- 1State Key Laboratory of Medical Neurobiology, Department of Otorhinolaryngology, Eye and ENT Hospital, MOE Frontiers Center for Brain Science, ENT Institute, Fudan University, Shanghai, China
- 2NHC Key Laboratory of Hearing Medicine, Fudan University, Shanghai, China
The vestibular system is a critical part of the human balance system, malfunction of this system will lead to balance disorders, such as vertigo. Mammalian vestibular hair cells, the mechanical receptors for vestibular function, are sensitive to ototoxic drugs and virus infection, and have a limited restorative capacity after damage. Considering that no artificial device can be used to replace vestibular hair cells, promoting vestibular hair cell regeneration is an ideal way for vestibular function recovery. In this manuscript, the development of human vestibular hair cells during the whole embryonic stage and the latest research on human vestibular hair cell regeneration is summarized. The limitations of current studies are emphasized and future directions are discussed.
Introduction
Vestibular sensory epithelia are composed of hair cells (HCs) and supporting cells (SCs). HCs of the vestibular sensory epithelia, which are surrounded by supporting cells, convert mechanical signals, such as head movement or tilt, into electrical signals (Gao et al., 2019; Liu et al., 2019; Qi et al., 2019, 2020; Tan et al., 2019). These signals are then transmitted by afferent fibers to the vestibular nuclei that send out fibers projected to the corresponding neural structures to control eye movement, posture, and balance (Cullen, 2012; Guo et al., 2019, 2020, 2021b,c; Hu et al., 2021; Wei et al., 2021).
Hair cells are easily injured by ototoxic drugs (Li et al., 2018; Zhang et al., 2019; Zhong et al., 2020; Fu et al., 2021b), aging (Cheng et al., 2019; Guo et al., 2021a; He et al., 2021), genetic factors (Qian et al., 2020; Cheng et al., 2021; Fu et al., 2021a; Lv et al., 2021; Zhang S. et al., 2021) and infections (Han et al., 2020; He et al., 2020; Zhang Y. et al., 2021). The loss of human vestibular HCs is closely related to balance dysfunction (Tsuji et al., 2000; Ishiyama et al., 2015). It has been stated that the annual incidence of vertigo is about 11% (Corrales and Bhattacharyya, 2016), and the lifetime prevalence of moderate to severe vertigo and dizziness is about 30% (Strupp et al., 2020). However, our current understanding of the development and generation of vestibular HCs is mainly derived from rodent models. Here, we review the current information on the development of human vestibular epithelia, as well as the latest progress made in restoring human vestibular HCs upon damage.
Structure of Human Vestibular Sensory Epithelia
The human vestibular sensory epithelia, like that of other mammals, are composed of three crista ampullaris perpendicular to each other for sensing rotational motion of the head, and the utricular and saccular maculae, which detect linear acceleration (Angelaki and Cullen, 2008). In the mature state, the average surface areas of human cristae, utricular maculae and saccular maculae are around 0.9, 3.6, and 2.2 mm2, respectively (Watanuki and Schuknecht, 1976).
Hair cells are vestibular receptors located on sensory epithelia and surrounded by supporting cells. According to the different afferent synaptic terminals, human vestibular HCs can be further classified into two types: Type I HCs innervated by flask-shaped calyces and Type II HCs innervated by boutons (Wersall, 1956). There are several other morphological and functional differences between the two types which have been widely discussed in rodents (Rüsch et al., 1998), and the characteristics of these two types of HCs are similar in the human vestibule (Oghalai et al., 1998; Lim et al., 2014).
The human vestibular sensory epithelia can be divided into central and peripheral regions according to different characteristics. In the cristae, the central regions account for 46% of the total surface area. Type I HCs account for 70% of the central region HCs, while type II HCs account for 50% of the peripheral region HCs in the human cristae (Rosenhall, 1972a). In the utricular maculae, the central striola region accounts for about 8.6% of the surface area. The proportion of type I HCs in the striola region is higher and type II HCs show a high density in the peripheral region. The HC distribution in saccule maculae is similar to that of the utricle maculae (Rosenhall, 1972b).
Interestingly, the polarity of the hair bundle, which is determined by the position of kinocilia of HCs, varies between the human utricular and saccular maculae. The orientation of the utricular kinocilia is directed from the periphery toward the striola, while the kinocilia orientation is opposite in the saccule. Moreover, the striolar region of the utricular maculae is crescent, while the saccular maculae are “S” shaped (Rosenhall, 1972b).
Both the vestibular hair cell distribution and cilia polarity of humans are similar to those of mice. However, the number and differentiation time of vestibular HCs are significantly different between the two species, as will be discussed below.
Development of Human Vestibular Epithelia
Morphological Development of Human Vestibular Organs
Anatomical studies have shown many details of human vestibular development (Figure 1). The formation of the otic placode is regarded as the first sign of inner ear development, which is the result of the ectoderm’s inner layer thickening at gestational week (GW) 3 (O’Rahilly, 1963). The otic placode then invaginates to form the otic cup that in turn pinches off the surrounding ectoderm and converts into the otic vesicle, composed of a dorsal (vestibular) and a ventral (cochlear) pouch, at the rhombomere 5 level by GW 4 (Streeter, 1906). From GW 4–5, the dorsal pouch expands into a triangular-shaped region forming the base of the three semicircular canals. The development of human anterior and posterior semicircular canals starts at embryonic days 41–43 with the depression of vestibular pouch wall, while the development of lateral semicircular canals begins a little later at embryonic days 44–46 (Yasuda et al., 2007). All semicircular canals are discernible at embryonic day (E) 47–E48 (Toyoda et al., 2015). Meanwhile, the atrium, which is the primordium of the utricle and saccule, can be observed in the ventral part of the vestibular pouch. Subsequently, a horizontal cleft that separates the atrium into an upper and lower compartment appears and the utricle and saccule are clearly detectable at E49–E51 (Streeter, 1906; Yasuda et al., 2007). By the end of the 5th month of the embryo, the bony labyrinth has been formed and the vestibular system is intact in morphology (Jeffery and Spoor, 2004), after which there is only a modest increase in the distance between the semicircular canals (Johnson Chacko et al., 2019).
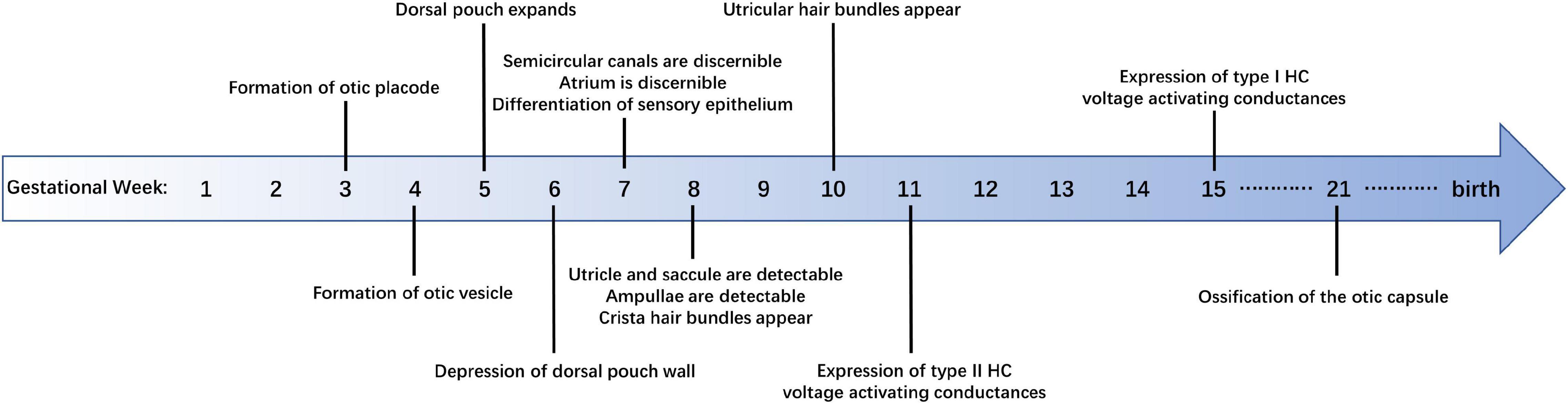
Figure 1. Summary of developmental milestones in the development of human vestibular sensory epithelia.
