- Department of Biological Sciences, Tata Institute of Fundamental Research, Mumbai, India
Early adversity is an important risk factor that influences brain aging. Diverse animal models of early adversity, including gestational stress and postnatal paradigms disrupting dam-pup interactions evoke not only persistent neuroendocrine dysfunction and anxio-depressive behaviors, but also perturb the trajectory of healthy brain aging. The process of brain aging is thought to involve hallmark features such as mitochondrial dysfunction and oxidative stress, evoking impairments in neuronal bioenergetics. Furthermore, brain aging is associated with disrupted proteostasis, progressively defective epigenetic and DNA repair mechanisms, the build-up of neuroinflammatory states, thus cumulatively driving cellular senescence, neuronal and cognitive decline. Early adversity is hypothesized to evoke an “allostatic load” via an influence on several of the key physiological processes that define the trajectory of healthy brain aging. In this review we discuss the evidence that animal models of early adversity impinge on fundamental mechanisms of brain aging, setting up a substratum that can accelerate and compromise the time-line and nature of brain aging, and increase risk for aging-associated neuropathologies.
Introduction
Early adversity is a potent risk factor for adult psychopathology (Gee, 2021; Teicher et al., 2021). Early stressors such as physical, sexual and emotional abuse, parental neglect/loss, parental/caregiver substance abuse and incarceration disrupt physiological and psychological functioning driving maladaptive health outcomes (Brown et al., 2009; Brenhouse et al., 2019). Animal models attempt to capture the molecular, cellular, neuroendocrine, structural, functional and behavioral changes that arise due to early stress, to gain a mechanistic insight into how early adversity programs psychiatric vulnerability (Tzanoulinou and Sandi, 2017; Blaisdell et al., 2019; Torres-Berrío et al., 2019). While the impact of early stress is experienced by multiple physiological systems, the brain remains the central player as a target of stress and in the top-down control over stress-response pathways (McEwen, 2007). Prior reviews have discussed the influence of early stress on anxio-depressive behaviors and disrupted cognition, accompanied by transcriptional, cytoarchitectural, neuroendocrine and functional changes in diverse limbic brain regions (Chen and Baram, 2016; Teicher et al., 2016; Pervanidou and Chrousos, 2018). Amongst the hallmark features of early stress is that it evokes enduring consequences (Miller et al., 2011; Szyf, 2019). Early adversity exacerbates aging-induced telomere erosion, establishing a pathophysiological basis for enhanced morbidity and mortality (Epel and Prather, 2018; Colich et al., 2020). Clinical literature also indicates that individuals exposed to early stress are more likely to suffer a premature death (Brown et al., 2009). In this review, we critically discuss the evidence that early stress accelerates brain aging.
Animal Models of Early Adversity
Animal models of early adversity involve stress exposure in utero, or during early postnatal time-windows, which can evoke persistent alterations in mood-related behavior, noted long after the cessation of stress (Figure 1A; Schmidt et al., 2011). Gestational stress which involves administration of chronic stress to the dam, or exposure to an inflammatory milieu in utero, such as in the maternal immune activation (MIA) model, results in persistent changes in anxio-depressive behaviors in the progeny (Brown and Conway, 2019). During the postnatal temporal window, most rodent models of early adversity capitalize on perturbation of dam-pup interactions and fragmented caregiving behavior from the dam (Orso et al., 2019). These include perturbed licking, grooming, and arched back nursing behavior (LGABN), maternal deprivation (MD), maternal separation (MS), maternal separation combined with unpredictable stress to the dam (MSUS), or limited access to bedding and nesting (LBN) (Caldji et al., 2000; Ladd et al., 2000; Molet et al., 2014; Walker et al., 2017). Common across these models are enhanced anxio-depressive behaviors in the progeny, often accompanied by perturbed cognitive, reward and social behavior (Syed and Nemeroff, 2017; Tzanoulinou and Sandi, 2017; Birnie et al., 2020). Juvenile stress models are usually initiated post-weaning from the dam during the peripubertal window, and for the purposes of this review we have restricted our discussion to early adversity models that involve time-windows prior to juvenile life. Early adversity disrupts stress-responsive neuroendocrine pathways, drives neuroinflammatory states, evokes epigenetic changes, and results in structural and functional changes in neurocircuits that regulate anxio-depressive behaviors, including the hippocampus, prefrontal cortex (PFC), amygdala, and the brain-stem monoaminergic nuclei (Babenko et al., 2015; Teicher et al., 2016; Agorastos et al., 2019; Brenhouse et al., 2019). In this review, we discuss the evidence of an altered brain aging trajectory as a consequence of early adversity, focusing predominantly on studies from animal models.
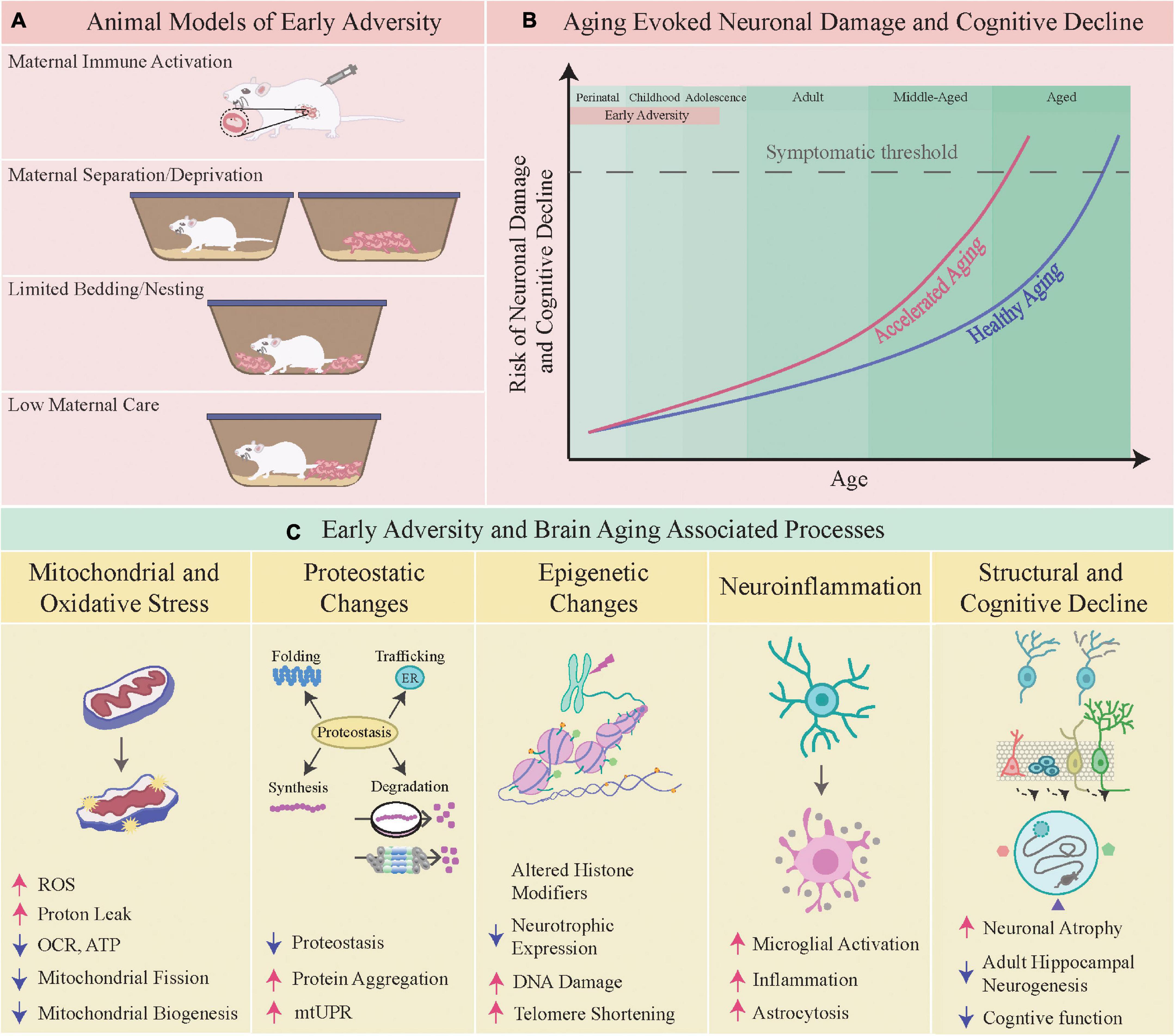
Figure 1. Early adversity and brain aging-associated processes. (A) Shown here are specific animal models of early adversity viz. maternal immune activation, maternal separation/deprivation, limited bedding and nesting, and low maternal care. (B) The schematic depicts the hastening of brain aging and an earlier onset of neuronal damage and cognitive decline following early adversity. (C) Depicted below are some of the aging-associated processes that are perturbed by early adversity, namely the following physiological mechanisms viz. mitochondrial homeostasis, proteostasis, epigenetics, neuroinflammation, structural and cognitive function.
