- 1Medical Plants Research Center, Basic Health Sciences Institute, Shahrekord University of Medical Sciences, Shahrekord, Iran
- 2Department of Orthopedics, Faculty of Medicine, Shahrekord University of Medical Sciences, Shahrekord, Iran
- 3Department of Microbiology and Immunology, Faculty of Medicine, Kashan University of Medical Sciences, Kashan, Iran
- 4Department of Microbiology, Faculty of Biological Sciences, Islamic Azad University, North Tehran Branch, Tehran, Iran
- 5Department of Molecular Genetics, Parand Branch, Islamic Azad University, Tehran, Iran
- 6Natural Products and Medicinal Plants Research Center, North Khorasan University of Medical Sciences, Bojnurd, Iran
- 7Applied Cellular and Molecular Research Center, Kerman University of Medical Sciences, Kerman, Iran
- 8Department of Nursing, School of Nursing and Midwifery, Bam University of Medical Sciences, Bam, Iran
- 9Cellular and Molecular Research Center, Sabzevar University of Medical Science, Sabzevar, Iran
- 10Autoimmune Diseases Research Center, Shahid Beheshti Hospital, Kashan University of Medical Sciences, Kashan, Iran
- 11Research Center for Biochemistry and Nutrition in Metabolic Diseases, Kashan University of Medical Sciences, Kashan, Iran
The result of infection of bone with microorganisms is osteomyelitis and septic arthritis. Methicillin-resistant Staphylococcus aureus (MRSA) is responsible for most of its cases (more than 50%). Since MRSA is resistant to many treatments, it is accompanied by high costs and numerous complications, necessitating more effective new treatments. Recently, development of gelatin nanoparticles have attracted the attention of scientists of biomedicine to itself, and have been utilized as a delivery vehicle for antibiotics because of their biocompatibility, biodegradability, and cost-effectiveness. Promising results have been reported with gelatin modification and combinations with chemical agents. Although these findings have been suggested that gelatin has the potential to be a suitable option for continuous release of antibiotics in osteomyelitis and septic arthritis treatment, they still have not become routine in clinical practices. The most deliver antibiotic using gelatin-derived composites is vancomycin which is showed the good efficacy. To date, a number of pre-clinical studies evaluated the utility of gelatin-based composites in the management of osteomyelitis. Gelatin-based composites were found to have satisfactory performance in the control of infection, as well as the promotion of bone defect repair in chronic osteomyelitis models. This review summarized the available evidence which provides a new insight into gelatin-derived composites with controlled release of antibiotics.
1 Introduction
Chronic osteomyelitis treatment is considered to be challenging, since elimination of the main responsible pathogen has remained a problem for orthopedic surgeons (Kavanagh et al., 2018). Various bacteria could cause chronic osteomyelitis such as Staphylococcus aureus, staphylococci, Propionibacterium species, Enterobacteriaceae species, Pseudomonas aeruginosa, Salmonella species, and Streptococcus pneumoniae (Hatzenbuehler and Pulling, 2011). Chronic osteomyelitis is accompanied by high rates of morbidity and mortality (particularly in older patients), and disrupts the quality of life of those affected with this disease. Similar to other infections, antibiotic therapy is generally employed to manage osteomyelitis (Schwarz, 2020).
Osteomyelitis represents a significant bone infection, presenting in either acute or chronic forms. This condition entails an inflammatory reaction affecting the bone and its associated structures, triggered by pyogenic microorganisms disseminated via the bloodstream, fractures, or surgical interventions. Chronic osteomyelitis is recognized with the development of low grade inflammation, presence of bacteria and/or other microorganisms in the affected area along with pus, sequestrate, and even fistula (Lew and Waldvogel, 1997).
Mostly, osteomyelitis cause is a microorganism which arrived to the bone from adjacent infected tissue, blood or even direct inoculation due to trauma. Usually, hematogenous infections are resulted from a single microorganism and other types (direct inoculation and adjacent tissue) resulted in polymicrobial infection. The challenge in the treatment of osteomyelitis is the ability of microorganisms to live in the necrotic tissues of the bone for a substantial period of time especially if the surgical debridement has not been occurred properly (Lima et al., 2014).
Debridement of necrotic tissues and using antimicrobial agents are the common methods for treatment of osteomyelitis. Choosing the best antibiotic against the osteomyelitis-causing microorganism should be based on primary evaluations including staging, culture, and determination of susceptibility. Early initiation of antibiotics have led to the more favorable results (Cierny III, 2011; Hatzenbuehler and Pulling, 2011; Walter et al., 2012; Lima et al., 2014).
Initiation of osteomyelitis is by establishment of bacteria via different routes such as direct inoculation, hematogenous seeding or from airborne infection. By reaching to the bone, bacteria produce biofilm to protect themselves against antimicrobial agents and immune system activity such as phagocytosis. Moreover, the metabolic activity of bacteria is reduced and they change from motile forms to sessile ones. These changes increase the resistance of bacteria against different antimicrobial agents as the effective dose for killing bacteria in biofilms are about 10–100 times the standard dose which make the antibiotic therapy to the dangerous and ineffective procedure (Gogia et al., 2009). The antibiotic might not be delivered well enough, at the required concentration to remove all bacteria. Persisted cells or biofilm will therefore remain and despite surgical debridement, therapies fail in ∼20% of subjects.
The complications of osteomyelitis treatment can be attributed to various reasons, some of which are i) antimicrobial resistance is widely observed, ii) biofilm production or metabolic alterations can lead to antibiotic tolerance, iii) antibiotics are unable to penetrate damaged and infected bone, and iv) antibiotic-protected reservoirs colonize in the bony substructure. For example, Figure 1 shows the failure of treatment of osteomyelitis due to S. aureus through multiple mechanisms outside of previously known antibiotic resistance (Gimza and Cassat, 2021). Several studies have reported multidrug-resistant strains of S. aureus in various types of samples (Sharafati-chaleshtori and Karimi, 2010; Silago et al., 2020; Zelmer et al., 2022).
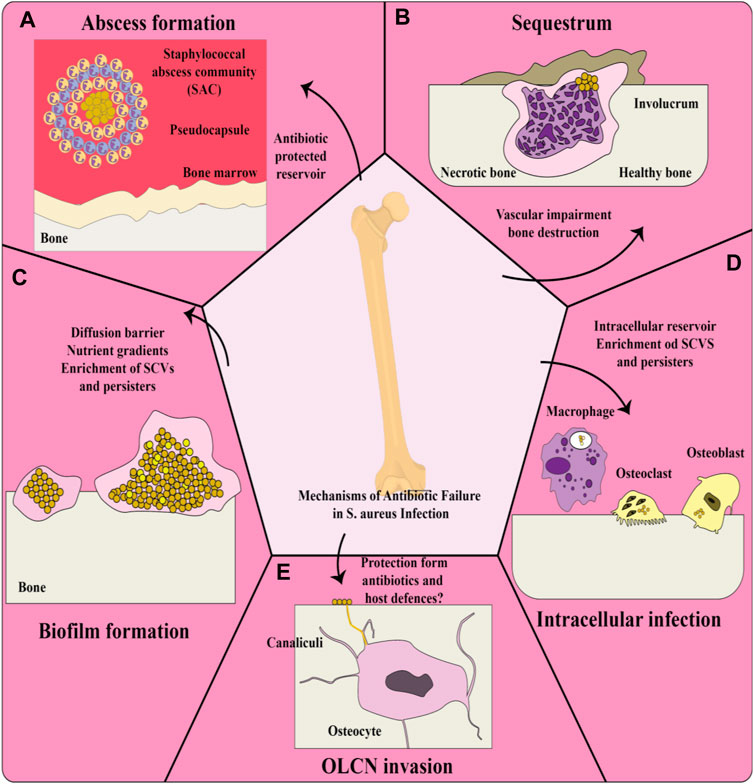
Figure 1. Treatment failure of Staphylococcus aureus osteomyelitis through multiple mechanisms; (A) Invasive staphylococcal infections are characterized by abscesses. Staphylococcal abscess community (SAC) is composed of bacteria inside the abscess core enclosed by a pseudocapsule containing fibrin and other extracellular matrix proteins of host, which subsequently lead to the recruitment of immune cells such as viable and non-viable neutrophils. Strong antibiotic resistance can be observed for bacteria inside a SAC. (B) Invading bacteria form an abscess and severe inflammation in osteomyelitis, which endangers the blood supply to the bone and therefore develops necrosis. This necrosis results in sequestra tissue lesions in chronic osteomyelitis, as a nidus during continuous infection. New bone is formed in reaction to the sequestrum, thereby forming the pathological lesion of the involucrum. The efficacy of systemic antibiotic therapy is greatly reduced due to infection-caused vascular disorders. (C) Bacteria achieve significantly longer persistence as a result of biofilm formation on bone within bone infection and show greater tolerance to antibiotics. The formed biofilm can prevent the diffusion and subsequent penetration of antibiotics into deeper layers. The environment of biofilm, which contains large amounts of nutrients and oxygen, intensifies the development of antibiotic-resistant bacteria, such as small colony variants [SCVs]: “pink cocci,” and persisters: “organ cocci.” (D) S. aureus invading resident bone cells (such as osteoblasts and osteoclasts) and professional phagocytes (such as macrophages) can survive inside these cells, leading to increased antibiotic tolerance because the majority of antibiotics have an extracellular action, so that the evidence showed that the intracellular host environment promotes the construction of persisters and SCVs. (E) Osteocytes are the main cells in the bone matrix found in lacunae structures and connected to each other through canaliculi, a 3D network of canals. Chronicity of osteomyelitis caused by S. aureus occurs through colonization of the osteocyte lacuno-canalicular network (OLCN) due to the absence of the antibiotic concentration required to eradicate the bacteria. Bacteria inside the OLCN may also remain hidden from the host response (Gimza and Cassat, 2021).
