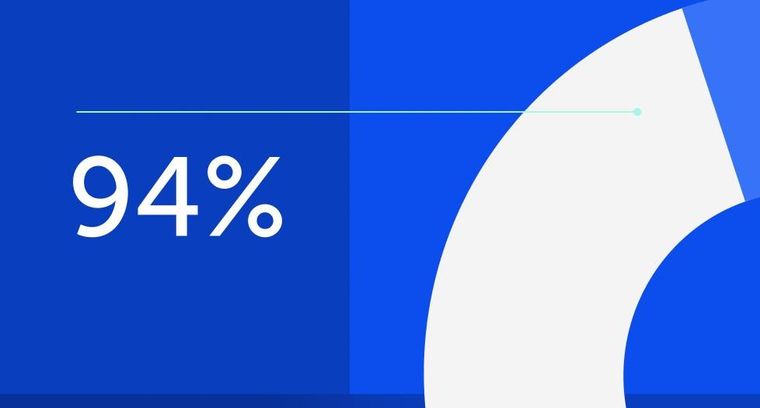
94% of researchers rate our articles as excellent or good
Learn more about the work of our research integrity team to safeguard the quality of each article we publish.
Find out more
REVIEW article
Front. Mol. Neurosci., 15 June 2022
Sec. Molecular Signalling and Pathways
Volume 15 - 2022 | https://doi.org/10.3389/fnmol.2022.919773
This article is part of the Research TopicEndogenous Opioids in Systems NeuroscienceView all 10 articles
Opioids mediate their effects via opioid receptors: mu, delta, and kappa. At the neuronal level, opioid receptors are generally inhibitory, presynaptically reducing neurotransmitter release and postsynaptically hyperpolarizing neurons. However, opioid receptor-mediated regulation of neuronal function and synaptic transmission is not uniform in expression pattern and mechanism across the brain. The localization of receptors within specific cell types and neurocircuits determine the effects that endogenous and exogenous opioids have on brain function. In this review we will explore the similarities and differences in opioid receptor-mediated regulation of neurotransmission across different brain regions. We discuss how future studies can consider potential cell-type, regional, and neural pathway-specific effects of opioid receptors in order to better understand how opioid receptors modulate brain function.
Opioid drugs, which include both prescription painkillers, such as morphine and oxycodone, and illicit substances, such as heroin, are widely used and frequently misused (Kosten and George, 2002; Von Korff, 2013). An increase in prescription of opioid analgesics has precipitated an opioid crisis characterized by widespread opioid misuse, related complications, and opioid overdose (Kosten and George, 2002; Von Korff, 2013; Dahlhamer et al., 2016). This crisis presents a severe health exigency and makes salient a crucial scientific initiative to better understand the effects of opioid drugs and the mechanisms and opioid receptor systems on which these drugs act.
Classically, opioid receptors can be categorized into one of three subtypes: mu (MOR), delta (DOR), and kappa (KOR) (Le Merrer et al., 2009). Endogenous signaling peptides activate opioid receptors: endorphins (MOR), enkephalins (primarily DOR, MOR), and dynorphins (KOR). Opioid peptides or synthetic opioid peptide derivatives are often utilized as selective opioid receptor agonists and antagonists in research. The pharmacology of these diverse ligands is reviewed elsewhere (Rasakham and Liu-Chen, 2011; Gendron et al., 2016; De Neve et al., 2021). Some commonly studied opioid receptor agonists include DAMGO (MOR), DPDPE (DOR), U69,593 or U50,488 (KOR), and the endogenous opioid peptides, met-enkephalin (MetEnk), leu-enkephalin (LeuEnk) (DOR, MOR), and dynorphin (KOR). Commonly used opioid receptor antagonists include CTAP/CTOP (MOR), naltrindole (DOR), and nor-binaltorphimine (KOR) or less selective antagonists such as naloxone. Many opioid drugs, including morphine, fentanyl, and heroin primarily activate MORs (Pasternak, 2012). Opioid receptors are Class A G protein coupled receptors (GPCRs) that couple to inhibitory Gi/o proteins (Figure 1; Stein et al., 2003; Allouche et al., 2014). These receptors transduce extracellular messages using G protein (Gαi and Gβγ), mitogen-activated protein kinase (MAPK), and arrestin signaling pathways (Rosenbaum et al., 2009; Al-Hasani and Bruchas, 2011). Opioid receptors generally decrease neurotransmission through inhibiting voltage-gated calcium channels and activating inwardly rectifying potassium channels (Yamada et al., 1998; Al-Hasani and Bruchas, 2011). Opioid receptors can be located postsynaptically in neuronal soma and presynaptically in axon terminals (Olive et al., 1997). Postsynaptic opioid receptors inhibit neurotransmission by directly hyperpolarizing neurons, while presynaptic opioid receptors can indirectly reduce or enhance neural activity by reducing excitatory or inhibitory neurotransmission, respectively. The opioid receptors and their endogenous ligands are differentially expressed throughout the brain (Le Merrer et al., 2009; Erbs et al., 2015). Because of their widespread expression, opioid receptors are involved in a diverse array of physiological and behavioral functions, including nociception, drug reward and consumptive behavior, social memory, fear learning, stress and emotion, immune activation, and various physiological processes, such as respiration and gastrointestinal tract motility (Shippenberg et al., 1998; Drews and Zimmer, 2010; Van’t Veer and Carlezon, 2013; Leroy et al., 2017; Eisenstein, 2019; Patel et al., 2019; Toubia and Khalife, 2019; van Steenbergen et al., 2019; Robble et al., 2020; Galaj and Xi, 2021).
Figure 1. Summary of potential mechanisms of opioid receptor-mediated modulation of neurotransmission. Opioid receptor activation enhances potassium channel (KV) and inhibits calcium channel (CaV) function, reducing neurotransmitter release or producing changes in postsynaptic excitability. Opioid receptors may modulate adenylyl cyclase (AC) function to reduce cAMP levels, thereby impacting protein kinase A (PKA) and type 1 hyperpolarization-activated cyclic nucleotide-gated (HCN1) channel activity. Beta-arrestin2 (Barr2), phospholipase A2 (PLA2), as well as kinases such as p38, ERK, protein kinase C (PKC), and cSrc have been implicated in mediating opioid receptor effects on neurotransmission. Opioid receptor-mediated G protein signaling could also directly affect neurotransmitter release machinery. Figure created with BioRender.com.
The expanding understanding of opioid receptor functionality, distribution, and modulation of neurotransmission has demonstrated an important role for opioids in modulating neuroplasticity. Neuroplasticity refers to the ability of the brain to change structure and function across life and in response to experience (Voss et al., 2017). The phenomenon is multi-level and can occur across networks, isolated circuits, and amongst cell populations (Citri and Malenka, 2008; Voss et al., 2017). This manifests as changes in functional and structural connectivity, the formation, migration, and elimination of neurons and glia, alterations of neuronal processes, and through synaptic plasticity (Kays et al., 2012; Kelly and Castellanos, 2014). Synaptic plasticity may be persistent with activity-dependent strengthening (long-term potentiation, LTP) and weakening (long-term depression, LTD) of connections between neurons, although there are abundant forms of short-term plasticity as well (Citri and Malenka, 2008; Atwood et al., 2014a; Motanis et al., 2018). Activity-dependent neuroplasticity is mediated by endogenous neurotransmitter systems (Viveros et al., 2007; Bliss and Cooke, 2011; Pitchers et al., 2014). Exposure to exogenous substances (e.g., neurotransmitter receptor agonists, antagonists) can also induce “chemical” plasticity (Atwood et al., 2014a). Neuroplasticity underlies many crucial processes, including learning, cognition, and neurodevelopment, and is implicated in the development of neuropathology, including mood disorders, addiction, and neurodegenerative diseases. Therefore, it is important to elucidate the role of opioid receptors in neuroplasticity (Johansson, 2004; O’Brien, 2009; Kays et al., 2012; Schaefers and Teuchert-Noodt, 2016; Voss et al., 2017). Due to their ability to modulate different neurotransmitter systems, as well as directly influencing cellular function, opioid receptors are positioned to modulate both activity-dependent plasticity and opioid drug-induced chemical plasticity (Lüscher and Malenka, 2011; Beltrán-Campos et al., 2015; Hearing et al., 2018; Hearing, 2019; Puryear et al., 2020).
The goal of this review is to demonstrate how opioid receptors modulate neurotransmission. While opioid receptors modulate a variety of neurotransmitter systems, we have limited the scope of this review to excitatory (glutamatergic) and inhibitory (often GABAergic) transmission and postsynaptic modulation of neuronal excitability. We have focused on brain regions where much work on opioid receptor-mediated regulation of neurotransmission has been performed. A summary of the literature reviewed below is provided in Table 1 and illustrated in Figure 1 as a reference for the reader. Figure 1 also illustrates how opioid receptors differentially impact neurotransmission pre- and postsynaptically. In this review, we focus on the role of opioid receptors themselves, rather than the impact of opioid drugs on general synapse and brain function. The studies reviewed herein utilized electrophysiology techniques in combination with pharmacological manipulation of opioid receptors. Studies investigating subpopulations within brain regions (i.e., input regions, cell types, projection targets) have utilized many techniques, including targeted expression of optogenetic tools, tracing strategies, and reporter animal models. We will discuss potential generalizable principles regarding opioid receptor-mediated neuroplasticity, point out broad knowledge gaps, and suggest areas of future research to advance the field, especially as it relates to cell type- and synapse-specific explorations of opioid receptor function.
Table 1. Summary of effects of mu (MOR), delta (DOR), and kappa (KOR) opioid receptor activation on neuronal excitability (postsynaptic effects), presynaptic GABA release, and presynaptic glutamate release.
The amygdaloid complex is involved with emotional processing and consists of 13 nuclei, categorized as basolateral (basal, lateral, and accessory basal nuclei; BLA), cortical-like (cortical and lateral olfactory tract nuclei, periamygdaloid complex), centromedial [medial (CeM) and central nuclei (CeA)], bed nucleus of stria terminalis (BNST), or other (intercalated nuclei, anterior amygdala area, amygdalohippocampal area) (Sah et al., 2003). The amygdaloid complex has extensive connectivity across the brain, including local connectivity between amygdala nuclei (Pitkänen et al., 1997). MORs in the amygdala are involved with analgesia, fear and anxiety responses, and social behavior (Good and Westbrook, 1995; Wilson and Junor, 2008; Zhang et al., 2013; Lebow and Chen, 2016). Amygdala DORs play a role in modulating ethanol’s effects; however, a functional role of amygdala DORs may not occur until after exposure to drugs of abuse, such as ethanol and morphine (Kang-Park et al., 2007; Bie et al., 2009a,b). Amygdala KORs are involved with anxiety and fear conditioning (Knoll et al., 2011). KOR activation in the amygdala increases anxiety-like behaviors and enhances the rewarding effects of nicotine, possibly due to nicotine’s anxiolytic effect (Smith et al., 2012).
The basolateral amygdala (BLA) is the primary input region of the amygdaloid complex and receives inputs from across the brain, including hippocampus, nucleus accumbens (NAc), prefrontal cortex (PFC), thalamus, and other amygdala nuclei (Huang et al., 2021). In the lateral nucleus, MORs hyperpolarize about 50% of neurons (Sugita and North, 1993). However, a later study found MORs do not directly hyperpolarize BLA neurons, but the activity of BLA neurons is modulated by presynaptic MORs (Blaesse et al., 2015). In the lateral nucleus, MORs and DORs presynaptically inhibit GABAergic input (Sugita and North, 1993). A later study found that MOR enhances voltage-gated potassium channel (Kv) 1.2 currents and enhances action potential (AP) spike adaptation via G protein PLA2 signaling in lateral amygdala (Faber and Sah, 2004). MetEnk inhibits GABAergic input to the BLA from intercalated cells, presumably through MORs (Gregoriou et al., 2019). It is unknown whether MORs regulate GABA transmission from other GABAergic inputs. MOR activation reduces GABAergic input to ∼75% of CeA-projecting BLA neurons via activation of Kv1.1/1.2 channels. Very few CeA-projecting BLA neurons have glutamate input that is inhibited by MOR activation (Finnegan et al., 2006). On the other hand, MOR activation produces a long-lasting depression of dorsal midline thalamic glutamatergic input to BLA neurons. MOR inhibition of midline thalamic input to BLA neurons is sufficient to reduce feedforward excitation of the CeM (Goedecke et al., 2019). These studies suggest MORs may primarily modulate BLA projections to the centromedial amygdaloid nuclei; however, additional studies are needed investigating MOR modulation of BLA projections to other regions.
Kappa activation in BLA enhances presynaptic GABA transmission in a tetrodotoxin (TTX)-sensitive manner with no effect on postsynaptic responses in adolescent, but not adult rats (Przybysz et al., 2017). KORs have no effect on glutamate transmission in BLA in rats. Further exploration of the effects of KOR activation on GABA transmission in adolescent rats showed that KOR activation has a variable effect on GABA transmission with subsets of cells showing potentiation, no responses, or depression (Varlinskaya et al., 2020). Further research is needed to determine if these subsets represent sub-populations with distinct afferents/efferents. In mice, KOR activation reduces synaptic transmission from the lateral amygdala to the BLA and blocks LTP induction in the BLA (Huge et al., 2009). Overall, these studies demonstrate KORs modulate neurotransmission in the BLA and these effects demonstrate species, age, input, and output specificity.
MORs presynaptically inhibit GABAergic transmission to Ventral Tegmental Area (VTA)-projecting neurons in the ventrolateral BNST (Dumont and Williams, 2004). It is unknown whether MORs inhibit GABA transmission to non-VTA-projecting BNST neurons. MOR’s effect on glutamate transmission in the BNST is also unknown. KORs presynaptically inhibit GABAergic input from CeA to BNST via extracellular signal-related kinases (ERK), but not p38 (Li et al., 2012). KOR activation induces presynaptic LTD via p38 (not PKA or MAPK) and calcium signaling in BNST at BLA, but not PFC inputs. Despite KOR-mediated inhibition of GABA transmission, the net effect of KOR activation is to reduce AP firing of BNST neurons. This may be caused by KOR-induced inhibition of glutamate transmission in the BNST. KOR inhibits glutamate onto both dynorphin-positive and dynorphin-negative neurons but has a larger effect on dynorphin-positive neurons (Crowley et al., 2016). Overall, presynaptic MORs and KORs modulate neurotransmission in the BNST; however, while KORs inhibit both GABA and glutamate transmission, MORs have only been shown to inhibit GABA transmission.
MORs inhibit about 60% of CeA neurons, particularly those with bipolar morphology (Chieng et al., 2006). CeA neurons can be characterized as Type A or B based on the absence or presence of spike accommodation in response to prolonged depolarization current. MORs hyperpolarize a subset of Type A neurons through activation of potassium currents, whereas KORs only hyperpolarize Type B neurons (Zhu and Pan, 2004). Separate subpopulations of MOR-inhibited neurons were also inhibited by KORs or DORs. When the investigators looked at projection targets they found that MORs hyperpolarize parabrachial nucleus (PBN)-projecting neurons (Chieng et al., 2006). It is possible MOR-sensitive Type A neurons may specifically project to the CeA, although additional studies are needed to confirm this.
MORs appear to play a role in tonically inhibiting GABA release from synaptic terminals in the CeA. In vivo opioid exposure can also induce postsynaptic MOR-mediated inhibition of GABA current amplitudes (Kang-Park et al., 2009; Bajo et al., 2011). Specifically, periaqueductal gray (PAG)-projecting CeA neurons receive MOR-sensitive GABAergic input (Finnegan et al., 2005). Additional studies are needed to identify MOR-sensitive GABAergic inputs in the CeA. Like MORs, KOR activation inhibits GABA release in CeA in rats (Przybysz et al., 2017) and KORs may also tonically inhibit GABA release (Gilpin et al., 2014; Bloodgood et al., 2021; Khom et al., 2021). Similarly, DORs also inhibit GABA release in the CeA, but there is evidence for species differences. In one study in mice, DOR activation was shown to reduce GABA release; whereas, in another study in rats, DORs did not have an effect on GABA transmission under normal conditions, but gained the ability to do so in ethanol-treated rats (Kang-Park et al., 2007; Bie et al., 2009a). Similar to the CeA, MORs inhibit GABA transmission in the CeM; MetEnk, presumably through MORs, inhibits GABergic input from the nearby intercalated cell region of the amygdala (Gregoriou et al., 2019). MORs on the intercalated cells prevent feedforward inhibition from the BLA to the CeM (Blaesse et al., 2015). Future studies are needed to determine whether KORs or DORs inhibit GABA transmission in the CeM.
In contrast to opioid receptor-mediated effects on GABA transmission, MOR, but not DOR or KOR, activation reduces glutamate input in the CeA but not CeM (Zhu and Pan, 2005; Blaesse et al., 2015). Specifically, a small subpopulation of PAG-projecting neurons in the CeA receive MOR-sensitive glutamate input (Finnegan et al., 2005). A later study determined that MORs inhibit glutamate input to CeA neurons from the parabrachial nucleus and BLA (Kissiwaa et al., 2020), but another study found MORs do not inhibit BLA inputs to CeM neurons (Blaesse et al., 2015). Another study found MOR activation produces a transient depression of dorsal midline thalamic glutamatergic input to CeA neurons (Goedecke et al., 2019). Similar to some studies of CeA GABA transmission, DOR-mediated inhibition of glutamate release may be inducible (Bie et al., 2009a,b). A subset of BLA inputs are dually regulated by KORs and DORs, suggesting that there may be some CeA synapses that are sensitive to KORs and DORs that may not be distinguished when glutamate transmission is probed more broadly, as done previously (Zhu and Pan, 2005; Kissiwaa et al., 2020). In CeA neurons, direct parabrachial glutamatergic input to corticotropin-releasing factor (CRF) neurons is insensitive to KORs; however, KOR activation presynaptically inhibits local GABA neurons that receive parabrachial glutamatergic input, resulting in disinhibition of the CRF neurons (Hein et al., 2021).
GABAergic neurons of the medial island of intercalated cells send inhibitory projections to the BLA and CeM. MORs hyperpolarize these neurons in both rats and mice (Blaesse et al., 2015; Winters et al., 2017). In rats, both MOR and DOR, but not KOR, activation can reduce glutamate release from BLA inputs to intercalated neurons. Endogenous opioid peptide release in the intercalated cell region produces presynaptic inhibition of glutamate release via DORs and postsynaptic hyperpolarization via MORs (Winters et al., 2017). On the other hand, one study found that MORs do not inhibit glutamate input from BLA to the medial intercalated cell region in mice, suggesting possible species differences (Blaesse et al., 2015). MORs also inhibit GABA transmission to intercalated neurons in rats. Direct MOR activation via exogenous agonist application greatly decreases local GABA transmission, although endogenous opioid peptide release has only a minor effect on this inhibitory transmission (Winters et al., 2017).
The brainstem connects the cerebrum to the spinal cord and cerebellum. It regulates respiration, consciousness, blood pressure, heart rate, and sleep (Angeles Fernández-Gil et al., 2010). The midbrain plays key roles in sensory and motor control and has received much attention for its role in reward processing and decision making (Ruchalski and Hathout, 2012). Brainstem and midbrain express the three opioid receptors (Mansour et al., 1987; Le Merrer et al., 2009) and play major roles in drug reward, pain, and respiration (Le Merrer et al., 2009; Dahan et al., 2018; Bagley and Ingram, 2020).
MOR activation presynaptically inhibits glutamate input, but not GABAergic input, consistent with MOR expression in terminals of glutamate, but not GABA neurons of the Dorsal Motor Nucleus of the Vagus (DVM) (Browning et al., 2002). Under normal conditions, opioid agonists fail to influence GABAergic input to these neurons; however, when cAMP signaling is engaged, MOR is trafficked to the synapse and inhibits GABA transmission. This is inhibited by disrupting cAMP and PKA signaling, suggesting that the cAMP-PKA pathway regulates trafficking of MORs into the cell surface of GABAergic nerve terminals (Browning et al., 2004). Conversely, another study found that MOR activation reduces both AP-dependent glutamate and GABA transmission in rat and mouse DVM GABA neurons. MOR activation reduces GABAergic input to DVM neurons from the nucleus of the solitary tract (NTS), potentially due to MORs on the NTS neurons (Glatzer et al., 2007). These data suggest that opioid actions may depend on the state of activation of vagal circuits.
The Locus Coeruleus (LC) has a long history of studies of the impact of opioid receptor-mediated regulation of cellular function due to its high expression of MORs that inhibit LC neuron excitability (Bird and Kuhar, 1977). Recording opioid effects on ion channel function in these neurons is a common methodology for exploring opioid receptor signaling and testing hypotheses regarding receptor desensitization and opioid tolerance (for review, see Allouche et al., 2014). However, a detailed discussion of the many studies of opioid receptor desensitization and tolerance in the LC are beyond the scope of this review. In addition to MOR-mediated regulation of LC neuron excitability, KORs also function in the LC to inhibit glutamate input to LC neurons without affecting postsynaptic currents/membrane potential (McFadzean et al., 1987; Pinnock, 1992b). Local KORs within the LC are targeted by dynorphinergic neurons from other brain regions (Al-Hasani et al., 2013). LC neurons that project to the spinal cord are excited by DOR agonists via inhibition of presynaptic DORs on GABAergic inputs, but without an effect on glutamate input (Pan et al., 2002).
MOR, but not KOR or DOR, agonists hyperpolarize neurons in the medial, dorsomedial and dorsolateral regions of the NTS through increasing potassium conductance (Rhim et al., 1993; Glatzer et al., 2007; Poole et al., 2007). In addition to increasing potassium conductance in these neurons, MORs are able to inhibit N- and P/Q-type voltage-gated calcium channels (VGCCs) in NTS neurons (Rhim et al., 1996; Endoh, 2006). While KORs were not found to hyperpolarize neurons, KORs and MORs were found to inhibit N- and P/Q-type, but not L-type VGCCs via Gβγ, but not PKA signaling (Rhim et al., 1993; Endoh, 2006). These data suggest that opioid receptors use different pathways to induce inhibition in the NTS.
MORs also inhibit synaptic transmission in the NTS. Presynaptic MORs reduce inhibitory input to NTS GABA neurons from solitary tract stimulation (Glatzer et al., 2007). Within the medial NTS, MOR activation blocks tonic GABA currents and reduces GABA release (Herman et al., 2012). Another study found that MOR-mediated local inhibition of GABA transmission was AP-dependent, suggesting MORs on cell bodies may modulate local GABA neurons (Glatzer and Smith, 2005).
Solitary tract glutamatergic input to NTS neurons is inhibited strongly by MOR and weakly by DOR and KOR agonists (Rhim et al., 1993; Glatzer and Smith, 2005; Poole et al., 2007; Boxwell et al., 2013). MOR inhibition is presynaptically localized (Glatzer and Smith, 2005). MORs equally inhibit solitary tract glutamate input to both GABAergic and non-GABAergic NTS neurons (Boxwell et al., 2013). Interestingly, MOR activation is less efficacious when GABA and glycine receptors are blocked (Boxwell et al., 2013). One study specifically recorded from NTS pro-opiomelanocortin (POMC) neurons and found that glutamate input was presynaptically regulated by MORs (Appleyard et al., 2005). On the other hand, in recordings from NTS neurons that project specifically to the PBN, DORs, but not MORs, inhibited solitary tract glutamatergic inputs (Zhu et al., 2009). One study specifically looked at tyrosine hydroxylase (TH)-positive and TH-negative neurons of the NTS (Cui et al., 2012). Like other studies they found that MORs presynaptically inhibited solitary tract input to both of these classes of neurons, but the effect was larger in TH-positive neurons. These data suggest that presynaptically expressed opioid receptors may differentially affect neurotransmitter release.
The PAG is a hot spot for opioid signaling in the brain. MORs hyperpolarize and activate G protein-couple inwardly rectifying potassium channels (GIRKs) in a subpopulation of neurons within the PAG, mostly in lateral and dorsal regions of ventrolateral PAG (vlPAG) (Chieng and Christie, 1994; Vaughan and Christie, 1997; Chiou and Huang, 1999; Vaughan et al., 2003; Chen et al., 2016). Some report that KORs have no effect on GIRK in rat PAG, while the same group report that they do in mice (Chieng and Christie, 1994; Vaughan et al., 2003), suggesting that the animal model used for studying the opioid receptor effects is important. MOR inhibits about half of lateral rostral ventromedial medulla (RVM)-projecting PAG neurons and less than a quarter of RVM-projecting vlPAG neurons through activating an outward current (Osborne et al., 1996). An investigation of the specific responses within different types of PAG neurons shows that MOR activation hyperpolarizes ventral PAG GABA neurons and reduces AP firing (Chen et al., 2016). In serotonergic (5-HT) neurons however, MOR activation hyperpolarizes the neurons but enhances AP firing. In addition to their effects on GIRKs, MORs, but not DORs or KORs, inhibit calcium channels in PAG neurons (Kim et al., 1997; Connor et al., 1999). Some CeA inputs to ventrolateral PAG are sensitive to MOR and DOR activation, responding with both excitation (20% of responses) and inhibition (25% of responses). The identities and types of responses are not clear from this study (da Costa Gomez and Behbehani, 1995). This could be due to changes in neuronal excitability described above or changes in synaptic function described below.
