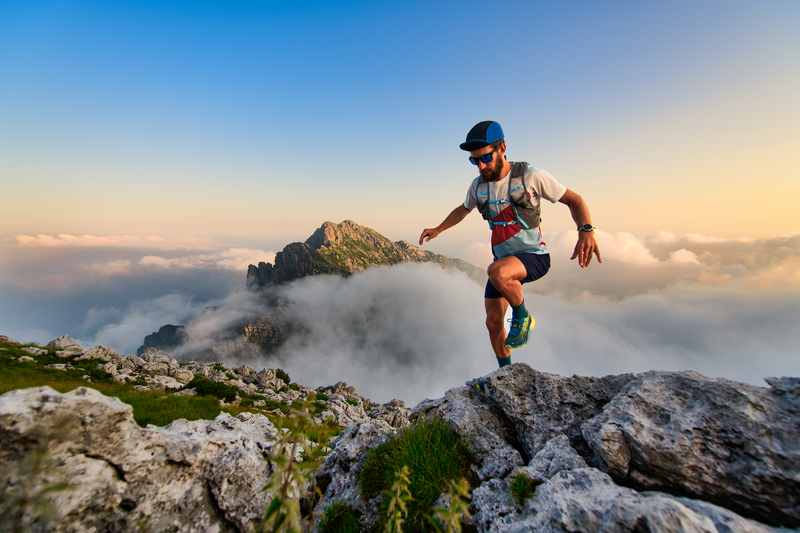
94% of researchers rate our articles as excellent or good
Learn more about the work of our research integrity team to safeguard the quality of each article we publish.
Find out more
ORIGINAL RESEARCH article
Front. Mol. Biosci. , 20 June 2024
Sec. Molecular Diagnostics and Therapeutics
Volume 11 - 2024 | https://doi.org/10.3389/fmolb.2024.1419213
Introduction: Nucleic acid tests for blood donor screening have improved the safety of the blood supply; however, increasing numbers of emerging pathogen tests are burdensome. Multiplex testing platforms are a potential solution.
Methods: The Blood Borne Pathogen Resequencing Microarray Expanded (BBP-RMAv.2) can perform multiplex detection and identification of 80 viruses, bacteria and parasites. This study evaluated pathogen detection in human blood or plasma. Samples spiked with selected pathogens, each with one of 6 viruses, 2 bacteria and 5 protozoans were tested on this platform. The nucleic acids were extracted, amplified using multiplexed sets of primers, and hybridized to a microarray. The reported sequences were aligned to a database to identify the pathogen. To directly compare the microarray to an emerging molecular approach, the amplified nucleic acids were also submitted to nanopore next generation sequencing (NGS).
Results: The BBP-RMAv.2 detected viral pathogens at a concentration as low as 100 copies/ml and a range of concentrations from 1,000 to 100,000 copies/ml for all the spiked pathogens. Coded specimens were identified correctly demonstrating the effectiveness of the platform. The nanopore sequencing correctly identified most samples and the results of the two platforms were compared.
Discussion: These results indicated that the BBP-RMAv.2 could be employed for multiplex detection with potential for use in blood safety or disease diagnosis. The NGS was nearly as effective at identifying pathogens in blood and performed better than BBP-RMAv.2 at identifying pathogen-negative samples.
Safety of blood and blood products is the priority for transfusion and tissue transplantation. The risk of transfusion-transmitted viral infections is significantly reduced by the implementation of nucleic acid tests (NATs) in parallel with conventional methods such as serological tests and questionnaires for blood donor screening (Coste et al., 2005; Roth et al., 2012). Currently, NATs are the routine approach for testing blood components and tissue transplants for HIV, HCV, HBV, and West Nile Virus (WNV) in the US using the mini-pool strategy (Chamberland et al., 2001; Coste et al., 2005). Emerging viral infections have caused blood safety responses to Zika virus. Recently, NAT testing has been applied to protozoan parasites (Tonnetti et al., 2022).
Although these tests have successfully reduced the risk and diagnostic window periods, single-donor testing could further improve sensitivity, which is important for pathogens at low concentration. The goal is to enable the detection, all in one single test, of additional organisms that threaten the blood supply, of which there are many (Stramer et al., 2009). Viruses like chikungunya and dengue as well as new strains of bacteria and parasites (Plasmodium falciparum and Babesia microti), other variants of already known viruses (HIV, HCV, and HBV) that could potentially escape detection with the existing blood donor screening tests, and many other non-routine blood-transmissible agents are all causes of concern. Therefore, the development of alternative blood screening tests detecting multiple pathogens from one single specimen, without losing the sensitivity and accuracy of conventional techniques, is now a priority for improving blood safety.
Research studies propose that microarrays can resolve a large number of targets usually amplified from the specimen by PCR, (Tomioka et al., 2005; Hsia et al., 2007; Lung et al., 2017; De Giorgi et al., 2019), showing promise in pathogen detection. Other platforms have used spatially multiplexed real-time PCR (Grigorenko et al., 2014).
Unlike the printed oligonucleotide arrays used mostly for gene expression experiments (Palmer et al., 2006; Wong et al., 2007), the Affymetrix GeneChips have been adapted for sequencing by hybridization of target DNA fragments. We previously published a study utilizing the in situ-synthesized GeneChips with high-density arrayed oligonucleotide probes to detect and identify blood-borne pathogens (Kourout et al., 2016). The previous study was conducted to assess the feasibility of the resequencing microarray (RMA) platform in testing blood donors.
The current study expands the platform to achieve low concentration detection and the unmatched specificity of determining the actual sequence of the targeted pathogens. There are new probes on the chip for segments of pathogen genomes that target more blood-borne pathogens with higher discrimination of species, strains, and genotypes. The blood-borne pathogen resequencing microarray version 2 (BBP-RMAv.2) was evaluated for its ability to detect and identify viral, bacterial, and protozoan pathogens spiked in blood or plasma that were selected because they are of concern for blood safety and were not tested on the previous version.
The current molecular detection methods are increasingly turning to next-generation sequencing (NGS) for accurate identification of multiple pathogens on one platform (Chiu et al., 2015). To compare the results of NGS to those of the BBP-RMAv.2, PCR products prepared in the microarray workflow were also used for library preparation and sequencing on the Oxford Nanopore MinION (Lin et al., 2021). This allowed evaluating the strengths and weaknesses of each method and side-by-side comparison.
The BBP-RMAv.2 was designed to identify 80 different pathogens categorized in 16 groups (Figure 1). Each pathogen sequence is represented by one or more locations on the microarray. The locations, called tile detectors, are prototype sequences that correspond to the targeted regions in the pathogens (Supplementary Table S1). Each tile detector comprises a series of overlapping oligonucleotide probes that are 25 bases long. Sets of four probes (one perfect-match probe and three probes with one of the other three mismatch bases in the 13th position) were generated for each of the bases in the prototype sequences (Gingeras et al., 1998), which range in length from 45 to 876 bases along the length of the pathogen genome. There are 177 detector tiles that cover a total of 47,973 nucleotides. Sequencing by hybridization requires a total of 383,784 oligonucleotide probes (including both strands) on the microarray to interrogate all the bases. For target regions in pathogens that are highly variable, multiple detector tiles were designed for the same sequence region, with each tile composed of probes corresponding to a particular strain or variant. For example, the 17 HIV-1 LTR tiles cover the same gene sequence, with each one designed to match the sequence of a different variant. The tile detector design was sent to Affymetrix, Inc. (Santa Clara, CA) for manufacturing, as previously described (Lin et al., 2006).
