- 1Department of Anesthesiology, Chinese Academy of Medical Sciences & Peking Union Medical College Hospital, Beijing, China
- 2Department of Pain Management, Anesthesiology Institute, Cleveland, OH, United States
- 3Department of Inflammation and Immunity, Lerner Research Institute, Cleveland, OH, United States
- 4Cleveland Clinic, Case Western Reserve University, Cleveland, OH, United States
Keratinocytes are the predominant block-building cells in the epidermis. Emerging evidence has elucidated the roles of keratinocytes in a wide range of pathophysiological processes including cutaneous nociception, pruritus, and inflammation. Intraepidermal free nerve endings are entirely enwrapped within the gutters of keratinocyte cytoplasm and form en passant synaptic-like contacts with keratinocytes. Keratinocytes can detect thermal, mechanical, and chemical stimuli through transient receptor potential ion channels and other sensory receptors. The activated keratinocytes elicit calcium influx and release ATP, which binds to P2 receptors on free nerve endings and excites sensory neurons. This process is modulated by the endogenous opioid system and endothelin. Keratinocytes also express neurotransmitter receptors of adrenaline, acetylcholine, glutamate, and γ-aminobutyric acid, which are involved in regulating the activation and migration, of keratinocytes. Furthermore, keratinocytes serve as both sources and targets of neurotrophic factors, pro-inflammatory cytokines, and neuropeptides. The autocrine and/or paracrine mechanisms of these mediators create a bidirectional feedback loop that amplifies neuroinflammation and contributes to peripheral sensitization.
Introduction
Keratinocytes (KCs) are the predominant cells that make up about 95% of cells in the epidermis. The epidermis is divided into four layers: stratum basale, spinosum, granulosum and corneum (Roger et al., 2019). KCs in stratum basale are mitotically active cells (Houben et al., 2007; Roger et al., 2019). Their proliferation is responsible for epidermis turnover (Houben et al., 2007; Roger et al., 2019). As KCs migrate superficially, they lose replicative potential and undergo differentiation (Houben et al., 2007). During this process, they deposit keratin, which adds to skin mechanical strength (Vidal Yucha et al., 2019). In the stratum corneum, KCs lose nuclei, release lipids, and terminally differentiate into squamous corneocytes (Roger et al., 2019). Keratin-filled corneocytes and densely packed intercellular lipids combine to form the skin barrier (Roger et al., 2019).
The proliferation, differentiation, and migration of KCs are crucial for the maintenance of skin homeostasis (Gallegos-Alcala et al., 2021). However, KCs are not just the structural backbone of the epidermis. Mounting evidence has showed the wide versatility of KCs. KCs have emerged as a key player in a wide range of pathophysiological processes including cutaneous nociception, pruritus, and inflammation.
In this review, we aim to present available evidence on the roles of KCs in nociceptive transduction and regulation. Nociceptive transduction is defined as peripheral terminals of nociceptive C fibers and A-delta (Aδ) fibers depolarized (to generate action potential) and activated by noxious mechanical, thermal, or chemical stimuli. KCs express various sensory receptors that can convert noxious stimuli into an action potential to get activated (Talagas et al., 2020a). The activated KCs contribute to nociceptive transduction by triggering action potentials in peripheral nociceptors in intraepidermal free nerve endings (FNEs) (Baumbauer et al., 2015; Moehring et al., 2018). We first elucidate the anatomical relationship and neurotransmission between KCs and intraepidermal nerve fibers. Next, we summarize the sensory and neurotransmitter receptors expressed on KCs, with a special focus on their contributions to nociceptive transduction. KCs can also be a source of cytokines, neurotrophic factors, neuropeptides, and neurotransmitters, which enhance their interaction with neighboring nerve fibers and immune cells (Vidal Yucha et al., 2019). In this way, KCs participate in nociceptive regulation. We also review the pivotal role of KCs in neuroinflammation and peripheral sensitization.
Anatomical relationship between keratinocytes and intraepidermal free nerve endings
The epidermis is highly innervated with intraepidermal FNEs expressing peripheral nociceptors whose cell bodies are localized in dorsal root ganglion (DRG) and trigeminal ganglion (TG) (Figure 1) (Talagas et al., 2018). The central projections of these sensory neurons transduce nociception (Basbaum et al., 2009). Passing tortuously between KCs, FNEs are able to detect mechanical, thermal, and chemical noxious stimuli, and are thus called “nociceptors” (Basbaum et al., 2009). Nociceptive FENs are generally divided into two categories: unmyelinated small-diameter C-fibers transducing slow and poorly localized pain, and thinly myelinated medium-diameter Aδ-fibers transducing fast and well localized pain (Basbaum et al., 2009).
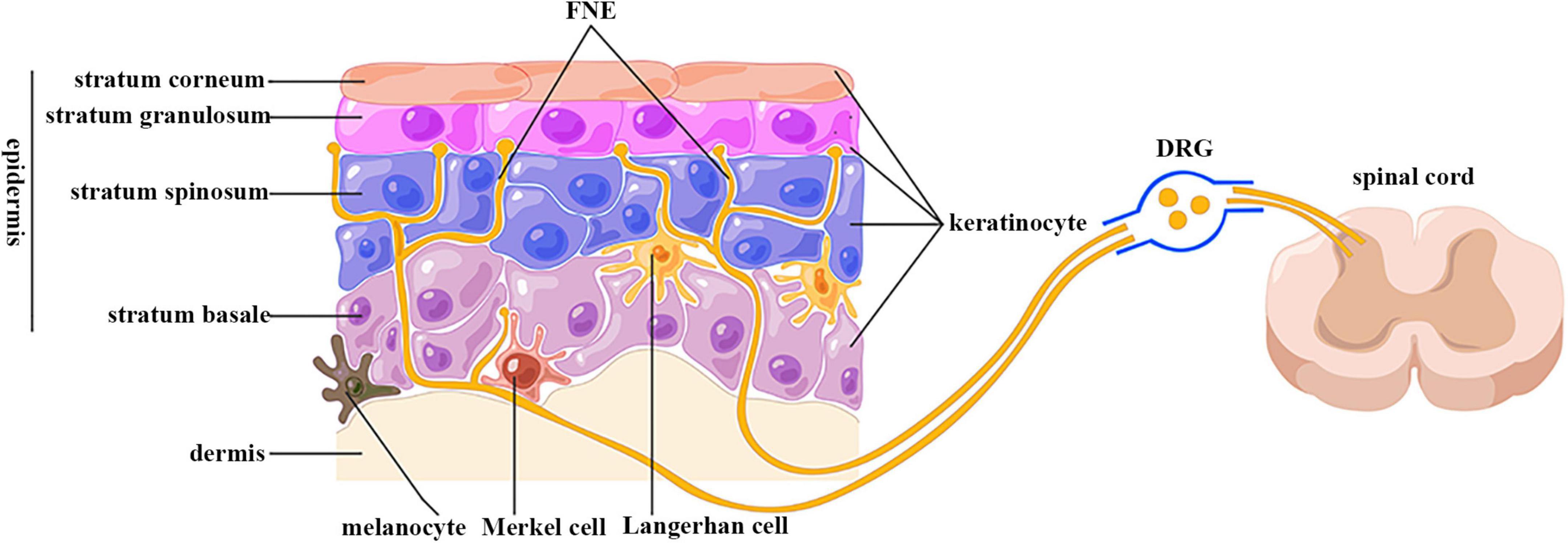
Figure 1. Anatomical relationship between keratinocytes and sensory nerve endings. FNE, free nerve ending; DRG, dorsal root ganglion. Epidermis is divided into four layers: stratum basale, spinosum, granulosum, and corneum. Keratinocytes are the predominant cells in epidermis. The epidermis is highly innervated with intraepidermal free nerve endings, which are enwrapped but also ensheathed by keratinocyte cytoplasm. Their cell bodies are located in dorsal root ganglion, and are centrally projected to spinal cord dorsal horn. (By Figdraw).
Wrapping of free nerve endings within keratinocyte cytoplasm
The utilization of microscopy and immunolabeling techniques has made it possible to investigate the anatomical relationship between FNEs and KCs. Electron microscopy data showed that FNEs are enwrapped within the gutters of KC cytoplasm over their entire circumference in biopsies of human glabrous and hairy skin (Chouchkov, 1974; Cauna, 1980; Hilliges et al., 1995; Talagas et al., 2020c). Immunostaining results indicated these FNEs were almost exclusively Aδ and C fibers in a KCs–sensory neuron coculture model (Talagas et al., 2020c). This neuroanatomical relation was also observed by the confocal laser scanning microscopy (Talagas et al., 2020b). Also in this coculture model, KCs stretch outgrowths that embrace FNEs (Talagas et al., 2020c). FNE ensheathment by KCs contributes to the morphogenesis and function of nociceptive sensory neurons in Drosophila; this evolutionarily conserved mechanism also exists in humans (Jiang et al., 2019; Talagas et al., 2020b).
En passant synaptic-like contact
Several studies provided ultrastructural and functional evidence on the presence of en passant synaptic-like contacts between KCs and FNEs. In human hairy skin, FNEs are closely apposed to the cell bodies or cilia of KCs without any specialized structures between their plasma membrane (Hilliges et al., 1995). This close contact was also visualized by the atomic force microscopy (AFM) in nanoscale in cocultured KCs and sensory nerve endings (Klusch et al., 2013). The adjacent KC membrane is slightly thickened, resembling a synaptic membrane (Chateau and Misery, 2004). However, using in situ correlative light electron microscopy (CLEM), Talagas et al. (2020c) did not observe membrane thickening. Instead, they identified presynaptic vesicle markers in a pearl necklace pattern in KC cytoplasm in the immediate vicinity of FNEs, but not at a distance from FNEs (Talagas et al., 2020c). The density of these vesicles is increased in skin biopsies from small fiber neuropathy (SFN) (Talagas et al., 2020c). A key molecule for exocytosis (syntaxin 1A) is also present in KC cytoplasmic gutters around FNEs, and is required for the communication with sensory neurons (Talagas et al., 2020c). Although the percentage of FNE enwrapped by KCs or forming a synaptic-like structure with KCs has not been reported, the integrated anatomical relation between KCs and FNEs forms the basis of connection and communication between them.
Sensory and neurotransmitter receptors expressed on keratinocytes
From conventional point of view, intraepidermal FNEs are the exclusive cutaneous nociceptors (Talagas et al., 2020a). However, accumulating data have highlighted the role of KCs in detecting and transducing noxious stimuli through their expression of a variety of sensory and neurotransmitter receptors. These sensory receptors expressed by KCs also contribute to the maintenance of skin hemostasis. The in vivo evidence on the roles of individual ion channels/receptors expressed on KCs in nociception was summarized in Table 1.
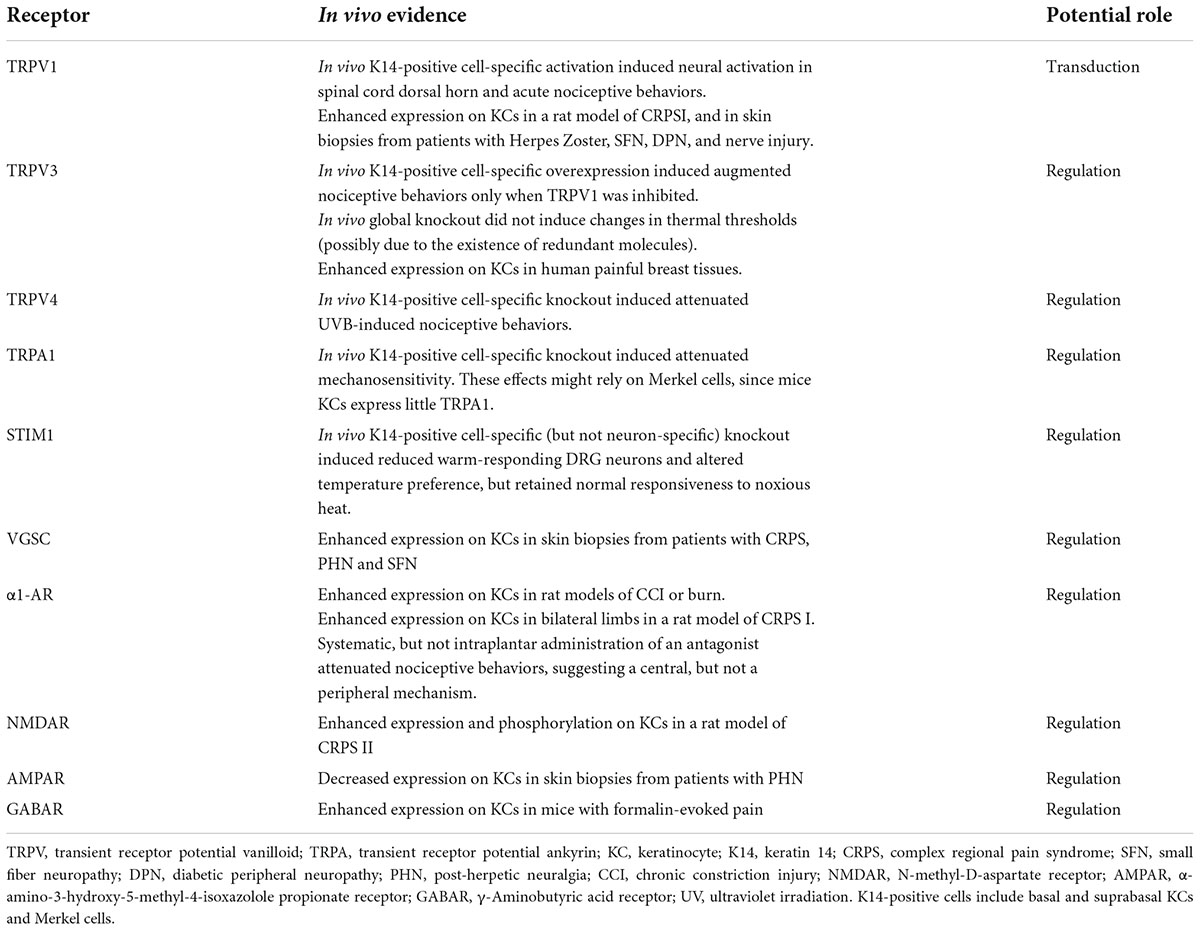
Table 1. Summary of in vivo evidence on the roles of sensory and neurotransmitter receptors expressed on keratinocytes in nociceptive transduction and regulation.
