- 1Department of Medicine (Marion Bessin Liver Research Center), Department of Pathology, Albert Einstein College of Medicine, Bronx, NY, United States
- 2Einstein Aging Research Center, Montefiore Einstein Comprehensive Cancer Center, Albert Einstein College of Medicine, Bronx, NY, United States
Aging and cancer are intricately linked through shared molecular processes that influence both the onset of malignancy and the progression of age-related decline. As organisms age, cellular stress, genomic instability, and an accumulation of senescent cells create a pro-inflammatory environment conducive to cancer development. Autophagy, a cellular process responsible for degrading and recycling damaged components, plays a pivotal role in this relationship. While autophagy acts as a tumor-suppressive mechanism by preventing the accumulation of damaged organelles and proteins, cancer cells often exploit it to survive under conditions of metabolic stress and treatment resistance. The interplay between aging, cancer, and autophagy reveals key insights into tumorigenesis, cellular senescence, and proteostasis dysfunction. This review explores the molecular connections between these processes, emphasizing the potential for autophagy-targeted therapies as strategies that could be further explored in both aging and cancer treatment. Understanding the dual roles of autophagy in suppressing and promoting cancer offers promising avenues for therapeutic interventions aimed at improving outcomes for elderly cancer patients while addressing age-related deterioration.
1 Introduction
Aging is a progressive, multifaceted process characterized by evolving cellular and molecular deterioration that leads to a decline in physiological function and an increase in susceptibility to diseases, including cancer.
The intersection of aging and oncology has gained significant attention as cancer is predominantly an aging-associated disease especially in individuals over 65 years. The molecular mechanisms of aging are closely linked to tumorigenesis, influencing both age-related decline and cancer development.
Understanding the connections between aging and cancer, opens new avenues for therapeutic interventions. Recent advances in molecular biology show how aging-related changes in the microenvironment, immune surveillance, or systemic inflammation, promote cancer. In addition, cancer and its treatments can accelerate aging, shortening health and lifespan, also in cancer survivors.
This review explores the intricate relationship between aging and cancer -with a focus on autophagy, highlighting key molecular insights and therapeutic advances that hold promise for improving outcomes in elderly and older cancer patients.
2 Mechanisms of aging
Aging is characterized by the gradual loss of homeostasis due to the decline physiological functions and biological integrity (Aunan et al., 2016; Hunt et al., 2019). It involves changes at molecular, cellular, organ, and body levels. Extensive research has identified key drivers of aging, initially defined by nine hallmarks (López-Otín et al., 2013) and recently expanded to twelve (López-Otín et al., 2023). These hallmarks of aging are categorized into: primary, antagonistic, and integrative (López-Otín et al., 2013).
Primary hallmarks include genomic instability, which refers to accumulation of DNA damage causing cellular dysfunction. Telomere attrition, the shortening of telomeres during cell division, leads to cellular senescence or apoptosis (López-Otín et al., 2023). Epigenetic alterations, such as changes in DNA methylation and chromatin remodeling, contribute to age-related diseases (López-Otín et al., 2023). The loss of proteostasis is another crucial hallmark, linked to age-related disorders through the accumulation of damaged and misfolded proteins (Tsakiri et al., 2013; Gordon et al., 2014; Bobkova et al., 2015; Munkácsy et al., 2019; Dong et al., 2021). Autophagy, essential for maintaining proteostasis, is a significant hallmark of aging. Studies shows that impaired protein homeostasis and reduced autophagy accelerate aging. Conversely, interventions that enhance proteostasis and boost autophagic activity can slow the aging process (Rangel et al., 2022). Studies highlight that promoting protein quality control and autophagic flux helps mitigate the progression of aging and its associated pathologies (Pyo et al., 2013; Fernández et al., 2018; Wang et al., 2022).
Antagonistic hallmarks of aging include deregulated nutrient-sensing pathways, such as insulin/IGF-1 signaling, PI3K-AKT, mTOR, AMPK, and Ras-MEK-ERK. While these pathways promote growth during youth, their prolonged activation contributes to aging by promoting excessive anabolism, inflammation, and inhibiting autophagy (López-Otín et al., 2023). Reduced pathway activity has been linked to extended lifespan and improved health span (López-Otín et al., 2013). Mitochondrial dysfunction, marked by declining bioenergetics and increased reactive oxygen species (ROS), contributes to inflammation and cell death as aging progresses (Amorim et al., 2022). Cellular senescence triggered by telomere shortening, or other stressors, results in stable cell cycle arrest (Campisi and d'Adda di Fagagna, 2007; Collado et al., 2007; Kuilman et al., 2010). Although senescence reduces oncogenesis risks, the accumulation of senescent cells with age impairs tissue function (Tuttle et al., 2020).
Integrative hallmarks include stem cell exhaustion, altered intercellular communication, chronic inflammation, and dysbiosis. Stem cell exhaustion leads to reduced tissue regeneration due to depletion and functional decline accelerating aging (López-Otín et al., 2023). Altered intercellular communication disrupts homeostasis and stress response, promoting chronic inflammation and impaired immune surveillance (López-Otín et al., 2023). Chronic inflammation, or “inflammaging” is linked to arteriosclerosis, neuroinflammation, osteoarthritis, and intervertebral disc degeneration (López-Otín et al., 2023). Aging is associated with an increase in circulating proinflammatory cytokines, which promote inflammation as immune function declines (Hirata et al., 2020; Mogilenko et al., 2021). Research suggests that modulating immune pathways and using anti-inflammatory treatments may improve health span (D'Souza et al., 2021; Sciorati et al., 2020; Marín-Aguilar et al., 2020). Dysbiosis, an imbalance in the gut microbiome, impairs communication with the nervous system and organs (López-Otín and Kroemer, 2021), contributing to diseases as obesity, type 2 diabetes, and cancer (Zmora et al., 2019).
3 Cancer development
Cancer involves diseases characterized by uncontrolled cell growth, which can invade nearby tissues and metastasize. It affects almost any tissue, presenting as solid tumors or blood malignancies.
Cancer, similarly to aging, is defined by key hallmarks. Initially, six hallmarks were identified, later expanded to eight: self-sufficiency in growth signals, insensitivity to anti-growth signals, evasion of apoptosis, limitless replicative potential, sustained angiogenesis, tissue invasion and metastasis, reprogrammed energy metabolism, and immune evasion (Hanahan, 2022). Cancer transformation mechanisms differ due to specific mutations.
Cancer cells differ from normal cells in their autonomous growth. They achieve this through autocrine signaling, receptor overexpression, and altered pathways. Additionally, cancer cells modify their microenvironment to support growth (Hanahan, 2022; Hanahan and Weinberg, 2000).
Cancer cells resistance to antiproliferative signaling occurs through mutations in pro-apoptotic genes like p53 and activation of survival pathways such as PI3K-AKT/PKB (Hanahan, 2022; Hanahan and Weinberg, 2000). Their limitless replicative potential allows continuous division (Hanahan, 2022; Hanahan and Weinberg, 2011), often supported by upregulated telomerase or alternative telomere maintenance mechanisms (Blasco, 2005; Shay and Wright, 2000). Angiogenesis is vital for tumor growth, as it supplies nutrients and oxygen. Tumors achieve this by tipping the balance toward pro-angiogenic factors, like VEGF, over inhibitors (Hanahan, 2022; Hanahan and Weinberg, 2000).
Altered energy metabolism is another feature. Many cancer cells shift from oxidative phosphorylation to glycolysis (the Warburg effect) (Hanahan, 2022; Hanahan and Weinberg, 2011), boosting glucose uptake for biosynthesis and growth. Hypoxic conditions in tumors further promote glycolysis, where hypoxic cells generate lactate used by others through the TCA cycle (Semenza, 2008; Feron, 2009; Kennedy and Dewhirst, 2010). This shift, driven by oncogenes such as RAS, MYC, and TP53, is a hallmark of cancer (DeBerardinis et al., 2008; Jones and Thompson, 2009). Proteostasis and autophagy manage cancer cells’ metabolic demands, and their dysregulation is linked to tumor progression and treatment resistance, positioning autophagy as a potential therapeutic target.
