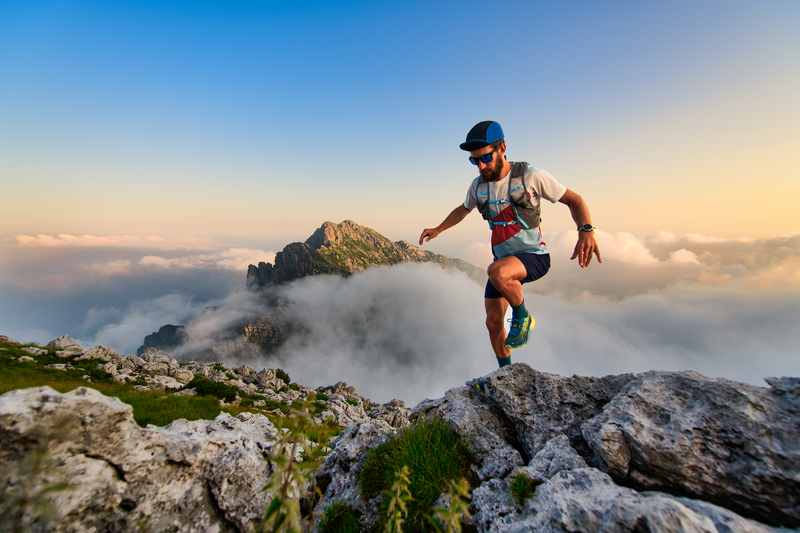
95% of researchers rate our articles as excellent or good
Learn more about the work of our research integrity team to safeguard the quality of each article we publish.
Find out more
REVIEW article
Front. Mol. Neurosci. , 08 January 2024
Sec. Brain Disease Mechanisms
Volume 16 - 2023 | https://doi.org/10.3389/fnmol.2023.1332348
This article is part of the Research Topic Molecular Mechanisms of Neuropsychiatric Diseases - Volume II View all 5 articles
The sympathoadrenal medullary system and the hypothalamic-pituitary-adrenal axis are both activated upon stressful events. The release of catecholamines, such as dopamine, norepinephrine (NE), and epinephrine (EPI), from sympathetic autonomic nerves participate in the adaptive responses to acute stress. Most theories suggest that activation of peripheral β-adrenoceptors (β-ARs) mediates catecholamines-induced memory enhancement. These include direct activation of β-ARs in the vagus nerve, as well as indirect responses to catecholamine-induced glucose changes in the brain. Excessive sympathetic activity is deeply associated with memories experienced during strong emotional stressful conditions, with catecholamines playing relevant roles in fear and traumatic memories consolidation. Recent findings suggest that EPI is implicated in fear and traumatic contextual memories associated with post-traumatic stress disorder (PTSD) by increasing hippocampal gene transcription (e.g., Nr4a) downstream to cAMP response-element protein activation (CREB). Herein, we reviewed the literature focusing on the molecular mechanisms underlying the pathophysiology of memories associated with fear and traumatic experiences to pave new avenues for the treatment of stress and anxiety conditions, such as PTSD.
Homeostasis comprises a set of coordinated physiological processes to sustain the steady state of an organism. Regulation of involuntary physiologic processes by the autonomic nervous system is a typical example of such processes (Walter, 1934). The “freeze, fight or flight” response, often referred to as the acute stress reaction, is an animal’s reaction to threats. This response is believed to prepare the organism to become capable of dealing with a stressor (Bracha, 2004; Lipov, 2014). The sympathoadrenal medullary system and the hypothalamic-pituitary-adrenocortical system have emerged as crucial pathways to stress responses in mammals (Selye, 1946; McEwen et al., 1974; Schommer et al., 2003). Consolidation of emotional memories is enhanced by neurotransmitters generated and released in response to stress (Matthews et al., 2012; McLaughlin et al., 2015). While these systems are adaptive in the context of acute stressful events, ongoing stress can produce chronic overactivation of the sympathoadrenal medullary system and of the hypothalamic-pituitary-adrenocortical axis. This prolonged activation is associated with the development and progression of anxiety, depression, and/or post-traumatic stress disorder (PTSD) (VanItallie, 2002).
The risk of suicide death is twice as high for people with PTSD compared to those without the condition (Fox et al., 2021). When people have symptoms of additional conditions like anxiety and depression, this association is stronger (Bantjes et al., 2016). Therefore, investigation of the variables involved in the resolution of stressful responses is deeply needed (Buckner et al., 2017; Brådvik, 2018; Twenge et al., 2018). This review provides an overview of the literature about the molecular mechanisms underlying fear memory physiology and traumatic memory pathophysiology. The involvement of catecholamines, namely norepinephrine (NE) and epinephrine (EPI), and adrenoceptors (ARs) in the development of stress and anxiety disorders will be detailed here, given that our hypothesis is that a thorough understanding of this interplay may pave the way for novel therapies to address stress and anxiety diseases, such as PTSD.
The hypothalamic-pituitary-adrenocortical system and the sympathoadrenal medullary system are both activated during a stressful scenario and work together to regulate the “freeze, fight, or flight” response (Bracha, 2004) by releasing stress hormones (corticosteroids and catecholamines) into the bloodstream (Figure 1; Timio et al., 1979; Floriou-Servou et al., 2021). According to preclinical research, hypothalamic-pituitary-adrenocortical and sympathoadrenal medullary systems cooperate to regulate the consolidation and retrieval of fear memories (Eiden, 2013; Hauer et al., 2014).
Figure 1. Activation of the hypothalamic-pituitary-adrenal system and the sympathoadrenal medullary system. Stress activates the limbic system in the midbrain. The amygdala stimulates the hypothalamus to release the corticotropin-releasing factor (CRF), thus inducing the production of adrenocorticotropic hormone (ACTH) by the pituitary gland. ACTH is released into the bloodstream and can act on the adrenal gland, triggering the cortical area of the gland to produce corticosteroids (Smith and Vale, 2006; Tank and Lee Wong, 2015). On the other hand, the hypothalamus also activates the Sympathetic Nervous System and through autonomic nerves triggers the release of norepinephrine and epinephrine from the adrenal medulla into the bloodstream (Smith and Vale, 2006; Tank and Lee Wong, 2015).
Upon a stressful event, the limbic system in the midbrain becomes active. The hypothalamus is activated by various systems, including immune, hormonal, and neural systems. Amygdala stimulation is a possible way of activating the hypothalamus and, consequently, two pathways may concur (Figure 1; Matheson et al., 1971; Beaulieu et al., 1988). The amygdala stimulates the hypothalamus and the anterior hypothalamus releases corticotropin-releasing factor (CRF), inducing the production of adrenocorticotropic hormone (ACTH) by the pituitary gland (Antoni et al., 1983; Gibbs et al., 1983). ACTH is released into the bloodstream and acts on the adrenal gland triggering the cortical region of the gland to produce corticosteroids, which then allows the organism to boost its metabolism in response to freeze, fight, or flight responses (Selye, 1956; Seaward, 2011). The hypothalamus also participates in intermediate and prolonged responses to aversive events by triggering the release of NE and EPI from the adrenal medulla into the bloodstream, therefore regulating the physiologic response to stressors (Smith and Vale, 2006; Tank and Lee Wong, 2015). The immune system is another mechanism of activation of the hypothalamus, through cytokines, such as IL-10, resulting in altered levels of ACTH and glucocorticoids (Petrowski et al., 2018). Therefore, the hypothalamus is a crucial part of the stress system, collaborating with other important brain regions and peripheral tissues and organs to mobilize an effective adaptive response against stressors (Li et al., 2021).
As mentioned, catecholamines, in particular EPI and NE, are two of the most important stress hormones (Romero and Butler, 2007), which together with glucocorticoids, are the main mediators of “freeze, fight or flight” responses (Bracha, 2004). Synergy between β2-ARs and glucocorticoid receptors has been reported. For instance, the β2-AR signaling pathway regulates the glucocorticoid receptor nuclear translocation and increases their affinity to steroids, as shown by the in vivo interaction of corticosteroids and inhaled long-acting β2-agonists on nuclear translocation of glucocorticoid receptors in human airway cells using immunocytochemistry (Usmani et al., 2005). This is also true in the opposite direction, since corticosteroids upregulate β 2-AR transcription and regulate both their number and binding to adenylate cyclase (Yates et al., 1996; Roth et al., 2002). Furthermore, adrenal glucocorticoids directly stimulate phenylethanolamine-N-methyltransferase (Pnmt), the enzyme that catalyzes the conversion of NE to EPI in the adrenal medulla among other tissues of the body. Sharara-Chami et al. (2010) demonstrated that high dosages of an exogenous corticosteroid increase Pnmt and catecholamine synthesis in the absence of stress when adrenocorticotropic hormone is low, probably independently of adrenal corticosterone concentration.
Adrenomedullary responses are extremely rapid because the sympathetic nervous system (SNS) directly innervates the adrenal medulla. Adrenomedullary responses can occur before the onset of actual stress, due to the involvement of several central nervous system (CNS) regions, specifically the hippocampus (Cattane et al., 2022). NE is released from postganglionic sympathetic neurons: the second neurons in the sympathetic pathway that are part of the autonomic nervous system. Through this release, the adrenomedullary output (80% EPI and 20% NE) is followed in a coordinated manner by SNS (White and Porterfield, 2012).
Catecholamines are hydrophilic and do not cross the blood-brain barrier (BBB) which prevents it from acting directly in the CNS (Weil-Malherbe et al., 1959). Catecholamines may indirectly act on brain areas responsible for learning and memory. Mounting evidence suggests the effects of stress hormones and, consequently peripheral and central β-AR activation, in memory consolidation and reconsolidation (Akirav and Maroun, 2013). The resulting effects of β-blockage with propranolol suggest a role of catecholamines in the consolidation of emotional memories (Cahill et al., 1994). This and other studies (van Stegeren et al., 1998) indicate that β-ARs activation influences long-term declarative memory consolidation for stressful emotional events triggering the release of adrenergic hormones.