Hallmark Signatures of Brain Aging
Aging is characterized by a time-dependent loss of molecular, cellular, structural and functional integrity leading to impaired homeostasis (López-Otín et al., 2013). Accompanying the aging-evoked attrition in all organ systems, “brain aging” also exhibits hallmark features with steady and cumulative decrements noted in structure and function, spanning from atrophy-associated cognitive decline to motor deficits (Mattson and Arumugam, 2018; Oschwald et al., 2019). The characteristic signatures of “brain aging” include mitochondrial dysfunction, oxidative stress, compromised neuronal bioenergetics, impaired proteostasis, perturbed DNA repair, altered intracellular signaling, and a cumulative buildup of neuroinflammatory states (Mattson and Arumugam, 2018). Distinct brain regions show individual variation in the extent of their vulnerability to aging-associated neuronal loss, with the hippocampus, cerebral cortex and cerebellum reported to exhibit both synaptic and cellular attrition, accompanied by impaired synaptic plasticity (Fjell and Walhovd, 2010; Morrison and Baxter, 2012; Bartsch and Wulff, 2015; Fan et al., 2018; Mattson and Arumugam, 2018). A key driver of these changes is thought to be aging-associated neuroinflammation, which also appears to differentially impact specific vulnerable cell populations in the brain (Sparkman and Johnson, 2008; Simen et al., 2011; Mattson and Arumugam, 2018). Correlated with these changes is the compromised structural/functional integrity of mitochondria and impaired neuronal bioenergetics, cumulative buildup of dysfunctional proteins and an unfolded protein response, markers of endoplasmic reticulum (ER) stress, failure to effectively scavenge reactive oxygen species (ROS) and oxidative damage, overlaid on a baseline substratum of neuroinflammatory changes, namely a disrupted cytokine milieu and microglial activation (Wyss-Coray, 2016; Mattson and Arumugam, 2018; Webb and Sideris, 2020; Uddin et al., 2021). While these changes overlap and correlate with each other, the causal association between these events still remains unclear. However, several studies highlight that these changes, namely enhanced oxidative stress and neuroinflammatory states, accompanied by impaired DNA repair and mitochondrial dysfunction may play a vital role in driving the synaptic, structural and functional impairments associated with aging (Raz and Rodrigue, 2006; Dröge and Schipper, 2007; Paradies et al., 2011; Regnell et al., 2012; Green and Nolan, 2014; Pluvinage and Wyss-Coray, 2020). While these molecular and cellular changes are vital drivers of determining the aging trajectory, they are further impacted by both genetic background and life-course factors (Yuan et al., 2009; Kõks et al., 2016; Zannas, 2018; Marini et al., 2020; Ancelin et al., 2021). In this review, we focus on the vital life-course factor of early life experience, which can exert a long-lasting impact in determining an organism’s aging trajectory and health-span, in particular impacting the quality and nature of brain aging. A working hypothesis suggests that early adversity sets up an underlying “allostatic load” which impacts the physiology of normal aging creating fertile conditions that hasten and compromise the brain aging trajectory (Figure 1B; Danese and McEwen, 2012; Epel and Prather, 2018).
Given that most of the literature addressing the impact of early adversity on the brain aging trajectory is based on rodent models, it is worth considering a comparative scale of the equivalent age stages between rodents and humans. We have described in Figure 1B the distinct stages of perinatal, childhood, adolescence, adult, middle-aged and aged, as the major epochs of life. The perinatal stage encompasses the window of life from 23 weeks of gestation onward till about 2 years of human age. While it is challenging to draw direct parallels, the emergence of developmental milestones suggest that postnatal day 1–10 for rodents is equivalent to 23–40 weeks of human gestation, and postnatal day 10–21 is comparable to the window from birth till 3 years of human age. The childhood window comprising of 2–11 years of age for humans is thought to have an equivalence based on developmental indices to postnatal day 20–35 in rodents. The adolescent phase in humans (12–18 years) is thought to be comparable to postnatal day 35–49 in rodent models, with adulthood (20 years onward) compared to rodent models commencing from postnatal day 60 onward. The middle-aged and aged windows are generally thought to commence from 40 and 60 years of age respectively in humans, which has been suggested to compare to 9–15 months for middle-aged and 18 months upward as aged in rodent models (Flurkey et al., 2007; Semple et al., 2013; Dutta and Sengupta, 2016; Agoston, 2017; Wang et al., 2020).
Early Adversity, Mitochondrial Dysfunction and Oxidative Stress
Mitochondria are an integrative hub that sense, adapt to and drive cellular stress responses, shaping the homeostatic adaptations to stress (Eisner et al., 2018; Picard et al., 2018). Mitochondria respond dynamically to stress signaling cues and mitokines, adjusting both architecture and function to rapidly adapt to altered energetic demands (Picard et al., 2015; Daniels et al., 2020). This ability of mitochondria to orchestrate effective cellular stress responses is a key component of the “resilient” phenotype (Hoffmann and Spengler, 2018), and a decline in this buffering capacity is linked to cellular senescence (Correia-Melo et al., 2016; Vasileiou et al., 2019). Early stress is speculated to deteriorate in the stress-buffering capacity of mitochondria, via a disrupted mitostasis, and thus accelerate senescence and neuronal damage, a cumulative consequence of brain aging (Figure 1C; Tyrka et al., 2016; Hoffmann and Spengler, 2018; Zitkovsky et al., 2021).
Studies using models of fragmented maternal care indicate both short (postnatal day 9) and long-term (10–12 months) changes in mitochondrial function within limbic brain regions and the periphery (Ruigrok et al., 2021). Adult progeny with a history of LBN exhibit perturbed electron transport chain (ETC) activity in the hypothalamus, and altered mitochondrial fusion/fission associated gene expression in the hippocampus upto 1 year of age (Ruigrok et al., 2021). MS evokes dysregulation of mitochondrial sirtuins within the PFC that persist well into middle-aged life (15 months) (Pusalkar et al., 2016), and robust decreases in mitochondrial mass in the periphery, namely the muscle, noted 8 months post the cessation of MS (Ghosh et al., 2016). Further, MS animals exhibit enhanced sensitivity to oxidative stress in peripheral mononuclear cells, noted until 18 months of age, and also reported in gut epithelial cells when examined in adulthood (2 months) in MS animals (Grigoruta et al., 2020; Khorjahani et al., 2020). Impaired calcium homeostasis, enhanced ROS and a decrease in oxygen consumption rate (OCR) or ATP production is also reported in the PFC, raphe and hippocampus of adult (2–6 month) MS animals, suggestive of a broad mitochondrial dysfunction in multiple systems (Della et al., 2013; Amini-Khoei et al., 2017; Masrour et al., 2018; Nold et al., 2019). Proteomic studies in diverse early stress models, spanning analysis from 12–24 weeks of age, indicate a dysregulation of proteins associated with mitochondrial energy metabolism in the PFC and hippocampus (Marais et al., 2009; Mairesse et al., 2012; Föcking et al., 2014; van Zyl et al., 2016; Nold et al., 2019), with a specific study suggesting a temporal variation in these effects noted at postnatal day 21 and 3–4 months following LBN, accompanied by a sex-specific differential expression of the hippocampal proteome at these timepoints (Eagleson et al., 2020). Furthermore, MS regulated glyoxalase enzymatic machinery at around 3 months of age that could result in a build-up of the pro-oxidant, methylglyoxal, which is a precursor of advanced glycation end-products implicated in neurodegeneration (Marais et al., 2009; Allaman et al., 2015). Early stress of MD also reduced superoxide dismutase and catalase activity in the hippocampus and PFC observed as early as postnatal day 20 and persisting into young adulthood (2 months), which could exacerbate oxidative stress in vulnerable limbic neurocircuits (Réus et al., 2017; Talukdar et al., 2020; Abelaira et al., 2021). Collectively, most reports of mitochondrial dysfunction following early stress restrict analysis to young adulthood (2–4 months of age) (Della et al., 2013; Masrour et al., 2018; Eagleson et al., 2020; Lapp et al., 2020), with few exceptions examining the consequences either in early postnatal life or well into middle-aged life ranging from 8–15 months (Ghosh et al., 2016; Pusalkar et al., 2016; Ruigrok et al., 2021). A careful analysis of the impact of early stress on the ontogeny of mitochondria within neuronal circuits, interaction with variables such as sex and genetic background remains to be extensively explored. Such studies are vital because a single-snapshot cannot capture the continuum of mitochondrial functional changes following early adversity, and it is likely that organ systems and brain regions will exhibit distinct timelines with different inflection points when adaptive attempts tip into maladaptive outcomes (Suri and Vaidya, 2015). Thus far the emerging picture raises the intriguing possibility that cumulative mitochondrial allostatic load following early adversity could sow the seeds for the hastening of age-associated impairments (Daniels et al., 2020).
Early Adversity, Impaired Proteostasis and Autophagy
Early stress is speculated to alter proteostasis, trigger abnormal unfolded protein responses (UPR), and drive impaired autophagy thus establishing a substrate for aging-associated neuropathology (Figure 1C; Pinto et al., 2016; Liu et al., 2018; Criado-Marrero et al., 2019; Saulnier et al., 2021; Sierra-Fonseca et al., 2021). Maintaining effective protein quality is a multistep process spanning from synthesis, appropriate folding and conformational stability to turnover, and is vital in neurons that do not have the scope of cellular replacement to maintain the proteome (Muñoz-Carvajal and Sanhueza, 2020; Giandomenico et al., 2021; Saulnier et al., 2021). The proteostasis network, consisting of proteasome-dependent degradation machinery and autophagic processes is critical to maintaining the integrity of the functional proteome. Aging-dependent progressive decline in the efficiency of the proteostatic network is implicated in the establishment of neurodegeneration (Giandomenico et al., 2021). MS evokes significant disruption in expression of components of both the ubiquitin-proteasome system and the autophagy-lysosomal pathway in the hippocampus with changes noted in young adulthood (3 months), and specific alterations persisting well into middle-aged life (16 months). It is interesting that these changes appear to be restricted to the hippocampus and are not observed in the neocortex, suggesting differential vulnerability of neuronal circuits (Sierra-Fonseca et al., 2021). A recent report indicates that MIA evokes a sex-specific integrated stress response, evoking disrupted proteostasis in the cortex of embryonic 14.5 and 18.5 day old male fetuses, that is linked to the emergence of perturbed social and stereotypic behavior via a cytokine-dependent mechanism (Kalish et al., 2021). Early adversity could aggravate the aging-evoked UPR, in particular in the context of mitochondrial proteins, and serve as an early molecular signature that accelerates neuronal impairment (Muñoz-Carvajal and Sanhueza, 2020). Postnatal metabolic stress results in a perturbed UPR in the hippocampus and hypothalamus at 3 months of age, raising the intriguing possibility that the toxic combination of early adversity and metabolic insults could be a potent insult that disrupts healthy brain aging in animals as early as young adulthood (Pinto et al., 2016; Chen et al., 2021). Early adversity is a risk factor for neurodegeneration, which is linked to a disruption of proteostasis and perturbed amyloidogenic processing in 6–12 month old genetic mouse models of Alzheimer’s disease subjected to LBN (Sarter and Bruno, 2004; Dallé and Mabandla, 2018; Lesuis et al., 2018b,a). LBN enhances hippocampal Aβ40 and Aβ42 levels, primary components of amyloid plaques, in 6–12 months old male animals (Lesuis et al., 2018b). Further, gestational stress, MS and LBN enhance plaque burden, hasten cognitive decline noted at 9–12 months of age and shorten life expectancy in genetic mouse models of Alzheimer’s disease (Lesuis et al., 2016, 2018b; Hui et al., 2017; Jafari et al., 2019). However, there are also contradictory reports, wherein LBN does not alter the course of cognitive or neurogenic decline in 8–10 month old genetic Alzheimer’s disease animal models (Hoeijmakers et al., 2018). Whilst several reports link early adversity to mitochondrial dysfunction, there is still a paucity of detailed reports examining the influence of early adversity on proteostasis, UPR and autophagy in the brain, in particular across the life-span.