The role of immune evasion as a core hallmark is debated, though evidence from human cancers and studies in immunodeficient mice indicates the immune system’s role in tumor control (Hanahan, 2022; Hanahan and Weinberg, 2011). Cancer’s hallmarks are facilitated by genomic instability and tumor-promoting inflammation (Teng et al., 2008; Kim et al., 2007).
These hallmarks in cancer overlap with aging’s hallmarks, including genetic mutations, epigenetic changes, telomere shortening, cellular senescence, proteostasis disruption, and chronic inflammation (Hanahan, 2022), all contributing to cancer development and progression (Figure 1A).
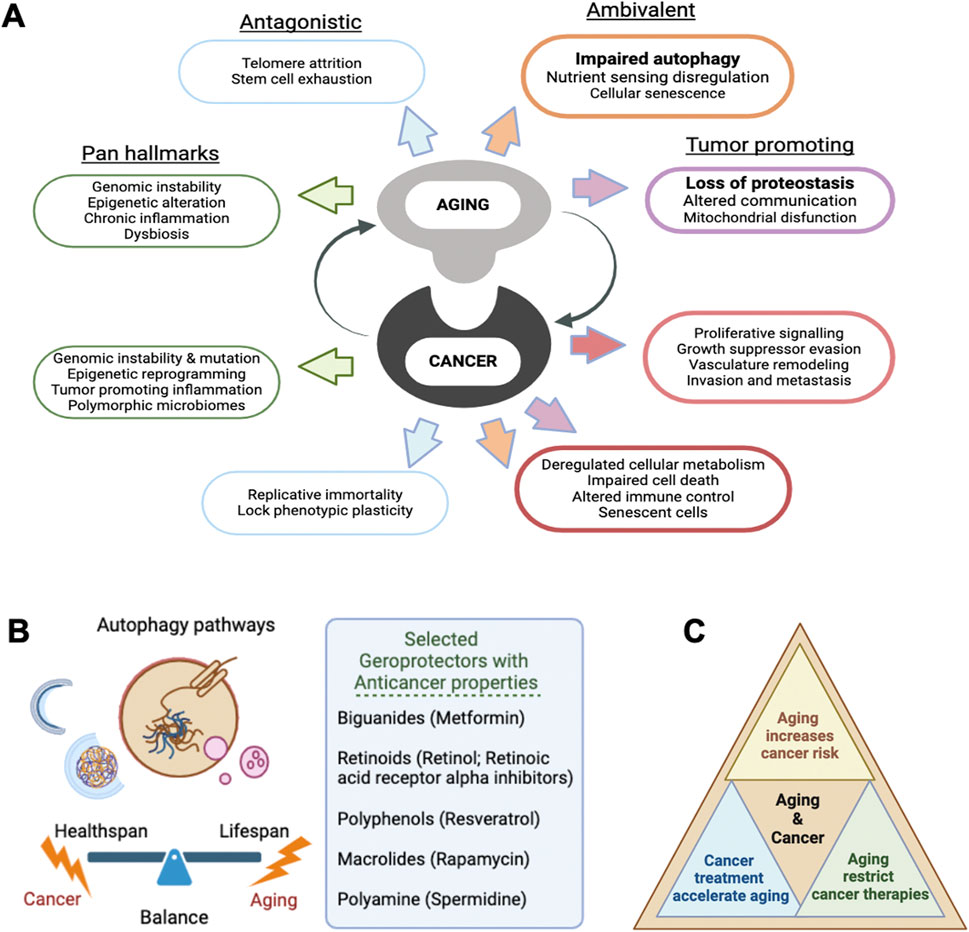
Figure 1. (A) Schematic illustration highlighting the close relationship between aging and cancer, summarizing key hallmarks of aging in four established groups alongside the related hallmarks of cancer. Both aging and cancer share multiple biological mechanisms that influence each other, including proteostasis and autophagy, highlighted on the right side of the scheme. (B) Illustration of the role of autophagy pathways in maintaining cellular balance, highlighting their impact on healthspan and lifespan, with implications for combating aging and cancer. (C) Age and frailty profoundly affect cancer risk, tumor biology. Factors such as treatment options, toxicity, tolerability, and effectiveness are heavily shaped by age, with frailty playing an even more significant role.
4 Autophagy: a crucial link between aging and cancer
One key process that connects both aging and cancer is autophagy. Autophagy is an essential mechanism by which cells degrade and recycle cellular components, thus maintaining homeostasis and controlling a variety of essential cellular processes. This term encompasses three well-differentiated processes: macroautophagy (including selective types), microautophagy, and chaperone-mediated autophagy (CMA) (Schneider and Cuervo, 2014).
All three types of autophagy coexist in mammal cells and can compensate for each other when one is impaired. However, data from different studies suggest that the crosstalk between the different autophagic processes is complex and likely context-dependent (Kaushik et al., 2021). As we age, the efficiency of autophagy tends to decline, contributing to the aging process and increasing susceptibility to cancer. We explore here the complex role of autophagy as a bridge between aging and cancer, highlighting its impact on cellular homeostasis with aging-related decline and tumor development.
4.1 Autophagy’s influence on the aging process
Age-related decline in autophagy leads to expansion of the lysosomal compartment, an increase in the levels of certain lysosomal proteases -but a decline in their proteolytic activity, and accumulation of undegraded material within lysosomes.
4.1.1 Macroautophagy and aging
There is a well-established link between macroautophagy and lifespan extension. Studies show that loss-of-function mutations in ATGs shorten lifespan, while increased macroautophagy, particularly under dietary restriction, extends it (Hansen et al., 2008). However, age-related macroautophagy decline varies by organ, and life-extending interventions are often organ-specific (Chang et al., 2017).
In mammals, aged rodents display a reduced autophagic flux (Del Roso et al., 2003; Donati et al., 2001; Terman, 1995), but interestingly, long-lived species and centenarians tend to maintain robust autophagic function even at advanced ages (Pérez et al., 2009; Raz et al., 2017). Age-related macroautophagy decline may stem from defective autophagosome-lysosome trafficking, hindering their fusion. Moreover, this autophagic dysfunction is characterized by downregulation of key autophagy effectors like Beclin-1, Atg5, Atg7 (Lipinski et al., 2010; Shibata et al., 2006), LC3 and Atg7 (Kaushik et al., 2012), and an increase in negative regulators like Rubicon (Nakamura et al., 2019). Altogether renders in the accumulation of defective mitochondria, increased oxidative stress, and neurodegeneration, contributing to age-related disorders.
It is also relevant to mention the selective processes, including mitophagy -used as a mechanism to repair mitochondrial DNA and proteins to maintain the mitochondrial network; its efficiency has been shown to decline with age (D'Arcy, 2024), as Parkin and PINK1 encompassing BNIP3/NIX, FUNDC1, and Bcl2-L-13 prompts the accumulation of dysfunctional mitochondria, which contributes to carcinogenesis (Denisenko et al., 2021).
Currently, research efforts are focused on strategies to upregulate autophagy genes or prevent the decline of autophagy, to extend lifespan and improve health span in mammalian models like mice.
4.1.2 CMA in aging
Chaperone-mediated autophagy activity has been reported to decline with age in nearly all cell types and tissues in both rodents and humans (Kaushik et al., 2021; Schneider et al., 2015; Valdor et al., 2014; Zhang and Cuervo, 2008). This decline is primarily attributed to reduced LAMP2A levels in aging organisms (Kaushik et al., 2021). However, genetic restoration of LAMP2A in aged mice has been shown to effectively reduce proteotoxicity and preserve cellular function (Dong et al., 2021; Zhang and Cuervo, 2008), by maintaining protein quality control (Dong et al., 2021; Zhang and Cuervo, 2008; Bourdenx et al., 2021), and regulating specific cellular processes like glycolysis or endocytosis (Dong et al., 2021; Bourdenx et al., 2021).
