- Centre for Haemato-Oncology, Barts Cancer Institute, Queen Mary University of London, London, United Kingdom
Mammalian hematopoietic stem cells (HSCs) emerge from the hemogenic endothelium in the major embryonic arteries. HSCs undergo a complex journey first migrating to the fetal liver (FL) and from there to the fetal bone marrow (FBM), where they mostly remain during adult life. In this process, a pool of adult HSCs is produced, which sustains lifelong hematopoiesis. Multiple cellular components support HSC maturation and expansion and modulate their response to environmental and developmental cues. While the adult HSC niche has been extensively studied over the last two decades, the niches present in the major embryonic arteries, FL, FBM and perinatal bone marrow (BM) are poorly described. Recent investigations highlight important differences among FL, FBM and adult BM niches and emphasize the important role that inflammation, microbiota and hormonal factors play regulating HSCs and their niches. We provide a review on our current understanding of these important cellular microenvironments across ontogeny. We mainly focused on mice, as the most widely used research model, and, when possible, include relevant insights from other vertebrates including birds, zebrafish, and human. Developing a comprehensive picture on these processes is critical to understand the earliest origins of childhood leukemia and to achieve multiple goals in regenerative medicine, such as mimicking HSC development in vitro to produce HSCs for broad transplantation purposes in leukemia, following chemotherapy, bone marrow failure, and in HSC-based gene therapy.
Introduction
In mammals at least three embryonic developmental waves of hematopoiesis produce hematopoietic cells with different contribution in the life of an organism. The first two waves emerge in the yolk sac (YS) produce primitive nucleated erythroid cells and erythroid-myeloid progenitor cells (EMPs) (Medvinsky et al., 2011) (Figure 1). Most YS-derived hematopoietic cells will serve to cope with embryonic needs, including oxygen distribution in growing embryos, and will have little contribution to adulthood. Yet, some YS-derived cells such as tissue-resident macrophages, with self-renewing activity, will persist during adult life (Medvinsky et al., 2011). Hematopoietic stem cells (HSCs), which sustain life-long hematopoiesis, emerge in a third wave of hematopoiesis (known as definitive hematopoiesis) (Medvinsky et al., 2011) when a fraction of endothelial cells (the hemogenic endothelium) located in the major arteries of the embryo, including the aorta and vitelline and umbilical arteries in mammals, undergo endothelial-to-hemogenic transition (EHT) (Ganuza et al., 2020; Ottersbach, 2019; Simic et al., 2020). Newly formed HSCs and hematopoietic stem and progenitor cells (HSPCs) migrate via blood circulation to the fetal liver (FL) where they mature and expand in number, although recent breakthroughs indicate that this expansion is modest (only ∼2 fold) and HSCs divide asymmetrically (Ganuza et al., 2020; Ganuza et al., 2022a; Patel et al., 2022; Ganuza et al., 2022b) (Figure 1). Later in gestation, HSCs relocate to the bone marrow (BM) where, under healthy conditions, remain during adult life (Figure 1). In the BM, the HSC pool expands in number before undergoing a “quiescence shift” that HSCs will only abandon to maintain or reinstate the homeostasis of the hematopoietic system following acute insults (e.g., bleeding, bacterial and viral infections…) or more stable and continuous damages such as cell turnover and chronic inflammation (Ganuza et al., 2022b; Bowie et al., 2006; Calvanese et al., 2014; Johnson et al., 2020; Fernandez Sanchez et al., 2024; Bogeska et al., 2022; Ganuza and McKinney-Freeman, 2017).
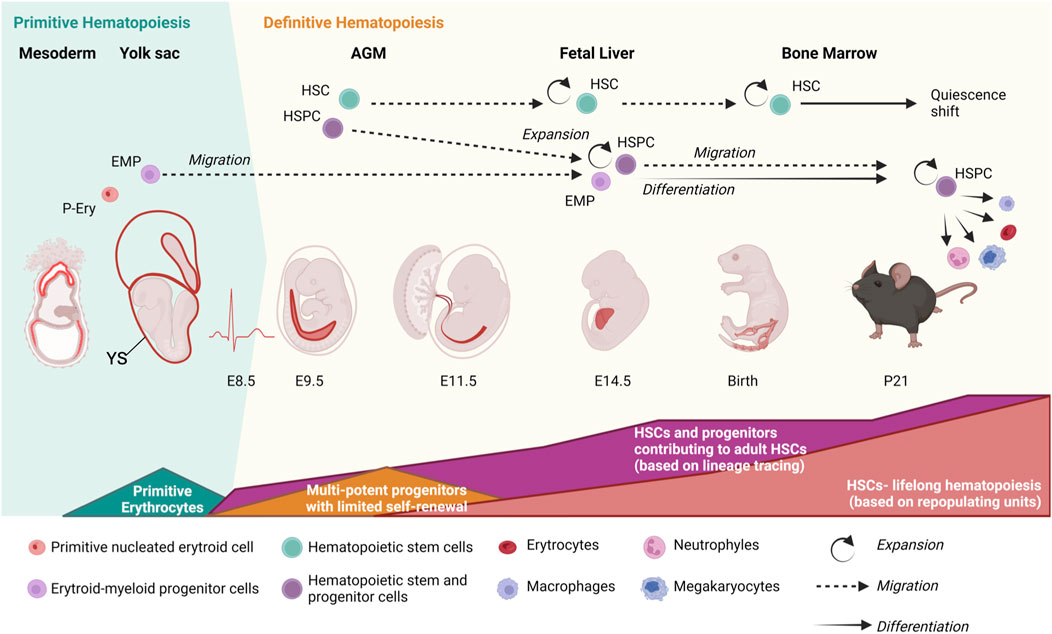
Figure 1. Developmental waves of hematopoiesis. Primitive erythroid cells (P-Ery) and erythroid-myeloid progenitors (EMPs) with limited contribution to adult hematopoiesis emerge in the yolk sac (YS). The presence of hematopoietic stem cells (HSCs), characterized by their ability to repopulate lethally irradiated recipients, is first detected around embryonic day of development (E) 10.5 in the embryo once circulation is stablished (∼E8.5). HSCs emerge from the hemogenic endothelium in the major arteries of the embryo (including aorta, located in the aorta-gonad-mesonephros region, AGM, and umbilical and vitelline arteries). Lineage-tracing studies detected a much higher number of HSC precursors in the embryo than assumed by classical estimates based on repopulating units. Shapes at the bottom indicate the duration and magnitude of each developmental wave. HSCs later migrate to the fetal liver (starting around E12.5), where they mature and modestly expand in numbers according to lineage tracing studies. From the fetal liver, HSCs start to migrate to the bone marrow around E15.5, where they will mostly remain during adulthood. Around 3–4 weeks post- birth, HSCs undergo a quiescence shift to preserve their cellular and molecular integrity and population size. Most adult HSCs are quiescent and only abandon this state to reinstate homeostasis in a controlled manner following insults (e.g., bleeding, inflammation, infections, cellular turnover). Embryonic days of development refer to mouse ontogeny. Processes seem preserved in other mammals. Figure created with Biorender.
Over these various stages of development, HSCs and their progenitors receive critical cues including cytokines, chemokines, growth factors and ligand-receptor interactions that first trigger their specification and later regulate their proliferative state and behavior (e.g., quiescence versus cell division and differentiation) (Clements and Khoury, 2024; Mikkola and Orkin, 2006; Calvanese and Mikkola, 2023; Agrawal et al., 2024). The cellular microenvironment where HSCs locate and that provide specific supporting signals constitutes the HSC-niche. Thus, the HSC-niche integrates cues received from the organism to maintain the homeostasis of the hematopoietic system. The HSC-niche dramatically changes during development, especially considering the journey of HSCs across multiple embryonic locations (Mikkola and Orkin, 2006). Although the adult HSC-niche has been widely studied and numerous reviews are available on the topic (Sanchez-Lanzas et al., 2022; Morrison and Scadden, 2014; Pinho and Frenette, 2019; Lucas, 2017), HSC-niches including those related to the specification of HSCs in the major embryonic arteries (Clements and Khoury, 2024; Jaffredo et al., 2013) and the FL-(Agrawal et al., 2024), fetal BM (FBM)-, perinatal- and infant-BM are much less characterized (Gao et al., 2018). In this Review Article we provide a comprehensive overview on the current knowledge of the various HSC-niches during ontogeny, with a focus on pre-adult HSC niches. We describe the major biological and developmental events taking place at each stage and the cellular and molecular niche components that constitute those microenvironments and how they are believed toshape HSC behavior. We focus mostly on the mouse as the best characterized mammalian system and provide additional insights on humans and other non-mammalian vertebrates as zebrafish and birds when possible. The reader can find key terms described in Table 1.
The HSC-specification niche: aorta-gonad-mesonephros
HSCs are mesodermal in origin and derive from a subset of endothelial cells (ECs) known as the hemogenic endothelium (HE) in the major embryonic arteries, including vitelline and umbilical arteries and the aorta (Medvinsky et al., 2011). The specific requirement of a supportive niche in HSC specification has been most directly demonstrated in avian embryos by the use of quail-chick chimeras (Pardanaud and Dieterlen-Lievre, 1999), in mice via in vitro explant-reaggregates cultures (Matsuoka et al., 2001; Peeters et al., 2009; Souilhol et al., 2016a; Taoudi and Medvinsky, 2007; Ivanovs et al., 2014) and in Xenopus via transplantation (Turpen et al., 1997). Table 2 summarizes the methods that have been employed to identify hematopoietic niches during development. We also refer the reader to our previous review article focused on classic and novel approaches to study the bone marrow niche (Sanchez-Lanzas et al., 2022). HSC specification mainly concentrates to the ventral aspect of the dorsal aorta (Jaffredo et al., 2013) (Figure 2). The establishment of a dorsoventral polarity in the dorsal aorta is key in HE commitment (Yvernogeau et al., 2023). It has been shown that the subaortic mesenchyme contributes to this process and during HSC specification (Jaffredo et al., 2013; Ivanovs et al., 2014; Chandrakanthan et al., 2022; Damm and Clements, 2017; Gonzalez Galofre et al., 2024; Kapeni et al., 2022; Miladinovic et al., 2024; Richard et al., 2013; Sa da Bandeira et al., 2022; Crosse et al., 2023; Crosse et al., 2020). The subaortic mesenchyme derives from the splanchnopleural mesoderm, as shown by studies on avian embryos, and contains mesenchymal cells harboring various differentiation potentials including the ability to generate osteoblasts, adipocytes and ECs (Jaffredo et al., 2013; Richard et al., 2013; Mendes et al., 2005; Durand et al., 2006; Pardanaud et al., 1996; Jaffredo et al., 2010). Cells with other embryonic origin also migrate to the subaortic mesenchyme and have a role on HSC specification (Figure 2). Particularly, migratory ectoderm-derived neural crest cells associate with the ventral aspect of the dorsal aorta before HSC specification in zebrafish embryos (Damm and Clements, 2017). Their migration requires platelet-derived growth factor receptor α (PDGFRα) signaling and blocking this migration leads to lack of HSC specification (Damm and Clements, 2017). Additionally, catecholamines produced by the sympathetic nervous system neurons (derived from a fraction neural crest cells) are required to maintain HSCs in the mouse embryo after HSC specification (Damm and Clements, 2017; Kapeni et al., 2022; Fitch et al., 2012). While in chick embryos somite-derived ECs migrate to the aorta to replace HE cells post-EHT (Pouget et al., 2006), in zebrafish dermomyotome-derived ECs were recently shown to travel to the dorsal aorta to support EHT from neighboring HE cells. It would be interesting to determine the timing and relevance of this migration in mammalian embryos (Sahai-Hernandez et al., 2023).
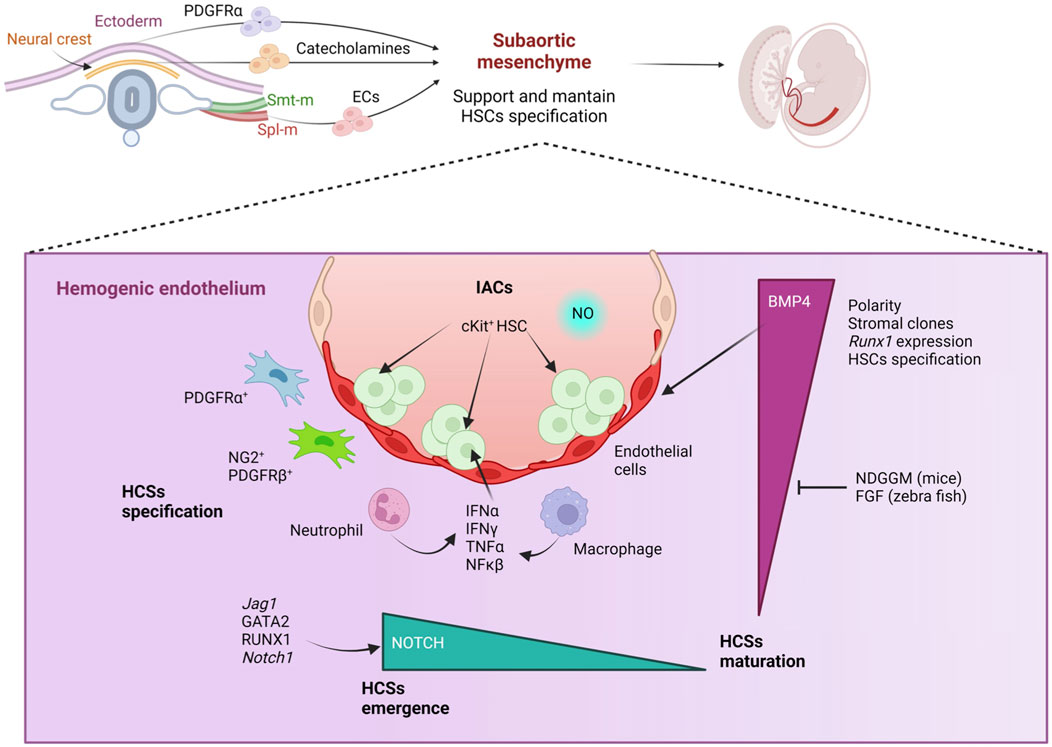
Figure 2. The hematopoietic stem cell (HSC) niche during HSC specification. The subaortic mesenchyme derived from the splachnopleural mesoderm, plays a key role in the establishment of a dorsoventral polarity in the aorta, which eventually leads to the emergence of HSCS from the hemogenic endothelium, located in the ventral aspect of the dorsal aorta, through a process known as endothelial-to-hematopoietic transition (EHT). This can be visualized as intra-aortic clusters (IACS) of c-Kit+ cells budding into the lumen of the aorta in midgestation mammalian embryos. Migratory ectoderm-derived neural crest cells guided to the ventral aspect of the aorta by platelet-derived growth factor receptor a (PDGFRα) are also required during EHT. Catecholamines produced by neural crest-derived neurons, supports HSCs maintenance post-specification. Bone morphogenic protein-4 (BMP4), GATA2 and NOTCH signaling play a key role in EHT and modulate RUNX1 expression. Both BMP and NOTCH activity need to be downregulated to allow HSC maturation following specification. Sterile inflammation, mediated by various cytokines produced by endothelial and myeloid cells (such as macrophages) also promotes HSC specification. Blood circulation triggering shear stress activating nitric oxide (NO) pathway in the hemogenic endothelium is also critical in EHT. Figure created with Biorender.
Within the subaortic mesenchyme, mesodermal-derived PDGFRα+ cells (Chandrakanthan et al., 2022) and NG2+ PDGFRβ+ cells (overlapping with NG2+RUNX1LOWCD146+ cells) (Gonzalez Galofre et al., 2024; Sa da Bandeira et al., 2022) have been shown recently to support HSC specification, are proximal to the hemogenic endothelium and required for optimal specification (Clements and Khoury, 2024; Fadlullah et al., 2022). The EHT supportive ability of mesodermal-derived PDGFRα+ stromal cells seems to rely on PDGFRα, BMP, WNT and NOTCH signaling pathways (Chandrakanthan et al., 2022). Interestingly, mesodermal-derived PDGFRα+ stromal cells are present in the AGM during E10.5-E11.5, i.e., during EHT, and by E13.5 are replaced by neural crest-derived PDGFRα+ stromal cells (Chandrakanthan et al., 2022). In contrast to mesodermal-derived PDGFRα+, neural crest-derived PDGFRα+ stromal cells do not support EHT, as shown in reaggregate co-cultures (Chandrakanthan et al., 2022). As suggested by Chandrakanthan et al., this opens up the intriguing possibility that the replacement of mesodermal-derived with neural crest-derived PDGFRα+ stromal cells constitutes a regulatory mechanism selected to actively control HSC specification confining it to a very defined developmental window (Chandrakanthan et al., 2022). Interestingly, in zebrafish, the PDGFβ/PDGFRβ axis mediates HIF1α-regulated signaling axis leading to IL-6/IL-6R activation and controls embryonic HSPC production (Lim et al., 2017).
The generation of stromal clones from the surrounding aortic tissues uncovered the presence of critical factors as bone morphogenic protein-4 (BMP4) (Oostendorp et al., 2002; Oostendorp et al., 2005; Ohneda et al., 2000; Renstrom et al., 2009; Istvanffy et al., 2011), highly expressed in the aortic ventral mesenchyme of avian, mouse, zebrafish and human embryos (Pimanda et al., 2007; Marshall et al., 2000) and active in transplantable HSCs in mouse embryos at embryonic day of development (E) 11 (Crisan et al., 2015). Moreover, BMP signaling contributes to AGM polarity in zebrafish (Wilkinson et al., 2009). The use of explant cultures derived from the aorta-gonad-mesonephros region (AGM), developed by the laboratory of Alexander Medvinsky (Taoudi and Medvinsky, 2007; Taoudi et al., 2008; McGarvey et al., 2017), allowed to show that BMP4 is required in HSC specification as HSC potential was devoid in AGM explants treated with gremlin (a BMP antagonist) (Durand et al., 2007). Analysis of gene regulatory networks supported that the BMP signaling pathway and the SCL transcriptional network regulate RUNX1 activity (Pimanda et al., 2007). Importantly, the subaortic mesenchyme triggers RUNX1 expression on aortic ECs. RUNX1 marks the hemogenic endothelium and is critical for HSC specification and dispensable in later hematopoietic stages (Chen et al., 2009; North et al., 2002; Lancrin et al., 2009). Furthermore, GATA3 binds to enhancer elements in the Runx1 locus in the HSC microenvironment, suggesting a role for RUNX1 also in the stromal cells that regulate EHT (Fitch et al., 2020).
Interestingly, BMP signaling must be downregulated following EHT to allow further HSC maturation and mediated at least in part by NOGGIN in mice (Souilhol et al., 2016a) and FGF signaling in zebrafish (Pouget et al., 2014). Additional work in avian, zebrafish and mouse embryos unveiled that the Hedgehog (Hh) signaling pathway regulated by ventral tissues is key in HSC specification (Pardanaud and Dieterlen-Lievre, 1999; Peeters et al., 2009; Wilkinson et al., 2009; Gering and Patient, 2005) while dorsal tissues exert an opposing effect, dorsoventrally polarizing the aorta (Jaffredo et al., 2013). The subaortic mesenchyme would be the source for ventral BMP4 (Marshall et al., 2000; Wilkinson et al., 2009; Durand et al., 2007), while Sonic Hedgehog (Shh) would be produced in the developing gut (Peeters et al., 2009) and both responsible for HSC specification in the ventral aspect of the dorsal aorta. Interestingly, in zebrafish embryos, the Hh pathway inhibits the hematopoietic program later during development in the dorsal aspect of the aorta, which highlights the fine-regulation of HSC specification (Wilkinson et al., 2009).
Furthermore, WNT16-dependent somite patterning and NOTCH signaling activation by DeltaC and DeltaD contributes to HSC specification (Clements et al., 2011). The intermediate mechanistic steps are still unknown (Clements and Khoury, 2024; Clements et al., 2011). The urogenital ridges in the AGM in mice also promote HSC specification although the exact involved mechanisms remain unclear (Clements and Khoury, 2024; Souilhol et al., 2016a).
Before the fusion of the paired aortas, all the aortic ECs present at that stage derive from the splanchnopleural mesoderm (Jaffredo et al., 2013). Later during development there is a replacement of the dorsal ECs with somitic mesoderm derived ECs (Pardanaud et al., 1996; Pouget et al., 2006). Particularly, in seminal experiments, Pardanaud and Dieterlen-Lievre showed in avian embryos that dorsal ECs derive from the somitic mesoderm, while ventral ECs derive from the lateral mesoderm ECs, which contributes to the dorsoventral asymmetry (Pardanaud et al., 1996). In zebrafish and mice, the EC replacement precedes HSC specification (Chandrakanthan et al., 2022; Sahai-Hernandez et al., 2023; Nguyen et al., 2014) contributing to dorsoventral polarity and influencing HSC specification.
The hemogenic endothelium (HE) undergoes an EHT to render the first HSCs defined by their ability to engraft and repopulate the hematopoietic system of a lethally irradiated recipient. The first functional HSC is detected at E11 in mouse embryos and EHT can be visualized as c-Kit+ clusters budding into the lumen of the aorta forming intra-aortic clusters, IACs (Medvinsky et al., 2011) (Figure 2). IACs emerge from the ventral aspect of the dorsal aorta (Medvinsky et al., 2011; Medvinsky and Dzierzak, 1996). The lack of IACs in Runx1−/− mouse embryos (Chen et al., 2009; North et al., 1999; Yokomizo et al., 2001) and the block in EHT observed in zebrafish embryos following runx1 inhibition by morpholino (Kissa and Herbomel, 2010) demonstrated a key role of Runx1 during EHT. NOTCH signaling is also required in EHT as shown via morpholino knockdown in zebrafish embryos (Bertrand et al., 2010). All components in the NOTCH signaling pathway including Notch ligands (Jag1 and Jag2), Notch1 receptor and NOTCH transcriptional targets (including Hes1 and Gata2) are expressed in the ventral aspect of the aorta (Robert-Moreno et al., 2005; Robert-Moreno et al., 2008; Hadland et al., 2022). GATA2 is critical in HSC emergence and regulates Runx1 expression (Nottingham et al., 2007), directly linking NOTCH activation with EHT. Furthermore, enforced Gata2 and Runx1 expression partially rescues in vitro the hematopoiesis defects driven by Notch1 and Jagged1 deficiency (Robert-Moreno et al., 2008; Nakagawa et al., 2006). Importantly, HSC specification is tightly connected with the arterial endothelial identity and suppression of NOTCH signaling via CoupTFII deficiency leads to the acquisition of arterial characteristics by the venous endothelium and emergence of venous hematopoietic clusters (You et al., 2005). In addition, JAG1 was shown to be required in embryonic hematopoiesis both in zebrafish and mice (Robert-Moreno et al., 2008). Similar to BMP signaling, NOTCH signaling needs to be downregulated to allow further HSC maturation (Richard et al., 2013; Souilhol et al., 2016b; Porcheri et al., 2020; Lizama et al., 2015; Ditadi et al., 2015; Kobayashi et al., 2014; Gama-Norton et al., 2015; Sacilotto et al., 2013; Thambyrajah and Bigas, 2022). Interestingly, the downregulation of NOTCH signaling may be driven by JAG1/NOTCH1 cis interaction (Ghersi et al., 2023).