Maturation of the human vestibular sensory epithelia includes lengthening of the cristae and thinning of the maculae. The length of cristae increases rapidly from GW 8–9 but slows down and changes in shape during GW 9–12. The anterior crista undergoes a second rapid growth during GW 12–14, reaching approximately 55% of adult size (Dechesne and Sans, 1985). The reduction of utricular epithelial thickness can be divided into two stages: GW 7–8 and GW 11–13, during which the number of supporting cell layers decreases significantly, while the thickness remains unchanged during GW 8–12. Finally, the supporting cell nuclei tend to be arranged in a single layer and the hair cell nuclei migrate to the cell base (Dechesne and Sans, 1985).
The morphogenesis of vestibular organs in humans is similar to that in rodents, but the human vestibular organs have been distinct in the early embryonic stage (by the end of GW 7, Figure 1; Streeter, 1906), while the mouse counterparts do not attain its mature shape until the late embryonic stage. The vestibular organs of mice become distinguishable on E15 and the membranous labyrinth morphology approaches maturity as late as E17 (Morsli et al., 1998).
Differentiation of Vestibular Hair Cells
Cilia
Differentiation of human vestibular sensory epithelium does not occur until GW 7. The differentiation level of vestibular HCs is usually judged by their cilia, stereocilia and kinocilia.
Crista stereocilia are detectable at GW 8, during which time cristae are covered by short hair bundles with the putative kinocilia, the latter are longer than stereocilia in most cases and located at the edge of the bundle. Subsequently, there is a lengthening of cristae stereocilia, which leads to the longer and more mature hair bundles at GW10–11. From GW 12–14, the length of the hair bundles increases significantly, and the number of growing hair bundles decreased (Dechesne and Sans, 1985).
In the case of the utricle, the hair bundles observed at GW 10 are very short, and some kinocilia are shorter than the adjacent stereocilia. At GW 12, some hair bundles in the protruding utricle are close to maturity, while some are still growing. At GW 14, the hair bundles are morphologically mature and the number of newborn bundles decreases, while the microvilli are still abundant (Rosenhall and Engström, 1974; Dechesne and Sans, 1985), which indicates the HCs have not been fully developed so far.
From GW 14–18, hair bundles of HCs further mature in both human utricle and cristae. Stereocilia of the HCs in GW 18 fetus seem to be thicker than those in the GW 14 fetus. However, the number and diameter of stereocilia do not change significantly after GW 14, with about 80–100 stereocilia per HC (Rosenhall and Engström, 1974; Hoshino, 1982).
Molecular Marker
The differentiation of mammalian HCs is accompanied by the expression of cell-specific molecular markers, such as MyosinVIIa and Sox2. In the human vestibule, MyosinVIIa and Sox2 staining can be observed as early as GW 9. Moreover, the expression of MyosinVIIa is restricted to the vestibular HCs, while Sox2 is expressed in the supporting cells and a few of the HCs (Chacko et al., 2020).
In order to distinguish different cell types, recent work on mouse utricles has identified specific molecular markers for Type II HCs (Calretinin, Anxa4, and Mapt) and Type I HCs (Spp1 and Ocm) (McInturff et al., 2018). However, it remains to be investigated whether these vestibular hair cell markers are applicable to human specimens.
Electrophysiology
Evidences in electrophysiology demonstrate the functional similarities between human and rodent HCs. Whole-cell conductances of human vestibular HCs from GW 11–14 fetus are similar to those of mature type II HCs from rodents. The peak outward conductances obtained from human type II HCs increase from GW 15–18. Moreover, the rodent type I HC specific low-voltage activated K+ conductance, which is called GK, L, can also be detected in GW 15 human cristae, although relatively small (Lim et al., 2014). The similarities were further confirmed by the voltage-dependent currents that are expressed in vestibular HCs of both adult humans and rodents (Oghalai et al., 1998). However, so far there are few studies on the electrophysiological differences of vestibular HCs between humans and other mammals.
Hair Cell Number
There are no reports about the accurate time point at which the progenitors of human vestibular sensory epithelium begin to differentiate into hair cells since the spatio-temporal expression patterns of atonal homolog 1 (ATOH1), which is critical to HC formation, has not been investigated in the human vestibule so far. The distinct high expression of the HC marker, MyosinVIIa, is first observed in the crista as early as GW 9, indicating that some vestibular HCs have been formed at GW 9 (Johnson Chacko et al., 2020). But how these immature hair cells differentiate into type I and type II vestibular hair cells is not clear.
There is no significant difference in the number of cristae HCs between adults and the 4th–6th month fetuses: an average of 7,800 HCs per cristae at GW 16–23 and 7,500 HCs per cristae after birth (Rosenhall, 1972a). Another study observed an average of 8,005 HCs (type I 4,119 and type II 3,886) per lateral cristae of adults aged 26–67 years (Lopez et al., 2005).
It is reported that the number of HCs in the utricular and saccular maculae is about 2–4 times that in the cristae (Watanuki and Schuknecht, 1976). This study divided the specimens by age into the embryonic group (GW 14–23) and the postnatal group (<40 years old), and the average number of HCs was comparable between the two groups. As for the utricle, the average number is 33,100 (2,300 in the central area), with 32,900 HCs in the embryonic group and 33,200 in the postnatal group. The average number of saccular maculae HCs is 18,800 (1,600 in the central area), with 19,100 in the embryonic group and 18,400 in the postnatal group. Another study about utricles shows that the average number of utricular HCs at GW 16 is about 36,000, not significantly different from that at the age of 15, but significantly higher than the 13,000 at GW 10–12 (Severinsen et al., 2010).
Overall, the time point of HC differentiation is remarkably earlier in humans than that in rodents. As mentioned above, the number of human vestibular HCs reaches the adult level no later than the 5th month of gestation. In contrast, over half the mouse HC population is formed after birth (Burns et al., 2012).
Factors Related to the Development of Human Vestibular Sensory Epithelia
Several reviews have summarized the relevant regulatory factors in hair cell development in animal models. In general, mammalian hair cell development involves the emergence of Sox2-labeled pro-sensory areas, the expression of transcription factor Atoh1, the regulation of cell cycle by factors such as p27Kip1, and the manipulation by signaling pathways such as Notch, Fgf, Wnt, Shh, and Bmp (Wu and Kelley, 2012; Atkinson et al., 2015; Whitfield, 2015). However, there haven’t been many studies on the regulation of human hair cell development so far.
Proliferation and apoptosis are essential processes during human inner ear development. It is demonstrated by Ki-67 staining that the percentages of proliferating cells in the utricle and semicircular canal are 43 and 38%, respectively, at GW 6, but decrease to 24 and 30% at GW 9. However, the trend of Bcl-2 expression in the vestibular epithelium is opposite to that of Ki67 during GW 7–10. Moreover, cysteine aspartate-specific protease-3 (caspase-3) and insulin-like growth factor-1 (IGF-1) are also expressed during vestibular epithelial development (Tafra et al., 2014). These results suggest that factors related to proliferation and apoptosis may contribute to the morphogenesis and differentiation of vestibular sensory epithelia.
Brain-derived neurotrophic factor (BDNF) is a neurotrophic protein. Previous studies have shown that BDNF plays an important role in vestibular nerve development in animals (Fritzsch et al., 1997). In the human vestibule, BDNF is firstly expressed in the entire utricular sensory epithelium, but its expression decreases from GW 9–12 and is restricted in the extrastriola at GW 12. In adult human utricles, BDNF is only present in the apical part of HCs. The expression of p75NTR in vestibular organs and TrkB and C in nerve fibers increase with development, suggesting an essential role of neurotrophic receptors in the survival of vestibular neurons during early embryonic stages (Johnson Chacko et al., 2017).
Another study reported the expression of several key transcription factors during human inner ear development. For the vestibular sensory epithelia, LGR5 expression increased from GW 8–12 and was broad in the apical poles of the vestibular HCs. Another transcription factor, GATA3, was expressed in the striola of the utricular and saccular maculae at GW 11. Expression for SOX2 was primarily restricted to the utricular supporting cells at GW 9, suggesting its function in regulating the differentiation of supporting cells (Johnson Chacko et al., 2020). These results indicate that the active transcription factors during the development of the mammalian inner ear may also play a critical role in the development of human vestibular sensory epithelia. However, the spatio-temporal expression patterns of other genes essential for hair cell formation, such as Math1, Six1, Gfi1, and Pou4f3, are not explored in the human vestibule up to now.
