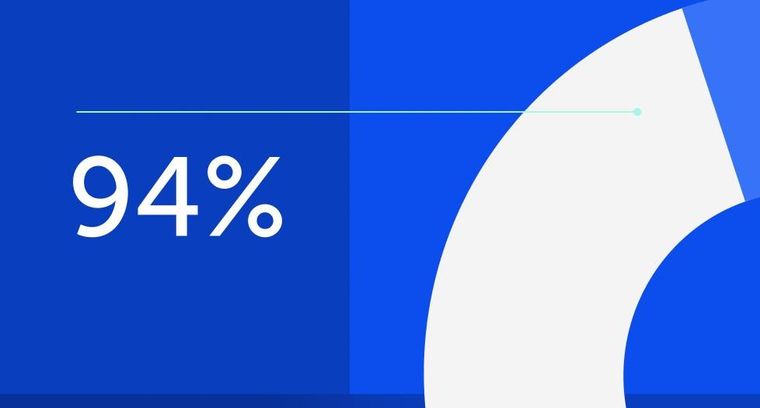
94% of researchers rate our articles as excellent or good
Learn more about the work of our research integrity team to safeguard the quality of each article we publish.
Find out more
REVIEW article
Front. Mol. Neurosci., 11 October 2022
Sec. Molecular Signalling and Pathways
Volume 15 - 2022 | https://doi.org/10.3389/fnmol.2022.1006419
This article is part of the Research TopicAstrocytic Synaptic Plasticity in Epilepsy: From Synapses to CircuitsView all 10 articles
Epilepsy is a common symptom of many neurological disorders and can lead to neuronal damage that plays a major role in seizure-related disability. The peptidyl-prolyl isomerase Pin1 has wide-ranging influences on the occurrence and development of neurological diseases. It has also been suggested that Pin1 acts on epileptic inhibition, and the molecular mechanism has recently been reported. In this review, we primarily focus on research concerning the mechanisms and functions of Pin1 in neurons. In addition, we highlight the significance and potential applications of Pin1 in neuronal diseases, especially epilepsy. We also discuss the molecular mechanisms by which Pin1 controls synapses, ion channels and neuronal signaling pathways to modulate epileptic susceptibility. Since neurotransmitters and some neuronal signaling pathways, such as Notch1 and PI3K/Akt, are vital to the nervous system, the role of Pin1 in epilepsy is discussed in the context of the CaMKII-AMPA receptor axis, PSD-95-NMDA receptor axis, NL2/gephyrin-GABA receptor signaling, and Notch1 and PI3K/Akt pathways. The effect of Pin1 on the progression of epilepsy in animal models is discussed as well. This information will lead to a better understanding of Pin1 signaling pathways in epilepsy and may facilitate development of new therapeutic strategies.
Epilepsy is a common, chronic, and severe neurological disorder. Recurrent and unpredictable interruptions of normal brain function are the characteristic symptoms of epileptic seizures (Fisher et al., 2005; Moshé et al., 2015), which result from transient abnormal synchronization of neurons in the brain (Moshé et al., 2015). Many studies have demonstrated electrophysiological abnormalities in epilepsy. High-frequency oscillations (HFOs), repetitive HFOs and spikes (rHFOSs) and sleep spindles are typical electrophysiological biomarkers of epileptogenesis (Perucca et al., 2019). Neurotransmitters and neuroendocrine axes are critical in the onset of epilepsy (Camfield et al., 2017). Although the molecular mechanism of epilepsy has not been fully elucidated, there is growing evidence for synaptic transmission in the pathological process of epilepsy (Amador et al., 2020; Puhahn-Schmeiser et al., 2021), and altered dynamics of inhibitory synapses and excitatory synapses may be a critical signature of epileptic networks (Vannini et al., 2020).
The peptidyl prolyl cis-trans isomerase (PPIase) superfamily consists of four families characterized by their structural differences: cyclophilins, FK506-binding proteins (FKBPs), parvulins, and the protein phosphatase (PPase) 2A phosphatase activator (PTPA) (Li et al., 2021). Discovered in 1996 as a substrate of NIMA (never in mitosis, gene A), PPIase NIMA-interacting 1 (Pin1) protein is associated with mitosis (Lu et al., 1996; Fagiani et al., 2021). It consists of 163 amino acid residues with a relative molecular mass of 18 kDa and contains 1 nuclear localization signal and 2 functional domains (Li et al., 2021). Pin1-catalyzed isomerization, which is detected by cis and trans proline isomer-specific antibodies (Nakamura et al., 2012a; Kondo et al., 2015), has the ability to function as a molecular timer to synchronize cellular processes (Lu et al., 2007; Liou et al., 2011; Zhou and Lu, 2016).
Recently, emerging evidence has demonstrated that Pin1 is closely related to many cellular processes, such as the cell cycle, cell proliferation, cell motility, and apoptosis, by its isomerization phosphorylated substrates and postphosphorylation regulation (Ranganathan et al., 1997; Hilton et al., 2015; Lin et al., 2015; Feng et al., 2017; Risal et al., 2017). In addition, Pin1 also exerts a pivotal effect on multiple physiological processes, including the immune response, neuronal differentiation, and tumorigenesis (Sacktor, 2010; Tun-Kyi et al., 2011; Daza-Martin et al., 2019). Pin1 activity is tightly regulated through finely tuned cellular pathways downstream of phosphorylation signaling, and its dysregulation under pathological conditions is related to multiple diseases (Fagiani et al., 2021; Li et al., 2021). In particular, Pin1 regulates several neuronal proteins, such as Tau, amyloid precursor protein (APP), and α-synuclein (Li et al., 2021) and thus has a significant impact on the development of many neurodegenerative diseases.
Spontaneous seizures have been observed in Pin1-knockout mice without any induction, suggesting that Pin1 may be a neuroprotective gene in the development of epilepsy, and its function may be associated with the regulation of excitatory and inhibitory synapses (Antonelli et al., 2014, 2016; Tang et al., 2017). Specifically, Pin1 may be associated with excitatory glutamate receptors N-methyl-D-aspartic acid [(NMDA) receptors and alpha-amino-3-hydroxy-5-methyl-4-isoxazolepropionic acid (AMPA) receptors] as well as inhibitory neurotransmitter receptors gamma-aminobutyric acid [(GABA) receptors and glycine receptors], which can effectively regulate the release of neurotransmitters and are significant in the progression of epilepsy. Electrophysiological studies of ion channels have also revealed some of the pathophysiological mechanisms underlying Pin1 and epilepsy (Antonelli et al., 2014, 2016; Hu J. H. et al., 2020; Hou et al., 2021).
Given the critical role of Pin1 in neurological diseases, we discuss in this review the recent findings of the dysregulation, mechanisms, and biological functions of Pin1 in epilepsy.
Pin1 was first found to be an essential PPIase that regulates mitosis, presumably by interacting with NIMA and attenuating its mitosis-promoting activity (Lu et al., 1996). Pin1 can specifically catalyze the isomerization of phosphorylated proline-directed serine or threonine (pS/T-P) (Wang et al., 2020; Li et al., 2021). The N-terminal WW domain (residues 1–39) and C-terminal PPIase domain (residues 50–163) are two main protein functional domains of Pin1. The WW domain performs the function of recognition and binding to the pSer/Thr-Pro motif of the substrate, while the PPIase domain is responsible for cis-trans isomerization and catalytic activity (Lu et al., 1999; Wang et al., 2020; Fagiani et al., 2021; Li et al., 2021). Since the WW domain specifically interacts with pSer-Pro or pThr-Pro motifs, which are the key phosphorylation sites of the substrates, Pin1 regulates diverse cellular processes in cell signaling pathways, such as cell cycle progression, cellular stress responses, development, neuronal function, immune responses, and cell death (Zhou et al., 2000; Lu and Zhou, 2007; Wang et al., 2020). Notably, Pin1 is expressed at very high levels in neurons (Nakamura et al., 2012b). Moreover, Pin1 function is inhibited in human central nervous system (CNS) disorders, including Alzheimer’s disease, Parkinson’s disease and schizophrenia, by multiple mechanisms (Driver et al., 2015; Fagiani et al., 2021), which supports the idea that it is an essential protective gene in the nervous system. The selected Pin1 substrates associated with the nervous system are summarized in Table 1.
Although many signaling pathways have been reported to be involved in the pathology of epilepsy by participating in seizure-induced cognitive dysfunction or neuronal apoptosis (Cendes et al., 2005; Henshall and Engel, 2013), the exact molecular mechanism of epilepsy is still unclear. In addition, Pin1 is involved in many cellular processes because of its plentiful protein targets (Liou et al., 2011; Yuan et al., 2011; Tang et al., 2017). Pin1 interacts with many neuronal proteins, and its deficiency is implicated in neurodegeneration (Balastik et al., 2007; Driver and Lu, 2010; Rudrabhatla and Pant, 2010; Tang et al., 2017). Pin1 can inhibit the protein synthesis required to sustain the late phase of long-term potentiation (LTP) (Keeney et al., 2012; Tang et al., 2017). In addition, Pin1 regulates conformational changes in the neuronal protein gephyrin, which is involved in the development of temporal lobe epilepsy (TLE) (Fang et al., 2011), and it modulates glycine receptor function as well (Zita et al., 2007). Therefore, it is reasonable to hypothesize that Pin1 is associated with the development of epilepsy.
Pin1-deficient mice have significantly upregulated seizure susceptibility in chemically induced models and develop age-dependent spontaneous epilepsy without any induction, strongly suggesting that Pin1 may be an important neuroprotective gene and that deletion of Pin1 may contribute to the occurrence of epilepsy (Hou et al., 2021). Recent studies have shown that the expression of Pin1 is remarkably downregulated in epileptic patients as well as experimental epileptic mouse models (Tang et al., 2017). The expression of Pin1 is dramatically decreased in the neocortex of epilepsy patients. Additionally, a pilocarpine-induced epileptic mouse model has also been used to verify the expression of Pin1 in epilepsy, and the results are consistent with those in humans (Tang et al., 2017), which supports the antiepileptic effect of Pin1. This study provided direct evidence that Pin1 is essential in regulating epileptic seizures.
The imbalance of excitability and inhibition of a large number of neurons is a significant cause of epileptic seizures. Inhibitory and excitatory neurotransmitters are critical regulators of hyperexcitability in epilepsy (Van Liefferinge et al., 2013). Abnormal increases in excitability, inadequate inhibitory mechanisms, or a combination of both lead to the excitability of the neurons in the epileptic foci, resulting in the uncontrolled spontaneous abnormal synchronous discharge of these neurons, followed by repeated seizures (Moshé et al., 2015; Camfield et al., 2017). Among them, excitatory glutamate receptor NMDA receptors, AMPA receptors and inhibitory neurotransmitter receptor GABA receptors, which are all regulated by Pin1, are involved in the regulation of epileptic activity (He et al., 2011; Rogawski, 2013; Lippman-Bell et al., 2016). The proposed relationship between Pin1 and synapses is presented as a conceptual model in Figure 1.