Figure 1. BBP-RMAv.2 microarray composition and pathogens detected. The BBP-RMAv.2 chip design contains 179 tile detectors of target sequence regions. The chip was designed to detect and identify 80 different organisms. The chip allocates two control tile detectors (NAC1 and TIM) and 177 pathogen tile detectors interrogating a total of 47,973 nucleotides of target pathogen genomic sequences; these cover a wide range of pathogens distributed among viruses, bacteria, and protozoans. The pie chart is scaled to the number of tiled bases in each category of pathogen (pie wedge). This microarray assay was evaluated for the detection and identification of 13 pathogens, indicated in the boxes positioned at the perimeter outside the category they belong to along with minimum concentrations detected, illustrating to what extent testing covered the range of pathogen types.
The complete description of the microarray is available at ArrayExpress (https://www.ebi.ac.uk/biostudies/arrayexpress/arrays/A-MTAB-670) with the accession number A-MTAB-670. Note that the archive is labeled as Ebolavirus Resequencing Array, however, the array contains probes for the blood-borne pathogens as well.
Specific primers were designed to enable PCR amplification of genome regions matching the target tiles that are on the array (Supplementary Table S2). Primers were extended with a primer–linker sequence at the 5′ end (Supplementary Table S2), and a linker–primer was added to the amplification mix to minimize dimer formation and enhance PCR amplification of the selected targets (Brownie et al., 1997). All steps of primer design, selection, and pool assembly were carried out as previously described (Kourout et al., 2016). Primers that were amplified in a single PCR were checked for efficient function in the multiplex PCR. Mixtures of primer pairs were assembled into four separate primer pools, with 8–12 pairs in each, using the “Multiplex All Sets” tool in the software OLIGO, version 7.60 (Molecular Biology Insights, Inc., Cascade, CO). Primer pools were tested under multiplex PCR conditions.
Chikungunya virus, hepatitis C virus genotype 1b, and HIV-1 group M samples were prepared from the CBER Lot Release panel members with copies/mL extensively validated by CBER (Grigorenko et al., 2014). The hepatitis C virus genotype 2a (HCV2a) is a virus stock of the strain J6/JfH1 (Duan et al., 2010). HCV genome copies/mL was established by quantitative PCR (Applied Biosystems 7500 RT-PCR Guide, PN 4347825). Dengue virus serotype 2 (DENV2) and Sindbis virus were laboratory stocks quantified by qPCR as PCR detectable units (PDU) per mL. To calculate PDUs, the nucleic acid was extracted from the original virus stock, and then 10-fold serial dilutions were made in the buffer. Each dilution was used as a template in singleplex real-time PCRs utilizing the same primers that were added to the multiplex pools. The lowest dilution that gave a Ct value at least two cycles less than the no-template control was assigned the value of one PCR detectable unit. The inverse of that dilution was the number of PDUs in the undiluted sample, and from that, the PDUs/mL was calculated.
Babesia microti (ATCC PRA-99) parasite cells were collected from an infected DBA/2 mouse at a level of microscopically determined parasitemia of 10%–15%. The Babesia cells/mL was calculated by microscopic determination of the percent of infected red blood cells (RBCs) and the determination of the number of RBCs per mL with a particle counter (Beckman/Coulter), and then the Babesia cells/mL was calculated by the product of these two numbers. Subsequently, infected mouse red cells were diluted in whole human blood to the desired concentrations. Leishmania aethiopica was purchased from ATCC (Manassas, VA), catalog number 50119, lot 125497, at a concentration of 1.1 × 106 cells/mL. Leishmania donovani 1S2D promastigote form (Debrabant et al., 2004) and Trypanosoma brucei as epimastigotes (Selvapandiyan et al., 2007) were cultured as previously described and quantified by automated microscopic image analysis (Vision 286 Cellometer, Nexcelom Bioscience, Lawrence, MA). Plasmodium vivax was obtained from ATCC (Manassas, VA) catalog number 30152, lot 3714526, labeled 5.0 × 106 parasites per 0.5-mL vial.
Bacterial species Yersinia pseudotuberculosis and Staphylococcus epidermidis were cultured in super broth (Quality Biological Inc., Gaithersburg, MD) and quantified by measuring OD600 and converting to cells/mL with the formula 5.0 × 108 cell/mL = 1 absorbance unit in a spectrophotometer (SmartSpec 3000, Bio-Rad Laboratories, Inc., Hercules, CA). This spectrophotometric method was shown to result in a cell count 100 times higher than a plate count for colony-forming units. However, the copy number measured by quantitative PCR is closer to the spectrophotometric measurement, reflecting the enumeration of cells that are not viable (Dong et al., 2016). The spiking range used for the molecular detection method in this study was best standardized to the spectrophotometric method.
All bacterial and protozoan pathogens were similarly diluted and spiked into whole heparinized pathogen-negative human blood. Viral pathogens were diluted and spiked into pathogen-negative human plasma.
The studies involving humans were approved by FDA Research Involving Human Subject Committee and the National Institutes of Health Institutional Review Board. The studies were conducted in accordance with the local legislation and institutional requirements. The participants provided their written informed consent to participate in this study. No potentially identifiable images or data are presented in this study.
The BBP-RMAv.2 assay protocol is a series of steps described in our previous publication (Kourout et al., 2016) and briefly summarized in the following paragraphs.
Nucleic acids from plasma spiked with viral pathogens and DNA from whole blood specimens were extracted, and the appropriate volume was added to a reverse transcription (RT) reaction. The products from the RT reaction were divided among the four PCRs, each containing one of the primer pools with internal positive controls and the primer–linker oligonucleotide. After several rounds of specific amplification, the primer–linker continues the amplification of the existing PCR products. The PCR products were combined and purified with a DNA clean-up system (Wizard SV Gel and PCR Clean-Up System, A9282, Promega, Madison, WI).
Microarray hybridization and processing were carried out according to the manufacturer’s protocol (Affymetrix Inc., Thermo Fisher Scientific, Carlsbad, CA). The scanned microarray image allowed sequence data to be generated as base calls using the GeneChip Sequence Analysis Software (GSEQ, Affymetrix, Inc.). The output is a FASTA file compiling all the detector tiles with the called bases for each tile.
The details of the microarray results including the image file (CEL files), base-calling file (CHP), the final called base sequence (txt files), and the pipeline output “blast.report” of each microarray are available at ArrayExpress (https://www.ebi.ac.uk/arrayexpress/) under the accession number E-MTAB-13972 with the title: “Blood borne pathogen detection in blood and plasma by Microarray” at the link: https://www.ebi.ac.uk/biostudies/arrayexpress/studies?query=E-MTAB-13972+.
