- Department of Pharmacology and Toxicology, University of Toronto, Toronto, ON, Canada
Inhibition of Glycogen synthase kinase 3 (GSK3) is a popular explanation for the effects of lithium ions on mood regulation in bipolar disorder and other mental illnesses, including major depression, cyclothymia, and schizophrenia. Contribution of GSK3 is supported by evidence obtained from animal and patient derived model systems. However, the two GSK3 enzymes, GSK3α and GSK3β, have more than 100 validated substrates. They are thus central hubs for major biological functions, such as dopamine-glutamate neurotransmission, synaptic plasticity (Hebbian and homeostatic), inflammation, circadian regulation, protein synthesis, metabolism, inflammation, and mitochondrial functions. The intricate contributions of GSK3 to several biological processes make it difficult to identify specific mechanisms of mood stabilization for therapeutic development. Identification of GSK3 substrates involved in lithium therapeutic action is thus critical. We provide an overview of GSK3 biological functions and substrates for which there is evidence for a contribution to lithium effects. A particular focus is given to four of these: the transcription factor cAMP response element-binding protein (CREB), the RNA-binding protein FXR1, kinesin subunits, and the cytoskeletal regulator CRMP2. An overview of how co-regulation of these substrates may result in shared outcomes is also presented. Better understanding of how inhibition of GSK3 contributes to the therapeutic effects of lithium should allow for identification of more specific targets for future drug development. It may also provide a framework for the understanding of how lithium effects overlap with those of other drugs such as ketamine and antipsychotics, which also inhibit brain GSK3.
Introduction
Lithium is the most mysterious drug used in psychiatry. Chemically, lithium ions can affect several biochemical functions. But how they regulate mood in bipolar disorder and other conditions remains poorly understood. This is problematic since lithium can be both very effective as a therapy and ultimately toxic for patients when administered over a lifetime (Volkmann et al., 2020). Inhibition of enzymes from the glycogen synthase kinase 3 (GSK3) family is a popular explanation for lithium’s therapeutic effects (O’Brien and Klein, 2009; Freland and Beaulieu, 2012; Alda, 2015; Haggarty et al., 2021). However, both GSK3α and GSK3β are regulatory hubs for several biological processes. It is thus unclear how inhibition of GSK3 can provide a solid heuristic foundation for the development of better alternatives to lithium therapy (Doble and Woodgett, 2003; Kaidanovich-Beilin and Woodgett, 2011; Sutherland, 2011; Kaidanovich-Beilin et al., 2012). Further understanding of mechanisms by which lithium acts beyond GSK3 inhibition is thus necessary.
Here, we present an overview of lithium therapy and its association with GSK3 inhibition. Afterward, we cover biological processes that are affected by lithium and GSK3 in relation to bipolar disorder. Finally, we focus on specific GSK3 direct substrates that are presumably important for lithium’s therapeutic effects. In doing so, we considered four main questions: (1)Does the substrate contribute to biological functions that are affected by lithium treatment? (2) Is the activity or expression of the substrate affected by lithium and GSK3 inhibition? (3) Is the regulation of the substrate by lithium and GSK3 inhibition compatible with therapy? and (4) Is the effect of lithium on the substrate dependent upon a direct or indirect inhibition of GSK3?
Lithium therapy
Lithium salts—of citrate or carbonate—are commonly prescribed as mood stabilizers to manage symptoms of bipolar disorder, a mental illness affecting up to 2.5% of the population (Merikangas et al., 2011; Iria et al., 2016). Bipolar disorder is characterized by a cycling of mood between depressive episodes and episodes of mania or hypomania (American Psychiatric Association and DSM-5 Task Force, 2013). Lithium maintenance therapy has been demonstrated to reduce the risk for both types of episodes in adult populations (Schou et al., 1954; Burgess et al., 2001;Volkmann et al., 2020). Lithium can also be effective as part of therapies to address depressive or manic/hypomanic symptoms in cyclothymia (Barroilhet and Ghaemi, 2020), major unipolar depression (De Montigny, 1994; Barroilhet and Ghaemi, 2020; Price et al., 2021), and schizophrenia (Leucht et al., 2015). Furthermore, lithium therapies have been shown to reduce suicidality (Kessing et al., 2005; Del Matto et al., 2020) and improve sleep (Billiard, 1987; Geoffroy et al., 2016).
Despite its demonstrated efficacy, lithium is not always a first-choice medication for treating bipolar disorder. Alternative pharmacotherapies mostly involve second generation antipsychotics and a handful of “mood stabilizing” anticonvulsant drugs (e.g., valproic acid, lamotrigine, and carmabazepine). However, it should be noted that in contrast to lithium, these pharmacological agents do not address the full spectrum of bipolar disorder symptoms and lack prophylactic action (Fountoulakis and Vieta, 2008; Zhihan et al., 2022). Hesitancy toward lithium may be explained by its various serious long-term side effects, narrow therapeutic window, and by a lack of clear mechanisms explaining its mood regulating actions (Volkmann et al., 2020). Uncovering how lithium affects mood to exert therapeutic action in bipolar disorder would not only improve clinical practice using lithium, but also allow for the development of more effective and safer therapeutic alternatives.
Direct and indirect inhibition of GSK3 by lithium
Several mechanisms have been proposed over the years for the regulation of mood by lithium (for review see: Freland and Beaulieu, 2012; Alda, 2015; Haggarty et al., 2021). Inhibition of GSK3α and GSK3β is presently one of the best supported mechanisms explaining the neuro-psychiatric effects of lithium. Indeed, multiple investigation using pharmacological inhibitors or genetic inactivation of GSK3 (by homologous recombination or CRISPR/Cas9 somatic approaches) have replicated correlates of lithium treatments in animal models and patient derived systems (Beaulieu et al., 2009; O’Brien and Klein, 2009; Freland and Beaulieu, 2012; Paul et al., 2020; Haggarty et al., 2021).
Direct inhibition of GSK3 by lithium (Figure 1A) has originally been uncovered in studies of development and differentiation in Xenopus, Dictyostelium, and Drosophila (Klein and Melton, 1996; Stambolic et al., 1996). This effect of lithium was later explained by a competition between Li+ and Mg2+ ions, preventing the association of Mg2+ to GSK3 kinases for which it is a co-factor (Ryves and Harwood, 2001; Sun et al., 2011). Lithium treatment resulting in therapeutic lithium serum levels (0.8–1.5 mM) also increases the inhibitory phosphorylation of GSK3 isoforms by Akt, thus providing an indirect mechanism (Figure 1A) for the inhibition of GSK3 by lithium (Chalecka-Franaszek and Chuang, 1999; Beaulieu et al., 2008). This indirect inhibition can be explained in part by an action of lithium ions on D2 dopamine receptor (DrD2) signaling. Activation of DrD2 results in the formation of a signaling complex in which Akt interacts with β-arrestin 2 and PP2A, ensuing in Akt dephosphorylation and inhibition (Beaulieu et al., 2004, 2005; O’Brien et al., 2011). Lithium has been shown to interfere with the interaction of Akt and β-arrestin 2, possibly via displacement of Mg2+ ions (Beaulieu et al., 2008). Disruption of the Akt/β-arrestin 2 signaling complex by lithium results in increased Akt activity and subsequent indirect inhibition of GSK3, therefore antagonizing the action of DrD2 on this pathway.
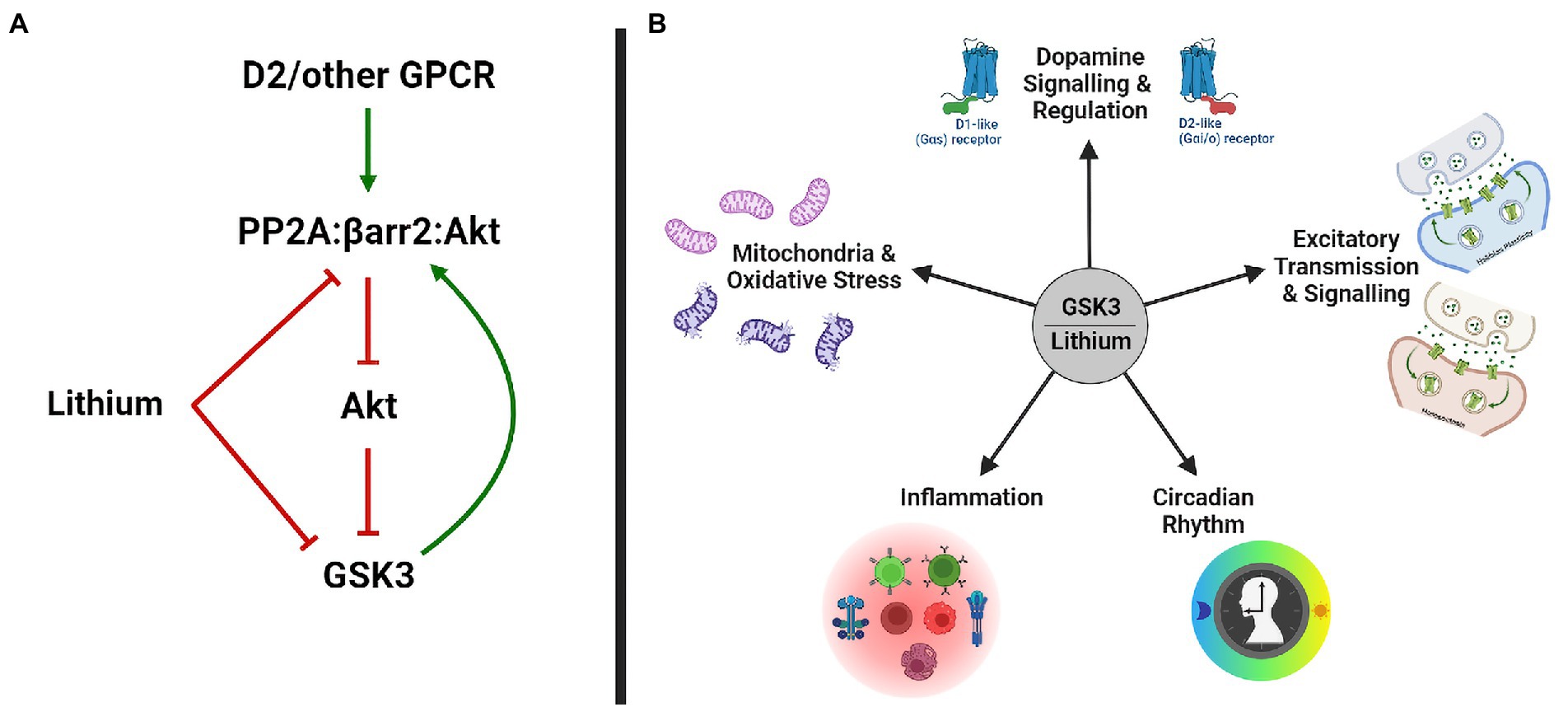
Figure 1. GSK3 and Lithium. (A) Lithium inhibits GSK3 directly and indirectly, by disrupting the PP2A/βarr2/Akt complex and increasing Akt activity. (B) Biological functions modulated by GSK3 and lithium in bipolar disorder. GSK3, glycogen synthase kinase-3; GPCR, g-protein coupled receptor; and PP2A, protein phosphatase 2A.
Biological functions modulated by GSK3 and lithium
The two GSK3 kinases are ubiquitously expressed enzymes, which have partially overlapping functions (Kaidanovich-Beilin and Woodgett, 2011; Ahmad and Woodgett, 2020). Not surprisingly these kinases also have about 100 validated substrates (Kaidanovich-Beilin and Woodgett, 2011) and several more predicted ones (Taelman et al., 2010). Furthermore, the central role of GSK3 in myriads of biological functions keeps their genes under strong selective pressure. This probably explains why few rare and no common variants of GSK3A or GSK3B genes have been associated with enhanced risk for bipolar disorder (Benedetti et al., 2013; Stahl et al., 2019; Li et al., 2021; Mullins et al., 2021). Identification of GSK3 as a probable mediator of the effects of lithium is thus rather unsatisfactory, as it does not point toward a clear process by which it can stabilize mood. Attempts to better understand the contribution of GSK3 inhibition to lithium effects have focused mostly on biological functions that are affected both by GSK3 and lithium (Figure 1B). However, this is not a simple task since GSK3 activity is involved in the regulation of gene expression, cytoskeletal organization, metabolism, mRNA regulation, inflammation, and neurotransmission, to name a few (Beaulieu et al., 2009; Kaidanovich-Beilin and Woodgett, 2011; Freland and Beaulieu, 2012; Beurel and Jope, 2014; Beurel et al., 2015; Snitow et al., 2021).
Dopamine signaling and regulation
Dopamine neurotransmission is suspected to play a role in the pathophysiology of bipolar disorder (van Enkhuizen et al., 2015). The dopamine hypothesis suggests a faulty homeostatic mechanism in which there is hyperdopaminergic tone in the manic phase, leading to overcompensation and a rapid decline into the depressive phase characterized by hypodopaminergic tone, which switches back to mania during a failed compensation (Tissot, 1975).
Some support for this hypothesis in humans comes from the efficacy of antipsychotic drugs in correcting manic symptoms of bipolar disorder (Gelenberg and Hopkins, 1996; Buoli et al., 2014). While, targeting several receptors, these drugs are all antagonists or partial agonists of DrD2 (Miyamoto et al., 2005). In schizophrenia, it has been demonstrated that clinical efficacy of antipsychotics is directly correlated with DrD2 antagonism, and long-term treatment results in diminished dopamine cell firing as well as decreased basal dopamine levels (Creese et al., 1976; Seeman et al., 1976; Amato et al., 2011). It is thus probable that antipsychotics also exert their effects on mania by regulating the efficacy of dopamine transmission. In addition, imaging studies point toward changes of dopamine homeostasis or receptor expression in the striatum and prefrontal cortex of people expressing different symptomatic phases of bipolar disorder (Berk et al., 2007; Ashok et al., 2017; Jauhar et al., 2017). Altered expression of dopamine receptors in post-mortem brains has also been associated with bipolar disorder (Zhan et al., 2011; Kaalund et al., 2014).
Animal models having elevated dopamine tone resulting from reduced expression of the dopamine transporter (DAT), or administration of pharmacological DAT inhibitors, replicate several motor and cognitive endophenotypes of bipolar disorder, which can be corrected by lithium and other mood stabilizers (Beaulieu et al., 2004; van Enkhuizen et al., 2015; Milienne-Petiot et al., 2017). Furthermore, pharmacological inhibition of GSK3 or inactivation of GSK3β, using recombinant alleles or intersectional somatic CRISPR/Cas9 approaches in neuronal populations expressing dopamine receptors, replicates behavioral outcomes of lithium treatment in mice (Beaulieu et al., 2004, 2009; Urs et al., 2012; Khlghatyan and Beaulieu, 2020).
Lithium has been shown to affect both dopamine release by neurons of the mesolimbic system and signaling response to dopamine downstream of its receptors (Beaulieu, 2016). Chronic administration of lithium has been shown to prevent excessive and maladaptive release of dopamine, without reducing basal dopamine tone (Can et al., 2016). The contribution of GSK3 inhibition to this effect has not been fully elucidated. However, it is notable that mice expressing a dominant negative GSK3β transgene display reduced DrD2 auto-receptor functions, and thus release more dopamine (Gomez-Sintes et al., 2014).
In neurons receiving dopamine innervation, acute lithium and chronic lithium have been shown to antagonize β-arrestin 2-mediated DrD2 signaling. Activation of DrD2 leads to an inhibition of Akt and concomitant relief of GSK3 inhibition. In contrast, antagonism of this pathway by lithium results in an indirect inhibition of GSK3, resulting from increased Akt activity (Beaulieu et al., 2004, 2005, 2008). Interestingly, mice lacking β-arrestin 2 expression displayed blunted responsiveness to lithium in behavioral tests having predict validity for anti-manic and antidepressant drug actions. This deficiency of responsiveness was correlated with an absence of indirect GSK3 inhibition both in β-arrestin 2 and DrD2 congenital knockout animals (Beaulieu et al., 2008; Del' Guidice and Beaulieu, 2015; Del'Guidice et al., 2015).
In addition to DrD2 signaling, lithium may also affect cyclic AMP driven signaling downstream of the D1 dopamine receptor (DrD1). Indeed, the transcription factor cAMP response element-binding protein (CREB) is a major target on which both DrD1 and GSK3 signaling can converge (Wang et al., 1994; Beaulieu and Gainetdinov, 2011; Rana et al., 2022). To our knowledge, the possibility of an interaction between DrD1 and GSK3 modulation of CREB activity has not been directly tested in the context of a contribution to the full scope of lithium effects in rodents. However, conditional knockout of GSK3β specifically in DrD1 expressing neurons of the striatum did not replicate inhibitory effects of lithium on amphetamine induced hyperactivity (Urs et al., 2012).
Excitatory transmission and signaling
Increased glutamate transmission and loss of inhibitory regulation leading to an excitatory/inhibitory neurotransmission imbalance and excitotoxic insults have been reported in post-mortem studies of bipolar disorder (Hashimoto et al., 2007; Rao et al., 2010). Furthermore, altered expression of vesicular glutamate transporter 1 (vGLUT1) that could lead to increased quantal glutamate release have also been observed (Uezato et al., 2009; Eastwood and Harrison, 2010).