Transient receptor potential ion channel
Transient receptor potential (TRP) proteins are non-selective ion channels permeable to Na+, Ca2+, and Mg2+ (Shirolkar and Mishra, 2022). TRPs are sensors of thermal, mechanical, and chemical stimuli, participating in nociception, pruritus, inflammation, and cell proliferation (Shirolkar and Mishra, 2022). Mammalian TRPs are classified into two main groups depending on their similarity to Drosophila TRPs (Shirolkar and Mishra, 2022). Group 1 includes TRP canonical (TRPC), TRP vanilloid (TRPV), TRP melastatin (TRPM), TRP ankyrin (TRPA), and TRPN (no mechanoreceptor potential C) (Shirolkar and Mishra, 2022). Among them, TRPV1, TRPV3, TRPV4, TRPA1, and TRPM8 have been found on human KCs, evoking a Ca2+ influx upon activation (Figure 2) (Inoue et al., 2002; Radtke et al., 2011; Bidaux et al., 2015; Luostarinen et al., 2021).
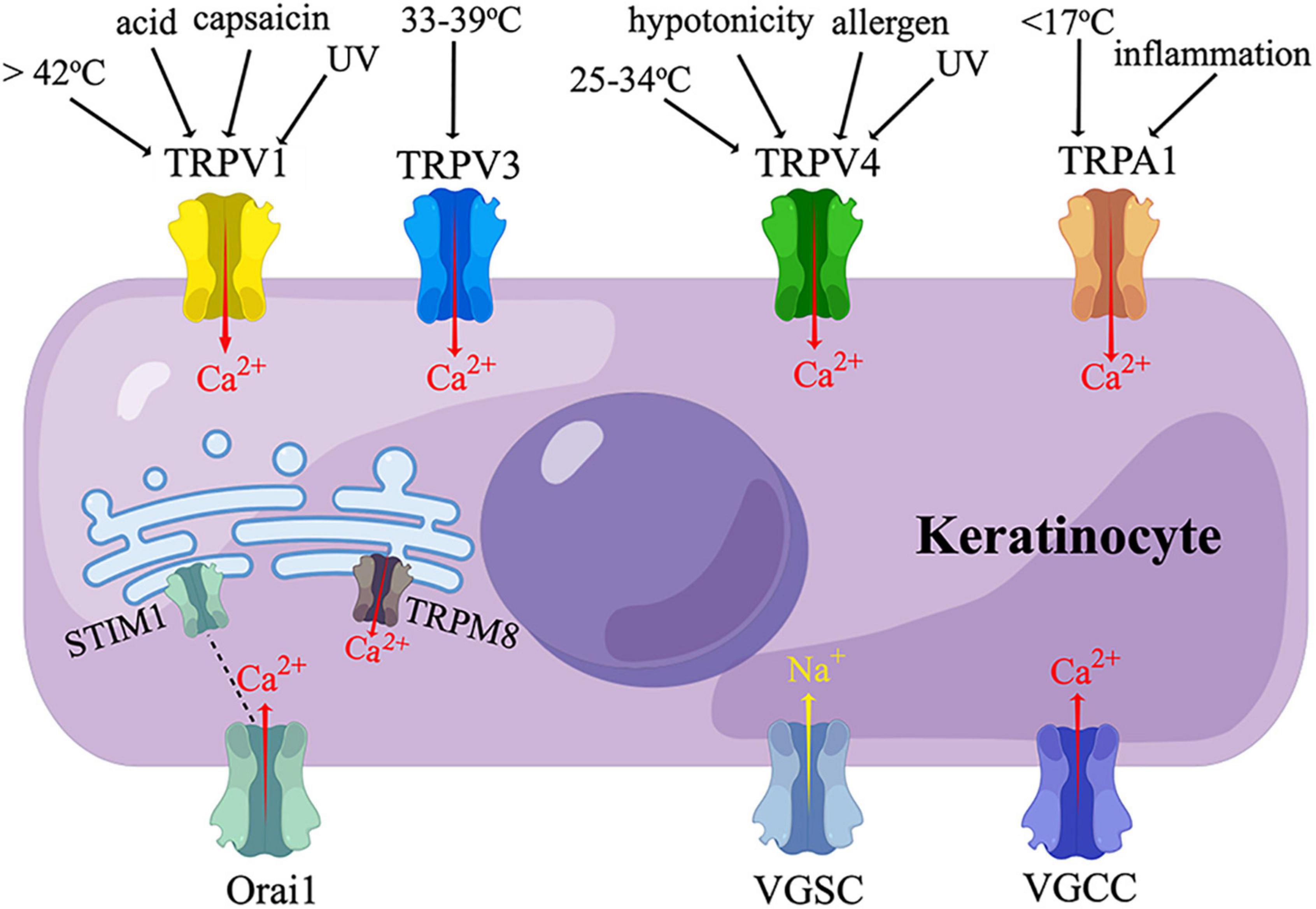
Figure 2. Sensory receptors expressed on keratinocytes. TRPV, transient receptor potential vanilloid; TRPA, transient receptor potential ankyrin; TRPM, transient receptor potential melastatin; VGSC, voltage-gated sodium channel; VGCC, voltage-gated calcium channel; UV, ultraviolet irradiation. Human keratinocytes express TRPV1, TRPV3, TRPV4, and TRPA1, which are activated by thermal, cold, chemical, or ultraviolet stimuli, and mediate a calcium influx. TRPM8 and STIM1 are temperature-sensitive endoplasmic reticulum transmembrane channels expressed in human keratinocytes. STIM1 is coupled to its plasma membrane subunit, Orail, which also mediate a calcium influx. VGSC and VGCC have also been found in human keratinocytes. (By Figdraw).
TRPV1 is activated by a temperature above 42°C, capsaicin, low pH, ultraviolet (UV) irradiation, or shear stress (Caterina et al., 1997; Inoue et al., 2002; Graham et al., 2013; Masumoto et al., 2013). TRPV1 is widely distributed in primary afferent neurons, and is also expressed in other tissues at a low level, including epidermal KCs (Lee and Caterina, 2005). Enhanced expression of TRPV1 on KCs was observed in a rat cast immobilization model of complex regional pain syndrome I (CRPS I) (Sekino et al., 2014). The expression of TRPV1 was also increased in the epidermal KCs of patients with Herpes Zoster, SFN, diabetic peripheral neuropathy (DPN), and nerve injury; with the expression level correlated with pain intensity (Facer et al., 2007; Wilder-Smith et al., 2007; Han et al., 2016). It is not clear whether the KCs selectively or broadly expressing TPRV1 are in direct contact with TRPV1-expressing or all the FNEs.
Keratin 14 (K14) is a marker of mitotically active cells in stratified squamous epithelia, including skin, hair follicle, tongue, mouth, esophagus, forestomach, and thymus (Vassar et al., 1989). By crossing mice with a target gene and mice with Cre under the control of the K14 promoter, investigators created transgenic mice that expressed the target gene predominantly in basal and suprabasal KCs along with other K14-expressing cells, such as Merkel cells (Hafner et al., 2004; Baumbauer et al., 2015; Moehring et al., 2018). In a mouse line exclusively expressing TRPV1 in K14-positive cells, a subcutaneous injection of capsaicin was sufficient to induce significant expression of c-fos, a marker of neural activation, in laminae I and II of the ipsilateral spinal cord dorsal horn and acute nociceptive behaviors (Pang et al., 2015). These findings indicated that selective stimulation of TRPV1 on KCs (in the absence of TRPV1 on nociceptors) is sufficient to trigger nociceptive transduction. Future in vivo KC-selective TRPV1 knockout studies are valuable to confirm the essential role of KCs TRPV1 in nociceptive transduction. Furthermore, KCs TRPV1 participates in the development of cutaneous inflammation. TRPV1 activation induced a production of pro-inflammatory mediators, including cyclooxygenase-2 (COX-2), prostaglandin E2 (PGE2), interleukin (IL)-8, IL-1β, IL-2, IL-4, and tumor necrosis factor (TNF)-α in KCs (Southall et al., 2003; Lee et al., 2011; Yoo et al., 2020). The expression of TRPV1 is upregulated by heat, UV irradiation, and blue light in human KCs, where TRPV1 mediated the expression of metalloproteinase-1 (MMP-1), MMP-2, MMP-3, MMP-9, and MMP-13 via a Ca2+-dependent protein kinase C-α (PKC-α) pathway (Li et al., 2007; Lee et al., 2008, 2009, 2011; Yoo et al., 2020). There is also evidence on the role of KC TRPV1 in regulating cell cycles (Radtke et al., 2011; Graham et al., 2013; Yoo et al., 2020). TRPV1 suppressed KC proliferation by promoting the degradation of epidermal growth factor receptor (EGFR) and inhibiting the downstream AKT/GSK3β/FoxO3a pathway (Yoo et al., 2020). Cell death of human KCs induced by heat at 42°C was relieved by a TRPV1 antagonist (Radtke et al., 2011). Cultured zebrafish KCs lost lamellipodia and demonstrated reduced motility when treated with TRPV1 antagonists (Graham et al., 2013).
TRPV3 is a sensor of a warm temperature (> 33-39°C) mainly expressed on KCs (Lee and Caterina, 2005; Moqrich et al., 2005). Activation of TRPV3 on KCs could excite sensory neurons by stimulating the release of Adenosine-5’-triphosphate (ATP) (Mandadi et al., 2009; Bang et al., 2010). Intraplantar injection of TRPV3 agonist triggered nociceptive behaviors in inflamed mice (Bang et al., 2010). Mice selectively overexpressing TRPV3 in K14-positive cells displayed augmented withdrawal behaviors to noxious heat only when TRPV1 was inhibited; this TRPV3-induced hyperalgesia is mediated by the production of COX-1 and PGE2 in KCs (Huang et al., 2008). Conversely, Huang et al. showed trpv3 knockout mice, trpv3/trpv4 double knockout mice, and wide-type mice had similar thermal sensitivity in both normal and complete Freund’s adjuvant (CFA)-induced inflammatory conditions, indicating the possible existence of redundant molecules that compensate for the functions of TRPV3 and TRPV4 (Huang et al., 2011). TRPV3 has also been identified on KCs in human skin biopsies. The expression of KCs TRPV3 was elevated in painful breast tissues, pruritic hypertrophic burn scars, and atopic dermatitis skin, but decreased in diabetic skin (Gopinath et al., 2005; Facer et al., 2007; Kim et al., 2016; Seo et al., 2020; Zhao et al., 2020). Additionally, TRPV3 promoted KC proliferation through an EGFR-dependent pathway (Wang et al., 2021).
TRPV4, which can be activated by a temperature between 25 and 34°C, extracellular hypotonicity, shear stress, or UVB, is also expressed on KCs (Chung et al., 2003; Lee and Caterina, 2005; Moore et al., 2013). When activated by UVB, TRPV4 upregulated the expression of endothelin-1 (ET-1) in KCs, which amplified TRPV4-dependent Ca2+ influx in a paracrine and/or autocrine manner (Moore et al., 2013). Topical application of a TRPV4 inhibitor or Trpv4 ablation in K14-expressing cells attenuated UVB-induced nociceptive behaviors and tissue damage in mice (Moore et al., 2013). These findings demonstrated that KC TRPV4 mediated UVB-induced pain via ET-1 signaling. Activation of TRPV4 by intraplantar injection of its agonist also elicited acute inflammatory reactions and hypersensitivity to noxious mechanical stimuli (Bang et al., 2012). TRPV4 could be activated by histaminergic pruritogens to evoke itch behaviors by stimulating the phosphorylation of mitogen-activated protein kinase (MAPK) and extracellular signal-regulated kinase (ERK) in KCs (Chen et al., 2016).
TRPA1 is localized in a variety of tissues, including sensory neurons and epidermis (Atoyan et al., 2009). The functions of KCs TRPA1 are controversial. TRPA1 was reported to be activated by noxious cold (< 17°C) in human KCs in vitro (Nagata et al., 2005; Tsutsumi et al., 2010), whereas global trpa1-knockout mice and rats exhibited normal cold sensations (Bautista et al., 2006; Reese et al., 2020). In contrast, others found that trpa1-knockout mice showed behavioral deficits in response to cold (0°C) and mechanical stimuli (Kwan et al., 2006). Interestingly, although mouse KCs express little TRPA1 (Zappia et al., 2016; Sadler et al., 2020), mice with a selective deletion of trpa1 in K14-expressing cells exhibited attenuated mechanosensitivity and decreased ATP release in both normal and CFA-induced inflammatory conditions (Zappia et al., 2016). The authors argued these effects might rely on other K14-expressing cells than adult KCs, such as Merkel cells (Zappia et al., 2016). TRPA1 can be activated by environmental irritants, and is involved in cutaneous inflammatory diseases, including allergic dermatitis, UVB-induced injury, and atopic dermatitis; but evidence specific to KCs TRPA1 was limited (Oh et al., 2013; Kang et al., 2017; Camponogara et al., 2020). The expression level of TRPA1 was very low in non-stimulated human KCs, but could be upregulated by TNF through nuclear factor κB (NFκB) and MAPK pathways (Luostarinen et al., 2021). TRPA1 in turn intensified inflammation by inducing the synthesis of monocyte chemoattractant protein 1 (MCP-1) and the production of IL-1α and IL-1β in KCs (Atoyan et al., 2009; Luostarinen et al., 2021). Topical application of a TRPA1 agonist induced the secretion of PGE2 and leukotriene B4 (LTB4) from human KCs in a pattern different from that induced by TRPV1 activation (Jain et al., 2011).
TRPM8 is a Ca2+-permeable ion channel located in the endoplasmic reticulum (ER) of human KCs (Bidaux et al., 2015). In vitro study showed, when activated by mild cold (24 to 33°C), epidermal TRPM8 coupled Ca2+ release from ER and Ca2+ uptake by mitochondria, which further modulated the synthesis of ATP and superoxide in mitochondria (Bidaux et al., 2015). In this way, epidermal TRPM8 regulated the proliferation and differentiation of KCs in a temperature-dependent manner (Bidaux et al., 2015).
In addition to the TRP family, KCs also express a temperature-sensitive ER transmembrane Ca2+ release-activated Ca2+ (CRAC) channel named STIM1, which is coupled to a plasma membrane pore-forming subunit named Orai1 (Figure 2) (Liu et al., 2019). When cultured KCs are heated to 42°C (heat-on) or cooled down to 25°C (heat-off), STIM1 is activated, and Orai1 mediates a Ca2+ influx (Liu et al., 2019). K14-positive cell-specific (but not neuron-specific) stim1 knockout mice showed reduced warm-responding DRG neurons and altered temperature preference behaviors. However, both DRG and behavioral responsiveness to noxious heat remained unchanged in this mouse line. The above evidence suggested the role of KC STIM1/Orai1 in normal thermal sensation, but not noxious heat sensation (Liu et al., 2019).
