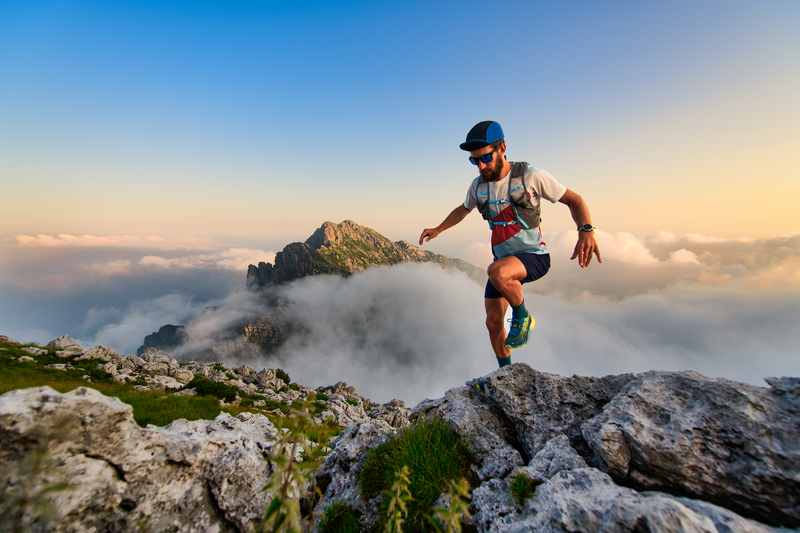
95% of researchers rate our articles as excellent or good
Learn more about the work of our research integrity team to safeguard the quality of each article we publish.
Find out more
REVIEW article
Front. Mol. Neurosci. , 26 July 2022
Sec. Pain Mechanisms and Modulators
Volume 15 - 2022 | https://doi.org/10.3389/fnmol.2022.935918
This article is part of the Research Topic Molecular Mechanisms Underlying Pain Relief and Drug Tolerance View all 6 articles
In addition to being studied for their exceptional regeneration abilities, planarians (i.e., flatworms) have also been extensively used in the context of pharmacological experiments during the past century. Many researchers used planarians as a model system for the study of drug abuse because they display high similarities with the nervous system of vertebrates at cellular and molecular levels (e.g., neuronal morphology, neurotransmitter ligands, and receptor function). This research field recently led to the discovery of causal relationships between the expression of Transient Receptor Potential ion channels in planarians and their behavioral responses to noxious stimuli such as heat, cold or pharmacological analogs such as TRP agonists, among others. It has also been shown that some antinociceptive drugs modulate these behaviors. However, among the few authors that tried to implement a full behavior analysis, none reached a consensual use of the terms used to describe planarian gaits yet, nor did they establish a comprehensive description of a potential planarian nociceptive system. The aim of this review is therefore to aggregate the ancient and the most recent evidence for a true nociceptive behavior in planarians. It also highlights the convenience and relevance of this invertebrate model for nociceptive tests and suggests further lines of research. In regards to past pharmacological studies, this review finally discusses the opportunities given by the model to extensively screen for novel antinociceptive drugs.
The term planaria (or planarians) encompasses flatworms (platyhelminthes phylum) within the Tricladida order. The other three out of the four major classes of flatworms – Trematoda, Cestoda, and Monogenea – contain mostly parasitic species but Tricladida are free-living organisms. They used to be classified as Turbellaria due to their gliding movement using ventral cilia but this class is now considered paraphyletic (de Vries and Sluys, 1991). Triclads include marine (Maricola), land (Geoplanidae) and freshwater flatworms. Most of them might be called planarians (e.g., “land planarians” for Geoplanidae species), but the term planaria or planarians in research models mostly refers to freshwater species, especially from the Dugesiidae family. Common species used as model animals come from the three Dugesiidae genus Girardia, Dugesia, and Schmidtea, such as Girardia tigrina, Girardia dorotocephala, Dugesia japonica, or Schmidtea mediterranea (Figure 1F). Note that tigrina and dorotocephala are still sometimes referred as from the “Dugesia” or “Dugesia (Girardia)” genus by common practice, but Girardia is not classified as a subgenus of Dugesia anymore (de Vries and Sluys, 1991).
Figure 1. Common planarian representations. (A) Dorsal exterior view. aur, auricles; oc, ocelli. (B) Ventral exterior view with extruded pharynx. exc, excretory pores; ph, pharynx; mo, mouth; geni, genital pore. (C) Nervous system. cer, cerebral ganglions; tra, transversal nerve; me, pharyngal mesh of neurons; lat, lateral nerve cord; gg, lateral ganglions. (D) Gastrovascular system. ga, gastrovascular cavity; di, diverticulum; ph, pharynx; ra, posterior ramus. (E) Reproductive system, female is represented on the left and male on the right but both are present symmetrically. ov, ovary; gl, yolk glands; bu, bursa copulatrix; at, genital atrium; tes, testis; pen, penis. (F) Photos of common planaria species from our laboratory (scale: 1 mm).
As they do not have a body cavity, planarians are considered as acoelomates. Their digestive system consists of a three-branched gastrovascular cavity, hence the term triclad (Figure 1D). It is shaped as an inverted Y that opens in the center of their ventral side with a muscular appendage called the pharynx. The pharynx is dedicated to food intake, to initiate digestion and finally to expel the leftovers. This structure possesses a dedicated mesh of neurons that guide the animal for food-localization movements (Miyamoto et al., 2020). After crossing the pharynx, food is then distributed throughout the gastrovascular cavity to provide nutrients by diffusion during the digestion. Outside lab conditions, they mostly feed on other small invertebrates (some are even cannibalistic) and debris. Regarding their reproductive behavior, all planarians are true hermaphrodites (Figure 1E). Despite having functional male and female reproductive systems, they can usually switch between sexual and asexual reproduction but some others only reproduce asexually (Ramm, 2017). Asexual reproduction is usually done by fragmentation or fissioning of the animal, resulting in further regeneration of missing parts. Planarians mostly perform fissioning when the population is decreasing and cease fissioning when the population is crowded (Best et al., 1969). They also have a basic excretory system composed of flame cells and mucus-producing cells called rhabdites – hence their classification in the Rhabditophora class (Figure 1B). This system is not only crucial for locomotion (Sugimoto, 2010) but may also participates to defense mechanisms against infections or predators as in other species (Cochet-Escartin et al., 2015).
Their nervous system consists of a “brain” located in the anterior part of the animal from which originates two ventral longitudinal nerve cords running on the entire length of the animal (Figure 1C; Agata, 2008). These nerve cords also branch out in transversal nerves, giving their central nervous system the appearance of a grid or a ladder. The so-called brain is characterized by two cerebral ganglions linked by a cerebral commissure. The term “brain” in planaria is often used as a shortcut for cerebral ganglions, even though there are arguments for actually using the term “brain” as an accurate depiction of its structure (Sarnat and Netsky, 2002). Sensory neurons branch out from the nerve cords all along the body. The brain also extends nine branches out of each lobe to the head margin that primarily serve as sensory neurons, especially as chemical and mechanical sensors, present in the “auricles” in some species (ears-like structure particularly visible in G. dorotocephala). Above the brain extend the eye spots, actually called ocelli (Figure 1A), which typically look cross-eyed because of their two cell type compositions: photoreceptors (white cupola) and pigmented cells (dark spots) (Saló et al., 2002; Inoue et al., 2004). Planarians are mostly nocturnal animals, hence showing more activity in the dark period (Lombardo et al., 2011; Hinrichsen et al., 2019). These photoreceptors sense photoperiodic information but also allow typical defensive photophobic reactions to light (Paskin et al., 2014). However, planarians also show behavioral responses mediated by extraocular photoreception, especially to UV light (Birkholz and Beane, 2017).
One of the key features of this model is that planarians display huge regenerative capacities. They possess populations of pluripotent stem cells called neoblasts that can be triggered by lesions and form a regenerative area called blastema (Reddien and Alvarado, 2004; Reddien, 2018). They are able to regenerate every tissue, thus allowing the complete regeneration of the animal’s missing part, to the extent of complete nervous system regeneration and even neural networks reconstruction (Cebrià, 2007; Nishimura et al., 2007). Even small parts from almost any tissue can regrow into a complete individual from limited number of cells and signaling gradients (Reddien and Alvarado, 2004; Ivankovic et al., 2019). From a comparative neurobiology point of view, it is quite striking that morphogenetic signals initiating planarian regeneration are very similar to the intrinsic factors shaping up the human brain during development (Currie et al., 2016; Reddien, 2018).
