- 1Institute of Structural Biology, Rudolf Virchow Center for Experimental Biomedicine, University of Würzburg, Würzburg, Germany
- 2Department of Biotechnology and Biophysics, Biocenter, University of Würzburg, Würzburg, Germany
γ-aminobutyric acid type A receptors (GABAARs) are the major mediators of synaptic inhibition in the brain. Aberrant GABAAR activity or regulation is observed in various neurodevelopmental disorders, neurodegenerative diseases and mental illnesses, including epilepsy, Alzheimer’s and schizophrenia. Benzodiazepines, anesthetics and other pharmaceutics targeting these receptors find broad clinical use, but their inherent lack of receptor subtype specificity causes unavoidable side effects, raising a need for new or adjuvant medications. In this review article, we introduce a new strategy to modulate GABAeric signaling: targeting the intracellular protein interactors of GABAARs. Of special interest are scaffolding, anchoring and supporting proteins that display high GABAAR subtype specificity. Recent efforts to target gephyrin, the major intracellular integrator of GABAergic signaling, confirm that GABAAR-associated proteins can be successfully targeted through diverse molecules, including recombinant proteins, intrabodies, peptide-based probes and small molecules. Small-molecule artemisinins and peptides derived from endogenous interactors, that specifically target the universal receptor binding site of gephyrin, acutely affect synaptic GABAAR numbers and clustering, modifying neuronal transmission. Interference with GABAAR trafficking provides another way to modulate inhibitory signaling. Peptides blocking the binding site of GABAAR to AP2 increase the surface concentration of GABAAR clusters and enhance GABAergic signaling. Engineering of gephyrin binding peptides delivered superior means to interrogate neuronal structure and function. Fluorescent peptides, designed from gephyrin binders, enable live neuronal staining and visualization of gephyrin in the post synaptic sites with submicron resolution. We anticipate that in the future, novel fluorescent probes, with improved size and binding efficiency, may find wide application in super resolution microscopy studies, enlightening the nanoscale architecture of the inhibitory synapse. Broader studies on GABAAR accessory proteins and the identification of the exact molecular binding interfaces and affinities will advance the development of novel GABAAR modulators and following in vivo studies will reveal their clinical potential as adjuvant or stand-alone drugs.
Introduction
γ-aminobutyric acid type A receptors (GABAARs) are the principal mediators of phasic and tonic inhibition in the human brain, being a vital part of the molecular machinery that creates cognition, behavior, and consciousness (Fritschy and Panzanelli, 2014). Dysfunctional GABAARs are involved in the pathogenesis of neurodevelopmental disorders (Ali Rodriguez et al., 2018), schizophrenia (de Jonge et al., 2017), postpartum depression (Mody, 2019), epilepsy (Palma et al., 2017; Hines et al., 2018), Alzheimer’s disease (Govindpani et al., 2017), autism (Vien et al., 2015) and stroke (Darmani et al., 2016; Wang et al., 2018). Structurally, these receptors belong to the pentameric ligand-gated ion channels harboring an extracellular domain (ECD), followed by four helical transmembrane domains (TMDs) and loops connecting these helices. GABAARs display a highly subtype-specific cellular and sub-cellular distribution and exhibit distinct physiological properties, making them very attractive pharmaceutical targets.
First GABAAR targeting compounds have been discovered more than a century ago. In 1904, Bayer marketed barbital, the first barbiturate and positive allosteric modulator of GABAARs (Löscher and Rogawski, 2012). In the 1960s, benzodiazepines, a new class of GABAAR allosteric modulators (Sancar and Czajkowski, 2011), became commercially available. Today, modulators of GABAAR activity find broad clinical use as anesthetics (Propofol; Olsen, 2018), anticonvulsants (Gabapentin) or as hypnotics, muscle-relaxants and anxiolytics (Clonazepam, Diazepam), and new experimental medicines are developed. Nonetheless, wider application of these classical GABAAR modulators is limited by their lack of receptor subtype specificity, due to the fundamental structural and functional constraints: pharmacologically exploited sites are small hydrophobic pockets with high subunit sequence homology located at the folded ECDs and TMDs of the ion channels (Figure 1; Miller et al., 2017; Kasaragod and Schindelin, 2018; Masiulis et al., 2019). Additionally, binding sites on the interface between two subunits, such as the benzodiazepine binding site, are shared among different synaptic receptor subtypes. Consequently, the action of classical clinically relevant GABAAR ligands can be unspecific and provoke unavoidable side effects.
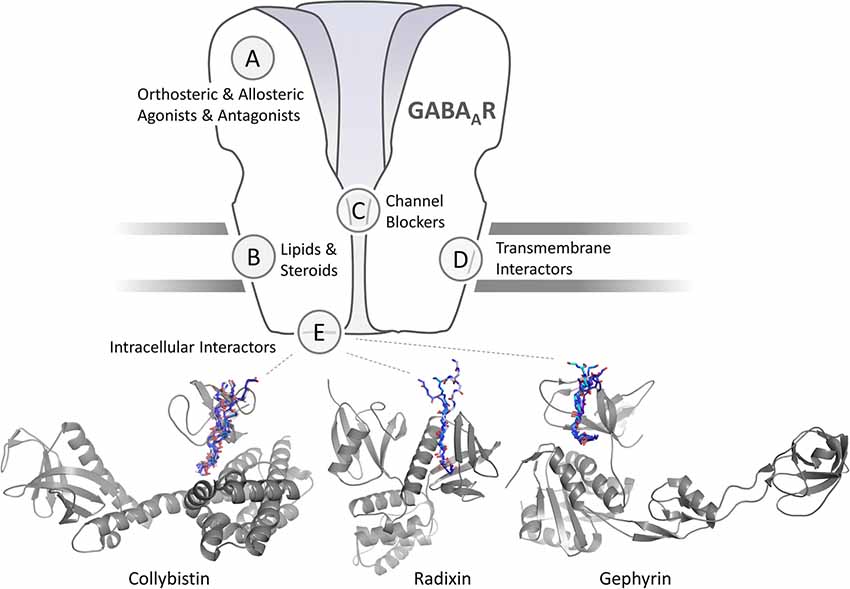
Figure 1. Schematic representation of a γ-aminobutyric acid type A receptors (GABAARs) and sites for pharmaceutical intervention. Orthosteric and allosteric agonists and antagonist are classical activity modulators that bind directly to the extracellular domain (ECD) or, in case of lipids and neurosteroids to the transmembrane domain (TMD) of GABAARs. Each of the sites could occur in five subunits or at five interfaces, or only in distinct subunits and specific interfaces. Channel blockers bind within the ion pore formed by the GABAAR pentamer. Intracellular interactors such as Collybistin (CB; Hines et al., 2018) and gephyrin (Maric et al., 2014) interact with distinct intracellular regions of a subset of GABAAR subunits. Transmembrane interactors such as LHFPL4 interact with the TMDs of γ2 subunit containing GABAARs (Davenport et al., 2017; Yamasaki et al., 2017). Cartoon representation of structurally characterized and predicted scaffold-GABAAR complexes. CB is shown in gray in its extended conformation (PDB-ID 4mt7) with its SH3 domain (PDB-ID 4mt6; Soykan et al., 2014) binding to a fragment of the GABAAR α2 subunit (Hines et al., 2018). Peptide backbones of resolved SH3 domain ligands (PDB-IDs 2df6, 4hvu, 4hvv, 4hvw, 4j9f, 4ln2 and 4rtz) are superimposed to indicate the putative GABAAR α2 binding site. The radixin FERM domain is shown in gray. Peptide backbones of resolved radixin FERM domain ligands (PDB-IDs 1j19, 2ems, 2d2q) are superimposed to indicate the putative GABAAR α5 binding site. Cartoon representation of the gephyrin E domain in complex with short linear GABAAR (PDB-IDs 4tk1, 4tk2, 4tk3, 4tk4) and GlyR derived peptides (PDB-IDs 2fts, 4u90, 4u91).
Molecules modulating receptor signaling through accessory proteins in the central nervous system (CNS; Figure 1) emerged as a new class of pharmaceuticals with superior receptor specificity and potential to treat epilepsy, neuropathic pain, fibromyalgia, migraines, and other diseases (Maher et al., 2017). Therefore, targeting GABAAR-associated proteins might be a superior pharmacological strategy compared to the classical approaches. This rational approach, however, requires detailed knowledge and advanced understanding of the intracellular signaling of distinct GABAAR subtypes. The large number of post-synaptic candidate proteins that directly or indirectly associate with GABAARs is still increasing (Krueger-Burg et al., 2017), with functional studies exploring some of their physiological roles and organization (Uezu et al., 2016; Lu et al., 2017), yet, the specific molecular details of these interactions remain largely unknown. We hypothesize that the identification of the exact molecular binding interfaces and binding affinities of known and newly identified GABAAR associated proteins will not only greatly expand our basic understanding of CNS function, but also provide new pharmaceutical opportunities.
