- World-Class Research Centre for Personalized Medicine, Almazov National Medical Research Centre, Saint Petersburg, Russia
This review describes the heterogeneity of peripheral glial cell populations, from the emergence of Schwann cells (SCs) in early development, to their involvement, and that of their derivatives in adult glial populations. We focus on the origin of the first glial precursors from neural crest cells (NCCs), and their ability to differentiate into several cell types during development. We also discuss the heterogeneity of embryonic glia in light of the latest data from genetic tracing and transcriptome analysis. Special attention has been paid to the biology of glial populations in adult animals, by highlighting common features of different glial cell types and molecular differences that modulate their functions. Finally, we consider the communication of glial cells with axons of neurons in normal and pathological conditions. In conclusion, the present review details how information available on glial cell types and their functions in normal and pathological conditions may be utilized in the development of novel therapeutic strategies for the treatment of patients with neurodiseases.
Embryonic Schwann Cells: Origin, Development, and Stemness
Schwann cells (SCs), or neurolemmocytes, are a type of glial cells that originate from neural crest cells (NCCs). Post delamination from the dorsal neural tube, these cells migrate to the embryo’s body and give rise to multiple cell lineages during early vertebrate development (Le Douarin and Kalcheim, 1999; Bronner and LeDouarin, 2012). NCCs give rise to a wide range of types of differentiated cells, such as craniofacial cartilage and bones, cardiac outflow septum, mesenchyme, pigment cells, and peripheral nervous system (Le Douarin and Kalcheim, 1999). According to the classical view, NCCs specialize into terminally differentiated glia (mature SCs) via a series of intermediate stages. These include, the differentiation of NCCs into Schwann cell precursors (S), followed by the differentiation of SCPs into immature SCs (iSCs), which, in turn, specialize into myelinating (mySCs) or non-myelinating mature SCs (nmSCs) (see review Jessen and Mirsky, 2019). The advent of genetic tracing methods, that allow accurate determination of the hierarchy of crest cells during development, has revealed the complexities associated with the various stages of NCCs differentiation, and that of their descendants. Using Cre-Lox genetic constructions and model animals, have revealed significant insights into the nature of SCPs and their capability to differentiate into a wide range of terminally differentiated cells in early development over the past 10 years (Figure 1; Adameyko et al., 2009; Dyachuk et al., 2014; Kaukua et al., 2014; Furlan et al., 2017; Hockman et al., 2018; Kastriti et al., 2019; Xie et al., 2019; Kamenev et al., 2021). The distinguishing feature that sets SCPs apart from iSCP and mSCPs, is that they retain the ability to differentiate into other cell types (Adameyko et al., 2009; Dyachuk et al., 2014; Kaukua et al., 2014; Furlan et al., 2017; Kastriti et al., 2019; Xie et al., 2019; Kamenev et al., 2021). This characteristic that SCPs partially share with NCCs, may possibly allow them to be exploited for the development of novel therapeutic strategies. Confirmatory evidence of SCPs’ ability to specialize into other cell types was obtained by the demonstration of their differentiation into skin melanocytes (Adameyko et al., 2009) as well as extracutaneous melanocytes to the heart, inner ear, supraorbital locations and brain meninges (Kaucka et al., 2021). Another example of the multipotency of SCPs is their ability to give rise to parasympathetic neurons. Genetic tracing experiments by two independent research groups on transgenic mice, have convincingly demonstrated that parasympathetic neurons in the cranial ganglia, intramular (interstitial) ganglia of the heart, and sacral parasympathetic ganglia after E12.5 are derived from nerve-associated SCPs (Dyachuk et al., 2014; Espinosa-Medina et al., 2014). Genetic tracing has convincingly shown the involvement of local SCPs in origin of the glomus cells of the carotid body oxygen-sensing organ that are primary oxygen-sensing cells (Hockman et al., 2018). Interesting data have been found on the differentiation of SCPs into enteric neurons of the enteric nervous system (ENS). SCPs are capable of differentiating into neurons in the gut during postnatal neurogenesis (Uesaka et al., 2015). Zebrafish lineage tracing performed using lipophilic dyes or the inducible Sox10-Cre system, recently revealed that post-embryonic enteric neurons arise from trunk neural crest-derived SCPs that migrate from the spinal cord into the intestines of these organisms (El-Nachef and Bronner, 2020). Additionally, SCPs might function as a source of mesenchymal cells that produce pulp cells and odontoblasts, as has been observed in a mouse growing tooth model (Kaukua et al., 2014). Besides the above-mentioned cell types, neuroendocrine cells of the adrenal medulla (chromaffin cells) have also been shown to be derived from SCPs in mouse embryos and zebrafish larvae (Furlan et al., 2017; Kamenev et al., 2021). Moreover, chromaffin cells of the organ of Zuckerkandl, and a portion of sympathetic neurons of the posterior paraganglia are known to be largely of SCP origin (Kastriti et al., 2019). Recent genetic lineage tracing has revealed that certain SCPs detach from nerve fibers to become mesenchymal cells, which further differentiate into chondrocytes and mature osteocytes during murine embryonic development. Moreover, the development of chondrocytes from SCPs is also known to occur in zebrafish, thus indicating evolutionary conservation (Xie et al., 2019).
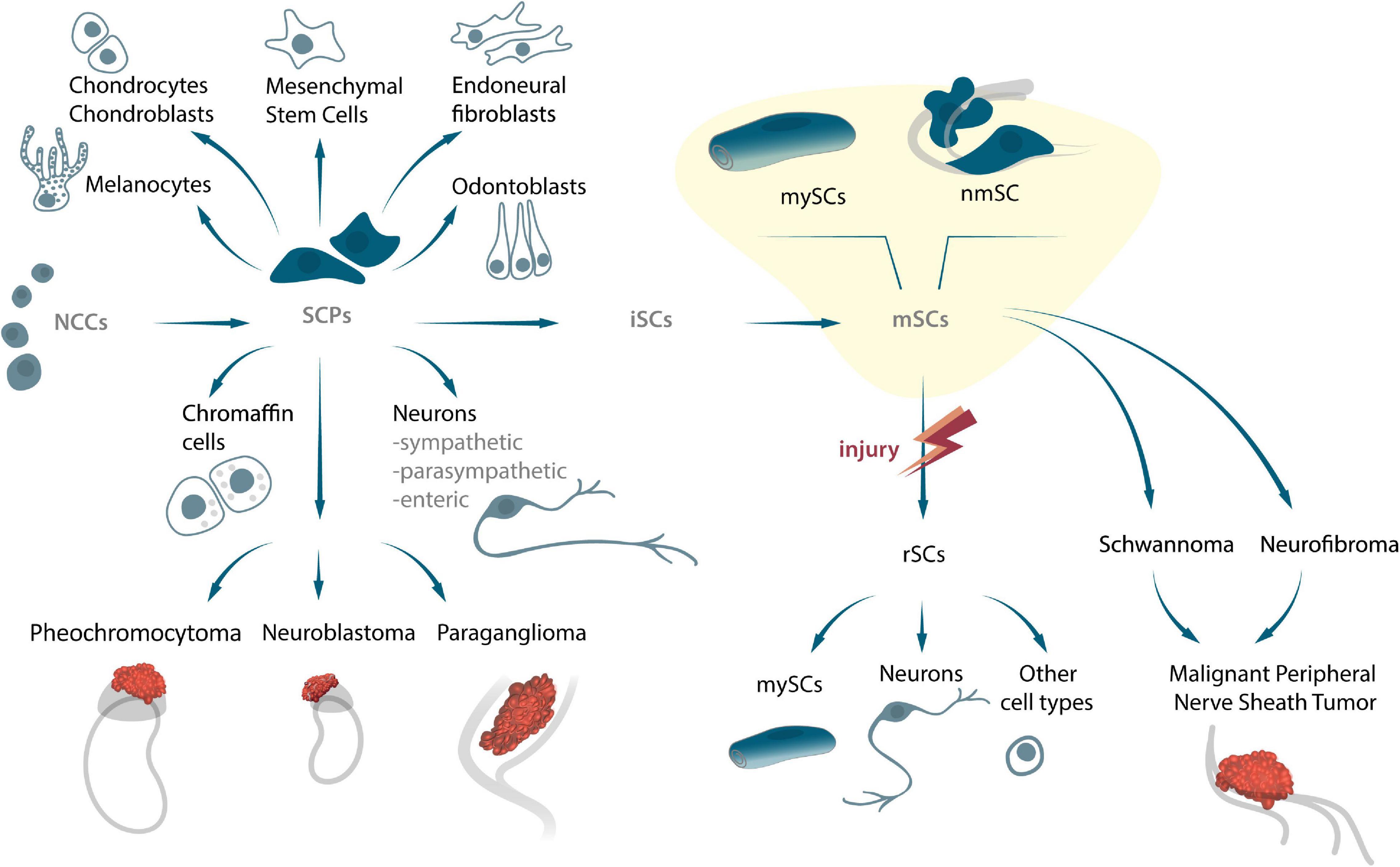
Figure 1. The origin of SCPs and SCs, their potency to differentiate into several cell types, and their capability to transform into peripheral nerve tumors during embryonic and adult stages.
One of the reasons that precluded elucidation of the properties of SCs was the similar expression patterns of key transcriptional factors (TF) including Sox9/10, FoxD3, and Tfap2a/b, as well as membrane molecules such as Plp1 and Erbb2/3 in both SCPs and NCCs (Stewart et al., 2001; Cheung and Briscoe, 2003; Nitzan et al., 2013; Balakrishnan et al., 2016). Despite the molecular similarities between these two cell types, newer data has revealed significant distinguishing characteristics, that are specific to SCPs in the context of cellular and molecular signatures (Lignell et al., 2017; Soldatov et al., 2019).
Several tumors originate from SC lines, which include schwannomas, neurofibromas and malignant tumors of the peripheral nerve membranes (MPNST) (Giovannini et al., 1999; Zhu et al., 2002; Evans et al., 2012; Figure 1). Given the wide potential of SCPs, and the fact that they are direct precursors of these cells under normal circumstances, it is very likely that they are involved in the development of embryonal tumors. For instance, NCCs and SCPs are both the original source of the sympatho-adrenal (sympathetic neurons and chromaffin cells) nervous systems, and can consequently also be responsible for the occurrence of pheochromocytes, neuroblastoma, and paragangliomas during sympatho-adrenal differentiation (Figure 1). The diversity of progenitors and cell transitions during early development might be recapitulated in some solid tumors associated with sympathetic ganglia and the adrenal gland (Lee et al., 2005; Scriba et al., 2020). Recently, using a combination of single cell transcriptomics and lineage tracing it was shown that human intra-adrenal sympathoblasts are directly derived from SCPs and can transit into local neuroendocrine chromaffin cells. Authors suggest, that in humans, this process persists during several weeks of development within the large intra-adrenal ganglia-like structures, which may also serve as reservoirs of originating cells in neuroblastoma (Kameneva et al., 2021). Obviously, new lineage connections might have important implications for understanding of neuroblastoma origin and cell heterogeneity Since multiple tumor cells share key genetic and signaling mechanisms associated with normal development and cancer, an in-depth analysis of the cellular and molecular mechanisms of embryonic cell-cancer cell transitions is of exceeding importance in understanding biological processes and developing personalized medicine.
