- 1Laboratory of Translational Epilepsy, Department of Pharmacology and Experimental Therapeutics, School of Medicine, Boston University, Boston, MA, United States
- 2Department of Biomedical Engineering, College of Engineering, Boston University, Boston, MA, United States
- 3Department of Pediatrics, Division of Neurology, School of Medicine, University of Colorado, Aurora, CO, United States
- 4Department of Pharmaceutical Sciences, Skaggs School of Pharmacy and Pharmaceutical Sciences, University of Colorado Anschutz Medical Campus, Aurora, CO, United States
- 5Department of Biology, Boston University, Boston, MA, United States
While the exact role of β1 subunit-containing GABA-A receptors (GABARs) in brain function is not well understood, altered expression of the β1 subunit gene (GABRB1) is associated with neurological and neuropsychiatric disorders. In particular, down-regulation of β1 subunit levels is observed in brains of patients with epilepsy, autism, bipolar disorder and schizophrenia. A pathophysiological feature of these disease states is imbalance in energy metabolism and mitochondrial dysfunction. The transcription factor, nuclear respiratory factor 1 (NRF-1), has been shown to be a key mediator of genes involved in oxidative phosphorylation and mitochondrial biogenesis. Using a variety of molecular approaches (including mobility shift, promoter/reporter assays, and overexpression of dominant negative NRF-1), we now report that NRF-1 regulates transcription of GABRB1 and that its core promoter contains a conserved canonical NRF-1 element responsible for sequence specific binding and transcriptional activation. Our identification of GABRB1 as a new target for NRF-1 in neurons suggests that genes coding for inhibitory neurotransmission may be coupled to cellular metabolism. This is especially meaningful as binding of NRF-1 to its element is sensitive to the kind of epigenetic changes that occur in multiple disorders associated with altered brain inhibition.
Introduction
The type A γ-aminobutyric acid receptor (GABA-A receptors, GABAR) is a ligand-gated Cl− ion channel that mediates inhibitory neurotransmission in the adult mammalian central nervous system. The majority of GABARs are composed of two α and two β subunits, and either a γ2 or δ subunit (Farrar et al., 1999; Barrera et al., 2008; Patel et al., 2014). For each receptor, there is the binding of two molecules of GABA, one molecule at each α and β subunit interface (Rabow et al., 1995; Connolly and Wafford, 2004; Olsen and Sieghart, 2009). In the mature neuron, activation of GABARs leads to hyperpolarization. Depending on its subunit composition, GABARs may contain binding sites for barbiturates, benzodiazepines, ethanol, and/or neuroactive steroids. There are nineteen different subunit genes to date, grouped into eight classes (i.e., α1–6, β1–3, γ1–3, δ, ε, θ, π, ρ1–3) that contribute to the diversity and differential assembly of receptor subtypes. The β subunits, which contain the domains that interact with mediators of receptor trafficking and endocytosis (for reviews see Jacob et al., 2008; Vithlani et al., 2011), play an important role in the expression of GABARs at the cell surface.
The human GABA receptor subtype A β1 subunit gene (GABRB1), located on chromosome 4, is part of a GABAR gene cluster that contains the genes that encode the α2, α4 and γ1 subunits. A dysregulation of GABAR-mediated neurotransmission has been implicated in various neurological disorders (Hines et al., 2012) that show altered levels of GABAR subunits, including β1. Through linkage studies, GABRB1 has been associated with alcohol dependance (Parsian and Zhang, 1999; Sun et al., 1999; Zinn-Justin and Abel, 1999; Song et al., 2003); and more recently, specific mutations in mouse Gabrb1 have been shown to produce increased alcohol consumption that is linked to increased tonic inhibition (Anstee et al., 2013). Interestingly, single nucleotide polymorphisms in GABRB1 are also associated with altered brain responses in human adolescents susceptible to addictive behaviors (Duka et al., 2017).
GABRB1 expression is also reduced in the lateral cerebella of subjects with bipolar disorder, major depression, and schizophrenia compared to healthy subjects (Fatemi et al., 2013). Particularly in schizophrenia, a significant association of GABRB1 has been identified by genome-wide association studies that were coupled to a protein-interaction-network-based analysis (Yu et al., 2014). As GABRB1 and GABRA4 lie within the same GABAR gene cluster and their promoters are head-to-head, it is interesting to note that the association of GABRA4 with autism risk increases with a GABRB1 interaction (Ma et al., 2005; Collins et al., 2006), suggesting that these genes may be coordinately regulated. Further support for an association of GABRB1 with autism is evidenced by a decrease in β1 subunit levels in the brains of autistic subjects (Fatemi et al., 2009, 2010). In addition, the levels of both β1 and β2 subunit mRNAs are reduced in a Fragile X mental retardation mouse model, where the gene Fragile X mental retardation 1 (fmr1) was removed (D’Hulst et al., 2006). Finally, down-regulation of β1 subunit mRNAs and protein are observed in the rat pilocarpine model of epilepsy (Brooks-Kayal et al., 1998). Yet, despite its prevalent association with brain disorders, there is still little known about the function and/or regulation of β1 in neurons.
The TATA-less GABRB1/Gabrb1 promoter (GABRB1-p (human)/Gabrb1-p (rodent)) contains multiple transcriptional start sites that lie within a CpG island (Russek et al., 2000; Saha et al., 2013). In unraveling the molecular determinants of GABAR β1 subunit gene regulation, our laboratory demonstrated that the minimal GABRB1-p lies within the first 500 bp of the 5’ flanking region. Within this region, there is a conserved initiator element (Inr) that mediates down-regulation in response to chronic GABA exposure, implicating an autologous mechanism of transcriptional control.
Nuclear respiratory factor 1 (NRF-1) is a transcription factor that functions primarily as a positive regulator of nuclear genes involved in mitochondrial biogenesis and oxidative phosphorylation, such as Tfam, which moves into the mitochondria and regulates mitochondrial DNA transcription (Scarpulla, 2006, 2008). However, it has also been shown that the binding of NRF-1 to a co-factor, such as SIRT7, can influence its polarity (from activator to repressor; Mohrin et al., 2015). In addition, binding of NRF-1 to DNA is regulated by the methylation state of its regulatory element (Domcke et al., 2015), suggesting that its role in neuronal gene expression will be sensitive to the epigenetic changes that occur in neurological and neuropsychiatric disorders.
It is well known that increased neuronal activity results in a parallel change in cellular metabolism, as orchestrated by the synthesis of NRF-1 and its control over mitochondrial biogenesis. Moreover, it has been reported that NRF-1 is a transcriptional activator of glutamate receptor subunit genes under conditions of depolarizing stimulation in neurons (Dhar and Wong-Riley, 2009) suggesting that in addition to its role in cellular metabolism, via regulation of the mitochondrial genome, NRF-1 coordinates activities in the nucleus to couple neuronal excitability with energy demands of synaptic neurotransmission.
Here, we ask whether NRF-1 may control the transcription of GABAR subunit genes (GABRs), and in particular the human β1 subunit gene (GABRB1), a gene that has been associated with neuronal developmental disorders, the pathophysiology of epilepsy, and alcohol dependance. In this study, we have uncovered a functional regulatory element within GABRB1 that demonstrates sequence specificity and is responsible for the majority of GABRB1 promoter-reporter activity, as well as a role for NRF-1 in the activity dependent transcription of endogenous Gabrb1 in rat primary cortical neurons.
Materials and Methods
Cell Culture and Drug Treatment
This study was carried out in accordance with the recommendations of the Boston University Institutional Animal Care and Use Committee (IACUC) that oversees and routinely evaluates the activities conducted by and at BU that involve animals. All animal care and use protocols must be approved by the IACUC before animals are procured and prior to performing any work with them in or out of the laboratory.
Primary neocortical neurons were isolated from embryonic day 18 Sprague-Dawley rat embryos (Charles River Laboratories). Isolated embryonic brains and the subsequently dissected cortices were maintained in ice-cold modified calcium-magnesium free Hank’s Balanced Salt Solution (HBSS; 4.2 mM sodium bicarbonate, 1 mM sodium pyruvate and 20 mM HEPES, 3 mg/ml BSA) buffering between pH range 7.25–7.3. Tissues were then separated from HBSS dissection solution and trypsinized (0.05% trypsin-EDTA) for 10 min in 37°C and 5% CO2. The trypsin reaction was stopped with serum inactivation using plating medium (Neural Basal Medium, 10% FBS, 10 U/ml penicillin/streptomycin, 2 mM L-glutamine). Tissues were triturated with a 1,000 mL micropipette and diluted to a concentration of 0.5 × 106 cells/mL in plating media for plating. Cells were allowed to adhere onto Poly-L-lysine coated culturing surface for 1 h prior to changing to serum-free feeding medium (2% B-27, 2 mM glutamine, 10 U/ml penicillin/streptomycin supplemented neurobasal medium). Neuronal cultures were maintained at 37°C in a 5% CO2 incubator. Primary cortical neurons (DIV7–8) were treated with either Vehicle or 20 mM KCl for 6 h before harvesting for analysis.
