- 1Department of Neuroscience, Northwestern University Feinberg School of Medicine, Chicago, IL, United States
- 2Mesulam Center for Cognitive Neurology and Alzheimer's Disease, Northwestern University Feinberg School of Medicine, Chicago, IL, United States
- 3Department of Psychiatry and Behavioral Sciences, Northwestern University Feinberg School of Medicine, Chicago, IL, United States
Dendritic spines are highly dynamic and changes in their density, size, and shape underlie structural synaptic plasticity in cognition and memory. Fine membranous protrusions of spines, termed dendritic spinules, can contact neighboring neurons or glial cells and are positively regulated by neuronal activity. Spinules are thinner than filopodia, variable in length, and often emerge from large mushroom spines. Due to their nanoscale, spinules have frequently been overlooked in diffraction-limited microscopy datasets. Until recently, our knowledge of spinules has been interpreted largely from single snapshots in time captured by electron microscopy. We summarize herein the current knowledge about the molecular mechanisms of spinule formation. Additionally, we discuss possible spinule functions in structural synaptic plasticity in the context of development, adulthood, aging, and psychiatric disorders. The literature collectively implicates spinules as a mode of structural synaptic plasticity and suggests the existence of morphologically and functionally distinct spinule subsets. A recent time-lapse, enhanced resolution imaging study demonstrated that the majority of spinules are small, short-lived, and dynamic, potentially exploring their environment or mediating retrograde signaling and membrane remodeling via trans-endocytosis. A subset of activity-enhanced, elongated, long-lived spinules is associated with complex PSDs, and preferentially contacts adjacent axonal boutons not presynaptic to the spine head. Hence, long-lived spinules can form secondary synapses with the potential to alter synaptic connectivity. Published studies further suggest that decreased spinules are associated with impaired synaptic plasticity and intellectual disability, while increased spinules are linked to hyperexcitability and neurodegenerative diseases. In summary, the literature indicates that spinules mediate structural synaptic plasticity and perturbations in spinules can contribute to synaptic dysfunction and psychiatric disease. Additional studies would be beneficial to further delineate the molecular mechanisms of spinule formation and determine the exact role of spinules in development, adulthood, aging, and psychiatric disorders.
1. Introduction
Dendritic spines are small, actin-rich, membrane protrusions of neuronal dendrites that form functional contacts with nearby axonal boutons to receive electrical or chemical input signals (Hering and Sheng, 2001). Spines represent a specialized compartment for most excitatory synaptic transmission in the mature mammalian brain, with a mature spine typically forming a single synapse at its head (Hering and Sheng, 2001). While spines are often 0.5–2 microns in length, they can extend up to 6 microns, and larger spines contain more diverse organelles and larger synapses (Harris and Kater, 1994). Their composition includes an electron-dense thickening of the postsynaptic membrane at the synaptic junction, termed the postsynaptic density (PSD), which contains many proteins involved in the regulation of synaptic function (Sheng and Kim, 2011). The PSD is aligned with the presynaptic active zone and can be continuous and simple or discontinuous and complex in shape, i.e., fenestrated, horseshoe, perforated, or segmented (Hering and Sheng, 2001). Preceding the formation of spines during early development, neuronal dendrites extend long, thin processes, termed dendritic filopodia, which are highly dynamic and transient (Fiala et al., 1998). Dendritic filopodia sample their environment for new axonal partners and can facilitate synaptogenesis by initiating contact with presynaptic terminals, eventually transitioning into spines (Fiala et al., 1998; Mao et al., 2018). Four basic classes of spines have been proposed: Thin, stubby, mushroom, and branched (Harris et al., 1992). Thin spines concentrate calcium ions (Ca2+) and respond to rapid changes in synaptic activity, suggesting they are “learning” spines, while more mature and stable mushroom spines exhibit greater complexity in their molecular architecture and are thought of as “memory spines” (Bourne and Harris, 2007).
Synaptic plasticity underlies cognitive processes and memory and can be mediated by functional alterations at synapses, i.e., changes in synaptic strength, or structural changes at synapses (Citri and Malenka, 2008). Spines are highly dynamic, and modifications in their density, shape, and/or size serve as the basis for structural synaptic plasticity (Hering and Sheng, 2001; Holtmaat and Svoboda, 2009; Kasai et al., 2010). It has been postulated that intrinsic fluctuations in spine volume are necessary for synapse maintenance over time, while fast synaptic activity-induced plasticity is important for cognitive processes (Kasai et al., 2010). Structural spine dynamics can mediate short- and long-term plasticity at excitatory synapses, and result in changes to local network organization (Kasai et al., 2010). Impairments in structural synaptic plasticity play a role in major psychiatric disorders, including intellectual disability, schizophrenia, and autism spectrum disorder (Citri and Malenka, 2008; Bernardinelli et al., 2014). Yet, the mechanisms underlying experience-dependent structural plasticity in cognition are not fully understood.
This review focuses on fine membranous protrusions at synapses, termed spinules, with an emphasis on the most widely studied spinule type, dendritic spinules, which emerge from spines. Spinules can invaginate nearby spines, axonal boutons, or glial cells, and potentially serve as a mode of localized communication to mediate structural synaptic plasticity (Petralia et al., 2015, 2018). Spinules can originate from postsynaptic spines, presynaptic terminals, or glial cell projections, and the range of small invaginating projections has been reviewed extensively (Petralia et al., 2015, 2017). While invaginating projections are present in invertebrates, such as cnidarians (Westfall, 1970; Case et al., 1972), they appear to have evolved in complexity in mammals that demonstrate a greater degree of synaptic plasticity (Petralia et al., 2015, 2018). Dendritic spinules, referred to herein simply as “spinules,” are finer than filopodia, variable in length, often emerge from large mushroom spines, and can be positively regulated by synaptic activity (Petralia et al., 2015). In a 1962 study by Westrum and Blackstad (1962) of the adult rat hippocampal stratum radiatum, spinules were first observed projecting from pyramidal cell spines and invaginating presynaptic terminal membranes. The tips of spinules are often surrounded by clathrin-coated pits at the end of presynaptic membrane invaginations, indicating a potential role in neuronal communication (Westrum and Blackstad, 1962; Tarrant and Routtenberg, 1977). An increase in spinule density following the induction of long-term potentiation (LTP) has been well documented in the rat dentate gyrus (Schuster et al., 1990), and an increase in spinule length and size has similarly been reported in rat hippocampal organotypic cultures (Toni et al., 1999). Since their discovery, spinules have been observed in the hippocampus, cerebellum, and cerebral cortex (Petralia et al., 2015). An EM study of the basal state adult rat hippocampus revealed that 91% of mushroom spines and 24% of thin spines form one or more spinules (Spacek and Harris, 2004), implying that spinules occur frequently in the mature healthy brain. Spinules can arise from perforations or edges of complex PSDs (Toni et al., 2001; Dhanrajan et al., 2004; Spacek and Harris, 2004), or emerge from non-perforated PSD edges or spine necks (Sorra et al., 1998; Tao-Cheng et al., 2009). The observed differences in spinules in relation to PSD complexity suggest the possibility of structurally distinct spinule subtypes, necessitating further investigation.
The diffraction limits of light microscopy techniques have hindered researchers ability to assess nanoscale spinules in experimental datasets of spines (Zaccard et al., 2021). In one key investigation of spinules in dissociated hippocampal rat neurons, ~70% of spines that appeared to be globular or cup shaped at low resolution were revealed to be more complex structures composed of multiple spinule-like protrusions at super-resolution (Chazeau et al., 2014). Because of these limitations, much of our knowledge of spinule structure, regulation, and function has been deduced from single snapshots in time acquired using electron microscopy (EM) of fixed samples. However, recent advances in enhanced resolution confocal imaging techniques have yielded new insights into spinule dynamics, regulation, and functions (Zaccard et al., 2020, 2021). Here we summarize the current knowledge about the molecular mechanisms of mammalian spinule formation. We discuss investigations of spinules in development, adulthood, and aging, highlighting recent investigations that reveal surprising structural and functional diversity of spinules. Finally, we survey the evidence for underlying changes in spinules in psychiatric disorders, including intellectual disability and neurodegenerative diseases.