During the recent years the role of sterile inflammatory signals on HSC specification have gained much attention (Hou et al., 2020; Espin-Palazon et al., 2014; Li et al., 2014; Mariani et al., 2019). Endothelial and myeloid cells (such as macrophages and primitive neutrophils) produced cytokines (including IFNα, IFNγ, NFκβ and TNFα) and affect HSC specification. Some of these cytokines (e.g., NFκβ and TNFα) converge on modulating NOTCH signaling in HSPCs in mice and zebrafish (Clements and Khoury, 2024; He et al., 2015) and are required in HSC specification. Additionally, myeloid populations contribute with matrix metalloproteases (Mmp2 and 9) that facilitate EHT and the migration of HSCs out from the dorsal aorta, as shown in zebrafish (Theodore et al., 2017). Interestingly, YS-derived macrophages, whose migration to the aorta depends on the chemokine receptor CX3CR1, constitute the largest hematopoietic cell population in the AGM and interact with IACs (Mariani et al., 2019). Notably, macrophage depletion in E10.5 AGM explant cultures blocks HSC specification in vitro (Mariani et al., 2019), supporting their role as part of the AGM HSC-specification niche.
Although it cannot be defined as a specific niche effect, the presence of blood circulation induces shear stress on the hemogenic endothelium, which activates the nitric oxide (NO) signaling pathway and plays an important role in EHT (Niranjan et al., 1995; Adamo et al., 2009; North et al., 2009). Blocking NO signaling pathway in NO synthase3 deficient mice and by the administration of L-NAME in vivo results in the lack of IACs (Adamo et al., 2009; North et al., 2009; Murayama et al., 2006). The absence of Runx1+ cells in zebrafish with no blood flow can be rescued via the administration of an NO donor (S-nitroso-N-acetylpenicillamine) further demonstrating that shear stress works through NO signaling pathway (North et al., 2009; Murayama et al., 2006). Moreover, compound BF170 hydrochloride has been shown to promote HSPC induction in vitro in zebrafish blastomere cell cultures and from mouse embryoid bodies by activating the NO signaling pathway (Liu et al., 2024).
Fetal liver: HSC maturation and moderate expansion
FL-HSC expansion vs. maturation
Following EHT, recently specified HSCs and immature HSC precursors migrate to the FL (Medvinsky et al., 2011). During this migration, Integrin-β1 (ITGB1) is critically required for FL colonization as Itgb1 deficient HSCs and progenitors are not able to home into the FL. Interestingly, the role of ITGB1 is conserved in later developmental stages and also necessary for seeding adult hematopoietic tissues (Potocnik et al., 2000). Upon colonization, the increase in repopulating units (RUs) from ∼1-2RU in the E11.5 AGM to ∼60 RUs in the E12.5 FL (Rybtsov et al., 2014) and ∼1,000 RUs by E15.5 (Ganuza et al., 2020; Ema and Nakauchi, 2000), together with the fact that HSPCs are actively cycling in the FL (Bowie et al., 2006; Bowie et al., 2007; Morrison et al., 1995; Harrison et al., 1997; Rebel et al., 1996; Pietras et al., 2011; Bonkhofer et al., 2019) and the ability of FL-HSCs to outcompete BM-HSCs following transplantation into conditioned mouse recipients (Bowie et al., 2007; Rebel et al., 1996), led to the generally accepted view that HSCs rapidly expand in numbers in the FL based on symmetric cell division and that the FL constitutes and expansion niche (Ganuza et al., 2020; Ganuza et al., 2022b) (Figure 1). Challenging this view recent investigations, including ours, have disputed this dogma (Ganuza et al., 2020; Ganuza et al., 2022a; Patel et al., 2022; Ganuza et al., 2022b). Classical investigations only took into consideration the potential of transplanted embryonic tissues to repopulate conditioned recipients (Ganuza et al., 2020; Ganuza et al., 2022b). These studies did not investigate the actual fate of those HSCs and only measured their engraftment potential as a readout. Lineage-tracing studies employing multicolor Confetti allele and CRISPR/Cas9-based DNA barcoding allele to label hematopoietic progenitors during mouse ontogeny revealed that the number of hematopoietic progenitors with actual contribution into the adult HSC pool is much larger prior to EHT than previously assumed and that this pool modestly expands in the FL (Ganuza et al., 2022a; Patel et al., 2022; Ganuza et al., 2017) (Figure 1). This indicates that in the early FL (E12.5-E14.5), HSC precursors fated to contribute to the adult HSC pool are just not mature enough to repopulate the hematopoietic system of a myeloablated recipient at the moment of transplantation. But if they were to stay in the embryo they would have matured and acquired engraftment ability (Kieusseian et al., 2012). Thus, the increase in RUs observed from E12.5 to E15.5 is likely a result of the maturation of immature FL-HSC precursors rather than of a dramatic HSC symmetric expansion (Ganuza et al., 2020; Ganuza et al., 2022b). Further supporting this maturation process, employing explant-reaggregate cultures paired with limiting dilution transplantation, the Medvinsky’s laboratory reported that the number of HSC precursors (pre-HSC) in the AGM expands from E9.5 to E11.5 leading to 60 pre-HSCs at E11.5. This matches the total number of RUs in the E12.5 FL (Rybtsov et al., 2014), strongly indicating that the initial increase in RUs detected in the FL from E11.5 to E12.5 is due to HSC maturation rather than based on symmetrical HSC cell division. This further supports the role of the FL as a maturation niche (Ganuza et al., 2020; Ganuza et al., 2022b).
FL-HSCs and progenitors are actively dividing. Particularly the c-Kit+ HSPC population divides four times as a bulk population from E12.5 to E14.5 in vivo based on H2B-GFP-based tracking of cell divisions (Ganuza et al., 2022a). Yet, E14.5 FL-HSCs are biased to differentiate and to undergo asymmetric cell division in vitro rather than symmetrically dividing (Ganuza et al., 2022a). Thus, it is likely that in vivo most of these cell divisions do not result in the expansion of the FL-HSC pool with actual contribution to adult HSCs. Particularly, HSC and progenitors with contribution to the adult HSC pool only duplicates from E12.5-E15.5 as seen by multicolor-Confetti lineage tracing (Ganuza et al., 2022a). Interestingly, adult-fated HSCs minimally contribute to fetal hematopoiesis, which instead is mostly sustained by HSC-independent progenitors that establish a hierarchical hematopoietic structure in the FL (Yokomizo et al., 2022; Ulloa et al., 2021). In particular, lineage tracing in Tg(drl:creERT2;ubi:lox-GFP-lox-mCherry) reporter zebrafish indicated that definitive HSPCs negligibly participates in embryonic lymphomyelopoiesis (Ulloa et al., 2021) and depletion of HSCs via nitroreductase/metronidazole administration did not affect embryonic lymphomyelopoiesis (Ulloa et al., 2021) as previously observed in mice (Ganuza et al., 2022b; Chen et al., 2011). Likewise, lineage tracing in HlfcreERT2;ROSA26tdTomato mice also showed limited contribution of HSC to FL hematopoiesis (Yokomizo et al., 2022). Additionally, transcriptional profiling of the HSC ontogeny also supports the presence of important differences among FL and adult hematopoiesis (McKinney-Freeman et al., 2012). Overall, these new body of data is important to interpret the actual role of FL-HSC niche on HSC behavior and suggests that the FL HSC-niche only supports a moderate expansion of HSCs fated to contribute to the adult-HSC pool.
FL-HSPC niche components and experimental approaches
The adult liver is mainly composed of hepatocytes, cholangiocytes (biliary epithelial cells), ECs and multiple hematopoietic cell lineages (Fomin et al., 2017). In the FL, hematopoietic cells constitute the major cellular compartment, are scattered in the parenchyma and their presence strongly decrease during adulthood (Agrawal et al., 2024; Fomin et al., 2017). The human FL is also enriched in hepatoblasts (hepatocytic precursors that generate hepatocytes and cholangiocytes) (Wauthier et al., 2008). Fetal and adult hepatocytes exhibit many functional differences (Turner et al., 2007; Schmelzer et al., 2006; Schmelzer et al., 2007). FL-ECs include ECs lining lymphatic vessels, large blood vessels (i.e., portal veins and hepatic artery) and mostly sinusoidal ECs (Elvevold et al., 2008). Anatomically, FL-erythroid precursors localize close to hepatocytes and sinusoids (Orlic et al., 1982), while myeloid precursors associate to portal vascular structures (Kamps et al., 1989), and B-cell progenitors are found more dispersed in the parenchyma (Kamps and Cooper, 1982). Although mouse FL-HSPCs seem to associate with pericytes in portal vessels (Khan et al., 2016), the location of human HSPCs is incompletely understood (Fomin et al., 2017) (Figure 3).
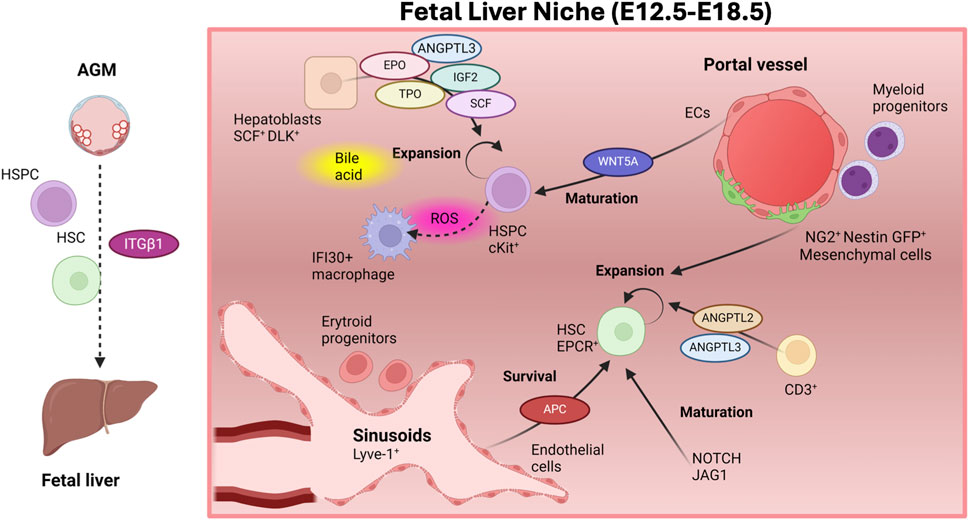
Figure 3. The fetal liver niche. Integrin-ẞ1 facilitates the migration of HSCs and immature HSC precursors from the AGM to the fetal liver (FL). In the FL, HSCs mature and expand in numbers, although the pool of HSCs with contribution to adult hematopoiesis moderately increases. HSCs are found close to sinusoids, where ECS sustain their survival by producing activated protein C (APC). Other FL niche components as CD3+ and NG2+ Nestin+ mesenchymal cells promote their expansion, while NOTCH activation is important in FL-HSC maturation. Endothelial cells in the portal vessels are involved in HSPCs maturation via activation of the WNT pathway. Many growth factors secreted by hepatocytes along with bile acid mediate the expansion of HSPCs. HSPCs are also found in close proximity to macrophages, which contribute to the scavenging of reactive oxygen species (ROS), produced by expanding HSPCs. Figure created with Biorender.
To identify specific cellular and molecular components of the FL-HSC niche, pioneering investigations derived FL stromal cell lines and assessed their ability on supporting HSC repopulating activity following their co-culture with HSCs as an indirect measurement of their role as HSC-niche components (Gao et al., 2018). FL-derived immortalized stromal cell lines show high heterogeneity in their hematopoietic supportive ability. Some of them, such as mesenchymal and epithelial cells, sustain the repopulating activity of human and mouse HSPCs, indirectly supporting their role in the FL-HSC niche (Moore et al., 1997a; Chagraoui et al., 2003; Moore et al., 1997b; Wineman et al., 1996; Nolta et al., 2002; Hackney et al., 2002). While FL stromal cells do not trigger HSC expansion, SCF+ DLK+ FL hepatoblasts yield a ∼20-fold expansion of mouse HSPCs in vitro after 2–3 weeks of co-culture. Hepatoblasts express many growth factors that support HSCs including angiopoietin-like 3 (ANGPTL3), insulin-like growth factor 2 (IGF2), thrombopoietin (TPO), erythropoietin (EPO) and stem cell factor (SCF or C-KIT-ligand) (Chou and Lodish, 2010; Chou et al., 2013; Zhang and Lodish, 2004; Sugiyama et al., 2011). SCF receptor (c-KIT) is required in FL hematopoiesis as shown in c-Kit deficient mice (Agrawal et al., 2024; Fantin et al., 2021). Additionally, FL sinusoidal ECs promote the survival of FL-HSCs via activated protein C (APC) production, which exerts an antiapoptotic effect on EPCR+ HSCs inducing protease-activated receptor 1 (PAR-1) mRNA expression in HSCs(Iwasaki et al., 2010). EPCR+ HSCs rapidly lose in vitro their long-term repopulation ability unless co-cultured with FL-Lyve-1+ endothelial cells, further implying a role of the sinusoidal niche in FL-HSC self-renewal activity (Iwasaki et al., 2010).
Investigations in the caudal hematopoietic tissue (CHT) in zebrafish, organ equivalent to the mammalian FL and where HSCs expand, uncovered various cellular components and chemokines in HSPC proliferation and CHT colonization, including ccl25b (Xue et al., 2017) (homolog to ccl21) and CXC chemokine ligand cxcl8 (Blaser et al., 2017). ccl25b expression in CHT endothelial cells (regulated by klf6a transcription factor) facilitates HSPC lodgement and proliferation via ccr7 (Xue et al., 2017). The CCL21/CCR7 interaction also allows mammalian HSPC expansion in vitro (Xue et al., 2017). cxcl18 and its receptor cxcr1 are both produced by CHT endothelial cells. Interestingly, cxcl18 activates cxcr1, which stimulates cxcl12 production on CHT ECs, promoting HSPC to colonize the CHT via increased “endothelial cuddling” of HSPCs (Blaser et al., 2017). In this process, ECs surround a single HSPC bound to a mesenchymal stromal cell. This orients the cell division of the HSPCs as shown by live imaging (Tamplin et al., 2015). Likewise, CXCL12 is critical in many aspects of adult HSC biology such as migration, niche retention and proliferation/quiescence (Zhang et al., 2016).
More recently, the use of algorithms aimed to detect cell interactions based on the expression of ligand-receptors among interacting cells and multiplexed fluorescence in situ hybridization methods further exposed the interaction among ECs and HSPCs both in the zebrafish CHT (Xue et al., 2019) and in the mouse FL (Lu et al., 2021). Molecularly, these studies highlight that integrin signaling and Smchd1 co-regulate both HSPCs and neighboring cells in the CHT (Xue et al., 2019), whose relevance will need to be evaluated. Additionally, at least one EC seems to be in direct contact with each HSC in the mouse FL (Lu et al., 2021). Among these mouse FL ECs, both arterial ECs and sinusoidal ECs were found as part of the HSC-niche (Lu et al., 2021). The role of ECs in the human FL-HSPC niche seems preserved as co-culture of human FL-ECs with FL-derived immature CD43+CD45− HSPCs support their maturation into CD45+CD34+ HSPCs. Meanwhile, ECs derived from other tissues do not support HSPC maturation, suggesting a specific role for the FL-endothelium, which is mediated by endothelial-produced WNT5A (Choi et al., 2021). In this context, ATF4 transcription factor was shown to play a role in the FL-niche both in stromal and ECs as Atf4−/− FL stromal and ECs lack the ability to sustain HSC repopulating activity in vitro. This may be mediated by their inability to trigger Angptl3 cytokine expression, a positive regulator of HSC activity (Zhao et al., 2015).
In vitro co-culture experiments also exposed a role for CD3+ hematopoietic FL cells in the HSPC-niche as they facilitate HSC expansion (Zhang et al., 2006). CD3+ cells produce high levels of Angptl2 and Angptl3 (Zhang et al., 2006). Culture of HSCs with ANGPTL2 and ANGPTL3 together with other saturating factors allows a ∼20–30-fold expansion of LT-HSCs (Zhang et al., 2006) reinforcing the role of FL-CD3+ hematopoietic cells in HSC expansion.
Overall, in vitro co-culture studies indirectly implicated multiple FL HSPC-niche components. Yet, this approach fails to provide information on actual cell-contact or proximity. Here, other methods allowing spatial analyses, such as immunohistochemistry (IHC) and immunofluorescence (IF), offer key information on niche composition. Spatially, mouse EPCR+ HSCs and CD150+CD48−Lin− lie close to Lyve-1+ FL sinusoids (enriched for APC and extracellular matrix, ECM), indicating that FL-HSCs reside in a perisinusoidal FL niche (Gao et al., 2018; Iwasaki et al., 2010; Lee et al., 2021) (Figure 3). Analogously, in zebrafish, HSPCs localize with vascular ECs as shown by time-lapse imaging (Xue et al., 2017). More recently, rare perivascular NG2+ Nestin-GFP+ mesenchymal stem cells, which associate with the portal vessel in the FL, were shown to support HSCs in the FL-niche (Khan et al., 2016). These FL Nestin-GFP+ cells, transcriptionally similar to adult BM Nestin-GFP+ but more proliferative, expand in number from E12 to E14.5. In vivo depletion of these cells, via specific diphtheria toxin expression restricted to NG2+ cells in NG2-Cre/iDTA mice, decreases HSC numbers in E14.5 FL demonstrating a regulatory role of Nestin+ cells at this developmental stage (Khan et al., 2016).
As a key approach to investigate HSC niche components (Sanchez-Lanzas et al., 2022), knockout murine models perturbing the numbers of candidate niche components and deleting critical factors (e.g., SCF, critical regulator of adult HSC activity), from candidate niche cells have supported the relevance of various FL components as integral part of the FL-HSC niche. Particularly, loss of hepatoblasts in Map2k4 null embryos reduced the levels of HSC-supportive cytokines and compromised HSPC proliferation (Sugiyama et al., 2013). Additionally, deletion of Scf from hepatic stellate cells and simultaneous deletion of Scf from ECs and hepatic stellate cells reduced or completely depleted HSCs from the FL, respectively (Lee et al., 2021), supporting their role in the FL-perisinusoidal vascular HSC niche. In contrast, conditional deletion of Scf from hepatocytes, hematopoietic cells, NG2+ cells or ECs did not perturb the number and function of HSCs (Lee et al., 2021). Reinforcing the role of FL-ECs in the FL HSPC-niche, depletion of membrane-bound SCF from the FL niche by the specific inactivation of Ezh2 in FL-ECs results in embryonic lethality of Ezh2 null embryos (Neo et al., 2018). Mechanistically, Ezh2 deletion triggers MMP9 expression depleting membrane-bound SCF (Neo et al., 2018). Furthermore, in Itgav and Postn-deficient mice FL-HSCs proliferate faster yielding an enlarged pool of FL-HSCs. POSTN expression is mainly localized to the FL vascular endothelium, supporting its role in controlling the HSC pool size (Biswas et al., 2020). These data also highlight an inhibitory role of the Periostin/Integrin-αv pathway on the proliferation of FL-HSCs.
In the FL, HSPCs exhibit an increased protein synthesis rate (Agrawal et al., 2024). In this scenario, properly regulating endoplasmic reticulum (ER) stress is critical to maintain HSC function as ER stress is triggered by the accumulation of misfolded proteins (Miharada et al., 2014). In the FL, HSPCs take advantage of the presence of FL bile acids (BAs, synthesized from cholesterol) to act as chemical chaperones and inhibit protein aggregation (Sigurdsson et al., 2016). Accordingly, reduced BA levels in Cyp27a1−/− mice (compromised in their ability to synthesize cholesterol) trigger ER stress and reduces the number of FL-HSCs in vivo, demonstrating that BAs are critical in the expansion of the FL-HSPC population (Sigurdsson et al., 2016). These data highlight an indirect role of the FL cells implicated in BA metabolism, including hepatoblasts and hepatocytes, in HSC maintenance.
Additionally among FL hematopoietic cells, FL macrophages, which promote erythrocyte maturation in the developing FL (Saffarzadeh et al., 2020), were recently shown to interact with HSPCs in the FL via spatial proteomics coupled with single-omics (Kayvanjoo et al., 2024). In the human FL, HSPCs are also found in close proximity to IFI30+ macrophages (Cacialli et al., 2021) (Figure 3). Interestingly, ifi30, expressed by the vascular CHT niche in zebrafish, contributes to recycling reduced glutathione (GSH) (Cacialli et al., 2021). GSH is critical to scavenge reactive oxygen species (ROS) produced by HSPCs expanding in the CHT (Cacialli et al., 2021). Connexin channels (e.g., Cx41.8) in HSPCs enable this process (Cacialli et al., 2021). Additionally, CCR4 ligands CCL17 and CCL22 chemokines are highly expressed in hematopoietic cells in the E12.5 FL in mice (Konno et al., 2020). They likely play a role in the migration and retention of HSPCs in the FL as in utero administration of anti-CCR4 neutralizing antibody in pregnant mice compromises the number of FL-HSPCs (Konno et al., 2020). More recently, single-cell analyses combined with spatial transcriptomics identified a mouse FL-HSC niche composed of hepatoblasts, stromal cells, ECs, and macrophages supporting HSC/MPPs (Agrawal et al., 2024; Gao et al., 2022). Furthermore, many hematopoietic cells in the FL, including HSCs, produce JAG1, a major NOTCH ligand whose expression is lost in adult BM. Jag1 deficient FL-HSCs exhibit compromised engraftment ability and downregulation of important hematopoietic factors (e.g., Gata2, Mllt3, and HoxA7) indicating that JAG1 mediated NOTCH activation is important in FL-HSC maturation (Shao et al., 2023). Additionally, mice lacking the Notch1 transcriptional activation domain and Rbpj deficient mice show lower numbers of HSCs at E14.5 even though they exhibit no defects during specification and migration to the FL (Gerhardt et al., 2014). Thus, in the FL HSPC-niche, NOTCH signaling pathway, which it is required iteratively at different stages of developmental hematopoiesis, also plays a role in FL-HSPC expansion and/or maturation.
Fetal spleen: limited supportive niche activity for HSCs
Synchronously to the FBM, the fetal spleen starts to receive HSCs from the FL at E15.5 as shown by repopulating activity detected at E15.5 which increases until E17.5 and remains detectable over the first couple of weeks post-birth (Christensen et al., 2004; Morita et al., 2011; Ikuta and Weissman, 1992; Wolber et al., 2002). The specific role of these HSC reservoir in the spleen is unclear but splenectomy in neonatal mouse pups does not result in a reduction of HSC numbers in the perinatal BM, indicating that the spleen is not an intermediate migration location for HSCs before moving to the BM (Bertrand et al., 2006). Furthermore, organ culture systems of fetal spleens are not capable of sustaining HSC repopulating activity following in vitro culture (for 4 days) while FL can (Bertrand et al., 2006). Additionally, spleen-derived stromal cell lines support myeloid differentiation but not HSC repopulating activity indirectly indicating that the fetal spleen niche is less supportive than the FL niche (Bertrand et al., 2006).
