- Department of Molecular Genetics, Faculty of Science and Engineering, Faculty of Health, Medicine and Life Sciences, Maastricht University, Maastricht, Netherlands
Heart disease represents one of the main challenges in modern medicine with insufficient treatment options. Whole genome sequencing allowed for the discovery of several classes of non-coding RNA (ncRNA) and widened our understanding of disease regulatory circuits. The intrinsic ability of long ncRNAs (lncRNAs) and circular RNAs (circRNAs) to regulate gene expression by a plethora of mechanisms make them candidates for conceptually new treatment options. However, important questions remain to be addressed before we can fully exploit the therapeutic potential of these molecules. Increasing our knowledge of their mechanisms of action and refining the approaches for modulating lncRNAs expression are just a few of the challenges we face. The accurate identification of novel lncRNAs is hampered by their relatively poor cross-species sequence conservation and their low and context-dependent expression pattern. Nevertheless, progress has been made in their annotation in recent years, while a few experimental studies have confirmed the value of lncRNAs as new mechanisms in the development of cardiac hypertrophy and other cardiovascular diseases. Here, we explore cardiac lncRNA biology and the evidence that this class of molecules has therapeutic benefit to treat cardiac hypertrophy.
Introduction
Heart failure (HF) is a highly prevalent disease and a leading cause of hospitalization and death that affects 23 million patients worldwide (Ziaeian and Fonarow, 2016). From a clinical point of view, HF is defined as the state of deterioration of the heart where it can no longer supply sufficient blood to meet the circulatory demands of the organism. Heart transplantations are still the only genuine curative interventions for patients with advanced forms of HF as contemporary pharmacotherapy is largely palliative and merely aimed to slow the progression of the disease (Ziaeian and Fonarow, 2016). The disease is typically preceded by structural remodeling of the heart where heart muscle cells undergo maladaptive growth without an increase in cell number in response to sustained stress or injury such as pressure- or volume overload to temporally sustain cardiac output, resulting in a measurable thickening of heart muscle walls. However, cardiac hypertrophy is also accompanied by a plethora of biochemical, molecular, metabolic and extracellular changes that provoke a decrease of pump function over time rather than preserving it, resulting in overt heart failure and a propensity for the occurrence of lethal arrhythmias (Dirkx et al., 2013). Accordingly, a better understanding of the molecular underpinnings of cardiac hypertrophy will help to clarify the maladaptive nature of this disease and may open new therapeutic targets for future treatment of hypertrophic heart diseases to reduce the number of HF patients.
The Encyclopedia of DNA Elements (ENCODE) and the Functional Annotation Of Mouse (FANTOM) consortiums reveal that there are much more transcripts than originally predicted. Over 80% of the genome is transcribed in various classes of RNA and, surprisingly, coding transcripts account for just up to 3% of the genome, while the vast majority of other transcripts have no coding ability (Figure 1) (Consortium, 2012). For a long time, the presence of a large number of noncoding transcripts was dismissed as evidence for junk DNA or transcriptional noise, but more recent research reveals that a substantial proportion of these noncoding transcripts are functionally active RNA molecules that can be subdivided into small noncoding RNAs (<200 nt), such as microRNAs (miRs), transfer RNAs, and small nucleolar RNAs, on the one hand, and longer noncoding RNAs (>200 nt) that include ribosomal RNAs, natural antisense transcripts and other long noncoding RNAs (lncRNAs) (Engreitz et al., 2016; Quinn and Chang, 2016; O'Brien et al., 2018). While our knowledge of lncRNAs is still in its infancy, here we will summarize examples of lncRNAs that are involved in cardiac hypertrophy as it may provide useful insights how lncRNAs are functionally involved in the heart.
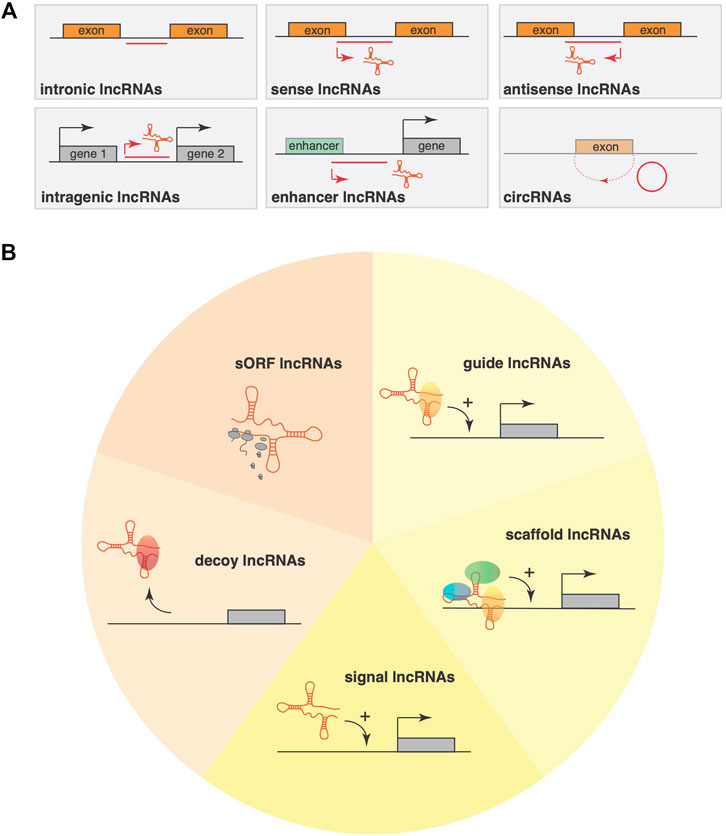
FIGURE 1. Classification systems for lncRNAs (A) LncRNA classification using purely their genomic location as input information allows a classification into six main categories: sense, antisense, bidirectional, intronic, intergenic and circRNAs. This classification mainly considers the position and orientation compared to the nearest gene or genomic features (promoters, enhancers). (B) An alternative classification system takes lncRNA mode of action into account, where lncRNAs have the ability to interact with DNA, other RNAs, with proteins or perform their function by pervasive transcription. Based on structural motifs within lncRNAs, an alternative classification divides lncRNAs into five classes, including short open reading frame (sORF) containing sORF lncRNAs that often encode functional, small peptide sequences; decoy lncRNAs that can either be located cytoplasmatic or nuclear and interact physically with RNAs or transcription factors to block their interaction with their cofactors or inhibit their function; signal lncRNAs that regulate gene expression in a time- and space dependent manner in response to cellular stimuli; guide lncRNAs that are predominantly nuclear and recruit chromatin-modifying complexes to specific loci either near the gene encoding the lncRNA; scaffold lncRNAs that can be either in the nucleus or cytoplasm and facilitate the assembling of multiple proteins, affecting histone modifications in the nucleus or that facilitate the assembling of ribonucleoproteins.
Classification Systems of Lncrnas
LncRNAs are often polyadenylated and often devoid of evident open reading frames (ORFs). The human genome is estimated to contain 16,000 lncRNA genes (Sun et al., 2018), mostly transcribed by RNA polymerase II and only a small fraction experimentally investigated (Marchese et al., 2017). Unfortunately, studying lncRNAs represents a challenge for molecular biology as they are evolutionarily poorly conserved, show a relatively low expression level that—interestingly - is more restricted in a tissue- and time-specific manner, and mechanisms of action that remain incompletely understood (Bhat et al., 2016).