In general, research on vestibules of human embryos is quite limited and the previous work mainly focused on the expression of specific molecules. Further experiments are required for demonstrating the similarities and differences of vestibular hair cell development between human and animal models, and the underlying mechanisms as well.
Regeneration of Human Vestibular Hair Cells
Discovery of Hair Cells Regeneration in the Mammalian Vestibular Epithelium
Considering that no artificial device can be used to replace vestibular function, recovery from vestibular dysfunction mainly depends on the compensation of central vestibular function, which can hardly lead to a full recovery. Promoting vestibular HC regeneration is an ideal way for vestibular function recovery.
Many studies have shown differences in the regeneration ability of vestibular sensory epithelium between different species. Non-mammalian vertebrates such as birds are able to produce HCs throughout their lives (Balak et al., 1990; Roberson et al., 1992; Weisleder and Rubel, 1992). In contrast, the restoration of vestibular HCs is relatively limited in mammals (Forge et al., 1993; Wang et al., 2015; Wu et al., 2016; You et al., 2018; Zhang et al., 2020), which can be realized through two processes, namely, mitosis or trans-differentiation (Rubel et al., 1995).
Loss of vestibular HCs, whether induced by aminoglycoside antibiotics (Kawamoto et al., 2009), IDPN (Zeng et al., 2020) or other injury methods (Golub et al., 2012; González-Garrido et al., 2021), significantly enhances spontaneous regenerative proliferation. However, compared to the complete recovery of vestibular function in most non-mammalian vertebrates (Jones and Nelson, 1992; Carey et al., 1996), both the number and the function of the newborn HCs are limited in the mammalian vestibular epithelium (Forge et al., 1993; Kawamoto et al., 2009; Golub et al., 2012; Zeng et al., 2020; González-Garrido et al., 2021). As a result, techniques to boost the regeneration of mammalian vestibular HCs are needed.
Manipulation of Vestibular Hair Cell Regeneration in Mammals
Considering the important role of growth factors in the development of the mammalian inner ear, many studies want to reveal whether they could also initiate the generation of vestibular HCs. Through in vitro culture, transforming growth factor alpha (TGF-α) is first found to be capable of restoring HCs in adult mouse vestibular organs after injury (Lambert, 1994). Subsequently, epidermal growth factor (EGF) (Yamashita and Oesterle, 1995), fibroblast growth factor (FGF) family members, IGF-1 and IGF-2 (Zheng et al., 1997) are demonstrated to trigger the proliferation of rat vestibular epithelial cells together with TGF-α. Recombinant human glial growth factor 2 (rhGGF2) and insulin are also capable of evoking great cell proliferation in the utricular epithelium of neonatal rats (Gu et al., 2007). Compared to TGF-α alone, simultaneous infusion of TGF-α and insulin into the rat inner ear shows combined effects in producing HCs (Kuntz and Oesterle, 1998a,b). Furthermore, combined utilization of TGF-α, IGF-1, and retinoic acid (RA) and BDNF performs well in restoring type I vestibular HCs in vivo and suggests application value (Kopke et al., 2001).
Regulation of intracellular signals is another method of promoting regeneration. Activation of phosphatidylinositol-3 kinase (PI-3K), mammalian target of rapamycin (mTOR), protein kinase C (PKC), mitogen-activated protein kinase (MAPK) and increased intracellular calcium enhance the proliferation of cells in murine vestibular epithelia. All of these signals are closely associated with the S-phase entry (Montcouquiol and Corwin, 2001).
The basic helix-loop-helix (bHLH) transcription factor, atonal homolog 1 or Atoh1, is critical for the differentiation of HCs (Bermingham et al., 1999). Atoh1 overexpression activates the HC differentiation in murine vestibular epithelia both in vitro (Zheng and Gao, 2000; Huang et al., 2009; Qian et al., 2021) and in vivo (Staecker et al., 2007; Schlecker et al., 2011; Gao et al., 2016; Sayyid et al., 2019), which can be enhanced by injury (Staecker et al., 2007; Schlecker et al., 2011; Sayyid et al., 2019; Hicks et al., 2020; Qian et al., 2021) and depressed by aging (Gao et al., 2016). On the other hand, Atoh1 deletion inhibits the spontaneous returning of HCs significantly (Hicks et al., 2020).
Disrupting the lateral inhibition established by Notch signaling is another classic strategy for producing new HCs. After adding DAPT and TAPI-1, two inhibitors of the Notch signaling pathway, to the explanted utricles of adult mice, enhanced hair cell regeneration was observed, especially in the striolar/juxtastriolar region (Lin et al., 2011). DAPT treatment also leads to extensive HC generation in cristae explants of adult mice (Slowik and Bermingham-McDonogh, 2013). Moreover, downregulation of the Notch target gene Hes5 through siRNA can also induce the trans-differentiation of supporting cells and boost hair cell regeneration in the damaged mouse utricles (Jung et al., 2013).
Apart from the traditional means mentioned above, recent studies have revealed some new targets for regulating HC regeneration. Several studies connected the ability of supporting cells to reenter the cell cycle during murine utricular development or after injury to nuclear Yap signaling (Gnedeva et al., 2017, 2020; Kastan et al., 2021). Collado et al. (2011) found the accumulation of E-Cadherin as an inhibitor for trans-differentiation of supporting cells. Knockdown of Foxg1 in supporting cells was revealed to be another viable method of enhancing HC regeneration in the neonatal mouse utricle (Zhang et al., 2020). In order to achieve better regenerative effects, attempts have been made to simultaneously promote supporting cells proliferation and hair cell differentiation through a combined regulation of multiple signaling pathways, such as Wnt and Notch (Wu et al., 2016).
Although vestibular hair cell regeneration in mammals still faces many hurdles, the good news is that murine vestibular function, under certain modulations, has been partially restored after injury (Kopke et al., 2001; Staecker et al., 2007; Schlecker et al., 2011). However, whether the regeneration phenomenon can also be discovered in humans and whether it can be regulated in the same manner as in rodents are questions that must be addressed for the clinical application of regenerative techniques.
Regenerative Potential of Human Vestibular Hair Cells
Studies on the regenerative potential of human vestibular HCs started almost at the same time as that of other mammals. Through in vitro experiments, Warchol et al. (1993) found proliferating supporting cells after neomycin injury in the human utricle. After 25 days of culture, some labeled nuclei in the lumenal stratum that was normally occupied by the nuclei of HCs appeared, which suggested the restoration of HCs (Warchol et al., 1993). In another study, 22 patients with Meniere’s disease were tested on their vestibular function 1–2 years after gentamicin treatment. The horizontal semicircular canal afferent nerve restored its excitability to warm and cold water in 38% of them, which was regarded as functional evidence for HC regeneration (De Waele et al., 2002). However, the evidence here is insufficient, in which the study could not confirm the direct cause of the functional recovery, since vestibular inhibition and central compensation could also lead to functional restoration. The morphological evidence came in 2015. Immature hair bundles were observed in human vestibular specimens harvested from elderly patients (Taylor et al., 2015). Given that no evidence of the hair bundle restoration has been detected on surviving “bald” HCs, there is likely to be spontaneous HC regeneration occurring in human utricles.
Another approach to investigate the stemness of vestibular sensory epithelial cells is to extract progenitors or stem cells from the human inner ear. It was first realized by Chen et al. (2009) who extracted stem cells capable of differentiating into HCs from the fetal cochlea in 2009. Since then, attempts have been made in vestibular organs. Hu et al. (2012) isolated sensory epithelial cells from postoperative human utricular specimens and cultured them in vitro. The proliferated cells expressed genes in pre-sensory cells or stem cells such as SOX2 and P27KIP1 and showed characteristics of mesenchymal cells when cultured on 2D substrates (Hu et al., 2012). A recent study demonstrated that the human vestibular epithelial cells have a strong sphere-forming ability through in vitro culture. Furthermore, the clonal spheres were able to produce cells expressing markers of HCs and displayed differentiation ability (Senn et al., 2020). The existence of multipotent progenitor cells in the adult human vestibule indicates the capability of sensory epithelial cells to re-enter the cell cycle and provides a promising source for neonatal HCs.
In conclusion, the regenerative potential of human vestibular HCs has been revealed from different aspects, reflecting the similarities between human and rodent vestibules. Therefore, the theoretical basis for the regulation of human hair cell regeneration following the example of other mammals has been established.