Increased glutamatergic tone in bipolar disorder could result from a dysregulation of both Hebbian [long-term potentiation (LTP) and long-term depression (LTD)] as well as non-Hebbian (homeostatic) plasticity, to which dysregulated GSK3 activity can contribute (Peineau et al., 2007; Bradley et al., 2012; Machado-Vieira, 2018; Tatavarty et al., 2020). Overall, activation of GSK3 promotes LTD, while its inhibition promotes LTP, although this is not always the case (Hooper et al., 2007; Peineau et al., 2007; Zhu et al., 2007). GSK3 is also important for the homeostatic regulation of glutamatergic transmission involved in cell autonomous synaptic scaling and system level sleep homeostasis (Khlghatyan et al., 2020). Moreover, lithium rescues SHANK3-deficiency-induced deficits of homeostatic synaptic scaling as a result of its inhibitory effect on GSK3 (Tatavarty et al., 2020).
Investigations in animals indicate that both acute and chronic lithium treatments affect glutamate synaptic concentration, glutamate reuptake, and ionotropic NMDA/AMPA receptor signaling, albeit with variable results across experimental systems (Dixon and Hokin, 1998; Nonaka et al., 1998; Du et al., 2004). Furthermore, chronic lithium treatment of cortical neurons shifts excitatory/inhibitory equilibrium of synaptic networks toward inhibition (Khayachi et al., 2021). This is supporting a model in which lithium reduces excitatory neurotransmission to correct an imbalance of excitatory/inhibitory neurotransmission in bipolar disorder. However, direct modulation of components of glutamate synapses by lithium and GSK3 are probably not the only contributing factors since lithium can also modulate neuronal morphology in response to allostatic pressure such as chronic stress (Wood et al., 2004; Gray and Mcewen, 2013).
Circadian rhythm and sleep
Disruptions of circadian rhythm and sleep are established endophenotypes of bipolar disorder. Patients with bipolar disorder are sensitive to effects of sleep deprivation, have aberrant sleep–wake cycles, alterations in sleep patterns, REM sleep, and sleep duration that are accompanied by increased number of arousals and decreased sleep efficiency (Moody and Allsopp, 1969; Kripke et al., 1978; Mansour et al., 2005; Rock et al., 2014).
The primary circadian transcription factors (CLOCK and BMAL1) heterodimerize and control rhythmic expression of clock genes Per and Cry. CLOCK/BMAL1 increases levels of PER/CRY proteins, which then translocate to the nucleus to implement a negative feedback loop by repressing CLOCK/BMAL1 (Jin et al., 1999; Lee et al., 2001; Lowrey and Takahashi, 2004). Expression of a dominant negative variant of Clock (ClockΔ19) in mice results in a mix of manic and depressive like phenotypes that are reminiscent of bipolar disorder (Mcclung, 2007; Roybal et al., 2007; Mukherjee et al., 2010). Interestingly, ClockΔ19 mice display decreased AMPA-mediated excitatory synaptic responses and GluA1 AMPA subunit levels in the nucleus accumbens. Overexpressing GluA1 normalized mania-like behavior in these mice (Parekh et al., 2018). Furthermore, ClockΔ19 mice also display exacerbated striatal dopamine release, altered expression of dopamine receptors, and increased DrD2 to DrD1 ratio (Spencer et al., 2012).
Circadian activity is regulated by a master pacemaker located in the suprachiasmatic nucleus (SCN) of the hypothalamus (Weaver, 1998). GSK3β activity in the SCN oscillates over a 24-h period, where inhibition is highest during the night. Furthermore, GSK3β regulates the phosphorylation and stability of circadian proteins by regulating CLOCK/BMAL1 and PER/CRY (Iwahana et al., 2004; Paul et al., 2012; Jaworski, 2020). Accordingly, GSK3β haploinsufficiency affects circadian rhythm by lengthening circadian locomotor activity period, and inhibiting GSK3β mitigates ClockΔ19 mice hyperactivity (Kozikowski et al., 2011; Lavoie et al., 2013).
Lithium modulates clock gene expression, lengthens circadian period, and resynchronizes circadian rhythms (Hofmann et al., 1978; Johnsson et al., 1979; Mccarthy et al., 2012, 2013). In a paradoxical sleep deprivation model of mania, lithium was able to reverse behavioral alterations, and bipolar disorder patients that do not respond to lithium treatment have significantly increased activity of GSK3β and decreased expression of circadian clock genes (Geoffroy et al., 2018; Dal-Pont et al., 2019). Additionally, lithium rescued phase-signaling dysfunction in the nucleus accumbens of ClockΔ19 mice (Dzirasa et al., 2010).
Inflammation
Increased inflammation has been implicated in multiple brain pathologies, including bipolar disorder, schizophrenia, major depression, and suicidal behavior (Nässberger and Träskman-Bendz, 1993; Dowlati et al., 2010; Miller et al., 2011; Munkholm et al., 2013; Beurel and Jope, 2014). In bipolar disorder, there are several increased inflammatory markers including tumor necrosis factor-α (TNFα), interleukin (IL)-1β, IL-6, IL-4, and IL-10 (Modabbernia et al., 2013). This inflammatory reaction can be modulated by GSK3. Activated GSK3 stimulates the production of pro-inflammatory cytokines (e.g., IL-6, IL-1β, and TNFα) and decreases the production of anti-inflammatory cytokines (e.g., IL-10; Martin et al., 2005; Huang et al., 2009; Jope et al., 2017). Whereas, inhibiting GSK3 reversed these effects, especially in microglia and astrocytes, in turn preventing inflammation-induced neural toxicity (Martin et al., 2005; Beurel and Jope, 2009; Yuskaitis and Jope, 2009). Moreover, GSK3 has been shown to promote inflammation in relation to aggression- and depressive-like behaviors in mice (Martin et al., 2005; Sengupta et al., 2007; Garcia et al., 2008; Beurel and Jope, 2014; Cheng et al., 2016, 2018; Pavlov et al., 2019).
Lithium exerts anti-inflammatory properties by reducing pro-inflammatory cytokines (TNFα, IL-1β, and IL-6) and increasing anti-inflammatory cytokines (IL-2 and IL-10). These effects have been correlated with diminished aggression, impulsivity, and depressive symptoms in both human and model systems (Kishter et al., 1985; Maes et al., 1999; Martin et al., 2005; Knijff et al., 2007; Beurel and Jope, 2014; Nassar and Azab, 2014). However, a study did show that TNFα was increased in lithium-treated bipolar disorder patients compared to non-medicated patients and healthy controls (Guloksuz et al., 2010).
Mitochondria and oxidative stress
Several studies have found a relationship between mitochondrial dysfunction and bipolar disorder, where there is impaired energy production and higher levels of oxidative stress, which can lead to inflammation (Kato and Kato, 2000; Andreazza et al., 2010, 2013; Machado et al., 2016; Jeong et al., 2020). Additionally, in bipolar disorder, mitochondrial gene expression is reduced and accelerated telomere shortening is suggested to be highly influenced by oxidative stress damage (Konradi et al., 2004; Simon et al., 2006). This mitochondrial dysregulation has been supported to be influenced by GSK3 directly and indirectly. For example, GSK3 has been shown to affect mitochondrial motility via effects involving disrupted-in-schizophrenia 1 (DISC1) and microtubule stability, thus perturbing mitochondrial transport to the dendrites and axons via motor proteins (Lipina et al., 2011; Dolma et al., 2014; Ogawa et al., 2014).
In addition, there is a separate mitochondrial pool of GSK3 (mGSK3) that modulates the Krebs cycle and oxidative phosphorylation, to maintain homeostatic redox equilibrium (Hoshi et al., 1995, 1996; King et al., 2008). Dysregulation of mGSK3 can result in various disorders. Inactivating mGSK3 can revert mitochondrial pathology by promoting mitochondrial biogenesis and dynamics, as well as attenuating mitochondrial permeability and mitochondrial apoptosis [for review see: Yang et al., 2017]. mGSK3 has also been suggested to be important for regulating oxidative stress (King et al., 2001), a function that it would share with non-mitochondrial GSK3 (Potz et al., 2018). Accordingly, lithium has been shown to have anti-oxidative effects by reducing mitochondrial dysfunction, increasing anti-oxidants, decreasing lipid peroxidation levels, and reversing complex I dysfunction (Macêdo et al., 2013; De Sousa et al., 2014; Scola et al., 2014; Kim et al., 2016).
Autism spectrum disorder related pathways
Lithium and GSK3 also appear to affect different cell signaling mechanisms that have been associated with the development of subtypes of autism spectrum disorders (ASD). This may not be that surprising since bipolar disorder associated risk genes such as SHANK2 and ANK3 are shared between these disorders (Bi et al., 2012; Stahl et al., 2019; Zaslavsky et al., 2019; Kushima et al., 2022).
One interesting point of interaction involves the effect of lithium in attenuating symptoms of fragile X syndrome (Berry-Kravis et al., 2008; Yuskaitis et al., 2010; Liu et al., 2012; King and Jope, 2013; Liu and Smith, 2014). This disorder is classified as a subtype of ASD and is associated with a loss of function of the FMR1 gene encoded on the human X chromosome. Lack of the encoded protein Fragile X messenger ribonucleoprotein 1 (FMRP; Herring et al., 2022) causes an array of cognitive, motor, mood, and anxiety symptoms (Arsenault et al., 2016; Hagerman et al., 2017). FMRP is a ribosome binding protein that has important roles in regulating mRNA functions (Oostra and Hoogeveen, 1997; Richter and Zhao, 2021). Several mRNA, including SHANK2, have been shown to be targets for FMRP (Dalal et al., 2021).
One consistent finding in fragile X syndrome is the increased activity of the mechanistic target of rapamycin (mTOR) signaling pathway (Sharma et al., 2010; Hoeffer et al., 2012). mTOR is itself involved in regulating mRNA translation and protein synthesis, as well as synaptic plasticity, cell growth, autophagy, and mitochondrial activity (Gingras et al., 2004; Hay and Sonenberg, 2004; Sarbassov et al., 2005; Morita et al., 2013). mTOR dysfunction is implicated in various disorders, including bipolar disorder where studies have found its activity to be decreased in brain and blood samples (Machado-Vieira et al., 2015; Vanderplow et al., 2021). Intriguingly, lithium treatment in a clinical trial and models of fragile X syndrome results in amelioration of behavioral abnormalities, decreased aberrant protein synthesis rates, and substantial decreases in mTOR activity (Berry-Kravis et al., 2008; Liu et al., 2012; Liu and Smith, 2014).
Mechanistic target of rapamycin is an upstream regulator of FMRP. However, the translational regulation of FMRP causes indirect regulation of mTOR itself via a feedback loop (Bagni and Zukin, 2019). What is of high interest is the crosstalk between GSK3, mTOR, and FMRP (Figure 2). Evidence indicates that GSK3 regulates and is regulated by mTOR. GSK3 can either inhibit mTOR by phosphorylating its upstream effector tuberous sclerosis 2 (TSC2) or activate it by phosphorylating Raptor and Rictor and increasing their association to mTOR (Inoki et al., 2006; Stretton et al., 2015; Evangelisti et al., 2020). Alternatively, mTOR is capable of inhibiting GSK3 and limiting its nuclear translocation, thus constraining the activation/repression of transcription factor targets of GSK3 (Ka et al., 2014; Bautista et al., 2018; Evangelisti et al., 2020). Finally, lack of FMRP has been reported to activate GSK3 by reducing its inhibition by phosphorylation (Min et al., 2009). The intricacy of GSK3, mTOR, and FMRP signaling, as well as their shared lithium sensitivity and functional overlaps, suggest that this signaling module may play a role in the effects of lithium in both fragile X syndrome and bipolar disorder.
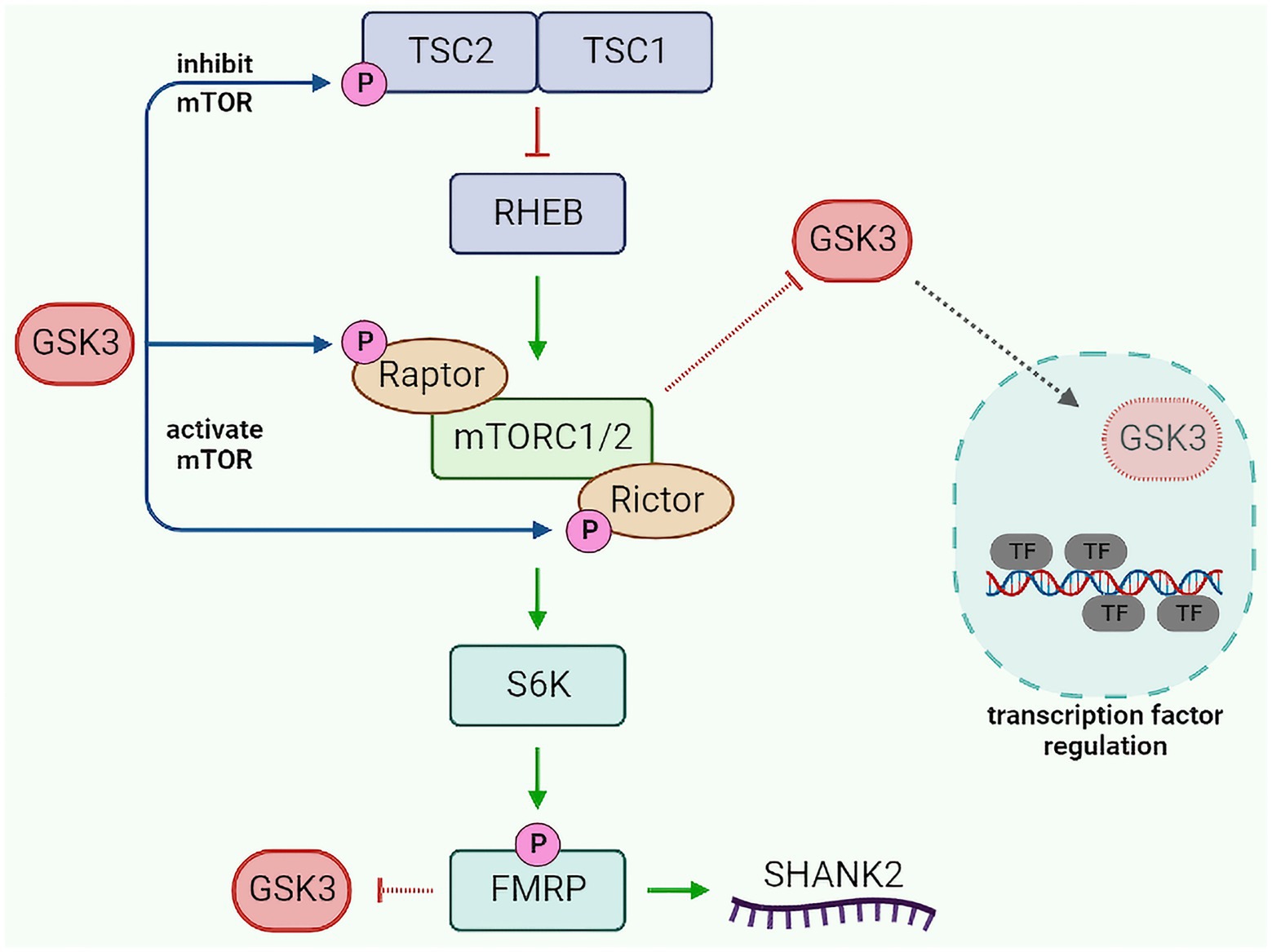
Figure 2. GSK3-mTOR-FMRP pathway crosstalk. GSK3 modulates mTOR activity by phosphorylating TSC2 (inhibit mTOR) or Raptor/Rictor (activate mTOR). Activated mTOR can inhibit GSK3 translocation to the nucleus, resulting in decreased GSK3-dependent regulation of transcription factors. Additionally, mTOR can increase FMRP activity (via S6K), which in turn can inhibit GSK3 and regulate SHANK2. GSK3, glycogen synthase kinase 3; TSC1/2, tuberous sclerosis 1/2; RHEB, ras homolog enriched in brain; mTORC1/2, mechanistic target of rapamycin complex 1/2; S6K, S6 kinase; FMRP, fragile X messenger ribonucleoprotein 1; SHANK2, SH3 and multiple ankyrin repeat domains protein 2; and TF, transcription factor.
Direct substrates of GSK3 regulated by lithium
While lithium can affect several biological functions, much less is known about the direct GSK3 substrates, by which it may mediate its therapeutic effects (Sutherland, 2011). It is important to note that GSK can act as a coincidence detector. Indeed, most GSK3 substrates need to first be primed (via phosphorylation) at a “priming sequence (in bold)”--Ser/Thr-X-X-X-Ser/Thr-P. GSK3 will recognize the primed Ser/Thr and further phosphorylate the substrate (Fiol et al., 1987). Phosphorylation by GSK3 often results in substrate inactivation or degradation via ubiquitination. While casein kinase II (CKII) was originally identified as a priming kinase for GSK3 substrates (Fiol et al., 1987), several other kinases have been shown to exert this function (Fiol et al., 1994; Del'Guidice et al., 2015). Interestingly, some priming kinases like PKA and ERK1/2 are regulated by extracellular signals, which support a role for GSK3 as a “coincidence detector” phosphorylating some of its substrates only under specific conditions. Consequently, inhibition of GSK3 may not result into specific biological outcomes if substrates are not primed to be modulated by GSK3 (Del’Guidice et al., 2015). We have already presented a few substrates including CLOCK, BMAL1, Raptor, and Rictor. In the remaining section of this review, the focus will be on a handful of substrates for which there is evidence of possible effects on bipolar disorder symptoms.
cAMP response element-binding protein
cAMP response element-binding protein is one of the most characterized substrates of GSK3. It is a transcription factor that regulates an array of cellular activity including proliferation, growth, survival, differentiation, and inflammatory responses (Gonzalez and Montminy, 1989; Wen et al., 2010). Importantly, CREB regulates brain derived neurotrophic factor (BDNF) at promoter IV, and CREB is in turn activated via BDNF–TrkB signaling (Esvald et al., 2020). Thus, activating CREB leads to increased growth and plasticity. CREB is activated by phosphorylation on Ser133 by PKA, which is also used as a priming site for subsequent phosphorylation of Ser129 by GSK3, inactivating CREB (Gonzalez and Montminy, 1989; Fiol et al., 1994).
