- 1College of Chemistry, Zhengzhou University, Zhengzhou, Henan, China
- 2Department of tobacco flavor, Zhengzhou Tobacco Research Institute of CNTC, Zhengzhou, Henan, China
- 3Food Laboratory of Zhongyuan, Flavour Science Research Center of Zhengzhou University, Zhengzhou, Henan, China
- 4Medical Laboratory, General Hospital of Ningxia Medical University, Yinchuan, Ningxia, China
Olfactory receptors, classified as G-protein coupled receptors (GPCRs), have been a subject of scientific inquiry since the early 1950s. Historically, investigations into the sensory mechanisms of olfactory receptors were often confined to behavioral characteristics in model organisms or the expression of related proteins and genes. However, with the development of cryo-electron microscopy techniques, it has gradually become possible to decipher the specific structures of olfactory receptors in insects and humans. This has provided new insights into the binding mechanisms between odor molecules and olfactory receptors. Furthermore, due to the rapid advancements in related fields such as computer simulations, the prediction and exploration of odor molecule binding to olfactory receptors have been progressively achieved through molecular dynamics simulations. Through this comprehensive review, we aim to provide a thorough analysis of research related to the binding mechanisms between odor molecules and olfactory receptors from the perspectives of structural biology and molecular dynamics simulations. Finally, we will provide an outlook on the future of research in the field of olfactory receptor sensory mechanisms.
1 Introduction
Olfactory receptors are classified as a subset of G-protein coupled receptors (GPCRs), which are integral to the transduction of olfactory signals, the earliest accessible literature can be traced back to the 1950s of the previous century (Sviridenko, 1951; Skouby and Zilstorff-Pedersen, 1954). Early attention was devoted to the potential presence of olfactory receptors within the nasal cavities of both animals and humans, leading to a series of related investigations. Linda Buck and Richard Axel first cloned and identified the olfactory receptor GPCR gene family from rats, discovering a large gene family comprising approximately 1,000 distinct genes, which give rise to a corresponding number of olfactory receptor types. Their work illuminated how animals or humans perceive a wide array of odors, suggesting that the mechanism involves the initial binding of odorants to odor receptors located within the olfactory receptor neurons of the nasal epithelium (Buck and Axel, 1991). Following activation by odor molecules, these receptors generate electrical signals within the olfactory receptor neurons. These signals are subsequently transmitted to discrete regions within the olfactory bulb of the brain, and from there, relayed to other brain regions for further processing, ultimately leading to the perception of these odors by animals or humans. The recognition of this study with the Nobel Prize in 2004 instigated a substantial redirection of research efforts towards elucidating the functional mechanisms of olfactory receptors (Firestein, 2005). For example, in 2010, researchers directed their attention to two proteins produced within insects, or83 and or67d, which play pivotal roles in guiding mosquito odor recognition and aggressive behaviors (Carey Et Al., 2010; Wang and Anderson, 2009); In 2012, scientists at the Rowland Institute at Harvard University uncovered how the asymmetry in neurotransmitter release aids fruit flies in rapid odor recognition. Additionally, subtle differences in the excitation timing and rate of olfactory receptor neurons (ORNs) were found to variations in olfactory behavior (Gaudry et al., 2012); In 2019, researchers at Columbia University in the United States, utilizing in situ Hi-C technology and focusing on mice as their study subjects, revealed the extensive formation of specific trans-interactions within the three-dimensional genomic space of each olfactory neuron, these interactions serve to regulate the expression of various OR genes, thereby giving rise to the diverse combinations of olfactory receptors necessary for odor recognition (Monahan et al., 2019).
The advent of cryo-electron microscopy technology dates back to 1970s (Taylor and Glaeser, 1974), culminating in a significant milestone in late 1981 when scientists Alasdair McDowall and Jacques Dubochet reported the successful outcomes of their cryo-electron microscopy observations and, subsequently, the mastery of cryo-preservation techniques (Dubochet et al., 1981). With the onset of the 21st century, marked by remarkable advancements in computer technology and hardware capabilities, the resolution of cryo-electron microscopy has experienced remarkable enhancements. Notably, in 2013, researchers at the University of California, San Francisco, achieved near-atomic-level resolution in the visualization of membrane protein structures, heralding the commencement of a new era in protein structural analysis through the application of cryo-electron microscopy technology (Cheng, 2018; Liao et al., 2013). These pioneering strides in the field culminated in the joint recognition of Jacques Dubochet, Joachim Frank, and Richard Henderson with the Nobel Prize in Chemistry in 2017 for their substantial contributions to the development of cryo-electron microscopy technology (Dubochet, 2018). The swift progress in cryo-electron microscopy technology has concurrently ushered in novel avenues for the investigation of human olfactory receptors. In the year 2023, an unprecedented revelation emerged as the three-dimensional architecture of odorant molecules, responsible for activating olfactory receptors, was unveiled at the molecular scale (Billesbolle et al., 2023). This momentous achievement stands as a pivotal milestone in unraveling the mysteries of the sense of smell. The pioneering study illuminated the intricate binding mechanisms between odorant molecules and olfactory receptors, thereby providing unprecedented insights into the human perception of odors.
In this study, the activation mechanisms of olfactory receptors were delineated by integrating cryo-electron microscopy with molecular dynamics simulations. Olfactory receptor proteins exhibit the canonical structure of G protein-coupled receptors (GPCRs), featuring seven transmembrane domains (7TM) and encompassing three Intracellular loops (ICL) along with three Extracellular loops (ECL). Researchers have discerned that the structural alterations induced in Extracellular Loop 3 (ECL3) by the fatty acid propionate, functioning as an odorant molecule, have the capacity to trigger the activation of the human olfactory receptor OR51E2 (Billesbolle et al., 2023). This underscores the profound significance of molecular dynamics simulations as an innovative tool for unraveling the intricacies governing the interaction between small molecular ligands and receptor proteins. Molecular dynamics simulation stands as a potent and indispensable tool for delving into the intricate dynamics exhibited by biomolecules, including proteins and DNA. This technique empowers researchers to meticulously simulate the three-dimensional motions of biomolecules, facilitating the dissection of the fundamental mechanisms governing their physiological functions and the intricate interactions with potential ligands (Hollingsworth and Dror, 2018; Rydzewski and Nowak, 2017). While contemporary cryo-electron microscopy technology may still encounter limitations in deciphering the structural details of all olfactory receptor proteins, molecular dynamics simulation assumes a pivotal role in elucidating the intricate binding kinetics of odorant molecules to receptors. The combination of AlphaFold2’s 3D protein structure prediction with molecular dynamics simulations has significantly broadened their applications. This has propelled progress in deciphering molecular mechanisms, protein design, and drug development (Jumper et al., 2021a; Tunyasuvunakool et al., 2021).
The undeniable truth lies in the swift strides made within the domains of cryo-electron microscopy and computational technologies, profoundly catalyzing human endeavors in the realm of olfactory receptor research. Experimental inquiries have transcended the conventional boundaries of gene or protein functions, venturing into the intricacies of molecular mechanisms at a more microscopic scale. Table 1 and Figure 1 chronicle the pivotal research milestones pertinent to olfactory receptor investigations since 2004. This paradigm shift has transformed biological inquiry into research based on structure mechanisms. This review endeavors to accentuate the deorphaning process of olfactory receptors, along with the noteworthy advancements in elucidating olfactory receptor structures and employing molecular dynamics simulations within this field. Our aspiration is that this review will foster a deeper comprehension and foresight regarding the prospective trends in olfactory receptor research.
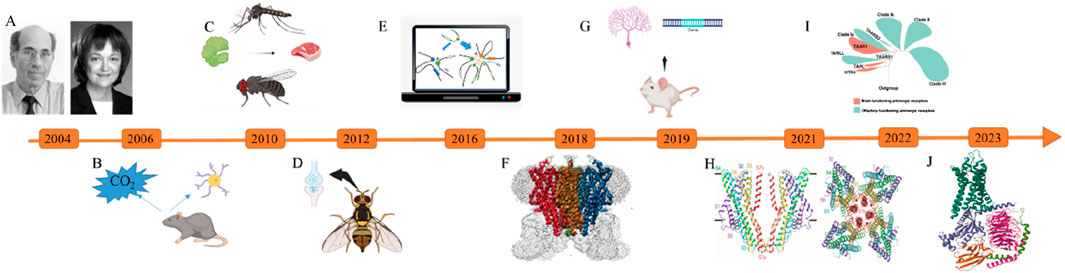
Figure 1. Significant research breakthroughs in olfactory receptors from 2004 to 2024. (A) The Nobel Prize in Physiology or Medicine (Buck and Axel, 1991); (B) Mice have special olfactory neurons that can sense carbon dioxide in the air (Hu et al., 2007); (C) Protein-Mediated Odor Recognition in Mosquitoes and Drosophila melanogaster (Wang and Anderson, 2009); (D) Asymmetric Neurotransmitter Release Facilitates Rapid Odor Recognition in Fruit Flies (Gaudry et al., 2012); (E) Through computational simulations, the study elucidates how olfactory neurons achieve monoallelic expression of olfactory receptors while maintaining diversity in their expression (Tian et al., 2016); (F) Structural Analysis of the Insect Olfactory Receptor Orco Using Cryo-Electron Microscopy (Butterwick et al., 2018); (G) The LDB1 Protein Governs the Expression of Olfactory Genes (Monahan et al., 2019); (H) Structural Analysis of the OR5 Receptor and Odor Molecule Binding Mechanisms in Machilis hrabei (Del Mármol et al., 2021); (I) The study elucidates the evolutionary origins, history, and co-evolutionary process of ligand recognition for a family of olfactory receptors known as trace amine-associated receptors (Guo et al., 2022); (J) The Inaugural Precision Three-Dimensional Structural Mapping of the Human Odor Receptor (OR51E2) Has Been Accomplished (Billesbolle et al., 2023).
