- ShanXi Key Laboratory of Stem Cells for Immunological Dermatosis, State Key Breeding Laboratory of Stem Cells for Immunological Dermatosis, Taiyuan Central Hospital, Taiyuan, China
The differentiation of vascular endothelial cells and the formation of new blood vessels are inseparable from the energy supply and regulation of metabolism. The budding of blood vessels is a starting point of glycolysis pathway in angiogenesis. Phosphofructokinase-2/fructose 2,6-biophosphatase 3 (PFKFB3), a key rate-limiting enzyme in glycolysis, exhibits strong kinase activity. Inhibition of PFKFB3 can reduce the rate of glycolysis, thereby inhibiting the budding of blood vessels, resulting in inhibition of pathological angiogenesis. In this review, the role of PFKFB3 in the angiogenesis of inflammatory diseases was summarized, and the endothelial inflammatory diseases associated with PFKFB3 were reviewed.
Introduction
Glycolysis is a metabolic pathway that converts glucose into pyruvate (Ganapathy-Kanniappan and Geschwind, 2013). Free energy released during this process is used to form the high-energy compounds, ATP and NADH (Luengo et al., 2021). Cancer cells tend to rely on glycolysis rather than oxidative phosphorylation, even when oxygen supplies are plentiful. This phenomenon is known as the “Warburg effect” (Vaupel et al., 2019; Kang et al., 2023). The rate of glycolysis flux is controlled by various mechanisms at different levels. One of the rate-limiting checkpoints for glycolytic flux is the conversion of fructose-6-phosphate (F6P) to fructose-1, 6-diphosphate (F1, 6P2) by fructose-6-phosphate kinase (PFK-1) (Kim et al., 2017; Chesney et al., 2005; Kanai et al., 2019a; Kanai et al., 2019b; Atsumi et al., 2005). The intracellular allosteric regulator, fructose 2, 6-bisphosphate (F2, 6P2), is an effective activator of PFK-1 (Atsumi et al., 2005; Cordero-Espinoza and Hagen, 2013; Hue and Rider, 1987; Pilkis et al., 1990; Minchenko et al., 2003). F2, 6P2 increases the affinity of PFK-1 for F6P and overcomes the steric inhibition of ATP on PFK-1, allowing glycolysis flux to pass through the PFK-1 checkpoint and to enter F1, 6P2 synthesis (Abuelgassim and Khoja, 1988). F2, 6P2 intracellular homeostasis is controlled by homodimers of the pfK-2/FBPase (PFKFB) family. Although the core catalytic domain of PFKFB (Ganapathy-Kanniappan and Geschwind, 2013; Luengo et al., 2021; Vaupel et al., 2019; Kang et al., 2023) has high sequence homology (85%), their four isoenzymes display different characteristics, including tissue expression profile, kinase/phosphatase activity ratio, and their response to protein kinase, hormone, and growth factor. The relationships among them is shown in Figure 1 (Minchenko et al., 2003; Okar et al., 2001; Heydasch et al., 2021).
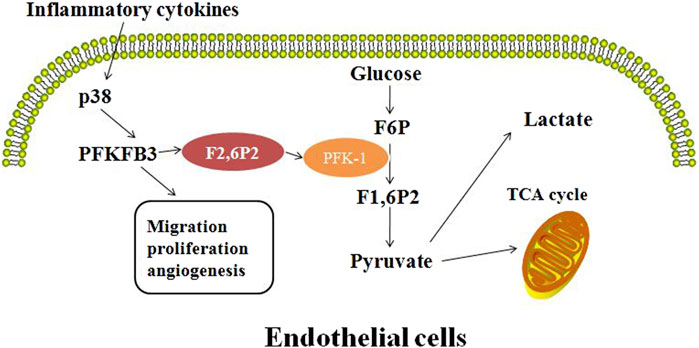
Figure 1. Inflammation affects the angiogenic profile of endothelial cells by upregulation of PFKFB3 expression and regulation of fructose-6-phosphate substrate cycle.
The Pfkfb3 gene was first cloned from fetal brain cDNA libraries, and PFKFB3 protein is widely expressed in all tissues, especially in proliferating tissues, transformed cells, solid tumors and leukemia cells (Long et al., 2019; Jiang et al., 2022). The bifunctional enzyme encoded by the Pfkfb3 gene has the highest kinase-phosphatase activity ratio (710 times), thereby controlling the rate of glycolysis by maintaining intracellular F2,6-P2 (Kotowski et al., 2021; Chesney, 2006; Calvo et al., 2006). In addition, there are different segmentation variants of PFKFB3 (Heydasch et al., 2021; Bando et al., 2005; Duran et al., 2008). So far, at least six splicing variants of PFKFB3 have been identified in the human brain (Bando et al., 2005). The activity and localization of these splicing variants may contribute to the regulation and function of PFKFB3 in glycolysis of tumor cells, as well as its requirement for tumor growth. The Pfkfb3 gene is located on chromosome 10P15.1 (Fleischer et al., 2011) and contains multiple copies of the AUUUA unstable element in its three untranslated regions (3 UTR) (Kanai et al., 2019b). The Pfkfb3 gene spans 109,770 bp, and contains at least 19 exons (Mahlknecht et al., 2003). The COOH terminal variable splicing results in at least six structural isomers, known as UBI2K1-6 in humans (Zscharnack et al., 2009). PFKFB3 protein is a homodimer (Min et al., 2021). The monomer structure is divided into two functional domains within the same peptide. The C-terminal domain contains the bisphosphatase activity. This domain catalyzes the hydrolysis and degradation of F2, 6P2 to F6P and inorganic phosphate. The N-terminal domain is responsible for synthesis of F2,6P2 from F6P and ATP (Kim et al., 2006).
Expression levels of PFKFB3 are upregulated during cell mitosis, inflammation and under hypoxic stimulation (Doménech et al., 2015; Yang et al., 2021; Zhang et al., 2021; Cao et al., 2019; Wang et al., 2021). Various stimuli, including hypoxia, progesterone, estrogen and stress can induce expression of the Pfkfb3 gene (Alvarez et al., 2021; Chédeville et al., 2020; Hamilton et al., 1997; Trenti et al., 2017; Novellasdemunt et al., 2013). These factors bind to specific sequences in the PFKFB promoter, and are the consensus hypoxia response element, progesterone response element, estrogen response element, and serum response element. The Pfkfb3 gene expression can also be upregulated by other factors, such as IL-6, lipopolysaccharides (LPS), adenosine, and different stress stimuli (NaCl, H2O2, UV radiation or anisomycin) (Zhou et al., 2022). Inflammatory cytokines and stress stimuli increase PFKFB3 production through the P38/MK2/SRF pathway. On the other hand, PFKFB3 can upregulate expression levels of insulin, IL-6, LPS, adenosine, and mitogenic lectins such as concanavin, plant lectins and transforming growth factor-β1 (TGF-β1) (Lu et al., 2020; Zou et al., 2017; Wang et al., 2019; Ruiz-García et al., 2011).