The FASTA file was the input for a custom bioinformatic pipeline, bbp_i2o. The code for the bbp_i2o pipeline and construction of TVRSD is freely available at: https://github.com/FDA/Resequencing-Microarray-Pipeline/blob/main/bbp_i2o_2. As previously described (Tiper et al., 2022), a very simple graphic user interface allows the choice of the pipeline and the choice of the FASTA file. With a click on the “run” button, the bbp_i2o executes a series of PERL-based scripts that link together some minor calculations and open-source software: BLAST for database searching (Altschul et al., 1990) and MUSCLE for multiple sequence alignment (Edgar, 2004), all unseen by the operator. The bbp_i2o pipeline is loaded on a Dell Precision Tower 7910 workstation with Dual Intel Xeon Processor E5-2 650 v3, 64 GB memory, and two hard drives (1 TB and 0.5 TB). As a first step, the pipeline determines the C3 score for each tile. The C3 score is the total number of nucleotides that occur in runs of three or more consecutive (non-N) base calls, expressed as a percentage of the length of the tile sequence (in bases) (Metzgar et al., 2010). The C3 score is a measure of the quantity of hybridized DNA and the quality of hybridization. Tile sequences with C3 scores greater than or equal to 20.0 are considered to represent true positive hybridization events; tile sequences with lower scores are postulated to be products of cross-hybridization or assay noise.
Tile sequences that qualify are searched for homologous sequences in a local Validated Reference Sequence Database (TVRSD) (Metzgar et al., 2010) that is stored on the Dell Precision Tower 7910 workstation using the BLAST alignment algorithm. BLAST results for each tile with C3 score ≥20 are placed in a report (blast.report), which contains the top three database hits, i.e., their Bit-score, E-value, accession number, and scientific name, along with the C3 score for each tile. This “blast.report” is deposited into a date and time-stamped folder along with a text file with the original FASTA file of all the tiles and a “blast.html” file that shows all the significant alignments of the top hit tile that is displayed in an internet browser window upon opening.
Samples were processed through extraction, reverse transcription, multiplex PCR reactions, pooling, and cleanup in preparation for the microarray, as described in the “BBP-RMAv.2 Processing” section above. These procedures generate enough nucleic acids to hybridize the microarray and prepare a library for MinION sequencing. Approximately 300 ng of DNA from the pooled PCR product sample was used to prepare a library with the Oxford Nanopore Amplicons-by-Ligation kit (SQK-LSK112) (Oxford_Nanopore_Technologies, 2023) or ligation sequencing amplicons-native barcoding kit (SQKNBD112.24) (Oxford_Nanopore_Technologies, 2021) following the manufacturer’s instructions.
The prepared libraries were loaded onto a MinION Mk1b with a SpotON Flow Cell, version R9 (FLO-MIN106D, Oxford Nanopore Technologies, Oxford, United Kingdom). The MinKNOW software, version 22.03.5 (Oxford Nanopore Technologies, Oxford, United Kingdom) collects the reads, performs base-calling, and sorts the reads into “failed reads” and “passed reads.” After 4 h of read collection, followed by several additional hours of base-calling, the passed reads as fastq.gz files were deposited on the hard drive of a Mac Pro computer. Fastq.gz files were uploaded to the FDA High-Performance Integrated Virtual Environment (CBER_HIVE, 2023), which is a specialized platform developed by CBER’s HIVE staff containing a sequence read archive with direct access to the high-performance computer cluster. Reads from a sequencing run were quality control-checked and aligned against a unique reference file with the alignment tool Minimap2 (Li, 2018), an advanced tool optimized for longer reads typical of nanopore sequencing. The number of reads aligning (mapping quality ≥20) with each pathogen sequence in the reference file is reported.
The reference file is composed of a select group of the sequences present in the tiles arrayed on the BBP-RMAv.2 microarray, listed in Supplementary Table S1. The sequences selected for the reference file are marked with an asterisk (*) in Supplementary Table S1. The reference file also has a human 18S rRNA sequence file, accession number NR_146117.1, available from the NCBI nucleotide database, to reduce false alignment of human sequence reads with protozoan 18S rRNA reference sequences. The sequences for the B. microti 18S rRNA tile and the Leishmania sp. 18S rRNA were excluded from the reference to reduce cross-alignment.
We evaluated the BBP-RMAv.2 assay using 13 high-priority blood-borne pathogens to evaluate the detection of nearly all the types of pathogens represented on the microarray (Figure 1). The detection of these pathogens at the species, subtype, and genotype level is assured by a total of 177 tile detectors on the microarray.
The target prototype sequences were designed and manufactured as an Affymetrix GeneChip (Figure 1). Tile sequences (Supplementary Table S1) were chosen to optimize sensitivity and discrimination between pathogens. A series of primer testing and optimizations were performed first in single-primer pair reactions. Representative optimization results are shown in Table 1. Once single-primer pair detection of purified nucleic acids was achieved, multiplexed primer pools were tested with purified nucleic acids. For example, the Chikungunya NSP4 primer pair yielded a high-quality sequence on the BBP-RMAv.2 first with purified RNA and then with virus particles in plasma at 1,000 copies/mL. Subsequent testing with virus particles in the plasma at two concentrations with the full multiplex assay is shown in the next section. DENV2, HIV-1, HCV genotype 2a, and HCV genotype 1b virus assays were optimized in a similar fashion (Table 1) with varying sensitivity.
Optimization of detection of bacteria species was initiated with purified DNA at a low concentration by testing two primer pairs for targets in Y. pseudotuberculosis. Of the two, the SUCA primer pair accomplished near-complete base calls, though the BLAST search showed an identical match in Y. pseudotuberculosis and Yersinia pestis, which is an undesired suggestion of the presence of this bio-threat agent (see Discussion). SUCA primers were added to the pools due to their sensitivity of detection. The updated pools (Supplementary Table S2) achieved near-perfect sequencing of purified DNA from the Gram-positive bacterium, S. epidermidis.
Optimization of detection of protozoan parasites that are found in the blood during an infection began with primer pairs that amplified the 18S ribosomal RNA segments. Earlier multiplex primer pools and individual primer pairs successfully detected purified DNA from B. microti, L. donovani, and T. brucei (Table 1). The same pools detected L. donovani diluted in whole blood at 100,000 cells/mL, though the result suggested that amplification needed to be improved. Therefore, additional L. donovani primers were added in the final primer pools, resulting in better detection in the final assay.
Once the primer pools were adjusted so that these tests were successful, full assay testing with updated multiplex primer pools was used to detect pathogens in blood or plasma.
The analytical detection capability of the BBP-RMAv.2 assay was evaluated using pathogens spiked in blood and plasma samples. Chikungunya virus, hepatitis C virus genotype 1b, and HIV-1 group M specimens were CBER Lot Release Panel members comprising human plasma containing the virus. The Lot Release Panels were diluted in human plasma at 10,000, 1,000, and 100 copies/mL. HCV2a specimens were created from a recombinant virus culture supernatant at 1,000 copies/mL (Table 2). The final optimized (2023) primer pools (Supplementary Table S2) effectively amplified all the virus samples diluted in human plasma, though there were differences in sensitivity. The chikungunya primers and detector tile were effective, though at 100 copies/mL, and the base calls on the NSP4 tile did not align with any database files using the automated pipeline default parameters. No other tiles in the microarray output FASTA file showed base calls, so additional, manual analysis was performed: the C3 score was manually calculated (Table 2), and the sequence of the CHIK_NSP4 tile was submitted to an NCBI BLAST search. BLAST parameters set to “somewhat similar sequence,” “allow six matches in the query range,” word size of seven, and match/mismatch score = 4/−5 resulted in hits on only chikungunya files with bit scores of 56 and E values of 0.004, which was sufficient to interpret the result as a positive detection. The least effective virus detection was of HCV 1b at 10,000 copies/mL (Table 2), suggesting that additional optimization of primers and tile is warranted. The Sindbis virus primers and tile were particularly effective, resulting in all the bases on the SINDBIS_NSP4-1 tile called correctly down to 100 copies/mL.