2 Olfactory receptor deorphanization and current research status
2.1 The importance of olfactory receptor deorphaning
Within the realm of scientific exploration, olfactory receptors constitute a prominent segment of the G-protein-coupled receptor (GPCR) superfamily, ranking among the most extensive protein families in the mammalian world (Peterlin et al., 2014). These receptors shoulder the responsibility of detecting and discerning a myriad of odorant molecules. Nevertheless, the current landscape reveals that more than 80% of olfactory receptors remain enigmatic orphan receptors while their ligands shrouded in mystery (March et al., 2015).
The pursuit of natural ligands for orphan olfactory receptors stands as a substantial domain of investigation. On one front, deorphaning empowers us with insights into the mechanisms through which the olfactory system deciphers and processes specific aromas (Peterlin et al., 2014; Reisert and Restrepo, 2009). Through the alignment of receptors with their corresponding ligands, we gain profound understanding of the molecular underpinnings and binding dynamics governing odor perception, ultimately unveiling the enigma of human sensory mechanisms. On another front, the deorphaning of olfactory receptors bears significant practical applications. For instance, within the realms of fragrance and food industries, a meticulous grasp of interactions among odor receptors can engender the creation of novel scents, particularly serving as substitutes for food additives in the evolving landscape of artificial food production (Zeng et al., 2023).
A compelling dataset underscores that in 2015, among the nearly 400 complete human olfactory receptors (ORs), only 49 ligands have been published (Mainland et al., 2015). Nevertheless, during the same year, an alternative dataset revealed that the count of human olfactory receptors that had been successfully deorphaned was also a modest 57 (March et al., 2015). At present, based on a comprehensive review of the available literature, the cumulative count of orphan olfactory receptors is estimated to exceed eighty (Table 1).
2.2 The research status of olfactory receptors and odor molecule ligands
Within the scientific literature, a significant corpus of research has been devoted to the pursuit of ligands for odorant molecules that trigger the activation of their respective olfactory receptors. A noteworthy development emerged as early as 2015 when a literature introduced a high-throughput screening technique tailored for olfactory receptors, effectively identifying agonists for 27 distinct odor receptors (Mainland et al., 2015). Notably, during that period, 18 of these olfactory receptors remained orphan receptors.
In 2016, Dietmar Krautwurst and his research team made a significant breakthrough by uncovering 14 novel crucial food odorant agonists for OR1A1 and 18 for OR2W1, respectively (Geithe et al., 2017). This study use dual-screening strategy, entailed the comprehensive test of a diverse spectrum of food odorant molecules with individual ORs. Subsequently, a single odor compound was tested the complete human OR, leading to the effective revelation of the intricate olfactory attributes associated with odorant molecules.
Moreover, recent years have witnessed a substantial body of research dedicated to the deorphaning of individual olfactory receptors. Pyrazine compounds, recognized as odorant molecules contributing to food enhancement, have undergone thorough investigation, leading to the revelation that OR5K1 stands as the exclusive receptor responsive to pyrazine compounds. Interestingly, homologous receptors of OR5K1 in mice have exhibited analogous activation functions (Marcinek et al., 2021). In parallel, furaneol and sotolone, essential flavoring furanones, have also undergone scrutiny. The outcomes have illuminated their distinct abilities to selectively activate the human olfactory receptors OR5M3 and OR8D1, respectively. Notably, both odorant molecules exhibited conspicuous concentration-dependent activation profiles throughout the investigation (Haag et al., 2021). Another illustrative instance pertains to 4-methylphenol, distinguished by its odor reminiscent of a stable’s fecal notes. It was discerned that OR9Q2 manifested an elevated response to this specific odorant molecule, unequivocally establishing OR9Q2 as the primary sensor for a spectrum of aromas, encompassing food odors, foul scents, and the chemical pheromone 4-methylpheno (Haag et al., 2023).
In the natural world, odorant molecules are frequently encountered in complex mixtures rather than in isolation. Consequently, the interactions among various odorant molecules remain largely uncharted. These interactions can yield diverse outcomes, ranging from the inhibition or potentiation of specific odor perceptions to the emergence of entirely novel and unpredictable olfactory sensations (Poupon et al., 2018; Thomas-Danguin et al., 2014). For example, the response of all odorant receptors to a moderate concentration of the malodorous compound indole, reminiscent of fecal odor, is effectively suppressed by the presence of a high concentration of the floral odorant α-ionone (Breheny et al., 2020). When present in mixtures with a high concentration of whisky lactone, olfactory receptors responsible for detecting the fruity aroma of isoamyl acetate undergo inhibition (Chaput et al., 2012). Additionally, it has been observed that undecanal can effectively diminish the sensitivity of olfactory receptors to bourgeonal (Brodin et al., 2009; De march et al., 2020).
Within well-known complex odor amalgamations, cigarette smoke stands as a prevalent and intricate olfactory ensemble, consisting of more than 400 distinct odorant molecules (Cortese et al., 2015). Experimental observations in mice have unveiled that the scent of cigarette smoke activates a remarkable response from 144 olfactory receptors (ORs) and 3 trace amine-associated receptors (TAARs). Moreover, sensory evaluation studies, have underscored the pivotal role of 1-pentanethiol as a significant constituent contributing to the distinctive aroma profile of synthetic cigarette smoke (Mcclintock et al., 2020).
2.3 The limitations of research on olfactory receptors
While there has been a surge in research on olfactory receptors and their deorphaning in recent years, the exploration of binding mechanisms between odorant molecules and olfactory receptors has remained relatively limited. This research gap becomes particularly evident when considering molecular ligands that exhibit stereoisomerism, such as chiral isomers or functional group isomers. Fundamental questions about the varying activation abilities of these isomers, the migration of optimal binding sites, and the binding conformations between odorant molecule ligands and olfactory receptors have largely remained unaddressed (Behrens et al., 2018). Additionally, comprehending the intermolecular forces at play between functional groups of ligands and amino acid residues in olfactory receptors has posed significant challenges.
Furthermore, olfactory receptors are dynamic proteins, undergoing conformational changes between active and inactive states (García-Nafría and Tate, 2021). Investigating these dynamic changes at the microscale level presents formidable challenges in deorphaning studies.
In light of these complexities, the growing interest in olfactory receptors underscores the imperative need for further research into the intricate details of odorant molecule and olfactory receptor interactions, particularly when confronted with structural isomerism and conformational dynamics.
3 Olfactory receptor and structural biology
3.1 Olfactory receptor and cryo-electron microscopy technology
Structural biology involves elucidating the three-dimensional arrangements of biological macromolecules at the atomic level and employing these structures to decipher the chemical underpinnings of their biological functions (Moore, 2017). In the early 20th century, X-ray crystallography gained widespread popularity as a technique for studying the structures of proteins and nucleic acids (Gaubert et al., 2020). By the mid-20th century, nuclear magnetic resonance (NMR) and protein crystallography emerged as an equally potent tool for elucidating the structures of biological molecules (Koehler leman and Künze, 2023).
Recent years, the advancement of cryo-electron microscopy technology has brought about a revolutionary change in the field of structural biology, offering scientists a pioneering instrument. This technique empowers researchers to scrutinize protein structures, including those of olfactory receptors, at remarkably high resolutions (Yip et al., 2020; Danev et al., 2019). With cryo-electron microscopy, investigators can unveil the intricate three-dimensional architecture of olfactory receptors, thereby facilitating a more profound comprehension of their mechanisms for recognizing odorant molecules.
In 2018, scientists from Rockefeller University’s Ruta Lab in the United States achieved a groundbreaking milestone by unveiling the single-particle cryo-electron microscopy structure of the odorant co-receptor Orco in a parasitic wasp, boasting an impressive resolution of approximately 3.5 Å (Butterwick et al., 2018). Insect odorant receptors diverge from their mammalian counterparts in their classification as ligand-gated ion channels rather than G-protein-coupled receptors (GPCRs). These receptors assemble into heteromeric ion channel complexes, consisting of ORs and the exceptionally conserved co-receptor, Orco. This ion channel functions akin to a conduit, permitting the flow of charged particles solely when the receptor encounters its intended odorant molecule, thereby initiating the activation of olfactory sensory cells.
In 2021, a separate research endeavor conducted by the Ruta Lab brought to light the cryo-electron microscopy structure of an odorant receptor found in Machilis hrabei, a species of stonefly. These terrestrial insects, whose genome has been recently sequenced, possess only five odorant receptors. The research team delved deeper into the examination of the binding sites and mechanisms of two chemically distinct molecules with the OR5 receptor. Furthermore, they conducted a comparative analysis of the structural alterations in OR5 induced by the binding of various odorant molecules. The study demonstrates that upon binding with eugenol, the receptor’s structure exhibits pore dilation, providing a channel for ion flow. Additionally, it was found that amino acids connected to the “pocket” do not form strong, selective chemical bonds with the odorant, but rather form weak bonds (Del mármol et al., 2021).