PFKFB3 in endothelial cells
The lumen of blood vessels is lined with a single layer of endothelial cells (ECs), including tip cells and stalk cells, each with a specific function. Endothelial cells normally lie dormant in the adult body. But during normal physiological processes or upon injury, these cells can be activated rapidly to form new vascular branches (Stapor et al., 2014). The three main metabolic pathways of energy and biomass produced by endothelial cells are glucose, fatty acid and amino acid metabolism. Because EC has a relatively low mitochondrial content (Stapor et al., 2014) and is primarily dependent on glycolysis, vascular endothelial cells rarely utilize the oxidative phosphorylation metabolic pathway, even when oxygen is abundant, but instead use glycolysis for energy (Potente and Carmeliet, 2017; Vander Heiden et al., 2009), possibly because glycolysis produces ATP more quickly than the oxidative metabolic pathway to meet the energy requirements of ECs.
Glycolysis provides energy for and regulates vascular growth (De Bock et al., 2013a; Eelen et al., 2018). PFKFB3 (PFK2) is a highly expressed glycolytic enzyme in vascular ECs (Wang et al., 2019). PFKFB3 converts F6P to F2, 6P2, which is an allosteric activator of PFK-1 (Yalcin et al., 2009; Gao et al., 2020; Pegoraro et al., 2013). Therefore, inhibition of PFKFB3 enzyme can only partially inhibit the glycolytic pathway and enable cells to enter the resting phase. Angiogenesis is a dynamic process that requires the involvement of a variety of endothelial cells. The tip cells, which have many threadlike pseudopodia, act as “pioneers” during angiogenesis, first migrating to the site where the new blood vessels will grow, and then being followed by the highly proliferative stalk cells to form the new blood vessels. Vascular endothelial growth factor (VEGF) activates tip cells (Melincovici et al., 2018; Ferrara et al., 2005). Angiogenesis is the process of growing new small blood vessels from existing ones (Huang and Nan, 2019). VEGF induces PFKFB3 expression to promote angiogenesis and EC migration by regulating the formation and directional migration of tubular and lamellar feet. Silencing of PFKFB3 in ECs reduces vascular germination by reducing cell apical migration and stalk cell proliferation (Figure 2).
ECs rely more on glycolysis than oxidative phosphorylation for ATP synthesis (Eelen et al., 2015) and loss of PFKFB3 in ECs impairs blood vessel formation (De Bock et al., 2013a; Stapor et al., 2014). Inhibition of glycolysis activity suppresses invasive angiogenesis in eye diseases and inflammatory disease (Liu et al., 2024; Feng et al., 2021). Targeting PFKFB3 in ECs normalizes tumor vascularity and significantly prevents metastasis (Teuwen et al., 2017). The nuclear factor (erythrocyte derived 2) -like2 (Nrf2) regulates endodermal glycolysis and proliferation through transcriptional regulation of PFKFB3, VEGFRA, FOXO1 and MYC (Kuosmanen et al., 2018). Silencing PFKFB3 expression decreases phosphorylation of Akt (Xu et al., 2014) and disrupts CtBP1 oligomerization (Guo et al., 2024), an NADH-sensitive transcriptional co-repressor.
PFKFB3 in inflammatory diseases
Glycolytic metabolism plays an important role in inflammation, including endothelial inflammation (Yang et al., 2021). Endothelial cells are highly glycolytic, with glycolytic rates equal to or exceeding those of some cancer cells (De Bock et al., 2013a). PFKFB3-mediated glycolysis in endothelial cells is critical in angiogenesis and the development of inflammatory diseases, such as retinopathy (Min et al., 2021), pulmonary hypertension (Cao et al., 2019), and metastatic cancers (Yang et al., 2018). Blocking Pfkfb3 reduces proliferation and migration of endothelial cells in inflammatory diseases to prevent pathological angiogenesis (Schoors et al., 2014; Cruys et al., 2016). In addition, inhibition of PFKFB3 was shown to put proliferating endothelial cells in a quiescent state to maintain phenotypic homeostasis. In tumor microvessels, PFKFB3 inhibition via 3PO reduces the expression of EC adhesion molecules by inhibiting the NF-κB signaling pathway (Cantelmo et al., 2016). Glycolysis is increased in vascular ECs in areas prone to atherosclerotic lesions and in ECs exposed to blood flow disturbances in vitro, accompanied by enhanced inflammatory signaling (Yang et al., 2018). Downregulation of glycolytic enzymes in ECs decreases NF-κB activity and inflammation (Wang et al., 2019). The PFKFB3-associated and endothelial inflammatory diseases is summarized in Table 1.
Pulmonary hypertension is a serious lung disease characterized by remodeling of small pulmonary vessels, associated with adverse vascular remodeling, including pulmonary vessel obstruction, hardening, and vasoconstriction (Mocumbi et al., 2024), resulting in a gradual increase in pulmonary vascular resistance, ultimately leading to right ventricular failure and death. The pathological changes in pulmonary hypertension include increased proliferation and resistance to apoptosis of pulmonary artery endothelial and smooth muscle cells, increased production and accumulation of extracellular matrix (Cao et al., 2019), increased local expression of proinflammatory cytokines and chemokines, and leukocyte infiltration into the perivascular region of the lung (Hu et al., 2020). The important pathogenic mechanism of pulmonary hypertension is abnormal metabolism, especially aerobic glycolysis or Warburg effect (Cao et al., 2019). PFKFB3-mediated glycolysis in ECs can increase the production of growth factors and proinflammatory cytokines in lung ECs (Cao et al., 2019). Through autocrine and paracrine pathways, these factors promote inflammatory response and pulmonary artery smooth muscle cells proliferation of lung ECs in animal models of pulmonary hypertension. It has been suggested that the ability of PFKFB3 to promote endothelial dysfunction is caused by increased expression of hypoxia-induced factor (HIF), which is stabilized by higher levels of glycolytic metabolites (Cao et al., 2019). Studies on transgenic mice have shown that hypoxia-inducible faction-2α (HIF-2α, HIF2A) and prolyl hydroxylase domain protein 2 (PHD2) are critical for Pulmonary hypertension development (Tang et al., 2018; Dai et al., 2016). These molecules play an important role in cell energy production, and endothelial cells HIF-2α and PHD2 are likely to promote PH by altering endothelial metabolism. Cao et al. (2019) revealed the key role of glycolytic regulatory factor PFKFB3 in the development of Pulmonary hypertension in the rodent Pulmonary hypertension model, and found that the inhibition of cellular PFKFB3 reduced the level of HIF2A, resulting in reduced production of growth factors, pro-inflammatory cytokines and chemokines, inhibition of adhesion molecules and attenuation of Pulmonary hypertension.
