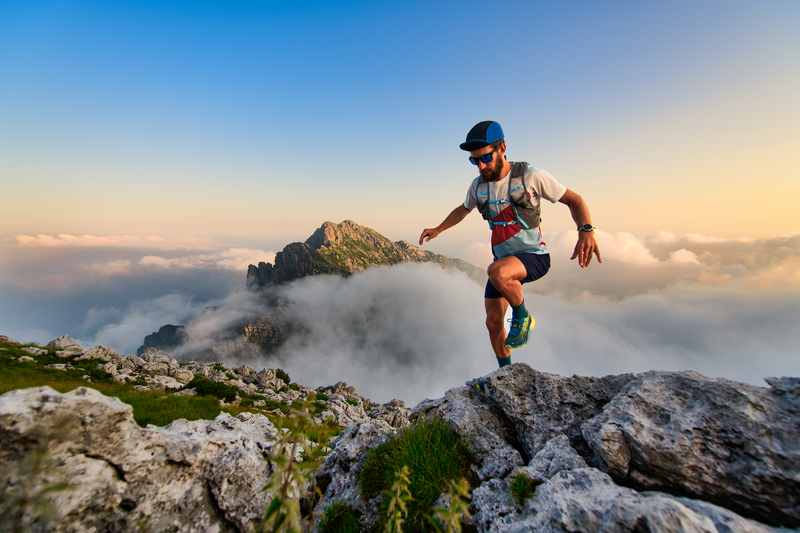
94% of researchers rate our articles as excellent or good
Learn more about the work of our research integrity team to safeguard the quality of each article we publish.
Find out more
ORIGINAL RESEARCH article
Front. Mol. Neurosci. , 29 April 2014
Sec. Molecular Signalling and Pathways
Volume 7 - 2014 | https://doi.org/10.3389/fnmol.2014.00034
This article is part of the Research Topic Ca2+ and Ca2+-interlocked Membrane Guanylate Cyclase Modulation of Neuronal and Cardiovascular Signal Transduction View all 17 articles
ROS-GC1 belongs to the Ca2+-modulated sub-family of membrane guanylate cyclases. It primarily exists and is linked with signaling of the sensory neurons – sight, smell, taste, and pinealocytes. Exceptionally, it is also present and is Ca2+-modulated in t he non-neuronal cells, the sperm cells in the testes, where S100B protein serves as its Ca2+ sensor. The present report demonstrates the identification of an additional Ca2+ sensor of ROS-GC1 in the testes, neurocalcin δ. Through mouse molecular genetic models, it compares and quantifies the relative input of the S100B and neurocalcin δ in regulating the Ca2+ signaling of ROS-GC1 transduction machinery, and via immunochemistry it demonstrates the co-presence of neurocalcin δ and ROS-GC1 in the spermatogenic cells of the testes. The suggestion is that in more ways than one the Ca2+-modulated ROS-GC1 transduction system is linked with the testicular function. This non-neuronal transduction system may represent an illustration of the ROS-GC1 expanding role in the trans-signaling of the neural and non-neural systems.
Rod outer segment membrane guanylate cyclase, ROS-GC1 (known also as Ret-GC1 or GC-E), belongs to the family of membrane guanylate cyclases. Its discovery and molecular characterization was a land mark event in the field of phototransduction (reviewed in Pugh et al., 1997; Koch et al., 2010) as it identified the source of cyclic GMP that serves as a second messenger of the LIGHT signal. It also impacted the entire membrane guanylate cyclase field by dividing the guanylate cyclase family into two subfamilies, one comprising the hormone receptor cyclases, and the other, cyclases modulated by intracellular [Ca2+]i signals (reviewed in: Sharma, 2010). ROS-GC1 belongs to the second subfamily together with another rod outer segment guanylate cyclase ROS-GC2 (Ret-GC2 or GC-F), and the olfactory neuroepithelial guanylate cyclase, ONE-GC (GC-D; reviewed in: Sharma, 2010).
The best-documented physiological function of ROS-GC1 and ROS-GC2 is in the recovery phase of phototransduction, to return the illuminated photoreceptors to the dark, resting state. Illumination of photoreceptors leads to activation of cyclic GMP phosphodiesterase, depletion of cyclic GMP, closure of the cyclic GMP gated (CNG) channels, lowering the free Ca2+ concentration, and hyperpolarization of the plasma membrane (reviewed in: Pugh et al., 1997; Koch et al., 2010). The ROS-GCs task is to restore the dark-level of cyclic GMP allowing opening of the CNG channels, increase of Ca2+ influx, and depolarization of plasma membrane. Ca2+ concentration, thus, determines the activities of ROS-GCs but in an indirect way. Guanylate cyclase activating proteins (GCAPs; GCAP1 and GCAP2) sense the post-illumination fall in Ca2+ and stimulate ROS-GCs to synthesize cyclic GMP at a faster rate and restore its dark level (reviewed in: Detwiler, 2000; Koch et al., 2010).
GCAPs, however, are not the only Ca2+ sensing modulators of ROS-GC activity. While increasing Ca2+ concentrations inhibit ROS-GCs activity through GCAPs, two other Ca2+ sensors, S100B and neurocalcin δ stimulate ROS-GC1 in a Ca2+-dependent fashion (Pozdnyakov et al., 1995; Margulis et al., 1996; Duda et al., 1996a; Kumar et al., 1999). The Ca2+-dependent S100B-mediated activation of ROS-GC1 operates in cones including their outer segments and pedicles (Duda et al., 2002; Wen et al., 2012). Its role in photo- and visual transductions remains to be established, but existing data indicate its involvement in transmission of the visual signal from cone ON-bipolar cells (Wen et al., 2012). Ca2+ signaling of ROS-GC1 activity mediated by neurocalcin δ is operative in retinal ganglion cells (Krishnan et al., 2004); and of ONE-GC in the olfactory neuroepithelium (Duda et al., 2001a, 2004).
Beyond the retina, ROS-GC1 is expressed in the pineal gland where in one subset of pinealocytes it co-localizes with GCAP1 and in another, with S100B (Venkataraman et al., 2000); in the mitral cells of the olfactory bulb where it co-localizes with GCAP1 (Duda et al., 2001b); and it co-immunoprecipitates with S100B in the gustatory epithelium (Duda and Sharma, 2004).