Expression Constructs
pCDNA 3.1 hygro hNRF-1 herpes simplex virus virion protein 16 (VP16) was generously provided by Dr. Tod Gulick (Ramachandran et al., 2008; Sanford-Burnham Medical Research Institute, Orlando, FL, USA). pcDNA 3.1 hygro hNRF-1 VP16 encodes a constitutively active form of NRF-1, consisting of the full-length human NRF-1 and the herpes simplex virus VP16 transactivation domain. The pcDNA3.0-NRF-1 DN expresses amino acid residues 1–304 of human NRF-1, which encodes the DNA-binding, dimerization and nuclear localization domains of NRF-1, but lacks the transactivation domain (amino acids 305–503). With the exception of a single conservative mutation at amino acid residue 293 (A→T), the 304 amino acid residues of NRF-1 are conserved between human and rat. The construct was created using PCR with the forward primer sequence 5’-CGGGGTACCACCATGGAGGAACACGGAGTGACCCAAAC-3’, containing the underlined Kpn1 restriction site and the kozak sequence on the 5’ end, and the reverse primer 5’GCTCTAGATCACTGTGATGGTACAAGATGAGCTATACTATGTGTGGCTGTGGC-3’, containing stop codon and Xba1 restriction site. PCR products were digested with restriction enzymes Kpn1 and Xba1, and ligated into pcDNA3.0 vector (Invitrogen).
Electrophoretic Mobility Shift (EMSA) and Supershift Assays
Briefly, 30 bp DNA probes containing the putative NRF-1 binding sequence were incubated with 25 μg of neocortical nuclear extracts for electrophoresis under non-denaturing conditions. Following electrophoresis, the protein-DNA complexes were detected by autoradiography. The DNA probes were created from annealing synthesized oligonucleotides1 and 5’ end labeling using [γ−32P] ATP (PerkinElmer) in a T4 polynucleotide kinase (NEB) reaction. Nuclear extracts were prepared from DIV7 primary neocortical neurons grown on 10-cm plates in the presence of protease inhibitor cocktail. Protein-DNA binding specificity was determined by adding poly (dI-dC; Roche) or/and 100-fold excess unlabeled DNA probe prior to the addition of labeled probe during the room temperature binding reaction. To generate a supershift complex, NRF-1 antibody (AbCam ab34682) was added to the reaction mixture for 15 min. The binding reactions were loaded onto a 5% polyacrylamide gel in 0.5× TBE buffer and run at 200V for 2 h at 4°C. The positive control probe consisted of a functional NRF-1 sequence (Evans and Scarpulla, 1990) found in the Rat cytochrome c (rCycs) gene (Evans and Scarpulla, 1990). Probe and competitor oligonucleotide sequences were: GABRB1 NRF-1, 5’-agcgcgcTCTGCGCATGCGCAggtccattc-3’ and 5’-gaatggaccTGCGCATGCGCAGAgcgcgct-3’. GABRB1 NRF-1 mutant, 5’-agcgcgcTCTGCcCATGgGCAggtccattc -3’ and 5’-gaatggaccTGCcCATGgGCAGAgcgcgct-3’. rCycs NRF-1, 5’-ctgctaGCCCGCATGCGCgcgcacctta-3’and 5’-taaggtgcgcGCGCATGCGGGCtagcag-3’.
Reporter Plasmids and Promoter Mutagenesis
The GABRB1p-Luc (pGL2-GABRB1) promoter construct containing the 5’ flanking region of the human β1 subunit gene was previously cloned by our laboratory and contains 436 bp upstream of the initiator sequence and 105 bp downstream (Russek et al., 2000). The promoter containing a mutated NRF-1 element (TCTGCcCATGgGCA) within the GABRB1p-Luc was created by PCR-driven overlap extension. Using wild-type GABRB1p-Luc as PCR template, two PCR fragments were amplified using the GL1 primer (Promega) and the antisense mutant NRF-1 oligonucleotide from electrophoretic mobility shift (EMSA), and sense mutant NRF-1 oligonucleotide and the GL2 primer (Promega), resulting in fragments with 30 bp overlapping sequences that contain the mutant NRF-1 element. A second PCR step using GL1, GL2 primers and both initial PCR products produced the mutant GABRB1 promoter insert.
Luciferase Reporter Assays
Magnetofection of DNA into primary neuron cultures was achieved with the NeuroMag transfection reagent according to the manufacturer’s protocol. Here, 2 ml of resuspended E18 primary cortical neurons at 0.5 × 106 cells/ml were plated in each well of a 6-well plate. On DIV7, neurons were transfected with 1 μg of expression construct, 2 μg of promoter reporter construct, and 3 μl of NeuroMag transfection reagent (1:1 DNA to reagent ratio). 24 h after transfection, neurons were actively lysed by scraping. Cell lysates were cleared of precipitates by centrifugation and then assayed for luciferase activity using a luciferase assay system (Promega). Luciferase activity was normalized to total protein as determined using a protein assay kit (ThermoScientific Pierce). All transfections were performed in sister dishes from three or more plating sessions to produce true N’s.
Chromatin Immunoprecipitation (ChIP)
Chromatin-immunoprecipitation (ChIP) was performed according to the Magna ChIP A protocol (Millipore). Briefly, primary neurons in 100 mm dishes were fixed with a final concentration of 1% formaldehyde in culturing media. The remaining unreacted formaldehyde was quenched with Glycine. Genomic DNA and protein complexes were extracted from cells using nuclear lysis buffers supplemented with protease and phosphatase inhibitors. The lysates containing DNA-protein complexes were sonicated (nine times, 5 min each at a 30 s on/off interval) in an ice-cold water bath with a Bioruptor (Diagenode) in order to generate fragments predominantly in the range of 200–500 bp in size. The sheared chromatin was immunoprecipitated with either anti-NRF-1 antibody (Abcam ab34682 ChIP grade antibody) or normal rabbit IgG overnight at 4°C with constant rotation. The antibody/transcription factor bound chromatin was separated from unbound chromatin using Protein A conjugated magnetic beads and magnetic pull-down. The isolated complexes were washed with a series of salt buffer solutions prior to eluting. DNA fragments were separated from complexes using Proteinase K and heating, and recovered through column purification. The co-precipitated DNA fragments were identified by quantitative PCR (qPCR) using specific primers and TaqMan probes that flank putative responsive elements in gene promoters using the FastStart Universal Probe Master (Roche) PCR reagent. PCR cycling was performed using the ABI7900HT Fast Real-Time PCR system. The Gabrb1 promoter fragment (114 bp) was amplified using: forward primer 5’- TGTTTGCAAGGCACAAGGTGTC-3’, reverse primer 5’- TCTGCGAAGATTCAAGGAATGCAACT, TaqMan® MGB probe 5’- GCGCATGCGCAGGTCCATTCGGGAAT-3’.
Western Blot Analysis
Total cellular proteins were extracted from primary neuronal cultures after KCl treatment with standard procedures and the use of RIPA lysis buffer (Tris, pH 7.4, 10 mM; Nonidet P-40 1%; NaCl 150 mM; SDS 0.1%; protease inhibitor mixture (Roche Applied Science) 1×; EDTA 1 mM; sodium orthovanadate 1 mM; sodium deoxycholate 0.1%; phenylmethylsulfonyl fluoride 1 mM). Thirty microgram of whole cell extracts were separated by SDS-PAGE under reducing conditions on either 10% or 4%–20% Tris-glycine gel according to mass/size. The electrophoresed samples were transferred to nitrocellulose membranes. Western blot analysis was performed using antibodies against NRF-1 (AbCam ab34682, 1:2,000 in 1× TBS-T). Membranes were incubated with peroxidase-conjugated goat anti-rabbit secondary antibody (Santa Cruz Biotechnology, 1:5,000) in TBS-T and visualized using the ECL enhanced chemiluminescence reagent (GE Healthcare Life Sciences). Data are presented as mean ± SEM. Significance was determined at p < 0.05 using the paired Student’s t-test (two-tailed).
