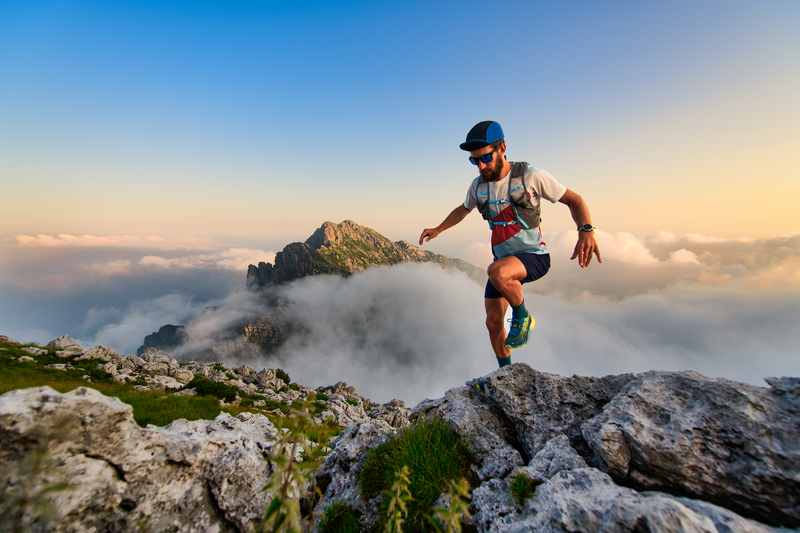
95% of researchers rate our articles as excellent or good
Learn more about the work of our research integrity team to safeguard the quality of each article we publish.
Find out more
REVIEW article
Front. Mol. Neurosci. , 22 July 2022
Sec. Molecular Signalling and Pathways
Volume 15 - 2022 | https://doi.org/10.3389/fnmol.2022.910543
This article is part of the Research Topic Molecular Mechanisms in Neural Development, Related Disorders, and Therapeutic Treatments View all 5 articles
As is known to all, glioma, a global difficult problem, has a high malignant degree, high recurrence rate and poor prognosis. We analyzed and summarized signal pathway of the Hippo/YAP, PI3K/AKT/mTOR, miRNA, WNT/β-catenin, Notch, Hedgehog, TGF-β, TCS/mTORC1 signal pathway, JAK/STAT signal pathway, MAPK signaling pathway, the relationship between BBB and signal pathways and the mechanism of key enzymes in glioma. It is concluded that Yap1 inhibitor may become an effective target for the treatment of glioma in the near future through efforts of generation after generation. Inhibiting PI3K/Akt/mTOR, Shh, Wnt/β-Catenin, and HIF-1α can reduce the migration ability and drug resistance of tumor cells to improve the prognosis of glioma. The analysis shows that Notch1 and Sox2 have a positive feedback regulation mechanism, and Notch4 predicts the malignant degree of glioma. In this way, notch cannot only be treated for glioma stem cells in clinic, but also be used as an evaluation index to evaluate the prognosis, and provide an exploratory attempt for the direction of glioma treatment. MiRNA plays an important role in diagnosis, and in the treatment of glioma, VPS25, KCNQ1OT1, KB-1460A1.5, and CKAP4 are promising prognostic indicators and a potential therapeutic targets for glioma, meanwhile, Rheb is also a potent activator of Signaling cross-talk etc. It is believed that these studies will help us to have a deeper understanding of glioma, so that we will find new and better treatment schemes to gradually conquer the problem of glioma.
Glioma is the most common primary malignant tumor. It is characterized by a high recurrence rate. There is a lack of effective treatment strategies, resulting in high mortality and short survival time. Only 5.5% of patients usually survive for 5 years after diagnosis (Ostrom et al., 2020). The median overall survival is approximately 14.5–16.6 months (Wen and Reardon, 2016). According to the classification of the tumors of the central nervous system (by WHO and CBTRUS), brain gliomas are divided into four levels (grade I–IV). Grade IV is characterized by the highest degree of malignancy, the strongest invasiveness, the worst prognosis, and the highest proportion of malignancy. The tumors belonging to this grade are known as glioblastoma or pleomorphic glioblastoma. Glioblastomas, based on gene expression, can be classified into anterior neural type, neural type, classical type, and mesenchymal type (Omuro and DeAngelis, 2013). Although different subtypes have been discovered over the years, there is no precise and effective targeted therapy for glioma that can improve its prognosis (Chen et al., 2017). Moreover, most low-grade gliomas recur, and gene mutations are observed post operation (Fangusaro et al., 2019). The tumor heterogeneity changes, the biological morphology worsens, and abnormalities are observed (Dentro et al., 2021). Inevitably, the generation of pleomorphic glioblastoma linked with high-grade gliomas is observed (Claus et al., 2015).
Despite the tremendous advances in the area of surgery, radiotherapy, and chemotherapy, but the prognosis of glioma still remained considerably unsatisfactory, which could be attributed to its complex and variability of pathogenesis and cellular origin (Xu S. et al., 2020). Therefore, to develop and find efficient treatment methods for glioma, it is significant important to further understand the pathogenesis associated with glioma. Due to the complexity of genetic and environmental initiation events and the lack of clarity of primitive cells or tissues, the initial origin of glioma and the specific correlation and crosstalk of gene mutations and signal pathways in various links (Johnson et al., 2014). Since the beginning of mRNA translation, there have been more complex and variable progress mechanisms (Sellars et al., 2020). Researchers associated with the sphere of basic research and clinical trials have reported that polygenic mutation is the primary mechanism responsible for the occurrence of glioma (Han S. et al., 2020). It is also linked with the processes of development, mutation superposition, and multi-step formation (Zhang et al., 2019). Tumor suppressor genes such as TP53, phosphatase, p16, and phosphatase tensin homolog (PTEN) control the progression of the cell growth cycle, proliferation, and invasion (Suwala et al., 2021). The mutation or deletion of tumor suppressor genes are conducive to regulate the microenvironment of glioma (Calvo et al., 2021). The “6” expression and modification of proteins (phosphorylation, ubiquitination, methylation, acetoxylation, glycosylation, and nitrosylation) can enable them to occur or further deform (Van Meir et al., 2010; Urulangodi and Mohanty, 2020). It has been widely reported that it is the key regulator of numerous GBM cell lines (Ishii et al., 1999). Oncogenes or anticancer genes usually control the malignant behavior of cancer cells by regulating different signal pathways (Liu et al., 2021). This affects the treatment efficiency and prognosis of glioma (Asad et al., 2021). This review describes the key signaling pathways and the associated regulatory mechanisms that affect the prognosis of glioma. The recent findings have been presented herein.
The Hippo signaling pathway was first identified in Drosophila melanogaster (Moloudizargari et al., 2022). This pathway plays a considerable important role in regulating the homeostasis of the tissue environment. The pathway primarily affects the processes of organ development and tumor promotion (Qiao et al., 2018). The key core kinases are Salvador (Sav), Hippo (Hpo), Warts (Wts), and Mob as the tumor suppressors (Mats) (Shimizu et al., 2008). The Hpo–Sav complex can activate the Wts–Mats complex, thereby the downstream effector of the Hippo signaling pathway and the transcription coactivator Yorkie (Yki) can be gradually phosphorylated (Sun X. et al., 2019). Through the study and analysis of evolutionary processes, researchers have found and confirmed that most of the core kinases belong to families of homologous genes, These studies shown that the Hippo signal pathway had not changed in multistage, multi overlap, and multi genes in the evolutionary process, but presents a highly conserved condition (Kim D. H. et al., 2019). According to research findings that the core kinases present in Drosophila melanogaster and mammals are highly homologous (Hong and Guan, 2012). Four types of core kinases, MST1/2, SAV1, LATS1/2, and MOB1A/B, are related with the Hippo signaling pathway in mammals (Juan and Hong, 2016). During the process of regulation, when various upstream signals are received, three types of core kinases (SAV1, LATS1/2, and MOB1) are activated by the activated MST1/2 system, subsequently, they also are phosphorylated (Zhou and Zhao, 2018). The bioactivity of the MST1/2 kinase is promoted under the conditions of the mutual crosstalk between MST1/2 and SAV1 (Bae et al., 2020). Under the above conditions, the expression and function of LATS1/2 are further enhanced. The activated Hippo signaling pathway facilitates the phosphorylation of Yap/TAZ on multiple serine residues, enables it to be degraded independently (Luo et al., 2020), All the above processes are mediated by proteasome. Through the negative feedback, the Hippo signaling pathway regulates the Yes-associated protein (Yap), which is a major of importance transcriptional coactivator (Figure 1; Yan F. et al., 2020). The Hippo signaling pathway is widely correlative a variety of biological processes, such as cell proliferation, apoptosis, tissue repair, and regeneration (Fan et al., 2016). The incidence and progression of varieties of tumors, including glioma, can be potentially traced when the Hippo signaling pathway is abnormally regulated (Johnson and Halder, 2014). Recently, the function of CKAP4 (Cytoskeleton-associated protein 4) on glioma was investigated in vitro and in an orthotopic brain tumor model in mice. The results shown that CKAP4 is highly upregulated in glioma and high CKAP4 expressing tumors were associated with poor patient survival. And CKAP4 promotes malignant progression of gliomas via inhibiting Hippo signaling (Luo et al., 2021). It has been widely reported that it plays a rather of importance inhibitory role in human cancer and the core key enzymes MST1/2 and LASTS1/2 are involved in the advancement of the Hippo signaling pathway (Xia et al., 2017). It has also been demonstrated that MST1 had a certain impact on glioma, including the process of progression and occurrence of the tumors. Chao et al. (2015) had indicated that the down-regulation of Mst1 can accelerate the proliferation and growth of glioma cells, can also help to inhibit the course of apoptosis. The Akt/mTOR pathway is able to inhibit the proliferation of glioma cells when Mst1 is in over-expression condition (Chao et al., 2015). In vitro studies of glioma cells showed that the levels of mRNA and proteins which associated with LATS1decreased significantly (Ji et al., 2012). The migration, proliferation, and aggression of cells were suppressed by the over-expression of LATS1. Liu et al. (2019) indicated that when SOCE was activated, the phosphorylation of LATS1/2 was subsequently triggered, meanwhile, the growth of glioblastoma was also restrained. Other researchers have verified that the proliferation of glioma cells could be potentially stifled by LATS2 (Guo et al., 2019). The path might enable to proceed via the reduction in the expression levels of cyclin D1, CDK4, and CDK6. A perspective has also been raised that the way of G1/s transformation is influenced by CDK4, CDK6, and cyclin D1 (Guo et al., 2019).
Yes-associated protein (YAP) plays a key regulatory role in the mediation of numerous malignant tumors (Vargas et al., 2020). It also provided an appropriate and suitable cellular environment for the progression of tumors (Panciera et al., 2020). YAP1 is an oncogene which is over-expressed during the invasion and infiltration of glioblastoma. Guichet et al. (2018) studied 117 glioma samples to analyze the expression of YAP protein in detail. They drawn a conclusion that the expression of YAP was closely relevant to the molecular subtype of glioma and was capable of affecting the prognosis of patients. Some researchers came to conclusion that YAP might be a potentially prognostic indicator of overall survival in patients suffering from gliomas. It is a particular of importance finding for patients suffering from low-grade gliomas. The upregulation of YAP has been widely studied in gliomas (Zhao et al., 2021). Unfortunately, it is not complete clear that whether the specific mechanism is associated with the process. The track of cell transformation can be possibly induced under the conditions of overexpression of YAP (Zhang C. et al., 2021). This way can be beneficial to prevent cell contact inhibition. Attack on host tissues and metastasis may also be fostered under these conditions (Zhao et al., 2007). The role of YAP1 in the process of proliferation of glioma cells was deeply studied by analyzing shRNA and using over-expression-based methods (Zhang et al., 2016). YAP1 is a major carcinogen that affects tumorigenesis (Hatterschide et al., 2022). As YAP1 is such an important regulator of tumor progression, its role in glioma expressing IDH1 with an R132H mutation was reported (Patrick et al., 2021). Attenuated nuclear levels of YAP1 in IDH1 mutant glioma tissues and cell lines were concomitant with decreased levels of mitochondrial transcription factor A (TFAM). It is of importance that these findings indicated that bosutinib treatment also aggrandized ROS levels and induced apoptosis in IDH1 wild-type cells when YAP1 was simultaneously exhausted. These findings emphasize the participation of YAP1 in coupling mitochondrial dysfunction with mitochondrial shuttling of TERT to constitute an essential non-canonical function of YAP1 in the regulation of REDOX homeostasis (Patrick et al., 2021). In multiple different types of tumors, including glioma, the amplification of the chromosome zone in the Yap1 gene (11q22) has been discovered (Modena et al., 2006). The over-expression of YAP1 leads to an imbalance in the Hippo signal transduction pathway (Hayashi et al., 2015). Under the above conditions, it is extremely difficult to control the processes of cell proliferation and tumor growth (Liu and Wang, 2015). An increased extent of nuclear localization and overexpression of YAP1 has been inspected in numerous cancer tissues (Zhao et al., 2007). Epigenetic changes or mutations result in the inactivation of the Hippo signaling pathway in cancer cells (Cavalli and Heard, 2019; Wu et al., 2019). Under all the conditions, YAP1 is activated and then is transferred to the nucleus. Cancer cells will be transformed into cancer stem cells when YAP1 is activated (Schaal et al., 2018). The activation of YAP1 also promotes occurrence of tumors and aggravates the deterioration and metastasis of tumors (Maehama et al., 2021). In addition, some findings suggested that AMOTL1 (Angiomotin-like 1) may exert a tumor-promoting function in glioma by enhancing the activation of YAP1 signaling. Thus, AMOTL1 may be a potential target for the development of antiglioma therapy (Xu et al., 2021).
To sum up, according to the above results, we speculated that CKAP4 has potential as a promising biomarker and can predict the prognosis of patients with gliomas. And targeting CKAP4 expression may be an effective therapeutic strategy for the treatment of human gliomas. YAP1’s up-regulated molecular pathway in glioma is the most feasible molecular target that needs to be broken in the future to improve the treatment processes of glioma. It can also help to promote the development of the area of medical research and provide a point of penetration and breach for the emergence of a new field. The results can potentially help the realm to reach a new peak, meanwhile More clinical patients are benefit from the process. With the further growth in YAP1 expression, the invasive trait of glioma also raises. The down-regulation of YAP1 can be potentially helpful to develop new treatment methods for glioma. Furthermore deeper researches, including basal studies and clinical trials, need to be testified if the YAP1 inhibitor can be a promising therapeutic target. Hence, it is so profound of importance to identify YAP inhibitors that to develop new and efficient treatment methods for glioma. Recently, researchers have been focusing on this aspect. It has been recently reported that metformin is a YAP inhibitor, and it can be used to treat glioma (Yuan et al., 2018). We believe that efforts are able to accomplish arduous tasks.
Serine/threonine and lipid kinases, including phosphomembrane-bound phosphatidylinositol-3 (PIP3) kinases, constitute the phosphatidylinositol-3-kinase (PI3K) family (Stefani et al., 2021; Yoshioka, 2021). These enzymes, along with the downstream Akt and rapamycin targets (mTOR), play considerable important roles in numerous key cellular processes such as growth, differentiation, metabolism, survival, and proliferation and so on (Miricescu et al., 2020; Xu Z. et al., 2020). PI3K enzymes can be classified into class I, class II, and class III enzymes (Yoshioka, 2021). Class I PI3K is one of the most widest considered as aberrant class of enzyme which is associated with cancer (Fruman et al., 2017; Koundouros et al., 2020). It consists of heterodimers of a regulatory subunit and a catalytic subunit (Canaud et al., 2021). Based on the activation mode, it is divided into the 1A and 1B categories (Sun and Meng, 2020; Yue et al., 2021). Class 1A PI3K is activated by many cell-surface tyrosine kinases and consists of catalytic p110 and regulated p85 subunits (Clayton et al., 2022). Class II and III PI3Ks are necessary to maintain normal cell functions, up to now, Both are not yet shown to have carcinogenic effects in human (Bilanges et al., 2019). Acetylgalactosaminyltransferase 2 (GALNT2), the enzyme that regulates the initial step of mucin O-glycosylation, has been reported to play a role in influencing the malignancy of various cancers. However, the mechanism through which it influences gliomas is still unknown. Overall, GALNT2 facilitates the malignant characteristics of glioma by influencing the O-glycosylation and phosphorylation of EGFR and the subsequent downstream PI3K/Akt/mTOR axis. Therefore, GALNT2 may serve as a novel biomarker and a potential target for future therapy of glioma (Sun Z. et al., 2019).
A dominant product of PI3K is PIP3, which is the phosphorylation form of membrane-bound phosphatidylinositol (Lien et al., 2017). It can initiate a wide range of active signal cascades. Serine/threonine kinase Akt is the major downstream target of PI3K (Liu R. et al., 2020). This is also the primary carcinogenic effector of the PI3K/Akt pathway. The phosphatase and the tensin homolog (PTEN) are mainly associated with the dephosphorylation of PIP3 (Lee and Pandolfi, 2020; Chauhan et al., 2021). All mentioned above are Akt’s the main negative regulators. The tumor suppressor gene PTEN is lost via the processes of somatic mutation or epigenetic silencing, and this process is widely observed in various types of cancers (Álvarez-Garcia et al., 2019). Membrane-bound PIP3 can promote Akt to dock with various kinase targets (Lien et al., 2017). Akt is involved in the indirect activation of mTOR, which is a complex cell growth checkpoint and is affected by growth factor signal transduction and the adenosine monophosphate levels (Kim S. W. et al., 2019). When TSC2 is phosphorylated by activated Akt at ser939 (Moore et al., 2014; Miyazaki and Takemasa, 2017). It dissociates from TSC1, resulting in the activation of mTORC1 (Carroll et al., 2016). A heterodimeric protein complex TSC1/TSC2 was considered as a key hub of signal transduction through which various environmental cues were merged to determine the functional activity of mTORC1 signaling. Growth factor-induced activation of the PI3K/Akt pathway led to the TSC2 phosphorylation at numerous residues (two key points, Ser939 and Thr1462), which are considered as be targeted by Akt. About the mechanism of interaction between TSC1, TSC2, and mTORC1. Some researchers have found that VEPH1 is frequently decreased in cancer, an event associated with poor prognosis of cancer patients. VEPH1 forms a stable trimeric complex with TSC1/TSC2. Loss of VEPH1 decreases TSC2 GAP activity, and relieves Rheb to activate mTORC1, which promotes cancer cell proliferation, invasion, and EMT process. Rapamycin can suppress VEPH1 deficiency induced mTORC1 activation and hepatocellular carcinogenesis (Inoki et al., 2002; Manning et al., 2002; Huang and Manning, 2008). MTORC1 activates translation inhibitors-eukaryotic translation initiation factors 4EBP1 and S6K1 and is sensitive to rapamycin such as mTOR inhibitors (Hua et al., 2019). These are also sensitive to rapamycin-like mTOR inhibitors. With increase the activity of MTORC1, an increase in the extent of mRNA translation, protein synthesis, and cell proliferation was observed (Cho et al., 2021). MTORC2 is the “upstream” of Akt, which directly phosphorylates Akt and participates in the regulation of the cytoskeleton (Cirone, 2021). Inhibition of mTORC1 can result in the activation of the PI3K pathway (Cai et al., 2021). This can be attributed to the negative feedback from mTORC2 that results in the phosphorylation of Akt (Amin et al., 2021). The researchers found that Akt/FoxM1 (Forkhead box M1) signaling pathway-mediated up-regulation of MYBL2 (MYB-related protein B2) fosters progression of human glioma. Down regulation of FoxM1 and MYBL2 by siRNAs induced the cell cycle blocking, apoptosis and EMT (Epithelial-mesenchymal transition) of glioma cells. Furthermore, inactivations of Akt/FoxM1 signaling by Akt inhibitor and siRNA-FoxM1 diminish the expression of MYBL2 in glioma cells. So we concluded that MYBL2 is of importance downstream factor of Akt/FoxM1 signaling to stimulate advancement of glioma, and could be considered as a promising gene for molecular targeting therapy and biomarker for radiotherapy of glioma (Zhang et al., 2017).