There are two single-nucleotide polymorphisms (SNPs) in the CREB1 gene that are associated with increased risk of bipolar disorder. These SNPs result in decreased expression and activity of both CREB protein and mRNA (Ren et al., 2014; Xiao et al., 2018). Analysis of protein interaction networks indicate that CREB can interact with several proteins encoded by GWAS-identified psychiatric disorder risk genes (Xiao et al., 2018). Furthermore, chronic lithium treatment increases CREB Ser133 phosphorylation and BDNF levels, preventing cognitive impairments and unregulated stress response (Grimes and Jope, 2001; Kopnisky et al., 2003; Miller et al., 2007; Fan et al., 2015; Rana et al., 2022).
Regulation of CREB activity by GSK3 may contribute to the regulation of several lithium responsive biological functions. For example, disrupted CREB activation results in highly fragmented bouts of activity during the night phase, elevated daytime activity, and disruption in cellular clock phase synchrony (Wheaton et al., 2018). This suggests an implication of the CREB pathway in regulating the circadian clock. However, the nature of the interaction between the effects of CREB, GSK3, and lithium to circadian regulation has not been characterized.
Another possible contribution of CREB to the effects of lithium and GSK3 inhibition can involve the regulation of Hebbian synaptic plasticity. Indeed, mice with loss of CREB function display impaired LTP and memory formation (Bourtchuladze et al., 1994; Kida, 2012). It is thus possible that increased GSK3 activity would inhibits CREB (leading to impaired LTP and strengthened LTD), whereas inactivation of GSK3 would restore CREB activity and induces LTP. Paradoxically, studies of chronic lithium treatment impact on CREB found decreased pSer133 CREB, as well as increased GluA1 internalization and decreased memory consolidation (Chen et al., 1999; Tardito et al., 2007; Mavrikaki et al., 2014; Amiri et al., 2020). However, lithium can also increase CREB activity (by increasing pSer133; Grimes and Jope, 2001; Kopnisky et al., 2003; Miller et al., 2007; Fan et al., 2015; Rana et al., 2022). This suggests that lithium has both inhibitory and disinhibitory effects on CREB in different neuronal populations or experimental systems.
Another important function of CREB is the regulation of anti-inflammatory pathways. Activated GSK3β causes decreased CREB translocation to the nucleus, resulting in decreased transcription of anti-inflammatory cytokines such as IL-10 (Platzer et al., 1999; Grimes and Jope, 2001; Wen et al., 2010). Inhibiting GSK3β has also been reported to modulate the activity of CREB to decrease the expression of pro-inflammatory cytokines IL-1β and TNFα (Song et al., 2003; Avni et al., 2010; Maixner and Weng, 2013). In addition, CREB can directly inhibit nuclear factor kappa-light-chain-enhancer of activated B cells (NF-κB) and prevent apoptotic and pro-inflammatory signals (Ollivier et al., 1996; Parry and Mackman, 1997). Taken together these observations suggest several mechanisms by which lithium could exert its anti-inflammatory actions via a modulation of GSK3 and CREB activity.
Kinesins
Kinesins are a superfamily of motor enzymes. These proteins use energy from ATP hydrolysis to produce mechanical force and movement along microtubule tracks. There are several members of this family involved in a variety of functions including microtubule dynamics, cargo transport, chromosome segregation, cellular shape regulation, and mechanical integrity (Vale et al., 1986; Sheetz, 1987; Schroer et al., 1988; Ali and Yang, 2020). Kinesins are formed by combining two kinesin heavy chains and two light chains and are essential for anterograde axonal transport and the delivery of cargos to dendrites (Karasmanis et al., 2018; Ali and Yang, 2020). Kinesin’s light chain (KLC) and heavy chain (KHC) domains are phosphorylated by CKII, priming it for GSK3 phosphorylation (Morfini et al., 2002).
Phosphorylation of kinesins subunits on different sites by GSK3 can lead to different outcomes. For example, GSK3β phosphorylation of KIF1A at S402 impairs transport but does not regulate mobility, whereas phosphorylation of kinesin-1 motor domain at S314 halts motility without detaching from microtubules (Gan et al., 2020; Banerjee et al., 2021). In SH-SY5Y cells and neuronal hippocampal cultures, GSK3 phosphorylation of KLC1 and KLC2 results in decreased association of cargoes from the kinesin cargo system, indicating failed delivery of cargos to their target locations (Du et al., 2010; Morel et al., 2012). GSK3β also disrupts axonal transport by blocking translocation on microtubules, and this disruption is enhanced if kinesin-1 is reduced (Dolma et al., 2014). Interestingly, excessive GSK3β activity decreases the anterograde and retrograde segmental velocities of mitochondria, suggesting interrupted mitochondrial transport to dendrites and axons (Dolma et al., 2014).
Kinesin and GSK3 also play an important role in the trafficking of AMPA receptor subunits (Morfini et al., 2002; Setou et al., 2002; Nakata and Hirokawa, 2003; Du et al., 2010; Hirokawa et al., 2010). Specifically, phosphorylation of KLC2 by GSK3 results in a dissociation of membrane bound vesicles from kinesin, causing an internalization of AMPA-containing vesicles. Conversely, GSK3 inhibition results in increased surface GluA1 levels, which could be due to both less internalization and recycling inhibition (Du et al., 2010). This increased surface GluA1 was associated with improved acute mania-related and depression-related behaviors in mice, thus suggesting a possible therapeutic contribution (Du et al., 2010).
There is also evidence for a role of kinesin dysregulation in bipolar disorder. A post-mortem study has reported a reduction of kinesin-1 protein expression in the prefrontal cortex of subjects with bipolar disorder (Shao et al., 2016). Moreover, GSK3β inhibits neuronal transport by phosphorylating the kinesin light/heavy chains in an ANK3 deficiency model of bipolar disorder (Gottschalk et al., 2017). Decreased levels of heavy chain KIF5A and light chain KLC2, KLC3 and KLC4 in this model were rescued by lithium treatment. Lithium also rescued AMPA receptor availability and ameliorated bipolar disorder-related phenotypes in this same mouse model.
Collapsin response mediator protein 2
The collapsin response mediator protein family contains five phosphoproteins (CRMP1-5). These proteins have important roles in axon formation, growth cone guidance, and cytoskeleton collapse via interactions with microtubules. CRMP2 is most studied out of the five proteins and seems to have additional roles other than regulating microtubule network, including axonal transport, vesicle trafficking, and neurotransmitter release (Gu and Ihara, 2000; Ip et al., 2014; Lee et al., 2019; Khanna et al., 2020). CRMP2 is phosphorylated by GSK3 at Thr509 and Thr514 sites (Cole et al., 2004; Yoshimura et al., 2005). GSK3-mediated phosphorylation of CRMP2 (pCRMP2) reduces polymerization of tubulin into microtubules, thus decreasing neurite outgrowth (Yoshimura et al., 2005). Non-phosphorylated CRMP2 promotes neurite outgrowth and microtubule stability. GSK3β has also been reported to modulate CRMP2 protein stability, as ablating GSK3β increased total levels of CRMP2 (Kim et al., 2019).
Dysregulations and increased phosphorylation of CRMP2 can result in dendritic spine defects or impaired microtubule dynamics, leading to smaller brain volume, which can contribute to pathology such as bipolar disorder (Crews et al., 2011; Zhang et al., 2016; Tobe et al., 2017). This is especially interesting as pCRMP2 is elevated in bipolar disorder stem cells, but only in lithium responsive patients (Tobe et al., 2017). In addition, increasing pCRMP2 or deleting the CRMP2 gene results in diminished mitochondrial trafficking, as well as alterations in mitochondrial morphology (Brustovetsky et al., 2021). Moreover, inhibiting CRMP2 phosphorylation reduces inflammatory response, via regulation of activated microglia and reactive astrocytes (Nagai et al., 2016).
Phosphorylation of CRMP2 can be regulated by lithium. Eliminating CRMP2 caused diminished spine density and loss of lithium responsiveness in neurons. Likewise, adding lithium decreased pCRMP2. These results were confirmed in human post-mortem brain samples and derived induced pluripotent cells that showed elevated pCRMP2 in bipolar disorder samples, which was normalized by lithium treatment (Tobe et al., 2017). Furthermore, increased pCRMP2 resulted in elevated soluble to polymerized tubulin ratio, which indicates enhanced microtubule dynamics. This increased ratio was also rescued by lithium treatment in cellular models of ANK3 deficiency (Garza et al., 2018). Additionally, pCRMP2 suppressors mimicked the inhibitory effects of lithium in the amphetamine-induced-locomotor-hyperactivity model of mania, suggesting regulation of CRMP2 by dopamine receptors (Zhao et al., 2020). Interestingly, lithium causes a complete loss of pCRMP2 even in GSK3β ablated cells, suggesting that lithium targets GSK3α or other regulators of CRMP2 (Kim et al., 2019).
Fragile X related protein 1
Fragile X related protein 1 (FXR1) is a member of the RNA binding protein family, which includes FMRP, FXR1, and Fragile X related protein 2 (FXR2). This family of proteins is involved in regulating mRNA stability, transport, splicing, and translation (Siomi et al., 1995). The Fragile X proteins are enriched in the brain, especially in neurons, with lower expression in glial cells (Tamanini, 1997; Zhang et al., 2014). Proteins from this family are known to interact with each other and often share similar target RNAs (Majumder et al., 2020; Taha et al., 2021). However, their functions are not always redundant (Winograd and Ceman, 2011). The functions of fragile X family proteins have been studied primarily in the context of fragile X syndrome and ASD. However, variants in the FXR1 locus have been genetically associated with bipolar disorder, schizophrenia, insomnia, and emotional regulation (Ripke et al., 2014; Liu et al., 2016; Bureau et al., 2017; Takata et al., 2017; Jansen et al., 2019). A study showed that FXR1 levels were decreased in a “post-traumatic stress disorder” mouse model (Nie et al., 2021). Moreover, regulation of FXR1 in mouse parvalbumin interneurons leads to the expression of schizophrenia-like behaviors (Shen et al., 2021).
Fragile X related protein 1 is primed by ERK1/2 phosphorylation, which then facilitates GSK3β phosphorylation and subsequent downregulation of FXR1, probably via ubiquitination (Del'Guidice et al., 2015; Qie et al., 2017). Targeting GSK3β or overexpressing FXR1 in the mouse prefrontal cortex led to behavioral effects similar to those of lithium and other mood stabilizers on anxiety-like behavioral dimensions in mice (Latapy et al., 2012; Del'Guidice et al., 2015; Khlghatyan and Beaulieu, 2020). Furthermore, human SNPs affecting the expression of GSK3B and FXR1 genes in the human prefrontal cortex were shown to interact and affect emotional stability in controls, negative symptoms and antipsychotic drug responsiveness in schizophrenia, as well as symptom severity in bipolar disorder (Del'Guidice et al., 2015; Bureau et al., 2017; Rampino et al., 2021).
Chronic treatments with lithium, valproic acid, or lamotrigine increase FXR1 expression in the mouse striatum and prefrontal cortex. This increase is absent in β-arrestin 2 knockout mice, suggesting the βArr2/Akt/GSK3 pathway could be involved in regulating levels of FXR1 following these treatment (Del' Guidice and Beaulieu, 2015; Del'Guidice et al., 2015). Several biological functions that are relevant to the effects of lithium in bipolar disorder can potentially be affected by FXR1. For example, FXR1 has been shown to affect mitochondrial functions, oxidative stress, neurogenesis, and cell proliferation (Jagannathan et al., 2015; Patzlaff et al., 2017; Qie et al., 2017; Cao et al., 2019; Qi et al., 2020), which can be pertinent to phenotypes observed in studies of people with bipolar disorder and patient derived systems (Madison et al., 2015; Duong et al., 2021).
One major focus of recent studies has been the regulation of AMPA receptor transcription and surface expression by FXR1. Conditional knockout of FXR1 in excitatory neurons of the hippocampus exhibits increased protein synthesis-dependent LTP (Cook et al., 2014). FXR1 was shown to negatively regulate the translation of GluA2-5’UTR fluorescent protein reporters, thus potentially explaining this effect (Cook et al., 2014). Furthermore, somatic CRISPR/Cas9 GSK3β knockout or increased FXR1 expression led to decreased AMPA-mediated excitatory postsynaptic currents, and resulted in anxiolytic-like responses (Khlghatyan et al., 2018). Overexpression of FXR1 and GSK3β knockout also resulted in changes in the expression of pre-synaptic vGLUT1 and synaptic AMPA receptors subunits without affecting NMDA or GABA-A receptors (Khlghatyan et al., 2018). Together these observations indicate that GSK3β and FXR1 have multiple antagonistic effects in regulating basal AMPA-mediated excitatory neurotransmission and GluA1 and GluA2 surface expression, at least in the prefrontal cortex.
In addition to basal excitatory transmission, there is evidence for a role of GSK3β/FXR1 signaling in regulating homeostatic synaptic plasticity. FXR1 is downregulated during in vitro homeostatic scaling, or as a result of acute sleep deprivation, in the mouse prefrontal cortex (Khlghatyan et al., 2020). Downregulation of FXR1 was necessary for the homeostatic modulation of surface AMPA receptors under both conditions and preventing this downregulation during sleep deprivation altered electroencephalographic signatures. Interestingly, regulation of FXR1 in sleep deprivation and synaptic scaling was lithium sensitive and prevented by CRISPR/Cas9-mediated GSK3β inactivation (Khlghatyan et al., 2020).
Additional transcriptomic analysis using a ribotag reporter in the sleep deprivation model (Khlghatyan et al., 2020) revealed changes in the regulation of the Rho family GTPase Rac1, the Rho-associated serine/threonine kinase p21-activated kinase 3 (PAK3), and Microtubule-associated protein 2 (MAP 2). These proteins are involved in AMPA receptor trafficking and the regulation of LTP to LTD ratio. For example, PAK3 modulates GluA1 surface expression by phosphorylation, and supressing PAK3 activity results in altered AMPA receptor expression and impaired LTP (Boda, 2004; Hussain et al., 2015). Similarly, MAP 2 is involved in LTP response, and silencing MAP 2 blocks surface delivery of AMPA receptors (Fukunaga et al., 1996; Kim et al., 2020). This regulation of MAP 2 might work in conjunction with CRMP2 to stabilize microtubules and eventually affect trafficking via kinesins. Furthermore, various studies suggest that activated Rac1 is necessary for both AMPA receptor endocytosis during LTD, as well as increased surface AMPA receptors during LTP (Wiens, 2005; Xie et al., 2007; Benoist et al., 2013; Li et al., 2015; Guo et al., 2021).
Fragile X related protein 1 also regulates inflammatory responses by inhibiting the translation of TNFα mRNA, and knockdown of FXR1 removes the ability to inhibit LPS-induced TNFα protein production (Garnon et al., 2005; Khera et al., 2010). Moreover, Herman et al. (2018) found that FXR1 destabilizes pro-inflammatory transcripts. On the other hand, FXR1 could also increase pro-inflammatory responses in selected situations (Vasudevan and Steitz, 2007). Additionally, FXR1 has protective effects against mitochondrial oxidative stress and reactive oxygen species (ROS) production (Qi et al., 2020).
Unfortunately, no published studies have assessed the contribution of FXR1 to circadian rhythms, but Fmr1; Fxr2 double knockout mice have arrhythmic activity, and either Fmr1 or Fxr2 knockout mice demonstrate shorter periods of free-running activity (Zhang et al., 2008). This suggests that FXR1 could potentially regulate circadian rhythms.
Conclusion
The apparent non-pharmacological selectivity of lithium has impaired the development of better approaches for the management of bipolar disorder symptoms. Identification of GSK3 as a potential important target raised little hope since these kinases are involved in numerous biological functions. Furthermore, while some consequences of GSK3 inhibition correlates with biological outcomes of lithium treatment, this overlap has not been thoroughly characterized (Table 1). Identification of biological processes and related direct GSK3 substrates affected by bipolar disorder and lithium can provide a more mechanistic picture and point toward overlapping key biological functions mediating therapeutic effects (Figure 3A).
