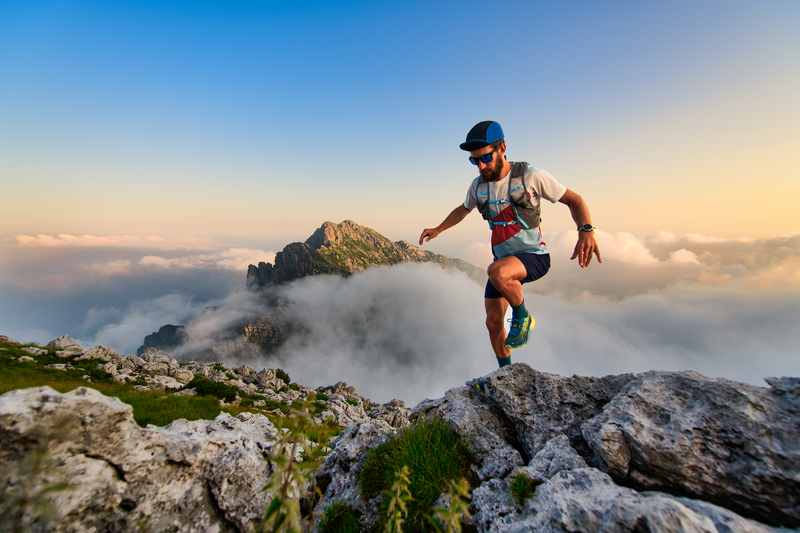
94% of researchers rate our articles as excellent or good
Learn more about the work of our research integrity team to safeguard the quality of each article we publish.
Find out more
REVIEW article
Front. Mol. Neurosci. , 13 November 2020
Sec. Brain Disease Mechanisms
Volume 13 - 2020 | https://doi.org/10.3389/fnmol.2020.577622
This article is part of the Research Topic Beta Amyloid: From Physiology to Pathogenesis View all 15 articles
Amyloid-β (Aβ) peptides can form protease-resistant aggregates within and outside of neurons. Accumulation of these aggregates is a hallmark of Alzheimer’s disease (AD) neuropathology and contributes to devastating cognitive deficits associated with this disorder. The primary etiological factor for Aβ aggregation is either an increase in Aβ production or a decrease in its clearance. Aβ is produced by the sequential activity of β- and γ-secretase on the amyloid precursor protein (APP) and the clearance is mediated by chaperone-mediated mechanisms. The Aβ aggregates vary from soluble monomers and oligomers to insoluble senile plaques. While excess intraneuronal oligomers can transduce neurotoxic signals into neurons causing cellular defects like oxidative stress and neuroepigenetic mediated transcriptional dysregulation, extracellular senile plaques cause neurodegeneration by impairing neural membrane permeabilization and cell signaling pathways. Paradoxically, senile plaque formation is hypothesized to be an adaptive mechanism to sequester excess toxic soluble oligomers while leaving native functional Aβ levels intact. This hypothesis is strengthened by the absence of positive outcomes and side effects from immunotherapy clinical trials aimed at complete Aβ clearance, and support beneficial physiological roles for native Aβ in cellular function. Aβ has been shown to modulate synaptic transmission, consolidate memory, and protect against excitotoxicity. We discuss the current understanding of beneficial and detrimental roles for Aβ in synaptic function and epigenetic gene control and the future promising prospects of early therapeutic interventions aimed at mediating Aβ induced neuroepigenetic and synaptic dysfunctions to delay AD onset.
Alzheimer’s disease (AD) affects 5.8 million Americans aged 65 and older and is estimated to grow to 13.8 million by mid-century. AD is the most common cause of dementia, presenting with hallmarks such as amyloid-β (Aβ) plaques, tau neurofibrillary tangles, neuronal cell death, cognitive dysfunction, and altered brain morphology. Aβ-plaques comprise of aggregated Aβ, a cleaved product of the glycoprotein amyloid precursor protein (APP). According to the amyloid cascade hypothesis, it is these plaques that are responsible for AD pathology.
Newly generated Aβ released into the extracellular space remain in soluble form or aggregate into insoluble Aβ-plaques. Soluble Aβ species can bind to various neuronal cell receptors and transduce neurotoxic signals causing cellular defects that include oxidative stress and epigenetic-mediated transcriptional dysregulation (Chen et al., 2017). However, recent evidence demonstrates that soluble Aβ shows beneficial physiological roles such as regulating cellular signaling pathways and synaptic function as well as possessing antimicrobial and antioxidant properties (Brothers et al., 2018). In this review, we summarize recent progress in understanding the beneficial and detrimental aspects of Aβ in mediating processes underlying synaptic and cognitive function and epigenetic neuronal gene control. We further discuss therapeutic interventions aimed at synaptic plasticity and epigenetic regulation to delay AD progression.
Synaptic plasticity mediated changes in neuronal connections have long been established as the primary mechanism of learning and memory (Martin et al., 2000; Ramirez and Arbuckle, 2016). Accordingly, loss of synaptic connections is an early event in AD pathogenesis and cognitive impairment (Selkoe, 2002; Scheff et al., 2006; Kashyap et al., 2019). Although the precise mechanisms underlying synaptic dysfunction in AD are obscure, emerging studies have uncovered a feedback regulation between Aβ and synaptic plasticity.