As of March 2023, an article published in Nature has, for the very first time, elucidated the enigmas shrouding the structure of human olfactory receptors. This study provides intricate insights into an olfactory receptor denoted as OR51E2, elucidating its ability to “discern” the aroma of cheese by engaging in precise molecular interactions, thus initiating receptor activation (Billesbolle et al., 2023).
3.2 Human olfactory receptor structures and the mechanisms olfactory sensation
We already know, human odorant receptors are G-protein-coupled receptors (GPCRs) (Gaillard et al., 2004). A common feature of these receptors is that they all possess seven transmembrane α-helices in their three-dimensional structure (Figure 2).
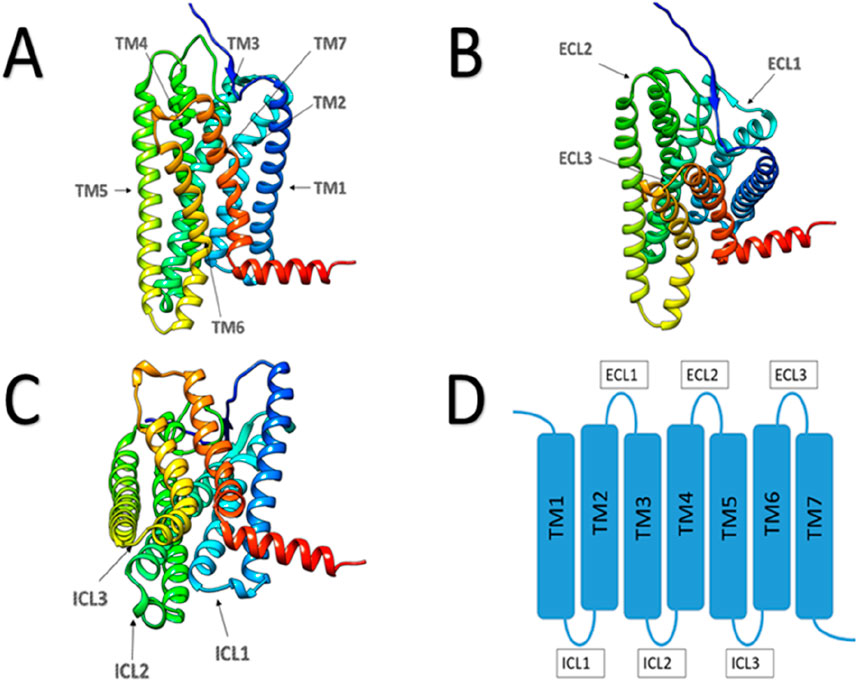
Figure 2. Schematic diagram of GPCR protein structure. (A) Main view of GPCR protein, with arrows indicating the seven transmembrane regions. (B) Top view of GPCR protein, with arrows pointing to three extracellular loops. (C) Bottom view of GPCR protein, with arrows pointing to three intracellular loops. (D) Plan view of GPCR protein structure.
Structural determination of human odorant receptor proteins poses a significant challenge for several reasons. Firstly, the sheer diversity of odorant receptors means that each possesses its distinct structural traits; secondly, the relatively low expression levels of these receptors in sensory cells introduce technical obstacles when trying to elucidate their protein structures through cryo-electron microscopy techniques; moreover, odorant receptors exhibit dynamic properties, with their conformations capable of altering upon binding different odorant molecules (Ikegami et al., 2020; Saito et al., 2004; Cook et al., 2009; Katada et al., 2004). This intricacy adds an additional layer of complexity to structural analysis under various conditions.
To tackle these formidable challenges, researchers opted to investigate the human olfactory receptor OR51E2 for specific reasons. Their choice was motivated by the receptor’s expression not only in olfactory nerve cells but also in non-olfactory organs such as the prostate. This dual expression pattern suggested that it would be more feasible to express the receptor in heterologous systems, making it easier to produce sufficient quantities of the protein. Previous studies had already demonstrated that this receptor could bind to and elicit responses from water-soluble short-chain fatty acid odorant molecules, particularly propionic acid (Saito et al., 2009). Through these strategic decisions, researchers adeptly navigated the challenges presented by the typically low expression levels of most odorant receptors, the low solubility of many volatile odorants, and the inherent instability of purified olfactory receptor proteins.
Structural examination of OR51E2 unveiled a fascinating mechanism: the receptor protein effectively entraps the odorant molecule propionic acid within a compact, enclosed binding pocket. Within this minute enclosure, propionic acid forms two types of interactions with OR51E2: polar interactions involving hydrogen and ionic bonds, as well as non-specific hydrophobic interactions (Billesbolle et al., 2023). Consequently, the way OR51E2 binds to odorant molecules differs significantly from that of insect odorant-gated ion channels, implying a heightened level of selectivity.
This discovery also elucidates why OR51E2 exclusively binds to short-chain fatty acids (Pluznick et al., 2013; Pronin and Slepak, 2021). The binding pocket’s limited volume, measuring 31 Å3, accommodates short-chain fatty acids like acetic and propionic acid, while effectively preventing the binding of longer fatty acid chains. Mutation of phenylalanine and leucine residues adjacent to the fatty acid propionate to the smaller alanine residue enlarges the binding pocket, facilitating the activation of OR51E2 by long-chain fatty acids. Thus, this revelation underscores that the volume of the binding pocket plays a pivotal role in determining the receptor’s selectivity for odorant molecules. This study has undeniably offered groundbreaking insights into the atomic-level structure and mechanisms governing OR51E2 function.
3.3 Limitations of olfactory receptors in the structural biology
Certainly, cryo-electron microscopy technology has ventured into the microscopic realm of olfactory receptors and sensory mechanisms, reshaping traditional biological inquiries into chemical explorations. Nevertheless, the comprehensive structural characterization of numerous human olfactory receptor proteins remains an arduous undertaking today. This challenge stems from multiple factors, including the limited expression levels of olfactory receptor proteins, their structural heterogeneity, and their dynamic conformational alterations. Furthermore, the intricacies of the interactions between odorant molecules and olfactory receptors should not be underestimated, encompassing hydrophobic interactions, hydrogen bonds, ionic bonds, and more. Consequently, it is essential to employ a diverse array of approaches to scrutinize these multifaceted binding mechanisms.
4 Olfactory receptor and molecular dynamic simulation
4.1 Molecular dynamic simulation (MD) and AlphaFold
Over the past few decades, the determination of protein structures has predominantly relied on various experimental techniques, including early methodologies such as X-ray crystallography and nuclear magnetic resonance (NMR) (Faraggi et al., 2017), as well as more recent innovations like cryo-electron microscopy (Yip et al., 2020). Nevertheless, these approaches are often laborious and time-intensive, involving extensive trial and error. Unraveling the structure of a single protein can span several years and necessitates the use of specialized equipment that comes with a multi-million-dollar price tag.
In 2020, the emergence of the AlphaFold2 (AF2) program marked a pivotal moment, demonstrating remarkable accuracy in predicting the three-dimensional structures of a substantial number of proteins (Bryant et al., 2022). Furthermore, in July 2021, the AlphaFold2 system unveiled a database containing protein structures it had predicted (https://alphafold.ebi.ac.uk/) (Jones and Thornton, 2022). On 15 May 2024, the Google DeepMind team in collaboration with Isomorphic Labs reported a groundbreaking model, AlphaFold 3 (Abramson et al., 2024), which is capable of predicting the complex structures composed of DNA, small molecules, ions, and proteins, as well as forecasting the structures and interactions of all biomolecules. The advent of AlphaFold 3 marks a significant leap forward in the field of structural biology, with profound implications for understanding the molecular basis of life. It is foreseen that AI-driven protein structure prediction will continue to achieve rapid breakthroughs over the next 2–5 years, holding significant implications for the field of drug discovery in the foreseeable future.
In the realm of computational chemistry and biophysics, Molecular Dynamics Simulation (MD) stands as a ubiquitous computational tool revered for its prowess in probing the intricate dynamics of atoms and molecules, tracing their evolution over time (Hildebrand et al., 2019). MD simulations find widespread applications across various domains, encompassing biophysics (Collier et al., 2020) (for the study of the structure and function of proteins and biomolecules), materials science (Xie et al., 2022) (for the exploration of material properties and phase transitions), and chemistry (Park, 2016) (for the examination of chemical reaction kinetics), among others. Equally paramount is MD’s role in the exploration of olfaction mechanisms, where it assumes a central position in unraveling the nuanced interplay between olfactory receptors and the molecules that tantalize our senses. Moreover, it is noteworthy that an intimate nexus exists between MD and AlphaFold. Prior to the advent of AF2, researchers predominantly relied upon homology modeling to construct the three-dimensional structures of olfactory receptor proteins, complemented by virtual screening techniques to unearth ligands that would unlock the mysteries of these receptors (De march et al., 2018; Cong et al., 2022). However, it has come to light in comparative studies that there exist disparities between the olfactory receptor protein structures derived from homology modeling and those obtained through AF2. For example, OR5K1, a major difference between HM and AF2 models is in the Extracellular Loop 2 (ECL2) folding. The ECL2 predicted by AF2 seems unique and was found to be rather stable in MD simulations (Nicoli et al., 2023).