Neurocalcin δ (NCδ) belongs to a subfamily of neuronal calcium sensor (NCS) proteins called visinin like proteins (VSNLs). Similar to other, but not all NCS proteins, it is acylated at the N-terminus by myristic acid and undergoes a classical calcium-myristoyl switch (Ladant, 1995) e.g., it buries the myristoyl group in a hydrophobic pocket in a Ca2+ free form and exposes it in Ca2+-bound form, a phenomenon first observed for recoverin (Zozulya and Stryer, 1992). Myristoylation of neurocalcin δ allows its membrane association in response to changes in the intracellular Ca2+ concentration. However, once NCδ binds to the cellular membranes in a Ca2+-dependent fashion, part of it remains membrane associated even after removing Ca2+ by the addition of ethylene glycol tetraacetic acid (EGTA; Krishnan et al., 2004). Although the highest level of NCδ has been detected in neuronal tissues, its expression in the periphery is also observed. Functionally, NCδ has been linked to a wide variety of processes such as receptor endocytosis through interaction with α- and β-clathrin and β-adaptin (Ivings et al., 2002), trafficking and membrane delivery of glutamate receptors of the kainate type (Coussen and Mulle, 2006), and with microtubule assembly (Iino et al., 1995). The presence of NCδ has been found in the inner plexiform layer of the retina, e.g., in the amacrine and ganglion cells (Krishnan et al., 2004), olfactory sensory neurons (Duda et al., 2001b, 2004) and in type II cells of mouse circumvallate taste papillae (Rebello et al., 2011).
In the inner retinal layer NCδ acts as Ca2+-dependent modulator of membrane guanylate cyclase ROS-GC1 (Krishnan et al., 2004) and in the olfactory neuroepithelium, of ONE-GC (Duda et al., 2001a, 2004). The exact physiological significance of the ROS-GC1-NCδ signaling system in the retina is not known yet, it has, however, been proposed that the system may be involved in synaptic processes (Krishnan et al., 2004). In the olfactory neuroepithelium neurocalcin δ has been proposed as a Ca2+ sensor component of the two-step odorant uroguanylin signaling machinery (Duda and Sharma, 2009).
Outside the neuronal system, NCδ is expressed in the adrenal glomerulosa cells (Duda et al., 2012a,b). There it co-localizes with the member of receptor guanylate cyclase sub-family, atrial natriuretic factor receptor guanylate cyclase (ANF-RGC), and has been proposed to be involved in the inhibition of aldosterone synthesis (Duda et al., 2012a,b). Accordingly, a mouse model in which one copy of NCδ gene is deleted is inflicted with hyperaldosteronism and hypertension (Duda et al., 2012b).
Preliminary studies localize also ROS-GC1 outside the neuronal system, in male gonads, where it co-localizes with GCAP1 and S100B (Jankowska et al., 2007). It remains to be determined, whether, and how ROS-GC1 and its companion calcium binding proteins are involved in the physiology of the testis. Considering, however, that cyclic GMP and Ca2+ are genuine mediators of signal transduction in the testes in the physiology of fertilization (reviewed in: Garbers, 1989; Jankowska and Warchol, 2010) it is highly possible that ROS-GC1, stimulated or inhibited as needed, in a Ca2+-dependent fashion by Ca2+-binding proteins, is the provider of the physiologically necessary quantities of cyclic GMP. We now present new observations on co-presence of NCδ and ROS-GC1 in the spermatogenic cells and Ca2+-dependent modes of NCδ and S100B in modulating ROS-GC1 signaling the mammalian testes.
The study group consists of testes from human (obtained from consenting organ donors, n = 5), bovine (purchased from local slaughter house, n = 3), and Wistar rat (obtained form the Vivarium of Poznan University of Medical Sciences, Poznan, Poland, n = 12). The study was approved by the IACUC and Ethics Review Boards of all the involved Institutions.
Care of the experimental animals conformed to the protocols approved by the IACUC at Salus University and was in strict compliance with the NIH guidelines. Construction of heterozygous NCδ-KO (NCδ+/-) mice is described in (Duda et al., 2012b). The S100B-KO mice are described in (Wen et al., 2012).
Rabbit ROS-GC1 and NCδ antibodies were produced, characterized and affinity purified as in (Venkataraman et al., 2003; Krishnan et al., 2004). Secondary antibodies, AP-conjugated goat anti-rabbit IgG, preimmune rabbit serum, NBT/BCIP, and Cy3-conjugated sheep anti-rabbit IgG were purchased from Sigma-Aldrich.
Total RNA was isolated from seminiferous tubules and interstitial tissue using TriPure isolation reagent (Roche Diagnostics), according to the manufacturer’s protocols. The cDNA library was constructed using Advantage RT for PCR kit (BD-Bioscience) and used for the amplification of ROS-GC1 and neurocalcin δ fragments. Sequences of primers used for the amplification are listed in Table 1. The amplified fragments were purified on agarose gel and sequenced to confirm their identities.
The procedure was carried out according to the previously published protocols (Venkataraman et al., 2000; Duda and Sharma, 2004; Jankowska et al., 2007). 150 mg of membrane fraction proteins were denatured in gel-loading buffer [62.5 mM Tris-HCl (pH 7.5), 2% SDS, 5% glycerol, 1 mM β-mercaptoethanol, and 0.005% bromophenol blue] in 95°C for 10 min. Samples were then subjected to SDS-PAGE in a buffer containing 0.025 mM Tris-HCl (pH 8.3), 0.192 M glycine, and 0.1% SDS. Afterwards the resolved proteins were transferred to nitrocellulose membranes. To avoid non-specific interactions membranes were incubated in Tris buffered saline containing 0.05% Tween 20 (TBS-T), 5% non-fat milk (blocking buffer) overnight at 4°C. ROS-GC1 and NCδ proteins were detected with specific rabbit polyclonal antibodies diluted 1:1500 and 1:1000, respectively. After 1 h incubation the blot was rinsed three times with TBS-T and incubated with anti-rabbit secondary antibodies conjugated with horseradish peroxidase (1:10 000).
To visualize the immunoreactive bands SuperSignal blaze chemiluminescent substrate (Pierce) was used according to the manufacturer’s protocol. Signal was detected by exposing the blot to Kodak X-ray film for 15 s. Then the X-ray film was scanned and processed using Photoshop 6.0 software.
Paraffin sections of the testes fixed in 4% paraformaldehyde were used for immunohistochemical detecting of ROS-GC1 and NCδ. To block the non-specific binding the sections were first washed with TBS (100 mM Tris-HCl, 0.9% NaCl) and incubated in blocking solution consisting of TBS buffer containing 0.05% Tween 20 (TBS-T) and 1% BSA for 1 h at room temperature. After washing with TBS-T the sections were incubated with the respective primary antibodies for 60 min at 37°C and washed four times (15 min each) in TBS-T. Anti ROS-GC1 antibodies were diluted 1:200 and anti neurocalcin δ, 1:100. AP-conjugated anti-rabbit IgG, diluted 1:200 and NBT/BCIP as the substrate were used for detection. Controls included detection reactions carried out under identical conditions except that the primary antibodies were replaced by preimmune serum.
Membrane fraction of the testes was isolated according to the protocol described previously (Marala and Sharma, 1988). The tissue was homogenized in a buffer containing 250 mM sucrose, 10 mM Tris-HCl (pH 7.4) and protease inhibitors. The homogenate was centrifuged at 400 g and then the supernatant at 10,000 g and finally, at 40,000 g. The resulting pellet represented the membrane fraction.
