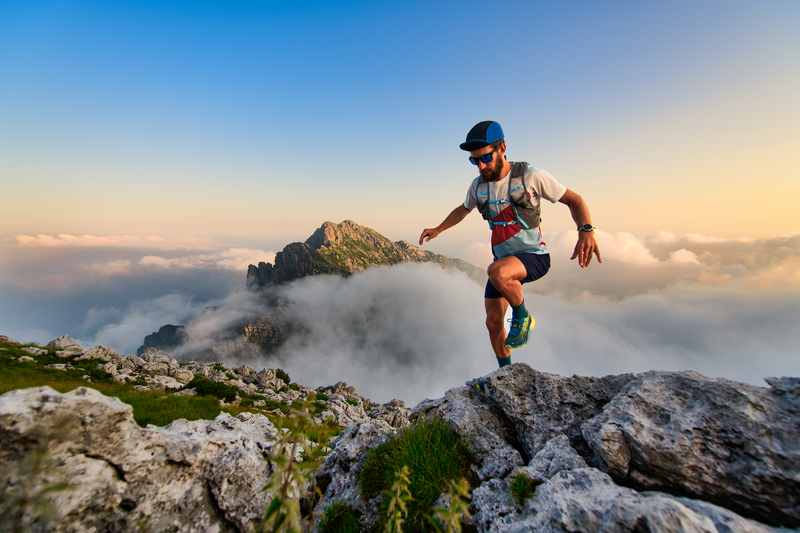
95% of researchers rate our articles as excellent or good
Learn more about the work of our research integrity team to safeguard the quality of each article we publish.
Find out more
ORIGINAL RESEARCH article
Front. Mol. Neurosci. , 05 September 2022
Sec. Brain Disease Mechanisms
Volume 15 - 2022 | https://doi.org/10.3389/fnmol.2022.954928
This article is part of the Research Topic RNA at a Breaking Point? Cytoplasmic Cleavage and other Post-Transcriptional RNA Processing in Neurodevelopment and Disease View all 18 articles
We describe the characterisation of a variable number tandem repeat (VNTR) domain within intron 1 of the amyotrophic lateral sclerosis (ALS) risk gene CFAP410 (Cilia and flagella associated protein 410) (previously known as C21orf2), providing insight into how this domain could support differential gene expression and thus be a modulator of ALS progression or risk. We demonstrated the VNTR was functional in a reporter gene assay in the HEK293 cell line, exhibiting both the properties of an activator domain and a transcriptional start site, and that the differential expression was directed by distinct repeat number in the VNTR. These properties embedded in the VNTR demonstrated the potential for this VNTR to modulate CFAP410 expression. We extrapolated these findings in silico by utilisation of tagging SNPs for the two most common VNTR alleles to establish a correlation with endogenous gene expression. Consistent with in vitro data, CFAP410 isoform expression was found to be variable in the brain. Furthermore, although the number of matched controls was low, there was evidence for one specific isoform being correlated with lower expression in those with ALS. To address if the genotype of the VNTR was associated with ALS risk, we characterised the variation of the CFAP410 VNTR in ALS cases and matched controls by PCR analysis of the VNTR length, defining eight alleles of the VNTR. No significant difference was observed between cases and controls, we noted, however, the cohort was unlikely to contain sufficient power to enable any firm conclusion to be drawn from this analysis. This data demonstrated that the VNTR domain has the potential to modulate CFAP410 expression as a regulatory element that could play a role in its tissue-specific and stimulus-inducible regulation that could impact the mechanism by which CFAP410 is involved in ALS.
Amyotrophic lateral sclerosis (ALS) is a fatal neurodegenerative disease primarily of the motor system, characterised by upper and lower motor neuron death, muscle atrophy and paralysis (Hardiman et al., 2017). ALS heritability has previously been estimated to be as high as 61%, indicating a significant genetic contribution to the variation in ALS risk and susceptibility (Al-Chalabi et al., 2010; Hardiman et al., 2017), and now there are over 40 genes associated with the disease, of varying effect sizes (Wroe et al., 2009; Abel et al., 2012; Shatunov and Al-Chalabi, 2021). Occasionally, patients can harbour multiple mutations, highlighting that ALS is a complex disease (van Blitterswijk et al., 2012a,b; Al-Chalabi and Visscher, 2014; Cady et al., 2015; Bury et al., 2016; Zou et al., 2017; Nguyen et al., 2018; Goldstein et al., 2019; Yilmaz et al., 2022). There is also evidence that ALS pathogenesis is a multistep process, with both genetic and environmental risk factors working in concert via gene–environment interaction (Al-Chalabi et al., 2014; Chio et al., 2018; Vucic et al., 2019; Garton et al., 2021). While genome-wide association studies have helped to uncover regions associated with ALS, it has been shown that single nucleotide polymorphisms (SNPs) only account for a small fraction of ALS heritability (van Rheenen et al., 2016). Furthermore, it has been shown that structural variants could be a key source of this missing heritability (Theunissen et al., 2020), with the hexameric repeat expansion in the first intron of the C9orf72 gene the most commonly reported mutation in European ALS patients (Zou et al., 2017). Since the discovery of the C9orf72 repeat expansion, there have been numerous studies investigating tandem repeat DNA and structural variation in ALS (DeJesus-Hernandez et al., 2011; Renton et al., 2011; Blauw et al., 2012; Lattante et al., 2018; Tazelaar et al., 2019, 2020; Course et al., 2020; Al Khleifat et al., 2022).
Tandem repeats are DNA sequence motifs that are recurrent and found contiguously in a region of the genome. Such tandem repeats constitute ~3% of the human genome (Bakhtiari et al., 2018; Hannan, 2018). They are often found to be polymorphic in the general population and are thus termed variable number tandem repeats (VNTRs); furthermore, specific alleles can be risk factors for diseases, which are often neurological in nature (Lam et al., 2018; Roeck et al., 2018; Pacheco et al., 2019). Non-coding VNTRs can be functional in a number of ways: they can act as transcription factor binding sites (Hsieh et al., 2007; Ali et al., 2010; Zukic et al., 2010), drive gene expression on the basis of repeat number (Warburton et al., 2015), work in concert with other genetic variants to induce combinatorial regulation (Warburton et al., 2016), regulate splicing (Roeck et al., 2018) and modulate gene expression and CpG methylation levels at the genome-wide level (Gymrek et al., 2016; Quilez et al., 2016). Thus, VNTRs are key transcriptional regulatory domains within the human genome, with repeat copy numbers driving differential gene expression profiles, altering affinity for transcription factors at gene promoters and modifying the secondary structure of DNA, all of which would modify gene expression both in a tissue specific and stimulus inducible manner (Marshall, 2021).
