenberg, L. (1875). Über das verhalten der metalle bei wiederholten anstrengungen, etc. United States: Ernst and Korn.
Stamenović, D., and Wang, N. (2011). Stress transmission within the cell. Compr. Physiol. 1:499. doi: 10.1002/cphy.c100019
Subach, F. V., Patterson, G. H., Renz, M., Lippincott-Schwartz, J., and Verkhusha, V. V. (2010). Bright monomeric photoactivatable red fluorescent protein for two-color super-resolution sptPALM of live cells. J. Am. Chem. Soc. 132, 6481–6491. doi: 10.1021/ja100906g
Swiatlowska, P., Sanchez-Alonso, J. L., Wright, P. T., Novak, P., and Gorelik, J. (2020). Microtubules regulate cardiomyocyte transversal Young’s modulus. Proc. Natl. Acad. Sci. 117, 2764–2766. doi: 10.1073/pnas.1917171117
Tang-Schomer, M. D., Johnson, V. E., Baas, P. W., Stewart, W., and Smith, D. H. (2012). Partial interruption of axonal transport due to microtubule breakage accounts for the formation of periodic varicosities after traumatic axonal injury. Exp. Neurol. 233, 364–372. doi: 10.1016/j.expneurol.2011.10.030
Tang-Schomer, M. D., Patel, A. R., Baas, P. W., and Smith, D. H. (2010). Mechanical breaking of microtubules in axons during dynamic stretch injury underlies delayed elasticity, microtubule disassembly, and axon degeneration. FASEB J. 24, 1401–1410. doi: 10.1096/fj.09-142844
Tao, L., Porto, D., Li, Z., Fechner, S., Lee, S. A., Goodman, M. B., et al. (2019). Parallel processing of two mechanosensory modalities by a single neuron in C. elegans. Dev. Cell 51, 617–631.e3. doi: 10.1016/j.devcel.2019.10.008
Tas, R. P., Chazeau, A., Cloin, B. M. C., Lambers, M. L. A., Hoogenraad, C. C., and Kapitein, L. C. (2017). Differentiation between oppositely oriented microtubules controls polarized neuronal transport. Neuron 96, 1264–1271.e5. doi: 10.1016/j.neuron.2017.11.018
Taylor, D. (1998). Fatigue of bone and bones: an analysis based on stressed volume. J. Orthop. Res. 16, 163–169. doi: 10.1002/jor.1100160203
Théry, M., and Blanchoin, L. (2021). Microtubule self-repair. Curr. Opin. Cell Biol. 68, 144–154. doi: 10.1016/j.ceb.2020.10.012
Thompson, A. J., Pillai, E. K., Dimov, I. B., Foster, S. K., Holt, C. E., and Franze, K. (2019). Rapid changes in tissue mechanics regulate cell behaviour in the developing embryonic brain. eLife 8:e39356. doi: 10.7554/eLife.39356
Tinevez, J.-Y., Perry, N., Schindelin, J., Hoopes, G. M., Reynolds, G. D., Laplantine, E., et al. (2017). TrackMate: an open and extensible platform for single-particle tracking. Methods 115, 80–90. doi: 10.1016/j.ymeth.2016.09.016
Tondon, A., and Kaunas, R. (2014). The direction of stretch-induced cell and stress fiber orientation depends on collagen matrix stress. PLoS One 9:e89592. doi: 10.1371/journal.pone.0089592
Tong, Z., Beuzer, P., Ye, Q., Axelrod, J., Hong, Z., and Cang, H. (2016). Ex-STORM: Expansion Single Molecule Super-resolution. Microscopy 2016:49403. doi: 10.1101/049403
Topp, K. S., and Boyd, B. S. (2006). Structure and biomechanics of peripheral nerves: nerve responses to physical stresses and implications for physical therapist practice. Phys. Ther. 86, 92–109. doi: 10.1093/ptj/86.1.92
Topp, K. S., and Boyd, B. S. (2012). Peripheral nerve: from the microscopic functional unit of the axon to the biomechanically loaded macroscopic structure. J. Hand Ther. 25, 142–152. doi: 10.1016/j.jht.2011.09.002
Torrino, S., Grasset, E. M., Audebert, S., Belhadj, I., Lacoux, C., Haynes, M., et al. (2021). Mechano-induced cell metabolism promotes microtubule glutamylation to force metastasis. Cell Metab. 33, 1342–1357.e10. doi: 10.1016/j.cmet.2021.05.009
Trushko, A., Schäffer, E., and Howard, J. (2013). The growth speed of microtubules with XMAP215-coated beads coupled to their ends is increased by tensile force. Proc. Natl. Acad. Sci. 110, 14670–14675. doi: 10.1073/pnas.1218053110
Uchida, K., Nakajima, H., Hirai, T., Roberts, S., Johnson, W. E. B., and Baba, H. (2014). “Microarray analysis of expression of cell death-associated genes in spinal cord cells with cyclic tensile strain” in Neuroprotection and Regeneration of the Spinal Cord. ed. K. Uchida (Berlin, Heidelberg: Springer), 119–127.