The particulate fraction was assayed for guanylate cyclase activity as described previously (Paul et al., 1987; Jankowska et al., 2007, 2008). Briefly, membranes were preincubated on an ice-bath with or without NCδ in an assay system containing 10 mM theophylline, 15 mM phosphocreatine, 20 μg creatine kinase, and 50 mM Tris-HCl (pH 7.5), adjusted to the appropriate free Ca2+ concentration with precalibrated Ca2/EGTA solutions (Molecular Probes). The reaction was initiated by the addition of a substrate solution (4 mM MgCl2 and 1 mM GTP, final concentration) and continued by incubation at 37°C for 10 min. The reaction was terminated by the addition of 50 mM sodium acetate (pH 6.2) followed by heating in a boiling water-bath for 3 min. The amount of cyclic GMP formed was determined by radioimmunoassay (Nambi et al., 1982; Jankowska et al., 2007, 2008).
The activity of guanylate cyclase was calculated as mean of at least six separate values ± SD.
General consensus has been that ROS-GC1 is expressed exclusively in the sensory as well as in the second order neurons of the retina and in the neurons of the pineal gland and the olfactory bulb (Hayashi and Yamazaki, 1991; Goraczniak et al., 1994; Liu et al., 1994; Yang et al., 1995; Venkataraman et al., 2000; Duda et al., 2001a). However, earlier results of these investigators provided the first indication that ROS-GC1 is also expressed outside the neuronal system, in bovine testes and sperm (Jankowska et al., 2007, 2008). In the sperm, it is co-expressed with calcium sensor S100B and neuronal calcium sensor proteins GCAP1 and NCδ (Jankowska et al., 2008). These results were striking enough to warrant more detailed studies. Present investigation represents a step in that direction.
The presence of ROS-GC1 and NCδ transcripts was analyzed in human, bovine, and rat testes. Using total RNA isolated from these tissues individual cDNA libraries were constructed and used for amplification of specific fragments of ROS-GC1 and NCδ cDNAs by polymerase chain reaction. For the amplification, distinct, species-specific primers for ROS-GC1 or NCδ were designed. These primers corresponded to the sequences located within so called “kinase-like domain” of ROS-GC1 cDNA and for NCδ they overlapped with the 5′- and 3′- ends of its coding sequence. The amplification yielded fragments of 553, 548, and 566 bp of the human, bovine, and rat ROS-GC1, respectively, and 526 bp fragment of neurocalcin δ (Table 1). Sequencing of all amplified products confirmed their identities with ROS-GC1 or NCδ cDNAs. The ROS-GC1 fragments represented indeed sequence coding for the kinase-like domain, and the NCδ fragment had sequence identical with the predicted part of NCδ coding region. Amplification of uninterrupted coding sequences for ROS-GC1 and NCδ documented that the RNA used was not contaminated with genomic DNA. Thus both, ROS-GC1 and NCδ transcripts are present in human, bovine, and rat testes.
At the protein level, the expression of ROS-GC1 and NCδ in mammalian testes was tested in two ways. First, a rudimentary assessment of the expression of both types of proteins was obtained by Western blotting; this was followed by immunocytochemical localization of ROS-GC1 and NCδ in human and rat testes. In both types of analyses appropriate affinity purified antibodies, anti-ROS-GC1, or anti-NCδ were used. Immunostaining of bovine testis was performed in parallel as positive control.
After establishing that ROS-GC1 and NCδ transcripts are present in mammalian testes, their protein identity was scrutinized by Western blotting. The mobility of the ROS-GC1 or NCδ immunoreactive band, ~116 kDa and ~20 kDa, respectively, (Figures 1A,B) was identical to that observed previously for ROS-GC1 expressed in photoreceptor outer segments (Duda et al., 1996b) and NCδ expressed in the inner retina (Krishnan et al., 2004). These results clearly confirm that both proteins, ROS-GC1 and NCδ are expressed in human, rat, and bovine testes.
FIGURE 1. Expression of ROS-GC1 and NCδ in the testes analyzed by Western blot. Membrane proteins from human, rat, and bovine testes were isolated and subjected to Western blot analyses with specific antibodies against ROS-GC1 (A), and NCδ (B) as described in “Materials and Methods.” Both types of analyzed proteins expressed in human, rat, and bovine testis are shown in lanes 2, 3, and 4, respectively. Western blotting of bovine retinal membranes was performed as a positive control and is shown in lane 1 in (A) and (B). The positions of the molecular size markers are given alongside.
Detailed analyses of the localization of ROS-GC1 and NCδ in the mammalian testes were performed by immunocytochemistry. In human and rat testes ROS-GC1 immunostaining was randomly distributed in the seminiferous tubules (Figures 2A,B). The pattern of this staining was identical to that observed for bovine testes (Figure 2C). Visual analysis allowed localizing the staining to spermatogenic cells, especially to primary spermatocytes and spermatids. The majority of primary spermatocytes (Figure 2; indicated as Sc), spermatids (Figure 2; indicated as Sd) and single spermatogonias (Figure 2; indicated as Sg) were immunostained in all species tested, human, rat, and bovine. To verify specificity of the ROS-GC1 immunostaining a control reaction was performed in which ROS-GC1 antibody was omitted and substituted with the preimmune serum. Under these conditions no labeling was observed (Figures 2A’–C’).
FIGURE 2. Immunolocalization of ROS-GC1 in the testes. Immunohistochemistry was performed using specific antibodies against ROS-GC1 on paraffin sections of human (A), rat (B), and bovine (C) testes. The ROS-GC1 was localized in spermatogenic cells. In the testes positive staining was observed in spermatogonias (Sg), spermatocytes (Sc) and spermatids (Sd), as indicated by the arrows. Control staining was performed according to the procedure for ROS-GC1 detection, except that preimmune serum was used instead of the primary antibody (A’–C’). Original magnification 400X.
In a similar manner the expression of NCδ in the human and rat testes was analyzed (Figures 3A,B) and compared with that in bovine testis (Figure 3C). NCδ immunoreactivity was observed in all types of germinal cells: spermatogonias (Figure 3: Sg), spermatocytes (Figure 3: Sc) and spermatids (Figure 3: Sd). Extremely strong staining was observed in spermatids localized close to seminiferous tubule lumen. No labeling was observed when the sections were incubated with preimmune rabbit serum instead of the NCδ antibody, attesting to the specificity of the staining (Figures 3A’–C’).
FIGURE 3. Immunolocalization of NCδ in the testes. Paraffin sections of the testes were incubated with primary antibodies against NCδ. NCδ immunoreactivity was observed in all spermatogenic cells of human (A), rat (B), and bovine (C) testes. Particularly strong signal was detected in spermatocytes and spermatids localized close to lumen of the seminiferous tubule (indicated as Sc and Sd, respectively). Control staining was performed according to the procedure for NCδ detection, except that preimmune serum was used instead of the primary antibody (A’–C’). Original magnification 400X.