To date, five genes linked to ALS have been identified to contain tandem repeat domains (C9orf72, ATXN1, ATXN2, NIPA1 and WDR7) demonstrating that VNTRs are a strong candidate for genetic risk for this disease (Sproviero et al., 2017; Iacoangeli et al., 2019; Tazelaar et al., 2019, 2020; Course et al., 2020). More recent global analysis to prioritise causal genes within ALS-risk loci has utilised not only rare pathogenic variants but also analysis of short tandem repeat domains of six bases or less (van Rheenen et al., 2021). This study highlighted the association of SNP rs75087725 within Cilia and flagella-associated protein 410 (CFAP410) as a missense variant associated with ALS but found no evidence for other variants including short-tandem repeat expansions within this locus associated with ALS. However, the bioinformatic pipelines used would not be able to accurately address larger VNTRs. Our analysis of transcriptional regulation of the CFAP410 gene highlighted six tandem repeats at this locus, five of which were not variable in repeat copy number. However, there was a VNTR in intron 1 of CFAP410 with a complex repeat domain of 22 bp or 35 bp extending to 500–600+bp in length (Marshall, 2021) (Figure 1, Supplementary Figure 2B). We therefore tested the hypothesis that the CFAP410 VNTR within intron 1 of the most 5' transcriptional start site is a transcriptional regulatory domain and that tandem repeat variation could be associated with both differential gene expression of CFAP410 and risk for ALS.
Figure 1. Location of multiple transcripts at the CFAP410 gene locus relative to the intron 1 VNTR. (A) The four main isoforms of CFAP410 overlaid with the Simple Tandem Repeats track and histone marks from the ENCODE database. The ALS GWAS SNP (rs75087725) and identified tagging SNP (rs56212056) are also shown. The VNTR identified in this study is boxed in red. (B) CFAP410 VNTR location overlaid with RefSeq genes from NCBI and predicted transcripts from Ensembl database. (C) PCR amplification and gel electrophoresis of the VNTR within the CFAP410 locus. This region was confirmed to be polymorphic and eight alleles were identified (numbered 1–8 to reflect increasing length). Variant 7 was found only in a single ALS case. All samples were run at 100 V on 2% agarose for 3.5 h. All samples shown are from the cohort of ALS cases and controls that are genotyped in Table 1.
Genomic DNA purified from the blood of ALS cases and controls was obtained from the UK Motor Neuron Disease (MND) Collection DNA and Cell Bank, which was used for PCR-based genotyping of the CFAP410 VNTR. A total of 500 ALS samples were provided, 456 from people fulfilling the El Escorial criteria, 21 with progressive muscular atrophy, 12 with ALS restricted to progressive bulbar palsy and 11 with primary lateral sclerosis. A total of 333 people with ALS were male and 167 were female, with an age range of 24–91 years old and a disease age of onset range of 23–88 years old. A panel of 499 controls was also obtained: 188 were male and 311 were female with an age range of 27–84 years old. The genotyping analysis was performed using this gender ratio because this was the total cohort size available to us in this database. We therefore did not generate a gender risk bias on the VNTR.
Tandem repeats in the CFAP410 locus were identified on UCSC HG19 (https://genome.ucsc.edu/) using the Simple Tandem Repeats track from Tandem Repeat Finder (https://tandem.bu.edu/trf/trf.submit.options.html) (Benson, 1999). A total of six distinct tandem repeats were identified over this locus; we focused on the repeat within intron 1, which is the only one we found to be variable in repeat number.
The VNTR was amplified by PCR, forward 5′ AACCCCAGACAACAGACCC 3′ and reverse 5′ CTGACGCGGAAGATGGTTC 3′ primers were designed to amplify the full-length VNTR sequence (584bp). Amplification reactions used KOD Hot Start DNA polymerase (Merck) with 1 × hot start buffer and the recommended protocol. The products were analysed on 2% agarose gels stained with ethidium bromide. Association analysis was performed comparing allele frequencies between controls and ALS cases using the software programme CLUMP with 100,000 simulations (Sham and Curtis, 1995).
The human embryonic kidney cell line, HEK293 (ATCC® CRL-1573™), was cultured in the Dulbecco's Modified Eagle's Media (DMEM) (Gibco) containing 4.5 g/L D-glucose and 200 mM L-glutamine (Gibco), supplemented with 10% foetal bovine serum (Gibco), penicillin/streptomycin (100 U/ml, 100 mg/ml; Sigma) and 1% (v/v) 100 mM sodium pyruvate (Sigma). Cells were incubated at 37°C in 5% CO2.
The CFAP410 VNTR was amplified from genomic DNA by PCR using the KOD Hot Start DNA polymerase following the recommended protocol. The VNTR was subcloned into the intermediate Zero Blunt PCR Vector (Invitrogen). This intermediate plasmid was then digested with restriction enzymes and the VNTR insert was cloned within the multiple cloning site of the reporter gene vectors either pGL3-promoter (pGL3P) or pGL3-basic (pGL3B) (Promega) in both orientations (forward and reverse) [restriction digests, vector maps and sequencing available at Marshall (2021)]. In pGL3P the VNTR was located upstream of the SV40 minimal promoter to address action as an activator, and cloned in pGL3B to address putative function as an inherent transcriptional start site.
HEK293 cells were seeded at approximately 100,000 cells per well in 24-well-plates. After 48 h of incubation, cells were co-transfected with 1 μg reporter gene plasmid (firefly luciferase) containing the VNTR sequences and 20 ng pRL control vector for normalisation (Renilla luciferase; Novagen) using TurboFect™ transfection reagent (ThermoScientific/Fermentas), according to the manufacturer's protocol. TurboFect™ was removed after 4 h of incubation and replaced with fresh media. Luciferase activity of reporter constructs was measured 48 h post-transfection using a dual luciferase reporter assay system (Promega) according to the manufacturer's instructions.
Samples from the UK MND Collections DNA bank had previously been analysed as part of Project MinE (van der Spek et al., 2019), and therefore, SNP genotype data were available for individuals that were part of this study. The genotypes of SNPs located within 500 kb of the CFAP410 gene were extracted from 232 individuals who were either homozygous or heterozygous for the two commonest alleles (4 and 5) of the VNTR. Analysis was performed using plink (v1.07) (Purcell et al., 2007) to identify SNPs in linkage disequilibrium (r2>0.7) with alleles 4 and 5 of the VNTR.
RNA-seq data from the Target ALS cohort obtained from the New York Genome Centre Consortium were analysed (https://www.targetals.org/research/resources-for-scientists/). Data were available from different tissues of 211 people, including 178 with ALS, 5 with other neurological diseases (frontotemporal dementia, Alzheimer's disease and multiple system atrophy), 2 with other motor neuron diseases (spinal bulbar muscular atrophy and polio) and 26 non-neurological controls (NNC). RNA-seq data from the following tissues (total 1,170) were included: cerebellum (161), frontal cortex (169), medial motor cortex (148), lateral motor cortex (147), unspecified motor cortex (9), occipital cortex (81), temporal cortex (10), sensory cortex (2), lumbar spinal cord (147), cervical spinal cord (161), thoracic spinal cord (76), iPS cell line (4), motor neuron cell line (4), choroid plexus (35), medulla (1) and liver (15) (Supplementary Table 1). Isoform quantification of RNA-seq data was performed using the Salmon tool (https://salmon.readthedocs.io). Salmon-generated quant files were imported into R using the tximport function from the tximport package of R (Soneson et al., 2015). Counts were extracted with the DESeqDataSetFromTximport function and raw counts were normalised using the median-of-ratios method, implemented in the DESeq2 package (Love et al., 2014). The DESeq2 package in R was also used to detect statistically significant differences in the CFAP410 isoform expression profiles between different subject groups (ALS vs. NNC). The ggplot2 package in R containing the geom_boxplot function was used to visualise the data specifying the stat_summary function to mean. The unpaired Wilcoxon test was used to compare two independent groups of samples and to demonstrate statistical significance (p-values and effect size can be found in Supplementary Figure 2A, and a histogram plot for the distribution of data for CFAP410 ENST00000462742 is shown in Supplementary Figure 5).