Unlike other classes of RNA, a universally accepted classification system is lacking for lncRNAs. Using purely the genomic location as input information allows a classification into five main categories: sense, antisense, bidirectional, intronic, and intergenic. This classification mainly considers the position and orientation compared to the nearest gene or genomic features (promoters, enhancers) (Cabili et al., 2011). Similarly, based on genomic position and features, alternative classifications have also been proposed. LncRNAs that are encoded at the promoter region or originate near the transcription start site (uaRNAs) represent up to 60% of all lncRNAs (Sigova et al., 2013), while those encoded from enhancer regions (eRNAs) amount to ∼20% (Kim et al., 2010). The remainder derive from coding genes or gene bodies can be subdivided into sense (gsRNAs) or antisense (gaRNAs) and amount to ∼5% of all lncRNAs (Modarresi et al., 2012), while those encoded in intragenic spaces are referred to as iRNAs or lincRNAs (Guttman et al., 2009). For instance, another widely used system is based on the cellular localization of lncRNAs, where they are subdivided into cytoplasmatic- or nuclear lncRNAs. Unfortunately, this system is not fully reliable either because only 30% of lncRNAs are found exclusively in the nucleus, 15% are found exclusively in the cytoplasm, while the remainder ∼50% show both nuclear and cytoplasmic localization (Kwon, 2013). To make matters worse, some lncRNAs can translocate between the nucleus and cytoplasm after stress or stimulation (van Heesch et al., 2014).
In terms of mode of action, lncRNAs can interact with other RNAs (e.g., mRNAs, miRNAs, circRNAs, and rRNAs), with proteins (mainly but not exclusively transcriptional factors and chromatin-remodeling complexes) or perform their function by pervasive transcription (Fatica and Bozzoni, 2014). One characteristic of lncRNAs is their ability to interact with proteins and is determined by their architecture and three dimensional folding. LncRNAs are single-strand RNAs that will try to reach a folded state with the lowest or most stable energy state. The form it takes will be determined from the primary sequence but also from its length, the electrical charges and interaction with molecular chaperones (Novikova et al., 2013). Indeed, the folding and 3D shape seems an evolutionary conserved characteristic since lncRNAs are less evolutionary conserved on the primary sequence, but more often maintain their 3D structure throughout species (Lai et al., 2018). Specific RNA motifs have been shown to interact with proteins, allowing lncRNA to sequester or interact with various proteins simultaneously. Finally, by being RNA molecules, lncRNAs can create RNA:RNA duplex which allow them to interact with other RNA classes (e.g., microRNAs) or in other circumstances they can form RNA:DNA duplexes (Trembinski et al., 2020), which allow them to interact to specific target sequences on the DNA.
Based on these molecular specifics, an alternative and comprehensive classification divides lncRNAs into four classes. The first class is represented as decoy lncRNAs that can either be located cytoplasmatic or nuclear and interact physically with transcription factors to block their interaction with their cofactors or inhibit their function (Wang and Chang, 2011). LncRNA ROR is an example of a decoy lncRNA, which activates the TESC promoter by repelling the histone G9A methyltransferase (Fan et al., 2015). Additionally, decoy lncRNAs can also sequester microRNAs such as linc-MD1 that sponges miR-133 and miR-135 to regulate the expression of MAML1 and MEF2C, two transcription factors that activate muscle-specific gene expression (Cesana et al., 2011).
Signal lncRNAs can also be localized either in the cytoplasm or nucleus and regulate gene expression in a time- and space dependent manner in response to cellular stimuli and often are only expressed at specific times during development (Fatica and Bozzoni, 2014). Their task is to modulate gene expression of key genes by reshaping the chromatin in a specific locus as, for instance, KCNQ1OT1 is expressed only during early development where it interacts with chromatin-modifying enzymes to trigger lineage-specific transcriptional profiles (Pandey et al., 2008).
Guide lncRNAs are predominantly nuclear and can recruit chromatin-modifying complexes to specific loci either near the gene encoding the lncRNA (cis-action), or distant target genes from the production site of the lncRNA (trans-action). HOTAIR is an example of a guide lncRNA that functions in trans to direct the chromatin modifier Polycomb Repressive Complex 2 (PRC2) to the developmental HOXD locus (Rinn et al., 2007). Instead, HOTTIP is a guide lncRNA encoded in the HOXA locus that binds WDR5 and the histone methyltransferase protein MLL and directs the WDR5/MLL complex towards activation of the HOXA locus (Wang et al., 2011).
Finally, scaffold lncRNAs can be either in the nucleus or cytoplasm and facilitate the assembling of multiple proteins, which may act on chromatin, affecting histone modifications in the nucleus or facilitate the assembling of ribonucleoproteins. Telomerase RNA TERRA is a classic example of an RNA scaffold that assembles the telomerase complex to maintain the ends of telomeres (Oliva-Rico and Herrera, 2017).
Even this classification system is not always adequate to unequivocally differentiate classes of lncRNA as some can simultaneously belong to multiple classes. For example, lncRNA KCNQ1OT1 functions both as a scaffold and guide lncRNA at the same time. For this reason, some authors have decreased the above classification system to only three: guides, decoy and a combined class called a dynamic scaffold. And yet, even with this adaptation, not all lncRNAs would still fall in any category. Some lncRNAs for instance can influence proximal genes (in cis) just by the effect of their own transcription in a process called transcriptional interference. Indeed, Airn can modulate the Igf2r promoter as long as only a small fraction of Airn is transcribed as this lncRNA is antisense to Igf2r and its transcription influences the state of chromatin in the surrounding area with an inability of RNA Polymerase II to interact freely with the promoter of Igf2r (Latos et al., 2012).
Finally, although it is not often included in lncRNA classification systems, several studies revealed that a proportion of lncRNAs contain short open reading frames (sORFs) that encode for small proteins or micropeptides with largely overlooked but fundamental biological importance (Galindo et al., 2007; Kondo et al., 2010; Andrews and Rothnagel, 2014; Bazzini et al., 2014; Pauli et al., 2014; Anderson et al., 2015; Anderson D. M. et al., 2016; Nelson et al., 2016). For example, Myoregulin (MLN) is a micropeptide encoded by LINC00948, an important regulator of skeletal muscle physiology. This micropeptide peptide controls calcium re-uptake in the sarcoplasmic reticulum (SR) by Serca2a inhibition (Anderson et al., 2015). Recently, van Heesch and colleagues (van Heesch et al., 2019) used ribosome profiling to capture ribosomal footprints on human cardiac transcripts and inferred actively translated sORFs that encode previously unknown microproteins in over 169 lncRNAs and 40 circular RNAs that are expressed in the heart. Dozens of microproteins are expressed even from previously non-coding roles for lncRNAs, such as DANCR (also known as ANCR (Kretz et al., 2012)), TUG1 (Young et al., 2005), JPX (Tian et al., 2010), Myheart (Han et al., 2014), and UPPERHAND (Anderson K. M. et al., 2016). This finding suggests that a number of translated lncRNAs that could be separately classified as sORF lncRNAs, likely have dual coding and noncoding roles and this duality should be considered when deciphering the function of this class of “non-coding” transcripts.
Next-generation sequencing (NGS) techniques such as deep RNA-seq or CAGE-seq and 3P-seq have greatly aided to provide genome-wide identification of relatively low abundantly expressed transcripts such as lncRNAs (Oliva-Rico and Herrera, 2017). The results of these profiling experiments are now incorporated in databases with collections of hundreds of lncRNAs with possible differential expression in pathological conditions in a variety of species (Table 1) (Latos et al., 2012).