Most theories on how catecholamines strengthen emotional memory have been focusing on the activation of peripheral β-ARs. One such hypothesis implicates the activation of β-ARs present in the vagus nerve (Miyashita and Williams, 2006; Figure 2A). As a matter of fact, EPI intraperitoneal injections increase vagal nerve firing, an effect that may be blocked by β-AR antagonists, such as sotalol or propranolol (Sternberg et al., 1985; Miyashita and Williams, 2006). The peripheral administration of β2-AR antagonist, ICI 118,551, induces amnesia in passive avoidance memory (Davies and Payne, 1989), as well as impairment of the contextual fear memory (Oliveira et al., 2018). In addition, ICI 118,551 along with the β1-AR antagonist, betaxolol, reduced the facilitation of field excitatory synaptic potentials induced by AR agonists in the basolateral amygdala (Abraham et al., 2008). Likewise, increases in endogenous amounts of NE in the basolateral amygdala improved memory formation after emotionally arousing experiences (Chen and Williams, 2012; Rudy, 2020).
Figure 2. The vagus nerve and glucose as mediators of catecholamine effects in strengthening emotional memories. Stress activates the limbic system, and amygdala stimulates the hypothalamus which activates the Sympathetic Nervous System and thus triggers catecholamines release in the adrenal gland and may follow one or both of the following pathways. (A) Catecholamines may activate the β-adrenoceptors present in peripheral vagus nerve afferents (Schreurs et al., 1986; Gordan et al., 2015). The nucleus tractus solitarius receives the afferent vagal input (Sumal et al., 1983) and its neurons may release norepinephrine (NE) onto the locus coeruleus, which in turn may release NE in the basolateral amygdala and hippocampus (Clark et al., 1999; Miyashita and Williams, 2004, 2006; Chen and Williams, 2012). On the other hand, catecholamines released in the adrenal gland (B) may activate the hepatic β-adrenoceptors, causing a release of glucose into the bloodstream (John et al., 1990). Blood glucose, through glucose transporters, can cross the blood-brain barrier and potentiate the synthesis of neuromodulators that will activate signaling pathways in the basolateral amygdala and hippocampus (McNay and Gold, 2002). Both paths activate signaling mechanisms that may affect the process of consolidation of fear and traumatic memory formation.
Another theory considers that increased glucose release by the liver could be a mediator of catecholamine effects in the brain (Gold, 2014; Figure 2B). According to this theory, glucose may potentiate the synthesis of neuroactive mediators participating in the process of memory formation and/or consolidation (Durkin et al., 1992). Behavioral tests performed in food-deprived rats show that these animals have difficulties in memory retention contrary to the rats that had normal access to food exhibiting high glucose reserves (Talley et al., 2000).
The effect of catecholamines was initially evaluated in adrenal medullectomy studies (Kvetnansky et al., 1979). Adrenal medullectomy involves surgically removing the medullar zone of the adrenal gland. Putative damage of the cortex and modification of corticosteroid, NE, chromogranin A, and neuropeptide Y release are limitations of this technique (Harrison and Seaton, 1966). By using this approach, it is also left unclear whether the reported effects are caused by EPI alone or by EPI plus NE combined. Therefore, we should investigate whether EPI and NE play different roles regarding the influence of catecholamines on emotional memory. This can be achieved using inhibitors of the Pnmt enzyme, thus preventing EPI formation from NE (Bondinell et al., 1983). Notwithstanding this, usage of Pnmt inhibitors still has disadvantages due to inhibition of α-ARs (Feder et al., 1989) and monoamine oxidase (Mefford et al., 1981), resulting in the disruption of normal physiological responses. This can be overcome by decreasing the expression of the enzyme. The EPI-deficient mouse model developed by Ebert et al. (2004) and Bao et al. (2007) is unable to convert NE to EPI since Pnmt is not expressed. These EPI-deficient mice are viable, fertile, and have no gross developmental impairments (Ebert et al., 2004), thus offering greater advantages over other methodological approaches to investigate the physiological influence of EPI among other catecholamines (Ebert et al., 2004; Bao et al., 2007; Sun et al., 2008).
Fear conditioning is a behavioral paradigm by which animals acquire abilities to predict aversive events by associating the stimulus to a specific context or tone (Curzon et al., 2009). Toth et al. (2013) were the first to use mice lacking Pnmt to evaluate EPI deficiency in contextual fear memory. Toth et al. (2013) and Alves et al. (2016) concluded that EPI-deficient mice exhibited reduced contextual memory after fear conditioning compared with wild-type mice, thus suggesting that contextual fear memory requires EPI synthesis. Data from these experimental studies also showed that EPI deficiency affects both fear memory retrieval and consolidation, but was devoid of effect on fear memory acquisition (Toth et al., 2013; Alves et al., 2016). Even though EPI strengthens fear contextual memory when the animals are in an aversive context, no differences were observed when the context was changed but the same auditory cue was present. Indeed, it is believed that hippocampus is not implicated in auditory fear conditioning memory, but it is involved in contextual fear conditioning (Rudy et al., 2004). Although auditory and contextual fear memories involve some common brain regions, such as amygdala (Goosens and Maren, 2001), these findings suggest that strengthening of contextual fear memory by EPI is hippocampal-dependent (Toth et al., 2013; Alves et al., 2016).
Deficits in contextual fear memory observed in EPI-deficient mice were restored by treating the animals with EPI, isoprenaline (a non-selective β-AR agonist), or fenoterol (a selective β2-AR agonist) (Alves et al., 2016). Interestingly, EPI strengthening of fear contextual memory was long-lasting, as it was still observed 1 month after the training of control mice compared to EPI-deficient mice (Oliveira et al., 2018). This effect seems to be mediated by peripheral β2-AR activation considering that intraperitoneal administration of sotalol (non-selective β-AR antagonist) and ICI 118,551 (selective β2-AR antagonist) reversed the EPI memory strengthening effect (Alves et al., 2016; Oliveira et al., 2018). Neither NE nor the selective β1-AR agonist, dobutamine, restored the contextual fear memory deficits observed in EPI-deficient mice (Alves et al., 2016).
On the other hand, the glucose effect in improving memory was characterized by an inverted U-dose response becoming detrimental above a given hyperglycemic threshold in humans [50 g, PO (Parsons and Gold, 1992)], mice [5 g/kg, PO (Messier and Destrade, 1988)], and rats [500 mg/kg, SC injection (Gold, 1986)], which is similar to EPI. The rise in glucose levels in the bloodstream during a stressful event may foster the production/release of certain neuromodulators affecting memory formation and consolidation in the inhibitory passive avoidance task (Sandusky et al., 2013). However, passive avoidance task does not allow for an accurate investigation of fear associative memory, contrary to fear conditioning (Ögren and Stiedl, 2010).
Interestingly, wild-type mice experienced higher range of glycemic variations following fear conditioning in comparison to that observed in EPI-deficient mice, which might presumably be caused by EPI release (Alves et al., 2016; Oliveira et al., 2018). The fact that glucose contributes to glycogen storage in astrocytes might explain its action as a mediator of contextual fear memory strengthening. Increased glycogen stores may contribute to sustain glucose supply to the brain and facilitate the synthesis of pyruvate or lactate (Newman et al., 2011). Pyruvate, lactate, and glucose may provide the necessary energy for processes triggered during fear memory formation (Brown et al., 2004). Glucose availability may also directly affect the membrane potential of glucose-sensing neurons in the hippocampus (de Araujo, 2014). In a more recent study, contextual fear memory in EPI-deficient mice was enhanced after glucose (30 mg/kg) administration, suggesting that moderate blood glucose levels during contextual fear memory acquisition and retrieval are necessary. Along with these findings, simultaneous administration of sub-effective doses of EPI (0.01 mg/kg) and glucose (10 mg/kg) enhanced contextual fear memory in EPI-deficient mice, whereas separate administration of these sub-effective doses was insufficient to enhance contextual fear memory, suggesting that Ad and glucose may act in synergy to strengthen contextual fear memory. These findings may reinforce the theory that glucose may be an important part of the peripheral to central pathway of contextual fear memory strengthening by EPI (Oliveira et al., 2023a). In this sense, peripheral EPI and subsequent glucose supply to the brain may promote hippocampal-dependent contextual fear memory (Alves et al., 2016; Oliveira et al., 2018, 2023a). In addition, it was observed enhanced contextual fear memory after insulin treatment, even in adrenaline absence, which may indicate a key role of insulin in contextual fear memory, possibly by increasing local cerebral energy use (Oliveira et al., 2023b). Insulin may facilitate glucose entry in neurons and astrocytes through insulin receptors activation and promotion of Glut-4 translocation to the hippocampus cell membrane (Pearson-Leary and McNay, 2016). In addition, in insulin administration group there was an increase in plasma catecholamines and a possible increase in the uptake of dopamine to hippocampus cells (Oliveira et al., 2023b). The influence of glucose and insulin in fear memory modulation may be important in diabetes. In fact, insulin plays a role in the regulation of the oxidative state and neuronal apoptosis in the CNS. These mechanisms might be responsible for the association between insulin activity changes and neuronal degeneration in the diabetic brain. In this context, it was observed resistance to fear extinction, increased fear generalization, along with increases in anxiety-like behaviors in a type 1 diabetes animal model (Lin et al., 2018; de Souza et al., 2019).
Long-term memory (LTM) consolidation and persistence involves synaptic remodeling as a consequence of long-lasting changes in gene expression (Josselyn et al., 2001; Dudai, 2002) and protein synthesis (Schafe and LeDoux, 2000; Kim et al., 2022). Glucose consumption increases contextual fear memory and results from a hippocampal-dependent associative learning mechanisms (Glenn et al., 2014), which may be mediated by phosphorylation (activation) of the cAMP response-element protein (CREB) (Impey et al., 1998). Because CREB is a transcription factor linked to LTM formation in various systems (Alberini, 2009), studying CREB-mediated signaling pathways in the hippocampus may be useful to understand the mechanisms underlying fear memory formation. The role of CREB in fear memory consolidation has been demonstrated in genetically altered mice (Kida et al., 2002). Phosphorylated CREB (pCREB) promotes the synthesis of immediate-early genes, like the nuclear receptor 4A (NR4A) transcription factor family (Darragh et al., 2005). The CREB interaction domain of the histone acetyltransferase CREB-binding protein with pCREB is needed for Nr4a gene expression after learning (Bridi et al., 2017). Learning-induced Nr4a expression is upregulated by histone deacetylase (HDAC) inhibition, which seems crucial for LTM improvement (Bridi and Abel, 2013).