Tagging SNP analysis identified that genotypes (AA, GA and GG) of rs56212056 are correlated with the two commonest alleles 4 (A) and 5 (G). The association of the three different SNP alleles with differential CFAP410 isoform expression was analysed. The unpaired Wilcoxon test was used to compare two independent groups of samples and to demonstrate statistical significance between different cases and controls and tagging SNP genotype, respectively (p-values and effect size can be found in Supplementary Figure 2B). LD analysis of the CFAP410 locus including the analysis of 31 SNPs from 243 individuals within Project MinE shows no evidence for significant LD with any of the tested SNPs across the region other than the associations made for alleles 4 and 5 of the CFAP410 VNTR (Supplementary Figure 3).
The CFAP410 gene contains an SNP (rs75087725) that is associated with ALS risk (p = 3.08 × 10−10) (van Rheenen et al., 2016). Analysis of the CFAP410 gene using the tandem repeat track on UCSC (https://genome.ucsc.edu/) led to the identification of six distinct tandem repeat domains at this locus. We focus in this communication on the VNTR in intron 1 (Figure 1A), which was the only one that was variable in repeat number. We noted its location relative to the transcriptional start sites and its primary sequence had high GC content (84%) and sequences related to those found in the C9orf72 VNTR (Supplementary Figure 1 and Figure 1B). It is difficult for global analysis such as ChIPseq to correctly assign ENCODE data over VNTRs themselves; however, when the VNTR was overlaid with ENCODE data, it was found to be adjacent to the markers H3K4Me1 (a marker of regulatory domains associated with enhancers), H3K4Me3 (a marker of regulatory domains associated with promoters) and H3K12Ac (a marker of active regulatory domains associated with active enhancer elements) (Supplementary Figure 1). On RefSeq analysis, this VNTR was found within intron 1 of isoforms 1–3 of CFAP410 and it could be a component of a promoter region for isoforms that initiate 3' of the VNTR. Extending RefSeq analysis to include Ensembl transcript data increased the number of isoforms identified at this locus (shown in red in Figure 1A). Specifically, this identified a predicted transcript initiating immediately 5' of the VNTR, Ensembl transcript (ENST00000462742), and an anti-sense transcript relative to CFAP410, which was annotated as a long non-coding RNA on Ensembl (ENST00000426029).
Transcriptomic data from the Target ALS cohort was utilised to assess the expression of CFAP410 isoforms in the CNS. Isoform expression analysis showed that five CFAP410 isoforms are expressed in the CNS (Figure 5A). The anti-sense transcript (ENST00000426029) relative to CFAP410 showed no expression in this RNA-seq dataset (Figure 5A). We next analysed the differential expression of CFAP410 isoforms in case and control subjects (Figure 2). The isoform ENST00000462742 showed significantly reduced expression in cases compared to controls (Figure 2D). This isoform was annotated as a non-coding retained intron transcript. The other two predominantly expressed isoforms (ENST00000339818 and ENST00000325223) demonstrated a similar pattern of expression to ENST00000462742; however, these associations did not reach statistical significance (Figures 2A,B). The remaining CFAP410 isoforms (ENST00000397956 and ENST00000496321) showed very low expression levels, therefore the data for these isoforms did not give us sufficient power to identify evidence for differential expression between case and control subjects (Figures 2C,E).
Figure 2. Differential expression of CFAP410 in Case and Controls in the CNS using the NYG transcriptomic data. Expression analysis of CFAP410 isoforms in ALS and control subjects. RNAseq data from the NYGC ALS cohort were used to compare expression of CFAP410 isoforms ENST00000339818 (A), ENST00000325223 (B), ENST00000397956 (C), ENST00000462742 (D) and ENST00000496321 (E). Wilcoxon test was applied to demonstrate statistical significance indicated as asterisks. **P ≤ 0.01.
PCR amplification and gel electrophoresis of the VNTR within the CFAP410 locus was performed in a subset of the cohort from the UK MND Collection DNA Bank (n = 983). This region was confirmed to be polymorphic and eight alleles were identified (numbered 1–8 to reflect increasing length) (Figure 1C). The sequence of allele 5 of the VNTR (Supplementary Figure 1B) was found to be comprised of two distinct repeat lengths, 35 and 22bp long, respectively. Furthermore, four alleles were sequenced (Supplementary Figure 1B) and were found to contain the same two repeat lengths in varying patterns. From PCR of this limited number of genomes, the most common alleles were those termed 4 and 5, which contained 9 and 10 repeats, respectively (Supplementary Figure 1B).
To determine the potential ability of the CFAP410 VNTR to regulate transcription, in vitro reporter gene assays were used. The most common alleles (alleles 4 and 5) were cloned in both orientations of the VNTR into the pGL3-P vector upstream of the SV40 minimal promoter and luciferase activity was compared to the activity of the pGL3-P vector alone. Both alleles demonstrated transcriptional regulatory properties (Figure 3). Alleles 4 and 5 in the endogenous orientation demonstrated a 2.64- and 1.71-fold increase in expression of luciferase over pGL3p alone, respectively, with both being statistically significant (Allele 5, 1.71 ± 0.13, T-test, p = 1.08 × 10−6 and Allele 4, 2.64 ± 0.18, T-test, p = 3.54 × 10−6). Furthermore, there was a significant difference in luciferase activity when cloned in this direction between alleles 4 and 5 (T-test, p = 4.39 × 10−7), with allele 4 showing a larger enhancement of reporter gene activity. In the reverse orientation, allele 5 alone demonstrated a mild decrease in expression compared to empty pGL3P (0.82±0.03, T-test, p = 1.49 × 10−7), but no change from empty pGL3P was observed for allele 4 in the reverse orientation (1.04 ± 0.06, T-test, p = 3.70 × 10−1).
Figure 3. The CFAP410 VNTR shows functional properties in the pGL3-P vector in the HEK293 cell line. (A) Schematic for VNTR containing constructs and pGL3 vectors (Promega) used in the luciferase assay. (B) The fold activity of alleles 4 and 5 of the CFAP410 VNTR in the endogenous (forward) orientation within the pGL3P vector, normalised to the internal control Renilla luciferase (Biological replicate n = 3, technical replicate per assay n = 4). The promoter-less vector (pGL3-B) was included as a negative control. T-test was used to compare VNTR-containing constructs to SV40 unmodified vector (pGL3-P) and to compare all VNTR containing constructs against each other. ***P = <0.001.
