- 1National Centre for Biological Sciences, Tata Institute of Fundamental Research, GKVK Campus, Bengaluru, India
- 2Department of Neurobiology, Harvard Medical School, Boston, MA, United States
- 3Stanley Center at the Broad, Cambridge, MA, United States
SK, HCN, and M channels are medium afterhyperpolarization (mAHP)-mediating ion channels. The three channels co-express in various brain regions, and their collective action strongly influences cellular excitability. However, significant diversity exists in the expression of channel isoforms in distinct brain regions and various subcellular compartments, which contributes to an equally diverse set of specific neuronal functions. The current review emphasizes the collective behavior of the three classes of mAHP channels and discusses how these channels function together although they play specialized roles. We discuss the biophysical properties of these channels, signaling pathways that influence the activity of the three mAHP channels, various chemical modulators that alter channel activity and their therapeutic potential in treating various neurological anomalies. Additionally, we discuss the role of mAHP channels in the pathophysiology of various neurological diseases and how their modulation can alleviate some of the symptoms.
Introduction
The correct regulation of neuronal excitability is crucial for healthy brain functioning. The cooperative activity of depolarizing and hyperpolarizing ion channels shape a neuron’s firing activity. Potassium channels are the primary ion channels which mediate outward potassium currents to repolarize/hyperpolarize the membrane potential, thereby limiting neuronal excitability (Ranjan et al., 2019). Afterhyperopolarization mediated by diverse types of potassium channels repolarizes the membrane, limits spike width and amplitude, and controls firing activity, thus preventing neurotoxicity due to excessive firing (Zhang and McBain, 1995). Based on their activation kinetics, different potassium channels mediate afterhyperpolarization at distinct time scales and are categorized as fast afterhyperpolarization (activated in 1–5 s), medium afterhyperpolarization (mAHP, activated between 10–300 ms), and slow afterhyperpolarization (sAHP, activated between 0.5-multiple seconds) (Storm, 1990; Zhang and McBain, 1995). Multiple studies have indicated that hyperpolarization activated cyclic nucleotide (HCN) channels, voltage gated K+ channel 7 (Kv7) and small conductance calcium activated potassium (SK) channels contribute to mAHP in neurons (Gu et al., 2005; Mateos-Aparicio et al., 2014). In the present review, we provide a detailed description of these mAHP-mediating ion channels. These channels control cellular excitability, and the anomalies associated with them can cause seizures, hyperactivity, and multiple neurological disorders. Thus, the pharmacological manipulation of these channels has therapeutic potential. The current review provides an insight into the biophysical properties of the channels, the kinetics of their various isoforms, their regional expression levels in the brain, and advancements in their therapeutic application for treating multiple neurological disorders.
mAHP Channels and Their Isoforms
Medium afterhyperpolarization channels exhibit a diverse distribution throughout the nervous system. Herein, we discuss the individual properties of the three main categories of mAHP channels in detail.
Based on phylogenetic analysis, the SK channel family comprises SK1 (KCa2.1), SK2 (KCa2.2), and SK3 (KCa2.2) as well as a fourth channel, SK4 (KCa3.1, IK1), which performs a function that is comparable but unrelated to the functions of the other SK channels (Wei et al., 2005; Kuiper et al., 2012). SK channels are voltage insensitive and are activated solely by an increase of 0.5–1 μM in intracellular calcium (Ca2+) levels (Blatz and Magleby, 1986; Köhler et al., 1996; Sah, 1996; Hirschberg et al., 1999). An individual channel has a conductance of 10 pS and achieves its half activation at an intracellular calcium level of approximately 0.6 μM (Hirschberg et al., 1999). The time constant of channel activation is 5–15 ms, and the deactivation time is 30 ms (Xia et al., 1998; Oliver et al., 2000). Many channel isoforms are generated by alternative splicing. Among the three SK channels, SK1 undergoes maximum alternative splicing to yield at least 16 SK1 isoforms (Shmukler et al., 2001). The presence of multiple SK1 variants indicates the diversity of the roles of this channel. A study by Strassmaier et al., 2005 reported SK2-L (the longer isoform weighing 78 kDa) and SK2-S (the smaller isoform weighing 49KDa) as two novel isoforms. Additionally, Murthy et al., 2008 discovered a cytoplasmic variant of SK2, which lacks the transmembrane fractions S3, 4, 5, and 6 and mediates the downstream effects of cytokine activation. Another splice variant of the SK2 channel that carries three extra amino acids at the 3’ terminus, couples with α9/10 nicotinic acetylcholine receptors (nAchRs) at olivocochlear synapses in the cochlea and controls their activity (Scholl et al., 2014). Lastly, SK3-1B is a truncated isoform of SK3 that is known to represent 20–60% of the total SK3 present in the brain (Tomita et al., 2003; Shakkottai et al., 2004; Villalobos et al., 2004). These findings demonstrate the isoform-dependent heterogeneity of the SK channels. Different channel isoforms also exhibit distinct localization in various neuronal compartments or brain regions, thereby imparting specialized neuronal functions to different brain regions (Table 1).
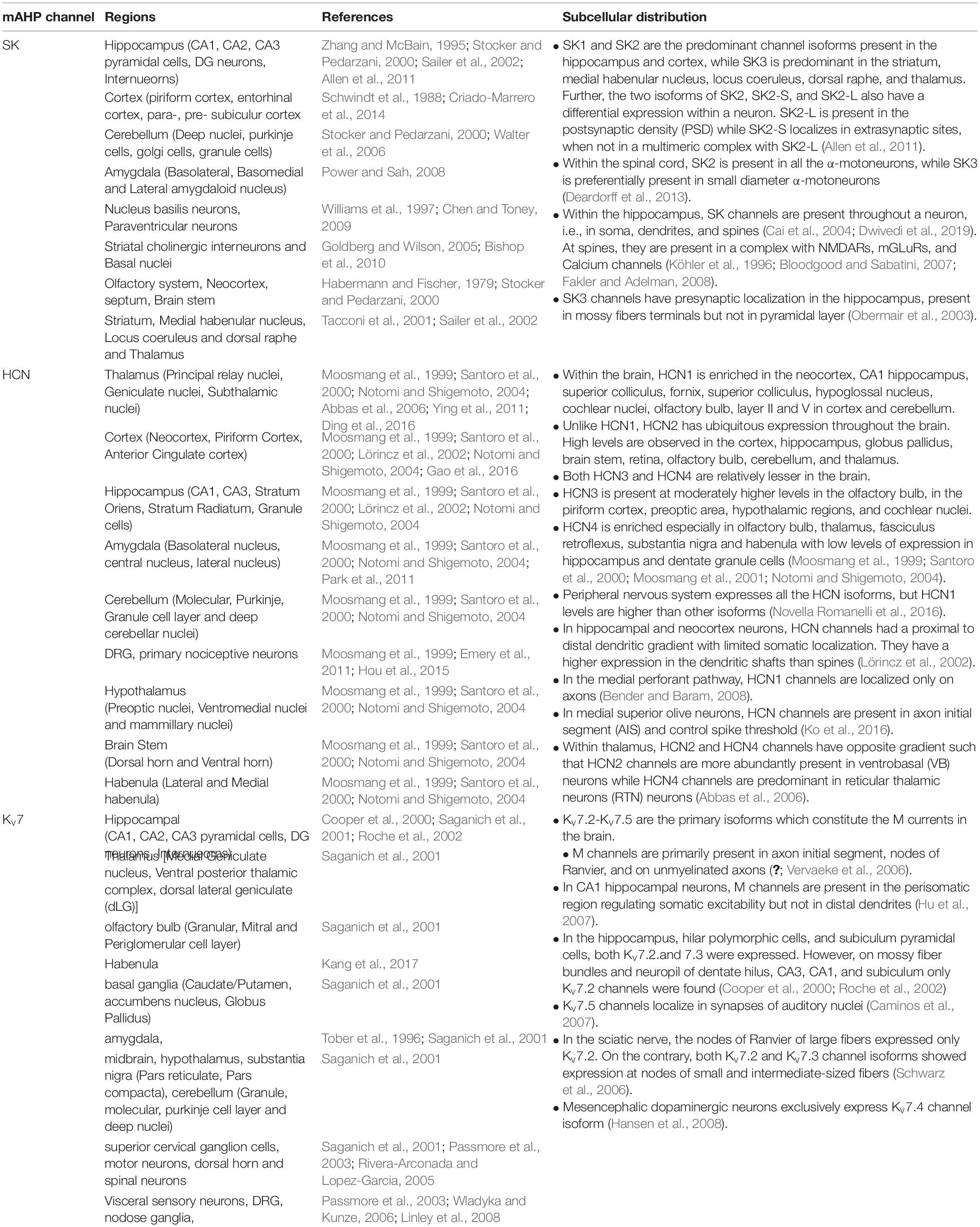
Table 1. Summary of the regional expression of different mAHP channels in distinct brain regions and the specific subcellular and region-specific distribution of various channel isoforms.
Hyperpolarization-activated cation (Ih) currents are mediated by HCN channels. These channels comprise four α subunits, encoded by four related genes, HCN 1, 2, 3, and 4 (Biel et al., 2009). All four isoforms of HCN channels have distinct activation kinetics. The HCN1 isoform exhibits the fastest activation, its V1/2 for activation lies between -90 and -70 mV, and tau for activation lies between 30 and 300 ms. The HCN2 isoform exhibits activation between 150 ms and 1 s while the HCN3 isoform exhibits activation between 250 and 400 ms. Both HCN2 and HCN4 are activated between -70 and -100 mV. HCN4 is the slowest to activate; its activation time is hundreds of milliseconds, and activation occurs at an extremely hyperpolarized potential of −140 mV (Biel et al., 2009). However, for human HCN channels, HCN2 and 3 have similar activation kinetics with HCN1 being fastest and HCN4 the slowest (Stieber et al., 2005). The four HCN isoforms exhibit a structural homology of approximately 60%. The major heterogeneity among the different isoforms originates from differences in the N and C termini of the channels (Santoro et al., 1998; Kaupp and Seifert, 2001). Different HCN isoforms assemble to form homotetramers or heterotetramers, thus conferring a range of neuronal functions that vary with the constituent subunits. In vivo, HCN1 and HCN2, but not HCN2 and HCN3, can form functional heteromers (Much et al., 2003). HCN channel activity is strongly regulated by changes in the levels of cyclic nucleotide monophosphates (cAMP/cGMP). HCN2 and HCN4 are strongly affected by cAMP levels, with moderate effect on HCN1 and none on HCN3 (Stieber et al., 2005). HCN isoforms can also assemble with different auxiliary subunits, such as KCNE2/minK-related peptide 1 (MiRP1), at the C terminus to form functional channels (Yu et al., 2001; Decher et al., 2003). In addition to KCNE, tetratricopeptide repeat-containing Rab8b–interacting protein (TRIP8b) is another regulatory subunit on HCN channels that binds to the CNBD and the N bundle loop on the C terminus. TRIP8b assists in surface targeting of the channel and regulation of activation kinetics (Porro et al., 2020). Phosphorylation of Ser237 on TRIP8B helps in its binding to the HCN channels (Foote et al., 2019). Furthermore, HCN channels undergo several post-translational modifications, which contribute to their functional heterogeneity and expression dynamics. For example, HCN channels (HCN1, HCN2, and HCN4 but not HCN3) undergo S-palmitoylation, which enhances their ability to form heteromeric channels with other isoforms and accessory proteins (Itoh et al., 2016). Under inflammation and chronic pain, HCN2 channels in dorsal root ganglion (DRG) neurons can undergo SUMOlytion, which increases their surface expression (Forster et al., 2020). N-linked glycosylation of HCN channels is also known to assist in the trafficking of channel proteins to the membrane (Much et al., 2003) (Table 1).
Voltage gated K+ channel 7 channels mediate voltage-activated potassium currents called M currents. These channels are encoded by the Kv7.1–Kv7.5 family of genes and exhibit ionic conductance in the range 1–8 pS, which varies among channel isoforms (Barrese et al., 2018). The channels exhibit slow activation (in the range 100–300 ms) and deactivation (in the range 100–450 ms) kinetics and remain open for prolonged periods (Chen and Johnston, 2004; Delmas and Brown, 2005; Hansen et al., 2008; Barrese et al., 2018). Like SK and HCN channels, different Kv7 isoforms (except Kv7.1) can also form homo or heteromers resulting in ion channels with different kinetics and regional expression, which mediate different regulatory functions (Jentsch, 2000). In the central and peripheral nervous system, Kv7.2–7.5 isoforms are the primary mediators of M current, and Kv7.2/7.3 heteromers constitute the predominant M current–mediating channels in neurons (Wang et al., 1998; Jentsch, 2000). Among all the Kv7 isoforms, Kv7.2 has the most splice variants with significantly different C termini but identical N termini (Nakamura et al., 1998). Two Kv7.2 splice variants, namely Q2L and Q2S, have also been found. Q2S forms non-functional M channels in underdeveloped fetal brains, while Q2L forms functional M channels in developed neurons (Smith et al., 2001). Furthermore, homologous Kv7.2 channel splice variants have been reported in the human brain. The variants named K2ΔL, K2KL, K2ΔKΔL, and K2ΔLMP. Among these K2KL and K2ΔL form functional channels in COS cells (Tinel et al., 1998).
Structure of mAHP Channels
The three mAHP channels have a high structural similarity with major structural differences in their N and C termini. All three channels are tetramers where each subunit has six transmembrane fragments. Different subunits assemble to form homo or heteromeric channels to create channels with varying kinetics and functions in distinct brain regions, thus imparting specialized neuronal activity (Ishii et al., 1997; Sailer et al., 2002; Monaghan et al., 2004; Strassmaier et al., 2005). Subunit (S) 4 of HCN and Kv7 channels has positively charged lysine and arginine residues, which confers voltage sensitivity to the channels. S5 and S6 of the channels form the channel pore (Kaupp and Seifert, 2001). Both the N and C termini of the channels are cytoplasmic. The C termini of the three channels have binding sites for regulatory proteins. The C termini of SK and Kv7 channels have calmodulin (CaM)-binding domain (CaMBD), while the HCN channels have a cyclic nucleotide-binding domain (CNBD) (Kaupp and Seifert, 2001; Barrese et al., 2018; Kshatri et al., 2018; Figure 1). CaM attached to SK and Kv7 channels makes them sensitive to changes in intracellular calcium levels (Wainger et al., 2001). CNBD in HCN channels can bind to cAMP/cGMP, making HCN channels responsive to signaling pathways that modulate these secondary molecules. Binding of cAMP/cGMP to CNBD removes a conformational strain, which favors the activation of HCN channels (Wainger et al., 2001). CaMBD has positively charged and hydrophobic residues that facilitate CaM binding (Fanger et al., 1999; Zhang Y. et al., 2014). An intrinsically disordered region between S6 and CaMBD (R396–M412) on the SK channels assists in the binding of Ca2+ to CaM and causes channel activation (Zhang M. et al., 2013). CaM can achieve different conformations upon binding to different SK2 splice variants, thereby imparting varying calcium sensitivity to different channel isoforms (Zhang et al., 2012). The C termini of Kv7 channels comprise four α-helices, which serve as a binding site for multiple regulatory molecules. The CaMBD of the Kv7 channels is on helices A and B and serves as a binding site for both CaM and phosphatidylinositol 4,5-bisphosphate (PIP2) (Haitin and Attali, 2008; Barrese et al., 2018; Figure 1). Unlike most K+ channels (other than the inward rectifying potassium channels), HCN channels exhibit reverse polarity, making them capable of conducting cationic current in response to hyperpolarization instead of depolarization (Lee and MacKinnon, 2017). Cryo-electron microscopy has assisted in resolving the gating mechanism of HCN channels. A long S4 linker helix, in association with S4, S5, and S6, assists in stabilizing the closed state of the channel upon cellular depolarization (Lee and MacKinnon, 2017). The S4C–term and S5N–term mediate the interaction between the voltage-sensitive and pore domains of the channel, which keeps the channel closed during depolarization. However, upon hyperpolarization, the long S4 helix undergoes displacement, which opens the HCN channel (Lee and MacKinnon, 2017; Flynn and Zagotta, 2018).
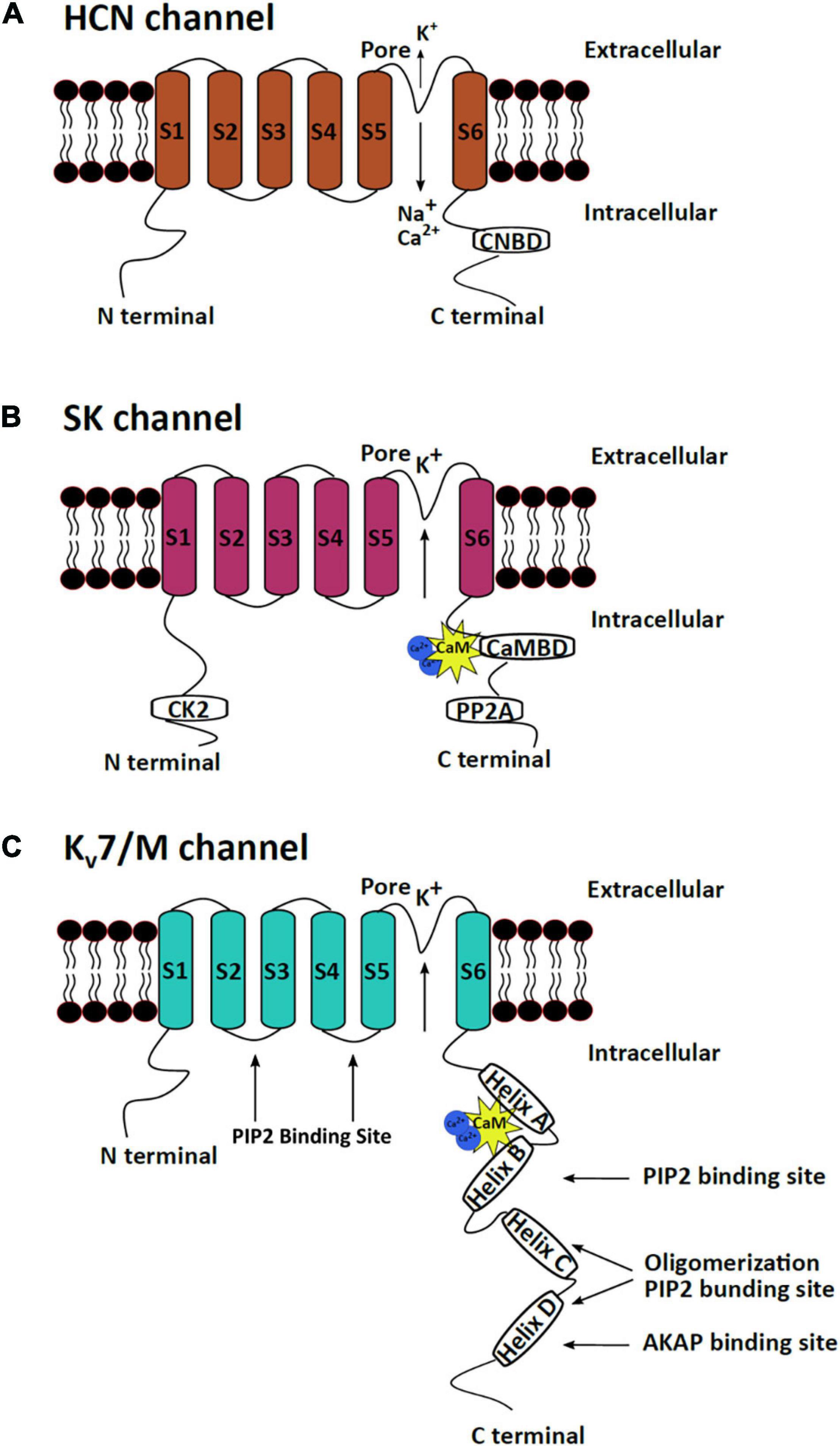
Figure 1. mAHP channel structures. The three mAHP channel subunits have a similar basic structure, which consist of six transmembrane segments, but significant heterogeneity is observed at the N and C termini. (A) HCN channels open in response to hyperpolarization and conduct a net inward current through the influx of Na+ and Ca+ and efflux of K+. The CNBD at the C terminus serves as the binding site for cAMP or cGMP, which regulates HCN channel activity. (B) SK channels are calcium-dependent potassium-conducting channels regulated by bound protein kinases and phosphatases at the N and C termini. (C) Kv7/M channels are voltage-sensitive potassium channels that are regulated by PIP2 and several protein kinases that bind to the C terminus of the protein.
The C termini of all the M channel isoforms exhibit a conserved A domain, which along with a proximal part of the B domain at the C terminal, assists in subunit assembly (Schwake et al., 2000, 2003; Robbins, 2001; Maljevic et al., 2003). The N termini of all M channel isoforms exhibit a high level of homology, while the C termini vary in lengths (Haitin and Attali, 2008). One CaM molecule per subunit is constitutively bound to the proximal C terminus of Kv7 channels and is crucial for proper channel folding (Wiener et al., 2008). The binding of CaM on helix A and B also assists in the heteromeric assembly of Kv7.2 and 3 (Liu and Devaux, 2014). In addition to the CaMBD, the C termini of Kv7 channels also contain sites for binding other modulatory proteins such as kinases and scaffolding proteins (Delmas and Brown, 2005). Phosphorylation of specific serine residues in the PIP2 binding sites on Kv7 channels alters the PIP2-binding efficiencies of the sites and modulates channel activity (Salzer et al., 2017; Figure 1).
Expression and kinetics of mAHP channels are additionally regulated by some auxiliary/accessory proteins. Both Kv7 and HCN channels interact with specific auxiliary proteins of the KCNE family, which alter their activation kinetics (Decher et al., 2003; Roura-Ferrer et al., 2009). KCNEs are single-subunit transmembrane proteins that can modulate both HCN and Kv7 channels. KCNE 1, 3, and 4 interact with Kv7 channels; however, only KCNE2 enhances the activity of HCN1, HCN2, and HCN4 (Decher et al., 2003). Deletion of KCNE2 reduces neuronal HCN1 and HCN2 levels, indicating that it also assists in surface targeting (Ying et al., 2012). Interaction with KCNE1 leads to slower inactivation and increased M current amplitudes (Jentsch, 2000). Furthermore, a single KCNE protein can have differential effects on different Kv7 isoforms. For example, when KCNE3 interacts with Kv7.2 channels, it can cause constitutive activation. By contrast, when KCNE3 binds to Kv7.4 channels, it causes inhibition (Jentsch, 2000). Multiple KCNE proteins can complex with Kv7 channels and exhibit intricate regulation of the activity of the channels (Lundby et al., 2010; Wrobel et al., 2012).
Therefore, as explained, the three mAHP channels have a similar basic structure. However, because of differences in their N and C termini, they can form heteromers with different channel isoforms and can be regulated by multiple regulatory elements. Together, these differences contribute significantly to heterogeneity in the kinetics and functions of the three mAHP channels.
Endogenous Regulators of the mAHP Channel Activity
Calcium
Calcium directly or indirectly regulates all three mAHP channels. CaM bound to the SK and Kv7 channels sensitizes them to intracellular calcium levels (Fanger et al., 1999; Schumacher et al., 2001; Adelman, 2016). Intracellular calcium levels increase because of calcium influx through voltage-dependent calcium channels, through ionotropic glutamate receptors, such as NMDARs and AMPARs, and calcium-induced calcium release (CICR) from intracellular calcium reserves (Brennan et al., 2008). In CA1 hippocampal pyramidal neurons, L-type calcium channels in the soma and R-type calcium channels in the dendrites are structurally coupled to SK channels (Bloodgood and Sabatini, 2007). By contrast, P/Q-type calcium channels in Purkinje cells are functionally, but not structurally, coupled to SK channels. Calcium entry through P/Q-type Ca2+ channels leads to CICR, which activates SK channels (Marrion and Tavalin, 1998; Bloodgood and Sabatini, 2007). Furthermore, in outer hair cells, nAChRs are also coupled to SK channels. Ca2+ influx through nAChRs causes SK channel activation, which hyperpolarizes the outer hair cells (Oliver et al., 2000, 2001). Additionally, in the dendritic spines of hippocampal neurons, metabotropic glutamate receptor (mGluR5) and NMDARs are coupled to SK channels (Lin et al., 2008; Ngo-Anh et al., 2008; García-Negredo et al., 2014). Such functional coupling between ionotropic and metabotropic channels to SK channels has led researchers to propose the presence of microdomains on the cellular membrane. In these microdomains, these channels, along with different membranal and cytoplasmic effector molecules, are in proximity with each other and jointly regulate neuronal functioning (Blackstone and Sheng, 2002; Fakler and Adelman, 2008). Increased intracellular calcium via CaM can increase the SK channel activity with a tau of ∼5–10 ms. Such fast Ca2+ regulation is possible as CaM is constitutively bound to the channel (Adelman, 2016). Using cryo electron microscopy Lee and MacKinnon (2018) have shown that four CaM molecules can bind to one SK channel tetramer (one CaM/subunit). The C lobe of CaM is attached to the channel in the absence of Ca2+. However, Ca2+ binding to the N lobe of CaM produces a conformational change, which results in rearrangement of the S6 of SK channel further opening the channel pore (Lee and MacKinnon, 2018).
Increased intracellular calcium levels can also upregulate adenylyl cyclase activity, which increases intracellular cAMP and augments HCN channel activity (Halls and Cooper, 2011; Neymotin et al., 2016). Contrary to its effect on SK and HCN channels, increased intracellular calcium inhibits Kv7 channels (Tobelaim et al., 2017). Calcium-free CaM is bound to both helices A and B at the C terminus of the Kv7 channels. In this configuration, it assists in PIP2 binding, which is crucial for Kv7 activation. An increase in intracellular calcium levels causes a structural rearrangement such that the calcium-bound CaM remains bound to helix B only. This rearrangement reduces PIP2 binding affinity, which reduces the opening probability of Kv7.2–Kv7.5 but not of Kv7.1 channels (Barrese et al., 2018). In the case of Kv7.1 channel, PIP2 and calcium-bound CaM share an overlying binding site. Thus, upon PIP2 depletion, calcium-bound CaM can bind to Kv7.1, mimic the PIP2 binding effect, and assist in channel activation (Tobelaim et al., 2017). Neurotransmitters, such as bradykinin, are known to regulate M channels via the CaM pathway (Gamper and Shapiro, 2003; Gomis-Perez et al., 2017) (Figure 2). Additionally, the βγ subunits of G proteins are also known to regulate Kv7.4 channel activity by increasing its opening probability. They stabilize the coupling between PIP2 and Kv7.4, thereby mediating their increased activation (Povstyan et al., 2017). Thus, calcium, through CaM directly activates SK channels and inhibits Kv7 channels. Increased intracellular calcium levels increase HCN channel function by increasing cAMP levels.