Fetal bone marrow: colonizing the final destination
The adult BM has been widely investigated over the last two decades. A large body of studies have shown that HSCs reside mostly in perivascular niches, both arteriolar and sinusoidal, during homeostasis in the adult BM (Sanchez-Lanzas et al., 2022; Morrison and Scadden, 2014; Lucas, 2017). Adult HSC niche components include sympathetic nerves, osteoblasts, stromal cells such perivascular stromal cells and sinusoidal endothelium, and hematopoietic cells including macrophages, megakaryocytes, T-regulatory cells and neutrophils (Sanchez-Lanzas et al., 2022; Casanova-Acebes et al., 2013; Nakamura-Ishizu et al., 2015; Fujisaki et al., 2011). Among stromal cells, leptin receptor LepR+ reticular cells and vascular ECs produce and secrete SCF which activates the c-KIT tyrosine kinase receptor in HSCs, key in regulating the self-renewal and maintenance of HSCs (Pinho and Frenette, 2019; Crane et al., 2017; Ramasamy et al., 2016; Mendez-Ferrer et al., 2020; Batsivari et al., 2020; Chen Q. et al., 2019; Liu et al., 2021; Ding et al., 2012; Comazzetto et al., 2019). Additionally, LepR+ cells are a source for stromal derived factor-1 (SDF1), also known as CXCL12, a chemokine critically required for homing, trafficking and maintenance of HSCs by signaling through the G-protein coupled receptor CXCR4 in HSCs (Greenbaum et al., 2013; Sugiyama et al., 2006; Lo et al., 2009). Other relevant signals and cytokines produced in the adult perivascular BM microenvironment include NOTCH ligands, Interleukin-7 (IL7), vascular endothelial growth factor (VEGF) and Angiopoietin-1 (AGPT1) (Fang et al., 2020; Tikhonova et al., 2019; Kusumbe et al., 2016; Poulos et al., 2013; Cordeiro Gomes et al., 2016; Zhou et al., 2015).
The FBM has been much less examined. Importantly, recent studies underline the presence of substantial differences among the adult and FBM both on the cellular components and at the molecular level (Hall et al., 2022; Langen et al., 2017) which have major functional implications on the behavior of the HSPCs.
The early FBM: migration into an “inhibitory” environment
The FBM stroma contains osteoprogenitors, chondrocytes, fibroblasts, pericytes and endothelial cells (Hall et al., 2022). But in contrast to the adult BM, the E16.5-P0 FBM (i.e., between E16.5 and day 0 post-birth, P0) does not seem to harbor PDGFRα+SCA-1+ mesenchymal stem cells (MSCs) and CXCL12-abundant reticular (CAR) MSCs, critical components in the adult HSC-niche which also produce ANGPT1 and SCF (Hall et al., 2022) (Figure 4).
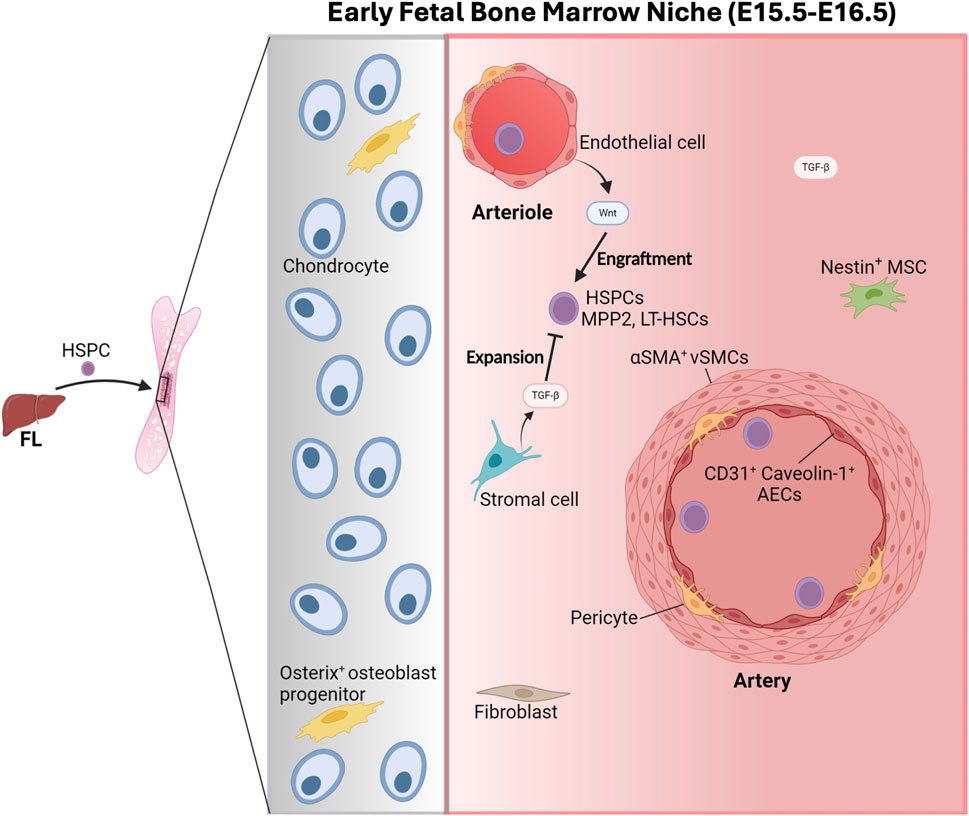
Figure 4. The early fetal bone marrow niche. At E15.5, the hematopoietic stem and progenitor cells (HSPCs) initiate the colonization of the recently vascularized cartilaginous femurs from the fetal liver (FL). The fetal bone marrow (FBM) is composed of chondrocytes, Osterix+ osteoblast progenitors, fibroblasts, Nestin+ mesenchymal stem cells (MSCs), pericytes and endothelial cells that support and regulate the functions of the HSPCs, mainly encompassing multipotent progenitor 2 (MPP2) and long-term hematopoietic stem cells (LT-HSCs). The secretion of WNT factors by arteriolar endothelial cells promotes the engraftment of HSPCs in the FBM. At E16.5, HSPCs are located preferentially with CD31+ Caveolin+ arterial endothelial cells (AEC), which, together with a-smooth muscle Actin+ vascular smooth muscle cells (aSMA+ VSMCs) originate arteries. The production of transforming growth factor beta (TGF-B) by stromal cells inhibits HSPC expansion. Figure created with Biorender.
Developmentally, coinciding with the vascularization of the femurs at E15 (Langen et al., 2017), HSPCs start to migrate from the FL and colonizing the BM around E15.5, as shown by the presence of HSC clonogenic potential (Gekas et al., 2005) and long-term multilineage repopulating activity, although at very low frequency (Christensen et al., 2004; Hall et al., 2022; Coskun et al., 2014). Arteriolar ECs facilitate the engraftment of HSPCs in the FBM by the secretion of WNT factors (Liu et al., 2022) (Figure 4). HSPCs keep translocating from the FL to the FBM until birth concomitant with a sustained decrease in the number of HSPCs in the FL from E15.5 to birth (Khan et al., 2016), while in the FBM the number of phenotypic long-term HSCs increases from ∼60 at E15.5 to ∼4,800 at P2 (Hall et al., 2022). In parallel, the number of Endomucin+ (EMCN+) vessels in the primary ossification centre of fetal femurs expands quickly from E15 (Langen et al., 2017) together with the emergence of CD31+ Caveolin-1+ arterial endothelial cells (AEC) and α-Smooth Muscle Actin+ (αSMA+) vascular smooth muscle cells leading to artery formation (Langen et al., 2017; Liu et al., 2022).
Intriguingly, recent investigations highlight critical differences among the HSPCs located in the FL and those in the FBM (Hall et al., 2022). The FL is mostly occupied by multipotent progenitors (MPP) type 3 (MPP3) and MPP4 until P6, when MPP3 accumulate; while MPP2s dominate in the FBM until birth when MPP3/MPP4s become prevalent (Hall et al., 2022). FBM HSPCs, including MPP2s and LT-HSCs, are vastly devoid of functional hematopoietic activity based on the lack of hematopoietic progeny following transplantation or in vitro clonogenic activity (Hall et al., 2022), which it is in sharp contrast with developmentally similar FL-HSPCs, suggesting the presence of “extrinsic” specific niche signals modulating HSC activity in the BM (Hall et al., 2022). Interestingly, these differences are present until birth (Hall et al., 2022). Accordingly, in contrast to FL-HSPCS, FBM HSPCs do not express stem cell programs which only become active perinatally. Moreover, the E16.5 FBM is devoid of niche cells that produce factors supportive of HSPCs (Hall et al., 2022). For instance, Nestin+ MSC cells present in the FBM produce lower levels of CXCL12 and other factors than adult BM stromal cells (Isern et al., 2014).
RNA-magnet based predictions (which consider the expression of known ligand-receptor pairs on interacting cells) of the HSC-niche interactome at E16.5 failed to identify significant cellular interactions, suggesting that LT-HSCs does not occupy a physically defined niche (Hall et al., 2022). However, immunostainings revealed that LT-HSCS and c-KIT+ HSPCs locate preferentially with Caveolin+ AECs at E16.5 (Liu et al., 2022), indicating a role of AECs in the colonization of the FBM.
Additionally, Osterix+ osteoblast progenitor cells, which are key in the adult HSC niches, are likely also important in the early FBM niche. Particularly, Osterix+ cells (also expressing Pdgfrb and Foxc1) are present in the E16.5 BM (Omatsu et al., 2014). Importantly, the E17.5 Osterix −/− FBM exhibits normal vasculature but does not harbor osteolineage cells and lacks ossification and long-term HSC repopulating activity, supporting the role of Osterix+ cells in the early FBM niche (Coskun et al., 2014).
Interestingly, three subclusters of LT-HSCs were identified at E16.5 including: migrating, T-cell producing, and inflamed-LT-HSCs which may comprise early thymic progenitors (ETPs) (Hall et al., 2022; Cumano et al., 2019). Although technically challenging it would be interesting to know if they occupy different specific niches in the E16.5 FBM that may determine their fate. Notably, the E16.5 FBM stromal cells are enriched for TGF-β production, with inhibitory effects on HSPC expansion (Hall et al., 2022; Blank and Karlsson, 2015). The presence of these inhibitory signals to LT-HSC and MPP2 proliferation likely hinders the function of E16.5 HSPCs (Hall et al., 2022).
The late FBM
Even though at E15.5, HSCs already started migrating to the FBM, only a small fraction of the phenotypic FBM-HSC are transplantable at E18.5 (∼%5 of HSCs in the long bones) (Medvinsky et al., 2011; Bowie et al., 2006; Mikkola and Orkin, 2006; Lessard et al., 2004; Benz et al., 2012; Qian et al., 2007). By E18.5 the FBM still harbors lower number of myeloid and megakaryocyte progenitors than the adult BM and although it contains osteolineage cell populations, it lacks LepR+ cells (Liu et al., 2022) (Figure 5). Osteolineage cells are initially detected perinatally (E18.5-P0) when the BM starts to transition from a cartilaginous BM (Hall et al., 2022; Hall and Miyake, 2000; Olsen et al., 2000; Matsushita et al., 2021). LepR+ BM cells initially locate to the metaphysis until they expand and distribute all over the adult BM (Zhou et al., 2014; Mizoguchi et al., 2014; Pineault et al., 2019; Shu et al., 2021; Matsushita et al., 2022). Various studies place quiescent adult LT-HSCs in the proximity of LepR+ cells in sinusoids vessels in the adult BM (Christodoulou et al., 2020) (Figure 5). Thus, the lack of these cells in the FBM likely has functional effects on LT-HSCs. As suggested by Liu and colleagues it would be interesting to unveil the molecular and cellular mechanisms that drive the migration of LT-HSCs from their arterial position in the FBM to sinusoid locations in the adult BM (Liu et al., 2022). A COL3A1high BM stromal cell population (also expressing Decorin, Gsn/Clec3b and Col1a1 and Pdgfra mesenchymal markers) constitutes the predominant stromal population in the E18.5 FBM (Liu et al., 2022). STAB2high sinusoidal ECs, with a role in adult hematopoiesis (Tikhonova et al., 2019), were also detected in E18.5 FBM. The expression of classical niche supportive factors in E18.5 FBM is low in stromal cells and equivalent in AECs compared to the adult BM (Liu et al., 2022). At E18.5, c-KIT+ HSPCs still concentrate in the diaphysis of the FBM and actively proliferate in proximity to AECs (Liu et al., 2022). AECs in the E18.5 FBM produce WNT2 which can induce “β-catenin-dependent proliferation of fetal HSPCs” (Liu et al., 2022). Importantly, genetic inactivation of WNT secretion in Wntless knockout mice blocks HSPC expansion, as shown by reduced numbers of HSCs in E18.5 Wntless−/− embryos (Liu et al., 2022). E18.5 AECs also produce Cxcl12, Kitl, Jag1, Jag2, Dll4, Vegfc and Tgfb2, all of them classic adult BM HSC-niche factors. Overall, available data supports a role for AECs in the E18.5 FBM (Pinho and Frenette, 2019; Fang et al., 2020; Tikhonova et al., 2019; Xu et al., 2018; Guo et al., 2017). Additionally, the E18.5 FBM contains an osteochondral progenitor population (characterized by high expression of Sox9, Col2a1 and Sparc). This population lacking expression of classical HSC maintenance factors, was predicted to interact with LT-HSCs based on RNAmagnet cell-to-cell interactions (Hall et al., 2022). By P0, both ECs and osteochondral populations were also predicted to be part of the HSC-niche (Hall et al., 2022). Interestingly these osteochondral progenitor populations and AECs produce IGF1 and IGF2, which can bind IGF1R receptors expressed by HSPCs at this developmental stage. Importantly, IGF1 is a critical regulator of adult HSC function (Hall et al., 2022; Young et al., 2021). Supporting the role of IGF1 in the perinatal HSC-niche, P0 BM-derived mesenchymal stromal cells are more efficient in supporting LT-HSCs repopulating activity in vitro than those derived from E16.5 and E18.5 (Hall et al., 2022). Other additional niche factors reported in the E18.5 FBM include fibroblast growth factor 2 (FGF2) and TNFSF9 which support HSPC expansion and stem cell self-renewal, respectively (Itkin et al., 2012; Kirouac et al., 2010) (Figure 5). Interestingly, a late gestational type I interferon pulse has been reported to promote the gradual transition of HSCs from fetal to adult state (Li et al., 2020). This pulse promotes the proliferation of HSPCs, enhances Major Histocompatibility I gene expression, potentially masking HSPCs from T cell-mediated destruction, and sensitizes them to FLT3ITD mediated transformation (Li et al., 2020). The progressive and asynchronous nature of this transition results in cellular heterogeneity among HSPCs (Li et al., 2020).
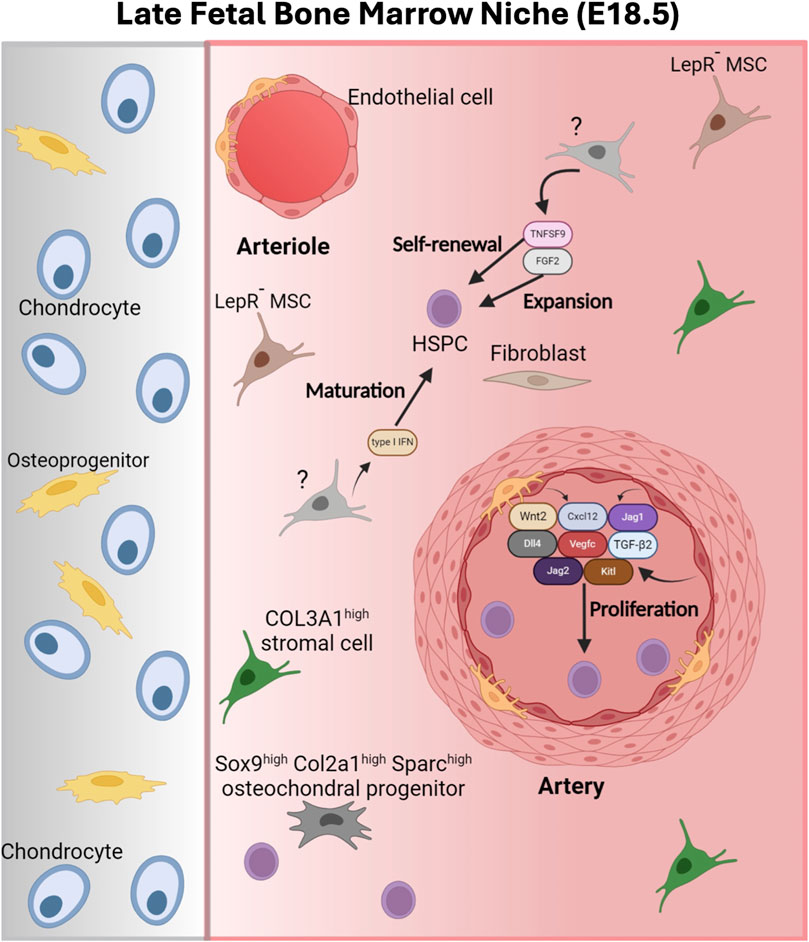
Figure 5. The late fetal bone marrow niche. The E18.5 FBM is characterized by the presence of Col3a1high stromal cells, Sox9high Col2a1high Sparchigh osteochondral progenitors and the absence of leptin receptor+ (Lepr+) MSCs. HSPCs are found in the proximity of AECS that produce supportive BM niche factors such as C-X-C motif chemokine 12 (Cxcl12), Delta-like canonical Notch ligand 4 (DI14), Jagged canonical Notch ligand 1 and 2 (Jag1/Jag2), Kit ligand (Kit), transforming growth factor beta 2 (Tgfb2), vascular endothelial growth factor (Vegfc) and Wnt2. Additional niche factors found in the E18.5 FBM include fibroblast growth factor 2 (FGF2) and tumor necrosis factor ligand superfamily member 9 (TNFSF9), which support HSPC expansion and stem cell self-renewal, respectively. A pulse of type I interferon (IFN) promotes the maturation of HSPCs to the adult state. Figure created with Biorender.
Facing a “new world”: changes in the bone marrow during birth
Around birth, the downregulation of several niche factors from FL stellate cells coincides with enhanced migration of HSCs to the BM (Lee et al., 2021). Intriguingly, there is a burst in the number of FL-HSPCs at birth, which declines by P2 in the mouse embryo (Hall et al., 2022). By P14, HSPCs are basically absent in the liver (Hall et al., 2022). Simultaneously, it is only near birth, by E19, when FBM HSPCs become fully functional and exhibit a molecular identity close to adult BM HSCs (Hall et al., 2022). This correlates with a shift in the relative frequencies and numbers of the various HSPCs and the development of a supportive BM niche, as revealed by changes in the ability of mesenchymal stromal cell derived from P0 BM to sustain LT-HSCs expansion (Hall et al., 2022), as mentioned above. However, even though the neonatal BM starts to contain niche cells expressing factors that support HSPCs, these factors are different from those present in the adult BM, emphasizing critical differences among perinatal and adult BM(185) (Figure 6). Particularly, although Cxcl12+ cells are present in the perinatal BM, they produce lower CXCL12 levels than the adult BM Cxcl12+ cells (Omatsu et al., 2014). Likewise adult-like CXCL12-abundant reticular (CAR) cells are absent from the perinatal BM (Hall et al., 2022). As aforementioned, a subtype of osteochondral progenitors supports perinatal HSPC functions by producing IGF1/2 (Hall et al., 2022) (Figure 6). It would be interesting to understand the molecular mechanisms that drive and defines these changes in the postnatal bone marrow.
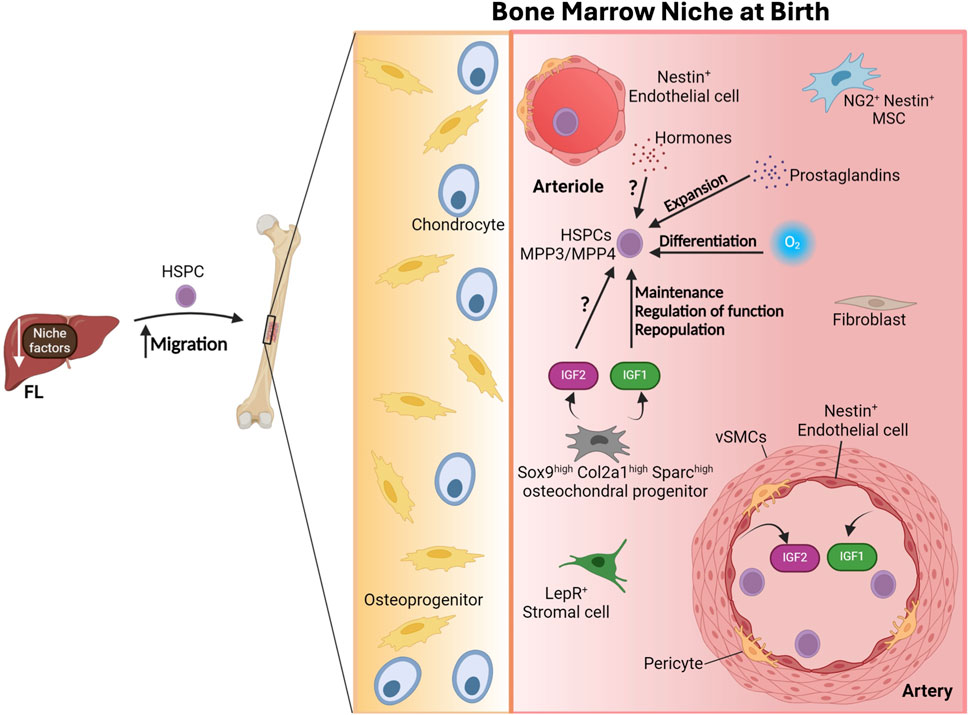
Figure 6. The bone marrow niche at birth. The downregulation of niche factors from the fetal liver (FL) results in the enhanced migration of hematopoietic stem and progenitor cells (HSPCs) to the bone marrow at birth. Multipotent progenitor type 3 and 4 (MPP3/MPP4) become the prevalent population of HSPCs. Neurogenin-2 (NG2+) Nestin+ mesenchymal stem cells (MSCs), leptin receptor (LepR+) stromal cells and Nestin+ endothelial cells are present in the natal bone marrow. Sox9high Col2a1high Sparchigh osteochondral progenitors and arterial endothelial cells (AECs) produce insulin-like growth factor 1 and 2 (IGF1 and IGF2) crucial in regulating HSPC function. Other environmental factors that impact and modulate HSPC dynamics include exposure to hormone fluctuations, prostaglandins and different oxygen levels. vSMC, vascular smooth muscle cell. Figure created with Biorender.
