- 1Gene Editing Technology Center of Guangdong Province, School of Medicine, Foshan University, Foshan, China
- 2School of Biology and Biological Engineering, South China University of Technology, Guangzhou, China
- 3Beijing Mercer United International Education Consulting Co., Ltd., Guangzhou, China
- 4State Key Laboratory of Respiratory Disease, Guangzhou Institute of Respiratory Health, The First Affiliated Hospital of Guangzhou Medical University, Guangzhou, China
Varying from other identified cell death pathways, cuproptosis is a new type of regulated cell death characterized by excess Cu ions, abnormal aggregation of lipoylated proteins in TCA cycle, loss of Fe-S cluster proteins, upregulation of HSP70, leading to proteotoxic and oxidative stress. Cuproptosis is highly concerned by scientific community and as the field of cuproptosis further develops, remarkable progress has been made in the verification and mechanism of cuproptosis, and methods used to detect cuproptosis have been continuously improved. According to the characteristic changes of cuproptosis, techniques based on cell death verification, Cu content, morphology, molecular biology of protein levels of cuproptosis-related molecules and biochemical pathways of cuproptosis-related enzyme activity and metabolites of oxidative stress, lipoic acid, TCA cycle, Fe-S cluster proteins, oxidative phosphorylation, cell respiration intensity have been subject to cuproptosis verification and research. In order to further deepen the understanding of detecting cuproptosis, the principle and application of common cuproptosis detection methods are reviewed and categorized in cellular phenomena and molecular mechanism in terms of cell death, Cu content, morphology, molecular biology, biochemical pathways with a flow chart. All the indicating results have been displayed in response to the markers of cuproptosis, their advantages and limitations are summaried, and comparison of cuproptosis and ferroptosis detection is performed in this study. Our collection of methods for cuproptosis detection will provide a great basis for cuproptosis verification and research in the future.
1 Introduction
Copper (Cu) is an essential micronutrient that acts as a vital catalytic cofactor to activate the target enzymes in a wide range of physiological process, including protein processing (Perkal et al., 2020), oxidative balance (Pan et al., 2024), mitochondrial respiration (Garza et al., 2023) and intranuclear transcriptional regulation (Zhong et al., 2023). Our body maintains the concentration of Cu ions at a very low level through some active internal environmental balancing mechanisms: the absorption of Cu ions occurs mainly in gastrointestinal tract, where Cu2+ are reduced to Cu+ by metal reductase prostate six-transmembrane epithelial antigen (STEAP), and reach to liver via the bloodstream; the uptake of Cu+ is mediated by solute carrier family 31 member 1 (SLC31A1) and Cu ions are stored by metallothionein (MT) in hepatocytes; Cu-atpase ATP7B in liver delivers Cu ions to the secretory pathway for ceruloplasmin metalation and Cu ions are carried to tissues and organs, in which ATP7A functions; homeostasis regulation of intracellular Cu content is influenced by a complex Cu-dependent network, including Cu enzymes, Cu chaperones and membrane transporters; they work together to mediate the input, output, and intracellular utilization of Cu ions, which maintain intracellular Cu levels within a specific range, and prevent the cell damage of Cu deficiency and overload (Figure 1) (Chen et al., 2022; Garza et al., 2023; Bartee and Lutsenko, 2007). More and more evidence shows that the imbalance of Cu homeostasis is related to the occurrence and development of adverse health effects, such as Menke’s disease (Fujisawa et al., 2022), Wilson’s disease (Stalke et al., 2023), neurodegenerative diseases (Alzheimer’s disease, amyotrophic lateral sclerosis, Huntington’s disease) (Shen et al., 2023; Yang Y. et al., 2023; Cordeiro et al., 2023), cardiovascular diseases (Yang L. et al., 2023) and cancers (Xie et al., 2023).
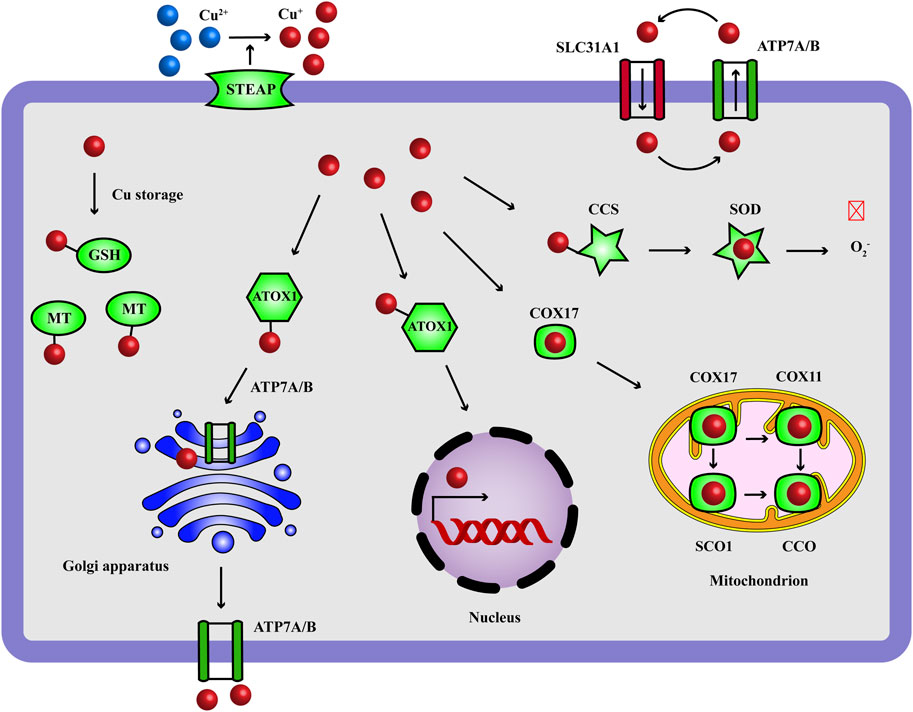
Figure 1. Copper homeostasis: the transport and functions of Cu ions. Cu ions are transported to target cells through blood circulation and the intracellular input and output of Cu+ (reduced by STEAP) are mediated by SLC31A1 and ATP7A/7B. GSH is a Cu chaperone and the intracellular Cu ions are stored with MT. Cu ions act as a catalytic cofactor to activate the target enzymes in oxidative balance (SOD), mitochondrial respiration (ETC.), transcriptional complex (nucleus) and protein processing (Golgi apparatus). CCS carries Cu+ to SOD, ATOX1 carries Cu+ to nucleus and Golgi apparatus, COX17 carries Cu+ to SCO1 and COX11 in mitochondrion for the assembly of CCO. Red and crossed symbol indicates inhibitory effect. ATOX1, antioxidant-1; CCO, cytochrome c oxidase; CCS, Cu chaperone for superoxide dismutase; COX, cytochrome c oxidase; GSH, glutathione; MT, metallothionein; SCO, synthesis of cytochrome c oxidase; SLC31A1, solute carrier family 31 member 1; SOD, superoxide dismutase; STEAP, metal reductase prostate six-transmembrane epithelial antigen.
Recently, Golub TR et al. reported a novel form of cell death caused by excess Cu ions, named cuproptosis (Tsvetkov et al., 2022). Cuproptosis mainly shows a series of characteristic changes: the excess Cu ions delivered by Cu ionophores (Elesclomol, Disulfiram, etc.) directly bind to the ferredoxin 1 (FDX1)/lipoyl synthase (LIAS)-lipoylated target dihydrolipoamide S-acetyltransferase (DLAT) in mitochondrial tricarboxylic acid (TCA) cycle and induce abnormal aggregation of DLAT, loss of Fe-S cluster proteins and upregulation of heat shock protein 70 (HSP70), leading to proteotoxic stress, oxidative stress and eventually cell death (Figure 2). Cuproptosis is a new type of regulated cell death (RCD) impairing mitochondrial respiration and highly concerned by scientific community, varying from other identified programmed cell death such as apoptosis (Ren Y. et al., 2023), necrosis (Sui et al., 2023), pyroptosis (Liu et al., 2022), autophagy (Tao et al., 2023) and ferroptosis (Wang et al., 2023b). Specifically, from the discovery of cuproptosis, 10 genes have been verified as cuproptosis-related genes, including positive regulatory factors: FDX1, lipoylation proteins [lipoic acid pathway lipoacyltransferase 1 (LIPT1), LIAS, dihydroacylamide dehydrogenase (DLD)], lipoylated targets [DLAT, pyruvate dehydrogenase E1 subunit α 1 (PDHA1), pyruvate dehydrogenase E1 subunit β (PDHB)] and negative regulatory factors: metal regulatory transcription factor 1 (MTF1), cyclin-dependent kinase inhibitor 2A (CDKN2A), glutaminase (GLS) (Tsvetkov et al., 2022).
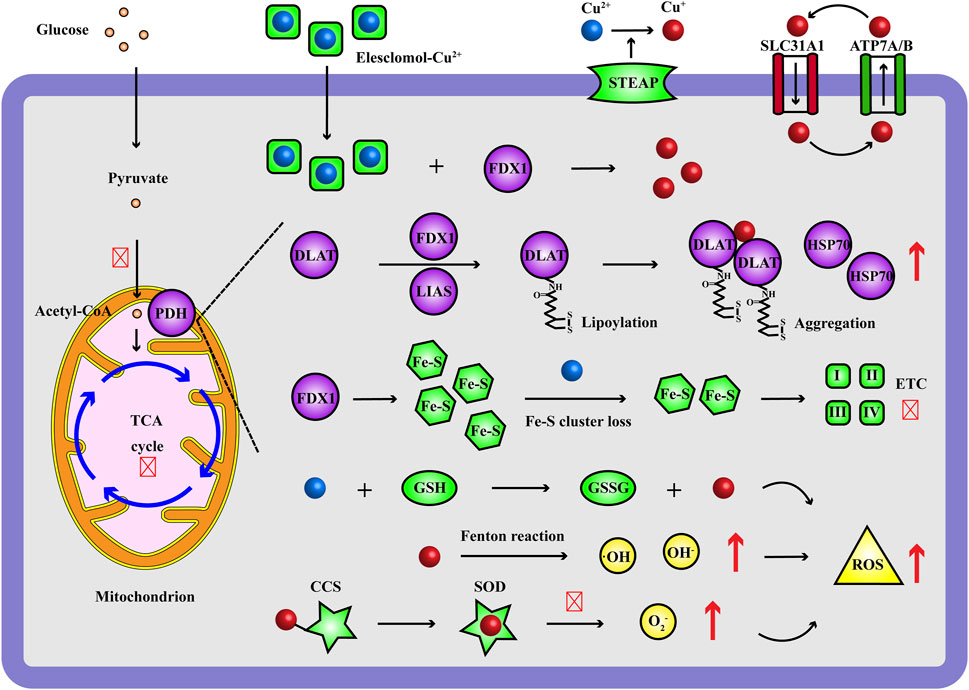
Figure 2. Occurrence and mechanism of Cu ions inducing cell death. During cuproptosis, the Elesclomol-delivered Cu2+ target FDX1 and are reduced to Cu+, which bind to the lipoylated DLAT of PDH complex in mitochondrial TCA cycle and induce aggregation of DLAT, up-regulation of HSP70, loss of Fe-S cluster proteins, ETC disruption; moreover, excess Cu ions improve Fenton reaction, impair GSH and SOD antioxidant system, resulting in increasing ROS levels (·OH, OH−, O2−). Red and up arrow indicates increasing effect, red and crossed symbol indicates inhibitory effect. CCS, Cu chaperone for superoxide dismutase; DLAT, dihydrolipoamide S-acetyltransferase; ETC, electron transport chain; FDX1, ferredoxin 1; GSH, glutathione; GSSG, glutathione; HSP70, heat shock protein 70; LIAS, lipoyl synthase; PDH, pyruvate dehydrogenase; ROS, reactive oxygen species; SLC31A1, solute carrier family 31 member 1; SOD, superoxide dismutase; STEAP, metal reductase prostate six-transmembrane epithelial antigen; TCA, tricarboxylic acid.