RNA Extraction and qRT-PCR
Total RNA was isolated from cultured primary neocortical using the RNeasy Micro Kit (Qiagen). For each reaction, 20 ng of total RNA was reverse-transcribed to cDNA and PCR amplified in a single reaction mixture using the TaqMan® One-Step RT-PCR Master Mix Reagents Kit (Applied Biosystems). Incubation and thermal cycling conditions were performed using the ABI7900HT in a 384-well PCR plate format (Applied Biosystems). The RT reaction was held at 48°C for 30 min, followed by 95°C for 10 min to activate the polymerase. The PCR reaction conditions were: 15 s denaturation at 95°C and coupled annealing and extension for 1 min at 60°C for 40 cycles. Co-detection of rat peptidylprolyl isomerase A (cyclophilin A) gene served as an internal control for normalization. Cyclophilin A expression has been shown to be stable in response to neuronal stimulation in culture (Santos and Duarte, 2008), which is consistent with our previous studies. Relative gene expression was quantified using 2(−ΔΔCT) and a standard curve was generated based on the amplification of total RNA extracted from untreated cultured neurons. The qRT-PCR primers and probes for rat mRNAs were: NRF-1, 57 bp amplicon (Assay ID: Rn01455958_m1, ThermoFisher Scientific); Gabrb1, 81 bp amplicon (Assay ID: Rn00564146_m1, ThermoFisher Scientific); Ppia, 60 bp amplicon, forward primer: 5’- TGCAGACATGGTCAACCCC-3’, reverse primer: 5’- CCCAAGGGCTCGCCA-3’, TaqMan probe with TAMARA quencher: 5’- CCGTGTTCTTCGACATCACGGCTG-3’.
Statistical Analysis
Data analysis was performed using the statistical package included with Microsoft Excel (V16.11.1) or Prism software (Version 7.0d). Experimental values were expressed as a percentage of or fold change from the mean of control values, where control was defined at 100% or 1. Values were reported with a standard error of the mean, which is the standard deviation divided by the square root of the number of observations per group. Statistical significance was evaluated using a 95% confidence interval. For comparison of two sets of experimental data normalized to the same control values, statistical analysis was performed using the Student’s t-test. The “95% range check” was used to test for normalcy as described in Limpert and Stahel (2011). Each N was defined as a set of data collected from the average of 2–4 sister dishes of vehicle or drug treated primary cultured neurons that are plated at a single culturing session with embryonic cells (E18) obtained from the litter of an individual pregnant rat. 2–4 replicates (sister dishes) are prepared for each condition within each N. (See legend of each figure to obtain experimental N, choice of statistical test, and meaning of error bars.) A two-way analysis of variance (ANOVA) was performed to determine statistical significance across two independent variables (Treatment (water/KCl) vs. Transfected DNA (empty vector/NRF-1 DN); Figure 5). Values were considered statistically different if the 95% confidence interval did not overlap with control values. Differences between all pairs of means were further compared through post hoc analysis using the Bonferroni multiple comparison’s test.
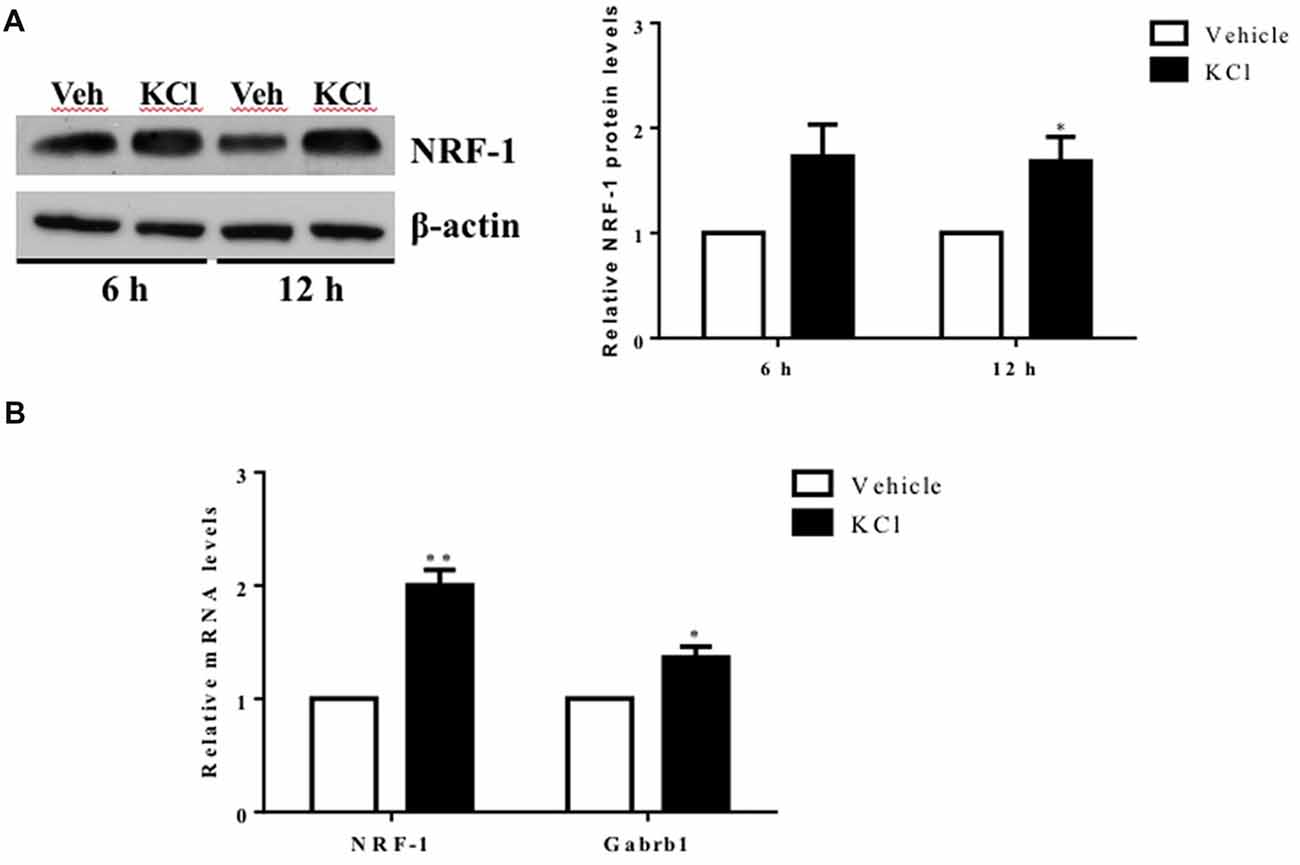
Figure 1. Activity-dependent regulation of nuclear respiratory factor 1 (NRF-1) and GABA receptor subtype A β1 subunit gene (Gabrb1) in primary cortical neurons. Primary cortical neurons (DIV7–8) were treated with either Vehicle or 20 mM KCl for 6 h. (A) Total protein was extracted from neurons and probed for the presence of NRF-1 and β-actin. A representative western blot is shown (left) for comparison. NRF-1 levels were quantified by densitometry and normalized to levels of β-actin. Levels of NRF-1 are expressed relative to vehicle (right; n = 6, paired Student’s t-test, p < 0.05 as significant. (B) Levels of mRNAs were quantified by TaqMan qRT-PCR. Transcripts specific to NRF-1 and Gabrb1 were normalized to Cyclophilin A. Messenger RNA levels are expressed relative to vehicle treated neurons plated in a six well dish. Data represent the average ± SEM of n = 6 independent neuronal cultures with neurons extracted from different animals and plated on different days (replicates N = 4). *p < 0.05; **p < 0.01, paired Student’s t-test. This dataset was originally published in the thesis of Li (2015).
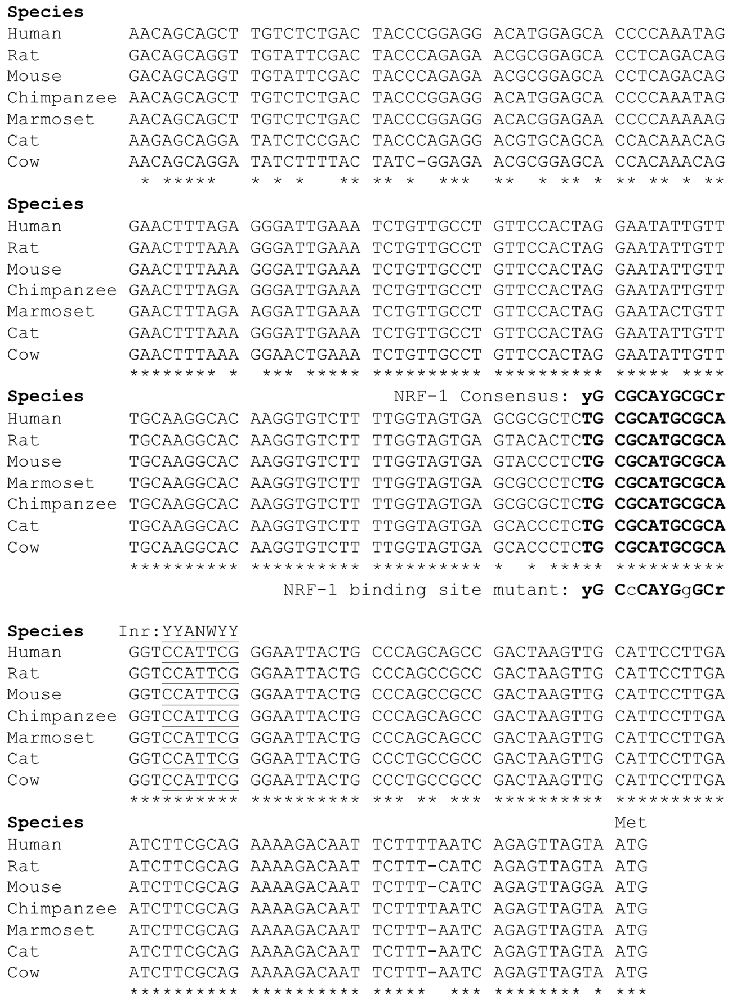
Figure 2. Sequence alignment of the 5’ promoter regions of β1 subunit genes. The β1 subunit promoters in mammals contain a conserved NRF-1 element, indicated in bold type upstream of the major initiator element (Inr) specific to each gene, underlined for reference. Sequences were aligned using ClustalW, where conserved nucleotides are as indicated “*”. Modified from the figure originally published in the thesis of Li (2015).
