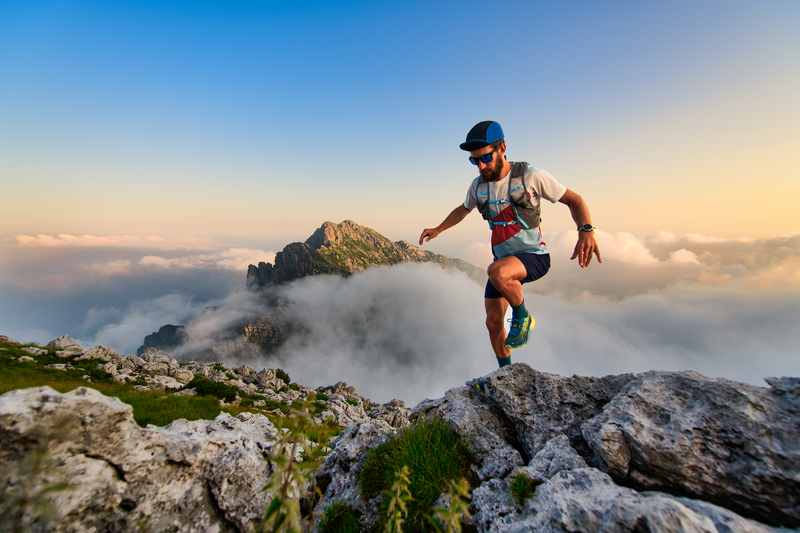
95% of researchers rate our articles as excellent or good
Learn more about the work of our research integrity team to safeguard the quality of each article we publish.
Find out more
ORIGINAL RESEARCH article
Front. Mol. Biosci. , 07 December 2023
Sec. Molecular Biophysics
Volume 10 - 2023 | https://doi.org/10.3389/fmolb.2023.1308693
Disrupted-in-schizophrenia-1 (DISC1) is a scaffolding protein that plays a pivotal role in orchestrating signaling pathways involved in neurodevelopment, neural migration, and synaptogenesis. Among those, it has recently been reported that the role of DISC1 in the Akt/mTOR pathway can shift from a global translational repressor to a translational activator in response to oxidative stress induced by arsenic. In this study we provide evidence that DISC1 can directly bind arsenic via a C-terminal cysteine motif (C-X-C-X-C). A series of fluorescence-based binding assays were conducted with a truncated C-terminal domain construct of DISC1 and a series of single, double, and triple cysteine mutants. We found that arsenous acid, a trivalent arsenic derivative, specifically binds to the C-terminal cysteine motif of DISC1 with low micromolar affinity. All three cysteines of the motif are required for high-affinity binding. Electron microscopy experiments combined with in silico structural predictions reveal that the C-terminal of DISC1 forms an elongated tetrameric complex. The cysteine motif is consistently predicted to be located within a loop, fully exposed to solvent, providing a simple molecular framework to explain the high-affinity of DISC1 toward arsenous acid. This study sheds light on a novel functional facet of DISC1 as an arsenic binding protein and highlights its potential role as both a sensor and translational modulator within Akt/mTOR pathway.
Disrupted-in-schizophrenia-1 (DISC1) was first identified at the breakpoint of a balanced chromosomal translocation (1; 11) (q42.1; q14.3) in a Scottish family with a history of schizophrenia and related psychiatric disorders (Millar et al., 2000; Millar et al., 2001). Subsequent studies have shown that several genetic variations of DISC1 are linked to increased risks of mental disorders and neuronal diseases (Tomppo et al., 2009; Hotta et al., 2011; Tomppo et al., 2012). The product of DISC1 is an 854 amino acid-long protein that serves as a scaffolding hub at the center stage of major neuronal signaling pathways (Yerabham et al., 2013). A growing wealth of information on the physiological role of DISC1 describes its importance in cellular functions such as proliferation, neuronal development, and synaptogenesis (Mackie et al., 2007; Ishizuka et al., 2011; Hikida et al., 2012; Tropea et al., 2018). Notably, accumulating evidence supports the participation of DISC1 in translational processes associated with the Akt/mTOR signaling pathway (Kim et al., 2009; Enomoto et al., 2009). It has for instance been reported that DISC1 directly binds and inhibits GSK3β, which is itself inhibited by Akt (Mao et al., 2009). DISC1 is known to interact with the actin-binding protein girdin (also known as KIAA1212), a key activator of the Akt/mTOR pathway (Enomoto et al., 2009; Kim et al., 2009). DISC1 has also been reported to directly associate with polyribosomes as a translational activator (Ogawa et al., 2005). Furthermore, a recent study by Fuentes-Villalobos et al. has highlighted the role of DISC1 in maintaining homeostatic control of protein synthesis during oxidative stress (Fuentes-Villalobos et al., 2019). In non-stress conditions DISC1 acts as an overall inhibitor of the Akt/mTOR pathway by sequestering the activator girdin, therefore exerting a negative control on the translation processes associated with this pathway (Figure 1A). (Enomoto et al., 2009; Kim et al., 2009) Interestingly, the role of DISC1 as a translational inhibitor is reversed when cells are treated with sodium arsenite, inducing oxidative stress (Fuentes-Villalobos et al., 2019). Indeed, in oxidative stress conditions, DISC1 appears to act as a general enhancer of protein synthesis by interacting with eiF3, a key component of the translational machinery that recruits the small ribosomal subunit 40S (Figure 1B). (Ogawa et al., 2005; Fuentes-Villalobos et al., 2019) The study by Fuentes-Villalobos et al. therefore suggests that DISC1 is required for rescuing protein synthesis under oxidative stress conditions (Fuentes-Villalobos et al., 2019). Yet the molecular mechanisms underlying the switch of DISC1 function from a general inhibitor to an activator of protein synthesis remain elusive.