MORs, but not KORs or DORs, presynaptically inhibit glutamate transmission to some degree in all regions of the PAG (Vaughan and Christie, 1997; Chiou and Huang, 1999). Looking at identified cellular targets, MOR decreases glutamate input to both GABA and 5-HT neurons (Chen et al., 2016). MORs presynaptically regulate GABA in all regions of the PAG to both GABAergic and 5-HT neurons, through presynaptic activation of potassium channels and PLA2 (Vaughan and Christie, 1997; Vaughan et al., 1997; Chen et al., 2016). MORs also inhibit GABA input to ventral PAG TH-expressing neurons that project to the BNST and co-release dopamine and glutamate (Li et al., 2016). Interestingly, this involves a short-term reduction in GABA release accompanied by a more persistent inhibition of GABA transmission via a postsynaptic mechanism. Regarding other opioid receptors that modulate GABA transmission, there may be species differences. In rats, only MORs inhibit GABA release; whereas, in mice, KORs, but not DORs, also inhibit GABA release (Vaughan et al., 2003; Li and Kash, 2019). MORs inhibit GABA input to a greater extent than glutamate input in the PAG. The greater inhibition of GABA input overcomes MOR’s effects on glutamate input, as well as hyperpolarization, to increase AP firing of ventral PAG neurons (Chiou and Huang, 1999). The ability of DORs to inhibit GABA release in the PAG is plastic. DOR agonists have no effect on PAG GABAergic transmission in naïve mice but may be induced to do so with chronic morphine treatment (Vaughan et al., 2003; Hack et al., 2005). DOR activation may also inhibit GABA reuptake via GABA transporter type 1 in the PAG (Pu et al., 2012). Overall, presynaptic opioid receptors modulate neurotransmission in the PAG; however, while MORs and KORs inhibit GABA, only MORs inhibit glutamate transmission. DORs have only been shown to inhibit GABA transmission, likely using a different mechanism.
There are two types of cells in the nucleus raphe magnus (NRM) that have differential responses to opioids. Primary 5-HT neurons are hyperpolarized via KOR-mediated GIRK activation (Pan et al., 1997; Li and Wang, 2001). Secondary GABAergic neurons are hyperpolarized by MORs also via GIRK activation (Pan et al., 1997; Li and Wang, 2001). MORs disinhibit primary cells through inhibiting GABA input to these KOR-sensitive cells (Pan et al., 1997). KORs also presynaptically inhibit glutamate input to both primary and secondary NRM cells (Bie and Pan, 2003).
MOR activation hyperpolarizes around 80% of non-5-HT DRN neurons and around 30% of 5-HT neurons, likely through enhancing potassium conductance. MOR activation reduces spontaneous GABA release and NMDA-induced activation of GABA release from local neurons, as well as neurons in the PAG onto 5-HT DRN neurons. As in the PAG, MORs also inhibit GABAergic input to DRN TH-expressing dopaminergic/glutamatergic neurons that project to BNST (Li et al., 2016). DOR and KOR activation have no effect on GABA transmission in these cells. One study found that MOR activation has no effect on glutamate input to 5-HT cells; whereas, a later study found that MORs are able to inhibit glutamate release and suggested this was due to experimental conditions (Pinnock, 1992a; Jolas and Aghajanian, 1997). In the positive study, they found that MORs were able to inhibit local glutamate release as well as glutamate input from the PAG (Jolas and Aghajanian, 1997). KORs are also able to inhibit glutamate input to DRN 5-HT neurons (Pinnock, 1992a). Therefore, MOR and KOR are capable of inhibiting both GABA and glutamate release, however up to the present time there is no evidence that DORs have a role in the Raphe nuclei.
In RVM there are three different cell types that show differential responses to noxious stimuli: ON cells increase firing, OFF cells decrease firing, and NEUTRAL cells show no responses (Sikandar and Dickenson, 2011). MORs and DORs inhibit ON cell responses, increase activity of OFF cells, and have no effect on NEUTRAL cells (Cheng et al., 1986; Harasawa et al., 2000). MOR activation in RVM directly inhibits ON cells. In OFF cells, there are no effects of direct MOR agonist application, suggesting that opioid-mediated excitation of OFF cells is indirect (Heinricher et al., 1992, 1994).
In measures of direct cellular responses, there are two major cell types in RVM that respond to opioids: primary cells and secondary cells. Primary cells have a wider action potential, more negative resting membrane potential, and are not inhibited by MOR agonists. Secondary cells are generally presumed to be inhibitory interneurons that serve only to regulate the activity of the output neurons, have a shorter action potential, are often firing spontaneously, and are mostly hyperpolarized by MOR agonists (Pan et al., 2000; Cleary et al., 2008). Also, primary cells are responsive to KOR activation, producing outward currents (Pan et al., 1990, 2000). Subpopulations of secondary cells are responsive to MOR activation, also producing outward currents. Almost all spinally projecting RVM neurons respond to opioids in some fashion. Subpopulations of these neurons show outward current responses to either only MOR, only KOR, or both receptor activations (Marinelli et al., 2002). Interestingly, MOR responsive secondary cells are similar to ON cells in vivo, and KOR responsive primary cells are similar to OFF cells (Pan et al., 1990). Non-5-HT spinally projecting neurons are almost exclusively MOR responders; whereas, 5-HT neurons have equal proportions of MOR, KOR, and MOR/KOR responders (Marinelli et al., 2002; Zhang et al., 2006; Zhang and Hammond, 2010). About two thirds of TH-expressing and TH-negative bulbospinal neurons are hyperpolarized by MOR via GIRK activation (Hayar and Guyenet, 1998). DORs produce outward currents in subpopulations of RVM neurons (Marinelli et al., 2005). They specifically act in a subpopulation of MOR-regulated non-5-HT spinal cord-projecting neurons, as well as subpopulations of 5-HT spinal cord-projecting neurons that have differential sensitivities to MOR and KOR activation.
MOR activation reduces GABA, but not glutamate input to primary cells (Pan et al., 1990, 2000). MOR activation reduces GABA input likely via inhibition of presynaptic calcium channels, but not glutamate input to RVM neurons; however, it is not clear whether these are primary or secondary neurons due to the recording conditions (Vaughan et al., 2001). Glutamatergic input to secondary cells is presynaptically inhibited by KORs (Ackley et al., 2001). MORs inhibit GABA and glutamate input to bulbospinal TH-expressing and TH-negative neurons through presynaptic mechanisms (Hayar and Guyenet, 1998). In spinal cord-projecting rat RVM neurons MORs inhibit evoked glutamate inputs in ∼50% of cells, miniature excitatory postsynaptic currents (mEPSCs) in 55% of cells, evoked inhibitory inputs in about 70% of cells, and miniature inhibitory postsynaptic currents (mIPSCs) in 100% of cells (Finnegan et al., 2004). MORs agonists frequently activate output neurons in the brain via disinhibition. Thus, direct inhibition of “secondary cells” disinhibits “primary cells” or output neurons, allowing them to become active (Cleary et al., 2008).
MORs, DORs, and KORs, have all been reported to modulate substantia nigra GABA release (Starr, 1985). KOR activation presynaptically inhibits glutamate transmission in Substantia Nigra (SN) pars reticulata (Maneuf et al., 1995). KORs can inhibit type-2 dopamine receptor (D2R)-mediated IPSCs in dopamine neurons of the SN pars compacta (Ford et al., 2007). The mechanism is unclear, given that KORs can both hyperpolarize and prevent IPSCs in the same neuron and this is not due to modulation of cAMP, kinases, calcium, or potassium channels. Overall, opioid receptors may play a role in regulating neurotransmitter release, however, more research is needed to clarify the specific actions of each of the different opioid receptors.
MORs hyperpolarize local GABA neurons within VTA, but not dopamine neurons, leading to greater excitation of dopamine neurons (Johnson and North, 1992). MORs can hyperpolarize secondary VTA cells, that are largely GABAergic as well as tertiary VTA cells that are NAc-projection neurons (Cameron et al., 1997). MOR-induced hyperpolarization of local GABAergic neurons rapidly desensitizes (Lowe and Bailey, 2015). In the Rostromedial Tegmental Nucleus (RMTg), also known as the tail of the VTA, neuron firing rate is reduced by MOR activation and RMTg neurons are hyperpolarized by MOR agonists, but not DOR or KOR (Lecca et al., 2011; Matsui and Williams, 2011). Contrary to other studies that find that MORs do not hyperpolarize dopamine neurons, there may be some dopamine neurons that express MORs. MORs can hyperpolarize some VTA dopamine neurons via increasing potassium conductance or exciting them via P/Q type calcium channel (Cav2.1) inhibition (Margolis et al., 2014, 2017). DPDPE-sensitive and deltorphin II-sensitive DORs are differentially expressed in different types of VTA neurons and produce a heterogeneous response: hyperpolarizing neurons via increasing potassium conductance or exciting neurons via Cav2.1, similar to MOR (Margolis et al., 2017). Interactions between the two different functional forms of DOR and MOR is not consistent between neurons, although receptor antagonist experiments reveal that functional interactions between the two different receptors do occur. KORs hyperpolarize VTA dopamine neurons via increasing potassium conductance (Margolis et al., 2003; Ford et al., 2007). Interestingly, only a subset of these neurons are disinhibited by MOR activation. KORs hyperpolarize VTA neurons that project to medial PFC (mPFC), but not to NAc (Margolis et al., 2006). Consistent with this, infusion of KOR agonist into VTA decreases dopamine levels in the mPFC, but not the NAc. Amygdala-projecting dopamine neurons within the VTA are also hyperpolarized by KOR activation (Margolis et al., 2008b). VTA dopaminergic neurons that project to NAc are more inhibited by KOR activation that produces outward currents (Ford et al., 2006). In contrast, VTA neurons that project to BLA (which are mostly dopaminergic) are more inhibited by MOR activation, also producing outward currents (Ford et al., 2006).
MORs reduce GABA transmission in VTA via inhibition of GABA release (Bergevin et al., 2002; Xiao and Ye, 2008; Matsui et al., 2014; Bull et al., 2017). MOR activation silences GABAergic VTA neuron firing and reduces evoked and spontaneous TTX-sensitive GABA release (Xiao and Ye, 2008). Knockout of MORs from NAc medium spiny neurons (MSN) reduces the ability of MORs to inhibit GABA input to local VTA GABA interneurons in VTA (Charbogne et al., 2017). Mechanisms for MOR-mediated GABA release inhibition implicate presynaptic potassium channels, beta-arrestin2, and proto-oncogene tyrosine-protein kinase Src (Bergevin et al., 2002; Bull et al., 2017). Contrary to postsynaptic MOR effects, presynaptic MORs on GABA terminals are resistant to desensitization, except when PKC is activated (Lowe and Bailey, 2015). MetEnk, presumably though MOR activation, reduces GABAergic input equally onto NAc- and BLA-projecting dopamine neurons (Ford et al., 2006). MOR regulates VTA GABAergic transmission at local interneuron synapses as well as at GABAergic inputs from the NAc, PAG, RMTg, and ventral pallidum (Matsui and Williams, 2011; Xia et al., 2011; Matsui et al., 2014; St Laurent et al., 2020). Comparing inputs to VTA dopamine neurons, one study found that MOR activation produces the greatest inhibition RMTg inputs, with very low inhibition of local interneuron input and moderate inhibition of NAc inputs (Matsui et al., 2014). A different study however concluded that MOR-modulated NAc inputs to VTA targeted VTA GABA neurons and not VTA dopamine neurons (Xia et al., 2011). MORs inhibit GABAergic input from the ventral pallidum onto both dopamine and non-dopamine neurons (Hjelmstad et al., 2013). Various forms of GABAergic plasticity occur at many of these synapses. Inhibitory LTD at RMTg-VTA dopamine neuron synapses occurs independently of MOR activation, however LTP at PAG-VTA neuron synapses is blocked by MOR activation (St Laurent et al., 2020). A variety of in vivo drug exposures and painful conditions shift the ability of MORs to regulate VTA GABA transmission (Shoji et al., 1999; Margolis et al., 2008a; Xiao and Ye, 2008; Guan and Ye, 2010; Madhavan et al., 2010; Graziane et al., 2013; Polter et al., 2014; Hipolito et al., 2015).
MORs and KORs non-occlusively reduce GABA input to VTA dopamine neurons (Shoji et al., 1999). GABAergic inputs from RMTg to VTA dopamine neurons are insensitive to KOR activation (Matsui and Williams, 2011). KOR activation has little effect on fast, GABAA-mediated IPSCs recorded in NAc-projecting cells, but inhibits fast, GABAA-mediated IPSCs in BLA-projecting cells (Ford et al., 2006). On the other hand, KOR activation inhibits GABAB-mediated slow IPSCs: KORs inhibit GABAergic input to both BLA- and NAc-projecting cells, but this effect is stronger in NAc-projecting cells.
There is a minor role for DORs in regulating VTA GABA transmission under normal conditions, but as in other brain regions, DOR-mediated inhibition of GABA transmission is inducible by in vivo drug exposure (Margolis et al., 2008a; Mitchell et al., 2012; Bull et al., 2017). Following stress exposure, DORs gain the ability to produce postsynaptic insertion of GABAA receptors in a subset of neurons, via phosphoinositide 3-kinase (PI3K) and Akt signaling (Margolis et al., 2011). DORs do not regulate RMTg GABA synaptic inputs (Matsui and Williams, 2011).
Presynaptic MOR activation in VTA reduces glutamate transmission onto dopamine and non-dopamine neurons (Bonci and Malenka, 1999; Manzoni and Williams, 1999). In principal VTA neurons, which are primarily dopaminergic, KOR activation produces a small inhibition of glutamate input, whereas MORs produce a larger inhibition; these are non-occlusive indicating inhibition of separate populations of inputs (Margolis et al., 2005). In secondary neurons, KORs and MORs produce similar inhibition of glutamate input and the responses to each receptor activation are positively correlated. In tertiary neurons, of which a small percentage are dopaminergic, KOR and MORs similarly inhibit glutamate input, but the magnitudes of inhibition are not correlated when dually tested in each cell. These effects are largely presynaptic, although neurons with postsynaptic KOR effects are more sensitive to MOR inhibition of glutamate input and vice versa (Margolis et al., 2005). MORs also inhibit glutamate input to RMTg neurons (Lecca et al., 2011). The LTPGABA described above can be acutely blocked by glutamatergic presynaptic MOR activation, removing the glutamate necessary for plasticity induction (Nugent et al., 2007). The role of MOR-mediated regulation of glutamate as part of the local VTA microcircuit is important to not overlook. For example, in order for morphine to activate VTA dopamine neurons, there must be a VTA glutamatergic tone for MOR-mediated inhibition of RMTg inputs to have an effect (Jalabert et al., 2011).
Altogether, these studies indicate that opioid receptor activation has a broad effect on the VTA, targeting GABA, glutamate and dopamine transmission. Therefore, VTA opioid receptors have a key clinical relevance on the control of dopamine modulation. Although there has been much investigation of opioid receptor function in VTA, there is certainly more discover regarding the cell type- and synapse-specific function of the different opioid receptors in the VTA.
The cortex is involved with many higher functions, including planning, processing sensory information, memory, decision making, and emotional processing (Lamotte et al., 2021; Nadeau, 2021; Kolk and Rakic, 2022). All three opioid receptors are found in the cortex; the presence, modulation of neural activity, and behavioral role of cortical opioid receptors varies across different cortical areas, and these are involved with analgesia, morphine-induced locomotor sensitization, reducing anxiety, and with the rewarding and locomotor stimulation effects of opioids (Saitoh et al., 2018; Wang et al., 2020; Jiang et al., 2021).
Many early studies of opioid receptor responses in cortex failed to identify which specific cortical regions were being explored or looked across regions non-specifically. In rat cortical brain slices MOR, DOR, and KOR agonists inhibit evoked glutamate and GABA release (Bradford et al., 1986). In addition, extracellular recordings show that MOR, DOR, and KOR agonists reduce glutamate-evoked neuronal firing (Janiri et al., 1988). However, in contrast, potassium-evoked glutamate release in rat cerebral cortex brain slices is inhibited by MOR and KOR agonists, but not DOR agonists (Nicol et al., 1996). Cultured mouse neocortical neurons express postsynaptic MORs that co-localize with AMPARs (Liao et al., 2005). Activation of these MORs inhibits glutamate transmission and induces dendritic spine retraction. Similarly, morphine inhibits glutamate release from cortical synaptosomes via inhibition of voltage-gated calcium channels (Yang et al., 2004). GABAergic cortical interneurons are inhibited by MORs via membrane hyperpolarization through increased potassium conductance (Ferezou et al., 2007). Unlike cortical GABAergic interneurons, MOR mRNA was not found in pyramidal neurons and MOR activation had no postsynaptic effects in these neurons. There was nearly a complete overlap in interneurons that responded to DAMGO and to nicotinic acetylcholine receptor (nAChR) agonist, DMPP. nAChR activation induced AP firing in interneurons and IPSCs in pyramidal neurons that were both inhibited by MOR activation. nAChR-induced GABAergic input to pyramidal cells was multiphasic, with an initial increase in IPSCs and a subsequent decrease below baseline levels. The decrease was blocked by a MOR antagonist, suggesting that nAChR activation induces enkephalin release as a form of feedback control.
The Anterior Cingulate Cortex (ACC) is involved with emotion and reward processing, learning, and memory (Rolls, 2019). Met-Enk inhibits spontaneous, acetylcholine-evoked, and glutamate-evoked neuronal activity in the ACC (Palmer et al., 1978). In a subset of rat layer 5 ACC pyramidal neurons, DOR, but not MOR, activation produces direct hyperpolarization, presumably through a postsynaptic increase in potassium conductance (Tanaka and North, 1994). In comparison, MOR, but not DOR, activation hyperpolarizes a subset of non-pyramidal neurons. Met-Enk inhibits glutamate and GABA transmission in ACC neurons. This effect is mimicked by DOR, but not MOR agonist, suggesting the effect is mediated by DORs. However, a later study found MORs specifically inhibit midline thalamus inputs to layers 2/3 and layer 5 anterior cingulate cortex pyramidal neurons and parvalbumin (PV)-expressing interneurons. DORs inhibit interneurons that receive MOR-positive medial thalamic input to regulate feedforward inhibition to pyramidal neurons. Ultimately, DORs function to disinhibit thalamocortical circuits (Birdsong et al., 2019).
The insular cortex is involved with interoception, emotion, cognition, and motivation (Namkung et al., 2017). Anterior agranular insular cortex GABAergic neurons express KORs that function to disinhibit L5 pyramidal cell inputs to the SN (Pina et al., 2020). Dynorphin decreases GABA release, but increases glutamate release, leading to disinhibition. In L5 of rat insular cortex, paired recordings between nearby GABA neurons and other GABA neurons or pyramidal cells revealed the role of MOR in regulating these synapses (Yokota et al., 2016). MOR activation reduces fast-spiking interneurons (FSI) input to other FSIs, but not to pyramidal neurons. MOR activation also reduced GABAergic input to FSIs from non-FSI neurons. In contrast, DOR activation reduced FSI input to both other FSIs and pyramidal neurons but had no effect on inhibitory transmission from non-FSI GABA neurons. All inhibition is presynaptically localized. KOR activation has no impact on FSI inputs to other insular cortex neurons.
The Medial Prefrontal Cortex (mPFC) is involved with many cognitive functions and is comprised primarily of excitatory pyramidal neurons and a smaller population of inhibitory interneurons (Xu et al., 2019). MORs inhibit both non-pyramidal and pyramidal mPFC neurons, but through different mechanisms. In non-pyramidal neurons, MORs inhibit sodium conductance through a G protein, PKA, and PKC pathway (Witkowski and Szulczyk, 2006). In pyramidal neurons, MORs inhibit N-type VGCCs through a cAMP-PKA pathway (Rola et al., 2008). DORs can both inhibit and disinhibit pyramidal neuron activation. Presynaptic DORs inhibit prelimbic mPFC principal neurons through inhibiting glutamate release onto these neurons (Yamada et al., 2021). On the other hand, DORs increase GABA transmission from somatostatin-expressing interneurons to PV-expressing interneurons, which disinhibits pyramidal neurons, which MORs do not do (Jiang et al., 2021). KORs also inhibit neurotransmission in mPFC. KOR activation reduces glutamate release onto mPFC pyramidal neurons (Tejeda et al., 2013). Specifically, BLA glutamatergic inputs to mPFC are inhibited by KOR activation in in vivo extracellular recordings in anesthetized rodents (Tejeda et al., 2015).
MORs presynaptically inhibit GABA release onto pyramidal neurons of the rat ventrolateral Orbitofrontal Cortex (OFC) (Qu et al., 2015), consistent with identified expression of MOR in these GABA cells (Huo et al., 2005). MOR-LTD of presynaptic FSI PV-expressing neurons inhibit GABAergic input to pyramidal neurons of medial, but not lateral OFC. Stimulating cAMP production shifts MOR activation to produce short-term depression rather than LTD. Endogenous opioid LTD can be induced via moderate frequency stimulation in the presence of peptidase inhibitors, but not low frequency stimulation (Lau et al., 2020).
MetEnk and LeuEnk inhibit a subset of sensorimotor cortical neurons, some of which are hyperpolarized by MOR agonists (Stanzione et al., 1989). In the somatosensory cortex, MORs and DORs inhibit spontaneous neuronal firing and glutamate-induced firing activity. In a subset of cells, dynorphin inhibits firing and in some recordings where dynorphin had little effect alone, it attenuated the effects of MOR and DOR activation (Janiri et al., 1988).
Overall, these studies indicate opioid receptor effects on neurotransmission and neural activity within cortical areas show great diversity across region, cell type, and neural pathways. As discussed, in some cortical regions, opioid receptor effects have been shown to occur via different mechanisms than in other regions. Additional studies are needed to evaluate circuit-specific opioid receptor regulation of neurotransmission throughout the cortex in order to more fully understand the impact of opioids on higher brain function.
The hippocampus is a brain region crucial to facilitating memory, learning, and spatial processing (Bird and Burgess, 2008). All three opioid receptors are heterogeneously distributed throughout the entire hippocampus and are regulated by the endogenous opioids dynorphin and enkephalin (Simmons and Chavkin, 1996).
In measures of population spike (PS) amplitudes in CA1, both MOR and DOR enhance amplitudes in CA1 (Lee, 1978; Dunwiddie et al., 1980; French and Zieglgansberger, 1982; Valentino and Dingledine, 1982; Dingledine et al., 1983; Bostock et al., 1984; Vidal et al., 1984; Dunwiddie and Su, 1988; Neumaier et al., 1988; Moudy et al., 1989; Wimpey et al., 1989; Pieretti et al., 1994). Morphine increases hippocampal activity in CA1 in slice and in freely moving animals (Linseman and Corrigall, 1982). MORs, but not DORs or KORs, increase the duration of CA1 field potentials (Pieretti et al., 1994). The timing of MOR activation can also determine whether it can enhance CA1 function. MOR activation prevents the inhibitory effects of temporo-ammonic pathway stimulation on Schaffer collateral inputs to CA1 when the timing of stimulation of the two pathways was further apart than one theta cycle, but had no effect when timing was less than one theta cycle (McQuiston, 2011).