The mechanisms of attachment NCCs to axons, and their subsequent transformation into SCPs, that either remain attached or detach from nerves to undergo further differentiation into diverse cell types is not fully understood. However, multiple studies on the specialization of glia into different cell types, report a downregulation of the glial program, concomitant with an upregulation of the said cell type program (for instance, neuronal, pigment, or mesodermal programs) (Adameyko et al., 2009; Dyachuk et al., 2014; Xie et al., 2019; Kamenev et al., 2021). The NRG1-ERBB2/3 signaling pathway plays a key role in NCCs and SCP migration/survival in mice and zebrafish (Birchmeier and Nave, 2008; Honjo et al., 2008; Kamenev et al., 2021). The transition from NCCs to SCPs is consequent to the interaction of the former with axons (Figure 2A). The molecular mechanisms that direct a part of the population of NCCs to settle on peripheral nerves, and the underlying reasons responsible for the selection of certain types of nerves are not entirely clear. The transition from SCPs to iSCs is accompanied by an important mechanistic step that involves the selection of axons for myelination (radial sorting) by iSCs, which in turn differentiate into a pro-myelinating SCs (Feltri et al., 2016). The remaining iSCs connect with axons of smaller diameter, and differentiate into non-myelinating SCs (Remak cells) (Monk et al., 2015; Gomez-Sanchez et al., 2017).
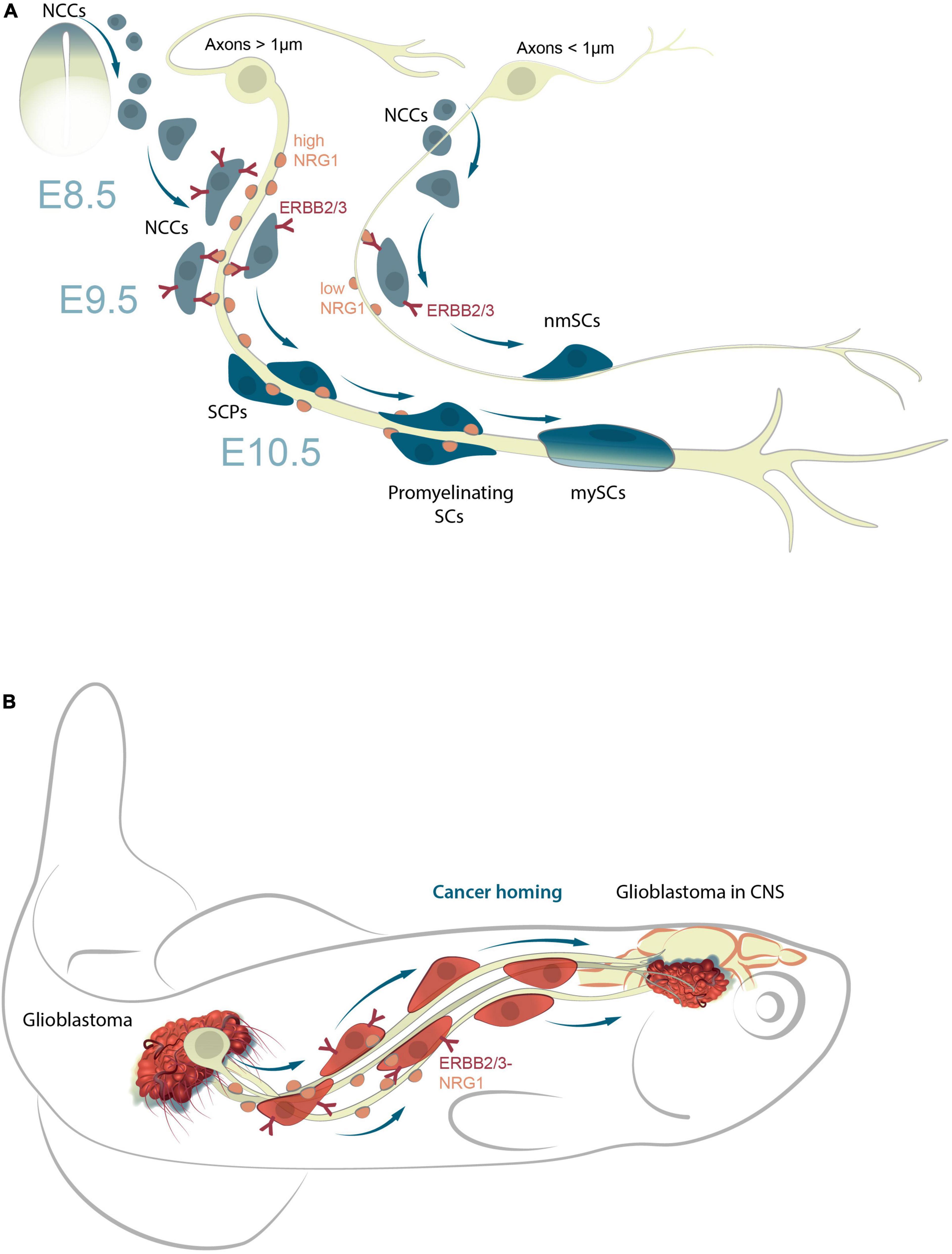
Figure 2. NRG1-ERBB2/3 signal pathway responsible for the maturation of SCPs into mnSCs during development and in cancer homing. (A) NRG1-ERBB2/3 interactions are essential for cellular attachment to axons and migration of SCPs along peripheral nerves; SCP survival is dependent on NRG1 concentrations. Those that receive insufficient NRG1 signals undergo apoptosis, or remain NCCs. Those that receive sufficient NRG1 signals differentiate into iSCs and then mySC, or detach from axons on reaching target-tissues and differentiate into other cell types (neurons, chromaffin cells, odontoblasts, or pigment cells). (B) A model of NRG1-ERBB2/3 signaling in cancer homing from periphery to CNS (zebrafish model system). NRG1 and ERBB induce the epithelial-mesenchymal transition (EMT) in certain cancers (e.g., breast) by modulating expression of proteins involved in invasion and metastasis. Eventually, the cancer-axonal molecular machinery triggers cancer cell dissemination and homing (e.g., glioblastoma migration from periphery to CNS) via nerve signals.
The decision of iSCs to differentiate into either myelinating or non-myelinating SCs is dictated by the expression levels of neuregulin 1 (NRG1). While lower levels of NRG1, released by a comparatively smaller axon leads to the maturation of iSCs into non-myelinating SCs, higher levels, result in the development of myelinating SCs (Figure 2A; Michailov et al., 2004; Taveggia et al., 2005; Gomez-Sanchez et al., 2017). Notably, this NRG1-ERBB2/3 signal pathway in human glioma cells, also promotes their migration in malignancy (Zhao and Schachner, 2013). It is likely that this mechanism, is also involved in the migration of cancer cells, for example, when human glioblastomas cells where transplanted into zebrafish (Pudelko et al., 2018; Figure 2B).
Despite the discovery of the ability of SCPs to transform into various cell types, the underlying molecular mechanisms responsible for these transitions remain unelucidated. While the overall pattern of molecular changes evidently involves the downregulation of glial genes and upregulation of cell-specific genes during early development, detailed information on the accompanying changes in gene cascades are not well understood. However, single cell trancriptomic analysis, has led to the revelation of changes in expression patterns of TFs and other molecules, that occur during the transition of NCCS and/or SCPs to specialized cells of the autonomic nervous system, sensory neurons, mesenchymal cells, and chromaffin cells (Dyachuk et al., 2014; Espinosa-Medina et al., 2014; Furlan et al., 2017; Soldatov et al., 2019). This data on the genetic basis of cellular transitions in early development might contribute immensely to the development of novel technologies, which may be exploited to regulate the processes of differentiation during development, or to correct human pathological states. Currently, several issues pertaining to the heterogeneity of SCPs and iSCs during developmental processes need to be addressed. These include unraveling the extent of heterogeneity among SCPs located on different nerves. Further, questions regarding how the positions of nerves, and glia attached to nerves, might affect the molecular signatures of SCPs need to be answered.
A secondary embryonic glial cell population, referred to as boundary cap cells (BCCs) is a subpopulation of multipotent cells that originate from NCCs, and give rise to cells of different lineages (Golding and Cohen, 1997). BCCs contribute with all SCPs occupying the dorsal roots, the progenitors of neurons, mainly nociceptive afferents, and satellite cells (Maro et al., 2004). Besides, BCC migrate along peripheral nerves to reach the skin, where they give rise to terminal glia associated with dermal nerve endings and neurogenic stem cells in the skin (Gresset et al., 2015). BCCs are localized to the dorsal root entry zone and motor exit point of the embryonic spinal cord, at the border between the central and peripheral nervous systems (Niederländer and Lumsden, 1996; Radomska and Topilko, 2017). Microarrays, genetic deletion assays, and single cell transcriptomic analysis have revealed sets of TFs that are expressed in both NCCs and BCCs (FOXD3 and SOX10), along with BCCs -specific TFs (KROX-20, NTRN5, WIF1, and HEY2) (Coulpier et al., 2009; Garrett et al., 2016; Faure et al., 2020). Supportive evidence for the multipotency of BCCs has been provided by genetic tracing and mice knockout models. These studies have determined that the functions of these cells include the production of glia and neurons of the PNS, as well as skin melanocytes and pericytes in cutaneous vasculature. Additionally, they also serve as gatekeepers that prevent motor neurons from escaping the CNS (Radomska and Topilko, 2017).
Adult Peripheral Glial Cells: Heterogeneity, Proliferation, Stemness, and Repair Capacity
Neural crest cells generate a diverse set of specialized non-myelinating cells during prenatal development. These include satellite glial cells of sensory and autonomic ganglia (Zirlinger et al., 2002; Dyachuk et al., 2014; Furlan et al., 2017), olfactory ensheathing cells (OSNs) (Barraud et al., 2010), perisynaptic Schwann cells (PSCs) (Armati, 2007), enteric glia (see review Bronner and LeDouarin, 2012), and other uncharacterized subtypes of adult glial cells (Figure 3). The following section of this review focuses on the biology and function of those populations of adult glia cells that may be potentially exploited for the regeneration of axons and other different cell types, and thereby aid the development of cell-based therapies to treat various pathologies.
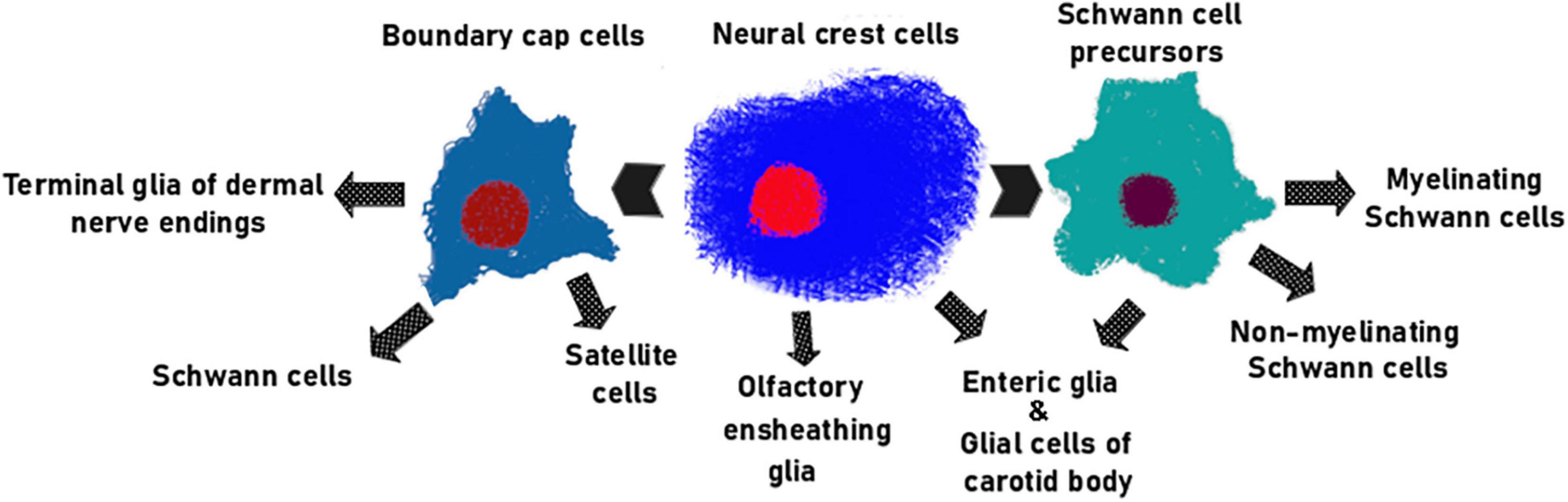
Figure 3. The main glial lineages originating from NC. Olfactory ensheathing cells (Barraud et al., 2010; Murdoch et al., 2010; Forni et al., 2011; Katoh et al., 2011) and majority of enteric glia (Espinosa-Medina et al., 2017) are direct descendants of NCCs. SCPs generate both myelinating and non-myelinating SCs (Jessen and Mirsky, 2019) along with portion of enteric glial cells (Uesaka et al., 2021). BCCs give rise to satellite glia (Maro et al., 2004), glomus cells of the carotid body (Hockman et al., 2018), terminal glial cells of the dermal nerve endings (Gresset et al., 2015) and mature Schwann cells of the nerve roots (Coulpier et al., 2009).