The results presented clearly demonstrate that both ROS-GC1 and NCδ are expressed in the mammalian testes and in the germinal cells. Importantly, the patterns of ROS-GC1 and NCδ immunoreactivities are identical in the species analyzed (compare Figures 2 and 3) strongly indicating that they co-localize with each other. Notably, the intensities of the immunostaining for ROS-GC1 or NCδ increase with the maturation of the spermatogenic cells.
Since the increase in the staining is observed across the cells in different stages of spermatogenesis process – the highest accumulation of analyzed proteins was observed in spermatocytes and spermatids – it indicates that ROS-GC1 and NCδ expression correlates with the stage of the seminiferous cycle.
To demonstrate that neurocalcin δ stimulates ROS-GC1 expressed in the testes, their membranes were analyzed for guanylate cyclase activity in the presence of NCδ. Membranes of human, bovine, and rat testes were isolated and incubated with increasing concentrations of NCδ and constant 100 μM Ca2+. The amount of cyclic GMP formed was determined as a measure of guanylate cyclase activity. NCδ stimulated the cyclase activity in a dose-dependent fashion (Figure 4). Half-maximal stimulation (EC50) was observed at ~0.8 μM NCδ in all three species tested. The maximal stimulation of ~2.2-fold above the basal value was at 2 μM NCδ. Importantly, the stimulatory profiles of guanylate cyclase in the analyzed membranes were very similar to those observed for the recombinant ROS-GC1 (Kumar et al., 1999) and ROS-GC1 native to the retinal photoreceptor outer segments and inner plexiform layer (Krishnan et al., 2004). These results show that NCδ indeed stimulates ROS-GC1 activity in the testes.
FIGURE 4. Effect of NCδ on the guanylate cyclase activity in the membranes of testes. Membranes of human, rat, and bovine testes were isolated as described in “Materials and Methods”. They were assayed for guanylate cyclase activity in the presence of indicated concentrations of NCδ and 100 μM Ca2+. Each experiment was done in triplicate, and repeated two times. The results shown (mean ± SD) are from these experiments.
Because the experiments described above were conducted on the native membranes of the testes but with exogenously supplied NCδ, they prove the ability of ROS-GC1 to respond to NCδ but they do not prove that these two together form a functional unit. To determine this, the mouse model with deletion of one copy of the NCδ gene, NCδ+/-, was used [mice with deletion of both copies of NCδ are not born (Duda et al., 2012b)]. It was reasoned that if NCδ is indeed the Ca2+-sensor modulator of ROS-GC1 in the testes of these mice the NCδ-modulated Ca2+ signaling pathway should be half as active as in those of the wild type mice [wt (NCδ+/+)]. To test this prediction, the particulate fractions of the testes from wild type and NCδ+/- mice were isolated in the presence of 100 μM Ca2+ and tested for guanylate cyclase activity in the presence and absence of Ca2+. The reason for isolating the membranes in the presence of Ca2+ was that NCδ exhibits the property of “calcium-myristoyl switch” which means that in the presence of Ca2+ it is membrane bound and therefore would be present in the membrane fraction (Ladant, 1995; Krishnan et al., 2004). The guanylate cyclase activity assayed in the absence of Ca2+ (1 mM EGTA was present in the assay mixture) was 58 ± 7 pmol cyclic GMP min-1 (mg prot)-1 for the wt and 63 ± 8 pmol cyclic GMP min-1 (mg prot)-1 for the NCδ+/- mice (Figure 5: panel “Ca2+”). However, the cyclase activity determined in the presence of Ca2+ was strongly dependent on the mice genotype. With 1 μM Ca2+ present in the assay mixture the activity was 248 ± 17 pmol cyclic GMP min-1 (mg prot)-1 for the wild type mice and 148 ± 16 pmol cyclic GMP min-1 (mg prot)-1 for the NCδ+/- mice (Figure 5: panel “+Ca2+ – basal”). These results, as predicted, demonstrate that the Ca2+-dependent NCδ-modulated ROS-GC1 signaling pathway in the mice with one copy of NCδ gene deleted (NCδ+/-) is about half as active as in the wild type mice. To further validate that the lowering of the Ca2+-dependent cyclase activity in the membranes of NCδ+/- mice is the exclusive consequence of lower NCδ expression, 4 μM exogenous NCδ was added to the wild type and NCδ+/- membranes and the cyclase activity was assessed in the presence of 1 μM Ca2+. The cyclase activity in the wild type membranes increased only minimally, from 248 to 273 ± 25 pmol cyclic GMP min-1 (mg prot)-1but in the NCδ+/- membranes, from 148 to 258 ± 28 (Figure 5: panel “+Ca2+ + 4 μM NCδ”). Thus, the activity achieved was practically the same for both types of membranes. These results demonstrate that addition of exogenous NCδ to the NCδ+/- membranes restores the guanylate cyclase catalytic activity and brings it to the level of activity in the wild type membranes. The slight activity increase in the wild type membranes can be explained by a partial loss of the native NCδ during the membrane preparation procedure. Together these results demonstrate that functional ROS-GC1-NCδ-Ca2+ transduction system is operative and is the functional transduction element in the testes.
FIGURE 5. NCδ modulates ROS-GC1 activity in mouse testes. Testes were removed from the wild type (WT) and NCδ+/- mice and their particulate fractions were isolated in the presence of 100 μM Ca2+. These were assayed for guanylate cyclase activity in the presence of 1 mM EGTA or 10 μM Ca2+ (panel “-Ca2+”- and “+Ca2+”-basal). 4 μM myristoylated NCδ was added to the membranes and the guanylate cyclase activity was assessed in the presence of 10 μM Ca2+. The experiment was done in triplicate and repeated with separate membrane preparations. The results are mean ± SD of these experiments.
Our previous investigation has shown that S100B is expressed in the testes (Jankowska et al., 2007) and the present results demonstrate that NCδ is also expressed there. Thus, two Ca2+-dependent modulators of ROS-GC1 activity are present in the testes. Taking advantage of the availability of two types of genetically modified mice, NCδ+/- and S100B-/- we attempted comparing the relative inputs of NCδ and S100B into regulation of ROS-GC1 transduction machinery.