FIGURE 1. (A, B) Schematic representation of the role of DISC1 as translational modulator within the Akt/mTOR pathway, in (A) normal conditions and in (B) situation of oxidative stress. In normal conditions, DISC1 indirectly modulates the translational activity of the eiF4 complex by inhibiting the Akt-mediated activation of mTOR through sequestration of actin-binding protein girdin. In oxidative stress conditions, the Akt/mTOR pathway is globally inhibited but DISC1 can rescue translational activity by activating eiF3. (C) Domain organization of DISC1 depicting the predominantly disordered N-terminal domain (residues 1-351) in blue that harbors known binding sites to GSK3β and PDE4B1. The C-terminal domain predicted to be rich in helical and coil motifs and containing the cysteine motif composed of C680, C682, and C684, is shown in yellow. The C-terminal construct used in this study (598-854) is highlighted in brown.
Bioinformatics analysis suggest that DISC1 is composed of a predominantly disordered N-terminal domain (residues 1-350) and a helical C-terminal domain (residues 351-854) (Sanchez-Pulido and Ponting, 2011). The N-terminal domain contains two nuclear-localization signals as well as the binding sites to key protein partners, including the phosphodiesterase PDE4B1 and GSK3β (Figure 1C). (Sanchez-Pulido and Ponting, 2011; Soares et al., 2011) As often observed with large scaffolding proteins, structural disorder appears to be tightly connected to DISC1 function. Coarse-grained molecular simulations have indeed shown that the conformational ensemble of DISC1 is predominantly shaped by transient “fuzzy” interactions mediated by the disordered regions of the protein (Roche and Potoyan, 2019). The C-terminal domain is predicted to be more helical with several coiled-coil regions and helical hairpins known as UVR motifs (Sanchez-Pulido and Ponting, 2011). Structural properties of short fragments of the C-terminal domain have been characterized via various biophysical techniques, including NMR spectroscopy and SAXS (Ye et al., 2017; Yerabham et al., 2018; Wang et al., 2019). The C-terminal domain harbors several mutations that are linked to increased risks of mental illness, including the polymorphism S704C (Hashimoto et al., 2006) and a frameshift mutation downstream of L807 that leads to pathological aggregation of DISC1 (Sachs et al., 2005). Moreover, the balanced chromosomal translocation discovered in the Scottish family results in the truncation of a large C-terminal fragment (residues 598-854) (Figure 1C).
Interestingly, analysis of the C-terminal domain sequence reveals the presence of a cysteine motif (C-X-C-X-C) at position 680-684 (Figure 1C), which is typically found in arsenic-binding proteins. It is indeed well-known that arsenic can bind to the sulfhydryl group of cysteines (Shen et al., 2013). This led us to investigate whether DISC1 can directly bind arsenic derivatives. We hypothesize that the switch of function from a global inhibitor to an activator of protein synthesis in oxidative stress conditions may be triggered by direct binding of arsenic. To test this hypothesis, we designed a fluorescence-based binding assay and measured the binding affinity of the C-terminal domain of DISC1 to arsenic using a series of single, double, and triple cysteine mutants. We found that arsenous acid specifically binds to the C-terminal cysteine motif of DISC1 (C680, C682, and C684) with low micromolar binding affinity. All three cysteines of the motif are required for high-affinity binding. These results highlight the potential role of DISC1 as a cellular sensor for toxic metals such as arsenic.
