- 1Department of Clinical and Experimental Medicine, University of Messina, Messina, Italy
- 2Department of Biomedical and Biotechnological Sciences, University of Catania, Catania, Italy
- 3Oasi Research Institute, IRCCS, Troina, Italy
- 4Institute of Neurological Sciences (ISN), National Research Council (CNR), Catania, Italy
- 5Department of Pharmacy — Drug Sciences, University of Bari, Bari, Italy
We have previously demonstrated that activation of serotonin 5-HT7 receptors (5-HT7R) reverses metabotropic glutamate receptor-mediated long term depression (mGluR-LTD) in the hippocampus of wild-type (WT) and Fmr1 Knockout (KO) mice, a model of Fragile X Syndrome (FXS) in which mGluR-LTD is abnormally enhanced. Here, we have investigated intracellular mechanisms underlying the effect of 5-HT7R activation using patch clamp on hippocampal slices. Furthermore, we have tested whether in vivo administration of LP-211, a selective 5-HT7R agonist, can rescue learning and behavior in Fmr1 KO mice. In the presence of an adenylate cyclase blocker, mGluR-LTD was slightly enhanced in WT and therefore the difference between mGluR-LTD in WT and Fmr1 KO slices was no longer present. Conversely, activation of adenylate cyclase by either forskolin or Pituitary Adenylate Cyclase Activating Polypeptide (PACAP) completely reversed mGluR-LTD in WT and Fmr1 KO. 5-HT7R activation reversed mGluR-LTD in WT and corrected exaggerated mGluR-LTD in Fmr1 KO; this effect was abolished by blockade of either adenylate cyclase or protein kinase A (PKA). Exposure of hippocampal slices to LP-211 caused an increased phosphorylation of extracellular signal regulated kinase (ERK), an intracellular effector involved in mGluR-LTD, in WT mice. Conversely, this effect was barely detectable in Fmr1 KO mice, suggesting that 5-HT7R-mediated reversal of mGluR-LTD does not require ERK stimulation. Finally, an acute in vivo administration of LP-211 improved novel object recognition (NOR) performance in WT and Fmr1 KO mice and reduced stereotyped behavior in Fmr1 KO mice. Our results indicate that mGluR-LTD in WT and Fmr1 KO slices is bidirectionally modulated in conditions of either reduced or enhanced cAMP formation. Activation of 5-HT7 receptors reverses mGluR-LTD by activation of the cAMP/PKA intracellular pathway. Importantly, a systemic administration of a 5-HT7R agonist to Fmr1 KO mice corrected learning deficits and repetitive behavior. We suggest that selective 5-HT7R agonists might become novel pharmacological tools for FXS therapy.
Introduction
Fragile X Syndrome (FXS), the most common single-gene cause of intellectual disability, autism and epilepsy (Garber et al., 2008), is caused by transcriptional silencing of the FMR1 gene coding for Fragile X Mental Retardation Protein (FMRP), an mRNA-binding protein that regulates translation of several synaptic proteins (Pfeiffer and Huber, 2009). An abnormal morphology and density of dendritic spines was observed in the brain cortex of FXS patients (Irwin et al., 2001) and in the cortex and hippocampus of Fmr1 knockout (KO) mice (Comery et al., 1997; Nimchinsky et al., 2001; Grossman et al., 2010), a model of FXS, suggesting a dysfunction of excitatory synaptic transmission in several brain regions. Accordingly, studies on the mouse model of FXS revealed altered synaptic plasticity mediated by metabotropic glutamate receptors (mGluRs; Pfeiffer and Huber, 2009). Metabotropic glutamate receptor-mediated long term depression (mGluR-LTD), a form of plasticity playing a crucial role in cognition and in behavioral flexibility (Luscher and Huber, 2010; Sanderson et al., 2016), is pathologically enhanced in the hippocampus of Fmr1 KO mice (Huber et al., 2002) and is regarded as the electrophysiological readout of synaptic malfunction in the mouse model of FXS (Bear et al., 2004; Waung and Huber, 2009).
In the last few years, a decrease of cyclic AMP (cAMP) has been proposed to be involved in FXS pathogenesis (Kelley et al., 2008). Different data support a “cAMP theory” of FXS: early observations indicated a reduction of basal cAMP levels in blood platelets from FXS patients and a decrease in cAMP production following adenylate cyclase stimulation (Berry-Kravis and Huttenlocher, 1992; Berry-Kravis and Sklena, 1993). Reduced cAMP production was later detected in the brain of dfmr1 null drosophila, in brain and blood platelets of Fmr1 KO mice and in neural precursor cells from human FXS fetal tissues (Kelley et al., 2007). Besides, pharmacological manipulation with agents that potentially increase cAMP, i.e., inhibitors of group II mGluRs and phosphodiesterase IV inhibitors (PDE4-Is), reversed the mGluR-LTD alteration in FXS mouse models (Choi et al., 2011, 2015, 2016), leading to the hypothesis that increasing cAMP formation might become a potential therapeutic strategy to rescue FXS phenotype.
We have previously demonstrated that exaggerated mGluR-LTD in Fmr1 KO mice was reversed by activation of 5-HT7 receptors (5-HT7Rs) for serotonin (Costa et al., 2012a, 2015). 5-HT7Rs are positively coupled to adenylate cyclase and are highly expressed in the hippocampus, where they are believed to regulate learning and memory (Matthys et al., 2011; Ciranna and Catania, 2014).
In the present work, we have tested the hypothesis that a dysregulation of cAMP pathway might play a role in abnormal mGluR-LTD in Fmr1 KO hippocampal neurons. In this perspective, we evaluated whether the activation of 5-HT7Rs, coupled to Gs, rescues mGluR-LTD by increasing cAMP levels. We also tested whether in vivo administration of a 5-HT7R agonist could rescue learning ability and the behavioral phenotype in a mouse model of FXS.
Materials and Methods
Animals
All experiments were performed in mice obtained from a breeding colony kept at the University of Catania. We used Fmr1 KO mice and wild-type (WT) littermates from C57BL/6J strain for electrophysiology and behavioral experiments; both FVB and C57BL/6J strains were used for Western blotting. We crossed homozygous Fmr1 KO females with hemizygous Fmr1 KO males and both male and female pups were used in our experiments. Mice were maintained with a controlled temperature (21°C ± 1°C) and humidity (50%) on a 12 h light/dark cycle, with ad libitum food and water. All animal experimentation was conducted in accordance with the European Community Council guidelines (2010/63/EU) and was approved by the University Institutional Animal Care and Use Committee (Projects #181; 250—approval number: 352/2016-PR, Project #286—approval number: 174/2017-PR).
Electrophysiology
Acute hippocampal slices were prepared as previously described (Costa et al., 2012b) from WT and Fmr1 KO mice on a C57BL/6J background [postnatal (PN) age 14–23 days]. The brains were removed, placed in oxygenated ice-cold artificial cerebrospinal fluid (ACSF; in mM NaCl 124; KCl 3.0; NaH2PO4 1.2; MgSO4 1.2; CaCl2 2.0; NaHCO3 26; D-glucose 10, pH 7.3) and cut into 300 μm slices with a vibratome (Leica VT 1200S). Slices were continually perfused with oxygenated ACSF and viewed with infrared microscopy (Leica DMLFS). Schaffer collaterals were stimulated with negative current pulses (duration 0.3 ms, delivered every 15 s by A310 Accupulser, WPI, USA). Evoked excitatory post synaptic currents (EPSCs) were recorded under whole-cell from CA1 pyramidal neurons (holding potential −70 mV; EPC7-plus amplifier HEKA, Germany). Stimulation intensity was set to induce half-maximal EPSC amplitude. Series resistance (Rs) was continuously monitored by 10 mV hyperpolarizing pulses; recordings were discarded from analysis if Rs changed by more than 20%. EPSC traces were filtered at 3 kHz and digitized at 10 kHz. Data were acquired and analyzed using Signal software (CED, England). The recording micropipette (resistance 1.5–3 MΩ) was filled with intracellular solution (in mM: K-gluconate 140; HEPES 10; NaCl 10; MgCl2 2; EGTA 0.2; Mg-ATP 3.5; Na-GTP 1; pH 7.3).
To isolate AMPA receptor-mediated EPSCs, bath solution (ACSF; flow rate of 1.5 ml/min) routinely contained (-)-bicuculline methiodide (5 μM, Hello Bio) and D-(-)-2-Amino-5-phosphonopentanoic acid (D-AP5, 50 μM, Hello Bio).
(S)-3,5-dihydroxyphenylglycine (DHPG; 100 μM; Hello Bio), forskolin (20 μM; Tocris), PACAP-38 (PACAP, 10 nM, Tocris) and LP-211 (10 nM) were dissolved in ACSF and applied by bath perfusion. LP-211 was synthesized and provided by the research group of Prof. Leopoldo (University of Bari, Italy). SQ-22536 (10 μM, Tocris), protein kinase A (PKA) inhibitor fragment 6–22 (PKI; 20 μM, Tocris) and PD-98059 (40 μM, Tocris) were included in the intracellular solution.
LTD Data Analysis
Peak amplitude values of EPSCs were averaged over 1 min and expressed as % of baseline EPSC amplitude (calculated from EPSCs recorded during at least 15 min before DHPG application). % EPSC values from groups of neurons were pooled (mean ± standard error of mean, SEM) and graphically represented as a function of time. For each neuron studied, the amount of mGluR-LTD was calculated by averaging EPSC values recorded during 5 min between 40 min and 45 min after LTD induction and was expressed as percentage of baseline (% EPSC amplitude). Cumulative bar graphs indicate % EPSC amplitude (mean ± SEM from groups of neurons) after application of DHPG alone (control LTD) or DHPG with the 5-HT7 receptor agonist under different experimental conditions. EPSC amplitude values from two groups of neurons were compared using unpaired Student’s t-test, with n indicating the number of neurons tested in each condition; several groups of data were compared by one-way ANOVA followed by Tukey’s multiple comparisons test (GraphPad Prism 6, La Jolla, CA, USA).
Western Blotting
We used brains of WT (FVB and C57BL6J strains) and Fmr1 KO (C57BL6J strains) mice for stimulation assay. Hippocampi from mice at PN age 14–23 days were dissected, quickly cut into 350 μm slices using a McIlwain tissue chopper and transferred to oxygenated ice-cold artificial cerebrospinal fluid (ACSF; in mM NaCl 124, KCl 3, CaCl2 2, NaHCO3 25, NaH2PO4 1.1, MgSO4 2, D-glucose 10, pH 7.4) containing protease inhibitor cocktails EDTA free (Roche) and (+)-MK 801 maleate (1 μM; Tocris). Thereafter, slices were pre-incubated with ACSF containing protease inhibitor cocktails EDTA free (Roche) and (+)-MK 801 maleate (1 μM; Tocris) for 35 min at 32°C. Then, slices were exposed to LP-211 (10 nM) for 5 min; when present, H-89 dihydrochloride (1 μM; Tocris) was added 5 min before stimulation with LP-211 and maintained during LP-211 stimulation. Then, slices were washed three times in ice-cold ACSF and immediately frozen at −80°C until use.
Frozen slices were homogenized in ice-cold extraction buffer [Tris 50 mM pH 8; NaCl 150 mM; MgCl2 1.5 mM; NP40 1%; protease inhibitor cocktail EDTA free and phosphatase inhibitor cocktail (Roche)], and centrifuged for 30 min at 18,000× g at 4°C. The supernatant was collected and one aliquot was used for protein determination using the BCA methods (Pierce BCA protein assay kit). 80–100 μg of proteins were eluted with SDS sample buffer and separated onto 9% SDS-PAGE, as previously described (Bonaccorso et al., 2015). The following primary antibodies were used for Western blotting: polyclonal rabbit p44/42 MAP-kinase antibody (1:1,000, Cell Signaling), polyclonal rabbit phospho-p44/42 MAP-kinase antibody (1:1,000, Cell Signaling), while polyclonal rabbit GAPDH antibody (1:1,000, Cell Signaling) was used as a loading control.
