- 1Department of Nursing, School of Nursing, Shandong Xiehe University, Jinan, China
- 2Department of Pharmacy, Shandong Rongjun General Hospital, Jinan, China
- 3Department of Endocrinology, Shandong Rongjun General Hospital, Jinan, China
- 4Department of Transfusion Medicine, Shandong Provincial Hospital Affiliated to Shandong First Medical University, Jinan, China
- 5Department of Pharmacy, Taishan Vocational College of Nursing, Taian, China
Prior studies have demonstrated a close association between brain insulin resistance and Alzheimer’s disease (AD), while selenium supplementation was shown to improve insulin homeostasis in AD patients and to exert neuroprotective effects in a mouse model of AD. However, the mechanisms underlying the neuroprotective actions of selenium remain incompletely understood. In this study, we performed a label-free liquid chromatography-tandem mass spectrometry (LC–MS/MS) quantitative proteomics approach to analyze differentially expressed proteins (DEPs) in the hippocampus and cerebral cortex of Aβ precursor protein (APP)/presenilin-1 (PS1) mice following 2 months of treatment with sodium selenate. A total of 319 DEPs (205 upregulated and 114 downregulated proteins) were detected after selenium treatment. Functional enrichment analysis revealed that the DEPs were mainly enriched in processes affecting axon development, neuron differentiation, tau protein binding, and insulin/insulin-like growth factor type 1 (IGF1)-related pathways. These results demonstrate that a number of insulin/IGF1 signaling pathway-associated proteins are differentially expressed in ways that are consistent with reduced central insulin resistance, suggesting that selenium has therapeutic value in the treatment of neurodegenerative and metabolic diseases such as AD and non-alcoholic fatty liver disease (NAFLD).
Introduction
Growing evidence implicates abnormal insulin signaling in the pathogenesis of Alzheimer’s disease (AD), a neurodegenerative condition whose fundamental feature is β-amyloid (Aβ) and tau protein deposition in the brain (De La Monte and Wands, 2008; Ahmad, 2012; Jahangir et al., 2014; Sridhar, 2015; Ribes-Navarro et al., 2018). AD represents a global public health problem; a recent study indicated that its prevalence in Chinese adults aged 60 years and older is ∼3.9% (Jia et al., 2020). AD is characterized by progressive cognitive decline, memory loss, sleep disturbance, and neuropsychiatric symptoms. It is the leading cause of dementia worldwide and is associated with significant reductions in lifespan and quality of life (Greer et al., 2013). Both genetic and environmental factors contribute to AD neuropathology; this is exacerbated by the interaction between Aβ precursor protein (APP) and fibrillar Aβ, which enhances cytotoxicity and triggers neuroinflammation by activating microglia and astrocytes (Lorenzo et al., 2000; Ozben and Ozben, 2019).
A number of clinical and experimental studies indicated that both central and peripheral insulin resistance influence the development of AD (Neth and Craft, 2017; Chow et al., 2019). It has been shown that the brain response to peripheral insulin declines in older people, and that low cerebrospinal fluid and high plasma insulin levels are characteristically found in AD patients (Craft et al., 1998; Deli et al., 2015). In this regard, impairment of insulin transport into the central nervous system, as well as abnormal expression and dysfunction of insulin receptor substrate 1 (Irs1), insulin receptor (InsR), insulin-like growth factor type 1 (IGF1), IGF1 receptor (IGFR), and insulin-degrading enzyme (IDE), were proposed to contribute to brain insulin resistance and AD development (Steen et al., 2005; Jahangir et al., 2014; Ribes-Navarro et al., 2018; Taguchi et al., 2019; Kellar and Craft, 2020). Based on the above evidence, and although results were modest, clinical trials evaluating the efficacy of insulin sensitizers such as metformin and rosiglitazone suggested improvement in insulin sensitivity and cognitive impairment in AD patients (Kellar and Craft, 2020). Along these lines, liraglutide (a glucagon-like peptide-1 receptor agonist) was shown to increase peripheral insulin sensitivity, attenuate memory impairment, and reduce amyloid plaque deposition in aged transgenic APP/presenilin-1 (PS1) mice, a widely used animal model of AD (Mcclean and Hölscher, 2014).
Selenium is an essential micronutrient in mammals and exerts multiple biological (e.g., antioxidant, anti-inflammatory, and immunomodulatory) activities (Mangiapane et al., 2014; Rayman, 2019). The impact of selenate on cellular physiology is concentration dependent, and its deficiency or excess is involved in pathophysiological processes related to multiple conditions and diseases, including aging, infertility, viral infection, thyroid disorders, and AD (Loef et al., 2011; Mintziori et al., 2019; Gheorghiu and Badiu, 2020; Kieliszek, 2021). Thus, the therapeutic potential of selenium supplementation is of great clinical interest (Wang et al., 2017). Clinical evidence indicates that selenium levels are decreased in the hippocampal, temporal, and cortical regions of AD patients, which is consistent with reduced antioxidant capacity and increased oxidative stress (Varikasuvu et al., 2018). A few clinical trials evaluating the effect of selenium supplementation on AD symptoms showed promising results, evidenced by lowered insulin and triglyceride levels, increased antioxidant capacity, and improvements in some markers of cognitive function (Tamtaji et al., 2019; Ashraf and So, 2020). Consistent with clinical findings, a recent study demonstrated that sodium selenate treatment improved cognitive impairment and neuropsychiatric symptoms by reducing the accumulation of tau protein in the hippocampus of PS1/APP/tau triple transgenic AD model (3xTg-AD) mice (Van Der Jeugd et al., 2018).
Despite the evidence summarized above, the specific impact of selenium on the expression of insulin signaling-related proteins in the brains of AD patients and animal models remains unknown. To address this issue, we performed label-free liquid chromatography-tandem mass spectrometry (LC–MS/MS) quantitative proteomics analysis of the hippocampus and cerebral cortex of transgenic APP/PS1 mice treated with sodium selenate. Our findings may provide new directions for the improved treatment or prevention of AD and other neurodegenerative disorders.
Materials and Methods
Mice
Transgenic APP/PS1 mice were purchased from Nanjing Junke Bioengineering Co., Ltd. (Nanjing, China). Mice were housed in standard laboratory conditions with constant humidity (55%) and temperature (22 ± 2°C) under a 12-h light-dark cycle with ad libitum access to water and food. Twelve-month-old male APP/PS1 transgenic mice were randomly divided into treatment and control groups (n = 10 in each group) and treated with 12 μg/ml sodium selenate (Sigma–Aldrich/Merck, St. Louis, MO, United States) or without sodium selenate in drinking water for 2 months (Van Der Jeugd et al., 2018). The experimental procedures were performed and approved by the Animal Care and Use Committee of Shandong Xiehe University (Jinan, China). After treatment, the mice were euthanized, and the hippocampus and cerebral cortex were immediately excised for subsequent proteomic analysis.
Proteomics
Sample Preparation and Liquid Chromatography Tandem Mass Spectrometry
The brain tissue was homogenized with SDT buffer (4% SDS, 100 mM Tris–HCl, 1 mM DTT, pH 7.6) for sample lysis and protein extraction (Zhu et al., 2014). Protein was quantified with a bicinchoninic acid (BCA) Protein Assay Kit (Bio-Rad, Laboratories, Hercules, CA, United States).
Protein samples were digested into peptides using the filter-aided sample preparation (FASP) method as previously described (Wiśniewski, 2018). LC–MS/MS analysis was performed on a timsTOFPro mass spectrometer coupled to a nanoElute UHPLC system (Bruker Daltonics, Germany) with a run time of 60 min. The peptides were loaded onto a reversed-phase trap column (Thermo Scientific Acclaim PepMap100, 100 μm × 2 cm, nanoViper C18) connected to a C18 reversed-phase analytical column (Thermo Scientific Easy Column, 10 cm long, 75 μm inner diameter, 3 μm resin) in buffer A (0.1% formic acid) and separated with a linear gradient of buffer B (84% acetonitrile and 0.1% formic acid) at a flow rate of 300 nl/min controlled by IntelliFlow technology. The mass spectrometer was operated in positive ion mode, collected ion mobility MS spectra over a mass range of m/z 100–1,700 and 1/k0 of 0.6 to 1.6, and then performed 10 cycles of PASEF MS/MS with a target intensity of 1.5k and a threshold of 2,500. Active exclusion was enabled with a release time of 0.4 min.
Protein Identification and Quantification
For identification and quantification analysis, raw MS sample data were combined and analyzed using MaxQuant 1.5.3.17 software (Chen et al., 2019). MS data were matched against the UniProt_MusMusculus_17027_20200226 database. The label-free quantification algorithm was used for quantitative analysis. Proteins with an adjusted P-value < 0.05 and fold change >1.5 were considered as significant.
Gene Ontology Annotation
The sequences of the selected DEPs were locally aligned using NCBI BLAST+ client software (ncbi-blast-2.2.28+-win32.exe) and InterProScan to find homologous sequences. Then, Gene Ontology (GO) terms were mapped, and sequences were annotated using Blast2GO software. GO annotation results were plotted with R scripts.
Kyoto Encyclopedia of Genes and Genomes Annotation
Following annotation steps, the studied proteins were blasted against the online Kyoto Encyclopedia of Genes and Genomes (KEGG) database1 to retrieve their orthology identifications and corresponding pathways. GO and KEGG pathway enrichment analyses were applied based on Fisher’s exact test, considering all quantified proteins as the background dataset.