Multiple studies demonstrate that soluble Aβ oligomers in pre- and post-synaptic compartments can disrupt synaptic morphology and inhibit long-term potentiation (LTP) that trigger cognitive dysfunction. Intriguingly, insoluble Aβ-plaques are less active in promoting such alterations (Lambert et al., 1998; Takahashi et al., 2002; Walsh et al., 2002; Shankar et al., 2008; Figure 1). For example, studies in amyloid mice reveal that reduction of synaptophysin puncta correlates with soluble Aβ and not plaque load (Mucke et al., 2000). Further, AD-associated apolipoprotein E4 has been implicated in facilitating the transport of soluble Aβ species to synapses elucidating toxic effects (Koffie et al., 2012). Aberrant activation of neuronal signal transduction pathways can arise via Aβ directly binding to Aβ receptors or competing with essential ligands to bind their receptors (Xia et al., 2016). For example, soluble Aβ dimers cause glutamate excitotoxicity via blockage of glutamate reuptake in the synaptic cleft, activating glutamate receptors and ion channels like N-Methyl-D-aspartate (NMDA) receptors that trigger downstream cell signaling transduction cascades to pathologically alter gene expression profiles (Li et al., 2009). Additionally, accumulation of extracellular Aβ42 triggers the loss of synaptic mushroom spines via hyperactivation of metabotropic glutamate receptor type 5 (mGluR5) receptors, resulting in elevated endoplasmic reticulum Ca2+ levels and downregulation of the Ca2+/calmodulin kinase II signaling pathway (Zhang et al., 2015). Interestingly, soluble APP has also been shown to directly modulate synaptic plasticity by binding to the gamma-aminobutyric acid (GABA) receptor and inducing a conformational change that facilitates reduced neurotransmitter release and neuronal activity (Rice et al., 2019; Figure 1). Together, these findings support the concept that soluble APP and Aβ oligomers promote synaptic impairment and cognitive deficits during the early stages of AD, followed by neurodegeneration in the later stages (Ferreira and Klein, 2011).
Figure 1. The interplay of amyloid precursor protein (APP) and amyloid-β (Aβ) impact on synaptic activity and neuroepigenetic gene control. Synaptic activity module. APP endocytosis and cleavage yield Aβ peptides and APP intracellular domain (AICD). Soluble APP regulates GABA receptor function to modulate synaptic transmission and plasticity. At physiological levels, Aβ peptides promote glutamate recycling keeping glutamate levels in check. In contrast, at pathological Aβ levels, impaired glutamate recycling increases post-synaptic uptake, leading to excitotoxicity, synaptic depression, and eventually neural cell death. Epigenetic module. Soluble Aβ monomer and dimer competition for binding of essential ligands to receptors can alter signal transduction pathways causing global changes in neuroepigenetic gene control. Such alterations include DNA methylation changes that alter the expression of genes involved in APP processing and Aβ degradation pathways to favor increased Aβ levels and disruption of Tip60/HDAC balance causing hypoacetylation of chromatin with concomitant repression of associated critical synaptic plasticity genes.
Conversely, synaptic activity positively modulates Aβ production to promote synaptic function (Kamenetz et al., 2003; Cirrito et al., 2005). Increased synaptic activity promotes APP endocytosis, and β-secretase 1 (BACE1) mediated Aβ production (Cirrito et al., 2008). Since Aβ depresses synaptic activity, the activity-dependent modulation of endogenous Aβ production has been suggested to be a finely tuned negative feedback loop that regulates the neuronal activity and appropriate function by preventing hyperactivation (Kamenetz et al., 2003). Perturbation in this homeostatic mechanism may interfere with synaptic activity and contribute to cognitive decline, as seen in AD. These studies support the premise that physiological levels of Aβ are critical for optimal synaptic activity (Kamenetz et al., 2003; Parihar and Brewer, 2010; Jang and Chung, 2016).
Epigenetic modifications of DNA and histone proteins regulate gene expression profiles via controlling chromatin accessibility. The neuroepigenome has been proven to be critical in memory formation and consolidation through dynamic control of neural genes essential for these functions (Feng et al., 2007; Sultan and Day, 2011). Neuroepigenetic imbalance in the brain causes transcriptional dysregulation, a pivotal step in AD etiology (Esposito and Sherr, 2019). Here, we summarize primary epigenetic alterations that affect or are affected by Aβ production.
DNA methylation: DNA methylation occurs at cytosine bases in CpG repeats and primarily controls gene repression (Saxonov et al., 2006; Miranda and Jones, 2007). Reports on DNA methylation and AD are conflicting with several studies reporting global DNA hypermethylation in the AD brain (Rao et al., 2012; Di Francesco et al., 2015; Liu et al., 2019), while other studies show reduction (Chen et al., 2009; Chouliaras et al., 2013; Li et al., 2019) or no alterations in global DNA methylation (Lashley et al., 2015). Common AD-associated methylation alterations often increase Aβ production. For example, AD-associated genes APP, Apolipoprotein E, and BACE1 are hypomethylated in AD brains with concomitant BACE1 activation increasing Aβ levels via the amyloidogenic processing pathway (West et al., 1995; Tulloch et al., 2018; Li et al., 2019). Conversely, the neprilysin gene that encodes for an Aβ degrading enzyme is hypermethylated and repressed in AD, also leading to increased Aβ levels (Chen et al., 2009).
Histone acetylation: Histone modifications, including acetylation, methylation, and phosphorylation on histone protein tails, modulate chromatin accessibility to control gene expression. Of these modifications, histone acetylation is best characterized for its role in learning and memory and contribution to AD when altered (Saha and Pahan, 2006; Sharma, 2010; Peixoto and Abel, 2013). Histone acetylation homeostasis is regulated by the antagonistic activity of histone acetyltransferases (HATs) and histone deacetylases (HDACs). Evidence from our group and others shows that neural histone acetylation dysregulation, caused by an imbalance between specific HATs and HDACs, is a crucial early step in AD pathology. Downregulation of the HAT Tip60 (KAT5) and upregulation of HDAC2 causes epigenetic repression of critical neuroplasticity genes in multiple types of AD animal models and patients (Graff et al., 2012; Panikker et al., 2018). Further, alteration of Tip60 epigenetic mediated control in the brain by either APP or Aβ driven Alzheimer’s disease pathology leads to repression of a set of neuronal genes critical for synaptic function (Panikker et al., 2018). Restoring such alterations in Tip60/HDAC2 balance protects against AD-associated pathologies in the AD Drosophila model expressing APP.