Figure 1. Pin1 as regulator of synaptic activity. The function of AMPAR could be inhibited by the interaction between Pin1 and CaMKII. Pin1 could down regulate the NMDA-mediated synaptic transmission by binding to PSD95. And the transmission of GABAergic will be modulated by Pin1 interfering the function of neuroligin2-gephyrin complex.
Ionotropic glutamate NMDA receptors are important regulators of glutamatergic synaptic transmission in the CNS and modulate the maturation and plasticity of glutamate synapses (Ladépêche et al., 2014). Recent studies have suggested the possible role of lateral diffusion in the surface distribution of NMDA receptors, which provides a powerful way to rapidly affect the content and composition of synaptic receptors (Peng et al., 2010; Ladépêche et al., 2014). The increased number of cell surface NMDARs is responsible for cell injury and neuronal death during epileptogenesis, and therapies that directly antagonize NMDA receptors may prevent excitotoxicity, which develops after various pro-epileptogenic brain injuries (Frasca et al., 2011; Naylor et al., 2013; Tang et al., 2017).
The relationship between Pin1 and NMDA receptors has recently been recognized. PSD-95 is a membrane-associated guanylate kinase with a catalytically inactive guanylate kinase domain, a Src homology 3 (SH3) domain and three PDZ (PSD-95/Disks large/zona occludens-1) domains, which allow it to anchor the NMDA receptors GluN2 and activate intracellular signaling complexes (Opazo et al., 2012). One study showed that Pin1 triggers structural changes in PSD-95 by interacting with PSD-95 at the T287, S290, and S295 consensus motifs and then negatively regulates its ability to interact with NMDARs (Antonelli et al., 2016). To be precise, Pin1 can bind with PSD-95 and then triggers its structural changes. Pin1-driven conformational rearrangements mainly impact on PDZ2, the domain involved in NMDAR recruitment. Larger NMDA-mediated synaptic currents in hippocampal slices were obtained from Pin1(–/–) mice compared with controls, and similar results were obtained in cultured hippocampal cells expressing a PSD-95 mutant unable to undergo prolyl-isomerization, further indicated the action of Pin1 on PSD-95 negatively regulates the ability of NMDARs. Furthermore, an increasing of dendritic spines was observed in Golgi-stained pyramidal neurons lacking Pin1 expression. Altogether, Pin1 could affect plasticity by determining the number of NMDARs initiating plasticity via PSD-95 prolyl-isomerization (Antonelli et al., 2016). Pin1 can also bind via its WW domain with phosphorylated threonine (T19) and serine (S25) in the N-terminal domain of PSD-95, and the binding reduces the palmitoylation of cysteine 3 and cysteine 5 in PSD-95 and then decreases its stability at excitatory synapses (Delgado, 2020; Delgado et al., 2020). This result indicates that Pin1 can modulate NMDARs via PSD-95 prolyl isomerization, suggesting that Pin1 plays a key role in synaptic transmission.
AMPA receptors (AMPARs) are major regulators of excitatory synaptic transmission (Traynelis et al., 2010), and the expression of AMPARs at synapses is very dynamic (Shepherd and Huganir, 2007). AMPA receptors consist of four subunits (GluA1–GluA4), and any of them can form both homo and heteromers (Traynelis et al., 2010) and possess multiple phosphorylation sites (He et al., 2011). The phosphorylation of its subunits can regulate AMPAR function effectively (Kittler and Moss, 2006; Lee, 2006b), which is significant for modulating different forms of synaptic plasticity (Turrigiano and Nelson, 2004). Research has highlighted the role of the GluA1 subunit on serine 845 (S845) in various forms of synaptic plasticity (Lee, 2006a). The phosphorylation of GluA1-S845 is a necessary prerequisite step for in vivo sensory experience-dependent homeostatic synaptic plasticity of visual cortical neurons (Goel et al., 2011). GluA1-S845 phosphorylation is critical for maintaining the perisynaptic population of AMPARs by trafficking AMPARs to extrasynaptic sites and subsequent delivery to synapses during LTP (Oh et al., 2006). In addition, the phosphorylation of Ser831 and Ser845 are also considered key factors in LTP (Shepherd and Huganir, 2007). Additional studies have shown the potential utility of AMPA receptors as targets for seizure protection and validated AMPA receptors as novel targets for epilepsy therapy (Rogawski and Donevan, 1999; French et al., 2012, 2013; Krauss et al., 2012; Rogawski, 2013).
Recent studies have indicated that Pin1 may negatively regulate AMPA receptors. Pin1 KO upregulates epileptic susceptibility in mice, which is associated with an increase in AMPA receptors (Hou et al., 2021). The specific mechanism may involve calcium/calmodulin-dependent protein kinase II (CaMKII), protein kinase A (PKA) and protein kinase C isoenzymes (PKCs), the phosphorylation of which can effectively alter the excitatory function of AMPA receptors and provides a novel mechanism for channel modulation through a variety of protein signaling cascades (Jenkins and Traynelis, 2012; Wang et al., 2013).
CaMKII is a key mediator of AMPA receptors through its GluA1 subunit (Hayashi et al., 2000) and plays a critical role in the process of epilepsy. The phosphorylation of GluA1 at Ser831 via CaMKII could increase the conductance of AMPA receptors (Derkach et al., 1999; Kristensen et al., 2011). The activity of CaMKII is modulated by its regulatory domains. The regulatory domain blocks the substrate binding site of the CaMKII kinase domain in its basal state structures (Krapivinsky et al., 2004; Rellos et al., 2010; Chao et al., 2011; Coultrap and Bayer, 2012), while Ca2+/calmodulin can bind to the regulatory domain and then autophosphorylate CaMKII at Thr286 to keep the catalyst active (Partida et al., 2018). Recent studies using pull-down and immunoprecipitation assays have identified that Pin1 binds CaMKII in a phosphorylation-specific manner, which indicates the role of Pin1 in the phosphorylation-dependent signals involving CaMKII (Tatara et al., 2010). Additional research has demonstrated that the activity of CaMKII is significantly increased in Pin1 KO mice, and Pin1 can bind to phosphorylated CaMKII and decrease its activity (Shimizu et al., 2018; Hou et al., 2021). Moreover, restoration of the expression of Pin1 can reduce the phosphorylation of CaMKII and GluA1 (Hou et al., 2021), and Pin1 may regulate the phosphorylation of GluA1 Ser831 by acting on CaMKII, which supports the view that Pin1 effectively controls seizure susceptibility via the Pin1-CaMKII-AMPA receptor pathway.
Protein kinase C acts on a multitude of signal transduction pathways (Newton, 2001; Steinberg, 2008; Yang and Igumenova, 2013), and the relationship between PKC and AMPA receptors is significant in excitatory transmission. Some studies observed an increasing activity of PKC in the hippocampus of amygdala-kindled and hippocampus-kindled rats (Akiyama et al., 1995). PKC expression has also been detected to be decreased in pilocarpine induced epileptic rats, and further research has demonstrated that PKC can phosphorylate the GluA1 subunit of AMPA receptors at its S831 site (Roche et al., 1996; Kim et al., 2019). In addition, the C-terminal V5 domain is one of the most variable domains in PKCs due to cis-trans isomerization of the peptidyl-prolyl bonds (Yang and Igumenova, 2013). It has been shown that Pin1 binds to the hydrophobic motif of V5 and isomerizes the phosphorylated turn motif of conventional PKC, which primes it for subsequent ubiquitin-mediated degradation (Abrahamsen et al., 2012; Yang and Igumenova, 2013), to be specific, the study demonstrated that Pin1 could down regulate the conventional isozymes of PKC family. These studies highlight the role of the V5a-Pin1 interaction in neural function. Although PKC showed no change in Pin1 KO mice (Hou et al., 2021), studies have shown the effect of Pin1 on the PKCα subunit (Abrahamsen et al., 2012). Thus, the interaction between PKC and Pin1 and its function and molecular mechanism in the development of epilepsy is urgently needed.
Protein kinase A is crucial for both postsynaptic and presynaptic mechanisms underlying LTD and LTP (Kameyama et al., 1998; Banke et al., 2000), which supports its role in epilepsy. Previous studies have demonstrated that PKA can enhance neuronal AMPA receptor equilibrium response amplitude and upregulate channel opening frequency (Knapp et al., 1990; Greengard et al., 1991; Banke et al., 2000). Moreover, phosphorylation of AMPA is regulated by PKA, which increases the peak response open probability of the AMPA receptor by phosphorylating GluA1 Ser845 (Banke et al., 2000; Kim et al., 2019). In addition, the ratios of the expression of pPKA and pERK1/2 are decreased in epileptic rats, which further supports the phosphorylation function of activated PKA in epileptic rats (Kim et al., 2019). In terms of the relationship between PKA and Pin1, recent studies have supported the idea that PKA can modulate the phosphorylation of the WW domain of Pin1 at Ser16. The phosphorylation would reduce conformational heterogeneity and flexibility of the phospho-binding loop upon S16 phosphorylation and abrogates the binding capacity of Pin1 afterward, which would definitely affect the neuronal signaling in epilepsy (Luh et al., 2013; Smet-Nocca et al., 2013). Although there was no change in PKA in Pin1 knockout mice (Hou et al., 2021), the underlying mechanism of mutual regulation between Pin1 and PKA is still worth further study.
As previously discussed, synaptic inhibition is essential for shaping the dynamics of neuronal networks, and abnormal inhibition is closely associated with epilepsy (Macha et al., 2022). GABA receptors represent the most significant inhibitory system in the CNS (Barki and Xue, 2021). The receptors can be categorized as GABAA and GABAB. GABAA can modulate ligand-gated (or receptor-operated) ion channels, and GABAB can operate through second messengers. Both contribute to seizure-like discharge control when repetitive electrical stimulation is delivered to the limbic structure (Avoli and Lévesque, 2022). It has also been demonstrated that aberrant alternative GPHN splicing, leading to curtailed protein and diminished GABA receptors, is related to temporal lope lobe epilepsy (Förstera et al., 2010). Thus, GABAergic signaling is closely linked to the onset of epilepsy.
The glycine receptor (GlyR) is closely related to GABAA in the ‘gene superfamily’ of ligand-gated ion channels. It plays an essential role in mediating inhibitory neurotransmission in the spinal cord and brain stem, yet it also works as a coagonist with NMDARs in the CNS (Zafra et al., 2017). The malfunctions of GlyR are linked to temporal lobe epilepsy (Lynch et al., 2017), and it may contribute to the neuropsychiatric symptoms of the disease in a neuron type-specific way (Kankowski et al., 2017). Neuron type-specific expression of a gain-of-function variant of GlyR has been observed in the hippocampus of patients with temporal lobe epilepsy (Winkelmann et al., 2014). In addition, preclinical studies have shown that inhibition of its substrate selective transporter, glycine transporter-1 (GlyT-1), can promote neuroprotection and provide a pharmacotherapeutic strategy for epilepsy (Cioffi and Guzzo, 2016). These studies support the link between GlyR and epilepsy.
