- 1Department of Pharmacology and Pharmacotherapy, Medical School and Centre for Neuroscience, University of Pécs, Pécs, Hungary
- 2National Laboratory for Drug Research and Development, Budapest, Hungary
- 3Hungarian Research Network, PTE HUN-REN Chronic Research Group, Budapest, Hungary
- 4Szentágothai Research Centre, Bioinformatics Research Group, Genomics and Bioinformatics Core Facility, University of Pécs, Pécs, Hungary
- 5PharmInVivo Ltd., Pécs, Hungary
The tachykinin hemokinin-1 (HK-1) is involved in immunological processes, inflammation, and pain. Although the neurokinin 1 receptor (NK1R) is described as its main target, several effects are mediated by currently unidentified receptor(s). The role of HK-1 in pain is controversial, depending on the involvement of peripheral and central sensitization mechanisms in different models. We earlier showed the ability of HK-1 to activate the trigeminovascular system, but the mechanisms need to be clarified. Therefore, in this study, we investigated HK-1-induced transcriptomic alterations in cultured rat trigeminal ganglion (TRG) primary sensory neurons. HK-1 was applied for 6 or 24 h in 1 μM causing calcium-influx in these neurons, 500 nM not inducing calcium-entry was used for comparison. Next-generation sequencing was performed on the isolated RNA, and transcriptomic changes were analyzed to identify differentially expressed (DE) genes. Functional analysis was performed for gene annotation using the Gene Ontology (GO), Kyoto Encyclopedia of Genes and Genomes (KEGG), and Reactome databases. NK1R and Neurokinin receptor 2 (NK2R) were not detected. Neurokinin receptor 3 (NK3R) was around the detection limit, which suggests the involvement of other NKR isoforms or other receptors in HK-1-induced sensory neuronal activation. We found protease-activated receptor 1 (PAR1) and epidermal growth factor receptor (EGFR) as DE genes in calcium signaling. The transmembrane protein anthrax toxin receptor 2 (ANTXR2), a potential novel pain-related target, was upregulated. Acid-sensing ion channel 1; 3 (Asic1,3), N-methyl-D-aspartate (NMDA) and alpha-amino-3-hydroxy-5-methyl-4-isoxazolepropionic acid (AMPA) glutamate receptors decreased, myelin production and maintenance related genes (Mbp, Pmp2, Myef2, Mpz) and GNDF changed by HK-1 treatment. Our data showed time and dose-dependent effects of HK-1 in TRG cell culture. Result showed calcium signaling as altered event, however, we did not detect any of NK receptors. Presumably, the activation of TRG neurons is independent of NK receptors. ANTXR2 is a potential new target, PAR-1 has also important role in pain, however their connection to HK-1 is unknown. These findings might highlight new targets or key mediators to solve how HK-1 acts on TRG.
1. Introduction
Tachykinins represent a neuropeptide family widely distributed in the body. The first members of this family were substance P (SP), neurokinin A (NKA), derived from the preprotachykinin A/Tac1 gene, and neurokinin B (NKB), encoded by the preprotachykinin B/Tac3 gene (Borbély and Helyes, 2017). Other members of this family have also been identified: neuropeptide K (NPK) (Roth et al., 1985), neuropeptide γ (NPγ) (Kage et al., 1988), hemokinin-1 (HK-1), and endokinins (EKs) (Kurtz et al., 2002). HK-1 encoded by the preprotachykinin C/Tac4 gene was discovered 20 years ago in B lymphocytes (Zhang et al., 2000), and similar to SP, it was shown in several immune cell types, including myeloid (polymorphonuclear granulocytes and eosinophils), lymphoid, dendritic cells, neurons, and microglia (Borbély and Helyes, 2017). This widespread expression of HK-1 in diverse cell types might be related to a broad range of physiological and pathophysiological functions by activating a multitude of signaling pathways, which are currently not understood. HK-1 induces a variety of physiological and pathophysiological functions in the immune and hematopoietic systems, gastrointestinal tract, airways, cardiovascular, endocrine, and neural systems, bone, joints, and cancer. The role of HK-1 in pain is contradictory and likely to be concentration/dose dependent (Borbély and Helyes, 2017). In mice, centrally administered HK-1 caused scratching (Borbély and Helyes, 2017), licking, and biting at low doses (Watanabe et al., 2010). Intrathecally injected HK-1 was analgesic in nanomolar concentrations in an NK1- or opioid receptor-dependent manner, but hyperalgesic in picomolar concentrations (Fu et al., 2005).
Tachykinins possess a differential affinity for the three tachykinin receptors. HK and EKs have the highest affinity for NK1R, similar to SP (Satake et al., 2013; Steinhoff et al., 2014). They are all G protein-coupled receptors of the rhodopsin family. Signaling through the NK receptors is complex with multiple directions: (1) Gq-related: activation of phospholipase C, resulting in inositol trisphosphate and diacylglycerol formation, mobilization of Ca2+ from intracellular stores, and activation of protein kinase C; (2) Gs-related activation of adenylyl cyclase, resulting in the cAMP formation and protein kinase A (PKA) activation; or (3) activation of phospholipase A2 and arachidonic acid production (Steinhoff et al., 2014; Garcia-Recio and Gascón, 2015). The existence of a specific receptor for HK-1 is currently intensively investigated (Duffy et al., 2003). It was shown that an NK1R antagonist did not inhibit pain caused by HK-1 in different concentrations, suggesting a specific HK-1 target/receptor (Watanabe et al., 2010). NK1R antagonists were developed as analgesic drug candidates, but they failed in human pain conditions. This might be due to different NK1R splice variants linked to distinct binding and activation mechanisms and/or a currently unidentified receptor (Zhang et al., 2000; Borbély and Helyes, 2017; Hunyady et al., 2019; Borbély et al., 2020). Our research group earlier showed that HK-1 mediated chronic adjuvant-induced inflammation and related pain (Borbély et al., 2013) and neuropathic and neurogenic inflammatory hyperalgesia via NK1 receptor activation (Hunyady et al., 2019). Interestingly, we recently demonstrated that unlike SP, HK-1 activates primary sensory neurons by inducing calcium influx via an NK1R-independent mechanism (Borbély et al., 2020). NK1R was shown to interact with the Mas-related G protein receptors (Mrgr) in certain pain-related mechanisms (Zhou et al., 2015). The human Mrgprx2, which is an ortholog of the rat Mrgprb5, was described as a potential target for HK-1 in mast cells to cause degranulation (Manorak et al., 2018). However, naturally occurring variants of Mrgprx2 lose HK-1 binding ability, showing the high importance of receptor variants (Alkanfari et al., 2018).
Based on a broad range of data demonstrating proinflammatory and pronociceptive functions of HK-1 in different organs partially independently of NK1 receptor activation (Hunyady et al., 2019; Borbély et al., 2020), our main aim was to identify the molecular mechanisms responsible for these actions. Our recent results demonstrated NK1R-independent calcium influx in cultured trigeminal ganglion cells induced by 1 μM, but not 500 nM HK-1 (Borbély et al., 2020). In the present study, we investigated the concentration- and treatment duration-dependent intracellular signaling mechanisms and pathways in these primary sensory neurons using an unbiased transcriptomic approach.
2. Materials and methods
2.1. Primary cultures of TG neurons and treatment protocols
Primary cell cultures of TG neuron cells were extracted from 1–4-day-old Wistar rat pups as described earlier (Szoke et al., 2000). TG cells were dissected and washed in several steps. Afterward, TG cells were plated on poly-D-lysin-coated glass coverslips and grown in a nutrient-supplemented medium for the experiment. The earlier washing steps and the cell culture medium recipe are detailed elsewhere (Takács-Lovász et al., 2022). According to an earlier calcium influx, cell cultures were treated with HK-1 (Sigma Aldrich, solved in culture media) in two concentrations: 500 nM (no evoked calcium influx) and 1 μM [evoked calcium influx in an earlier study (Takács-Lovász et al., 2022)]. Untreated cultures were used as controls. After 6 h and 24 h of HK-1 administration, samples were collected for RNA isolation. Except for the HK-1 500 nM 24 h condition, which was repeated in duplicates, other conditions were repeated in triplicate.
2.2. Illumina library preparation and sequencing
In order to provide a complete gene expression profile, RNA sequencing was performed (Fang and Cui, 2011). The library for Illumina sequencing was made using the QuantSeq 30 mRNA-Seq Library Prep Kit FWD for Illumina (Lexogen, Vienna, Austria). A total of 400 ng of total RNA was used for first-strand cDNA generation using an oligodT primer followed by RNA removal. The second strand synthesis was followed by random priming, and the products were purified with magnetic beads. Finally, the libraries were amplified and barcoded using PCR. All libraries were quality-checked on the TapeStation 4200 (Agilent Technologies, Santa Clara, CA, USA) to examine if adapter dimers formed during PCR. The QuantSeq libraries were sequenced using the Illumina NextSeq550 platform to produce 75 bp single-end reads.
2.3. Bioinformatics
The sequencing reads were aligned against the Rattus norvegicus reference genome (Rnor 6.0 Ensembl release) with STAR v2.5.3a (Dobin et al., 2013). Following alignment, reads were associated with known protein-coding genes, and the number of reads aligned within each gene was counted using HTSeq library v0.11.1 (Anders et al., 2015). Gene count data were normalized using the trimmed mean of M values (TMM) normalization method of the edgeR R/Bioconductor package (v3.28, R v3.6.0, Bioconductor v3.9) (Robinson et al., 2010). Data were further log-transformed using the voom approach for statistical evaluation (Law et al., 2014) in the limma package (Ritchie et al., 2015). Fold change (FC) values between the compared groups resulting from the linear modeling process and moderated t-test p-values relative to a minimum required fold change threshold were calculated by the limma package. When determining differentially expressed (DE) genes, filtering thresholds were set to at least FC 1,2 and p-value 0.05 when the HK-1 1 μM 24 h and HK-1 500 nM 6 h treatments were compared to the untreated control group, and to FC 1.3 and p-value 0.05 for the HK-1 1 μM 6 h vs. untreated control, and to FC 2 and p-value 0.05 for HK-1 500 nM 24 h vs. untreated control group comparison. Normalized counts were represented as transcripts per million (TPM) values. Functional analysis (annotations of genes) was prepared using the Gene Ontology (GO), Kyoto Encyclopedia of Genes and Genomes (KEGG), and Reactome databases. Detection of functional enrichment was performed in the differentially expressed gene list (DE list enrichment: Fisher's exact test for GO, hypergeometric test for KEGG and Reactome) and toward the top of the list when all genes have been ranked according to the evidence for being differentially expressed (ranked list enrichment: non-parametric Kolmogorov–Smirnov test for GO and KEGG, hypergeometric test for Reactome) applying the topGO v2.37.0, ReactomePA v1.30.0, and gage v2.36.0 packages. The pathview package v1.26.0 (Luo and Brouwer, 2013) was used to visualize mapping data to KEGG pathways. GO terms were merged using the Revigo tool (tiny resulting list set up) for extracting the most relevant terms.
2.4. Validation of gene expression fold changes by RT-qPCR
To validate certain up and downregulated genes together with unaltered ones determined by RNA sequencing, 200 ng RNA per sample was used for reverse transcription with the High-Capacity cDNA Reverse Transcription Kit (Thermo Fisher Scientific). Primers were designed with Primer-BLAST (https://www.ncbi.nlm.nih.gov/tools/primer-blast/) for the genes selected on the basis of the transcriptomics results [nuclear receptor subfamily 4 group A member 1 (Nr4a1), solute carrier family 25 member 5 (Slc25a5), fibroblast growth factor receptor 1 (Fgfr1), heat shock protein HSP 90-alpha (Hsp90aa1), integrin subunit alpha 4 (Itga4), NADH: ubiquinone oxidoreductase subunit B6 (Ndufb6), F2r/PAR1, G protein subunit beta 2 (Gnb2), G protein subunit alpha I1 (Gnai1), fibroblast growth factor 5 (Fgf5)], and they are demonstrated in Supplementary Table S1. The reactions were carried out with SensiFAST SYBR® Lo-ROX mix (Meridian BioScience) reagents in triplicate using 1 μl of cDNA per well in a Bio-Rad CFX96 real-time cycler. The amplification program was as follows: 95 °C for 120 s, 40 cycles at 95 °C for 5 s, 58 °C for 10s, and 60 °C for 30 s. The geometric mean of the CT values for the two housekeeping genes was determined to obtain the ΔCT for each gene of interest. The ΔΔCT values were calculated by subtracting the ΔCT value of the control sample from the ΔCT of the treated sample. Relative fold changes in gene expression were calculated using the comparative 2–ΔΔCt method (Livak and Schmittgen, 2001).