Testes were removed from the wild type, NCδ+/- and S100B-/- mice, their membrane fractions were isolated in the presence of 100 μM Ca2+ and assessed for guanylate cyclase activity. The results of this experiment are shown in Figure 6. In the absence of Ca2+ all three types of membranes exhibited comparable activity of ~60 pmol cyclic GMP min-1 (mg prot)-1 (Figure 6A: panel “basal”) and, as expected, the activity was not affected by the addition of NCδ or S100B (Figure 6A: panels “+ncalc δ” and “+S100B”). However, when the cyclase activity was assayed in the presence of Ca2+ the values differed between the genotypes: 250 pmol cyclic GMP min-1 (mg prot)-1 for the wild type, 150 pmol cyclic GMP min-1 (mg prot)-1 for the NCδ+/- and 200 pmol cyclic GMP min-1 (mg prot)-1 for the S100B-/- (Figure 6B: panel “basal”). These values describe the ROS-GC1 activity stimulated together by NCδ and S100B in the case of the wild type membranes; stimulated by half of wild type amount of NCδ and S100B in the case of NCδ+/- membranes; and stimulated by NCδ only in the case of S100B-/- membranes. Thus, the difference between ROS-GC1 activity in testes of the wild type mouse and the activity in the genetically modified mice (100 and 50 pmol pmol cyclic GMP min-1 (mg prot)-1 for the NCδ+/- and S100B-/-, respectively) describes the Ca2+-dependent stimulatory effects lost due to the absence of half of the NCδ present in the wild type testes or to the total absence of S100B. Higher loss of activity resulting from deletion of one copy of NCδ gene than resulting from deletion of two copies of S100B gene indicates that NCδ is more potent than S100B in modulating the Ca2+-dependent ROS-GC1 activity. One could argue, however, that this comparison might not be totally reflective of the in vivo contributions of NCδ and/or S100B, because there is a possibility that some of these proteins were lost during the membrane preparation process. To settle this possibility the ROS-GC1 activity was measured in the presence of individually added NCδ or S100B.
FIGURE 6. Comparison of NCδ and S100B stimulatory effects on ROS-GC1 activity in mouse testes. Testes were removed from the wild type (WT), NCδ+/-, and S100-/- mice and their particulate fractions were isolated in the presence of 100 μM Ca2+. (A) The membranes were assayed for guanylate cyclase activity in the presence of 1 mM EGTA in the absence (panel “basal”) or presence of 4 μM myristoylated NCδ (panel (“+ 4 μM ncalc δ”) or 4 μM S100B (panel “+S100B”). (B) The membranes were assayed for guanylate cyclase activity in the presence of 10 μM Ca2+(“basal”) or with the addition of 4 μM NCδ (panel “+ 4 μM ncalc δ”) or 4 μM S100B (panel “+ 4 μM S100B”). The experiment was done in triplicate and repeated with separate membrane preparations. The results are mean ± SD of these experiments.
Addition of 4 μM NCδ (Figure 6B: panel “+ncalc δ”) resulted in a small increase of the cyclase activity exhibited by the membranes isolated from the wild type testes (from ~250 to ~270 pmol cyclic GMP min-1 (mg prot)-1 and isolated from the S100B-/- testes [from ~200 to ~220 pmol cyclic GMP min-1 (mg prot)-1]. This non-significant to minimal increase of ROS-GC1 catalytic activity in these membranes indicates that if any NCδ was lost during the membrane preparation, it was only the insignificant amount. For the NCδ+/- membranes the increase was significant, from ~150 to ~260 pmol cyclic GMP min-1 (mg prot)-1. Importantly, addition of 4 μM NCδ brought the ROS-GC1 activity in these membranes very close to the ROS-GC1 activity in the wild type membranes. Because only residual amount of NCδ was lost during membrane preparation, as concluded from the wild type and S100B-/- membranes, practically all the increase of activity in the NCδ+/- membranes accounts for the activity lost due to the expression of only half of the NCδ present in the membranes from wild type testes.
When the ROS-GC1 activity was assayed with 4 μM S100B added to the membranes (Figure 6B: panel “+S100B”) it increased by ~40 pmol cyclic GMP min-1 (mg prot)-1 in the wild type and by 25 pmol cyclic GMP min-1 (mg prot)-1in the NCδ+/- membranes indicating that some S100B was lost in membranes preparation. The increase was ~80 pmol cyclic GMP min-1 (mg prot)-1for the S100B-/- membranes [from 205 to 282 pmol cyclic GMP min-1 (mg prot)-1] and this increase of activity in the S100B-/- membranes accounts for the activity lost due to the loss of S100B expression in the testes. Comparing the activity loss because of the absence of half of NCδ with the activity loss due to the total absence of S100B expression shows that neurocalcin δ is more significant contributor to ROS-GC1 Ca2+-dependent activity than S100B. It could be estimated that NCδ contributes about 75% to ROS-GC1 Ca2+-dependent activity and S100B, about 25%.
The core finding of this study is that it establishes the presence and molecular nature of a Ca2+- modulated ROS-GC transduction system in all types of the germinal cells of the testes: spermatogonias, spermatocytes, and spermatids. Because abundance of this transduction system is present in the spermatids residing close to the seminiferous tubule lumen, it hints that the system is linked with the processes of spermatogenesis.
This Ca2+-dependent cyclic GMP generating transduction system is composed of a transducer component ROS-GC1 and two Ca2+ sensor components, NCδ and S100B. NCδ is the major signal contributor responsible for about 75% of the guanylate cyclase stimulated catalytic activity, and S100B the relatively minor contributor, about 25% of signaling activity.
Cyclic GMP appears to be a critical second messenger in the physiology of the testes. In particular, it has been shown to influence motility in spermatozoa, development of testicular germ cells, relaxation of peritubular lamina propia cells, testosterone synthesis in Leydig cells, and dilatation of testicular blood vessels (Singh et al., 1988; Armstrong et al., 1994; Middendorff et al., 1997, 2000). Our immunostaining results demonstrate that both ROS-GC1 and NCδ immunoreactivity is present in all germ cells but its intensity is lowest in spermatogonias and highest in spermatids, hence, it increases with maturation, indicating that development of these cells may be directed by the activity of ROS-GC1.
The present study, of basic biochemical nature, brings to the fore several issues for future research. (1) What is the exact composition of the transduction system: does it represent a single pathway, composed of one ROS-GC1 and two Ca2+ sensors, NCδ, and S100B operating simultaneously? Or, two independent, but complementary pathways, each composed of one ROS-GC1 and one Ca2+ sensor, NCδ, or S100B? (2) What is the precise morphological residence of any of these transduction systems in the testicular cells? (3) What is the exact stoichiometry of these components in the given cell types? (4) What are the mechanistic details of the operational modes of this/these ROS-GC transduction pathways? (5) What is the physiological significance of these pathways to the testicular function, endocrine, fertility, including spermatogenesis; (6) what is the interplay between the Ca2+-modulated ROS-GC1 signaling pathway and ANF-dependent ANF-RGC pathway, another cyclic GMP generating machinery functioning in the mammalian testes (Marala and Sharma, 1988; Pandey and Singh, 1990; Pandey et al., 1999; Mourdjeva et al., 2001) and finally, (7) how this information can be translated to the clinical level to search for the pathway/s-linked diseases and finding their cures?