Application of Gene Therapy in Human Vestibular Sensory Epithelia
As is mentioned above, overexpression of Atoh1 serves as a classic approach to triggering regeneration of vestibular HCs in rodents. In 2003, Shou et al. (2003) upregulated HATH1 (a human homolog of Atoh1) in cultured adult rat utricular maculae through local adenoviral treatment and robust production of new HCs was observed in normal and gentamicin-injured utricles as a result of supporting cell conversion, implying the conserved function of the atonal homologs during the revolution. Moreover, considering the similarity between human and murine homologs of atonal in giving rise to HCs, overexpressing HATH1 may be a promising target for gene therapy in human balance organs.
Choosing a suitable transduction vector is essential for inner ear gene therapy. Adenovirus has been demonstrated to efficiently transfect both HCs and supporting cells, thus becoming a competitive candidate. In 2007, Kesser et al. (2007) developed a multi-gene deletion and replication-free adenovirus vector (AD2) to test its transfection efficacy in human tissues, which drove the expression of the green fluorescent protein GFP gene (AD2-GFP) by cytomegalovirus (CMV) promoter. Results indicated that both supporting cells and HCs were transfected and the transfection rate was higher in supporting cells and varied with viral titer and transfection time. Furthermore, the adenovirus vector also performed well when GFP and wild-type potassium channel gene KCNQ4 were transfected simultaneously into the human inner ear, with 17.3% hair cell transfection rate for GFP and 10% for KCNQ4 (Kesser et al., 2007). Adeno-associated virus (AAV) is another promising vector that has enabled efficient gene transfer to several organs. Recently, an AAV variant (AAV-ie) has been designed for inner ear gene delivery. It was shown that AAV-ie infected about 93% of SCs and 76% of HCs in human utricle. In addition, the saccular macula and cristae could be transduced as well (Tan et al., 2019).
Based on the maturity of the transduction vector, Taylor et al. (2018) made the first attempt to generate HCs in human utricle. Taylor et al. (2018) collected utricles from patients undergoing excision of vestibular schwannoma and HCs were ablated through gentamicin. Ad2-GFP-Atoh1 was used here to transfect utricular maculae and supporting cells were efficiently transduced. Compared with the control group, transfection successfully increased the HC number in the maculae. Moreover, Notch signaling pathway inhibitor TAPI-1 also induced regeneration in human utricles, while the newborn HCs were fewer than those in the Atoh1 transfection group. Finally, no synergistic effect of the two treatments was observed, implying Atoh1 overexpression as a more effective solution. This study creates a precedent for gene therapy targeting the human inner ear.
Future Perspectives
Although gene therapy targeting human vestibular epithelium has triggered HC regeneration successfully, many problems remain to be addressed before more mature and functional HCs can be generated. Considering the similarities between vestibular and auditory sensory epithelium, recent studies on the cochlea may offer some inspiration.
Atoh1-induced HC regeneration still has some limitations in both mouse and human vestibules. First, the number of hair cells could not be fully restored after injury. Second, nearly all the new hair cells converted from supporting cells were Sox2 + type II hair cells, the lack of new type I hair cells is not conducive to vestibular rehabilitation. Moreover, the regenerated hair cells were immature, as demonstrated by immature cilia (Schlecker et al., 2011; Taylor et al., 2018; Sayyid et al., 2019; Qian et al., 2021). Recent studies report some progresses in cochlear hair cell regeneration by manipulating multiple signaling pathways or transcriptional factors (Walters et al., 2017; Lee et al., 2020; Menendez et al., 2020; Chen et al., 2021; Sun et al., 2021). In order to improve both the quality and quantity of new vestibular hair cells, regulating multiple factors which can promote the maturation and subtype differentiation of vestibular hair cells may be an important strategy. In addition, it might be beneficial to combine epigenetic regulatory factors with Atoh1 overexpression to promote hair cell regeneration in the human vestibule.
Generating organoids provides ideal models for screening drug candidates for the treatment of inner ear diseases. Recent studies have succeeded in producing otic organoids from human pluripotent stem cells. Some of them shared many characteristics with human vestibular epithelia (Koehler et al., 2017; Jeong et al., 2018; van der Valk et al., 2021). In the future, exploring the regulatory mechanisms underlying hair cell formation using organoids and the latest techniques, such as single-cell and single-nuclear sequencing, may help to identify regulatory pathways and key factors which play important roles in the maturation and subtype differentiation of vestibular hair cells. Progresses in this area will contribute to the study of hair cell regeneration in human vestibule and balance function reconstruction.
Chen et al. (2012) transplanted otic neuroprogenitors derived from human embryonic stem cells (hESCs) into ouabain-treated gerbils (an auditory neuropathy model) through the round window, and restoration of auditory evoked response was observed. The protocol of transplanting progenitor cells discussed here offers another promising strategy for generating functional HCs in the human vestibule.
Trans-differentiation of Lgr5+ or Plp1+ supporting cells is traditionally regarded as the main source of postnatal hair cell generation in utricles (Wang et al., 2015, 2019). However, the latest studies suggested transitional epithelial cells (TECs) located at the border between sensory and non-sensory regions to be another reliable source of HCs (Huang et al., 2009; Burns et al., 2015; Gao et al., 2016; Jan et al., 2021; Qian et al., 2021). How to achieve effective trans-differentiation from TECs into supporting cells or HCs could be another future direction.
Author Contributions
YH, YC, and HM wrote and revised the manuscript. All authors contributed to the article and approved the submitted version.
Funding
This work was supported by the National Natural Science Foundation of China (No. 82071049).
Conflict of Interest
The authors declare that the research was conducted in the absence of any commercial or financial relationships that could be construed as a potential conflict of interest.
Publisher’s Note
All claims expressed in this article are solely those of the authors and do not necessarily represent those of their affiliated organizations, or those of the publisher, the editors and the reviewers. Any product that may be evaluated in this article, or claim that may be made by its manufacturer, is not guaranteed or endorsed by the publisher.
Abbreviations
AAV, adeno-associated virus; AD, adenovirus; Anxa4, annexin A4; Atoh1, atonal homolog 1; BDNF, brain-derived neurotrophic factor; bHLH, basic helix-loop-helix; caspase-3, cysteine aspartate-specific protease-3; CMV, cytomegalovirus; E, embryonic day; EGF, epidermal growth factor; FGF, fibroblast growth factor; GATA3: GATA binding protein 3; GW, gestational week; HATH1, human homolog of Atoh1; HC, hair cell; hESCs, human embryonic stem cells; IDPN: 3,3′-iminodiproprionitrile; IGF-1, insulin-like growth factor-1; IGF-2, insulin-like growth factor-1; LGR5: leucine-rich repeat-containing G protein-coupled receptor 5; MAPK, mitogen-activated protein kinase; Mapt, microtubule associated protein; mTOR, mammalian target of rapamycin; Ocm, oncomodulin; PI-3K, activation of phosphatidylinositol-3 kinase; PKC, protein kinase C; RA, retinoic acid; rhGGF2, recombinant human glial growth factor 2; SC, supporting cell; SOX2: SRY (sex-determining region Y)-box 2; Spp1, secreted phosphoprotein 1; TGF-α, transforming growth factor alpha.
References
Angelaki, D. E., and Cullen, K. E. (2008). Vestibular system: the many facets of a multimodal sense. Annu. Rev. Neurosci. 31, 125–150. doi: 10.1146/annurev.neuro.31.060407.125555
Atkinson, P. J., Huarcaya Najarro, E., Sayyid, Z. N., and Cheng, A. G. (2015). Sensory hair cell development and regeneration: similarities and differences. Development 142, 1561–1571. doi: 10.1242/dev.114926
Balak, K. J., Corwin, J. T., and Jones, J. E. (1990). Regenerated hair cells can originate from supporting cell progeny: evidence from phototoxicity and laser ablation experiments in the lateral line system. J. Neurosci. 10, 2502–2512. doi: 10.1523/jneurosci.10-08-02502.1990
Bermingham, N. A., Hassan, B. A., Price, S. D., Vollrath, M. A., Ben-Arie, N., Eatock, R. A., et al. (1999). Math1: an essential gene for the generation of inner ear hair cells. Science 284, 1837–1841. doi: 10.1126/science.284.5421.1837
Burns, J. C., Kelly, M. C., Hoa, M., Morell, R. J., and Kelley, M. W. (2015). Single-cell RNA-Seq resolves cellular complexity in sensory organs from the neonatal inner ear. Nat. Commun. 6:8557. doi: 10.1038/ncomms9557
Burns, J. C., On, D., Baker, W., Collado, M. S., and Corwin, J. T. (2012). Over half the hair cells in the mouse utricle first appear after birth, with significant numbers originating from early postnatal mitotic production in peripheral and striolar growth zones. J. Assoc. Res. Otolaryngol. 13, 609–627. doi: 10.1007/s10162-012-0337-0
Carey, J. P., Fuchs, A. F., and Rubel, E. W. (1996). Hair cell regeneration and recovery of the vestibuloocular reflex in the avian vestibular system. J. Neurophysiol. 76, 3301–3312. doi: 10.1152/jn.1996.76.5.3301
Chacko, L. J., Sergi, C., Eberharter, T., Dudas, J., Rask-Andersen, H., Hoermann, R., et al. (2020). Early appearance of key transcription factors influence the spatiotemporal development of the human inner ear. Cell Tissue Res. 379, 459–471.