Therefore, the introduction of AlphaFold has greatly enhanced the precision of predicting protein structures, even in scenarios where there is no apparent homologous or low homologous protein available. This feature stands out as superior to the homology modeling approach that relies on known protein structures as templates.
4.2 MD and olfactory mechanisms
MD can be employed in various aspects of olfactory receptor mechanism studies. For instance, MD can replicate the structural dynamics of olfactory receptors, encompassing transitions between different conformations. This aids in comprehending the structural characteristics of receptors in different states, such as active and inactive states. Additionally, it can elucidate the binding modes and affinities between olfactory receptors and odorant molecules (ligands), shedding light on how receptors identify and interact with different odor molecules. Furthermore, MD can simulate the activation process of olfactory receptors, including their interaction with G proteins and the intricate details of signal transduction mechanisms. This contributes to revealing how receptors transduce external signals into intracellular biological responses. Lastly, by leveraging the known features of ligands and olfactory receptors, it becomes possible to predict novel antagonists and agonists.
GPCRs serve as the molecular conduits for transmitting chemical signals, orchestrating an intricate ballet that transitions between active and inactive states upon ligand binding, thereby bridging the chasm from extracellular to intracellular domains (Weis and Kobilka, 2018; Alhadeff et al., 2018). Yet, it is notable that AF3, in its current form, falls short by primarily predicting a solitary state and exhibiting a propensity to favor either active or inactive conformations contingent upon the specific GPCR class under scrutiny (Jumper et al., 2021b; Kinch et al., 2021). MD empowers researchers to transcend the constraints of AF3’s singular state predictions and venture into the modeling of both active and inactive states. In doing so, MD gracefully captures the intricate and pivotal structural transformations that transpire within receptors during the delicate interplay of activation and deactivation (Heo and Feig, 2022).
The investigation into how odor molecules engage with olfactory receptors stands as a notable focal point within the domain of MD research. The scientific literature has delved into the mechanisms governing the interactions between 18 caramel-like odorants and receptors, unveiling a distinct preference for odor molecules to establish bonds with the transmembrane regions TM-3, TM-5, and TM-6 of olfactory receptors (Katada et al., 2005). Through meticulous analysis and computational simulations of caramel-like odors, it has come to light that hydrogen bonding and π-π stacking assume pivotal roles in conferring stability upon these aromatic compounds. Incorporating the paradigm of molecular field-based similarity analysis has yielded two noteworthy ligands: 4-hydroxy-5-methylfuran-3(2H)-one and methylglyoxal. These compounds exhibit a pronounced affinity for binding to receptors OR1G1 and OR52H1, respectively, thereby eliciting sensory perceptions akin to the enticing aroma of caramel (Zeng et al., 2023).
In our previous discussion, we highlighted that olfactory receptors are part of the G protein-coupled receptor family, characterized by their transmembrane structure comprising seven α-helices. In addition to these transmembrane regions, olfactory receptors feature three extracellular loops (ECLs) and three intracellular loops (ICLs) (Venkatakrishnan et al., 2013). A specific study has illuminated the pivotal role played by ECL2 in shaping and regulating the volume of the odorant-binding pocket. ECL2 also maintains the pocket’s hydrophobic properties and serves as a gatekeeper for odorant binding (Yu et al., 2022). This underscores the paramount significance of Olfactory Receptor ECL2 in influencing both the diversity and specificity of olfactory receptor responses. In the investigation of OR51E2, conformational alterations within ECL3 play an equally pivotal role in the activation of OR51E2. The authors hypothesize that ECL3 in olfactory receptors plays a role in stabilizing odorants, which is conducive to the further activation of olfactory receptors by the odorants. This stabilization is essential for the diverse activation of olfactory receptors necessary for odor recognition (Billesbolle et al., 2023) (Figure 3).
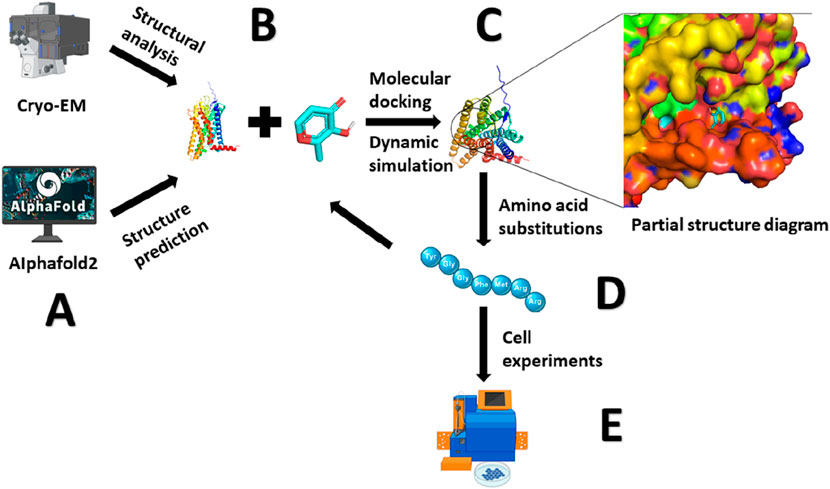
Figure 3. Methods for Studying the Olfactory Receptor - Odorant Binding Mechanism. (A) Resolving protein structures through cryo-electron microscopy (Cryo-EM) or predicting protein conformations using AlphaFold2. (B) Using molecular docking and molecular dynamics simulations to bind odorants with olfactory receptors. (C) Analyzing the interactions forces between protein and ligand. (D) Identifying crucial amino acid residues, inducing mutations, molecular docking and molecular dynamics simulations, and further analyzing the binding mechanism. (E) Cellular experiments are conducted to detect the activation of olfactory receptors, validating the proposed mechanism.
To aid in deorphanization of ORs, Jérôme Golebiowski and his team have developed a Protein Chemistry Metric (PCM) model based on OR sequence similarity and the physicochemical characteristics of ligands. This model employs supervised machine learning to predict odor responses by ORs. Starting from a dataset of OR-odorant pairs and considering the surrounding 60 residues of the binding pocket, this model forecasts changes in OR responses to odors. Remarkably, it accomplishes these predictions with less than 20% of the residue sequence. The model achieves an impressive hit rate of 58%, uncovering 64 novel odorant-OR pairs (Cong et al., 2022). Another study have revealed that, through the utilization of molecular docking and virtual screening techniques, novel antagonists or agonists for mOR256-3 have been successfully identified. Remarkably, these findings have been substantiated by cell-based assays, demonstrating an impressive 70% success rate (Yu et al., 2022).
4.3 Prospects of olfactory receptors in the field of MD
While computational simulation techniques have undeniably facilitated the investigation of olfactory mechanisms, the exploration of sensory processes associated with olfactory receptors is a relatively nascent field, spanning just over two decades (Coppola, 2022). Numerous pressing inquiries beckon researchers across the globe to delve deeper into this intriguing domain.
To begin, it is crucial to acknowledge that receptor protein activation constitutes a dynamic journey. Hence, the thorough examination of structural dynamics within olfactory receptors, encompassing the transitions between diverse conformations, assumes paramount significance. This endeavor serves as a foundational pillar for achieving a more comprehensive comprehension of their functionality and activation mechanisms. Furthermore, a more exhaustive exploration into the intricate interplay between olfactory receptors and odor molecules is imperative. This includes a meticulous scrutiny of binding modes and affinities, for it is within these specifics that the enigma of odor recognition truly lies. Lastly, leveraging the power of MD simulations to anticipate interactions between olfactory receptors and novel compounds stands as a linchpin. This pursuit holds immense potential in unearthing new pharmaceutical agents or aromatic compounds, thus advancing the frontiers of the pharmaceutical and food industries.
In summation, while MD have eased the path of exploration, the realm of olfactory receptor-based sensory mechanisms remains in its infancy. It is a realm ripe with myriad questions, awaiting the diligent investigations of researchers worldwide.
5 Summary and outlook
In conclusion, the research prospects pertaining to olfactory receptors are replete with promise. This article has provided an overview of recent investigations into the deorphanization of olfactory receptors, delved into the advancements within the field of structural biology, and explored pertinent findings from the domain of molecular dynamics. Nevertheless, the overarching objective within each of these domains remains the clarification of the binding mechanism governing the interaction between odor molecules and olfactory receptors.
Moreover, as cryo-electron microscopy technology continues to advance, it is likely to surmount challenges such as the low expression and instability of olfactory receptor proteins. This opens the door to a future where the structures of numerous human olfactory receptor proteins will be systematically elucidated. The amalgamation of these structural revelations with MD will undoubtedly propel our comprehension of the mechanisms underpinning olfactory perception. This, in turn, holds the promise of catalyzing fresh innovations and opportunities across an array of domains, encompassing pharmaceuticals, food science, and the fragrance industry.
Author contributions statement
JW: Conceptualization, Data curation, Formal Analysis, Methodology, Software, Validation, Visualization, Writing–original draft, Writing–review and editing. QZ: Conceptualization, Data curation, Methodology, Software, Validation, Visualization, Writing–original draft. WF: Conceptualization, Methodology, Writing–original draft. QS: Data curation, Methodology, Writing–original draft. JM: Data curation, Software, Writing–original draft. JX: Investigation, Supervision, Writing–review and editing. GC: Conceptualization, Methodology, Supervision, Writing–review and editing. CZ: Conceptualization, Resources, Supervision, Writing–review and editing.