Voltage-gated cation channels
Voltage-gated sodium channel (VGSC, Nav) is widely distributed in neurons and KCs (Figure 2) (Wang et al., 2017). Ten subtypes of VGSC have been discovered to date, named Nav1.1-Nav1.9 and Nav X. Nav1.1, Nav1.6, and Nav1.8 were responsible for the release of ATP from rat KCs (Wijaya et al., 2020a). Compared with control skin biopsies, painful skin from patients with CRPS or post-herpetic neuralgia (PHN) exhibited an enhanced expression of Nav1.5, Nav1.6, Nav1.7, and an additional expression of Nav1.1, Nav1.2, and Nav1.8 (Wijaya et al., 2020a). A higher expression of Nav 1.7 was also detected in patients with SFN (Karl et al., 2021). These studies showed KCs VGSCs participate in neuropathic pain by promoting ATP release. VGSC also mediated ciguatoxin-induced activation and internalization of protease-activated receptor-2 (PAR2), leading to an increase in intracellular Ca2+ concentration [(Ca2+)i] in KCs (L’Herondelle et al., 2021). This finding provided an insight into the mechanisms of ciguatoxin-induced cutaneous sensory disturbance (L’Herondelle et al., 2021).
In addition to VGSC, L-type voltage-gated calcium channel (VGCC) subunit α1C has been found to be present in mouse and human KCs (Figure 2). The activation of L-type VGCC stimulates Ca2+ influx and delays the skin barrier recovery (Denda et al., 2006).
Adrenoceptors
Adrenoceptors (AR) are activated by catecholamines released by the sympathetic nervous system during injury or stress (Wijaya et al., 2020a). Although α1- and β2-ARs are mainly expressed in smooth muscles, growing evidence has shown their expression in KCs (Li et al., 2013; Wijaya et al., 2020a). Expression of α1-AR was increased on KCs and nerve fibers around the injury site after a chronic constriction injury (CCI) or burn (Drummond et al., 2014a,2015). Interestingly, in a distal tibia fracture and cast immobilization model of CRPS I, the upregulation of KC α1-AR was also observed in the plantar skin of the contralateral limb (Drummond et al., 2014b). Moreover, a systemic, but not intraplantar, administration of an α1-AR antagonist relieved pain behaviors, suggesting the α1-AR upregulation is mediated by a central, but not a peripheral mechanism (Drummond et al., 2014b).
KCs ARs reinforce inflammation and pain sensitization by forming a positive feedback loop with pro-inflammatory cytokines. Application of TNF-α increased expression of the α1-AR subtype B in cultured KCs (Wijaya et al., 2020a,b). α1-AR activation further amplified the release of IL-6 from KCs (Drummond, 2014; Wijaya et al., 2020a). Similarly, KC β2-AR activation induced upregulation of IL-6 expression, along with the phosphorylation of MAPK, ERK, and c-Jun N-terminal kinase (JNK) (Li et al., 2013).
Acetylcholine receptor
Acetylcholine (ACh) is a main neurotransmitter of the cholinergic system that acts through muscarinic and nicotinic receptors (mAChRs and nAChRs) (Zoli et al., 2018). There are five subtypes of mAChRs named M1-M5, and all of them are expressed in human KCs (Grando, 2012). Mammalian nAChRs are pentamers composed of 12 subunits named α2 to α10 and β2-β4 (Zoli et al., 2018). To date, α3, α5, α6, α7, α9, α10, β1, β2, and β4 subunits have been identified in human KCs (Grando, 1997; Nguyen et al., 2004; Zoli et al., 2018). The mAChR is a member of GCRPs that inhibits Ca2+ influx, while ionotropic nAChR stimulates Ca2+ influx (Grando, 1997). Human KCs are capable of synthesizing, secreting, and degrading ACh, indicating ACh works in a paracrine and/or autocrine manner in the epidermis (Grando et al., 1993). Previous studies have shown KCs AChRs mediated cell migration, differentiation, proliferation, adhesion, and apoptosis, and were responsible for wound healing and skin barrier recovery (Nguyen et al., 2000, 2003, 2004; Zia et al., 2000; Chernyavsky et al., 2004; Curtis et al., 2012; Ockenga et al., 2014; Sloniecka et al., 2015; Uberti et al., 2017, 2018). However, the roles of KCs AChRs in neurotransmission have not been illustrated. Topical administration of M1 receptor antagonist reversed mechanical allodynia and thermal hypoalgesia in a mouse model of DPN, whereas this study did not demonstrate whether these neuroprotective effects were attributed to KCs M1 receptors (Jolivalt et al., 2020).
Glutamate receptor
As an important excitatory neurotransmitter, glutamate is a target for fast-acting ionotropic (iGluRs) and slow-acting metabotropic receptors (mGluRs) (Pereira and Goudet, 2018). Three major classes of iGluR have been discovered and named after their preferred ligands: N-methyl-D-aspartate (NMDA), α-amino-3-hydroxy-5-methyl-4-isoxazolole propionate (AMPA), and kainate acid (KA). KCs express NMDA receptors, AMPA receptors, and mGluRs (Genever et al., 1999; Cabanero et al., 2016; Xu et al., 2020). Intraplantar injection of NMDA, AMPA, or KA induced nociceptive behaviors in rats, which were alleviated by their antagonists (Zhou et al., 1996; Davidson et al., 1997). However, these studies did not elucidate whether the agents acted at their receptors in KCs or in FNEs.
N-methyl-D-aspartate (NMDA) receptors are heterotetramers consisting of two obligatory GluN1 subunits in combination with two GluN2 and/or GluN3 subunits (Vyklicky et al., 2014). GluN2B and subunit 2D have been identified in human KCs (Fischer et al., 2006; Xu et al., 2020). Using a rat chronic post-ischemia pain model of CRPS II, we reported upregulation and phosphorylation of GluN2B in KCs, which were associated with nociceptive behaviors (Xu et al., 2020). Moreover, GluN2B mediated activation of ERK and NF-κB in epidermis and DRGs, activation of astrocyte and microglia in spinal cord dorsal horns to initiate and maintain nociceptive behaviors, indicating the important role of KCs GluN2B in peripheral and central sensitization (Xu et al., 2020). The activation of NMDA receptors triggers Ca2+ influx, which regulates cell cycles of KCs (Genever et al., 1999; Fischer et al., 2004). NMDA receptors were found to be colocalized with a glutamate receptor, excitatory amino acid carrier type 1 (EAAC1) in stratum basale, and their distributions were significantly altered during wound re-epithelialization (Genever et al., 1999). Inhibition of KCs NMDA receptors led to a decrease in cell proliferation and differentiation, and an increase in apoptosis (Fischer et al., 2004; Morhenn et al., 2004). After barrier disruption with tape stripping, topical application of an NMDA receptor agonist or antagonist delayed or accelerated the barrier repair in hairless mice, respectively (Fuziwara et al., 2003).
α-amino-3-hydroxy-5-methyl-4-isoxazolole propionate (AMPA) receptors are tetramers composed of dimers assembled from 4 possible subunits, including GluA1-GluA4 (Cabanero et al., 2016). GluA4 has been found in human and mouse KCs (Cabanero et al., 2016). Ipsilateral skin biopsies exhibited a significantly lower KC GluA4 expression compared with contralateral skin from patients with PHN (Cabanero et al., 2016).
γ-aminobutyric acid receptor
γ-Aminobutyric acid (GABA) is a main inhibitory neurotransmitter that acts on ionotropic (GABAA or GABAC) and metabotropic (GABAB) receptors (Jiang et al., 2012). Ionotropic receptors are ligand-gated Cl– channels that mediate fast GABA responses, while metabotropic receptors are G-protein-coupled receptors (GPCRs) that mediate slow responses (Jiang et al., 2012).
Cultured human KCs express functional GABA receptors that are able to induce an increase in intracellular Cl– concentration, which was blocked by a GABAA receptor antagonist (Denda et al., 2002). GABAA receptors have also been identified in hairless mouse epidermis, where they mediate epidermal hyperplasia and skin barrier recovery after disruption (Denda et al., 2002).
GABAB receptors are heterodimers containing GABAB1 and GABAB2 subunits (Jiang et al., 2012). When formalin was injected subcutaneously in mouse hindpaws to evoke pain, a co-localization of GABAB1 and GABAB2 was found on KCs and FNEs in the stratum spinosum around the injection site (Whitehead et al., 2012). The activation of KC GABAB receptors elicits antinociceptive effects. Intraplantar injection of GABAB receptor agonist alleviated PGE2-injection-induced allodynia (Whitehead et al., 2012). Application of GABA caused a decreased release of IL1-α and nerve growth factor (NGF) from cultured human KCs in neuroinflammatory conditions (Scandolera et al., 2018).
Neurotransmission between keratinocytes and sensory neurons
The expression of neurotransmitter receptors on KCs and the en passant synaptic-like contacts between KCs and FNEs imply the possible signal transmission between them. Keratinocyte-sensory neuron coculture study and use of opto- or chemogenetic techniques to exclusively activate KCs have shed new light on the interaction between KCs and sensory neurons or nerve fibers (Talagas et al., 2020a).
The ability of keratinocytes to excite sensory neurons
In vivo studies on KCs on peripheral nociceptive transduction are limited by the small diameter of the peripheral nociceptive nerve endings and the difficult experimental accessibility (Klusch et al., 2013). Nevertheless, in vitro studies have shed some lights on the role of KCs in nociception. Using a compartmented chamber coculture combined with live-cell imaging and atomic force microscopy, Klusch et al. (2013) reported structurally nanoscaled close contact between porcine KCs and sensory nerve endings in vitro. They found functionally direct cross talk between KCs and neurites. Mechanically stimulated KCs caused a transient increase in calcium concentration in nearby neuritis (Klusch et al., 2013). In cocultures of human KCs and rat DRG sensory neurons, neuronal currents and conductances recorded by patch clamp significantly increased only after the application of capsaicin to the KCs contacting with neurons, indicating sensory neuron activation and AP generation subsequent to nociceptive activation of KCs (Talagas et al., 2020c). In a coculture system of KCs and mouse DRG neurons, mechanical stimuli evoked an increase in [Ca2+]i in KCs, followed by an [Ca2+]i increase in adjacent DRG neurons after a time lag, indicating DRG neurons might be excited by KCs (Koizumi et al., 2004). Similarly, mechanical stimulation of a single KC cell induced a propagation of [Ca2+]i increase in neighboring KCs and rat DRG neurons (Tsutsumi et al., 2011; Shindo et al., 2021). This phenomenon was observed when they were seeded together at the same time, but not when KCs were seeded first and then DRG neurons were seeded on KCs (Tsutsumi et al., 2011). Exposure to the odorant chemicals induced [Ca2+]i increase in monocultured KCs and TG neurons cocultured with KCs, but not in monocultured TG neurons (Sondersorg et al., 2014). These findings suggest the necessity of contacts between KCs and sensory neurons in neurotransmission.
Cell-specific optogenetic approaches have allowed researchers to study the specific roles of KCs in vivo. In a mouse line that selectively expresses a light-sensitive cation channel (channelrhodopsin-2) in K14-expressing cells, exposure to light could depolarize and activate KCs (Baumbauer et al., 2015; Moehring et al., 2018). In contrast, when a light-driven inward chloride pump (halorhodopsin) or outward proton pump (archaerhodopsin-3, Arch) was exclusively expressed in K14-expressing cells, KCs could be hyperpolarized and inhibited by light (Baumbauer et al., 2015; Moehring et al., 2018; Sadler et al., 2020). The optogenetic activation of KCs was sufficient to trigger action potentials in Aδ- and C-fibers, and initiated nociceptive behaviors (Baumbauer et al., 2015; Moehring et al., 2018). Conversely, an optogenetic silence of KCs reduced action potentials of nociceptors, as well as behavioral responses to both noxious and innoxious mechanical stimuli (Baumbauer et al., 2015; Moehring et al., 2018). Furthermore, an optogenetic inhibition of KCs also attenuated normal cold and heat sensation (Sadler et al., 2020).
Keratinocytes (KCs) were also associated with the hyperexcitability of sensory neurons in neuropathic conditions. In a rat model of sciatic nerve neuroma, human KCs were transplanted into the ligated and transected sciatic nerve; they assembled in a structure resembling stratum spinosum. The transplanted rats displayed spontaneous pain behaviors, and their DRG neurons fired spontaneously at resting potential (Radtke et al., 2010).
Adenosine-5′-triphosphate signaling
Adenosine-5′-triphosphate (ATP) is a key extracellular signaling molecule participating in neurotransmission and neuromodulation (Suurvali et al., 2017). ATP can bind to and activate two main classes of purinergic cell surface receptors: ionotropic P2X receptors and metabotropic P2Y receptors (Suurvali et al., 2017). The fast-acting P2X receptors are ligand-gated cation channels, depolarizing the cell membrane upon activation (Alves et al., 2018). The slow-acting P2Y receptors are GPCRs, regulating the release of Ca2+ from an intracellular store (Alves et al., 2018).
Recent in vitro and in vivo data have indicated neurotransmission between KCs and sensory neurons depended on the release of ATP from KCs and the activation of P2 receptors on sensory neurons (Figure 3). Cultured human KCs constitutively released ATP at a low level (Dixon et al., 1999). Application of a depolarizing stimulus (10mM K+) led to a significant increase in ATP release over baseline levels in cultured KCs (Zhao et al., 2008). When KCs were cultured with HEK293 cells expressing P2X2 receptors (sniffer cells), sniffer cells exhibited inward currents when an adjacent KC was mechanically stimulated, and the current was abolished by an ATP-degrading enzyme, apyrase (Moehring et al., 2018). In a coculture model of sensory neurons and KCs, ATP was released from the mechanically or chemically stimulated KCs, and ATP levels were also elevated on the neuron cell surface closer to the stimulated KCs (Koizumi et al., 2004; Sondersorg et al., 2014; Shindo et al., 2021). KC-initiated propagation of Ca2+ wave in the coculture was at least partially blocked by apyrase or a P2 receptor antagonist (Tsutsumi et al., 2011; Sondersorg et al., 2014; Sadler et al., 2020). The amount of ATP release correlated with the intensity of cutaneous mechanical stimuli in glabrous hindpaw skin of mice (Moehring et al., 2018). Subcutaneous injection of apyrase decreased mechanical responsiveness and action potentials fired from nociceptors in naive mice, and also abolished behavioral responses evoked by optogenetic activation of KCs (Moehring et al., 2018). However, the treatment of apyrase did not produce additional effects during optogenetic inhibition of KCs, suggesting the KCs is a major source of ATP (Moehring et al., 2018). Furthermore, behavioral responses were reduced either by a subcutaneous injection of a P2X4 receptor inhibitor or by a neuron-specific deletion of p2x4, indicating ATP acts through P2X4 receptors on sensory neurons (Moehring et al., 2018; Sadler et al., 2020). Additionally, both KCs and FNEs expressed ecto-5’-nucleotidase (NT5E), a membrane-anchored protein hydrolyzing adenosine 5’-monophosphate (AMP) to adenosine (Sowa et al., 2010). Inflammation or nerve injury induced nociceptive behaviors were intensified in Nt5e–/– mice, implying the role of purinergic signaling in cutaneous nociception (Sowa et al., 2010).
