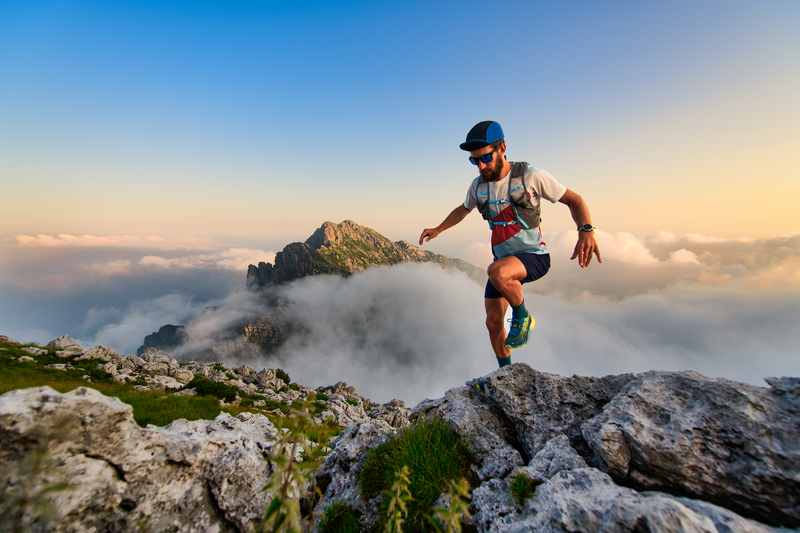
95% of researchers rate our articles as excellent or good
Learn more about the work of our research integrity team to safeguard the quality of each article we publish.
Find out more
REVIEW article
Front. Mol. Neurosci. , 04 January 2023
Sec. Pain Mechanisms and Modulators
Volume 15 - 2022 | https://doi.org/10.3389/fnmol.2022.1081288
This article is part of the Research Topic Rising Stars in Molecular Neuroscience - Pain Mechanisms and Modulators: 2022 View all 4 articles
Since the discovery of STING in 2008, numerous studies have investigated its functions in immunity, inflammation, and cancer. STING activates downstream molecules including IFN-I, NLRP3, and NF-κB. The STING–IFN-I pathway plays a vital role in nociception. After receiving the upstream signal, STING is activated and induces the expression of IFN-I, and after paracrine and autocrine signaling, IFN-I binds to IFN receptors. Subsequently, the activity of ion channels is inhibited by TYK2, which induces an acute antinociceptive effect. JAK activates PIK3 and MAPK–MNK–eIF4E pathways, which sensitize nociceptors in the peripheral nervous system. In the mid-late stage, the STING–IFN-I pathway activates STAT, increases pro-inflammatory and anti-inflammatory cytokines, inhibits ER-phagy, and promotes microglial M1-polarization in the central nervous system, leading to central sensitization. Thus, the STING–IFN-I pathway may exert complex effects on nociception at various stages, and these effects require further comprehensive elucidation. Therefore, in this review, we systematically summarized the mechanisms of the STING–IFN-I pathway and discussed its function in nociception.
Pain is defined by the International Association for the Study of Pain as an unpleasant sensory and emotional experience associated with actual or potential tissue or other damage (Merskey and Spear, 1967). After sensing physical and chemical stimuli, nociceptors produce and transmit information to the central nervous system(CNS). Notably, the brain can generate pain without a message from nociceptors or the spinal cord, such as in phantom limb pain (Loeser and Melzack, 1999; Julius and Basbaum, 2001). Multiple molecules are involved in the production of pain, such as G protein-coupled receptors, cyclic nucleotides, capsaicin, and acid (Julius and Basbaum, 2001).
Stimulator of interferon genes (also called STING, MITA, MPYS, ERIS, and TMEM173) was first discovered in 2008 (Ishikawa and Barber, 2008; Zhong et al., 2008). STING could regulate antimicrobial response, autoimmune disease, and cancer progression (Ahn et al., 2012; Li et al., 2013; Zheng et al., 2020). The stimulator of interferon genes (STING)–interferon-I (IFN-I) pathway can control nociception (Donnelly et al., 2021; Wang et al., 2021). In neuropathic pain models including bone cancer pain, chemotherapy-induced peripheral neuropathy, and nerve injury, administration of STING agonists activates STING, increases the expression of IFN-I, and inhibits the excitability of nociceptors in the peripheral nervous system (PNS) (Wang et al., 2021). These effects induce transient, short-term, and dose-dependent antinociception at an early stage (Donnelly et al., 2021). However, the antinociceptive effect was not substantial 11 days after the injection (Wang et al., 2021). Similarly, activation of the STING–IFN-I pathway induces nociception or neuropathic pain at a late stage (Liu et al., 2022; Wu et al., 2022). The exact effects of STING–IFN-I remain controversial, and its differential role in different sexes, neuropathic pain models, cells, and stages requires further research. Previous studies hypothesized that this pathway might be a potential therapeutic target for pain management.
In this review, we systematically summarize the mechanisms of the STING–IFN-I pathway and discuss its function in nociception.
STING is located in the endoplasmic reticulum (ER) (Ishikawa and Barber, 2008; Zhong et al., 2008). In human cells, STING comprises 379 amino acids and contains five putative transmembrane regions (Ishikawa and Barber, 2008). The N-terminal of STING, consisting of four transmembrane regions, is responsible for membrane anchoring. The C-terminal protrudes into the cytoplasm and contains a domain that binds with cyclic dinucleotides (CDNs) (Zhong et al., 2008; Sun et al., 2009; Landman et al., 2020). STING can directly detect bacterial CDNs and activate immune responses (Burdette et al., 2011; Ablasser et al., 2013). In addition, it can detect cytosolic double-stranded DNA (dsDNA) released by tumor and dead cells via cyclic guanosine monophosphate–adenosine monophosphate (cyclic GMP–AMP or cGAMP) synthase (cGAS) activity (Chen et al., 2016). Moreover, leakage of mitochondrial DNA can activate STING in adjacent phagocytic cells (West et al., 2015). After STING activation, the expression of IFN-I, NOD-like receptor protein 3 (NLRP3), and nuclear factor-κB (NF-κB) increases (Zhong et al., 2008; Abe and Barber, 2014; Liu et al., 2015).
IFN-I was first discovered in 1957 and is composed of IFN-α, IFN-β, IFN-δ, IFN-ε, IFN-κ, IFN-τ, and IFN-ω (Lindenmann et al., 1957; Tan et al., 2021). IFN-I participates in the antiviral response, cell proliferation, apoptosis, inflammation, and adaptive immunity (Lindenmann et al., 1957; Stark et al., 1998; Randall and Goodbourn, 2008).