How might Aβ influence early HAT/HDAC disruption? Our findings reveal that APP expression results in a reduction of Tip60 protein levels but not Tip60 mRNA levels (Panikker et al., 2018), suggesting a mechanism of post-transcriptional regulation. Thus far, there is no evidence to demonstrate a direct Aβ and Tip60 interaction underlying reduced Tip60 levels, but a potential mechanism is via ubiquitin-mediated Aβ-induced Tip60 degradation. Another consideration is soluble Aβ monomer and dimer competition for the binding of essential ligands to receptors (Xia et al., 2016). Such interactions possibly alter signal transduction pathways that disrupt Tip60/HDAC balance and acetylation levels, inducing altered gene expression profiles contributing to AD.
Currently, the Food and Drug Administration (FDA) approved drugs for AD are limited to palliative medications: cholinesterase inhibitors and a non-competitive NMDA antagonist (National Institute on Aging, 2018, April 01). As Aβ-plaques are considered as primary effector molecules in AD pathogenesis, therapeutic strategies are focused on developing agents that can block Aβ production or clear Aβ-plaques. The clinical trials are ongoing, but the initial results thus far are not encouraging. The β- and γ-secretase inhibitors, aimed to block Aβ production, were discontinued due to unfavorable risk/benefit profile and cognitive worsening. Also, Aβ immunotherapies, intended to clear the Aβ-plaques, were terminated due to toxicity and cognitive worsening. Efforts are in progress to refine the approaches to these trials (reviewed in Panza et al., 2019). Further, it is hypothesized that the complete reduction of Aβ as a principal reason for these failures, underscoring the necessity to understand the physiological roles of Aβ.
The U-shaped natural course of cerebrospinal fluid Aβ levels in aging suggests it as physiologically active (Shoji and Kanai, 2001). One of the main reasons for clinical trial failures is the toxicity resulting from reducing Aβ, supporting a critical role for Aβ in neuronal survival and function. In support of this concept, synthetic Aβ42 monomers (30–100 nM) have been shown to promote survival in developing neurons deprived with trophic factors (Giuffrida et al., 2009). Further, in different neuronal cell types, exogenous Aβ40 had a neuroprotective effect on cells dying from Aβ immunodepletion, while the same levels of exogenous Aβ42 oligomers proved to be toxic (Plant et al., 2003). These studies demonstrate a hormetic effect of Aβ in neuroprotection and the neurotoxicity of soluble oligomeric forms over insoluble aggregates. Considering the physiological importance of monomeric Aβ, monoclonal antibody Aducanumab was developed with a much greater affinity to Aβ-aggregates versus monomeric forms. Currently, Phase 3 trials have been discontinued based on futility analysis but not on safety concerns (U.S. National Library of Medicine, 2020a,b). In the future, the predicted aggregate-specific N-terminal binding motif of Aducanumab could potentially serve as a basis to re-engineer Aducanumab for further enhanced preference to bind Aβ -aggregates versus monomers (Frost and Zacharias, 2020).
Another disappointing outcome from clinical trials focused on Aβ depletion is the failure to alleviate cognitive decline. Studies show that Aβ affects memory by regulating synaptic vesicle dynamics and synaptic plasticity with physiological levels increasing recycling and supraphysiological levels decreasing recycling (Lazarevic et al., 2017). Similarly, exogenously applied Aβ42 shows a biphasic dose-response curve on hippocampal LTP and reference memory (Puzzo et al., 2012). Additional studies carried out to understand the synaptic plasticity and memory formation by different isoforms (Aβ40 and Aβ42) and aggregation status (monomer and oligomer) revealed that lower levels of oligomeric Aβ42 enhanced LTP and spatial memory while higher concentrations of oligomeric Aβ40, oligomeric Aβ42 & monomeric Aβ42 impaired LTP and spatial memory (Gulisano et al., 2018). In addition to memory formation, Aβ is required for memory consolidation and stability. Intrahippocampal administration of picomolar concentrations of exogenous Aβ42, following training, enhances memory retention (Garcia-Osta and Alberini, 2009). Elevated soluble Aβ42 in the amygdala of adult rats, during the formation of auditory fear memories, is required for memory consolidation and stability (Finnie and Nader, 2020). These studies signify the importance of physiological concentrations of Aβ on memory formation and retention and substantiate the hypothesis that cognitive deficits increase due to Aβ depletion.
The two major histopathological hallmarks of AD are extracellular Aβ-plaques and intracellular neurofibrillary tangles. These changes predominantly occur in the later stages of AD. In contrast, synaptic dysfunction typically appears early in prodromal or mild cognitive impairment (MCI) stages of the disease, thus serving as a potent target for early stage therapeutic intervention to slow AD progression. Soluble Aβ42 oligomers can interact with proteins participating in the regulation of the synaptic vesicle life cycle that includes Syntaxin1a, Synaptophysin, and Synapsin1 (Snp1), causing aberrant glutamate release and reduction in synaptic vesicle recycling (reviewed in Marsh and Alifragis, 2018). Currently, there are three publicly disclosed drug trials with endpoints that specifically inform on synapse density and/or function (reviewed in Jackson et al., 2019). First, Elayta (CT1812) is a small-molecule that prevents and displaces beta-amyloid binding to the sigma-2 receptor on the nerve cells and interferes with its toxicity. Elayta lowered the neurogranin and synaptotagmin-1, markers of synaptic damage, in AD patients (U.S. National Library of Medicine, 2020d). A second trial is using imaging techniques and cognitive performance testing to assess the efficiency of LMTX (methylthioninium chloride), a tau aggregation inhibitor, to elicit changes in brain function (U.S. National Library of Medicine, 2020c). Finally, Saracatinib inhibition of Fyn is another potential synaptic specific therapeutic intervention in AD. Fyn is a non-receptor tyrosine kinase that is activated by Aβ oligomers and alters synaptic plasticity (U.S. National Library of Medicine, 2019). These studies have moved the field forward toward clinical trials testing therapuetic drugs designed specifically for synaptic plasticity enhancement (Figure 2). Indeed, recent years have shown an increase in the number of drugs/biologics targeting pathways other than amyloid or tau (Cummings et al., 2020).