Prohypertrophic Cardiac Lncrnas
H19 is a lncRNA that was first associated with genomic imprinting of the H19/Igf2 locus (Kaffer et al., 2001; Table 2). Subsequent studied demonstrated that H19 is upregulated in pathological forms of cardiac hypertrophy and heart failure. Interestingly, H19 also encodes for the small ncRNA miR-675 that is embedded in the H19 locus and that can target Ca2+/calmodulin-dependent protein kinase IIδ (CaMKIIδ), a powerful inducer of cardiac hypertrophy (Liu et al., 2016). CTBP1 Antisense RNA 2 (CTBP1-AS2) is a novel lncRNA that can trigger the hypertrophic response as it is upregulated in hearts that undergo hypertrophy after transverse aortic constriction (TAC) surgery in mice, while, conversely, silencing of CTBP1-AS2 attenuates hypertrophy in AngII agonist stimulation of cardiomyocytes in culture. Mechanistically, this lncRNA can stabilize the mRNA encoding Toll-like receptor four by recruiting the RNA-binding protein FUS/TLS, thereby triggering cardiac inflammation, a commonly observed phenomenon associated with cardiac hypertrophy (Luo et al., 2019). Cardiac Hypertrophy-Related Factor (CHRF) is upregulated in both hypertrophic mouse hearts and human biopsies of patients with heart failure and functions as a sponge for miR-489, regulating Myeloid differentiation primary response gene 88 (Myd88) as downstream target of miR-489. Myd88, a signal transduction adaptor involved in immune modulation, was demonstrated to suppress cardiomyocyte hypertrophy. Other studies have shown that mir-489 is not the only target of CHRF (Wang et al., 2014), as miR-93 can also bind CHRF and influence AKT3 activation status (Wo et al., 2018). LncRNA ROR acts as a decoy lncRNA that can trap miR-133, thereby influencing RhoA and Cdc42 downstream of Gαq/Gα11 signaling (Jiang et al., 2016). Cardiac Hypertrophy-Associated Transcript (CHAST) is a signal lncRNA that show temporal regulation of expression in cardiac hypertrophy in mice and silencing of Chast with a GapmeR in vivo attenuated the hypertrophic response. Conversely, Chast overexpression triggers cardiomyocyte hypertrophy. Further studies revealed that Chast negatively regulates Plekhm1, a multivalent endocytic adaptor involved in controlling selective and nonselective autophagy pathways resulting in adverse cardiac remodeling (Viereck et al., 2016).
Anti-Hypertrophic Cardiac Lncrnas
Myosin Heavy Chain Associated RNA Transcripts (MHRT) was first identified as the antisense transcript of Myosin heavy chain 7 (Myh7). MHRT is highly expressed in the mouse and human adult heart and MHRT lentiviral overexpression reduced hypertrophic stress markers (Table 3). RNA immunoprecipitation (RIP) revealed that MHRT interacts with Brahma-related gene 1 (Brg1) to remodel chromatin and regulates gene expression such as Myh6 (Han et al., 2014) and can influence the acetylation of the Myocardin protein in an HDAC5-dependent fashion (Miano, 2015; Luo et al., 2018). LncRNA TINCR is downregulated in hypertrophic hearts and lentiviral TINCR overexpression attenuates cardiac hypertrophy by interacting with EZH2, a functional enzymatic component of the Polycomb Repressive Complex 2 (PRC2). Chromatin-immunoprecipitation (ChIP) pull-down assays against EZH2 demonstrated that the TINCR-EZH2 complex binds directly to the CaMKII promoter and induces H3K27me3 histone modification (Shao et al., 2017). Lnc-Plscr4 is a lncRNA is significantly increased in hearts from mice that underwent pressure overload-induced cardiac hypertrophy, where it reduces the expression and activity of miR-214, a prohypertrophic microRNA (Aurora et al., 2012; Azzouzi et al., 2013; Lv et al., 2018). The responsible downstream target of miR-214 is Mitofusin 2 (Mfn2), a protein that regulates mitochondrial fusion (Chen et al., 2011). Accordingly, reduced expression of Mfn2 attenuates protein synthesis in cardiac hypertrophy (Yu et al., 2018). HOTAIR is a lncRNA that has been extensively studied in embryonic development and cancer, but recently it was observed that it is substantially decreased in cardiac hypertrophy. Overexpression of HOTAIR suppresses angiotensin II-stimulated cardiomyocyte hypertrophy and following pressure overload surgery in mice. Mechanistically, HOTAIR was proposed to act as a ceRNA for miR-19, where miR-19 regulates Phosphatase and tensin homolog (PTEN) expression and indirectly regulates hypertrophy by influencing the activation status of PI3K/phospho-Akt signaling cascade in cardiomyocytes (Lai et al., 2017; Zhang et al., 2020).
CircRNAs
Circular RNAs are a class of non-coding RNAs with a continuous closed loop where the 3 and 5′ ends of exons of protein-coding genes or lncRNA genes are joined through back-splicing (Pamudurti et al., 2017; Table 4). CircRNAs are predominantly found in the cytoplasm and are highly stable. Moreover, they are evolutionarily conserved across the eukaryotic (Wang L et al., 2018). Based on their origin, circRNAs can be divided into four main classes: exonic circRNAs (ecircRNA), produced with the removal of each intron and generated from the back-spliced exons, while in exon-intron circRNAs (EIciRNA) the black-splicing takes place by including the intron inside the mature circRNA. The circularization of only introns produces circular intron RNAs (ciRNAs), while intergenic circRNAs are produced when two fragments from different genomic regions called intergenic circRNA fragments or ICFs are merged together and circularized (Wang F et al., 2016). CircRNAs can interact with proteins, or they can serve as decoys for microRNAs. For example, Circ-Foxo3 inhibits proliferation by direct interaction with p21 and CDK2 and subsequent interference of the cell cycle (Du et al., 2016). CircACTA2 instead acts as a microRNAs sponge in vascular smooth muscle cell where it interacts with miR-548f-5p, which in turn modulates the expression of smooth muscle α-actin (α-SMA) (Sun et al., 2017).
Similarly, circRNA_000203 can modulate the expression in Gata4 by binding microRNA miR-26b-5p and miR-140-3p (Li et al., 2020). Occasionally, circRNAs can encode for micropeptides by sORFs, an example is Circ-ZNF609 that controls myoblast proliferation the production of a new small peptide generated by the back-splicing event (Legnini et al., 2017). Heart-related circRNA (HRCR) is a circRNAs that regulates cardiac homeostasis by modulating the expression of miR-223 in vivo, a well-known microRNA that induces cardiac hypertrophy (Wang K et al., 2016). Conversely, CircSlc8a1 is highly expressed in heart failure, and can bind miR-133 to induce cardiac hypertrophy (Carè et al., 2007; Wang K et al., 2016). On the other hand, cirRNAs can act as early biomarkers of cardiac hypertrophy, circTMEM56 and circDNAJC6 could serve as indicators of disease severity in patients with hypertrophic obstructive cardiomyopathy (Sonnenschein et al., 2019)
Experimental and Therapeutic Considerations
One widely employed strategy to silence endogenous lncRNAs involves the introduction of double or single stranded antisense oligonucleotides in the form of siRNAs or antisense locked nucleic acid (LNA) containing oligos (GapmeRs), respectively. For short hairpins RNA or siRNAs, it is observed that those molecules seem more suitable for cytoplasmic lncRNAs rather than nuclear lncRNAs (Berezhna et al., 2006), although this might depend on various other parameters in siRNA design as well. GapmeRs are very potent antisense oligonucleotides with two LNA-modified linkers at 3′ and 5’ end of the molecule to resist exo-and endonucleases once introduced in the cell or in the extracellular space. Once bound with the lncRNA by full base pair complementarity, the dsRNA complex is degraded in an RNAse H dependent manner (Vickers et al., 2003). These molecules are highly efficient in silencing both cytoplasmatic and nuclear lncRNAs (Figure 2).