Chen, W., Johnson, S. L., Marcotti, W., Andrews, P. W., Moore, H. D., and Rivolta, M. N. (2009). Human fetal auditory stem cells can be expanded in vitro and differentiate into functional auditory neurons and hair cell-like cells. Stem Cells 27, 1196–1204. doi: 10.1002/stem.62
Chen, W., Jongkamonwiwat, N., Abbas, L., Eshtan, S. J., Johnson, S. L., Kuhn, S., et al. (2012). Restoration of auditory evoked responses by human ES-cell-derived otic progenitors. Nature 490, 278–282. doi: 10.1038/nature11415
Chen, Y., Gu, Y., Li, Y., Li, G. L., Chai, R., Li, W., et al. (2021). Generation of mature and functional hair cells by co-expression of Gfi1, Pou4f3, and Atoh1 in the postnatal mouse cochlea. Cell Rep. 35:109016. doi: 10.1016/j.celrep.2021.109016
Cheng, C., Hou, Y., Zhang, Z., Wang, Y., Lu, L., Zhang, L., et al. (2021). Disruption of the autism-related gene Pak1 causes stereocilia disorganization, hair cell loss, and deafness in mice. J. Genet. Genomics 48, 324–332. doi: 10.1016/j.jgg.2021.03.010
Cheng, C., Wang, Y., Guo, L., Lu, X., Zhu, W., Muhammad, W., et al. (2019). Age-related transcriptome changes in Sox2+ supporting cells in the mouse cochlea. Stem Cell Res. Ther. 10:365. doi: 10.1186/s13287-019-1437-0
Collado, M. S., Thiede, B. R., Baker, W., Askew, C., Igbani, L. M., and Corwin, J. T. (2011). The postnatal accumulation of junctional E-cadherin is inversely correlated with the capacity for supporting cells to convert directly into sensory hair cells in mammalian balance organs. J. Neurosci. 31, 11855–11866. doi: 10.1523/jneurosci.2525-11.2011
Corrales, C. E., and Bhattacharyya, N. (2016). Dizziness and death: an imbalance in mortality. Laryngoscope 126, 2134–2136. doi: 10.1002/lary.25902
Cullen, K. E. (2012). The vestibular system: multimodal integration and encoding of self-motion for motor control. Trends Neurosci. 35, 185–196. doi: 10.1016/j.tins.2011.12.001
De Waele, C., Meguenni, R., Freyss, G., Zamith, F., Bellalimat, N., Vidal, P. P., et al. (2002). Intratympanic gentamicin injections for Meniere disease: vestibular hair cell impairment and regeneration. Neurology 59, 1442–1444. doi: 10.1212/wnl.59.9.1442
Dechesne, C. J., and Sans, A. (1985). Development of vestibular receptor surfaces in human fetuses. Am. J. Otolaryngol. 6, 378–387. doi: 10.1016/s0196-0709(85)80016-8
Forge, A., Li, L., Corwin, J. T., and Nevill, G. (1993). Ultrastructural evidence for hair cell regeneration in the mammalian inner ear. Science 259, 1616–1619. doi: 10.1126/science.8456284
Fritzsch, B., Silos-Santiago, I., Bianchi, L. M., and Fariñas, I. (1997). The role of neurotrophic factors in regulating the development of inner ear innervation. Trends Neurosci. 20, 159–164. doi: 10.1016/s0166-2236(96)01007-7
Fu, X., Wan, P., Li, P., Wang, J., Guo, S., Zhang, Y., et al. (2021b). Mechanism and Prevention of Ototoxicity Induced by Aminoglycosides. Front. Cell. Neurosci. 15:692762. doi: 10.3389/fncel.2021.692762
Fu, X., An, Y., Wang, H., Li, P., Lin, J., Yuan, J., et al. (2021a). Deficiency of Klc2 Induces Low-Frequency Sensorineural Hearing Loss in C57BL/6 J Mice and Human. Mol. Neurobiol. 58, 4376–4391. doi: 10.1007/s12035-021-02422-w
Gao, S., Cheng, C., Wang, M., Jiang, P., Zhang, L., Wang, Y., et al. (2019). Blebbistatin Inhibits Neomycin-Induced Apoptosis in Hair Cell-Like HEI-OC-1 Cells and in Cochlear Hair Cells. Front. Cell. Neurosci. 13:590. doi: 10.3389/fncel.2019.00590
Gao, Z., Kelly, M. C., Yu, D., Wu, H., Lin, X., Chi, F. L., et al. (2016). Spatial and Age-Dependent Hair Cell Generation in the Postnatal Mammalian Utricle. Mol. Neurobiol. 53, 1601–1612. doi: 10.1007/s12035-015-9119-0
Gnedeva, K., Jacobo, A., Salvi, J. D., Petelski, A. A., and Hudspeth, A. J. (2017). Elastic force restricts growth of the murine utricle. Elife 6:e25681. doi: 10.7554/eLife.25681
Gnedeva, K., Wang, X., McGovern, M. M., Barton, M., Tao, L., Trecek, T., et al. (2020). Organ of Corti size is governed by Yap/Tead-mediated progenitor self-renewal. Proc. Natl. Acad. Sci. U. S. A. 117, 13552–13561. doi: 10.1073/pnas.2000175117
Golub, J. S., Tong, L., Ngyuen, T. B., Hume, C. R., Palmiter, R. D., Rubel, E. W., et al. (2012). Hair cell replacement in adult mouse utricles after targeted ablation of hair cells with diphtheria toxin. J. Neurosci. 32, 15093–15105. doi: 10.1523/jneurosci.1709-12.2012
González-Garrido, A., Pujol, R., López-Ramírez, O., Finkbeiner, C., Eatock, R. A., and Stone, J. S. (2021). The Differentiation Status of Hair Cells That Regenerate Naturally in the Vestibular Inner Ear of the Adult Mouse. J. Neurosci. 41, 7779–7796. doi: 10.1523/jneurosci.3127-20.2021
Gu, R., Montcouquiol, M., Marchionni, M., and Corwin, J. T. (2007). Proliferative responses to growth factors decline rapidly during postnatal maturation of mammalian hair cell epithelia. Eur. J. Neurosci. 25, 1363–1372. doi: 10.1111/j.1460-9568.2007.05414.x
Guo, R., Li, J., Chen, C., Xiao, M., Liao, M., Hu, Y., et al. (2021b). Biomimetic 3D bacterial cellulose-graphene foam hybrid scaffold regulates neural stem cell proliferation and differentiation. Colloids Surf. B Biointerfaces 200:111590. doi: 10.1016/j.colsurfb.2021.111590
Guo, R., Liao, M., Ma, X., Hu, Y., Qian, X., Xiao, M., et al. (2021c). Cochlear implant-based electric-acoustic stimulation modulates neural stem cell-derived neural regeneration. J. Mater. Chem. B 9, 7793–7804. doi: 10.1039/d1tb01029h
Guo, L., Cao, W., Niu, Y., He, S., Chai, R., and Yang, J. (2021a). Autophagy Regulates the Survival of Hair Cells and Spiral Ganglion Neurons in Cases of Noise, Ototoxic Drug, and Age-Induced Sensorineural Hearing Loss. Front. Cell. Neurosci. 15:760422. doi: 10.3389/fncel.2021.760422
Guo, R., Ma, X., Liao, M., Liu, Y., Hu, Y., Qian, X., et al. (2019). Development and Application of Cochlear Implant-Based Electric-Acoustic Stimulation of Spiral Ganglion Neurons. ACS Biomater. Sci. Eng. 5, 6735–6741. doi: 10.1021/acsbiomaterials.9b01265
Guo, R., Xiao, M., Zhao, W., Zhou, S., Hu, Y., Liao, M., et al. (2020). 2D Ti(3)C(2)T(x)MXene couples electrical stimulation to promote proliferation and neural differentiation of neural stem cells. Acta Biomater. 139, 105–117. doi: 10.1016/j.actbio.2020.12.035
Han, S., Xu, Y., Sun, J., Liu, Y., Zhao, Y., Tao, W., et al. (2020). Isolation and analysis of extracellular vesicles in a Morpho butterfly wing-integrated microvortex biochip. Biosens. Bioelectron. 154:112073. doi: 10.1016/j.bios.2020.112073
He, Z. H., Li, M., Fang, Q. J., Liao, F. L., Zou, S. Y., Wu, X., et al. (2021). FOXG1 promotes aging inner ear hair cell survival through activation of the autophagy pathway. Autophagy 17, 4341–4362. doi: 10.1080/15548627.2021.1916194