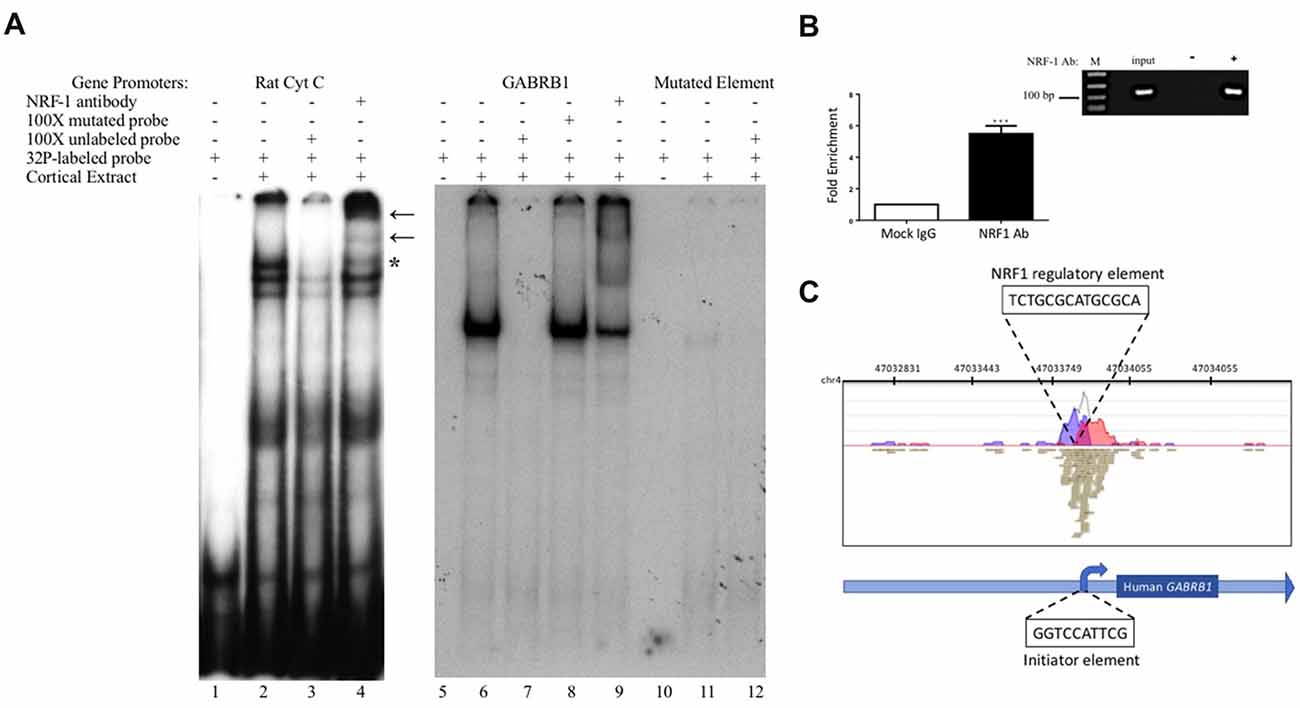
Figure 3. In vitro and in vivo binding of NRF-1 to the putative NRF-1 site in GABRB1. (A) 32P-labeled probes encompassing the NRF-1 binding site were incubated with 20 μg of DIV7 primary rat cortical nuclear extracts. 100-fold excess of unlabeled probe was added to the binding reaction to assess specificity. NRF-1 Abs were pre-incubated with nuclear extracts and radiolabeled probe to test for “supershift” and protein identification. (Left Panel) The NRF-1 element in the rat cytochrome c (Cyt C) promoter displays NRF-1 specific binding (lane 2) and “supershift” (lane 4). (Right Panel) The proposed NRF-1 element in the human GABRB1 promoter displays a probe specific shift (lane 6; note that excess probe was run off of the gel to provide room for the detection of the shifted probe), competition of complex formation with cold competitor (lane 7), lack of competition with mutant cold competitor (lane 8), and supershift upon addition of NRF-1 specific Ab (lane 9). In contrast, binding to radiolabeled probe for NRF-1 mutant GABRB1 shows markedly reduced signal strength (lanes 11 and 12). “*” Indicates specific interaction between labeled probe and nuclear extract, “←” indicates location of supershift. (B) Chromatin Immunoprecipitation (ChIP) assays were performed using sonicated genomic DNA from DIV7 primary rat cortical neurons and either ChIP grade NRF-1 polyclonal antibody (Abcam, ab34682) that recognizes the full length protein or rabbit IgG (Vector Laboratories, I-1,000). Co-precipitated GABRB1 gene promoter fragments were detected with specific quantitative PCR (qPCR) primers and probe. Data represent the average ± SEM of n = 4 independent primary cultures and co-precipitations. ***p < 0.001, student t-test. (C) Representative ChIP-seq track from the Strand NGS software platform for GABRB1 in H1-hESC cells after peak detection (MACS version 2.0). Read density profile plots of forward reads (blue) and reverse reads (red) aligned to the UCSC transcript model are depicted; each brown box represents a single 27-bp sequencing read. The NRF-1 motif sequence is shown in black text above its position. Relative position of the Inr in GABRB1 is shown for reference. Chr, chromosome. (A,B) Datasets were originally published in the thesis of Li (2015).
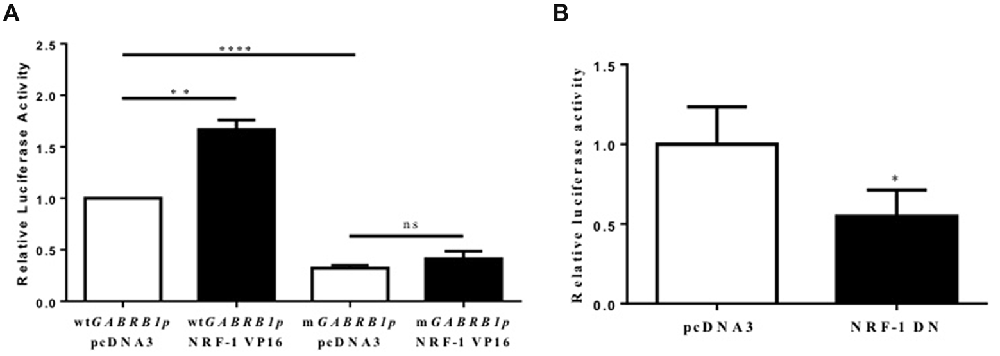
Figure 4. Evidence for the regulation of the GABAR β1 promoter by NRF-1. (A) Primary cortical neurons were co-transfected with 2 μg of wild type GABRB1p (wtGABRB1p) or the NRF-1 binding site mutant (mGABRB1p) and 1 μg of empty vector pcDNA3 or the NRF-1:VP16 fusion construct. Cells were assayed for luciferase activity 24 h after transfection. Data represent the average ± SEM (n = 5 independent transfections) of luciferase activity relative to wild type GABRB1p in the absence of NRF-1:VP16. **p < 0.01, ****p < 0.0001, and “ns” (non-specific) represent presence or absence respectively of significance according to one-way ANOVA with Tukey’s multiple comparisons test. (B) Primary cortical neurons were co-transfected with either pcDNA3 or the dominant negative variant of NRF-1 (NRF-1 DN) and GABRB1p reporter (2 μg). Twenty-four hours after transfection, cells were assayed for luciferase activity. Data represent the average ± SEM of n = 6 independent transfections (see above), normalized to wtGABRB1p and pcDNA3. *p < 0.05, Student’s t-test. This dataset was originally published in the thesis of Li (2015).