Microbial DNA invasion triggers a series of immune responses. STING is essential for detecting exogenous microbial DNA (Li et al., 2013). Activation of STING consequently activates the transcription factors NF-κB and interferon regulatory factor 3 (IRF3) to induce cytokines and IFN-I expression (Ishikawa and Barber, 2011). STING is required by fibroblasts, macrophages, dendritic cells, and myeloid cells to induce IFN-I production against vaccinia virus (VACV), cytomegalovirus (HCMV), baculovirus, several strains of herpes simplex virus-1 (HSV1), and Listeria monocytogenes (Ishikawa and Barber, 2008; Ishikawa et al., 2009).
In addition to exogenous DNA, STING can detect self-DNA. Undigested DNA from apoptotic cells triggers DNA sensors, which increase the expression of cytokines and result in autoimmune diseases (Nagata, 2010; Ahn et al., 2012). The exonuclease, three prime repair exonuclease 1 (TREX1), degrades cytosolic DNA (Mazur and Perrino, 1999; Crow et al., 2006) and its deficiency leads to multiple inflammatory and autoimmune diseases such as systemic lupus erythematosus, Aicardi–Goutieres syndrome, and familial chilblain lupus (Rice et al., 2015). In a TREX1-deficient rat model, cGAS activated STING through cGAMP production and mediated inflammatory disease and death in mice (Gao et al., 2015). Similarly, STING triggered by apoptotic or necrotic DNA promoted the expression of cytokines, whereas its deficiency abrogated the production of cytokines activated by self-DNA in a DNase II-deficient model (Ahn et al., 2012).
Nuclear and mitochondrial DNA are easily damaged in tumor cells, inducing IFN-I through the cGAS–STING–IRF3-dependent pathway (Woo et al., 2014; Chen Y. A. et al., 2017; Mackenzie et al., 2017). IFN-I is a mediator of STING and exerts adaptive antitumor effects (Zheng et al., 2020). It can promote cross-presentation by stimulating the maturation of DCs, slowing down the endosome–lysosome acidification process to prevent phagocytic tumor antigen clearance, and increasing the expression of cell surface MHC I molecules, which accelerates DC migration to lymph nodes to cross-trigger tumor-specific CD8+ T cells (Reboulet et al., 2010; Diamond et al., 2011; Lorenzi et al., 2011; Zheng et al., 2020). In addition, IFN-I can induce the expression of multiple chemokines (Padovan et al., 2002; Takashima et al., 2016). For instance, CXCL9 and CXCL10 are involved in cytotoxic T lymphocyte transfer and infiltration, whereas CCL5 and CXCL10 promote the recruitment and activation of NK cells and T cells in tumors (Padovan et al., 2002; Takashima et al., 2016). By contrast, the cGAS–STING pathway can induce the senescence-associated secretory phenotype (SASP) (Loo et al., 2020). The SASP factor induces immune surveillance and acts as a tumor suppressor. However, continuous exposure to SASP may cause tissue damage and chronic inflammation associated with tumor growth (Loo et al., 2020). Nevertheless, long-term activation of STING may promote tumor growth and metastasis, and this effect is associated with tumor stage, CIN status, and the degree of STING activation (Zheng et al., 2020). STING agonists including cyclic dinucleotides and their derivatives, DMXAA and its analogs, and small-molecule agonists are widely studied as cancer treatment agents (Corrales and Gajewski, 2015; Ablasser and Chen, 2019; Zheng et al., 2020).
Cell bodies of nociceptors are distributed in the dorsal root ganglia (DRG) and trigeminal ganglion (Basbaum et al., 2009). Most nociceptors contain unmyelinated C fibers (Woolf and Ma, 2007). However, initial and acute pain is mediated by nociceptors with A fibers (Djouhri and Lawson, 2004). After sensing physical and chemical stimuli, peripheral nociceptors are activated to produce pain through different signal transduction pathways (Julius and Basbaum, 2001; Donnelly et al., 2020). Particularly, TRP channels recognize noxious heat, and the ENaC/DEG channel family senses mechanical stimuli (Lingueglia et al., 1997; Tominaga et al., 1998). Nociceptors can convert receptor potentials into action potentials through voltage-gated channels (including sodium, calcium, and potassium channels) (Dubin and Patapoutian, 2010). Primary nociceptors transmit noxious stimuli to projection neurons located in the cornu dorsalis medullae spinalis (Basbaum et al., 2009). Harmful information is transmitted to the somatosensory cortex through the thalamus, indicating the location and intensity of the pain (Basbaum et al., 2009). Other projection neurons contact the cingulate gyrus and insular cortex through the brain stem and amygdala, forming emotional elements of pain experiences (Basbaum et al., 2009).
Pattern recognition receptors (PRRs) recognize pathogen-associated molecular patterns (PAMPs) and damage-associated molecular patterns (DAMPs) to induce the transcription of genes involved in inflammatory responses (Takeuchi and Akira, 2010). PRRs include toll-like, RIG-I-like, NOD-like, and DNA receptors (cytosolic sensors for DNA) (Takeuchi and Akira, 2010; Kumar et al., 2011). Both immune cells and peripheral nociceptors express PRRs (Usoskin et al., 2015; Zeisel et al., 2018; Zheng et al., 2019). Cytosolic DNA sensors such as the cGAS–STING pathway are highly expressed in nociceptive neurons (Usoskin et al., 2015; Zeisel et al., 2018; Donnelly et al., 2020). PRRs on immune cells recognize DAMPs/PAMPs and release cytokines/chemokines and inflammatory mediators to react with nociceptor terminals (Donnelly et al., 2020). Meanwhile, the terminals of nociceptors can directly detect PAMPs/DAMPs and danger signals (Donnelly et al., 2020). The indirect and direct pathways can regulate the function of sodium (e.g., Nav1.7, Nav1.8, and Nav1.9), calcium, and transient receptor potential channels. Thus, the excitability and activity of nociceptors are altered (Jin, 2006; Gold and Gebhart, 2010; Ji et al., 2014; Figure 1).
Figure 1. Pattern recognition receptors (PRRs) in nociception. PRRs play a vital role in nociception through indirect and direct pathways. (1) Indirect pathway: After PRRs on immune cells detect DAMPs/PAMPs, immune cells release cytokines, chemokines, and inflammatory mediators to react with the terminals of nociceptors. (2) Direct pathway: Terminals of nociceptors express PRRs to detect DAMPs/PAMPs directly. Indirect and direct pathways can alter the excitability and activity of ion channels to regulate nociceptors.
cGAS, a cytosolic sensor for DNA, can activate STING through cGAMP production (Ablasser et al., 2013; Gao et al., 2013; Li et al., 2013; Sun et al., 2013). STING directly detects bacterial cytoplasmic CDNs including cyclic-di-GMP, cyclic-di-AMP, and 3′,3′-cGAMP (Burdette et al., 2011; Jin et al., 2011; Ablasser et al., 2013; Yi et al., 2013). Aside from cGAS, DNA-dependent activators of interferon regulatory factors, IFN-γ-inducible protein 16, and DEAD box polypeptide 41 can also recognize cytosolic DNA and activate STING (Tanaka and Chen, 2012; Wu and Chen, 2014; Cheng et al., 2020; Figure 2). Intracellular dsDNA and dsRNA can induce IFN-I-dependent antinociception; however, only the dsDNA-dependent pathway requires the cGAS–STING pathway (Donnelly et al., 2021).