Gelatin is a natural, biocompatible, and biodegradable biopolymer, which can perform multiple functions. It has been broadly used in the food, pharmaceutical, cosmetic, and medical industries, thanks to its beneficial mechanical and technological properties (Su and Wang, 2015). In the medical and pharmaceutical areas, gelatin has been employed for both implants and as a matrix for device coatings. However, there some disadvantages associated with the use of gelatin, such as weak mechanical properties, thermal instability, and having a slow rate of degradation. When gelatin is used in studies involving longer periods in a biological environment, such as controlled drug release, cellular adhesion and division, or wound regeneration, it is advisable that gelatin-based materials do not remain intact over the long term (Gorgieva and Kokol, 2011). In comparison to collagen, gelatin, in its hydrolyzed form, is more prone to degradation by various protease enzymes, resulting in a faster breakdown. Gelatin is formed as a denatured product of collagen under specific conditions, constituting an uneven protein mixture. Some drawbacks of gelatin could be simply avoided by gelatin modification, and the production of gelatin composites to improve the mechanical stability, biocompatibility, and bioactivity. The drawbacks of gelatin used in biomedical approaches have become less important owing to progress in manufacturing technology and our understanding of material chemistry (Bello et al., 2020). In the following sections, we discuss a variety of biomaterials based on gelatin and their use in biomedical applications such as scaffolds, drug delivery, and bone substitutes for the healing and regeneration of bones.
2 Gelatin for drug delivery
Extensive investigations have been carried out into the use of gelatin as a drug delivery carrier for various drug types, based on its properties as an organic biomaterial and its track record of safety in a number of medical and pharmaceutical applications. Antibacterial agents, antineoplastic cytotoxic drugs, anti-inflammatory drugs, and most recently nucleic acids and hydrophobic materials have all been reported in the literature to be advantageously delivered by gelatin-based materials (Kumar P. S. et al., 2011; Lee et al., 2013; Santoro, Tatara and Mikos, 2014). Adjusting the properties of gelatin can result in optimized drug-loading efficiency. Remarkably, the isoelectric point for gelatin can be aligned with the electrostatic properties of the drug molecule, which allows it to be employed in either alkaline or acidic conditions (Tabata and Ikada, 1998).
3 Preparation of gelatin nanoparticles
In the literature, gelatin nanoparticles (GNPs) have recently been described as a carrier system for drug delivery as well as gene delivery (Zwiorek et al., 2004; Changez et al., 2005; Jiang et al., 2012; Elzoghby, 2013; Zhou J. et al., 2018; Zhou X. et al, 2018). Since the first description in 1978 (Marty & RC, 1978), various methods for preparing GNPs have been described, including the techniques described below. Some advantages and disadvantages of several different preparation techniques for gelatin nanoparticles are shown in Table 1.
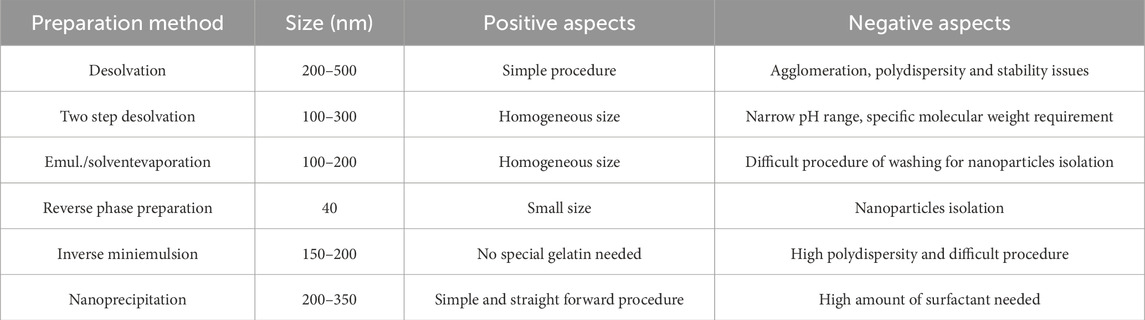
Table 1. Some advantages and disadvantages of several different preparation techniques for GNPs (Khan, 2020).
3.1 Two-step desolvation
Two-step desolvation is a common method used during GNPs development. Desolvation is a thermodynamically driven process for self-assembly of polymeric materials to prepare nanoparticles. Coester et al., in 2008 (Coester et al., 2000) described a method involving the addition of a desolvating agent (acetone) to an aqueous gelatin solution in order to dehydrate the gelatin molecules and produce triple-helix coiled polymeric nanoparticles. In the first desolvation step low molecular weight (LMW < 65 kDa) gelatin components remained in solution, and the HMW precipitate was then redissolved in water. This solution was subjected to a second desolvation step involving careful addition of drops of acetone at monitored pH (2.3–4.0) to avoid the isoelectric point. Small solid nanoparticles with an identical spherical shape were formed by adding a cross-linker and stirring for 12 h. After centrifuging at 10.000 g for 30 min in acetone:water (30:70 ratio), the GNPs were lyophilized 3 times at 2 mbar over the course of 24 h. As shown in Figure 2, the size of the GNPs produced by this method was 110–257 nm (Sahoo et al., 2015).
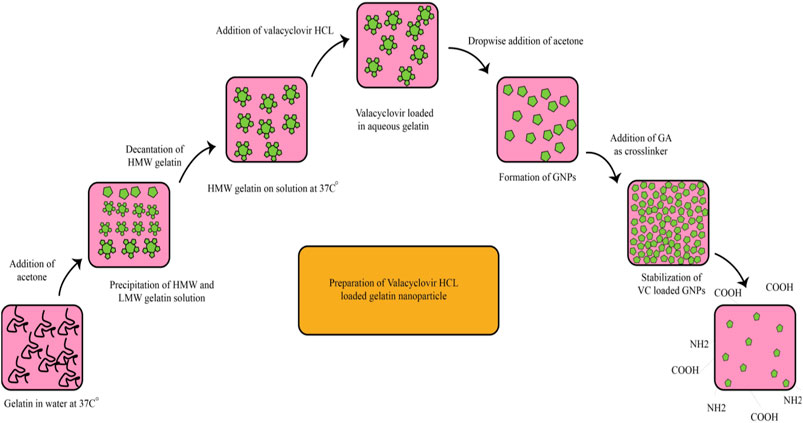
Figure 2. The preparation of valacyclovir (VC)-loaded GNPs via a two-step desolvation process (Sahoo et al., 2015).
3.2 Simple coacervation
Stable and small-scale particles can be prepared by a simple coacervation method. Coacervation involves the separation of an aqueous solution of a macromolecular polymer into two non-miscible liquid phases, with the lower denser phase containing the macromolecules. To prepare the NPs, salts (sodium chloride or sodium sulfate), or alcohols (ethanol) can be added. Macromolecules with a pronounced charge (like proteins or polyelectrolytes), can undergo complex coacervation. Gelatin molecules are dehydrated at the end and the GNPs are subsequently cross-linked with cross-linking agents like glutaraldehyde (GA) (Yasmin et al., 2017).
3.3 Solvent evaporation
This approach involves single or double emulsions, such as oil-in-water (w/o) or double emulsions, water-in-oil-in-water (w/o/w). A high-speed homogenization or ultrasonic mixing technique is used to mix an aqueous phase containing both gelatin and the drug with an oil phase (such as an organic solution of polymethyl methacrylate or paraffin oil), which is then crosslinked with GA or possibly genipin. The solvent can then be evaporated either under reduced pressure or by constant magnetic stirring at room temperature. Then, to remove additives like surfactants, the solidified nanoparticles are aggregated by ultracentrifugation and washed with distilled water. In the final step the material is lyophilized (Sahoo et al., 2015).
3.4 Microemulsions
In this technique GNPs were developed using sodium bis (2-ethylhexyl) sulfosuccinate redispersed in n-hexane (AOT) as a surfactant and soaked gelatin solution. Nanoparticles crosslinking by GA and further evaporation of the n-hexane were the final steps for production of GNPs. N-hexane dissolvent of AOT resulted in inverted micelles in which the hydrophobic tails pointed to the outward surface while the hydrophilic head groups are oriented on the inner side surrounding an aqueous core, within which the gelatin and cross-linker are dissolved. As a result, the GNPs are produced inside the inner aqueous core of the inverted micelles due to the cross-linking (Elzoghby, 2013).
3.5 Nanoprecipitation
In the nanoprecipitation method, an aqueous solution gelatin and the drug is gradually added to ethanol, which contains poloxamer acting as a stabilizer. Afterwards the cross-linker GA is added. Then, a spatially confined distribution occurred due to the miscibility between the solvents. The solvent droplets were disrupted on the nanoscale, and were then stabilized by the stabilizer. When the solvent diffusion was terminated the condensation of the protein took place (Elzoghby, 2013). Figure 3 shows the preparation of GNPs by the nanoprecipitation method.
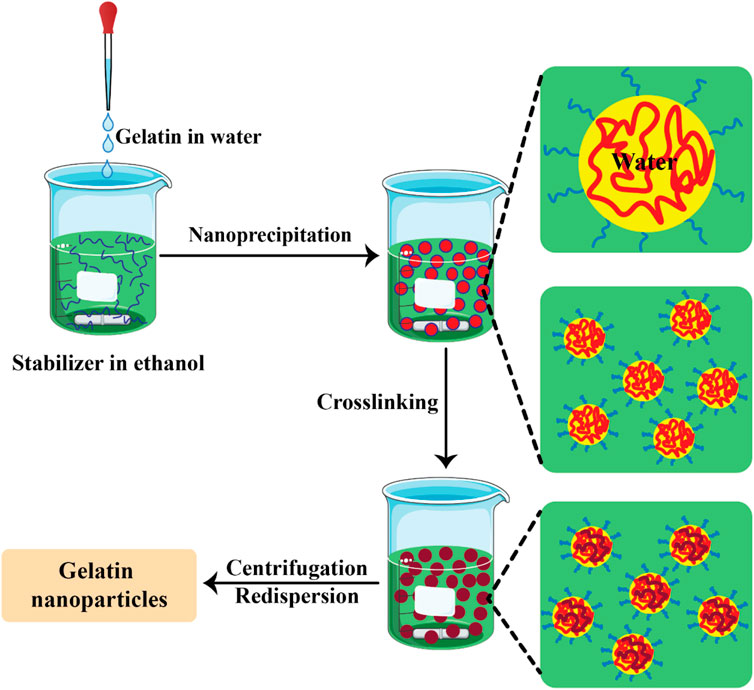
Figure 3. The preparation of gelatin nanoparticles by the nanoprecipitation method (Khan and Schneider, 2013).