Blots were developed by using the specific Western Breeze Chemiluminescent Immunodetection Kit (Invitrogen). Band densities were measured using ImageJ 1.49 software (Figure 5; Supplementary Figure S2) or the VersaDoc 4000 Imaging System (Bio-Rad, Figure 5; Supplementary Figure S3). The expression levels of phospho-extracellular signal regulated kinase (ERK)1/2 and ERK1/2 were normalized first against the levels of respective GAPDH and then calculated as ratio of total ERK signal.
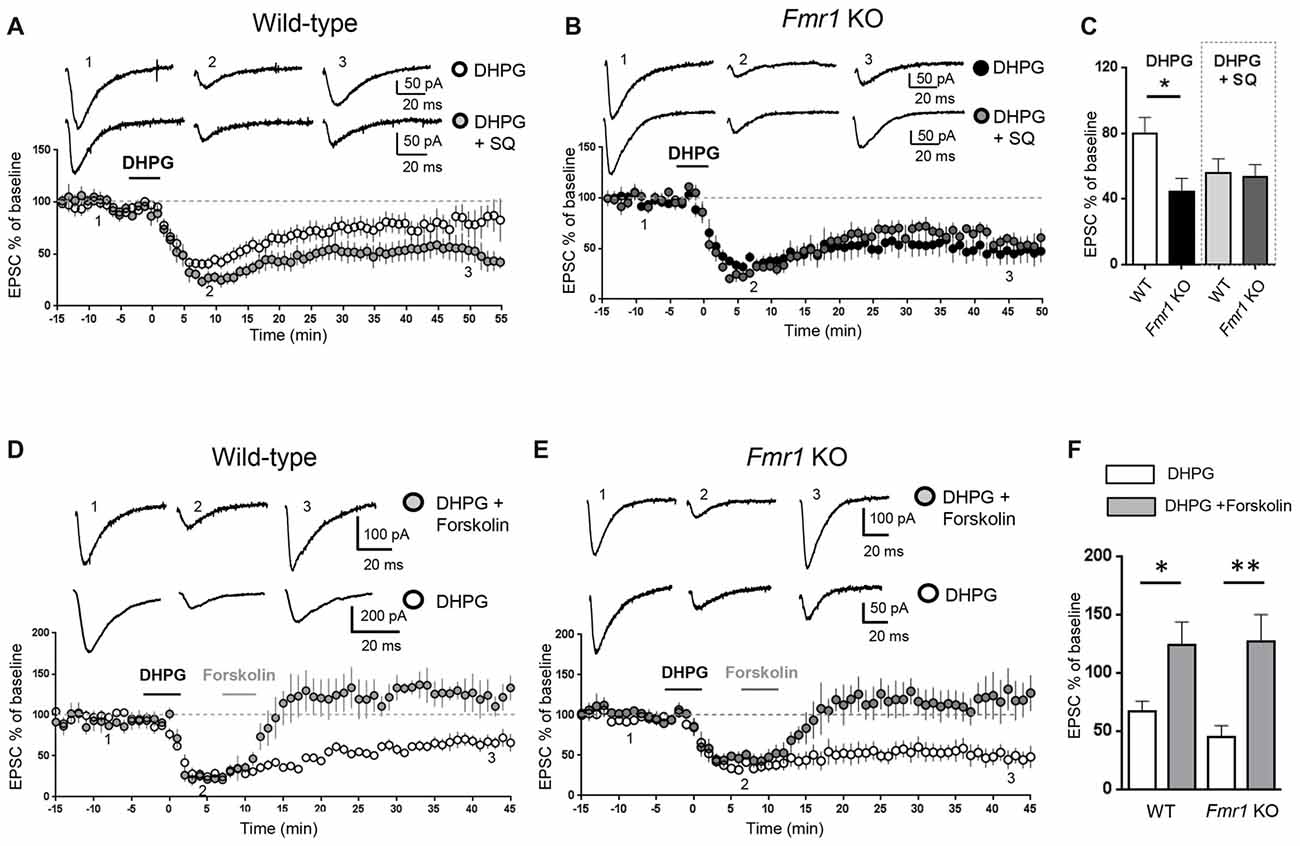
Figure 1. Modulation of adenylate cyclase activity modified metabotropic glutamate receptor-mediated long term depression (mGluR-LTD) in wild-type (WT) and Fmr1 Knockout (KO) slices. AMPAR-mediated excitatory post-synaptic currents (EPSCs) were recorded in the presence of D-AP5 (50 μM) and bicuculline (5 μM) under whole-cell patch clamp in the CA3–CA1 synapse in hippocampal slices from WT and Fmr1 KO mice. (A) In WT slices, bath application of the group I mGluR agonist (S)-3,5-dihydroxyphenylglycine (DHPG; 100 μM, 5 min) induced a long-term depression (mGluR-LTD) of EPSC amplitude (white dots, n = 11). When the adenylate cyclase blocker SQ 22536 (SQ, 10 μM) was added to intracellular medium, DHPG-induced mGluR-LTD was slightly enhanced (gray dots, n = 8) with respect to control. Individual representative EPSC traces are shown on top of the graph (1: baseline; 2: acute EPSC reduction; 3: mGluR-LTD). (B) In Fmr1 KO slices, DHPG-induced mGluR-LTD (black dots, n = 9) was enhanced with respect to WT (A, white dots) and was not further enhanced in the presence of intracellular SQ 22536 (SQ, 10 μM; dark gray dots, n = 6). (C) The bar graph shows the amount of mGluR-LTD (mean EPSC amplitude in all tested neurons, expressed as % of baseline EPSC amplitude). The amount of mGluR-LTD was compared by one-way ANOVA followed by Tukey’s multiple comparisons test in the four experimental conditions illustrated in (A,B) (*P = 0.03). In control conditions (DHPG), mGluR-LTD in Fmr1 KO was significantly enhanced with respect to WT (*P = 0.025, by unpaired t-test). In the presence of intracellular SQ 22536 (DHPG+SQ), the amount of mGluR-LTD in WT was not significantly different from Fmr1 KO (P = 0.83, by unpaired t-test) and was also comparable to that observed in Fmr1 KO in control conditions (P = 0.35 by unpaired t-test). (D) When DHPG application was followed by bath application of forskolin, a direct activator of adenylate cyclase (20 μM, 5 min), mGluR-LTD was reduced (gray dots, n = 4) with respect to control conditions (white dots; n = 5). (E) The same result was observed in Fmr1 KO slices: DHPG-induced mGluR-LTD (white dots; n = 9) was completely reversed by application of forskolin (20 μM, 5 min; gray dots, n = 5). (F) Reversal of mGluR-LTD by forskolin was statistically significant both in WT (*P = 0.025; by unpaired t-test) and in Fmr1 KO (**P = 0.0026).
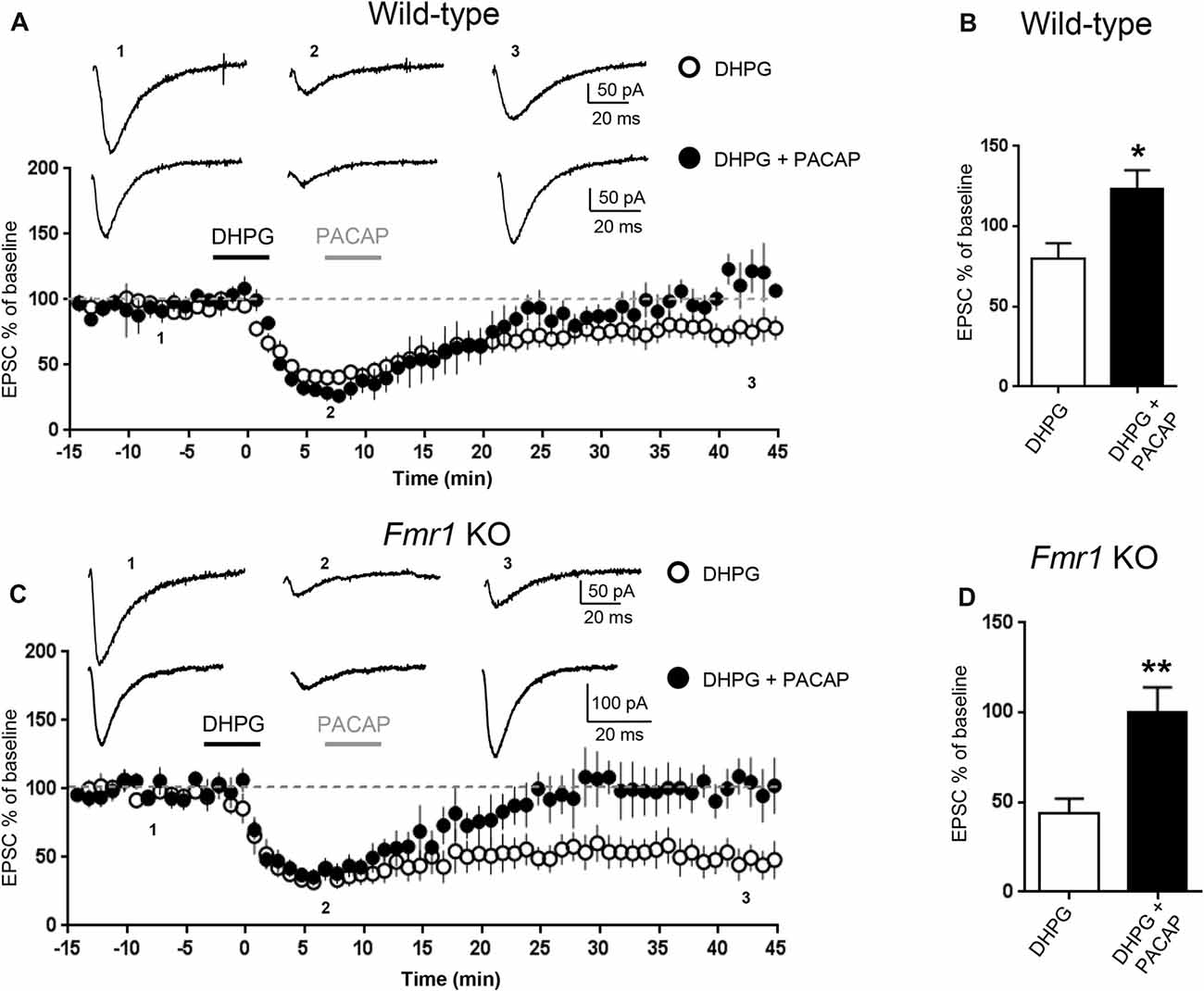
Figure 2. Pituitary adenylate cyclase activating peptide (PACAP) reversed mGluR-LTD in WT and in Fmr1 KO hippocampus. mGluR-LTD was induced by application of DHPG (100 μM, 5 min). Application of PACAP (10 nM, 5 min) fully reversed DHPG-induced mGluR-LTD in WT (A) and in Fmr1 KO slices (C). (B,D) Reversal of mGluR-LTD by PACAP was statistically significant both in WT (*P = 0.04, by unpaired t-test) and in Fmr1 KO slices (**P = 0.0025, unpaired t-test).