Funding
The author(s) declare that no financial support was received for the research, authorship, and/or publication of this article.
Conflict of interest
The authors declare that the research was conducted in the absence of any commercial or financial relationships that could be construed as a potential conflict of interest.
Publisher’s note
All claims expressed in this article are solely those of the authors and do not necessarily represent those of their affiliated organizations, or those of the publisher, the editors and the reviewers. Any product that may be evaluated in this article, or claim that may be made by its manufacturer, is not guaranteed or endorsed by the publisher.
References
Abramson, J., Adler, J., Dunger, J., Evans, R., Green, T., Pritzel, A., et al. (2024). Accurate structure prediction of biomolecular interactions with AlphaFold 3. Nature 630 (8016), 493–500. doi:10.1038/s41586-024-07487-w
Alhadeff, R., Vorobyov, I., Yoon, H. W., and Warshel, A. (2018). Exploring the free-energy landscape of GPCR activation. Proc. Natl. Acad. Sci. 115 (41), 10327–10332. doi:10.1073/pnas.1810316115
Behrens, M., Briand, L., De March, C. A., Matsunami, H., Yamashita, A., Meyerhof, W., et al. (2018). Structure–function relationships of olfactory and taste receptors. Chem. senses 43 (2), 81–87. doi:10.1093/chemse/bjx083
Ben Khemis, I., Aouaini, F., Ben, HADJ HASSINE S., and Ben Lamine, A. (2022). Theoretical study of the olfactory perception of floral odorant on OR10J5 and Olfr16 using the grand canonical ensemble in statistical physics approach. Int. J. Biol. Macromol. 223 (Pt B), 1667–1673. doi:10.1016/j.ijbiomac.2022.10.201
Ben Khemis, I., Aouaini, F., Smati, H., Zouidi, F., and Ben Lamine, A. (2023b). Advanced investigation of the olfactory perception of semiochemical TMT on OR5K1 and Olfr175 by statistical physics approach. Int. J. Biol. Macromol. 235, 123824. doi:10.1016/j.ijbiomac.2023.123824
Ben Khemis, I., and Ben Lamine, A. (2021). Physico-chemical investigations of human olfactory receptors OR10G4 and OR2B11 activated by vanillin, ethyl vanillin, coumarin and quinoline molecules using statistical physics method. Int. J. Biol. Macromol. 193, 915–922. doi:10.1016/j.ijbiomac.2021.10.155
Ben Khemis, I., Noureddine, O., Smati, H., Aouaini, F., Ben Hadj Hassine, S., and Ben Lamine, A. (2023a). Advanced investigation of a putative adsorption process of nine non key food odorants (non-KFOs) on the broadly tuned human olfactory receptor OR2W1: statistical physics modeling and molecular docking study. Int. J. Biol. Macromol. 233, 123548. doi:10.1016/j.ijbiomac.2023.123548
Ben Khemis, I., Sagaama, A., Issaoui, N., and Ben Lamine, A. (2021). Steric and energetic characterizations of mouse and human musk receptors activated by nitro musk smelling compounds at molecular level: statistical physics treatment and molecular docking analysis. Int. J. Biol. Macromol. 188, 333–342. doi:10.1016/j.ijbiomac.2021.08.042
Billesbolle, C. B., de March, C. A., van der Velden, W. J. C., Ma, N., Tewari, J., Del Torrent, C. L., et al. (2023). Structural basis of odorant recognition by a human odorant receptor. Nature 615 (7953), 742–749. doi:10.1038/s41586-023-05798-y
Breheny, P., Titlow, W. B., Sengoku, T., and Breheny, P. (2020). Mixture and concentration effects on odorant receptor response patterns in vivo. Chem. senses 45 (6), 429–438. doi:10.1093/chemse/bjaa032
Brodin, M., Laska, M., and Olsson, M. J. (2009). Odor interaction between bourgeonal and its antagonist undecanal. Chem. senses 34 (7), 625–630. doi:10.1093/chemse/bjp044
Bryant, P., Pozzati, G., and Elofsson, A. (2022). Improved prediction of protein-protein interactions using AlphaFold2. Nat. Commun. 13 (1), 1265–1276. doi:10.1038/s41467-022-28865-w
Buck, L., and Axel, R. (1991). A novel multigene family may encode odorant receptors: a molecular basis for odor recognition. Cell 65 (1), 175–187. doi:10.1016/0092-8674(91)90418-x
Bushdid, C., De March, C. A., Fiorucci, S., Matsunami, H., and Golebiowski, J. (2018). Agonists of G-protein-coupled odorant receptors are predicted from chemical features. J. Phys. Chem. Lett. 9 (9), 2235–2240. doi:10.1021/acs.jpclett.8b00633
Butterwick, J. A., Del MáRMOL, J., Kim, K. H., Kahlson, M. A., Rogow, J. A., Walz, T., et al. (2018). Cryo-EM structure of the insect olfactory receptor Orco. Nature 560 (7719), 447–452. doi:10.1038/s41586-018-0420-8
Carey, A. F., Wang, G., Su, C.-Y., Zwiebel, L. J., and Carlson, J. R. (2010). Odorant reception in the malaria mosquito Anopheles gambiae. Nature 464 (7285), 66–71. doi:10.1038/nature08834
Chaput, M. A., El Mountassir, F., Atanasova, B., Thomas-Danguin, T., Le Bon, A. M., Perrut, A., et al. (2012). Interactions of odorants with olfactory receptors and receptor neurons match the perceptual dynamics observed for woody and fruity odorant mixtures. Eur. J. Neurosci. 35 (4), 584–597. doi:10.1111/j.1460-9568.2011.07976.x
Cheng, Y. (2018). Single-particle cryo-EM—how did it get here and where will it go. Science 361 (6405), 876–880. doi:10.1126/science.aat4346
Choi, Y. R., Shim, J., Park, J. H., Kim, Y. S., and Kim, M. J. (2021). Discovery of orphan olfactory receptor 6M1 as a new anticancer target in MCF-7 cells by a combination of surface plasmon resonance-based and cell-based systems. Sensors (Basel) 21 (10), 3468–3515. doi:10.3390/s21103468
Choi, Y., and Yoon, M. (2021). The expression of androstenone receptor (OR7D4) in vomeronasal organ and olfactory epithelium of horses. Domest. Anim. Endocrinol. 74, 106535. doi:10.1016/j.domaniend.2020.106535
Collier, T., Piggot, T., and Allison, J. (2020). Molecular dynamics simulation of proteins. Methods Mol. Biol. Clift. NJ 2073, 311–327. doi:10.1007/978-1-4939-9869-2_17
Cong, X., Ren, W., Pacalon, J., Xu, R., Xu, L., Li, X., et al. (2022). Large-scale G protein-coupled olfactory receptor–ligand pairing. ACS central Sci. 8 (3), 379–387. doi:10.1021/acscentsci.1c01495
Cook, B. L., Steuerwald, D., Kaiser, L., Graveland-Bikker, J., Vanberghem, M., Berke, A. P., et al. (2009). Large-scale production and study of a synthetic G protein-coupled receptor: human olfactory receptor 17-4. Proc. Natl. Acad. Sci. 106 (29), 11925–11930. doi:10.1073/pnas.0811089106
Coppola, D. M. (2022). The sorption/chromatography hypothesis of olfactory discrimination: the rise, fall, and rebirth of a Phoenix. BioEssays 44 (3), e2100263. doi:10.1002/bies.202100263
Cortese, B., Uhde, T., Brady, K., McClernon, F. J., Yang, Q. X., Collins, H. R., et al. (2015). The fMRI BOLD response to unisensory and multisensory smoking cues in nicotine-dependent adults. Psychiatry Res. 234 (3), 321–327. doi:10.1016/j.pscychresns.2015.10.008
Curtis, T. M., Nilon, A. M., Greenberg, A. J., Besner, M., Scibek, J. J., Nichols, J. A., et al. (2023). Odorant binding causes cytoskeletal rearrangement, leading to detectable changes in endothelial and epithelial barrier function and micromotion. Biosens. (Basel) 13 (3), 329–415. doi:10.3390/bios13030329
Danev, R., Yanagisawa, H., and Kikkawa, M. (2019). Cryo-electron microscopy methodology: current aspects and future directions. Trends Biochem. Sci. 44 (10), 837–848. doi:10.1016/j.tibs.2019.04.008
Del MáRMOL, J., Yedlin, M. A., and Ruta, V. (2021). The structural basis of odorant recognition in insect olfactory receptors. Nature 597 (7874), 126–131. doi:10.1038/s41586-021-03794-8
De March, C. A., Titlow, W. B., Sengoku, T., Breheny, P., Matsunami, H., and McClintock, T. S. (2020). Modulation of the combinatorial code of odorant receptor response patterns in odorant mixtures. Mol. Cell. Neurosci. 104, 103469. doi:10.1016/j.mcn.2020.103469
De March, C. A., Topin, J., Bruguera, E., Novikov, G., Ikegami, K., Matsunami, H., et al. (2018). Odorant receptor 7D4 activation dynamics. Angew. Chem. Int. Ed. 57 (17), 4554–4558. doi:10.1002/anie.201713065
Dubochet, J. (2018). On the development of electron cryo-microscopy (Nobel lecture). Angew. Chem. Int. Ed. 57 (34), 10842–10846. doi:10.1002/anie.201804280