Sepsis is caused by infection or injury that adversely affects microvascular metabolism and immune-inflammatory homeostasis (Deutschman and Tracey, 2014; Angus and van der Poll, 2013; Xiao et al., 2023). Excessive inflammatory responses can cause severe cellular and tissue damages and organ dysfunction, such as acute lung injury (Imam et al., 2015; Shen et al., 2023), where ECs in the lining of blood vessels mediate vascular tone and the balance of proinflammatory and anti-inflammatory responses (Galley and Webster, 2004). Endothelial activation and dysfunction, characterized by upregulation of adhesion molecules and increased leukocyte adhesion, are characteristics of sepsis and can cause multiple organ dysfunctions (Aird, 2003). Studies have found that the expression of glycolytic regulatory factor PFKFB3 is significantly upregulated in sepsis lung, especially in lung endothelial cells (Wang et al., 2019), and experimental data show that endothelium-specific PFKFB3-mediated endothelial inflammation, including leukocyte infiltration mediated by increased expression of adhesion molecules, leads to the development of acute lung injury. In addition, NF-κB is highly activated in patients with sepsis and plays a key role in acute lung injury. Therefore, in a mouse model of LPS-induced sepsis, endothelium-specific PFKFB3 deficiency or 3-(3-pyridyl)-1-(4-pyridyl) -2-propenyl-1-one (3PO) inhibition of PFKFB3 significantly reduced pulmonary edema and improved survival. This is mainly due to the inhibition of the activity of NF-κB signaling pathway, which can inhibit the expression of LPS-induced inflammatory cytokines and adhesion molecules, reduce neutrophil influx, and thus reduce leukocyte infiltration and endothelial permeability (Wang et al., 2019). In addition, Zinc fingers and homeoboxes 2 (Zhx2) increases glycolytic metabolism in macrophages in a PFKFB3-dependent manner, resulting in enhanced inflammation during sepsis, whereas bone marrow-specific Zhx2 null mice are more resistant to sepsis induced by either LPS or cecal ligation and puncture (Wang et al., 2020).
Preeclampsia is a heterogeneous disease, affecting 3%–5% of pregnant women (Mol et al., 2016). Preeclampsia includes two major subtypes, i.e., early-onset preeclampsia and late-onset preeclampsia. Proper formation of blood vessels in the placenta ensures an adequate supply of oxygenated and nutritious blood to the foetus, a prerequisite for a successful pregnancy (Harris et al., 2019). Abnormal fetal vascular development can lead to placental vascular disease and affect fetal intrauterine development. ECs are a key determinant in angiogenesis in both healthy and diseased conditions (De Bock et al., 2013a; Breier et al., 2017). Vascular EC dysfunction reduces placental angiogenesis and promotes preeclampsia (Roberts et al., 1989; Chaiworapongsa et al., 2014). Expression levels of PFKFB3 and metastasis-associated lung adenocarcinoma transcript-1 (MALAT1) are reduced in placental tissues of early-onset preeclampsia patients (Li et al., 2021). In vitro, MALAT1 functions as a ceRNA of miR-26a and miR-26b and inhibits the expression of PFKFB3 in ECs. Knocking down MALAT1 can inhibit the expression of PFKFB3, thereby reducing glycolytic activity and ultimately leading to abnormal angiogenesis in early-onset preeclampsia. Knockdown of MALAT1 inhibits EC proliferation by inducing cell cycle arrest in the G0/G1 phase, and reduces EC motility by inhibiting cell process formation in a PFKFB3-dependent manner. Overexpression of PFKFB3 ameliorates angiogenesis caused by decreased MALAT1 expression in ECs. Collectively, the MALAT1/miR-26/PFKFB3 axis regulates EC angiogenesis by regulating glycolysis and plays a key role in the pathogenesis of early-onset preeclampsia (Li et al., 2021).
Ocular neovascular diseases, specifically posterior segment neovascularization, are the main cause for vision impairment and irreversible blindness in developed and developing countries (Campochiaro, 2013; Zhang and Ma, 2007). Increased EC activation, such as abnormal proliferation and migration stimulated by hypoxia and ischemia, is a major cause of vascular germination (Carmeliet and Jain, 2011). Hypoxia promotes YAP expression and nuclear translocation in human umbilical vein ECs. YAP acts as a transcriptional coactivator and binds to the PFKFB3 promoter with TEAD1, thereby increasing the expression of PFKFB3 (Feng et al., 2021). Silencing of YAP inhibits hypoxia-enhanced endodermal glycolysis and activation (such as cell proliferation and blood vessel germination), which all can be reversed by overexpression of PFKFB3. Additionally, intravitreal injection of either YAP or PFKFB3 siRNA significantly inhibits the neovasculargrowth (Feng et al., 2021). This line of evidence provides new insights into the targeting of the YAP/PFKFB3 axis for the treatment of ocular neovascularization treatment.
Diabetic kidney disease is a chronic, progressive disease (Koye et al., 2018). Diabetes-induced glomerular EC dysfunction is associated with the acquisition of pro-inflammatory and pre-thrombotic phenotypes, which facilitate the adhesion and infiltration of immune cells, and damage the integrity of fenestrated ECs, and increased endothelium-to-mesenchymal transformation (Zeng et al., 2022; Song et al., 2022; Fu et al., 2015). Activation of endothelial inflammatory phenotypes and synthesis of pro-inflammatory factors are all energy-dependent processes, and metabolic pathways in ECs can independently reprogram EC phenotypes (De Bock et al., 2013a; De Bock et al., 2013b). Glucose-induced expression of EGR1 is associated with the pathological changes of diabetic retinopathy (Ao et al., 2019). IGFBP5 can increase expression of EGR1, while the binding of EGR1 to the PFKFB3 promoter induces the expression of PFKFB3, accompanied by enhanced glycolysis (Song et al., 2022). Several studies have shown that PFKFB3-driven glycolysis in ECs increases inflammation. EC activation is a hallmark of diabetes, and glomerular EC dysfunction plays a key role in the development and progression of diabetic kidney disease (Yu et al., 2023). Diabetic nephropathy shows elevated expression levels of PFKFB3, which promotes glycolysis and inflammatory responses of ECs. Inhibition of PFKFB3 not only reverses the increase in glycolytic activity, but also inhibits endothelial inflammation and monocyte migration (Immanuel and Yun, 2023). Taken together, this line of evidence suggests that the glycolytic pathway of vascular ECs can serve as target for the treatment of angiogenesis-related diseases. This speculation is supported by that inhibition of PFKFB3 activity with 3PO inhibits angiogenesis in murine models of psoriasis and colitis (Schoors et al., 2014). Interestingly, in animal models of macular degeneration and retinopathy, which both are caused by blood vessel hyperproliferation, targeting PFKFB3 can also enhance the effect of VEGF signaling blocking on angiogenesis (Zhou et al., 2021).