According to these characteristic changes of cuproptosis, detection methods involving in cell death verification, Cu content, morphology, molecular biology of protein levels of cuproptosis-related molecules and biochemical pathways of cuproptosis-related enzyme activity and metabolites of oxidative stress, lipoic acid, TCA cycle, Fe-S cluster proteins, oxidative phosphorylation, cell respiration intensity, have been widely applied in exploring the verification and molecular mechanism of cuproptosis. For further enhancing the understanding of cuproptosis detection methods, we categorize the principle and application of common cuproptosis detection methods with a flow chart, summarize their advantages and limitations, and perform comparison of cuproptosis and ferroptosis detection in this study, hoping to provide a great basis for verifying and studying cuproptosis in the future.
2 Detection based on cell death
The Cu homeostasis imbalance will induce Cu toxicity to cells through aggravating Cu import and reducing Cu efflux. And it has been demonstrated that Cu ionophores (Elesclomol, Disulfiram, etc.) deliver excess Cu2+ into cells and result in a new type of RCD, which can be rescued by pretreatment of Cu chelators (Tsvetkov et al., 2022). Firstly, we should identify the induction of cell death effects which may be mediated by excess Cu ions. Assays of CCK8/MTT (indicated by absorbance), clone formation, Annexin V/PI (flow cytometry) and xenograft (in vivo test) are common methods to verify cell viability and cell death effects in Cu stress, with the results of lower optical density (OD), less cell quantity, higher apoptosis rate and smaller xenograft, respectively. Since cell death pathways are very complex, cell death inhibitors based on sensitivity are recognized regents to exclude classic cell death pathways during cuproptosis study, involving in apoptosis [Z-VAD-FMK (Yan et al., 2021), Z-DEVD-FMK (Deng et al., 2021)], necrosis [Necrostatin-1 (Sun et al., 2023), Necrosulfonamide (Gao et al., 2023)], pyroptosis [BAY 11-7082 (Wu et al., 2021), Ac-YVAD-cmk (Lu et al., 2024)], autophagy [Bafilomycin A1 (Zhang et al., 2023), MHY1485 (Lin et al., 2020)] and ferroptosis [Ferrostatin-1 (Yu et al., 2022), Cycloheximide (Zheng et al., 2023)]. To preliminarily identify the cell death triggered by Cu ions, inhibitory effects on cell viability should be measured, which are not sensitive to inhibitors of classic cell death pathways but pretreatment of Cu chelators.
3 Detection based on Cu content
Cu ions should be kept in a narrow range for normal cellular metabolic activities. After the preliminary verification of cell death effects in Cu stress, next, it’s essential to measure the intracellular Cu ion levels, a key procedure used to cuproptosis. Researchers found that Elesclomol as low as 40 nM increased tenfold intracellular Cu ion levels (indicated by inductively coupled plasma mass spectrometry, ICP-MS) and triggered cell death after 24 h, but the inhibitory effects could be reversed when pretreating with Cu chelators or in the absence of Cu ions, indicating that the cell death was induced by the accumulation of Cu ions, not the Cu ionophore itself (Tsvetkov et al., 2022). Herein, the intracellular Cu ion levels should be significantly increased during cuproptosis so that the Cu content awaits measurement when the cell death effects have been observed in Cu stress. ICP-MS is one of the most accurate and sensitive technologies for quantifying metal ion content, but limited by complex testing preparation and instrument. Thus, some other effective methods are required to accurately and quickly measure Cu content in serum and cell lysate, including colorimetric, fluorescent probe, and electrochemistry methods (Table 1).
3.1 Colorimetric
Chromogen specifically forms color complex with Cu ions and the intensity proportional to Cu content can be measured using a microplate reader, such as copper assay kits from MesGen (Tong et al., 2023), Elabscience (Li et al., 2023) and BioAssay Systems (Parmar et al., 2018). They are simple, sensitive, accurate and high-throughput, but will be interfered by metal chelators in samples, and microplate reader needed (Figure 3A).
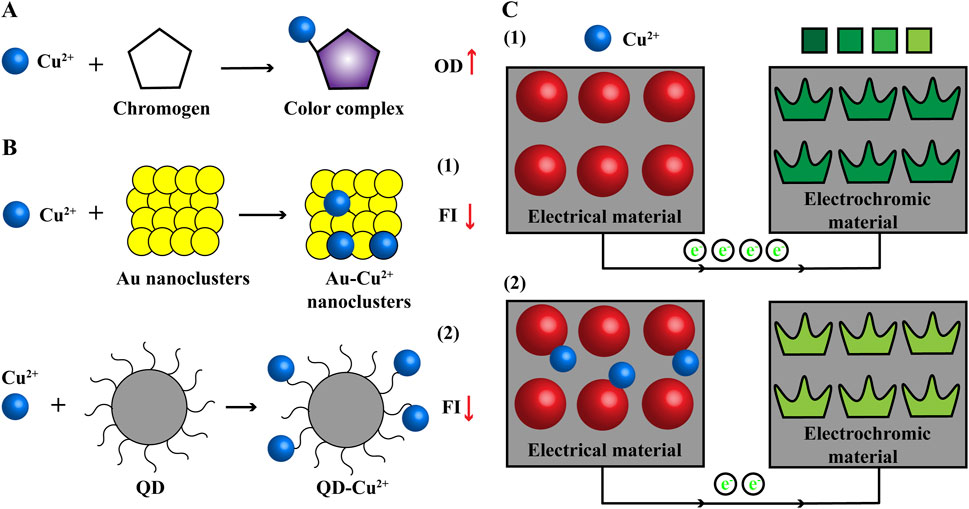
Figure 3. Common methods used to detect Cu content (A) Colorimetric. Cu ions and chromogen form color complex with increasing OD. (B) Fluorescent probe. The FI of noble metallic nanoclusters (Au) and QD will be quenched when combined with Cu ions. (C) Electrochemistry. The electrical signal of electrical material will be changed and recorded in the presence of Cu ions and signal can be visual with combination of electrochromic material. e−: electron; FI, fluorescence intensity; OD, optical density; QD, quantum dot.
3.2 Fluorescent probe
Fluorescent probe is a kind of fluorescence signal nanomaterial with high sensitivity and short reaction time, which is widely used in chemical biological sensing, biomedical imaging and so on. The fluorescence signal of noble metallic nanoclusters and quantum dots will be quenched in the presence of Cu ions (Figure 3B). Thus, they have been developed to detect Cu content, with advantages of more sensitive, accurate and high-throughput, but fluorescent reader needed (Ou et al., 2018; Liu and Sun, 2023).
3.3 Electrochemistry
Scientists use the highly electrical material (Ti3C2Tx/MWNTs-Au) as the working electrode and the electrical signal will be changed and recorded when binding to Cu ions (Hui et al., 2020). But electrochemistry technique has high background signal and the results are unvisualized. The electrochromic material has stable and reversed color change in electric field. Further, the electrochemistry technique and electrochromic material are combined that electrochromic material is used as the counter electrode (CdS QDs/WO3 nanoflakes); Cu ions bind to the electrical material and reduce the electrical signal, which causes the color change of electrochromic material from dark to light. Visual signal of Cu ions can be obtained from color change and used for fast semiquantitative field detection; electrical signal can provide more accurate data for Cu content (Figure 3C) (Zhang N. et al., 2022). Electrochemical workstation is needed for this method of detecting Cu content.
4 Detection based on morphology
In the first step and second step, the cell death effects rescued by pretreatment of Cu chelators and Cu deposition can indicate the induction of cuproptosis. Since some specific morphological properties will appear during cuproptosis, morphological detection can intuitively distinguish cuproptosis. Then we can check the morphology properties of cell membrane and organelles for adjuvant estimation. According to the characteristics of cuproptosis, Yang L. et al. summarized that the morphological features of cuproptosis involve cell membrane rupture, mitochondrial contraction, endoplasmic reticulum and chromatin damage (Yang et al., 2023a). These morphological changes of subcellular structure and organelles can be intuitively examined by transmission electron microscope (TEM). Morphology method has intuitive and clear results, but the sample preparation is complicated and there are subjective biases in the observation of subcellular morphology, so it is often combined with quantitative detection methods based on cell death and Cu content we describe above to preliminarily verify cuproptosis. By far methods based on cell death effects with inhibitors, Cu ions overload and morphological features may thus be promising tools for detecting the cellular phenomena of cuproptosis, further investigation about molecular mechanism is needed to gain comprehensive evaluation of cuproptosis. Therefore, addressing the detection methods of Cu-induced cell death based on molecular biology of protein levels of cuproptosis-related molecules, biochemical pathways of cuproptosis-related enzyme activity and metabolites presents a challenging and inevitable undertaking.
5 Detection based on molecular biology
According to the discovery of cuproptosis, it is proved to be related to the collapse of Cu homeostasis and mitochondrial respiration mediated by Cu ionophores, and the specific changes of protein levels of cuproptosis-related molecules including lipoylation process, electron transport chain (ETC), HSP70 are involved in this new type of cell death (Figure 2). Beyond that, abnormal gene expression of Cu transport and Cu chaperones may also lead to the Cu homeostasis disruption and more seriously the progress of genetic, neurodegenerative, cardiovascular, tumoral diseases (Chen et al., 2022; Tsvetkov et al., 2022). Evaluating these molecular biology of protein levels of cuproptosis-related molecules are essential to quantitatively verify the occurrence of cuproptosis, which include Cu ionophore-Cu-induced cell death (5.1–5.3) and endogenous Cu homeostasis imbalance (5.4–5.5) in this section.