3. Results
3.1. DE genes
Extracting the most important and relevant DE genes helps to understand the effects and intracellular signaling mechanisms of HK-1. In average rank, the p and FC values were taken into account shows the most relevant ranked gene list for all groups (Table 1). After 24 h of response to 1 μM HK-1, which we previously showed induces calcium influx in trigeminal primary sensory neurons, acid-sensing ion channel subunit 3 (Asic3), Glutamate ionotropic receptor NMDA type subunit 1 (Grin1), and C-C motif chemokine ligand 7 (Ccl7) were downregulated. At the earlier, 6 h timepoint, 1 μM HK-1 downregulated Slc25a5, while upregulating myelin-associated glycoprotein (Mag). For 6 h results, Mag, Itga4, and the LBH regulator of the Wnt signaling pathway (Lbb) were at the top of the list and upregulated independently of concentration.
The 500 nM HK-1 concentration, which did not evoke calcium influx in our earlier studies, downregulated Slc25a5 at 24 h and upregulated Ndufb6. Additionally, mitochondrially encoded NADH dehydrogenase 6 (Mt-nd6) was downregulated in the HK-1 500 nM 6 h group. Supplementary Images I1–I4 show heatmaps of all DE genes for different concentrations at different sampling times.
Similarly, altered DE genes over time (both at the 6 h and 24 h timepoints) in response to the same HK-1 concentration may yield insight into the key mechanisms and effects. Figure 1 shows the numerical representation of DE genes across all groups and a summary of different group comparisons. Most DE genes were detected in response to 500 nM HK-1 treatment after 24h, where Nr4a1, Slc25a5, F2r, Ndufb6, and Gnb2 were upregulated, which were confirmed by qPCR (FC: 1.726-; 1.783; 2.205; 2.105; 1.445, respectively). Itga4, Fgf5, and Gnai1 were upregulated, and Ndufb6, Gnb2, and F2r were downregulated 6h after 1 μM HK-1 treatment, as determined both by sequencing and qPCR (FC: 2.942; 3.652; 1.14;−1.15;−1.01;−1.08). Unaltered genes were Fgfr1 and Gnai1 according to RNA sequencing and qPCR (FC: 1.385; 1.365) 24h after 1 μM HK-1 treatment. Although qPCR confirmed all these alterations obtained with sequencing, 24 h after 500 nM HK-1 treatment, Fgfr1 changes were different with the two techniques: it was downregulated according to RNA sequencing but upregulated by PCR (FC: 1.18). The qPCR results are shown in Supplementary Table S2.
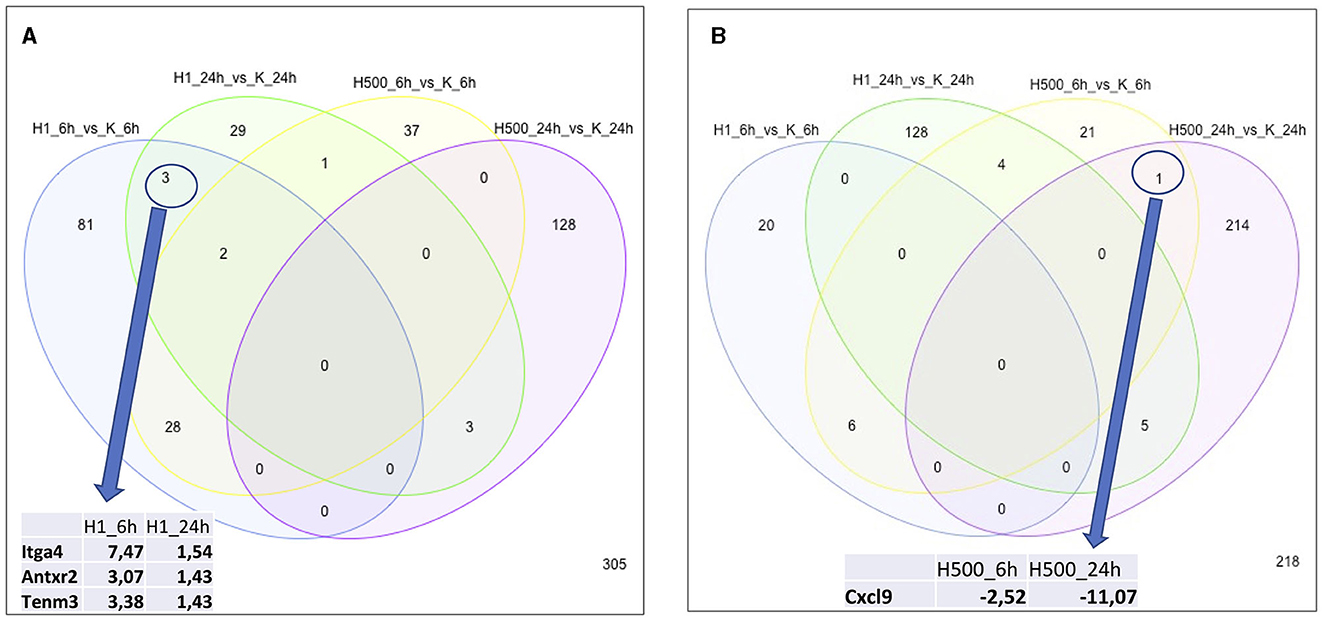
Figure 1. Number of DE genes for all groups. Insert tables show the FC values of the DE genes for the respective comparisons. (A) Panel demonstrates the upregulated genes and (B) shows the numbers of downregulated genes.
In response to 1 μM HK-1, the expression of three genes, Itga4, ANTXR cell adhesion molecule 2 (Antxr2), and teneurin transmembrane protein 3 (Tenm3), changed similarly (Figure 1). There was only one DE gene after 500 nM HK-1, Cxcl9 (C-X-C motif chemokine ligand 9), which was downregulated. Results for 24 h include less common DE genes, showing a greater concentration-dependent effect of HK-1. The common DE genes expressed in differently treated groups at the same timepoints showed 30 upregulated and 6 downregulated genes at 6 h and 3 upregulated and 5 downregulated genes at 24 h.
Common DE genes independent of the HK-1 concentration (and the calcium influx-inducing potential) at both timepoints are shown in Tables 2, 3. Antxr2 and Itga4 at 6 h were concentration-independently upregulated. Serine protease 12 (Prss12), T cell differentiation protein (Mal), and Mag were also upregulated, but the expression level of voltage-gated sodium channel beta subunit 4 (Scn4b) was decreased at 6 h after both concentrations. Regarding concentration-independent results at 24 h, frizzled class receptor 1 (Fzd1) and 3-hydroxyacyl-CoA dehydratase 2 (Hacd2) were upregulated, and rabphilin 3A (Rph3a), gamma-aminobutyric acid type A receptor alpha2 subunit (Gabra2), ryanodine receptor 2 (Ryr2) Mag, and voltage-gated sodium channel alpha subunit 1 (Scn1a) were downregulated. There were significant DE genes with potential importance in neuronal and inflammatory functions, but they are not in the top 30 ranked gene lists (Table 4). In response to 1 μM HK-1 potassium voltage-gated channel-interacting protein 4 (Kcnip4), myelin basic protein (Mbp) was downregulated and Gpr108 was upregulated at 24 h, while fibroblast growth factor 9 (Fgf9), Ndufb6, Myelin expression factor 2 (Myef2), myelin protein zero (Mpzc), and GDNF were upregulated at 6 h. Interestingly, 500 nM HK-1 downregulated PACAP, while upregulating peripheral myelin protein 2 (Pmp2), glial cell-derived neurotrophic factor (GDNF) at 6 h, transmembrane protein 128 (Tmem128), and integrin subunit alpha V (Itgav) at 24 h.
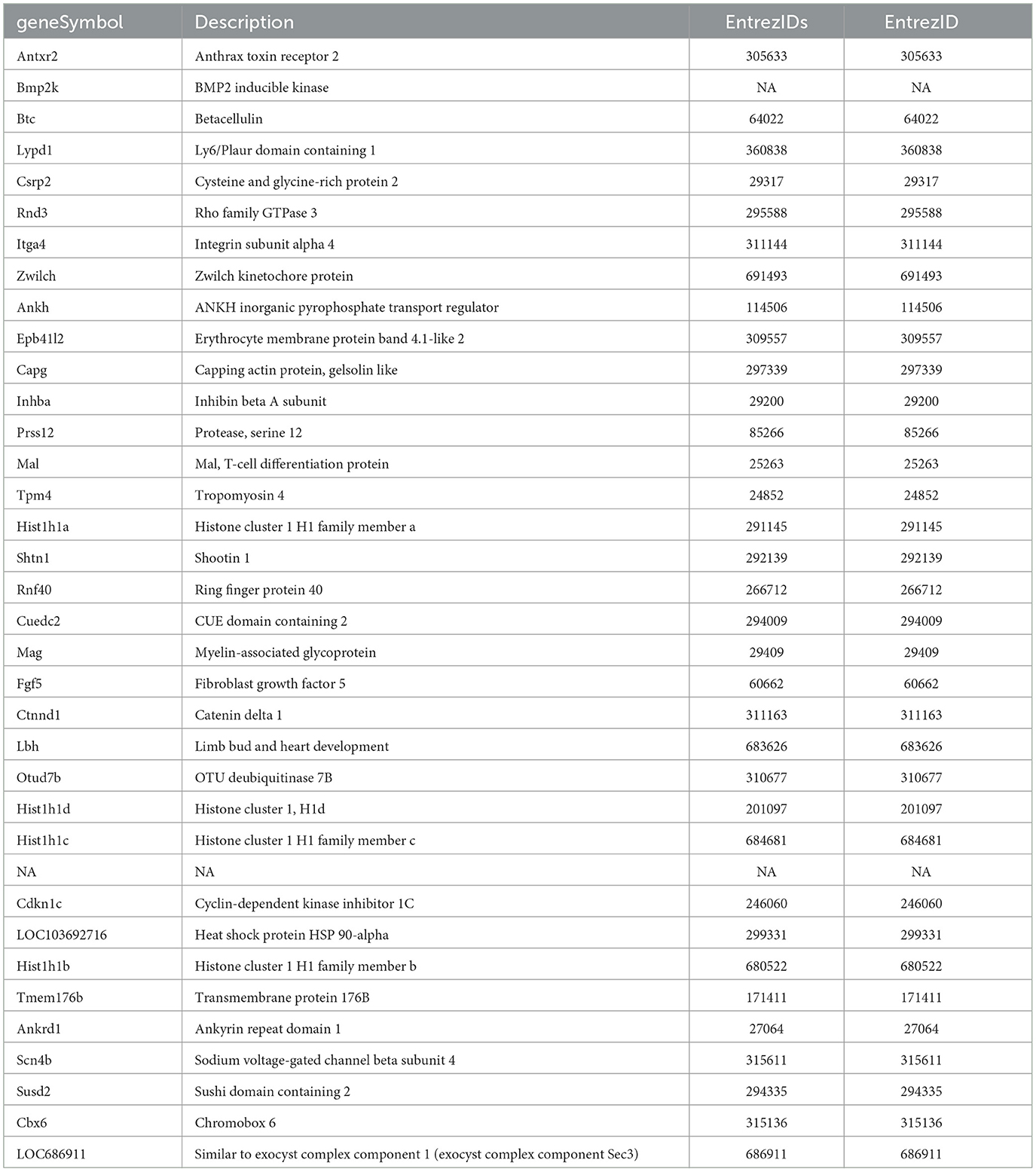
Table 2. Common DE genes for the 1μM and 500 nM HK-1 concentrations at 6 h. Red means upregulated, while green shows downregulated DE genes.