Figure 2. Clinical Trials for therapeutic drugs targeting synaptic activity and neuroepigenetic mechanisms for Alzheimer’s disease treatment. The mechanism of action of the drug is classified using Common Alzheimer’s and Related Dementias Research Ontology (CADRO) (Cummings et al., 2020). SV2A, Synaptic vesicle glycoprotein 2A; PDE, Phosphodiesterase; HDAC, Histone deacetylases; hTERT, human telomerase reverse transcriptase.
Compelling evidence demonstrates that repression of histone acetylation mediated epigenetic gene control involving an increase in HDAC2 and a reduction of Tip60 are early contributors to AD (Panikker et al., 2018). Thus, epigenetic therapeutic approaches that involve increasing acetylation levels using HDAC inhibitors (HDACi) and HAT activators is a promising therapeutic approach. At present, there are two HDACi at clinical trials targeting AD pathology (Figure 2; Cummings et al., 2020). Nicotinamide is at Phase 2 testing to assess the reduction of phosphorylated tau in patients that display MCI or mild AD dementia. Another HDACi vorinostat is in Phase 1b to determine the maximal tolerable dose in AD patients between (including) 55 and 90 years with mild symptoms. The epigenetic drug valproic acid restores the physiological regulation of Snp1, a pre-synaptic protein that regulates the availability of synaptic vesicles, in Aβ42 treated primary rat hippocampal neurons (Marsh et al., 2017). Mithramycin A (FDA approved antineoplastic antibiotic) significantly upregulates the synaptic plasticity gene expression and downregulates HDAC2 in SH-SY5Y cells overexpressing APP (Atluri et al., 2019).
HDACi can be either multitargeting like M344, an inhibitor of class I and IIB HDACs, or specific like CM-695 and RGFP966 selectively inhibiting HDAC6 and HDAC3, respectively. M344 regulated multiple AD-related genes and exhibited significant cognitive benefits in vivo (Volmar et al., 2017). CM-695 inhibits HDAC6 and phosphodiesterase 9 (PDE9) and ameliorates memory impairment, and reduces Aβ42 levels in vivo (Cuadrado-Tejedor et al., 2019). RGFP966 inhibits HDAC3 and reverse the attenuation of LTP by Aβ oligomers in rat CA1 pyramidal neurons (Krishna et al., 2016). Selective inhibition by specific HDACi can reduce the side effects and serves as a viable therapeutic strategy. An alternative approach to improve target specificity is to target the binding partners in the HDAC complex rather than the HDAC. Utilizing weighted gene co-expression network analysis (WGCNA), transcription factor Sp3 identified as a putative HDAC2 co-regulator, and its expression was also elevated in AD patients. The knockdown of Sp3 reduced the HDAC2 occupancy and reversed the HDAC2 associated synaptic gene repression (Yamakawa et al., 2017). Therefore, targeting the HDAC2-Sp3 complex may be a feasible approach for AD therapy.
Many HATs as opposed to HDACs have non-redundant physiological functions as different HATs exhibit a specialized preference for site-specific chromatin marks that regulate synaptic gene expression and cognitive function. Thus, HAT activators are a potentially powerful epigenetic therapeutic tool for the treatment of neurodegenerative diseases. As such, chemical modifications are being made to existing drugs to increase their cell permeability in the brain. For example, TTK21, an activator of the HAT CBP/p300, is conjugated to a glucose-based carbon nanosphere enabling it to cross the blood-brain barrier (Chatterjee et al., 2013). It promotes neurogenesis and extends memory duration in vivo. A patent publication (US20180050982A1) covers the use of HAT activators to enhance learning and memory and to treat AD (Francis et al., 2018). Alternatively, downstream molecules/pathways regulated by HATs can also be targeted for therapeutic effects.
A recent growing interest among many researchers is moving toward exploration of non-coding RNA (ncRNA) related neuroepigenetic alterations in AD and its relationship with synaptic dysfunction. Notably, some microRNAs (miRNAs) are particularly enriched in presynaptic and postsynaptic compartments. For example, miR-34a can target the synaptic proteins synaptotagmin-1 and syntaxin-1A to regulate neurite outgrowth and dendritic spine morphology and function (Agostini et al., 2011). Further, in AD-associated HDAC2-induced tauopathy, 5′ AMP-activated protein kinase (AMPK) activation is correlated with the loss of spine density. AMPK expression is under the control of the miR-101b promoter and as such, miR-101b mimics have been shown to block dendritic impairments in vitro (Liu et al., 2017). Thus, understanding the various ncRNAs in AD pathology should lead to new pharmacological interventions. Interestingly, non-pharmacological approaches like an enriched environment (EE) and non-invasive brain stimulation techniques can be utilized to attenuate early stage synaptic dysfunction and appear to act via neuroepigenetic mechanisms. For example, EE triggers hippocampal induction of histone acetylation at specific sites linked to synaptic plasticity and learning and memory enhancement and also ameliorates soluble Aβ oligomer induced synaptic dysfunction by upregulating miRNA-132 and reducing HDAC3 signaling (Wei et al., 2020). Currently, transcranial magnetic stimulation (TMS) and transcranial direct current stimulation (tDCS) usage have been shown to be beneficial for stroke and Parkinson’s patients to positively modulate brain plasticity (reviewed in Schulz et al., 2013). Thus, exciting new avenues involving these types of non-invasive treatment methods likely hold promise for AD patients as well.