Although inhibition of CMA and macroautophagy generates similar outcomes, both pathways are complementary and non-redundant, as they target distinct subsets of proteins and cellular components for degradation (Kaushik et al., 2021).
4.1.3 Microautphagy and aging
Microautophagy has been less extensively studied compared to other forms of autophagy, so its role in the aging process remains largely unknown. However, evidence suggests that with age, there is an accumulation of carbonylated proteins and lipid peroxidation products in multivesicular bodies (MVBs), which would indicate a deterioration in endosomal microautophagy (eMI) (Cannizzo et al., 2012).
In summary, autophagy and aging are tightly interconnected, with autophagy playing a critical role in many hallmarks of aging, and preventing physiological and developmental problems like neurodegeneration, liver failure and cancer. Defective autophagy leads to accumulation of damaged proteins, causing mitochondrial dysfunction, oxidative stress and inflammation. In addition, autophagy regulates metabolism, prevents excessive apoptosis and modulates the immune system (Schneider et al., 2014; Tabibzadeh, 2023).
For a broader and extended review related the role of autophagy in aging-related disorders there has been recently published (Wu et al., 2024; Cassidy and Narita, 2022).
4.2 The impact of autophagy in cancer
Autophagy plays a dual and complex role in cancer, acting both as a tumor suppressor and as a promoter of tumor growth depending on the context. This role varies with the specific type of cancer, the stage of tumor development, and the tumor microenvironment.
4.2.1 Autophagy as a tumor suppressor
Aging impairs the protective role of autophagy against cancer development. As a tumor suppressor, autophagy regulates key factors such as cell proliferation, genomic instability (Mathew et al., 2009), necrosis, inflammation (Tang et al., 2010), and oxidative stress (Tabibzadeh, 2023). Proteins such as Beclin-1, ATG5, ATG7, BNIP3, and BNIP3L, are essential in tumor suppression (Tabibzadeh, 2023; Yang Z. J. et al., 2011; Debnath et al., 2023). Additionally, accumulation of p62/SQSTM1, in autophagy-deficient cells increases. DNA damage and genomic instability, and its removal protects against carcinoma development (Mathew et al., 2009; Duran et al., 2008). Autophagy also limits necrosis and chronic inflammation reinforcing its tumor-suppressive role (Tang et al., 2010).
Moreover, CMA -a selective type of autophagy, is key in cancer, with increased activity in several tumors and cell lines. Blocking CMA reduces survival, tumorigenicity and tumor growth (Gomez-Sintes and Arias, 2021).
4.2.2 Autophagy as a tumor growth promoter
Autophagy can act as a promoter of tumor growth under certain conditions. Aging promotes a proinflammatory environment, in which cancer cells often emerge. Once transformed, they may upregulate autophagy as an adaptative mechanism to overcome stressors such as accumulation of senescent cells and oxidative stress, supporting their growth and survival.
In established tumors, autophagy is essential for cancer cell survival, particularly by enabling cells to tolerate cytotoxic and metabolic stressors, such as hypoxia -via HIF-1α (Semenza, 2010), and nutrient deprivation. Tumor cells often have high metabolic demands, and autophagy allows them to recycle ATP and maintain crucial biosynthetic processes for growth and survival (Yang Z. J. et al., 2011).
Additionally, autophagy may support tumor recurrence and progression by promoting dormancy in cancer cells that survive chemotherapy and/or radiotherapy (Lu et al., 2008). Elevated basal levels of autophagy have been detected in different cancer cells, including pancreatic cancer and tumors with H-ras or K-ras mutations (Yang Z. J. et al., 2011). Inhibition of autophagy in those tumors leads to tumor regression and improve survival, suggesting that targeting autophagy could improve the efficiency of cancer therapies (Yang S. et al., 2011; Guo et al., 2011).
Following malignant transformation, cancer cells frequently upregulate CMA, contributing to tumor growth and proliferation by sustaining the Warburg effect, and providing protection against cytotoxic and chemical agents, modulating immune cell populations in the tumor microenvironment, and degrading tumor suppressors, pro-apoptotic and anti-proliferative factors (Gomez-Sintes and Arias, 2021).
Other selective types as mitophagy also show a pro-tumorigenic role that can vary depending on the cancer type and stage (Song et al., 2022). In glioblastoma and triple-negative breast cancer, for example, it contributes to enhance tumor cell proliferation and metastasis (Dong and Zhang, 2024).
While we have growing understanding of how autophagy promotes tumor suppression, survival and progression, further research is necessary across different tumor types to fully elucidate how this process can either inhibit or promote cancer development. This knowledge is crucial for designing targeted therapies tailored to specific cancers.
4.3 Non-canonical autophagy processes
Research on alternative mechanisms of autophagy is rapidly expanding. These mechanisms, collectively referred to as non-canonical autophagy (NCA), have been identified under specific cellular conditions and hold particular relevance in cancer (Debnath et al., 2023). Unsurprisingly, our understanding of how tumors and associated cells adapt NCA pathways to support tumor growth and progression, especially in the context of aging, is also advancing. Among these alternative pathways, notable examples include: (I) LC3-associated processes: The finding of phagocytic vesicles decorated with LC3 revealed a non-classical function of ATG proteins beyond autophagosome formation (Sanjuan et al., 2007). Posterior studies further expanded this process of LC3-associated phagocytosis (LAP) and identified LAP-like LC3 conjugation on endosomes (Jacquin et al., 2017), LC3-associated endocytosis (LANDO) (Heckmann et al., 2019) and LDELS [(LC3)-dependent extracellular vesicle (EV) loading and secretion] (Leidal et al., 2020). (II) Autophagic membranes as signaling platforms: autophagy-deficient mice show reduced oncogenic signaling through well-known pathways as the AKT–PI3K and MAPK-ERK (Karsli-Uzunbas et al., 2014; Fraser et al., 2017; Martinez-Lopez et al., 2013). This may be due to the tumor-promoting roles of autophagy, or interactions between autophagy components and growth factor signaling pathways. (III) Autophagy-Independent Functions of ATG Proteins in Tumorigenesis: ATG proteins can exert non-autophagy-related functions that significantly influence immune response, vesicular trafficking, cell death and tumorigenesis (Galluzzi and Green, 2019), a critical consideration when targeting autophagy in cancer therapy.
Not all cargoes from NCA undergo lysosomal degradation. Due to the diverse outcomes of cargo processing, NCA can function either as a degradative or a secretory pathway. Recent studies illustrating the importance of autophagy in the host stroma have coincided with a growing appreciation in the field that autophagy controls extracellular secretion (Deretic et al., 2012; Ponpuak et al., 2015).
4.4 Therapeutic advances
Given the dual role of autophagy in both aging and cancer, researchers are now exploring therapies that modulate autophagy in a more detailed strategy. In healthy individuals, different studies have shown that restoring autophagy levels in aged organisms can reduce tumor formation. On the other hand, for aging population with cancer, maintaining a delicate balance between activating autophagy to support healthy aging and inhibiting it to prevent tumor growth in the tissues affected is a central challenge. Precision medicine approaches that tailor autophagy modulation based on an individual’s age, cancer type, and overall health status are emerging as a promising area of therapeutic innovation.
When focusing on aged cancer patients, different strategies have been explored, summarized in Table 1. Among them, several compounds considered geroprotectants, such as biguanides (metformin) (Zajda et al., 2020), retinoids (Hałubiec et al., 2021), polyphenols (resveratrol) (Raj et al., 2021; Mundo Rivera et al., 2024), rapamycin (Juricic et al., 2022), and polyamines (spermidine) (Hofer et al., 2022; Zimmermann et al., 2023), are currently being studied for their ability to activate autophagy, making them exciting compounds for their potential use in cancer treatment (Figure 1B).