The Nr4a gene family seems to be important for contextual fear memory formation and consolidation (Malkani and Rosen, 2000; von Hertzen and Giese, 2005; Hawk et al., 2012). The three members of Nr4a transcription factors gene family, namely Nr4a1 (NGF-B/Nur77), Nr4a2 (NURR1), and Nr4a3 (NOR1), belong to the immediate-early genes category (Milbrandt, 1988; Law et al., 1992; Ohkura et al., 1994). Interestingly, the deficits in contextual fear memory observed in EPI-deficient mice occur along with decreases in Nr4a1, Nr4a2, and Nr4a3 mRNA gene transcripts in the hippocampus compared to the levels observed in wild-type mice. At the mRNA level, the Nr4a2 gene transcription increased significantly in the hippocampus after administration of EPI to EPI-deficient mice (Oliveira et al., 2018). In addition, glucose administration in EPI-deficient mice increased hippocampus Nr4a3 gene transcription after contextual fear conditioning (Oliveira et al., 2023a). These findings strengthen our conclusion that the EPI-glucose pathway fosters the transcription of immediate-early genes in the hippocampus to promote contextual fear memory consolidation (Oliveira et al., 2018, 2023a).
Expression of Nr4a immediate early genes increases after a few hours of the stimulus and may influence other target genes such as brain-derived neurotrophic factor (Bdnf) gene. The BDNF peptide is also mainly generated in areas associated with learning and memory, as is the case of the hippocampal formation (Dugich-Djordjevic et al., 1995; Kawamoto et al., 1996). Overexpression of this neurotrophin participates in contextual fear conditioning (Hall et al., 2000; Mizuno et al., 2012). Hippocampal mRNA expression of Bdnf was increased in glucose-treated or insulin-treated EPI-deficient mice compared to vehicle-treated mice, thus suggesting that up-regulation of this gene associated with exogenous glucose or insulin results in increased contextual fear memory (Oliveira et al., 2023a,b). This situation may be due to insulin’s action on cerebral energy expenditure since it is known that insulin promotes glucose transporter Glut-4 translocation to the plasma membrane of hippocampus cells which increases glucose availability inside the cell (Pearson-Leary and McNay, 2016; Oliveira et al., 2023b).
Emotions can affect memory under certain circumstances, such as during acute or prolonged extreme stressful conditions (LaBar, 2007). Indeed, stress responses are crucial for preserving homeostasis when threatening situations occur (Cannon, 1939; McEwen, 1998). Nevertheless, serious anxiety and stress disorders can emerge whenever stress responses are inadequately triggered or become dysregulated. Severe dreadful experiences, such as death threats, violent crimes, warfare experience, sexual assault, especially if causing acute or chronic pain may predispose some individuals to psychiatric stress-related disorders, such as PTSD (North et al., 2012). In addition, adverse experiences may affect the maturation of the brain (van der Bij et al., 2020). PTSD affects nearly 6.8% of adults in the USA, with a significant women vs. men predisposition (Gradus, 2007). In fact, women are more predisposed to symptoms of several psychological diseases including stress, anxiety, depression, and PTSD (Gao et al., 2020; Xiong et al., 2020). The risk rate of lifetime PTSD could rise to 30% in populations that are highly exposed to stress, namely during armed conflicts and natural disasters (Breslau et al., 1998; Grinage, 2003).
Post-traumatic stress disorder patients suffer from three main conditions leading to specific symptomatology: re-experiencing, avoidance, and hyperarousal (North et al., 2012). Patients with PTSD constantly avoid any social environment or circumstance that may trigger memories of the event. Moreover, the hyperarousal state affects the ability to concentrate and sleep and causes a heightened startle response. Furthermore, hyperactivation of the SNS is a characteristic of PTSD patients (Shalev et al., 1992; Sherin and Nemeroff, 2011). In fact, increased stress hormones in plasma and urine, namely NE and EPI, have been observed in PTSD patients (Yehuda et al., 1992, 1998; Lemieux and Coe, 1995; Delahanty et al., 2005); this was also observed in a systematic meta-analysis (Pan et al., 2018).
Notwithstanding, the aforementioned considerations, PTSD is not a homogeneous disorder. The dissociative PTSD subtype has been associated with clinical severity, early life trauma and comorbid psychiatric disorders. This subtype exhibits the opposite pattern of the conventional amygdala hyperactivity with low prefrontal cortex (PFC) activation which is defined by low amygdala activation with a hyperactive prefrontal area (Lanius et al., 2010). In dissociative PTSD, the relationship between hyperactive prefrontal areas and reduced amygdala activation is complicated and requires further elucidation. The hypoactivity of the amygdala may contribute to emotional detachment and to reduce the capacity to understand and integrate emotional experiences in these patients (Forster et al., 2017). A negative correlation between EPI and NE levels and symptoms of peritraumatic dissociation exists (Delahanty et al., 2003). This suggests that highly dissociative individuals may not react physiologically to the initial traumatic event, thus dissociating and distancing themselves from trauma. On the other hand, activation of the prefrontal cortex may function as a compensatory mechanism. Individuals afflicted by dissociative PTSD may undertake excessive cognitive control processes as a coping mechanism for intense emotions or traumatic experiences. The PFC, particularly the medial PFC, has a role in executive tasks, such as emotional regulation and cognitive control (Waugh et al., 2014; Alexandra Kredlow et al., 2022). Therefore, a dissociative state may occasionally be exacerbated by increased PFC activity as a protective mechanism to suppress or control trauma. This polarity between hyper- and hypo-arousal may be just one of many facets of emotion-regulation mechanisms. However, there are many exceptions to what is considered the general rule and the clinical reality scenarios may be even more complex. Certain patients have unpredictable and unstable responses, meaning that their subjective emotions and physiological parameters do not correspond with each other (Kozlowska, 2007; González et al., 2017). Together, these findings suggest that the unique symptomatology of PTSD might be derived from hyper- and hypo-arousal of the amygdala and the PFC, which may explain the putative differences in catecholamines levels in these patients.
The simultaneous occurrence of PTSD with various mental disorders, such as depression and anxiety, is relatively common. This gives rise to comorbid PTSD conditions associated to a wide anxiety/depression spectrum (Koenig et al., 2018; Malgaroli et al., 2018), which may be correlated to disturbances in the functioning of hypothalamic-pituitary-adrenal axis (Pan et al., 2018).
To overcome environmental and inter-individual variability, animal models of PTSD have been instrumental to understand the neurobiological aspects of PTSD in terms of individual susceptibility, clinical response to stress, and prediction of therapeutic outcomes. Previous reviews have corroborated several animal models for stress paradigms, including physical stress, predator stress, and social defeat stress (Verbitsky et al., 2020). Since the traumatic experience necessary for the onset of PTSD can be considered an unconditioned stimulus associated with a conditioned stimulus (the context), variations of contextual fear conditioning are described as PTSD animal models (VanElzakker et al., 2014). One of these models uses multiple electric shocks as the unconditioned stimulus to simulate the unpleasant traumatic event that might trigger PTSD (Verma et al., 2016). This model combines prolonged exposure time with high-intensity currents, to produce long-lasting symptoms. Animals normally exhibit increased respiratory rate and freezing, as well as anxiety-like behaviors in response to foot shocks. This is also consistent with the pathological nature of PTSD and its symptoms (Li et al., 2006; Zhang et al., 2012). Moreover, animals exposed to repeated foot shocks may acquire phobia as a result of the traumatic experience (Delahanty et al., 2005), which fits the phobia occurrence in PTSD patients (Orsillo et al., 1996). The fear contextual memory that arises from the training days might be a representation of trauma reminders in PTSD patients (Li et al., 2006). The electric shock model may be explored to develop in-context treatments since repeated exposure to the environments and cues associated with stressors is the clinical counterpart of exposure therapies (Verbitsky et al., 2020). Thus, a physical stressor, such as electric foot shocks, might be a valuable resource to access the full range of signs (and symptoms) presented in this disorder.
Using the latter animal model, Martinho et al. (2020) investigated the role of catecholamines in PTSD (Moreira-Rodrigues and Grubisha, 2022; Abumaria et al., 2023); data indicate that mice with PTSD display higher contextual traumatic memory, along with increased levels of NE and EPI in adrenal glands and higher levels of EPI in the plasma compared to controls. The persistence of contextual traumatic memories led to an anxiety-like behavior and resistance to traumatic memory extinction with a possible involvement of hippocampal Nr4a2 and Nr4a3 genes (Martinho et al., 2020; Moreira-Rodrigues and Grubisha, 2022; Abumaria et al., 2023).
Selective serotonin reuptake inhibitors (SSRIs), like paroxetine and sertraline, are the only drugs approved by FDA and EMA for treating PTSD in human patients (Davidson et al., 2001; McRae and Brady, 2001; Friedman et al., 2007). SSRIs have only marginal effects on the severity and progression of PTSD symptoms compared to a control group (Bowers and Ressler, 2015; Hoskins et al., 2015). A meta-analysis of 55 studies found a 29% average dropout rate, thus indicating that most individuals do not tolerate or respond to existing PTSD treatments, including SSRIs (Lee et al., 2016). The fact that the majority of these drugs either fail to reach their maximal effect or cause severe adverse side effects agrees with the view that there is still an unmet clinical need in the treatment of PTSD. There is a potential suitability of molecules involved in inflammatory, immune, and hypothalamic-pituitary-adrenal axis responses to diagnose and treat PTSD. One studied molecule was matrix metalloproteinase 9 (MMP9), an extracellular matrix-degrading enzyme (Chevalier et al., 2021).
In the catecholamine biosynthesis pathway, dopamine-β-hydroxylase (DBH) is responsible for the conversion of DA to NE. Mice lacking DBH exhibit diminished contextual fear memory, which is restored by the administration of isoprenaline (a non-selective β-AR agonist) (Murchison et al., 2004). Nepicastat, a highly active reversible DBH inhibitor, effectively reduces NE in peripheral and central tissues both in rats (Bonifacio et al., 2015; Loureiro et al., 2015) and dogs (Stanley et al., 1997). By effectively modulating SNS hyperactivation, nepicastat may be a useful strategy to treat PTSD. As a matter of fact, Martinho et al. (2021) demonstrated that nepicastat significantly decreased DBH activity in the adrenal glands, which led to a gradual decrease in NE and EPI over 24 h (Moreira-Rodrigues and Grubisha, 2022; Abumaria et al., 2023). Nepicastat-treated PTSD mice showed reduced traumatic contextual memory and reduced anxiety-like behavior compared to control litter mates (Martinho et al., 2021; Moreira-Rodrigues and Grubisha, 2022; Abumaria et al., 2023).