Figure 2. Mechanism of the STING–IFN-I pathway in nociception. After detecting both foreign and autologous cytosolic DNAs, STING exposes its C terminal and recruits TBK1. Activated STING transfers from the ER to the Golgi complex and recruits IRF3. IRF3 phosphorylated by TBK1 forms dimers and accesses the nucleus to activate the transcription of IFN. Secretion of IFN-I increases. Then, IFN-I binds with IFN receptors. TYK2 associated with IFNAR1 promotes the acute antinociceptive effect. JAK associated with IFNAR2 activates the MAPK–MNK–eIF4E pathway and PIK3 at a later stage, causing nociceptor sensitization in DRG. In the mid-late stage, IFN-I induces a delayed effect in the CNS.
After binding with CDNs, STING is transferred from the ER to the Golgi complex via perinuclear vesicles (Ishikawa et al., 2009). STING forms oligomers in the ER–Golgi membrane and exposes its C terminal (Tanaka and Chen, 2012; Ergun et al., 2019). The C terminal of STING recruits TANK-binding kinase 1 (TBK1), and the STING dimer accesses the active site of TBK1 for its phosphorylation (Barber, 2011; Zhang et al., 2019). In addition, two TBK1 dimers can be mutually activated via transautophosphorylation (Zhang et al., 2019). The phosphorylated tail of STING recruits IRF3 and transports it to TBK1 for phosphorylation (Tanaka and Chen, 2012; Zhang et al., 2019). Notably, the interaction between STING and TBK1 enhances the binding of TBK1 and IRF3 (Zhong et al., 2008; Tanaka and Chen, 2012; Zhang et al., 2019). Phosphorylated IRF3 dimers access the nucleus and activate the transcription of IFN and inflammatory factor genes (Liu et al., 2015; Zhang H. et al., 2020). Thus, IFN-I synthesis increases notably. After paracrine and autocrine signaling, IFN-I binds with IFN receptors on sensory neurons to generate nociceptive effects (Donnelly et al., 2021; Tan et al., 2021). IFN receptors comprise IFNAR1 and IFNAR2 (Wang et al., 2021). IFNAR1 plays a vital role in acute nociceptive functions (Donnelly et al., 2021; Wang et al., 2021). Inhibition of tyrosine kinase (TYK2) eliminates the analgesic effect of IFN-β (Donnelly et al., 2021). Thus, TYK2 associated with IFNAR1 inhibits the activity of sodium (Nav1.7) and calcium channels (Donnelly et al., 2021; Tan et al., 2021). Furthermore, the low excitability of nociceptors is attributed to the loss of function of sodium (Nav1.7) and calcium channels (Binshtok et al., 2007; McDermott et al., 2019). Therefore, IFN-I can induce acute and short-term antinociception via TYK2.
Conversely, IFNAR2 is associated with Janus-activated kinases (JAKs) (Michalska et al., 2018; Tan et al., 2021). After activation of JAKs, the mitogen-activated protein kinase (MAPK)–interacting kinase (MNK)–eukaryotic translation initiation factor 4E (eIF4E) pathway and PIK3 are activated, causing nociceptor sensitization in the PNS at a later stage (Tan et al., 2021). In the mid-late stage, IFN-I activates STAT to induce the expression of pro-inflammatory and anti-inflammatory cytokines, inhibits ER-phagy, and promotes microglial M1-polarization, which generates delayed nociceptive effects in the CNS (Figure 2; Ivashkiv and Donlin, 2014; Michalska et al., 2018; Tan et al., 2021; Wu et al., 2022).
Regulation of the STING–IFN-I pathway mostly depends on STING activity. Posttranslational modifications including phosphorylation, ubiquitination, and palmitoylation play vital roles in regulating STING activity (Zhang H. et al., 2020). Activation of STING requires palmitoylation in the Golgi complex (Mukai et al., 2016; Haag et al., 2018). After recruiting TBK1 and activating IRF3, negative feedback is triggered. STING is subsequently phosphorylated by serine/threonine UNC-51-like kinase, and IRF3 activity is inhibited (Konno et al., 2013).
Among posttranslational modifications, ubiquitination is essential for STING activity. Some molecules have been shown to play essential roles in STING regulation. AMFR facilitates K27-linked polyubiquitination of STING through the ER membrane protein INSIG1 and promotes the recruitment and activation of TBK1 (Wang et al., 2014). EIF3S5, OTUD5, CYLD, and ubiquitin-specific protease (USP) 44 (USP44) are deubiquitinases that remove K48-linked polyubiquitination to maintain the stability of STING (Luo et al., 2016; Zhang et al., 2018; Zhang H. Y. et al., 2020; Guo et al., 2021). TRIM32 promotes K63-linked polyubiquitination of STING and increases the production of IFN-I (Cui et al., 2017). iRhom2 recruits the translocon-associated protein (TRAPβ) and the deubiquitination enzyme (EIF3S5) to promote STING trafficking from the ER to perinuclear microsomes (Luo et al., 2016). USP13 deconjugates polyubiquitin chains on STING to prevent recruitment of TBK1 (Sun et al., 2017), while USP21 hydrolyzes the K27/63-linked polyubiquitin chain on STING to negatively regulate the production of IFN-I (Table 1; Chen Y. et al., 2017). The regulatory mechanism of STING is complex and warrants further investigation.
Table 1. Summary of molecules associated with ubiquitination of STING and regulation of STING activity.
Limited studies indicated that the STING–IFN-I pathway has dual effects on nociception (Table 2).
The STING–IFN-I pathway is associated with acute antinociceptive effects. In a rat model, deficiency of the STING–IFN-I pathway increased the excitability of nociceptors (Donnelly et al., 2021). In the chronic constriction injury model of rats, knockdown of the D-type protein tyrosine phosphatase receptor increased the expression of STING and IFN-α, which attenuated pain (Sun et al., 2022). STING agonists can relieve neuropathic pain in peripheral neuropathy induced by paclitaxel chemotherapy (Donnelly et al., 2021) and pain induced by nerve injury (Donnelly et al., 2021). Similarly, they may inhibit bone cancer pain and maintain motor function by reducing tumor burden and inhibiting cancer-induced osteoclast generation (Donnelly et al., 2021; Wang et al., 2021). Moreover, STING agonists can attenuate fracture-induced pain in tumor-free mice (Wang et al., 2021). Notably, after injecting STING agonists in rats, IFN-I levels in serum, DRG tissues, and bone marrow lysates were significantly upregulated 1000-fold in 4 h and maintained for up to 24 h (Donnelly et al., 2021; Wang et al., 2021; Sun et al., 2022). Therefore, the STING–IFN-I pathway may promote short-term antinociception.