Dubochet, J., Booy, F. P., Freeman, R., Jones, A. V., and Walter, C. A. (1981). Low temperature electron microscopy. Annu. Rev. biophysics Bioeng. 10 (1), 133–149. doi:10.1146/annurev.bb.10.060181.001025
Duroux, R., Mandeau, A., Guiraudie-Capraz, G., Quesnel, Y., and Loing, E. (2020). A rose extract protects the skin against stress mediators: a potential role of olfactory receptors. Molecules 25 (20), 4743–4758. doi:10.3390/molecules25204743
Edelkamp, J., Lousada, M. B., Pinto, D., et al. (2023). Management of the human hair follicle microbiome by a synthetic odorant. J. dermatological Sci. 1811 (23), 221–229. doi:10.1016/j.jdermsci.2023.09.006
Faraggi, E., Dunker, A. K., Sussman, J. L., and Kloczkowski, A. (2017). Comparing NMR and X-ray protein structure: lindemann-like parameters and NMR disorder. J. Biomol. Struct. Dyn. 36 (9), 2331–2341. doi:10.1080/07391102.2017.1352539
Firestein, S. (2005). A Nobel nose: the 2004 Nobel prize in Physiology and medicine. Neuron 45 (3), 333–338. doi:10.1016/j.neuron.2005.01.021
Gaillard, I., Rouquier, S., and Giorgi, D. (2004). Olfactory receptors. Cell. Mol. Life Sci. (CMLS) 61 (4), 456–469. doi:10.1007/s00018-003-3273-7
GarcíA-NafríA, J., and Tate, C. G. (2021). Structure determination of GPCRs: cryo-EM compared with X-ray crystallography. Biochem. Soc. Trans. 49 (5), 2345–2355. doi:10.1042/BST20210431
Gaubert, A., Amigues, B., Spinelli, S., and Cambillau, C. (2020). Structure of odorant binding proteins and chemosensory proteins determined by X-ray crystallography. Methods Enzym. 642, 151–167. doi:10.1016/bs.mie.2020.04.070
Gaudry, Q., Hong, E. J., Kain, J., de Bivort, B. L., and Wilson, R. I. (2012). Asymmetric neurotransmitter release enables rapid odour lateralization in Drosophila. Nature 493 (7432), 424–428. doi:10.1038/nature11747
Geithe, C., Noe, F., Kreissl, J., and Krautwurst, D. (2017). The broadly tuned odorant receptor OR1A1 is highly selective for 3-Methyl-2,4-nonanedione, a key food odorant in aged wines, tea, and other foods. Chem. Senses 42 (3), 181–193. doi:10.1093/chemse/bjw117
Gonzalez-Kristeller, D. C., Do Nascimento, J. O. B. P., Galante, P. A. F., and Malnic, B. (2015). Identification of agonists for a group of human odorant receptors. Front. Pharmacol. 6, 35–43. doi:10.3389/fphar.2015.00035
Guo, L., Dai, W., Xu, Z., Liang, Q., Miller, E. T., Li, S., et al. (2022). Evolution of brain-expressed biogenic amine receptors into olfactory trace amine-associated receptors. Mol. Biol. Evol. 39 (3), msac006–16. doi:10.1093/molbev/msac006
Haag, F., Ahmed, L., Reiss, K., Block, E., Batista, V. S., and Krautwurst, D. (2019). Copper-mediated thiol potentiation and mutagenesis-guided modeling suggest a highly conserved copper-binding motif in human OR2M3. Cell. Mol. Life Sci. 77 (11), 2157–2179. doi:10.1007/s00018-019-03279-y
Haag, F., Frey, T., Hoffmann, S., Kreissl, J., Stein, J., Kobal, G., et al. (2023). The multi-faceted food odorant 4-methylphenol selectively activates evolutionary conserved receptor OR9Q2. Food Chem. 426, 136492. doi:10.1016/j.foodchem.2023.136492
Haag, F., Hoffmann, S., and Krautwurst, D. (2021). Key food furanones furaneol and sotolone specifically activate distinct odorant receptors. J. Agric. Food Chem. 69 (37), 10999–11005. doi:10.1021/acs.jafc.1c03314
Hartmann, C., Triller, A., Spehr, M., Dittrich, R., Hatt, H., and Buettner, A. (2013). Sperm-activating odorous substances in human follicular fluid and vaginal secretion: identification by gas chromatography-olfactometry and Ca(2+) imaging. ChemPlusChem 78 (7), 695–702. doi:10.1002/cplu.201300008
Heo, L., and Feig, M. (2022). Multi-state modeling of G-protein coupled receptors at experimental accuracy. Proteins Struct. Funct. Bioinforma. 90 (11), 1873–1885. doi:10.1002/prot.26382
Hildebrand, P. W., Rose, A. S., and Tiemann, J. K. S. (2019). Bringing molecular dynamics simulation Data into view. Trends Biochem. Sci. 44 (11), 902–913. doi:10.1016/j.tibs.2019.06.004
Hollingsworth, S. A., and Dror, R. O. (2018). Molecular dynamics simulation for all. Neuron 99 (6), 1129–1143. doi:10.1016/j.neuron.2018.08.011
Huang, J., Lam, H., Koziol-White, C., Limjunyawong, N., Kim, D., Kim, N., et al. (2020). The odorant receptor OR2W3 on airway smooth muscle evokes bronchodilation via a cooperative chemosensory tradeoff between TMEM16A and CFTR. Proc. Natl. Acad. Sci. U. S. A. 117 (45), 28485–28495. doi:10.1073/pnas.2003111117
Hu, J., Zhong, C., Ding, C., Chi, Q., Walz, A., Mombaerts, P., et al. (2007). Detection of near-atmospheric concentrations of CO2 by an olfactory subsystem in the mouse. Science 317 (5840), 953–957. doi:10.1126/science.1144233
Ikegami, K., De March, C. A., Nagai, M. H., Ghosh, S., Do, M., Sharma, R., et al. (2020). Structural instability and divergence from conserved residues underlie intracellular retention of mammalian odorant receptors. Proc. Natl. Acad. Sci. 117 (6), 2957–2967. doi:10.1073/pnas.1915520117
Jaeger, S. R., Mcrae, J. F., Bava, C. M., Beresford, M. K., Hunter, D., Jia, Y., et al. (2013). A Mendelian trait for olfactory sensitivity affects odor experience and food selection. Curr. Biol. 23 (16), 1601–1605. doi:10.1016/j.cub.2013.07.030
Jones, D. T., and Thornton, J. M. (2022). The impact of AlphaFold2 one year on. Nat. methods 19 (1), 15–20. doi:10.1038/s41592-021-01365-3
Jumper, J., Evans, R., Pritzel, A., Green, T., Figurnov, M., Ronneberger, O., et al. (2021a). Highly accurate protein structure prediction with AlphaFold. Nature 596 (7873), 583–589. doi:10.1038/s41586-021-03819-2
Jumper, J., Evans, R., Pritzel, A., Green, T., Figurnov, M., Ronneberger, O., et al. (2021b). Applying and improving AlphaFold at CASP14. Proteins Struct. Funct. Bioinforma. 89 (12), 1711–1721. doi:10.1002/prot.26257
Kalbe, B., Knobloch, J., Schulz, V. M., Wecker, C., Schlimm, M., Scholz, P., et al. (2016). Olfactory receptors modulate physiological processes in human airway smooth muscle cells. Front. physiology 7, 339–354. doi:10.3389/fphys.2016.00339
Kalbe, B., Schulz, V. M., Schlimm, M., Philippou, S., Jovancevic, N., Jansen, F., et al. (2017). Helional-induced activation of human olfactory receptor 2J3 promotes apoptosis and inhibits proliferation in a non-small-cell lung cancer cell line. Eur. J. cell Biol. 96 (1), 34–46. doi:10.1016/j.ejcb.2016.11.004
Kang, W., Choi, D., Son, B., Park, S., and Park, T. (2022). Activation of OR10A3 by suberic acid promotes collagen synthesis in UVB-irradiated dermal fibroblasts via the cAMP-akt pathway. Cells 11 (24), 3961–4016. doi:10.3390/cells11243961
Katada, S., Hirokawa, T., Oka, Y., Suwa, M., and Touhara, K. (2005). Structural basis for a broad but selective ligand spectrum of a mouse olfactory receptor: mapping the odorant-binding site. J. Neurosci. 25 (7), 1806–1815. doi:10.1523/JNEUROSCI.4723-04.2005
Katada, S., Tanaka, M., and Touhara, K. (2004). Structural determinants for membrane trafficking and G protein selectivity of a mouse olfactory receptor. J. Neurochem. 90 (6), 1453–1463. doi:10.1111/j.1471-4159.2004.02619.x
Kim, J. M., Dziobaka, S., Yoon, Y. E., Lee, H. L., Jeong, J. H., Lee, I. R., et al. (2023). OR2H2 activates camkkβ–AMPK–autophagy signaling Axis and suppresses senescence in VK2/e6e7 cells. Pharmaceuticals 16 (9), 1221–1241. doi:10.3390/ph16091221
Kinch, L. N., Schaeffer, R. D., Kryshtafovych, A., and Grishin, N. V. (2021). Target classification in the 14th round of the critical assessment of protein structure prediction (CASP14). Proteins Struct. Funct. Bioinforma. 89 (12), 1618–1632. doi:10.1002/prot.26202
Koehler Leman, J., and KüNZE, G. (2023). Recent advances in NMR protein structure prediction with ROSETTA. Int. J. Mol. Sci. 24 (9), 7835–7857. doi:10.3390/ijms24097835
Liao, M., Cao, E., Julius, D., and Cheng, Y. (2013). Structure of the TRPV1 ion channel determined by electron cryo-microscopy. Nature 504 (7478), 107–112. doi:10.1038/nature12822
