- Department of Surgery and Biomedical Sciences, Marshall University Joan C. Edwards School of Medicine, Huntington, WV, United States
There has been a progressive increase in the prevalence of obesity and its comorbidities such as type 2 diabetes and cardiovascular diseases worldwide. Recent studies have suggested that the crosstalk between adipose tissue and central nervous system (CNS), through cellular mediators and signaling pathways, may causally link obesity with cognitive decline and give rise to neurodegenerative disorders. Several mechanisms have been proposed in obesity, including inflammation, oxidative stress, insulin resistance, altered lipid and cholesterol homeostasis, which may result in neuroinflammation, altered brain insulin signaling, amyloid-beta (Aβ) deposition and neuronal cell death. Since obesity is associated with functional and morphological alterations in the adipose tissues, the resulting peripheral immune response augments the development and progression of cognitive decline and increases susceptibility of neurodegenerative disorders, such as Alzheimer’s Disease (AD) and Parkinson’s Disease (PD). Studies have also elucidated an important role of high fat diet in the exacerbation of these clinical conditions. However, the underlying factors that propel and sustain this obesity associated cognitive decline and neurodegeneration, remains highly elusive. Moreover, the mechanisms linking these phenomena are not well-understood. The cumulative line of evidence have demonstrated an important role of microRNAs (miRNAs), a class of small non-coding RNAs that regulate gene expression and transcriptional changes, as biomarkers of pathophysiological conditions. Despite the lack of utility in current clinical practices, miRNAs have been shown to be highly specific and sensitive to the clinical condition being studied. Based on these observations, this review aims to assess the role of several miRNAs and aim to elucidate underlying mechanisms that link obesity with cognitive decline and neurodegenerative disorders. Furthermore, this review will also provide evidence for the effect of dietary modulation which can potentially ameliorate cognitive decline and neurodegenerative diseases associated with obesity.
Introduction
Obesity has become a growing public health concern and it is estimated that by 2025, one out of every five adults will be obese worldwide (Mohammed et al., 2018). Furthermore, it is estimated that approximately 300,000 people die from obesity related causes every year (Mokdad et al., 2000). It has been approximated that before the year 2030, there will be a 30% increase in obesity prevalence and a 133% increase in the prevalence of severe obesity (Finkelstein et al., 2012). The current prevalence and an increase in the incidence of obesity will undoubtedly give rise to obesity associated comorbidities. Previous studies in a large cohort have demonstrated that body mass index (BMI) significantly correlates with risk of mortality, as well as the development of comorbidities such as type 2 diabetes and cardiovascular diseases (Berrington de Gonzalez et al., 2010).
Obesity is characterized by an increase in lipid accumulation and central adiposity, causing a significant risk of developing type 2 diabetes and cardiovascular disease (Pulgaron and Delamater, 2014; Tchang et al., 2021). The mechanism underlying this association is related to the dysregulation of glucose metabolism that results from lipotoxicity, consequently resulting in an increased insulin resistance or decreased insulin sensitivity (Kahn et al., 2006; Yazici and Sezer, 2017). Furthermore, cytokines secreted from adipose tissue, called adipokines, have been shown to be altered in obesity (Mattu and Randeva, 2013; Fasshauer and Bluher, 2015), which plays an important role in the development of adipose tissue induced inflammation and oxidative stress (Kahn et al., 2006). While these adipokines are under homeostatic control in normal physiological conditions, there is excessive production of reactive oxygen species (ROS) and dysregulation of inflammatory factors in an obese state which leads to a metabolic crisis (Kwon and Pessin, 2013). This inflammatory state and oxidant stress in obesity is mediated by dyslipidemia, insulin resistance and unmetabolized glucose substrate which leads to mitochondrial dysfunction (Barazzoni et al., 2018). It is also known that the ROS are generated through excessive substrates provided by a high calorie diet that directly impair insulin signaling in obesity (Rains and Jain, 2011). Apart from that, the dysregulation of adipokines and excessive generation of ROS has also been indicated to mediate the pathogenesis of cardiovascular diseases (Kwon and Pessin, 2013). It is also thought that obesity provides the environment necessary to mediate phenotypic changes in the adipocytes that result in macrophage recruitment and a subsequent increase in the macrophage to adipocyte ratio within the adipose tissue (Nakamura et al., 2014). Obesity has also been linked with localized hypoxia in adipose tissue through vascular changes (Ye, 2009; Nakamura et al., 2014).
The cumulative line of evidence shows that altered levels of adipokines as well as subsequent oxidative stress in obesity also has deleterious effects in the central nervous system (CNS) which has been linked to increased incidence of neurodegenerative disorders (Procaccini et al., 2016; Salim, 2017). Studies have demonstrated that midlife obesity was associated with an increased risk for the development of Alzheimer’s Disease (AD) (Ronnemaa et al., 2011), Parkinson’s Disease (PD) (Chen et al., 2004), and decline in cognitive function in the general population (Nguyen et al., 2014). Specifically, a large population based study showed that obesity at midlife, along with associated risk factors such as high cholesterol levels and high blood pressure, each almost doubled the risk for dementia with an even higher risk (odds ratio of 6.2) among the patient having all risk factors combined (Kivipelto et al., 2005). One of the pioneering epidemiological evidences, the Rotterdam Study, have shown that the risk of dementia and AD almost doubles in patients with type 2 diabetes (Ott et al., 1999). Interestingly, the Rotterdam study also showed that the risk of dementia and AD was significantly higher in patients with severe diabetes at baseline. A summary of clinical evidence from literature that suggests obesity is associated with cognitive decline and neurodegenerative disorders in illustrated in Table 1. Neurodegenerative diseases are characterized by toxic accumulation of proteins in the brain as well as consequential loss of neuronal cell number and function. Some commonly found proteins that accumulate in the brain are Aβ, prion protein, tau, α-synuclein, TARDBP, and FUS protein (Kovacs, 2017). The development and progression of neurodegenerative disorders relies primarily on the altered expression of these protein. The Aβ hypothesis is a well-established explanation for the deposition of Aβ in AD brains. The evidence of Aβ deposition alone is the first Alzheimer’s pathologic change of AD (Jack et al., 2018). Studies have shown that Aβ starts accumulating in brain regions such as precuneus, medial orbitofrontal and posterior cingulate cortices in pre-clinical AD patients (Palmqvist et al., 2017). Another important process in AD is associated with hyperphosphorylation of tau in neurofibrillary tangles, with new evidence from human AD brain samples demonstrating phosphorylation of Tau at multiple serine and tyrosine residues in different brain regions (Neddens et al., 2018). The cumulative line of evidence suggests that Aβ peptide for amyloid plaques and tau for neurofibrillary tangles work synergistically to alter neuronal function in AD, further demonstrating that Aβ triggers Tau pathology (Gotz et al., 2001; Lewis et al., 2001; Hurtado et al., 2010). While these studies suggest that Aβ functions upstream of tau, evidence shows that tau may also influences Aβ (Leroy et al., 2012). This raises the possibility that Aβ may trigger a pathological feedback loop with tau and characterize biological changes associated with AD (Bloom, 2014; Quiroz et al., 2018). The hyperphosphorylation of tau, along with aggregation of α-synuclein protein, is also known to play a mechanistic role in the development and progression of PD, as evidenced by murine models of parkinsonism (Duka et al., 2006; Haggerty et al., 2011). The synergistic action of tau and α-synuclein results in the formation of Lewy bodies, leading to the degeneration and loss of dopaminergic neurons, a hallmark in progression of PD (Haik et al., 2008). Further evidence from murine models suggests that aggregation of α-synuclein leads to nuclear factor erythroid 2–related factor 2 (Nrf2) deficiency that aggravate neuronal death in PD (Lastres-Becker et al., 2012). The overexpression of α-synuclein also contributes to the modulation of vascular pathology and causes blood brain barrier (BBB) leakage in PD (Elabi et al., 2021). Extensive research in murine models and clinical studies have shown that the aggregation of these toxic proteins, through their respective degenerative pathological processes, result in excessive production of inflammatory factors, such as TNFα, which are then transported across “leaky” BBB (Lastres-Becker et al., 2012; Maphis et al., 2015; Lee et al., 2021). These inflammatory factors alter and cross BBB through either active transport across BBB, through protein specific receptors on endothelial cells of BBB or passive transport via circumventricular organs, through simple diffusion (Pan et al., 2007; Fu, 2018). The transport of inflammatory factors, such as TNFα, across BBB allows these protein to be freely secreted in neuronal tissue, which induces neuroinflammation (Kim et al., 2012). In addition to harmful generation of ROS and other intermediates, adipokine dysfunction has also been indicated in the development of neuroinflammation, cognitive decline, and neurodegenerative diseases (Forny-Germano et al., 2018; Lee et al., 2019). Leptin, an important adipokine, produced primarily by adipose tissue, have been extensively shown to regulate hippocampal synaptic function (Dumon et al., 2018; McGregor et al., 2018), hippocampal neurogenesis (Garza et al., 2008) and neuronal morphology (O’Malley et al., 2007). Since adipokine leptin has free access to circumventricular organs, leptin is actively transported across BBB through leptin receptor (Di Spiezio et al., 2018; Harrison et al., 2019) and alters hippocampal function. Another important adipokine, adiponectin, have been shown to have neuroprotective effects in brain including regulation of hippocampal neurogenesis (Yau et al., 2014), neuronal excitability (Qiu et al., 2011), and protection from neuroinflammation (Chen et al., 2009; Chabry et al., 2015). While some studies suggest that adiponectin does not cross BBB (Pan et al., 2006; Spranger et al., 2006), contrary evidence suggests that adiponectin crosses BBB and induces neuronal alterations under diseased state (Qi et al., 2004; Yau et al., 2014). The adipokines, such as TNFα and interleukin 6 (IL6), that act as proinflammatory molecules are also able to cross the BBB and augment their function once localized to neuronal tissue (Banks et al., 1994; Pan et al., 1999; Pan and Kastin, 2002; Geng et al., 2018). It has even been proposed that these adipokines, that do not originate from neuronal tissue and enter the CNS by crossing the BBB, can work in unison with local factors that are produced by microglia within the brain to induce neuronal inflammation (Forny-Germano et al., 2018). Hence, these adipokines, such as adipokine, leptin, TNFα, IL6 and mediators of microglial activation also contribute to neuronal insulin resistance (Andre et al., 2017; Maldonado-Ruiz et al., 2017). Neurodegenerative states associated with neuronal protein deposition are also highly associated with obesity and comorbidities such as type 2 diabetes and cardiovascular disease due to increased amount of proinflammatory markers that inflict damage on the CNS (Forny-Germano et al., 2018; Surguchov, 2020). This indicates that there is a growing field to investigate new therapeutic targets and preventative measures for obesity associated with cognitive decline and neurodegeneration.
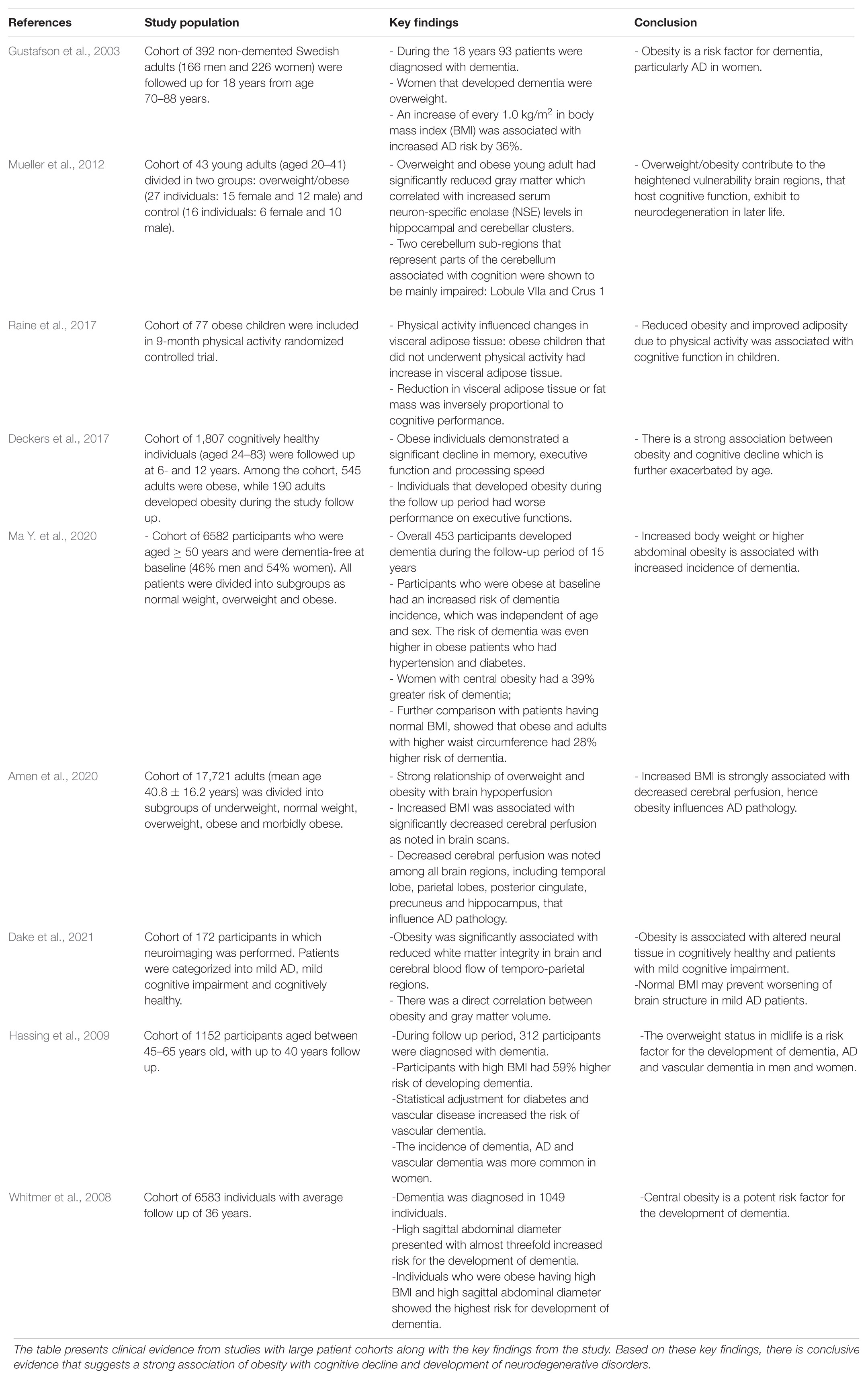
Table 1. Summary of clinical evidence from literature for obesity associated cognitive decline and neurodegeneration.
Although obesity and neurodegeneration are distinct pathological conditions, there are important molecular markers of each that may link the two together, such as miRNAs. miRNAs are small, non-coding RNAs that help regulate gene expression by silencing or activating mRNA transcripts (O’Brien et al., 2018). While there is an extensive evidence demonstrating the mechanistic action of miRNAs in exacerbating obese phenotype (McGregor and Choi, 2011; Mentzel et al., 2015; Iacomino and Siani, 2017), as well as recent evidence in literature demonstrating the role of circulating levels of miRNAs in neurodegenerative disease (Sharma and Lu, 2018; Salama et al., 2019; Yuen et al., 2021). However, there have been limited research that links the underlying mechanisms operant with transcriptional regulation of miRNAs in cognitive decline and neurodegeneration caused by obesity and associated comorbidities. The study of miRNAs in obesity associated neurodegenerative disorders and their potential role as biomarkers has high translational applicability, since miRNAs are highly stable in various body fluids and the sequence of most miRNAs are conserved among different species (Lakhani et al., 2018). Furthermore, the expression of some miRNAs are specific to individual tissues and biological states (Lakhani et al., 2021). Hence, this review will study the role of several miRNAs and aim to elucidate underlying mechanisms that link obesity with neurodegenerative disorders in light of increased oxidative stress and dysfunctional adipokine signaling. These underlying mechanisms, associated with the transcriptional regulation of miRNAs, may allow for new therapeutic targets for obesity associated cognitive decline. In addition to investigating possible mechanisms using miRNA, this review will also provide evidence for effect of dietary modulation in potentially ameliorating cognitive decline and neurodegenerative diseases associated with obesity.
Obesity Associated Cognitive Decline and Neurodegeneration
Obesity and associated metabolic disorders can affect not only metabolic homeostasis but also brain function, resulting in onset of non-genetic cognitive decline and neurodegenerative disorders (Lee et al., 2018; Graham et al., 2019). Recent studies have extensively investigated the anthropometric association of obesity and cognitive decline, however the mechanisms linking these pathological conditions remain highly elusive. One of the well-studied notions is the presence of oxidant stress caused by inflammation in obesity (Betteridge, 2000; Preiser, 2012). Previous studies have shown that mechanisms operant within adipocytes, under obese state, produce proinflammatory markers that are able to cross the BBB, resulting in oxidant stress which directly affect neuronal processes (Klein and Ackerman, 2003). Evidence suggests that these cytokines may cross BBB through active transport across BBB or through passive transport through areas in the brain where BBB in incomplete and the cytokines may cross through simple diffusion (Yarlagadda et al., 2009). Furthermore, studies have also shown that these inflammatory factors may also alter the BBB integrity by damaging the tight junctions of microvascular endothelial cells that form the BBB, resulting in an increased permeability (Yarlagadda et al., 2009). Mitochondrial dysfunction is thought to play a large role in the process of oxidative damage to the brain as well (Albers and Beal, 2000; Picca et al., 2020). Dysfunctional mitochondria produces ROS that interact with intermediates involved in neuronal processes and disturb normal homeostatic function of these cells (Onyango and Khan, 2006). Studies have extensively shown that excessive production of ROS damages mitochondrial DNA as well as causes lipid peroxidation and oxidative phosphorylation (Guo et al., 2013). The diet induced obesity significantly alters the expression of peroxisome proliferator-activated receptor gamma coactivator 1-alpha (PGC1α), a master regulator of mitochondrial biogenesis, also affecting the expression of its transcriptional complexes in hippocampus (Rius-Perez et al., 2020). Since, neuronal mitochondria regulates synaptic plasticity, the alteration of PGC1α expression directly affects hippocampal neuronal function (Cheng et al., 2012; Choi and Han, 2021). This obesity mediated release of inflammatory factors directly results in neuroinflammation, while subsequent oxidative damage caused by this inflammation may significantly damage the CNS, alter synaptic plasticity of the neurons, affect the development of neuronal synaptic activity and function and may lead to neuronal death (Khan and Hegde, 2020).