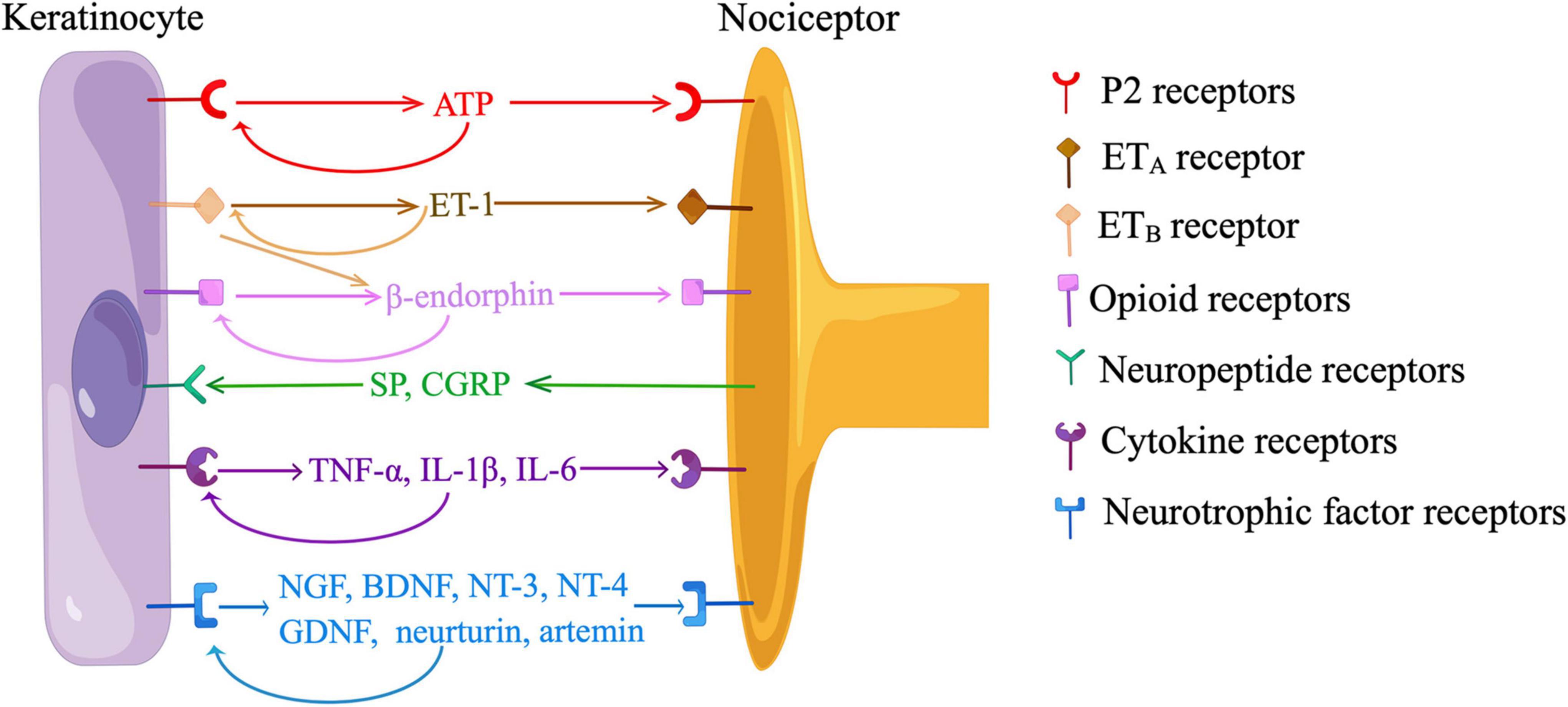
Figure 3. Communications between keratinocytes and nociceptors. ATP, adenosine-5’-triphosphate; ET-1, endothelin-1; ETA, endothelin-1 receptor A; ETB, endothelin-1 receptor B; SP, substance P; CGRP, calcitonin gene-related peptide; TNF-α, tumor necrosis factor-α; IL, interleukin; NGF, nerve growth factor; BDNF, brain-derived neurotrophic factor; NT, neurotrophin; GDNF, glia-derived neurotrophic factor. Neurotransmission between keratinocytes and sensory neurons is dependent on the release of ATP from keratinocytes and the activation of P2 receptors on nociceptors. Keratinocytes produce ET-1, which binds to ETA on nociceptors to evoke pain. ET-1 also activates ETB on keratinocytes, stimulating the release of β-endorphin, which binds to opioid receptors on nociceptors to relieve pain. Keratinocytes participate in neuroinflammation by producing neurotrophic factors and pro-inflammatory cytokines in response to neuropeptides released from nociceptors. Keratinocytes also express receptors of ATP, β-endorphin, neurotrophic factors, and pro-inflammatory cytokines, indicating the existence of paracrine and/or autocrine mechanisms. (By Figdraw).
Multiple subtypes of P2 receptors are expressed on KCs. In rat epidermis, P2Y1 and P2Y2 receptors are expressed in stratum basale, controlling cell proliferation; P2X5 receptors are predominately expressed in stratum basale and stratum spinosum, where they promote differentiation; P2X7 regulates terminal differentiation and death of KCs in stratum corneum (Greig et al., 2003a,b; Dussor et al., 2009). P2X1, P2X2, P2X3, P2X7, P2Y4, and P2Y6 receptors have also been identified in KCs (Burrell et al., 2003; Inoue et al., 2005). Exogenous application of ATP produced an increase in [Ca2+]i, and enhanced the expression of P2X receptors in KCs, indicating a possible paracrine and/or autocrine of ATP (Koizumi et al., 2004; Inoue et al., 2005). ATP release and the expression of P2X and P2Y receptors were also increased in response to inflammation, UVB irradiation, and skin injury in KCs, possibly resulting in increased nociceptor sensitivity (Greig et al., 2003a; Inoue et al., 2005; Dussor et al., 2009).
Endogenous opioid system
The endogenous opioid system consists of neuropeptides (endorphins, enkephalins, endomorphins, and dynorphins) and opioid receptors (ORs, μ, δ, and κ) (Cirillo, 2021). δ-OR, κ-OR, μ-OR, and nociceptin/orphanin FQ peptide receptor (NOP) are expressed on KCs (Bigliardi et al., 1998, 2015; Andoh et al., 2004; Tominaga et al., 2007). KCs are able to secrete neuropeptide precursor forms, including proenkephalin (PENK), proopiomelanocortin (POMC), and prodynorphin, as well as their mature proteins (Bigliardi et al., 2016).
β-endorphin, a POMC-derived neuropeptide plays an important role in the endogenous analgesic circuit. Immunohistochemistry showed KCs clustered around unmyelinated FNEs were positive for β-endorphin, suggesting KCs can communicate with FNEs by secreting β-endorphin (Bigliardi-Qi et al., 2004). An elevated level of circulating β-endorphin was associated with increased nociceptive thresholds in mice (Fell et al., 2014). The analgesic effects were abolished by a selective silence of p53-mediated POMC induction in K14-positive cells, indicating β-endorphin was mainly produced by KCs (Fell et al., 2014). β-endorphin-induced antinociception was also inhibited by the functional deletion of μ-OR or G-protein-coupled inwardly rectifying potassium channel 2 (GIRK2), or a subcutaneous injection of a κ-OR antagonist, suggesting β-endorphin acts at μ and κ-ORs on nociceptors and inhibits membrane excitability by activating GIRK (Khodorova et al., 2003; Ibrahim et al., 2005).
β-endorphin signaling was modulated by cell membrane receptors on KCs, such as cannabinoid receptor 2 (CB2) and ET-1 receptor B (ETB) (Khodorova et al., 2003; Ibrahim et al., 2005; Katsuyama et al., 2013; Gao et al., 2016). The activation of CB2 receptor stimulated the release of β-endorphin from KCs through the Gi/o-Gβγ-MAPK-Ca2+ pathway, and attenuated nociceptive behaviors in rodent models of inflammatory pain and capsaicin-induced pain (Ibrahim et al., 2005; Katsuyama et al., 2013; Gao et al., 2016). KCs secreted ET-1, a pain mediator that acts in a paracrine and/or autocrine manner by binding to ETA and ETB on KCs and FNEs (Figure 3) (Khodorova et al., 2003). The activation of ETA on FNEs evoked nociceptive behaviors, which were alleviated by the activation of ETB in KCs (Khodorova et al., 2003). This ETB-dependent analgesia was blocked by a subcutaneous injection of antiserum to β-endorphin, and ETB and β-endorphin were co-localized in KCs adjacent to FNEs (Khodorova et al., 2003). These findings, along with in vitro data in cultured KCs, showed ETB mediates the release of β-endorphin from KCs (Figure 3).
Roles of keratinocytes in neuroinflammation
“Neuroinflammation” refers to a mechanism by which sensory nerves contribute to inflammation (Choi and Di Nardo, 2018). Classically, KCs participate in neuroinflammation by producing NGF, glia-derived neurotrophic factor (GDNF), and pro-inflammatory cytokines in response to neuropeptides released from FNEs. These mediators in turn stimulate FNEs and immune cells, creating a bidirectional feedback loop that amplifies inflammation and contributes to peripheral sensitization (Figure 3) (Choi and Di Nardo, 2018).
Stimulation of keratinocytes by neuropeptides
Intraepidermal nerve fibers can be classified as peptidergic and nonpeptidergic fibers, which terminate in the stratum spinosum and granulosum, respectively, providing an opportunity of layer-specific nociceptive transduction (Zylka et al., 2005; Basbaum et al., 2009). Peptidergic fibers release either substance P (SP) and/or calcitonin gene-related peptide (CGRP) and respond to NGF, while nonpeptidergic fibers are sensitive to GDNF (Basbaum et al., 2009). As a member of the tachykinin family, SP acts on neurokinin 1 (NK1) receptors with a high affinity, as well as NK2 and NK3 receptors with a relatively low affinity (Steinhoff et al., 2014). There are two distinctive forms of CGRP: α-CGRP and β-CGRP (Hou et al., 2011). The CGRP receptor complex consists of a ligand-binding GPCR named calcitonin-like receptor (CLR) and two accessory proteins, receptor activity modifying protein 1 (RAMP1) and CGRP-receptor component protein (RCP) (Hou et al., 2011).
Previous studies have indicated human KCs express NK1 receptor, CLR, RAMP1, and RCP, while mouse KCs additionally express NK2 receptors (Song et al., 2000; Liu et al., 2006; Hou et al., 2011). Human KCs were able to release endogenous SP when stimulated by exogenous SP (Bae et al., 1999). A strong and widespread expression of CGRP (predominantly β-CGRP) was discovered on KCs in the skin biopsies of patients with PHN or CRPS I, monkeys infected with simian immunodeficiency virus, and rat models of spinal nerve ligation, CCI, or CFA-induced inflammation (Hou et al., 2011). Exposure to SP and CGRP induced an increased expression of these peptides and an upregulation of their receptors in KCs, indicating a paracrine and/or autocrine mechanism (Paus et al., 1995; Hou et al., 2011; Shi et al., 2013). SP and CGRP could also promote the proliferation and nitric oxide (NO) production of KCs (Paus et al., 1995; Yaping et al., 2006; Roggenkamp et al., 2013; Shi et al., 2013).
Substance P (SP) and/or CGPR induced an enhanced production of NGF and pro-inflammatory cytokines as well as an upregulation of IL-1 receptor on KCs (Li et al., 2009; Shi et al., 2011, 2013; Wei et al., 2012). These effects are mediated by NK1 and CLR receptors, both of which regulate the phosphorylation of p38 and ERK (Shi et al., 2013). NK1 could also activate JNK, cathepsin B, caspase-1, and Nacht, leucine-rich repeat and pyrin domain containing protein (NALP1) inflammasome (Li et al., 2009; Shi et al., 2011, 2013). In a tibia fracture and immobilization rat model of CRPS I, an increased expression of SP and CGPR was observed in the sciatic nerve, while KCs in hindpaw skin were activated and underwent rapid proliferation with an enhanced expression of NK1 (Li et al., 2009, 2010; Wei et al., 2009a,2012, 2016; Kingery, 2010; Guo et al., 2014). Fracture and/or immobilization induced mechanical allodynia, unweighting, warmth, and edema, in associations with an elevated release of TNF-α, IL-1β, IL-6, and NGF from KCs (Li et al., 2009, 2010; Wei et al., 2009a,b; Whitehead et al., 2012; Kingery, 2010; Guo et al., 2012, 2014). These effects were also created by an intraplantar injection of SP in normal rats, and were attenuated in SP or CGPR receptor deficient mice, or by systemic treatments of NK1, IL-1, or IL-6 receptor antagonists, or anti-NGF antibody (Li et al., 2009; Wei et al., 2009a,2012, 2016; Kingery, 2010; Guo et al., 2012, 2014). Skin biopsies and blisters from patients with acute CRPS displayed an elevated expression of TNF-α and IL-6 in KCs (Huygen et al., 2004; Birklein et al., 2014). In addition to CRPS, a proliferation of KCs and an increased release of IL-1β, IL-6, and TNFα were observed in a murine incisional model for postsurgical pain (Clark et al., 2007; Guo et al., 2020). These changes were more persistent in diabetic mice, in associations with a prolonged postoperative pain hypersensitivity (Guo et al., 2020).
In addition to SP and CGPR, other neuropeptides, such as neurokinin A, vasoactive intestinal polypeptide (VIP), and galanin (GAL), have similar effects in stimulating the release of pro-inflammatory mediators from KCs (Burbach et al., 2001; Dallos et al., 2006).
Release of neurotrophic factors from keratinocytes
NGF is a main neurotrophic factor participating in neuroinflammation. As a member of the family of neurotrophins, NGF regulates the survival and differentiation of peripheral sensory and sympathetic neurons, and the synthesis of neuropeptides and neurotransmitters (Yaping et al., 2006). NGF acts on a low-affinity p75 receptor and a high-affinity tropomyosin-related kinase receptor A (TrkA) (Pincelli and Marconi, 2000).