Summary
The pathogenic roles of PFKFB3 in inflammatory diseases include increasing glucose glycolysis, enhancing angiogenesis and stimulating proliferation of ECs. PFKFB3 can be a promising therapeutic target. Undoubtedly, development of an effective and safe PFKFB3 inhibitor can benefit some inflammatory disorders. Although PFKFB3 inhibitors 3PO and 7, 8-dihydroxy-3 -(4-hydroxyphenyl) -Chromen4-one (YN1) decreased angiogenic germination and HUVECs glycolysis rates both in vitro and in vivo (Schoors et al., 2014). However, it is worth noting that 3PO has a narrow therapeutic index, and the dose mediating vascular normalization and improving barrier function is only slightly lower than the dose causing toxicity and barrier destruction (Conradi et al., 2017). The maximum inhibitory effect of 3PO on glycolysis (Schoors et al., 2014) depends on high doses of 3PO (40 μM), which also has great potential to disrupt endothelial connections (Conradi et al., 2017). In addition, it was reported that 3PO is inactive in the PFKFB3 kinase assay, and there was no crystal structure to confirm 3PO binding to PFKFB3 kinase (Boyd et al., 2015). The anti-glycolytic activity of 3PO depends on its ability to interfere with the acidification of the intracellular environment, rather than directly binding to PFKFB3 (Emini et al., 2020). Therefore, there is still a long way to go to develop PFKFB3 inhibitors for clinical application.
Author contributions
LZ: Writing–original draft, Conceptualization. JaL: Writing–original draft. JW: Investigation, Writing–original draft. XN: Funding acquisition, Writing–review and editing. JnL: Writing–review and editing. KZ: Funding acquisition, Writing–review and editing.
Funding
The author(s) declare that financial support was received for the research, authorship, and/or publication of this article. This project was supported by the National Natural Science Foundation of China (grant no. 82273539), Taiyuan Bureau of Science and Technology, Science, Technology, and Innovation Program of National Regional Medical Center (grant no. 202214) and the Nature Science Foundation of Shanxi Province (grant nos. 202203021211009 and 202403021212278).
Conflict of interest
The authors declare that the research was conducted in the absence of any commercial or financial relationships that could be construed as a potential conflict of interest.
Publisher’s note
All claims expressed in this article are solely those of the authors and do not necessarily represent those of their affiliated organizations, or those of the publisher, the editors and the reviewers. Any product that may be evaluated in this article, or claim that may be made by its manufacturer, is not guaranteed or endorsed by the publisher.
Abbreviations
PFKFB3, phosphofructokinase-2/fructose 2,6-bisphosphatase 3; PFK-1, phosphofructokinase-1; PFKFB, PFK-2/FBPase; 3PO, 3-(3-Pyridinyl)-1-(4-Pyridinyl)-2-Propen-1-One; F6P, fructose 6-phosphate; F1, 6P2, fructose 1, 6-bisphosphate; F2, 6P2, fructose 2, 6-bisphosphate; PFK-1, fructose-6-phosphate 1-kinase; PI, inorganic phosphate; VEGF, Vascular endothelial growth factor; ECs, Endothelial cells; Nrf2, nuclear factor (erythrocyte derived 2) -like2; LPS, lipopolysaccharides.
References
Abuelgassim, A. O., and Khoja, S. M. (1988). Fructose-2,6-bisphosphate in rat mesenteric lymph nodes. Int. J. Biochem. 20 (10), 1185–1188. doi:10.1016/0020-711x(88)90266-2
Aird, W. C. (2003). The role of the endothelium in severe sepsis and multiple organ dysfunction syndrome. Blood 101 (10), 3765–3777. doi:10.1182/blood-2002-06-1887
Alvarez, R., Mandal, D., and Chittiboina, P. (2021). Canonical and non-canonical roles of PFKFB3 in brain tumors. Cells 10 (11), 2913. doi:10.3390/cells10112913
Angus, D. C., and van der Poll, T. (2013). Severe sepsis and septic shock. N. Engl. J. Med. 369 (9), 840–851. doi:10.1056/NEJMra1208623
Ao, H., Liu, B., Li, H., and Lu, L. (2019). Egr1 mediates retinal vascular dysfunction in diabetes mellitus via promoting p53 transcription. J. Cell Mol. Med. 23 (5), 3345–3356. doi:10.1111/jcmm.14225
Atsumi, T., Nishio, T., Niwa, H., Takeuchi, J., Bando, H., Shimizu, C., et al. (2005). Expression of inducible 6-phosphofructo-2-kinase/fructose-2,6-bisphosphatase/PFKFB3 isoforms in adipocytes and their potential role in glycolytic regulation. Diabetes 54 (12), 3349–3357. doi:10.2337/diabetes.54.12.3349
Bando, H., Atsumi, T., Nishio, T., Niwa, H., Mishima, S., Shimizu, C., et al. (2005). Phosphorylation of the 6-phosphofructo-2-kinase/fructose 2,6-bisphosphatase/PFKFB3 family of glycolytic regulators in human cancer. Clin. Cancer Res. 11 (16), 5784–5792. doi:10.1158/1078-0432.CCR-05-0149
Boyd, S., Brookfield, J. L., Critchlow, S. E., Cumming, I. A., Curtis, N. J., Debreczeni, J., et al. (2015). Structure-based design of potent and selective inhibitors of the metabolic kinase PFKFB3. J. Med. Chem. 58 (8), 3611–3625. doi:10.1021/acs.jmedchem.5b00352
Breier, G., Chavakis, T., and Hirsch, E. (2017). Angiogenesis in metabolic-vascular disease. Thromb. Haemost. 117 (7), 1289–1295. doi:10.1160/TH17-05-0325
Calvo, M. N., Bartrons, R., Castaño, E., Perales, J. C., Navarro-Sabaté, A., and Manzano, A. (2006). PFKFB3 gene silencing decreases glycolysis, induces cell-cycle delay and inhibits anchorage-independent growth in HeLa cells. FEBS Lett. 580 (13), 3308–3314. doi:10.1016/j.febslet.2006.04.093
Campochiaro, P. A. (2013). Ocular neovascularization. J. Mol. Med. Berl. 91 (3), 311–321. doi:10.1007/s00109-013-0993-5
Cantelmo, A. R., Conradi, L. C., Brajic, A., Goveia, J., Kalucka, J., Pircher, A., et al. (2016). Inhibition of the glycolytic activator PFKFB3 in endothelium induces tumor vessel normalization, impairs metastasis, and improves chemotherapy. Cancer Cell 30 (6), 968–985. doi:10.1016/j.ccell.2016.10.006