5.1 Lipoylation: FDX1, DLAT
Scientists proved that mitochondrial respiration was required for cuproptosis and metabolite analysis showed a time-dependent increase in the dysregulation of TCA cycle-related metabolites in cuproptosis cells; genome-wide CRISPR/Cas9 function loss screening identified relevant genes reducing cuproptosis: FDX1, lipoylation proteins (LIPT1; LIAS; DLD) and lipoylated targets (PDH complex: DLAT, PDHA1, PDHB) (Tsvetkov et al., 2022; Solmonson and DeBerardinis, 2018). Thus the cells with high levels of FDX1 and DLAT are more sensitive to Cu cell death effects. FDX1 encodes a reductase that reduces Cu2+ to more toxic form Cu+ and its content in cells and tissues can be determined using real-time quantitative PCR (qRT-PCR), western blot, immunohistochemistry (IHC), ELISA assays (Zulkifli et al., 2023). In addition, FDX1 was proved to be an upstream regulator of protein lipoylation and regulated the lipoylation of DLAT cooperated with LIAS, essential for PDH complex and TCA cycle (Tsvetkov et al., 2022). qRT-PCR, western blot, IHC, immunoprecipitation, mass spectrometry assays can be performed to measure the expression and lipoylation (indicated by DLAT antibody and lipoic acid antibody) of DLAT (Nowinski et al., 2020). Cu can bind free lipoic acid with a considerable affinity so that the lipoyl moiety of DLAT is required for Cu binding.
One of the markers of cuproptosis is triggering the aggregation of lipoylated target DLAT due to the binding to Cu+, leading to the reduction of free monomer of DLAT (Tsvetkov et al., 2022). The aggregation of Cu+-DLAT can be captured by immunofluorescence (IF), nondenaturing gel electrophoresis/western blot, high performance liquid chromatography (HPLC), and alternative methods include dynamic light scattering (DLS), Taylor dispersion analysis (TDA), electrospray differential mobility analysis (ES-DMA) (Wang and Roberts, 2018).
5.2 Fe-S cluster proteins
NADH and FADH2 generated from TCA cycle transfer electrons to the Fe-S cluster proteins in ETC complex I/II and start the electron transport in ETC I-IV (Tran et al., 2019; Hsu et al., 2019). Another important marker of cuproptosis is triggering the instability of Fe-S cluster proteins (Tsvetkov et al., 2022). The Fe-S cluster proteins expression can be determined by Western blot, IHC (indicated by Fe-S cluster protein antibody) and qRT-PCR assays. The assembly and transfer proteins of Fe-S cluster proteins involve: disulfurase complex NFS1-ISD11, frataxin, ferredoxin, ferredoxin reductase, mitochondrial carrier Mrs3/4, iron-sulfur cluster assembly 1/2 (Isa1/2), ironsulfur cluster assembly factor for biotin synthase and aconitase like mitochondrial proteins (57 kDa, Iba57), nucleoside hydrolase Ind1, assembly factor Nfu1, Fe-S protein subunits (NDUFS1, NDUFV1, SDHA, UQCRFS1), and so on (Sheftel et al., 2010; Terali et al., 2013; Schmucker et al., 2011; Moore et al., 2018; Sheftel et al., 2012; Muehlenhoff et al., 2011; Camponeschi et al., 2022; Da Vela et al., 2023; Chipuk, 2019; Ren L. et al., 2023; Maio et al., 2016; Sato et al., 2018). Herein, the expression of Fe-S cluster-related assembly and transfer proteins are significant for evaluating cuproptosis, and based on the instability of Fe-S cluster proteins, the detection results are suggested for negative trend.
5.3 HSP70
Heat shock proteins (HSPs), as molecular chaperons, play a protective role against the misfolding, aggregation and complex assembly disorder of cellular proteins under stress (Abd El-Fattah and Zakaria, 2022). HSP70, one of key HSPs, is low expressed and difficult to be detected in normal cells, but significantly upregulated under stress such as heat shock, oxidative stress, hypoxia and metal overload, which improves proteins misfolding, aggregation and sends them to ubiquitin-proteasome degradation or lysosome autophagy degradation pathways to avoid cell damage (Gráf et al., 2018). Importantly, during cuproptosis, HSP70 was the third marker and found to be upregulated in response to Cu overload and DLAT aggregation (Tsvetkov et al., 2022). Thus, the expression of HSP70 should be involved in cuproptosis verification using Western blot, IHC (indicated by HSP70 antibody) and qRT-PCR assays.
5.4 Cu transport and storage: ceruloplasmin, SLC31A1, ATP7A/7B, MT
Cu ions are absorbed from gastrointestinal tract and delivered to target cells by blood ceruloplasmin; SLC31A1, a membrane Cu importer, is internalized when there are sufficient intracellular Cu ions; ATP7A/B are present in Golgi apparatus network to pump in Cu ions for cuproenzyme metalation, but these proteins migrate to efflux Cu ions out of cells in Cu overload; the plasma protein metallothionein (MT) modulates the storage system of Cu ions, increasing expression level of MT suggests increasing metal levels and Cu toxicity in Cu stress (Nishito et al., 2024). Additionally, it has been revealed that it’s the mutation of ATP7B gene that results in Cu deposition in Wilson’s disease, accompanied with low levels of ceruloplasmin and Cu ions in plasma (Kirk et al., 2024; Bartee and Lutsenko, 2007). Lower expression level of ceruloplasmin in plasma suggests increasing level of Cu ions in hepatocytes, while higher expression level of MT suggests increasing intracellular Cu ions, which can be detected by Western blot, IHC (indicated by ceruloplasmin antibody and MT antibody) and qRT-PCR assays; IF assay can indicate the localization of SLC31A1 and ATP7A/B, the overexpression of SLC31A1 in membrane and low levels of ATP7A/B suggest more Cu import and less Cu efflux in cells; and the mutation of ATP7A/B measured by sequence alignment will also result in Cu deposition. Therefore, ceruloplasmin, SLC31A1, ATP7A/7B, MT are endogenous factors of Cu transport and storage to detect Cu toxicity. However, changing the expression status of ceruloplasmin, SLC31A1, ATP7A/7B, MT do not mean the induction of Cu cell death, these types of endogenous changes of Cu levels still need complete detection to verify the cell death effects and mechanisms existed through the flow chart of “Methods for cuproptosis detection” (Figure 4).
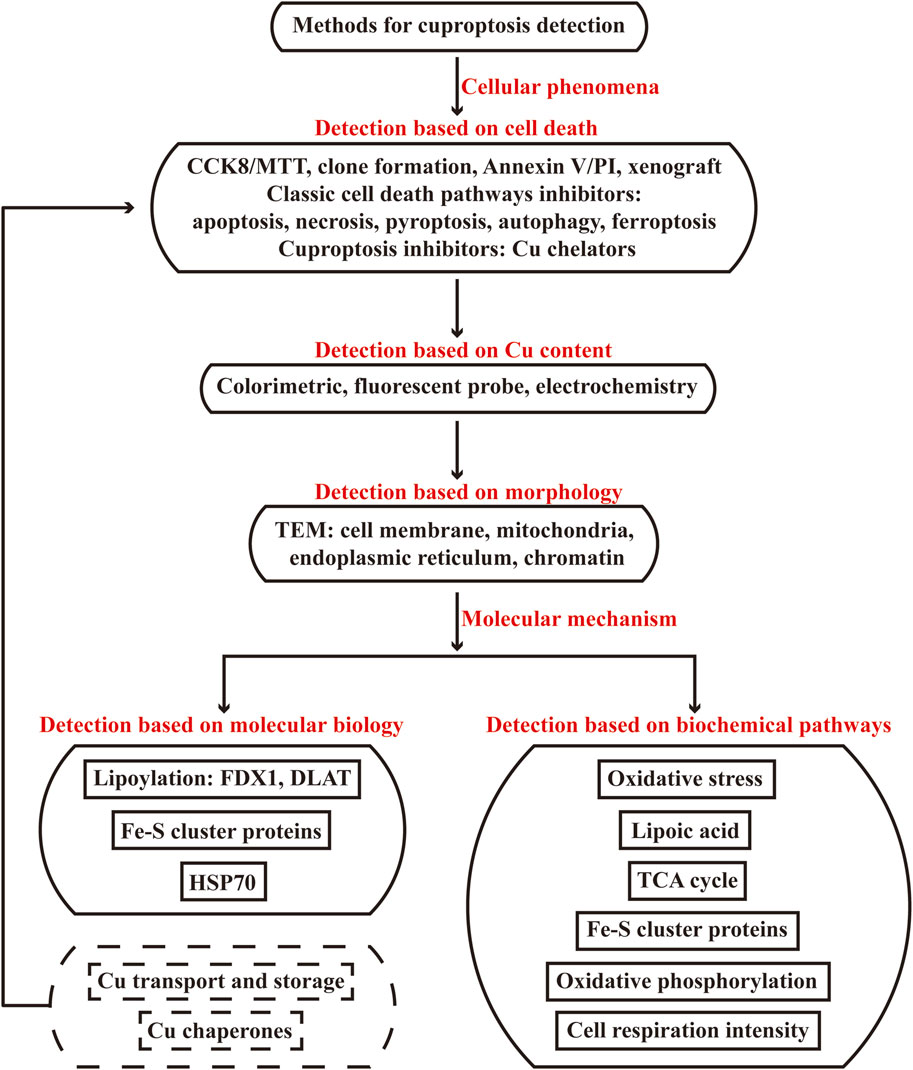
Figure 4. Flow chart of methods used to detect cuproptosis. The flow chart is categorized in two parts of cellular phenomena and molecular mechanism methods used to detect cuproptosis. The first part includes detection based on cell death, Cu content and morphology, the second part includes detection based on molecular biology of protein levels of cuproptosis-related molecules, biochemical pathways of cuproptosis-related enzyme activity and metabolites of oxidative stress, lipoic acid pathway, TCA cycle, Fe-S cluster proteins, oxidative phosphorylation, cell respiration intensity. The endogenous changes of protein levels of Cu transport and storage, Cu chaperones belong to molecular biology section, but such changes do not mean the induction of Cu cell death and need complete detection to verify the cell death effects and mechanisms existed through the flow chart.