3.6 Microfluidic methods
Development of microfluidic approaches and devices are possible due to the collaboration between different fields including physics, chemistry, material science, microelectronics, and fluid dynamics. Increase of products quality along with reduction of cost and time by enhancing different biological and chemical processes are some of the advantages of these devices (Chen and Lv, 2022). These abilities of these devices increase the attention to nanomaterial products. Mixture of metal salt with an agent with reduction ability is a common method for production of metal nanoparticles. The microfluidic method allowed convenient optimization of parameters to obtain a narrower size distribution compared to conventional batch synthesis (Niculescu et al., 2021). Commonly employed microfluidic technologies encompass the T-junction, hydrodynamic flow focusing, staggered herringbone, toroidal mixer, multi-inlet vortex mixers, and others (Xia et al., 2023). The flow behavior within the microfluidic device operates within the laminar regime, where viscous forces predominate. In this regime, laminar flow entails a velocity distribution contingent upon boundary conditions and mass transfer primarily driven by diffusion within the microchannel. Typically, in a hydrodynamic flow-focusing apparatus, a core fluid with a lower flow rate is enveloped by an outer sheath fluid flowing at a higher rate. The heightened flow rate of the outer sheath fluid causes compression within the central flow, thereby diminishing mixing duration. Consequently, this facilitates diffusive mass transfer within the focused stream within microchannels. The reaction occurs predominantly within the centrally focused stream rather than along the channel walls, resulting in a homogeneous distribution of particles. The combination of reproducibility and cost-effectiveness renders microfluidic flow-focusing technology an appealing method for nanoparticle production (Joseph et al., 2022) (Figure 4).
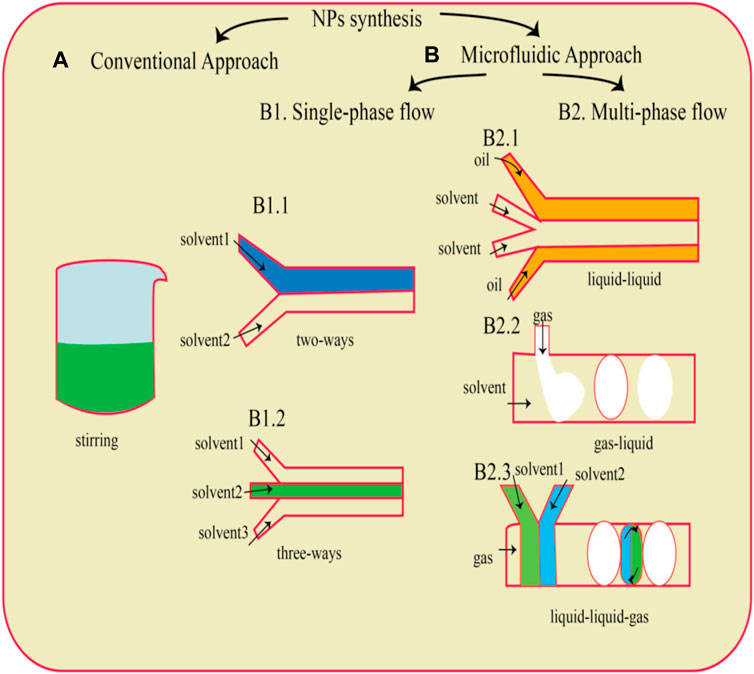
Figure 4. A schematic depiction of a widely utilized traditional technique for nanoparticle (NP) generation namely, the dropwise method (A), is presented. Microfluidic chips (B) with diverse designs can be employed for NP production, depending on the type of flow utilized. This includes single-phase flow systems (B1) featuring either two-way (B1.1) or three-way channels (B1.2), as well as multiphase flow configurations (B2) such as liquid–liquid (B2.1), gas–liquid (B2.2), and liquid–liquid-gas (B2.3) systems (Gimondi et al., 2023).
4 Self-assembly
Some procedures encourage the self-assembly of gelatin molecules to form nanoparticles.
4.1 Chemical modification
The hydrophilic gelatin molecules can be conjugated to various hydrophobic molecules to produce amphiphilic polymers. Hydrophobically modified gelatin can be dissolved in an aqueous solvent, leading to self-assembly into micelle-like nanospheres, where the hydrophobic regions are located in the central part, forming a hydrophobic core which can incorporate hydrophobic drug molecules, along with an external hydrophilic shell.
In another modification of hydrophilic gelatin, hexanoyl anhydride, and alpha-tocopheryl succinate (TOS) were used as hydrophobic groups to be attached to recombinant human gelatin (rHG) (Figure 5) (Li et al., 2011). The nanoparticle core could be loaded with the lipophilic drugs, camptothecin or 17-AAG (17-allylamino-17-demethoxygeldanamycin) by copolymer dilution and sonication. Any free drug was eliminated after centrifugation and dialysis, followed by lyophilization. However, the hexanoyl-modified GNPs demonstrated some instability in aqueous solution, suggesting they may not survive in the bloodstream.
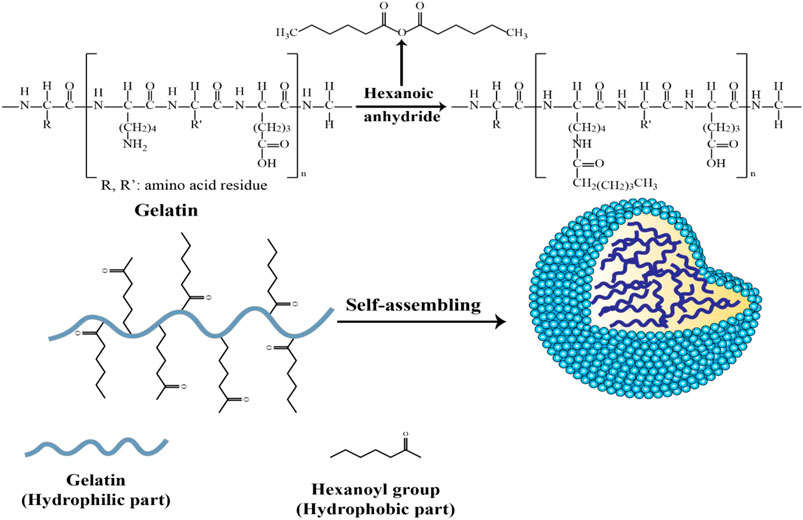
Figure 5. Schematic of the synthesis of self-assembled hexanoyl-modified GNPs (Li et al., 2011).
Tanigo et al. (2010) reported that simvastatin could be incorporated into L-lactic acid oligomer (LAo)-grafted gelatin micelles, thus providing water solubility. After gelatin was chemically crosslinked to generate gelatin hydrogels, the micelles were produced. In the presence of collagenase, the hydrogels were enzymatically cleaved to produce water-soluble gelatin leading to the release of simvastatin. In another study using double emulsion or nanoprecipitation methods, the hydrophobic drug, doxorubicin hydrochloride (DOX) was incorporated into amphiphilic gelatin-co-poly (lactide)-1,2-dipalmitoyl-sn-glycero-3-phosphoethanolamine copolymer nanoparticles (Elzoghby, 2013).
4.2 Simple mixing
The solutions of gelatin and drugs could be simply blended to produce nanoparticles without the gelatin being chemically modified. Self-assembled GNPs could be formed from mixtures with tea catechins or fractionally purified ellagitannins (PPE) (collectively called tannins) by simple mixing relying on the formation of hydrogen bonds. It has been established that some proteins have tertiary structures with fewer hydrophobic regions that force interactions with the tannin molecules. Gelatin is enriched with proline residues, producing an extensive random coil conformation. Therefore gelatin has regions which can interact with tannin molecules (Elzoghby, 2013).