Insulin resistance is also associated with diet induced obesity and frequently develops as a comorbidity of obesity (Castro et al., 2014). Studies have shown that impaired insulin signaling within insulin resistance directly affects the ability of neuronal glucose uptake (Holscher, 2020). This perturbance has been demonstrated to participate in the genesis of some neurodegenerative diseases due to the necessary role that insulin plays in providing neurons with glucose for metabolic processes (de la Monte, 2009; Femminella et al., 2021). The build-up of neuronal plaques and tau proteins within the brain is also potentiated by insulin resistance (Rad et al., 2018). This leads to devascularization within the cerebral cortex and cerebellum which can cause heightened levels of decline in cognitive function and the development of neurodegenerative diseases (Arnold et al., 2018; Rad et al., 2018). Since hippocampus plays an important role in regulating spatial learning and memory processes, several studies have shown obesity associated altered insulin signaling leads to altered hippocampal function. Specifically, studies have shown that diabetes induces structural and synaptic deficits in hippocampus which results in learning and memory deficits (Lee and Yau, 2020). Furthermore, under physiological condition of obesity and diabetes, murine models have been demonstrated to have significantly decreased cell proliferation, neuronal differentiation and cell survival in hippocampus (Ho et al., 2013). Subsequently, there is significant reduction in dendritic complexity and synaptogenesis in the hippocampus in obese and diabetic states, suggesting that hippocampal activity and function is highly affected by the metabolic imbalance caused by obesity (Ho et al., 2013). Studies have also demonstrated that insulin resistance leads to reduced phosphoinositide 3-kinase (PI3K) signaling and increased mitogen-activated protein kinase signaling, which results in decreased nitrogen oxide (NO) production (Boucher et al., 2014). The decrease in NO production has been shown to be associated with endothelial dysfunction. The altered production of NO in obesity and associated insulin resistance impairs neurovascular coupling which has been shown to cause cognitive impairment and neurodegeneration (Buie et al., 2019). Apart from that, obesity may also exacerbate the development and progression of cognitive decline and neurodegeneration through metabolic imbalance. Studies have shown that obesity may impair cognitive function through increased basal levels of corticosteroids and glucocorticoids. Furthermore, evidence from murine model suggests that microglial activation in diet-induced obesity is associated with reduction in dendritic spines and memory impairments (Bocarsly et al., 2015; Cope et al., 2018). Studies have also demonstrated that microglia plays an active role in synaptic degeneration when in a high calorie state (Cope et al., 2018). Neurogenesis and the maintenance of synaptic connections is also thought to be a targeted process in obesity. The cumulative line of evidence in murine models have demonstrated that diet induced obesity results in neurogenesis in the hippocampus which is directly correlated with the weight and adiposity (Bracke et al., 2019). A schematic representation of mechanisms operant is obesity associated cognitive decline is shown in Figure 1.
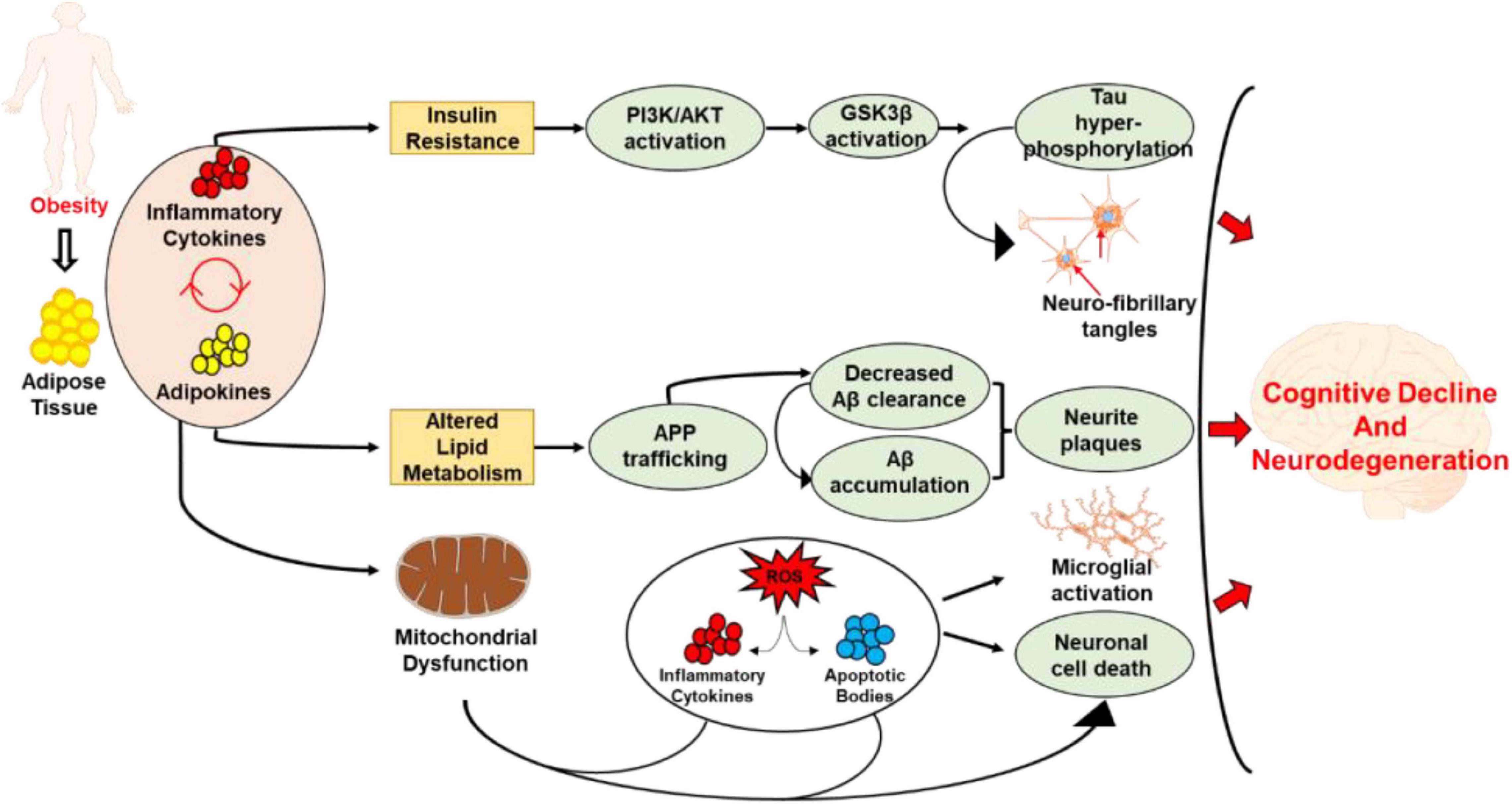
Figure 1. Schematic representation demonstrating the mechanisms related to obesity associated cognitive decline and neurodegeneration. The excess accumulation of adipose tissue and the resulting release of adipokines and inflammatory cytokines leads to insulin resistance, altered lipid metabolism, and mitochondrial dysfunction. While the insulin resistance leads to tau hyperphosphorylation and development of neurofibrillary tangles, altered lipid metabolism caused Aβ accumulation, and development of neurite plaques. On the other hand, mitochondrial dysfunction causes excessive production of ROS, inflammatory cytokines and apoptotic bodies which lead to neural cell death. The inflammation resulting from mitochondrial dysfunction also leads to microglial activation. Collectively, these processes lead to cognitive decline and the development and progression of neurodegenerative disorders.
miRNAs
From the perspective of neurodegeneration, the mechanistic insight into the impact of miRNA signaling have been investigated as significant biomarkers for AD diagnosis, disease prediction, prognosis and therapeutic purposes (Sharma and Lu, 2018; Zhao et al., 2020; Siedlecki-Wullich et al., 2021). The presence and persistence of various miRNAs in brain tissues and biofluids during neurodegenerative diseases suggest that miRNAs are involved in the regulation of multiple gene expression pathways throughout the human brain and CNS (Lukiw et al., 2012; Lukiw, 2020; Lukiw and Pogue, 2020; Ma F. et al., 2020). Detailed investigations based on fluorescent miRNA-array and RNA sequencing analysis, demonstrate that various miRNAs, and their enrichment in different sources of AD samples including patient blood serum, post-mortem brain tissues, etc. are highly sensitive to alterations in the biochemical, neurochemical, neuropathological, and/or cellular environment (Alexandrov et al., 2012; Lukiw, 2013a, b; Kumar and Reddy, 2020). As discussed in the previous section, growing evidences suggest that, obesity and other diet-induced metabolic dysfunctions can trigger and aggravate the development of neurodegenerative diseases (Ashrafian et al., 2013; Mazon et al., 2017; Lee and Yau, 2020). Hence, the exploration of some major miRNA, which are having possible role in obesity associated neurodegenerative disorders, may help to highlight perspectives of studying microRNA regulation in multiple brain signaling pathways.
miR-26a
miR-26a is a well conserved miRNA whose overexpression or inhibition has been implicated in the pathogenesis of multiple disease, including obesity associated comorbidities such as reduced insulin sensitivity (Xu et al., 2020) and atherosclerosis (Feng et al., 2018). The cumulative line of evidence also suggests an important role of miR-26a in neurodegenerative disorders like AD (Liu et al., 2020) and PD (Su et al., 2019). Various mechanisms of gene regulation have been implicated in these diseased states, the pathophysiological consequences of which are exacerbated by the decreased expression of miR-26a. This suggests a potential link between low miR-26a levels and obesity associated cognitive decline and neurodegeneration.
Previous studies with in vivo models of obesity have demonstrated that reduced expression of miR-26a was associated with both insulin and glucose (Fu et al., 2015). Moreover, murine models of type 2 diabetes have shown that decreased expression of miR-26a was associated with decreased insulin sensitivity in pancreatic β cells (Xu et al., 2020). Further evidence from literature suggests that increased expression of miR-26a, decreases glucose stimulated insulin secretion (GSIS), thereby reducing hyperinsulinemia, a contributor in diabetes and obesity (Xu et al., 2020). GSIS is mediated in phases, relies on both actin remodeling for insulin granule release and focal adhesion, processes which are mediated by a focal adhesion kinase (FAK) and an extracellular signal-regulated kinase (ERK) (Xu et al., 2020). Hence, evidence suggests that an increase in circulating levels of miR-26a resulted in downregulation of phosphorylation of these kinases, resulting in less downstream effects and thus less GSIS (Xu et al., 2020). miR-26 family has been studied for regulating the human adipocyte differentiation by promoting characteristics of energy-dissipating thermogenic adipocytes (Karbiener et al., 2014). The potential role of miR-26a in the regulation of fatty acid and sterol metabolism has also been elucidated in experimental models of Non-alcoholic Fatty Liver Disease (Ali et al., 2018). Another study shows that miR-26a suppresses adipocyte progenitor differentiation and fat production by targeting Fbxl19 and highlights the critical role in regulating adipose tissue formation (Acharya et al., 2019).
The role of miR-26a have also been implicated in AD, which is a hallmark disease in the realm of neurodegeneration and the most leading form of dementia. Studies have shown that under expression of miR-26a is associated with the mechanisms underlying AD (Liu et al., 2020). By contrast, overexpression of miR-26a has been shown to be potentially neuroprotective. One mechanism behind the protective effect of miR-26a in AD is its downregulation of a target gene DYRK1A, which leads to decreased Tau phosphorylation and less Aβ build-up (Liu et al., 2020). This mechanism of upregulated miR-26a expression leading to less downstream phosphorylation is similar to the effect noted in obesity and GSIS in type 2 diabetes.
The common mechanisms of miR-26a and its effect on phosphorylation of Tau and kinases, have also been shown to play a similar role in PD. Studies in murine model of PD have shown a significant correlation between reduced expression of miR-26a and an increased expression of serine/threonine kinase called DAPK1 (Su et al., 2019). DAPK1 functions in part to phosphorylate alpha-synuclein, which is hallmark to Lewy Bodies, pathologic in PD (Su et al., 2019). By contrast, increased expression of miR-26a expression resulted in less DAPK1 expression (Su et al., 2019). Taken together, in both AD and PD, miR-26a expression and upregulation has been shown to use similar mechanisms to downregulate kinases which play a role in phosphorylation of pathological proteins in these diseases. The knockdown studies using cultured microglia demonstrate the role of miR-26a as a negative regulator of TLR4 triggered inflammatory response (Kumar et al., 2015). In addition, the miRNA expression profiling studies on the peripheral blood samples of patients with Amyotrophic lateral sclerosis and PD showed significant downregulation of miR-26a (Martins et al., 2011; Liguori et al., 2018). Furthermore, miR-26a has been included in a non-invasive miRNA-panel to improve the diagnosis of AD (Guo et al., 2017).
Apart from that, studies have also noted upregulation of miR-26a expression in budding neurites, and even higher expression in mature neurons, implicating its role in neural growth (Li and Sun, 2013). The underlying mechanism of this is suggested to be due to miR-26a downregulating PTEN, a molecule which suppresses neurite outgrowth (Li and Sun, 2013). Collectively, evidence from literature suggests a protective role of miR-26a and an association between low miR-26a expression and mechanisms underlying obesity associated neurodegenerative disorders (Absalon et al., 2013; Fu et al., 2015; Konovalova et al., 2019). This relays the importance of miR-26a as a critical marker, or even as a potential therapeutic target, in obesity associated cognitive decline and neurodegeneration.
miR-29a
miR-29a is a member of the miR-29 family, which have been shown to target a diverse range of genes throughout the body (Kriegel et al., 2012; Wang et al., 2018). The involvement of miR-29a in several physiological and pathological processes has been extensively investigated, including its role in fibrosis (Harmanci et al., 2017), metastasis (Ngankeu et al., 2018), apoptosis (Wang et al., 2018), as well as a potential biomarker of non-alcoholic fatty liver disease (NAFLD) (Jampoka et al., 2018), highlighting its important role in metabolic dysfunction.
The role of miR-29a has been implicated in lipid metabolism and insulin resistance. A recent study has demonstrated the important role of miR-29a in fatty acid oxidation through regulation of PGC1α, an important marker of mitochondrial biogenesis (Cheng C.F. et al., 2018) which is intricately linked to obesity and type 2 diabetes (Liang and Ward, 2006; Massart et al., 2017). Overexpression of miR-29a was found to downregulate PGC1α, demonstrating miR-29a’s potential contribution to the development of metabolic disease (Massart et al., 2017). Apart from that, studies have also shown that miR-29a downregulates the expression of genes directly involved in fatty acid oxidation, including expression of pyruvate dehydrogenase kinase isoform (PDK4), fatty acid transport protein 2 (FATP2), and long chain specific acyl-CoA dehydrogenase (LCAD) (Wu et al., 2018).
In addition to lipid metabolism, miR-29a also plays a role in glucose homeostasis. One study found that increased levels of miR-29a in the anterior tibialis muscle of mice caused a decrease in glucose uptake and glycogen content, which was attributed to decreased levels of glucose transporter-4 (GLUT4) in the plasma membrane (Massart et al., 2017). The importance of miR-29a in glucose regulation have been further corroborated by other studies demonstrating that silencing of miR-29a expression increases insulin-induced glucose uptake in mice (Wu et al., 2018). This occurs through mechanistic action of miR-29a which downregulates peroxisome proliferator-activated receptor δ (PPARδ) (Wu et al., 2018) at the post-translational level (Zhou et al., 2016). PPARδ functions by increasing the transportation of GLUT4 to the plasma membrane of skeletal muscle (Wu et al., 2018), allowing for the uptake of glucose into cells. The role of PPARδ have also been implicated as a metabolic regulator and have been shown to enhance fatty acid catabolism, cardiac contractility, and lowers triglycerides (Barish et al., 2006). Together, these data suggest that miR-29a may play a role in impaired glucose uptake and obesity via downregulation of PPARδ. miR-29a mediated negative feedback loop regulation of peripheral glucocorticoid receptor signaling and its possible role in metabolic disorders such as insulin resistance, obesity and hyperglycemia has already been studied in detail (Glantschnig et al., 2019).
In addition to its role in metabolism, there is a cumulative line of evidence supporting miR-29a’s role in neurodegeneration. Specifically, expression of miR-29a have been shown to be increase by a factor of 2.2 in the CSF of patients with AD (Muller et al., 2016). Another study found that in the post-mortem human brain, miR-29a was upregulated in the anterior cingulate gyri of patients with PD (Goh et al., 2019). A recent study further demonstrated that miR-29a targets PGC1α and mitochondrial biogenesis and plays a significant role in maintaining neural cell function by preserving and promoting mitochondrial function (Li et al., 2017). Furthermore, expression of PPARδ, another target of miR-29a, has been found to be decreased in the brains of patients with AD (Strosznajder et al., 2021). This is not surprising because PPARδ is known to be a potent anti-inflammatory agent and acts to stabilize the myelin sheath as well as decreased Aβ deposits (Strosznajder et al., 2021). Other studies have also shown that a decline in the expression of PPARδ increases neuroinflammation, oxidative stress, and Aβ42 deposition, which highlights the protective role PPARδ serves in the brain (Strosznajder et al., 2021). The regulation of PPARδ and PGC1α through overexpression of miR-29a may serve as a potential mechanism for obesity associated neurodegenerative diseases. Microarray studies conducted on the frontal cortex derived from amyotrophic lateral sclerosis patients suggest that underexpression of miR-29a affects neurodegenerative processes by enhancing neuronal NAV3 expression in AD brains (Shioya et al., 2010). miRNA expression profiles of sporadic AD patients found that miR-29a is potentially involved in the regulation of APP and BACE1 gene expression which cause Aβ accumulation and appeared to be decreased in diseased brain (Hebert et al., 2008). miR-29a can also be a candidate biomarker for AD in cell-free CSF (Muller et al., 2016). The reverse transcription-quantitative real-time PCR studies followed by cloning and sequencing on blood serum of patients with PD also showed the significant reduction of miR-29a, which warrants further investigation of its potential serving as biomarkers for PD (Bai et al., 2017).
miR-425
miR-425 is another miRNA that have been implicated not only in obesity related pathogenesis but also via various mechanisms in the brain and neurodegeneration (Olivo-Marston et al., 2014; de la Rocha et al., 2020). Although under-expression of miR-425 has a documented association with PD, evidence from literature suggests that overexpression of this miRNA is associated with multiple neurodegenerative disorders (Yuan et al., 2020). Furthermore, in vivo models of diet induced obesity have been shown to have increased inflammatory cytokine release and significantly increased expression of miR-425 (Olivo-Marston et al., 2014). Apart from that, miR-425 was also found to increase fat deposition and synthesis and have been shown to inhibit expression of proteins which play direct a role in negating adipogenesis (Qi et al., 2019). These lines of evidence suggest miR-425 overexpression as a possible culprit in obesity associated cognitive decline and neurodegeneration.