2. Mechanisms of spinule formation
Numerous studies have linked spinule formation to neuronal activity and structural LTP. In hippocampal slice cultures, EM revealed spinules 80–500 nm in length with varying morphologies that were induced by high potassium (K+) depolarization and N-methyl-D-aspartate (NMDA) treatment (Tao-Cheng et al., 2009). Similarly, spinules have been induced by theta burst stimulation for the induction of LTP (Toni et al., 1999). Live confocal imaging of hippocampal slice cultures revealed long spinule-like protrusions of spines induced by exogenous glutamate application, with orientation directed toward the glutamate (Richards et al., 2005). Further, LTP induction by two-photon glutamate uncaging can induce spinule formation (Ueda and Hayashi, 2013).
Published studies implicate central signaling molecules and pathways underlying synaptic transmission and plasticity in spinule formation. Ca2+ is a key signaling ion in synaptic transmission and regulates spinule-dependent synaptic plasticity in fish horizontal cells (Country and Jonz, 2017). Actin regulatory pathways may be involved in spinule formation, as one study noted F-actin-driven spinules induced by electrical stimulation (Colicos et al., 2001), and another study described “spine head protrusions” formed by Rac1-dependent elongation of F-actin barbed ends away from the PSD (Chazeau et al., 2014). The lipid second messenger, phosphatidylinositol-3,4,5-trisphosphate (PIP3), supports a range of neuronal functions, including maintenance of AMPA receptor clustering during LTP (Arendt et al., 2010). Local PIP3 has been shown to positively regulate spinules during structural LTP (Ueda and Hayashi, 2013). Kalirin, a guanine nucleotide exchange factor, plays an essential role in structural spine plasticity downstream of NMDA receptors by inducing Ca2+/calmodulin-dependent protein kinase II (CaMKII)-mediated activation of the Rho-GTPase Rac-1, which in turn mediates actin polymerization and spine head enlargement (Xie et al., 2007). Importantly, the Sec14p-like domain of kalirin, which contains the CaMKII phosphorylation site, binds directly to PIP3 (Miller et al., 2015). Studies show that kalirin is essential for activity-dependent synaptic plasticity, as well as learning and memory (Ma et al., 2001; Xie et al., 2007).
We recently investigated the relationship between spinules and the PSD, and the roles of local Ca2+ and kalirin-7, the most common isoform in the adult brain, during spinule formation in dissociated cortical pyramidal mouse neurons (Zaccard et al., 2020). We used live, time-lapse, rapid structured illumination microscopy and enhanced resolution confocal microscopy to show that smaller, dynamic, short-lived spinules (<60 s) emerged from near the edges of simple, discrete PSDs of mushroom spines, while more stable, long-lived spinules (≥60 s) were associated with complex PSDs. Our results indicated that Ca2+ is essential for spinule formation and that Ca2+ nanodomains localize to long-lived spinules, typically isolated from, but in synchrony with spine head Ca2+ transients. Interestingly, spines with frequent high-amplitude Ca2+ transients displayed both short-lived and long-lived spinules, while spines with sporadic peaks developed only short-lived spinules. Short-lived spinule number per spine was positively correlated with Ca2+ peak mean and maxima, while long-lived spinule number was correlated with Ca2+ peak frequency and duration. Further, kalirin-7 promoted spinule formation, elongation, and recurrence, which was defined as the appearance, disappearance, and reappearance of a spinule at one topographical spine head location. Although kalirin-independent mechanisms likely exist, we hypothesize that kalirin-7 modulates activity-dependent spinule formation downstream of PIP3 and Ca2+ signaling, and that perturbations in this pathway contribute to spinule dysregulation and disease.
3. Emerging functions of spinules in localized structural synaptic plasticity
3.1. Spinules may be trans-endocytosed or participate in synapse formation and maintenance
Many EM studies have reported spinules invaginating presynaptic terminals, and their proposed functions include facilitating communication with opposing presynaptic terminals, the formation of new synapses, and increasing the stability or complexity of existing synapses. Here, we focus on the role of spinules in synapse formation and stability, as well as consider evidence for morphologically and functionally distinct spinule subsets. For reference, we compiled a table of key mammalian spinule studies that includes spinule origination and contacts, the mode of induction or developmental state, spinule morphology, and proposed spinule functions (Table 1). A serial section EM study of the developing rat hippocampus at postnatal day 15 revealed that the majority of spinules emerge from the edges of nonperforated PSDs, or from spine necks, and extend into distal axonal boutons (Sorra et al., 1998). A similar study of the adult male rat hippocampus showed that 84% of the spinules emerging from mushroom spines are engulfed by presynaptic axons and 16% by neighboring axons, while only 17% of spinules from thin spines are engulfed by presynaptic axons, 67% by neighboring axons, and 14% by astrocytic processes (Spacek and Harris, 2004). The presence of coated pits on the engulfing membrane at most spinule tips and the presence of double-membrane structures in the cytoplasm of axons and astrocytes suggests postsynaptic membrane remodeling and retrograde signaling via trans-endocytosis. Another investigation described the outgrowth of fine membrane projections toward nascent presynaptic actin puncta, implicating spinules in new synapse formation (Colicos et al., 2001).
Recent studies have demonstrated that microglia are essential for normal brain wiring, in addition to their established role in innate responses to immunological stimuli and injury (Eyo and Wu, 2013; Wu et al., 2015; Hong et al., 2016). Microglia survey the local environment in the steady-state by rapidly extending and retracting finger-like membrane processes, which mediate transient contacts with synapses (Tremblay et al., 2011; Eyo and Wu, 2013; Wake et al., 2013). In vivo studies of the mouse lateral geniculate nucleus revealed that interactions between microglial processes and extranumerary presynaptic terminals trigger their elimination by phagocytosis, termed “pruning” (Schafer et al., 2012; Stephan et al., 2012). These data support the concept that microglia regulate synapses during early developmental synaptic refinement and experience-dependent plasticity, impacting neural circuit structure and function, as well as cognition and behavior (Stephan et al., 2012; Wu et al., 2015; Hong et al., 2016). However, the underlying molecular mechanisms are still being elucidated and the role of spinules is unclear. One study used EM to characterize microglia-synapse interactions in the visual cortex of juvenile mice and found that microglia processes simultaneously contact synaptic elements at multiple synapses (Tremblay et al., 2010). Furthermore, clathrin-coated pits reminiscent of spinules were observed at the interfaces between microglia and synaptic elements, suggesting they function in cell signaling via clathrin-mediated trans-endocytosis of membrane-bound receptors and ligands. In a more recent study, Gross and colleagues used time-lapse, light sheet microscopy to investigate microglia-driven synapse remodeling in developing organotypic hippocampal cultures (Weinhard et al., 2018). Their results showed that microglia frequently contact mature, persistent spines, which subsequently stretch and extend “spine head filopodia” toward the microglia. Roughly one quarter of these spines relocated to the site of the spine head filopodia tip and were significantly more stable than spines without spine head filopodia. In several examples, the spine bearing these spinule-like structures switched to associate with an adjacent axonal bouton. The prevalence of spine head filopodia on microglia-contacted mature spines was confirmed using EM of fixed brain tissue. To a lesser degree, microglia processes also contacted transient spines, which formed via filopodia extending from the microglia contact point, or near the dendritic shaft. These data together suggest a role for microglia-induced spinules in synaptic switching and/or stability of mature spines and the formation of new synapses. Additional investigations are needed to assess whether these observations represent a wide-spread phenomenon, and to determine the molecular underpinnings of microglia-mediated spinule induction. Together, published studies suggest a range of spinule functions from retrograde signaling and membrane remodeling to initiation and/or maintenance of synaptic connections.