Planarians have been a useful model in numerous domains of biology for the past three centuries. Their first known mentions date back to the late 18th century (Müller, 1776; Pagán, 2014). The phenomenon that brought researchers’ attention was their extraordinary regeneration abilities. In the early 19th century, Dalyell (1814) already wrote the now famous citation “It may almost be called immortal under the edge of the knife.” Later, at the turn of the 20th century, multiple scientists were publishing extensive descriptions of the worm’s morphology, physiology and behavior (Bardeen, 1901; Pearl, 1903). By the middle of the century, research on planarians ranged as far as tropism (Viaud, 1948), magnetobiology (Brown, 1962), conditioning and learning (Corning and Kelly, 1973), phylogenetics (Minelli, 1977), and behavior (Ogren, 1956), as only a few non-exhaustive examples. Regeneration studies also continued to thrive from planarians, elucidating the cellular nature of regeneration and the fundamental properties of stem cells (Elliott and Alvarado, 2018). In the meantime, their use in pharmacology and toxicology studies has increased steadily (Pagán, 2017). Indeed, planarians’ cerebral ganglions can actually constitute a brain analog (Sarnat and Netsky, 2002). An idea popularized by Pagán (2014) in “The First Brain,” where the author described flatworms as the “simplest” animals with a central nervous system. Yet, they share a lot of common characteristics with vertebrates, such as multipolar neurons with a unique axon, extensive dendritic branching with developed dendritic spines, a majority of chemical synapses and a low-frequency spontaneous electrical activity (Sarnat and Netsky, 1985, 2002). These characteristics are not shared with most higher-grade invertebrates. Besides, they possess many of the neurotransmitters that are present in vertebrates (Ribeiro et al., 2006; Buttarelli et al., 2008), as well as similar interactions between their pathways (Carolei et al., 1975). They also serve as a great model for a wide range of drugs and for poly-drug testing, as they allow the study of multiple compounds at once and with higher accuracy of pharmacokinetic factors (absorption, distribution, metabolism, excretion; i.e., ADME) than with higher models like rodents or primates (Raffa, 2008). Hence, planaria has been a model animal for hundreds of pharmacological studies with a particular focus on substances of abuse like cocaine, amphetamines or nicotine, as they display signs of withdrawal and tolerance in the same way more evolved animals do (Pagán, 2017).
Planarians glide on surfaces using ciliated cells located on their ventral side, on a layer of mucus that they constantly produce (Rompolas et al., 2010; Sugimoto, 2010). It has been long known that when exposed to a harmful stimulus (either mechanical, chemical, or electrical), planarians display clear behavior changes (Pearl, 1903). When disrupted, the animals will usually produce more mucus, stop gliding smoothly and adopt various body shapes, movements, and postures. These reactions highly resemble nocifensive behavior seen in other invertebrates or even vertebrates, including mammals (Kavaliers, 1988). Yet, planarians are still not considered as animal models for nociception testing, nor clearly presented as having a nociceptive system or pathway. In the next sections, we will discuss why there are many reasons to consider that planarians may actually present a nociceptive system and that many experiments can be done to decipher more precisely its mechanisms, once again supporting the usefulness and the ethical relevance of this invertebrate model in the nociception and pain research domain.
The vast majority of nociception and pain research has been and is done in mammals, and rodents in particular (Mogil, 2009). In this context, nociceptors represent the free nerve endings that specialize in the detection of noxious stimuli and forward this information to the spinal cord before being sent to higher cortical regions. Thus, nociception is defined as “the neural process of encoding noxious stimuli” (Terminology | International Association for the Study of Pain [IASP], 2022). Nociceptors are usually defined by the composition of their axon, either myelinated (fast-conducting “Aδ” fibers) or unmyelinated (slower-conducting “C” fibers) and express multiple ion channels specialized in detecting noxious compounds or stimuli. These ion channel receptors include mainly the transient receptor potential (TRP) ion channels among others, such as ASICs or P2X channels (Tracey, 2017). Pain, on the other hand, is defined as “an unpleasant sensory and emotional experience associated with, or resembling that associated with, actual or potential tissue damage” (Terminology | International Association for the Study of Pain [IASP], 2022). Thus, as an over-simplification, pain is usually the result of sufficient nociceptive input that is integrated in the cortex as emotionally unpleasant. This definition is particularly relevant for humans that communicate but lacks inclusivity toward animal pain as it involves conscious representations that we cannot yet interpret from animal behavior. The animal pain research field tries to decipher whether animals experience pain in similar ways as humans do through lists of criteria, and even though so-called pain testing paradigms exist (e.g., place preference test or analgesia self-administration), they cannot conclude on certainty that they experience pain the same way humans do (Sneddon et al., 2014). Conclusions mostly revolves around ethical considerations and humane use of animals in research as a precautionary principle. This should also be valid toward the invertebrates’ pain and nociception research field. Indeed, while vertebrates’ and especially mammalians’ pain is mostly accepted as a highly probable concept, invertebrates’ nociception is unfortunately way less regarded (Elwood, 2019).
Looking back at nociceptors, invertebrates do not possess such fibers classification and their sensory neurons might not be as specialized. Nonetheless, some of their neurons also express nociceptive ion channels. Indeed, TRP ion channels were initially discovered in Drosophila photoreceptors (Cosens and Manning, 1969; Katz et al., 2017). They are highly conserved along the animal kingdom and seem to be at the base of many noxious sensing systems (Peng et al., 2015; Arenas et al., 2017). Moreover, the strict definition of nociception previously discussed does not apply exclusively to vertebrates. Many animals, including very low species on the phylogenetic tree of life, show signs of what seems to be defense mechanisms when exposed to unusual environmental conditions or predators (Kavaliers, 1988). Many other “pain perception” criteria have been experimentally tested on multiple invertebrates models, such as protective, avoidance or trade-off behaviors (Sneddon et al., 2014; Elwood, 2019). However, only a few invertebrate models actually have a complete or even partial description of their nociceptive system, usually involving more or less specialized neurons (Burrell, 2017; Tracey, 2017).
In one of the most well studied invertebrate model Drosophila, sensory neurons are classified as class I to IV based on their dendritic arborescence. The class IV neurons are particularly involved in noxious heat, mechanical and chemical detection and project to the nerve cords of the CNS (Im and Galko, 2012). In the nematode Caenorhabditis elegans, nociception is based on behavioral responses following aversive cues. It involves the polymodal ASH and the mechanosensory PVD neurons (Tobin and Bargmann, 2004). In the leech Hirudo verbana, sensory neurons are classified as N cells (Nociceptive), T cells (Touch), and P cells (Pressure) based on their threshold of activation following mechanical stimuli (Blackshaw et al., 1982). In the mollusk Aplysia, its so-called LE sensory neurons are particularly involved in noxious mechanical stimuli detection (Illich and Walters, 1997). In the squid Loligo, mechanosensitive nociceptors are located in its fins (Crook et al., 2013). Other models have been studied, mostly less extensively, and can be used to compare the phylogeny of nociceptors, such as other mollusks, fish, amphibians, or reptilians. These studies include different sets of tests, sometimes only partially carried out, such as behavioral responses to noxious stimuli, electrophysiological responses of sensory afferent fibers in teased nerve preparations or whole cell patch clamp of sensory neurons (Smith and Lewin, 2009).
These specialized neurons in each species have been identified as nociceptive because they display all, or a part of, characteristics similar to those of vertebrates: an implication in behavioral noxious responses, a specific expression of TRP-like ion channels or other nociceptive-related receptors, a high threshold to induce an action potential, a slow adaptation, a specific activation following a noxious stimuli and a potential modulation. Indeed, while nociceptors usually display specific physiological characteristics, they also display high plasticity. For example, a common modulation process is sensitization, which lowers the activation threshold and/or augments the action potential frequency, induced by continuous stimuli, inflammatory soup (e.g., ATP, cytokines, acidic compounds, etc.) or phosphorylation of TRPs’ intracellular segments (Smith and Lewin, 2009).