Keratinocytes (KCs) is a major source of NGF in the epidermis (Di Marco et al., 1991; Pincelli et al., 1994; Pincelli and Marconi, 2000). In addition to neuropeptides, TNF-α and histamine could stimulate the production of NGF from KCs via the Raf/MEK/ERK pathway (Woolf et al., 1997; Takaoka et al., 2009; Wijaya et al., 2020a,b). Human KCs expressed both p75 and TrkA receptors, suggesting the existence of an autocrine and/or paracrine system (Pincelli et al., 1994; Pincelli and Marconi, 2000). TrKA receptors were primarily located in stratum basale, where they stimulated proliferation and prevented apoptosis of KCs upon activation (Pincelli et al., 1994; Bronzetti et al., 1995; Marconi et al., 1999; Pincelli and Marconi, 2000). Conversely, p75 has a pro-apoptotic role in KCs (Truzzi et al., 2011). In a coculture model of DRG neurons and KCs, NGF level was enhanced as compared to the monocultured neurons or KCs, leading to a stimulation of axonal outgrowth and KC proliferation (Ulmann et al., 2007; Radtke et al., 2013).
Keratinocytes (KCs)-derived NGF was able to increase epidermal innervation and the excitability of sensory neurons, KCs were thus involved in a variety of neuropathic conditions (Barker et al., 2020). CCI induced an increased production of the precursor form of NGF (proNGF) in KCs in rats (Peleshok and Ribeiro-da-Silva, 2012). Intraplantar injection of CFA triggered a long-last upregulation of NGF and p75 expression in KCs, which led to an upregulation of CGRP in primary sensory neurons (Woolf et al., 1997; Sivilia et al., 2008). Blockade of p75 by a neutralizing antibody or decomposition of proNGF by plasmin ameliorated CFA-induced inflammatory thermal hyperalgesia in rats (Watanabe et al., 2008). Muscle incision caused an increased production of NGF in KCs in rats; and pretreatments of anti-NGF antibody decreased incision-induced hyperalgesia behaviors (Wu et al., 2009). Transgenic mice that selectively overexpress NGF in K14-positive cells displayed a hypertrophy of the peripheral nervous system and a hyperalgesia to noxious mechanical stimuli, while mice with NGF antisense in KCs displayed hypotrophy (Davis et al., 1993; Albers et al., 1994). A subcutaneous or intradermal injection of NGF evoked long-lasting local hyperalgesia in healthy humans (Barker et al., 2020). Patients with diabetic polyneuropathy or leprosy exhibited a decreased level of NGF in skin and a compensatory increased TrKA expression in KCs, which contributed to hypoalgesia (Anand et al., 1996; Terenghi et al., 1997; Facer et al., 2000).
In addition to NGF, KCs could produce other neurotrophin family members, including brain-derived neurotrophic factor (BDNF), neurotrophin-3 (NT-3), and neurotrophin-4 (NT-4), as well as GDNF family members, including GDNF, neurturin and artemin (Botchkarev et al., 1999; Malin et al., 2006; Wang et al., 2013). Expression of artemin was enhanced after intraplantar injection of CFA (Malin et al., 2006; Ikeda-Miyagawa et al., 2015). Selective overexpression of BDNF, neurturin, or artemin in K14-positive cells induced an increased behavioral sensitivity and nociceptor responses to mechanical, thermal, and cold stimuli (Albers et al., 2006; Elitt et al., 2006; Wang et al., 2013; Jankowski et al., 2017).
Conclusion and prospects
In summary, recent literature has indicated that KCs are not just the block builder in the skin but also able to sense and transduce noxious and innoxious stimuli to sensory neurons. KCs express a variety of receptors for ion channels and neurotransmitters. At this time, only TRPV1 on KCs has been implicated in nociceptive transduction. Other ion channels/receptors seem to contribute to more regulation than transduction of nociceptive signals. KCs serve as both sources and targets of neuroactive and inflammatory mediators. The emerging roles of KCs in nociception has extended the knowledge in peripheral sensitization of pain. Understanding of the pivotal roles of KCs could provide a solid foundation for targeting KCs to treat pain.
Author contributions
JX: conceptualization. XX and LX: literature review and analysis. XX: writing – original draft preparation. CY, LX, and JX: writing – review and editing. LX and JX: supervision and funding acquisition. All authors contributed to manuscript revision, read, and approved the submitted version.
Funding
This research was funded by Beijing Natural Science Foundation [grant number: 7182136] to LX and National Institutes of Health grant [grant number: K08 CA228039] to JX. JX also received research support from Steve and Melody Golding Foundation.
Conflict of interest
The authors declare that the research was conducted in the absence of any commercial or financial relationships that could be construed as a potential conflict of interest.
Publisher’s note
All claims expressed in this article are solely those of the authors and do not necessarily represent those of their affiliated organizations, or those of the publisher, the editors and the reviewers. Any product that may be evaluated in this article, or claim that may be made by its manufacturer, is not guaranteed or endorsed by the publisher.
References
Albers, K. M., Woodbury, C. J., Ritter, A. M., Davis, B. M., and Koerber, H. R. (2006). Glial cell-line-derived neurotrophic factor expression in skin alters the mechanical sensitivity of cutaneous nociceptors. J. Neurosci. 26, 2981–2990.
Albers, K. M., Wright, D. E., and Davis, B. M. (1994). Overexpression of nerve growth factor in epidermis of transgenic mice causes hypertrophy of the peripheral nervous system. J. Neurosci. 14, 1422–1432.
Alves, M., Beamer, E., and Engel, T. (2018). The metabotropic purinergic P2Y receptor family as novel drug target in epilepsy. Front. Pharmacol. 9:193. doi: 10.3389/fphar.2018.00193
Anand, P., Terenghi, G., Warner, G., Kopelman, P., Williams-Chestnut, R. E., and Sinicropi, D. V. (1996). The role of endogenous nerve growth factor in human diabetic neuropathy. Nat. Med. 2, 703–707.
Andoh, T., Yageta, Y., Takeshima, H., and Kuraishi, Y. (2004). Intradermal nociceptin elicits itch-associated responses through leukotriene B(4) in mice. J. Invest. Dermatol. 123, 196–201. doi: 10.1111/j.0022-202X.2004.22704.x
Atoyan, R., Shander, D., and Botchkareva, N. V. (2009). Non-neuronal expression of transient receptor potential type A1 (TRPA1) in human skin. J. Invest. Dermatol. 129, 2312–2315. doi: 10.1038/jid.2009.58
Bae, S., Matsunaga, Y., Tanaka, Y., and Katayama, I. (1999). Autocrine induction of substance P mRNA and peptide in cultured normal human keratinocytes. Biochem. Biophys. Res. Commun. 263, 327–333. doi: 10.1006/bbrc.1999.1285
Bang, S., Yoo, S., Yang, T. J., Cho, H., and Hwang, S. W. (2010). Farnesyl pyrophosphate is a novel pain-producing molecule via specific activation of TRPV3. J. Biol. Chem. 285, 19362–19371. doi: 10.1074/jbc.M109.087742
Bang, S., Yoo, S., Yang, T. J., Cho, H., and Hwang, S. W. (2012). Nociceptive and pro-inflammatory effects of dimethylallyl pyrophosphate via TRPV4 activation. Br. J. Pharmacol. 166, 1433–1443. doi: 10.1111/j.1476-5381.2012.01884.x
Barker, P. A., Mantyh, P., Arendt-Nielsen, L., Viktrup, L., and Tive, L. (2020). Nerve growth factor signaling and its contribution to pain. J. Pain Res. 13, 1223–1241.
Basbaum, A. I., Bautista, D. M., Scherrer, G., and Julius, D. (2009). Cellular and molecular mechanisms of pain. Cell 139, 267–284. doi: 10.1177/0022034515612022
Baumbauer, K. M., DeBerry, J. J., Adelman, P. C., Miller, R. H., Hachisuka, J., Lee, K. H., et al. (2015). Keratinocytes can modulate and directly initiate nociceptive responses. eLife 4:e09674.
Bautista, D. M., Jordt, S. E., Nikai, T., Tsuruda, P. R., Read, A. J., Poblete, J., et al. (2006). TRPA1 mediates the inflammatory actions of environmental irritants and proalgesic agents. Cell 124, 1269–1282.
Bidaux, G., Borowiec, A. S., Gordienko, D., Beck, B., Shapovalov, G. G., Lemonnier, L., et al. (2015). Epidermal TRPM8 channel isoform controls the balance between keratinocyte proliferation and differentiation in a cold-dependent manner. Proc. Natl. Acad. Sci. U S A. 112, E3345–E3354. doi: 10.1073/pnas.1423357112
Bigliardi, P. L., Bigliardi-Qi, M., Buechner, S., and Rufli, T. (1998). Expression of mu-opiate receptor in human epidermis and keratinocytes. J. Invest. Dermatol. 111, 297–301.
Bigliardi, P. L., Dancik, Y., Neumann, C., and Bigliardi-Qi, M. (2016). Opioids and skin homeostasis, regeneration and ageing - What’s the evidence? Exp. Dermatol. 25, 586–591. doi: 10.1111/exd.13021
Bigliardi, P. L., Neumann, C., Teo, Y. L., Pant, A., and Bigliardi-Qi, M. (2015). Activation of the delta-opioid receptor promotes cutaneous wound healing by affecting keratinocyte intercellular adhesion and migration. Br. J. Pharmacol. 172, 501–514. doi: 10.1111/bph.12687
Bigliardi-Qi, M., Sumanovski, L. T., Buchner, S., Rufli, T., and Bigliardi, P. L. (2004). Mu-opiate receptor and Beta-endorphin expression in nerve endings and keratinocytes in human skin. Dermatology 209, 183–189.
Birklein, F., Drummond, P. D., Li, W., Schlereth, T., Albrecht, N., Finch, P. M., et al. (2014). Activation of cutaneous immune responses in complex regional pain syndrome. J. Pain 15, 485–495.
Botchkarev, V. A., Metz, M., Botchkareva, N. V., Welker, P., Lommatzsch, M., Renz, H., et al. (1999). Brain-derived neurotrophic factor, neurotrophin-3, and neurotrophin-4 act as “epitheliotrophins” in murine skin. Lab. Invest. 79, 557–572.
Bronzetti, E., Ciriaco, E., Germana, G., and Vega, J. A. (1995). Immunohistochemical localization of neurotrophin receptor proteins in human skin. Ital. J. Anat. Embryol. 100(Suppl. 1), 565–571.
Burbach, G. J., Kim, K. H., Zivony, A. S., Kim, A., Aranda, J., Wright, S., et al. (2001). The neurosensory tachykinins substance P and neurokinin a directly induce keratinocyte nerve growth factor. J. Invest. Dermatol. 117, 1075–1082. doi: 10.1046/j.0022-202x.2001.01498.x
Burrell, H. E., Bowler, W. B., Gallagher, J. A., and Sharpe, G. R. (2003). Human keratinocytes express multiple P2Y-receptors: evidence for functional P2Y1. P2Y2, and P2Y4 receptors. J. Invest. Dermatol. 120, 440–447. doi: 10.1046/j.1523-1747.2003.12050.x
Cabanero, D., Irie, T., Celorrio, M., Trousdale, C., Owens, D. M., Virley, D., et al. (2016). Identification of an epidermal keratinocyte AMPA glutamate receptor involved in dermatopathies associated with sensory abnormalities. Pain Rep. 1:e573. doi: 10.1097/PR9.0000000000000573
Camponogara, C., Brum, E. S., Pegoraro, N. S., Brusco, I., Rocha, F. G., Brandenburg, M. M., et al. (2020). Neuronal and non-neuronal transient receptor potential ankyrin 1 mediates UVB radiation-induced skin inflammation in mice. Life Sci. 262:118557. doi: 10.1016/j.lfs.2020.118557
Caterina, M. J., Schumacher, M. A., Tominaga, M., Rosen, T. A., Levine, J. D., and Julius, D. (1997). The capsaicin receptor: a heat-activated ion channel in the pain pathway. Nature 389, 816–824.
Cauna, N. (1980). Fine morphological characteristics and microtopography of the free nerve endings of the human digital skin. Anat. Rec. 198, 643–656. doi: 10.1002/ar.1091980409
Chateau, Y., and Misery, L. (2004). Connections between nerve endings and epidermal cells: are they synapses? Exp. Dermatol. 13, 2–4.
Chen, Y., Fang, Q., Wang, Z., Zhang, J. Y., MacLeod, A. S., Hall, R. P., et al. (2016). Transient receptor potential vanilloid 4 ion channel functions as a pruriceptor in epidermal keratinocytes to evoke histaminergic itch. J. Biol. Chem. 291, 10252–10262. doi: 10.1074/jbc.M116.716464
Chernyavsky, A. I., Arredondo, J., Marubio, L. M., and Grando, S. A. (2004). Differential regulation of keratinocyte chemokinesis and chemotaxis through distinct nicotinic receptor subtypes. J. Cell Sci. 117, 5665–5679. doi: 10.1242/jcs.01492
Choi, J. E., and Di Nardo, A. (2018). Skin neurogenic inflammation. Semin. Immunopathol. 40, 249–259.
Chouchkov, C. N. (1974). An electron microscopic study of the intraepidermal innervation of human glabrous skin. Acta Anat. 88, 84–92. doi: 10.1159/000144226
Chung, M. K., Lee, H., and Caterina, M. J. (2003). Warm temperatures activate TRPV4 in mouse 308 keratinocytes. J. Biol. Chem. 278, 32037–32046. doi: 10.1074/jbc.M303251200
Clark, J. D., Shi, X., Li, X., Qiao, Y., Liang, D., Angst, M. S., et al. (2007). Morphine reduces local cytokine expression and neutrophil infiltration after incision. Mol. Pain 3:28. doi: 10.1186/1744-8069-3-28
Curtis, B. J., Plichta, J. K., Blatt, H., Droho, S., Griffin, T. M., and Radek, K. A. (2012). Nicotinic acetylcholine receptor stimulation impairs epidermal permeability barrier function and recovery and modulates cornified envelope proteins. Life Sci. 91, 1070–1076. doi: 10.1016/j.lfs.2012.08.02
Dallos, A., Kiss, M., Polyanka, H., Dobozy, A., Kemeny, L., and Husz, S. (2006). Effects of the neuropeptides substance P, calcitonin gene-related peptide, vasoactive intestinal polypeptide and galanin on the production of nerve growth factor and inflammatory cytokines in cultured human keratinocytes. Neuropeptides 40, 251–263. doi: 10.1016/j.npep.2006.06.002
Davidson, E. M., Coggeshall, R. E., and Carlton, S. M. (1997). Peripheral NMDA and non-NMDA glutamate receptors contribute to nociceptive behaviors in the rat formalin test. Neuroreport 8, 941–946.