Of particular note is the concept of “the autophagic switch”, where autophagy shifts from cytoprotective to cytotoxic, opening the possibility of more effective treatments (Frentzel et al., 2017). The complexity and specificity of autophagy pathways emphasizes the need for further research on these cellular mechanisms.
In metastatic cancer cells, autophagy often becomes upregulated, helping these cells survive under the harsh conditions they encounter during invasion and colonization, such as nutrient deprivation, oxidative stress, and immune surveillance. This ability to use autophagy to adapt and thrive in new environments makes it a key driver of metastasis. However, autophagy also has a dual role in metastasis that is stage specific, and in later stages, it can suppress metastatic colonization (Marsh et al., 2021). Therapeutically, targeting autophagy has become a promising approach in the treatment of metastatic cancers. Inhibitors are currently being tested in combination with other cancer therapies to limit not only the survival but also the dissemination of metastatic cells.
5 Concluding remarks
The relationship between aging and cancer is thus highly complex and ambivalent, with certain mechanisms, like autophagy, that can both hinder and fuel cancer progression. Understanding the interactions would lead to better therapeutic strategies that balance cancer treatment with managing the effects of aging.
Future research should explore how geroprotective measures, including intervention of autophagy processes, might be integrated with cancer therapies without compromising their efficacy. The collaboration between oncology and geriatric medicine is essential, particularly as population is reaching older ages, cancer is most often diagnosed in older adults, and oncological treatments accelerate aging (Figure 1C). Clinical trials exploring biological aging markers could also provide more personalized treatment strategies by considering a patient’s biological rather than chronological age. This could pave the way for safer, more effective treatment approaches that account for the interplay between aging and cancer.
Author contributions
BZ: Writing–original draft. EA: Writing–review and editing.
Funding
The author(s) declare that financial support was received for the research, authorship, and/or publication of this article. Work in our laboratory is supported by the National Institutes of Health DK124308, P30AG038072 and the generous support of The Ceriale Foundation.
Acknowledgments
The authors express their gratitude to the insightful feedback and constructive suggestions during the preparation of this manuscript. Unfortunately, due to space constraints, we may not have been able to include the work of all esteemed colleagues.
Conflict of interest
The authors declare that the research was conducted in the absence of any commercial or financial relationships that could be construed as a potential conflict of interest.
Generative AI statement
The author(s) declare that no Generative AI was used in the creation of this manuscript.
Publisher’s note
All claims expressed in this article are solely those of the authors and do not necessarily represent those of their affiliated organizations, or those of the publisher, the editors and the reviewers. Any product that may be evaluated in this article, or claim that may be made by its manufacturer, is not guaranteed or endorsed by the publisher.
References
Akin, D., Wang, S. K., Habibzadegah-Tari, P., Law, B., Ostrov, D., Li, M., et al. (2014). A novel ATG4B antagonist inhibits autophagy and has a negative impact on osteosarcoma tumors. Autophagy 10 (11), 2021–2035. doi:10.4161/auto.32229
Amaravadi, R. K., Kimmelman, A. C., and Debnath, J. (2019). Targeting autophagy in cancer: recent advances and future directions. Cancer Discov. 9 (9), 1167–1181. doi:10.1158/2159-8290.CD-19-0292
Amorim, J. A., Coppotelli, G., Rolo, A. P., Palmeira, C. M., Ross, J. M., and Sinclair, D. A. (2022). Mitochondrial and metabolic dysfunction in ageing and age-related diseases. Nat. Rev. Endocrinol. 18 (4), 243–258. doi:10.1038/s41574-021-00626-7
Aunan, J. R., Watson, M. M., Hagland, H. R., and Søreide, K. (2016). Molecular and biological hallmarks of ageing. Br. J. Surg. 103 (2), e29–e46. doi:10.1002/bjs.10053
Bago, R., Malik, N., Munson, M. J., Prescott, A. R., Davies, P., Sommer, E., et al. (2014). Characterization of VPS34-IN1, a selective inhibitor of Vps34, reveals that the phosphatidylinositol 3-phosphate-binding SGK3 protein kinase is a downstream target of class III phosphoinositide 3-kinase. Biochem. J. 463 (3), 413–427. doi:10.1042/BJ20140889
Behroozaghdam, M., Dehghani, M., Zabolian, A., Kamali, D., Javanshir, S., Hasani Sadi, F., et al. (2022). Resveratrol in breast cancer treatment: from cellular effects to molecular mechanisms of action. Cell. Mol. Life Sci. 79 (11), 539. doi:10.1007/s00018-022-04551-4
Blagosklonny, M. V. (2023). Cancer prevention with rapamycin. Oncotarget 14, 342–350. doi:10.18632/oncotarget.28410
Blasco, M. A. (2005). Telomeres and human disease: ageing, cancer and beyond. Nat. Rev. Genet. 6 (8), 611–622. doi:10.1038/nrg1656
Bobkova, N. V., Evgen'ev, M., Garbuz, D. G., Kulikov, A. M., Morozov, A., Samokhin, A., et al. (2015). Exogenous Hsp70 delays senescence and improves cognitive function in aging mice. Proc. Natl. Acad. Sci. U. S. A. 112 (52), 16006–16011. doi:10.1073/pnas.1516131112
Bourdenx, M., Martín-Segura, A., Scrivo, A., Rodriguez-Navarro, J. A., Kaushik, S., Tasset, I., et al. (2021). Chaperone-mediated autophagy prevents collapse of the neuronal metastable proteome. Cell. 184 (10), 2696–2714.e25. doi:10.1016/j.cell.2021.03.048
Campisi, J., and d'Adda di Fagagna, F. (2007). Cellular senescence: when bad things happen to good cells. Nat. Rev. Mol. Cell. Biol. 8 (9), 729–740. doi:10.1038/nrm2233
Cannizzo, E. S., Clement, C. C., Morozova, K., Valdor, R., Kaushik, S., Almeida, L. N., et al. (2012). Age-related oxidative stress compromises endosomal proteostasis. Cell. Rep. 2 (1), 136–149. doi:10.1016/j.celrep.2012.06.005
Cassidy, L. D., and Narita, M. (2022). Autophagy at the intersection of aging, senescence, and cancer. Mol. Oncol. 16 (18), 3259–3275. doi:10.1002/1878-0261.13269
Chang, J. T., Kumsta, C., Hellman, A. B., Adams, L. M., and Hansen, M. (2017). Spatiotemporal regulation of autophagy during Caenorhabditis elegans aging. Elife 6, e18459. doi:10.7554/eLife.18459