Several studies have also reported contradictory results, wherein the STING–IFN-I pathway exerted negative effects. A case series reported that IFN-I induced by STING causes neuropathic pain in young patients (Papa et al., 2021). Similarly, in patients with hepatitis C virus infection, the use of IFN-α leads to somatic pain (Lin et al., 2020).
Intraplantar administration of IFN-α (300 U/25 μL) or IFN-β (300 U/25 μL) can activate the MNK-eIF4E pathway via the STING–IFN-I pathway (Barragán-Iglesias et al., 2020). Subsequently, this pathway induces nociceptor hyperexcitability and mechanical pain sensitization at the DRG level for a short period of time (Barragán-Iglesias et al., 2020). Pain induction was not significant 3 days after peripheral injection (Barragán-Iglesias et al., 2020). Thus, the effects of IFN-I may be acute or transient.
In the spared nerve injury (SNI) model, inhibiting the cGAS–STING pathway can restrain microglial M1-polarization and attenuate neuropathic pain (Wu et al., 2022). M1-polarization microglia express CD16 and induce TNF-α and IL-1β synthesis, which may cause central sensitization (Mesquida-Veny et al., 2021). In a rat SNL model, ketamine and dexmedetomidine induced ER-phagy and alleviated ER stress to provide antianxiety and antinociceptive effects by inhibiting the STING–TBK pathway in the spinal cord (Liu et al., 2022).
There are several possible explanations for these contradictions. First, the sex of the animals may have caused this discrepancy. This pathway more likely has a negative effect on male rats (Wu et al., 2022). Second, animal experiments were used to create different neuropathic pain models to explore its effects. However, different animal models may exhibit various neuropathies. Third, different injection methods may also have caused bias. Peripheral administration of IFN-I induced pain behavior in rat models (Barragán-Iglesias et al., 2020). However, intrathecal injection of IFN-α inhibited mechanical hypersensitivity caused by intraplantar (Donnelly et al., 2021). Fourth, the different effective times influenced the results. Short-term activation of this pathway led to transient and acute antinociception, which was maintained for up to 24 h (Wang et al., 2021). However, consecutive and repeated administration of STING agonists caused central sensitization and nociception (Wu et al., 2022). Fifth, the STING–IFN-I pathway does not participate in the physiological regulation of pain sensitivity and is only involved in the regulation of pain after nerve injury (Sun et al., 2022; Wu et al., 2022). Therefore, observing a positive effect in normal rat models injected with STING agonists or IFN-I is challenging. Finally, the STING–IFN-I pathway may play distinct roles in different parts of the PNS and CNS. A study has demonstrated that after STING agonist DMXAA treatment in mouse models, bone cancer-induced cold and mechanical allodynia were reduced at an early stage but not at the mid-late stage (Donnelly et al., 2021). Therefore, at an early stage, it induces antinociception and reduces pain by restraining the activity of ion channels and the excitability of nociceptors in the PNS. Subsequently, this pathway may induce nociceptor sensitization via the MAPK–MNK–eIF4E pathway and PIK3. At the mid-late stage, it can cause central sensitization in several ways (Figure 3).
Figure 3. Different roles of the STING–IFN-I pathway in the peripheral nerve system (PNS) and central nerve system (CNS). In the PNS, the pathway inhibits the excitability of nociceptors at an early stage. Subsequently, it may cause nociceptor sensitization. At the mid-late stage, it plays a dominant role in the CNS, which leads to central sensitization. Under normal physiological conditions, antinociception and nociception of the STING–IFN-I pathway maintain homeostasis. A nerve injury disrupts the balance and leads to antinociception or nociception.
Since the isolation of morphine in 1805, opioids have been widely used for pain management (Pasternak, 2014). Opioids, including morphine, interact with μ, κ, and δ receptors to produce analgesic effects, respiratory depression, and euphoria addiction. After binding with opioid receptors, opioids cause antinociception through the same mechanism as enkephalin, which involves hyperpolarization of interneurons and reduction of transmitters associated with pain (Haigler, 1987; Lipp, 1991). In addition, morphine can react with opioid receptors in supraspinal structures to activate the supraspinal system (Lipp, 1991). By contrast, STING agonists produce acute and short-term antinociception via the STING–IFN-I pathway in the PNS. Furthermore, opioids are highly addictive, which is caused by a reduction in the inhibitory function of GABAergic synapses in the neurons of the central amygdala and brain reward/motivational mesocorticolimbic circuitry (Navratilova and Porreca, 2014; Zhang et al., 2014). In contrast, the repeated use of STING agonists does not cause addiction and attenuates SNI-induced astrogliosis (Donnelly et al., 2021). In non-human primates, intrathecal administration of STING agonists produces longer lasting analgesic effects at lower doses than morphine (3 vs. 100 nmol) (Sjöström et al., 1987; Donnelly et al., 2021; Wang et al., 2021). Naloxone, a nonselective and short-acting opioid receptor antagonist, can reverse the analgesic effect of morphine (Drug and the Therapeutics Bulletin, 1981; van Dorp et al., 2007). By contrast, STING agonist-mediated analgesia is not affected by naloxone (Donnelly et al., 2021).
Previous studies have suggested that STING agonists have potential advantages including strong efficacy at low doses, a longer lasting effect, and non-addictive. However, the exact effects of STING–IFN-I on nociception remain unclear and require further investigation.
Apart from inducing antimicrobial response, mediating autoimmune disease, and regulating tumor growth, the STING–IFN-I pathway can induce acute antinociception for a short period of time. Therefore, the STING–IFN-I pathway may be a potential therapeutic target for pain management.
However, the effects of STING on nociception have several issues that need to be discussed. First, Donnelly et al. (2021) demonstrated that STING agonists reduced bone cancer pain. However, Zhang et al. (2022) suggested that mitochondrial DNA triggers the STING pathway, leading to peripheral neuroinflammation and sensitization (Zhang et al., 2022). In the early stage, the STING–IFN-I pathway was dominant, which reduced bone cancer pain. In the mid-late stage, the MAPK–MNK–eIF4E pathway was activated, and the STING–NF-κB pathway increased bone cancer pain via IL-1β, IL-6, and TNF-α (Barragán-Iglesias et al., 2020; Zhang et al., 2022). In addition, STING agonists have been shown to reduce bone cancer pain through immune and neuronal modulation, reducing tumor burden and inhibiting osteoclastogenesis (Amouzegar et al., 2021; Wang et al., 2021). Therefore, it is difficult to determine the true effects of STING agonists in bone cancer pain models. Second, the STING–IFN-I pathway may influence central sensitization through ER-phagy and microglial M1-polarization. Further studies are needed to confirm this hypothesis and to determine how the STING–IFN-I pathway regulates ER-phagy and microglial M1-polarization. Third, previous studies have only discussed one downstream pathway in a neuropathic pain model. However, STING has various downstream signaling components, including IFN-I, NF-κB, and NLPRS. Studies that include all downstream signals of STING are still lacking. Lastly, STING–IFN-I exists not only in peripheral and central neurons but also in immune cells. Whether STING agonists interact with these cell types to cause nociception requires further studies (Wang et al., 2021).