Handicapped with these informational gaps, it is difficult to construct a proper Ca2+-modulated signal transduction model for any of the specific physiological function/s of the testes. However, a preliminary signal transduction scheme is conceptualized where [Ca2+]i modulation of ROS-GC1 activity can occur through three modes. MODE 1 involves interaction of ROS-GC1 with its Ca2+ sensors, NCδ and S100B. The ROS-GC is stimulated in [Ca2+]i – dependent manner and generates cyclic GMP. MODE 2 involves interaction of the ROS-GC1 with GCAP1 which is also present in the testes (Jankowska et al., 2007). It is recalled that GCAP1 signaling is opposite to that of NCδ or S100B; it stimulates ROS-GC1 catalytic activity in the absence of Ca2+ and progressively inhibits it with increasing Ca2+ concentration (Duda et al., 1996b). Thus, through GCAP1 [Ca2+]i keeps the ROS-GC activity in the suppressed state. MODE 3, it is the most interesting scenario. ROS-GC1 is present together with the Ca2+-dependent guanylate cyclase activators (CD-GCAPs)–NCδ, S100B – and with GCAP1. The ROS-GC activity oscillates between the GCAP1-dependent state and CD-GCAPs-dependent state. In these states the cyclase is a bimodal Ca2+ transduction switch. And it functions according to the principles established for the synapse region between the photoreceptor and ON-bipolar cells (Duda et al., 2002; Venkataraman et al., 2003). This mode of ROS-GC1 activity regulation is possible because each of the three modulators targets its individual domain in ROS-GC1. These domains have been mapped and are schematically shown in Figure 7. Neurocalcin δ targets the ROS-GC1 region V836–D856 (Venkataraman et al., 2008); S100B, G962–N981and I1030–Q1041 (Duda et al., 2002); GCAP1, M445–L456 and L503–1522(Lange et al., 1999).
FIGURE 7. Topography of ROS-GC1. A schematic diagram of ROS-GC1, with its multiple domains is provided. The extracellular (ECD), the transmembrane (TM), the juxtamembrane (JMD), the kinase homology (KHD), the signalling helix (SHD), the catalytic (CD), and the C-terminal extension (CTE) domains have been identified on the left-hand side; the GCAP1, NCδ, and S100B binding domains are indicated to the right.
Basal state – low Ca2+: Ca2+-free GCAP1 stimulates ROS-GC1 catalytic activity. Cyclic GMP formed in response to this stimulation opens some of the CNG channels present in the sperm membranes (Wiesner et al., 1998; Revelli et al., 2002). At least some of the Ca2+ ions entering the sperm through the open CNG channels are bound by neurocalcin δ and/or S100B. It is important to note that the half-maximal activation of ROS-GC1 by GCAP1 occurs at 707 nM Ca2+ (Hwang et al., 2003), the Ca2+ concentration at which neurocalcin δ and S100B are able to activate ROS-GC1 with approximately half-maximal effectiveness. With increasing Ca2+ concentrations GCAP1 inhibits ROS-GC1 activity while neurocalcin δ and S100B stimulate it. Active state – Ca2+-bound NCδ and/or S100B further stimulate ROS-GC1 and cyclic GMP is formed in higher quantities leading to massive opening of CNG channels and influx of Ca2+ (Shapiro et al., 1990; Revelli et al., 2002). In this phase, the ROS-GC1 activity is resultant of two opposing effects, inhibitory through GCAP1 and stimulatory, through NCδ and S100B. Signal termination: when cyclic GMP and [Ca2+]i reach the optimal level necessary for sperm membrane hyperpolarization and physiological function (e.g., capacitation and/or acrosome reaction; Galinado et al., 2000) combined effects of protein phosphorylation and phosphodiesterase activity (Su and Vacquier, 2006) lead to lowering of cyclic GMP levels, closure of the CNG channels and cessation of the Ca2+ influx.
Co-expression of NCδ, S100B, and GCAP1 with ROS-GC1 in mammalian spermatogenic cells confers bimodal Ca2+ sensitivity to cyclic GMP synthesis. The stimulatory arm of the switch operated by NCδ and S100B is essential for timely opening of the CNG channels by cyclic GMP produced and vast influx of Ca2+ necessary for the acrosome reaction. In this stimulatory arm NCδ appears to be a significantly more important player than S100B. The inhibitory arm operated by GCAP1 stops the cyclic GMP synthesis and therefore Ca2+ influx. Our findings demonstrate that ROS-GC1 transduction system and its bimodal Ca2+ modulation is not unique to neurosensory and neurosensory-linked systems and has a more widespread role in general Ca2+-dependent signaling and may be a part of the fertilization machinery.
The authors declare that the research was conducted in the absence of any commercial or financial relationships that could be construed as a potential conflict of interest.
Armstrong, V. L., Clulow, J., Murdoch, R. N., and Jones, R. C. (1994). Intracellular signal transduction mechanisms of rat epididymal spermatozoa and their relationship to motility and metabolism. Mol. Reprod. Dev. 38, 77–84. doi: 10.1002/mrd.1080380113
Coussen, F., and Mulle, C. (2006). Kainate receptor-interacting proteins and membrane trafficking. Biochem. Soc. Trans. 34, 927–930. doi: 10.1042/BST0340927
Detwiler, P. (2000). Open the loop: dissecting feedback regulation of a second messenger transduction cascade. Neuron 36, 3–4. doi: 10.1016/S0896-6273(02)00940-6
Duda, T., Fik-Rymarkiewicz, E., Venkataraman, V., Krishnan, A., and Sharma, R. K. (2004). Calcium-modulated ciliary membrane guanylate cyclase transduction machinery: constitution and operational principles. Mol. Cell. Biochem. 267, 107–122. doi: 10.1023/B:MCBI.0000049372.33965.4f
Duda, T., Goraczniak, R. M., and Sharma, R. K. (1996a). Molecular characterization of S100A1-S100B protein in retina and its activation mechanism of bovine photoreceptor guanylate cyclase. Biochemistry 35, 6263–6266. doi: 10.1021/bi960007m
Duda, T., Goraczniak, R., Surgucheva, I., Rudnicka-Nawrot, M., Gorczyca, W. A., Palczewski, K., et al. (1996b). Calcium modulation of bovine photoreceptor guanylate cyclase. Biochemistry 35, 8478–8482. doi: 10.1021/bi960752z
Duda, T., Jankowska, A., Venkataraman, V., Nagele, R. G., and Sharma, R. K. (2001a). A novel calcium-regulated membrane guanylate cyclase transduction system in the olfactory neuroepithelium. Biochemistry 40, 12067–12077. doi: 10.1021/bi0108406
Duda, T., Venkataraman, V., Krishnan, A., Nagele, R. G., and Sharma, R. K. (2001b). Negatively calcium-modulated membrane guanylate cyclase signaling system in the rat olfactory bulb. Biochemistry 40, 4654–4662. doi: 10.1021/bi0027985
Duda, T., Koch, K. W., Venkataraman, V., Lange, C., Beyermann, M., and Sharma, R. K. (2002). Ca2+ sensor S100beta-modulated sites of membrane guanylate cyclase in the photoreceptor-bipolar synapse. EMBO J. 21, 2547–2556. doi: 10.1093/emboj/21.11.2547
Duda, T., Pertzev, A., Koch, K. W., and Sharma, R. K. (2012a). Antithetical modes of and the Ca2+ sensors targeting in ANF-RGC and ROS-GC1 membrane guanylate cyclases. Front. Mol. Neurosci. 5:44. doi: 10.3389/fnmol.2012.00044
Duda, T., Pertzev, A., and Sharma, R. K. (2012b). Ca2+ modulation of ANF-RGC: new signaling paradigm interlocked with blood pressure regulation. Biochemistry 51, 9394–9405. doi: 10.1021/bi301176c
Duda, T., and Sharma, R. K. (2004). S100B-modulated Ca2+-dependentROS-GC1 transduction machinery in the gustatory epithelium: a new mechanism in gustatory transduction. FEBS Lett. 577, 393–398. doi: 10.1016/j.febslet.2004.09.089
Duda, T., and Sharma, R. K. (2009). Ca2+-modulated ONE-GC odorant signal transduction. FEBS Lett. 583, 1327–1330. doi: 10.1016/j.febslet.2009.03.036
Galinado, B. E., Beltrán, C., Cragoe, E. J. Jr., and Darszon, A. (2000). Participation of K+ channel modulated directly by cGMP in the speract-induced signaling cascade of Strongylocentrotus purpuratus sea urchin sperm. Dev. Biol. 221, 285–294. doi: 10.1006/dbio.2000.9678
Garbers, D. L. (1989). Molecular basis of signalling in the spermatozoon. J. Androl. 10, 99–107 doi: 10.1002/j.1939-4640.1989.tb00068.x
Goraczniak, R. M., Duda, T., Sitaramayya, A., and Sharma, R. K. (1994). Structural and functional characterization of the rod outer segment membrane guanylate cyclase. Biochem. J. 302, 455–461.