Based on previous observations about invertebrate models of nociception, we suggest here that planarians show sufficient arguments for the model to be considered for nociceptive tests.
First and foremost, planarians seem to display a variety of reproductible behaviors in response to noxious stimuli. As planarians do not have movable ocelli or facial expressions, almost everything we know about planarian behavior comes from their locomotion. Therefore, we can only observe the way they move or the body shape they take. Planarians also display negative phototaxis, which allows for locomotion inducing and tests such as place preference or place conditioning experiments (Paskin et al., 2014; Dziedowiec et al., 2018; Zewde et al., 2018). Further description of planarian behavior studies will be discussed in section “Planarian behavioral metrics.”
Secondly, the TRP ion channels present in many sensory and nociceptive pathways are highly conserved among the animal kingdom so they were the main target to identify a possible nociceptive pathway in planarians. TRPs are membrane ion channels that have a non-selective permeability to cations and represent the main ion channel family responsible for the detection of noxious stimuli on nociceptors (González-Ramírez et al., 2017). They form as tetramers and are regrouped into different families based on their amino acid sequence and structure. The main TRP families involved in nociception are TRPA (ankyrin), TRPV (vanilloid), and TRPM (melastatin). The presence of TRP channels in planarians were first assumed from the reactions of the animals to TRP agonists (Rawls et al., 2007a), but the expression of planarian TRP family genes have since then been observed throughout the whole body of the animals by in situ hybridization (Inoue et al., 2014). Notably, TRPAa and TRPMa displayed a scattered expression on the periphery and a denser expression in the head suggesting their particular involvement in sensory neurons, while TRPVa pattern of expression is more homogenously distributed throughout the body in mesenchymal cells (Inoue et al., 2014).
On the other hand, in planarians, protein knockdown can be achieved by interference RNA technology (RNAi) using double-stranded (ds) RNA microinjected directly in the host tissue (Sánchez Alvarado and Newmark, 1999) or, in a broader way, using food infected with dsRNA-producing bacteria (Newmark et al., 2003) or supplemented with in vitro synthetized dsRNA (Rouhana et al., 2013). After injection or ingestion, RNA segments will diffuse throughout the animal and hybridize with the target protein’s transcript, effectively blocking its translation and thus the formation of the protein of interest. Hence, multiple gene expression marking and repressing of TRP channels have been done in planarians thanks to the continuous efforts in the sequencing of their genome and its availability (Rozanski et al., 2019).
Performing such knockdown on planarians’ TRPA1 channels, commonly involved in noxious heat sensing, successfully inhibited noxious heat avoidance and chemical avoidance of irritants, such as allyl isothiocyanate (AITC), a TRPA1 agonist commonly found in mustard oil (Arenas et al., 2017). Heat avoidance experiments consisted of opposing floor tiles of two different temperatures: noxious 32°C tiles that planarians mostly avoid and 24°C tiles that planarians mostly glide on. Inhibiting the expression of TRPA1 made planarians spend as much time on hot tiles as on room-temperature tiles. Planarians thus seem to detect noxious heat through TRPA1 but, in fact, further experiments suggested that heat does not directly activate TRPA1’s opening. The suggested cellular mechanism is rather an induction of reactive oxygen species (ROS) production that in turn can activate the TRPA1 ion channel (Arenas et al., 2017). This idea also corroborates with experiments on extraocular UV-light expositions in planarians, which induced noxious behavioral responses (by means of cellular ROS production) that were reduced in TRPA1-knockdown animals (Birkholz and Beane, 2017). Other examples of TRP knockdowns included targeting of planarian TRPM8 that resulted in a defect in thermotaxis for a wide range of cold temperatures (Inoue et al., 2014). Noxious cold avoidance experiments were done in similar conditions as for heat avoidance, but instead of tiles of different temperatures, a gradient was used from the side (non-noxious 25°C) to the center (noxious 17°C to 0°C) of a circular arena. Similarly, inhibition of the expression of TRPM8 induced more time spent in noxious cold areas than for wild-type animals. Moreover, genetic targeting of planarian TRPV1 reduced the noxious responses to the TRPV1 agonist and endocannabinoid anandamide. It did not, however, reduce capsaicin-induced responses, another well-known TRPV1 agonist, suggesting other receptors may be involved (Sabry et al., 2019).
Lately, many antinociceptive compounds have been shown to modulate planarians’ responses in a similar way as in other animals. The first observation of opioids’ (i.e., morphine) effects on planarians behavior dates back to 1967 and has shown both tolerance and dependence characteristics (Needleman, 1967). By measuring the time an individual worm took to travel from the center of an illuminated petri dish to the side, Needleman demonstrated that when exposed to a high acute dose of morphine, the worm increased its transit time because of slow gliding or erratic movements. He then showed that this hypokinesia phenomenon could be gradually reduced to normal travel time after continuous exposure to morphine and that it could appear again after a withdrawal from chronic exposure (showing tolerance and dependence characteristic behaviors). Since then, other opioid agonists have been tested on planarians (e.g., DAMGO, a strong selective Mu opioid receptor agonist) with similar results and reversible effects using opioid antagonists (e.g., Naltrexone, a strong selective Mu opioid receptor antagonist) (Dziedowiec et al., 2018). However, to our knowledge, equivalents to opioid receptors still have not been specifically identified in planarians. In contrast, the presence of endogenous opioids (i.e., met-enkephalin) has been shown (Venturini et al., 1983). Many other synthetic opioid agonists and antagonists, as well as pro- and antinociceptive drugs have also been used in planarians. Examples include non-steroidal anti-inflammatory drugs such as meloxicam (Kim and Rawls, 2022), the polypeptide nociceptin (Rawls et al., 2008a), cannabinoid agonists or many other drugs of abuse mainly acting on monoamine and/or glutamatergic neurotransmission (Supplementary Table 1).
For further insights into behavior-modulating drugs in planarians, we listed in Supplementary Table 1 references that used behavioral tests or behavioral metrics in planarians and the stimuli they used. Supplementary Table 1 includes all references listed in section “Evidence for nociception in planarians” and “Planarian behavioral metrics” that modulated planarians’ behavior via a wide range of stimuli: chemical, mechanical, electric, thermic, or light. All chemicals are sorted based on the main known effect they have on nervous systems. Supplementary Table 1 is not expected to be an exhaustive list of the literature but rather a big entry point and a display of the wide variety of chemicals and stimuli used to modulate planarian behavior.
Looking back into behavior, we noticed that the methods used in the literature to measure planarian locomotion can fall into two types of metrics: the quantitative and the qualitative metrics.
The quantitative metrics simply compared the distance traveled by planarians in a given time, either by counting the crossing of squares on a grid (Raffa et al., 2000, 2001, 2003, 2008, 2013; Raffa and Valdez, 2001; Rawls et al., 2006, 2007a,b, 2008a,b,c) or by measuring the total distance traveled by video tracking (Nishimura et al., 2007, 2011). The first one is usually noted as planarian LocoMotor Velocity (pLMV) or planarian LocoMotor Activity (pLMA).
The qualitative metrics are the observations of the body shape and the way planarians move. It is usually done either by identifying the main locomotion type (Carolei et al., 1975; Venturini et al., 1989), by grading the amount and intensity of multiple observed behaviors (Passarelli et al., 1999; Buttarelli et al., 2000) or by counting the occurrences of abnormal behaviors in a set time (Rawls et al., 2009, 2010, 2011; Pagán et al., 2012, 2015; Tallarida et al., 2014; Kim and Rawls, 2022). The latter was sometimes named planarian Seizure-Like Activity (pSLA) or planarian Seizure-Like Movements (pSLM) or simply “activity counts.”
Quantitative metrics, such as pLMV, had been introduced to propose and develop an objective measurement, independent of any experimenter bias. Indeed, those that have tried and analyzed the movements of planarians may have realized that they often display movements that are hard to characterize, often betwixt and between two types of locomotion that we want to identify, thus creating possible bias in the subjective interpretation of the movement and body shapes. However, if planarians sustain a clear type of locomotion for long enough, then the interpretation biases become negligible and the reaction to different compounds or concentrations can be compared more easily. The goal is to select the relevant properties of their movements to distinguish every possible form of reaction to heat, cold, irritants, narcotics, mechanical stimuli, etc. The best outcome would be to precisely characterize each movement with different criteria so that errors would be limited. At present time, only a few papers have tried to implement such classification of locomotion, and none of them seem to be used as a common baseline for further studies, even if some behaviors are commonly identified and used.