Adjusting GABAergic Signaling Through Intracellular Modulation
The majority of GABAARs assemble as heteropentamers to form GABA-gated chloride channels. Different subunit combinations possess unique pharmacology (Olsen and Sieghart, 2009), divergent brain region distribution (Wisden and Seeburg, 1992), cell-type specific expression (Lee and Maguire, 2014), and varying subcellular localization between synaptic and extra-synaptic sites (Mody and Pearce, 2004). Thus, subtype-specific modulators of GABAAR signaling should affect distinct circuits, brain regions or subcellular populations with improved accuracy and more selective pharmacology. Combined structural and functional studies have revealed the molecular details of the interplay of the ECD and TMDs in channel gating (Miller and Aricescu, 2014; Lu et al., 2017; Kasaragod and Schindelin, 2018; Zhu et al., 2018; Laverty et al., 2019). Structural studies of the receptors could, so far, not resolve most of the presumably intrinsically disordered intracellular regions of GABAARs. Short intracellular receptor regions, however, do adopt defined conformations when engaged with structured intracellular interactors, such as gephyrin (Maric et al., 2014) and the AP2 complex (Kittler et al., 2008; Table 1). Functional studies validated that distinct motifs within these unstructured regions exert tight control over channel biosynthesis, recycling, diffusion and synaptic recruitment (Tretter et al., 2012; Nakamura et al., 2015; Groeneweg et al., 2018; Lorenz-Guertin and Jacob, 2018). Remarkably, these intracellular regions display the highest level of sequence heterogeneity among receptor subunits, thereby enabling subtype-specific modulation of GABAergic signaling. Agents targeting these discrete regions will probably be highly selective and could affect GABAAR subtypes with distinct functional and pharmacological properties. It is noteworthy that, so far, all intracellular GABAAR interactions that displayed sufficient affinity and specificity ended up being exploited to modulate neuronal communication (Table 1).
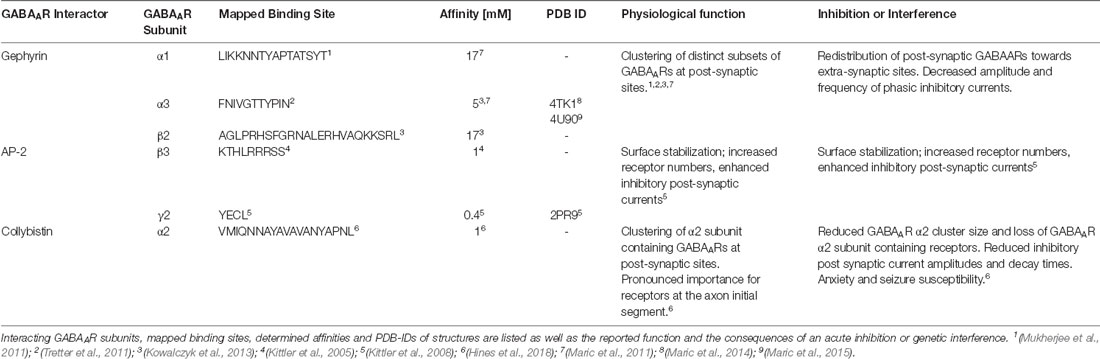
Table 1. List of all known intracellular GABAAR-associated proteins that display micromolar or better binding affinity.
Affecting Postsynaptic GABAAR Accumulation by Targeting Intracellular Scaffolds
The concept of neurotransmission modulation through targeting receptor-scaffolding protein interactions originated from studies investigating PSD-95/Discs-large/ZO-1 (PDZ) domain carrying proteins. These showed that through modulation of receptor-scaffolding protein interactions a variety of responses could be achieved, ranging from disruption of glutamate signaling to neuroprotective effects in ischemic brain damage (Hammond et al., 2006; Sainlos et al., 2011; Bach et al., 2012; Figure 2A). These results suggested that modulation of the inhibitory neurotransmission could be accomplished in a similar way, a concept recently proved with the inhibitory scaffold protein gephyrin (Maric et al., 2017).
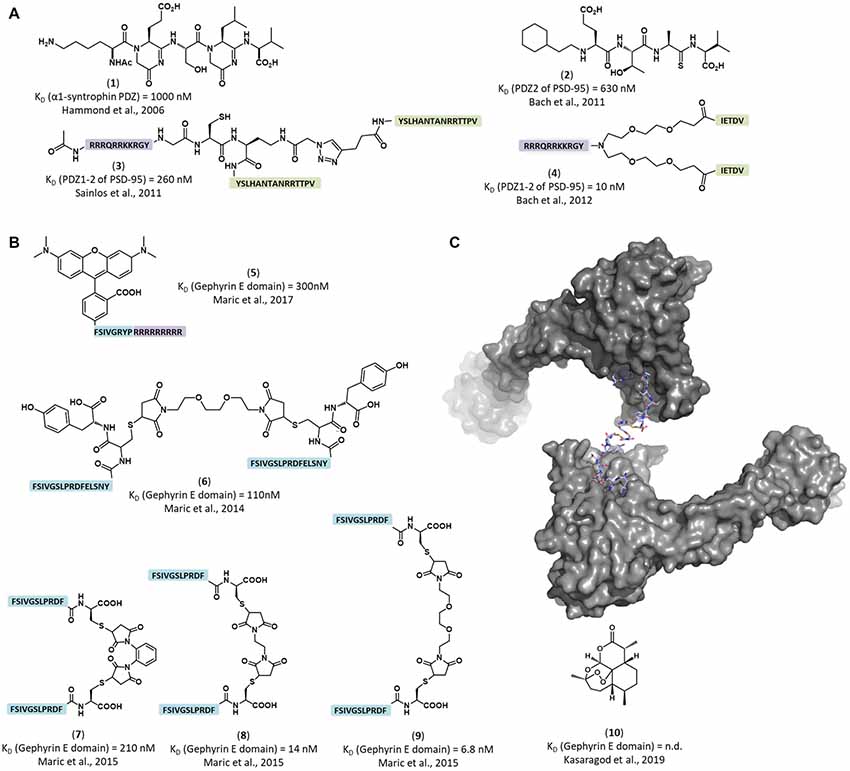
Figure 2. Structure of representative inhibitors and labels of the inhibitory post-synaptic scaffold gephyrin and the excitatory post-synaptic scaffold PSD-95. (A) Peptidomimetic and dimeric PSD-95/Discs-large/ZO-1 (PDZ) domain binders. PSD-95 binding peptides (green) were dimerized and combined with cell-penetrating moieties (violet). (1) Peptidomimetic ligand for a α-1 syntrophin PDZ domain (Hammond et al., 2006). (2) Peptidomimetic inhibitor of the PSD-95 PDZ2 domain (Bach et al., 2011) (3) Dimeric inhibitor of PSD-95 PDZ domains, showed strong inhibition of AMPA receptor synaptic currents (Sainlos et al., 2011). (4) Dimeric inhibitor of PSD-95 PDZ domains, showed neuroprotective properties in mice with cerebral ischemia (Bach et al., 2012). (B) Engineered peptide-based gephyrin inhibitors and fluorescent labels. (5) Peptide-based fluorescent gephyrin label (Maric et al., 2017). Tamra dye was directly conjugated to gephyrin binding sequence (blue) combined with cell penetrating peptide (in purple). (6–9) Nanomolar affinity dimerized gephyrin peptide binders (blue; Maric et al., 2015). (10) The small molecule inhibitor artemisinin competes with the universal engineered peptide-based molecules for receptor binding pocket (Kasaragod et al., 2019). (C) Representative crystal structure of a peptide dimer engaging with a gephyrin E domain dimer (PDB-ID 4U90; Maric et al., 2015).
Scaffolding proteins, such as gephyrin (Specht et al., 2013), radixin (Loebrich et al., 2006; Hausrat et al., 2015) and (collybistin, CB; Mayer et al., 2013; Hines et al., 2018), dynamically regulate the cell membrane distribution of postsynaptic and extrasynaptic GABAARs. Interestingly, their scaffolding functions are highly receptor specific, potentially allowing a fine tuning of neurotransmission.