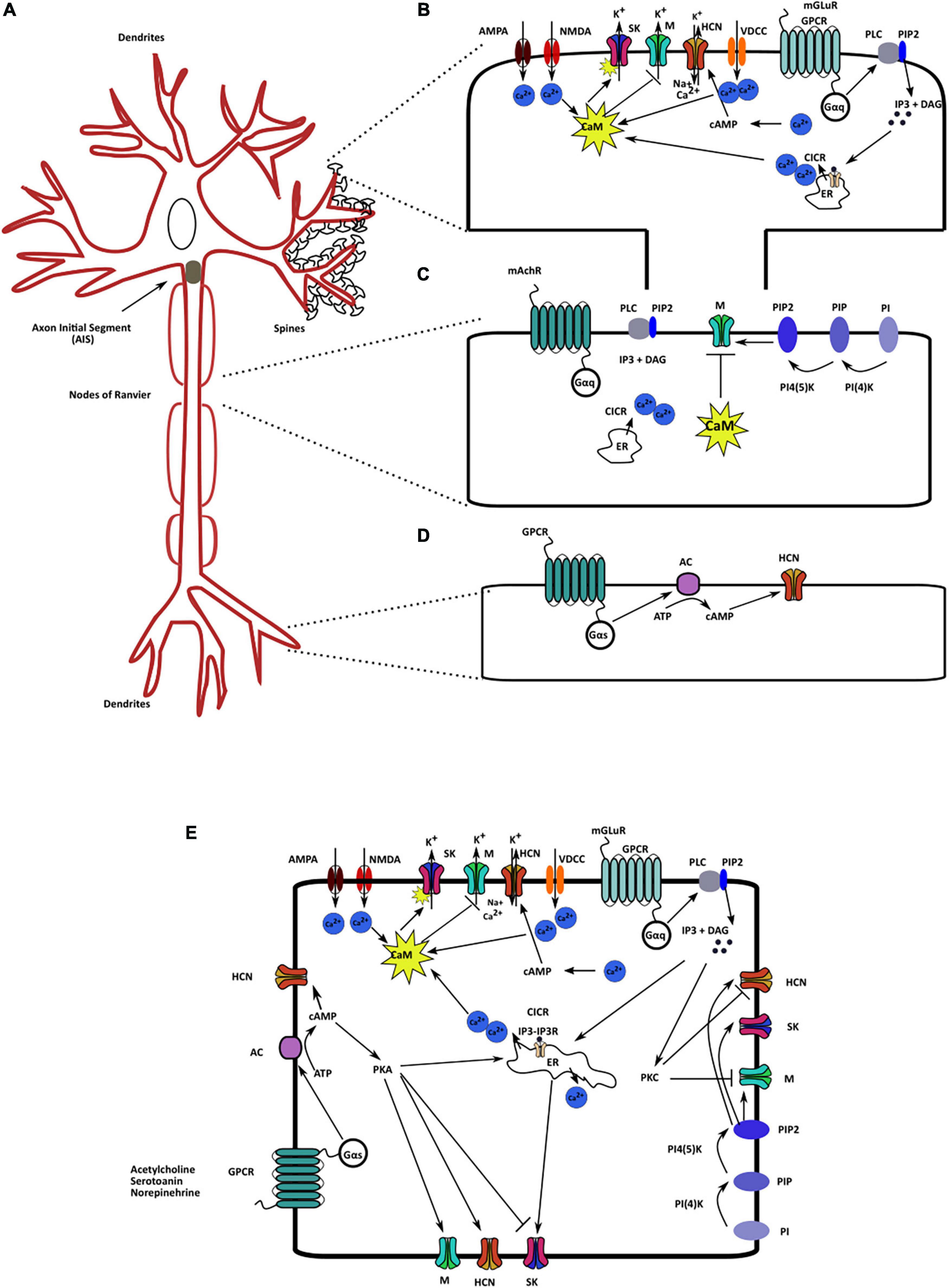
Figure 2. Localization and regulatory pathways of the mAHP channels. (A) A neuron schematic illustrating the localization of the three mAHP channels. SK channels are localized on the spines and modulate synaptic response. Kv7/M channels present in the axon initial segment and node of Ranvier control cellular resting membrane potential (RMP) and input resistance (IR). HCN channels in hippocampal neurons are present as an increasing gradient from proximal to distal dendrites. (B) SK channels present on the spines are regulated by calcium influx from multiple sources; the primary sources include voltage-dependent calcium channels, ionotropic and metabotropic glutamate receptors, and CICR. (C). PIP2 levels and intracellular calcium via CaM serve as the most critical Kv7/M channel regulators. (D) Changes in intracellular cAMP levels augment HCN channel activity through cAMP binding on the CNBD on the channel. (E) Various signaling and regulatory proteins co-exist in the intracellular milieu, modulating mAHP channels and regulating cellular excitability.
cAMP/cGMP
Similar to calcium, cyclic nucleotide monophosphates are also critical regulatory molecules for mAHP channels. Binding of cAMP/cGMP to the CNBD on HCN channels is one of the primary mechanisms underlying the regulation of HCN channel activity. The binding of cAMP to HCN channels is activity-dependent; hence, an increase in channel activity increases cAMP affinity to the channel (Wang et al., 2002). The binding of cAMP to the CNBD on HCN channels stabilizes the open channel conformation, which provides prolonged activation of HCN channels and facilitates neuronal excitability and rhythmic activity (Wang et al., 2002). Binding of cAMP to HCN channel can produce a 20 mV shift in their activation curve. However, cAMP binding exhibits variable effects on different HCN channel isoforms. cAMP binding does not affect HCN3, moderately affects HCN1 (shifts the activation curve by 2–4 mV), and strongly activates HCN2 and HCN4 channels (Stieber et al., 2005; Biel et al., 2009). Notably, because of the differential distribution of channel isoforms in various brain regions, changes in cAMP levels exhibit differential effects on the neuronal activity of distinct brain regions. Additionally, other cyclic nucleotides such as cUMP and cCMP (activates HCN2 and HCN4, but not HNC1 and HCN3) can also activate HCN channels (Zong et al., 2012; He et al., 2014).
G protein-coupled receptor activity causes cAMP production through the catalytic action of adenylyl cyclase. cGMP is produced downstream of the nitric oxide signaling pathway. Nitric oxide binds to and increases the catalytic activity of soluble guanylyl cyclase (Wilson and Garthwaite, 2010). Both cAMP and cGMP have the same binding site on HCN channels although they are produced through different signaling pathways. However, binding affinity of cAMP to HCN channels is ∼10–100 folds greater than that of cGMP (Biel et al., 2009). Neuromodulators such as acetylcholine, noradrenaline, serotonin, and epinephrine increase HCN channel activity by increasing intracellular cAMP levels (McCormick and Pape, 1990; Wilson and Garthwaite, 2010). However, changes in intracellular cAMP levels also increase Kv7 channel activity (Schroeder et al., 1998).
Cyclic nucleotide monophosphates levels positively affect Kv7 channel opening. Increased cAMP levels cause PKA-dependent phosphorylation of Kv7.2 channels at the N terminus, which increases the channel conductance (Schroeder et al., 1998). Increasing cAMP levels also increase calcium influx, which in turn affects all the three mAHP channels (Konieczny et al., 2017; Figure 2). Thus, increased intracellular cAMP levels can produce larger mAHPs through their positive effects on the three mAHP channels. The aforementioned effect can function as a feedback mechanism to control neuronal excitability.
PIP2
Like cAMP and cGMP, an increase in PIP2 levels also activates the three mAHP channels. PIP2 binding stabilizes the channels’ open configuration, which leads to increased conductance (Pian et al., 2006; Brown et al., 2007; Zhang Y. et al., 2014). This interaction between PIP2 and HCN/Kv7 channels results from an electrostatic interaction between PIP2 head groups and the channel (Oliver et al., 2004; Zolles et al., 2006). Biological processes, such as the activation of muscarinic receptors that results in Gq signaling and increased PLC activity, consume PIP2 to produced IP3 and DAG. This causes PIP2 depletion, which results in a reduction in M and SK currents (Brown et al., 2007; Zhang M. et al., 2014). However, as a feedback mechanism, increased production of IP3 also increases calcium influx by CICR. Neuronal Ca2+ sensor I can sense an increase in calcium influx, which then stimulates the activity of PI4K and replenishes depleted PIP2 levels (Hernandez et al., 2008; Carver and Shapiro, 2018). Increased metabotropic Ach receptor (mAChR) stimulation in the dentate gyrus cells increases PIP2 synthesis as well as M currents. By contrast, in CA1 cells, the same mechanism causes PIP2 depletion and reduces M currents. Such opposing actions highlight the complexity and pleiotropic effects of the intracellular signaling cascade on M channels (Carver and Shapiro, 2018). Both mAChr and bradykinin cause a reduction in M and SK currents by the direct depletion of PIP2 levels. PIP2 binding affinity for Kv7 is different for different Kv7 isoforms. The affinity is highest for Kv7.3, followed by that of Kv7.2 and Kv7.4 (Li et al., 2005). Different heteromers of different Kv7 isoforms have affinities that are intermediate to those of their constitutive channels (Li et al., 2005). The PIP2 binding site on SK channels is in the vicinity of the CaMBD, and CK2-dependent phosphorylation of the CaMBD also reduces PIP2 binding affinity and SK channel activity (Zhang Y. et al., 2014). However, the PIP2 binding domain on HCN channels is not in proximity with the CNBD; consequently, both PIP2 and cAMP can separately regulate HCN activity (Zolles et al., 2006). PIP2 can produce a 20mV positive shift in the activation curve of HCN channels (He et al., 2014; Figure 2). Therefore, PIP2, in addition to being crucial for Kv7 channel activation, is a positive regulator for the other mAHP channels.
Protein Kinases
Protein kinases and phosphatases coupled to SK and Kv7 can serve as additional channel activity regulators. CK2 bound to the C termini of SK channels phosphorylates CaM and reduces its calcium sensitivity, which favors reduced SK channel activity. By contrast, CK2 bound to the N terminus of Kv7 phosphorylates CaM and strengthens its binding to the channel, causing increased channel activity (Bildl et al., 2004). CK2 kinase functioning is checked by coupled phosphatases, namely PP2A for SK and PP1 for Kv7 channels (Maingret et al., 2008; Adelman et al., 2012; Kang et al., 2014). Kinase- and phosphatase-dependent regulation of SK channels modulate mGluR long-term potentiation (LTP) in hippocampal CA1 (Sourdet et al., 2003). Identical phosphoregulation of SK channels modulates LTP between parallel fibers and Purkinje cells in the cerebellum (Belmeguenai et al., 2010). Additionally, CK2 is enriched in the post synaptic density (PSD), where it can regulate NMDAR and AMPAR, which are also functionally coupled to SK channels (Castello et al., 2017). Thus, CK2 can directly (as bound to SK channel) and indirectly (via NMDAR and AMPAR) modulate SK channels, thereby regulating synaptic receptor functions.
In addition to CK2, PKA is another critical protein kinase that can regulate all the three mAHP channels. cAMP-PKA signaling can regulate the surface expression of SK channels. A high level of PKA activity reduces SK channels surface expression, while reduced PKA activity increases expression levels (Abiraman et al., 2016). A PKA-dependent decrease in SK channels surface expression levels facilitates NMDAR-mediated LTP induction in CA1 hippocampal neurons (Lin et al., 2008; Abiraman et al., 2016). In smooth muscle cells, increased cAMP-PKA activity facilitates Kv7.5 and Kv7.4 isoform activity (Mani et al., 2016). Similarly, in DRG neurons and hippocampal mossy fibers, PKA augments HCN channel activity (Mellor et al., 2002; Cheng and Zhou, 2013; Figure 2). PKC dependent phosphorylation of M channels increases their activity. However, under mGluR stimulation, A-kinase anchoring protein (AKAP) binds to PKC and reduces the accessibility of the PKC kinase site, resulting in a decrease in M current (Delmas and Brown, 2005; Kreir et al., 2019). In hippocampal and anterior cingulate cortex neurons, an increase in PLC–PKC activity via the mGluR signaling pathway also reduces HCN1 channel expression and HCN currents (Williams et al., 2015; Gao et al., 2016). Additionally, other signaling pathways that affect the above mentioned protein kinases can modulate the mAHP channels and regulate synaptic and cellular functions.
We have described the key regulatory molecules for the mAHP channels. Some of these regulators have a more significant impact on the activity of one type mAHP channel than on the activities of the other types; however, considerable overlap and common signaling pathways can affect all three mAHP channels. The isoform-specific effects of these regulators and the differential distribution of the mAHP channels can provide specialized neuronal functions in distinct brain regions.
Functions of mAHP Channels in Regulating Intrinsic Cellular and Network Properties
Intrinsic Cellular Properties
The diverse functionality of mAHP channels make them critical factors in various neurological diseases. mAHP channels play a crucial role in controlling neuronal excitability. Increased mAHP channel activity reduces the firing threshold for a cell, stabilizes RMP, and limits firing activity, thus controlling intrinsic neuronal excitability. Increased intracellular calcium (SK channels) and voltage change (HCN and M channels) cause channel activation. M and HCN channels, but not SK channels, are active at the RMP of the cell; hence, they strongly influence the RMP and IR of the cell. HCN channels specifically play a key role in stabilizing cellular RMP (Lupica et al., 2001). However, because of differences in the localization of the channels, M channels affect axonal RMP most significantly, while HCN channels control somatic and dendritic RMPs (Hu and Bean, 2018). Activation of M and SK channels also leads to spike frequency adaptation, which reduces net spiking output from a neuron (Ha and Cheong, 2017). Spike frequency adaptation is a progressive decline in the interspike interval in a spike train produced under sustained depolarization. Thus, the combined effects of the three mAHP channels control various intrinsic cellular properties and neuronal responses to input stimuli.
Spike Generation in Soma and Dendrites
Specialized localization of M, HCN, and SK channels in different neuronal compartments regulate local neuronal properties. In general, activation of mAHP channels reduces excitability. However, in Layer 5 cortical cells, SK channels have a differential effect on excitability in the soma compared with that on apical dendrites (Bock and Stuart, 2016). SK channel activation in the soma reduces action potential output, while SK channel activation in dendrites increases dendritic spike generation. This differential effect is a result of the functional coupling of SK channels in apical dendrites to R-type calcium channels. Consequently, SK channel activation increases Ca2+ influx through R-type calcium channels, reduces the NMDAR-mediated spike threshold, and increases dendritic excitability (Bock and Stuart, 2016; Bock et al., 2019). M channels specifically regulate axonal properties because M channels are localized in axon initial segment (AIS), which enables them to regulate axonal excitability, axonal plasticity, and interneuronal signal transmission (Lezmy et al., 2017). In hippocampal neurons, HCN channels exhibit a proximal to distal dendritic gradient. Consequently, they strongly influence proximal to distal dendritic computation and hippocampal-specific learning and memory tasks (Berger et al., 2003). HCN1 channel activity is also crucial for Purkinje cell dendritic integration and assists in motor-dependent memory formation and coordination (Nolan et al., 2003).
Synaptic and Network Activity Patterning
In addition to controlling intrinsic cellular properties, mAHP channels regulate neuronal synaptic activity, oscillatory activity of various neurons and network rhythms (Llinas and Jahnsen, 1982; Ludwig et al., 2003). Dendritic M and HCN activation produce a shunt inhibition on incoming excitatory post synaptic currents (EPSCs), thus reducing their amplitude and duration. This causes an increased threshold to spike and reduces EPSC integration (Berger et al., 2003). Presynaptic M and HCN channel activation control the paired-pulse ratio in the calyx of Held and sIPSC frequency in the amygdala, respectively (Martire et al., 2004; Huang and Trussell, 2011; Park et al., 2011). In Layer 3 EC neurons, presynaptic HCN channels reduce exocytosis of glutamate; hence, blocking presynaptic HCN channels produces an increase in miniature excitatory postsynaptic current frequency (Huang et al., 2018). SK channels in nucleus reticularis thalamic neurons and locus coeruleus (LC) control their pacemaker activity, thus driving network oscillations (Matschke et al., 2018). Rhythmic burst firing in nucleus reticularis thalamic neurons dependent on SK channels were reported to generate spindle waves (7–12 Hz) (Bal and Mccormick, 1993). SK channels, with T-type calcium channels in nucleus reticularis thalamic neurons, are responsible for sleep-related oscillations. SK2 channel knock outs exhibit a reduction in low-frequency rhythms in non–rapid-eye-movement sleep and disrupted sleep (Cueni et al., 2008). HCN channels control the circadian rhythm in suprachiasmatic neurons (Akasu et al., 1993; Atkinson et al., 2011), modulate hippocampal theta rhythms by controlling the firing activity of septohippocampal GABAergic neurons and stellate neurons of the entorhinal cortex (Dickson et al., 2000; Fransén et al., 2004; Xu et al., 2004); furthermore, HCN channels control the pace-making activity of globus pallidus neurons (Chen et al., 2015). Neurotransmitters such as noradrenaline and serotonin can further modulate these oscillatory activities through their effect on HCN channels (McCormick and Pape, 1990; Maingret et al., 2008; Giessel and Sabatini, 2010). Thus, mAHP channels play a significant role in shaping network rhythms, which affects the cognitive state and functioning of animals.
Therapeutic Potentials of mAHP Modulators
As discussed, mAHP channels are crucial in regulating essential neuronal functioning. Consequently, mutations that directly or indirectly affect these ion channels lead to severe neurological defects. Herein, we provide an in-depth account of various clinical disorders where altered functioning of mAHP channels contributes to multiple symptoms.
Ischemia
Ischemia is a commonly occurring form of brain damage, where the brain experiences a partial reduction or complete stoppage in blood supply because of cardiac arrest or stroke. Alterations in mAHP channel activity can either aggravate or circumvent ischemic damage. During an ischemic episode, the brain undergoes excitotoxic damage because of elevated extracellular glutamate levels that lead to increased calcium influx through NMDARs (Lo et al., 2003). Additionally, studies have shown that an ischemic attack causes a reduction in SK and an increase in HCN channel activity. This change further increases neuronal activity, thereby aggravating neuronal damage. A specific example of this is CA1 hippocampal neurons, where after an ischemic episode, SK2 channels decouple from NMDARs in the PSD, aggravating increased extracellular glutamate-induced damage to neurons (Allen et al., 2011). These neurons also undergo an increase in HCN1 and HCN2 activity levels after a transient ischemic insult. The increase in HCN channel activity decays after 4 days of the ischemic incidence, which contributes to the initial excitotoxic damage associated with ischemia. Both SK channel activators and HCN channel antagonists have shown neuroprotective action against ischemic attacks (Table 2). In CA3 cells, as a natural defense mechanism, transient ischemic insult activates SK currents, which provide a neuroprotective effect (Tanabe et al., 1999). In HT22 cells, glutamate-mediated oxidative stress increases mitochondrial SK2 channel activity, which exhibits a neuroprotective effect on these cells (Honrath et al., 2017; Krabbendam et al., 2018). Increasing SK channel activity by using 1-EBIO (SK channel agonist) increases the effect of Mg2+ blocking on NMDARs and prevents glutamate-mediated excitotoxic damage (Allen et al., 2011). The use of the HCN blocker ZD7288 and the M channel activator retigabine soon after ischemic damage (within 0–6 h) prevents excessive activation of postsynaptic NMDARs, prevents LTP deficits, and provides neuroprotection against oxygen or glucose deprivation in organotypic hippocampal cultures and in vivo ischemic models (Boscia et al., 2006; He et al., 2014; Bierbower et al., 2015; Diao et al., 2019; Chen et al., 2020; Table 2). Astrocytes also respond to ischemia to prevent neurotoxicity. HCN1 and HCN2 levels increase in astrocytes after 4 days and up to 2 weeks, contributing to long-term compensatory or neuroprotective effects (Honsa et al., 2014; Park et al., 2019). Thus, SK and M activators and HCN channel inhibitors are promising therapeutic targets for neuroprotection against ischemic insult (Table 3).
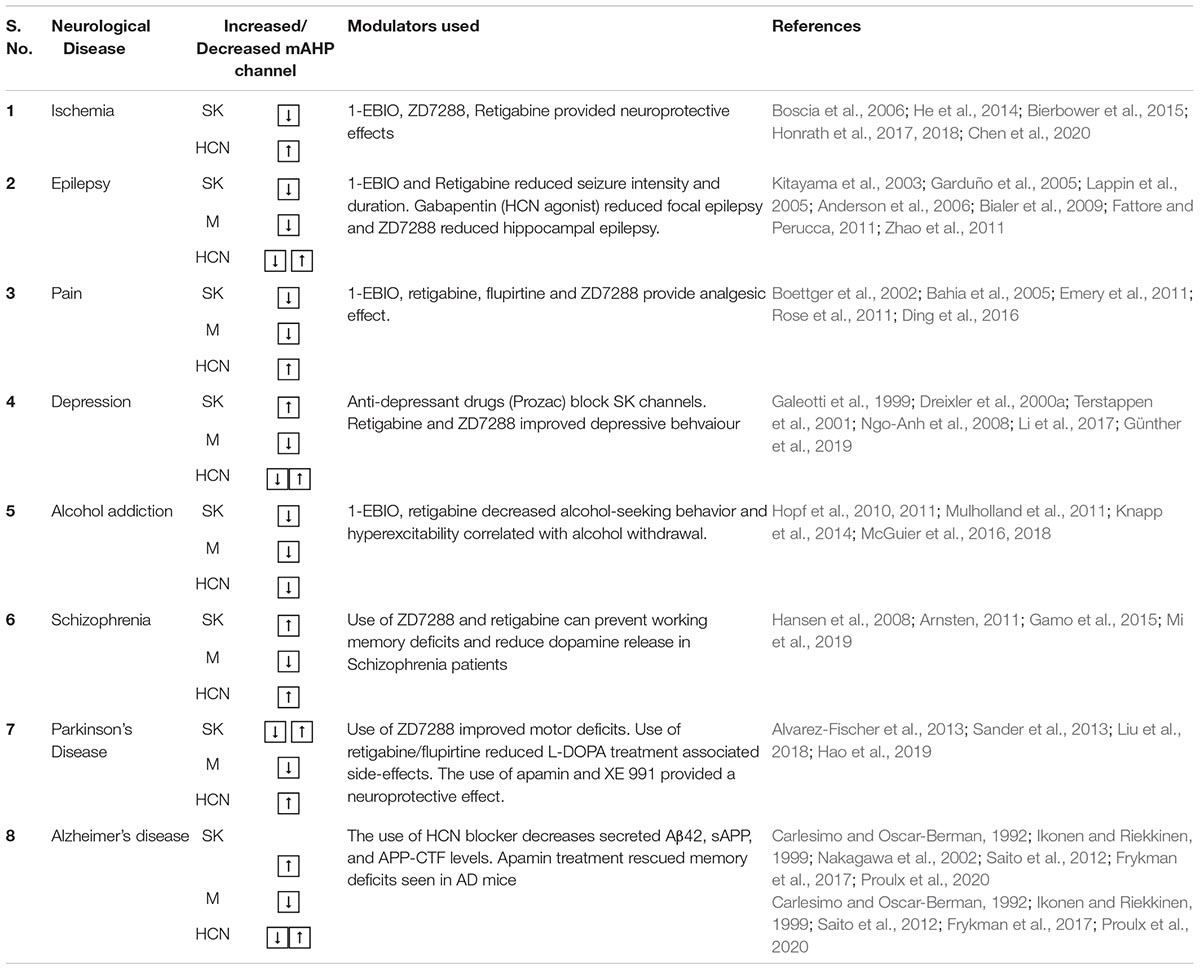
Table 3. The table summarizes various neurological anomalies dependent on the altered activity of the mAHP channels and their chemical modulators, which can alleviate the symptoms along with corresponding references.
Epilepsy
Epilepsy is a common neurological disorder caused by bursts of increased neuronal activity in different brain regions, which is characterized by jerking body movements. Because mAHP channels play a crucial role in controlling neuronal excitability, mutations in each of these channels can cause or aggravate epilepsy. Numerous studies have reported that a decrease in the activity of SK and M channels and an increase in the activity of HCN channels reduce neuronal mAHPs, which result in seizure phenotypes. Thus, mAHP channel modulators are potential therapeutic targets for epilepsy treatment.
In CA3 hippocampal cells, SK channels control epileptic bursts and spontaneous interictal discharge activity (Alger and Nicoll, 1980; Chamberlin and Dingledine, 1989; Fernández De Sevilla et al., 2006; Bakker et al., 2007). Ca2+ entry facilitated by L-type Ca2+ channels lead to the activation of these SK channels, which control burst duration, frequency, and intensity (Huang et al., 2018). In pilocarpine-treated chronic epilepsy rat models, a reduction in SK channel levels and currents has been observed (Schulz et al., 2012). Epileptic activity may cause different SK channel isoforms to undergo transient downregulation, further propagating acute seizures. In medial temporal lobe epilepsy (TLE) models, SK1 and SK2 channels and SK3 channel are downregulated acutely and chronically, respectively (Oliveira et al., 2010). Loss-of-function mutations in Kv7.2 and 7.3 cause benign familial neonatal seizures, and G271V mutations in the pore region of Kv7.2 cause familial epilepsy (Biervert et al., 1998; Wang et al., 2015). Increasing PIP2 levels by increasing PIP5K can rescue reduced Kv7.2 function, thus suggesting that decreased “sensitivity” to PIP2 is responsible for impaired Kv7.2 currents (Soldovieri et al., 2016). In specific benign familial neonatal seizures mutations, loss of preferential targeting of Kv7.2/Kv7.3 channels on AIS (due to mutation in the ankyrin G binding motif at the C terminal) and axons can cause losses in their function and epilepsy (Chung et al., 2006). Blocking of M channels increases ADP levels, which produces burst firing in hippocampal neurons, which start or propagate seizure waves and cause neurodegeneration (Vervaeke et al., 2006; Greene et al., 2018).