It is worth highlighting that at birth mammalian newborns are exposed to major environmental changes including significant hormonal fluctuations during labor and exposure to microbes and higher oxygen levels when they abandon sterile conditions in the uterus (Hall et al., 2022). Importantly, all these factors are known to impact hematopoiesis. Particularly, ambient oxygen levels cause HSC differentiation in vitro (Mantel et al., 2015). Furthermore, the composition of the gut microbiota plays a role in hematopoiesis (Dominguez-Bello et al., 2010; Josefsdottir et al., 2017), thus it is likely that the changes in the microbiome of the newborn shapes the HSC-niche. Moreover, as noted by Hall and colleagues (Hall et al., 2022), prostaglandins are upregulated during birth and they have been shown to modulate HSC expansion (Ratajczak and Muglia, 2008; North et al., 2007; Porter et al., 2013). Hence, it is very conceivable that they also influence HSC biology and the BM niche at this stage.
HSC expansion in the postnatal bone marrow and quiescence shift
As FL-HSCs migrate to the FBM, HSC numbers increase until they reach a plateau around 3–4 weeks post-birth in the mouse. Importantly, HSCs are highly proliferative during the first 3 weeks post-birth in the neonatal BM before they undergo a quiescence shift that characterizes the adult state (Bowie et al., 2006). By 4 weeks, considering that the total number of BM nucleated cells is ∼2 × 108 in a mouse and that HSC frequency is 1/10,000 per nucleated BM cells (Kiel et al., 2005; Trevisan et al., 1996; Sudo et al., 2000; Sieburg et al., 2006; Szilvassy et al., 1990; Boggs et al., 1982), a mouse harbors a total of ∼20,000 HSCs. The cellular mechanisms driving this increase in the pool of HSCs are incompletely understood and relevant to regenerative medicine. The specific weight that maturation, symmetric versus asymmetric cell division, differentiation and apoptosis have in this process requires further investigation (Ganuza et al., 2020).
Lineage tracing studies tracking the global clonal complexity dynamics of hematopoietic progenitors exposed that the number of HSCs with lifelong contribution to the HSC pool expands by ∼2 fold from birth to P21 when the quiescence shift happens in mice (Ganuza et al., 2022a; Bowie et al., 2006) (Figure 1).
Similar dynamics have been detected in human based studies. Analysis of the effect of age in X-chromosome inactivation patterns in healthy women (Catlin et al., 2011) and in telomere lengths in granulocytes and lymphocytes in healthy individuals (Werner et al., 2015; Busque et al., 1996; Busque et al., 2012), showed that HSC numbers mostly expand from birth until adolescence and divide once every 40 weeks (Catlin et al., 2011) with a progressive bias towards the accumulation of HSPCs with myeloid potential with age (Werner et al., 2015). Whole genome sequencing of clones of expanded human BM-HSPCs and reconstruction of phylogenetic trees based on the analysis of the natural accumulation of somatic mutations allowed to investigate the clonal complexity origins and lineage relationships in the hematopoiesis system in humans (Lee-Six et al., 2018; Osorio et al., 2018). These studies revealed that between 50,000 and 2,00,000 clones participate in adult steady-state hematopoiesis in humans (Lee-Six et al., 2018), compatible with clonal dynamics observed in mice (Ganuza et al., 2019). Furthermore, these analyses indicated that most of the expansion in the HSC pool occurs during childhood and adolescence (Lee-Six et al., 2018). In agreement with a quiescence shift at that stage, HSC numbers would stabilize in the adulthood (Lee-Six et al., 2018). These studies also predict the presence of HSPC clones with persistent myeloid and B-lymphocyte in life, while dynamics on T-lymphoid potential were not as conclusive (Lee-Six et al., 2018; Osorio et al., 2018).
The expansion of the HSC pool may be allowed by “the development of the BM vasculature and adult niches” (Gao et al., 2018; Arai and Suda, 2007; Wilson et al., 2008) (Figure 7). In this regard, scRNA sequencing revealed that the frequencies of LepR+ stromal cells and ECs (arteriolar and sinusoidal) increase during the first weeks post-birth (analyzed by Kara et al. perinatally at P4, P14 and at 8 weeks post-birth) (Kara et al., 2023). These changes are accompanied by variations in the HSC-supportive ability of various populations (Kara et al., 2023). Functionally, confirming a role of Nestin+ cells and CXCL12 in the early postnatal BM niche, deletion of Cxcl12 in Nestin-CRE-ERT Cxcl12 floxed mice at P7 depletes HSCs (Isern et al., 2014), while Cxcl12 and Scf deletion from Nestin+ cells during adulthood does not perturb HSC numbers (Ding et al., 2012; Ding and Morrison, 2013). Likewise, Cxcl12 deletion from NG2+ cells at P14 depletes the HSC pool while its deletion during adulthood does not impact HSCs (Acar et al., 2015; Asada et al., 2017). Interestingly, Scf deletion from perinatal Nestin+ cells does not carry any defect (Kara et al., 2023). In the postnatal BM the patterns of expression of key adult BM factors are similar to those found in adult BM (Tikhonova et al., 2019; Baryawno et al., 2019; Baccin et al., 2020; Zhong et al., 2020; Matsushita et al., 2020). Particularly, perinatal BM LepR+ cells express the highest levels of Cxcl12, and both perinatal LepR+ and ECs exhibit the highest Scf levels, while Nestin+ and NG2+ cells do not constitute a main source of SCF (Kara et al., 2023). Interestingly, most ECs express Nestin in the perinatal BM (Mizoguchi et al., 2014; Ono et al., 2014) which becomes restricted to periarteriolar ECs and other stromal cells in the adult (Asada et al., 2017; Kunisaki et al., 2013). Notably, perinatal LepR+ cells-produced SCF promotes myelopoiesis and erythropoiesis but they are not required in HSC maintenance as shown by inducible conditional mouse models (Kara et al., 2023). Meanwhile, the use of Tie2-cre; Scf-Ex7fl/fl mice, which enables to delete the transmembrane domain of SCF from ECs (Buono et al., 2016), showed that membrane-bound SCF in ECs is required to maintain the HSC pool in the perinatal BM (Kara et al., 2023). Still, this does not exclude that soluble SCF-produced by ECs plays also a role (Kara et al., 2023). Among adult BM-ECs, SCF is mostly expressed by arteriolar ECs (Tikhonova et al., 2019; Liu et al., 2022; Xu et al., 2018; Baryawno et al., 2019), whereas at P4, sinusoidal ECs also produce it (Kara et al., 2023). Confocal imaging of α-CatulinGFP/+ mice showed that, like in the adult BM, HSCs concentrate mostly in sinusoidal blood vessels in the perinatal BM (P4 and P14) (Kara et al., 2023). A small fraction of HSCs also locates to transition zone vessels, which exhibit “intermediate properties between sinusoids and arterioles” (Kara et al., 2023) (Figure 7). This may be reminiscent of the ongoing migration of HSCs from their arterial location in the FBM to sinusoids during adulthood (Liu et al., 2022).
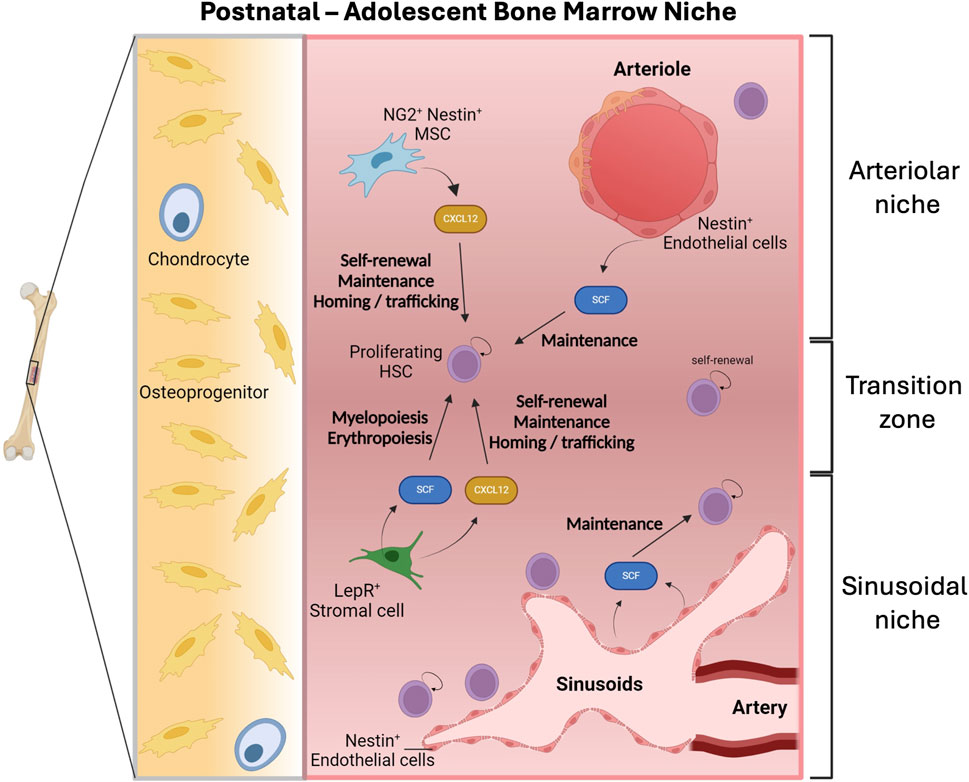
Figure 7. The postnatal adolescent bone marrow niche. Hematopoietic stem cells (HSCs) are highly proliferative in the neonatal bone marrow (BM) and their expansion might be associated with the development of the BM vasculature. Post-natal HSCs concentrate predominantly in sinusoidal blood vessels where Nestin+ endothelial cells produce stem cell factor (SCF). Additionally, the production of SCF by leptin receptor (LepR+) stroma cells promotes myelopoiesis and erythropoiesis. Both neurogenin-2 (NG2+) Nestin+ mesenchymal stem cells (MSC) and LepR+ stroma cells express C-X-C motif chemokine 12 (CXCL12) that is involved in processes such as self-renewal, maintenance, homing and trafficking of HSCs. Figure created with Biorender.
Molecularly, important metabolic, transcriptional and epigenetic differences have been reported among FL-HSC an adult-BM HSCs which are likely linked to their proliferative stage and transition into quiescence (Manesia et al., 2015; Jassinskaja et al., 2017; Roy et al., 2021; Manesia et al., 2017). FL-HSCs contain more mitochondria, express higher levels of genes implicated in oxidative phosphorylation (OxPhos) and citric acid cycle (TCA), consume higher oxygen and produce increased ROS levels than BM HSCs (Agrawal et al., 2024; Manesia et al., 2015). Interestingly, adult HSCs display a larger collection of proteins involved in the protection against ROS-induced protein oxidation that help to preserve HSC stemness in the long-term (Jassinskaja et al., 2017). Inflammatory signaling is also upregulated in adult HSCs (Roy et al., 2021). Remarkably, Sox17 is expressed in fetal and perinatal HSCs until ∼4 weeks post-birth concomitant with the quiescence shift, and conditional knockout of Sox17 leads to the loss of fetal HSCs but does not impact adult HSCs when deleted later in development. This underscores the critical role of Sox17 in fetal HSCs (Kim et al., 2007).
Epigenomic analyses revealed that even though chromosomal compartments and topologically associating domains (TADs) are broadly conserved between fetal and adult HSCs, there is still an increased compartmentalization in the chromosomal compartments and more dynamic chromatin interactions within TADs in adult HSCs (Chen C. et al., 2019). This affects thousands of interactions among gene promoters and enhancers. These changes are driven by particular transcription factors, including TCF3 and MAFB in fetal HSCs and NR4A1 and GATA3 in adult HSCs (Chen C. et al., 2019).
Overall, these investigations emphasized major molecular and functional differences among fetal and adult HSCs which are highly determined and regulated by developmentally distinct HSPC niches.
Discussion and future perspectives
From their emergence in the major embryonic arteries, HSCs and their precursors travel over multiple locations that initially allow their specification and later shape their maturation and expansion. Those HSC microenvironments provide a variety of signals that enable the fine-tuning of HSC development by temporally and spatially confining specific developmental stages, for instance by activating BMP and NOTCH signaling before EHT and blocking it afterwards to allow HSC maturation (Souilhol et al., 2016a; Richard et al., 2013; Pouget et al., 2014; Souilhol et al., 2016b; Porcheri et al., 2020; Lizama et al., 2015; Ditadi et al., 2015; Kobayashi et al., 2014; Gama-Norton et al., 2015; Sacilotto et al., 2013). Interestingly, similar pathways are iteratively employed at different developmental stages. NOTCH signaling, required during HSC specification in the AGM and HSC expansion in the FL, constitutes a clear example (Clements and Khoury, 2024; He et al., 2015; Gerhardt et al., 2014). Importantly, during the migration of HSCs and progenitors between embryonic/fetal locations, the presence of adequate niche space to allocate migrating HSCs can constitute a developmental bottleneck shaping the ability of migrating cells to realize their cellular potential and a factor to be taken into consideration (Ganuza et al., 2020).
Over the recent years, the advent of novel non-invasive lineage tracing approaches coupled with single-cell technologies are dramatically changing our view of hematopoiesis and exposing unexpected findings (Sanchez-Lanzas et al., 2022), some of which await functional validation. These techniques have revealed the presence of an unexpected degree of cellular heterogeneity at multiple developmental stages of hematopoiesis, including the detection of both transient and persistent embryonic hematopoietic populations with an HSC-dependent or independent origin (Patel et al., 2022; Yokomizo et al., 2022; Ulloa et al., 2021; Beaudin et al., 2016). This suggests the possibility of specific developmental niches facilitating their emergence and supporting these different hematopoietic populations and/or the presence of cell-intrinsic differences among precursors that make them to behave differently under the same niche inputs.
Among others, recent lineage-tracing studies provocatively indicated the presence of an HSC-independent population of embryonic multipotent progenitors (eMPPs) which was suggested to predominantly contribute to hematopoiesis in young mice with a lymphoid bias, and which also contributes to lifelong hematopoiesis (Patel et al., 2022).
Additionally, lineage-tracing analyses, next generation sequencing of naturally occurring mutations, telomere lengths and X-chromosome inactivation patterns revealed unexpected clonal dynamics on the expansion of the HSC pool with age, which affects the way we used to understand the FL as an expansion niche and rather support a major role of the FL as a maturation niche (Ganuza et al., 2022a; Catlin et al., 2011; Werner et al., 2015; Lee-Six et al., 2018). This calls for caution on focusing on the FL in a search for signals driving HSC symmetric expansion. Likewise, the lack of contribution of adult-fated HSCs to fetal hematopoiesis (Ulloa et al., 2021) and the presence of a hierarchical hematopoietic structure independent from adult-fated HSCs (Yokomizo et al., 2022) further complicates the overall picture of hematopoiesis during fetal stages, suggesting the presence of specific FL niches for each of those populations and/or cell-intrinsic differences among HSCs and other HSPCs as aforementioned.
Furthermore, lineage-tracing studies also unveiled additional layers of complexity in developmental immunity with an embryonic Flk2+ transient HSC population (Beaudin et al., 2016). This population exhibits multilineage potential and contributes mostly to B1a B-cells and γδ T-lymphocytes (Beaudin et al., 2016). This Flk2+ transient HSC population is not detected normally in the adulthood. However, the induction of inflammation in utero increases its persistence in the adult HSC compartment and affects postnatal immunity (Lopez et al., 2022). The effect of inflammation and infection on adult hematopoiesis has been well-established (Johnson et al., 2020) but their impact on fetal hematopoiesis and their long-term consequences on postnatal immunity is only now starting to be explored (Apostol et al., 2020; Tseng and Beaudin, 2023). In the same line, future investigations on the effect of extrinsic and environmental factors such as the establishment of gut microbiota during birth (Fernandez Sanchez et al., 2024), congenital infections (Lopez et al., 2022), hormonal changes during delivery (Calvanese et al., 2014) and inflammation, in developmental hematopoiesis, including changes in the HSC niche composition, will surely uncover important biological insights.
Importantly, over the recent years our understanding on the molecular regulation that the various HSC niches exert on HSC emergence and maturation has rendered several breakthroughs in regenerative medicine related to HSC expansion and HSC generation from other cellular sources (i.e., induced pluripotent stem cells, iPSCs) (Ng et al., 2024). HSCs have historically proved very difficult to expand and maintain in culture. Unfortunately, this implies the continuous need to search for compatible bone marrow donors to cope with the thousands of patients in dramatic need of this life saving therapy every year. Intense research on culture conditions supportive of HSC expansion recently led to protocols that significantly improve HSC expansion while maintaining their engraftment ability over long periods of time (Wilkinson et al., 2019; Sakurai et al., 2023). In parallel, several approaches have been taken to derive HSCs from other cell types. For instance, enforced expression of a cocktail of transcriptional factors supportive of HSC specification combined with their co-culture with engineered niche cells allowed to derive HSC-like cells from human iPSCs and mouse endothelial cells (Hadland et al., 2022; Sugimura et al., 2017; Lis et al., 2017; Hadland et al., 2015). Additionally, various stepwise differentiation protocols based on the subsequent and timely administration of specific developmental cues (including BMP4, ActivinA, bFGF, VEGF…) have been developed to in vitro mimic the transition from pluripotent stem cells to embryoid bodies, mesoderm and hemogenic endothelium and have rendered hematopoietic products close to adult mature HSCs, but with limited lymphoid production (Ditadi et al., 2015; Ditadi et al., 2017; Sturgeon et al., 2014). Very recently, a stepwise protocol has been optimized to produce long-term engrafting multilineage HSCs from human iPSCs (Ng et al., 2024). Although this work awaits to be replicated by others and to be evaluated in clinical trials, it holds the promise to provide unlimited numbers of clinically applicable HSCs for autologous transplantation avoiding serious problems related with graft-versus-host disease.
Our knowledge on the HSC niches and how they change during leukemogenesis is also being exploited to develop novel anti-leukemia therapies. Particularly, the role of the BM niche in adult leukemia is a matter of intense research, and a large body of data has exposed a critical role of the BM microenvironment in leukemia emergence, progression and development of chemoresistance. For instance, this includes how acute myeloid leukemia (AML) cells dramatically modify and highjack the BM niche to survive (Lane et al., 2009; Schepers et al., 2015; Duarte et al., 2018; Krevvata et al., 2014; Hanoun et al., 2014; Passaro et al., 2021) and the way the adipocyte niche changes following therapy promoting quiescence and chemoresistance of adult acute lymphoblastic leukemia (ALL) cells (Dander et al., 2021; Heydt et al., 2021). Likewise, in chronic myeloid leukemia (CML), CXCL12 was shown to maintain the quiescence of tyrosine kinase inhibitor (TKI)-resistant leukemia stem cells. Remarkably CXCL12 depletion from MSCs improves TKI efficacy in this context (Agarwal et al., 2019; Zhang et al., 2012; Kvasnicka and Thiele, 2004).
In contrast to adult leukemia, the specific role of cellular components supporting the transformation of HSCs and hematopoietic progenitors into preleukemic clones during embryogenesis and fetal development is widely unknown. Childhood leukemia encompass a heterogenous group of diseases. The evolution of preleukemic clones into the same type of leukemia in both monozygotic twins sharing clonal markers robustly supports a fetal origin (Greaves et al., 2003). The presence of fusion genes typically detected in childhood leukemia (e.g., ETV6-RUNX1) in mesenchymal stem cells of leukemia infant patients indicates that these mutations are acquired before HSCs emerge (Shalapour et al., 2010). The way how these mutations affect HSC development, their interactions with the FL- and BM-niches and childhood leukemia development is vastly unknown. Future investigations will surely address these critical questions in leukemogenesis. For instance, the initial steps leading to B-cell acute lymphoblastic leukemia (B-ALL) development are usually unnoticed in children. It has been recently shown that “immune stress suppresses innate immune signaling in preleukemic precursor B-cells” leading to leukemia in predisposed mice harboring pre-malignant Pax5+/− B-cell precursors (Isidro-Hernandez et al., 2023). Thus, understanding the molecular changes that the hematopoietic niches undergo during the earliest stages of childhood leukemia will offer new therapeutic intervention options in children.
Overall, the use of novel technologies has uncovered new insights in developmental hematopoiesis and highlighted the importance of reevaluating commonly accepted dogmas to reassign specific properties to particular developmental stages and niches. The implementation of new research tools combined with their functional validation will keep shaping our understanding on the various HSC niches. The multiple sequential anatomic locations that form the HSC niche during development provides a paradigmatic example on the highly complex and exquisitely regulated processes required to yield a functional tissue and maintain homeostasis. Furthermore, recent breakthroughs in regenerative medicine highlight the critical importance on understanding embryonic and fetal developmental processes to implement and improve life-saving cellular therapies and its potential to provide alternative anti-leukemia strategies.
Author contributions
RS-L: Writing–original draft, Writing–review and editing. AJ-P: Writing–original draft, Writing–review and editing. MG: Conceptualization, Funding acquisition, Supervision, Writing–original draft, Writing–review and editing.
Funding
The author(s) declare that financial support was received for the research, authorship, and/or publication of this article. M. G. is funded by the American Society of Hematology (Global Research Award, ASH GRA 2021), Barts Charity (The Rising Stars Programme, MGU0459), Greg Wolf Fund, Kay Kendall Leukaemia Fund (KKL1444), Leukaemia UK (John Goldman Fellowship, 2020/JGF/001), and Medical Research Council (MRC Career Development Award, MR/V009222/1).
Acknowledgments
We thank Trent Hall (St. Jude Children’s Research Hospital, Memphis, United States) for critical discussions and reading of the manuscript.
Conflict of interest
The authors declare that the research was conducted in the absence of any commercial or financial relationships that could be construed as a potential conflict of interest.
Publisher’s note
All claims expressed in this article are solely those of the authors and do not necessarily represent those of their affiliated organizations, or those of the publisher, the editors and the reviewers. Any product that may be evaluated in this article, or claim that may be made by its manufacturer, is not guaranteed or endorsed by the publisher.
Abbreviations
AGM, aorta-gonad-mesonephros; AECs, aortic endothelial cells; BA, bile acid; BM, bone marrow; E11, embryonic day of development 11; CHT, caudal hematopoietic tissue; CAR, CXCL12-abundant reticular cells; ER, endoplasmic reticulum; ECs, endothelial cells; EHT, endothelial to hematopoietic transition; EMPs, erythroid-myeloid progenitor cells; FBM, fetal bone marrow; FL, fetal liver; HSCs, hematopoietic stem cells; HSPCs, hematopoietic stem and progenitor cells; HE, hemogenic endothelium; IHC, immunohistochemistry; IF, immunofluorescence; IACs, intra-aortic clusters; RUs, repopulating units; MSCs, mesenchymal stem cells; YS, yolk sac.