Accumulating evidence has demonstrated the interaction of Pin1 with GABA receptors and GlyR. It is known that Gephyrin is a central component of GABAergic signaling and plays a key role in α2 and γ2 subunit-containing GABAAR clustering (Kneussel et al., 1999; Tretter et al., 2012; Antonelli et al., 2014). The NL2 isoform is a kind of adhesion molecule that is present in GABAergic PSDs (Varoqueaux et al., 2004). The interaction of NL2 and gephyrin can modulate NL-scaffolding protein interactions and regulate excitatory and inhibitory synaptic transmission (Antonelli et al., 2014). Pin1 has been shown to interact with gephyrin and alter its overall structure, thereby enhancing its ability to bind GlyR (Zita et al., 2007; Antonelli et al., 2014). Additionally, Pin1 can mediate propyl isomerization of phosphorylated serine 714. Further studies have shown that Pin1 negatively regulates the interaction of the gephyrin–NL2 complex formation, which in turn downregulates GABAergic synaptic transmission (Antonelli et al., 2014), suggesting the existence of a Pin1/NL2/gephyrin signaling pathway in the regulation of GABAergic synapses. Moreover, ten putative Pin1 consensus motifs have been identified, mostly concentrated in the C-domain of gephyrin. The C-domain’s cluster was responsible for Pin1 recruitment, following by Pin1-driven conformational changes of gephyrin substrate. Such structural remodeling of gephyrin could affect its binding affinity for the β subunit of the GlyR without affecting its oligomerization properties. Consistent with these findings, hippocampal neurons derived from Pin1 knockout mice show a loss in the number of GlyR immunoreactive puncta, corresponding to a reduction in amplitude of glycine-induced current (Zacchi et al., 2014). These studies further demonstrate the mechanism by which Pin1 regulates GlyR function.
Ion channels also participate in the occurrence of epilepsy. Approximately 25% of genes identified in epilepsy encode ion channels (Oyrer et al., 2018), and electrophysiological studies have shown not only pathophysiological mechanisms underlying epilepsy but also the mechanism of action of several antiepileptic drugs involve ion channels (Sugiura and Ugawa, 2017). Research has shown that when AMPA receptors are activated, they allow Na+ to enter or leave, and extracellular Na + influx causes postsynaptic membrane depolarization and induces EPSP to participate in excitatory synaptic transmission. At the same time, membrane depolarization induced by the AMPA receptor causes Mg2+ to move away from the NMDA receptor channel and open the NMDA receptor channel. Activated NMDA receptors result in excessive Ca2+ influx and intracellular calcium overload, leading to cell death. In addition, activated GABA receptors can induce the opening of chloride ion channels, and the rapid influx of Cl– causes hyperpolarization of the postsynaptic membrane and generates inhibitory postsynaptic potentials that inhibit neuronal excitation (Lippman-Bell et al., 2016).
In terms of the relationship between Pin1 and ion channels, a Pin1-dependent mechanism has been reported to regulate the association of the A-type K+ channel subunit Kv4.2, suggesting a Pin1-mediated mechanism in the regulation of reversal learning (Hu J. H. et al., 2020). Recent studies have also hypothesized that hypoxia-induced changes in the flavoprotein (Fp)-mediated redox ratio of the carotid body (CB) modulate the Pin1/p47phox tandem to alter type I cell potassium channels and therefore chemoreceptor discharge (CND) (Bernardini et al., 2020). Pin1 also regulates Ca2+ influx by binding to salt-induced kinase 2 (SIK2) and decreasing the expression of p35, a negative regulator of Ca2+ influx (Nakatsu et al., 2017), yet the effect is induced by high glucose, which is not the same as the microenvironment of epilepsy. The association of Na(+)/H(+) exchanger regulatory factor (NHERF)-1 with Pin1 may also indicate the role of Pin1 in the Na(+)/H(+) channel (He et al., 2001). The mechanism by which Pin1 regulates epileptic ion channels may illuminate the underlying roles of Pin1 in epilepsy.
Notch1 is a membrane receptor in the Notch family (Arumugam et al., 2018). Notch1 is tightly connected to cell proliferation, differentiation, and developmental fate switching (Arumugam et al., 2018). For neurons, Notch1 plays various roles in the development of the CNS, participating in the proliferation, survival, self-renewal and differentiation of neural stem cells (NSCs) (Lathia et al., 2008; Arumugam et al., 2018). In addition, Notch1 signaling may also affect synaptic plasticity and learning and memory in the adult brain (Lathia et al., 2008; Alberi et al., 2013; Sargin et al., 2013; Arumugam et al., 2018). In terms of epilepsy, Notch1 signaling has the ability to induce astrogliosis in glioma, which is critical in the occurrence of epilepsy. Notch signaling has been observed to be downregulated in a status epilepticus model, and the decrease may be associated with the disruption of the stem cell niche (Sibbe et al., 2012). Another study suggested that the Notch1 signaling pathway is activated in TLE mouse models and that its function is closely related to microglial phenotypic transformation (Deng et al., 2020). In addition, Notch1 was observed to increase in rats with status epilepticus and spontaneous recurrent seizures (Liu et al., 2014). Overall, the Notch1 signaling pathway is significant in the pathogenesis of epilepsy. Notch1 was found to modulate hippocampal plasticity via interaction with the reelin pathway, glutamatergic transmission and CREB signaling (Brai et al., 2015). CaMKII is closely related to Notch signaling activation by accelerating the degradation of the silencing mediator of retinoic acid and thyroid hormone receptor (SMRT), a corepressor of Notch signaling (Ann et al., 2012). In addition, the coaction of the PKA pathway and Notch1 expression is essential for the differentiation of C6 astrocytes (Angulo-Rojo et al., 2013). These studies highlight the interaction of CaMKII and PKA with the Notch1 signaling pathway.
Furthermore, Pin1 interacts with the Notch1 pathway. A strong correlation between Pin1 overexpression and high levels of activated Notch1 has been observed in breast cancer patients, and some studies have suggested that Notch1 may directly induce transcription of Pin1 (Rustighi et al., 2009). Resveratrol downregulates Pin1 and Notch1 intracellular domain (NICD1) at the same time and reverses cell dysfunction by inhibiting the Pin1/Notch1 signaling pathway (Yu and Fang, 2022). The relationship between NIC and Pin1 has also been analyzed in Pin1/p53 double-knockout mice, Pin1 deficiency might affect NIC stabilization, which is associated with the activity of presenilin1, a component of the gsecretase complex that would affect the releasing of NIC (Takahashi et al., 2008). Pin1 can also mediate cell death through the p53-NICD complex in ischemic stroke (Arumugam et al., 2018). The results indicated that the Pin1-p53-NIC system might be essential for preventing the pathogenesis and progression of epilepsy. Further research has demonstrated that Pin1 could bind with the crucial phosphorylated sites within the Ser-Thr-rich (STR) region of Notch1, then the conformational changes would lead to cleavage by γ-secretase and increased release of the active intracellular domain of Notch1, which explain the role of Pin1 in Notch1 pathway (Rustighi et al., 2009). Pin1 may promote proapoptotic function in neuronal death by promoting NICD1 stability (Baik et al., 2015), while other argue that Notch1 may increase the expression level of anti-apoptotic gene in rats with cerebral infarction and act an anti-apoptotic function in neuron (Zhao et al., 2019). Besides, Pin1 is closely associated with anti-apoptosis protein BCL-2 (Sultana et al., 2006; Li et al., 2007) and depict an anti-apoptotic effect in AD neurons (LaFerla et al., 1996; Rong et al., 2017; Wang et al., 2023). These findings strongly point to an anti-epileptic effect of Pin1 by antagonizing neuronal apoptosis in epilepsy, thus Pin1-Nocth1 complex may play anti-epileptic role.
Therefore, we can hypothesize that the interaction between Pin1 and Notch1 pathway may play a role in epilepsy and that Notch1 may participate in epileptogenesis via the function of CaMKII and PKA. The relevant characteristics of the Notch1 pathway are summarized in Figure 2.
Figure 2. Pin1 may regulate Notch1 signaling and PI3K/Akt signaling pathways in epilepsy. Pin1 has an interaction with Notch1 pathways, and the function may associated with CaMKII. AMPAR and NMDAR can both be mediated by PI3K/Akt pathway, whose activation will be regulated by PKA, PKC, and Pin1. The relationship between Pin1 and PKA, PKC and CaMKII deserved further research.
The PI3K/Akt pathway is significant for growth and survival and participates in many biological processes. It has been demonstrated that the PI3K/Akt pathway is associated with early ischemia/reperfusion injury (Fu et al., 2014), and it can regulate neuronal apoptosis and neuroinflammation in Alzheimer’s disease (Liu and Li, 2020). Recent studies have shown that PI3K/Akt signaling improves the survival of neurons and axonal growth as well (Huang et al., 2017). For epilepsy, the PI3K/Akt signaling pathway can effectively inhibit the occurrence of epilepsy by attenuating neuronal apoptosis and autophagy (Duan et al., 2018; Wei et al., 2018; Hu F. et al., 2020). Moreover, the PI3K/Akt signaling pathway synergistically enhances adipogenesis and glucose uptake in porcine bone marrow mesenchymal stem cells (pBMSCs) through CaMKII (Zhang et al., 2018). Although few data describe the interaction between CaMKII and the PI3K/Akt pathway in neurons, it is logical to infer their role in epilepsy. In addition, fluoxetine elicited neuroprotection via PKA and PI3K/Akt (Zeng et al., 2016), which suggests the interaction of the PI3K/Akt signaling pathway and PKA in neurons. Furthermore, PKA-PI3K-Akt signaling has the ability to modulate the AMPA receptor GluA1 subunit to assist in learning and memory. These data demonstrate the coaction of PKA and PI3K/AKT and demonstrate their function on the AMPA receptor, which has a critical role in the progression of epilepsy (Chen M. W. et al., 2020). The relationship between PKC and PI3K/AKT has also been studied. PKC can regulate the activation of PKA-PI3K-Akt signaling, which effectively broadens the knowledge of the downstream signaling of GluA1 (Chen M. W. et al., 2020). Recent studies have also shown that the PI3K/Akt pathway may affect NMDA receptors via TrKB and BDNF (Srivastava et al., 2018), and activation of the PI3K-AKT-mTOR pathway can block NMDA-induced autophagy in neurons (Wang et al., 2014). The studies above have identified the interaction of PI3K/AKT and synaptic receptors and highlighted the effects of the PI3K/AKT pathway in epilepsy.
Pin1 can facilitate the activation of PI3K/Akt signaling. Some research has suggested that the expression of Pin1 is positively associated with activation of PI3K/Akt signaling (Yang et al., 2020), and that Pin1 can phosphorylate Akt at Ser434 and then increase Akt stability and subsequently activate PI3K/Akt (Nakatsu et al., 2011). The deletion of Pin1 can block the function of PI3K/Akt and suppress the growth of cells (Zhang et al., 2019). However, some studies have indicated an inhibitory effect of Pin1 on the PI3K/Akt pathway. Pin1 may downregulate the action of the PI3K/Akt/mTOR pathway and play a protective role in senescent cells (Zhang et al., 2021). The detailed mechanisms by which Pin1 acts on PI3K/Akt signaling may be useful to explain the role of Pin1 in neurons. Notably, Pin1 enables phosphatase PP2A to further mediate tau dephosphorylation in the AD brain (Ramakrishnan et al., 2003). PP2A is a special protein phosphatase that can dephosphorylate Akt. This function further supports the role of the Pin1/PI3K/Akt interaction in neurons. Therefore, it is valuable to study the mechanism by which Pin1 acts on the PI3K/Akt pathway, and PI3K/Akt may be another potential target for epilepsy treatment. The function of the PI3K/Akt pathway is shown in Figure 2.