The effects of MOR and DOR activation are likely not due to effects on pyramidal cells themselves, although KORs might have some effects on pyramidal cell potassium currents (Madamba et al., 1999). Rather, opioid receptor-induced enhancement of population spike amplitudes is due to disinhibitory mechanisms (Zieglgansberger et al., 1979; Corrigall and Linseman, 1980; Dunwiddie et al., 1980; Neumaier et al., 1988; Lupica and Dunwiddie, 1991; Miller and Lupica, 1994; McQuiston, 2007, 2008; Tian et al., 2015). Specifically, opioids hyperpolarize GABAergic interneurons within CA1 and reduce GABA input to pyramidal neurons (Madison and Nicoll, 1988; Lupica and Dunwiddie, 1991; Lupica et al., 1992; Lupica, 1995; Capogna et al., 1996; Lafourcade and Alger, 2008; Krook-Magnuson et al., 2011; Banghart et al., 2018; Fan et al., 2019). Although DORs can inhibit GABA transmission, they do not appear to be the primary mediators of these effects (Watson and Lanthorn, 1993; Lupica, 1995). MORs can reduce feedforward and feedback inhibition, whereas DORs do not. However, both MORs and DORs are able to inhibit spontaneous GABA transmission, but not monosynaptic inhibitory postsynaptic potentials (IPSPs) (Lupica et al., 1992). However, some of the complexity may be attributable to how MORs and DORs individually regulate GABA transmission in local circuits. In CA1, MORs inhibit interneuron input to the soma, whereas DORs inhibit input to dendrites of pyramidal neurons (Svoboda et al., 1999). In support of this, one study showed that MORs inhibit FSI GABA, but not regular spiking GABA basket cell input to CA1 pyramidal neurons (Glickfeld et al., 2008; Shao et al., 2020). However, a very recent study showed that both MORs and DORs independently activate GIRK in PV neurons as well as inhibit GABA release on to pyramidal neurons (He et al., 2021). MORs hyperpolarize FSI basket cell neurons, but not regular spiking basket cell neurons. FSIs typically synapse on to somas, whereas regular spiking neurons synapse on to dendrites (Straub et al., 2016). MORs also inhibit neuropeptide Y (NPY)-expressing neurogliaform interneurons through membrane hyperpolarization (Krook-Magnuson et al., 2011). In addition, MOR specifically reduces tonic firing of the Ivy class of neurogliaform cells in CA1, reducing GABAergic input to pyramidal neurons (Krook-Magnuson et al., 2011).
The ability of MORs and DORs to disinhibit CA1 pyramidal cell function can be pathway and layer specific and may explain some of the confusing results regarding DOR activation and the broader effect of MOR activation. MORs, but not DORs, mediate feedforward inhibition from Schaffer collateral input (Rezai et al., 2013). However, DORs are expressed in interneurons within CA1 that receive input from the temporo-ammonic pathway, but not the Schaffer collateral pathway. Both MOR and DOR mediate feedforward inhibition from the temporo-ammonic pathway. While MOR enhances excitatory transmission in all layers, it is most effective at enhancing propagation through CA1 output layers (McQuiston, 2007, 2008). Stimulating CA2 pyramidal neuron input to CA1, MOR activation prevented feedforward inhibition of CA1 pyramidal neurons in deep layer and excitatory radiatum giant cells layers, but not pyramidal neurons in superficial layers through its inhibition of FSI interneurons (Nasrallah et al., 2019). MORs can also enhance excitation of pyramidal cells through enhancing excitatory responses to acetylcholine receptor activation (Kearns et al., 2001). Along with this, MOR activation can inhibit cholinergic receptor-induced cholecystokinin-expressing basket cell-mediated theta oscillations in CA1 (Nagode et al., 2014).
Opioid receptors can also have an inhibitory effect on CA1 function. LTD in CA1 is blocked by naloxone and enhanced by MOR, but not DOR or KOR activation (Francesconi et al., 1997; Wagner et al., 2001). Prior fentanyl exposure enhances LTD expression in CA1 as well (Tian et al., 2015).
KOR and DOR activation in CA2 increases the PS following stratum radiatum stimulation (Vidal et al., 1984). Presynaptic DORs produce GABAergic LTD at FSI PV-expressing basket cell inputs to pyramidal neurons of CA2, but only short-term depression in CA1 (Piskorowski and Chevaleyre, 2013). The DOR effects enable long-lasting potentiation of CA2 transmission following high frequency stimulation of Schaffer collateral inputs that prevents the strong feedforward inhibition of CA3-CA2 transmission through DOR-mediated inhibitory LTD (iLTD) (Nasrallah et al., 2015). DOR-mediated iLTD acts as a gate for feedforward inhibition in CA2 to allow for greater activation of CA2 pyramidal neurons in response to both distal and proximal glutamatergic synaptic drive (Nasrallah et al., 2017). DOR antagonists block input timing-dependent plasticity in CA2, likely preventing the iLTD of PV-expressing inputs to pyramidal neurons (Leroy et al., 2017).
Mossy fiber stimulation induces a potentiation of glutamate transmission in stimulated pathway of guinea pig CA3, but inhibition of nearby mossy fiber synapses (Weisskopf et al., 1993). Dynorphin presynaptically inhibits these other mossy fiber pathways; inhibiting KOR signaling allows for LTP induction in this other pathway. Dynorphin is more effective at inhibiting synapses that had undergone LTP induction than those that did not. KOR effects on CA3 LTP are mediated by a non-voltage-gated channel, calcium-dependent process (Castillo et al., 1996). KORs inhibit NMDAR-mediated currents in CA3 of guinea pig hippocampus, but DORs and MORs do not (Caudle et al., 1994). KOR modulation of mossy fiber signaling within CA3 does not occur in Sprague-Dawley rats, but does occur in other rodents. MORs equally inhibit mossy fiber transmission in rats and guinea pig (Salin et al., 1995). Species differences could be due to differential KOR expression. KOR activation enhances the voltage-dependent potassium current known as the M-current [I(M)] in rat CA3 pyramidal neurons, whereas DOR activation reduces I(M) (Moore et al., 1994). DOR antagonists inhibit IPSCs in CA3, but do not block LTP (Krug et al., 2001; Leroy et al., 2019).
MOR activation has no effect on excitatory postsynaptic potentials, but instead reduces IPSPs (Capogna et al., 1993). Activation of DORs and KORs does not inhibit IPSPs. MOR-mediated presynaptic inhibition of GABA transmission produces disinhibition that is G protein mediated and blocked by PKC activation but does not involve potassium or calcium conductance changes (Capogna et al., 1993, 1996). Later studies show that opioid analgesics that activate MORs can inhibit glutamate transmission in CA3, contrary to earlier studies (Lu et al., 2020, 2021).
Morphine increases hippocampal activity in dentate gyrus in slice and in freely moving animals (Linseman and Corrigall, 1982). Within the dentate gyrus, MOR activation enhances LTP induction and naloxone prevents LTP induction of the lateral, but not medial perforant pathway (Bramham et al., 1991; Xie and Lewis, 1991; Sagratella et al., 1996; Ito et al., 2001). Interestingly, electrophysiological studies of MOR knockout mice demonstrated an inability to form LTP in the DG but not in CA1, indicating that MOR activation was crucial to LTP in the DG, but not in CA1 (Matthies et al., 2000). LTP of synaptic transmission is blocked by a DOR antagonist, without affecting potentiation of the population spike (Bramham et al., 1991; Krug et al., 2001). Perforant pathway stimulation-induced opioid peptide release with a resultant MOR- and DOR-mediated disinhibition is crucial to facilitating LTP in the dentate gyrus (Bramham and Sarvey, 1996; Ito et al., 2001). In contrast to their lack of effect in CA1, KOR activation in dentate gyrus prevents LTP induction, in contrast to MOR-induced enhancement of LTP (Sagratella et al., 1996).
MORs and DORs hyperpolarize granule cells in the dentate gyrus (Piguet and North, 1993). A study showed that activation of KORs in dentate gyrus produces hyperexcitable granule cells through a postsynaptic G protein-Kv4.2 A-type potassium current mechanism, but without a change in resting membrane potential or input resistance (McDermott and Schrader, 2011).
As in CA1–CA3 areas of hippocampus, opioid receptors in the dentate gyrus also produce disinhibition via their actions on GABAergic neurons; although, it appears that this disinhibition has less of an effect on LTP induction at dentate gyrus synapses. Consistent with this, MOR, DOR, and KOR activation enhance excitatory transmission in dentate gyrus granule cells, likely due to disinhibition. MOR activation is the most efficacious (Neumaier et al., 1988). MORs and DORs inhibit GABA transmission in the dentate gyrus (Piguet and North, 1993). In granule cells, MORs inhibit GABAA and GABAB-mediated IPSCs (Shao et al., 2020). Dentate gyrus population spikes are potentiated by morphine through disinhibition, but morphine does not affect LTP induction itself (Akaishi et al., 2000).
While some studies show that KORs can enhance excitatory transmission in dentate gyrus, other studies demonstrate that KOR has more of an inhibitory effect due to effects on glutamate transmission (Neumaier et al., 1988). In guinea pig dentate gyrus, KOR activation reduces PS amplitude, while DOR and MOR had no effect. KOR activation inhibits glutamate transmission from perforant path inputs, without affecting GABA transmission (Wagner et al., 1992). A combination of brain slice electrophysiology, pharmacological probing, and anatomical lesioning revealed that KOR activation in dentate gyrus presynaptically inhibits glutamate release (Simmons et al., 1994). Activation of KORs inhibits LTP formation between the perforant path and granule cells of the guinea pig dentate gyrus (Terman et al., 1994). KORs inhibit hilar mossy fiber collateral-based LTP of guinea pig dentate gyrus granule cells, the latter of which likely occurs in a GABAA-dependent mechanism (Terman et al., 2000). A recent study showed MORs can inhibit glutamate transmission in dentate gyrus, specifically, NMDAR-mediated, but not AMPAR-mediated, EPSCs (Shao et al., 2020).
The hypothalamus coordinates the neuroendocrine system (Swaab et al., 1993) and regulates metabolism, reproduction, and parental behavior (Travaglio and Ebling, 2019; Evans et al., 2021; Orikasa, 2021). Hypothalamic neurons release several neurotransmitters and peptides, including GABA, glutamate, dopamine, growth hormone-releasing hormone, gonadotropin-releasing hormone, oxytocin, and vasopressin (Kim et al., 2020). All three opioid receptors are expressed in the hypothalamus (Tavakoli-Nezhad and Arbogast, 2010; Chu Sin Chung and Kieffer, 2013).
In the Arcuate Nucleus (AN), MORs most likely inhibit only oxytocin cells, not vasopressin cells (Wakerley et al., 1983). MOR activation hyperpolarizes a subset of neurons by inducing outward current with inward rectification with no effect of TTX. Some of these MOR-sensitive cells are POMC neurons (Loose et al., 1991; Pennock and Hentges, 2011). MOR activation induces outward potassium currents in POMC neurons within the AN (Ibrahim et al., 2003). MORs act as autoreceptors, having direct effects and reducing AP firing within the recorded neuron, but can have similar effects in non-POMC neurons (Kelly et al., 1990, 1992; Lagrange et al., 1994). MORs also inhibit gonadotropin-releasing hormone-expressing neurons (Lagrange et al., 1995). DORs specifically hyperpolarize non-POMC AN neurons, while KORs do not appear to hyperpolarize AN neurons (Loose and Kelly, 1990; Pennock and Hentges, 2011). Interestingly, POMC neurons are directly inhibited by dynorphin A through activation of potassium conductance (Zhang and van den Pol, 2013; Pennock and Hentges, 2014). Previously it was considered that was due to KOR activation (Zhang and van den Pol, 2013). However, follow up studies found that this was likely due to actions of dynorphin A on MORs (Pennock and Hentges, 2014). Later studies determined KORs do hyperpolarize a subset of AN neurons, specifically NPY neurons (Zhang and van den Pol, 2013). In the AN, KOR activation reduces AP firing of neurons that express dynorphin, indicating that these receptors serve as autoreceptors (Ruka et al., 2013, 2016). Looking at synaptic transmission, in AN, MORs and KORs, but not DORs, presynaptically reduce glutamate input (Emmerson and Miller, 1999). Presynaptic MORs and KORs inhibit glutamate and GABA input to POMC neurons (Pennock and Hentges, 2011; Zhang and van den Pol, 2013). In comparison, a DOR agonist was unable to inhibit evoked GABA release but had a modest inhibitory effect on basal GABA transmission; although, it was not clear what the cause of this discrepancy was (Pennock and Hentges, 2011). MOR-mediated inhibition of GABA input is more sensitive than that of postsynaptic hyperpolarization, suggesting there may be opioid peptide concentration-dependent local circuit dynamics at play (Pennock and Hentges, 2011).
The preoptic hypothalamus plays a role in thermoregulation, where the neurons can be characterized by their thermosensitivity (impulses s–1°C–1) by the thermal coefficient (TC). Preoptic area neurons are hyperpolarized by MOR activation (Wagner et al., 2000). MOR activation-induced hyperpolarization reduces tonic firing activity of all types of neurons and reduces the temperature sensitivity of warm-sensitive neurons (neurons with a TC ≥ 0.8 impulses s–1°C–1) (Yakimova, 2006). In the ventrolateral preoptic area, morphine reduces the firing rate and hyperpolarizes sleep-promoting neurons (as assessed by sensitivity to norephinephrine treatment) but has no effect on non-sleep-promoting interneurons (Wang et al., 2013). The investigators found that this was due to dually activated MORs and KORs.
In the Paraventricular Nucleus (PVN), LTD of glutamate input to vasopressin neurons is induced by paired stimulation that combines metabotropic glutamate receptor (mGluR) 1/5 activation with postsynaptic activity to cause somatodendritic dynorphin release that acts at presynaptic KORs (Iremonger et al., 2011). Presynaptic KOR activation mediates synaptic depression via inhibition of glutamate release downstream of calcium channel opening that the investigators predict is due to actions on release machinery (Iremonger and Bains, 2009). PVN parvocellular neurons can undergo LTD of GABAergic input via mGluR5-driven L-type calcium channel-dependent somatodendritic enkephalin release to act on presynaptic MORs. This iLTD requires ongoing MOR activation, as it is reversible by naloxone (Wamsteeker Cusulin et al., 2013). The released enkephalin can spread to other nearby GABA and glutamate synapses to produce pathway-independent LTD as well.
KORs inhibit both oxytocin and vasopressin neurons of the Supraoptic Nucleus (SON), whereas MORs and DORs primarily inhibit oxytocin neurons (Inenaga et al., 1990). KORs inhibit neuron function by limiting calcium entry to reduce AP firing (Inenaga et al., 1994). In magnocellular neurons of the SON, MORS, but not KORs or DORs, inhibit postsynaptic N- and P/Q-type voltage-gated calcium channels (Soldo and Moises, 1998). In oxytocin neurons of the SON, naloxone treatment increases post spike excitability in vivo, suggesting an endogenous MOR tonic activation. The authors discovered that morphine treatment likely engages potassium conductances that are relieved during naloxone-precipitated opioid withdrawal, resulting in hyperexcitable oxytocin neurons, with no effects in nearby vasopressin neurons (Brown et al., 2005). MOR effects on magnocellular neurons are weak, due to inhibition of glutamate input (presynaptic), with no effects on GABA or postsynaptic effects (Liu et al., 1999). Glutamatergic and GABAergic input to magnocellular neurons is decreased presynaptically by MOR activation, with no apparent postsynaptic effects. MOR-mediated inhibition appears to be independent of inhibition of calcium channels or activation of potassium channels. KORs are also able to inhibit GABAergic input to a subpopulation of magnocellular neurons (Honda et al., 2004). Vasopressin magnocellular SON neurons were recorded in organotypic slice cultures to measure rhythmic firing patterns. KOR-mediated inhibition of glutamate release is part of the mechanism that governs the rhythmic firing of these neurons (Israel et al., 2010). This is supported by in vivo measures that show that KOR activation influences rhythmic firing of vasopressin, but not oxytocin, neurons of the SON (Brown et al., 1998). Dynorphin is co-released with vasopressin from the dendrites of these neurons (Brown and Bourque, 2004).
The hypothalamus is a region of great cell-type heterogeneity across hypothalamic nuclei. Both presynaptic and postsynaptic MORs and KORs have been shown to regulate hypothalamus neurons; although, the effect and mechanism varies across nuclei and cell-type. The role of DORs in the hypothalamus is less clear, as studies have found conflicting results. This may be due to a limited effect of DORs in subpopulations of hypothalamic neurons, but additional studies are needed to understand how DORs regulate neurotransmission in the hypothalamus. Most research of opioid receptor regulation of neurotransmission in the hypothalamus has focused on only a handful of hypothalamic nuclei, leaving much to be discovered. Interestingly, MORs and KORs have been shown to act as autoreceptors in multiple hypothalamic nuclei. Future studies will reveal if these opioid receptors also act as autoreceptors in other hypothalamic nuclei.
The lateral habenula (LHb) regulates reward, aversion, motor and cognitive function, sleep and circadian rhythms, pain, navigation, and maternal behaviors (Hu et al., 2020). It is not clear if DOR is expressed in this area, however, MORs and KORs are expressed, suggesting a role in reward, analgesic and stress responses (Gardon et al., 2014; Simmons et al., 2020). In the LHb, MOR activation has subpopulation effects: some neurons show hyperpolarization, some neurons show reduced glutamate synaptic input, and some neurons show reduced GABA input (Margolis and Fields, 2016). KOR activation in LHb presynaptically inhibits glutamate transmission, but has both inhibitory and enhancing effects on GABA transmission (Simmons et al., 2020). The net impact of KOR on regulating glutamate and GABA transmission produces KOR-mediated hyperexcitability of neurons that express hyperpolarization-activated cation currents (Ih) and decreases the excitability of Ih-negative neurons. Additional studies are needed to identify which specific LHb inputs are regulated by MORs and KORs.
The pallidum is composed of the globus pallidus, entopeduncular nucleus, and ventral pallidum. Together, the pallidum has important roles in hedonic actions, motivation, and cognition (Smith et al., 2009; Saga et al., 2017). All three opioid receptor are highly expressed in the pallidum (Le Merrer et al., 2009).
Presynaptic MORs inhibit GABA input from dorsal striatum and from local GABAergic neurons (Stanford and Cooper, 1999). In contrast, DORs inhibit evoked local GABA transmission, but do not inhibit striatal inputs. DOR activation has no effect on AP-dependent spontaneous IPSCs, but inhibits mIPSCs. MORs, but not DORs or KORs, postsynaptically inhibit N-type VGCCs in dissociated Globus Pallidus (GP) neurons (Stefani et al., 2001). Similar to MOR, KOR activation in GP hyperpolarizes about 25% of cells and presynaptically inhibits GABAergic input from striatum and local GABAergic collaterals (Ogura and Kita, 2000). KORs have no effect on glutamate transmission, and it is unknown if DORs or KORs regulate glutamate transmission in GP.
A subpopulation of Entopeduncular Nucleus (EPN) neurons were hyperpolarized by dynorphin-mediated KOR activation via increasing potassium conductance. Electrical stimulation of the (GP) evokes GABA release from striatal and pallidal inputs to the EPN. Dynorphin equally inhibited IPSCs from both sources (short- and medium-latency IPSCs) presynaptically. Dynorphin released from striatal inputs could be an autofeedback mechanism, heterosynaptic (targeting pallidal input), or directly inhibit EPN neurons (Ogura and Kita, 2002).
MORs hyperpolarize a subpopulation of Ventral Pallidum (VP) neurons, presumably through activation of potassium currents (Napier and Mitrovic, 1999). Looking at specific regional targets of VP neurons, MORs hyperpolarize GABAergic VP neurons that project to the VTA (Hjelmstad et al., 2013). In vivo electrophysiological recordings reveal that MOR activation reduces inhibitory GABAergic input, and excitatory substance P input from the NAc within the VP and enhances glutamate input from amygdala (Napier and Mitrovic, 1999). MOR activation produces LTD of GABA release in VP (Kupchik et al., 2014). In in vivo electrophysiological recordings, stimulation of VTA inputs to VP reduces firing of VP neurons. KOR and MOR activation block this, either due to direct inhibition of dopamine inputs or inhibition of non-dopaminergic VTA input (Napier and Mitrovic, 1999; Mitrovic and Napier, 2002). MORs also antagonize NAc-induced inhibitory transmission in VP (Chrobak and Napier, 1993). KORs postsynaptically inhibit GABAergic transmission from both direct pathway MSN (dMSN) and indirect pathway MSN (iMSN) inputs to VP GABA neurons. KORs generally increase GABAergic input to VP vGluT2-expressing neurons, but they could not determine if this was pre- or postsynaptically mediated and did not test specific GABAergic synaptic inputs (Inbar et al., 2020).
In summary, subpopulations of pallidal neurons are hyperpolarized by postsynaptic MORs and KORs. Presynaptic opioid receptors also modulate neural activity of pallidal neurons by inhibiting GABA release from striatal terminals and local GABAergic collaterals; although, the effect varies across opioid receptor and neurocircuit. Excitatory neurotransmission in VP is regulated by MORs and KORs, but excitatory transmission in other pallidal areas has not been shown to be modulated by opioid receptors. Most studies investigated circuit and subpopulation effects of opioid receptors in pallidum have focused on VP, therefore future studies are needed to identify specific subpopulation effects in GP and EPN.
The striatum is divided into dorsal and ventral regions. The dorsal striatum (DS) is heavily involved in motor control, learning, reward, and decision making (Balleine et al., 2007). The dorsal striatum is further divided into the dorsolateral (DLS) and dorsomedial striatum (DMS). The DMS is involved with goal-directed behaviors, while the DLS is involved with habitual behaviors (Lovinger, 2010; Corbit and Janak, 2016). The ventral striatum, also known as the nucleus accumbens (NAc) plays a critical role in establishing reward-associated memories to the effects of drugs and natural cues (Hyman et al., 2006). All 3 opioid receptors are highly expressed in the striatum and regulate synaptic plasticity (Le Merrer et al., 2009; Atwood et al., 2014b).
Aside from an early study of opioid effects on neuronal function in dorsal striatum, there is very little indication that opioid receptors alter membrane properties of the principal dorsal striatal MSNs. One early study found that MORs slightly hyperpolarize a subset of MSNs (Jiang and North, 1992). They also found that DORs hyperpolarize a subset of non-MSN, tonically active neurons, ablating AP firing. Later studies suggest that these are likely tonically active interneurons that release acetylcholine and glutamate and their firing is inhibited by both MORs and DORs (Ponterio et al., 2013; Laurent et al., 2014). MORs reduce the firing of these cholinergic interneurons through postsynaptic G protein signaling (Ponterio et al., 2013, 2018). MOR modulation of these neurons may be circadian (Jabourian et al., 2005).
It was initially thought that opioid receptors do not inhibit GABA release in dorsal striatum(Jiang and North, 1992). However, later work found opioid receptors regulate GABA transmission in a subregion and synapse-specific manner that could be missed using more non-specific measures. MORs only inhibit GABAergic transmission within striosome subcompartments. MOR-mediated inhibition of GABA transmission within striosomes is mediated by presynaptic cAMP-PKA signaling, likely modulating presynaptic potassium channel function, and MOR inhibition is enhanced by PKC inhibition (Miura et al., 2007; Inoue et al., 2012). MORs inhibit spontaneous and TTX-insensitive GABAergic inputs in both cell types (dMSN and iMSN) (Ma et al., 2012). An elegant dissection of specific GABAergic synapses within striosomes that MORs and DORs regulate found that MORs inhibit dMSN and iMSN input to dMSNs, although inhibition of dMSN-dMSN transmission is stronger than iMSN-dMSN transmission (Banghart et al., 2015). DORs selectively inhibit iMSN input to dMSNs. Neither MOR nor DOR inhibit somatostatin-expressing interneuron input to dMSNs. DOR-mediated disinhibition of dMSNs is slightly more efficacious than MOR. MOR and DOR have little effect on GABA transmission in matrix of dorsal striatum. DOR activation produces iLTD at FSI-MSN synapses (Patton et al., 2016).
It has been known for some time that MORs and DORs inhibit glutamate release in dorsal striatum (Jiang and North, 1992). Despite MORs being enriched in striosome subcompartments of striatum, MORs equally inhibit glutamate transmission in both striosomes and matrix (Miura et al., 2007). One study that explored differences in MOR effects in dMSNs and iMSNs found that MORs reduce spontaneous glutamate release onto iMSNs in DLS, but not dMSNs (Ma et al., 2012). However, these data do not align with data from other laboratories that found more widespread MOR-mediated inhibition of glutamate release (Atwood et al., 2014b). They also reported that MORs have minimal effect on TTX-insensitive glutamate transmission in either type of MSNs in the DLS (Ma et al., 2012). MOR and DOR activation in the DLS and DMS produce antagonist-irreversible LTD in young rats and mice as well as adult mice (Atwood et al., 2014b; Fritz et al., 2018; Munoz et al., 2018, 2020). In the DLS, MOR and DOR LTD are not mutually occlusive, indicating that they inhibit different inputs. In the presence of peptidase inhibitors, electrical stimulation of glutamate release produces opioid receptor antagonist-sensitive LTD that is mGluR5 dependent. Antagonists for both MOR and DOR each partially prevent this LTD, while naloxone fully prevents this LTD. KORs may also play a role in this LTD (see below). Others have found that antidromic stimulation within the globus pallidus induces opioid peptide release (presumably enkephalins) within dorsal striatum that is sufficient to inhibit glutamate input from cortex. This was mediated by MORs, but not DORs. Paired recordings showed MSN firing could produce corticostriatal inhibition in a nearby MSN with a subpopulation showing reciprocal inhibition of cortical input (Blomeley and Bracci, 2011).