Radixin
Radixin is involved in the anchoring of numerous membrane proteins to the actin cytoskeleton (Kawaguchi et al., 2017). Its C-terminal domain mediates actin binding, while the N-terminal FERM domain functions as a universal protein-binding module that directly interacts with receptors, extracellular matrix components, transmembrane and adhesion proteins (Kitano et al., 2006; Takai et al., 2007; Terawaki et al., 2007, 2008; Yogesha et al., 2011; Figure 1). Radixin also harbors a central α-helical domain, which either adopts a closed or elongated conformation to allow its auto-inhibitory module to mask the FERM domain. In neurons, radixin is activated through phosphorylation, which enables its simultaneous binding to cytoskeletal elements and transmembrane proteins, including α5 subunit containing GABAARs (Loebrich et al., 2006; Hausrat et al., 2015). In primary hippocampal neuronal cultures, the association of radixin with α5-containing GABAARs at extrasynaptic sites decreases upon maturation, in contrast, the number of α5-containing GABAARs, that associate with gephyrin at post-synaptic sites remains constant (Brady and Jacob, 2015). Structural and thermodynamic details of the radixin-GABAAR α5 complex will reveal whether modulation can be achieved without simultaneously affecting the binding of other ligands.
Collybistin
Collybistin (CB) is a guanine nucleotide exchange factor for Cdc42, a gephyrin binding partner (Kins et al., 2000) and an important determinant of inhibitory postsynaptic membrane formation and plasticity (Harvey et al., 2004; Tyagarajan et al., 2011a). Robust evidence supports the role of CB in GABAAR scaffolding with yeast three-hybrid studies (Saiepour et al., 2010) and proteomic studies (Nakamura et al., 2016) suggesting the presence of a tripartite complex between gephyrin, CB and α2 subunit containing GABAARs. Recently, a thermodynamic analysis revealed that CB binds GABAAR α2-subunits with high selectivity and affinity (Hines et al., 2018; Figure 1 and Table 1). CB is targeted to the neuronal surface membrane through phosphoinositides (Reddy-Alla et al., 2010; Ludolphs et al., 2016) and interfering human mutations result in cognitive deficits (Long et al., 2016; Chiou et al., 2019). Deficiency in CB reduces gephyrin and GABAAR clustering and impairs spatial learning (Papadopoulos et al., 2007, 2008). Moreover, mice with a mutation in the α2-subunit binding region of CB display a loss of a distinct subset of inhibitory synapses and a decreased amplitude of inhibitory synaptic currents, which results in a phenotype with increased susceptibility to seizures and early mortality (Hines et al., 2018). Notably, treatment with the α2/α3-selective positive modulator AZD7325 improves the conditions of affected mice, suggesting that compounds targeting the CB-GABAAR α2 complex could provide an alternative route to specifically affect GABAARs containing the α2 subunit.
Neuroligin 2
Proteomic studies (Kang et al., 2014; Nakamura et al., 2016) revealed that the synapse-specific adhesion molecule neuroligin 2 (NL2; Varoqueaux et al., 2004) strongly associates with a subset of GABAAR subtypes and GABAAR scaffolds. Neuroligin dysfunction has been implicated in autism (Pettem et al., 2013) and specific intracellular residues in NL1 (Nguyen et al., 2016; Letellier et al., 2018) and NL2 (Poulopoulos et al., 2009; Kang et al., 2014) are critical for proper GABAergic signaling. Yet, the exact molecular interfaces, that mediate the direct or indirect gephyrin or CB dependent (Soykan et al., 2014) interactions of neuroligin with GABAAR, remain uncharacterized. These molecular insights could greatly contribute to our understanding of the development of the inhibitory synapse, as well as the underlying molecular causes of developmental diseases. Neuroligin family members exert distinct roles in the formation and stabilization of inhibitory and excitatory synapses and display distinct cellular and subcellular distributions. Accordingly, molecules that interfere with their isoform-specific interactions could act as highly cell-type selective modulators of neurotransmission.
Gephyrin
Gephyrin is a prime candidate for the role of master regulator of neuronal function at inhibitory sites (Tyagarajan and Fritschy, 2014) and specifically the GABAergic synapses (Choii and Ko, 2015). Initially identified as a glycine receptor binding (Pfeiffer et al., 1982) and scaffolding protein (Feng et al., 1998), gephyrin was later found to be responsible for the post-synaptic accumulation of GABAARs. The loss of gephyrin clusters following the loss of the GABAAR γ2 subunit (Essrich et al., 1998) and the loss of GABAAR clusters upon gephyrin deficiency (Kneussel et al., 1999) substantiates their critical mutual dependency. Direct binding of gephyrin to α and β GABAAR subunits could be confirmed (Tretter et al., 2008, 2011; Maric et al., 2011; Mukherjee et al., 2011; Kowalczyk et al., 2013; Brady and Jacob, 2015), but the exact binding interfaces and affinities are still largely undefined. Structural, thermodynamic and high-end microscopic approaches elucidated the complex structure-function relationships between gephyrin and a distinct subgroup of inhibitory neurotransmitter receptors (Kasaragod and Schindelin, 2018) and indicated an important role of the nanoscale arrangement of gephyrin and the associated receptors at post-synaptic sites (Groeneweg et al., 2018; Specht, 2019). Further functional and in-silico studies (Pennacchietti et al., 2017) confirmed that gephyrin organizes the receptors in distinct nanoscale structures, which shape GABAergic synaptic potentiation and reduce current variability. The stability, oligomerization and receptor binding of gephyrin are highly regulated and exert tight control over receptor numbers at post-synaptic sites, affecting synaptic strength (Alvarez, 2017; Groeneweg et al., 2018). Biophysical (Maric et al., 2011) and structural (Maric et al., 2014, 2015) studies provided first insights into the structure and function of the gephyrin-GABAAR complexes and demonstrated that at least the GABAAR α1–3 and GlyR β subunits bind to an overlapping site within gephyrin in a mutually exclusive fashion (Maric et al., 2011, 2014; Figure 1 and Table 1). Microscopy studies substantiated that gephyrin acts as a dynamic post-synaptic platform for both, GABAARs and GlyRs (Specht et al., 2013), and that receptor residence times at the postsynapse depend on binding affinities and distinct post-translational modifications (Mukherjee et al., 2011; Specht et al., 2011). The concept of ligand competition for gephyrin binding, therefore, lends an elegant explanation for the comparably high diffusion dynamics of high-affinity gephyrin binding receptors. This phenomenon may be the natural solution to the biological requirement to maintain distinct subsets of receptor subtypes in high density at post-synaptic sites, while at the same time allowing for the rapid exchange of these receptors and scalability through diffusion dynamics (Specht, 2019). This model is also consistent with the observation of reciprocal stabilization of receptors, and the underlying protein scaffold, at inhibitory synapses (Essrich et al., 1998).
Gephyrin itself is dynamically regulated, affecting GABAAR diffusion and contributing to input-specific adaptations at postsynaptic sites (Chen et al., 2012; Villa et al., 2016; Battaglia et al., 2018). Gephyrin phosphorylation at Ser268 and Ser270, located in the intrinsically disordered central region of the protein, directly affects GABAergic signaling (Tyagarajan et al., 2011b, 2013) and induce gephyrin-mediated remodeling of GABAergic synapses in specific neuronal cell-types (Flores et al., 2015). Despite its major functional relevance only a few of the molecular interfaces that engage with the central region of gephyrin could be identified (Groeneweg et al., 2018). The underlying molecular mechanisms for these gephyrin phosphorylation-induced GABAAR synapse dynamics remain to be explored in a comprehensive approach that includes an extensive alternative splicing and complex post-translational modification patterns of this region. Identification of the targeted binding pockets and insights into the binding affinities of the modified and unmodified peptide regions within the central region of gephyrin could shed light on the enigmatic molecular mechanisms of gephyrin multimerization, degradation and the tuning of its ligand binding affinities. Additionally, gephyrin isoforms are tissue-specific (Paarmann et al., 2006), therefore, molecules targeting distinct gephyrin splice variants may display pronounced effects in distinct tissues or brain regions.