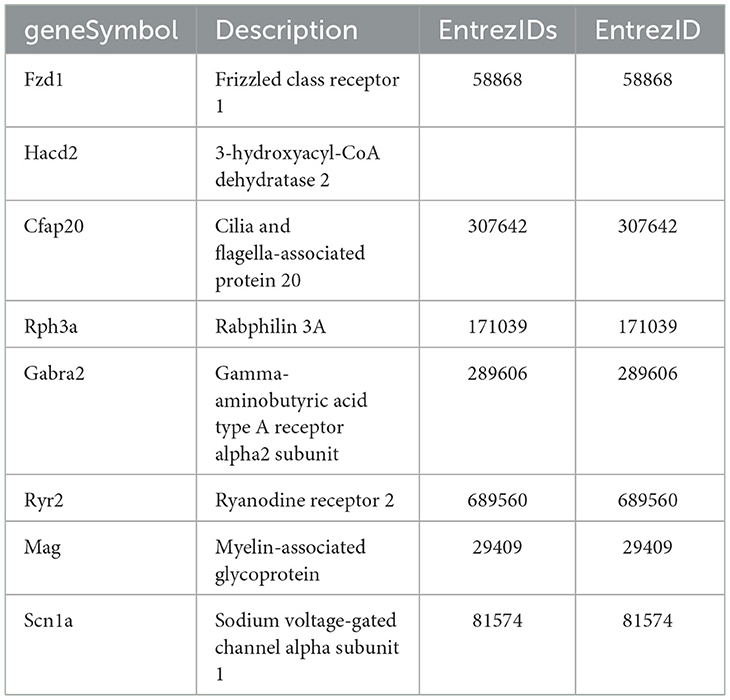
Table 3. Common DE genes for both doses at 24 h. Red means upregulated DEs, whereas green shows downregulated Des.
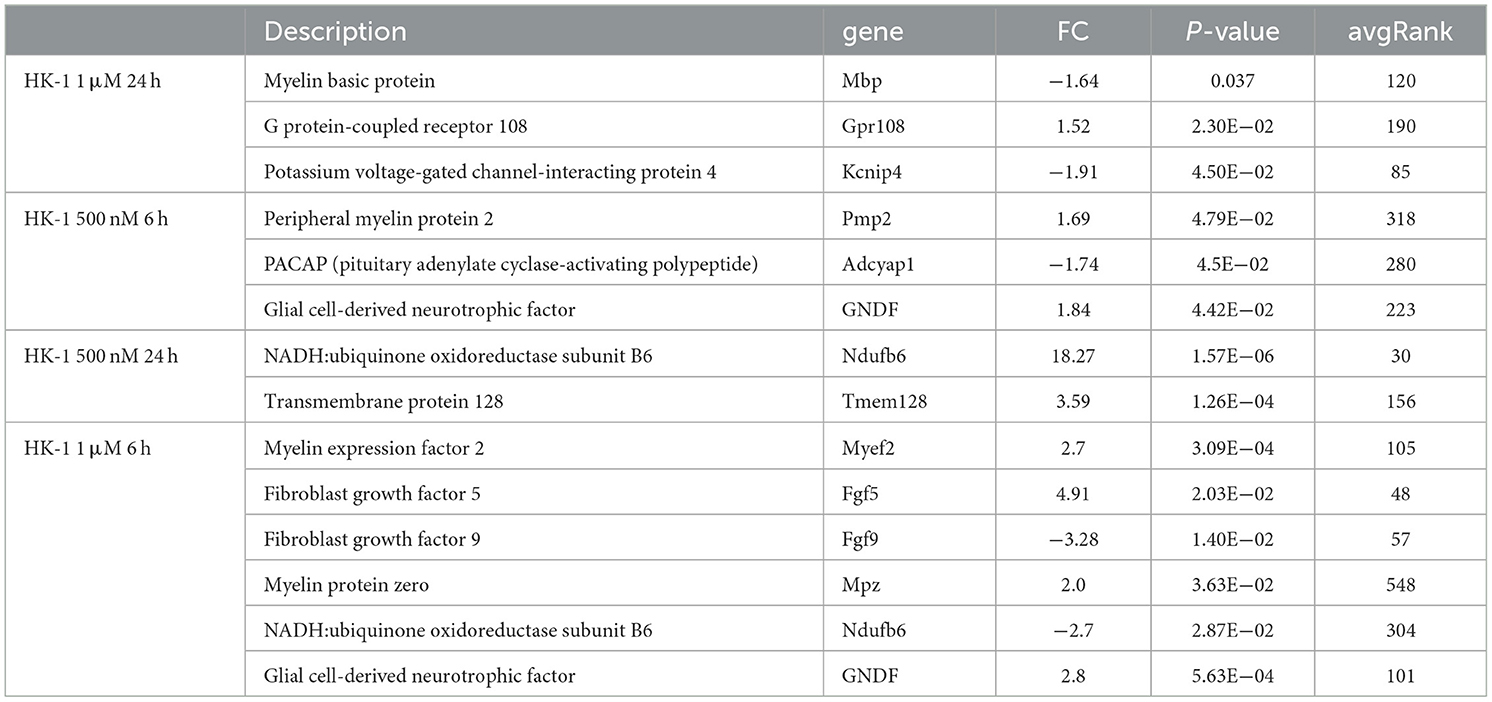
Table 4. FC, p, and avgRank values for other possible relevant and significant DE genes for all groups.
3.2. Potential targets for HK-1
The mRNA level of the Tacr3 receptor was detected around the detection limit, showing that low expression of Tacr1 and Tacr2 was not found in these primary cell cultures. This result was confirmed by earlier, unpublished findings with primary cell cultures from adult rat trigeminal ganglia showing similar expression patterns of these receptors. The TPM values of these receptors in different primary cultures are shown in Supplementary Table S3. No significant difference was detected between the HK-1-treated and control groups; the overlapping receptors are shown in Figure 2. To identify potential target molecules, we collected relevant receptors related to neural and/or inflammatory mechanisms based on different gene databases. Notably, the MAS-related G protein-coupled receptor, member B5 (Mrgprb5), was detected in all groups, however, with transcripts per million (TPM) below 2. Figure 3 shows receptors present in control primary sensory neuronal cultures at 6 h and 24 h. Abbreviations for receptors can be found in Supplementary Table S4. The expressions of the receptors were similar at 6 h and 24 h. Rack1, Ngfr, and Ednrb were detected at very high TPM at both sampling timepoints. Tnfrsf12a, Adipor1, and Adipor2 were also present in both concentrations. Ntrk1, P2rx3, and F2r were also expressed in both cases, highlighting the possible impact on pain transmission and calcium ion flow. Cxcr4 was found at 6 h. Figure 2 shows the DE receptors. Adipor2 at 500 nM 24 h was downregulated; F2r at 1 μM 6 h was, however, upregulated at 500 nM 24 h. Not only F2r but also Egfr expression was upregulated at 1 μM 6 h. An interesting result was the expression change in transient receptor potential melastatin 3,7,8 (Trpm3,7,8) cation channels at 500 nM 24 h. Supplementary Image I5 shows important receptors for all treatment conditions.
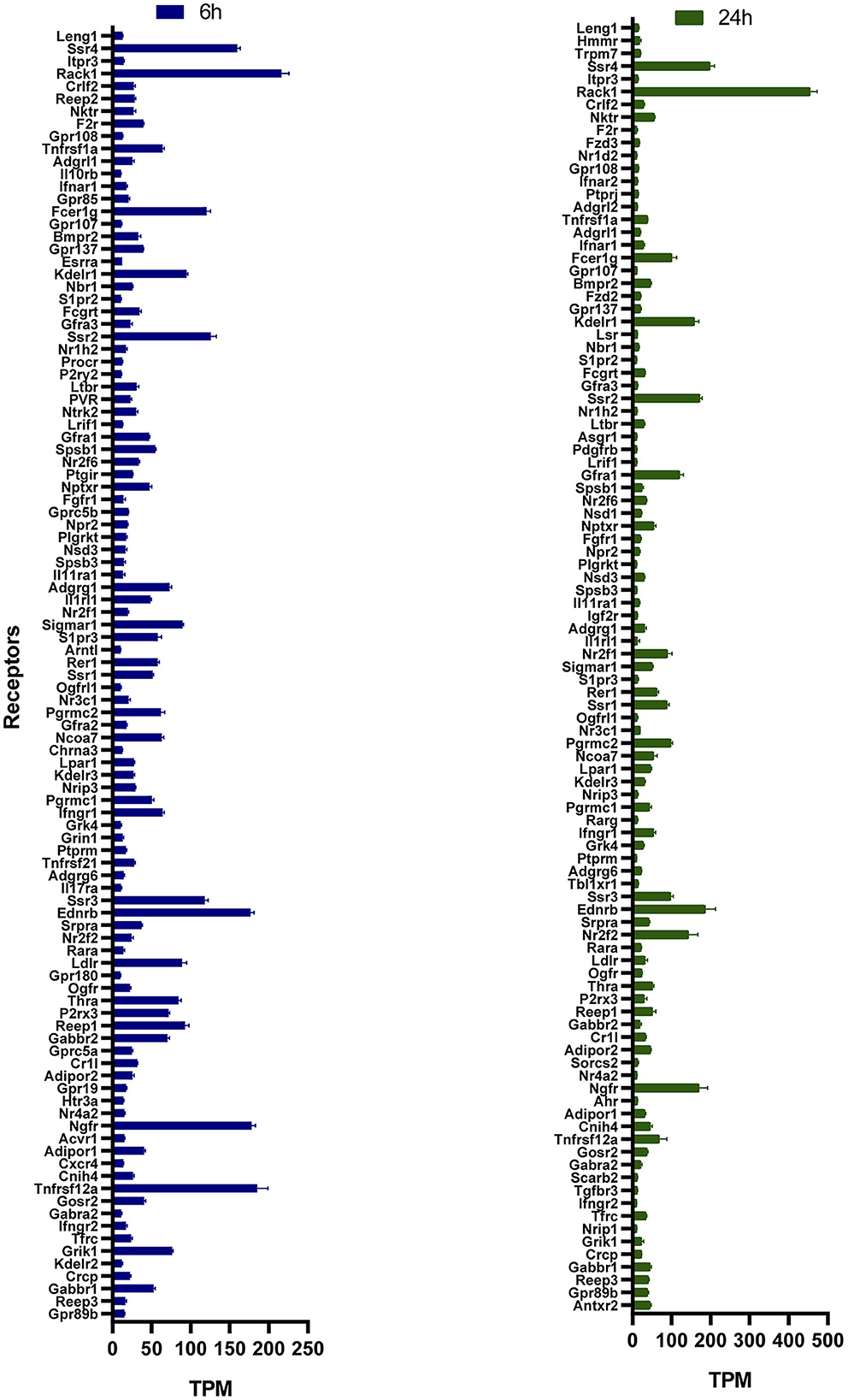
Figure 2. TPM values of receptors in control primary sensory neuronal cultures 6 h and 24 h. All data are shown in the Supplementary Image I1. Receptors having a TPM >10 with specific roles in neural mechanisms are demonstrated here. Functions of receptors were filtered based on public databases (https://rgd.mcw.edu/, https://www.genecards.org/, and https://www.ncbi.nlm.nih.gov).