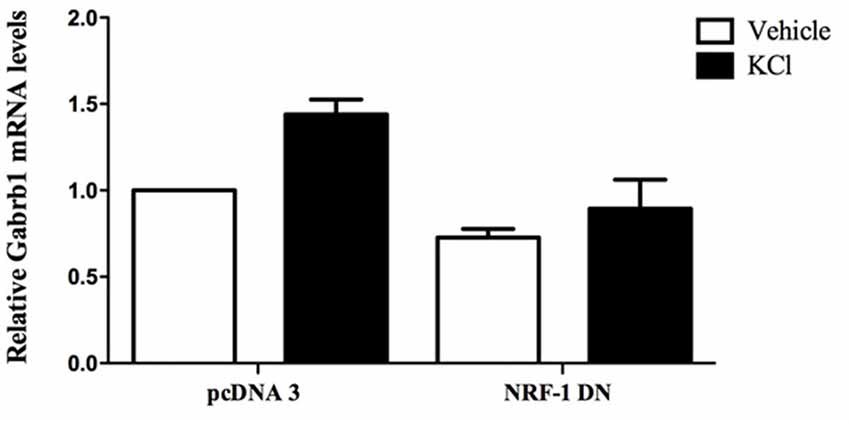
Figure 5. Overexpression of dominant negative NRF-1 attenuates the increase in β1 subunit mRNA levels in response to neuronal stimulation of primary cortical neurons. Primary cortical neurons were transfected with empty vector (pcDNA3) or dominant negative NRF-1 (NRF-1 DN; using Nucleofection™) and plated in 6-well plates. DIV7 cells were treated with either vehicle or 20 mM KCl for 6 h. Total mRNA was isolated from cells and quantified by TaqMan qRT-PCR. Gabrb1 mRNA expression was normalized to Cyclophilin A mRNA levels and is presented relative to its levels in pcDNA3 transfected neurons that were treated with vehicle (expressed as 1). Data represent the average ± SEM of n = 3 independent primary neuronal cultures. Two-way ANOVA was performed (comparison between treatments, p = 0.0148; comparison between transfected DNA conditions, p = 0.0031) with Bonferroni post hoc analysis (adjusted p value = 0.0263 for KCl vs. water with pcDNA transfection, not significant for NRF-1 DN).
Results
Neuronal Depolarization Increases NRF-1 and GABARβ1 Subunit Gene Transcription
To determine whether Gabrb1 is activity dependent, primary cortical neurons were treated with KCl. Both NRF-1 protein and NRF-1 mRNA levels have been previously shown to increase with KCl-stimulated depolarization (Dhar and Wong-Riley, 2009). We asked whether under conditions where NRF-1 levels increase in response to neuronal activity, is it accompanied by increased levels of Gabrb1 transcripts. As shown in Figures 1A,B, there is a 2-fold increase in NRF-1 mRNA levels (1.982 ± 0.445, n = 5, paired Student’s t-test, **p < 0.01) upon KCl stimulation for 6 h that is accompanied by a ~30% increase in the levels of NRF-1 protein (fold change: 1.285 ± 0.330, n = 6, *p < 0.05) when compared to vehicle control. In parallel to changes in NRF-1, we now report a ~40% increase in levels of Gabrb1 transcripts (fold change: 1.424 ± 0.324, n = 5, *p < 0.05).
Identification of a Conserved NRF-1 Element in the GABRB1 Promoter
Our laboratory previously defined the 5’-regulatory region of the human β1 subunit gene GABRB1, identifying transcriptional start sites (TSSs) within a 10 bp functional Inr that mediates the response of the gene to chronic GABA exposure (Russek et al., 2000; Saha et al., 2013). Now we report that directly upstream of this Inr is a canonical NRF-1 element spanning −11/+1 relative to the major TSS for the rat homolog Gabrb1 in neocortical neurons. As shown in Figure 2, the location of the NRF-1 element within the promoter region is conserved across multiple species. Given the ubiquitous expression of NRF-1, its conservation across species, and its established role in cellular respiration and mitochondrial biogenesis, the sequence comparison presented in Figure 2 strongly suggests that the NRF-1 element is functionally relevant to β1 subunit expression in the mammalian brain.
NRF-1 Recognizes the Cis-Element in the Human GABRB1 Promoter
To determine the specific binding site within GABRB1-p that binds to NRF-1, we performed an electrophoretic mobility shift assay (EMSA) with a 32P-labeled probe specific to its NRF-1 consensus element in a binding reaction with nuclear protein extracts from E18 primary cortical neurons. To validate the specificity of the NRF-1 antibody for EMSA analysis, nuclear extracts were incubated with a positive control probe (Dhar et al., 2008) containing the NRF-1 binding site of the rat cytochrome c promoter. As shown in lane 2 of Figure 3A, the control radiolabeled probe (rat Cyt C) displays specific DNA recognition from nuclear extracts of cortical neurons that is confirmed by supershift with the addition of an NRF-1 specific antibody (Figure 3A, lane 4). Next, specific binding to the putative NRF-1 consensus site in GABRB1 was confirmed using the same nuclear extracts, with sequence specificity defined by competition with an unlabeled double stranded oligonucleotide that was identical to the probe sequence (competitor; Figure 3A, lanes 6 and 7). Addition of an unlabeled competitor mutant probe, containing substitutions within the GC core, failed to compete for complex formation (Figure 3A, lane 8). Presence of endogenous NRF-1 at the GABRB1 NRF-1 consensus site was further confirmed by supershift analysis using the NRF-1 specific antibody (Figure 3A, lane 9). Finally, a radiolabeled probe containing the sequence of the mutant NRF-1 site in GABRB1 shows little or no complex formation (Figure 3A, lanes 10–12).
To determine whether the endogenous β1 promoter in neurons is occupied by NRF-1, ChIP was performed using genomic DNA derived from E18 rat primary cortical cultures (DIV7) that was precipitated with NRF-1 antibodies. Precipitated fragments were detected using PCR primers that specifically amplify DNA encompassing the putative NRF-1 binding site in rat Gabrb1. As can be seen in Figure 3B, there is a 5-fold increase (5.045 ± 0.981, n = 4, *p < 0.001) in PCR detection of the NRF-1 site in Gabrb1 when precipitated using an NRF-1 Ab, as compared to rabbit IgG. Moreover, NRF-1 is also present at the core promoter of GABRB1 in human embryonic stem cells (H1-hESC) as detected in ChIP-sequencing (ChIP-seq) datasets of the ENCODE project2 using our bioinformatic analysis algorithm (in the Strand NGS pipeline, Model-based Analysis for ChIP-Seq (MACS, version 2.0, Zhang et al., 2008)) with a p-value cutoff set to 1.0E-05, quality threshold ≥30, 99% match to the sequence, and all duplicates removed (Figure 3C). We also found coincident peak detection using ENCODE datasets from NRF-1 ChIP-seq with genomic DNA from immortalized cell lines (K562, HepG2, CH12.LX, GM 12878 and HeLa-S3; data not shown). Note that the detected peak in H1-hESC is identical to that predicted by Figure 2 and within the wildtype oligonucleotide sequence that bound nuclear extracts from rat primary neurons (Figure 3).
Overexpression of NRF-1 Induces GABRB1 Promoter Activity in Transfected Primary Cortical Neurons
To evaluate whether there is a functional consequence to NRF-1 binding to its consensus site in GABRB1-p, primary cortical neurons were transfected with the GABRB1p-luciferase construct containing the 541 bp 5’ flanking region upstream of the human β1 subunit gene (Russek et al., 2000). We chose this approach to study functional relevance of the NRF-1 site to GABRB1 transcription in neurons because NRF-1’s influence on the genome is difficult to detect by siRNA knockdown due to its robust expression at baseline and protein stability (Baar et al., 2003; Scarpulla, 2006; Ramachandran et al., 2008).
As the expression of the NRF-1:VP16 fusion protein has been shown to induce the promoter activity of NRF-1 responsive genes in cell lines (Ramachandran et al., 2008; Gonen and Assaraf, 2010), we transfected primary cortical neurons with NRF-1:VP16 along with the GABRB1p-luciferase reporter and found a marked increase (~70%, fold change: 1.671 ± 0.404, n = 5, **p < 0.01) above baseline (when compared to co-transfection with empty vector control, 1.00 ± 0.225, n = 5; Figure 4A). Mutations were introduced into GABRB1-p using site-directed mutagenesis (based on the loss of specific binding of NRF-1 as identified in EMSA (see Figure 3, lane 8)). As can be seen in Figure 4A for mGABRB1-p, with and without NRF-1:VP16 overexpression, mutation of the NRF-1 regulatory element in GABRB1-p reduces basal activity to around 30% (fold change: 0.314 ± 0.067, n = 5, ****p < 0.0001) of wild type. Overexpression of NRF-1:VP16 has no effect on mGABRB1-p (0.358 ± 0.057, n = 5, ns) showing that increased GABRB1 promoter activity directed by NRF-1 is sequence specific; and, moreover, that NRF-1 may be an important positive regulator of β1 subunit expression in developing neurons, especially interesting because β1 is found in the germinal zones and associated with pre-migrating neurons (Ma and Barker, 1995). Furthermore, increased mitochondrial biogenesis has also been associated with neuronal differentiation (Vayssière et al., 1992; Cheng et al., 2010).