Van Essen, D. C. (1997). A tension-based theory of morphogenesis and compact wiring in the central nervous system. Nature 385, 313–318. doi: 10.1038/385313a0
Van Liedekerke, P., Neitsch, J., Johann, T., Alessandri, K., Nassoy, P., and Drasdo, D. (2019). Quantitative cell-based model predicts mechanical stress response of growing tumor spheroids over various growth conditions and cell lines. PLoS Comput. Biol. 15:e1006273. doi: 10.1371/journal.pcbi.1006273
Vandenburgh, H., and Kaufman, S. (1979). In vitro model for stretch-induced hypertrophy of skeletal muscle. Science 203, 265–268. doi: 10.1126/science.569901
Ventura Santos, C., Rogers, S. L., and Carter, A. P. (2023). CryoET shows cofilactin filaments inside the microtubule lumen. EMBO Rep. 2023:e57264. doi: 10.1101/2023.03.31.535077
Verma, D., Ye, N., Meng, F., Sachs, F., Rahimzadeh, J., and Hua, S. Z. (2012). Interplay between cytoskeletal stresses and cell adaptation under chronic flow. PLoS One 7:e44167. doi: 10.1371/journal.pone.0044167
Walker, M., Rizzuto, P., Godin, M., and Pelling, A. E. (2020). Structural and mechanical remodeling of the cytoskeleton maintains tensional homeostasis in 3D microtissues under acute dynamic stretch. Sci. Rep. 10:7696. doi: 10.1038/s41598-020-64725-7
Wang, L., and Brown, A. (2002). Rapid movement of microtubules in axons. Curr. Biol. 12, 1496–1501. doi: 10.1016/S0960-9822(02)01078-3
Wang, N., Butler, J. P., and Ingber, D. E. (1993). Mechanotransduction across the cell surface and through the cytoskeleton. Science 260, 1124–1127. doi: 10.1126/science.7684161
Wang, J. H. C., Goldschmidt-Clermont, P., Wille, J., and Yin, F. C.-P. (2001). Specificity of endothelial cell reorientation in response to cyclic mechanical stretching. J. Biomech. 34, 1563–1572. doi: 10.1016/S0021-9290(01)00150-6
Wang, N., Naruse, K., Stamenović, D., Fredberg, J. J., Mijailovich, S. M., Tolić-Nørrelykke, I. M., et al. (2001). Mechanical behavior in living cells consistent with the tensegrity model. Proc. Natl. Acad. Sci. 98, 7765–7770. doi: 10.1073/pnas.141199598
Wang, Y., Schnitzbauer, J., Hu, Z., Li, X., Cheng, Y., Huang, Z.-L., et al. (2014). Localization events-based sample drift correction for localization microscopy with redundant cross-correlation algorithm. Opt. Express 22, 15982–15991. doi: 10.1364/OE.22.015982
Watson, P. A. (1991). Function follows form: generation of intracellular signals by cell deformation. FASEB J. 5, 2013–2019. doi: 10.1096/fasebj.5.7.1707019
Wen, Q., Po, M. D., Hulme, E., Chen, S., Liu, X., Kwok, S. W., et al. (2012). Proprioceptive coupling within motor neurons drives C. elegans forward locomotion. Neuron 76, 750–761. doi: 10.1016/j.neuron.2012.08.039
Werner, C., Sauer, M., and Geis, C. (2021). Super-resolving microscopy in neuroscience. Chem. Rev. 121, 11971–12015. doi: 10.1021/acs.chemrev.0c01174
Williamson, R. E. (1990). Alignment of cortical microtubules by anisotropic wall stresses. Funct. Plant Biol. 17, 601–613. doi: 10.1071/PP9900601
Willig, K. I., Keller, J., Bossi, M., and Hell, S. W. (2006). STED microscopy resolves nanoparticle assemblies. New J. Phys. 8:106. doi: 10.1088/1367-2630/8/6/106
Wortman, J. C., Shrestha, U. M., Barry, D. M., Garcia, M. L., Gross, S. P., and Clare, C. Y. (2014). Axonal transport: how high microtubule density can compensate for boundary effects in small-caliber axons. Biophys. J. 106, 813–823. doi: 10.1016/j.bpj.2013.12.047
Wright, T. W., Glowczewskie, F. Jr., Cowin, D., and Wheeler, D. L. (2001). Ulnar nerve excursion and strain at the elbow and wrist associated with upper extremity motion. J. Hand Surg. Am. 26, 655–662. doi: 10.1053/jhsu.2001.26140
Wu, Y.-T., and Adnan, A. (2018). Damage and failure of axonal microtubule under extreme high strain rate: an in-silico molecular dynamics study. Sci. Rep. 8:12260. doi: 10.1038/s41598-018-29804-w
Wu, T., Nieminen, T. A., Mohanty, S., Miotke, J., Meyer, R. L., Rubinsztein-Dunlop, H., et al. (2012). A photon-driven micromotor can direct nerve fibre growth. Nat. Photonics 6, 62–67. doi: 10.1038/nphoton.2011.287
Xu, Z., Schaedel, L., Portran, D., Aguilar, A., Gaillard, J., Marinkovich, M. P., et al. (2017). Microtubules acquire resistance from mechanical breakage through intralumenal acetylation. Science 356, 328–332. doi: 10.1126/science.aai8764