A great portion of the research using behavioral locomotion of planarians is presented in Table 1, where similar movement descriptions were associated to their respective designations by the authors. Table 1 shows that a few terms were used multiple times by independent research teams, such as “C-like position” or “Screw-like hyperkinesia.” Unfortunately, to the best of our knowledge, none of them properly defined these movements: they are mere descriptive terms of what the movement of the animal looked like. It is probable that different interpretations of the movement were made, such as for the term “headswing” which was used for two different gaits. All other terms mostly appeared only once. Some studies have implemented completely new descriptions of movements, but unfortunately none of them reached a consensual use in the field.
The latest description of the “crawling” movement firstly observed more than a century ago and now referred to as “scrunching,” is so far the most detailed description of planarian noxious behavior (Cochet-Escartin et al., 2015). Scrunching is an almost-ubiquitous muscular type of gait induced by noxious stimuli. It is thought to be a defense mechanism triggered when normal gliding is impaired, such as from lesions, with distinct body movement characteristics (Figure 2). Authors have not only described the movements of this “scrunching” behavior but also characterized, for different species, the frequency, velocity and inducers of this behavior, among others. This study also proposed a disambiguation from another type of gait present in worm species: peristalsis. Peristalsis is another form of muscular locomotion that arises from a disruption of the planarian ciliated cells. It can be recognized by a short contraction of the body from the head to the tail. As the planarian moves forward, it looks as if the contraction bulge stays immobile relative to the substrate (Rompolas et al., 2010; Tanaka et al., 2012). Hence, we would suggest using the terms “gliding,” “scrunching,” and “peristalsis” in further studies of such behaviors. Concerning other types of locomotion in response to noxious stimuli, precise descriptions and disambiguations are awaited.
Figure 2. Normal “gliding” and noxious “scrunching” behavior representation. (A) Typical gliding behavior of planarians. They produce mucus and glide at a constant rate on a surface using ciliated cells located on their ventral side. Gliding speed is usually about 1–5 mm/s. (B) Typical noxious “scrunching” behavior. Scrunching is a muscular gait. The head is first anchored to the surface (t1), the body contracts (t2), anchor is switched from the head to the tail (t3 to t4), then the body elongates forward (t5), and loops to the first step (t6). The frequency of one loop varies between 0.2 and 0.8 Hz depending mostly on the species (Cochet-Escartin et al., 2015). Scrunching sometimes happen in reverse order, making the worm scrunch backward for a few seconds only.
While many arguments can already be found for planarian nociception, it should be noted that further experiments could be pursued to fully defend this concept.
To further describe nociceptive pathways in planaria, sensory neurons could be precisely traced. Fluorescent dye tracing of neural projections has already been done: it demonstrated, for example, that chemosensory neurons from the lateral branches project to the peripheral part of the brain (Okamoto et al., 2005; Agata, 2008). Precise tracing of neurons of interest for nociception, such as those that highly express TRPA1 or TRPV1 for example, has not been done yet, in part due to the lack of antibodies against planarian homologs of TRP channels. However, cells that express TRP channels genes can be marked by whole-mount in situ hybridization (Inoue et al., 2014). This technique allows precise mapping of cells that express specific families of TRP channels. One more step in nervous cells tracing is to be able to do it in vivo to facilitate electrophysiology recordings, which seems to be a hard task to accomplish (Koopowitz et al., 1996). We believe that nociceptive pathways could be traced using one or a combination of these techniques to further describe planarian sensory neurons.
With regards to electrophysiological properties of planarian nerve cells, most studies were performed by the Koopowitz team which used the polyclad flatworms Freemania litoricola (Koopowitz, 1975a,b) and Notoplana acticola (Bernardo et al., 1977; Keenan et al., 1979; Koopowitz et al., 1979a,b). These species are marine planarians, uncommon models that were solely used by this team. They performed extracellular recordings of both spontaneous and evoked activity mostly around the ventral nerve cords, from which results were discussed previously in section “Planarian research throughout centuries” (Sarnat and Netsky, 1985, 2002). More recently, one team has performed EEG-like recordings of the planarian brain (an electrode was inserted in the cerebral ganglions) which showed a low-frequency spontaneous activity, as well as short and loosely coupled neural feedback loops in D. japonica (Aoki et al., 2009). Hence, while new data is very scarce, it is still promising as it already replicated some of the older experimental results using common freshwater planarian models. The model could benefit from new replications of electrophysiological recordings, both to confirm the data in common lab planarians, and to make the most of the current technology and advances in the field. For example, simple patch-clamp recordings of single sensory neurons have not been done in planarians to the best of our knowledge. These recordings could eventually lead to the description of different functional families of sensory neurons, similar to those of other invertebrates that have been described. This would be a necessary step to confirm the physiological properties of nociceptors in planarian sensory neurons, such as sensitization, slow adaptation, high threshold, etc.
Moreover, multiple types of stimuli could be used to precisely decipher their nociceptive mechanisms. Indeed, planaria is especially convenient for chemically induced nociceptive behavior testing (Sabry et al., 2019), but nociceptive behavior has shown to be induced by many types of stimuli. They include amputation or electrical shocks (Cochet-Escartin et al., 2015), UV light (Birkholz and Beane, 2017) and noxious heat (Arenas et al., 2017) or cold (Inoue et al., 2014). Hence, electrophysiological recordings and screening of antinociceptive drugs could be adapted to multiple stimuli of interest (i.e., mechanical, thermal, inflammatory, etc.).
The high practicability of the planaria model led us, and others, to believe that planarians could be used in screening fields that need a high number of animals, such as environmental toxicology (Wu and Li, 2018) or high throughput screenings (Ireland et al., 2020). Toxicology studies have also previously showed that the lethality of dozens of different chemicals on hundreds of animals could be tested in parallel (Li, 2013). Later studies showed that not only lethality but also regeneration capacities and behavior (such as velocity or thermotaxis) could be analyzed in large quantities for neurotoxicology screening (Hagstrom et al., 2015). As previously discussed, the planaria model is also particularly adapted to poly-drug applications (Raffa, 2008). These ideas could lead to further large quantity screenings of compounds from chemotheques waiting for first-line in vivo testing, such as new analgesics, anti-inflammatory drugs, metabolites or other synthetic compounds.
We should note that, even if planaria is a particularly useful model for high throughput screening, there is a need for replication of results in the field. As we discussed, the term planaria represents at least 3 to 4 common species used in research and even more including less common ones. Japanese research teams would work mostly on D. japonica, American teams on G. dorotocephala or G. tigrina and European teams on S. mediterranea, for example. However, there is evidence that species react differently to some stimuli. G. dorotocephala seemed to be less photophobic than G. tigrina (Debold et al., 1965; Reynierse, 1967). D. japonica had higher locomotion velocities and frequency of “scrunching” behavior following any type of stimuli than S. mediterranea (Cochet-Escartin et al., 2015). D. japonica showed less lethality and more motility than S. mediterranea and G. tigrina after extensive storage, making them particularly adapted to high-throughput screening (Ireland et al., 2020). The need for standardization is obvious, but when one specie is chosen over the others for practical or scientific purposes, results should be interpreted carefully and conclusions should emphasize the specificities of the model.
If we want to look back at other nociceptive receptors, there is little to no data available. Of course, as we have seen in Supplementary Table 1, many pro- or antinociceptive compounds have been tested on planarians’ behavior. Beyond the scope of behavior, other compounds involved in the vertebrates’ nociception system have also been tested on planarians, such as substance P, which seemed to be a potent mitogen for planarian regeneration (Saló and Baguñà, 1986). However, still little is known about the presence of receptors of such compounds in planarians apart from TRPs. Examining available transcriptomes shows that ASIC4 and PIEZO receptors, respectively, acid-sensing and mechano-sensitive receptors, may be present, indicated by in silico gene prediction in multiple S. mediterranea transcriptomes (Rozanski et al., 2019). Homologies for P2X channels, P substance receptor NK1R or even opioid receptors are yet, on the other hand, unknown.