In contrast to SK and M channels, where reduced activity leads to epilepsy, an increase as well as a decrease in the activity of HCN channels are known to cause seizures. Increased HCN activity can reduce the mAHP of the neurons and produce hyperexcitability. Furthermore, reduced HCN levels increase cellular input resistance and dendritic excitability, which also causes hyperexcitability. This causes faster dendritic EPSC integration, which elicits spiking with fewer EPSCs. Increased dendritic excitability also increases EPSCs in the network, which increases network excitability (Biel et al., 2009). Genetic analysis of epilepsy patients revealed that the loss-of-function mutations in the HCN1 gene is responsible for various epilepsies, such as febrile seizures, genetic generalized epilepsies, and neonatal epileptic encephalopathy. Mutations in HCN2 and HCN3 can also contribute to epilepsy in some cases (Marini et al., 2018; DiFrancesco et al., 2019). In CA1 hippocampal neurons, 1–2 days after a single epileptic event, before the onset of spontaneous seizures, HCN1 channels exhibit an increase in surface expression. However, after 28 days, when spontaneous seizures have begun, the expression levels of HCN channel levels decrease. These bidirectional changes in HCN levels are responsible for the development of long-term epilepsy symptoms (Shin et al., 2008). Febrile seizures caused by fever are the most common type of seizures in children. A gain-of-function mutation in HCN2 is responsible for these seizures in patients (Dibbens et al., 2010). A hyperthermic event also produces an increase in incoming inhibitory post synaptic potentials (IPSPs), to reduce increased neural excitability during an epileptic activity. However, contrary to expectations, a burst of IPSPs, which is hyperpolarizing in nature, activates HCN channels, thereby causing hyperexcitability and increased seizure attacks (Chen et al., 2001). Contrary to the aforementioned studies, a single seizure episode can reduce HCN1 and HCN2 channel currents after 24 h for up to 1 week in EC layer III and hippocampal neurons (Shah et al., 2004; Jung et al., 2007; Powell et al., 2008). Additionally, loss of function in HCN2 and loss of TRIP8b protein (an auxiliary protein to HCN channels) can lead to the emergence of spontaneous absence seizures (Ludwig et al., 2003; Heuermann et al., 2016). TRIP8b deletion mice have reduced Ih currents which can cause loss of HCN-mediated inhibition of T-type calcium currents in the thalamocortical relay and cortical neurons. This leads to increased spontaneous oscillatory activity in the thalamocortical neuronal networks and subsequent spontaneous absence seizures in mice (Ludwig et al., 2003; Ramírez et al., 2018; Hammelmann et al., 2019; Zobeiri et al., 2019). In the TLE model of epilepsy, reduced phosphorylation of Ser237 in TRIP8b proteins has also been found to be a contributing factor to epilepsy (Foote et al., 2019). Loss of KCNE2 can also lead to reduced levels and slowed activation kinetics of HCN channels (Ying et al., 2012).
Because a decrease in SK and M channel activity and an increase or a decrease in HCN channel activity causes epilepsy symptoms, their modulators are useful therapeutic targets. The use of SK and M activators, 1-EBIO, and retigabine reduce epileptic activity. Additionally, they delay seizure initiation; increase the threshold; reduce seizure intensity in the kindling model of epilepsy, audiogenic seizures, and focal epilepsy; and reduce seizure intensity in genetically epilepsy-prone mice. However, they also cause side effects such as reduced locomotor activity, dizziness, fatigue, loss in motor coordination, and impairment in exploratory behavior (Xing and Hu, 1999; Garduño et al., 2005; Lappin et al., 2005; Anderson et al., 2006; Bialer et al., 2009; Fattore and Perucca, 2011; Weisenberg and Wong, 2011). Augmenting Kv7 activity after an epileptic event also prevents neurodegeneration (Greene et al., 2018). In genetically epilepsy-prone rat models, acoustic seizures were reported to reduce the activity of SK1 and SK3 channels in the inferior colliculus, and the use of 1-EBIO was reported to reduce seizure incidences in these models (Khandai et al., 2020; Table 2). D-23129, a derivative of flupirtine, is also effective against amygdala kindling seizure models (Tober et al., 1996). HCN1 channels limit the spread of seizures from the forebrain to the hindbrain (Huang et al., 2009; Santoro et al., 2010). Because an increase/decrease in the levels or activity of HCN channels can contribute to epilepsy, both blockers and activators of HCN channels are possible therapeutic agents. Gabapentin, an HCN channel agonist, is reported to be effective against focal epilepsy, while the HCN channel blocker ZD 7288 reduces hippocampal epileptic activity in rabbits (Kitayama et al., 2003; Table 2). In the VB nucleus, loss of HCN2, but not HCN4, alters underlying thalamic oscillations, thereby causing absence seizures (Ludwig et al., 2003; Hammelmann et al., 2019; Zobeiri et al., 2019). By contrast, David et al. (2018) reported a decrease in Ih levels in the neocortical neurons in absence seizure models and an increase in Ih currents in the thalamocortical neurons. Microdialysis of ZD7288 in the VB nucleus or knockdown of HCN channels in the VB nucleus abolishes absence seizures (David et al., 2018). Bidirectional changes in HCN levels in different brain regions negates the possibility of treating epilepsy symptoms by using a general HCN blocker. Additionally, SK channels contribute to alterations in thalamocortical oscillations. In a bicuculline methiodide epilepsy model, the SK channel antagonist apamin was reported to produce epileptic thalamocortical oscillations, which are the underlying causes of absence seizures. Thus, both HCN and SK channels are involved in the etiology of absence seizures and their dual modulation is likely an effective treatment strategy (Ludwig et al., 2003; Kleiman-weiner et al., 2009; Hammelmann et al., 2019).
As explained previously, mAHP channels are critical molecules contributing to epilepsy. The levels of SK and HCN channels increase and decrease in an isoform-specific and a time-dependent manner, which result in epilepsy initiation and progression. Loss-of-function mutations in Kv7 channels can also lead to epilepsy. Additional work to elucidate region-dependent isoform-specific alterations in the levels of the mAHP channels can be effectively used in clinical practice (Table 3).
Pain
Dorsal root ganglion, peripheral neurons, sensory neurons, and nociceptor neurons express all the three mAHP channels (Akins and McCleskey, 1993; Villière and McLachlan, 1996; Amir and Devor, 1997; Boettger et al., 2002; Passmore et al., 2003; Linley et al., 2008; Hou et al., 2015). These channels regulate the firing activity of the aforementioned neurons, thereby modulating pain responses. Pain or nerve damage reduces mAHP channel activity prompting hyperactivity in these neurons; thus, mAHP channel activators can be used as pain-relieving agents.
Injury to a peripheral neuron or its nearest DRG leads to ectopic spontaneous discharges on account of diminished extracellular potassium or calcium (Xing and Hu, 1999). Xing and Hu (1999) showed that increasing extracellular calcium increases calcium influx, activates SK currents, and reduces firing in DRG neurons. Thus, activating SK channels eases pain perception. Reduction in SK1 and Kv7.2, 7.3, and 7.4 currents and increased HCN activity in small and large nuclei DRG neurons after injury contribute to increased neuronal activity and pain sensation (Boettger et al., 2002; Emery et al., 2011; Rose et al., 2011; Yu et al., 2018). Elevated HCN currents in rat thalamus and spinal cord present chronic neuropathic pain. Similarly, HCN2 knockout mice showed a reduction in pain response to thermal or mechanical pain stimuli (Emery et al., 2011). In the spared nerve injury pain model, the medial prefrontal cortex (mPFC) neurons showed reduced cAMP levels and loss in PKA activity leading to a hyperpolarization shift in HCN activity (Matos et al., 2015). Although not examined in this study, loss in PKA-dependent regulation will also reduce M channel activity and SK channel surface expression (Lin et al., 2008; Abiraman et al., 2016; Mani et al., 2016). The combined loss of activity of mAHP channels can give rise to increased IR and hyperexcitability in these neurons, thereby increasing pain sensation (Matos et al., 2015). Neuropeptide S is a naturally occurring anxiolytic that inhibits HCN channels on medial amygdala cells. Reducing HCN activity by using neuropeptide S enhances glutamatergic inputs on GABAergic interneurons, which in turn suppresses pyramidal cells and relieve pain (Zhang et al., 2016). Neuropeptide S also possesses weak capacity to bind to SK channels (Liegeois et al., 2005). Mimicking neuropeptide S action and inhibiting HCN and SK channels by targeting amygdalar nuclei is a possible pain-relieving mechanism.
Additionally, blocking HCN channels by injection of ZD7288 in the thalamus has shown to attenuate chronic pain and sensitivity to pain in a dose-dependent manner (Ding et al., 2016; Zhang et al., 2016) (Table 2). Propofol (2,6-di-isopropyl phenol) and its derivatives, 2,6- and 2,4-di-tertbutylphenol are potent, selective HCN1 channel blockers and can reduce mechanical and thermal hyperalgesia (Tibbs et al., 2013). Furthermore, chronic pain also contributes to anxiety. Koga et al. (2015) found that an increase in the surface expression of HCN channels on the anterior cingulate cortex neurons increases both pain and anxiety. The use of ZD7288 reduces pain perception. HCN blockers, such as ZD7288, loperamide, and clonidine, also inhibit spontaneous neuronal discharge and ectopic spontaneous firing activity from DRG neurons in the event of a nerve injury, thus producing analgesic effects (Yagi and Sumino, 1998; Vasilyev et al., 2007; Bernal and Roza, 2018) (Table 2). Ivabradine is an FDA-approved drug that blocks all HCN channel isoforms. The drug can provide prolonged relief from trigeminal neuropathic pain by acting on the peripheral nervous system (Young et al., 2014; Ding et al., 2018). However, a clinical trial conducted by Lee et al. (2019) showed ivabradine reduces the heart rate without significant analgesic effects in human capsaicin-induced pain models.
In the spinal nerve ligation pain model, reducing SK channel activity in the central amygdala output nuclei was reported to increase pain sensitivity. SK channel activation by riluzole or 1-EBIO can reduce sensory inputs to the spinal cord and produce analgesic effects (Bahia et al., 2005; Thompson et al., 2018). M channel blockers, such as retigabine and flupirtine, can also relieve pain symptoms, as seen in chronic constriction injury, spinal cord injury, and nerve damage pain models (Blackburn-Munro and Jensen, 2003; Devulder, 2010; Rose et al., 2011; Wu et al., 2017; Yu et al., 2018; Table 2). Non-steroidal anti-inflammatory drugs, such as meclofenamate, diclofenac, NH6, and NH29 and acetaminophen (paracetamol), relieve migraine and neuropathic pains by activating M channels. These drugs produce a hyperpolarization shift in M channel activation and reduce their inactivation (Peretz et al., 2005, 2007, 2010; Ray et al., 2019). Artificially synthesized M channel activators, such as pyrazolopyrimidines, benzimidazole, and pyrazolo[1,5-a] pyrimidine-7(4H)-one compounds, have enhanced potency and diminished side effects (Wickenden et al., 2009; Zhang et al., 2011; Zhang F. et al., 2013; Osuma et al., 2019). QO-58 and QO-lysine are the pyrazolo[1,5-a] pyrimidine-7(4H)-one compounds that can alleviate pain symptoms in the chronic constriction injury pain model by activating all Kv7 isoforms except for Kv7.3 (Zhang M. et al., 2013; Teng et al., 2016). Paclitaxel treatment in patients with cancer produces hyperexcitability in nociceptive neurons, which contributes to chronic pain. Early treatment with retigabine can avert chronic pain (Li et al., 2018). Future investigations are focusing on finding Kv7 channel activators, targeting the peripheral nervous system and averting the side effects caused by their action on the central nervous system (Hayashi et al., 2014). Reduced activity of SK, M and increased activity of HCN channels causes hyperexcitability in the sensory or nociceptive neurons, which increases pain sensation. Specific targeting and increasing the activity of the mAHP channels in the pain perception neurons can produce analgesic effects (Table 3).
Depression
An imbalance in the dopamine (DA) levels in the ventral tegmental area (VTA)–Nucleus accumbens (NAc) circuit in the underlying cause of depression and anxiety behavior (Polter and Kauer, 2014). DA neurons exhibit a wide range of firing patterns from tonic spiking pace-making activity to a bursting response. These diverse firing activities or patterns control dopamine release in the brain (Paladini et al., 2003). Stress and depression can increase or reduce firing in the VTA DA neurons, depending on the severity of the stress. VTA DA neurons exhibit an increase in firing under severe stress conditions and a decrease in firing when subjected to weak stress (Valenti et al., 2012).
Alterations in SK, HCN, and M channel activity cause altered neuronal firing activity, leading to significant stress or depression symptoms. An increase in SK activity, a reduction in M channel activity, and an increase or decrease in HCN activity conductance can produce depression symptoms in mice. Hippocampal-specific HCN4 knockdown causes increased anxiety, whereas an increase in perisomatic HCN1 channels in CA1 hippocampal neurons contribute to chronic stress. These findings demonstrate the isoform-specific but opposing effects on a given phenotype within the same brain region (Kim et al., 2017; Kim and Johnston, 2018; Günther et al., 2019). Chronic stress causes an increase in L-type Ca2+ channels and increased intracellular calcium through CICR (Kim et al., 2017; Kim and Johnston, 2018). Increased calcium levels augment both SK and HCN channel activity and reduce M channel activity. Enhanced SK and HCN channel activity and reduced M channel activity contribute to increased tonic firing activity in VTA DA neurons, which is associated with depressive behavior. SK channel antagonists can transform tonic firing activity to phasic firing activity in DA neurons, which can rescue antidepressive behavior in mice (Van Der Staay et al., 1999; Oster et al., 2015). In the social defeat stress model, VTA neurons exhibit increased excitability due to a reduction in the expression levels of M channel and an increase in HCN activity. The overexpression of Kv7.2, retigabine injection in VTA, chronic treatment with fluoxetine (HCN and SK channel antagonist), or local infusion of ZD7288 have been reported to improve social behavior, reduce anxiety, increase sucrose preference, and produce antidepressive behavior in mice (Cao et al., 2010; Friedman et al., 2016; Li et al., 2017) (Table 2).
Multiple antidepressant drugs, including tricyclic antidepressants, such as desipramine, imipramine, and nortriptyline, as well as phenothiazines, such as fluphenazine, promethazine, chlorpromazine and trifluoperazine, and apamin, can block SK2 and SK3 channels, thereby reducing immobility time in the forced swim test, which is a model for depression (Galeotti et al., 1999; Dreixler et al., 2000b; Terstappen et al., 2001) (Table 2). Fluoxetine (prozac) is a common serotonin reuptake inhibitor that is used to treat depression, anxiety, and obsessive–compulsive disorders. It can block all three isoforms of SK channels to varying degrees (Terstappen et al., 2003). In addition to the VTA DA circuit, increased activity in the amygdala in response to fear stimuli also contributes to anxiety in animals. The use of the M channel agonist, BMS-204352, and retigabine exhibits an anxiolytic effect on these mice (Rauch et al., 2003; Korsgaard et al., 2005) (Table 2).
Additionally, alterations in HCN channels, but not in SK and M channels, can increase resilience to depression and facilitate coping with stress (Fisher et al., 2018). Contrary to expectations, mice that are resilient to depression were reported to exhibit higher HCN currents than those with depression, but the resilient mice did not exhibit increased firing in VTA neurons. This increased HCN channel activity probably activates some other K+ conductance, which restores the firing activity in resilient mice to a level comparable to that of the control mice. Mice with TRIP8b deletion, which causes reduced neuronal expression of HCN channels, and those with HCN1 knockdown exhibit resilience to depressive behavior (Lewis et al., 2011; Kim et al., 2012; Han et al., 2017). Thus, mAHP channels strongly affect the net dopamine level in the brain and control the activity of amygdala neurons, which control emotions in an animal. Hence, mAHP channel modulators potentially exhibit both antidepressive action and can cause an increase in resilience to depression (Table 3).
Alcohol Addiction
Altered neurotransmission, abnormal molecular pathways, and aberrant ion channel activity can give rise to alcohol addiction and withdrawal relapse. Alcohol addiction involves alcohol-seeking behavior, anxiety during alcohol withdrawal, alcohol-seeking behavior during withdrawal, and alcohol extinction memory. The aforementioned behavioral decisions are controlled by different brain regions. Altered neuronal excitability due to the mAHP channels affects the functioning of these regions.
Ethanol consumption increases DA neuronal activity in the substantia nigra (SN) and VTA because of the alteration of HCN, M, and SK channel activity (Hopf et al., 2007; Cannady et al., 2017; Rinker et al., 2017). SK channels coupled to NMDA channels, protect against NMDA-dependent excitotoxicity. Chronic ethanol consumption produces NMDA–SK functional uncoupling causing hyperexcitability in CA1 cells (Mulholland, 2012), reduced HCN currents in VTA neurons, and reduced Kv7.2/7.3 surface expression in nucleus accumbens (NAc) neurons (Hopf et al., 2007; McGuier et al., 2016). VTA DA neurons undergo a transition in firing activity from their regular pace-making activity to burst firing during the withdrawal phase, which is correlated with relapse. A reduction in SK2 levels, which causes NMDAR hyperactivity, was reported to be responsible for this transformation in firing patterns (Hopf et al., 2007; Mulholland, 2012). NAc is correlated with drug-induced addiction and reward systems (Janak et al., 1999). After alcohol abstinence, the NAc core neurons become hyperexcitable, which increases alcohol-seeking behavior. This hyperexcitability results from reduced activity of SK3 channels (but not SK2 channels) and reduced Kv7.2/7.3 surface expression levels in NAc neurons (Hopf et al., 2011; McGuier et al., 2016). Systemic retigabine, a microinfusion of retigabine in NAc and VTA DA neurons, retigabine administration in rats, and the use of chlorzoxone, an SK agonist, have been reported to reduce firing in NAc neurons as well as ethanol consumption (Hopf et al., 2011; Knapp et al., 2014; McGuier et al., 2016, 2018). The use of an SK activator (1-EBIO) is also reported to reduce motivation for alcohol (Hopf et al., 2010). Furthermore, 1-EBIO reduced network hyperexcitability and neurotoxicity associated with ethanol withdrawal in mice (Mulholland, 2012; Table 2).
Increased lateral habenula activity due to a decrease in Kv7.2/7.3 channel activity causes alcohol withdrawal–driven anxiety. Inhibition of habenula by the M channel agonist retigabine rescued anxiety phenotypes and alcohol preference in tested animals (Kang et al., 2017). The inhibition of serotonergic pathways can also treat alcohol withdrawal–associated anxiety. Inhibiting serotonergic pathway increases Kv7.2/7.3 channel expression, which reduces firing in lateral habenula neurons and helps treat alcohol addiction (Fu et al., 2020). In addition to the use of SK activators, which can restore regular firing activity in VTA, NAc, and hippocampal neurons and assist in alcohol abstinence, SK blocker treatment in the infralimbic prefrontal cortex causes alcohol extinction memory (Cannady et al., 2017). mGluR5 and SK2 are coupled functionally and act collectively to regulate neural excitability (Sourdet et al., 2003). Increasing mGluR activity aids in alcohol and cocaine-seeking extinction learning (Gass and Olive, 2009; Cleva et al., 2011; Cannady et al., 2017). Increased mGluR activity downregulates SK2 channels in the infralimbic prefrontal cortex, contributing to extinction learning. Microinfusion of the SK blocker apamin in the infralimbic prefrontal cortex aids in the process of extinction learning (Cannady et al., 2017). Thus, the use of an SK activator can control neuronal hyperexcitability due to alcohol withdrawal, and SK inhibitors can facilitate extinction learning. The dual effect of SK channel activators and inhibitors highlight the heterogeneity of SK channel operating in diverse brain regions, which further emphasizes the need for directed channel inhibition and activation in various brain regions to alleviate diverse disease symptoms (Table 2). In summary, excessive alcohol consumption leads to addiction, and withdrawal causes anxiety owing to changes in the firing pattern of VTA, SN, NAc, and habenula neurons, which in turn affect the dopamine levels in the brain. The use of SK and M activators can restore regular firing activity and help overcome addiction (Table 3).
Schizophrenia
Schizophrenia is a degenerative mental disorder characterized by hallucinations, anxiety, and eccentric behavior. Like depression, alterations in brain dopamine levels contribute to the pathophysiology of schizophrenia. Genetic mutations and environmental components can trigger and aggravate schizophrenia symptoms.
Midbrain DA neurons exhibit high levels of expression of SK3 and Kv7.2/7.4 homomeric channels, which control their firing patterns and hence dopamine release (Rimini et al., 2000; Stocker and Pedarzani, 2000; Hansen et al., 2008). Alterations in SK and M channel activity can cause an imbalance in dopamine levels in the brain, thereby contributing to schizophrenia symptoms such as hallucinations (Hansen et al., 2008). An increase in CAG repeats in the SK3 gene with a higher polymorphism in the repeat length of CAG increases susceptibility to schizophrenia (Bates and Davies, 1997; Chandy et al., 1998; Wittekindt et al., 1998; Cardno et al., 1999). Increased glutamine (because of increased CAG numbers) contributes to a gain-in-function mutation in SK channels by either enhancing the channel’s calcium sensitivity or modifying its kinetic properties. In maternal immune activation and juvenile social isolation mouse models of schizophrenia, layer 5 PFC neurons had higher expression levels of SK3 channel (with no change in SK1 and SK2 expression levels), which cause depolarized cellular RMP, higher levels mAHP expression, and schizophrenia-related behavioral deficits (Mi et al., 2019). By contrast, some other studies have either reported a lack of association between SK3 gene CAG repeat polymorphism and schizophrenia or have observed loss-of-function variants in the SK3 gene. A frameshift mutation in exon 1 of the SK3 gene produced a truncated form of the protein that did not exhibit transmembrane segments, was accumulated in the nucleus, and was not express on the cell surface (Bowen et al., 2001; Miller et al., 2001; Laurent et al., 2003; Ritsner et al., 2003; Tomita et al., 2003). Linkage studies have also demonstrated an association between SK3 gene polymorphism and mutations in phosphatidylinositol-4-phosphate 5-kinase II alpha in patients with schizophrenia (Wittekindt et al., 1998; Brzustowicz et al., 2000; Bakker et al., 2007). Phosphatidylinositol-4-phosphate 5-kinase II alpha mediates PIP2 synthesis. Loss of function of phosphatidylinositol-4-phosphate 5-kinase II alpha, as observed in patients with schizophrenia, reduces PIP2 levels, which reduces M channel activity (Bakker et al., 2007; Fedorenko et al., 2008). Disrupted M channel activity in neurons in the SN and VTA DA alters brain dopamine levels. Kv7.4 channel enhancers, such as retigabine, can increase M channel activity in DA neurons and can alleviate schizophrenia symptoms (Hansen et al., 2008; Peng et al., 2017).
Neuronal NMDAR hypofunction is one of the most promising theories explaining the pathophysiology of schizophrenia. In support of the NMDAR hypofunction theory, the use of phencyclidine, an NMDAR blocker, was reported to produce psychotic schizophrenia-like symptoms in experimental animals (Lodge and Anis, 1982; Davies et al., 1988; Javitt, 1991). Increased SK activity can cause NMDAR hypofunction (Bates and Davies, 1997; Chandy et al., 1998; Gargus et al., 1998). Reduced SK2 channel activity in DA neurons produces bursting firing activity, which increases dopamine levels in the brain, contributing to schizophrenia symptoms. Hence, blocking dopamine receptors is an approved remedy for patients with schizophrenia (Olney and Farber, 1995; Farde, 1998; Lam et al., 2013). SK2 activators also reduces dopamine release and might alleviate some symptoms. However, the use of SK2 activators will increase NMDAR hypofunction and aggravate the symptoms. Thus, additional meticulous investigation to assess the role of SK channels in schizophrenia is crucial for understanding its therapeutic potential. However, selective blocking or activating specific SK isoforms in distinct brain regions, while sparing others, is a potential therapeutic strategy.
Altered neuronal activity in the PFC, which regulates the working memory, also contributes to schizophrenia symptoms. Disrupted in schizophrenia 1 (DISC1) protein is a cAMP effector protein; low levels of DISC1 are correlated with schizophrenia (El-Hassar et al., 2014). In PFC pyramidal neurons, diminished levels of DISC1 cause an increase in mGluR-dependent increase in intracellular calcium influx, which augments SK channel activity (El-Hassar et al., 2014). In PFC neuronal spines, DISC1, along with other cAMP effector proteins, such as PDE4 and D1Rs, are also co-localized with HCN channels. DISC1 regulates cAMP levels; hence, its loss of function, as observed in schizophrenia, causes increased cAMP levels, which in turn increase HCN channel activity and lead to spine loss (Arnsten, 2011; Paspalas et al., 2013). Stress is another factor, which can act as a stimulant or exaggerator of schizophrenia symptoms. Increased stress can elevate cAMP and PKA levels through D1R activation, which further increases HCN, and probably M channel activity; both cooperatively reduce firing activity and the number of spines in PFC neurons (Arnsten, 2011). A specific HCN blocker, ZD7288, can rescue this reduced firing response in PFC and prevent working memory deficits (Gamo et al., 2015; Table 2). Positron emission tomography imaging studies in patients with schizophrenia have shown increased D1R binding in PFC neurons, and the use of effective antipsychotic drugs has been shown to reduce D1R expression (Abi-Dargham et al., 2002; Hirvonen et al., 2006). Thereby, region-specific gain-of-function mutations of SK channels and reduced PIP2 levels that diminish M channel function cause abnormal dopamine levels in the brain circuits. Additionally, increased HCN channel activity contributes to hyperactivity in DA neurons. Thus, use of mAHP channel modulators can restore dopamine levels and firing activity of specific neurons, thereby alleviating schizophrenia symptoms (Table 3).