Inhibition of NRF-1 Function in Neurons
To evaluate the specific effect of endogenous NRF-1 on GABRB1 transcription, a dominant negative form of NRF-1 was utilized that contains the DNA binding domain but lacks the NRF-1 trans-activation domain (Gugneja et al., 1996). Co-expression of this dominant negative NRF-1 represses GABRB1 promoter activity by 45% (fold change: 0.549 ± 0.164, n = 6, Students t-test, *p < 0.05, Figure 4B) compared to empty-vector control (1.000 ± 0.235, n = 6). Most importantly, overexpression of dominant negative NRF-1 blocks the activity dependent increase of endogenous Gabrb1 mRNA levels in response to KCl treatment (N = 3, two-way ANOVA, effect of treatment p = 0.0148, effect of transfected DNA p = 0.0031; Bonferroni adjusted p value for effect of pcDNA3 vs. NRF-1 DN transfected constructs on the response to KCl treatment is 0.0263, Figure 5).
When taken together with the fact that the NRF-1 element in the β1 subunit gene is completely conserved across species (Figure 2), and that there is a mutation-induced loss of binding (Figure 3) and function (Figure 4), our results strongly suggest that NRF-1 is an essential feature of β1 subunit expression in neurons and that it couples transcription to the activity pattern of individual cells.
Discussion
We now report that the GABAR β1 subunit gene (GABRB1/Gabrb1) is regulated by NRF-1, a crucial transcription factor involved in oxidative phosphorylation and mitochondrial biogenesis. While it is believed that NRF-1 coordinates synaptic activity and energy metabolism by regulating excitatory neurotransmission via genes that code for subunits of the N-methyl-D-aspartate (NMDA) receptor (Dhar and Wong-Riley, 2009, 2011; Dhar et al., 2009), it is clear that the this regulatory program is more complex than originally expected given our observation that elements of inhibitory neurotransmission may be coordinately regulated with excitation. This possibility is especially important as a variety of brain disorders present with a decrease in GABAR β1 subunit levels, including epilepsy where there is also aberrant hyperactivity.
Previously, our laboratory mapped the 5’ flanking region of the human β1 subunit promoter. Within this TATA-less promoter, we identified the major TSS and described an Inr that senses the presence of prolonged GABA to mediate the autologous downregulation of β1 subunit expression (Russek et al., 2000). Our recent studies have discovered that such decreases in β1 subunit RNA levels may reflect a change in the chromatin state as mediated by PhF1b, a polycomb-like protein (Saha et al., 2013). In our present work, we have found a conserved canonical NRF-1 binding element (Figure 2) that interacts with NRF-1 in vitro as verified by mobility shift assays (Figure 3). Interestingly, our results in primary rat neurons are consistent with a peak of NRF-1 binding over the core promoter of GABRB1 in human embryonic stem cells, as displayed in Figure 3C, using our bioinformatic analysis of ENCODE project datasets (Gerstein et al., 2012; Wang et al., 2012, 2013) with the Strand NGS pipeline (MACS V2 peak detection). We identified the same peak of binding in additional NRF-1 ChIP-seq ENCODE datasets from immortalized cell lines. Interestingly, we did not detect any additional NRF-1 peaks on the genes that code for other β subunit genes, suggesting that NRF-1 regulation may be unique to β1.
It is thought that NRF-1 binds as a homodimer to the consensus binding sequence (T/C)GCGCA(C/T)GCGC(A/G), making contact with DNA at the guanine nucleotides (Virbasius et al., 1993). This model is supported by the results of our mutational studies which show that a single mutation of G > C eliminates the ability of a cold double stranded oligonucleotide to compete for complex formation as assayed by mobility shift. The location of the NRF-1 element in GABRB1 centers at -12 relative to the major TTS in neocortical neurons. The GC-rich NRF-1 binding motif is often associated with TATA-less promoters and found within 100 bp DNA regions around transcriptional start sites in the human genome (Virbasius et al., 1993; Xi et al., 2007). The proximity of the NRF-1 element in GABRB1 to the Inr that binds polycomb-like proteins associated with chromatin remodeling and DNA methylation (Vire et al., 2006) may underlie its major role in controlling basal levels of β1 subunit mRNAs in neurons. Whether GABRB1 is epigenetically regulated in vivo remains to be determined and could be a feature of why its transcription decreases in disease, especially since NRF-1 binding is blocked by DNA methylation (Gebhard et al., 2010).
Using the sensitivity of the luciferase reporter system, we have overexpressed NRF-1:VP16 in living neurons and shown that it indeed regulates the GABRB1p-luciferase reporter construct and that such regulation is lost upon mutation of the GABRB1 NRF-1 regulatory element (Figure 4A) and upon competition for endogenous NRF-1 binding to the promoter by overexpression of a dominant negative NRF-1 expression construct (Figure 4B). We have also shown that the same mutation in the NRF-1 regulatory element of GABRB1p removes binding of endogenous NRF-1 to neuronal extracts in a mobility shift assay, as seen in Figure 3. Finally, and perhaps most importantly, we have shown that overexpression of dominant negative NRF-1 protein blocks the activity dependent increase in endogenous Gabrb1 mRNA levels identifying a key molecular determinant of β1 subunit gene expression within cells (Figure 5).
Our results are consistent with previous studies from the Russek laboratory, using the same wild type GABRB1p-luciferase reporter construct, where promoter truncation and/or deletion that removes the Inr and at the same time disrupts the element for NRF-1 results in a 75%–90% decrease in luciferase gene transcription (Russek et al., 2000; Figure 4). Given that GABAR blockade by bicuculine has also been shown to drive NRF-1 dependent transcription (Delgado and Owens, 2012) and that bicuculine reverses GABA-induced downregulation of β1 mRNA levels (Russek et al., 2000), presumably through PhF1b binding to the Inr, our new results suggest that the NRF-1 responsive element and Inr may act synergistically to regulate β1 subunit levels as neurons adapt to changes in their activity state.
The direct regulation of NRF-1 in GABRB1/Gabrb1 gene expression in the brain may also have implications in the initiation of sleep. The use of fragrant dioxane derivatives that show a 6-fold preference for β1-containing GABARs (Sergeeva et al., 2010) suggest that the β1 subunit is required for the modulation of wakefulness that is mediated by the histaminergic neurons of the posterior hypothalamus (tubermamillary nucleus-TMN; Yanovsky et al., 2012). Given that energy metabolism is sensitive to restoration during the sleep cycle and that NRF-1 levels rise with sleep deprivation (Nikonova et al., 2010), it is interesting that β1-containing GABARs are the major source of inhibitory control over sleep.
Although differential expression of α subunits in relationship to brain disorders has clearly been associated with their region-specific control over changes in tonic and phasic inhibition, it is only recently that the importance of differential β subunit expression to GABAR function has been noted. This selective property of GABAR function ascribed to the assembly of particular β subunits, however, has been limited to β2 and β3, with β1 present in only a limited population of receptors in the brain. However, of all β subunits, β1 has been most associated with both neurological and neuropsychiatric disorders. The reason for this functional relationship remains to be described and is an active area of investigation in our laboratories.
Author Contributions
SR conceived the project, funding and supervision. AB-K: key collaborator and funding. ZL carried out most experiments while as a graduate student, helped to interpret datasets, wrote first draft of the manuscript. MC carried out additional experiments, edited manuscript with Russek into final form, reproduced datasets collected by ZL for rigors in science. KH: graduate student, did bioinformatic analysis, made experimental contribution to Figure 3.
Funding
This work was supported by grants from the National Institutes of Health (NIH/NINDS R01 NS4236301 to SR and AB-K, T32 GM00854 to ZL, MC and KH).
Conflict of Interest Statement
The authors declare that the research was conducted in the absence of any commercial or financial relationships that could be construed as a potential conflict of interest.
Acknowledgments
We thank Dr. Tod Gulick (Sanford-Burnham Medical Research Institute, Orlando, FL, USA) for his helpful discussion and providing us with expression constructs that were invaluable for our experiments. We also thank Ms. Allison Tipton for her efforts in the laboratory to provide datasets to better understand the interaction of endogenous NRF-1 at Gabrb1 during her laboratory rotation.