Targeting the Gephyrin-GABAAR Complex
Gephyrin’s crucial role in glycinergic and GABAergic transmission made it a major pharmacological target. The modulation of synaptic responses via gephyrin was achieved more than a decade ago using intrabodies (Zacchi et al., 2008), and a related approach turned out to be useful for acutely removing inhibitory synapses (Gross et al., 2016). Since then, several studies made an impressive progress in the development of agents affecting the intracellular interplay of GABAARs. One such example is artemisinins [Figure 2B(10)]. Li et al. (2017) found that artemisinins, lactones derived from the Qinghao plant, affect pancreatic cells by binding gephyrin and modifying GABAAR signaling. Kasaragod et al. (2019) identified the artemisinin binding site within gephyrin and showed that application of artemisinins reduces gephyrin and GABAARs clustering, making artemisinins the first small molecule lead compounds for a new class of inhibitory neurotransmission modulators. Strikingly, the druggable artemisinin-binding pocket overlaps with the universal receptor binding region of gephyrin, which is critical for the interaction with GABAA and glycine receptors (Kasaragod et al., 2019). Thermodynamic and structural studies (Maric et al., 2011, 2014) identified the “hotspot” fragments of GABAA and glycine receptors that bind to gephyrin. Biomimetic optimization of the “hotspots” amino acid sequence, enhanced the affinity of the resulting peptide ligands 46,000-fold compared to the corresponding native peptides (Maric et al., 2015, 2017; Figures 2B,C). Further in vitro applications of these new super binder peptide reduced GABAAR α2 conductivity and clustering, providing evidence that GABAAR-associated proteins can be successfully targeted with modified peptides to modulate fast synaptic inhibition (Maric et al., 2017).
Targeting Non-scaffold GABAAR Associated Proteins
GABAAR trafficking is pivotal for the plasticity (Luscher et al., 2011) and the development (Lorenz-Guertin and Jacob, 2018) of inhibitory synapses, consequently, dysfunction of the GABAAR cycling is involved in various neurological disorders (Smith and Kittler, 2010; Mele et al., 2019). Noteworthy, phosphorylation of the intracellular GABAAR sites, that are involved in the trafficking of the receptors, has been identified to control receptor numbers and their concentration at synaptic sites (Comenencia-Ortiz et al., 2014; Nakamura et al., 2015), a mechanism that proves to be critical for the physiological function of inhibitory synapses (Vien et al., 2015). Therefore, targeting protein-protein interactions (PPIs) that mediate GABAAR trafficking, endocytosis, degradation or recycling, is a promising pharmacological strategy. The proposed direct protein interactors are numerous, among them are muskelin (Heisler et al., 2011), GABARAP (Wang et al., 1999), the brefeldin-A inhibited GDP/GTP exchange factor 2 (Charych et al., 2004), phospholipase C-related catalytically inactive proteins 1 and 2 (Mizokami et al., 2007), N-ethylmaleimide sensitive factor (Goto et al., 2005), neurobeachin (Nair et al., 2013), Huntingtin-associated protein 1, calcium-modulating cyclophilin ligand (Kittler et al., 2004; Yuan et al., 2008) and the clathrin adaptor protein AP2 (Kittler et al., 2005).
The AP2-GABAAR interaction rapidly modulates synaptic GABAAR numbers, inhibitory synaptic strength, neuronal excitability, and notably, affects animal behavior (Kittler et al., 2000, 2005, 2008; Tretter et al., 2009). The μ2 subunit of the clathrin adaptor protein AP2 binds with high affinity to linear and short peptide motifs within the intracellular regions of specific GABAAR subunits (Table 1). Short GABAAR derived peptides, that effectively compete with AP2 binding, were successfully used to block the receptor internalization in hippocampal neurons, increasing surface concentration of GABAAR clusters by 50% (Smith et al., 2012) and enhancing the strength of inhibitory synapses (Kittler et al., 2008). AP2 antagonists demonstrate that the modulation of GABAAR interactions with its intracellular trafficking partners is an alternative way to influence GABAergic signaling.
Perspectives
Ongoing research uncovered original, seemingly contrasting, strategies of GABAergic signaling modulation. On the one hand, ligands disrupting gephyrin-GABAAR clustering, like artemisinins or “super binding peptides,” could reduce the GABAAR synaptic concentration and function. On the other hand, peptides hampering receptor interaction with AP2 trafficking protein increased the synaptic receptor levels. In theory, these approaches could be applied together to achieve bi-directional modulation of inhibitory neurotransmission, promoting a shift in the dynamic equilibrium from phasic to tonic neuronal response.
Those new strategies of GABAergic neurotransmission modulation possess an untapped clinical potential. Agents targeting GABAAR associated scaffold or trafficking proteins could be applied wherever abnormal GABAergic activity or regulation is involved in pathogenesis. In status epilepticus patients develop a time-dependent pharmacoresistance to GABAergic agents, probably, due to GABAAR internalization (Naylor et al., 2005). In benzodiazepine tolerance linked to prolonged benzodiazepine use, neurons continuously exposed to diazepam lose postsynaptic GABAARs (Nicholson et al., 2018). Both pathologies are related to the reduction of available postsynaptic GABAARs and both could potentially be alleviated by targeting GABAAR-associated proteins. Stabilization of the gephyrin-receptor scaffolds at inhibitory postsynapses with molecules that mimic the stabilizing action of CB (Saiepour et al., 2010) could help prevent GABAAR loss and preserve inhibitory neurotransmission, alternatively, applying AP2 inhibitors could reduce GABAAR internalization and reverse the loss of postsynaptic GABAARs. Those examples illustrate the potential of GABAergic modulators as adjuvants ameliorating the effect of existing potent drugs, whereas in epilepsy or other diseases involving deregulation of inhibitory neurotransmission they could be applied as stand-alone therapeutics.
We expect that the study of GABAAR intracellular interactors, accelerated by in-silico predictions and high throughput approaches, will lead to the discovery of novel GABAergic modulators. Affinity, selectivity, bioavailability and immunogenicity of these compounds would have to be optimized for clinical applications, where peptide-based ligands could be further evolved by the introduction of unnatural amino acids, cyclization and other chemical modifications.
Microscopy is an additional intriguing application of these molecules. The enhanced affinity and specificity of the engineered peptide-based compounds allowed to pioneer their use as fluorescent probes [Figure 2B(5)], enabling live neuronal staining and visualization of inhibitory post synaptic sites with submicron resolution (Maric et al., 2017). Compact fluorescent peptides, developed from these super binding peptides, bring several advantages over conventional staining agents, namely the antibodies. In contrast to antibodies, peptide probes are live cell compatible and could provide better resolution and localization precision, since the fluorophore, owing to its small size, stays close to the target surface, reducing the linkage error. Moreover, highly affine and selective peptides could achieve stoichiometric labeling, enabling quantification of the target protein.
Here, we discussed how the targeting of GABAAR associated proteins could prove to be a versatile pharmacological strategy with clinical potential. Further, we suggested that when combined with state-of-the-art super-resolution microscopy methods, the peptide-based fluorescent probes may resolve the nanoscale architecture of synapses in unprecedented detail. We anticipate that the discovery of additional GABAAR interactors could open the way for the development of new imaging tools and alternative pharmacological approaches.
Author Contributions
VK and HM wrote the manuscript and prepared the figures.
Funding
This work was supported by Association of the Chemical Industry (Verband der Chemischen Industrie).
Conflict of Interest Statement
The authors declare that the research was conducted in the absence of any commercial or financial relationships that could be construed as a potential conflict of interest.
Acknowledgments
We thank Drs Vikram Babu Kasaragod and Hermann Schindelin for helpful suggestions, discussions and editing.