The location of the VNTR upstream of the Ensembl transcripts ENST00000462742 and ENS00000496321 (equivalent position to the RefSeq short isoform) and the anti-sense transcript (ENST00000426029) (Figures 1A,B) suggested that it could elicit promoter activity. This might be especially true for ENST00000462742, which is directly adjacent to the VNTR, as it has been known for some time that GC-rich sequences can act to initiate transcription in those promoters that lack a TATA box (Kageyama et al., 1989). The VNTR primary sequence demonstrated high GC content (84%), which was consistent with such a model. To address this hypothesis, alleles 4 and 5 of the VNTR were cloned in both orientations into pGL3-B (Promega) containing a firefly luciferase reporter without a promoter, which is a well-characterised model to test if a region of DNA can act as a transcriptional start site in reporter gene studies. The unmodified pGL3-P vector (Figure 4) was included in this assay as it contains the minimal SV40 early promoter and therefore serves as a positive control for the level of expression directed by a well-characterised promoter in this cell line model. Both orientations of the VNTR demonstrated promoter activity which would require these domains to act as a transcriptional start site to allow expression of the luciferase reporter. This was especially true in the reverse orientation; VNTR orientations are given as endogenous and reverse to reflect that found in relation to the CFAP410 gene. VNTR allele 4 in the endogenous orientation led to a 17.02-fold increase in luciferase expression (17.02 ± 0.67, T-test, p = 1.81 × 10−13), while the reverse orientation induced a 100.60-fold increase in reporter gene activity (100.60 ± 1.83, T-test, p =2.38 × 10−17) (Figure 4). Similarly, a 20.37-fold increase in luciferase expression was observed in the allele 5 endogenous construct (20.37±0.72, T-test, p = 4.88 × 10−14), while a 113.25-fold increase in luciferase activity in the allele 5 reverse orientation VNTR construct was observed (113.25 ± 4.16, T-test, p = 5.27 × 10−14). For comparison, the pGL3-P vector demonstrated a 46.98-fold increase in luciferase expression when compared to empty pGL3-B (46.98±1.28, T-test, p = 1.09 × 10−15). Thus, overall, a moderate but statistically significant increase in luciferase activity was observed in both endogenous orientation VNTR constructs when compared to empty pGL3-B, but this was significantly less than the SV40 minimal promoter activity seen with pGL3-P (T-test, p = 1.09 × 10−15). However, in the reverse orientation, a statistically significant increase compared to pGL3-P was observed: a 2.1- and a 2.4-fold increase in luciferase expression by alleles 4 and 5, respectively. Overall, both alleles of the VNTR induced a significant increase in reporter gene expression compared to empty pGL3-B, but allele 5 drove the largest effect in this model. This data suggests the possibility that the VNTR has promoter activity to initiate and direct expression at the CFAP410 locus.
Figure 4. The CFAP410 VNTR shows promoter activity in the pGL3-B vector in HEK293. (A) Schematic for VNTR containing constructs and pGL3 vectors (Promega) used in luciferase assay. (B) The fold activity of the CFAP410 VNTR in the endogenous and reverse orientation within the pGL3-B vector normalised to the internal control Renilla luciferase (Biological replicate n = 3, technical replicate per assay n = 4). The pGL3-P vector was included as a positive control as this contains an SV40 promoter. T-test was used to compare VNTR containing constructs to each other (allele 4 vs. allele 5) and promoterless vector alone (pGL3-B). T-test was also used to compare VNTR containing constructs to the SV40 promoter vector (pGL3-P). ***P = <0.001.
We identified SNP rs56212056 to be in moderate linkage disequilibrium (r2 = 0.75, D' = 0.949) with the two most common alleles (termed alleles 4 and 5) of the VNTR. It was not possible to identify tagging SNPs for the rarer VNTR alleles in this study given their frequency in the population and the size of our cohort. The A allele of the SNP was found to be correlated with allele 4 of the VNTR and the G allele with allele 5. We used the Target ALS brain expression data to correlate these variants with CFAP410 isoform expression. The association of three different SNP genotypes (AA, GA and GG) based on the correlation with allele 4 (A) or allele 5 (G) was analysed (Figure 5). We observed differential levels of isoform expression correlated with distinct G or A genotypes. Three CFAP410 isoforms (ENST00000339818, ENST00000325223 and ENST00000462742) showed significantly reduced expression with GG genotype compared to both GA and AA genotype (Figures 5B-E). This was in contrast with the levels of expression of the isoform ENST00000496321 where the opposite pattern was observed (Figure 5E). Isoform ENST00000397956 correlated with significantly reduced expression in subjects with two copies of allele 5 (GG genotype) compared to subjects with the GA genotype only indicating one allele 4 and one allele 5 are present (Figure 5D). The data indicate that distinct VNTRs are able to support differential gene expression in the brain.
Figure 5. The expression of CFAP410 isoforms is associated with tagging the SNP rs56212056 genotype. (A) CFAP410 isoform expression using RNAseq data from the NYGC Target ALS cohort. (B–F) Association of three different SNP genotypes (AA, GA, and GG) with an expression of isoforms ENST00000339818, ENST00000325223, ENST00000397956Q24, ENST00000462742, and ENST00000496321 was analysed based on the correlation with allele 4 (A) or allele 5 (G) using transcriptomic data from the NYGC ALS cohort. Wilcoxon test was applied to demonstrate statistical significance indicated as asterisks. *P = <0.05, **P = <0.01, ***P = <0.001, ****P = <0.0001, ns > 0.05.
To assess if specific VNTRs in this region were associated with ALS, the CFAP410 VNTR was genotyped in a cohort of cases and controls (Table 1). The VNTR was amplified by PCR from blood-derived genomic DNA from patients (n = 490) and controls (n = 493) and a total of eight alleles of the CFAP410 VNTR were identified and numbered according to increasing length. Allele 5 (reference genome variant, 584 bp) was the most common allele and was present in 74.49% of cases and 74.75% of controls; no significant difference in frequency was found between the two populations (Fisher's exact test, p = 0.75). The next most common variant was allele 4, which was identified in 22.86% of cases and 22.31% of controls: no significant difference in allele frequency was observed between the two groups (Fisher's exact test, p = 0.93). The other six alleles were infrequent in the population, with no statistically significant differences being observed (Table 1). There was also no significant difference in the allele frequencies of the eight alleles of the CFAP410 VNTR when comparing controls and cases using CLUMP software (T1, p = 0.91 and T4, p = 0.94).
To determine the repeat composition and length of the VNTR alleles, the two alleles found most commonly in this cohort (alleles 4 and 5) and two of the rare variants (alleles 2 and 8) were cloned and sequence validated. All alleles were found to be a composite of various numbers of both a 22 bp and 35 bp repeat unit (Supplementary Figures 1A,B). The primary sequence of the VNTR demonstrated variation in the actual sequence of both the 22 or 35 bp repeat unit and the repeating structure indicated a more complex evolution of variation than simply separating into two blocks. It may be of interest to note that the most 5' repeat element had only 34 bp.
We have demonstrated that the VNTR in intron 1 of the CFAP410 gene was functional as a transcriptional regulator in vitro. It served as a transcriptional regulatory domain in reporter gene constructs in the HEK293 cell line and directed differential gene expression on the basis of repeat number as both an activator domain and transcriptional start site/promoter. These data were extended to in silico analysis in which tagging SNP analysis for the two most common alleles of this VNTR was associated with differential gene expression of CFAP410 in the brain. This VNTR was found to be highly variable in the general population, with at least eight distinct variants, but there was no significant association of specific genotype with ALS risk. Nevertheless, our data indicated the regulatory properties of this VNTR could support differential regulation of CFAP410 based on the copy number of the repeat. The ability of the VNTR to direct such expression could be one parameter that works in conjunction with other mechanisms at this locus that could lead to the progression or severity of ALS to specific challenges in the CNS.