Tau is an intrinsically disordered protein which has a closely relationship with microtubules. Its interaction with microtubules is characterized by a rapid “kiss-and-hop” process. It is most abundant in neuronal axons and the rapid interaction may explain how tau binding to microtubules without disturb axonal transport (Janning et al., 2014; Wang and Mandelkow, 2016; Chang et al., 2021b). Tau is also present in neuronal cell bodies, nuclei, synaptic specializations, glia and other cell types in the brain and peripheral organs (Wang and Mandelkow, 2016; Ittner and Ittner, 2018), which indicated that it may be involved in a variety of common and disabling brain diseases, including epilepsy. Reducing levels of endogenous tau has protective effects in epilepsy experimental models (DeVos et al., 2013; Gheyara et al., 2014). Tau deficit mice have an increased frequency of inhibitory postsynaptic currents in dentate granule cells (Roberson Halabisky et al., 2011), which may explain the beneficial effects of decreasing tau in epilepsy. Further study observed that Tau ablation could increase the excitability of inhibitory neurons, and structurally alters their axon initial segments, thus promote inhibition and suppress hypersynchrony (Chang et al., 2021a). Together, tau reduction prevents the occurrence of epilepsy. Hyperphosphorylated Tau protein might contribute to vulnerability to epileptogenesis, for excessive post-translational modifications of Tau creates a pathologic environment promoting epileptogenesis (Paudel et al., 2019). Therefore, therapy targeting Tau protein and p-tau anti-may be a viable epileptogenic strategy.
The interaction between Pin1 and tau has been demonstrated. Pin1 binds to pT231-tau and promotes tau dephosphorylation, and further study confirmed that the binding of Pin1 and p-tau could in turn isomerize phosphorylated tau proteins so that their conformation changes from cis to trans, thus favoring its recognition by the phosphatase PP2A. PP2A has conformational specificity and dephosphorylates only the trans pS/T-P motif (Zhou et al., 2000; Luna-Muñoz et al., 2007; Wang et al., 2020). Cis pT231-tau is associated with neuronal apoptosis under neuronal stress, thus is a key role in neurodegeneration, while Pin1 has the ability to decrease the level of abnormal p-tau and the regulation is a critical mechanism to protect against tau-related pathology (Wang et al., 2020).
The onset of seizure is mainly due to sudden abnormal discharge of neurons in the brain leading to temporary brain dysfunction. Thus, controlling or significantly reducing the abnormal charging of neurons is the main treatment for epilepsy. As mentioned above, Pin1 plays a critical role in preventing pathologies in epilepsy by regulating synapses; thus, it is reasonable to speculate that the upregulation and/or activation of Pin1 may be helpful for epilepsy treatment. However, Pin1 is a well-known oncogene, and overexpression of Pin1 has been reported to be associated with several cancers (Zhou and Lu, 2016). It can effectively activate a number of oncogenes and inactivate various tumor suppressors by modulating their functions to promote the development of cancer (Cheng and Tse, 2019; Yu et al., 2020). Therefore, treatment with Pin1 should be carried out with caution. Delivering the Pin1 activator to neurons specifically or therapies targeting the upstream regulators of Pin1 may be optional therapeutic strategies (Wang et al., 2020). In addition, although no drug candidates targeting Pin1 for neuronal diseases have been reported, specific antibodies targeting Thr231 of tau, which is the Pin1 binding site, have been developed, and such monoclonal antibodies could effectively prevent tau-related pathology development in AD and TBI patients by entering neurons and blocking the induction of pathological cis p-tau (Shahpasand et al., 2018; Chen D. et al., 2020; Wang et al., 2020), which is closely related to cognitive impairment and dementia (Albayram et al., 2017; Qiu et al., 2021). Moreover, aberrant p-tau aggregation has been reported in the epileptic human brain and in animal models of epilepsy (Machado et al., 2019; Liu et al., 2021; Canet et al., 2022), highlighting the role of pathological p-tau in the onset of epilepsy, suggesting that a therapy targeting the Pin1 binding site, especially p-tau, may also be a potential anti-epileptogenic therapy (Liu et al., 2016). Brivaracetam and the non-competitive antagonist of the AMPA glutamate receptor perampanel are the more recent antiseizure medications with a low risk of interaction, and have recently been approved for monotherapy and/or adjunctive treatment in the USA and Europe (Rohracher et al., 2021). Because of the close relationship between the AMPA receptor and Pin1, this novel therapy may broaden the spectrum of Pin1 in the treatment of epilepsy. More research in animal models and technological innovation would be helpful to further boost the success of Pin1 in epilepsy treatment.
The evidence discussed in this review supports the idea that Pin1 is implicated in the onset and progression of epilepsy. Pin1 is identified as a unique enzyme and a crucial regulator that precisely regulates the cis-trans isomerization of a wide variety of phosphorylated proteins and alters the activity of its target proteins. Regulation of the phosphorylation and transcriptional landscapes by Pin1 makes this enzyme a central effector of many physiological and pathological activities related to neuronal cells (Zannini et al., 2019). In line with this hypothesis, Pin1 has been reported to take part in neuro-associated diseases (Fagiani et al., 2021), including epilepsy. In addition, emerging evidence supports the notion that Pin1 is a key molecule in epileptogenesis. Pin1 has strong neuroprotective effects in the progression of epilepsy, and the Pin1/NMDAR complex, Pin1-CaMKII-AMPA receptor axis and Pin1-NL2/gephyrin- GABAergic signaling may all be involved in the mechanism (Antonelli et al., 2014, 2016; Tang et al., 2017; Hou et al., 2021). The data strongly suggest the potential utility of Pin1 as a target for seizure protection (Hou et al., 2021). The function of Pin1 in epilepsy may be associated with some classical signaling pathways, such as the Notch1 signaling and PI3K/Akt signaling pathways. However, more evidence is needed to clarify the potential role of Pin1 in neuronal signaling, particularly in the occurrence of epilepsy. Finally, Pin1-related treatment is promising, and more research and technological innovation are needed to overcome its limitations in direct administration. We anticipate that the specific molecular mechanisms will be elucidated in the coming years, pushing Pin1 toward true clinical application.
All authors: conceptualization, substantive supervision, revision over the article, read and agreed to the published version of the manuscript. YC: collection the literature and writing—review and editing the manuscript. HL: project administration and funding acquisition.
This study was funded by Natural Science Foundation of Fujian Province (2020J01602).
We are grateful to Kun Ping Lu and Xiao Zhen Zhou for the guidance and valuable suggestions of our epilepsy-related projects.
The authors declare that the research was conducted in the absence of any commercial or financial relationships that could be construed as a potential conflict of interest.
All claims expressed in this article are solely those of the authors and do not necessarily represent those of their affiliated organizations, or those of the publisher, the editors and the reviewers. Any product that may be evaluated in this article, or claim that may be made by its manufacturer, is not guaranteed or endorsed by the publisher.
HFOs, high-frequency oscillations; rHFOSs, repetitive HFOs and spikes; PPIases, the peptidyl prolyl cis-trans isomerases; PPase, protein phosphatase; NIMA, never in mitosis, gene A; APP, amyloid precursor protein; Pin1, PPIase NIMA-interacting 1; LTP, long-term potentiation; TLE, temporal lobe epilepsy; CNS, central nervous system; SH3, Src homology 3; PDZ, PSD-95/Disks large/zona occludens-1; CaMKII, calcium/calmodulin-dependent protein kinase II; PKA, protein kinase A; PKCs, protein kinase C isoenzymes; GlyT-1, glycine transporter-1; NL2, Neuroligin2; CB, carotid body; CND, chemoreceptor discharge; SIK2, salt-induced kinase 2; NHERF, Na(+)/H(+) exchanger regulatory factor; NSC, neural stem cell; SMRT, silencing mediator of retinoic acid and thyroid hormone receptor; NICD1, Notch1 intracellular domain; pBMSCs, porcine bone marrow mesenchymal stem cells.
Abrahamsen, H., O’Neill, A. K., Kannan, N., Kruse, N., Taylor, S. S., Jennings, P. A., et al. (2012). Peptidyl-prolyl isomerase Pin1 controls down-regulation of conventional protein kinase C isozymes. J. Biol. Chem. 287, 13262–13278. doi: 10.1074/jbc.M112.349753
Akiyama, K., Ono, M., Kohira, I., Daigen, A., Ishihara, T., and Kuroda, S. (1995). Long-lasting increase in protein kinase C activity in the hippocampus of amygdala-kindled rat. Brain Res. 679, 212–220. doi: 10.1016/0006-8993(95)00221-b
Albayram, O., Kondo, A., Mannix, R., Smith, C., Tsai, C. Y., Li, C., et al. (2017). Cis P-tau is induced in clinical and preclinical brain injury and contributes to post-injury sequelae. Nat. Commun. 8:1000. doi: 10.1038/s41467-017-01068-4
Alberi, L., Hoey, S. E., Brai, E., Scotti, A. L., and Marathe, S. (2013). Notch signaling in the brain: In good and bad times. Ageing Res. Rev. 12, 801–814. doi: 10.1016/j.arr.2013.03.004
Amador, A., Bostick, C. D., Olson, H., Peters, J., Camp, C. R., Krizay, D., et al. (2020). Modelling and treating GRIN2A developmental and epileptic encephalopathy in mice. Brain 143, 2039–2057.
Angulo-Rojo, C., Manning-Cela, R., Aguirre, A., Ortega, A., and López-Bayghen, E. (2013). Involvement of the Notch pathway in terminal astrocytic differentiation: Role of PKA. ASN neuro 5:e00130. doi: 10.1042/AN20130023
Ann, E. J., Kim, H. Y., Seo, M. S., Mo, J. S., Kim, M. Y., Yoon, J. H., et al. (2012). Wnt5a controls Notch1 signaling through CaMKII-mediated degradation of the SMRT corepressor protein. J. Biol. Chem. 287, 36814–36829. doi: 10.1074/jbc.M112.356048
Antonelli, R., De Filippo, R., Middei, S., Stancheva, S., Pastore, B., Ammassari-Teule, M., et al. (2016). Pin1 modulates the synaptic content of NMDA receptors via prolyl-isomerization of PSD-95. J. Neurosci. 36, 5437–5447. doi: 10.1523/JNEUROSCI.3124-15.2016
Antonelli, R., Pizzarelli, R., Pedroni, A., Fritschy, J. M., Del Sal, G., Cherubini, E., et al. (2014). Pin1-dependent signalling negatively affects GABAergic transmission by modulating neuroligin2/gephyrin interaction. Nat. Commun. 5:5066. doi: 10.1038/ncomms6066
Arumugam, T. V., Baik, S. H., Balaganapathy, P., Sobey, C. G., Mattson, M. P., and Jo, D. G. (2018). Notch signaling and neuronal death in stroke. Prog. Neurobiol. 16, 103–116.