Perisynaptic Schwann Cells
Perisynaptic Schwann cells (PSCs) are localized at neuromuscular junctions and play a key role in synapse formation during development (Herrera et al., 2000). Although growing motor axons successfully reach target muscles and inhibition of muscle activity allows synapse formation even in the complete absence of PSCs (Lin et al., 2000; Liu et al., 2019), they are essential to prevent the degeneration of nerve terminals after initial contact (Riethmacher et al., 1997). PSCs participate in the remodeling of motor units during early postnatal development. The process involves the initial formation of multiple connections between each muscle fiber with several motor neurons, and the subsequent transition to mononeural innervation by the separation and elimination of competing nerve terminals is mediated by PSCs. The decoding of presynaptic activity by PSCs has been attributed to purinergic signaling (Darabid et al., 2013). Thus, both the synaptic efficacy of competing terminals and postsynaptic activity play key roles in the regulation of synapse elimination by PSCs.
The function of the PSC is not limited only to clearing neuronal debris during early development. PSCs have been demonstrated to function as major phagocytic cells such disease as Guillain-Barre syndrome (Cunningham et al., 2020). After denervation, PSCs formed “bridge-like” structures with undamaged synapses (Love and Thompson, 1999), which facilitate the growth of nerve terminals to denervated endplates. The sprouting of PSC is dependent on NRG1-ErbB2 signaling, since both the expression of the constitutively active ErbB2 receptors (Hayworth et al., 2006), and the presence of the soluble form of neurogenin (GGF2), enhance the migratory potential of these cells (Trachtenberg and Thompson, 1997). Further, the treatment of chicken embryo with the NRG antagonist HBD-S-H4, has been shown to significantly attenuate the encapsulation of synapses by PSCs (Wang et al., 2017).
In the early stages of synaptogenesis, PSCs connect with pre-patterned acetylcholine receptor clusters that are localized near nerve endings (Barik et al., 2016). Several factors, including TGF-β1 (Feng and Ko, 2008a), BDNF (Feng and Ko, 2008b), as well as the B11 and B19 isoform of agrins (Yang et al., 2001), that are produced by PSCs support the formation of presynaptic terminals. PSCs are also capable of argins cleavage via the secretion of matrix metalloproteinase 3 (VanSaun et al., 2007). MMP3 KO mice have been observed to develop a larger number of junctional folds, and a higher density of the acetylcholine receptor clusters in comparison to those of the wild-type (VanSaun et al., 2003). These factors also play a role in long-term synapse maintenance. While acute ablation of PSCs in adult mice did not induce neuromuscular dysfunction, it was found to cause a severe reduction in neurotransmitter release, and axon viability on day 6 after loss of glial support (Todd et al., 2010).
Abnormal functioning of PSCs has been observed in SOD1G37R mice, which are a model for slow-onset amyotrophic lateral sclerosis. In normal physiological conditions, PSCs detect synaptic activity due to an increase in intracellular Ca2+ concentration. SOD1G37R mice have abnormally enhanced intracellular Ca2+ concentrations, even before the onset of denervation (Martineau et al., 2020). The loss of S100-positive cells that is induced by nerve crush injury, is more pronounced in this strain, and is consequently associated with delayed reinnervation and functional recovery (Carrasco et al., 2016). Furthermore, the intrusion of morphologically abnormal PSCs in the synaptic cleft has been identified in muscle biopsies from patients with amyotrophic lateral sclerosis (Bruneteau et al., 2015).
Olfactory Ensheathing Cells
Postnatal neurogenesis is known to occur in the olfactory neuroepithelium. Given that olfactory sensory neurons (OSNs) have an average lifespan of 1–3 months (Ekberg et al., 2012), extensive proliferation and differentiation of neural stem cells are required to match the turnover rate. A unique type of cells referred to as olfactory ensheathing cells (OECs), that cannot unambiguously be attributed to any major glial type, are known to play a crucial role in axon extension and guidance during this process (Boyd et al., 2005).
The majority of cell types that populate the peripheral part of the olfactory system are descendants of the olfactory placode (Forni et al., 2011). Although OECs migrate along with nascent olfactory fibers as part of a migratory mass, their neural crest origin was determined by genetic lineage tracing by using Pax7Cre (Murdoch et al., 2010), Wnt1Cre (Barraud et al., 2010; Forni et al., 2011; Katoh et al., 2011), and P0Cre (Katoh et al., 2011) mice. Although the expression profile of OECs shares some similarities with that of SCs (Perera et al., 2020), functionally however, these cells encase bundles of multiple axons rather than form myelin sheaths (Murtaza et al., 2019). Another distinctive characteristic of OECs, is their ability to actively migrate from the PNS into the CNS (Reshamwala et al., 2019). During development, as well as in continuous postnatal remodeling, these cells are known to migrate ahead of a growing axon toward the glomerular layer of the olfactory bulb (Ekberg et al., 2012). While direct contact between the growth cone and OEC processes is believed to be critical, their reparative potential may be also be attributed in part to distant intercellular interactions, and the maintenance of a suitable microenvironment. In fact, OECs-conditioned media have been shown to induce axonal growth in vitro and in vivo (Gu et al., 2016).
Extracellular matrix proteins and various neurotrophic factors are potential mediators of the above-mentioned effect. OECs express a variety of neurotrophic factors, including that referred to as axogenic NGF (Cao et al., 2007), BDNF (Pastrana et al., 2007), CNTF (Wewetzer et al., 2001), NT-4, and GDNF (Cao et al., 2004). Their actions, however, are not limited only to their effect on axons, since these cells simultaneously also express a range of receptors for the same factors. GDNF has been shown to enhance the motility of OECs by the activation of JNK and Src via the GFRα1 and RET receptors (Cao et al., 2006). An experimental GDNF over-secreting cell line was found to be superior to wild-type OECs in promoting axonal extension (Cao et al., 2004). Additionally, FZD1 and NRP1 may be potential scavengers of WNT1 and SEMA3A, that are recognized inhibitors of axonal growth, after spinal cord lesions (Perera et al., 2020). Extracellular vesicles isolated from human OECs are known to increase proliferation of neural progenitors, inhibit oxidative stress-induced neuronal toxicity in vitro (Tu and Hsueh, 2020), and promote regeneration of peripheral nerves in vivo (Xia et al., 2019).
Olfactory ensheathing cells are have been shown to modulate axonal regeneration via microenvironment modification. These cells function as major phagocytic cells in the olfactory epithelium and olfactory bulb, to remove neuronal debris (Nazareth et al., 2014). Further, they retain this property even at their site of transplantation (He et al., 2014). High-throughput proteomics has identified at least 168 extracellular proteins that are secreted by cultured rat OECs (Liu et al., 2010), which include collagen type IV, fibronectin, and laminin amongst others. OECs also express a range of adhesion proteins, including E-NCAM (Barnett, 2004), L1, β2-laminin, and N-cadherin (Fairless et al., 2005), that are essential for their migration and axonal guidance. Knockdown of genes LEPRE1 and NID2 encoding collagen and laminin-binding proteins, resulted in suppression of neurite outgrowth in a co-culture of OECs and DRG neurons (Wassenhove-McCarthy and McCarthy, 1999). Further, these cells reduce the expression of chondroitin sulfate proteoglycans in excessively proliferating glial cells (Wang G. Y. et al., 2021).
Olfactory ensheathing cells are also known to possess immunomodulatory properties. After grafting them into the sub-retinal space, they have been shown to reduce the rate of retinal degeneration via downregulation of the Notch signaling pathway in Müller cells (Xie et al., 2017). Moreover, their anti-inflammatory effect after intravenous transplantation in injured spinal cord has been conclusively demonstrated (Zhang et al., 2021).
In recent decades, the unique properties of OECs that promote axonal growth have driven the development of OEC-based cell therapies for CNS trauma. Pre-clinical studies on OEC transplantation, for the treatment of spinal cord injury has been summarized in several systematic reviews and meta-analyses (Liu et al., 2014; Li et al., 2015; Watzlawick et al., 2016; Nakhjavan-Shahraki et al., 2018). Overall, the process seems to effectively restore motor function, however, the recovery of sensory function has been less successful. The inconsistent outcomes may be accounted for in part, by differential purities of cell preparations and the variabilities introduced by different sources of OECs. Nevertheless, preparations from the olfactory bulb typically have more stable cell composition (Barnett and Chang, 2004), and OECs derived from the mucosa have been found to be most beneficial for this application (Jani and Raisman, 2004), especially since obtaining olfactory mucosal biopsies is a simple and non-invasive technique. Various preparations have been used thus far for transplantation in clinical trials, that range from OECs that have been purified by selective media (Bianco et al., 2004) to whole tissue pieces of undetermined cellular composition (Chhabra et al., 2009).
Olfactory ensheathing cell transplantation has remarkable potential to enhance peripheral nerve regeneration and remyelination after transection. These cells were found to form myelin sheaths on regenerated axons distal to the transection site after microsurgical reparation (Radtke et al., 2008). Further, reparation with OECs embedded in a polycaprolactone conduit was found to be as effective as an autologous nerve graft (Donoghue et al., 2013).
However, the bridging of large nerve defects requires the utilization of different materials for the preparation of artificial nerve grafts that are seeded with OECs. Examples of these include, PLGA (Li et al., 2010), acellularized vein filled with spider silk (Radtke et al., 2011), muscle-stuffed vein (Lokanathan et al., 2014), collagen (Goulart et al., 2016), as well as silicone and nanofibrous CNT/PLLA (Kabiri et al., 2015).