5.5 Cu chaperones: ATOX1, CCS, COX17
For intracellular Cu functions, Cu+ shuttle and activate the target enzymes through different protein carriers and mediate different biological functions (Figure 1). Antioxidant-1 (ATOX1) carries Cu+ to nucleus, where Cu+ bind to transcription complex and drive the expression of target genes associated with growth, metastasis, angiogenesis and so on (Blockhuys et al., 2020; Wang et al., 2023a). ATOX1 carries Cu+ to Golgi apparatus in which Cu+ are pumped in by ATP7A/B to activate enzyme activity of protein processing; when Cu ions become excess, ATP7A/B move from Golgi apparatus to export them out (Garza et al., 2023; Magistrato et al., 2019). Cu chaperone for superoxide dismutase (CCS) carries Cu+ to superoxide dismutase (SOD), where Cu+ participate in SOD eliminating free radicals for redox homeostasis (Luchinat and Banci, 2018). Cytochrome c oxidase 17 (COX17) carries Cu+ to synthesis of cytochrome c oxidase 1 (SCO1) and COX11 for cytochrome c oxidase (CCO) assembly in mitochondrion, where Cu+ activate enzyme activity of ETC (Zhu et al., 2023). The expression status of Cu chaperones indicate Cu utilization, which can be determined via Western blot, IHC (indicated by ATOX1 antibody, CCS antibody, COX17 antibody) and qRT-PCR assays. If ATOX1, CCS, COX17 decrease, it may suggests Cu accumulation. Thus ATOX1, CCS, COX17 are another endogenous factors of Cu chaperones to detect Cu overload. Similarly, changing the expression status of ATOX1, CCS, COX17 also do not mean the induction of Cu cell death, they need complete detection in response to the flow chart of “Methods for cuproptosis detection” (Figure 4).
6 Detection based on biochemical pathways
Cuproptosis initiates when excess Cu ions induce massive reactive oxygen species (ROS), bind to lipoylated DLAT, cause the aggregation of DLAT and the instability of Fe-S cluster proteins, which in turn disrupt the normal biochemical pathways of oxidative stress, lipoic acid, TCA cycle, Fe-S cluster proteins, oxidative phosphorylation, cell respiration intensity, and ultimately lead to cell death (Figure 2) (Tsvetkov et al., 2022). Herein, these biochemical pathways are vital indicators for evaluating the cell death effects in response to excess Cu ions. We focus on the enzyme activity (indicated by substrate consumption and product generation) and metabolites involved in these biochemical pathways, using ELISA, colorimetric, fluorescent, fluorometric, luminescent methods, respectively (Table 2).
6.1 Oxidative stress: GSH, SOD, ROS
Glutathione (GSH) is one of the cellular Cu chelators and excess Cu2+ oxidize the reductive GSH into oxidized glutathione (GSSG), which makes exhaustion of GSH and impairs GSH antioxidant system (Li et al., 2022). Fenton reaction mediated by excess Cu+ catalyzes the synthesis of active hydroxyl radicals (·OH, OH−) (Jomova et al., 2022). Moreover, SOD with cofactor Cu+ eliminates free radicals and excess Cu+ will destroy the redox homeostasis (O2−) modulated by SOD (Magistrato et al., 2019). GSH/SOD antioxidant system failure and Cu Fenton reaction will result in increasing ROS levels (·OH, OH−, O2−) and cell toxic damage. Herein, in Cu stress, GSH level and SOD activity are decreased, accompanied with increasing ROS levels, fluorescent and colorimetric methods listed in “Oxidative stress” can indicate the quantitative results, respectively (Wang et al., 2022; Zhang W. et al., 2022; Mursaleen et al., 2021; Scur et al., 2022).
6.2 Lipoic acid: SAM, PDH, KGDH, pyruvate, α-ketoglutarate, acetyl-CoA, succinate
Lipoic acid is an eight-carbon saturated fatty acid that connects two sulfur atoms. It has strong antioxidant properties to directly remove ROS and can chelate heavy metal ions (Tsvetkov et al., 2022; Bera et al., 2023). Lipoic acid connects with target enzyme proteins through an amide bond and lipoylated modification after translation to maintain the catalytic activity, such as DLAT in PDH complex and dihydrolipoamide succinyltransferase (DLST) in α-ketoglutarate dehydrogenase (KGDH complex) (Koufaki et al., 2004; Cronan, 2016; Cronan, 2018). Lipoic acid transfers intermediate products between the active center of multi-enzyme complex, for example acyl group is transferred from lipoic acid to coenzyme A (CoA) (Cronan, 2020). FDX1 cooperated with LIAS are upstream regulators of protein lipoylation and their enzyme activities are impaired during cuproptosis, leading to the increase of S-adenosylmethionine (SAM, the substrate for LIAS), the inactivation of PDH complex and KGDH complex, the accumulation of pyruvate and α-ketoglutarate, the consumption of acetyl-CoA and succinate (Tsvetkov et al., 2022). Common methods to detect these enzyme activity, substrate levels and product levels are listed in “Lipoic acid pathway,” using ELISA, colorimetric, fluorometric and fluorescent methods, respectively (Shen et al., 2021; Yang et al., 2021; Atlante et al., 2018; Obaid et al., 2022; Wang Y. et al., 2020; Puca et al., 2021; Stifel et al., 2022).
6.3 TCA cycle: citrate synthase, IDH, KGDH, NADH, FADH2
In TCA cycle, pyruvate is converted to acetyl-CoA under the action of PDH complex, and acetyl-CoA plus oxaloacetate produce citrate, which initiates TCA cycle and subsequently generates isocitrate, cis-aconitate, oxalosuccinate, α-ketoglutarate, succinyl-CoA, succinate, fumarate, malate, oxaloacetate. Citrate synthase (Ren et al., 2020), isocitrate dehydrogenase (IDH) (Huang et al., 2019) and KGDH (Hansen and Gibson, 2022) catalyze the rate-limiting steps in TCA cycle. NADH and FADH2 are generated in TCA cycle and transported to ETC for electron transfer (Tran et al., 2019; Hsu et al., 2019). Cuproptosis induces the aggregation of lipoylated enzyme proteins (DLAT in PDH complex and DLST in KGDH complex) that involve in pyruvate converting to acetyl-CoA and the progress of TCA cycle, which inactivate the rate-limiting enzyme proteins of citrate synthase, IDH, KGDH and reduce the product NADH, FADH2 of TCA cycle. The enzyme protein activity and product quantitative methods are listed in “TCA cycle”, using colorimetric and ELISA methods, respectively (Meng et al., 2021; Vaziri-Gohar et al., 2022; Atlante et al., 2018; Ch et al., 2021).
6.4 Fe-S cluster proteins: prosthetic groups (LIAS, aconitase, mitochondrial complex I-III) and downstream targets (PDH, KGDH, mitochondrial complex IV)
Fe-S cluster proteins are prosthetic groups of LIAS (indicated by SAM consumption), aconitase, mitochondrial complex I-III, the instability of Fe-S cluster proteins during cuproptosis will decrease their enzyme activity, leading to low activity of LIAS-dependent PDH, LIAS-dependent KGDH and ETC (indicated by membrane potential and mitochondrial complex I-IV) (Tan et al., 2009; Navarro-Sastre et al., 2011; Tong et al., 2003; Cameron et al., 2011). Common methods to detect these enzyme activity of prosthetic groups and downstream targets in response to Fe-S cluster proteins instability are listed in “Fe-S cluster proteins,” using ELISA, colorimetric and fluorescent methods, respectively (Shen et al., 2021; Bailey et al., 2019; Yang et al., 2021; Atlante et al., 2018; Xiao et al., 2023).
6.5 Oxidative phosphorylation: mitochondrial complex V, ATP
H+ potential energy and free energy released by electrons transfer are used to produce ATP in mitochondrial complex V (Manoj et al., 2023). The reduction of TCA cycle and ETC activity induced by cuproptosis will decrease the enzyme activity of mitochondrial complex V and ATP production. Their detection methods are listed in “Oxidative phosphorylation,” using colorimetric and luminescent methods, respectively (Ge et al., 2021; Ma et al., 2021).
6.6 Cell respiration intensity: oxygen consumption rate, LDH
Cells highly dependent on mitochondrial respiration are much more sensitive to Cu toxicity than glycolytic cells, indicating that cuproptosis is mitochondrial respiration dependent (Tsvetkov et al., 2022). But the cell respiration may favor glycolysis from mitochondrial respiration due to excess Cu ions disrupting lipoylated TCA enzymes and ETC. Thus, the cell respiration intensity can be auxiliary indicators to evaluate the induction of cuproptosis. If cuproptosis predominates, the oxygen consumption rate will decrease from a high level and the enzyme activity of lactic dehydrogenase (LDH) will increase from a low level, which can be determined by Seahorse technology and LDH activity assay kit, respectively (“Cell respiration intensity”) (Cinquegrani et al., 2022; O'Day et al., 2013; Seki et al., 2022).
7 Comparison of cuproptosis and ferroptosis detection
In 2012, Stockwell BR et al. discovered a Fe-dependent form of nonapoptotic cell death named ferroptosis, characterizing by lipid peroxidation and excess ROS (Dixon et al., 2012). In our previous study, we demonstrated that the morpholine derivative N-(4-morpholinomethylene)ethanesulfonamide (MESA) could induce ferroptosis effect via targeting nuclear factor erythroid 2-related factor 2 (NRF2) signal pathways and suppress the viability of tumor cells (Sun et al., 2024). Thus the disorders of Fe and Cu ions metabolism have vital research value for the exploration of disease mechanisms and the development of therapeutic targets. In the past 10 years, ferroptosis has received great interests in the field of cell death and well-established detection methods have been continuously developed for verifying ferroptosis, which can also be categorized into cell death, Fe content, morphology, molecular biology and biochemical pathways according to the characteristic changes of ferroptosis. Techniques based on detecting the cell death effects are familiar with cuproptosis, accompanied with ferroptosis inhibitors; fluorescent probe (FerroOrange) is a common and specific technique for Fe content detection (Tian et al., 2021); morphological properties of ferroptosis include cell rounding cell membrane rupture, chromatin damage and more mitochondrial changes: small size, increased membrane density, outer membrane rupture, vanished crista, reduced membrane potential (Dixon et al., 2012); molecular biology can investigate the protein levels of ferroptosis-related molecules such as glutathione peroxidase 4 (GPX4) solute carrier family 7 member 12 (SLC7A12), acyl-CoA synthetase long-chain family member 4 (ACSL4), NRF2, Kelch-like ECH-associated protein 1 (KEAP1), P62 and so on (Wang L. et al., 2020); biochemical pathways of ferroptosis involve in GPX4, mevalonatepath, GSH, lipid peroxidation, oxidative stress and so on (Stockwell et al., 2017). Meanwhile, detection methods of cuproptosis are also summarized in Table 3 compared with that of ferroptosis, and they have some similarities of cell death effects, morphology, biochemical pathways (GSH, oxidative stress). Herein, we should focus our investigation on cell death specific inhibitors, ion content, cuproptosis-related molecules and cuproptosis specific biochemical pathways when verifying the occurrence of cuproptosis and distinguishing ferroptosis and other classic cell death pathways.