Avoli, M., and Lévesque, M. (2022). GABAB receptors: Are they missing in action in focal epilepsy research? Curr. Neuropharmacol. 20, 1704–1716. doi: 10.2174/1570159X19666210823102332
Baik, S. H., Fane, M., Park, J. H., Cheng, Y. L., Yang-Wei Fann, D., Yun, U. J., et al. (2015). Pin1 promotes neuronal death in stroke by stabilizing Notch intracellular domain. Ann. Neurol. 77, 504–516.
Balastik, M., Lim, J., Pastorino, L., and Lu, K. P. (2007). Pin1 in Alzheimer’s disease: Multiple substrates, one regulatory mechanism? Biochim. Biophys. Acta 1772, 422–429. doi: 10.1016/j.bbadis.2007.01.006
Banke, T. G., Bowie, D., Lee, H., Huganir, R. L., Schousboe, A., and Traynelis, S. F. (2000). Control of GluR1 AMPA receptor function by cAMP-dependent protein kinase. J. Neurosci. 20, 89–102.
Barki, M., and Xue, H. (2021). GABRB2, a key player in neuropsychiatric disorders and beyond. Gene 809:146021. doi: 10.1016/j.gene.2021.146021
Bernardini, A., Wolf, A., Brockmeier, U., Riffkin, H., Metzen, E., Acker-Palmer, A., et al. (2020). Carotid body type I cells engage flavoprotein and Pin1 for oxygen sensing. Am. J. Physiol. Cell Physiol. 318:C719–C731. doi: 10.1152/ajpcell.00320.2019
Brai, E., Marathe, S., Astori, S., Fredj, N. B., Perry, E., Lamy, C., et al. (2015). Notch1 regulates hippocampal plasticity through interaction with the reelin pathway, glutamatergic transmission and CREB signaling. Front. Cell. Neurosci. 9:447. doi: 10.3389/fncel.2015.00447
Camfield, P., Camfield, C., Busiah, K., Cohen, D., Pack, A., and Nabbout, R. (2017). The transition from pediatric to adult care for youth with epilepsy: Basic biological, sociological, and psychological issues. Epilepsy Behav. 69, 170–176. doi: 10.1016/j.yebeh.2016.11.009
Canet, G., Zub, E., Zussy, C., Hernandez, C., Blaquiere, M., Garcia, V., et al. (2022). Seizure activity triggers tau hyperphosphorylation and amyloidogenic pathways. Epilepsia 63, 919–935. doi: 10.1111/epi.17186
Cendes, F., Kobayashi, E., and Lopes-Cendes, I. (2005). Familial temporal lobe epilepsy with auditory features. Epilepsia 46, 59–60.
Chang, C. W., Shao, E., and Mucke, L. (2021b). Tau: Enabler of diverse brain disorders and target of rapidly evolving therapeutic strategies. Science 371:eabb8255.
Chang, C. W., Evans, M. D., Yu, X., Yu, G. Q., and Mucke, L. (2021a). Tau reduction affects excitatory and inhibitory neurons differently, reduces excitation/inhibition ratios, and counteracts network hypersynchrony. Cell Rep. 37:109855. doi: 10.1016/j.celrep.2021.109855
Chao, L. H., Stratton, M. M., Lee, I. H., Rosenberg, O. S., Levitz, J., Mandell, D. J., et al. (2011). A mechanism for tunable autoinhibition in the structure of a human Ca2+/calmodulin- dependent kinase II holoenzyme. Cell 146, 732–745. doi: 10.1016/j.cell.2011.07.038
Chen, D., Wang, L., and Lee, T. H. (2020). Post-translational Modifications of the peptidyl-prolyl isomerase Pin1. Front. Cell Dev. Biol. 8:129. doi: 10.3389/fcell.2020.00129
Chen, M. W., Zhu, H., Xiong, C. H., Li, J. B., Zhao, L. X., Chen, H. Z., et al. (2020). PKC and Ras are involved in M1 muscarinic receptor-mediated modulation of AMPA receptor GluA1 subunit. Cell. Mol. Neurobiol. 40, 547–554. doi: 10.1007/s10571-019-00752-x
Cheng, C. W., and Tse, E. (2019). Targeting PIN1 as a therapeutic approach for hepatocellular carcinoma. Front. Cell Dev. Biol. 7:369. doi: 10.3389/fcell.2019.00369
Cioffi, C. L., and Guzzo, P. R. (2016). Inhibitors of Glycine Transporter-1: Potential therapeutics for the treatment of CNS disorders. Curr. Topics Med. Chem. 16, 3404–3437.
Coultrap, S. J., and Bayer, K. U. (2012). CaMKII regulation in information processing and storage. Trends Neurosci. 35, 607–618.
Daza-Martin, M., Starowicz, K., Jamshad, M., Tye, S., Ronson, G. E., MacKay, H. L., et al. (2019). Isomerization of BRCA1-BARD1 promotes replication fork protection. Nature 571, 521–527. doi: 10.1038/s41586-019-1363-4
Delgado, J. Y. (2020). An alternative Pin1 binding and isomerization Site in the N-terminus domain of PSD-95. Front. Mol. Neurosci. 13:31. doi: 10.3389/fnmol.2020.00031
Delgado, J. Y., Nall, D., and Selvin, P. R. (2020). Pin1 binding to phosphorylated PSD-95 regulates the number of functional excitatory synapses. Front. Mol. Neurosci. 13:10. doi: 10.3389/fnmol.2020.00010
Deng, X. L., Feng, L., Wang, Z. X., Zhao, Y. E., Zhan, Q., Wu, X. M., et al. (2020). The Runx1/Notch1 signaling pathway participates in M1/M2 microglia polarization in a mouse model of temporal lobe epilepsy and in BV-2 Cells. Neurochem. Res. 45, 2204–2216. doi: 10.1007/s11064-020-03082-3
Derkach, V., Barria, A., and Soderling, T. R. (1999). Ca2+/calmodulin-kinase II enhances channel conductance of alpha-amino-3-hydroxy-5-methyl-4-isoxazolepropionate type glutamate receptors. Proc. Natl. Acad. Sci. U.S.A. 96, 3269–3274. doi: 10.1073/pnas.96.6.3269
DeVos, S. L., Goncharoff, D. K., Chen, G., Kebodeaux, C. S., Yamada, K., Stewart, F. R., et al. (2013). Antisense reduction of tau in adult mice protects against seizures. J. Neurosci. 33, 12887–12897. doi: 10.1523/JNEUROSCI.2107-13.2013
Driver, J. A., and Lu, K. P. (2010). Pin1: A new genetic link between Alzheimer’s disease, cancer and aging. Curr. Aging Sci. 3, 158–165.
Driver, J. A., Zhou, X. Z., and Lu, K. P. (2015). Pin1 dysregulation helps to explain the inverse association between cancer and Alzheimer’s disease. Biochim. Biophys. Acta 1850, 2069–2076. doi: 10.1016/j.bbagen.2014.12.025
Duan, W., Chen, Y., and Wang, X. R. (2018). MicroRNA-155 contributes to the occurrence of epilepsy through the PI3K/Akt/mTOR signaling pathway. Int. J. Mol. Med. 42, 1577–1584. doi: 10.3892/ijmm.2018.3711
Fagiani, F., Govoni, S., Racchi, M., and Lanni, C. (2021). The peptidyl-prolyl isomerase Pin1 in neuronal signaling: From neurodevelopment to neurodegeneration. Mol. Neurobiol. 58, 1062–1073. doi: 10.1007/s12035-020-02179-8
Fang, M., Shen, L., Yin, H., Pan, Y. M., Wang, L., Chen, D., et al. (2011). Downregulation of gephyrin in temporal lobe epilepsy neurons in humans and a rat model. Synapse 65, 1006–1014. doi: 10.1002/syn.20928
Feng, D., Yao, J., Wang, G., Li, Z., Zu, G., Li, Y., et al. (2017). Inhibition of p66Shc-mediated mitochondrial apoptosis via targeting prolyl-isomerase Pin1 attenuates intestinal ischemia/reperfusion injury in rats. Clin. Sci. 131, 759–773. doi: 10.1042/CS20160799
Fisher, R. S., van Emde Boas, W., Blume, W., Elger, C., Genton, P., Lee, P., et al. (2005). epileptic seizures and epilepsy: Definitions proposed by the international league against epilepsy (ILAE) and the International Bureau for Epilepsy (IBE). Epilepsia 46, 470–472.
Förstera, B., Belaidi, A. A., Jüttner, R., Bernert, C., Tsokos, M., Lehmann, T. N., et al. (2010). Irregular RNA splicing curtails postsynaptic gephyrin in the cornu ammonis of patients with epilepsy. Brain 133, 3778–3794. doi: 10.1093/brain/awq298
Frasca, A., Aalbers, M., Frigerio, F., Fiordaliso, F., Salio, M., Gobbi, M., et al. (2011). Misplaced NMDA receptors in epileptogenesis contribute to excitotoxicity. Neurobiol. Dis. 43, 507–515. doi: 10.1016/j.nbd.2011.04.024
French, J. A., Krauss, G. L., Biton, V., Squillacote, D., Yang, H., Laurenza, A., et al. (2012). Adjunctive perampanel for refractory partial-onset seizures: Randomized phase III study 304. Neurology 79, 589–596.
French, J. A., Krauss, G. L., Steinhoff, B. J., Squillacote, D., Yang, H., Kumar, D., et al. (2013). Evaluation of adjunctive perampanel in patients with refractory partial-onset seizures: Results of randomized global phase III study 305. Epilepsia 54, 117–125. doi: 10.1111/j.1528-1167.2012.03638.x
Fu, H., Xu, H., Chen, H., Li, Y., Li, W., Zhu, Q., et al. (2014). Inhibition of glycogen synthase kinase 3 ameliorates liver ischemia/reperfusion injury via an energy-dependent mitochondrial mechanism. J. Hepatol. 61, 816–824. doi: 10.1016/j.jhep.2014.05.017
Gheyara, A. L., Ponnusamy, R., Djukic, B., Craft, R. J., Ho, K., Guo, W., et al. (2014). Tau reduction prevents disease in a mouse model of Dravet syndrome. Ann. Neurol. 76, 443–456. doi: 10.1002/ana.24230
Goel, A., Xu, L. W., Snyder, K. P., Song, L., Goenaga-Vazquez, Y., Megill, A., et al. (2011). Phosphorylation of AMPA receptors is required for sensory deprivation-induced homeostatic synaptic plasticity. PLoS One 6:e18264. doi: 10.1371/journal.pone.0018264
Greengard, P., Jen, J., Nairn, A. C., and Stevens, C. F. (1991). Enhancement of the glutamate response by cAMP-dependent protein kinase in hippocampal neurons. Science 253, 1135–1138.