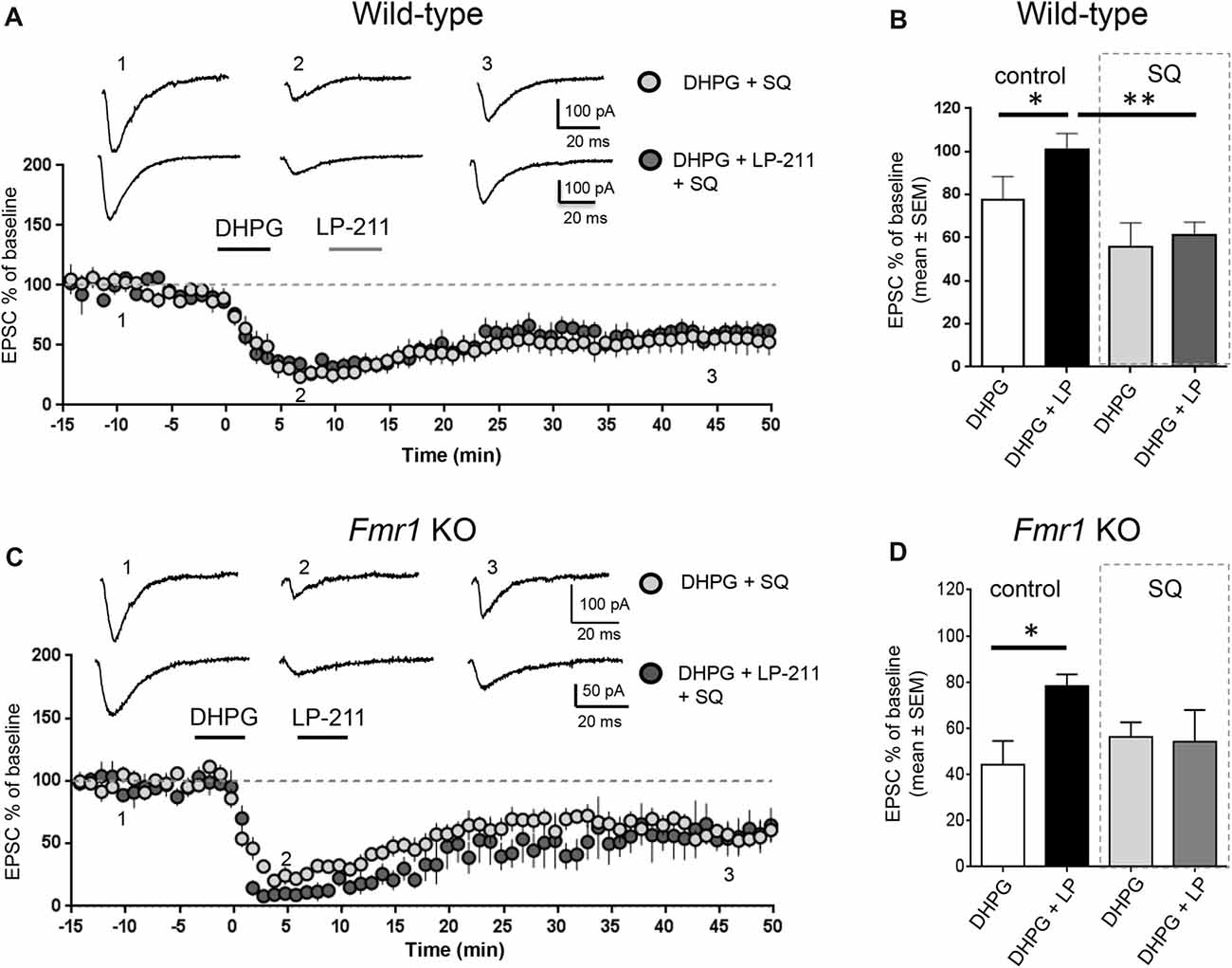
Figure 3. Activation of 5-HT7 receptors reversed mGluR-LTD by stimulation of adenylate cyclase. (A) In hippocampal WT slices, application of LP-211 did not modify mGluR-LTD in the presence of the adenylate cyclase blocker SQ 22536 (10 μM, included in intracellular pipette solution; dark gray dots, n = 6). (B) The bar graph shows the amount of mGluR-LTD measured 45 min after LTD induction (mean EPSC amplitude in all tested neurons, expressed as % of baseline EPSC amplitude). In WT slices, bath applications of DHPG-induced mGluR-LTD (white column; n = 11) that was completely reversed when DHPG application was followed by application of the 5-HT7R agonist LP-211 (10 nM, 5 min; black column, n = 7; *P = 0.03 by unpaired t-test). In the presence of intracellular SQ 22536 (10 μM; gray column, n = 6), the amount of mGluR-LTD was slightly increased with respect to control and was not reversed by application of LP-211 (10 nM, 5 min; dark gray column, n = 6; significantly different from the effect of LP-211 in control conditions, **P = 0.0026 by unpaired t-test). (C) Similarly, in Fmr1 KO slices application of LP-211 (10 nM, 5 min) had no effect on mGluR-LTD in the presence of intracellular SQ 22536 (10 μM; dark gray column, n = 6). (D) In Fmr1 KO slices, application of LP-211 reversed DHPG-induced mGluR-LTD only in control conditions (black column, n = 6; *P = 0.02 by unpaired t-test) but not in the presence of SQ 22536 (SQ, 10 μM; gray column, n = 6), showing that 5-HT7R-mediated effect required stimulation of adenylate cyclase also in Fmr1 KO slices.
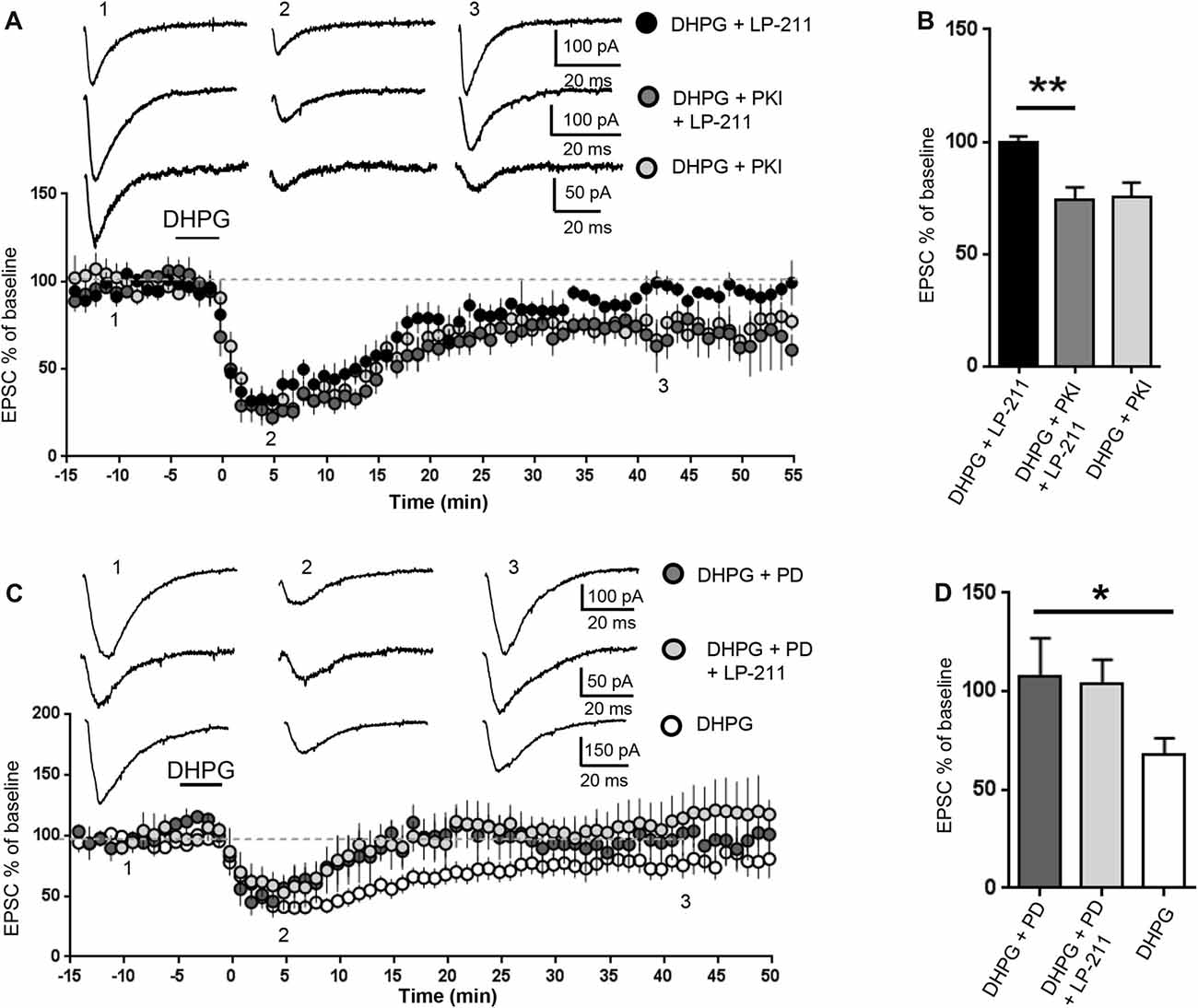
Figure 4. 5-HT7 receptor-mediated reversal of mGluR-LTD required protein kinase A (PKA) and was occluded by inhibition of extracellular signal regulated kinase (ERK). mGluR-LTD was induced by bath application of DHPG (100 μM, 5 min) in WT slices in control conditions and in the presence of peptide fragment 6–22 (PKI, added to intracellular solution), an inhibitor of PKA. (A) Application of LP-211 (10 nM, 5 min) reversed mGluR-LTD in control conditions (black dots, n = 7) but had no effect in the presence of PKI (dark gray dots, n = 5). (B) In the presence of PKI, the effect of LP-211 was significantly reduced (**P = 0.0013, by unpaired t-test), indicating that PKA activation was necessary for 5-HT7R-mediated reversal of mGluR-LTD. (C) mGluR-LTD was induced by bath application of DHPG (100 μM, 5 min) in WT slices in control conditions (white dots, n = 11) and in the presence of the mitogen-activated protein kinase (MAPK)/ERK blocker PD-98059 (PD, 40 μM, added to intracellular solution). Intracellular PD-98059 completely reversed mGluR-LTD (dark gray dots, n = 5) and occluded the effect of LP-211 (10 nM, 5 min; gray dots, n = 5). (D) mGluR-LTD was significantly reversed by PD-98059 (*P = 0.03) and was not further modified by LP-211 (P = 0.52).
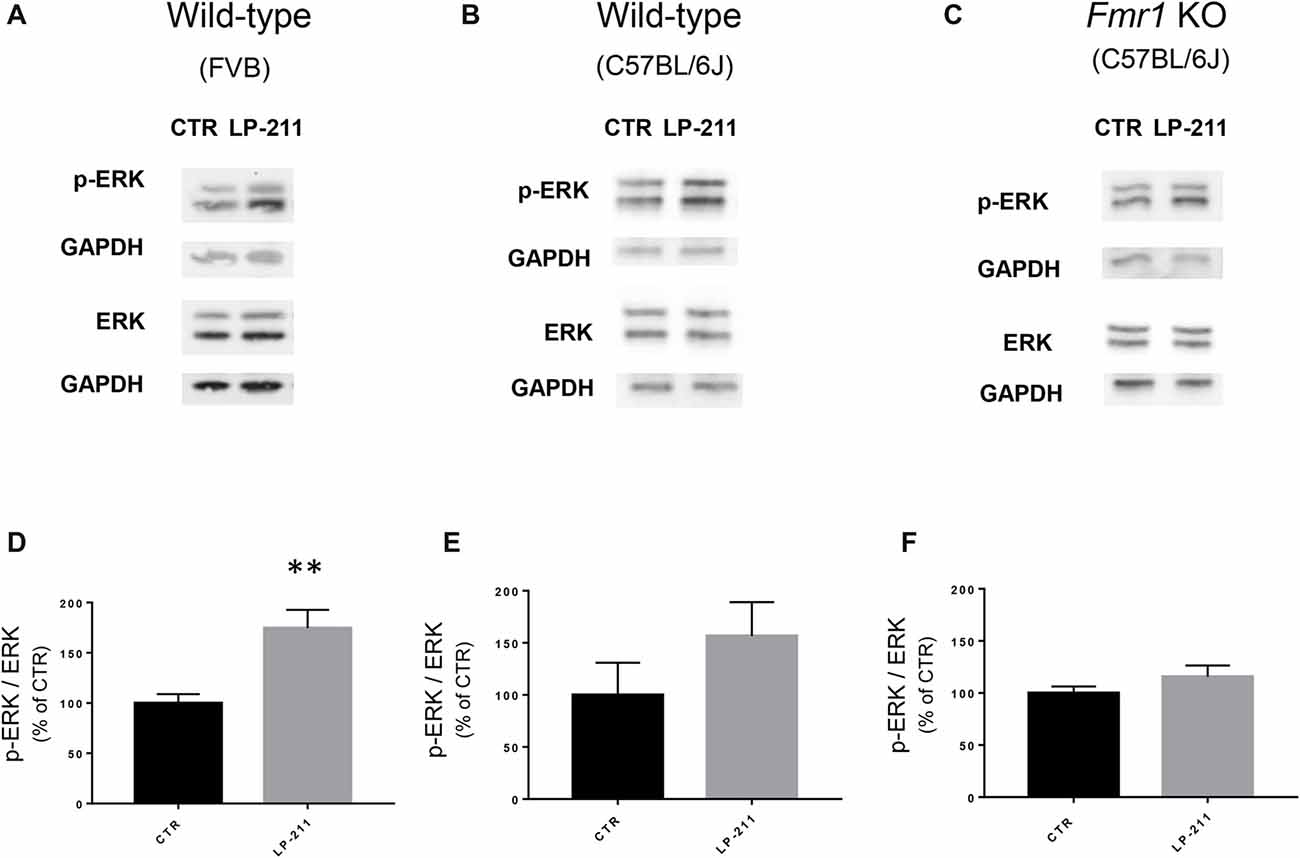
Figure 5. Activation of 5-HT7 receptors stimulated ERK phosphorylation. (A–C) Representative immunoblots showing the levels of phosphorylated and total ERK1/2 in control and LP-211 treated (10 nM, 5 min) hippocampal slices from WT (FVB strain; A), WT (C57BL/6J strain; B), and Fmr1 KO (C57BL/6J strain; C) mice. (D–F) Semi-quantitative analysis of phosphorylated ERK1/2 vs. total ERK1/2 in control and LP-211 treated hippocampal slices from WT (FVB strain; D), WT (C57BL/6J strain; E), and Fmr1 KO (C57BL/6J; F) mice. GAPDH was used as loading control. Relative optical density is presented as percentage of control. Data represent mean ± SEM of five (D), four (E,F) separate experiments, each performed on a pool of three mice. **p = 0.0064 by unpaired t-test. Full length immunoblots are shown in Supplementary Figures S2, S3.