Parkinson Disease
Parkinson disease (PD) is a neurodegenerative disease characterized by loss of DA neurons in the substantia nigra (SN) and basal ganglia because of α-synuclein aggregates called Lewy bodies. This loss of function of DA neurons reduces brain dopamine levels and causes ataxia, tremors, and motor disability, the common symptoms of PD. PD is associated with the dysfunction of multiple ion channels (Pedarzani and Stocker, 2008; Wang et al., 2008). SK, HCN, and M channels present in DA neurons and striatal cholinergic interneurons control the firing activity of DA neurons. The single spiking tonic firing mode of these neurons is reported to control baseline dopamine levels, while their transition to burst firing activity increases their postsynaptic dopamine release (Pedarzani and Stocker, 2008; Carbone et al., 2017). SK, HCN, and M channel blockers can alleviate PD symptoms and provide neuroprotective effects, while M activators reduce the side effects associated with levodopa (L-DOPA) treatment.
In a rat model of PD, SK3, and SK2 exhibited opposite trends of change. In the SN, downregulation of SK3 channels is observed with disease progression, while in the subthalamic nucleus (STN), a delayed increase in SK2 levels (21 days after PD induction) was reported. Furthermore, differential effects of blocking these SK channels were reported in the SN and STN. Blocking SK channels by using apamin in the substantia nigra pars compacta (SNc) alleviated PD symptoms, while the symptoms were aggravated when the channels were blocked in the STN (Mourre et al., 2017). Initial PD symptoms were reported to correlate with changes in beta frequency oscillations in globus pallidus (GPe) neurons. In healthy brains, the activities of different GPe neurons are unsynchronized. However, upon dopamine depletion in PD GPe neurons are unable to maintain their pacemaking activity leading to increased intraneuronal oscillations within basal ganglia with a sharp peak in the beta frequency range. Alteration in HCN and SK channels have been reported to be responsible for GPe neuron synchronization and changes in network properties (Chan et al., 2011; Schwab et al., 2013). Microinjection of the HCN blocker ZD7288 in vivo in the GPe increases the firing activity of some neurons and reduces firing in some others. Overall, ZD7288 improves motor deficits observed in 1-methyl-4-phenyl-1,2,3,6-tetrahydropyridine) PD mouse models (Hao et al., 2019). In PD rat models, many DA neurons were reported to undergo degeneration, and the surviving DA neurons exhibited a reduction in HCN channels, which altered their firing pattern (Guatteo et al., 2017). Downregulation of HCN channels by multiple mechanisms contribute to various PD symptoms.
SUMOlytation of α-synuclein helps in correct protein folding and produces soluble protein. By contrast, deSUMOlytation of α-synuclein causes their aggregation leading to PD. Impaired SUMOlytation can also lead to a reduction in surface levels of HCN channels in PD (Parker et al., 2017). Progression of PD also correlates with mitochondrial dysfunction, which in turn increases oxidative stress on the cell and reduces ATP levels in the cell. This produces low cAMP levels and reduced HCN functions (Schapira and Gegg, 2011; Carbone et al., 2017). Reduction in HCN channel transcription produces a spike-timing impairment in Ach-releasing striatal interneurons (Chl), which reduces the net Ach release. Although both Ach and DA levels are low in PD brains, surviving Chl neurons increase their Ach release, which leads to an increase in the Ach/DA ratio and contributes to PD symptoms (McKinley et al., 2019). Kv7.2, 7.3, and 7.5 expressed on striatal neurons and Chls can regulate their activity and excitability.
The use of artificial L-DOPA is a long-term treatment strategy for PD (Dunnett and Björklund, 1999). It compensates for the lack of DA and mitigates motor deficit. However, its chronic use leads to impairment of voluntary movement (dyskinesia) and increased severity of involuntary movement (Sander et al., 2012). The administration of Kv7 channel openers retigabine and flupirtine along with L-DOPA has been reported to reduce the symptoms of dyskinesia without influencing the effect of L-DOPA (Sander et al., 2012; Pérez-Ramírez et al., 2015). A specific activator of Kv7.2/7.3, ICA27243, exhibits a substantial antidyskinetic effect, without the side effects of sedation and ataxia, which are observed with the use of retigabine. The beneficial effects were because of specific targeting of striatal neurons only, which spared other channel isoforms (Sander et al., 2013).
SK and M channel blockers have shown neuroprotective activity in different PD models (Salthun-Lassalle et al., 2004; Doo et al., 2010; Alvarez-Fischer et al., 2013; Paz et al., 2018; Table 2). Blocking M channels by using XE 991 prevents the loss of DA neurons, increases the activity of surviving striatal DA neurons, restores dopamine levels, and improves motor coordination (Liu et al., 2018). Thus, where M channel activators can facilitate circumventing the side-effects of L-DOPA treatment, M channel blockers serve a neuroprotective role. Both M and SK blockers cause an increase in intracellular Ca2+ levels, which increases DA neuron excitability. In the 1-methyl-4-phenyl-1,2,3,6-tetrahydropyridine-induced PD model, bee venom (apamin) acupuncture in limbs or intraperitoneal administration of apamin prevented the loss of DA neurons in the SN and striatum, thus evidencing its neuroprotective action. Moreover, in an in vitro mesencephalic neuronal culture, the use of apamin prevented neuronal degeneration (Salthun-Lassalle et al., 2004). The use of bee venom to block SK activity has also been reported to cause an increase in basal dopamine levels in the striatum, which reduces motor deficits, prevents catalepsy symptoms, and causes a general increase in motor activity in PD mouse models (Alvarez and Sabatini, 2007; Chen et al., 2014; Maurice et al., 2015). Bee venom exhibits neuroprotective effects because it downregulates Jun N-kinase activity, which is responsible for the degenerative effects observed in 1-methyl-4-phenyl-1,2,3,6-tetrahydropyridine-induced PD models (Doo et al., 2010; Alvarez-Fischer et al., 2013). Other studies have also shown that Jun N-kinase activity causes neurodegenerative effects in PD animal models and reducing its activity can be a therapeutic strategy. Patients with PD and animal models exhibit an increase in Jun N-kinase activity (Hunot et al., 2004).
Contrary to the aforementioned results, where SK blockers were reported to provide a neuroprotective effect and increase in basal DA levels, an organotypic PD culture of the SN was reported to exhibit rescue effects in the presence of 1-EBIO, an SK channel activator. The SN cells exhibited a change in firing behavior from their regular pace-making to an irregular bursting activity. Furthermore, the cells exhibited an increase in resting membrane potential and neurotoxic death. 1-EBIO reduced burst firing and prevented the 6-OHDA mediated death of these neurons (Wang et al., 2014). Thus, contradictory information is available to answer the question of whether a blocker or an enhancer of SK channels has therapeutic potential. Possibly, SK channels influence both excitability and spiking patterns; consequently, they have a complex effect on the disease pathophysiology. Therefore, a detailed study is necessary to understand the contribution of SK channels to PD and how their modulation might alleviate PD symptoms. A clinical trial that used bee venom, patients with PD did not show improvement. However, the authors believe that the use of higher levels of the toxin at an increased frequency might result in an improvement in the symptoms (Hartmann et al., 2016).
As with other neurological disorders, region-dependent alterations in SK channel expression levels are observed, such that blocking SK channels in the SNc but not in STN alleviates PD symptoms. An M channel opener can circumvent side effects of L-DOPA treatment, and an HCN channel opener can be used to restore dopamine/Ach ratios. A drug cocktail modulating all mAHP channels can potentially be used to treat multiple PD symptoms (Table 3).
Alzheimer’s Disease
Aggregation of insoluble Aβ fibrils in the brain is the principal underlying cause of Alzheimer’s Disease (AD). Amyloid precursor protein (APP) cleaved by β secretase yields a carboxy-terminal fragment (APP-CTFβ) and an amino-terminal soluble APP fragment (sAPPβ). Furthermore, abnormal APP-CTFβ cleavage by γ secretase (presenilin) yields Aβ42 and APP intracellular domain (AICD). Aβ42 undergoes fibril aggregation, causing progressive neurodegeneration, while AICD modulates intracellular calcium signaling assisting in aggregate formation (Leissring et al., 2002; Vassar et al., 2014; Frykman et al., 2017). Inhibition of β and γ secretase is the most promising contender for AD treatment. However, β and γ secretase inhibition can also influence other cellular pathways, producing severe side effects. For example, β secretase augments M channel activity. Reducing β secretase activity will also inhibit M currents, which might aggravate seizure phenotypes in patients with AD (Vossel et al., 2013; Vassar et al., 2014; Hessler et al., 2015).
According to the calcium hypothesis proposed by Khachaturian (1989), intracellular calcium dysregulation contributes to the phenotypes associated with AD. Studies have demonstrated that changes in calcium dynamics assist in Aβ fibril formation, and Aβ fibril aggregation further increases calcium influx, which increase neurological deterioration. A study by Yoo et al. (2000) showed that reduced calcium entry is an early event in the Presenilin AD model and facilitates Aβ fibril formation. Furthermore, fibril aggregation increases G protein-coupled receptor-mediated CICR, which increases intracellular calcium levels and contributes to neurotoxicity (Leissring et al., 2002; Stutzmann, 2005). Human studies have shown that an increase in intracellular calcium influx induced by Aβ fibril aggregation increases the vulnerability of cortical cells to calcium-mediated neurotoxicity (Mattson et al., 1992). Increased intracellular Ca2+ levels can cause increase SK channel activity, leading to memory deficit, which is observed in AD mouse models and patients with AD (Carlesimo and Oscar-Berman, 1992). A study provided evidence of an age-dependent increment in SK channel activity, which can lead to further aggravation of AD symptoms (Blank et al., 2003). Thus, apamin treatment has been reported to reduce deficits in spatial learning and working memory in AD mice (Ikonen and Riekkinen, 1999; Proulx et al., 2020). Increased calcium influx also reduces PIP2 binding to M channels and diminishes their kinetics. Additionally, reduced expression levels of KCNE5 (auxiliary protein to Kv7 channels, which assist their activity) reduces the activity of Kv7.3 and Kv7.5 channels in cerebral arteries in the AD mouse model. Consequently, blood flow in the cerebral areas decreases, promoting fibril aggregation (de Jong and Jepps, 2018).
The effect of HCN channels on AD pathophysiology is not well understood. Both HCN1 upregulation and HCN2 blocking can be useful in treating AD pathophysiology. In AD mouse models and in the brains of patients with AD, HCN1 channel expression is reduced (Saito et al., 2012). In AD mouse models, aging causes HCN1 channels to get sequestered in the endoplasmic reticulum, thereby reducing net HCN channel expression on the distal dendrites of CA1 neurons and resulting in CA1 hyperexcitability and seizure phenotypes (Musial et al., 2018). X11 and X11L are the proteins that assist in Aβ trafficking. HCN channels can form a complex with X11, X11L, and APP. Mutant mice lacking X11 and X11L were reported to exhibit a higher incidence of seizure because of reduced HCN channel conductance. HCN1 knockout mice and mice with HCN1 blocking were reported to exhibit increased Aβ aggregation (Saito et al., 2012). Thus, reduced HCN channel expression contributes to AD symptoms. Consistent with the aforementioned results, treatment of Aβ aggregation in CA1 hippocampal cells caused increased HCN1 expression and reduced neuronal excitability (Eslamizade et al., 2015). However, contrary results were obtained in another study; blocking HCN2 channels in HEK cells reduced secretion of Aβ42, sAPP, and APP-CTF by reducing APP maturation or β action (Frykman et al., 2017). The differential effects may be isoform-specific effects or part of underlying signaling changes, the details of which warrant examination.
AD involves complex changes in intracellular calcium dynamics, which directly or indirectly affect all three mAHP channels. Increased SK currents, reduced M currents and increased or decreased HCN currents in neurons and cerebral arteries control fibril aggregation, seizure incidence and memory deficits. Thus, mAHP channel modulators can potentially alleviate some AD symptoms (Table 3).
Conclusion
The therapeutic potential of mAHP channels is being explored recently for treating various neurological anomalies. Decades of research have provided us with extensive information about the heterogeneity of channel isoforms and their expression in various brain regions. The current review highlights the commonalities and specializations of the three mAHP channels. Although the three channels work together to reduce excessive neuronal firing, share similar subcellular and regional localization, are controlled by similar regulatory pathways, and exhibit similar activation and inactivation kinetics, they have region-specific isoforms that are responsible for specialized neuronal functions. The concept of isoforms is an essential point of consideration for future research because it stresses the point that an indiscriminate modulation of an ion channel might produce undesirable effects. For example, in schizophrenia, M channel activity decreases in DA neurons and increases in PFC neurons, causing reduced spiking response and spine loss, respectively. The use of an M channel activator to rescue firing activity in DA neurons aggravates the spine loss phenotype of PFC neurons. Similarly, an M channel blocker rescues the spine loss phenotype in PFC neurons, but it further reduces DA firing activity. Similarly, in the PD model, SK channels in SNc and STN neurons exhibit opposite trends. In SNc neurons, a reduction in the levels of SK3 is observed, while in STN neurons, a delayed increase in SK2 levels is observed. Thus, the use of a general activator or blocker may have limited clinical potential. In the worst case, the general activator or blocker may aggravate disease symptoms. Consequently, isoform- and region-specific modulation is needed to provide an effective treatment strategy. Multiple other examples cited in this review clearly illustrate isoform- and region-specific alteration in the activity of various mAHP channel, which demands the identification, synthesis, and use of isoform- and region-specific modulators. Research in the past few years appears to be focused on the synthesis of several isoform-specific modulators. Additionally, intervention timing might be another crucial factor in treating various neurological diseases. Studies have shown transient as well as long-term changes in mAHP channel levels, emphasizing the need for extensive information on age-dependent and region-dependent alterations to identify the correct intervention that can be used to treat disease symptoms. In addition to isoform-specific modulators, region-specific gene targeting to remedy gain- or loss-of-function mutations in mAHP channels can also be used to treat abnormal neurological functions. With the advancement of region-specific targeting techniques, we might witness a revolution in treatment methodology in the near future.
Author Contributions
DD wrote the manuscript. Both authors structured and refined the manuscript.
Funding
DD and USB received support from NCBS-TIFR and the Department of Atomic Energy, Government of India, under project no. 12-R&D-TFR-5.04-0800. USB was a JC Bose Fellow.
Conflict of Interest
The authors declare that the research was conducted in the absence of any commercial or financial relationships that could be construed as a potential conflict of interest.
Acknowledgments
We would like to thank Dr. Vinu Varghese for providing useful inputs to the manuscript.
References
Abbas, S. Y., Ying, S. W., and Goldstein, P. A. (2006). Compartmental distribution of hyperpolarization-activated cyclic-nucleotide-gated channel 2 and hyperpolarization-activated cyclic-nucleotide-gated channel 4 in thalamic reticular and thalamocortical relay neurons. Neuroscience 141, 1811–1825. doi: 10.1016/j.neuroscience.2006.05.034
Abi-Dargham, A., Mawlawi, O., Lombardo, I., Gil, R., Martinez, D., Huang, Y., et al. (2002). Prefrontal dopamine D1 receptors and working memory in schizophrenia. J. Neurosci. 22, 3708–3719.
Abiraman, K., Sah, M., Walikonis, R. S., Lykotrafitis, G., and Tzingounis, A. V. (2016). Tonic PKA activity regulates SK channel nanoclustering and somatodendritic distribution. J. Mol. Biol. 428, 2521–2537. doi: 10.1016/j.jmb.2016.04.014
Adelman, J. P. (2016). SK channels and calmodulin. Channels 10, 1–6. doi: 10.1080/19336950.2015.1029688
Adelman, J. P., Maylie, J., and Sah, P. (2012). Small-conductance Ca 2+-activated K + channels: form and function. Annu. Rev. Physiol. 74, 245–269. doi: 10.1146/annurev-physiol-020911-153336
Akasu, T., Shoji, S., and Hasuo, H. (1993). Inward rectifier and low-threshold calcium currents contribute to the spontaneous firing mechanism in neurons of the rat suprachiasmatic nucleus. Pflügers Arch. Eur. J. Physiol. 425, 109–116. doi: 10.1007/BF00374510
Akins, P. T., and McCleskey, E. W. (1993). Characterization of potassium currents in adult rat sensory neurons and modulation by opioids and cyclic AMP. Neuroscience 56, 759–769. doi: 10.1016/0306-4522(93)90372-M
Alger, B. E., and Nicoll, R. A. (1980). Epileptiform burst afterhyperpolarization: calcium-dependent potassium potential in hippocampal CA1 pyramidal cells. Science 210, 1122–1124. doi: 10.1126/science.7444438
Allen, D., Nakayama, S., Kuroiwa, M., Nakano, T., Palmateer, J., Kosaka, Y., et al. (2011). SK2 channels are neuroprotective for ischemia-induced neuronal cell death. J. Cereb. Blood Flow Metab. 31, 2302–2312. doi: 10.1038/jcbfm.2011.90
Alvarez, V. A., and Sabatini, B. L. (2007). Anatomical and physiological plasticity of dendritic spines. Annu. Rev. Neurosci. 30, 79–97. doi: 10.1146/annurev.neuro.30.051606.094222
Alvarez-Fischer, D., Noelker, C., Vulinoviæ, F., Grünewald, A., Chevarin, C., Klein, C., et al. (2013). Bee venom and its component apamin as neuroprotective agents in a Parkinson disease mouse model. PLoS One 8:e61700. doi: 10.1371/journal.pone.0061700
Amir, R., and Devor, M. (1997). Spike-evoked suppression and burst patterning in dorsal root ganglion neurons of the rat. J. Physiol. 501, 183–196. doi: 10.1111/j.1469-7793.1997.183bo.x
Anderson, N. J., Slough, S., and Watson, W. P. (2006). In vivo characterisation of the small-conductance KCa (SK) channel activator 1-ethyl-2-benzimidazolinone (1-EBIO) as a potential anticonvulsant. Eur. J. Pharmacol. 546, 48–53. doi: 10.1016/j.ejphar.2006.07.007
Arnsten, A. F. T. (2011). Prefrontal cortical network connections: key site of vulnerability in stress and schizophrenia. Int. J. Dev. Neurosci. 29, 215–223. doi: 10.1016/j.ijdevneu.2011.02.006
Atkinson, S. E., Maywood, E. S., Chesham, J. E., Wozny, C., Colwell, C. S., Hastings, M. H., et al. (2011). Cyclic AMP signaling control of action potential firing rate and molecular circadian pacemaking in the suprachiasmatic nucleus. J. Biol. Rhythms 26, 210–220. doi: 10.1177/0748730411402810
Bahia, P. K., Suzuki, R., Benton, D. C. H., Jowett, A. J., Chen, M. X., Trezise, D. J., et al. (2005). A functional role for small-conductance calcium-activated potassium channels in sensory pathways including nociceptive processes. J. Neurosci. 25, 3489–3498. doi: 10.1523/JNEUROSCI.0597-05.2005
Bakker, S. C., Hoogendoorn, M. L. C., Hendriks, J., Verzijlbergen, K., Caron, S., Verduijn, W., et al. (2007). The PIP5K2A and RGS4 genes are differentially associated with deficit and non-deficit schizophrenia. Genes Brain Behav. 6, 113–119. doi: 10.1111/j.1601-183X.2006.00234.x
Bal, B. Y. T., and Mccormick, D. A. (1993). Mechanisms of oscillatory activity in guinea-pig nucleus reticularis thalami in vitro: a mammalian pacemaker. J. Physiol. 468, 669–691. doi: 10.1113/jphysiol.1993.sp019794
Barrese, V., Stott, J. B., and Greenwood, I. A. (2018). KCNQ-encoded potassium channels as therapeutic targets. Annu. Rev. Pharmacol. Toxicol. 58, 625–673. doi: 10.1146/annurev-pharmtox
Bates, G. P., and Davies, S. W. (1997). Transgenic mouse models of neurodegenerative disease caused by CAG/polyglutamine expansions. Mol. Med. Today 3, 508–515. doi: 10.1016/S1357-4310(97)01142-8
Belmeguenai, A., Hosy, E., Bengtsson, F., Pedroarena, C. M., Piochon, C., Teuling, E., et al. (2010). Intrinsic plasticity complements long-term potentiation in parallel fiber input gain control in cerebellar Purkinje cells. J. Neurosci. 30, 13630–13643. doi: 10.1523/JNEUROSCI.3226-10.2010
Bender, R. A., and Baram, T. Z. (2008). Hyperpolarization activated cyclic-nucleotide gated (HCN) channels in developing neuronal networks. Prog. Neurobiol. 86, 129–140. doi: 10.1016/j.pneurobio.2008.09.007
Berger, T., Senn, W., and Lüscher, H. R. (2003). Hyperpolarization-activated current Ih disconnects somatic and dendritic spike initiation zones in layer V pyramidal neurons. J. Neurophysiol. 90, 2428–2437. doi: 10.1152/jn.00377.2003
Bernal, L., and Roza, C. (2018). Hyperpolarization-activated channels shape temporal patterns of ectopic spontaneous discharge in C-nociceptors after peripheral nerve injury. Eur. J. Pain 22, 1377–1387. doi: 10.1002/ejp.1226
Bialer, M., Johannessen, S. I., Levy, R. H., Perucca, E., Tomson, T., and White, H. S. (2009). Progress report on new antiepileptic drugs: a summary of the Ninth Eilat Conference (EILAT IX). Epilepsy Res. 83, 1–43. doi: 10.1016/j.eplepsyres.2008.09.005
Biel, M., Wahl-Schott, C., Michalakis, S., and Zong, X. (2009). Hyperpolarization-activated cation channels: from genes to function. Physiol. Rev. 89, 847–885. doi: 10.1152/physrev.00029.2008
Bierbower, S. M., Choveau, F. S., Lechleiter, J. D., and Shapiro, M. S. (2015). Augmentation of M-type (KCNQ) potassium channels as a novel strategy to reduce stroke-induced brain injury. J. Neurosci. 35, 2101–2111. doi: 10.1523/JNEUROSCI.3805-14.2015
Biervert, C., Schroeder, B. C., Kubisch, C., Berkovic, S. F., Propping, P., Jentsch, T. J., et al. (1998). A potassium channel mutation in neonatal human epilepsy. Science 279, 403–406. doi: 10.1126/science.279.5349.403
Bildl, W., Strassmaier, T., Thurm, H., Andersen, J., Eble, S., Oliver, D., et al. (2004). Protein kinase CK2 is coassembled with small conductance Ca 2+-activated K+ channels and regulates channel gating. Neuron 43, 847–858. doi: 10.1016/j.neuron.2004.08.033
Bishop, M. W., Chakraborty, S., Matthews, G. A. C., Dougalis, A., Wood, N. W., Festenstein, R., et al. (2010). Hyperexcitable substantia nigra dopamine neurons in PINK1- and HtrA2/omi-deficient mice. J. Neurophysiol. 104, 3009–3020. doi: 10.1152/jn.00466.2010
Blackburn-Munro, G., and Jensen, B. S. (2003). The anticonvulsant retigabine attenuates nociceptive behaviours in rat models of persistent and neuropathic pain. Eur. J. Pharmacol. 460, 109–116. doi: 10.1016/S0014-2999(02)02924-2
Blackstone, C., and Sheng, M. (2002). Postsynaptic calcium signaling microdomains in neurons. Front. Biosci. 7, d872–d885. doi: 10.2741/blacksto
Blank, T., Nijholt, I., Kye, M. J., Radulovic, J., and Spiess, J. (2003). Small-conductance, Ca2+-activated K+ channel SK3 generates age-related memory and LTP deficits. Nat. Neurosci. 6, 911–912. doi: 10.1038/nn1101
Blatz, A. L., and Magleby, K. L. (1986). Single apamin-blocked Ca-activated K+ channels of small conductance in cultured rat skeletal muscle. Nature 323, 718–720. doi: 10.1038/323718a0
Bloodgood, B. L., and Sabatini, B. L. (2007). Nonlinear regulation of unitary synaptic signals by CaV2.3 voltage-sensitive calcium channels located in dendritic spines. Neuron 53, 249–260. doi: 10.1016/j.neuron.2006.12.017