Characterization of DISC1 at a molecular level has historically been hampered by its low solubility and high aggregation propensity when expressed recombinantly. For this study, we focused on a truncated C-terminal construct of the human DISC1 corresponding to residues 598-854 (Figure 1C). This construct encompasses the structured regions S (residues 635–738) and C (residues 691–836) identified by Korth and coworkers (Yerabham et al., 2017). Importantly, it also contains the cysteine motif (C680, C682, and C684) that constitutes the main focus of the present study. When co-expressed with an N-terminal hexa-histidine tag, we were able to express and purify the C-terminal construct of DISC1 with high-yield. The purified protein elutes predominantly as a tetrameric species (Supplementary Figure S1) and shows no sign of aggregation at the concentration employed in our assays (2 μM). For sake of simplicity, this C-terminal construct (598-854) will be referred as “WT” throughout the manuscript.
1. Arsenous acid binds to the C-terminal region of DISC1. To investigate whether DISC1 could act as a cellular sensor for toxic heavy metals such as arsenic, we designed a fluorescence assay for monitoring potential binding to the C-terminal DISC1 (WT) construct in an in-vitro environment. We observed a significant decrease in tryptophan fluorescence intensity when the WT construct was titrated with trivalent arsenous acid (As(OH)3), a reactive species known to bind with high affinity to cysteine thiol groups of arsenic binding proteins. These assays revealed that the WT construct binds with much lower affinity to pentavalent arsenate compared to trivalent arsenous acid (Supplementary Figure S2), which is typically observed in arsenic binding proteins (Shen et al., 2013). We then compared the binding properties of WT to a series of variants to decipher the role played by the cysteine motif (C680, C682, and C684) in mediating interaction with arsenous acid (Table 1). All variants show similar retention time by size exclusion chromatography, indicating that the engineered mutations did not affect the quaternary structure of the DISC1 C-terminal domain. Binding curves were collectively fitted for all variants (including WT) to a simple binding saturation model to extract the specific and non-specific dissociation constants (Kd (high) and Kd (low) respectively, Figure 2) and maximum binding capacity (Table 1).
TABLE 1. List of constructs with corresponding mutations used in this study. The amino acid numbers refer to the human sequence of DISC1 (Figure 1C). Location of the cysteine-to-serine mutations is highlighted in bold. Maximum binding capacity reported for each variant was obtained via a collective fit of all the titration profiles to a two-site specific binding model.
FIGURE 2. Titration of DISC1 WT construct (598-854) and single (C680S*), double (C680S/C684S*), and triple (C680S/C684S/C682S*) cysteine mutants with arsenous acid. The plot depicts overall binding saturation as a function of arsenous acid concentration derived from fluorescence-based assays. Error bars represents standard deviations from three independent measurements. Lines correspond to a collective fit of all data presented in Table 1 using a simple two-site specific binding model. The dissociation constants calculated from the global fit (Kd (high) and Kd (low) are shown in insert.
We first examined a variant for which all cysteines, beside C680, C682, and C684, were mutated to serine. This variant named WT* displayed strong binding to arsenous acid, with a maximum binding capacity yet significantly lower than WT (75% compared to 95%, Table 1, Supplementary Figure S3A). These results suggest that non-specific binding of arsenous acid to cysteines outside the cysteine motif C680, C682, and C684 accounts for nearly 20% of the total binding capacity measured for the WT construct. Next, we consider a series of single, double, and triple mutants engineered in the WT* background (i.e., all cysteines beside C680, C682, and C684 mutated to serine, Table 1). All three single-point mutants show drastic reduction in binding capacity indicating that all three cysteines of the cysteines motif are required for optimal binding to trivalent arsenic (Figure 2 and Supplementary Figure S3B). Interestingly, we found that C680S* retains a higher binding capacity than C682S* and C684S* (Table 1), suggesting a difference in solvent exposure or conformational flexibility among the three cysteines of the motif. The binding capacity of double cysteine-to-serine mutants did not show further decrease, confirming that optimal binding to arsenous acid require all three cysteines of the motif (Figure 2, Supplementary Figure S3C; Table 1). Finally, we examined a variant for which all cysteines have been mutated to serine (C680S/C6802S/C684S*). This variant displays a very low but non-zero binding capacity (Figure 2), suggesting that polar amino acids other than cysteines provide a small but non-negligeable contribution to arsenous acid binding. All together these results indicate that the trivalent arsenous acid binds with high affinity (Kd (high) = 0.88 ± 0.13 μM, Figure 2) to a cysteine motif composed of C680S, C682S, and C684S, found in the C-terminal domain of DISC1. We found that all three cysteines within the motif are required to achieve optimal binding to arsenous acid.
