- Unitat d’Histologia i Neurobiologia, Departament de Ciències Mèdiques Bàsiques, Facultat de Medicina i Ciències de la Salut, Universitat Rovira i Virgili, Reus, Spain
At the neuromuscular junction (NMJ), motor neurons and myocytes maintain a bidirectional communication that guarantees adequate functionality. Thus, motor neurons’ firing pattern, which is influenced by retrograde muscle-derived neurotrophic factors, modulates myocyte contractibility. Myocytes can be fast-twitch fibers and become easily fatigued or slow-twitch fibers and resistant to fatigue. Extraocular muscles (EOM) show mixed properties that guarantee fast contraction speed and resistance to fatigue and the degeneration caused by Amyotrophic lateral sclerosis (ALS) disease. The TrkB signaling is an activity-dependent pathway implicated in the NMJ well-functioning. Therefore, it could mediate the differences between fast and slow myocytes’ resistance to fatigue. The present study elucidates a specific protein expression profile concerning the TrkB signaling that correlates with higher resistance to fatigue and better neuroprotective capacity through time. The results unveil that Extra-ocular muscles (EOM) express lower levels of NT-4 that extend TrkB signaling, differential PKC expression, and a higher abundance of phosphorylated synaptic proteins that correlate with continuous neurotransmission requirements. Furthermore, common molecular features between EOM and slow soleus muscles including higher neurotrophic consumption and classic and novel PKC isoforms balance correlate with better preservation of these two muscles in ALS. Altogether, higher resistance of Soleus and EOM to fatigue and ALS seems to be associated with specific protein levels concerning the TrkB neurotrophic signaling.
1 Introduction
Motor neurons (MNs) interact with myocytes through the neuromuscular junction (NMJ) to guarantee their adequate functionality in a feedback loop mode through the neuromotor and neurotrophic control mechanisms. In brief, MNs regulate the properties of the myocytes they innervate through their firing activity pattern, determined by their intrinsic properties and by the inputs that they receive. In their turn, myocytes influence MNs through the retrograde activity-dependent secretion of neurotrophic factors (Baldwin et al., 2013; Cisterna et al., 2014; Hurtado et al., 2017b).
One MN innervates several myocytes to form a motor unit (MU). At their time, several MU innervate all the myocytes in one muscle to generate a complete functional contraction. Consequently, fast and slow skeletal muscles are differently innervated and combine their intrinsic characteristics to organize the functionality of a whole limb. MNs are named fast-twitch fatigable (FF), fast-twitch fatigue-resistant (FR), and slow-twitch fatigue-resistant (S) in accordance with the properties of the myocytes they innervate (Kanning et al., 2010). In their turn, myocytes are classified by their contraction speed and strength, which depend on myosin heavy chain expression (Ausoni et al., 1990) and mitochondrial activity, which is higher in slow-twitching fibers (Leary et al., 2003; Hakamata et al., 2018). Slow-twitching muscles present a more oxidative and fatigue-resistant metabolism than fast-twitching ones, which are preferentially glycolytic (Delp and Duan, 1996; Schiaffino and Reggiani, 2011). Finally, extraocular muscles (EOM), which are among the fastest muscles in mammals, have unique characteristics, including the expression of specific MHC isoforms to guarantee both fast contraction speed and vast resistance to fatigue (Porter et al., 1995). Furthermore, EOMs are less vulnerable to Amyotrophic lateral sclerosis (ALS) neurodegenerative disease, like slow and fatigue-resistant muscles.
Among the muscles used in the present study, Extensor Digitorum Longus (EDL) and Tibialis Anterior (TA) are fast extensor and flexor muscles, respectively, mainly composed of fast-twitching fibers. On the other hand, Soleus (SOL) is composed of slow-twitching fibers (Novák et al., 2010). Type I and IIA NMJ nerve terminals are smaller and less branched (Mech et al., 2020). Also, they have deeper postsynaptic gutters and a more efficient synaptic vesicle recycling mechanism in comparison with fiber IIB (Tomas et al., 1992; Mantilla et al., 2007), which is consistent with an enlarged pool of releasable synaptic vesicles found in the slower fibers (Reid et al., 1999). Indeed, the EDL vesicle pool is ∼33% smaller than SOL one despite that recycling times are equal (Reid et al., 1999). Consequently, EDL’s ability to sustain neuromuscular transmission during repeated activation is minor (Miyata et al., 1995; Prakash et al., 1999). Therefore, fiber composition in EDL is prepared to support relatively short bursts of strong contractions and it is easily fatigued after a short time of activity. In contrast, SOL is resistant to fatigue as MN are able to release ACh for extended periods of time at a lower frequency and myocytes smoothly contract in response. During stimulation, the initial quantal content to generate the end-plate potentials (EPPs) is significantly higher in EDL than in SOL (Gertler and Robbins, 1978; Wood and Slater, 2001) while EPP amplitude further decreases in EDL than in SOL (Reid et al., 1999). However, under continuous stimulation, motor units are able to adapt from faster to slower activity patterns and gain resistance to fatigue, both molecularly (Just-Borràs et al., 2021) and functionally (Windisch et al., 1998). Altogether resistance to fatigue during tonic stimulation is mainly sustained by larger vesicle pools and lower quantal content in which SNARE-SM proteins are involved. In opposition to limb muscles, EOMs combine fast, slow, embryonic, and EOM-specific MHC isoforms to guarantee fast contraction speed and vast resistance to fatigue (Zhou et al., 2011).
The Brain-derived neurotrophic factor (BDNF)/Tropomyosin receptor kinase B (TrkB) signaling is a retrograde neurotrophic signaling tightly related to NMJ stability and neurotransmission. In brief, nerve-induced muscle contraction regulates the pathway to retrogradely modulate the activity of presynaptic protein kinases PKC and PKA on SNARE-SM targets (Hurtado et al., 2017a,b; Simó et al., 2018, 2019), directly involved in the control of the ACh release. Consequently, TrkB regulates both the postsynaptic and presynaptic components to adapt the neurotransmission mechanism to the requirements of each synapse (Lu, 2003; Mantilla et al., 2004, 2014; Matthews et al., 2009; Garcia et al., 2010a; Santafé et al., 2014; Hurtado et al., 2017a,b; Simó et al., 2018, 2019).
Here, we hypothesize that BDNF signaling pathway expression and activity should be different in slow and fast limb muscles and in the EOMs, in accordance with their activity patterns. Furthermore, it could have an impact on their resistance to fatigue and the ALS effect. Indeed, Delezie et al. (2019) reported that reducing BDNF expression in fast muscles was correlated with a slower metabolism acquisition in fast muscles and neurturin expression, which is related to the capillary density and oxidative capacity, was also related to slower action patterns (Correia et al., 2021). Furthermore, previous works reported that the TrkB pathway was disturbed in fast muscles affected by ALS (Just-Borràs et al., 2019; Tosolini et al., 2022), while exercise, that improved mice phenotype (Deforges et al., 2009), reduced the molecular alterations (Just-Borràs et al., 2019, 2020). Also, BDNF administration reduced the endosome traffic reduction in vivo in SOD1-G93A mice (Tosolini et al., 2022). Thus, the higher resistance to ALS neurodegenerative disease of the EOM and the slow limb soleus muscle could be associated with well-adapted BDNF/TrkB neurotrophic signaling. Consequently, understanding how the TrkB pathway works under physiological conditions in different skeletal muscles will help to understand the preferential affection of the myocytes in relation to different neuroprotection capacities. Altogether the present study compares the molecular phenotype of the TrkB signaling pathway in two fast and one slow limb muscles and EOM to find the different protein expressions of these last ones that could result in their specific resistance to fatigue and disease. In brief, the results of this article elucidate the relation between differential protein expression in each muscle and its physiological demands. Thus, EOMs express lower levels of NT-4 that extend TrkB signaling through time and a higher abundance of phosphorylated synaptic proteins, that correlate with continuous synaptic activity. Furthermore, common features between EOM and soleus muscles, including classic and novel PKC isoforms balance, also correlate with better preservation of these two muscles in ALS (Figure 1).
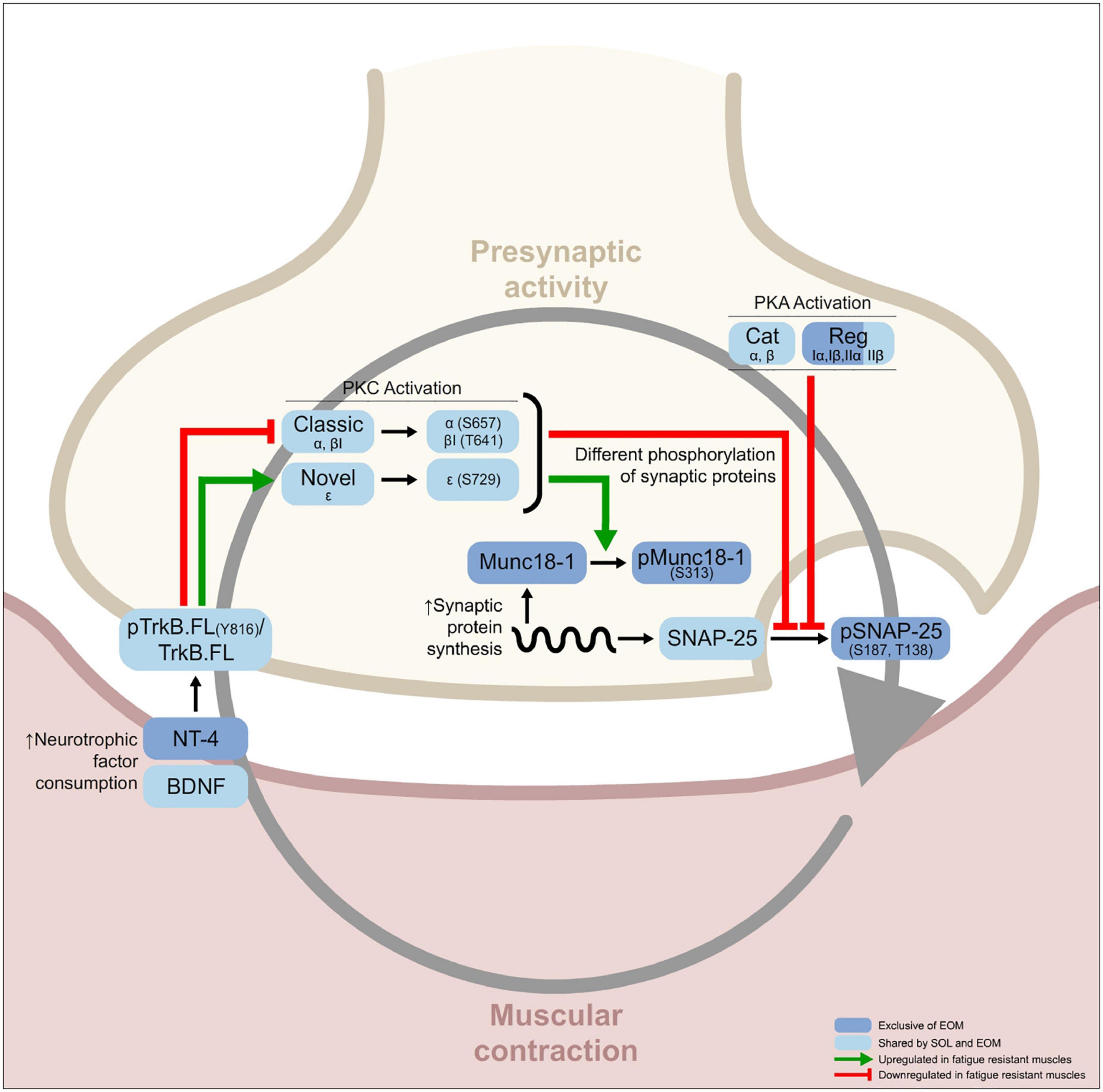
Figure 1. Graphical abstract showing the differential molecular features of fatigue resistant muscles [soleus (SOL) and extraocular muscles (EOM)] in comparison with fatigable muscles [extensor digitorum longus (EDL) and tibialis anterior (TA)]. Following the circular relation between the presynaptic and postsynaptic components of the neuromuscular junction (NMJ) gray arrow characterized by previous work done in Sprague Dawley rat diaphragms stimulated through the phrenic nerve ex vivo and activating or inhibiting each one of the proteins in the pathway (Obis et al., 2015a,b; Hurtado et al., 2017a,b; Simó et al., 2018, 2019; Cilleros-Mañé et al., 2020, 2021), one can identify that muscular resistance to fatigue is mediated by constant neurotrophic consumption that modulates tropomyosin receptor kinase B (TrkB) phosphorylation ratio. Consequently, PKC activity in both SOL and EOM muscles is modified, which results in a differential balance of synaptic protein phosphorylation between fatigable and fatigue resistant muscles. Also, PKA activity and synaptic protein abundance are differential between fatigue resistant and fatigable muscles. Many protein levels are shared between SOL and EOM, indicated in pale blue squares. However, there are specific differences between the two fatigue resistant muscles SOL and EOM indicated in dark blue squares.