It has been established that VNTRs can be both biomarkers for disease risk and also functional regulatory domains affecting both transcription and post-transcriptional mechanisms as reviewed recently (Marshall et al., 2021). These latter transcriptional properties can be exhibited together for the same VNTR as in the case of the monoamine oxidase A (MAOA) gene (Manca et al., 2018) and a VNTR in the mir137 gene (Warburton et al., 2016). Indeed, we have previously demonstrated that a human-specific intron 2 VNTR within the serotonin transporter gene can direct both tissue-specific and differential levels of reporter gene expression in a mouse transgenic model (MacKenzie and Quinn, 1999). We hypothesise that similar mechanisms may operate on the CFAP410 VNTR, which could lead to distinct levels of expression from different alleles in response to the same cellular challenge. Specifically, we theorise that the association of the two most common alleles with differential CFAP410 expression can be extrapolated to such properties being embedded in the other minor VNTR alleles.
The genomic location of the CFAP410 gene is a complex region with multiple isoforms that can be either coding or non-coding in nature, indeed one of the isoforms ENST00000462742 has the hallmarks of a retained intron (Monteuuis et al., 2019) (Figure 1). Interestingly, this isoform was significantly downregulated in the cases relative to the controls. Notably, retained introns have higher GC content compared to introns that are not and this is true for the large GC-rich VNTR found in this intron of CFAP410, similarly the difference in the size of the VNTR and thus relative GC content could affect the efficiency of retention. This is consistent with a model in which the presence of RNA structures induced by the GC-rich microsatellite expansions has an inhibitory effect on splicing (Sznajder et al., 2018). New techniques and improved sequencing protocols have demonstrated as much as 95% of the genome can be transcribed, hence alternative splicing and intron retention are emerging areas of importance for gene regulation and disease progression (Monteuuis et al., 2019). In addition to the aforementioned CFAP410 isoforms, there is also a non-coding RNA that is antisense to the CFAP410 gene (Figure 1); however, we could find no evidence for measurable levels of this antisense message in the CNS transcriptomic data.
Genotyping the CFAP410 VNTR led to the discovery of eight alleles in the cases and controls used for this study (n = 983) (Figure 1C). The frequencies of all alleles and genotypes were not found to be significantly different between cases and controls (Table 1); however, our limited cohort size would likely to be insufficient to identify rare variants associated with ALS. ALS is a complex disease and given the small sample size we were unable to address many of the different metrics, which contribute to this disease, including disease severity, the onset of disease, disease pathology, age, ethnicity, and known ALS genetic mutations in these patients: the VNTR in question could be important in any one of these metrics. However, we do pose a potential mechanism as to how such variants could contribute to disease risk and show that tandem repeat numbers can drive differential gene expression. Furthermore, the original CFAP410 ALS association was from a rare variant (van Rheenen et al., 2016), so such rare VNTR variants discovered here could be part of a yet unidentified polygenic risk score.
Unfortunately, expansion of the cohort size is both time- and resource consuming due to the requirement for PCR analysis of all samples. However, the properties of the VNTR to modulate gene expression in vitro and correlation with differential CFAP410 expression in silico allowed us to hypothesise that this domain could regulate the expression of this locus in response to the same challenge, which could result in distinct levels of protein expression that could modulate risk and progression of ALS. The primary sequence of the CFAP410 VNTR also contains consensus sequence binding sites for proteins predicted to bind the C9ORF72 intronic VNTR (Supplementary Figure 4), potentially inferring contribution to the same signalling pathways. Similarly, modulation of the levels of CFAP410 could have a significant effect on the function of other ALS risk genes, such as functional interactions with NEK1 (Fang et al., 2015; Watanabe et al., 2020). These studies demonstrate the increased fusion of genetic risk with an environmental challenge to modulate ALS risk and progression.
The original contributions presented in the study are included in the article/Supplementary material, further inquiries can be directed to the corresponding author.
The studies involving human participants were reviewed and approved by Trent Research Ethics Committee in February 2003 ref MREC/02/4/107 and in July 2009, ref 09/HO405/32. Please see https://www.ncbi.nlm.nih.gov/pmc/articles/PMC4501191/ for more details. The patients/participants provided their written informed consent to participate in this study.
JM, AF, VB, and JQ: study idea and design. JM, AF, LL, BM, TS, AP, SK, VB, and JQ: data analysis and interpretation. JM: manuscript drafting. All authors reviewed and approved the final manuscript.
JM was funded by a Medical Research Council Doctoral Training Studentship [Grant Number MR/N013840/1]. AF is a recipient of a Andrzej Wlodarski Memorial Research Ph.D. scholarship. Motor Neurone Disease Association (Grant Number: 41/523) supports the work of VB and JQ. AF, VB, and JQ by the Andrzej Wlodarski Memorial Research. BM, VB, and JQ are supported by the Darby Rimmer MND Foundation. LL was supported by the Chinese Scholarship Council 201806370099. SK and AP were supported by MSWA and Perron Institute. AA-C is an NIHR Senior Investigator (NIHR202421), also supported by an EU Joint Programme—Neurodegenerative Disease Research (JPND) project grant through the following funding organisations under the aegis of JPND–www.jpnd.eu[United Kingdom, Medical Research Council (MR/L501529/1; MR/R024804/1) and Economic and Social Research Council (ES/L008238/1)] and through the Motor Neurone Disease Association, My Name'5 Doddie Foundation and Alan Davidson Foundation. This study represents an independent research part funded by the National Institute for Health Research (NIHR) Biomedical Research Centre at South London and Maudsley NHS Foundation Trust and King's College London. This work was supported by resources provided by the Pawsey Supercomputing Centre with funding from the Australian Government and the Government of Western Australia.
Samples used in this research were obtained from the UK MND DNA Bank for ALS Research, funded by the MND Association and the Wellcome Trust. We would like to thank people with ALS and their families for their participation in this project. We acknowledge the Target ALS Human Postmortem Tissue Core, New York Genome Centre for Genomics of Neurodegenerative Disease, Amyotrophic Lateral Sclerosis Association and TOW Foundation for RNA-seq data of human ALS patients.
The authors declare that the research was conducted in the absence of any commercial or financial relationships that could be construed as a potential conflict of interest.
All claims expressed in this article are solely those of the authors and do not necessarily represent those of their affiliated organizations, or those of the publisher, the editors and the reviewers. Any product that may be evaluated in this article, or claim that may be made by its manufacturer, is not guaranteed or endorsed by the publisher.
The Supplementary Material for this article can be found online at: https://www.frontiersin.org/articles/10.3389/fnmol.2022.954928/full#supplementary-material
Supplementary Figure 1. (A) CFAP410 VNTR repeat unit breakdown. Number of 22 bp and 35 bp repeat units per allele of the CFAP410 VNTR (confirmed through Sanger sequencing). (B) CFAP410 VNTR alleles primary sequence and repeat order. Variants 2, 4, 5 and 8 of the CFAP410 VNTR aligned and split into respective 22 bp and 35 bp repeat units. Allele 2 = 262 bp, allele 4 = 297 bp, allele 5 = 319 bp, allele 8 = 468 bp. Boxes indicate exemplars of the 35p and 22 bp repeat unit. All repeats have been aligned by eye and are therefore arbitrary.