He, Z. H., Zou, S. Y., Li, M., Liao, F. L., Wu, X., Sun, H. Y., et al. (2020). The nuclear transcription factor FoxG1 affects the sensitivity of mimetic aging hair cells to inflammation by regulating autophagy pathways. Redox Biol. 28:101364. doi: 10.1016/j.redox.2019.101364
Hicks, K. L., Wisner, S. R., Cox, B. C., and Stone, J. S. (2020). Atoh1 is required in supporting cells for regeneration of vestibular hair cells in adult mice. Hear. Res. 385:107838. doi: 10.1016/j.heares.2019.107838
Hoshino, T. (1982). Scanning electron microscopic observation of the foetal labyrinthine vestibule. Acta Otolaryngol. 93, 349–354. doi: 10.3109/00016488209130892
Hu, Y., Li, D., Wei, H., Zhou, S., Chen, W., Yan, X., et al. (2021). Neurite Extension and Orientation of Spiral Ganglion Neurons Can Be Directed by Superparamagnetic Iron Oxide Nanoparticles in a Magnetic Field. Int. J. Nanomedicine 16, 4515–4526. doi: 10.2147/ijn.S313673
Hu, Z., Luo, X., Zhang, L., Lu, F., Dong, F., Monsell, E., et al. (2012). Generation of human inner ear prosensory-like cells via epithelial-to-mesenchymal transition. Regen. Med. 7, 663–673. doi: 10.2217/rme.12.53
Huang, Y., Chi, F., Han, Z., Yang, J., Gao, W., and Li, Y. (2009). New ectopic vestibular hair cell-like cells induced by Math1 gene transfer in postnatal rats. Brain Res. 1276, 31–38. doi: 10.1016/j.brainres.2009.04.036
Ishiyama, G., Lopez, I. A., Sepahdari, A. R., and Ishiyama, A. (2015). Meniere’s disease: histopathology, cytochemistry, and imaging. Ann. N. Y. Acad. Sci. 1343, 49–57. doi: 10.1111/nyas.12699
Jan, T. A., Eltawil, Y., Ling, A. H., Chen, L., Ellwanger, D. C., Heller, S., et al. (2021). Spatiotemporal dynamics of inner ear sensory and non-sensory cells revealed by single-cell transcriptomics. Cell Rep. 36:109358. doi: 10.1016/j.celrep.2021.109358
Jeffery, N., and Spoor, F. (2004). Prenatal growth and development of the modern human labyrinth. J. Anat. 204, 71–92. doi: 10.1111/j.1469-7580.2004.00250.x
Jeong, M., O’Reilly, M., Kirkwood, N. K., Al-Aama, J., Lako, M., Kros, C. J., et al. (2018). Generating inner ear organoids containing putative cochlear hair cells from human pluripotent stem cells. Cell Death Dis. 9:922. doi: 10.1038/s41419-018-0967-1
Johnson Chacko, L., Blumer, M. J. F., Pechriggl, E., Rask-Andersen, H., Dietl, W., Haim, A., et al. (2017). Role of BDNF and neurotrophic receptors in human inner ear development. Cell Tissue Res. 370, 347–363. doi: 10.1007/s00441-017-2686-9
Johnson Chacko, L., Sergi, C., Eberharter, T., Dudas, J., Rask-Andersen, H., Hoermann, R., et al. (2020). Early appearance of key transcription factors influence the spatiotemporal development of the human inner ear. Cell Tissue Res. 379, 459–471. doi: 10.1007/s00441-019-03115-6
Johnson Chacko, L., Wertjanz, D., Sergi, C., Dudas, J., Fischer, N., Eberharter, T., et al. (2019). Growth and cellular patterning during fetal human inner ear development studied by a correlative imaging approach. BMC Dev. Biol. 19:11. doi: 10.1186/s12861-019-0191-y
Jones, T. A., and Nelson, R. C. (1992). Recovery of vestibular function following hair cell destruction by streptomycin. Hear. Res. 62, 181–186. doi: 10.1016/0378-5955(92)90184-o
Jung, J. Y., Avenarius, M. R., Adamsky, S., Alpert, E., Feinstein, E., and Raphael, Y. (2013). siRNA targeting Hes5 augments hair cell regeneration in aminoglycoside-damaged mouse utricle. Mol. Ther. 21, 834–841. doi: 10.1038/mt.2013.18
Kastan, N., Gnedeva, K., Alisch, T., Petelski, A. A., Huggins, D. J., Chiaravalli, J., et al. (2021). Small-molecule inhibition of Lats kinases may promote Yap-dependent proliferation in postmitotic mammalian tissues. Nat. Commun. 12:3100. doi: 10.1038/s41467-021-23395-3
Kawamoto, K., Izumikawa, M., Beyer, L. A., Atkin, G. M., and Raphael, Y. (2009). Spontaneous hair cell regeneration in the mouse utricle following gentamicin ototoxicity. Hear. Res. 247, 17–26. doi: 10.1016/j.heares.2008.08.010
Kesser, B. W., Hashisaki, G. T., Fletcher, K., Eppard, H., and Holt, J. R. (2007). An in vitro model system to study gene therapy in the human inner ear. Gene Ther. 14, 1121–1131. doi: 10.1038/sj.gt.3302980
Koehler, K. R., Nie, J., Longworth-Mills, E., Liu, X. P., Lee, J., Holt, J. R., et al. (2017). Generation of inner ear organoids containing functional hair cells from human pluripotent stem cells. Nat. Biotechnol. 35, 583–589. doi: 10.1038/nbt.3840
Kopke, R. D., Jackson, R. L., Li, G., Rasmussen, M. D., Hoffer, M. E., Frenz, D. A., et al. (2001). Growth factor treatment enhances vestibular hair cell renewal and results in improved vestibular function. Proc. Natl. Acad. Sci. U. S. A. 98, 5886–5891. doi: 10.1073/pnas.101120898
Kuntz, A. L., and Oesterle, E. C. (1998a). Transforming growth factor-alpha with insulin induces proliferation in rat utricular extrasensory epithelia. Otolaryngol. Head Neck Surg. 118, 816–824. doi: 10.1016/s0194-5998(98)70275-x
Kuntz, A. L., and Oesterle, E. C. (1998b). Transforming growth factor alpha with insulin stimulates cell proliferation in vivo in adult rat vestibular sensory epithelium. J. Comp. Neurol. 399, 413–423. doi: 10.1002/(sici)1096-9861(19980928)399:3<413::aid-cne9>3.0.co;2-3
Lambert, P. R. (1994). Inner ear hair cell regeneration in a mammal: identification of a triggering factor. Laryngoscope 104, 701–718. doi: 10.1288/00005537-199406000-00010
Lee, S., Song, J. J., Beyer, L. A., Swiderski, D. L., Prieskorn, D. M., Acar, M., et al. (2020). Combinatorial Atoh1 and Gfi1 induction enhances hair cell regeneration in the adult cochlea. Sci. Rep. 10:21397. doi: 10.1038/s41598-020-78167-8
Li, A., You, D., Li, W., Cui, Y., He, Y., Li, W., et al. (2018). Novel compounds protect auditory hair cells against gentamycin-induced apoptosis by maintaining the expression level of H3K4me2. Drug Deliv. 25, 1033–1043. doi: 10.1080/10717544.2018.1461277
Lim, R., Drury, H. R., Camp, A. J., Tadros, M. A., Callister, R. J., and Brichta, A. M. (2014). Preliminary characterization of voltage-activated whole-cell currents in developing human vestibular hair cells and calyx afferent terminals. J. Assoc. Res. Otolaryngol. 15, 755–766. doi: 10.1007/s10162-014-0471-y
Lin, V., Golub, J. S., Nguyen, T. B., Hume, C. R., Oesterle, E. C., and Stone, J. S. (2011). Inhibition of Notch activity promotes nonmitotic regeneration of hair cells in the adult mouse utricles. J. Neurosci. 31, 15329–15339. doi: 10.1523/jneurosci.2057-11.2011
Liu, Y., Qi, J., Chen, X., Tang, M., Chu, C., Zhu, W., et al. (2019). Critical role of spectrin in hearing development and deafness. Sci. Adv. 5:eaav7803. doi: 10.1126/sciadv.aav7803