Concerning the molecular pathways involved in the formation and consolidation of contextual fear and traumatic memories, besides Nr4a gene products, NPAS4 may also have a role. NPAS4 encodes for the activity-dependent transcription factor known as neuronal PAS domain protein 4 expressed in the CA3 hippocampal region and may play a role in neuronal regulation (Yun et al., 2010; Ploski et al., 2011). In fact, Npas4 has been associated with fear memory and contextual fear conditioning (Ramamoorthi et al., 2011). According to some, the amount of hippocampal NPAS4 mRNA gene transcripts is positively correlated with hippocampal activation (Drouet et al., 2018) and is implicated in the control of a transcriptional program that includes the Bdnf gene (Hall et al., 2000; Lin et al., 2008; Mizuno et al., 2012). Interestingly, nepicastat increased Npas4 and Bdnf genes transcription in the hippocampus, thus strengthening the hypothesis that these gene products play a crucial role in the weakening of traumatic contextual memories by replacing these with neutral contextual memories (Martinho et al., 2021; Moreira-Rodrigues and Grubisha, 2022; Abumaria et al., 2023). Since nepicastat application was made after the traumatic event but before contextual tests, catecholamines may strengthen traumatic memory at first, but afterward, this drug decreases catecholamines and, thus, the contextual memory may become neutral upon repetitive application of the DBH inhibitor before contextual exposition days. This approach mimics the combination of pharmacotherapy (DBH inhibitor) with psychotherapy (context exposition in a safe environment) for the treatment of PTSD, which may contribute to resilience and coping with the trauma.
Since no DBH inhibitor has received marketing approval due to poor DBH selectivity, low potency, and/or significant side effects, DBH gene silencing with small interference RNA technology may be a potential new therapeutic alternative for PTSD, particularly in patients with increased sympathetic activity. There are already several FDA-approved agents for metabolic diseases that are small interfering RNA (siRNA) based therapies and exert their effects by RNA interference (RNAi) of their target mRNA (Padda et al., 2023).
Furthermore, the reduction of the aforementioned sympathetic autonomic overshooting in PTSD patients may be achieved through inhibition of β-ARs activity (Murchison et al., 2004; Ramos et al., 2005). In fact, administration of propranolol (a peripheral and central β-AR antagonist) actually prevents the onset and progression of PTSD symptoms in human subjects exposed to traumatic situations, in particular when administered prior to trauma memory reactivation (Pitman et al., 2002; Brunet et al., 2008; Young and Butcher, 2020). Systemic propranolol disrupts the consolidation and reconsolidation of traumatic memories, but these putative beneficial effects are undermined due to unwanted side effects (e.g., gastrointestinal disturbances, bradycardia, fatigue, and sleep disorders) (Shukla and McGowan, 2009), along with memory deficits (e.g., decreased memory consolidation in non-aversive tasks and impairments in memory reconsolidation in least aversive tasks) (Villain et al., 2016).
Considering these facts, administration of the peripheral β-AR antagonist, sotalol, could overcome the unwanted central effects of propranolol. In fact, PTSD animals treated with sotalol exhibited decreased traumatic contextual memories, anxiety-like behaviors, and plasma catecholamines compared to vehicle-treated animals (Martinho et al., 2022). These effects of sotalol most probably result from a reduction of catecholamine effects in peripheral β-ARs leading to attenuation of the sympathetic autonomic overactivity, which indirectly down-modulates traumatic contextual memories (Martinho et al., 2022). With the data available so far, one can only speculate about the involvement of the sympathetic-induced vagal nerve drive and/or glucose release theories (Clark et al., 1999; Miyashita and Williams, 2004, 2006; Chen and Williams, 2012; Gold, 2014) to explain the results showing decreases in Nr4a1 mRNA transcripts in the hippocampus of sotalol-treated mice (Martinho et al., 2022). Our hypothesis is that sotalol may be repurposed as a possible alternative to SSIRs for PTSD treatment, especially in patients with peripheral sympathetic hyperactivity. Sotalol is currently used in ventricular and supra-ventricular arrhythmias, despite the associated risk of unwanted pro-arrhythmic effects due to prolongation of the QT interval (Somberg et al., 2010). Upon increasing the dosage of sotalol it abruptly interrupts the response to adrenergic stimuli by competitively blocking β-ARs. While the sotalol dosage to treat PTSD may be lower, the reversible inhibition of DBH may be a better option for this disease condition due to slower installation yet a more sustained decrease of adrenergic responses.
These data show that hippocampus genes may play a role in the modulation of traumatic contextual memories in PTSD animals: Nr4a family genes in sotalol treatment (Martinho et al., 2022), and Npas4 and Bdnf genes in nepicastat treatment (Martinho et al., 2021; Moreira-Rodrigues and Grubisha, 2022; Abumaria et al., 2023). This difference may also be related to different treatment regimens. Nepicastat treatment may have affected formation, expression, retrieval, consolidation or reconsolidation processes of contextual traumatic memory, whereas treatment with sotalol does not affect memory formation since no treatment with sotalol was applied in the traumatic event days, contrary to that occurring with nepicastat.
Furthermore, data indicate that EPI may trigger persistence of traumatic contextual memories in PTSD, possibly by increasing the transcription of Nr4a2 and Nr4a3 genes in the hippocampus (Martinho et al., 2020; Moreira-Rodrigues and Grubisha, 2022; Abumaria et al., 2023). Therefore, therapeutic options aiming at specifically blocking EPI effects could decrease unwanted side effects compared to other broader effect options, as could be the case for PNMT gene silencing in PTSD.
Catecholamines play a role in the consolidation of fear memories, which are crucial for developing an adaptive defensive mechanism and responding to adverse environmental stressors (Romero and Butler, 2007). Catecholamines are released upon activation of the sympathoadrenal medullary system after stressful events, thus initiating the important homeostasis response of “freeze, fight or flight” (Timio et al., 1979; Bracha, 2004). When fear responses are inaccurately triggered and/or regulated, homeostasis is compromised and traumatic memories can emerge leading to pathological conditions, such as anxiety and PTSD. Some, but not all, PTSD patients exhibit sympathetic autonomic overshooting (Shalev et al., 1992; Sherin and Nemeroff, 2011) with increased plasma and urinary levels of catecholamines (Yehuda et al., 1992; Lemieux and Coe, 1995; Delahanty et al., 2005).
Through peripheral β2-ARs activation of vagal nerve inputs to the CNS and/or by triggering glucose release from the liver, EPI may play a crucial role in strengthening contextual fear and traumatic memories (Miyashita and Williams, 2006; Chen and Williams, 2012; Toth et al., 2013; Gold, 2014; Alves et al., 2016; Oliveira et al., 2018, 2023a,b; Martinho et al., 2022; Moreira-Rodrigues and Grubisha, 2022; Abumaria et al., 2023). Subsequently, the transcription of immediate-early genes of the Nr4a family results in specific protein synthesis in the hippocampus to allow contextual fear and traumatic memories formation and consolidation (Oliveira et al., 2018, 2023a,b; Martinho et al., 2020, 2022). In this sense, our hypothesis is that sotalol (a peripheral non-selective β-ARs blocker) (Martinho et al., 2022) and nepicastat (a DBH inhibitor) (Martinho et al., 2021; Moreira-Rodrigues and Grubisha, 2022; Abumaria et al., 2023) might help to decrease PTSD occurrence by weakening abnormal traumatic memories formation and consolidation, and may support in the formation of neutral contextual memories. From the molecular point of view, we show that hippocampus genes may play a role in the modulation of traumatic contextual memories in PTSD animals: Nr4a family genes in sotalol treatment (Martinho et al., 2022), and Npas4 and Bdnf genes in nepicastat treatment (Martinho et al., 2021; Moreira-Rodrigues and Grubisha, 2022; Abumaria et al., 2023). Thus, the take-home message of this review is that PTSD patients with sympathetic autonomic overshooting may benefit from downregulating dysregulated catecholamine effects and DBH or PNMT gene silencing may be a therapeutic option. An approach that mimics the combination of pharmacotherapy with psychotherapy may contribute to resilience and coping with the trauma.
MA: Writing – original draft, Writing – review and editing. RM: Writing – original draft. AO: Writing – original draft. PC-d-S: Funding acquisition, Resources, Writing – review and editing. MM-R: Conceptualization, Funding acquisition, Resources, Supervision, Writing – original draft, Writing – review and editing.
The author(s) declare financial support was received for the research, authorship, and/or publication of this article. This work was partially supported by Foundation for Science and Technology (FCT) (projects UIDB/04308/2020 and UIDP/04308/2020, doi: 10.54499/UIDB/04308/2020). RM (SFRH/BD/133860/2017) and AO (SFRH/BD/138984/2018 and COVID/BD/152954/2022) received Ph.D. studentships from FCT.
Foundation for Science and Technology (FCT) and ICBAS, University of Porto.
The authors declare that the research was conducted in the absence of any commercial or financial relationships that could be construed as a potential conflict of interest.
The author(s) declared that they were an editorial board member of Frontiers, at the time of submission. This had no impact on the peer review process and the final decision.
All claims expressed in this article are solely those of the authors and do not necessarily represent those of their affiliated organizations, or those of the publisher, the editors and the reviewers. Any product that may be evaluated in this article, or claim that may be made by its manufacturer, is not guaranteed or endorsed by the publisher.
Abraham, P. A., Xing, G., Zhang, L., Eric, Z. Y., Post, R., Gamble, E. H., et al. (2008). β1-and β2-adrenoceptor induced synaptic facilitation in rat basolateral amygdala. Brain Res. 1209, 65–73.
Abumaria, N., Grubisha, M., and Moreira-Rodrigues, M. I. (2023). Molecular mechanisms of neuropsychiatric diseases. Lausanne: Frontiers Media SA.
Akirav, I., and Maroun, M. (2013). Stress modulation of reconsolidation. Psychopharmacology 226, 747–761. doi: 10.1007/s00213-012-2887-6
Alberini, C. M. (2009). Transcription factors in long-term memory and synaptic plasticity. Physiol. Rev. 89, 121–145. doi: 10.1152/physrev.00017.2008
Alexandra Kredlow, M., Fenster, R. J., Laurent, E. S., Ressler, K. J., and Phelps, E. A. (2022). Prefrontal cortex, amygdala, and threat processing: Implications for PTSD. Neuropsychopharmacology 47, 247–259. doi: 10.1038/s41386-021-01155-7
Alves, E., Lukoyanov, N., Serrao, P., Moura, D., and Moreira-Rodrigues, M. (2016). Epinephrine increases contextual learning through activation of peripheral beta2-adrenoceptors. Psychopharmacology 233, 2099–2108. doi: 10.1007/s00213-016-4254-5
Antoni, F. A., Holmes, M. C., and Jones, M. T. (1983). Oxytocin as well as vasopressin potentiate ovine CRF in vitro. Peptides 4, 411–415. doi: 10.1016/0196-9781(83)90041-4
Bantjes, J. R., Kagee, A., McGowan, T., and Steel, H. (2016). Symptoms of posttraumatic stress, depression, and anxiety as predictors of suicidal ideation among South African university students. J. Am. Coll. Health 64, 429–437.