Supplementary Figure 2. (A) P-values and effect size of analysis of CFAP410 isoform expression using NYGC ALS dataset. (B) P-values and effect size of tagging SNP analysis of CFAP410 VNTR.
Supplementary Figure 3. LD plot over CFAP410 locus. LD plot over a 45kb region using 31 SNPs from 243 individuals from the Project MinE encompassing the CFAP410 gene. Two blocks of LD are located 5' and 3' of the CFAP410 gene. The * indicates rs56212056 which is the SNP in moderate LD with allele 4 and 5 of the VNTR located in CFAP410. There is minimal LD between rs56212056 and the other SNPs located in the region. Intensity of each square represents the r2 between the two SNPs; darker the colour the larger the r2.
Supplementary Figure 4. Alignment of CFAP410 and C9ORF72 intronic VNTRs. Both VNTR sequences are polymorphic and contained within intron 1 of the most 5' start site. The hexamer sequence within C9orf72 from the UCSC browser (A) contains the sequence CCCCGG and GCCCCG identified in red and green respectively which are also found in the CFAP410 sequence (B).
Supplementary Figure 5. Histogram plot of the distribution of data in the Target ALS cohort for the CFAP410 ENST00000462742 transcript.
Supplementary Table 1. Available tissues from the NYGC Target ALS cohort stratified by controls and ALS subjects.
ALS, Amyotrophic lateral sclerosis; ENCODE, The Encyclopaedia of DNA Elements; CFAP410, Cilia and flagella associated protein 410; MAF, Minor allele frequency; MND, Motor neuron disease; NNC, Non-neurological controls; NYGC ALS, New York Genome Centre Consortium Target ALS; SNP, Single nucleotide polymorphism; UCSC, University of California, Santa Cruz; VNTR, Variable number tandem repeat.
Abel, O., Powell, J. F., Andersen, P. M., and Al-Chalabi, A. (2012). ALSoD: a user-friendly online bioinformatics tool for amyotrophic lateral sclerosis genetics. Hum. Mutat. 33, 1345–1351. doi: 10.1002/humu.22157
Al Khleifat, A., Iacoangeli, A., van Vugt, J., Bowles, H., Moisse, M., Zwamborn, R. A. J., et al. (2022). Structural variation analysis of 6,500 whole genome sequences in amyotrophic lateral sclerosis. NPJ. Genom. Med. 7, 8. doi: 10.1038/s41525-021-00267-9
Al-Chalabi, A., Calvo, A., Chio, A., Colville, S., Ellis, C. M., Hardiman, O., et al. (2014). Analysis of amyotrophic lateral sclerosis as a multistep process: a population-based modelling study. Lancet Neurol. 13, 1108–1113. doi: 10.1016/S1474-4422(14)70219-4
Al-Chalabi, A., Fang, F., Hanby, M. F., Leigh, P. N., Shaw, C. E., Ye, W., et al. (2010). An estimate of amyotrophic lateral sclerosis heritability using twin data. J. Neurol. Neurosurg. Psychiatry 81, 1324–1326. doi: 10.1136/jnnp.2010.207464
Al-Chalabi, A., and Visscher, P. M. (2014). Common genetic variants and the heritability of ALS. Nat. Rev. Neurol. 10, 549–550. doi: 10.1038/nrneurol.2014.166
Ali, F. R., Vasiliou, S. A., Haddley, K., Paredes, U. M., Roberts, J. C., Miyajima, F., et al. (2010). Combinatorial interaction between two human serotonin transporter gene variable number tandem repeats and their regulation by CTCF. J. Neurochem. 112, 296–306. doi: 10.1111/j.1471-4159.2009.06453.x
Bakhtiari, M., Shleizer-Burko, S., Gymrek, M., Bansal, V., and Bafna, V. (2018). Targeted genotyping of variable number tandem repeats with adVNTR. Genome Res. 28, 1709–1719. doi: 10.1101/gr.235119.118
Benson, G. (1999). Tandem repeats finder: a program to analyze DNA sequences. Nucleic Acids Res. 27, 573–580. doi: 10.1093/nar/27.2.573
Blauw, H. M., van Rheenen, W., Koppers, M., Van Damme, P., Waibel, S., Lemmens, R., et al. (2012). NIPA1 polyalanine repeat expansions are associated with amyotrophic lateral sclerosis. Hum. Mol. Genet. 21, 2497–2502. doi: 10.1093/hmg/dds064
Bury, J. J., Highley, J. R., Cooper-Knock, J., Goodall, E. F., Higginbottom, A., McDermott, C. J., et al. (2016). Oligogenic inheritance of optineurin (OPTN) and C9ORF72 mutations in ALS highlights localisation of OPTN in the TDP-43-negative inclusions of C9ORF72-ALS. Neuropathology 36, 125–134. doi: 10.1111/neup.12240
Cady, J., Allred, P., Bali, T., Pestronk, A., Goate, A., Miller, T. M., et al. (2015). Amyotrophic lateral sclerosis onset is influenced by the burden of rare variants in known amyotrophic lateral sclerosis genes. Ann. Neurol. 77, 100–113. doi: 10.1002/ana.24306
Chio, A., Mazzini, L., D'Alfonso, S., Corrado, L., Canosa, A., Moglia, C., et al. (2018). The multistep hypothesis of ALS revisited: The role of genetic mutations. Neurology 91, e635–e642. doi: 10.1212/WNL.0000000000005996
Course, M. M., Gudsnuk, K., Smukowski, S. N., Winston, K., Desai, D., Ross, J. P., et al. (2020). Evolution of a human-specific tandem repeat associated with ALS. Am. J. Hum. Genet. 107, 445–460. doi: 10.1016/j.ajhg.2020.07.004
DeJesus-Hernandez, M., Mackenzie, I. R., Boeve, B. F., Boxer, A. L., Baker, M., Rutherford, N. J., et al. (2011). Expanded GGGGCC hexanucleotide repeat in non-coding region of C9ORF72 causes chromosome 9p-linked frontotemporal dementia and amyotrophic lateral sclerosis. Neuron 72, 245–256. doi: 10.1016/j.neuron.2011.09.011
Fang, X., Lin, H., Wang, X., Zuo, Q., Qin, J., and Zhang, P. (2015). The NEK1 interactor, C21ORF2, is required for efficient DNA damage repair. Acta Biochim Biophys Sin. 47, 834–841. doi: 10.1093/abbs/gmv076
Garton, F. C., Trabjerg, B. B., Wray, N. R., and Agerbo, E. (2021). Cardiovascular disease, psychiatric diagnosis and sex differences in the multistep hypothesis of amyotrophic lateral sclerosis. Eur. J. Neurol. 28, 421–429. doi: 10.1111/ene.14554
Goldstein, O., Kedmi, M., Gana-Weisz, M., Twito, S., Nefussy, B., Vainer, B., et al. (2019). Rare homozygosity in amyotrophic lateral sclerosis suggests the contribution of recessive variants to disease genetics. J. Neurol. Sci. 402, 62–68. doi: 10.1016/j.jns.2019.05.006