Early Adversity, Epigenetic and Transcriptional Dysregulation
Amongst the foremost candidates for mediating the persistent effects of early adversity is a perturbed epigenetic landscape, thus driving transcriptional changes that hasten aging-evoked changes (Szyf, 2009; Doherty and Roth, 2018; Zannas, 2019; Palma-Gudiel et al., 2020). In animals exposed to early adversity, cognitive decline emerges as early as 12 months, and has been correlated with an altered epigenome in the hippocampus and PFC (Brunson et al., 2005; McClelland et al., 2011; Suri et al., 2013, 2014; Short et al., 2020). Several studies indicate altered expression of epigenetic machinery and of epigenetic modifications in the promoter regions of stress-responsive genes, such as the glucocorticoid receptor (GR) and brain derived neurotrophic factor (BDNF), with only a few reports examining these changes across the life-span (Roth and Sweatt, 2011; Suri et al., 2013; Pusalkar et al., 2016; Seo et al., 2016, 2020; Liu and Nusslock, 2018; Mourtzi et al., 2021). Several of the epigenetic and transcriptional changes evoked by early adversity are sex-dependent (Parel and Peña, 2022). MS is associated with dysregulated expression of the “writer” and “eraser” class of histone modifying enzymes, as well as DNA modifying enzymes, which in specific cases persist across the life-span, well into middle-aged life (15 months) (Pusalkar et al., 2016). This could contribute to global transcriptional changes in limbic brain regions, in particular within the hippocampus as observed at 15 months of age by Suri et al. (2014) (Marrocco et al., 2019; Peña et al., 2019; Usui et al., 2021). 15 month old MS animals exhibit perturbed expression of genes associated with calcium homeostasis, neuroinflammation, synaptogenesis, autophagy, proteasomal function, and cellular responses to stress (Suri et al., 2014). The nature of transcriptional dysregulation evoked by early adversity varies based on age, highlighting the importance of life-span studies (Suri et al., 2014). Amongst the key genes targeted by early adversity is GR, which plays a key role in mediating stress responses and HPA axis regulation. Diverse models of early adversity exhibit enhanced CpG methylation at the GR promoter in the hippocampus in young adults, driving reduced GR expression and disrupting the negative feedback regulation of the HPA axis (Weaver, 2007; Smart et al., 2015). This would enhance circulating corticosteroid levels, thus impacting neuronal atrophy and cognitive decline (McEwen, 2007). Aging is associated with enhanced circulating corticosteroid that negatively impact hippocampal neuron structure and function (Yau and Seckl, 2012). Following early adversity, animals have enhanced baseline, circadian and stress-evoked corticosteroid levels, compromising hippocampal cytoarchitecture/function and enhancing cognitive decline (McEwen, 2007). GRs and BDNF, both of which are dysregulated by early adversity, profoundly influence mitochondria (Daskalakis et al., 2015). GRs translocate into mitochondria, can regulate oxidative phosphorylation associated nuclear-encoded and mitochondrial gene expression, and influence bioenergetics (Psarra and Sekeris, 2009; Picard et al., 2014). BDNF, which is shown to exhibit a robust decline in the hippocampus and PFC of 15 month old animals subjected to early stress, can influence mitochondrial biogenesis and transport (Roth et al., 2009; Suri et al., 2013; Markham et al., 2014). The disrupted dyad of BDNF-GR signaling could influence cellular changes spanning from altered mitochondrial structure/function to dendritic atrophy, and at the organismal level perturb the neuroendocrine milieu and drive neurodegenerative decline (Rothman and Mattson, 2013; Suri and Vaidya, 2013; Daskalakis et al., 2015). A prior study indicates a marked reduction in expression of genes linked to antioxidant responses and DNA repair in the aging human neocortex after 40 years of age with enhanced oxidative DNA damage associated with the promoters of these downregulated genes likely due to attenuated base-excision repair mechanisms (Lu et al., 2004). Though speculative, one can envisage that early adversity could cumulatively enhance oxidative damage to DNA, RNA, proteins, and lipids (Karanikas et al., 2021). This is supported by evidence of hastened telomere attrition noted in 4–5 year old children that experienced childhood maltreatment, and phenocopied in animal models of early adversity (Drury et al., 2012; Price et al., 2013; Ridout et al., 2018). Amongst the implicated mediators of such DNA damage and telomere shortening following early adversity are the toxic cocktail of glucocorticoid-evoked oxidative stress, mitochondrial dysfunction, enhanced proton leak and neuroinflammation (Swaab et al., 2005; Casagrande et al., 2020).
Early Adversity, Neuroinflammation, Structural and Cognitive Decline
Early stress triggers neuroimmune responses that drive prolonged, pathological and maladaptive neuroinflammation (Ganguly and Brenhouse, 2015; Nettis et al., 2020). Neuroinflammatory states evoked by early stress have been reviewed extensively, with evidence pointing to an induction of inflammatory cytokines, astrogliosis, and microglial activation (Ganguly and Brenhouse, 2015; Desplats et al., 2020). Most studies examine consequences of early adversity in postnatal or adult life, and do not address the long-term consequences on neuroinflammation (Delpech et al., 2016; Réus et al., 2019; Desplats et al., 2020; Dutcher et al., 2020; Reshetnikov et al., 2020; Kim et al., 2021). One of the reports indicates that MS increases microglial numbers/activation in 10 month old animals (Criado-Marrero et al., 2020), but few studies have actually followed animals with a history of early adversity across the life-span, to address the temporal and circuit-specific emergence of neuroinflammatory signatures (Ganguly and Brenhouse, 2015; Tay et al., 2018; Andersen, 2022). However, it is noteworthy that in some models of early stress (MIA), neuroinflammatory changes do not appear to contribute to synaptic atrophy and cognitive decline, with no changes reported in microglia or reactive astrocytes in 22 month old animals with a history of MIA (Giovanoli et al., 2015). Adult female, but not male, mice (2–3 months of age) with a life history of being subjected to fragmented maternal care showed deficits in reversal learning, suggesting a sex-specific influence of early adversity on cognition (Goodwill et al., 2018). This raises the possibility that while neuroinflammation is a consequence of early adversity, it remains poorly understood whether it is a causal contributor to accelerated aging-evoked neuronal and functional decline (Merz and Turner, 2021). It also highlights the critical importance of addressing potential sex differences in the pattern, onset and magnitude of neuroinflammatory changes evoked by early adversity, as neurohormones may exert a profound impact in modifying the trajectory of neuroinflammatory signatures (Ganguly and Brenhouse, 2015; González-Pardo et al., 2020).
The aging brain has several hallmark features, including atrophy of vulnerable neuronal populations and marked cognitive impairments (Fjell and Walhovd, 2010). Amongst the brain regions most extensively studied in this regard are the PFC and hippocampus, with dendritic atrophy, reduced spine density, decreased hippocampal neurogenesis, cellular shrinkage and volumetric loss being the key reported features (Figure 1C; McEwen and Morrison, 2013; Bartsch and Wulff, 2015). Several of these changes evoked by early adversity have been shown to be sex-dependent. In rats exposed to pre-pubertal stress, adult hippocampal neurogenesis is altered in adulthood in males, but not in females (Brydges et al., 2018). Further, in rats exposed to MS, females exhibit a more elaborate dendritic morphology and reduced thin spine density in infralimbic pyramidal neurons of the mPFC, which is not observed in male rats when examined at postnatal day 40 (Farrell et al., 2016). Several of these changes arise in a milieu associated with enhanced oxidative stress, mitochondrial dysfunction, disrupted proteostasis, neuroinflammatory signatures and an epigenetic milieu that drives reduced growth factor and enhanced inflammatory cytokine expression (Mattson and Arumugam, 2018). Early stress is associated with a long-lasting BDNF dysregulation in the hippocampus and/or PFC noted well into aged life reported at the age of 15 months (Suri et al., 2013) and 22 months (Giovanoli et al., 2015), along with a robust decline in hippocampal neurogenesis reported at the age of 10 months (Ruiz et al., 2018) and 15 months (Suri et al., 2013). The effects of early adversity on neurotransmitters and growth factors are suggested to be sex-dependent, which has been reviewed extensively by Perry et al. (2021). Neural stem cells shift to quiescence with aging, but continue to show similar proliferative capacity upon activation. Early stress is suggested to impair this proliferative capacity in aging neural stem cells, dampening the capacity for repair (Suri et al., 2013; Kalamakis et al., 2019). Several early stress models (MS, LBN, MIA, and MD) exhibit significant cognitive impairments in middle-aged and aged life (Brunson et al., 2005; Sterlemann et al., 2010; Suri et al., 2013; Sousa et al., 2014; Giovanoli et al., 2015; Yajima et al., 2018). The preponderance of literature using early stress models reporting changes at the epigenetic, transcriptional, mitochondrial, proteostatic, neuroinflammatory, cytoarchitectural and behavioral level is at best correlative, but thus far does not provide a clear causal relationship between changes at distinct levels of organization that mechanistically drive the accelerated aging phenotype evoked by early adversity.