Ultimately, it has to be reported that a majority of experiments discussed in this review exposed planarians only to single condition, or to only single stimulus (one chemical, one wavelength, one electric shock, etc.). In the context of nociception, it would be relevant to apply multiple stimuli. Indeed, as we discussed, nociceptive systems usually show great plasticity, which was partially confirmed by electrophysiology recordings in marine planarian models. Adaptation, sensitization and desensitization are such examples of responses that need multiple sets of tests to appear and that are relevant in a drug screening context, especially for chronic drug exposure.
To briefly conclude, we would stress that, beyond the field of regeneration, planarians constitute a promising model to study the molecular mechanisms behind nociception in invertebrates and beyond.
GR performed the literature search. GR and HC wrote the manuscript. HC designed the figures. VL contributed to the review process of the manuscript and handed out valuable feedback and relevant revisions. All authors contributed to the article and approved the submitted version.
GR was a first-year Ph.D. candidate from EURIDOL Graduate School of Pain, University of Strasbourg [French National Research Agency (ANR) through the Programme d’Investissement d’Avenir, contract ANR-17-EURE-0022, EURIDOL Graduate School of Pain]. HC was supported by the French National Research Agency (Grant NOCRYMAGSENS).
We thank Prof. Pierrick Poisbeau for his input on nociception and its implication in planarians.
The authors declare that the research was conducted in the absence of any commercial or financial relationships that could be construed as a potential conflict of interest.
All claims expressed in this article are solely those of the authors and do not necessarily represent those of their affiliated organizations, or those of the publisher, the editors and the reviewers. Any product that may be evaluated in this article, or claim that may be made by its manufacturer, is not guaranteed or endorsed by the publisher.
The Supplementary Material for this article can be found online at: https://www.frontiersin.org/articles/10.3389/fnmol.2022.935918/full#supplementary-material
Supplementary Table 1. List of the chemicals or stimuli used to modulate planarians’ behavior from references discussed in this review. Chemicals are sorted based on their known main action on nervous systems. Attached is a list of succinct description of each chemical from the table to help apprehend their role in its corresponding study.
TRPs, Transient Receptor Potential ion channels; G., Girardia; D., Dugesia; S., Schmidtea; ROS, Reactive Oxygen Species; pLMV, planarian LocoMotor Velocity; pLMA, planarian LocoMotor Activity; pSLA, planarian Seizure-Like Activity; pSLM, planarian Seizure-Like Movements; AITC, Allyl IsoThioCyanate; dsRNA, double-stranded RNA; RNAi, interference RNA technology.
Algeri, S., Carolei, A., Ferretti, P., Gallone, C., Palladini, G., and Venturini, G. (1983). Effects of dopaminergic agents on monoamine levels and motor behaviour in planaria. Comp. Biochem. Physiol. C. Comp. Pharmacol. Toxicol. 74, 27–29. doi: 10.1016/0742-8413(83)90142-1
Aoki, R., Wake, H., Sasaki, H., and Agata, K. (2009). Recording and spectrum analysis of the planarian electroencephalogram. Neuroscience 159, 908–914. doi: 10.1016/j.neuroscience.2008.11.011
Arenas, O. M., Zaharieva, E. E., Para, A., Vásquez-Doorman, C., Petersen, C. P., and Gallio, M. (2017). Activation of planarian TRPA1 by reactive oxygen species reveals a conserved mechanism for animal nociception. Nat. Neurosci. 20, 1686–1693. doi: 10.1038/s41593-017-0005-0
Bardeen, C. R. (1901). On the physiology of the planaria maculata, with especial reference to the phenomena of regeneration. Am. J. Physiol. 5, 1–55. doi: 10.1152/ajplegacy.1901.5.1.1
Bernardo, K., Stone, G. C., and Koopowitz, H. (1977). Primitive nervous systems: peripheral habituation in decerebrate polyclad flatworms. J. Neurobiol. 8, 141–150. doi: 10.1002/neu.480080206
Best, J. B., Goodman, A. B., and Pigon, A. (1969). Fissioning in planarians: control by the brain. Science 164, 565–566. doi: 10.1126/science.164.3879.565
Birkholz, T. R., and Beane, W. S. (2017). The planarian TRPA1 homolog mediates extraocular behavioral responses to near-ultraviolet light. J. Exp. Biol. 220, 2616–2625. doi: 10.1242/jeb.152298
Blackshaw, S. E., Nicholls, J. G., and Parnas, I. (1982). Physiological responses, receptive fields and terminal arborizations of nociceptive cells in the leech. J. Physiol. 326, 251–260. doi: 10.1113/jphysiol.1982.sp014189
Brown, F. (1962). Response of the planarian, dugesia, to very weak horizontal electrostatic fields. Biol. Bull. 123, 282–294. doi: 10.2307/1539274
Burrell, B. D. (2017). Comparative biology of pain: what invertebrates can tell us about how nociception works. J. Neurophysiol. 117, 1461–1473. doi: 10.1152/jn.00600.2016
Buttarelli, F. R., Pellicano, C., and Pontieri, F. E. (2008). Neuropharmacology and behavior in planarians: translations to mammals. Comp. Biochem. Physiol. C. Toxicol. Pharmacol. 147, 399–408. doi: 10.1016/j.cbpc.2008.01.009
Buttarelli, F. R., Pontieri, F. E., Margotta, V., and Palladini, G. (2000). Acetylcholine/dopamine interaction in planaria. Comp. Biochem. Physiol. C. Pharmacol. Toxicol. Endocrinol. 125, 225–231. doi: 10.1016/S0742-8413(99)00111-5
Carolei, A., Margotta, V., and Palladini, G. (1975). Proposal of a new model with dopaminergic-cholinergic interactions for neuropharmacological investigations. Neuropsychobiology 1, 355–364. doi: 10.1159/000117512
Cebrià, F. (2007). Regenerating the central nervous system: how easy for planarians! Dev. Genes Evol. 217, 733–748. doi: 10.1007/s00427-007-0188-6
Cochet-Escartin, O., Mickolajczyk, K. J., and Collins, E. M. S. (2015). Scrunching: a novel escape gait in planarians. Phys. Biol. 12:056010.
Corning, W. C., and Freed, S. (1968). Planarian behaviour and biochemistry. Nature 219, 1227–1229. doi: 10.1038/2191227a0
Corning, W. C., and Kelly, S. (1973). “Platyhelminthes: the turbellarians,” in Invertebrate Learning: Volume 1 Protozoans Through Annelids, eds W. C. Corning, J. A. Dyal, and A. O. D. Willows (Berlin: Springer Science & Business Media), 171–224.