Cao, Y., Zhang, X., Wang, L., Yang, Q., Ma, Q., Xu, J., et al. (2019). PFKFB3-mediated endothelial glycolysis promotes pulmonary hypertension. Proc. Natl. Acad. Sci. U. S. A. 116 (27), 13394–13403. doi:10.1073/pnas.1821401116
Carmeliet, P., and Jain, R. K. (2011). Molecular mechanisms and clinical applications of angiogenesis. Nature 473 (7347), 298–307. doi:10.1038/nature10144
Chaiworapongsa, T., Chaemsaithong, P., Yeo, L., and Romero, R. (2014). Pre-eclampsia part 1: current understanding of its pathophysiology. Nat. Rev. Nephrol. 10 (8), 466–480. doi:10.1038/nrneph.2014.102
Chédeville, A. L., Lourdusamy, A., Monteiro, A. R., Hill, R., and Madureira, P. A. (2020). Investigating glioblastoma response to hypoxia. Biomedicines 8 (9), 310. doi:10.3390/biomedicines8090310
Chesney, J. (2006). 6-phosphofructo-2-kinase/fructose-2, 6-bisphosphatase and tumor cell glycolysis. Curr. Opin. Clin. Nutr. Metab. Care 9 (5), 535–539. doi:10.1097/01.mco.0000241661.15514.fb
Chesney, J., Telang, S., Yalcin, A., Clem, A., Wallis, N., and Bucala, R. (2005). Targeted disruption of inducible 6-phosphofructo-2-kinase results in embryonic lethality. Biochem. Biophys. Res. Commun. 331 (1), 139–146. doi:10.1016/j.bbrc.2005.02.193
Conradi, L. C., Brajic, A., Cantelmo, A. R., Bouché, A., Kalucka, J., Pircher, A., et al. (2017). Tumor vessel disintegration by maximum tolerable PFKFB3 blockade. Angiogenesis 20 (4), 599–613. doi:10.1007/s10456-017-9573-6
Cordero-Espinoza, L., and Hagen, T. (2013). Increased concentrations of fructose 2,6-bisphosphate contribute to the Warburg effect in phosphatase and tensin homolog (PTEN)-deficient cells. J. Biol. Chem. 288 (50), 36020–36028. doi:10.1074/jbc.M113.510289
Cruys, B., Wong, B. W., Kuchnio, A., Verdegem, D., Cantelmo, A. R., Conradi, L. C., et al. (2016). Glycolytic regulation of cell rearrangement in angiogenesis. Nat. Commun. 7, 12240. doi:10.1038/ncomms12240
Dai, Z., Li, M., Wharton, J., Zhu, M. M., and Zhao, Y. Y. (2016). Prolyl-4 hydroxylase 2 (PHD2) deficiency in endothelial cells and hematopoietic cells induces obliterative vascular remodeling and severe pulmonary arterial hypertension in mice and humans through hypoxia-inducible factor-2α. Circulation 133 (24), 2447–2458. doi:10.1161/CIRCULATIONAHA.116.021494
De Bock, K., Georgiadou, M., and Carmeliet, P. (2013b). Role of endothelial cell metabolism in vessel sprouting. Cell Metab. 18 (5), 634–647. doi:10.1016/j.cmet.2013.08.001
De Bock, K., Georgiadou, M., Schoors, S., Kuchnio, A., Wong, B. W., Cantelmo, A. R., et al. (2013a). Role of PFKFB3-driven glycolysis in vessel sprouting. Cell 154 (3), 651–663. doi:10.1016/j.cell.2013.06.037
Deutschman, C. S., and Tracey, K. J. (2014). Sepsis: current dogma and new perspectives. Immunity 40 (4), 463–475. doi:10.1016/j.immuni.2014.04.001
Doménech, E., Maestre, C., Esteban-Martínez, L., Partida, D., Pascual, R., Fernández-Miranda, G., et al. (2015). AMPK and PFKFB3 mediate glycolysis and survival in response to mitophagy during mitotic arrest. Nat. Cell Biol. 17 (10), 1304–1316. doi:10.1038/ncb3231
Duran, J., Gómez, M., Navarro-Sabate, A., Riera-Sans, L., Obach, M., Manzano, A., et al. (2008). Characterization of a new liver- and kidney-specific pfkfb3 isozyme that is downregulated by cell proliferation and dedifferentiation. Biochem. Biophys. Res. Commun. 367 (4), 748–754. doi:10.1016/j.bbrc.2008.01.005
Eelen, G., de Zeeuw, P., Simons, M., and Carmeliet, P. (2015). Endothelial cell metabolism in normal and diseased vasculature. Circ. Res. 116 (7), 1231–1244. doi:10.1161/CIRCRESAHA.116.302855
Eelen, G., de Zeeuw, P., Treps, L., Harjes, U., Wong, B. W., and Carmeliet, P. (2018). Endothelial cell metabolism. Physiol. Rev. 98 (1), 3–58. doi:10.1152/physrev.00001.2017
Emini, V. B., Perrotta, P., Van Wielendaele, P., Lambeir, A. M., Abdali, A., Bellosta, S., et al. (2020). Small molecule 3PO inhibits glycolysis but does not bind to 6-phosphofructo-2-kinase/fructose-2,6-bisphosphatase-3 (PFKFB3). FEBS Lett. 594 (18), 3067–3075. doi:10.1002/1873-3468.13878
Feng, Y., Zou, R., Zhang, X., Shen, M., Chen, X., Wang, J., et al. (2021). YAP promotes ocular neovascularization by modifying PFKFB3-driven endothelial glycolysis. Angiogenesis 24 (3), 489–504. doi:10.1007/s10456-020-09760-8
Ferrara, N., Hillan, K. J., and Novotny, W. (2005). Bevacizumab (Avastin), a humanized anti-VEGF monoclonal antibody for cancer therapy. Biochem. Biophys. Res. Commun. 333 (2), 328–335. doi:10.1016/j.bbrc.2005.05.132
Fleischer, M., Kessler, R., Klammer, A., Warnke, J. P., and Eschrich, K. (2011). LOH on 10p14-p15 targets the PFKFB3 gene locus in human glioblastomas. Genes Chromosom. Cancer 50 (12), 1010–1020. doi:10.1002/gcc.20914
Fu, J., Lee, K., Chuang, P. Y., Liu, Z., and He, J. C. (2015). Glomerular endothelial cell injury and cross talk in diabetic kidney disease. Am. J. Physiol. Ren. Physiol. 308 (4), F287–F297. doi:10.1152/ajprenal.00533.2014
Galley, H. F., and Webster, N. R. (2004). Physiology of the endothelium. Br. J. Anaesth. 93 (1), 105–113. doi:10.1093/bja/aeh163
Ganapathy-Kanniappan, S., and Geschwind, J. F. (2013). Tumor glycolysis as a target for cancer therapy: progress and prospects. Mol. Cancer 12, 152. doi:10.1186/1476-4598-12-152
Gao, L., Wang, C., Qin, B., Li, T., Xu, W., Lenahan, C., et al. (2020). 6-phosphofructo-2-kinase/fructose-2,6-bisphosphatase suppresses neuronal apoptosis by increasing glycolysis and cyclin-dependent kinase 1-mediated phosphorylation of p27 after traumatic spinal cord injury in rats. Cell Transpl. 29, 963689720950226. doi:10.1177/0963689720950226
Guo, S., Wang, L., Cao, K., Li, Z., Song, M., Huang, S., et al. (2024). Endothelial nucleotide-binding oligomerization domain-like receptor protein 3 inflammasome regulation in atherosclerosis. Cardiovasc Res. 120 (8), 883–898. doi:10.1093/cvr/cvae071