Table 2. Analytic performance of the RMA-BBPv.2 (2023 primer pools) using spiked human plasma samples.
The tile detector C3 scores were high enough to identify the six plasma-spiked viruses and determine the lowest detected concentration of each based on the concentrations tested (Table 2). Plasma samples negative for pathogens were tested with the BBP-RMAv.2 system. One tile for Babesia 18S rRNA showed a low number of base calls in the result with this sample. The Babesia 18S rRNA is not shown in Table 2 because this consistent false-positive outcome was avoided by eliminating the B. microti 18S rRNA tile from the analysis. Babesia was adequately detected with the BABE_BAMI_CCT7 tile. Low levels of base calls for Leishmania 18S rRNA or HCV in two tested pathogen-negative samples were interpreted as false positives. Detailed analysis of the origin of these undesirable base calls is presented in the Discussion section.
Gram-positive bacteria species S. epidermidis and Gram-negative Y. pseudotuberculosis, cultured in the laboratory and diluted in whole human blood at 100,000 and 10,000 cells/mL were detected by BBP-RMAv.2, though S. epidermidis at 10,000 cells/mL was very close to the cutoff of C3 = 24 (Table 3). Five protozoan parasites (B. microti, L. aethiopica, L. donovani, P. vivax, and T. brucei) were diluted in whole human blood at 10,000, 1,000, and 100 cells/mL. All were detected at 10,000 cells/mL, and some at 1,000, but only L. donovani was detected at the lowest concentration of 100 cells/mL. The lowest concentration of L. donovani gave an initial pipeline BLAST report that selected L. infantum for identification of the pathogen in the sample. Further examination of the alignments revealed the tile result was identical in the L. infantum genome and the L. donovani genome. Thus, at this parasite load, the two closely related species are not distinguished by the microarray sequence result.
Each pathogen was detected and identified by at least one target sequence tiled onto the BBP-RMAv.2 chip (Table 3). The assay results reveal the actual nucleotide sequences of the detected targets accounting for the usual high level of specificity. The specificity of the BBP-RMAv.2 is also indicated by the lack of cross-reactivity among the seven pathogen-containing whole blood samples and one pathogen-negative whole blood sample that showed no tiles with base calls (Table 3).
With further performance evaluation, the BBP-RMAv.2 was challenged with coded blood and plasma specimens. The coded specimen’s nucleic acids were extracted at the CBER/FDA laboratory, then re-aliquoted into identical tubes, coded, and returned to the lab for testing. The sample identities were revealed only after the results had been finalized.
Three coded plasma nucleic acid samples including the pathogen-negative plasma resulted in two true positives and one false positive (Table 4). One coded blood sample was detected as a true positive (Table 4). Correct identification of unknown samples demonstrates the potential for clinical use of a platform such as the BBP-RMAv.2.
To evaluate the comparative effectiveness of a microarray platform to targeted next-generation sequencing for detection of pathogens in blood, identical samples were subject to analysis by each system.
PCR products pooled from the multiplex amplifications of 16 of the samples, indicated by the hashtag numbers in Tables 2, 3, were each used to prepare a library that was loaded in a MinION nanopore sequencing device. The passed reads were aligned against a database or reference file and the number of reads aligning with each pathogen reported. Initial attempts searching the NCBI nucleotide database or a custom-assembled reference file containing the genomes of the 30 pathogens that our primer set was able to amplify quickly demonstrated that there is substantial cross-alignment among these sequences, particularly considering the large proportion of the reads that match the human sequences.
A fuller understanding of the source of cross-alignment and false positives arose from the joint analysis of the sequence results from the BBP-RMAv.2 and the MinION sequencer for any one sample. Alignment to human sequences was avoided by eliminating the human target sequences from the reference file, except one human 18S rRNA sequence. Due to the high identity between human 18S rRNA reads and the protozoan 18S rRNA sequences in the reference, false-positive cross-alignment occurred. Including a human 18S rRNA sequence in the reference file provided a higher identity target for human reads and significantly reduced the cross-alignment of human reads to pathogen targets. To further improve the specificity, the target sequences included in the reference file were reduced to the exact length of the tiles on the BBP-RMAv.2 microarray. Finally, restricting the search parameters in Minimap2 to MAPQ≥20 achieved high specificity with acceptable sensitivity (Table 5). Each NGS dataset was derived from a library prepared with the multiplex PCR products indicated by the hashtag (#) number shown in Table 5 and Tables 2, 3, indicating the microarray results for the sample with the same hashtag number. This proof-of-concept study was not extensive enough to establish a cutoff value for the alignment score that indicates a positive detection. The datasets for which Minimap2 identified the same pathogen as the microarray with alignment scores greater than or equal to 5 are likely to represent true positives. The low score and ambiguous score for dataset #13 make it uncertain whether T. brucei or Leishmania braziliensis has been detected. For dataset #14, there is no indication of a pathogen other than S. epidermidis; however, the low score does not permit a certainty of detection. Thus, these two results are interpreted as “possible positive.” Dataset #15 clearly failed to detect Y. pseudotuberculosis. The clean results with pathogen-negative plasma and whole blood (datasets #5, #6, and #16) suggest that the analysis algorithm has achieved the desired specificity.
Table 5. Pathogen identification with MinION nanopore sequencing of nucleic acid that was multiplex-amplified from pathogen-spiked human blood or plasma samples.
To facilitate the comparison of the BBP-RMAv.2 results to the MinION results, the outcomes for each sample are arranged side by side in Table 6. The two false negatives (datasets #2 and #15) with the MinION that were positive detections with the BBP-RMAv.2 suggest that the microarray platform is more sensitive. The Babesia 1,000 cells/mL result with the microarray is FN, though there were base calls on the 18SrRNA tile and FN on the MinION. We have shown from the MinION results that there is an abundant amount of human 18S rRNA in the sample that is nearly identical to Babesia 18S rRNA. The human fragments could hybridize to the Babesia 18S rRNA tile on the microarray, generating a false positive. Without confirming base calls on the Babesia CCT7 tile, the #8 interpretation is FN. The two “possible positives” in the MinION interpretation, where the result was too weak for certainty, that were both positive detections with the BBP-RMAv.2, further indicate that the BBP-RMAv.2 is more sensitive. Two false-positive results with the microarray that were interpreted as true negatives by the MinION platform suggest that NGS with the analysis algorithm that we followed is more specific than the BBP-RMAv.2. The raw MinION NGS passed reads are available at ArrayExpress at the link: (https://www.ebi.ac.uk/biostudies/) under accession number E-MTAB-14011.
High-density DNA resequencing microarray technology has great potential in blood safety and diagnostics. It has been shown to be effective for high-throughput detection of microorganisms in clinical, animal, and environmental samples (Leski et al., 2011; Leski et al., 2012; Berthet et al., 2013; Hans et al., 2015; Brockmann et al., 2023). The RMA platform has been validated for detection of influenza and other respiratory viruses using a large number of specimens with high sensitivity and specificity (Wang et al., 2008; Metzgar et al., 2010) and genetic disease screening (Tanner et al., 2014).