3.2. Spinules in development, adulthood, and aging, and evidence of distinct spinule subsets
Two recent studies investigated spinule prevalence and structure in development and adulthood. In an EM-based investigation of the ferret visual cortex, Nahmani and colleagues assessed spinules enveloped by excitatory presynaptic boutons, termed spinule bearing boutons (SSBs), at key developmental time points (Campbell et al., 2020). Notably, spinules engulfed by boutons typically originated from either postsynaptic spines, or nearby axons and boutons. The prevalence of SBBs increased during postnatal development, with ∼25% of excitatory boutons classified as SSBs in late adolescence. Another recent report utilized focused ion beam scanning EM (FIB-SEM) in the adult male mouse hippocampus to analyze individual synapses by identifying the postsynaptic neurite, the presence of SBBs, origins of spinules within SBBs, and perforated PSDs (Gore et al., 2022). In this study, 74% of excitatory boutons bore spinules and these SBBs were found to be 2.5 times larger than non-SBBs. Spinules within SBBs were often associated with perforated PSDs, an indicator of increased activity, and originated from adjacent axons and boutons, postsynaptic spines, or adjacent non-synaptic spines. Importantly, two subtypes of spinules were detected within SBBs, including smaller clathrin-coated spinules and larger non-clathrin-coated spinules. These results strongly suggest differences in spinule prevalence throughout development and adulthood, as well as the existence of structurally and functionally distinct spinule subsets.
Very few studies have directly investigated the role of spinules in aging. Given that they are generally associated with perforated synapses, leading investigators have proposed that changes in spinules accompany changes in perforated synapses during aging (Petralia et al., 2015). Age-related changes in the prefrontal cortex typically involve loss of thin spines, which are linked to synaptic plasticity and cognitive function (Petralia et al., 2014). On the other hand, age-related changes in the hippocampus often involve loss of large, perforated mushroom spines, which can underly memory and learning (Petralia et al., 2014). For example, perforated synapse loss has been reported in the dentate gyrus of aged, memory-impaired rats (Geinisman et al., 1986). One investigation of the rat parietal cortex assessed perforated synapses at nine ages, ranging from 0.5 to 22 months, from youth to old-age, and non-perforated synapses at 0.5, 12, and 22 months (Jones and Calverley, 1991). In perforated synapses, PSD size and complexity increased with age. At 0.5 and 1 month, perforations tended to be discrete and small with a negatively curved PSD, while small segments of the PSD were sometimes positively curved and associated with small spinules. At 4 months, discrete perforations were larger, with short broad spinules, and this trend continued at 7 and 10 months of age. At 12 months, the majority of PSDs were large, segmented, and associated with large spinules, which projected into presynaptic terminals. Overall, perforated synapses and spinules peaked in middle-aged rats, and these spinules were distinctly larger and projected deeper into presynaptic terminals. These data suggest differences in spinule prevalence and functions in development, adulthood, and aging, as well as the presence of unique spinule subsets.
Our recent, time-lapse rapid SIM and enhanced resolution confocal imaging study of dissociated cortical mouse neurons supports the existence of at least two functionally and morphologically distinct spinule subsets (Zaccard et al., 2020). In the basal state, most spinules emerging from mushroom spines were dynamic and short-lived (<60 s), extending and retracting repeatedly at the same topographical spine head locations, while subset of elongated spinules was more stable and long-lived (≥60 s). Short-lived spinules typically emerged from the edges of simple PSDs, while long-lived spinules were associated with more complex PSDs. Exclusively in long-lived spinules, we often observed PSD95 fragments trafficking to spinule tips and Ca2+ nanodomains, in synchrony with, but isolated from spine head Ca2+ transients. Additionally, neuronal activity increased the number and length of spinules, and elongated spinules preferentially contacted adjacent boutons, which were not presynaptic to the spine head. Long-lived mushroom-shaped spinules sustained dynamic interactions with large spine head distal presynaptic terminals, and PSD95 puncta colocalized with presynaptic markers at the bulbous spinule tips. We confirmed our findings of dynamic, short-lived spinules and elongated, stable, long-lived spinules in the somatosensory cortex in acute mouse brain slices from one-month-old mice. We propose that spinule morphology and dynamics are on a continuum echoing that of spine classes (Figure 1). Long-lived filopodia- and thin-shaped spinules can traffic PSD fragments or interact with distal presynaptic terminals, suggesting their role in new synapse formation, while mushroom spinules mediate sustained contact with presynaptic terminals. These data together suggest that long-lived spinules can facilitate the formation of secondary synapses, potentially resulting in multi-contact synapse formation and altered connectivity.
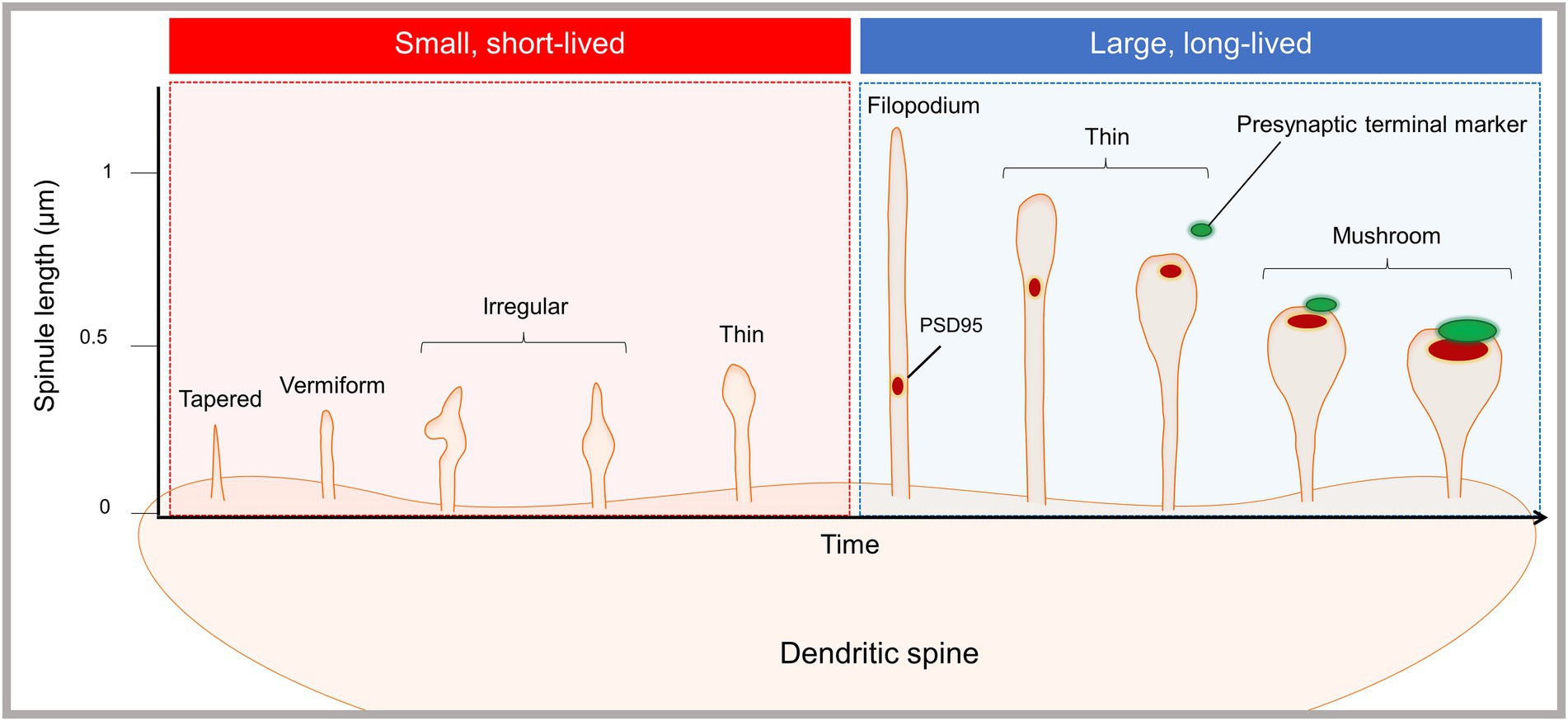
Figure 1. Emergence of morphologically and functionally distinct spinule subsets. The proposed spinule classification system is based on our recent live-cell, time-lapse, enhanced resolution imaging study of spinules in cortical mouse neurons (Zaccard et al., 2020). The majority of spinules emerging from mushroom spines were dynamic and short-lived, existing for sec and up to 1 min, while a minority of spinules were larger and long-lived. Short-lived spinules were typically less than 0.5 microns in length, and displayed diverse morphologies, e.g., tapered, vermiform, irregular, and thin shapes. Long-lived spinules existed for ≥60 s, in some cases exceeding imaging durations up to 10 min, and were typically between 0.5 and 1 microns in length. Additionally, a subset of filopodia-shaped spinules extended up to several microns. Both filopodia and thin spinules often trafficked PSD fragments or contacted presynaptic terminals, but not both. Mushroom spinules were the most stable, with colocalized presynaptic and postsynaptic markers at enlarged spinule tips. Synaptic activity enhanced the number of spinules and the proportion of elongated, long-lived spinules.