Bock, T., and Stuart, G. J. (2016). The impact of BK channels on cellular excitability depends on their subcellular location. Front. Cell. Neurosci. 10:206. doi: 10.3389/fncel.2016.00206
Bock, T., Honnuraiah, S., and Stuart, G. J. (2019). Paradoxical excitatory impact of SK channels on dendritic excitability. J. Neurosci. 39, 7826–7839. doi: 10.1523/JNEUROSCI.0105-19.2019
Boettger, M. K., Till, S., Chen, M. X., Anand, U., Otto, W. R., Plumpton, C., et al. (2002). Calcium-activated potassium channel SK1- and IK1-like immunoreactivity in injured human sensory neurones and its regulation by neurotrophic factors. Brain 125, 252–263. doi: 10.1093/brain/awf026
Boscia, F., Annunziato, L., and Taglialatela, M. (2006). Retigabine and flupirtine exert neuroprotective actions in organotypic hippocampal cultures. Neuropharmacology 51, 283–294. doi: 10.1016/j.neuropharm.2006.03.024
Bowen, T., Williams, N., Norton, N., Spurlock, G., Wittekindt, O. H., Morris-Rosendahl, D. J., et al. (2001). Mutation screening of the KCNN3 gene reveals a rare frameshift mutation [3]. Mol. Psychiatry 6, 259–260. doi: 10.1038/sj.mp.4000128
Brennan, A. R., Dolinsky, B., Vu, M. A. T., Stanley, M., Yeckel, M. F., and Arnsten, A. F. T. (2008). Blockade of IP3-mediated SK channel signaling in the rat medial prefrontal cortex improves spatial working memory. Learn. Mem. 15, 93–96. doi: 10.1101/lm.767408
Brown, B. M., Shim, H., Zhang, M., Yarov-Yarovoy, V., and Wulff, H. (2017). Structural determinants for the selectivity of the positive KCa3.1 gating modulator 5-methylnaphtho[2,1-d]oxazol-2-amine (SKA-121). Mol. Pharmacol. 92, 469–480. doi: 10.1124/mol.117.109421
Brown, D. A., Hughes, S. A., Marsh, S. J., and Tinker, A. (2007). Regulation of M(Kv7.2/7.3) channels in neurons by PIP2 and products of PIP2 hydrolysis: Significance for receptor-mediated inhibition. J. Physiol. 582, 917–925. doi: 10.1113/jphysiol.2007.132498
Brzustowicz, L. M., Hodgkinson, K. A., Chow, E. W. C., Honer, W. G., and Bassett, A. S. (2000). Location of a major susceptibility locus for familial schizophrenia on chromosome 1q21-q22. Science 288, 678–682. doi: 10.1126/science.288.5466.678
Bucchi, A., Baruscotti, M., and Difrancesco, D. (2002). Current-dependent block of rabbit sino-atrial node I f channels by ivabradine. J. Gen. Physiol. 120, 1–13. doi: 10.1085/jgp.20028593
Cai, X., Liang, C. W., Muralidharan, S., Kao, J. P., Tang, C. M., and Thompson, S. M. (2004). Unique roles of SK and Kv4.2 potassium channels in dendritic integration. Neuron 44, 351–364. doi: 10.1016/j.neuron.2004.09.026
Caminos, E., Garcia-Pino, E., Martinez-Galan, J. R., and Juiz, J. M. (2007). The potassium channel KCNQ5/Kv7.5 is localized in synaptic endings of auditory brainstem nuclei of the rat. J. Comp. Neurol. 505, 363–378. doi: 10.1002/cne.21497
Cannady, R., McGonigal, J. T., Newsom, R. J., Woodward, J. J., Mulholland, P. J., and Gass, J. T. (2017). Prefrontal cortex KCa2 channels regulate mglu5-dependent plasticity and extinction of alcohol-seeking behavior. J. Neurosci. 37, 4359–4369. doi: 10.1523/JNEUROSCI.2873-16.2017
Cao, J. L., Covington, H. E., Friedman, A. K., Wilkinson, M. B., Walsh, J. J., Cooper, D. C., et al. (2010). Mesolimbic dopamine neurons in the brain reward circuit mediate susceptibility to social defeat and antidepressant action. J. Neurosci. 30, 16453–16458. doi: 10.1523/JNEUROSCI.3177-10.2010
Carbone, C., Costa, A., Provensi, G., Mannaioni, G., and Masi, A. (2017). The hyperpolarization-activated current determines synaptic excitability, calcium activity and specific viability of Substantia Nigra dopaminergic neurons. Front. Cell. Neurosci. 11:187. doi: 10.3389/fncel.2017.00187
Cardno, A. G., Bowen, T., Guy, C. A., Jones, L. A., Mccarthy, G., Williams, N. M., et al. (1999). CAG repeat length in the hKCa3 gene and symptom dimensions in schizophrenia. Biol. Psychiatry 45, 1592–1596. doi: 10.1016/s0006-3223(99)00033-5
Carlesimo, G. A., and Oscar-Berman, M. (1992). Memory deficits in Alzheimer’s patients: a comprehensive review. Neuropsychol. Rev. 3, 119–169. doi: 10.1007/BF01108841
Carlson, A. E., Rosenbaum, J. C., Brelidze, T. I., Klevit, R. E., and Zagotta, W. N. (2013). Flavonoid regulation of HCN2 channels. J. Biol. Chem. 288, 33136–33145. doi: 10.1074/jbc.M113.501759
Carver, C. M., and Shapiro, M. S. (2018). Gq-coupled muscarinic receptor enhancement of KCNQ2 / 3 channels and activation of TRPC channels in multimodal control of excitability in dentate gyrus granule cells Gq-coupled muscarinic receptor enhancement of KCNQ2 / 3 channels and activation of TRPC. J. Neurosci. 39, 1566–1587. doi: 10.1523/JNEUROSCI.1781-18.2018
Castello, J., Ragnauth, A., Friedman, E., and Rebholz, H. (2017). CK2—an emerging target for neurological and psychiatric disorders. Pharmaceuticals 10:7. doi: 10.3390/ph10010007
Chamberlin, N. L., and Dingledine, R. (1989). Control of epileptiform burst rate by CA3 hippocampal cell afterhyperpolarizations in vitro. Brain Res. 492, 337–346. doi: 10.1016/0006-8993(89)90917-7
Chan, C. S., Glajch, K. E., Gertler, T. S., Guzman, J. N., Mercer, J. N., Lewis, A. S., et al. (2011). HCN channelopathy in external globus pallidus neurons in models of Parkinson’s disease. Nat. Neurosci. 14, 85–94. doi: 10.1038/nn.2692
Chandy, K. G., Fantino, E., Wittekindt, O., Kalman, K., Tong, L.-L., Ho, T.-H., et al. (1998). Isolation of a novel potassium channel gene hSKCa3 containing a polymorphic CAG repeat: a candidate for schizophrenia and bipolar disorder? Mol. Psychiatry 3, 32–37. doi: 10.1038/sj.mp.4000353
Chen, C., Liu, L., Shu, Y. Q., Jing, P., Lu, Y., Zhang, X. X., et al. (2020). Blockade of HCN2 channels provides neuroprotection against ischemic injury via accelerating autophagic degradation in hippocampal neurons. Neurosci. Bull. 36, 875–894. doi: 10.1007/s12264-020-00513-7
Chen, K., Aradi, I., Thon, N., Eghbal-Ahmadi, M., Baram, T. Z., and Soltesz, I. (2001). Persistently modified h-channels after complex febrile seizures convert the seizure-induced enhancement of inhibition to hyperexcitability. Nat. Med. 7, 331–337. doi: 10.1038/85480
Chen, L., Deltheil, T., Turle-Lorenzo, N., Liberge, M., Rosier, C., Watabe, I., et al. (2014). SK channel blockade reverses cognitive and motor deficits induced by nigrostriatal dopamine lesions in rats. Int. J. Neuropsychopharmacol. 17, 1295–1306. doi: 10.1017/S1461145714000236
Chen, L., Xu, R., Sun, F. J., Xue, Y., Hao, X. M., Liu, H. X., et al. (2015). Hyperpolarization-activated cyclic nucleotide-gated (HCN) channels regulate firing of globus pallidus neurons in vivo. Mol. Cell. Neurosci. 68, 46–55. doi: 10.1016/j.mcn.2015.04.001
Chen, Q. H., and Toney, G. M. (2009). Excitability of paraventricular nucleus neurones that project to the rostral ventrolateral medulla is regulated by small-conductance Ca2+-activated K+ channels. J. Physiol. 587, 4235–4247. doi: 10.1113/jphysiol.2009.175364
Chen, X., and Johnston, D. (2004). Properties of single voltage-dependent K+ channels in dendrites of CA1 pyramidal neurones of rat hippocampus. J. Physiol. 559, 187–203. doi: 10.1113/jphysiol.2004.068114
Cheng, L., Kinard, K., Rajamani, R., and Sanguinetti, M. C. (2007). Molecular mapping of the binding site for a blocker of pacemaker channels. J. Pharm. Exp. Ther. 322, 931–939. doi: 10.1124/jpet.107.121467.four
Cheng, Q., and Zhou, Y. (2013). Novel role of KT5720 on regulating hyperpolarization-activated cyclic nucleotide-gated channel activity and dorsal root ganglion neuron excitability. DNA Cell Biol. 32, 320–328. doi: 10.1089/dna.2013.2021
Cho, L. T. Y., Alexandrou, A. J., Torella, R., Knafels, J., Hobbs, J., Taylor, T., et al. (2018). An intracellular allosteric modulator binding pocket in SK2 ion channels is shared by multiple chemotypes. Structure 26, 533–544.e3. doi: 10.1016/j.str.2018.02.017
Christophersen, P., and Wulff, H. (2015). Pharmacological gating modulation of small- and intermediate-conductance Ca2+ -activated K+ channel (KCa2.x and KCa3.1). Channels 9, 336–343. doi: 10.1080/19336950.2015.1071748
Chung, H. J., Jan, Y. N., and Jan, L. Y. (2006). Polarized axonal surface expression of neuronal KCNQ channels is mediated by multiple signals in the KCNQ2 and KCNQ3 C-terminal domains. Proc. Natl. Acad. Sci. U.S.A. 103, 8870–8875. doi: 10.1073/pnas.0603376103
Cleva, R. M., Hicks, M. P., Gass, J. T., Wischerath, K. C., Plasters, E. T., Widholm, J. J., et al. (2011). MGluR5 positive allosteric modulation enhances extinction learning following cocaine self-administration. Behav. Neurosci. 125, 10–19. doi: 10.1037/a0022339
Cooper, E. C., Aldape, K. D., Abosch, A., Barbaro, N. M., Berger, M. S., Peacock, W. S., et al. (2000). Colocalization and coassembly of two human brain M-type potassium channel subunits that are mutated in epilepsy. Proc. Natl. Acad. Sci. U.S.A. 97, 4914–4919. doi: 10.1073/pnas.090092797
Corbin-Leftwich, A., Mossadeq, S. M., Ha, J., Ruchala, I., Le, A. H. N., and Villalba-Galea, C. A. (2016). Retigabine holds KV7 channels open and stabilizes the resting potential. J. Gen. Physiol. 147, 229–241. doi: 10.1085/jgp.201511517
Criado-Marrero, M., Santini, E., and Porter, J. T. (2014). Modulating fear extinction memory by manipulating SK potassium channels in the infralimbic cortex. Front. Behav. Neurosci. 8:96. doi: 10.3389/fnbeh.2014.00096
Cueni, L., Canepari, M., Luján, R., Emmenegger, Y., Watanabe, M., Bond, C. T., et al. (2008). T-type Ca2+ channels, SK2 channels and SERCAs gate sleep-related oscillations in thalamic dendrites. Nat. Neurosci. 11, 683–692. doi: 10.1038/nn.2124
David, F., Çarçak, N., Furdan, S., Onat, F., Gould, T., and Mészáros, Á, et al. (2018). Suppression of hyperpolarization-activated cyclic nucleotide-gated channel function in thalamocortical neurons prevents genetically determined and pharmacologically induced absence seizures. J. Neurosci. 38, 6615–6627. doi: 10.1523/JNEUROSCI.0896-17.2018
Davies, S. N., Martin, D., Millar, J. D., Aram, J. A., Church, J., and Lodge, D. (1988). Differences in results from in vivo and in vitro studies on the use-dependency of N-methylaspartate antagonism by MK-801 and other phencyclidine receptor ligands. Eur. J. Pharmacol. 145, 141–151. doi: 10.1016/0014-2999(88)90225-7
de Jong, I. E. M., and Jepps, T. A. (2018). Impaired Kv7 channel function in cerebral arteries of a tauopathy mouse model (rTg4510). Physiol. Rep. 6, 1–10. doi: 10.14814/phy2.13920
Deardorff, A. S., Romer, S. H., Deng, Z., Bullinger, K. L., Nardelli, P., Cope, T. C., et al. (2013). Expression of postsynaptic Ca2+-activated K+ (SK) channels at C-bouton synapses in mammalian lumbar -motoneurons. J Physiol. 591, 875–897. doi: 10.1113/jphysiol.2012.240879
Decher, N., Bundis, F., Vajna, R., and Steinmeyer, K. (2003). KCNE2 modulates current amplitudes and activation kinetics of HCN4: Influence of KCNE family members on HCN4 currents. Pflugers Arch. Eur. J. Physiol. 446, 633–640. doi: 10.1007/s00424-003-1127-7
Delmas, P., and Brown, D. A. (2005). Pathways modulating neural KCNQ / M Kv7 potassium channels. Nat. Rev. Neurosci. 6, 850–862. doi: 10.1038/nrn1785
Devaux, J. J., Kleopa, K. A., Cooper, E. C., and Scherer, S. S. (2004). KCNQ2 is a nodal K+ channel. J. Neurosci. 24, 1236–1244. doi: 10.1523/JNEUROSCI.4512-03.2004
Devulder, J. (2010). Flupirtine in pain management pharmacological properties and clinical use. CNS Drugs 24, 867–881. doi: 10.2165/11536230-000000000-00000
Diao, Y., Yan, W., Sun, W., Luo, Y., Li, J., and Yin, Y. (2019). The dual role of KCNQ/M channels upon OGD or OGD/R insults in cultured cortical neurons of mice: timing is crucial in targeting M-channels against ischemic injuries. J. Cell. Physiol. 234, 12714–12726. doi: 10.1002/jcp.27889
Dibbens, L. M., Reid, C. A., Hodgson, B., Thomas, E. A., Phillips, A. M., Gazina, E., et al. (2010). Augmented currents of an HCN2 variant in patients with febrile seizure syndromes. Ann. Neurol. 67, 542–546. doi: 10.1002/ana.21909
Dickson, C. T., Magistretti, J., Shalinsky, M. H., Fransé, E. N., Hasselmo, M. E., Alonso, A., et al. (2000). Properties and role of I h in the pacing of subthreshold oscillations in entorhinal cortex layer II neurons. J. Neurophysiol. 83, 2562–2579. doi: 10.1152/jn.2000.83.5.2562
DiFrancesco, J. C., Castellotti, B., Milanesi, R., Ragona, F., Freri, E., Canafoglia, L., et al. (2019). HCN ion channels and accessory proteins in epilepsy: genetic analysis of a large cohort of patients and review of the literature. Epilepsy Res. 153, 49–58. doi: 10.1016/j.eplepsyres.2019.04.004
Ding, W., You, Z., Shen, S., Chen, L., Zhu, S., and Mao, J. (2016). Inhibition of HCN channel activity in the thalamus attenuates chronic pain in rats. Neurosci. Lett. 631, 97–103. doi: 10.1016/j.neulet.2016.08.021
Ding, W., You, Z., Shen, S., Yang, J., Lim, G., Doheny, J. T., et al. (2018). Increased HCN channel activity in the gasserian ganglion contributes to trigeminal neuropathic pain. J. Pain 19, 626–634. doi: 10.1016/j.jpain.2018.01.003
Doo, A. R., Kim, S. T., Kim, S. N., Moon, W., Yin, C. S., Chae, Y., et al. (2010). Neuroprotective effects of bee venom pharmaceutical acupuncture in acute 1-methyl-4-phenyl-1,2,3,6-tetrahydropyridineinduced mouse model of Parkinson’s disease. Neurol. Res. 32(Suppl. 1), 4–7. doi: 10.1179/016164109X
Dreixler, J. C., Bian, J.-T., Cao, Y.-J., Roberts, M. T., Roizen, J. D., and Houamed, K. M. (2000a). Block of rat brain recombinant SK channels by tricyclic antidepressants and related compounds. Eur. J. Pharmacol. 401, 1–7. doi: 10.1016/s0014-2999(00)00401-5
Dreixler, J. C., Jenkins, A., Cao, Y.-J., Roizen, J. D., and Houamed, K. M. (2000b). Patch-clamp analysis of anesthetic interactions with recombinant SK2 subtype neuronal calcium-activated potassium channels. Anesth. Analg. 90, 727–732. doi: 10.1097/00000539-200003000-00040
Dunnett, S. B., and Björklund, A. (1999). Prospects for new restorative and neuroprotective treatments in Parkinson’s disease. Nature 399(6738 Suppl.), A32–A39. doi: 10.1038/399a032
Dwivedi, D., Chattarji, S., and Bhalla, U. S. (2019). Impaired reliability and precision of spiking in adults but not juveniles in a mouse model of Fragile X syndrome. eNeuro 6:ENEURO.0217-19.2019. doi: 10.1523/ENEURO.0217-19.2019
El-Hassar, L., Simen, A. A., Duque, A., Patel, K. D., Kaczmarek, L. K., Arnsten, A. F. T., et al. (2014). Disrupted in schizophrenia 1 modulates medial prefrontal cortex pyramidal neuron activity through cAMP regulation of transient receptor potential c and small-conductance k+ channels. Biol. Psychiatry 76, 476–485. doi: 10.1016/j.biopsych.2013.12.019
Emery, E. C., Young, G. T., Berrocoso, E. M., Chen, L., and McNaughton, P. A. (2011). HCN2 ion channels play a central role in inflammatory and neuropathic pain. Science 333, 1462–1466. doi: 10.1126/science.1206243
Eslamizade, M. J., Saffarzadeh, F., Mousavi, S. M. M., Meftahi, G. H., Hosseinmardi, N., Mehdizadeh, M., et al. (2015). Alterations in ca1 pyramidal neuronal intrinsic excitability mediated by iH channel currents in a rat model of amyloid beta pathology. Neuroscience 305, 279–292. doi: 10.1016/j.neuroscience.2015.07.087
Fakler, B., and Adelman, J. P. (2008). Control of KCa channels by calcium nano/microdomains. Neuron 59, 873–881. doi: 10.1016/j.neuron.2008.09.001
Fanger, C. M., Ghanshani, S., Logsdon, N. J., Rauer, H., Kalman, K., Zhou, J., et al. (1999). Calmodulin mediates calcium-dependent activation of the intermediate conductance K(Ca) channel, IKCa1. J. Biol. Chem. 274, 5746–5754. doi: 10.1074/jbc.274.9.5746
Fanger, C. M., Rauer, H., Neben, A. L., Miller, M. J., Rauer, H., Wulff, H., et al. (2001). Calcium-activated potassium channels sustain calcium signaling in T lymphocytes. Selective blockers and manipulated channel expression levels. J. Biol. Chem. 276, 12249–12256. doi: 10.1074/jbc.M011342200
Farde, L. (1998). Brain imaging of schizophrenia – the dopamine hypothesis. Schizophr. Res. 28, 157–162. doi: 10.1016/s0920-9964(97)00121-7
Fattore, C., and Perucca, E. (2011). Novel medications for epilepsy. Drugs 71, 2151–2178. doi: 10.2165/11594640-000000000-00000
Fedorenko, O., Strutz-Seebohm, N., Henrion, U., Ureche, O. N., Lang, F., Seebohm, G., et al. (2008). A schizophrenia-linked mutation in PIP5K2A fails to activate neuronal M channels. Psychopharmacology 199, 47–54. doi: 10.1007/s00213-008-1095-x
Fernández De Sevilla, D., Garduño, J., Galván, E., and Buño, W. (2006). Calcium-activated after hyperpolarizations regulate synchronization and timing of epileptiform bursts in hippocampal CA3 pyramidal neurons. J. Neurophysiol. 96, 3028–3041. doi: 10.1152/jn.00434.2006
Fisher, D. W., Han, Y., Lyman, K. A., Heuermann, R. J., Bean, L. A., Ybarra, N., et al. (2018). HCN channels in the hippocampus regulate active coping behavior. J. Neurochem. 146, 753–766. doi: 10.1111/jnc.14539
Flynn, G. E., and Zagotta, W. N. (2018). Insights into the molecular mechanism for hyperpolarization-dependent activation of HCN channels. Proc. Natl. Acad. Sci. U.S.A. 115, E8086–E8095. doi: 10.1073/pnas.1805596115
Foote, K. M., Lyman, K. A., Han, Y., Michailidis, I. E., Heuermann, R. J., Mandikian, D., et al. (2019). Phosphorylation of the HCN channel auxiliary subunit TRIP8b is altered in an animal model of temporal lobe epilepsy and modulates channel function. J. Biol. Chem. 294, 15743–15758. doi: 10.1074/jbc.RA119.010027
Forster, L. A., Jansen, L. A. R., Rubaharan, M., Murphy, A. Z., and Baro, D. J. (2020). Alterations in SUMOylation of the hyperpolarization-activated cyclic nucleotide-gated ion channel 2 during persistent inflammation. Eur. J. Pain 24, 1517–1536. doi: 10.1002/ejp.1606
Fransén, E., Alonso, A. A., Dickson, C. T., Magistretti, J., and Hasselmo, M. E. (2004). Ionic mechanisms in the generation of subthreshold oscillations and action potential clustering in entorhinal layer II stellate neurons. Hippocampus 14, 368–384. doi: 10.1002/hipo.10198
Friedman, A. K., Juarez, B., Ku, S. M., Zhang, H., Calizo, R. C., Walsh, J. J., et al. (2016). KCNQ channel openers reverse depressive symptoms via an active resilience mechanism. Nat. Commun. 7:11671. doi: 10.1038/ncomms11671
Frykman, S., Inoue, M., Ikeda, A., Teranishi, Y., Kihara, T., Lundgren, J. L., et al. (2017). Maturation and processing of the amyloid precursor protein is regulated by the potassium/sodium hyperpolarization-activated cyclic nucleotide-gated ion channel 2 (HCN2). Biochem. Biophys. Res. Commun. 483, 352–358. doi: 10.1016/j.bbrc.2016.12.140
Fu, R., Mei, Q., Shiwalkar, N., Zuo, W., Zhang, H., Gregor, D., et al. (2020). Anxiety during alcohol withdrawal involves 5-HT2C receptors and M-channels in the lateral habenula. Neuropharmacology 163:107863. doi: 10.1016/j.neuropharm.2019.107863
Galeotti, N., Ghelardini, C., Caldari, B., and Bartolini, A. (1999). Effect of potassium channel modulators in mouse forced swimming test. Br. J. Pharmacol. 126, 1653–1659. doi: 10.1038/sj.bjp.0702467
Gamo, N. J., Lur, G., Higley, M. J., Wang, M., Paspalas, C. D., Vijayraghavan, S., et al. (2015). Stress impairs prefrontal cortical function via D1 dopamine receptor interactions with hyperpolarization-activated cyclic nucleotide-gated channels. Biol. Psychiatry 78, 860–870. doi: 10.1016/j.biopsych.2015.01.009
Gamper, N., and Shapiro, M. S. (2003). Calmodulin mediates Ca2+-dependent modulation of M- type K+ channels. J. Gen. Physiol. 122, 17–31. doi: 10.1085/jgp.200208783
Gao, S. H., Wen, H. Z., Shen, L. L., Zhao, Y. D., and Ruan, H. Z. (2016). Activation of mGluR1 contributes to neuronal hyperexcitability in the rat anterior cingulate cortex via inhibition of HCN channels. Neuropharmacology 105, 361–377. doi: 10.1016/j.neuropharm.2016.01.036
García-Negredo, G., Soto, D., Llorente, J., Morató, X., Galenkamp, K. M. O., Gómez-Soler, M., et al. (2014). Coassembly and coupling of SK2 channels and mGlu5 receptors. J. Neurosci. 34, 14793–14802. doi: 10.1523/JNEUROSCI.2038-14.2014
Garduño, J., Galván, E., Fernández De Sevilla, D., and Buño, W. (2005). 1-Ethyl-2-benzimidazolinone (EBIO) suppresses epileptiform activity in in vitro hippocampus. Neuropharmacology 49, 376–388. doi: 10.1016/j.neuropharm.2005.03.021
Gargus, J. J., Fantino, E., and Gutman, G. A. (1998). A piece in the puzzle: an ion channel candidate gene for schizophrenia. Mol. Med. Today 4, 518–524. doi: 10.1016/S1357-4310(98)01358-6
Gass, J. T., and Olive, M. F. (2009). Positive allosteric modulation of mGluR5 receptors facilitates extinction of a cocaine contextual memory. Biol. Psychiatry 65, 717–720. doi: 10.1016/j.biopsych.2008.11.001
Giessel, A. J., and Sabatini, B. L. (2010). M1 muscarinic receptors boost synaptic potentials and calcium influx in dendritic spines by inhibiting postsynaptic SK channels. Neuron 68, 936–947. doi: 10.1016/j.neuron.2010.09.004
Gill, C. H., Randall, A., Bates, S. A., Hill, K., Owen, D., Larkman, P. M., et al. (2004). Characterization of the human HCN1 channel and its inhibition by capsazepine. Br. J. Pharmacol. 143, 411–421. doi: 10.1038/sj.bjp.0705945
Goldberg, J. A., and Wilson, C. J. (2005). Control of spontaneous firing patterns by the selective coupling of calcium currents to calcium-activated potassium currents in striatal cholinergic interneurons. J. Neurosci. 25, 10230–10238. doi: 10.1523/JNEUROSCI.2734-05.2005
Gomis-Perez, C., Soldovieri, M. V., Malo, C., Ambrosino, P., Taglialatela, M., Areso, P., et al. (2017). Differential regulation of pi(4,5)p2 sensitivity of kv7.2 and kv7.3 channels by calmodulin. Front. Mol. Neurosci. 10:117. doi: 10.3389/fnmol.2017.00117
Greene, D. L., Kosenko, A., and Hoshi, N. (2018). Attenuating M-current suppression in vivo by a mutant Kcnq2 gene knock-in reduces seizure burden and prevents status epilepticus–induced neuronal death and epileptogenesis. Epilepsia 59, 1908–1918. doi: 10.1111/epi.14541
Gu, N., Vervaeke, K., Hu, H., and Storm, J. F. (2005). Kv7/KCNQ/M and HCN/h, but not KCa2/SK channels, contribute to the somatic medium after-hyperpolarization and excitability control in CA1 hippocampal pyramidal cells. J. Physiol. 566(Pt 3), 689–715. doi: 10.1113/jphysiol.2005.086835m
Guatteo, E., Rizzo, F. R., Federici, M., Cordella, A., Ledonne, A., Latini, L., et al. (2017). Functional alterations of the dopaminergic and glutamatergic systems in spontaneous α-synuclein overexpressing rats. Exp. Neurol. 287, 21–33. doi: 10.1016/j.expneurol.2016.10.009
Günther, A., Luczak, V., Gruteser, N., Abel, T., and Baumann, A. (2019). HCN4 knockdown in dorsal hippocampus promotes anxiety-like behavior in mice. Genes Brain Behav. 18:e12550. doi: 10.1111/gbb.12550
Ha, G. E., and Cheong, E. (2017). Spike frequency adaptation in neurons of the central nervous system. Exp. Neurobiol. 26, 179–185. doi: 10.5607/en.2017.26.4.179
Habermann, E., and Fischer, K. (1979). Bee venom neurotoxin (Apamin): Iodine Labeling and characterization of binding sites. Eur. J. Biochem. 94, 355–364. doi: 10.1111/j.1432-1033.1979.tb12901.x
Haitin, Y., and Attali, B. (2008). The C-terminus of Kv7 channels: a multifunctional module. J. Physiol. 586, 1803–1810. doi: 10.1113/jphysiol.2007.149187
Halls, M. L., and Cooper, D. M. F. (2011). Regulation by Ca2+-signaling pathways of adenylyl cyclases. Cold Spring Harb. Perspect. Biol. 3, 1–22. doi: 10.1101/cshperspect.a004143
Hammelmann, V., Stieglitz, M. S., Hülle, H., Le Meur, K., Kass, J., Brümmer, M., et al. (2019). Abolishing cAMP sensitivity in HCN2 pacemaker channels induces generalized seizures. JCI Insight 4:e126418. doi: 10.1172/jci.insight.126418
Han, Y., Heuermann, R. J., Lyman, K. A., Fisher, D., Ismail, Q. A., and Chetkovich, D. M. (2017). HCN-channel dendritic targeting requires bipartite interaction with TRIP8b and regulates antidepressant-like behavioral effects. Mol. Psychiatry 22, 458–465. doi: 10.1038/mp.2016.99
Hansen, H. H., Waroux, O., Seutin, V., Jentsch, T. J., Aznar, S., and Mikkelsen, J. D. (2008). Kv7 channels: interaction with dopaminergic and serotonergic neurotransmission in the CNS. J. Physiol. 586, 1823–1832. doi: 10.1113/jphysiol.2007.149450
Hao, X. M., Xu, R., Chen, A. Q., Sun, F. J., Wang, Y., Liu, H. X., et al. (2019). Endogenous HCN channels modulate the firing activity of globus pallidus neurons in Parkinsonian animals. Front. Aging Neurosci. 10:190. doi: 10.3389/fnagi.2019.00190
Hartmann, A., Müllner, J., Meier, N., Hesekamp, H., Van Meerbeeck, P., Habert, M. O., et al. (2016). Bee venom for the treatment of Parkinson disease – a randomized controlled clinical trial. PLoS One 11:e0158235. doi: 10.1371/journal.pone.0158235
Hayashi, H., Iwata, M., Tsuchimori, N., and Matsumoto, T. (2014). Activation of peripheral KCNQ channels attenuates inflammatory pain. Mol. Pain 10, 1–9. doi: 10.1186/1744-8069-10-15