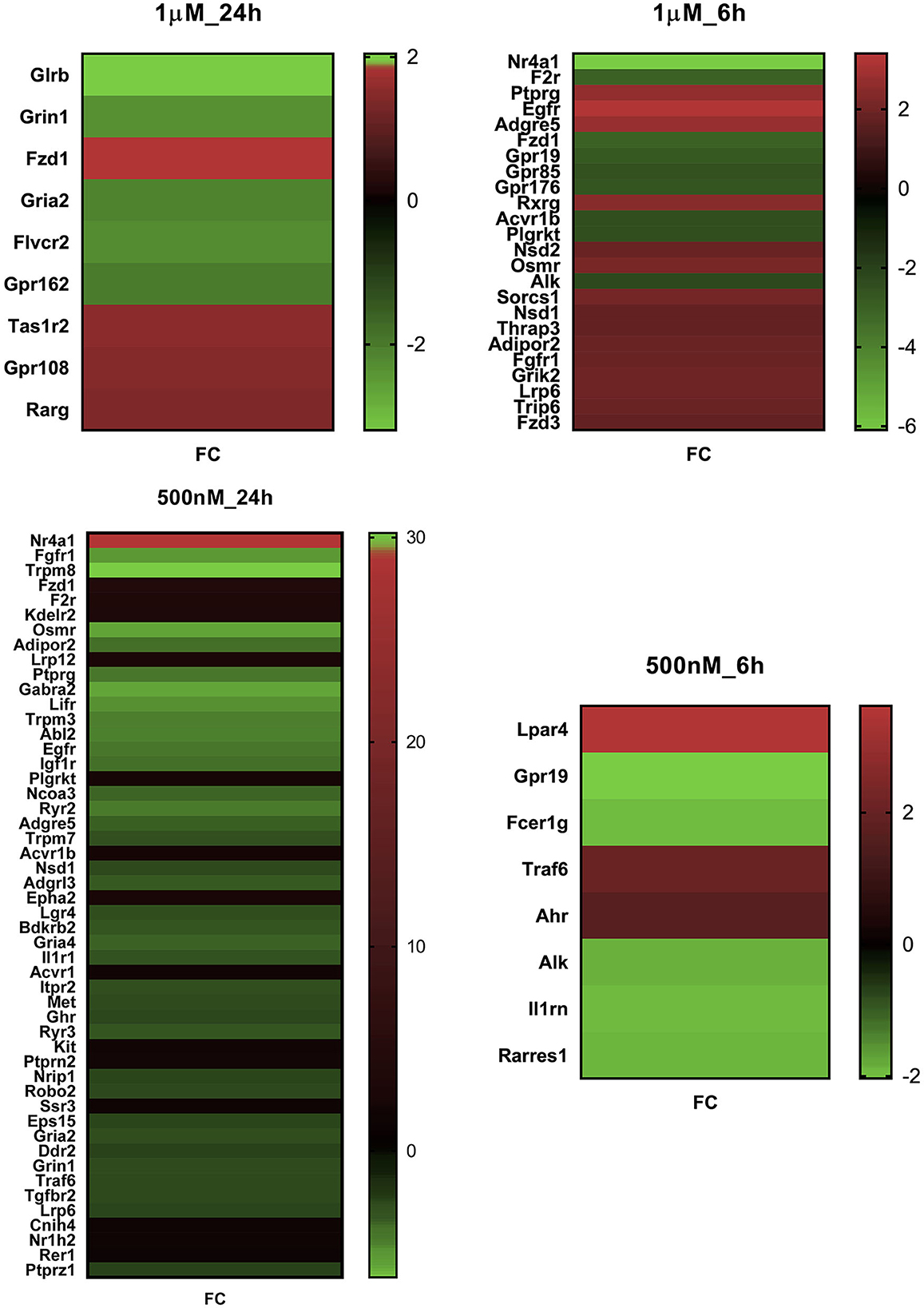
Figure 3. FC value of significant differentially expressed genes in primary sensory neuron cultures of the trigeminal ganglia in response to two HK-1 concentrations after 6 h and 24 h treatment durations. Abbreviations are shown in Supplementary Table S4. All DE genes can be found in Supplementary Images I2–I5.
3.3. Signaling pathways influenced by HK-1 treatments
Significantly altered pathways 6 h after 500 nM and 1 μM HK-1 treatment determined by the Kyoto Encyclopedia of Genes and Genomes (KEGG) database were predominantly related to calcium and Wnt signaling (Table 5). Figure 4 shows calcium signaling pathways with significant DE genes and represents the FC values of DE genes involved in this process. Among receptors, F2r had shown down-, Egfr upregulation. The transcriptomic level of Slc25a5 and Prkaca (protein kinase CAMP-activated catalytic subunit alpha) has changed negatively, and that of Gna11 (G protein subunit Alpha 11) and Prkacb (protein kinase cAMP-activated catalytic subunit beta) has changed positively.
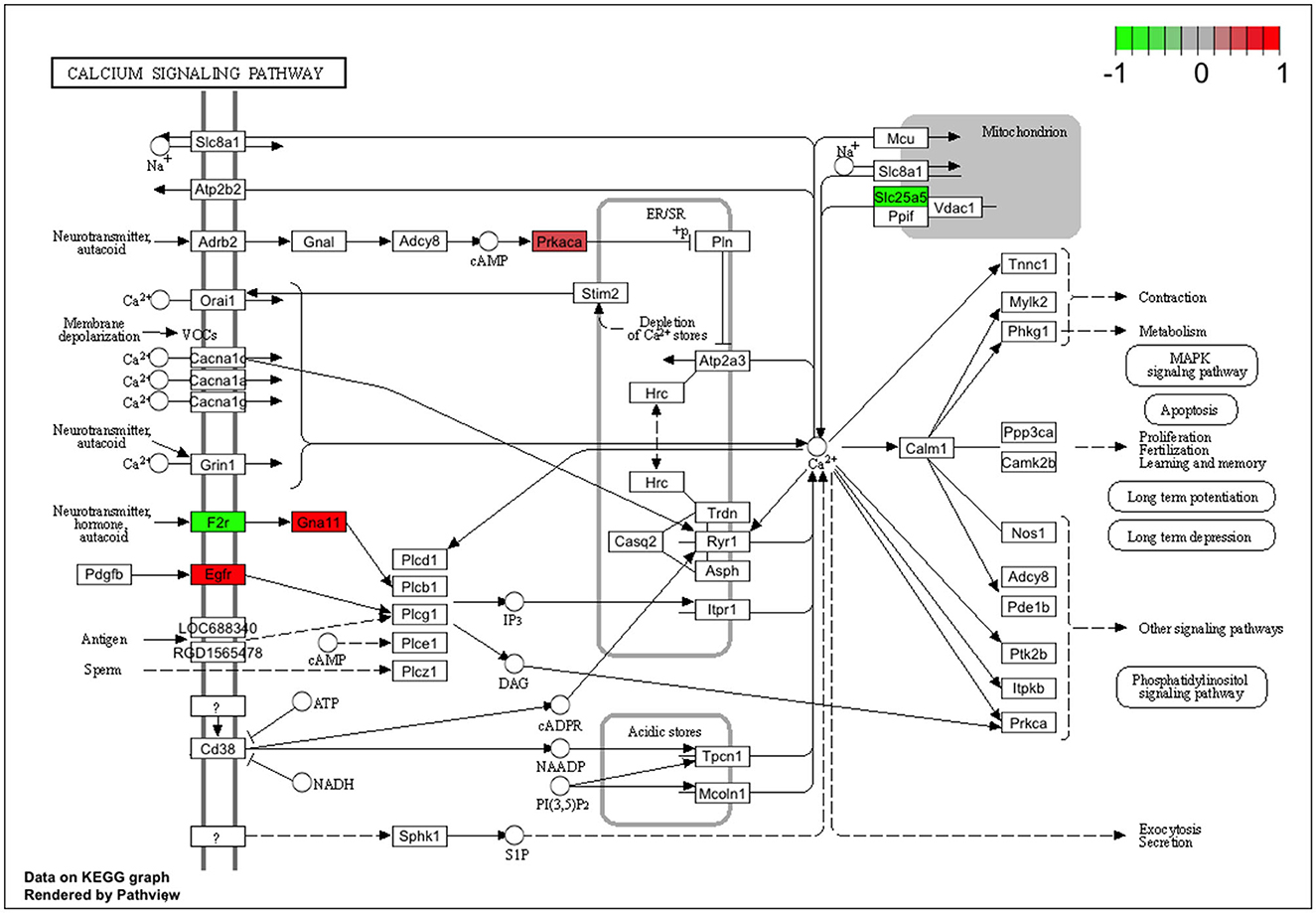
Figure 4. Genes involved in the calcium signaling pathway from the KEGG database. Colored rectangle is for the altered expression of genes at 6 h of HK-1 1 μM. Color means Z-score calculated from fold change value; green means downregulated, and red means upregulated.
The Gene Ontology (GO) database provides information on the functions of genes. Tables 6A–D shows the collected GO terms for all treated groups, simplified with the Revigo tool. Table 6A shows GO results for the HK-1 1 μM 6 h group. Protein kinase inhibitor activity, protein kinase A regulatory subunit binding, and thyroid hormone receptor binding are present in this list. Synaptic cleft was found to be a significant GO term in the 6 h results independent of the HK-1 concentration. Interestingly, biological processes altered by 500 nM HK-1 at 6 h (Table 6B) included the adenylate cyclase-activating G protein-coupled receptor signaling pathway, positive regulation of T cell-mediated immunity, neutrophil chemotaxis, positive regulation of leukocyte adhesion to vascular endothelial cells, and highlighting possible immunological functions. Notable cellular components were the synaptic cleft, T-tubule, and myelin sheath, and remarkable molecular functions were adrenergic receptor binding, NADH dehydrogenase activity, and cAMP binding. GO results revealed that 1 μM HK-1 treatment significantly altered the glutamate and insulin receptor signaling pathways at the 24-h timepoint, which are intracellular responses to calcium ions, as shown in Table 6C. A notable affected cellular component was the synaptic vesicle. Among modified molecular functions, we found ligand-gated ion channel activity, postsynaptic neurotransmitter receptor activity, and sodium channel activity (Table 6C). Meanwhile, 500 nM HK-1 at the same timepoint regulated presynapse assembly, cGMP-mediated signaling, mitochondrial ATP synthesis-coupled electron transport, Schwann cell proliferation, cell fate specification, positive regulation of dendritic spine development, positive regulation of cold-induced thermogenesis, and ceramide biosynthetic processes. As significant molecular functions, palmitoyltransferase activity, fibroblast growth factor binding, oxidoreductase activity, and acting on peroxide as an acceptor were found (Table 6D).
3.4. HK-1-induced signaling pathways
Table 7 shows results gained from the pathway database Reactome with DE genes for HK-1 1 μM 6 h, 500 nM 6 h, and 24 h groups. There was no significant finding for the HK-1 1 μM 24 h group. Noteworthy terms for HK-1 1 μM 6 h were apoptosis, signal amplification, programmed cell death, G alpha (s) signaling events, chaperone-mediated autophagy, and opioid signaling. ADP signaling through P2Y purinoreceptor 12 and opioid signaling were also important results for HK-1 500 nM 6 h group. Additional relevant terms for HK-1 500 nM 6 h treatment group were oxidative stress-induced senescence and GABA receptor activation. Common results for two concentrations at 6 h are the G alpha (s) signaling events, showing potential concentration- and calcium influx-independent effects of HK-1. Moreover, GPCR, GPCR ligand binding, and glycosphingolipid metabolism are important terms for HK-1 500 nM 6 h.
4. Discussion
We demonstrate here the first transcriptomic data on the signaling pathways of HK-1 in rat primary sensory neurons, focusing on potential HK-1 targets, mechanisms of action, and DE genes involved in pain transmission and inflammation. The effect of HK-1 is complex, concentration dependent, and time dependent. Our findings support the well-described nociceptive actions of HK-1 and might explain the divergent neuronal activation processes. Since HK-1 is likely to act both on the primary sensory neurons and satellite glia cells, in this study we extract knowledge on these interactions to mimic in vivo conditions (Sakai et al., 2012; Theoharides et al., 2019).
Peripheral inflammatory and neuroinflammatory mechanisms resulting from complex interactions of immune cells, glial cells, and neurons play crucial roles in several diseases, including chronic pain (Siiskonen and Harvima, 2019). Itga4 and Antxr2 were upregulated for both concentrations, Tenm3 for 1 μM and Cxcl9 was downregulated after 500 nM at both timepoints. Itga4 encodes CD49d (alpha 4), one chain of very late antigen 4 (VLA-4), which belongs to adhesive molecules that activate the inflammatory process by facilitating the migration of immune cells into the central nervous system. The role of VLA-4 in genetic predisposition to chronic neuroinflammatory diseases has been demonstrated by several studies (Andreoli et al., 2007; Odoherty et al., 2007; Correia et al., 2009), supporting our findings in sensory neurons. Anthrax toxin components binding to ANTXR2, highly expressed on primary sensory neurons, were shown to inhibit inflammatory and neuropathic mechanical and thermal hyperalgesia (Yang et al., 2022). Tenm3 encodes a transmembrane protein also related to pain (Rouillard et al., 2016). Chemokines (CXCL4, CXCL9, CXCL10, and CXCL11) are important nociceptive mediators produced by neurons, microglia, and/or astroglia (Colvin et al., 2004). The CXCR3 receptor is expressed predominantly on T cells activated by CXCL9, CXCL10, and CXCL11 (Ransohoff, 2009). A CXCR3 receptor antagonist was recently shown to inhibit glia activation and neuropathic pain and enhance the effectiveness of morphine (Piotrowska et al., 2018). We showed the upregulation of Prss12, Mal, and Mag, but the downregulation of the Nav beta subunit 4 (Scn4b) 6 h after both HK-1 concentrations. Motopsyn/Prss12 can activate the PAR receptor in astrocytes and trigger glutamate release to activate neuronal NMDA receptors (Lee et al., 2007; Wójtowicz et al., 2015). MAL is a protein predominantly expressed by oligodendrocytes and Schwann cells and inhibits peripheral nerve myelination (Buser et al., 2009). Voltage-gated sodium channels (Nav) initiate the action potential in excitable cells, and their mutations are implicated in chronic pain (Namadurai et al., 2015).