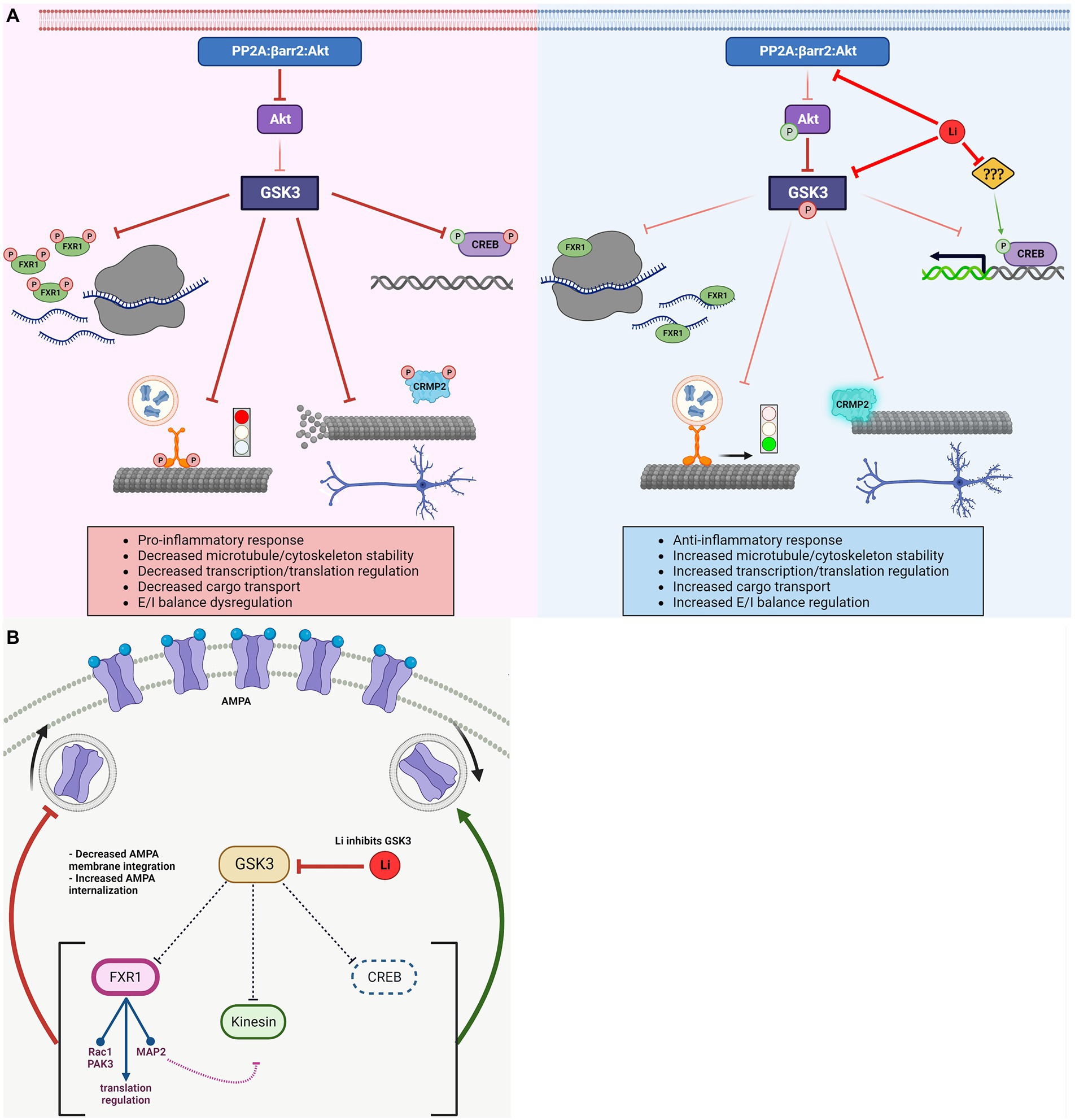
Figure 3. Regulation of GSK3 substrates and associated biological effects. (A) Activated GSK3 results in: decreased FXR1 mediated translational regulation, halted Kinesin and dissociated cargo, depolymerizing microtubule due to detached CRMP2, and inhibition of CREB-dependent transcription. Lithium inactivates GSK3 (directly and indirectly) resulting in: increased FXR1 mediated translation regulation, Kinesin mobility and delivery of cargo, CRMP2 association with (and increased stability of) microtubules, and activation of CREB-dependent transcription. Lithium has other targets, which may regulate CREB activity independently of GSK3. (B) GSK3 has various reported roles in regulating AMPA receptor trafficking and/or cell surface expression via modulation of different substrates. FXR1 decreases surface AMPA expression by regulating translational of AMPA receptor subunits mRNAs directly (mRNA, ribosomes) or indirectly by regulating expression of signaling molecules (Rac1, PAK3, and MAP2) involved in the regulation of receptor trafficking. CREB activity increases surface AMPA. Active kinesin also increases surface AMPA, but might be impeded by regulation of MAP2 and Rho GTPases by FXR1 (e.g., decreased MAP2 leads to unstable microtubules, preventing kinesin movement). Net result could depend on the relative activation/inhibition of specific pathways. GSK3, glycogen synthase kinase-3; FXR1, fragile X related protein 1; CRMP2, collapsin response mediator protein 2; CREB, cAMP response element-binding protein; Li, lithium; AMPA, α-amino-3-hydroxy-5-methyl-4-isoxazolepropionic acid; PAK3, p21-activated kinase 3; and MAP2, microtubule-associated protein 2.
For example, CREB, CRMP2, and FXR1 contribute to the regulation of glutamatergic and dopaminergic signaling, inflammation, cellular morphology, microtubule stability, and mitochondrial functions (Figure 3). FXR1 may also be affected by mTOR signaling just as FMRP (Figure 2). Furthermore, kinesins contribute to the trafficking of glutamate receptors, mitochondria, and mRNAs implicated in local protein synthesis. Interestingly, these biological processes are often intertwined as cytokine signaling, mitochondrial regulation of inflammation, oxidative stress, and excitatory/inhibitory transmission balance have been shown to affect each other in multiple systems (Galic et al., 2012; Pandey et al., 2021).
However, divergences in the known contributions of some of these GSK3 substrates also exist. For instance, following inhibition of GSK3, FXR1 provides a block to increased AMPA cell surface expression while other substrates reported here enhance AMPA functions in response to lithium (Figure 3B). These effects could be dependent of experimental systems or may contribute to regulatory mechanisms involved in neuronal circuit associated to specific behavioral outcomes. A more systematic characterization of the impacts of GSK3 inhibition in relation to its various substrates may thus be needed to clarify contradictory observations and provide mechanistic basis for drug development.
Still, contradictions between the biological effects of lithium involving different GSK3 substrates may also point toward a more intriguing mechanism of action. One possibility is that several GSK3 substrates are affected by lithium to provide a homeostatic buffer, preventing extreme functional variation, and limiting maladaptive imbalance (Gray and Mcewen, 2013; Khlghatyan et al., 2020). In this perspective, GSK3 inhibition would enhance the function of substrates that oppose cellular plasticity in response to allostatic load. These various “breaks” would thus be working in concert to maintain brain functions within an optimal working equilibrium. Differential substrate priming may provide context-dependent flexibility for this action (Del’Guidice et al., 2015). Interestingly, this type of mechanism is compatible with the clinical effects of lithium as a mood stabilizer.
Finally, an important remaining frontier is the relationship between the various GSK3 substrates and the cellular mechanisms responsible for bipolar disorder. Is it that lithium reverses core changes induced by bipolar disorder? In which case, identifying key mechanisms would be simple! Alternatively, lithium may not be addressing causative mechanisms. It may just remediate symptoms by overriding the effects of bipolar disorder on brain functions. This latter scenario may be the most probable since GSK3 enzymes are also inhibited by several other drugs used in psychiatry, including antipsychotics and ketamine (Beaulieu et al., 2009). Identification of a link between GSK3 substrates and the effects of lithium on bipolar disorder symptoms could at best provide a better understanding of disease mechanisms, or at least better drug targets and significant biomarkers of treatment efficacy.
Author contributions
JB and DC conducted research and wrote the manuscript. All authors contributed to the article and approved the submitted version.
Funding
JB holds a Canada Research Chair (Tier1) in Molecular Psychiatry. DC is supported by an Ontario Graduate Scholarship. This work was also supported by a Project Grant from the Canada Institutes of Health Research (CIHR) Grant#: PJT-148568.
Conflict of interest
The authors declare that the research was conducted in the absence of any commercial or financial relationships that could be construed as a potential conflict of interest.
Publisher’s note
All claims expressed in this article are solely those of the authors and do not necessarily represent those of their affiliated organizations, or those of the publisher, the editors and the reviewers. Any product that may be evaluated in this article, or claim that may be made by its manufacturer, is not guaranteed or endorsed by the publisher.
References
Ahmad, F., and Woodgett, J. R. (2020). Emerging roles of GSK-3alpha in pathophysiology: emphasis on cardio-metabolic disorders. Biochim. Biophys. Acta, Mol. Cell Res. 1867:118616. doi: 10.1016/j.bbamcr.2019.118616
Alda, M. (2015). Lithium in the treatment of bipolar disorder: pharmacology and pharmacogenetics. Mol. Psychiatry 20, 661–670. doi: doi: 10.1038/mp.2015.4
Ali, I., and Yang, W.-C. (2020). The functions of kinesin and kinesin-related proteins in eukaryotes. Cell Adhes. Migr. 14, 139–152. doi: doi: 10.1080/19336918.2020.1810939
Amato, D., Natesan, S., Yavich, L., Kapur, S., and Müller, C. P. (2011). Dynamic regulation of dopamine and serotonin responses to salient stimuli during chronic haloperidol treatment. Int. J. Neuropsychopharmacol. 14, 1327–1339. doi: doi: 10.1017/S1461145711000010
American Psychiatric Association and DSM-5 Task Force (2013). Diagnostic and Statistical Manual of Mental Disorders: DSM-5. Washington, D.C.: American Psychiatric Association. Available at: https://cdn.website-editor.net/30f11123991548a0af708722d458e476/files/uploaded/DSM%2520V.pdf
Amiri, S., Jafari-Sabet, M., Keyhanfar, F., Falak, R., Shabani, M., and Rezayof, A. (2020). Hippocampal and prefrontal cortical NMDA receptors mediate the interactive effects of olanzapine and lithium in memory retention in rats: the involvement of CAMKII-CREB signaling pathways. Psychopharmacology 237, 1383–1396. doi: 10.1007/s00213-020-05465-4
Andreazza, A. C., Shao, L., Wang, J.-F., and Young, L. T. (2010). Mitochondrial complex I activity and oxidative damage to mitochondrial proteins in the prefrontal cortex of patients with bipolar disorder. Arch. Gen. Psychiatry 67, 360–368. doi: 10.1001/archgenpsychiatry.2010.22
Andreazza, A. C., Wang, J.-F., Salmasi, F., Shao, L., and Young, L. T. (2013). Specific subcellular changes in oxidative stress in prefrontal cortex from patients with bipolar disorder. J. Neurochem. 127, 552–561. doi: 10.1111/jnc.12316
Arsenault, J., Gholizadeh, S., Niibori, Y., Pacey, L. K., Halder, S. K., Koxhioni, E., et al. (2016). FMRP expression levels in mouse central nervous system neurons determine behavioral phenotype. Hum. Gene Ther. 27, 982–996. doi: 10.1089/hum.2016.090
Ashok, A. H., Marques, T. R., Jauhar, S., Nour, M. M., Goodwin, G. M., Young, A. H., et al. (2017). The dopamine hypothesis of bipolar affective disorder: the state of the art and implications for treatment. Mol. Psychiatry 22, 666–679. doi: 10.1038/mp.2017.16
Avni, D., Ernst, O., Philosoph, A., and Zor, T. (2010). Role of CREB in modulation of TNFalpha and IL-10 expression in LPS-stimulated RAW264.7 macrophages. Mol. Immunol. 47, 1396–1403. doi: 10.1016/j.molimm.2010.02.015
Bagni, C., and Zukin, R. S. (2019). A synaptic perspective of fragile X syndrome and autism Spectrum disorders. Neuron 101, 1070–1088. doi: 10.1016/j.neuron.2019.02.041
Banerjee, R., Chakraborty, P., Yu, M. C., and Gunawardena, S. (2021). A stop or go switch: glycogen synthase kinase 3β phosphorylation of the kinesin 1 motor domain at Ser314 halts motility without detaching from microtubules. Development 148, 1–10. doi: 10.1242/dev.199866
Barroilhet, S. A., and Ghaemi, S. N. (2020). When and how to use lithium. Acta Psychiatr. Scand. 142, 161–172. doi: 10.1111/acps.13202
Bautista, S. J., Boras, I., Vissa, A., Mecica, N., Yip, C. M., Kim, P. K., et al. (2018). mTOR complex 1 controls the nuclear localization and function of glycogen synthase kinase 3β. J. Biol. Chem. 293, 14723–14739. doi: 10.1074/jbc.RA118.002800
Beaulieu, J. M. (2016). Converging evidence for regulation of dopamine neurotransmission by lithium: an editorial highlight for 'Chronic lithium treatment rectifies maladaptive dopamine release in the nucleus accumbens'. J. Neurochem. 139, 520–522. doi: 10.1111/jnc.13846
Beaulieu, J. M., and Gainetdinov, R. R. (2011). The physiology, signaling, and pharmacology of dopamine receptors. Pharmacol. Rev. 63, 182–217. doi: 10.1124/pr.110.002642
Beaulieu, J. M., Gainetdinov, R. R., and Caron, M. G. (2009). Akt/GSK3 signaling in the action of psychotropic drugs. Annu. Rev. Pharmacol. Toxicol. 49, 327–347. doi: 10.1146/annurev.pharmtox.011008.145634
Beaulieu, J. M., Marion, S., Rodriguiz, R. M., Medvedev, I. O., Sotnikova, T. D., Ghisi, V., et al. (2008). A beta-arrestin 2 signaling complex mediates lithium action on behavior. Cells 132, 125–136. doi: 10.1016/j.cell.2007.11.041
Beaulieu, J. M., Sotnikova, T. D., Marion, S., Lefkowitz, R. J., Gainetdinov, R. R., and Caron, M. G. (2005). An Akt/beta-arrestin 2/PP2A signaling complex mediates dopaminergic neurotransmission and behavior. Cells 122, 261–273. doi: 10.1016/j.cell.2005.05.012
Beaulieu, J. M., Sotnikova, T. D., Yao, W. D., Kockeritz, L., Woodgett, J. R., Gainetdinov, R. R., et al. (2004). Lithium antagonizes dopamine-dependent behaviors mediated by an AKT/glycogen synthase kinase 3 signaling cascade. Proc. Natl. Acad. Sci. U. S. A. 101, 5099–5104. doi: 10.1073/pnas.0307921101
Benedetti, F., Bollettini, I., Barberi, I., Radaelli, D., Poletti, S., Locatelli, C., et al. (2013). Lithium and GSK3-beta promoter gene variants influence white matter microstructure in bipolar disorder. Neuropsychopharmacology 38, 313–327. doi: 10.1007/s00213-014-3770-4
Benoist, M., Palenzuela, R., Rozas, C., Rojas, P., Tortosa, E., Morales, B., et al. (2013). MAP1B-dependent Rac activation is required for AMPA receptor endocytosis during long-term depression. EMBO J. 32, 2287–2299. doi: 10.1038/emboj.2013.166
Berk, M., Dodd, S., Kauer-Sant Anna, M., Malhi, G. S., Bourin, M., Kapczinski, F., et al. (2007). Dopamine dysregulation syndrome: implications for a dopamine hypothesis of bipolar disorder. Acta Psychiatr. Scand. 116, 41–49. doi: 10.1111/j.1600-0447.2007.01058.x
Berry-Kravis, E., Sumis, A., Hervey, C., Nelson, M., Porges, S. W., Weng, N., et al. (2008). Open-label treatment trial of lithium to target the underlying defect in fragile X syndrome. J. Dev. Behav. Pediatr. 29, 293–302. doi: 10.1097/DBP.0b013e31817dc447
Beurel, E., Grieco, S. F., and Jope, R. S. (2015). Glycogen synthase kinase-3 (GSK3): regulation, actions, and diseases. Pharmacol. Ther. 148, 114–131. doi: 10.1016/j.pharmthera.2014.11.016
Beurel, E., and Jope, R. S. (2009). Lipopolysaccharide-induced interleukin-6 production is controlled by glycogen synthase kinase-3 and STAT3 in the brain. J. Neuroinflammation 6:9. doi: 10.1186/1742-2094-6-9
Beurel, E., and Jope, R. S. (2014). Inflammation and lithium: clues to mechanisms contributing to suicide-linked traits. Transl. Psychiatry 4:e488
Bi, C., Wu, J., Jiang, T., Liu, Q., Cai, W., Yu, P., et al. (2012). Mutations of ANK3 identified by exome sequencing are associated with autism susceptibility. Hum. Mutat. 33, 1635–1638. doi: 10.1002/humu.22174
Billiard, M. (1987). Lithium carbonate: effects on sleep patterns of normal and depressed subjects and its use in sleep-wake pathology. Pharmacopsychiatry 20, 195–196. doi: 10.1055/s-2007-1017102
Boda, B. (2004). The mental retardation protein PAK3 contributes to synapse formation and plasticity in hippocampus. J. Neurosci. 24, 10816–10825. doi: 10.1523/JNEUROSCI.2931-04.2004
Bourtchuladze, R., Frenguelli, B., Blendy, J., Cioffi, D., Schutz, G., and Silva, A. (1994). Deficient long-term memory in mice with a targeted mutation of the cAMP-responsive element-binding protein. Cells 79, 59–68.
Bradley, C. A., Peineau, S., Taghibiglou, C., Nicolas, C. S., Whitcomb, D. J., Bortolotto, Z. A., et al. (2012). A pivotal role of GSK-3 in synaptic plasticity. Front. Mol. Neurosci. 5:13. doi: 10.3389/fnmol.2012.00013
Brustovetsky, T., Khanna, R., and Brustovetsky, N. (2021). CRMP2 is involved in regulation of mitochondrial morphology and motility in neurons. Cells 10:2781. doi: 10.3390/cells10102781
Buoli, M., Serati, M., and Altamura, A. C. (2014). Is the combination of a mood stabilizer plus an antipsychotic more effective than mono-therapies in long-term treatment of bipolar disorder? A systematic review. J. Affect. Disord. 152-154, 12–18. doi: 10.1016/j.jad.2013.08.024
Bureau, A., Beaulieu, J. M., Paccalet, T., Chagnon, Y. C., and Maziade, M. (2017). The interaction of GSK3B and FXR1 genotypes may influence the mania and depression dimensions in mood disorders. J. Affect. Disord. 213, 172–177.