Cosens, D. J., and Manning, A. (1969). Abnormal electroretinogram from a drosophila mutant. Nature 224, 285–287. doi: 10.1038/224285a0
Crook, R. J., Hanlon, R. T., and Walters, E. T. (2013). Squid have nociceptors that display widespread long-term sensitization and spontaneous activity after bodily injury. J. Neurosci. 33, 10021–10026. doi: 10.1523/JNEUROSCI.0646-13.2013
Currie, K. W., Molinaro, A. M., and Pearson, B. J. (2016). Neuronal sources of hedgehog modulate neurogenesis in the adult planarian brain. Elife 5:e19735. doi: 10.7554/eLife.19735
Dalyell, J. G. (1814). Observations on Some Interesting Phenomena in Animal Physiology, Exhibited by Several Species of Planariæ. Edinburgh: Archibald Constable & Co
de Vries, E. J., and Sluys, R. (1991). Phylogenetic relationships of the genus Dugesia (Platyhelminthes. Tricladida, Paludicola). J. Zool. 223, 103–116. doi: 10.1111/j.1469-7998.1991.tb04752.x
Debold, R. C., Thompson, W. R., and Landraitis, C. (1965). Differences in responses to light between two species of planaria: Dugesis tigrina and D. dorotocephala. Psychon. Sci. 2, 79–80. doi: 10.3758/BF03343339
Dziedowiec, E., Nayak, S. U., Gruver, K. S., Jennings, T., Tallarida, C. S., and Rawls, S. M. (2018). Mu opioid receptor agonist DAMGO produces place conditioning. abstinence-induced withdrawal, and naltrexone-dependent locomotor activation in planarians. Neuroscience 386, 214–222. doi: 10.1016/j.neuroscience.2018.06.029
Elliott, S. A., and Alvarado, A. S. (2018). “Planarians and the history of animal regeneration: paradigm shifts and key concepts in biology,” in Planarian Regeneration: Methods and Protocols Methods in Molecular Biology, ed. J. C. Rink (New York, NY: Springer), 207–239. doi: 10.1007/978-1-4939-7802-1_4
Elwood, R. W. (2019). “Assessing the potential for pain in crustaceans and other invertebrates,” in The Welfare of Invertebrate Animals Animal Welfare, eds C. Carere and J. Mather (Cham: Springer International Publishing), 147–177. doi: 10.1007/978-3-030-13947-6_7
Farrell, M. S., Gilmore, K., Raffa, R. B., and Walker, E. A. (2008). Behavioral characterization of serotonergic activation in the flatworm Planaria. Behav. Pharmacol. 19, 177–182. doi: 10.1097/FBP.0b013e3282fe885e
González-Ramírez, R., Chen, Y., Liedtke, W. B., and Morales-Lázaro, S. L. (2017). “TRP channels and pain,” in Neurobiology of TRP Channels, ed. T. L. R. Emir (Boca Raton: CRC Press), 125–148. doi: 10.4324/9781315152837
Hagstrom, D., Cochet-Escartin, O., Zhang, S., Khuu, C. D., and Collins, E.-M. S. (2015). freshwater planarians as an alternative animal model for neurotoxicology. Toxicol. Sci. Off. J. Soc. Toxicol. 147, 270–285. doi: 10.1093/toxsci/kfv129
Hinrichsen, R. D., Fabi, J. L., Craig, S. E., Rovins, P. S., Cerwensky, A. J., Major, R. J., et al. (2019). Photoresponsivity and motility in the planarian Schmidtea mediterranea vary diurnally. Chronobiol. Int. 36, 1789–1793. doi: 10.1080/07420528.2019.1683023
Illich, P. A., and Walters, E. T. (1997). Mechanosensory neurons innervating aplysia siphon encode noxious stimuli and display nociceptive sensitization. J. Neurosci. 17, 459–469. doi: 10.1523/JNEUROSCI.17-01-00459.1997
Im, S. H., and Galko, M. J. (2012). Pokes, sunburn, and hot sauce: drosophila as an emerging model for the biology of nociception. Dev. Dyn. 241, 16–26. doi: 10.1002/dvdy.22737
Inoue, T., Kumamoto, H., Okamoto, K., Umesono, Y., Sakai, M., Sánchez Alvarado, A., et al. (2004). Morphological and functional recovery of the planarian photosensing system during head regeneration. Zoolog. Sci. 21, 275–283. doi: 10.2108/zsj.21.275
Inoue, T., Yamashita, T., and Agata, K. (2014). Thermosensory signaling by TRPM is processed by brain serotonergic neurons to produce planarian thermotaxis. J. Neurosci. 34, 15701–15714. doi: 10.1523/JNEUROSCI.5379-13.2014
International Association for the Study of Pain [IASP] (2022). Terminology. Available online at: https://www.iasp-pain.org/resources/terminology/ (Accessed March 21, 2022).
Ireland, D., Bochenek, V., Chaiken, D., Rabeler, C., Onoe, S., Soni, A., et al. (2020). Dugesia japonica is the best suited of three planarian species for high-throughput toxicology screening. Chemosphere 253:126718. doi: 10.1016/j.chemosphere.2020.126718
Ivankovic, M., Haneckova, R., Thommen, A., Grohme, M. A., Vila-Farré, M., Werner, S., et al. (2019). Model systems for regeneration: planarians. Development 146:dev167684. doi: 10.1242/dev.167684
Katz, B., Payne, R., and Minke, B. (2017). “TRP channels in vision,” in Neurobiology of TRP Channels, ed. T. L. R. Emir (Boca Raton: CRC Press), 27–64.
Kavaliers, M. (1988). Evolutionary and comparative aspects of nociception. Brain Res. Bull. 21, 923–931. doi: 10.1016/0361-9230(88)90030-5
Keenan, L., Koopowitz, H., and Bernardo, K. (1979). Primitive nervous systems: action of aminergic drugs and blocking agents on activity in the ventral nerve cord of the flatworm Notoplana acticola. J. Neurobiol. 10, 397–407. doi: 10.1002/neu.480100406
Kim, A., and Rawls, S. M. (2022). Nicotine-induced C-shape movements in planarians are reduced by antinociceptive drugs: implications for pain in planarian paroxysm etiology? Brain Res. 1778:147770. doi: 10.1016/j.brainres.2021.147770
Kimmel, H. D., and Carlyon, W. D. (1990). Persistent effects of a serotonin depletor (p-chlorophenylalanine) in regenerated planaria (Dugesia dorotocephala). Behav. Neurosci. 104, 127–134. doi: 10.1037//0735-7044.104.1.127
Koopowitz, H. (1975a). Activity and habituation in the brain of the polyclad flatworm Freemania litoricola. J. Exp. Biol. 62, 455–467. doi: 10.1242/jeb.62.2.455
Koopowitz, H. (1975b). Electrophysiology of the peripheral nerve net in the polyclad flatworm Freemania litoricola. J. Exp. Biol. 62, 469–479. doi: 10.1242/jeb.62.2.469
Koopowitz, H., Bernardo, K., and Keenan, L. (1979a). Primitive nervous systems: electrical activity in ventral nerve cords of the flatworm. Notoplana acticola. J. Neurobiol. 10, 367–381. doi: 10.1002/neu.480100404
Koopowitz, H., Elvin, M., and Keenan, L. (1996). In vivo visualization of living flatworm neurons using intracellular injections. J. Neurosci. Methods 69, 83–89. doi: 10.1016/S0165-0270(96)00023-4
Koopowitz, H., Keenan, L., and Bernardo, K. (1979b). Primitive nervous system: electrophysiology of inhibitory events in flatworm nerve cords. J. Neurobiol. 10, 383–395. doi: 10.1002/neu.480100405
Li, M.-H. (2013). Acute toxicity of 30 pharmaceutically active compounds to freshwater planarians. Dugesia japonica. Toxicol. Environ. Chem. 95, 1157–1170. doi: 10.1080/02772248.2013.857671
Lombardo, P., Giustini, M., Miccoli, F., and Cicolani, B. (2011). Fine-scale differences in diel activity among nocturnal freshwater planarias (Platyhelminthes: Tricladida). J. Circadian Rhythms 9:2. doi: 10.1186/1740-3391-9-2
Minelli, A. (1977). A taxonomic review of the terrestrial planarians of Europe. Bolletino Zool. 44, 399–419. doi: 10.1080/11250007709429278
Miyamoto, M., Hattori, M., Hosoda, K., Sawamoto, M., Motoishi, M., Hayashi, T., et al. (2020). The pharyngeal nervous system orchestrates feeding behavior in planarians. Sci. Adv. 6:eaaz0882. doi: 10.1126/sciadv.aaz0882
Mogil, J. S. (2009). Animal models of pain: progress and challenges. Nat. Rev. Neurosci. 10, 283–294. doi: 10.1038/nrn2606
Müller, O. F. (1776). Zoologiae Danicae Prodromus : Seu Animalium Daniae et Norvegiae Indigenarum; Characteres, Nomina, et Synonyma Imprimis Popularium. Havniae : Typis Hallageriis. Available online at: https://www.biodiversitylibrary.org/item/47550 (accessed January 18, 2022).