Figure 5 represents a summary of DE genes in different conditions. Both HK-1 concentrations resulted in more downregulated than upregulated genes at 24 h. Rph3a, Gabra2, Ryr2, Mag, and Scn1a were downregulated, while Hacd2 was upregulated. Hacd2 plays an important role in long-chain fatty acid biogenesis involved in neuronal membrane functions. Tachykinin receptors interact with Ryr2 in the endoplasmic reticulum, releasing calcium (Lin et al., 2005). The interaction of Rph3A with the NMDA receptor in hippocampal neurons plays a crucial role in synaptic retention and long-term potentiation. Moreover, Rph3A can also interact with AMPA receptors (Franchini et al., 2022). Notably, we have found remarkable upregulation of Fzd1 expressed by astrocytes and involved in their cross-talks (L'Episcopo et al., 2011, 2018). Increased levels of myelin-associated proteins, including Mag and Mbp proteins, were demonstrated through Activin A oligodendroglial ACVR1B-mediated white matter remyelination after ischemic stroke in mice (Zheng et al., 2021).
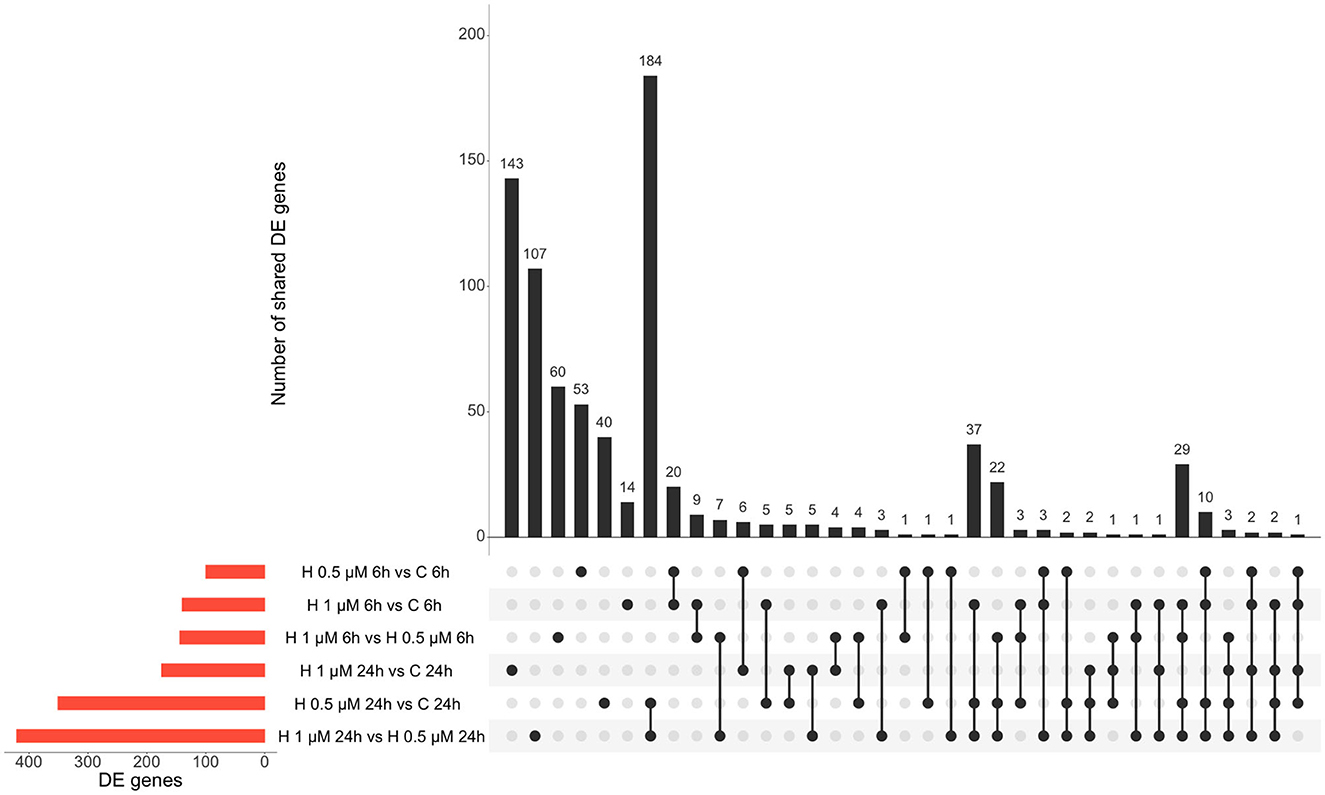
Figure 5. Summary of DE genes found in different treatment conditions: red columns refer to the respective group comparisons and the black columns demonstrate the shared DE genes of two or more groups. H: HK-1.
One of the most interesting issues is assigning potential targets for HK-1. Interestingly, the Tacr genes encoding tachykinin receptors were expressed around the detection limit in the primary sensory neuron cultures. These results are similar to the dorsal root ganglia data from Linnarson and co-workers (http://linnarssonlab.org/drg/). In human primary sensory neurons, Tac receptors were also detected at a low level (LaPaglia et al., 2018). Tachykinin receptors with three NK1R isoforms are expressed in primary sensory neurons of the rat trigeminal system (Beaujouan et al., 1999, 2002; Page, 2005; Garcia-Recio and Gascón, 2015; Edvinsson et al., 2021), which we could not detect by our sequencing technique in the cultures derived from neonatal rats. The lack of a functionally active NK1 receptor in our system is supported by no calcium influx in response to SP treatment (Borbély et al., 2020).
HK-1 at 1 μM induced calcium signaling-related transcriptomic alterations 6 h later, which is supported by our earlier fluorescent calcium influx data, which was not inhibited either by the NK1 receptor antagonist CP99994 or NK1R deletion (Borbély et al., 2020). In calcium signaling processes, F2r and EGFR receptors were DEs (Figures 6, 7). F2r/PAR1 was downregulated, while the EGFR was upregulated. NK1R signaling by SP can activate tyrosine kinase receptors such as EGFR, and PAR1 can activate EGFR (Castagliuolo et al., 2000; Arora et al., 2008). This is supported by our earlier in vivo findings that in HK-1-deficient mice, the PAR agonist mast cell tryptase-induced arthritic pain was decreased (Borbély et al., 2020). Mast cells were shown to be involved in fibromyalgia-related pain (Theoharides et al., 2019). Interestingly, Nr4a1 was downregulated 6 h after 1 μM and upregulated 24 h after 500 nM HK-1. Nr4a1 is an orphan nuclear receptor (Safe et al., 2016) involved in innate and adaptive immune responses in leukocytes (Shaked et al., 2015), which is downregulated and inactivated during neuroinflammation (Palumbo-Zerr et al., 2015; Shaked et al., 2015; Xiong et al., 2020). NR4a1 suppresses inflammatory responses driven by interferon (IFN) and nuclear factor kappa-light-chain-enhancer of activated B cells (NF-κB) signaling (Freire and Conneely, 2018) and microglia polarization (Rothe et al., 2017). Calcium influx might inhibit CXCL9 production and consequently decrease T cell activation (Mishra and Lal, 2021). Downregulated Slc25a5 might lead to mitochondrial dysfunction (Babenko et al., 2018), which is involved in several chronic pain conditions (Meeus et al., 2013; van den Ameele et al., 2020; Yousuf et al., 2020) related to reactive oxygen species generation and nociceptor sensitization (Salvemini et al., 2011). Intracellular calcium homeostasis, regulated mainly by the mitochondria, endoplasmic reticulum, and nucleus, determines neuronal excitability. Nuclear calcium sensor 1 (NCS-1) was suggested to modulate the expression of mitochondrial genes (Simons et al., 2019) together with the ATP sensor transporter Slc25a5 (Zhang et al., 2009). Dysfunction of Grin1, an NMDA receptor subunit, has been associated with several neurological disorders (Intson et al., 2019). Gria 2 is an AMPA receptor subunit having a major role in calcium permeation and voltage rectification; its abnormal functions also play a role in neural pathophysiologies (Salpietro et al., 2019). Downregulation of Grin1 and Gria 2 might decrease calcium concentration after a longer time, influencing neuronal survival (Guo and Ma, 2021). Several TRP channels are involved in sensory functions (Nilius et al., 2003; Voets et al., 2004, 2005; Nilius and Sage, 2005), and their expression or functional changes alter neuronal responsiveness (Nilius and Owsianik, 2011). TRPM 3,7,8 were downregulated 24 h after 500 nM HK-1, which is the opposite of what we earlier found in response to another sensory neuropeptide, PACAP (Takács-Lovász et al., 2022). Notably, PACAP was downregulated 6 h after 500 nM HK-1, suggesting a potential link between the effects of these two peptides on TRP channel expression. The mitochondrial complex I Ndufb6 subunit was significantly downregulated, similar to PACAP (Takács-Lovász et al., 2022). Figure 6 demonstrates these findings in network.
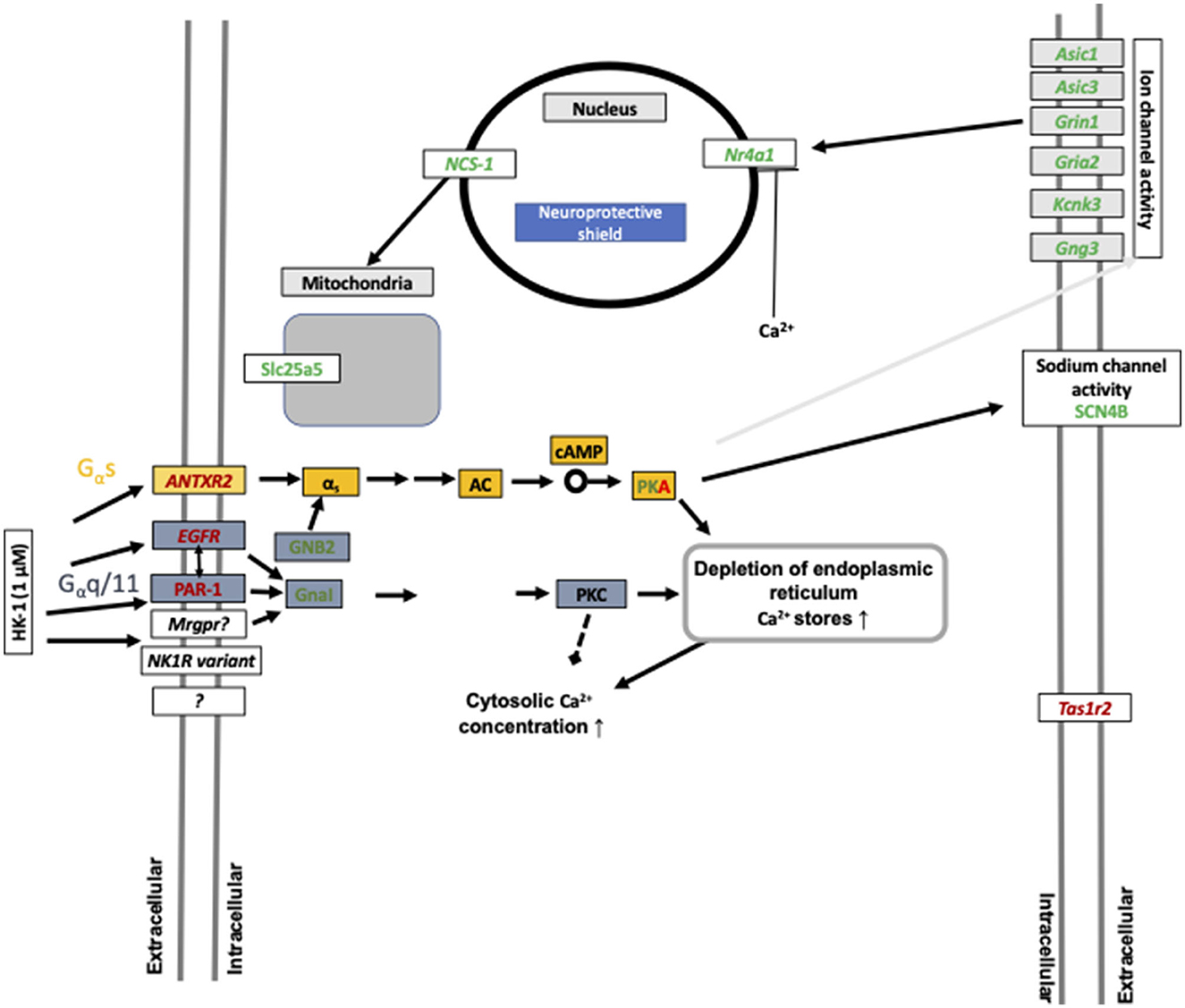
Figure 6. Summary of calcium influx-related gene expression changes after 1 μM HK-1 treatment. Red means upregulated, green means downregulated genes, and the gray arrow shows the 24-h effect. Protein kinase A (PKA) was up and downregulated in different concentrations. Yellow rectangular means G α s signaling, and blue means G α q/11 signaling. Gray rectangular demonstrates 24 h result. Abbreviations: G protein subunit alpha I1 (GnaI), G protein subunit beta 2 (GNB2), acid-sensing ion channel subunit 1,3 (Asic1; Asic3), potassium two pore domain channel subfamily K member 3 (Kcnk3), taste 1 receptor member (Tas1r2), adenylyl cyclase (AC), cyclic adenosine monophosphate (cAMP), G protein subunit gamma 3 (Gng3), and sodium voltage-gated channel beta subunit 4 (SCN4B) having a potential role in nociceptive sensation.