Burgess, S. S. A., Geddes, J., Hawton, K. K. E., Taylor, M. J., Townsend, E., Jamison, K., et al. (2001). Lithium for maintenance treatment of mood disorders. Cochrane Database Syst. Rev. doi: 10.1002/14651858.CD003013
Can, A., Frost, D. O., Cachope, R., Cheer, J. F., and Gould, T. D. (2016). Chronic lithium treatment rectifies maladaptive dopamine release in the nucleus accumbens. J. Neurochem. 139, 576–585. doi: 10.1111/jnc.13769
Cao, S., Zheng, J., Liu, X., Liu, Y., Ruan, X., Ma, J., et al. (2019). FXR1 promotes the malignant biological behavior of glioma cells via stabilizing MIR17HG. J. Exp. Clin. Cancer Res. 38:37. doi: 10.1186/s13046-018-0991-0
Chalecka-Franaszek, E., and Chuang, D. M. (1999). Lithium activates the serine/threonine kinase Akt-1 and suppresses glutamate-induced inhibition of Akt-1 activity in neurons. Proc. Natl. Acad. Sci. U. S. A. 96, 8745–8750.
Chen, B., Wang, J., Hill, B., and Young, L. (1999). Lithium and valproate differentially regulate brain regional expression of phosphorylated CREB and c-Fos. Mol. Brain Res. 70, 45–53. doi: 10.1016/S0169-328X(99)00125-4
Cheng, Y., Desse, S., Martinez, A., Worthen, R. J., Jope, R. S., and Beurel, E. (2018). TNFα disrupts blood brain barrier integrity to maintain prolonged depressive-like behavior in mice. Brain Behav. Immun. 69, 556–567. doi: 10.1016/j.bbi.2018.02.003
Cheng, Y., Pardo, M., Armini, R. D. S., Martinez, A., Mouhsine, H., Zagury, J.-F., et al. (2016). Stress-induced neuroinflammation is mediated by GSK3-dependent TLR4 signaling that promotes susceptibility to depression-like behavior. Brain Behav. Immun. 53, 207–222. doi: 10.1016/j.bbi.2015.12.012
Cole, A. R., Knebel, A., Morrice, N. A., Robertson, L. A., Irving, A. J., Connolly, C. N., et al. (2004). GSK-3 phosphorylation of the Alzheimer epitope within Collapsin response mediator proteins regulates axon elongation in primary neurons. J. Biol. Chem. 279, 50176–50180. doi: 10.1074/jbc.C400412200
Cook, D., Nuro, E., Jones, E. V., Altimimi, H. F., Farmer, W. T., Gandin, V., et al. (2014). FXR1P limits long-term memory, long-lasting synaptic potentiation, and de novo GluA2 translation. Cell Rep. 9, 1402–1416. doi: 10.1016/j.celrep.2014.10.028
Creese, I., Burt, D. R., and Snyder, S. H. (1976). Dopamine receptor binding predicts clinical and pharmacological potencies of antischizophrenic drugs. Science 192, 481–483. doi: 10.1126/science.3854
Crews, L., Ruf, R., Patrick, C., Dumaop, W., Trejo-Morales, M., Achim, C. L., et al. (2011). Phosphorylation of collapsin response mediator protein-2 disrupts neuronal maturation in a model of adult neurogenesis: implications for neurodegenerative disorders. Mol. Neurodegener. 6:67. doi: 10.1186/1750-1326-6-67
Dalal, J. S., Winden, K. D., Salussolia, C. L., Sundberg, M., Singh, A., Pham, T. T., et al. (2021). Loss of Tsc1 in cerebellar Purkinje cells induces transcriptional and translation changes in FMRP target transcripts. elife 10. doi: 10.7554/eLife.67399
Dal-Pont, G. C., Jório, M. T. S., Resende, W. R., Gava, F. F., Aguiar-Geraldo, J. M., Possamai-Della, T., et al. (2019). Effects of lithium and valproate on behavioral parameters and neurotrophic factor levels in an animal model of mania induced by paradoxical sleep deprivation. J. Psychiatr. Res. 119, 76–83. doi: 10.1016/j.jpsychires.2019.09.003
De Montigny, C. (1994). Lithium addition in treatment-resistant depression. Int. Clin. Psychopharmacol. 9, 31–36. doi: 10.1097/00004850-199406002-00006
De Sousa, R. T., Zarate, C. A., Zanetti, M. V., Costa, A. C., Talib, L. L., Gattaz, W. F., et al. (2014). Oxidative stress in early stage bipolar disorder and the association with response to lithium. J. Psychiatr. Res. 50, 36–41. doi: 10.1016/j.jpsychires.2013.11.011
Del' Guidice, T., and Beaulieu, J. M. (2015). Selective disruption of dopamine D2-receptors/beta-arrestin2 signaling by mood stabilizers. J. Recept. Signal Transduct. Res. 35, 224–232. doi: 10.3109/10799893.2015.1072976
Del’Guidice, T., Latapy, C., Rampino, A., Khlghatyan, J., Lemasson, M., Gelao, B., et al. (2015). FXR1P is a GSK3beta substrate regulating mood and emotion processing. Proc. Natl. Acad. Sci. U. S. A. 112, E4610–E4619. doi: 10.1073/pnas.1506491112
Del Matto, L., Muscas, M., Murru, A., Verdolini, N., Anmella, G., Fico, G., et al. (2020). Lithium and suicide prevention in mood disorders and in the general population: a systematic review. Neurosci. Biobehav. Rev. 116, 142–153. doi: 10.1016/j.neubiorev.2020.06.017
Dixon, J. F., and Hokin, L. E. (1998). Lithium acutely inhibits and chronically up-regulates and stabilizes glutamate uptake by presynaptic nerve endings in mouse cerebral cortex. Proc. Natl. Acad. Sci. 95, 8363–8368. doi: 10.1073/pnas.95.14.8363
Doble, B. W., and Woodgett, J. R. (2003). GSK-3: tricks of the trade for a multi-tasking kinase. J. Cell Sci. 116, 1175–1186. doi: 10.1242/jcs.00384
Dolma, K., Iacobucci, G. J., Hong Zheng, K., Shandilya, J., Toska, E., White, J. A., et al. (2014). Presenilin influences glycogen synthase kinase-3 β (GSK-3β) for kinesin-1 and dynein function during axonal transport. Hum. Mol. Genet. 23, 1121–1133. doi: 10.1093/hmg/ddt505
Dowlati, Y., Herrmann, N., Swardfager, W., Liu, H., Sham, L., Reim, E. K., et al. (2010). A meta-analysis of cytokines in major depression. Biol. Psychiatry 67, 446–457. doi: 10.1016/j.biopsych.2009.09.033
Du, J., Gray, N. A., Falke, C. A., Chen, W., Yuan, P., Szabo, S. T., et al. (2004). Modulation of synaptic plasticity by Antimanic agents: the role of AMPA glutamate receptor subunit 1 synaptic expression. J. Neurosci. 24, 6578–6589. doi: 10.1523/JNEUROSCI.1258-04.2004
Du, J., Wei, Y., Liu, L., Wang, Y., Khairova, R., Blumenthal, R., et al. (2010). A kinesin signaling complex mediates the ability of GSK-3β to affect mood-associated behaviors. Proc. Natl. Acad. Sci. 107, 11573–11578. doi: 10.1073/pnas.0913138107
Duong, A., Evstratova, A., Sivitilli, A., Hernandez, J. J., Gosio, J., Wahedi, A., et al. (2021). Characterization of mitochondrial health from human peripheral blood mononuclear cells to cerebral organoids derived from induced pluripotent stem cells. Sci. Rep. 11:4523. doi: 10.1038/s41598-021-84071-6
Dzirasa, K., Coque, L., Sidor, M. M., Kumar, S., Dancy, E. A., Takahashi, J. S., et al. (2010). Lithium ameliorates nucleus Accumbens phase-signaling dysfunction in a genetic mouse model of mania. J. Neurosci. 30, 16314–16323. doi: 10.1523/JNEUROSCI.4289-10.2010
Eastwood, S. L., and Harrison, P. J. (2010). Markers of glutamate synaptic transmission and plasticity are increased in the anterior cingulate cortex in bipolar disorder. Biol. Psychiatry 67, 1010–1016. doi: 10.1016/j.biopsych.2009.12.004
Esvald, E.-E., Tuvikene, J., Sirp, A., Patil, S., Bramham, C. R., and Timmusk, T. (2020). CREB family transcription factors are major mediators of BDNF transcriptional autoregulation in cortical neurons. J. Neurosci. 40, 1405–1426. doi: 10.1523/JNEUROSCI.0367-19.2019
Evangelisti, C., Chiarini, F., Paganelli, F., Marmiroli, S., and Martelli, A. M. (2020). Crosstalks of GSK3 signaling with the mTOR network and effects on targeted therapy of cancer. Biochim. Biophys. Acta 1867:118635. doi: 10.1016/j.bbamcr.2019.118635
Fan, M., Jin, W., Zhao, H., Xiao, Y., Jia, Y., Yin, Y., et al. (2015). Lithium chloride administration prevents spatial learning and memory impairment in repeated cerebral ischemia-reperfusion mice by depressing apoptosis and increasing BDNF expression in hippocampus. Behav. Brain Res. 291, 399–406. doi: 10.1016/j.bbr.2015.05.047
Fiol, C. J., Mahrenholz, A. M., Wang, Y., Roeske, R. W., and Roach, P. J. (1987). Formation of protein kinase recognition sites by covalent modification of the substrate. Molecular mechanism for the synergistic action of casein kinase II and glycogen synthase kinase 3. J. Biol. Chem. 262, 14042–14048. doi: 10.1016/S0021-9258(18)47901-X
Fiol, C., Williams, J., Chou, C., Wang, Q., Roach, P., and Andrisani, O. (1994). A secondary phosphorylation of CREB341 at Ser129 is required for the cAMP-mediated control of gene expression. A role for glycogen synthase kinase-3 in the control of gene expression. J. Biol. Chem. 269, 32187–32193. doi: 10.1016/S0021-9258(18)31619-3
Fountoulakis, K. N., and Vieta, E. (2008). Treatment of bipolar disorder: a systematic review of available data and clinical perspectives. Int. J. Neuropsychopharmacol. 11, 999–1029. doi: 10.1017/S1461145708009231
Freland, L., and Beaulieu, J. M. (2012). Inhibition of GSK3 by lithium, from single molecules to signaling networks. Front. Mol. Neurosci. 5:14. doi: 10.3389/fnmol.2012.00014
Fukunaga, K., Muller, D., and Miyamoto, E. (1996). CaM kinase II in long-term potentiation. Neurochem. Int. 28, 343–358. doi: 10.1016/0197-0186(95)00097-6
Galic, M. A., Riazi, K., and Pittman, Q. J. (2012). Cytokines and brain excitability. Front. Neuroendocrinol. 33, 116–125. doi: 10.1016/j.yfrne.2011.12.002
Gan, K. J., Akram, A., Blasius, T. L., Ramser, E. M., Budaitis, B. G., Gabrych, D. R., et al. (2020). GSK3β impairs KIF1A transport in a cellular model of Alzheimer’s disease but does not regulate motor motility at S402. Eneuro 7:120. doi: 10.1523/ENEURO.0176-20.2020
Garcia, C. A., Benakanakere, M. R., Alard, P., Kosiewicz, M. M., Kinane, D. F., and Martin, M. (2008). Antigenic experience dictates functional role of glycogen synthase Kinase-3 in human CD4+ T cell responses. J. Immunol. 181, 8363–8371. doi: 10.4049/jimmunol.181.12.8363
Garnon, J., Lachance, C., Di Marco, S., Hel, Z., Marion, D., Ruiz, M. C., et al. (2005). Fragile X-related protein FXR1P regulates proinflammatory cytokine tumor necrosis factor expression at the post-transcriptional level. J. Biol. Chem. 280, 5750–5763. doi: 10.1074/jbc.M401988200
Garza, J. C., Qi, X., Gjeluci, K., Leussis, M. P., Basu, H., Reis, S. A., et al. (2018). Disruption of the psychiatric risk gene Ankyrin 3 enhances microtubule dynamics through GSK3/CRMP2 signaling. Transl. Psychiatry 8:135. doi: 10.1038/s41398-018-0182-y
Gelenberg, A., and Hopkins, H. (1996). Antipsychotics in bipolar disorder. J. Clin. Psychiatry 57, 49–52.