Needleman, H. L. (1967). Tolerance and dependence in the planarian after continuous exposure to morphine [63]. Nature 215:784. doi: 10.1038/215784b0
Newmark, P. A., Reddien, P. W., Cebrià, F., and Alvarado, A. S. (2003). Ingestion of bacterially expressed double-stranded RNA inhibits gene expression in planarians. Proc. Natl. Acad. Sci. U.S.A. 100, 11861–11865. doi: 10.1073/pnas.1834205100
Nishimura, K., Inoue, T., Yoshimoto, K., Taniguchi, T., Kitamura, Y., and Agata, K. (2011). Regeneration of dopaminergic neurons after 6-hydroxydopamine-induced lesion in planarian brain. J. Neurochem. 119, 1217–1231. doi: 10.1111/j.1471-4159.2011.07518.x
Nishimura, K., Kitamura, Y., Inoue, T., Umesono, Y., Sano, S., Yoshimoto, K., et al. (2007). Reconstruction of dopaminergic neural network and locomotion function in planarian regenerates. Dev. Neurobiol. 67, 1059–1078. doi: 10.1002/dneu.20377
Ogren, R. E. (1956). Physiological observations on movement and behavior of the land Planarian Rhynchodemus Sylvaticus (Leidy). Proc. Pa. Acad. Sci. 30, 218–225.
Okamoto, K., Takeuchi, K., and Agata, K. (2005). Neural projections in planarian brain revealed by fluorescent dye tracing. Zoolog. Sci. 22, 535–546. doi: 10.2108/zsj.22.535
Pagán, O. R. (2014). The First Brain: The Neuroscience of Planarians, 1st Edn. Oxford: Oxford University Press.
Pagán, O. R. (2017). Planaria: an animal model that integrates development, regeneration and pharmacology. Int. J. Dev. Biol. 61, 519–529. doi: 10.1387/ijdb.160328op
Pagán, O. R., Baker, D., Deats, S., Montgomery, E., Tenaglia, M., Randolph, C., et al. (2012). Planarians in pharmacology: parthenolide is a specific behavioral antagonist of cocaine in the planarian Girardia tigrina. Int. J. Dev. Biol. 56, 193–196. doi: 10.1387/ijdb.113486op
Pagán, O. R., Montgomery, E., Deats, S., Bach, D., and Baker, D. (2015). Evidence of nicotine-induced. curare-insensitive, behavior in planarians. Neurochem. Res. 40, 2087–2090. doi: 10.1007/s11064-015-1512-6
Palladini, G., Margotta, V., Medolago-Albani, L., Hernandez, M. C., Carolei, A., and De Michele, T. (1981). Photosensitization and the nervous system in the planarian Dugesia gonocephala: a histochemical, ultrastructural and behavioral investigation. Cell Tissue Res. 215, 271–279. doi: 10.1007/BF00239114
Palladini, G., Ruggeri, S., Stocchi, F., De Pandis, M. F., Venturini, G., and Margotta, V. (1996). A pharmacological study of cocaine activity in planaria. Comp. Biochem. Physiol. C. Pharmacol. Toxicol. Endocrinol. 115, 41–45. doi: 10.1016/s0742-8413(96)00053-9
Paskin, T. R., Jellies, J., Bacher, J., and Beane, W. S. (2014). Planarian phototactic assay reveals differential behavioral responses based on wavelength. PLoS One 9:e114708. doi: 10.1371/journal.pone.0114708
Passarelli, F., Merante, A., Pontieri, F. E., Margotta, V., Venturini, G., and Palladini, G. (1999). Opioid-dopamine interaction in planaria: a behavioral study. Comp. Biochem. Physiol. C. Pharmacol. Toxicol. Endocrinol. 124, 51–55. doi: 10.1016/s0742-8413(99)00048-1
Pearl, R. (1903). The movements and reactions of freshwater planarians: a study in animal behaviour. Q. J. Microsc. Sci. 46, 509–714.
Peng, G., Shi, X., and Kadowaki, T. (2015). Evolution of TRP channels inferred by their classification in diverse animal species. Mol. Phylogenet. Evol. 84, 145–157. doi: 10.1016/j.ympev.2014.06.016
Raffa, R. B. (2008). “Planaria as model in drug abuse research,” in Planaria: A Model for Drug Action and Abuse, eds R. B. Raffa and S. M. Rawls (Boca Raton: CRC Press), 53–62. doi: 10.1201/9781498713597
Raffa, R. B., and Desai, P. (2005). Description and quantification of cocaine withdrawal signs in Planaria. Brain Res. 1032, 200–202. doi: 10.1016/j.brainres.2004.10.052
Raffa, R. B., and Valdez, J. M. (2001). Cocaine withdrawal in Planaria. Eur. J. Pharmacol. 430, 143–145. doi: 10.1016/s0014-2999(01)01358-9
Raffa, R. B., Baron, S., Bhandal, J. S., Brown, T., Song, K., Tallarida, C. S., et al. (2013). Opioid receptor types involved in the development of nicotine physical dependence in an invertebrate (Planaria) model. Pharmacol. Biochem. Behav. 112, 9–14. doi: 10.1016/j.pbb.2013.09.012
Raffa, R. B., Holland, L. J., and Schulingkamp, R. J. (2001). Quantitative assessment of dopamine D2 antagonist activity using invertebrate (Planaria) locomotion as a functional endpoint. J. Pharmacol. Toxicol. Methods 45, 223–226. doi: 10.1016/S1056-8719(01)00152-6
Raffa, R. B., Stagliano, G. W., and Umeda, S. (2003). kappa-Opioid withdrawal in Planaria. Neurosci. Lett. 349, 139–142. doi: 10.1016/s0304-3940(03)00814-0
Raffa, R. B., Stagliano, G. W., Ross, G., Powell, J. A., Phillips, A. G., Ding, Z., et al. (2008). The kappa-opioid receptor antagonist nor-BNI inhibits cocaine and amphetamine, but not cannabinoid (WIN 52212-2), abstinence-induced withdrawal in planarians: an instance of “pharmacologic congruence.” Brain Res. 1193, 51–56. doi: 10.1016/j.brainres.2007.12.001
Raffa, R. B., Valdez, J. M., Holland, L. J., and Schulingkamp, R. J. (2000). Energy-dependent UV light-induced disruption of (-) sulpiride antagonism of dopamine. Eur. J. Pharmacol. 406, R11–R12. doi: 10.1016/S0014-2999(00)00730-5
Ramm, S. A. (2017). Exploring the sexual diversity of flatworms: ecology, evolution, and the molecular biology of reproduction. Mol. Reprod. Dev. 84, 120–131. doi: 10.1002/mrd.22669
Rawls, S. M., Baron, S., Ding, Z., Roth, C., Zaveri, N., and Raffa, R. B. (2008a). Nociceptin attenuates methamphetamine abstinence-induced withdrawal-like behavior in planarians. Neuropeptides 42, 229–237. doi: 10.1016/j.npep.2008.03.005
Rawls, S. M., Cavallo, F., Capasso, A., Ding, Z., and Raffa, R. B. (2008b). The β-lactam antibiotic ceftriaxone inhibits physical dependence and abstinence-induced withdrawal from cocaine, amphetamine, methamphetamine, and clorazepate in planarians. Eur. J. Pharmacol. 584, 278–284. doi: 10.1016/j.ejphar.2008.02.018
Rawls, S. M., Gerber, K., Ding, S., Roth, C., and Raffa, R. B. (2008c). Agmatine: identification and inhibition of methamphetamine, kappa opioid, and cannabinoid withdrawal in planarians. Synapse 62, 927–934. doi: 10.1002/syn.20571
Rawls, S. M., Gomez, T., and Raffa, R. B. (2007b). An NMDA antagonist (LY 235959) attenuates abstinence-induced withdrawal of planarians following acute exposure to a cannabinoid agonist (WIN 52212-2). Pharmacol. Biochem. Behav. 86, 499–504. doi: 10.1016/j.pbb.2007.01.010
Rawls, S. M., Gomez, T., Ding, Z., and Raffa, R. B. (2007a). Differential behavioral effect of the TRPM8/TRPA1 channel agonist icilin (AG-3-5). Eur. J. Pharmacol. 575, 103–104. doi: 10.1016/j.ejphar.2007.07.060
Rawls, S. M., Patil, T., Tallarida, C. S., Baron, S., Kim, M., Song, K., et al. (2011). Nicotine behavioral pharmacology: clues from planarians. Drug Alcohol Depend. 118, 274–279. doi: 10.1016/j.drugalcdep.2011.04.001