In gliomas, the PI3K/Akt/mTOR signaling pathway is Anomalously regulated (Liu Z. et al., 2017; Fan et al., 2018; Xu F. et al., 2020; Figure 2). Shortly survival time of patients and strongly invasive features of tumor were associated with decreased PTEN levels and increased Akt activity (Zhu and Wei, 2020). In cases of extremely aggressive high-grade gliomas, mutations and the loss of functions of PTEN are observed (Mattoo et al., 2019). Cell growth and the expression of PI3K are inhibited by PTEN, which is the prime tumor suppressor gene. PTEN depletes the PIP3 level, and its loss is directly related with invasive phenotype (Huang W. et al., 2018). The origin of approximately 20–40% of malignant gliomas can be attributed to PTEN mutations (Mattoo et al., 2019). PTEN mutations are also observed in approximately 65% of high-grade gliomas and 15–40% of primary glioblastomas (Xia and Xu, 2015). It has been previously shown that malignant glioma cell lines containing wild-type PTEN gene can significantly reduce the invasion characteristics cell and migration ability of transfected cells. Rapamycin is a classic mTOR inhibitor (Huang et al., 2019). When these alternative drugs bind to mTOR, they can inhibit kinase activity, and prevent the G1 period of cell cycle to further progress the next step. Preclinical trials were conducted to study and confirm the cell inhibiting effects of rapamycin on glioblastoma and medulloblastoma xenografts (Kuger et al., 2013). However, compared to PTEN-positive tumors, the PTEN negative tumors are more sensitive to the inhibiting effects of rapamycin (Zeng et al., 2020). Clinical study results revealed that the mTOR and PI3K inhibitors were the candidate drugs which could be used to treat solid tumors in mice (LoRusso, 2016). Highly activated PI3K/Akt/mTOR and the occurrence of the Sonic Hedgehog (SHH) signaling pathways were detected in original cells of glioblastoma. The recent research shown that the combined with inhibitors of PI3K/Akt/mTOR and SHH is obviously better therapeutic effect than the single inhibitor for glioma (Nanta et al., 2019; Zhang et al., 2020).
The tuberous sclerosis complex (TSC) complex suppresses mechanistic target of rapamycin complex 1 (mTORC1) (Tee, 2018; Kim and Guan, 2019; Liu and Sabatini, 2020; Prentzell et al., 2021), a central driver of anabolism (Mossmann et al., 2018; Hoxhaj and Manning, 2020). mTORC1 hyperactivity causes diseases related to cellular overgrowth, migration, and neuronal excitability and (Condon and Sabatini, 2019) often arises from disturbances of the TSC complex, consisting of TSC complex subunit 1 (TSC1, 9q34), TSC2 (16p13.3) (van Veelen et al., 2011), and TBC1 domain family member 7 (TBC1D7) (Dibble et al., 2012). mTORC1, consisting of mTOR, raptor and mLST8, controls cell growth mainly through the regulation of protein translation (van Veelen et al., 2011). Activated TSC1:TSC2 complex expresses GTPase activating protein activity toward Rheb, thereby inducing conversion of active GTP-bound Rheb to inactive GDP-bound Rheb. Active Rheb promotes mTORC1 activation, controlling protein translation by activating the ribosomal protein S6 kinase (S6K), inhibiting inhibition eukaryotic initiation factor 4E binding proteins (4E-BP) (Hara et al., 2002; Kim et al., 2002) and inhibiting the RNA polymerase III (PolIII) repressor MAF1 (Kantidakis et al., 2010; Michels et al., 2010). In addition, mTORC1 induces angiogenesis through induction of hypoxia-inducible factor 1a (HIF1a)-dependent expression of vascular endothelial growth factor (VEGF), and inhibits autophagy by phosphorylating ATG13 and ULK1/2 (Hosokawa et al., 2009; Jung et al., 2009). In addition to TSC-dependent regulation of mTORC1 activity, the complex is directly activated by sufficient levels of energy (ATP) and nutrients (amino acids), as well as through phosphorylation of mTOR by PKB and of raptor by AMPK (Vander Haar et al., 2007; Gwinn et al., 2008; van Veelen et al., 2011; Figure 3). Another some researches that Plk1-mediated phosphorylation of TSC1 enhances the efficacy of rapamycin (Li et al., 2018), the results indicates Plk1 activates mTORC1. The researches elucidated that mTOR activity is controlled by two different pathways during cell cycle. In interphase, the PI3K/AKT pathway plays a key role to activate the mTOR pathway by AKT-mediated phosphorylation of TSC2 in response to intracellular signaling. However, in mitosis, Plk1 is the major kinase to activate the mTOR pathway by targeting TSC1. Instead of activation of the mTOR pathway, Plk1 phosphorylation of TSC1 also leads to mitotic defects in an mTOR-independent manner. A novel signaling pathway where Plk1 regulates mTOR independently of AKT in mitosis. The latest researcher indicated that in the context of tumors, low G3BP1 levels enhance mTORC1-driven cancer cell motility and correlate with adverse outcomes in patients. G3BPs are not only core components of SGs (stress granules) but also a key element of lysosomal TSC-mTORC1 signaling (Prentzell et al., 2021).
Rheb (Ras homolog enriched in brain) is a member of the Ras superfamily that appears to be conserved in all eukaryotes and, despite the term “brain” in its name, is in fact ubiquitously expressed in mammals (Huang and Manning, 2008). A Rheb homologue exists, named Rhebl1 (Rheb like-1) or Rheb2, that appears to have overlapping functions with Rheb in controlling mTORC1 signaling downstream of TSC1 and TSC2 (Tabancay et al., 2003; Tee et al., 2005). Rheb is a potent activator of mTORC1 (Gromov et al., 1995; Inoki et al., 2003; Yuan et al., 2005). It can stimulate mTORC1, but some researchers suggest that Rheb does not stimulate the activity of mTORC2 kinase (Yang et al., 2006; Huang et al., 2008; Sato et al., 2009). However, active Rheb can indirectly suppress PI3K and mTORC2 signaling by eliciting stimulus varieties of mTORC1-dependent negative feedback circuit systems. Genetic and biochemical studies unified some pathways through identification of two missing links between Akt and mTORC1: the small GTPase Rheb and its negative regulator, the TSC complex, and Rheb is the main mechanism through which PI3K signaling activatesmTORC1 (Goncharova et al., 2002; Castro et al., 2003; Garami et al., 2003; Zhang et al., 2003). Akt inhibits the tuberous sclerosis complex (TSC), the specific GTPase activating protein (GAP) for the small GTPase Once mTORC1 is activated by Rheb, the coinstantaneous phosphorylation of its inhibitory subunit 40-kDa proline-rich Akt substrate (PRAS40) by Akt and mTORC1 itself causes PRAS40 to dissociate from mTORC1. This is thought to increase substrate access to the complex. Glucose, oxygen, and energy levels are also sensed upstream of the TSC complex. Amino acids (and glucose) are sensed upstream of mTORC1 via pathways that regulate the Rag GTPases, which do not directly activate mTORC1 but serve to bring it in proximity to Rheb in cells. mTORC1 directly phosphorylates many substrates including ribosomal protein S6 kinase (S6K) and eukaryotic translation initiation factor 4E-binding protein (4E-BP), which mediate its control of anabolic metabolism, cellular growth, and proliferation (Dibble and Cantley, 2015).
The induction of hypoxia can activate the PI3K/Akt/mTOR pathway and impulse the migration, expansion, and invasion of the U87 cells (Huang W. et al., 2018). When the inhibitor acts on the PI3K/Akt/mTOR pathway and hypoxia-inducible factor-1α, the migration, expansion, and invasion of the U87 cells were significantly inhibited. Under the same conditions, the expression of HIF-1α can be inhibited by siRNA or the PI3K/Akt/mTOR pathway inhibitors (Xia and Xu, 2015). It can be inferred that tumors exposed to hypoxic environments are often more invasive than those not exposed to such environments. These may evolve into highly malignant tumors (Kim and Lee, 2017). Therefore, these tumors are potentially more resistant to clinical postoperative chemotherapy and radiotherapy. They also exhibit the potential of self-renewal, proliferation, and multidirectional differentiation. It can be further inferred that hypoxia may be related to HIF-1α (Domènech et al., 2021). The combined inhibition of PI3K/Akt/mTOR and the Shh dual pathways and knockout or inhibition of HIF-1α can help reduce the migration ability of tumor cells and prolong the postoperative survival of patients.
In conclusion, the development of drug-resistant tumors presents a significant challenge to the success of conventional treatment approaches. Activation of the PTEN/PI3K/Akt/mTOR pathway is implicated both in the pathogenesis of malignancies and development of resistance to anticancer therapies. Therefore, PI3K/Akt/mTOR inhibitors appear as a promising therapeutic option in association with cytotoxic and other targeted therapies to circumvent mechanisms of resistance. Novel therapeutic strategies could be tailored according to appropriate biomarkers and patient-specific mutation profiles to maximize clinical efficacy and benefit of combination therapies. This will enable administration of selective therapies based on the expression of molecular targets, more appropriately individualizing cancer treatment for patients.
Glioma is characterized by a variety of regulation modes at the RNA level (Lu et al., 2020). Such as miRNA, lncRNAs, and snoRNA, etc. LncRNA is a molecule that also affects the survival ability and various functions of tumor cells (Wang et al., 2020). It is composed of a non-protein-coding unit, the length of which ranges from approximately 200 nucleotides (NT) to > 100 kb (KB) (Hsiao et al., 2016). Thus, LncRNA is a short non-coding RNA. Although IncRNA is emerging in glioma genetics research, it is also a hotspot of current research such as lncRNAOR, lncRNHoxA11-AS and lncRNTUG1. Based on the different genomic positions and backgrounds, lncRNAs is classify as follows: (Jarroux et al., 2017; Lin et al., 2020): sense, antisense, intron, intergenic, and bidirectional (transcribed near the transcription starting point toward the sense and antisense direction) (Ma et al., 2013). As transcriptional regulators, lncRNAs can alter gene transcription through transcriptional interference and chromatin remodeling (that follows the processes of chromatin remodeling and transcriptional interference) (Sarkar et al., 2015). In addition, lncRNA can also regulate and guide translation through the processes of base pairing. It can also regulate the process of splicing by getting combined with splicing factors (Cech and Steitz, 2014). This way can help to change the levels of gene expression after transcription. Results from research conducted in the last 5 years revealed that LncRNAs play an important role in tissue homeostasis and biological processes, including the process of cancer progression (Tan et al., 2021). Recent studies have shown that the epigenetic inheritance, regulation of cell differentiation and cycle of lncRNAs presented abnormal biological behavior, which may affect the occurrence and development of glioma (Plasek and Valadkhan, 2021). Therefore, lncRNAs can be a promising biomarker for the diagnosis, prognosis assessment and targeted therapy of glioma (Bian et al., 2015).
Long non-coding RNAs (lncRNAs) have been shown to be closely related to cancer progression and therapy. Recent demonstrated that lncRNA KB-1460A1.5 inhibits glioma tumorigenesis via miR-130a-3p/TSC1/mTOR/YY1 feedback loop. The researchers shown that the lncRNA KB-1460A1.5 is downregulated and positively correlated with prognosis in glioma, and further manifested that over-expression of KB-1460A1.5 inhibits glioma cell proliferation, migration and invasion in vitro and in vivo, while down-regulation of KB-1460A1.5 has the opposite effects (Xu et al., 2022). Another some studies have shown that the expressions of HOTAIR1 and CRNDE are positively correlated with the degree of malignancy of tumors, and CRNDE is the most up-regulated lncRNA in gliomas (Seo et al., 2021; Zhang F. et al., 2021). (CRNDE is a long non-coding RNA with alternative splicing and is implicated in the pathogenesis of several cancers) (Jing et al., 2016; Ma et al., 2019). Kaplan-meier analysis revealed that the up-down regulation of BC002811 XLOC-010967 and NR-002809 was significantly correlated with the prognostic survival cycle (Zhi et al., 2015). Another study confirmed that the expression of CRNDE promotes or inhibits the proliferation and migration of cell by phosphorylation of p70S6K (Lu et al., 2018). This suggests that CRNDE can affect the process of mTOR signal transduction (Wang et al., 2015). It has been reported that the expression of MALAT1 in glioma tissue is lesser than normal brain tissue. In the human glioma cell line and glioma xenotransplantation model, the knockout of the MALAT1 gene promotes cell proliferation and invasion (Su et al., 2021). On the contrary, the over-expression of MALAT1 plays a dominant role in the processes of tumor cell proliferation and invasion (Xiao et al., 2020). It can neutralize the significant inhibition of cell proliferation and invasion in vitro and in vivo. These results indicated that MALAT1 exhibits tumor-inhibiting properties (Han et al., 2016). In addition, inhibition of the ERK signal (U0126) can simulate the levels of phosphorylated ERK1/2 and MMP2 (induced by MALAT1 over-expression). The results indicate that the tumor inhibitory property of MALAT1 can be attributed to the inhibition of the ERK/MAPK-mediated cell growth and MMP2/9-mediated invading processes (Han et al., 2016).
MicroRNA (miR)-mediated mRNA and multiple signaling pathway dysregulations have been extensively implicated in several cancer types, including gliomas. Long non-coding RNA FAM66C regulates glioma growth via the miRNA/LATS1 signaling pathway. The luciferase activity of FAM66C was block by miR15a/miR15b, and the promotion of cell growth effects caused by FAM66C deficiency was attenuated by miR15a/miR15b mimics, further proved that FAM66C functioned as a competing endogenous RNA to regulate glioma growth via the miRNA/LATS1 signaling pathway (Xiao and Peng, 2021). Based on The Cancer Genome Atlas and Chinese Glioma Genome Atlas databases. The study identified that miR-301a was significantly upregulated in gliomas and was associated with a poor prognosis. Moreover, zinc and ring finger 3 (ZNRF3) exerted a critical role in the miR-301a-mediated effects on the malignant phenotype, such as by affecting proliferation and apoptosis. Mechanistically, ZNRF3 was a direct functional target of miR-301a (Sun et al., 2021). Another study shown that lncRNA KCNQ1OT1 promotes proliferation and invasion of glioma cells by targeting the miR-375/YAP pathway. The results indicated that KCNQ1OT1 was upregulated in glioma tissues compared with adjacent tissues, which was associated with poor prognosis (Ding et al., 2020).
MiRNAs are small non-coding RNA molecules that silence target mRNA through post-transcriptional mechanisms and can leapfrog regulate multiple mRNAs (Mishra et al., 2016). For example, miRNA-21 is highly expressed in GBM, after being inhibited, it can promote the apoptosis of tumor cells (Labib et al., 2020). It plays an important regulatory role in normal cells and tumor cells. Following the processes of transcription, shearing, assembly, silencing, and recombination, they finally recognize the target mRNAs by base complementation. These can degrade the silencing complex or block the further translation of the target mRNA (Kim H. et al., 2017). These can also regulate the expression levels. The miRNAs helps to promote the incidence and development of glioma (Zhang et al., 2017). They also act as a cancer-promoting factor/tumor suppressor factor. It has been reported that miRNAs are characterized by different expression patterns and functional significances in different types of cancers (including glioma) (Godlewski et al., 2015). MiRNAs regulate GSCs, invasive properties, pathogenesis, epigenetic, and signaling pathways (Vinchure et al., 2019; Jiang et al., 2020). MiRNAs have different expression patterns and functional implications in many cancers, including gliomas. MiRNAs are important regulators of GSCs in maintaining aggressive pathogenesis and epigenetic and signaling pathways. Evidences from the new study also indicates that miRNA and lncRNA are associated in controlling the occurrence and development of glioma, and proposes that the mirNA-lncrNA interaction may provide new insights into the therapeutic targets of glioma (Mu et al., 2020; Figure 4). The expression of Mir-148b-3p, a member of the mir148/152 family, is poor in several tumor cell lines. In addition, the research shown that Mir-148B-3p binds to HOTAIR in a sequence-specific manner (Wang et al., 2016a). The down-regulated expression of Mir-326 in glioma specimens and glioma cell lines (U87 and U251) was negatively correlated with the pathological grade of glioma (Ke et al., 2015). Studies have shown that HOTAIR promotes development of glioma by inhibiting Mir-326 and further promoting the expression of fibroblast growth factor 1 (FGF1), which plays a significant carcinogenic role in tumorigenesis by activating the MEK1/2 and PT3K/AKT pathways (Hadari et al., 2001; Ke et al., 2015). It has been recently reported that miRNA and lncRNA control the occurrence and development of glioma, and it is proposed that the interaction of miRNA-lncRNA may provide new insights into the identification of therapeutic targets of glioma (Angelopoulou et al., 2020; Wang et al., 2021). The level of expression of miR-148b-3p, belonging to the miR148/152 family, decreased in several tumor cell lines. In addition, miR-148b-3p was also demonstrated to bind to HOTAIR in a sequence-specific manner (Sa et al., 2017). Downregulation of the expression of miR-326 was observed in glioma specimens and glioma cell lines (U87 and U251), which correlated negatively with the pathological grade of glioma Studies have shown that HOTAIR inhibits miR-326 and promotes the expression of the fibroblast growth factor 1 (FGF1) to promote the development of glioma. FGF1 plays a significant carcinogenic role in tumorigenesis by activating the MEK1/2 and PT3K/AKT pathways (Wang et al., 2016a).
To sum up, miRNA can be potentially used for the diagnosis of glioma. MiRNA-21 has been proved to be the most reliable marker for the diagnosis of glioma. A novel miR-301a/ZNRF3/wnt/β-catenin signaling feedback loop that serves critical roles in glioma tumorigenesis, and that may represent a potential therapeutic target. Targeting KCNQ1OT1 may represent a promising strategy in glioma therapeutics. However, the treatment applicability of miRNA is limited as it exhibits poor stability and low efficiency under conditions of targeted delivery. However, progress has been made in recent years in the field of the development of nano carriers. MiRNAs can be enclosed inside nano capsules to improve their stability and achieve targeted delivery. This can block the delivery of nutrients to the tumors and effectively inhibit the growth of tumors. Thus, new ideas for the identification of therapeutic targets of gliomas can be obtained. MiRNA can effectively help in the diagnosis and treatment of glioma. However, to date, preliminary experiments have been conducted. However, with the progress of scientific research, the accuracy of the targeted delivery method and the drug loading rate or organic or inorganic nanoparticle carriers can be improved. It is noteworthy that the drug-releasing property is controllable. The process of nano drug loading can also block the key factor activation pathway associated with the signal pathway to limit the further occurrence and development of tumors. We believe that the results can help us gain a deeper understanding of gliomas. The results can also help to identify new treatment methods to gradually address the global problem posed by tumors.
More research has shown that MET and its ligand HGF play an important role in proliferation, survival, migration, invasion of angiogenic stem cells, drug resistance and recurrence of glioblastoma cells (Guo et al., 2017). The loci of human MET proto-oncogene is located on chromosome 7q31, and HGF on chromosome 7q21.1 (Saccone et al., 1992; Parikh et al., 2014). Analysis of TCGA data demonstrated that about 30% of glioblastomas showed over-expression of HGF and MET (Mulcahy et al., 2020). Immunohistochemical staining showed that MET was located in cytoplasm and cell membrane, and there was a high expression of MET in tumor cells, blood vessels and necrotic regions of glioma samples, and the high expression intensity of MET was correlated with WHO grade of glioblastoma, shorter PFS and OS of patients suffering from glioblastoma (Petterson et al., 2015; Yu et al., 2021). Analysis of MET gene 7Q31.2 indicated that, high expression was found in 47% of primary GBM and 44% of secondary GBM (Pierscianek et al., 2013), the above data suggest that genetic alteration plays a certain role in the pathogenesis of both glioblastoma subtypes. In addition, the activation mutation of MET is an important promoter of the transformation of from low-grade glioma to secondary glioblastoma (Hu et al., 2018). Increased MET in diffuse astrocytoma was associated with shorter OS time. MET was over-expressed in 23% of unamplified glioblastomas, and the range of staining intensity was mainly from weak to moderate (Burel-Vandenbos et al., 2017). In addition to autocrine HGF, paracrine HGF can also promote the invasion of glioma and enhance the chemotactic invasion and proliferation of the MET-positive cells (Yamamoto et al., 1997). In the meantime, HGF also acts as a chemokine of microglia and may be related with the infiltration of glioma (Badie et al., 1999). All of the above mechanisms may stimulate the highly invasive progression of gliomas.