In AD patients, Aβ accumulation and associated neuroepigenetic transcriptional alterations contribute to synaptic dysfunction and cognitive impairment (Panikker et al., 2018). However, failure to attenuate or reverse the cognitive decline by anti-amyloid therapeutics in clinical trials raises concerns toward these strategies. Intriguingly, recent studies demonstrate roles for Aβ in neuroprotection, synaptic function, and memory consolidation (Giuffrida et al., 2009; Lazarevic et al., 2017; Finnie and Nader, 2020). These beneficial roles are Aβ concentration- and species-specific. Picomolar concentrations and monomers proved to be beneficial, while higher concentrations and soluble oligomers proved to be detrimental. These findings underscore the necessity to understand the physiological and pathological roles of Aβ for refining the current amyloid-based therapeutic strategies. As AD is a multifactorial disease, targeting AD-associated processes like tau-associated pathology, inflammatory responses, synaptic activity, and neuroepigenetic regulation of AD-related genes may provide alternative therapeutic strategies during early AD progression. Additionally, exploring the synergistic effects of HDACi and HAT activators to restore histone acetylation homeostasis, opens new less invasive and early avenues for treatment. Recent studies utilizing methodological improvements to specifically target toxic Aβ species demonstrate encouraging results. Thus, the development of early therapeutic interventions aimed at mediating Aβ induced neuroepigenetic and synaptic dysfunctions while simultaneously maintaining beneficial physiological levels and forms of Aβ provide exciting new avenues for preventing or treating AD.
BK, AB, EMA, FE, and HZ contributed to writing and reviewing the manuscript. MB and BK contributed to figures and reviewing the manuscript. All authors contributed to the article and approved the submitted version.
Funding for this work was provided by National Institutes of Health Grant NINDS/NIA 1R01NS095799 to FE.
The authors declare that the research was conducted in the absence of any commercial or financial relationships that could be construed as a potential conflict of interest.
Agostini, M., Tucci, P., Steinert, J. R., Shalom-Feuerstein, R., Rouleau, M., Aberdam, D., et al. (2011). microRNA-34a regulates neurite outgrowth, spinal morphology, and function. Proc. Natl. Acad. Sci. U.S.A. 108, 21099–21104. doi: 10.1073/pnas.1112063108
Atluri, V. S. R., Tiwari, S., Rodriguez, M., Kaushik, A., Yndart, A., Kolishetti, N., et al. (2019). Inhibition of amyloid-beta production, associated neuroinflammation, and histone deacetylase 2-mediated epigenetic modifications prevent neuropathology in alzheimer’s disease in vitro model. Front. Aging Neurosci. 11:342. doi: 10.3389/fnagi.2019.00342
Brothers, H. M., Gosztyla, M. L., and Robinson, S. R. (2018). The physiological roles of amyloid-beta peptide hint at new ways to treat Alzheimer’s disease. Front. Aging Neurosci. 10:118. doi: 10.3389/fnagi.2018.00118
Chatterjee, S., Mizar, P., Cassel, R., Neidl, R., Selvi, B. R., Mohankrishna, D. V., et al. (2013). A novel activator of CBP/p300 acetyltransferases promotes neurogenesis and extends memory duration in adult mice. J. Neurosci. 33, 10698–10712. doi: 10.1523/JNEUROSCI.5772-12.2013
Chen, G. F., Xu, T. H., Yan, Y., Zhou, Y. R., Jiang, Y., Melcher, K., et al. (2017). Amyloid beta: structure, biology and structure-based therapeutic development. Acta Pharmacol. Sin. 38, 1205–1235. doi: 10.1038/aps.2017.28
Chen, K. L., Wang, S. S., Yang, Y. Y., Yuan, R. Y., Chen, R. M., and Hu, C. J. (2009). The epigenetic effects of amyloid-beta(1-40) on global DNA and neprilysin genes in murine cerebral endothelial cells. Biochem. Biophys. Res. Commun. 378, 57–61. doi: 10.1016/j.bbrc.2008.10.173
Chouliaras, L., Mastroeni, D., Delvaux, E., Grover, A., Kenis, G., Hof, P. R., et al. (2013). Consistent decrease in global DNA methylation and hydroxymethylation in the hippocampus of Alzheimer’s disease patients. Neurobiol. Aging 34, 2091–2099. doi: 10.1016/j.neurobiolaging.2013.02.021
Cirrito, J. R., Kang, J.-E., Lee, J., Stewart, F. R., Verges, D. K., Silverio, L. M., et al. (2008). Endocytosis is required for synaptic activity-dependent release of amyloid-β in vivo. Neuron 58, 42–51. doi: 10.1016/j.neuron.2008.02.003
Cirrito, J. R., Yamada, K. A., Finn, M. B., Sloviter, R. S., Bales, K. R., May, P. C., et al. (2005). Synaptic activity regulates interstitial fluid amyloid-β levels in vivo. Neuron 48, 913–922. doi: 10.1016/j.neuron.2005.10.028
Cuadrado-Tejedor, M., Perez-Gonzalez, M., Garcia-Munoz, C., Muruzabal, D., Garcia-Barroso, C., Rabal, O., et al. (2019). Taking advantage of the selectivity of histone deacetylases and phosphodiesterase inhibitors to design better therapeutic strategies to treat Alzheimer’s disease. Front. Aging Neurosci. 11:149. doi: 10.3389/fnagi.2019.00149
Cummings, J., Lee, G., Ritter, A., Sabbagh, M., and Zhong, K. J. A. S. (2020). Alzheimer’s disease drug development pipeline: 2020. Alzheimers Dement (N. Y.) 6:e12050.