The current clinical use of STING agonists focuses on cancer immunotherapy. Several combination therapies are currently available in clinical trials (Zheng et al., 2020). Few studies have indicated the effectiveness of STING in nociception; however, the use of STING for nociception remains controversial and warrants further extensive and comprehensive studies.
At an early stage, the STING–IFN-I pathway can induce short-term antinociceptive effects by activating TYK2, restraining the activity of calcium and sodium channels, and inhibiting the excitability of nociceptors in the PNS. Subsequently, it activates the JAK–MAPK–MNK–eIF4E pathway and PIK3, which cause nociceptor sensitization. At the mid-late stage, it promotes microglial M1-polarization, inhibits ER-phagy, activates STAT, and increases the expression of pro-inflammatory and anti-inflammatory cytokines in the CNS, which leads to central sensitization. Thus, the STING–IFN-I pathway at various stages has a dual effect on nociception.
JY wrote the manuscript and made illustrations. HD, BS, and YZ (Fifth author) provided advice for the manuscript. YZ (Fourth author) provided the supervision and comments on the manuscript. All the authors read and approved the final manuscript.
The work was supported by the National Natural Science Foundation of China (grant no. 81901144).
The authors declare that the research was conducted in the absence of any commercial or financial relationships that could be construed as a potential conflict of interest.
All claims expressed in this article are solely those of the authors and do not necessarily represent those of their affiliated organizations, or those of the publisher, the editors and the reviewers. Any product that may be evaluated in this article, or claim that may be made by its manufacturer, is not guaranteed or endorsed by the publisher.
The Supplementary Material for this article can be found online at: https://www.frontiersin.org/articles/10.3389/fnmol.2022.1081288/full#supplementary-material
Supplementary Data Sheet 1 | Search process for cited articles. Forty-seven articles were found initially. After excluding ineligible articles, only eight articles were included.
Abe, T., and Barber, G. N. (2014). Cytosolic-DNA-mediated, sting-dependent proinflammatory gene induction necessitates canonical NF-κB activation through TBK1. J. Virol. 88, 5328–5341. doi: 10.1128/JVI.00037-14
Ablasser, A., and Chen, Z. J. (2019). CGAS in action: Expanding roles in immunity and inflammation. Science 363:eaat8657. doi: 10.1126/science.aat8657
Ablasser, A., Goldeck, M., Cavlar, T., Deimling, T., Witte, G., Röhl, I., et al. (2013). Cgas produces a 2′-5′-linked cyclic dinucleotide second messenger that activates sting. Nature 498, 380–384. doi: 10.1038/nature12306
Ahn, J., Gutman, D., Saijo, S., and Barber, G. N. (2012). Sting manifests self DNA-dependent inflammatory disease. Proc. Natl. Acad. Sci. U. S. A. 109, 19386–19391. doi: 10.1073/pnas.1215006109
Amouzegar, A., Chelvanambi, M., Filderman, J. N., Storkus, W. J., and Luke, J. J. (2021). Sting agonists as cancer therapeutics. Cancers 13:2695.
Barragán-Iglesias, P., Franco-Enzástiga, Ú., Jeevakumar, V., Shiers, S., Wangzhou, A., Granados-Soto, V., et al. (2020). Type I interferons act directly on nociceptors to produce pain sensitization: Implications for viral infection-induced pain. J. Neurosci. 40, 3517–3532. doi: 10.1523/JNEUROSCI.3055-19.2020
Basbaum, A. I., Bautista, D. M., Scherrer, G., and Julius, D. (2009). Cellular and molecular mechanisms of pain. Cell 139, 267–284. doi: 10.1177/0022034515612022
Binshtok, A. M., Bean, B. P., and Woolf, C. J. (2007). Inhibition of nociceptors by TRPV1-mediated entry of impermeant sodium channel blockers. Nature 449, 607–610. doi: 10.1038/nature06191
Burdette, D. L., Monroe, K. M., Sotelo-Troha, K., Iwig, J. S., Eckert, B., Hyodo, M., et al. (2011). Sting is a direct innate immune sensor of cyclic di-GMP. Nature 478, 515–518.
Chen, Q., Sun, L., and Chen, Z. J. (2016). Regulation and function of the Cgas-sting pathway of cytosolic DNA sensing. Nat. Immunol. 17, 1142–1149.
Chen, Y., Wang, L., Jin, J., Luan, Y., Chen, C., Li, Y., et al. (2017). P38 inhibition provides anti-DNA virus immunity by regulation of USP21 phosphorylation and sting activation. J. Exp. Med. 214, 991–1010. doi: 10.1084/jem.20161387
Chen, Y. A., Shen, Y. L., Hsia, H. Y., Tiang, Y. P., Sung, T. L., and Chen, L. Y. (2017). Extrachromosomal telomere repeat DNA is linked to ALT development via Cgas-sting DNA sensing pathway. Nat. Struct. Mol. Biol. 24, 1124–1131. doi: 10.1038/nsmb.3498
Cheng, Z., Dai, T., He, X., Zhang, Z., Xie, F., Wang, S., et al. (2020). The interactions between Cgas-sting pathway and pathogens. Signal. Transduct. Target. Ther. 5:91.
Corrales, L., and Gajewski, T. F. (2015). Molecular pathways: Targeting the stimulator of interferon genes (sting) in the immunotherapy of cancer. Clin. Cancer Res. 21, 4774–4779.
Crow, Y. J., Hayward, B. E., Parmar, R., Robins, P., Leitch, A., Ali, M., et al. (2006). Mutations in the gene encoding the 3′-5′ DNA exonuclease TREX1 cause Aicardi-Goutières syndrome at the AGS1 locus. Nat. Genet. 38, 917–920. doi: 10.1038/ng1845
Cui, H., Liu, Y., and Huang, Y. (2017). Roles of TRIM32 in corneal epithelial cells after infection with herpes simplex virus. Cell. Physiol. Biochem. 43, 801–811. doi: 10.1159/000481563
Diamond, M. S., Kinder, M., Matsushita, H., Mashayekhi, M., Dunn, G. P., Archambault, J. M., et al. (2011). Type I interferon is selectively required by dendritic cells for immune rejection of tumors. J. Exp. Med. 208, 1989–2003.
Djouhri, L., and Lawson, S. N. (2004). Abeta-fiber nociceptive primary afferent neurons: A review of incidence and properties in relation to other afferent A-fiber neurons in mammals. Brain Res. Brain Res. Rev. 46, 131–145. doi: 10.1016/j.brainresrev.2004.07.015
Donnelly, C. R., Chen, O., and Ji, R. R. (2020). How do sensory neurons sense danger signals? Trends Neurosci. 43, 822–838.