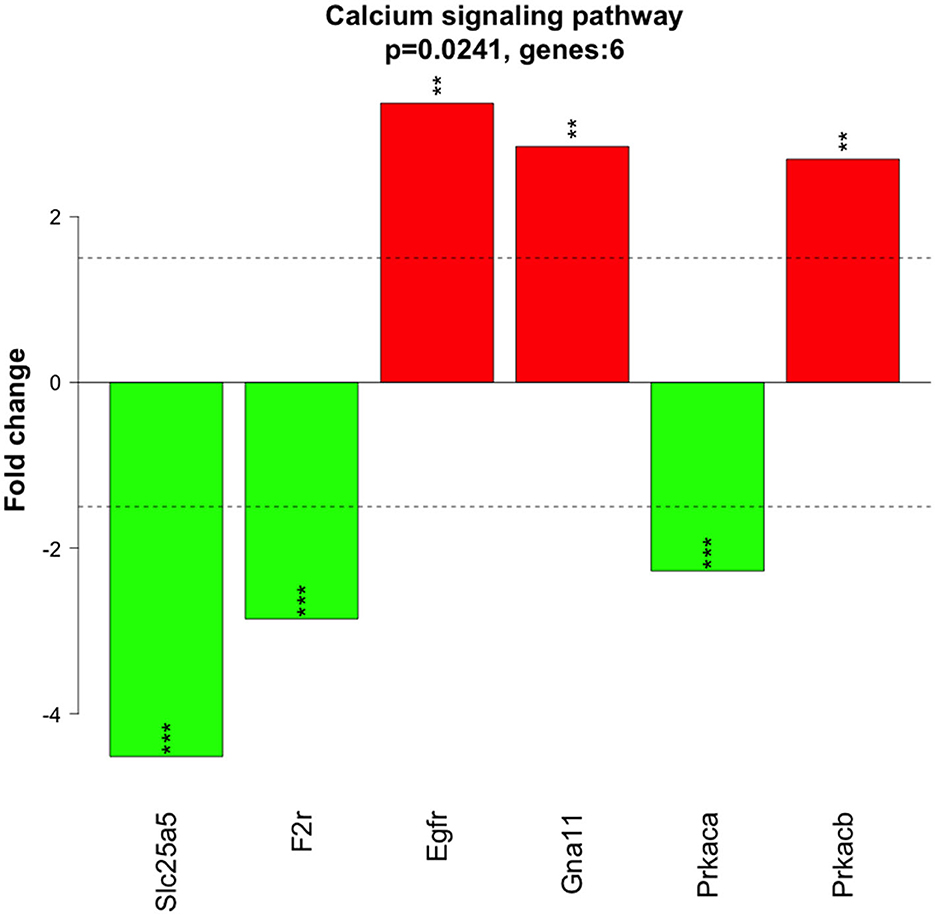
Figure 7. FC value of significant DE genes at 6 h of HK-1 1 μM involved in calcium signaling pathways. Red means upregulated genes and green shows downregulated genes. Abbreviations can be found in the Supplementary material. Asterisks denote these genes were found significant DE genes (*p < 0,05; **p < 0,01; ***p < 0,001) as analyzed by moderated t-test.
Our previous in vivo data demonstrated the pain-mediating role of HK-1 in several acute and chronic orofacial, neuropathic, and inflammatory pain models (partial sciatic nerve ligation, acute, and chronic CFA and mast cell tryptase-induced, as well as K/BxN serum-transfer arthritis models) (Borbély et al., 2013, 2020; Aczél et al., 2018; Hunyady et al., 2019). Although it is difficult to directly compare the present results with in vivo conditions, several similarities can be determined between gene expressions in the mouse TRG after adjuvant-induced orofacial inflammation and the present TRG culture experiment following HK-1 treatment. We earlier showed that the HK-1 encoding Tac4 gene was upregulated in the TRG in the adjuvant-induced model. Meanwhile, fibroblast growth factor 13 (Fgf13, also termed as glia activating factor) was downregulated in wild-type mice, but upregulated in HK-1-deficient ones (Aczél et al., 2020). This well correlates with the Fgf9 downregulation we observed in the present study in response to 1 μM 6 h later. Fgfs are involved in neuron–glia interactions and glia proliferation, mediating neuroinflammatory mechanisms (Stork et al., 2014). Furthermore, an orphan G protein-coupled receptor, Gpr62, playing a role in axo-myelinic signaling, was upregulated in the TRG of adjuvant-treated wild-type mice (Hay et al., 2021) similar to Gpr108 upregulation after 1 μM HK-1 in the present experiment to inhibit Toll-like receptor-triggered inflammatory responses (Dong et al., 2018). The voltage-gated K+ channel-interacting protein 9 (Kcnj9) was also downregulated in the TRG after adjuvant injection (Aczél et al., 2020), which supports our findings on the Kcnip4 downregulations in the present model. Inward-rectifying K+ currents have been shown to change in the trigeminal ganglia during peripheral inflammation (Takeda et al., 2011). Transmembrane protein 100 (Tmem100) was upregulated in the TRG in the in vivo mouse model, while in our cell system the expressions of related members of this protein family, Tmem128, significantly increased. Integrin subunit alpha 7 (Itga7) involved in glia proliferation (Tan et al., 2022), was also downregulated both in the mouse model (Aczél et al., 2020) and in the present cell culture study Itgav both are expressed on glial cells (Mapps et al., 2022). RNA-Seq literature data demonstrated Kcnj9, F2rl2 (PAR3) upregulation in the DRG in a neuropathic pain model similar to what we found for F2r and PAR1 (Stevens et al., 2020). Validation of the RNA sequencing data with qPCR showed a good correlation for all investigated genes except Fgfr1. However, such discrepancies are not unusual based on the literature data; others described that these two techniques provide similar results in 87.9% (Protasio et al., 2013) and 95.2% of genes (Liu et al., 2014), which is in agreement with the present findings.
In conclusion, this is the first approach to determining transcriptomic alterations induced by HK-1 in primary sensory neurons that might be related to their activation mechanisms and pain. We showed concentration- and time-dependent actions and identified potential pain-related processes such as microglial–neuron or GPCR interactions, myelin-associated gene expression, and orphan GPCRs having roles in GABAergic pathways by modifying NMDA and AMPA receptor subunits, demonstrating that not just neurons, but mast cells and glia cells might be affected by HK-1. The effects specific to the 1 μM (sodium channel activity, neurotransmitter receptor activity, cytokine activity) were potentially calcium influx-dependent, as we earlier showed that the 1 μM, but not the 500 nM concentration, induced a calcium signal in this experimental paradigm. Although the target(s) of HK-1 cannot be determined on the basis of the present results, it is not likely to be the NK1 tachykinin receptor. Identifying the receptor might open novel perspectives in analgesic research.
Data availability statement
The datasets presented in this study can be found in online repositories. The names of the repository/repositories and accession number(s) can be at: https://www.ebi.ac.uk/ena, PRJEB47291.
Ethics statement
The animal studies were approved by the Ethical Committee on Use of Laboratory Animals at the University of Pécs (permission no: BA02/2000-51/2017). The studies were conducted in accordance with the local legislation and institutional requirements. Written informed consent was obtained from the owners for the participation of their animals in this study.
Author contributions
ÉB, ÉS, KB, and ZH: conceptualization. TA and KB: methodology. JK: software. JK, KT-L, LC, and PU: validation. KT-L and JK: formal analysis, data curation, and visualization. ZH: investigation and supervision. AG and ZH: resources and funding acquisition. KT-L: writing—original draft preparation. KT-L, ÉB, ÉS, KB, and ZH: writing—review and editing. All authors contributed to the article and approved the submitted version.
Funding
This research was supported by the National Brain Research Program 3.0 (NAP-3; Chronic Pain Research Group) and the National Research Development and Innovation Office grants OTKA FK132587 and FK137951. AG and JK were supported by the grants GINOP-2.3.4-15-2020-00010, GINOP-2.3.1-20-2020-00001, and Educating Experts of the Future: Developing Bioinformatics and Biostatistics Competencies of European Biomedical Students (BECOMING, 2019-1-HU01-KA203-061251). Bioinformatics infrastructure was supported by ELIXIR Hungary (https://www.bioinformatics.hu/). Project no. TKP 2021-EGA-16 has been implemented with the support provided by the National Research, Development, and Innovation Fund of Hungary, financed under the EGA 16 funding scheme. The research was performed in collaboration with the Genomics and Bioinformatics Core Facility at the Szentágothai Research Centre of the University of Pécs. This project was supported by the National Research, Development, and Innovation Office (PharmaLab, RRF-2.3.1-21-2022-00015). RRF-2.3.1-21-2022-00015 has been implemented with the support of the European Union. ÉB was supported by the János Bolyai Research Scholarship of the Hungarian Academy of Sciences (BO/00592/19/5).
Acknowledgments
The authors wish to thank Cecília Disztl for expert technical assistance and the Genomics and Bioinformatics Core Facility at the Szentágothai Research Centre of the University of Pécs and the Eötvös Lóránd Research Network (ELKH-PTE changed to HUN-REN Eötvös Lóránd Research Network to Hungarian Research Network Chronic Pain Research Group). The authors wish to thank Prof. Dr. Balázs Gyorffy and his Oncological Biomarker Research Group, ELKH Research Centre for Natural Sciences, for their contribution to the development of the bioinformatics pipelines.
Conflict of interest
ZH was employed by the company PharmInVivo Ltd.
The remaining authors declare that the research was conducted in the absence of any commercial or financial relationships that could be construed as a potential conflict of interest.
Publisher's note
All claims expressed in this article are solely those of the authors and do not necessarily represent those of their affiliated organizations, or those of the publisher, the editors and the reviewers. Any product that may be evaluated in this article, or claim that may be made by its manufacturer, is not guaranteed or endorsed by the publisher.