Hayashi, Y., Shi, S. H., Esteban, J. A., Piccini, A., Poncer, J. C., and Malinow, R. (2000). Driving AMPA receptors into synapses by LTP and CaMKII: Requirement for GluR1 and PDZ domain interaction. Science 287, 2262–2267. doi: 10.1126/science.287.5461.2262
He, J., Lau, A. G., Yaffe, M. B., and Hall, R. A. (2001). Phosphorylation and cell cycle-dependent regulation of Na+/H+ exchanger regulatory factor-1 by Cdc2 kinase. J. Biol. Chem. 276, 41559–41565. doi: 10.1074/jbc.M106859200
He, K., Goel, A., Ciarkowski, C. E., Song, L., and Lee, H. K. (2011). Brain area specific regulation of synaptic AMPA receptors by phosphorylation. Commun. Integr. Biol. 4, 569–572.
Henshall, D. C., and Engel, T. (2013). Contribution of apoptosis-associated signaling pathways to epileptogenesis: Lessons from Bcl-2 family knockouts. Front. Cell. Neurosci. 7:110. doi: 10.3389/fncel.2013.00110
Hilton, B. A., Li, Z., Musich, P. R., Wang, H., Cartwright, B. M., Serrano, M., et al. (2015). ATR plays a direct antiapoptotic role at mitochondria, which is regulated by prolyl isomerase Pin1. Mol. Cell 60, 35–46.
Hou, X., Yang, F., Li, A., Zhao, D., Ma, N., Chen, L., et al. (2021). The Pin1-CaMKII-AMPA receptor axis regulates epileptic susceptibility. Cereb. Cortex 31, 3082–3095. doi: 10.1093/cercor/bhab004
Hu, F., Shao, L., Zhang, J., Zhang, H., Wen, A., and Zhang, P. (2020). Knockdown of ZFAS1 inhibits hippocampal neurons apoptosis and autophagy by activating the PI3K/AKT Pathway via Up-regulating miR-421 in Epilepsy. Neurochem. Res. 45, 2433–2441. doi: 10.1007/s11064-020-03103-1
Hu, J. H., Malloy, C., Tabor, G. T., Gutzmann, J. J., Liu, Y., Abebe, D., et al. (2020). Activity-dependent isomerization of Kv4.2 by Pin1 regulates cognitive flexibility. Nat. Commun. 11:1567. doi: 10.1038/s41467-020-15390-x
Huang, H., Liu, H., Yan, R., and Hu, M. (2017). PI3K/Akt and ERK/MAPK signaling promote different aspects of neuron survival and axonal regrowth following rat facial nerve axotomy. Neurochem. Res. 42, 3515–3524. doi: 10.1007/s11064-017-2399-1
Janning, D., Igaev, M., Sündermann, F., Brühmann, J., Beutel, O., Heinisch, J. J., et al. (2014). Single-molecule tracking of tau reveals fast kiss-and-hop interaction with microtubules in living neurons. Mol. Biol. Cell 25, 3541–3551. doi: 10.1091/mbc.E14-06-1099
Jenkins, M. A., and Traynelis, S. F. (2012). PKC phosphorylates GluA1-Ser831 to enhance AMPA receptor conductance. Channels 6, 60–64. doi: 10.4161/chan.18648
Kameyama, K., Lee, H. K., Bear, M. F., and Huganir, R. L. (1998). Involvement of a postsynaptic protein kinase A substrate in the expression of homosynaptic long-term depression. Neuron 21, 1163–1175. doi: 10.1016/s0896-6273(00)80633-9
Kankowski, S., Förstera, B., Winkelmann, A., Knauff, P., Wanker, E. E., You, X. A., et al. (2017). A novel RNA editing sensor tool and a specific agonist determine neuronal protein expression of RNA-edited glycine receptors and identify a genomic APOBEC1 dimorphism as a new genetic risk factor of epilepsy. Front. Mol. Neurosci. 10:439. doi: 10.3389/fnmol.2017.00439
Keeney, J. T., Swomley, A. M., Harris, J. L., Fiorini, A., Mitov, M. I., Perluigi, M., et al. (2012). Cell cycle proteins in brain in mild cognitive impairment: Insights into progression to Alzheimer disease. Neurotox. Res. 22, 220–230.
Kim, J. E., Choi, H. C., Song, H. K., and Kang, T. C. (2019). Perampanel affects up-stream regulatory signaling pathways of glua1 phosphorylation in normal and epileptic rats. Front. Cell. Neurosci. 13:80. doi: 10.3389/fncel.2019.00080
Kittler, J. T., and Moss, S. J. (eds) (2006). The Dynamic Synapse: Molecular Methods in Ionotropic Receptor Biology. Boca Raton, FL: CRC Press.
Knapp, A. G., Schmidt, K. F., and Dowling, J. E. (1990). Dopamine modulates the kinetics of ion channels gated by excitatory amino acids in retinal horizontal cells. Proc. Natl. Acad. Sci. U.S.A. 87, 767–771.
Kneussel, M., Brandstätter, J. H., Laube, B., Stahl, S., Müller, U., and Betz, H. (1999). Loss of postsynaptic GABA(A) receptor clustering in gephyrin-deficient mice. J. Neurosci. 19, 9289–9297.
Kondo, A., Shahpasand, K., Mannix, R., Qiu, J., Moncaster, J., Chen, C. H., et al. (2015). Antibody against early driver of neurodegeneration cis P-tau blocks brain injury and tauopathy. Nature 523, 431–436. doi: 10.1038/nature14658
Krapivinsky, G., Medina, I., Krapivinsky, L., Gapon, S., and Clapham, D. E. (2004). SynGAP-MUPP1-CaMKII synaptic complexes regulate p38 MAP kinase activity and NMDA receptor-dependent synaptic AMPA receptor potentiation. Neuron 43, 563–574. doi: 10.1016/j.neuron.2004.08.003
Krauss, G. L., Serratosa, J. M., Villanueva, V., Endziniene, M., Hong, Z., French, J., et al. (2012). Randomized phase III study 306: Adjunctive perampanel for refractory partial-onset seizures. Neurology 78, 1408–1415.
Kristensen, A. S., Jenkins, M. A., Banke, T. G., Schousboe, A., Makino, Y., Johnson, R. C., et al. (2011). Mechanism of Ca2+/calmodulin-dependent kinase II regulation of AMPA receptor gating. Nat. Neurosci. 14, 727–735. doi: 10.1038/nn.2804
Ladépêche, L., Dupuis, J. P., and Groc, L. (2014). Surface trafficking of NMDA receptors: Gathering from a partner to another. Semin. Cell Dev. Biol. 27, 3–13. doi: 10.1016/j.semcdb.2013.10.005
LaFerla, F. M., Hall, C. K., Ngo, L., and Jay, G. (1996). Extracellular deposition of beta-amyloid upon p53-dependent neuronal cell death in transgenic mice. J. Clin. Invest. 98, 1626–1632. doi: 10.1172/JCI118957
Lathia, J. D., Mattson, M. P., and Cheng, A. (2008). Notch: From neural development to neurological disorders. J. Neurochem. 107, 1471–1481.
Lee, H. K. (2006a). “Frontiers in Neuroscience AMPA Receptor Phosphorylation in Synaptic Plasticity: Insights from Knockin Mice,” in The Dynamic Synapse: Molecular Methods in Ionotropic Receptor Biology, eds J. T. Kittler and S. J. Moss (Boca Raton, FL: CRC Press).
Li, J., Mo, C., Guo, Y., Zhang, B., Feng, X., Si, Q., et al. (2021). Roles of peptidyl-prolyl isomerase Pin1 in disease pathogenesis. Theranostics 11, 3348–3358.
Li, Q. M., Tep, C., Yune, T. Y., Zhou, X. Z., Uchida, T., Lu, K. P., et al. (2007). Opposite regulation of oligodendrocyte apoptosis by JNK3 and Pin1 after spinal cord injury. J. Neurosci. 27, 8395–8404.
Lin, C. H., Li, H. Y., Lee, Y. C., Calkins, M. J., Lee, K. H., Yang, C. N., et al. (2015). Landscape of Pin1 in the cell cycle. Exp. Biol. Med. 240, 403–408. doi: 10.1177/1535370215570829
Liou, Y. C., Zhou, X. Z., and Lu, K. P. (2011). Prolyl isomerase Pin1 as a molecular switch to determine the fate of phosphoproteins. Trends Biochem. Sci. 36, 501–514.
Lippman-Bell, J. J., Zhou, C., Sun, H., Feske, J. S., and Jensen, F. E. (2016). Early-life seizures alter synaptic calcium-permeable AMPA receptor function and plasticity. Mol. Cell. Neurosci. 76, 11–20. doi: 10.1016/j.mcn.2016.08.002
Liu, K., Zhu, J., Chang, Y., Lin, Z., Shi, Z., Li, X., et al. (2021). Attenuation of cerebral edema facilitates recovery of glymphatic system function after status epilepticus. JCI Insight 6:e151835. doi: 10.1172/jci.insight.151835
Liu, N. X., and Li, Q. H. (2020). LncRNA BC200 regulates neuron apoptosis and neuroinflammation via PI3K/AKT pathway in Alzheimer’s disease. J. Biol. Regul. Homeost. Agents 34, 2255–2261. doi: 10.23812/20-498-L
Liu, S. J., Zheng, P., Wright, D. K., Dezsi, G., Braine, E., Nguyen, T., et al. (2016). Sodium selenate retards epileptogenesis in acquired epilepsy models reversing changes in protein phosphatase 2A and hyperphosphorylated tau. Brain 139, 1919–1938. doi: 10.1093/brain/aww116
Liu, X., Yang, Z., Yin, Y., and Deng, X. (2014). Increased expression of Notch1 in temporal lobe epilepsy: Animal models and clinical evidence. Neural Regene. Res. 9, 526–533. doi: 10.4103/1673-5374.130083
Lu, K. P., and Zhou, X. Z. (2007). The prolyl isomerase PIN1: A pivotal new twist in phosphorylation signalling and disease. Nat. Rev. Mol. Cell Biol. 8, 904–916. doi: 10.1038/nrm2261
Lu, K. P., Finn, G., Lee, T. H., and Nicholson, L. K. (2007). Prolyl cis-trans isomerization as a molecular timer. Nat. Chem. Biol. 3, 619–629.
Lu, K. P., Hanes, S. D., and Hunter, T. (1996). A human peptidyl-prolyl isomerase essential for regulation of mitosis. Nature 380, 544–547.
Lu, P. J., Zhou, X. Z., Shen, M., and Lu, K. P. (1999). Function of WW domains as phosphoserine- or phosphothreonine-binding modules. Science 283, 1325–1328.
Luh, L. M., Hänsel, R., Löhr, F., Kirchner, D. K., Krauskopf, K., Pitzius, S., et al. (2013). Molecular crowding drives active Pin1 into nonspecific complexes with endogenous proteins prior to substrate recognition. J. Am. Chem. Soc. 135, 13796–13803. doi: 10.1021/ja405244v
Luna-Muñoz, J., Chávez-Macías, L., García-Sierra, F., and Mena, R. (2007). Earliest stages of tau conformational changes are related to the appearance of a sequence of specific phospho-dependent tau epitopes in Alzheimer’s disease. J. Alzheimers Dis. 12, 365–375. doi: 10.3233/jad-2007-12410
Lynch, J. W., Zhang, Y., Talwar, S., and Estrada-Mondragon, A. (2017). Glycine receptor drug discovery. Adv. Pharmacol. 79, 225–253.