Recent studies have found that the interaction between the HGF/MET signaling pathway and other signaling pathways play a profound and significant roles in the pathogenesis of glioblastoma (Cheng and Guo, 2019; Moosavi et al., 2019). The Wnt/β-catenin RAS/MAPK PI3K/Akt and STAT pathways which belong to downstream signal transduction medium of HGF/MET signal can enable to mediate a variety of behaviors of glioblastoma cells, including the progression of the cell cycle, invasion, dryness, angiogenesis, migration, drug-resistant, and recurrence (Zhang Y. et al., 2018; Figure 5). Both Wnt/β-catenin and HGF/MET signaling pathways regulate proliferation, migration and stem cell behavior of glioblastoma cells via increasing phosphorylation of β-conjunction (Y142) and Snail/Slug expression (Mimeault and Batra, 2011; Náger et al., 2015). In addition, the COX-2/PGE2 signaling pathway can influenced most markers of cancer and activate the PGE2-dependent downstream pathways (such as Ras-MAPK) (Majchrzak-Celiñska et al., 2021). It has been shown that HGF/MET signaling can promote growth and migration of gliomas cells via up-regulating COX-2 expression and stimulating the release of PGE2 (Zhao et al., 2015). Heat shock protein 90 (HSP90) plays a key role in protein folding, stabilization and degradation. A cancer study by Greenall et al. (2015) demonstrated that the expression of MET receptor is dependent on HSP90 protein. Therefore, inhibitors of HSP90 can prevent the growth and migration of glioma cells though inhibiting the expression of MET receptors. HGF/MET signaling is also involved in crosstalk with EGFR HER3 and EGFRvIII, which can lead to enhance activation of oncogenic signaling in glioblastoma. In addition, EGFRvIII is able to induce trans-activation of JNK2 in glioblastoma cells and then to promote increase of invasion of cell by stimulating the HGF/MET signaling circuit (Saunders et al., 2015).
Dysregulation of MET signal is closely associated with the tissue grade, treatment resistance, tumor recurrence and poor prognosis (Vengoji et al., 2019). Hence, the receptor of MET signal is considered as an potentially attractive target for treatment. The humanized anti-HGF monoclonal antibody YYB101 can inhibit the growth of tumors in vitro and in situ mouse model of human glioblastoma (Kim and Heo, 2021), Importantly, it can downregulate effective factors of cell molecular including such as p-FAK, p-met, Ki-67, pGab1, MMP2, and uPA/plasminogen (Kim H. K. et al., 2017). Onartuzumab is an anti-MET antibody, preclinical trials shown that it can inhibit the growth of glioblastoma. Similarly Crizotinib can inhibit structure-dependent tyrosine kinases, such as ROS proto-oncogenes 1 and ALK (Junca et al., 2017). Crizotinib can also effectively inhibit the survival and proliferation of MET-positive glioma stem cells, meanwhile, and can prolong survival of mice with MET-positive GSCs. However, for MET -negative GSCs, it is invalid (Tasaki et al., 2016).
Thus, we speculate that the inhibitors of the MET receptor could be a potentially promising target for treatment of glioma. At present, there are many studies on interference of HGF/MET signal in pre-clinical experiments. It is a key factor that we need to pay much closer attention to the crosstalk between the signals and then to bit by bit conquer every aspect of glioma, including mechanism, signal pathway, mutation, BBB, BTB, immune system, drug-resistant, exosome, and so on.
Wnt is a member of a large family of cysteine-rich secretory glycoproteins. At least, 19 members are found in humans (Jhanwar-Uniyal et al., 2013). During the process of development, wingless/integrated (Wnt) is involved with the processes of migration, cell differentiation and proliferation (Bahmad et al., 2018). The process of Wnt signal transduction is carried out in extracellular and intracellular systems (Roussel and Hatten, 2011). The Wnt/β-catenin pathway is a classic pathway that proceeds via the activation of the Wnt target genes which can regulate the processes of cell differentiation and proliferation and can control stress reaction (Sheng et al., 2021). The procedures are mediated by β-catenin/T cytokine systems. The Wnt/β-catenin pathway is associated with tumorigenesis (Liu M. et al., 2017). It has been reported that the downregulation of the Wnt inhibitor-1 is observed in the abnormal Wnt signaling pathways in 75% of glioma cells (Wang et al., 2018). To date, a minimum of 11 crimp protein (Fz)-based transmembrane proteins have been identified as the receptors of the Wnt ligands (Bai et al., 2018). The pathway of Wnt signaling is initiated by after the Wnt ligand binds to the target FZ, the co-receptor, and the low-density lipoprotein receptor associated with the protein LRP5 (or LPR6) (Dai et al., 2018). Nuclear β-catenin has been reported to be a marker of the active Wnt pathway, and it can supervise the gene expression of MGMT. β-catenin is the primary mediator of the Wnt signal transduction process (Dai et al., 2018). It can activate the downstream target genes (such as c-Jun, c-Myc, c-fos, cyclin D1, fra-1, and those belonging to the AP1 family members) (Koch et al., 2005), which are primarily associated with the regulation of cell attribution and proliferation. The downstream target genes are activated by the interaction of β-catenin and T cytokine/LEF transcription factors in the nucleus (Dai et al., 2018; Figure 6).
In glioma tissues, the expression of β-catenin was significantly higher than normal tissues (Du et al., 2020). The primary signal pathway associated with the process of glioblastoma cell invasion (induced by ionizing radiation) is the Wnt/β-catenin pathway (Dong et al., 2015). Inhibition and stimulation of the Wnt/β-catenin signaling pathway can affect the cell cycle (Gao et al., 2017). Some experiments indicated that inhibitors can reduce the proliferation and survival extent of the U87 glioma cells (Gao et al., 2017). The over-expression of Frizzled2, Wnt2, β-Catenin, and Wnt5a are observed in gliomas (Pu et al., 2009). Wnt2 and β-catenin (the key mediator) were knocked down by siRNA in the human glioma U251 cells. Under these conditions, cell proliferation and invasion were obviously inhibited, and apoptotic cell death was induced (Pu et al., 2009). In addition, the growth of tumor was delayed when nude mice which be subcutaneously inoculated with U251 glioma were treated with siRNA targeting Wnt2 and β-catenin. In vitro and in vivo studies were conducted, and the results revealed that the down-regulation of Wnt2 and β-catenin could be attributed to the decrease in the PI3K/p-Akt expression levels. The finding indicated that Wnt/β-catenin was interacted with the PI3K/Akt signal (Pu et al., 2009). The intracranial transplantation model of glioblastoma mice was studied, it was observed that the inhibition of Wnt5a activity could prevent the process of brain invasion and enhance the survival rate of the host (Li et al., 2021).
Temozolomide (TMZ) is a first-line key clinical drug for postoperative glioma. The significant drug-resistant could be attributed to the existence of MGMT (Oldrini et al., 2020). It has been confirmed that MGMT methylation combined with TMZ can be effectively served as the treatment of glioma. The postoperative survival time can also be significantly prolonged. It has also been found that Wnt/β-catenin can regulate the expression of the MGMT gene and reduce the drug-resistant of the tumor cells (toward chemotherapeutic drugs) (Bi et al., 2018). The approach provides a new treatment idea for clinical treatment.
Therefore, we believe that a combination of drugs which are used in chemotherapy (TMZ and Wnt/β-catenin inhibitor) can enable to prolong the survival time of postoperative of glioma. This may be a promising target for the treatment of glioma.
Notch1, Notch2, Notch3, and Notch4 are the four homologous proteins that are displayed in mammals (Katoh and Katoh, 2020), and these can bind to Delta-like (Dll1-3 and -4) and serrated proteins (Jagged 1 and -2) (two ligand families). These ligands and receptors belong to the category of unidirectional transmembrane proteins (Fulop et al., 2019). The maintenance and development of the central nervous system depend on the proper functioning of these ligands and receptors. The origin of neurogenesis and other neural functions can be ascribed to neural stem cells (Bond et al., 2015). These stem cells are beneficial to maintain the homeostasis of the central nervous system. Therefore, genetic changes and functional features of neural stem cells can potentially cause occurrence of brain tumors, such as glioma (Achanta et al., 2010). The failure of treatment methods of glioma and the recurrence of glioma can be attributed to the existence of a small number of tumor cells which are known as glioma stem cells, in the microenvironment. According to reports, the glioma stem cells are identical in characteristics and invasive phenotype characterize to stem cells (Ma et al., 2018). A highly active Notch signal is observed in GSCs (Yi et al., 2019). Inhibition of the process of differentiation is observed, and stem cell-like properties are maintained. It is a of importance reason that glioma can enable to occur and resist to conventional treatment.
In the course of evolution, Notch signal pathway always presents a relatively conserved and stable condition. But, it plays a certain significant role in cell proliferation, apoptosis, stress regulation, differentiation, and angiogenesis. It can stimulate a cascade reaction and generate a series of biological effects (McIntyre et al., 2020). The notch can promote its proliferation by inhibiting the differentiation process associated with neural stem cells (An et al., 2020). During cell interaction period, notch can be transcribed when it combined with ligands.
The levels of proteins and mRNA in Notch1, Dll1, Notch4, Dll4, Hey1, Jagged1, CBF1, Hey2, and Hes1 in glioma cells are higher expression than those in healthy brain cells. These phenomena could be attributed to the increased expression levels of pAKT and VEGF and the decreased PTEN level (Bazzoni and Bentivegna, 2019). The low overall survival could be ascribed to the over-expression of Notch1 (Han et al., 2017). It was also observed that the expression of Notch1 exist in GSCs around the tumors (Biswas and Rao, 2017). At the same time, the neural stem cell transcription factor Sox2 was up-regulated to reduce the methylation level of Notch1 promoter and enhance the expression of Notch1 in GSCs (Wang C. et al., 2019). The Notch4 and Notch1 levels were, respectively, associated with vimentin and GFAP (Dell’Albani et al., 2014). The expression level of Notch4 increases with the increase of the grade and primary tumor (Dell’Albani et al., 2014). The level of expression of Notch2 (in glioblastoma) could be positively correlate with the neural stem cell gene (SOX2), glial fibrillary acidic protein, vimentin, and anti-apoptotic protein (Wang J. et al., 2019). However, the expression level is negatively correlate with the pro-apoptotic protein (Dell’Albani et al., 2014; Figure 7).
Based on the transduction and regulation mechanism of the Notch signal pathway associated with extracellular and intracellular processes, we can speculate that Notch 1 and Sox2 are positively regulated by a feedback loop. It can also be hypothesized that these are related to the poor prognosis of glioma. It can further inhibit the invasion of GSCs. At the same time, based on the characteristics of over-expression of Notch4 (associated with the increase in the degree of tumor malignancy), it can be considered as the basis of classification for glioma. Thus, not only we can pay much closer attention to clinically treat glioma stem cells but also regard notch as an effective index of evaluation prognosis. A valuable indicator is of importance, which can assist us to formulate the future direction of glioma treatment.
The hedgehog (HH) signal significantly influences the process of development of organs and tissues (Finco et al., 2015). It was prove to be pivotal in a mitogen and (or) Morphological characteristics. It also plays a significant role in differentiation factor. Except it can help to maintain tissue conditions. The HH pathway presents significant lower-activity state in adults (Petrova and Joyner, 2014). The abnormal activation of the protein cause the development of several malignancies in humans, including glioma. Hence, it is a very effective therapeutic target for the treatment of cancer (Skoda et al., 2018). The major participants associated with the HH pathway include HH ligands sonic (SHH), Patched transmembrane receptors (PTCH1 and 2), Indian (IHH) or Desert Hedgehog (DHH), glioma-associated oncogene transcription factors (GLI1, GLI2, and GLI3), and G protein-coupled receptors, such as Smoothened (SMO) (Asiabi et al., 2021). Typically, HH signal is activated when the HH ligand binds with PTCH1, then cause the release of SMO. Subsequently, the SMO receptor is transposed to the primary cilia and then initiates the signal cascade reaction, which eventually results in the dissociation of GLI from the negative regulator SUFU. It also causes its subsequent nucleation. The activated form of the GLI factor raises the transcription of the Hh target genes (i.e., CCND2, BMI1, MYCN, and VEGF) (Huang D. et al., 2018). Thereby, the path of cell survival, invasion, and angiogenesis are regulate throughout the entire sequential procedures. The processes correlative with the self-renewal of stem cell and the process of epithelial mesenchymal transformation (EMT) are also regulated and controlled (Figure 8; Infante et al., 2018).
An abnormal HH signaling pathway is observed in glioma cells. It is especially true for the Shh signaling pathway (Gampala and Yang, 2021). Somatic mutations and changes in the copy number in key genes associated with the Shh signaling pathway are observed in most of the patients suffering from glioma (Garcia-Lopez et al., 2021). These mutations are accompanied by the deletion or loss of function of PTCH1 or SUFU. These are also accompanied by mutations that activate GLI1 and SMO, or GLI2 amplification (Northcott et al., 2017). In some cases, the MYC/MYCN gene which is responsible for transcriptional regulation is repeatedly amplified (Infante et al., 2018). The changes in the genetic characteristic result in the ligand-independent activation of the HH pathway. This promotes tumorigenesis and further worsens the condition. A number of experimental investigation have manifested that the inhibition of the Shh pathway can enhance the chemotherapeutic effect of TMZ, reduce GSCs, and even prevent the growth of GSCs, and cause tumor proliferation, differentiation, and migration (Ming et al., 2017).
Based on the recent findings of many studies, we believe that the inhibition of the HH pathway, especially the Shh signaling pathway (attributable to Shh inhibitors), can reduce the extent of tumor proliferation and then promote apoptosis. It can also strengthen the chemotherapeutic effect of the first-line clinical drug TMZ. Multi-targeted drug combination can control and reduce the recurrence and deterioration of tumors, and then prolong the survival time of patients in the near future. To be sure, this therapeutic effect is positive and worth looking forward to.
TGF-β is a type of cytokine with a molecular weight of 25 kDa, which regulates the processes of cell proliferation and differentiation (Morikawa et al., 2016). It can be secreted by various types of cells, such as immune cells and nausea tumor cells. It also can regulate various biological functions, such as cell proliferation, migration, embryonic stem cell differentiation, and immune surveillance (Yang et al., 2010). The binding of TGF-β to its receptor can initiate the SAMD signal cascade (Held-Feindt et al., 2003). In many malignant tumors, such as intracranial tumors, gastrointestinal tumors, respiratory tumors, and urinary tumors, the expression of TGF-β is associated with the grade and degree of malignancy (Kjellman et al., 2000). It has been clinically proven that the TGF-β signal pathway which is interrupted is an effective strategy which can restore the antitumor immune function of glioma. LY2109761, an inhibitor of TGF-βR1 kinase, can improve the sensitivity of glioma cells toward radiotherapy. The most promising drug is Trabedersen, which is an oligoclonal nucleotide that can antagonizes TGF-βmRNA (Infante et al., 2018). It can play an inhibitory role by down-regulating TGF-β2 mRNA and can enhance the 2-year survival rate of patients suffering from late-stage glioma (Zhang et al., 2011).
Over-expressed and activated mitogen-activated protein kinase interacting kinase 1 (MNK1) is a potentially attractive and promising therapeutic target (Hou et al., 2012). This can increase the extent of translation of SMAD2 to promote the TGF-β pathway through the SMAD2/3/4 complex (Wang et al., 2016b; Mao et al., 2020). The TGF-β pathway interacts with the transcription factors (TF). This interaction induces the expression of various genes which are interrelated with the movement, proliferation, and survival of malignant GBM cells (Northcott et al., 2017). Atypical pathways can also be activated by the overactivated TGF-β receptors (TβRI and TβRII). This can lead to the phosphorylation of ERKs and p38 kinases. MNK1can further increase the extent of translation of specific mRNAs which are closely correlated with the process of cancer progression and can also be activated under these conditions (Northcott et al., 2017). It has been shown and reported that primary glioblastoma and glioma cell lines contain overexpressed MNK1 (Grzmil et al., 2011). The mRNA related with the regulation of the TGF-β pathway was identified by conducting microarray analysis of total RNA and polychromatic RNA obtained from MNK1-depleted GBM cells (Grzmil et al., 2011). After activating SMAD2/3/4, TGF-β promotes LIF transcription, thus activates the JAK/STAT3 signal pathway and then improves the proliferation ability of the stem cells. TGF-β can also induce the expression of the DNA binding inhibitory protein 1 (ID1) in GSCs and enhance the malignant proliferation of cells (Infante et al., 2018). The MNK1 signaling pathway affects and controls mRNA translation, TGF-β-induced cell movement, and vimentin expression. Results from tissue microarray analysis revealed a positive correlation between MNK1 and SMAD2 immunohistochemical staining. Grzmil et al. (2011) reported that SMAD2 is a of importance component associated with the TGF-β signaling pathway. They provided an insight into the MKN1 pathway and the mode of control of the translation process associated with the cancer-related mRNA, including SMAD2. In addition, they also suggested that the MNK1-controlled translation pathway should be used to develop targeting strategies which can even be used to effectively treat GBM (Figure 9).
Figure 9. TGF-β signal pathway. TGF-β pathway interacts with TF to induce the expression of GBM cell proliferation, motility and survival genes, and participates in the translation of tumor mRNA.
A series of biological behaviors (such as over-expression of MNK1) and the increase of the translation properties of MNK1 were deeply studied. The proliferation of malignant GBM cells was also studied, and based on the results, we hypothesized that MNK1 could be used as a marker for predicting tumors. At present, eFT508 is considered as an efficient and highly selective inhibitor of MNK1 during experiments (Jin et al., 2021). But further deeper researches and more trails are needed to conduct and confirm whether inhibitors of MNK1 can be applied to the stage of clinical experiment. We believe that MNK1 is an attractive and promising therapeutic target in the near future.
The Janus kinase/signal transducer and activator of transcription (JAK/STAT) signaling pathway is considered as one of the central communication nodes in the cell function (Darnell, 1997). The JAK/STAT signaling pathway has profoundly influenced recent understanding attained of human health and disease. The JAK/STAT signaling pathway is evolutionarily conserved. It is composed of ligand-receptor complexes, JAKs, and STATs. There are 4 members in the JAK family: JAK1, JAK2, JAK3, and TYK2. The STAT family comprises seven members: STAT1, STAT2, STAT3, STAT4, STAT5a, STAT5b, and STAT6 (Xin et al., 2020). The composition of the JAK/STAT pathway is as follows: one is the classical JAK/STAT signaling pathway, another one is the non-classical JAK/STAT signaling pathway. Many researches have reported the importance of this pathway in malignancies (Aaronson and Horvath, 2002; O’Shea and Plenge, 2012; Ivashkiv and Donlin, 2014; O’Shea et al., 2015; Banerjee et al., 2017; Villarino et al., 2017). Thus, inhibiting the JAK/STAT pathway is promising for treating various diseases. Currently, many JAK inhibitors have achieved efficacy in many clinical settings, and more medications are currently being studied, such as STA-21, LLL-3, LLL12, and curcumin etc. (Ashrafizadeh et al., 2020; Xin et al., 2020; Shi et al., 2021). There are two ways of the regulation in JAK/STAT signaling pathways, that are positive and negative (Hu et al., 2021). The resent research shown that YTHDC1-mediated VPS25 regulates cell cycle by targeting JAK-STAT signaling in human glioma cells. VPS25 was upregulated in glioma tissues, which was correlated with poor prognosis in glioma patients. Furthermore, VPS25 knockdown inhibited the proliferation, blocked the cell cycle, and promoted apoptosis in glioma cells. Meanwhile, VPS25 induced a G0/G1 phase arrest of the cell cycle in glioma cells by directly mediating p21, CDK2, and cyclin E expression, and JAK-signal transducer and activator of transcription (STAT) activation. These results suggest that VPS25 is a promising prognostic indicator and a potential therapeutic target for glioma (Zhu et al., 2021).
Signaling cross-talk between JAK/STAT and other pathways is an intricate and colossal network, happens at different levels, and covers varieties of molecules, such as a ligand, receptor, phosphorylation, JAK, STAT, and gene transcription factors etc. (Zhu et al., 2021). These cross-talk activities play crucial roles in pluripotency and differentiation transcription program, immune regulation, and tumorigenesis. For example, TGF-β hedges IL-12 mediated JAK2 and TYK2 tyrosine phosphorylation (Bright and Sriram, 1998). Notch signaling inhibits JAK/STAT activation by interfering with STAT translocation to the DNA domain, and signals of JAK/STAT inhibited Notch signaling conversely (Assa-Kunik et al., 2007). SMAD3 inhibits STAT3 activation via recruiting PIAS3 to STAT3 and so on (Wang et al., 2016a; Hu et al., 2021; Figure 10).
According to the above analysis, the future studies should offer innovative insights into the potential mechanisms of the JAK/STAT pathway effects and cancer development. Moreover, we should concentrate all efforts on maximize efficacy and minimize side effects in patients in different stages of certain tumors and to explore more biomarkers that predict efficacy and offer prognoses.