Conclusion
Early adversity disrupts the functioning of key physiological processes that facilitate adaptive stress responses, setting in motion a cumulative “allostatic load” that alters the nature and time-line of healthy brain aging. Further, early adversity could also impact key neurodevelopmental milestones, which could alter the optimal functioning of neurocircuits thus setting up a substratum for a disruption of the trajectory for brain aging. The interim duration between exposure to early stressors and eventual brain aging outcomes provides a substantial temporal window for interventional approaches, including life-course factors such as exercise, diet, environmental enrichment, epigenetic and pharmacological interventions that may serve to reverse or ameliorate the negative impacts of early adversity on brain aging. Here we have provided an overview of the key brain aging-associated processes targeted by early adversity, and highlighted gaps in knowledge that require future investigation.
Author Contributions
PC, AS, and VV jointly wrote the manuscript. All authors contributed to the article and approved the submitted version.
Funding
We acknowledge funding to VV from the Sree Ramakrishna Paramahamsa Research Grant for Translational Biomedical Research of the Sree Padmavathi Venkateswara Foundation (SreePVF/G/BS/19/1) and intramural support from the Tata Institute of Fundamental Research and Department of Atomic Energy, Mumbai (Grant reference number: RTI4003). PC received funding support from the India Alliance Early Career Fellowship (IA/E/18/1/504310) from the Department of Biotechnology, Government of India and the Wellcome Trust, United Kingdom.
Conflict of Interest
The authors declare that the research was conducted in the absence of any commercial or financial relationships that could be construed as a potential conflict of interest.
Publisher’s Note
All claims expressed in this article are solely those of the authors and do not necessarily represent those of their affiliated organizations, or those of the publisher, the editors and the reviewers. Any product that may be evaluated in this article, or claim that may be made by its manufacturer, is not guaranteed or endorsed by the publisher.
Abbreviations
MIA, maternal immune activation; LGABN, licking, grooming, arched back nursing; MD, maternal deprivation; MS, maternal separation; MSUS, maternal separation and unpredictable stress; LBN, limited bedding and nesting; PFC, prefrontal cortex; DNA, Deoxyribonucleic acid; ETC, electron transport chain; ROS, reactive oxygen species; OCR, oxygen consumption rate; ATP, Adenosine triphosphate; UPR, unfolded protein response; GR, glucocorticoid receptor; BDNF, brain derived neurotrophic factor; HPA, Hypothalamo-pituitary-adrenal axis; RNA, Ribonucleic acid.
References
Abelaira, H. M., Veron, D. C., de Moura, A. B., Carlessi, A. S., Borba, L. A., Botelho, M. E. M., et al. (2021). Sex differences on the behavior and oxidative stress after ketamine treatment in adult rats subjected to early life stress. Brain Res. Bull. 172, 129–138. doi: 10.1016/J.BRAINRESBULL.2021.04.021
Agorastos, A., Pervanidou, P., Chrousos, G. P., and Baker, D. G. (2019). Developmental Trajectories of Early Life Stress and Trauma: a Narrative Review on Neurobiological Aspects Beyond Stress System Dysregulation. Front. Psychiatry 10:118. doi: 10.3389/FPSYT.2019.00118
Agoston, D. V. (2017). How to Translate Time? The Temporal Aspect of Human and Rodent Biology. Front. Neurol. 8:92. doi: 10.3389/FNEUR.2017.00092
Allaman, I., Bélanger, M., and Magistretti, P. J. (2015). Methylglyoxal, the dark side of glycolysis. Front. Neurosci. 9:23. doi: 10.3389/FNINS.2015.00023
Amini-Khoei, H., Mohammadi-Asl, A., Amiri, S., Hosseini, M. J., Momeny, M., Hassanipour, M., et al. (2017). Oxytocin mitigated the depressive-like behaviors of maternal separation stress through modulating mitochondrial function and neuroinflammation. Prog. Neuropsychopharmacology Biol. Psychiatry 76, 169–178. doi: 10.1016/J.PNPBP.2017.02.022
Ancelin, M. L., Carrière, I., Artero, S., Maller, J. J., Meslin, C., Dupuy, A. M., et al. (2021). Structural brain alterations in older adults exposed to early-life adversity. Psychoneuroendocrinology 129:105272. doi: 10.1016/J.PSYNEUEN.2021.105272
Andersen, S. L. (2022). Neuroinflammation, Early-Life Adversity, and Brain Development. Harvard Rev. Psychiatry 30, 24–39. doi: 10.1097/HRP.0000000000000325
Babenko, O., Kovalchuk, I., and Metz, G. A. S. (2015). Stress-induced perinatal and transgenerational epigenetic programming of brain development and mental health. Neurosci. Biobehav. Rev. 48, 70–91. doi: 10.1016/J.NEUBIOREV.2014.11.013
Bartsch, T., and Wulff, P. (2015). The hippocampus in aging and disease: from plasticity to vulnerability. Neuroscience 309, 1–16. doi: 10.1016/J.NEUROSCIENCE.2015.07.084
Birnie, M. T., Kooiker, C. L., Short, A. K., Bolton, J. L., Chen, Y., and Baram, T. Z. (2020). Plasticity of the Reward Circuitry After Early-Life Adversity: mechanisms and Significance. Biol. Psychiatry 87, 875–884. doi: 10.1016/J.BIOPSYCH.2019.12.018
Blaisdell, K. N., Imhof, A. M., and Fisher, P. A. (2019). Early adversity, child neglect, and stress neurobiology: from observations of impact to empirical evaluations of mechanisms. Int. J. Dev. Neurosci. 78, 139–146. doi: 10.1016/J.IJDEVNEU.2019.06.008
Brenhouse, H. C., Danese, A., and Grassi-Oliveira, R. (2019). Neuroimmune Impacts of Early-Life Stress on Development and Psychopathology. Curr. Topics Behav. Neurosci. 43, 423–447. doi: 10.1007/7854_2018_53
Brown, A. S., and Conway, F. (2019). Maternal Immune Activation and Related Factors in the Risk of Offspring Psychiatric Disorders. Front. Psychiatry 10:430. doi: 10.3389/FPSYT.2019.00430
Brown, D. W., Anda, R. F., Tiemeier, H., Felitti, V. J., Edwards, V. J., Croft, J. B., et al. (2009). Adverse childhood experiences and the risk of premature mortality. Am. J. Prevent. Med. 37, 389–396. doi: 10.1016/J.AMEPRE.2009.06.021
Brunson, K. L., Kramár, E., Lin, B., Chen, Y., Colgin, L. L., Yanagihara, T. K., et al. (2005). Mechanisms of late-onset cognitive decline after early-life stress. J. Neurosci: 25, 9328–9338. doi: 10.1523/JNEUROSCI.2281-05.2005
Brydges, N. M., Moon, A., Rule, L., Watkin, H., Thomas, K. L., and Hall, J. (2018). Sex specific effects of pre-pubertal stress on hippocampal neurogenesis and behaviour. Trans. Psychiatry 8:271. doi: 10.1038/S41398-018-0322-4
Caldji, C., Diorio, J., and Meaney, M. J. (2000). Variations in maternal care in infancy regulate the development of stress reactivity. Biol. Psychiatry 48, 1164–1174. doi: 10.1016/S0006-3223(00)01084-2
Casagrande, S., Stier, A., Monaghan, P., Loveland, J. L., Boner, W., Lupi, S., et al. (2020). Increased glucocorticoid concentrations in early life cause mitochondrial inefficiency and short telomeres. J. Exp. Biol. 223:jeb222513. doi: 10.1242/JEB.222513
Chen, N., Zhang, Y., Wang, M., Lin, X., Li, J., Li, J., et al. (2021). Maternal obesity interrupts the coordination of the unfolded protein response and heat shock response in the postnatal developing hypothalamus of male offspring in mice. Mol. Cell. Endocrinol. 527:111218. doi: 10.1016/J.MCE.2021.111218
Chen, Y., and Baram, T. Z. (2016). Toward Understanding How Early-Life Stress Reprograms Cognitive and Emotional Brain Networks. Neuropsychopharmacology: 41, 197–206. doi: 10.1038/NPP.2015.181
Colich, N. L., Rosen, M. L., Williams, E. S., and McLaughlin, K. A. (2020). Biological aging in childhood and adolescence following experiences of threat and deprivation: a systematic review and meta-analysis. Psychol. Bull. 146, 721–764. doi: 10.1037/BUL0000270
Correia-Melo, C., Marques, F. D., Anderson, R., Hewitt, G., Hewitt, R., Cole, J., et al. (2016). Mitochondria are required for pro-ageing features of the senescent phenotype. EMBO J. 35, 724–742. doi: 10.15252/EMBJ.201592862
Criado-Marrero, M., Gebru, N. T., Gould, L. A., Smith, T. M., Kim, S., Blackburn, R. J., et al. (2019). Early Life Stress and High FKBP5 Interact to Increase Anxiety-Like Symptoms through Altered AKT Signaling in the Dorsal Hippocampus. Int. J. Mol. Sci. 20:2738. doi: 10.3390/IJMS20112738