Donnelly, C. R., Jiang, C., Andriessen, A. S., Wang, K., Wang, Z., Ding, H., et al. (2021). Sting controls nociception via type I interferon signalling in sensory neurons. Nature 591, 275–280. doi: 10.1038/s41586-020-03151-1
Drug and the Therapeutics Bulletin (1981). Naloxone–opiate antagonist. Drug Ther. Bull. 19, 83–84. doi: 10.1136/dtb.19.21.83
Dubin, A. E., and Patapoutian, A. (2010). Nociceptors: The sensors of the pain pathway. J. Clin. Investig. 120, 3760–3772.
Ergun, S. L., Fernandez, D., Weiss, T. M., and Li, L. (2019). Sting polymer structure reveals mechanisms for activation, hyperactivation, and inhibition. Cell 178, 290–301.e10. doi: 10.1016/j.cell.2019.05.036
Gao, D., Li, T., Li, X. D., Chen, X., Li, Q. Z., Wight-Carter, M., et al. (2015). Activation of cyclic GMP-AMP synthase by self-DNA causes autoimmune diseases. Proc. Natl. Acad. Sci. U. S. A. 112, E5699–E5705.
Gao, P., Ascano, M., Wu, Y., Barchet, W., Gaffney, B. L., Zillinger, T., et al. (2013). Cyclic [G(2′,5′)pA(3′,5′)p] is the metazoan second messenger produced by DNA-activated cyclic GMP-AMP synthase. Cell 153, 1094–1107. doi: 10.1016/j.cell.2013.04.046
Gold, M. S., and Gebhart, G. F. (2010). Nociceptor sensitization in pain pathogenesis. Nat. Med. 16, 1248–1257.
Guo, Y., Jiang, F., Kong, L., Wu, H., Zhang, H., Chen, X., et al. (2021). OTUD5 promotes innate antiviral and antitumor immunity through deubiquitinating and stabilizing sting. Cell. Mol. Immunol. 18, 1945–1955. doi: 10.1038/s41423-020-00531-5
Haag, S. M., Gulen, M. F., Reymond, L., Gibelin, A., Abrami, L., Decout, A., et al. (2018). Targeting sting with covalent small-molecule inhibitors. Nature 559, 269–273.
Haigler, H. J. (1987). Neurophysiological effects of opiates in the CNS. Monogr. Neural Sci. 13, 132–160.
Ishikawa, H., and Barber, G. N. (2008). Sting is an endoplasmic reticulum adaptor that facilitates innate immune signalling. Nature 455, 674–678.
Ishikawa, H., and Barber, G. N. (2011). The sting pathway and regulation of innate immune signaling in response to DNA pathogens. Cell. Mol. Life Sci. 68, 1157–1165.
Ishikawa, H., Ma, Z., and Barber, G. N. (2009). Sting regulates intracellular DNA-mediated, type I interferon-dependent innate immunity. Nature 461, 788–792. doi: 10.1038/nature08476
Ivashkiv, L. B., and Donlin, L. T. (2014). Regulation of type I interferon responses. Nat. Rev. Immunol. 14, 36–49.
Ji, R. R., Xu, Z. Z., and Gao, Y. J. (2014). Emerging targets in neuroinflammation-driven chronic pain. Nat. Rev. Drug Discov. 13, 533–548. doi: 10.1038/nrd4334
Jin, L., Hill, K. K., Filak, H., Mogan, J., Knowles, H., Zhang, B., et al. (2011). MPYS is required for IFN response factor 3 activation and type I IFN production in the response of cultured phagocytes to bacterial second messengers cyclic-di-AMP and cyclic-di-GMP. J. Immunol. 187, 2595–2601. doi: 10.4049/jimmunol.1100088
Jin, X. (2006). Gereau RWt. Acute p38-mediated modulation of tetrodotoxin-resistant sodium channels in mouse sensory neurons by tumor necrosis factor-alpha. J. Neurosci. 26, 246–255. doi: 10.1523/JNEUROSCI.3858-05.2006
Konno, H., Konno, K., and Barber, G. N. (2013). Cyclic dinucleotides trigger ULK1 (ATG1) phosphorylation of STING to prevent sustained innate immune signaling. Cell 155, 688–698. doi: 10.1016/j.cell.2013.09.049
Kumar, H., Kawai, T., and Akira, S. (2011). Pathogen recognition by the innate immune system. Int. Rev. Immunol. 30, 16–34.
Landman, S. L., Ressing, M. E., and van der Veen, A. G. (2020). Balancing sting in antimicrobial defense and autoinflammation. Cytokine Growth Factor Rev. 55, 1–14. doi: 10.1016/j.cytogfr.2020.06.004
Li, X. D., Wu, J., Gao, D., Wang, H., Sun, L., and Chen, Z. J. (2013). Pivotal roles of cGAS-cGAMP signaling in antiviral defense and immune adjuvant effects. Science 341, 1390–1394. doi: 10.1126/science.1244040
Lin, C. Y., Guu, T. W., Lai, H. C., Peng, C. Y., Chiang, J. Y., Chen, H. T., et al. (2020). Somatic pain associated with initiation of interferon-alpha (IFN-α) plus ribavirin (RBV) therapy in chronic HCV patients: A prospective study. Brain Behav. Immun. Health. 2:100035. doi: 10.1016/j.bbih.2019.100035
Lindenmann, J., Burke, D. C., and Isaacs, A. (1957). Studies on the production, mode of action and properties of interferon. Br. J. Exp. Pathol. 38, 551–562.
Lingueglia, E., de Weille, J. R., Bassilana, F., Heurteaux, C., Sakai, H., Waldmann, R., et al. (1997). A modulatory subunit of acid sensing ion channels in brain and dorsal root ganglion cells. J. Biol. Chem. 272, 29778–29783. doi: 10.1074/jbc.272.47.29778
Liu, S., Cai, X., Wu, J., Cong, Q., Chen, X., Li, T., et al. (2015). Phosphorylation of innate immune adaptor proteins MAVS, STING, and TRIF induces IRF3 activation. Science 347:aaa2630.
Liu, Y., Kuai, S., Ding, M., Wang, Z., Zhao, L., and Zhao, P. (2022). Dexmedetomidine and ketamine attenuated neuropathic pain related behaviors via sting pathway to induce ER-Phagy. Front. Synapt. Neurosci. 14:891803. doi: 10.3389/fnsyn.2022.891803
Loo, T. M., Miyata, K., Tanaka, Y., and Takahashi, A. (2020). Cellular senescence and senescence-associated secretory phenotype via the cgas-sting signaling pathway in cancer. Cancer Sci. 111, 304–311.