The upregulated expression of miR-425 have been supported by previous studies with in vivo models of diet induced obesity (Olivo-Marston et al., 2014). Studies in humans have found miR-425 levels to be significantly increased in diabetic patients versus control patients, providing further evidence that the expression of this miRNA has a substantial role in obesity (Luo et al., 2020). The underlying mechanism that supports this effect of miR-425 in diabetes suggests that it downregulates expression of monocarboxylate transporter 4 (MCT4), a lactate transporter, which when dysfunctional results in lactate build-up in endothelial cells, leading to apoptosis (Luo et al., 2020). Endothelial cell damage has been linked to diabetes, and as miR-425 expression has been shown to induce deleterious effects and endothelial cell death, there is clear indication of its role in obesity related disorders (Luo et al., 2020). Other studies in murine models of obesity revealed that miR-425 plays a direct role in the synthesis of fat, and that its inhibition prevents development and progression of obesity (Qi et al., 2019). This mechanism has been investigated and has revealed that miR-425 expression inhibits p38a, a gene that inhibits lipogenesis (Qi et al., 2019). miR-425 is closely associated with dysregulation of insulin/PI3K-AKT signaling in liver (Kwon et al., 2014). miR-425 is also reported to have an important role in the regulation of intestinal lipid metabolism (Ruiz-Roso et al., 2020).
In neurogenerative processes, upregulated expression of miR-425 was found in AD patients, which corresponded with increased tau phosphorylation in these patients (Yuan et al., 2020). In vivo models suggest the causative mechanism to be upregulation of miR-425 leading to decreased expression of heat shock protein 8, and as a result, increased tau phosphorylation at multiple residues and apoptosis of neural cells (Yuan et al., 2020). In other processes in the brain, miR-425 expression at basal and high levels have been shown to promote phosphorylated forms of AKT survival pathway in glioblastoma multiforme, revealing its role in neural related pathways (de la Rocha et al., 2020). Glioblastoma multiforme is well documented to cause cognitive deficits and neurodegeneration (Hanif et al., 2017; de la Rocha et al., 2020). The inhibition of miR-425 in these mice subsequently resulted in decreased phosphorylation of this pathway, and less brain tumor survival (de la Rocha et al., 2020). miR-425 deficiency triggers necroptosis of dopaminergic neurons in PD and the study propose miR-425 supplements as a probable therapeutic approach for neurodegenerative disease with neuron loss (Hu et al., 2019). Also another study identifies miR-425 as a biomarkers to discriminate mild Traumatic brain injury from severe (Di Pietro et al., 2017). Hence, miR-425 have a potential role in obesity and neurodegenerative disorders and may serve as potential biomarker of this clinical condition.
miR-33
miR-33 is a family of miRNA that is well known to regulate lipid metabolism, cholesterol homeostasis, and fatty acid metabolism as well (Cirera-Salinas et al., 2012). While these processes may be involved more directly in obesity, emerging studies have begun to reveal the function that miR-33 has to play within neurodegeneration as well.
Studies have shown that the antagonism of miR-33 induces plaque regression and an increase in activity of the reverse cholesterol transport pathway in murine model of obesity (Rayner et al., 2011). The mechanism that miR-33 controls the regulation of cholesterol is through downregulation of the protein ATP-binding cassette transporter protein 1 (ABCA1), which is involved in the production of high-density lipoproteins (HDL) (Medvinskii et al., 1990). A proposed model states that ABCA1 assists in the migration of phospholipids from the cytoplasm to the extracellular fluid, or cholesterol efflux, and the binding of apoA1 to the plasma membrane of ABCA1-expressing cells to create nascent HDL (Wang and Smith, 2014). This is related to the topic at hand because lower HDL levels are associated with obesity (Rashid and Genest, 2007). miR-33 is involved in the down regulation of several genes encoding key enzymes involved in cholesterol efflux, fatty acid metabolism, and insulin signaling (Goedeke et al., 2013). These studies suggest that miR-33 plays a role in obesity through the regulation of cholesterol. Report shows that the genetic ablation of miR-33 increases food intake, enhances adipose tissue expansion, and promotes obesity and insulin resistance (Price et al., 2018). In addition, a study using mouse model of diet-induced obesity have shown that the therapeutic inhibition of miR-33 may promote whole-body oxidative metabolism but does not affect metabolic dysregulation (Karunakaran et al., 2015).
The role of miR-33 has also been implicated in the neurodegenerative processes. Studies have demonstrated that the knockdown of miR-33 improved synaptic plasticity, reduced inflammation, oxidative stress, and cell apoptosis (Wang et al., 2020). The direct intracerebral delivery of a miR-33 antisense oligonucleotide into mouse brain was shown to be an effective strategy for increasing brain ABCA1 expression/activity, which can have beneficial effects on various neurodegenerative and cardiovascular diseases (Jan et al., 2015). Evidence have also shown that miR-33 in the brain contributes to the maintenance of adaptive thermogenesis and whole-body metabolism via enhanced sympathetic nerve tone through suppressing GABAergic inhibitory neurotransmission (Horie et al., 2021). A proposed mechanism by which miR-33 regulates neurodegeneration is through the inhibition of ABCA1, a protein that is downregulated by the presence of miR-33. Accordingly, mice that were deficient in miR-33 expressed higher levels of ABCA1 in the brain (Kim J. et al., 2015). Furthermore, one particular study demonstrated that cells expressing high levels of miR-33 demonstrated higher levels of Aβ, a distinctive feature of AD, and lower levels of ABCA1 (Jaouen and Gascon, 2016), further supporting the argument that miR-33 is involved with the aggregation of Aβ through cholesterol homeostasis (Jaouen and Gascon, 2016). Accordingly, elevated levels of cholesterol have been found to increase the likelihood of developing AD as well (Shepardson et al., 2011), due to excessive accumulation of Aβ in the brain, which can lead to a disruption in neuronal function (Huang and Liu, 2020). It is suggested that altered cholesterol homeostasis through ABCA1, downregulated by the expression of miR-33, may lead to an increase in APP cleavage, thus causing an increase of Aβ in the brain (Montesinos et al., 2020). Aβ, in turn, propagates hyperphosphorylation of tau proteins, which can cause the aggregation of this protein and lead to the disruption of synapses. The mechanism of tau hyperphosphorylation and an excessive accumulation of Aβ is associated with the activation of the enzyme GSK3β (Huang and Liu, 2020). Hence, the potential role of miR-33 in altering cholesterol homeostasis and excessive Aβ accumulation may implicate a causal mechanistic link in obesity associated neurodegenerative disorder.
miR-21
miRNA-21 has been known to be linked to the physiology of cancer, inflammation and cardiac disease (Sheedy, 2015; Yang et al., 2018). However, recent evidence from literature suggests its potential role in both the promotion of obesity as well as neurodegeneration. The overexpression of miR-21 has been shown in vitro to promote adipocyte differentiation through adipogenesis (Kang et al., 2013). Furthermore, in vivo models of miR-21 knockdown mice exhibited a reduction in obesity and an increase in weight loss (Seeger et al., 2014), suggesting that higher expressions of miR-21 may be correlated with obesity and weight gain. Additionally, levels of phosphatase and tensin homolog (PTEN) were restored to a in miR-21 deficient mice (Seeger et al., 2014). The role of PTEN have been implicated as a tumor suppressor (Hopkins et al., 2014), but has implications within cognitive decline (Zhang et al. 2006b) as well as insulin resistance (Pal et al., 2012). One particular study found that carriers of mutated PTEN were more overweight and had higher insulin resistance than control subjects (Pal et al., 2012). This is most likely due to the fact that it prevents the effect of the PI3K-AKT pathway, which is involved in insulin signaling (Pal et al., 2012). This pathway is also crucial in the normal function of β-cells, which produce insulin (Elghazi and Bernal-Mizrachi, 2009). Additionally, mice that had higher levels of PTEN expression had an increase in the use of energy and less prevalence of adiposity (Seeger et al., 2014), suggesting that the repression of this protein could cause an increase in the chance of developing obesity. Hence, overexpression of miR-21 and subsequent decrease in the levels of PTEN may promote obesity associated insulin resistance. The progressive increase in the expression of miR-21 in the serum samples of a study population with obesity and diabetes with or without fatty infiltration, shows its predictive efficacy as a biomarker (Pillai et al., 2020).
The role of miR-21 has also been implicated in neurodegenerative processes. Studies in AD patients, have shown that the expression of miR-21 was significantly upregulated as compared to control subjects (Giuliani et al., 2021). MicroRNA 21 is reported to be up-regulated in adipose tissue of obese diabetic subjects undergoing bariatric surgery (Guglielmi et al., 2017). The effect of miR-21 has been reported against ischemic neuronal death, possibly by targeting a tumor necrosis factor family cell death-inducing ligand (Fas ligand gene) (Buller et al., 2010). The regulatory effect of miR-21 on α-synuclein expression in neurons has been exploited as a protective mechanism of some drugs against Parkinson’s disease (Buller et al., 2010). Furthermore, a deficiency or mutation in PTEN, a target of miR-21, may cause aggregation of tau protein (Zhang et al. 2006b), which is a fundamental component of AD disrupting neuronal function by forming tangles (Gendron and Petrucelli, 2009). This evidence suggests that miR-21 can inhibit the expression of PTEN, thus causing the aggregation of tau. Additionally, PTEN may have an effect in the phosphatidylinositol 3,4,5 trisphosphate (PIP3) survival signaling pathway, which is involved in the exacerbation of tau toxicity (Zhang et al. 2006b). Hence, the role of miR-21 through regulation of PTEN may provide a mechanistic link in obesity associated neurodegeneration can may be a crucial biomarker for this condition.
miR-34a
As a member of the miR-34 family of microRNAs, miR-34a has been extensively investigated in a number of biological processes including cell development, metabolism, and differentiation (Imani et al., 2018). Most recently, miR-34a has been found to be upregulated in both metabolic and neurodegenerative disorders. Specifically, one study found that miR-34a was one of the most highly elevated genes during the development of diet induced obesity (Pan et al., 2019). Furthermore, the study demonstrated that high levels of miR-34a in adipose tissue increased obesity-induced inflammation by regulating Krüppel-like factor 4 (klf4). The gene klf4, which is responsible for polarization of anti-inflammatory M2 macrophages, was found to be significantly decreased in the adipose tissue of obese individuals (Pan et al., 2019). Because miR-34a significantly represses klf4, mice with increased miR-34a expression experienced significantly increased transition to the pro-inflammatory M1 macrophage type, exacerbating the systemic inflammation and metabolic dysregulation, strongly associated with obesity (Pan et al., 2019). Evidence from other studies suggests that miR-34a directly targets and downregulates Sirtuin1 (SIRT1), an NAD + -dependent deacetylase, known to be protective against obesity (Choi et al., 2013). An important location of SIRT1 is in the hypothalamus, where it plays a vital role in regulating energy homeostasis and suppressing metabolic disease such as obesity and type 2 diabetes (Xu et al., 2018). Expression of SIRT1 stimulates mitochondrial biogenesis, fatty acid oxidation, and ketogenesis (Xu et al., 2018), further demonstrating the importance of miR-34a in the regulation of obesity associated metabolic processes. A study conducted in the plasma samples of obese children and adolescents showed that miR-34a is associated with insulin and HOMA-IR and thus have significant role in IR (Ahmadpour et al., 2018). A significantly upregulated expression of miR-34a has been observed in patients with obesity, diabetes and fatty infiltration, suggesting the possible role as a biomarker for disease progression (Pillai et al., 2020).
A recent study has also demonstrated the highly beneficial role SIRT1 plays in the brain, protecting against neurological disorders and cerebral ischemia (Xu et al., 2018). It was found that SIRT1 is expressed at low levels in the brains of patients with AD, where it acts to deacetylate AD-affected neurons causing repression of p53 and inhibition of apoptosis (Manjula et al., 2020). The levels of SIRT1 were also highly correlated to Braak stage, a system used to determine the severity of dysfunction in neurological pathologies (Burke et al., 2008; Manjula et al., 2020). Further, elevated levels of SIRT1 were shown to provide protection against the development of Huntington’s Disease 30532738 (Xu et al., 2018). The regulation of SIRT1 by miR-34a serves as a potential mechanism for the neurological dysfunction observed in these patients. In addition to SIRT1, the gene klf4 was also found to be protective against the development of cerebral injury 30297986 (Cheng Z. et al., 2018). Specifically, it was found that klf4 is involved in the processes of anti-inflammation, anti-apoptosis, and axon regeneration in the brain via the JAK-STAT3 pathway (Cheng Z. et al., 2018). The study performed for the microRNA abundance in CSF and brain tissue-derived extracellular fluid (ECF) from AD neocortex also showed dysregulation in the expression of miR-34a along with some other microRNAs (Alexandrov et al., 2012; Zhao et al., 2020). The differential profile of circulating miR−34a has also been explored in PD patients in comparison with age-matched control subjects (Grossi et al., 2021). Evidence shows that miR-34a-mediated autophagy, abnormal mitochondrial dynamics and SIRT1/mTOR signaling pathway has important role in the functional complications of brain associated with AD (Kou et al., 2017; Chen et al., 2019).
There is a cumulative line of evidence supporting the role of miR-34a in neurodegeneration. A recent study found that overexpression of miR-34a resulted in rapid cognitive decline in conjunction with intracellular Aβ deposits and tau hyperphosphorylation in mice (Sarkar et al., 2019). Furthermore, evidence suggests that overexpression of miR-34a downregulates the expression of neuroprotective proteins including SIRT1, ADAM10, and NMDAR2B (Sarkar et al., 2019). Another study demonstrated that miR-34a elevation is linked to numerous neurodegenerative processes including hearing loss and age-related macular degeneration (Chua and Tang, 2019). It was found that miR-34a significantly contributes to neuronal cell death during epileptic seizures and ischemic stroke via its regulation of downstream targets such as SIRT1 (Chua and Tang, 2019). Regulation of the genes SIRT1 and klf4 may serve as a mechanism through which miR-34a promotes obesity associated neurodegenerative disorders, implicating its importance as a biomarker.
miR-200
The miR-200 family of micro-RNAs consists of miR-200a, 200b, 200c, 141, and 429 (Humphries and Yang, 2015). The family has been well-studied in cancer initiation and metastasis (Humphries and Yang, 2015), while some of its members have been implicated in metabolic (Belgardt et al., 2015) and neurological dysfunction (Fu et al., 2019). Evidence suggests that overexpression of the miR-200 family in mice induces pancreatic beta cell apoptosis, subsequently decreasing insulin production and inducing lethal type 2 diabetes (Belgardt et al., 2015; Magenta et al., 2017). Furthermore, these miRNAs induce apoptosis through positive regulation of the tumor suppressor Trp53 (Belgardt et al., 2015). The study in murine model of diabetes showed that miR-200 levels were elevated in the pancreatic islets of diabetic mice (Belgardt et al., 2015), further demonstrating the significance of the miR-200 family in metabolism.
In addition to inducing apoptosis, another study found that the miR-200 family is involved in metabolic processes through promoting insulin resistance. Mechanistically, miR-200 acts by targeting Zinc Finger Protein Family Member 2 (FOG2), a transcription factor that blocks insulin signaling. The miR-200 family also downregulates insulin receptor substrate-2 (IRS2), an important regulator of hypothalamic leptin and peripheral insulin sensitivity (Brady, 2004; Dong et al., 2016; Magenta et al., 2017). Silencing of mir-200a resulted in increased expression of leptin receptor and IRS2, decreased weight gain, and increased responsiveness to insulin in the liver (Crepin et al., 2014). Another mechanism through which the miR-200 family is involved in metabolic dysfunction is through promoting inflammation (Reddy et al., 2012). Both miR-200b and miR-200c have been found to downregulate ZEB1, contributing to the pro-inflammatory state associated with obesity (Reddy et al., 2012; Magenta et al., 2017). It was also found that miR-200c directly downregulates SIRT1 (Magenta et al., 2017), which has a significant role in protection from obesity and associated comorbidities (Mariani et al., 2018). A study conducted in the serum samples from obese preschoolers (aged 2–6 years) identified miR-200 as a s biomarkers of insulin resistance in obese condition (Masotti et al., 2017). Evidence shows that, miR-200c exerts its pathophysiological effects of obesity on endothelial function via oxidative stress (Ait-Aissa et al., 2020).