Hayashi, F., and Yamazaki, A. (1991). Polymorphism in purified guanylate cyclase from vertebrate rod photoreceptors. Proc. Natl. Acad. Sci. U.S.A. 88, 4746–4770. doi: 10.1073/pnas.88.11.4746
Hwang, J. Y., Lange, C., Helten, A., Höppner-Heitmann, D., Duda, T., Sharma, R. K., et al. (2003). Regulatory modes of rod outer segment membrane guanylate cyclase differ in catalytic efficiency and Ca2+-sensitivity. Eur. J. Biochem. 270, 3814–3821. doi: 10.1046/j.1432-1033.2003.03770.x
Iino, S., Kobayashi, S., Okazaki, K., and Hidaka, H. (1995). Immunohistochemical localization of neurocalcin in the rat inner ear. Brain Res. 680, 128–134. doi: 10.1016/0006-8993(95)00253-M
Ivings, L., Pennington, S. R., Jenkins, R., Weiss, J. L., and Burgoyne, R. D. (2002). Identification of Ca2+-dependent binding partners for the neuronal calcium sensor protein neurocalcin delta: interaction with actin, clathrin and tubulin. Biochem. J. 363, 599–608. doi: 10.1042/0264-6021:3630599
Jankowska, A., Burczynska, B., Duda, T., and Warchol, J. B. (2008). Rod outer segment membrane guanylate cyclase type 1, ROS-GC1, calcium-modulated transduction system in the sperm. Fertil. Steril. 93, 904–912. doi: 10.1016/j.fertnstert.2008.10.048
Jankowska, A., Burczynska, B., Duda, T., Warchol, J. B., and Sharma, R. K. (2007). Calcium-modulated rod outer segment membrane guanylate cyclase type 1 transduction machinery in the testes. J. Androl. 1, 50–58.
Jankowska, A., and Warchol, J. B. (2010). Ca2+-modulated membrane guanylate cyclase in the testes. Mol. Cell. Biochem. 334, 169–179. doi: 10.1007/s11010-009-0329-5
Koch, K. W., Duda, T., and Sharma, R. K. (2010). Ca2+-modulated vision-linked ROS-GC guanylate cyclase transduction machinery. Mol. Cell. Biochem. 334, 105–115. doi: 10.1007/s11010-009-0330-z
Krishnan, A., Venkataraman, V., Fik-Rymarkiewicz, E., Duda, T., and Sharma, R. K. (2004). Structural, biochemical and functional characterization of the calcium sensor neurocalcin δ in the inner retinal neurons and its linkage with the rod outer segment membrane guanylate cyclase transudation system. Biochemistry 43, 2708–2723. doi: 10.1021/bi035631v
Kumar, V. D., Vijay-Kumar, S., Krishnan, A., Duda, T., and Sharma, R. K. (1999). A second calcium regulator of rod outer segment membrane guanylate cyclase, ROS-GC1: neurocalcin. Biochemistry 38, 12614–12620. doi: 10.1021/bi990851n
Ladant, D. (1995). Calcium and membrane binding properties of bovine neurocalcin delta expressed in Escherichia coli. J. Biol. Chem. 270, 3179–3185.
Lange, C., Duda, T., Beyermann, M., Sharma, R. K., and Koch, K.-W. (1999). Regions in vertebrate photoreceptor guanylyl cyclase ROS-GC1 involved in Ca2+-dependent regulation by guanylyl cyclase-activating protein GCAP-1. FEBS Lett. 460, 27–31. doi: 10.1016/S0014-5793(99)01312-5
Liu, X., Seno, K., Nishizawa, Y., Hayashi, F., Yamazaki, A., Matsumoto, H., et al. (1994). Ultrastructural localization of retinal guanylate cyclase in human and monkey retinas. Exp. Eye Res. 59, 761–768. doi: 10.1006/exer.1994.1162
Marala, R. B., and Sharma, R. K. (1988). Characterization of atrial-natriuretic-factor-receptor-coupled membrane guanylate cyclase from rat and mouse testes. Biochem. J. 251, 301–304.
Margulis, A., Pozdnyakov, N., and Sitaramayya, A. (1996). Activation of bovine photoreceptor guanylate cyclase by S100 proteins. Biochem. Biophys. Res. Commun. 218, 243–247. doi: 10.1006/bbrc.1996.0043
Middendorff, R., Davidoff, M. S., Behrends, S., Mewe, M., Miethens, A., and Muller, D. (2000). Multiple roles of the messenger molecule cGMP in testicular function. Andrologia 32, 55–59.
Middendorff, R., Muller, D., Wichers, S., Holstein, A. F., and Davidoff, M. S. (1997). Evidence for production and functional activity of nitric oxide in seminiferous tubules and blood vessels of the human testes. J. Clin. Endocrinol. Metab. 82, 4154–4161.