8 Discussion
Normal and stable Cu homeostasis plays an important role in a wide range of physiological process, while Cu overload will lead to cell damage and diseases of Cu toxicity. Cuproptosis is a newly discovered RCD in 2022 caused by excess Cu ions, which will induce cell damage due to aggregation of lipoylated DLAT, loss of Fe-S cluster proteins, upregulation of HSP70, proteotoxic and oxidative stress. Varying from other classic RCD, cuproptosis is highly concerned in many research fields nowadays, thereby effective detection methods to verify cuproptosis are an essential premise for cuproptosis studies. For this purpose, the principle and application of common cuproptosis detection methods are reviewed in this study according to the characteristic changes of Cu-dependent cell death, including cell death verification, Cu content, morphology, molecular biology of protein levels of cuproptosis-related molecules and biochemical pathways of cuproptosis-related enzyme activity and metabolites of oxidative stress, lipoic acid, TCA cycle, Fe-S cluster proteins, oxidative phosphorylation, cell respiration intensity. According to the markers of cuproptosis, we offer a flow chart of “Methods for cuproptosis detection” that categorizes into two part of cellular phenomena and molecular mechanism. The former including detection based on cell death, Cu content, morphology are employed to preliminarily verify the occurrence of cuproptosis, while the latter including detection based on molecular biology and biochemical pathways are employed to further gain comprehensive evaluation of cellular changes induced by cuproptosis with quantitative methods. All the indicating results have been displayed in the detection of cellular phenomena and molecular mechanism in response to the markers of cuproptosis, accompanied with the advantages and limitations.
Importantly, cuproptosis should be identified step by step in Cu stress. For cell death verification, the inhibitory effects on cell viability should not be sensitive to other classic cell death inhibitors (apoptosis, necrosis, autophagy, ferroptosis, etc.) but can be rescued by pretreatment of Cu chelators. For Cu content, ICP-MS is advanced for detecting Cu2+ and Cu+ but limited for application. We introduce three simple, sensitive, accurate and high-throughput methods of colorimetric, fluorescent probe and electrochemistry assays to effectively measure the Cu2+ content in serum, cell lysate and other samples. These three methods can be applied to readily detect Cu content according to the sensitivity for samples and the instrument for manipulation. For morphology, TEM can suggest the morphological features of cuproptosis with complicated sample preparation and subjective results of cell membrane rupture, mitochondrial contraction, endoplasmic reticulum and chromatin damage. Therefore, detection of cellular phenomena based on cell death effects sensitive to pretreatment of Cu chelators, Cu overload and specific morphological characteristics can only preliminarily indicate the induction of cuproptosis, further detection of molecular mechanism is needed to gain comprehensive evaluation of cellular changes induced by cuproptosis.
More quantitative detection methods are collected to completely evaluate the cellular changes in protein levels of cuproptosis-related molecules and in cuproptosis-related enzyme activity and metabolites in response to the markers of Cu-dependent cell death. For molecular biology, the characteristic changes of cuproptosis are abnormal aggregation of DLAT, loss of Fe-S cluster proteins and upregulation of HSP70, and the aggregation of DLAT involves the lipoylation regulated by DLAT/LIAS and the Cu binding to lipoic acid. The results of qRT-PCR assay in transcriptional level and Western blot, IHC assays can indicate the protein level changes of FDX1, DLAT, Fe-S cluster proteins, HSP70 in cells and tissues; the results of immunoprecipitation, mass spectrometry assays can indicate the lipoylation of DLAT; the aggregation of Cu+-DLAT can be captured by IF, nondenaturing gel electrophoresis/Western blot, HPLC, DLS, TDA, ES-DMA. Although the endogenous changes of protein levels of Cu transport and storage including increasing SLC31A1, MT, decreasing ceruloplasmin, decreasing/mutated ATP7A/7B and Cu chaperones including decreasing ATOX1, CCS, COX17 may lead to Cu accumulation, such changes do not mean the induction of cuproptosis and still need complete detection to verify the cell death effects and mechanisms existed through the flow chart we offer for cuproptosis detection (Figure 4). Similarly, the protein levels involved can be measured by qRT-PCR, Western blot, IHC assays, the localization of SLC31A1 and ATP7A/B can be measured by IF assay, and the mutation of ATP7A/B should be measured by sequence alignment. For biochemical pathways, cuproptosis has been revealed to disrupt the normal cellular metabolic pathways of oxidative stress, lipoic acid, TCA cycle, Fe-S cluster proteins, oxidative phosphorylation and cell respiration intensity (Figures 2, 4). Thus these biochemical pathways of cuproptosis-related enzyme activity and metabolites are vital indicators for cuproptosis verification and evaluation. We collect methods of ELISA, colorimetric, fluorescent, fluorometric and luminescent assays to measure the cuproptosis-related enzyme activity and metabolites in Cu stress, including GSH, SOD, ROS in oxidative stress, SAM, PDH, KGDH, pyruvate, α-ketoglutarate, acetyl-CoA, succinate in lipoic acid pathway, citrate synthase, IDH, KGDH, NADH, FADH2 in TCA cycle, LIAS, aconitase, mitochondrial complex I-III, PDH, KGDH, mitochondrial complex IV in Fe-S cluster protein pathway, mitochondrial complex V, ATP in oxidative phosphorylation and oxygen consumption rate, LDH in cell respiration intensity. Most of the cuproptosis-related enzyme activity are decreased, but the enzyme activity of LDH increases in Cu stress because the cell respiration favors glycolysis instead of oxidative phosphorylation due to the Cu toxicity on TCA cycle and ETC. Notably, the detection based on molecular biology of protein levels of cuproptosis-related molecules and biochemical pathways of cuproptosis-related enzyme activity and metabolites are employed to evaluate the characteristic changes of cuproptosis in molecular level, after the preliminary verification of cuproptosis based on cell death effects, Cu content and morphological features. But only detecting these changes of molecular biology and biochemical pathways can not suggest the occurrence of cuproptosis so that the type of cell death may be identified as cuproptosis followed by our flow chart of methods based on cellular phenomena and molecular mechanism detection (Figure 4).
The detection methods of cuproptosis we offer are mainly based on the characteristic changes of cell death caused by excess Cu ions, and the proper methods applied to verify the occurrence of cuproptosis depend on the reagents and instruments in laboratory. In addition, considering the prospective significance of cuproptosis and ferroptosis, the metabolic disorder of other metal ions also have specific research value for pathology exploration and therapeutic target development (Tsvetkov et al., 2022; Sun et al., 2024). Both cuproptosis and ferroptosis are involved in mitochondrial metabolism, other reported metal ions related to mitochondrial function are calcium, potassium, sodium, magnesium, zinc, and the reported metal elements involved in cell death are zinc (Du et al., 2021), manganese (Zhao et al., 2019) and calcium (Bai et al., 2022). However, the action mode of cell death of these metal ions are not clear enough compared with cuproptosis and ferroptosis. We will keep going through the progress of metal elements-mediated cell death and the principle and application of detection methods involved, hoping to provide technical support for cell death verification and exploration based on metal elements.
9 Conclusion
Collectively, the new type of cell death cuproptosis can be verified and evaluated based on the characteristic markers in terms of cell death, Cu content, morphology, molecular biology of protein levels of cuproptosis-related molecules, biochemical pathways of cuproptosis-related enzyme activity and metabolites, distinguishing from ferroptosis and other classic cell death. The cellular phenomena and molecular mechanism detection methods of cuproptosis we collect would contribute to verifying and exploring cuproptosis in future studies.
Author contributions
LZ: Writing–review and editing, Writing–original draft, Software, Investigation, Conceptualization. RD: Writing–original draft, Software, Investigation. RG: Writing–original draft, Software, Investigation. YJ: Writing–original draft, Software, Investigation. YG: Writing–original draft, Investigation. CC: Writing–original draft, Investigation. WZ: Writing–original draft, Investigation. GH: Writing–original draft, Investigation. LL: Writing–review and editing, Supervision, Project administration, Funding acquisition. HD: Writing–review and editing, Supervision, Project administration, Conceptualization. DT: Writing–review and editing, Supervision, Project administration, Funding acquisition, Conceptualization.
Funding
The authors declare that financial support was received for the research, authorship, and/or publication of this article. This work was supported by research grants from the National Key Research Development Plan of China (2021YFA0805900), National Natural Science Foundation of China (82070199) and Scientific Research Project of Department of Education of Guangdong Province (2023ZDZX2057).
Conflict of interest
Author RD was employed by Beijing Mercer United International Education Consulting Co., Ltd.
The remaining authors declare that the research was conducted in the absence of any commercial or financial relationships that could be construed as a potential conflict of interest.
Publisher’s note
All claims expressed in this article are solely those of the authors and do not necessarily represent those of their affiliated organizations, or those of the publisher, the editors and the reviewers. Any product that may be evaluated in this article, or claim that may be made by its manufacturer, is not guaranteed or endorsed by the publisher.