Lorenzi, S., Mattei, F., Sistigu, A., Bracci, L., Spadaro, F., Sanchez, M., et al. (2011). Type I IFNs control antigen retention and survival of CD8α(+) dendritic cells after uptake of tumor apoptotic cells leading to cross-priming. J. Immunol. 186, 5142–5150. doi: 10.4049/jimmunol.1004163
Luo, W. W., Li, S., Li, C., Lian, H., Yang, Q., Zhong, B., et al. (2016). Irhom2 is essential for innate immunity to DNA viruses by mediating trafficking and stability of the adaptor sting. Nat. Immunol. 17, 1057–1066. doi: 10.1038/ni.3510
Mackenzie, K. J., Carroll, P., Martin, C. A., Murina, O., Fluteau, A., Simpson, D. J., et al. (2017). cGAS surveillance of micronuclei links genome instability to innate immunity. Nature 548, 461–465. doi: 10.1038/nature23449
Mazur, D. J., and Perrino, F. W. (1999). Identification and expression of the TREX1 and TREX2 cDNA sequences encoding mammalian 3′–>5′ exonucleases. J. Biol. Chem. 274, 19655–19660. doi: 10.1074/jbc.274.28.19655
McDermott, L. A., Weir, G. A., Themistocleous, A. C., Segerdahl, A. R., Blesneac, I., Baskozos, G., et al. (2019). Defining the functional role of Na(V)1.7 in human nociception. Neuron 101, 905–919. doi: 10.1016/j.neuron.2019.01.047
Merskey, H., and Spear, F. G. (1967). The concept of pain. J. Psychosom. Res. 11, 59–67. doi: 10.1016/0022-3999(67)90057-8
Mesquida-Veny, F., Del Río, J. A., and Hervera, A. (2021). Macrophagic and microglial complexity after neuronal injury. Progress Neurobiol. 200:101970.
Michalska, A., Blaszczyk, K., Wesoly, J., and Bluyssen, H. A. R. (2018). A positive feedback amplifier circuit that regulates interferon (IFN)-stimulated gene expression and controls Type I and Type II IFN responses. Front. Immunol. 9:1135. doi: 10.3389/fimmu.2018.01135
Mukai, K., Konno, H., Akiba, T., Uemura, T., Waguri, S., Kobayashi, T., et al. (2016). Activation of STING requires palmitoylation at the Golgi. Nat. Commun. 7:11932. doi: 10.1038/ncomms11932
Navratilova, E., and Porreca, F. (2014). Reward and motivation in pain and pain relief. Nat. Neurosci. 17, 1304–1312.
Padovan, E., Spagnoli, G. C., Ferrantini, M., and Heberer, M. (2002). IFN-alpha2a induces IP-10/CXCL10 and MIG/CXCL9 production in monocyte-derived dendritic cells and enhances their capacity to attract and stimulate CD8+ effector T cells. J. Leukocyte Biol. 71, 669–676.
Papa, A., Salzano, A. M., Di Dato, M. T., Lo Bianco, G., Tedesco, M., Salzano, A., et al. (2021). COVID-19 related acro-ischemic neuropathic-like painful lesions in pediatric patients: A case series. Anesthesiol. Pain Med. 11:e113760. doi: 10.5812/aapm.113760
Pasternak, G. W. (2014). Opioids and their receptors: Are we there yet? Neuropharmacology 76, 198–203.
Randall, R. E., and Goodbourn, S. (2008). Interferons and viruses: An interplay between induction, signalling, antiviral responses and virus countermeasures. J. General Virol. 89, 1–47. doi: 10.1099/vir.0.83391-0
Reboulet, R. A., Hennies, C. M., Garcia, Z., Nierkens, S., and Janssen, E. M. (2010). Prolonged antigen storage endows merocytic dendritic cells with enhanced capacity to prime anti-tumor responses in tumor-bearing mice. J. Immunol. 185, 3337–3347. doi: 10.4049/jimmunol.1001619
Rice, G. I., Rodero, M. P., and Crow, Y. J. (2015). Human disease phenotypes associated with mutations in TREX1. J. Clin. Immunol. 35, 235–243.
Sjöström, S., Tamsen, A., Persson, M. P., and Hartvig, P. (1987). Pharmacokinetics of intrathecal morphine and meperidine in humans. Anesthesiology 67, 889–895.
Stark, G. R., Kerr, I. M., Williams, B. R., Silverman, R. H., and Schreiber, R. D. (1998). How cells respond to interferons. Annu. Rev. Biochem. 67, 227–264.
Sun, C., Wu, G., Zhang, Z., Cao, R., and Cui, S. (2022). Protein tyrosine phosphatase receptor type d regulates neuropathic pain after nerve injury via the sting-IFN-I pathway. Front. Mol. Neurosci. 15:859166. doi: 10.3389/fnmol.2022.859166
Sun, H., Zhang, Q., Jing, Y. Y., Zhang, M., Wang, H. Y., Cai, Z., et al. (2017). USP13 negatively regulates antiviral responses by deubiquitinating sting. Nat. Commun. 8:15534. doi: 10.1038/ncomms15534
Sun, L., Wu, J., Du, F., Chen, X., and Chen, Z. J. (2013). Cyclic GMP-AMP synthase is a cytosolic DNA sensor that activates the type I interferon pathway. Science 339, 786–791. doi: 10.1126/science.1232458
Sun, W., Li, Y., Chen, L., Chen, H., You, F., Zhou, X., et al. (2009). ERIS, an endoplasmic reticulum IFN stimulator, activates innate immune signaling through dimerization. Proc. Natl. Acad. Sci. U. S. A. 106, 8653–8658. doi: 10.1073/pnas.0900850106
Takashima, K., Takeda, Y., Oshiumi, H., Shime, H., Okabe, M., Ikawa, M., et al. (2016). Sting in tumor and host cells cooperatively work for NK cell-mediated tumor growth retardation. Biochem. Biophys. Res. Commun. 478, 1764–1771. doi: 10.1016/j.bbrc.2016.09.021
Takeuchi, O., and Akira, S. (2010). Pattern recognition receptors and inflammation. Cell 140, 805–820. doi: 10.1016/j.cell.2010.01.022
Tan, P. H., Ji, J., Yeh, C. C., and Ji, R. R. (2021). Interferons in pain and infections: Emerging roles in neuro-immune and neuro-glial interactions. Front. Immunol. 12:783725. doi: 10.3389/fimmu.2021.783725
Tanaka, Y., and Chen, Z. J. (2012). Sting specifies IRF3 phosphorylation by TBK1 in the cytosolic DNA signaling pathway. Sci. Signal. 5:ra20. doi: 10.1126/scisignal.2002521
Tominaga, M., Caterina, M. J., Malmberg, A. B., Rosen, T. A., Gilbert, H., Skinner, K., et al. (1998). The cloned capsaicin receptor integrates multiple pain-producing stimuli. Neuron 21, 531–543. doi: 10.1016/S0896-6273(00)80564-4
Usoskin, D., Furlan, A., Islam, S., Abdo, H., Lönnerberg, P., Lou, D., et al. (2015). Unbiased classification of sensory neuron types by large-scale single-cell RNA sequencing. Nat. Neurosci. 18, 145–153. doi: 10.1038/nn.3881