Davis, B. M., Lewin, G. R., Mendell, L. M., Jones, M. E., and Albers, K. M. (1993). Altered expression of nerve growth factor in the skin of transgenic mice leads to changes in response to mechanical stimuli. Neuroscience 56, 789–792. doi: 10.1016/0306-4522(93)90127-2
Denda, M., Fujiwara, S., and Hibino, T. (2006). Expression of voltage-gated calcium channel subunit alpha1C in epidermal keratinocytes and effects of agonist and antagonists of the channel on skin barrier homeostasis. Exp. Dermatol. 15, 455–460. doi: 10.1111/j.0906-6705.2006.00430.x
Denda, M., Inoue, K., Inomata, S., and Denda, S. (2002). gamma-Aminobutyric acid (A) receptor agonists accelerate cutaneous barrier recovery and prevent epidermal hyperplasia induced by barrier disruption. J. Invest. Dermatol. 119, 1041–1047. doi: 10.1046/j.1523-1747.2002.19504.x
Di Marco, E., Marchisio, P. C., Bondanza, S., Franzi, A. T., Cancedda, R., and De Luca, M. (1991). Growth-regulated synthesis and secretion of biologically active nerve growth factor by human keratinocytes. J. Biol. Chem. 266, 21718–21722.
Dixon, C. J., Bowler, W. B., Littlewood-Evans, A., Dillon, J. P., Bilbe, G., Sharpe, G. R., et al. (1999). Regulation of epidermal homeostasis through P2Y2 receptors. Br. J. Pharmacol. 127, 1680–1686.
Drummond, E. S., Dawson, L. F., Finch, P. M., Bennett, G. J., and Drummond, P. D. (2014a). Increased expression of cutaneous alpha1-adrenoceptors after chronic constriction injury in rats. J. Pain 15, 188–196. doi: 10.1016/j.jpain.2013.10.010
Drummond, E. S., Dawson, L. F., Finch, P. M., Li, W., Guo, T. Z., Kingery, W. S., et al. (2014b). Increased bilateral expression of alpha1-adrenoceptors on peripheral nerves, blood vessels and keratinocytes does not account for pain or neuroinflammatory changes after distal tibia fracture in rats. Neuroscience 281, 99–109. doi: 10.1016/j.neuroscience.2014.09.046
Drummond, P. D. (2014). Neuronal changes resulting in up-regulation of alpha-1 adrenoceptors after peripheral nerve injury. Neural Regen. Res. 9, 1337–1340.
Drummond, P. D., Dawson, L. F., Finch, P. M., Drummond, E. S., Wood, F. M., and Fear, M. W. (2015). Up-regulation of cutaneous alpha1-adrenoceptors after a burn. Burns 41, 1227–1234. doi: 10.1016/j.burns.2014.12.015
Dussor, G., Koerber, H. R., Oaklander, A. L., Rice, F. L., and Molliver, D. C. (2009). Nucleotide signaling and cutaneous mechanisms of pain transduction. Brain Res. Rev. 60, 24–35.
Elitt, C. M., McIlwrath, S. L., Lawson, J. J., Malin, S. A., Molliver, D. C., Cornuet, P. K., et al. (2006). Artemin overexpression in skin enhances expression of TRPV1 and TRPA1 in cutaneous sensory neurons and leads to behavioral sensitivity to heat and cold. J. Neurosci. 26, 8578–8587. doi: 10.1523/JNEUROSCI.2185-06.2006
Facer, P., Casula, M. A., Smith, G. D., Benham, C. D., Chessell, I. P., Bountra, C., et al. (2007). Differential expression of the capsaicin receptor TRPV1 and related novel receptors TRPV3, TRPV4 and TRPM8 in normal human tissues and changes in traumatic and diabetic neuropathy. BMC Neurol. 7:11. doi: 10.1186/1471-2377-7-11
Facer, P., Mann, D., Mathur, R., Pandya, S., Ladiwala, U., Singhal, B., et al. (2000). Do nerve growth factor-related mechanisms contribute to loss of cutaneous nociception in leprosy? Pain 85, 231–238. doi: 10.1016/s0304-3959(99)00273-0
Fell, G. L., Robinson, K. C., Mao, J., Woolf, C. J., and Fisher, D. E. (2014). Skin beta-endorphin mediates addiction to UV light. Cell 157, 1527–1534.
Fischer, M., Fiedler, E., Seidel, C., Meiss, F., Klapperstuck, T., Helmbold, P., et al. (2006). Cultivated keratinocytes express N-methyl-D-aspartate receptors of the NMDAR2D type. Arch. Dermatol. Res. 297, 316–318. doi: 10.1007/s00403-005-0625-1
Fischer, M., Glanz, D., William, T., Klapperstuck, T., Wohlrab, J., and Marsch, W. (2004). N-methyl-D-aspartate receptors influence the intracellular calcium concentration of keratinocytes. Exp. Dermatol. 13, 512–519.
Fuziwara, S., Inoue, K., and Denda, M. (2003). NMDA-type glutamate receptor is associated with cutaneous barrier homeostasis. J. Invest. Dermatol. 120, 1023–1029. doi: 10.1046/j.1523-1747.2003.12238.x
Gallegos-Alcala, P., Jimenez, M., Cervantes-Garcia, D., and Salinas, E. (2021). The keratinocyte as a crucial cell in the predisposition, onset, progression, therapy and study of the atopic dermatitis. Int. J. Mol. Sci. 22:10661. doi: 10.3390/ijms221910661
Gao, F., Zhang, L. H., Su, T. F., Li, L., Zhou, R., Peng, M., et al. (2016). Signaling mechanism of cannabinoid Receptor-2 Activation-induced beta-endorphin release. Mol. Neurobiol. 53, 3616–3625. doi: 10.1007/s12035-015-9291-2
Genever, P. G., Maxfield, S. J., Kennovin, G. D., Maltman, J., Bowgen, C. J., Raxworthy, M. J., et al. (1999). Evidence for a novel glutamate-mediated signaling pathway in keratinocytes. J. Invest. Dermatol. 112, 337–342. doi: 10.1046/j.1523-1747.1999.00509.x
Gopinath, P., Wan, E., Holdcroft, A., Facer, P., Davis, J. B., Smith, G. D., et al. (2005). Increased capsaicin receptor TRPV1 in skin nerve fibres and related vanilloid receptors TRPV3 and TRPV4 in keratinocytes in human breast pain. BMC Womens Health 5:2. doi: 10.1186/1472-6874-5-2
Graham, D. M., Huang, L., Robinson, K. R., and Messerli, M. A. (2013). Epidermal keratinocyte polarity and motility require Ca(2)(+) influx through TRPV1. J. Cell Sci. 126, 4602–4613. doi: 10.1242/jcs.122192
Grando, S. A. (1997). Biological functions of keratinocyte cholinergic receptors. J. Investig. Dermatol. Symp. Proc. 2, 41–48.
Grando, S. A., Kist, D. A., Qi, M., and Dahl, M. V. (1993). Human keratinocytes synthesize, secrete, and degrade acetylcholine. J. Invest. Dermatol. 101, 32–36.
Grando, S. A. (2012). Muscarinic receptor agonists and antagonists: effects on keratinocyte functions. Handb. Exp. Pharmacol. 208, 429–450.
Greig, A. V., James, S. E., McGrouther, D. A., Terenghi, G., and Burnstock, G. (2003a). Purinergic receptor expression in the regeneration epidermis in a rat model of normal and delayed wound healing. Exp. Dermatol. 12, 860–871. doi: 10.1111/j.0906-6705.2003.00110.x
Greig, A. V., Linge, C., Terenghi, G., McGrouther, D. A., and Burnstock, G. (2003b). Purinergic receptors are part of a functional signaling system for proliferation and differentiation of human epidermal keratinocytes. J. Invest. Dermatol. 120, 1007–1015.
Guo, R., Hao, J., Ma, D., Li, H., Liao, K., and Wang, Y. (2020). Persistent proliferation of keratinocytes and prolonged expression of pronociceptive inflammatory mediators might be associated with the postoperative pain in KK mice. Mol. Pain 16:1744806920927284. doi: 10.1177/1744806920927284
Guo, T. Z., Wei, T., Li, W. W., Li, X. Q., Clark, J. D., and Kingery, W. S. (2014). Immobilization contributes to exaggerated neuropeptide signaling, inflammatory changes, and nociceptive sensitization after fracture in rats. J. Pain 15, 1033–1045. doi: 10.1016/j.jpain.2014.07.004
Guo, T. Z., Wei, T., Shi, X., Li, W. W., Hou, S., Wang, L., et al. (2012). Neuropeptide deficient mice have attenuated nociceptive, vascular, and inflammatory changes in a tibia fracture model of complex regional pain syndrome. Mol. Pain 8:85. doi: 10.1186/1744-8069-8-85
Hafner, M., Wenk, J., Nenci, A., Pasparakis, M., Scharffetter-Kochanek, K., Smyth, N., et al. (2004). Keratin 14 Cre transgenic mice authenticate keratin 14 as an oocyte-expressed protein. Genesis 38, 176–181. doi: 10.1002/gene.20016
Han, S. B., Kim, H., Cho, S. H., Lee, J. D., Chung, J. H., and Kim, H. S. (2016). Transient receptor potential vanilloid-1 in epidermal keratinocytes may contribute to acute pain in herpes zoster. Acta Derm. Venereol. 96, 319–322. doi: 10.2340/00015555-2247
Hilliges, M., Wang, L., and Johansson, O. (1995). Ultrastructural evidence for nerve fibers within all vital layers of the human epidermis. J. Invest. Dermatol. 104, 134–137. doi: 10.1111/1523-1747.ep12613631
Hou, Q., Barr, T., Gee, L., Vickers, J., Wymer, J., Borsani, E., et al. (2011). Keratinocyte expression of calcitonin gene-related peptide beta: implications for neuropathic and inflammatory pain mechanisms. Pain 152, 2036–2051. doi: 10.1016/j.pain.2011.04.033
Houben, E., De Paepe, K., and Rogiers, V. (2007). A keratinocyte’s course of life. Skin Pharmacol. Physiol. 20, 122–132.
Huang, S. M., Lee, H., Chung, M. K., Park, U., Yu, Y. Y., Bradshaw, H. B., et al. (2008). Overexpressed transient receptor potential vanilloid 3 ion channels in skin keratinocytes modulate pain sensitivity via prostaglandin E2. J. Neurosci. 28, 13727–13737. doi: 10.1523/JNEUROSCI.5741-07.2008
Huang, S. M., Li, X., Yu, Y., Wang, J., and Caterina, M. J. (2011). TRPV3 and TRPV4 ion channels are not major contributors to mouse heat sensation. Mol. Pain 7:37.
Huygen, F. J., Ramdhani, N., van Toorenenbergen, A., Klein, J., and Zijlstra, F. J. (2004). Mast cells are involved in inflammatory reactions during complex regional pain syndrome type 1. Immunol. Lett. 91, 147–154.
Ibrahim, M. M., Porreca, F., Lai, J., Albrecht, P. J., Rice, F. L., Khodorova, A., et al. (2005). CB2 cannabinoid receptor activation produces antinociception by stimulating peripheral release of endogenous opioids. Proc. Natl. Acad. Sci. U S A. 102, 3093–3098.
Ikeda-Miyagawa, Y., Kobayashi, K., Yamanaka, H., Okubo, M., Wang, S., Dai, Y., et al. (2015). Peripherally increased artemin is a key regulator of TRPA1/V1 expression in primary afferent neurons. Mol. Pain 11:8. doi: 10.1186/s12990-015-0004-7
Inoue, K., Denda, M., Tozaki, H., Fujishita, K., Koizumi, S., and Inoue, K. (2005). Characterization of multiple P2X receptors in cultured normal human epidermal keratinocytes. J. Invest. Dermatol. 124, 756–763. doi: 10.1111/j.0022-202X.2005.23683.x
Inoue, K., Koizumi, S., Fuziwara, S., Denda, S., Inoue, K., and Denda, M. (2002). Functional vanilloid receptors in cultured normal human epidermal keratinocytes. Biochem. Biophys. Res. Commun. 291, 124–129.
Jain, A., Bronneke, S., Kolbe, L., Stab, F., Wenck, H., and Neufang, G. (2011). TRP-channel-specific cutaneous eicosanoid release patterns. Pain 152, 2765–2772. doi: 10.1016/j.pain.2011.08.025
Jankowski, M. P., Baumbauer, K. M., Wang, T., Albers, K. M., Davis, B. M., and Koerber, H. R. (2017). Cutaneous neurturin overexpression alters mechanical, thermal, and cold responsiveness in physiologically identified primary afferents. J. Neurophysiol. 117, 1258–1265. doi: 10.1152/jn.00731.2016
Jiang, N., Rasmussen, J. P., Clanton, J. A., Rosenberg, M. F., Luedke, K. P., Cronan, M. R., et al. (2019). A conserved morphogenetic mechanism for epidermal ensheathment of nociceptive sensory neurites. eLife 8:e42455. doi: 10.7554/eLife.42455
Jiang, X., Su, L., Zhang, Q., He, C., Zhang, Z., Yi, P., et al. (2012). GABAB receptor complex as a potential target for tumor therapy. J. Histochem. Cytochem. 60, 269–279.
Jolivalt, C. G., Frizzi, K. E., Han, M. M., Mota, A. J., Guernsey, L. S., Kotra, L. P., et al. (2020). Topical delivery of muscarinic receptor antagonists prevents and reverses peripheral neuropathy in female diabetic mice. J. Pharmacol. Exp. Ther. 374, 44–51. doi: 10.1124/jpet.120.265447
Kang, J., Ding, Y., Li, B., Liu, H., Yang, X., and Chen, M. (2017). TRPA1 mediated aggravation of allergic contact dermatitis induced by DINP and regulated by NF-kappaB activation. Sci. Rep. 7:43586. doi: 10.1038/srep43586
Karl, F., Bischler, T., Egenolf, N., Evdokimov, D., Heckel, T., and Uceyler, N. (2021). Fibromyalgia vs small fiber neuropathy: diverse keratinocyte transcriptome signature. Pain 162, 2569–2577. doi: 10.1097/j.pain.0000000000002249
Katsuyama, S., Mizoguchi, H., Kuwahata, H., Komatsu, T., Nagaoka, K., Nakamura, H., et al. (2013). Involvement of peripheral cannabinoid and opioid receptors in beta-caryophyllene-induced antinociception. Eur. J. Pain 17, 664–675. doi: 10.1002/j.1532-2149.2012.00242.x
Khodorova, A., Navarro, B., Jouaville, L. S., Murphy, J. E., Rice, F. L., Mazurkiewicz, J. E., et al. (2003). Endothelin-B receptor activation triggers an endogenous analgesic cascade at sites of peripheral injury. Nat. Med. 9, 1055–1061. doi: 10.1038/nm885
Kim, H. O., Cho, Y. S., Park, S. Y., Kwak, I. S., Choi, M. G., Chung, B. Y., et al. (2016). Increased activity of TRPV3 in keratinocytes in hypertrophic burn scars with postburn pruritus. Wound Repair Regen. 24, 841–850. doi: 10.1111/wrr.12469
Kingery, W. S. (2010). Role of neuropeptide, cytokine, and growth factor signaling in complex regional pain syndrome. Pain. Med. 11, 1239–1250.