Macha, A., Liebsch, F., Fricke, S., Hetsch, F., Neuser, F., Johannes, L., et al. (2022). Biallelic gephyrin variants lead to impaired GABAergic inhibition in a patient with developmental and epileptic encephalopathy. Hum. Mol. Genet. 31, 901–913. doi: 10.1093/hmg/ddab298
Machado, R. A., Benjumea-Cuartas, V., Zapata Berruecos, J. F., Agudelo-Flóres, P. M., and Salazar-Peláez, L. M. (2019). Reelin, tau phosphorylation and psychiatric complications in patients with hippocampal sclerosis and structural abnormalities in temporal lobe epilepsy. Epilepsy Behav. 96, 192–199. doi: 10.1016/j.yebeh.2019.04.052
Moshé, S. L., Perucca, E., Ryvlin, P., and Tomson, T. (2015). Epilepsy: New advances. Lancet 385, 884–898.
Nakamura, K., Greenwood, A., Binder, L., Bigio, E. H., Denial, S., Nicholson, L., et al. (2012a). Proline isomer-specific antibodies reveal the early pathogenic tau conformation in Alzheimer’s disease. Cell 149, 232–244. doi: 10.1016/j.cell.2012.02.016
Nakamura, K., Kosugi, I., Lee, D. Y., Hafner, A., Sinclair, D. A., Ryo, A., et al. (2012b). Prolyl isomerase Pin1 regulates neuronal differentiation via β-catenin. Mol. Cell. Biol. 32, 2966–2978. doi: 10.1128/MCB.05688-11
Nakatsu, Y., Mori, K., Matsunaga, Y., Yamamotoya, T., Ueda, K., Inoue, Y., et al. (2017). The prolyl isomerase Pin1 increases β-cell proliferation and enhances insulin secretion. J. Biol. Chem. 292, 11886–11895. doi: 10.1074/jbc.M117.780726
Nakatsu, Y., Sakoda, H., Kushiyama, A., Zhang, J., Ono, H., Fujishiro, M., et al. (2011). Peptidyl-prolyl cis/trans isomerase NIMA-interacting 1 associates with insulin receptor substrate-1 and enhances insulin actions and adipogenesis. J. Biol. Chem. 286, 20812–20822. doi: 10.1074/jbc.M110.206904
Naylor, D. E., Liu, H., Niquet, J., and Wasterlain, C. G. (2013). Rapid surface accumulation of NMDA receptors increases glutamatergic excitation during status epilepticus. Neurobiol. Dis. 54, 225–238. doi: 10.1016/j.nbd.2012.12.015
Newton, A. C. (2001). Protein kinase C: Structural and spatial regulation by phosphorylation, cofactors, and macromolecular interactions. Chem. Rev. 101, 2353–2364. doi: 10.1021/cr0002801
Oh, M. C., Derkach, V. A., Guire, E. S., and Soderling, T. R. (2006). Extrasynaptic membrane trafficking regulated by GluR1 serine 845 phosphorylation primes AMPA receptors for long-term potentiation. J. Biol. Chem. 281, 752–758. doi: 10.1074/jbc.M509677200
Opazo, P., Sainlos, M., and Choquet, D. (2012). Regulation of AMPA receptor surface diffusion by PSD-95 slots. Curr. Opin. Neurobiol. 22, 453–460. doi: 10.1016/j.conb.2011.10.010
Oyrer, J., Maljevic, S., Scheffer, I. E., Berkovic, S. F., Petrou, S., and Reid, C. A. (2018). Ion channels in genetic epilepsy: From genes and mechanisms to disease-targeted therapies. Pharmacol. Rev. 70, 142–173. doi: 10.1124/pr.117.014456
Partida, G. J., Fasoli, A., Fogli Iseppe, A., Ogata, G., Johnson, J. S., Thambiaiyah, V., et al. (2018). Autophosphorylated CaMKII facilitates spike propagation in rat optic nerve. J. Neurosci. 38, 8087–8105. doi: 10.1523/JNEUROSCI.0078-18.2018
Paudel, Y. N., Angelopoulou, E., Jones, N. C., O’Brien, T. J., Kwan, P., Piperi, C., et al. (2019). Tau related pathways as a connecting link between epilepsy and Alzheimer’s disease. ACS Chem. Neurosci. 10, 4199–4212. doi: 10.1021/acschemneuro.9b00460
Peng, Y., Zhao, J., Gu, Q. H., Chen, R. Q., Xu, Z., Yan, J. Z., et al. (2010). Distinct trafficking and expression mechanisms underlie LTP and LTD of NMDA receptor-mediated synaptic responses. Hippocampus 20, 646–658. doi: 10.1002/hipo.20654
Perucca, P., Smith, G., Santana-Gomez, C., Bragin, A., and Staba, R. (2019). Electrophysiological biomarkers of epileptogenicity after traumatic brain injury. Neurobiol. Dis. 123, 69–74.
Puhahn-Schmeiser, B., Leicht, K., Gessler, F., and Freiman, T. M. (2021). Aberrant hippocampal mossy fibers in temporal lobe epilepsy target excitatory and inhibitory neurons. Epilepsia 62, 2539–2550. doi: 10.1111/epi.17035
Qiu, C., Albayram, O., Kondo, A., Wang, B., Kim, N., Arai, K., et al. (2021). Cis P-tau underlies vascular contribution to cognitive impairment and dementia and can be effectively targeted by immunotherapy in mice. Sci. Transl. Med. 13:eaaz7615. doi: 10.1126/scitranslmed.aaz7615
Ramakrishnan, P., Dickson, D. W., and Davies, P. (2003). Pin1 colocalization with phosphorylated tau in Alzheimer’s disease and other tauopathies. Neurobiol. Dis. 14, 251–264. doi: 10.1016/s0969-9961(03)00109-8
Ranganathan, R., Lu, K. P., Hunter, T., and Noel, J. P. (1997). Structural and functional analysis of the mitotic rotamase Pin1 suggests substrate recognition is phosphorylation dependent. Cell 89, 875–886. doi: 10.1016/s0092-8674(00)80273-1
Rellos, P., Pike, A. C., Niesen, F. H., Salah, E., Lee, W. H., von Delft, F., et al. (2010). Structure of the CaMKIIdelta/calmodulin complex reveals the molecular mechanism of CaMKII kinase activation. PLoS Biol. 8:e1000426. doi: 10.1371/journal.pbio.1000426
Risal, P., Shrestha, N., Chand, L., Sylvester, K. G., and Jeong, Y. J. (2017). Involvement of prolyl isomerase PIN1 in the cell cycle progression and proliferation of hepatic oval cells. Pathol. Res. Pract. 213, 373–380. doi: 10.1016/j.prp.2017.01.005
Roberson, Halabisky, B., Yoo, J. W., Yao, J., Chin, J., Yan, F., et al. (2011). Amyloid-β/Fyn-induced synaptic, network, and cognitive impairments depend on tau levels in multiple mouse models of Alzheimer’s disease. J. Neurosci. 31, 700–711. doi: 10.1523/JNEUROSCI.4152-10.2011
Roche, K. W., O’Brien, R. J., Mammen, A. L., Bernhardt, J., and Huganir, R. L. (1996). Characterization of multiple phosphorylation sites on the AMPA receptor GluR1 subunit. Neuron 16, 1179–1188. doi: 10.1016/s0896-6273(00)80144-0
Rogawski, M. A. (2013). AMPA receptors as a molecular target in epilepsy therapy. Acta Neurol. Scand. Suppl. 9–18. doi: 10.1111/ane.12099
Rogawski, M. A., and Donevan, S. D. (1999). AMPA receptors in epilepsy and as targets for antiepileptic drugs. Adv. Neurol. 79, 947–963.
Rohracher, A., Kalss, G., Kuchukhidze, G., Neuray, C., Leitinger, M., Höfler, J., et al. (2021). New anti-seizure medication for elderly epilepsy patients - a critical narrative review. Expert Opin. Pharmacother. 22, 621–634. doi: 10.1080/14656566.2020.1843636
Rong, X. F., Sun, Y. N., Liu, D. M., Yin, H. J., Peng, Y., Xu, S. F., et al. (2017). The pathological roles of NDRG2 in Alzheimer’s disease, a study using animal models and APPwt-overexpressed cells. CNS Neurosci. Ther. 23, 667–679.
Rudrabhatla, P., and Pant, H. C. (2010). Phosphorylation-specific peptidyl-prolyl isomerization of neuronal cytoskeletal proteins by Pin1: Implications for therapeutics in neurodegeneration. J. Alzheimers Dis. 19, 389–403. doi: 10.3233/JAD-2010-1243
Rustighi, A., Tiberi, L., Soldano, A., Napoli, M., Nuciforo, P., Rosato, A., et al. (2009). The prolyl-isomerase Pin1 is a Notch1 target that enhances Notch1 activation in cancer. Nat. Cell Biol. 11, 133–142.
Sargin, D., Botly, L. C., Higgs, G., Marsolais, A., Frankland, P. W., Egan, S. E., et al. (2013). Disrupting Jagged1-Notch signaling impairs spatial memory formation in adult mice. Neurobiol. Learn. Mem. 103, 39–49.
Shahpasand, K., Sepehri Shamloo, A., Nabavi, S. M., Ping Lu, K., and Zhen Zhou, X. (2018). Tau immunotherapy: Hopes and hindrances. Hum. Vaccines Immunother. 14, 277–284. doi: 10.1080/21645515.2017.1393594
Shepherd, J. D., and Huganir, R. L. (2007). The cell biology of synaptic plasticity: AMPA receptor trafficking. Annu. Rev. Cell Dev. Biol. 23, 613–643.
Shimizu, T., Kanai, K., Sugawara, Y., Uchida, C., and Uchida, T. (2018). Prolyl Isomerase Pin1 directly regulates calcium/calmodulin-dependent protein kinase II activity in mouse brains. Front. Pharmacol. 9:1351. doi: 10.3389/fphar.2018.01351
Sibbe, M., Häussler, U., Dieni, S., Althof, D., Haas, C. A., and Frotscher, M. (2012). Experimental epilepsy affects Notch1 signalling and the stem cell pool in the dentate gyrus. Eur. J. Neurosci. 36, 3643–3652. doi: 10.1111/j.1460-9568.2012.08279.x
Smet-Nocca, C., Launay, H., Wieruszeski, J. M., Lippens, G., and Landrieu, I. (2013). Unraveling a phosphorylation event in a folded protein by NMR spectroscopy: Phosphorylation of the Pin1 WW domain by PKA. J. Biomol. NMR 55, 323–337. doi: 10.1007/s10858-013-9716-z
Srivastava, P., Dhuriya, Y. K., Kumar, V., Srivastava, A., Gupta, R., Shukla, R. K., et al. (2018). PI3K/Akt/GSK3β induced CREB activation ameliorates arsenic mediated alterations in NMDA receptors and associated signaling in rat hippocampus: Neuroprotective role of curcumin. Neurotoxicology 67, 190–205. doi: 10.1016/j.neuro.2018.04.018
Steinberg, S. F. (2008). Structural basis of protein kinase C isoform function. Physiol. Rev. 88, 1341–1378.