References
Abd El-Fattah, E. E., and Zakaria, A. Y. (2022). Targeting HSP47 and HSP70: promising therapeutic approaches in liver fibrosis management. J. Transl. Med. 20, 544. doi:10.1186/s12967-022-03759-z
Atlante, S., Visintin, A., Marini, E., Savoia, M., Dianzani, C., Giorgis, M., et al. (2018). α-ketoglutarate dehydrogenase inhibition counteracts breast cancer-associated lung metastasis. Cell Death Dis. 9, 756. doi:10.1038/s41419-018-0802-8
Bai, S., Lan, Y., Fu, S., Cheng, H., Lu, Z., and Liu, G. (2022). Connecting calcium-based nanomaterials and cancer: from diagnosis to therapy. Nanomicro. Lett. 14, 145. doi:10.1007/s40820-022-00894-6
Bailey, J. D., Diotallevi, M., Nicol, T., McNeill, E., Shaw, A., Chuaiphichai, S., et al. (2019). Nitric oxide modulates metabolic remodeling in inflammatory macrophages through TCA cycle regulation and itaconate accumulation. Cell Rep. 28, 218–230. doi:10.1016/j.celrep.2019.06.018
Bartee, M. Y., and Lutsenko, S. (2007). Hepatic copper-transporting ATPase ATP7B: function and inactivation at the molecular and cellular level. Biometals 20, 627–637. doi:10.1007/s10534-006-9074-3
Bera, A., Ghosh, P., Ghosh, S., Mukherjee, A., and De, P. (2023). Antioxidant polymers with enhanced neuroprotection against insulin fibrillation. Macromol. Biosci. 23, e2300100. doi:10.1002/mabi.202300100
Blockhuys, S., Brady, D. C., and Wittung-Stafshede, P. (2020). Evaluation of copper chaperone ATOX1 as prognostic biomarker in breast cancer. Breast Cancer 27, 505–509. doi:10.1007/s12282-019-01044-4
Cameron, J. M., Janer, A., Levandovskiy, V., Mackay, N., Rouault, T. A., Tong, W. H., et al. (2011). Mutations in iron-sulfur cluster scaffold genes NFU1 and BOLA3 cause a fatal deficiency of multiple respiratory chain and 2-oxoacid dehydrogenase enzymes. Am. J. Hum. Genet. 89, 486–495. doi:10.1016/j.ajhg.2011.08.011
Camponeschi, F., Ciofi-Baffoni, S., Calderone, V., and Banci, L. (2022). Molecular basis of rare diseases associated to the maturation of mitochondrial [4Fe-4S]-Containing proteins. Biomolecules 12, 1009. doi:10.3390/biom12071009
Ch, R., Rey, G., Ray, S., Jha, P. K., Driscoll, P. C., Dos Santos, M. S., et al. (2021). Rhythmic glucose metabolism regulates the redox circadian clockwork in human red blood cells. Nat. Commun. 12, 377. doi:10.1038/s41467-020-20479-4
Chen, L., Min, J., and Wang, F. (2022). Copper homeostasis and cuproptosis in health and disease. Signal Transduct. Target Ther. 7, 378. doi:10.1038/s41392-022-01229-y
Chipuk, J. E. (2019). Complex I and MDM2: hit me baby one more time. Mol. Cell Oncol. 6, 1607457. doi:10.1080/23723556.2019.1607457
Cinquegrani, G., Spigoni, V., Fantuzzi, F., Bonadonna, R. C., and Dei Cas, A. (2022). Empagliflozin does not reverse lipotoxicity-induced impairment in human myeloid angiogenic cell bioenergetics. Cardiovasc. Diabetol. 21, 27. doi:10.1186/s12933-022-01461-4
Cordeiro, L. M., Soares, M. V., da Silva, A. F., Dos Santos, L. V., de Souza, L. I., da Silveira, T. L., et al. (2023). Toxicity of copper and zinc alone and in combination in Caenorhabditis elegans model of Huntington’s disease and protective effects of rutin. Neurotoxicology 97, 120–132. doi:10.1016/j.neuro.2023.06.005
Cronan, J. E. (2016). Assembly of lipoic acid on its cognate enzymes: an extraordinary and essential biosynthetic pathway. Microbiol. Mol. Biol. Rev. 80, 429–450. doi:10.1128/MMBR.00073-15
Cronan, J. E. (2018). Advances in synthesis of biotin and assembly of lipoic acid. Curr. Opin. Chem. Biol. 47, 60–66. doi:10.1016/j.cbpa.2018.08.004
Cronan, J. E. (2020). Progress in the enzymology of the mitochondrial diseases of lipoic acid requiring enzymes. Front. Genet. 11, 510. doi:10.3389/fgene.2020.00510
Da Vela, S., Saudino, G., Lucarelli, F., Banci, L., Svergun, D. I., and Ciofi-Baffoni, S. (2023). Structural plasticity of NFU1 upon interaction with binding partners: insights into the mitochondrial [4Fe-4S] cluster pathway. J. Mol. Biol. 435, 168154. doi:10.1016/j.jmb.2023.168154
Deng, B., Lin, Y., Chen, Y., Ma, S., Cai, Q., Wang, W., et al. (2021). Plasmacytoid dendritic cells promote acute kidney injury by producing interferon-α. Cell Mol. Immunol. 18, 219–229. doi:10.1038/s41423-019-0343-9
Dixon, S. J., Lemberg, K. M., Lamprecht, M. R., Skouta, R., Zaitsev, E. M., Gleason, C. E., et al. (2012). Ferroptosis: an iron-dependent form of nonapoptotic cell death. Cell 149, 1060–1072. doi:10.1016/j.cell.2012.03.042
Du, W., Gu, M., Hu, M., Pinchi, P., Chen, W., Ryan, M., et al. (2021). Lysosomal Zn2+ release triggers rapid, mitochondria-mediated, non-apoptotic cell death in metastatic melanoma. Cell Rep. 37, 109848. doi:10.1016/j.celrep.2021.109848
Fujisawa, C., Kodama, H., Sato, Y., Mimaki, M., Yagi, M., Awano, H., et al. (2022). Early clinical signs and treatment of Menkes disease. Mol. Genet. Metab. Rep. 31, 100849. doi:10.1016/j.ymgmr.2022.100849
Gao, P., Cao, M., Jiang, X., Wang, X., Zhang, G., Tang, X., et al. (2023). Cannabinoid receptor 2-centric molecular feedback loop drives necroptosis in diabetic heart injuries. Circulation 147, 158–174. doi:10.1161/CIRCULATIONAHA.122.059304
Garza, N. M., Swaminathan, A. B., Maremanda, K. P., Zulkifli, M., and Gohil, V. M. (2023). Mitochondrial copper in human genetic disorders. Trends Endocrinol. Metab. 34, 21–33. doi:10.1016/j.tem.2022.11.001
Ge, Q., Jia, D., Cen, D., Qi, Y., Shi, C., Li, J., et al. (2021). Micropeptide ASAP encoded by LINC00467 promotes colorectal cancer progression by directly modulating ATP synthase activity. J. Clin. Invest. 131, e152911. doi:10.1172/JCI152911
Gráf, L., Barabás, L., Madaras, B., Garam, N., Maláti, É., Horváth, L., et al. (2018). High serum Hsp70 level predicts poor survival in colorectal cancer: results obtained in an independent validation cohort. Cancer Biomark. 23, 539–547. doi:10.3233/CBM-181683
Hansen, G. E., and Gibson, G. E. (2022). The α-ketoglutarate dehydrogenase complex as a hub of plasticity in neurodegeneration and regeneration. Int. J. Mol. Sci. 23, 12403. doi:10.3390/ijms232012403
Hsu, C. C., Tsai, H. H., Fu, T. C., and Wang, J. S. (2019). Exercise training enhances platelet mitochondrial bioenergetics in stroke patients: a randomized controlled trial. J. Clin. Med. 8, 2186. doi:10.3390/jcm8122186
Huang, J., Yu, J., Tu, L., Huang, N., Li, H., and Luo, Y. (2019). Isocitrate dehydrogenase mutations in glioma: from basic discovery to therapeutics development. Front. Oncol. 9, 506. doi:10.3389/fonc.2019.00506
Hui, X., Sharifuzzaman, M., Sharma, S., Xuan, X., Zhang, S., Ko, S. G., et al. (2020). High-performance flexible electrochemical heavy metal sensor based on layer-by-layer assembly of Ti3C2tx/MWNTs nanocomposites for noninvasive detection of copper and zinc ions in human biofluids. ACS Appl. Mat. Interfaces 12, 48928–48937. doi:10.1021/acsami.0c12239
Jomova, K., Makova, M., Alomar, S. Y., Alwasel, S. H., Nepovimova, E., Kuca, K., et al. (2022). Essential metals in health and disease. Chem. Biol. Interact. 367, 110173. doi:10.1016/j.cbi.2022.110173
Kirk, F. T., Munk, D. E., Swenson, E. S., Quicquaro, A. M., Vendelbo, M. H., Larsen, A., et al. (2024). Effects of tetrathiomolybdate on copper metabolism in healthy volunteers and in patients with Wilson disease. J. Hepatol. 80, 586–595. doi:10.1016/j.jhep.2023.11.023
Koufaki, M., Detsi, A., Theodorou, E., Kiziridi, C., Calogeropoulou, T., Vassilopoulos, A., et al. (2004). Synthesis of chroman analogues of lipoic acid and evaluation of their activity against reperfusion arrhythmias. Bioorg. Med. Chem. 12, 4835–4841. doi:10.1016/j.bmc.2004.07.012
Li, D., Shi, Z., Liu, X., Jin, S., Chen, P., Zhang, Y., et al. (2023). Identification and development of a novel risk model based on cuproptosis-associated RNA methylation regulators for predicting prognosis and characterizing immune status in hepatocellular carcinoma. Hepatol. Int. 17, 112–130. doi:10.1007/s12072-022-10460-2
Li, Q., Wang, F., Shi, L., Tang, Q., Li, B., Wang, X., et al. (2022). Nanotrains of DNA copper nanoclusters that triggered a cascade fenton-like reaction and glutathione depletion to doubly enhance chemodynamic therapy. ACS Appl. Mat. Interfaces 14, 37280–37290. doi:10.1021/acsami.2c05944
Lin, J. Z., Wang, W. W., Hu, T. T., Zhu, G. Y., Li, L. N., Zhang, C. Y., et al. (2020). FOXM1 contributes to docetaxel resistance in castration-resistant prostate cancer by inducing AMPK/mTOR-mediated autophagy. Cancer Lett. 469, 481–489. doi:10.1016/j.canlet.2019.11.014
Liu, W., Peng, J., Xiao, M., Cai, Y., Peng, B., Zhang, W., et al. (2022). The implication of pyroptosis in cancer immunology: current advances and prospects. Genes Dis. 10, 2339–2350. doi:10.1016/j.gendis.2022.04.019
Liu, X., and Sun, B. (2023). One-pot synthesis of nitrogen-doped graphene quantum dots and their applications in bioimaging and detecting copper ions in living cells. ACS Omega 8, 27333–27343. doi:10.1021/acsomega.3c02705