Satellite Glia
Satellite glial cells (SGCs) are found in ganglia (sensory, sympathetic, and parasympathetic ganglia) of the peripheral nervous system (PNS) (see review Hanani, 2010). These cells surround neuronal cell bodies. SGCs express several markers including cadherin 19 (George et al., 2018), potassium channel Kir4.1 (Vit et al., 2008), glutamine synthetase (GS) (Miller et al., 2002), and GFAP (Wang et al., 2019). Each neuron is usually isolated from those surrounding it by several enveloping glial cells that create a suitable microenvironment (Cece et al., 1995). Neuroglial communication in sensory ganglia occurs predominantly via chemical messengers. SCs express a plethora of functional receptors that are involved in the regulation of pain sensitivity, including purinergic receptors (Villa et al., 2010), the NMDA receptor (Castillo et al., 2013), TRPA1 (Shin et al., 2020), and CGRP receptors (Edvinsson et al., 2020). However, communication between cells in ganglia is also mediated via direct contact. In normal physiological conditions, satellite cells express Cx26, Cx47 (Garrett and Durham, 2008), and Panx1 (Retamal et al., 2014; Zhang et al., 2015). These proteins form gap junctions and membrane channels that allow direct diffusion of small molecules between the intracellular compartments of neighboring cells. It has been previously suggested, that the formation of interconnected cell clusters may play a crucial role in hyperalgesia and its associated pathologies. This notion is supported by the observation of abnormally increased coupling of SCs near gap junctions in various models of chronic pain and inflammation (Zhang et al., 2015; Blum et al., 2017; Komiya et al., 2018; Yamakita et al., 2018). The inhibition of SC-SC and SC-neuron coupling has been demonstrated to be a potential target for pain management. Knockdown of the most abundant connexin in mouse SCs, Cx43, has been shown to reduce pain post nerve injury (Ohara et al., 2008). The gap-junction blocker carbenoxolone has also demonstrated analgesic properties in acute pain models (Lemes et al., 2018). Direct electrical coupling between SCs and neurons has been observed in cultured (Suadicani et al., 2010) and freshly dissected sensory ganglia (Spray et al., 2019), and the association between coupled activation of DRG neurons and the upregulation of gap junctions has been shown in a mouse model of inflammation and nerve injury (Kim et al., 2016). Neuronal activity leads to the release of cytokines (TNF, IL-1β, and IL-6) by SCs, which increases neuronal excitability (Dubový et al., 2006). An instance of such altered secretion was observed as a consequence of pancreatic cell destruction, which contributed to the pathogenesis of diabetic neuropathic pain (Gonçalves et al., 2018).
While little is known about the biology of SGCs, it is becoming increasingly obvious that these cells on account of being localized in morphologically different neuro-structures, possess distinct molecular, morphological, and functional signatures. Moreover, recent data from a single cell RNA sequencing (scRNAseq) study has permitted the analysis of the molecular profiles of these cells in various physiological processes, including the development of somatosensory dorsal root ganglia and auditory spiral ganglia (Tasdemir-Yilmaz et al., 2021), in individual types of adult ganglia (Avraham et al., 2020; van Weperen et al., 2021), in nerve regeneration (Avraham et al., 2020), and pain (Wang K. et al., 2021). scRNAseq data analysis of several types of ganglia convincingly demonstrated a high level of SGCs heterogeneity in sympathetic stellate ganglia (van Weperen et al., 2021) and also in both naïve and injured conditions (Avraham et al., 2020). The transcriptomic profiles of SGCs in the stellate ganglia revealed the presence of five subpopulations (by degree of maturity) of SGCs, that shared many characteristics, including common signaling and metabolic pathways (van Weperen et al., 2021). scRNAseq data of adult L4-L5 DRG (in control mice) identified 13 distinct cell clusters among which SGCs, with high expression of Kir4.1, Cdh19, and Bfabp, but not the classical marker Glul/GS, accounted for 28% of the total cells. In the same analysis, SCs with high expression of Mpz, Mbp, Plp1, Mag, Prx, and Ncmap accounted for 10% of the adult DRG (Avraham et al., 2020). Despite the fact that the scRNAseq data obtained from different ganglia of the PNS, is insufficient for in-depth comparison and identification of the extent of glia heterogeneity, data from sympathetic ganglia and DRG have revealed that glia are highly heterogeneous, and are characterized by both unique and common molecular markers.
Enteric Glia
Enteric glial cells (EGCs) comprise a large population of peripheral glia of the enteric nervous system (ENS), that are part of ganglionated myenteric and submucosal plexus (myenteric/Auerbach and submucosal/Meissner) and extra ganglionic spaces, within the intestinal mucosa and muscle layers (Gershon and Rothman, 1991). These cells have been classified into four subgroups based on differences in cellular morphology (Hanani, 1994). They have also been classified based on their locations in the intestinal wall (mucosal, intraamniotic, and intramuscular intestinal glia) (Gulbransen and Sharkey, 2012). EGCs express proteins that are characteristic of other types of glia. For instance, they express SOX10, GFAP, PLP1, and S100beta I / I as has been observed in astrocytes (Jessen and Mirsky, 1983; Boesmans et al., 2015). Their TFs expression profile however, is similar to that of myelinating glia, and not of astrocytes (Rao et al., 2015). SOX10 and PLP1 are expressed in myelinating glia (Schwann cells) and oligodendrocytes, and in conjunction with S100B are common markers that are used to characterize EGCs (Hoff et al., 2008; Rao et al., 2015). Given that EGCs and SCs share some TFs, it is difficult to distinguish between them in the ENS (Rao et al., 2015). Specialized functions and specific locations in the tissues/organs of the digestive system add a heterogeneity imprint on EGCs, leading to the appearance of individual subpopulations with unique characteristics. However, little is known about the local heterogeneity of EGCs and the functional aspects managed by these subpopulations in the regulation of digestion and homeostasis.
Recent transcriptional profiling data revealed that EGCs diversity differs between regions of the digestive tract, and that the ENS glial diversity of humans, is greater than that of mice (Zeisel et al., 2018; Drokhlyansky et al., 2020). A scRNA-seq study of the Wnt1Cre;R26RTomato transgenic mouse nervous system identified seven EGCs subtypes in the small intestinal myenteric plexus (Zeisel et al., 2018). The problem of separating SCs from EGCs was tackled by adopting the single-cell transcriptional approach, which revealed that the expression patterns of individual genes, including Dhh, Mal, and Mpz can distinguish two cell types that express similar TFs (Zeisel et al., 2018; Morarach et al., 2021). Several enteric glial populations with unique transcriptional profiles have been described in the adult mouse and human ENS using newer methods that isolate cell nuclei from the gut for RNA expression analysis (Drokhlyansky et al., 2020). Three transcriptionally distinct populations of colonic EGCs have been identified based on the expression of Gfra2, Slc18a2, and Ntsr1 in mice, while the human colon was found to contain six glial subsets (Drokhlyansky et al., 2020). Despite greater understanding of the diversification of glial cell populations of the ENS, the role of transcriptional molecules in the spatial and morphological diversity of EGC groups, remains unknown. Further research on the comparative analysis of EGC with other populations of peripheral glia, including satellite glia, sympathetic and parasympathetic glia composed of ganglia, intramular ganglia, and regional myelinized and non-myelinized SCs will aid comprehension of cellular diversity and function.
Repair Schwann Cells
Repair Schwann cells (rSCs) are a separate population of adult SCs, that lose contact with and demyelinate the distal stump after axonal injury. These cells undergo reprogramming that involves the upregulation of several genes and activation of multiple transcriptional mechanisms. For instance, rSCs are known to upregulate c-Jun, mitogen-activated protein kinase (MAPK) pathway, Sonic Hedgehog (Shh) pathway, secrete trophic factors that support the survival of damaged neurons, and promote axonal regrowth (Arthur-Farraj et al., 2012; Nocera and Jacob, 2020). This process occurs via a transcriptional program orchestrated by c-JUN that culminates in the generation of “repair” glial cells with a specific molecular signature (Arthur-Farraj et al., 2012). The TFs expression patterns of these cells share commonalities with that of epithelial-mesenchymal transition (EMT) (Arthur-Farraj et al., 2017; Clements et al., 2017; Jessen and Arthur-Farraj, 2019).
Potency of Adult Peripheral Glial Cells to Differentiate Into Another Cell Types in Normal and Pathological Conditions
Investigations into the ability of embryonic glia to differentiate into different cell types, has led to further studies that explore the possibility of similar properties in adult glia (Falk and Götz, 2017). While multiple data on the ability of differentiated glia to transform into various cell types are available, the range of such transformations is severely limited in comparison to that of embryonic glia. Despite the presence of different subpopulations of glial cells in the PNS, not all are capable of active proliferation and differentiation. For instance, the major cell types of peripheral glia, namely, myelinating SCs (mySCs) and non-myelinating SCs (nmySCs) are inert cell that rarely undergo division in normal circumstances (Nocera and Jacob, 2020). After injury, however, all mySCs are capable of dedifferentiating into proliferative progenitor-like SCs, that subsequently transform into mSCs cells, which orchestrate regenerative responses. These processes occur without any contribution from regional stem cells, and consequently the recovery process is limited by its own capacity and by neuro-glial signals. Dedifferentiated glial cells, such as repair SCs (rSCs) are poorly understood despite the fact that their specific molecular signatures are well defined. Evidence suggests that a significant expansion of Sox2+, S100b+ dedifferentiated SCs within the regenerating digit coincident with blastema growth in the model of Sox2-CreERT2/+; R26-lsl–TdTomato mice (Arnold et al., 2011) is necessary for normal nail and bone regeneration (Johnston et al., 2016), and for skin regeneration via the forceful proliferation of mesenchymal cells (Johnston et al., 2013). Peripheral glial cells are known to be activated in response to injury, and promote wound healing in the skin of adult mice. The process is initiated by the detachment of SCs from injured nerves, which then proceed to move into the granulation tissue, where they undergo reprogramming into invasive mesenchymal-like cells, and ultimately drive peripheral nerve regeneration (Clements et al., 2017; Parfejevs et al., 2018). All of the above-mentioned examples describe the indirect effects of glia on other cell types and tissues in response to damage.
Recent data on zebrafish reveals that enteric neurons do not originate from resident neuronal progenitors or enteric glia that are absent in adult zebrafish, but from trunk neural crest-derived SCPs that migrate into the intestine, and differentiate into neurons. This indicates a new role for SCPs in the context of adult neurogenesis, where they function as a source of enteric neurons in adult fish and mouse (Uesaka et al., 2015; Espinosa-Medina et al., 2017; El-Nachef and Bronner, 2020; Kamenev et al., 2021). These are the only examples of post-embryonic SCPs that differentiate into distinct types of neuronal or non-neuronal cells that have been recorded till date.
Future Perspectives
Despite documentation of the distinguishing characteristics of SCPs, the mechanisms that operate to select their fate, and direct them to specialize into certain cell types (neurons, neuroendocrine cells, odontoblasts, pigment cells, osteoblasts, and osteoclasts) are poorly understood. Some of the questions that need to be answered include: (1) What causes a cell to detach from a nerve and undergo differentiation? (2) Do the transforming signals arrive from peripheral nerves (positional information) on which glia are located? (3) Could they possibly arise from the internal heterogeneity of SCPs, from neighboring tissues, or all of the above? A second aspect that need to be studied is the application of the multipotency of SCPs in clinical scenarios. Disturbances in the migration and directed differentiation mechanisms of SCPs can result in a wide range of disorders associated with lack of sufficient cells required for proper functioning. This further leads to abnormalities in the development of tissues/organs, including the parasympathetic ganglia and their innervations, neurogenesis of the enteric system, adrenal glands, skeletal structures, teeth, and pigmentation. For instance, more than 20 types of autonomic dysfunctions currently known, cause symptoms including heartburn, intestinal gas, flatulence, diarrhea, constipation, colitis, dry mouth, cardiac disorders, dysuria, and sexual dysfunction. The mechanisms that underlie these disorders have not been elucidated, but may possibly involve SCPs and their ability to differentiate into autonomic neurons. The immense potencies of different glia types in adults are just beginning to be discovered. Cells of several peripheral glia types have been found to be capable of differentiating into neurons under both normal and pathological conditions, participating in perineural invasion, and playing important roles in carcinogenesis, apart from their involvement in axon regeneration.
Author Contributions
AS, DA, NB, MV, LM, and VD planned the study, and wrote the manuscript. MV and LM participated in data acquisition. VD supervised the study. All authors read and approved the final version of the manuscript.
Funding
This work was financially supported by the Ministry of Science and Higher Education of the Russian Federation (Agreement No. 075-15-2020-901). Open access funding was provided by the Almazov National Medical Research Centre, Saint Petersburg, Russia.