He, C., Chen, F., Li, B., and Hu, Z. (2014). Neurophysiology of HCN channels: from cellular functions to multiple regulations. Prog. Neurobiol. 112, 1–23. doi: 10.1016/j.pneurobio.2013.10.001
Hernandez, C. C., Zaika, O., Tolstykh, G. P., and Shapiro, M. S. (2008). Regulation of neural KCNQ channels: signalling pathways, structural motifs and functional implications. J. Physiol. 586, 1811–1821. doi: 10.1113/jphysiol.2007.148304
Hessler, S., Zheng, F., Hartmann, S., Rittger, A., Lehnert, S., Völkel, M., et al. (2015). β-secretase BACE1 regulates hippocampal and reconstituted M-currents in a β-subunit-like fashion. J. Neurosci. 35, 3298–3311. doi: 10.1523/JNEUROSCI.3127-14.2015
Heuermann, R. J., Jaramillo, T. C., Ying, S. W., Suter, B. A., Lyman, K. A., Han, Y., et al. (2016). Reduction of thalamic and cortical Ih by deletion of TRIP8b produces a mouse model of human absence epilepsy. Neurobiol. Dis. 85, 81–92. doi: 10.1016/j.nbd.2015s.10.005
Hirschberg, B., Maylie, J., Adelman, J. P., and Marrion, N. V. (1999). Gating properties of single SK channels in hippocampal CA1 pyramidal neurons. Biophys. J. 77, 1905–1913. doi: 10.1016/S0006-3495(99)77032-3
Hirvonen, J., van Erp, T. G., Jukka Huttunen, M., Aalto, S., Någren, K., Huttunen, M., et al. (2006). Brain dopamine D 1 receptors in twins discordant for schizophrenia. Am. J. Psychiatry 163, 1747–1753. doi: 10.1176/ajp.2006.163.10.1747
Honrath, B., Krabbendam, I. E., Ijsebaart, C., Pegoretti, V., Bendridi, N., Rieusset, J., et al. (2018). SK channel activation is neuroprotective in conditions of enhanced ER-mitochondrial coupling article. Cell Death Dis. 9, 1–11. doi: 10.1038/s41419-018-0590-1
Honrath, B., Matschke, L., Meyer, T., Magerhans, L., Perocchi, F., Ganjam, G. K., et al. (2017). SK2 channels regulate mitochondrial respiration and mitochondrial Ca2+ uptake. Cell Death Differ. 24, 761–773. doi: 10.1038/cdd.2017.2
Honsa, P., Pivonkova, H., Harantova, L., Butenko, O., Kriska, J., Dzamba, D., et al. (2014). Increased expression of hyperpolarization-activated cyclic nucleotide-gated (HCN) channels in reactive astrocytes following ischemia. Glia 62, 2004–2021. doi: 10.1002/glia.22721
Hopf, F. W., Bowers, M. S., Chang, S. J., Chen, B. T., Martin, M., Seif, T., et al. (2010). Reduced nucleus accumbens SK channel activity enhances alcohol seeking during abstinence. Neuron 65, 682–694. doi: 10.1016/j.neuron.2010.02.015
Hopf, F. W., Martin, M., Chen, B. T., Bowers, M. S., Mohamedi, M. M., and Bonci, A. (2007). Withdrawal from intermittent ethanol exposure increases probability of burst firing in VTA neurons in vitro. J. Neurophysiol. 98, 2297–2310. doi: 10.1152/jn.00824.2007
Hopf, F. W., Simms, J. A., Chang, S. J., Seif, T., Bartlett, S. E., and Bonci, A. (2011). Chlorzoxazone, an SK-type potassium channel activator used in humans, reduces excessive alcohol intake in rats. Biol. Psychiatry 69, 618–624. doi: 10.1016/j.biopsych.2010.11.011
Hou, B., Chen, H., Qu, X., Lin, X., Luo, F., and Li, C. (2015). Characteristics of hyperpolarization-activated cyclic nucleotide-gated channels in dorsal root ganglion neurons at different ages and sizes. NeuroReport 26, 981–987. doi: 10.1097/WNR.0000000000000455
Hougaard, C., Eriksen, B. L., Jørgensen, S., Johansen, T. H., Dyhring, T., Madsen, L. S., et al. (2007). Selective positive modulation of the SK3 and SK2 subtypes of small conductance Ca 2+-activated K + channels. Br. J. Pharmacol. 151, 655–665. doi: 10.1038/sj.bjp.0707281
Hu, H., Vervaeke, K., and Storm, J. F. (2007). M-channels (Kv7/KCNQ channels) that regulate synaptic integration, excitability, and spike pattern of CA1 pyramidal cells are located in the perisomatic region. J. Neurosci. 27, 1853–1867. doi: 10.1523/JNEUROSCI.4463-06.2007
Hu, W., and Bean, B. P. (2018). Differential control of axonal and somatic resting potential by voltage-dependent conductances in cortical layer 5 pyramidal neurons. Neuron 97, 1315–1326.e3. doi: 10.1016/j.neuron.2018.02.016
Huang, H., and Trussell, L. O. (2011). KCNQ5 channels control resting properties and release probability of a synapse. Nat. Neurosci. 14, 840–847. doi: 10.1038/nn.2830
Huang, Y., Liu, X., Wang, G., and Wang, Y. (2018). SK channels participate in the formation of after burst hyperpolarization and partly inhibit the burst strength of epileptic ictal discharges. Mol. Med. Rep. 17, 1762–1774. doi: 10.3892/mmr.2017.8068
Huang, Z., Walker, M. C., and Shah, M. M. (2009). Loss of dendritic HCN1 subunits enhances cortical excitability and epileptogenesis. J. Neurosci. 29, 10979–10988. doi: 10.1523/JNEUROSCI.1531-09.2009
Hunot, S., Vila, M., Teismann, P., Davis, R. J., Hirsch, E. C., Przedborski, S., et al. (2004). JNK-mediated induction of cyclooxygenase 2 is required for neurodegeneration in a mouse model of Parkinson’s disease. Proc. Natl. Acad. Sci. U.S.A. 101, 665–670. doi: 10.1073/pnas.0307453101
Ikonen, S., and Riekkinen, P. (1999). Effects of apamin on memory processing of hippocampal-lesioned mice. Eur. J. Pharmacol. 382, 151–156. doi: 10.1016/S0014-2999(99)00616-0
Ishii, T. M., Maylie, J., and Adelman, J. P. (1997). Determinants of apamin and d -tubocurarine block in SK potassium channels. J. Biol. Chem. 272, 23195–23200. doi: 10.1074/jbc.272.37.23195
Itoh, M., Ishihara, K., Nakashima, N., and Takano, M. (2016). The hyperpolarization-activated cyclic nucleotide-gated (HCN) channels contain multiple S-palmitoylation sites. J. Physiol. Sci. 66, 241–248. doi: 10.1007/s12576-015-0420-5
Janak, P. H., Chang, J. Y., and Woodward, D. J. (1999). Neuronal spike activity in the nucleus accumbens of behaving rats during ethanol self-administration. Brain Res. 817, 172–184. doi: 10.1016/S0006-8993(98)01245-1
Javitt, C. (1991). Recent advances in the phencyclidine model of schizophrenia. Am. J. Psychiatry 148, 1301–1308. doi: 10.1176/ajp.148.10.1301
Jentsch, T. J. (2000). Neuronal KCNQ potassium channels: physislogy and role in disease. Nat. Rev. Neurosci. 1, 21–30. doi: 10.1038/35036198
Jung, S., Jones, T. D., Lugo, J. N., Sheerin, A. H., Miller, J. W., D’Ambrosio, R., et al. (2007). Progressive dendritic HCN channelopathy during epileptogenesis in the rat pilocarpine model of epilepsy. J. Neurosci. 27, 13012–13021. doi: 10.1523/JNEUROSCI.3605-07.2007
Kang, S., Li, J., Zuo, W., Fu, R., Gregor, D., Krnjevic, K., et al. (2017). Ethanol withdrawal drives anxiety-related behaviors by reducing m-type potassium channel activity in the lateral habenula. Neuropsychopharmacology 42, 1813–1824. doi: 10.1038/npp.2017.68
Kang, S., Xu, M., Cooper, E. C., and Hoshi, N. (2014). Channel-anchored protein kinase CK2 and protein phosphatase 1 reciprocally regulate KCNQ2-containing M-channels via phosphorylation of calmodulin. J. Biol. Chem. 289, 11536–11544. doi: 10.1074/jbc.M113.528497
Kaupp, U. B., and Seifert, R. (2001). Molecular diversity of pacemaker ion channels. Annu. Rev. Physiol. 63, 235–257. doi: 10.1146/annurev.physiol.63.1.235
Khachaturian, Z. S. (1989). Calcium, membranes, aging and Alzheimer’s disease: Introduction and overview. Ann. N. Y. Acad. Sci. 568, 1–4. doi: 10.1111/j.1749-6632.1989.tb12485.x
Khandai, P., Forcelli, P. A., and N’Gouemo, P. (2020). Activation of small conductance calcium-activated potassium channels suppresses seizure susceptibility in the genetically epilepsy-prone rats. Neuropharmacology 163:107865. doi: 10.1016/j.neuropharm.2019.107865
Kim, C. S., and Johnston, D. (2018). A possible link between HCN channels and depression. Chronic Stress 2, 1–6. doi: 10.1177/2470547018787781
Kim, C. S., Brager, D. H., and Johnston, D. (2017). Perisomatic changes in h-channels regulate depressive behaviors following chronic unpredictable stress. Mol. Psychiatry 23, 892–903. doi: 10.1038/mp.2017.28
Kim, C. S., Chang, P. Y., and Johnston, D. (2012). Enhancement of dorsal hippocampal activity by knockdown of hcn1 channels leads to anxiolytic- and antidepressant-like behaviors. Neuron 75, 503–516. doi: 10.1016/j.neuron.2012.05.027
Kitayama, M., Miyata, H., Yano, M., Saito, N., Matsuda, Y., Yamauchi, T., et al. (2003). Ih blockers have a potential of antiepileptic effects. Epilepsia 44, 20–24. doi: 10.1046/j.1528-1157.2003.22702.x
Kleiman-weiner, M., Beenhakker, M. P., Segal, W. A., and Huguenard, J. R. (2009). Synergistic roles of GABAA receptors and SK channels in regulating thalamocortical oscillations. J. Neurophysiol. 102, 203–213. doi: 10.1152/jn.91158.2008
Knapp, C. M., O’Malley, M., Datta, S., and Ciraulo, D. A. (2014). The Kv7 potassium channel activator retigabine decreases alcohol consumption in rats. Am. J. Drug Alcohol Abuse 40, 244–250. doi: 10.3109/00952990.2014.892951
Ko, K. W., Rasband, M. N., Meseguer, V., Kramer, R. H., and Golding, N. L. (2016). Serotonin modulates spike probability in the axon initial segment through HCN channels. Nat. Neurosci. 19, 826–834. doi: 10.1038/nn.4293
Koga, K., Descalzi, G., Chen, T., Ko, H. G., Lu, J., Li, S., et al. (2015). Coexistence of two forms of LTP in ACC provides a synaptic mechanism for the interactions between anxiety and chronic pain. Neuron 85, 377–389. doi: 10.1016/j.neuron.2014.12.021
Köhler, A. M., Hirschberg, B., Bond, C. T., Kinzie, J. M., Marrion, N. V., Maylie, J., et al. (1996). Small-Conductance, Calcium-Activated Potassium Channels from Mammalian Brain. Science 273, 1709–1714. doi: 10.1126/science.273.5282.1709
Konieczny, V., Tovey, S. C., Mataragka, S., Prole, D. L., and Taylor, C. W. (2017). Cyclic AMP recruits a discrete intracellular Ca2+ store by unmasking hypersensitive IP3 receptors. Cell Rep. 18, 711–722. doi: 10.1016/j.celrep.2016.12.058
Korsgaard, M. P. G., Hartz, B. P., Brown, W. D., Ahring, P. K., Strøbæk, D., and Mirza, N. R. (2005). Anxiolytic effects of maxipost (BMS-204352) and retigabine via activation of neuronal Kv7 channels. J. Pharmacol. Exp. Ther. 314, 282–292. doi: 10.1124/jpet.105.083923
Krabbendam, I. E., Honrath, B., Culmsee, C., and Dolga, A. M. (2018). Mitochondrial Ca2+-activated K+ channels and their role in cell life and death pathways. Cell Calcium 69, 101–111. doi: 10.1016/j.ceca.2017.07.005
Kreir, M., De Bondt, A., Van den Wyngaert, I., Teuns, G., Lu, H. R., and Gallacher, D. J. (2019). Role of Kv7.2/Kv7.3 and M1 muscarinic receptors in the regulation of neuronal excitability in hiPSC-derived neurons. Eur. J. Pharmacol. 858:172474. doi: 10.1016/j.ejphar.2019.172474
Kshatri, A. S., Gonzalez-Hernandez, A., and Giraldez, T. (2018). Physiological roles and therapeutic potential of Ca2+ activated potassium channels in the nervous system. Front. Mol. Neurosci. 11:258. doi: 10.3389/fnmol.2018.00258
Kuiper, E. F. E., Nelemans, A., Luiten, P., Nijholt, I., Dolga, A., and Eisel, A. D. (2012). Kca2 and Kca3 channels in learning and memory processes, and neurodegeneration. Front. Pharmacol. 3:107. doi: 10.3389/fphar.2012.00107
Lam, J., Coleman, N., Garing, A. L. A., and Wulff, H. (2013). The therapeutic potential of small-conductance KCa2 channels in neurodegenerative and psychiatric diseases. Expert Opin. Ther. Targets 17, 1203–1220. doi: 10.1517/14728222.2013.823161
Lappin, S. C., Dale, T. J., Brown, J. T., Trezise, D. J., and Davies, C. H. (2005). Activation of SK channels inhibits epileptiform bursting in hippocampal CA3 neurons. Brain Res. 1065, 37–46. doi: 10.1016/j.brainres.2005.10.024
Laurent, C., Niehaus, D., Bauché, S., Levinson, D. F., Soubigou, S., Pimstone, S., et al. (2003). CAG repeat polymorphisms in KCNN3 (HSKCa3) and PPP2R2B show no association or linkage to schizophrenia. Am. J. Med. Genet. B Neuropsychiatr. Genet. 116B, 45–50. doi: 10.1002/ajmg.b.10797
Lee, C. H., and MacKinnon, R. (2017). Structures of the human HCN1 hyperpolarization-activated channel. Cell 168, 111–120.e11. doi: 10.1016/j.cell.2016.12.023
Lee, C. H., and MacKinnon, R. (2018). Activation mechanism of a human SK-calmodulin channel complex elucidated by cryo-EM structures. Science 360, 508–513. doi: 10.1126/science.aas9466
Lee, M. C., Bond, S., Wheeler, D., Scholtes, I., Armstrong, G., McNaughton, P., et al. (2019). randomised, double-blind, placebo-controlled crossover trial of the influence of the HCN channel blocker ivabradine in a healthy volunteer pain model: an enriched population trial. Pain 160, 2554–2565. doi: 10.1097/j.pain.0000000000001638
Leissring, M. A., Murphy, M. P., Mead, T. R., Akbari, Y., Sugarman, M. C., Jannatipour, M., et al. (2002). A physiologic signaling role for the γ-secretase-derived intracellular fragment of APP. Proc. Natl. Acad. Sci. U.S.A. 99, 4697–4702. doi: 10.1073/pnas.072033799
Lewis, A. S., Vaidya, S. P., Blaiss, C. A., Liu, Z., Stoub, T. R., Brager, D. H., et al. (2011). Deletion of the hyperpolarization-activated cyclic nucleotide-gated channel auxiliary subunit TRIP8b impairs hippocampal Ih localization and function and promotes antidepressant behavior in mice. J. Neurosci. 31, 7424–7440. doi: 10.1523/JNEUROSCI.0936-11.2011
Lezmy, J., Lipinsky, M., Khrapunsky, Y., Patrich, E., Shalom, L., Peretz, A., et al. (2017). M-current inhibition rapidly induces a unique CK2-dependent plasticity of the axon initial segment. Proc. Natl. Acad. Sci. U.S.A. 114, E10234–E10243. doi: 10.1073/pnas.1708700114
Li, L., Sun, H., Ding, J., Niu, C., Su, M., Zhang, L., et al. (2017). Selective targeting of M-type potassium Kv7.4 channels demonstrates their key role in the regulation of dopaminergic neuronal excitability and depression-like behaviour. Br. J. Pharmacol. 174, 4277–4294. doi: 10.1111/bph.14026
Li, Y., Gamper, N., Hilgemann, D. W., and Shapiro, M. S. (2005). Regulation of Kv7 (KCNQ) K+ channel open probability by phosphatidylinositol 4,5-bisphosphate. J. Neurosci. 25, 9825–9835. doi: 10.1523/JNEUROSCI.2597-05.2005
Li, Y., Xu, J., Xu, Y., Zhao, X. Y., Liu, Y., Wang, J., et al. (2018). Regulatory effect of general anesthetics on activity of potassium channels. Neurosci. Bull. 34, 887–900. doi: 10.1007/s12264-018-0239-1
Liegeois, J., Mercier, F., Graulich, A., Graulich-Lorge, F., Scuvee-Moreau, J., and Seutin, V. (2005). Modulation of small conductance calcium-activated potassium (SK) channels: a new challenge in medicinal chemistry. Curr. Med. Chem. 10, 625–647. doi: 10.2174/0929867033457908
Lin, M. T., Luján, R., Watanabe, M., Adelman, J. P., and Maylie, J. (2008). SK2 channel plasticity contributes to LTP at Schaffer collateral-CA1 synapses. Nat. Neurosci. 11, 170–177. doi: 10.1038/nn2041
Linley, J. E., Rose, K., Patil, M., Robertson, B., Akopian, A. N., and Gamper, N. (2008). Inhibition of M current in sensory neurons by exogenous proteases: a signaling pathway mediating inflammatory nociception. J. Neurosci. 28, 11240–11249. doi: 10.1523/JNEUROSCI.2297-08.2008
Liu, H., Jia, L., Chen, X., Shi, L., and Xie, J. (2018). The Kv7/KCNQ channel blocker XE991 protects nigral dopaminergic neurons in the 6-hydroxydopamine rat model of Parkinson’s disease. Brain Res. Bull. 137, 132–139. doi: 10.1016/j.brainresbull.2017.11.011
Liu, W., and Devaux, J. J. (2014). Calmodulin orchestrates the heteromeric assembly and the trafficking of KCNQ2/3 (Kv7.2/3) channels in neurons. Mol. Cell. Neurosci. 58, 40–52. doi: 10.1016/j.mcn.2013.12.005
Llinas, R., and Jahnsen, H. (1982). Electrophysiology of mammalian thalamic neurones in vitro. Nature 297, 406–408. doi: 10.1038/297406a0
Lo, E. H., Dalkara, T., and Moskowitz, M. A. (2003). Neurological diseases: mechanisms, challenges and opportunities in stroke. Nat. Rev. Neurosci. 4, 399–414. doi: 10.1038/nrn1106
Lodge, D., and Anis, N. A. (1982). Effects of phencyclidine on excitatory amino acid activation of spinal interneurones in the cat. Eur. J. Pharmacol. 77, 203–204. doi: 10.1016/0014-2999(82)90022-x
Lörincz, A., Notomi, T., Tamás, G., Shigemoto, R., and Nusser, Z. (2002). Polarized and compartment-dependent distribution of HCN1 in pyramidal cell dendrites. Nat. Neurosci. 5, 1185–1193. doi: 10.1038/nn962
Ludwig, A., Budde, T., Stieber, J., Moosmang, S., Wahl, C., Holthoff, K., et al. (2003). Absence epilepsy and sinus dysrhythmia in mice lacking the pacemaker channel HCN2. EMBO J. 22, 216–224. doi: 10.1093/emboj/cdg032
Lundby, A., Tseng, G. N., and Schmitt, N. (2010). Structural basis for KV7.1-KCNEx interactions in the IKs channel complex. Heart Rhythm 7, 708–713. doi: 10.1016/j.hrthm.2009.12.017
Lupica, C. R., Bell, J. A., Hoffman, A. F., and Watson, P. L. (2001). Contribution of the hyperpolarization-activated current (Ih) to membrane potential and GABA release in hippocampal interneurons. J. Neurophysiol. 86, 261–268. doi: 10.1152/jn.2001.86.1.261
Main, M. J., Cryan, J. E., Dupere, J. R. B., Cox, B., Clare, J. J., and Burbidge, S. A. (2000). Modulation of KCNQ2/3 potassium channels by the novel anticonvulsant retigabine. Mol. Pharmacol. 58, 253–262. doi: 10.1124/mol.58.2.253
Maingret, F., Coste, B., Hao, J., Giamarchi, A., Allen, D., Crest, M., et al. (2008). Neurotransmitter modulation of small-conductance Ca2+- activated K+ channels by regulation of Ca2+ gating. Neuron 59, 439–449. doi: 10.1016/j.neuron.2008.05.026
Maljevic, S., Lerche, C., Seebohm, G., Alekov, A. K., Busch, A. E., and Lerche, H. (2003). C-terminal interaction of KCNQ2 and KCNQ3 K+ channels. J. Physiol. 548, 353–360. doi: 10.1113/jphysiol.2003.040980
Mani, B. K., Robakowski, C., Brueggemann, L. I., Cribbs, L. L., Tripathi, A., Majetschak, M., et al. (2016). Kv7.5 Potassium channel subunits are the primary targets for PKA-dependent enhancement of vascular smooth muscle Kv7 currents. Mol. Pharmacol. 89, 323–334. doi: 10.1124/mol.115.101758
Manville, R. W., and Abbott, G. W. (2018). Ancient and modern anticonvulsants act synergistically in a KCNQ potassium channel binding pocket. Nat. Commun. 9:3845. doi: 10.1038/s41467-018-06339-2
Marini, C., Porro, A., Rastetter, A., Dalle, C., Rivolta, I., Bauer, D., et al. (2018). HCN1 mutation spectrum: From neonatal epileptic encephalopathy to benign generalized epilepsy and beyond. Brain 141, 3160–3178. doi: 10.1093/brain/awy263
Marrion, N. V., and Tavalin, S. J. (1998). Selective activation of Ca2+-activated K+ channels by co-localized Ca2+ channels in hippocampal neurons. Nature 395, 900–905. doi: 10.1038/27674
Martire, M., Castaldo, P., D’Amico, M., Preziosi, P., Annunziato, L., and Taglialatela, M. (2004). M channels containing KCNQ2 subunits modulate norepinephrine, aspartate, and GABA release from hippocampal nerve terminals. J. Neurosci. 24, 592–597. doi: 10.1523/JNEUROSCI.3143-03.2004
Mateos-Aparicio, P., Murphy, R., and Storm, J. F. (2014). Complementary functions of SK and Kv7/M potassium channels in excitability control and synaptic integration in rat hippocampal dentate granule cells. J. Physiol. 592, 669–693. doi: 10.1113/jphysiol.2013.267872
Matos, S. C., Zhang, Z., and Séguéla, P. (2015). Peripheral neuropathy induces HCN channel dysfunction in pyramidal neurons of the medial prefrontal cortex. J. Neurosci. 35, 13244–13256. doi: 10.1523/jneurosci.0799-15.2015
Matschke, L. A., Rinné, S., Snutch, T. P., Oertel, W. H., Dolga, A. M., and Decher, N. (2018). Calcium-activated SK potassium channels are key modulators of the pacemaker frequency in locus coeruleus neurons. Mol. Cell. Neurosci. 88, 330–341. doi: 10.1016/j.mcn.2018.03.002
Mattson, M. P., Cheng, B., Davis, D., Bryant, K., Lieberburg, I., and Rydel, R. E. (1992). β-Amyloid peptides destabilize calcium homeostasis and render human cortical neurons vulnerable to excitotoxicity. J. Neurosci. 12, 376–389. doi: 10.1523/jneurosci.12-02-00376.1992
Maurice, N., Deltheil, T., Melon, C., Degos, B., Mourre, C., Amalric, M., et al. (2015). Bee venom alleviates motor deficits and modulates the transfer of cortical information through the basal ganglia in rat models of Parkinson’s disease. PLoS One 10:e0142838. doi: 10.1371/journal.pone.0142838
McCormick, D. A., and Pape, H. C. (1990). Noradrenergic and serotonergic modulation of a hyperpolarization-activated cation current in thalamic relay neurones. J. Physiol. 431, 319–342. doi: 10.1113/jphysiol.1990.sp018332
McGuier, N. S., Griffin, W. C., Gass, J. T., Padula, A. E., Chesler, E. J., and Mulholland, P. J. (2016). Kv7 channels in the nucleus accumbens are altered by chronic drinking and are targets for reducing alcohol consumption. Addict. Biol. 21, 1097–1112. doi: 10.1111/adb.12279
McGuier, N. S., Rinker, J. A., Cannady, R., Fulmer, D. B., Jones, S. R., Hoffman, M., et al. (2018). Identification and validation of midbrain Kcnq4 regulation of heavy alcohol consumption in rodents. Neuropharmacology 138, 10–19. doi: 10.1016/j.neuropharm.2018.05.020
McKinley, J. W., Shi, Z., Kawikova, I., Hur, M., Bamford, I. J., Sudarsana Devi, S. P., et al. (2019). Dopamine deficiency reduces striatal cholinergic interneuron function in models of Parkinson’s disease. Neuron 103, 1056–1072.e6. doi: 10.1016/j.neuron.2019.06.013
Mellor, J., Nicoll, R. A., and Schmitz, D. (2002). Mediation of hippocampal mossy fiber long-term potentiation by presynaptic Ih channels. Science 295, 143–147. doi: 10.1126/science.1064285
Mi, Z., Yang, J., He, Q., Zhang, X., Xiao, Y., and Shu, Y. (2019). Alterations of electrophysiological properties and ion channel expression in prefrontal cortex of a mouse model of schizophrenia. Front. Cell. Neurosci. 13:554. doi: 10.3389/fncel.2019.00554
Miller, M. J., Rauer, H., Tomita, H., Rauer, H., Gargus, J. J., Gutman, G. A., et al. (2001). Nuclear localization and dominant-negative suppression by a mutant SKCa3 N-terminal channel fragment identified in a patient with schizophrenia. J. Biol. Chem. 276, 27753–27756. doi: 10.1074/jbc.C100221200
Monaghan, A. S., Benton, D. C. H., Bahia, P. K., Hosseini, R., Shah, Y. A., Haylett, D. G., et al. (2004). The SK3 subunit of small conductance Ca2+ -activated K + channels interacts with both SK1 and SK2 subunits in a heterologous expression system. J. Biol. Chem. 279, 1003–1009. doi: 10.1074/jbc.M308070200
Moosmang, S., Biel, M., Hofmann, F., and Ludwig, A. (1999). Differential distribution of four hyperpolarization-activated cation channels in mouse brain. Biol. Chem. 380, 975–980. doi: 10.1515/BC.1999.121
Moosmang, S., Stieber, J., Zong, X., Biel, M., Hofmann, F., and Ludwig, A. (2001). Cellular expression and functional characterization of four hyperpolarization-activated pacemaker channels in cardiac and neuronal tissues. Eur. J. Biochem. 268, 1646–1652. doi: 10.1046/j.1432-1327.2001.02036.x
Mourre, C., Manrique, C., Camon, J., Aidi-Knani, S., Deltheil, T., Turle-Lorenzo, N., et al. (2017). Changes in SK channel expression in the basal ganglia after partial nigrostriatal dopamine lesions in rats: functional consequences. Neuropharmacology 113, 519–532. doi: 10.1016/j.neuropharm.2016.11.003
Much, B., Wahl-Schott, C., Zong, X., Schneider, A., Baumann, L., Moosmang, S., et al. (2003). Role of subunit heteromerization and N-linked glycosylation in the formation of functional hyperpolarization-activated cyclic nucleotide-gated channels. J. Biol. Chem. 278, 43781–43786. doi: 10.1074/jbc.M306958200
Mulholland, P. J. (2012). KCa2 channels: novel therapeutic targets for treating alcohol withdrawal and escalation of alcohol consumption. Alcohol 46, 309–315. doi: 10.1016/j.alcohol.2011.11.002
Mulholland, P. J., Becker, H. C., Woodward, J. J., and Chandler, L. J. (2011). Small conductance calcium-activated potassium type 2 channels regulate alcohol-associated plasticity of glutamatergic synapses. Biol. Psychiatry 69, 625–632. doi: 10.1016/j.biopsych.2010.09.025
Murthy, S. R. K., Teodorescu, G., Nijholt, I. M., Dolga, A. M., Grissmer, S., Spiess, J., et al. (2008). Identification and characterization of a novel, shorter isoform of the small conductance Ca2+-activated K+ channel SK2. J. Neurochem. 106, 2312–2321. doi: 10.1111/j.1471-4159.2008.05557.x
Musial, T. F., Molina-Campos, E., Bean, L. A., Ybarra, N., Borenstein, R., Russo, M. L., et al. (2018). Store depletion-induced h-channel plasticity rescues a channelopathy linked to Alzheimer’s disease. Neurobiol. Learn. Mem. 154, 141–157. doi: 10.1016/j.nlm.2018.06.004
Nakagawa, S., Kim, J., Lee, R., Malberg, J. E., Chen, J., Steffen, C., et al. (2002). Regulation of neurogenesis in adult mouse hippocampus by cAMP and the cAMP response element-binding protein. J. Neurosci. 22, 3673–3682. doi: 10.1523/JNEUROSCI.22-09-03673.2002
Nakamura, M., Watanabe, H., Kubo, Y., Yokoyama, M., Matsumoto, T., Sasai, H., et al. (1998). KQT2, a new putative potassium channel family produced by alternative splicing. Isolation, genomic structure, and alternative splicing of the putative potassium channels. Recept. Channels 5, 255–271.