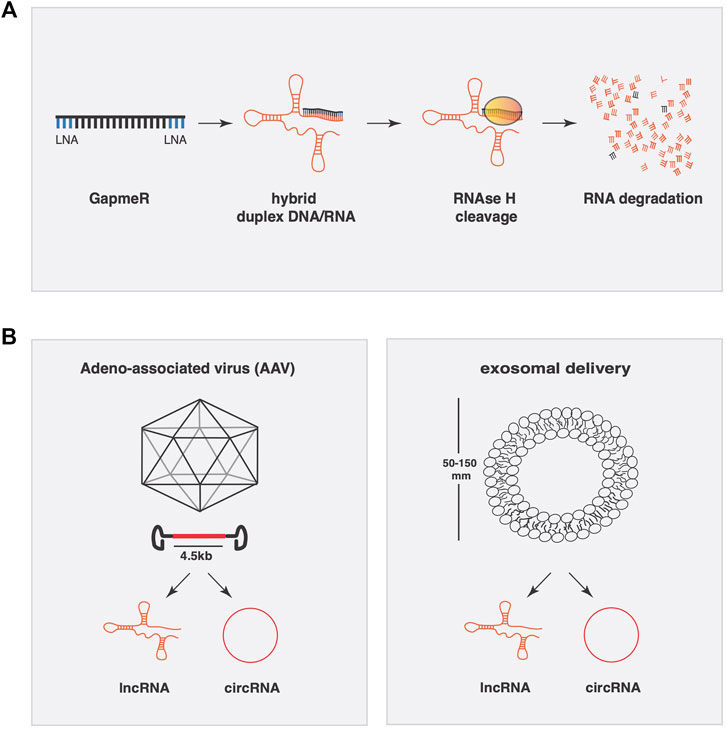
FIGURE 2. Conceptual approaches to manipulate lncRNAs expression for therapeutic applications. (A) Gapmer oligonucleotides have two LNA-modified linkers at the 3′ and 5′ end of the molecule to resist exo-and endonucleases once introduced into the cell or inside the extracellular space. Once bound to the lncRNA by full base pair complementarity, the dsRNA complex is degraded in an RNAse H dependent manner. (B) Adeno-associated viruses (AAV) have a packaging limit of 4.5 kb and can be engineered to transcribe lncRNAs or circRNAs. Exosomes are a specialized subgroup of extracellular vesicles ranging from 50–150 nm capable of carrying a variable composition of RNAs (lncRNA, microRNA, and circRNA).
To achieve the opposite, i.e. supplementing or overexpressing a lncRNA, viral-mediated gene delivery remains the most reliable system (Figure 2). Viral vectors enhance cardiac delivery of polyanions, such as RNA, across cell membranes. One oftenly used viral vector is the non-pathogenic human parvovirus adeno-associated virus (AAV) for its genomic simplicity, possibility of generating high-titre vector preparations, and their capacity to deliver genes into postmitotic cells. The specific tropism of AAV serotypes drives their selectively for different tissues in vivo, where AAV9 stands as the most cardiotropic serotype in gene transfer studies in rodents. A limitation of AAV vectors is their packaging limit (∼4.5 kb), which may be exceeded by certain lncRNAs. Another viral vector is based on human adenovirus (HAdV). The best studied member of the HAdV species is serotype 5 (HAdV-5). HAdV-5 infects many cell types, including low-replicative or quiescent cell populations such as cardiomyocytes. The HAdV-5 genome is easy to engineer with large foreign DNA cloning capacity and can be produced on an industrial scale. All these attributes make HAdV-5 vectors the most preferred vector type used to date in vaccine, cancer, and gene therapy trials (Appaiahgari and Vrati, 2015).
Other systems of delivery are engineered nanoparticles and extracellular vesicles such as exosomes (Figure 2). Nanoparticles have demonstrated their potential in the oncological field as delivery systems for microRNAs, very efficiently and in general with relatively low toxicity. Furthermore, effective cardiac-specific nanoparticle delivery systems for in vivo use remain to be developed. In contrast, exosomes are a specialized subgroup of extracellular vesicles ranging from 50–150 nm and released from cells to the extracellular microenvironment where they can be found in various extracellular fluids including the systemic circulation. Naturally occurring exosomes carry a variable composition of proteins, lipids, RNA (mRNA, lncRNA, microRNA, and circRNA), and DNA, where they influence cell migration, angiogenesis, the immune response and tumor cell growth (Boriachek et al., 2018). Engineered extracellular vesicles could be used as engineered vehicles to temporally deliver lncRNAs. Proof of principle exist in cancer research, where exosomes that carry different lncRNAs to influence tumor development. MALAT-1, BCAR4, and LncRNA-p21 are LncRNAs found in exosomes that traveled in the bloodstream derived from different types of cancers. Previous work has demonstrated the efficacy of exosomes as delivery vehicle for microRNAs in cardiac pathologies. Exosomes from cardiac progenitor cells enriched with miR-451/144 promote cardiomyocyte survival in a myocardial ischemia/reperfusion model. To tackle the problem of cellular tropism, recently, exosomes derived from cardiosphere-derived cells (CDCs) engineered to express Lamp2b, an exosomal membrane protein, fused to a cardiomyocyte-specific peptide (CMP), resulted in an increased efficiency of exosomal uptake by cardiomyocytes (Mentkowski and Lang, 2019).
Conclusion
The development of whole genome sequencing technologies allowed for the discovery of several classes of ncRNA and has strongly influenced our understanding of disease-regulatory circuits. LncRNAs represent one of the classes of ncRNA which has aroused more interest in the last decade. Several lncRNAs and circRNAs have been linked to cardiovascular physiology, providing new targets for the treatment of cardiovascular diseases. Additionally, the intrinsic ability of lncRNAs and circRNAs to regulate gene expression through various mechanisms makes them one of the best candidates for the development of more accurate and efficient therapies. However, important questions remain to be addressed before we can fully exploit the therapeutic potential of these molecules. Increasing our knowledge of their mechanisms of action and refining the approaches for modulating lncRNAs expression in vivo are two of the main challenges we still must face. Another aspect, that should be addressed, is the identification of novel lncRNAs involved in cardiovascular disease. Although the action of these RNAs is remarkably strong in the regulation of several molecular aspects, the low and time-related expression combined with a poor sequence conservation trough species makes the identification of novel lncRNAs difficult. Nevertheless, significant progress has been made in recent years, confirming the value of ncRNA as a new tool for the treatment of numerous diseases, including cardiac hypertrophy.
Author Contributions
NM wrote the manuscript; LD wrote the manuscript and obtained funding.
Funding
LD is further supported by a VICI award 918-156-47 from NWO. This project received funding from the European Union’s Horizon 2020 research and innovation programme under the Marie Sklodowska-Curie grant agreement No. 813716.
Conflict of Interest
LD is co-founder and stockholder of Mirabilis Therapeutics BV.