5 Gelatin-based nanoparticles and osteomyelitis
A primary challenge in osteomyelitis therapy is the prolonged presence of the infectious agent within the bone tissue. Inflammation predominantly occurs in avascular regions, leading to spatially diminished bactericidal effectiveness of systemic antibiotic administration and the host immune response (El Zein et al., 2023). The prevailing treatment protocol for both acute and chronic osteomyelitis necessitates a comprehensive approach involving appropriate antimicrobial treatment, surgical intervention, bone reconstruction, and rehabilitation measures (Barakat et al., 2019). Penicillin continues to be the primary antibiotic of choice. For resistant microorganisms, alternatives such as clindamycin, metronidazole, ticarcillin and clavulanic acid, cephalosporins, carbapenems, and vancomycin, often in combination with other antibiotics, may be considered. Empirically, a full course of parenteral antibiotic treatment spanning 4–6 weeks is typically prescribed. Alternatively, initial parenteral therapy may be followed by a 3-week course of oral antibiotics, such as ciprofloxacin and levofloxacin, known for their excellent oral bioavailability and ability to penetrate bone tissue (Bury et al., 2021). In light of this, localized delivery of antibacterial agents emerges as an attractive option for managing chronic osteomyelitis. Historically, antibiotic-loaded acrylic cement (ALAC), polymethylmethacrylate (PMMA) beads, and calcium sulfate pellets have surpassed systemic antibiotic therapy in Europe (Aiken et al., 2024; Jiang et al., 2024; Restrepo et al., 2024). Nevertheless, there are drawbacks associated with the utilization of PMMA and ALAC, notably their nonbiodegradable nature. For instance, during polymerization the PMMA reaction is exothermic, which could result in the destruction of several drugs. Moreover, the antibiotic elution from PMMA could be sub-optimal causing the bacteria to become drug-resistant. Furthermore, patients often undergo secondary surgical procedures for the extraction of the polymeric devices post-antibiotic release, leading to elevated costs and prolonged hospital stays. Failure to remove these devices surgically can result in the formation of foreign bodies, potentially triggering subsequent inflammation and infection (Gibon et al., 2017). Therefore, an ideal device should offer sustained release of local antimicrobial agents. Additionally, it should not hinder the process of new bone ingrowth and ideally promote bone regeneration. Consequently, the integration of resorbable osteoconductive biomaterials with antimicrobial agents appears promising for one-stage surgery in the treatment of osteomyelitis. Therefore, biodegradable scaffolds are preferred for sustained release of drugs to prevent revision surgery. These scaffolds can not only reduce infection, but also boost the regeneration of the bone. Some biodegradable biomaterials, such as bioglass, calcium sulfate, HA-collagen or hydroxyapatite (HAP) are considered to be osteoconductive, and have a chemical composition making them compatible with native bone (Cierny III, 2011; Walter et al., 2012; Lima et al., 2014; Luo et al., 2016; Parent et al., 2016). These materials can be used for the release of antibiotics to reduce infection. For instance, it was shown that the mixture of calcium sulfate beads and antibiotics was useful in osteomyelitis treatment (Papagelopoulos et al., 2006). Nevertheless, the efficiency of these materials is limited by the volume of debrided bone (above a critical size) that needs to be regenerated in osteomyelitis. Regarding infected bone defects, scaffold biodegradation speed should be in line with the time needed for the removal of the bacteria, and then to allow tissue regeneration (typically 1–4 months). Furthermore, neovascularization should be promoted by the scaffold. Neovascularization required several sub-processes including osteoblast movement and stem cell boosting at the site of the bone defect. Studies also showed that adding elements such as silicon or boron in to these bioceramic materials have increased the bone regeneration rate. The antibiotic teicoplanin was released over 20–30 days from particles of borate-containing bioactive glass prepared from ammonium phosphate and chitosan, and this preparation resulted in increased bone regeneration in a in vivo model of osteomyelitis over 12 weeks of implantation (Zhang et al., 2010).
Controlled infection offered one-step structural support for the ingrowth of bone tissue (Krishnan et al., 2020). After 3 months, the stimulant was rapidly absorbed, and there was still a vacancy in the bone at the defect site. Therefore, the vancomycin-containing nanocomposite fibrous scaffold acted as a bi-functional graft, both decreasing bacterial infection, and regenerating the bone in osteomyelitis.
There have been studies on strontium-incorporated hydroxyapatite (Sr-HAP) in orthopedics, dentistry, and bone tissue engineering. HAP has a Ca:P ratio of 1.67, and can act as a useful replacement for the apatite naturally found in bones. Different studies have been conducted on HAP combined with organic or inorganic polymer systems, as well as for drug delivery methods including hydro-gels, scaffolds, coatings, thin films, etc. The extensive adaptability of HAP and its compatibility with many systems, allows it to act as an appropriate niche for bone mineralization. Additionally, HAP have favorable features including its bio-degradability, and -compatibility along with osteoconductive nature, supporting cell attachment via adhesion and proliferation (Kumar R. et al., 2011; Olad and Azhar, 2014). Chitosan (deacetylated chitin) and gelatin (the denatured form of collagen) are widely used in bone reengineering as these proteins have high similarity with bone collagens (Olad and Azhar, 2014). Moreover, when gelatin and HAP are mixed at the ratio of 65:35 they imitate the natural bone composition. In addition to the eco-friendly and non-toxic properties of chitosan and gelatin, no immunologic reactions are stimulated (Jiang et al., 2012; Yao et al., 2015). In comparison to synthetic polymers, natural polymers degrade rapidly leading to loss of their mechanical properties. Porous co-polymer systems composed of chitosan and gelatin combined with HAP have been used in tissue repair as well as drug delivery systems. Extensive research has been conducted on their ability to deliver antibacterial and anti-inflammatory drugs, carrier systems for gene delivery, molecular mechanics, anti-cancer agents, etc. (Bose and Tarafder, 2012; Zhang et al., 2012; Kalil et al., 2014). Their high surface energy and bioinertness have led them to be used as coatings for titanium metallic implants (Oldani and Dominguez, 2012). Due to their inert behavior, titanium implants are renowned for having a prolonged lifespan of at least 15 years with no negative or hazardous reactions. However due to the surface inconsistency, a fibrous capsule will be produced surrounding the metal, which protects infections from attack by the host immune response. As a result, the delivery of antibacterial drugs is difficult, which prevents the bone from being regenerated (Dawes et al., 2010). Sun et al. (2023) illustrated that manganese-doped albumin-gelatin composite nanogels loaded with berberine exhibited the capability to target inflammatory joints. This targeting ability stems from albumin’s inherent high affinity for secreted protein acidic and rich in cysteine (SPARC), which is notably overexpressed at the inflammatory sites of gouty arthritis. Both in vitro and in vivo experimental findings demonstrated that this composite formulation yielded superior therapeutic outcomes, effectively alleviating oxidative stress and dampening inflammation (Sun et al., 2023). Another study documented the efficacy of injectable vancomycin (Van)-loaded gelatin/nanohydroxyapatite (Gel/n-HA) composite microspheres (VM) in modulating inflammation in a rabbit model of osteomyelitis. Injectable VM demonstrated a successful treatment outcome by virtue of its targeted antibacterial action, inflammation modulatory effects, recruitment of osteoblasts, and promotion of bone regeneration, thus effectively addressing osteomyelitis (Zhang et al., 2023). In a recent study, a novel hyaluronic acid/gelatin nanocomposite hydrogel coating was applied to titanium-based implants in a rat model of implant-associated infection. This coating demonstrated the ability to remove biofilms and regulate oxidative stress and inflammatory responses, thereby promoting osseointegration (Ding et al., 2023). Figure 6 provides an overview of the mechanisms of nanoparticle (NP) treatment for rheumatoid arthritis, highlighting its role in addressing the inflammatory aspects of the disease.
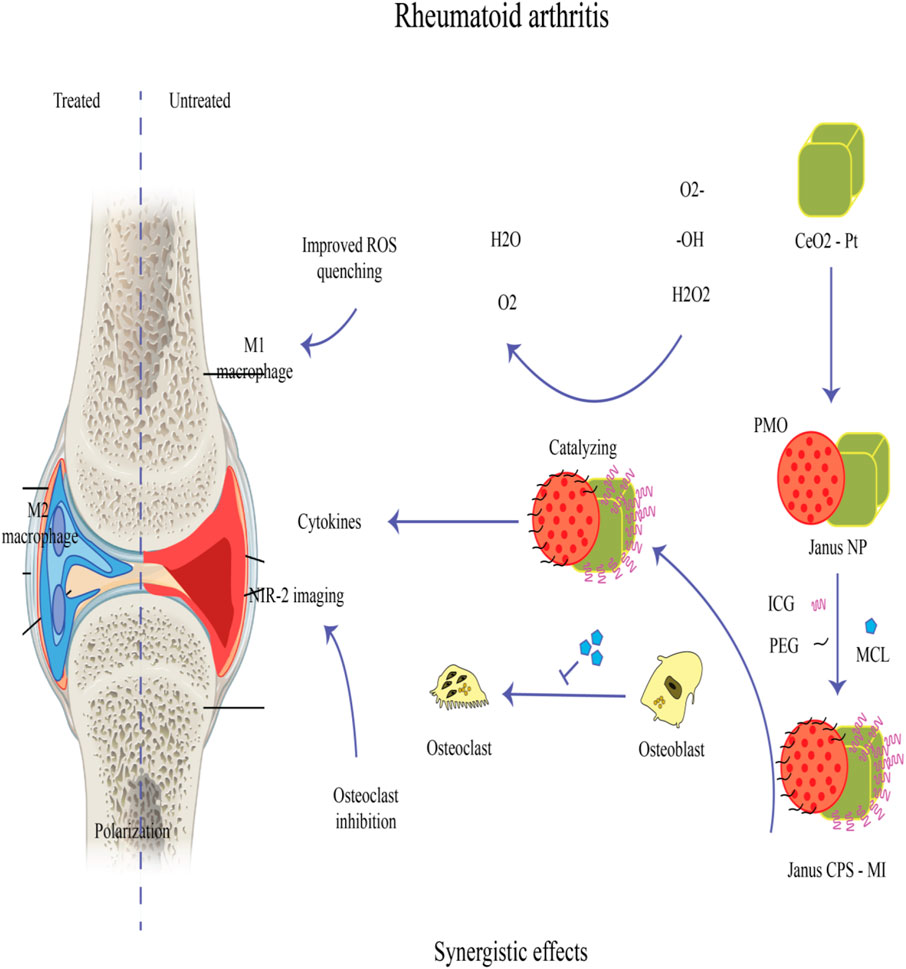
Figure 6. A Janus nanoplatform (Janus-CPS) has been developed for the simultaneous early detection and combined treatment of rheumatoid arthritis (RA). This platform consists of CeO2-Pt nanozyme on one side and periodic mesoporous organosilica (PMO) on the other. Micheliolide (MCL), known for its anti-osteoclastogenesis properties, is encapsulated within the mesopores of PMO to synergistically complement the soothing properties of nanozymes, thereby effectively managing RA. To achieve the desired efficacy in early RA detection, Janus-CPS loaded with indocyanine green (ICG) utilizes NIR-II fluorescence imaging (Huang et al., 2024).
Encouraging potential in the management of patients with persistent osteomyelitis infection a possible solution for non-union fractures to stimulate bone regeneration (David and Nallaiyan, 2018). David et al. prepared vancomycin-loaded chitosan–gelatin polyelectrolyte complex combined with gelatin-SrHAP to produce HV scaffolds at different concentrations HV1-0.5% and HV2-1%. Scaffolds of HG, HV1, and HV2 were efficiently formed on Cp-Ti by attachment using dopamine linkers, which formed bidentate coordination by NH bonds. In addition, the HV2 scaffold greatly outperformed the Cp-Ti, HG, and HV1 scaffolds in terms of cell viability. Vancomycin did not have any harmful effect on the cells; on the contrary, it increased cell proliferation and spreading.