These studies collectively suggest the presence of spinule subsets that are divergent in structure, dynamics, and function. Small, clathrin-coated spinules observed by EM may be involved in clathrin-mediated trans-endocytosis, while large non-coated spinules may be involved in forming and/or maintaining complex synapses. We propose that small, short-lived spinules mediate communication with presynaptic partners or glia via trans-endocytosis, while elongated long-lived spinules facilitate the formation, stabilization, or modification of synaptic connections (Figure 2). Additionally, some small spinules may serve as precursors to larger, elongated spinules, which are enhanced by synaptic activity. The synapse has long been presented as a one-to-one presynaptic and postsynaptic connection. However, “multi-synaptic boutons,” wherein multiple spines contact one presynaptic terminal to form one-to-many connections, have been reported in the hippocampus, cortex, and cerebellum in association with sensory experience, brain lesions, and learning (Yang et al., 2018). Additionally, “multi-synaptic spines,” which receive inputs from multiple presynaptic boutons to form many-to-one connections, have been observed in complex motor learning in rats, fear conditioning in mice, and epilepsy (Jones et al., 1999; Kuwajima et al., 2013; Yang et al., 2016). We hypothesize that contact between spinules and distal presynaptic terminals can lead to the formation of secondary synapses and multi-synaptic spines, providing an additional modality for altering dendritic spine connectivity (Figure 2). Hence, spinules can represent a mode of structural synaptic plasticity that can modulate pre-existing synapse structure and downstream function or facilitate the formation of connectivity-altering secondary synapses.
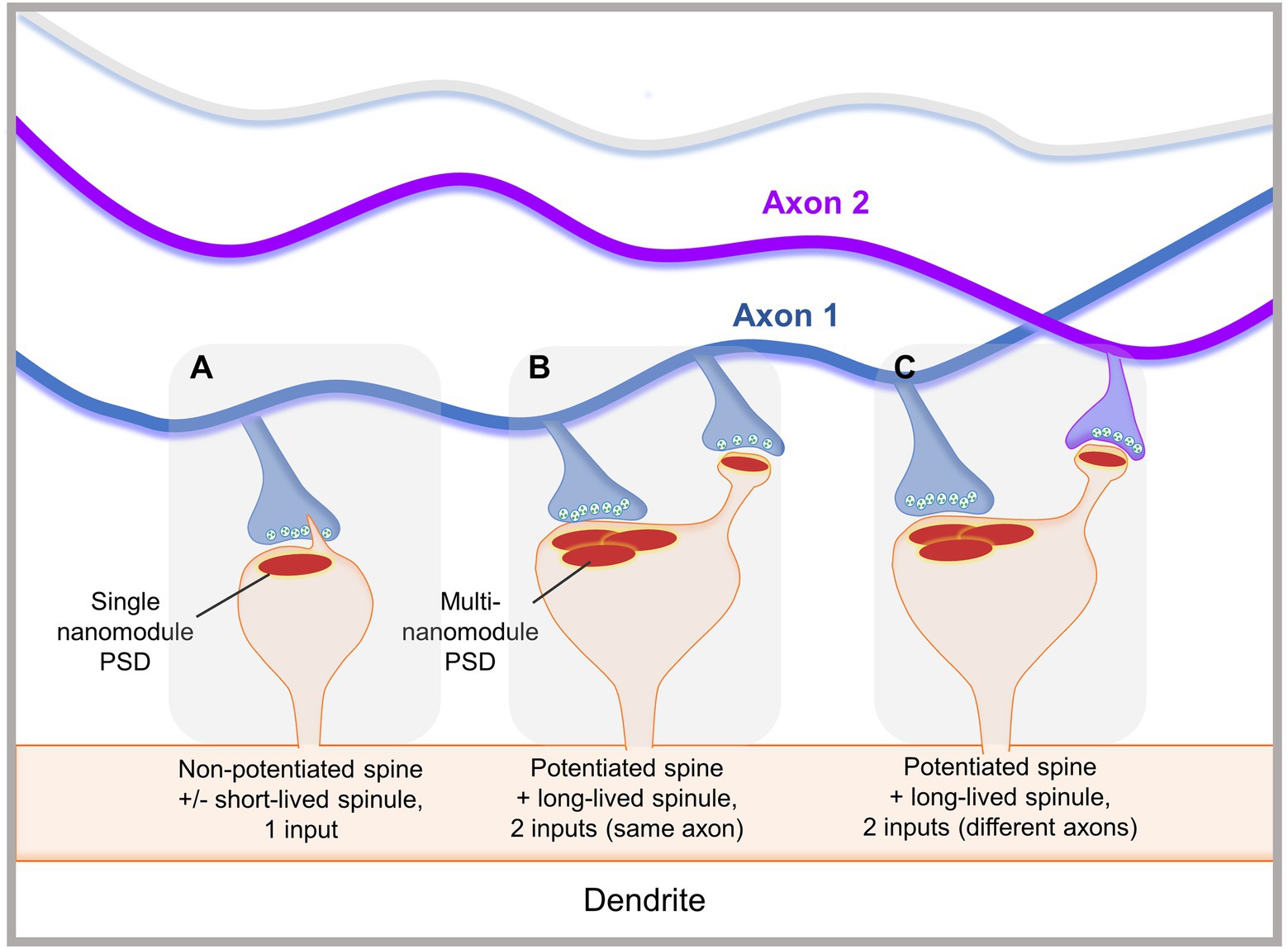
Figure 2. Proposed functions of spinules in mediating localized structural synaptic plasticity. (A) A smaller, non-potentiated spine with a simple PSD occasionally forms small, dynamic short-lived spinules, which emerge from close to the PSD edge. Small spinules may be trans-endocytosed or explore their environment. (B) A large, potentiated spine displays a complex PSD, with multiple nanomodules, and an elongated long-lived spinule. The spinule forms a secondary synaptic connection with a bouton from the same axon as the one forming a synapse with the spine head. (C) A large, potentiated spine with multiple PSD nanomodules displays a long-lived spinule that contacts an adjacent bouton originating from a different axon than the one synapsing with the spine head. These interactions may facilitate secondary synapse formation, potentially leading to alterations in synaptic plasticity, connectivity, and down-stream brain function.