There is a cumulative line of evidence demonstrating the role of the mir-200 family in neurodegeneration. Similar to the role of miR-200 family in regulating SIRT1 in metabolic disease, it also plays a role in the regulation of SIRT1 in cognitive decline. Studies have shown that miR-200a was upregulated in AD and caused Aβ-induced neuronal apoptosis through downregulation of SIRT1 (Zhang et al., 2017; Fu et al., 2019). Additionally, the role that the miR-200 family plays in inducing inflammation in obesity through regulation of ZEB1 may also serve as a mechanism for inducing inflammation in neurodegenerative disorders. A large line of evidence supports enhanced inflammatory responses in the development of many neurological disorders including PD (Stojkovska et al., 2015) and Huntington’s disease (Valadao et al., 2020). The protein ZEB1 has been found to play a protective role in the brain, specifically against cerebral ischemia (Bui et al., 2009). Just as miR-200b and miR-200c downregulate ZEB1 in obesity, they may also play a role in its regulation during periods of ischemia. Another study reported that the miR-200 family is involved in multiple pathological processes including Aβ secretion, alpha-synuclein aggregation, and DNA repair (Fu et al., 2019). The expression studies of miR-200c in the serum of both AD mice and human AD patients demonstrate the role of miR-200c in the neuronal cell-intrinsic adaptive machinery, neuronal survival, and differentiation in response to Aβ induced ER-stress (Wu et al., 2016). miR-200b/c is reported to have role in reducing Aβ secretion and Aβ-induced cognitive impairment by promoting insulin signaling (Higaki et al., 2018). Furthermore, it has been established that miR-200 family along with other miRNAs can modulate energy homeostasis and body fat content by affecting leptin transduction pathway in pro-opiomelanocortin, Agouti-related peptide/Neuropeptide Y and melanocortin receptors-expressing neurons (Derghal et al., 2017). Given its significant roles in metabolism and cognition, the miR-200 family may serve as an important biomarker for obesity-associated neurodegeneration.
miR-144
The role of miR-144 have been implicated in multiple pathologies, including its role as a tumor suppressor (Kooshkaki et al., 2020) and in erythropoiesis (Rasmussen et al., 2010). However, evidence from literature have also linked this miRNA to obesity as well as neurodegeneration. Recent studies have showed that miR-144 was overexpressed in the pancreas, adipose tissue, and liver of diabetic rats, further targeting insulin receptor substrate 1 (IRS1), which is crucial in insulin signaling (Patterson, 1990). Evidence have also shown that expression of miR-144 was upregulated in the adipose tissue of obese mice (Shen et al., 2018). The overexpression of miR-144 increased lipid accumulation and promoted adipogenesis while levels of CCAAT-enhancer binding protein alpha (C/EBPα) were also upregulated in the murine model of obesity. This is relevant because C/EBPα is known to promote adipogenesis and is also involved in positively regulating PPARγ, a protein involved in glucose metabolism (Shen et al., 2018). Other studies have also supported the role of miR-144 in regulating levels of C/EBPα suggesting a positive correlation (Chen et al., 2020). Additionally, it has also been demonstrated that miR-144 downregulates hydroxymethylglutaryl-CoA reductase (hmgcr), cholesterol 7a-monooxygenase A1 (cyp7a1), and ABCA1 in a model of diet induced obesity (Chen et al., 2020). These proteins have been shown to be involved in the regulation of cholesterol homeostasis (Chen et al., 2020), suggesting the role of miR-144 in cholesterol metabolism (Chen et al., 2020). In addition, levels of A Disintegrin and metalloproteinase domain-containing protein 10 (ADAM10), a target of miR-144 (Sun et al., 2017), have been demonstrated to be significantly decreased in obese subjects and which was associated with an increase an oxidative stress (Elsworthy and Aldred, 2020). The studies on the liver biopsies from morbidly obese subjects undergoing bariatric surgery showed that miR-33a/144 and their target gene ABCA1 may contribute to the pathogenesis of NASH in morbidly obese subjects (Vega-Badillo et al., 2016). Recently, a novel mechanism of miR-144 action in modulating the activity of Nrf2 through fumarate production, has been demonstrated for the impaired antioxidant response in obesity (Azzimato et al., 2021).
Apart from the role of miR-144 in obesity and associated metabolic dysregulation, studies have shown that miR-144 was upregulated in the cortex and cerebellum of patients with AD (Persengiev et al., 2011). Another study found that miR-144 downregulate the levels of ADAM10, inhibiting its protective effects against Aβ accumulation in AD (Cheng et al., 2013). On the other hand, the expression of miR-144 significantly decreased the levels of C/EBPα, in a model of Huntington Disease (Chiang et al., 2007). Additionally, the suppression of C/EBPα caused a decrease in the activity of the urea cycle, a hallmark of Huntington’s Disease (Chiang et al., 2007). A qRT-PCR study conducted using the brain samples of postmortem PD patients showed that downregulation of miR-144 in the brain is related to PD via activation of NF-κB signaling (Xing et al., 2020). miR-144 has been identified as a nuclear respiratory factor 2 (Nrf2)-regulating miR, which is reported to have role in responsiveness to insulin and insulin-like growth factor stimulation, which will have possible effect on brain glucose utilization in AD (Karolina et al., 2011; Paladino et al., 2018). Along with the inhibition of APP, miR-144 increased the expression of key genes involved in maintaining mitochondrial function, including peroxisome proliferator-activated receptor γ coactivator-1α (PGC-1α), nuclear respiratory factor 1 (Nrf-1) and mitochondrial transcription factor A (TFAM) in SH-SY5Y cells (Li et al., 2016). These evidence may further explore the importance of miR-144 in connection with metabolic function and neurodegenerative diseases including PD. In addition, the involvement of miR-144 in inducing cholinergic degeneration by impairing the maturation of nerve growth factor in Alzheimer’s Disease has been revealed in experimental models (Zhou et al., 2021). Although more research is necessary to understand the role of miR-144, evidence from literature provides a mechanistic link of miR-144 in obesity associated neurodegenerative disorders.
Effect of Dietary Modulation in Obesity Associated Cognitive Decline and Neurodegeneration
This review highlights the role of several miRNAs that have been in implicated in both obesity and cognitive decline. While there is room for exploration of developing therapies targeted to these specific miRNAs in obesity associated cognitive decline, such model requires additional research and testing. However, one therapeutic approach that is currently available in the fight against obesity associated cognitive decline is preventing its development and progression through dietary intervention. As obesity and associated comorbidities have been linked to cognitive decline and neurodegenerative disorders, there is growing evidence that supports the idea that dietary measures such as fat reduction and calorie restriction can lead to prevention of obesity and also neuroprotective effects (Schafer et al., 2015; Tan and Norhaizan, 2019).
A high fat diet has been shown to lead to obesity and related health consequences such as insulin resistance, which have now been demonstrated to cause insulin resistance in the brain as well, playing a role in neurodegeneration (Kothari et al., 2017). Insulin resistance in the body has been shown to lead to metabolic syndrome, which is associated with multiple obesity associated pathologies such as cardiovascular disease and NAFLD (Kothari et al., 2017). A previous study have demonstrated decreased tyrosine phosphorylation and increased serine phosphorylation of insulin receptor substrate 1 (IRS1) in the whole brain tissues of mice fed a high fat and high sugar diet, resulting in neuronal insulin resistance and an increased production of inflammatory factors in the brain (Kothari et al., 2017). The study also observed increased levels of pSer473, associated with AKT inhibition and peripheral insulin resistance, in the whole brain tissue of these high fat diet fed mice. Evidence from in vivo models have also suggested that high fat diets increase the neuroinflammatory markers which play a role in hippocampal deficits in memory loss (Duffy et al., 2019). Other studies supported these results, showing high fat fed mice had consistently increased weight compared to those which were chow fed, and also that offspring of high fat fed mice had a statistically significant decline in memory exercises like maze escapes compared to the offspring of those on chow fed diets (Cordner et al., 2019). Moreover, the offspring of the high fat fed mice also showed differential expression of genes within the hippocampus, including significantly decreased expression of INSR, a gene which plays a role in insulin signaling and is thought to be involved in neuron maturation (Cordner et al., 2019). All these results taken together should provide meaningful evidence to link high fat diets not only to obesity, but also to the processes that modulate cognitive decline and impaired neuronal function.
Therapeutic measures that have been shown to prevent obesity associated disorders and diseases of cognitive decline includes implementation of Mediterranean diet and caloric restriction (Schafer et al., 2015; van den Brink et al., 2019). Mediterranean diet comprises of a higher than usual fruit and vegetable intake, increased olive oil usage as the hallmark fat included, and otherwise decreased fat intake by limiting items like processed and red meat (McGrattan et al., 2019). This diet has shown antioxidant properties and an array of decreased proinflammatory factors such as decreases in IL-6, IL-1, and TNF-alpha, all factors which mediate inflammation (McGrattan et al., 2019). These inflammatory markers, especially IL-6 have been positively linked with neurodegeneration, decreased cognitive decline, and decreased brain volume (McGrattan et al., 2019). In patients with metabolic syndrome, a condition interconnected to obesity, starting on a Mediterranean diet resulted in decreased in pro-inflammatory markers like IL-6 (McGrattan et al., 2019). Studies with murine models fed with Mediterranean diet had elevated levels of polyphenol, which come in most part from the olive oil, hallmark to the Mediterranean diet (Reutzel et al., 2018). These mice were found to have increased ATP levels in the brain, along with improved cognitive function in memory tasks compared to their control counterparts not fed with polyphenol heavy Mediterranean diet (Reutzel et al., 2018). Another therapeutic approach worthy of consideration is that of caloric restriction. Studies in mice models predisposed to Aβ plaque build-up, characteristic of AD, revealed that a caloric restriction of even 30% resulted in downregulation of complex required for cleavage of APP and subsequent build-up of Aβ protein in mice (Schafer et al., 2015). These results show that calorie restriction as a means of dietary intervention can potentially play a role in protection against neurodegeneration and cognitive decline as it can result in reduced Aβ. Caloric restriction has been clearly shown to play a role in prevention of obesity and weight loss as well, and so its role as a potentially preventative therapy against obesity associated cognitive decline should not go understated (Zubrzycki et al., 2018). Taken all together, the limited intake of high fat diets as well as caloric restriction and a dietary focus on the foods in the Mediterranean diet could provide protection against obesity and associated cognitive decline and neurodegenerative disorders.
Conclusion
This review aims to highlight the mechanistic role of eight microRNAs involved in the pathogenic link between obesity and its associated comorbidities, such as type 2 diabetes and cardiovascular disease, and neurodegenerative disorders characterized by cognitive decline. It has been demonstrated that obesity is characterized with increased levels of oxidative stress and inflammation that is the direct result of increased lipid accumulation. This state of metabolic dysregulation favors pathophysiological mechanisms that may lead to CNS neurodegeneration and cognitive decline (Fischer and Maier, 2015). These pathophysiological mechanisms propagate the development of neurodegenerative diseases through the generation of oxidative stress and inflammation that is damaging to neuronal tissue. There may be a myriad of mechanisms, but the one this review aims to isolate and further identify is through the generation of aberrant levels of miRNAs involved in key cellular functions. Some of these functions are glucose and lipid metabolism, molecular signaling pathways, and apoptotic pathways. When these functions are perturbed, it can lead to further development of oxidative stress, inflammation, and apoptosis (Figure 2). This continual cycle promotes the further development of pro-inflammatory markers that wreak cellular havoc on physiologically homeostatic systems. We also investigated the efficacy of dietary interventions on the disruption of these cyclic pathways that favor the pro-inflammatory state. As a non-invasive and inexpensive remedy, dietary intervention plays an important role in the amelioration of the obese-phenotype and development of its associated comorbidities. The regression and termination of the development of the obese phenotype through dietary interventions may even have important ramifications in light of CNS pathologies that are directly linked to the obese state. It is thought that the modification of diet can slow pro-inflammatory pathways to the point that the CNS is not receiving any harmful overflow that may lead to the development of neurodegenerative associated cognitive decline.
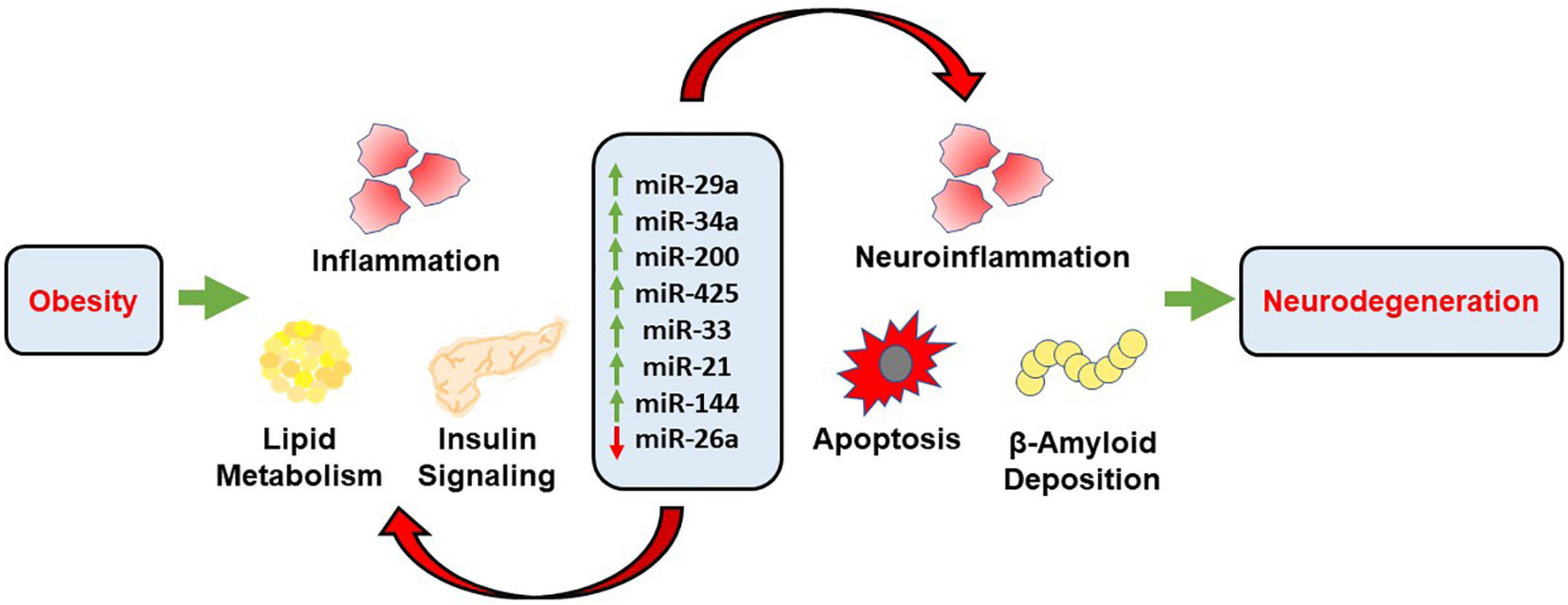
Figure 2. Schematic representation demonstrating the mechanistic role of miRNAs in obesity associated neurodegeneration. Obesity propagates inflammation, lipid accumulation and alters insulin signaling through the transcriptional role of respective miRNAs. These miRNAs further mediate neuroinflammation, apoptosis and accumulation of Aβ through distinct pathways, along with alterations associated with obesity which further results in cognitive decline and neurodegeneration.
The microRNAs that were investigated showed insight into the development and continuation of pro-inflammatory states that favor oxidative stress, and thus may be linked with the severity of neurodegenerative diseases affiliated with the obese phenotype. The role of these microRNAs is variable, with involvement in a few pathways rather than widespread involvement with minimal role in several pathways. One of the roles is the inhibition of glucose metabolism when levels of the corresponding miRNA are perturbed. The microRNAs that are thought to have involvement in this are miR-29a, miR-200 family, miR-26a, miR-21, and miR-144. The contribution to the disruption of glucose metabolism is variable between each miRNA, but the basis for disruption includes some involvement in either insulin resistance or metabolic glucose pathways (Ono, 2011; Williams and Mitchell, 2012). Another way that miRNAs encourage the development and continuation of the obese phenotype associated with neurodegeneration is through the disruption of lipid metabolism. The role of miR-29a, miR-33, miR-21, and miR-144 have been implicated in lipogenic pathways as well as cholesterol metabolism. Furthermore, another major pathway that plays an important role in the development of neurodegenerative diseases associated with the obese state is the propagation of pro-inflammatory markers, the mechanism of which are targeted by miR-200 family.
The disruption of glucose metabolism, insulin signaling, lipid metabolism, and the inflammatory state is directly linked to the development of oxidative stress associated with the obese phenotype (Kilic et al., 2016) (as summarized in Table 2). The continued propagation of these harmful cycles causes positive feedback on cellular pathways that generate inflammatory markers, ROS, pro-apoptotic factors, and metabolic pathway intermediates. These intermediates are then able to cross the BBB and cause oxidative damage to neuronal tissue (Roediger and Armati, 2003; Salim, 2017). Increased oxidative damage in the obese state can encourage the development of neuronal disorders characterized by neurodegeneration as well as cognitive decline later in life (Kim G.H. et al., 2015). The role of miRNAs in the proliferation of obese phenotype associated oxidative stress that causes neurodegenerative diseases cannot be overlooked, as there is evidence to suggest that these molecules do play a role. Aberrant levels of microRNAs cause these homeostatic functions to be disturbed, and further continues the progression of oxidative and inflammatory damage on neuronal tissue.
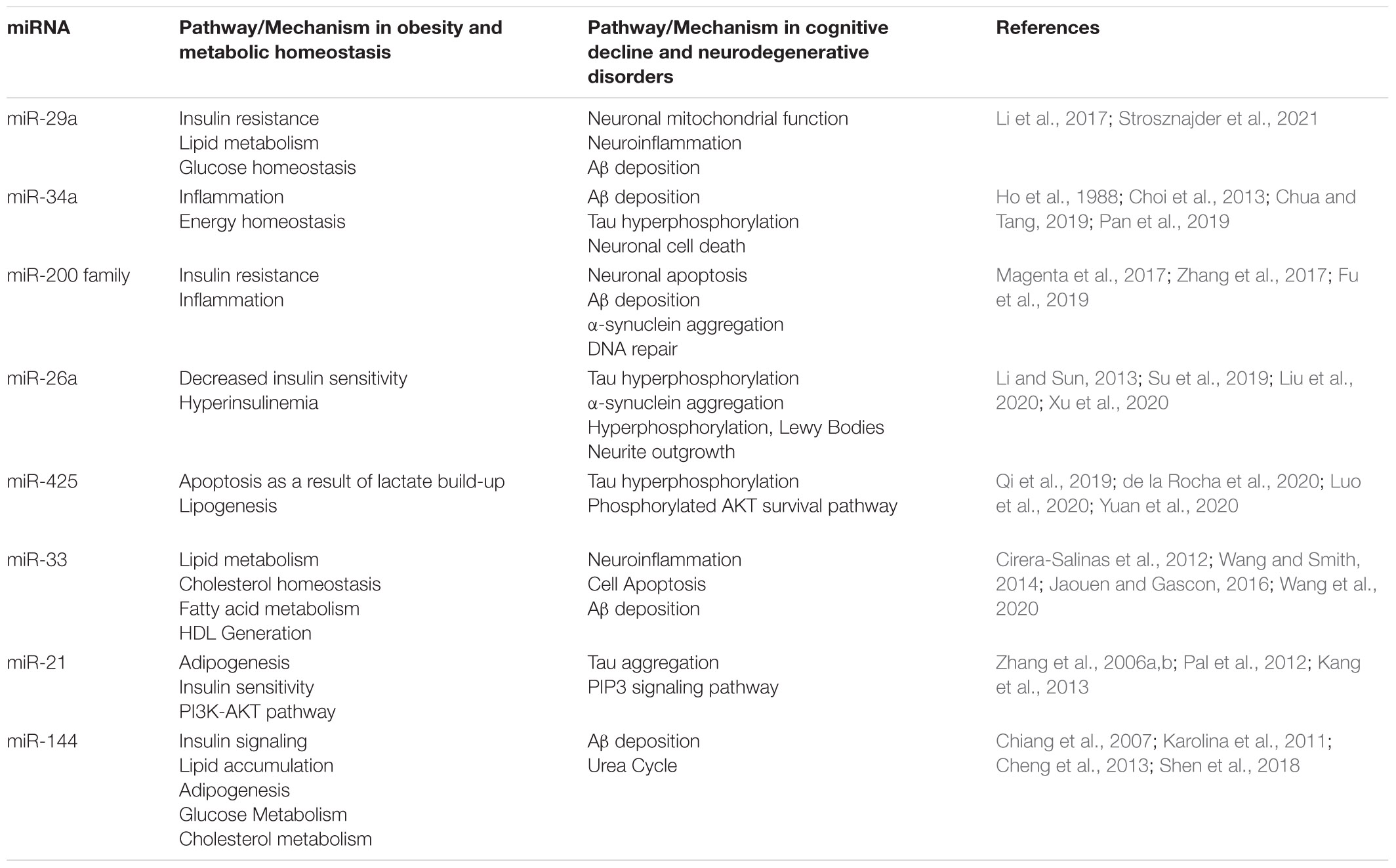
Table 2. Summary of mechanistic action of miRNAs in obesity associated cognitive decline and neurodegenerative disorders.