Xu, K., Zhong, G., and Zhuang, X. (2013). Actin, spectrin, and associated proteins form a periodic cytoskeletal structure in axons. Science 339, 452–456. doi: 10.1126/science.1232251
Yamada, K. M., Spooner, B. S., and Wessells, N. K. (1971). Ultrastructure and function of growth cones and axons of cultured nerve cells. J. Cell Biol. 49, 614–635. doi: 10.1083/jcb.49.3.614
Yan, C., Wang, F., Peng, Y., Williams, C. R., Jenkins, B., Wildonger, J., et al. (2018). Microtubule acetylation is required for mechanosensation in Drosophila. Cell Rep. 25, 1051–1065.e6. doi: 10.1016/j.celrep.2018.09.075
Yang, Y., Bauer, C., Strasser, G., Wollman, R., Julien, J.-P., and Fuchs, E. (1999). Integrators of the cytoskeleton that stabilize microtubules. Cell 98, 229–238. doi: 10.1016/S0092-8674(00)81017-X
Ye, Z., Wang, Y., Quan, X., Li, J., Hu, X., Huang, J., et al. (2012). Effects of mechanical force on cytoskeleton structure and calpain-induced apoptosis in rat dorsal root ganglion neurons in vitro. PLoS One 7:e52183. doi: 10.1371/journal.pone.0052183
Yeon, J., Kim, J., Kim, D.-Y., Kim, H., Kim, J., Du, E. J., et al. (2018). A sensory-motor neuron type mediates proprioceptive coordination of steering in C. elegans via two TRPC channels. PLoS Biol. 16:e2004929. doi: 10.1371/journal.pbio.2004929
Yun, X., and Gardner, L. (2017). Stress-strain curves for hot-rolled steels. J. Constr. Steel Res. 133, 36–46. doi: 10.1016/j.jcsr.2017.01.024
Zandomeni, K., and Schopfer, P. (1994). Mechanosensory microtubule reorientation in the epidermis of maize coleoptiles subjected to bending stress. Protoplasma 182, 96–101. doi: 10.1007/BF01403471
Zhang, Y., Chang, H., Gu, L., Zhao, Y., Xu, T., and Ji, W. (2013). Super-resolution microscopy of live cells using single molecule localization. Chin. Sci. Bull. 58, 4519–4527. doi: 10.1007/s11434-013-6088-4
Zheng, J., Buxbaum, R. E., and Heidemann, S. R. (1993). Investigation of microtubule assembly and organization accompanying tension-induced neurite initiation. J. Cell Sci. 104, 1239–1250. doi: 10.1242/jcs.104.4.1239
Zheng, J., Lamoureux, P., Santiago, V., Dennerll, T., Buxbaum, R. E., and Heidemann, S. R. (1991). Tensile regulation of axonal elongation and initiation. J. Neurosci. 11, 1117–1125. doi: 10.1523/JNEUROSCI.11-04-01117.1991
Keywords: neuron, axon, cytoskeleton, mechanical fatigue, mechanobiology
Citation: Coppini A, Falconieri A, Mualem O, Nasrin SR, Roudon M, Saper G, Hess H, Kakugo A, Raffa V and Shefi O (2024) Can repetitive mechanical motion cause structural damage to axons? Front. Mol. Neurosci. 17:1371738. doi: 10.3389/fnmol.2024.1371738
Received: 16 January 2024; Accepted: 23 May 2024;
Published: 07 June 2024.
Edited by:
Jean-Michel Peyrin, Laboratoire Neuroscience Paris Seine (IBPS), FranceReviewed by:
Devrim Kilinc, Institut Pasteur de Lille, FranceCopyright © 2024 Coppini, Falconieri, Mualem, Nasrin, Roudon, Saper, Hess, Kakugo, Raffa and Shefi. This is an open-access article distributed under the terms of the Creative Commons Attribution License (CC BY). The use, distribution or reproduction in other forums is permitted, provided the original author(s) and the copyright owner(s) are credited and that the original publication in this journal is cited, in accordance with accepted academic practice. No use, distribution or reproduction is permitted which does not comply with these terms.
*Correspondence: Henry Hess, aGhlc3NAY29sdW1iaWEuZWR1; Akira Kakugo, a2FrdWdvLmFraXJhLjhuQGt5b3RvLXUuYWMuanA=; Vittoria Raffa, dml0dG9yaWEucmFmZmFAdW5pcGkuaXQ=; Orit Shefi, b3JpdC5zaGVmaUBiaXUuYWMuaWw=
Disclaimer: All claims expressed in this article are solely those of the authors and do not necessarily represent those of their affiliated organizations, or those of the publisher, the editors and the reviewers. Any product that may be evaluated in this article or claim that may be made by its manufacturer is not guaranteed or endorsed by the publisher.
Research integrity at Frontiers
Learn more about the work of our research integrity team to safeguard the quality of each article we publish.