4. Spinules in psychiatric disorders
Structural synaptic plasticity is important for key neurological functions, such as memory and cognition, and aberrant spine dynamics have been associated with many psychiatric disorders. In vivo studies have demonstrated that spines are altered in response to environmental factors impacting brain function. For example, visual stimulation increases spine density in the rat visual cortex, while visual deprivation results in abnormal spine shape and reduced spine number in the rabbit visual cortex (Hering and Sheng, 2001). Additionally, spines are typically found to be abnormally small and immature in human patients and most animal models of intellectual disability (Ramakers, 2002; Kasai et al., 2010). We propose that pathological changes in spines can include not only differences in size, shape, and density, but also differences in spinule formation. To date, a limited number of published studies have reported on the presence or absence of spinules in psychiatric disorders. Herein, we review those studies noting differences in spinules in human patients and disease models associated with altered cognitive function and memory (Table 2). The current literature suggests a link between decreased spinules and impaired synaptic plasticity and/or intellectual disability and between increased spinules and hyper-excitability and/or neurodegenerative diseases.
4.1. Decreased spinules are associated with impaired synaptic plasticity and intellectual disability
The single nucleotide polymorphism in the KIBRA (KIdney/BRAin) gene has been linked to individual differences in human memory performance, which become more prominent in aging (Witte et al., 2016). Additionally, deletion of the gene encoding the KIBRA protein results in impaired long-term synaptic plasticity (Papassotiropoulos et al., 2006; Makuch et al., 2011). In an EM study of the neocortex and hippocampus in KIBRA knock-out mice, researchers found that synapse ultrastructure and density were generally normal, but they displayed fewer spinules and perforations (Blanque et al., 2015). Another EM study examined the synaptic ultrastructure of the hippocampus dentate gyrus and area CA3 in the Ts65Dn mouse model for Down Syndrome (DS), which is associated with learning and memory deficits (Popov et al., 2011). Large and complex spines, termed thorny excrescences (TEs), receive inputs from dentate gyrus mossy fibers, and 3D reconstructions revealed a reduced number and volume of TEs, as well as a decrease in spinule-like protrusions. These morphological changes of TEs in Ts65Dn mice suggest a role for spinules in TE connectivity, synaptic transmission, and down-stream cognitive and memory function. While the presence of spinules in human DS models is not well documented, abnormal dendritic spine structure and function is one of the most prominent features of the disease and is directly linked to intellectual disability (Torres et al., 2018). Thus, these studies together suggest that a decrease in spinules may represent a structural correlate for reduced synaptic plasticity and/or diseases of intellectual disability.
4.2. Increased spinules are associated with hyperexcitability and neurodegenerative diseases
Temporal lobe epilepsy (TLE) is a common localized epilepsy characterized by complex partial seizures, epileptic discharges stemming from temporal lobe limbic structures, and hippocampal neuron loss and gliosis (Sutula, 1990). Humans with medically intractable TLE typically display loss of hippocampal neurons and reorganization of granule cell axons and their collaterals, termed mossy fibers, to the inner molecular layer of the dentate gyrus (Zhang and Houser, 1999). This “sprouting” of mossy fibers often precedes the onset of seizures in animal models and may contribute to increased spontaneous seizure susceptibility observed in TLE (Tauck and Nadler, 1985; Ben-Ari and Represa, 1990; Babb et al., 1992; Buckmaster and Dudek, 1997). An examination of mossy fiber ultrastructure in TLE patients revealed that mossy fiber terminals contact postsynaptic spines, often forming complex, multi-spine connections (Zhang and Houser, 1999). In some cases, postsynaptic spines displayed perforations and spinules, which were sometimes elongated and protruded into mossy fiber terminals. These results suggest an abundance of highly active synapses in the inner molecular layer of the dentate gyrus in TLE. Additionally, structural changes in mossy fiber connections, including spinules, could play a significant role in multi-synaptic bouton formation and granule cell hyperexcitability in TLE.
Parkinson’s disease (PD) is the second most common age-related neurodegenerative disease and is associated with cognitive and memory impairment (Aarsland et al., 2021). In an EM study of asymmetrical synapses in the caudate nucleus of PD patients, dendritic spines displayed increased PSD length and number of perforated PSDs, suggesting hyperactivity, as well as increased spinule-like structures, compared to age-matched controls (Anglade et al., 1996). Importantly, the size and density of spines and the size of PSD perforations were unchanged. These results suggest that dynamic changes in spinules may contribute to altered synaptic plasticity and transmission in the corticostriatal synapses of PD patients in response to hyperactivity. Researchers investigated synaptic plasticity in Betz cells in an EM study of the motor cortex of amyotrophic lateral sclerosis (ALS) patients (Sasaki and Iwata, 1999). Presynaptic terminals displayed increased spinule-like structures, accompanied by a range of changes indicative of hyperexcitability and neurodegeneration, such as aggregated presynaptic vesicles and increased mitochondria (Sasaki and Iwata, 1999; Kann and Kovacs, 2007). These studies suggest that increased spinules and changes in spinule dynamics may be a feature of synaptic changes in hyperexcitability and progressive neurodegenerative disorders. However, the role of spinules in neuronal hyperactivity and in altered synaptic transmission and plasticity underlying neurodegenerative diseases requires further investigation.
Research on axon degradation has largely focused on experimental models of Wallerian degeneration, a process in which axonal injury at a defined site and time simultaneously affects all axons in a nerve, leading to sensory and motor deficits (Conforti et al., 2014). Originally arising spontaneously in C57Bl/6 mice, the Wallerian degeneration slow (Wlds) gene mutation reverses this process, prolonging the viability of “orphaned axons,” i.e., axons that survive separated from their parental cell body, to delay the onset of Wallerian degeneration (Lunn et al., 1989; Perry et al., 1990). A recent EM study of the Wlds mutation examined spines in granule cells of the hippocampal dentate gyrus following lesions of perforant path inputs from the entorhinal cortex (Steward et al., 2021). Spines contacted by orphaned axons displayed drastic morphological changes, including hypertrophy of spine heads, enlargement of PSDs, and the formation of spinules extending into presynaptic terminals. While the signaling mechanisms between the postsynaptic neuron and orphaned axons are largely unknown, the observed increased spine complexity and spinules was similar to that seen following synaptic activity and induction of LTP (Petralia et al., 2015). These results suggest one possible future direction to explore the role of spinules in facilitating trans-synaptic signaling between orphaned axons and their postsynaptic targets to delay axonal degeneration.
5. Discussion
A growing body of evidence demonstrates that spinules are a feature of increased neuronal activity and LTP and suggests their function in mediating localized structural synaptic plasticity. Mechanistic studies have revealed that PIP3, local Ca2+ signaling, and kalirin-7 are involved in the regulation of spinules. However, the field could benefit from additional studies focused on connecting the known molecular players and further delineating the molecular pathways underlying spinule formation. Multiple studies suggest that spinules can participate in cell signaling via trans-endocytosis, or mediate formation of new synapses and maintenance of stable complex synapses. Additionally, microglia-driven contacts with spines can induce spinule formation potentially to facilitate synaptic switching, stability, or complexity of mature spines, and to form new synapses at sites of transient spines (Weinhard et al., 2018). A few key studies have provided insights into the prevalence and possible functions of spinules in development, adulthood, and aging. One developmental study showed that spinules peak in late adolescence, implicating spinules in synaptic plasticity during key periods of brain development (Campbell et al., 2020). In general, the literature suggests that spinule number and size increase with age, peaking in middle-age, and that spinules are a prominent feature of complex, active synapses in adulthood. We propose that spinules mediate structural plasticity during key developmental periods and, most prominently, structural plasticity of complex spines in adulthood, followed by a slow decline of spinules in aging. Future studies would be beneficial for further delineating the structure and functions of spinules in development, adulthood, and aging.