Klusch, A., Ponce, L., Gorzelanny, C., Schafer, I., Schneider, S. W., Ringkamp, M., et al. (2013). Coculture model of sensory neurites and keratinocytes to investigate functional interaction: chemical stimulation and atomic force microscope-transmitted mechanical stimulation combined with live-cell imaging. J. Invest. Dermatol. 133, 1387–1390. doi: 10.1038/jid.2012.471
Koizumi, S., Fujishita, K., Inoue, K., Shigemoto-Mogami, Y., Tsuda, M., and Inoue, K. (2004). Ca2+ waves in keratinocytes are transmitted to sensory neurons: the involvement of extracellular ATP and P2Y2 receptor activation. Biochem. J. 380, 329–338. doi: 10.1042/BJ20031089
Kwan, K. Y., Allchorne, A. J., Vollrath, M. A., Christensen, A. P., Zhang, D. S., Woolf, C. J., et al. (2006). TRPA1 contributes to cold, mechanical, and chemical nociception but is not essential for hair-cell transduction. Neuron 50, 277–289.
Lee, H., and Caterina, M. J. (2005). TRPV channels as thermosensory receptors in epithelial cells. Pflugers. Arch. 451, 160–167.
Lee, Y. M., Kang, S. M., Lee, S. R., Kong, K. H., Lee, J. Y., Kim, E. J., et al. (2011). Inhibitory effects of TRPV1 blocker on UV-induced responses in the hairless mice. Arch. Dermatol. Res. 303, 727–736. doi: 10.1007/s00403-011-1153-9
Lee, Y. M., Kim, Y. K., Kim, K. H., Park, S. J., Kim, S. J., and Chung, J. H. (2009). A novel role for the TRPV1 channel in UV-induced matrix metalloproteinase (MMP)-1 expression in HaCaT cells. J. Cell. Physiol. 219, 766–775. doi: 10.1002/jcp.21729
Lee, Y. M., Li, W. H., Kim, Y. K., Kim, K. H., and Chung, J. H. (2008). Heat-induced MMP-1 expression is mediated by TRPV1 through PKCalpha signaling in HaCaT cells. Exp. Dermatol. 17, 864–870. doi: 10.1111/j.1600-0625.2008.00738.x
L’Herondelle, K., Pierre, O., Fouyet, S., Leschiera, R., Le Gall-Ianotto, C., Philippe, R., et al. (2021). PAR2, keratinocytes, and cathepsin S mediate the sensory effects of ciguatoxins responsible for ciguatera poisoning. J. Invest. Dermatol. 141:e643. doi: 10.1016/j.jid.2020.07.020
Li, W., Shi, X., Wang, L., Guo, T., Wei, T., Cheng, K., et al. (2013). Epidermal adrenergic signaling contributes to inflammation and pain sensitization in a rat model of complex regional pain syndrome. Pain 154, 1224–1236. doi: 10.1016/j.pain.2013.03.033
Li, W. H., Lee, Y. M., Kim, J. Y., Kang, S., Kim, S., Kim, K. H., et al. (2007). Transient receptor potential vanilloid-1 mediates heat-shock-induced matrix metalloproteinase-1 expression in human epidermal keratinocytes. J. Invest. Dermatol. 127, 2328–2335. doi: 10.1038/sj.jid.5700880
Li, W. W., Guo, T. Z., Li, X. Q., Kingery, W. S., and Clark, D. J. (2010). Fracture induces keratinocyte activation, proliferation, and expression of pro-nociceptive inflammatory mediators. Pain 151, 843–852. doi: 10.1016/j.pain.2010.09.026
Li, W. W., Guo, T. Z., Liang, D., Shi, X., Wei, T., Kingery, W. S., et al. (2009). The NALP1 inflammasome controls cytokine production and nociception in a rat fracture model of complex regional pain syndrome. Pain 147, 277–286. doi: 10.1016/j.pain.2009.09.032
Liu, J. Y., Hu, J. H., Zhu, Q. G., Li, F. Q., and Sun, H. J. (2006). Substance P receptor expression in human skin keratinocytes and fibroblasts. Br. J. Dermatol. 155, 657–662.
Liu, X., Wang, H., Jiang, Y., Zheng, Q., Petrus, M., Zhang, M., et al. (2019). STIM1 thermosensitivity defines the optimal preference temperature for warm sensation in mice. Cell Res. 29, 95–109. doi: 10.1038/s41422-018-0129-0
Luostarinen, S., Hamalainen, M., and Moilanen, E. (2021). Transient receptor potential ankyrin 1 (TRPA1)-an inflammation-induced factor in human HaCaT keratinocytes. Int. J. Mol. Sci. 22:3322. doi: 10.3390/ijms22073322
Malin, S. A., Molliver, D. C., Koerber, H. R., Cornuet, P., Frye, R., Albers, K. M., et al. (2006). Glial cell line-derived neurotrophic factor family members sensitize nociceptors in vitro and produce thermal hyperalgesia in vivo. J. Neurosci. 26, 8588–8599. doi: 10.1523/JNEUROSCI.1726-06.2006
Mandadi, S., Sokabe, T., Shibasaki, K., Katanosaka, K., Mizuno, A., Moqrich, A., et al. (2009). TRPV3 in keratinocytes transmits temperature information to sensory neurons via ATP. Pflugers. Arch. 458, 1093–1102. doi: 10.1007/s00424-009-0703-x
Marconi, A., Vaschieri, C., Zanoli, S., Giannetti, A., and Pincelli, C. (1999). Nerve growth factor protects human keratinocytes from ultraviolet-B-induced apoptosis. J. Invest. Dermatol. 113, 920–927.
Masumoto, K., Tsukimoto, M., and Kojima, S. (2013). Role of TRPM2 and TRPV1 cation channels in cellular responses to radiation-induced DNA damage. Biochim. Biophys. Acta 1830, 3382–3390. doi: 10.1016/j.bbagen.2013.02.020
Moehring, F., Cowie, A. M., Menzel, A. D., Weyer, A. D., Grzybowski, M., Arzua, T., et al. (2018). Keratinocytes mediate innocuous and noxious touch via ATP-P2X4 signaling. eLife 7:e31684. doi: 10.7554/eLife.31684
Moore, C., Cevikbas, F., Pasolli, H. A., Chen, Y., Kong, W., Kempkes, C., et al. (2013). UVB radiation generates sunburn pain and affects skin by activating epidermal TRPV4 ion channels and triggering endothelin-1 signaling. Proc. Natl. Acad. Sci. U S A. 110, E3225–E3234. doi: 10.1073/pnas.1312933110
Moqrich, A., Hwang, S. W., Earley, T. J., Petrus, M. J., Murray, A. N., Spencer, K. S., et al. (2005). Impaired thermosensation in mice lacking TRPV3, a heat and camphor sensor in the skin. Science 307, 1468–1472. doi: 10.1126/science.1108609
Morhenn, V. B., Murakami, M., O’Grady, T., Nordberg, J., and Gallo, R. L. (2004). Characterization of the expression and function of N-methyl-D-aspartate receptor in keratinocytes. Exp. Dermatol. 13, 505–511.
Nagata, K., Duggan, A., Kumar, G., and Garcia-Anoveros, J. (2005). Nociceptor and hair cell transducer properties of TRPA1, a channel for pain and hearing. J. Neurosci. 25, 4052–4061. doi: 10.1523/JNEUROSCI.0013-05.2005
Nguyen, V. T., Arredondo, J., Chernyavsky, A. I., Kitajima, Y., and Grando, S. A. (2003). Keratinocyte acetylcholine receptors regulate cell adhesion. Life Sci. 72, 2081–2085.
Nguyen, V. T., Chernyavsky, A. I., Arredondo, J., Bercovich, D., Orr-Urtreger, A., Vetter, D. E., et al. (2004). Synergistic control of keratinocyte adhesion through muscarinic and nicotinic acetylcholine receptor subtypes. Exp. Cell Res. 294, 534–549. doi: 10.1016/j.yexcr.2003.12.010
Nguyen, V. T., Ndoye, A., and Grando, S. A. (2000). Novel human alpha9 acetylcholine receptor regulating keratinocyte adhesion is targeted by Pemphigus vulgaris autoimmunity. Am. J. Pathol. 157, 1377–1391. doi: 10.1016/s0002-9440(10)64651-2
Ockenga, W., Kuhne, S., Bocksberger, S., Banning, A., and Tikkanen, R. (2014). Epidermal growth factor receptor transactivation is required for mitogen-activated protein kinase activation by muscarinic acetylcholine receptors in HaCaT keratinocytes. Int. J. Mol. Sci. 15, 21433–21454. doi: 10.3390/ijms151121433
Oh, M. H., Oh, S. Y., Lu, J., Lou, H., Myers, A. C., Zhu, Z., et al. (2013). TRPA1-dependent pruritus in IL-13-induced chronic atopic dermatitis. J. Immunol. 191, 5371–5382. doi: 10.4049/jimmunol.1300300
Pang, Z., Sakamoto, T., Tiwari, V., Kim, Y. S., Yang, F., Dong, X., et al. (2015). Selective keratinocyte stimulation is sufficient to evoke nociception in mice. Pain 156, 656–665. doi: 10.1097/j.pain.0000000000000092
Paus, R., Heinzelmann, T., Robicsek, S., Czarnetzki, B. M., and Maurer, M. (1995). Substance P stimulates murine epidermal keratinocyte proliferation and dermal mast cell degranulation in situ. Arch. Dermatol. Res. 287, 500–502. doi: 10.1007/BF00373436
Peleshok, J. C., and Ribeiro-da-Silva, A. (2012). Neurotrophic factor changes in the rat thick skin following chronic constriction injury of the sciatic nerve. Mol. Pain 8:1. doi: 10.1186/1744-8069-8-1
Pereira, V., and Goudet, C. (2018). Emerging trends in pain modulation by metabotropic glutamate receptors. Front. Mol. Neurosci. 11:464. doi: 10.3389/fnmol.2018.00464
Pincelli, C., and Marconi, A. (2000). Autocrine nerve growth factor in human keratinocytes. J. Dermatol. Sci. 22, 71–79.
Pincelli, C., Sevignani, C., Manfredini, R., Grande, A., Fantini, F., Bracci-Laudiero, L., et al. (1994). Expression and function of nerve growth factor and nerve growth factor receptor on cultured keratinocytes. J. Invest. Dermatol. 103, 13–18.
Radtke, C., Rennekampff, H. O., Reimers, K., Vogt, P. M., and Kocsis, J. D. (2013). Paracrine loop of keratinocyte proliferation and directed neuritic outgrowth in a neuroepithelial coculture. Ann. Plast. Surg. 70, 162–167. doi: 10.1097/SPA.0b013e318276d946
Radtke, C., Sinis, N., Sauter, M., Jahn, S., Kraushaar, U., Guenther, E., et al. (2011). TRPV channel expression in human skin and possible role in thermally induced cell death. J. Burn Care Res. 32, 150–159. doi: 10.1097/BCR.0b013e318203350c
Radtke, C., Vogt, P. M., Devor, M., and Kocsis, J. D. (2010). Keratinocytes acting on injured afferents induce extreme neuronal hyperexcitability and chronic pain. Pain 148, 94–102. doi: 10.1016/j.pain.2009.10.014
Reese, R. M., Dourado, M., Anderson, K., Warming, S., Stark, K. L., Balestrini, A., et al. (2020). Behavioral characterization of a CRISPR-generated TRPA1 knockout rat in models of pain, itch, and asthma. Sci. Rep. 10:979. doi: 10.1038/s41598-020-57936-5
Roger, M., Fullard, N., Costello, L., Bradbury, S., Markiewicz, E., O’Reilly, S., et al. (2019). Bioengineering the microanatomy of human skin. J. Anat. 234, 438–455.
Roggenkamp, D., Kopnick, S., Stab, F., Wenck, H., Schmelz, M., and Neufang, G. (2013). Epidermal nerve fibers modulate keratinocyte growth via neuropeptide signaling in an innervated skin model. J. Invest. Dermatol. 133, 1620–1628. doi: 10.1038/jid.2012.464
Sadler, K. E., Moehring, F., and Stucky, C. L. (2020). Keratinocytes contribute to normal cold and heat sensation. eLife 9:e58625. doi: 10.7554/eLife.58625
Scandolera, A., Hubert, J., Humeau, A., Lambert, C., De Bizemont, A., Winkel, C., et al. (2018). GABA and GABA-Alanine from the red microalgae Rhodosorus marinus exhibit a significant neuro-soothing activity through inhibition of neuro-inflammation mediators and positive regulation of TRPV1-Related skin sensitization. Mar. Drugs 16:96. doi: 10.3390/md16030096
Sekino, Y., Nakano, J., Hamaue, Y., Chuganji, S., Sakamoto, J., Yoshimura, T., et al. (2014). Sensory hyperinnervation and increase in NGF. TRPV1 and P2X3 expression in the epidermis following cast immobilization in rats. Eur. J. Pain 18, 639–648. doi: 10.1002/j.1532-2149.2013.00412.x
Seo, S. H., Kim, S., Kim, S. E., Chung, S., and Lee, S. E. (2020). Enhanced thermal sensitivity of TRPV3 in keratinocytes underlies heat-induced pruritogen release and pruritus in atopic dermatitis. J. Invest. Dermatol. 140, 2199–2209.e6.. doi: 10.1016/j.jid.2020.02.028
Shi, X., Wang, L., Clark, J. D., and Kingery, W. S. (2013). Keratinocytes express cytokines and nerve growth factor in response to neuropeptide activation of the ERK1/2 and JNK MAPK transcription pathways. Regul. Pept. 186, 92–103. doi: 10.1016/j.regpep.2013.08.001
Shi, X., Wang, L., Li, X., Sahbaie, P., Kingery, W. S., and Clark, J. D. (2011). Neuropeptides contribute to peripheral nociceptive sensitization by regulating interleukin-1beta production in keratinocytes. Anesth. Analg. 113, 175–183. doi: 10.1213/ANE.0b013e31821a0258
Shindo, Y., Fujita, K., Tanaka, M., Fujio, H., Hotta, K., and Oka, K. (2021). Mechanical stimulus-evoked signal transduction between keratinocytes and sensory neurons via extracellular ATP. Biochem. Biophys. Res. Commun. 582, 131–136. doi: 10.1016/j.bbrc.2021.10.046
Shirolkar, P., and Mishra, S. K. (2022). Role of TRP ion channels in pruritus. Neurosci. Lett. 768:136379.