Behavior
Novel object recognition (NOR) test was performed as previously described (Puzzo et al., 2013) on sex-balanced WT and Fmr1 KO mice (C57BL/6J background; age 3–4 months). After 3 days of habituation (10 min/day), mice underwent the training session (T1). They were placed in the arena for 10 min, a time sufficient to learn the task, and allowed to explore two identical objects, i.e., two glass beakers upside-down placed in the central part of the box, equally distant from the perimeter. Thirty minutes before T1 they received a i.p. injection of LP-211 (3 mg/Kg) or vehicle. Twenty-four hours after T1 mice underwent the second trial (T2) where a “familiar” (i.e., the one used for T1) and a “novel” object (ceramic cup) were presented to test memory retention. The novel object was placed on the left or the right side of the box in a randomly but balanced manner, to minimize potential biases because of a preference for particular locations or objects. To avoid olfactory cues, the objects and the apparatus were cleaned with 70% ethanol after each trial. Animal exploration, defined as the mouse pointing its nose toward the object from a distance not >2 cm (as marked by a reference circle), was evaluated in T2. We analyzed: (i) % exploration of the novel and % exploration of the familiar object; (ii) discrimination (D) index calculated as “exploration of novel object minus exploration of familiar object/total exploration time”; (iii) latency to first approach to novel object; and (iv) total exploration time.
Marble burying test was performed as previously described (Thomas et al., 2009), under standard room lighting and noise conditions. Twenty green glass marbles (15 mm in diameter) were arranged in a clean standard cage filled with a sani-chips bedding in a 4 × 5-cm pattern. Each mouse was gently placed into the cage and allowed to explore for 20 min. The number of marbles buried (covered by >50% bedding) was recorded.
Open field (OF) was performed as previously described (Palmeri et al., 2016). Each mouse was gently put in the arena (a white plastic bow divided into sectors by black lines) and was allowed to freely explore the environment for 5 min. The test was performed in a quiet, darkened room and one light bulb provided a bright illumination. We scored the following parameters: (i) % time spent into the center; (ii) number of entries into the center; (iii) “horizontal activity”, time spent moving into the arena; (iv) rearing or “vertical activity”, time spent erected on its hind legs; (v) grooming (time spent scratching itself with the forepaws); (vi) freezing (time of immobility); and (vii) defecation (number of fecal boli produced).
Results
Modulation of Adenylate Cyclase Activity Modified the Amount of mGluR-LTD in WT and Fmr1 KO Hippocampus
Excitatory post-synaptic currents (EPSCs) mediated by AMPA receptors were recorded in the CA3-CA1 synapse on hippocampal slices from WT and Fmr1 KO mice. mGluR-LTD of EPSCs was chemically induced by bath application of the mGluR agonist DHPG (100 μM, 5 min). We have previously confirmed (Costa et al., 2012a) that mGluR-LTD in Fmr1 KO slices is enhanced compared to WT, consistent with previous findings (Huber et al., 2002). To test if changes in intracellular cAMP levels might affect mGluR-LTD, we measured the amount of mGluR-LTD in hippocampal slices from WT and Fmr1 KO mice under experimental conditions reducing or enhancing cAMP levels in the recorded neuron. When the adenylate cyclase inhibitor SQ 22536 (10 μM) was included in the intracellular pipette solution (Figure 1A), the amount of mGluR-LTD in WT slices showed a trend towards an enhancement compared to control conditions, although not statistically significant (EPSC % amplitude measured 45 min after LTD induction: 79.5 ± 10, vs. 56 ± 9 comparing control vs. SQ 22536, n = 11/7; unpaired t-test: t(16) = 1.56; P = 0.07).
In Fmr1 KO slices, the amount of mGluR-LTD in control conditions was significantly higher than in WT (EPSC %: 44 ± 8 vs. 79.5 ± 10, comparing Fmr1 KO vs. WT, n = 9/11; t(18) = 2.44; P = 0.025; Figure 1C) and was not further enhanced by the adenylate cyclase blocker (EPSC %: 44 ± 8 vs. 53 ± 7, comparing Fmr1 KO control vs. Fmr1 KO + SQ 22536, n = 9/6; t(13) = 0.76; P = 0.45; Figures 1B,C).
When comparing the amount of mGluR-LTD in all the different experimental conditions (Figure 1C), a significant difference was found only between WT and Fmr1 KO in control conditions (P = 0.03 by one-way ANOVA followed by Tukey’s multiple comparisons). In the presence of SQ 22536, the amount of mGluR-LTD was comparable in WT and Fmr1 KO slices (EPSC %: 55 ± 10 vs. 53 ± 7 comparing WT + SQ 22536 vs. Fmr1 KO + SQ 22536, n = 7/6; t(11) = 0.21; P = 0.83) and was not significantly different from that measured in Fmr1 KO slices without SQ 22536 (t(14) = 0.96; P = 0.35).
Conversely, a direct stimulation of adenylate cyclase by bath application of forskolin (20 μM, 5 min) completely abolished DHPG-induced mGluR-LTD in both WT (EPSC %: 67 ± 8 vs. 124 ± 20, n = 5/4; t(7) = 2.8; P = 0.025, comparing DHPG vs. DHPG + forskolin, Figures 1D,F) and Fmr1 KO neurons (EPSC %: 45 ± 10 vs. 127 ± 23, n = 9/5; t(12) = 3.7; P = 0.0026, comparing DHPG vs. DHPG + forskolin, Figures 1E,F), showing that mGluR-LTD was reversed by increasing cAMP levels.
To further study the effect of increasing cAMP levels on mGluR-LTD, we tested the effects of Pituitary Adenylate Cyclase Activating Polyeptide (PACAP), a potent endogenous stimulator of adenylate cyclase activity (Harmar et al., 2012). Application of PACAP (10 nM, 5 min) reversed DHPG-induced mGluR-LTD both in WT (EPSC% 79.5 ± 10 vs. 123 ± 11, DHPG vs. DHPG + PACAP, n = 11/6, t(15) = 2.72, P = 0.015, Figures 2A,B) and in Fmr1 KO hippocampus (EPSC% 44 ± 8 vs. 100 ± 14, DHPG vs. DHPG + PACAP, n = 9/7, t(13) = 3.66, P = 0.0025, Figures 2C,D).
Activation of 5-HT7 Receptors Reversed mGluR-LTD by Stimulation of Adenylate Cyclase and Protein Kinase A
We tested the effect of LP-211, a 5-HT7R agonist, on mGluR-LTD in the presence of pharmacological modulators of the cAMP/PKA pathway. LP-211 was applied at 10 nM dose in order to activate selectively 5-HT7 receptors without activating the 5-HT1A subtype, based on its reported binding affinity for 5-HT7 and 5-HT1A receptors (Ki 0.58 and 188 nM respectively, see compound 25 in Leopoldo et al. (2008). In the presence of intracellular SQ 22536 (10 μM), application of LP-211 did not modify the amount of mGluR-LTD (EPSC %: 55 ± 10 vs. 61 ± 6, n = 7/6; t(11) = 0.39, P = 0.69 comparing DHPG + SQ 22536 vs. DHPG + SQ 22536 + LP-211; Figures 3A,B). Therefore, in the presence of SQ 22536 we did not observe the 5-HT7R-mediated reversal of mGluR-LTD that we described in control conditions (Costa et al., 2012a, 2015).
Similar to WT, in Fmr1 KO 5-HT7R-mediated reversal of mGluR-LTD was abolished by SQ-22536 (EPSC %: 53 ± 7 vs. 54 ± 13, n = 6/5; t(9) = 0.04; P = 0.96, comparing DHPG + SQ22536 vs. DHPG + SQ22536 + LP-211; Figures 3C,D), showing that reversal of mGluR-LTD by 5-HT7R activation was mediated by cAMP.
We then tested a possible involvement of PKA, one of the main cAMP effector targets (Taylor et al., 1990). The PKA inhibitor peptide fragment 6–22 (PKI, 20 μM) was added to the intracellular solution (Figures 4A,B): in this condition, the amount of DHPG-induced mGluR-LTD was not significantly different from control (EPSC % amplitude: 75 ± 6 vs. 79.5 ± 10, n = 10/11; t(19) = 0.32; P = 0.75, comparing PKI vs. control) but the effect of LP-211 was significantly reduced (EPSC %: 74 ± 5 vs. 99.5 ± 3, n = 6/7; t(11) = 4.26; P = 0.0013, comparing LP-211+PKI vs. LP-211; Figures 4A,B), indicating an involvement of PKA in 5-HT7R-mediated reversal of mGluR-LTD.
5-HT7R-Activation Stimulated Extracellular Signal-Regulated Kinase (ERK)
The cAMP pathway can also interact with the RAS/MEK/ERK signaling cascade (Dumaz and Marais, 2005), which is required for mGluR-LTD and dysregulated in the mouse model of FXS (Hou et al., 2006; Kim et al., 2008; Osterweil et al., 2010; Sawicka et al., 2016). Intracellular PD-98059 (40 μM), a mitogen-activated protein kinase (MAPK)/ERK blocker, reversed mGluR-LTD in WT hippocampal slices: the amount of mGluR-LTD was significantly decreased compared to control conditions (EPSC% 25 min after LTD induction: 68±8 vs. 108±19, n = 11/5; t(14) = 2.27; P = 0.03; control vs. PD-98059; Figures 4A,B). This result is consistent with previous data showing that ERK activation is necessary for mGluR-LTD (Gallagher et al., 2004).
mGluR-LTD was equally reversed by LP-211 (10 nM, 5 min) in the presence of PD-98059 (40 μM) and by PD-98059 alone (EPSC % amplitude: 112 ± 20 vs. 96 ± 11; n = 5/5; t(8) = 0.66; P = 0.52, comparing LP-211+PD-98059 vs. PD-98059; Figures 4C,D), indicating that PD-98059 occluded the effect of LP-211. Therefore, using mGluR-LTD as readout of 5-HT7R-mediated effect, we could not test if 5-HT7R activation was modulating ERK activity.