Although there is evidence to suggest that miRNAs play important functions in the development of oxidative stress in the obese state that directly impacts neuronal tissue, more research is required to determine the exact role of where these miRNAs play their part. The eight miRNAs that this review investigates did show therapeutic potential as possible targets of treatment. However, the mechanism in which this would be done remains to be further uncovered. In order to investigate the efficacy of miRNAs as therapeutic targets, more defined mechanisms need to be determined. The viability of dietary intervention in the role of improving health outcomes related to obesity and neurodegenerative disorders remains to be important. Increased research in this area could lead to new treatment alternatives that can impact patients worldwide and use miRNA technologies in order to inhibit the progression of the obese state and possible development of neurodegenerative disorders associated with resulting cellular and tissue specific phenotypic alterations.
Author Contributions
KS: conceptualization. HL and KS: validation and project administration. MP, AK, JW, SL, SP, and DP: writing—original draft preparation. HL: writing—review and editing. KS: supervision and funding. All authors read and agreed to the published version of the manuscript.
Funding
This research was supported by the National Institutes of Health Grants 1R15HL150721 (to KS) and NIH Bench-to-Bedside award made possible by the Office of Research on Women’s Health (ORWH) 736214 (to KS).
Conflict of Interest
The authors declare that the research was conducted in the absence of any commercial or financial relationships that could be construed as a potential conflict of interest.
Publisher’s Note
All claims expressed in this article are solely those of the authors and do not necessarily represent those of their affiliated organizations, or those of the publisher, the editors and the reviewers. Any product that may be evaluated in this article, or claim that may be made by its manufacturer, is not guaranteed or endorsed by the publisher.
References
Absalon, S., Kochanek, D. M., Raghavan, V., and Krichevsky, A. M. (2013). MiR-26b, upregulated in Alzheimer’s disease, activates cell cycle entry, tau-phosphorylation, and apoptosis in postmitotic neurons. J. Neurosci. 33, 14645–14659. doi: 10.1523/JNEUROSCI.1327-13.2013
Acharya, A., Berry, D. C., Zhang, H., Jiang, Y., Jones, B. T., Hammer, R. E., et al. (2019). miR-26 suppresses adipocyte progenitor differentiation and fat production by targeting Fbxl19. Genes Dev. 33, 1367–1380. doi: 10.1101/gad.328955.119
Ahmadpour, F., Nourbakhsh, M., Razzaghy-Azar, M., Khaghani, S., Alipoor, B., Abdolvahabi, Z., et al. (2018). The association of plasma levels of miR-34a AND miR-149 with obesity and insulin resistance in obese children and adolescents. Acta Endocrinol. (Buchar) 14, 149–154. doi: 10.4183/aeb.2018.149
Ait-Aissa, K., Nguyen, Q. M., Gabani, M., Kassan, A., Kumar, S., Choi, S. K., et al. (2020). MicroRNAs and obesity-induced endothelial dysfunction: key paradigms in molecular therapy. Cardiovasc. Diabetol. 19:136. doi: 10.1186/s12933-020-01107-3
Albers, D. S., and Beal, M. F. (2000). Mitochondrial dysfunction and oxidative stress in aging and neurodegenerative disease. J. Neural. Transm. Suppl. 59, 133–154.
Alexandrov, P. N., Dua, P., Hill, J. M., Bhattacharjee, S., Zhao, Y., and Lukiw, W. J. (2012). microRNA (miRNA) speciation in Alzheimer’s disease (AD) cerebrospinal fluid (CSF) and extracellular fluid (ECF). Int. J. Biochem. Mol. Biol. 3, 365–373.
Ali, O., Darwish, H. A., Eldeib, K. M., and Abdel Azim, S. A. (2018). miR-26a potentially contributes to the regulation of fatty acid and sterol metabolism in vitro human HepG2 cell model of nonalcoholic fatty liver disease. Oxid. Med. Cell Longev. 2018:8515343. doi: 10.1155/2018/8515343
Amen, D. G., Wu, J., George, N., and Newberg, A. (2020). Patterns of regional cerebral blood flow as a function of obesity in adults. J. Alzheimers Dis. 77, 1331–1337.
Andre, C., Guzman-Quevedo, O., Rey, C., Remus-Borel, J., Clark, S., Castellanos-Jankiewicz, A., et al. (2017). Inhibiting Microglia Expansion Prevents Diet-Induced Hypothalamic and Peripheral Inflammation. Diabetes 66, 908–919. doi: 10.2337/db16-0586
Arnold, S. E., Arvanitakis, Z., Macauley-Rambach, S. L., Koenig, A. M., Wang, H. Y., Ahima, R. S., et al. (2018). Brain insulin resistance in type 2 diabetes and Alzheimer disease: concepts and conundrums. Nat. Rev. Neurol. 14, 168–181. doi: 10.1038/nrneurol.2017.185
Ashrafian, H., Harling, L., Darzi, A., and Athanasiou, T. (2013). Neurodegenerative disease and obesity: what is the role of weight loss and bariatric interventions? Metab. Brain Dis. 28, 341–353.
Azzimato, V., Chen, P., Barreby, E., Morgantini, C., Levi, L., Vankova, A., et al. (2021). Hepatic miR-144 drives fumarase activity preventing NRF2 activation during obesity. Gastroenterology doi: 10.1053/j.gastro.2021.08.030 [Epub Online ahead of print].
Bai, X., Tang, Y., Yu, M., Wu, L., Liu, F., Ni, J., et al. (2017). Downregulation of blood serum microRNA 29 family in patients with Parkinson’s disease. Sci. Rep. 7:5411.
Banks, W. A., Kastin, A. J., and Gutierrez, E. G. (1994). Penetration of interleukin-6 across the murine blood-brain barrier. Neurosci. Lett. 179, 53–56.
Barazzoni, R., Gortan Cappellari, G., Ragni, M., and Nisoli, E. (2018). Insulin resistance in obesity: an overview of fundamental alterations. Eat Weight Disord. 23, 149–157. doi: 10.1007/s40519-018-0481-6
Barish, G. D., Narkar, V. A., and Evans, R. M. (2006). PPAR delta: a dagger in the heart of the metabolic syndrome. J. Clin. Invest. 116, 590–597. doi: 10.1172/JCI27955
Belgardt, B. F., Ahmed, K., Spranger, M., Latreille, M., Denzler, R., Kondratiuk, N., et al. (2015). The microRNA-200 family regulates pancreatic beta cell survival in type 2 diabetes. Nat. Med. 21, 619–627. doi: 10.1038/nm.3862
Berrington de Gonzalez, A., Hartge, P., Cerhan, J. R., Flint, A. J., Hannan, L., Macinnis, R. J., et al. (2010). Body-mass index and mortality among 1.46 million white adults. N. Engl. J. Med. 363, 2211–2219. doi: 10.1056/NEJMoa1000367
Bloom, G. S. (2014). Amyloid-beta and tau: the trigger and bullet in Alzheimer disease pathogenesis. JAMA Neurol. 71, 505–508.
Bocarsly, M. E., Fasolino, M., Kane, G. A., Lamarca, E. A., Kirschen, G. W., Karatsoreos, I. N., et al. (2015). Obesity diminishes synaptic markers, alters microglial morphology, and impairs cognitive function. Proc. Natl. Acad. Sci. U. S. A. 112, 15731–15736. doi: 10.1073/pnas.1511593112
Boucher, J., Kleinridders, A., and Kahn, C. R. (2014). Insulin receptor signaling in normal and insulin-resistant states. Cold Spring Harb. Perspect. Biol. 6:a009191.
Bracke, A., Domanska, G., Bracke, K., Harzsch, S., Van Den Brandt, J., Broker, B., et al. (2019). Obesity Impairs Mobility and Adult Hippocampal Neurogenesis. J. Exp. Neurosci. 13:1179069519883580. doi: 10.1177/1179069519883580
Brady, M. J. (2004). IRS2 takes center stage in the development of type 2 diabetes. J. Clin. Invest. 114, 886–888. doi: 10.1172/JCI23108
Bui, T., Sequeira, J., Wen, T. C., Sola, A., Higashi, Y., Kondoh, H., et al. (2009). ZEB1 links p63 and p73 in a novel neuronal survival pathway rapidly induced in response to cortical ischemia. PLoS One 4:e4373. doi: 10.1371/journal.pone.0004373
Buie, J. J., Watson, L. S., Smith, C. J., and Sims-Robinson, C. (2019). Obesity-related cognitive impairment: the role of endothelial dysfunction. Neurobiol. Dis. 132:104580. doi: 10.1016/j.nbd.2019.104580
Buller, B., Liu, X., Wang, X., Zhang, R. L., Zhang, L., Hozeska-Solgot, A., et al. (2010). MicroRNA-21 protects neurons from ischemic death. FEBS J. 277, 4299–4307.
Burke, R. E., Dauer, W. T., and Vonsattel, J. P. (2008). A critical evaluation of the Braak staging scheme for Parkinson’s disease. Ann. Neurol. 64, 485–491.
Castro, A. V., Kolka, C. M., Kim, S. P., and Bergman, R. N. (2014). Obesity, insulin resistance and comorbidities? Mechanisms of association. Arq. Bras. Endocrinol. Metabol. 58, 600–609.
Chabry, J., Nicolas, S., Cazareth, J., Murris, E., Guyon, A., Glaichenhaus, N., et al. (2015). Enriched environment decreases microglia and brain macrophages inflammatory phenotypes through adiponectin-dependent mechanisms: relevance to depressive-like behavior. Brain Behav. Immun. 50, 275–287. doi: 10.1016/j.bbi.2015.07.018
Chen, B., Liao, W. Q., Xu, N., Xu, H., Wen, J. Y., Yu, C. A., et al. (2009). Adiponectin protects against cerebral ischemia-reperfusion injury through anti-inflammatory action. Brain Res. 1273, 129–137. doi: 10.1016/j.brainres.2009.04.002
Chen, G., Wu, K., Zhao, T., Ling, S., Liu, W., and Luo, Z. (2020). miR-144 Mediates high fat-induced changes of cholesterol metabolism via direct regulation of C/EBPalpha in the liver and isolated hepatocytes of yellow catfish. J. Nutr. 150, 464–474. doi: 10.1093/jn/nxz282
Chen, H., Zhang, S. M., Schwarzschild, M. A., Hernan, M. A., Willett, W. C., and Ascherio, A. (2004). Obesity and the risk of Parkinson’s disease. Am. J. Epidemiol. 159, 547–555.
Chen, P., Chen, F., Lei, J., Li, Q., and Zhou, B. (2019). Activation of the miR-34a-Mediated SIRT1/mTOR Signaling Pathway by Urolithin A Attenuates D-Galactose-Induced Brain Aging in Mice. Neurotherapeutics 16, 1269–1282. doi: 10.1007/s13311-019-00753-0
Cheng, A., Wan, R., Yang, J. L., Kamimura, N., Son, T. G., Ouyang, X., et al. (2012). Involvement of PGC-1alpha in the formation and maintenance of neuronal dendritic spines. Nat. Commun. 3:1250. doi: 10.1038/ncomms2238
Cheng, C., Li, W., Zhang, Z., Yoshimura, S., Hao, Q., Zhang, C., et al. (2013). MicroRNA-144 is regulated by activator protein-1 (AP-1) and decreases expression of Alzheimer disease-related a disintegrin and metalloprotease 10 (ADAM10). J. Biol. Chem. 288, 13748–13761. doi: 10.1074/jbc.M112.381392
Cheng, C. F., Ku, H. C., and Lin, H. (2018). PGC-1alpha as a pivotal factor in lipid and metabolic regulation. Int. J. Mol. Sci. 19:3447.
Cheng, Z., Zou, X., Jin, Y., Gao, S., Lv, J., Li, B., et al. (2018). The Role of KLF4 in Alzheimer’s disease. Front. Cell Neurosci. 12:325. doi: 10.3389/fncel.2018.00325
Chiang, M. C., Chen, H. M., Lee, Y. H., Chang, H. H., Wu, Y. C., Soong, B. W., et al. (2007). Dysregulation of C/EBPalpha by mutant Huntingtin causes the urea cycle deficiency in Huntington’s disease. Hum. Mol. Genet. 16, 483–498. doi: 10.1093/hmg/ddl481
Choi, G. E., and Han, H. J. (2021). Glucocorticoid impairs mitochondrial quality control in neurons. Neurobiol. Dis. 152:105301.
Choi, S. E., Fu, T., Seok, S., Kim, D. H., Yu, E., Lee, K. W., et al. (2013). Elevated microRNA-34a in obesity reduces NAD+ levels and SIRT1 activity by directly targeting NAMPT. Aging Cell 12, 1062–1072. doi: 10.1111/acel.12135
Chua, C. E. L., and Tang, B. L. (2019). miR-34a in Neurophysiology and Neuropathology. J. Mol. Neurosci. 67, 235–246. doi: 10.1007/s12031-018-1231-y
Cirera-Salinas, D., Pauta, M., Allen, R. M., Salerno, A. G., Ramirez, C. M., Chamorro-Jorganes, A., et al. (2012). Mir-33 regulates cell proliferation and cell cycle progression. Cell Cyc. 11, 922–933.
Cope, E. C., Lamarca, E. A., Monari, P. K., Olson, L. B., Martinez, S., Zych, A. D., et al. (2018). Microglia play an active role in obesity-associated cognitive decline. J. Neurosci. 38, 8889–8904. doi: 10.1523/JNEUROSCI.0789-18.2018
Cordner, Z. A., Khambadkone, S. G., Boersma, G. J., Song, L., Summers, T. N., Moran, T. H., et al. (2019). Maternal high-fat diet results in cognitive impairment and hippocampal gene expression changes in rat offspring. Exp. Neurol. 318, 92–100. doi: 10.1016/j.expneurol.2019.04.018
Crepin, D., Benomar, Y., Riffault, L., Amine, H., Gertler, A., and Taouis, M. (2014). The over-expression of miR-200a in the hypothalamus of ob/ob mice is linked to leptin and insulin signaling impairment. Mol. Cell Endocrinol. 384, 1–11. doi: 10.1016/j.mce.2013.12.016
Dake, M. D., De Marco, M., Blackburn, D. J., Wilkinson, I. D., Remes, A., Liu, Y., et al. (2021). Obesity and brain vulnerability in normal and abnormal aging: a multimodal MRI study. J. Alzheimers Dis. Rep. 5, 65–77. doi: 10.3233/ADR-200267
de la Rocha, A. M. A., Gonzalez-Huarriz, M., Guruceaga, E., Mihelson, N., Tejada-Solis, S., Diez-Valle, R., et al. (2020). miR-425-5p, a SOX2 target, regulates the expression of FOXJ3 and RAB31 and promotes the survival of GSCs. Arch. Clin. Biomed. Res. 4, 221–238. doi: 10.26502/acbr.50170100
Deckers, K., Van Boxtel, M. P. J., Verhey, F. R. J., and Kohler, S. (2017). Obesity and cognitive decline in adults: effect of methodological choices and confounding by age in a longitudinal study. J. Nutr. Health Aging 21, 546–553. doi: 10.1007/s12603-016-0757-3
Derghal, A., Djelloul, M., Trouslard, J., and Mounien, L. (2017). The Role of MicroRNA in the Modulation of the Melanocortinergic System. Front. Neurosci. 11:181. doi: 10.3389/fnins.2017.00181
Di Pietro, V., Ragusa, M., Davies, D., Su, Z., Hazeldine, J., Lazzarino, G., et al. (2017). MicroRNAs as novel biomarkers for the diagnosis and prognosis of mild and severe traumatic brain injury. J. Neurotrauma 34, 1948–1956.
Di Spiezio, A., Sandin, E. S., Dore, R., Muller-Fielitz, H., Storck, S. E., Bernau, M., et al. (2018). The LepR-mediated leptin transport across brain barriers controls food reward. Mol. Metab. 8, 13–22. doi: 10.1016/j.molmet.2017.12.001
Dong, S., Meng, X., Xue, S., Yan, Z., Ren, P., and Liu, J. (2016). microRNA-141 inhibits thyroid cancer cell growth and metastasis by targeting insulin receptor substrate 2. Am. J. Transl. Res. 8, 1471–1481.
Duffy, C. M., Hofmeister, J. J., Nixon, J. P., and Butterick, T. A. (2019). High fat diet increases cognitive decline and neuroinflammation in a model of orexin loss. Neurobiol. Learn Mem. 157, 41–47. doi: 10.1016/j.nlm.2018.11.008
Duka, T., Rusnak, M., Drolet, R. E., Duka, V., Wersinger, C., Goudreau, J. L., et al. (2006). Alpha-synuclein induces hyperphosphorylation of Tau in the MPTP model of parkinsonism. FASEB J. 20, 2302–2312.