The remaining author declares that the research was conducted in the absence of any commercial or financial relationships that could be construed as a potential conflict of interest.
Publisher’s Note
All claims expressed in this article are solely those of the authors and do not necessarily represent those of their affiliated organizations, or those of the publisher, the editors and the reviewers. Any product that may be evaluated in this article, or claim that may be made by its manufacturer, is not guaranteed or endorsed by the publisher.
Acknowledgments
LD acknowledges support from the Netherlands CardioVascular Research Initiative: The Dutch Heart Foundation, Dutch Federation of University Medical Centers, ZonMW, and the Royal Netherlands Academy of Sciences.
References
Anderson, D. M., Anderson, K. M., Chang, C.-L., Makarewich, C. A., Nelson, B. R., McAnally, J. R., et al. (2015). A Micropeptide Encoded by a Putative Long Noncoding RNA Regulates Muscle Performance. Cell 160, 595–606. doi:10.1016/j.cell.2015.01.009
Anderson, D. M., Makarewich, C. A., Anderson, K. M., Shelton, J. M., Bezprozvannaya, S., Bassel-Duby, R., et al. (2016). Widespread Control of Calcium Signaling by a Family of SERCA-Inhibiting Micropeptides. Sci. Signal. 9, ra119. doi:10.1126/scisignal.aaj1460
Anderson, K. M., Anderson, D. M., McAnally, J. R., Shelton, J. M., Bassel-Duby, R., and Olson, E. N. (2016). Transcription of the Non-Coding RNA Upperhand Controls Hand2 Expression and Heart Development. Nature 539, 433–436. doi:10.1038/nature20128
Andrews, S. J., and Rothnagel, J. A. (2014). Emerging Evidence for Functional Peptides Encoded by Short Open reading Frames. Nat. Rev. Genet. 15, 193–204. doi:10.1038/nrg3520
Appaiahgari, M. B., and Vrati, S. (2015). Adenoviruses as Gene/Vaccine Delivery Vectors: Promises and Pitfalls. Expert Opin. Biol. Ther. 15, 337–351. doi:10.1517/14712598.2015.993374
Aurora, A. B., Mahmoud, A. I., Luo, X., Johnson, B. A., van Rooij, E., Matsuzaki, S., et al. (2012). MicroRNA-214 Protects the Mouse Heart from Ischemic Injury by Controlling Ca2+ Overload and Cell Death. J. Clin. Invest. 122, 1222–1232. doi:10.1172/jci59327
Azzouzi, H., Leptidis, S., Dirkx, E., Hoeks, J., van Bree, B., Brand, K., et al. (2013). The Hypoxia-Inducible microRNA Cluster miR-199a∼214 Targets Myocardial PPARδ and Impairs Mitochondrial Fatty Acid Oxidation. Cell Metab 18, 341–354. doi:10.1016/j.cmet.2013.08.009
Bao, Z., Yang, Z., Huang, Z., Zhou, Y., Cui, Q., and Dong, D. (2019). LncRNADisease 2.0: An Updated Database of Long Non-coding RNA-Associated Diseases. Nucleic Acids Res. 47, D1034–d1037. doi:10.1093/nar/gky905
Bazzini, A. A., Johnstone, T. G., Christiano, R., Mackowiak, S. D., Obermayer, B., Fleming, E. S., et al. (2014). Identification of Small ORFs in Vertebrates Using Ribosome Footprinting and Evolutionary Conservation. EMBO J. 33, 981–993. doi:10.1002/embj.201488411
Berezhna, S. Y., Supekova, L., Supek, F., Schultz, P. G., and Deniz, A. A. (2006). siRNA in Human Cells Selectively Localizes to Target RNA Sites. Proc. Natl. Acad. Sci. 103, 7682–7687. doi:10.1073/pnas.0600148103
Bhat, S. A., Ahmad, S. M., Mumtaz, P. T., Malik, A. A., Dar, M. A., Urwat, U., et al. (2016). Long Non-coding RNAs: Mechanism of Action and Functional Utility. Non-coding RNA Res. 1, 43–50. doi:10.1016/j.ncrna.2016.11.002
Boriachek, K., Islam, M. N., Möller, A., Salomon, C., Nguyen, N. T., Hossain, M. S. A., et al. (2018). Biological Functions and Current Advances in Isolation and Detection Strategies for Exosome Nanovesicles. Small 14. doi:10.1002/smll.201702153
Cabili, M. N., Trapnell, C., Goff, L., Koziol, M., Tazon-Vega, B., Regev, A., et al. (2011). Integrative Annotation of Human Large Intergenic Noncoding RNAs Reveals Global Properties and Specific Subclasses. Genes Dev. 25, 1915–1927. doi:10.1101/gad.17446611
Carè, A., Catalucci, D., Felicetti, F., Bonci, D., Addario, A., Gallo, P., et al. (2007). MicroRNA-133 Controls Cardiac Hypertrophy. Nat. Med. 13, 613–618. doi:10.1038/nm1582
Cesana, M., Cacchiarelli, D., Legnini, I., Santini, T., Sthandier, O., Chinappi, M., et al. (2011). A Long Noncoding RNA Controls Muscle Differentiation by Functioning as a Competing Endogenous RNA. Cell 147, 358–369. doi:10.1016/j.cell.2011.09.028
Chen, Y., Liu, Y., and Dorn, G. W. (2011). Mitochondrial Fusion Is Essential for Organelle Function and Cardiac Homeostasis. Circ. Res. 109, 1327–1331. doi:10.1161/circresaha.111.258723
Consortium, E. P. (2012). An Integrated Encyclopedia of DNA Elements in the Human Genome. Nature 489, 57–74. doi:10.1038/nature11247
Dirkx, E., da Costa Martins, P. A., and De Windt, L. J. (2013). Regulation of Fetal Gene Expression in Heart Failure. Biochim. Biophys. Acta (Bba) - Mol. Basis Dis. 1832, 2414–2424. doi:10.1016/j.bbadis.2013.07.023
Du, W. W., Yang, W., Liu, E., Yang, Z., Dhaliwal, P., and Yang, B. B. (2016). Foxo3 Circular RNA Retards Cell Cycle Progression via Forming Ternary Complexes with P21 and CDK2. Nucleic Acids Res. 44, 2846–2858. doi:10.1093/nar/gkw027
Engreitz, J. M., Ollikainen, N., and Guttman, M. (2016). Long Non-Coding RNAs: Spatial Amplifiers that Control Nuclear Structure and Gene Expression. Nat. Rev. Mol. Cel Biol 17, 756–770. doi:10.1038/nrm.2016.126
Fan, J., Xing, Y., Wen, X., Jia, R., Ni, H., He, J., et al. (2015). Long Non-Coding RNA ROR Decoys Gene-Specific Histone Methylation to Promote Tumorigenesis. Genome Biol. 16, 139. doi:10.1186/s13059-015-0705-2
Fatica, A., and Bozzoni, I. (2014). Long Non-Coding RNAs: New Players in Cell Differentiation and Development. Nat. Rev. Genet. 15, 7–21. doi:10.1038/nrg3606
Frankish, A., Diekhans, M., Ferreira, A.-M., Johnson, R., Jungreis, I., Loveland, J., et al. (2019). GENCODE Reference Annotation for the Human and Mouse Genomes. Nucleic Acids Res. 47, D766–d773. doi:10.1093/nar/gky955