Bao, X., Lu, C. M., Liu, F., Gu, Y., Dalton, N. D., Zhu, B. Q., et al. (2007). Epinephrine is required for normal cardiovascular responses to stress in the phenylethanolamine N-methyltransferase knockout mouse. Circulation 116, 1024–1031. doi: 10.1161/CIRCULATIONAHA.107.696005
Beaulieu, S., Gagné, B., and Barden, N. (1988). Glucocorticoid regulation of proopiomelanocortin messenger ribonucleic acid content of rat hypothalamus. Molecular Endocrinology 2, 727–731.
Bondinell, W. E., Chapin, F. W., Frazee, J. S., Girard, G. R., Holden, K. G., Kaiser, C., et al. (1983). Inhibitors of phenylethanolamine N-methyltransferase and epinephrine biosynthesis: A potential source of new drugs. Drug Metab. Rev. 14, 709–721. doi: 10.3109/03602538308991406
Bonifacio, M. J., Sousa, F., Neves, M., Palma, N., Igreja, B., Pires, N. M., et al. (2015). Characterization of the interaction of the novel antihypertensive etamicastat with human dopamine-beta-hydroxylase: Comparison with nepicastat. Eur. J. Pharmacol. 751, 50–58. doi: 10.1016/j.ejphar.2015.01.034
Bowers, M. E., and Ressler, K. J. (2015). An overview of translationally informed treatments for posttraumatic stress disorder: Animal models of Pavlovian fear conditioning to human clinical trials. Biol. Psychiatry 78, E15–E27.
Bracha, H. S. (2004). Freeze, flight, fight, fright, faint: Adaptationist perspectives on the acute stress response spectrum. CNS Spectr. 9, 679–685. doi: 10.1017/s1092852900001954
Brådvik, L. (2018). Suicide risk and mental disorders. Int. J. Environ. Res. Public Health 15:2028. doi: 10.3390/ijerph15092028
Breslau, N., Kessler, R. C., Chilcoat, H. D., Schultz, L. R., Davis, G. C., and Andreski, P. (1998). Trauma and posttraumatic stress disorder in the community: The 1996 Detroit Area Survey of Trauma. Arch. Gen. Psychiatry 55, 626–632. doi: 10.1001/archpsyc.55.7.626
Bridi, M. S., and Abel, T. (2013). The NR4A orphan nuclear receptors mediate transcription-dependent hippocampal synaptic plasticity. Neurobiol. Learn. Mem. 105, 151–158. doi: 10.1016/j.nlm.2013.06.020
Bridi, M. S., Hawk, J. D., Chatterjee, S., Safe, S., and Abel, T. (2017). Pharmacological activators of the NR4A nuclear receptors enhance LTP in a CREB/CBP-dependent manner. Neuropsychopharmacology 42, 1243–1253.
Brown, A. M., Tekkök, S. B., and Ransom, B. R. (2004). Energy transfer from astrocytes to axons: The role of CNS glycogen. Neurochem. Int. 45, 529–536.
Brunet, A., Orr, S. P., Tremblay, J., Robertson, K., Nader, K., and Pitman, R. K. (2008). Effect of post-retrieval propranolol on psychophysiologic responding during subsequent script-driven traumatic imagery in post-traumatic stress disorder. J. Psychiatr. Res. 42, 503–506. doi: 10.1016/j.jpsychires.2007.05.006
Buckner, J. D., Lemke, A. W., Jeffries, E. R., and Shah, S. M. (2017). Social anxiety and suicidal ideation: Test of the utility of the interpersonal-psychological theory of suicide. J. Anxiety Disord. 45, 60–63. doi: 10.1016/j.janxdis.2016.11.010
Cahill, L., Prins, B., Weber, M., and McGaugh, J. L. (1994). β-Adrenergic activation and memory for emotional events. Nature 371, 702–704.
Cattane, N., Vernon, A. C., Borsini, A., Scassellati, C., Endres, D., Capuron, L., et al. (2022). Preclinical animal models of mental illnesses to translate findings from the bench to the bedside: Molecular brain mechanisms and peripheral biomarkers associated to early life stress or immune challenges. Eur. Neuropsychopharmacol. 58, 55–79.
Chen, C. C., and Williams, C. L. (2012). Interactions between epinephrine, ascending vagal fibers, and central noradrenergic systems in modulating memory for emotionally arousing events. Front. Behav. Neurosci. 6:35. doi: 10.3389/fnbeh.2012.00035
Chevalier, C. M., Krampert, L., Schreckenbach, M., Schubert, C. F., Reich, J., Novak, B., et al. (2021). MMP9 mRNA is a potential diagnostic and treatment monitoring marker for PTSD: Evidence from mice and humans. Eur. Neuropsychopharmacol. 51, 20–32.
Clark, K. B., Naritoku, D. K., Smith, D. C., Browning, R. A., and Jensen, R. A. (1999). Enhanced recognition memory following vagus nerve stimulation in human subjects. Nat. Neurosci. 2, 94–94. doi: 10.1038/4600
Curzon, P., Rustay, N. R., and Browman, K. E. (2009). Cued and contextual fear conditioning for rodents, 2nd Edn. Boca Raton, FL: CRC Press/Taylor & Francis.
Darragh, J., Soloaga, A., Beardmore, V. A., Wingate, A. D., Wiggin, G. R., Peggie, M., et al. (2005). MSKs are required for the transcription of the nuclear orphan receptors Nur77, Nurr1 and Nor1 downstream of MAPK signalling. Biochem. J. 390, 749–759. doi: 10.1042/BJ20050196
Davidson, J. R., Rothbaum, B. O., van der Kolk, B. A., Sikes, C. R., and Farfel, G. M. (2001). Multicenter, double-blind comparison of sertraline and placebo in the treatment of posttraumatic stress disorder. Arch. Gen. Psychiatry 58, 485–492. doi: 10.1001/archpsyc.58.5.485
Davies, D., and Payne, J. (1989). Amnesia of a passive avoidance task due to the β2-adrenoceptor antagonist ICI 118,551. Pharmacol. Biochem. Behav. 32, 187–190. doi: 10.1016/0091-3057(89)90231-1
de Araujo, I. E. (2014). Contextual fear conditioning: Connecting brain glucose sensing and hippocampal-dependent memories. Biol. Psychiatry 75, 834–835. doi: 10.1016/j.biopsych.2014.03.024
de Souza, C. P., Gambeta, E., Stern, C. A. J., and Zanoveli, J. M. (2019). Posttraumatic stress disorder-type behaviors in streptozotocin-induced diabetic rats can be prevented by prolonged treatment with vitamin E. Behav. Brain Res. 359, 749–754.
Delahanty, D. L., Nugent, N. R., Christopher, N. C., and Walsh, M. (2005). Initial urinary epinephrine and cortisol levels predict acute PTSD symptoms in child trauma victims. Psychoneuroendocrinology 30, 121–128. doi: 10.1016/j.psyneuen.2004.06.004
Delahanty, D. L., Royer, D. K., Raimonde, A. J., and Spoonster, E. (2003). Peritraumatic dissociation is inversely related to catecholamine levels in initial urine samples of motor vehicle accident victims. Journal of Trauma Dissociation 4, 65–80. doi: 10.1300/J229v04n01_05
Drouet, J.-B., Peinnequin, A., Faure, P., Denis, J., Fidier, N., Maury, R., et al. (2018). Stress-induced hippocampus Npas4 mRNA expression relates to specific psychophysiological patterns of stress response. Brain Res. 1679, 75–83. doi: 10.1016/j.brainres.2017.11.024
Dudai, Y. (2002). Molecular bases of long-term memories: A question of persistence. Curr. Opin. Neurobiol. 12, 211–216. doi: 10.1016/S0959-4388(02)00305-7
Dugich-Djordjevic, M. M., Peterson, C., Isono, F., Ohsawa, F., Widmer, H. R., Denton, T. L., et al. (1995). Immunohistochemical visualization of brain-derived neurotrophic factor in the rat brain. Eur. J. Neurosci. 7, 1831–1839. doi: 10.1111/j.1460-9568.1995.tb00703.x
Durkin, T. P., Messier, C., de Boer, P., and Westerink, B. H. (1992). Raised glucose levels enhance scopolamine-induced acetylcholine overflow from the hippocampus: An in vivo microdialysis study in the rat. Behav. Brain Res. 49, 181–188. doi: 10.1016/S0166-4328(05)80163-9
Ebert, S. N., Rong, Q., Boe, S., Thompson, R. P., Grinberg, A., and Pfeifer, K. (2004). Targeted insertion of the Cre-recombinase gene at the phenylethanolamine n-methyltransferase locus: A new model for studying the developmental distribution of adrenergic cells. Dev. Dyn. 231, 849–858. doi: 10.1002/dvdy.20188
Eiden, L. E. (2013). Neuropeptide-catecholamine interactions in stress. Adv. Pharmacol. 68, 399–404. doi: 10.1016/b978-0-12-411512-5.00018-x
Feder, H. H., Crowley, W. R., and Nock, B. (1989). Inhibition of guinea pig lordosis behavior by the phenylethanolamine N-methyltransferase (PNMT) inhibitor SKF-64139: Mediation by alpha noradrenergic receptors. Horm. Behav. 23, 106–117. doi: 10.1016/0018-506X(89)90078-0
Floriou-Servou, A., von Ziegler, L., Waag, R., Schläppi, C., Germain, P.-L., and Bohacek, J. (2021). The acute stress response in the multiomic era. Biol. Psychiatry 89, 1116–1126.
Forster, G. L., Simons, R. M., and Baugh, L. A. (2017). “Revisiting the role of the amygdala in posttraumatic stress disorder,” in The amygdala-where emotions shape perception, learning memories, ed. B. Ferry (London: IntechOpen), 67585. doi: 10.5772/67585
Fox, V., Dalman, C., Dal, H., Hollander, A.-C., Kirkbride, J. B., and Pitman, A. (2021). Suicide risk in people with post-traumatic stress disorder: A cohort study of 3.1 million people in Sweden. J. Affect. Disord. 279, 609–616.