Hamilton, J. A., Callaghan, M. J., Sutherland, R. L., and Watts, C. K. (1997). Identification of PRG1, a novel progestin-responsive gene with sequence homology to 6-phosphofructo-2-kinase/fructose-2,6-bisphosphatase. Mol. Endocrinol. 11 (4), 490–502. doi:10.1210/mend.11.4.9909
Harris, L. K., Benagiano, M., D'Elios, M. M., Brosens, I., and Benagiano, G. (2019). Placental bed research: II. Functional and immunological investigations of the placental bed. Am. J. Obstet. Gynecol. 221 (5), 457–469. doi:10.1016/j.ajog.2019.07.010
Heydasch, U., Kessler, R., Warnke, J. P., Eschrich, K., Scholz, N., and Bigl, M. (2021). Functional diversity of PFKFB3 splice variants in glioblastomas. PLoS One 16 (7), e0241092. doi:10.1371/journal.pone.0241092
Hu, Y., Chi, L., Kuebler, W. M., and Goldenberg, N. M. (2020). Perivascular inflammation in pulmonary arterial hypertension. Cells 9 (11), 2338. doi:10.3390/cells9112338
Huang, Y. J., and Nan, G. X. (2019). Oxidative stress-induced angiogenesis. J. Clin. Neurosci. 63, 13–16. doi:10.1016/j.jocn.2019.02.019
Hue, L., and Rider, M. H. (1987). Role of fructose 2,6-bisphosphate in the control of glycolysis in mammalian tissues. Biochem. J. 245 (2), 313–324. doi:10.1042/bj2450313
Imam, F., Al-Harbi, N. O., Al-Harbi, M. M., Ansari, M. A., Zoheir, K. M., Iqbal, M., et al. (2015). Diosmin downregulates the expression of T cell receptors, pro-inflammatory cytokines and NF-κB activation against LPS-induced acute lung injury in mice. Pharmacol. Res. 102, 1–11. doi:10.1016/j.phrs.2015.09.001
Immanuel, J., and Yun, S. (2023). Vascular inflammatory diseases and endothelial phenotypes. Cells 12 (12), 1640. doi:10.3390/cells12121640
Jiang, Y. X., Siu, M. K. Y., Wang, J. J., Leung, T. H. Y., Chan, D. W., Cheung, A. N. Y., et al. (2022). PFKFB3 regulates chemoresistance, metastasis and stemness via IAP proteins and the NF-κB signaling pathway in ovarian cancer. Front. Oncol. 12, 748403. doi:10.3389/fonc.2022.748403
Kanai, S., Shimada, T., Narita, T., and Okabayashi, K. (2019a). Phosphofructokinase-1 and fructose bisphosphatase-1 in canine liver and kidney. J. Vet. Med. Sci. 81 (10), 1515–1521. doi:10.1292/jvms.19-0361
Kanai, S., Shimada, T., Narita, T., and Okabayashi, K. (2019b). Phosphofructokinase-1 subunit composition and activity in the skeletal muscle, liver, and brain of dogs. J. Vet. Med. Sci. 81 (5), 712–716. doi:10.1292/jvms.19-0049
Kang, H., Kim, B., Park, J., Youn, H., and Youn, B. (2023). The Warburg effect on radioresistance: survival beyond growth. Biochim. Biophys. Acta Rev. Cancer 1878 (6), 188988. doi:10.1016/j.bbcan.2023.188988
Kim, N. H., Cha, Y. H., Lee, J., Lee, S. H., Yang, J. H., Yun, J. S., et al. (2017). Snail reprograms glucose metabolism by repressing phosphofructokinase PFKP allowing cancer cell survival under metabolic stress. Nat. Commun. 8, 14374. doi:10.1038/ncomms14374
Kim, S. G., Manes, N. P., El-Maghrabi, M. R., and Lee, Y. H. (2006). Crystal structure of the hypoxia-inducible form of 6-phosphofructo-2-kinase/fructose-2,6-bisphosphatase (PFKFB3): a possible new target for cancer therapy. J. Biol. Chem. 281 (5), 2939–2944. doi:10.1074/jbc.M511019200
Kotowski, K., Rosik, J., Machaj, F., Supplitt, S., Wiczew, D., Jabłońska, K., et al. (2021). Role of PFKFB3 and PFKFB4 in cancer: genetic basis, impact on disease development/progression, and potential as therapeutic targets. Cancers (Basel) 13 (4), 909. doi:10.3390/cancers13040909
Koye, D. N., Magliano, D. J., Nelson, R. G., and Pavkov, M. E. (2018). The global epidemiology of diabetes and kidney disease. Adv. Chronic Kidney Dis. 25 (2), 121–132. doi:10.1053/j.ackd.2017.10.011
Kuosmanen, S. M., Kansanen, E., Kaikkonen, M. U., Sihvola, V., Pulkkinen, K., Jyrkkänen, H. K., et al. (2018). NRF2 regulates endothelial glycolysis and proliferation with miR-93 and mediates the effects of oxidized phospholipids on endothelial activation. Nucleic Acids Res. 46 (3), 1124–1138. doi:10.1093/nar/gkx1155
Li, Q., Liu, X., Liu, W., Zhang, Y., Wu, M., Chen, Z., et al. (2021). MALAT1 sponges miR-26a and miR-26b to regulate endothelial cell angiogenesis via PFKFB3-driven glycolysis in early-onset preeclampsia. Mol. Ther. Nucleic Acids 23, 897–907. doi:10.1016/j.omtn.2021.01.005
Liu, P., Sun, D., Zhang, S., Chen, S., Wang, X., Li, H., et al. (2024). PFKFB3 in neovascular eye disease: unraveling mechanisms and exploring therapeutic strategies. Cell Biosci. 14 (1), 21. doi:10.1186/s13578-024-01205-9
Long, Q., Zou, X., Song, Y., Duan, Z., and Liu, L. (2019). PFKFB3/HIF-1α feedback loop modulates sorafenib resistance in hepatocellular carcinoma cells. Biochem. Biophys. Res. Commun. 513 (3), 642–650. doi:10.1016/j.bbrc.2019.03.109
Lu, Y., Zhang, L., Zhu, R., Zhou, H., Fan, H., and Wang, Q. (2020). PFKFB3, a key glucose metabolic enzyme regulated by pathogen recognition receptor TLR4 in liver cells. Ther. Adv. Endocrinol. Metab. 11, 2042018820923474. doi:10.1177/2042018820923474
Luengo, A., Li, Z., Gui, D. Y., Sullivan, L. B., Zagorulya, M., Do, B. T., et al. (2021). Increased demand for NAD+ relative to ATP drives aerobic glycolysis. Mol. Cell 81 (4), 691–707.e6. doi:10.1016/j.molcel.2020.12.012
Mahlknecht, U., Chesney, J., Hoelzer, D., and Bucala, R. (2003). Cloning and chromosomal characterization of the 6-phosphofructo-2-kinase/fructose-2,6-bisphosphatase-3 gene (PFKFB3, iPFK2). Int. J. Oncol. 23 (4), 883–891. doi:10.3892/ijo.23.4.883
Melincovici, C. S., Boşca, A. B., Şuşman, S., Mărginean, M., Mihu, C., Istrate, M., et al. (2018). Vascular endothelial growth factor (VEGF) - key factor in normal and pathological angiogenesis. Rom. J. Morphol. Embryol. 59 (2), 455–467.