Pediatric low-grade glioma (the most common glioma is the WHO grade I pilocytic astrocytoma PA) is the most common CNS tumor of childhood. Although overall survival is good, disease often recurs (Fangusaro et al., 2019). Abnormal MAPK pathway activation is the most common genetic aberration in pediatric low-grade glioma (Hargrave, 2009; Jones et al., 2013, 2018). Several drugs that target the MAPK pathway have been developed (Zhang et al., 2013). Selumetinib is a selective and potent orally available non-ATP-competitive small molecule inhibitor of MEK1/2 (Fangusaro et al., 2019). To our knowledge, aside from the use of mTOR inhibitors in tuberous-sclerosis-associated subependymal giant cell astrocytoma, 33 which is rare in the pediatric population, selumetinib is one of the first prospectively tested and active molecularly targeted agents in pediatric low-grade glioma (Fangusaro et al., 2019). MAPK (mitogen-activated protein kinase) signaling pathways regulate a variety of biological processes through multiple cellular mechanisms (Yue and López, 2020). MAPK cascade is a critical pathway for human cancer cell survival, dissemination, and resistance to drug therapy (De Luca et al., 2012; Elbaz et al., 2012). The MAPK/extracellular signal-regulated kinase (ERK) pathway is a convergent signaling node that receives input from numerous stimuli, including internal metabolic stress and DNA damage pathways and altered protein concentrations, as well as through signaling from external growth factors, cell-matrix interactions, and communication from other cells (Morrison, 2012; Yang et al., 2013). Mutated genes responsible for the regulation of cell fate, genome integrity, and survival can lead to increased protein amplification and alter the tumor microenvironment, thus overactivating the pathway (Birkbak and McGranahan, 2020). These mutations can occur upstream in membrane receptor genes, such as epithelial growth factor receptor (EGFR) (Wang et al., 2020) in signal transducers (RAS) (Malumbres and Barbacid, 2003) regulatory partners (Sprouty) (Muram-Zborovski et al., 2010; Yan W. et al., 2020) and downstream kinases that belong to the MAPK/ERK pathway itself (BRAF) (Fang and Richardson, 2005; Burotto et al., 2014; Moon and Ro, 2021). Low-grade glioma growth control pathways. LGGs arise from mutations in genes whose protein products regulate RAS pathway signaling. In this manner, mutations in genes encoding receptor tyrosine kinases (RTK), which transduce extracellular signals, as well as the downstream effectors PTPN11, RAS, BRAF/RAF, lead to hyperactivation of mitogenic signaling downstream through the MAP kinase and PI3-kinase pathways. In addition, mutational inactivation of the neurofibromatosis type 1 (NF1) tumor suppressor gene results in increased RAS activity. MEK and AKT can shorten G1 transition through mammalian target of rapamycin (mTOR)-dependent and -independent signaling. The presence of stromal growth factors and chemokines in the tumor microenvironment generate growth-promoting signals through tumor cell receptors and further enhance glioma expansion in a paracrine fashion (Baker et al., 2016; Figure 11).
The blood–brain barrier (BBB) is an extremely of significance factor to consider when determining treatments for various neurological diseases, both because disruption of the BBB can result in grievous pathology observed in many different diseases, especially brain tumors, but also because crossing the BBB is a basic element in the development of Central nervous system therapy (Daneman and Prat, 2015). BBB, Genetic and transcriptomic studies have proved activation of signaling pathways, such as WNT–β-catenin and sonic hedgehog (SHH)-dependent signaling in brain ECs (endothelial cells) within the BBB (Daneman and Prat, 2015). Intriguingly, some pathways including the G protein-coupled receptor (GPCR), GPR124 and WNT-β-catenin axis also regulate additional characteristics of vascular structure and function (Kuhnert et al., 2010; Umans et al., 2017; Griveau et al., 2018) CNS (central nervous system) ECs (endothelial cells) signaling pathways can be directly regulated by pericytes and astrocytes.
Neuronal and non-neuronal cells regulate the expression of transport and tight junction proteins in ECs, which in turn may “loosen” or “tighten” the BBB (Arvanitis et al., 2020). We introduce ways of key signaling pathways connecting astrocytes, pericytes and neurons to ECs (Sweeney et al., 2019). These pathways will modify transcellular transport by changing the expression of transporters and the paracellular path by tangling junctional protein complexes. Importantly, ECs mutually adjust and control components of the NVU (neurovascular unit). For instance, cognate receptor on pericytes are stimulated by EC-secreted TGF-β (Umans et al., 2017; Griveau et al., 2018). During development and maturation, glial cells, pericytes and neurons regulate EC behavior via numerous ligands and receptors, which in turn activate downstream signaling cascades that instruct expression of junctional and transcytosis proteins and control CNS homeostasis. Astrocytes directly modulate NVU demands such as water content in the neuroparenchymal space via the major water channel protein AQP4 (aquaporin 4) (Vanlandewijck et al., 2018) regulate immune cell and cancer cell (Arvanitis et al., 2020; Figure 12).
In conclusion, the brain microenvironment can thwart the effectiveness of drugs against primary brain cancer as well as brain metastases. It is a tremendous challenges that posed by the BBB and BTB for drug delivery, how multiple cell types, signaling pathways, and signaling cross-talk dictate BBB function and the role of the BTB in tumors progression and treatment. We believe emerging signal pathway targeting molecular to improve drug delivery across the BBB and BTB (blood–tumor barrier) and improve Prognosis of giloma, such as immune checkpoint inhibitors, Nanotechology, and engineered T cells. Common key signal pathways inhibitors glioma, Table 1.
In recent years, researchers have focused on signal pathways and glioma. This paper discusses the mechanisms associated with the Hippo/YAP, PI3K/AKT/Mtor, miRNA, Hedgehog, WnT/β-catenin, Notch, and TGF-β signal pathways and the key enzymes associated with glioma. The results can potentially help explore new diagnostic and prognostic biomarkers for the identification of new and efficient molecular therapeutic targets. However, further research should be conducted to clarify a few points. Significant levels of heterogeneity are observed in tumor cells (attributable to the recruitment of various cells), resulting in the generation of a tumor microenvironment. There are few reports on signal pathways and tumor heterogeneity. Though the relationship between signal pathways and glioma progression has been reported in the literature, the crosstalk between multiple signal pathways makes it difficult to determine the effective molecular targets associated with glioma treatment. We believe that the application of organic or inorganic nano carriers or exosomes (combined with clinical medication and adjuvant immunotherapy, such as monoclonal antibody-targeted therapy, polypeptide vaccine, DC vaccine, adoptive immunotherapy, and cytokine therapy), can potentially help in effectively treating glioma. Large-scale cohort studies and related molecular experiments should be conducted to find more reliable molecular therapeutic targets and address the existing problems.
HW: design of the work. MW: the acquisition analysis. QM: interpretation of data. YL: the creation of new software used in the work. HZ: have drafted the work or substantively revised it. All authors read and approved the final manuscript.
This work was supported by Fund Program “Nanoparticle Drug Carrier System for Glioma” of the Northern Jiangsu People’s Hospital of Jiangsu Province (No. SBJC21009), by “Improvement and clinical application of cervical 7 nerve transposition via cervical posterior approach in the treatment of central upper limb paralysis” of Project 333, The Fifth Phase of Yangzhou in 2019 (No. BRA2019026), by “The role of Neurod1 in neuronal reprogramming of NG2 cells in the repair of neural function in hemorrhagic stroke” of Yangzhou commission of health china (No. H2018064).
The authors declare that the research was conducted in the absence of any commercial or financial relationships that could be construed as a potential conflict of interest.
All claims expressed in this article are solely those of the authors and do not necessarily represent those of their affiliated organizations, or those of the publisher, the editors and the reviewers. Any product that may be evaluated in this article, or claim that may be made by its manufacturer, is not guaranteed or endorsed by the publisher.
We give thanks to all those who have helped with this issue.
PTEN, phosphatase and tension homolog deleted on chromosome ten; GBM, glioblastomas; Sav, salvador; Mats, mob as the tumor suppressors; Yki, yorkie; Yap, yes-associated protein; PI3K, phosphatidylinositol-3-kinase; PIP3, phosphomembrane-bound phosphatidylinositol-3; PI3K, phosphatidylinositol-3-kinase; Mtor, mammalian target of rapamycin; SHH, sonic hedgehog; LATS1, large tumor suppressor homolog 1; MGMT, O6-methylguanine-DNA methyltransferase; CKAP4, cytoskeleton-associated protein 4; TFA, transcription factor A; AMOTL1, Angiomotin-like 1; TMZ, temozolomide; Mst1, mammalian sterile 20-like 1; P, phosphorylation; SMC, stromal Mesenchymal Cells; CBL, casitas B lineage lymphoma proto-oncogene; JM, juxtamembrane; ADAM, a disintegrin and metallo_x0002_proteinase family; STAT3, signal transducer and activator of transcription 3; FAK, focal adhesion kinase; MAPK, mitogen-activated protein kinase; PI3K, phosphoinositide 3-kinase; RTK, receptor tyrosine kinase; RISC, RNA-induced silencing complex; FGF1, fibroblast growth factor-1; HOTAIR, hox transcript antisense RN; Sox2, sex determining region Y-box 2; MNK1, mitogen-activated protein kinase-interacting kinase 1; ID1, inhibitor of DNA binding protein-1; GALNT2, galactosaminyl transferase 2; Akt/FoxM1, Forkhead box M 1; MYBL2, MYB-related protein B2; EMT, Epithelial-mesenchymal transition; TSC, tuberous sclerosis complex; S6K, protein S6 kinase; 4E-BP, 4E binding proteins; HIF1a, hypoxia-inducible factor 1a; VEGF, vascular endothelial growth factor; SGs, stress granules; Rheb, Ras homolog enriched in brain; lncRNAs, long non-coding RNAs; ZNRF3, zinc and ring finger 3; TF, transcription factors; JAK/STAT, Janus kinase/signal transducer and activator of transcription; LGG, low-grade glioma; MAPK, mitogen-activated protein kinase; ERK, extracellular signal-regulated kinase; EGFR, epithelial growth factor receptor; NF1, neurofibromatosis type 1; BBB, blood–brain barrier; ECs, endothelial cells; CNS, central nervous system; GPCR, G protein-coupled receptor; NVU, neurovascular unit; AQP4, aquaporin 4; BTB, blood–tumor barrier.
Aaronson, D. S., and Horvath, C. M. (2002). A road map for those who don’t know JAK-STAT. Science 296, 1653–1655. doi: 10.1126/science.1071545
Achanta, P., Sedora Roman, N. I., and Quiñones-Hinojosa, A. (2010). Gliomagenesis and the use of neural stem cells in brain tumor treatment. Anti-cancer Agents Med. Chem. 10, 121–130. doi: 10.2174/187152010790909290
Álvarez-Garcia, V., Tawil, Y., Wise, H. M., and Leslie, N. R. (2019). Mechanisms of PTEN loss in cancer: it’s all about diversity. Sem. Cancer Biol. 59, 66–79. doi: 10.1016/j.semcancer.2019.02.001
Amin, A. G., Jeong, S. W., Gillick, J. L., Sursal, T., Murali, R., Gandhi, C. D., et al. (2021). Targeting the mTOR pathway using novel ATP-competitive inhibitors, Torin1, Torin2 and XL388, in the treatment of glioblastoma. Int. J. Oncol. 59:83. doi: 10.3892/ijo.2021.5263
An, B., Ma, Y., Xu, Y., Liu, X., Zhang, X., Zhang, J., et al. (2020). Crocin regulates the proliferation and migration of neural stem cells after cerebral ischemia by activating the Notch1 pathway. Folia Neuropathol. 58, 201–212. doi: 10.5114/fn.2020.100063
Angelopoulou, E., Paudel, Y. N., and Piperi, C. (2020). Critical role of HOX transcript antisense intergenic RNA (HOTAIR) in gliomas. J. Mol. Med. (Berlin, Germany) 98, 1525–1546. doi: 10.1007/s00109-020-01984-x
Arvanitis, C. D., Ferraro, G. B., and Jain, R. K. (2020). The blood-brain barrier and blood-tumour barrier in brain tumours and metastases. Nat. Rev. Cancer 20, 26–41. doi: 10.1038/s41568-019-0205-x
Asad, A. S., Asad, A. S., Candia, A. J. N., González, N., Zuccato, C. F., Seilicovich, A., et al. (2021). Current non-viral gene therapy strategies for the treatment of glioblastoma. Curr. Med. Chem. 28, 7729–7748. doi: 10.2174/0929867328666210525141243
Ashrafizadeh, M., Rafiei, H., Mohammadinejad, R., Afshar, E. G., Farkhondeh, T., and Samarghandian, S. (2020). Potential therapeutic effects of curcumin mediated by JAK/STAT signaling pathway: a review. Phytotherapy Res. : PTR 34, 1745–1760. doi: 10.1002/ptr.6642
Asiabi, P., David, C., Camboni, A., Marbaix, E., Dolmans, M. M., and Amorim, C. A. (2021). New insights into the GDF9-Hedgehog-GLI signaling pathway in human ovaries: from fetus to postmenopause. J. Assist. Reprod. Genet. 38, 1387–1403. doi: 10.1007/s10815-021-02161-w
Assa-Kunik, E., Torres, I. L., Schejter, E. D., Johnston, D. S., and Shilo, B. Z. (2007). Drosophila follicle cells are patterned by multiple levels of Notch signaling and antagonism between the Notch and JAK/STAT pathways. Development (Cambridge, England) 134, 1161–1169. doi: 10.1242/dev.02800
Badie, B., Schartner, J., Klaver, J., and Vorpahl, J. (1999). In vitro modulation of microglia motility by glioma cells is mediated by hepatocyte growth factor/scatter factor. Neurosurgery 44, 1077–1083. doi: 10.1097/00006123-199905000-00075
Bae, S. J., Ni, L., and Luo, X. (2020). STK25 suppresses Hippo signaling by regulating SAV1-STRIPAK antagonism. eLife 9:e54863. doi: 10.7554/eLife.54863
Bahmad, H. F., Mouhieddine, T. H., Chalhoub, R. M., Assi, S., Araji, T., Chamaa, F., et al. (2018). The Akt/mTOR pathway in cancer stem/progenitor cells is a potential therapeutic target for glioblastoma and neuroblastoma. Oncotarget 9, 33549–33561. doi: 10.18632/oncotarget.26088
Bai, Z. L., Tay, V., Guo, S. Z., Ren, J., and Shu, M. G. (2018). Silibinin induced human glioblastoma cell apoptosis concomitant with autophagy through simultaneous inhibition of mTOR and YAP. Biomed. Res. Int. 2018:6165192. doi: 10.1155/2018/6165192
Baker, S. J., Ellison, D. W., and Gutmann, D. H. (2016). Pediatric gliomas as neurodevelopmental disorders. Glia 64, 879–895. doi: 10.1002/glia.22945
Banerjee, S., Biehl, A., Gadina, M., Hasni, S., and Schwartz, D. M. (2017). JAK-STAT signaling as a target for inflammatory and autoimmune diseases: current and future prospects. Drugs 77, 521–546. doi: 10.1007/s40265-017-0701-709
Bao, Z., Xu, X., Liu, Y., Chao, H., Lin, C., Li, Z., et al. (2017). CBX7 negatively regulates migration and invasion in glioma via Wnt/β-catenin pathway inactivation. Oncotarget 8, 39048–39063. doi: 10.18632/oncotarget.16587
Bazzoni, R., and Bentivegna, A. (2019). Role of notch signaling pathway in glioblastoma pathogenesis. Cancers 11:292. doi: 10.3390/cancers11030292
Bi, Y., Li, H., Yi, D., Bai, Y., Zhong, S., Liu, Q., et al. (2018). β-catenin contributes to cordycepin-induced MGMT inhibition and reduction of temozolomide resistance in glioma cells by increasing intracellular reactive oxygen species. Cancer Lett. 435, 66–79. doi: 10.1016/j.canlet.2018.07.040
Bian, E. B., Li, J., Xie, Y. S., Zong, G., Li, J., and Zhao, B. (2015). LncRNAs: new players in gliomas, with special emphasis on the interaction of lncRNAs With EZH2. J. Cell. Physiol. 230, 496–503. doi: 10.1002/jcp.24549
Bilanges, B., Posor, Y., and Vanhaesebroeck, B. (2019). PI3K isoforms in cell signalling and vesicle trafficking. Nat. Rev. Mol. Cell Biol. 20, 515–534. doi: 10.1038/s41580-019-0129-z
Birkbak, N. J., and McGranahan, N. (2020). Cancer genome evolutionary trajectories in metastasis. Cancer Cell 37, 8–19. doi: 10.1016/j.ccell.2019.12.004
Biswas, S., and Rao, C. M. (2017). Epigenetics in cancer: fundamentals and beyond. Pharmacol. Ther. 173, 118–134. doi: 10.1016/j.pharmthera.2017.02.011
Bond, A. M., Ming, G. L., and Song, H. (2015). Adult mammalian neural stem cells and neurogenesis: five decades later. Cell Stem Cell 17, 385–395. doi: 10.1016/j.stem.2015.09.003
Bright, J. J., and Sriram, S. (1998). TGF-beta inhibits IL-12-induced activation of Jak-STAT pathway in T lymphocytes. J. Immunol. 161, 1772–1777.
Burel-Vandenbos, F., Ngo-Mai, M., Dadone, B., Di Mauro, I., Gimet, S., Saada-Bouzid, E., et al. (2017). MET immunolabelling is a useful predictive tool for MET gene amplification in glioblastoma. Neuropathol. Appl. Neurobiol. 43, 252–266. doi: 10.1111/nan.12320
Burotto, M., Chiou, V. L., Lee, J. M., and Kohn, E. C. (2014). The MAPK pathway across different malignancies: a new perspective. Cancer 120, 3446–3456. doi: 10.1002/cncr.28864
Cai, Y., Xu, G., Wu, F., Michelini, F., Chan, C., Qu, X., et al. (2021). Genomic alterations in PIK3CA-Mutated breast cancer result in mTORC1 activation and limit the sensitivity to PI3Kα inhibitors. Cancer Res. 81, 2470–2480. doi: 10.1158/0008-5472.CAN-20-3232
Calvo, J. A., Fritchman, B., Hernandez, D., Persky, N. S., Johannessen, C. M., Piccioni, F., et al. (2021). Comprehensive mutational analysis of the BRCA1-Associated DNA helicase and tumor-suppressor FANCJ/BACH1/BRIP1. Mol. Cancer Res. MCR 19, 1015–1025. doi: 10.1158/1541-7786.MCR-20-0828
Canaud, G., Hammill, A., Adams, D. M., Vikkula, M., and Keppler-Noreuil, K. M. (2021). A review of mechanisms of disease across PIK3CA-related disorders with vascular manifestations. Orphanet J. Rare Dis. 16:306. doi: 10.1186/s13023-021-01929-8
Carroll, B., Maetzel, D., Maddocks, O. D., Otten, G., Ratcliff, M., Smith, G. R., et al. (2016). Control of TSC2-Rheb signaling axis by arginine regulates mTORC1 activity. eLife 5:e11058. doi: 10.7554/eLife.11058
Castro, A. F., Rebhun, J. F., Clark, G. J., and Quilliam, L. A. (2003). Rheb binds tuberous sclerosis complex 2 (TSC2) and promotes S6 kinase activation in a rapamycin- and farnesylation-dependent manner. J. Biol. Chem. 278, 32493–32496. doi: 10.1074/jbc.C300226200
Cavalli, G., and Heard, E. (2019). Advances in epigenetics link genetics to the environment and disease. Nature 571, 489–499. doi: 10.1038/s41586-019-1411-0
Cech, T. R., and Steitz, J. A. (2014). The noncoding RNA revolution-trashing old rules to forge new ones. Cell 157, 77–94. doi: 10.1016/j.cell.2014.03.008
Chao, Y., Wang, Y., Liu, X., Ma, P., Shi, Y., Gao, J., et al. (2015). Mst1 regulates glioma cell proliferation via the AKT/mTOR signaling pathway. J. Neurooncol. 121, 279–288. doi: 10.1007/s11060-014-1654-4
Chauhan, A., Sah, D. K., Kumari, N., Kalra, N., Soni, R., Narayan Bhatt, A., et al. (2021). PTEN inhibitor bpV(HOpic) confers protection against ionizing radiation. Sci. Rep. 11:1720. doi: 10.1038/s41598-020-80754-8
Chen, R., Smith-Cohn, M., Cohen, A. L., and Colman, H. (2017). Glioma subclassifications and their clinical significance. Neurotherapeutics 14, 284–297. doi: 10.1007/s13311-017-0519-x
Cheng, F., and Guo, D. (2019). MET in glioma: signaling pathways and targeted therapies. J. Exp. Clin. Cancer Res. CR 38:270. doi: 10.1186/s13046-019-1269-x
Cho, S., Lee, G., Pickering, B. F., Jang, C., Park, J. H., He, L., et al. (2021). mTORC1 promotes cell growth via m6A-dependent mRNA degradation. Mol. Cell. 81, 2064–2075.e8. doi: 10.1016/j.molcel.2021.03.010.