Criado-Marrero, M., Smith, T. M., Gould, L. A., Kim, S., Penny, H. J., Sun, Z., et al. (2020). FKBP5 and early life stress affect the hippocampus by an age-dependent mechanism. Brain Behav. Immun. Health 9:100143. doi: 10.1016/J.BBIH.2020.100143
Dallé, E., and Mabandla, M. V. (2018). Early Life Stress, Depression And Parkinson’s Disease: a New Approach. Mol. Brain 11:18. doi: 10.1186/S13041-018-0356-9
Danese, A., and McEwen, B. S. (2012). Adverse childhood experiences, allostasis, allostatic load, and age-related disease. Physiol. Behav. 106, 29–39. doi: 10.1016/J.PHYSBEH.2011.08.019
Daniels, T. E., Olsen, E. M., and Tyrka, A. R. (2020). Stress and Psychiatric Disorders: The Role of Mitochondria. Annu. Rev. Clin. Psychol. 16, 165–186. doi: 10.1146/ANNUREV-CLINPSY-082719-104030
Daskalakis, N. P., Kloet, E. R., de Yehuda, R., Malaspina, D., and Kranz, T. M. (2015). Early Life Stress Effects on Glucocorticoid-BDNF Interplay in the Hippocampus. Front. Mol. Neurosci. 8:68. doi: 10.3389/FNMOL.2015.00068
Della, F. P., Abelaira, H. M., Réus, G. Z., dos Santos, M. A. B., Tomaz, D. B., Antunes, A. R., et al. (2013). Treatment with tianeptine induces antidepressive-like effects and alters the neurotrophin levels, mitochondrial respiratory chain and cycle Krebs enzymes in the brain of maternally deprived adult rats. Metab. Brain Dis. 28, 93–105. doi: 10.1007/S11011-012-9375-X
Delpech, J. C., Wei, L., Hao, J., Yu, X., Madore, C., Butovsky, O., et al. (2016). Early life stress perturbs the maturation of microglia in the developing hippocampus. Brain Behav. Immun. 57, 79–93. doi: 10.1016/J.BBI.2016.06.006
Desplats, P., Gutierrez, A. M., Antonelli, M. C., and Frasch, M. G. (2020). Microglial memory of early life stress and inflammation: susceptibility to neurodegeneration in adulthood. Neurosci. Biobehav. Rev. 117, 232–242. doi: 10.1016/J.NEUBIOREV.2019.10.013
Doherty, T. S., and Roth, T. L. (2018). Epigenetic Landscapes of the Adversity-Exposed Brain. Prog. Mol. Biol. Trans. Sci. 157, 1–19. doi: 10.1016/BS.PMBTS.2017.11.025
Dröge, W., and Schipper, H. M. (2007). Oxidative stress and aberrant signaling in aging and cognitive decline. Aging Cell 6, 361–370. doi: 10.1111/J.1474-9726.2007.00294.X
Drury, S. S., Theall, K., Gleason, M. M., Smyke, A. T., de Vivo, I., Wong, J. Y. Y., et al. (2012). Telomere length and early severe social deprivation: linking early adversity and cellular aging. Mol. Psychiatry 17, 719–727. doi: 10.1038/MP.2011.53
Dutcher, E. G., Pama, E. A. C., Lynall, M.-E., Khan, S., Clatworthy, M. R., Robbins, T. W., et al. (2020). Early-life stress and inflammation: a systematic review of a key experimental approach in rodents. Brain Neurosci. Adv. 4:239821282097804. doi: 10.1177/2398212820978049
Dutta, S., and Sengupta, P. (2016). Men and mice: relating their ages. Life Sci. 152, 244–248. doi: 10.1016/J.LFS.2015.10.025
Eagleson, K. L., Villaneuva, M., Southern, R. M., and Levitt, P. (2020). Proteomic and mitochondrial adaptations to early-life stress are distinct in juveniles and adults. Neurobiol. Stress 13:100251. doi: 10.1016/J.YNSTR.2020.100251
Eisner, V., Picard, M., and Hajnóczky, G. (2018). Mitochondrial dynamics in adaptive and maladaptive cellular stress responses. Nat. Cell Biol. 20, 755–765. doi: 10.1038/S41556-018-0133-0
Epel, E. S., and Prather, A. A. (2018). Stress, Telomeres, and Psychopathology: toward a Deeper Understanding of a Triad of Early Aging. Annu. Rev. Clin. Psychol. 14, 371–397. doi: 10.1146/ANNUREV-CLINPSY-032816-045054
Fan, W. J., Yan, M. C., Wang, L., Sun, Y. Z., Deng, J. B., and Deng, J. X. (2018). Synaptic aging disrupts synaptic morphology and function in cerebellar Purkinje cells. Neural Regen. Res. 13, 1019–1025. doi: 10.4103/1673-5374.233445
Farrell, M. R., Holland, F. H., Shansky, R. M., and Brenhouse, H. C. (2016). Sex-specific effects of early life stress on social interaction and prefrontal cortex dendritic morphology in young rats. Behav. Brain Res. 310, 119–125. doi: 10.1016/J.BBR.2016.05.009
Fjell, A. M., and Walhovd, K. B. (2010). Structural brain changes in aging: courses, causes and cognitive consequences. Rev. Neurosci. 21, 187–221. doi: 10.1515/REVNEURO.2010.21.3.187
Flurkey, K., Currer, J. M., and Harrison, D. E. (2007). Mouse Models in Aging Research. Mouse Biomed. Res. 3, 637–672. doi: 10.1016/B978-012369454-6/50074-1
Föcking, M., Opstelten, R., Prickaerts, J., Steinbusch, H. W. M., Dunn, M. J., van den Hove, D. L. A., et al. (2014). Proteomic investigation of the hippocampus in prenatally stressed mice implicates changes in membrane trafficking, cytoskeletal, and metabolic function. Dev. Neurosci. 36, 432–442. doi: 10.1159/000365327
Ganguly, P., and Brenhouse, H. C. (2015). Broken or maladaptive? Altered trajectories in neuroinflammation and behavior after early life adversity. Dev. Cogn. Neurosci. 11, 18–30. doi: 10.1016/J.DCN.2014.07.001
Gee, D. G. (2021). Early Adversity and Development: parsing Heterogeneity and Identifying Pathways of Risk and Resilience. Am. J. Psychiatry 178, 998–1013. doi: 10.1176/APPI.AJP.2021.21090944
Ghosh, S., Banerjee, K. K., Vaidya, V. A., and Kolthur-Seetharam, U. (2016). Early Stress History Alters Serum Insulin-Like Growth Factor-1 and Impairs Muscle Mitochondrial Function in Adult Male Rats. J. Neuroendocrinol. 28, doi: 10.1111/JNE.12397
Giandomenico, S. L., Alvarez-Castelao, B., and Schuman, E. M. (2021). Proteostatic regulation in neuronal compartments. Trends Neurosci. 45, 41–52. doi: 10.1016/J.TINS.2021.08.002
Giovanoli, S., Notter, T., Richetto, J., Labouesse, M. A., Vuillermot, S., Riva, M. A., et al. (2015). Late prenatal immune activation causes hippocampal deficits in the absence of persistent inflammation across aging. J. Neuroinflamm. 12:221. doi: 10.1186/S12974-015-0437-Y
González-Pardo, H., Arias, J. L., Gómez-Lázaro, E., Taboada, I. L., and Conejo, N. M. (2020). Sex-Specific Effects of Early Life Stress on Brain Mitochondrial Function. Monoamine Levels Neuroinflamm. Brain Sci. 10, 1–17. doi: 10.3390/BRAINSCI10070447
Goodwill, H. L., Manzano-Nieves, G., LaChance, P., Teramoto, S., Lin, S., Lopez, C., et al. (2018). Early Life Stress Drives Sex-Selective Impairment in Reversal Learning by Affecting Parvalbumin Interneurons in Orbitofrontal Cortex of Mice. Cell Rep. 25, 2299.e–2307.e. doi: 10.1016/J.CELREP.2018.11.010
Green, H. F., and Nolan, Y. M. (2014). Inflammation and the developing brain: consequences for hippocampal neurogenesis and behavior. Neurosci. Biobehav. Rev. 40, 20–34. doi: 10.1016/J.NEUBIOREV.2014.01.004
Grigoruta, M., Chavez-Solano, M., Varela-Ramirez, A., Sierra-Fonseca, J. A., Orozco-Lucero, E., Hamdan, J. N., et al. (2020). Maternal separation induces retinal and peripheral blood mononuclear cell alterations across the lifespan of female rats. Brain Res. 1749:147117. doi: 10.1016/J.BRAINRES.2020.147117
Hoeijmakers, L., Amelianchik, A., Verhaag, F., Kotah, J., Lucassen, P. J., and Korosi, A. (2018). Early-Life Stress Does Not Aggravate Spatial Memory or the Process of Hippocampal Neurogenesis in Adult and Middle-Aged APP/PS1 Mice. Front. Aging Neurosci. 10:61. doi: 10.3389/FNAGI.2018.00061
Hoffmann, A., and Spengler, D. (2018). The Mitochondrion as Potential Interface in Early-Life Stress Brain Programming. Front. Behav. Neurosci. 12:306. doi: 10.3389/FNBEH.2018.00306
Hui, J., Feng, G., Zheng, C., Jin, H., and Jia, N. (2017). Maternal separation exacerbates Alzheimer’s disease-like behavioral and pathological changes in adult APPswe/PS1dE9 mice. Behav. Brain Res. 318, 18–23. doi: 10.1016/J.BBR.2016.10.030
Jafari, Z., Mehla, J., Kolb, B. E., and Mohajerani, M. H. (2019). Gestational Stress Augments Postpartum β-Amyloid Pathology and Cognitive Decline in a Mouse Model of Alzheimer’s Disease. Cereb. Cortex 29, 3712–3724. doi: 10.1093/CERCOR/BHY251
Kalamakis, G., Brüne, D., Ravichandran, S., Bolz, J., Fan, W., Ziebell, F., et al. (2019). Quiescence Modulates Stem Cell Maintenance and Regenerative Capacity in the Aging Brain. Cell 176, 1407.e–1419.e. doi: 10.1016/J.CELL.2019.01.040