Di Francesco, A., Arosio, B., Falconi, A., Micioni Di Bonaventura, M. V., Karimi, M., Mari, D., et al. (2015). Global changes in DNA methylation in Alzheimer’s disease peripheral blood mononuclear cells. Brain Behav. Immun. 45, 139–144. doi: 10.1016/j.bbi.2014.11.002
Esposito, M., and Sherr, G. L. (2019). Epigenetic modifications in Alzheimer’s neuropathology and therapeutics. Front. Neurosci. 13:476. doi: 10.3389/fnins.2019.00476
Feng, J., Fouse, S., and Fan, G. (2007). Epigenetic regulation of neural gene expression and neuronal function. Pediatric Res. 61, 58–63. doi: 10.1203/pdr.0b013e3180457635
Ferreira, S. T., and Klein, W. L. (2011). The Aβ oligomer hypothesis for synapse failure and memory loss in Alzheimer’s disease. Neurobiol. Learn. Memory 96, 529–543. doi: 10.1016/j.nlm.2011.08.003
Finnie, P. S. B., and Nader, K. (2020). Amyloid beta secreted during consolidation prevents memory malleability. Curr. Biol. 30, 1934–1940.e1934. doi: 10.1016/j.cub.2020.02.083
Francis, Y., Arancio, O., and Fa, M. (2018). Uses of Histone Acetyltransferase Activators. New York: Google Patents.
Frost, C. V., and Zacharias, M. J. (2020). From monomer to fibril: abeta−amyloid binding to Aducanumab antibody studied by molecular dynamics simulation. Protiens Struct. Func. Bioinform. 88, 1592–1606. doi: 10.1002/prot.25978
Garcia-Osta, A., and Alberini, C. M. (2009). Amyloid beta mediates memory formation. Learn. Mem. 16, 267–272. doi: 10.1101/lm.1310209
Giuffrida, M. L., Caraci, F., Pignataro, B., Cataldo, S., De Bona, P., Bruno, V., et al. (2009). Beta-amyloid monomers are neuroprotective. J. Neurosci. 29, 10582–10587. doi: 10.1523/JNEUROSCI.1736-09.2009
Graff, J., Rei, D., Guan, J. S., Wang, W. Y., Seo, J., Hennig, K. M., et al. (2012). An epigenetic blockade of cognitive functions in the neurodegenerating brain. Nature 483, 222–226. doi: 10.1038/nature10849
Gulisano, W., Melone, M., Li Puma, D. D., Tropea, M. R., Palmeri, A., Arancio, O., et al. (2018). The effect of amyloid-beta peptide on synaptic plasticity and memory is influenced by different isoforms, concentrations, and aggregation status. Neurobiol. Aging 71, 51–60. doi: 10.1016/j.neurobiolaging.2018.06.025
Jackson, J., Jambrina, E., Li, J., Marston, H., Menzies, F., Phillips, K., et al. (2019). Targeting the synapse in Alzheimer’s disease. Front. Neurosci. 13:735. doi: 10.3389/fnins.2019.00735
Jang, S.-S., and Chung, H. J. (2016). Emerging link between Alzheimer’s disease and homeostatic synaptic plasticity. Neural. Plasticity 2016:7969272. doi: 10.1155/2016/7969272
Kamenetz, F., Tomita, T., Hsieh, H., Seabrook, G., Borchelt, D., Iwatsubo, T., et al. (2003). APP processing and synaptic function. Neuron 37, 925–937. doi: 10.1016/S0896-6273(03)00124-7
Kashyap, G., Bapat, D., Das, D., Gowaikar, R., Amritkar, R. E., Rangarajan, G., et al. (2019). Synapse loss and progress of Alzheimer’s disease -A network model. Sci. Rep. 9:6555. doi: 10.1038/s41598-019-43076-y
Koffie, R. M., Hashimoto, T., Tai, H.-C., Kay, K. R., Serrano-Pozo, A., Joyner, D., et al. (2012). Apolipoprotein E4 effects in Alzheimer’s disease are mediated by synaptotoxic oligomeric amyloid-β. Brain 135, 2155–2168. doi: 10.1093/brain/aws127
Krishna, K., Behnisch, T., and Sajikumar, S. J. (2016). Inhibition of histone deacetylase 3 restores amyloid-β oligomer-induced plasticity deficit in hippocampal CA1 pyramidal neurons. J. Alzheimers Dis. 51, 783–791. doi: 10.3233/jad-150838
Lambert, M. P., Barlow, A. K., Chromy, B. A., Edwards, C., Freed, R., Liosatos, M., et al. (1998). Diffusible, nonfibrillar ligands derived from Abeta1-42 are potent central nervous system neurotoxins. Proc. Natl. Acad. Sci. U.S.A. 95, 6448–6453. doi: 10.1073/pnas.95.11.6448
Lashley, T., Gami, P., Valizadeh, N., Li, A., Revesz, T., and Balazs, R. (2015). Alterations in global DNA methylation and hydroxymethylation are not detected in Alzheimer’s disease. Neuropathol. Appl. Neurobiol. 41, 497–506. doi: 10.1111/nan.12183
Lazarevic, V., Fienko, S., Andres-Alonso, M., Anni, D., Ivanova, D., Montenegro-Venegas, C., et al. (2017). Physiological concentrations of amyloid beta regulate recycling of synaptic vesicles via alpha7 acetylcholine receptor and CDK5/calcineurin signaling. Front. Mol. Neurosci. 10:221. doi: 10.3389/fnmol.2017.00221
Li, P., Marshall, L., Oh, G., Jakubowski, J. L., Groot, D., He, Y., et al. (2019). Epigenetic dysregulation of enhancers in neurons is associated with Alzheimer’s disease pathology and cognitive symptoms. Nat. Commun. 10:2246. doi: 10.1038/s41467-019-10101-7
Li, S., Hong, S., Shepardson, N. E., Walsh, D. M., Shankar, G. M., and Selkoe, D. (2009). Soluble oligomers of amyloid Beta protein facilitate hippocampal long-term depression by disrupting neuronal glutamate uptake. Neuron 62, 788–801. doi: 10.1016/j.neuron.2009.05.012
Liu, D., Tang, H., Li, X.-Y., Deng, M.-F., Wei, N., Wang, X., et al. (2017). Targeting the HDAC2/HNF-4A/miR-101b/AMPK pathway rescues tauopathy and dendritic abnormalities in Alzheimer’s disease. Mol. Ther. 25, 752–764. doi: 10.1016/j.ymthe.2017.01.018
Liu, Y., Wang, M., Marcora, E. M., Zhang, B., and Goate, A. M. (2019). Promoter DNA hypermethylation – Implications for Alzheimer’s disease. Neurosci. Lett. 711:134403. doi: 10.1016/j.neulet.2019.134403
Marsh, J., and Alifragis, P. J. N. (2018). Synaptic dysfunction in Alzheimer’s disease: the effects of amyloid beta on synaptic vesicle dynamics as a novel target for therapeutic intervention. Neural. Regen Res. 13:616. doi: 10.4103/1673-5374.230276