Protein–Protein Interaction Network
The protein–protein interaction (PPI) network of the identified differentially expressed proteins (DEPs) was retrieved from the IntAct database.2 Cytoscape software (version 3.6.1) was utilized to visualize and analyze potential correlations between DEPs (Shannon et al., 2003).
RNA Isolation and Real-Time Quantitative Reverse Transcribed Polymerase Chain Reaction
Total RNA was isolated from the hippocampus and cortex of mice using a TRIzol kit (Invitrogen, Carlsbad, CA, United States) and reverse-transcribed to cDNA using PrimeScript reagent (TaKaRa, Kusatsu, Japan) following the manufacturer’s instructions. SYBR Green Premix Ex TaqII (TaKaRa, Kusatsu, Japan) and the Roche 480 detection system were used to analyze the relative mRNA expression of target genes. GAPDH was used as an internal control for normalization. The relative gene expression levels were calculated according to the 2–ΔΔCt method.
The primer sequences were as follows:
mouse Irs1 forward primer 5′-TCTACACCCGAGACGAAC ACT-3′, Irs1 reverse primer 5′-TGGGCCTTTGCCCGATTATG-3′; InsR forward primer 5′-TCAAGACCAGACCCGAAGATT-3′, InsR reverse primer 5′-TCTCGAAGATAACCAGGGCATAG-3′; serine/arginine-rich splicing factor (Srsf3) forward primer 5′-CTGTGTGGCTGCCGTGTAA-3′, Srsf3 reverse primer, 5′-TCCTCCTGCGGTAATCATCTC-3′; growth factor receptor-bound protein 2 (Grb2) forward primer 5′-ACGGAGCCGGGAAGTATTTC-3′, Grb2 reverse primer, 5′-GGTTCCTGGACACGGATGTTG-3′; GAPDH forward primer 5′-GTCGGTGTGAACGGATTTG-3′, and GAPDH reverse primer 5′-GAATTTGCCGTGAGTGGAG-3′.
Immunohistochemistry
The immunohistochemistry protocol was conducted as described previously (Eraky et al., 2021). Briefly, paraffin sections (5 μM thick) of the hypothalamus were deparaffinized, rehydrated, and then immunostained with rabbit Irs-1 (Servicebio, Wuhan, China) polyclonal and Srsf3 monoclonal antibodies (Thermo Fisher Scientific, Runcorn, Cheshire, United Kingdom). After three washes in phosphate-buffered saline (pH 7.4), slides were subsequently incubated with corresponding species of horseradish peroxidase-labeled secondary antibody. Diaminobenzidine (Servicebio, Wuhan, China) was incubated as a chromogenic reagent. The staining time was controlled under the microscope. Positive staining was brownish yellow. Hematoxylin was used for counterstaining and visualized by a Nikon optical microscope (Nikon, Tokyo, Japan). Average optical density (AOD) value was used to semi-quantify the protein expression on immunohistochemical staining by ImageJ software (version 1.45) (NIH, Bethesda, MD, United States) (Liu et al., 2015).
Statistical Analysis
All data were expressed as the mean ± SEM and analyzed by Prism v 6.0 (GraphPad Software Inc., San Diego, CA, United States). A two-tailed, unpaired Student’s t-test was used to examine the significant difference between two groups. P < 0.05 was considered as statistically significant. All experiments were conducted three times.
Results
Selenium Supplementation Induces Differential Protein Expression in the Aβ Precursor Protein/Presenilin-1 Mouse Brain
There is a close association between brain insulin resistance and AD (Sridhar, 2015). Because selenium supplementation was shown to improve insulin homeostasis in AD patients and to exert neuroprotective effects in a mouse model of AD (Sridhar, 2015; Van Der Jeugd et al., 2018; Tamtaji et al., 2019), we performed label-free LC–MS/MS quantitative proteomics to identify DEPs in the hippocampus and cerebral cortex of APP/PS1 mice—an AD animal model—following 2 months of treatment with sodium selenate. A total of 2,389 proteins were identified by proteomics analysis; among these, 319 DEPs (>1.5-fold change), including 205 upregulated and 114 downregulated proteins, were detected in the brains of selenate-treated mice compared to control mice (Figure 1). These data indicate that sodium selenate treatment has a distinct impact on brain protein expression in AD mice.
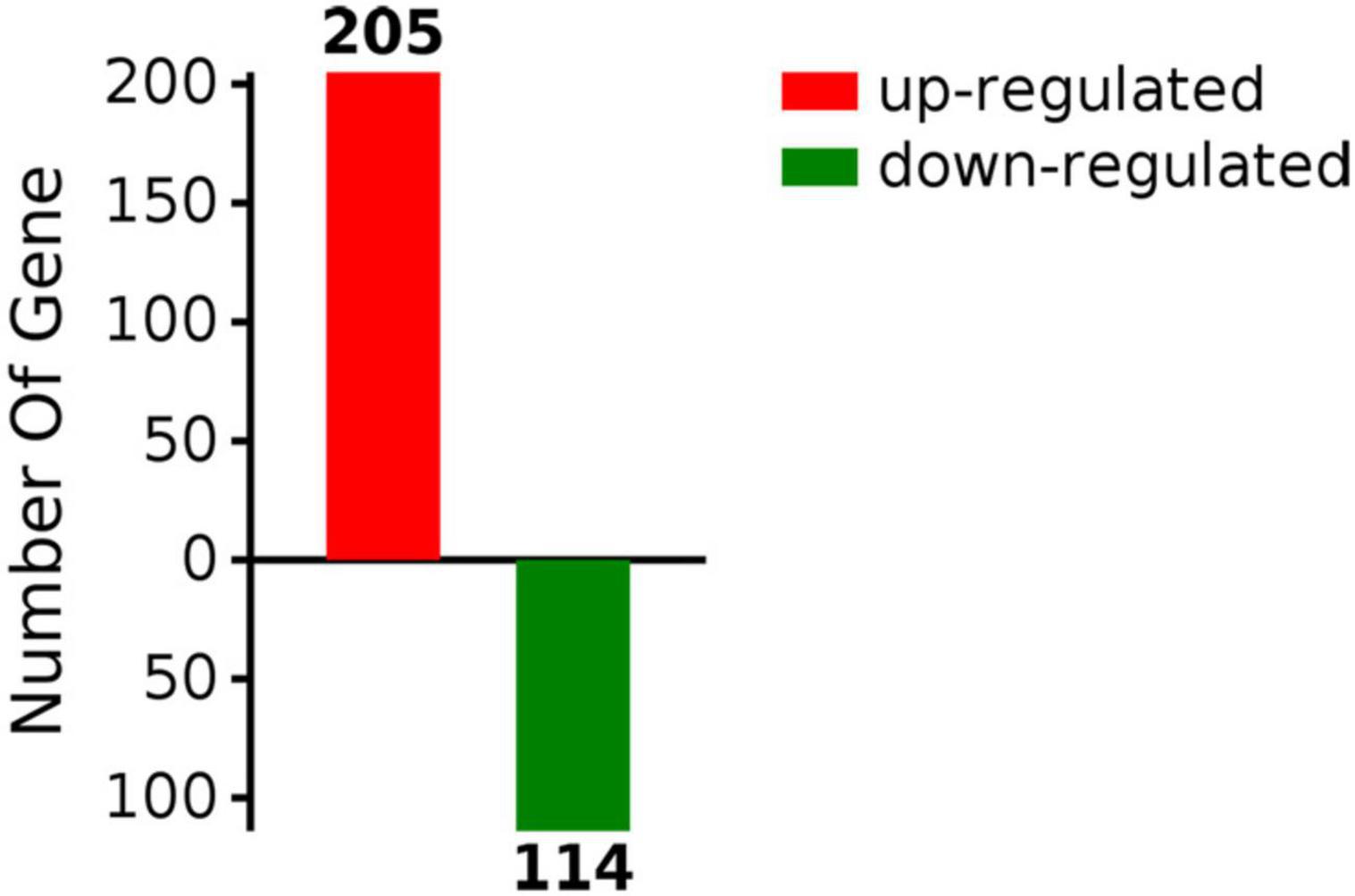
Figure 1. Differentially expressed proteins in brain tissue of sodium selenate-treated APP/PS1 mice.
Gene Ontology Analysis of Differentially Expressed Proteins
To investigate the functions of the DEPs influenced by sodium selenate treatment, GO and KEGG enrichment analyses were conducted. In the molecular function (MF) category, the DEPs were mainly enriched in “RNA binding,” “protein homodimerization activity,” “cadherin binding,” “insulin-like growth factor receptor binding,” and “tau protein binding” (Figures 2A,B). The latter term suggests a differential impact of selenate in AD pathophysiology by influencing tau dynamics (Jouanne et al., 2017). Within the cellular component (CC) category, the DEPs were mainly enriched in “cytoplasmic part” (71%), “membrane-bounded organelle” (14%), and “cytoplasm” (8%) (Figures 3A,B).