Kalish, B. T., Kim, E., Finander, B., Duffy, E. E., Kim, H., Gilman, C. K., et al. (2021). Maternal immune activation in mice disrupts proteostasis in the fetal brain. Nat. Neurosci. 24, 204–213. doi: 10.1038/S41593-020-00762-9
Karanikas, E., Daskalakis, N. P., and Agorastos, A. (2021). Oxidative Dysregulation in Early Life Stress and Posttraumatic Stress Disorder: a Comprehensive Review. Brain Sci. 11:723. doi: 10.3390/BRAINSCI11060723
Khorjahani, A., Peeri, M., and Azarbayjani, M. A. (2020). The Therapeutic Effect of Exercise on Anxiety and Bowel Oxidative Stress in the Maternal Separation Animal Model. Basic Clin. Neurosci. 11, 69–78. doi: 10.32598/BCN.9.10.450
Kim, J., Suh, Y. H., and Chang, K. A. (2021). Interleukin-17 induced by cumulative mild stress promoted depression-like behaviors in young adult mice. Mol. Brain 14:11. doi: 10.1186/S13041-020-00726-X
Kõks, S., Dogan, S., Tuna, B. G., González-Navarro, H., Potter, P., and Vandenbroucke, R. E. (2016). Mouse models of ageing and their relevance to disease. Mech. Ageing Dev. 160, 41–53. doi: 10.1016/J.MAD.2016.10.001
Ladd, C. O., Huot, R. L., Thrivikraman, K. V., Nemeroff, C. B., Meaney, M. J., and Plotsky, P. M. (2000). Long-term behavioral and neuroendocrine adaptations to adverse early experience. Prog. Brain Res. 122, 81–103. doi: 10.1016/S0079-6123(08)62132-9
Lapp, H. E., Mueller, I., and Moore, C. L. (2020). Limited bedding and nesting material changes indices of cellular metabolism and behavioral thermal regulation in Long-Evans rats during the first two weeks of life. Physiol. Behav. 222:112957. doi: 10.1016/J.PHYSBEH.2020.112957
Lesuis, S. L., Hoeijmakers, L., Korosi, A., de Rooij, S. R., Swaab, D. F., Kessels, H. W., et al. (2018a). Vulnerability and resilience to Alzheimer’s disease: early life conditions modulate neuropathology and determine cognitive reserve. Alzheimers Res. Ther. 10:95. doi: 10.1186/S13195-018-0422-7
Lesuis, S. L., Weggen, S., Baches, S., Lucassen, P. J., and Krugers, H. J. (2018b). Targeting glucocorticoid receptors prevents the effects of early life stress on amyloid pathology and cognitive performance in APP/PS1 mice. Trans. Psychiatry 8:53. doi: 10.1038/S41398-018-0101-2
Lesuis, S. L., Maurin, H., Borghgraef, P., Lucassen, P. J., van Leuven, F., and Krugers, H. J. (2016). Positive and negative early life experiences differentially modulate long term survival and amyloid protein levels in a mouse model of Alzheimer’s disease. Oncotarget 7, 39118–39135. doi: 10.18632/ONCOTARGET.9776
Liu, C., Hao, S., Zhu, M., Wang, Y., Zhang, T., and Yang, Z. (2018). Maternal Separation Induces Different Autophagic Responses in the Hippocampus and Prefrontal Cortex of Adult Rats. Neuroscience 374, 287–294. doi: 10.1016/J.NEUROSCIENCE.2018.01.043
Liu, P. Z., and Nusslock, R. (2018). How Stress Gets Under the Skin: Early Life Adversity and Glucocorticoid Receptor Epigenetic Regulation. Curr. Genom. 19, 653–664. doi: 10.2174/1389202919666171228164350
López-Otín, C., Blasco, M. A., Partridge, L., Serrano, M., and Kroemer, G. (2013). The hallmarks of aging. Cell 153:1194. doi: 10.1016/J.CELL.2013.05.039
Lu, T., Pan, Y., Kao, S. Y., Li, C., Kohane, I., Chan, J., et al. (2004). Gene regulation and DNA damage in the ageing human brain. Nature 429, 883–891. doi: 10.1038/NATURE02661
Mairesse, J., Vercoutter-Edouart, A. S., Marrocco, J., Zuena, A. R., Giovine, A., Nicoletti, F., et al. (2012). Proteomic characterization in the hippocampus of prenatally stressed rats. J. Proteom. 75, 1764–1770. doi: 10.1016/J.JPROT.2011.12.017
Marais, L., Hattingh, S. M., Stein, D. J., and Daniels, W. M. U. (2009). A proteomic analysis of the ventral hippocampus of rats subjected to maternal separation and escitalopram treatment. Metab. Brain Dis. 24, 569–586. doi: 10.1007/S11011-009-9156-3
Marini, S., Davis, K. A., Soare, T. W., Zhu, Y., Suderman, M. J., Simpkin, A. J., et al. (2020). Adversity exposure during sensitive periods predicts accelerated epigenetic aging in children. Psychoneuroendocrinology 113:104484. doi: 10.1016/J.PSYNEUEN.2019.104484
Markham, A., Bains, R., Franklin, P., and Spedding, M. (2014). Changes in mitochondrial function are pivotal in neurodegenerative and psychiatric disorders: how important is BDNF? Br. J. Pharmacol. 171, 2206–2229. doi: 10.1111/BPH.12531
Marrocco, J., Gray, J. D., Kogan, J. F., Einhorn, N. R., O’Cinneide, E. M., Rubin, T. G., et al. (2019). Early Life Stress Restricts Translational Reactivity in CA3 Neurons Associated With Altered Stress Responses in Adulthood. Front. Behav. Neurosci. 13:157. doi: 10.3389/FNBEH.2019.00157
Masrour, F. F., Peeri, M., Azarbayjani, M. A., and Hosseini, M. J. (2018). Voluntary Exercise During Adolescence Mitigated Negative the Effects of Maternal Separation Stress on the Depressive-Like Behaviors of Adult Male Rats: role of NMDA Receptors. Neurochem. Res. 43, 1067–1074. doi: 10.1007/s11064-018-2519-6
Mattson, M. P., and Arumugam, T. V. (2018). Hallmarks of Brain Aging: adaptive and Pathological Modification by Metabolic States. Cell Metab. 27, 1176–1199. doi: 10.1016/J.CMET.2018.05.011
McClelland, S., Korosi, A., Cope, J., Ivy, A., and Baram, T. Z. (2011). Emerging roles of epigenetic mechanisms in the enduring effects of early-life stress and experience on learning and memory. Neurobiol. Learn. Memory 96, 79–88. doi: 10.1016/J.NLM.2011.02.008
McEwen, B. S. (2007). Physiology and neurobiology of stress and adaptation: central role of the brain. Physiol. Rev. 87, 873–904. doi: 10.1152/PHYSREV.00041.2006
McEwen, B. S., and Morrison, J. H. (2013). The brain on stress: vulnerability and plasticity of the prefrontal cortex over the life course. Neuron 79, 16–29. doi: 10.1016/J.NEURON.2013.06.028
Merz, M. P., and Turner, J. D. (2021). Is early life adversity a trigger towards inflammageing? Exp. Gerontol. 150:111377. doi: 10.1016/J.EXGER.2021.111377
Miller, G. E., Chen, E., and Parker, K. J. (2011). Psychological stress in childhood and susceptibility to the chronic diseases of aging: moving toward a model of behavioral and biological mechanisms. Psychol. Bull. 137, 959–997. doi: 10.1037/A0024768
Molet, J., Maras, P. M., Avishai-Eliner, S., and Baram, T. Z. (2014). Naturalistic rodent models of chronic early-life stress. Dev. Psychobiol. 56, 1675–1688. doi: 10.1002/DEV.21230
Morrison, J. H., and Baxter, M. G. (2012). The ageing cortical synapse: hallmarks and implications for cognitive decline. Nat. Rev. Neurosci. 13, 240–250. doi: 10.1038/NRN3200
Mourtzi, N., Sertedaki, A., and Charmandari, E. (2021). Glucocorticoid Signaling and Epigenetic Alterations in Stress-Related Disorders. Int. J. Mol. Sci. 22:5964. doi: 10.3390/IJMS22115964
Muñoz-Carvajal, F., and Sanhueza, M. (2020). The Mitochondrial Unfolded Protein Response: a Hinge Between Healthy and Pathological Aging. Front. Aging Neurosci. 12:581849. doi: 10.3389/FNAGI.2020.581849
Nettis, M. A., Pariante, C. M., and Mondelli, V. (2020). Early-Life Adversity, Systemic Inflammation and Comorbid Physical and Psychiatric Illnesses of Adult Life. Curr. Topics Behav. Neurosci. 44, 207–225. doi: 10.1007/7854_2019_89
Nold, V., Sweatman, C., Karabatsiakis, A., Böck, C., Bretschneider, T., Lawless, N., et al. (2019). Activation of the kynurenine pathway and mitochondrial respiration to face allostatic load in a double-hit model of stress. Psychoneuroendocrinology 107, 148–159. doi: 10.1016/J.PSYNEUEN.2019.04.006
Orso, R., Creutzberg, K. C., Wearick-Silva, L. E., Wendt Viola, T., Tractenberg, S. G., Benetti, F., et al. (2019). How Early Life Stress Impact Maternal Care: a Systematic Review of Rodent Studies. Front. Behav. Neurosci. 13:197. doi: 10.3389/FNBEH.2019.00197
Oschwald, J., Guye, S., Liem, F., Rast, P., Willis, S., Röcke, C., et al. (2019). Brain structure and cognitive ability in healthy aging: a review on longitudinal correlated change. Rev. Neurosci. 31, 1–57. doi: 10.1515/REVNEURO-2018-0096
Palma-Gudiel, H., Fañanás, L., Horvath, S., and Zannas, A. S. (2020). Psychosocial stress and epigenetic aging. Int. Rev. Neurobiol. 150, 107–128. doi: 10.1016/BS.IRN.2019.10.020