Lopez, I., Ishiyama, G., Tang, Y., Tokita, J., Baloh, R. W., and Ishiyama, A. (2005). Regional estimates of hair cells and supporting cells in the human crista ampullaris. J. Neurosci. Res. 82, 421–431. doi: 10.1002/jnr.20652
Lv, J., Fu, X., Li, Y., Hong, G., Li, P., Lin, J., et al. (2021). Deletion of Kcnj16 in Mice Does Not Alter Auditory Function. Front. Cell. Dev. Biol. 9:630361. doi: 10.3389/fcell.2021.630361
McInturff, S., Burns, J. C., and Kelley, M. W. (2018). Characterization of spatial and temporal development of Type I and Type II hair cells in the mouse utricle using new cell-type-specific markers. Biol. Open 7:bio038083. doi: 10.1242/bio.038083
Menendez, L., Trecek, T., Gopalakrishnan, S., Tao, L., Markowitz, A. L., Yu, H. V., et al. (2020). Generation of inner ear hair cells by direct lineage conversion of primary somatic cells. Elife 9:e55249. doi: 10.7554/eLife.55249
Montcouquiol, M., and Corwin, J. T. (2001). Intracellular signals that control cell proliferation in mammalian balance epithelia: key roles for phosphatidylinositol-3 kinase, mammalian target of rapamycin, and S6 kinases in preference to calcium, protein kinase C, and mitogen-activated protein kinase. J. Neurosci. 21, 570–580. doi: 10.1523/jneurosci.21-02-00570.2001
Morsli, H., Choo, D., Ryan, A., Johnson, R., and Wu, D. K. (1998). Development of the mouse inner ear and origin of its sensory organs. J. Neurosci. 18, 3327–3335. doi: 10.1523/jneurosci.18-09-03327.1998
Oghalai, J. S., Holt, J. R., Nakagawa, T., Jung, T. M., Coker, N. J., Jenkins, H. A., et al. (1998). Ionic currents and electromotility in inner ear hair cells from humans. J. Neurophysiol. 79, 2235–2239. doi: 10.1152/jn.1998.79.4.2235
O’Rahilly, R. (1963). The early development of the otic vesicle in staged human embryos. J. Embryol. Exp. Morphol. 11, 741–755. doi: 10.1242/dev.11.4.741
Qi, J., Liu, Y., Chu, C., Chen, X., Zhu, W., Shu, Y., et al. (2019). A cytoskeleton structure revealed by super-resolution fluorescence imaging in inner ear hair cells. Cell Discov. 5:12. doi: 10.1038/s41421-018-0076-4
Qi, J., Zhang, L., Tan, F., Liu, Y., Chu, C., Zhu, W., et al. (2020). Espin distribution as revealed by super-resolution microscopy of stereocilia. Am. J. Transl. Res. 12, 130–141.
Qian, F., Wang, X., Yin, Z., Xie, G., Yuan, H., Liu, D., et al. (2020). The slc4a2b gene is required for hair cell development in zebrafish. Aging 12, 18804–18821. doi: 10.18632/aging.103840
Qian, X., Ma, R., Wang, X., Xu, X., Yang, J., Chi, F., et al. (2021). Simultaneous gentamicin-mediated damage and Atoh1 overexpression promotes hair cell regeneration in the neonatal mouse utricle. Exp. Cell Res. 398:112395. doi: 10.1016/j.yexcr.2020.112395
Roberson, D. F., Weisleder, P., Bohrer, P. S., and Rubel, E. W. (1992). Ongoing production of sensory cells in the vestibular epithelium of the chick. Hear. Res. 57, 166–174. doi: 10.1016/0378-5955(92)90149-h
Rosenhall, U. (1972a). Mapping of the cristae ampullares in man. Ann. Otol. Rhinol. Laryngol. 81, 882–889. doi: 10.1177/000348947208100622
Rosenhall, U. (1972b). Vestibular macular mapping in man. Ann. Otol. Rhinol. Laryngol. 81, 339–351. doi: 10.1177/000348947208100305
Rosenhall, U., and Engström, B. (1974). Surface Structures of the Human Vestibular Sensory Regions. Acta Otolaryngol. 77, 3–18. doi: 10.1080/16512251.1974.11675749
Rubel, E. W., Dew, L. A., and Roberson, D. W. (1995). Mammalian vestibular hair cell regeneration. Science 267, 701–707. doi: 10.1126/science.7839150
Rüsch, A., Lysakowski, A., and Eatock, R. A. (1998). Postnatal development of type I and type II hair cells in the mouse utricle: acquisition of voltage-gated conductances and differentiated morphology. J. Neurosci. 18, 7487–7501. doi: 10.1523/jneurosci.18-18-07487.1998
Sayyid, Z. N., Wang, T., Chen, L., Jones, S. M., and Cheng, A. G. (2019). Atoh1 Directs Regeneration and Functional Recovery of the Mature Mouse Vestibular System. Cell Rep. 28, 312–324.e4. doi: 10.1016/j.celrep.2019.06.028
Schlecker, C., Praetorius, M., Brough, D. E., Presler, R. G. Jr., Hsu, C., Plinkert, P. K., et al. (2011). Selective atonal gene delivery improves balance function in a mouse model of vestibular disease. Gene Ther. 18, 884–890. doi: 10.1038/gt.2011.33
Senn, P., Mina, A., Volkenstein, S., Kranebitter, V., Oshima, K., and Heller, S. (2020). Progenitor Cells from the Adult Human Inner Ear. Anat. Rec. 303, 461–470. doi: 10.1002/ar.24228
Severinsen, S. A., Sørensen, M. S., Kirkegaard, M., and Nyengaard, J. R. (2010). Stereological estimation of total cell numbers in the young human utricular macula. Acta Otolaryngol. 130, 773–779. doi: 10.3109/00016480903397694
Shou, J., Zheng, J. L., and Gao, W. Q. (2003). Robust generation of new hair cells in the mature mammalian inner ear by adenoviral expression of Hath1. Mol. Cell. Neurosci. 23, 169–179. doi: 10.1016/s1044-7431(03)00066-6
Slowik, A. D., and Bermingham-McDonogh, O. (2013). Hair cell generation by notch inhibition in the adult mammalian cristae. J. Assoc. Res. Otolaryngol. 14, 813–828. doi: 10.1007/s10162-013-0414-z
Staecker, H., Praetorius, M., Baker, K., and Brough, D. E. (2007). Vestibular hair cell regeneration and restoration of balance function induced by math1 gene transfer. Otol. Neurotol. 28, 223–231. doi: 10.1097/MAO.0b013e31802b3225
Streeter, G. L. (1906). On the development of the membranous labyrinth and the acoustic and facial nerves in the human embryo. Am. J. Anat. 6, 139–165. doi: 10.1002/aja.1000060103
Strupp, M., Dlugaiczyk, J., Ertl-Wagner, B. B., Rujescu, D., Westhofen, M., and Dieterich, M. (2020). Vestibular Disorders. Dtsch. Arztebl. Int. 117, 300–310. doi: 10.3238/arztebl.2020.0300
Sun, S., Li, S., Luo, Z., Ren, M., He, S., Wang, G., et al. (2021). Dual expression of Atoh1 and Ikzf2 promotes transformation of adult cochlear supporting cells into outer hair cells. Elife 10:e66547. doi: 10.7554/eLife.66547
Tafra, R., Brakus, S. M., Vukojevic, K., Kablar, B., Colovic, Z., and Saraga-Babic, M. (2014). Interplay of proliferation and proapoptotic and antiapoptotic factors is revealed in the early human inner ear development. Otol. Neurotol. 35, 695–703. doi: 10.1097/mao.0000000000000210
Tan, F., Chu, C., Qi, J., Li, W., You, D., Li, K., et al. (2019). AAV-ie enables safe and efficient gene transfer to inner ear cells. Nat. Commun. 10:3733. doi: 10.1038/s41467-019-11687-8
Taylor, R. R., Filia, A., Paredes, U., Asai, Y., Holt, J. R., Lovett, M., et al. (2018). Regenerating hair cells in vestibular sensory epithelia from humans. Elife 7:e34817. doi: 10.7554/eLife.34817