Min, J., Zeng, T., Roux, M., Lazar, D., Chen, L., and Tudzarova, S. (2021). The role of HIF1α-PFKFB3 pathway in diabetic retinopathy. J. Clin. Endocrinol. Metab. 106 (9), 2505–2519. doi:10.1210/clinem/dgab362
Minchenko, O., Opentanova, I., and Caro, J. (2003). Hypoxic regulation of the 6-phosphofructo-2-kinase/fructose-2,6-bisphosphatase gene family (PFKFB-1-4) expression in vivo. FEBS Lett. 554 (3), 264–270. doi:10.1016/s0014-5793(03)01179-7
Mocumbi, A., Humbert, M., Saxena, A., Jing, Z. C., Sliwa, K., Thienemann, F., et al. (2024). Pulmonary hypertension. Nat. Rev. Dis. Prim. 10 (1), 1. Erratum in: Nat Rev Dis Primers. 2024 Jan 17;10(1):5. 10.1038/s41572-024-00493-2. doi:10.1038/s41572-023-00486-7
Mol, B. W. J., Roberts, C. T., Thangaratinam, S., Magee, L. A., de Groot, C. J. M., and Hofmeyr, G. J. (2016). Pre-eclampsia. Lancet 387 (10022), 999–1011. doi:10.1016/S0140-6736(15)00070-7
Novellasdemunt, L., Bultot, L., Manzano, A., Ventura, F., Rosa, J. L., Vertommen, D., et al. (2013). PFKFB3 activation in cancer cells by the p38/MK2 pathway in response to stress stimuli. Biochem. J. 452 (3), 531–543. doi:10.1042/BJ20121886
Okar, D. A., Manzano, A., Navarro-Sabatè, A., Riera, L., Bartrons, R., and Lange, A. J. (2001). PFK-2/FBPase-2: maker and breaker of the essential biofactor fructose-2,6-bisphosphate. Trends Biochem. Sci. 26 (1), 30–35. doi:10.1016/s0968-0004(00)01699-6
Pegoraro, C., Maczkowiak, F., and Monsoro-Burq, A. H. (2013). Pfkfb (6-phosphofructo-2-kinase/fructose-2,6-bisphosphatase) isoforms display a tissue-specific and dynamic expression during Xenopus laevis development. Gene Expr. Patterns 13 (7), 203–211. doi:10.1016/j.gep.2013.04.002
Pilkis, S. J., el-Maghrabi, M. R., and Claus, T. H. (1990). Fructose-2,6-bisphosphate in control of hepatic gluconeogenesis. From metabolites to molecular genetics. Diabetes Care 13 (6), 582–599. doi:10.2337/diacare.13.6.582
Potente, M., and Carmeliet, P. (2017). The link between angiogenesis and endothelial metabolism. Annu. Rev. Physiol. 79, 43–66. doi:10.1146/annurev-physiol-021115-105134
Roberts, J. M., Taylor, R. N., Musci, T. J., Rodgers, G. M., Hubel, C. A., and McLaughlin, M. K. (1989). Preeclampsia: an endothelial cell disorder. Am. J. Obstet. Gynecol. 161 (5), 1200–1204. doi:10.1016/0002-9378(89)90665-0
Ruiz-García, A., Monsalve, E., Novellasdemunt, L., Navarro-Sabaté, A., Manzano, A., Rivero, S., et al. (2011). Cooperation of adenosine with macrophage Toll-4 receptor agonists leads to increased glycolytic flux through the enhanced expression of PFKFB3 gene. J. Biol. Chem. 286 (22), 19247–19258. doi:10.1074/jbc.M110.190298
Schoors, S., De Bock, K., Cantelmo, A. R., Georgiadou, M., Ghesquière, B., Cauwenberghs, S., et al. (2014). Partial and transient reduction of glycolysis by PFKFB3 blockade reduces pathological angiogenesis. Cell Metab. 19 (1), 37–48. doi:10.1016/j.cmet.2013.11.008
Shen, K., Wang, X., Wang, Y., Jia, Y., Zhang, Y., Wang, K., et al. (2023). miR-125b-5p in adipose derived stem cells exosome alleviates pulmonary microvascular endothelial cells ferroptosis via Keap1/Nrf2/GPX4 in sepsis lung injury. Redox Biol. 62, 102655. doi:10.1016/j.redox.2023.102655
Song, C., Wang, S., Fu, Z., Chi, K., Geng, X., Liu, C., et al. (2022). IGFBP5 promotes diabetic kidney disease progression by enhancing PFKFB3-mediated endothelial glycolysis. Cell Death Dis. 13 (4), 340. doi:10.1038/s41419-022-04803-y
Stapor, P., Wang, X., Goveia, J., Moens, S., and Carmeliet, P. (2014). Angiogenesis revisited - role and therapeutic potential of targeting endothelial metabolism. J. Cell Sci. 127 (Pt 20), 4331–4341. doi:10.1242/jcs.153908
Tang, H., Babicheva, A., McDermott, K. M., Gu, Y., Ayon, R. J., Song, S., et al. (2018). Endothelial HIF-2α contributes to severe pulmonary hypertension due to endothelial-to-mesenchymal transition. Am. J. Physiol. Lung Cell Mol. Physiol. 314 (2), L256–L275. doi:10.1152/ajplung.00096.2017
Teuwen, L. A., Draoui, N., Dubois, C., and Carmeliet, P. (2017). Endothelial cell metabolism: an update anno 2017. Curr. Opin. Hematol. 24 (3), 240–247. doi:10.1097/MOH.0000000000000335
Trenti, A., Tedesco, S., Boscaro, C., Ferri, N., Cignarella, A., Trevisi, L., et al. (2017). The glycolytic enzyme PFKFB3 is involved in estrogen-mediated angiogenesis via GPER1. J. Pharmacol. Exp. Ther. 361 (3), 398–407. doi:10.1124/jpet.116.238212
Vander Heiden, M. G., Cantley, L. C., and Thompson, C. B. (2009). Understanding the Warburg effect: the metabolic requirements of cell proliferation. Science 324 (5930), 1029–1033. doi:10.1126/science.1160809
Vaupel, P., Schmidberger, H., and Mayer, A. (2019). The Warburg effect: essential part of metabolic reprogramming and central contributor to cancer progression. Int. J. Radiat. Biol. 95 (7), 912–919. doi:10.1080/09553002.2019.1589653