Rawls, S. M., Patil, T., Yuvasheva, E., and Raffa, R. B. (2010). First evidence that drugs of abuse produce behavioral sensitization and cross sensitization in planarians. Behav. Pharmacol. 21, 301–313. doi: 10.1097/FBP.0b013e32833b0098
Rawls, S. M., Rodriguez, T., Baron, D. A., and Raffa, R. B. (2006). A nitric oxide synthase inhibitor (l-NAME) attenuates abstinence-induced withdrawal from both cocaine and a cannabinoid agonist (WIN 55212-2) in Planaria. Brain Res. 1099, 82–87. doi: 10.1016/j.brainres.2006.04.103
Rawls, S. M., Thomas, T., Adeola, M., Patil, T., Raymondi, N., Poles, A., et al. (2009). Topiramate antagonizes NMDA- and AMPA-induced seizure-like activity in planarians. Pharmacol. Biochem. Behav. 93, 363–367. doi: 10.1016/j.pbb.2009.05.005
Reddien, P. W. (2018). The cellular and molecular basis for planarian regeneration. Cell 175, 327–345. doi: 10.1016/j.cell.2018.09.021
Reddien, P. W., and Alvarado, A. S. (2004). Fundamentals of planarian regeneration. Annu. Rev. Cell Dev. Biol. 20, 725–757. doi: 10.1146/annurev.cellbio.20.010403.095114
Reynierse, J. H. (1967). Reactions to light in four species of planaria. J. Comp. Physiol. Psychol. 63, 366–368. doi: 10.1037/h0024360
Ribeiro, P., El-Shehabi, F., and Patocka, N. (2006). Classical transmitters and their receptors in flatworms. Parasitology 131, S19–S40. doi: 10.1017/S0031182005008565
Rompolas, P., Patel-King, R. S., and King, S. M. (2010). An outer arm dynein conformational switch is required for metachronal synchrony of motile cilia in planaria. Mol. Biol. Cell 21, 3669–3679. doi: 10.1091/mbc.e10-04-0373
Rouhana, L., Weiss, J. A., Forsthoefel, D. J., Lee, H., King, R. S., Inoue, T., et al. (2013). RNA interference by feeding in vitro–synthesized double-stranded RNA to planarians: Methodology and dynamics. Dev. Dyn. 242, 718–730. doi: 10.1002/dvdy.23950
Rozanski, A., Moon, H., Brandl, H., Martín-Durán, J. M., Grohme, M. A., Hüttner, K., et al. (2019). PlanMine 3.0—improvements to a mineable resource of flatworm biology and biodiversity. Nucleic Acids Res. 47, D812–D820. doi: 10.1093/nar/gky1070
Sabry, Z., Ho, A., Ireland, D., Rabeler, C., Cochet-Escartin, O., and Collins, E. M. S. (2019). Pharmacological or genetic targeting of Transient Receptor Potential (TRP) channels can disrupt the planarian escape response. PLoS One 14:e0226104. doi: 10.1371/journal.pone.0226104
Saló, E., and Baguñà, J. (1986). Stimulation of cellular proliferation and differentiation in the intact and regenerating planarian Dugesia(G) tigrina by the neuropeptide substance P. J. Exp. Zool. 237, 129–135. doi: 10.1002/jez.1402370117
Saló, E., Pineda, D., Marsal, M., Gonzalez, J., Gremigni, V., and Batistoni, R. (2002). Genetic network of the eye in Platyhelminthes: expression and functional analysis of some players during planarian regeneration. Gene 287, 67–74. doi: 10.1016/S0378-1119(01)00863-0
Sánchez Alvarado, A., and Newmark, P. A. (1999). Double-stranded RNA specifically disrupts gene expression during planarian regeneration. Proc. Natl. Acad. Sci. U.S.A. 96, 5049–5054. doi: 10.1073/pnas.96.9.5049
Sarnat, H. B., and Netsky, M. G. (1985). The brain of the planarian as the ancestor of the human brain. Can. J. Neurol. Sci. 12, 296–302. doi: 10.1017/S031716710003537X
Sarnat, H. B., and Netsky, M. G. (2002). When does a ganglion become a brain? Evolutionary origin of the central nervous system. Semin. Pediatr. Neurol. 9, 240–253. doi: 10.1053/spen.2002.32502
Smith, E. S. J., and Lewin, G. R. (2009). Nociceptors: a phylogenetic view. J. Comp. Physiol. A. 195, 1089–1106. doi: 10.1007/s00359-009-0482-z
Sneddon, L. U., Elwood, R. W., Adamo, S. A., and Leach, M. C. (2014). Defining and assessing animal pain. Anim. Behav. 97, 201–212. doi: 10.1016/j.anbehav.2014.09.007
Sugimoto, T. (2010). A theory for ciliary gliding in freshwater planarians. J. Aero. Aqua. Bio Mech. 1, 57–63. doi: 10.5226/jabmech.1.57
Tallarida, C. S., Bires, K., Avershal, J., Tallarida, R. J., Seo, S., and Rawls, S. M. (2014). Ethanol and cocaine: environmental place conditioning, stereotypy, and synergism in planarians. Alcohol Fayettev. N. 48, 579–586. doi: 10.1016/j.alcohol.2014.07.006
Tanaka, Y., Ito, K., Nakagaki, T., and Kobayashi, R. (2012). Mechanics of peristaltic locomotion and role of anchoring. J. R. Soc. Interface 9, 222–233. doi: 10.1098/rsif.2011.0339
Tobin, D. M., and Bargmann, C. I. (2004). Invertebrate nociception: behaviors, neurons and molecules. J. Neurobiol. 61, 161–174. doi: 10.1002/neu.20082
Venturini, G., Carolei, A., Palladini, G., Margotta, V., and Lauro, M. G. (1983). Radioimmunological and immunocytochemical demonstration of Met-enkephalin in planaria. Comp. Biochem. Physiol. C 74, 23–25. doi: 10.1016/0742-8413(83)90141-x
Venturini, G., Stocchi, F., Margotta, V., Ruggieri, S., Bravi, D., Bellantuono, P., et al. (1989). A pharmacological study of dopaminergic receptors in planaria. Neuropharmacology 28, 1377–1382. doi: 10.1016/0028-3908(89)90013-0
Viaud, G. (1948). Recherches expérimentales sur le phototropisme des planaires. Le signe primaire positif et la polarité tropistique. Année. Psychol. 49, 175–221.
Welsh, J. H., and Williams, L. D. (1970). Monoamine-containing neurons in planaria. J. Comp. Neurol. 138, 103–115. doi: 10.1002/cne.901380108
Wu, J.-P., and Li, M.-H. (2018). The use of freshwater planarians in environmental toxicology studies: advantages and potential. Ecotoxicol. Environ. Sa.f 161, 45–56. doi: 10.1016/j.ecoenv.2018.05.057
Keywords: planarians, nociception, drug screening, animal model, opioids, TRP ion channels, behavior, invertebrate
Citation: Reho G, Lelièvre V and Cadiou H (2022) Planarian nociception: Lessons from a scrunching flatworm. Front. Mol. Neurosci. 15:935918. doi: 10.3389/fnmol.2022.935918
Received: 04 May 2022; Accepted: 08 July 2022;
Published: 26 July 2022.
Edited by:
Ana Reynders, UMR 7288 Institut de Biologie du Développement de Marseille (IBDM), FranceReviewed by:
Nigel Atkinson, University of Texas at Austin, United StatesCopyright © 2022 Reho, Lelièvre and Cadiou. This is an open-access article distributed under the terms of the Creative Commons Attribution License (CC BY). The use, distribution or reproduction in other forums is permitted, provided the original author(s) and the copyright owner(s) are credited and that the original publication in this journal is cited, in accordance with accepted academic practice. No use, distribution or reproduction is permitted which does not comply with these terms.
*Correspondence: Hervé Cadiou, Y2FkaW91QGluY2ktY25ycy51bmlzdHJhLmZy
Disclaimer: All claims expressed in this article are solely those of the authors and do not necessarily represent those of their affiliated organizations, or those of the publisher, the editors and the reviewers. Any product that may be evaluated in this article or claim that may be made by its manufacturer is not guaranteed or endorsed by the publisher.
Research integrity at Frontiers
Learn more about the work of our research integrity team to safeguard the quality of each article we publish.