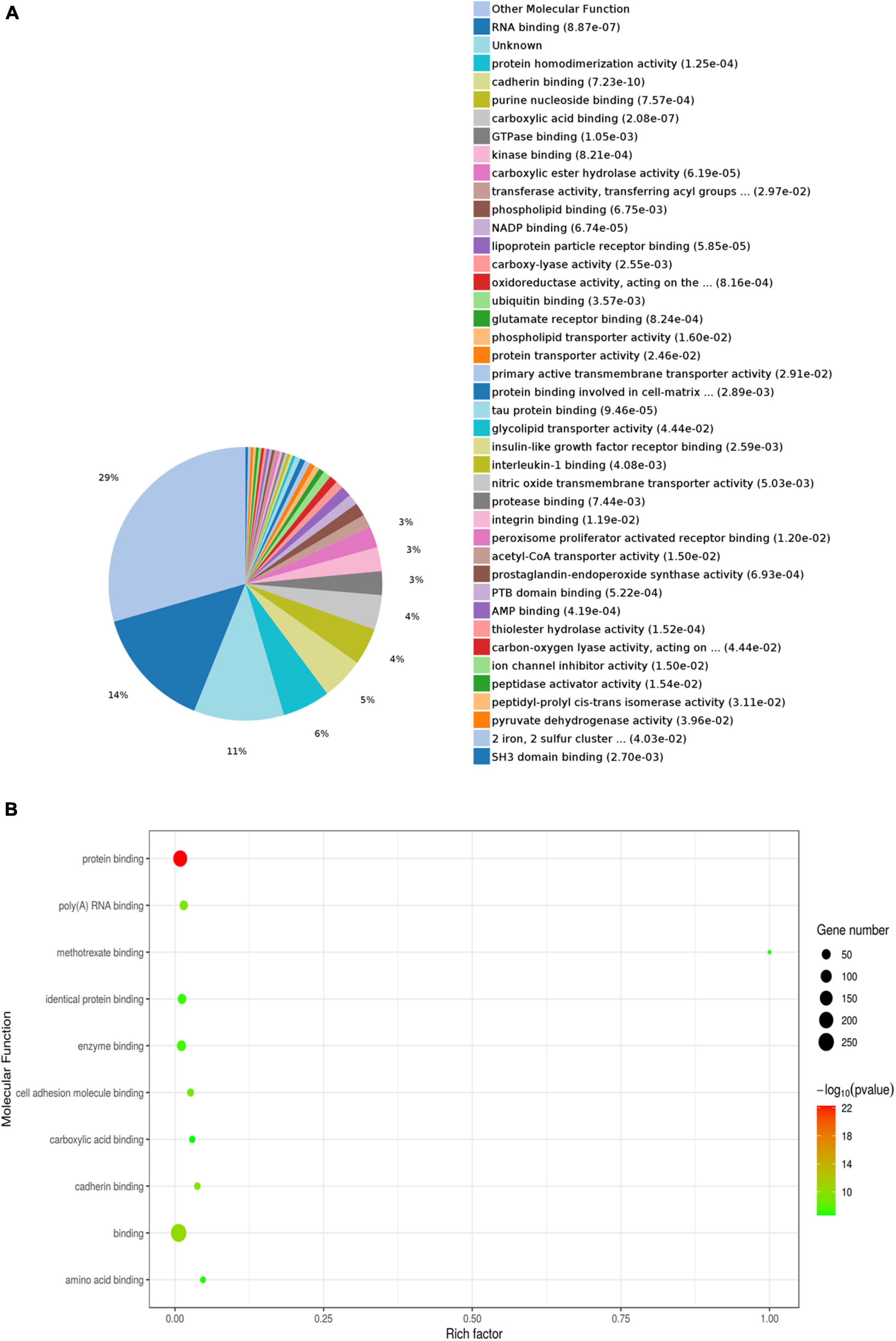
Figure 2. Gene Ontology (GO) enrichment analysis of molecular function for selenium-influenced DEPs. (A) Pie chart of DEP-enriched GO terms for molecular function (MF). (B) Enriched GO terms for MF.
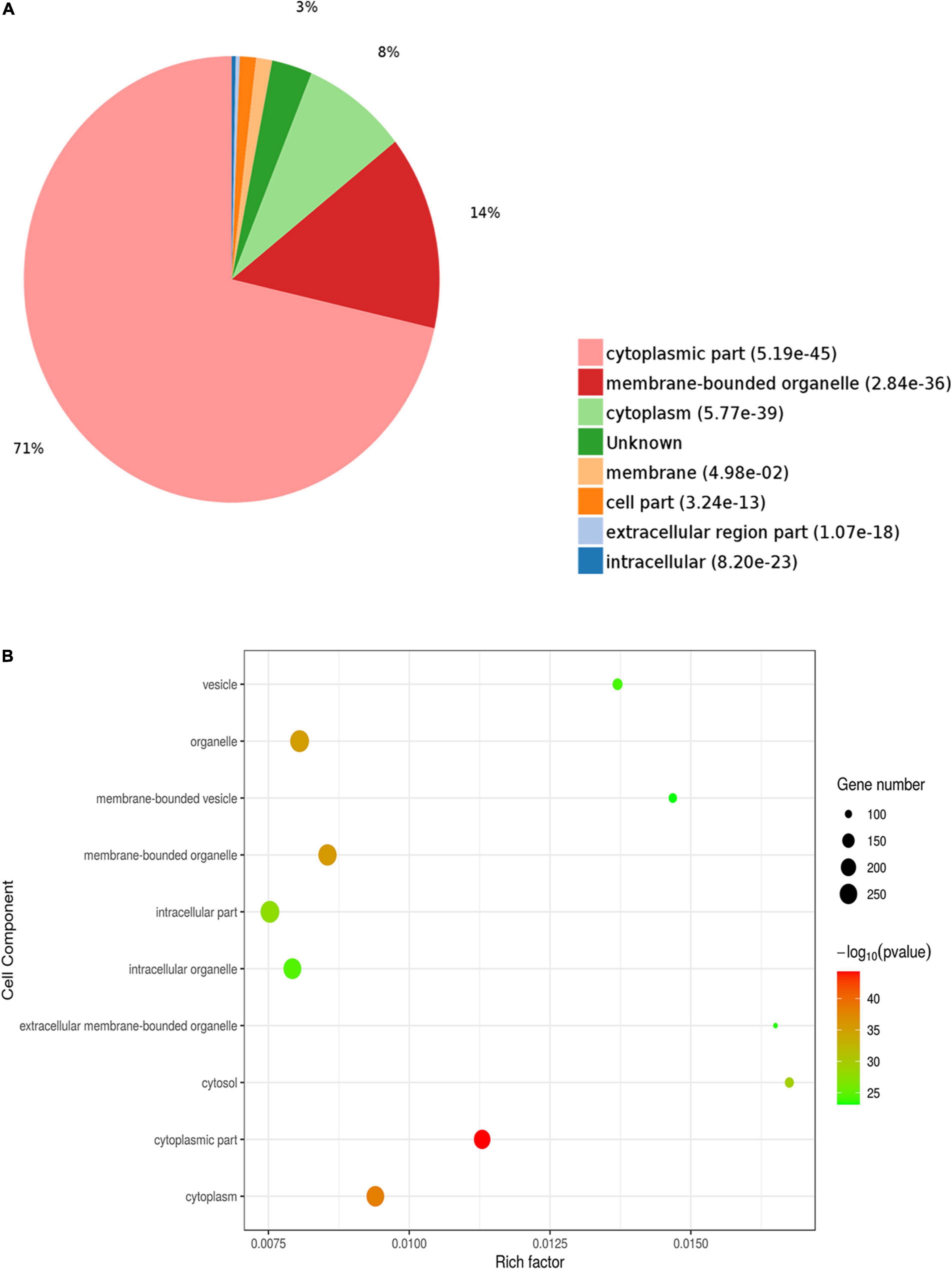
Figure 3. Gene Ontology (GO) enrichment analysis of cellular components for selenium-influenced DEPs. (A) Pie chart of DEP-enriched GO terms for cellular component (CC). (B) Enriched GO terms for CC.
In the biological process (BP) category, the identified DEPs showed enrichment in axon development (8%) and neuron differentiation (6%) (Figure 4). In addition, 3, 3, and 2% of DEPs were associated with “response to insulin,” “cellular response to insulin stimulus,” and “insulin receptor (IR) signaling pathway,” respectively, whereas other DEPs were implicated in “cellular response to insulin-like growth factor stimulus,” “insulin-like growth factor receptor signaling pathway,” and “regulation of insulin secretion” (Figure 5A). In relation to the response to insulin, eight DEPs, including the candidate phosphatase LPIN1 (Lpin1), Irs1, and InsR, were upregulated, while two DEPs (Srsf3 and Grb2) were downregulated after sodium selenate treatment (Figure 5B and Table 1). Validation of significantly DEPs by real-time quantitative reverse transcribed polymerase chain reaction (RT-PCR) assay were consistent with the results in the proteomic approach (Figure 5C). Similarly, there were a significant increased expression of Irs1 and a decreased expression of Srsf3 by immunohistochemistry staining in the hippocampus of APP/PS1 mice after selenium treatment compared with the untreated control mice (Figure 6). In turn, PPI network analysis revealed that Grb2 may interact with InsR, Irs1, and IGF1 (Figure 7). These results suggest that selenium supplementation exerts a major effect on the regulation of central insulin sensitivity.