Ciprofloxacin (CFX) hydrochloride (1-cyclopropyl-6-fluoro-4-oxo-7-piperazin-1-yl-1,4-dihydroquinoline-3-carboxylic acid hydrochloride) is from second-generation fluoroquinolones and is a wide spectrum antibiotic influence on both gram negative and positive bacteria. It has received approval to treat respiratory tract infections, different bone and joint infections, urinary tract infections and some topical infections (Tjan-Heijnen et al., 2001). Pandey et al. (2016) designed a system composed of gelatin-based chemically cross-linked cryogel, CaCO3 microspheres, and CFX for treatment of osteomyelitis and osteoporosis. The porosity, pore volume, swelling ratio, swelling kinetics, compression strength, and in vitro degradation rate of the produced cryogel, were all correlated with the amount of gelatin present, the time of the freezing process, and the number of freeze-thaw cycles. After an initial burst release of CFX, sustained release continued over 21 days, and the concentration was maintained higher than the MIC for the entire research phase. The in vitro antibacterial activity measurement against S. aureus and Escherichia coli showed the following zones of inhibition on Mueller-Hinton agar plates on days 1, 3, 5, and 7: 33, 30, 28, and 27 mm for S aureus; 43, 37, 37, and 36 mm for E. coli, respectively. As opposed to a 2D surface, the cell viability, the number of cells within the growth phase, and the alkaline phosphatase activity in rat osteoblasts cultured in the cryogel were considerably higher. They concluded that this microsphere incorporated, CFX-loaded, industrially amenable cryogel system could be beneficial in osteomyelitis and osteoporosis treatment.
A number of organic and inorganic particles, including silica nanoparticles, nano-hydroxyapatite, and poly (lactic-co-glycolic acid) (PLGA) microparticles, have been investigated as the drug carriers (Gentile et al., 2016; Qiu et al., 2016). Mesoporous silica nanoparticles (MSNs) have been explored in tissue engineering as potential nano-additives. This is because they provide many advantages, including adjustable particle and pore sizes, a considerable drug loading capacity, and superb biocompatibility (Zhou et al., 2015; Chen et al., 2016).
Zhou X. et al. (2018) developed Van@MSNs which was composed of both vancomycin and MSNs, in combination with a gelatin matrix to create a composite scaffold. The gelatin-based composite scaffolds were found to have a very porous microscopic structure. Improvement of compression property of composite structures occurred using MSNs. Additionally, in vitro studies revealed that the Van was released from Van@MSN-incorporated composite scaffolds continuously almost without an initial burst. This efficiently prevented S. aureus growth bone mesenchymal stem cells (BMSCs) homeostasis functions along with their differentiation and promotion were not adversely affected by the drug-loaded composite scaffold, indicating acceptable biocompatibility. In vivo study indicated increased bone regeneration along with reduction of bacterial contamination following utilizing of these antibiotic-loaded agents. The synthetic Van@MSNs/Gelatin composite scaffold could be a suitable biomaterial for treating infected bone by providing a localized and sustained-release of antibiotics. Another study demonstrated that tannic acid–mineral nanoparticles embedded within a gelatin-based cryogel notably improved both the quality and quantity of newly formed bone (Song et al., 2024).
For the controlled release of vancomycin, Zhou et al. created gelatin scaffolds with varying concentrations of β-TCP (0%, 10%, 30%, and 50%). These scaffolds were denoted G-TCP0, G-TCP1, G-TCP3, and G-TCP5, respectively (Jiang et al., 2012). The Van release profile was examined using the Kirby-Bauer method. They then tested them in rabbit models of chronic osteomyelitis. The infected bone defects were implanted with scaffolds following complete debridement. The effectiveness of infection reduction and the repair of bone defects were investigated using radiographs and histological analyses. The gelatin/β-TCP scaffolds revealed a homogeneously interconnected 3D porous structure. The G-TCP0 scaffold had the longest Van release period of 8 weeks. The Van release time of the composite scaffolds containing more β-TCP was shorter as its content increased. Within 3 weeks, Van was fully released from the G-TCP5 scaffold. The G-TCP3 scaffold was found to be the most effective in reducing infection and healing bone defects in rabbits with osteomyelitis. The G-TCP3 scaffold performed well in terms of porosity, connectivity, and controlled release. As a result, this scaffold could be employed to treat chronic osteomyelitis lesions. Table 2 lists some different gelatin composites that have been investigated in the treatment of osteomyelitis. Furthermore, Figure 7 illustrates the potential mechanisms of antibacterial drugs delivered via gelatin nanoparticles, which can be beneficial in treating various infectious diseases. These mechanisms encompass four aspects: antibiotic delivery, targeting bacterial toxins, impairing bacterial cell walls and membranes, and disrupting bacterial DNA, proteins, and enzymes (Huang et al., 2024).
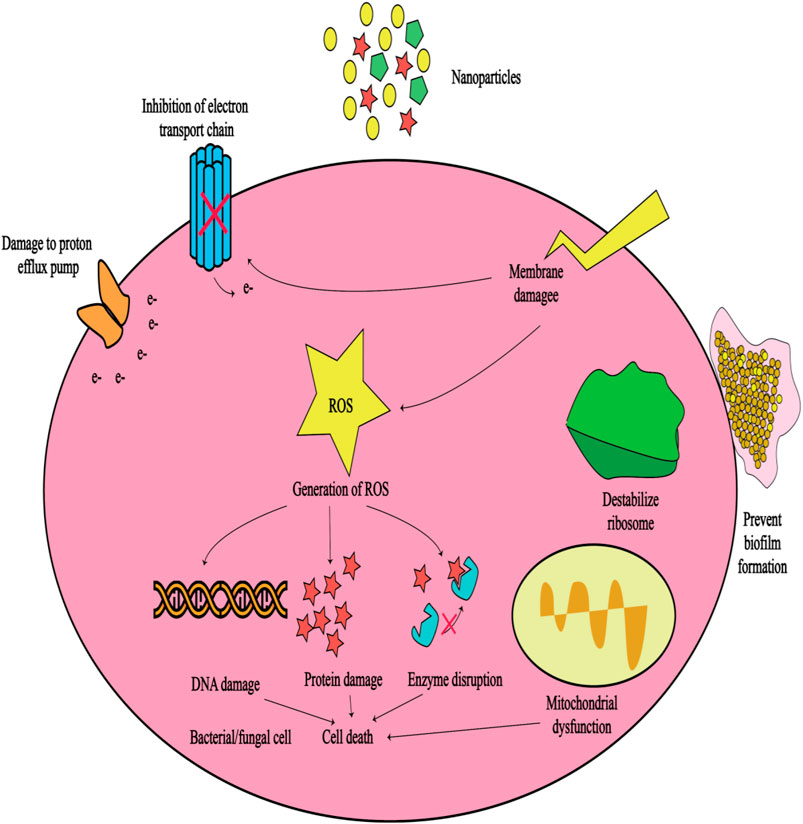
Figure 7. Schematic of the potential mechanisms of antibacterial drugs delivered by gelatin nanoparticles that can be useful in the treatment of infectious diseases (Madkhali, 2023).
Although nanoparticles offer innovative approaches to combat bacterial infections and may potentially mitigate antibiotic resistance, it is crucial to acknowledge that resistance mechanisms can still emerge. For example, bacteria may evolve mechanisms to hinder the attachment of nanoparticles or to efflux them (Hetta et al., 2023). Hence, GNPs leveraging their aforementioned characteristics, can serve as carriers to encapsulate metal nanoparticles like silver or gold nanoparticles, renowned for their antimicrobial efficacy. The resultant hybrid nanoparticles offer heightened antibacterial activity and have the potential to diminish antibiotic resistance by offering an alternative to conventional antibiotic therapies (Khan and Rasool, 2023). Finally, GNPs have been widely employed for delivering diverse antibiotics. They serve the dual purpose of safeguarding these drugs from degradation in the physiological milieu and facilitating targeted, controlled release, thereby potentially augmenting their efficacy while minimizing side effects (Figure 7). Numerous studies (Table 2) have successfully loaded antibiotics such as vancomycin, rifampicin, levofloxacin, ciprofloxacin, and gentamicin into GNPs for targeted delivery to sites of osteomyelitis infection, resulting in enhanced antibacterial activity.
6 Conclusion
Gelatin is an optimal delivery system for sustained release of different biomolecules used widely in regenerative medicine researches. This macromolecule is a suitable system for drug delivery due to its low cast, availability, biodegradability, and biocompatibility. However, induction immune response due to their potential antigenicity and the risk of contamination of gelatin with different pathogens especially in animal-origin forms along with their low batch-to-batch reproducibility have challenged the use of these molecules in medicine. Development of GNPs increases the hopes for using gelatin in medicine with more efficacy and less side effects as these unfavorable effects have not been seen in studies yet. But safety of starting material and cross-linking agents should be studied more in further researches. Moreover, the efficacy of these particles could be improved significantly by conjugation of other materials to cover these particles limitations. Despite these promising findings, using these agents have not become a routine in clinic yet. Due to the favorable release profile of these agents, delivery of antibiotics such as vancomycin to the infection site via this module have increased the succession rate of osteomyelitis eradication (Zhou et al., 2012a). Considering that gelatin can be chemically modified, and combined with different growth factors and several antibiotics, gelatin-derived composites could be promising candidates for bone tissue engineering and therapeutics. With the recent development of 3D printing technology, substantial improvements in the biomaterial field have already been made. To date, a number of in vivo research studies have been conducted to evaluate the utility of gelatin-based composites in the management of osteomyelitis. Modification of GNPs surface via different methods such as coating with ligands and polyethyl glycols along with using amine derivatives on the surface of these particles are used for recruitment of these particles in different fields of medicine. GNPs also have better stability in biological fluids which provide better controlled drug release in the targeted sites compared to other colloidal carriers. Gelatin-based composites were found to have satisfactory performance in the control of infection, as well as the promotion of bone defect repair in chronic osteomyelitis models. Therefore, this review of the available evidence provides a new insight into gelatin-derived composites with controlled release of antibiotics. When combined with the stimulated repair of bone defects, these may be a sign of the future development of osteomyelitis treatment.