Marsh, J., Bagol, S. H., Williams, R. S. B., Dickson, G., and Alifragis, P. (2017). Synapsin I phosphorylation is dysregulated by beta-amyloid oligomers and restored by valproic acid. Neurobiol. Dis. 106, 63–75. doi: 10.1016/j.nbd.2017.06.011
Martin, S. J., Grimwood, P. D., and Morris, R. G. (2000). Synaptic plasticity and memory: an evaluation of the hypothesis. Ann. Rev. Neurosci. 23, 649–711. doi: 10.1146/annurev.neuro.23.1.649
Miranda, T. B., and Jones, P. A. (2007). DNA methylation: the nuts and bolts of repression. J. Cell. Physiol. 213, 384–390. doi: 10.1002/jcp.21224
Mucke, L., Masliah, E., Yu, G.-Q., Mallory, M., Rockenstein, E. M., Tatsuno, G., et al. (2000). High-level neuronal expression of Aβ1–42 in wild-type human amyloid protein precursor transgenic mice: synaptotoxicity without plaque formation. J. Neurosci. 20, 4050–4058. doi: 10.1523/jneurosci.20-11-04050.2000
National Institute on Aging (2018). How Is Alzheimer’s Disease Treated? [Online]. Available online at: https://www.nia.nih.gov/health/how-alzheimers-disease-treated (accessed April 1, 2018).
Panikker, P., Xu, S.-J., Zhang, H., Sarthi, J., Beaver, M., Sheth, A., et al. (2018). Restoring Tip60 HAT/HDAC2 balance in the neurodegenerative brain relieves epigenetic transcriptional repression and reinstates cognition. J. Neurosci. 38, 4569–4583. doi: 10.1523/jneurosci.2840-17.2018
Panza, F., Lozupone, M., Logroscino, G., and Imbimbo, B. P. (2019). A critical appraisal of amyloid-beta-targeting therapies for Alzheimer disease. Nat. Rev. Neurol. 15, 73–88. doi: 10.1038/s41582-018-0116-6
Parihar, M. S., and Brewer, G. J. (2010). Amyloid-β as a modulator of synaptic plasticity. J. Alzheimers Dis. 22, 741–763. doi: 10.3233/JAD-2010-101020
Peixoto, L., and Abel, T. (2013). The role of histone acetylation in memory formation and cognitive impairments. Neuropsychopharmacology 38, 62–76. doi: 10.1038/npp.2012.86
Plant, L. D., Boyle, J. P., Smith, I. F., Peers, C., and Pearson, H. A. J. J. (2003). The production of amyloid β peptide is a critical requirement for the viability of central neurons. J. Neurosci. 23, 5531–5535. doi: 10.1523/jneurosci.23-13-05531.2003
Puzzo, D., Privitera, L., and Palmeri, A. (2012). Hormetic effect of amyloid-beta peptide in synaptic plasticity and memory. Neurobiol. Aging 33, e1415–e1424. doi: 10.1016/j.neurobiolaging.2011.12.020
Ramirez, A., and Arbuckle, M. R. (2016). Synaptic plasticity: the role of learning and unlearning in addiction and beyond. Biol. Psychiatry 80, e73–e75. doi: 10.1016/j.biopsych.2016.09.002
Rao, J. S., Keleshian, V. L., Klein, S., and Rapoport, S. I. (2012). Epigenetic modifications in frontal cortex from Alzheimer’s disease and bipolar disorder patients. Transl. Psychiatry 2:e132. doi: 10.1038/tp.2012.55
Rice, H. C., De Malmazet, D., Schreurs, A., Frere, S., Van Molle, I., Volkov, A. N., et al. (2019). Secreted amyloid-β precursor protein functions as a GABABR1a ligand to modulate synaptic transmission. Science 363:6423.
Saha, R., and Pahan, K. (2006). HATs and HDACs in neurodegeneration: a tale of disconcerted acetylation homeostasis. Cell Death Different. 13:539. doi: 10.1038/sj.cdd.4401769
Saxonov, S., Berg, P., and Brutlag, D. L. (2006). A genome-wide analysis of CpG dinucleotides in the human genome distinguishes two distinct classes of promoters. Proc. Natl. Acad. Sci. U.S.A. 103, 1412–1417. doi: 10.1073/pnas.0510310103
Scheff, S. W., Price, D. A., Schmitt, F. A., and Mufson, E. J. (2006). Hippocampal synaptic loss in early Alzheimer’s disease and mild cognitive impairment. Neurobiol. Aging 27, 1372–1384. doi: 10.1016/j.neurobiolaging.2005.09.012
Schulz, R., Gerloff, C., and Hummel, F. C. J. N. (2013). Non-invasive brain stimulation in neurological diseases. Neuropharmacology 64, 579–587. doi: 10.1016/j.neuropharm.2012.05.016
Shankar, G. M., Li, S., Mehta, T. H., Garcia-Munoz, A., Shepardson, N. E., Smith, I., et al. (2008). Amyloid-β protein dimers isolated directly from Alzheimer’s brains impair synaptic plasticity and memory. Nat. Med. 14:837. doi: 10.1038/nm1782
Sharma, S. K. (2010). Protein acetylation in synaptic plasticity and memory. Neurosci. Biobehav. Rev. 34, 1234–1240. doi: 10.1016/j.neubiorev.2010.02.009
Shoji, M., and Kanai, M. J. J. (2001). Cerebrospinal fluid Aβ40 and Aβ42: natural course and clinical usefulness. J. Alzheimers Dis. 3, 313–321. doi: 10.3233/jad-2001-3306
Sultan, F. A., and Day, J. J. (2011). Epigenetic mechanisms in memory and synaptic function. Epigenomics 3, 157–181. doi: 10.2217/epi.11.6
Takahashi, R. H., Milner, T. A., Li, F., Nam, E. E., Edgar, M. A., Yamaguchi, H., et al. (2002). Intraneuronal Alzheimer abeta42 accumulates in multivesicular bodies and is associated with synaptic pathology. Am. J. Pathol. 161, 1869–1879. doi: 10.1016/s0002-9440(10)64463-x
Tulloch, J., Leong, L., Thomson, Z., Chen, S., Lee, E.-G., Keene, C. D., et al. (2018). Glia-specific APOE epigenetic changes in the Alzheimer’s disease brain. Brain Res. 1698, 179–186. doi: 10.1016/j.brainres.2018.08.006
U.S. National Library of Medicine (2019). NCT02167256 A Phase IIa Multi-Center Study of 18F-FDG PET, Safety, and Tolerability of AZD0530 in Mild Alzheimer’s Disease. Available online at: https://ClinicalTrials.gov/show/NCT02167256 (accessed August 14, 2019).