Wang, L., Cao, Y., Gorshkov, B., Zhou, Y., Yang, Q., Xu, J., et al. (2019). Ablation of endothelial Pfkfb3 protects mice from acute lung injury in LPS-induced endotoxemia. Pharmacol. Res. 146, 104292. doi:10.1016/j.phrs.2019.104292
Wang, L., Zhang, X., Cao, Y., Ma, Q., Mao, X., Xu, J., et al. (2021). Mice with a specific deficiency of Pfkfb3 in myeloid cells are protected from hypoxia-induced pulmonary hypertension. Br. J. Pharmacol. 178 (5), 1055–1072. doi:10.1111/bph.15339
Wang, Z., Kong, L., Tan, S., Zhang, Y., Song, X., Wang, T., et al. (2020). Zhx2 accelerates sepsis by promoting macrophage glycolysis via PFKFB3. J. Immunol. 204 (8), 2232–2241. doi:10.4049/jimmunol.1901246
Xiao, M., Liu, D., Xu, Y., Mao, W., and Li, W. (2023). Role of PFKFB3-driven glycolysis in sepsis. Ann. Med. 55 (1), 1278–1289. doi:10.1080/07853890.2023.2191217
Xu, Y., An, X., Guo, X., Habtetsion, T. G., Wang, Y., Xu, X., et al. (2014). Endothelial PFKFB3 plays a critical role in angiogenesis. Arterioscler. Thromb. Vasc. Biol. 34 (6), 1231–1239. doi:10.1161/ATVBAHA.113.303041
Yalcin, A., Clem, B. F., Simmons, A., Lane, A., Nelson, K., Clem, A. L., et al. (2009). Nuclear targeting of 6-phosphofructo-2-kinase (PFKFB3) increases proliferation via cyclin-dependent kinases. J. Biol. Chem. 284 (36), 24223–24232. doi:10.1074/jbc.M109.016816
Yang, Q., Xu, J., Ma, Q., Liu, Z., Sudhahar, V., Cao, Y., et al. (2018). PRKAA1/AMPKα1-driven glycolysis in endothelial cells exposed to disturbed flow protects against atherosclerosis. Nat. Commun. 9 (1), 4667. doi:10.1038/s41467-018-07132-x
Yang, Q., Xu, J., Ma, Q., Liu, Z., Zhou, Y., Cai, Y., et al. (2021). Disruption of endothelial Pfkfb3 ameliorates diet-induced murine insulin resistance. J. Endocrinol. 250 (3), 93–104. doi:10.1530/JOE-20-0524
Yu, B., Shen, K., Li, T., Li, J., Meng, M., Liu, W., et al. (2023). Glycolytic enzyme PFKFB3 regulates sphingosine 1-phosphate receptor 1 in proangiogenic glomerular endothelial cells under diabetic condition. Am. J. Physiol. Cell Physiol. 325 (5), C1354–C1368. doi:10.1152/ajpcell.00261.2023
Zeng, H., Pan, T., Zhan, M., Hailiwu, R., Liu, B., Yang, H., et al. (2022). Suppression of PFKFB3-driven glycolysis restrains endothelial-to-mesenchymal transition and fibrotic response. Signal Transduct. Target Ther. 7 (1), 303. doi:10.1038/s41392-022-01097-6
Zhang, S. X., and Ma, J. X. (2007). Ocular neovascularization: implication of endogenous angiogenic inhibitors and potential therapy. Prog. Retin Eye Res. 26 (1), 1–37. doi:10.1016/j.preteyeres.2006.09.002
Zhang, Y., Liu, W., Wu, M., Li, Q., Liu, Y., Yang, L., et al. (2021). PFKFB3 regulates lipopolysaccharide-induced excessive inflammation and cellular dysfunction in HTR-8/Svneo cells: implications for the role of PFKFB3 in preeclampsia. Placenta 106, 67–78. doi:10.1016/j.placenta.2021.02.014
Zhou, Z., Plug, L. G., Patente, T. A., de Jonge-Muller, E. S. M., Elmagd, A. A., van der Meulen-de Jong, A. E., et al. (2022). Increased stromal PFKFB3-mediated glycolysis in inflammatory bowel disease contributes to intestinal inflammation. Front. Immunol. 13, 966067. doi:10.3389/fimmu.2022.966067
Zhou, Z. Y., Wang, L., Wang, Y. S., and Dou, G. R. (2021). PFKFB3: a potential key to ocular angiogenesis. Front. Cell Dev. Biol. 9, 628317. doi:10.3389/fcell.2021.628317
Zou, Y., Zeng, S., Huang, M., Qiu, Q., Xiao, Y., Shi, M., et al. (2017). Inhibition of 6-phosphofructo-2-kinase suppresses fibroblast-like synoviocytes-mediated synovial inflammation and joint destruction in rheumatoid arthritis. Br. J. Pharmacol. 174 (9), 893–908. doi:10.1111/bph.13762
Keywords: PFKFB3, endothelial cells, glycolysis, inflammatory, 3PO
Citation: Zhou L, Li J, Wang J, Niu X, Li J and Zhang K (2024) Pathogenic role of PFKFB3 in endothelial inflammatory diseases. Front. Mol. Biosci. 11:1454456. doi: 10.3389/fmolb.2024.1454456
Received: 25 June 2024; Accepted: 30 August 2024;
Published: 10 September 2024.
Edited by:
Venkaiah Betapudi, United States Department of Health and Human Services, United StatesReviewed by:
Carlotta Boscaro, University of Padua, ItalyJonas Aakre Wik, Oslo University Hospital, Norway
Maria I. Bokarewa, University of Gothenburg, Sweden
Mithlesh Kumar Temre, National Institute on Aging (NIH), United States
Copyright © 2024 Zhou, Li, Wang, Niu, Li and Zhang. This is an open-access article distributed under the terms of the Creative Commons Attribution License (CC BY). The use, distribution or reproduction in other forums is permitted, provided the original author(s) and the copyright owner(s) are credited and that the original publication in this journal is cited, in accordance with accepted academic practice. No use, distribution or reproduction is permitted which does not comply with these terms.
*Correspondence: Kaiming Zhang, emhhbmdrYWltaW5nQHNpbmEuY29t