Conflict of Interest
The authors declare that the research was conducted in the absence of any commercial or financial relationships that could be construed as a potential conflict of interest.
Publisher’s Note
All claims expressed in this article are solely those of the authors and do not necessarily represent those of their affiliated organizations, or those of the publisher, the editors and the reviewers. Any product that may be evaluated in this article, or claim that may be made by its manufacturer, is not guaranteed or endorsed by the publisher.
Acknowledgments
We thank Olga Kharchenko for the drawings presented in Figures.
References
Adameyko, I., Lallemend, F., Aquino, J. B., Pereira, J. A., Topilko, P., Müller, T., et al. (2009). Schwann cell precursors from nerve innervation are a cellular origin of melanocytes in skin. Cell 139, 366–379. doi: 10.1016/j.cell.2009.07.049
Arnold, K., Sarkar, A., Yram, M. A., Polo, J. M., Bronson, R., Sengupta, S., et al. (2011). Sox2(+) adult stem and progenitor cells are important for tissue regeneration and survival of mice. Cell Stem Cell 9, 317–329. doi: 10.1016/j.stem.2011.09.001
Arthur-Farraj, P. J., Latouche, M., Wilton, D. K., Quintes, S., Chabrol, E., Banerjee, A., et al. (2012). c-Jun reprograms Schwann cells of injured nerves to generate a repair cell essential for regeneration. Neuron 75, 633–647. doi: 10.1016/j.neuron.2012.06.021
Arthur-Farraj, P. J., Morgan, C. C., Adamowicz, M., Gomez-Sanchez, J. A., Fazal, S. V., Beucher, A., et al. (2017). Changes in the coding and non-coding transcriptome and DNA methylome that define the Schwann cell repair phenotype after nerve injury. Cell Rep. 20, 2719–2734. doi: 10.1016/j.celrep.2017.08.064
Avraham, O., Deng, P. Y., Jones, S., Kuruvilla, R., Semenkovich, C. F., Klyachko, V. A., et al. (2020). Satellite glial cells promote regenerative growth in sensory neurons. Nat. Commun. 11:4891. doi: 10.1038/s41467-020-18642-y
Balakrishnan, A., Stykel, M. G., Touahri, Y., Stratton, J. A., Biernaskie, J., and Schuurmans, C. (2016). Temporal analysis of gene expression in the murine Schwann cell lineage and the acutely injured postnatal nerve. PLoS One 11:e0153256. doi: 10.1371/journal.pone.0153256
Barik, A., Li, L., Sathyamurthy, A., Xiong, W. C., and Mei, L. (2016). Schwann cells in neuromuscular junction formation and maintenance. J. Neurosci. 36, 9770–9781. doi: 10.1523/JNEUROSCI.0174-16.2016
Barnett, S. C. (2004). Olfactory ensheathing cells: unique glial cell types? J. Neurotrauma 21, 375–382. doi: 10.1089/089771504323004520
Barnett, S. C., and Chang, L. (2004). Olfactory ensheathing cells and CNS repair: going solo or in need of a friend? Trends Neurosci. 27, 54–60. doi: 10.1016/j.tins.2003.10.011
Barraud, P., Seferiadis, A. A., Tyson, L. D., Zwart, M. F., Szabo-Rogers, H. L., Ruhrberg, C., et al. (2010). Neural crest origin of olfactory ensheathing glia. Proc. Natl. Acad. Sci. U.S.A. 107, 21040–21045. doi: 10.1073/pnas.1012248107
Bianco, J. I., Perry, C., Harkin, D. G., Mackay-Sim, A., and Féron, F. (2004). Neurotrophin 3 promotes purification and proliferation of olfactory ensheathing cells from human nose. Glia 45, 111–123. doi: 10.1002/glia.10298
Birchmeier, C., and Nave, K.-A. (2008). Neuregulin-1, a key axonal signal that drives Schwann cell growth and differentiation. Glia 56, 1491–1497. doi: 10.1002/glia.20753
Blum, E., Procacci, P., Conte, V., Sartori, P., and Hanani, M. (2017). Long term effects of lipopolysaccharide on satellite glial cells in mouse dorsal root ganglia. Exp. Cell Res. 350, 236–241. doi: 10.1016/j.yexcr.2016.11.026
Boesmans, W., Lasrado, R., Vanden Berghe, P., and Pachnis, V. (2015). Heterogeneity and phenotypic plasticity of glial cells in the mammalian enteric nervous system. Glia 63, 229–241. doi: 10.1002/glia.22746
Boyd, J. G., Doucette, R., and Kawaja, M. D. (2005). Defining the role of olfactory ensheathing cells in facilitating axon remyelination following damage to the spinal cord. FASEB J. 19, 694–703. doi: 10.1096/fj.04-2833rev
Bronner, M. E., and LeDouarin, N. M. (2012). Development and evolution of the neural crest: an overview. Dev. Biol. 366, 2–9. doi: 10.1016/j.ydbio.2011.12.042
Bruneteau, G., Bauché, S., Gonzalez de Aguilar, J. L., Brochier, G., Mandjee, N., Tanguy, M. L., et al. (2015). Endplate denervation correlates with Nogo-A muscle expression in amyotrophic lateral sclerosis patients. Ann. Clin. Transl. Neurol. 2, 362–372. doi: 10.1002/acn3.179
Cao, L., Liu, L., Chen, Z. Y., Wang, L. M., Ye, J. L., Qiu, H. Y., et al. (2004). Olfactory ensheathing cells genetically modified to secrete GDNF to promote spinal cord repair. Brain 127(Pt. 3), 535–549. doi: 10.1093/brain/awh072
Cao, L., Su, Z., Zhou, Q., Lv, B., Liu, X., Jiao, L., et al. (2006). Glial cell line-derived neurotrophic factor promotes olfactory ensheathing cells migration. Glia 54, 536–544. doi: 10.1002/glia.20403
Cao, L., Zhu, Y. L., Su, Z., Lv, B., Huang, Z., Mu, L., et al. (2007). Olfactory ensheathing cells promote migration of Schwann cells by secreted nerve growth factor. Glia 55, 897–904. doi: 10.1002/glia.20511
Carrasco, D. I., Bahr, B. A., Seburn, K. L., and Pinter, M. J. (2016). Abnormal response of distal Schwann cells to denervation in a mouse model of motor neuron disease. Exp. Neurol. 278, 116–126. doi: 10.1016/j.expneurol.2016.02.002
Castillo, C., Norcini, M., Martin Hernandez, L. A., Correa, G., Blanck, T. J., and Recio-Pinto, E. (2013). Satellite glia cells in dorsal root ganglia express functional NMDA receptors. Neuroscience 240, 135–146. doi: 10.1016/j.neuroscience.2013.02.031
Cece, R., Petruccioli, M. G., Pizzini, G., Cavaletti, G., and Tredici, G. (1995). Ultrastructural aspects of DRG satellite cell involvement in experimental cisplatin neuronopathy. J. Submicrosc. Cytol. Pathol. 27, 417–425.
Cheung, M., and Briscoe, J. (2003). Neural crest development is regulated by the transcription factor Sox9. Development 130, 5681–5693. doi: 10.1242/dev.00808
Chhabra, H. S., Lima, C., Sachdeva, S., Mittal, A., Nigam, V., Chaturvedi, D., et al. (2009). Autologous olfactory [corrected] mucosal transplant in chronic spinal cord injury: an Indian Pilot Study. Spinal Cord 47, 887–895. doi: 10.1038/sc.2009.54
Clements, M. P., Byrne, E., Camarillo Guerrero, L. F., Cattin, A. L., Zakka, L., Ashraf, A., et al. (2017). The wound microenvironment reprograms Schwann cells to invasive mesenchymal-like cells to drive peripheral nerve regeneration. Neuron 96, 98–114.e7. doi: 10.1016/j.neuron.2017.09.008
Coulpier, F., Le Crom, S., Maro, G. S., Manent, J., Giovannini, M., Maciorowski, Z., et al. (2009). Novel features of boundary cap cells revealed by the analysis of newly identified molecular markers. Glia 57, 1450–1457. doi: 10.1002/glia.20862
Cunningham, M. E., Meehan, G. R., Robinson, S., Yao, D., McGonigal, R., and Willison, H. J. (2020). Perisynaptic Schwann cells phagocytose nerve terminal debris in a mouse model of Guillain-Barré syndrome. J. Peripher. Nerv. Syst. 25, 143–151. doi: 10.1111/jns.12373
Darabid, H., Arbour, D., and Robitaille, R. (2013). Glial cells decipher synaptic competition at the mammalian neuromuscular junction. J. Neurosci. 33, 1297–1313. doi: 10.1523/JNEUROSCI.2935-12.2013
Donoghue, P. S., Lamond, R., Boomkamp, S. D., Sun, T., Gadegaard, N., Riehle, M. O., et al. (2013). The development of a ε-polycaprolactone scaffold for central nervous system repair. Tissue Eng. Part A. 19, 497–507. doi: 10.1089/ten.tea.2012.0382
Drokhlyansky, E., Smillie, C. S., Van Wittenberghe, N., Ericsson, M., Griffin, G. K., Eraslan, G., et al. (2020). The human and mouse enteric nervous system at single-cell resolution. Cell 182, 1606–1622.e23. doi: 10.1016/j.cell.2020.08.003
Dubový, P., Jancálek, R., Klusáková, I., Svízenská, I., and Pejchalová, K. (2006). Intra- and extraneuronal changes of immunofluorescence staining for TNF-alpha and TNFR1 in the dorsal root ganglia of rat peripheral neuropathic pain models. Cell. Mol. Neurobiol. 26, 1205–1217. doi: 10.1007/s10571-006-9006-3
Dyachuk, V., Furlan, A., Shahidi, M. K., Giovenco, M., Kaukua, N., Konstantinidou, C., et al. (2014). Neurodevelopment. Parasympathetic neurons originate from nerve-associated peripheral glial progenitors. Science 345, 82–87. doi: 10.1126/science.1253281
Edvinsson, L., Grell, A. S., and Warfvinge, K. (2020). Expression of the CGRP family of neuropeptides and their receptors in the trigeminal ganglion. J. Mol. Neurosci. 70, 930–944. doi: 10.1007/s12031-020-01493-z
Ekberg, J. A., Amaya, D., Mackay-Sim, A., and St John, J. A. (2012). The migration of olfactory ensheathing cells during development and regeneration. Neurosignals 20, 147–158. doi: 10.1159/000330895
El-Nachef, W. N., and Bronner, M. E. (2020). De novo enteric neurogenesis in post-embryonic zebrafish from Schwann cell precursors rather than resident cell types. Development 147:dev186619. doi: 10.1242/dev.186619
Espinosa-Medina, I., Jeans, B., Boismoreau, F., Chettouh, Z., Enomoto, H., Müller, T., et al. (2017). Dual origin of enteric neurons in vagal Schwann cell precursors and the sympathetic neural crest. Proc. Natl. Acad. Sci. U.S.A.? 114, 11980–11985. doi: 10.1073/pnas.1710308114
Espinosa-Medina, I., Outin, E., Picard, C. A., Chettouh, Z., Dymecki, S., Consalez, G. G., et al. (2014). Neurodevelopment. Parasympathetic ganglia derive from Schwann cell precursors. Science 345, 87–90. doi: 10.1126/science.1253286
Evans, D. G., Huson, S. M., and Birch, J. M. (2012). Malignant peripheral nerve sheath tumours in inherited disease. Clin. Sarcoma Res. 2:17. doi: 10.1186/2045-3329-2-17