Supplementary material
The Supplementary Material for this article can be found online at: https://www.frontiersin.org/articles/10.3389/fnmol.2023.1186279/full#supplementary-material
References
Aczél, T., Kecskés, A., Kun, J., Szenthe, K., Bánáti, F., Szathmary, S., et al. (2020). Hemokinin-1 gene expression is upregulated in trigeminal ganglia in an inflammatory orofacial pain model: potential role in peripheral sensitization. IJMS 21, 2938. doi: 10.3390/ijms21082938
Aczél, T., Kun, J., Szoke, É., Rauch, T., Junttila, S., Gyenesei, A., et al. (2018). Transcriptional alterations in the trigeminal ganglia, nucleus and peripheral blood mononuclear cells in a rat orofacial pain model. Front. Mol. Neurosci. 11, 219. doi: 10.3389/fnmol.2018.00219
Alkanfari, I., Gupta, K., Jahan, T., and Ali, H. (2018). Naturally occurring missense MRGPRX2 variants display loss of function phenotype for mast cell degranulation in response to substance P, hemokinin-1, human β-defensin-3, and icatibant. J. Immunol. 201, 343–349. doi: 10.4049/jimmunol.1701793
Anders, S., Pyl, P. T., and Huber, W. (2015). HTSeq–a Python framework to work with high-throughput sequencing data. Bioinformatics 31, 166–169. doi: 10.1093/bioinformatics/btu638
Andreoli, V., Cittadella, R., Valentino, P., Condino, F., Larussa, A., Liguori, M., et al. (2007). The role of VLA4 polymorphisms in multiple sclerosis: an association study. J. Neuroimmunol. 189, 125–128. doi: 10.1016/j.jneuroim.2007.06.015
Arora, P., Cuevas, B. D., Russo, A., Johnson, G. L., and Trejo, J. (2008). Persistent transactivation of EGFR and ErbB2/HER2 by protease-activated receptor-1 promotes breast carcinoma cell invasion. Oncogene 27, 4434–4445. doi: 10.1038/onc.2008.84
Babenko, V. N., Smagin, D. A., Galyamina, A. G., Kovalenko, I. L., and Kudryavtseva, N. N. (2018). Altered Slc25 family gene expression as markers of mitochondrial dysfunction in brain regions under experimental mixed anxiety/depression-like disorder. BMC Neurosci. 19, 79. doi: 10.1186/s12868-018-0480-6
Beaujouan, J.-C., Saffroy, M., Torrens, Y., and Glowinski, J. (2002). Different subtypes of tachykinin NK1 receptor binding sites are present in the rat brain. J. Neurochem. 75, 1015–1026. doi: 10.1046/j.1471-4159.2000.0751015.x
Beaujouan, J.-C., Saffroy, M., Torrens, Y., Sagan, S., and Glowinski, J. (1999). Pharmacological characterization of tachykinin septide-sensitive binding sites in the rat submaxillary gland. Peptides 20, 1347–1352. doi: 10.1016/S0196-9781(99)00140-0
Borbély, É., Hajna, Z., Sándor, K., Kereskai, L., Tóth, I., Pintér, E., et al. (2013). Role of tachykinin 1 and 4 gene-derived neuropeptides and the neurokinin 1 receptor in adjuvant-induced chronic arthritis of the mouse. PLoS ONE 8, e61684. doi: 10.1371/journal.pone.0061684
Borbély, É., and Helyes, Z. (2017). Role of hemokinin-1 in health and disease. Neuropeptides 64, 9–17. doi: 10.1016/j.npep.2016.12.003
Borbély, É., Hunyady, Á., Pohóczky, K., Payrits, M., Botz, B., Mócsai, A., et al. (2020). Hemokinin-1 as a mediator of arthritis-related pain via direct activation of primary sensory neurons. Front. Pharmacol. 11, 594479. doi: 10.3389/fphar.2020.594479
Buser, A. M., Schmid, D., Kern, F., Erne, B., Lazzati, T., and Schaeren-Wiemers, N. (2009). The myelin protein MAL affects peripheral nerve myelination: a new player influencing p75 neurotrophin receptor expression. Eur. J. Neurosci. 29, 2276–2290. doi: 10.1111/j.1460-9568.2009.06785.x
Castagliuolo, I., Valenick, L., Liu, J., and Pothoulakis, C. (2000). Epidermal growth factor receptor transactivation mediates substance p-induced mitogenic responses in U-373 MG cells. J. Biol. Chem. 275, 26545–26550. doi: 10.1074/jbc.M003990200
Colvin, R. A., Campanella, G. S. V., Sun, J., and Luster, A. D. (2004). Intracellular domains of CXCR3 that mediate CXCL9, CXCL10, and CXCL11 function. J. Biol. Chem. 279, 30219–30227. doi: 10.1074/jbc.M403595200
Correia, C., Coutinho, A. M., Almeida, J., Lontro, R., Lobo, C., Miguel, T. S., et al. (2009). Association of the α4 integrin subunit gene (ITGA4) with autism. Am. J. Med. Genet. 150B, 1147–1151. doi: 10.1002/ajmg.b.30940
Dobin, A., Davis, C. A., Schlesinger, F., Drenkow, J., Zaleski, C., Jha, S., et al. (2013). STAR: ultrafast universal RNA-seq aligner. Bioinformatics 29, 15–21. doi: 10.1093/bioinformatics/bts635
Dong, D., Zhou, H., Na, S.-Y., Niedra, R., Peng, Y., Wang, H., et al. (2018). GPR108, an NF-κB activator suppressed by TIRAP, negatively regulates TLR-triggered immune responses. PLoS ONE 13, e0205303. doi: 10.1371/journal.pone.0205303
Duffy, R. A., Hedrick, J. A., Randolph, G., Morgan, C. A., Cohen-Williams, M. E., Vassileva, G., et al. (2003). Centrally administered hemokinin-1 (HK-1), a neurokinin NK1 receptor agonist, produces substance P-like behavioral effects in mice and gerbils. Neuropharmacology 45, 242–250. doi: 10.1016/S0028-3908(03)00150-3
Edvinsson, J. C., Reducha, P. V., Sheykhzade, M., Warfvinge, K., Haanes, K. A., and Edvinsson, L. (2021). Neurokinins and their receptors in the rat trigeminal system: differential localization and release with implications for migraine pain. Mol. Pain 17, 174480692110594. doi: 10.1177/17448069211059400
Fang, Z., and Cui, X. (2011). Design and validation issues in RNA-seq experiments. Briefings in Bioinformatics 12, 280–287. doi: 10.1093/bib/bbr004
Franchini, L., Stanic, J., Barzasi, M., Zianni, E., Mauceri, D., Diluca, M., et al. (2022). Rabphilin-3A drives structural modifications of dendritic spines induced by long-term potentiation. Cells 11, 1616. doi: 10.3390/cells11101616
Freire, P. R., and Conneely, O. M. (2018). NR4A1 and NR4A3 restrict HSC proliferation via reciprocal regulation of C/EBPα and inflammatory signaling. Blood 131, 1081–1093. doi: 10.1182/blood-2017-07-795757
Fu, C. -Y., Kong, Z. -Q., Wang, K.- R., Yang, Q., Zhai, K., Chen, Q., et al. (2005). Effects and mechanisms of supraspinal administration of rat/mouse hemokinin-1, a mammalian tachykinin peptide, on nociception in mice. Brain Res. 1056, 51–58. doi: 10.1016/j.brainres.2005.07.020
Garcia-Recio, S., and Gascón, P. (2015). Biological and pharmacological aspects of the NK1-receptor. Bio.Med. Res. Int. 2015, 1–14. doi: 10.1155/2015/495704
Guo, C., and Ma, Y.-Y. (2021). Calcium permeable-AMPA receptors and excitotoxicity in neurological disorders. Front. Neural. Circuits 15, 711564. doi: 10.3389/fncir.2021.711564
Hay, C. M., Jackson, S., Mitew, S., Scott, D. J., Koenning, M., Bensen, A. L., et al. (2021). The oligodendrocyte-enriched orphan G protein-coupled receptor Gpr62 is dispensable for central nervous system myelination. Neural. Dev. 16, 6. doi: 10.1186/s13064-021-00156-y
Hunyady, Á., Hajna, Z., Gubányi, T., Scheich, B., Kemény, Á., Gaszner, B., et al. (2019). Hemokinin-1 is an important mediator of pain in mouse models of neuropathic and inflammatory mechanisms. Brain Res. Bullet. 147, 165–173. doi: 10.1016/j.brainresbull.2019.01.015
Intson, K., van Eede, M. C., Islam, R., Milenkovic, M., Yan, Y., Salahpour, A., et al. (2019). Progressive neuroanatomical changes caused by Grin1 loss-of-function mutation. Neurobiol. Dis. 132, 104527. doi: 10.1016/j.nbd.2019.104527
Kage, R., McGregor, G. P., Thim, L., and Conlon, J. M. (1988). Neuropeptide-gamma: a peptide isolated from rabbit intestine that is derived from gamma-preprotachykinin. J. Neurochem. 50, 1412–1417. doi: 10.1111/j.1471-4159.1988.tb03024.x
Kurtz, M. M., Wang, R., Clements, M. K., Cascieri, M. A., Austin, C. P., Cunningham, B. R., et al. (2002). Identification, localization and receptor characterization of novel mammalian substance P-like peptides. Gene 296, 205–212. doi: 10.1016/S0378-1119(02)00861-2
LaPaglia, D. M., Sapio, M. R., Burbelo, P. D., Thierry-Mieg, J., Thierry-Mieg, D., Raithel, S. J., et al. (2018). RNA-Seq investigations of human post-mortem trigeminal ganglia. Cephalalgia 38, 912–932. doi: 10.1177/0333102417720216
Law, C. W., Chen, Y., Shi, W., and Smyth, G. K. (2014). voom: precision weights unlock linear model analysis tools for RNA-seq read counts. Genome Biol. 15, R29. doi: 10.1186/gb-2014-15-2-r29
Lee, C. J., Mannaioni, G., Yuan, H., Woo, D. H., Gingrich, M. B., and Traynelis, S. F. (2007). Astrocytic control of synaptic NMDA receptors: ASTROCYTIC CONTROL OF SYNAPTIc NMDA receptors. J. Physiol. 581, 1057–1081. doi: 10.1113/jphysiol.2007.130377
L'Episcopo, F., Serapide, M. F., Tirolo, C., Testa, N., Caniglia, S., Morale, M. C., et al. (2011). A Wnt1 regulated Frizzled-1/β-Cateninsignaling pathway as a candidate regulatory circuit controlling mesencephalic dopaminergic neuron-astrocyte crosstalk: therapeutical relevance for neuron survival and neuroprotection. Mol. Neurodegeneration 6, 49. doi: 10.1186/1750-1326-6-49
L'Episcopo, F., Tirolo, C., Serapide, M. F., Caniglia, S., Testa, N., Leggio, L., et al. (2018). Microglia polarization, gene-environment interactions and Wnt/β-Catenin signaling: emerging roles of glia-neuron and glia-stem/neuroprogenitor crosstalk for dopaminergic neurorestoration in aged parkinsonian brain. Front. Aging Neurosci. 10, 12. doi: 10.3389/fnagi.2018.00012
Lin, Y. -R., Kao, P. -C., and Chan, M.- H. (2005). Involvement of Ca2+ signaling in tachykinin-mediated contractile responses in swine trachea. J. Biomed. Sci. 12, 547–558. doi: 10.1007/s11373-005-6796-0
Liu, S., Gao, G., Palti, Y., Cleveland, B. M., Weber, G. M., and Rexroad, C. E. (2014). RNA-seq analysis of early hepatic response to handling and confinement stress in rainbow trout. PLoS ONE 9, e88492. doi: 10.1371/journal.pone.0088492
Livak, K. J., and Schmittgen, T. D. (2001). Analysis of relative gene expression data using real-time quantitative PCR and the 2–ΔΔCT method. Methods 25, 402–408. doi: 10.1006/meth.2001.1262
Luo, W., and Brouwer, C. (2013). Pathview: an R/Bioconductor package for pathway-based data integration and visualization. Bioinformatics 29, 1830–1831. doi: 10.1093/bioinformatics/btt285
Manorak, W., Idahosa, C., Gupta, K., Roy, S., Panettieri, R., and Ali, H. (2018). Upregulation of mas-related G protein coupled receptor X2 in asthmatic lung mast cells and its activation by the novel neuropeptide hemokinin-1. Respir. Res. 19, 1. doi: 10.1186/s12931-017-0698-3
Mapps, A. A., Thomsen, M. B., Boehm, E., Zhao, H., Hattar, S., and Kuruvilla, R. (2022). Diversity of satellite glia in sympathetic and sensory ganglia. Cell Rep. 38, 110328. doi: 10.1016/j.celrep.2022.110328
Meeus, M., Nijs, J., Hermans, L., Goubert, D., and Calders, P. (2013). The role of mitochondrial dysfunctions due to oxidative and nitrosative stress in the chronic pain or chronic fatigue syndromes and fibromyalgia patients: peripheral and central mechanisms as therapeutic targets? Exp. Opin. Ther. Target. 17, 1081–1089. doi: 10.1517/14728222.2013.818657
Mishra, A., and Lal, G. (2021). Neurokinin receptors and their implications in various autoimmune diseases. Curr. Res. Immunol. 2, 66–78. doi: 10.1016/j.crimmu.2021.06.001
Namadurai, S., Yereddi, N. R., Cusdin, F. S., Huang, C. L.-H., Chirgadze, D. Y., and Jackson, A. P. (2015). A new look at sodium channel β subunits. Open Biol. 5, 140192. doi: 10.1098/rsob.140192