To check for a possible coupling of 5-HT7Rs to the ERK pathway, we measured ERK phosphorylation levels by Western blotting. Exposure of hippocampal slices to LP-211 (10 nM) for 5 min increased ERK1/2 phosphorylation, showing that 5-HT7R activation stimulates ERK signaling (Figures 5A,B,D,E). Stimulation of ERK phosphorylation by LP-211 was observed in hippocampal slices from WT mice, although it resulted statistically significant only in WT of FVB strain (Figure 5D, FVB; CTR: 100 ± 14, LP-211: 182 ± 17, n = 5, P = 0.0064 by unpaired t-test; Figure 5E, C57BL/6J: CTR: 100 ± 31, LP-211: 157 ± 32.5, n = 4, P = 0.254 by unpaired t-test). An increased phosphorylation of both ERK1 and ERK2 was detected after LP-211 exposure (Supplementary Figure S1). Interestingly, LP-211 caused only a negligible increase of ERK1/2 phosphorylation levels in hippocampal slices from Fmr1 KO mice (Figures 5C,F; CTR:100 ± 6; LP-211: 116 ± 11, n = 4, P = 0.255 by unpaired t-test). Overall, our data suggest that LP-211-mediated reversal of mGluR-LTD does not operate through stimulation of ERK signaling.
Acute in vivo Administration of a 5-HT7R Agonist Improved Object Recognition Memory in WT and Fmr1 KO Mice
Fmr1 KO mice show a cognitive impairment when evaluated by Novel object recognition (NOR) tasks based on the natural tendency of rodents to explore unfamiliar objects (Ventura et al., 2004; King and Jope, 2013; Franklin et al., 2014; Gomis-González et al., 2016). Here we first confirmed that Fmr1 KO presented a damage of recognition memory, since their D index did not significantly differ from zero (t(11) = 1.864, P = 0.089, n = 12; Figures 6A,B). Interestingly, Fmr1 KO spent a higher amount of time exploring the old vs. the new object (55.18 ± 2.78 vs. 44.81 ± 2.78 s of exploration time familiar vs. novel object; paired t-test: t(23) = 21.446, P < 0.0001; Figures 6A,B). WT littermates showed a normal recognition memory, as demonstrated by the higher time spent exploring the new object (39.61 ± 3.47 vs. 60.61 ± 3.47 s of exploration time familiar vs. novel object; n = 11; paired t-test: t(21) = 15.039, P < 0.0001; Figure 6A) and D index different than zero (t(10) = 2.99, P = 0.014; Figure 6B).
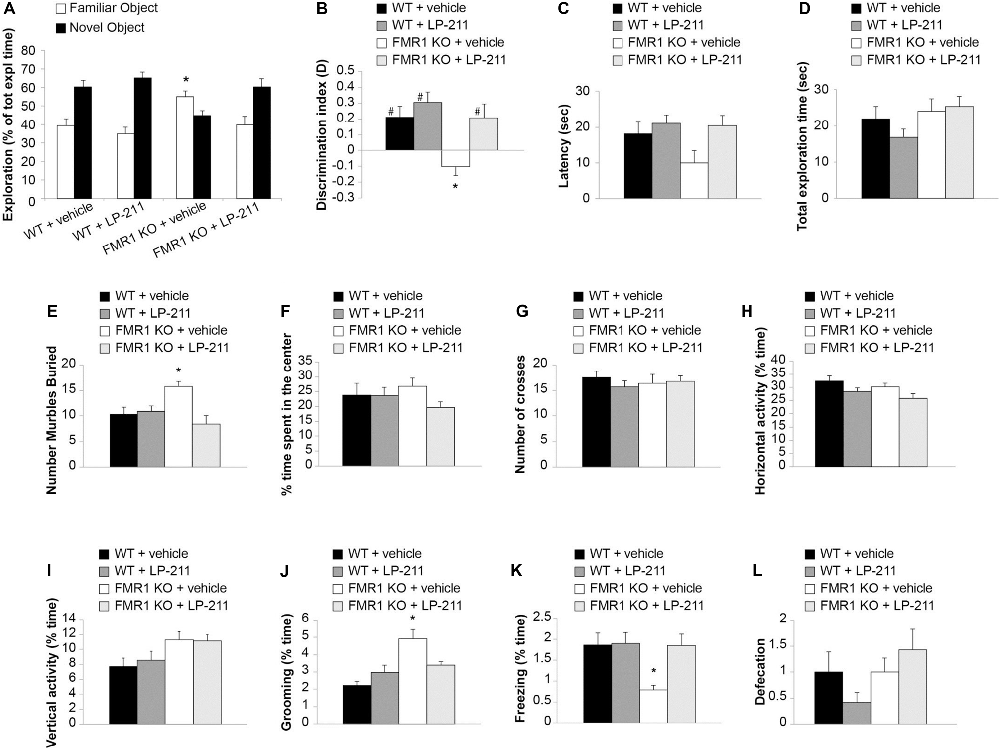
Figure 6. Acute in vivo administration of LP-211 improved memory and reduced stereotyped behavior in Fmr1 KO mice. (A) Exploration times of familiar and novel object during T2 (after a 24-h retention interval) show that Fmr1 KO mice treated with vehicle present an impairment of memory (higher amount of time exploring the familiar vs. the novel object; p < 0.0001) that is rescued by treatment with LP-211 (3 mg/kg 30 min before T1). WT + vehicle = 11; Fmr1 KO + vehicle = 12; WT + LP-211 = 10; Fmr1 KO + LP-211 = 10. (B) Analysis of the discrimination (D) index confirms that the impairment of recognition memory in Fmr1 KO (P = 0.015 vs. WT) is rescued by LP-211 (P = 0.02 vs. Fmr1 KO + LP-211). A difference from 0 is depicted with hashes (#p < 0.05) (C) Latency to first approach to the novel object and (D) total exploration time are comparable in the 4 groups of mice. (E) Fmr1 KO treated with vehicle buried a higher number of marbles compared to WT (P = 0.002). This stereotypic behavior was rescued by treatment with LP-211 (3 mg/kg 30 min before test). WT + vehicle = 11; WT + LP-211 = 10; Fmr1 KO + vehicle = 12; Fmr1 KO + LP-211 = 10 for novel object recognition (NOR; A–D) and Marble Burying (E) tasks. (F) Time spent in the center of the arena and (G) the number of crosses into the center is comparable in the four groups of mice. (H–I) General locomotor activity analyzed as horizontal activity and vertical activity (rearing) is not modified by genotype and treatment. (J) The increase in stereotyped behavior such as grooming in Fmr1 KO mice (P < 0.0001) is rescued by treatment with LP-211. (K) Fmr1 KO mice show a decrease of spontaneous freezing behavior compared to WT littermates (P = 0.036) that is rescued by LP-211. (L) Defecation is comparable in the four groups of mice. WT + vehicle = 10; WT + LP-211 = 12; Fmr1 KO + vehicle = 12; Fmr1 KO + LP-211 = 14 for Open field (OF) test. Data are expressed as mean ± SEM. *Significant difference (p < 0.05 by one-way ANOVA with Bonferroni’s multiple comparisons test); #difference with zero (one-sample t-test).
LP-211 is a brain-penetrant molecule, reaching the brain within 30 min after intraperitoneal injection in mice (Hedlund et al., 2010). Intraperitoneal treatment with LP-211 (3 mg/kg) 30 min before training rescued memory in Fmr1 KO mice (39.74 ± 4.55 vs. 60.25 ± 4.44 s of exploration time familiar vs. novel object; n = 10; paired t-test: t(19) = 12.804, P < 0.0001; Figure 6A; D: t(9) = 2.308, P = 0.046; Figure 6B), without modifying cognitive performances in WT animals (34.93 ± 3.39 vs. 65.06 ± 3.39 s of exploration time familiar vs. novel object; n = 10; paired t-test: t(19) = 14.344, P < 0.0001; D: t(9) = 4.437, P = 0.002; Figures 6A,B). These findings were confirmed by the analyses of D among groups (one-way ANOVA with Bonferroni’s: F(3,39) = 6.71, P = 0.001; WT vs. Fmr1 KO p = 0.015; Fmr1 KO vs. Fmr1 KO + LP-211 p = 0.02). The 4 groups of mice did not show differences in the latency to first approach to the novel object (one-way ANOVA: F(3,39) = 1.103, P = 0.360; Figure 6C) nor in total exploration time (one-way ANOVA: F(3,39) = 1.394, P = 0.259; Figure 6D).
Acute in vivo Administration of a 5-HT7R Agonist Reversed Stereotyped Behavior in Fmr1 KO Mice
FXS patients present perseverative or stereotypic behaviors that can be studied in rodents through a marble burying task (Thomas et al., 2009). Here, we observed that Fmr1 KO mice buried a higher number of marbles compared to WT littermates (Bonferroni’s P = 0.043; Figure 6E; Supplementary Figure S4), confirming previous data (Veeraragavan et al., 2012; Gholizadeh et al., 2014). An acute treatment with LP-211 (3 mg/kg, 30 min before trial) rescued this stereotypic behavior since it induced a reduction of marble burying in Fmr1 KO mice (Bonferroni’s P = 0.002), whereas it did not affect WT behavior (Bonferroni’s P = 1; Figure 6E). ANOVA among all: F(3,39) = 5.680, P = 0.003.
Then, we performed the OF task, which allows to study locomotor activity, anxiety-like and stereotyped behaviors (Kelley, 2001; Prut and Belzung, 2003). No differences were found in the % time spent in the center (F(3,44) = 1.144, P = 0.342), the number of crosses (F(3,44) = 6.656, P = 0.308), the horizontal activity (F(3,44) = 2.679, P = 0.059), the vertical activity (F(3,44) = 2.769, P = 0.053), suggesting that locomotor activity and anxiety-like behavior were not influenced by genotype and treatment (Figures 6F–I). However, Fmr1 KO mice showed an increase of grooming (Bonferroni’s P < 0.0001; Figure 6J) and a decrease of freezing (Bonferroni’s P = 0.036; Figure 6K) compared to WT littermates that were rescued by treatment with LP-211 (Bonferroni’s P = 0.245 and P = 1). No differences were detected in defecation (Figure 6L), considered as an indirect index of anxiety.
Discussion
We have studied the intracellular action mechanisms underlying 5-HT7R-mediated reversal of mGluR-LTD in WT and Fmr1 KO mice, a model of FXS. mGluR-LTD plays a fundamental role in learning and memory (Luscher and Huber, 2010; Sanderson et al., 2016) and is abnormally enhanced in the hippocampus of Fmr1 KO mice (Huber et al., 2002). Exaggerated hippocampal mGluR-LTD has been confirmed by several studies (Hou et al., 2006; Zhang et al., 2009; Choi et al., 2011; Costa et al., 2012a; Till et al., 2015), and is considered as a reliable readout of synaptic dysfunction in animal models of FXS and a cause of learning impairment and behavioral alterations (Sanderson et al., 2016). FMRP, the protein lacking in FXS, is most highly expressed at PN 7–12 (Davidovic et al., 2011; Bonaccorso et al., 2015). In the absence of FMRP, dendritic spine morphology and synapse formation are impaired in cortex (Comery et al., 1997) and hippocampus (Grossman et al., 2010) of Fmr1 KO mice. We have studied the effect of 5-HT7R activation on mGluR-LTD at PN 14–23, a developmental stage during which physiological synaptogenesis (Semple et al., 2013) and synaptic pruning (Jawaid et al., 2018) reach the highest levels in the brain of rodents.
We have previously shown that activation of 5-HT7 receptors reverses mGluR-LTD in WT and Fmr1 KO mouse hippocampus (Costa et al., 2012a). Different effects of the 5-HT7R agonist LP-211 were observed in mouse cerebellar cortex, where application of LP-211 induced a long-term depression of basal glutamatergic transmission in parallel fibers—Purkinje cells synapses (Lippiello et al., 2016). In the hippocampal CA3-CA1 synapse, instead, we never observed any long-term effect of LP-211 on basal synaptic transmission: application of LP-211 induced a transient enhancement of EPSC amplitude that fully recovered within 20 min (Costa et al., 2012b), indicating that 5-HT7 receptors activate different mechanisms in distinct brain areas.
Here, we show that the amount of hippocampal mGluR-LTD, which is abnormally enhanced in Fmr1 KO neurons, is reduced by 5-HT7 receptors through an increase in intracellular cAMP levels. Our conclusion is supported by data showing that 5-HT7R-mediated reversal of mGluR-LTD was: (1) mimicked by forskolin, a direct stimulator of adenylate cyclase; (2) mimicked by PACAP, a potent endogenous stimulator of adenylate cyclase; (3) completely abolished by SQ 22536, an adenylate cyclase inhibitor; and (4) fully blocked by an inhibitor of PKA, one of the main cAMP target enzymes.