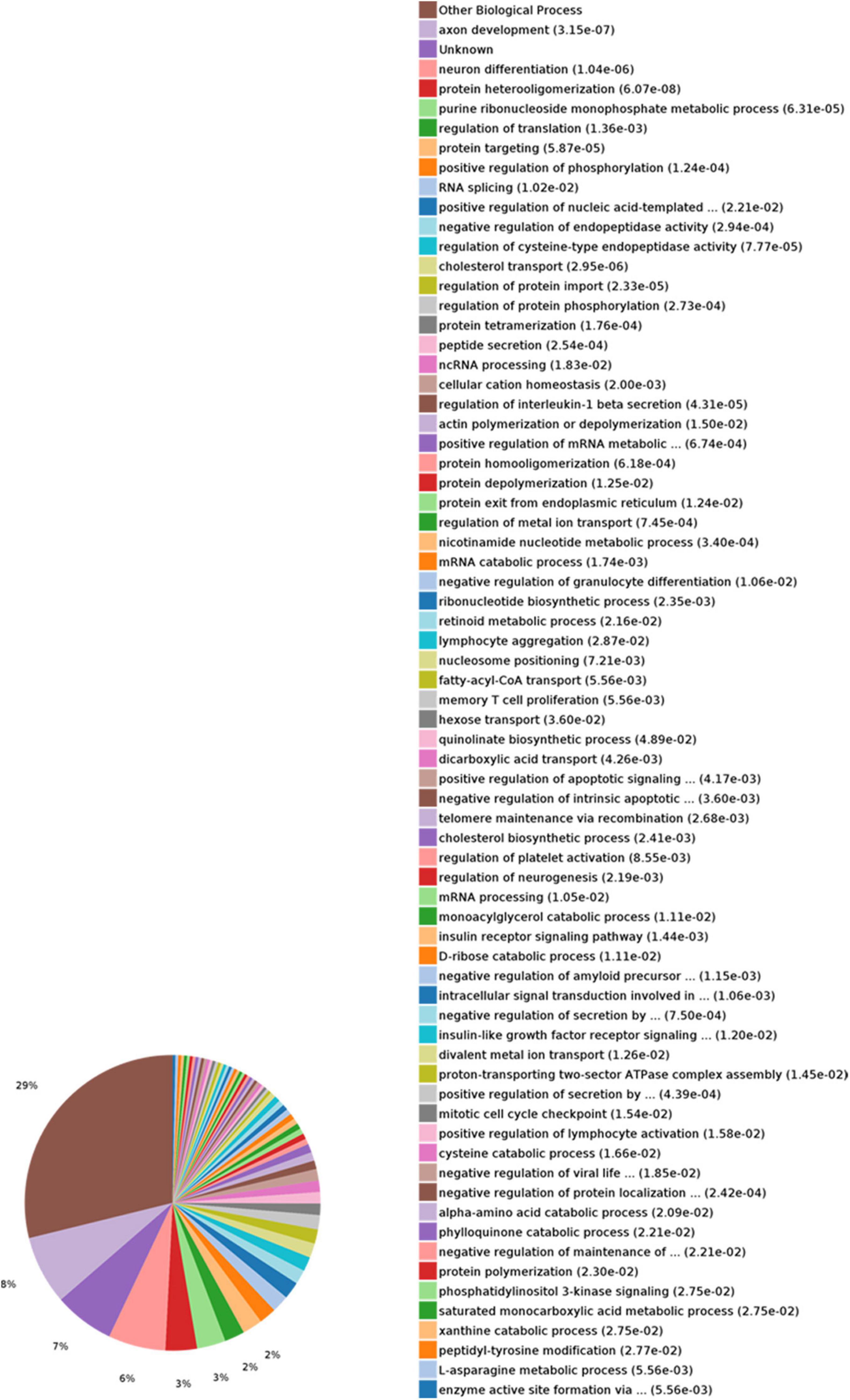
Figure 4. Gene Ontology (GO) enrichment analysis of biological processes for selenium-influenced DEPs.
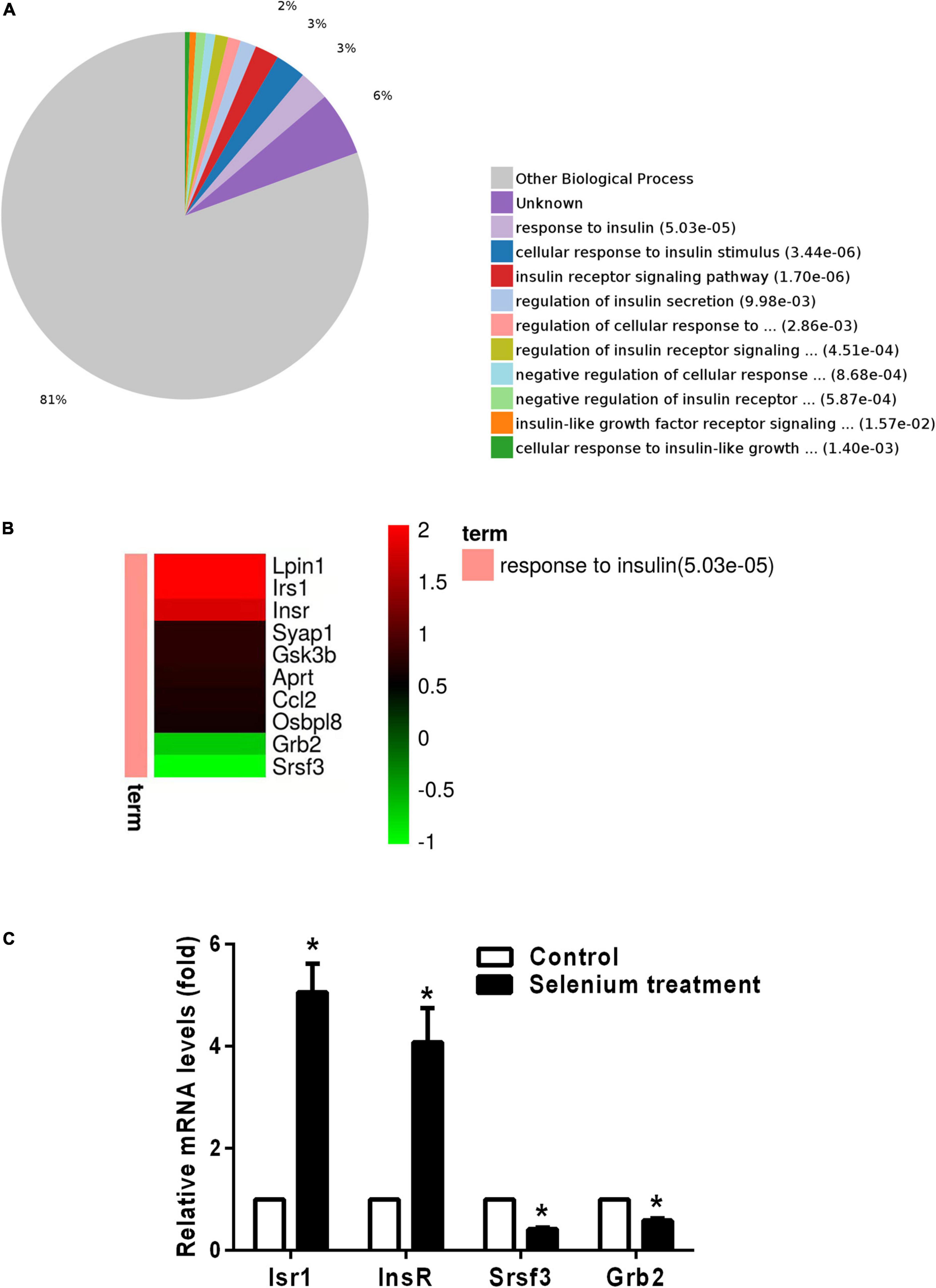
Figure 5. Gene Ontology (GO) enrichment analysis of biological processes for DEPs involved in insulin signaling pathways. (A) Pie chart of DEP-enriched GO terms for biological processes related to the insulin/IGF signaling pathway. (B) Heatmap of upregulated and downregulated DEPs involved in the response to insulin. (C) The relative mRNA levels of Irs1, InsR, Srsf3, and Grb2 in the cortex of APP/PS1 mice treated with or without selenium. GAPDH was used for normalization, and the control was set to 1 in the RT-PCR data. All experiments were performed in duplicate. *P < 0.05 compared with control (untreated).