2 Materials and methods
2.1 Animal care
Adult male Sprague Dawley rats (50 days of age; Criffa, Barcelona, Spain; RRID: RGD_5508397) were kept in the animal facility under standard conditions: constant temperature (22 ± 2°C), relative humidity (50 ± 10%), a 12-h light/dark schedule and unrestricted access to food and water. Experiments were performed under the approval of the Ethics Committee of Animal Experimentation of the Universitat Rovira i Virgili, in accordance with the European Union Directive 2010/63/EU guidelines for the humane treatment of laboratory animals. Muscles were obtained from six animals from the colony (n = 6) to be used as biological replicates.
2.2 Western blotting
Animals were euthanized and immediately afterward Extensor Digitorum Longs (EDL), Tibialis Anterior (TA), Soleus (SOL), and the four rectus EOM muscles were dissected from their insertions and frozen in liquid nitrogen. Homogenization was performed with an overhead stirrer (VWR International, Clarksburg, MD) in ice-cold lysis buffer [in mM: NaCl 150, Tris–HCl 50 (pH 7.4), EDTA 1, NaF 50, PMSF 1, Na3VO4 1; NP-40 1%, Triton X-100 0.1%, and protease inhibitor cocktail 1% (Sigma, Saint Louis, MO, USA)]. Insoluble materials were removed with two centrifugations at 4°C: 1,000 g for 10 min and 15,000 g for 20 min. The final supernatant contained the whole-cell fraction lysate. Protein concentrations were determined by DC protein assay (Bio-Rad, Hercules, CA, IL, USA).
The western blotting procedure was performed as previously described (Just-Borràs et al., 2019). Protein samples of 30 μg were separated by 8 or 12% SDS-polyacrylamide electrophoresis and electro-transferred to a polyvinylidene difluoride (PVDF) membrane (Hybond™-P; Amersham, GE Healthcare, Marlborough, MA, USA) using Trans-Blot Turbo Transfer System (Bio-Rad, Hercules, CA, USA). For immunodetection, membranes were blocked with TBST containing 5% (w/v) phosphoblocker or bovine serum albumin (BSA) for phosphorylated proteins and non-fat dry milk for non-phosphorylated proteins for an hour. Then, membranes were incubated in the primary antibody overnight and with the corresponding horseradish peroxidase-conjugated secondary antibody for 1 h (Table 1). Membranes were revealed with Bio-Rad ECL kid on the ChemiDoc XRS + machine (Bio-Rad, Hercules, CA, USA). The bands’ optical density was normalized in relation to (1) the background values and to (2) the total protein transferred on PVDF membranes, measured by total protein analysis [Sypro Ruby protein blot stain, Bio-Rad (Aldridge et al., 2008)]. The relative variations between samples were calculated from the same membrane image. Data were taken from densitometry measurements made in at least three biological replicates. Primary antibodies were omitted from some samples during the procedure as controls, and they never revealed bands of the expected molecular weight. Furthermore, their specificity has been proved in previous publications from the group (Obis et al., 2015b; Hurtado et al., 2017a,b; Simó et al., 2018, 2019; Cilleros-Mañé et al., 2020, 2021).
2.3 Statistical analysis
All values are represented as mean ± standard deviation (SEM) within each muscle. Also, each circle represents the value of one animal, normalized in relation to EDL muscle, whose value was transformed to 1, to visualize proportional data distribution. The statistical significance of the differences between the experimental groups was evaluated under a non-parametric Kruskal–Wallis test followed by Dunn’s post-hoc test (GraphPad Prism software, San Diego, CA, USA). The criterion for statistical significance was: *p < 0.05, **p < 0.01, and ***p < 0.001.
3 Results
To determine the expression of the presynaptic TrkB neurotrophic signaling pathway that regulates synaptic vesicles exocytosis at the NMJ, we analyzed the entire TrkB pathway at the synapses of EDL, TA, SOL, and EOM muscles in its three molecular levels: (i) the neurotrophic factors BDNF and NT-4 and their receptors TrkB full length (TrkB.FL) and truncated (TrkB.T) and p75NTR, (ii) the coupled serine-threonine kinases C (PKC isoforms) and their priming kinase phosphoinositide-dependent kinase 1 (PDK1) and the different subunits of the cAMP-dependent kinase (PKA), and (iii) PKC and PKA targets related with ACh release (Munc18-1 and SNAP-25).
3.1 Neurotrophic factors and receptors expression is lower in fatigue-resistant muscles
Figure 2A shows that proBDNF is uniformly expressed in 3 of the 4 studied muscles, being upregulated in TA. On the other hand, mature BDNF (mBDNF) decreases in SOL and EOM (Figure 2A). Consequently, the proportion between mature and pro BDNF detected (mBDNF/proBDNF ratio) is significantly lower in both EOM and SOL than in EDL and TA. On the other hand, NT-4 expression is significantly lower in EOM than in limb muscles.
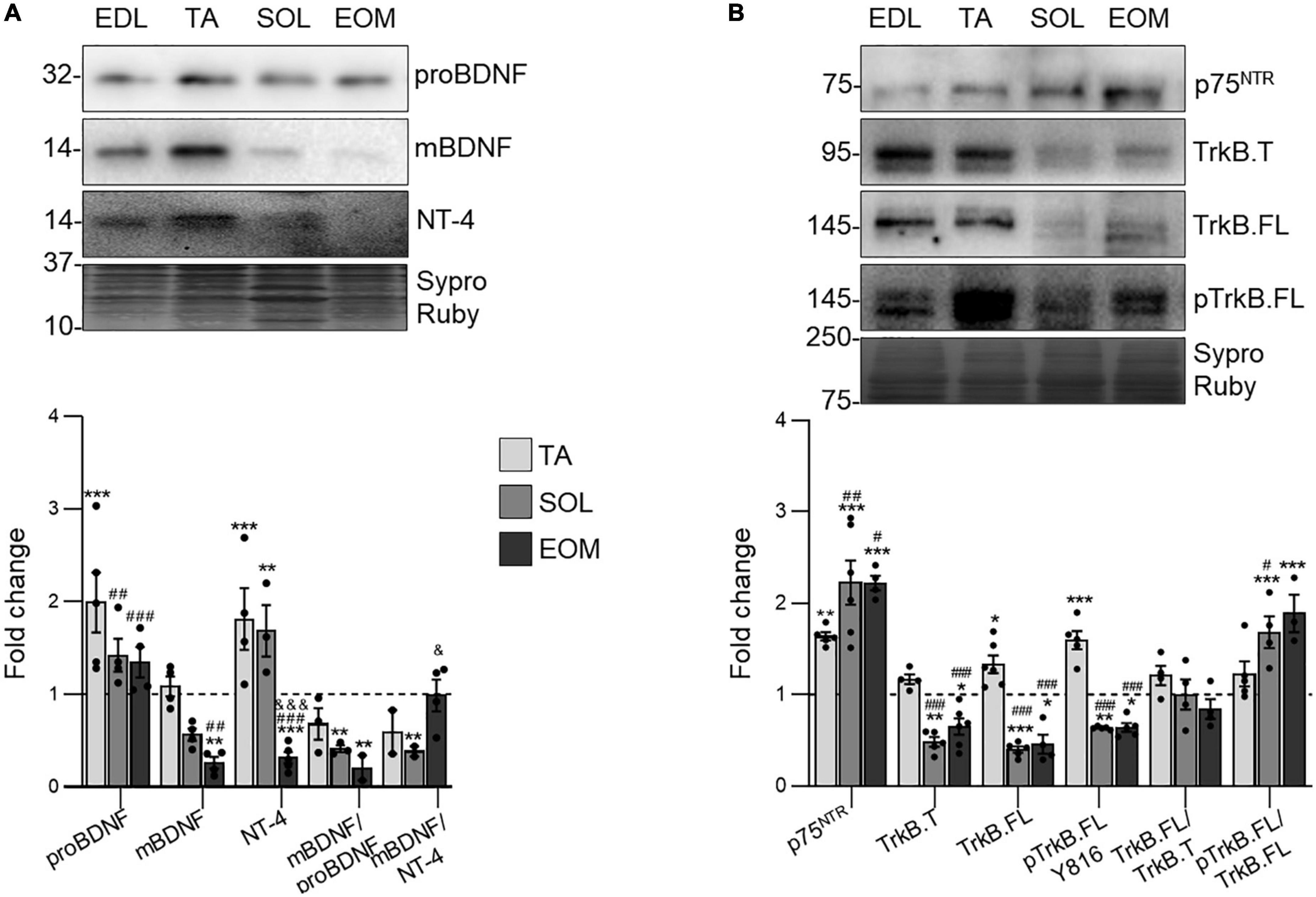
Figure 2. NTFs and receptors expression changes in EDL, TA, SOL, and EOM of Sprague Dawley rats. Western blot analysis and data quantification of (A) neurotrophic factors and (B) neurotrophic factor receptors protein levels in different muscles. Sypro Ruby images show the region surrounding each protein of interest despite the bands’ optical densities being normalized using the full length of the lanes. Data are presented as mean ± SEM. Each circle in the bars is the mean result of one animal. *P ≤ 0.05; **P ≤ 0.01; ***P ≤ 0.001; *against EDL, # against TA, & against SOL (Kruskal–Wallis test and Dunn’s post-hoc test). EDL, Extensor Digitorum Longus; TA, Tibialis Anterior; SOL, Soleus; EOM, Extraocular Muscles. Graphs done with GraphPad Prism software, San Diego, USA.
Understanding neurotrophic factor consumption requires the analysis of the receptors that trigger their signaling. Thus, Figure 2B shows that p75NTR expression is proportional to muscular fatigue resistance, being less expressed in EDL muscles and upregulated in SOL and EOM. On the other hand, TrkB is more expressed and phosphorylated in the fast, fatigable EDL and TA than in the fatigue resistant muscles SOL and EOM in healthy young adult Sprague Dawley rats. However, results reveal that TrkB.FL is proportionally more phosphorylated in SOL and EOM muscles, as the proportion between phosphorylated and total TrkB.FL detected (pTrkB.FL/TrkB.FL ratio) increases in these muscles. Furthermore, the proportion between full and truncated TrkB detected (TrkB.FL/TrkB.T) ratio is kept through all the studied muscles.