Cirone, M. (2021). Cancer cells dysregulate PI3K/AKT/mTOR pathway activation to ensure their survival and proliferation: mimicking them is a smart strategy of gammaherpesviruses. Crit. Rev. Biochem. Mol. Biol. 56, 500–509. doi: 10.1080/10409238.2021.1934811
Claus, E. B., Walsh, K. M., Wiencke, J. K., Molinaro, A. M., Wiemels, J. L., Schildkraut, J. M., et al. (2015). Survival and low-grade glioma: the emergence of genetic information. Neurosurg. Focus 38:E6. doi: 10.3171/2014.10.FOCUS12367
Clayton, N. S., Fox, M., Vicenté-Garcia, J. J., Schroeder, C. M., Littlewood, T. D., Wilde, J. I., et al. (2022). Assembly of nuclear dimers of PI3K regulatory subunits is regulated by the Cdc42-activated tyrosine kinase ACK. J. Biol. Chem. 298:101916. doi: 10.1016/j.jbc.2022.101916
Condon, K. J., and Sabatini, D. M. (2019). Nutrient regulation of mTORC1 at a glance. J. Cell Sci. 132:jcs222570. doi: 10.1242/jcs.222570
Dai, Z., Wang, L., Wang, X., Zhao, B., Zhao, W., Bhardwaj, S. S., et al. (2018). Oxymatrine induces cell cycle arrest and apoptosis and suppresses the invasion of human glioblastoma cells through the EGFR/PI3K/Akt/mTOR signaling pathway and STAT3. Oncol. Rep. 40, 867–876. doi: 10.3892/or.2018.6512
Daneman, R., and Prat, A. (2015). The blood-brain barrier. Cold Spring Harb. Perspect. Biol. 7:a020412. doi: 10.1101/cshperspect.a020412
Darnell, J. E. Jr. (1997). STATs and gene regulation. Science 277, 1630–1635. doi: 10.1126/science.277.5332.1630
De Luca, A., Maiello, M. R., D’Alessio, A., Pergameno, M., and Normanno, N. (2012). The RAS/RAF/MEK/ERK and the PI3K/AKT signalling pathways: role in cancer pathogenesis and implications for therapeutic approaches. Expert Opin. Ther. Targets 16(Suppl. 2), S17–S27. doi: 10.1517/14728222.2011.639361
Delen, E., and Doğanlar, O. (2020). The dose dependent effects of ruxolitinib on the invasion and tumorigenesis in gliomas cells via inhibition of interferon gamma-depended JAK/STAT signaling pathway. J. Korean Neurosurg. Soc. 63, 444–454. doi: 10.3340/jkns.2019.0252
Dell’Albani, P., Rodolico, M., Pellitteri, R., Tricarichi, E., Torrisi, S. A., D’Antoni, S., et al. (2014). Differential patterns of NOTCH1-4 receptor expression are markers of glioma cell differentiation. Neuro Oncol. 16, 204–216. doi: 10.1093/neuonc/not168
Deng, Y. W., Shu, Y. G., and Sun, S. L. (2022). miR-376a inhibits glioma proliferation and angiogenesis by regulating YAP1/VEGF signalling via targeting of SIRT1. Trans. Oncol. 15:101270. doi: 10.1016/j.tranon.2021.101270
Dentro, S. C., Leshchiner, I., Haase, K., Tarabichi, M., Wintersinger, J., Deshwar, A. G., et al. (2021). Characterizing genetic intra-tumor heterogeneity across 2,658 human cancer genomes. Cell 184, 2239–2254.e39. doi: 10.1016/j.cell.2021.03.009.
Dibble, C. C., and Cantley, L. C. (2015). Regulation of mTORC1 by PI3K signaling. Trends Cell Biol. 25, 545–555. doi: 10.1016/j.tcb.2015.06.002
Dibble, C. C., Elis, W., Menon, S., Qin, W., Klekota, J., Asara, J. M., et al. (2012). TBC1D7 is a third subunit of the TSC1-TSC2 complex upstream of mTORC1. Mol. Cell. 47, 535–546. doi: 10.1016/j.molcel.2012.06.009
Ding, P., Liang, B., Shou, J., and Wang, X. (2020). lncRNA KCNQ1OT1 promotes proliferation and invasion of glioma cells by targeting the miR-375/YAP pathway. Int. J. Mol. Med. 46, 1983–1992. doi: 10.3892/ijmm.2020.4760
Domènech, M., Hernández, A., Plaja, A., Martínez-Balibrea, E., and Balañà, C. (2021). Hypoxia: the cornerstone of glioblastoma. Int. J. Mol. Sci. 22:12608. doi: 10.3390/ijms222212608
Dong, Z., Zhou, L., Han, N., Zhang, M., and Lyu, X. (2015). Wnt/β-catenin pathway involvement in ionizing radiation-induced invasion of U87 glioblastoma cells. Strahlenther. Onkol. 191, 672–680. doi: 10.1007/s00066-015-0858-7
Du, L., Lee, J. H., Jiang, H., Wang, C., Wang, S., Zheng, Z., et al. (2020). β-Catenin induces transcriptional expression of PD-L1 to promote glioblastoma immune evasion. J. Exp. Med. 217:e20191115. doi: 10.1084/jem.20191115
Elbaz, H. A., Stueckle, T. A., Tse, W., Rojanasakul, Y., and Dinu, C. Z. (2012). Digitoxin and its analogs as novel cancer therapeutics. Exp. Hematol. Oncol. 1:4. doi: 10.1186/2162-3619-1-4
Fan, F., He, Z., Kong, L. L., Chen, Q., Yuan, Q., Zhang, S., et al. (2016). Pharmacological targeting of kinases MST1 and MST2 augments tissue repair and regeneration. Sci. Trans. Med. 8:352ra108. doi: 10.1126/scitranslmed.aaf2304
Fan, Q. W., Nicolaides, T. P., and Weiss, W. A. (2018). Inhibiting 4EBP1 in glioblastoma. Clin. Cancer Res. 24, 14–21. doi: 10.1158/1078-0432.CCR-17-0042
Fang, J. Y., and Richardson, B. C. (2005). The MAPK signalling pathways and colorectal cancer. Lancet Oncol. 6, 322–327. doi: 10.1016/S1470-2045(05)70168-6
Fangusaro, J., Onar-Thomas, A., Young Poussaint, T., Wu, S., Ligon, A. H., Lindeman, N., et al. (2019). Selumetinib in paediatric patients with BRAF-aberrant or neurofibromatosis type 1-associated recurrent, refractory, or progressive low-grade glioma: a multicentre, phase 2 trial. Lancet Oncol. 20, 1011–1022. doi: 10.1016/S1470-2045(19)30277-3
Finco, I., LaPensee, C. R., Krill, K. T., and Hammer, G. D. (2015). Hedgehog signaling and steroidogenesis. Annu. Rev. Physiol. 77, 105–129. doi: 10.1146/annurev-physiol-061214-111754
Fruman, D. A., Chiu, H., Hopkins, B. D., Bagrodia, S., Cantley, L. C., Abraham, R. T., et al. (2017). The PI3K pathway in human disease. Cell 170, 605–635. doi: 10.1016/j.cell.2017.07.029
Fulop, B. D., Sandor, B., Szentleleky, E., Karanyicz, E., Reglodi, D., Gaszner, B., et al. (2019). Altered notch signaling in developing molar teeth of pituitary adenylate cyclase-activating polypeptide (PACAP)-Deficient mice. J. Mol. Neurosci. MN 68, 377–388. doi: 10.1007/s12031-018-1146-7
Gampala, S., and Yang, J. Y. (2021). Hedgehog pathway inhibitors against tumor microenvironment. Cells 10:3135. doi: 10.3390/cells10113135
Gao, L., Chen, B., Li, J., Yang, F., Cen, X., Liao, Z., et al. (2017). Wnt/β-catenin signaling pathway inhibits the proliferation and apoptosis of U87 glioma cells via different mechanisms. PLoS One 12:e0181346. doi: 10.1371/journal.pone.0181346
Garami, A., Zwartkruis, F. J., Nobukuni, T., Joaquin, M., Roccio, M., Stocker, H., et al. (2003). Insulin activation of Rheb, a mediator of mTOR/S6K/4E-BP signaling, is inhibited by TSC1 and 2. Mol. Cell. 11, 1457–1466. doi: 10.1016/s1097-2765(03)00220-x
Garcia-Lopez, J., Kumar, R., Smith, K. S., and Northcott, P. A. (2021). Deconstructing sonic hedgehog medulloblastoma: molecular subtypes, drivers, and beyond. Trends Genet. TIG 37, 235–250. doi: 10.1016/j.tig.2020.11.001
Godlewski, J., Krichevsky, A. M., Johnson, M. D., Chiocca, E. A., and Bronisz, A. (2015). Belonging to a network–microRNAs, extracellular vesicles, and the glioblastoma microenvironment. Neuro Oncol. 17, 652–662. doi: 10.1093/neuonc/nou292
Goncharova, E. A., Goncharov, D. A., Eszterhas, A., Hunter, D. S., Glassberg, M. K., Yeung, R. S., et al. (2002). Tuberin regulates p70 S6 kinase activation and ribosomal protein S6 phosphorylation. a role for the TSC2 tumor suppressor gene in pulmonary lymphangioleiomyomatosis (LAM). J. Biol. Chem. 277, 30958–30967. doi: 10.1074/jbc.M202678200
Greenall, S. A., Donoghue, J. F., Van Sinderen, M., Dubljevic, V., Budiman, S., Devlin, M., et al. (2015). EGFRvIII-mediated transactivation of receptor tyrosine kinases in glioma: mechanism and therapeutic implications. Oncogene 34, 5277–5287. doi: 10.1038/onc.2014.448
Griveau, A., Seano, G., Shelton, S. J., Kupp, R., Jahangiri, A., Obernier, K., et al. (2018). A glial signature and Wnt7 signaling regulate glioma-vascular interactions and tumor microenvironment. Cancer Cell 33, 874–889.e7. doi: 10.1016/j.ccell.2018.03.020.
Gromov, P. S., Madsen, P., Tomerup, N., and Celis, J. E. (1995). A novel approach for expression cloning of small GTPases: identification, tissue distribution and chromosome mapping of the human homolog of rheb. FEBS Lett. 377, 221–226. doi: 10.1016/0014-5793(95)01349-0
Grzmil, M., Morin, P. Jr., Lino, M. M., Merlo, A., Frank, S., Wang, Y., et al. (2011). MAP kinase-interacting kinase 1 regulates SMAD2-dependent TGF-β signaling pathway in human glioblastoma. Cancer Res. 71, 2392–2402. doi: 10.1158/0008-5472.CAN-10-3112
Guichet, P. O., Masliantsev, K., Tachon, G., Petropoulos, C., Godet, J., Larrieu, D., et al. (2018). Fatal correlation between YAP1 expression and glioma aggressiveness: clinical and molecular evidence. J. Pathol. 246, 205–216. doi: 10.1002/path.5133
Guo, C., Liang, C., Yang, J., Hu, H., Fan, B., and Liu, X. (2019). LATS2 inhibits cell proliferation and metastasis through the Hippo signaling pathway in glioma. Oncol. Rep. 41, 2753–2761. doi: 10.3892/or.2019.7065
Guo, G., Narayan, R. N., Horton, L., Patel, T. R., and Habib, A. A. (2017). The role of EGFR-Met interactions in the pathogenesis of glioblastoma and resistance to treatment. Curr. Cancer Drug. Targets 17, 297–302. doi: 10.2174/1568009616666161215162515
Gwinn, D. M., Shackelford, D. B., Egan, D. F., Mihaylova, M. M., Mery, A., Vasquez, D. S., et al. (2008). AMPK phosphorylation of raptor mediates a metabolic checkpoint. Mol. Cell 30, 214–226. doi: 10.1016/j.molcel.2008.03.003
Hadari, Y. R., Gotoh, N., Kouhara, H., Lax, I., and Schlessinger, J. (2001). Critical role for the docking-protein FRS2 alpha in FGF receptor-mediated signal transduction pathways. Proc. Natl. Acad. Sci. U S A. 98, 8578–8583. doi: 10.1073/pnas.161259898
Han, M., Wang, S., Fritah, S., Wang, X., Zhou, W., Yang, N., et al. (2020). Interfering with long non-coding RNA MIR22HG processing inhibits glioblastoma progression through suppression of Wnt/β-catenin signalling. Brain 143, 512–530. doi: 10.1093/brain/awz406
Han, N., Hu, G., Shi, L., Long, G., Yang, L., Xi, Q., et al. (2017). Notch1 ablation radiosensitizes glioblastoma cells. Oncotarget 8, 88059–88068. doi: 10.18632/oncotarget.21409
Han, S., Liu, Y., Cai, S. J., Qian, M., Ding, J., Larion, M., et al. (2020). IDH mutation in glioma: molecular mechanisms and potential therapeutic targets. Br. J. Cancer 122, 1580–1589. doi: 10.1038/s41416-020-0814-x
Han, Y., Wu, Z., Wu, T., Huang, Y., Cheng, Z., Li, X., et al. (2016). Tumor-suppressive function of long noncoding RNA MALAT1 in glioma cells by downregulation of MMP2 and inactivation of ERK/MAPK signaling. Cell Death Dis. 7:e2123. doi: 10.1038/cddis.2015.407
Hara, K., Maruki, Y., Long, X., Yoshino, K., Oshiro, N., Hidayat, S., et al. (2002). Raptor, a binding partner of target of rapamycin (TOR), mediates TOR action. Cell 110, 177–189. doi: 10.1016/s0092-8674(02)00833-4
Hargrave, D. (2009). Paediatric high and low grade glioma: the impact of tumour biology on current and future therapy. Br. J. Neurosurg. 23, 351–363. doi: 10.1080/02688690903158809
Hatterschide, J., Castagnino, P., Kim, H. W., Sperry, S. M., Montone, K. T., Basu, D., et al. (2022). YAP1 activation by human papillomavirus E7 promotes basal cell identity in squamous epithelia. eLife 11:e75466. doi: 10.7554/eLife.75466
Hayashi, H., Higashi, T., Yokoyama, N., Kaida, T., Sakamoto, K., Fukushima, Y., et al. (2015). An imbalance in TAZ and YAP expression in hepatocellular carcinoma confers cancer stem cell-like behaviors contributing to disease progression. Cancer Res. 75, 4985–4997. doi: 10.1158/0008-5472.CAN-15-0291
Held-Feindt, J., Lütjohann, B., Ungefroren, H., Mehdorn, H. M., and Mentlein, R. (2003). Interaction of transforming growth factor-beta (TGF-beta) and epidermal growth factor (EGF) in human glioma cells. J. Neurooncol. 63, 117–127. doi: 10.1023/a:1023943405292
Herrera-Rios, D., Li, G., Khan, D., Tsiampali, J., Nickel, A. C., Aretz, P., et al. (2020). A computational guided, functional validation of a novel therapeutic antibody proposes Notch signaling as a clinical relevant and druggable target in glioma. Sci. Rep. 10:16218. doi: 10.1038/s41598-020-72480-y
Hong, W., and Guan, K.-L. (2012). The YAP and TAZ transcription co-activators: key downstream effectors of the mammalian Hippo pathway. Sem. Cell Dev. Biol. 23, 785–793. doi: 10.1016/j.semcdb.2012.05.004
Hosokawa, N., Hara, T., Kaizuka, T., Kishi, C., Takamura, A., Miura, Y., et al. (2009). Nutrient-dependent mTORC1 association with the ULK1-Atg13-FIP200 complex required for autophagy. Mol. Biol. Cell 20, 1981–1991. doi: 10.1091/mbc.e08-12-1248
Hou, J., Lam, F., Proud, C., and Wang, S. (2012). Targeting Mnks for cancer therapy. Oncotarget 3, 118–131. doi: 10.18632/oncotarget.453
Hoxhaj, G., and Manning, B. D. (2020). The PI3K-AKT network at the interface of oncogenic signalling and cancer metabolism. Nat. Rev. Cancer 20, 74–88. doi: 10.1038/s41568-019-0216-7
Hsiao, J., Yuan, T. Y., Tsai, M. S., Lu, C. Y., Lin, Y. C., Lee, M. L., et al. (2016). Upregulation of haploinsufficient gene expression in the brain by targeting a long non-coding RNA improves seizure phenotype in a model of dravet syndrome. EBioMedicine 9, 257–277. doi: 10.1016/j.ebiom.2016.05.011
Hu, H., Mu, Q., Bao, Z., Chen, Y., Liu, Y., Chen, J., et al. (2018). Mutational landscape of secondary glioblastoma guides MET-Targeted trial in brain tumor. Cell 175, 1665–1678.e18. doi: 10.1016/j.cell.2018.09.038.
Hu, X., Li, J., Fu, M., Zhao, X., and Wang, W. (2021). The JAK/STAT signaling pathway: from bench to clinic. Signal Trans. Targeted Therapy 6:402. doi: 10.1038/s41392-021-00791-1
Hua, H., Kong, Q., Zhang, H., Wang, J., Luo, T., and Jiang, Y. (2019). Targeting mTOR for cancer therapy. J. Hematol. Oncol. 12:71. doi: 10.1186/s13045-019-0754-1
Huang, J., and Manning, B. D. (2008). The TSC1-TSC2 complex: a molecular switchboard controlling cell growth. Biochem. J. 412, 179–190. doi: 10.1042/BJ20080281
Huang, J., Dibble, C. C., Matsuzaki, M., and Manning, B. D. (2008). The TSC1-TSC2 complex is required for proper activation of mTOR complex 2. Mol. Cell. Biol. 28, 4104–4115. doi: 10.1128/MCB.00289-08
Huang, T., Wan, X., Alvarez, A. A., James, C. D., Song, X., and Yang, Y. (2019). MIR93 (microRNA -93) regulates tumorigenicity and therapy response of glioblastoma by targeting autophagy. Autophagy 15, 1100–1111. doi: 10.1080/15548627.2019.1569947
Huang, D., Wang, Y., Xu, L., Chen, L., Cheng, M., Shi, W., et al. (2018). GLI2 promotes cell proliferation and migration through transcriptional activation of ARHGEF16 in human glioma cells. J. Exp. Clin. Cancer Res. CR 37:247. doi: 10.1186/s13046-018-0917-x
Huang, W., Ding, X., Ye, H., Wang, J., Shao, J., and Huang, T. (2018). Hypoxia enhances the migration and invasion of human glioblastoma U87 cells through PI3K/Akt/mTOR/HIF-1α pathway. Neuroreport 29, 1578–1585. doi: 10.1097/WNR.0000000000001156
Huo, L., Wang, B., Zheng, M., Zhang, Y., Xu, J., Yang, G., et al. (2019). miR-128-3p inhibits glioma cell proliferation and differentiation by targeting NPTX1 through IRS-1/PI3K/AKT signaling pathway. Exp. Therapeutic Med. 17, 2921–2930. doi: 10.3892/etm.2019.7284
Infante, P., Faedda, R., Bernardi, F., Bufalieri, F., Lospinoso Severini, L., Alfonsi, R., et al. (2018). Itch/β-arrestin2-dependent non-proteolytic ubiquitylation of SuFu controls Hedgehog signalling and medulloblastoma tumorigenesis. Nat. Commun. 9:976. doi: 10.1038/s41467-018-03339-0
Inoki, K., Li, Y., Xu, T., and Guan, K. L. (2003). Rheb GTPase is a direct target of TSC2 GAP activity and regulates mTOR signaling. Genes Dev. 17, 1829–1834. doi: 10.1101/gad.1110003
Inoki, K., Li, Y., Zhu, T., Wu, J., and Guan, K. L. (2002). TSC2 is phosphorylated and inhibited by Akt and suppresses mTOR signalling. Nat. Cell Biol. 4, 648–657. doi: 10.1038/ncb839
Ishii, N., Maier, D., Merlo, A., Tada, M., Sawamura, Y., Diserens, A. C., et al. (1999). Frequent co-alterations of TP53, p16/CDKN2A, p14ARF, PTEN tumor suppressor genes in human glioma cell lines. Brain Pathol. 9, 469–479. doi: 10.1111/j.1750-3639.1999.tb00536.x
Ivashkiv, L. B., and Donlin, L. T. (2014). Regulation of type I interferon responses. Nat. Rev. Immunol. 14, 36–49. doi: 10.1038/nri3581
Jane, E. P., Premkumar, D. R., Sutera, P. A., Cavaleri, J. M., and Pollack, I. F. (2017). Survivin inhibitor YM155 induces mitochondrial dysfunction, autophagy, DNA damage and apoptosis in Bcl-xL silenced glioma cell lines. Mol. Carcinog. 56, 1251–1265. doi: 10.1002/mc.22587
Jarroux, J., Morillon, A., and Pinskaya, M. (2017). History, Discovery, and classification of lncRNAs. Adv. Exp. Med. Biol. 1008, 1–46. doi: 10.1007/978-981-10-5203-3_1
Jhanwar-Uniyal, M., Jeevan, D., Neil, J., Shannon, C., Albert, L., and Murali, R. (2013). Deconstructing mTOR complexes in regulation of glioblastoma multiforme and its stem cells. Adv. Biol. Regul. 53, 202–210. doi: 10.1016/j.jbior.2012.10.001
Ji, T., Liu, D., Shao, W., Yang, W., Wu, H., and Bian, X. (2012). Decreased expression of LATS1 is correlated with the progression and prognosis of glioma. J. Exp. Clin. Cancer Res. CR 31:67. doi: 10.1186/1756-9966-31-67
Jiang, Y., Zhou, J., Zhao, J., Zhang, H., Li, L., Li, H., et al. (2020). The U2AF2/circRNA ARF1/miR-342-3p/ISL2 feedback loop regulates angiogenesis in glioma stem cells. J. Exp. Clin. Cancer Res. CR 39:182. doi: 10.1186/s13046-020-01691-y
Jin, X., Yu, R., Wang, X., Proud, C. G., and Jiang, T. (2021). Progress in developing MNK inhibitors. Eur. J. Med. Chem. 219:113420. doi: 10.1016/j.ejmech.2021.113420
Jing, S. Y., Lu, Y. Y., Yang, J. K., Deng, W. Y., Zhou, Q., and Jiao, B. H. (2016). Expression of long non-coding RNA CRNDE in glioma and its correlation with tumor progression and patient survival. Eur. Rev. Med. Pharmacol. Sci. 20, 3992–3996.