Geoffroy, P. A., Curis, E., Courtin, C., Moreira, J., Morvillers, T., Etain, B., et al. (2018). Lithium response in bipolar disorders and core clock genes expression. World J. Biol. Psychiatry 19, 619–632. doi: 10.1080/15622975.2017.1282174
Geoffroy, P. A., Samalin, L., Llorca, P. M., Curis, E., and Bellivier, F. (2016). Influence of lithium on sleep and chronotypes in remitted patients with bipolar disorder. J. Affect. Disord. 204, 32–39. doi: 10.1016/j.jad.2016.06.015
Gingras, A., Raught, B., and Sonenberg, N. (2004). mTOR signaling to translation. Curr. Top. Microbiol. Immunol. 279, 169–197. doi: 10.1007/978-3-642-18930-2_11
Gomez-Sintes, R., Bortolozzi, A., Artigas, F., and Lucas, J. J. (2014). Reduced striatal dopamine DA D2 receptor function in dominant-negative GSK-3 transgenic mice. Eur. Neuropsychopharmacol. 24, 1524–1533. doi: 10.1016/j.euroneuro.2014.07.004
Gonzalez, G., and Montminy, M. (1989). Cyclic AMP stimulates somatostatin gene transcription by phosphorylation of CREB at serine 133. Cells 59, 675–680. doi: 10.1016/0092-8674(89)90013-5
Gottschalk, M. G., Leussis, M. P., Ruland, T., Gjeluci, K., Petryshen, T. L., and Bahn, S. (2017). Lithium reverses behavioral and axonal transport-related changes associated with ANK3 bipolar disorder gene disruption. Eur. Neuropsychopharmacol. 27, 274–288. doi: 10.1016/j.euroneuro.2017.01.001
Gray, J. D., and Mcewen, B. S. (2013). Lithium's role in neural plasticity and its implications for mood disorders. Acta Psychiatr. Scand. 128, 347–361. doi: 10.1111/acps.12139
Grimes, C. A., and Jope, R. S. (2001). CREB DNA binding activity is inhibited by glycogen synthase kinase-3β and facilitated by lithium. J. Neurochem. 78, 1219–1232. doi: 10.1046/j.1471-4159.2001.00495.x
Gu, Y., and Ihara, Y. (2000). Evidence that collapsin response mediator protein-2 is involved in the dynamics of microtubules. J. Biol. Chem. 275, 17917–17920. doi: 10.1074/jbc.C000179200
Guloksuz, S., Cetin, E. A., Cetin, T., Deniz, G., Oral, E. T., and Nutt, D. J. (2010). Cytokine levels in euthymic bipolar patients. J. Affect. Disord. 126, 458–462. doi: 10.1016/j.jad.2010.04.027
Guo, D., Peng, Y., Wang, L., Sun, X., Wang, X., Liang, C., et al. (2021). Autism-like social deficit generated by Dock4 deficiency is rescued by restoration of Rac1 activity and NMDA receptor function. Mol. Psychiatry 26, 1505–1519. doi: 10.1038/s41380-019-0472-7
Hagerman, R. J., Berry-Kravis, E., Hazlett, H. C., Bailey, D. B., Moine, H., Kooy, R. F., et al. (2017). Fragile X syndrome. Nat. Rev. Dis. Primers. 3:17065. doi: 10.1038/nrdp.2017.65
Haggarty, S. J., Karmacharya, R., and Perlis, R. H. (2021). Advances toward precision medicine for bipolar disorder: mechanisms & molecules. Mol. Psychiatry 26, 168–185. doi: 10.1038/s41380-020-0831-4
Hashimoto, K., Sawa, A., and Iyo, M. (2007). Increased levels of glutamate in brains from patients with mood disorders. Biol. Psychiatry 62, 1310–1316. doi: 10.1016/j.biopsych.2007.03.017
Hay, N., and Sonenberg, N. (2004). Upstream and downstream of mTOR. Genes Dev. 18, 1926–1945. doi: 10.1101/gad.1212704
Herman, A. B., Vrakas, C. N., Ray, M., Kelemen, S. E., Sweredoski, M. J., Moradian, A., et al. (2018). FXR1 is an IL-19-responsive RNA-binding protein that destabilizes pro-inflammatory transcripts in vascular smooth muscle cells. Cell Rep. 24, 1176–1189. doi: 10.1016/j.celrep.2018.07.002
Herring, J., Johnson, K., and Richstein, J. (2022). The use of "retardation" in FRAXA, FMRP, FMR1 and other designations. Cells 11:1044. doi: 10.3390/cells11061044
Hirokawa, N., Niwa, S., and Tanaka, Y. (2010). Molecular Motors in Neurons: transport mechanisms and roles in brain function, development, and disease. Neuron 68, 610–638. doi: 10.1016/j.neuron.2010.09.039
Hoeffer, C. A., Sanchez, E., Hagerman, R. J., Mu, Y., Nguyen, D. V., Wong, H., et al. (2012). Altered mTOR signaling and enhanced CYFIP2 expression levels in subjects with fragile X syndrome. Genes Brain Behav. 11, 332–341. doi: 10.1111/j.1601-183X.2012.00768.x
Hofmann, K., Günderoth-Palmowski, M., Wiedenmann, G., and Engelmann, W. (1978). Further evidence for period lengthening effect of Li+ on circadian rhythms. Zeitschr. Naturforsch. Sec. C J. Biosci. 33, 231–234. doi: 10.1515/znc-1978-3-411
Hooper, C., Markevich, V., Plattner, F., Killick, R., Schofield, E., Engel, T., et al. (2007). Glycogen synthase kinase-3 inhibition is integral to long-term potentiation. Eur. J. Neurosci. 25, 81–86. doi: 10.1111/j.1460-9568.2006.05245.x
Hoshi, M., Sato, M., Kondo, S., Takashima, A., Noguchi, K., Takahashi, M., et al. (1995). Different localization of tau protein kinase I/glycogen synthase kinase-3 beta from glycogen synthase kinase-3 alpha in cerebellum mitochondria. J. Biochem. 118, 683–685. doi: 10.1093/oxfordjournals.jbchem.a124965
Hoshi, M., Takashima, A., Noguchi, K., Murayama, M., Sato, M., Kondo, S., et al. (1996). Regulation of mitochondrial pyruvate dehydrogenase activity by tau protein kinase I/glycogen synthase kinase 3beta in brain. Proc. Natl. Acad. Sci. 93, 2719–2723. doi: 10.1073/pnas.93.7.2719
Huang, W.-C., Lin, Y.-S., Wang, C.-Y., Tsai, C.-C., Tseng, H.-C., Chen, C.-L., et al. (2009). Glycogen synthase kinase-3 negatively regulates anti-inflammatory interleukin-10 for lipopolysaccharide-induced iNOS/NO biosynthesis and RANTES production in microglial cells. Immunology 128, e275–e286. doi: 10.1111/j.1365-2567.2008.02959.x
Hussain, N. K., Thomas, G. M., Luo, J., and Huganir, R. L. (2015). Regulation of AMPA receptor subunit GluA1 surface expression by PAK3 phosphorylation. Proc. Natl. Acad. Sci. 112, E5883–E5890. doi: 10.1073/pnas.1518382112
Inoki, K., Ouyang, H., Zhu, T., Lindvall, C., Wang, Y., Zhang, X., et al. (2006). TSC2 integrates Wnt and energy signals via a coordinated phosphorylation by AMPK and GSK3 to regulate cell growth. Cells 126, 955–968. doi: 10.1016/j.cell.2006.06.055
Ip, J. P. K., Fu, A. K. Y., and Ip, N. Y. (2014). CRMP2: functional roles in neural development and therapeutic potential in neurological diseases. Neuroscientist 20, 589–598. doi: 10.1177/1073858413514278
Iria, G., Michael, B., Boris, B., and Eduard, V. (2016). Bipolar disorder. Lancet 387, 1561–1572. doi: 10.1016/S0140-6736(15)00241-X
Iwahana, E., Akiyama, M., Miyakawa, K., Uchida, A., Kasahara, J., Fukunaga, K., et al. (2004). Effect of lithium on the circadian rhythms of locomotor activity and glycogen synthase kinase-3 protein expression in the mouse suprachiasmatic nuclei. Eur. J. Neurosci. 19, 2281–2287. doi: 10.1111/j.0953-816X.2004.03322.x
Jagannathan, R., Thapa, D., Nichols, C. E., Shepherd, D. L., Stricker, J. C., Croston, T. L., et al. (2015). Translational regulation of the mitochondrial genome following redistribution of mitochondrial MicroRNA in the diabetic heart. Circ. Cardiovasc. Genet. 8, 785–802. doi: 10.1161/CIRCGENETICS.115.001067
Jansen, P. R., Watanabe, K., Stringer, S., Skene, N., Bryois, J., Hammerschlag, A. R., et al. (2019). Genome-wide analysis of insomnia in 1,331,010 individuals identifies new risk loci and functional pathways. Nat. Genet. 51, 394–403. doi: 10.1038/s41588-018-0333-3
Jauhar, S., Nour, M. M., Veronese, M., Rogdaki, M., Bonoldi, I., Azis, M., et al. (2017). A test of the Transdiagnostic dopamine hypothesis of psychosis using positron emission tomographic imaging in bipolar affective disorder and schizophrenia. JAMA Psychiat. 74, 1206–1213. doi: 10.1001/jamapsychiatry.2017.2943
Jaworski, T. (2020). Control of neuronal excitability by GSK-3beta: epilepsy and beyond. Biochimica et Biophysica Acta (BBA) - molecular. Cell Res. 1867:118745. doi: 10.1016/j.bbamcr.2020.118745
Jeong, H., Dimick, M. K., Sultan, A., Duong, A., Park, S. S., El Sabbagh, D. E. S., et al. (2020). Peripheral biomarkers of mitochondrial dysfunction in adolescents with bipolar disorder. J. Psychiatr. Res. 123, 187–193. doi: 10.1016/j.jpsychires.2020.02.009
Jin, X., Shearman, L. P., Weaver, D. R., Zylka, M. J., De Vries, G. J., and Reppert, S. M. (1999). A molecular mechanism regulating rhythmic output from the Suprachiasmatic circadian clock. Cells 96, 57–68. doi: 10.1016/S0092-8674(00)80959-9
Johnsson, A., Pflug, B., Engelmann, W., and Klemke, W. (1979). Effect of lithium carbonate on circadian periodicity in humans. Pharmacopsychiatry 12, 423–425. doi: 10.1055/s-0028-1094638
Jope, R. S., Cheng, Y., Lowell, J. A., Worthen, R. J., Sitbon, Y. H., and Beurel, E. (2017). Stressed and inflamed, Can GSK3 be blamed? Trends Biochem. Sci. 42, 180–192. doi: 10.1016/j.tibs.2016.10.009
Ka, M., Condorelli, G., Woodgett, J. R., and Kim, W.-Y. (2014). mTOR regulates brain morphogenesis by mediating GSK3 signaling. Development 141, 4076–4086. doi: 10.1242/dev.108282
Kaalund, S. S., Newburn, E. N., Ye, T., Tao, R., Li, C., Deep-Soboslay, A., et al. (2014). Contrasting changes in DRD1 and DRD2 splice variant expression in schizophrenia and affective disorders, and associations with SNPs in postmortem brain. Mol. Psychiatry 19, 1258–1266. doi: 10.1038/mp.2013.165
Kaidanovich-Beilin, O., Beaulieu, J. M., Jope, R. S., and Woodgett, J. R. (2012). Neurological functions of the masterswitch protein kinase - gsk-3. Front. Mol. Neurosci. 5:48. doi: 10.3389/fnmol.2012.00048
Kaidanovich-Beilin, O., and Woodgett, J. R. (2011). GSK-3: functional insights from cell biology and animal models. Front. Mol. Neurosci. 4:40. doi: 10.3389/fnmol.2011.00040
Karasmanis, E. P., Phan, C.-T., Angelis, D., Kesisova, I. A., Hoogenraad, C. C., Mckenney, R. J., et al. (2018). Polarity of neuronal membrane traffic requires sorting of Kinesin motor cargo during entry into dendrites by a microtubule-associated Septin. Dev. Cell 46, 204–218.e7. doi: 10.1016/j.devcel.2018.06.013
Kato, T., and Kato, N. (2000). Mitochondrial dysfunction in bipolar disorder. Bipolar Disord. 2, 180–190. doi: 10.1034/j.1399-5618.2000.020305.x
Kessing, L. V., Sondergard, L., Kvist, K., and Andersen, P. K. (2005). Suicide risk in patients treated with lithium. Arch. Gen. Psychiatry 62, 860–866. doi: 10.1001/archpsyc.62.8.860
Khanna, R., Moutal, A., Perez-Miller, S., Chefdeville, A., Boinon, L., and Patek, M. (2020). Druggability of CRMP2 for neurodegenerative diseases. ACS Chem. Neurosci. 11, 2492–2505. doi: 10.1021/acschemneuro.0c00307
Khayachi, A., Ase, A., Liao, C., Kamesh, A., Kuhlmann, N., Schorova, L., et al. (2021). Chronic lithium treatment alters the excitatory/inhibitory balance of synaptic networks and reduces mGluR5–PKC signalling in mouse cortical neurons. J. Psychiatry Neurosci. 46, E402–E414. doi: 10.1503/jpn.200185
Khera, T. K., Dick, A. D., and Nicholson, L. B. (2010). FragileX-related protein FXR1 controls post-transcriptional suppression of lipopolysaccharide-induced tumor necrosis factor-α production by transforming growth factor-β1. FEBS J. 277, 2754–2765. doi: 10.1111/j.1742-4658.2010.07692.x
Khlghatyan, J., and Beaulieu, J. M. (2020). CRISPR-Cas9-mediated intersectional knockout of glycogen synthase kinase 3beta in D2 receptor-expressing medial prefrontal cortex neurons reveals contributions to emotional regulation. CRISPR J. 3, 198–210. doi: 10.1089/crispr.2019.0075
Khlghatyan, J., Evstratova, A., Bozoyan, L., Chamberland, S., Chatterjee, D., Marakhovskaia, A., et al. (2020). Fxr1 regulates sleep and synaptic homeostasis. EMBO J. 39:e103864. doi: 10.15252/embj.2019103864
Khlghatyan, J., Evstratova, A., Chamberland, S., Marakhovskaia, A., Bahremand, A., Toth, K., et al. (2018). Mental illnesses-associated Fxr1 and its negative regulator Gsk3β are modulators of anxiety and Glutamatergic neurotransmission. Front. Mol. Neurosci. 11:119. doi: 10.3389/fnmol.2018.00119
Kida, S. (2012). A functional role for CREB as a positive regulator of memory formation and LTP. Exp. Neurobiol. 21, 136–140. doi: 10.5607/en.2012.21.4.136
Kim, H. K., Isaacs-Trepanier, C., Elmi, N., Rapoport, S. I., and Andreazza, A. C. (2016). Mitochondrial dysfunction and lipid peroxidation in rat frontal cortex by chronic NMDA administration can be partially prevented by lithium treatment. J. Psychiatr. Res. 76, 59–65. doi: 10.1016/j.jpsychires.2016.02.001
Kim, Y., Jang, Y. N., Kim, J. Y., Kim, N., Noh, S., Kim, H., et al. (2020). Microtubule-associated protein 2 mediates induction of long-term potentiation in hippocampal neurons. FASEB J. 34, 6965–6983. doi: 10.1096/fj.201902122RR
Kim, W., Won, S.-Y., and Yoon, B.-J. (2019). CRMP2 mediates GSK3β actions in the striatum on regulating neuronal structure and mania-like behavior. J. Affect. Disord. 245, 1079–1088. doi: 10.1016/j.jad.2018.10.371
King, T., Bijur, G., and Jope, R. (2001). Caspase-3 activation induced by inhibition of mitochondrial complex I is facilitated by glycogen synthase kinase-3beta and attenuated by lithium. Brain Res. 919, 106–114. doi: 10.1016/S0006-8993(01)03005-0
King, T. D., Clodfelder-Miller, B., Barksdale, K. A., and Bijur, G. N. (2008). Unregulated mitochondrial GSK3β activity results in NADH:ubiquinone oxidoreductase deficiency. Neurotox. Res. 14, 367–382. doi: 10.1007/BF03033861
King, M. K., and Jope, R. S. (2013). Lithium treatment alleviates impaired cognition in a mouse model of fragile X syndrome. Genes Brain Behav. 12, 723–731. doi: 10.1111/gbb.12071
Kishter, S., Hoffman, F., and Pizzo, P. (1985). Production of and response to interleukin-2 by cultured T cells: effects of lithium chloride and other putative modulators. J. Biol. Response Mod. 4, 185–194.
Klein, P. S., and Melton, D. A. (1996). A molecular mechanism for the effect of lithium on development. Proc. Natl. Acad. Sci. U. S. A. 93, 8455–8459. doi: 10.1073/pnas.93.16.8455
Knijff, E. M., Nadine Breunis, M., Kupka, R. W., De Wit, H. J., Ruwhof, C., Akkerhuis, G. W., et al. (2007). An imbalance in the production of IL-1β and IL-6 by monocytes of bipolar patients: restoration by lithium treatment. Bipolar Disord. 9, 743–753. doi: 10.1111/j.1399-5618.2007.00444.x
Konradi, C., Eaton, M., Macdonald, M. L., Walsh, J., Benes, F. M., and Heckers, S. (2004). Molecular evidence for mitochondrial dysfunction in bipolar disorder. Arch. Gen. Psychiatry 61:300. doi: 10.1001/archpsyc.61.3.300
Kopnisky, K., Chalecka-Franaszek, E., and Gonzalez-Zulueta, M. D. M. C. (2003). Chronic lithium treatment antagonizes glutamate-induced decrease of phosphorylated CREB in neurons via reducing protein phosphatase 1 and increasing MEK activities. Neuroscience 116, 425–435. doi: 10.1016/S0306-4522(02)00573-0
Kozikowski, A. P., Gunosewoyo, H., Guo, S., Gaisina, I. N., Walter, R. L., Ketcherside, A., et al. (2011). Identification of a glycogen synthase kinase-3β inhibitor that attenuates hyperactivity in CLOCK mutant mice. ChemMedChem 6, 1593–1602. doi: 10.1002/cmdc.201100188
Kripke, D., Mullaney, D., Atkinson, M., and Wolf, S. (1978). Circadian rhythm disorders in manic-depressives. Biol. Psychiatry 13, 335–351.