3.2 Classic and novel PKC isoforms differential expression determines muscle phenotype
Next, we studied three relevant PKC isoforms involved in neurotransmission at the NMJ that are activated following the downstream TrkB signaling (Santafé et al., 2014; Obis et al., 2015a,b; Hurtado et al., 2017b; Simó et al., 2018, 2019). As shown in Figure 3, classical cPKCα and cPKCβI isoforms are less expressed and phosphorylated in fatigue-resistant muscles, SOL, and EOM. However, while the proportion between phosphorylated and total cPKCα detected (the phosphorylation ratio) in EOM is decreased, the ratio increases in both SOL and EOM for cPKCβI (Figures 3A,B), pointing to a differential consumption pattern through the isoforms.
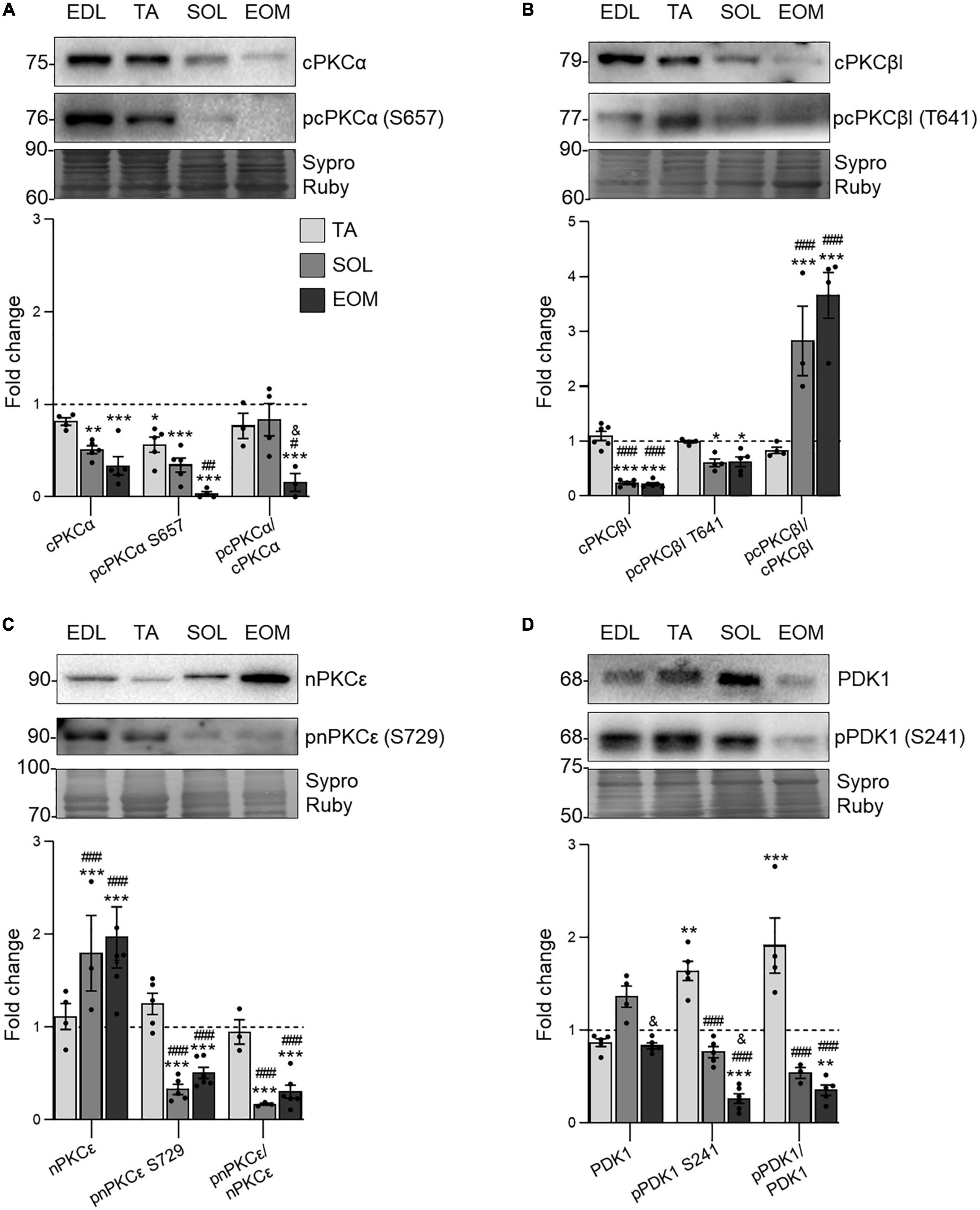
Figure 3. PKC isoforms and PDK1 expression changes in EDL, TA, SOL, and EOM of Sprague Dawley rats. Western blot analysis and data quantification of (A) PDK1, (B) cPKCα, (C) cPKCβI, and (D) nPKCε protein levels in different muscles. Sypro Ruby images show the region surrounding each protein of interest despite the bands’ optical densities being normalized using the full length of the lanes. Data are presented as mean ± SEM. Each circle in the bars is the mean result of one animal. *P ≤ 0.05; **P ≤ 0.01; ***P ≤ 0.001; *against EDL, # against TA, & against SOL (Kruskal–Wallis test and Dunn’s post-hoc test). EDL, Extensor Digitorum Longus; TA, Tibialis Anterior; SOL, Soleus; EOM, Extraocular Muscles. Graphs done with GraphPad Prism software, San Diego, USA.
On the contrary, novel nPKCε abundance is higher in SOL and EOM than in EDL and TA but it is less phosphorylated (Figure 3C). Finally, PDK1 expression, which is responsible for PKCs priming for activation (Dutil and Newton, 2000; Biondi, 2004), is sustained through the studied muscles. However, the phosphorylation ratio is lower in fatigue resistant muscles, pointing to higher consumption of them (Figure 3D).
3.3 Catalytic and regulatory PKA subunits are differentially expressed through muscle type
Since PKC and PKA are closely related to regulating ACh release at the NMJ (Santafé et al., 2009), we also analyzed PKA subunits in the selected muscles. The present results show that the catalytic subunits Cα and Cβ are more abundant in fatigue-resistant muscles than in fast, fatigable ones (Figure 4A). On the other hand, SOL and EOM differ in regulatory subunits expression. RI subunits are more expressed in SOL while barely detectable in EOMs, which preferentially express RII subunits (Figure 4B).
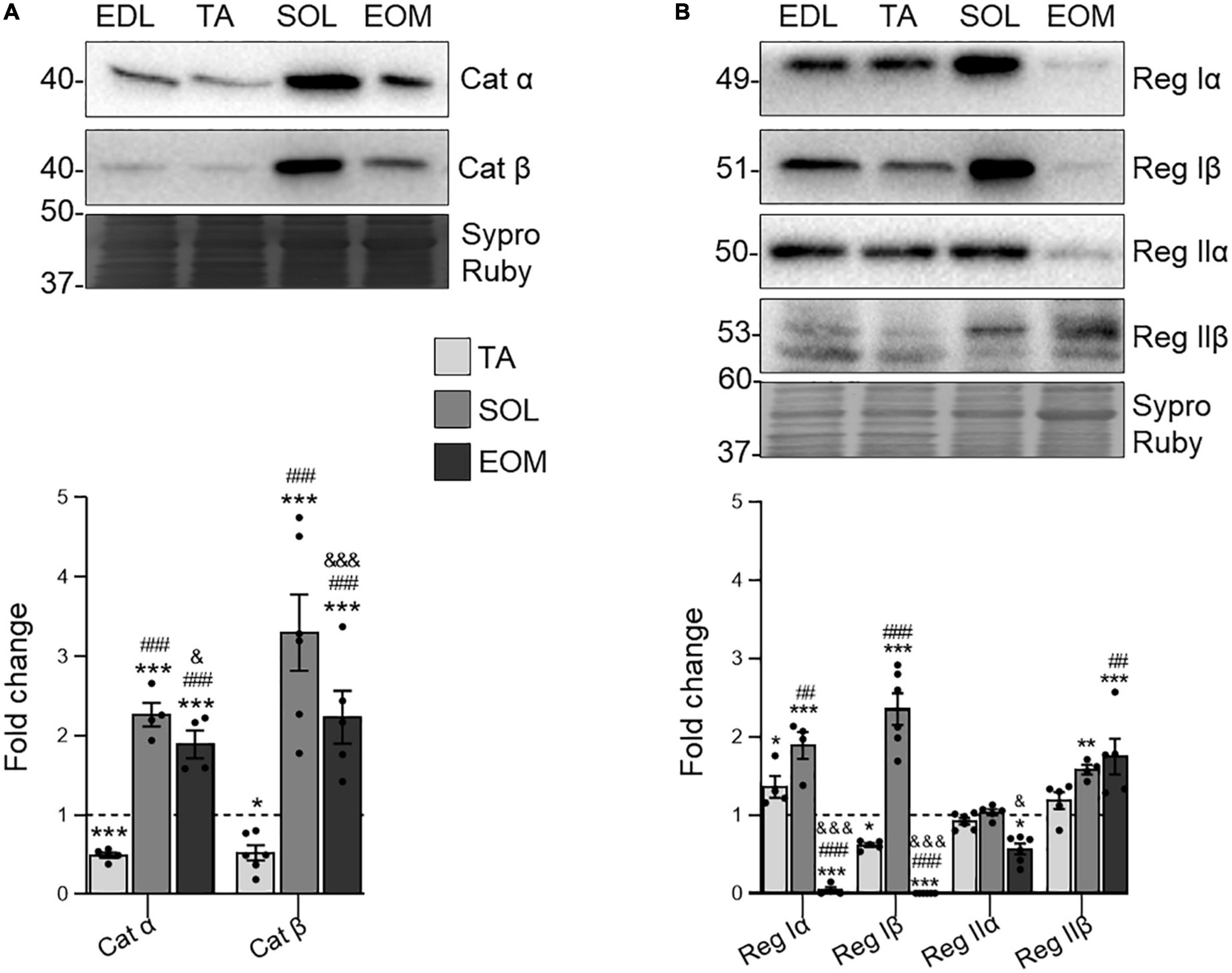
Figure 4. Catalytic and regulatory PKA subunits expression changes in EDL, TA, SOL, and EOM of Sprague Dawley rats. Western blot analysis and data quantification of (A) catalytic and (B) regulatory PKA isoforms protein levels in different muscles. Sypro Ruby images show the region surrounding each protein of interest despite the bands’ optical densities being normalized using the full length of the lanes. Data are presented as mean ± SEM. Each circle in the bars is the mean result of one animal. *P ≤ 0.05; **P ≤ 0.01; ***P ≤ 0.001; *against EDL, # against TA, & against SOL (Kruskal–Wallis test and Dunn’s post-hoc test). EDL, Extensor Digitorum Longus; TA, Tibialis Anterior; SOL, Soleus; EOM, Extraocular Muscles. Graphs done with GraphPad Prism software, San Diego, USA.
3.4 EOMs abundantly express the exocytotic proteins Munc18-1 and SNAP-25
Both PKC and PKA phosphorylate targets are keys in the exocytosis of ACh synaptic vesicles. Munc18-1 is an essential, neuron-specific protein involved in neurotransmitter release (Südhof and Rothman, 2009), specifically expressed at the nerve terminal of the NMJ (Simó et al., 2018). The S313 phosphorylation is modulated by cPKCβI and nPKCε at the NMJ (Simó et al., 2018) and regulates Munc18-1 function (Südhof, 2013). Here, the present results show an unchanged phosphorylation ratio through the studied muscles. However, both total and phosphorylated levels of Munc18-1 are upregulated in EOM in relation to limb muscles (Figure 5A), pointing to higher requirements concerning Munc18-1 in EOM.