Abbreviations
ChIP, Chromatin-immunoprecipitation; DIV, Days in vitro; EMSA, Electrophoretic mobility shift assay; GABAR, GABA type A receptors; GABRB1, GABA receptor subtype A β1 subunit gene; HRP, Horseradish peroxidase; Inr, Initiator element; NRF-1, Nuclear respiratory factor 1; PhF1, Polycomb-like protein; TSS, Transcriptional start site; VP16, Herpes simplex virus virion protein 16.
Footnotes
References
Anstee, Q. M., Knapp, S., Maguire, E. P., Hosie, A. M., Thomas, P., Mortensen, M., et al. (2013). Mutations in the Gabrb1 gene promote alcohol consumption through increased tonic inhibition. Nat. Commun. 4:2816. doi: 10.1038/ncomms3816
Baar, K., Song, Z., Semenkovich, C. F., Jones, T. E., Han, D. H., Nolte, L. A., et al. (2003). Skeletal muscle overexpression of nuclear respiratory factor 1 increases glucose transport capacity. FASEB J. 17, 1666–1673. doi: 10.1096/fj.03-0049com
Barrera, N. P., Betts, J., You, H., Henderson, R. M., Martin, I. L., Dunn, S. M., et al. (2008). Atomic force microscopy reveals the stoichiometry and subunit arrangement of the α4β3δ GABAA receptor. Mol. Pharmacol. 73, 960–967. doi: 10.1124/mol.107.042481
Brooks-Kayal, A. R., Shumate, M. D., Jin, H., Rikhter, T. Y., and Coulter, D. A. (1998). Selective changes in single cell GABAA receptor subunit expression and function in temporal lobe epilepsy. Nat. Med. 4, 1166–1172. doi: 10.1038/2661
Cheng, A., Hou, Y., and Mattson, M. P. (2010). Mitochondria and neuroplasticity. ASN Neuro 2:e00045. doi: 10.1042/AN20100019
Collins, A. L., Ma, D., Whitehead, P. L., Martin, E. R., Wright, H. H., Abramson, R. K., et al. (2006). Investigation of autism and GABA receptor subunit genes in multiple ethnic groups. Neurogenetics 7, 167–174. doi: 10.1007/s10048-006-0045-1
Connolly, C. N., and Wafford, K. A. (2004). The Cys-loop superfamily of ligand-gated ion channels: the impact of receptor structure on function. Biochem. Soc. Trans. 32, 529–534. doi: 10.1042/bst0320529
Delgado, J. Y., and Owens, G. C. (2012). The cytochrome c gene proximal enhancer drives activity-dependent reporter gene expression in hippocampal neurons. Front. Mol. Neurosci. 5:31. doi: 10.3389/fnmol.2012.00031
Dhar, S. S., Liang, H. L., and Wong-Riley, M. T. (2009). Nuclear respiratory factor 1 co-regulates AMPA glutamate receptor subunit 2 and cytochrome c oxidase: tight coupling of glutamatergic transmission and energy metabolism in neurons. J. Neurochem. 108, 1595–1606. doi: 10.1111/j.1471-4159.2009.05929.x
Dhar, S. S., Ongwijitwat, S., and Wong-Riley, M. T. (2008). Nuclear respiratory factor 1 regulates all ten nuclear-encoded subunits of cytochrome c oxidase in neurons. J. Biol. Chem. 283, 3120–3129. doi: 10.1074/jbc.M707587200
Dhar, S. S., and Wong-Riley, M. T. (2009). Coupling of energy metabolism and synaptic transmission at the transcriptional level: role of nuclear respiratory factor 1 in regulating both cytochrome c oxidase and NMDA glutamate receptor subunit genes. J. Neurosci. 29, 483–492. doi: 10.1523/JNEUROSCI.3704-08.2009
Dhar, S. S., and Wong-Riley, M. T. (2011). The kinesin superfamily protein KIF17 is regulated by the same transcription factor (NRF-1) as its cargo NR2B in neurons. Biochim. Biophys. Acta 1813, 403–411. doi: 10.1016/j.bbamcr.2010.12.013
D’Hulst, C., De Geest, N., Reeve, S. P., Van Dam, D., De Deyn, P. P., Hassan, B. A., et al. (2006). Decreased expression of the GABAA receptor in fragile X syndrome. Brain Res. 1121, 238–245. doi: 10.1016/j.brainres.2006.08.115
Duka, T., Nikolaou, K., King, S. L., Banaschewski, T., Bokde, A. L. W., Büchel, C., et al. (2017). GABRB1 single nucleotide polymorphism associated with altered brain responses (but not Performance) during measures of impulsivity and reward sensitivity in human adolescents. Front. Behav. Neurosci. 11:24. doi: 10.3389/fnbeh.2017.00024
Domcke, S., Bardet, A. F., Adrian Ginno, P., Hartl, D., Burger, L., and Schübeler, D. (2015). Competition between DNA methylation and transcription factors determines binding of NRF1. Nature 528, 575–579. doi: 10.1038/nature16462
Evans, M. J., and Scarpulla, R. C. (1990). NRF-1: a trans-activator of nuclear-encoded respiratory genes in animal cells. Genes Dev. 4, 1023–1034. doi: 10.1101/gad.4.6.1023
Farrar, S. J., Whiting, P. J., Bonnert, T. P., and McKernan, R. M. (1999). Stoichiometry of a ligand-gated ion channel determined by fluorescence energy transfer. J. Biol. Chem. 274, 10100–10104. doi: 10.1074/jbc.274.15.10100
Fatemi, S. H., Folsom, T. D., Rooney, R. J., and Thuras, P. D. (2013). mRNA and protein expression for novel GABAA receptors θ and rho2 are altered in schizophrenia and mood disorders; relevance to FMRP-mGluR5 signaling pathway. Transl. Psychiatry 3:e271. doi: 10.1038/tp.2013.46
Fatemi, S. H., Reutiman, T. J., Folsom, T. D., Rooney, R. J., Patel, D. H., and Thuras, P. D. (2010). mRNA and protein levels for GABAAα4, α5, β1 and GABABR1 receptors are altered in brains from subjects with autism. J. Autism Dev. Disord. 40, 743–750. doi: 10.1007/s10803-009-0924-z
Fatemi, S. H., Reutiman, T. J., Folsom, T. D., and Thuras, P. D. (2009). GABAA receptor downregulation in brains of subjects with autism. J. Autism Dev. Disord. 39, 223–230. doi: 10.1007/s10803-008-0646-7
Gebhard, C., Benner, C., Ehrich, M., Schwarzfischer, L., Schilling, E., Klug, M., et al. (2010). General transcription factor binding at CpG islands in normal cells correlates with resistance to de novo DNA methylation in cancer cells. Cancer Res. 70, 1398–1407. doi: 10.1158/0008-5472.CAN-09-3406
Gerstein, M. B., Kundaje, A., Hariharan, M., Landt, S. G., Yan, K. K., Cheng, C., et al. (2012). Architecture of the human regulatory network derived from ENCODE data. Nature 489, 91–100. doi: 10.1038/nature11245
Gonen, N., and Assaraf, Y. G. (2010). The obligatory intestinal folate transporter PCFT (SLC46A1) is regulated by nuclear respiratory factor 1. J. Biol. Chem. 285, 33602–33613. doi: 10.1074/jbc.M110.135640
Gugneja, S., Virbasius, C. M., and Scarpulla, R. C. (1996). Nuclear respiratory factors 1 and 2 utilize similar glutamine-containing clusters of hydrophobic residues to activate transcription. Mol. Cell. Biol. 16, 5708–5716. doi: 10.1128/mcb.16.10.5708
Hines, R. M., Davies, P. A., Moss, S. J., and Maguire, J. (2012). Functional regulation of GABAA receptors in nervous system pathologies. Curr. Opin. Neurobiol. 22, 552–558. doi: 10.1016/j.conb.2011.10.007
Jacob, T. C., Moss, S. J., and Jurd, R. (2008). GABAA receptor trafficking and its role in the dynamic modulation of neuronal inhibition. Nat. Rev. Neurosci. 9, 331–343. doi: 10.1038/nrn2370
Li, Z. (2015). Activity-Dependent Gene Regulation in Neurons: Energy Coupling and a Novel Biosensor [Dissertation]. Boston, MA: Boston University.