Chen, Y., Zhuang, H., Chen, X., Shi, Z., and Wang, X. (2018). Spermidine-induced growth inhibition and apoptosis via autophagic activation in cervical cancer. Oncol. Rep. 39 (6), 2845–2854. doi:10.3892/or.2018.6377
Collado, M., Blasco, M. A., and Serrano, M. (2007). Cellular senescence in cancer and aging. Cell. 130 (2), 223–233. doi:10.1016/j.cell.2007.07.003
D'Arcy, M. S. (2024). Mitophagy in health and disease. Molecular mechanisms, regulatory pathways, and therapeutic implications. Apoptosis 29 (9-10), 1415–1428. doi:10.1007/s10495-024-01977-y
DeBerardinis, R. J., Lum, J. J., Hatzivassiliou, G., and Thompson, C. B. (2008). The biology of cancer: metabolic reprogramming fuels cell growth and proliferation. Cell. Metab. 7 (1), 11–20. doi:10.1016/j.cmet.2007.10.002
Debnath, J., Gammoh, N., and Ryan, K. M. (2023). Autophagy and autophagy-related pathways in cancer. Nat. Rev. Mol. Cell. Biol. 24 (8), 560–575. doi:10.1038/s41580-023-00585-z
Del Roso, A., Vittorini, S., Cavallini, G., Donati, A., Gori, Z., Masini, M., et al. (2003). Ageing-related changes in the in vivo function of rat liver macroautophagy and proteolysis. Exp. Gerontol. 38 (5), 519–527. doi:10.1016/s0531-5565(03)00002-0
Denisenko, T. V., Gogvadze, V., and Zhivotovsky, B. (2021). Mitophagy in carcinogenesis and cancer treatment. Discov. Oncol. 12 (1), 58. doi:10.1007/s12672-021-00454-1
Deretic, V., Jiang, S., and Dupont, N. (2012). Autophagy intersections with conventional and unconventional secretion in tissue development, remodeling and inflammation. Trends Cell. Biol. 22 (8), 397–406. doi:10.1016/j.tcb.2012.04.008
Donati, A., Cavallini, G., Paradiso, C., Vittorini, S., Pollera, M., Gori, Z., et al. (2001). Age-related changes in the autophagic proteolysis of rat isolated liver cells: effects of antiaging dietary restrictions. J. Gerontol. A Biol. Sci. Med. Sci. 56 (9), B375–B383. doi:10.1093/gerona/56.9.b375
Dong, S., Wang, Q., Kao, Y. R., Diaz, A., Tasset, I., Kaushik, S., et al. (2021). Chaperone-mediated autophagy sustains haematopoietic stem-cell function. Nature 591 (7848), 117–123. doi:10.1038/s41586-020-03129-z
Dong, Y., and Zhang, X. (2024). Targeting cellular mitophagy as a strategy for human cancers. Front. Cell. Dev. Biol. 12, 1431968. doi:10.3389/fcell.2024.1431968
D'Souza, S. S., Zhang, Y., Bailey, J. T., Fung, I. T. H., Kuentzel, M. L., Chittur, S. V., et al. (2021). Type I Interferon signaling controls the accumulation and transcriptomes of monocytes in the aged lung. Aging Cell. 20 (10), e13470. doi:10.1111/acel.13470
Duran, A., Linares, J. F., Galvez, A. S., Wikenheiser, K., Flores, J. M., Diaz-Meco, M. T., et al. (2008). The signaling adaptor p62 is an important NF-kappaB mediator in tumorigenesis. Cancer Cell. 13 (4), 343–354. doi:10.1016/j.ccr.2008.02.001
Egan, D. F., Chun, M. G., Vamos, M., Zou, H., Rong, J., Miller, C. J., et al. (2015). Small molecule inhibition of the autophagy kinase ULK1 and identification of ULK1 substrates. Mol. Cell. 59 (2), 285–297. doi:10.1016/j.molcel.2015.05.031
El-Gowily, A. H., Loutfy, S. A., Ali, E. M. M., Mohamed, T. M., and Mansour, M. A. (2021). Tioconazole and chloroquine act synergistically to combat doxorubicin-induced toxicity via inactivation of PI3K/AKT/mTOR signaling mediated ROS-dependent apoptosis and autophagic flux inhibition in MCF-7 breast cancer cells. Pharm. (Basel) 14 (3), 254. doi:10.3390/ph14030254
Fernández, Á. F., Sebti, S., Wei, Y., Zou, Z., Shi, M., McMillan, K. L., et al. (2018). Author Correction: disruption of the beclin 1-BCL2 autophagy regulatory complex promotes longevity in mice. Nature 561 (7723), E30. doi:10.1038/s41586-018-0270-4
Feron, O. (2009). Pyruvate into lactate and back: from the Warburg effect to symbiotic energy fuel exchange in cancer cells. Radiother. Oncol. 92 (3), 329–333. doi:10.1016/j.radonc.2009.06.025
Fraser, J., Cabodevilla, A. G., Simpson, J., and Gammoh, N. (2017). Interplay of autophagy, receptor tyrosine kinase signalling and endocytic trafficking. Essays Biochem. 61 (6), 597–607. doi:10.1042/EBC20170091
Frentzel, J., Sorrentino, D., and Giuriato, S. (2017). Targeting autophagy in ALK-associated cancers. Cancers (Basel) 9 (12), 161. doi:10.3390/cancers9120161
Fu, Y., Huang, Z., Hong, L., Lu, J. H., Feng, D., Yin, X. M., et al. (2019). Targeting ATG4 in cancer therapy. Cancers (Basel) 11 (5), 649. doi:10.3390/cancers11050649
Galluzzi, L., and Green, D. R. (2019). Autophagy-independent functions of the autophagy machinery. Cell. 177 (7), 1682–1699. doi:10.1016/j.cell.2019.05.026
Gammoh, N., Lam, D., Puente, C., Ganley, I., Marks, P. A., and Jiang, X. (2012). Role of autophagy in histone deacetylase inhibitor-induced apoptotic and nonapoptotic cell death. Proc. Natl. Acad. Sci. U. S. A. 109 (17), 6561–6565. doi:10.1073/pnas.1204429109
Gomez-Sintes, R., and Arias, E. (2021). Chaperone-mediated autophagy and disease: implications for cancer and neurodegeneration. Mol. Asp. Med. 82, 101025. doi:10.1016/j.mam.2021.101025
Gordon, L. B., Rothman, F. G., López-Otín, C., and Misteli, T. (2014). Progeria: a paradigm for translational medicine. Cell. 156 (3), 400–407. doi:10.1016/j.cell.2013.12.028
Guo, J. Y., Chen, H. Y., Mathew, R., Fan, J., Strohecker, A. M., Karsli-Uzunbas, G., et al. (2011). Activated Ras requires autophagy to maintain oxidative metabolism and tumorigenesis. Genes. Dev. 25 (5), 460–470. doi:10.1101/gad.2016311
Hałubiec, P., Łazarczyk, A., Szafrański, O., Bohn, T., and Dulińska-Litewka, J. (2021). Synthetic retinoids as potential therapeutics in prostate cancer-an update of the last decade of research: a review. Int. J. Mol. Sci. 22 (19), 10537. doi:10.3390/ijms221910537
Hanahan, D. (2022). Hallmarks of cancer: new dimensions. Cancer Discov. 12 (1), 31–46. doi:10.1158/2159-8290.CD-21-1059
Hanahan, D., and Weinberg, R. A. (2000). The hallmarks of cancer. Cell. 100 (1), 57–70. doi:10.1016/s0092-8674(00)81683-9
Hanahan, D., and Weinberg, R. A. (2011). Hallmarks of cancer: the next generation. Cell. 144 (5), 646–674. doi:10.1016/j.cell.2011.02.013
Hansen, M., Chandra, A., Mitic, L. L., Onken, B., Driscoll, M., and Kenyon, C. (2008). A role for autophagy in the extension of lifespan by dietary restriction in C. elegans. PLoS Genet. 4 (2), e24. doi:10.1371/journal.pgen.0040024
Hassan, AMIA, Zhao, Y., Chen, X., and He, C. (2024). Blockage of autophagy for cancer therapy: a comprehensive review. Int. J. Mol. Sci. 25 (13), 7459. doi:10.3390/ijms25137459