In the presence of an adenylate cyclase blocker or of a PKA blocker, mGluR-LTD persisted but 5-HT7R-mediated effect was abolished. These results indicate that the cAMP/PKA pathway is not required for mGluR-LTD induction and/or expression, in agreement with previous studies (Camodeca et al., 1999; Schnabel et al., 2001), but stimulation of this pathway by 5-HT7 receptors modulates the amount of mGluR-LTD.
In WT neurons, following blockade of adenylate cyclase the amount of mGluR-LTD became comparable to that observed in Fmr1 KO slices, suggesting that exaggerated mGluR-LTD in Fmr1 KO mice might be related to reduced cAMP production. This hypothesis is in line with different studies supporting the view that the cAMP cascade is impaired in FXS. The hypothesis of low cAMP levels in FXS was suggested by early studies showing reduced basal cAMP levels and reduced cAMP production in blood platelets from FXS patients (Berry-Kravis and Huttenlocher, 1992; Berry-Kravis and Sklena, 1993). Another study shows that forskolin-induced cAMP production was reduced in blood platelets and brain tissues from different FXS animal models, but basal cAMP levels were unchanged (Kelley et al., 2007). Comparable total cAMP levels were also found in WT and Fmr1 KO mouse hippocampus homogenates (Sethna et al., 2017). Furthermore, a very recent report reveals that the mRNA encoding phosphodiesterase 2A (PDE2A), the main cAMP degradative enzyme, is a prominent target of FMRP and the absence of FMRP leads to PDE2A overexpression in cortical and hippocampal Fmr1 KO neurons (Maurin et al., 2018a). Overall these data strongly support the hypothesis of unbalanced cAMP production/degradation potentially leading to reduced cAMP levels in FXS.
In the present work, we show that activation of 5-HT7Rs reversed mGluR-LTD through an increase of intracellular cAMP levels and activation of PKA, one of the main effectors of cAMP.
5-HT7R activation also stimulated ERK, a subclass of the MAPKs that can interact with the cAMP pathway (Dumaz and Marais, 2005). In the hippocampus, ERK phosphorylation is stimulated by activation of group I mGluRs and is required for mGluR-LTD (Gallagher et al., 2004; Banko et al., 2006). Our electrophysiology data confirm that mGluR-LTD was completely abolished by intracellular inclusion of a MAPK/ERK blocker, thus required ERK activation. Using Western blotting, we found that activation of 5-HT7Rs by LP-211 also stimulated ERK phosphorylation in WT mice, with a major effect on the FVB background, in line with previous reports (Errico et al., 2001; Lin et al., 2003; Norum et al., 2003). Interestingly, we detected a much lower and not significant 5-HT7R-mediated ERK phosphorylation in Fmr1 KO mice, in line with evidence showing that ERK phosphorylation after receptor activation is reduced or blunted in Fragile X mouse (Hou et al., 2006; Hu et al., 2008; Kim et al., 2008; Shang et al., 2009). Future experiments will be aimed at clarifying the mechanisms underlying the reduced/absent ERK phosphorylation after 5-HT7 receptor stimulation in Fmr1 KO mice. For the purpose of the present study, since mGluR-LTD was significantly reversed by 5-HT7R activation in both WT and Fmr1 KO mice, the lack of phospho-ERK activation in Fmr1 KO mice suggests that LP-211-mediated reversal of mGluR-LTD operates through a distinct mechanism that does not involve stimulation of ERK signaling.
Other intracellular proteins are crucially involved in mGluR-LTD among which the activity-regulated cytoskeletal-associated protein (Arc; Arc/Arg3.1). Arc/Arg3.1 belongs to the intracellular signaling machinery that is necessary for mGluR-LTD, as a rapid synthesis of Arc/Arg3.1 is triggered by mGluR activation and induces mGluR-dependent endocytosis of AMPA receptors (Park et al., 2008). Several other intracellular molecules are necessary for mGluR-LTD (Luscher and Huber, 2010), thus modulation of mGluR-LTD may occur at many different steps. We do not exclude that 5-HT7R activation might modulate translation/transcription of proteins playing a key role in mGluR-LTD; we are currently investigating which “LTD proteins” might be regulated by 5-HT7 receptors.
Our present data indicate that 5-HT7 receptors reverse mGluR-LTD through the cAMP/PKA pathway, which is not required for mGluR-LTD induction and/or expression as shown by previous data and confirmed by our results. As a matter of fact, inhibition of adenylate cyclase or PKA did not block mGluR-LTD but completely blocked 5-HT7-mediated reversal of mGluR-LTD. Thus, the 5-HT7R-activated pathway is not necessary for mGluR-LTD induction but is able to modulate the final amount of synaptic inhibition.
We have previously speculated that activation of 5-HT7 receptors may indeed affect AMPA receptor trafficking. Interestingly, 5-HT7R activation was recently shown to phosphorylate AMPA receptors in rat hippocampal neurons, increasing their membrane insertion and conductance, thus enhancing AMPAR-mediated synaptic currents (Andreetta et al., 2016), consistent with data from our laboratory (Costa et al., 2012b). We have also shown that 5-HT7 receptor activation prevented DHPG-induced internalization of AMPA receptors in WT and Fmr1 KO (Costa et al., 2012a). In view of these data, the 5-HT7R-activated cAMP/PKA pathway might ultimately phosphorylate AMPA receptors, reducing their internalization and increasing their conductance, thus reducing the amount of mGluR-LTD.
Our result that increasing cAMP levels rescues abnormal synaptic plasticity in Fmr1 KO hippocampus suggests that other molecules acting on Gs-coupled receptors might be used for FXS therapy. Among these, we show that PACAP is a promising candidate since this neurotrophic peptide modulates hippocampal synaptic transmission (Costa et al., 2009) and plasticity, correcting abnormal mGluR-LTD in Fmr1 KO neurons (present results).
In accordance with cAMP involvement, exaggerated mGluR-LTD in Fmr1 KO neurons was reversed by mGluR2 blockade (Choi et al., 2011, 2016) or by PDE4 inhibition (Choi et al., 2015, 2016), both virtually increasing cAMP levels. Altered mechanisms leading to reduced cAMP production in FXS are under investigation. Over-expression of FMRP causes an increased production of cAMP in transfected cell lines, leading to the conclusion that FMRP might directly regulate the translation of mRNA(s) coding for cAMP cascade proteins (Berry-Kravis and Ciurlionis, 1998). Indeed, several mRNAs encoding components of cAMP signaling cascade are target of FMRP (Darnell et al., 2011) among which, as above discussed, PDE2A is a major FMRP target (Maurin et al., 2018a). Importantly, very recent data demonstrate that PDE2A is overactivated in Fmr1 KO mouse brain, leading to reduced cAMP levels, and pharmacological inhibition of PDE2A reverses exaggerated mGluR-LTD in Fmr1 KO hippocampus (Maurin et al., 2018b).
Impaired signaling through dopamine Gs-coupled receptors was also evidenced in Fmr1 KO cultured cortical neurons, where D1 receptor-stimulated cAMP formation was decreased with respect to WT, due to reduced coupling of D1 receptors to adenylate cyclase (Wang et al., 2008). Interestingly, the same work also shows that AS-19, a selective 5-HT7R agonist, instead stimulated adenylate cyclase comparably in WT and in Fmr1 KO neurons and we show that 5-HT7R activation corrects excessive mGluR-LTD in Fmr1 KO mice. These results have important therapeutic implications indicating that, unlike D1 receptors, 5-HT7Rs are fully functional in Fmr1 KO mice, thus 5-HT7R agonists might become new pharmacological tools.
In this perspective, in this manuscript we also investigated whether systemic administration of LP-211 to Fmr1 KO might rescue learning and behavioral deficits that mirror cognitive impairment and autistic-like behavior in FXS patients. We used the NOR test to study cortex- and hippocampus-dependent novelty detection ability (Broadbent et al., 2010), which is known to involve hippocampal mGluR-LTD (reviewed by Sanderson et al., 2016). We first confirmed that recognition memory tested by NOR is impaired in Fmr1 KO mice (Ventura et al., 2004; King and Jope, 2013; Franklin et al., 2014; Gomis-González et al., 2016), since discrimination index is impaired compared to WT littermates and less time is spent exploring the novel object. Interestingly, Fmr1 KO mice showed a preference for the familiar compared to the novel object, consistent with previous studies demonstrating alterations of novelty preferences, with stereotyped behavior and restricted interests, in autism spectrum disorders (Jacob et al., 2009). Here we show that an acute systemic administration of LP-211 rescued recognition memory impairment in Fmr1 KO mice.
About one third of FXS patients display autistic behavior, including gaze and touch avoidance and repetitive behavior (Garber et al., 2008). Using the marble burying and the OF tasks, two protocols revealing stereotyped behavior in rodents (Thomas et al., 2009), Fmr1 KO mice showed increased repetitive behavior, i.e., marble burying and grooming, with respect to WT, as previously demonstrated (Veeraragavan et al., 2012; Gholizadeh et al., 2014; Kazdoba et al., 2014). Interestingly, Fmr1 KO mice presented less spontaneous freezing behavior compared to WT, consistent with previous studies in GAP43 mice model of autism spectrum disorder (Zaccaria et al., 2010) and probably reflecting some aspects of maladaptive behavior to stress and catatonia in patients. This phenotype was completely rescued by systemic administration of LP-211.
In conclusion, we show that selective activation of 5-HT7Rs corrects abnormal intracellular signaling and synaptic plasticity in newborn Fmr1 KO mice and rescues learning and behavior in young adult Fmr1 KO mice. The latter result has important implications for therapy, indicating that a rescue of FXS phenotypes by pharmacological treatment can also be possible at adult age. Therefore, selective 5-HT7 receptor agonists might represent a new pharmacological strategy for FXS therapy.
Author Contributions
LCosta: electrophysiology data collection, analysis and interpretation; final approval of manuscript. LS: electrophysiology data collection; animal care, final approval of manuscript. MS, CB and SD’A: western blotting data collection, analysis and interpretation; animal care, final approval of manuscript. WG and MT: behavioral data collection, analysis and interpretation; final approval of manuscript. ML and EL: design and synthesis of 5-HT7R agonists; data interpretation; final approval of manuscript. LCiranna, MVC and DP: conception and design; data analysis and interpretation; manuscript writing; final approval of manuscript.
Funding
The present work was financed by FRAXA Research Foundation (call 2013), Telethon Foundation (Fondazione Telethon; grant GGP13145) and Ricerca Finalizzata of Ministry of Health PE-2013-02355126.
Conflict of Interest Statement
The authors declare that the research was conducted in the absence of any commercial or financial relationships that could be construed as a potential conflict of interest.
Acknowledgments
We wish to thank Dr. Marco Abbate for veterinary assistance, Mrs. Elisa Giuffrida for animal care and technical assistance, Mrs. Giuseppina Barrancotto (Oasi IRCCS, Troina), Dr.ssa Marisa Raciti (Oasi IRCCS, Troina) and Mr. Samuele Giuffrida (a student of the University of Catania under an undergraduate training program) for their technical support in performing experimental work.
Supplementary Material
The Supplementary Material for this article can be found online at: https://www.frontiersin.org/articles/10.3389/fnmol.2018.00353/full#supplementary-material
FIGURE S1 | LP-211 treatment increased levels of both phospho-ERK1 and phospho-ERK2 in hippocampal slices of wild-type (WT) mice. (A) Semi-quantitative analysis of phosphorylated ERK1 vs. total ERK1 in control and LP-211 (10 nM, 5 min) treated hippocampal slices from WT mice (FVB strain). Relative optical density is presented as percentage of control. Data represent mean ± SEM of four separate experiments, each performed on a pool of three mice. *p = 0.0286 by Mann-Whitney Rank Sum Test. (B) Semi-quantitative analysis of phosphorylated ERK2 vs. total ERK2 in control and LP-211 treated hippocampal slices from WT mice (FVB strain). Relative optical density is presented as percentage of control. Data represent mean + SEM of four separate experiments, each performed on a pool of three mice. *p = 0.0412 by unpaired t-test.