Mourdjeva, M., Russinova, A., Kyurkchiev, S., and Kehayov, I. (2001). Spatial and temporal distribution of atrial natriuretic factor in the rat testis. Biol. Cell 93, 301–307. doi: 10.1016/S0248-4900(01)01119-4
Nambi, P., Aiyar, N. V., and Sharma, R. K. (1982). Adrenocorticotropin-dependent particulate guanylate cyclase in rat adrenal and adrenocortical carcinoma: comparison of its properties with soluble guanylate cyclase and its relationship with ACTH-induced steroidogenesis. Arch. Biochem. Biophys. 217, 638–646. doi: 10.1016/0003-9861(82)90545-8
Pandey, K. N., Oliver, P. M., Maeda, N., and Smithies, O. (1999). Hypertension associated with decreased testosterone levels in natriuretic peptide receptor-A gene-knockout and gene-duplicated mutant mouse models. Endocrinology 140, 5112–5119. doi: 10.1210/endo.140.11.7121
Pandey, K. N., and Singh, S. (1990). Molecular cloning and expression of murine guanylate cyclase/atrial natriuretic factor receptor cDNA. J. Biol. Chem. 265, 12342–12348.
Paul, A. K., Marala, R. B., Jaiswal, R. K., and Sharma, R. K. (1987). Coexistence of guanylate cyclase and atrial natriuretic factor receptor in a 180-kD protein. Science 235, 1224–1226. doi: 10.1126/science.2881352
Pozdnyakov, N., Yoshida, A., Cooper, N. G., Margulis, A., Duda, T., Sharma, R. K., et al. (1995). A novel calcium-dependent activator of retinal rod outer segment membrane guanylate cyclase. Biochemistry 34, 14279–14283. doi: 10.1021/bi00044a002
Pugh, E. N. Jr., Duda, T., Sitaramayya, A., and Sharma, R. K. (1997). Photoreceptor guanylate cyclases: a review. Biosci. Rep. 17, 429–473. doi: 10.1023/A:1027365520442
Rebello, M. R., Atkas, A., and Medle, K. F. (2011). Expression of calcium binding proteins in mouse type II taste cells. J. Histochem. Cytochem. 59, 530–539. doi: 10.1369/0022155411402352
Revelli, A., Ghigo, D., Moffa, F., Massobrio, M., and Tur-Kaspa, I. (2002). Guanylate cyclase activity and sperm function. Endocr. Rev. 23, 484–494. doi: 10.1210/er.2001-0020
Singh, S., Lowe, D. G., Thorpe, D. S., Rodriguez, H., Kuang, W. J., Dangott, L. J., et al. (1988). Membrane guanylate cyclase is a cell-surface receptor with homology to protein kinases. Nature 334, 708–712. doi: 10.1038/334708a0
Shapiro, B. M., Cook, S., Quest, A. F., Oberdorf, J., and Wothe, D. J. (1990). Molecular mechanisms of sea-urchin sperm activation before fertilization. Reprod. Fertil. 42, 3–8.
Sharma, R. K. (2010). Membrane guanylate cyclase is a beautiful signal transduction machine: overview. Mol. Cell. Biochem. 334, 3–6. doi: 10.1007/s11010-009-0336-6
Su, Y.-H., and Vacquier, V. D. (2006). Cyclic GMP-specific phosphodiesterase-5 regulates motility of sea urchin spermatozoa. Mol. Biol. Cell. 17, 114–121. doi: 10.1091/mbc.E05-08-0820
Venkataraman, V., Duda, T., Ravichandran, S., and Sharma, R. K. (2008). Neurocalcin delta modulation of ROS-GC1, a new model of Ca(2+) signaling. Biochemistry 47, 6590–6601. doi: 10.1021/bi800394s
Venkataraman, V., Duda, T., Vardi, N., Koch, K. W., and Sharma, R. K. (2003). Calcium-modulated guanylate cyclase transduction machinery in the photoreceptor – bipolar synaptic region. Biochemistry 40, 5640–5648. doi: 10.1021/bi034025x
Venkataraman, V., Nagele, R., Duda, T., and Sharma, R. K. (2000). Rod outer segment membrane guanylate cyclase type 1-linked stimulatory and inhibitory calcium signaling systems in the pineal gland: biochemical, molecular, and immunohistochemical evidence. Biochemistry 39, 6042–6052. doi: 10.1021/bi9929960
Wen, X. H., Duda, T., Pertzev, A., Venkataraman, V., Makino, C. L., and Sharma, R. K. (2012). S100B serves as a Ca(2+) sensor for ROS-GC1 guanylate cyclase in cones but not in rods of the murine retina. Cell. Physiol. Biochem. 29, 417–430. doi: 10.1159/000338496
Wiesner, B., Weiner, J., Middendorff, R., Hagen, V., Kaupp, U. B., and Weyand, I. (1998). Cyclic nucleotide-gated channels on the flagellum control Ca2+ entry into sperm. J. Cell Biol. 142, 473–484. doi: 10.1083/jcb.142.2.473
Yang, R. B., Foster, D. C., Garbers, D. L., and Fulle, H. J. (1995). Two membrane forms of guanylyl cyclase found in the eye. Proc. Natl. Acad. Sci. U.S.A. 92, 602–606. doi: 10.1073/pnas.92.2.602
Keywords: testes, neurocalcin δ, membrane guanylate cyclase, ROS-GC1, calcium ions
Citation: Jankowska A, Sharma RK and Duda T (2014) Ca2+-modulated ROS-GC1 transduction system in testes and its presence in the spermatogenic cells. Front. Mol. Neurosci. 7:34. doi: 10.3389/fnmol.2014.00034
Received: 27 February 2014; Accepted: 08 April 2014;
Published online: 29 April 2014.
Edited by:
Clint Lawrence Makino, Massachusetts Eye and Ear Infirmary and Harvard Medical School, USAReviewed by:
Rosario Donato, University of Perugia, ItalyCopyright © 2014 Jankowska, Sharma and Duda. This is an open-access article distributed under the terms of the Creative Commons Attribution License (CC BY). The use, distribution or reproduction in other forums is permitted, provided the original author(s) or licensor are credited and that the original publication in this journal is cited, in accordance with accepted academic practice. No use, distribution or reproduction is permitted which does not comply with these terms.
*Correspondence: Anna Jankowska, The Unit of Molecular Biology, Department of Cell Biology, Poznan University of Medical Sciences, 5D Rokietnicka Street, 60-781 Poznan, Poland e-mail:YWphbmtvQHVtcC5lZHUucGw=;
Teresa Duda, The Unit of Regulatory and Molecular Biology, Research Divisions of Biochemistry and Molecular Biology, Salus University, 8360 Old York Road, Elkins Park, PA 19027, USA e-mail:dGR1ZGFAc2FsdXMuZWR1
Disclaimer: All claims expressed in this article are solely those of the authors and do not necessarily represent those of their affiliated organizations, or those of the publisher, the editors and the reviewers. Any product that may be evaluated in this article or claim that may be made by its manufacturer is not guaranteed or endorsed by the publisher.
Research integrity at Frontiers
Learn more about the work of our research integrity team to safeguard the quality of each article we publish.