Fairless, R., Frame, M. C., and Barnett, S. C. (2005). N-cadherin differentially determines Schwann cell and olfactory ensheathing cell adhesion and migration responses upon contact with astrocytes. Mol. Cell. Neurosci. 28, 253–263. doi: 10.1016/j.mcn.2004.09.009
Falk, S., and Götz, M. (2017). Glial control of neurogenesis. Curr. Opin. Neurobiol. 47, 188–195. doi: 10.1016/j.conb.2017.10.025
Faure, L., Wang, Y., Kastriti, M. E., Fontanet, P., Cheung, K. K., Petitpré, C., et al. (2020). Single cell RNA sequencing identifies early diversity of sensory neurons forming via bi-potential intermediates. Nat. Commun. 11:4175. doi: 10.1038/s41467-020-17929-4
Feltri, M. L., Poitelon, Y., and Previtali, S. C. (2016). How Schwann cells sort axons: new concepts. Neuroscientist 22, 252–265. doi: 10.1177/1073858415572361
Feng, Z., and Ko, C. P. (2008a). Schwann cells promote synaptogenesis at the neuromuscular junction via transforming growth factor-beta1. J. Neurosci. 28, 9599–9609. doi: 10.1523/JNEUROSCI.2589-08.2008
Feng, Z., and Ko, C. P. (2008b). The role of glial cells in the formation and maintenance of the neuromuscular junction. Ann. N. Y. Acad. Sci. 1132, 19–28. doi: 10.1196/annals.1405.016
Forni, P. E., Taylor-Birds, C., Melvin, V. S., Williams, T., and Wray, S. (2011). Neural crest and ectodermal cells intermix in the nasal placode to give rise to GnRH1 neurons, sensory neurons, and olfactory ensheathing cells. J. Neurosci. J. Soc. Neurosci. 31, 6915–6927. doi: 10.1523/JNEUROSCI.6087-10.2011
Furlan, A., Dyachuk, V., Kastriti, M. E., Calvo-Enrique, L., Abdo, H., Hadjab, S., et al. (2017). Multipotent peripheral glial cells generate neuroendocrine cells of the adrenal medulla. Science 357:eaal3753. doi: 10.1126/science.aal3753
Garrett, A. M., Jucius, T. J., Sigaud, L. P., Tang, F. L., Xiong, W. C., Ackerman, S. L., et al. (2016). Analysis of expression pattern and genetic deletion of netrin5 in the developing mouse. Front. Mol. Neurosci. 9:3. doi: 10.3389/fnmol.2016.00003
Garrett, F. G., and Durham, P. L. (2008). Differential expression of connexins in trigeminal ganglion neurons and satellite glial cells in response to chronic or acute joint inflammation. Neuron Glia Biol. 4, 295–306. doi: 10.1017/S1740925X09990093
George, D., Ahrens, P., and Lambert, S. (2018). Satellite glial cells represent a population of developmentally arrested Schwann cells. Glia 66, 1496–1506. doi: 10.1002/glia.23320
Gershon, M. D., and Rothman, T. P. (1991). Enteric glia. Glia 4, 195–204. doi: 10.1002/glia.440040211
Giovannini, M., Robanus-Maandag, E., Niwa-Kawakita, M., van der Valk, M., Woodruff, J. M., Goutebroze, L., et al. (1999). Schwann cell hyperplasia and tumors in transgenic mice expressing a naturally occurring mutant NF2 protein. Genes Dev. 13, 978–986. doi: 10.1101/gad.13.8.978
Golding, J. P., and Cohen, J. (1997). Border controls at the mammalian spinal cord: late-surviving neural crest boundary cap cells at dorsal root entry sites may regulate sensory afferent ingrowth and entry zone morphogenesis. Mol. Cell. Neurosci. 9, 381–396. doi: 10.1006/mcne.1997.0647
Gomez-Sanchez, J. A., Pilch, K. S., van der Lans, M., Fazal, S. V., Benito, C., Wagstaff, L. J., et al. (2017). After nerve injury, lineage tracing shows that Myelin and Remak Schwann cells elongate extensively and branch to form repair Schwann cells, which shorten radically on remyelination. J. Neurosci. 37, 9086–9099. doi: 10.1523/JNEUROSCI.1453-17.2017
Gonçalves, N. P., Vægter, C. B., and Pallesen, L. T. (2018). Peripheral glial cells in the development of diabetic neuropathy. Front. Neurol. 9:268. doi: 10.3389/fneur.2018.00268
Goulart, C. O., Ângelo Durço, D. F., de Carvalho, L. A., Oliveira, J. T., Alves, L., Cavalcante, L. A., et al. (2016). Olfactory ensheathing glia cell therapy and tubular conduit enhance nerve regeneration after mouse sciatic nerve transection. Brain Res. 1650, 243–251. doi: 10.1016/j.brainres.2016.09.021
Gresset, A., Coulpier, F., Gerschenfeld, G., Jourdon, A., Matesic, G., Richard, L., et al. (2015). Boundary caps give rise to neurogenic stem cells and terminal glia in the skin. Stem Cell Rep. 5, 278–290. doi: 10.1016/j.stemcr.2015.06.005
Gu, M., Gao, Z., Li, X., Guo, L., Lu, T., Li, Y., et al. (2016). Conditioned medium of olfactory ensheathing cells promotes the functional recovery and axonal regeneration after contusive spinal cord injury. Brain Res. 1654(Pt. A), 43–54. doi: 10.1016/j.brainres.2016.10.023
Gulbransen, B. D., and Sharkey, K. A. (2012). Novel functional roles for enteric glia in the gastrointestinal tract. Nat. Rev. Gastroenterol. Hepatol. 9, 625–632. doi: 10.1038/nrgastro.2012.138
Hanani, M. (1994). Morphology of horseradish peroxidase (HRP)-injected glial cells in the myenteric plexus of the guinea-pig. Cell Tissue Res. 278, 153–160. doi: 10.1007/BF00305787
Hanani, M. (2010). Satellite glial cells in sympathetic and parasympathetic ganglia: in search of function. Brain Res. Rev. 64, 304–327. doi: 10.1016/j.brainresrev.2010.04.009
Hayworth, C. R., Moody, S. E., Chodosh, L. A., Krieg, P., Rimer, M., and Thompson, W. J. (2006). Induction of neuregulin signaling in mouse Schwann cells in vivo mimics responses to denervation. J. Neurosci. 26, 6873–6884. doi: 10.1523/JNEUROSCI.1086-06.2006
He, B. R., Xie, S. T., Wu, M. M., Hao, D. J., and Yang, H. (2014). Phagocytic removal of neuronal debris by olfactory ensheathing cells enhances neuronal survival and neurite outgrowth via p38MAPK activity. Mol. Neurobiol. 49, 1501–1512. doi: 10.1007/s12035-013-8588-2
Herrera, A. A., Qiang, H., and Ko, C. P. (2000). The role of perisynaptic Schwann cells in development of neuromuscular junctions in the frog (Xenopus laevis). J. Neurobiol. 45, 237–254. doi: 10.1002/1097-4695(200012)45:4<237::aid-neu5gt;3.0.co;2-j
Hockman, D., Adameyko, I., Kaucka, M., Barraud, P., Otani, T., Hunt, A., et al. (2018). Striking parallels between carotid body glomus cell and adrenal chromaffin cell development. Dev. Biol. 444(Suppl. 1), S308–S324. doi: 10.1016/j.ydbio.2018.05.016
Hoff, S., Zeller, F., Von Weyhern, C. W., Wegner, M., Schemann, M., Michel, K., et al. (2008). Quantitative assessment of glial cells in the human and Guinea pig enteric nervous system with an anti-Sox8/9/10 antibody. J. Comp. Neurol. 509, 356–371. doi: 10.1002/cne.21769
Honjo, Y., Kniss, J., and Eisen, J. S. (2008). Neuregulin-mediated ErbB3 signaling is required for formation of zebrafish dorsal root ganglion neurons. Development 135, 2615–2625. doi: 10.1242/dev.022178
Jani, H. R., and Raisman, G. (2004). Ensheathing cell cultures from the olfactory bulb and mucosa. Glia. 47, 130–137. doi: 10.1002/glia.20038
Jessen, K. R., and Arthur-Farraj, P. (2019). Repair Schwann cell update: adaptive reprogramming, EMT, and stemness in regenerating nerves. Glia 67, 421–437. doi: 10.1002/glia.23532
Jessen, K. R., and Mirsky, R. (1983). Astrocyte-like glia in the peripheral nervous system: an immunohistochemical study of enteric glia. J. Neurosci. 3, 2206–2218. doi: 10.1523/JNEUROSCI.03-11-02206.1983
Jessen, K. R., and Mirsky, R. (2019). Schwann cell precursors; multipotent glial cells in embryonic nerves. Front. Mol. Neurosci. 12:69. doi: 10.3389/fnmol.2019.00069
Johnston, A. P. W., Naska, S., Jones, K., Jinno, H., Kaplan, D. R., and Miller, F. D. (2013). Sox2-mediated regulation of adult neural crest precursors and skin repair. Stem Cell Rep. 1, 38–45. doi: 10.1016/j.stemcr.2013.04.004
Johnston, A. P. W., Yuzwa, S. A., Carr, M. J., Mahmud, N., Storer, M. A., Krause, M. P., et al. (2016). Dedifferentiated Schwann cell precursors secreting paracrine factors are required for regeneration of the mammalian digit tip. Cell Stem Cell 19, 433–448. doi: 10.1016/j.stem.2016.06.002
Kabiri, M., Oraee-Yazdani, S., Shafiee, A., Hanaee-Ahvaz, H., Dodel, M., Vaseei, M., et al. (2015). Neuroregenerative effects of olfactory ensheathing cells transplanted in a multi-layered conductive nanofibrous conduit in peripheral nerve repair in rats. J. Biomed. Sci. 22:35. doi: 10.1186/s12929-015-0144-0
Kamenev, D., Sunadome, K., Shirokov, M., Chagin, A. S., Singh, A., Irion, U., et al. (2021). Schwann cell precursors generate sympathoadrenal system during zebrafish development. J. Neurosci. Res. 99, 2540–2557. doi: 10.1002/jnr.24909
Kameneva, P., Artemov, A. V., Kastriti, M. E., Faure, L., Olsen, T. K., Otte, J., et al. (2021). Single-cell transcriptomics of human embryos identifies multiple sympathoblast lineages with potential implications for neuroblastoma origin. Nat. Genet. 53, 694–706. doi: 10.1038/s41588-021-00818-x
Kastriti, M. E., Kameneva, P., Kamenev, D., Dyachuk, V., Furlan, A., Hampl, M., et al. (2019). Schwann cell precursors generate the majority of chromaffin cells in zuckerkandl organ and some sympathetic neurons in Paraganglia. Front. Mol. Neurosci. 12:6. doi: 10.3389/fnmol.2019.00006
Katoh, H., Shibata, S., Fukuda, K., Sato, M., Satoh, E., Nagoshi, N., et al. (2011). The dual origin of the peripheral olfactory system: placode and neural crest. Mol. Brain 4:34. doi: 10.1186/1756-6606-4-34
Kaucka, M., Szarowska, B., Kavkova, M., Kastriti, M. E., Kameneva, P., Schmidt, I., et al. (2021). Nerve-associated Schwann cell precursors contribute extracutaneous melanocytes to the heart, inner ear, supraorbital locations and brain meninges. Cell. Mol. Life Sci. 78, 6033–6049. doi: 10.1007/s00018-021-03885-9
Kaukua, N., Shahidi, M. K., Konstantinidou, C., Dyachuk, V., Kaucka, M., Furlan, A., et al. (2014). Glial origin of mesenchymal stem cells in a tooth model system. Nature 513, 551–554. doi: 10.1038/nature13536
Kim, Y. S., Anderson, M., Park, K., Zheng, Q., Agarwal, A., Gong, C., et al. (2016). Coupled activation of primary sensory neurons contributes to chronic pain. Neuron 91, 1085–1096. doi: 10.1016/j.neuron.2016.07.044