References
Acar, M., Kocherlakota, K. S., Murphy, M. M., Peyer, J. G., Oguro, H., Inra, C. N., et al. (2015). Deep imaging of bone marrow shows non-dividing stem cells are mainly perisinusoidal. Nature 526 (7571), 126–130. doi:10.1038/nature15250
Adamo, L., Naveiras, O., Wenzel, P. L., McKinney-Freeman, S., Mack, P. J., Gracia-Sancho, J., et al. (2009). Biomechanical forces promote embryonic haematopoiesis. Nature 459 (7250), 1131–1135. doi:10.1038/nature08073
Agarwal, P., Isringhausen, S., Li, H., Paterson, A. J., He, J., Gomariz, A., et al. (2019). Mesenchymal niche-specific expression of Cxcl12 controls quiescence of treatment-resistant leukemia stem cells. Cell Stem Cell. 24 (5), 769–784.e6. doi:10.1016/j.stem.2019.02.018
Agrawal, H., Mehatre, S. H., and Khurana, S. (2024). The hematopoietic stem cell expansion niche in fetal liver: current state of the art and the way forward. Exp. Hematol. 136, 104585. doi:10.1016/j.exphem.2024.104585
Apostol, A. C., Jensen, K. D. C., and Beaudin, A. E. (2020). Training the fetal immune system through maternal inflammation-a layered hygiene hypothesis. Front. Immunol. 11, 123. doi:10.3389/fimmu.2020.00123
Arai, F., and Suda, T. (2007). Maintenance of quiescent hematopoietic stem cells in the osteoblastic niche. Ann. N. Y. Acad. Sci. 1106, 41–53. doi:10.1196/annals.1392.005
Asada, N., Katayama, Y., Sato, M., Minagawa, K., Wakahashi, K., Kawano, H., et al. (2013). Matrix-embedded osteocytes regulate mobilization of hematopoietic stem/progenitor cells. Cell Stem Cell 12 (6), 737–747. doi:10.1016/j.stem.2013.05.001
Asada, N., Kunisaki, Y., Pierce, H., Wang, Z., Fernandez, N. F., Birbrair, A., et al. (2017). Differential cytokine contributions of perivascular haematopoietic stem cell niches. Nat. Cell Biol. 19 (3), 214–223. doi:10.1038/ncb3475
Baccin, C., Al-Sabah, J., Velten, L., Helbling, P. M., Grunschlager, F., Hernandez-Malmierca, P., et al. (2020). Combined single-cell and spatial transcriptomics reveal the molecular, cellular and spatial bone marrow niche organization. Nat. Cell Biol. 22 (1), 38–48. doi:10.1038/s41556-019-0439-6
Bandyopadhyay, S., Duffy, M. P., Ahn, K. J., Sussman, J. H., Pang, M., Smith, D., et al. (2024). Mapping the cellular biogeography of human bone marrow niches using single-cell transcriptomics and proteomic imaging. Cell 187 (12), 3120–3140.e29. doi:10.1016/j.cell.2024.04.013
Baryawno, N., Przybylski, D., Kowalczyk, M. S., Kfoury, Y., Severe, N., Gustafsson, K., et al. (2019). A cellular taxonomy of the bone marrow stroma in homeostasis and leukemia. Cell. 177 (7), 1915–1932. doi:10.1016/j.cell.2019.04.040
Batsivari, A., Haltalli, M. L. R., Passaro, D., Pospori, C., Lo Celso, C., and Bonnet, D. (2020). Dynamic responses of the haematopoietic stem cell niche to diverse stresses. Nat. Cell Biol. 22 (1), 7–17. doi:10.1038/s41556-019-0444-9
Beaudin, A. E., Boyer, S. W., Perez-Cunningham, J., Hernandez, G. E., Derderian, S. C., Jujjavarapu, C., et al. (2016). A transient developmental hematopoietic stem cell gives rise to innate-like B and T cells. Cell Stem Cell 19 (6), 768–783. doi:10.1016/j.stem.2016.08.013
Benz, C., Copley, M. R., Kent, D. G., Wohrer, S., Cortes, A., Aghaeepour, N., et al. (2012). Hematopoietic stem cell subtypes expand differentially during development and display distinct lymphopoietic programs. Cell Stem Cell 10 (3), 273–283. doi:10.1016/j.stem.2012.02.007
Bertrand, J. Y., Cisson, J. L., Stachura, D. L., and Traver, D. (2010). Notch signaling distinguishes 2 waves of definitive hematopoiesis in the zebrafish embryo. Blood 115 (14), 2777–2783. doi:10.1182/blood-2009-09-244590
Bertrand, J. Y., Desanti, G. E., Lo-Man, R., Leclerc, C., Cumano, A., and Golub, R. (2006). Fetal spleen stroma drives macrophage commitment. Development 133 (18), 3619–3628. doi:10.1242/dev.02510
Biswas, A., Roy, I. M., Babu, P. C., Manesia, J., Schouteden, S., Vijayakurup, V., et al. (2020). The periostin/integrin-αv axis regulates the size of hematopoietic stem cell pool in the fetal liver. Stem Cell Rep. 15 (2), 340–357. doi:10.1016/j.stemcr.2020.06.022
Blank, U., and Karlsson, S. (2015). TGF-β signaling in the control of hematopoietic stem cells. Blood 125 (23), 3542–3550. doi:10.1182/blood-2014-12-618090
Blaser, B. W., Moore, J. L., Hagedorn, E. J., Li, B., Riquelme, R., Lichtig, A., et al. (2017). CXCR1 remodels the vascular niche to promote hematopoietic stem and progenitor cell engraftment. J. Exp. Med. 214 (4), 1011–1027. doi:10.1084/jem.20161616
Bogeska, R., Mikecin, A.-M., Kaschutnig, P., Fawaz, M., Büchler-Schäff, M., Le, D., et al. (2022). Inflammatory exposure drives long-lived impairment of hematopoietic stem cell self-renewal activity and accelerated aging. Cell Stem Cell 29 (8), 1273–1284. e8. doi:10.1016/j.stem.2022.06.012
Boggs, D. R., Boggs, S. S., Saxe, D. F., Gress, L. A., and Canfield, D. R. (1982). Hematopoietic stem cells with high proliferative potential. Assay of their concentration in marrow by the frequency and duration of cure of W/Wv mice. J. Clin. Invest 70 (2), 242–253. doi:10.1172/jci110611
Boisset, J. C., van Cappellen, W., Andrieu-Soler, C., Galjart, N., Dzierzak, E., and Robin, C. (2010). In vivo imaging of haematopoietic cells emerging from the mouse aortic endothelium. Nature 464 (7285), 116–120. doi:10.1038/nature08764
Bonkhofer, F., Rispoli, R., Pinheiro, P., Krecsmarik, M., Schneider-Swales, J., Tsang, I. H. C., et al. (2019). Blood stem cell-forming haemogenic endothelium in zebrafish derives from arterial endothelium. Nat. Commun. 10 (1), 3577. doi:10.1038/s41467-019-11423-2
Bowie, M. B., Kent, D. G., Dykstra, B., McKnight, K. D., McCaffrey, L., Hoodless, P. A., et al. (2007). Identification of a new intrinsically timed developmental checkpoint that reprograms key hematopoietic stem cell properties. Proc. Natl. Acad. Sci. U. S. A. 104 (14), 5878–5882. doi:10.1073/pnas.0700460104
Bowie, M. B., McKnight, K. D., Kent, D. G., McCaffrey, L., Hoodless, P. A., and Eaves, C. J. (2006). Hematopoietic stem cells proliferate until after birth and show a reversible phase-specific engraftment defect. J. Clin. Invest 116 (10), 2808–2816. doi:10.1172/JCI28310
Browaeys, R., Saelens, W., and Saeys, Y. (2020). NicheNet: modeling intercellular communication by linking ligands to target genes. Nat. Methods 17 (2), 159–162. doi:10.1038/s41592-019-0667-5
Bruns, I., Lucas, D., Pinho, S., Ahmed, J., Lambert, M. P., Kunisaki, Y., et al. (2014). Megakaryocytes regulate hematopoietic stem cell quiescence through CXCL4 secretion. Nat. Med. 20 (11), 1315–1320. doi:10.1038/nm.3707
Buch, T., Heppner, F. L., Tertilt, C., Heinen, T. J., Kremer, M., Wunderlich, F. T., et al. (2005). A Cre-inducible diphtheria toxin receptor mediates cell lineage ablation after toxin administration. Nat. Methods 2 (6), 419–426. doi:10.1038/nmeth762
Buono, M., Facchini, R., Matsuoka, S., Thongjuea, S., Waithe, D., Luis, T. C., et al. (2016). A dynamic niche provides kit ligand in a stage-specific manner to the earliest thymocyte progenitors. Nat. Cell Biol. 18 (2), 157–167. doi:10.1038/ncb3299
Busque, L., Mio, R., Mattioli, J., Brais, E., Blais, N., Lalonde, Y., et al. (1996). Nonrandom X-inactivation patterns in normal females: lyonization ratios vary with age. Blood 88 (1), 59–65. doi:10.1182/blood.v88.1.59.bloodjournal88159
Busque, L., Patel, J. P., Figueroa, M. E., Vasanthakumar, A., Provost, S., Hamilou, Z., et al. (2012). Recurrent somatic TET2 mutations in normal elderly individuals with clonal hematopoiesis. Nat. Genet. 44 (11), 1179–1181. doi:10.1038/ng.2413
Cacialli, P., Mahony, C. B., Petzold, T., Bordignon, P., Rougemont, A. L., and Bertrand, J. Y. (2021). A connexin/ifi30 pathway bridges HSCs with their niche to dampen oxidative stress. Nat. Commun. 12 (1), 4484. doi:10.1038/s41467-021-24831-0
Calaminus, S. D., Guitart, A. V., Sinclair, A., Schachtner, H., Watson, S. P., Holyoake, T. L., et al. (2012). Lineage tracing of Pf4-Cre marks hematopoietic stem cells and their progeny. PLoS One 7 (12), e51361. doi:10.1371/journal.pone.0051361
Calvanese, V., Lee, L. K., and Mikkola, H. K. (2014). Sex hormone drives blood stem cell reproduction. EMBO J. 33 (6), 534–535. doi:10.1002/embj.201487976
Calvanese, V., and Mikkola, H. K. A. (2023). The genesis of human hematopoietic stem cells. Blood 142 (6), 519–532. doi:10.1182/blood.2022017934
Calvi, L. M., Adams, G. B., Weibrecht, K. W., Weber, J. M., Olson, D. P., Knight, M. C., et al. (2003). Osteoblastic cells regulate the haematopoietic stem cell niche. Nature 425 (6960), 841–846. doi:10.1038/nature02040
Casanova-Acebes, M., Pitaval, C., Weiss, L. A., Nombela-Arrieta, C., Chevre, R., A-González, N., et al. (2013). Rhythmic modulation of the hematopoietic niche through neutrophil clearance. Cell 153 (5), 1025–1035. doi:10.1016/j.cell.2013.04.040
Catlin, S. N., Busque, L., Gale, R. E., Guttorp, P., and Abkowitz, J. L. (2011). The replication rate of human hematopoietic stem cells in vivo. Blood 117 (17), 4460–4466. doi:10.1182/blood-2010-08-303537
Chagraoui, J., Lepage-Noll, A., Anjo, A., Uzan, G., and Charbord, P. (2003). Fetal liver stroma consists of cells in epithelial-to-mesenchymal transition. Blood 101 (8), 2973–2982. doi:10.1182/blood-2002-05-1341
Chan, C. K., Chen, C. C., Luppen, C. A., Kim, J. B., DeBoer, A. T., Wei, K., et al. (2009). Endochondral ossification is required for haematopoietic stem-cell niche formation. Nature 457 (7228), 490–494. doi:10.1038/nature07547
Chandrakanthan, V., Rorimpandey, P., Zanini, F., Chacon, D., Olivier, J., Joshi, S., et al. (2022). Mesoderm-derived PDGFRA(+) cells regulate the emergence of hematopoietic stem cells in the dorsal aorta. Nat. Cell Biol. 24 (8), 1211–1225. doi:10.1038/s41556-022-00955-3
Chen, C., Yu, W., Tober, J., Gao, P., He, B., Lee, K., et al. (2019). Spatial genome Re-organization between fetal and adult hematopoietic stem cells. Cell Rep. 29 (12), 4200–4211. doi:10.1016/j.celrep.2019.11.065
Chen, D., Ji, X., Harris, M. A., Feng, J. Q., Karsenty, G., Celeste, A. J., et al. (1998). Differential roles for bone morphogenetic protein (BMP) receptor type IB and IA in differentiation and specification of mesenchymal precursor cells to osteoblast and adipocyte lineages. J. Cell Biol. 142 (1), 295–305. doi:10.1083/jcb.142.1.295
Chen, J. Y., Miyanishi, M., Wang, S. K., Yamazaki, S., Sinha, R., Kao, K. S., et al. (2016). Hoxb5 marks long-term haematopoietic stem cells and reveals a homogenous perivascular niche. Nature 530 (7589), 223–227. doi:10.1038/nature16943
Chen, M. J., Li, Y., De Obaldia, M. E., Yang, Q., Yzaguirre, A. D., Yamada-Inagawa, T., et al. (2011). Erythroid/myeloid progenitors and hematopoietic stem cells originate from distinct populations of endothelial cells. Cell Stem Cell 9 (6), 541–552. doi:10.1016/j.stem.2011.10.003
Chen, M. J., Yokomizo, T., Zeigler, B. M., Dzierzak, E., and Speck, N. A. (2009). Runx1 is required for the endothelial to haematopoietic cell transition but not thereafter. Nature 457 (7231), 887–891. doi:10.1038/nature07619
Chen, Q., Liu, Y., Jeong, H. W., Stehling, M., Dinh, V. V., Zhou, B., et al. (2019). Apelin(+) endothelial niche cells control hematopoiesis and mediate vascular regeneration after myeloablative injury. Cell Stem Cell 25 (6), 768–783. doi:10.1016/j.stem.2019.10.006
Choi, Y. J., Heck, A. M., Hayes, B. J., Lih, D., Rayner, S. G., Hadland, B., et al. (2021). WNT5A from the fetal liver vascular niche supports human fetal liver hematopoiesis. Stem Cell Res. Ther. 12 (1), 321. doi:10.1186/s13287-021-02380-z
Chou, S., Flygare, J., and Lodish, H. F. (2013). Fetal hepatic progenitors support long-term expansion of hematopoietic stem cells. Exp. Hematol. 41 (5), 479–490. doi:10.1016/j.exphem.2013.02.003
Chou, S., and Lodish, H. F. (2010). Fetal liver hepatic progenitors are supportive stromal cells for hematopoietic stem cells. Proc. Natl. Acad. Sci. U. S. A. 107 (17), 7799–7804. doi:10.1073/pnas.1003586107
Christensen, J. L., Wright, D. E., Wagers, A. J., and Weissman, I. L. (2004). Circulation and chemotaxis of fetal hematopoietic stem cells. PLoS Biol. 2 (3), E75. doi:10.1371/journal.pbio.0020075
Christodoulou, C., Spencer, J. A., Yeh, S. A., Turcotte, R., Kokkaliaris, K. D., Panero, R., et al. (2020). Live-animal imaging of native haematopoietic stem and progenitor cells. Nature 578 (7794), 278–283. doi:10.1038/s41586-020-1971-z
Clements, W. K., and Khoury, H. (2024). The molecular and cellular hematopoietic stem cell specification niche. Exp. Hematol. 136, 104280. doi:10.1016/j.exphem.2024.104280
Clements, W. K., Kim, A. D., Ong, K. G., Moore, J. C., Lawson, N. D., and Traver, D. (2011). A somitic Wnt16/Notch pathway specifies haematopoietic stem cells. Nature 474 (7350), 220–224. doi:10.1038/nature10107
Comazzetto, S., Murphy, M. M., Berto, S., Jeffery, E., Zhao, Z., and Morrison, S. J. (2019). Restricted hematopoietic progenitors and erythropoiesis require SCF from leptin receptor+ niche cells in the bone marrow. Cell Stem Cell 24 (3), 477–486. doi:10.1016/j.stem.2018.11.022
Cordeiro Gomes, A., Hara, T., Lim, V. Y., Herndler-Brandstetter, D., Nevius, E., Sugiyama, T., et al. (2016). Hematopoietic stem cell niches produce lineage-instructive signals to control multipotent progenitor differentiation. Immunity 45 (6), 1219–1231. doi:10.1016/j.immuni.2016.11.004
Coskun, S., Chao, H., Vasavada, H., Heydari, K., Gonzales, N., Zhou, X., et al. (2014). Development of the fetal bone marrow niche and regulation of HSC quiescence and homing ability by emerging osteolineage cells. Cell Rep. 9 (2), 581–590. doi:10.1016/j.celrep.2014.09.013
Crane, G. M., Jeffery, E., and Morrison, S. J. (2017). Adult haematopoietic stem cell niches. Nat. Rev. Immunol. 17 (9), 573–590. doi:10.1038/nri.2017.53
Crisan, M., Kartalaei, P. S., Vink, C. S., Yamada-Inagawa, T., Bollerot, K., van, I. W., et al. (2015). BMP signalling differentially regulates distinct haematopoietic stem cell types. Nat. Commun. 6, 8040. doi:10.1038/ncomms9040
Crosse, E. I., Binagui-Casas, A., Gordon-Keylock, S., Rybtsov, S., Tamagno, S., Olofsson, D., et al. (2023). An interactive resource of molecular signalling in the developing human haematopoietic stem cell niche. Development 150 (23), dev201972. doi:10.1242/dev.201972
Crosse, E. I., Gordon-Keylock, S., Rybtsov, S., Binagui-Casas, A., Felchle, H., Nnadi, N. C., et al. (2020). Multi-layered spatial transcriptomics identify secretory factors promoting human hematopoietic stem cell development. Cell Stem Cell 27 (5), 822–839. doi:10.1016/j.stem.2020.08.004
Cumano, A., Berthault, C., Ramond, C., Petit, M., Golub, R., Bandeira, A., et al. (2019). New molecular insights into immune cell development. Annu. Rev. Immunol. 37, 497–519. doi:10.1146/annurev-immunol-042718-041319
Damm, E. W., and Clements, W. K. (2017). Pdgf signalling guides neural crest contribution to the haematopoietic stem cell specification niche. Nat. Cell Biol. 19 (5), 457–467. doi:10.1038/ncb3508
Dander, E., Palmi, C., D’Amico, G., and Cazzaniga, G. (2021). The bone marrow niche in B-cell acute lymphoblastic leukemia: the role of microenvironment from pre-leukemia to overt leukemia. Int. J. Mol. Sci. 22 (9), 4426. doi:10.3390/ijms22094426
Ding, L., and Morrison, S. J. (2013). Haematopoietic stem cells and early lymphoid progenitors occupy distinct bone marrow niches. Nature 495 (7440), 231–235. doi:10.1038/nature11885
Ding, L., Saunders, T. L., Enikolopov, G., and Morrison, S. J. (2012). Endothelial and perivascular cells maintain haematopoietic stem cells. Nature 481 (7382), 457–462. doi:10.1038/nature10783
Ditadi, A., Sturgeon, C. M., and Keller, G. (2017). A view of human haematopoietic development from the Petri dish. Nat. Rev. Mol. Cell Biol. 18 (1), 56–67. doi:10.1038/nrm.2016.127
Ditadi, A., Sturgeon, C. M., Tober, J., Awong, G., Kennedy, M., Yzaguirre, A. D., et al. (2015). Human definitive haemogenic endothelium and arterial vascular endothelium represent distinct lineages. Nat. Cell Biol. 17 (5), 580–591. doi:10.1038/ncb3161
Dominguez-Bello, M. G., Costello, E. K., Contreras, M., Magris, M., Hidalgo, G., Fierer, N., et al. (2010). Delivery mode shapes the acquisition and structure of the initial microbiota across multiple body habitats in newborns. Proc. Natl. Acad. Sci. U. S. A. 107 (26), 11971–11975. doi:10.1073/pnas.1002601107
Duarte, D., Hawkins, E. D., Akinduro, O., Ang, H., De Filippo, K., Kong, I. Y., et al. (2018). Inhibition of endosteal vascular niche remodeling rescues hematopoietic stem cell loss in AML. Cell Stem Cell 22 (1), 64–77. doi:10.1016/j.stem.2017.11.006
Durand, C., Robin, C., Bollerot, K., Baron, M. H., Ottersbach, K., and Dzierzak, E. (2007). Embryonic stromal clones reveal developmental regulators of definitive hematopoietic stem cells. Proc. Natl. Acad. Sci. U. S. A. 104 (52), 20838–20843. doi:10.1073/pnas.0706923105
Durand, C., Robin, C., and Dzierzak, E. (2006). Mesenchymal lineage potentials of aorta-gonad-mesonephros stromal clones. Haematologica 91 (9), 1172–1179.
Efremova, M., Vento-Tormo, M., Teichmann, S. A., and Vento-Tormo, R. (2020). CellPhoneDB: inferring cell-cell communication from combined expression of multi-subunit ligand-receptor complexes. Nat. Protoc. 15 (4), 1484–1506. doi:10.1038/s41596-020-0292-x
El-Badri, N. S., Wang, B. Y., and Cherry, G. R. A. (1998). Osteoblasts promote engraftment of allogeneic hematopoietic stem cells. Exp. Hematol. 26 (2), 110–116.