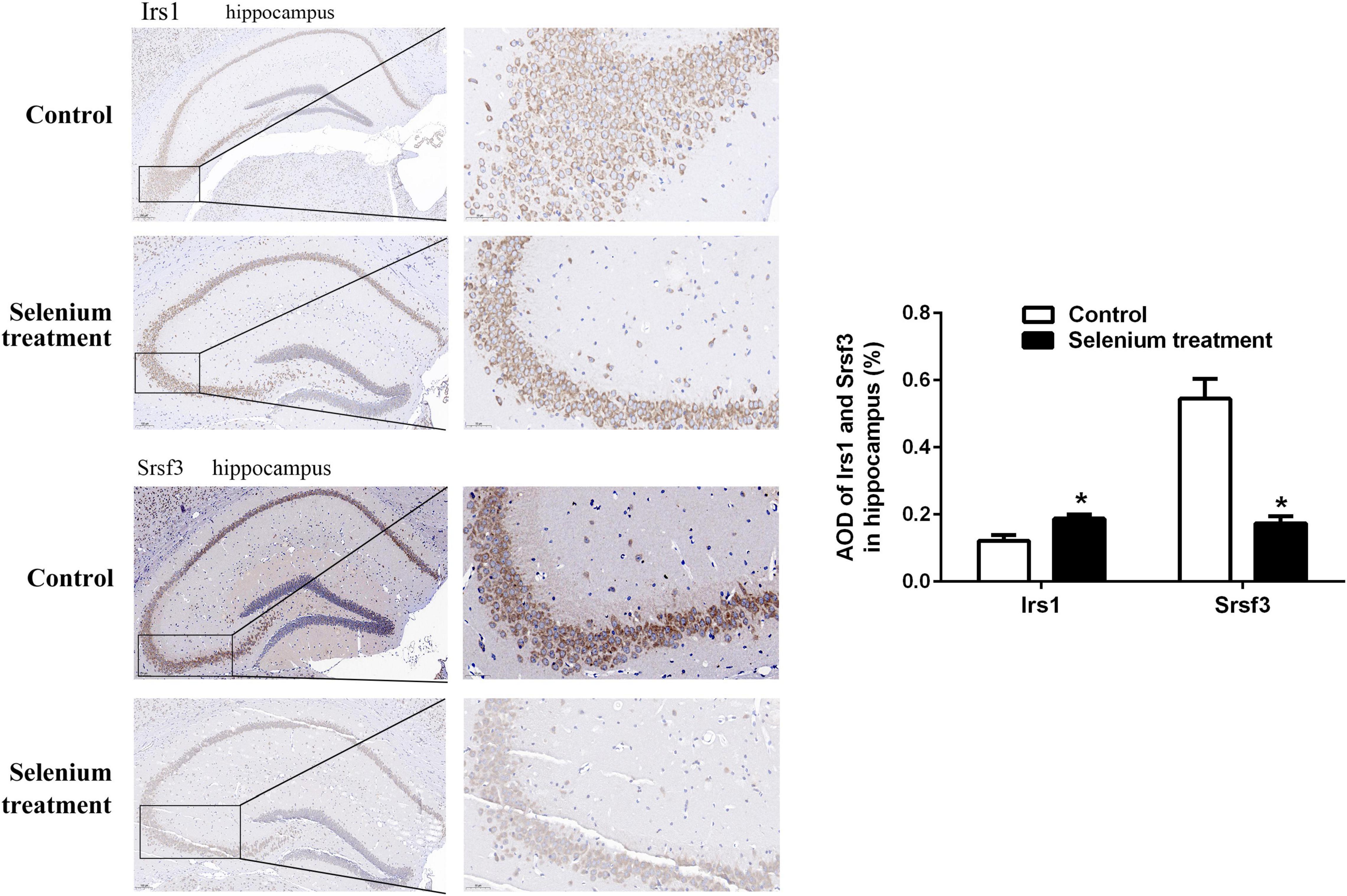
Figure 6. Immunohistochemistry staining (at higher magnification) of Irs1 and Srsf3 expression in the hippocampus of APP/PS1 mice treated with or without selenium. AOD value is calculated by ImageJ. *P < 0.05 compared with control (n = 4 per group). The positive staining is brownish yellow. Magnification 200×, scale bar = 100 μM.
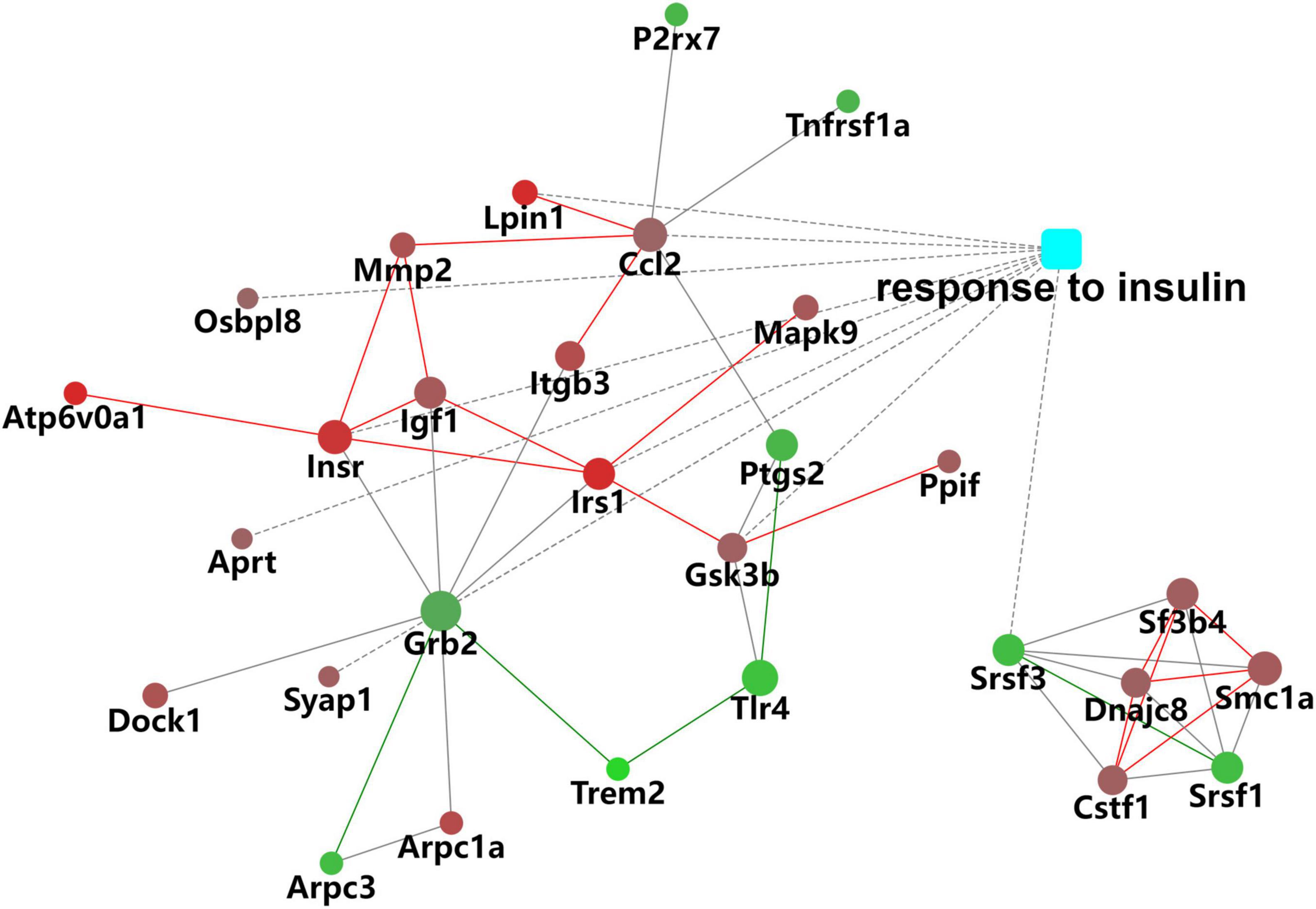
Figure 7. Protein–protein interaction (PPI) network of DEPs associated with the response to insulin.
Kyoto Encyclopedia of Genes and Genomes Pathway Analysis of Differentially Expressed Proteins
The functions of the identified DEPs were further explored by KEGG pathway enrichment analysis. The results showed enrichment of DEPs in pathways related to several pathological conditions, including AD, non-alcoholic fatty liver disease (NAFLD), Parkinson’s disease, insulin resistance, amyotrophic lateral sclerosis, and type II diabetes mellitus (Figures 8A,B). These data further support the influence of selenium supplementation on the expression of proteins closely associated with neurodegenerative and metabolic diseases.
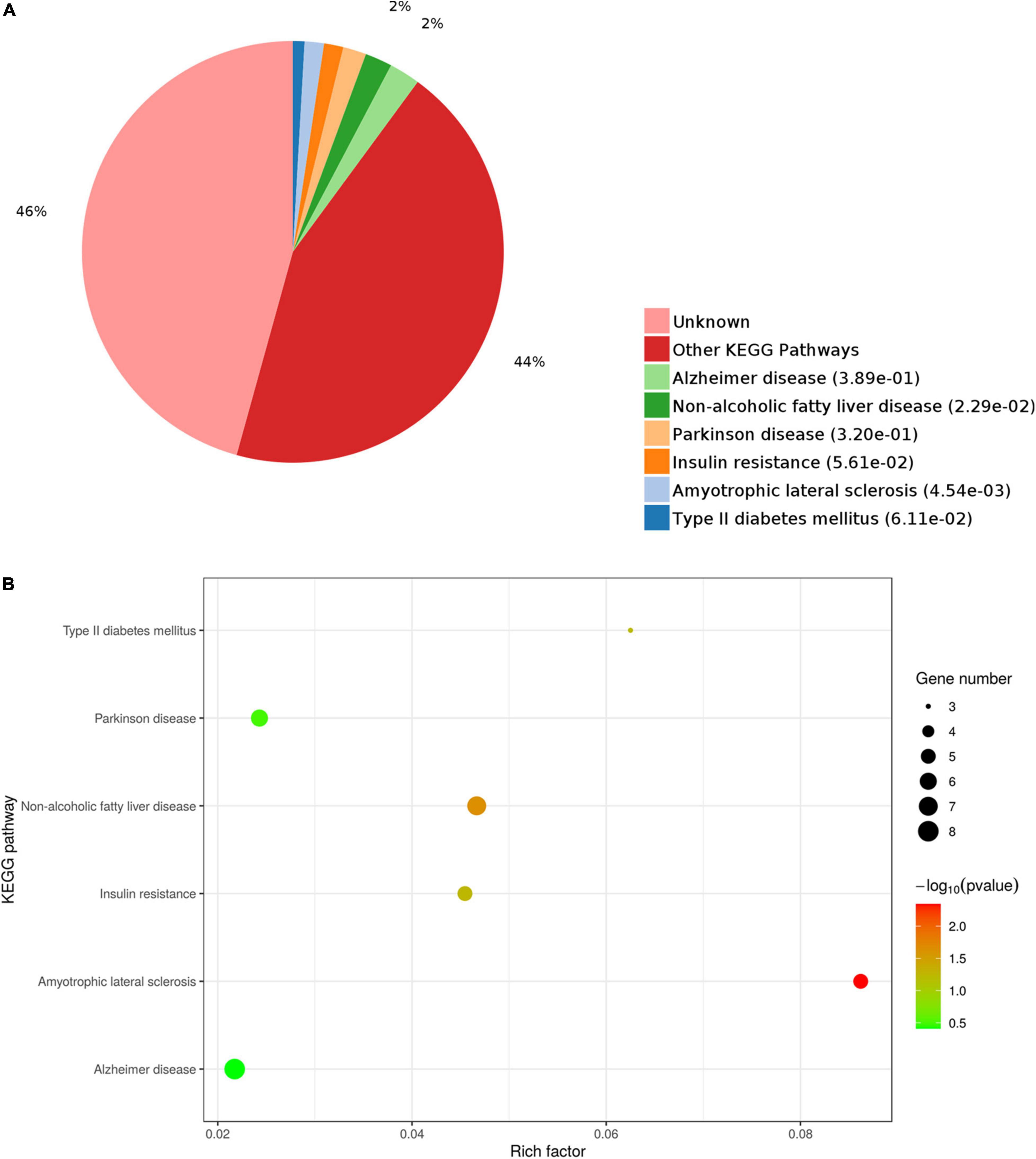
Figure 8. Kyoto Encyclopedia of Genes and Genomes (KEGG) pathway analysis of DEPs. (A) Pie chart of DEP-enriched KEGG pathways. (B) KEGG pathway enrichment distribution of DEPs.