Gymrek, M., Willems, T., Guilmatre, A., Zeng, H., Markus, B., Georgiev, S., et al. (2016). Abundant contribution of short tandem repeats to gene expression variation in humans. Nat. Genet. 48, 22–29. doi: 10.1038/ng.3461
Hannan, A. J. (2018). Tandem repeats and repeatomes: Delving deeper into the ‘dark matter' of genomes. EBioMedicine. 31, 3–4. doi: 10.1016/j.ebiom.2018.04.004
Hardiman, O., Al-Chalabi, A., Chio, A., Corr, E. M., Logroscino, G., Robberecht, W., et al. (2017a). Amyotrophic lateral sclerosis. Nat. Rev. Dis. Primers 3, 1–19. doi: 10.1038/nrdp.2017.71
Hsieh, T. Y., Shiu, T. Y., Huang, S. M., Lin, H. H., Lee, T. C., Chen, P. J., et al. (2007). Molecular pathogenesis of Gilbert's syndrome: decreased TATA-binding protein binding affinity of UGT1A1 gene promoter. Pharmacogenet. Genomics 17, 229–236. doi: 10.1097/FPC.0b013e328012d0da
Iacoangeli, A., Al Khleifat, A., Jones, A. R., Sproviero, W., Shatunov, A., Opie-Martin, S., et al. (2019). C9orf72 intermediate expansions of 24-30 repeats are associated with ALS. Acta. Neuropathol. Commun. 7, 115. doi: 10.1186/s40478-019-0724-4
Kageyama, R., Merlino, G. T., and Pastan, I. (1989). Nuclear factor ETF specifically stimulates transcription from promoters without a TATA box. J. Biol. Chem. 264, 15508–15514. doi: 10.1016/S0021-9258(19)84859-7
Lam, D., Ancelin, M.-L., Ritchie, K., Freak-Poli, R., Saffery, R., and Ryan, J. (2018). Genotype-dependent associations between serotonin transporter gene (SLC6A4) DNA methylation and late-life depression. BMC Psychiatry 18, 1–10. doi: 10.1186/s12888-018-1850-4
Lattante, S., Pomponi, M., Conte, A., Marangi, G., Bisogni, G., Patanella, A., et al. (2018). ATXN1 intermediate-length polyglutamine expansions are associated with amyotrophic lateral sclerosis. Neurobiol. Aging. 64, 157.e151–157.e155. doi: 10.1016/j.neurobiolaging.2017.11.011
Love, M. I., Huber, W., and Anders, S. (2014). Moderated estimation of fold change and dispersion for RNA-seq data with DESeq2. Genome Biol. 15, 1–21. doi: 10.1186/s13059-014-0550-8
MacKenzie, A., and Quinn, J. (1999). A serotonin transporter gene intron 2 polymorphic region, correlated with affective disorders, has allele-dependent differential enhancer-like properties in the mouse embryo. Proc. Natl. Acad. Sci. USA. 96, 15251–15255. doi: 10.1073/pnas.96.26.15251
Manca, M., Pessoa, V., Lopez, A. I., Harrison, P. T., Miyajima, F., Sharp, H., et al. (2018). The regulation of monoamine oxidase a gene expression by distinct variable number tandem repeats. J. Mol. Neurosci. 64, 459–470. doi: 10.1007/s12031-018-1044-z
Marshall, J. (2021). The role of non-coding tandem repeat DNA and non-LTR retrotransposons in Amyotrophic Lateral Sclerosis risk loci (Ph. D. thesis). University of Liverpool, England, United Kingdom. Available online at: https://livrepository.liverpool.ac.uk/3116866/1/200883864_March2021.pdf
Marshall, J. N., Lopez, A. I., Pfaff, A. L., Koks, S., Quinn, J. P., and Bubb, V. J. (2021). Variable number tandem repeats – their emerging role in sickness and health. Exp. Biol. Med. 246, 1368–1376. doi: 10.1177/15353702211003511
Monteuuis, G., Wong, J. J. L., Bailey, C. G., Schmitz, U, and Rasko, J. E. J. (2019). The changing paradigm of intron retention: regulation, ramifications and recipes. Nucleic Acids Res. 47, 11497–11513. doi: 10.1093/nar/gkz1068
Nguyen, H. P., Van Mossevelde, S., Dillen, L., De Bleecker, J. L., Moisse, M., Van Damme, P., et al. (2018). NEK1 genetic variability in a Belgian cohort of ALS and ALS-FTD patients. Neurobiol Aging 61 255.e251–255.e257. doi: 10.1016/j.neurobiolaging.2017.08.021
Pacheco, A., Berger, R., Freedman, R., and Law, A. J. (2019). A VNTR regulates miR-137 expression through novel alternative splicing and contributes to risk for schizophrenia. Sci. Rep. 9, 11793. doi: 10.1038/s41598-019-48141-0
Purcell, S., Neale, B., Todd-Brown, K., Thomas, L., Ferreira, M. A., Bender, D., et al. (2007). PLINK: a tool set for whole-genome association and population-based linkage analyses. Am. J. Hum. Genet. 81, 559–575. doi: 10.1086/519795
Quilez, J., Guilmatre, A., Garg, P., Highnam, G., Gymrek, M., Erlich, Y., et al. (2016). Polymorphic tandem repeats within gene promoters act as modifiers of gene expression and DNA methylation in humans. Nucleic Acids Res. 44, 3750–3762. doi: 10.1093/nar/gkw219
Renton, A. E., Majounie, E., Waite, A., Simón-Sánchez, J., Rollinson, S., Gibbs, J. R., et al. (2011). A hexanucleotide repeat expansion in C9ORF72 is the cause of chromosome 9p21-linked ALS-FTD. Neuron 72, 257–268. doi: 10.1016/j.neuron.2011.09.010
Roeck, A. D., Duchateau, L., Dongen, J. V., Cacace, R., Bjerke, M., Bossche, T. V., et al. (2018). An intronic VNTR affects splicing of ABCA7 and increases risk of Alzheimer's disease. Acta Neuropathol. 135, 827–837. doi: 10.1007/s00401-018-1841-z
Sham, P. C., and Curtis, D. (1995). Monte Carlo tests for associations between disease and alleles at highly polymorphic loci. Ann. Hum. Genet. 59, 97–105. doi: 10.1111/j.1469-1809.1995.tb01608.x
Shatunov, A., and Al-Chalabi, A. (2021). The genetic architecture of ALS. Neurobiol. Dis. 147, 105156. doi: 10.1016/j.nbd.2020.105156
Soneson, C., Love, M. I., and Robinson, M. D. (2015). Differential analyses for RNA-seq: transcript-level estimates improve gene-level inferences. F1000Res 4, 1521. doi: 10.12688/f1000research.7563.1
Sproviero, W., Shatunov, A., Stahl, D., Shoai, M., van Rheenen, W., Jones, A. R., et al. (2017). ATXN2 trinucleotide repeat length correlates with risk of ALS. Neurobiol Aging 51, 178.e1–178.e9. doi: 10.1016/j.neurobiolaging.2016.11.010
Sznajder, L. J., Thomas, J. D., Carrell, E. M., Reid, T., McFarland, K. N., Cleary, J. D., et al. (2018). Intron retention induced by microsatellite expansions as a disease biomarker. Proc. Natl. Acad. Sci. USA. 115, 4234–4239. doi: 10.1073/pnas.1716617115
Tazelaar, G. H. P., Boeynaems, S., De Decker, M., van Vugt, J., Kool, L., Goedee, H. S., et al. (2020). ATXN1 repeat expansions confer risk for amyotrophic lateral sclerosis and contribute to TDP-43 mislocalization. Brain Commun. 2, fcaa064. doi: 10.1093/braincomms/fcaa064