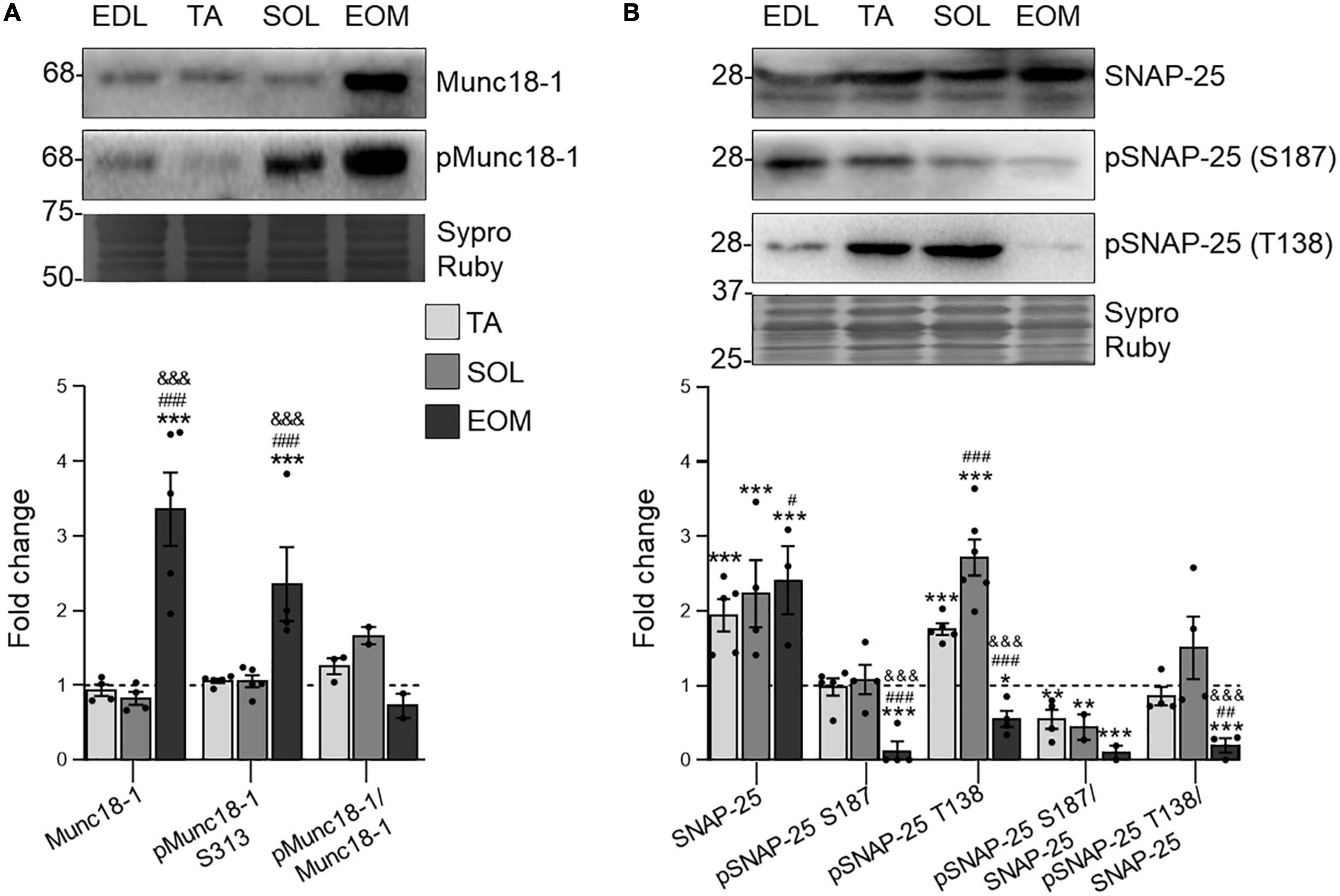
Figure 5. The SNARE/SM Munc18-1 and SNAP-25 expression changes in EDL, TA, SOL, and EOM of Sprague Dawley rats. Western blot analysis and data quantification of (A) Munc18-1 and (B) SNAP-25 protein levels in different muscles. Sypro Ruby images show the region surrounding each protein of interest despite the bands’ optical densities being normalized using the full length of the lanes. Data are presented as mean ± SEM. Each circle in the bars is the mean result of one animal. *P ≤ 0.05; **P ≤ 0.01; ***P ≤ 0.001; *against EDL, # against TA, & against SOL (Kruskal–Wallis test and Dunn’s post-hoc test). EDL, Extensor Digitorum Longus; TA, Tibialis Anterior; SOL, Soleus; EOM, Extraocular Muscles. Graphs done with GraphPad Prism software, San Diego, USA.
SNAP-25 is a component of the SNARE core complex that is essential for functional neurotransmission (Katayama et al., 2017). Thus, it is localized at the nerve terminal component of the NMJ (Simó et al., 2019). On the one hand, non-phosphorylated SNAP-25 strongly interacts with Syntaxin-1, forming the SNARE complex necessary for ACh exocytosis. On the other hand, once SNAP-25 is phosphorylated, exocytosis ends (Horváth et al., 2017) and SNAP-25 switches from forming the SNARE complex to promoting vesicle recycling and refilling, in a process that depends on PKC for the S187 residue and PKA for the T138 residue phosphorylation (Nagy et al., 2002, 2004; Leenders and Sheng, 2005). Our results show that SNAP-25 expression levels are proportional to fatigue resistance in the analyzed muscles. On the other hand, both phosphorylation residues S187 and T138 are uniquely low in EOMs. Finally, SNAP-25 S187 phosphorylation does not change through limb muscles and, therefore, the pSNAP-25 S187/SNAP-25 ratio decreases with fatigue resistance. On the other hand, pSNAP-25 T138 levels are higher in TA and SOL than in EDL and, consequently, the pSNAP-25 T138/SNAP-25 ratio does not change (Figure 5B).
4 Discussion
Skeletal muscles differ from one specie to the other, with activity patterns, disease conditions, and aging, among others and despite sharing functional characteristics. In consequence, skeletal muscle tissue is very plastic and its small molecular variations can induce significative differences despite being in the same generic group (i.e., fast and fatigable or slow and fatigue resistant). Until now, it was unknown whether the TrkB signaling is differently expressed in fast and slow limb muscles and in EOM, which have specific functional characteristics, resistance to fatigue, and different vulnerability to ALS neurodegenerative disease (Tjust et al., 2012). Here, we report that EOM shares molecular features of the slow fatigue-resistant soleus muscle but also particular details that correlate with EOM activity patterns. A summary of the main findings is represented in Figures 1, 6.
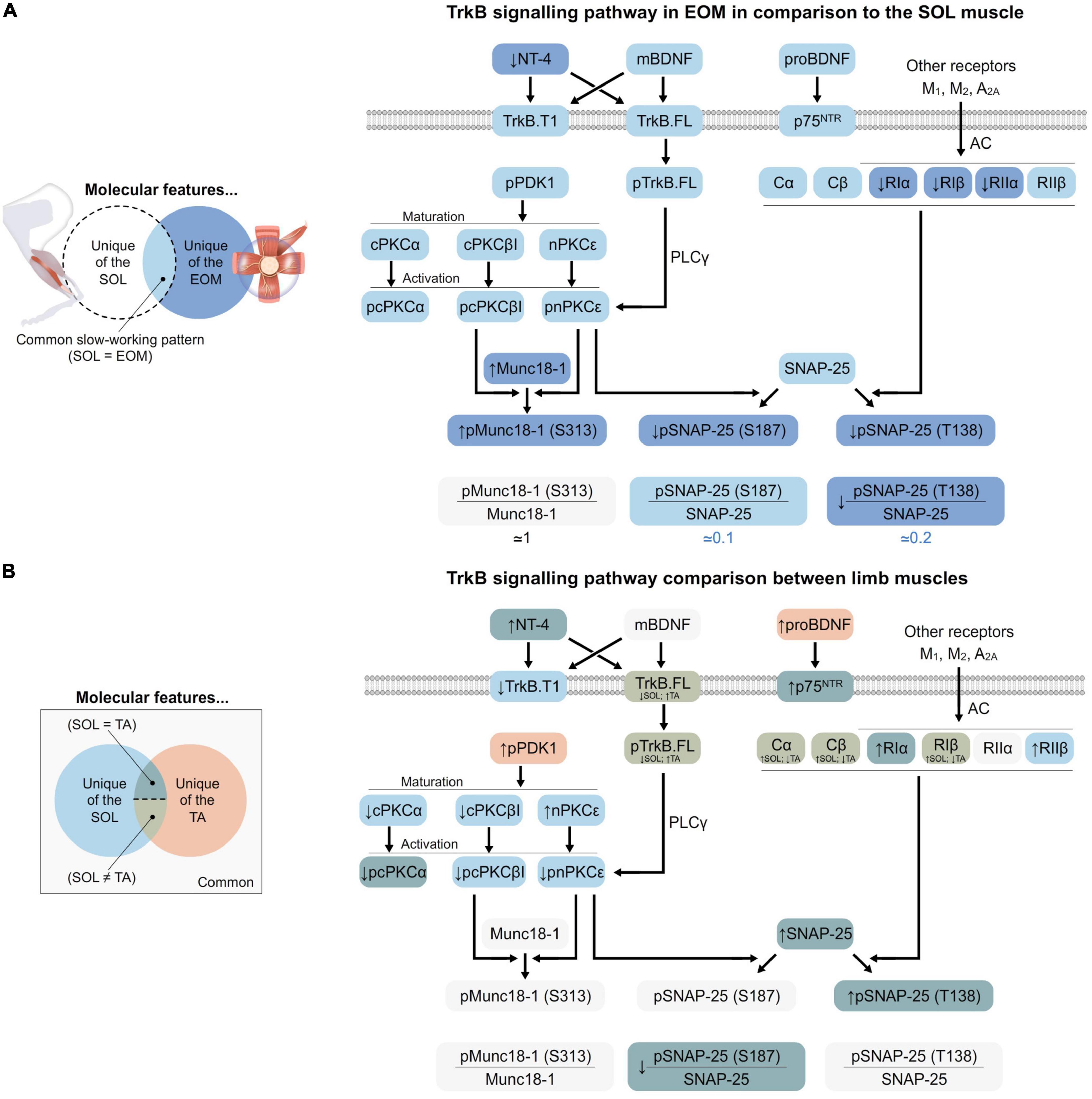
Figure 6. Graphical representation of the TrkB signaling pathway at the NMJ. (A) EOM in comparison with SOL. EOM muscles share several molecular features with SOL, represented in pale blue. However, they also have some unique features (dark blue), whose relationship with SOL is indicated by arrows (↑ for increase and ↓ for decrease). These characteristics are possibly involved in the ability of EOM to contract fast without being fatigued despite continuous usage. (B) The different limb muscles. Limb muscles share a few molecular features (pale gray). Also, the TA muscle has some unique features (orange). On the other hand, SOL’s unique features are always coincident with EOM (pale blue; like in panel A). Finally, some proteins equally change in SOL and TA in relation to EDL (dark green). Thus, two levels of adaptations to resistance to fatigue can be spotted depending on if they are exclusive of SOL or shared with TA. Finally, TA also shows some characteristics that could be involved in the differences between flexor and extensor muscles as they are different from SOL and EDL (pale green). The BDNF/TrkB downstream signaling is always the same at the NMJ, but the different proportions of proteins allow the adaptation to the precise requirements of each muscle and vice versa. In brief, proBDNF preferentially binds p75NTR while mBDNF and NT-4 selectively bind and activate either full length or truncated TrkB. When TrkB.FL is not inhibited by heterodimerization with truncated isoforms, it activates PKCs in the membrane, which has been previously phosphorylated by PDK1. This is mediated by PLCγ, which enhances intracellular Ca2+ and DAG concentration. At the plasma membrane, PKCs modulate ACh release by phosphorylating exocytotic machinery proteins such as Munc18-1 and SNAP-25. Furthermore, SNAP-25 is also phosphorylated by PKA, whose activity depends on muscarinic ACh receptors, among others.