FIGURE S2 | Original images of immunoblots shown in Figure 5A.
FIGURE S3 | (A) Original images of immunoblots shown in Figure 5B. (B) Original images of immunoblots shown in Figure 5C.
FIGURE S4 | Experimental procedures for marble burying test. Marbles distribution before testing shows twenty marbles equidistantly distributed. Mice were left in the cage for 20 min and number of buried marbles was analyzed in four different groups (vehicle-treated WT; WT treated with LP-211; vehicle-treated Fmr1 KO; Fmr1 KO treated with LP-211).
References
Andreetta, F., Carboni, L., Grafton, G., Jeggo, R., Whyment, A. D., van den Top, M., et al. (2016). Hippocampal 5-HT7 receptors signal phosphorylation of the GluA1 subunit to facilitate AMPA receptor mediated-neurotransmission in vitro and in vivo. Br. J. Pharmacol. 173, 1438–1451. doi: 10.1111/bph.13432
Banko, J. L., Hou, L., Poulin, F., Sonenberg, N., and Klann, E. (2006). Regulation of eukaryotic initiation factor 4E by converging signaling pathways during metabotropic glutamate receptor-dependent long-term depression. J. Neurosci. 26, 2167–2173. doi: 10.1523/JNEUROSCI.5196-05.2006
Bear, M. F., Huber, K. M., and Warren, S. T. (2004). The mGluR theory of fragile X mental retardation. Trends Neurosci. 27, 370–377. doi: 10.1016/j.tins.2004.04.009
Berry-Kravis, E., and Ciurlionis, R. (1998). Overexpression of fragile X gene (FMR-1) transcripts increases cAMP production in neural cells. J. Neurosci. Res. 51, 41–48. doi: 10.1002/(SICI)1097-4547(19980101)51:1<41::AID-JNR4>3.0.CO;2-L
Berry-Kravis, E., and Huttenlocher, P. R. (1992). Cyclic AMP metabolism in fragile X syndrome. Ann. Neurol. 31, 22–26. doi: 10.1002/ana.410310105
Berry-Kravis, E., and Sklena, P. (1993). Demonstration of abnormal cyclic AMP production in platelets from patients with fragile X syndrome. Am. J. Med. Genet. 45, 81–87. doi: 10.1002/ajmg.1320450120
Bonaccorso, C. M., Spatuzza, M., Di Marco, B., Gloria, A., Barrancotto, G., Cupo, A., et al. (2015). Fragile X mental retardation protein (FMRP) interacting proteins exhibit different expression patterns during development. Int. J. Dev. Neurosci. 42, 15–23. doi: 10.1016/j.ijdevneu.2015.02.004
Broadbent, N. J., Gaskin, S., Squire, L. R., and Clark, R. E. (2010). Object recognition memory and the rodent hippocampus. Learn. Mem. 17, 5–11. doi: 10.1101/lm.1650110
Camodeca, N., Breakwell, N. A., Rowan, M. J., and Anwyl, R. (1999). Induction of LTD by activation of group I mGluR in the dentate gyrus in vitro. Neuropharmacology 38, 1597–1606. doi: 10.1016/s0028-3908(99)00093-3
Choi, C. H., Schoenfeld, B. P., Bell, A. J., Hinchey, P., Kollaros, M., Gertner, M. J., et al. (2011). Pharmacological reversal of synaptic plasticity deficits in the mouse model of fragile X syndrome by group II mGluR antagonist or lithium treatment. Brain Res. 1380, 106–119. doi: 10.1016/j.brainres.2010.11.032
Choi, C. H., Schoenfeld, B. P., Bell, A. J., Hinchey, J., Rosenfelt, C., Gertner, M. J., et al. (2016). Multiple drug treatments that increase cAMP signaling restore long-term memory and aberrant signaling in fragile X syndrome models. Front. Behav. Neurosci. 10:136. doi: 10.3389/fnbeh.2016.00136
Choi, C. H., Schoenfeld, B. P., Weisz, E. D., Bell, A. J., Chambers, D. B., Hinchey, J., et al. (2015). PDE-4 inhibition rescues aberrant synaptic plasticity in Drosophila and mouse models of fragile X syndrome. J. Neurosci. 35, 396–408. doi: 10.1523/JNEUROSCI.1356-12.2015
Ciranna, L., and Catania, M. V. (2014). 5-HT7 receptors as modulators of neuronal excitability, synaptic transmission and plasticity: physiological role and possible implications in autism spectrum disorders. Front. Cell. Neurosci. 8:250. doi: 10.3389/fncel.2014.00250
Comery, T. A., Harris, J. B., Willems, P. J., Oostra, B. A., Irwin, S. A., Weiler, I. J., et al. (1997). Abnormal dendritic spines in fragile X knockout mice: maturation and pruning deficits. Proc. Natl. Acad. Sci. U S A 94, 5401–5404. doi: 10.1073/pnas.94.10.5401
Costa, L., Santangelo, F., Li Volsi, G., and Ciranna, L. (2009). Modulation of AMPA receptor-mediated ion current by pituitary adenylate cyclase-activating polypeptide (PACAP) in CA1 pyramidal neurons from rat hippocampus. Hippocampus 19, 99–109. doi: 10.1002/hipo.20488
Costa, L., Sardone, L. M., Lacivita, E., Leopoldo, M., and Ciranna, L. (2015). Novel agonists for serotonin 5-HT7 receptors reverse metabotropic glutamate receptor-mediated long-term depression in the hippocampus of wild-type and Fmr1 KO mice, a model of Fragile X Syndrome. Front. Behav. Neurosci. 9:65. doi: 10.3389/fnbeh.2015.00065
Costa, L., Spatuzza, M., D’Antoni, S., Bonaccorso, C. M., Trovato, C., Musumeci, S. A., et al. (2012a). Activation of 5-HT7 serotonin receptors reverses metabotropic glutamate receptor-mediated synaptic plasticity in wild-type and Fmr1 knockout mice, a model of Fragile X syndrome. Biol. Psychiatry 72, 924–933. doi: 10.1016/j.biopsych.2012.06.008
Costa, L., Trovato, C., Musumeci, S. A., Catania, M. V., and Ciranna, L. (2012b). 5-HT1A and 5-HT7 receptors differently modulate AMPA receptor-mediated hippocampal synaptic transmission. Hippocampus 22, 790–801. doi: 10.1002/hipo.20940
Darnell, J. C., Van Driesche, S. J., Zhang, C., Hung, K. Y., Mele, A., Fraser, C. E., et al. (2011). FMRP stalls ribosomal translocation on mRNAs linked to synaptic function and autism. Cell 146, 247–261. doi: 10.1016/j.cell.2011.06.013
Davidovic, L., Navratil, V., Bonaccorso, C. M., Catania, M. V., Bardoni, B., and Dumas, M. E. (2011). A metabolomic and systems biology perspective on the brain of the fragile X syndrome mouse model. Genome Res. 21, 2190–2202. doi: 10.1101/gr.116764.110
Dumaz, N., and Marais, R. (2005). Integrating signals between cAMP and the RAS/RAF/MEK/ERK signalling pathways. Based on the anniversary prize of the Gesellschaft fur Biochemie und Molekularbiologie Lecture delivered on 5 July 2003 at the Special FEBS Meeting in Brussels. FEBS J. 272, 3491–3504. doi: 10.1111/j.1742-4658.2005.04763.x
Errico, M., Crozier, R. A., Plummer, M. R., and Cowen, D. S. (2001). 5-HT7 receptors activate the mitogen activated protein kinase extracellular signal related kinase in cultured rat hippocampal neurons. Neuroscience 102, 361–367. doi: 10.1016/s0306-4522(00)00460-7
Franklin, A. V., King, M. K., Palomo, V., Martinez, A., McMahon, L. L., and Jope, R. S. (2014). Glycogen synthase kinase-3 inhibitors reverse deficits in long-term potentiation and cognition in fragile X mice. Biol. Psychiatry 75, 198–206. doi: 10.1016/j.biopsych.2013.08.003
Gallagher, S. M., Daly, C. A., Bear, M. F., and Huber, K. M. (2004). Extracellular signal-regulated protein kinase activation is required for metabotropic glutamate receptor-dependent long-term depression in hippocampal area CA1. J. Neurosci. 24, 4859–4864. doi: 10.1523/JNEUROSCI.5407-03.2004
Garber, K. B., Visootsak, J., and Warren, S. T. (2008). Fragile X syndrome. Eur. J. Hum. Genet. 16, 666–672. doi: 10.1038/ejhg.2008.61
Gholizadeh, S., Arsenault, J., Xuan, I. C., Pacey, L. K., and Hampson, D. R. (2014). Reduced phenotypic severity following adeno-associated virus-mediated Fmr1 gene delivery in fragile X mice. Neuropsychopharmacology 39, 3100–3111. doi: 10.1038/npp.2014.167
Gomis-González, M., Busquets-Garcia, A., Matute, C., Maldonado, R., Mato, S., and Ozaita, A. (2016). Possible therapeutic doses of cannabinoid type 1 receptor antagonist reverses key alterations in fragile X syndrome mouse model. Genes 7:E56. doi: 10.3390/genes7090056
Grossman, A. W., Aldridge, G. M., Lee, K. J., Zeman, M. K., Jun, C. S., Azam, H. S., et al. (2010). Developmental characteristics of dendritic spines in the dentate gyrus of Fmr1 knockout mice. Brain Res. 1355, 221–227. doi: 10.1016/j.brainres.2010.07.090
Harmar, A. J., Fahrenkrug, J., Gozes, I., Laburthe, M., May, V., Pisegna, J. R., et al. (2012). Pharmacology and functions of receptors for vasoactive intestinal peptide and pituitary adenylate cyclase-activating polypeptide: IUPHAR review 1. Br. J. Pharmacol. 166, 4–17. doi: 10.1111/j.1476-5381.2012.01871.x
Hedlund, P. B., Leopoldo, M., Caccia, S., Sarkisyan, G., Fracasso, C., Martelli, G., et al. (2010). LP-211 is a brain penetrant selective agonist for the serotonin 5-HT7 receptor. Neurosci Lett 481, 12–16. doi: 10.1016/j.neulet.2010.06.036
Hou, L., Antion, M. D., Hu, D., Spencer, C. M., Paylor, R., and Klann, E. (2006). Dynamic translational and proteasomal regulation of fragile X mental retardation protein controls mGluR-dependent long-term depression. Neuron 51, 441–454. doi: 10.1016/j.neuron.2006.07.005
Hu, H., Qin, Y., Bochorishvili, G., Zhu, Y., van Aelst, L., and Zhu, J. J. (2008). Ras signaling mechanisms underlying impaired GluR1-dependent plasticity associated with fragile X syndrome. J. Neurosci. 28, 7847–7862. doi: 10.1523/JNEUROSCI.1496-08.2008
Huber, K. M., Gallagher, S. M., Warren, S. T., and Bear, M. F. (2002). Altered synaptic plasticity in a mouse model of fragile X mental retardation. Proc. Natl. Acad. Sci. U S A 99, 7746–7750. doi: 10.1073/pnas.122205699
Irwin, S. A., Patel, B., Idupulapati, M., Harris, J. B., Crisostomo, R. A., Larsen, B. P., et al. (2001). Abnormal dendritic spine characteristics in the temporal and visual cortices of patients with fragile-X syndrome: a quantitative examination. Am. J. Med. Genet. 98, 161–167. doi: 10.1002/1096-8628(20010115)98:2<161::aid-ajmg1025>3.0.co;2-b
Jacob, S., Landeros-Weisenberger, A., and Leckman, J. F. (2009). Autism spectrum and obsessive-compulsive disorders: OC behaviors, phenotypes and genetics. Autism Res. 2, 293–311. doi: 10.1002/aur.108
Jawaid, S., Kidd, G. J., Wang, J., Swetlik, C., Dutta, R., and Trapp, B. D. (2018). Alterations in CA1 hippocampal synapses in a mouse model of fragile X syndrome. Glia 66, 789–800. doi: 10.1002/glia.23284