Heckmann, B. L., Teubner, B. J. W., Tummers, B., Boada-Romero, E., Harris, L., Yang, M., et al. (2019). LC3-Associated endocytosis facilitates β-amyloid clearance and mitigates neurodegeneration in murine alzheimer's disease. Cell. 178 (3), 536–551.e14. doi:10.1016/j.cell.2019.05.056
Hirata, T., Arai, Y., Yuasa, S., Abe, Y., Takayama, M., Sasaki, T., et al. (2020). Associations of cardiovascular biomarkers and plasma albumin with exceptional survival to the highest ages. Nat. Commun. 11 (1), 3820. doi:10.1038/s41467-020-17636-0
Hofer, S. J., Simon, A. K., Bergmann, M., Eisenberg, T., Kroemer, G., and Madeo, F. (2022). Mechanisms of spermidine-induced autophagy and geroprotection. Nat. Aging 2 (12), 1112–1129. doi:10.1038/s43587-022-00322-9
Hunt, N. J., Kang, S. W. S., Lockwood, G. P., Le Couteur, D. G., and Cogger, V. C. (2019). Hallmarks of aging in the liver. Comput. Struct. Biotechnol. J. 17, 1151–1161. doi:10.1016/j.csbj.2019.07.021
Jacquin, E., Leclerc-Mercier, S., Judon, C., Blanchard, E., Fraitag, S., and Florey, O. (2017). Pharmacological modulators of autophagy activate a parallel noncanonical pathway driving unconventional LC3 lipidation. Autophagy 13 (5), 854–867. doi:10.1080/15548627.2017.1287653
Jones, R. G., and Thompson, C. B. (2009). Tumor suppressors and cell metabolism: a recipe for cancer growth. Genes. Dev. 23 (5), 537–548. doi:10.1101/gad.1756509
Juricic, P., Lu, Y. X., Leech, T., Drews, L. F., Paulitz, J., Lu, J., et al. (2022). Long-lasting geroprotection from brief rapamycin treatment in early adulthood by persistently increased intestinal autophagy. Nat. Aging 2 (9), 824–836. doi:10.1038/s43587-022-00278-w
Karsli-Uzunbas, G., Guo, J. Y., Price, S., Teng, X., Laddha, S. V., Khor, S., et al. (2014). Autophagy is required for glucose homeostasis and lung tumor maintenance. Cancer Discov. 4 (8), 914–927. doi:10.1158/2159-8290.CD-14-0363
Kaushik, S., Arias, E., Kwon, H., Lopez, N. M., Athonvarangkul, D., Sahu, S., et al. (2012). Loss of autophagy in hypothalamic POMC neurons impairs lipolysis. EMBO Rep. 13 (3), 258–265. doi:10.1038/embor.2011.260
Kaushik, S., Tasset, I., Arias, E., Pampliega, O., Wong, E., Martinez-Vicente, M., et al. (2021). Autophagy and the hallmarks of aging. Ageing Res. Rev. 72, 101468. doi:10.1016/j.arr.2021.101468
Kemp Bohan, P. M., Chick, R. C., O'Shea, A. E., Vreeland, T. J., Hickerson, A. T., Cindass, J. L., et al. (2021). Phase I trial of encapsulated rapamycin in patients with prostate cancer under active surveillance to prevent progression. Cancer Prev. Res. (Phila) 14 (5), 551–562. doi:10.1158/1940-6207.CAPR-20-0383
Kennedy, K. M., and Dewhirst, M. W. (2010). Tumor metabolism of lactate: the influence and therapeutic potential for MCT and CD147 regulation. Future Oncol. 6 (1), 127–148. doi:10.2217/fon.09.145
Kim, R., Emi, M., and Tanabe, K. (2007). Cancer immunoediting from immune surveillance to immune escape. Immunology 121 (1), 1–14. doi:10.1111/j.1365-2567.2007.02587.x
Kuilman, T., Michaloglou, C., Mooi, W. J., and Peeper, D. S. (2010). The essence of senescence. Genes. Dev. 24 (22), 2463–2479. doi:10.1101/gad.1971610
Kurdi, A., Cleenewerck, M., Vangestel, C., Lyssens, S., Declercq, W., Timmermans, J. P., et al. (2017). ATG4B inhibitors with a benzotropolone core structure block autophagy and augment efficiency of chemotherapy in mice. Biochem. Pharmacol. 138, 150–162. doi:10.1016/j.bcp.2017.06.119
Lee, M. J., Park, J. S., Jo, S. B., and Joe, Y. A. (2023). Enhancing anti-cancer therapy with selective autophagy inhibitors by targeting protective autophagy. Biomol. Ther. Seoul. 31 (1), 1–15. doi:10.4062/biomolther.2022.153
Leidal, A. M., Huang, H. H., Marsh, T., Solvik, T., Zhang, D., Ye, J., et al. (2020). The LC3-conjugation machinery specifies the loading of RNA-binding proteins into extracellular vesicles. Nat. Cell. Biol. 22 (2), 187–199. doi:10.1038/s41556-019-0450-y
Levy, J. M. M., Towers, C. G., and Thorburn, A. (2017). Targeting autophagy in cancer. Nat. Rev. Cancer 17 (9), 528–542. doi:10.1038/nrc.2017.53
Lim, J., and Murthy, A. (2020). Targeting autophagy to treat cancer: challenges and opportunities. Front. Pharmacol. 11, 590344. doi:10.3389/fphar.2020.590344
Lipinski, M. M., Zheng, B., Lu, T., Yan, Z., Py, B. F., Ng, A., et al. (2010). Genome-wide analysis reveals mechanisms modulating autophagy in normal brain aging and in Alzheimer's disease. Proc. Natl. Acad. Sci. U. S. A. 107 (32), 14164–14169. doi:10.1073/pnas.1009485107
Liu, P. F., Tsai, K. L., Hsu, C. J., Tsai, W. L., Cheng, J. S., Chang, H. W., et al. (2018). Drug repurposing screening identifies tioconazole as an ATG4 inhibitor that suppresses autophagy and sensitizes cancer cells to chemotherapy. Theranostics 8 (3), 830–845. doi:10.7150/thno.22012
López-Otín, C., Blasco, M. A., Partridge, L., Serrano, M., and Kroemer, G. (2013). The hallmarks of aging. Cell. 153 (6), 1194–1217. doi:10.1016/j.cell.2013.05.039
López-Otín, C., Blasco, M. A., Partridge, L., Serrano, M., and Kroemer, G. (2023). Hallmarks of aging: an expanding universe. Cell. 186 (2), 243–278. doi:10.1016/j.cell.2022.11.001
López-Otín, C., and Kroemer, G. (2021). Hallmarks of health. Cell. 184 (7), 1929–1939. doi:10.1016/j.cell.2021.03.033
Lu, Z., Luo, R. Z., Lu, Y., Zhang, X., Yu, Q., Khare, S., et al. (2008). The tumor suppressor gene ARHI regulates autophagy and tumor dormancy in human ovarian cancer cells. J. Clin. Investig. 118 (12), 3917–3929. doi:10.1172/JCI35512
Marín-Aguilar, F., Lechuga-Vieco, A. V., Alcocer-Gómez, E., Castejón-Vega, B., Lucas, J., Garrido, C., et al. (2020). NLRP3 inflammasome suppression improves longevity and prevents cardiac aging in male mice. Aging Cell. 19 (1), e13050. doi:10.1111/acel.13050
Markowska, A., Stanislawiak-Rudowicz, J., Kasprzak, T., Markowska, J., and Szarszewska, M. (2022). Metformin in selected malignancies in women. Ginekol. Pol. 93 (5), 416–421. doi:10.5603/GP.a2021.0222
Marsh, T., Tolani, B., and Debnath, J. (2021). The pleiotropic functions of autophagy in metastasis. J. Cell. Sci. 134 (2), jcs247056. doi:10.1242/jcs.247056
Martin, K. R., Celano, S. L., Solitro, A. R., Gunaydin, H., Scott, M., O'Hagan, R. C., et al. (2018). A potent and selective ULK1 inhibitor suppresses autophagy and sensitizes cancer cells to nutrient stress. iScience 8, 74–84. doi:10.1016/j.isci.2018.09.012
Martinez-Lopez, N., Athonvarangkul, D., Mishall, P., Sahu, S., and Singh, R. (2013). Autophagy proteins regulate ERK phosphorylation. Nat. Commun. 4, 2799. doi:10.1038/ncomms3799