Tazelaar, G. H. P., Dekker, A. M., van Vugt, J., van der Spek, R. A., Westeneng, H. J., Kool, L., et al. (2019). Association of NIPA1 repeat expansions with amyotrophic lateral sclerosis in a large international cohort. Neurobiol Aging 74, 234.e239–234.e215. doi: 10.1016/j.neurobiolaging.2018.09.012
Theunissen, F., Flynn, L. L., Anderton, R.S., Mastaglia, F., Pytte, J., Jiang, L., et al. (2020). Structural variants may be a source of missing heritability in sALS. Front. Neurosci. 14, 47. doi: 10.3389/fnins.2020.00047
van Blitterswijk, M., van Es, M. A., Hennekam, E. A., Dooijes, D., van Rheenen, W., Medic, J., et al. (2012a). Evidence for an oligogenic basis of amyotrophic lateral sclerosis. Hum. Mol. Genet. 21, 3776–3784. doi: 10.1093/hmg/dds199
van Blitterswijk, M., van Es, M. A., Koppers, M., van Rheenen, W., Medic, J., Schelhaas, H. J., et al. (2012b). VAPB and C9orf72 mutations in 1 familial amyotrophic lateral sclerosis patient. Neurobiol Aging 33, 2950.e2951–2954. doi: 10.1016/j.neurobiolaging.2012.07.004
van der Spek, R. A. A., van Rheenen, W., Pulit, S. L., Kenna, K. P., van den Berg, L. H., Veldink, J. H., et al. (2019). The project MinE databrowser: bringing large-scale whole-genome sequencing in ALS to researchers and the public. Amyotroph. Lateral Scler. Frontotemporal Degener. 20, 432–440. doi: 10.1080/21678421.2019.1606244
van Rheenen, W., Shatunov, A., Dekker, A. M., McLaughlin, R. L., Diekstra, F. P., Pulit, S. L., et al. (2016). Genome-wide association analyses identify new risk variants and the genetic architecture of amyotrophic lateral sclerosis. Nat. Genet. 48, 1043–1048. doi: 10.1038/ng.3622
van Rheenen, W., van der Spek, R. A. A., Bakker, M. K., van Vugt, J. J. F. A., Hop, P. J., Zwamborn, R. A. J., et al. (2021). Common and rare variant association analyses in amyotrophic lateral sclerosis identify 15 risk loci with distinct genetic architectures and neuron-specific biology. Nat. Genet. 53, 1636–1648. doi: 10.1038/s41588-021-00973-1
Vucic, S., Westeneng, H. J., Al-Chalabi, A., Van Den Berg, L. H., Talman, P., and Kiernan, M. C. (2019). Amyotrophic lateral sclerosis as a multi-step process: an Australia population study. Amyotroph. Lateral Scler. Frontotemporal Degener. 20, 532–537. doi: 10.1080/21678421.2018.1556697
Warburton, A., Breen, G., Bubb, V. J., and Quinn, J. P. (2016). A GWAS SNP for Schizophrenia is linked to the internal mir137 promoter and supports differential allele-specific expression. Schizophr. Bull. 42, 1003–1008. doi: 10.1093/schbul/sbv144
Warburton, A., Breen, G., Rujescu, D., Bubb, V. J., and Quinn, J. P. (2015). Characterization of a REST-regulated internal promoter in the schizophrenia genome-wide associated gene MIR137. Schizophr. Bull. 41, 698–707. doi: 10.1093/schbul/sbu117
Watanabe, Y., Nakagawa, T., Akiyama, T., Nakagawa, M., Suzuki, N., Warita, H., et al. (2020). An amyotrophic lateral sclerosis-associated mutant of C21ORF2 is stabilized by NEK1-mediated hyperphosphorylation and the inability to bind FBXO3. iScience 23, 101491. doi: 10.1016/j.isci.2020.101491
Wroe, R., Butler, A. W.-L., Andersen, P. M., Powell, J. F., and Al-Chalabi, A. (2009). ALSOD: the amyotrophic lateral sclerosis online database. Amyotrophic Lateral Sclerosis 9, 249–250. doi: 10.1080/17482960802146106
Yilmaz, R., Weishaupt, K., Valkadinov, I., Knehr, A., Brenner, D., and Weishaupt, J.H. (2022). Quadruple genetic variants in a sporadic ALS patient. Mol. Genet. Genomic Med. 10, e1953. doi: 10.1002/mgg3.1953
Zou, Z.-Y., Zhou, Z.-R., Che, C.-H., Liu, C.-Y., He, R.-L., and Huang, H.-P. (2017). Genetic epidemiology of amyotrophic lateral sclerosis: a systematic review and meta-analysis. J. Neurol. Neurosurg. Psychiatry. 88, 540–549. doi: 10.1136/jnnp-2016-315018
Keywords: CFAP410, ALS, VNTR, gene expression, transcriptional regulation
Citation: Marshall JNG, Fröhlich A, Li L, Pfaff AL, Middlehurst B, Spargo TP, Iacoangeli A, Lang B, Al-Chalabi A, Koks S, Bubb VJ and Quinn JP (2022) A polymorphic transcriptional regulatory domain in the amyotrophic lateral sclerosis risk gene CFAP410 correlates with differential isoform expression. Front. Mol. Neurosci. 15:954928. doi: 10.3389/fnmol.2022.954928
Received: 27 May 2022; Accepted: 22 July 2022;
Published: 05 September 2022.
Edited by:
Detlev Boison, Rutgers, The State University of New Jersey, United StatesReviewed by:
Philip Wing-Lok Ho, The University of Hong Kong, Hong Kong SAR, ChinaCopyright © 2022 Marshall, Fröhlich, Li, Pfaff, Middlehurst, Spargo, Iacoangeli, Lang, Al-Chalabi, Koks, Bubb and Quinn. This is an open-access article distributed under the terms of the Creative Commons Attribution License (CC BY). The use, distribution or reproduction in other forums is permitted, provided the original author(s) and the copyright owner(s) are credited and that the original publication in this journal is cited, in accordance with accepted academic practice. No use, distribution or reproduction is permitted which does not comply with these terms.
*Correspondence: John P. Quinn, anF1aW5uQGxpdmVycG9vbC5hYy51aw==
†Present Address: Jack N. G. Marshall, Section of Infections of the Nervous System, National Institute of Neurological Disorders and Stroke, National Institutes of Health, Bethesda, MD, United States
‡These authors share first authorship
Disclaimer: All claims expressed in this article are solely those of the authors and do not necessarily represent those of their affiliated organizations, or those of the publisher, the editors and the reviewers. Any product that may be evaluated in this article or claim that may be made by its manufacturer is not guaranteed or endorsed by the publisher.
Research integrity at Frontiers
Learn more about the work of our research integrity team to safeguard the quality of each article we publish.