Discussion
A growing body of research indicates that impaired insulin/IGF signaling contributes to brain insulin resistance and plays a causal role in AD pathogenesis (De La Monte and Wands, 2008; Ahmad, 2012; Sridhar, 2015; Neth and Craft, 2017; Denver et al., 2018; Song et al., 2018; Komleva et al., 2021). Insulin resistance promotes metabolic dysfunction, oxidative stress, and inflammation and is a main determinant of the proven association between diabetes and AD development (De La Monte and Wands, 2008; Chow et al., 2019; Spinelli et al., 2019, 2020). Regarding the relevance of the APP/PS1 transgenic mouse as an experimental AD model, a previous study showed that impaired glucose tolerance and reduced insulin sensitivity precede amyloid plaque deposition and cognitive decline in these mice (Macklin et al., 2017).
Growing research indicates that selenium supplementation may have beneficial effects on AD progression and symptoms (Tamtaji et al., 2019). Importantly, clinical trials showed that selenium supplementation regimens were well tolerated, improved insulin homeostasis, and slowed disease progression in AD patients (Malpas et al., 2016; Tamtaji et al., 2019; Vivash et al., 2021). However, the molecular mechanisms underlying the neuroprotective actions of selenium remain incompletely understood.
In this study, we performed a label-free LC–MS/MS quantitative proteomics approach to analyze selenium-induced changes in protein expression in the hippocampus and cerebral cortex of APP/PS1 mice. Suggestive of the extensive effects of selenium on AD pathophysiology, 319 DEPs (205 upregulated and 114 downregulated proteins) were detected after sodium selenate treatment. Importantly, functional enrichment analysis revealed that the DEPs were mainly enriched in processes affecting tau protein binding and insulin-related pathways, which are significantly related to the onset and progression of AD (Oddo et al., 2003; Jouanne et al., 2017).
Specifically, GO analysis showed the involvement of the identified DEPs in BPs such as “axon development,” “neuron differentiation,” “response to insulin,” “cellular response to insulin stimulus,” “insulin receptor signaling pathway,” “IGFR signaling pathway,” and “regulation of insulin secretion.” Several DEPs related to the insulin response, including Lpin1, Irs1, and InsR, were significantly upregulated in the brains of AD mice following sodium selenate treatment.
Phosphatidate phosphatase LPIN1 plays an important role in adipocyte differentiation and lipid metabolism; decreased Lpin1 expression is correlated with insulin resistance, and mutations in Lpin1 determine cognitive impairment in mice with fatty liver dystrophy (Ryu et al., 2009; Chen et al., 2012; Shang et al., 2020; Xie et al., 2020). Lpin1 expression is decreased in the hippocampus of a rat model of type I diabetic encephalopathy. In this model, lentivirus-mediated lipin1 overexpression was able to improve cognitive deficits and exert neuroprotective effects by inhibiting the protein kinase D/limk1/cofilin signaling pathway (Xie et al., 2020). However, because the association between Lpin1 and AD remains uncertain, further work is needed to assess whether Lpin1 upregulation contributes to the beneficial effect of selenium against AD.
Decreased expression of both InsR and IGFR was observed in the cortex and hippocampus of AD patients (Steen et al., 2005). A recent study demonstrated that sodium selenate supplementation exerted a beneficial effect on healthspan and metabolism by inhibiting IGF1 signaling and other hormones (Plummer et al., 2021). Moreover, in an AD mouse model, IGF1 overexpression led to decreased cortical and hippocampal expression of amyloid β protein (Aβ1–40) (Song et al., 2018). Hence, both human and animal research strongly suggest that strategies aimed at restoring insulin sensitivity may be effective in decreasing amyloid deposition (Han et al., 2017; Khandelwal et al., 2020). In this regard, our findings suggest that the upregulation of Irs1 and InsR and the improvement of insulin/IGF signaling are likely important determinants of the beneficial effects of selenium-based therapies targeting AD.
Our study also showed that the levels of Srsf3 and Grb2, two proteins also linked to insulin responses, were significantly downregulated after treatment with sodium selenate. Srsf3 is critical for mRNA splicing and mRNA nuclear export (Xiao et al., 2016; Nakura et al., 2021). A recent study demonstrated that Srsf3 was involved in alternative splicing of the InsR gene to produce two variants, InsR-A and InsR-B, which display different tissue specificity and regulatory roles (Nakura et al., 2021). Another study revealed that Srsf3 expression is increased in the hippocampus and temporal cortex of AD patients and suggested that its activity modulates tropomyosin receptor kinase B (TrkB) pre-mRNA splicing to promote neurodegeneration (Wong et al., 2012). Furthermore, Srsf3 was also shown to affect alternative splicing of the tau gene, one of the main biomarkers of AD (Yu et al., 2004; Peyron et al., 2021). The above observations are in line with our study in suggesting that inhibition of Srsf3 expression mediates, at least in part, the beneficial effect of selenium on AD pathology.
Growth factor receptor-bound protein 2 is a key adaptor protein in the insulin signaling pathway (Kasuga, 1993; Liu et al., 2009). Previous studies reported that Grb2 expression is upregulated in the brains of AD patients and model mice and suggested that Grb2 contributes to brain insulin resistance and AD pathogenesis through interactions with APP and NADPH oxidase 4 (Nox4) (Bruce-Keller et al., 2011; Wu and Williams, 2012; Roy et al., 2014; Majumder et al., 2017, 2021; Park et al., 2021). Notably, downregulation of Grb2 in skeletal muscle was suggested to mediate the insulin-sensitizing effect of caloric restriction, a notion supported by data from both Grb2± heterozygous knockout mice and muscle cells in vitro (Liu et al., 2009). Therefore, downregulation of Grb2 may represent another mechanism by which selenium helps improve insulin signaling in AD.
There were several limitations in our study. However, one of the most important limitations is that the study protocol lacked the inclusion of healthy controls and a group treated with selenium alone. In the absence of these groups, the generalizability of these results is challenging and warrants further investigation.
Taken together, our present study demonstrates that several insulin/IGF1 signaling pathway-associated proteins are differentially expressed in ways that are consistent with reduced central insulin resistance after treatment of APP/PS1 mice with sodium selenate. Although risk-assessment principles should guide the clinical administration of selenium-based therapies, our findings support their therapeutic value in the treatment of neurodegenerative and metabolic diseases such as AD and NAFLD.
Data Availability Statement
The datasets presented in this study can be found in online repositories. The name of the repository and accession number can be found below: iProX, https://www.iprox.cn/, IPX0004556000. The mass spectrometry proteomics data have been deposited to the ProteomeXchange Consortium (http://proteomecentral.proteomexchange.org) via the iProX partner repository (Ma et al., 2019) with the dataset identifier PXD034613.
Ethics Statement
The animal study was reviewed and approved by the Animal Care and Use Committee of Shandong Xiehe University (Jinan, China).
Author Contributions
WS and SL conceived the idea and designed the study. XX, PQ, and YY carried out the animal experiments. YZ and HS performed the proteomics work. XX conducted the formal analysis and prepared the manuscript. SL was responsible for the review and revision of the manuscript. All authors have read and agreed to the published version of the manuscript.
Funding
This study was supported by the Taian Technology Innovation Development Project (Grant No. 2021NS196).
Conflict of Interest
The authors declare that the research was conducted in the absence of any commercial or financial relationships that could be construed as a potential conflict of interest.
Publisher’s Note
All claims expressed in this article are solely those of the authors and do not necessarily represent those of their affiliated organizations, or those of the publisher, the editors and the reviewers. Any product that may be evaluated in this article, or claim that may be made by its manufacturer, is not guaranteed or endorsed by the publisher.