References
Ali Rodriguez, R., Joya, C., and Hines, R. M. (2018). Common ribs of inhibitory synaptic dysfunction in the umbrella of neurodevelopmental disorders. Front. Mol. Neurosci. 11:132. doi: 10.3389/fnmol.2018.00132
Alvarez, F. J. (2017). Gephyrin and the regulation of synaptic strength and dynamics at glycinergic inhibitory synapses. Brain Res. Bull. 129, 50–65. doi: 10.1016/j.brainresbull.2016.09.003
Bach, A., Clausen, B. H., Moller, M., Vestergaard, B., Chi, C. N., Round, A., et al. (2012). A high-affinity, dimeric inhibitor of PSD-95 bivalently interacts with PDZ1–2 and protects against ischemic brain damage. Proc. Natl. Acad. Sci. U S A 109, 3317–3322. doi: 10.1073/pnas.1113761109
Bach, A., Eildal, J. N., Stuhr-Hansen, N., Deeskamp, R., Gottschalk, M., Pedersen, S. W., et al. (2011). Cell-permeable and plasma-stable peptidomimetic inhibitors of the postsynaptic density-95/N-methyl-D-aspartate receptor interaction. J. Med. Chem. 54, 1333–1346. doi: 10.1021/jm1013924
Battaglia, S., Renner, M., Russeau, M., Come, E., Tyagarajan, S. K., and Lévi, S. (2018). Activity-dependent inhibitory synapse scaling is determined by gephyrin phosphorylation and subsequent regulation of GABAA receptor diffusion. eNeuro 5:ENEURO.0203-17.2017. doi: 10.1523/eneuro.0203-17.2017
Brady, M. L., and Jacob, T. C. (2015). Synaptic localization of α5 GABAA receptors via gephyrin interaction regulates dendritic outgrowth and spine maturation. Dev. Neurobiol. 75, 1241–1251. doi: 10.1002/dneu.22280
Charych, E. I., Yu, W., Li, R., Serwanski, D. R., Miralles, C. P., Li, X., et al. (2004). A four PDZ domain-containing splice variant form of GRIP1 is localized in GABAergic and glutamatergic synapses in the brain. J. Biol. Chem. 279, 38978–38990. doi: 10.1074/jbc.m405786200
Chen, J. L., Villa, K. L., Cha, J. W., So, P. T., Kubota, Y., and Nedivi, E. (2012). Clustered dynamics of inhibitory synapses and dendritic spines in the adult neocortex. Neuron 74, 361–373. doi: 10.1016/j.neuron.2012.02.030
Chiou, T.-T., Long, P., Schumann-Gillett, A., Kanamarlapudi, V., Haas, S. A., Harvey, K., et al. (2019). Mutation p.R356Q in the collybistin phosphoinositide binding site is associated with mild intellectual disability. Front. Mol. Neurosci. 12:60. doi: 10.3389/fnmol.2019.00060
Choii, G., and Ko, J. (2015). Gephyrin: a central GABAergic synapse organizer. Exp. Mol. Med. 47:e158. doi: 10.1038/emm.2015.5
Comenencia-Ortiz, E., Moss, S. J., and Davies, P. A. (2014). Phosphorylation of GABAA receptors influences receptor trafficking and neurosteroid actions. Psychopharmacology 231, 3453–3465. doi: 10.1007/s00213-014-3617-z
Darmani, G., Zipser, C. M., Bohmer, G. M., Deschet, K., Muller-Dahlhaus, F., Belardinelli, P., et al. (2016). Effects of the selective α5-GABAAR antagonist S44819 on excitability in the human brain: a TMS-EMG and TMS-EEG phase I study. J. Neurosci. 36, 12312–12320. doi: 10.1523/JNEUROSCI.1689-16.2016
Davenport, E. C., Pendolino, V., Kontou, G., Mcgee, T. P., Sheehan, D. F., López-Doménech, G., et al. (2017). An essential role for the tetraspanin LHFPL4 in the cell-type-specific targeting and clustering of synaptic GABAA receptors. Cell Rep. 21, 70–83. doi: 10.1016/j.celrep.2017.09.025
de Jonge, J. C., Vinkers, C. H., Hulshoff Pol, H. E., and Marsman, A. (2017). GABAergic mechanisms in schizophrenia: linking postmortem and in vivo studies. Front. Psychiatry 8:118. doi: 10.3389/fpsyt.2017.00118
Essrich, C., Lorez, M., Benson, J. A., Fritschy, J. M., and Luscher, B. (1998). Postsynaptic clustering of major GABAA receptor subtypes requires the γ 2 subunit and gephyrin. Nat. Neurosci. 1, 563–571. doi: 10.1038/2798
Feng, G., Tintrup, H., Kirsch, J., Nichol, M. C., Kuhse, J., Betz, H., et al. (1998). Dual requirement for gephyrin in glycine receptor clustering and molybdoenzyme activity. Science 282, 1321–1324. doi: 10.1126/science.282.5392.1321
Flores, C. E., Nikonenko, I., Mendez, P., Fritschy, J. M., Tyagarajan, S. K., and Muller, D. (2015). Activity-dependent inhibitory synapse remodeling through gephyrin phosphorylation. Proc. Natl. Acad. Sci. U S A 112, E65–E72. doi: 10.1073/pnas.1411170112
Fritschy, J. M., and Panzanelli, P. (2014). GABA receptors and plasticity of inhibitory neurotransmission in the central nervous system. Eur. J. Neurosci. 39, 1845–1865. doi: 10.1111/ejn.12534
Goto, H., Terunuma, M., Kanematsu, T., Misumi, Y., Moss, S. J., and Hirata, M. (2005). Direct interaction of N-ethylmaleimide-sensitive factor with GABAA receptor β subunits. Mol. Cell. Neurosci. 30, 197–206. doi: 10.1016/j.mcn.2005.07.006
Govindpani, K., Calvo-Flores Guzman, B., Vinnakota, C., Waldvogel, H. J., Faull, R. L., and Kwakowsky, A. (2017). Towards a better understanding of GABAergic remodeling in Alzheimer’s disease. Int. J. Mol. Sci. 18:E1813. doi: 10.3390/ijms18081813
Groeneweg, F. L., Trattnig, C., Kuhse, J., Nawrotzki, R. A., and Kirsch, J. (2018). Gephyrin: a key regulatory protein of inhibitory synapses and beyond. Histochem. Cell Biol. 150, 489–508. doi: 10.1007/s00418-018-1725-2
Gross, G. G., Straub, C., Perez-Sanchez, J., Dempsey, W. P., Junge, J. A., Roberts, R. W., et al. (2016). An E3-ligase-based method for ablating inhibitory synapses. Nat. Methods 13, 673–678. doi: 10.1038/nmeth.3894
Hammond, M. C., Harris, B. Z., Lim, W. A., and Bartlett, P. A. (2006). β strand peptidomimetics as potent PDZ domain ligands. Chem. Biol. 13, 1247–1251. doi: 10.1016/j.chembiol.2006.11.010
Harvey, K., Duguid, I. C., Alldred, M. J., Beatty, S. E., Ward, H., Keep, N. H., et al. (2004). The GDP-GTP exchange factor collybistin: an essential determinant of neuronal gephyrin clustering. J. Neurosci. 24, 5816–5826. doi: 10.1523/JNEUROSCI.1184-04.2004
Hausrat, T. J., Muhia, M., Gerrow, K., Thomas, P., Hirdes, W., Tsukita, S., et al. (2015). Radixin regulates synaptic GABAA receptor density and is essential for reversal learning and short-term memory. Nat. Commun. 6:6872. doi: 10.1038/ncomms7872
Heisler, F. F., Loebrich, S., Pechmann, Y., Maier, N., Zivkovic, A. R., Tokito, M., et al. (2011). Muskelin regulates actin filament- and microtubule-based GABAA receptor transport in neurons. Neuron 70, 66–81. doi: 10.1016/j.neuron.2011.03.008
Hines, R. M., Maric, H. M., Hines, D. J., Modgil, A., Panzanelli, P., Nakamura, Y., et al. (2018). Developmental seizures and mortality result from reducing GABAA receptor α2-subunit interaction with collybistin. Nat. Commun. 9:3130. doi: 10.1038/s41467-018-05481-1
Kang, Y., Ge, Y., Cassidy, R. M., Lam, V., Luo, L., Moon, K. M., et al. (2014). A combined transgenic proteomic analysis and regulated trafficking of neuroligin-2. J. Biol. Chem. 289, 29350–29364. doi: 10.1074/jbc.m114.549279
Kasaragod, V. B., Hausrat, T. J., Schaefer, N., Kuhn, M., Christensen, N. R., Tessmer, I., et al. (2019). Elucidating the molecular basis for inhibitory neurotransmission regulation by artemisinins. Neuron 101, 673.e11–689.e11. doi: 10.1016/j.neuron.2019.01.001