4.1 Fatigue-resistant muscles have a higher consumption of mature neurotrophic factors
NT-4 is an activity-dependent neurotrophic factor that mediates NMJ growth and remodeling (Funakoshi et al., 1995). Previous results show that in healthy fast muscles, NT-4 increases after physical exercise proportionally to the training intensity, following a fast-to-slow molecular transition (Just-Borràs et al., 2021). In accordance, the present results show that NT-4 is upregulated in the slow fatigue resistant limb muscle soleus. On the other hand, NT-4 is downregulated in EOM, which was previously related to EOM resistance to ALS (Harandi et al., 2016), pointing that NT-4 low levels in EOM could be key to confer EOMs-specific properties, understood together with neurotrophic receptor balance.
Concerning BDNF, results show a differential consumption of mBDNF among the different studied muscles, which is higher in fatigue-resistant muscles, where TrkB isoforms expression is lower. TrkB can follow the degradative pathway after binding to its ligand (Chen et al., 2005) and activating the corresponding intracellular responses. Therefore, it seems that TrkB signaling could be more intense in SOL and EOM than TrkB. FL levels are reduced, in accordance with activity-dependent consumption (González-Gutiérrez et al., 2020) and accumulated due to ALS (Just-Borràs et al., 2019). Thus, because of canonical TrkB.FL neuroprotective effect (Reichardt, 2006; Akil et al., 2016), these results correlate with EOM and SOL being more resistant to neurodegenerative diseases such as ALS, suggesting that this is an advantageous molecular phenotype at the same time that these muscles benefit from a reduced truncated TrkB expression. Indeed, the present results show that EDL and TA present higher TrkB and mBDNF levels, showing a correlation between their accumulation and decreased neuroprotective function. Indeed, these proteins are reduced in ALS-trained animals that show a better phenotype than non-trained ALS mice (Grondard et al., 2008; Just-Borràs et al., 2020).
Thus, fatigue-resistant muscles present a sustained activity of the TrkB.FL signaling pathway correlated with their stability through disease and supported by a higher TrkB.FL phosphorylation ratio in SOL and EOM. Thus, the higher TrkB.FL phosphorylation ratio observed in SOL and EOM seems to be related to the maintenance of neurotransmission. Indeed, several studies related TrkB signaling with mitochondrial function maintenance and promotion (Ahuja et al., 2022; Cheng and Lee, 2022; Li et al., 2022; Wang et al., 2022). Therefore, TrkB.FL activity seems to be proportional to mitochondrial activity, related to slower, fatigue resistant fibers. In accordance, BDNF reduction results in slower muscle metabolism and more resistance to fatigue, while BDNF accumulation induces a faster, glycolytic metabolism (Delezie et al., 2019). Altogether, mBDNF levels are proportional to the contraction speed of the muscles and inversely proportional to fatigue resistance due to consumption.
On the other hand, the neurotrophic factor receptor p75NTR (Friedman and Greene, 1999) is widely expressed in the nervous system, including the NMJ (Garcia et al., 2010b), where it regulates different cellular functions depending on its interaction with co-receptors and neurotrophins (Hempstead, 2002). Particularly, during development, proBDNF promotes synaptic plasticity and refinement through p75NTR (Garcia et al., 2011). Similarly, exercise promotes p75NTR-mediated plasticity mechanisms to refine and optimize mature adult NMJ connections (Eslami et al., 2018; Zhan et al., 2018). Indeed, mature NMJ in p75NTR–/– mice showed impaired structural complexity correlated with altered synaptic function and increased susceptibility to fatigue (Pérez et al., 2019). These results correlate with decreased p75NTR expression in fast plantaris muscles of ALS mice (Just-Borràs et al., 2019) while it is maintained in the EOMs despite ALS (Harandi et al., 2016). Furthermore, exercise recovers p75NTR in the limb muscles of these animals (Just-Borràs et al., 2020). In accordance, the present results show that p75NTR levels are proportional to fatigue resistance. Indeed, p75NTR is upregulated in EOM myogenic progenitor cells, in relation to higher proliferative and fusion rates and better regenerative properties (Carrero-Rojas et al., 2020). Thus, p75NTR seems to be related to selective muscle resistance.
4.2 PKC isoforms differential expression identifies myocyte metabolic profile
As a result of TrkB.FL-mediated PLCγ1 activation, mature PKCs are anchored to the membrane (Colón-González and Kazanietz, 2006; Griner and Kazanietz, 2007). PKC maturation and correct folding require a priming phosphorylation step by PDK1 (Dutil and Newton, 2000; Biondi, 2004), which is promoted by synaptic activity at the NMJ (Hurtado et al., 2017b). In mammalian NMJ, PDK1 is self-phosphorylated in the activation loop residue S241 to be constitutively active (Casamayor et al., 1999; Hurtado et al., 2017a). The present results show a decrease in phosphorylated PDK1 (S241) in EOMs, which could be related to consumption and degradation after its function (Hurtado et al., 2017a), pointing to more intense activity in relation to the studied limb muscles.
Once PKCs are phosphorylated by PDK1, they can sense intracellular second messengers and bind to the membrane for substrate phosphorylation (Colón-González and Kazanietz, 2006). Afterward, PKCs are degraded by the proteasome in an activation-dependent manner (Gould and Newton, 2008). Studies have proposed that classic cPKC might be activated by fast-twitching patterns of activity (Pedersen et al., 2009). Here, we add new evidence that cPKCα and cPKCβI are reduced in fatigue-resistant muscles. Thus, in rodents, like in avian models (Gundersen, 2011), cPKC activity is higher in fatigable muscles than in fatigue-resistant ones. Indeed, cPKCα elevation was correlated with downregulated slow MHC isoforms in slow muscles (DiMario, 2001). In accordance, the ratio of phosphorylated cPKCβI in fatigue-resistant muscles triplicates the one in fatigable muscles, which could be understood as an accumulation, showing that cPKCβI is less active in SOL and EOM than in TA and EDL. These differences are not evident for cPKCα, probably in relation to its cellular distribution, as cPKCα is ubiquitously located while cPKCβI is confined to the presynaptic component (Besalduch et al., 2010).
On the other hand, novel nPKC isoforms are more active in slow fibers (Yuan et al., 2002; Rykx et al., 2003) in correlation with increased myoglobin levels, reporting a slower metabolism in these muscles (Kim et al., 2008). In the present results, nPKCε abundance, which is only expressed in the presynaptic component (Obis et al., 2015a) is accompanied by pnPKCε consumption in SOL and EOM, which could be correlated with constant and higher activity of this isoform guaranteed by a pool of available protein. Altogether SOL and EOM share PKC expression levels, being a potential reason for the resistance to fatigue in these muscles (Santafé et al., 2009; Hurtado et al., 2017b; Simó et al., 2018, 2019). On the other hand, TA and EDL also share PKC expression levels, making a strong difference between fast, fatigable, and fatigue-resistant muscles. Thus, in accordance with previous results (Gundersen, 2011), and due to their exclusive presence in the presynaptic terminal, cPKCβI and nPKCε (Besalduch et al., 2010; Obis et al., 2015a) could be considered as candidates to identify fast and slow NMJ in limb muscles, respectively.
4.3 PKA activity is higher in fatigue resistance than in fast fatigable muscles
cAMP-dependent kinase regulatory isoforms have a specific tissue and subcellular distribution (Colledge and Scott, 1999). RIα and RIIα are widely expressed through tissues; RIβ is highly expressed in the nervous tissue and RIIβ is especially abundant in adipose and hepatic tissues (Cadd and McKnight, 1989; Brandon et al., 1997; Skalhegg and Tasken, 2000; Fagerberg et al., 2014). As previously reported (Hoover et al., 2001), our results show that PKA subunits are always higher in SOL than EDL, probably in relation to the functional differences between fast and slow muscles. In addition, following RI major affinity to release catalytic subunits, their scarcity in EOM could indicate higher PKA activity in EOM than in SOL. On the other hand, RIIβ levels were proportional to fatigue resistance, which correlates with RIIβ expression increase in the fast plantaris after endurance training due to a fast-to-slow molecular transition (Just-Borràs et al., 2021). Furthermore, slow fibers have been reported to accumulate more lipids than fast fibers with age in human subjects (Gueugneau et al., 2015; Choi et al., 2016), which could explain the prevalence of RIIβ in EOM and SOL since RIIβ is abundant in the adipose tissue (Cadd and McKnight, 1989; Brandon et al., 1997; Skalhegg and Tasken, 2000; Fagerberg et al., 2014), maybe in association with the lipids that are in there.
4.4 EOM fatigue-resistant muscles show increased phosphorylation and synthesis of Munc18-1 and SNAP-25 exocytotic proteins
PKCs phosphorylate several proteins that are essential for the exocytotic mechanism. On the one hand, Munc18-1, which favors membrane fusion when associated with the SNARE complex (Südhof, 2013), depends on two PKC phosphorylation sites S306 and S313 (Fujita et al., 1996; Barclay et al., 2003; Morgan et al., 2004; Snyder et al., 2006). Particularly, at the NMJ, S313 phosphorylation depends on the coordinated action of presynaptic nPKCε and cPKCβI and the retrograde TrkB signaling (Simó et al., 2018).
The present results show that Munc18-1 and pMunc18-1 levels are higher in EOM than in EDL, TA, and SOL. However, despite PKC activity differences, the Munc18-1 phosphorylation ratio is maintained through the four muscles. In SOD1-G93A mice, the amount of Munc18-1 able to interact with the SNARE complex is reduced due to over-phosphorylation (Just-Borràs et al., 2019) and in relation to neurotransmission failure (Eisen, 2001; Rocha et al., 2013). On the other hand, exercise normalizes pMunc18-1 accumulation in ALS mice (Just-Borràs et al., 2020), in correlation with an improvement of the NMJ function (Genç et al., 2014) evidenced by a better motor function (Deforges et al., 2009). Thus, the maintenance of the pMunc18-1/Munc18-1 ratio through muscles, as in trained ALS mice, seems to be essential for a correct neuromuscular function. Furthermore, the high availability in the EOM could be related to the specific release properties of their MNs.
On the other hand, SNAP-25, a member of the exocytotic SNARE complex, is phosphorylated by PKC at S187 in response to synaptic activity and elevated intracellular calcium levels, which induces its translocation to the membrane. At the NMJ, nPKCε mediates this process especially during synaptic activity, while muscle contraction exerts negative feedback to return S187 phosphorylation to basal levels (Simó et al., 2019). In particular, pSNAP-25 S187 negatively modulates neuronal activity during intense activation (Verderio et al., 2004; Pozzi et al., 2008). Consequently, the absence of SNAP-25 blocks fast calcium-triggered exocytosis, as the primed pool of synaptic vesicles becomes empty. This is in accordance with S187 phosphorylation downregulation resulting in decreased vesicle secretion (Sørensen et al., 2002, 2003) while phosphorylated S187 mutant overexpression accelerates vesicle recruitment after the emptying of the releasable vesicle pool (Nagy et al., 2002). Here, results show that SNAP-25 total levels are upregulated in all but EDL muscle, indicating that it could be a limiting factor for continuous neurotransmission in accordance with its function. Surprisingly, S187 phosphorylation is uniquely very low in EOMs, which could be understood as a result of continued consumption that is guaranteed by nPKCε availability. In accordance, the SNAP-25 S187/SNAP-25 ratio gradually decreases, being inversely proportional to fatigue resistance.