Dumon, C., Diabira, D., Chudotvorova, I., Bader, F., Sahin, S., Zhang, J., et al. (2018). The adipocyte hormone leptin sets the emergence of hippocampal inhibition in mice. Elife 7:e36726. doi: 10.7554/eLife.36726
Elabi, O., Gaceb, A., Carlsson, R., Padel, T., Soylu-Kucharz, R., Cortijo, I., et al. (2021). Human alpha-synuclein overexpression in a mouse model of Parkinson’s disease leads to vascular pathology, blood brain barrier leakage and pericyte activation. Sci. Rep. 11:1120. doi: 10.1038/s41598-020-80889-8
Elghazi, L., and Bernal-Mizrachi, E. (2009). Akt and PTEN: beta-cell mass and pancreas plasticity. Trends Endocrinol. Metab. 20, 243–251. doi: 10.1016/j.tem.2009.03.002
Elsworthy, R. J., and Aldred, S. (2020). The effect of age and obesity on platelet amyloid precursor protein processing and plasma markers of oxidative stress and inflammation. Exp. Gerontol. 132:110838. doi: 10.1016/j.exger.2020.110838
Fasshauer, M., and Bluher, M. (2015). Adipokines in health and disease. Trends Pharmacol. Sci. 36, 461–470.
Femminella, G. D., Livingston, N. R., Raza, S., Van Der Doef, T., Frangou, E., Love, S., et al. (2021). Does insulin resistance influence neurodegeneration in non-diabetic Alzheimer’s subjects? Alzheimers Res. Ther. 13:47. doi: 10.1186/s13195-021-00784-w
Feng, M., Xu, D., and Wang, L. (2018). miR-26a inhibits atherosclerosis progression by targeting TRPC3. Cell Biosci. 8:4. doi: 10.1186/s13578-018-0203-9
Finkelstein, E. A., Khavjou, O. A., Thompson, H., Trogdon, J. G., Pan, L., Sherry, B., et al. (2012). Obesity and severe obesity forecasts through 2030. Am. J. Prev. Med. 42, 563–570.
Fischer, R., and Maier, O. (2015). Interrelation of oxidative stress and inflammation in neurodegenerative disease: role of TNF. Oxid. Med. Cell Longev. 2015:610813.
Forny-Germano, L., De Felice, F. G., and Vieira, M. (2018). The Role of Leptin and Adiponectin in Obesity-Associated Cognitive Decline and Alzheimer’s Disease. Front. Neurosci. 12:1027. doi: 10.3389/fnins.2018.01027
Fu, J., Peng, L., Tao, T., Chen, Y., Li, Z., and Li, J. (2019). Regulatory roles of the miR-200 family in neurodegenerative diseases. Biomed. Pharmacother. 119:109409.
Fu, X., Dong, B., Tian, Y., Lefebvre, P., Meng, Z., Wang, X., et al. (2015). MicroRNA-26a regulates insulin sensitivity and metabolism of glucose and lipids. J. Clin. Invest. 125, 2497–2509.
Garza, J. C., Guo, M., Zhang, W., and Lu, X. Y. (2008). Leptin increases adult hippocampal neurogenesis in vivo and in vitro. J. Biol. Chem. 283, 18238–18247.
Gendron, T. F., and Petrucelli, L. (2009). The role of tau in neurodegeneration. Mol. Neurodegener. 4:13.
Geng, J., Wang, L., Zhang, L., Qin, C., Song, Y., Ma, Y., et al. (2018). Blood-brain barrier disruption induced cognitive impairment is associated with increase of inflammatory cytokine. Front. Aging Neurosci. 10:129. doi: 10.3389/fnagi.2018.00129
Giuliani, A., Gaetani, S., Sorgentoni, G., Agarbati, S., Laggetta, M., Matacchione, G., et al. (2021). Circulating Inflamma-miRs as potential biomarkers of cognitive impairment in patients affected by Alzheimer’s disease. Front. Aging Neurosci. 13:647015. doi: 10.3389/fnagi.2021.647015
Glantschnig, C., Koenen, M., Gil-Lozano, M., Karbiener, M., Pickrahn, I., Williams-Dautovich, J., et al. (2019). A miR-29a-driven negative feedback loop regulates peripheral glucocorticoid receptor signaling. FASEB J. 33, 5924–5941. doi: 10.1096/fj.201801385RR
Goedeke, L., Vales-Lara, F. M., Fenstermaker, M., Cirera-Salinas, D., Chamorro-Jorganes, A., Ramirez, C. M., et al. (2013). A regulatory role for microRNA 33∗ in controlling lipid metabolism gene expression. Mol. Cell Biol. 33, 2339–2352.
Goh, S. Y., Chao, Y. X., Dheen, S. T., Tan, E. K., and Tay, S. S. (2019). Role of MicroRNAs in Parkinson’s Disease. Int. J. Mol. Sci. 20:5649.
Gotz, J., Chen, F., Van Dorpe, J., and Nitsch, R. M. (2001). Formation of neurofibrillary tangles in P301l tau transgenic mice induced by Abeta 42 fibrils. Science 293, 1491–1495. doi: 10.1126/science.1062097
Graham, L. C., Grabowska, W. A., Chun, Y., Risacher, S. L., Philip, V. M., Saykin, A. J., et al. (2019). Exercise prevents obesity-induced cognitive decline and white matter damage in mice. Neurobiol. Aging 80, 154–172. doi: 10.1016/j.neurobiolaging.2019.03.018
Grossi, I., Radeghieri, A., Paolini, L., Porrini, V., Pilotto, A., Padovani, A., et al. (2021). MicroRNA34a5p expression in the plasma and in its extracellular vesicle fractions in subjects with Parkinson’s disease: an exploratory study. Int. J. Mol. Med. 47, 533–546. doi: 10.3892/ijmm.2020.4806
Guglielmi, V., D’adamo, M., Menghini, R., Cardellini, M., Gentileschi, P., Federici, M., et al. (2017). MicroRNA 21 is up-regulated in adipose tissue of obese diabetic subjects. Nutr. Healthy Aging 4, 141–145. doi: 10.3233/NHA-160020
Guo, C., Sun, L., Chen, X., and Zhang, D. (2013). Oxidative stress, mitochondrial damage and neurodegenerative diseases. Neural. Regen. Res. 8, 2003–2014.
Guo, R., Fan, G., Zhang, J., Wu, C., Du, Y., Ye, H., et al. (2017). A 9-microRNA signature in serum serves as a noninvasive biomarker in early diagnosis of Alzheimer’s disease. J. Alzheimers Dis. 60, 1365–1377. doi: 10.3233/JAD-170343
Gustafson, D., Rothenberg, E., Blennow, K., Steen, B., and Skoog, I. (2003). An 18-year follow-up of overweight and risk of Alzheimer disease. Arch. Intern. Med. 163, 1524–1528. doi: 10.1001/archinte.163.13.1524
Haggerty, T., Credle, J., Rodriguez, O., Wills, J., Oaks, A. W., Masliah, E., et al. (2011). Hyperphosphorylated Tau in an alpha-synuclein-overexpressing transgenic model of Parkinson’s disease. Eur. J. Neurosci. 33, 1598–1610. doi: 10.1111/j.1460-9568.2011.07660.x
Haik, K. L., Shear, D. A., Hargrove, C., Patton, J., Mazei-Robison, M., Sandstrom, M. I., et al. (2008). 7-nitroindazole attenuates 6-hydroxydopamine-induced spatial learning deficits and dopamine neuron loss in a presymptomatic animal model of Parkinson’s disease. Exp. Clin. Psychopharmacol. 16, 178–189. doi: 10.1037/1064-1297.16.2.178
Hanif, F., Muzaffar, K., Perveen, K., Malhi, S. M., and Simjee Sh, U. (2017). Glioblastoma multiforme: a review of its epidemiology and pathogenesis through clinical presentation and treatment. Asian Pac. J. Cancer Prev. 18, 3–9.
Harmanci, D., Erkan, E. P., Kocak, A., and Akdogan, G. G. (2017). Role of the microRNA-29 family in fibrotic skin diseases. Biomed. Rep. 6, 599–604. doi: 10.3892/br.2017.900
Harrison, L., Schriever, S. C., Feuchtinger, A., Kyriakou, E., Baumann, P., Pfuhlmann, K., et al. (2019). Fluorescent blood-brain barrier tracing shows intact leptin transport in obese mice. Int. J. Obes. (Lond) 43, 1305–1318. doi: 10.1038/s41366-018-0221-z
Hassing, L. B., Dahl, A. K., Thorvaldsson, V., Berg, S., Gatz, M., Pedersen, N. L., et al. (2009). Overweight in midlife and risk of dementia: a 40-year follow-up study. Int. J. Obes. (Lond) 33, 893–898.
Hebert, S. S., Horre, K., Nicolai, L., Papadopoulou, A. S., Mandemakers, W., Silahtaroglu, A. N., et al. (2008). Loss of microRNA cluster miR-29a/b-1 in sporadic Alzheimer’s disease correlates with increased BACE1/beta-secretase expression. Proc. Natl. Acad. Sci. U. S. A. 105, 6415–6420. doi: 10.1073/pnas.0710263105
Higaki, S., Muramatsu, M., Matsuda, A., Matsumoto, K., Satoh, J. I., Michikawa, M., et al. (2018). Defensive effect of microRNA-200b/c against amyloid-beta peptide-induced toxicity in Alzheimer’s disease models. PLoS One 13:e0196929. doi: 10.1371/journal.pone.0196929
Ho, C. Y., Hageman, W. E., Mohrbacher, R. J., and End, D. W. (1988). Inhibition of leukotriene and thromboxane biosynthesis by a 9-(4-chlorophenyl) analogue of arachidonic acid. J. Pharm. Sci. 77, 149–152. doi: 10.1002/jps.2600770211
Ho, N., Sommers, M. S., and Lucki, I. (2013). Effects of diabetes on hippocampal neurogenesis: links to cognition and depression. Neurosci. Biobehav. Rev. 37, 1346–1362.
Holscher, C. (2020). Brain insulin resistance: role in neurodegenerative disease and potential for targeting. Expert. Opin. Investig. Drugs 29, 333–348.
Hopkins, B. D., Hodakoski, C., Barrows, D., Mense, S. M., and Parsons, R. E. (2014). PTEN function: the long and the short of it. Trends Biochem. Sci. 39, 183–190. doi: 10.1016/j.tibs.2014.02.006
Horie, T., Nakao, T., Miyasaka, Y., Nishino, T., Matsumura, S., Nakazeki, F., et al. (2021). microRNA-33 maintains adaptive thermogenesis via enhanced sympathetic nerve activity. Nat. Commun. 12:843. doi: 10.1038/s41467-021-21107-5
Hu, Y. B., Zhang, Y. F., Wang, H., Ren, R. J., Cui, H. L., Huang, W. Y., et al. (2019). miR-425 deficiency promotes necroptosis and dopaminergic neurodegeneration in Parkinson’s disease. Cell Death Dis. 10:589. doi: 10.1038/s41419-019-1809-5
Huang, Y. R., and Liu, R. T. (2020). The Toxicity and Polymorphism of beta-Amyloid Oligomers. Int. J. Mol. Sci. 21:4477.
Humphries, B., and Yang, C. (2015). The microRNA-200 family: small molecules with novel roles in cancer development, progression and therapy. Oncotarget 6, 6472–6498. doi: 10.18632/oncotarget.3052
Hurtado, D. E., Molina-Porcel, L., Iba, M., Aboagye, A. K., Paul, S. M., Trojanowski, J. Q., et al. (2010). A{beta} accelerates the spatiotemporal progression of tau pathology and augments tau amyloidosis in an Alzheimer mouse model. Am. J. Pathol. 177, 1977–1988. doi: 10.2353/ajpath.2010.100346
Iacomino, G., and Siani, A. (2017). Role of microRNAs in obesity and obesity-related diseases. Genes Nutr. 12:23.
Imani, S., Wu, R. C., and Fu, J. (2018). MicroRNA-34 family in breast cancer: from research to therapeutic potential. J. Cancer 9, 3765–3775. doi: 10.7150/jca.25576
Jack, C. R. Jr., Bennett, D. A., Blennow, K., Carrillo, M. C., Dunn, B., Haeberlein, S. B., et al. (2018). NIA-AA Research framework: toward a biological definition of Alzheimer’s disease. Alzheimers Dement. 14, 535–562. doi: 10.1016/j.jalz.2018.02.018
Jampoka, K., Muangpaisarn, P., Khongnomnan, K., Treeprasertsuk, S., Tangkijvanich, P., and Payungporn, S. (2018). Serum miR-29a and miR-122 as Potential Biomarkers for Non-Alcoholic Fatty Liver Disease (NAFLD). Microrna 7, 215–222. doi: 10.2174/2211536607666180531093302
Jan, A., Karasinska, J. M., Kang, M. H., De Haan, W., Ruddle, P., Kaur, A., et al. (2015). Direct intracerebral delivery of a miR-33 antisense oligonucleotide into mouse brain increases brain ABCA1 expression. [Corrected]. Neurosci. Lett. 598, 66–72. doi: 10.1016/j.neulet.2015.05.007
Jaouen, F., and Gascon, E. (2016). Understanding the Role of miR-33 in Brain Lipid Metabolism: implications for Alzheimer’s Disease. J. Neurosci. 36, 2558–2560. doi: 10.1523/JNEUROSCI.4571-15.2016
Kahn, S. E., Hull, R. L., and Utzschneider, K. M. (2006). Mechanisms linking obesity to insulin resistance and type 2 diabetes. Nature 444, 840–846.
Kang, M., Yan, L. M., Zhang, W. Y., Li, Y. M., Tang, A. Z., and Ou, H. S. (2013). Role of microRNA-21 in regulating 3T3-L1 adipocyte differentiation and adiponectin expression. Mol. Biol. Rep. 40, 5027–5034. doi: 10.1007/s11033-013-2603-6
Karbiener, M., Pisani, D. F., Frontini, A., Oberreiter, L. M., Lang, E., Vegiopoulos, A., et al. (2014). MicroRNA-26 family is required for human adipogenesis and drives characteristics of brown adipocytes. Stem Cells 32, 1578–1590. doi: 10.1002/stem.1603
Karolina, D. S., Armugam, A., Tavintharan, S., Wong, M. T., Lim, S. C., Sum, C. F., et al. (2011). MicroRNA 144 impairs insulin signaling by inhibiting the expression of insulin receptor substrate 1 in type 2 diabetes mellitus. PLoS One 6:e22839. doi: 10.1371/journal.pone.0022839
Karunakaran, D., Richards, L., Geoffrion, M., Barrette, D., Gotfrit, R. J., Harper, M. E., et al. (2015). Therapeutic inhibition of miR-33 promotes fatty acid oxidation but does not ameliorate metabolic dysfunction in diet-induced obesity. Arterioscler. Thromb. Vasc. Biol. 35, 2536–2543. doi: 10.1161/ATVBAHA.115.306404
Khan, M. S. H., and Hegde, V. (2020). Obesity and diabetes mediated chronic inflammation: a potential biomarker in Alzheimer’s disease. J. Pers. Med. 10:42.
Kilic, E., Ozer, O. F., Erek Toprak, A., Erman, H., Torun, E., Kesgin Ayhan, S., et al. (2016). Oxidative stress status in childhood obesity: a potential risk predictor. Med. Sci. Monit. 22, 3673–3679. doi: 10.12659/msm.897965
Kim, G. H., Kim, J. E., Rhie, S. J., and Yoon, S. (2015). The Role of oxidative stress in neurodegenerative diseases. Exp. Neurobiol. 24, 325–340.
Kim, J., Yoon, H., Horie, T., Burchett, J. M., Restivo, J. L., Rotllan, N., et al. (2015). microRNA-33 regulates ApoE lipidation and amyloid-beta metabolism in the brain. J. Neurosci. 35, 14717–14726.
Kim, S. Y., Buckwalter, M., Soreq, H., Vezzani, A., and Kaufer, D. (2012). Blood-brain barrier dysfunction-induced inflammatory signaling in brain pathology and epileptogenesis. Epilepsia 53, 37–44. doi: 10.1111/j.1528-1167.2012.03701.x
Kivipelto, M., Ngandu, T., Fratiglioni, L., Viitanen, M., Kareholt, I., Winblad, B., et al. (2005). Obesity and vascular risk factors at midlife and the risk of dementia and Alzheimer disease. Arch. Neurol. 62, 1556–1560.
Klein, J. A., and Ackerman, S. L. (2003). Oxidative stress, cell cycle, and neurodegeneration. J. Clin. Invest. 111, 785–793.
Konovalova, J., Gerasymchuk, D., Parkkinen, I., Chmielarz, P., and Domanskyi, A. (2019). Interplay between MicroRNAs and oxidative stress in neurodegenerative diseases. Int. J. Mol. Sci. 20:6055.
Kooshkaki, O., Rezaei, Z., Rahmati, M., Vahedi, P., Derakhshani, A., Brunetti, O., et al. (2020). MiR-144: a new possible therapeutic target and diagnostic/prognostic tool in cancers. Int. J. Mol. Sci. 21:2578. doi: 10.3390/ijms21072578
Kothari, V., Luo, Y., Tornabene, T., O’neill, A. M., Greene, M. W., Geetha, T., et al. (2017). High fat diet induces brain insulin resistance and cognitive impairment in mice. Biochim. Biophys. Acta. Mol. Basis Dis. 1863, 499–508.
Kou, X., Li, J., Liu, X., Chang, J., Zhao, Q., Jia, S., et al. (2017). Swimming attenuates d-galactose-induced brain aging via suppressing miR-34a-mediated autophagy impairment and abnormal mitochondrial dynamics. J. Appl. Physiol. 122, 1462–1469. doi: 10.1152/japplphysiol.00018.2017
Kovacs, G. G. (2017). Concepts and classification of neurodegenerative diseases. Handb. Clin. Neurol. 145, 301–307.
Kriegel, A. J., Liu, Y., Fang, Y., Ding, X., and Liang, M. (2012). The miR-29 family: genomics, cell biology, and relevance to renal and cardiovascular injury. Physiol. Genomics 44, 237–244. doi: 10.1152/physiolgenomics.00141.2011
Kumar, A., Bhatia, H. S., De Oliveira, A. C., and Fiebich, B. L. (2015). microRNA-26a modulates inflammatory response induced by toll-like receptor 4 stimulation in microglia. J. Neurochem. 135, 1189–1202. doi: 10.1111/jnc.13364
Kumar, S., and Reddy, P. H. (2020). The role of synaptic microRNAs in Alzheimer’s disease. Biochim. Biophys. Acta. Mol. Basis Dis. 1866:165937.