Supplementary Material
The Supplementary Material for this article can be found online at: https://www.frontiersin.org/articles/10.3389/fnmol.2022.931788/full#supplementary-material
Footnotes
References
Ahmad, W. (2012). Overlapped Metabolic and Therapeutic Links between Alzheimer and Diabetes. Mole. Neurobiol. 47, 399–424. doi: 10.1007/s12035-012-8352-z
Ashraf, A., and So, P.-W. (2020). Spotlight on Ferroptosis: iron-Dependent Cell Death in Alzheimer’s Disease. Front. Aging Neurosci. 2020:12. doi: 10.3389/fnagi.2020.00196
Bruce-Keller, A. J., Gupta, S., Knight, A. G., Beckett, T. L., Mcmullen, J. M., Davis, P. R., et al. (2011). Cognitive impairment in humanized APP×PS1 mice is linked to Aβ1–42 and NOX activation. Neurobiol. Dis. 44, 317–326. doi: /10.1016/j.nbd.2011.07.012
Chen, D., Li, X., Zhao, X., Qin, Y., Wang, J., and Wang, C. (2019). Comparative proteomics of goat milk during heated processing. Food Chem. 275, 504–514. doi: 10.1016/j.foodchem.2018.09.129
Chen, S., Zhuang, X., Liu, Y., Sun, A., and Chen, C. (2012). Expression and Significance of Lipin1 and AMPKα in Hepatic Insulin Resistance in Diet-Induced Insulin Resistance Rats. Exp. Clin. Endocrinol. Diab. 120, 84–88. doi: 10.1055/s-0031-1298013
Chow, H.-M., Shi, M., Cheng, A., Gao, Y., Chen, G., Song, X., et al. (2019). Age-related hyperinsulinemia leads to insulin resistance in neurons and cell-cycle-induced senescence. Nat. Neurosci. 22, 1806–1819. doi: 10.1038/s41593-019-0505-1
Craft, S., Peskind, E., Schwartz, M. W., Schellenberg, G. D., Raskind, M., and Porte, D. (1998). Cerebrospinal fluid and plasma insulin levels in Alzheimer’s disease. Neurology 50, 164–168. doi: 10.1212/WNL.50.1.164
De La Monte, S. M., and Wands, J. R. (2008). Alzheimer’s Disease is Type 3 Diabetes—Evidence Reviewed. J. Diab. Sci. Technol. 2, 1101–1113. doi: 10.1177/193229680800200619
Deli, M. A., Sartorius, T., Peter, A., Heni, M., Maetzler, W., Fritsche, A., et al. (2015). The Brain Response to Peripheral Insulin Declines with Age: a Contribution of the Blood-Brain Barrier? PLoS One 10:e0126804. doi: 10.1371/journal.pone.0126804
Denver, P., English, A., and Mcclean, P. L. (2018). Inflammation, insulin signaling and cognitive function in aged APP/PS1 mice. Brain, Behav. Immun. 70, 423–434. doi: 10.1016/j.bbi.2018.03.032
Eraky, S. M., Ramadan, N. M., and Abo El-Magd, N. F. (2021). Antidiabetic effects of quercetin and liraglutide combination through modulation of TXNIP/ IRS-1/ PI3K pathway. Cell Biochem. Funct. 40, 90–102. doi: 10.1002/cbf.3678
Gheorghiu, M. L., and Badiu, C. (2020). Selenium involvement in mitochondrial function in thyroid disorders. Hormones 19, 25–30. doi: 10.1007/s42000-020-00173-2
Greer, C., Seifan, A., Glazer, H., Oboudiyat, C., and Isaacson, R. (2013). Alzheimer’s Disease. Semin. Neurol. 33, 313–329. doi: 10.1055/s-0033-1359319
Han, K., Jia, N., Zhong, Y., and Shang, X. (2017). S14G-humanin alleviates insulin resistance and increases autophagy in neurons of APP/PS1 transgenic mouse. J. Cell. Biochem. 119, 3111–3117. doi: 10.1002/jcb.26452
Jahangir, Z., Ahmad, W., and Shabbiri, K. (2014). Alternate Phosphorylation/O-GlcNAc Modification on Human Insulin IRSs: a Road towards Impaired Insulin Signaling in Alzheimer and Diabetes. Adv. Bioinform. 2014, 1–18. doi: 10.1155/2014/324753
Jia, L., Du, Y., Chu, L., Zhang, Z., Li, F., Lyu, D., et al. (2020). Prevalence, risk factors, and management of dementia and mild cognitive impairment in adults aged 60 years or older in China: a cross-sectional study. Lancet Public Health 5, e661–e671. doi: 10.1016/S2468-2667(20)30185-7
Jouanne, M., Rault, S., and Voisin-Chiret, A.-S. (2017). Tau protein aggregation in Alzheimer’s disease: an attractive target for the development of novel therapeutic agents. Eur. J. Med. Chem. 139, 153–167. doi: 10.1016/j.ejmech.2017.07.070
Kasuga, M. (1993). Mechanism of Insulin Action. Folia Endocrinol. Jap. 69, 1029–1034. doi: 10.1507/endocrine1927.69.10_1029
Kellar, D., and Craft, S. (2020). Brain insulin resistance in Alzheimer’s disease and related disorders: mechanisms and therapeutic approaches. Lancet Neurol. 19, 758–766. doi: 10.1016/S1474-4422(20)30231-3
Khandelwal, M., Manglani, K., Gupta, S., and Tiku, A. B. (2020). Gamma radiation improves AD pathogenesis in APP/PS1 mouse model by potentiating insulin sensitivity. Heliyon 6:e04499. doi: 10.1016/j.heliyon.2020.e04499
Komleva, Y., Chernykh, A., Lopatina, O., Gorina, Y., Lokteva, I., Salmina, A., et al. (2021). Inflamm-Aging and Brain Insulin Resistance: new Insights and Role of Life-style Strategies on Cognitive and Social Determinants in Aging and Neurodegeneration. Front. Neurosci. 2021:14. doi: 10.3389/fnins.2020.618395
Liu, K., Shen, C., and Chen, X. (2015). Expression of androgen receptor in coronary artery in the cases of sudden coronary death. Internat. J. Clin. Exp. Pathol. 8, 3742–3747.
Liu, X., Liu, M., Zhang, J., Bai, X., Ramos, F., Van Remmen, H., et al. (2009). Downregulation of Grb2 contributes to the insulin-sensitizing effect of calorie restriction. Am. J. Physiol. Endocrinol. Metabol. 296, E1067–E1075. doi: 10.1152/ajpendo.90714.2008
Loef, M., Schrauzer, G. N., and Walach, H. (2011). Selenium and Alzheimer’s Disease: a Systematic Review. J. Alzheimer’s Dis. 26, 81–104. doi: 10.3233/JAD-2011-110414
Lorenzo, A., Yuan, M., Zhang, Z., Paganetti, P. A., Sturchler-Pierrat, C., Staufenbiel, M., et al. (2000). Amyloid β interacts with the amyloid precursor protein: a potential toxic mechanism in Alzheimer’s disease. Nat. Neurosci. 3, 460–464. doi: 10.1038/74833
Ma, J., Chen, T., Wu, S., Yang, C., Bai, M., Shu, K., et al. (2019). iProX: an integrated proteome resource. Nucleic Acids Res. 47, D1211–D1217. doi: 10.1093/nar/gky869
Macklin, L., Griffith, C. M., Cai, Y., Rose, G. M., Yan, X.-X., and Patrylo, P. R. (2017). Glucose tolerance and insulin sensitivity are impaired in APP/PS1 transgenic mice prior to amyloid plaque pathogenesis and cognitive decline. Exp. Gerontol. 88, 9–18.
Majumder, P., Chanda, K., Das, D., Singh, B. K., Chakrabarti, P., Jana, N. R., et al. (2021). A nexus of miR-1271, PAX4 and ALK/RYK influences the cytoskeletal architectures in Alzheimer’s Disease and Type 2 Diabetes. Biochem. J. 478, 3297–3317. doi: 10.1042/BCJ20210175
Majumder, P., Roy, K., Singh, B. K., Jana, N. R., and Mukhopadhyay, D. (2017). Cellular levels of growth factor receptor bound protein 2 (Grb2) and cytoskeleton stability are correlated in a neurodegenerative scenario. Dis. Mod. Mech. 10, 655–669. doi: 10.1242/dmm.027748
Malpas, C. B., Vivash, L., Genc, S., Saling, M. M., Desmond, P., Steward, C., et al. (2016). A Phase IIa Randomized Control Trial of VEL015 (Sodium Selenate) in Mild-Moderate Alzheimer’s Disease. J. Alzheimer’s Dis. 54, 223–232. doi: 10.3233/JAD-160544
Mangiapane, E., Pessione, A., and Pessione, E. (2014). Selenium and Selenoproteins: an Overview on Different Biological Systems. Curr. Prot. Pept. Sci. 15, 598–607.
Mcclean, P. L., and Hölscher, C. (2014). Liraglutide can reverse memory impairment, synaptic loss and reduce plaque load in aged APP/PS1 mice, a model of Alzheimer’s disease. Neuropharmacology 76, 57–67. doi: 10.1016/j.neuropharm.2013.08.005
Mintziori, G., Mousiolis, A., Duntas, L. H., and Goulis, D. G. (2019). Evidence for a manifold role of selenium in infertility. Hormones 19, 55–59. doi: 10.1007/s42000-019-00140-6
Nakura, T., Ozoe, A., Narita, Y., Matsuo, M., Hakuno, F., Kataoka, N., et al. (2021). Rbfox2 mediates exon 11 inclusion in insulin receptor pre-mRNA splicing in hepatoma cells. Biochimie 187, 25–32. doi: 10.1016/j.biochi.2021.05.007
Neth, B. J., and Craft, S. (2017). Insulin Resistance and Alzheimer’s Disease: Bioenergetic Linkages. Front. Aging Neurosci. 2017:9.