Limpert, E., and Stahel, W. A. (2011). Problems with using the normal distribution—and ways to improve quality and efficiency of data analysis. PLoS One 6:e21403. doi: 10.1371/journal.pone.0021403
Ma, W., and Barker, J. L. (1995). Complementary expressions of transcripts encoding GAD67 and GABAA receptor α-4, β-1, and γ-1 subunits in the proliferative zone of the embryonic rat central nervous system. J. Neurosci. 15, 2547–2560. doi: 10.1523/JNEUROSCI.15-03-02547.1995
Ma, D. Q., Whitehead, P. L., Menold, M. M., Martin, E. R., Ashley-Koch, A. E., Mei, H., et al. (2005). Identification of significant association and gene-gene interaction of GABA receptor subunit genes in autism. Am. J. Hum. Genet. 77, 377–388. doi: 10.1086/433195
Mohrin, M., Shin, J., Liu, Y., Brown, K., Luo, H., Xi, Y., et al. (2015). A mitochondrial UPR-mediated metabolic checkpoint regulates hematopoietic stem cell aging. Science 347, 1374–1377. doi: 10.1126/science.aaa2361
Nikonova, E. V., Naidoo, N., Zhang, L., Romer, M., Cater, J. R., Scharf, M. T., et al. (2010). Changes in components of energy regulation in mouse cortex with increases in wakefulness. Sleep 33, 889–900. doi: 10.1093/sleep/33.7.889
Olsen, R. W., and Sieghart, W. (2009). GABAA receptors: subtypes provide diversity of function and pharmacology. Neuropharmacology 56, 141–148. doi: 10.1016/j.neuropharm.2008.07.045
Parsian, A., and Zhang, Z. H. (1999). Human chromosomes 11p15 and 4p12 and alcohol dependence: possible association with the GABRB1 gene. Am. J. Med. Genet. 88, 533–538. doi: 10.1002/(sici)1096-8628(19991015)88:5<533::aid-ajmg18>3.0.co;2-c
Patel, B., Mortensen, M., and Smart, T. G. (2014). Stoichiometry of δ subunit containing GABAA receptors. Br. J. Pharmacol. 171, 985–994. doi: 10.1111/bph.12514
Rabow, L. E., Russek, S. J., and Farb, D. H. (1995). From ion currents to genomic analysis: recent advances in GABAA receptor research. Synapse 21, 189–274. doi: 10.1002/syn.890210302
Ramachandran, B., Yu, G., and Gulick, T. (2008). Nuclear respiratory factor 1 controls myocyte enhancer factor 2A transcription to provide a mechanism for coordinate expression of respiratory chain subunits. J. Biol. Chem. 283, 11935–11946. doi: 10.1074/jbc.M707389200
Russek, S. J., Bandyopadhyay, S., and Farb, D. H. (2000). An initiator element mediates autologous downregulation of the human type A γ -aminobutyric acid receptor β 1 subunit gene. Proc. Natl. Acad. Sci. U S A 97, 8600–8605. doi: 10.1073/pnas.97.15.8600
Saha, S., Hu, Y., Martin, S. C., Bandyopadhyay, S., Russek, S. J., and Farb, D. H. (2013). Polycomblike protein PHF1b: a transcriptional sensor for GABA receptor activity. BMC Pharmacol. Toxicol. 14:37. doi: 10.1186/2050-6511-14-37
Santos, A. R., and Duarte, C. B. (2008). Validation of internal control genes for expression studies: effects of the neurotrophin BDNF on hippocampal neurons. J. Neurosci. Res. 86, 3684–3692. doi: 10.10.1002/jnr.21796
Scarpulla, R. C. (2006). Nuclear control of respiratory gene expression in mammalian cells. J. Cell. Biochem. 97, 673–683. doi: 10.1002/jcb.20743
Scarpulla, R. C. (2008). Nuclear control of respiratory chain expression by nuclear respiratory factors and PGC-1-related coactivator. Anna. N Y Acad. Sci. 1147, 321–334. doi: 10.1196/annals.1427.006
Sergeeva, O. A., Kletke, O., Kragler, A., Poppek, A., Fleischer, W., Schubring, S. R., et al. (2010). Fragrant dioxane derivatives identify β1-subunit-containing GABAA receptors. J. Biol. Chem. 285, 23985–23993. doi: 10.1074/jbc.M110.103309
Song, J., Koller, D. L., Foroud, T., Carr, K., Zhao, J., Rice, J., et al. (2003). Association of GABAA receptors and alcohol dependence and the effects of genetic imprinting. Am. J. Med. Genet. B Neuropsychiatr. Genet. 117B, 39–45. doi: 10.1002/ajmg.b.10022
Sun, F., Cheng, R., Flanders, W. D., Yang, Q., and Khoury, M. J. (1999). Whole genome association studies for genes affecting alcohol dependence. Genet. Epidemiol. 17, S337–S342. doi: 10.1002/gepi.1370170757
Vayssière, J. L., Cordeau-Lossouarn, L., Larcher, J. C., Basseville, M., Gros, F., and Croizat, B. (1992). Participation of the mitochondrial genome in the differentiation of neuroblastoma cells. In Vitro Cell. Dev. Biol. 28A, 763–772. doi: 10.1007/bf02631065
Virbasius, C. A., Virbasius, J. V., and Scarpulla, R. C. (1993). NRF-1, an activator involved in nuclear-mitochondrial interactions, utilizes a new DNA-binding domain conserved in a family of developmental regulators. Genes Dev. 7, 2431–2445. doi: 10.1101/gad.7.12a.2431
Vire, E., Brenner, C., Deplus, R., Blanchon, L., Fraga, M., Didelot, C., et al. (2006). The Polycomb group protein EZH2 directly controls DNA methylation. Nature 439, 871–874. doi: 10.1038/nature04431
Vithlani, M., Terunuma, M., and Moss, S. J. (2011). The dynamic modulation of GABAA receptor trafficking and its role in regulating the plasticity of inhibitory synapses. Physiol. Rev. 91, 1009–1022. doi: 10.1152/physrev.00015.2010
Wang, J., Zhuang, J., Iyer, S., Lin, X. Y., Greven, M. C., Kim, B. H., et al. (2013). Factorbook.org: a Wiki-based database for transcription factor-binding data generated by the ENCODE consortium. Nucleic Acids Res. 41, D171–D176. doi: 10.1093/nar/gks1221
Wang, J., Zhuang, J., Iyer, S., Lin, X., Whitfield, T. W., Greven, M. C., et al. (2012). Sequence features and chromatin structure around the genomic regions bound by 119 human transcription factors. Genome Res. 22, 1798–1812. doi: 10.1101/gr.139105.112
Xi, H., Yu, Y., Fu, Y., Foley, J., Halees, A., and Weng, Z. (2007). Analysis of overrepresented motifs in human core promoters reveals dual regulatory roles of YY1. Genome Res. 17, 798–806. doi: 10.1101/gr.5754707
Yanovsky, Y., Schubring, S., Fleischer, W., Gisselmann, G., Zhu, X. R., Lübbert, H., et al. (2012). GABAA receptors involved in sleep and anaesthesia: β1- versus β3-containing assemblies. Pflugers Arch. 463, 187–199. doi: 10.1007/s00424-011-0988-4
Yu, H., Bi, W., Liu, C., Zhao, Y., Zhang, J. F., Zhang, D., et al. (2014). Protein-interaction-network-based analysis for genome-wide association analysis of schizophrenia in Han Chinese population. J. Psychiatr. Res. 50, 73–78. doi: 10.1016/j.jpsychires.2013.11.014
Zinn-Justin, A., and Abel, L. (1999). Genome search for alcohol dependence using the weighted pairwise correlation linkage method: interesting findings on chromosome 4. Genet. Epidemiol. 17, S421–S426. doi: 10.1002/gepi.1370170771
Keywords: GABA-A receptor, GABRB1, NRF-1, cortical neurons, activity-dependent, mitochondrial biogenesis
Citation: Li Z, Cogswell M, Hixson K, Brooks-Kayal AR and Russek SJ (2018) Nuclear Respiratory Factor 1 (NRF-1) Controls the Activity Dependent Transcription of the GABA-A Receptor Beta 1 Subunit Gene in Neurons. Front. Mol. Neurosci. 11:285. doi: 10.3389/fnmol.2018.00285
Received: 21 March 2018; Accepted: 27 July 2018;
Published: 21 August 2018.
Edited by:
Gregg E. Homanics, University of Pittsburgh, United StatesReviewed by:
Deep R. Sharma, SUNY Downstate Medical Center, United StatesPeter Vanhoutte, Centre National de la Recherche Scientifique (CNRS), France
Copyright © 2018 Li, Cogswell, Hixson, Brooks-Kayal and Russek. This is an open-access article distributed under the terms of the Creative Commons Attribution License (CC BY). The use, distribution or reproduction in other forums is permitted, provided the original author(s) and the copyright owner(s) are credited and that the original publication in this journal is cited, in accordance with accepted academic practice. No use, distribution or reproduction is permitted which does not comply with these terms.
*Correspondence: Shelley J. Russek, c3J1c3Nla0BidS5lZHU=
† These authors have contributed equally to this work