Author contributions
AS: Writing–review and editing, Writing–original draft, Validation, Methodology, Investigation, Data curation. ZM: Writing–review and editing, Writing–original draft, Investigation. NS: Writing–review and editing, Writing–original draft, Validation. RA: Writing–review and editing, Writing–original draft, Investigation. SM: Writing–review and editing, Writing–original draft, Validation, Investigation. HP: Writing–review and editing, Writing–original draft, Validation. ES: Writing–review and editing, Writing–original draft, Software, Investigation. KA-G: Writing–review and editing, Writing–original draft, Validation, Investigation. AH-A: Writing–review and editing, Writing–original draft, Investigation. RS: Writing–review and editing, Writing–original draft, Validation, Supervision, Project administration, Investigation.
Funding
The author(s) declare that no financial support was received for the research, authorship, and/or publication of this article.
Acknowledgments
The authors acknowledge the generous scientific support of Clinical Research Development Unit of Shahid Beheshti Hospital in Kashan university of Medical sciences.
Conflict of interest
The authors declare that the research was conducted in the absence of any commercial or financial relationships that could be construed as a potential conflict of interest.
Publisher’s note
All claims expressed in this article are solely those of the authors and do not necessarily represent those of their affiliated organizations, or those of the publisher, the editors and the reviewers. Any product that may be evaluated in this article, or claim that may be made by its manufacturer, is not guaranteed or endorsed by the publisher.
References
Aiken, K. T., Elliott, L., and Da Costa, M. (2024). Acute osteomyelitis: how to recognize, diagnose, and treat—a narrative review. J. Nurse Pract. 20 (2), 104899. doi:10.1016/j.nurpra.2023.104899
Ak, G., Bozkaya, Ü. F., Yılmaz, H., Sarı Turgut, Ö., Bilgin, İ., Tomruk, C., et al. (2021). An intravenous application of magnetic nanoparticles for osteomyelitis treatment: an efficient alternative. Int. J. Pharm. 592, 119999. doi:10.1016/j.ijpharm.2020.119999
Aksoy, E. A., Yagci, B. S., Manap, G., Eroglu, I., Ozturk, S., Ekizoglu, M., et al. (2019). Vancomycin loaded gelatin microspheres containing wet spun poly (ε-caprolactone) fibers and films for osteomyelitis treatment. Fibers Polym. 20 (11), 2236–2246. doi:10.1007/s12221-019-9271-7
Ali, A. F., Ahmed, M. M., El-Kady, A. M., Abd El-Hady, B. M., and Ibrahim, A. M. (2020). Synthesis of gelatin-agarose scaffold for controlled antibiotic delivery and its modification by glass nanoparticles addition as a potential osteomyelitis treatment. Silicon 13, 2011–2028. doi:10.1007/s12633-020-00576-1
Barakat, A., Schilling, W. H., Sharma, S., Guryel, E., and Freeman, R. (2019). Chronic osteomyelitis: a review on current concepts and trends in treatment. Orthop. Trauma 33 (3), 181–187. doi:10.1016/j.mporth.2019.03.005
Bello, A. B., Kim, D., Kim, D., Park, H., and Lee, S.-H. (2020). Engineering and functionalization of gelatin biomaterials: from cell culture to medical applications. Tissue Eng. Part B Rev. 26 (2), 164–180. doi:10.1089/ten.TEB.2019.0256
Bose, S., and Tarafder, S. (2012). Calcium phosphate ceramic systems in growth factor and drug delivery for bone tissue engineering: a review. Acta biomater. 8 (4), 1401–1421. doi:10.1016/j.actbio.2011.11.017
Budiatin, A. S., Zainuddin, M., and Khotib, J. (2014). Biocompatable composite as gentamicin delivery system for osteomyelitis and bone regeneration. Int. J. Pharm. Pharm. Sci. 6 (3), 223–226.
Bury, D. C., Rogers, T. S., and Dickman, M. M. (2021). Osteomyelitis: diagnosis and treatment. Am. Fam. Physician 104 (4), 395–402.
Changez, M., Koul, V., and Dinda, A. K. (2005). Efficacy of antibiotics-loaded interpenetrating network (IPNs) hydrogel based on poly (acrylic acid) and gelatin for treatment of experimental osteomyelitis: in vivo study. Biomaterials 26 (14), 2095–2104. doi:10.1016/j.biomaterials.2004.06.008
Chen, L., Zhou, X., Nie, W., Zhang, Q., Wang, W., Zhang, Y., et al. (2016). Multifunctional redox-responsive mesoporous silica nanoparticles for efficient targeting drug delivery and magnetic resonance imaging. ACS Appl. Mater. Interfaces 8 (49), 33829–33841. doi:10.1021/acsami.6b11802
Chen, X., and Lv, H. (2022). Intelligent control of nanoparticle synthesis on microfluidic chips with machine learning. NPG Asia Mater. 14 (1), 69. doi:10.1038/s41427-022-00416-1
Cierny III, G. (2011). Surgical treatment of osteomyelitis. Plastic Reconstr. Surg. 127, 190S-204S–204S. doi:10.1097/PRS.0b013e3182025070
Coester, C., Kreuter, J., Von Briesen, H., and Langer, K. (2000). Preparation of avidin-labelled gelatin nanoparticles as carriers for biotinylated peptide nucleic acid (PNA). Int. J. Pharm. 196 (2), 147–149. doi:10.1016/s0378-5173(99)00409-3
David, N., and Nallaiyan, R. (2018). Biologically anchored chitosan/gelatin-SrHAP scaffold fabricated on Titanium against chronic osteomyelitis infection. Int. J. Biol. Macromol. 110, 206–214. doi:10.1016/j.ijbiomac.2017.11.174
Dawes, G., Fratila-Apachitei, L., Necula, B., Apachitei, I., Witkamp, G., and Duszczyk, J. (2010). Release of PLGA–encapsulated dexamethasone from microsphere loaded porous surfaces. J. Mater. Sci. Mater. Med. 21 (1), 215–221. doi:10.1007/s10856-009-3846-6
Ding, Y., Ma, R., Liu, G., Li, X., Xu, K., Liu, P., et al. (2023). Fabrication of a new hyaluronic acid/gelatin nanocomposite hydrogel coating on titanium-based implants for treating biofilm infection and excessive inflammatory response. ACS Appl. Mater. Interfaces 15 (10), 13783–13801. doi:10.1021/acsami.2c23320
El Zein, S., Berbari, E. F., Passerini, M., Petri, F., Maamari, J., Murad, M. H., et al. (2023). Rifampin based therapy for patients with Staphylococcus aureus native vertebral osteomyelitis: a systematic review and meta-analysis. Clin. Infect. Dis. 78 (1), 40–47. doi:10.1093/cid/ciad560
Elzoghby, A. O. (2013). Gelatin-based nanoparticles as drug and gene delivery systems: reviewing three decades of research. J. Control. release 172 (3), 1075–1091. doi:10.1016/j.jconrel.2013.09.019
Gentile, P., Nandagiri, V. K., Daly, J., Chiono, V., Mattu, C., Tonda-Turo, C., et al. (2016). Localised controlled release of simvastatin from porous chitosan–gelatin scaffolds engrafted with simvastatin loaded PLGA-microparticles for bone tissue engineering application. Mater. Sci. Eng. C 59, 249–257. doi:10.1016/j.msec.2015.10.014
Gibon, E., Córdova, L. A., Lu, L., Lin, T. H., Yao, Z., Hamadouche, M., et al. (2017). The biological response to orthopedic implants for joint replacement. II: polyethylene, ceramics, PMMA, and the foreign body reaction. J. Biomed. Mater Res. B Appl. Biomater. 105 (6), 1685–1691. doi:10.1002/jbm.b.33676
Gimondi, S., Ferreira, H., Reis, R. L., and Neves, N. M. (2023). Microfluidic devices: a tool for nanoparticle synthesis and performance evaluation. ACS Nano 17 (15), 14205–14228. doi:10.1021/acsnano.3c01117
Gimza, B. D., and Cassat, J. E. (2021). Mechanisms of antibiotic failure during Staphylococcus aureus osteomyelitis. Front. Immunol. 12, 638085. doi:10.3389/fimmu.2021.638085
Gogia, J. S., Meehan, J. P., Di Cesare, P. E., and Jamali, A. A. (2009). Local antibiotic therapy in osteomyelitis. Semin. Plast. Surg. 23 (2), 100–107. doi:10.1055/s-0029-1214162
Gorgieva, S., and Kokol, V. (2011). Collagen-vs. gelatine-based biomaterials and their biocompatibility: review and perspectives. Biomaterials Appl. nanomedicine 2, 17–52. doi:10.5772/24118
Hatzenbuehler, J., and Pulling, T. J. (2011). Diagnosis and management of osteomyelitis. Am. Fam. physician 84 (9), 1027–1033.