More recent work has attempted to dissect which specific glutamate synapses in the dorsal striatum are sensitive to MOR and DOR activation. In the DLS, the only cortical input that is sensitive to MOR activation are those that arise from anterior insular cortex in a mechanism that involve the activation of presynaptic HCN1 channels (Munoz et al., 2018, 2021). MORs also produce LTD in the DMS, but in this subregion the LTD is mediated by inputs from BLA, mPFC, and ACC (Munoz et al., 2020). In contrast, another recent study concluded that MORs do not inhibit ACC or mPFC inputs to DMS MSNs (Birdsong et al., 2019). The two studies were both done in mice, so it is not clear why the results are not aligned. DOR inhibits prelimbic mPFC input to DMS MSNs and motor cortex inputs to DLS MSNs (Atwood et al., 2014b; Birdsong et al., 2019). There has not been an exhaustive study of DORs effects on other cortical inputs to date. Interestingly, MORs also produce LTD of glutamate release from tonically active “cholinergic” interneurons in the DLS (Munoz et al., 2018). MORs also inhibit glutamatergic inputs from thalamus, albeit with a transient suppression rather than LTD in both DLS and DMS (Atwood et al., 2014b; Munoz et al., 2018; Birdsong et al., 2019; Reeves et al., 2021). It does not appear that DORs inhibit glutamate input from thalamus (Atwood et al., 2014b; Birdsong et al., 2019).
The early study of opioid effects on neurotransmission in dorsal striatum concluded that KORs have no effect on glutamate release (Jiang and North, 1992). However, a more recent study found that KORs can inhibit glutamate release in brain slices from young rats, specifically in dorsolateral striatum (DLS) (Atwood et al., 2014b). Activation of KORs produces an irreversible, long-lasting synaptic depression, which is similar to plasticity produced by DORs, but not MORs, in dorsal striatum. A KOR antagonist could also fully block endogenous opioid LTD in DLS, similar to the effects of naloxone, whereas MOR and DOR antagonists individually only partially blocked LTD (Atwood et al., 2014b). Given that KORs also inhibit dopamine release in dorsal striatum, it will be important to disambiguate in the future if this is due to direct activation of KORs on glutamate terminals or due to its actions on dopamine terminals which could account for the KOR antagonist on endogenous opioid LTD (Schoffelmeer et al., 1997; Szabo et al., 1999; Mamaligas et al., 2016; Hawes et al., 2017). For example, activation of Pdyn-containing dMSNs in DMS induces release of dynorphin that acts on presynaptic KORs on dopamine terminals to prevent theta burst stimulation-induced glutamatergic LTP in MSNs (Hawes et al., 2017). Similar mechanisms could account for the effects of KOR on inhibiting glutamate transmission under certain conditions.
The NAc can be subdivided into shell and core regions. Many studies specifically state whether measures were made in shell or core, and some provide even greater specificity. However, plenty of other studies make no distinction. Therefore, in this section where there is no specific subregion mentioned we are only able to generalize the role of opioid receptors on the specific measures discussed. There is very little evidence that opioid receptors have postsynaptic effects that influence AP firing in NAc, but much evidence that they do modulate synaptic transmission (Yuan et al., 1992; Martin et al., 1997).
As in dorsal striatum, there are some discrepant data regarding the role of MOR in regulating GABA transmission in NAc. One report demonstrates that MORs inhibit GABAergic transmission in both NAc shell and core, however, MORs have a larger effect on GABA transmission in the shell (Brundege and Williams, 2002). Another study shows that MORs inhibit GABA release in NAc shell equally in control and in forskolin-enhanced GABA release conditions (Chieng and Williams, 1998). A third study shows that MORs inhibit spontaneous GABA release similarly in D1 and D2 MSNs of the NAc core and NAc shell. Measures of TTX-insensitive GABA release show that GABA input is only inhibited in D1 MSNs in the core and D2 MSNs of the shell (Ma et al., 2012). However, a different study of the NAc shell showed that MOR activation has no effect on GABAergic input (Hoffman and Lupica, 2001). In contrast, about 50% of MSNs received input that was presumably regulated by DOR as a mixed DOR/MOR agonist was effective at blocking GABA transmission, but a MOR agonist was ineffective in these neurons. KOR activation strongly inhibits GABAergic output from D1 MSNs, but more weakly inhibits GABA output from D2 MSNs (Tejeda et al., 2017). KORs also inhibit GABA release, but with a different mechanism. KOR-mediated inhibition of GABA release is at the level of calcium entry through N-type VGCCs (Hjelmstad and Fields, 2003). Potassium channel blockade had no effect on KOR actions. Due to KOR expression on VTA dopamine neuron inputs, KORs could theoretically inhibit CIN-driven GABA co-release from VTA dopamine inputs (Britt and McGehee, 2008; Nelson et al., 2014).
MORs presynaptically inhibit glutamate release in NAc core and shell (Martin et al., 1997; Hoffman and Lupica, 2001; Brundege and Williams, 2002; Hoffman et al., 2003; James et al., 2013). Postsynaptic MOR activation was reported to enhance NMDAR, but reduce AMPAR currents (Martin et al., 1997). Regarding spontaneous glutamate release, MORs equally inhibit glutamate input to D1 MSNs in NAc core and NAc shell, but have a much larger effect on D2 MSNs of the NAc shell. MORs inhibit TTX-insensitive glutamate inputs to D1 and D2 MSNs in NAc core and NAc shell, although the effect is most robust in D1 MSNs of the shell (Ma et al., 2012). MOR’s effects on glutamate transmission in NAc may not always be neuronal in origin. MOR activation on astrocytes in NAc core induces glutamate release, producing slow inward currents via extrasynaptic NMDARs in nearby neurons (Corkrum et al., 2019). DOR has a minor effect on inhibiting glutamate transmission in NAc shell, perhaps only in a subset of glutamate inputs (Brundege and Williams, 2002). KORs presynaptically inhibit glutamate release on to MSNs of NAc shell without having any postsynaptic effects (Hjelmstad and Fields, 2001). KOR inhibition of glutamate transmission persist in the presence of N- and P/Q-type calcium and potassium channel blockers (Hjelmstad and Fields, 2003). Strong PFC input to NAc can produce heterosynaptic inhibition of weaker ventral hippocampal inputs, a process that is in part mediated by KORs (Brooks and O’Donnell, 2017). KOR inhibits glutamate input from BLA, but not ventral hippocampus, to D1 MSNs of the NAc shell and core, but not D2 MSNs (Tejeda et al., 2017). This effect was stronger in NAc shell than core, but was independent of D1 MSN projection target. The net effect of KOR activation at the GABA and glutamate synapses allows for KORs to decrease D1 MSN firing and disinhibit D2 MSN firing in response to BLA input. In contrast, KOR has no effect on ventral hippocampal drive of D1 MSNs, but still allows for disinhibition of D2 MSNs. The authors conclude that KOR acts as a pathway-specific filtering mechanism for BLA versus ventral hippocampal control of NAc function. KOR inhibition of glutamate transmission in NAc MSNs is lost in animals with 5 days of repeated cocaine exposure with at least up to 2 weeks of withdrawal (Mu et al., 2011). KORs also regulate glutamatergic input to PV-expressing FSIs in NAc, however, this is specific to thalamic, but not cortical inputs (Coleman et al., 2021). In addition, activation of KORs produces a postsynaptic LTD plasticity, wherein AMPARs are internalized via a PKA-calcineurin signaling pathway.
Altogether, these studies indicate that opioid receptor activation has little effect on membrane properties of both dorsal and ventral striatal neurons, with the exception of cholinergic interneurons. A growing body of evidence indicates that each type of opioid receptor is capable of inhibiting glutamate transmission and MORs and DORs regulate GABA transmission, although not universally at all striatal synapses. The biological relevance of synapse- and opioid receptor subtype-specific regulation of striatal excitatory and inhibitory transmission is currently unclear. Refined approaches for manipulating the expression of these receptors at specific synapses will help decipher the interplay between receptors in controlling striatal-mediated behaviors and circuit function.
The thalamus acts as a relay hub for cortical sensory and motor functions, controlling perception, action and mentation (Schmitt and Halassa, 2017). MORs are highly expressed, but DORs and KORs are sparsely expressed, throughout the thalamus (Le Merrer et al., 2009; Chu Sin Chung and Kieffer, 2013; Chen et al., 2015; Bengoetxea et al., 2020).
In the thalamic reticular nucleus there are two predominant types of neurons that are both GABAergic, but display different firing properties (bursting and non-bursting). MOR activation, but not DOR or KOR, hyperpolarizes subpopulations of each class of neurons, revealing further subpopulations of neurons in this nucleus. The mechanism of hyperpolarization is due to increased potassium conductance (Brunton and Charpak, 1997). MOR activation hyperpolarizes dorsal midline thalamus neurons that project to the BLA and CeA (Goedecke et al., 2019). In the centrolateral thalamus, MOR activation, but not DOR or KOR, hyperpolarized neurons via increased GIRK function, independent of synaptic input. The investigators explored MOR hyperpolarization of other thalamic neurons (principal relay, midline, and intralaminar nuclei) and found widespread MOR-mediated inhibition of thalamic neurons, suggesting that the thalamus is a highly sensitive region to MOR-mediated neuronal hyperpolarization (Brunton and Charpak, 1998).
In contrast to MORs, KOR effects in the thalamus appear to be restricted to specific thalamic nuclei. KOR activation produces direct hyperpolarization of anterior paraventricular thalamic neurons through GIRKs that peak around the ages of puberty and then decrease at later ages. MOR activation hyperpolarizes these neurons; although, the effect of the MOR agonist desensitizes and produces heterologous desensitization of KOR responses (KOR responses do not desensitize independent of MOR activation) (Chen et al., 2015). Additional studies are needed to investigate potential KOR effects in other thalamic nuclei.
In the above sections, we opted to review brain regions that have received the most attention. However, in our survey of the literature there are, in the context of opioid receptor-mediated regulation of neurotransmission, some other less studied brain regions or subregions that deserve further investigation and we briefly review them here.
In the Lateral Hypothalamus (LH), local GABA neurons within the perifornical region inhibit the activity of orexin neurons. KOR activation specifically reduces this GABAergic input, as revealed by optical probing of these local GABA neurons (Ferrari et al., 2018). No studies to date have investigated MOR or DOR effects on neurons in the LH.
Also known as the nucleus of Schwalbe is located in the brainstem (Highstein and Holstein, 2006). DORs, but not MORs or KORs inhibit Medial Vestibular Nucleus (MVN) neurons. DOR inhibition is via activation of an outward potassium current (Sulaiman and Dutia, 1998).
Located in the brainstem, parabrachial nucleus neurons are hyperpolarized by MORs, but not DORs or KORs, likely through enhancing potassium currents (Christie and North, 1988; Cramer et al., 2021). Pontine Kölliker-Fuse nucleus neurons are hyperpolarized by MOR activation via GIRK activation (Levitt et al., 2015; Levitt and Williams, 2018). MORs and KORs inhibit GABA release in PBN, but only MORs regulate glutamate release (Cramer et al., 2021).
Very few studies have investigated the role of opioid receptors in modulating neural function in the Ventromedial Hypothalamus (VMH) or LH. In the VMH, MORs hyperpolarize neurons to reduce cellular excitability, including those that express the leptin receptor, via enhancing GIRK currents (Emmerson and Miller, 1999). DORs and KORs do not appear to hyperpolarize neurons in the VMH. Presynaptic MORs strongly inhibit glutamate input to VMH neurons, whereas DORs have no effect, and KORs have only a small effect on glutamate release (Emmerson and Miller, 1999; Devidze et al., 2008). It is unknown which glutamatergic inputs to the VMH are modulated by MORs and KORs.
Across brain regions opioid receptors play major roles in regulating glutamate and GABA release through presynaptic mechanisms and neuronal excitability through postsynaptic mechanisms. There is heterogeneity in the precise mechanisms whereby opioid receptors regulate neurotransmitter release, even within any given brain region (Figure 1). At some synapses this appears to involve inhibition of calcium channels, while at others it involves activating potassium channels. There is also evidence that diverse kinase signaling pathways may be involved at distinct synapses. These divergent mechanisms do not appear to be due to the specific identity of the opioid receptors, but rather due to the specific synaptic terminals on which the receptors are expressed. On the other hand, all three opioid receptor types appear to generally modulate neuronal excitability through their actions on potassium channels, such as GIRKs. However, local circuit effects must be considered when deciphering pre- versus postsynaptic localization of opioid receptor actions, as postsynaptic hyperpolarization can reduce local circuit neurotransmitter release (Figures 2A,B).
Figure 2. Summary of opioid receptor-mediated modulation of neurotransmission. Opioid receptor activation-mediated modulation of neurotransmission can have differential effects on neurocircuit function depending on the localization of the receptors. (A) Opioid receptors found on glutamatergic terminals will reduce glutamate release upon activation, thus inhibiting a postsynaptic neuron. Opioid receptors on postsynaptic neurons will generally reduce neuronal excitability. (B) Opioid receptors found on inhibitory neuron (e.g., GABAergic) terminals or postsynaptically will reduce inhibitory transmission, disinhibiting a postsynaptic neuron. Alternatively, opioid receptors on glutamate neurons that impinge on inhibitory neurons will reduce excitatory drive of these neurons, thus reducing inhibitory transmission and producing disinhibition through a polysynaptic mechanism. (C) Opioid receptors localized to different synaptic terminals can produce differential outcomes upon activation. As an example from our own work, MORs are localized to cortical and thalamic glutamatergic inputs to dorsal striatum (DS). (D) Upon activation by the MOR agonist, DAMGO, MORs reduce the amplitude of glutamate-mediated excitatory postsynaptic currents (EPSCs). Activation MORs on glutamate inputs from cortex produces a long-lasting reduction in EPSC amplitudes. However, activation of MORs on thalamic inputs only produces a transient reduction, despite also being a glutamatergic input to the same neurons that express the long-lasting reduction in glutamate transmission from cortical inputs. Adapted from data from Munoz et al. (2018). Figure created with BioRender.com.
In order to better understand how opioid receptors modulate neurocircuit function, there is a need to identify the specific cell types that express these receptors and the subcellular localization of the receptors. Conditional knockout and fluorescent reporter transgenic mice are useful for identifying the cell types that express the various opioid receptors and how the expression of receptors within those cell types affects neurotransmission (Gaveriaux-Ruff et al., 2011; Weibel et al., 2013; Ehrich et al., 2015; Erbs et al., 2015; Chen et al., 2020). Another important consideration is identifying specific circuits that are modulated by opioid receptors. In many brain regions, opioid receptor effects on neurotransmission differ according to localization within the region, projection targets, or input regions. Optogenetic methods are increasingly accessible and are useful for identifying the specific synapses at which opioid receptors reside and how they specifically modulate neurotransmission.
It is not common for assessments of long-term opioid receptor-mediated synaptic plasticity to be performed. For many investigators, it is sufficient to determine whether a synapse is regulated by opioid receptors. However, there are missed opportunities to observe the diversity in ways in which opioid receptors modulate neurotransmission. At some synapses, activation of opioid receptors produces long-lasting effects on neurotransmission that persist even once opioid receptor antagonists are applied, which argues against persistent receptor activation. At other synapses, opioid receptor activation only produces transient responses, only lasting while the receptors are engaged (Figures 2C,D). Opioid receptors display desensitization at some synapses, while other synapses appear to be resistant to receptor desensitization. Whether a particular type of receptor in a given synapse or cell type produces long-lasting or short-term effects upon activation or desensitizes or not is a fascinating area of study that will yield rich insights into how opioids affect cognition, behavioral output, and physiological functions. Comparisons between mechanisms of synapse- and cell type-specific opioid receptor modulation of neurotransmission could also reveal novel opportunities for targeted combinatorial therapeutics. There is clearly much left to discover regarding how opioid receptors can utilize such a diverse array of mechanisms to precisely modulate neurotransmission.
All authors contributed to the conceptualization, writing, and review of this manuscript and approved it for publication.
This work was supported by funding from the National Institutes of Health (grant numbers: R01 AA027214 and T32 AA07462), Indiana University, Indiana University Health, and Stark Neurosciences Research Institute.
The authors declare that the research was conducted in the absence of any commercial or financial relationships that could be construed as a potential conflict of interest.
All claims expressed in this article are solely those of the authors and do not necessarily represent those of their affiliated organizations, or those of the publisher, the editors and the reviewers. Any product that may be evaluated in this article, or claim that may be made by its manufacturer, is not guaranteed or endorsed by the publisher.
Ackley, M. A., Hurley, R. W., Virnich, D. E., and Hammond, D. L. (2001). A cellular mechanism for the antinociceptive effect of a kappa opioid receptor agonist. Pain 91, 377–388. doi: 10.1016/S0304-3959(00)00464-4
Akaishi, T., Saito, H., Ito, Y., Ishige, K., and Ikegaya, Y. (2000). Morphine augments excitatory synaptic transmission in the dentate gyrus through GABAergic disinhibition. Neurosci. Res. 38, 357–363. doi: 10.1016/s0168-0102(00)00177-2
Al-Hasani, R., McCall, J. G., Foshage, A. M., and Bruchas, M. R. (2013). Locus coeruleus kappa-opioid receptors modulate reinstatement of cocaine place preference through a noradrenergic mechanism. Neuropsychopharmacology 38, 2484–2497. doi: 10.1038/npp.2013.151
Al-Hasani, R., and Bruchas, M. R. (2011). Molecular mechanisms of opioid receptor-dependent signaling and behavior. Anesthesiology 115, 1363–1381. doi: 10.1097/ALN.0b013e318238bba6
Allouche, S., Noble, F., and Marie, N. (2014). Opioid receptor desensitization: mechanisms and its link to tolerance. Front. Pharmacol. 5:280. doi: 10.3389/fphar.2014.00280
Angeles Fernández-Gil, M., Palacios-Bote, R., Leo-Barahona, M., and Mora-Encinas, J. P. (2010). Anatomy of the brainstem: a gaze into the stem of life. Semin. Ultrasound. CT MR 31, 196–219. doi: 10.1053/j.sult.2010.03.006
Appleyard, S. M., Bailey, T. W., Doyle, M. W., Jin, Y. H., Smart, J. L., Low, M. J., et al. (2005). Proopiomelanocortin neurons in nucleus tractus solitarius are activated by visceral afferents: regulation by cholecystokinin and opioids. J. Neurosci. Off. J. Soc. Neurosci. 25, 3578–3585. doi: 10.1523/JNEUROSCI.4177-04.2005
Atwood, B. K., Kupferschmidt, D. A., and Lovinger, D. M. (2014b). Opioids induce dissociable forms of long-term depression of excitatory inputs to the dorsal striatum. Nature neuroscience 17, 540–548. doi: 10.1038/nn.3652
Atwood, B. K., Lovinger, D. M., and Mathur, B. N. (2014a). Presynaptic long-term depression mediated by Gi/o-coupled receptors. Trends Neurosci. 37, 663–673. doi: 10.1016/j.tins.2014.07.010
Bagley, E. E., and Ingram, S. L. (2020). Endogenous opioid peptides in the descending pain modulatory circuit. Neuropharmacology 173:108131. doi: 10.1016/j.neuropharm.2020.108131
Bajo, M., Roberto, M., Madamba, S. G., and Siggins, G. R. (2011). Neuroadaptation of GABAergic transmission in the central amygdala during chronic morphine treatment. Addict. Biol. 16, 551–564. doi: 10.1111/j.1369-1600.2010.00269.x
Balleine, B. W., Delgado, M. R., and Hikosaka, O. (2007). The role of the dorsal striatum in reward and decision-making. J. Neurosci. Offic. J. Soc. Neurosci. 27, 8161–8165. doi: 10.1523/JNEUROSCI.1554-07.2007
Banghart, M. R., He, X. J., and Sabatini, B. L. (2018). A caged enkephalin optimized for simultaneously probing mu and delta opioid receptors. ACS Chem. Neurosci. 9, 684–690. doi: 10.1021/acschemneuro.7b00485
Banghart, M. R., Neufeld, S. Q., Wong, N. C., and Sabatini, B. L. (2015). Enkephalin disinhibits mu opioid receptor-rich striatal patches via delta opioid receptors. Neuron 88, 1227–1239. doi: 10.1016/j.neuron.2015.11.010
Beltrán-Campos, V., Silva-Vera, M., García-Campos, M. L., and íaz-Cintra, S. D. (2015). Effects of morphine on brain plasticity. Neurol. (Engl. Ed.) 30, 176–180. doi: 10.1016/j.nrl.2014.08.004
Bengoetxea, X., Goedecke, L., Blaesse, P., Pape, H. C., and Jüngling, K. (2020). The μ-opioid system in midline thalamic nuclei modulates defence strategies towards a conditioned fear stimulus in male mice. J. Psychopharmacol. 34, 1280–1288. doi: 10.1177/0269881120940919
Bergevin, A., Girardot, D., Bourque, M. J., and Trudeau, L. E. (2002). Presynaptic mu-opioid receptors regulate a late step of the secretory process in rat ventral tegmental area GABAergic neurons. Neuropharmacology 42, 1065–1078. doi: 10.1016/s0028-3908(02)00061-8
Bie, B., and Pan, Z. Z. (2003). Presynaptic mechanism for anti-analgesic and anti-hyperalgesic actions of kappa-opioid receptors. J. Neurosci. Offic. J. Soc. Neurosci. 23, 7262–7268. doi: 10.1523/JNEUROSCI.23-19-07262.2003
Bie, B., Zhu, W., and Pan, Z. Z. (2009a). Ethanol-induced delta-opioid receptor modulation of glutamate synaptic transmission and conditioned place preference in central amygdala. Neuroscience 160, 348–358. doi: 10.1016/j.neuroscience.2009.02.049
Bie, B., Zhu, W., and Pan, Z. Z. (2009b). Rewarding morphine-induced synaptic function of delta-opioid receptors on central glutamate synapses. J. Pharmacol. Exp. Ther. 329, 290–296. doi: 10.1124/jpet.108.148908
Bird, C. M., and Burgess, N. (2008). The hippocampus and memory: insights from spatial processing. Nat. Rev. Neurosci. 9, 182–194. doi: 10.1038/nrn2335
Bird, S. J., and Kuhar, M. J. (1977). Iontophoretic application of opiates to the locus coeruleus. Brain Res. 122, 523–533. doi: 10.1016/0006-8993(77)90462-0
Birdsong, W. T., Jongbloets, B. C., Engeln, K. A., Wang, D., Scherrer, G., and Mao, T. (2019). Synapse-specific opioid modulation of thalamo-cortico-striatal circuits. Elife 8:2548. doi: 10.7554/eLife.45146
Blaesse, P., Goedecke, L., Bazelot, M., Capogna, M., Pape, H. C., and Jungling, K. (2015). mu-Opioid receptor-mediated inhibition of intercalated neurons and effect on synaptic transmission to the central amygdala. J. Neurosci. Offic. J. Soc. Neurosci. 35, 7317–7325. doi: 10.1523/JNEUROSCI.0204-15.2015
Bliss, T. V. P., and Cooke, S. F. (2011). Long-term potentiation and long-term depression: a clinical perspective. Clinics (Sao Paulo Brazil) 66(Suppl. 1), 3–17. doi: 10.1590/s1807-59322011001300002
Blomeley, C. P., and Bracci, E. (2011). Opioidergic interactions between striatal projection neurons. J. Neurosci. Offic. J. Soc. Neurosci. 31, 13346–13356. doi: 10.1523/JNEUROSCI.1775-11.2011
Bloodgood, D. W., Hardaway, J. A., Stanhope, C. M., Pati, D., Pina, M. M., Neira, S., et al. (2021). Kappa opioid receptor and dynorphin signaling in the central amygdala regulates alcohol intake. Mol. Psychiatry 26, 2187–2199. doi: 10.1038/s41380-020-0690-z
Bonci, A., and Malenka, R. C. (1999). Properties and plasticity of excitatory synapses on dopaminergic and GABAergic cells in the ventral tegmental area. J. Neurosci. Offic. J. Soc. Neurosci. 19, 3723–3730. doi: 10.1523/JNEUROSCI.19-10-03723.1999
Bostock, E., Dingledine, R., Xu, G., and Chang, K. J. (1984). Mu opioid receptors participate in the excitatory effect of opiates in the hippocampal slice. J. Pharmacol. Exp. Ther. 231, 512–517.