Li, B., Kamarck, M. L., Peng, Q., Lim, F. L., Keller, A., Smeets, M. A. M., et al. (2022). From musk to body odor: decoding olfaction through genetic variation. PLoS Genet. 18 (2), e1009564. doi:10.1371/journal.pgen.1009564
Li, S., Ahmed, L., Zhang, R., Pan, Y., Matsunami, H., Burger, J. L., et al. (2016). Smelling sulfur: copper and silver regulate the response of human odorant receptor OR2T11 to low-molecular-weight thiols. J. Am. Chem. Soc. 138 (40), 13281–13288. doi:10.1021/jacs.6b06983
Mainland, J. D., Keller, A., Li, Y. R., Zhou, T., Trimmer, C., Snyder, L. L., et al. (2014). The missense of smell: functional variability in the human odorant receptor repertoire. Nat. Neurosci. 17 (1), 114–120. doi:10.1038/nn.3598
Mainland, J. D., Li, Y. R., Zhou, T., Liu, W. L. L., and Matsunami, H. (2015). Human olfactory receptor responses to odorants. Sci. data 2 (1), 150002. doi:10.1038/sdata.2015.2
Manteniotis, S., Wojcik, S., Gothert, J. R., Dürig, J., Dührsen, U., Gisselmann, G., et al. (2016). Deorphanization and characterization of the ectopically expressed olfactory receptor OR51B5 in myelogenous leukemia cells. Cell Death Discov. 2, 16010. doi:10.1038/cddiscovery.2016.10
March, C. A. D., Ryu, S. E., Sicard, G., Moon, C., and Golebiowski, J. (2015). Structure–odour relationships reviewed in the postgenomic era. Flavour Fragr. J. 30 (5), 342–361. doi:10.1002/ffj.3249
Marcinek, P., Haag, F., Geithe, C., and Krautwurst, D. (2021). An evolutionary conserved olfactory receptor for foodborne and semiochemical alkylpyrazines. FASEB J. 35 (6), e21638. doi:10.1096/fj.202100224R
Massberg, D., Jovancevic, N., Offermann, A., Simon, A., Baniahmad, A., Perner, S., et al. (2016). The activation of OR51E1 causes growth suppression of human prostate cancer cells. Oncotarget 7 (30), 48231–48249. doi:10.18632/oncotarget.10197
Mcclintock, T. S., Khan, N., Alimova, Y., Aulisio, M., Han, D. Y., and Breheny, P. (2020). Encoding the odor of cigarette smoke. J. Neurosci. 40 (37), 7043–7053. doi:10.1523/JNEUROSCI.1144-20.2020
Mcrae, J. F., Mainland, J. D., Jaeger, S. R., Adipietro, K. A., Matsunami, H., and Newcomb, R. D. (2012). Genetic variation in the odorant receptor OR2J3 is associated with the ability to detect the “grassy” smelling odor, cis-3-hexen-1-ol. Chem. senses 37 (7), 585–593. doi:10.1093/chemse/bjs049
Mei, S., Ding, J., and Chen, X. (2023). Identification of differential volatile and non-volatile compounds in coffee leaves prepared from different tea processing steps using HS-SPME/GC–MS and HPLC-Orbitrap-MS/MS and investigation of the binding mechanism of key phytochemicals with olfactory and taste receptors using molecular docking. Food Res. Int. 168, 112760. doi:10.1016/j.foodres.2023.112760
Menashe, I., Abaffy, T., Hasin, Y., Goshen, S., Yahalom, V., Luetje, C. W., et al. (2007). Genetic elucidation of human hyperosmia to isovaleric acid. PLoS Biol. 5 (11), e284–e2468. doi:10.1371/journal.pbio.0050284
Monahan, K., Horta, A., and Lomvardas, S. (2019). LHX2-and LDB1-mediated trans interactions regulate olfactory receptor choice. Nature 565 (7740), 448–453. doi:10.1038/s41586-018-0845-0
Moore, P. B. (2017). Structural biology: past, present, and future. New Biotechnol. 38, 29–35. doi:10.1016/j.nbt.2016.07.010
Murayama, N., Miyaki, T., Okuzaki, D., Shibata, Y., Koji, T., Inoue, A., et al. (2023). Transcriptome profiling of anhidrotic eccrine sweat glands reveals that olfactory receptors on eccrine sweat glands regulate perspiration in a ligand-dependent manner. JID Innov. 3 (4), 100196. doi:10.1016/j.xjidi.2023.100196
Nakanishi, S., Tsutsui, T., Itai, N., and Denda, M. (2023). Distinct sets of olfactory receptors highly expressed in different human tissues evaluated by meta-transcriptome analysis: association of OR10A6 in skin with keratinization. Front. Cell Dev. Biol. 11, 1102585. doi:10.3389/fcell.2023.1102585
Nicoli, A., Haag, F., Marcinek, P., He, R., Kreißl, J., Stein, J., et al. (2023). Modeling the orthosteric binding site of the G protein-coupled odorant receptor OR5K1. J. Chem. Inf. Model. 63 (7), 2014–2029. doi:10.1021/acs.jcim.2c00752
Noe, F., Polster, J., Geithe, C., Kotthoff, M., Schieberle, P., and Krautwurst, D. (2017). OR2M3: a highly specific and narrowly tuned human odorant receptor for the sensitive detection of onion key food odorant 3-Mercapto-2-methylpentan-1-ol. Chem. Senses 42 (3), 195–210. doi:10.1093/chemse/bjw118
Oh, S. J. (2021). Computational evaluation of interactions between olfactory receptor OR2W1 and its ligands. Genomics Inf. 19 (1), e9. doi:10.5808/gi.21026
Orecchioni, M., Matsunami, H., and Ley, K. (2022). Olfactory receptors in macrophages and inflammation. Front. Immunol. 13, 1029244. doi:10.3389/fimmu.2022.1029244
Ovali, M. A., and Bozgeyik, I. (2022). Asprosin, a C-terminal cleavage product of fibrillin 1 encoded by the FBN1 gene, in health and disease. Mol. Syndromol. 13 (3), 175–183. doi:10.1159/000520333
Park, K.-L. (2016). Emergence of hydrogen bonds from molecular dynamics simulation of substituted N-phenylthiourea–catechol oxidase complex. Archives pharmacal Res. 40 (1), 57–68. doi:10.1007/s12272-016-0866-x
Peterlin, Z., Firestein, S., and Rogers, M. E. (2014). The state of the art of odorant receptor deorphanization: a report from the orphanage. J. General Physiology 143 (5), 527–542. doi:10.1085/jgp.201311151
Pluznick, J. L., Protzko, R. J., Gevorgyan, H., Peterlin, Z., Sipos, A., Han, J., et al. (2013). Olfactory receptor responding to gut microbiota-derived signals plays a role in renin secretion and blood pressure regulation. Proc. Natl. Acad. Sci. 110 (11), 4410–4415. doi:10.1073/pnas.1215927110
Poupon, D., Fernandez, P., Archambault, BOISVERT S., Migneault-Bouchard, C., and Frasnelli, J. (2018). Can the identification of odorants within a mixture Be trained? Chem. senses 43 (9), 721–726. doi:10.1093/chemse/bjy060
Pronin, A., and Slepak, V. (2021). Ectopically expressed olfactory receptors OR51E1 and OR51E2 suppress proliferation and promote cell death in a prostate cancer cell line. J. Biol. Chem. 296, 100475. doi:10.1016/j.jbc.2021.100475
Reisert, J., and Restrepo, D. (2009). Molecular tuning of odorant receptors and its implication for odor signal processing. Chem. senses 34 (7), 535–545. doi:10.1093/chemse/bjp028
Rydzewski, J., and Nowak, W. (2017). Ligand diffusion in proteins via enhanced sampling in molecular dynamics. Phys. life Rev. 22-23, 58–74. doi:10.1016/j.plrev.2017.03.003
Saito, H., Chi, Q., Zhuang, H., Matsunami, H., and Mainland, J. D. (2009). Odor coding by a mammalian receptor repertoire. Sci. Signal. 2 (60), ra9. doi:10.1126/scisignal.2000016
Saito, H., Kubota, M., Roberts, R. W., Chi, Q., and Matsunami, H. (2004). RTP family members induce functional expression of mammalian odorant receptors. Cell 119 (5), 679–691. doi:10.1016/j.cell.2004.11.021
Sanz, G., Thomas-Danguin, T., Hamdani, E. H., Le Poupon, C., Briand, L., Pernollet, J. C., et al. (2008). Relationships between molecular structure and perceived odor quality of ligands for a human olfactory receptor. Chem. senses 33 (7), 639–653. doi:10.1093/chemse/bjn032
Silva Teixeira, C. S., Silva Ferreira, A. C., and Cerqueira, N. M. F. S. A. (2016). Studying haloanisoles interaction with olfactory receptors. ACS Chem. Neurosci. 7 (7), 870–885. doi:10.1021/acschemneuro.5b00335
Skouby, A., and Zilstorff-Pedersen, K. (1954). The influence of acetylcholine-like substances, menthol and strychnine on olfactory receptors in man. Acta physiol. Scand. 32, 252–258. doi:10.1111/j.1748-1716.1954.tb01172.x
Son, M., Cho, D. G., Lim, J. H., Park, J., Hong, S., Ko, H. J., et al. (2015). Real-time monitoring of geosmin and 2-methylisoborneol, representative odor compounds in water pollution using bioelectronic nose with human-like performance. Biosens. Bioelectron. 74, 199–206. doi:10.1016/j.bios.2015.06.053
Sviridenko, P. (1951). Theoretical differences on the rôle of olfactory receptors in rodents and their practical deflection. Zool. zhurnal 30 (4), 344–351.