Recent advances in microscopy techniques have enabled live, time-lapse imaging studies of spinules to enhance our current knowledge of their structure and function. Importantly, standard light microcopy techniques cannot adequately detect smaller spinules. Electron microscopy provides the necessary resolution, but captures only frozen snap-shots in time, yielding little information about spinule dynamics. Newer studies provide convincing evidence of structurally and functionally distinct spinule subsets (Zaccard et al., 2020; Gore et al., 2022). In general, spinule shape, dynamics, and functions may echo that of dendritic spine classes. Small, dynamic, short-lived spinules are associated with simple PSDs and may serve to explore their environment or mediate retrograde signaling and/or membrane remodeling via trans-endocytosis. Larger, elongated, long-lived spinules are associated with complex PSDs, are enhanced by activity, and preferentially contact spine head adjacent boutons as opposed to the spine’s presynaptic partner. These data implicate large, long-lived spinules in altering synaptic connectivity and stability, including the formation of multi-contact synapses. Hence, distinct spinule subtypes likely mediate structural synaptic plasticity, which is known to underlie cognition, memory, and many psychiatric disorders, via divergent mechanisms. Additional investigations are needed to further advance our understanding of spinule structural and functional diversity in health and psychiatric disease.
While spinules remain understudied in the context of disease, a number of investigations have reported changes in spinules in psychiatric disorders. Generally, decreased spinules are associated with impaired synaptic plasticity and intellectual disability, while increased spinules accompany hyperexcitability and neurodegenerative disease. The main caveat to these inferences remains the limited number of published studies that have reported the presence or absence of spinules in human disease. Hence, additional studies are necessary to delineate the molecular mechanisms underlying spinule dysfunction and determine the role of spinules in development, adulthood, aging, and psychiatric disorders. An improved understanding of spinule regulation, dynamics, function, and dysfunction could reveal spinules as therapeutic targets, or as markers for diseases that involve altered structural synaptic plasticity.
Author contributions
PP and CG: project supervision. CZ, IG, and AS: conceptualization and manuscript preparation and editing. All authors contributed to the article and approved the submitted version.
Funding
This study was supported by the NIH grants R01MH107182, 2R56MH071316-16, and P30AG072977.
Conflict of interest
The authors declare that the research was conducted in the absence of any commercial or financial relationships that could be construed as a potential conflict of interest.
Publisher’s note
All claims expressed in this article are solely those of the authors and do not necessarily represent those of their affiliated organizations, or those of the publisher, the editors and the reviewers. Any product that may be evaluated in this article, or claim that may be made by its manufacturer, is not guaranteed or endorsed by the publisher.
References
Aarsland, D., Batzu, L., Halliday, G. M., Geurtsen, G. J., Ballard, C., Ray Chaudhuri, K., et al. (2021). Parkinson disease-associated cognitive impairment. Nat. Rev. Dis. Primers 7:47. doi: 10.1038/s41572-021-00280-3
Anglade, P., Mouatt-Prigent, A., Agid, Y., and Hirsch, E. C. (1996). Synaptic plasticity in the caudate nucleus of patients with Parkinson's disease. Neurodegeneration 5, 121–128. doi: 10.1006/neur.1996.0018
Arendt, K. L., Royo, M., Fernández-Monreal, M., Knafo, S., Petrok, C. N., Martens, J. R., et al. (2010). PIP3 controls synaptic function by maintaining AMPA receptor clustering at the postsynaptic membrane. Nat. Neurosci. 13, 36–44. doi: 10.1038/nn.2462
Babb, T. L., Pretorius, J. K., Mello, L. E., Mathern, G. W., and Levesque, M. F. (1992). Synaptic reorganizations in epileptic human and rat kainate hippocampus may contribute to feedback and feedforward excitation. Epilepsy Res. Suppl. 9, 193–202.
Ben-Ari, Y., and Represa, A. (1990). Brief seizure episodes induce long-term potentiation and mossy fibre sprouting in the hippocampus. Trends Neurosci. 13, 312–318. doi: 10.1016/0166-2236(90)90135-W
Bernardinelli, Y., Nikonenko, I., and Muller, D. (2014). Structural plasticity: mechanisms and contribution to developmental psychiatric disorders. Front. Neuroanat. 8:123. doi: 10.3389/fnana.2014.00123
Blanque, A., Repetto, D., Rohlmann, A., Brockhaus, J., Duning, K., Pavenstädt, H., et al. (2015). Deletion of KIBRA, protein expressed in kidney and brain, increases filopodial-like long dendritic spines in neocortical and hippocampal neurons in vivo and in vitro. Front. Neuroanat. 9:13. doi: 10.3389/fnana.2015.00013
Bourne, J., and Harris, K. M. (2007). Do thin spines learn to be mushroom spines that remember? Curr. Opin. Neurobiol. 17, 381–386. doi: 10.1016/j.conb.2007.04.009
Buckmaster, P. S., and Dudek, F. E. (1997). Neuron loss, granule cell axon reorganization, and functional changes in the dentate gyrus of epileptic kainate-treated rats. J. Comp. Neurol. 385, 385–404. doi: 10.1002/(SICI)1096-9861(19970901)385:3<385::AID-CNE4>3.0.CO;2-%23
Campbell, C., Lindhartsen, S., Knyaz, A., Erisir, A., and Nahmani, M. (2020). Cortical presynaptic Boutons progressively engulf spinules as they mature. eNeuro 7:ENEURO.0426-19.2020.
Case, N. M., Gray, E. G., and Young, J. Z. (1972). Ultrastructure and synaptic relations in the optic lobe of the brain of Eledone and octopus. J. Ultrastruct. Res. 39, 115–123. doi: 10.1016/S0022-5320(72)80012-1
Chazeau, A., Mehidi, A., Nair, D., Gautier, J. J., Leduc, C., Chamma, I., et al. (2014). Nanoscale segregation of actin nucleation and elongation factors determines dendritic spine protrusion. EMBO J. 33, 2745–2764. doi: 10.15252/embj.201488837
Citri, A., and Malenka, R. C. (2008). Synaptic plasticity: multiple forms, functions, and mechanisms. Neuropsychopharmacology 33, 18–41. doi: 10.1038/sj.npp.1301559
Colicos, M. A., Collins, B. E., Sailor, M. J., and Goda, Y. (2001). Remodeling of synaptic actin induced by photoconductive stimulation. Cells 107, 605–616. doi: 10.1016/S0092-8674(01)00579-7
Conforti, L., Gilley, J., and Coleman, M. P. (2014). Wallerian degeneration: an emerging axon death pathway linking injury and disease. Nat. Rev. Neurosci. 15, 394–409. doi: 10.1038/nrn3680
Country, M. W., and Jonz, M. G. (2017). Calcium dynamics and regulation in horizontal cells of the vertebrate retina: lessons from teleosts. J. Neurophysiol. 117, 523–536. doi: 10.1152/jn.00585.2016
Dhanrajan, T. M., Lynch, M. A., Kelly, A., Popov, V. I., Rusakov, D. A., and Stewart, M. G. (2004). Expression of long-term potentiation in aged rats involves perforated synapses but dendritic spine branching results from high-frequency stimulation alone. Hippocampus 14, 255–264. doi: 10.1002/hipo.10172