Hetta, H. F., Ramadan, Y. N., Al-Harbi, A. I., A. Ahmed, E., Battah, B., Abd Ellah, N. H., et al. (2023). Nanotechnology as a promising approach to combat multidrug resistant bacteria: a comprehensive review and future perspectives. Biomedicines 11 (2), 413. doi:10.3390/biomedicines11020413
Huang, Y., Guo, X., Wu, Y., Chen, X., Feng, L., Xie, N., et al. (2024). Nanotechnology’s frontier in combatting infectious and inflammatory diseases: prevention and treatment. Signal Transduct. Target. Ther. 9 (1), 34. doi:10.1038/s41392-024-01745-z
Hui-Zhong, Z., Yu-Fon, C., Ya-Chu, Y., Cheng-Rung, H., Yi-Sheng, J., Chang-Shi, C., et al. (2023). Gelatin composite gel particles comprised of in-situ formed zinc oxide and silver nanoparticles with enhanced antibacterial activities via enzymatic degradation. Colloids Surfaces A Physicochem. Eng. Aspects 678, 132509. doi:10.1016/j.colsurfa.2023.132509
Jiang, C., Zhu, G., and Liu, Q. (2024). Current application and future perspectives of antimicrobial degradable bone substitutes for chronic osteomyelitis. Front. Bioeng. Biotechnol. 12, 1375266. doi:10.3389/fbioe.2024.1375266
Jiang, J.-L., Li, Y.-F., Fang, T.-L., Zhou, J., Li, X.-L., Wang, Y.-C., et al. (2012). Vancomycin-loaded nano-hydroxyapatite pellets to treat MRSA-induced chronic osteomyelitis with bone defect in rabbits. Inflamm. Res. 61 (3), 207–215. doi:10.1007/s00011-011-0402-x
Joseph, X., Akhil, V., Arathi, A., and Mohanan, P. V. (2022). Microfluidic synthesis of gelatin nanoparticles conjugated with nitrogen-doped carbon dots and associated cellular response on A549 cells. Chemico-Biological Interact. 351, 109710. doi:10.1016/j.cbi.2021.109710
Kalil, A. C., Van Schooneveld, T. C., Fey, P. D., and Rupp, M. E. (2014). Association between vancomycin minimum inhibitory concentration and mortality among patients with Staphylococcus aureus bloodstream infections: a systematic review and meta-analysis. Jama 312 (15), 1552–1564. doi:10.1001/jama.2014.6364
Kavanagh, N., Ryan, E. J., Widaa, A., Sexton, G., Fennell, J., O’Rourke, S., et al. (2018). Staphylococcal osteomyelitis: disease progression, treatment challenges, and future directions. Clin. Microbiol. Rev. 31 (2), e00084-17–e00017. doi:10.1128/CMR.00084-17
Khan, R. T., and Rasool, S. (2023). “Nanotechnology: a new strategy to combat bacterial infections and antibiotic resistant bacteria,” in Nanotechnology and human health (Germany: Elsevier), 167–190.
Khan, S. A. (2020). Mini-Review: opportunities and challenges in the techniques used for preparation of gelatin nanoparticles. Pak J. Pharm. Sci. 33 (1), 221–228. doi:10.1016/B978-0-323-90750-7.00012-0
Khan, S. A., and Schneider, M. (2013). Improvement of nanoprecipitation technique for preparation of gelatin nanoparticles and potential macromolecular drug loading. Macromol. Biosci. 13 (4), 455–463. doi:10.1002/mabi.201200382
Krishnan, A. G., Biswas, R., Menon, D., and Nair, M. B. (2020). Biodegradable nanocomposite fibrous scaffold mediated local delivery of vancomycin for the treatment of MRSA infected experimental osteomyelitis. Biomaterials Sci. 8 (9), 2653–2665. doi:10.1039/d0bm00140f
Krishnan, A. G., Jayaram, L., Biswas, R., and Nair, M. (2015). Evaluation of antibacterial activity and cytocompatibility of ciprofloxacin loaded Gelatin–Hydroxyapatite scaffolds as a local drug delivery system for osteomyelitis treatment. Tissue Eng. Part A 21 (7-8), 1422–1431. doi:10.1089/ten.TEA.2014.0605
Kumar, P. S., Srinivasan, S., Lakshmanan, V.-K., Tamura, H., Nair, S., and Jayakumar, R. (2011a). β-Chitin hydrogel/nano hydroxyapatite composite scaffolds for tissue engineering applications. Carbohydr. Polym. 85 (3), 584–591. doi:10.1016/j.carbpol.2011.03.018
Kumar, R., Nagarwal, R. C., Dhanawat, M., and Pandit, J. K. (2011b). In-vitro and in-vivo study of indomethacin loaded gelatin nanoparticles. J. Biomed. Nanotechnol. 7 (3), 325–333. doi:10.1166/jbn.2011.1290
Lee, S. J., Yhee, J. Y., Kim, S. H., Kwon, I. C., and Kim, K. (2013). Biocompatible gelatin nanoparticles for tumor-targeted delivery of polymerized siRNA in tumor-bearing mice. J. Control. release 172 (1), 358–366. doi:10.1016/j.jconrel.2013.09.002
Lew, D. P., and Waldvogel, F. A. (1997). Osteomyelitis. N. Engl. J. Med. 336 (14), 999–1007. doi:10.1056/NEJM199704033361406
Li, W.-M., Liu, D.-M., and Chen, S.-Y. (2011). Amphiphilically-modified gelatin nanoparticles: self-assembly behavior, controlled biodegradability, and rapid cellular uptake for intracellular drug delivery. J. Mater. Chem. 21 (33), 12381–12388. doi:10.1039/c1jm10188a
Lima, A. L. L., Oliveira, P. R., Carvalho, V. C., Cimerman, S., and Savio, E.Diretrizes Panamericanas para el Tratamiento de las Osteomielitis e Infecciones de Tejidos Blandos Group (2014). Recommendations for the treatment of osteomyelitis. Braz. J. Infect. Dis. 18, 526–534. doi:10.1016/j.bjid.2013.12.005
Llorente, J. J., Junquera, L., Gallego, L., Pérez-Basterrechea, M., Suárez, L. I., and Llorente, S. (2024). Design, in vitro evaluation and in vivo biocompatibility of additive manufacturing three-dimensional printing of β beta-tricalcium phosphate scaffolds for bone regeneration. Biomedicines 12 (5), 1049. doi:10.3390/biomedicines12051049
Luo, S., Jiang, T., Yang, Y., Yang, X., and Zhao, J. (2016). Combination therapy with vancomycin-loaded calcium sulfate and vancomycin-loaded PMMA in the treatment of chronic osteomyelitis. BMC Musculoskelet. Disord. 17 (1), 502–512. doi:10.1186/s12891-016-1352-9
Luo, Y., Chen, H., Chen, H., Xiu, P., Zeng, J., Song, Y., et al. (2024). Recent advances in nanotechnology-based strategies for bone tuberculosis management. Pharmaceuticals 17 (2), 170. doi:10.3390/ph17020170
Madkhali, O. A. (2023). Drug delivery of gelatin nanoparticles as a biodegradable polymer for the treatment of infectious diseases: perspectives and challenges. Polymers 15 (21), 4327. doi:10.3390/polym15214327
Nancy, D., and Rajendran, N. (2018). Vancomycin incorporated chitosan/gelatin coatings coupled with TiO2–SrHAP surface modified cp-titanium for osteomyelitis treatment. Int. J. Biol. Macromol. 110, 197–205. doi:10.1016/j.ijbiomac.2018.01.004
Niculescu, A. G., Chircov, C., Bîrcă, A. C., and Grumezescu, A. M. (2021). Nanomaterials synthesis through microfluidic methods: an updated overview. Nanomater. (Basel) 11 (4), 864. doi:10.3390/nano11040864
Olad, A., and Azhar, F. F. (2014). The synergetic effect of bioactive ceramic and nanoclay on the properties of chitosan–gelatin/nanohydroxyapatite–montmorillonite scaffold for bone tissue engineering. Ceram. Int. 40 (7), 10061–10072. doi:10.1016/j.ceramint.2014.04.010
Oldani, C., and Dominguez, A. (2012). Titanium as a biomaterial for implants. Recent Adv. arthroplasty 218, 149–162. doi:10.5772/27413
Pandey, G., Mittapelly, N., Pant, A., Sharma, S., Singh, P., Banala, V. T., et al. (2016). Dual functioning microspheres embedded crosslinked gelatin cryogels for therapeutic intervention in osteomyelitis and associated bone loss. Eur. J. Pharm. Sci. 91, 105–113. doi:10.1016/j.ejps.2016.06.008
Papagelopoulos, P., Mavrogenis, A., Tsiodras, S., Vlastou, C., Giamarellou, H., and Soucacos, P. (2006). Calcium sulphate delivery system with tobramycin for the treatment of chronic calcaneal osteomyelitis. J. Int. Med. Res. 34 (6), 704–712. doi:10.1177/147323000603400618
Parent, M., Magnaudeix, A., Delebassee, S., Sarre, E., Champion, E., Viana Trecant, M., et al. (2016). Hydroxyapatite microporous bioceramics as vancomycin reservoir: antibacterial efficiency and biocompatibility investigation. J. Biomaterials Appl. 31 (4), 488–498. doi:10.1177/0885328216653108
Qiu, K., Chen, B., Nie, W., Zhou, X., Feng, W., Wang, W., et al. (2016). Electrophoretic deposition of dexamethasone-loaded mesoporous silica nanoparticles onto poly (L-lactic acid)/poly (ε-caprolactone) composite scaffold for bone tissue engineering. ACS Appl. Mater. Interfaces 8 (6), 4137–4148. doi:10.1021/acsami.5b11879
Restrepo, R., Park, H. J., Karakas, S. P., Cervantes, L. F., Rodriguez-Ruiz, F. G., Zahrah, A. M., et al. (2024). Bacterial osteomyelitis in pediatric patients: a comprehensive review. Skelet. Radiol. doi:10.1007/s00256-024-04639-x
Sahoo, N., Sahoo, R. K., Biswas, N., Guha, A., and Kuotsu, K. (2015). Recent advancement of gelatin nanoparticles in drug and vaccine delivery. Int. J. Biol. Macromol. 81, 317–331. doi:10.1016/j.ijbiomac.2015.08.006
Santoro, M., Tatara, A. M., and Mikos, A. G. (2014). Gelatin carriers for drug and cell delivery in tissue engineering. J. Control. release 190, 210–218. doi:10.1016/j.jconrel.2014.04.014
Schrade, S., Ritschl, L., Süss, R., Schilling, P., and Seidenstuecker, M. (2022). Gelatin nanoparticles for targeted dual drug release out of alginate-di-aldehyde-gelatin gels. Gels 8 (6), 365. doi:10.3390/gels8060365
Schwarz, E. M. (2020). CORR Insights®: does the alpha defensin elisa test perform better than the alpha defensin lateral flow test for pji diagnosis? A systematic review and meta-analysis of prospective studies. Clin. Orthop. Relat. Res. 478 (6), 1345–1347. doi:10.1097/CORR.0000000000001238
Sharafati-chaleshtori, R., and Karimi, A. (2010). Antibiotic resistance pattern of staphylococcus strains isolated from orange and apple juices in Shahre-kord, Iran. Pak J. Med. Sci. July-September 26 (3), 615–618.