Taylor, K. A., and Glaeser, R. M. (1974). Electron diffraction of frozen, hydrated protein crystals. Science 186 (4168), 1036–1037. doi:10.1126/science.186.4168.1036
Tham, E. H., Dyjack, N., Kim, B. E., Rios, C., Seibold, M. A., Leung, D. Y. M., et al. (2019). Expression and function of the ectopic olfactory receptor OR10G7 in patients with atopic dermatitis. J. Allergy Clin. Immunol. 143 (5), 1838–1848. doi:10.1016/j.jaci.2018.11.004
Thomas-Danguin, T., Sinding, C., Romagny, S. B., El Mountassir, F., Atanasova, B., Le Berre, E., et al. (2014). The perception of odor objects in everyday life: a review on the processing of odor mixtures. Front. Psychol. 5, 504–523. doi:10.3389/fpsyg.2014.00504
Tian, X.-J., Zhang, H., Sannerud, J., and Xing, J. (2016). Achieving diverse and monoallelic olfactory receptor selection through dual-objective optimization design. Proc. Natl. Acad. Sci. 113 (21), 2889–2898. doi:10.1073/pnas.1601722113
Topin, J., De March, C. A., Charlier, L., Ronin, C., Antonczak, S., and Golebiowski, J. (2014). Discrimination between olfactory receptor agonists and non-agonists. Chem. – A Eur. J. 20 (33), 10227–10230. doi:10.1002/chem.201402486
Trimmer, C., Arroyave, R., Vuilleumier, C., Wu, L., Dumer, A., DeLaura, C., et al. (2023). Allosteric modulation of a human odorant receptor. Curr. Biol. 33 (8), 1523–1534.e4. doi:10.1016/j.cub.2023.03.016
Tsai, T., Veitinger, S., Peek, I., Busse, D., Eckardt, J., Vladimirova, D., et al. (2016). Two olfactory receptors—OR2A4/7 and OR51B5—differentially affect epidermal proliferation and differentiation. Exp. Dermatol. 26 (1), 58–65. doi:10.1111/exd.13132
Tunyasuvunakool, K., Adler, J., Wu, Z., Green, T., Zielinski, M., Žídek, A., et al. (2021). Highly accurate protein structure prediction for the human proteome. Nature 596 (7873), 590–596. doi:10.1038/s41586-021-03828-1
Venkatakrishnan, A. J., Deupi, X., Lebon, G., Tate, C. G., Schertler, G. F., and Babu, M. M. (2013). Molecular signatures of G-protein-coupled receptors. Nature 494 (7436), 185–194. doi:10.1038/nature11896
Wang, L., and Anderson, D. J. (2009). Identification of an aggression-promoting pheromone and its receptor neurons in Drosophila. Nature 463 (7278), 227–231. doi:10.1038/nature08678
Weber, L., Al-Refae, K., Ebbert, J., Jägers, P., Altmüller, J., Becker, C., et al. (2017). Activation of odorant receptor in colorectal cancer cells leads to inhibition of cell proliferation and apoptosis. PloS one 12 (3), e0172491. doi:10.1371/journal.pone.0172491
Weber, L., Schulz, W. A., Philippou, S., Eckardt, J., Ubrig, B., Hoffmann, M. J., et al. (2018). Characterization of the olfactory receptor OR10H1 in human urinary bladder cancer. Front. Physiol. 9, 456–471. doi:10.3389/fphys.2018.00456
Weidinger, D., Jovancevic, N., Zwanziger, D., Theurer, S., Hönes, J., Führer, D., et al. (2021). Functional characterization of olfactory receptors in the thyroid gland. Front. physiology 12, 676907. doi:10.3389/fphys.2021.676907
Weis, W. I., and Kobilka, B. K. (2018). The molecular basis of G protein–coupled receptor activation. Annu. Rev. Biochem. 87 (1), 897–919. doi:10.1146/annurev-biochem-060614-033910
Xie, B.-B., Jia, P.-K., Wang, K.-X., Chen, W. K., Liu, X. Y., and Cui, G. (2022). Generalized ab initio nonadiabatic dynamics simulation methods from molecular to extended systems. J. Phys. Chem. A 126 (11), 1789–1804. doi:10.1021/acs.jpca.1c10195
Xu, J., and Pluznick, J. L. (2022). Key amino acids alter activity and trafficking of a well-conserved olfactory receptor. Am. J. Physiol. Cell Physiol. 322 (6), 1279–1288. doi:10.1152/ajpcell.00440.2021
Xu, X., Khater, M., and Wu, G. (2022). The olfactory receptor OR51E2 activates ERK1/2 through the Golgi-localized Gβγ-PI3Kγ-ARF1 pathway in prostate cancer cells. Front. Pharmacol. 13, 1009380. doi:10.3389/fphar.2022.1009380
Yasi, E. A., Eisen, S. L., Wang, H., Sugianto, W., Minniefield, A. R., Hoover, K. A., et al. (2019). Rapid deorphanization of human olfactory receptors in yeast. Biochemistry 58 (16), 2160–2166. doi:10.1021/acs.biochem.8b01208
Yasunaga, M., Takai, E., Hattori, S., Tatematsu, K., and Kuroda, S. (2022). Effects of 3-octen-2-one on human olfactory receptor responses to vanilla flavor. Biosci. Biotechnol. Biochem. 86 (11), 1562–1569. doi:10.1093/bbb/zbac147
Yip, K. M., Fischer, N., Paknia, E., Chari, A., and Stark, H. (2020). Atomic-resolution protein structure determination by cryo-EM. Nature 587 (7832), 157–161. doi:10.1038/s41586-020-2833-4
Yoshikawa, K., Deguchi, J., Hu, J., Lu, H. Y., and Matsunami, H. (2022). An odorant receptor that senses four classes of musk compounds. Curr. Biol. 32 (23), 5172–5179.e5. doi:10.1016/j.cub.2022.10.038
Yuan, X., Wang, Y., Ali, M. A., Qin, Z., Guo, Z., Zhang, Y., et al. (2023). Odorant receptor OR2C1 is an essential modulator of boar sperm capacitation by binding with heparin. Int. J. Mol. Sci. 24 (2), 1664–1679. doi:10.3390/ijms24021664
Yu, Y., Ma, Z., Pacalon, J., Xu, L., Li, W., Belloir, C., et al. (2022). Extracellular loop 2 of G protein–coupled olfactory receptors is critical for odorant recognition. J. Biol. Chem. 298 (9), 102331. doi:10.1016/j.jbc.2022.102331
Zeng, S., Zhang, L., Li, P., Pu, D., Fu, Y., Zheng, R., et al. (2023). Molecular mechanisms of caramel-like odorant-olfactory receptor interactions based on a computational chemistry approach. Food Res. Int. 171, 113063. doi:10.1016/j.foodres.2023.113063
Zhou, C., Liu, Y., Zhao, G., Liu, Z., Chen, Q., Yue, B., et al. (2023). Comparative analysis of olfactory receptor repertoires sheds light on the diet adaptation of the bamboo-eating giant panda based on the chromosome-level genome. Animals 13 (6), 979–994. doi:10.3390/ani13060979
Keywords: olfactory receptors, odor molecules, sensory mechanisms, structural biology, molecular dynamics simulations
Citation: Wang J, Zhang Q, Fan W, Shi Q, Mao J, Xie J, Chai G and Zhang C (2025) Deciphering olfactory receptor binding mechanisms: a structural and dynamic perspective on olfactory receptors. Front. Mol. Biosci. 11:1498796. doi: 10.3389/fmolb.2024.1498796
Received: 20 September 2024; Accepted: 23 December 2024;
Published: 08 January 2025.
Edited by:
Ramin Ekhteiari Salmas, King’s College London, United KingdomReviewed by:
Ozge Sensoy, Istanbul Medipol University, TürkiyeIsmail Erol, Gebze Technical University, Türkiye
Copyright © 2025 Wang, Zhang, Fan, Shi, Mao, Xie, Chai and Zhang. This is an open-access article distributed under the terms of the Creative Commons Attribution License (CC BY). The use, distribution or reproduction in other forums is permitted, provided the original author(s) and the copyright owner(s) are credited and that the original publication in this journal is cited, in accordance with accepted academic practice. No use, distribution or reproduction is permitted which does not comply with these terms.
*Correspondence: Chenglei Zhang, YXJpZXMxNDBhYUAxNjMuY29t; Guobi Chai, Y2hhaWdiQHp6dS5lZHUuY24=