Eyo, U. B., and Wu, L. J. (2013). Bidirectional microglia-neuron communication in the healthy brain. Neural Plast. 2013:456857. doi: 10.1155/2013/456857
Fiala, J. C., Feinberg, M., Popov, V., and Harris, K. M. (1998). Synaptogenesis via dendritic filopodia in developing hippocampal area CA1. J. Neurosci. 18, 8900–8911. doi: 10.1523/JNEUROSCI.18-21-08900.1998
Geinisman, Y., de Toledo-Morrell, L., and Morrell, F. (1986). Loss of perforated synapses in the dentate gyrus: morphological substrate of memory deficit in aged rats. Proc. Natl. Acad. Sci. U. S. A. 83, 3027–3031. doi: 10.1073/pnas.83.9.3027
Gore, A., Yurina, A., Yukevich-Mussomeli, A., and Nahmani, M. (2022). Synaptic spinules are reliable indicators of excitatory presynaptic Bouton size and strength and are ubiquitous components of excitatory synapses in CA1 hippocampus. Front. Synaptic Neurosci. 14:968404. doi: 10.3389/fnsyn.2022.968404
Harris, K. M., Jensen, F. E., and Tsao, B. (1992). Three-dimensional structure of dendritic spines and synapses in rat hippocampus (CA1) at postnatal day 15 and adult ages: implications for the maturation of synaptic physiology and long-term potentiation. J. Neurosci. 12, 2685–2705. doi: 10.1523/JNEUROSCI.12-07-02685.1992
Harris, K. M., and Kater, S. B. (1994). Dendritic spines: cellular specializations imparting both stability and flexibility to synaptic function. Annu. Rev. Neurosci. 17, 341–371. doi: 10.1146/annurev.ne.17.030194.002013
Hering, H., and Sheng, M. (2001). Dendritic spines: structure, dynamics and regulation. Nat. Rev. Neurosci. 2, 880–888. doi: 10.1038/35104061
Holtmaat, A., and Svoboda, K. (2009). Experience-dependent structural synaptic plasticity in the mammalian brain. Nat. Rev. Neurosci. 10, 647–658. doi: 10.1038/nrn2699
Hong, S., Dissing-Olesen, L., and Stevens, B. (2016). New insights on the role of microglia in synaptic pruning in health and disease. Curr. Opin. Neurobiol. 36, 128–134. doi: 10.1016/j.conb.2015.12.004
Jones, D. G., and Calverley, R. K. (1991). Perforated and non-perforated synapses in rat neocortex: three-dimensional reconstructions. Brain Res. 556, 247–258. doi: 10.1016/0006-8993(91)90312-J
Jones, T. A., Chu, C. J., Grande, L. A., and Gregory, A. D. (1999). Motor skills training enhances lesion-induced structural plasticity in the motor cortex of adult rats. J. Neurosci. 19, 10153–10163. doi: 10.1523/JNEUROSCI.19-22-10153.1999
Kann, O., and Kovacs, R. (2007). Mitochondria and neuronal activity. Am. J. Physiol. Cell Physiol. 292, C641–C657. doi: 10.1152/ajpcell.00222.2006
Kasai, H., Fukuda, M., Watanabe, S., Hayashi-Takagi, A., and Noguchi, J. (2010). Structural dynamics of dendritic spines in memory and cognition. Trends Neurosci. 33, 121–129. doi: 10.1016/j.tins.2010.01.001
Kuwajima, M., Spacek, J., and Harris, K. M. (2013). Beyond counts and shapes: studying pathology of dendritic spines in the context of the surrounding neuropil through serial section electron microscopy. Neuroscience 251, 75–89. doi: 10.1016/j.neuroscience.2012.04.061
Lunn, E. R., Perry, V. H., Brown, M. C., Rosen, H., and Gordon, S. (1989). Absence of Wallerian degeneration does not hinder regeneration in peripheral nerve. Eur. J. Neurosci. 1, 27–33. doi: 10.1111/j.1460-9568.1989.tb00771.x
Ma, X. M., Johnson, R. C., Mains, R. E., and Eipper, B. A. (2001). Expression of kalirin, a neuronal GDP/GTP exchange factor of the trio family, in the central nervous system of the adult rat. J. Comp. Neurol. 429, 388–402. doi: 10.1002/1096-9861(20010115)429:3<388::AID-CNE3>3.0.CO;2-I
Makuch, L., Volk, L., Anggono, V., Johnson, R. C., Yu, Y., Duning, K., et al. (2011). Regulation of AMPA receptor function by the human memory-associated gene KIBRA. Neuron 71, 1022–1029. doi: 10.1016/j.neuron.2011.08.017
Mao, Y. T., Zhu, J. X., Hanamura, K., Iurilli, G., Datta, S. R., and Dalva, M. B. (2018). Filopodia conduct target selection in cortical neurons using differences in signal kinetics of a single kinase. Neuron 98, 767.e8–782.e8. doi: 10.1016/j.neuron.2018.04.011
Miller, M. B., Vishwanatha, K. S., Mains, R. E., and Eipper, B. A. (2015). An N-terminal amphipathic helix binds Phosphoinositides and enhances Kalirin Sec14 domain-mediated membrane interactions. J. Biol. Chem. 290, 13541–13555. doi: 10.1074/jbc.M115.636746
Papassotiropoulos, A., Stephan, D. A., Huentelman, M. J., Hoerndli, F. J., Craig, D. W., Pearson, J. V., et al. (2006). Common Kibra alleles are associated with human memory performance. Science 314, 475–478. doi: 10.1126/science.1129837
Perry, V. H., Lunn, E. R., Brown, M. C., Cahusac, S., and Gordon, S. (1990). Evidence that the rate of Wallerian degeneration is controlled by a single autosomal dominant gene. Eur. J. Neurosci. 2, 408–413. doi: 10.1111/j.1460-9568.1990.tb00433.x
Petralia, R. S., Mattson, M. P., and Yao, P. J. (2014). Communication breakdown: the impact of ageing on synapse structure. Ageing Res. Rev. 14, 31–42. doi: 10.1016/j.arr.2014.01.003
Petralia, R. S., Wang, Y. X., Mattson, M. P., and Yao, P. J. (2015). Structure, distribution, and function of neuronal/synaptic Spinules and related Invaginating projections. NeuroMolecular Med. 17, 211–240. doi: 10.1007/s12017-015-8358-6
Petralia, R. S., Wang, Y. X., Mattson, M. P., and Yao, P. J. (2017). Invaginating presynaptic terminals in neuromuscular junctions, photoreceptor terminals, and other synapses of animals. NeuroMolecular Med. 19, 193–240. doi: 10.1007/s12017-017-8445-y
Petralia, R. S., Wang, Y. X., Mattson, M. P., and Yao, P. J. (2018). Invaginating structures in mammalian synapses. Front. Synaptic Neurosci. 10:4. doi: 10.3389/fnsyn.2018.00004
Popov, V. I., Kleschevnikov, A. M., Klimenko, O. A., Stewart, M. G., and Belichenko, P. V. (2011). Three-dimensional synaptic ultrastructure in the dentate gyrus and hippocampal area CA3 in the Ts65Dn mouse model of down syndrome. J. Comp. Neurol. 519, 1338–1354. doi: 10.1002/cne.22573
Ramakers, G. J. (2002). Rho proteins, mental retardation and the cellular basis of cognition. Trends Neurosci. 25, 191–199. doi: 10.1016/S0166-2236(00)02118-4
Richards, D. A., Mateos, J. M., Hugel, S., de Paola, V., Caroni, P., Gähwiler, B. H., et al. (2005). Glutamate induces the rapid formation of spine head protrusions in hippocampal slice cultures. Proc. Natl. Acad. Sci. U. S. A. 102, 6166–6171. doi: 10.1073/pnas.0501881102
Sasaki, S., and Iwata, M. (1999). Ultrastructural change of synapses of Betz cells in patients with amyotrophic lateral sclerosis. Neurosci. Lett. 268, 29–32. doi: 10.1016/S0304-3940(99)00374-2
Schafer, D. P., Lehrman, E. K., Kautzman, A. G., Koyama, R., Mardinly, A. R., Yamasaki, R., et al. (2012). Microglia sculpt postnatal neural circuits in an activity and complement-dependent manner. Neuron 74, 691–705. doi: 10.1016/j.neuron.2012.03.026
Schuster, T., Krug, M., and Wenzel, J. (1990). Spinules in axospinous synapses of the rat dentate gyrus: changes in density following long-term potentiation. Brain Res. 523, 171–174. doi: 10.1016/0006-8993(90)91654-Y