Kasaragod, V. B., and Schindelin, H. (2018). Structure-function relationships of glycine and GABAA receptors and their interplay with the scaffolding protein gephyrin. Front. Mol. Neurosci. 11:317. doi: 10.3389/fnmol.2018.00317
Kawaguchi, K., Yoshida, S., Hatano, R., and Asano, S. (2017). Pathophysiological roles of ezrin/radixin/moesin proteins. Biol. Pharm. Bull. 40, 381–390. doi: 10.1248/bpb.b16-01011
Kins, S., Betz, H., and Kirsch, J. (2000). Collybistin, a newly identified brain-specific GEF, induces submembrane clustering of gephyrin. Nat. Neurosci. 3, 22–29. doi: 10.1038/71096
Kitano, K., Yusa, F., and Hakoshima, T. (2006). Structure of dimerized radixin FERM domain suggests a novel masking motif in C-terminal residues 295-304. Acta Crystallogr. Sect. F Struct. Biol. Cryst. Commun. 62, 340–345. doi: 10.1107/s1744309106010062
Kittler, J. T., Arancibia-Carcamo, I. L., and Moss, S. J. (2004). Association of GRIP1 with a GABAA receptor associated protein suggests a role for GRIP1 at inhibitory synapses. Biochem. Pharmacol. 68, 1649–1654. doi: 10.1016/j.bcp.2004.07.028
Kittler, J. T., Chen, G., Honing, S., Bogdanov, Y., Mcainsh, K., Arancibia-Carcamo, I. L., et al. (2005). Phospho-dependent binding of the clathrin AP2 adaptor complex to GABAA receptors regulates the efficacy of inhibitory synaptic transmission. Proc. Natl. Acad. Sci. U S A 102, 14871–14876. doi: 10.1073/pnas.0506653102
Kittler, J. T., Chen, G., Kukhtina, V., Vahedi-Faridi, A., Gu, Z., Tretter, V., et al. (2008). Regulation of synaptic inhibition by phospho-dependent binding of the AP2 complex to a YECL motif in the GABAA receptor γ2 subunit. Proc. Natl. Acad. Sci. U S A 105, 3616–3621. doi: 10.1073/pnas.0707920105
Kittler, J. T., Delmas, P., Jovanovic, J. N., Brown, D. A., Smart, T. G., and Moss, S. J. (2000). Constitutive endocytosis of GABAA receptors by an association with the adaptin AP2 complex modulates inhibitory synaptic currents in hippocampal neurons. J. Neurosci. 20, 7972–7977. doi: 10.1523/jneurosci.20-21-07972.2000
Kneussel, M., Brandstatter, J. H., Laube, B., Stahl, S., Muller, U., and Betz, H. (1999). Loss of postsynaptic GABAA receptor clustering in gephyrin-deficient mice. J. Neurosci. 19, 9289–9297. doi: 10.1523/jneurosci.19-21-09289.1999
Kowalczyk, S., Winkelmann, A., Smolinsky, B., Forstera, B., Neundorf, I., Schwarz, G., et al. (2013). Direct binding of GABAA receptor β2 and β3 subunits to gephyrin. Eur. J. Neurosci. 37, 544–554. doi: 10.1111/ejn.12078
Krueger-Burg, D., Papadopoulos, T., and Brose, N. (2017). Organizers of inhibitory synapses come of age. Curr. Opin. Neurobiol. 45, 66–77. doi: 10.1016/j.conb.2017.04.003
Laverty, D., Desai, R., Uchański, T., Masiulis, S., Stec, W. J., Malinauskas, T., et al. (2019). Cryo-EM structure of the human α1β3γ2 GABAA receptor in a lipid bilayer. Nature 565, 516–520. doi: 10.1038/s41586-018-0833-4
Lee, V., and Maguire, J. (2014). The impact of tonic GABAA receptor-mediated inhibition on neuronal excitability varies across brain region and cell type. Front. Neural Circuits 8:3. doi: 10.3389/fncir.2014.00003
Letellier, M., Sziber, Z., Chamma, I., Saphy, C., Papasideri, I., Tessier, B., et al. (2018). A unique intracellular tyrosine in neuroligin-1 regulates AMPA receptor recruitment during synapse differentiation and potentiation. Nat. Commun. 9:3979. doi: 10.1038/s41467-018-06220-2
Li, J., Casteels, T., Frogne, T., Ingvorsen, C., Honore, C., Courtney, M., et al. (2017). Artemisinins target GABAA receptor signaling and impair α cell identity. Cell 168, 86.e15–100.e15. doi: 10.1016/j.cell.2016.11.010
Loebrich, S., Bahring, R., Katsuno, T., Tsukita, S., and Kneussel, M. (2006). Activated radixin is essential for GABAA receptor α5 subunit anchoring at the actin cytoskeleton. EMBO J. 25, 987–999. doi: 10.1038/sj.emboj.7600995
Long, P., May, M. M., James, V. M., Granno, S., Johnson, J. P., Tarpey, P., et al. (2016). Missense mutation R338W in ARHGEF9 in a family with X-linked intellectual disability with variable macrocephaly and macro-orchidism. Front. Mol. Neurosci. 8:83. doi: 10.3389/fnmol.2015.00083
Lorenz-Guertin, J. M., and Jacob, T. C. (2018). GABA type a receptor trafficking and the architecture of synaptic inhibition. Dev. Neurobiol. 78, 238–270. doi: 10.1002/dneu.22536
Löscher, W., and Rogawski, M. A. (2012). How theories evolved concerning the mechanism of action of barbiturates. Epilepsia 53, 12–25. doi: 10.1111/epi.12025
Lu, W., Bromley-Coolidge, S., and Li, J. (2017). Regulation of GABAergic synapse development by postsynaptic membrane proteins. Brain Res. Bull. 129, 30–42. doi: 10.1016/j.brainresbull.2016.07.004
Ludolphs, M., Schneeberger, D., Soykan, T., Schafer, J., Papadopoulos, T., Brose, N., et al. (2016). Specificity of collybistin-phosphoinositide interactions: impact of the individual protein domains. J. Biol. Chem. 291, 244–254. doi: 10.1074/jbc.m115.673400
Luscher, B., Fuchs, T., and Kilpatrick, C. L. (2011). GABAA receptor trafficking-mediated plasticity of inhibitory synapses. Neuron 70, 385–409. doi: 10.1016/j.neuron.2011.03.024
Maher, M. P., Matta, J. A., Gu, S., Seierstad, M., and Bredt, D. S. (2017). Getting a handle on neuropharmacology by targeting receptor-associated proteins. Neuron 96, 989–1001. doi: 10.1016/j.neuron.2017.10.001
Maric, H. M., Hausrat, T. J., Neubert, F., Dalby, N. O., Doose, S., Sauer, M., et al. (2017). Gephyrin-binding peptides visualize postsynaptic sites and modulate neurotransmission. Nat. Chem. Biol. 13, 153–160. doi: 10.1038/nchembio.2246
Maric, H. M., Kasaragod, V. B., Haugaard-Kedstrom, L., Hausrat, T. J., Kneussel, M., Schindelin, H., et al. (2015). Design and synthesis of high-affinity dimeric inhibitors targeting the interactions between gephyrin and inhibitory neurotransmitter receptors. Angew. Chem. Int. Ed Engl. 54, 490–494. doi: 10.1002/anie.201409043
Maric, H. M., Kasaragod, V. B., Hausrat, T. J., Kneussel, M., Tretter, V., Stromgaard, K., et al. (2014). Molecular basis of the alternative recruitment of GABAA versus glycine receptors through gephyrin. Nat. Commun. 5:5767. doi: 10.1038/ncomms6767
Maric, H. M., Mukherjee, J., Tretter, V., Moss, S. J., and Schindelin, H. (2011). Gephyrin-mediated γ-aminobutyric acid type A and glycine receptor clustering relies on a common binding site. J. Biol. Chem. 286, 42105–42114. doi: 10.1074/jbc.m111.303412
Masiulis, S., Desai, R., Uchański, T., Serna Martin, I., Laverty, D., Karia, D., et al. (2019). GABAA receptor signalling mechanisms revealed by structural pharmacology. Nature 565, 454–459. doi: 10.1038/s41586-018-0832-5
Mayer, S., Kumar, R., Jaiswal, M., Soykan, T., Ahmadian, M. R., Brose, N., et al. (2013). Collybistin activation by GTP-TC10 enhances postsynaptic gephyrin clustering and hippocampal GABAergic neurotransmission. Proc. Natl. Acad. Sci. U S A 110, 20795–20800. doi: 10.1073/pnas.1309078110