Many existing microarray-based detection assays have been developed for highly parallel pathogen screening. Most published microarrays depend on hybridization to a probe yielding a result suggesting presence/absence (Wang et al., 2002). In contrast, the BBP-RMA uses specific primers to accomplish discriminating amplification without sacrificing the sensitivity of detection, and the result of hybridization is the determination of the nucleotide sequence of the pathogen across the targeted region. The RMA represents substantially more information about the pathogen detected and achieves greater discrimination (Kourout et al., 2016).
In this study, we evaluated the BBP-RMAv.2 prototype device for detection and identification of viral, bacterial, and protozoan parasite pathogens known to pose a risk for transmission in blood and blood component transfusion. Furthermore, we developed a computer-based analysis of the microarray image to interpret the sequence revealed by the overlapping probes, score the quality of the sequences, and search high scoring sequences for matching pathogens in the sequence databases. The BBP-RMAv.2 performance was evaluated with whole human blood and plasma spiked with the infectious agents at various concentrations, challenging the system to perform with the clinical matrix.
Currently, blood safety has been improved with FDA-licensed multiplex nucleic acid tests (NATs) to screen donor plasma specimens (Dodd et al., 2020). Compared to commercial assays, the BBP-RMAv.2 has superior multiplicity to detect several gene targets from many target pathogens in one single specimen and one procedure. In addition, the results demonstrate that the BBP-RMAv.2 can detect some spiked viral pathogens at concentrations as low as 100 copies/mL, which is comparable to the limit of detection of the licensed commercial multiplex tests (which are also labeled for IU/mL with the equivalence of 1 copy per 0.56 IU (Holmes et al., 2001)) and at the viral load found in blood donor specimens. In addition, the bacterial and protozoan pathogens, for which NAT assays were recently licensed or are in development, were all detected at concentrations of 10,000 cells/mL and some as low as 100 cells/mL. Currently licensed tests for blood-borne protozoan parasites include B. microti (Stanley et al., 2021) and Trypanosoma cruzi, with a malaria test recently being licensed (FDA/CBER, 2024). However, these are all individual tests that must be applied to blood units one at a time. In contrast, BBP-RMAv.2 has been demonstrated to screen for four parasites in every sample. Furthermore, none of the available commercial assays provide pathogen-specific gene sequence information that could be used for more detailed discrimination of detected pathogen strains. The BBP-RMAv.2 would be an improvement in blood safety platforms because its high multiplicity allows low prevalence or emerging infectious agents to be included in a test panel. For example, Plasmodium and Babesia, agents whose known presence in donor blood is restricted to certain at-risk donors, could be easily included in the panel of pathogens tested more broadly with the BBP-RMAv.2.
The BBP-RMAv.2 continues the high level of multiplicity of BBP-RMAv.1 with multiple targets per pathogen genome, assuring that a mutation in a primer-binding site does not cause a failure in detection. The major improvement of BBP-RMAv.2 is the expanded list of blood-borne pathogens and tiles to achieve more discrimination of species and genotypes that can cause very different disease consequences. Version 2 also solves specific problems that were identified in the testing of version 1 (Kourout et al., 2016). For example, the limited effectiveness of the HIV-1 tile on version 1 was corrected on version 2 by 33 tiles, which can detect four major groups and eleven genotypes of HIV-1 group M.
The results of testing the BBP-RMAv.2 with 12 different pathogens led to discovery of the components of the platform needing improvement. Among viruses, HIV-1 was readily identified, but the sensitivity will need to be improved. The LTR tiles, intended to increase sensitivity, were too short, which caused a lack of specificity, suggesting that longer tiles should be designed. The Chikungunya virus was detected at the low concentration of 100 copies/mL. However, this was only possible after a manual analysis, which suggests that improvements need to be made in the bioinformatic pipeline, allowing more flexibility in the search parameters. False positives resulted from base calls just above the C3 score cutoff on two pathogen-negative plasma samples. The LESH_18SRRNA sequence result reported in the pathogen-negative plasma sample showed 24.6% identity with the tile sequence. The likely explanation is amplicon contamination, even though steps have been taken, including UTP incorporation into PCR products and strict isolation of post-PCR manipulation from the location of PCR preparation. The bases called on another negative plasma sample that matched a small segment of HCV conserved in many genotypes. The sequence result is identical to that of a primer, HCV 1A 5utr_f, with six additional 3’ bases that match the HCV genome. The interpretation of this result is not definitive, and either the HCV 1A 5utr_f primer mis-primed on the abundant human DNA in the nucleic acid sample, resulting in addition of this small fragment to the hybridization mix, or there was a very minor contamination with an HCV PCR product. These two false-positive results can be distinguished from true positives because if Leishmania cells or HCV particles were present, more than one of the tiles present for these species would have had base calls.
An additional component that needs improvement is the blast report for identification of the pathogen. In some cases, with low pathogen load, the sequence revealed by the microarray leads to a blast alignment that is identical in multiple organisms. The blast.report only lists the top three hits to the database. If there are more than three hits in the database that are all identical, there are no criteria for the order in which they are displayed. The best example of this unwanted result is the blast.report for Y. pseudotuberculosis that listed Y. pestis as the top hit. The two bacterial species had identical matches to the microarray base calls, but the database search arbitrarily listed Y. pestis first. As a minimum correction, the user must be aware to check the blast.html file, which displays all the database hits for the top scoring tile, as well as the blast.report, which only displays the top three hits. Another solution to this problem would be to re-design the Y. pseudotuberculosis tile to a segment that discriminates between the two bacterial species.
The microarray was manufactured with 177 independent pathogen gene sequences of 80 organisms. The 12 pathogens tested in this study covered the diverse types of pathogens, but the full capability of the BBP-RMAv.2 has not been demonstrated. The primer development process will need to continue for the BBP-RMAv.2 to reach its full final capability.
Though the BBP-RMAv.2 is a research technology, other studies have suggested that once optimized for turnaround time and cost, it can be implemented in clinical use (Davignon et al., 2005; Lin et al., 2006; Berthet et al., 2013; Hoff et al., 2021). Recognizing the limitations to throughput for the BBP-RMAv.2 suggests that using it as a supplemental test for many different screening tests may be its initial role. Modifications to the platform can increase throughput and reduce cost (Shimada et al., 2021), such that the primers and tiles that were demonstrated effective here could be more practically applied.
Understanding the current value of the BBP-RMAv.2 is also revealed by a comparison to the potential for NGS to perform similar clinical functions. To address this question directly, we performed targeted NGS with the same PCR products that were prepared for hybridization to the microarray and compared the outcomes. The Oxford Nanopore Technologies (ONT) MinION is an attractive platform due to its compact size and low initial price compared to other NGS platforms.
Beginning at the time the PCR products are produced until the platform provides an answer regarding the identity of a pathogen present in the sample, both platforms reached the answer within 24 h. However, for a fully unknown sample, the automated pipeline for the BBP-RMAv.2 was faster and simpler for the operator than the analysis pipeline for the MinION. The analysis procedure for the NGS reads required high-performance computing at a remote location and a skilled bioinformatician to reach pathogen identification. Furthermore, our results suggested that additional optimization of the sequencing procedure, read filtering, the alignment tools, and the reference file will be required to achieve the level of performance already possible with the BBP-RMAv.2. Both platforms have limitations for sample throughput. The ONT MinION flow cell, a reusable, consumable component of the MinION, processes one sample at a time, as does the Affymetrix GeneChip. If the flow cell can be washed and reused three times, the cost per sample is similar to the Affymetrix microarray. However, upon reuse, the flow cell sometimes does not have the required number of active pores. The sample throughput on the MinION is improved with barcoding, a process of ligating unique tags on the molecules in each DNA sample so that the barcoded samples can be pooled for sequencing and the reads sorted by the ONT software at the time of base calling. Thus, the number of samples per run on the MinION can be higher than that on the BBP-RMAv.2 but still lower than desired for clinical use.