Lu, W., Yan, J., Wang, C., Qin, W., Han, X., Qin, Z., et al. (2024). Interorgan communication in neurogenic heterotopic ossification: the role of brain-derived extracellular vesicles. Bone Res. 12, 11. doi:10.1038/s41413-023-00310-8
Luchinat, E., and Banci, L. (2018). In-cell NMR in human cells: direct protein expression allows structural studies of protein folding and maturation. Acc. Chem. Res. 51, 1550–1557. doi:10.1021/acs.accounts.8b00147
Ma, X., Mao, Z., Zhu, J., Liu, H., and Chen, F. (2021). lncRNA PANTR1 upregulates BCL2A1 expression to promote tumorigenesis and warburg effect of hepatocellular carcinoma through restraining miR-587. J. Immunol. Res. 2021, 1736819. doi:10.1155/2021/1736819
Magistrato, A., Pavlin, M., Qasem, Z., and Ruthstein, S. (2019). Copper trafficking in eukaryotic systems: current knowledge from experimental and computational efforts. Curr. Opin. Struct. Biol. 58, 26–33. doi:10.1016/j.sbi.2019.05.002
Maio, N., Ghezzi, D., Verrigni, D., Rizza, T., Bertini, E., Martinelli, D., et al. (2016). Disease-causing SDHAF1 mutations impair transfer of Fe-S clusters to SDHB. Cell Metab. 23, 292–302. doi:10.1016/j.cmet.2015.12.005
Manoj, K. M., Bazhin, N. M., Tamagawa, H., Jaeken, L., and Parashar, A. (2023). The physiological role of complex V in ATP synthesis: murzyme functioning is viable whereas rotary conformation change model is untenable. J. Biomol. Struct. Dyn. 41, 3993–4012. doi:10.1080/07391102.2022.2060307
Meng, L. B., Hu, G. F., Shan, M. J., Zhang, Y. M., Yu, Z. M., Liu, Y. Q., et al. (2021). Citrate synthase and OGDH as potential biomarkers of atherosclerosis under chronic stress. Oxid. Med. Cell Longev. 2021, 9957908. doi:10.1155/2021/9957908
Moore, M. J., Wofford, J. D., Dancis, A., and Lindahl, P. A. (2018). Recovery of mrs3Δmrs4Δ Saccharomyces cerevisiae cells under iron-sufficient conditions and the role of Fe580. Biochemistry 57, 672–683. doi:10.1021/acs.biochem.7b01034
Muehlenhoff, U., Richter, N., Pines, O., Pierik, A. J., and Lill, R. (2011). Specialized function of yeast Isa1 and Isa2 proteins in the maturation of mitochondrial 4Fe-4S proteins. J. Biol. Chem. 286, 41205–41216. doi:10.1074/jbc.M111.296152
Mursaleen, L., Noble, B., Somavarapu, S., and Zariwala, M. G. (2021). Micellar nanocarriers of hydroxytyrosol are protective against Parkinson’s related oxidative stress in an in vitro hCMEC/D3-SH-SY5Y Co-culture system. Antioxidants (Basel) 10, 887. doi:10.3390/antiox10060887
Navarro-Sastre, A., Tort, F., Stehling, O., Uzarska, M. A., Arranz, J. A., Del Toro, M., et al. (2011). A Fatal mitochondrial disease is associated with defective NFU1 function in the maturation of a subset of mitochondrial Fe-S proteins. Am. J. Hum. Genet. 89, 656–667. doi:10.1016/j.ajhg.2011.10.005
Nishito, Y., Kamimura, Y., Nagamatsu, S., Yamamoto, N., Yasui, H., and Kambe, T. (2024). Zinc and manganese homeostasis closely interact in mammalian cells. FASEB J. 38, e23605. doi:10.1096/fj.202400181R
Nowinski, S. M., Solmonson, A., Rusin, S. F., Maschek, J. A., Bensard, C. L., Fogarty, S., et al. (2020). Mitochondrial fatty acid synthesis coordinates oxidative metabolism in mammalian mitochondria. Elife 9, e58041. doi:10.7554/eLife.58041
Obaid, Q. A., Al-Shammari, A. M., and Khudair, K. K. (2022). Glucose deprivation induced by acarbose and oncolytic newcastle disease virus promote metabolic oxidative stress and cell death in a breast cancer model. Front. Mol. Biosci. 9, 816510. doi:10.3389/fmolb.2022.816510
O'Day, S. J., Eggermont, A. M., Chiarion-Sileni, V., Kefford, R., Grob, J. J., Mortier, L., et al. (2013). Final results of phase III SYMMETRY study: randomized, double-blind trial of elesclomol plus paclitaxel versus paclitaxel alone as treatment for chemotherapy-naive patients with advanced melanoma. J. Clin. Oncol. 31, 1211–1218. doi:10.1200/JCO.2012.44.5585
Ou, G., Zhao, J., Chen, P., Xiong, C., Dong, F., Li, B., et al. (2018). Fabrication and application of noble metal nanoclusters as optical sensors for toxic metal ions. Anal. Bioanal. Chem. 410, 2485–2498. doi:10.1007/s00216-017-0808-6
Pan, Z., Deng, C., Shui, L., Yin, H., and Liu, B. (2024). Copper deficiency induces oxidative stress in liver of mice by blocking the Nrf2 pathway. Biol. Trace Elem. Res. 202, 1603–1611. doi:10.1007/s12011-023-03769-y
Parmar, A., Pascali, G., Voli, F., Lerra, L., Yee, E., Ahmed-Cox, A., et al. (2018). In vivo [64Cu]CuCl2 PET imaging reveals activity of Dextran-Catechin on tumor copper homeostasis. Theranostics 8, 5645–5659. doi:10.7150/thno.29840
Perkal, O., Qasem, Z., Turgeman, M., Schwartz, R., Gevorkyan-Airapetov, L., Pavlin, M., et al. (2020). Cu(I) controls conformational States in human Atox1 metallochaperone: an EPR and multiscale simulation study. J. Phys. Chem. B 124, 4399–4411. doi:10.1021/acs.jpcb.0c01744
Puca, F., Yu, F., Bartolacci, C., Pettazzoni, P., Carugo, A., Huang-Hobbs, E., et al. (2021). Medium-chain acyl-CoA dehydrogenase protects mitochondria from lipid peroxidation in glioblastoma. Cancer Discov. 11, 2904–2923. doi:10.1158/2159-8290.CD-20-1437
Ren, L., Meng, L., Gao, J., Lu, M., Guo, C., Li, Y., et al. (2023). PHB2 promotes colorectal cancer cell proliferation and tumorigenesis through NDUFS1-mediated oxidative phosphorylation. Cell Death Dis. 14, 44. doi:10.1038/s41419-023-05575-9
Ren, M., Yang, X., Bie, J., Wang, Z., Liu, M., Li, Y., et al. (2020). Citrate synthase desuccinylation by SIRT5 promotes colon cancer cell proliferation and migration. Biol. Chem. 401, 1031–1039. doi:10.1515/hsz-2020-0118
Ren, Y., Wang, R., Weng, S., Xu, H., Zhang, Y., Chen, S., et al. (2023). Multifaceted role of redox pattern in the tumor immune microenvironment regarding autophagy and apoptosis. Mol. Cancer 22, 130. doi:10.1186/s12943-023-01831-w
Sato, T., Chang, H. C., Bayeva, M., Shapiro, J. S., Ramos-Alonso, L., Kouzu, H., et al. (2018). mRNA-binding protein tristetraprolin is essential for cardiac response to iron deficiency by regulating mitochondrial function. Proc. Natl. Acad. Sci. U. S. A. 115, E6291–E6300. doi:10.1073/pnas.1804701115
Schmucker, S., Martelli, A., Colin, F., Page, A., Wattenhofer-Donzé, M., Reutenauer, L., et al. (2011). Mammalian frataxin: an essential function for cellular viability through an interaction with a preformed ISCU/NFS1/ISD11 iron-sulfur assembly complex. PLoS One 6, e16199. doi:10.1371/journal.pone.0016199
Scur, M., Mahmoud, A. B., Dey, S., Abdalbarri, F., Stylianides, I., Medina-Luna, D., et al. (2022). Alveolar macrophage metabolic programming via a C-type lectin receptor protects against lipo-toxicity and cell death. Nat. Commun. 13, 7272. doi:10.1038/s41467-022-34935-w
Seki, T., Yang, Y., Sun, X., Lim, S., Xie, S., Guo, Z., et al. (2022). Brown-fat-mediated tumour suppression by cold-altered global metabolism. Nature 608, 421–428. doi:10.1038/s41586-022-05030-3
Sheftel, A. D., Stehling, O., Pierik, A. J., Elsässer, H. P., Mühlenhoff, U., Webert, H., et al. (2010). Humans possess two mitochondrial ferredoxins, Fdx1 and Fdx2, with distinct roles in steroidogenesis, heme, and Fe/S cluster biosynthesis. Proc. Natl. Acad. Sci. U. S. A. 107, 11775–11780. doi:10.1073/pnas.1004250107
Sheftel, A. D., Wilbrecht, C., Stehling, O., Niggemeyer, B., Elsässer, H. P., Mühlenhoff, U., et al. (2012). The human mitochondrial ISCA1, ISCA2, and IBA57 proteins are required for 4Fe-4S protein maturation. Mol. Biol. Cell 23, 1157–1166. doi:10.1091/mbc.E11-09-0772
Shen, H., Dou, Y., Wang, X., Wang, X., Kong, F., and Wang, S. (2023). Guluronic acid can inhibit copper(II) and amyloid - β peptide coordination and reduce copper-related reactive oxygen species formation associated with Alzheimer’s disease. J. Inorg. Biochem. 245, 112252. doi:10.1016/j.jinorgbio.2023.112252
Shen, L., Hu, P., Zhang, Y., Ji, Z., Shan, X., Ni, L., et al. (2021). Serine metabolism antagonizes antiviral innate immunity by preventing ATP6V0d2-mediated YAP lysosomal degradation. Cell Metab. 33, 971–987.e6. doi:10.1016/j.cmet.2021.03.006
Solmonson, A., and DeBerardinis, R. J. (2018). Lipoic acid metabolism and mitochondrial redox regulation. J. Biol. Chem. 293, 7522–7530. doi:10.1074/jbc.TM117.000259
Stalke, A., Behrendt, A., Hennig, F., Gohlke, H., Buhl, N., Reinkens, T., et al. (2023). Functional characterization of novel or yet uncharacterized ATP7B missense variants detected in patients with clinical Wilson’s disease. Clin. Genet. 104, 174–185. doi:10.1111/cge.14352
Stifel, U., Wolfschmitt, E. M., Vogt, J., Wachter, U., Vettorazzi, S., Tews, D., et al. (2022). Glucocorticoids coordinate macrophage metabolism through the regulation of the tricarboxylic acid cycle. Mol. Metab. 57, 101424. doi:10.1016/j.molmet.2021.101424
Stockwell, B. R., Friedmann Angeli, J. P., Bayir, H., Bush, A. I., Conrad, M., Dixon, S. J., et al. (2017). Ferroptosis: a regulated cell death nexus linking metabolism, redox biology, and disease. Cell 171, 273–285. doi:10.1016/j.cell.2017.09.021
Sui, X., Zhang, X., Zhao, J., Liu, J., Li, S., Zhang, X., et al. (2023). Establishment of a prognostic model for melanoma based on necroptosis-related genes. Funct. Integr. Genomics 23, 202. doi:10.1007/s10142-023-01129-6