Boxwell, A. J., Yanagawa, Y., Travers, S. P., and Travers, J. B. (2013). The mu-opioid receptor agonist DAMGO presynaptically suppresses solitary tract-evoked input to neurons in the rostral solitary nucleus. J. Neurophysiol. 109, 2815–2826. doi: 10.1152/jn.00711.2012
Bradford, H. F., Crowder, J. M., and White, E. J. (1986). Inhibitory actions of opioid compounds on calcium fluxes and neurotransmitter release from mammalian cerebral cortical slices. Br. J. Pharmacol. 88, 87–93. doi: 10.1111/j.1476-5381.1986.tb09474.x
Bramham, C. R., Milgram, N. W., and Srebro, B. (1991). Delta opioid receptor activation is required to induce LTP of synaptic transmission in the lateral perforant path in vivo. Brain Res. 567, 42–50. doi: 10.1016/0006-8993(91)91433-2
Bramham, C. R., and Sarvey, J. M. (1996). Endogenous activation of μ and δ-1 opioid receptors is required for long-term potentiation induction in the lateral perforant path: dependence on GABAergic inhibition. J. Neurosci. 16, 8123–8131. doi: 10.1523/JNEUROSCI.16-24-08123.1996
Britt, J. P., and McGehee, D. S. (2008). Presynaptic opioid and nicotinic receptor modulation of dopamine overflow in the nucleus accumbens. J. Neurosci. Offic. J. Soc. Neurosci. 28, 1672–1681. doi: 10.1523/JNEUROSCI.4275-07.2008
Brooks, J. M., and O’Donnell, P. (2017). Kappa opioid receptors mediate heterosynaptic suppression of hippocampal inputs in the rat ventral striatum. J. Neurosci. Offic. J. Soc. Neurosci. 37, 7140–7148. doi: 10.1523/JNEUROSCI.0876-17.2017
Brown, C. H., and Bourque, C. W. (2004). Autocrine feedback inhibition of plateau potentials terminates phasic bursts in magnocellular neurosecretory cells of the rat supraoptic nucleus. J. Physiol. 557, 949–960. doi: 10.1113/jphysiol.2004.063818
Brown, C. H., Ludwig, M., and Leng, G. (1998). kappa-opioid regulation of neuronal activity in the rat supraoptic nucleus in vivo. J. Neurosci. Offic. J. Soc. Neurosci. 18, 9480–9488. doi: 10.1523/JNEUROSCI.18-22-09480.1998
Brown, C. H., Stern, J. E., Jackson, K. L., Bull, P. M., Leng, G., and Russell, J. A. (2005). Morphine withdrawal increases intrinsic excitability of oxytocin neurons in morphine-dependent rats. Eur. J. Neurosci. 21, 501–512. doi: 10.1111/j.1460-9568.2005.03885.x
Browning, K. N., Kalyuzhny, A. E., and Travagli, R. A. (2002). Opioid peptides inhibit excitatory but not inhibitory synaptic transmission in the rat dorsal motor nucleus of the vagus. J. Neurosci. Offic. J. Soc. Neurosci. 22, 2998–3004. doi: 10.1523/JNEUROSCI.22-08-02998.2002
Browning, K. N., Kalyuzhny, A. E., and Travagli, R. A. (2004). Mu-opioid receptor trafficking on inhibitory synapses in the rat brainstem. J. Neurosci. Offic. J. Soc. Neurosci. 24, 7344–7352. doi: 10.1523/JNEUROSCI.1676-04.2004
Brundege, J. M., and Williams, J. T. (2002). Differential modulation of nucleus accumbens synapses. J. Neurophysiol. 88, 142–151.
Brunton, J., and Charpak, S. (1997). Heterogeneity of cell firing properties and opioid sensitivity in the thalamic reticular nucleus. Neuroscience 78, 303–307. doi: 10.1016/s0306-4522(97)00028-6
Brunton, J., and Charpak, S. (1998). mu-Opioid peptides inhibit thalamic neurons. J. Neurosci. Offic. J. Soc. Neurosci. 18, 1671–1678. doi: 10.1523/JNEUROSCI.18-05-01671.1998
Bull, F. A., Baptista-Hon, D. T., Lambert, J. J., Walwyn, W., and Hales, T. G. (2017). Morphine activation of mu opioid receptors causes disinhibition of neurons in the ventral tegmental area mediated by beta-arrestin2 and c-Src. Sci. Rep. 7:9969. doi: 10.1038/s41598-017-10360-8
Cameron, D. L., Wessendorf, M. W., and Williams, J. T. (1997). A subset of ventral tegmental area neurons is inhibited by dopamine, 5-hydroxytryptamine and opioids. Neuroscience 77, 155–166. doi: 10.1016/s0306-4522(96)00444-7
Capogna, M., Gahwiler, B. H., and Thompson, S. M. (1993). Mechanism of mu-opioid receptor-mediated presynaptic inhibition in the rat hippocampus in vitro. J. Physiol. 470, 539–558. doi: 10.1113/jphysiol.1993.sp019874
Capogna, M., Gahwiler, B. H., and Thompson, S. M. (1996). Presynaptic inhibition of calcium-dependent and -independent release elicited with ionomycin, gadolinium, and alpha-latrotoxin in the hippocampus. J. Neurophysiol. 75, 2017–2028. doi: 10.1152/jn.1996.75.5.2017
Castillo, P. E., Salin, P. A., Weisskopf, M. G., and Nicoll, R. A. (1996). Characterizing the site and mode of action of dynorphin at hippocampal mossy fiber synapses in the guinea pig. J. Neurosci. Offic. J. Soc. Neurosci. 16, 5942–5950. doi: 10.1523/JNEUROSCI.16-19-05942.1996
Caudle, R. M., Chavkin, C., and Dubner, R. (1994). Kappa 2 opioid receptors inhibit NMDA receptor-mediated synaptic currents in guinea pig CA3 pyramidal cells. J. Neurosci. Offic. J. Soc. Neurosci. 14, 5580–5589. doi: 10.1523/JNEUROSCI.14-09-05580.1994
Charbogne, P., Gardon, O., Martin-Garcia, E., Keyworth, H. L., Matsui, A., Mechling, A. E., et al. (2017). Mu opioid receptors in gamma-aminobutyric acidergic forebrain neurons moderate motivation for heroin and palatable food. Biol. Psychiatry 81, 778–788. doi: 10.1016/j.biopsych.2016.12.022
Chen, C., Willhouse, A. H., Huang, P., Ko, N., Wang, Y., Xu, B., et al. (2020). Characterization of a knock-in mouse line expressing a fusion protein of kappa opioid receptor conjugated with tdTomato: 3-dimensional brain imaging via CLARITY. eNeuro 7:2554. doi: 10.1523/ENEURO.0028-20.2020
Chen, T., Li, J., Feng, B., Hui, R., Dong, Y. L., Huo, F. Q., et al. (2016). Mechanism underlying the analgesic effect exerted by endomorphin-1 in the rat ventrolateral periaqueductal gray. Mol. Neurobiol. 53, 2036–2053. doi: 10.1007/s12035-015-9159-5
Chen, Z., Tang, Y., Tao, H., Li, C., Zhang, X., and Liu, Y. (2015). Dynorphin activation of kappa opioid receptor reduces neuronal excitability in the paraventricular nucleus of mouse thalamus. Neuropharmacology 97, 259–269. doi: 10.1016/j.neuropharm.2015.05.030
Cheng, Z. F., Fields, H. L., and Heinricher, M. M. (1986). Morphine microinjected into the periaqueductal gray has differential effects on 3 classes of medullary neurons. Brain Res. 375, 57–65. doi: 10.1016/0006-8993(86)90958-3
Chieng, B., and Christie, M. J. (1994). Hyperpolarization by opioids acting on mu-receptors of a sub-population of rat periaqueductal gray neurones in vitro. Br. J. Pharmacol. 113, 121–128. doi: 10.1111/j.1476-5381.1994.tb16183.x
Chieng, B., and Williams, J. T. (1998). Increased opioid inhibition of GABA release in nucleus accumbens during morphine withdrawal. J. Neurosci. Offic. J. Soc. Neurosci. 18, 7033–7039. doi: 10.1523/JNEUROSCI.18-17-07033.1998
Chieng, B. C., Christie, M. J., and Osborne, P. B. (2006). Characterization of neurons in the rat central nucleus of the amygdala: cellular physiology, morphology, and opioid sensitivity. J. Comp. Neurol. 497, 910–927. doi: 10.1002/cne.21025
Chiou, L. C., and Huang, L. Y. (1999). Mechanism underlying increased neuronal activity in the rat ventrolateral periaqueductal grey by a mu-opioid. J. Physiol. 518(Pt 2), 551–559. doi: 10.1111/j.1469-7793.1999.0551p.x
Christie, M. J., and North, R. A. (1988). Agonists at mu-opioid, M2-muscarinic and GABAB-receptors increase the same potassium conductance in rat lateral parabrachial neurones. Br. J. Pharmacol. 95, 896–902. doi: 10.1111/j.1476-5381.1988.tb11719.x
Chrobak, J. J., and Napier, T. C. (1993). Opioid and GABA modulation of accumbens-evoked ventral pallidal activity. J. Neural. Transm. Gen. Sect. 93, 123–143. doi: 10.1007/BF01245342
Chu Sin Chung, P., and Kieffer, B. L. (2013). Delta opioid receptors in brain function and diseases. Pharmacol. Therap. 140, 112–120. doi: 10.1016/j.pharmthera.2013.06.003
Citri, A., and Malenka, R. C. (2008). Synaptic plasticity: multiple forms, functions, and mechanisms. Neuropsychopharmacology 33, 18–41. doi: 10.1038/sj.npp.1301559
Cleary, D. R., Neubert, M. J., and Heinricher, M. M. (2008). Are opioid-sensitive neurons in the rostral ventromedial medulla inhibitory interneurons? Neuroscience 151, 564–571. doi: 10.1016/j.neuroscience.2007.10.023
Coleman, B. C., Manz, K. M., and Grueter, B. A. (2021). Kappa opioid receptor modulation of excitatory drive onto nucleus accumbens fast-spiking interneurons. Neuropsychopharmacology 46, 2340–2349. doi: 10.1038/s41386-021-01146-8
Connor, M., Schuller, A., Pintar, J. E., and Christie, M. J. (1999). Mu-opioid receptor modulation of calcium channel current in periaqueductal grey neurons from C57B16/J mice and mutant mice lacking MOR-1. Br. J. Pharmacol. 126, 1553–1558. doi: 10.1038/sj.bjp.0702457
Corbit, L. H., and Janak, P. H. (2016). Habitual alcohol seeking: neural bases and possible relations to alcohol use disorders. Alcohol. Clin. Exp. Res. 40, 1380–1389. doi: 10.1111/acer.13094
Corkrum, M., Rothwell, P. E., Thomas, M. J., Kofuji, P., and Araque, A. (2019). Opioid-mediated astrocyte-neuron signaling in the nucleus accumbens. Cells 8:5774. doi: 10.3390/cells8060586
Corrigall, W. A., and Linseman, M. A. (1980). A specific effect of morphine on evoked activity in the rat hippocampal slice. Brain Res. 192, 227–238. doi: 10.1016/0006-8993(80)91022-7
Cramer, N., Silva-Cardoso, G., Masri, R., and Keller, A. (2021). Control of synaptic transmission and neuronal excitability in the parabrachial nucleus. Neurobiol. Pain 9:100057. doi: 10.1016/j.ynpai.2020.100057
Crowley, N. A., Bloodgood, D. W., Hardaway, J. A., Kendra, A. M., McCall, J. G., Al-Hasani, R., et al. (2016). Dynorphin controls the gain of an amygdalar anxiety circuit. Cell Rep. 14, 2774–2783. doi: 10.1016/j.celrep.2016.02.069
Cui, R. J., Roberts, B. L., Zhao, H., Andresen, M. C., and Appleyard, S. M. (2012). Opioids inhibit visceral afferent activation of catecholamine neurons in the solitary tract nucleus. Neuroscience 222, 181–190. doi: 10.1016/j.neuroscience.2012.07.010
da Costa Gomez, T. M., and Behbehani, M. M. (1995). An electrophysiological characterization of the projection from the central nucleus of the amygdala to the periaqueductal gray of the rat: the role of opioid receptors. Brain Res. 689, 21–31. doi: 10.1016/0006-8993(95)00525-u
Dahan, A., van der Schrier, R., Smith, T., Aarts, L., van Velzen, M., and Niesters, M. (2018). Averting opioid-induced respiratory depression without affecting analgesia. Anesthesiology 128, 1027–1037. doi: 10.1097/ALN.0000000000002184
Dahlhamer, J., Lucas, J., Zelaya, C., Nahin, R., Mackey, S., DeBar, L., et al. (2016). Prevalence of chronic pain and high-impact chronic pain among adults. MMWR Morb. Mortal. Wkly. Rep. 2018, 1001–1006. doi: 10.15585/mmwr.mm6736a2
De Neve, J., Barlow, T. M. A., Tourwe, D., Bihel, F., Simonin, F., and Ballet, S. (2021). Comprehensive overview of biased pharmacology at the opioid receptors: biased ligands and bias factors. RSC Med. Chem. 12, 828–870. doi: 10.1039/d1md00041a
Devidze, N., Zhang, Q., Zhou, J., Lee, A. W., Pataky, S., Kow, L. M., et al. (2008). Presynaptic actions of opioid receptor agonists in ventromedial hypothalamic neurons in estrogen- and oil-treated female mice. Neuroscience 152, 942–949. doi: 10.1016/j.neuroscience.2008.01.033
Dingledine, R., Valentino, R. J., Bostock, E., King, M. E., and Chang, K. J. (1983). Down-regulation of delta but not mu opioid receptors in the hippocampal slice associated with loss of physiological response. Life Sci. 33(Suppl. 1), 333–336. doi: 10.1016/0024-3205(83)90510-6
Drews, E., and Zimmer, A. (2010). Modulation of alcohol and nicotine responses through the endogenous opioid system. Progr. Neurobiol. 90, 1–15. doi: 10.1016/j.pneurobio.2009.09.004
Dumont, E. C., and Williams, J. T. (2004). Noradrenaline triggers GABAA inhibition of bed nucleus of the stria terminalis neurons projecting to the ventral tegmental area. J. Neurosci. Offic. J. Soc. Neurosci. 24, 8198–8204. doi: 10.1523/JNEUROSCI.0425-04.2004
Dunwiddie, T., Mueller, A., Palmer, M., Stewart, J., and Hoffer, B. (1980). Electrophysiological interactions of enkephalins with neuronal circuitry in the rat hippocampus. I. Effects on pyramidal cell activity. Brain Res. 184, 311–330. doi: 10.1016/0006-8993(80)90801-x
Dunwiddie, T. V., and Su, M. T. (1988). Pertussis toxin pretreatment antagonizes the actions of mu- and delta-opiate agonists in hippocampal slices. Neurosci. Lett. 95, 329–334. doi: 10.1016/0304-3940(88)90680-5
Ehrich, J. M., Messinger, D. I., Knakal, C. R., Kuhar, J. R., Schattauer, S. S., Bruchas, M. R., et al. (2015). Kappa opioid receptor-induced aversion requires p38 MAPK activation in VTA dopamine neurons. J. Neurosci. Offic. J. Soc. Neurosci. 35, 12917–12931. doi: 10.1523/JNEUROSCI.2444-15.2015
Eisenstein, T. K. (2019). The role of opioid receptors in immune system function. Front. Immunol. 10:2904. doi: 10.3389/fimmu.2019.02904
Emmerson, P. J., and Miller, R. J. (1999). Pre- and postsynaptic actions of opioid and orphan opioid agonists in the rat arcuate nucleus and ventromedial hypothalamus in vitro. J. Physiol. 517(Pt 2), 431–445. doi: 10.1111/j.1469-7793.1999.0431t.x
Endoh, T. (2006). Pharmacological characterization of inhibitory effects of postsynaptic opioid and cannabinoid receptors on calcium currents in neonatal rat nucleus tractus solitarius. Br. J. Pharmacol. 147, 391–401. doi: 10.1038/sj.bjp.0706623
Erbs, E., Faget, L., Scherrer, G., Matifas, A., Filliol, D., Vonesch, J.-L., et al. (2015). A mu-delta opioid receptor brain atlas reveals neuronal co-occurrence in subcortical networks. Brain Struct. Fun. 220, 677–702. doi: 10.1007/s00429-014-0717-9
Evans, M. C., Hill, J. W., and Anderson, G. M. (2021). Role of insulin in the neuroendocrine control of reproduction. J. Neuroendocrinol. 33:e12930. doi: 10.1111/jne.12930
Faber, E. S., and Sah, P. (2004). Opioids inhibit lateral amygdala pyramidal neurons by enhancing a dendritic potassium current. J. Neurosci. Offic. J. Soc. Neurosci. 24, 3031–3039. doi: 10.1523/JNEUROSCI.4496-03.2004
Fan, K. M., Qiu, L. J., Ma, N., Du, Y. N., Qian, Z. Q., Wei, C. L., et al. (2019). Acute stress facilitates LTD induction at glutamatergic synapses in the hippocampal CA1 region by activating mu-opioid receptors on GABAergic neurons. Front. Neurosci. 13:71. doi: 10.3389/fnins.2019.00071
Ferezou, I., Hill, E. L., Cauli, B., Gibelin, N., Kaneko, T., Rossier, J., et al. (2007). Extensive overlap of mu-opioid and nicotinic sensitivity in cortical interneurons. Cereb. Cortex 17, 1948–1957. doi: 10.1093/cercor/bhl104
Ferrari, L. L., Park, D., Zhu, L., Palmer, M. R., Broadhurst, R. Y., and Arrigoni, E. (2018). Regulation of lateral hypothalamic orexin activity by local GABAergic neurons. J. Neurosci. Offic. J. Soc. Neurosci. 38, 1588–1599. doi: 10.1523/JNEUROSCI.1925-17.2017
Finnegan, T. F., Chen, S. R., and Pan, H. L. (2005). Effect of the mu opioid on excitatory and inhibitory synaptic inputs to periaqueductal gray-projecting neurons in the amygdala. J. Pharmacol. Exp. Ther. 312, 441–448. doi: 10.1124/jpet.104.074633
Finnegan, T. F., Chen, S. R., and Pan, H. L. (2006). Mu opioid receptor activation inhibits GABAergic inputs to basolateral amygdala neurons through Kv1.1/1.2 channels. J. Neurophysiol. 95, 2032–2041.
Finnegan, T. F., Li, D. P., Chen, S. R., and Pan, H. L. (2004). Activation of mu-opioid receptors inhibits synaptic inputs to spinally projecting rostral ventromedial medulla neurons. J. Pharmacol. Exp. Ther. 309, 476–483. doi: 10.1124/jpet.103.064808
Ford, C. P., Beckstead, M. J., and Williams, J. T. (2007). Kappa opioid inhibition of somatodendritic dopamine inhibitory postsynaptic currents. J. Neurophysiol. 97, 883–891. doi: 10.1152/jn.00963.2006
Ford, C. P., Mark, G. P., and Williams, J. T. (2006). Properties and opioid inhibition of mesolimbic dopamine neurons vary according to target location. J. Neurosci. Offic. J. Soc. Neurosci. 26, 2788–2797. doi: 10.1523/JNEUROSCI.4331-05.2006
Francesconi, W., Berton, F., Demuro, A., Madamba, S. G., and Siggins, G. R. (1997). Naloxone blocks long-term depression of excitatory transmission in rat CA1 hippocampus in vitro. Arch. Ital. Biol. 135, 37–48.
French, E. D., and Zieglgansberger, W. (1982). The excitatory response of in vitro hippocampal pyramidal cells to normorphine and methionine-enkephalin may be mediated by different receptor populations. Exp. Brain Res. 48, 238–244. doi: 10.1007/BF00237219
Fritz, B. M., Munoz, B., Yin, F., Bauchle, C., and Atwood, B. K. (2018). A high-fat, high-sugar western diet alters dorsal striatal glutamate, opioid, and dopamine transmission in mice. Neuroscience 372, 1–15. doi: 10.1016/j.neuroscience.2017.12.036
Galaj, E., and Xi, Z. X. (2021). Progress in opioid reward research: from a canonical two-neuron hypothesis to two neural circuits. Pharmacol. Biochem. Behav. 200:173072. doi: 10.1016/j.pbb.2020.173072
Gardon, O., Faget, L., Chu Sin Chung, P., Matifas, A., Massotte, D., and Kieffer, B. L. (2014). Expression of mu opioid receptor in dorsal diencephalic conduction system: new insights for the medial habenula. Neuroscience 277, 595–609. doi: 10.1016/j.neuroscience.2014.07.053
Gaveriaux-Ruff, C., Nozaki, C., Nadal, X., Hever, X. C., Weibel, R., Matifas, A., et al. (2011). Genetic ablation of delta opioid receptors in nociceptive sensory neurons increases chronic pain and abolishes opioid analgesia. Pain 152, 1238–1248. doi: 10.1016/j.pain.2010.12.031
Gendron, L., Cahill, C. M., von Zastrow, M., Schiller, P. W., and Pineyro, G. (2016). Molecular pharmacology of delta-opioid receptors. Pharmacol. Rev. 68, 631–700. doi: 10.1124/pr.114.008979
Gilpin, N. W., Roberto, M., Koob, G. F., and Schweitzer, P. (2014). Kappa opioid receptor activation decreases inhibitory transmission and antagonizes alcohol effects in rat central amygdala. Neuropharmacology 77, 294–302. doi: 10.1016/j.neuropharm.2013.10.005
Glatzer, N. R., Derbenev, A. V., Banfield, B. W., and Smith, B. N. (2007). Endomorphin-1 modulates intrinsic inhibition in the dorsal vagal complex. J. Neurophysiol. 98, 1591–1599. doi: 10.1152/jn.00336.2007
Glatzer, N. R., and Smith, B. N. (2005). Modulation of synaptic transmission in the rat nucleus of the solitary tract by endomorphin-1. J. Neurophysiol. 93, 2530–2540. doi: 10.1152/jn.00429.2004
Glickfeld, L. L., Atallah, B. V., and Scanziani, M. (2008). Complementary modulation of somatic inhibition by opioids and cannabinoids. J. Neurosci. Offic. J. Soc. Neurosci. 28, 1824–1832. doi: 10.1523/JNEUROSCI.4700-07.2008
Goedecke, L., Bengoetxea, X., Blaesse, P., Pape, H. C., and Jungling, K. (2019). micro-opioid receptor-mediated downregulation of midline thalamic pathways to basal and central amygdala. Sci. Rep. 9:17837. doi: 10.1038/s41598-019-54128-8
Good, A. J., and Westbrook, R. F. (1995). Effects of a microinjection of morphine into the amygdala on the acquisition and expression of conditioned fear and hypoalgesia in rats. Behav. Neurosci. 109, 631–641. doi: 10.1037//0735-7044.109.4.631
Graziane, N. M., Polter, A. M., Briand, L. A., Pierce, R. C., and Kauer, J. A. (2013). Kappa opioid receptors regulate stress-induced cocaine seeking and synaptic plasticity. Neuron 77, 942–954. doi: 10.1016/j.neuron.2012.12.034
Gregoriou, G. C., Kissiwaa, S. A., Patel, S. D., and Bagley, E. E. (2019). Dopamine and opioids inhibit synaptic outputs of the main island of the intercalated neurons of the amygdala. Eur. J. Neurosci. 50, 2065–2074. doi: 10.1111/ejn.14107
Guan, Y. Z., and Ye, J. H. (2010). Ethanol blocks long-term potentiation of GABAergic synapses in the ventral tegmental area involving mu-opioid receptors. Neuropsychopharmacology 35, 1841–1849. doi: 10.1038/npp.2010.51
Hack, S. P., Bagley, E. E., Chieng, B. C., and Christie, M. J. (2005). Induction of delta-opioid receptor function in the midbrain after chronic morphine treatment. J. Neurosci. Offic. J. Soc. Neurosci. 25, 3192–3198. doi: 10.1523/JNEUROSCI.4585-04.2005
Harasawa, I., Fields, H. L., and Meng, I. D. (2000). Delta opioid receptor mediated actions in the rostral ventromedial medulla on tail flick latency and nociceptive modulatory neurons. Pain 85, 255–262. doi: 10.1016/s0304-3959(99)00280-8
Hawes, S. L., Salinas, A. G., Lovinger, D. M., and Blackwell, K. T. (2017). Long-term plasticity of corticostriatal synapses is modulated by pathway-specific co-release of opioids through kappa-opioid receptors. J. Physiol. 595, 5637–5652. doi: 10.1113/JP274190
Hayar, A., and Guyenet, P. G. (1998). Pre- and postsynaptic inhibitory actions of methionine-enkephalin on identified bulbospinal neurons of the rat RVL. J. Neurophysiol. 80, 2003–2014. doi: 10.1152/jn.1998.80.4.2003
He, X. J., Patel, J., Weiss, C. E., Ma, X., Bloodgood, B. L., and Banghart, M. R. (2021). Convergent, functionally independent signaling by mu and delta opioid receptors in hippocampal parvalbumin interneurons. Elife 10:e69746. doi: 10.7554/eLife.69746
Hearing, M. (2019). Prefrontal-accumbens opioid plasticity: implications for relapse and dependence. Pharmacol. Res. 139, 158–165. doi: 10.1016/j.phrs.2018.11.012
Hearing, M., Graziane, N., Dong, Y., and Thomas, M. J. (2018). Opioid and psychostimulant plasticity: targeting overlap in nucleus accumbens glutamate signaling. Trends Pharmacol. Sci. 39, 276–294. doi: 10.1016/j.tips.2017.12.004
Hein, M., Ji, G., Tidwell, D., DSouza, P., Kiritoshi, T., Yakhnitsa, V., et al. (2021). Kappa opioid receptor activation in the amygdala disinhibits CRF neurons to generate pain-like behaviors. Neuropharmacology 185:108456. doi: 10.1016/j.neuropharm.2021.108456
Heinricher, M. M., Morgan, M. M., and Fields, H. L. (1992). Direct and indirect actions of morphine on medullary neurons that modulate nociception. Neuroscience 48, 533–543. doi: 10.1016/0306-4522(92)90400-v
Heinricher, M. M., Morgan, M. M., Tortorici, V., and Fields, H. L. (1994). Disinhibition of off-cells and antinociception produced by an opioid action within the rostral ventromedial medulla. Neuroscience 63, 279–288. doi: 10.1016/0306-4522(94)90022-1
Herman, M. A., Gillis, R. A., Vicini, S., Dretchen, K. L., and Sahibzada, N. (2012). Tonic GABAA receptor conductance in medial subnucleus of the tractus solitarius neurons is inhibited by activation of mu-opioid receptors. J. Neurophysiol. 107, 1022–1031. doi: 10.1152/jn.00853.2011
Highstein, S. M., and Holstein, G. R. (2006). The anatomy of the vestibular nuclei. Prog. Brain Res. 151, 157–203.