Komiya, H., Shimizu, K., Ishii, K., Kudo, H., Okamura, T., Kanno, K., et al. (2018). Connexin 43 expression in satellite glial cells contributes to ectopic tooth-pulp pain. J. Oral Sci. 60, 493–499. doi: 10.2334/josnusd.17-0452
Le Douarin, N., and Kalcheim, C. (1999). The Neural Crest. Cambridge: Cambridge University Press, doi: 10.1017/cbo9780511897948
Lee, S., Nakamura, E., Yang, H., Wei, W., Linggi, M. S., Sajan, M. P., et al. (2005). Neuronal apoptosis linked to EglN3 prolyl hydroxylase and familial pheochromocytoma genes: developmental culling and cancer. Cancer Cell 8, 155–167. doi: 10.1016/j.ccr.2005.06.015
Lemes, J. B. P., de Campos Lima, T., Santos, D. O., Neves, A. F., de Oliveira, F. S., Parada, C. A., et al. (2018). Participation of satellite glial cells of the dorsal root ganglia in acute nociception. Neurosci. Lett. 676, 8–12. doi: 10.1016/j.neulet.2018.04.003
Li, B. C., Jiao, S. S., Xu, C., You, H., and Chen, J. M. (2010). PLGA conduit seeded with olfactory ensheathing cells for bridging sciatic nerve defect of rats. J. Biomed. Mater. Res. A 94, 769–780. doi: 10.1002/jbm.a.32727
Li, L., Adnan, H., Xu, B., Wang, J., Wang, C., Li, F., et al. (2015). Effects of transplantation of olfactory ensheathing cells in chronic spinal cord injury: a systematic review and meta-analysis. Eur. Spine J. 24, 919–930. doi: 10.1007/s00586-014-3416-6
Lignell, A., Kerosuo, L., Streichan, S. J., Cai, L., and Bronner, M. E. (2017). Identification of a neural crest stem cell niche by Spatial Genomic Analysis. Nat. Commun. 8:1830. doi: 10.1038/s41467-017-01561-w
Lin, W., Sanchez, H. B., Deerinck, T., Morris, J. K., Ellisman, M., and Lee, K. F. (2000). Aberrant development of motor axons and neuromuscular synapses in erbB2-deficient mice. Proc. Natl. Acad. Sci. U.S.A. 97, 1299–1304. doi: 10.1073/pnas.97.3.1299
Liu, J., Chen, P., Wang, Q., Chen, Y., Yu, H., Ma, J., et al. (2014). Meta analysis of olfactory ensheathing cell transplantation promoting functional recovery of motor nerves in rats with complete spinal cord transection. Neural Regen. Res. 9, 1850–1858. doi: 10.4103/1673-5374.143434
Liu, Y., Sugiura, Y., Chen, F., Lee, K. F., Ye, Q., and Lin, W. (2019). Blocking skeletal muscle DHPRs/Ryr1 prevents neuromuscular synapse loss in mutant mice deficient in type III Neuregulin 1 (CRD-Nrg1). PLoS Genet. 15:e1007857. doi: 10.1371/journal.pgen.1007857
Liu, Y., Teng, X., Yang, X., Song, Q., Lu, R., Xiong, J., et al. (2010). Shotgun proteomics and network analysis between plasma membrane and extracellular matrix proteins from rat olfactory ensheathing cells. Cell Transplant. 19, 133–146. doi: 10.3727/096368910X492607
Lokanathan, Y., Ng, M. H., Hasan, S., Ali, A., Mahmod, M., Htwe, O., et al. (2014). Olfactory ensheathing cells seeded muscle-stuffed vein as nerve conduit for peripheral nerve repair: a nerve conduction study. J. Biosci. Bioeng. 118, 231–234. doi: 10.1016/j.jbiosc.2014.02.002
Love, F. M., and Thompson, W. J. (1999). Glial cells promote muscle reinnervation by responding to activity-dependent postsynaptic signals. J. Neurosci. 19, 10390–10396. doi: 10.1523/JNEUROSCI.19-23-10390.1999
Maro, G., Vermeren, M., Voiculescu, O., Melton, L., Cohen, J., Charnay, P., et al. (2004). Neural crest boundary cap cells constitute a source of neuronal and glial cells of the PNS. Nat. Neurosci. 7, 930–938. doi: 10.1038/nn1299
Martineau, É, Arbour, D., Vallée, J., and Robitaille, R. (2020). Properties of glial cell at the neuromuscular junction are incompatible with synaptic repair in the SOD1G37R ALS mouse model. J. Neurosci. 40, 7759–7777. doi: 10.1523/JNEUROSCI.1748-18.2020
Michailov, G. V., Sereda, M. W., Brinkmann, B. G., Fischer, T. M., Haug, B., Birchmeier, C., et al. (2004). Axonal neuregulin-1 regulates myelin sheath thickness. Science 304, 700–703. doi: 10.1126/science.1095862
Miller, K. E., Richards, B. A., and Kriebel, R. M. (2002). Glutamine-, glutamine synthetase-, glutamate dehydrogenase- and pyruvate carboxylase-immunoreactivities in the rat dorsal root ganglion and peripheral nerve. Brain Res. 945, 202–211. doi: 10.1016/s0006-8993(02)02802-0
Monk, K. R., Feltri, M. L., and Taveggia, C. (2015). New insights on Schwann cell development. Glia 63, 1376–1393. doi: 10.1002/glia.22852
Morarach, K., Mikhailova, A., Knoflach, V., Memic, F., Kumar, R., Li, W., et al. (2021). Diversification of molecularly defined myenteric neuron classes revealed by single-cell RNA sequencing. Nat. Neurosci. 24, 34–46. doi: 10.1038/s41593-020-00736-x
Murdoch, B., DelConte, C., and García-Castro, M. I. (2010). Embryonic Pax7-expressing progenitors contribute multiple cell types to the postnatal olfactory epithelium. J. Neurosci. 30, 9523–9532. doi: 10.1523/JNEUROSCI.0867-10.2010
Murtaza, M., Chacko, A., Delbaz, A., Reshamwala, R., Rayfield, A., McMonagle, B., et al. (2019). Why are olfactory ensheathing cell tumors so rare? Cancer Cell Int. 19:260. doi: 10.1186/s12935-019-0989-5
Nakhjavan-Shahraki, B., Yousefifard, M., Rahimi-Movaghar, V., Baikpour, M., Nasirinezhad, F., Safari, S., et al. (2018). Transplantation of olfactory ensheathing cells on functional recovery and neuropathic pain after spinal cord injury; systematic review and meta-analysis. Sci. Rep. 8:325. doi: 10.1038/s41598-017-18754-4
Nazareth, L., Lineburg, K. E., Chuah, M. I., Tello Velasquez, J., Chehrehasa, F., St John, J. A., et al. (2014). Olfactory ensheathing cells are the main phagocytic cells that remove axon debris during early development of the olfactory system. J. Comp. Neurol. 523, 479–494. doi: 10.1002/cne.23694
Niederländer, C., and Lumsden, A. (1996). Late emigrating neural crest cells migrate specifically to the exit points of cranial branchiomotor nerves. Development 122, 2367–2374. doi: 10.1242/dev.122.8.2367
Nitzan, E., Pfaltzgraff, E. R., Labosky, P. A., and Kalcheim, C. (2013). Neural crest and Schwann cell progenitor derived melanocytes are two spatially segregated populations similarly regulated by Foxd3. Proc. Natl. Acad. Sci. U.S.A. 110, 12709–12714. doi: 10.1073/pnas.1306287110
Nocera, G., and Jacob, C. (2020). Mechanisms of Schwann cell plasticity involved in peripheral nerve repair after injury. Cell. Mol. Life Sci. 77, 3977–3989. doi: 10.1007/s00018-020-03516-9
Ohara, P. T., Vit, J. P., Bhargava, A., and Jasmin, L. (2008). Evidence for a role of connexin 43 in trigeminal pain using RNA interference in vivo. J. Neurophysiol. 100, 3064–3073. doi: 10.1152/jn.90722.2008
Parfejevs, V., Debbache, J., Shakhova, O., Schaefer, S. M., Glausch, M., Wegner, M., et al. (2018). Injury-activated glial cells promote wound healing of the adult skin in mice. Nat. Commun. 9:236. doi: 10.1038/s41467-017-01488-2
Pastrana, E., Moreno-Flores, M. T., Avila, J., Wandosell, F., Minichiello, L., and Diaz-Nido, J. (2007). BDNF production by olfactory ensheathing cells contributes to axonal regeneration of cultured adult CNS neurons. Neurochem. Int. 50, 491–498. doi: 10.1016/j.neuint.2006.10.004
Perera, S. N., Williams, R. M., Lyne, R., Stubbs, O., Buehler, D. P., Sauka-Spengler, T., et al. (2020). Insights into olfactory ensheathing cell development from a laser-microdissection and transcriptome-profiling approach. Glia 68, 2550–2584. doi: 10.1002/glia.23870
Pudelko, L., Edwards, S., Balan, M., Nyqvist, D., Al-Saadi, J., Dittmer, J., et al. (2018). An orthotopic glioblastoma animal model suitable for high-throughput screenings. Neuro Oncol. 20, 1475–1484. doi: 10.1093/neuonc/noy071
Radomska, K. J., and Topilko, P. (2017). Boundary cap cells in development and disease. Curr. Opin. Neurobiol. 47, 209–215. doi: 10.1016/j.conb.2017.11.003
Radtke, C., Aizer, A. A., Agulian, S. K., Lankford, K. L., Vogt, P. M., and Kocsis, J. D. (2008). Transplantation of olfactory ensheathing cells enhances peripheral nerve regeneration after microsurgical nerve repair. Brain Res. 1254, 10–17. doi: 10.1016/j.brainres.2008.11.036
Radtke, C., Allmeling, C., Waldmann, K. H., Reimers, K., Thies, K., Schenk, H. C., et al. (2011). Spider silk constructs enhance axonal regeneration and remyelination in long nerve defects in sheep. PLoS One 6:e16990. doi: 10.1371/journal.pone.0016990
Rao, M., Nelms, B. D., Dong, L., Salinas-Rios, V., Rutlin, M., Gershon, M. D., et al. (2015). Enteric glia express proteolipid protein 1 and are a transcriptionally unique population of glia in the mammalian nervous system. Glia 63, 2040–2057. doi: 10.1002/glia.22876
Reshamwala, R., Shah, M., St John, J., and Ekberg, J. (2019). Survival and integration of transplanted olfactory ensheathing cells are crucial for spinal cord injury repair: insights from the last 10 years of animal model studies. Cell Transplant. 28(Suppl. 1), 132S–159S. doi: 10.1177/0963689719883823.7
Retamal, M. A., Alcayaga, J., Verdugo, C. A., Bultynck, G., Leybaert, L., Sáez, P. J., et al. (2014). Opening of pannexin- and connexin-based channels increases the excitability of nodose ganglion sensory neurons. Front. Cell. Neurosci. 8:158. doi: 10.3389/fncel.2014.00158
Riethmacher, D., Sonnenberg-Riethmacher, E., Brinkmann, V., Yamaai, T., Lewin, G. R., and Birchmeier, C. (1997). Severe neuropathies in mice with targeted mutations in the ErbB3 receptor. Nature 389, 725–730. doi: 10.1038/39593
Scriba, L. D., Bornstein, S. R., Santambrogio, A., Mueller, G., Huebner, A., Hauer, J., et al. (2020). Cancer stem cells in pheochromocytoma and paraganglioma. Front. Endocrinol. (Lausanne) 11:79. doi: 10.3389/fendo.2020.00079
Shin, S. M., Itson-Zoske, B., Cai, Y., Qiu, C., Pan, B., Stucky, C. L., et al. (2020). Satellite glial cells in sensory ganglia express functional transient receptor potential ankyrin 1 that is sensitized in neuropathic and inflammatory pain. Mol. Pain 16:1744806920925425. doi: 10.1177/1744806920925425