Sheng, M., and Kim, E. (2011). The postsynaptic organization of synapses. Cold Spring Harb. Perspect. Biol. 3:a005678. doi: 10.1101/cshperspect.a005678
Sorra, K. E., Fiala, J. C., and Harris, K. M. (1998). Critical assessment of the involvement of perforations, spinules, and spine branching in hippocampal synapse formation. J. Comp. Neurol. 398, 225–240. doi: 10.1002/(SICI)1096-9861(19980824)398:2<225::AID-CNE5>3.0.CO;2-2
Spacek, J., and Harris, K. M. (2004). Trans-endocytosis via spinules in adult rat hippocampus. J. Neurosci. 24, 4233–4241. doi: 10.1523/JNEUROSCI.0287-04.2004
Stephan, A. H., Barres, B. A., and Stevens, B. (2012). The complement system: an unexpected role in synaptic pruning during development and disease. Annu. Rev. Neurosci. 35, 369–389. doi: 10.1146/annurev-neuro-061010-113810
Steward, O., Yonan, J. M., and Falk, P. M. (2021). The curious anti-pathology of the Wld (s) mutation: paradoxical postsynaptic spine growth accompanies delayed presynaptic Wallerian degeneration. Front. Mol. Neurosci. 14:735919. doi: 10.3389/fnmol.2021.735919
Sutula, T. P. (1990). Experimental models of temporal lobe epilepsy: new insights from the study of kindling and synaptic reorganization. Epilepsia 31, S45–S54. doi: 10.1111/j.1528-1157.1990.tb05859.x
Tao-Cheng, J. H., Dosemeci, A., Gallant, P. E., Miller, S., Galbraith, J. A., Winters, C. A., et al. (2009). Rapid turnover of spinules at synaptic terminals. Neuroscience 160, 42–50. doi: 10.1016/j.neuroscience.2009.02.031
Tarrant, S. B., and Routtenberg, A. (1977). The synaptic spinule in the dendritic spine: electron microscopic study of the hippocampal dentate gyrus. Tissue Cell 9, 461–473. doi: 10.1016/0040-8166(77)90006-4
Tauck, D. L., and Nadler, J. V. (1985). Evidence of functional mossy fiber sprouting in hippocampal formation of kainic acid-treated rats. J. Neurosci. 5, 1016–1022. doi: 10.1523/JNEUROSCI.05-04-01016.1985
Toni, N., Buchs, P. A., Nikonenko, I., Bron, C. R., and Muller, D. (1999). LTP promotes formation of multiple spine synapses between a single axon terminal and a dendrite. Nature 402, 421–425. doi: 10.1038/46574
Toni, N., Buchs, P. A., Nikonenko, I., Povilaitite, P., Parisi, L., and Muller, D. (2001). Remodeling of synaptic membranes after induction of long-term potentiation. J. Neurosci. 21, 6245–6251. doi: 10.1523/JNEUROSCI.21-16-06245.2001
Torres, M. D., Garcia, O., Tang, C., and Busciglio, J. (2018). Dendritic spine pathology and thrombospondin-1 deficits in down syndrome. Free Radic. Biol. Med. 114, 10–14. doi: 10.1016/j.freeradbiomed.2017.09.025
Tremblay, M. E., Lowery, R. L., and Majewska, A. K. (2010). Microglial interactions with synapses are modulated by visual experience. PLoS Biol. 8:e1000527. doi: 10.1371/journal.pbio.1000527
Tremblay, M. E., Stevens, B., Sierra, A., Wake, H., Bessis, A., and Nimmerjahn, A. (2011). The role of microglia in the healthy brain. J. Neurosci. 31, 16064–16069. doi: 10.1523/JNEUROSCI.4158-11.2011
Ueda, Y., and Hayashi, Y. (2013). PIP(3) regulates spinule formation in dendritic spines during structural long-term potentiation. J. Neurosci. 33, 11040–11047. doi: 10.1523/JNEUROSCI.3122-12.2013
Wake, H., Moorhouse, A. J., Miyamoto, A., and Nabekura, J. (2013). Microglia: actively surveying and shaping neuronal circuit structure and function. Trends Neurosci. 36, 209–217. doi: 10.1016/j.tins.2012.11.007
Weinhard, L., di Bartolomei, G., Bolasco, G., Machado, P., Schieber, N. L., Neniskyte, U., et al. (2018). Microglia remodel synapses by presynaptic trogocytosis and spine head filopodia induction. Nat. Commun. 9:1228. doi: 10.1038/s41467-018-03566-5
Westfall, J. A. (1970). Ultrastructure of synapses in a primitive coelenterate. J. Ultrastruct. Res. 32, 237–246. doi: 10.1016/S0022-5320(70)80004-1
Westrum, L. E., and Blackstad, T. W. (1962). An electron microscopic study of the stratum radiatum of the rat hippocampus (regio superior, CA 1) with particular emphasis on synaptology. J. Comp. Neurol. 119, 281–309. doi: 10.1002/cne.901190303
Witte, A. V., Köbe, T., Kerti, L., Rujescu, D., and Flöel, A. (2016). Impact of KIBRA polymorphism on memory function and the hippocampus in older adults. Neuropsychopharmacology 41, 781–790. doi: 10.1038/npp.2015.203
Wu, Y., Dissing-Olesen, L., MacVicar, B. A., and Stevens, B. (2015). Microglia: dynamic mediators of synapse development and plasticity. Trends Immunol. 36, 605–613. doi: 10.1016/j.it.2015.08.008
Xie, Z., Srivastava, D. P., Photowala, H., Kai, L., Cahill, M. E., Woolfrey, K. M., et al. (2007). Kalirin-7 controls activity-dependent structural and functional plasticity of dendritic spines. Neuron 56, 640–656. doi: 10.1016/j.neuron.2007.10.005
Yang, Y., Liu, D. Q., Huang, W., Deng, J., Sun, Y., Zuo, Y., et al. (2016). Selective synaptic remodeling of amygdalocortical connections associated with fear memory. Nat. Neurosci. 19, 1348–1355. doi: 10.1038/nn.4370
Yang, Y., Lu, J., and Zuo, Y. (2018). Changes of synaptic structures associated with learning. Mem. Dis. Brain Sci. Adv. 4, 99–117. doi: 10.26599/BSA.2018.2018.9050012
Zaccard, C. R., Kirchenbuechler, D., Yoon, S., Arvanitis, C., and Penzes, P. (2021). Protocol for live enhanced resolution confocal imaging of dendritic spinule dynamics in primary mouse cortical neuron culture. STAR Protoc. 2:100427. doi: 10.1016/j.xpro.2021.100427
Zaccard, C. R., Shapiro, L., Martin-de-Saavedra, M. D., Pratt, C., Myczek, K., Song, A., et al. (2020). Rapid 3D enhanced resolution microscopy reveals diversity in dendritic Spinule dynamics, regulation, and function. Neuron 107, 522.e6–537.e6. doi: 10.1016/j.neuron.2020.04.025
Keywords: dendritic spines, memory and cognition, synaptic activity, synaptic connectivity, electron microscopy, enhanced resolution microscopy, neurodegenerative disease, postsynaptic density
Citation: Zaccard CR, Gippo I, Song A, Geula C and Penzes P (2023) Dendritic spinule-mediated structural synaptic plasticity: Implications for development, aging, and psychiatric disease. Front. Mol. Neurosci. 16:1059730. doi: 10.3389/fnmol.2023.1059730
Edited by:
Clive R. Bramham, University of Bergen, NorwayReviewed by:
Pirta Elina Hotulainen, Minerva Foundation Institute for Medical Research, FinlandWon Chan Oh, University of Colorado Anschutz Medical Campus, United States
Richard J. Weinberg, University of North Carolina at Chapel Hill, United States
Copyright © 2023 Zaccard, Gippo, Song, Geula and Penzes. This is an open-access article distributed under the terms of the Creative Commons Attribution License (CC BY). The use, distribution or reproduction in other forums is permitted, provided the original author(s) and the copyright owner(s) are credited and that the original publication in this journal is cited, in accordance with accepted academic practice. No use, distribution or reproduction is permitted which does not comply with these terms.
*Correspondence: Peter Penzes, ✉ cC1wZW56ZXNAbm9ydGh3ZXN0ZXJuLmVkdQ==