Elvevold, K., Smedsrod, B., and Martinez, I. (2008). The liver sinusoidal endothelial cell: a cell type of controversial and confusing identity. Am. J. Physiol. Gastrointest. Liver Physiol. 294 (2), G391–G400. doi:10.1152/ajpgi.00167.2007
Ema, H., and Nakauchi, H. (2000). Expansion of hematopoietic stem cells in the developing liver of a mouse embryo. Blood 95 (7), 2284–2288. doi:10.1182/blood.v95.7.2284
Espin-Palazon, R., Stachura, D. L., Campbell, C. A., Garcia-Moreno, D., Del Cid, N., Kim, A. D., et al. (2014). Proinflammatory signaling regulates hematopoietic stem cell emergence. Cell 159 (5), 1070–1085. doi:10.1016/j.cell.2014.10.031
Fadlullah, M. Z. H., Neo, W. H., Lie, A. L. M., Thambyrajah, R., Patel, R., Mevel, R., et al. (2022). Murine AGM single-cell profiling identifies a continuum of hemogenic endothelium differentiation marked by ACE. Blood 139 (3), 343–356. doi:10.1182/blood.2020007885
Fang, S., Chen, S., Nurmi, H., Leppanen, V. M., Jeltsch, M., Scadden, D., et al. (2020). VEGF-C protects the integrity of the bone marrow perivascular niche in mice. Blood 136 (16), 1871–1883. doi:10.1182/blood.2020005699
Fantin, A., Tacconi, C., Villa, E., Ceccacci, E., Denti, L., and Ruhrberg, C. (2021). KIT is required for fetal liver hematopoiesis. Front. Cell Dev. Biol. 9, 648630. doi:10.3389/fcell.2021.648630
Fernandez Sanchez, J., Maknojia, A. A., and King, K. Y. (2024). Blood and guts: how the intestinal microbiome shapes hematopoiesis and treatment of hematologic disease. Blood 143 (17), 1689–1701. doi:10.1182/blood.2023021174
Fitch, S. R., Kapeni, C., Tsitsopoulou, A., Wilson, N. K., Gottgens, B., de Bruijn, M. F., et al. (2020). Gata3 targets Runx1 in the embryonic haematopoietic stem cell niche. IUBMB Life 72 (1), 45–52. doi:10.1002/iub.2184
Fitch, S. R., Kimber, G. M., Wilson, N. K., Parker, A., Mirshekar-Syahkal, B., Gottgens, B., et al. (2012). Signaling from the sympathetic nervous system regulates hematopoietic stem cell emergence during embryogenesis. Cell Stem Cell 11 (4), 554–566. doi:10.1016/j.stem.2012.07.002
Fomin, M. E., Beyer, A. I., and Muench, M. O. (2017). Human fetal liver cultures support multiple cell lineages that can engraft immunodeficient mice. Open Biol. 7 (12), 170108. doi:10.1098/rsob.170108
Friedenstein, A. J., Chailakhyan, R. K., Latsinik, N. V., Panasyuk, A. F., and Keiliss-Borok, I. V. (1974). Stromal cells responsible for transferring the microenvironment of the hemopoietic tissues. Cloning in vitro and retransplantation in vivo. Transplantation 17 (4), 331–340. doi:10.1097/00007890-197404000-00001
Friedenstein, A. J., Petrakova, K. V., Kurolesova, A. I., and Frolova, G. P. (1968). Heterotopic of bone marrow. Analysis of precursor cells for osteogenic and hematopoietic tissues. Transplantation 6 (2), 230–247. doi:10.1097/00007890-196803000-00009
Fujioka, M., Tokano, H., Fujioka, K. S., Okano, H., and Edge, A. S. (2011). Generating mouse models of degenerative diseases using Cre/lox-mediated in vivo mosaic cell ablation. J. Clin. Invest 121 (6), 2462–2469. doi:10.1172/JCI45081
Fujisaki, J., Wu, J., Carlson, A. L., Silberstein, L., Putheti, P., Larocca, R., et al. (2011). In vivo imaging of Treg cells providing immune privilege to the haematopoietic stem-cell niche. Nature 474 (7350), 216–219. doi:10.1038/nature10160
Gama-Norton, L., Ferrando, E., Ruiz-Herguido, C., Liu, Z., Guiu, J., Islam, A. B., et al. (2015). Notch signal strength controls cell fate in the haemogenic endothelium. Nat. Commun. 6, 8510. doi:10.1038/ncomms9510
Ganuza, M., Clements, W., and McKinney-Freeman, S. (2022b). Specification of hematopoietic stem cells in mammalian embryos: a rare or frequent event? Blood 140 (4), 309–320. doi:10.1182/blood.2020009839
Ganuza, M., Hall, T., Finkelstein, D., Chabot, A., Kang, G., and McKinney-Freeman, S. (2017). Lifelong haematopoiesis is established by hundreds of precursors throughout mammalian ontogeny. Nat. Cell Biol. 19 (10), 1153–1163. doi:10.1038/ncb3607
Ganuza, M., Hall, T., Finkelstein, D., Wang, Y. D., Chabot, A., Kang, G., et al. (2019). The global clonal complexity of the murine blood system declines throughout life and after serial transplantation. Blood 133 (18), 1927–1942. doi:10.1182/blood-2018-09-873059
Ganuza, M., Hall, T., Myers, J., Nevitt, C., Sanchez-Lanzas, R., Chabot, A., et al. (2022a). Murine foetal liver supports limited detectable expansion of life-long haematopoietic progenitors. Nat. Cell Biol. 24 (10), 1475–1486. doi:10.1038/s41556-022-00999-5
Ganuza, M., Hall, T., Obeng, E. A., and McKinney-Freeman, S. (2020). Clones assemble! The clonal complexity of blood during ontogeny and disease. Exp. Hematol. 83, 35–47. doi:10.1016/j.exphem.2020.01.009
Ganuza, M., and McKinney-Freeman, S. (2017). Hematopoietic stem cells under pressure. Curr. Opin. Hematol. 24 (4), 314–321. doi:10.1097/MOH.0000000000000347
Gao, S., Shi, Q., Zhang, Y., Liang, G., Kang, Z., Huang, B., et al. (2022). Identification of HSC/MPP expansion units in fetal liver by single-cell spatiotemporal transcriptomics. Cell Res. 32 (1), 38–53. doi:10.1038/s41422-021-00540-7
Gao, X., Xu, C., Asada, N., and Frenette, P. S. (2018). The hematopoietic stem cell niche: from embryo to adult. Development 145 (2), dev139691. doi:10.1242/dev.139691
Gekas, C., Dieterlen-Lievre, F., Orkin, S. H., and Mikkola, H. K. (2005). The placenta is a niche for hematopoietic stem cells. Dev. Cell 8 (3), 365–375. doi:10.1016/j.devcel.2004.12.016
Gerhardt, D. M., Pajcini, K. V., D’Altri, T., Tu, L., Jain, R., Xu, L., et al. (2014). The Notch1 transcriptional activation domain is required for development and reveals a novel role for Notch1 signaling in fetal hematopoietic stem cells. Genes Dev. 28 (6), 576–593. doi:10.1101/gad.227496.113
Gering, M., and Patient, R. (2005). Hedgehog signaling is required for adult blood stem cell formation in zebrafish embryos. Dev. Cell 8 (3), 389–400. doi:10.1016/j.devcel.2005.01.010
Ghersi, J. J., Baldissera, G., Hintzen, J., Luff, S. A., Cheng, S., Xia, I. F., et al. (2023). Haematopoietic stem and progenitor cell heterogeneity is inherited from the embryonic endothelium. Nat. Cell Biol. 25 (8), 1135–1145. doi:10.1038/s41556-023-01187-9
Gonzalez Galofre, Z. N., Kilpatrick, A. M., Marques, M., Sa da Bandeira, D., Ventura, T., Gomez Salazar, M., et al. (2024). Runx1+ vascular smooth muscle cells are essential for hematopoietic stem and progenitor cell development in vivo. Nat. Commun. 15 (1), 1653. doi:10.1038/s41467-024-44913-z
Greaves, M. F., Maia, A. T., Wiemels, J. L., and Ford, A. M. (2003). Leukemia in twins: lessons in natural history. Blood 102 (7), 2321–2333. doi:10.1182/blood-2002-12-3817
Greenbaum, A., Hsu, Y. M., Day, R. B., Schuettpelz, L. G., Christopher, M. J., Borgerding, J. N., et al. (2013). CXCL12 in early mesenchymal progenitors is required for haematopoietic stem-cell maintenance. Nature 495 (7440), 227–230. doi:10.1038/nature11926
Guo, P., Poulos, M. G., Palikuqi, B., Badwe, C. R., Lis, R., Kunar, B., et al. (2017). Endothelial jagged-2 sustains hematopoietic stem and progenitor reconstitution after myelosuppression. J. Clin. Invest 127 (12), 4242–4256. doi:10.1172/JCI92309
Hackney, J. A., Charbord, P., Brunk, B. P., Stoeckert, C. J., Lemischka, I. R., and Moore, K. A. (2002). A molecular profile of a hematopoietic stem cell niche. Proc. Natl. Acad. Sci. U. S. A. 99 (20), 13061–13066. doi:10.1073/pnas.192124499
Hadland, B., Varnum-Finney, B., Dozono, S., Dignum, T., Nourigat-McKay, C., Heck, A. M., et al. (2022). Engineering a niche supporting hematopoietic stem cell development using integrated single-cell transcriptomics. Nat. Commun. 13 (1), 1584. doi:10.1038/s41467-022-28781-z
Hadland, B. K., Varnum-Finney, B., Poulos, M. G., Moon, R. T., Butler, J. M., Rafii, S., et al. (2015). Endothelium and NOTCH specify and amplify aorta-gonad-mesonephros-derived hematopoietic stem cells. J. Clin. Invest 125 (5), 2032–2045. doi:10.1172/JCI80137
Hall, B. K., and Miyake, T. (2000). All for one and one for all: condensations and the initiation of skeletal development. Bioessays 22 (2), 138–147. doi:10.1002/(SICI)1521-1878(200002)22:2<138::AID-BIES5>3.0.CO;2-4
Hall, T. D., Kim, H., Dabbah, M., Myers, J. A., Crawford, J. C., Morales-Hernandez, A., et al. (2022). Murine fetal bone marrow does not support functional hematopoietic stem and progenitor cells until birth. Nat. Commun. 13 (1), 5403. doi:10.1038/s41467-022-33092-4
Hanoun, M., Zhang, D., Mizoguchi, T., Pinho, S., Pierce, H., Kunisaki, Y., et al. (2014). Acute myelogenous leukemia-induced sympathetic neuropathy promotes malignancy in an altered hematopoietic stem cell niche. Cell Stem Cell 15 (3), 365–375. doi:10.1016/j.stem.2014.06.020
Harrison, D. E., Zhong, R. K., Jordan, C. T., Lemischka, I. R., and Astle, C. M. (1997). Relative to adult marrow, fetal liver repopulates nearly five times more effectively long-term than short-term. Exp. Hematol. 25 (4), 293–297.
He, Q., Zhang, C., Wang, L., Zhang, P., Ma, D., Lv, J., et al. (2015). Inflammatory signaling regulates hematopoietic stem and progenitor cell emergence in vertebrates. Blood 125 (7), 1098–1106. doi:10.1182/blood-2014-09-601542
Heydt, Q., Xintaropoulou, C., Clear, A., Austin, M., Pislariu, I., Miraki-Moud, F., et al. (2021). Adipocytes disrupt the translational programme of acute lymphoblastic leukaemia to favour tumour survival and persistence. Nat. Commun. 12 (1), 5507. doi:10.1038/s41467-021-25540-4
Hou, S., Li, Z., Zheng, X., Gao, Y., Dong, J., Ni, Y., et al. (2020). Embryonic endothelial evolution towards first hematopoietic stem cells revealed by single-cell transcriptomic and functional analyses. Cell Res. 30 (5), 376–392. doi:10.1038/s41422-020-0300-2
Ikuta, K., and Weissman, I. L. (1992). Evidence that hematopoietic stem cells express mouse c-kit but do not depend on steel factor for their generation. Proc. Natl. Acad. Sci. U. S. A. 89 (4), 1502–1506. doi:10.1073/pnas.89.4.1502
Isern, J., Garcia-Garcia, A., Martin, A. M., Arranz, L., Martin-Perez, D., Torroja, C., et al. (2014). The neural crest is a source of mesenchymal stem cells with specialized hematopoietic stem cell niche function. Elife 3, e03696. doi:10.7554/eLife.03696
Isidro-Hernandez, M., Casado-Garcia, A., Oak, N., Aleman-Arteaga, S., Ruiz-Corzo, B., Martinez-Cano, J., et al. (2023). Immune stress suppresses innate immune signaling in preleukemic precursor B-cells to provoke leukemia in predisposed mice. Nat. Commun. 14 (1), 5159. doi:10.1038/s41467-023-40961-z
Istvanffy, R., Kroger, M., Eckl, C., Gitzelmann, S., Vilne, B., Bock, F., et al. (2011). Stromal pleiotrophin regulates repopulation behavior of hematopoietic stem cells. Blood 118 (10), 2712–2722. doi:10.1182/blood-2010-05-287235
Itkin, T., Ludin, A., Gradus, B., Gur-Cohen, S., Kalinkovich, A., Schajnovitz, A., et al. (2012). FGF-2 expands murine hematopoietic stem and progenitor cells via proliferation of stromal cells, c-Kit activation, and CXCL12 down-regulation. Blood 120 (9), 1843–1855. doi:10.1182/blood-2011-11-394692
Ivanovs, A., Rybtsov, S., Anderson, R. A., Turner, M. L., and Medvinsky, A. (2014). Identification of the niche and phenotype of the first human hematopoietic stem cells. Stem Cell Rep. 2 (4), 449–456. doi:10.1016/j.stemcr.2014.02.004
Iwasaki, H., Arai, F., Kubota, Y., Dahl, M., and Suda, T. (2010). Endothelial protein C receptor-expressing hematopoietic stem cells reside in the perisinusoidal niche in fetal liver. Blood 116 (4), 544–553. doi:10.1182/blood-2009-08-240903
Jaffredo, T., Lempereur, A., Richard, C., Bollerot, K., Gautier, R., Canto, P. Y., et al. (2013). Dorso-ventral contributions in the formation of the embryonic aorta and the control of aortic hematopoiesis. Blood Cells Mol. Dis. 51 (4), 232–238. doi:10.1016/j.bcmd.2013.07.004
Jaffredo, T., Richard, C., Pouget, C., Teillet, M. A., Bollerot, K., Gautier, R., et al. (2010). Aortic remodelling during hemogenesis: is the chicken paradigm unique? Int. J. Dev. Biol. 54 (6-7), 1045–1054. doi:10.1387/ijdb.103062tj
Jassinskaja, M., Johansson, E., Kristiansen, T. A., Akerstrand, H., Sjoholm, K., Hauri, S., et al. (2017). Comprehensive proteomic characterization of ontogenic changes in hematopoietic stem and progenitor cells. Cell Rep. 21 (11), 3285–3297. doi:10.1016/j.celrep.2017.11.070
Johnson, C. B., Zhang, J., and Lucas, D. (2020). The role of the bone marrow microenvironment in the response to infection. Front. Immunol. 11, 585402. doi:10.3389/fimmu.2020.585402
Josefsdottir, K. S., Baldridge, M. T., Kadmon, C. S., and King, K. Y. (2017). Antibiotics impair murine hematopoiesis by depleting the intestinal microbiota. Blood 129 (6), 729–739. doi:10.1182/blood-2016-03-708594
Kamps, W. A., and Cooper, M. D. (1982). Microenvironmental studies of pre-B and B cell development in human and mouse fetuses. J. Immunol. 129 (2), 526–531. doi:10.4049/jimmunol.129.2.526
Kamps, W. A., Timens, W., De Boer, G. J., Spanjer, H. H., and Poppema, S. (1989). In situ study of haemopoiesis in human fetal liver. Scand. J. Immunol. 30 (4), 399–408. doi:10.1111/j.1365-3083.1989.tb02443.x
Kapeni, C., Nitsche, L., Kilpatrick, A. M., Wilson, N. K., Xia, K., Mirshekar-Syahkal, B., et al. (2022). p57Kip2 regulates embryonic blood stem cells by controlling sympathoadrenal progenitor expansion. Blood 140 (5), 464–477. doi:10.1182/blood.2021014853
Kara, N., Xue, Y., Zhao, Z., Murphy, M. M., Comazzetto, S., Lesser, A., et al. (2023). Endothelial and Leptin Receptor(+) cells promote the maintenance of stem cells and hematopoiesis in early postnatal murine bone marrow. Dev. Cell 58 (5), 348–360.e6. doi:10.1016/j.devcel.2023.02.003
Kayvanjoo, A. H., Splichalova, I., Bejarano, D. A., Huang, H., Mauel, K., Makdissi, N., et al. (2024). Fetal liver macrophages contribute to the hematopoietic stem cell niche by controlling granulopoiesis. Elife 13, e86493. doi:10.7554/eLife.86493
Khan, J. A., Mendelson, A., Kunisaki, Y., Birbrair, A., Kou, Y., Arnal-Estape, A., et al. (2016). Fetal liver hematopoietic stem cell niches associate with portal vessels. Science 351 (6269), 176–180. doi:10.1126/science.aad0084
Kiel, M. J., Yilmaz, O. H., Iwashita, T., Yilmaz, O. H., Terhorst, C., and Morrison, S. J. (2005). SLAM family receptors distinguish hematopoietic stem and progenitor cells and reveal endothelial niches for stem cells. Cell 121 (7), 1109–1121. doi:10.1016/j.cell.2005.05.026
Kieusseian, A., Brunet de la Grange, P., Burlen-Defranoux, O., Godin, I., and Cumano, A. (2012). Immature hematopoietic stem cells undergo maturation in the fetal liver. Development 139 (19), 3521–3530. doi:10.1242/dev.079210
Kim, I., Saunders, T. L., and Morrison, S. J. (2007). Sox17 dependence distinguishes the transcriptional regulation of fetal from adult hematopoietic stem cells. Cell 130 (3), 470–483. doi:10.1016/j.cell.2007.06.011
Kirouac, D. C., Ito, C., Csaszar, E., Roch, A., Yu, M., Sykes, E. A., et al. (2010). Dynamic interaction networks in a hierarchically organized tissue. Mol. Syst. Biol. 6, 417. doi:10.1038/msb.2010.71
Kissa, K., and Herbomel, P. (2010). Blood stem cells emerge from aortic endothelium by a novel type of cell transition. Nature 464 (7285), 112–115. doi:10.1038/nature08761
Kobayashi, I., Kobayashi-Sun, J., Kim, A. D., Pouget, C., Fujita, N., Suda, T., et al. (2014). Jam1a-Jam2a interactions regulate haematopoietic stem cell fate through Notch signalling. Nature 512 (7514), 319–323. doi:10.1038/nature13623
Kohler, A., Schmithorst, V., Filippi, M. D., Ryan, M. A., Daria, D., Gunzer, M., et al. (2009). Altered cellular dynamics and endosteal location of aged early hematopoietic progenitor cells revealed by time-lapse intravital imaging in long bones. Blood 114 (2), 290–298. doi:10.1182/blood-2008-12-195644
Kokkaliaris, K. D., Kunz, L., Cabezas-Wallscheid, N., Christodoulou, C., Renders, S., Camargo, F., et al. (2020). Adult blood stem cell localization reflects the abundance of reported bone marrow niche cell types and their combinations. Blood 136 (20), 2296–2307. doi:10.1182/blood.2020006574
Konno, K., Sasaki, T., Kulkeaw, K., and Sugiyama, D. (2020). Paracrine CCL17 and CCL22 signaling regulates hematopoietic stem/progenitor cell migration and retention in mouse fetal liver. Biochem. Biophys. Res. Commun. 527 (3), 730–736. doi:10.1016/j.bbrc.2020.04.045
Krevvata, M., Silva, B. C., Manavalan, J. S., Galan-Diez, M., Kode, A., Matthews, B. G., et al. (2014). Inhibition of leukemia cell engraftment and disease progression in mice by osteoblasts. Blood 124 (18), 2834–2846. doi:10.1182/blood-2013-07-517219
Kunisaki, Y., Bruns, I., Scheiermann, C., Ahmed, J., Pinho, S., Zhang, D., et al. (2013). Arteriolar niches maintain haematopoietic stem cell quiescence. Nature 502 (7473), 637–643. doi:10.1038/nature12612
Kusumbe, A. P., Ramasamy, S. K., Itkin, T., Mae, M. A., Langen, U. H., Betsholtz, C., et al. (2016). Age-dependent modulation of vascular niches for haematopoietic stem cells. Nature 532 (7599), 380–384. doi:10.1038/nature17638
Kvasnicka, H. M., and Thiele, J. (2004). Bone marrow angiogenesis: methods of quantification and changes evolving in chronic myeloproliferative disorders. Histol. Histopathol. 19 (4), 1245–1260. doi:10.14670/HH-19.1245
Lancrin, C., Sroczynska, P., Stephenson, C., Allen, T., Kouskoff, V., and Lacaud, G. (2009). The haemangioblast generates haematopoietic cells through a haemogenic endothelium stage. Nature 457 (7231), 892–895. doi:10.1038/nature07679
Lane, S. W., Scadden, D. T., and Gilliland, D. G. (2009). The leukemic stem cell niche: current concepts and therapeutic opportunities. Blood 114 (6), 1150–1157. doi:10.1182/blood-2009-01-202606
Langen, U. H., Pitulescu, M. E., Kim, J. M., Enriquez-Gasca, R., Sivaraj, K. K., Kusumbe, A. P., et al. (2017). Cell-matrix signals specify bone endothelial cells during developmental osteogenesis. Nat. Cell Biol. 19 (3), 189–201. doi:10.1038/ncb3476
Lee, Y., Leslie, J., Yang, Y., and Ding, L. (2021). Hepatic stellate and endothelial cells maintain hematopoietic stem cells in the developing liver. J. Exp. Med. 218 (3), e20200882. doi:10.1084/jem.20200882
Lee-Six, H., Obro, N. F., Shepherd, M. S., Grossmann, S., Dawson, K., Belmonte, M., et al. (2018). Population dynamics of normal human blood inferred from somatic mutations. Nature 561 (7724), 473–478. doi:10.1038/s41586-018-0497-0
Lessard, J., Faubert, A., and Sauvageau, G. (2004). Genetic programs regulating HSC specification, maintenance and expansion. Oncogene 23 (43), 7199–7209. doi:10.1038/sj.onc.1207940
Li, Y., Esain, V., Teng, L., Xu, J., Kwan, W., Frost, I. M., et al. (2014). Inflammatory signaling regulates embryonic hematopoietic stem and progenitor cell production. Genes Dev. 28 (23), 2597–2612. doi:10.1101/gad.253302.114
Li, Y., Kong, W., Yang, W., Patel, R. M., Casey, E. B., Okeyo-Owuor, T., et al. (2020). Single-cell analysis of neonatal HSC ontogeny reveals gradual and uncoordinated transcriptional reprogramming that begins before birth. Cell Stem Cell 27 (5), 732–747. doi:10.1016/j.stem.2020.08.001
Lim, S. E., Esain, V., Kwan, W., Theodore, L. N., Cortes, M., Frost, I. M., et al. (2017). HIF1alpha-induced PDGFRbeta signaling promotes developmental HSC production via IL-6 activation. Exp. Hematol. 46, 83–95 e6. doi:10.1016/j.exphem.2016.10.002
Lis, R., Karrasch, C. C., Poulos, M. G., Kunar, B., Redmond, D., Duran, J. G. B., et al. (2017). Conversion of adult endothelium to immunocompetent haematopoietic stem cells. Nature 545 (7655), 439–445. doi:10.1038/nature22326