Friedman, M. J., Marmar, C. R., Baker, D. G., Sikes, C. R., and Farfel, G. M. (2007). Randomized, double-blind comparison of sertraline and placebo for posttraumatic stress disorder in a department of veterans affairs setting. J. Clin. Psychiatry 68, 711–720. doi: 10.4088/jcp.v68n0508
Gao, J., Zheng, P., Jia, Y., Chen, H., Mao, Y., Chen, S., et al. (2020). Mental health problems and social media exposure during COVID-19 outbreak. PLoS One 15:e0231924. doi: 10.1371/journal.pone.0231924
Gibbs, D., Stewart, R., Vale, W., Rivier, J., and Yen, S. (1983). Synthetic corticotropin-releasing factor stimulates secretion of immunoreactive β-endorphin/β-lipotropin and ACTH by human fetal pituitaries in vitro. Life Sci. 32, 547–550. doi: 10.1016/0024-3205(83)90150-9
Glenn, D. E., Minor, T. R., Vervliet, B., and Craske, M. G. (2014). The effect of glucose on hippocampal-dependent contextual fear conditioning. Biol. Psychiatry 75, 847–854.
Gold, P. E. (1986). Glucose modulation of memory storage processing. Behav. Neural Biol. 45, 342–349. doi: 10.1016/s0163-1047(86)80022-x
Gold, P. E. (2014). Regulation of memory – From the adrenal medulla to liver to astrocytes to neurons. Brain Res. Bull. 105, 25–35. doi: 10.1016/j.brainresbull.2013.12.012
González, A., del Río-Casanova, L., and Justo-Alonso, A. (2017). Integrating neurobiology of emotion regulation and trauma therapy: Reflections on EMDR therapy. Rev. Neurosci. 28, 431–440. doi: 10.1515/revneuro-2016-0070
Goosens, K. A., and Maren, S. (2001). Contextual and auditory fear conditioning are mediated by the lateral, basal, and central amygdaloid nuclei in rats. Learn. Mem. 8, 148–155.
Gordan, R., Gwathmey, J. K., and Xie, L. H. (2015). Autonomic and endocrine control of cardiovascular function. World J. Cardiol. 7, 204–214. doi: 10.4330/wjc.v7.i4.204
Grinage, B. D. (2003). Diagnosis and management of post-traumatic stress disorder. Am. Fam. Phys. 68, 2401–2409.
Hall, J., Thomas, K. L., and Everitt, B. J. (2000). Rapid and selective induction of BDNF expression in the hippocampus during contextual learning. Nat. Neurosci. 3, 533–535.
Harrison, T. S., and Seaton, J. F. (1966). Tissue content of epinephrine and norepinephrine following adrenal medullectomy. Am. J. Physiol. Legacy Content 210, 599–600. doi: 10.1152/ajplegacy.1966.210.3.599
Hauer, D., Kaufmann, I., Strewe, C., Briegel, I., Campolongo, P., and Schelling, G. (2014). The role of glucocorticoids, catecholamines and endocannabinoids in the development of traumatic memories and posttraumatic stress symptoms in survivors of critical illness. Neurobiol. Learn. Mem. 112, 68–74. doi: 10.1016/j.nlm.2013.10.003
Hawk, J. D., Bookout, A. L., Poplawski, S. G., Bridi, M., Rao, A. J., Sulewski, M. E., et al. (2012). NR4A nuclear receptors support memory enhancement by histone deacetylase inhibitors. J. Clin. Investig. 122, 3593–3602. doi: 10.1172/JCI64145
Hoskins, M., Pearce, J., Bethell, A., Dankova, L., Barbui, C., Tol, W. A., et al. (2015). Pharmacotherapy for post-traumatic stress disorder: Systematic review and meta-analysis. Br. J. Psychiatry 206, 93–100. doi: 10.1192/bjp.bp.114.148551
Impey, S., Smith, D. M., Obrietan, K., Donahue, R., Wade, C., and Storm, D. R. (1998). Stimulation of cAMP response element (CRE)-mediated transcription during contextual learning. Nat. Neurosci. 1, 595–601.
John, G. W., Doxey, J. C., Walter, D. S., and Reid, J. L. (1990). The role of alpha- and beta-adrenoceptor subtypes in mediating the effects of catecholamines on fasting glucose and insulin concentrations in the rat. Br. J. Pharmacol. 100, 699–704. doi: 10.1111/j.1476-5381.1990.tb14078.x
Josselyn, S. A., Shi, C., Carlezon, W. A., Neve, R. L., Nestler, E. J., and Davis, M. (2001). Long-term memory is facilitated by cAMP response element-binding protein overexpression in the amygdala. J. Neurosci. 21, 2404–2412. doi: 10.1523/JNEUROSCI.21-07-02404.2001
Kawamoto, Y., Nakamura, S., Nakano, S., Oka, N., Akiguchi, I., and Kimura, J. (1996). Immunohistochemical localization of brain-derived neurotrophic factor in adult rat brain. Neuroscience 74, 1209–1226.
Kida, S., Josselyn, S. A., de Ortiz, S. P., Kogan, J. H., Chevere, I., Masushige, S., et al. (2002). CREB required for the stability of new and reactivated fear memories. Nat. Neurosci. 5, 348–355.
Kim, D., Jung, H., Shirai, Y., Kim, H., Kim, J., Lim, D., et al. (2022). IQSEC3 deletion impairs fear memory through upregulation of ribosomal S6K1 signaling in the hippocampus. Biol. Psychiatry 91, 821–831.
Koenig, H. G., Youssef, N. A., Oliver, R. J. P., Ames, D., Haynes, K., Volk, F., et al. (2018). Religious involvement, anxiety/depression, and PTSD symptoms in US veterans and active duty military. J. Relig. Health 57, 2325–2342. doi: 10.1007/s10943-018-0692-1
Kozlowska, K. (2007). The developmental origins of conversion disorders. Clin. Child Psychol. Psychiatry 12, 487–510. doi: 10.1177/1359104507080977
Kvetnansky, R., Weise, V. K., Thoa, N. B., and Kopin, I. J. (1979). Effects of chronic guanethidine treatment and adrenal medullectomy on plasma levels of catecholamines and corticosterone in forcibly immobilized rats. J. Pharmacol. Exp. Therapeut. 209, 287–291.
LaBar, K. S. (2007). Beyond fear: Emotional memory mechanisms in the human brain. Curr. Direct. Psychol. Sci. 16, 173–177.
Lanius, R., Frewen, P., Vermetten, E., and Yehuda, R. (2010). Fear conditioning and early life vulnerabilities: Two distinct pathways of emotional dysregulation and brain dysfunction in PTSD. Eur. J. Psychotraumatol. 1:5467. doi: 10.3402/ejpt.v1i0.5467
Law, S. W., Conneely, O. M., DeMayo, F. J., and O’Malley, B. W. (1992). Identification of a new brain-specific transcription factor, NURR1. Mol. Endocrinol. 6, 2129–2135. doi: 10.1210/mend.6.12.1491694
Lee, D. J., Schnitzlein, C. W., Wolf, J. P., Vythilingam, M., Rasmusson, A. M., and Hoge, C. W. (2016). Psychotherapy versus pharmacotherapy for post-traumatic stress disorder: Systemic review and meta-analyses to determine first-line treatments. Depress Anxiety 33, 792–806. doi: 10.1002/da.22511
Lemieux, A. M., and Coe, C. L. (1995). Abuse-related posttraumatic stress disorder: Evidence for chronic neuroendocrine activation in women. Psychoso. Med. 57, 105–115. doi: 10.1097/00006842-199503000-00002
Li, L., Xu, Z., Chen, L., Suo, X., Fu, S., Wang, S., et al. (2021). Dysconnectivity of the amygdala and dorsal anterior cingulate cortex in drug-naive post-traumatic stress disorder. Eur. Neuropsychopharmacol. 52, 84–93.
Li, S., Murakami, Y., Wang, M., Maeda, K., and Matsumoto, K. (2006). The effects of chronic valproate and diazepam in a mouse model of posttraumatic stress disorder. Pharmacol. Biochem. Behav. 85, 324–331. doi: 10.1016/j.pbb.2006.08.015
Lin, L.-W., Tsai, F.-S., Yang, W.-T., Lai, S.-C., Shih, C.-C., Lee, S.-C., et al. (2018). Differential change in cortical and hippocampal monoamines, and behavioral patterns in streptozotocin-induced type 1 diabetic rats. Iran. J. Basic Med. Sci. 21, 1026.
Lin, Y., Bloodgood, B. L., Hauser, J. L., Lapan, A. D., Koon, A. C., Kim, T.-K., et al. (2008). Activity-dependent regulation of inhibitory synapse development by Npas4. Nature 455, 1198–1204. doi: 10.1038/nature07319
Lipov, E. (2014). Post Traumatic Stress Disorder (PTSD) as an over activation of sympathetic nervous system: An alternative view. Trauma Treatment 3, 1–3. doi: 10.4172/2167-1222.1000181
Loureiro, A. I., Bonifacio, M. J., Fernandes-Lopes, C., Pires, N., Igreja, B., Wright, L. C., et al. (2015). Role of P-glycoprotein and permeability upon the brain distribution and pharmacodynamics of etamicastat: A comparison with nepicastat. Xenobiotica 45, 828–839. doi: 10.3109/00498254.2015.1018985
Malgaroli, M., Maccallum, F., and Bonanno, G. A. (2018). Symptoms of persistent complex bereavement disorder, depression, and PTSD in a conjugally bereaved sample: A network analysis. Psychol. Med. 48, 2439–2448. doi: 10.1017/S0033291718001769
Malkani, S., and Rosen, J. B. (2000). Induction of NGFI-B mRNA following contextual fear conditioning and its blockade by diazepam. Mol. Brain Res. 80, 153–165. doi: 10.1016/s0169-328x(00)00130-3
Martinho, R., Correia, G., Seixas, R., Oliveira, A., Silva, S., Serrão, P., et al. (2021). Treatment with nepicastat decreases contextual traumatic memories persistence in post-traumatic stress disorder. Front. Mol. Neurosci. 14:745219. doi: 10.3389/fnmol.2021.745219
Martinho, R., Oliveira, A., Correia, G., Marques, M., Seixas, R., Serrão, P., et al. (2020). Epinephrine may contribute to the persistence of traumatic memories in a post-traumatic stress disorder animal model. Front. Mol. Neurosci. 13:588802. doi: 10.3389/fnmol.2020.588802
Martinho, R., Seixas, R., Azevedo, M., Oliveira, A., Serrão, P., and Moreira-Rodrigues, M. (2022). Sotalol treatment may interfere with retrieval, expression, and/or reconsolidation processes thus disrupting traumatic memories in a post-traumatic stress disorder mice model. Front. Pharmacol. 12:809271. doi: 10.3389/fphar.2021.809271
Matheson, G. K., Branch, B., and Taylor, A. N. (1971). Effects of amygdaloid stimulation on pituitary-adrenal activity in conscious cats. Brain Res. 32, 151–167.