Oddo, S., Caccamo, A., Shepherd, J. D., Murphy, M. P., Golde, T. E., Kayed, R., et al. (2003). Triple-Transgenic Model of Alzheimer’s Disease with Plaques and Tangles. Neuron 39, 409–421.
Ozben, T., and Ozben, S. (2019). Neuro-inflammation and anti-inflammatory treatment options for Alzheimer’s disease. Clin. Biochem. 72, 87–89.
Park, M. W., Cha, H. W., Kim, J., Kim, J. H., Yang, H., Yoon, S., et al. (2021). NOX4 promotes ferroptosis of astrocytes by oxidative stress-induced lipid peroxidation via the impairment of mitochondrial metabolism in Alzheimer’s diseases. Redox Biol. 41:101947. doi: 10.1016/j.redox.2021.101947
Peyron, P.-A., Hirtz, C., Baccino, E., Ginestet, N., Tiers, L., Martinez, A. Y., et al. (2021). Tau protein in cerebrospinal fluid: a novel biomarker of the time of death? Internat. J. Legal Med. 135, 2081–2089.
Plummer, J. D., Postnikoff, S. D. L., Tyler, J. K., and Johnson, J. E. (2021). Selenium supplementation inhibits IGF-1 signaling and confers methionine restriction-like healthspan benefits to mice. eLife 2021:10. doi: 10.7554/eLife.62483
Rayman, M. P. (2019). Selenium intake, status, and health: a complex relationship. Hormones 19, 9–14. doi: 10.1007/s42000-019-00125-5
Ribes-Navarro, A., Atef, M., S Nchez-Saras, A. S., Beltr N-Bretones, M. T., Olucha-Bordonau, F., and S Nchez-P Rez, A. M. (2018). Abscisic Acid Supplementation Rescues High Fat Diet-Induced Alterations in Hippocampal Inflammation and IRSs Expression. Mole. Neurobiol. 56, 454–464.
Roy, K., Chakrabarti, O., and Mukhopadhyay, D. (2014). Interaction of Grb2 SH3 domain with UVRAG in an Alzheimer’s disease–like scenario. Biochem. Cell Biol. 92, 219–225. doi: 10.1139/bcb-2014-0001
Ryu, D., Oh, K.-J., Jo, H.-Y., Hedrick, S., Kim, Y.-N., Hwang, Y.-J., et al. (2009). TORC2 Regulates Hepatic Insulin Signaling via a Mammalian Phosphatidic Acid Phosphatase, LIPIN1. Cell Metabol. 9, 240–251. doi: 10.1016/j.cmet.2009.01.007
Shang, P., Zheng, F., Han, F., Song, Y., Pan, Z., Yu, S., et al. (2020). Lipin1 mediates cognitive impairment in fld mice via PKD-ERK pathway. Biochem. Biophys. Res. Comm. 525, 286–291. doi: 10.1016/j.bbrc.2020.02.070
Shannon, P., Markiel, A., Ozier, O., Baliga, N. S., Wang, J. T., Ramage, D., et al. (2003). Cytoscape: a Software Environment for Integrated Models of Biomolecular Interaction Networks. Gen. Res. 13, 2498–2504. doi: 10.1101/gr.1239303
Song, F., Liu, T., Meng, S., Li, F., Zhang, Y., and Jiang, L. (2018). Insulin-Like Growth Factor-1 Alleviates Expression of Aβ1–40 and α-, β-, and γ-Secretases in the Cortex and Hippocampus of APP/PS1 Double Transgenic Mice. J. Mole. Neurosci. 66, 595–603. doi: 10.1007/s12031-018-1201-4
Spinelli, M., Fusco, S., and Grassi, C. (2019). Brain Insulin Resistance and Hippocampal Plasticity: Mechanisms and Biomarkers of Cognitive Decline. Front. Neurosci. 2019:13. doi: 10.3389/fnins.2019.00788
Spinelli, M., Fusco, S., and Grassi, C. (2020). Brain insulin resistance impairs hippocampal plasticity. Vit. Horm. 114, 281–306.
Sridhar, G. R. (2015). Emerging links between type 2 diabetes and Alzheimer’s disease. World J. Diab. 6:744.
Steen, E., Terry, B. M., J. Rivera, E., Cannon, J. L., Neely, T. R., Tavares, R., et al. (2005). Impaired insulin and insulin-like growth factor expression and signaling mechanisms in Alzheimer’s disease – is this type 3 diabetes? J. Alzheimer’s Dis. 7, 63–80.
Taguchi, A., Tanokashira, D., and Fukuokaya, W. (2019). Involvement of insulin receptor substrates in cognitive impairment and Alzheimer’s disease. Neur. Regen. Res. 14:1330.
Tamtaji, O. R., Heidari-Soureshjani, R., Mirhosseini, N., Kouchaki, E., Bahmani, F., and Aghadavod, E. (2019). Probiotic and selenium co-supplementation, and the effects on clinical, metabolic and genetic status in Alzheimer’s disease: a randomized, double-blind, controlled trial. Clin. Nutr. 38, 2569–2575. doi: 10.1016/j.clnu.2018.11.034
Van Der Jeugd, A., Parra-Damas, A., Baeta-Corral, R., Soto-Fagu, S. C. M., Ahmed, T., Laferla, F. M., et al. (2018). Reversal of memory and neuropsychiatric symptoms and reduced tau pathology by selenium in 3xTg-AD mice. Sci. Rep. 2018:8. doi: 10.1038/s41598-018-24741-0
Varikasuvu, S. R., Prasad, V. S., Kothapalli, J., and Manne, M. (2018). Brain Selenium in Alzheimer’s Disease (BRAIN SEAD Study): a Systematic Review and Meta-Analysis. Biolog. Trace Element Res. 189, 361–369. doi: 10.1007/s12011-018-1492-x
Vivash, L., Malpas, C. B., Hovens, C. M., Brodtmann, A., Collins, S., Macfarlane, S., et al. (2021). Sodium selenate as a disease-modifying treatment for mild–moderate Alzheimer’s disease: an open-label extension study. BMJ Neurol. Open 3:e000223. doi: 10.1136/bmjno-2021-000223
Wang, N., Tan, H.-Y., Li, S., Xu, Y., Guo, W., and Feng, Y. (2017). Supplementation of Micronutrient Selenium in Metabolic Diseases: its Role as an Antioxidant. Oxid. Med. Cell. Long. 2017, 1–13. doi: 10.1155/2017/7478523
Wiśniewski, J. R. (2018). Filter-Aided Sample Preparation for Proteome Analysis. Methods Mole. Biol. 1841, 3–10. doi: 10.1007/978-1-4939-8695-8_1
Wong, J., Garner, B., Halliday, G. M., and Kwok, J. B. J. (2012). Srp20 regulates TrkB pre-mRNA splicing to generate TrkB-Shc transcripts with implications for Alzheimer’s disease. J. Neurochem. 123, 159–171. doi: 10.1111/j.1471-4159.2012.07873.x
Wu, X., and Williams, K. J. (2012). NOX4 Pathway as a Source of Selective Insulin Resistance and Responsiveness. Arterioscl. Thromb. Vasc. Biol. 32, 1236–1245. doi: 10.1161/ATVBAHA.111.244525
Xiao, W., Adhikari, S., Dahal, U., Chen, Y.-S., Hao, Y.-J., Sun, B.-F., et al. (2016). Nuclear m 6 A Reader YTHDC1 Regulates mRNA Splicing. Mole. Cell 61, 507–519. doi: 10.1016/j.molcel.2016.01.012
Xie, M., Wang, M., Liu, W., Xu, M., Shang, P., Jiang, D., et al. (2020). Lipin1 Is Involved in the Pathogenesis of Diabetic Encephalopathy through the PKD/Limk/Cofilin Signaling Pathway. Oxidat. Med. Cell. Long. 2020, 1–14. doi: 10.1155/2020/1723423
Yu, Q., Guo, J., and Zhou, J. (2004). A minimal length between tau exon 10 and 11 is required for correct splicing of exon 10. J. Neurochem. 90, 164–172. doi: 10.1111/j.1471-4159.2004.02477.x
Keywords: Alzheimer’s disease (AD), selenium, insulin sensitivity, proteomic analysis, neurodegenerative disease, metabolic diseases
Citation: Xu X, Qi P, Zhang Y, Sun H, Yan Y, Sun W and Liu S (2022) Effect of Selenium Treatment on Central Insulin Sensitivity: A Proteomic Analysis in β-Amyloid Precursor Protein/Presenilin-1 Transgenic Mice. Front. Mol. Neurosci. 15:931788. doi: 10.3389/fnmol.2022.931788
Received: 29 April 2022; Accepted: 21 June 2022;
Published: 07 July 2022.
Edited by:
Jose A. Vega, Universidad de Oviedo Mieres, SpainReviewed by:
Saadia Zahid, National University of Sciences and Technology (NUST), PakistanZehra Batool, University of Karachi, Pakistan
Copyright © 2022 Xu, Qi, Zhang, Sun, Yan, Sun and Liu. This is an open-access article distributed under the terms of the Creative Commons Attribution License (CC BY). The use, distribution or reproduction in other forums is permitted, provided the original author(s) and the copyright owner(s) are credited and that the original publication in this journal is cited, in accordance with accepted academic practice. No use, distribution or reproduction is permitted which does not comply with these terms.
*Correspondence: Wenxiu Sun, c2R3ZW54aXVAMTYzLmNvbQ==; Shudong Liu, bHNkMDA4QDEyNi5jb20=