Soldatov, R., Kaucka, M., Kastriti, M. E., Petersen, J., Chontorotzea, T., Englmaier, L., et al. (2019). Spatiotemporal structure of cell fate decisions in murine neural crest. Science 364:eaas9536. doi: 10.1126/science.aas9536
Spray, D. C., Iglesias, R., Shraer, N., Suadicani, S. O., Belzer, V., Hanstein, R., et al. (2019). Gap junction mediated signaling between satellite glia and neurons in trigeminal ganglia. Glia 67, 791–801. doi: 10.1002/glia.23554
Stewart, H. J., Brennan, A., Rahman, M., Zoidl, G., Mitchell, P. J., Jessen, K. R., et al. (2001). Developmental regulation and overexpression of the transcription factor AP-2, a potential regulator of the timing of Schwann cell generation. Eur. J. Neurosci. 14, 363–372. doi: 10.1046/j.0953-816x.2001.01650.x
Suadicani, S. O., Cherkas, P. S., Zuckerman, J., Smith, D. N., Spray, D. C., and Hanani, M. (2010). Bidirectional calcium signaling between satellite glial cells and neurons in cultured mouse trigeminal ganglia. Neuron Glia Biol. 6, 43–51. doi: 10.1017/S1740925X09990408
Tasdemir-Yilmaz, O. E., Druckenbrod, N. R., Olukoya, O. O., Dong, W., Yung, A. R., Bastille, I., et al. (2021). Diversity of developing peripheral glia revealed by single-cell RNA sequencing. Dev Cell. 56, 2516–2535.e8. doi: 10.1016/j.devcel.2021.08.005
Taveggia, C., Zanazzi, G., Petrylak, A., Yano, H., Rosenbluth, J., Einheber, S., et al. (2005). Neuregulin-1 type III determines the ensheathment fate of axons. Neuron 47, 681–694. doi: 10.1016/j.neuron.2005.08.017
Todd, K. J., Darabid, H., and Robitaille, R. (2010). Perisynaptic glia discriminate patterns of motor nerve activity and influence plasticity at the neuromuscular junction. J. Neurosci. 30, 11870–11882. doi: 10.1523/JNEUROSCI.3165-10.2010
Trachtenberg, J. T., and Thompson, W. J. (1997). Nerve terminal withdrawal from rat neuromuscular junctions induced by neuregulin and Schwann cells. J. Neurosci. 17, 6243–6255. doi: 10.1523/JNEUROSCI.17-16-06243.1997
Tu, Y. K., and Hsueh, Y. H. (2020). Extracellular vesicles isolated from human olfactory ensheathing cells enhance the viability of neural progenitor cells. Neurol. Res. 42, 959–967. doi: 10.1080/01616412.2020.1794371
Uesaka, T., Nagashimada, M., and Enomoto, H. (2015). Neuronal differentiation in Schwann cell lineage underlies postnatal neurogenesis in the enteric nervous system. J. Neurosci. 35, 9879–9888. doi: 10.1523/jneurosci.1239-15.2015
Uesaka, T., Okamoto, M., Nagashimada, M., Tsuda, Y., Kihara, M., Kiyonari, H., et al. (2021). Enhanced enteric neurogenesis by Schwann cell precursors in mouse models of Hirschsprung disease. Glia 69, 2575–2590. doi: 10.1002/glia.24059
van Weperen, V. Y. H., Littman, R. J., Arneson, D. V., Contreras, J., Yang, X., and Ajijola, O. A. (2021). Single-cell transcriptomic profiling of satellite glial cells in stellate ganglia reveals developmental and functional axial dynamics. Glia 69, 1281–1291. doi: 10.1002/glia.23965
VanSaun, M., Herrera, A. A., and Werle, M. J. (2003). Structural alterations at the neuromuscular junctions of matrix metalloproteinase 3 null mutant mice. J. Neurocytol. 32, 1129–1142. doi: 10.1023/B:NEUR.0000021907.68461.9c
VanSaun, M., Humburg, B. C., Arnett, M. G., Pence, M., and Werle, M. J. (2007). Activation of Matrix Metalloproteinase-3 is altered at the frog neuromuscular junction following changes in synaptic activity. Dev. Neurobiol. 67, 1488–1497. doi: 10.1002/dneu.20523
Villa, G., Fumagalli, M., Verderio, C., Abbracchio, M. P., and Ceruti, S. (2010). Expression and contribution of satellite glial cells purinoceptors to pain transmission in sensory ganglia: an update. Neuron Glia Biol. 6, 31–42. doi: 10.1017/S1740925X10000086
Vit, J. P., Ohara, P. T., Bhargava, A., Kelley, K., and Jasmin, L. (2008). Silencing the Kir4.1 potassium channel subunit in satellite glial cells of the rat trigeminal ganglion results in pain-like behavior in the absence of nerve injury. J. Neurosci. 28, 4161–4171. doi: 10.1523/JNEUROSCI.5053-07.2008
Wang, G. Y., Cheng, Z. J., Yuan, P. W., Li, H. P., and He, X. J. (2021). Olfactory ensheathing cell transplantation alters the expression of chondroitin sulfate proteoglycans and promotes axonal regeneration after spinal cord injury. Neural Regen. Res. 16, 1638–1644. doi: 10.4103/1673-5374.301023
Wang, J., Song, F., and Loeb, J. A. (2017). Neuregulin1 fine-tunes pre-, post-, and perisynaptic neuromuscular junction development. Dev. Dyn. 246, 368–380. doi: 10.1002/dvdy.24494
Wang, K., Wang, S., Chen, Y., Wu, D., Hu, X., Lu, Y., et al. (2021). Single-cell transcriptomic analysis of somatosensory neurons uncovers temporal development of neuropathic pain. Cell Res. 31, 904–918. doi: 10.1038/s41422-021-00479-9
Wang, X.-B., Ma, W., Luo, T., Yang, J. W., Wang, X. P., Dai, Y. F., et al. (2019). A novel primary culture method for high-purity satellite glial cells derived from rat dorsal root ganglion. Neural Regen. Res. 14, 339–345. doi: 10.4103/1673-5374.244797
Wassenhove-McCarthy, D. J., and McCarthy, K. J. (1999). Molecular characterization of a novel basement membrane-associated proteoglycan, leprecan. J. Biol. Chem. 274, 25004–25017. doi: 10.1074/jbc.274.35.25004
Watzlawick, R., Rind, J., Sena, E. S., Brommer, B., Zhang, T., Kopp, M. A., et al. (2016). Olfactory ensheathing cell transplantation in experimental spinal cord injury: effect size and reporting bias of 62 experimental treatments: a systematic review and meta-analysis. PLoS Biol. 14:e1002468. doi: 10.1371/journal.pbio.1002468
Wewetzer, K., Grothe, C., and Claus, P. (2001). In vitro expression and regulation of ciliary neurotrophic factor and its alpha receptor subunit in neonatal rat olfactory ensheathing cells. Neurosci. Lett. 306, 165–168. doi: 10.1016/s0304-3940(01)01891-2
Xia, B., Gao, J., Li, S., Huang, L., Ma, T., Zhao, L., et al. (2019). Extracellular vesicles derived from olfactory ensheathing cells promote peripheral nerve regeneration in rats. Front. Cell. Neurosci. 13:548. doi: 10.3389/fncel.2019.00548
Xie, J., Huo, S., Li, Y., Dai, J., Xu, H., and Yin, Z. Q. (2017). Olfactory ensheathing cells inhibit gliosis in retinal degeneration by downregulation of the Müller cell notch signaling pathway. Cell Transplant. 26, 967–982. doi: 10.3727/096368917X694994
Xie, M., Kamenev, D., Kaucka, M., Kastriti, M. E., Zhou, B., Artemov, A. V., et al. (2019). Schwann cell precursors contribute to skeletal formation during embryonic development in mice and zebrafish. Proc. Natl. Acad. Sci. U.S.A. 116, 15068–15073. doi: 10.1073/pnas.1900038116
Yamakita, S., Horii, Y., Takemura, H., Matsuoka, Y., Yamashita, A., Yamaguchi, Y., et al. (2018). Synergistic activation of ERK1/2 between A-fiber neurons and glial cells in the DRG contributes to pain hypersensitivity after tissue injury. Mol. Pain 14:1744806918767508. doi: 10.1177/1744806918767508
Yang, J. F., Cao, G., Koirala, S., Reddy, L. V., and Ko, C. P. (2001). Schwann cells express active agrin and enhance aggregation of acetylcholine receptors on muscle fibers. J. Neurosci. 21, 9572–9584. doi: 10.1523/JNEUROSCI.21-24-09572.2001
Zeisel, A., Hochgerner, H., Lönnerberg, P., Johnsson, A., Memic, F., van der Zwan, J., et al. (2018). Molecular architecture of the mouse nervous system. Cell 174, 999–1014.e22. doi: 10.1016/j.cell.2018.06.021
Zhang, L., Zhuang, X., Kotitalo, P., Keller, T., Krzyczmonik, A., Haaparanta-Solin, M., et al. (2021). Intravenous transplantation of olfactory ensheathing cells reduces neuroinflammation after spinal cord injury via interleukin-1 receptor antagonist. Theranostics 11, 1147–1161. doi: 10.7150/thno.52197
Zhang, Y., Laumet, G., Chen, S. R., Hittelman, W. N., and Pan, H. L. (2015). Pannexin-1 Up-regulation in the dorsal root ganglion contributes to neuropathic pain development. J. Biol. Chem. 290, 14647–14655. doi: 10.1074/jbc.M115.650218
Zhao, W. J., and Schachner, M. (2013). Neuregulin 1 enhances cell adhesion molecule l1 expression in human glioma cells and promotes their migration as a function of malignancy. J. Neuropathol. Exp. Neurol. 72, 244–255. doi: 10.1097/NEN.0b013e3182863dc5
Zhu, Y., Ghosh, P., Charnay, P., Burns, D. K., and Parada, L. F. (2002). Neurofibromas in NF1: Schwann cell origin and role of tumor environment. Science 296, 920–922. doi: 10.1126/science.1068452
Keywords: heterogeneity, pluripotency, cellular hierarchy, Schwann cells, neural crest, peripheral glia
Citation: Sinegubov A, Andreeva D, Burzak N, Vasyutina M, Murashova L and Dyachuk V (2022) Heterogeneity and Potency of Peripheral Glial Cells in Embryonic Development and Adults. Front. Mol. Neurosci. 15:737949. doi: 10.3389/fnmol.2022.737949
Received: 07 July 2021; Accepted: 08 February 2022;
Published: 25 March 2022.
Edited by:
Dezhi Liao, University of Minnesota Twin Cities, United StatesReviewed by:
Shen-Ju Chou, Academia Sinica, TaiwanJorge B. Aquino, Instituto de Investigaciones en Medicina Traslacional, Universidad Austral, Argentina
Copyright © 2022 Sinegubov, Andreeva, Burzak, Vasyutina, Murashova and Dyachuk. This is an open-access article distributed under the terms of the Creative Commons Attribution License (CC BY). The use, distribution or reproduction in other forums is permitted, provided the original author(s) and the copyright owner(s) are credited and that the original publication in this journal is cited, in accordance with accepted academic practice. No use, distribution or reproduction is permitted which does not comply with these terms.
*Correspondence: Vyacheslav Dyachuk, ZHlhY2h1a192YUBhbG1hem92Y2VudHJlLnJ1