Kwon, D. N., Chang, B. S., and Kim, J. H. (2014). MicroRNA dysregulation in liver and pancreas of CMP-Neu5Ac hydroxylase null mice disrupts insulin/PI3K-AKT signaling. Biomed. Res. Int. 2014:236385. doi: 10.1155/2014/236385
Kwon, H., and Pessin, J. E. (2013). Adipokines mediate inflammation and insulin resistance. Front. Endocrinol. (Lausanne) 4:71. doi: 10.3389/fendo.2013.00071
Lakhani, H. V., Khanal, T., Gabi, A., Yousef, G., Alam, M. B., Sharma, D., et al. (2018). Developing a panel of biomarkers and miRNA in patients with myocardial infarction for early intervention strategies of heart failure in West Virginian population. PLoS One 13:e0205329. doi: 10.1371/journal.pone.0205329
Lakhani, H. V., Pillai, S. S., Zehra, M., Dao, B., Tirona, M. T., Thompson, E., et al. (2021). Detecting early onset of anthracyclines-induced cardiotoxicity using a novel panel of biomarkers in West-Virginian population with breast cancer. Sci. Rep. 11:7954. doi: 10.1038/s41598-021-87209-8
Lastres-Becker, I., Ulusoy, A., Innamorato, N. G., Sahin, G., Rabano, A., Kirik, D., et al. (2012). alpha-Synuclein expression and Nrf2 deficiency cooperate to aggravate protein aggregation, neuronal death and inflammation in early-stage Parkinson’s disease. Hum. Mol. Genet. 21, 3173–3192. doi: 10.1093/hmg/dds143
Lee, H. J., Jeon, S. G., Kim, J., Kang, R. J., Kim, S. M., Han, K. M., et al. (2021). Ibrutinib modulates Abeta/tau pathology, neuroinflammation, and cognitive function in mouse models of Alzheimer’s disease. Aging Cell 20:e13332. doi: 10.1111/acel.13332
Lee, S., Kim, J. Y., Kim, E., Seo, K., Kang, Y. J., Kim, J. Y., et al. (2018). Assessment of Cognitive Impairment in a Mouse Model of High-Fat Diet-Induced Metabolic Stress with Touchscreen-Based Automated Battery System. Exp. Neurobiol. 27, 277–286. doi: 10.5607/en.2018.27.4.277
Lee, T. H., Cheng, K. K., Hoo, R. L., Siu, P. M., and Yau, S. Y. (2019). The novel perspectives of adipokines on brain health. Int. J. Mol. Sci. 20:5638. doi: 10.3390/ijms20225638
Lee, T. H., and Yau, S. Y. (2020). From obesity to hippocampal neurodegeneration: pathogenesis and non-pharmacological interventions. Int. J. Mol. Sci. 22:201. doi: 10.3390/ijms22010201
Leroy, K., Ando, K., Laporte, V., Dedecker, R., Suain, V., Authelet, M., et al. (2012). Lack of tau proteins rescues neuronal cell death and decreases amyloidogenic processing of APP in APP/PS1 mice. Am. J. Pathol. 181, 1928–1940. doi: 10.1016/j.ajpath.2012.08.012
Lewis, J., Dickson, D. W., Lin, W. L., Chisholm, L., Corral, A., Jones, G., et al. (2001). Enhanced neurofibrillary degeneration in transgenic mice expressing mutant tau and APP. Science 293, 1487–1491.
Li, B., and Sun, H. (2013). MiR-26a promotes neurite outgrowth by repressing PTEN expression. Mol. Med. Rep. 8, 676–680. doi: 10.3892/mmr.2013.1534
Li, K., Zhang, J., Ji, C., and Wang, L. (2016). MiR-144-3p and Its Target Gene beta-Amyloid Precursor Protein Regulate 1-Methyl-4-Phenyl-1,2-3,6-Tetrahydropyridine-Induced Mitochondrial Dysfunction. Mol. Cells 39, 543–549. doi: 10.14348/molcells.2016.0050
Li, P. A., Hou, X., and Hao, S. (2017). Mitochondrial biogenesis in neurodegeneration. J. Neurosci. Res. 95, 2025–2029.
Liang, H., and Ward, W. F. (2006). PGC-1alpha: a key regulator of energy metabolism. Adv. Physiol. Educ. 30, 145–151.
Liguori, M., Nuzziello, N., Introna, A., Consiglio, A., Licciulli, F., D’errico, E., et al. (2018). Corrigendum: dysregulation of micrornas and target genes networks in peripheral blood of patients with sporadic amyotrophic lateral sclerosis. Front. Mol. Neurosci. 11:488. doi: 10.3389/fnmol.2018.00488
Liu, Y., Wang, L., Xie, F., Wang, X., Hou, Y., Wang, X., et al. (2020). Overexpression of miR-26a-5p Suppresses Tau Phosphorylation and Abeta Accumulation in the Alzheimer’s Disease Mice by Targeting DYRK1A. Curr. Neurovasc. Res. 17, 241–248. doi: 10.2174/1567202617666200414142637
Lukiw, W. J. (2013a). Circular RNA (circRNA) in Alzheimer’s disease (AD). Front. Genet. 4:307. doi: 10.3389/fgene.2013.00307
Lukiw, W. J. (2013b). Variability in micro RNA (miRNA) abundance, speciation and complexity amongst different human populations and potential relevance to Alzheimer’s disease (AD). Front. Cell Neurosci. 7:133. doi: 10.3389/fncel.2013.00133
Lukiw, W. J. (2020). Gastrointestinal (GI) Tract Microbiome-Derived Neurotoxins-Potent Neuro-Inflammatory Signals From the GI Tract via the Systemic Circulation Into the Brain. Front. Cell Infect. Microbiol. 10:22. doi: 10.3389/fcimb.2020.00022
Lukiw, W. J., Alexandrov, P. N., Zhao, Y., Hill, J. M., and Bhattacharjee, S. (2012). Spreading of Alzheimer’s disease inflammatory signaling through soluble micro-RNA. Neuroreport 23, 621–626.
Lukiw, W. J., and Pogue, A. I. (2020). Vesicular Transport of Encapsulated microRNA between Glial and Neuronal Cells. Int. J. Mol. Sci. 21:5078. doi: 10.3390/ijms21145078
Luo, E., Wang, D., Yan, G., Qiao, Y., Zhu, B., Liu, B., et al. (2020). The NF-kappaB/miR-425-5p/MCT4 axis: a novel insight into diabetes-induced endothelial dysfunction. Mol. Cell Endocrinol. 500:110641. doi: 10.1016/j.mce.2019.110641
Ma, F., Zhang, X., and Yin, K. J. (2020). MicroRNAs in central nervous system diseases: a prospective role in regulating blood-brain barrier integrity. Exp. Neurol. 323:113094. doi: 10.1016/j.expneurol.2019.113094
Ma, Y., Ajnakina, O., Steptoe, A., and Cadar, D. (2020). Higher risk of dementia in English older individuals who are overweight or obese. Int. J. Epidemiol. 49, 1353–1365.
Magenta, A., Ciarapica, R., and Capogrossi, M. C. (2017). The Emerging Role of miR-200 Family in Cardiovascular Diseases. Circ. Res. 120, 1399–1402. doi: 10.1161/CIRCRESAHA.116.310274
Maldonado-Ruiz, R., Montalvo-Martinez, L., Fuentes-Mera, L., and Camacho, A. (2017). Microglia activation due to obesity programs metabolic failure leading to type two diabetes. Nutr. Diabetes 7:e254. doi: 10.1038/nutd.2017.10
Manjula, R., Anuja, K., and Alcain, F. J. (2020). SIRT1 and SIRT2 activity control in neurodegenerative diseases. Front. Pharmacol. 11:585821. doi: 10.3389/fphar.2020.585821
Maphis, N., Xu, G., Kokiko-Cochran, O. N., Cardona, A. E., Ransohoff, R. M., Lamb, B. T., et al. (2015). Loss of tau rescues inflammation-mediated neurodegeneration. Front. Neurosci. 9:196. doi: 10.3389/fnins.2015.00196
Mariani, S., Di Giorgio, M. R., Martini, P., Persichetti, A., Barbaro, G., Basciani, S., et al. (2018). Inverse association of circulating sirt1 and adiposity: a study on underweight, normal weight, and obese patients. Front Endocrinol (Lausanne) 9:449. doi: 10.3389/fendo.2018.00449
Martins, M., Rosa, A., Guedes, L. C., Fonseca, B. V., Gotovac, K., Violante, S., et al. (2011). Convergence of miRNA expression profiling, alpha-synuclein interacton and GWAS in Parkinson’s disease. PLoS One 6:e25443. doi: 10.1371/journal.pone.0025443
Masotti, A., Baldassarre, A., Fabrizi, M., Olivero, G., Loreti, M. C., Giammaria, P., et al. (2017). Oral glucose tolerance test unravels circulating miRNAs associated with insulin resistance in obese preschoolers. Pediatr. Obes. 12, 229–238. doi: 10.1111/ijpo.12133
Massart, J., Sjogren, R. J. O., Lundell, L. S., Mudry, J. M., Franck, N., O’gorman, D. J., et al. (2017). Altered miR-29 Expression in Type 2 Diabetes Influences Glucose and Lipid Metabolism in Skeletal Muscle. Diabetes 66, 1807–1818. doi: 10.2337/db17-0141
Mattu, H. S., and Randeva, H. S. (2013). Role of adipokines in cardiovascular disease. J. Endocrinol. 216, T17–T36.
Mazon, J. N., De Mello, A. H., Ferreira, G. K., and Rezin, G. T. (2017). The impact of obesity on neurodegenerative diseases. Life Sci. 182, 22–28.
McGrattan, A. M., Mcguinness, B., Mckinley, M. C., Kee, F., Passmore, P., Woodside, J. V., et al. (2019). Diet and inflammation in cognitive ageing and Alzheimer’s disease. Curr. Nutr. Rep. 8, 53–65.
McGregor, G., Clements, L., Farah, A., Irving, A. J., and Harvey, J. (2018). Age-dependent regulation of excitatory synaptic transmission at hippocampal temporoammonic-CA1 synapses by leptin. Neurobiol. Aging 69, 76–93. doi: 10.1016/j.neurobiolaging.2018.05.007
McGregor, R. A., and Choi, M. S. (2011). microRNAs in the regulation of adipogenesis and obesity. Curr. Mol. Med. 11, 304–316.
Medvinskii, A. B., Tsyganov, M. A., Fishov, I. L., Karpov, V. A., Ponomareva, V. M., and Ivanitskii, G. R. (1990). [Does the age heterogeneity of a bacterial population influence the rate of chemotactic ring expansion in Escherichia coli?]. Dokl. Akad. Nauk SSSR 314, 1495–1499.
Mentzel, C. M., Anthon, C., Jacobsen, M. J., Karlskov-Mortensen, P., Bruun, C. S., Jorgensen, C. B., et al. (2015). Gender and obesity specific microrna expression in adipose tissue from lean and obese pigs. PLoS One 10:e0131650. doi: 10.1371/journal.pone.0131650
Mohammed, M. S., Sendra, S., Lloret, J., and Bosch, I. (2018). Systems and WBANs for controlling obesity. J. Healthc. Eng. 2018:1564748. doi: 10.1155/2018/1564748
Mokdad, A. H., Serdula, M. K., Dietz, W. H., Bowman, B. A., Marks, J. S., and Koplan, J. P. (2000). The continuing epidemic of obesity in the United States. JAMA 284, 1650–1651.
Montesinos, J., Pera, M., Larrea, D., Guardia-Laguarta, C., Agrawal, R. R., Velasco, K. R., et al. (2020). The Alzheimer’s disease-associated C99 fragment of APP regulates cellular cholesterol trafficking. EMBO J. 39:e103791. doi: 10.15252/embj.2019103791
Mueller, K., Sacher, J., Arelin, K., Holiga, S., Kratzsch, J., Villringer, A., et al. (2012). Overweight and obesity are associated with neuronal injury in the human cerebellum and hippocampus in young adults: a combined MRI, serum marker and gene expression study. Transl. Psychiatry 2:e200. doi: 10.1038/tp.2012.121
Muller, M., Jakel, L., Bruinsma, I. B., Claassen, J. A., Kuiperij, H. B., and Verbeek, M. M. (2016). MicroRNA-29a Is a Candidate Biomarker for Alzheimer’s Disease in Cell-Free Cerebrospinal Fluid. Mol. Neurobiol. 53, 2894–2899. doi: 10.1007/s12035-015-9156-8
Nakamura, K., Fuster, J. J., and Walsh, K. (2014). Adipokines: a link between obesity and cardiovascular disease. J. Cardiol. 63, 250–259.
Neddens, J., Temmel, M., Flunkert, S., Kerschbaumer, B., Hoeller, C., Loeffler, T., et al. (2018). Phosphorylation of different tau sites during progression of Alzheimer’s disease. Acta. Neuropathol. Commun. 6:52.
Ngankeu, A., Ranganathan, P., Havelange, V., Nicolet, D., Volinia, S., Powell, B. L., et al. (2018). Discovery and functional implications of a miR-29b-1/miR-29a cluster polymorphism in acute myeloid leukemia. Oncotarget 9, 4354–4365. doi: 10.18632/oncotarget.23150
Nguyen, J. C., Killcross, A. S., and Jenkins, T. A. (2014). Obesity and cognitive decline: role of inflammation and vascular changes. Front. Neurosci. 8:375. doi: 10.3389/fnins.2014.00375
O’Brien, J., Hayder, H., Zayed, Y., and Peng, C. (2018). Overview of MicroRNA biogenesis, mechanisms of actions, and circulation. Front Endocrinol (Lausanne) 9:402. doi: 10.3389/fendo.2018.00402
Olivo-Marston, S. E., Hursting, S. D., Perkins, S. N., Schetter, A., Khan, M., Croce, C., et al. (2014). Effects of calorie restriction and diet-induced obesity on murine colon carcinogenesis, growth and inflammatory factors, and microRNA expression. PLoS One 9:e94765. doi: 10.1371/journal.pone.0094765
O’Malley, D., Macdonald, N., Mizielinska, S., Connolly, C. N., Irving, A. J., and Harvey, J. (2007). Leptin promotes rapid dynamic changes in hippocampal dendritic morphology. Mol. Cell Neurosci. 35, 559–572. doi: 10.1016/j.mcn.2007.05.001
Onyango, I. G., and Khan, S. M. (2006). Oxidative stress, mitochondrial dysfunction, and stress signaling in Alzheimer’s disease. Curr. Alzheimer Res. 3, 339–349.
Ott, A., Stolk, R. P., Van Harskamp, F., Pols, H. A., Hofman, A., and Breteler, M. M. (1999). Diabetes mellitus and the risk of dementia: the Rotterdam study. Neurology 53, 1937–1942.
Pal, A., Barber, T. M., Van De Bunt, M., Rudge, S. A., Zhang, Q., Lachlan, K. L., et al. (2012). PTEN mutations as a cause of constitutive insulin sensitivity and obesity. N. Engl. J. Med. 367, 1002–1011.
Paladino, S., Conte, A., Caggiano, R., Pierantoni, G. M., and Faraonio, R. (2018). Nrf2 pathway in age-related neurological disorders: insights into MicroRNAs. Cell Physiol. Biochem. 47, 1951–1976. doi: 10.1159/000491465
Palmqvist, S., Scholl, M., Strandberg, O., Mattsson, N., Stomrud, E., Zetterberg, H., et al. (2017). Earliest accumulation of beta-amyloid occurs within the default-mode network and concurrently affects brain connectivity. Nat. Commun. 8:1214. doi: 10.1038/s41467-017-01150-x
Pan, W., and Kastin, A. J. (2002). TNFalpha transport across the blood-brain barrier is abolished in receptor knockout mice. Exp. Neurol. 174, 193–200.
Pan, W., Kastin, A. J., Bell, R. L., and Olson, R. D. (1999). Upregulation of tumor necrosis factor alpha transport across the blood-brain barrier after acute compressive spinal cord injury. J. Neurosci. 19, 3649–3655. doi: 10.1523/JNEUROSCI.19-09-03649.1999
Pan, W., Kastin, A. J., Daniel, J., Yu, C., Baryshnikova, L. M., and Von Bartheld, C. S. (2007). TNFalpha trafficking in cerebral vascular endothelial cells. J. Neuroimmunol. 185, 47–56.
Pan, W., Tu, H., and Kastin, A. J. (2006). Differential BBB interactions of three ingestive peptides: obestatin, ghrelin, and adiponectin. Peptides 27, 911–916. doi: 10.1016/j.peptides.2005.12.014
Pan, Y., Hui, X., Hoo, R. L. C., Ye, D., Chan, C. Y. C., Feng, T., et al. (2019). Adipocyte-secreted exosomal microRNA-34a inhibits M2 macrophage polarization to promote obesity-induced adipose inflammation. J. Clin. Invest. 129, 834–849. doi: 10.1172/JCI123069
Persengiev, S., Kondova, I., Otting, N., Koeppen, A. H., and Bontrop, R. E. (2011). Genome-wide analysis of miRNA expression reveals a potential role for miR-144 in brain aging and spinocerebellar ataxia pathogenesis. Neurobiol. Aging 32, 2316.e17–27. doi: 10.1016/j.neurobiolaging.2010.03.014
Picca, A., Calvani, R., Coelho-Junior, H. J., Landi, F., Bernabei, R., and Marzetti, E. (2020). Mitochondrial Dysfunction, Oxidative Stress, and Neuroinflammation: intertwined Roads to Neurodegeneration. Antioxidants (Basel) 9:647. doi: 10.3390/antiox9080647
Pillai, S. S., Lakhani, H. V., Zehra, M., Wang, J., Dilip, A., Puri, N., et al. (2020). Predicting nonalcoholic fatty liver disease through a panel of plasma biomarkers and micrornas in female west virginia population. Int. J. Mol. Sci. 21:6698. doi: 10.3390/ijms21186698
Price, N. L., Singh, A. K., Rotllan, N., Goedeke, L., Wing, A., Canfran-Duque, A., et al. (2018). Genetic Ablation of miR-33 increases food intake, enhances adipose tissue expansion, and promotes obesity and insulin resistance. Cell Rep. 22, 2133–2145. doi: 10.1016/j.celrep.2018.01.074
Procaccini, C., Santopaolo, M., Faicchia, D., Colamatteo, A., Formisano, L., De Candia, P., et al. (2016). Role of metabolism in neurodegenerative disorders. Metabolism 65, 1376–1390.