Kushima, I., Nakatochi, M., Aleksic, B., Okada, T., Kimura, H., Kato, H., et al. (2022). Cross-disorder analysis of genic and regulatory copy number variations in bipolar disorder, schizophrenia, and autism Spectrum disorder. Biol. Psychiatry 92, 362–374. doi: 10.1016/j.biopsych.2022.04.003
Latapy, C., Rioux, V., Guitton, M. J., and Beaulieu, J. M. (2012). Selective deletion of forebrain glycogen synthase kinase 3beta reveals a central role in serotonin-sensitive anxiety and social behavior. Philos. Trans. R. Soc. Lond. B. Biol. Sci. 367, 2460–2474. doi: 10.1098/rstb.2012.0094
Lavoie, J., Hébert, M., and Beaulieu, J.-M. (2013). Glycogen synthase kinase-3β haploinsufficiency lengthens the circadian locomotor activity period in mice. Behav. Brain Res. 253, 262–265. doi: 10.1016/j.bbr.2013.08.001
Lee, C., Etchegaray, J.-P., Cagampang, F. R. A., Loudon, A. S. I., and Reppert, S. M. (2001). Posttranslational mechanisms regulate the mammalian circadian clock. Cells 107, 855–867. doi: 10.1016/S0092-8674(01)00610-9
Lee, J. Y., Kim, M. J., Thomas, S., Oorschot, V., Ramm, G., Aui, P. M., et al. (2019). Limiting neuronal Nogo receptor 1 signaling during experimental autoimmune encephalomyelitis preserves axonal transport and abrogates inflammatory demyelination. J. Neurosci. 39, 5562–5580. doi: 10.1523/JNEUROSCI.1760-18.2019
Leucht, S., Helfer, B., Dold, M., Kissling, W., and Mcgrath, J. J. (2015). Lithium for schizophrenia. Cochrane Database Syst. Rev. 2015:CD003834. doi: 10.1002/14651858.CD003834.pub3
Li, J., Chai, A., Wang, L., Ma, Y., Wu, Z., Yu, H., et al. (2015). Synaptic P-Rex1 signaling regulates hippocampal long-term depression and autism-like social behavior. Proc. Natl. Acad. Sci. 112, E6964–E6972. doi: 10.1073/pnas.1512913112
Li, H. J., Zhang, C., Hui, L., Zhou, D. S., Li, Y., Zhang, C. Y., et al. (2021). Novel risk loci associated with genetic risk for bipolar disorder among Han Chinese individuals: a genome-wide association study and meta-analysis. JAMA Psychiat. 78, 320–330. doi: 10.1001/jamapsychiatry.2020.3738
Lipina, T. V., Kaidanovich-Beilin, O., Patel, S., Wang, M., Clapcote, S. J., Liu, F., et al. (2011). Genetic and pharmacological evidence for schizophrenia-related Disc1 interaction with GSK-3. Synapse 65, 234–248. doi: 10.1002/syn.20839
Liu, Z.-H., Huang, T., and Smith, C. B. (2012). Lithium reverses increased rates of cerebral protein synthesis in a mouse model of fragile X syndrome. Neurobiol. Dis. 45, 1145–1152. doi: 10.1016/j.nbd.2011.12.037
Liu, X., Kelsoe, J. R., and Greenwood, T. A. (2016). A genome-wide association study of bipolar disorder with comorbid eating disorder replicates the SOX2-OT region. J. Affect. Disord. 189, 141–149. doi: 10.1016/j.jad.2015.09.029
Liu, Z., and Smith, C. B. (2014). Lithium: a promising treatment for fragile X syndrome. ACS Chem. Neurosci. 5, 477–483. doi: 10.1021/cn500077p
Lowrey, P. L., and Takahashi, J. S. (2004). MAMMALIAN CIRCADIAN BIOLOGY: elucidating genome-wide levels of temporal organization. Annu. Rev. Genomics Hum. Genet. 5, 407–441. doi: 10.1146/annurev.genom.5.061903.175925
Macêdo, D. S., De Lucena, D. F., Queiroz, A. I. G., Cordeiro, R. C., Araújo, M. M., Sousa, F. C., et al. (2013). Effects of lithium on oxidative stress and behavioral alterations induced by lisdexamfetamine dimesylate: relevance as an animal model of mania. Prog. Neuro-Psychopharmacol. Biol. Psychiatry 43, 230–237. doi: 10.1016/j.pnpbp.2013.01.007
Machado, A. K., Pan, A. Y., Da Silva, T. M., Duong, A., and Andreazza, A. C. (2016). Upstream pathways controlling mitochondrial function in major psychosis. Can. J. Psychiatr. 61, 446–456. doi: 10.1177/0706743716648297
Machado-Vieira, R. (2018). Lithium, stress, and resilience in bipolar disorder: deciphering this key homeostatic synaptic plasticity regulator. J. Affect. Disord. 233, 92–99. doi: 10.1016/j.jad.2017.12.026
Machado-Vieira, R., Zanetti, M. V., Teixeira, A. L., Uno, M., Valiengo, L. L., Soeiro-De-Souza, M. G., et al. (2015). Decreased AKT1/mTOR pathway mRNA expression in short-term bipolar disorder. Eur. Neuropsychopharmacol. 25, 468–473. doi: 10.1016/j.euroneuro.2015.02.002
Madison, J. M., Zhou, F., Nigam, A., Hussain, A., Barker, D. D., Nehme, R., et al. (2015). Characterization of bipolar disorder patient-specific induced pluripotent stem cells from a family reveals neurodevelopmental and mRNA expression abnormalities. Mol. Psychiatry 20, 703–717. doi: 10.1038/mp.2015.7
Maes, M., Song, C., Lin, A.-H., Pioli, R., Kenis, G., Kubera, M., et al. (1999). In vitro immunoregulatory effects of lithium in healthy volunteers. Psychopharmacology 143, 401–407. doi: 10.1007/s002130050965
Maixner, D. W., and Weng, H.-R. (2013). The role of glycogen synthase kinase 3 Beta in Neuroinflammation and pain. J. Pharm. Pharmacol. 1:001. doi: 10.13188/2327-204X.1000001
Majumder, M., Johnson, R. H., and Palanisamy, V. (2020). Fragile X-related protein family: a double-edged sword in neurodevelopmental disorders and cancer. Crit. Rev. Biochem. Mol. Biol. 55, 409–424. doi: 10.1080/10409238.2020.1810621
Mansour, H. A., Monk, T. H., and Nimgaonkar, V. L. (2005). Circadian genes and bipolar disorder. Ann. Med. 37, 196–205. doi: 10.1080/078538905100073
Martin, M., Rehani, K., Jope, R. S., and Michalek, S. M. (2005). Toll-like receptor–mediated cytokine production is differentially regulated by glycogen synthase kinase 3. Nat. Immunol. 6, 777–784. doi: 10.1038/ni1221
Mavrikaki, M., Schintu, N., Kastellakis, A., Nomikos, G. G., Svenningsson, P., and Panagis, G. (2014). Effects of lithium and aripiprazole on brain stimulation reward and neuroplasticity markers in the limbic forebrain. Eur. Neuropsychopharmacol. 24, 630–638. doi: 10.1016/j.euroneuro.2013.10.014
Mccarthy, M. J., Nievergelt, C. M., Kelsoe, J. R., and Welsh, D. K. (2012). A survey of genomic studies supports Association of Circadian Clock Genes with bipolar disorder Spectrum illnesses and lithium response. PLoS One 7:e32091. doi: 10.1371/journal.pone.0032091
Mccarthy, M. J., Wei, H., Marnoy, Z., Darvish, R. M., Mcphie, D. L., Cohen, B. M., et al. (2013). Genetic and clinical factors predict lithium’s effects on PER2 gene expression rhythms in cells from bipolar disorder patients. Transl. Psychiatry 3:e318. doi: 10.1038/tp.2013.90
Mcclung, C. A. (2007). Role for the clock gene in bipolar disorder. Cold Spring Harb. Symp. Quant. Biol. 72, 637–644. doi: 10.1101/sqb.2007.72.031
Merikangas, K. R., Jin, R., He, J. P., Kessler, R. C., Lee, S., Sampson, N. A., et al. (2011). Prevalence and correlates of bipolar spectrum disorder in the world mental health survey initiative. Arch. Gen. Psychiatry 68, 241–251. doi: 10.1001/archgenpsychiatry.2011.12
Milienne-Petiot, M., Kesby, J. P., Graves, M., Van Enkhuizen, J., Semenova, S., Minassian, A., et al. (2017). The effects of reduced dopamine transporter function and chronic lithium on motivation, probabilistic learning, and neurochemistry in mice: modeling bipolar mania. Neuropharmacology 113, 260–270. doi: 10.1016/j.neuropharm.2016.07.030
Miller, B. J., Buckley, P., Seabolt, W., Mellor, A., and Kirkpatrick, B. (2011). Meta-analysis of cytokine alterations in schizophrenia: clinical status and antipsychotic effects. Biol. Psychiatry 70, 663–671. doi: 10.1016/j.biopsych.2011.04.013
Miller, J. C., Jiménez, P., and Mathé, A. A. (2007). Restraint stress influences AP-1 and CREB DNA-binding activity induced by chronic lithium treatment in the rat frontal cortex and hippocampus. Int. J. Neuropsychopharmacol. 10, 609–619. doi: 10.1017/S1461145706007279
Min, W. W., Yuskaitis, C. J., Yan, Q., Sikorski, C., Chen, S., Jope, R. S., et al. (2009). Elevated glycogen synthase kinase-3 activity in fragile X mice: key metabolic regulator with evidence for treatment potential. Neuropharmacology 56, 463–472. doi: 10.1016/j.neuropharm.2008.09.017
Miyamoto, S., Duncan, G. E., Marx, C. E., and Lieberman, J. A. (2005). Treatments for schizophrenia: a critical review of pharmacology and mechanisms of action of antipsychotic drugs. Mol. Psychiatry 10, 79–104. doi: 10.1038/sj.mp.4001556
Modabbernia, A., Taslimi, S., Brietzke, E., and Ashrafi, M. (2013). Cytokine alterations in bipolar disorder: a meta-analysis of 30 studies. Biol. Psychiatry 74, 15–25. doi: 10.1016/j.biopsych.2013.01.007
Moody, J. P., and Allsopp, M. N. E. (1969). Circadian rhythms of water and electrolyte excretion in manic-depressive psychosis. Br. J. Psychiatry 115, 923–928. doi: 10.1192/bjp.115.525.923
Morel, M., Héraud, C., Nicaise, C., Suain, V., and Brion, J.-P. (2012). Levels of kinesin light chain and dynein intermediate chain are reduced in the frontal cortex in Alzheimer’s disease: implications for axoplasmic transport. Acta Neuropathol. 123, 71–84. doi: 10.1007/s00401-011-0901-4
Morfini, G., Szebenyi, G., Elluru, R., Ratner, N., and Brady, S. T. (2002). Glycogen synthase kinase 3 phosphorylates kinesin light chains and negatively regulates kinesin-based motility. EMBO J. 21, 281–293. doi: 10.1093/emboj/21.3.281
Morita, M., Gravel, S.-P., Chénard, V., Sikström, K., Zheng, L., Alain, T., et al. (2013). mTORC1 controls mitochondrial activity and biogenesis through 4E-BP-dependent translational regulation. Cell Metab. 18, 698–711. doi: 10.1016/j.cmet.2013.10.001
Mukherjee, S., Coque, L., Cao, J.-L., Kumar, J., Chakravarty, S., Asaithamby, A., et al. (2010). Knockdown of clock in the ventral tegmental area through RNA interference results in a mixed state of mania and depression-like behavior. Biol. Psychiatry 68, 503–511. doi: 10.1016/j.biopsych.2010.04.031
Mullins, N., Forstner, A. J., O'connell, K. S., Coombes, B., Coleman, J. R. I., Qiao, Z., et al. (2021). Genome-wide association study of more than 40,000 bipolar disorder cases provides new insights into the underlying biology. Nat. Genet. 53, 817–829. doi: 10.1038/s41588-021-00857-4
Munkholm, K., Vestergaard Braüner, J., Vedel Kessing, L., and Vinberg, M. (2013). Cytokines in bipolar disorder vs. healthy control subjects: a systematic review and meta-analysis. J. Psychiatr. Res. 47, 1119–1133. doi: 10.1016/j.jpsychires.2013.05.018
Nagai, J., Owada, K., Kitamura, Y., Goshima, Y., and Ohshima, T. (2016). Inhibition of CRMP2 phosphorylation repairs CNS by regulating neurotrophic and inhibitory responses. Exp. Neurol. 277, 283–295. doi: 10.1016/j.expneurol.2016.01.015
Nakata, T., and Hirokawa, N. (2003). Microtubules provide directional cues for polarized axonal transport through interaction with kinesin motor head. J. Cell Biol. 162, 1045–1055. doi: 10.1083/jcb.200302175
Nassar, A., and Azab, A. N. (2014). Effects of lithium on inflammation. ACS Chem. Neurosci. 5, 451–458. doi: 10.1021/cn500038f
Nässberger, L., and Träskman-Bendz, L. (1993). Increased soluble interleukin-2 receptor concentrations in suicide attempters. Acta Psychiatr. Scand. 88, 48–52. doi: 10.1111/j.1600-0447.1993.tb03412.x
Nie, P.-Y., Ji, L.-L., Fu, C.-H., Peng, J.-B., Wang, Z.-Y., and Tong, L. (2021). miR-132 regulates PTSD-like behaviors in rats following single-prolonged stress through fragile X-related protein 1. Cell. Mol. Neurobiol. 41, 327–340. doi: 10.1007/s10571-020-00854-x
Nonaka, S., Hough, C. J., and Chuang, D.-M. (1998). Chronic lithium treatment robustly protects neurons in the central nervous system against excitotoxicity by inhibiting N-methyl-d-aspartate receptor-mediated calcium influx. Proc. Natl. Acad. Sci. 95, 2642–2647. doi: 10.1073/pnas.95.5.2642
O’Brien, W. T., Huang, J., Buccafusca, R., Garskof, J., Valvezan, A. J., Berry, G. T., et al. (2011). Glycogen synthase kinase-3 is essential for beta-arrestin-2 complex formation and lithium-sensitive behaviors in mice. J. Clin. Invest. 121, 3756–3762. doi: 10.1172/JCI45194
O’Brien, W. T., and Klein, P. S. (2009). Validating GSK3 as an in vivo target of lithium action. Biochem. Soc. Trans. 37, 1133–1138. doi: 10.1042/BST0371133
Ogawa, F., Malavasi, E. L. V., Crummie, D. K., Eykelenboom, J. E., Soares, D. C., Mackie, S., et al. (2014). DISC1 complexes with TRAK1 and Miro1 to modulate anterograde axonal mitochondrial trafficking. Hum. Mol. Genet. 23, 906–919. doi: 10.1093/hmg/ddt485
Ollivier, V., Parry, G. C. N., Cobb, R. R., De Prost, D., and Mackman, N. (1996). Elevated cyclic AMP inhibits NF-κB-mediated transcription in human Monocytic cells and endothelial cells. J. Biol. Chem. 271, 20828–20835. doi: 10.1074/jbc.271.34.20828
Oostra, B. A., and Hoogeveen, A. T. (1997). Animal Model for Fragile X Syndrome. Ann. Med. 29, 563–567. doi: 10.3109/07853899709007483
Pandey, A., Shen, C., Feng, S., and Man, S. M. (2021). Cell biology of inflammasome activation. Trends Cell Biol. 31, 924–939. doi: 10.1016/j.tcb.2021.06.010
Parekh, P. K., Becker-Krail, D., Sundaravelu, P., Ishigaki, S., Okado, H., Sobue, G., et al. (2018). Altered GluA1 (Gria1) function and Accumbal synaptic plasticity in the ClockΔ19 model of bipolar mania. Biol. Psychiatry 84, 817–826. doi: 10.1016/j.biopsych.2017.06.022
Parry, G., and Mackman, N. (1997). Role of cyclic AMP response element-binding protein in cyclic AMP inhibition of NF-kappaB-mediated transcription. J. Immunol. 159, 5450–5456.
Patzlaff, N. E., Nemec, K. M., Malone, S. G., Li, Y., and Zhao, X. (2017). Fragile X related protein 1 (FXR1P) regulates proliferation of adult neural stem cells. Hum. Mol. Genet. 26, 1340–1352. doi: 10.1093/hmg/ddx034
Paul, P., Iyer, S., Nadella, R. K., Nayak, R., Chellappa, A. S., Ambardar, S., et al. (2020). Lithium response in bipolar disorder correlates with improved cell viability of patient derived cell lines. Sci. Rep. 10:7428. doi: 10.1038/s41598-020-64202-1
Paul, J. R., Johnson, R. L., Jope, R. S., and Gamble, K. L. (2012). Disruption of circadian rhythmicity and suprachiasmatic action potential frequency in a mouse model with constitutive activation of glycogen synthase kinase 3. Neuroscience 226, 1–9. doi: 10.1016/j.neuroscience.2012.08.047
Pavlov, D., Bettendorff, L., Gorlova, A., Olkhovik, A., Kalueff, A. V., Ponomarev, E. D., et al. (2019). Neuroinflammation and aberrant hippocampal plasticity in a mouse model of emotional stress evoked by exposure to ultrasound of alternating frequencies. Prog. Neuro-Psychopharmacol. Biol. Psychiatry 90, 104–116. doi: 10.1016/j.pnpbp.2018.11.014
Peineau, S., Taghibiglou, C., Bradley, C., Wong, T. P., Liu, L., Lu, J., et al. (2007). LTP inhibits LTD in the hippocampus via regulation of GSK3beta. Neuron 53, 703–717. doi: 10.1016/j.neuron.2007.01.029
Platzer, C., Fritsch, E., Elsner, T., Lehmann, M., Volk, H., and Prösch, S. (1999). Cyclic adenosine monophosphate-responsive elements are involved in the transcriptional activation of the human IL-10 gene in monocytic cells. Eur. J. Immunol. 29, 3098–3104. doi: 10.1002/(SICI)1521-4141(199910)29:10<3098::AID-IMMU3098>3.0.CO;2-H
Potz, B. A., Scrimgeour, L. A., Sabe, S. A., Clements, R. T., Sodha, N. R., and Sellke, F. W. (2018). Glycogen synthase kinase 3β inhibition reduces mitochondrial oxidative stress in chronic myocardial ischemia. J. Thorac. Cardiovasc. Surg. 155, 2492–2503. doi: 10.1016/j.jtcvs.2017.12.127
Price, J. B., Yates, C. G., Morath, B. A., Van De Wakker, S. K., Yates, N. J., Butters, K., et al. (2021). Lithium augmentation of ketamine increases insulin signaling and antidepressant-like active stress coping in a rodent model of treatment-resistant depression. Transl. Psychiatry 11:598. doi: 10.1038/s41398-021-01716-w
Qi, F., Meng, Q., Hayashi, I., and Kobayashi, J. (2020). FXR1 is a novel MRE11-binding partner and participates in oxidative stress responses. J. Radiat. Res. 61, 368–375. doi: 10.1093/jrr/rraa011
Qie, S., Majumder, M., Mackiewicz, K., Howley, B. V., Peterson, Y. K., Howe, P. H., et al. (2017). Fbxo4-mediated degradation of Fxr1 suppresses tumorigenesis in head and neck squamous cell carcinoma. Nat. Commun. 8:1534. doi: 10.1038/s41467-017-01199-8