Sultana, R., Boyd-Kimball, D., Poon, H. F., Cai, J., Pierce, W. M., Klein, J. B., et al. (2006). Oxidative modification and down-regulation of Pin1 in Alzheimer’s disease hippocampus: A redox proteomics analysis. Neurobiol. Aging 27, 918–925. doi: 10.1016/j.neurobiolaging.2005.05.005
Takahashi, K., Uchida, C., Shin, R. W., Shimazaki, K., and Uchida, T. (2008). Prolyl isomerase, Pin1: New findings of post-translational modifications and physiological substrates in cancer, asthma and Alzheimer’s disease. Cell. Mol. Life Sci. 65, 359–375. doi: 10.1007/s00018-007-7270-0
Tang, L., Zhang, Y., Chen, G., Xiong, Y., Wang, X., and Zhu, B. (2017). Down-regulation of Pin1 in temporal lobe epilepsy patients and mouse model. Neurochem. Res. 42, 1211–1218. doi: 10.1007/s11064-016-2158-8
Tatara, Y., Terakawa, T., and Uchida, T. (2010). Identification of Pin1-binding phosphorylated proteins in the mouse brain. Biosci. Biotechnol. Biochem. 74, 2480–2483. doi: 10.1271/bbb.100580
Traynelis, S. F., Wollmuth, L. P., McBain, C. J., Menniti, F. S., Vance, K. M., Ogden, K. K., et al. (2010). Glutamate receptor ion channels: Structure, regulation, and function. Pharmacol. Rev. 62, 405–496.
Tretter, V., Mukherjee, J., Maric, H. M., Schindelin, H., Sieghart, W., and Moss, S. J. (2012). Gephyrin, the enigmatic organizer at GABAergic synapses. Front. Cell. Neurosci. 6:23. doi: 10.3389/fncel.2012.00023
Tun-Kyi, A., Finn, G., Greenwood, A., Nowak, M., Lee, T. H., Asara, J. M., et al. (2011). Essential role for the prolyl isomerase Pin1 in Toll-like receptor signaling and type I interferon-mediated immunity. Nat. Immunol. 12, 733–741. doi: 10.1038/ni.2069
Turrigiano, G. G., and Nelson, S. B. (2004). Homeostatic plasticity in the developing nervous system. Nat. Rev. Neurosci. 5, 97–107.
Van Liefferinge, J., Massie, A., Portelli, J., Di Giovanni, G., and Smolders, I. (2013). Are vesicular neurotransmitter transporters potential treatment targets for temporal lobe epilepsy? Front. Cell. Neurosci. 7:139. doi: 10.3389/fncel.2013.00139
Vannini, E., Restani, L., Dilillo, M., McDonnell, L. A., Caleo, M., and Marra, V. (2020). Synaptic vesicles dynamics in neocortical epilepsy. Front. Cell. Neurosci. 14:606142. doi: 10.3389/fncel.2020.606142
Varoqueaux, F., Jamain, S., and Brose, N. (2004). Neuroligin 2 is exclusively localized to inhibitory synapses. Eur. J. Cell Biol. 83, 449–456.
Wang, L., Zhou, Y., Chen, D., and Lee, T. H. (2020). peptidyl-prolyl Cis/trans isomerase Pin1 and Alzheimer’s disease. Front. Cell Dev. Biol. 8:355. doi: 10.3389/fcell.2020.00355
Wang, S. C., Hu, X. M., and Xiong, K. (2023). The regulatory role of Pin1 in neuronal death. Neural Regen. Res. 18, 74–80.
Wang, Y., Wang, W., Li, D., Li, M., Wang, P., Wen, J., et al. (2014). IGF-1 alleviates NMDA-induced excitotoxicity in cultured hippocampal neurons against autophagy via the NR2B/PI3K-AKT-mTOR pathway. J. Cell. Physiol. 229, 1618–1629. doi: 10.1002/jcp.24607
Wang, Y., Wu, J., Guo, R., Zhao, Y., Wang, Y., Zhang, M., et al. (2013). Surgical incision induces phosphorylation of AMPA receptor GluR1 subunits at Serine-831 sites and GluR1 trafficking in spinal cord dorsal horn via a protein kinase Cγ-dependent mechanism. Neuroscience 240, 361–370. doi: 10.1016/j.neuroscience.2013.02.051
Wei, H., Duan, G., He, J., Meng, Q., Liu, Y., Chen, W., et al. (2018). Geniposide attenuates epilepsy symptoms in a mouse model through the PI3K/Akt/GSK-3β signaling pathway. Exp. Therapeutic Med. 15, 1136–1142. doi: 10.3892/etm.2017.5512
Winkelmann, A., Maggio, N., Eller, J., Caliskan, G., Semtner, M., Häussler, U., et al. (2014). Changes in neural network homeostasis trigger neuropsychiatric symptoms. J. Clin. Investig. 124, 696–711. doi: 10.1172/JCI71472
Yang, H., Zhang, P., Li, J., Gao, Y., Zhao, L., Li, J., et al. (2020). Targeting PIN-1 attenuates GCB DLBCL cell proliferation through inhibition of PI3K/AKT Signaling. Onco Targets Ther. 13, 8593–8600. doi: 10.2147/OTT.S247429
Yang, Y., and Igumenova, T. I. (2013). The C-terminal V5 domain of protein kinase Cα is intrinsically disordered, with propensity to associate with a membrane mimetic. PLoS One 8:e65699. doi: 10.1371/journal.pone.0065699
Yu, G. H., and Fang, Y. (2022). Resveratrol attenuates atherosclerotic endothelial injury through the Pin1/Notch1 pathway. Toxicol. Appl. Pharmacol. 446:116047. doi: 10.1016/j.taap.2022.116047
Yu, J. H., Im, C. Y., and Min, S. H. (2020). Function of PIN1 in cancer development and its inhibitors as cancer therapeutics. Front. Cell Dev. Biol. 8:120. doi: 10.3389/fcell.2020.00120
Yuan, W. C., Lee, Y. R., Huang, S. F., Lin, Y. M., Chen, T. Y., Chung, H. C., et al. (2011). A Cullin3-KLHL20 Ubiquitin ligase-dependent pathway targets PML to potentiate HIF-1 signaling and prostate cancer progression. Cancer Cell 20, 214–228. doi: 10.1016/j.ccr.2011.07.008
Zacchi, P., Antonelli, R., and Cherubini, E. (2014). Gephyrin phosphorylation in the functional organization and plasticity of GABAergic synapses. Front. Cell. Neurosci. 8:103. doi: 10.3389/fncel.2014.00103
Zafra, F., Ibáñez, I., Bartolomé-Martín, D., Piniella, D., Arribas-Blázquez, M., and Giménez, C. (2017). Glycine transporters and its coupling with NMDA receptors. Adv. Neurobiol. 16, 55–83.
Zannini, A., Rustighi, A., Campaner, E., and Del Sal, G. (2019). Oncogenic hijacking of the PIN1 signaling network. Front. Oncol. 9:94. doi: 10.3389/fonc.2019.00094
Zeng, B., Li, Y., Niu, B., Wang, X., Cheng, Y., Zhou, Z., et al. (2016). Involvement of PI3K/Akt/FoxO3a and PKA/CREB signaling pathways in the protective effect of fluoxetine against corticosterone-induced cytotoxicity in PC12 cells. J. Mol. Neurosci. 59, 567–578. doi: 10.1007/s12031-016-0779-7
Zhang, F., Ye, J., Meng, Y., Ai, W., Su, H., Zheng, J., et al. (2018). calcium supplementation enhanced adipogenesis and improved glucose homeostasis through activation of camkii and PI3K/Akt signaling pathway in porcine bone marrow mesenchymal stem cells (pBMSCs) and mice fed high fat diet (HFD). Cell. Physiol. Biochem. 51, 154–172. doi: 10.1159/000495171
Zhang, Y., Lv, Z., Liu, Y., Cao, H., Yang, J., and Wang, B. (2021). PIN1 protects hair cells and auditory HEI-OC1 cells against senescence by inhibiting the PI3K/Akt/mTOR pathway. Oxid. Med. Cell. Longev. 2021:9980444. doi: 10.1155/2021/9980444
Zhang, Z., Yu, W., Zheng, M., Liao, X., Wang, J., Yang, D., et al. (2019). Pin1 inhibition potently suppresses gastric cancer growth and blocks PI3K/AKT and Wnt/β-catenin oncogenic pathways. Mol. Carcinog. 58, 1450–1464. doi: 10.1002/mc.23027
Zhao, J. H., Wang, B., Wang, X. H., and Xu, C. W. (2019). Effect of lncRNA GAS5 on the apoptosis of neurons via the notch1 signaling pathway in rats with cerebral infarction. Eur. Rev. Med. Pharmacol. Sci. 23, 10083–10091. doi: 10.26355/eurrev_201911_19576
Zhou, X. Z., Kops, O., Werner, A., Lu, P. J., Shen, M., Stoller, G., et al. (2000). Pin1-dependent prolyl isomerization regulates dephosphorylation of Cdc25C and tau proteins. Mol. Cell 6, 873–883. doi: 10.1016/s1097-2765(05)00083-3
Zhou, X., and Lu, K. (2016). The isomerase PIN1 controls numerous cancer-driving pathways and is a unique drug target. Nat. Rev. Cancer 16, 463–478.
Keywords: Pin1, neuronal signaling, neurodegeneration, epilepsy, synapses
Citation: Chen Y, Hou X, Pang J, Yang F, Li A, Lin S, Lin N, Lee TH and Liu H (2022) The role of peptidyl-prolyl isomerase Pin1 in neuronal signaling in epilepsy. Front. Mol. Neurosci. 15:1006419. doi: 10.3389/fnmol.2022.1006419
Received: 29 July 2022; Accepted: 20 September 2022;
Published: 11 October 2022.
Edited by:
Rochelle Marie Hines, University of Nevada, Las Vegas, United StatesReviewed by:
Yue Zou, University of Toledo, United StatesCopyright © 2022 Chen, Hou, Pang, Yang, Li, Lin, Lin, Lee and Liu. This is an open-access article distributed under the terms of the Creative Commons Attribution License (CC BY). The use, distribution or reproduction in other forums is permitted, provided the original author(s) and the copyright owner(s) are credited and that the original publication in this journal is cited, in accordance with accepted academic practice. No use, distribution or reproduction is permitted which does not comply with these terms.
*Correspondence: Hekun Liu, ZmpsaGtAMTYzLmNvbQ==
Disclaimer: All claims expressed in this article are solely those of the authors and do not necessarily represent those of their affiliated organizations, or those of the publisher, the editors and the reviewers. Any product that may be evaluated in this article or claim that may be made by its manufacturer is not guaranteed or endorsed by the publisher.
Research integrity at Frontiers
Learn more about the work of our research integrity team to safeguard the quality of each article we publish.