Kazdoba, T. M., Leach, P. T., Silverman, J. L., and Crawley, J. N. (2014). Modeling fragile X syndrome in the Fmr1 knockout mouse. Intractable Rare Dis. Res. 3, 118–133. doi: 10.5582/irdr.2014.01024
Kelley, A. E. (2001). Measurement of rodent stereotyped behavior. Curr. Protoc. Neurosci. Chapter 8:Unit 8.8. doi: 10.1002/0471142301.ns0808s04
Kelley, D. J., Bhattacharyya, A., Lahvis, G. P., Yin, J. C., Malter, J., and Davidson, R. J. (2008). The cyclic AMP phenotype of fragile X and autism. Neurosci. Biobehav. Rev. 32, 1533–1543. doi: 10.1016/j.neubiorev.2008.06.005
Kelley, D. J., Davidson, R. J., Elliott, J. L., Lahvis, G. P., Yin, J. C., and Bhattacharyya, A. (2007). The cyclic AMP cascade is altered in the fragile X nervous system. PLoS One 2:e931. doi: 10.1371/journal.pone.0000931
Kim, S. H., Markham, J. A., Weiler, I. J., and Greenough, W. T. (2008). Aberrant early-phase ERK inactivation impedes neuronal function in fragile X syndrome. Proc. Natl. Acad. Sci. U S A 105, 4429–4434. doi: 10.1073/pnas.0800257105
King, M. K., and Jope, R. S. (2013). Lithium treatment alleviates impaired cognition in a mouse model of fragile X syndrome. Genes Brain Behav. 12, 723–731. doi: 10.1111/gbb.12071
Leopoldo, M., Lacivita, E., De Giorgio, P., Fracasso, C., Guzzetti, S., Caccia, S., et al. (2008). Structural modifications of N-(1,2,3,4-tetrahydronaphthalen-1-yl)-4-aryl-1-piperazinehexanamides: influence on lipophilicity and 5-HT7 receptor activity: Part III. J. Med. Chem. 51, 5813–5822. doi: 10.1021/jm800615e
Lin, S. L., Johnson-Farley, N. N., Lubinsky, D. R., and Cowen, D. S. (2003). Coupling of neuronal 5-HT7 receptors to activation of extracellular-regulated kinase through a protein kinase A-independent pathway that can utilize Epac. J. Neurochem. 87, 1076–1085. doi: 10.1046/j.1471-4159.2003.02076.x
Lippiello, P., Hoxha, E., Speranza, L., Volpicelli, F., Ferraro, A., Leopoldo, M., et al. (2016). The 5-HT7 receptor triggers cerebellar long-term synaptic depression via PKC-MAPK. Neuropharmacology 101, 426–438. doi: 10.1016/j.neuropharm.2015.10.019
Luscher, C., and Huber, K. M. (2010). Group 1 mGluR-dependent synaptic long-term depression: mechanisms and implications for circuitry and disease. Neuron 65, 445–459. doi: 10.1016/j.neuron.2010.01.016
Matthys, A., Haegeman, G., Van Craenenbroeck, K., and Vanhoenacker, P. (2011). Role of the 5-HT7 receptor in the central nervous system: from current status to future perspectives. Mol. Neurobiol. 43, 228–253. doi: 10.1007/s12035-011-8175-3
Maurin, T., Lebrigand, K., Castagnola, S., Paquet, A., Jarjat, M., Popa, A., et al. (2018a). HITS-CLIP in various brain areas reveals new targets and new modalities of RNA binding by fragile X mental retardation protein. Nucleic Acids Res. 46, 6344–6355. doi: 10.1093/nar/gky267
Maurin, T., Melancia, F., Jarjat, M., Castro, L., Costa, L., Delhaye, S., et al. (2018b). Involvement of phosphodiesterase 2A activity in the pathophysiology of fragile X syndrome. Cereb. Cortex doi: 10.1093/cercor/bhy192 [Epub ahead of print].
Nimchinsky, E. A., Oberlander, A. M., and Svoboda, K. (2001). Abnormal development of dendritic spines in FMR1 knock-out mice. J. Neurosci. 21, 5139–5146. doi: 10.1523/JNEUROSCI.21-14-05139.2001
Norum, J. H., Hart, K., and Levy, F. O. (2003). Ras-dependent ERK activation by the human Gs-coupled serotonin receptors 5-HT4(b) and 5-HT7(a). J. Biol. Chem. 278, 3098–3104. doi: 10.1074/jbc.M206237200
Osterweil, E. K., Krueger, D. D., Reinhold, K., and Bear, M. F. (2010). Hypersensitivity to mGluR5 and ERK1/2 leads to excessive protein synthesis in the hippocampus of a mouse model of fragile X syndrome. J. Neurosci. 30, 15616–15627. doi: 10.1523/JNEUROSCI.3888-10.2010
Palmeri, A., Mammana, L., Tropea, M. R., Gulisano, W., and Puzzo, D. (2016). Salidroside, a bioactive compound of rhodiola rosea, ameliorates memory and emotional behavior in adult mice. J. Alzheimers Dis. 52, 65–75. doi: 10.3233/jad-151159
Park, S., Park, J. M., Kim, S., Kim, J. A., Shepherd, J. D., Smith-Hicks, C. L., et al. (2008). Elongation factor 2 and fragile X mental retardation protein control the dynamic translation of Arc/Arg3.1 essential for mGluR-LTD. Neuron 59, 70–83. doi: 10.1016/j.neuron.2008.05.023
Pfeiffer, B. E., and Huber, K. M. (2009). The state of synapses in fragile X syndrome. Neuroscientist 15, 549–567. doi: 10.1177/1073858409333075
Prut, L., and Belzung, C. (2003). The open field as a paradigm to measure the effects of drugs on anxiety-like behaviors: a review. Eur. J. Pharmacol. 463, 3–33. doi: 10.1016/s0014-2999(03)01272-x
Puzzo, D., Bizzoca, A., Privitera, L., Furnari, D., Giunta, S., Girolamo, F., et al. (2013). F3/Contactin promotes hippocampal neurogenesis, synaptic plasticity, and memory in adult mice. Hippocampus 23, 1367–1382. doi: 10.1002/hipo.22186
Sanderson, T. M., Hogg, E. L., Collingridge, G. L., and Corrêa, S. A. (2016). Hippocampal mGluR-LTD in health and disease: focus on the p38 MAPK and ERK1/2 pathways. J. Neurochem. 139, 200–214. doi: 10.1111/jnc.13592
Sawicka, K., Pyronneau, A., Chao, M., Bennett, M. V., and Zukin, R. S. (2016). Elevated ERK/p90 ribosomal S6 kinase activity underlies audiogenic seizure susceptibility in fragile X mice. Proc. Natl. Acad. Sci. U S A 113, E6290–E6297. doi: 10.1073/pnas.1610812113
Schnabel, R., Kilpatrick, I. C., and Collingridge, G. L. (2001). Protein phosphatase inhibitors facilitate DHPG-induced LTD in the CA1 region of the hippocampus. Br. J. Pharmacol. 132, 1095–1101. doi: 10.1038/sj.bjp.0703905
Semple, B. D., Blomgren, K., Gimlin, K., Ferriero, D. M., and Noble-Haeusslein, L. J. (2013). Brain development in rodents and humans: identifying benchmarks of maturation and vulnerability to injury across species. Prog. Neurobiol. 106–107, 1–16. doi: 10.1016/j.pneurobio.2013.04.001
Sethna, F., Feng, W., Ding, Q., Robison, A. J., Feng, Y., and Wang, H. (2017). Enhanced expression of ADCY1 underlies aberrant neuronal signalling and behaviour in a syndromic autism model. Nat. Commun. 8:14359. doi: 10.1038/ncomms14359
Shang, Y., Wang, H., Mercaldo, V., Li, X., Chen, T., and Zhuo, M. (2009). Fragile X mental retardation protein is required for chemically-induced long-term potentiation of the hippocampus in adult mice. J. Neurochem. 111, 635–646. doi: 10.1111/j.1471-4159.2009.06314.x
Taylor, S. S., Buechler, J. A., and Yonemoto, W. (1990). cAMP-dependent protein kinase: framework for a diverse family of regulatory enzymes. Annu. Rev. Biochem. 59, 971–1005. doi: 10.1146/annurev.biochem.59.1.971
Thomas, A., Burant, A., Bui, N., Graham, D., Yuva-Paylor, L. A., and Paylor, R. (2009). Marble burying reflects a repetitive and perseverative behavior more than novelty-induced anxiety. Psychopharmacology 204, 361–373. doi: 10.1007/s00213-009-1466-y
Till, S. M., Asiminas, A., Jackson, A. D., Katsanevaki, D., Barnes, S. A., Osterweil, E. K., et al. (2015). Conserved hippocampal cellular pathophysiology but distinct behavioural deficits in a new rat model of FXS. Hum. Mol. Genet. 24, 5977–5984. doi: 10.1093/hmg/ddv299
Veeraragavan, S., Graham, D., Bui, N., Yuva-Paylor, L. A., Wess, J., and Paylor, R. (2012). Genetic reduction of muscarinic M4 receptor modulates analgesic response and acoustic startle response in a mouse model of fragile X syndrome (FXS). Behav. Brain Res. 228, 1–8. doi: 10.1016/j.bbr.2011.11.018
Ventura, R., Pascucci, T., Catania, M. V., Musumeci, S. A., and Puglisi-Allegra, S. (2004). Object recognition impairment in Fmr1 knockout mice is reversed by amphetamine: involvement of dopamine in the medial prefrontal cortex. Behav. Pharmacol. 15, 433–442. doi: 10.1097/00008877-200409000-00018
Wang, H., Wu, L. J., Kim, S. S., Lee, F. J., Gong, B., Toyoda, H., et al. (2008). FMRP acts as a key messenger for dopamine modulation in the forebrain. Neuron 59, 634–647. doi: 10.1016/j.neuron.2008.06.027
Waung, M. W., and Huber, K. M. (2009). Protein translation in synaptic plasticity: mGluR-LTD, Fragile X. Curr. Opin. Neurobiol. 19, 319–326. doi: 10.1016/j.conb.2009.03.011
Zaccaria, K. J., Lagace, D. C., Eisch, A. J., and McCasland, J. S. (2010). Resistance to change and vulnerability to stress: autistic-like features of GAP43-deficient mice. Genes Brain Behav. 9, 985–996. doi: 10.1111/j.1601-183x.2010.00638.x
Keywords: serotonin, 5-HT7 receptor, fragile X syndrome, cyclic AMP, mGluR-LTD, learning, PACAP
Citation: Costa L, Sardone LM, Bonaccorso CM, D’Antoni S, Spatuzza M, Gulisano W, Tropea MR, Puzzo D, Leopoldo M, Lacivita E, Catania MV and Ciranna L (2018) Activation of Serotonin 5-HT7 Receptors Modulates Hippocampal Synaptic Plasticity by Stimulation of Adenylate Cyclases and Rescues Learning and Behavior in a Mouse Model of Fragile X Syndrome. Front. Mol. Neurosci. 11:353. doi: 10.3389/fnmol.2018.00353
Received: 23 November 2017; Accepted: 10 September 2018;
Published: 02 October 2018.
Edited by:
Regina Dahlhaus, Friedrich-Alexander-Universität Erlangen-Nürnberg, GermanyReviewed by:
Niraj S. Desai, University of Texas at Austin, United StatesJean-Martin Beaulieu, University of Toronto, Canada
Hansen Wang, University of Toronto, Canada
Copyright © 2018 Costa, Sardone, Bonaccorso, D’Antoni, Spatuzza, Gulisano, Tropea, Puzzo, Leopoldo, Lacivita, Catania and Ciranna. This is an open-access article distributed under the terms of the Creative Commons Attribution License (CC BY). The use, distribution or reproduction in other forums is permitted, provided the original author(s) and the copyright owner(s) are credited and that the original publication in this journal is cited, in accordance with accepted academic practice. No use, distribution or reproduction is permitted which does not comply with these terms.
*Correspondence: Lucia Ciranna, Y2lyYW5uYUB1bmljdC5pdA==