Liu, W., Ding, Y., Shen, Z., Xu, C., Yi, W., Wang, D., et al. (2024). BF170 hydrochloride enhances the emergence of hematopoietic stem and progenitor cells. Development 151 (13), dev202476. doi:10.1242/dev.202476
Liu, Y., Chen, Q., Jeong, H. W., Han, D., Fabian, J., Drexler, H. C. A., et al. (2021). Dopamine signaling regulates hematopoietic stem and progenitor cell function. Blood 138 (21), 2051–2065. doi:10.1182/blood.2020010419
Liu, Y., Chen, Q., Jeong, H. W., Koh, B. I., Watson, E. C., Xu, C., et al. (2022). A specialized bone marrow microenvironment for fetal haematopoiesis. Nat. Commun. 13 (1), 1327. doi:10.1038/s41467-022-28775-x
Lizama, C. O., Hawkins, J. S., Schmitt, C. E., Bos, F. L., Zape, J. P., Cautivo, K. M., et al. (2015). Repression of arterial genes in hemogenic endothelium is sufficient for haematopoietic fate acquisition. Nat. Commun. 6, 7739. doi:10.1038/ncomms8739
Lo, C. C., Fleming, H. E., Wu, J. W., Zhao, C. X., Miake-Lye, S., Fujisaki, J., et al. (2009). Live-animal tracking of individual haematopoietic stem/progenitor cells in their niche. Nature 457 (7225), 92–96. doi:10.1038/nature07434
Lopez, D. A., Apostol, A. C., Lebish, E. J., Valencia, C. H., Romero-Mulero, M. C., Pavlovich, P. V., et al. (2022). Prenatal inflammation perturbs murine fetal hematopoietic development and causes persistent changes to postnatal immunity. Cell Rep. 41 (8), 111677. doi:10.1016/j.celrep.2022.111677
Lu, Y., Liu, M., Yang, J., Weissman, S. M., Pan, X., Katz, S. G., et al. (2021). Spatial transcriptome profiling by MERFISH reveals fetal liver hematopoietic stem cell niche architecture. Cell Discov. 7 (1), 47. doi:10.1038/s41421-021-00266-1
Lucas, D. (2017). The bone marrow microenvironment for hematopoietic stem cells. Adv. Exp. Med. Biol. 1041, 5–18. doi:10.1007/978-3-319-69194-7_2
Manesia, J. K., Franch, M., Tabas-Madrid, D., Nogales-Cadenas, R., Vanwelden, T., Van Den Bosch, E., et al. (2017). Distinct molecular signature of murine fetal liver and adult hematopoietic stem cells identify novel regulators of hematopoietic stem cell function. Stem Cells Dev. 26 (8), 573–584. doi:10.1089/scd.2016.0294
Manesia, J. K., Xu, Z., Broekaert, D., Boon, R., van Vliet, A., Eelen, G., et al. (2015). Highly proliferative primitive fetal liver hematopoietic stem cells are fueled by oxidative metabolic pathways. Stem Cell Res. 15 (3), 715–721. doi:10.1016/j.scr.2015.11.001
Maniatis, A., Tavassoli, M., and Crosby, W. H. (1971). Origin of osteogenic precursor cells in extramedullary marrow implants. Blood 38 (5), 569–575. doi:10.1182/blood.v38.5.569.569
Mantel, C. R., O'Leary, H. A., Chitteti, B. R., Huang, X., Cooper, S., Hangoc, G., et al. (2015). Enhancing hematopoietic stem cell transplantation efficacy by mitigating oxygen shock. Cell 161 (7), 1553–1565. doi:10.1016/j.cell.2015.04.054
Mariani, S. A., Li, Z., Rice, S., Krieg, C., Fragkogianni, S., Robinson, M., et al. (2019). Pro-inflammatory aorta-associated macrophages are involved in embryonic development of hematopoietic stem cells. Immunity 50 (6), 1439–1452. doi:10.1016/j.immuni.2019.05.003
Marshall, C. J., Kinnon, C., and Thrasher, A. J. (2000). Polarized expression of bone morphogenetic protein-4 in the human aorta-gonad-mesonephros region. Blood 96 (4), 1591–1593. doi:10.1182/blood.v96.4.1591.h8001591_1591_1593
Matsuoka, S., Tsuji, K., Hisakawa, H., Xu, M., Ebihara, Y., Ishii, T., et al. (2001). Generation of definitive hematopoietic stem cells from murine early yolk sac and paraaortic splanchnopleures by aorta-gonad-mesonephros region-derived stromal cells. Blood 98 (1), 6–12. doi:10.1182/blood.v98.1.6
Matsushita, Y., Chu, A. K. Y., Tsutsumi-Arai, C., Orikasa, S., Nagata, M., Wong, S. Y., et al. (2022). The fate of early perichondrial cells in developing bones. Nat. Commun. 13 (1), 7319. doi:10.1038/s41467-022-34804-6
Matsushita, Y., Nagata, M., Kozloff, K. M., Welch, J. D., Mizuhashi, K., Tokavanich, N., et al. (2020). A Wnt-mediated transformation of the bone marrow stromal cell identity orchestrates skeletal regeneration. Nat. Commun. 11 (1), 332. doi:10.1038/s41467-019-14029-w
Matsushita, Y., Ono, W., and Ono, N. (2021). Bone regeneration via skeletal cell lineage plasticity: all hands mobilized for emergencies: quiescent mature skeletal cells can be activated in response to injury and robustly participate in bone regeneration through cellular plasticity. Bioessays 43 (1), e2000202. doi:10.1002/bies.202000202
McGarvey, A. C., Rybtsov, S., Souilhol, C., Tamagno, S., Rice, R., Hills, D., et al. (2017). A molecular roadmap of the AGM region reveals BMPER as a novel regulator of HSC maturation. J. Exp. Med. 214 (12), 3731–3751. doi:10.1084/jem.20162012
McKinney-Freeman, S., Cahan, P., Li, H., Lacadie, S. A., Huang, H. T., Curran, M., et al. (2012). The transcriptional landscape of hematopoietic stem cell ontogeny. Cell Stem Cell 11 (5), 701–714. doi:10.1016/j.stem.2012.07.018
Medvinsky, A., and Dzierzak, E. (1996). Definitive hematopoiesis is autonomously initiated by the AGM region. Cell 86 (6), 897–906. doi:10.1016/s0092-8674(00)80165-8
Medvinsky, A., Rybtsov, S., and Taoudi, S. (2011). Embryonic origin of the adult hematopoietic system: advances and questions. Development 138 (6), 1017–1031. doi:10.1242/dev.040998
Mendes, S. C., Robin, C., and Dzierzak, E. (2005). Mesenchymal progenitor cells localize within hematopoietic sites throughout ontogeny. Development 132 (5), 1127–1136. doi:10.1242/dev.01615
Mendez-Ferrer, S., Bonnet, D., Steensma, D. P., Hasserjian, R. P., Ghobrial, I. M., Gribben, J. G., et al. (2020). Bone marrow niches in haematological malignancies. Nat. Rev. Cancer 20 (5), 285–298. doi:10.1038/s41568-020-0245-2
Mendez-Ferrer, S., Michurina, T. V., Ferraro, F., Mazloom, A. R., Macarthur, B. D., Lira, S. A., et al. (2010). Mesenchymal and haematopoietic stem cells form a unique bone marrow niche. Nature 466 (7308), 829–834. doi:10.1038/nature09262
Miharada, K., Sigurdsson, V., and Karlsson, S. (2014). Dppa5 improves hematopoietic stem cell activity by reducing endoplasmic reticulum stress. Cell Rep. 7 (5), 1381–1392. doi:10.1016/j.celrep.2014.04.056
Mikkola, H. K., and Orkin, S. H. (2006). The journey of developing hematopoietic stem cells. Development 133 (19), 3733–3744. doi:10.1242/dev.02568
Miladinovic, O., Canto, P. Y., Pouget, C., Piau, O., Radic, N., Freschu, P., et al. (2024). A multistep computational approach reveals a neuro-mesenchymal cell population in the embryonic hematopoietic stem cell niche. Development 151 (7), dev202614. doi:10.1242/dev.202614
Mizoguchi, T., Pinho, S., Ahmed, J., Kunisaki, Y., Hanoun, M., Mendelson, A., et al. (2014). Osterix marks distinct waves of primitive and definitive stromal progenitors during bone marrow development. Dev. Cell 29 (3), 340–349. doi:10.1016/j.devcel.2014.03.013
Moore, K. A., Ema, H., and Lemischka, I. R. (1997b). In vitro maintenance of highly purified, transplantable hematopoietic stem cells. Blood 89 (12), 4337–4347. doi:10.1182/blood.v89.12.4337
Moore, K. A., Pytowski, B., Witte, L., Hicklin, D., and Lemischka, I. R. (1997a). Hematopoietic activity of a stromal cell transmembrane protein containing epidermal growth factor-like repeat motifs. Proc. Natl. Acad. Sci. U. S. A. 94 (8), 4011–4016. doi:10.1073/pnas.94.8.4011
Morita, Y., Iseki, A., Okamura, S., Suzuki, S., Nakauchi, H., and Ema, H. (2011). Functional characterization of hematopoietic stem cells in the spleen. Exp. Hematol. 39 (3), 351–359. doi:10.1016/j.exphem.2010.12.008
Morrison, S. J., Hemmati, H. D., Wandycz, A. M., and Weissman, I. L. (1995). The purification and characterization of fetal liver hematopoietic stem cells. Proc. Natl. Acad. Sci. U. S. A. 92 (22), 10302–10306. doi:10.1073/pnas.92.22.10302
Morrison, S. J., and Scadden, D. T. (2014). The bone marrow niche for haematopoietic stem cells. Nature 505 (7483), 327–334. doi:10.1038/nature12984
Murayama, E., Kissa, K., Zapata, A., Mordelet, E., Briolat, V., Lin, H. F., et al. (2006). Tracing hematopoietic precursor migration to successive hematopoietic organs during zebrafish development. Immunity 25 (6), 963–975. doi:10.1016/j.immuni.2006.10.015
Nakagawa, M., Ichikawa, M., Kumano, K., Goyama, S., Kawazu, M., Asai, T., et al. (2006). AML1/Runx1 rescues Notch1-null mutation-induced deficiency of para-aortic splanchnopleural hematopoiesis. Blood 108 (10), 3329–3334. doi:10.1182/blood-2006-04-019570
Nakamura-Ishizu, A., Takubo, K., Fujioka, M., and Suda, T. (2014). Megakaryocytes are essential for HSC quiescence through the production of thrombopoietin. Biochem. Biophys. Res. Commun. 454 (2), 353–357. doi:10.1016/j.bbrc.2014.10.095
Nakamura-Ishizu, A., Takubo, K., Kobayashi, H., Suzuki-Inoue, K., and Suda, T. (2015). CLEC-2 in megakaryocytes is critical for maintenance of hematopoietic stem cells in the bone marrow. J. Exp. Med. 212 (12), 2133–2146. doi:10.1084/jem.20150057
Neo, W. H., Booth, C. A. G., Azzoni, E., Chi, L., Delgado-Olguin, P., de Bruijn, M., et al. (2018). Cell-extrinsic hematopoietic impact of Ezh2 inactivation in fetal liver endothelial cells. Blood 131 (20), 2223–2234. doi:10.1182/blood-2017-10-811455
Ng, E. S., Sarila, G., Li, J. Y., Edirisinghe, H. S., Saxena, R., Sun, S., et al. (2024). Long-term engrafting multilineage hematopoietic cells differentiated from human induced pluripotent stem cells. Nat. Biotechnol. doi:10.1038/s41587-024-02360-7
Nguyen, P. D., Hollway, G. E., Sonntag, C., Miles, L. B., Hall, T. E., Berger, S., et al. (2014). Haematopoietic stem cell induction by somite-derived endothelial cells controlled by meox1. Nature 512 (7514), 314–318. doi:10.1038/nature13678
Niranjan, B., Buluwela, L., Yant, J., Perusinghe, N., Atherton, A., Phippard, D., et al. (1995). HGF/SF: a potent cytokine for mammary growth, morphogenesis and development. Development 121 (9), 2897–2908. doi:10.1242/dev.121.9.2897
Nolta, J. A., Thiemann, F. T., Arakawa-Hoyt, J., Dao, M. A., Barsky, L. W., Moore, K. A., et al. (2002). The AFT024 stromal cell line supports long-term ex vivo maintenance of engrafting multipotent human hematopoietic progenitors. Leukemia 16 (3), 352–361. doi:10.1038/sj.leu.2402371
North, T., Gu, T. L., Stacy, T., Wang, Q., Howard, L., Binder, M., et al. (1999). Cbfa2 is required for the formation of intra-aortic hematopoietic clusters. Development 126 (11), 2563–2575. doi:10.1242/dev.126.11.2563
North, T. E., de Bruijn, M. F., Stacy, T., Talebian, L., Lind, E., Robin, C., et al. (2002). Runx1 expression marks long-term repopulating hematopoietic stem cells in the midgestation mouse embryo. Immunity 16 (5), 661–672. doi:10.1016/s1074-7613(02)00296-0
North, T. E., Goessling, W., Peeters, M., Li, P., Ceol, C., Lord, A. M., et al. (2009). Hematopoietic stem cell development is dependent on blood flow. Cell 137 (4), 736–748. doi:10.1016/j.cell.2009.04.023
North, T. E., Goessling, W., Walkley, C. R., Lengerke, C., Kopani, K. R., Lord, A. M., et al. (2007). Prostaglandin E2 regulates vertebrate haematopoietic stem cell homeostasis. Nature 447 (7147), 1007–1011. doi:10.1038/nature05883
Nottingham, W. T., Jarratt, A., Burgess, M., Speck, C. L., Cheng, J. F., Prabhakar, S., et al. (2007). Runx1-mediated hematopoietic stem-cell emergence is controlled by a Gata/Ets/SCL-regulated enhancer. Blood 110 (13), 4188–4197. doi:10.1182/blood-2007-07-100883
Ohneda, O., Ohneda, K., Nomiyama, H., Zheng, Z., Gold, S. A., Arai, F., et al. (2000). WECHE: a novel hematopoietic regulatory factor. Immunity 12 (2), 141–150. doi:10.1016/s1074-7613(00)80167-3
Olsen, B. R., Reginato, A. M., and Wang, W. (2000). Bone development. Annu. Rev. Cell Dev. Biol. 16, 191–220. doi:10.1146/annurev.cellbio.16.1.191
Omatsu, Y., Seike, M., Sugiyama, T., Kume, T., and Nagasawa, T. (2014). Foxc1 is a critical regulator of haematopoietic stem/progenitor cell niche formation. Nature 508 (7497), 536–540. doi:10.1038/nature13071
Omatsu, Y., Sugiyama, T., Kohara, H., Kondoh, G., Fujii, N., Kohno, K., et al. (2010). The essential functions of adipo-osteogenic progenitors as the hematopoietic stem and progenitor cell niche. Immunity 33 (3), 387–399. doi:10.1016/j.immuni.2010.08.017
Ono, N., Ono, W., Mizoguchi, T., Nagasawa, T., Frenette, P. S., and Kronenberg, H. M. (2014). Vasculature-associated cells expressing nestin in developing bones encompass early cells in the osteoblast and endothelial lineage. Dev. Cell 29 (3), 330–339. doi:10.1016/j.devcel.2014.03.014
Oostendorp, R. A., Harvey, K. N., Kusadasi, N., de Bruijn, M. F., Saris, C., Ploemacher, R. E., et al. (2002). Stromal cell lines from mouse aorta-gonads-mesonephros subregions are potent supporters of hematopoietic stem cell activity. Blood 99 (4), 1183–1189. doi:10.1182/blood.v99.4.1183
Oostendorp, R. A., Robin, C., Steinhoff, C., Marz, S., Brauer, R., Nuber, U. A., et al. (2005). Long-term maintenance of hematopoietic stem cells does not require contact with embryo-derived stromal cells in cocultures. Stem Cells 23 (6), 842–851. doi:10.1634/stemcells.2004-0120
Orlic, D., Porcellini, A., and Rizzoli, V. (1982). Electron microscopy of human fetal erythroid cells before and after cryopreservation. Exp. Hematol. 10 (7), 628–636.
Osorio, F. G., Rosendahl Huber, A., Oka, R., Verheul, M., Patel, S. H., Hasaart, K., et al. (2018). Somatic mutations reveal lineage relationships and age-related mutagenesis in human hematopoiesis. Cell Rep. 25 (9), 2308–2316. doi:10.1016/j.celrep.2018.11.014
Ottersbach, K. (2019). Endothelial-to-haematopoietic transition: an update on the process of making blood. Biochem. Soc. Trans. 47 (2), 591–601. doi:10.1042/BST20180320
Pardanaud, L., and Dieterlen-Lievre, F. (1999). Manipulation of the angiopoietic/hemangiopoietic commitment in the avian embryo. Development 126 (4), 617–627. doi:10.1242/dev.126.4.617
Pardanaud, L., Luton, D., Prigent, M., Bourcheix, L. M., Catala, M., and Dieterlen-Lievre, F. (1996). Two distinct endothelial lineages in ontogeny, one of them related to hemopoiesis. Development 122 (5), 1363–1371. doi:10.1242/dev.122.5.1363
Passaro, D., Garcia-Albornoz, M., Diana, G., Chakravarty, P., Ariza-McNaughton, L., Batsivari, A., et al. (2021). Integrated OMICs unveil the bone-marrow microenvironment in human leukemia. Cell Rep. 35 (6), 109119. doi:10.1016/j.celrep.2021.109119
Patel, S. H., Christodoulou, C., Weinreb, C., Yu, Q., da Rocha, E. L., Pepe-Mooney, B. J., et al. (2022). Lifelong multilineage contribution by embryonic-born blood progenitors. Nature 606 (7915), 747–753. doi:10.1038/s41586-022-04804-z
Peeters, M., Ottersbach, K., Bollerot, K., Orelio, C., de Bruijn, M., Wijgerde, M., et al. (2009). Ventral embryonic tissues and Hedgehog proteins induce early AGM hematopoietic stem cell development. Development 136 (15), 2613–2621. doi:10.1242/dev.034728
Pietras, E. M., Warr, M. R., and Passegue, E. (2011). Cell cycle regulation in hematopoietic stem cells. J. Cell Biol. 195 (5), 709–720. doi:10.1083/jcb.201102131
Pimanda, J. E., Donaldson, I. J., de Bruijn, M. F., Kinston, S., Knezevic, K., Huckle, L., et al. (2007). The SCL transcriptional network and BMP signaling pathway interact to regulate RUNX1 activity. Proc. Natl. Acad. Sci. U. S. A. 104 (3), 840–845. doi:10.1073/pnas.0607196104
Pineault, K. M., Song, J. Y., Kozloff, K. M., Lucas, D., and Wellik, D. M. (2019). Hox11 expressing regional skeletal stem cells are progenitors for osteoblasts, chondrocytes and adipocytes throughout life. Nat. Commun. 10 (1), 3168. doi:10.1038/s41467-019-11100-4
Pinho, S., and Frenette, P. S. (2019). Haematopoietic stem cell activity and interactions with the niche. Nat. Rev. Mol. Cell Biol. 20 (5), 303–320. doi:10.1038/s41580-019-0103-9
Pinho, S., Lacombe, J., Hanoun, M., Mizoguchi, T., Bruns, I., Kunisaki, Y., et al. (2013). PDGFRα and CD51 mark human nestin+ sphere-forming mesenchymal stem cells capable of hematopoietic progenitor cell expansion. J. Exp. Med. 210 (7), 1351–1367. doi:10.1084/jem.20122252
Pinho, S., Marchand, T., Yang, E., Wei, Q., Nerlov, C., and Frenette, P. S. (2018). Lineage-biased hematopoietic stem cells are regulated by distinct niches. Dev. Cell 44 (5), 634–641. doi:10.1016/j.devcel.2018.01.016
Porcheri, C., Golan, O., Calero-Nieto, F. J., Thambyrajah, R., Ruiz-Herguido, C., Wang, X., et al. (2020). Notch ligand Dll4 impairs cell recruitment to aortic clusters and limits blood stem cell generation. EMBO J. 39 (8), e104270. doi:10.15252/embj.2019104270
Porter, R. L., Georger, M. A., Bromberg, O., McGrath, K. E., Frisch, B. J., Becker, M. W., et al. (2013). Prostaglandin E2 increases hematopoietic stem cell survival and accelerates hematopoietic recovery after radiation injury. Stem Cells 31 (2), 372–383. doi:10.1002/stem.1286
Potocnik, A. J., Brakebusch, C., and Fassler, R. (2000). Fetal and adult hematopoietic stem cells require beta1 integrin function for colonizing fetal liver, spleen, and bone marrow. Immunity 12 (6), 653–663. doi:10.1016/s1074-7613(00)80216-2
Pouget, C., Gautier, R., Teillet, M. A., and Jaffredo, T. (2006). Somite-derived cells replace ventral aortic hemangioblasts and provide aortic smooth muscle cells of the trunk. Development 133 (6), 1013–1022. doi:10.1242/dev.02269
Pouget, C., Peterkin, T., Simoes, F. C., Lee, Y., Traver, D., and Patient, R. (2014). FGF signalling restricts haematopoietic stem cell specification via modulation of the BMP pathway. Nat. Commun. 5, 5588. doi:10.1038/ncomms6588
Poulos, M. G., Guo, P., Kofler, N. M., Pinho, S., Gutkin, M. C., Tikhonova, A., et al. (2013). Endothelial Jagged-1 is necessary for homeostatic and regenerative hematopoiesis. Cell Rep. 4 (5), 1022–1034. doi:10.1016/j.celrep.2013.07.048
Qian, H., Georges-Labouesse, E., Nystrom, A., Domogatskaya, A., Tryggvason, K., Jacobsen, S. E., et al. (2007). Distinct roles of integrins alpha6 and alpha4 in homing of fetal liver hematopoietic stem and progenitor cells. Blood 110 (7), 2399–2407. doi:10.1182/blood-2006-10-051276
Ramasamy, S. K., Kusumbe, A. P., Itkin, T., Gur-Cohen, S., Lapidot, T., and Adams, R. H. (2016). Regulation of hematopoiesis and osteogenesis by blood vessel-derived signals. Annu. Rev. Cell Dev. Biol. 32, 649–675. doi:10.1146/annurev-cellbio-111315-124936
Ratajczak, C. K., and Muglia, L. J. (2008). Insights into parturition biology from genetically altered mice. Pediatr. Res. 64 (6), 581–589. doi:10.1203/PDR.0b013e31818718d2
Rebel, V. I., Miller, C. L., Eaves, C. J., and Lansdorp, P. M. (1996). The repopulation potential of fetal liver hematopoietic stem cells in mice exceeds that of their liver adult bone marrow counterparts. Blood 87 (8), 3500–3507. doi:10.1182/blood.v87.8.3500.bloodjournal8783500
Renstrom, J., Istvanffy, R., Gauthier, K., Shimono, A., Mages, J., Jardon-Alvarez, A., et al. (2009). Secreted frizzled-related protein 1 extrinsically regulates cycling activity and maintenance of hematopoietic stem cells. Cell Stem Cell 5 (2), 157–167. doi:10.1016/j.stem.2009.05.020
Richard, C., Drevon, C., Canto, P. Y., Villain, G., Bollerot, K., Lempereur, A., et al. (2013). Endothelio-mesenchymal interaction controls runx1 expression and modulates the notch pathway to initiate aortic hematopoiesis. Dev. Cell 24 (6), 600–611. doi:10.1016/j.devcel.2013.02.011
Robert-Moreno, A., Espinosa, L., de la Pompa, J. L., and Bigas, A. (2005). RBPjkappa-dependent Notch function regulates Gata2 and is essential for the formation of intra-embryonic hematopoietic cells. Development 132 (5), 1117–1126. doi:10.1242/dev.01660