Johnson, B. E., Mazor, T., Hong, C., Barnes, M., Aihara, K., McLean, C. Y., et al. (2014). Mutational analysis reveals the origin and therapy-driven evolution of recurrent glioma. Science 343, 189–193. doi: 10.1126/science.1239947
Johnson, R., and Halder, G. (2014). The two faces of Hippo: targeting the hippo pathway for regenerative medicine and cancer treatment. Nat. Rev. Drug Discov. 13, 63–79. doi: 10.1038/nrd4161
Jones, D. T., Hutter, B., Jäger, N., Korshunov, A., Kool, M., Warnatz, H. J., et al. (2013). Recurrent somatic alterations of FGFR1 and NTRK2 in pilocytic astrocytoma. Nat. Genet. 45, 927–932. doi: 10.1038/ng.2682
Jones, D., Kieran, M. W., Bouffet, E., Alexandrescu, S., Bandopadhayay, P., Bornhorst, M., et al. (2018). Pediatric low-grade gliomas: next biologically driven steps. Neuro-oncology 20, 160–173. doi: 10.1093/neuonc/nox141
Juan, W. C., and Hong, W. (2016). Targeting the hippo signaling pathway for tissue regeneration and cancer therapy. Genes (Basel) 7:55. doi: 10.3390/genes7090055
Junca, A., Villalva, C., Tachon, G., Rivet, P., Cortes, U., Guilloteau, K., et al. (2017). Crizotinib targets in glioblastoma stem cells. Cancer Med. 6, 2625–2634. doi: 10.1002/cam4.1167
Jung, C. H., Jun, C. B., Ro, S. H., Kim, Y. M., Otto, N. M., Cao, J., et al. (2009). ULK-Atg13-FIP200 complexes mediate mTOR signaling to the autophagy machinery. Mol. Biol. Cell 20, 1992–2003. doi: 10.1091/mbc.e08-12-1249
Kantidakis, T., Ramsbottom, B. A., Birch, J. L., Dowding, S. N., and White, R. J. (2010). mTOR associates with TFIIIC, is found at tRNA and 5S rRNA genes, and targets their repressor Maf1. Proc. Natl. Acad. Sci. U S A. 107, 11823–11828. doi: 10.1073/pnas.1005188107
Katoh, M., and Katoh, M. (2020). Precision medicine for human cancers with Notch signaling dysregulation (Review). Int. J. Mol. Med. 45, 279–297. doi: 10.3892/ijmm.2019.4418
Ke, J., Yao, Y. L., Zheng, J., Wang, P., Liu, Y. H., Ma, J., et al. (2015). Knockdown of long non-coding RNA HOTAIR inhibits malignant biological behaviors of human glioma cells via modulation of miR-326. Oncotarget 6, 21934–21949. doi: 10.18632/oncotarget.4290
Kierulf-Vieira, K. S., Sandberg, C. J., Waaler, J., Lund, K., Skaga, E., Saberniak, B. M., et al. (2020). A small-molecule tankyrase inhibitor reduces glioma stem cell proliferation and sphere formation. Cancers 12:1630. doi: 10.3390/cancers12061630
Kim, D. H., Choi, H. I., Park, J. S., Kim, C. S., Bae, E. H., Ma, S. K., et al. (2019). Src-mediated crosstalk between FXR and YAP protects against renal fibrosis. FASEB J. 33, 11109–11122. doi: 10.1096/fj.201900325R
Kim, D. H., Sarbassov, D. D., Ali, S. M., King, J. E., Latek, R. R., Erdjument-Bromage, H., et al. (2002). mTOR interacts with raptor to form a nutrient-sensitive complex that signals to the cell growth machinery. Cell 110, 163–175. doi: 10.1016/s0092-8674(02)00808-5
Kim, H. J., and Heo, K. (2021). YYB-101, a humanized antihepatocyte growth factor monoclonal antibody, inhibits ovarian cancer cell motility and proliferation. Anticancer. Res. 41, 671–678. doi: 10.21873/anticanres.14818
Kim, H. K., Fuchs, G., Wang, S., Wei, W., Zhang, Y., Park, H., et al. (2017). A transfer-RNA-derived small RNA regulates ribosome biogenesis. Nature 552, 57–62. doi: 10.1038/nature25005
Kim, H., Hong, S. H., Kim, J. Y., Kim, I. C., Park, Y. W., Lee, S. J., et al. (2017). Preclinical development of a humanized neutralizing antibody targeting HGF. Exp. Mol. Med. 49:e309. doi: 10.1038/emm.2017.21
Kim, J. Y., and Lee, J. Y. (2017). Targeting tumor adaption to chronic hypoxia: implications for drug resistance, and how it can be overcome. Int. J. Mol. Sci. 18:1854. doi: 10.3390/ijms18091854
Kim, J., and Guan, K. L. (2019). mTOR as a central hub of nutrient signalling and cell growth. Nat. Cell Biol. 21, 63–71. doi: 10.1038/s41556-018-0205-1
Kim, S. W., Kim, H. I., Thapa, B., Nuwormegbe, S., and Lee, K. (2019). Critical role of mTORC2-Akt signaling in TGF-β1-Induced myofibroblast differentiation of human pterygium fibroblasts. Invest. Ophthalmol. Visual Sci. 60, 82–92. doi: 10.1167/iovs.18-25376
Kjellman, C., Olofsson, S. P., Hansson, O., Von Schantz, T., Lindvall, M., Nilsson, I., et al. (2000). Expression of TGF-beta isoforms, TGF-beta receptors, and SMAD molecules at different stages of human glioma. Int. J. Cancer 89, 251–258. doi: 10.1002/1097-0215(20000520)89:3<251::aid-ijc7>3.0.co;2-5
Koch, A., Waha, A., Hartmann, W., Hrychyk, A., Schüller, U., Waha, A., et al. (2005). Elevated expression of Wnt antagonists is a common event in hepatoblastomas. Clin. Cancer Res. 11, 4295–4304. doi: 10.1158/1078-0432.CCR-04-1162
Kongkham, P. N., Northcott, P. A., Ra, Y. S., Nakahara, Y., Mainprize, T. G., Croul, S. E., et al. (2008). An epigenetic genome-wide screen identifies SPINT2 as a novel tumor suppressor gene in pediatric medulloblastoma. Cancer Res. 68, 9945–9953. doi: 10.1158/0008-5472.CAN-08-2169
Koundouros, N., Karali, E., Tripp, A., Valle, A., Inglese, P., and Perry, N. J. S. (2020). Metabolic fingerprinting links oncogenic PIK3CA with enhanced arachidonic acid-derived eicosanoids. Cell 181, 1596–1611.e27. doi: 10.1016/j.cell.2020.05.053.
Kuger, S., Graus, D., Brendtke, R., Günther, N., Katzer, A., Lutyj, P., et al. (2013). Radiosensitization of glioblastoma cell lines by the dual PI3K and mTOR inhibitor NVP-BEZ235 depends on drug-irradiation schedule. Trans. Oncol. 6, 169–179. doi: 10.1593/tlo.12364
Kuhnert, F., Mancuso, M. R., Shamloo, A., Wang, H. T., Choksi, V., Florek, M., et al. (2010). Essential regulation of CNS angiogenesis by the orphan G protein-coupled receptor GPR124. Science 330, 985–989. doi: 10.1126/science.1196554
Labib, E. M., Ezz, El Arab, L. R., Ghanem, H. M., Hassan, R. E., and Swellam, M. (2020). Relevance of circulating MiRNA-21 and MiRNA-181 in prediction of glioblastoma multiforme prognosis. Arch. Physiol. Biochem. Online ahead of print. doi: 10.1080/13813455.2020.1739716
Lee, Y.-R., and Pandolfi, P. P. (2020). PTEN mouse models of cancer initiation and progression. Cold Spring Harb. Perspect. Med. 10:a037283. doi: 10.1101/cshperspect.a037283
Li, H., Tong, F., Meng, R., Peng, L., Wang, J., Zhang, R., et al. (2021). E2F1-mediated repression of WNT5A expression promotes brain metastasis dependent on the ERK1/2 pathway in EGFR-mutant non-small cell lung cancer. Cell. Mol. Life Sci. CMLS 78, 2877–2891. doi: 10.1007/s00018-020-03678-6
Li, K., Wu, L., Chen, Y., Li, Y., Wang, Q., Li, M., et al. (2020). Cytotoxic and antiproliferative effects of β-Mangostin on rat C6 glioma cells depend on oxidative stress induction via PI3K/AKT/mTOR pathway inhibition. Drug Design Dev. Therapy 14, 5315–5324. doi: 10.2147/DDDT.S278414
Li, Z., Kong, Y., Song, L., Luo, Q., Liu, J., Shao, C., et al. (2018). Plk1-Mediated phosphorylation of TSC1 enhances the efficacy of rapamycin. Cancer Res. 78, 2864–2875. doi: 10.1158/0008-5472.CAN-17-3046
Lien, E. C., Dibble, C. C., and Toker, A. (2017). PI3K signaling in cancer: beyond AKT. Curr. Opin. Cell Biol. 45, 62–71. doi: 10.1016/j.ceb.2017.02.007
Lin, J., Jiang, L., Wang, X., Wei, W., Song, C., Cui, Y., et al. (2021). P4HA2 promotes epithelial-to-mesenchymal transition and glioma malignancy through the collagen-dependent PI3K/AKT pathway. J. Oncol. 2021:1406853. doi: 10.1155/2021/1406853
Lin, W., Zhou, Q., Wang, C. Q., Zhu, L., Bi, C., Zhang, S., et al. (2020). LncRNAs regulate metabolism in cancer. Int. J. Biol. Sci. 16, 1194–1206. doi: 10.7150/ijbs.40769
Liu, G. Y., and Sabatini, D. M. (2020). mTOR at the nexus of nutrition, growth, ageing and disease. Nat. Rev. Mol. Cell Biol. 21, 183–203. doi: 10.1038/s41580-019-0199-y
Liu, M., Lin, Y., Zhang, X. C., Tan, Y. H., Yao, Y. L., Tan, J., et al. (2017). Phosphorylated mTOR and YAP serve as prognostic markers and therapeutic targets in gliomas. Lab. Invest. 97, 1354–1363. doi: 10.1038/labinvest.2017.70
Liu, R., Chen, Y., Liu, G., Li, C., Song, Y., Cao, Z., et al. (2020). PI3K/AKT pathway as a key link modulates the multidrug resistance of cancers. Cell Death Dis. 11:797. doi: 10.1038/s41419-020-02998-6
Liu, X., Chen, J., Li, W., Hang, C., and Dai, Y. (2020). Inhibition of casein kinase II by CX-4945, but not yes-associated protein (YAP) by verteporfin, enhances the antitumor efficacy of temozolomide in glioblastoma. Trans. Oncol. 13, 70–78. doi: 10.1016/j.tranon.2019.09.006
Liu, Y. C., and Wang, Y. Z. (2015). Role of Yes-associated protein 1 in gliomas: pathologic and therapeutic aspects. Tumour Biol. 36, 2223–2227. doi: 10.1007/s13277-015-3297-2
Liu, Y., Lang, F., and Yang, C. (2021). NRF2 in human neoplasm: Cancer biology and potential therapeutic target. Pharmacol. Therapeut. 217:107664. doi: 10.1016/j.pharmthera.2020.107664
Liu, Z., Wang, F., Zhou, Z. W., Xia, H. C., Wang, X. Y., Yang, Y. X., et al. (2017). Alisertib induces G2/M arrest, apoptosis, and autophagy via PI3K/Akt/mTOR- and p38 MAPK-mediated pathways in human glioblastoma cells. Am. J. Transl. Res. 9, 845–873.
Liu, Z., Wei, Y., Zhang, L., Yee, P. P., Johnson, M., Zhang, X., et al. (2019). Induction of store-operated calcium entry (SOCE) suppresses glioblastoma growth by inhibiting the Hippo pathway transcriptional coactivators YAP/TAZ. Oncogene 38, 120–139. doi: 10.1038/s41388-018-0425-7
LoRusso, P. M. (2016). Inhibition of the PI3K/AKT/mTOR Pathway in Solid Tumors. J. Clin. Oncol. 34, 3803–3815. doi: 10.1200/JCO.2014.59.0018
Lu, C., Wei, Y., Wang, X., Zhang, Z., Yin, J., Li, W., et al. (2020). DNA-methylation-mediated activating of lncRNA SNHG12 promotes temozolomide resistance in glioblastoma. Mol. Cancer 19:28. doi: 10.1186/s12943-020-1137-5
Lu, Q. B., Wan, M. Y., Wang, P. Y., Zhang, C. X., Xu, D. Y., Liao, X., et al. (2018). Chicoric acid prevents PDGF-BB-induced VSMC dedifferentiation, proliferation and migration by suppressing ROS/NFκB/mTOR/P70S6K signaling cascade. Redox Biol. 14, 656–668. doi: 10.1016/j.redox.2017.11.012
Luo, M., Meng, Z., Moroishi, T., Lin, K. C., Shen, G., Mo, F., et al. (2020). Heat stress activates YAP/TAZ to induce the heat shock transcriptome. Nat. Cell Biol. 22, 1447–1459. doi: 10.1038/s41556-020-00602-9
Luo, T., Ding, K., Ji, J., Zhang, X., Yang, X., Chen, A., et al. (2021). Cytoskeleton-associated protein 4 (CKAP4) promotes malignant progression of human gliomas through inhibition of the Hippo signaling pathway. J. Neurooncol. 154, 275–283. doi: 10.1007/s11060-021-03831-6
Ma, L., Bajic, V. B., and Zhang, Z. (2013). On the classification of long non-coding RNAs. RNA Biol. 10, 925–933. doi: 10.4161/rna.24604
Ma, Q., Long, W., Xing, C., Chu, J., Luo, M., Wang, H. Y., et al. (2018). Cancer stem cells and immunosuppressive microenvironment in glioma. Front. Immunol. 9:2924. doi: 10.3389/fimmu.2018.02924
Ma, X., Zhang, W., Zhang, R., Li, J., Li, S., Ma, Y., et al. (2019). Overexpressed long noncoding RNA CRNDE with distinct alternatively spliced isoforms in multiple cancers. Front. Med. 13:330–343. doi: 10.1007/s11684-017-0557-0
Maehama, T., Nishio, M., Otani, J., Mak, T. W., and Suzuki, A. (2021). The role of Hippo-YAP signaling in squamous cell carcinomas. Cancer Sci. 112, 51–60. doi: 10.1111/cas.14725
Majchrzak-Celiñska, A., Misiorek, J. O., Kruhlenia, N., Przybyl, L., Kleszcz, R., Rolle, K., et al. (2021). COXIBs and 2,5-dimethylcelecoxib counteract the hyperactivated Wnt/β-catenin pathway and COX-2/PGE2/EP4 signaling in glioblastoma cells. BMC Cancer 21:493. doi: 10.1186/s12885-021-08164-1
Malumbres, M., and Barbacid, M. (2003). RAS oncogenes: the first 30 years. Nat. Rev. Cancer 3, 459–465. doi: 10.1038/nrc1097
Manning, B. D., Tee, A. R., Logsdon, M. N., Blenis, J., and Cantley, L. C. (2002). Identification of the tuberous sclerosis complex-2 tumor suppressor gene product tuberin as a target of the phosphoinositide 3-kinase/akt pathway. Mol. Cell. 10, 151–162. doi: 10.1016/s1097-2765(02)00568-3
Mao, J., Sun, Z., Cui, Y., Du, N., Guo, H., Wei, J., et al. (2020). PCBP2 promotes the development of glioma by regulating FHL3/TGF-β/Smad signaling pathway. J. Cell. Physiol. 235, 3280–3291. doi: 10.1002/jcp.29104
Mason, W. P., Belanger, K., Nicholas, G., Vallières, I., Mathieu, D., Kavan, P., et al. (2012). A phase II study of the Ras-MAPK signaling pathway inhibitor TLN-4601 in patients with glioblastoma at first progression. J. Neurooncol. 107, 343–349. doi: 10.1007/s11060-011-0747-6
Mattoo, A. R., Joun, A., and Jessup, J. M. (2019). Repurposing of mTOR complex inhibitors attenuates MCL-1 and sensitizes to PARP inhibition. Mol. Cancer Res. 17, 42–53. doi: 10.1158/1541-7786.MCR-18-0650
McIntyre, B., Asahara, T., and Alev, C. (2020). Overview of basic mechanisms of notch signaling in development and disease. Adv. Exp. Med. Biol. 1227, 9–27. doi: 10.1007/978-3-030-36422-9_2
Michels, A. A., Robitaille, A. M., Buczynski-Ruchonnet, D., Hodroj, W., Reina, J. H., Hall, M. N., et al. (2010). mTORC1 directly phosphorylates and regulates human MAF1. Mol. Cell. Biol. 30, 3749–3757. doi: 10.1128/MCB.00319-10
Mimeault, M., and Batra, S. K. (2011). Complex oncogenic signaling networks regulate brain tumor-initiating cells and their progenies: pivotal roles of wild-type EGFR, EGFRvIII mutant and hedgehog cascades and novel multitargeted therapies. Brain Pathol. 21, 479–500. doi: 10.1111/j.1750-3639.2011.00505.x
Ming, J., Sun, B., Li, Z., Lin, L., Meng, X., Han, B., et al. (2017). Aspirin inhibits the SHH/GLI1 signaling pathway and sensitizes malignant glioma cells to temozolomide therapy. Aging 9, 1233–1247. doi: 10.18632/aging.101224
Miricescu, D., Totan, A., Stanescu-Spinu, I. I., Badoiu, S. C., Stefani, C., Greabu, M., et al. (2020). PI3K/AKT/mTOR signaling pathway in breast cancer: from molecular landscape to clinical aspects. Int. J. Mol. Sci. 22:173. doi: 10.3390/ijms22010173
Mishra, S., Yadav, T., and Rani, V. (2016). Exploring miRNA based approaches in cancer diagnostics and therapeutics. Crit. Rev. Oncology/Hematol. 98, 12–23. doi: 10.1016/j.critrevonc.2015.10.003
Miyazaki, M., and Takemasa, T. (2017). TSC2/Rheb signaling mediates ERK-dependent regulation of mTORC1 activity in C2C12 myoblasts. FEBS Open Bio 7:424–433. doi: 10.1002/2211-5463.12195
Mo, J., Liu, F., Sun, X., Huang, H., Tan, K., Zhao, X., et al. (2021). Inhibition of the FACT complex targets aberrant hedgehog signaling and overcomes resistance to smoothened antagonists. Cancer Res. 81, 3105–3120. doi: 10.1158/0008-5472.CAN-20-3186
Modena, P., Lualdi, E., Facchinetti, F., Veltman, J., Reid, J. F., Minardi, S., et al. (2006). Identification of tumor-specific molecular signatures in intracranial ependymoma and association with clinical characteristics. J. Clin. Oncol. 24, 5223–5233. doi: 10.1200/JCO.2006.06.3701