Mathew, R., Karp, C. M., Beaudoin, B., Vuong, N., Chen, G., Chen, H. Y., et al. (2009). Autophagy suppresses tumorigenesis through elimination of p62. Cell. 137 (6), 1062–1075. doi:10.1016/j.cell.2009.03.048
Mogilenko, D. A., Shpynov, O., Andhey, P. S., Arthur, L., Swain, A., Esaulova, E., et al. (2021). Comprehensive profiling of an aging immune system reveals clonal GZMK(+) CD8(+) T cells as conserved hallmark of inflammaging. Immunity 54 (1), 99–115.e12. doi:10.1016/j.immuni.2020.11.005
Mohsen, S., Sobash, P. T., Algwaiz, G. F., Nasef, N., Al-Zeidaneen, S. A., and Karim, N. A. (2022). Autophagy agents in clinical trials for cancer therapy: a brief review. Curr. Oncol. 29 (3), 1695–1708. doi:10.3390/curroncol29030141
Mundo Rivera, V. M., Tlacuahuac Juárez, J. R., Murillo Melo, N. M., Leyva Garcia, N., Magaña, J. J., Cordero Martínez, J., et al. (2024). Natural autophagy activators to fight age-related diseases. Cells 13 (19), 1611. doi:10.3390/cells13191611
Munkácsy, E., Chocron, E. S., Quintanilla, L., Gendron, C. M., Pletcher, S. D., and Pickering, A. M. (2019). Neuronal-specific proteasome augmentation via Prosβ5 overexpression extends lifespan and reduces age-related cognitive decline. Aging Cell. 18 (5), e13005. doi:10.1111/acel.13005
Nakamura, S., Oba, M., Suzuki, M., Takahashi, A., Yamamuro, T., Fujiwara, M., et al. (2019). Suppression of autophagic activity by Rubicon is a signature of aging. Nat. Commun. 10 (1), 847. doi:10.1038/s41467-019-08729-6
Nawrocki, S. T., Han, Y., Visconte, V., Przychodzen, B., Espitia, C. M., Phillips, J., et al. (2019). The novel autophagy inhibitor ROC-325 augments the antileukemic activity of azacitidine. Leukemia 33 (England2019), 2971–2974. doi:10.1038/s41375-019-0529-2
Noman, M. Z., Parpal, S., Van Moer, K., Xiao, M., Yu, Y., Viklund, J., et al. (2020). Inhibition of Vps34 reprograms cold into hot inflamed tumors and improves anti-PD-1/PD-L1 immunotherapy. Sci. Adv. 6 (18), eaax7881. doi:10.1126/sciadv.aax7881
Pasquier, B. (2015). SAR405, a PIK3C3/Vps34 inhibitor that prevents autophagy and synergizes with MTOR inhibition in tumor cells. Autophagy 11 (4), 725–726. doi:10.1080/15548627.2015.1033601
Pérez, V. I., Buffenstein, R., Masamsetti, V., Leonard, S., Salmon, A. B., Mele, J., et al. (2009). Protein stability and resistance to oxidative stress are determinants of longevity in the longest-living rodent, the naked mole-rat. Proc. Natl. Acad. Sci. U. S. A. 106 (9), 3059–3064. doi:10.1073/pnas.0809620106
Petherick, K. J., Conway, O. J., Mpamhanga, C., Osborne, S. A., Kamal, A., Saxty, B., et al. (2015). Pharmacological inhibition of ULK1 kinase blocks mammalian target of rapamycin (mTOR)-dependent autophagy. J. Biol. Chem. 290 (18), 28726–28783. doi:10.1074/jbc.A114.627778
Petiot, A., Ogier-Denis, E., Blommaart, E. F., Meijer, A. J., and Codogno, P. (2000). Distinct classes of phosphatidylinositol 3'-kinases are involved in signaling pathways that control macroautophagy in HT-29 cells. J. Biol. Chem. 275 (2), 992–998. doi:10.1074/jbc.275.2.992
Phillips, J. D., Pooler, D. B., Ness, D. B., Fay, K., Tau, S., Demidenko, E., et al. (2023). Tumour, whole-blood, plasma and tissue concentrations of metformin in lung cancer patients. Br. J. Clin. Pharmacol. 89 (3), 1027–1035. doi:10.1111/bcp.15546
Pietrocola, F., Castoldi, F., Kepp, O., Carmona-Gutierrez, D., Madeo, F., and Kroemer, G. (2019). Spermidine reduces cancer-related mortality in humans. Autophagy 15 (2), 362–365. doi:10.1080/15548627.2018.1539592
Ponpuak, M., Mandell, M. A., Kimura, T., Chauhan, S., Cleyrat, C., and Deretic, V. (2015). Secretory autophagy. Curr. Opin. Cell. Biol. 35, 106–116. doi:10.1016/j.ceb.2015.04.016
Prasher, P., Sharma, M., Singh, S. K., Gulati, M., Chellappan, D. K., Rajput, R., et al. (2023). Spermidine as a promising anticancer agent: recent advances and newer insights on its molecular mechanisms. Front. Chem. 11, 1164477. doi:10.3389/fchem.2023.1164477
Pyo, J. O., Yoo, S. M., Ahn, H. H., Nah, J., Hong, S. H., Kam, T. I., et al. (2013). Overexpression of Atg5 in mice activates autophagy and extends lifespan. Nat. Commun. 4, 2300. doi:10.1038/ncomms3300
Raj, S. D., Fann, D. Y., Wong, E., and Kennedy, B. K. (2021). Natural products as geroprotectors: an autophagy perspective. Med. Res. Rev. 41 (6), 3118–3155. doi:10.1002/med.21815
Rangel, M., Kong, J., Bhatt, V., Khayati, K., and Guo, J. Y. (2022). Autophagy and tumorigenesis. FEBS J. 289 (22), 7177–7198. doi:10.1111/febs.16125
Raz, Y., Guerrero-Ros, I., Maier, A., Slagboom, P. E., Atzmon, G., Barzilai, N., et al. (2017). Activation-Induced autophagy is preserved in CD4+ T-cells in familial longevity. J. Gerontol. A Biol. Sci. Med. Sci. 72 (9), 1201–1206. doi:10.1093/gerona/glx020
Rebecca, V. W., Nicastri, M. C., Fennelly, C., Chude, C. I., Barber-Rotenberg, J. S., Ronghe, A., et al. (2019). PPT1 promotes tumor growth and is the molecular target of chloroquine derivatives in cancer. Cancer Discov. 9 (2), 220–229. doi:10.1158/2159-8290.CD-18-0706
Ronan, B., Flamand, O., Vescovi, L., Dureuil, C., Durand, L., Fassy, F., et al. (2014). A highly potent and selective Vps34 inhibitor alters vesicle trafficking and autophagy. Nat. Chem. Biol. 10 (12), 1013–1019. doi:10.1038/nchembio.1681
Roshani, M., Jafari, A., Loghman, A., Sheida, A. H., Taghavi, T., Tamehri Zadeh, S. S., et al. (2022). Applications of resveratrol in the treatment of gastrointestinal cancer. Biomed. Pharmacother. 153, 113274. doi:10.1016/j.biopha.2022.113274
Sanjuan, M. A., Dillon, C. P., Tait, S. W., Moshiach, S., Dorsey, F., Connell, S., et al. (2007). Toll-like receptor signalling in macrophages links the autophagy pathway to phagocytosis. Nature 450 (7173), 1253–1257. doi:10.1038/nature06421
Schneider, J. L., and Cuervo, A. M. (2014). Autophagy and human disease: emerging themes. Curr. Opin. Genet. Dev. 26, 16–23. doi:10.1016/j.gde.2014.04.003
Schneider, J. L., Suh, Y., and Cuervo, A. M. (2014). Deficient chaperone-mediated autophagy in liver leads to metabolic dysregulation. Cell. Metab. 20 (3), 417–432. doi:10.1016/j.cmet.2014.06.009
Schneider, J. L., Villarroya, J., Diaz-Carretero, A., Patel, B., Urbanska, A. M., Thi, M. M., et al. (2015). Loss of hepatic chaperone-mediated autophagy accelerates proteostasis failure in aging. Aging Cell. 14 (2), 249–264. doi:10.1111/acel.12310
Sciorati, C., Gamberale, R., Monno, A., Citterio, L., Lanzani, C., De Lorenzo, R., et al. (2020). Pharmacological blockade of TNFα prevents sarcopenia and prolongs survival in aging mice. Aging (Albany NY) 12 (23), 23497–23508. doi:10.18632/aging.202200