Hipolito, L., Wilson-Poe, A., Campos-Jurado, Y., Zhong, E., Gonzalez-Romero, J., Virag, L., et al. (2015). Inflammatory pain promotes increased opioid self-administration: role of dysregulated ventral tegmental area mu opioid receptors. J. Neurosci. Offic. J. Soc. Neurosci. 35, 12217–12231. doi: 10.1523/JNEUROSCI.1053-15.2015
Hjelmstad, G. O., and Fields, H. L. (2001). Kappa opioid receptor inhibition of glutamatergic transmission in the nucleus accumbens shell. J. Neurophysiol. 85, 1153–1158. doi: 10.1152/jn.2001.85.3.1153
Hjelmstad, G. O., and Fields, H. L. (2003). Kappa opioid receptor activation in the nucleus accumbens inhibits glutamate and GABA release through different mechanisms. J. Neurophysiol. 89, 2389–2395. doi: 10.1152/jn.01115.2002
Hjelmstad, G. O., Xia, Y., Margolis, E. B., and Fields, H. L. (2013). Opioid modulation of ventral pallidal afferents to ventral tegmental area neurons. J. Neurosci. Offic. J. Soc. Neurosci. 33, 6454–6459. doi: 10.1523/JNEUROSCI.0178-13.2013
Hoffman, A. F., and Lupica, C. R. (2001). Direct actions of cannabinoids on synaptic transmission in the nucleus accumbens: a comparison with opioids. J. Neurophysiol. 85, 72–83. doi: 10.1152/jn.2001.85.1.72
Hoffman, A. F., Oz, M., Caulder, T., and Lupica, C. R. (2003). Functional tolerance and blockade of long-term depression at synapses in the nucleus accumbens after chronic cannabinoid exposure. J. Neurosci. Offic. J. Soc. Neurosci. 23, 4815–4820. doi: 10.1523/JNEUROSCI.23-12-04815.2003
Honda, E., Ono, K., and Inenaga, K. (2004). DAMGO suppresses both excitatory and inhibitory synaptic transmission in supraoptic neurones of mouse hypothalamic slice preparations. J. Neuroendocrinol. 16, 198–207. doi: 10.1111/j.0953-8194.2004.01151.x
Hu, H., Cui, Y., and Yang, Y. (2020). Circuits and functions of the lateral habenula in health and in disease. Nat. Rev. Neurosci. 21, 277–295. doi: 10.1038/s41583-020-0292-4
Huang, L., Chen, Y., Jin, S., Lin, L., Duan, S., Si, K., et al. (2021). Organizational principles of amygdalar input-output neuronal circuits. Mol. Psychiatry 26, 7118–7129. doi: 10.1038/s41380-021-01262-3
Huge, V., Rammes, G., Beyer, A., Zieglgansberger, W., and Azad, S. C. (2009). Activation of kappa opioid receptors decreases synaptic transmission and inhibits long-term potentiation in the basolateral amygdala of the mouse. Eur. J. Pain 13, 124–129. doi: 10.1016/j.ejpain.2008.03.010
Huo, F. Q., Wang, J., Li, Y. Q., Chen, T., Han, F., and Tang, J. S. (2005). GABAergic neurons express mu-opioid receptors in the ventrolateral orbital cortex of the rat. Neurosci. Lett. 382, 265–268. doi: 10.1016/j.neulet.2005.03.070
Hyman, S. E., Malenka, R. C., and Nestler, E. J. (2006). Neural mechanisms of addiction: the role of reward-related learning and memory. Annu. Rev. Neurosci. 29, 565–598. doi: 10.1146/annurev.neuro.29.051605.113009
Ibrahim, N., Bosch, M. A., Smart, J. L., Qiu, J., Rubinstein, M., Ronnekleiv, O. K., et al. (2003). Hypothalamic proopiomelanocortin neurons are glucose responsive and express K(ATP) channels. Endocrinology 144, 1331–1340. doi: 10.1210/en.2002-221033
Inbar, K., Levi, L. A., Bernat, N., Odesser, T., Inbar, D., and Kupchik, Y. M. (2020). Cocaine dysregulates dynorphin modulation of inhibitory neurotransmission in the ventral pallidum in a cell-type-specific manner. J. Neurosci. Offic. J. Soc. Neurosci. 40, 1321–1331. doi: 10.1523/JNEUROSCI.1262-19.2019
Inenaga, K., Imura, H., Yanaihara, N., and Yamashita, H. (1990). Kappa-selective opioid receptor agonists leumorphin and dynorphin inhibit supraoptic neurons in rat hypothalamic slice preparations. J. Neuroendocrinol. 2, 389–395. doi: 10.1111/j.1365-2826.1990.tb00423.x
Inenaga, K., Nagatomo, T., Nakao, K., Yanaihara, N., and Yamashita, H. (1994). Kappa-selective agonists decrease postsynaptic potentials and calcium components of action potentials in the supraoptic nucleus of rat hypothalamus in vitro. Neuroscience 58, 331–340. doi: 10.1016/0306-4522(94)90039-6
Inoue, R., Aosaki, T., and Miura, M. (2012). Protein kinase C activity alters the effect of mu-opioid receptors on inhibitory postsynaptic current in the striosomes. Neuroreport 23, 184–188. doi: 10.1097/WNR.0b013e32834faab0
Iremonger, K. J., and Bains, J. S. (2009). Retrograde opioid signaling regulates glutamatergic transmission in the hypothalamus. J. Neurosci. Offic. J. Soc. Neurosci. 29, 7349–7358. doi: 10.1523/JNEUROSCI.0381-09.2009
Iremonger, K. J., Kuzmiski, J. B., Baimoukhametova, D. V., and Bains, J. S. (2011). Dual regulation of anterograde and retrograde transmission by endocannabinoids. J. Neurosci. Offic. J. Soc. Neurosci. 31, 12011–12020. doi: 10.1523/JNEUROSCI.2925-11.2011
Israel, J. M., Poulain, D. A., and Oliet, S. H. (2010). Glutamatergic inputs contribute to phasic activity in vasopressin neurons. J. Neurosci. Offic. J. Soc. Neurosci. 30, 1221–1232. doi: 10.1523/JNEUROSCI.2948-09.2010
Ito, Y., Tabata, K., Makimura, M., and Fukuda, H. (2001). Acute and chronic intracerebroventricular morphine infusions affect long-term potentiation differently in the lateral perforant path. Pharmacol. Biochem. Behav. 70, 353–358. doi: 10.1016/s0091-3057(01)00618-9
Jabourian, M., Venance, L., Bourgoin, S., Ozon, S., Perez, S., Godeheu, G., et al. (2005). Functional mu opioid receptors are expressed in cholinergic interneurons of the rat dorsal striatum: territorial specificity and diurnal variation. Eur. J. Neurosci. 21, 3301–3309. doi: 10.1111/j.1460-9568.2005.04154.x
Jalabert, M., Bourdy, R., Courtin, J., Veinante, P., Manzoni, O. J., Barrot, M., et al. (2011). Neuronal circuits underlying acute morphine action on dopamine neurons. Proc. Natl. Acad. Sci. U.S.A. 108, 16446–16450. doi: 10.1073/pnas.1105418108
James, A. S., Chen, J. Y., Cepeda, C., Mittal, N., Jentsch, J. D., Levine, M. S., et al. (2013). Opioid self-administration results in cell-type specific adaptations of striatal medium spiny neurons. Behav. Brain Res. 256, 279–283. doi: 10.1016/j.bbr.2013.08.009
Janiri, L., dAmato, R., and Zieglgansberger, W. (1988). Dynorphin1-17 reduces the inhibitory actions of mu- and delta-selective opioid agonists in cortical neurons of the rat in vivo. Neurosci. Lett. 84, 79–83. doi: 10.1016/0304-3940(88)90341-2
Jiang, C., Wang, X., Le, Q., Liu, P., Liu, C., Wang, Z., et al. (2021). Morphine coordinates SST and PV interneurons in the prelimbic cortex to disinhibit pyramidal neurons and enhance reward. Mol. Psychiatry 26, 1178–1193. doi: 10.1038/s41380-019-0480-7
Jiang, Z. G., and North, R. A. (1992). Pre- and postsynaptic inhibition by opioids in rat striatum. J. Neurosci. Offic. J. Soc. Neurosci. 12, 356–361. doi: 10.1523/JNEUROSCI.12-01-00356.1992
Johnson, S. W., and North, R. A. (1992). Opioids excite dopamine neurons by hyperpolarization of local interneurons. J. Neurosci. Offic. J. Soc. Neurosci. 12, 483–488. doi: 10.1523/JNEUROSCI.12-02-00483.1992
Jolas, T., and Aghajanian, G. K. (1997). Opioids suppress spontaneous and NMDA-induced inhibitory postsynaptic currents in the dorsal raphe nucleus of the rat in vitro. Brain Res. 755, 229–245. doi: 10.1016/s0006-8993(97)00103-0
Kang-Park, M. H., Kieffer, B. L., Roberts, A. J., Roberto, M., Madamba, S. G., Siggins, G. R., et al. (2009). Mu-opioid receptors selectively regulate basal inhibitory transmission in the central amygdala: lack of ethanol interactions. J. Pharmacol. Exp. Ther. 328, 284–293. doi: 10.1124/jpet.108.140749
Kang-Park, M. H., Kieffer, B. L., Roberts, A. J., Siggins, G. R., and Moore, S. D. (2007). Presynaptic delta opioid receptors regulate ethanol actions in central amygdala. J. Pharmacol. Exp. Ther. 320, 917–925. doi: 10.1124/jpet.106.112722
Kays, J. L., Hurley, R. A., and Taber, K. H. (2012). The dynamic brain: neuroplasticity and mental health. J. Neuropsych. Clin. Neurosci. 24, 118–124. doi: 10.1176/appi.neuropsych.24.1.118
Kearns, I. R., Morton, R. A., Bulters, D. O., and Davies, C. H. (2001). Opioid receptor regulation of muscarinic acetylcholine receptor-mediated synaptic responses in the hippocampus. Neuropharmacology 41, 565–573. doi: 10.1016/s0028-3908(01)00108-3
Kelly, C., and Castellanos, F. X. (2014). Strengthening connections: functional connectivity and brain plasticity. Neuropsychol. Rev. 24, 63–76. doi: 10.1007/s11065-014-9252-y
Kelly, M. J., Loose, M. D., and Ronnekleiv, O. K. (1990). Opioids hyperpolarize beta-endorphin neurons via mu-receptor activation of a potassium conductance. Neuroendocrinology 52, 268–275. doi: 10.1159/000125597
Kelly, M. J., Loose, M. D., and Ronnekleiv, O. K. (1992). Estrogen suppresses mu-opioid- and GABAB-mediated hyperpolarization of hypothalamic arcuate neurons. J. Neurosci. Offic. J. Soc. Neurosci. 12, 2745–2750. doi: 10.1523/JNEUROSCI.12-07-02745.1992
Khom, S., Nguyen, J. D., Vandewater, S. A., Grant, Y., Roberto, M., and Taffe, M. A. (2021). Self-administration of entactogen psychostimulants dysregulates gamma-aminobutyric acid (GABA) and kappa opioid receptor signaling in the central nucleus of the amygdala of female wistar rats. Front. Behav. Neurosci. 15:780500. doi: 10.3389/fnbeh.2021.780500
Kim, C. J., Rhee, J. S., and Akaike, N. (1997). Modulation of high-voltage activated Ca2+ channels in the rat periaqueductal gray neurons by mu-type opioid agonist. J. Neurophysiol. 77, 1418–1424. doi: 10.1152/jn.1997.77.3.1418
Kim, D. W., Washington, P. W., Wang, Z. Q., Lin, S. H., Sun, C., Ismail, B. T., et al. (2020). The cellular and molecular landscape of hypothalamic patterning and differentiation from embryonic to late postnatal development. Nat. Commun. 11:4360.
Kissiwaa, S. A., Patel, S. D., Winters, B. L., and Bagley, E. E. (2020). Opioids differentially modulate two synapses important for pain processing in the amygdala. Br. J. Pharmacol. 177, 420–431. doi: 10.1111/bph.14877
Knoll, A. T., Muschamp, J. W., Sillivan, S. E., Ferguson, D., Dietz, D. M., Meloni, E. G., et al. (2011). Kappa opioid receptor signaling in the basolateral amygdala regulates conditioned fear and anxiety in rats. Biol. Psychiatry 70, 425–433. doi: 10.1016/j.biopsych.2011.03.017
Kolk, S. M., and Rakic, P. (2022). Development of prefrontal cortex. Neuropsychopharmacology 47, 41–57.
Kosten, T. R., and George, T. P. (2002). The neurobiology of opioid dependence: implications for treatment. Sci. Pract. Perspect. 1, 13–20. doi: 10.1151/spp021113
Krook-Magnuson, E., Luu, L., Lee, S. H., Varga, C., and Soltesz, I. (2011). Ivy and neurogliaform interneurons are a major target of mu-opioid receptor modulation. J. Neurosci. Offic. J. Soc. Neurosci. 31, 14861–14870. doi: 10.1523/JNEUROSCI.2269-11.2011
Krug, M., Brodemann, R., and Wagner, M. (2001). Simultaneous activation and opioid modulation of long-term potentiation in the dentate gyrus and the hippocampal CA3 region after stimulation of the perforant pathway in freely moving rats. Brain Res. 913, 68–77. doi: 10.1016/s0006-8993(01)02401-5
Kupchik, Y. M., Scofield, M. D., Rice, K. C., Cheng, K., Roques, B. P., and Kalivas, P. W. (2014). Cocaine dysregulates opioid gating of GABA neurotransmission in the ventral pallidum. J. Neurosci. Offic. J. Soc. Neurosci. 34, 1057–1066. doi: 10.1523/JNEUROSCI.4336-13.2014
Lafourcade, C. A., and Alger, B. E. (2008). Distinctions among GABAA and GABAB responses revealed by calcium channel antagonists, cannabinoids, opioids, and synaptic plasticity in rat hippocampus. Psychopharmacology 198, 539–549. doi: 10.1007/s00213-007-1040-4
Lagrange, A. H., Ronnekleiv, O. K., and Kelly, M. J. (1994). The potency of mu-opioid hyperpolarization of hypothalamic arcuate neurons is rapidly attenuated by 17 beta-estradiol. J. Neurosci. Offic. J. Soc. Neurosci. 14, 6196–6204. doi: 10.1523/JNEUROSCI.14-10-06196.1994
Lagrange, A. H., Ronnekleiv, O. K., and Kelly, M. J. (1995). Estradiol-17 beta and mu-opioid peptides rapidly hyperpolarize GnRH neurons: a cellular mechanism of negative feedback? Endocrinology 136, 2341–2344. doi: 10.1210/endo.136.5.7720682
Lamotte, G., Shouman, K., and Benarroch, E. E. (2021). Stress and central autonomic network. Auton Neurosci. 235:102870.
Lau, B. K., Ambrose, B. P., Thomas, C. S., Qiao, M., and Borgland, S. L. (2020). Mu-opioids suppress GABAergic synaptic transmission onto orbitofrontal cortex pyramidal neurons with subregional selectivity. J. Neurosci. Offic. J. Soc. Neurosci. 40, 5894–5907. doi: 10.1523/JNEUROSCI.2049-19.2020
Laurent, V., Bertran-Gonzalez, J., Chieng, B. C., and Balleine, B. W. (2014). delta-opioid and dopaminergic processes in accumbens shell modulate the cholinergic control of predictive learning and choice. J. Neurosci. Offic. J. Soc. Neurosci. 34, 1358–1369. doi: 10.1523/JNEUROSCI.4592-13.2014
Le Merrer, J., Becker, J. A. J., Katia, B., and Kieffer, B. L. (2009). Reward processing by the opioid system in the brain. Physiol. Rev. 89, 1379–1412. doi: 10.1152/physrev.00005.2009
Lebow, M. A., and Chen, A. (2016). Overshadowed by the amygdala: the bed nucleus of the stria terminalis emerges as key to psychiatric disorders. Mol. Psychiatry 21, 450–463.
Lecca, S., Melis, M., Luchicchi, A., Ennas, M. G., Castelli, M. P., Muntoni, A. L., et al. (2011). Effects of drugs of abuse on putative rostromedial tegmental neurons, inhibitory afferents to midbrain dopamine cells. Neuropsychopharmacology 36, 589–602. doi: 10.1038/npp.2010.190
Lee, H. K. (1978). Iontophoresis of enkephalins potentiates evoked field potentials in the in vitro rat hippocampus. Chin. J. Physiol. 22, 165–170.
Leroy, F., Brann, D. H., Meira, T., and Siegelbaum, S. A. (2017). Input-timing-dependent plasticity in the hippocampal CA2 region and its potential role in social memory. Neuron 95, 1089–1102.e5.
Leroy, F., Brann, D. H., Meira, T., and Siegelbaum, S. A. (2019). Input-timing-dependent plasticity in the hippocampal CA2 region and its potential role in social memory. Neuron 102, 260–262.
Levitt, E. S., Abdala, A. P., Paton, J. F., Bissonnette, J. M., and Williams, J. T. (2015). mu opioid receptor activation hyperpolarizes respiratory-controlling Kolliker-Fuse neurons and suppresses post-inspiratory drive. J. Physiol. 593, 4453–4469. doi: 10.1113/JP270822
Levitt, E. S., and Williams, J. T. (2018). Desensitization and tolerance of mu opioid receptors on pontine kolliker-fuse neurons. Mol. Pharmacol. 93, 8–13. doi: 10.1124/mol.117.109603
Li, A. H., and Wang, H. L. (2001). G protein-coupled receptor kinase 2 mediates mu-opioid receptor desensitization in GABAergic neurons of the nucleus raphe magnus. J. Neurochem. 77, 435–444. doi: 10.1046/j.1471-4159.2001.00267.x
Li, C., and Kash, T. L. (2019). kappa-opioid receptor modulation of GABAergic inputs onto ventrolateral periaqueductal gray dopamine neurons. Mol. Neuropsychiatry 5, 190–199. doi: 10.1159/000496974
Li, C., Pleil, K. E., Stamatakis, A. M., Busan, S., Vong, L., Lowell, B. B., et al. (2012). Presynaptic inhibition of gamma-aminobutyric acid release in the bed nucleus of the stria terminalis by kappa opioid receptor signaling. Biol. Psychiatry 71, 725–732. doi: 10.1016/j.biopsych.2011.11.015
Li, C., Sugam, J. A., Lowery-Gionta, E. G., McElligott, Z. A., McCall, N. M., Lopez, A. J., et al. (2016). Mu opioid receptor modulation of dopamine neurons in the periaqueductal gray/dorsal raphe: a role in regulation of pain. Neuropsychopharmacology 41, 2122–2132. doi: 10.1038/npp.2016.12
Liao, D., Lin, H., Law, P. Y., and Loh, H. H. (2005). Mu-opioid receptors modulate the stability of dendritic spines. Proc. Natl. Acad. Sci. U.S.A. 102, 1725–1730. doi: 10.1073/pnas.0406797102
Linseman, M. A., and Corrigall, W. A. (1982). Effects of morphine on CA1 versus dentate hippocampal field potentials following systemic administration in freely-moving rats. Neuropharmacology 21, 361–366. doi: 10.1016/0028-3908(82)90101-0
Liu, Q. S., Han, S., Jia, Y. S., and Ju, G. (1999). Selective modulation of excitatory transmission by mu-opioid receptor activation in rat supraoptic neurons. J. Neurophysiol. 82, 3000–3005. doi: 10.1152/jn.1999.82.6.3000
Loose, M. D., and Kelly, M. J. (1990). Opioids act at mu-receptors to hyperpolarize arcuate neurons via an inwardly rectifying potassium conductance. Brain Res. 513, 15–23. doi: 10.1016/0006-8993(90)91084-t
Loose, M. D., Ronnekleiv, O. K., and Kelly, M. J. (1991). Neurons in the rat arcuate nucleus are hyperpolarized by GABAB and mu-opioid receptor agonists: evidence for convergence at a ligand-gated potassium conductance. Neuroendocrinology 54, 537–544. doi: 10.1159/000125979
Lovinger, D. M. (2010). Neurotransmitter roles in synaptic modulation, plasticity and learning in the dorsal striatum. Neuropharmacology 58, 951–961. doi: 10.1016/j.neuropharm.2010.01.008
Lowe, J. D., and Bailey, C. P. (2015). Functional selectivity and time-dependence of mu-opioid receptor desensitization at nerve terminals in the mouse ventral tegmental area. Br. J. Pharmacol. 172, 469–481. doi: 10.1111/bph.12605
Lu, C. W., Lin, T. Y., Huang, S. K., Chiu, K. M., Lee, M. Y., Huang, J. H., et al. (2021). Inhibition of glutamatergic transmission and neuronal excitability by oxycodone in the rat hippocampal CA3 neurons. Can J. Physiol. Pharmacol. 99, 737–743. doi: 10.1139/cjpp-2020-0469
Lu, S., Huang, K., Lin, T. Y., and Wang, S. J. (2020). Tapentadol suppresses glutamatergic transmission and neuronal firing in rat hippocampal CA3 pyramidal neurons. Pharmacology 105, 445–453. doi: 10.1159/000504886
Lupica, C. R. (1995). Delta and mu enkephalins inhibit spontaneous GABA-mediated IPSCs via a cyclic AMP-independent mechanism in the rat hippocampus. J. Neurosci. Offic. J. Soc. Neurosci. 15, 737–749. doi: 10.1523/JNEUROSCI.15-01-00737.1995
Lupica, C. R., and Dunwiddie, T. V. (1991). Differential effects of mu- and delta-receptor selective opioid agonists on feedforward and feedback GABAergic inhibition in hippocampal brain slices. Synapse 8, 237–248. doi: 10.1002/syn.890080402
Lupica, C. R., Proctor, W. R., and Dunwiddie, T. V. (1992). Dissociation of mu and delta opioid receptor-mediated reductions in evoked and spontaneous synaptic inhibition in the rat hippocampus in vitro. Brain Res. 593, 226–238. doi: 10.1016/0006-8993(92)91312-3
Lüscher, C., and Malenka, R. C. (2011). Drug-evoked synaptic plasticity in addiction: from molecular changes to circuit remodeling. Neuron 69, 650–663. doi: 10.1016/j.neuron.2011.01.017
Ma, Y. Y., Cepeda, C., Chatta, P., Franklin, L., Evans, C. J., and Levine, M. S. (2012). Regional and cell-type-specific effects of DAMGO on striatal D1 and D2 dopamine receptor-expressing medium-sized spiny neurons. ASN Neuro 4:554. doi: 10.1042/AN20110063
Madamba, S. G., Schweitzer, P., and Siggins, G. R. (1999). Dynorphin selectively augments the M-current in hippocampal CA1 neurons by an opiate receptor mechanism. J. Neurophysiol. 82, 1768–1775. doi: 10.1152/jn.1999.82.4.1768
Madhavan, A., Bonci, A., and Whistler, J. L. (2010). Opioid-Induced GABA potentiation after chronic morphine attenuates the rewarding effects of opioids in the ventral tegmental area. J. Neurosci. Offic. J. Soc. Neurosci. 30, 14029–14035. doi: 10.1523/JNEUROSCI.3366-10.2010
Madison, D. V., and Nicoll, R. A. (1988). Enkephalin hyperpolarizes interneurones in the rat hippocampus. J. Physiol. 398, 123–130. doi: 10.1113/jphysiol.1988.sp017033
Mamaligas, A. A., Cai, Y., and Ford, C. P. (2016). Nicotinic and opioid receptor regulation of striatal dopamine D2-receptor mediated transmission. Sci. Rep. 6:37834. doi: 10.1038/srep37834
Maneuf, Y. P. I., Mitchell, J., Crossman, A. R., Woodruff, G. N., and Brotchie, J. M. (1995). Functional implications of kappa opioid receptor-mediated modulation of glutamate transmission in the output regions of the basal ganglia in rodent and primate models of Parkinsons disease. Brain Res. 683, 102–108. doi: 10.1016/0006-8993(95)00358-w
Mansour, A., Khachaturian, H., Lewis, M. E., Akil, H., and Watson, S. J. (1987). Autoradiographic differentiation of mu, delta, and kappa opioid receptors in the rat forebrain and midbrain. J. Neurosci. Offic. J. Soc. Neurosci. 7, 2445–2464.