Paradies, G., Petrosillo, G., Paradies, V., and Ruggiero, F. M. (2011). Mitochondrial dysfunction in brain aging: role of oxidative stress and cardiolipin. Neurochem. Int. 58, 447–457. doi: 10.1016/J.NEUINT.2010.12.016
Parel, S. T., and Peña, C. J. (2022). Genome-wide Signatures of Early-Life Stress: influence of Sex. Biol. Psychiatry 91, 36–42. doi: 10.1016/J.BIOPSYCH.2020.12.010
Peña, C. J., Smith, M., Ramakrishnan, A., Cates, H. M., Bagot, R. C., Kronman, H. G., et al. (2019). Early life stress alters transcriptomic patterning across reward circuitry in male and female mice. Nat. Commun. 10:5098. doi: 10.1038/S41467-019-13085-6
Perry, C. J., Campbell, E. J., Drummond, K. D., Lum, J. S., and Kim, J. H. (2021). Sex differences in the neurochemistry of frontal cortex: impact of early life stress. J. Neurochem. 157, 963–981. doi: 10.1111/JNC.15208
Pervanidou, P., and Chrousos, G. P. (2018). Early-Life Stress: from Neuroendocrine Mechanisms to Stress-Related Disorders. Horm. Res. Paediatr. 89, 372–379. doi: 10.1159/000488468
Picard, M., Juster, R. P., and McEwen, B. S. (2014). Mitochondrial allostatic load puts the “gluc” back in glucocorticoids. Nat. Rev. Endocrinol. 10, 303–310. doi: 10.1038/NRENDO.2014.22
Picard, M., McEwen, B. S., Epel, E. S., and Sandi, C. (2018). An energetic view of stress: focus on mitochondria. Front. Neuroendocrinol. 49:72–85. doi: 10.1016/J.YFRNE.2018.01.001
Picard, M., McManus, M. J., Gray, J. D., Nasca, C., Moffat, C., Kopinski, P. K., et al. (2015). Mitochondrial functions modulate neuroendocrine, metabolic, inflammatory, and transcriptional responses to acute psychological stress. Proc. Natl. Acad. Sci. U.S.A. 112, E6614–E6623. doi: 10.1073/PNAS.1515733112
Pinto, B. A. S., Melo, T. M., Flister, K. F. T., França, L. M., Kajihara, D., Tanaka, L. Y., et al. (2016). Early and sustained exposure to high-sucrose diet triggers hippocampal ER stress in young rats. Metab. Brain Dis. 31, 917–927. doi: 10.1007/S11011-016-9830-1
Pluvinage, J. V., and Wyss-Coray, T. (2020). Systemic factors as mediators of brain homeostasis, ageing and neurodegeneration. Nat. Rev. Neurosci. 21, 93–102. doi: 10.1038/S41583-019-0255-9
Price, L. H., Kao, H. T., Burgers, D. E., Carpenter, L. L., and Tyrka, A. R. (2013). Telomeres and early-life stress: an overview. Biol. Psychiatry 73, 15–23. doi: 10.1016/J.BIOPSYCH.2012.06.025
Psarra, A. M. G., and Sekeris, C. E. (2009). Glucocorticoid receptors and other nuclear transcription factors in mitochondria and possible functions. Biochim. et Biophys. Acta 1787, 431–436. doi: 10.1016/J.BBABIO.2008.11.011
Pusalkar, M., Suri, D., Kelkar, A., Bhattacharya, A., Galande, S., and Vaidya, V. A. (2016). Early stress evokes dysregulation of histone modifiers in the medial prefrontal cortex across the life span. Dev. Psychobiol. 58, 198–210. doi: 10.1002/DEV.21365
Raz, N., and Rodrigue, K. M. (2006). Differential aging of the brain: patterns, cognitive correlates and modifiers. Neurosci. Biobehav. Rev. 30, 730–748. doi: 10.1016/J.NEUBIOREV.2006.07.001
Regnell, C. E., Hildrestrand, G. A., Sejersted, Y., Medin, T., Moldestad, O., Rolseth, V., et al. (2012). Hippocampal adult neurogenesis is maintained by Neil3-dependent repair of oxidative DNA lesions in neural progenitor cells. Cell Rep. 2, 503–510. doi: 10.1016/J.CELREP.2012.08.008
Reshetnikov, V., Ryabushkina, Y., Kovner, A., Lepeshko, A., and Bondar, N. (2020). Repeated and single maternal separation specifically alter microglial morphology in the prefrontal cortex and neurogenesis in the hippocampus of 15-day-old male mice. Neuroreport 31, 1256–1264. doi: 10.1097/WNR.0000000000001544
Réus, G. Z., Fernandes, G. C., de Moura, A. B., Silva, R. H., Darabas, A. C., de Souza, T. G., et al. (2017). Early life experience contributes to the developmental programming of depressive-like behaviour, neuroinflammation and oxidative stress. J. Psychiatric Res. 95, 196–207. doi: 10.1016/J.JPSYCHIRES.2017.08.020
Réus, G. Z., Silva, R. H., de Moura, A. B., Presa, J. F., Abelaira, H. M., Abatti, M., et al. (2019). Early Maternal Deprivation Induces Microglial Activation, Alters Glial Fibrillary Acidic Protein Immunoreactivity and Indoleamine 2,3-Dioxygenase during the Development of Offspring Rats. Mol. Neurobiol. 56, 1096–1108. doi: 10.1007/S12035-018-1161-2
Ridout, K. K., Levandowski, M., Ridout, S. J., Gantz, L., Goonan, K., Palermo, D., et al. (2018). Early life adversity and telomere length: a meta-analysis. Mol. Psychiatry 23, 858–871. doi: 10.1038/MP.2017.26
Roth, T. L., Lubin, F. D., Funk, A. J., and Sweatt, J. D. (2009). Lasting epigenetic influence of early-life adversity on the BDNF gene. Biol. Psychiatry 65, 760–769. doi: 10.1016/J.BIOPSYCH.2008.11.028
Roth, T. L., and Sweatt, J. D. (2011). Epigenetic marking of the BDNF gene by early-life adverse experiences. Horm. Behav. 59, 315–320. doi: 10.1016/J.YHBEH.2010.05.005
Rothman, S. M., and Mattson, M. P. (2013). Activity-dependent, stress-responsive BDNF signaling and the quest for optimal brain health and resilience throughout the lifespan. Neuroscience 239, 228–240. doi: 10.1016/J.NEUROSCIENCE.2012.10.014
Ruigrok, S. R., Yim, K., Emmerzaal, T. L., Geenen, B., Stöberl, N., den Blaauwen, J. L., et al. (2021). Effects of early-life stress on peripheral and central mitochondria in male mice across ages. Psychoneuroendocrinology 132:105346. doi: 10.1016/J.PSYNEUEN.2021.105346
Ruiz, R., Roque, A., Pineda, E., Licona-Limón, P., José Valdéz-Alarcón, J., and Lajud, N. (2018). Early life stress accelerates age-induced effects on neurogenesis, depression, and metabolic risk. Psychoneuroendocrinology 96, 203–211. doi: 10.1016/J.PSYNEUEN.2018.07.012
Sarter, M., and Bruno, J. P. (2004). Developmental origins of the age-related decline in cortical cholinergic function and associated cognitive abilities. Neurobiol. Aging 25, 1127–1139. doi: 10.1016/J.NEUROBIOLAGING.2003.11.011
Saulnier, R. J., Best, C., Kostyniuk, D. J., Gilmour, K. M., and Lamarre, S. G. (2021). Chronic social stress alters protein metabolism in juvenile rainbow trout, Oncorhynchus mykiss. J. Comp. Physiol. B Biochem. Syst. Environ. Physiol. 191, 517–530. doi: 10.1007/S00360-021-01340-6
Schmidt, M. V., Wang, X. D., and Meijer, O. C. (2011). Early life stress paradigms in rodents: potential animal models of depression? Psychopharmacology 214, 131–140. doi: 10.1007/S00213-010-2096-0
Semple, B. D., Blomgren, K., Gimlin, K., Ferriero, D. M., and Noble-Haeusslein, L. J. (2013). Brain development in rodents and humans: identifying benchmarks of maturation and vulnerability to injury across species. Prog. Neurobiol. 10, 1–16. doi: 10.1016/J.PNEUROBIO.2013.04.001
Seo, M. K., Kim, S. G., Seog, D. H., Bahk, W. M., Kim, S. H., Park, S. W., et al. (2020). Effects of Early Life Stress on Epigenetic Changes of the Glucocorticoid Receptor 1 7 Promoter during Adulthood. Int. J. Mol. Sci. 21, 1–12. doi: 10.3390/IJMS21176331
Seo, M. K., Ly, N. N., Lee, C. H., Cho, H. Y., Choi, C. M., Nhu, L. H., et al. (2016). Early life stress increases stress vulnerability through BDNF gene epigenetic changes in the rat hippocampus. Neuropharmacology 105, 388–397. doi: 10.1016/J.NEUROPHARM.2016.02.009
Short, A. K., Maras, P. M., Pham, A. L., Ivy, A. S., and Baram, T. Z. (2020). Blocking CRH receptors in adults mitigates age-related memory impairments provoked by early-life adversity. Neuropsychopharmacology 45, 515–523. doi: 10.1038/S41386-019-0562-X
Sierra-Fonseca, J. A., Hamdan, J. N., Cohen, A. A., Cardenas, S. M., Saucedo, S., Lodoza, G. A., et al. (2021). Neonatal Maternal Separation Modifies Proteostasis Marker Expression in the Adult Hippocampus. Front. Mol. Neurosci. 14:661993. doi: 10.3389/FNMOL.2021.661993
Simen, A. A., Bordner, K. A., Martin, M. P., Moy, L. A., and Barry, L. C. (2011). Cognitive dysfunction with aging and the role of inflammation. Ther. Adv. Chronic Dis. 2, 175–195. doi: 10.1177/2040622311399145
Smart, C., Strathdee, G., Watson, S., Murgatroyd, C., and McAllister-Williams, R. H. (2015). Early life trauma, depression and the glucocorticoid receptor gene–an epigenetic perspective. Psychol. Med. 45, 3393–3410. doi: 10.1017/S0033291715001555