Galindo, M. I., Pueyo, J. I., Fouix, S., Bishop, S. A., and Couso, J. P. (2007). Peptides Encoded by Short ORFs Control Development and Define a New Eukaryotic Gene Family. Plos Biol. 5, e106. doi:10.1371/journal.pbio.0050106
Guttman, M., Amit, I., Garber, M., French, C., Lin, M. F., Feldser, D., et al. (2009). Chromatin Signature Reveals over a Thousand Highly Conserved Large Non-Coding RNAs in Mammals. Nature 458, 223–227. doi:10.1038/nature07672
Han, P., Li, W., Lin, C.-H., Yang, J., Shang, C., Nurnberg, S. T., et al. (2014). A Long Noncoding RNA Protects the Heart from Pathological Hypertrophy. Nature 514, 102–106. doi:10.1038/nature13596
Hon, C.-C., Ramilowski, J. A., Harshbarger, J., Bertin, N., Rackham, O. J. L., Gough, J., et al. (2017). An Atlas of Human Long Non-coding RNAs with Accurate 5′ Ends. Nature 543, 199–204. doi:10.1038/nature21374
Jiang, F., Zhou, X., and Huang, J. (2016). Long Non-coding RNA-ROR Mediates the Reprogramming in Cardiac Hypertrophy. PLOS ONE 11, e0152767. doi:10.1371/journal.pone.0152767
Kaffer, C. R., Grinberg, A., and Pfeifer, K. (2001). Regulatory Mechanisms at the Mouse Igf2/H19 Locus. Mol. Cel Biol 21, 8189–8196. doi:10.1128/mcb.21.23.8189-8196.2001
Kim, T.-K., Hemberg, M., Gray, J. M., Costa, A. M., Bear, D. M., Wu, J., et al. (2010). Widespread Transcription at Neuronal Activity-Regulated Enhancers. Nature 465, 182–187. doi:10.1038/nature09033
Kondo, T., Plaza, S., Zanet, J., Benrabah, E., Valenti, P., Hashimoto, Y., et al. (2010). Small Peptides Switch the Transcriptional Activity of Shavenbaby during Drosophila Embryogenesis. Science 329, 336–339. doi:10.1126/science.1188158
Kretz, M., Webster, D. E., Flockhart, R. J., Lee, C. S., Zehnder, A., Lopez-Pajares, V., et al. (2012). Suppression of Progenitor Differentiation Requires the Long Noncoding RNA ANCR. Genes Dev. 26, 338–343. doi:10.1101/gad.182121.111
Kwon, S. (2013). Single-Molecule Fluorescence In Situ Hybridization: Quantitative Imaging of Single RNA Molecules. BMB Rep. 46, 65–72. doi:10.5483/bmbrep.2013.46.2.016
Lai, Y., He, S., Ma, L., Lin, H., Ren, B., Ma, J., et al. (2017). HOTAIR Functions as a Competing Endogenous RNA to Regulate PTEN Expression by Inhibiting miR-19 in Cardiac Hypertrophy. Mol. Cel Biochem 432, 179–187. doi:10.1007/s11010-017-3008-y
Lai, W.-J. C., Kayedkhordeh, M., Cornell, E. V., Farah, E., Bellaousov, S., Rietmeijer, R., et al. (2018). mRNAs and lncRNAs Intrinsically Form Secondary Structures with Short End-To-End Distances. Nat. Commun. 9, 4328. doi:10.1038/s41467-018-06792-z
Latos, P. A., Pauler, F. M., Koerner, M. V., Şenergin, H. B., Hudson, Q. J., Stocsits, R. R., et al. (2012). Airn Transcriptional Overlap, but Not its lncRNA Products, Induces Imprinted Igf2r Silencing. Science 338, 1469–1472. doi:10.1126/science.1228110
Legnini, I., Di Timoteo, G., Rossi, F., Morlando, M., Briganti, F., Sthandier, O., et al. (2017). Circ-ZNF609 Is a Circular RNA that Can Be Translated and Functions in Myogenesis. Mol. Cel 66, 22–37. e9. doi:10.1016/j.molcel.2017.02.017
Li, C., Zhou, G., Feng, J., Zhang, J., Hou, L., and Cheng, Z. (2018). Upregulation of lncRNA VDR/CASC15 Induced by Facilitates Cardiac Hypertrophy through Modulating miR-432-5p/TLR4 axis. Biochem. Biophysical Res. Commun. 503, 2407–2414. doi:10.1016/j.bbrc.2018.06.169
Li, H., Xu, J.-D., Fang, X.-H., Zhu, J.-N., Yang, J., Pan, R., et al. (2020). Circular RNA circRNA_000203 Aggravates Cardiac Hypertrophy via Suppressing miR-26b-5p and miR-140-3p Binding to Gata4. Cardiovasc. Res. 116, 1323–1334. doi:10.1093/cvr/cvz215
Lim, T. B., Aliwarga, E., Luu, T. D. A., Li, Y. P., Ng, S. L., Annadoray, L., et al. (2019). Targeting the Highly Abundant Circular RNA circSlc8a1 in Cardiomyocytes Attenuates Pressure Overload Induced Hypertrophy. Cardiovasc. Res. 115, 1998–2007. doi:10.1093/cvr/cvz130
Liu, L., An, X., Li, Z., Song, Y., Li, L., Zuo, S., et al. (2016). The H19 Long Noncoding RNA Is a Novel Negative Regulator of Cardiomyocyte Hypertrophy. Cardiovasc. Res. 111, 56–65. doi:10.1093/cvr/cvw078
Luo, Y., Xu, Y., Liang, C., Xing, W., and Zhang, T. (2018). The Mechanism of Myocardial Hypertrophy Regulated by the Interaction between Mhrt and Myocardin. Cell Signal. 43, 11–20. doi:10.1016/j.cellsig.2017.11.007
Luo, X., He, S., Hu, Y., Liu, J., and Chen, X. (2019). Sp1-Induced LncRNA CTBP1-AS2 Is a Novel Regulator in Cardiomyocyte Hypertrophy by Interacting with FUS to Stabilize TLR4. Cardiovasc. Pathol. 42, 21–29. doi:10.1016/j.carpath.2019.04.005
Lv, L., Li, T., Li, X., Xu, C., Liu, Q., Jiang, H., et al. (2018). The lncRNA Plscr4 Controls Cardiac Hypertrophy by Regulating miR-214. Mol. Ther. - Nucleic Acids 10, 387–397. doi:10.1016/j.omtn.2017.12.018
Marchese, F. P., Raimondi, I., and Huarte, M. (2017). The Multidimensional Mechanisms of Long Noncoding RNA Function. Genome Biol. 18, 206. doi:10.1186/s13059-017-1348-2
Mentkowski, K. I., and Lang, J. K. (2019). Exosomes Engineered to Express a Cardiomyocyte Binding Peptide Demonstrate Improved Cardiac Retention In Vivo. Sci. Rep. 9, 10041. doi:10.1038/s41598-019-46407-1
Miano, J. M. (2015). Myocardin in Biology and Disease. J. Biomed. Res. 29, 3–19. doi:10.7555/JBR.29.20140151
Modarresi, F., Faghihi, M. A., Lopez-Toledano, M. A., Fatemi, R. P., Magistri, M., Brothers, S. P., et al. (2012). Inhibition of Natural Antisense Transcripts In Vivo Results in Gene-Specific Transcriptional Upregulation. Nat. Biotechnol. 30, 453–459. doi:10.1038/nbt.2158
Nelson, B. R., Makarewich, C. A., Anderson, D. M., Winders, B. R., Troupes, C. D., Wu, F., et al. (2016). A Peptide Encoded by a Transcript Annotated as Long Noncoding RNA Enhances SERCA Activity in Muscle. Science 351, 271–275. doi:10.1126/science.aad4076