Moloudizargari, M., Asghari, M. H., Nabavi, S. F., Gulei, D., Berindan-Neagoe, I., Bishayee, A., et al. (2022). Targeting Hippo signaling pathway by phytochemicals in cancer therapy. Sem. Cancer Biol. 80, 183–194. doi: 10.1016/j.semcancer.2020.05.005
Moon, H., and Ro, S. W. (2021). MAPK/ERK signaling pathway in hepatocellular carcinoma. Cancers 13:3026. doi: 10.3390/cancers13123026
Moore, S. F., Hunter, R. W., and Hers, I. (2014). Protein kinase C and P2Y12 take center stage in thrombin-mediated activation of mammalian target of rapamycin complex 1 in human platelets. J. Thrombosis Haemostasis : JTH 12, 748–760. doi: 10.1111/jth.12552
Moosavi, F., Giovannetti, E., Saso, L., and Firuzi, O. (2019). HGF/MET pathway aberrations as diagnostic, prognostic, and predictive biomarkers in human cancers. Crit. Rev. Clin. Lab. Sci. 56, 533–566. doi: 10.1080/10408363.2019.1653821
Morikawa, M., Derynck, R., and Miyazono, K. (2016). TGF-β and the TGF-β family: context-dependent roles in cell and tissue physiology. Cold Spring Harb. Perspect. Biol. 8:a021873. doi: 10.1101/cshperspect.a021873
Morrison, D. K. (2012). MAP kinase pathways. Cold Spring Harb. Perspect. Biol. 4:a011254. doi: 10.1101/cshperspect.a011254
Mossmann, D., Park, S., and Hall, M. N. (2018). mTOR signalling and cellular metabolism are mutual determinants in cancer. Nat. Rev. Cancer 18, 744–757. doi: 10.1038/s41568-018-0074-8
Mu, M., Niu, W., Zhang, X., Hu, S., and Niu, C. (2020). LncRNA BCYRN1 inhibits glioma tumorigenesis by competitively binding with miR-619-5p to regulate CUEDC2 expression and the PTEN/AKT/p21 pathway. Oncogene 39, 6879–6892. doi: 10.1038/s41388-020-01466-x
Mulcahy, E., Colün, R. R., and Abounader, R. (2020). HGF/MET signaling in malignant brain tumors. Int. J. Mol. Sci. 21:7546. doi: 10.3390/ijms21207546
Muram-Zborovski, T. M., Stevenson, D. A., Viskochil, D. H., Dries, D. C., Wilson, A. R., and Rong Mao. (2010). SPRED 1 mutations in a neurofibromatosis clinic. J. Child Neurol. 25, 1203–1209. doi: 10.1177/0883073809359540
Náger, M., Santacana, M., Bhardwaj, D., Valls, J., Ferrer, I., Nogués, P., et al. (2015). Nuclear phosphorylated Y142 β-catenin accumulates in astrocytomas and glioblastomas and regulates cell invasion. Cell Cycle 14, 3644–3655. doi: 10.1080/15384101.2015.1104443
Nanta, R., Shrivastava, A., Sharma, J., Shankar, S., and Srivastava, R. K. (2019). Inhibition of sonic hedgehog and PI3K/Akt/mTOR pathways cooperate in suppressing survival, self-renewal and tumorigenic potential of glioblastoma-initiating cells. Mol. Cell. Biochem. 454, 11–23. doi: 10.1007/s11010-018-3448-z
Nawaz, Z., Patil, V., Arora, A., Hegde, A. S., Arivazhagan, A., Santosh, V., et al. (2016). Cbx7 is epigenetically silenced in glioblastoma and inhibits cell migration by targeting YAP/TAZ-dependent transcription. Sci. Rep. 6:27753. doi: 10.1038/srep27753
Northcott, P. A., Buchhalter, I., Morrissy, A. S., Hovestadt, V., Weischenfeldt, J., Ehrenberger, T., et al. (2017). The whole-genome landscape of medulloblastoma subtypes. Nature 547, 311–317. doi: 10.1038/nature22973
Oldrini, B., Vaquero-Siguero, N., Mu, Q., Kroon, P., Zhang, Y., Galán-Ganga, M., et al. (2020). MGMT genomic rearrangements contribute to chemotherapy resistance in gliomas. Nat. Commun. 11:3883. doi: 10.1038/s41467-020-17717-0
Omeljaniuk, W. J., Krêtowski, R., Ratajczak-Wrona, W., Jabłoñska, E., and Cechowska-Pasko, M. (2021). Novel dual PI3K/mTOR inhibitor, apitolisib (GDC-0980), inhibits growth and induces apoptosis in human glioblastoma cells. Int. J. Mol. Sci. 22:11511. doi: 10.3390/ijms222111511
Omuro, A., and DeAngelis, L. M. (2013). Glioblastoma and other malignant gliomas:a clinical review. JAMA. 310, 1842–1850. doi: 10.1001/jama.2013.280319
Oppermann, H., Faust, H., Yamanishi, U., Meixensberger, J., and Gaunitz, F. (2019). Carnosine inhibits glioblastoma growth independent from PI3K/Akt/mTOR signaling. PLoS One 14:e0218972. doi: 10.1371/journal.pone.0218972
O’Shea, J. J., and Plenge, R. (2012). JAK and STAT signaling molecules in immunoregulation and immune-mediated disease. Immunity 36, 542–550. doi: 10.1016/j.immuni.2012.03.014
O’Shea, J. J., Schwartz, D. M., Villarino, A. V., Gadina, M., McInnes, I. B., and Laurence, A. (2015). The JAK-STAT pathway: impact on human disease and therapeutic intervention. Annu. Rev. Med. 66, 311–328. doi: 10.1146/annurev-med-051113-024537
Ostrom, Q. T., Patil, N., Cioffi, G., Waite, K., Kruchko, C., Barnholtz-Sloan, J. S., et al. (2020). CBTRUS statistical report: primary brain and other central nervous system tumors diagnosed in the united states in 2013-2017. Neuro Oncol. 22(12 Suppl. 2), iv1–iv96. doi: 10.1093/neuonc/noaa200
Panciera, T., Citron, A., Di Biagio, D., Battilana, G., Gandin, A., Giulitti, S., et al. (2020). Reprogramming normal cells into tumour precursors requires ECM stiffness and oncogene-mediated changes of cell mechanical properties. Nat. Mater. 19, 797–806. doi: 10.1038/s41563-020-0615-x
Panza, S., Russo, U., Giordano, F., Leggio, A., Barone, I., Bonofiglio, D., et al. (2020). Leptin and notch signaling cooperate in sustaining glioblastoma multiforme progression. Biomolecules 10:886. doi: 10.3390/biom10060886
Parikh, R. A., Wang, P., Beumer, J. H., Chu, E., and Appleman, L. J. (2014). The potential roles of hepatocyte growth factor (HGF)-MET pathway inhibitors in cancer treatment. OncoTargets Therapy 7, 969–983. doi: 10.2147/OTT.S40241
Patrick, S., Gowda, P., Lathoria, K., Suri, V., and Sen, E. (2021). YAP1-mediated regulation of mitochondrial dynamics in IDH1 mutant gliomas. J. Cell Sci. 134:jcs259188. doi: 10.1242/jcs.259188
Perreault, S., Larouche, V., Tabori, U., Hawkin, C., Lippé, S., Ellezam, B., et al. (2019). A phase 2 study of trametinib for patients with pediatric glioma or plexiform neurofibroma with refractory tumor and activation of the MAPK/ERK pathway: TRAM-01. BMC Cancer 19:1250. doi: 10.1186/s12885-019-6442-2
Petrova, R., and Joyner, A. L. (2014). Roles for hedgehog signaling in adult organ homeostasis and repair. Development 141, 3445–3457. doi: 10.1242/dev.083691
Petterson, S. A., Dahlrot, R. H., Hermansen, S. K., Munthe, S., Gundesen, M. T., Wohlleben, H., et al. (2015). High levels of c-Met is associated with poor prognosis in glioblastoma. J. Neurooncol. 122, 517–527. doi: 10.1007/s11060-015-1723-3
Pham, K., Luo, D., Siemann, D. W., Law, B. K., Reynolds, B. A., Hothi, P., et al. (2015). VEGFR inhibitors upregulate CXCR4 in VEGF receptor-expressing glioblastoma in a TGFβR signaling-dependent manner. Cancer Lett. 360, 60–67. doi: 10.1016/j.canlet.2015.02.005
Pierscianek, D., Kim, Y. H., Motomura, K., Mittelbronn, M., Paulus, W., Brokinkel, B., et al. (2013). MET gain in diffuse astrocytomas is associated with poorer outcome. Brain Pathology 23, 13–18. doi: 10.1111/j.1750-3639.2012.00609.x
Plasek, L. M., and Valadkhan, S. (2021). lncRNAs in T lymphocytes: RNA regulation at the heart of the immune response. Am. J. Physiol. Cell Physiol. 320, C415–C427. doi: 10.1152/ajpcell.00069.2020
Prentzell, M. T., Rehbein, U., Cadena Sandoval, M., De Meulemeester, A. S., Baumeister, R., Brohée, L., et al. (2021). G3BPs tether the TSC complex to lysosomes and suppress mTORC1 signaling. Cell 184, 655–674.e27. doi: 10.1016/j.cell.2020.12.024.
Pu, P., Zhang, Z., Kang, C., Jiang, R., Jia, Z., Wang, G., et al. (2009). Downregulation of Wnt2 and beta-catenin by siRNA suppresses malignant glioma cell growth. Cancer Gene Ther. 16, 351–361. doi: 10.1038/cgt.2008.78
Qiao, Y., Li, T., Zheng, S., and Wang, H. (2018). The Hippo pathway as a drug target in gastric cancer. Cancer Lett. 420, 14–25. doi: 10.1016/j.canlet.2018.01.062
Qin, Y., Musket, A., Kou, J., Preiszner, J., Tschida, B. R., Qin, A., et al. (2020). Overexpression of HGF/MET axis along with p53 inhibition induces de novo glioma formation in mice. Neuro-oncology Adv. 2:vdaa067. doi: 10.1093/noajnl/vdaa067
Rimkus, T. K., Carpenter, R. L., Qasem, S., Chan, M., and Lo, H. W. (2016). Targeting the sonic hedgehog signaling pathway: review of smoothened and GLI inhibitors. Cancers 8:22. doi: 10.3390/cancers8020022
Roussel, M. F., and Hatten, M. E. (2011). Cerebellum development and medulloblastoma. Curr. Top. Dev. Biol. 94, 235–282. doi: 10.1016/B978-0-12-380916-2.00008-5
Ryall, S., Tabori, U., and Hawkins, C. (2020). Pediatric low-grade glioma in the era of molecular diagnostics. Acta Neuropathol. Commun. 8:30. doi: 10.1186/s40478-020-00902-z
Sa, L., Li, Y., Zhao, L., Liu, Y., Wang, P., Liu, L., et al. (2017). The role of HOTAIR/miR-148b-3p/USF1 on regulating the permeability of BTB. Front. Mol. Neurosci. 10:194. doi: 10.3389/fnmol.2017.00194
Saccone, S., Narsimhan, R. P., Gaudino, G., Dalprà, L., Comoglio, P. M., and Della Valle, G. (1992). Regional mapping of the human hepatocyte growth factor (HGF)-scatter factor gene to chromosome 7q21.1. Genomics 13, 912–914. doi: 10.1016/0888-7543(92)90191-t
Sarkar, D., Leung, E. Y., Baguley, B. C., Finlay, G. J., and Askarian-Amiri, M. E. (2015). Epigenetic regulation in human melanoma: past and future. Epigenetics 10, 103–121. doi: 10.1080/15592294.2014.1003746
Sato, T., Nakashima, A., Guo, L., and Tamanoi, F. (2009). Specific activation of mTORC1 by Rheb G-protein in vitro involves enhanced recruitment of its substrate protein. J. Biol. Chem. 284, 12783–12791. doi: 10.1074/jbc.M809207200
Saunders, J. T., Holmes, B., Benavides-Serrato, A., Kumar, S., Nishimura, R. N., and Gera, J. (2021). Targeting the YAP-TEAD interaction interface for therapeutic intervention in glioblastoma. J. Neurooncol. 152, 217–231. doi: 10.1007/s11060-021-03699-6
Saunders, V. C., Lafitte, M., Adrados, I., Quereda, V., Feurstein, D., Ling, Y., et al. (2015). Identification of an EGFRvIII-JNK2-HGF/c-met-signaling axis required for intercellular crosstalk and glioblastoma multiforme cell invasion. Mol. Pharmacol. 88, 962–969. doi: 10.1124/mol.115.097774
Schaal, C. M., Bora-Singhal, N., Kumar, D. M., and Chellappan, S. P. (2018). Regulation of Sox2 and stemness by nicotine and electronic-cigarettes in non-small cell lung cancer. Mol. Cancer 17:149. doi: 10.1186/s12943-018-0901-2
Sellars, E., Gabra, M., and Salmena, L. (2020). The complex landscape of PTEN mRNA regulation. Cold Spring Harb. Perspect. Med. 10:a036236. doi: 10.1101/cshperspect.a036236
Seo, S. I., Yoon, J. H., Byun, H. J., and Lee, S. K. (2021). HOTAIR induces methylation of PCDH10, a tumor suppressor gene, by regulating DNMT1 and sponging with miR-148b in gastric adenocarcinoma. Yonsei Med. J. 62, 118–128. doi: 10.3349/ymj.2021.62.2.118
Sheng, J., He, X., Yu, W., Chen, Y., Long, Y., Wang, K., et al. (2021). p53-targeted lncRNA ST7-AS1 acts as a tumour suppressor by interacting with PTBP1 to suppress the Wnt/β-catenin signalling pathway in glioma. Cancer Lett. 503, 54–68. doi: 10.1016/j.canlet.2020.12.039
Shi, G., Zhang, Z., Fang, Y., Bian, D., and Bai, Z. (2021). Curcumin combined with low-intensity ultrasound suppresses the growth of glioma cells via inhibition of the AKT pathway. Neoplasma 68, 290–297. doi: 10.4149/neo_2020_200605N604
Shimizu, T., Ho, L. L., and Lai, Z. C. (2008). The mob as tumor suppressor gene is essential for early development and regulates tissue growth in Drosophila. Genetics 178, 957–965. doi: 10.1534/genetics.107.081570
Skoda, A. M., Simovic, D., Karin, V., Kardum, V., Vranic, S., and Serman, L. (2018). The role of the hedgehog signaling pathway in cancer: a comprehensive review. Bosn. J. Basic Med. Sci. 18, 8–20. doi: 10.17305/bjbms.2018.2756
Stefani, C., Miricescu, D. Stanescu-Spinu, I. I., Nica, R. I., Greabu, M., Totan, A. R., et al. (2021). Growth factors, PI3K/AKT/mTOR and MAPK signaling pathways in colorectal cancer pathogenesis: where are we now? Int. J. Mol. Sci. 22:10260. doi: 10.3390/ijms221910260
Su, Y., Liang, C., and Yang, Q. (2021). LncRNA MALAT1 promotes glioma cell growth through sponge miR-613. J. B.U.ON. 26, 984–991.
Sun, J., Ma, Q., Shu, C., Xiong, J., Li, B., Wu, J., et al. (2021). MicroRNA-301a/ZNRF3/wnt/β-catenin signal regulatory crosstalk mediates glioma progression. Int. J. Oncol. 58, 45–56. doi: 10.3892/ijo.2020.5145
Sun, P., and Meng, L.-H. (2020). Emerging roles of class I PI3K inhibitors in modulating tumor microenvironment and immunity. Acta Pharmacol. Sin. 41, 1395–1402. doi: 10.1038/s41401-020-00500-8
Sun, X., Ding, Y., Zhan, M., Li, Y., Gao, D., Wang, G., et al. (2019). Usp7 regulates Hippo pathway through deubiquitinating the transcriptional coactivator Yorkie. Nat. Commun. 10:411. doi: 10.1038/s41467-019-08334-7
Sun, Z., Xue, H., Wei, Y., Wang, C., Yu, R., Wang, C., et al. (2019). Mucin O-glycosylating enzyme GALNT2 facilitates the malignant character of glioma by activating the EGFR/PI3K/Akt/mTOR axis. Clin. Sci. 133, 1167–1184. doi: 10.1042/CS20190145
Suwala, A. K., Stichel, D., Schrimpf, D., Maas, S. L. N., Sill, M., Dohmen, H., et al. (2021). Glioblastomas with primitive neuronal component harbor a distinct methylation and copy-number profile with inactivation of TP53, PTEN, and RB1. Acta Neuropathol. 142, 179–189. doi: 10.1007/s00401-021-02302-6
Sweeney, M. D., Zhao, Z., Montagne, A., Nelson, A. R., and Zlokovic, B. V. (2019). Blood-Brain barrier: from physiology to disease and back. Physiol. Rev. 99, 21–78. doi: 10.1152/physrev.00050.2017
Tabancay, A. P. Jr., Gau, C. L., Machado, I. M., Uhlmann, E. J., Gutmann, D. H., Guo, L., et al. (2003). Identification of dominant negative mutants of Rheb GTPase and their use to implicate the involvement of human Rheb in the activation of p70S6K. J. Biol. Chem. 278, 39921–39930. doi: 10.1074/jbc.M306553200
Tan, Y. T., Lin, J. F., Li, T., Li, J. J., Xu, R. H., and Ju, H. Q. (2021). LncRNA-mediated posttranslational modifications and reprogramming of energy metabolism in cancer. Cancer Commun. 41, 109–120. doi: 10.1002/cac2.12108
Tasaki, T., Fujita, M., Okuda, T., Yoneshige, A., Nakata, S., Yamashita, K., et al. (2016). MET expressed in glioma stem cells is a potent therapeutic target for glioblastoma multiforme. Anticancer. Res. 36, 3571–3577.
Tee, A. R. (2018). The target of rapamycin and mechanisms of cell growth. Int. J. Mol. Sci. 19:880. doi: 10.3390/ijms19030880
Tee, A. R., Blenis, J., and Proud, C. G. (2005). Analysis of mTOR signaling by the small G-proteins, Rheb and RhebL1. FEBS Lett. 579, 4763–4768. doi: 10.1016/j.febslet.2005.07.054
Trelinska, J., Dachowska, I., Baranska, D., Stawiski, K., Kotulska, K., Fendler, W., et al. (2017). Maintenance therapy with everolimus for subependymal giant cell astrocytoma in patients with tuberous sclerosis (the EMINENTS study). Pediatric Blood Cancer 64:10.1002/pbc.26347.