Mele, M., Costa, R. O., and Duarte, C. B. (2019). Alterations in GABAA-receptor trafficking and synaptic dysfunction in brain disorders. Front. Cell. Neurosci. 13:77. doi: 10.3389/fncel.2019.00077
Miller, P. S., and Aricescu, A. R. (2014). Crystal structure of a human GABAA receptor. Nature 512, 270–275. doi: 10.1038/nature13293
Miller, P. S., Scott, S., Masiulis, S., De Colibus, L., Pardon, E., Steyaert, J., et al. (2017). Structural basis for GABAA receptor potentiation by neurosteroids. Nat. Struct. Mol. Biol. 24, 986–992. doi: 10.1038/nsmb.3484
Mizokami, A., Kanematsu, T., Ishibashi, H., Yamaguchi, T., Tanida, I., Takenaka, K., et al. (2007). Phospholipase C-related inactive protein is involved in trafficking of γ2 subunit-containing GABAA receptors to the cell surface. J. Neurosci. 27, 1692–1701. doi: 10.1523/jneurosci.3155-06.2007
Mody, I. (2019). GABAAR modulator for postpartum depression. Cell 176:1. doi: 10.1016/j.cell.2018.12.016
Mody, I., and Pearce, R. A. (2004). Diversity of inhibitory neurotransmission through GABAA receptors. Trends Neurosci. 27, 569–575. doi: 10.1016/j.tins.2004.07.002
Mukherjee, J., Kretschmannova, K., Gouzer, G., Maric, H. M., Ramsden, S., Tretter, V., et al. (2011). The residence time of GABAARs at inhibitory synapses is determined by direct binding of the receptor α1 subunit to gephyrin. J. Neurosci. 31, 14677–14687. doi: 10.1523/jneurosci.2001-11.2011
Nair, R., Lauks, J., Jung, S., Cooke, N. E., De Wit, H., Brose, N., et al. (2013). Neurobeachin regulates neurotransmitter receptor trafficking to synapses. J. Cell Biol. 200, 61–80. doi: 10.1083/jcb.201207113
Nakamura, Y., Darnieder, L. M., Deeb, T. Z., and Moss, S. J. (2015). Regulation of GABAARs by phosphorylation. Adv. Pharmacol. 72, 97–146. doi: 10.1016/bs.apha.2014.11.008
Nakamura, Y., Morrow, D. H., Modgil, A., Huyghe, D., Deeb, T. Z., Lumb, M. J., et al. (2016). Proteomic characterization of inhibitory synapses using a novel pHluorin-tagged γ-aminobutyric acid receptor, type A (GABAA), α2 subunit knock-in mouse. J. Biol. Chem. 291, 12394–12407. doi: 10.1074/jbc.m116.724443
Naylor, D. E., Liu, H., and Wasterlain, C. G. (2005). Trafficking of GABAA receptors, loss of inhibition, and a mechanism for pharmacoresistance in status epilepticus. J. Neurosci. 25, 7724–7733. doi: 10.1523/jneurosci.4944-04.2005
Nguyen, Q. A., Horn, M. E., and Nicoll, R. A. (2016). Distinct roles for extracellular and intracellular domains in neuroligin function at inhibitory synapses. Elife 5:e19236. doi: 10.7554/elife.19236
Nicholson, M. W., Sweeney, A., Pekle, E., Alam, S., Ali, A. B., Duchen, M., et al. (2018). Diazepam-induced loss of inhibitory synapses mediated by PLCdelta/ Ca2+/calcineurin signalling downstream of GABAA receptors. Mol. Psychiatry 23, 1851–1867. doi: 10.1038/s41380-018-0100-y
Olsen, R. W. (2018). GABAA receptor: Positive and negative allosteric modulators. Neuropharmacology 136, 10–22. doi: 10.1016/j.neuropharm.2018.01.036
Olsen, R. W., and Sieghart, W. (2009). GABA A receptors: subtypes provide diversity of function and pharmacology. Neuropharmacology 56, 141–148. doi: 10.1016/j.neuropharm.2008.07.045
Paarmann, I., Schmitt, B., Meyer, B., Karas, M., and Betz, H. (2006). Mass spectrometric analysis of glycine receptor-associated gephyrin splice variants. J. Biol. Chem. 281, 34918–34925. doi: 10.1074/jbc.m607764200
Palma, E., Ruffolo, G., Cifelli, P., Roseti, C., Vliet, E. A. V., and Aronica, E. (2017). Modulation of GABAA receptors in the treatment of epilepsy. Curr. Pharm. Des. 23, 5563–5568. doi: 10.2174/1381612823666170809100230
Papadopoulos, T., Eulenburg, V., Reddy-Alla, S., Mansuy, I. M., Li, Y., and Betz, H. (2008). Collybistin is required for both the formation and maintenance of GABAergic postsynapses in the hippocampus. Mol. Cell. Neurosci. 39, 161–169. doi: 10.1016/j.mcn.2008.06.006
Papadopoulos, T., Korte, M., Eulenburg, V., Kubota, H., Retiounskaia, M., Harvey, R. J., et al. (2007). Impaired GABAergic transmission and altered hippocampal synaptic plasticity in collybistin-deficient mice. EMBO J. 26, 3888–3899. doi: 10.1038/sj.emboj.7601819
Pennacchietti, F., Vascon, S., Nieus, T., Rosillo, C., Das, S., Tyagarajan, S. K., et al. (2017). Nanoscale molecular reorganization of the inhibitory postsynaptic density is a determinant of GABAergic synaptic potentiation. J. Neurosci. 37, 1747–1756. doi: 10.1523/JNEUROSCI.0514-16.2016
Pettem, K. L., Yokomaku, D., Takahashi, H., Ge, Y., and Craig, A. M. (2013). Interaction between autism-linked MDGAs and neuroligins suppresses inhibitory synapse development. J. Cell Biol. 200, 321–336. doi: 10.1083/jcb.201206028
Pfeiffer, F., Graham, D., and Betz, H. (1982). Purification by affinity chromatography of the glycine receptor of rat spinal cord. J. Biol. Chem. 257, 9389–9393.
Poulopoulos, A., Aramuni, G., Meyer, G., Soykan, T., Hoon, M., Papadopoulos, T., et al. (2009). Neuroligin 2 drives postsynaptic assembly at perisomatic inhibitory synapses through gephyrin and collybistin. Neuron 63, 628–642. doi: 10.1016/j.neuron.2009.08.023
Reddy-Alla, S., Schmitt, B., Birkenfeld, J., Eulenburg, V., Dutertre, S., Bohringer, C., et al. (2010). PH-domain-driven targeting of collybistin but not Cdc42 activation is required for synaptic gephyrin clustering. Eur. J. Neurosci. 31, 1173–1184. doi: 10.1111/j.1460-9568.2010.07149.x
Saiepour, L., Fuchs, C., Patrizi, A., Sassoè-Pognetto, M., Harvey, R. J., and Harvey, K. (2010). Complex role of collybistin and gephyrin in GABAA receptor clustering. J. Biol. Chem. 285, 29623–29631. doi: 10.1074/jbc.M110.121368
Sainlos, M., Tigaret, C., Poujol, C., Olivier, N. B., Bard, L., Breillat, C., et al. (2011). Biomimetic divalent ligands for the acute disruption of synaptic AMPAR stabilization. Nat. Chem. Biol. 7, 81–91. doi: 10.1038/nchembio.498
Sancar, F., and Czajkowski, C. (2011). Allosteric modulators induce distinct movements at the GABA-binding site interface of the GABA-A receptor. Neuropharmacology 60, 520–528. doi: 10.1016/j.neuropharm.2010.11.009
Smith, K. R., and Kittler, J. T. (2010). The cell biology of synaptic inhibition in health and disease. Curr. Opin. Neurobiol. 20, 550–556. doi: 10.1016/j.conb.2010.06.001
Smith, K. R., Muir, J., Rao, Y., Browarski, M., Gruenig, M. C., Sheehan, D. F., et al. (2012). Stabilization of GABAA receptors at endocytic zones is mediated by an AP2 binding motif within the GABAA receptor β3 subunit. J. Neurosci. 32, 2485–2498. doi: 10.1523/JNEUROSCI.1622-11.2011
Soykan, T., Schneeberger, D., Tria, G., Buechner, C., Bader, N., Svergun, D., et al. (2014). A conformational switch in collybistin determines the differentiation of inhibitory postsynapses. EMBO J. 33, 2113–2133. doi: 10.15252/embj.201488143
Specht, C. G. (2019). Fractional occupancy of synaptic binding sites and the molecular plasticity of inhibitory synapses. Neuropharmacology doi: 10.1016/j.neuropharm.2019.01.008 [Epub ahead of print].