The BBP-RMAv.2 microarray faces challenges in complexity and flexibility. The high multiplicity would make validation of each tile or pathogen with a separate reference test particularly burdensome because a reference sample may need to be tested simultaneously with a comparator for each of the pathogens evaluated by the BBP-RMAv.2. This problem might be solved by “a clearly defined randomized approach” to comparator testing, as recommended by the FDA Center for Devices and Radiological Health (FDA/CDRH, 2014). Additionally, detection of non-infectious contaminant nucleic acid often found in clinical specimens could impact the result interpretation (Salter et al., 2014). A weakness in flexibility exists because the manufactured RMAs cannot be changed without synthesizing a new batch of microarrays. It is a problem that would be reduced by a more modular manufacturing technique than the one employed by Affymetrix. This property of the RMA emphasizes the importance of careful design before manufacture. NGS as an approach is far superior in terms of flexibility because the platform is completely agnostic to the input DNA, providing the readout of whatever sequence is in the prepared library. In our application, we restricted the input to the material present in the PCR products and restricted the reference file to the panel of pathogens of concern. These restrictions could be adjusted without the re-manufacturing that is required for the microarray platform.
In future studies, we plan to implement some of the lessons mentioned above. The microarray would be synthesized by an alternate method that increases throughput, simplifies processing, and reduces cost (Hoff et al., 2021). The tiles arrayed on the microarray would be optimized for length to achieve more sensitive detection without sacrificing specificity. Further evaluation of the variable effectiveness of PCR primers and target tiles will facilitate primer selection to improve sensitivity. The MinION NGS approach is a usable approach. Further studies will aim to streamline the analysis pipeline. Notably, there are other pipelines such as BugSeq (Fan et al., 2021) and TaxTriage (Merritt, 2022) that could be improvements. Increasing the throughput by using barcoded library preparation with up to 24 samples in a sequencing run will also improve the NGS approach. The success in detecting the 12 pathogens tested paves the way for further pathogen testing, including clinical specimens. We have established relationships with blood collection and testing organizations that archive frozen plasma specimens from pathogen-reactive blood donations that can be tested with the BBPv.2-RMA and MinION NGS.
The BBP-RMAv.2 demonstrated a high level of multiplicity and superior sensitivity compared to the MinION targeted next-generation sequencing. The sequence analysis with the custom pipeline for the BBP-RMAv.2 was simpler and sufficiently accurate, while the bioinformatic analysis of the NGS reads was more complex and contributed to the reduced sensitivity of the MinION approach.
Protecting the blood supply from known pathogens and detecting newly emerging infectious agents remain a high priority. Today, multiplex NATs and NGS have the potential to provide valuable tools for broad-range identification of microorganisms in clinical samples. The safety of blood and blood products as well as clinical diagnosis will benefit from the continued development of new technology multiplex testing devices like the BBP-RMAv.2 and nanopore sequencing.
The datasets presented in this study can be found in online repositories. The names of the repository/repositories and accession number(s) can be found below: EMBL-EBI Biostudies repository (https://www.ebi.ac.uk/biostudies/), accession numbers A-MTAB-670: https://www.ebi.ac.uk/biostudies/arrayexpress/arrays/A-MTAB-670, E-MTAB-13972: https://www.ebi.ac.uk/biostudies/arrayexpress/studies/E-MTAB-13972?query=E-MTAB-13972%20, E-MTAB-14011: https://www.ebi.ac.uk/biostudies/arrayexpress/studies/E-MTAB-14011
MK: writing–review and editing, investigation, and conceptualization. SE: writing–review and editing and investigation. CF: writing–review and editing and investigation. IT: writing–review and editing and investigation. AP: writing–review and editing, software, and conceptualization. SS: writing–review and editing, investigation, and formal analysis. LS-Q: writing–review and editing and supervision. RD: writing–review and editing, writing–original draft, supervision, project administration, investigation, funding acquisition, formal analysis, and conceptualization.
The author(s) declare that financial support was received for the research, authorship, and/or publication of this article. MK, IT and SE were supported in part by an appointment to the Oak Ridge Institute for Science and Education (ORISE) Research Fellowship Program at the Center for Biologics Evaluation and Research administered by the ORISE through an interagency agreement between the U.S. Department of Energy and the U.S. Food and Drug Administration (https://www.orau.org/). Funding for this research was partially provided by the FDA Medical Countermeasures Initiative (MCMi), grant #2015904. (https://www.fda.gov/emergency-preparedness-andresponse/mcm-regulatory-science/intramural-research). The funders had no role in study design, data collection and analysis, decision to publish, or preparation of the manuscript.
The authors thank Jiangqin Zhao, Indira Hewlett, Hong Zheng, Sanjai Kumar, Rana Nagarkatti, Alain Debrabant, and Dino Feigelstock (CBER, FDA, Silver Spring, MD) for the pathogen source material. They also thank David McGivern and Majid Lassri for reading and the useful comments on the manuscript.
Author AP was employed by the company Openbox Bio.
The remaining authors declare that the research was conducted in the absence of any commercial or financial relationships that could be construed as a potential conflict of interest.
All claims expressed in this article are solely those of the authors and do not necessarily represent those of their affiliated organizations, or those of the publisher, the editors, and the reviewers. Any product that may be evaluated in this article, or claim that may be made by its manufacturer, is not guaranteed or endorsed by the publisher.
The Supplementary Material for this article can be found online at: https://www.frontiersin.org/articles/10.3389/fmolb.2024.1419213/full#supplementary-material
COA, certificate of analysis; BBP-RMA, blood-borne pathogen resequencing microarray; FDA, Food and Drug Administration; CBER, Center for Biologics Evaluation and Research; NIH, National Institutes of Health; pg, picogram.
Altschul, S. F., Gish, W., Miller, W., Myers, E. W., and Lipman, D. J. (1990). Basic local alignment search tool. J. Mol. Biol. 215 (3), 403–410. doi:10.1016/s0022-2836(05)80360-2
Berthet, N., Paulous, S., Coffey, L. L., Frenkiel, M. P., Moltini, I., Tran, C., et al. (2013). Resequencing microarray method for molecular diagnosis of human arboviral diseases. J. Clin. Virol. 56 (3), 238–243. doi:10.1016/j.jcv.2012.10.022
Brockmann, S. J., Buck, E., Casoli, T., Meirelles, J. L., Ruf, W. P., Fabbietti, P., et al. (2023). Mitochondrial genome study in blood of maternally inherited ALS cases. Hum. Genomics 17 (1), 70. doi:10.1186/s40246-023-00516-1
Brownie, J., Shawcross, S., Theaker, J., Whitcombe, D., Ferrie, R., Newton, C., et al. (1997). The elimination of primer-dimer accumulation in PCR. Nucleic Acids Res. 25 (16), 3235–3241. doi:10.1093/nar/25.16.3235
CBER_HIVE (2023). High-performance integrated virtual environment (HIVE). Available at: https://researchcentral.fda.gov/ScientificComputing/HIVE.html (Accessed December 14, 2023).