Sivilia, S., Paradisi, M., D’Intino, G., Fernandez, M., Pirondi, S., Lorenzini, L., et al. (2008). Skin homeostasis during inflammation: a role for nerve growth factor. Histol. Histopathol. 23, 1–10.
Sloniecka, M., Backman, L. J., and Danielson, P. (2015). Acetylcholine enhances keratocyte proliferation through muscarinic receptor activation. Int. Immunopharmacol. 29, 57–62. doi: 10.1016/j.intimp.2015.05.039
Sondersorg, A. C., Busse, D., Kyereme, J., Rothermel, M., Neufang, G., Gisselmann, G., et al. (2014). Chemosensory information processing between keratinocytes and trigeminal neurons. J. Biol. Chem. 289, 17529–17540. doi: 10.1074/jbc.M113.499699
Song, I. S., Bunnett, N. W., Olerud, J. E., Harten, B., Steinhoff, M., Brown, J. R., et al. (2000). Substance P induction of murine keratinocyte PAM 212 interleukin 1 production is mediated by the neurokinin 2 receptor (NK-2R). Exp. Dermatol. 9, 42–52. doi: 10.1034/j.1600-0625.2000.009001042.x
Southall, M. D., Li, T., Gharibova, L. S., Pei, Y., Nicol, G. D., and Travers, J. B. (2003). Activation of epidermal vanilloid receptor-1 induces release of proinflammatory mediators in human keratinocytes. J. Pharmacol. Exp. Ther. 304, 217–222. doi: 10.1124/jpet.102.040675
Sowa, N. A., Taylor-Blake, B., and Zylka, M. J. (2010). Ecto-5’-nucleotidase (CD73) inhibits nociception by hydrolyzing AMP to adenosine in nociceptive circuits. J. Neurosci. 30, 2235–2244.
Steinhoff, M. S., von Mentzer, B., Geppetti, P., Pothoulakis, C., and Bunnett, N. W. (2014). Tachykinins and their receptors: contributions to physiological control and the mechanisms of disease. Physiol. Rev. 94, 265–301. doi: 10.1152/physrev.00031.2013
Suurvali, J., Boudinot, P., Kanellopoulos, J., and Ruutel Boudinot, S. (2017). P2X4: a fast and sensitive purinergic receptor. Biomed. J. 40, 245–256.
Takaoka, K., Shirai, Y., and Saito, N. (2009). Inflammatory cytokine tumor necrosis factor-alpha enhances nerve growth factor production in human keratinocytes. HaCaT cells. J. Pharmacol. Sci. 111, 381–391. doi: 10.1254/jphs.09143fp
Talagas, M., Lebonvallet, N., Berthod, F., and Misery, L. (2020a). Lifting the veil on the keratinocyte contribution to cutaneous nociception. Protein Cell 11, 239–250. doi: 10.1007/s13238-019-00683-9
Talagas, M., Lebonvallet, N., Leschiera, R., Elies, P., Marcorelles, P., and Misery, L. (2020b). Intra-epidermal nerve endings progress within keratinocyte cytoplasmic tunnels in normal human skin. Exp. Dermatol. 29, 387–392. doi: 10.1111/exd.14081
Talagas, M., Lebonvallet, N., Leschiera, R., Sinquin, G., Elies, P., Haftek, M., et al. (2020c). Keratinocytes communicate with sensory neurons via synaptic-like contacts. Ann. Neurol. 88, 1205–1219. doi: 10.1002/ana.25912
Talagas, M., Lebonvallet, N., Leschiera, R., Marcorelles, P., and Misery, L. (2018). What about physical contacts between epidermal keratinocytes and sensory neurons? Exp. Dermatol. 27, 9–13.
Terenghi, G., Mann, D., Kopelman, P. G., and Anand, P. (1997). trkA and trkC expression is increased in human diabetic skin. Neurosci. Lett. 228, 33–36. doi: 10.1016/s0304-3940(97)00350-9
Tominaga, M., Ogawa, H., and Takamori, K. (2007). Possible roles of epidermal opioid systems in pruritus of atopic dermatitis. J. Invest. Dermatol. 127, 2228–2235. doi: 10.1038/sj.jid.5700942
Truzzi, F., Marconi, A., Atzei, P., Panza, M. C., Lotti, R., Dallaglio, K., et al. (2011). p75 neurotrophin receptor mediates apoptosis in transit-amplifying cells and its overexpression restores cell death in psoriatic keratinocytes. Cell Death. Differ. 18, 948–958. doi: 10.1038/cdd.2010.162
Tsutsumi, M., Denda, S., Ikeyama, K., Goto, M., and Denda, M. (2010). Exposure to low temperature induces elevation of intracellular calcium in cultured human keratinocytes. J. Invest. Dermatol. 130, 1945–1948. doi: 10.1038/jid.2010.33
Tsutsumi, M., Goto, M., Denda, S., and Denda, M. (2011). Morphological and functional differences in coculture system of keratinocytes and dorsal-root-ganglion-derived cells depending on time of seeding. Exp. Dermatol. 20, 464–467. doi: 10.1111/j.1600-0625.2011.01276.x
Uberti, F., Bardelli, C., Morsanuto, V., Ghirlanda, S., Cochis, A., and Molinari, C. (2017). Stimulation of the nonneuronal cholinergic system by highly diluted acetylcholine in keratinocytes. Cells Tissues Organs 203, 215–230. doi: 10.1159/000451023
Uberti, F., Morsanuto, V., Ghirlanda, S., Ruga, S., Clemente, N., Boieri, C., et al. (2018). Highly diluted acetylcholine promotes wound repair in an in vivo model. Adv. Wound Care 7, 121–133. doi: 10.1089/wound.2017.0766
Ulmann, L., Rodeau, J. L., Danoux, L., Contet-Audonneau, J. L., Pauly, G., and Schlichter, R. (2007). Trophic effects of keratinocytes on the axonal development of sensory neurons in a coculture model. Eur. J. Neurosci. 26, 113–125. doi: 10.1111/j.1460-9568.2007.05649.x
Vassar, R., Rosenberg, M., Ross, S., Tyner, A., and Fuchs, E. (1989). Tissue-specific and differentiation-specific expression of a human K14 keratin gene in transgenic mice. Proc. Natl. Acad. Sci. U S A. 86, 1563–1567. doi: 10.1073/pnas.86.5.1563
Vidal Yucha, S. E., Tamamoto, K. A., and Kaplan, D. L. (2019). The importance of the neuro-immuno-cutaneous system on human skin equivalent design. Cell Prolif. 52:e12677.
Vyklicky, V., Korinek, M., Smejkalova, T., Balik, A., Krausova, B., Kaniakova, M., et al. (2014). Structure, function, and pharmacology of NMDA receptor channels. Physiol. Res. 63, S191–S203.
Wang, J., Ou, S. W., and Wang, Y. J. (2017). Distribution and function of voltage-gated sodium channels in the nervous system. Channels 11, 534–554.
Wang, T., Jing, X., DeBerry, J. J., Schwartz, E. S., Molliver, D. C., Albers, K. M., et al. (2013). Neurturin overexpression in skin enhances expression of TRPM8 in cutaneous sensory neurons and leads to behavioral sensitivity to cool and menthol. J. Neurosci. 33, 2060–2070. doi: 10.1523/JNEUROSCI.4012-12.2013
Wang, Y., Li, H., Xue, C., Chen, H., Xue, Y., Zhao, F., et al. (2021). TRPV3 enhances skin keratinocyte proliferation through EGFR-dependent signaling pathways. Cell Biol. Toxicol. 37, 313–330. doi: 10.1007/s10565-020-09536-2
Watanabe, T., Ito, T., Inoue, G., Ohtori, S., Kitajo, K., Doya, H., et al. (2008). The p75 receptor is associated with inflammatory thermal hypersensitivity. J. Neurosci. Res. 86, 3566–3574. doi: 10.1002/jnr.21808
Wei, T., Guo, T. Z., Li, W. W., Hou, S., Kingery, W. S., and Clark, J. D. (2012). Keratinocyte expression of inflammatory mediators plays a crucial role in substance P-induced acute and chronic pain. J. Neuroinflamm. 9:181. doi: 10.1186/1742-2094-9-181
Wei, T., Guo, T. Z., Li, W. W., Kingery, W. S., and Clark, J. D. (2016). Acute versus chronic phase mechanisms in a rat model of CRPS. J. Neuroinflamm. 13:14. doi: 10.1186/s12974-015-0472-8
Wei, T., Li, W. W., Guo, T. Z., Zhao, R., Wang, L., Clark, D. J., et al. (2009a). Post-junctional facilitation of substance P signaling in a tibia fracture rat model of complex regional pain syndrome type I. Pain 144, 278–286. doi: 10.1016/j.pain.2009.04.020
Wei, T., Sabsovich, I., Guo, T. Z., Shi, X., Zhao, R., Li, W., et al. (2009b). Pentoxifylline attenuates nociceptive sensitization and cytokine expression in a tibia fracture rat model of complex regional pain syndrome. Eur. J. Pain 13, 253–262. doi: 10.1016/j.ejpain.2008.04.014
Whitehead, R. A., Puil, E., Ries, C. R., Schwarz, S. K., Wall, R. A., Cooke, J. E., et al. (2012). GABA(B) receptor-mediated selective peripheral analgesia by the non-proteinogenic amino acid, isovaline. Neuroscience 213, 154–160. doi: 10.1016/j.neuroscience.2012.04.026
Wijaya, L. K., Stumbles, P. A., and Drummond, P. D. (2020a). A positive feedback loop between alpha1-adrenoceptors and inflammatory cytokines in keratinocytes. Exp. Cell Res. 391:112008. doi: 10.1097/j.pain.0000000000002764
Wijaya, L. K., Stumbles, P. A., and Drummond, P. D. (2020b). Tumor necrosis factor alpha induces alpha1B-adrenergic receptor expression in keratinocytes. Cytokine 125:154851. doi: 10.1016/j.cyto.2019.154851
Wilder-Smith, E. P., Ong, W. Y., Guo, Y., and Chow, A. W. (2007). Epidermal transient receptor potential vanilloid 1 in idiopathic small nerve fibre disease, diabetic neuropathy and healthy human subjects. Histopathology 51, 674–680. doi: 10.1111/j.1365-2559.2007.02851.x
Woolf, C. J., Allchorne, A., Safieh-Garabedian, B., and Poole, S. (1997). Cytokines, nerve growth factor and inflammatory hyperalgesia: the contribution of tumour necrosis factor alpha. Br. J. Pharmacol. 121, 417–424.
Wu, C., Erickson, M. A., Xu, J., Wild, K. D., and Brennan, T. J. (2009). Expression profile of nerve growth factor after muscle incision in the rat. Anesthesiology 110, 140–149.
Xu, X., Tao, X., Huang, P., Lin, F., Liu, Q., Xu, L., et al. (2020). N-methyl-d-aspartate receptor subunit 2B on keratinocyte mediates peripheral and central sensitization in chronic post-ischemic pain in male rats. Brain Behav. Immun. 87, 579–590.
Yaping, E., Golden, S. C., Shalita, A. R., Lee, W. L., Maes, D. H., and Matsui, M. S. (2006). Neuropeptide (calcitonin gene-related peptide) induction of nitric oxide in human keratinocytes in vitro. J. Invest. Dermatol. 126, 1994–2001. doi: 10.1038/sj.jid.5700349
Yoo, J. A., Yu, E., Park, S. H., Oh, S. W., Kwon, K., Park, S. J., et al. (2020). Blue light irradiation induces human keratinocyte cell damage via transient receptor potential vanilloid 1 (TRPV1) regulation. Oxid. Med. Cell Longev. 2020:8871745. doi: 10.1155/2020/8871745
Zappia, K. J., Garrison, S. R., Palygin, O., Weyer, A. D., Barabas, M. E., Lawlor, M. W., et al. (2016). Mechanosensory and ATP release deficits following Keratin14-Cre-Mediated TRPA1 deletion despite absence of TRPA1 in murine keratinocytes. PLoS One 11:e0151602. doi: 10.1371/journal.pone.0151602
Zhao, J., Munanairi, A., Liu, X. Y., Zhang, J., Hu, L., Hu, M., et al. (2020). PAR2 mediates itch via TRPV3 signaling in keratinocytes. J. Invest. Dermatol. 140, 1524–1532. doi: 10.1016/j.jid.2020.01.012
Zhao, P., Barr, T. P., Hou, Q., Dib-Hajj, S. D., Black, J. A., Albrecht, P. J., et al. (2008). Voltage-gated sodium channel expression in rat and human epidermal keratinocytes: evidence for a role in pain. Pain 139, 90–105. doi: 10.1016/j.pain.2008.03.016
Zhou, S., Bonasera, L., and Carlton, S. M. (1996). Peripheral administration of NMDA, AMPA or KA results in pain behaviors in rats. Neuroreport 7, 895–900.
Zia, S., Ndoye, A., Lee, T. X., Webber, R. J., and Grando, S. A. (2000). Receptor-mediated inhibition of keratinocyte migration by nicotine involves modulations of calcium influx and intracellular concentration. J. Pharmacol. Exp. Ther. 293, 973–981.
Zoli, M., Pucci, S., Vilella, A., and Gotti, C. (2018). Neuronal and extraneuronal nicotinic acetylcholine receptors. Curr. Neuropharmacol. 16, 338–349.
Keywords: keratinocyte, free nerve ending, peripheral sensitization, neurotransmitter, neuropeptide, neuroinflammation
Citation: Xu X, Yu C, Xu L and Xu J (2022) Emerging roles of keratinocytes in nociceptive transduction and regulation. Front. Mol. Neurosci. 15:982202. doi: 10.3389/fnmol.2022.982202
Received: 30 June 2022; Accepted: 23 August 2022;
Published: 09 September 2022.
Edited by:
Ildikó Rácz, University Hospital Bonn, GermanyReviewed by:
Zhiyong Tan, Indiana University, Purdue University Indianapolis, United StatesZili Xie, Washington University in St. Louis, United States
Matthieu Talagas, Université de Bretagne Occidentale, France
Copyright © 2022 Xu, Yu, Xu and Xu. This is an open-access article distributed under the terms of the Creative Commons Attribution License (CC BY). The use, distribution or reproduction in other forums is permitted, provided the original author(s) and the copyright owner(s) are credited and that the original publication in this journal is cited, in accordance with accepted academic practice. No use, distribution or reproduction is permitted which does not comply with these terms.
*Correspondence: Li Xu, cHVtY2h4dWxpQDE2My5jb20=; Jijun Xu, eHVqM0BjY2Yub3Jn
†These authors have contributed equally to this work