Specht, C. G., Grunewald, N., Pascual, O., Rostgaard, N., Schwarz, G., and Triller, A. (2011). Regulation of glycine receptor diffusion properties and gephyrin interactions by protein kinase C. EMBO J. 30, 3842–3853. doi: 10.1038/emboj.2011.276
Specht, C. G., Izeddin, I., Rodriguez, P. C., El Beheiry, M., Rostaing, P., Darzacq, X., et al. (2013). Quantitative nanoscopy of inhibitory synapses: counting gephyrin molecules and receptor binding sites. Neuron 79, 308–321. doi: 10.1016/j.neuron.2013.05.013
Takai, Y., Kitano, K., Terawaki, S., Maesaki, R., and Hakoshima, T. (2007). Crystallographic characterization of the radixin FERM domain bound to the cytoplasmic tails of adhesion molecules CD43 and PSGL-1. Acta Crystallogr. Sect. F Struct. Biol. Cryst. Commun. 63, 49–51. doi: 10.1107/s1744309106054145
Terawaki, S., Kitano, K., Aoyama, M., and Hakoshima, T. (2008). Crystallographic characterization of the radixin FERM domain bound to the cytoplasmic tail of membrane-type 1 matrix metalloproteinase (MT1-MMP). Acta Crystallogr. Sect. F Struct. Biol. Cryst. Commun. 64, 911–913. doi: 10.1107/s1744309108026869
Terawaki, S., Kitano, K., and Hakoshima, T. (2007). Structural basis for type II membrane protein binding by ERM proteins revealed by the radixin-neutral endopeptidase 24.11 (NEP) complex. J. Biol. Chem. 282, 19854–19862. doi: 10.1074/jbc.m609232200
Tretter, V., Jacob, T. C., Mukherjee, J., Fritschy, J. M., Pangalos, M. N., and Moss, S. J. (2008). The clustering of GABAA receptor subtypes at inhibitory synapses is facilitated via the direct binding of receptor α 2 subunits to gephyrin. J. Neurosci. 28, 1356–1365. doi: 10.1523/JNEUROSCI.5050-07.2008
Tretter, V., Kerschner, B., Milenkovic, I., Ramsden, S. L., Ramerstorfer, J., Saiepour, L., et al. (2011). Molecular basis of the γ-aminobutyric acid A receptor α3 subunit interaction with the clustering protein gephyrin. J. Biol. Chem. 286, 37702–37711. doi: 10.1074/jbc.m111.291336
Tretter, V., Mukherjee, J., Maric, H. M., Schindelin, H., Sieghart, W., and Moss, S. J. (2012). Gephyrin, the enigmatic organizer at GABAergic synapses. Front. Cell. Neurosci. 6:23. doi: 10.3389/fncel.2012.00023
Tretter, V., Revilla-Sanchez, R., Houston, C., Terunuma, M., Havekes, R., Florian, C., et al. (2009). Deficits in spatial memory correlate with modified γ-aminobutyric acid type A receptor tyrosine phosphorylation in the hippocampus. Proc. Natl. Acad. Sci. U S A 106, 20039–20044. doi: 10.1073/pnas.0908840106
Tyagarajan, S. K., and Fritschy, J. M. (2014). Gephyrin: a master regulator of neuronal function? Nat. Rev. Neurosci. 15, 141–156. doi: 10.1038/nrn3670
Tyagarajan, S. K., Ghosh, H., Harvey, K., and Fritschy, J. M. (2011a). Collybistin splice variants differentially interact with gephyrin and Cdc42 to regulate gephyrin clustering at GABAergic synapses. J. Cell Sci. 124, 2786–2796. doi: 10.1242/jcs.086199
Tyagarajan, S. K., Ghosh, H., Yevenes, G. E., Nikonenko, I., Ebeling, C., Schwerdel, C., et al. (2011b). Regulation of GABAergic synapse formation and plasticity by GSK3β-dependent phosphorylation of gephyrin. Proc. Natl. Acad. Sci. U S A 108, 379–384. doi: 10.1073/pnas.1011824108
Tyagarajan, S. K., Ghosh, H., Yevenes, G. E., Imanishi, S. Y., Zeilhofer, H. U., Gerrits, B., et al. (2013). Extracellular signal-regulated kinase and glycogen synthase kinase 3β regulate gephyrin postsynaptic aggregation and GABAergic synaptic function in a calpain-dependent mechanism. J. Biol. Chem. 288, 9634–9647. doi: 10.1074/jbc.m112.442616
Uezu, A., Kanak, D. J., Bradshaw, T. W., Soderblom, E. J., Catavero, C. M., Burette, A. C., et al. (2016). Identification of an elaborate complex mediating postsynaptic inhibition. Science 353, 1123–1129. doi: 10.1126/science.aag0821
Varoqueaux, F., Jamain, S., and Brose, N. (2004). Neuroligin 2 is exclusively localized to inhibitory synapses. Eur. J. Cell Biol. 83, 449–456. doi: 10.1078/0171-9335-00410
Vien, T. N., Modgil, A., Abramian, A. M., Jurd, R., Walker, J., Brandon, N. J., et al. (2015). Compromising the phosphodependent regulation of the GABAAR β3 subunit reproduces the core phenotypes of autism spectrum disorders. Proc. Natl. Acad. Sci. U S A 112, 14805–14810. doi: 10.1073/pnas.1514657112
Villa, K. L., Berry, K. P., Subramanian, J., Cha, J. W., Oh, W. C., Kwon, H. B., et al. (2016). Inhibitory synapses are repeatedly assembled and removed at persistent sites in vivo. Neuron 89, 756–769. doi: 10.1016/j.neuron.2016.01.010
Wang, H., Bedford, F. K., Brandon, N. J., Moss, S. J., and Olsen, R. W. (1999). GABAA-receptor-associated protein links GABAA receptors and the cytoskeleton. Nature 397, 69–72. doi: 10.1038/16264
Wang, Y. C., Dzyubenko, E., Sanchez-Mendoza, E. H., Sardari, M., Silva de Carvalho, T., Doeppner, T. R., et al. (2018). Postacute delivery of GABAA α5 antagonist promotes postischemic neurological recovery and peri-infarct brain remodeling. Stroke 49, 2495–2503. doi: 10.1161/strokeaha.118.021378
Wisden, W., and Seeburg, P. H. (1992). GABAA receptor channels: from subunits to functional entities. Curr. Opin. Neurobiol. 2, 263–269. doi: 10.1016/0959-4388(92)90113-y
Yamasaki, T., Hoyos-Ramirez, E., Martenson, J. S., Morimoto-Tomita, M., and Tomita, S. (2017). GARLH family proteins stabilize GABAA receptors at synapses. Neuron 93, 1138.e6–1152.e6. doi: 10.1016/j.neuron.2017.02.023
Yogesha, S. D., Sharff, A. J., Giovannini, M., Bricogne, G., and Izard, T. (2011). Unfurling of the band 4.1, ezrin, radixin, moesin (FERM) domain of the merlin tumor suppressor. Protein Sci. 20, 2113–2120. doi: 10.1002/pro.751
Yuan, X., Yao, J., Norris, D., Tran, D. D., Bram, R. J., Chen, G., et al. (2008). Calcium-modulating cyclophilin ligand regulates membrane trafficking of postsynaptic GABAA receptors. Mol. Cell. Neurosci. 38, 277–289. doi: 10.1016/j.mcn.2008.03.002
Zacchi, P., Dreosti, E., Visintin, M., Moretto-Zita, M., Marchionni, I., Cannistraci, I., et al. (2008). Gephyrin selective intrabodies as a new strategy for studying inhibitory receptor clustering. J. Mol. Neurosci. 34, 141–148. doi: 10.1007/s12031-007-9018-6
Keywords: GABAA receptors, gephyrin, collybistin, protein-protein interaction (PPI), super resolution microscopy, fluorescent probes, dimeric peptide, peptide inhibitor design
Citation: Khayenko V and Maric HM (2019) Targeting GABAAR-Associated Proteins: New Modulators, Labels and Concepts. Front. Mol. Neurosci. 12:162. doi: 10.3389/fnmol.2019.00162
Received: 31 March 2019; Accepted: 12 June 2019;
Published: 26 June 2019.
Edited by:
Andrea Barberis, Istituto Italiano di Tecnologia, ItalyReviewed by:
Christian G. Specht, Institut National de la Santé et de la Recherche Médicale (INSERM), FranceJosef Kittler, University College London, United Kingdom
Copyright © 2019 Khayenko and Maric. This is an open-access article distributed under the terms of the Creative Commons Attribution License (CC BY). The use, distribution or reproduction in other forums is permitted, provided the original author(s) and the copyright owner(s) are credited and that the original publication in this journal is cited, in accordance with accepted academic practice. No use, distribution or reproduction is permitted which does not comply with these terms.
*Correspondence: Hans Michael Maric, aGFucy5tYXJpY0B1bmktd3VlcnpidXJnLmRl