Manzoni, O. J., and Williams, J. T. (1999). Presynaptic regulation of glutamate release in the ventral tegmental area during morphine withdrawal. J. Neurosci. Offic. J. Soc. Neurosci. 19, 6629–6636. doi: 10.1523/JNEUROSCI.19-15-06629.1999
Margolis, E. B., and Fields, H. L. (2016). Mu opioid receptor actions in the lateral habenula. PLoS One 11:e0159097. doi: 10.1371/journal.pone.0159097
Margolis, E. B., Fields, H. L., Hjelmstad, G. O., and Mitchell, J. M. (2008a). Delta-opioid receptor expression in the ventral tegmental area protects against elevated alcohol consumption. J. Neurosci. Offic. J. Soc. Neurosci. 28, 12672–12681. doi: 10.1523/JNEUROSCI.4569-08.2008
Margolis, E. B., Fujita, W., Devi, L. A., and Fields, H. L. (2017). Two delta opioid receptor subtypes are functional in single ventral tegmental area neurons, and can interact with the mu opioid receptor. Neuropharmacology 123, 420–432. doi: 10.1016/j.neuropharm.2017.06.019
Margolis, E. B., Hjelmstad, G. O., Bonci, A., and Fields, H. L. (2003). Kappa-opioid agonists directly inhibit midbrain dopaminergic neurons. J. Neurosci. Offic. J. Soc. Neurosci. 23, 9981–9986. doi: 10.1523/JNEUROSCI.23-31-09981.2003
Margolis, E. B., Hjelmstad, G. O., Bonci, A., and Fields, H. L. (2005). Both kappa and mu opioid agonists inhibit glutamatergic input to ventral tegmental area neurons. J. Neurophysiol. 93, 3086–3093.
Margolis, E. B., Hjelmstad, G. O., Fujita, W., and Fields, H. L. (2014). Direct bidirectional mu-opioid control of midbrain dopamine neurons. J. Neurosci. Offic. J. Soc. Neurosci. 34, 14707–14716. doi: 10.1523/JNEUROSCI.2144-14.2014
Margolis, E. B., Lock, H., Chefer, V. I., Shippenberg, T. S., Hjelmstad, G. O., and Fields, H. L. (2006). Kappa opioids selectively control dopaminergic neurons projecting to the prefrontal cortex. Proc. Natl. Acad. Sci. U.S.A. 103, 2938–2942. doi: 10.1073/pnas.0511159103
Margolis, E. B., Mitchell, J. M., Hjelmstad, G. O., and Fields, H. L. (2011). A novel opioid receptor-mediated enhancement of GABAA receptor function induced by stress in ventral tegmental area neurons. J. Physiol. 589, 4229–4242. doi: 10.1113/jphysiol.2011.209023
Margolis, E. B., Mitchell, J. M., Ishikawa, J., Hjelmstad, G. O., and Fields, H. L. (2008b). Midbrain dopamine neurons: projection target determines action potential duration and dopamine D(2) receptor inhibition. J. Neurosci. Offic. J. Soc. Neurosci. 28, 8908–8913. doi: 10.1523/JNEUROSCI.1526-08.2008
Marinelli, S., Connor, M., Schnell, S. A., Christie, M. J., Wessendorf, M. W., and Vaughan, C. W. (2005). delta-opioid receptor-mediated actions on rostral ventromedial medulla neurons. Neuroscience 132, 239–244. doi: 10.1016/j.neuroscience.2005.01.015
Marinelli, S., Vaughan, C. W., Schnell, S. A., Wessendorf, M. W., and Christie, M. J. (2002). Rostral ventromedial medulla neurons that project to the spinal cord express multiple opioid receptor phenotypes. J. Neurosci. Offic. J. Soc. Neurosci. 22, 10847–10855. doi: 10.1523/JNEUROSCI.22-24-10847.2002
Martin, G., Nie, Z., and Siggins, G. R. (1997). mu-Opioid receptors modulate NMDA receptor-mediated responses in nucleus accumbens neurons. J. Neurosci. Offic. J. Soc. Neurosci. 17, 11–22. doi: 10.1523/JNEUROSCI.17-01-00011.1997
Matsui, A., Jarvie, B. C., Robinson, B. G., Hentges, S. T., and Williams, J. T. (2014). Separate GABA afferents to dopamine neurons mediate acute action of opioids, development of tolerance, and expression of withdrawal. Neuron 82, 1346–1356. doi: 10.1016/j.neuron.2014.04.030
Matsui, A., and Williams, J. T. (2011). Opioid-sensitive GABA inputs from rostromedial tegmental nucleus synapse onto midbrain dopamine neurons. J. Neurosci. Offic. J. Soc. Neurosci. 31, 17729–17735. doi: 10.1523/JNEUROSCI.4570-11.2011
Matthies, H., Schroeder, H., Becker, A., Loh, H., Höllt, V., and Krug, M. (2000). Lack of expression of long-term potentiation in the dentate gyrus but not in the CA1 region of the hippocampus of μ-opioid receptor-deficient mice. Neuropharmacology 39, 952–960.
McDermott, C. M., and Schrader, L. A. (2011). Activation of kappa opioid receptors increases intrinsic excitability of dentate gyrus granule cells. J. Physiol. 589, 3517–3532. doi: 10.1113/jphysiol.2011.211623
McFadzean, I., Lacey, M. G., Hill, R. G., and Henderson, G. (1987). Kappa opioid receptor activation depresses excitatory synaptic input to rat locus coeruleus neurons in vitro. Neuroscience 20, 231–239. doi: 10.1016/0306-4522(87)90015-7
McQuiston, A. R. (2007). Effects of mu-opioid receptor modulation on GABAB receptor synaptic function in hippocampal CA1. J. Neurophysiol. 97, 2301–2311. doi: 10.1152/jn.01179.2006
McQuiston, A. R. (2008). Layer selective presynaptic modulation of excitatory inputs to hippocampal cornu Ammon 1 by mu-opioid receptor activation. Neuroscience 151, 209–221. doi: 10.1016/j.neuroscience.2007.09.077
McQuiston, A. R. (2011). Mu opioid receptor activation normalizes temporo-ammonic pathway driven inhibition in hippocampal CA1. Neuropharmacology 60, 472–479. doi: 10.1016/j.neuropharm.2010.10.029
Miller, K. K., and Lupica, C. R. (1994). Morphine-induced excitation of pyramidal neurons is inhibited by cholecystokinin in the CA1 region of the rat hippocampal slice. J. Pharmacol. Exp. Ther. 268, 753–761.
Mitchell, J. M., Margolis, E. B., Coker, A. R., and Fields, H. L. (2012). Alcohol self-administration, anxiety, and cortisol levels predict changes in delta opioid receptor function in the ventral tegmental area. Behav. Neurosci. 126, 515–522. doi: 10.1037/a0029027
Mitrovic, I., and Napier, T. C. (2002). Mu and kappa opioid agonists modulate ventral tegmental area input to the ventral pallidum. Eur. J. Neurosci. 15, 257–268. doi: 10.1046/j.0953-816x.2001.01860.x
Miura, M., Saino-Saito, S., Masuda, M., Kobayashi, K., and Aosaki, T. (2007). Compartment-specific modulation of GABAergic synaptic transmission by mu-opioid receptor in the mouse striatum with green fluorescent protein-expressing dopamine islands. J. Neurosci. Offic. J. Soc. Neurosci. 27, 9721–9728. doi: 10.1523/JNEUROSCI.2993-07.2007
Moore, S. D., Madamba, S. G., Schweitzer, P., and Siggins, G. R. (1994). Voltage-dependent effects of opioid peptides on hippocampal CA3 pyramidal neurons in vitro. J. Neurosci. Offic. J. Soc. Neurosci. 14, 809–820. doi: 10.1523/JNEUROSCI.14-02-00809.1994
Motanis, H., Seay, M. J., and Buonomano, D. V. (2018). Short-term synaptic plasticity as a mechanism for sensory timing. Trends Neurosci. 41, 701–711. doi: 10.1016/j.tins.2018.08.001
Moudy, A. M., Coscia, C. J., and Laskowski, M. B. (1989). A mu-specific opioid peptide agonist increases excitability of pyramidal neurons in untreated and receptor up-regulated hippocampus. J. Pharmacol. Exp. Ther. 251, 536–542.
Mu, P., Neumann, P. A., Panksepp, J., Schluter, O. M., and Dong, Y. (2011). Exposure to cocaine alters dynorphin-mediated regulation of excitatory synaptic transmission in nucleus accumbens neurons. Biol. Psychiatry 69, 228–235. doi: 10.1016/j.biopsych.2010.09.014
Munoz, B., Brandon, M. F., and Atwood, B. K. (2021). HCN1 channels mediate mu opioid receptor long-term depression at insular cortex inputs to the dorsal striatum. bioRxiv [Preprint]. 2021.08.31.458358.
Munoz, B., Fritz, B. M., Yin, F., and Atwood, B. K. (2018). Alcohol exposure disrupts mu opioid receptor-mediated long-term depression at insular cortex inputs to dorsolateral striatum. Nat. Commun. 9:1318. doi: 10.1038/s41467-018-03683-1
Munoz, B., Haggerty, D. L., and Atwood, B. K. (2020). Synapse-specific expression of mu opioid receptor long-term depression in the dorsomedial striatum. Sci. Rep. 10:7234. doi: 10.1038/s41598-020-64203-0
Nadeau, S. E. (2021). Neural mechanisms of emotions, alexithymia, and depression. Handb. Clin. Neurol. 183, 299–313. doi: 10.1016/B978-0-12-822290-4.00014-1
Nagode, D. A., Tang, A. H., Yang, K., and Alger, B. E. (2014). Optogenetic identification of an intrinsic cholinergically driven inhibitory oscillator sensitive to cannabinoids and opioids in hippocampal CA1. J. Physiol. 592, 103–123. doi: 10.1113/jphysiol.2013.257428
Namkung, H., Kim, S. H., and Sawa, A. (2017). The insula: an underestimated brain area in clinical neuroscience, psychiatry, and neurology. Trends Neurosci. 40, 200–207.
Napier, T. C., and Mitrovic, I. (1999). Opioid modulation of ventral pallidal inputs. Ann. N. Y. Acad. Sci. 877, 176–201. doi: 10.1111/j.1749-6632.1999.tb09268.x
Nasrallah, K., Piskorowski, R. A., and Chevaleyre, V. (2015). Inhibitory plasticity permits the recruitment of CA2 pyramidal neurons by CA3. eNeuro 2:4795.
Nasrallah, K., Piskorowski, R. A., and Chevaleyre, V. (2017). Bi-directional interplay between proximal and distal inputs to CA2 pyramidal neurons. Neurobiol. Learn. Mem. 138, 173–181. doi: 10.1016/j.nlm.2016.06.024
Nasrallah, K., Therreau, L., Robert, V., Huang, A. J. Y., McHugh, T. J., Piskorowski, R. A., et al. (2019). Routing hippocampal information flow through parvalbumin interneuron plasticity in Area CA2. Cell Rep. 27, 86–98.e3. doi: 10.1016/j.celrep.2019.03.014
Nelson, A. B., Hammack, N., Yang, C. F., Shah, N. M., Seal, R. P., and Kreitzer, A. C. (2014). Striatal cholinergic interneurons Drive GABA release from dopamine terminals. Neuron 82, 63–70. doi: 10.1016/j.neuron.2014.01.023
Neumaier, J. F., Mailheau, S., and Chavkin, C. (1988). Opioid receptor-mediated responses in the dentate gyrus and CA1 region of the rat hippocampus. J. Pharmacol. Exp. Ther. 244, 564–570.
Nicol, B., Rowbotham, D. J., and Lambert, D. G. (1996). mu- and kappa-opioids inhibit K+ evoked glutamate release from rat cerebrocortical slices. Neurosci. Lett. 218, 79–82. doi: 10.1016/s0304-3940(96)13104-9
Nugent, F. S., Penick, E. C., and Kauer, J. A. (2007). Opioids block long-term potentiation of inhibitory synapses. Nature 446, 1086–1090. doi: 10.1038/nature05726
O’Brien, C. P. (2009). Neuroplasticity in addictive disorders. Dialog. Clin. Neurosci. 11, 350–353. doi: 10.31887/DCNS.2009.11.3/cpobrien
Ogura, M., and Kita, H. (2000). Dynorphin exerts both postsynaptic and presynaptic effects in the Globus pallidus of the rat. J. Neurophysiol. 83, 3366–3376. doi: 10.1152/jn.2000.83.6.3366
Ogura, M., and Kita, H. (2002). Effects of dynorphin on rat entopeduncular nucleus neurons in vitro. Neuroscience 114, 973–982. doi: 10.1016/s0306-4522(02)00355-x
Olive, M. F., Anton, B., Micevych, P., Evans, C. J., and Maidment, N. T. (1997). Presynaptic versus postsynaptic localization of mu and delta opioid receptors in dorsal and ventral striatopallidal pathways. J. Neurosci. 17, 7471–7479. doi: 10.1523/JNEUROSCI.17-19-07471.1997
Orikasa, C. (2021). Neural contributions of the hypothalamus to parental behaviour. Int. J. Mol. Sci. 22:6998. doi: 10.3390/ijms22136998
Osborne, P. B., Vaughan, C. W., Wilson, H. I., and Christie, M. J. (1996). Opioid inhibition of rat periaqueductal grey neurones with identified projections to rostral ventromedial medulla in vitro. J. Physiol. 490(Pt 2), 383–389. doi: 10.1113/jphysiol.1996.sp021152
Palmer, M. R., Morris, D. H., Taylor, D. A., Stewart, J. M., and Hoffer, B. J. (1978). Electrophysiological effects of enkephalin analogs in rat cortex. Life Sci. 23, 851–860. doi: 10.1016/0024-3205(78)90520-9
Pan, Y. Z., Li, D. P., Chen, S. R., and Pan, H. L. (2002). Activation of delta-opioid receptors excites spinally projecting locus coeruleus neurons through inhibition of GABAergic inputs. J. Neurophysiol. 88, 2675–2683. doi: 10.1152/jn.00298.2002
Pan, Z. N. Hirakawa, and H. L. Fields. (2000). A cellular mechanism for the bidirectional pain-modulating actions of orphanin FQ/nociceptin. Neuron 26, 515–22. doi: 10.1016/s0896-6273(00)81183-6
Pan, Z. Z., Tershner, S. A., and Fields, H. L. (1997). Cellular mechanism for anti-analgesic action of agonists of the kappa-opioid receptor. Nature 389, 382–385. doi: 10.1038/38730
Pan, Z. Z. J. T. Williams, and P. B. Osborne. (1990). Opioid actions on single nucleus raphe magnus neurons from rat and guinea-pig in vitro. J. Physiol. 427: 519–532. doi: 10.1113/jphysiol.1990.sp018185
Pasternak, G. W. (2012). Preclinical pharmacology and opioid combinations. Pain Med. 13(Suppl. 1), S4–S11. doi: 10.1111/j.1526-4637.2012.01335.x
Patel, D., Callaway, J., and Vaezi, M. (2019). Opioid-induced foregut dysfunction. Am. J. Gastroenterol. 114, 1716–1725.
Patton, M. H., Roberts, B. M., Lovinger, D. M., and Mathur, B. N. (2016). Ethanol disinhibits dorsolateral striatal medium spiny neurons through activation of a presynaptic delta opioid receptor. Neuropsychopharmacology 41, 1831–1840. doi: 10.1038/npp.2015.353
Pennock, R. L., and Hentges, S. T. (2011). Differential expression and sensitivity of presynaptic and postsynaptic opioid receptors regulating hypothalamic proopiomelanocortin neurons. J. Neurosci. Offic. J. Soc. Neurosci. 31, 281–288. doi: 10.1523/JNEUROSCI.4654-10.2011
Pennock, R. L., and Hentges, S. T. (2014). Direct inhibition of hypothalamic proopiomelanocortin neurons by dynorphin A is mediated by the mu-opioid receptor. J. Physiol. 592, 4247–4256. doi: 10.1113/jphysiol.2014.275339
Pieretti, S., Di Giannuario, A., Domenici, M. R., Sagratella, S., Capasso, A., Sorrentino, L., et al. (1994). Dexamethasone-induced selective inhibition of the central mu opioid receptor: functional in vivo and in vitro evidence in rodents. Br. J. Pharmacol. 113, 1416–1422. doi: 10.1111/j.1476-5381.1994.tb17155.x
Piguet, P., and North, R. A. (1993). Opioid actions at mu and delta receptors in the rat dentate gyrus in vitro. J. Pharmacol. Exp. Ther. 266, 1139–1149.
Pina, M. M., Pati, D., Hwa, L. S., Wu, S. Y., Mahoney, A. A., Omenyi, C. G., et al. (2020). The kappa opioid receptor modulates GABA neuron excitability and synaptic transmission in midbrainprojections from the insular cortex. Neuropharmacology 165:107831. doi: 10.1016/j.neuropharm.2019.107831
Pinnock, R. D. (1992a). Activation of kappa-opioid receptors depresses electrically evoked excitatory postsynaptic potentials on 5-HT-sensitive neurones in the rat dorsal raphé nucleus in vitro. Brain Res. 583, 237–246. doi: 10.1016/s0006-8993(10)80029-0
Pinnock, R. D. (1992b). A highly selective kappa-opioid receptor agonist, CI-977, reduces excitatory synaptic potentials in the rat locus coeruleus in vitro. Neuroscience 47, 87–94. doi: 10.1016/0306-4522(92)90123-j
Piskorowski, R. A., and Chevaleyre, V. (2013). Delta-opioid receptors mediate unique plasticity onto parvalbumin-expressing interneurons in area CA2 of the hippocampus. J. Neurosci. Offic. J. Soc. Neurosci. 33, 14567–14578. doi: 10.1523/JNEUROSCI.0649-13.2013
Pitchers, K. K., Coppens, C. M., Beloate, L. N., Fuller, J., Van Karla, S., Frohmader, S. R., et al. (2014). Endogenous opioid-induced neuroplasticity of dopaminergic neurons in the ventral tegmental area influences natural and opiate reward. J. Neurosci. Offic. J. Soc. Neurosci. 34, 8825–8836. doi: 10.1523/JNEUROSCI.0133-14.2014
Pitkänen, A., Savander, V., and LeDoux, J. E. (1997). Organization of intra-amygdaloid circuitries in the rat: an emerging framework for understanding functions of the amygdala. Trends Neurosci. 20, 517–523. doi: 10.1016/s0166-2236(97)01125-9
Polter, A. M., Bishop, R. A., Briand, L. A., Graziane, N. M., Pierce, R. C., and Kauer, J. A. (2014). Poststress block of kappa opioid receptors rescues long-term potentiation of inhibitory synapses and prevents reinstatement of cocaine seeking. Biol. Psychiatry 76, 785–793. doi: 10.1016/j.biopsych.2014.04.019
Ponterio, G., Tassone, A., Sciamanna, G., Riahi, E., Vanni, V., Bonsi, P., et al. (2013). Powerful inhibitory action of mu opioid receptors (MOR) on cholinergic interneuron excitability in the dorsal striatum. Neuropharmacology 75, 78–85. doi: 10.1016/j.neuropharm.2013.07.006
Ponterio, G., Tassone, A., Sciamanna, G., Vanni, V., Meringolo, M., Santoro, M., et al. (2018). Enhanced mu opioid receptor-dependent opioidergic modulation of striatal cholinergic transmission in DYT1 dystonia. Mov. Disord. 33, 310–320. doi: 10.1002/mds.27212
Poole, S. L., Deuchars, J., Lewis, D. I., and Deuchars, S. A. (2007). Subdivision-specific responses of neurons in the nucleus of the tractus solitarius to activation of mu-opioid receptors in the rat. J. Neurophysiol. 98, 3060–3071. doi: 10.1152/jn.00755.2007
Przybysz, K. R., Werner, D. F., and Diaz, M. R. (2017). Age-dependent regulation of GABA transmission by kappa opioid receptors in the basolateral amygdala of Sprague-Dawley rats. Neuropharmacology 117, 124–133. doi: 10.1016/j.neuropharm.2017.01.036
Pu, L., Xu, N., Xia, P., Gu, Q., Ren, S., Fucke, T., et al. (2012). Inhibition of activity of GABA transporter GAT1 by Delta-Opioid Receptor. Evid. Based Complem. Alternat. Med. 2012:818451. doi: 10.1155/2012/818451
Puryear, C. B., Brooks, J., Tan, L., Smith, K., Yan, L., Cunningham, J., et al. (2020). Opioid receptor modulation of neural circuits in depression: What can be learned from preclinical data? Neurosci. Biobehav. Rev. 108, 658–678. doi: 10.1016/j.neubiorev.2019.12.007
Qu, C. L., Huo, F. Q., Huang, F. S., and Tang, J. S. (2015). Activation of mu-opioid receptors in the ventrolateral orbital cortex inhibits the GABAergic miniature inhibitory postsynaptic currents in rats. Neurosci. Lett. 592, 64–69. doi: 10.1016/j.neulet.2015.02.045
Rasakham, K., and Liu-Chen, L. Y. (2011). Sex differences in kappa opioid pharmacology. Life Sci. 88, 2–16. doi: 10.1016/j.lfs.2010.10.007
Reeves, K. C., Kube, M. J., Grecco, G. G., Fritz, B. M., Munoz, B., Yin, F., et al. (2021). Mu opioid receptors on vGluT2-expressing glutamatergic neurons modulate opioid reward. Addict. Biol. 26:e12942. doi: 10.1111/adb.12942
Rezai, X., Kieffer, B. L., Roux, M. J., and Massotte, D. (2013). Delta opioid receptors regulate temporoammonic-activated feedforward inhibition to the mouse CA1 hippocampus. PLoS One 8:e79081. doi: 10.1371/journal.pone.0079081
Rhim, H., Glaum, S. R., and Miller, R. J. (1993). Selective opioid agonists modulate afferent transmission in the rat nucleus tractus solitarius. J. Pharmacol. Exp. Ther. 264, 795–800.
Rhim, H., Toth, P. T., and Miller, R. J. (1996). Mechanism of inhibition of calcium channels in rat nucleus tractus solitarius by neurotransmitters. Br. J. Pharmacol. 118, 1341–1350. doi: 10.1111/j.1476-5381.1996.tb15543.x
Robble, M. A., Bozsik, M. E., Wheeler, D. S., and Wheeler, R. A. (2020). Learned avoidance requires VTA KOR-mediated reductions in dopamine. Neuropharmacology 167:107996. doi: 10.1016/j.neuropharm.2020.107996
Rola, R., Jarkiewicz, M., and Szulczyk, P. (2008). Modulation of Ca2+ channel current by mu opioid receptors in prefrontal cortex pyramidal neurons in rats. Acta Neurobiol. Exp. (Wars) 68, 10–25.
Rolls, E. T. (2019). The cingulate cortex and limbic systems for emotion, action, and memory. Brain Struct. Funct. 224, 3001–3018.
Rosenbaum, D. M., Søren, G. F., and Kobilka, B. K. (2009). The structure and function of G-protein-coupled receptors. Nature 459, 356–363.