Silago, V., Mushi, M. F., Remi, B. A., Mwayi, A., Swetala, S., Mtemisika, C. I., et al. (2020). Methicillin resistant Staphylococcus aureus causing osteomyelitis in a tertiary hospital, Mwanza, Tanzania. J. Orthop. Surg. Res. 15 (1), 95. doi:10.1186/s13018-020-01618-5
Song, T., Zhao, F., Yan, L., Liu, P., Yang, J., Ruan, C., et al. (2024). Structure driven bio-responsive ability of injectable nanocomposite hydrogels for efficient bone regeneration. Biomaterials 122601. doi:10.1016/j.biomaterials.2024.122601
Su, K., and Wang, C. (2015). Recent advances in the use of gelatin in biomedical research. Biotechnol. Lett. 37 (11), 2139–2145. doi:10.1007/s10529-015-1907-0
Sun, J., Liu, X., Du, J., An, J., Li, Y., Hu, Y., et al. (2023). Manganese-doped albumin-gelatin composite nanogel loaded with berberine applied to the treatment of gouty arthritis in rats via a SPARC-dependent mechanism. Int. J. Biol. Macromol. 253, 126999. doi:10.1016/j.ijbiomac.2023.126999
Tabata, Y., and Ikada, Y. (1998). Protein release from gelatin matrices. Adv. drug Deliv. Rev. 31 (3), 287–301. doi:10.1016/s0169-409x(97)00125-7
Tanigo, T., Takaoka, R., and Tabata, Y. (2010). Sustained release of water-insoluble simvastatin from biodegradable hydrogel augments bone regeneration. J. Control. release 143 (2), 201–206. doi:10.1016/j.jconrel.2009.12.027
Tjan-Heijnen, V., Postmus, P., Ardizzoni, A., Manegold, C., Burghouts, J., Van Meerbeeck, J., et al. (2001). Reduction of chemotherapy-induced febrile leucopenia by prophylactic use of ciprofloxacin and roxithromycin in small-cell lung cancer patients: an EORTC double-blind placebo-controlled phase III study. Ann. Oncol. 12 (10), 1359–1368. doi:10.1023/a:1012545507920
Uskoković, V., Ghosh, S., and Wu, V. M. (2017). Antimicrobial hydroxyapatite–gelatin–silica composite pastes with tunable setting properties. J. Mater. Chem. B 5 (30), 6065–6080. doi:10.1039/C7TB01794D
Walter, G., Kemmerer, M., Kappler, C., and Hoffmann, R. (2012). Treatment algorithms for chronic osteomyelitis. Dtsch. Ärzteblatt Int. 109 (14), 257–264. doi:10.3238/arztebl.2012.0257
Wu, T., Zhang, Q., Ren, W., Yi, X., Zhou, Z., Peng, X., et al. (2013). Controlled release of gentamicin from gelatin/genipin reinforced beta-tricalcium phosphate scaffold for the treatment of osteomyelitis. J. Mater. Chem. B 1 (26), 3304–3313. doi:10.1039/c3tb20261e
Xia, Y., Xu, R., Ye, S., Yan, J., Kumar, P., Zhang, P., et al. (2023). Microfluidic formulation of curcumin-loaded multiresponsive gelatin nanoparticles for anticancer therapy. ACS Biomater. Sci. Eng. 9 (6), 3402–3413. doi:10.1021/acsbiomaterials.3c00318
Yao, Q., Li, W., Yu, S., Ma, L., Jin, D., Boccaccini, A. R., et al. (2015). Multifunctional chitosan/polyvinyl pyrrolidone/45S5 Bioglass® scaffolds for MC3T3-E1 cell stimulation and drug release. Mater. Sci. Eng. C 56, 473–480. doi:10.1016/j.msec.2015.06.046
Yasmin, R., Shah, M., Khan, S. A., and Ali, R. (2017). Gelatin nanoparticles: a potential candidate for medical applications. Nanotechnol. Rev. 6 (2), 191–207. doi:10.1515/ntrev-2016-0009
Yekani, M., Dizaj, S. M., Sedaghat, H., Nahand, J. S., Saffari, M., and Memar, M. Y. (2023). Preparation, biocompatibility, and antimicrobial effects of gelatin nanofibers scaffolds containing vancomycin and curcumin. J. Drug Deliv. Sci. Technol. 90, 105029. doi:10.1016/j.jddst.2023.105029
Zelmer, A. R., Nelson, R., Richter, K., and Atkins, G. J. (2022). Can intracellular Staphylococcus aureus in osteomyelitis be treated using current antibiotics? A systematic review and narrative synthesis. Bone Res. 10 (1), 53. doi:10.1038/s41413-022-00227-8
Zhang, J., Wang, C., Wang, J., Qu, Y., and Liu, G. (2012). In vivo drug release and antibacterial properties of vancomycin loaded hydroxyapatite/chitosan composite. Drug Deliv. 19 (5), 264–269. doi:10.3109/10717544.2012.704093
Zhang, R., Chen, L., Stehle, Y., Lin, M., Wang, C., Li, Y., et al. (2023). Injectable gelatin microspheres for osteomyelitis treatment: osteogenic and anti-inflammatory effect. Mater. Adv. 4 (19), 4349–4368. doi:10.1039/d3ma00279a
Zhang, X., Jia, W., Gu, Y., Xiao, W., Liu, X., Wang, D., et al. (2010). Teicoplanin-loaded borate bioactive glass implants for treating chronic bone infection in a rabbit tibia osteomyelitis model. Biomaterials 31 (22), 5865–5874. doi:10.1016/j.biomaterials.2010.04.005
Zhang, X., Song, J., Klymov, A., Zhang, Y., de Boer, L., Jansen, J. A., et al. (2018). Monitoring local delivery of vancomycin from gelatin nanospheres in zebrafish larvae. Int. J. nanomedicine 13, 5377–5394. doi:10.2147/IJN.S168959
Zhou, J., Fang, T., Wang, Y., and Dong, J. (2012a). The controlled release of vancomycin in gelatin/β-TCP composite scaffolds. J. Biomed. Mater. Res. Part A 100A (9), 2295–2301. doi:10.1002/jbm.a.34170
Zhou, J., Fang, T., Wang, Y., and Dong, J. (2012b). The controlled release of vancomycin in gelatin/β-TCP composite scaffolds. J. Biomed. Mater. Res. Part A 100 (9), 2295–2301. doi:10.1002/jbm.a.34170
Zhou, J., Zhou, X., Wang, J., Zhou, H., and Dong, J. (2018a). Treatment of osteomyelitis defects by a vancomycin-loaded gelatin/β-tricalcium phosphate composite scaffold. Bone and Jt. Res. 7 (1), 46–57. doi:10.1302/2046-3758.71.BJR-2017-0129.R2
Zhou, X., Feng, W., Qiu, K., Chen, L., Wang, W., Nie, W., et al. (2015). BMP-2 derived peptide and dexamethasone incorporated mesoporous silica nanoparticles for enhanced osteogenic differentiation of bone mesenchymal stem cells. ACS Appl. Mater. Interfaces 7 (29), 15777–15789. doi:10.1021/acsami.5b02636
Zhou, X., Weng, W., Chen, B., Feng, W., Wang, W., Nie, W., et al. (2018b). Mesoporous silica nanoparticles/gelatin porous composite scaffolds with localized and sustained release of vancomycin for treatment of infected bone defects. J. Mater. Chem. B 6 (5), 740–752. doi:10.1039/c7tb01246b
Keywords: gelatin-based nanoparticles, osteomyelitis, antibiotics, sustained release, biocompatibility
Citation: Sherafati Chaleshtori A, Marzhoseyni Z, Saeedi N, Azar Bahadori R, Mollazadeh S, Pourghadamyari H, Sajadimoghadam E, Abbaszadeh-Goudarzi K, Moradi Hasan-Abad A and Sharafati Chaleshtori R (2024) Gelatin-based nanoparticles and antibiotics: a new therapeutic approach for osteomyelitis?. Front. Mol. Biosci. 11:1412325. doi: 10.3389/fmolb.2024.1412325
Received: 04 April 2024; Accepted: 09 July 2024;
Published: 30 July 2024.
Edited by:
Yasunari Matsuzaka, The University of Tokyo, JapanReviewed by:
Maria Del Carmen Moran, University of Barcelona, SpainElena Marcello, Polytechnic University of Turin, Italy
Copyright © 2024 Sherafati Chaleshtori, Marzhoseyni, Saeedi, Azar Bahadori, Mollazadeh, Pourghadamyari, Sajadimoghadam, Abbaszadeh-Goudarzi, Moradi Hasan-Abad and Sharafati Chaleshtori. This is an open-access article distributed under the terms of the Creative Commons Attribution License (CC BY). The use, distribution or reproduction in other forums is permitted, provided the original author(s) and the copyright owner(s) are credited and that the original publication in this journal is cited, in accordance with accepted academic practice. No use, distribution or reproduction is permitted which does not comply with these terms.
*Correspondence: Reza Sharafati Chaleshtori, c2hhcmFmYXRpLnJlemFAZ21haWwuY29t; Amin Moradi Hasan-Abad, YW1pbi5tb3JhZGk2M0B5YWhvby5jb20=