Nam, Y. W., Orfali, R., Liu, T., Yu, K., Cui, M., Wulff, H., et al. (2017). Structural insights into the potency of SK channel positive modulators. Sci. Rep. 7:17178. doi: 10.1038/s41598-017-16607-8
Neymotin, S. A., McDougal, R. A., Bulanova, A. S., Zeki, M., Lakatos, P., Terman, D., et al. (2016). Calcium regulation of HCN channels supports persistent activity in a multiscale model of neocortex. Neuroscience 316, 344–366. doi: 10.1016/j.neuroscience.2015.12.043
Ngo-Anh, T. J., Bloodgood, B. L., Lin, M. M. T., Sabatini, B. L., Maylie, J., Adelman, J. P., et al. (2008). KCa channels as therapeutic targets in episodic ataxia type-2. Brain Res. 11, 170–177.
Nolan, M. F., Malleret, G., Lee, K. H., Gibbs, E., Dudman, J. T., Santoro, B., et al. (2003). The hyperpolarization-activated HCN1 channel is important for motor learning and neuronal integration by cerebellar Purkinje cells. Cell 115, 551–564. doi: 10.1016/S0092-8674(03)00884-5
Nolting, A., Ferraro, T., D’Hoedt, D., and Stocker, M. (2007). An amino acid outside the pore region influences apamin sensitivity in small conductance Ca2+-activated K+ channels. J. Biol. Chem. 282, 3478–3486. doi: 10.1074/jbc.M607213200
Notomi, T., and Shigemoto, R. (2004). Immunohistochemical localization of Ih channel subunits, HCN1-4, in the rat brain. J. Comp. Neurol. 471, 241–276. doi: 10.1002/cne.11039
Novella Romanelli, M., Sartiani, L., Masi, A., Mannaioni, G., Manetti, D., Mugelli, A., et al. (2016). HCN channels modulators: the need for selectivity. Curr. Top. Med. Chem. 16, 1764–1791. doi: 10.2174/1568026616999160315130832
Obermair, G. J., Kaufmann, W. A., Knaus, H. G., and Flucher, B. E. (2003). The small conductance Ca2+-activated K+ channel SK3 is localized in nerve terminals of excitatory synapses of cultured mouse hippocampal neurons. Eur. J. Neurosci. 17, 721–731. doi: 10.1046/j.1460-9568.2003.02488.x
Oliveira, M. S., Skinner, F., Arshadmansab, M. F., Garcia, I., Mello, C. F., Knaus, H. G., et al. (2010). Altered expression and function of small-conductance (SK) Ca 2+-activated K+ channels in pilocarpine-treated epileptic rats. Brain Res. 1348, 187–199. doi: 10.1016/j.brainres.2010.05.095
Oliver, D., Klöcker, N., Schuck, J., Baukrowitz, T., Ruppersberg, J. P., and Fakler, B. (2000). Gating of Ca2+-activated K+ channels controls fast inhibitory synaptic transmission at auditory outer hair cells. Neuron 26, 595–601. doi: 10.1016/S0896-6273(00)81197-6
Oliver, D., Lien, C. C., Soom, M., Baukrowitz, T., Jonas, P., and Fakler, B. (2004). Functional conversion between A-type and delayed rectifier K+ channels by membrane lipids. Science 304, 265–270. doi: 10.1126/science.1094113
Oliver, D., Ludwig, J., Reisinger, E., Zoellner, W., Ruppersberg, J. P., and Fakler, B. (2001). Memantine inhibits efferent cholinergic transmission in the cochlea by blocking nicotinic acetylcholine receptors of outer hair cells. Mol. Pharmacol. 60, 183–189. doi: 10.1124/mol.60.1.183
Olney, J. W., and Farber, N. B. (1995). Glutamate receptor dysfunction and schizophrenia. Arch. Gen. Psychiatry 52, 998–1007. doi: 10.1001/archpsyc.1995.03950240016004
Oster, A., Faure, P., and Gutkin, B. S. (2015). Mechanisms for multiple activity modes of VTA dopamine neurons. Front. Comput. Neurosci. 9:95. doi: 10.3389/fncom.2015.00095
Osuma, A. T., Xu, X., Wang, Z., Van Camp, J. A., and Freiberg, G. M. (2019). Design and evaluation of pyrazolopyrimidines as KCNQ channel modulators. Bioorg. Med. Chem. Lett. 29:126603. doi: 10.1016/j.bmcl.2019.08.007
Paladini, C. A., Robinson, S., Morikawa, H., Williams, J. T., and Palmiter, R. D. (2003). Dopamine controls the firing pattern of dopamine neurons via a network feedback mechanism. Proc. Natl. Acad. Sci. U.S.A. 100, 2866–2871. doi: 10.1073/pnas.0138018100
Park, J. H., Kim, D. W., Lee, T. K., Park, C. W., Park, Y. E., Ahn, J. H., et al. (2019). Improved HCN channels in pyramidal neurons and their new expression levels in pericytes and astrocytes in the gerbil hippocampal CA1 subfield following transient ischemia. Int. J. Mol. Med. 44, 1801–1810. doi: 10.3892/ijmm.2019.4353
Park, K., Yi, J. H., Kim, H., Choi, K., Kang, S. J., and Shin, K. S. (2011). HCN channel activity-dependent modulation of inhibitory synaptic transmission in the rat basolateral amygdala. Biochem. Biophys. Res. Commun. 404, 952–957. doi: 10.1016/j.bbrc.2010.12.087
Park, Y. B. (1994). Ion selectivity and gating of small conductance Ca(2+)-activated K+ channels in cultured rat adrenal chromaffin cells. J. Physiol. 481, 555–570. doi: 10.1113/jphysiol.1994.sp020463
Parker, A. R., Welch, M. A., Forster, L. A., Tasneem, S. M., Dubhashi, J. A., and Baro, D. J. (2017). SUMOylation of the hyperpolarization-activated cyclic nucleotide-gated channel 2 increases surface expression and the maximal conductance of the hyperpolarization-activated current. Front. Mol. Neurosci. 9:168. doi: 10.3389/fnmol.2016.00168
Paspalas, C. D., Wang, M., and Arnsten, A. F. T. (2013). Constellation of HCN channels and cAMP regulating proteins in dendritic spines of the primate prefrontal cortex: potential substrate for working memory deficits in schizophrenia. Cereb. Cortex 23, 1643–1654. doi: 10.1093/cercor/bhs152
Passmore, G. M., Selyanko, A. A., Mistry, M., Al-Qatari, M., Marsh, S. J., Matthews, E. A., et al. (2003). KCNQ/M currents in sensory neurons: significance for pain therapy. J. Neurosci. 23, 7227–7236. doi: 10.1523/jneurosci.23-18-07227.2003
Paz, R. M., Tubert, C., Stahl, A., Díaz, A. L., Etchenique, R., Murer, M. G., et al. (2018). Inhibition of striatal cholinergic interneuron activity by the Kv7 opener retigabine and the nonsteroidal anti-inflammatory drug diclofenac. Neuropharmacology 137, 309–321. doi: 10.1016/j.neuropharm.2018.05.010
Pedarzani, P., and Stocker, M. (2008). Molecular and cellular basis of small- and intermediate-conductance, calcium-activated potassium channel function in the brain. Cell. Mol. Life Sci. 65, 3196–3217. doi: 10.1007/s00018-008-8216-x
Peng, B. W., Justice, J. A., Zhang, K., He, X. H., and Sanchez, R. M. (2010). Increased basal synaptic inhibition of hippocampal area CA1 pyramidal neurons by an antiepileptic drug that enhances i H. Neuropsychopharmacology 35, 464–472. doi: 10.1038/npp.2009.150
Peng, H., Bian, X. L., Ma, F. C., and Wang, K. W. (2017). Pharmacological modulation of the voltage-gated neuronal Kv7/KCNQ/M-channel alters the intrinsic excitability and synaptic responses of pyramidal neurons in rat prefrontal cortex slices. Acta Pharmacol. Sin. 38, 1248–1256. doi: 10.1038/aps.2017.72
Peretz, A., Degani, N., Nachman, R., Uziyel, Y., Gibor, G., Shabat, D., et al. (2005). Meclofenamic acid and diclofenac, novel templates of KCNQ2/Q3 potassium channel openers, depress cortical neuron activity and exhibit anticonvulsant properties. Mol. Pharmacol. 67, 1053–1066. doi: 10.1124/mol.104.007112
Peretz, A., Pell, L., Gofman, Y., Haitin, Y., Shamgar, L., Patrich, E., et al. (2010). Targeting the voltage sensor of Kv7.2 voltage-gated K+ channels with a new gating-modifier. Proc. Natl. Acad. Sci. U.S.A. 107, 15637–15642. doi: 10.1073/pnas.0911294107
Peretz, A., Sheinin, A., Yue, C., Degani-Katzav, N., Gibor, G., Nachman, R., et al. (2007). Pre- and postsynaptic activation of M-channels by a novel opener dampens neuronal firing and transmitter release. J. Neurophysiol. 97, 283–295. doi: 10.1152/jn.00634.2006
Pérez-Ramírez, M. B., Laville, A., Tapia, D., Duhne, M., Lara-González, E., Bargas, J., et al. (2015). KV7 channels regulate firing during synaptic integration in GABAergic striatal neurons. Neural Plast. 2015:472676. doi: 10.1155/2015/472676
Pian, P., Bucchi, A., Robinson, R. B., and Siegelbaum, S. A. (2006). Regulation of gating and rundown of HCN hyperpolarization-activated channels by exogenous and endogenous PIP2. J. Gen. Physiol. 128, 593–604. doi: 10.1085/jgp.200609648
Polter, A. M., and Kauer, J. A. (2014). Stress and VTA synapses: implications for addiction and depression. Eur. J. Neurosci. 39, 1179–1188. doi: 10.1111/ejn.12490
Porro, A., Binda, A., Pisoni, M., Donadoni, C., Rivolta, I., and Saponaro, A. (2020). Rational design of a mutation to investigate the role of the brain protein TRIP8b in limiting the cAMP response of HCN channels in neurons. J. Gen. Physiol. 152:e202012596. doi: 10.1085/jgp.202012596
Povstyan, O. V., Barrese, V., Stott, J. B., and Greenwood, I. A. (2017). Synergistic interplay of Gβγ and phosphatidylinositol 4,5-bisphosphate dictates Kv7.4 channel activity. Pflugers Arch. Eur. J. Physiol. 469, 213–223. doi: 10.1007/s00424-016-1916-4
Powell, K. L., Ng, C., O’Brien, T., Xu, S. H., Williams, D. A., Foote, S., et al. (2008). Decreases in HCN mRNA expression in the hippocampus after kindling and status epilepticus in adult rats. Epilepsia 49, 1686–1695. doi: 10.1111/j.1528-1167.2008.01593.x
Power, J. M., and Sah, P. (2008). Competition between calcium-activated K+ channels determines cholinergic action on firing properties of basolateral amygdala projection neurons. J. Neurosci. 28, 3209–3220. doi: 10.1523/JNEUROSCI.4310-07.2008
Proulx, É, Power, S. K., Oliver, D. K., Sargin, D., McLaurin, J., and Lambe, E. K. (2020). Apamin improves prefrontal nicotinic impairment in mouse model of Alzheimer’s disease. Cereb. Cortex 30, 563–574. doi: 10.1093/cercor/bhz107
Ramírez, D., Zúñiga, R., Concha, G., and Zúñiga, L. (2018). HCN channels: new therapeutic targets for pain treatment. Molecules 23:2094. doi: 10.3390/molecules23092094
Ranjan, R., Logette, E., Marani, M., Herzog, M., Tâche, V., Scantamburlo, E., et al. (2019). A kinetic map of the homomeric voltage-gated potassium channel (Kv) family. Front. Cell. Neurosci. 13:358. doi: 10.3389/fncel.2019.00358
Rauch, S. L., Shin, L. M., and Wright, C. I. (2003). Neuroimaging studies of amygdala function in anxiety disorders. Ann. N. Y. Acad. Sci. 985, 389–410. doi: 10.1111/j.1749-6632.2003.tb07096
Ray, S., Salzer, I., Kronschläger, M. T., and Boehm, S. (2019). The paracetamol metabolite N-acetylp-benzoquinone imine reduces excitability in first- and second-order neurons of the pain pathway through actions on KV7 channels. Pain 160, 954–964. doi: 10.1097/j.pain.0000000000001474
Rimini, R., Rimland, J. M., and Terstappen, G. C. (2000). Quantitative expression analysis of the small conductance calcium-activated potassium channels, SK1, SK2 and SK3, in human brain. Mol. Brain Res. 85, 218–220. doi: 10.1016/S0169-328X(00)00255-2
Rinker, J. A., Fulmer, D. B., Trantham-Davidson, H., Smith, M. L., Williams, R. W., Lopez, M. F., et al. (2017). Differential potassium channel gene regulation in BXD mice reveals novel targets for pharmacogenetic therapies to reduce heavy alcohol drinking. Alcohol 58, 33–45. doi: 10.1016/j.alcohol.2016.05.007
Ritsner, M., Amir, S., Koronyo-Hamaoui, M., Gak, E., Ziv, H., Halperin, T., et al. (2003). Association study of CAG repeats in the KCNN3 gene in Israeli patients with major psychosis. Psychiatr. Genet. 13, 143–150. doi: 10.1097/00041444-200309000-00002
Rivera-Arconada, I., and Lopez-Garcia, J. A. (2005). Effects of M-current modulators on the excitability of immature rat spinal sensory and motor neurones. Eur. J. Neurosci. 22, 3091–3098. doi: 10.1111/j.1460-9568.2005.04507.x
Robbins, J. (2001). KCNQ potassium channels: physiology, pathophysiology, and pharmacology. Pharmacol. Ther. 90, 1–19. doi: 10.1016/S0163-7258(01)00116-4
Roche, J. P., Westenbroek, R., Sorom, A. J., Hille, B., Mackie, K., and Shapiro, M. S. (2002). Antibodies and a cysteine-modifying reagent show correspondence of M current in neurons to KCNQ2 and KCNQ3 K + channels. Br. J. Pharmacol. 137, 1173–1186. doi: 10.1038/sj.bjp.0704989
Rose, K., Ooi, L., Dalle, C., Robertson, B., Wood, I. C., and Gamper, N. (2011). Transcriptional repression of the M channel subunit Kv7.2 in chronic nerve injury. Pain 152, 742–754. doi: 10.1016/j.pain.2010.12.028
Roura-Ferrer, M., Etxebarria, A., Solé, L., Oliveras, A., Comes, N., Villarroel, A., et al. (2009). Functional implications of KCNE subunit expression for the Kv7.5 (KCNQ5) channel. Cell. Physiol. Biochem. 24, 325–334. doi: 10.1159/000257425
Saganich, M. J., Machado, E., and Rudy, B. (2001). Differential expression of genes encoding subthreshold-operating voltage-gated K+ channels in brain. J. Neurosci. 21, 4609–4624. doi: 10.1523/JNEUROSCI.21-13-04609.2001
Sah, P. (1996). Ca∗’-activated K+ currents in neurones: types, physiological roles and modulation. Trends Neurosci. 19, 150–154. doi: 10.1016/s0166-2236(96)80026-9
Sailer, C. A., Hu, H., Kaufmann, W. A., Trieb, M., Schwarzer, C., Storm, J. F., et al. (2002). Regional differences in distribution and functional expression of small-conductance Ca2+-activated K+ channels in rat brain. J. Neurosci. 22, 9698–9707. doi: 10.1523/JNEUROSCI.22-22-09698.2002
Saito, Y., Inoue, T., Zhu, G., Kimura, N., Okada, M., Nishimura, M., et al. (2012). Hyperpolarization-activated cyclic nucleotide gated channels: a potential molecular link between epileptic seizures and Aβ generation in Alzheimer’s disease. Mol. Neurodegener. 7:50. doi: 10.1186/1750-1326-7-50
Salthun-Lassalle, B., Hirsch, E. C., Wolfart, J., Ruberg, M., and Michel, P. P. (2004). Rescue of mesencephalic dopaminergic neurons in culture by low-level stimulation of voltage-gated sodium channels. J. Neurosci. 24, 5922–5930. doi: 10.1523/JNEUROSCI.5668-03.2004
Salzer, I., Erdem, F. A., Chen, W. Q., Heo, S., Koenig, X., Schicker, K. W., et al. (2017). Phosphorylation regulates the sensitivity of voltage-gated Kv7.2 channels towards phosphatidylinositol-4,5-bisphosphate. J. Physiol. 595, 759–776. doi: 10.1113/JP273274
Sander, S. E., Lambrecht, C., and Richter, A. (2013). The KV7.2/3 preferring channel opener ICA 27243 attenuates L-DOPA-induced dyskinesia in hemiparkinsonian rats. Neurosci. Lett. 545, 59–63. doi: 10.1016/j.neulet.2013.04.017
Sander, S. E., Lemm, C., Lange, N., Hamann, M., and Richter, A. (2012). Retigabine, a K V7 (KCNQ) potassium channel opener, attenuates l-DOPA-induced dyskinesias in 6-OHDA-lesioned rats. Neuropharmacology 62, 1052–1061. doi: 10.1016/j.neuropharm.2011.10.016
Santoro, B., Chen, S., Lüthi, A., Pavlidis, P., Shumyatsky, G. P., Tibbs, G. R., et al. (2000). Molecular and functional heterogeneity of hyperpolarization-activated pacemaker channels in the mouse CNS. J. Neurosci. 20, 5264–5275. doi: 10.1523/jneurosci.20-14-05264.2000
Santoro, B., Lee, J. Y., Englot, D. J., Gildersleeve, S., Piskorowski, R. A., Siegelbaum, S. A., et al. (2010). Increased seizure severity and seizure-related death in mice lacking HCN1 channels. Epilepsia 51, 1624–1627. doi: 10.1111/j.1528-1167.2010.02554.x
Santoro, B., Liu, D. T., Yao, H., Bartsch, D., Kandel, E. R., Siegelbaum, S. A., et al. (1998). Identification of a gene encoding a hyperpolarization-activated pacemaker channel of brain. Cell 93, 717–729. doi: 10.1016/S0092-8674(00)81434-8
Schapira, A. H. V., and Gegg, M. (2011). Mitochondrial contribution to Parkinson’s disease pathogenesis. Parkinson’s Dis. 2011:159160. doi: 10.4061/2011/159160
Scholl, E. S., Pirone, A., Cox, D. H., Duncan, R. K., and Jacob, M. H. (2014). Alternative splice isoforms of small conductance calcium-activated SK2 channels differ in molecular interactions and surface levels. Channels 8, 62–75. doi: 10.4161/chan.27470
Schroeder, B. C., Kubisch, C., Stein, V., and Jentsch, T. J. (1998). Moderate loss of function of cyclic-AMP-modulated KCNQ2/KCNQ3 K+ channels causes epilepsy. Nature 396, 687–690. doi: 10.1038/25367
Schulz, R., Kirschstein, T., Brehme, H., Porath, K., Mikkat, U., and Köhling, R. (2012). Network excitability in a model of chronic temporal lobe epilepsy critically depends on SK channel-mediated AHP currents. Neurobiol. Dis. 45, 337–347. doi: 10.1016/j.nbd.2011.08.019
Schumacher, M. A., Rivard, A. F., Bächinger, H. P., and Adelman, J. P. (2001). Structure of the gating domain of a Ca2+-activated K+ channel complexed with Ca2+/calmodulin. Nature 410, 1120–1124. doi: 10.1038/35074145
Schwab, B. C., Heida, T., Zhao, Y., Marani, E., van Gils, S. A., and van Wezel, R. J. A. (2013). Synchrony in Parkinson’s disease: importance of intrinsic properties of the external globus pallidus. Front. Syst. Neurosci. 7:60. doi: 10.3389/fnsys.2013.00060
Schwake, M., Jentsch, T. J., and Friedrich, T. (2003). A carboxy-terminal domain determines the subunit specificity of KCNQ K+ channel assembly. EMBO Rep. 4, 76–81. doi: 10.1038/sj.embor.embor715
Schwake, M., Pusch, M., Kharkovets, T., and Jentsch, T. J. (2000). Surface expression and single channel properties of KCNQ2/KCNQ3, M-type K+ channels involved in epilepsy. J. Biol. Chem. 275, 13343–13348. doi: 10.1074/jbc.275.18.13343
Schwarz, J. R., Glassmeier, G., Cooper, E. C., Kao, T. C., Nodera, H., Tabuena, D., et al. (2006). KCNQ channels mediate IKs, a slow K+ current regulating excitability in the rat node of Ranvier. J. Physiol. 573(Pt 1), 17–34. doi: 10.1113/jphysiol.2006.106815
Schwindt, P. C., Spain, W. J., Foehring, R. C., Stafstrom, C. E., Chubb, M. C., and Crill, W. E. (1988). Multiple potassium conductances and their functions in neurons from cat sensorimotor cortex in vitro. J. Neurophysiol. 59, 424–449. doi: 10.1152/jn.1988.59.2.424
Shah, M. M., Anderson, A. E., Leung, V., Lin, X., and Johnston, D. (2004). Seizure-induced plasticity of h channels in entorhinal cortical layer III pyramidal neurons. Neuron 44, 495–508. doi: 10.1016/j.neuron.2004.10.011
Shakkottai, V. G., Chou, C., Oddo, S., Sailer, C. A., Knaus, H.-G., Gutman, G. A., et al. (2004). Enhanced neuronal excitability in the absence of neurodegeneration induces cerebellar ataxia. J. Clin. Investig. 113, 582–590. doi: 10.1172/jci20216
Shang, Q., Xu, H., and Huang, L. (2012). Tanshinone IIA: a promising natural cardioprotective agent. Evid. Based Complement. Alternat. Med. 2012:716459. doi: 10.1155/2012/716459
Shin, M., Brager, D., Jaramillo, T. C., Johnston, D., and Chetkovich, D. M. (2008). Mislocalization of h channel subunits underlies h channelopathy in temporal lobe epilepsy. Neurobiol. Dis. 32, 26–36. doi: 10.1016/j.nbd.2008.06.013
Shmukler, B. E., Bond, C. T., Wilhelm, S., Bruening-Wright, A., Maylie, J., Adelman, J. P., et al. (2001). Structure and complex transcription pattern of the mouse SK1 KCa channel gene, KCNN1. Biochim. Biophys. Acta Gene Struct. Expr. 1518, 36–46. doi: 10.1016/S0167-4781(01)00166-X
Smith, J. S., Iannotti, C. A., Dargis, P., Christian, E. P., and Aiyar, J. (2001). Differential expression of KCNQ2 splice variants: implications to M current function during neuronal development. J. Neurosci. 21, 1096–1103. doi: 10.1523/jneurosci.21-04-01096.2001
Soldovieri, M. V., Ambrosino, P., Mosca, I., De Maria, M., Moretto, E., Miceli, F., et al. (2016). Early-onset epileptic encephalopathy caused by a reduced sensitivity of Kv7.2 potassium channels to phosphatidylinositol 4,5-bisphosphate. Sci. Rep. 6:38167. doi: 10.1038/srep38167
Sørensen, U. S., Strøbaek, D., Christophersen, P., Hougaard, C., Jensen, M. L., and Nielsen, E. Ø, et al. (2008). Synthesis and structure-activity relationship studies of 2-(N-substituted)-aminobenzimidazoles as potent negative gating modulators ofsmall conductance Ca2+-activated K+ channels. J. Med. Chem. 51, 7625–7634. doi: 10.1021/jm800809f