Chamberland, M. E., Alter, H. J., Busch, M. P., Nemo, G., and Ricketts, M. (2001). Emerging infectious disease issues in blood safety. Emerg. Infect. Dis. 7 (3 Suppl. l), 552–553. doi:10.3201/eid0707.010731
Chiu, C. Y., Bres, V., Yu, G., Krysztof, D., Naccache, S. N., Lee, D., et al. (2015). Genomic assays for identification of Chikungunya virus in blood donors, Puerto Rico, 2014. Emerg. Infect. Dis. 21 (8), 1409–1413. doi:10.3201/eid2108.150458
Coste, J., Reesink, H. W., Engelfriet, C. P., Laperche, S., Brown, S., Busch, M. P., et al. (2005). Implementation of donor screening for infectious agents transmitted by blood by nucleic acid technology: update to 2003. Vox Sang. 88 (4), 289–303. doi:10.1111/j.1423-0410.2005.00636_1.x
Davignon, L., Walter, E. A., Mueller, K. M., Barrozo, C. P., Stenger, D. A., and Lin, B. (2005). Use of resequencing oligonucleotide microarrays for identification of Streptococcus pyogenes and associated antibiotic resistance determinants. J. Clin. Microbiol. 43 (11), 5690–5695. doi:10.1128/JCM.43.11.5690-5695.2005
Debrabant, A., Joshi, M. B., Pimenta, P. F. P., and Dwyer, D. M. (2004). Generation of Leishmania donovani axenic amastigotes: their growth and biological characteristics. Intl J. Parasitol. 34 (2), 205–217. doi:10.1016/j.ijpara.2003.10.011
De Giorgi, V., Zhou, H., Alter, H. J., and Allison, R. D. (2019). A microarray-based pathogen chip for simultaneous molecular detection of transfusion-transmitted infectious agents. J. Transl. Med. 17 (1), 156. doi:10.1186/s12967-019-1905-4
Dodd, R. Y., Crowder, L. A., Haynes, J. M., Notari, E. P., Stramer, S. L., and Steele, W. R. (2020). Screening blood donors for HIV, HCV, and HBV at the American red cross: 10-year trends in prevalence, incidence, and residual risk, 2007 to 2016. Transfus. Med. Rev. 34 (2), 81–93. doi:10.1016/j.tmrv.2020.02.001
Dong, M., Fisher, C., Anez, G., Rios, M., Nakhasi, H. L., Hobson, J. P., et al. (2016). Standardized methods to generate mock (spiked) clinical specimens by spiking blood or plasma with cultured pathogens. J. Appl. Microbiol. 120 (4), 1119–1129. doi:10.1111/jam.13082
Duan, H., Struble, E., Zhong, L., Mihalik, K., Major, M., Zhang, P., et al. (2010). Hepatitis C virus with a naturally occurring single amino-acid substitution in the E2 envelope protein escapes neutralization by naturally-induced and vaccine-induced antibodies. Vaccine 28 (25), 4138–4144. doi:10.1016/j.vaccine.2010.04.024
Edgar, R. C. (2004). MUSCLE: multiple sequence alignment with high accuracy and high throughput. Nucleic Acids Res. 32 (5), 1792–1797. doi:10.1093/nar/gkh340
Fan, J., Huang, S., and Chorlton, S. D. (2021). BugSeq: a highly accurate cloud platform for long-read metagenomic analyses. BMC Bioinforma. 22 (1), 160. doi:10.1186/s12859-021-04089-5
FDA/CBER (2024). Cobas Malaria FDA website: FDA. Available at: https://www.fda.gov/vaccines-blood-biologics/approved-blood-products/cobas-malaria (Accessed April 16, 2024).
FDA/CDRH (2014). Highly multiplexed microbiological/medical countermeasure in vitro nucleic acid based diagnostic devices. Available at: www.fda.gov/downloads/medicaldevices/deviceregulationandguidance/guidancedocuments/ucm327294.pdf (Accessed January 16, 2024).
Gingeras, T. R., Ghandour, G., Wang, E., Berno, A., Small, P. M., Drobniewski, F., et al. (1998). Simultaneous genotyping and species identification using hybridization pattern recognition analysis of generic Mycobacterium DNA arrays. Genome Res. 8 (5), 435–448. doi:10.1101/gr.8.5.435
Grigorenko, E., Fisher, C., Patel, S., Chancey, C., Rios, M., Nakhasi, H. L., et al. (2014). Multiplex screening for blood-borne viral, bacterial, and protozoan parasites using an OpenArray platform. J. Mol. Diagn 16 (1), 136–144. doi:10.1016/j.jmoldx.2013.08.002
Hans, A., Gaudaire, D., Manuguerra, J. C., Leon, A., Gessain, A., Laugier, C., et al. (2015). Combination of an unbiased amplification method and a resequencing microarray for detecting and genotyping equine arteritis virus. J. Clin. Microbiol. 53 (1), 287–291. doi:10.1128/JCM.01935-14
Hoff, K., Ding, X., Carter, L., Duque, J., Lin, J. Y., Dung, S., et al. (2021). Highly accurate chip-based resequencing of SARS-CoV-2 clinical samples. Langmuir 37 (16), 4763–4771. doi:10.1021/acs.langmuir.0c02927
Holmes, H., Davis, C., Heath, A., Hewlett, I., and Lelie, N. (2001). An international collaborative study to establish the 1st international standard for HIV-1 RNA for use in nucleic acid-based techniques. J. Virol. Methods 92 (2), 141–150. doi:10.1016/s0166-0934(00)00283-4
Hsia, C. C., Chizhikov, V. E., Yang, A. X., Selvapandiyan, A., Hewlett, I., Duncan, R., et al. (2007). Microarray multiplex assay for the simultaneous detection and discrimination of hepatitis B, hepatitis C, and human immunodeficiency type-1 viruses in human blood samples. Biochem. Biophys. Res. Commun. 356 (4), 1017–1023. doi:10.1016/j.bbrc.2007.03.087
Kourout, M., Fisher, C., Purkayastha, A., Tibbetts, C., Winkelman, V., Williamson, P., et al. (2016). Multiplex detection and identification of viral, bacterial, and protozoan pathogens in human blood and plasma using a high-density resequencing pathogen microarray platform. Transfusion 56 (6 Pt 2), 1537–1547. doi:10.1111/trf.13524
Leski, T. A., Lin, B., Malanoski, A. P., and Stenger, D. A. (2012). Application of resequencing microarrays in microbial detection and characterization. Future Microbiol. 7 (5), 625–637. doi:10.2217/fmb.12.30
Leski, T. A., Malanoski, A. P., Gregory, M. J., Lin, B., and Stenger, D. A. (2011). Application of a broad-range resequencing array for detection of pathogens in desert dust samples from Kuwait and Iraq. Appl. Environ. Microbiol. 77 (13), 4285–4292. doi:10.1128/AEM.00021-11
Li, H. (2018). Minimap2: pairwise alignment for nucleotide sequences. Bioinformatics 34 (18), 3094–3100. doi:10.1093/bioinformatics/bty191
Lin, B., Hui, J., and Mao, H. (2021). Nanopore technology and its applications in gene sequencing. Biosens. Basel 11 (7), 214. doi:10.3390/bios11070214
Lin, B., Wang, Z., Vora, G. J., Thornton, J. A., Schnur, J. M., Thach, D. C., et al. (2006). Broad-spectrum respiratory tract pathogen identification using resequencing DNA microarrays. Genome Res. 16 (4), 527–535. doi:10.1101/gr.4337206
Lung, O., Ohene-Adjei, S., Buchanan, C., Joseph, T., King, R., Erickson, A., et al. (2017). Multiplex PCR and microarray for detection of swine respiratory pathogens. Transbound. Emerg. Dis. 64 (3), 834–848. doi:10.1111/tbed.12449
Merritt, B. (2022). jhuapl-bio/taxtriage. Available at: https://github.com/jhuapl-bio/taxtriage (Accessed May 15, 2024).