Ning, S., Yue, M., Wang, P., Liu, Y., Zhi, H., Zhang, Y., et al. (2016). LincSNP 2.0: an Updated Database for Linking Disease-Associated SNPs to Human Long Non-Coding RNAs and Their TFBSs. Nucleic Acids Res. 45, D74–D78. doi:10.1093/nar/gkw945
Novikova, I. V., Hennelly, S. P., Tung, C.-S., and Sanbonmatsu, K. Y. (2013). Rise of the RNA Machines: Exploring the Structure of Long Non-coding RNAs. J. Mol. Biol. 425, 3731–3746. doi:10.1016/j.jmb.2013.02.030
O'Brien, J., Hayder, H., Zayed, Y., and Peng, C. (2018). Overview of MicroRNA Biogenesis, Mechanisms of Actions, and Circulation. Front. Endocrinol. 9, 402. doi:10.3389/fendo.2018.00402
Oliva-Rico, D., and Herrera, L. A. (2017). Regulated Expression of the lncRNA TERRA and its Impact on Telomere Biology. Mech. Ageing Develop. 167, 16–23. doi:10.1016/j.mad.2017.09.001
Pamudurti, N. R., Bartok, O., Jens, M., Ashwal-Fluss, R., Stottmeister, C., Ruhe, L., et al. (2017). Translation of CircRNAs. Mol. Cel 66, 9–21. e7. doi:10.1016/j.molcel.2017.02.021
Pandey, R. R., Mondal, T., Mohammad, F., Enroth, S., Redrup, L., Komorowski, J., et al. (2008). Kcnq1ot1 Antisense Noncoding RNA Mediates Lineage-Specific Transcriptional Silencing through Chromatin-Level Regulation. Mol. Cel 32, 232–246. doi:10.1016/j.molcel.2008.08.022
Pauli, A., Norris, M. L., Valen, E., Chew, G.-L., Gagnon, J. A., Zimmerman, S., et al. (2014). Toddler: an Embryonic Signal that Promotes Cell Movement via Apelin Receptors. Science 343, 1248636. doi:10.1126/science.1248636
Piccoli, M.-T., Gupta, S. K., Viereck, J., Foinquinos, A., Samolovac, S., Kramer, F. L., et al. (2017). Inhibition of the Cardiac Fibroblast-Enriched lncRNA Meg3 Prevents Cardiac Fibrosis and Diastolic Dysfunction. Circ. Res. 121, 575–583. doi:10.1161/circresaha.117.310624
Quek, X. C., Thomson, D. W., Maag, J. L. V., Bartonicek, N., Signal, B., Clark, M. B., et al. (2015). lncRNAdb v2.0: Expanding the Reference Database for Functional Long Noncoding RNAs. Nucleic Acids Res. 43, D168–D173. doi:10.1093/nar/gku988
Quinn, J. J., and Chang, H. Y. (2016). Unique Features of Long Non-Coding RNA Biogenesis and Function. Nat. Rev. Genet. 17, 47–62. doi:10.1038/nrg.2015.10
Rinn, J. L., Kertesz, M., Wang, J. K., Squazzo, S. L., Xu, X., Brugmann, S. A., et al. (2007). Functional Demarcation of Active and Silent Chromatin Domains in Human HOX Loci by Noncoding RNAs. Cell 129, 1311–1323. doi:10.1016/j.cell.2007.05.022
Shao, M., Chen, G., Lv, F., Liu, Y., Tian, H., Tao, R., et al. (2017). LncRNA TINCR Attenuates Cardiac Hypertrophy by Epigenetically Silencing CaMKII. Oncotarget 8, 47565–47573. doi:10.18632/oncotarget.17735
Sigova, A. A., Mullen, A. C., Molinie, B., Gupta, S., Orlando, D. A., Guenther, M. G., et al. (2013). Divergent Transcription of Long Noncoding RNA/mRNA Gene Pairs in Embryonic Stem Cells. Proc. Natl. Acad. Sci. 110, 2876–2881. doi:10.1073/pnas.1221904110
Sonnenschein, K., Wilczek, A. L., de Gonzalo-Calvo, D., Pfanne, A., Derda, A. A., Zwadlo, C., et al. (2019). Serum Circular RNAs Act as Blood-Based Biomarkers for Hypertrophic Obstructive Cardiomyopathy. Sci. Rep. 9, 20350. doi:10.1038/s41598-019-56617-2
Sun, Y., Yang, Z., Zheng, B., Zhang, X.-H., Zhang, M.-L., Zhao, X.-S., et al. (2017). A Novel Regulatory Mechanism of Smooth Muscle α-Actin Expression by NRG-1/circACTA2/miR-548f-5p Axis. Circ. Res. 121, 628–635. doi:10.1161/CIRCRESAHA.117.311441
Sun, Q., Hao, Q., and Prasanth, K. V. (2018). Nuclear Long Noncoding RNAs: Key Regulators of Gene Expression. Trends Genet. 34, 142–157. doi:10.1016/j.tig.2017.11.005
Tian, D., Sun, S., and Lee, J. T. (2010). The Long Noncoding RNA, Jpx, Is a Molecular Switch for X Chromosome Inactivation. Cell 143, 390–403. doi:10.1016/j.cell.2010.09.049
Trembinski, D. J., Bink, D. I., Theodorou, K., Sommer, J., Fischer, A., van Bergen, A., et al. (2020). Aging-regulated Anti-apoptotic Long Non-coding RNA Sarrah Augments Recovery from Acute Myocardial Infarction. Nat. Commun. 11, 2039. doi:10.1038/s41467-020-15995-2
van Heesch, S., van Iterson, M., Jacobi, J., Boymans, S., Essers, P. B., de Bruijn, E., et al. (2014). Extensive Localization of Long Noncoding RNAs to the Cytosol and Mono- and Polyribosomal Complexes. Genome Biol. 15, R6. doi:10.1186/gb-2014-15-1-r6
van Heesch, S., Witte, F., Schneider-Lunitz, V., Schulz, J. F., Adami, E., Faber, A. B., et al. (2019). The Translational Landscape of the Human Heart. Cell 178, 242–260. doi:10.1016/j.cell.2019.05.010
Vickers, T. A., Koo, S., Bennett, C. F., Crooke, S. T., Dean, N. M., and Baker, B. F. (2003). Efficient Reduction of Target RNAs by Small Interfering RNA and RNase H-Dependent Antisense Agents. A Comparative Analysis. J. Biol. Chem. 278, 7108–7118. doi:10.1074/jbc.m210326200
Viereck, J., Kumarswamy, R., Foinquinos, A., Xiao, K., Avramopoulos, P., Kunz, M., et al. (2016). Long Noncoding RNA Chast Promotes Cardiac Remodeling. Sci. Transl Med. 8, 326ra22. doi:10.1126/scitranslmed.aaf1475
Volders, P.-J., Helsens, K., Wang, X., Menten, B., Martens, L., Gevaert, K., et al. (2013). LNCipedia: A Database for Annotated Human lncRNA Transcript Sequences and Structures. Nucleic Acids Res. 41, D246–D251. doi:10.1093/nar/gks915
Wang, K. C., and Chang, H. Y. (2011). Molecular Mechanisms of Long Noncoding RNAs. Mol. Cel 43, 904–914. doi:10.1016/j.molcel.2011.08.018
Wang, K. C., Yang, Y. W., Liu, B., Sanyal, A., Corces-Zimmerman, R., Chen, Y., et al. (2011). A Long Noncoding RNA Maintains Active Chromatin to Coordinate Homeotic Gene Expression. Nature 472, 120–124. doi:10.1038/nature09819
Wang, K., Liu, F., Zhou, L.-Y., Long, B., Yuan, S.-M., Wang, Y., et al. (2014). The Long Noncoding RNA CHRF Regulates Cardiac Hypertrophy by Targeting miR-489. Circ. Res. 114, 1377–1388. doi:10.1161/circresaha.114.302476
Wang, F., Nazarali, A. J., and Ji, S. (2016). Circular RNAs as Potential Biomarkers for Cancer Diagnosis and Therapy. Am. J. Cancer Res. 6, 1167–1176.