Nilius, B., and Owsianik, G. (2011). The transient receptor potential family of ion channels. Genome Biol. 12, 218. doi: 10.1186/gb-2011-12-3-218
Nilius, B., Prenen, J., Droogmans, G., Voets, T., Vennekens, R., Freichel, M., et al. (2003). Voltage dependence of the Ca2+-activated cation channel TRPM4. J. Biol. Chem. 278, 30813–30820. doi: 10.1074/jbc.M305127200
Nilius, B., and Sage, S. O. (2005). TRP channels: novel gating properties and physiological functions. J. Physiol. 567, 33–34. doi: 10.1113/jphysiol.2005.093245
Odoherty, C., Roos, I., Antiguedad, A., Aransay, A., Hillert, J., and Vandenbroeck, K. (2007). ITGA4 polymorphisms and susceptibility to multiple sclerosis. J. Neuroimmunol. 189, 151–157. doi: 10.1016/j.jneuroim.2007.07.006
Page, N. M. (2005). New challenges in the study of the mammalian tachykinins. Peptides 26, 1356–1368. doi: 10.1016/j.peptides.2005.03.030
Palumbo-Zerr, K., Zerr, P., Distler, A., Fliehr, J., Mancuso, R., Huang, J., et al. (2015). Orphan nuclear receptor NR4A1 regulates transforming growth factor-β signaling and fibrosis. Nat. Med. 21, 150–158. doi: 10.1038/nm.3777
Piotrowska, A., Rojewska, E., Pawlik, K., Kreiner, G., Ciechanowska, A., Makuch, W., et al. (2018). Pharmacological blockade of CXCR3 by (±)-NBI-74330 reduces neuropathic pain and enhances opioid effectiveness - Evidence from in vivo and in vitro studies. BBA Mol. Basis Dis. 1864, 3418–3437. doi: 10.1016/j.bbadis.2018.07.032
Protasio, A. V., Dunne, D. W., and Berriman, M. (2013). Comparative study of transcriptome profiles of mechanical- and skin-transformed schistosoma mansoni schistosomula. PLoS Negl. Trop. Dis. 7, e2091. doi: 10.1371/journal.pntd.0002091
Ransohoff, R. M. (2009). Chemokines and chemokine receptors: standing at the crossroads of immunobiology and neurobiology. Immunity 31, 711–721. doi: 10.1016/j.immuni.2009.09.010
Ritchie, M. E., Phipson, B., Wu, D., Hu, Y., Law, C. W., Shi, W., et al. (2015). limma powers differential expression analyses for RNA-sequencing and microarray studies. Nucleic Acids Res. 43, e47. doi: 10.1093/nar/gkv007
Robinson, M. D., McCarthy, D. J., and Smyth, G. K. (2010). edgeR: a Bioconductor package for differential expression analysis of digital gene expression data. Bioinformatics 26, 139–140. doi: 10.1093/bioinformatics/btp616
Roth, K. A., Makk, G., Beck, O., Faull, K., Tatemoto, K., Evans, C. J., et al. (1985). Isolation and characterization of substance P, substance P 5-11, and substance K from two metastatic ileal carcinoids. Regul. Pept. 12, 185–199. doi: 10.1016/0167-0115(85)90060-6
Rothe, T., Ipseiz, N., Faas, M., Lang, S., Perez-Branguli, F., Metzger, D., et al. (2017). The nuclear receptor Nr4a1 acts as a microglia rheostat and serves as a therapeutic target in autoimmune-driven central nervous system inflammation. J. Immunol. 198, 3878–3885. doi: 10.4049/jimmunol.1600638
Rouillard, A. D., Gundersen, G. W., Fernandez, N. F., Wang, Z., Monteiro, C. D., McDermott, M. G., et al. (2016). The harmonizome: a collection of processed datasets gathered to serve and mine knowledge about genes and proteins. Database 2016, baw100. doi: 10.1093/database/baw100
Safe, S., Jin, U.-H., Morpurgo, B., Abudayyeh, A., Singh, M., and Tjalkens, R. B. (2016). Nuclear receptor 4A (NR4A) family – orphans no more. J. Steroid Biochem. Mol. Biol. 157, 48–60. doi: 10.1016/j.jsbmb.2015.04.016
Sakai, A., Takasu, K., Sawada, M., and Suzuki, H. (2012). Hemokinin-1 gene expression is upregulated in microglia activated by lipopolysaccharide through NF-κB and p38 MAPK signaling pathways. PLoS ONE 7, e32268. doi: 10.1371/journal.pone.0032268
Salpietro, V., Dixon, C. L., Guo, H., Bello, O. D., Vandrovcova, J., Efthymiou, S., et al. (2019). AMPA receptor GluA2 subunit defects are a cause of neurodevelopmental disorders. Nat.Commun. 10, 3094. doi: 10.1038/s41467-019-10910-w
Salvemini, D., Little, J. W., Doyle, T., and Neumann, W. L. (2011). Roles of reactive oxygen and nitrogen species in pain. Free Rad. Biol. Med. 51, 951–966. doi: 10.1016/j.freeradbiomed.2011.01.026
Satake, H., Aoyama, M., Sekiguchi, T., and Kawada, T. (2013). Insight into molecular and functional diversity of tachykinins and their receptors. PPL 20, 615–627. doi: 10.2174/0929866511320060002
Shaked, I., Hanna, R. N., Shaked, H., Chodaczek, G., Nowyhed, H. N., Tweet, G., et al. (2015). Transcription factor Nr4a1 couples sympathetic and inflammatory cues in CNS-recruited macrophages to limit neuroinflammation. Nat. Immunol 16, 1228–1234. doi: 10.1038/ni.3321
Siiskonen, H., and Harvima, I. (2019). Mast cells and sensory nerves contribute to neurogenic inflammation and pruritus in chronic skin inflammation. Front. Cell. Neurosci. 13, 422. doi: 10.3389/fncel.2019.00422
Simons, C., Benkert, J., Deuter, N., Poetschke, C., Pongs, O., Schneider, T., et al. (2019). NCS-1 deficiency affects mRNA levels of genes involved in regulation of ATP synthesis and mitochondrial stress in highly vulnerable substantia nigra dopaminergic neurons. Front. Mol. Neurosci. 12, 252. doi: 10.3389/fnmol.2019.00252
Steinhoff, M. S., von Mentzer, B., Geppetti, P., Pothoulakis, C., and Bunnett, N. W. (2014). Tachykinins and their receptors: contributions to physiological control and the mechanisms of disease. Physiol. Rev. 94, 265–301. doi: 10.1152/physrev.00031.2013
Stevens, A. M., Saleem, M., Deal, B., Janjic, J., and Pollock, J. A. (2020). Targeted cyclooxygenase-2 inhibiting nanomedicine results in pain-relief and differential expression of the RNA transcriptome in the dorsal root ganglia of injured male rats. Mol. Pain 16, 174480692094330. doi: 10.1177/1744806920943309
Stork, T., Sheehan, A., Tasdemir-Yilmaz, O. E., and Freeman, M. R. (2014). Neuron-glia interactions through the heartless FGF receptor signaling pathway mediate morphogenesis of drosophila astrocytes. Neuron 83, 388–403. doi: 10.1016/j.neuron.2014.06.026
Szoke, É., Balla, Z., Csernoch, L., Czéh, G., and Szolcsányi, J. (2000). Interacting effects of capsaicin and anandamide on intracellular calcium in sensory neurones. Neuro Rep. 11, 1949–1952. doi: 10.1097/00001756-200006260-00028
Takács-Lovász, K., Kun, J., Aczél, T., Urbán, P., Gyenesei, A., Bölcskei, K., et al. (2022). PACAP-38 induces transcriptomic changes in rat trigeminal ganglion cells related to neuroinflammation and altered mitochondrial function presumably via PAC1/VPAC2 receptor-independent mechanism. IJMS 23, 2120. doi: 10.3390/ijms23042120
Takeda, M., Takahashi, M., Nasu, M., and Matsumoto, S. (2011). Peripheral inflammation suppresses inward rectifying potassium currents of satellite glial cells in the trigeminal ganglia. Pain 152, 2147–2156. doi: 10.1016/j.pain.2011.05.023
Tan, Z., Zhang, Z., Yu, K., Yang, H., Liang, H., Lu, T., et al. (2022). Integrin subunit alpha V is a potent prognostic biomarker associated with immune infiltration in lower-grade glioma. Front. Neurol. 13, 964590. doi: 10.3389/fneur.2022.964590
Theoharides, T. C., Tsilioni, I., and Bawazeer, M. (2019). Mast cells, neuroinflammation and pain in fibromyalgia syndrome. Front. Cell. Neurosci. 13, 353. doi: 10.3389/fncel.2019.00353
van den Ameele, J., Fuge, J., Pitceathly, R. D. S., Berry, S., McIntyre, Z., Hanna, M. G., et al. (2020). Chronic pain is common in mitochondrial disease. Neuromus. Dis. 30, 413–419. doi: 10.1016/j.nmd.2020.02.017
Voets, T., Droogmans, G., Wissenbach, U., Janssens, A., Flockerzi, V., and Nilius, B. (2004). The principle of temperature-dependent gating in cold- and heat-sensitive TRP channels. Nature 430, 748–754. doi: 10.1038/nature02732
Voets, T., Talavera, K., Owsianik, G., and Nilius, B. (2005). Sensing with TRP channels. Nat. Chem. Biol. 1, 85–92. doi: 10.1038/nchembio0705-85
Watanabe, C., Mizoguchi, H., Yonezawa, A., and Sakurada, S. (2010). Characterization of intrathecally administered hemokinin-1-induced nociceptive behaviors in mice. Peptides 31, 1613–1616. doi: 10.1016/j.peptides.2010.04.025
Wójtowicz, T., Brzdak, P., and Mozrzymas, J. W. (2015). Diverse impact of acute and long-term extracellular proteolytic activity on plasticity of neuronal excitability. Front. Cell. Neurosci. 9. doi: 10.3389/fncel.2015.00313
Xiong, Y., Ran, J., Xu, L., Tong, Z., Adel Abdo, M. S., Ma, C., et al. (2020). Reactivation of NR4A1 restrains chondrocyte inflammation and ameliorates osteoarthritis in rats. Front. Cell Dev. Biol. 8, 158. doi: 10.3389/fcell.2020.00158
Yang, N. J., Isensee, J., Neel, D. V., Quadros, A. U., Zhang, H.-X. B., Lauzadis, J., et al. (2022). Anthrax toxins regulate pain signaling and can deliver molecular cargoes into ANTXR2+ DRG sensory neurons. Nat. Neurosci. 25, 168–179. doi: 10.1038/s41593-021-00973-8
Yousuf, M. S., Maguire, A. D., Simmen, T., and Kerr, B. J. (2020). Endoplasmic reticulum–mitochondria interplay in chronic pain: the calcium connection. Mol. Pain 16, 174480692094688. doi: 10.1177/1744806920946889
Zhang, S. -J., Zou, M., Lu, L., Lau, D., Ditzel, D. A. W., Delucinge-Vivier, C., et al. (2009). Nuclear calcium signaling controls expression of a large gene pool: identification of a gene program for acquired neuroprotection induced by synaptic activity. PLoS Genet. 5, e1000604. doi: 10.1371/journal.pgen.1000604
Zhang, Y., Lu, L., Furlonger, C., Wu, G. E., and Paige, C. J. (2000). Hemokinin is a hematopoietic-specific tachykinin that regulates B lymphopoiesis. Nat. Immunol. 1, 392–397. doi: 10.1038/80826
Zheng, J., Zhang, T., Han, S., Liu, C., Liu, M., Li, S., et al. (2021). Activin A improves the neurological outcome after ischemic stroke in mice by promoting oligodendroglial ACVR1B-mediated white matter remyelination. Exp. Neurol. 337, 113574. doi: 10.1016/j.expneurol.2020.113574
Keywords: trigeminal ganglion, cell culture, transcriptomics, pain, migraine, Mrgpr-ANTXR2, tac4
Citation: Takács-Lovász K, Aczél T, Borbély É, Szőke É, Czuni L, Urbán P, Gyenesei A, Helyes Z, Kun J and Bölcskei K (2023) Hemokinin-1 induces transcriptomic alterations in pain-related signaling processes in rat primary sensory neurons independent of NK1 tachykinin receptor activation. Front. Mol. Neurosci. 16:1186279. doi: 10.3389/fnmol.2023.1186279
Received: 14 March 2023; Accepted: 18 September 2023;
Published: 27 October 2023.
Edited by:
Manuela Simonetti, Heidelberg University, GermanyReviewed by:
Temugin Berta, University of Cincinnati, United StatesBang Sangsu, Duke University, United States
Copyright © 2023 Takács-Lovász, Aczél, Borbély, Szőke, Czuni, Urbán, Gyenesei, Helyes, Kun and Bölcskei. This is an open-access article distributed under the terms of the Creative Commons Attribution License (CC BY). The use, distribution or reproduction in other forums is permitted, provided the original author(s) and the copyright owner(s) are credited and that the original publication in this journal is cited, in accordance with accepted academic practice. No use, distribution or reproduction is permitted which does not comply with these terms.
*Correspondence: Éva Borbély, ZXZhLmJvcmJlbHlAYW9rLnB0ZS5odQ==