Semenza, G. L. (2008). Tumor metabolism: cancer cells give and take lactate. J. Clin. Investig. 118 (12), 3835–3837. doi:10.1172/JCI37373
Semenza, G. L. (2010). HIF-1: upstream and downstream of cancer metabolism. Curr. Opin. Genet. Dev. 20 (1), 51–56. doi:10.1016/j.gde.2009.10.009
Shay, J. W., and Wright, W. E. (2000). Hayflick, his limit, and cellular ageing. Nat. Rev. Mol. Cell. Biol. 1 (1), 72–76. doi:10.1038/35036093
Shibata, M., Lu, T., Furuya, T., Degterev, A., Mizushima, N., Yoshimori, T., et al. (2006). Regulation of intracellular accumulation of mutant Huntingtin by Beclin 1. J. Biol. Chem. 281 (20), 14474–14485. doi:10.1074/jbc.M600364200
Song, C., Pan, S., Zhang, J., Li, N., and Geng, Q. (2022). Mitophagy: a novel perspective for insighting into cancer and cancer treatment. Cell. Prolif. 55 (12), e13327. doi:10.1111/cpr.13327
Tabibzadeh, S. (2023). Role of autophagy in aging: the good, the bad, and the ugly. Aging Cell. 22 (1), e13753. doi:10.1111/acel.13753
Tang, D., Kang, R., Livesey, K. M., Cheh, C. W., Farkas, A., Loughran, P., et al. (2010). Endogenous HMGB1 regulates autophagy. J. Cell. Biol. 190 (5), 881–892. doi:10.1083/jcb.200911078
Teng, M. W., Swann, J. B., Koebel, C. M., Schreiber, R. D., and Smyth, M. J. (2008). Immune-mediated dormancy: an equilibrium with cancer. J. Leukoc. Biol. 84 (4), 988–993. doi:10.1189/jlb.1107774
Terman, A. (1995). The effect of age on formation and elimination of autophagic vacuoles in mouse hepatocytes. Gerontology 41 (Suppl. 2), 319–326. doi:10.1159/000213753
Tsakiri, E. N., Iliaki, K. K., Höhn, A., Grimm, S., Papassideri, I. S., Grune, T., et al. (2013). Diet-derived advanced glycation end products or lipofuscin disrupts proteostasis and reduces life span in Drosophila melanogaster. Free Radic. Biol. Med. 65, 1155–1163. doi:10.1016/j.freeradbiomed.2013.08.186
Tuttle, C. S. L., Waaijer, M. E. C., Slee-Valentijn, M. S., Stijnen, T., Westendorp, R., and Maier, A. B. (2020). Cellular senescence and chronological age in various human tissues: a systematic review and meta-analysis. Aging Cell. 19 (2), e13083. doi:10.1111/acel.13083
U.S. Department of Health and Human Services (2024). National Institutes of health NLoM, and national, information CfB. ClinicalTrials.gov. Available at: https://clinicaltrials.gov/2024 (Accessed December, 2011).
Valdor, R., Mocholi, E., Botbol, Y., Guerrero-Ros, I., Chandra, D., Koga, H., et al. (2014). Chaperone-mediated autophagy regulates T cell responses through targeted degradation of negative regulators of T cell activation. Nat. Immunol. 15 (11), 1046–1054. doi:10.1038/ni.3003
Villa-Ruano, N., Anaya-Ruiz, M., Villafaña-Diaz, L., Barron-Villaverde, D., and Perez-Santos, M. (2023). Drug repurposing of mito-atovaquone for cancer treatment. Pharm. Pat. Anal. 12 (4), 143–149. doi:10.4155/ppa-2023-0015
Wang, C., Haas, M., Yeo, S. K., Sebti, S., Fernández, Á. F., Zou, Z., et al. (2022). Enhanced autophagy in F121A/F121A knockin mice counteracts aging-related neural stem cell exhaustion and dysfunction. Autophagy 18 (2), 409–422. doi:10.1080/15548627.2021.1936358
Wu, N., Zheng, W., Zhou, Y., Tian, Y., Tang, M., Feng, X., et al. (2024). Autophagy in aging-related diseases and cancer: principles, regulatory mechanisms and therapeutic potential. Ageing Res. Rev. 100, 102428. doi:10.1016/j.arr.2024.102428
Xin, R., Shen, B., Huang, Z. Y., Liu, J. B., Li, S., Jiang, G. X., et al. (2023). Research progress in elucidating the mechanisms underlying resveratrol action on lung cancer. Curr. Pharm. Biotechnol. 24 (3), 427–437. doi:10.2174/1389201023666220818085945
Xu, G., Jiang, Y., Xiao, Y., Liu, X. D., Yue, F., Li, W., et al. (2016). Fast clearance of lipid droplets through MAP1S-activated autophagy suppresses clear cell renal cell carcinomas and promotes patient survival. Oncotarget 7 (5), 6255–6265. doi:10.18632/oncotarget.6669
Yang, S., Wang, X., Contino, G., Liesa, M., Sahin, E., Ying, H., et al. (2011). Pancreatic cancers require autophagy for tumor growth. Genes. Dev. 25 (7), 717–729. doi:10.1101/gad.2016111
Yang, Z. J., Chee, C. E., Huang, S., and Sinicrope, F. A. (2011). The role of autophagy in cancer: therapeutic implications. Mol. Cancer Ther. 10 (9), 1533–1541. doi:10.1158/1535-7163.MCT-11-0047
Zajda, A., Huttunen, K. M., Sikora, J., Podsiedlik, M., and Markowicz-Piasecka, M. (2020). Is metformin a geroprotector? A peek into the current clinical and experimental data. Mech. Ageing Dev. 191, 111350. doi:10.1016/j.mad.2020.111350
Zhang, C., and Cuervo, A. M. (2008). Restoration of chaperone-mediated autophagy in aging liver improves cellular maintenance and hepatic function. Nat. Med. 14 (9), 959–965. doi:10.1038/nm.1851
Zhang, Q., Xiong, D., Pan, J., Wang, Y., Hardy, M., Kalyanaraman, B., et al. (2022). Chemoprevention of lung cancer with a combination of mitochondria-targeted compounds. Cancers (Basel) 14 (10), 2538. doi:10.3390/cancers14102538
Zhang, Y., Zhou, F., Guan, J., Zhou, L., and Chen, B. (2023). Action mechanism of metformin and its application in hematological malignancy treatments: a review. Biomolecules 13 (2), 250. doi:10.3390/biom13020250
Zimmermann, A., Hofer, S. J., and Madeo, F. (2023). Molecular targets of spermidine: implications for cancer suppression. Cell. Stress 7, 50–58. doi:10.15698/cst2023.07.281
Keywords: aging, cancer, autopaghy, proteostasis, therapeutics
Citation: Zapatería B and Arias E (2025) Aging, cancer, and autophagy: connections and therapeutic perspectives. Front. Mol. Biosci. 11:1516789. doi: 10.3389/fmolb.2024.1516789
Received: 24 October 2024; Accepted: 24 December 2024;
Published: 28 January 2025.
Edited by:
Dechao Feng, University College London, United KingdomReviewed by:
Ruheena Javed, University of New Mexico, United StatesSerena Castelli, University of Rome Tor Vergata, Italy
Copyright © 2025 Zapatería and Arias. This is an open-access article distributed under the terms of the Creative Commons Attribution License (CC BY). The use, distribution or reproduction in other forums is permitted, provided the original author(s) and the copyright owner(s) are credited and that the original publication in this journal is cited, in accordance with accepted academic practice. No use, distribution or reproduction is permitted which does not comply with these terms.
*Correspondence: Esperanza Arias, ZXNwZXJhbnphLmFyaWFzLXBlcmV6QGVpbnN0ZWlubWVkLmVkdQ==