Robert-Moreno, A., Guiu, J., Ruiz-Herguido, C., Lopez, M. E., Ingles-Esteve, J., Riera, L., et al. (2008). Impaired embryonic haematopoiesis yet normal arterial development in the absence of the Notch ligand Jagged1. EMBO J. 27 (13), 1886–1895. doi:10.1038/emboj.2008.113
Roy, A., Wang, G., Iskander, D., O'Byrne, S., Elliott, N., O'Sullivan, J., et al. (2021). Transitions in lineage specification and gene regulatory networks in hematopoietic stem/progenitor cells over human development. Cell Rep. 36 (11), 109698. doi:10.1016/j.celrep.2021.109698
Rybtsov, S., Batsivari, A., Bilotkach, K., Paruzina, D., Senserrich, J., Nerushev, O., et al. (2014). Tracing the origin of the HSC hierarchy reveals an SCF-dependent, IL-3-independent CD43(-) embryonic precursor. Stem Cell Rep. 3 (3), 489–501. doi:10.1016/j.stemcr.2014.07.009
Sacchetti, B., Funari, A., Michienzi, S., Di Cesare, S., Piersanti, S., Saggio, I., et al. (2007). Self-renewing osteoprogenitors in bone marrow sinusoids can organize a hematopoietic microenvironment. Cell 131 (2), 324–336. doi:10.1016/j.cell.2007.08.025
Sacilotto, N., Monteiro, R., Fritzsche, M., Becker, P. W., Sanchez-Del-Campo, L., Liu, K., et al. (2013). Analysis of Dll4 regulation reveals a combinatorial role for Sox and Notch in arterial development. Proc. Natl. Acad. Sci. U. S. A. 110 (29), 11893–11898. doi:10.1073/pnas.1300805110
Sa da Bandeira, D., Kilpatrick, A. M., Marques, M., Gomez-Salazar, M., Ventura, T., Gonzalez, Z. N., et al. (2022). PDGFRβ+ cells play a dual role as hematopoietic precursors and niche cells during mouse ontogeny. Cell Rep. 40 (3), 111114. doi:10.1016/j.celrep.2022.111114
Saffarzadeh, M., Grunz, K., Nguyen, T. S., Lee, Y. K., Kitano, M., Danckwardt, S., et al. (2020). Macrophage protease-activated receptor 2 regulates fetal liver erythropoiesis in mice. Blood Adv. 4 (22), 5810–5824. doi:10.1182/bloodadvances.2020003299
Sahai-Hernandez, P., Pouget, C., Eyal, S., Svoboda, O., Chacon, J., Grimm, L., et al. (2023). Dermomyotome-derived endothelial cells migrate to the dorsal aorta to support hematopoietic stem cell emergence. Elife 12, e58300. doi:10.7554/eLife.58300
Saito, M., Iwawaki, T., Taya, C., Yonekawa, H., Noda, M., Inui, Y., et al. (2001). Diphtheria toxin receptor-mediated conditional and targeted cell ablation in transgenic mice. Nat. Biotechnol. 19 (8), 746–750. doi:10.1038/90795
Sakurai, M., Ishitsuka, K., Ito, R., Wilkinson, A. C., Kimura, T., Mizutani, E., et al. (2023). Chemically defined cytokine-free expansion of human haematopoietic stem cells. Nature 615 (7950), 127–133. doi:10.1038/s41586-023-05739-9
Sanchez-Lanzas, R., Kalampalika, F., and Ganuza, M. (2022). Diversity in the bone marrow niche: classic and novel strategies to uncover niche composition. Br. J. Haematol. 199 (5), 647–664. doi:10.1111/bjh.18355
Schepers, K., Campbell, T. B., and Passegue, E. (2015). Normal and leukemic stem cell niches: insights and therapeutic opportunities. Cell Stem Cell 16 (3), 254–267. doi:10.1016/j.stem.2015.02.014
Schmelzer, E., Wauthier, E., and Reid, L. M. (2006). The phenotypes of pluripotent human hepatic progenitors. Stem Cells 24 (8), 1852–1858. doi:10.1634/stemcells.2006-0036
Schmelzer, E., Zhang, L., Bruce, A., Wauthier, E., Ludlow, J., Yao, H. L., et al. (2007). Human hepatic stem cells from fetal and postnatal donors. J. Exp. Med. 204 (8), 1973–1987. doi:10.1084/jem.20061603
Shalapour, S., Eckert, C., Seeger, K., Pfau, M., Prada, J., Henze, G., et al. (2010). Leukemia-associated genetic aberrations in mesenchymal stem cells of children with acute lymphoblastic leukemia. J. Mol. Med. (Berl). 88 (3), 249–265. doi:10.1007/s00109-009-0583-8
Shao, L., Paik, N. Y., Sanborn, M. A., Bandara, T., Vijaykumar, A., Sottoriva, K., et al. (2023). Hematopoietic Jagged1 is a fetal liver niche factor required for functional maturation and engraftment of fetal hematopoietic stem cells. Proc. Natl. Acad. Sci. U. S. A. 120 (20), e2210058120. doi:10.1073/pnas.2210058120
Shu, H. S., Liu, Y. L., Tang, X. T., Zhang, X. S., Zhou, B., Zou, W., et al. (2021). Tracing the skeletal progenitor transition during postnatal bone formation. Cell Stem Cell 28 (12), 2122–2136.e3. doi:10.1016/j.stem.2021.08.010
Sieburg, H. B., Cho, R. H., Dykstra, B., Uchida, N., Eaves, C. J., and Muller-Sieburg, C. E. (2006). The hematopoietic stem compartment consists of a limited number of discrete stem cell subsets. Blood 107 (6), 2311–2316. doi:10.1182/blood-2005-07-2970
Sigurdsson, V., Takei, H., Soboleva, S., Radulovic, V., Galeev, R., Siva, K., et al. (2016). Bile acids protect expanding hematopoietic stem cells from unfolded protein stress in fetal liver. Cell Stem Cell 18 (4), 522–532. doi:10.1016/j.stem.2016.01.002
Silberstein, L., Goncalves, K. A., Kharchenko, P. V., Turcotte, R., Kfoury, Y., Mercier, F., et al. (2016). Proximity-based differential single-cell analysis of the niche to identify stem/progenitor cell regulators. Cell Stem Cell 19 (4), 530–543. doi:10.1016/j.stem.2016.07.004
Simic, M., Manosalva, I., Spinelli, L., Gentek, R., Shayan, R. R., Siret, C., et al. (2020). Distinct waves from the hemogenic endothelium give rise to layered lymphoid tissue inducer cell ontogeny. Cell Rep. 32 (6), 108004. doi:10.1016/j.celrep.2020.108004
Souilhol, C., Gonneau, C., Lendinez, J. G., Batsivari, A., Rybtsov, S., Wilson, H., et al. (2016a). Inductive interactions mediated by interplay of asymmetric signalling underlie development of adult haematopoietic stem cells. Nat. Commun. 7, 10784. doi:10.1038/ncomms10784
Souilhol, C., Lendinez, J. G., Rybtsov, S., Murphy, F., Wilson, H., Hills, D., et al. (2016b). Developing HSCs become Notch independent by the end of maturation in the AGM region. Blood 128 (12), 1567–1577. doi:10.1182/blood-2016-03-708164
Sturgeon, C. M., Ditadi, A., Awong, G., Kennedy, M., and Keller, G. (2014). Wnt signaling controls the specification of definitive and primitive hematopoiesis from human pluripotent stem cells. Nat. Biotechnol. 32 (6), 554–561. doi:10.1038/nbt.2915
Sudo, K., Ema, H., Morita, Y., and Nakauchi, H. (2000). Age-associated characteristics of murine hematopoietic stem cells. J. Exp. Med. 192 (9), 1273–1280. doi:10.1084/jem.192.9.1273
Sugimura, R., Jha, D. K., Han, A., Soria-Valles, C., da Rocha, E. L., Lu, Y. F., et al. (2017). Haematopoietic stem and progenitor cells from human pluripotent stem cells. Nature 545 (7655), 432–438. doi:10.1038/nature22370
Sugiyama, D., Kulkeaw, K., and Mizuochi, C. (2013). TGF-beta-1 up-regulates extra-cellular matrix production in mouse hepatoblasts. Mech. Dev. 130 (2–3), 195–206. doi:10.1016/j.mod.2012.09.003
Sugiyama, D., Kulkeaw, K., Mizuochi, C., Horio, Y., and Okayama, S. (2011). Hepatoblasts comprise a niche for fetal liver erythropoiesis through cytokine production. Biochem. Biophys. Res. Commun. 410 (2), 301–306. doi:10.1016/j.bbrc.2011.05.137
Sugiyama, T., Kohara, H., Noda, M., and Nagasawa, T. (2006). Maintenance of the hematopoietic stem cell pool by CXCL12-CXCR4 chemokine signaling in bone marrow stromal cell niches. Immunity 25 (6), 977–988. doi:10.1016/j.immuni.2006.10.016
Szilvassy, S. J., Humphries, R. K., Lansdorp, P. M., Eaves, A. C., and Eaves, C. J. (1990). Quantitative assay for totipotent reconstituting hematopoietic stem cells by a competitive repopulation strategy. Proc. Natl. Acad. Sci. U. S. A. 87 (22), 8736–8740. doi:10.1073/pnas.87.22.8736
Tamplin, O. J., Durand, E. M., Carr, L. A., Childs, S. J., Hagedorn, E. J., Li, P., et al. (2015). Hematopoietic stem cell arrival triggers dynamic remodeling of the perivascular niche. Cell 160 (1-2), 241–252. doi:10.1016/j.cell.2014.12.032
Taoudi, S., Gonneau, C., Moore, K., Sheridan, J. M., Blackburn, C. C., Taylor, E., et al. (2008). Extensive hematopoietic stem cell generation in the AGM region via maturation of VE-cadherin+CD45+ pre-definitive HSCs. Cell Stem Cell 3 (1), 99–108. doi:10.1016/j.stem.2008.06.004
Taoudi, S., and Medvinsky, A. (2007). Functional identification of the hematopoietic stem cell niche in the ventral domain of the embryonic dorsal aorta. Proc. Natl. Acad. Sci. U. S. A. 104 (22), 9399–9403. doi:10.1073/pnas.0700984104
Tavassoli, M., and Crosby, W. H. (1968). Transplantation of marrow to extramedullary sites. Science 161 (3836), 54–56. doi:10.1126/science.161.3836.54
Thambyrajah, R., and Bigas, A. (2022). Notch signaling in HSC emergence: when, why and how. Cells 11 (3), 358. doi:10.3390/cells11030358
Theodore, L. N., Hagedorn, E. J., Cortes, M., Natsuhara, K., Liu, S. Y., Perlin, J. R., et al. (2017). Distinct roles for matrix metalloproteinases 2 and 9 in embryonic hematopoietic stem cell emergence, migration, and niche colonization. Stem Cell Rep. 8 (5), 1226–1241. doi:10.1016/j.stemcr.2017.03.016
Tikhonova, A. N., Dolgalev, I., Hu, H., Sivaraj, K. K., Hoxha, E., Cuesta-Dominguez, A., et al. (2019). The bone marrow microenvironment at single-cell resolution. Nature 569 (7755), 222–228. doi:10.1038/s41586-019-1104-8
Trevisan, M., Yan, X. Q., and Iscove, N. N. (1996). Cycle initiation and colony formation in culture by murine marrow cells with long-term reconstituting potential in vivo. Blood 88 (11), 4149–4158. doi:10.1182/blood.v88.11.4149.bloodjournal88114149
Tseng, N. A., and Beaudin, A. E. (2023). The impact of prenatal inflammation on hematopoietic development. Curr. Opin. Hematol. 30 (4), 130–136. doi:10.1097/MOH.0000000000000770
Turner, W. S., Schmelzer, E., McClelland, R., Wauthier, E., Chen, W., and Reid, L. M. (2007). Human hepatoblast phenotype maintained by hyaluronan hydrogels. J. Biomed. Mater Res. B Appl. Biomater. 82 (1), 156–168. doi:10.1002/jbm.b.30717
Turpen, J. B., Kelley, C. M., Mead, P. E., and Zon, L. I. (1997). Bipotential primitive-definitive hematopoietic progenitors in the vertebrate embryo. Immunity 7 (3), 325–334. doi:10.1016/s1074-7613(00)80354-4
Ulloa, B. A., Habbsa, S. S., Potts, K. S., Lewis, A., McKinstry, M., Payne, S. G., et al. (2021). Definitive hematopoietic stem cells minimally contribute to embryonic hematopoiesis. Cell Rep. 36 (11), 109703. doi:10.1016/j.celrep.2021.109703
Upadhaya, S., Krichevsky, O., Akhmetzyanova, I., Sawai, C. M., Fooksman, D. R., and Reizis, B. (2020). Intravital imaging reveals motility of adult hematopoietic stem cells in the bone marrow niche. Cell Stem Cell 27 (2), 336–345. doi:10.1016/j.stem.2020.06.003
Visnjic, D., Kalajzic, Z., Rowe, D. W., Katavic, V., Lorenzo, J., and Aguila, H. L. (2004). Hematopoiesis is severely altered in mice with an induced osteoblast deficiency. Blood 103 (9), 3258–3264. doi:10.1182/blood-2003-11-4011
Wauthier, E., Schmelzer, E., Turner, W., Zhang, L., LeCluyse, E., Ruiz, J., et al. (2008). Hepatic stem cells and hepatoblasts: identification, isolation, and ex vivo maintenance. Methods Cell Biol. 86, 137–225. doi:10.1016/S0091-679X(08)00008-3
Werner, B., Beier, F., Hummel, S., Balabanov, S., Lassay, L., Orlikowsky, T., et al. (2015). Reconstructing the in vivo dynamics of hematopoietic stem cells from telomere length distributions. Elife 4, e08687. doi:10.7554/eLife.08687
Wilkinson, A. C., Ishida, R., Kikuchi, M., Sudo, K., Morita, M., Crisostomo, R. V., et al. (2019). Long-term ex vivo haematopoietic-stem-cell expansion allows nonconditioned transplantation. Nature 571 (7763), 117–121. doi:10.1038/s41586-019-1244-x
Wilkinson, R. N., Pouget, C., Gering, M., Russell, A. J., Davies, S. G., Kimelman, D., et al. (2009). Hedgehog and Bmp polarize hematopoietic stem cell emergence in the zebrafish dorsal aorta. Dev. Cell 16 (6), 909–916. doi:10.1016/j.devcel.2009.04.014
Wilson, A., Laurenti, E., Oser, G., van der Wath, R. C., Blanco-Bose, W., Jaworski, M., et al. (2008). Hematopoietic stem cells reversibly switch from dormancy to self-renewal during homeostasis and repair. Cell 135 (6), 1118–1129. doi:10.1016/j.cell.2008.10.048
Wineman, J., Moore, K., Lemischka, I., and Muller-Sieburg, C. (1996). Functional heterogeneity of the hematopoietic microenvironment: rare stromal elements maintain long-term repopulating stem cells. Blood 87 (10), 4082–4090. doi:10.1182/blood.v87.10.4082.bloodjournal87104082
Winkler, I. G., Barbier, V., Wadley, R., Zannettino, A. C., Williams, S., and Levesque, J. P. (2010b). Positioning of bone marrow hematopoietic and stromal cells relative to blood flow in vivo: serially reconstituting hematopoietic stem cells reside in distinct nonperfused niches. Blood 116 (3), 375–385. doi:10.1182/blood-2009-07-233437
Winkler, I. G., Sims, N. A., Pettit, A. R., Barbier, V., Nowlan, B., Helwani, F., et al. (2010a). Bone marrow macrophages maintain hematopoietic stem cell (HSC) niches and their depletion mobilizes HSCs. Blood 116 (23), 4815–4828. doi:10.1182/blood-2009-11-253534
Wolber, F. M., Leonard, E., Michael, S., Orschell-Traycoff, C. M., Yoder, M. C., and Srour, E. F. (2002). Roles of spleen and liver in development of the murine hematopoietic system. Exp. Hematol. 30 (9), 1010–1019. doi:10.1016/s0301-472x(02)00881-0
Wolf, N. S. (1978). Dissecting the hematopoietic microenvironment. III. Evidence for a positive short range stimulus for cellular proliferation. Cell Tissue Kinet. 11 (4), 335–345. doi:10.1111/j.1365-2184.1978.tb00806.x
Wolock, S. L., Krishnan, I., Tenen, D. E., Matkins, V., Camacho, V., Patel, S., et al. (2019). Mapping distinct bone marrow niche populations and their differentiation paths. Cell Rep. 28 (2), 302–311. doi:10.1016/j.celrep.2019.06.031
Xie, Y., Yin, T., Wiegraebe, W., He, X. C., Miller, D., Stark, D., et al. (2009). Detection of functional haematopoietic stem cell niche using real-time imaging. Nature 457 (7225), 97–101. doi:10.1038/nature07639
Xu, C., Gao, X., Wei, Q., Nakahara, F., Zimmerman, S. E., Mar, J., et al. (2018). Stem cell factor is selectively secreted by arterial endothelial cells in bone marrow. Nat. Commun. 9 (1), 2449. doi:10.1038/s41467-018-04726-3
Xue, Y., Liu, D., Cui, G., Ding, Y., Ai, D., Gao, S., et al. (2019). A 3D atlas of hematopoietic stem and progenitor cell expansion by multi-dimensional RNA-seq analysis. Cell Rep. 27 (5), 1567–1578. doi:10.1016/j.celrep.2019.04.030
Xue, Y., Lv, J., Zhang, C., Wang, L., Ma, D., and Liu, F. (2017). The vascular niche regulates hematopoietic stem and progenitor cell lodgment and expansion via klf6a-ccl25b. Dev. Cell 42 (4), 349–362. doi:10.1016/j.devcel.2017.07.012
Yokomizo, T., Ideue, T., Morino-Koga, S., Tham, C. Y., Sato, T., Takeda, N., et al. (2022). Independent origins of fetal liver haematopoietic stem and progenitor cells. Nature 609 (7928), 779–784. doi:10.1038/s41586-022-05203-0
Yokomizo, T., Ng, C. E., Osato, M., and Dzierzak, E. (2011). Three-dimensional imaging of whole midgestation murine embryos shows an intravascular localization for all hematopoietic clusters. Blood 117 (23), 6132–6134. doi:10.1182/blood-2011-02-334037
Yokomizo, T., Ogawa, M., Osato, M., Kanno, T., Yoshida, H., Fujimoto, T., et al. (2001). Requirement of Runx1/AML1/PEBP2alphaB for the generation of haematopoietic cells from endothelial cells. Genes Cells 6 (1), 13–23. doi:10.1046/j.1365-2443.2001.00393.x
You, L. R., Lin, F. J., Lee, C. T., DeMayo, F. J., Tsai, M. J., and Tsai, S. Y. (2005). Suppression of Notch signalling by the COUP-TFII transcription factor regulates vein identity. Nature 435 (7038), 98–104. doi:10.1038/nature03511
Young, K., Eudy, E., Bell, R., Loberg, M. A., Stearns, T., Sharma, D., et al. (2021). Decline in IGF1 in the bone marrow microenvironment initiates hematopoietic stem cell aging. Cell Stem Cell 28 (8), 1473–1482.e7. doi:10.1016/j.stem.2021.03.017
Yvernogeau, L., Dainese, G., and Jaffredo, T. (2023). Dorsal aorta polarization and haematopoietic stem cell emergence. Development 150 (1), dev201173. doi:10.1242/dev.201173
Zhang, B., Ho, Y. W., Huang, Q., Maeda, T., Lin, A., Lee, S. U., et al. (2012). Altered microenvironmental regulation of leukemic and normal stem cells in chronic myelogenous leukemia. Cancer Cell 21 (4), 577–592. doi:10.1016/j.ccr.2012.02.018
Zhang, C. C., Kaba, M., Ge, G., Xie, K., Tong, W., Hug, C., et al. (2006). Angiopoietin-like proteins stimulate ex vivo expansion of hematopoietic stem cells. Nat. Med. 12 (2), 240–245. doi:10.1038/nm1342
Zhang, C. C., and Lodish, H. F. (2004). Insulin-like growth factor 2 expressed in a novel fetal liver cell population is a growth factor for hematopoietic stem cells. Blood 103 (7), 2513–2521. doi:10.1182/blood-2003-08-2955
Zhang, J., Niu, C., Ye, L., Huang, H., He, X., Tong, W. G., et al. (2003). Identification of the haematopoietic stem cell niche and control of the niche size. Nature 425 (6960), 836–841. doi:10.1038/nature02041
Zhang, Y., Depond, M., He, L., Foudi, A., Kwarteng, E. O., Lauret, E., et al. (2016). CXCR4/CXCL12 axis counteracts hematopoietic stem cell exhaustion through selective protection against oxidative stress. Sci. Rep. 6, 37827. doi:10.1038/srep37827
Zhao, M., Perry, J. M., Marshall, H., Venkatraman, A., Qian, P., He, X. C., et al. (2014). Megakaryocytes maintain homeostatic quiescence and promote post-injury regeneration of hematopoietic stem cells. Nat. Med. 20 (11), 1321–1326. doi:10.1038/nm.3706
Zhao, Y., Zhou, J., Liu, D., Dong, F., Cheng, H., Wang, W., et al. (2015). ATF4 plays a pivotal role in the development of functional hematopoietic stem cells in mouse fetal liver. Blood 126 (21), 2383–2391. doi:10.1182/blood-2015-03-633354
Zhong, L., Yao, L., Tower, R. J., Wei, Y., Miao, Z., Park, J., et al. (2020). Single cell transcriptomics identifies a unique adipose lineage cell population that regulates bone marrow environment. Elife 9, e54695. doi:10.7554/eLife.54695
Zhou, B. O., Ding, L., and Morrison, S. J. (2015). Hematopoietic stem and progenitor cells regulate the regeneration of their niche by secreting Angiopoietin-1. Elife 4, e05521. doi:10.7554/eLife.05521
Zhou, B. O., Yue, R., Murphy, M. M., Peyer, J. G., and Morrison, S. J. (2014). Leptin-receptor-expressing mesenchymal stromal cells represent the main source of bone formed by adult bone marrow. Cell Stem Cell 15 (2), 154–168. doi:10.1016/j.stem.2014.06.008
Keywords: hematopoietic stem cells, developmental hematopoiesis, hematopoietic niches, aorta-gonad-mesonephros, fetal liver, bone marrow
Citation: Sánchez-Lanzas R, Jiménez-Pompa A and Ganuza M (2024) The evolving hematopoietic niche during development. Front. Mol. Biosci. 11:1488199. doi: 10.3389/fmolb.2024.1488199
Received: 29 August 2024; Accepted: 20 September 2024;
Published: 02 October 2024.
Edited by:
Emanuele Azzoni, University of Milano Bicocca, ItalyReviewed by:
Virginia Actis Dato, University of California, San Diego, United StatesStefania Oliveto, University of Milan, Italy
Satish Khurana, Indian Institute of Science Education and Research, India
Copyright © 2024 Sánchez-Lanzas, Jiménez-Pompa and Ganuza. This is an open-access article distributed under the terms of the Creative Commons Attribution License (CC BY). The use, distribution or reproduction in other forums is permitted, provided the original author(s) and the copyright owner(s) are credited and that the original publication in this journal is cited, in accordance with accepted academic practice. No use, distribution or reproduction is permitted which does not comply with these terms.
*Correspondence: Miguel Ganuza, bS5nYW51emFAcW11bC5hYy51aw==
†These authors have contributed equally to this work and share first authorship
‡ORCID: Raúl Sánchez-Lanzas, orcid.org/0000-0002-6179-6546