Matthews, A. R., He, O. H., Buhusi, M., and Buhusi, C. V. (2012). Dissociation of the role of the prelimbic cortex in interval timing and resource allocation: Beneficial effect of norepinephrine and dopamine reuptake inhibitor nomifensine on anxiety-inducing distraction. Front. Integr. Neurosci. 6:111. doi: 10.3389/fnint.2012.00111
McEwen, B. S. (1998). “Stress, adaptation, and disease: Allostasis and allostatic load,” in Neuroimmunomodulation: Molecular aspects, integrative systems, and clinical advances, Vol. 840, ed. J. M. Lipton (New York, NY: New York Academy of Sciences), 33–44.
McEwen, B. S., Wallach, G., and Magnus, C. (1974). Corticosterone binding to hippocampus: Immediate and delayed influences of the absence of adrenal secretion. Brain Res. 70, 321–334.
McLaughlin, T., Blum, K., Oscar-Berman, M., Febo, M., Agan, G., Fratantonio, J. L., et al. (2015). Putative dopamine agonist (KB220Z) attenuates lucid nightmares in PTSD patients: Role of enhanced brain reward functional connectivity and homeostasis redeeming joy. J. Behav. Addict. 4, 106–115. doi: 10.1556/2006.4.2015.008
McNay, E. C., and Gold, P. E. (2002). Food for thought: Fluctuations in brain extracellular glucose provide insight into the mechanisms of memory modulation. Behav. Cogn. Neurosci. Rev. 1, 264–280. doi: 10.1177/153458230223833
McRae, A. L., and Brady, K. T. (2001). Review of sertraline and its clinical applications in psychiatric disorders. Expert Opin. Pharmacother. 2, 883–892. doi: 10.1517/14656566.2.5.883
Mefford, I. N., Roth, K. A., Gilberg, M., and Barchas, J. D. (1981). In vivo intraneuronal MAO inhibition in rat brain SKF 64139, comparison to other potent PNMT inhibitors. Eur. J. Pharmacol. 70, 345–353. doi: 10.1016/0014-2999(81)90168-0
Messier, C., and Destrade, C. (1988). Improvement of memory for an operant response by post-training glucose in mice. Behav. Brain Res. 31, 185–191. doi: 10.1016/0166-4328(88)90022-8
Milbrandt, J. (1988). Nerve growth factor induces a gene homologous to the glucocorticoid receptor gene. Behav. Brain Res. 1, 183–188.
Miyashita, T., and Williams, C. L. (2004). Peripheral arousal-related hormones modulate norepinephrine release in the hippocampus via influences on brainstem nuclei. Behav. Brain Res. 153, 87–95. doi: 10.1016/j.bbr.2003.11.005
Miyashita, T., and Williams, C. L. (2006). Epinephrine administration increases neural impulses propagated along the vagus nerve: Role of peripheral β-adrenergic receptors. Neurobiol. Learn. Mem. 85, 116–124. doi: 10.1016/j.nlm.2005.08.013
Mizuno, K., Dempster, E., Mill, J., and Giese, K. (2012). Long-lasting regulation of hippocampal Bdnf gene transcription after contextual fear conditioning. Genes Brain Behav. 11, 651–659. doi: 10.1111/j.1601-183X.2012.00805.x
Moreira-Rodrigues, M., and Grubisha, M. J. (2022). Editorial: Molecular mechanisms of neuropsychiatric diseases. Front. Mol. Neurosci. 15:1102296. doi: 10.3389/fnmol.2022.1102296
Murchison, C. F., Zhang, X.-Y., Zhang, W.-P., Ouyang, M., Lee, A., and Thomas, S. A. (2004). A distinct role for norepinephrine in memory retrieval. Cell 117, 131–143. doi: 10.1016/S0092-8674(04)00259-4
Newman, L. A., Korol, D. L., and Gold, P. E. (2011). Lactate produced by glycogenolysis in astrocytes regulates memory processing. PLoS One 6:e28427. doi: 10.1371/journal.pone.0028427
North, C. S., Oliver, J., and Pandya, A. (2012). Examining a comprehensive model of disaster-related posttraumatic stress disorder in systematically studied survivors of 10 disasters. Am. J. Public Health 102, e40–e48.
Ögren, S. O., and Stiedl, O. (2010). “Passive avoidance,” in Encyclopedia of psychopharmacology, ed. I. P. Stolerman (Berlin: Springer), 960–967.
Ohkura, N., Hijikuro, M., Yamamoto, A., and Miki, K. (1994). Molecular cloning of a novel thyroid/steroid receptor superfamily gene from cultured rat neuronal cells. Biochem. Biophys. Res. Commun. 205, 1959–1965.
Oliveira, A., Azevedo, M., Seixas, R., Martinho, R., Serrão, P., and Moreira-Rodrigues, M. (2023a). Glucose may contribute to retrieval and reconsolidation of contextual fear memory through hippocampal Nr4a3 and Bdnf mRNA expression and may act synergically with adrenaline. Mol. Neurobiol. doi: 10.1007/s12035-023-03745-6 [Epub ahead of print]
Oliveira, A., Martinho, R., Serrão, P., and Moreira-Rodrigues, M. (2018). Epinephrine released during traumatic events may strengthen contextual fear memory through increased hippocampus mRNA expression of Nr4a transcription factors. Front. Mol. Neurosci. 11:334–334. doi: 10.3389/fnmol.2018.00334
Oliveira, A., Seixas, R., Pereira, F., Azevedo, M., Martinho, R., Serrao, P., et al. (2023b). Insulin enhances contextual fear memory independently of its effect in increasing plasma adrenaline. Life Sci. 328:121881. doi: 10.1016/j.lfs.2023.121881
Orsillo, S. M., Heimberg, R. G., Juster, H. R., and Garrett, J. (1996). Social phobia and PTSD in Vietnam veterans. J. Traum. Stress 9, 235–252. doi: 10.1007/bf02110658
Padda, I. S., Mahtani, A. U., and Parmar, M. (2023). Small Interfering RNA (siRNA)-based therapy. Treasure Island, FL: StatPearls.
Pan, X., Kaminga, A. C., Wen, S. W., and Liu, A. (2018). Catecholamines in post-traumatic stress disorder: A systematic review and meta-analysis. Front. Mol. Neurosci. 11:450. doi: 10.3389/fnmol.2018.00450
Parsons, M. W., and Gold, P. E. (1992). Glucose enhancement of memory in elderly humans: An inverted-U dose-response curve. Neurobiol. Aging 13, 401–404. doi: 10.1016/0197-4580(92)90114-d
Pearson-Leary, J., and McNay, E. C. (2016). Novel roles for the insulin-regulated glucose transporter-4 in hippocampally dependent memory. J. Neurosci. 36, 11851–11864. doi: 10.1523/JNEUROSCI.1700-16.2016
Petrowski, K., Wichmann, S., and Kirschbaum, C. (2018). Stress-induced pro-and anti-inflammatory cytokine concentrations in panic disorder patients. Psychoneuroendocrinology 94, 31–37.
Pitman, R. K., Sanders, K. M., Zusman, R. M., Healy, A. R., Cheema, F., Lasko, N. B., et al. (2002). Pilot study of secondary prevention of posttraumatic stress disorder with propranolol. Biol. Psychiatry 51, 189–192. doi: 10.1016/s0006-3223(01)01279-3
Ploski, J. E., Monsey, M. S., Nguyen, T., DiLeone, R. J., and Schafe, G. E. (2011). The neuronal PAS domain protein 4 (Npas4) is required for new and reactivated fear memories. PLoS One 6:e23760. doi: 10.1371/journal.pone.0023760
Ramamoorthi, K., Fropf, R., Belfort, G. M., Fitzmaurice, H. L., McKinney, R. M., Neve, R. L., et al. (2011). Npas4 regulates a transcriptional program in CA3 required for contextual memory formation. Science 334, 1669–1675. doi: 10.1126/science.1208049
Ramos, B. P., Colgan, L., Nou, E., Ovadia, S., Wilson, S. R., and Arnsten, A. F. (2005). The beta-1 adrenergic antagonist, betaxolol, improves working memory performance in rats and monkeys. Biol. Psychiatry 58, 894–900.
Roth, M., Johnson, P. R., Rudiger, J. J., King, G. G., Ge, Q., Burgess, J. K., et al. (2002). Interaction between glucocorticoids and beta2 agonists on bronchial airway smooth muscle cells through synchronised cellular signalling. Lancet 360, 1293–1299. doi: 10.1016/S0140-6736(02)11319-5
Rudy, J., and Huff, N. Matus-Amat, P. (2004). Understanding contextual fear conditioning: Insights from a two-process model. Neurosci. Biobehav. Rev. 28, 675–685. doi: 10.1016/j.neubiorev.2004.09.004
Sandusky, L. A., Flint, R. W., and McNay, E. C. (2013). Elevated glucose metabolism in the amygdala during an inhibitory avoidance task. Behav. Brain Res. 245, 83–87. doi: 10.1016/j.bbr.2013.02.006
Schafe, G. E., and LeDoux, J. E. (2000). Memory consolidation of auditory pavlovian fear conditioning requires protein synthesis and protein kinase A in the amygdala. J. Neurosci. 20, Rc96. doi: 10.1523/JNEUROSCI.20-18-j0003.2000
Schommer, N. C., Hellhammer, D. H., and Kirschbaum, C. (2003). Dissociation between reactivity of the hypothalamus-pituitary-adrenal axis and the sympathetic-adrenal-medullary system to repeated psychosocial stress. Psychosom. Med. 65, 450–460. doi: 10.1097/01.PSY.0000035721.12441.17
Schreurs, J., Seelig, T., and Schulman, H. (1986). Beta 2-adrenergic receptors on peripheral nerves. J. Neurochem. 46, 294–296.
Seaward, B. (2011). Managing stress: Principles and strategies for health and well-being. Burlington, MA: Jones & Bartlett Learning.