van Dorp, E., Yassen, A., and Dahan, A. (2007). Naloxone treatment in opioid addiction: The risks and benefits. Exp. Opin. Drug Saf. 6, 125–132. doi: 10.1517/14740338.6.2.125
Wang, K., Donnelly, C. R., Jiang, C., Liao, Y., Luo, X., Tao, X., et al. (2021). STING suppresses bone cancer pain via immune and neuronal modulation. Nat. Commun. 12:4558. doi: 10.1038/s41467-021-24867-2
Wang, Q., Liu, X., Cui, Y., Tang, Y., Chen, W., Li, S., et al. (2014). The E3 ubiquitin ligase AMFR and INSIG1 bridge the activation of TBK1 kinase by modifying the adaptor STING. Immunity 41, 919–933. doi: 10.1016/j.immuni.2014.11.011
West, A. P., Khoury-Hanold, W., Staron, M., Tal, M. C., Pineda, C. M., Lang, S. M., et al. (2015). Mitochondrial DNA stress primes the antiviral innate immune response. Nature 520, 553–557. doi: 10.1038/nature14156
Woo, S. R., Fuertes, M. B., Corrales, L., Spranger, S., Furdyna, M. J., Leung, M. Y., et al. (2014). Sting-dependent cytosolic DNA sensing mediates innate immune recognition of immunogenic tumors. Immunity 41, 830–842. doi: 10.1016/j.immuni.2014.10.017
Woolf, C. J., and Ma, Q. (2007). Nociceptors–noxious stimulus detectors. Neuron 55, 353–364. doi: 10.1016/j.neuron.2007.07.016
Wu, J., and Chen, Z. J. (2014). Innate immune sensing and signaling of cytosolic nucleic acids. Annu. Rev. Immunol. 32, 461–488. doi: 10.1146/annurev-immunol-032713-120156
Wu, W., Zhang, X., Wang, S., Li, T., Hao, Q., Li, S., et al. (2022). Pharmacological inhibition of the cGAS-sting signaling pathway suppresses microglial M1-polarization in the spinal cord and attenuates neuropathic pain. Neuropharmacology 217:109206. doi: 10.1016/j.neuropharm.2022.109206
Yi, G., Brendel, V. P., Shu, C., Li, P., Palanathan, S., and Cheng Kao, C. (2013). Single nucleotide polymorphisms of human sting can affect innate immune response to cyclic dinucleotides. PLoS One 8:e77846. doi: 10.1371/journal.pone.0077846
Zeisel, A., Hochgerner, H., Lönnerberg, P., Johnsson, A., Memic, F., van der Zwan, J., et al. (2018). Molecular architecture of the mouse nervous system. Cell 174, 999–1014.e22. doi: 10.1016/j.cell.2018.06.021
Zhang, C., Shang, G., Gui, X., Zhang, X., Bai, X. C., and Chen, Z. J. (2019). Structural basis of sting binding with and phosphorylation by TBK1. Nature 567, 394–398. doi: 10.1038/s41586-019-1000-2
Zhang, H., You, Q. D., and Xu, X. L. (2020). Targeting stimulator of interferon genes (sting): A medicinal chemistry perspective. J. Med. Chem. 63, 3785–3816. doi: 10.1371/journal.ppat.1008178
Zhang, H. Y., Liao, B. W., Xu, Z. S., Ran, Y., Wang, D. P., Yang, Y., et al. (2020). USP44 positively regulates innate immune response to DNA viruses through deubiquitinating MITA. PLoS Pathog. 16:e1008178.
Zhang, L., Wei, N., Cui, Y., Hong, Z., Liu, X., Wang, Q., et al. (2018). The deubiquitinase CYLD is a specific checkpoint of the sting antiviral signaling pathway. PLoS Pathog. 14:e1007435. doi: 10.1371/journal.ppat.1007435
Zhang, Y., Wang, W., Gong, Z., Peng, Y., Li, X., Zhang, Z., et al. (2022). Activation of the sting pathway induces peripheral sensitization via neuroinflammation in a rat model of bone cancer pain. Inflammat. Res. [Epub ahead of print]. doi: 10.1007/s00011-022-01663-2
Zhang, Z., Tao, W., Hou, Y. Y., Wang, W., Lu, Y. G., and Pan, Z. Z. (2014). Persistent pain facilitates response to morphine reward by downregulation of central amygdala GABAergic function. Neuropsychopharmacology 39, 2263–2271. doi: 10.1038/npp.2014.77
Zheng, J., Mo, J., Zhu, T., Zhuo, W., Yi, Y., Hu, S., et al. (2020). Comprehensive elaboration of the cGAS-sting signaling axis in cancer development and immunotherapy. Mol. Cancer 19:133. doi: 10.1186/s12943-020-01250-1
Zheng, Y., Liu, P., Bai, L., Trimmer, J. S., Bean, B. P., and Ginty, D. D. (2019). Deep sequencing of somatosensory neurons reveals molecular determinants of intrinsic physiological properties. Neuron 103, 598–616.e7. doi: 10.1016/j.neuron.2019.05.039
Keywords: nociception, stimulator of interferon genes, interferon-I, peripheral nerve system (PNS), central nerve system (CNS)
Citation: Yang J, Ding H, Shuai B, Zhang Y and Zhang Y (2023) Mechanism and effects of STING–IFN-I pathway on nociception: A narrative review. Front. Mol. Neurosci. 15:1081288. doi: 10.3389/fnmol.2022.1081288
Received: 27 October 2022; Accepted: 05 December 2022;
Published: 04 January 2023.
Edited by:
Xiaodong Sheldon Liu, Beijing University of Chinese Medicine, ChinaReviewed by:
Qiuyi Lv, Beijing University of Chinese Medicine, ChinaCopyright © 2023 Yang, Ding, Shuai, Zhang and Zhang. This is an open-access article distributed under the terms of the Creative Commons Attribution License (CC BY). The use, distribution or reproduction in other forums is permitted, provided the original author(s) and the copyright owner(s) are credited and that the original publication in this journal is cited, in accordance with accepted academic practice. No use, distribution or reproduction is permitted which does not comply with these terms.
*Correspondence: Yan Zhang, ✉ eWFueml6aGFuZzkxN0BodXN0LmVkdS5jbg==
†These authors have contributed equally to this work and share first authorship
Disclaimer: All claims expressed in this article are solely those of the authors and do not necessarily represent those of their affiliated organizations, or those of the publisher, the editors and the reviewers. Any product that may be evaluated in this article or claim that may be made by its manufacturer is not guaranteed or endorsed by the publisher.
Research integrity at Frontiers
Learn more about the work of our research integrity team to safeguard the quality of each article we publish.