Umans, R. A., Henson, H. E., Mu, F., Parupalli, C., Ju, B., Peters, J. L., et al. (2017). CNS angiogenesis and barriergenesis occur simultaneously. Dev. Biol. 425, 101–108. doi: 10.1016/j.ydbio.2017.03.017
Urulangodi, M., and Mohanty, A. (2020). DNA damage response and repair pathway modulation by non-histone protein methylation: implications in neurodegeneration. J. Cell Commun. Signal. 14, 31–45. doi: 10.1007/s12079-019-00538-2
Van Meir, E. G., Hadjipanayis, C. G., Norden, A. D., Shu, H. K., Wen, P. Y., and Olson, J. J. (2010). Exciting new advances in neuro-oncology: the avenue to a cure for malignant glioma. CA Cancer J. Clin. 60, 166–193. doi: 10.3322/caac.20069
van Veelen, W., Korsse, S. E., van de Laar, L., and Peppelenbosch, M. P. (2011). The long and winding road to rational treatment of cancer associated with LKB1/AMPK/TSC/mTORC1 signaling. Oncogene 30, 2289–2303. doi: 10.1038/onc.2010.630
Vander Haar, E., Lee, S. I., Bandhakavi, S., Griffin, T. J., and Kim, D. H. (2007). Insulin signalling to mTOR mediated by the Akt/PKB substrate PRAS40. Nat. Cell Biol. 9, 316–323. doi: 10.1038/ncb1547
Vangala, V., Nimmu, N. V., Khalid, S., Kuncha, M., Sistla, R., Banerjee, R., et al. (2020). Combating glioblastoma by codelivering the small-molecule inhibitor of STAT3 and STAT3siRNA with α5β1 integrin receptor-selective liposomes. Mol. Pharmaceutics 17, 1859–1874. doi: 10.1021/acs.molpharmaceut.9b01271
Vanlandewijck, M., He, L., Mäe, M. A., Andrae, J., Ando, K., Del Gaudio, F., et al. (2018). A molecular atlas of cell types and zonation in the brain vasculature. Nature 554, 475–480. doi: 10.1038/nature25739
Vargas, R. E., Duong, V. T., Han, H., Ta, A. P., Chen, Y., Zhao, S., et al. (2020). Elucidation of WW domain ligand binding specificities in the Hippo pathway reveals STXBP4 as YAP inhibitor. EMBO J. 39:e102406. doi: 10.15252/embj.2019102406
Vengoji, R., Macha, M. A., Nimmakayala, R. K., Rachagani, S., Siddiqui, J. A., Mallya, K., et al. (2019). Afatinib and Temozolomide combination inhibits tumorigenesis by targeting EGFRvIII-cMet signaling in glioblastoma cells. J. Exp. Clin. Cancer Res. CR 38:266. doi: 10.1186/s13046-019-1264-2
Villarino, A. V., Kanno, Y., and O’Shea, J. J. (2017). Mechanisms and consequences of Jak-STAT signaling in the immune system. Nat. Immunol. 18, 374–384. doi: 10.1038/ni.3691
Vinchure, O. S., Sharma, V., Tabasum, S., Ghosh, S., Singh, R. P., Sarkar, C., et al. (2019). Polycomb complex mediated epigenetic reprogramming alters TGF-β signaling via a novel EZH2/miR-490/TGIF2 axis thereby inducing migration and EMT potential in glioblastomas. Int. J. Cancer 145, 1254–1269. doi: 10.1002/ijc.32360
Viswanathan, A., Kute, D., Musa, A., Konda Mani, S., Sipilä, V., Emmert-Streib, F., et al. (2019). 2-(2-(2,4-dioxopentan-3-ylidene)hydrazineyl)benzonitrile as novel inhibitor of receptor tyrosine kinase and PI3K/AKT/mTOR signaling pathway in glioblastoma. Eur. J. Med. Chem. 166, 291–303. doi: 10.1016/j.ejmech.2019.01.021
Wang, C., Chen, Y., Wang, Y., Liu, X., Liu, Y., Li, Y., et al. (2019). Inhibition of COX-2, mPGES-1 and CYP4A by isoliquiritigenin blocks the angiogenic Akt signaling in glioma through ceRNA effect of miR-194-5p and lncRNA NEAT1. J. Exp. Clin. Cancer Res. CR 38:371. doi: 10.1186/s13046-019-1361-2
Wang, F., Zhang, L., Luo, Y., Zhang, Q., Zhang, Y., Shao, Y., et al. (2021). The LncRNA RP11-279C4.1 enhances the malignant behaviour of glioma cells and glioma stem-like cells by regulating the miR-1273g-3p/CBX3 axis. Mol. Neurobiol. 58, 3362–3373. doi: 10.1007/s12035-021-02337-6
Wang, G., Li, Z., Tian, N., Han, L., Fu, Y., Guo, Z., et al. (2016a). miR-148b-3p inhibits malignant biological behaviors of human glioma cells induced by high HOTAIR expression. Oncol. Lett. 12, 879–886. doi: 10.3892/ol.2016.4743
Wang, G., Yu, Y., Sun, C., Liu, T., Liang, T., Zhan, L., et al. (2016b). STAT3 selectively interacts with Smad3 to antagonize TGF-β signalling. Oncogene 35, 4388–4398. doi: 10.1038/onc.2015.446
Wang, J., Liu, Z., Pang, Q., Zhang, T., Chen, X., Er, P., et al. (2020). Prognostic analysis of patients with non-small cell lung cancer harboring exon 19 or 21 mutation in the epidermal growth factor gene and brain metastases. BMC Cancer 20:837. doi: 10.1186/s12885-020-07249-7
Wang, J., Xu, S. L., Duan, J. J., Yi, L., Guo, Y. F., Shi, Y., et al. (2019). Invasion of white matter tracts by glioma stem cells is regulated by a NOTCH1-SOX2 positive-feedback loop. Nat. Neurosci. 22, 91–105. doi: 10.1038/s41593-018-0285-z
Wang, Y., Gao, S., Wang, W., Xia, Y., and Liang, J. (2018). Downregulation of N-Myc inhibits neuroblastoma cell growth via the Wnt/β-catenin signaling pathway. Mol. Med. Rep. 18, 377–384. doi: 10.3892/mmr.2018.8966
Wang, Y., Wang, Y., Li, J., Zhang, Y., Yin, H., and Han, B. (2015). CRNDE, a long-noncoding RNA, promotes glioma cell growth and invasion through mTOR signaling. Cancer Lett. 367, 122–128. doi: 10.1016/j.canlet.2015.03.027
Wei, L., Li, L., Liu, L., Yu, R., Li, X., and Luo, Z. (2021). Knockdown of Annexin-A1 inhibits growth, migration and invasion of glioma cells by suppressing the PI3K/Akt signaling pathway. ASN Neuro 13:17590914211001218. doi: 10.1177/17590914211001218
Weinberg, M. A. (2016). RES-529: a PI3K/AKT/mTOR pathway inhibitor that dissociates the mTORC1 and mTORC2 complexes. Anticancer. Drugs 27, 475–487. doi: 10.1097/CAD.0000000000000354
Wen, N., Guo, B., Zheng, H., Xu, L., Liang, H., Wang, Q., et al. (2019). Bromodomain inhibitor jq1 induces cell cycle arrest and apoptosis of glioma stem cells through the VEGF/PI3K/AKT signaling pathway. Int. J. Oncol. 55, 879–895. doi: 10.3892/ijo.2019.4863
Wen, P. Y., and Reardon, D. A. (2016). Neuro-oncology in 2015: progress in glioma diagnosis, classification and treatment. Nat. Rev. Neurol. 12, 69–70. doi: 10.1038/nrneurol.2015.242
Wu, J., Minikes, A. M., Gao, M., Bian, H., Li, Y., Stockwell, B. R., et al. (2019). Intercellular interaction dictates cancer cell ferroptosis via NF2-YAP signalling. Nature 572, 402–406. doi: 10.1038/s41586-019-1426-6
Wu, X., Ouyang, Y., Wang, B., Lin, J., and Bai, Y. (2020). Hypermethylation of the IRAK3-Activated MAPK signaling pathway to promote the development of glioma. Cancer Manag. Res. 12, 7043–7059. doi: 10.2147/CMAR.S252772
Xia, J., Zeng, M., Zhu, H., Chen, X., Weng, Z., and Li, S. (2017). Emerging role of hippo signalling pathway in bladder cancer. J. Cell Mol. Med. 22, 4–15. doi: 10.1111/jcmm.13293
Xia, P., and Xu, X. Y. (2015). PI3K/Akt/mTOR signaling pathway in cancer stem cells: from basic research to clinical application. Am. J. Cancer Res. 5, 1602–1609.
Xiao, K., and Peng, G. (2021). Long non-coding RNA FAM66C regulates glioma growth via the miRNA/LATS1 signaling pathway. Biol. Chem. 403, 679–689. doi: 10.1515/hsz-2021-0333
Xiao, L., Wang, W., Zhao, J., Xu, H., Li, S., and Yang, X. (2020). lncRNA MALAT1 promotes cell proliferation and invasion by regulating the miR-101/EZH2 axis in oral squamous cell carcinoma. Oncol. Lett. 20:164. doi: 10.3892/ol.2020.12024
Xin, P., Xu, X., Deng, C., Liu, S., Wang, Y., Zhou, X., et al. (2020). The role of JAK/STAT signaling pathway and its inhibitors in diseases. Int. Immunopharmacol. 80:106210. doi: 10.1016/j.intimp.2020.106210
Xu, F., Na, L., Li, Y., and Chen, L. (2020). Roles of the PI3K/AKT/mTOR signalling pathways in neurodegenerative diseases and tumours. Cell Biosci. 10:54. doi: 10.1186/s13578-020-00416-0
Xu, G., Seng, Z., Zhang, M., and Qu, J. (2021). Angiomotin-like 1 plays a tumor-promoting role in glioma by enhancing the activation of YAP1 signaling. Environ. Toxicol. 36, 2500–2511. doi: 10.1002/tox.23363
Xu, L., Wu, Q., Yan, H., Shu, C., Fan, W., Tong, X., et al. (2022). Long noncoding RNA KB-1460A1.5 inhibits glioma tumorigenesis via miR-130a-3p/TSC1/mTOR/YY1 feedback loop. Cancer Lett. 525, 33–45. doi: 10.1016/j.canlet.2021.10.033
Xu, R., Shimizu, F., Hovinga, K., Beal, K., Karimi, S., Droms, L., et al. (2016). Molecular and clinical effects of notch inhibition in glioma patients: a phase 0/I trial. Clin. Cancer Res. 22, 4786–4796. doi: 10.1158/1078-0432.CCR-16-0048
Xu, S., Tang, L., Li, X., Fan, F., and Liu, Z. (2020). Immunotherapy for glioma: current management and future application. Cancer Lett. 476, 1–12. doi: 10.1016/j.canlet.2020.02.002
Xu, Z., Han, X., Ou, D., Liu, T., Li, Z., Jiang, G., et al. (2020). Targeting PI3K/AKT/mTOR-mediated autophagy for tumor therapy. Appl. Microbiol. Biotechnol. 104, 575–587. doi: 10.1007/s00253-019-10257-8
Yamamoto, S., Wakimoto, H., Aoyagi, M., Hirakawa, K., and Hamada, H. (1997). Modulation of motility and proliferation of glioma cells by hepatocyte growth factor. Japanese J. Cancer Res. Gann 88, 564–577. doi: 10.1111/j.1349-7006.1997.tb00420.x
Yan, F., Qian, M., He, Q., Zhu, H., and Yang, B. (2020). The posttranslational modifications of Hippo-YAP pathway in cancer. Biochim Biophys. Acta Gen. Subj. 1864:129397. doi: 10.1016/j.bbagen.2019.07.006
Yan, W., Markegard, E., Dharmaiah, S., Urisman, A., Drew, M., Esposito, D., et al. (2020). Structural insights into the SPRED1-Neurofibromin-KRAS complex and disruption of SPRED1-neurofibromin interaction by oncogenic EGFR. Cell Rep. 32:107909. doi: 10.1016/j.celrep.2020.107909
Yang, K., Tang, X. J., Xu, F. F., Liu, J. H., Tan, Y. Q., Gao, L., et al. (2020). PI3K/mTORC1/2 inhibitor PQR309 inhibits proliferation and induces apoptosis in human glioblastoma cells. Oncol. Rep. 43, 773–782. doi: 10.3892/or.2020.7472
Yang, L., Pang, Y., and Moses, H. L. (2010). TGF-beta and immune cells: an important regulatory axis in the tumor microenvironment and progression. Trends Immunol. 31, 220–227. doi: 10.1016/j.it.2010.04.002
Yang, Q., Inoki, K., Kim, E., and Guan, K. L. (2006). TSC1/TSC2 and Rheb have different effects on TORC1 and TORC2 activity. Proc. Natl. Acad. Sci. U S A. 103, 6811–6816. doi: 10.1073/pnas.0602282103
Yang, R., Wang, M., Zhang, G., Li, Y., Wang, L., and Cui, H. (2021). POU2F2 regulates glycolytic reprogramming and glioblastoma progression via PDPK1-dependent activation of PI3K/AKT/mTOR pathway. Cell Death Dis. 12:433. doi: 10.1038/s41419-021-03719-3
Yang, S. H., Sharrocks, A. D., and Whitmarsh, A. J. (2013). MAP kinase signalling cascades and transcriptional regulation. Gene 513, 1–13. doi: 10.1016/j.gene.2012.10.033
Yang, Y., Song, Y., Nie, Q., Tian, R., Huang, J., and Mao, G. (2021). Baicalein inhibits invasion and promotes apoptosis in glioma cells through the PI3K/Akt pathway. J. B.U.ON. 26, 395–401.
Yap, T. A., Vieito, M., Baldini, C., Sepúlveda-Sánchez, J. M., Kondo, S., Simonelli, M., et al. (2021). First-In-Human phase I study of a next-generation, oral, TGFβ receptor 1 inhibitor, LY3200882, in patients with advanced cancer. Clin. Cancer Res. 27, 6666–6676. doi: 10.1158/1078-0432.CCR-21-1504
Yi, L., Zhou, X., Li, T., Liu, P., Hai, L., Tong, L., et al. (2019). Notch1 signaling pathway promotes invasion, self-renewal and growth of glioma initiating cells via modulating chemokine system CXCL12/CXCR4. J. Exp. Clin. Cancer Res. CR 38:339. doi: 10.1186/s13046-019-1319-4
Yin, W., Zhang, K., Deng, Q., Yu, Q., Mao, Y., Zhao, R., et al. (2021). AZD3759 inhibits glioma through the blockade of the epidermal growth factor receptor and Janus kinase pathways. Bioengineered 12, 8679–8689. doi: 10.1080/21655979.2021.1991160
Yoshioka, K. (2021). Class II phosphatidylinositol 3-kinase isoforms in vesicular trafficking. Biochem. Soc. Trans. 49, 893–901. doi: 10.1042/BST20200835
Yu, Y., Peng, X. D., Qian, X. J., Zhang, K. M., Huang, X., Chen, Y. H., et al. (2021). Fis1 phosphorylation by Met promotes mitochondrial fission and hepatocellular carcinoma metastasis. Signal Transduction Targeted Therapy 6:401. doi: 10.1038/s41392-021-00790-2
Yuan, J., Shan, Y., Chen, X., Tang, W., Luo, K., Ni, J., et al. (2005). Identification and characterization of RHEBL1, a novel member of Ras family, which activates transcriptional activities of NF-kappa B. Mol. Biol. Rep. 32, 205–214. doi: 10.1007/s11033-005-0984-x
Yuan, X., Wei, W., Bao, Q., Chen, H., Jin, P., and Jiang, W. (2018). Metformin inhibits glioma cells stemness and epithelial-mesenchymal transition via regulating YAP activity. Biomed. Pharmacother. 102, 263–270. doi: 10.1016/j.biopha.2018.03.031
Yue, J., and López, J. M. (2020). Understanding MAPK signaling pathways in apoptosis. Int. J. Mol. Sci. 21:2346. doi: 10.3390/ijms21072346
Yue, Q., Khojasteh, S. C., Cho, S., Ma, S., Mulder, T., Chen, J., et al. (2021). Absorption, metabolism and excretion of pictilisib, a potent pan-class I phosphatidylinositol-3-Kinase (PI3K) inhibitor, in rats, dogs, and humans. Xenobiotica 51, 796–810. doi: 10.1080/00498254.2021.1923859
Zeng, Q., Zhou, Z., Qin, S., Yao, Y., Qin, J., Zhang, H., et al. (2020). Rapamycin inhibits B-cell activating factor (BAFF)-stimulated cell proliferation and survival by suppressing Ca2+-CaMKII-dependent PTEN/Akt-Erk1/2 signaling pathway in normal and neoplastic B-lymphoid cells. Cell Calcium 87:102171. doi: 10.1016/j.ceca.2020.102171
Zhang, C., Cao, J., Lv, W., and Mou, H. (2021). CircRNA_100395 carried by exosomes from adipose-derived mesenchymal stem cells inhibits the malignant transformation of non-small cell lung carcinoma through the miR-141-3p-LATS2 axis. Front. Cell Dev. Biol. 9:663147. doi: 10.3389/fcell.2021.663147
Zhang, C., Wang, Q., Zhou, X., Zhang, L., Yao, Y., Gu, J., et al. (2020). MicroRNA-138 modulates glioma cell growth, apoptosis and invasion through the suppression of the AKT/mTOR signalling pathway by targeting CREB1. Oncol. Rep. 44, 2559–2568. doi: 10.3892/or.2020.7809
Zhang, D., Jing, Z., Qiu, B., Wu, A., and Wang, Y. (2011). Temozolomide decreases invasion of glioma stem cells by down-regulating TGF-β2. Oncol. Rep. 26, 901–908. doi: 10.3892/or.2011.1362
Zhang, F., Huang, Y., Wang, B., Zhong, C., Liu, X., and Ding, S. (2018). LINC00673 silencing inhibits cell migration and invasion by suppressing PI3K/AKT signaling in glioma. Neuroreport 29, 718–722. doi: 10.1097/WNR.0000000000001022
Zhang, F., Wang, H., Yu, J., Yao, X., Yang, S., Li, W., et al. (2021). LncRNA CRNDE attenuates chemoresistance in gastric cancer via SRSF6-regulated alternative splicing of PICALM. Mol. Cancer 20:6. doi: 10.1186/s12943-020-01299-y
Zhang, H., Geng, D., Gao, J., Qi, Y., Shi, Y., Wang, Y., et al. (2016). Expression and significance of Hippo/YAP signaling in glioma progression. Tumour Biol. Online ahead of print. doi: 10.1007/s13277-016-5318-1
Zhang, H., Liao, J., Zhang, X., Zhao, E., Liang, X., Luo, S., et al. (2019). Sex difference of mutation clonality in diffuse glioma evolution. Neuro-Oncology 21, 201–213. doi: 10.1093/neuonc/noy154
Zhang, J., Wu, G., Miller, C. P., Tatevossian, R. G., Dalton, J. D., Tang, B., et al. (2013). Whole-genome sequencing identifies genetic alterations in pediatric low-grade gliomas. Nat. Genet. 45, 602–612. doi: 10.1038/ng.2611
Zhang, X., Lv, Q. L., Huang, Y. T., Zhang, L. H., and Zhou, H. H. (2017). Akt/FoxM1 signaling pathway-mediated upregulation of MYBL2 promotes progression of human glioma. J. Exp. Clin. Cancer Res. CR 36:105.
Zhang, Y., Gao, X., Saucedo, L. J., Ru, B., Edgar, B. A., and Pan, D. (2003). Rheb is a direct target of the tuberous sclerosis tumour suppressor proteins. Nat. Cell Biol. 5, 578–581. doi: 10.1038/ncb999
Zhang, Y., Xia, M., Jin, K., Wang, S., Wei, H., Fan, C., et al. (2018). Function of the c-Met receptor tyrosine kinase in carcinogenesis and associated therapeutic opportunities. Mol. Cancer 17:45. doi: 10.1186/s12943-018-0796-y
Zhao, B., Wei, X., Li, W., Udan, R. S., Yang, Q., Kim, J., et al. (2007). Inactivation of YAP oncoprotein by the Hippo pathway is involved in cell contact inhibition and tissue growth control. Genes Dev. 21, 2747–2761. doi: 10.1101/gad.1602907
Zhao, M., Zhang, Y., Jiang, Y., Wang, K., Wang, X., Zhou, D., et al. (2021). YAP promotes autophagy and progression of gliomas via upregulating HMGB1. J. Exp. Clin. Cancer Res. CR 40:99. doi: 10.1186/s13046-021-01897-8
Zhao, Y., Sun, Y., Zhang, H., Liu, X., Du, W., Li, Y., et al. (2015). HGF/MET signaling promotes glioma growth via up-regulation of Cox-2 expression and PGE2 production. Int. J. Clin. Exp. Pathol. 8, 3719–3726.
Zhi, F., Wang, Q., Xue, L., Shao, N., Wang, R., Deng, D., et al. (2015). The use of three long non-coding RNAs as potential prognostic indicators of astrocytoma. PLoS One 10:e0135242. doi: 10.1371/journal.pone.0135242
Zhou, W., and Zhao, M. (2018). How hippo signaling pathway modulates cardiovascular development and diseases. J. Immunol. Res. 2018:3696914. doi: 10.1155/2018/3696914
Zhu, B., and Wei, Y. (2020). Antitumor activity of celastrol by inhibition of proliferation, invasion, and migration in cholangiocarcinoma via PTEN/PI3K/Akt pathway. Cancer Med. 9, 783–796. doi: 10.1002/cam4.2719
Keywords: glioma, gene expression, signal pathway, prognosis, neoplastic
Citation: Wu H, Wei M, Li Y, Ma Q and Zhang H (2022) Research Progress on the Regulation Mechanism of Key Signal Pathways Affecting the Prognosis of Glioma. Front. Mol. Neurosci. 15:910543. doi: 10.3389/fnmol.2022.910543
Received: 01 April 2022; Accepted: 30 May 2022;
Published: 22 July 2022.
Edited by:
Kausik Bishayee, Soonchunhyang University, South KoreaReviewed by:
Dinesh Upadhya, Manipal Academy of Higher Education, IndiaCopyright © 2022 Wu, Wei, Li, Ma and Zhang. This is an open-access article distributed under the terms of the Creative Commons Attribution License (CC BY). The use, distribution or reproduction in other forums is permitted, provided the original author(s) and the copyright owner(s) are credited and that the original publication in this journal is cited, in accordance with accepted academic practice. No use, distribution or reproduction is permitted which does not comply with these terms.
*Correspondence: Hengzhu Zhang, emhhbmdoZW5nemh1QHNpbmEuY29t
Disclaimer: All claims expressed in this article are solely those of the authors and do not necessarily represent those of their affiliated organizations, or those of the publisher, the editors and the reviewers. Any product that may be evaluated in this article or claim that may be made by its manufacturer is not guaranteed or endorsed by the publisher.
Research integrity at Frontiers
Learn more about the work of our research integrity team to safeguard the quality of each article we publish.