Rampino, A., Torretta, S., Gelao, B., Veneziani, F., Iacoviello, M., Marakhovskaya, A., et al. (2021). Evidence of an interaction between FXR1 and GSK3beta polymorphisms on levels of negative symptoms of schizophrenia and their response to antipsychotics. Eur. Psychiatry 64:e39. doi: 10.1192/j.eurpsy.2021.26
Rana, A. K., Sharma, S., Patial, V., and Singh, D. (2022). Lithium therapy subdues neuroinflammation to maintain pyramidal cells arborization and rescues neurobehavioral impairments in ovariectomized rats. Mol. Neurobiol. 59, 1706–1723. doi: 10.1007/s12035-021-02719-w
Rao, J. S., Harry, G. J., Rapoport, S. I., and Kim, H. W. (2010). Increased excitotoxicity and neuroinflammatory markers in postmortem frontal cortex from bipolar disorder patients. Mol. Psychiatry 15, 384–392. doi: 10.1038/mp.2009.47
Ren, X., Rizavi, H. S., Khan, M. A., Bhaumik, R., Dwivedi, Y., and Pandey, G. N. (2014). Alteration of cyclic-AMP response element binding protein in the postmortem brain of subjects with bipolar disorder and schizophrenia. J. Affect. Disord. 152–154, 326–333. doi: 10.1016/j.jad.2013.09.033
Richter, J. D., and Zhao, X. (2021). The molecular biology of FMRP: new insights into fragile X syndrome. Nat. Rev. Neurosci. 22, 209–222. doi: 10.1038/s41583-021-00432-0
Ripke, S., Neale, B. M., Corvin, A., Walters, J. T. R., Farh, K.-H., Holmans, P. A., et al. (2014). Biological insights from 108 schizophrenia-associated genetic loci. Nature 511, 421–427. doi: 10.1038/nature13595
Rock, P., Goodwin, G., Harmer, C., and Wulff, K. (2014). Daily rest-activity patterns in the bipolar phenotype: a controlled actigraphy study. Chronobiol. Int. 31, 290–296. doi: 10.3109/07420528.2013.843542
Roybal, K., Theobold, D., Graham, A., Dinieri, J. A., Russo, S. J., Krishnan, V., et al. (2007). Mania-like behavior induced by disruption of CLOCK. Proc. Natl. Acad. Sci. 104, 6406–6411. doi: 10.1073/pnas.0609625104
Ryves, W. J., and Harwood, A. J. (2001). Lithium inhibits glycogen synthase kinase-3 by competition for magnesium. Biochem. Biophys. Res. Commun. 280, 720–725. doi: 10.1006/bbrc.2000.4169
Sarbassov, D. D., Ali, S. M., and Sabatini, D. M. (2005). Growing roles for the mTOR pathway. Curr. Opin. Cell Biol. 17, 596–603. doi: 10.1016/j.ceb.2005.09.009
Schou, M., Juel-Nielsen, N., Stromgren, E., and Voldby, H. (1954). The treatment of manic psychoses by the administration of lithium salts. J. Neurol. Neurosurg. Psychiatry 17, 250–260. doi: 10.1136/jnnp.17.4.250
Schroer, T. A., Schnapp, B. J., Reese, T. S., and Sheetz, M. P. (1988). The role of kinesin and other soluble factors in organelle movement along microtubules. J. Cell Biol. 107, 1785–1792. doi: 10.1083/jcb.107.5.1785
Scola, G., Kim, H. K., Young, L. T., Salvador, M., and Andreazza, A. C. (2014). Lithium reduces the effects of rotenone-induced complex I dysfunction on DNA methylation and hydroxymethylation in rat cortical primary neurons. Psychopharmacology 231, 4189–4198. doi: 10.1007/s00213-014-3565-7
Seeman, P., Lee, T., Chau-Wong, M., and Wong, K. (1976). Antipsychotic drug doses and neuroleptic/dopamine receptors. Nature 261, 717–719. doi: 10.1038/261717a0
Sengupta, S., Jayaraman, P., Chilton, P. M., Casella, C. R., and Mitchell, T. C. (2007). Unrestrained glycogen synthase kinase-3β activity leads to activated T cell death and Can be inhibited by natural adjuvant. J. Immunol. 178, 6083–6091. doi: 10.4049/jimmunol.178.10.6083
Setou, M., Seog, D.-H., Tanaka, Y., Kanai, Y., Takei, Y., Kawagishi, M., et al. (2002). Glutamate-receptor-interacting protein GRIP1 directly steers kinesin to dendrites. Nature 417, 83–87. doi: 10.1038/nature743
Shao, L., Golbaz, K., Honer, W. G., and Beasley, C. L. (2016). Deficits in axon-associated proteins in prefrontal white matter in bipolar disorder but not schizophrenia. Bipolar Disord. 18, 342–351. doi: 10.1111/bdi.12395
Sharma, A., Hoeffer, C. A., Takayasu, Y., Miyawaki, T., Mcbride, S. M., Klann, E., et al. (2010). Dysregulation of mTOR signaling in fragile X syndrome. J. Neurosci. 30, 694–702. doi: 10.1523/JNEUROSCI.3696-09.2010
Sheetz, M. P. (1987). What are the functions of kinesin? BioEssays 7, 165–168. doi: 10.1002/bies.950070406
Shen, M., Guo, Y., Dong, Q., Gao, Y., Stockton, M. E., Li, M., et al. (2021). FXR1 regulation of parvalbumin interneurons in the prefrontal cortex is critical for schizophrenia-like behaviors. Mol. Psychiatry 26, 6845–6867. doi: 10.1038/s41380-021-01096-z
Simon, N. M., Smoller, J. W., Mcnamara, K. L., Maser, R. S., Zalta, A. K., Pollack, M. H., et al. (2006). Telomere shortening and mood disorders: preliminary support for a chronic stress model of accelerated aging. Biol. Psychiatry 60, 432–435. doi: 10.1016/j.biopsych.2006.02.004
Siomi, M. C., Siomi, H., Sauer, W. H., Srinivasan, S., Nussbaum, R. L., and Dreyfuss, G. (1995). FXR1, an autosomal homolog of the fragile X mental retardation gene. EMBO J. 14, 2401–2408. doi: 10.1002/j.1460-2075.1995.tb07237.x
Snitow, M. E., Bhansali, R. S., and Klein, P. S. (2021). Lithium and therapeutic targeting of GSK-3. Cells 10:255. doi: 10.3390/cells10020255
Song, K. S., Lee, W.-J., Chung, K. C., Koo, J. S., Yang, E. J., Choi, J. Y., et al. (2003). Interleukin-1β and tumor necrosis factor-α induce MUC5AC overexpression through a mechanism involving ERK/p38 mitogen-activated protein kinases-MSK1-CREB activation in human airway epithelial cells. J. Biol. Chem. 278, 23243–23250. doi: 10.1074/jbc.M300096200
Spencer, S., Torres-Altoro, M. I., Falcon, E., Arey, R., Marvin, M., Goldberg, M., et al. (2012). A mutation in CLOCK leads to altered dopamine receptor function. J. Neurochem. 123, 124–134. doi: 10.1111/j.1471-4159.2012.07857.x
Stahl, E. A., Breen, G., Forstner, A. J., Mcquillin, A., Ripke, S., Trubetskoy, V., et al. (2019). Genome-wide association study identifies 30 loci associated with bipolar disorder. Nat. Genet. 51, 793–803. doi: 10.1038/s41588-019-0397-8
Stambolic, V., Ruel, L., and Woodgett, J. R. (1996). Lithium inhibits glycogen synthase kinase-3 activity and mimics wingless signalling in intact cells. Curr. Biol. 6, 1664–1669. doi: 10.1016/S0960-9822(02)70790-2
Stretton, C., Hoffmann, M. T., Munson, M. J., Prescott, A., Taylor, M. P., Ganley, G. I., et al. (2015). GSK3-mediated raptor phosphorylation supports amino-acid-dependent mTORC1-directed signalling. Biochem. J. 470, 207–221. doi: 10.1042/BJ20150404
Sun, H., Jiang, Y. J., Yu, Q. S., Luo, C. C., and Zou, J. W. (2011). The effect of Li+ on GSK-3 inhibition: molecular dynamics simulation. J. Mol. Model. 17, 377–381. doi: 10.1007/s00894-010-0738-0
Sutherland, C. (2011). What are the bona fide GSK3 substrates? Int. J. Alzheimers Dis. 2011:505607. doi: 10.4061/2011/505607
Taelman, V. F., Dobrowolski, R., Plouhinec, J. L., Fuentealba, L. C., Vorwald, P. P., Gumper, I., et al. (2010). Wnt signaling requires sequestration of glycogen synthase kinase 3 inside multivesicular endosomes. Cells 143, 1136–1148. doi: 10.1016/j.cell.2010.11.034
Taha, M. S., Haghighi, F., Stefanski, A., Nakhaei-Rad, S., Kazemein Jasemi, N. S., Al Kabbani, M. A., et al. (2021). Novel FMRP interaction networks linked to cellular stress. FEBS J. 288, 837–860. doi: 10.1111/febs.15443
Takata, A., Matsumoto, N., and Kato, T. (2017). Genome-wide identification of splicing QTLs in the human brain and their enrichment among schizophrenia-associated loci. Nat. Commun. 8:14519. doi: 10.1038/ncomms14519
Tamanini, F. (1997). Differential expression of FMR1, FXR1 and FXR2 proteins in human brain and testis. Hum. Mol. Genet. 6, 1315–1322. doi: 10.1093/hmg/6.8.1315
Tardito, D., Tiraboschi, E., Kasahara, J., Racagni, G., and Popoli, M. (2007). Reduced CREB phosphorylation after chronic lithium treatment is associated with down-regulation of CaM kinase IV in rat hippocampus. Int. J. Neuropsychopharmacol. 10, 491–496. doi: 10.1017/S1461145706007140
Tatavarty, V., Pacheco, A. T., Kuhnle, C. G., Lin, H., Koundinya, P., Miska, N. J., et al. (2020). Autism-associated Shank3 is essential for homeostatic compensation in rodent V1. Neuron 106, 769–777.e4. doi: 10.1016/j.neuron.2020.02.033
Tissot, R. (1975). The common pathophysiology of monaminergic psychoses: a new hypothesis. Neuropsychobiology 1, 243–260. doi: 10.1159/000117498
Tobe, B. T. D., Crain, A. M., Winquist, A. M., Calabrese, B., Makihara, H., Zhao, W.-N., et al. (2017). Probing the lithium-response pathway in hiPSCs implicates the phosphoregulatory set-point for a cytoskeletal modulator in bipolar pathogenesis. Proc. Natl. Acad. Sci. 114, E4462–E4471. doi: 10.1073/pnas.1700111114
Uezato, A., Meador-Woodruff, J. H., and Mccullumsmith, R. E. (2009). Vesicular glutamate transporter mRNA expression in the medial temporal lobe in major depressive disorder, bipolar disorder, and schizophrenia. Bipolar Disord. 11, 711–725. doi: 10.1111/j.1399-5618.2009.00752.x
Urs, N. M., Snyder, J. C., Jacobsen, J. P., Peterson, S. M., and Caron, M. G. (2012). Deletion of GSK3beta in D2R-expressing neurons reveals distinct roles for beta-arrestin signaling in antipsychotic and lithium action. Proc. Natl. Acad. Sci. U. S. A. 109, 20732–20737. doi: 10.1073/pnas.1215489109
Vale, R. D., Scholey, J. M., and Sheetz, M. P. (1986). Kinesin: possible biological roles for a new microtubule motor. Trends Biochem. Sci. 11, 464–468. doi: 10.1016/0968-0004(86)90248-3
Van Enkhuizen, J., Janowsky, D. S., Olivier, B., Minassian, A., Perry, W., Young, J. W., et al. (2015). The catecholaminergic-cholinergic balance hypothesis of bipolar disorder revisited. Eur. J. Pharmacol. 753, 114–126. doi: 10.1016/j.ejphar.2014.05.063
Vanderplow, A. M., Eagle, A. L., Kermath, B. A., Bjornson, K. J., Robison, A. J., and Cahill, M. E. (2021). Akt-mTOR hypoactivity in bipolar disorder gives rise to cognitive impairments associated with altered neuronal structure and function. Neuron 109, 1479–1496.e6. doi: 10.1016/j.neuron.2021.03.008
Vasudevan, S., and Steitz, J. A. (2007). AU-rich-element-mediated Upregulation of translation by FXR1 and Argonaute 2. Cells 128, 1105–1118. doi: 10.1016/j.cell.2007.01.038
Volkmann, C., Bschor, T., and Kohler, S. (2020). Lithium treatment over the lifespan in bipolar disorders. Front. Psychol. 11:377. doi: 10.3389/fpsyt.2020.00377
Wang, Q. M., Roach, P. J., and Fiol, C. J. (1994). Use of a synthetic peptide as a selective substrate for glycogen synthase kinase 3. Anal. Biochem. 220, 397–402. doi: 10.1006/abio.1994.1356
Weaver, D. R. (1998). The suprachiasmatic nucleus: a 25-year retrospective. J. Biol. Rhythm. 13, 100–112. doi: 10.1177/074873098128999952
Wen, A. Y., Sakamoto, K. M., and Miller, L. S. (2010). The role of the transcription factor CREB in immune function. J. Immunol. 185, 6413–6419. doi: 10.4049/jimmunol.1001829
Wheaton, K. L., Hansen, K. F., Aten, S., Sullivan, K. A., Yoon, H., Hoyt, K. R., et al. (2018). The phosphorylation of CREB at serine 133 is a key event for circadian clock timing and entrainment in the Suprachiasmatic nucleus. J. Biol. Rhythm. 33, 497–514. doi: 10.1177/0748730418791713
Wiens, K. M. (2005). Rac1 induces the clustering of AMPA receptors during Spinogenesis. J. Neurosci. 25, 10627–10636. doi: 10.1523/JNEUROSCI.1947-05.2005
Winograd, C., and Ceman, S. (2011). Fragile X family members have important and non-overlapping functions. Biomol. Concepts 2, 343–352. doi: 10.1515/BMC.2011.033
Wood, G. E., Young, L. T., Reagan, L. P., Chen, B., and Mcewen, B. S. (2004). Stress-induced structural remodeling in hippocampus: prevention by lithium treatment. Proc. Natl. Acad. Sci. U. S. A. 101, 3973–3978. doi: 10.1073/pnas.0400208101
Xiao, X., Zhang, C., Grigoroiu-Serbanescu, M., Wang, L., Li, L., Zhou, D., et al. (2018). The cAMP responsive element-binding (CREB)-1 gene increases risk of major psychiatric disorders. Mol. Psychiatry 23, 1957–1967. doi: 10.1038/mp.2017.243
Xie, Z., Srivastava, D. P., Photowala, H., Kai, L., Cahill, M. E., Woolfrey, K. M., et al. (2007). Kalirin-7 controls activity-dependent structural and functional plasticity of dendritic spines. Neuron 56, 640–656. doi: 10.1016/j.neuron.2007.10.005
Yang, K., Chen, Z., Gao, J., Shi, W., Li, L., Jiang, S., et al. (2017). The key roles of GSK-3β in regulating mitochondrial activity. Cell. Physiol. Biochem. 44, 1445–1459. doi: 10.1159/000485580
Yoshimura, T., Kawano, Y., Arimura, N., Kawabata, S., Kikuchi, A., and Kaibuchi, K. (2005). GSK-3β regulates phosphorylation of CRMP-2 and neuronal polarity. Cells 120, 137–149. doi: 10.1016/j.cell.2004.11.012
Yuskaitis, C. J., and Jope, R. S. (2009). Glycogen synthase kinase-3 regulates microglial migration, inflammation, and inflammation-induced neurotoxicity. Cell. Signal. 21, 264–273. doi: 10.1016/j.cellsig.2008.10.014
Yuskaitis, C. J., Mines, M. A., King, M. K., Sweatt, J. D., Miller, C. A., and Jope, R. S. (2010). Lithium ameliorates altered glycogen synthase kinase-3 and behavior in a mouse model of fragile X syndrome. Biochem. Pharmacol. 79, 632–646. doi: 10.1016/j.bcp.2009.09.023
Zaslavsky, K., Zhang, W. B., Mccready, F. P., Rodrigues, D. C., Deneault, E., Loo, C., et al. (2019). SHANK2 mutations associated with autism spectrum disorder cause hyperconnectivity of human neurons. Nat. Neurosci. 22, 556–564. doi: 10.1038/s41593-019-0365-8
Zhan, L., Kerr, J. R., Lafuente, M. J., Maclean, A., Chibalina, M. V., Liu, B., et al. (2011). Altered expression and coregulation of dopamine signaling genes in schizophrenia and bipolar disorder. Neuropathol. Appl. Neurobiol. 37, 206–219. doi: 10.1111/j.1365-2990.2010.01128.x
Zhang, Y., Chen, K., Sloan, S. A., Bennett, M. L., Scholze, A. R., Okeeffe, S., et al. (2014). An RNA-sequencing Transcriptome and splicing database of glia, neurons, and vascular cells of the cerebral cortex. J. Neurosci. 34, 11929–11947. doi: 10.1523/JNEUROSCI.1860-14.2014
Zhang, J., Fang, Z., Jud, C., Vansteensel, M. J., Kaasik, K., Lee, C. C., et al. (2008). Fragile X-Related Proteins Regulate Mammalian Circadian Behavioral Rhythms. Am. J. Hum. Genet. 83, 43–52. doi: 10.1016/j.ajhg.2008.06.003
Zhang, H., Kang, E., Wang, Y., Yang, C., Yu, H., Wang, Q., et al. (2016). Brain-specific Crmp2 deletion leads to neuronal development deficits and behavioral impairments in mice. Nat. Commun. 7:11773. doi: 10.1038/ncomms11773
Zhao, W.-N., Tobe, B. T. D., Udeshi, N. D., Xuan, L. L., Pernia, C. D., Zolg, D. P., et al. (2020). Discovery of suppressors of CRMP2 phosphorylation reveals compounds that mimic the behavioral effects of lithium on amphetamine-induced hyperlocomotion. Translational. Psychiatry 10:76. doi: 10.1038/s41398-020-0753-6
Zhihan, G., Fengli, S., Wangqiang, L., Dong, S., and Weidong, J. (2022). Lamotrigine and lithium combination for treatment of rapid cycling bipolar disorder: results from meta-analysis. Front. Psychol. 13:913051. doi: 10.3389/fpsyt.2022.913051
Keywords: lithium, bipolar disorder, kinesin, CREB, glycogen synthase kinase 3, FXR1, collapsin response mediator protein 2
Citation: Chatterjee D and Beaulieu JM (2022) Inhibition of glycogen synthase kinase 3 by lithium, a mechanism in search of specificity. Front. Mol. Neurosci. 15:1028963. doi: 10.3389/fnmol.2022.1028963
Edited by:
Florian Plattner, Neuro-Research Strategies, United StatesReviewed by:
Miroslav Nenov, Temple University, United StatesChuang Wang, Ningbo University, China
Peter Klein, University of Pennsylvania, United States
Copyright © 2022 Chatterjee and Beaulieu. This is an open-access article distributed under the terms of the Creative Commons Attribution License (CC BY). The use, distribution or reproduction in other forums is permitted, provided the original author(s) and the copyright owner(s) are credited and that the original publication in this journal is cited, in accordance with accepted academic practice. No use, distribution or reproduction is permitted which does not comply with these terms.
*Correspondence: Jean Martin Beaulieu, bWFydGluLmJlYXVsaWV1QHV0b3JvbnRvLmNh