Selye, H. (1946). The general adaptation syndrome and the diseases of adaptation. J. Clin. Endocrinol. 6, 117–230.
Shalev, A. Y., Orr, S. P., Peri, T., Schreiber, S., and Pitman, R. K. (1992). Physiologic responses to loud tones in Israeli patients with posttraumatic stress disorder. Arch. Gen. Psychiatry 49, 870–875. doi: 10.1001/archpsyc.1992.01820110034005
Sharara-Chami, R. I., Joachim, M., Pacak, K., and Majzoub, J. A. (2010). Glucocorticoid treatment–effect on adrenal medullary catecholamine production. Shock 33, 213–217. doi: 10.1097/SHK.0b013e3181af0633
Sherin, J. E., and Nemeroff, C. B. (2011). Post-traumatic stress disorder: The neurobiological impact of psychological trauma. Dialogues Clin. Neurosci. 13, 263–278. doi: 10.31887/DCNS.2011.13.2/jsherin
Shukla, A. C., and McGowan, F. X. Jr. (2009). “Chapter 16 - Cardiac physiology and pharmacology A2 -,” in A Practice of anesthesia for infants and children, 4th Edn, eds C. J. Coté, I. D. Todres, N. G. Goudsouzian, and J. F. Ryanin (Philadelphia: W.B. Saunders).
Smith, S. M., and Vale, W. W. (2006). The role of the hypothalamic-pituitary-adrenal axis in neuroendocrine responses to stress. Dialogues Clin. Neurosci. 8, 383–395. doi: 10.31887/DCNS.2006.8.4/ssmith
Somberg, J. C., Preston, R. A., Ranade, V., and Molnar, J. (2010). QT prolongation and serum sotalol concentration are highly correlated following intravenous and oral sotalol. Cardiology 116, 219–225. doi: 10.1159/000316050
Stanley, W. C., Li, B., Bonhaus, D. W., Johnson, L. G., Lee, K., Porter, S., et al. (1997). Catecholamine modulatory effects of nepicastat (RS-25560-197), a novel, potent and selective inhibitor of dopamine-beta-hydroxylase. Br. J. Pharmacol. 121, 1803–1809. doi: 10.1038/sj.bjp.0701315
Sternberg, D., Isaacs, K., Gold, P., and McGaugh, J. (1985). Epinephrine facilitation of appetitive learning: Attenuation with adrenergic receptor antagonists. Behav. Neural Biol. 44, 447–453.
Sumal, K. K., Blessing, W. W., Joh, T. H., Reis, D. J., and Pickel, V. M. (1983). Synaptic interaction of vagal afferents and catecholaminergic neurons in the rat nucleus tractus solitarius. Brain Res. 277, 31–40. doi: 10.1016/0006-8993(83)90904-6
Sun, P., Bao, X., Elayan, H., Milic, M., Liu, F., and Ziegler, M. G. (2008). Epinephrine regulation of hemodynamics in catecholamine knockouts and the pithed mouse. Ann. N. Y. Acad. Sci. 1148, 325–330. doi: 10.1196/annals.1410.078
Talley, C. E. P., Kahn, S., Alexander, L. J., and Gold, P. E. (2000). Epinephrine fails to enhance performance of food-deprived rats on a delayed spontaneous alternation task. Neurobiol. Learn. Mem. 73, 79–86. doi: 10.1006/nlme.1999.3920
Tank, A. W., and Lee Wong, D. (2015). Peripheral and central effects of circulating catecholamines. Compr. Physiol. 5, 1–15. doi: 10.1002/cphy.c140007
Timio, M., Gentili, S., and Pede, S. (1979). Free adrenaline and noradrenaline excretion related to occupational stress. Br. Heart J. 42, 471–474.
Toth, M., Ziegler, M., Sun, P., Gresack, J., and Risbrough, V. (2013). Impaired conditioned fear response and startle reactivity in epinephrine deficient mice. Behav. Pharmacol. 24, 1–9. doi: 10.1097/FBP.0b013e32835cf408
Twenge, J. M., Joiner, T. E., Rogers, M. L., and Martin, G. N. (2018). Increases in depressive symptoms, suicide-related outcomes, and suicide rates among US adolescents after 2010 and links to increased new media screen time. Clin. Psychol. Sci. 6, 3–17. doi: 10.1177/2167702617723376
Usmani, O. S., Ito, K., Maneechotesuwan, K., Ito, M., Johnson, M., Barnes, P. J., et al. (2005). Glucocorticoid receptor nuclear translocation in airway cells after inhaled combination therapy. Am. J. Respir. Crit. Care Med. 172, 704–712.
van der Bij, J., den Kelder, R. O., Montagne, B., and Hagenaars, M. A. (2020). Inhibitory control in trauma-exposed youth: A systematic review. Neurosci. Biobehav. Rev. 118, 451–462.
van Stegeren, A. H., Everaerd, W., Cahill, L., McGaugh, J. L., and Gooren, L. J. (1998). Memory for emotional events: Differential effects of centrally versus peripherally acting β-blocking agents. Psychopharmacology 138, 305–310.
VanElzakker, M. B., Kathryn Dahlgren, M., Caroline Davis, F., Dubois, S., and Shin, L. M. (2014). From Pavlov to PTSD: The extinction of conditioned fear in rodents, humans, and anxiety disorders. Neurobiol. Learn. Mem. 113, 3–18. doi: 10.1016/j.nlm.2013.11.014
Verbitsky, A., Dopfel, D., and Zhang, N. (2020). Rodent models of post-traumatic stress disorder: Behavioral assessment. Transl. Psychiatry 10:132.
Verma, M., Bali, A., Singh, N., and Jaggi, A. S. (2016). Investigating the role of nisoldipine in foot-shock-induced post-traumatic stress disorder in mice. Fundament. Clin. Pharmacol. 30, 128–136. doi: 10.1111/fcp.12174
Villain, H., Benkahoul, A., Drougard, A., Lafragette, M., Muzotte, E., Pech, S., et al. (2016). Effects of propranolol, a β-noradrenergic antagonist, on memory consolidation and reconsolidation in mice. Front. Behav. Neurosci. 10:49. doi: 10.3389/fnbeh.2016.00049
von Hertzen, L. S., and Giese, K. P. (2005). Memory reconsolidation engages only a subset of immediate-early genes induced during consolidation. J. Neurosci. 25, 1935–1942. doi: 10.1523/jneurosci.4707-04.2005
Waugh, C. E., Lemus, M. G., and Gotlib, I. H. (2014). The role of the medial frontal cortex in the maintenance of emotional states. Soc. Cogn. Affect. Neurosci. 9, 2001–2009. doi: 10.1093/scan/nsu011
Weil-Malherbe, H., Axelrod, J., and Tomchick, R. (1959). Blood-brain barrier for adrenaline. Science 129, 1226–1227.
White, B., and Porterfield, S. (2012). Endocrine and reproductive physiology E-book: Mosby physiology monograph series. Amsterdam: Elsevier Health Sciences.
Xiong, J., Lipsitz, O., Nasri, F., Lui, L. M. W., Gill, H., Phan, L., et al. (2020). Impact of COVID-19 pandemic on mental health in the general population: A systematic review. J. Affect. Disord. 277, 55–64. doi: 10.1016/j.jad.2020.08.001
Yates, D. H., Kharitonov, S. A., and Barnes, P. J. (1996). An inhaled glucocorticoid does not prevent tolerance to the bronchoprotective effect of a long-acting inhaled beta 2-agonist. Am. J. Respirat. Crit. Care Med. 154(6 Pt. 1), 1603–1607. doi: 10.1164/ajrccm.154.6.8970342
Yehuda, R., Siever, L. J., Teicher, M. H., Levengood, R. A., Gerber, D. K., Schmeidler, J., et al. (1998). Plasma norepinephrine and 3-methoxy-4-hydroxyphenylglycol concentrations and severity of depression in combat posttraumatic stress disorder and major depressive disorder. Biol. Psychiatry 44, 56–63.
Yehuda, R., Southwick, S., Giller, E. L., Ma, X., and Mason, J. W. (1992). Urinary catecholamine excretion and severity of PTSD symptoms in Vietnam combat veterans. J. Nerv. Ment. Dis. 180, 321–325. doi: 10.1097/00005053-199205000-00006
Young, C., and Butcher, R. (2020). Propranolol for post-traumatic stress disorder: A review of clinical effectiveness. Ottawa, ON: CADTH.
Yun, J., Koike, H., Ibi, D., Toth, E., Mizoguchi, H., Nitta, A., et al. (2010). Chronic restraint stress impairs neurogenesis and hippocampus-dependent fear memory in mice: Possible involvement of a brain-specific transcription factor Npas4. J. Neurochem. 114, 1840–1851. doi: 10.1111/j.1471-4159.2010.06893.x
Keywords: physiology of fear, contextual fear memory, pathophysiology of fear, traumatic contextual memory, catecholamines, adrenoceptors
Citation: Azevedo M, Martinho R, Oliveira A, Correia-de-Sá P and Moreira-Rodrigues M (2024) Molecular pathways underlying sympathetic autonomic overshooting leading to fear and traumatic memories: looking for alternative therapeutic options for post-traumatic stress disorder. Front. Mol. Neurosci. 16:1332348. doi: 10.3389/fnmol.2023.1332348
Received: 02 November 2023; Accepted: 12 December 2023;
Published: 08 January 2024.
Edited by:
Jingqi Yan, Cleveland State University, United StatesReviewed by:
Jacob C. Nordman, Southern Illinois University Carbondale, United StatesCopyright © 2024 Azevedo, Martinho, Oliveira, Correia-de-Sá and Moreira-Rodrigues. This is an open-access article distributed under the terms of the Creative Commons Attribution License (CC BY). The use, distribution or reproduction in other forums is permitted, provided the original author(s) and the copyright owner(s) are credited and that the original publication in this journal is cited, in accordance with accepted academic practice. No use, distribution or reproduction is permitted which does not comply with these terms.
*Correspondence: Mónica Moreira-Rodrigues, bWlyb2RyaWd1ZXNAaWNiYXMudXAucHQ=
Disclaimer: All claims expressed in this article are solely those of the authors and do not necessarily represent those of their affiliated organizations, or those of the publisher, the editors and the reviewers. Any product that may be evaluated in this article or claim that may be made by its manufacturer is not guaranteed or endorsed by the publisher.
Research integrity at Frontiers
Learn more about the work of our research integrity team to safeguard the quality of each article we publish.