Ruchalski, K., and Hathout, G. M. (2012). A medley of midbrain maladies: a brief review of midbrain anatomy and syndromology for radiologists. Radiol. Res. Pract. 2012:258524. doi: 10.1155/2012/258524
Ruka, K. A., Burger, L. L., and Moenter, S. M. (2013). Regulation of arcuate neurons coexpressing kisspeptin, neurokinin B, and dynorphin by modulators of neurokinin 3 and kappa-opioid receptors in adult male mice. Endocrinology 154, 2761–2771. doi: 10.1210/en.2013-1268
Ruka, K. A., Burger, L. L., and Moenter, S. M. (2016). Both estrogen and androgen modify the response to activation of neurokinin-3 and kappa-opioid receptors in arcuate kisspeptin neurons from male mice. Endocrinology 157, 752–763. doi: 10.1210/en.2015-1688
Saga, Y., Hoshi, E., and Tremblay, L. (2017). Roles of multiple globus pallidus territories of monkeys and humans in motivation, cognition and action: an anatomical, physiological and pathophysiological review. Front. Neuroanat. 11:30. doi: 10.3389/fnana.2017.00030
Sagratella, S., Longo, R., and Domenici, M. R. (1996). Selective opposite modulation of dentate granule cells excitability by mu and kappa opioids in rat hippocampal slices. Neurosci. Lett. 205, 53–56. doi: 10.1016/0304-3940(96)12370-3
Saitoh, A., Suzuki, S., Soda, A., Ohashi, M., Yamada, M., Oka, J. I., et al. (2018). The delta opioid receptor agonist KNT-127 in the prelimbic medial prefrontal cortex attenuates veratrine-induced anxiety-like behaviors in mice. Behav. Brain Res. 336, 77–84. doi: 10.1016/j.bbr.2017.08.041
Sah, P., Faber, E. S. Lopez De Armentia, M., and Power, J. (2003). The amygdaloid complex: anatomy and physiology. Physiol. Rev. 83, 803–834.
Salin, P. A., Weisskopf, M. G., and Nicoll, R. A. (1995). A comparison of the role of dynorphin in the hippocampal mossy fiber pathway in guinea pig and rat. J. Neurosci. Offic. J. Soc. Neurosci. 15, 6939–6945. doi: 10.1523/JNEUROSCI.15-10-06939.1995
Schaefers, A. T., and Teuchert-Noodt, G. (2016). Developmental neuroplasticity and the origin of neurodegenerative diseases. World J. Biol. Psychiatry 17, 587–599. doi: 10.3109/15622975.2013.797104
Schmitt, L. I., and Halassa, M. M. (2017). Interrogating the mouse thalamus to correct human neurodevelopmental disorders. Mol. Psychiatry 22, 183–191. doi: 10.1038/mp.2016.183
Schoffelmeer, A. N., Hogenboom, F., and Mulder, A. H. (1997). Kappa1- and kappa2-opioid receptors mediating presynaptic inhibition of dopamine and acetylcholine release in rat neostriatum. Br. J. Pharmacol. 122, 520–524. doi: 10.1038/sj.bjp.0701394
Shao, C., Chen, P., Chen, Q., Zhao, M., Zhang, W. N., and Yang, K. (2020). Mu opioid receptors inhibit GABA release from parvalbumin interneuron terminals onto CA1 pyramidal cells. Biochem. Biophys. Res. Commun. 522, 1059–1062. doi: 10.1016/j.bbrc.2019.12.013
Shippenberg, T. S., LeFevour, A., and Thompson, A. C. (1998). Sensitization to the conditioned rewarding effects of morphine and cocaine: differential effects of the kappa-opioid receptor agonist U69593. Eur. J. Pharmacol. 345, 27–34. doi: 10.1016/s0014-2999(97)01614-2
Shoji, Y., Delfs, J., and Williams, J. T. (1999). Presynaptic inhibition of GABA(B)-mediated synaptic potentials in the ventral tegmental area during morphine withdrawal. J. Neurosci. Offic. J. Soc. Neurosci. 19, 2347–2355. doi: 10.1523/JNEUROSCI.19-06-02347.1999
Sikandar, S., and Dickenson, A. H. (2011). Pregabalin modulation of spinal and brainstem visceral nociceptive processing. Pain 152, 2312–2322. doi: 10.1016/j.pain.2011.06.020
Simmons, M. L., and Chavkin, C. (1996). Endogenous opioid regulation of hippocampal function. Int. Rev. Neurobiol. 39, 145–196.
Simmons, M. L., Terman, G. W., Drake, C. T., and Chavkin, C. (1994). Inhibition of glutamate release by presynaptic kappa 1-opioid receptors in the guinea pig dentate gyrus. J. Neurophysiol. 72, 1697–1705. doi: 10.1152/jn.1994.72.4.1697
Simmons, S. C., Shepard, R. D., Gouty, S., Langlois, L. D., Flerlage, W. J., Cox, B. M., et al. (2020). Early life stress dysregulates kappa opioid receptor signaling within the lateral habenula. Neurobiol. Stress 13:100267. doi: 10.1016/j.ynstr.2020.100267
Smith, J. S., Schindler, A. G., Martinelli, E., Gustin, R. M., Bruchas, M. R., and Chavkin, C. (2012). Stress-induced activation of the dynorphin/κ-opioid receptor system in the amygdala potentiates nicotine conditioned place preference. J. Neurosci. Offic. J. Soc. Neurosci. 32, 1488–1495. doi: 10.1523/JNEUROSCI.2980-11.2012
Smith, K. S., Tindell, A. J., Aldridge, J. W., and Berridge, K. C. (2009). Ventral pallidum roles in reward and motivation. Behav. Brain Res. 196, 155–167. doi: 10.1016/j.bbr.2008.09.038
Soldo, B. L., and Moises, H. C. (1998). mu-opioid receptor activation inhibits N- and P-type Ca2+ channel currents in magnocellular neurones of the rat supraoptic nucleus. J. Physiol. 513(Pt 3), 787–804. doi: 10.1111/j.1469-7793.1998.787ba.x
St Laurent, R., Martinez Damonte, V., Tsuda, A. C., and Kauer, J. A. (2020). Periaqueductal gray and rostromedial tegmental inhibitory afferents to VTA have distinct synaptic plasticity and opiate sensitivity. Neuron 106, 624–636.e4. doi: 10.1016/j.neuron.2020.02.029
Stanford, I. M., and Cooper, A. J. (1999). Presynaptic mu and delta opioid receptor modulation of GABAA IPSCs in the rat globus pallidus in vitro. J. Neurosci. Offic. J. Soc. Neurosci. 19, 4796–4803. doi: 10.1523/JNEUROSCI.19-12-04796.1999
Stanzione, P., Stefani, A., Calabresi, P., Mercuri, N. B., and Bernardi, G. (1989). Met- and leu-enkephalins inhibit rat cortical neurons intracellularly recorded in vivo while morphine excites them: evidence for naloxone-sensitive and naloxone-insensitive effects. Exp. Brain Res. 77, 302–308. doi: 10.1007/BF00274987
Starr, M. S. (1985). Multiple opiate receptors may be involved in suppressing gamma-aminobutyrate release in substantia nigra. Life Sci. 37, 2249–2255. doi: 10.1016/0024-3205(85)90015-3
Stefani, A., Spadoni, F., Giacomini, P., Lavaroni, F., and Bernardi, G. (2001). The activation of mu opioid receptors promotes a small modulation of calcium currents in rat pallidal neurons. Brain Res. 897, 207–212. doi: 10.1016/s0006-8993(01)02120-5
Stein, C., Schäfer, M., and Machelska, H. (2003). Attacking pain at its source: new perspectives on opioids. Nat. Med. 9, 1003–1008. doi: 10.1038/nm908
Straub, C., Saulnier, J. L., Bègue, A., Feng, D. D., Huang, K. W., and Sabatini, B. L. (2016). Principles of synaptic organization of GABAergic interneurons in the striatum. Neuron 92, 84–92. doi: 10.1016/j.neuron.2016.09.007
Sugita, S., and North, R. A. (1993). Opioid actions on neurons of rat lateral amygdala in vitro. Brain Res. 612, 151–155. doi: 10.1016/0006-8993(93)91655-c
Sulaiman, M. R., and Dutia, M. B. (1998). Opioid inhibition of rat medial vestibular nucleus neurones in vitro and its dependence on age. Exp. Brain Res. 122, 196–202. doi: 10.1007/s002210050507
Svoboda, K. R., Adams, C. E., and Lupica, C. R. (1999). Opioid receptor subtype expression defines morphologically distinct classes of hippocampal interneurons. J. Neurosci. Offic. J. Soc. Neurosci. 19, 85–95. doi: 10.1523/JNEUROSCI.19-01-00085.1999
Swaab, D. F., Hofman, M. A., Lucassen, P. J., Purba, J. S., Raadsheer, F. C., and Van de Nes, J. A. (1993). Functional neuroanatomy and neuropathology of the human hypothalamus. Anat. Embryol. (Berl) 187, 317–330. doi: 10.1007/BF00185889
Szabo, B., Muller, T., and Koch, H. (1999). Effects of cannabinoids on dopamine release in the corpus striatum and the nucleus accumbens in vitro. J. Neurochem. 73, 1084–1089. doi: 10.1046/j.1471-4159.1999.0731084.x
Tanaka, E., and North, R. A. (1994). Opioid actions on rat anterior cingulate cortex neurons in vitro. J. Neurosci. Offic. J. Soc. Neurosci. 14, 1106–1113. doi: 10.1523/JNEUROSCI.14-03-01106.1994
Tavakoli-Nezhad, M., and Arbogast, L. A. (2010). Mu and kappa opioid receptor expression in the mediobasal hypothalamus and effectiveness of selective antagonists on prolactin release during lactation. Neuroscience 166, 359–367. doi: 10.1016/j.neuroscience.2009.12.066
Tejeda, H. A., Counotte, D. S., Oh, E., Ramamoorthy, S., Schultz-Kuszak, K. N., Bäckman, C. M., et al. (2013). Prefrontal cortical kappa-opioid receptor modulation of local neurotransmission and conditioned place aversion. Neuropsychopharmacology 38, 1770–1779. doi: 10.1038/npp.2013.76
Tejeda, H. A., Hanks, A. N., Scott, L., Mejias-Aponte, C., Hughes, Z. A., and ODonnell, P. (2015). Prefrontal cortical kappa opioid receptors attenuate responses to amygdala inputs. Neuropsychopharmacology 40, 2856–2864. doi: 10.1038/npp.2015.138
Tejeda, H. A., Wu, J., Kornspun, A. R., Pignatelli, M., Kashtelyan, V., Krashes, M. J., et al. (2017). Pathway- and cell-specific kappa-opioid receptor modulation of excitation-inhibition balance differentially gates D1 and D2 accumbens neuron activity. Neuron 93, 147–163. doi: 10.1016/j.neuron.2016.12.005
Terman, G. W., Drake, C. T., Simmons, M. L., Milner, T. A., and Chavkin, C. (2000). Opioid modulation of recurrent excitation in the hippocampal dentate gyrus. J. Neurosci. 20:4379. doi: 10.1523/JNEUROSCI.20-12-04379.2000
Terman, G. W., Wagner, J. J., and Chavkin, C. (1994). Kappa opioids inhibit induction of long-term potentiation in the dentate gyrus of the guinea pig hippocampus. J. Neurosci. Offic. J. Soc. Neurosci. 14, 4740–4747. doi: 10.1523/JNEUROSCI.14-08-04740.1994
Tian, H., Xu, Y., Liu, F., Wang, G., and Hu, S. (2015). Effect of acute fentanyl treatment on synaptic plasticity in the hippocampal CA1 region in rats. Front. Pharmacol. 6:251. doi: 10.3389/fphar.2015.00251
Toubia, T., and Khalife, T. (2019). The endogenous opioid system: role and dysfunction caused by opioid therapy. Clin. Obstet. Gynecol. 62, 3–10. doi: 10.1097/GRF.0000000000000409
Travaglio, M., and Ebling, F. J. P. (2019). Role of hypothalamic tanycytes in nutrient sensing and energy balance. Proc. Nutr. Soc. 78, 272–278. doi: 10.1017/S0029665118002665
Valentino, R. J., and Dingledine, R. (1982). Pharmacological characterization of opioid effects in the rat hippocampal slice. J. Pharmacol. Exp. Ther. 223, 502–509.
van Steenbergen, H., Eikemo, M., and Leknes, S. (2019). The role of the opioid system in decision making and cognitive control: a review. Cogn. Affect. Behav. Neurosci. 19, 435–458. doi: 10.3758/s13415-019-00710-6
Van’t Veer, A., and Carlezon, W. A. Jr. (2013). Role of kappa-opioid receptors in stress and anxiety-related behavior. Psychopharmacology 229, 435–452. doi: 10.1007/s00213-013-3195-5
Varlinskaya, E. I., Johnson, J. M., Przybysz, K. R., Deak, T., and Diaz, M. R. (2020). Adolescent forced swim stress increases social anxiety-like behaviors and alters kappa opioid receptor function in the basolateral amygdala of male rats. Prog. Neuropsychopharmacol. Biol. Psychiatry 98:109812. doi: 10.1016/j.pnpbp.2019.109812
Vaughan, C. W., Bagley, E. E., Drew, G. M., Schuller, A., Pintar, J. E., Hack, S. P., et al. (2003). Cellular actions of opioids on periaqueductal grey neurons from C57B16/J mice and mutant mice lacking MOR-1. Br. J. Pharmacol. 139, 362–367. doi: 10.1038/sj.bjp.0705261
Vaughan, C. W., and Christie, M. J. (1997). Presynaptic inhibitory action of opioids on synaptic transmission in the rat periaqueductal grey in vitro. J. Physiol. 498(Pt 2), 463–472. doi: 10.1113/jphysiol.1997.sp021872
Vaughan, C. W., Connor, M., Jennings, E. A., Marinelli, S., Allen, R. G., and Christie, M. J. (2001). Actions of nociceptin/orphanin FQ and other prepronociceptin products on rat rostral ventromedial medulla neurons in vitro. J. Physiol. 534, 849–859. doi: 10.1111/j.1469-7793.2001.00849.x
Vaughan, C. W., Ingram, S. L., Connor, M. A., and Christie, M. J. (1997). How opioids inhibit GABA-mediated neurotransmission. Nature 390, 611–614. doi: 10.1038/37610
Vidal, C., Maier, R., and Zieglgansberger, W. (1984). Effects of dynorphin A (1-17), dynorphin A (1-13) and D-ala2-D-leu5-enkephalin on the excitability of pyramidal cells in CA1 and CA2 of the rat hippocampus in vitro. Neuropeptides 5, 237–240.
Viveros, M.-P., Marco, E.-M., Llorente, R., and López-Gallardo, M. (2007). Endocannabinoid system and synaptic plasticity: implications for emotional responses. Neur. Plasticity 2007, 52908–52908. doi: 10.1155/2007/52908
Von Korff, M. R. (2013). Long-term use of opioids for complex chronic pain, best practice & research. Clin. Rheumatol. 27, 663–672.
Voss, P. M. E., Thomas, J., Cisneros-Franco, M., and de Villers-Sidani, É (2017). Dynamic brains and the changing rules of neuroplasticity: implications for learning and recovery. Front. Psychol. 8:1657. doi: 10.3389/fpsyg.2017.01657
Wagner, E. J., Reyes-Vazquez, C., Ronnekleiv, O. K., and Kelly, M. J. (2000). The role of intrinsic and agonist-activated conductances in determining the firing patterns of preoptic area neurons in the guinea pig. Brain Res. 879, 29–41. doi: 10.1016/s0006-8993(00)02698-6
Wagner, J. J., Caudle, R. M., and Chavkin, C. (1992). Kappa-opioids decrease excitatory transmission in the dentate gyrus of the guinea pig hippocampus. J. Neurosci. Offic. J. Soc. Neurosci. 12, 132–141. doi: 10.1523/JNEUROSCI.12-01-00132.1992
Wagner, J. J., Etemad, L. R., and Thompson, A. M. (2001). Opioid-mediated facilitation of long-term depression in rat hippocampus. J. Pharmacol. Exp. Ther. 296, 776–781.
Wakerley, J. B., Noble, R., and Clarke, G. (1983). Effects of morphine and D-Ala, D-Leu enkephalin on the electrical activity of supraoptic neurosecretory cells in vitro. Neuroscience 10, 73–81. doi: 10.1016/0306-4522(83)90081-7
Wamsteeker Cusulin, J. I., Fuzesi, T., Inoue, W., and Bains, J. S. (2013). Glucocorticoid feedback uncovers retrograde opioid signaling at hypothalamic synapses. Nat. Neurosci. 16, 596–604. doi: 10.1038/nn.3374
Wang, L., Hou, K., Wang, H., Fu, F., and Yu, L. (2020). Role of mu-opioid receptor in nociceptive modulation in anterior cingulate cortex of rats. Mol. Pain 16:1744806920966144. doi: 10.1177/1744806920966144
Wang, Q., Yue, X. F., Qu, W. M., Tan, R., Zheng, P., Urade, Y., et al. (2013). Morphine inhibits sleep-promoting neurons in the ventrolateral preoptic area via mu receptors and induces wakefulness in rats. Neuropsychopharmacology 38, 791–801. doi: 10.1038/npp.2012.244
Watson, G. B., and Lanthorn, T. H. (1993). Electrophysiological actions of delta opioids in CA1 of the rat hippocampal slice are mediated by one delta receptor subtype. Brain Res. 601, 129–135. doi: 10.1016/0006-8993(93)91703-u
Weibel, R., Reiss, D., Karchewski, L., Gardon, O., Matifas, A., Filliol, D., et al. (2013). Mu opioid receptors on primary afferent nav1.8 neurons contribute to opiate-induced analgesia: insight from conditional knockout mice. PLoS One 8:e74706. doi: 10.1371/journal.pone.0074706
Weisskopf, M. G., Zalutsky, R. A., and Nicoll, R. A. (1993). The opioid peptide dynorphin mediates heterosynaptic depression of hippocampal mossy fibre synapses and modulates long-term potentiation. Nature 362, 423–427.
Wilson, M. A., and Junor, L. (2008). The role of amygdalar mu-opioid receptors in anxiety-related responses in two rat models. Neuropsychopharmacology 33, 2957–2968. doi: 10.1038/sj.npp.1301675
Wimpey, T. L., Opheim, K. E., and Chavkin, C. (1989). Effects of chronic morphine administration on the mu and delta opioid responses in the CA1 region of the rat hippocampus. J. Pharmacol. Exp. Ther. 251, 405–411.
Winters, B. L., Gregoriou, G. C., Kissiwaa, S. A., Wells, O. A., Medagoda, D. I., Hermes, S. M., et al. (2017). Endogenous opioids regulate moment-to-moment neuronal communication and excitability. Nat. Commun. 8:14611. doi: 10.1038/ncomms14611
Witkowski, G., and Szulczyk, P. (2006). Opioid mu receptor activation inhibits sodium currents in prefrontal cortical neurons via a protein kinase A- and C-dependent mechanism. Brain Res. 1094, 92–106. doi: 10.1016/j.brainres.2006.03.119
Xia, Y., Driscoll, J. R., Wilbrecht, L., Margolis, E. B., Fields, H. L., and Hjelmstad, G. O. (2011). Nucleus accumbens medium spiny neurons target non-dopaminergic neurons in the ventral tegmental area. J. Neurosci. Offic. J. Soc. Neurosci. 31, 7811–7816. doi: 10.1523/JNEUROSCI.1504-11.2011
Xiao, C., and Ye, J. H. (2008). Ethanol dually modulates GABAergic synaptic transmission onto dopaminergic neurons in ventral tegmental area: role of mu-opioid receptors. Neuroscience 153, 240–248. doi: 10.1016/j.neuroscience.2008.01.040
Xie, C. W., and Lewis, D. V. (1991). Opioid-mediated facilitation of long-term potentiation at the lateral perforant path-dentate granule cell synapse. J. Pharmacol. Exp. Ther. 256, 289–296.
Xu, P., Chen, A., Li, Y., Xing, X., and Lu, H. (2019). Medial prefrontal cortex in neurological diseases. Physiol. Genom. 51, 432–442. doi: 10.1152/physiolgenomics.00006.2019
Yakimova, K. S. (2006). Effects of GABAB-agonist on rat hypothalamic neurons: functional antagonism with mu-receptor agonist. Neurosci. Res. 54, 281–287. doi: 10.1016/j.neures.2005.12.010
Yamada, D., Takahashi, J., Iio, K., Nagase, H., and Saitoh, A. (2021). Modulation of glutamatergic synaptic transmission and neuronal excitability in the prelimbic medial prefrontal cortex via delta-opioid receptors in mice. Biochem. Biophys. Res. Commun. 560, 192–198. doi: 10.1016/j.bbrc.2021.05.002
Yamada, M., Inanobe, A., and Kurachi, Y. (1998). G protein regulation of potassium ion channels. Pharmacol. Rev. 50, 723–760.
Yang, T. T., Hung, C. F., Lee, Y. J., Su, M. J., and Wang, S. J. (2004). Morphine inhibits glutamate exocytosis from rat cerebral cortex nerve terminals (synaptosomes) by reducing Ca2+ influx. Synapse 51, 83–90. doi: 10.1002/syn.10290
Yokota, E., Koyanagi, Y., Yamamoto, K., Oi, Y., Koshikawa, N., and Kobayashi, M. (2016). Opioid subtype- and cell-type-dependent regulation of inhibitory synaptic transmission in the rat insular cortex. Neuroscience 339, 478–490. doi: 10.1016/j.neuroscience.2016.10.004
Yuan, X. R., Madamba, S., and Siggins, G. R. (1992). Opioid peptides reduce synaptic transmission in the nucleus accumbens. Neurosci. Lett. 134, 223–228. doi: 10.1016/0304-3940(92)90522-9
Zhang, L., and Hammond, D. L. (2010). Cellular basis for opioid potentiation in the rostral ventromedial medulla of rats with persistent inflammatory nociception. Pain 149, 107–116. doi: 10.1016/j.pain.2010.01.017
Zhang, L., Sykes, K. T., Buhler, A. V., and Hammond, D. L. (2006). Electrophysiological heterogeneity of spinally projecting serotonergic and nonserotonergic neurons in the rostral ventromedial medulla. J. Neurophysiol. 95, 1853–1863. doi: 10.1152/jn.00883.2005
Zhang, R. X., Zhang, M., Li, A., Pan, L., Berman, B. M., Ren, K., et al. (2013). DAMGO in the central amygdala alleviates the affective dimension of pain in a rat model of inflammatory hyperalgesia. Neuroscience 252, 359–366. doi: 10.1016/j.neuroscience.2013.08.030
Zhang, X., and van den Pol, A. N. (2013). Direct inhibition of arcuate proopiomelanocortin neurons: a potential mechanism for the orexigenic actions of dynorphin. J. Physiol. 591, 1731–1747. doi: 10.1113/jphysiol.2012.248385
Zhu, M., Cho, Y. K., and Li, C. S. (2009). Activation of delta-opioid receptors reduces excitatory input to putative gustatory cells within the nucleus of the solitary tract. J. Neurophysiol. 101, 258–268. doi: 10.1152/jn.90648.2008
Zhu, W., and Pan, Z. Z. (2004). Synaptic properties and postsynaptic opioid effects in rat central amygdala neurons. Neuroscience 127, 871–879. doi: 10.1016/j.neuroscience.2004.05.043
Zhu, W., and Pan, Z. Z. (2005). Mu-opioid-mediated inhibition of glutamate synaptic transmission in rat central amygdala neurons. Neuroscience 133, 97–103. doi: 10.1016/j.neuroscience.2005.02.004
Keywords: opioid, synaptic plasticity, receptor signal transduction, neurotransmission, glutamate, GABA
Citation: Reeves KC, Shah N, Muñoz B and Atwood BK (2022) Opioid Receptor-Mediated Regulation of Neurotransmission in the Brain. Front. Mol. Neurosci. 15:919773. doi: 10.3389/fnmol.2022.919773
Received: 13 April 2022; Accepted: 26 May 2022;
Published: 15 June 2022.
Edited by:
Hugo Tejeda, National Institute on Drug Abuse (NIH), United StatesReviewed by:
Brian McCool, Wake Forest School of Medicine, United StatesCopyright © 2022 Reeves, Shah, Muñoz and Atwood. This is an open-access article distributed under the terms of the Creative Commons Attribution License (CC BY). The use, distribution or reproduction in other forums is permitted, provided the original author(s) and the copyright owner(s) are credited and that the original publication in this journal is cited, in accordance with accepted academic practice. No use, distribution or reproduction is permitted which does not comply with these terms.
*Correspondence: Brady K. Atwood, YmthdHdvb2RAaXUuZWR1
Disclaimer: All claims expressed in this article are solely those of the authors and do not necessarily represent those of their affiliated organizations, or those of the publisher, the editors and the reviewers. Any product that may be evaluated in this article or claim that may be made by its manufacturer is not guaranteed or endorsed by the publisher.
Research integrity at Frontiers
Learn more about the work of our research integrity team to safeguard the quality of each article we publish.