Metzgar, D., Myers, C. A., Russell, K. L., Faix, D., Blair, P. J., Brown, J., et al. (2010). Single assay for simultaneous detection and differential identification of human and avian influenza virus types, subtypes, and emergent variants. PLoS One 5 (2), e8995. doi:10.1371/journal.pone.0008995
Oxford_Nanopore_Technologies (2021). Ligation sequencing amplicons - native barcoding (SQK-NBD112.24). Oxford, United Kingdom: ONT website. Available at: https://community.nanoporetech.com/docs/prepare/library_prep_protocols/native-barcoding-kit-24-with-amplicons-sqk-nbd112-24/v/nba_9135_v112_revl_01dec2021 (Accessed November 22, 2023).
Oxford_Nanopore_Technologies (2023). Ligation sequencing amplicons (SQK-LSK112). Oxford, United Kingdom. Available at: https://community.nanoporetech.com/docs/prepare/library_prep_protocols/amplicons-by-ligation-sqk-lsk112/v/acde_9142_v112_revi_01dec2021 (Accessed November 22, 2023).
Palmer, C., Bik, E. M., Eisen, M. B., Eckburg, P. B., Sana, T. R., Wolber, P. K., et al. (2006). Rapid quantitative profiling of complex microbial populations. Nucleic Acids Res. 34 (1), e5. doi:10.1093/nar/gnj007
Roth, W. K., Busch, M. P., Schuller, A., Ismay, S., Cheng, A., Seed, C. R., et al. (2012). International survey on NAT testing of blood donations: expanding implementation and yield from 1999 to 2009. Vox Sang. 102 (1), 82–90. doi:10.1111/j.1423-0410.2011.01506.x
Salter, S. J., Cox, M. J., Turek, E. M., Calus, S. T., Cookson, W. O., Moffatt, M. F., et al. (2014). Reagent and laboratory contamination can critically impact sequence-based microbiome analyses. BMC Biol. 12, 87. doi:10.1186/s12915-014-0087-z
Selvapandiyan, A., Kumar, P., Morris, J. C., Salisbury, J. L., Wang, C. C., and Nakhasi, H. L. (2007). Centrin1 is required for organelle segregation and cytokinesis in Trypanosoma brucei. Mol. Biol. Cell 18 (9), 3290–3301. doi:10.1091/mbc.e07-01-0022
Shimada, R., Alden, E. N., Hoff, K., Ding, X., Sun, J., Halasz, A. M., et al. (2021). SARS-CoV-2 variant identification using a genome tiling array and genotyping probes. Available at: https://www.biorxiv.org/content/10.1101/2021.05.11.443659v1 (Accessed January 12, 2024).
Stanley, J., Stramer, S. L., Erickson, Y., Cruz, J., Gorlin, J., Janzen, M., et al. (2021). Detection of Babesia RNA and DNA in whole blood samples from US blood donations. Transfusion 61 (10), 2969–2980. doi:10.1111/trf.16617
Stramer, S. L., Hollinger, F. B., Katz, L. M., Kleinman, S., Metzel, P. S., Gregory, K. R., et al. (2009). Emerging infectious disease agents and their potential threat to transfusion safety. Transfusion 49 (Suppl. 2), 1S–29S. doi:10.1111/j.1537-2995.2009.02279.x
Tanner, A. K., Valencia, C. A., Rhodenizer, D., Espirages, M., Da Silva, C., Borsuk, L., et al. (2014). Development and performance of a comprehensive targeted sequencing assay for pan-ethnic screening of carrier status. J. Mol. Diagn 16 (3), 350–360. doi:10.1016/j.jmoldx.2013.12.003
Tiper, I., Kourout, M., Fisher, C., Konduru, K., Purkayastha, A., Kaplan, G., et al. (2022). Tracking ebolavirus genomic drift with a resequencing microarray. PLoS One 17 (2), e0263732. doi:10.1371/journal.pone.0263732
Tomioka, K., Peredelchuk, M., Zhu, X., Arena, R., Volokhov, D., Selvapandiyan, A., et al. (2005). A multiplex polymerase chain reaction microarray assay to detect bioterror pathogens in blood. J. Mol. Diagn 7 (4), 486–494. doi:10.1016/S1525-1578(10)60579-X
Tonnetti, L., Dodd, R. Y., Foster, G., and Stramer, S. L. (2022). Babesia blood testing: the first-year experience. Transfusion 62 (1), 135–142. doi:10.1111/trf.16718
Wang, D., Coscoy, L., Zylberberg, M., Avila, P. C., Boushey, H. A., Ganem, D., et al. (2002). Microarray-based detection and genotyping of viral pathogens. Proc. Natl. Acad. Sci. U. S. A. 99 (24), 15687–15692. doi:10.1073/pnas.242579699
Wang, Z., Malanoski, A. P., Lin, B., Kidd, C., Long, N. C., Blaney, K. M., et al. (2008). Resequencing microarray probe design for typing genetically diverse viruses: human rhinoviruses and enteroviruses. BMC Genomics 9, 577. doi:10.1186/1471-2164-9-577
Keywords: microarray, blood-borne pathogens, multiplex detection, targeted sequencing, resequencing microarray
Citation: Kourout M, Espich S, Fisher C, Tiper I, Purkayastha A, Smith S, Santana-Quintero L and Duncan R (2024) Multiplex detection and identification of viral, bacterial, and protozoan pathogens in human blood and plasma using an expanded high-density resequencing microarray platform. Front. Mol. Biosci. 11:1419213. doi: 10.3389/fmolb.2024.1419213
Received: 17 April 2024; Accepted: 23 May 2024;
Published: 20 June 2024.
Edited by:
Frank J. Hernandez, Linköping University, SwedenReviewed by:
Sherry Dunbar, Luminex, United StatesCopyright © 2024 Kourout, Espich, Fisher, Tiper, Purkayastha, Smith, Santana-Quintero and Duncan. This is an open-access article distributed under the terms of the Creative Commons Attribution License (CC BY). The use, distribution or reproduction in other forums is permitted, provided the original author(s) and the copyright owner(s) are credited and that the original publication in this journal is cited, in accordance with accepted academic practice. No use, distribution or reproduction is permitted which does not comply with these terms.
*Correspondence: Irina Tiper, aXJpbmEudGlwZXJAZmRhLmhocy5nb3Y=
†Present address: Scott Espich, University of California, Berkeley, CA, United States
Disclaimer: All claims expressed in this article are solely those of the authors and do not necessarily represent those of their affiliated organizations, or those of the publisher, the editors and the reviewers. Any product that may be evaluated in this article or claim that may be made by its manufacturer is not guaranteed or endorsed by the publisher.
Research integrity at Frontiers
Learn more about the work of our research integrity team to safeguard the quality of each article we publish.