U.S. National Library of Medicine (2020a). NCT02477800 221AD301 Phase 3 Study of Aducanumab (BIIB037) in Early Alzheimer’s Disease. Availble online at: https://ClinicalTrials.gov/show/NCT02477800 (accessed August 14, 2020).
U.S. National Library of Medicine (2020b). NCT02484547 221AD302 Phase 3 Study of Aducanumab (BIIB037) in Early Alzheimer’s Disease. Available online at: https://ClinicalTrials.gov/show/NCT02484547 (August 7, 2020).
U.S. National Library of Medicine (2020c). NCT03446001 Safety and Efficacy of TRx0237 in Subjects With Alzheimer’s Disease Followed by Open-Label Treatment. Available online at: https://ClinicalTrials.gov/show/NCT03446001 (accessed September 1, 2020).
U.S. National Library of Medicine (2020d). NCT03493282 Effect of CT1812 Treatment on Brain Synaptic Density. Available online at: https://ClinicalTrials.gov/show/NCT03493282 (accessed February 5, 2020).
Volmar, C.-H., Salah-Uddin, H., Janczura, K. J., Halley, P., Lambert, G., Wodrich, A., et al. (2017). M344 promotes nonamyloidogenic amyloid precursor protein processing while normalizing Alzheimer’s disease genes and improving memory. Proc. Natl. Acad. Sci. U.S.A. 114, E9135–E9144. doi: 10.1097/00002093-199709000-00012
Walsh, D. M., Klyubin, I., Fadeeva, J. V., Cullen, W. K., Anwyl, R., Wolfe, M. S., et al. (2002). Naturally secreted oligomers of amyloid β protein potently inhibit hippocampal long-term potentiation in vivo. Nature 416, 535–539. doi: 10.1038/416535a
Wei, Z., Meng, X., El Fatimy, R., Sun, B., Mai, D., Zhang, J., et al. (2020). Environmental enrichment prevents Aβ oligomer-induced synaptic dysfunction through mirna-132 and hdac3 signaling pathways. Neurobiol. Dis. 134:104617. doi: 10.1016/j.nbd.2019.104617
West, R. L., Lee, J. M., and Maroun, L. E. (1995). Hypomethylation of the amyloid precursor protein gene in the brain of an alzheimer’s disease patient. J. Mol Neurosci. 6, 141–146. doi: 10.1007/BF02736773
Xia, M., Cheng, X., Yi, R., Gao, D., and Xiong, J. (2016). The binding receptors of aβ: an alternative therapeutic target for Alzheimer’s disease. Mol. Neurobiol. 53, 455–471. doi: 10.1007/s12035-014-8994-0
Yamakawa, H., Cheng, J., Penney, J., Gao, F., Rueda, R., Wang, J., et al. (2017). The transcription factor Sp3 cooperates with HDAC2 to regulate synaptic function and plasticity in neurons. Cell Rep. 20, 1319–1334. doi: 10.1016/j.celrep.2017.07.044
Keywords: neuroepigenetics, TIP60, synaptic function, therapeutics, amyloid beta, KAT5
Citation: Karisetty BC, Bhatnagar A, Armour EM, Beaver M, Zhang H and Elefant F (2020) Amyloid-β Peptide Impact on Synaptic Function and Neuroepigenetic Gene Control Reveal New Therapeutic Strategies for Alzheimer’s Disease. Front. Mol. Neurosci. 13:577622. doi: 10.3389/fnmol.2020.577622
Received: 29 June 2020; Accepted: 22 October 2020;
Published: 13 November 2020.
Edited by:
Daniela Puzzo, University of Catania, ItalyReviewed by:
Takashi Saito, Nagoya City University, JapanCopyright © 2020 Karisetty, Bhatnagar, Armour, Beaver, Zhang and Elefant. This is an open-access article distributed under the terms of the Creative Commons Attribution License (CC BY). The use, distribution or reproduction in other forums is permitted, provided the original author(s) and the copyright owner(s) are credited and that the original publication in this journal is cited, in accordance with accepted academic practice. No use, distribution or reproduction is permitted which does not comply with these terms.
*Correspondence: Felice Elefant, ZmUyMkBkcmV4ZWwuZWR1
†These authors have contributed equally to this work and share second authorship
Disclaimer: All claims expressed in this article are solely those of the authors and do not necessarily represent those of their affiliated organizations, or those of the publisher, the editors and the reviewers. Any product that may be evaluated in this article or claim that may be made by its manufacturer is not guaranteed or endorsed by the publisher.
Research integrity at Frontiers
Learn more about the work of our research integrity team to safeguard the quality of each article we publish.