On the other hand, SNAP-25 T138 is phosphorylated by PKA (Hepp et al., 2002). This phosphorylation is not essential for SNARE complex assembly and stability (Risinger and Bennett, 1999) but it is necessary to maintain the ready releasable and primed pool of vesicles (Nagy et al., 2004). Here, results show that the pSNAP-25 T138/SNAP-25 ratio only decreases in EOM. Furthermore, this occurs in parallel with low pSNAP-25 S187 in EOMs, which makes us hypothesize that SNAP-25 phosphorylated residues are quickly lost after their action which could be related to their non-stop activity. Thus, the exceptionally low level of pSNAP-25 (both S187 and T138) in the EOM may facilitate the SNARE-SM complex formation, stability, and vesicle docking. Indeed, in opposition to healthy conditions, both pSNAP-25 S187 and T138 and total SNAP-25 were accumulated in ALS mice (Just-Borràs et al., 2019), in correlation with neurotransmission failure, showing that SNAP-25 was not able to work properly for exocytosis. These, together with high pMunc18-1 levels point to a singular regulation of the vesicular release mechanism specially related to phosphorylation residues conservation time.
5 Concluding remarks
The TrkB signaling is crucial for the correct bidirectional communication between the MN and the muscle fibers that they innervate (Hurtado et al., 2017b; Simó et al., 2018, 2019). This allows a precise functionality of the NMJ and, therefore, the contractibility of the muscle. Here, the present results show that healthy muscles have an individualized molecular pattern of the TrkB pathway, involved in the neurotransmission, that guarantee the achievement of their precise functions even despite the fact of having very similar contraction velocities and fatigue resistance, such as TA and EDL. In brief, TrkB signaling seems to be more active in slow than in fast muscles, in accordance with higher neurotrophic factors consumption and following their resistance to fatigue and vulnerability to neurodegenerative diseases such as ALS. In accordance, BDNF reduction results in slower muscle metabolism and more resistance to fatigue, while its accumulation is related to a faster metabolism (Delezie et al., 2019). Furthermore, these differences allow us to understand and differentiate the phenotype of healthy muscles and their susceptibility to changes in activity patterns. Consequently, the relation between the synaptic and neurotrophic control at the NMJ is adapted to the precise characteristics of each motor unit. EOMs are of particular interest as they have distinctive characteristics that guarantee fast contraction but high resistance to fatigue and remarkable resistance to neurodegenerative diseases such as ALS. Here, we show that wild-type EOM has a specific molecular phenotype of the TrkB signaling pathway, including enhanced retrograde neurotrophic control and more robust machinery to maintain constant synaptic activity oriented to provide a slow performance of the muscles without compromising resistance to continuous usage, summarized in Figure 6.
Data availability statement
The raw data supporting the conclusions of this article will be made available by the authors, upon reasonable request.
Ethics statement
The animal study was reviewed and approved by Ethics Committee of Animal Experimentation of the Universitat Rovira i Virgili.
Author contributions
LJ-B: data collection and quantitative analysis and statistics. VC-M: graphical abstract design. LJ-B, JT, ML, and NG: literature search. LJ-B, JT, and ML: manuscript preparation. JT and ML: conception and design. All authors data interpretation, read, and approved the final manuscript.
Funding
This study was supported by Ministerio de Economía y Competitividad (MINECO); Agencia Estatal de Investigación (AEI); European Regional Development Fund (ERDF), PID2019-106332GB-I00; Universitat Rovira i Virgili (URV), 2021PFR-URV-107; Catalan Government, 2017SGR704; Spanish Ministerio de Ciencia Innovación y Universidades, PRE2020-092084; Universitat Rovira i Virgili (URV), Contractes de personal investigador predoctoral en formació, 2018PMF-PIPF-18; and Catalan Government, 2021-FI-B00755.
Conflict of interest
The authors declare that the research was conducted in the absence of any commercial or financial relationships that could be construed as a potential conflict of interest.
Publisher’s note
All claims expressed in this article are solely those of the authors and do not necessarily represent those of their affiliated organizations, or those of the publisher, the editors and the reviewers. Any product that may be evaluated in this article, or claim that may be made by its manufacturer, is not guaranteed or endorsed by the publisher.
References
Ahuja, P., Ng, C. F., Pang, B. P. S., Chan, W. S., Tse, M. C. L., Bi, X., et al. (2022). Muscle-generated BDNF (brain derived neurotrophic factor) maintains mitochondrial quality control in female mice. Autophagy 18, 1367–1384. doi: 10.1080/15548627.2021.1985257
Akil, H., Perraud, A., Jauberteau, M. O., and Mathonnet, M. (2016). Tropomyosin-related kinase B/brain derived-neurotrophic factor signaling pathway as a potential therapeutic target for colorectal cancer. World J. Gastroenterol. 22:500. doi: 10.3748/WJG.V22.I2.490
Aldridge, G. M., Podrebarac, D. M., Greenough, W. T., and Weiler, I. J. (2008). The use of total protein stains as loading controls: An alternative to high-abundance single-protein controls in semi-quantitative immunoblotting. J. Neurosci. Methods 172, 250–254. doi: 10.1016/j.jneumeth.2008.05.003
Ausoni, S., Gorza, L., Schiaffino, S., Gundersen, K., and Lomo, T. (1990). Expression of myosin heavy chain isoforms in stimulated fast and slow rat muscles. J. Neurosci. 10, 153–160. doi: 10.1523/jneurosci.10-01-00153.1990
Baldwin, K. M., Haddad, F., Pandorf, C. E., Roy, R. R., and Edgerton, V. R. (2013). Alterations in muscle mass and contractile phenotype in response to unloading models: Role of transcriptional/pretranslational mechanisms. Front. Physiol. 4:284. doi: 10.3389/fphys.2013.00284
Barclay, J. W., Craig, T. J., Fisher, R. J., Ciufo, L. F., Evans, G. J. O., Morgan, A., et al. (2003). Phosphorylation of Munc18 by protein kinase C regulates the kinetics of exocytosis. J. Biol. Chem. 278, 10538–10545. doi: 10.1074/jbc.M211114200
Besalduch, N., Tomàs, M., Santafé, M. M., Garcia, N., Tomàs, J., and Lanuza, M. A. (2010). Synaptic activity-related classical protein kinase C isoform localization in the adult rat neuromuscular synapse. J. Comp. Neurol. 518, 211–228. doi: 10.1002/cne.22220
Biondi, R. (2004). Phosphoinositide-dependent protein kinase 1, a sensor of protein conformation. Trends Biochem. Sci. 29, 136–142. doi: 10.1016/j.tibs.2004.01.005
Brandon, E. P., Idzerda, R. L., and McKnight, G. S. (1997). PKA isoforms, neural pathways, and behaviour: Making the connection. Curr. Opin. Neurobiol. 7, 397–403. doi: 10.1016/S0959-4388(97)80069-4
Cadd, G., and McKnight, G. S. (1989). Distinct patterns of cAMP-dependent protein kinase gene expression in mouse brain. Neuron 3, 71–79. doi: 10.1016/0896-6273(89)90116-5
Carrero-Rojas, G., Benítez-Temiño, B., Pastor, A. M., and Davis López de Carrizosa, M. A. (2020). Muscle progenitors derived from extraocular muscles express higher levels of neurotrophins and their receptors than other cranial and limb muscles. Cells 9:747. doi: 10.3390/cells9030747
Casamayor, A., Morrice, N. A., and Alessi, D. R. (1999). Phosphorylation of Ser-241 is essential for the activity of 3-phosphoinositide-dependent protein kinase-1: Identification of five sites of phosphorylation in vivo. Biochem. J. 342:287. doi: 10.1042/0264-6021:3420287
Chen, Z. Y., Ieraci, A., Tanowitz, M., and Lee, F. S. (2005). A novel endocytic recycling signal distinguishes biological responses of Trk neurotrophin receptors. Mol. Biol. Cell 16, 5761–5772. doi: 10.1091/mbc.E05-07-0651
Cheng, S.-M., and Lee, S.-D. (2022). Exercise training enhances BDNF/TrkB signaling pathway and inhibits apoptosis in diabetic cerebral cortex. Int. J. Mol. Sci. 23:6740. doi: 10.3390/IJMS23126740
Choi, S. J., Files, D. C., Zhang, T., Wang, Z.-M., Messi, M. L., Gregory, H., et al. (2016). Intramyocellular lipid and impaired myofiber contraction in normal weight and obese older adults. J. Gerontol. A Biol. Sci. Med. Sci. 71, 557–564. doi: 10.1093/gerona/glv169
Cilleros-Mañé, V., Just-Borràs, L., Polishchuk, A., Durán, M., Tomàs, M., Garcia, N., et al. (2021). M 1 and M 2 mAChRs activate PDK1 and regulate PKC βI and ε and the exocytotic apparatus at the NMJ. FASEB J. 35:e21724. doi: 10.1096/fj.202002213R
Cilleros-Mañé, V., Just-Borràs, L., Tomàs, M., Garcia, N., Tomàs, J. M., and Lanuza, M. A. (2020). The M2 muscarinic receptor, in association to M1, regulates the neuromuscular PKA molecular dynamics. FASEB J. 34, 4934–4955. doi: 10.1096/fj.201902113R
Cisterna, B. A., Cardozo, C., and Saez, J. C. (2014). Neuronal involvement in muscular atrophy. Front. Cell Neurosci. 8:405. doi: 10.3389/fncel.2014.00405
Colledge, M., and Scott, J. D. (1999). AKAPs: From structure to function. Trends Cell Biol. 9, 216–221. doi: 10.1016/S0962-8924(99)01558-5
Colón-González, F., and Kazanietz, M. G. (2006). C1 domains exposed: From diacylglycerol binding to protein-protein interactions. Biochim. Biophys. Acta 1761, 827–837. doi: 10.1016/j.bbalip.2006.05.001
Correia, J. C., Kelahmetoglu, Y., Jannig, P. R., Schweingruber, C., Shvaikovskaya, D., Zhengye, L., et al. (2021). Muscle-secreted neurturin couples myofiber oxidative metabolism and slow motor neuron identity. Cell Metab. 33, 2215–2230.e8. doi: 10.1016/j.cmet.2021.09.003
Deforges, S., Branchu, J., Biondi, O., Grondard, C., Pariset, C., Lécolle, S., et al. (2009). Motoneuron survival is promoted by specific exercise in a mouse model of amyotrophic lateral sclerosis. J. Physiol. 587, 3561–3571. doi: 10.1113/jphysiol.2009.169748
Delezie, J., Weihrauch, M., Maier, G., Tejero, R., Ham, D. J., Gill, J. F., et al. (2019). BDNF is a mediator of glycolytic fiber-type specification in mouse skeletal muscle. Proc. Natl. Acad. Sci. U. S. A. 116, 16111–16120. doi: 10.1073/pnas.1900544116
Delp, M. D., and Duan, C. (1996). Composition and size of type I, IIA, IID/X, and IIB fibers and citrate synthase activity of rat muscle. J. Appl. Physiol. 80, 261–270. doi: 10.1152/JAPPL.1996.80.1.261
DiMario, J. X. (2001). Protein kinase C signaling controls skeletal muscle fiber types. Exp. Cell Res. 263, 23–32. doi: 10.1006/excr.2000.5094
Dutil, E. M., and Newton, A. C. (2000). Dual role of pseudosubstrate in the coordinated regulation of protein kinase C by phosphorylation and diacylglycerol. J. Biol. Chem. 275, 10697–10701. doi: 10.1074/jbc.275.14.10697
Eisen, A. (2001). Clinical electrophysiology of the upper and lower motor neuron in amyotrophic lateral sclerosis. Semin. Neurol. 21, 141–154. doi: 10.1055/s-2001-15261
Eslami, R., Gharakhanlou, R., and Parnow, A.-H. (2018). The response of skeletal muscle-expressed neurotrophins to acute resistance exercise in male wistar rats. Ann. Appl. Sport Sci. 6, 45–53.