Wang, K., Long, B., Liu, F., Wang, J.-X., Liu, C.-Y., Zhao, B., et al. (2016). A Circular RNA Protects the Heart from Pathological Hypertrophy and Heart Failure by Targeting miR-223. Eur. Heart J. 37, 2602–2611. doi:10.1093/eurheartj/ehv713
Wang, Z., Zhang, X.-J., Ji, Y.-X., Zhang, P., Deng, K.-Q., Gong, J., et al. (2016). The Long Noncoding RNA Chaer Defines an Epigenetic Checkpoint in Cardiac Hypertrophy. Nat. Med. 22, 1131–1139. doi:10.1038/nm.4179
Wang, L., Meng, X., Li, G., Zhou, Q., and Xiao, J. (2018). Circular RNAs in Cardiovascular Diseases. Adv. Exp. Med. Biol. 1087, 191–204. doi:10.1007/978-981-13-1426-1_15
Wang, W. J., Wang, Y. M., Hu, Y., Lin, Q., Chen, R., Liu, H., et al. (2018). HDncRNA: a Comprehensive Database of Non-coding RNAs Associated with Heart Diseases. Database (Oxford) 2018, bay067. doi:10.1093/database/bay067
Wang, Y., Cao, R., Yang, W., and Qi, B. (2019). SP1‐SYNE1‐AS1‐miR‐525‐5p Feedback Loop Regulates Ang‐II‐induced Cardiac Hypertrophy. J. Cel Physiol 234, 14319–14329. doi:10.1002/jcp.28131
Wo, Y., Guo, J., Li, P., Yang, H., and Wo, J. (2018). Long Non-Coding RNA CHRF Facilitates Cardiac Hypertrophy through Regulating Akt3 via miR-93. Cardiovasc. Pathol. 35, 29–36. doi:10.1016/j.carpath.2018.04.003
Wu, H., Zhao, Z.-A., Liu, J., Hao, K., Yu, Y., Han, X., et al. (2018). Long Noncoding RNA Meg3 Regulates Cardiomyocyte Apoptosis in Myocardial Infarction. Gene Ther. 25, 511–523. doi:10.1038/s41434-018-0045-4
Xiao, L., Gu, Y., Sun, Y., Chen, J., Wang, X., Zhang, Y., et al. (2019). The Long Noncoding RNA XIST Regulates Cardiac Hypertrophy by Targeting miR‐101. J. Cel Physiol 234, 13680–13692. doi:10.1002/jcp.28047
Yang, M.-H., Wang, H., Han, S.-N., Jia, X., Zhang, S., Dai, F.-F., et al. (2020). Circular RNA Expression in Isoproterenol Hydrochloride-Induced Cardiac Hypertrophy. Aging 12, 2530–2544. doi:10.18632/aging.102761
Young, T. L., Matsuda, T., and Cepko, C. L. (2005). The Noncoding RNA Taurine Upregulated Gene 1 Is Required for Differentiation of the Murine Retina. Curr. Biol. 15, 501–512. doi:10.1016/j.cub.2005.02.027
Yu, F., Xu, T., Wang, M., Chang, W., Li, P., and Wang, J. (2018). Function and Regulation of Mitofusin 2 in Cardiovascular Physiology and Pathology. Eur. J. Cel Biol. 97, 474–482. doi:10.1016/j.ejcb.2018.07.003
Yuan, Y., Wang, J., Chen, Q., Wu, Q., Deng, W., Zhou, H., et al. (2019). Long Non-coding RNA Cytoskeleton Regulator RNA (CYTOR) Modulates Pathological Cardiac Hypertrophy through miR-155-Mediated IKKi Signaling. Biochim. Biophys. Acta (Bba) - Mol. Basis Dis. 1865, 1421–1427. doi:10.1016/j.bbadis.2019.02.014
Zhang, Q., Wang, F., Wang, F., and Wu, N. (2020). Long noncoding RNA MAGI1‐IT1 regulates cardiac hypertrophy by modulating miR‐302e/DKK1/Wnt/beta‐catenin signaling pathway. J. Cel Physiol 235, 245–253. doi:10.1002/jcp.28964
Zhao, Y., Li, H., Fang, S., Kang, Y., Wu, W., Hao, Y., et al. (2016). NONCODE 2016: an Informative and Valuable Data Source of Long Non-coding RNAs. Nucleic Acids Res. 44, D203–D208. doi:10.1093/nar/gkv1252
Zheng, L.-L., Li, J.-H., Wu, J., Sun, W.-J., Liu, S., Wang, Z.-L., et al. (2016). deepBase v2.0: Identification, Expression, Evolution and Function of Small RNAs, LncRNAs and Circular RNAs from Deep-Sequencing Data. Nucleic Acids Res. 44, D196–D202. doi:10.1093/nar/gkv1273
Zhou, K.-R., Liu, S., Sun, W.-J., Zheng, L.-L., Zhou, H., Yang, J.-H., et al. (2016). ChIPBase v2.0: Decoding Transcriptional Regulatory Networks of Non-coding RNAs and Protein-Coding Genes from ChIP-Seq Data. Nucleic Acids Res. 45, D43–D50. doi:10.1093/nar/gkw965
Zhu, X. H., Yuan, Y. X., Rao, S. L., and Wang, P. (2016). LncRNA MIAT Enhances Cardiac Hypertrophy Partly through Sponging miR-150. Eur. Rev. Med. Pharmacol. Sci. 20, 3653–3660.
Keywords: lncRNA, circRNAs, cardiac hypertrophy, heart, heart disease
Citation: Mangraviti N and De Windt LJ (2022) Long Non-Coding RNAs in Cardiac Hypertrophy. Front. Mol. Med. 2:836418. doi: 10.3389/fmmed.2022.836418
Received: 15 December 2021; Accepted: 08 February 2022;
Published: 08 March 2022.
Edited by:
Thierry Pedrazzini, Centre Hospitalier Universitaire Vaudois (CHUV), SwitzerlandReviewed by:
Simona Greco, IRCCS San Donato Polyclinic, ItalyShizuka Uchida, Aalborg University Copenhagen, Denmark
Copyright © 2022 Mangraviti and De Windt. This is an open-access article distributed under the terms of the Creative Commons Attribution License (CC BY). The use, distribution or reproduction in other forums is permitted, provided the original author(s) and the copyright owner(s) are credited and that the original publication in this journal is cited, in accordance with accepted academic practice. No use, distribution or reproduction is permitted which does not comply with these terms.
*Correspondence: Leon J. De Windt, bC5kZXdpbmR0QG1hYXN0cmljaHR1bml2ZXJzaXR5Lm5s