Sun, B., Zhang, L., Wu, B., and Luo, X. (2024). A morpholine derivative N-(4-Morpholinomethylene)ethanesulfonamide induces ferroptosis in tumor cells by targeting NRF2. Biol. Pharm. Bull. 47, 417–426. doi:10.1248/bpb.b23-00544
Sun, Y., Revach, O. Y., Anderson, S., Kessler, E. A., Wolfe, C. H., Jenney, A., et al. (2023). Targeting TBK1 to overcome resistance to cancer immunotherapy. Nature 615, 158–167. doi:10.1038/s41586-023-05704-6
Tan, G., Lu, J., Bitoun, J. P., Huang, H., and Ding, H. (2009). IscA/SufA paralogues are required for the 4Fe-4S cluster assembly in enzymes of multiple physiological pathways in Escherichia coli under aerobic growth conditions. Biochem. J. 420, 463–472. doi:10.1042/BJ20090206
Tao, T., Zhang, P., Zeng, Z., and Wang, M. (2023). Advances in autophagy modulation of natural products in cervical cancer. J. Ethnopharmacol. 314, 116575. doi:10.1016/j.jep.2023.116575
Terali, K., Beavil, R. L., Pickersgill, R. W., and van der Giezen, M. (2013). The effect of the adaptor protein Isd11 on the quaternary structure of the eukaryotic cysteine desulphurase Nfs1. Biochem. Biophys. Res. Commun. 440, 235–240. doi:10.1016/j.bbrc.2013.09.039
Tian, R., Abarientos, A., Hong, J., Hashemi, S. H., Yan, R., Dräger, N., et al. (2021). Genome-wide CRISPRi/a screens in human neurons link lysosomal failure to ferroptosis. Nat. Neurosci. 24, 1020–1034. doi:10.1038/s41593-021-00862-0
Tong, F., Hu, H., Xu, Y., Zhou, Y., Xie, R., Lei, T., et al. (2023). Hollow copper sulfide nanoparticles carrying ISRIB for the sensitized photothermal therapy of breast cancer and brain metastases through inhibiting stress granule formation and reprogramming tumor-associated macrophages. Acta Pharm. Sin. B 13, 3471–3488. doi:10.1016/j.apsb.2022.11.003
Tong, W. H., Jameson, G. N., Huynh, B. H., and Rouault, T. A. (2003). Subcellular compartmentalization of human Nfu, an iron-sulfur cluster scaffold protein, and its ability to assemble a [4Fe-4S] cluster. Proc. Natl. Acad. Sci. U. S. A. 100, 9762–9767. doi:10.1073/pnas.1732541100
Tran, K. N., Niu, S., and Ichiye, T. (2019). Reduction potential calculations of the Fe-S clusters in Thermus thermophilus respiratory complex I. J. Comput. Chem. 40, 1248–1256. doi:10.1002/jcc.25785
Tsvetkov, P., Coy, S., Petrova, B., Dreishpoon, M., Verma, A., Abdusamad, M., et al. (2022). Copper induces cell death by targeting lipoylated TCA cycle proteins. Science 375, 1254–1261. doi:10.1126/science.abf0529
Vaziri-Gohar, A., Cassel, J., Mohammed, F. S., Zarei, M., Hue, J. J., Hajihassani, O., et al. (2022). Limited nutrient availability in the tumor microenvironment renders pancreatic tumors sensitive to allosteric IDH1 inhibitors. Nat. Cancer 3, 852–865. doi:10.1038/s43018-022-00393-y
Wang, J., Fröhlich, H., Torres, F. B., Silva, R. L., Poschet, G., Agarwal, A., et al. (2022). Mitochondrial dysfunction and oxidative stress contribute to cognitive and motor impairment in FOXP1 syndrome. Proc. Natl. Acad. Sci. U. S. A. 119, e2112852119. doi:10.1073/pnas.2112852119
Wang, L., Chen, X., and Yan, C. (2020). Ferroptosis: an emerging therapeutic opportunity for cancer. Genes Dis. 9, 334–346. doi:10.1016/j.gendis.2020.09.005
Wang, W., and Roberts, C. J. (2018). Protein aggregation-Mechanisms, detection, and control. Int. J. Pharm. 550, 251–268. doi:10.1016/j.ijpharm.2018.08.043
Wang, X., Zhou, M., Liu, Y., and Si, Z. (2023a). Cope with copper: from copper linked mechanisms to copper-based clinical cancer therapies. Cancer Lett. 561, 216157. doi:10.1016/j.canlet.2023.216157
Wang, X., Zhou, Y., Min, J., and Wang, F. (2023b). Zooming in and out of ferroptosis in human disease. Front. Med. 17, 173–206. doi:10.1007/s11684-023-0992-z
Wang, Y., Deng, P., Liu, Y., Wu, Y., Chen, Y., Guo, Y., et al. (2020). Alpha-ketoglutarate ameliorates age-related osteoporosis via regulating histone methylations. Nat. Commun. 11, 5596. doi:10.1038/s41467-020-19360-1
Wu, Y., Ma, L., Cai, S., Zhuang, Z., Zhao, Z., Jin, S., et al. (2021). RNA-induced liquid phase separation of SARS-CoV-2 nucleocapsid protein facilitates NF-κB hyper-activation and inflammation. Signal Transduct. Target Ther. 6, 167. doi:10.1038/s41392-021-00575-7
Xiao, S., Zhang, Y., Liu, Z., Li, A., Tong, W., Xiong, X., et al. (2023). Alpinetin inhibits neuroinflammation and neuronal apoptosis via targeting the JAK2/STAT3 signaling pathway in spinal cord injury. CNS Neurosci. Ther. 29, 1094–1108. doi:10.1111/cns.14085
Xie, J., Yang, Y., Gao, Y., and He, J. (2023). Cuproptosis: mechanisms and links with cancers. Mol. Cancer 22, 46. doi:10.1186/s12943-023-01732-y
Yan, R., Cao, P., Song, W., Qian, H., Du, X., Coates, H., et al. (2021). A structure of human Scap bound to Insig-2 suggests how their interaction is regulated by sterols. Science 371, eabb2224. doi:10.1126/science.abb2224
Yang, L., Yang, P., Lip, G. Y. H., and Ren, J. (2023). Copper homeostasis and cuproptosis in cardiovascular disease therapeutics. Trends Pharmacol. Sci. 44, 573–585. doi:10.1016/j.tips.2023.07.004
Yang, W., Pang, D., Chen, M., Du, C., Jia, L., Wang, L., et al. (2021). Rheb mediates neuronal-activity-induced mitochondrial energetics through mTORC1-independent PDH activation. Dev. Cell 56, 811–825.e6. doi:10.1016/j.devcel.2021.02.022
Yang, Y., Rowe, D., McCann, H., Shepherd, C. E., Kril, J. J., Kiernan, M. C., et al. (2023). Treatment with the copper compound CuATSM has no significant effect on motor neuronal pathology in patients with ALS. Neuropathol. Appl. Neurobiol. 49, e12919. doi:10.1111/nan.12919
Yu, F., Zhang, Q., Liu, H., Liu, J., Yang, S., Luo, X., et al. (2022). Dynamic O-GlcNAcylation coordinates ferritinophagy and mitophagy to activate ferroptosis. Cell Discov. 8, 40. doi:10.1038/s41421-022-00390-6
Zhang, N., Dai, D., Hu, P., Guo, S., and Yang, H. (2022). Dual-modal photoelectrochemical and visualized detection of copper ions. ACS Omega 7, 5415–5420. doi:10.1021/acsomega.1c06673
Zhang, W., Zhang, Y., and Zhu, Q. (2022). Cigarette smoke extract-mediated FABP4 upregulation suppresses viability and induces apoptosis, inflammation and oxidative stress of bronchial epithelial cells by activating p38 MAPK/MK2 signaling pathway. J. Inflamm. (Lond) 19, 7. doi:10.1186/s12950-022-00304-z
Zhang, Y., Fang, Q., Wang, H., Qi, J., Sun, S., Liao, M., et al. (2023). Increased mitophagy protects cochlear hair cells from aminoglycoside-induced damage. Autophagy 19, 75–91. doi:10.1080/15548627.2022.2062872
Zhao, X., Liu, Y., Zhu, G., Liang, Y., Liu, B., Wu, Y., et al. (2019). SIRT1 downregulation mediated Manganese-induced neuronal apoptosis through activation of FOXO3a-Bim/PUMA axis. Sci. Total Environ. 646, 1047–1055. doi:10.1016/j.scitotenv.2018.07.363
Zheng, J., Zhang, Q., Zhao, Z., Qiu, Y., Zhou, Y., Wu, Z., et al. (2023). Epigenetically silenced lncRNA SNAI3-AS1 promotes ferroptosis in glioma via perturbing the m6A-dependent recognition of Nrf2 mRNA mediated by SND1. J. Exp. Clin. Cancer Res. 42, 127. doi:10.1186/s13046-023-02684-3
Zhong, C. C., Zhao, T., Hogstrand, C., Song, C. C., Zito, E., Tan, X. Y., et al. (2023). Copper induces liver lipotoxicity disease by up-regulating Nrf2 expression via the activation of MTF-1 and inhibition of SP1/Fyn pathway. Biochim. Biophys. Acta Mol. Basis Dis. 1869, 166752. doi:10.1016/j.bbadis.2023.166752
Zhu, S. Y., Zhou, W. Q., Niu, Y. Y., Zheng, C., Liu, X., Zhang, Y. Y., et al. (2023). COX17 restricts renal fibrosis development by maintaining mitochondrial copper homeostasis and restoring complex IV activity. Acta Pharmacol. Sin. 44, 2091–2102. doi:10.1038/s41401-023-01098-3
Keywords: cuproptosis, detection methods, cell death, Cu content, morphology, molecular biology, biochemical pathways
Citation: Zhang L, Deng R, Guo R, Jiang Y, Guan Y, Chen C, Zhao W, Huang G, Liu L, Du H and Tang D (2024) Recent progress of methods for cuproptosis detection. Front. Mol. Biosci. 11:1460987. doi: 10.3389/fmolb.2024.1460987
Received: 09 July 2024; Accepted: 21 August 2024;
Published: 04 September 2024.
Edited by:
Marie-Pierre Golinelli, UPR2301 Institut de Chimie des Substances Naturelles (ICSN CNRS), FranceReviewed by:
Naresh Singh, Indiana University, United StatesMohammad Zulkifli, Texas A and M University, United States
Copyright © 2024 Zhang, Deng, Guo, Jiang, Guan, Chen, Zhao, Huang, Liu, Du and Tang. This is an open-access article distributed under the terms of the Creative Commons Attribution License (CC BY). The use, distribution or reproduction in other forums is permitted, provided the original author(s) and the copyright owner(s) are credited and that the original publication in this journal is cited, in accordance with accepted academic practice. No use, distribution or reproduction is permitted which does not comply with these terms.
*Correspondence: Ligang Zhang, bGd6aGFuZ0Bmb3N1LmVkdS5jbg==; Hongli Du, aGxkdUBzY3V0LmVkdS5jbg==; Dongsheng Tang, dGFuZ2RzaEAxNjMuY29t
†These authors have contributed equally to this work