Pulgaron, E. R., and Delamater, A. M. (2014). Obesity and type 2 diabetes in children: epidemiology and treatment. Curr. Diab. Rep. 14:508.
Qi, R., Wang, J., Wang, Q., Qiu, X., Yang, F., Liu, Z., et al. (2019). MicroRNA-425 controls lipogenesis and lipolysis in adipocytes. Biochim. Biophys. Acta. Mol. Cell Biol. Lipids 1864, 744–755. doi: 10.1016/j.bbalip.2019.02.007
Qi, Y., Takahashi, N., Hileman, S. M., Patel, H. R., Berg, A. H., Pajvani, U. B., et al. (2004). Adiponectin acts in the brain to decrease body weight. Nat. Med. 10, 524–529.
Qiu, G., Wan, R., Hu, J., Mattson, M. P., Spangler, E., Liu, S., et al. (2011). Adiponectin protects rat hippocampal neurons against excitotoxicity. Age (Dordr) 33, 155–165. doi: 10.1007/s11357-010-9173-5
Quiroz, Y. T., Sperling, R. A., Norton, D. J., Baena, A., Arboleda-Velasquez, J. F., Cosio, D., et al. (2018). Association between amyloid and tau accumulation in young adults with autosomal dominant Alzheimer disease. JAMA Neurol. 75, 548–556.
Rad, S. K., Arya, A., Karimian, H., Madhavan, P., Rizwan, F., Koshy, S., et al. (2018). Mechanism involved in insulin resistance via accumulation of beta-amyloid and neurofibrillary tangles: link between type 2 diabetes and Alzheimer’s disease. Drug Des. Dev. Ther. 12, 3999–4021. doi: 10.2147/DDDT.S173970
Raine, L. B., Khan, N. A., Drollette, E. S., Pontifex, M. B., Kramer, A. F., and Hillman, C. H. (2017). Obesity, visceral adipose tissue, and cognitive function in childhood. J. Pediatr. 187:e133.
Rains, J. L., and Jain, S. K. (2011). Oxidative stress, insulin signaling, and diabetes. Free Radic. Biol. Med. 50, 567–575.
Rashid, S., and Genest, J. (2007). Effect of obesity on high-density lipoprotein metabolism. Obesity (Silver Spring) 15, 2875–2888.
Rasmussen, K. D., Simmini, S., Abreu-Goodger, C., Bartonicek, N., Di Giacomo, M., Bilbao-Cortes, D., et al. (2010). The miR-144/451 locus is required for erythroid homeostasis. J. Exp. Med. 207, 1351–1358.
Rayner, K. J., Sheedy, F. J., Esau, C. C., Hussain, F. N., Temel, R. E., Parathath, S., et al. (2011). Antagonism of miR-33 in mice promotes reverse cholesterol transport and regression of atherosclerosis. J. Clin. Invest. 121, 2921–2931. doi: 10.1172/JCI57275
Reddy, M. A., Jin, W., Villeneuve, L., Wang, M., Lanting, L., Todorov, I., et al. (2012). Pro-inflammatory role of microrna-200 in vascular smooth muscle cells from diabetic mice. Arterioscler. Thromb. Vasc. Biol. 32, 721–729. doi: 10.1161/ATVBAHA.111.241109
Reutzel, M., Grewal, R., Silaidos, C., Zotzel, J., Marx, S., Tretzel, J., et al. (2018). Effects of long-term treatment with a blend of highly purified olive secoiridoids on cognition and brain ATP Levels in aged NMRI mice. Oxid. Med. Cell Longev. 2018:4070935. doi: 10.1155/2018/4070935
Rius-Perez, S., Torres-Cuevas, I., Millan, I., Ortega, A. L., and Perez, S. (2020). PGC-1alpha, inflammation, and oxidative stress: an integrative view in metabolism. Oxid. Med. Cell Longev. 2020:1452696. doi: 10.1155/2020/1452696
Roediger, B., and Armati, P. J. (2003). Oxidative stress induces axonal beading in cultured human brain tissue. Neurobiol. Dis. 13, 222–229. doi: 10.1016/s0969-9961(03)00038-x
Ronnemaa, E., Zethelius, B., Lannfelt, L., and Kilander, L. (2011). Vascular risk factors and dementia: 40-year follow-up of a population-based cohort. Dement. Geriatr. Cogn. Disord. 31, 460–466.
Ruiz-Roso, M. B., Gil-Zamorano, J., Lopez De Las Hazas, M. C., Tome-Carneiro, J., Crespo, M. C., et al. (2020). Intestinal Lipid Metabolism Genes Regulated by miRNAs. Front. Genet. 11:707. doi: 10.3389/fgene.2020.00707
Salama, I. I., Salama, S. I., Elmosalami, D. M., Saleh, R. M., Rasmy, H., Ibrahim, M. H., et al. (2019). Risk factors associated with mild cognitive impairment among apparently healthy people and the role of microRNAs. Open Access Maced. J. Med. Sci. 7, 3253–3261. doi: 10.3889/oamjms.2019.834
Salim, S. (2017). Oxidative stress and the central nervous system. J. Pharmacol. Exp. Ther. 360, 201–205.
Sarkar, S., Engler-Chiurazzi, E. B., Cavendish, J. Z., Povroznik, J. M., Russell, A. E., Quintana, D. D., et al. (2019). Over-expression of miR-34a induces rapid cognitive impairment and Alzheimer’s disease-like pathology. Brain Res. 1721:146327. doi: 10.1016/j.brainres.2019.146327
Schafer, M. J., Alldred, M. J., Lee, S. H., Calhoun, M. E., Petkova, E., Mathews, P. M., et al. (2015). Reduction of beta-amyloid and gamma-secretase by calorie restriction in female Tg2576 mice. Neurobiol. Aging 36, 1293–1302. doi: 10.1016/j.neurobiolaging.2014.10.043
Seeger, T., Fischer, A., Muhly-Reinholz, M., Zeiher, A. M., and Dimmeler, S. (2014). Long-term inhibition of miR-21 leads to reduction of obesity in db/db mice. Obesity (Silver Spring) 22, 2352–2360. doi: 10.1002/oby.20852
Sharma, S., and Lu, H. C. (2018). microRNAs in Neurodegeneration: current findings and potential impacts. J. Alzheimers Dis. Parkinsonism 8:420.
Sheedy, F. J. (2015). Turning 21: induction of miR-21 as a key switch in the inflammatory response. Front. Immunol. 6:19. doi: 10.3389/fimmu.2015.00019
Shen, L., Li, Q., Wang, J., Zhao, Y., Niu, L., Bai, L., et al. (2018). miR-144-3p Promotes Adipogenesis Through Releasing C/EBPalpha From Klf3 and CtBP2. Front. Genet. 9:677. doi: 10.3389/fgene.2018.00677
Shepardson, N. E., Shankar, G. M., and Selkoe, D. J. (2011). Cholesterol level and statin use in Alzheimer disease: I. Review of epidemiological and preclinical studies. Arch. Neurol. 68, 1239–1244.
Shioya, M., Obayashi, S., Tabunoki, H., Arima, K., Saito, Y., Ishida, T., et al. (2010). Aberrant microRNA expression in the brains of neurodegenerative diseases: miR-29a decreased in Alzheimer disease brains targets neurone navigator 3. Neuropathol. Appl. Neurobiol. 36, 320–330. doi: 10.1111/j.1365-2990.2010.01076.x
Siedlecki-Wullich, D., Minano-Molina, A. J., and Rodriguez-Alvarez, J. (2021). microRNAs as early biomarkers of Alzheimer’s disease: a synaptic perspective. Cells 10:113.
Spranger, J., Verma, S., Gohring, I., Bobbert, T., Seifert, J., Sindler, A. L., et al. (2006). Adiponectin does not cross the blood-brain barrier but modifies cytokine expression of brain endothelial cells. Diabetes 55, 141–147.
Stojkovska, I., Wagner, B. M., and Morrison, B. E. (2015). Parkinson’s disease and enhanced inflammatory response. Exp. Biol. Med. (Maywood) 240, 1387–1395.
Strosznajder, A. K., Wojtowicz, S., Jezyna, M. J., Sun, G. Y., and Strosznajder, J. B. (2021). Recent insights on the role of PPAR-beta/delta in neuroinflammation and neurodegeneration, and its potential target for therapy. Neuromol. Med. 23, 86–98. doi: 10.1007/s12017-020-08629-9
Su, Y., Deng, M. F., Xiong, W., Xie, A. J., Guo, J., Liang, Z. H., et al. (2019). MicroRNA-26a/death-associated protein kinase 1 signaling induces synucleinopathy and dopaminergic neuron degeneration in Parkinson’s disease. Biol. Psychiatry 85, 769–781. doi: 10.1016/j.biopsych.2018.12.008
Sun, L., Zhao, M., Zhang, J., Liu, A., Ji, W., Li, Y., et al. (2017). MiR-144 promotes beta-amyloid accumulation-induced cognitive impairments by targeting ADAM10 following traumatic brain injury. Oncotarget 8, 59181–59203. doi: 10.18632/oncotarget.19469
Surguchov, A. (2020). Caveolin: a new link between diabetes and AD. Cell. Mol. Neurobiol. 40, 1059–1066. doi: 10.1007/s10571-020-00796-4
Tan, B. L., and Norhaizan, M. E. (2019). Effect of high-fat diets on oxidative stress, cellular inflammatory response and cognitive function. Nutrients 11:2579.
Tchang, B. G., Saunders, K. H., and Igel, L. I. (2021). Best practices in the management of overweight and obesity. Med. Clin. North. Am. 105, 149–174.
Valadao, P. A. C., Santos, K. B. S., Ferreira, E. V. T. H., Macedo, E. C. T., Teixeira, A. L., Guatimosim, C., et al. (2020). Inflammation in Huntington’s disease: a few new twists on an old tale. J. Neuroimmunol. 348:577380. doi: 10.1016/j.jneuroim.2020.577380
van den Brink, A. C., Brouwer-Brolsma, E. M., Berendsen, A. A. M., and Van De Rest, O. (2019). The Mediterranean, dietary approaches to stop hypertension (DASH), and mediterranean-DASH Intervention for neurodegenerative delay (MIND) diets are associated with less cognitive decline and a lower risk of Alzheimer’s disease-a review. Adv. Nutr. 10, 1040–1065. doi: 10.1093/advances/nmz054
Vega-Badillo, J., Gutierrez-Vidal, R., Hernandez-Perez, H. A., Villamil-Ramirez, H., Leon-Mimila, P., Sánchez-Muñoz, F., et al. (2016). Hepatic miR-33a/miR-144 and their target gene ABCA1 are associated with steatohepatitis in morbidly obese subjects. Liver Int. 36, 1383–1391. doi: 10.1111/liv.13109
Wang, J. Y., Zhang, Q., Wang, D. D., Yan, W., Sha, H. H., Zhao, J. H., et al. (2018). MiR-29a: a potential therapeutic target and promising biomarker in tumors. Biosci. Rep. 38:BSR20171265.
Wang, X., Li, X., Huang, B., Yang, L., Chen, K., Zhao, D., et al. (2020). Downregulation of miR-33 Has Protective Effect Against Abeta(2)(5)(-)(3)(5)-Induced Injury in SH-SH-SY5Y Cells. Med. Sci. Monit. 26:e921026. doi: 10.12659/MSM.921026
Whitmer, R. A., Gustafson, D. R., Barrett-Connor, E., Haan, M. N., Gunderson, E. P., and Yaffe, K. (2008). Central obesity and increased risk of dementia more than three decades later. Neurology 71, 1057–1064.
Williams, M. D., and Mitchell, G. M. (2012). MicroRNAs in insulin resistance and obesity. Exp. Diabetes Res. 2012:484696.
Wu, P., Wang, Q., Jiang, C., Chen, C., Liu, Y., Chen, Y., et al. (2018). MicroRNA29a is involved lipid metabolism dysfunction and insulin resistance in C2C12 myotubes by targeting PPARdelta. Mol. Med. Rep. 17, 8493–8501. doi: 10.3892/mmr.2018.8902
Wu, Q., Ye, X., Xiong, Y., Zhu, H., Miao, J., Zhang, W., et al. (2016). The Protective Role of microRNA-200c in Alzheimer’s disease pathologies is induced by beta amyloid-triggered endoplasmic reticulum stress. Front. Mol. Neurosci. 9:140. doi: 10.3389/fnmol.2016.00140
Xing, R. X., Li, L. G., Liu, X. W., Tian, B. X., and Cheng, Y. (2020). Down regulation of miR-218, miR-124, and miR-144 relates to Parkinson’s disease via activating NF-kappaB signaling. Kaohsiung J. Med. Sci. 36, 786–792. doi: 10.1002/kjm2.12241
Xu, H., Du, X., Xu, J., Zhang, Y., Tian, Y., Liu, G., et al. (2020). Pancreatic β cell microRNA-26a alleviates type 2 diabetes by improving peripheral insulin sensitivity and preserving beta cell function. PLoS Biol. 18:e3000603. doi: 10.1371/journal.pbio.3000603
Xu, J., Jackson, C. W., Khoury, N., Escobar, I., and Perez-Pinzon, M. A. (2018). Brain SIRT1 mediates metabolic homeostasis and neuroprotection. Front. Endocrinol. (Lausanne) 9:702. doi: 10.3389/fendo.2018.00702
Yang, L., Wang, B., Zhou, Q., Wang, Y., Liu, X., Liu, Z., et al. (2018). MicroRNA-21 prevents excessive inflammation and cardiac dysfunction after myocardial infarction through targeting KBTBD7. Cell Death Dis. 9:769. doi: 10.1038/s41419-018-0805-5
Yarlagadda, A., Alfson, E., and Clayton, A. H. (2009). The blood brain barrier and the role of cytokines in neuropsychiatry. Psychiatry (Edgmont) 6, 18–22.
Yau, S. Y., Li, A., Hoo, R. L., Ching, Y. P., Christie, B. R., Lee, T. M., et al. (2014). Physical exercise-induced hippocampal neurogenesis and antidepressant effects are mediated by the adipocyte hormone adiponectin. Proc. Natl. Acad. Sci. U. S. A. 111, 15810–15815.
Yazici, D., and Sezer, H. (2017). Insulin resistance, obesity and lipotoxicity. Adv. Exp. Med. Biol. 960, 277–304.
Ye, J. (2009). Emerging role of adipose tissue hypoxia in obesity and insulin resistance. Int. J. Obes. (Lond) 33, 54–66.
Yuan, J., Wu, Y., Li, L., and Liu, C. (2020). MicroRNA-425-5p promotes tau phosphorylation and cell apoptosis in Alzheimer’s disease by targeting heat shock protein B8. J. Neural. Transm. (Vienna) 127, 339–346. doi: 10.1007/s00702-019-02134-5
Yuen, S. C., Liang, X., Zhu, H., Jia, Y., and Leung, S. W. (2021). Prediction of differentially expressed microRNAs in blood as potential biomarkers for Alzheimer’s disease by meta-analysis and adaptive boosting ensemble learning. Alzheimers Res. Ther. 13:126. doi: 10.1186/s13195-021-00862-z
Zhang, Q. S., Liu, W., and Lu, G. X. (2017). miR-200a-3p promotes b-Amyloid-induced neuronal apoptosis through down-regulation of SIRT1 in Alzheimer’s disease. J. Biosci. 42, 397–404. doi: 10.1007/s12038-017-9698-1
Zhang, X., Li, F., Bulloj, A., Zhang, Y. W., Tong, G., Zhang, Z., et al. (2006a). Tumor-suppressor PTEN affects tau phosphorylation, aggregation, and binding to microtubules. FASEB J. 20, 1272–1274.
Zhang, X., Zhang, Y. W., Liu, S., Bulloj, A., Tong, G. G., Zhang, Z., et al. (2006b). Tumor suppressor PTEN affects tau phosphorylation: deficiency in the phosphatase activity of PTEN increases aggregation of an FTDP-17 mutant Tau. Mol. Neurodegener. 1:7. doi: 10.1186/1750-1326-1-7
Zhao, Y., Jaber, V., Alexandrov, P. N., Vergallo, A., Lista, S., Hampel, H., et al. (2020). microRNA-Based Biomarkers in Alzheimer’s Disease (AD). Front. Neurosci. 14:585432. doi: 10.3389/fnins.2020.585432
Zhou, L. T., Zhang, J., Tan, L., Huang, H. Z., Zhou, Y., Liu, Z. Q., et al. (2021). Elevated Levels of miR-144-3p Induce Cholinergic Degeneration by Impairing the Maturation of NGF in Alzheimer’s Disease. Front. Cell Dev. Biol. 9:667412. doi: 10.3389/fcell.2021.667412
Zhou, Y., Gu, P., Shi, W., Li, J., Hao, Q., Cao, X., et al. (2016). MicroRNA-29a induces insulin resistance by targeting PPARdelta in skeletal muscle cells. Int. J. Mol. Med. 37, 931–938.
Keywords: obesity, adipose tissue, cognitive decline, neurodegenerative disorders, microRNA
Citation: Perdoncin M, Konrad A, Wyner JR, Lohana S, Pillai SS, Pereira DG, Lakhani HV and Sodhi K (2021) A Review of miRNAs as Biomarkers and Effect of Dietary Modulation in Obesity Associated Cognitive Decline and Neurodegenerative Disorders. Front. Mol. Neurosci. 14:756499. doi: 10.3389/fnmol.2021.756499
Received: 10 August 2021; Accepted: 10 September 2021;
Published: 07 October 2021.
Edited by:
Andrei Surguchov, University of Kansas Medical Center, United StatesReviewed by:
Irina G. Sourgoutcheva, University of Kansas Medical Center, United StatesAndrea Vergallo, Sorbonne Universités, France
Copyright © 2021 Perdoncin, Konrad, Wyner, Lohana, Pillai, Pereira, Lakhani and Sodhi. This is an open-access article distributed under the terms of the Creative Commons Attribution License (CC BY). The use, distribution or reproduction in other forums is permitted, provided the original author(s) and the copyright owner(s) are credited and that the original publication in this journal is cited, in accordance with accepted academic practice. No use, distribution or reproduction is permitted which does not comply with these terms.
*Correspondence: Komal Sodhi, c29kaGlAbWFyc2hhbGwuZWR1
†These authors have contributed equally to this work