Fagerberg, L., Hallström, B. M., Oksvold, P., Kampf, C., Djureinovic, D., Odeberg, J., et al. (2014). Analysis of the human tissue-specific expression by genome-wide integration of transcriptomics and antibody-based proteomics. Mol. Cell. Proteomics 13, 397–406. doi: 10.1074/mcp.M113.035600
Friedman, W. J., and Greene, L. A. (1999). Neurotrophin signaling via trks and p75. Exp. Cell Res. 253, 131–142. doi: 10.1006/EXCR.1999.4705
Fujita, Y., Sasaki, T., Fukui, K., Kotani, H., Kimura, T., Hata, Y., et al. (1996). Phosphorylation of Munc-18/n-Sec1/rbSec1 by protein kinase C: Its implication in regulating the interaction of Munc-18/n-Sec1/rbSec1 with syntaxin. J. Biol. Chem. 271, 7265–7268. doi: 10.1074/jbc.271.13.7265
Funakoshi, H., Belluardo, N., Arenas, E., Yamamoto, Y., Casabona, A., Persson, H., et al. (1995). Muscle-derived neurotrophin-4 as an activity-dependent trophic signal for adult motor neurons. Science 268, 1495–1499. doi: 10.1126/science.7770776
Garcia, N., Santafe, M. M., Tomàs, M., Lanuza, M. A., Besalduch, N., and Tomàs, J. (2010a). Involvement of brain-derived neurotrophic factor (BDNF) in the functional elimination of synaptic contacts at polyinnervated neuromuscular synapses during development. J. Neurosci. Res. 88, 1406–1419. doi: 10.1002/jnr.22320
Garcia, N., Tomas, M., Santafe, M. M., Besalduch, N., Lanuza, M. A., and Tomas, J. (2010b). The interaction between tropomyosin-related kinase B receptors and presynaptic muscarinic receptors modulates transmitter release in adult rodent motor nerve terminals. J. Neurosci. 30, 16514–16522. doi: 10.1523/JNEUROSCI.2676-10.2010
Garcia, N., Tomàs, M., Santafe, M. M., Lanuza, M. A., Besalduch, N., and Tomàs, J. (2011). Blocking p75NTR receptors alters polyinnervationz of neuromuscular synapses during development. J. Neurosci. Res. 89, 1331–1341. doi: 10.1002/jnr.22620
Genç, O., Kochubey, O., Toonen, R. F., Verhage, M., and Schneggenburger, R. (2014). Munc18-1 is a dynamically regulated PKC target during short-term enhancement of transmitter release. Elife 3, 1715–1734. doi: 10.7554/eLife.01715
Gertler, R. A., and Robbins, N. (1978). Differences in neuromuscular transmission in red and white muscles. Brain Res. 142, 160–164. doi: 10.1016/0006-8993(78)90186-5
González-Gutiérrez, A., Lazo, O. M., and Bronfman, F. C. (2020). The Rab5-rab11 endosomal pathway is required for bdnf-induced CREB transcriptional regulation in hippocampal neurons. J. Neurosci. 40, 8042–8054. doi: 10.1523/JNEUROSCI.2063-19.2020
Gould, C., and Newton, A. (2008). The life and death of protein kinase C. Curr. Drug Targets 9, 614–625. doi: 10.2174/138945008785132411
Griner, E. M., and Kazanietz, M. G. (2007). Protein kinase C and other diacylglycerol effectors in cancer. Nat. Rev. Cancer 7, 281–294. doi: 10.1038/nrc2110
Grondard, C., Biondi, O., Pariset, C., Lopes, P., Deforges, S., Lécolle, S., et al. (2008). Exercise-induced modulation of calcineurin activity parallels thetime course of myofibretransitions. J. Cell Physiol. 2008, 126–135. doi: 10.1002/jcp.21168
Gueugneau, M., Coudy-Gandilhon, C., Théron, L., Meunier, B., Barboiron, C., Combaret, L., et al. (2015). Skeletal muscle lipid content and oxidative activity in relation to muscle fiber type in aging and metabolic syndrome. J. Gerontol. A Biol. Sci. Med. Sci. 70, 566–576. doi: 10.1093/gerona/glu086
Gundersen, K. (2011). Excitation-transcription coupling in skeletal muscle: The molecular pathways of exercise. Biol. Rev. 86, 564–600. doi: 10.1111/j.1469-185X.2010.00161.x
Hakamata, Y., Watanabe, K., Amo, T., Toyomizu, M., and Kikusato, M. (2018). Characterization of mitochondrial content and respiratory capacities of broiler chicken skeletal muscles with different muscle fiber compositions. J. Poult. Sci. 55:210. doi: 10.2141/JPSA.0170141
Harandi, V. M., Gaied, A. R. N., Brännström, T., Pedrosa Domellöf, F., and Liu, J.-X. (2016). Unchanged neurotrophic factors and their receptors correlate with sparing in extraocular muscles in amyotrophic lateral sclerosis. Investig. Opthalmol. Vis. Sci. 57, 6831–6842. doi: 10.1167/iovs.16-20074
Hempstead, B. L. (2002). The many faces of p75NTR. Curr. Opin. Neurobiol. 12, 260–267. doi: 10.1016/S0959-4388(02)00321-5
Hepp, R., Cabaniols, J.-P., and Roche, P. A. (2002). Differential phosphorylation of SNAP-25 in vivo by protein kinase C and protein kinase A. FEBS Lett. 532, 52–56. doi: 10.1016/S0014-5793(02)03629-3
Hoover, F., Mathiesen, I., Skålhegg, B. S., Lømo, T., and Taskén, K. (2001). Differential expression and regulation of the PKA signalling pathway in fast and slow skeletal muscle. Anat. Embryol. 203, 193–201. doi: 10.1007/s004290000155
Horváth, D., Tamás, I., Sipos, A., Darula, Z., Bécsi, B., Nagy, D., et al. (2017). Myosin phosphatase and RhoA-activated kinase modulate neurotransmitter release by regulating SNAP-25 of SNARE complex. PLoS One 12:e0177046. doi: 10.1371/journal.pone.0177046
Hurtado, E., Cilleros, V., Nadal, L., Simó, A., Obis, T., Garcia, N., et al. (2017b). Muscle contraction regulates BDNF/TrkB signaling to modulate synaptic function through presynaptic cPKCα and cPKCβI. Front. Mol. Neurosci. 10:147. doi: 10.3389/fnmol.2017.00147
Hurtado, E., Cilleros, V., Just, L., Simó, A., Nadal, L., Tomàs, M., et al. (2017a). Synaptic activity and muscle contraction increases PDK1 and PKCβI phosphorylation in the presynaptic membrane of the neuromuscular junction. Front. Mol. Neurosci. 10:270. doi: 10.3389/fnmol.2017.00270
Just-Borràs, L., Cilleros-Mañé, V., Hurtado, E., Biondi, O., Charbonnier, F., Tomàs, M., et al. (2021). Running and swimming differently adapt the BDNF/TrkB pathway to a slow molecular pattern at the NMJ. Int. J. Mol. Sci. 22:4577. doi: 10.3390/ijms22094577
Just-Borràs, L., Hurtado, E., Cilleros-Mañé, V., Biondi, O., Charbonnier, F., Tomàs, M., et al. (2019). Overview of impaired BDNF signaling, their coupled downstream serine-threonine kinases and SNARE/SM complex in the neuromuscular junction of the amyotrophic lateral sclerosis model SOD1-G93A mice. Mol. Neurobiol. 56, 6856–6872. doi: 10.1007/s12035-019-1550-1
Just-Borràs, L., Hurtado, E., Cilleros-Mañé, V., Biondi, O., Charbonnier, F., Tomàs, M., et al. (2020). Running and swimming prevent the deregulation of the BDNF/TrkB neurotrophic signalling at the neuromuscular junction in mice with amyotrophic lateral sclerosis. Cell. Mol. Life Sci. 77, 3027–3040. doi: 10.1007/s00018-019-03337-5
Kanning, K. C., Kaplan, A., and Henderson, C. E. (2010). Motor neuron diversity in development and disease. Annu. Rev. Neurosci. 33, 409–440. doi: 10.1146/annurev.neuro.051508.135722
Katayama, N., Yamamori, S., Fukaya, M., Kobayashi, S., Watanabe, M., Takahashi, M., et al. (2017). SNAP-25 phosphorylation at Ser187 regulates synaptic facilitation and short-term plasticity in an age-dependent manner. Sci. Rep. 7, 79–96. doi: 10.1038/s41598-017-08237-x
Kim, M.-S., Fielitz, J., McAnally, J., Shelton, J. M., Lemon, D. D., McKinsey, T. A., et al. (2008). Protein kinase D1 stimulates MEF2 activity in skeletal muscle and enhances muscle performance. Mol. Cell Biol. 28, 3600–3609. doi: 10.1128/mcb.00189-08
Leary, S. C., Lyons, C. N., Rosenberger, A. G., Ballantyne, J. S., Stillman, J., and Moyes, C. D. (2003). Fiber-type differences in muscle mitochondrial profiles. Am. J. Physiol. Regul. Integr. Comp. Physiol. 285, 817–826. doi: 10.1152/AJPREGU.00058.2003/ASSET/IMAGES/LARGE/H61031862004.JPEG
Leenders, A., and Sheng, Z. H. (2005). Modulation of neurotransmitter release by the second messenger-activated protein kinases: Implications for presynaptic plasticity. Pharmacol. Ther. 105, 69–84. doi: 10.1016/j.pharmthera.2004.10.012
Li, T., Li, X., Huang, X., Yu, H., Li, S., Zhang, Z., et al. (2022). Mitochondriomics reveals the underlying neuroprotective mechanism of TrkB receptor agonist R13 in the 5×FAD mice. Neuropharmacology 204:108899. doi: 10.1016/J.NEUROPHARM.2021.108899
Lu, B. (2003). BDNF and activity-dependent synaptic modulation. Learn. Mem. 10, 86–98. doi: 10.1101/lm.54603
Mantilla, C. B., Rowley, K. L., Zhan, W. Z., Fahim, M. A., and Sieck, G. C. (2007). Synaptic vesicle pools at diaphragm neuromuscular junctions vary with motoneuron soma, not axon terminal, inactivity. Neuroscience 146, 178–189. doi: 10.1016/j.neuroscience.2007.01.048
Mantilla, C. B., Stowe, J. M., Sieck, D. C., Ermilov, L. G., Greising, S. M., Zhang, C., et al. (2014). TrkB kinase activity maintains synaptic function and structural integrity at adult neuromuscular junctions. J. Appl. Physiol. 117, 910–920. doi: 10.1152/japplphysiol.01386.2013
Mantilla, C. B., Zhan, W.-Z., and Sieck, G. C. (2004). Neurotrophins improve neuromuscular transmission in the adult rat diaphragm. Muscle Nerve 29, 381–386. doi: 10.1002/mus.10558
Matthews, V. B., Åström, M.-B., Chan, M. H. S., Bruce, C. R., Krabbe, K. S., Prelovsek, O., et al. (2009). Brain-derived neurotrophic factor is produced by skeletal muscle cells in response to contraction and enhances fat oxidation via activation of AMP-activated protein kinase. Diabetologia 52, 1409–1418. doi: 10.1007/s00125-009-1364-1
Mech, A. M., Brown, A., Schiavo, G., and Sleigh, J. N. (2020). Morphological variability is greater at developing than mature mouse neuromuscular junctions. J. Anat. 237, 603–617. doi: 10.1111/joa.13228
Miyata, H., Zhan, W.-Z., Prakash, Y. S., and Sieck, G. C. (1995). Myoneural interactions affect diaphragm muscle adaptations to inactivity. J. Appl. Physiol. 79, 1640–1649. doi: 10.1152/JAPPL.1995.79.5.1640
Morgan, A., Craig, T. J., and Evans, G. J. O. (2004). Use of phospho-specific antibodies to demonstrate phosphorylation of Munc18 / nSec1 in chromaffin cells. Cell Biol. Chromaffin Cell 1, 65–70.