Sousa, V. C., Vital, J., Costenla, A. R., Batalha, V. L., Sebastião, A. M., Ribeiro, J. A., et al. (2014). Maternal separation impairs long term-potentiation in CA1-CA3 synapses and hippocampal-dependent memory in old rats. Neurobiol. Aging 35, 1680–1685. doi: 10.1016/J.NEUROBIOLAGING.2014.01.024
Sparkman, N. L., and Johnson, R. W. (2008). Neuroinflammation associated with aging sensitizes the brain to the effects of infection or stress. Neuroimmunomodulation 15, 323–330. doi: 10.1159/000156474
Sterlemann, V., Rammes, G., Wolf, M., Liebl, C., Ganea, K., Müller, M. B., et al. (2010). Chronic social stress during adolescence induces cognitive impairment in aged mice. Hippocampus 20, 540–549. doi: 10.1002/HIPO.20655
Suri, D., Bhattacharya, A., and Vaidya, V. A. (2014). Early stress evokes temporally distinct consequences on the hippocampal transcriptome, anxiety and cognitive behaviour. Int. J. Neuropsychopharmacol. 17, 289–301. doi: 10.1017/S1461145713001004
Suri, D., and Vaidya, V. A. (2013). Glucocorticoid regulation of brain-derived neurotrophic factor: relevance to hippocampal structural and functional plasticity. Neuroscience 239, 196–213. doi: 10.1016/J.NEUROSCIENCE.2012.08.065
Suri, D., and Vaidya, V. A. (2015). The adaptive and maladaptive continuum of stress responses - a hippocampal perspective. Rev. Neurosci. 26, 415–442. doi: 10.1515/REVNEURO-2014-0083
Suri, D., Veenit, V., Sarkar, A., Thiagarajan, D., Kumar, A., Nestler, E. J., et al. (2013). Early stress evokes age-dependent biphasic changes in hippocampal neurogenesis. BDNF Express. Cogn. Biol. Psychiatry 73, 658–666. doi: 10.1016/J.BIOPSYCH.2012.10.023
Swaab, D. F., Bao, A. M., and Lucassen, P. J. (2005). The stress system in the human brain in depression and neurodegeneration. Ageing Res. Rev. 4, 141–194. doi: 10.1016/J.ARR.2005.03.003
Syed, S. A., and Nemeroff, C. B. (2017). Early Life Stress, Mood, and Anxiety Disorders. Chron. Stress 1:2470547017694461. doi: 10.1177/2470547017694461
Szyf, M. (2009). The early life environment and the epigenome. Biochim. et Biophys. Acta 1790, 878–885. doi: 10.1016/J.BBAGEN.2009.01.009
Szyf, M. (2019). The epigenetics of perinatal stress. Dial. Clin. Neurosci. 21, 369–378. doi: 10.31887/DCNS.2019.21.4/MSZYF
Talukdar, P. M., Abdul, F., Maes, M., Binu, V., Venkatasubramanian, G., Kutty, B. M., et al. (2020). Maternal Immune Activation Causes Schizophrenia-like Behaviors in the Offspring through Activation of Immune-Inflammatory, Oxidative and Apoptotic Pathways, and Lowered Antioxidant Defenses and Neuroprotection. Mol. Neurobiol. 57, 4345–4361. doi: 10.1007/S12035-020-02028-8
Tay, T. L., Béchade, C., D’Andrea, I., St-Pierre, M. K., Henry, M. S., Roumier, A., et al. (2018). Microglia Gone Rogue: impacts on Psychiatric Disorders across the Lifespan. Front. Mol. Neurosci. 10:421. doi: 10.3389/FNMOL.2017.00421
Teicher, M. H., Gordon, J. B., and Nemeroff, C. B. (2021). Recognizing the importance of childhood maltreatment as a critical factor in psychiatric diagnoses, treatment, research, prevention, and education. Mol. Psychiatry 26, 1–8. doi: 10.1038/S41380-021-01367-9
Teicher, M. H., Samson, J. A., Anderson, C. M., and Ohashi, K. (2016). The effects of childhood maltreatment on brain structure, function and connectivity. Nat. Rev. Neurosci. 17, 652–666. doi: 10.1038/NRN.2016.111
Torres-Berrío, A., Issler, O., Parise, E. M., and Nestler, E. J. (2019). Unraveling the epigenetic landscape of depression: focus on early life stress. Dial. Clin. Neurosci. 21, 341–357. doi: 10.31887/DCNS.2019.21.4/ENESTLER
Tyrka, A. R., Parade, S. H., Price, L. H., Kao, H. T., Porton, B., Philip, N. S., et al. (2016). Alterations of Mitochondrial DNA Copy Number and Telomere Length With Early Adversity and Psychopathology. Biol. Psychiatry 79, 78–86. doi: 10.1016/J.BIOPSYCH.2014.12.025
Tzanoulinou, S., and Sandi, C. (2017). The Programming of the Social Brain by Stress During Childhood and Adolescence: from Rodents to Humans. Curr. Topics Behav. Neurosci. 30, 411–429. doi: 10.1007/7854_2015_430
Uddin, M. S., Yu, W. S., and Lim, L. W. (2021). Exploring ER stress response in cellular aging and neuroinflammation in Alzheimer’s disease. Ageing Res. Rev. 70:101417. doi: 10.1016/J.ARR.2021.101417
Usui, N., Ono, Y., Aramaki, R., Berto, S., Konopka, G., Matsuzaki, H., et al. (2021). Early Life Stress Alters Gene Expression and Cytoarchitecture in the Prefrontal Cortex Leading to Social Impairment and Increased Anxiety. Front. Genet. 12:754198. doi: 10.3389/FGENE.2021.754198
van Zyl, P. J., Dimatelis, J. J., and Russell, V. A. (2016). Behavioural and biochemical changes in maternally separated Sprague-Dawley rats exposed to restraint stress. Metab. Brain Dis. 31, 121–133. doi: 10.1007/S11011-015-9757-Y
Vasileiou, P., Evangelou, K., Vlasis, K., Fildisis, G., Panayiotidis, M., Chronopoulos, E., et al. (2019). Mitochondrial Homeostasis and Cellular Senescence. Cells 8:686. doi: 10.3390/CELLS8070686
Walker, C. D., Bath, K. G., Joels, M., Korosi, A., Larauche, M., Lucassen, P. J., et al. (2017). Chronic early life stress induced by limited bedding and nesting (LBN) material in rodents: critical considerations of methodology, outcomes and translational potential. Stress 20, 421–448. doi: 10.1080/10253890.2017.1343296
Wang, S., Lai, X., Deng, Y., and Song, Y. (2020). Correlation between mouse age and human age in anti-tumor research: significance and method establishment. Life Sci. 242:117242. doi: 10.1016/J.LFS.2019.117242
Weaver, I. C. G. (2007). Epigenetic programming by maternal behavior and pharmacological intervention. Nature versus nurture: let’s call the whole thing off. Epigenetics 2, 22–28. doi: 10.4161/EPI.2.1.3881
Webb, M., and Sideris, D. P. (2020). Intimate Relations-Mitochondria and Ageing. Int. J. Mol. Sci. 21, 1–48. doi: 10.3390/IJMS21207580
Wyss-Coray, T. (2016). Ageing, neurodegeneration and brain rejuvenation. Nature 539, 180–186. doi: 10.1038/NATURE20411
Yajima, H., Haijima, A., Khairinisa, M. A., Shimokawa, N., Amano, I., and Takatsuru, Y. (2018). Early-life stress induces cognitive disorder in middle-aged mice. Neurobiol. Aging 64, 139–146. doi: 10.1016/J.NEUROBIOLAGING.2017.12.021
Yau, J. L. W., and Seckl, J. R. (2012). Local amplification of glucocorticoids in the aging brain and impaired spatial memory. Front. Aging Neurosci. 4:24. doi: 10.3389/FNAGI.2012.00024
Yuan, R., Tsaih, S. W., Petkova, S. B., de Evsikova, C. M., Xing, S., Marion, M. A., et al. (2009). Aging in inbred strains of mice: study design and interim report on median lifespans and circulating IGF1 levels. Aging Cell 8, 277–287. doi: 10.1111/J.1474-9726.2009.00478.X
Zannas, A. S. (2018). Gene-environment Interactions in Late Life: linking Psychosocial Stress with Brain Aging. Curr. Neuropharmacol. 16, 327–333. doi: 10.2174/1570159X15666171109121452
Zannas, A. S. (2019). Epigenetics as a key link between psychosocial stress and aging: concepts, evidence, mechanisms. Dial. Clin. Neurosci. 21, 389–396. doi: 10.31887/DCNS.2019.21.4/AZANNAS
Keywords: maternal separation, early stress, hippocampus, proteostasis, mitochondria, neuronal survival, neuroinflammation, cognition
Citation: Chaudhari PR, Singla A and Vaidya VA (2022) Early Adversity and Accelerated Brain Aging: A Mini-Review. Front. Mol. Neurosci. 15:822917. doi: 10.3389/fnmol.2022.822917
Received: 26 November 2021; Accepted: 25 February 2022;
Published: 22 March 2022.
Edited by:
Parthiv Haldipur, Seattle Children’s Research Institute, United StatesReviewed by:
Weiwen Wang, Institute of Psychology (CAS), ChinaCopyright © 2022 Chaudhari, Singla and Vaidya. This is an open-access article distributed under the terms of the Creative Commons Attribution License (CC BY). The use, distribution or reproduction in other forums is permitted, provided the original author(s) and the copyright owner(s) are credited and that the original publication in this journal is cited, in accordance with accepted academic practice. No use, distribution or reproduction is permitted which does not comply with these terms.
*Correspondence: Vidita A. Vaidya, dnZhaWR5YUB0aWZyLnJlcy5pbg==
†These authors have contributed equally to this work and share first authorship