Sourdet, V., Russier, M., Daoudal, G., Ankri, N., and Debanne, D. (2003). Long-term enhancement of neuronal excitability and temporal fidelity mediated by metabotropic glutamate receptor subtype 5. J. Neurosci. 23, 10238–10248. doi: 10.1523/jneurosci.23-32-10238.2003
Stieber, J., Stöckl, G., Herrmann, S., Hassfurth, B., and Hofmann, F. (2005). Functional expression of the human HCN3 channel. J. Biol. Chem. 280, 34635–34643. doi: 10.1074/jbc.M502508200
Stocker, M., and Pedarzani, P. (2000). Differential distribution of three Ca2+-activated K2+ channel subunits, SK1, SK2, and SK3, in the adult rat central nervous system. Mol. Cell. Neurosci. 15, 476–493. doi: 10.1006/mcne.2000.0842
Storm, J. F. (1990). Potassium currents in hippocampal pyramidal cells. Prog Brain Res. 83, 161–187. doi: 10.1016/s0079-6123(08)61248-0
Strassmaier, T., Bond, C. T., Sailer, C. A., Knaus, H. G., Maylie, J., and Adelman, J. P. (2005). A novel isoform of SK2 assembles with other SK subunits in mouse brain. J. Biol. Chem. 280, 21231–21236. doi: 10.1074/jbc.M413125200
Stutzmann, G. E. (2005). Calcium dysregulation, IP3 signaling, and Alzheimer’s disease. Neuroscientist 11, 110–115. doi: 10.1177/1073858404270899
Surges, R., Freiman, T. M., and Feuerstein, T. J. (2003). Gabapentin increases the hyperpolarization-activated cation current Ih in rat CA1 pyramidal cells. Epilepsia 44, 150–156. doi: 10.1046/j.1528-1157.2003.36802.x
Tacconi, S., Carletti, R., Bunnemann, B., Plumpton, C., Merlo Pich, E., and Terstappen, G. C. (2001). Distribution of the messenger RNA for the small conductance calcium-activated potassium channel SK3 in the adult rat brain and correlation with immunoreactivity. Neuroscience 102, 209–215. doi: 10.1016/S0306-4522(00)00486-3
Tae, H. S., Smith, K. M., Phillips, A. M., Boyle, K. A., Li, M., Forster, I. C., et al. (2017). Gabapentin modulates HCN4 channel voltage-dependence. Front. Pharmacol. 8:554. doi: 10.3389/fphar.2017.00554
Tanabe, M., Mori, M., Gähwiler, B. H., and Gerber, U. (1999). Apamin-sensitive conductance mediates the K+ current response during chemical ischemia in CA3 pyramidal cells. J. Neurophysiol. 82, 2876–2882. doi: 10.1152/jn.1999.82.6.2876
Tanguay, J., Callahan, K. M., and D’Avanzo, N. (2019). Characterization of drug binding within the HCN1 channel pore. Sci. Rep. 9:465. doi: 10.1038/s41598-018-37116-2
Tatulian, L., and Brown, D. A. (2003). Effect of the KCNQ potassium channel opener retigabine on single KCNQ2/3 channels expressed in CHO cells. J. Physiol. 549, 57–63. doi: 10.1113/jphysiol.2003.039842
Tatulian, L., Delmas, P., Abogadie, F. C., and Brown, D. A. (2001). Activation of expressed KCNQ potassium currents and native neuronal M-type potassium currents by the anti-convulsant drug retigabine. J. Neurosci. 21, 5535–5545. doi: 10.1523/jneurosci.21-15-05535.2001
Teng, B. C., Song, Y., Zhang, F., Ma, T. Y., Qi, J. L., Zhang, H. L., et al. (2016). Activation of neuronal Kv7/KCNQ/M-channels by the opener QO58-lysine and its anti-nociceptive effects on inflammatory pain in rodents. Acta Pharmacol. Sin. 37, 1054–1062. doi: 10.1038/aps.2016.33
Terstappen, G. C., Pellacani, A., Aldegheri, L., Graziani, F., Carignani, C., Pula, G., et al. (2003). The antidepressant fluoxetine blocks the human small conductance calcium-activated potassium channels SK1, SK2 and SK3. Neurosci. Lett. 346, 85–88. doi: 10.1016/S0304-3940(03)00574-3
Terstappen, G. C., Pula, G., Carignani, C., Chen, M. X., and Roncarati, R. (2001). Pharmacological characterisation of the human small conductance calcium-activated potassium channel hSK3 reveals sensitivity to tricyclic antidepressants and antipsychotic phenothiazines. Neuropharmacology 40, 772–783. doi: 10.1016/S0028-3908(01)00007-7
Thompson, J. M., Yakhnitsa, V., Ji, G., and Neugebauer, V. (2018). Small conductance calcium activated potassium (SK) channel dependent and independent effects of riluzole on neuropathic pain-related amygdala activity and behaviors in rats. Neuropharmacology 138, 219–231. doi: 10.1016/j.neuropharm.2018.06.015
Tibbs, G. R., Rowley, T. J., Sanford, R. L., Herold, K. F., Proekt, A., Hemmings, H. C., et al. (2013). HCN1 channels as targets for anesthetic and nonanesthetic propofol analogs in the amelioration of mechanical and thermal hyperalgesia in a mouse model of neuropathic pains. J. Pharmacol. Exp. Ther. 345, 363–373. doi: 10.1124/jpet.113.203620
Tinel, N., Lauritzen, I., Chouabe, C., Lazdunski, M., Borsotto, M., and Antipolis, S. (1998). The KCnq2 potassium channel splice varinats functional and develpmental expression. FEBS Lett. 438, 171–176. doi: 10.1016/s0014-5793(98)01296-4
Tobelaim, W. S., Dvir, M., Lebel, G., Cui, M., Buki, T., Peretz, A., et al. (2017). Ca2+-Calmodulin and PIP2 interactions at the proximal C-terminus of Kv7 channels. Channels 11, 686–695. doi: 10.1080/19336950.2017.1388478
Tober, C., Rostock, A., Rundfeldt, C., and Bartsch, R. (1996). D-23129: a potent anticonvulsant in the amygdala kindling model of complex partial seizures. Eur. J. Pharmacol. 303, 163–169. doi: 10.1016/0014-2999(96)00073-8
Tomita, H., Shakkottai, V. G., Gutman, G. A., Sun, G., Bunney, W. E., Cahalan, M. D., et al. (2003). Novel truncated isoform of SK3 potassium channel is a potent dominant-negative regulator of SK currents: implications in schizophrenia. Mol. Psychiatry 8, 524–535. doi: 10.1038/sj.mp.4001271
Valenti, O., Gill, K. M., and Grace, A. A. (2012). Different stressors produce excitation or inhibition of mesolimbic dopamine neuron activity: response alteration by stress pre-exposure. Eur. J. Neurosci. 35, 1312–1321. doi: 10.1111/j.1460-9568.2012.08038.x
Van Der Staay, F. J., Fanelli, R. J., Blokland, A., and Schmidt, B. H. (1999). Behavioral effects of apamin, a selective inhibitor of the SK Ca -channel, in mice and rats. Neurosci. Biobehav. Rev. 23, 1087–1110. doi: 10.1016/s0149-7634(99)00043-3
Vasilyev, D. V., Shan, Q., Lee, Y., Mayer, S. C., Bowlby, M. R., Strassle, B. W., et al. (2007). Direct inhibition of Ih by analgesic loperamide in rat DRG neurons. J. Neurophysiol. 97, 3713–3721. doi: 10.1152/jn.00841.2006
Vassar, R., Kuhn, P. H., Haass, C., Kennedy, M. E., Rajendran, L., Wong, P. C., et al. (2014). Function, therapeutic potential and cell biology of BACE proteases: current status and future prospects. J. Neurochem. 130, 4–28. doi: 10.1111/jnc.12715
Vervaeke, K., Hu, H., Graham, L. J., and Storm, J. F. (2006). Contrasting effects of the persistent Na+ current on neuronal excitability and spike timing. Neuron 49, 257–270. doi: 10.1016/j.neuron.2005.12.022
Villalobos, C., Shakkottai, V. G., Chandy, K. G., Michelhaugh, S. K., and Andrade, R. (2004). SKCa channels mediate the medium but not the slow calcium-activated afterhyperpolarization in cortical neurons. J. Neurosci. 24, 3537–3542. doi: 10.1523/JNEUROSCI.0380-04.2004
Villière, V., and McLachlan, E. M. (1996). Electrophysiological properties of neurons in intact rat dorsal root ganglia classified by conduction velocity and action potential duration. J. Neurophysiol. 76, 1924–1941. doi: 10.1152/jn.1996.76.3.1924
Vossel, K. A., Beagle, A. J., Rabinovici, G. D., Shu, H., Lee, S. E., Naasan, G., et al. (2013). Seizures and epileptiform activity in the early stages of Alzheimer disease. JAMA Neurol. 70, 1158–1166. doi: 10.1001/jamaneurol.2013.136
Wainger, B. J., Degennaro, M., Santoro, B., Siegelbaum, S. A., and Tibbs, G. R. (2001). Molecular mechanism of cAMP modulation of HCN pacemaker channels. Nature 411, 805–810. doi: 10.1038/35081088
Walter, J. T., Alviña, K., Womack, M. D., Chevez, C., and Khodakhah, K. (2006). Decreases in the precision of Purkinje cell pacemaking cause cerebellar dysfunction and ataxia. Nat. Neurosci. 9, 389–397. doi: 10.1038/nn1648
Wang, H. S., Pan, Z., Shi, W., Brown, B. S., Wymore, R. S., Cohen, I. S., et al. (1998). KCNQ2 and KCNQ3 potassium channel subunits: molecular correlates of the M-channel. Science 282, 1890–1893. doi: 10.1126/science.282.5395.1890
Wang, J., Chen, S., Nolan, M. F., and Siegelbaum, S. A. (2002). Activity-dependent regulation of HCN pacemaker channels by cyclic AMP: signaling through dynamic allosteric coupling. Neuron 36, 451–461. doi: 10.1016/S0896-6273(02)00968-6
Wang, J., Li, Y., Hui, Z., Cao, M., Shi, R., Zhang, W., et al. (2015). Functional analysis of potassium channels in Kv7.2 G271V mutant causing early onset familial epilepsy. Brain Res. 1616, 112–122. doi: 10.1016/j.brainres.2015.04.060
Wang, Y., Qu, L., Wang, X. L., Gao, L., Li, Z. Z., Gao, G. D., et al. (2014). Firing pattern modulation through SK channel current increase underlies neuronal survival in an organotypic slice model of Parkinson’s disease. Mol. Neurobiol. 51, 424–436. doi: 10.1007/s12035-014-8728-3
Wang, Y., Yang, P. L., Tang, J. F., Lin, J. F., Cai, X. H., Wang, X. T., et al. (2008). Potassium channels: possible new therapeutic targets in Parkinson’s disease. Med. Hypotheses 71, 546–550. doi: 10.1016/j.mehy.2008.05.021
Wei, A. D., Gutman, G. A., Aldrich, R., Chandy, K. G., Grissmer, S., and Wulff, H. (2005). Nomenclature and molecular relationships of calcium-activated potassium channels. Pharmacol. Rev. 57, 463–472. doi: 10.1124/pr.57.4.9.1
Weisenberg, J. L., and Wong, M. (2011). Profile of ezogabine (retigabine) and its potential as an adjunctive treatment for patients with partial-onset seizures. Neuropsychiatr. Dis. Treat. 7, 409–414. doi: 10.2147/NDT.S14208
Wickenden, A. D., Universitario, I., Antonio, D. B. I., Astrofisica, A., and Sánchez, F. (2009). Kv7 channels as targets for the treatment of pain. Curr. Pharm. Des. 15, 1773–1798. doi: 10.2174/138161209788186326
Wiener, R., Haitin, Y., Shamgar, L., Ferna, M. C., Martos, A., Chomsky-hecht, O., et al. (2008). The KCNQ1 (Kv7. 1) COOH terminus, a multitiered scaffold for subunit assembly and protein interaction. J. Biol. Chem. 283, 5815–5830. doi: 10.1074/jbc.M707541200
Williams, A. D., Jung, S., and Poolos, N. P. (2015). Protein kinase C bidirectionally modulates Ih and hyperpolarization-activated cyclic nucleotide-gated (HCN) channel surface expression in hippocampal pyramidal neurons. J. Physiol. 593, 2779–2792. doi: 10.1113/JP270453
Williams, S., Serafin, M., Mühlethaler, M., and Bernheim, L. (1997). Distinct contributions of high- and low-voltage-activated calcium currents to afterhyperpolarizations in cholinergic nucleus basalis neurons of the guinea pig. J. Neurosci. 17, 7307–7315. doi: 10.1523/jneurosci.17-19-07307.1997
Wilson, G. W., and Garthwaite, J. (2010). Hyperpolarization-activated ion channels as targets for nitric oxide signalling in deep cerebellar nuclei. Eur. J. Neurosci. 31, 1935–1945. doi: 10.1111/j.1460-9568.2010.07226.x
Wittekindt, O., Jauch, A., Burgert, E., Schärer, L., Holtgreve-Grez, H., Yvert, G., et al. (1998). The human small conductance calcium-regulated potassium channel gene (hSKCa3) contains two CAG repeats in exon 1, is on chromosome 1q21.3, and shows a possible association with schizophrenia. Neurogenetics 1, 259–265. doi: 10.1007/s100480050038
Wladyka, C. L., and Kunze, D. L. (2006). KCNQ / M-currents contribute to the resting membrane potential in rat visceral sensory neurons. J. Physiol. 1, 175–189. doi: 10.1113/jphysiol.2006.113308
Won, J., Lee, P. R., and Oh, S. B. (2019). Alpha 2 adrenoceptor agonist guanabenz directly inhibits hyperpolarization-activated, cyclic nucleotide-modulated (HCN) channels in mesencephalic trigeminal nucleus neurons. Eur. J. Pharmacol. 854, 320–327. doi: 10.1016/j.ejphar.2019.04.036
Wrobel, E., Tapken, D., and Seebohm, G. (2012). The KCNE tango – how KCNE1 interacts with Kv7. 1. Front. Pharmacol. 3:142. doi: 10.3389/fphar.2012.00142
Wu, Z., Li, L., Xie, F., Du, J., Zuo, Y., Frost, J. A., et al. (2017). Activation of KCNQ channels suppresses spontaneous activity in dorsal root ganglion neurons and reduces chronic pain after spinal cord injury. J. Neurotrauma. 34, 1260–1270. doi: 10.1089/neu.2016.4789
Xia, X. M., Fakler, B., Rivard, A., Wayman, G., Johnson-Pais, T., Keen, J. E., et al. (1998). Mechanism of calcium gating in small-conductance calcium-activated potassium channels. Nature 395, 503–507. doi: 10.1038/26758
Xing, J. L., and Hu, S. J. (1999). Relationship between calcium-dependent potassium channel and ectopic spontaneous discharges of injured dorsal root ganglion neurons in the rat. Brain Res. 838, 218–221. doi: 10.1016/S0006-8993(99)01682-0
Xiong, Q., Sun, H., Zhang, Y., Nan, F., and Li, M. (2008). Combinatorial augmentation of voltage-gated KCNQ potassium channels by chemical openers. Proc. Natl. Acad. Sci. U.S.A. 105, 3128–3133. doi: 10.1073/pnas.0712256105
Xu, C., Datta, S., Wu, M., and Alreja, M. (2004). Hippocampal theta rhythm is reduced by suppression of the H-current in septohippocampal GABAergic neurons. Eur. J. Neurosci. 19, 2299–2309. doi: 10.1111/j.1460-9568.2004.03316.x
Yagi, J., and Sumino, R. (1998). Inhibition of a hyperpolarization-activated current by clonidine in rat dorsal root ganglion neurons. J. Neurophysiol. 80, 1094–1104. doi: 10.1152/jn.1998.80.3.1094
Ying, S. W., Kanda, V. A., Hu, Z., Purtell, K., King, E. C., Abbott, G. W., et al. (2012). Targeted deletion of Kcne2 impairs HCN channel function in mouse thalamocortical circuits. PLoS One 7:e42756. doi: 10.1371/journal.pone.0042756
Ying, S., Tibbs, G. R., Picollo, A., Abbas, S. Y., Sanford, R. L., Accardi, A., et al. (2011). PIP 2 -mediated HCN3 channel gating is crucial for rhythmic burst firing in thalamic intergeniculate leaflet neurons. J. Neurosci. 31, 10412–10423. doi: 10.1523/JNEUROSCI.0021-11.2011
Yoo, A. S., Cheng, I., Chung, S., Grenfell, T. Z., Lee, H., Pack-Chung, E., et al. (2000). Presenilin-mediated modulation of capacitative calcium entry. Neuron 27, 561–572. doi: 10.1016/S0896-6273(00)00066-0
Young, G. T., Emery, E. C., Mooney, E. R., Tsantoulas, C., and Mcnaughton, P. A. (2014). Inflammatory and neuropathic pain are rapidly suppressed by peripheral block of hyperpolarisation-activated cyclic nucleotide-gated ion channels. Pain 155, 1708–1719. doi: 10.1016/j.pain.2014.05.021
Yu, H., Wu, J., Potapova, I., Wymore, R. T., Holmes, B., Zuckerman, J., et al. (2001). MinK- related peptide 1: a beta subunit for the HCN ion channel subunit family enhances expression and speeds activation. Circ. Res. 88, E84–E87. doi: 10.1161/hh1201.093511
Yu, T., Li, L., Liu, H., Li, H., Liu, Z., and Li, Z. (2018). KCNQ2/3/5 channels in dorsal root ganglion neurons can be therapeutic targets of neuropathic pain in diabetic rats. Mol. Pain. 14:1744806918793229. doi: 10.1177/1744806918793229
Zhang, D., Thimmapaya, R., Zhang, X., Anderson, D. J., Baranowski, J. L., Scanio, M., et al. (2011). KCNQ2 / 3 openers show differential selectivity and site of action across multiple KCNQ channels. J. Neurosci. Methods 200, 54–62. doi: 10.1016/j.jneumeth.2011.06.014
Zhang, F., Liu, Y., Tang, F., Liang, B., Chen, H., Zhang, H., et al. (2019). Electrophysiological and pharmacological characterization of a novel and potent neuronal Kv7 channel opener SCR2682 for antiepilepsy. FASEB J. 33, 9154–9166. doi: 10.1096/fj.201802848RR
Zhang, F., Mi, Y., Qi, J. L., Li, J. W., Si, M., Guan, B. C., et al. (2013). Modulation of K v 7 potassium channels by a novel opener pyrazolo [1,5-a] pyrimidin-7 (4H) -one compound QO-58. Br. J. Pharmacol. 168, 1030–1042. doi: 10.1111/j.1476-5381.2012.02232.x
Zhang, L., and McBain, C. J. (1995). Potassium conductances underlying repolarization and after-hyperpolarization in rat CA1 hippocampal interneurones. J. Physiol. 488, 661–672. doi: 10.1113/jphysiol.1995.sp020998
Zhang, M., Abrams, C., Wang, L., Gizzi, A., He, L., Lin, R., et al. (2012). Structural basis for calmodulin as a dynamic calcium sensor. Structure 20, 911–923. doi: 10.1016/j.str.2012.03.019
Zhang, M., Meng, X. Y., Cui, M., Pascal, J. M., Logothetis, D. E., and Zhang, J. F. (2014). Selective phosphorylation modulates the PIP 2 sensitivity of the CaM-SK channel complex. Nat. Chem. Biol. 10, 753–759. doi: 10.1038/nchembio.1592
Zhang, M., Pascal, J. M., and Zhang, J. F. (2013). Unstructured to structured transition of an intrinsically disordered protein peptide in coupling Ca2+-sensing and SK channel activation. Proc. Natl. Acad. Sci. U.S.A. 110, 4828–4833. doi: 10.1073/pnas.1220253110
Zhang, S., You, Z., Wang, S., Yang, J., Yang, L., Sun, Y., et al. (2016). Neuropharmacology neuropeptide S modulates the amygdaloidal HCN activities (I h) in rats: implication in chronic pain. Neuropharmacology 105, 420–433. doi: 10.1016/j.neuropharm.2016.02.004
Zhang, Y., Bonnan, A., Bony, G., Ferezou, I., Pietropaolo, S., Ginger, M., et al. (2014). Dendritic channelopathies contribute to neocortical and sensory hyperexcitability in Fmr1-/y mice. Nat. Neurosci. 17, 1701–1709. doi: 10.1038/nn.3864
Zhao, W., Chuang, S.-C., Bianchi, R., and Wong, R. K. S. (2011). Dual regulation of fragile X mental retardation protein by group I metabotropic glutamate receptors controls translation-dependent epileptogenesis in the hippocampus. J. Neurosci. 31, 725–734. doi: 10.1523/JNEUROSCI.2915-10.2011
Zhao, W., Liang, P., Liu, J., Li, H., and Liao, D. (2019). Capsazepine prolongation of the duration of lidocaine block of sensory transmission in mice may be mediated by modulation of HCN channel currents. PeerJ 7:e7111. doi: 10.7717/peerj.7111
Zobeiri, M., Chaudhary, R., Blaich, A., Rottmann, M., Herrmann, S., Meuth, P., et al. (2019). The hyperpolarization-activated HCN4 channel is important for proper maintenance of oscillatory activity in the thalamocortical system. Cereb. Cortex 29, 2291–2304. doi: 10.1093/cercor/bhz047
Zolles, G., Klöcker, N., Wenzel, D., Weisser-Thomas, J., Fleischmann, B. K., Roeper, J., et al. (2006). Pacemaking by HCN channels requires interaction with phosphoinositides. Neuron 52, 1027–1036. doi: 10.1016/j.neuron.2006.12.005
Keywords: ion channels, neuropsychaitric disorders, therapeutic targets, advances and challenges, SK, HCN and M channels
Citation: Dwivedi D and Bhalla US (2021) Physiology and Therapeutic Potential of SK, H, and M Medium AfterHyperPolarization Ion Channels. Front. Mol. Neurosci. 14:658435. doi: 10.3389/fnmol.2021.658435
Received: 25 January 2021; Accepted: 13 April 2021;
Published: 03 June 2021.
Edited by:
Jianmin Cui, Washington University in St. Louis, United StatesReviewed by:
Nazzareno D’Avanzo, Université de Montréal, CanadaAlessio Masi, University of Florence, Italy
Copyright © 2021 Dwivedi and Bhalla. This is an open-access article distributed under the terms of the Creative Commons Attribution License (CC BY). The use, distribution or reproduction in other forums is permitted, provided the original author(s) and the copyright owner(s) are credited and that the original publication in this journal is cited, in accordance with accepted academic practice. No use, distribution or reproduction is permitted which does not comply with these terms.
*Correspondence: Deepanjali Dwivedi, ZGVlcGFuamFsaV9kd2l2ZWRpQGhtcy5oYXJ2YXJkLmVkdQ==; ZGR3aXZlZGlAYnJvYWRpbnN0aXR1dGUub3Jn