Nagy, G., Matti, U., Nehring, R. B., Binz, T., Rettig, J., Neher, E., et al. (2002). Protein kinase C-dependent phosphorylation of synaptosome-associated protein of 25 kDa at Ser 187 potentiates vesicle recruitment. J Neurosci. 22, 9278–9286. doi: 10.1523/JNEUROSCI.22-21-09278.2002
Nagy, G., Reim, K., Matti, U., Brose, N., Binz, T., Rettig, J., et al. (2004). Regulation of releasable vesicle pool sizes by protein kinase A-dependent phosphorylation of SNAP-25. Neuron 41, 417–429. doi: 10.1016/S0896-6273(04)00038-8
Novák, P., Zacharová, G., and Soukup, T. (2010). Individual, age and sex differences in fiber type composition of slow and fast muscles of adult lewis rats: Comparison with other rat strains. Physiol. Res. 59, 783–801.
Obis, T., Hurtado, E., Nadal, L., Tomàs, M., Priego, M., Simon, A., et al. (2015b). The novel protein kinase C epsilon isoform modulates acetylcholine release in the rat neuromuscular junction. Mol. Brain 8:80. doi: 10.1186/s13041-015-0171-5
Obis, T., Besalduch, N., Hurtado, E., Nadal, L., Santafe, M. M., Garcia, N., et al. (2015a). The novel protein kinase C epsilon isoform at the adult neuromuscular synapse: Location, regulation by synaptic activity-dependent muscle contraction through TrkB signaling and coupling to ACh release. Mol. Brain 8:8. doi: 10.1186/s13041-015-0098-x
Pedersen, T. H., Macdonald, W. A., de Paoli, F. V., Gurung, I. S., and Nielsen, O. B. (2009). Comparison of regulated passive membrane conductance in action potential–firing fast- and slow-twitch muscle. J. Gen. Physiol. 134, 323–337. doi: 10.1085/jgp.200910291
Pérez, V., Bermedo-Garcia, F., Zelada, D., Court, F. A., Pérez, M. Á, Fuenzalida, M., et al. (2019). The p75NTR neurotrophin receptor is required to organize the mature neuromuscular synapse by regulating synaptic vesicle availability. Acta Neuropathol. Commun. 7:147. doi: 10.1186/s40478-019-0802-7
Porter, J., Baker, R., Ragusa, R., and Brueckner, J. (1995). Extraocular muscles: Basic and clinical aspects of structure and function. Surv. Ophthalmol. 39, 451–484. doi: 10.1016/S0039-6257(05)80055-4
Pozzi, D., Condliffe, S., Bozzi, Y., Chikhladze, M., Grumelli, C., Proux-Gillardeaux, V., et al. (2008). Activity-dependent phosphorylation of Ser187 is required for SNAP-25-negative modulation of neuronal voltage-gated calcium channels. Proc. Natl. Acad. Sci. U. S. A. 105, 323–328. doi: 10.1073/pnas.0706211105
Prakash, Y., Miyata, H., Zhan, W.-Z., and Sieck, G. C. (1999). Inactivity-induced remodeling of neuromuscular junctions in rat diaphragmatic muscle. Muscle Nerve 22, 307–319. doi: 10.1002/(SICI)1097-4598(199903)22:3<307::AID-MUS3<3.0.CO;2-M
Reichardt, L. F. (2006). Neurotrophin-regulated signalling pathways. Philos. Trans. R. Soc. Lond. B Biol. Sci. 361, 1545–1564. doi: 10.1098/rstb.2006.1894
Reid, B., Slater, C. R., and Bewick, G. S. (1999). Synaptic vesicle dynamics in rat fast and slow motor nerve terminals. J Neurosci. 19, 2511–2521. doi: 10.1523/JNEUROSCI.19-07-02511.1999
Risinger, C., and Bennett, M. K. (1999). Differential phosphorylation of syntaxin and synaptosome-associated protein of 25 kDa (SNAP-25) isoforms. J. Neurochem. 72, 614–624. doi: 10.1046/j.1471-4159.1999.0720614.x
Rocha, M. C., Pousinha, P. A., Correia, A. M., Sebastião, A. M., and Ribeiro, J. A. (2013). Early changes of neuromuscular transmission in the SOD1(G93A) mice model of ALS start long before motor symptoms onset. PLoS One 8:e73846. doi: 10.1371/journal.pone.0073846
Rykx, A., De Kimpe, L., Mikhalap, S., Vantus, T., Seufferlein, T., Vandenheede, J. R., et al. (2003). Protein kinase D: A family affair. FEBS Lett. 546, 81–86. doi: 10.1016/S0014-5793(03)00487-3
Santafé, M. M., Garcia, N., Lanuza, M. A., Tomàs, M., and Tomàs, J. (2009). Interaction between protein kinase C and protein kinase A can modulate transmitter release at the rat neuromuscular synapse. J. Neurosci. Res. 87, 683–690. doi: 10.1002/jnr.21885
Santafé, M. M., Garcia, N., Tomàs, M., Obis, T., Lanuza, M. A., Besalduch, N., et al. (2014). The interaction between tropomyosin-related kinase B receptors and serine kinases modulates acetylcholine release in adult neuromuscular junctions. Neurosci. Lett. 561, 171–175. doi: 10.1016/j.neulet.2013.12.073
Schiaffino, S., and Reggiani, C. (2011). Fiber types in mammalian skeletal muscles. Physiol. Rev. 91, 1447–1531. doi: 10.1152/physrev.00031.2010
Simó, A., Cilleros-Mañé, V., Just-Borràs, L., Hurtado, E., Nadal, L., Tomàs, M., et al. (2019). nPKCε mediates SNAP-25 phosphorylation of Ser-187 in basal conditions and after synaptic activity at the neuromuscular junction. Mol. Neurobiol. 56, 5346–5364. doi: 10.1007/s12035-018-1462-5
Simó, A., Just-Borràs, L., Cilleros-Mañé, V., Hurtado, E., Nadal, L., Tomàs, M., et al. (2018). BDNF-TrkB signaling coupled to nPKCε and cPKCβI modulate the phosphorylation of the exocytotic protein Munc18-1 during synaptic activity at the neuromuscular junction. Front. Mol. Neurosci. 11:207. doi: 10.3389/fnmol.2018.00207
Skalhegg, B., and Tasken, K. (2000). Specificity in the cAMP/PKA signaling pathway. Differential expression, regulation, and subcellular localization of subunits of PKA. Front. Biosci. 5:D678–D693. doi: 10.2741/Skalhegg
Snyder, D. A., Kelly, M. L., and Woodbury, D. J. (2006). SNARE complex regulation by phosphorylation. Cell Biochem. Biophys. 45, 111–124. doi: 10.1385/CBB:45:1:111
Sørensen, J. B., Matti, U., Wei, S.-H., Nehring, R. B., Voets, T., Ashery, U., et al. (2002). The SNARE protein SNAP-25 is linked to fast calcium triggering of exocytosis. Proc. Natl. Acad. Sci. U. S. A. 99, 1627–1632. doi: 10.1073/pnas.251673298
Sørensen, J. B., Nagy, G., Varoqueaux, F., Nehring, R. B., Brose, N., Wilson, M. C., et al. (2003). Differential control of the releasable vesicle pools by SNAP-25 splice variants and SNAP-23. Cell 114, 75–86. doi: 10.1016/S0092-8674(03)00477-X
Südhof, T. C. (2013). Neurotransmitter release: The last millisecond in the life of a synaptic vesicle. Neuron 80, 675–690. doi: 10.1016/j.neuron.2013.10.022
Südhof, T. C., and Rothman, J. E. (2009). Membrane fusion: Grappling with SNARE and SM proteins. Science 323, 474–477. doi: 10.1126/science.1161748
Tjust, A. E., Brannstrom, T., and Pedrosa Domellof, F. (2012). Unaffected motor endplate occupancy in eye muscles of ALS G93A mouse model. Front. Biosci. 4:1547–1555. doi: 10.2741/s351
Tomas, J., Santafé, M., Fenoll, R., Mayayo, E., Batlle, J., Lanuza, A., et al. (1992). Pattern of arborization of the motor nerve terminals in the fast and slow mammalian muscles. Biol. Cell 74, 299–305. doi: 10.1016/0248-4900(92)90041-X
Tosolini, A. P., Sleigh, J. N., Surana, S., Rhymes, E. R., Cahalan, S. D., and Schiavo, G. (2022). BDNF-dependent modulation of axonal transport is selectively impaired in ALS. Acta Neuropathol. Commun. 10:121. doi: 10.1186/s40478-022-01418-4
Verderio, C., Pozzi, D., Pravettoni, E., Inverardi, F., Schenk, U., Coco, S., et al. (2004). SNAP-25 modulation of calcium dynamics underlies differences in GABAergic and glutamatergic responsiveness to depolarization. Neuron 41, 599–610. doi: 10.1016/S0896-6273(04)00077-7
Wang, Y. L., Chio, C. C., Kuo, S. C., Yeh, C. H., Ma, J. T., Liu, W. P., et al. (2022). Exercise rehabilitation and/or astragaloside attenuate amyloid-beta pathology by reversing BDNF/TrkB signaling deficits and mitochondrial dysfunction. Mol. Neurobiol. 59, 3091–3109. doi: 10.1007/S12035-022-02728-3
Windisch, A., Gundersen, K., Szabolcs, M. J., Gruber, H., and Lømo, T. (1998). Fast to slow transformation of denervated and electrically stimulated rat muscle. J. Physiol. 510, 623–632. doi: 10.1111/j.1469-7793.1998.623bk.x
Wood, S. J., and Slater, C. R. (2001). Safety factor at the neuromuscular junction. Prog. Neurobiol. 64, 393–429.
Yuan, J., Bae, D., Cantrell, D., Nel, A., and Rozengurt, E. (2002). Protein kinase D is a downstream target of protein kinase Ctheta. Biochem. Biophys. Res. Commun. 291, 444–452. doi: 10.1006/BBRC.2002.6469
Zhan, G., Huang, N., Li, S., Hua, D., Zhang, J., Fang, X., et al. (2018). PGC-1α-FNDC5-BDNF signaling pathway in skeletal muscle confers resilience to stress in mice subjected to chronic social defeat. Psychopharmacology 235, 3351–3358. doi: 10.1007/s00213-018-5041-2
Keywords: TrkB-BDNF, PKC, PKA, neuromuscular junction, skeletal muscle, EOM, fatigue-resistant
Citation: Just-Borràs L, Cilleros-Mañé V, Polishchuk A, Balanyà-Segura M, Tomàs M, Garcia N, Tomàs J and Lanuza MA (2022) TrkB signaling is correlated with muscular fatigue resistance and less vulnerability to neurodegeneration. Front. Mol. Neurosci. 15:1069940. doi: 10.3389/fnmol.2022.1069940
Received: 14 October 2022; Accepted: 29 November 2022;
Published: 22 December 2022.
Edited by:
Robert W. Burgess, Jackson Laboratory, United StatesReviewed by:
Zarife Sahenk, Abigail Wexner Research Institute, Nationwide Children’s Hospital, United StatesAndrew Paul Tosolini, University College London, United Kingdom
Copyright © 2022 Just-Borràs, Cilleros-Mañé, Polishchuk, Balanyà-Segura, Tomàs, Garcia, Tomàs and Lanuza. This is an open-access article distributed under the terms of the Creative Commons Attribution License (CC BY). The use, distribution or reproduction in other forums is permitted, provided the original author(s) and the copyright owner(s) are credited and that the original publication in this journal is cited, in accordance with accepted academic practice. No use, distribution or reproduction is permitted which does not comply with these terms.
*Correspondence: Josep Tomàs, ✉ am9zZXBtYXJpYS50b21hc0B1cnYuY2F0; Maria A. Lanuza, ✉ bWFyaWFhbmdlbC5sYW51emFAdXJ2LmNhdA==
†These authors have contributed equally to this work