Taylor, R. R., Jagger, D. J., Saeed, S. R., Axon, P., Donnelly, N., Tysome, J., et al. (2015). Characterizing human vestibular sensory epithelia for experimental studies: new hair bundles on old tissue and implications for therapeutic interventions in ageing. Neurobiol. Aging 36, 2068–2084. doi: 10.1016/j.neurobiolaging.2015.02.013
Toyoda, S., Shiraki, N., Yamada, S., Uwabe, C., Imai, H., Matsuda, T., et al. (2015). Morphogenesis of the inner ear at different stages of normal human development. Anat. Rec. 298, 2081–2090. doi: 10.1002/ar.23268
Tsuji, K., Velázquez-Villaseñor, L., Rauch, S. D., Glynn, R. J., Wall, C. III, and Merchant, S. N. (2000). Temporal bone studies of the human peripheral vestibular system. Meniere’s disease. Ann. Otol. Rhinol. Laryngol. Suppl. 181, 26–31. doi: 10.1177/00034894001090s505
van der Valk, W. H., Steinhart, M. R., Zhang, J., and Koehler, K. R. (2021). Building inner ears: recent advances and future challenges for in vitro organoid systems. Cell Death Differ. 28, 24–34. doi: 10.1038/s41418-020-00678-8
Walters, B. J., Coak, E., Dearman, J., Bailey, G., Yamashita, T., Kuo, B., et al. (2017). In Vivo Interplay between p27(Kip1), GATA3, ATOH1, and POU4F3 Converts Non-sensory Cells to Hair Cells in Adult Mice. Cell Rep. 19, 307–320. doi: 10.1016/j.celrep.2017.03.044
Wang, T., Chai, R., Kim, G. S., Pham, N., Jansson, L., Nguyen, D. H., et al. (2015). Lgr5+ cells regenerate hair cells via proliferation and direct transdifferentiation in damaged neonatal mouse utricle. Nat. Commun. 6:6613. doi: 10.1038/ncomms7613
Wang, T., Niwa, M., Sayyid, Z. N., Hosseini, D. K., Pham, N., Jones, S. M., et al. (2019). Uncoordinated maturation of developing and regenerating postnatal mammalian vestibular hair cells. PLoS Biol. 17:e3000326. doi: 10.1371/journal.pbio.3000326
Warchol, M. E., Lambert, P. R., Goldstein, B. J., Forge, A., and Corwin, J. T. (1993). Regenerative proliferation in inner ear sensory epithelia from adult guinea pigs and humans. Science 259, 1619–1622. doi: 10.1126/science.8456285
Watanuki, K., and Schuknecht, H. F. (1976). A morphological study of human vestibular sensory epithelia. Arch. Otolaryngol. 102, 853–858.
Wei, H., Chen, Z., Hu, Y., Cao, W., Ma, X., Zhang, C., et al. (2021). Topographically Conductive Butterfly Wing Substrates for Directed Spiral Ganglion Neuron Growth. Small 17:e2102062. doi: 10.1002/smll.202102062
Weisleder, P., and Rubel, E. W. (1992). Hair cell regeneration in the avian vestibular epithelium. Exp. Neurol. 115, 2–6. doi: 10.1016/0014-4886(92)90211-8
Wersall, J. (1956). Studies on the structure and innervation of the sensory epithelium of the cristae ampulares in the guinea pig; a light and electron microscopic investigation. Acta Otolaryngol. Suppl. 126, 1–85.
Whitfield, T. T. (2015). Development of the inner ear. Curr. Opin. Genet. Dev. 32, 112–118. doi: 10.1016/j.gde.2015.02.006
Wu, D. K., and Kelley, M. W. (2012). Molecular mechanisms of inner ear development. Cold Spring Harb. Perspect. Biol. 4:a008409. doi: 10.1101/cshperspect.a008409
Wu, J., Li, W., Lin, C., Chen, Y., Cheng, C., Sun, S., et al. (2016). Co-regulation of the Notch and Wnt signaling pathways promotes supporting cell proliferation and hair cell regeneration in mouse utricles. Sci. Rep. 6:29418. doi: 10.1038/srep29418
Yamashita, H., and Oesterle, E. C. (1995). Induction of cell proliferation in mammalian inner-ear sensory epithelia by transforming growth factor alpha and epidermal growth factor. Proc. Natl. Acad. Sci. U. S. A. 92, 3152–3155. doi: 10.1073/pnas.92.8.3152
Yasuda, M., Yamada, S., Uwabe, C., Shiota, K., and Yasuda, Y. (2007). Three-dimensional analysis of inner ear development in human embryos. Anat. Sci. Int. 82, 156–163. doi: 10.1111/j.1447-073X.2007.00176.x
You, D., Guo, L., Li, W., Sun, S., Chen, Y., Chai, R., et al. (2018). Characterization of Wnt and Notch-Responsive Lgr5+ Hair Cell Progenitors in the Striolar Region of the Neonatal Mouse Utricle. Front. Mol. Neurosci. 11:137. doi: 10.3389/fnmol.2018.00137
Zeng, S., Ni, W., Jiang, H., You, D., Wang, J., Lu, X., et al. (2020). Toxic Effects of 3,3′-Iminodipropionitrile on Vestibular System in Adult C57BL/6J Mice In Vivo. Neural Plast. 2020:1823454. doi: 10.1155/2020/1823454
Zhang, S., Dong, Y., Qiang, R., Zhang, Y., Zhang, X., Chen, Y., et al. (2021). Characterization of Strip1 Expression in Mouse Cochlear Hair Cells. Front. Genet. 12:625867. doi: 10.3389/fgene.2021.625867
Zhang, Y., Li, W., He, Z., Wang, Y., Shao, B., Cheng, C., et al. (2019). Pre-treatment With Fasudil Prevents Neomycin-Induced Hair Cell Damage by Reducing the Accumulation of Reactive Oxygen Species. Front. Mol. Neurosci. 12:264. doi: 10.3389/fnmol.2019.00264
Zhang, Y., Li, Y., Fu, X., Wang, P., Wang, Q., Meng, W., et al. (2021). The Detrimental and Beneficial Functions of Macrophages After Cochlear Injury. Front. Cell. Dev. Biol. 9:631904. doi: 10.3389/fcell.2021.631904
Zhang, Y., Zhang, S., Zhang, Z., Dong, Y., Ma, X., Qiang, R., et al. (2020). Knockdown of Foxg1 in Sox9+ supporting cells increases the trans-differentiation of supporting cells into hair cells in the neonatal mouse utricle. Aging 12, 19834–19851. doi: 10.18632/aging.104009
Zheng, J. L., and Gao, W. Q. (2000). Overexpression of Math1 induces robust production of extra hair cells in postnatal rat inner ears. Nat. Neurosci. 3, 580–586. doi: 10.1038/75753
Zheng, J. L., Helbig, C., and Gao, W. Q. (1997). Induction of cell proliferation by fibroblast and insulin-like growth factors in pure rat inner ear epithelial cell cultures. J. Neurosci. 17, 216–226. doi: 10.1523/jneurosci.17-01-00216.1997
Keywords: human, vestibule, hair cell, development, regeneration
Citation: Huang Y, Mao H and Chen Y (2022) Regeneration of Hair Cells in the Human Vestibular System. Front. Mol. Neurosci. 15:854635. doi: 10.3389/fnmol.2022.854635
Received: 14 January 2022; Accepted: 14 February 2022;
Published: 24 March 2022.
Edited by:
Yu Sun, Huazhong University of Science and Technology, ChinaCopyright © 2022 Huang, Mao and Chen. This is an open-access article distributed under the terms of the Creative Commons Attribution License (CC BY). The use, distribution or reproduction in other forums is permitted, provided the original author(s) and the copyright owner(s) are credited and that the original publication in this journal is cited, in accordance with accepted academic practice. No use, distribution or reproduction is permitted which does not comply with these terms.
*Correspondence: Yan Chen, Y2hlbnlhbjA1MjhAZnVkYW4uZWR1LmNu