2. Arsenic binding motif is exposed to solvent in DISC1 tetrameric state. To determine the structural and molecular basis for DISC1 binding to arsenic, we then collected a series of negative-contrast electron micrographs of the “WT” DISC1 C-terminal construct (598-854) in the absence and in the presence of arsenous acid. The micrographs collected in the absence of arsenous acid show two major populations: a heterogeneous ensemble of high-molecular species representing higher oligomers, fibrils, and amorphous aggregates, and a homogeneous population of oligomeric particles of apparent spheroidal shape (Figure 3A). 3D reconstruction of the smaller oligomeric species show a symmetrical spheroid with a major axis of 10–12 nm and shorter axis of 5–6 nm (Figure 3C, upper panel). The dimension of these particles are within with the range of expected dimensions for the tetrameric species observed by size-exclusion chromatography (Supplementary Figure S1). No significant change in the population distribution was observed upon incubation with arsenous acid, suggesting that arsenic binding does not induce any major dissociation of the oligomeric species present in solution (Figure 3B). Independent 3D reconstruction performed on the smaller oligomeric species reveals a spheroidal particle similar in shape and size to the reconstructed model obtained in the absence of arsenous acid (Figure 3D, middle panel).
FIGURE 3. (A, B) Micrographs collected by TEM on a solution of the C-terminal WT construct in (A) absence of arsenous acid, and (B) in the presence of arsenous acid (C) 3D models reconstructed from the smaller oligomeric species visible in the micrographs, with the upper panel (blue) showing the reconstructed model obtained in the absence of arsenous acid, the middle panel (pink) showing the model obtained in the presence arsenous panel, and the lower panel (orange) showing the envelop representation of the structural prediction obtained by Alphafold2 (D) Structural prediction of a tetrameric assembly of DISC1 C-terminal WT construct (WT) by Alphafold2, depicting the cysteine motif composed of C680, C682, and C684 in red. Insert shows that the cysteine motif is predicted to be located in a loop, fully exposed to solvent.
We then compare these low-resolution models with structural predictions from Alphafold2 (Jumper et al., 2021). We found that the best prediction for the tetrameric C-terminal DISC1 WT construct matches very well with the 3D reconstructed models calculated from the TEM experiments described above (Figure 3C, lower panel). The tetrameric model predicted by Alphafold2 consists of a head-to-toe assembly of two dimeric units (Figure 3D). Individual subunits are predominantly composed of coils and helix-turn-helix motifs, in good agreement with previous reports. The oligomerization interface is formed by a large helical bundle involving the C-terminal region of the domain (residues 689-834) while the N-terminal region forms a helix-turn-helix motif that is sticking out of the central core and gives the oligomer its spheroidal shape (Figure 3D). Importantly, the cysteine motif encompassing C680, C682, and C683 is predicted to be located in a loop at the edge of the central bundle, fully exposed to solvent, and therefore readily accessible to arsenic (insert Figure 3D). To complement the predictions by Alphafold2, we also performed a series of secondary structure analysis with RaptorX-SS8 (Wang et al., 2016), Jpred4 (Drozdetskiy et al., 2015), and s2D (Sormanni et al., 2015). All suggest that the cysteine motif is located within a loop predicted to be exposed to solvent.
The combination of SEC, TEM, and structural predictions, described above provide a molecular framework to explain the high affinity of DISC1 to arsenous acid. Altogether these results suggest indeed that the predominant species observed in our sample consist of a tetrameric complex of DISC1 C-terminal domain into which the arsenic-binding cysteine motif is fully exposed to solvent and readily accessible to arsenous acid.
An ever-growing wealth of data has firmly established DISC1 as a multi-faceted scaffold protein that plays a pivotal role in mediating enzyme activity and protein-protein interaction for multiple signaling pathways involved in neurodevelopment, neural migration, and synaptogenesis. The role of DISC1 as a translational activator within Akt/mTOR pathway in particular has attracted a lot of attention over the past decade (Enomoto et al., 2009; Kim et al., 2009). Yet a precise analysis of structure-function relationship has proven to be extremely challenging. Structural characterization of DISC1 has long been hampered by its partially disordered nature and high-propensity for aggregation of recombinantly expressed constructs. In addition, the low degree of conservation of DISC1 sequence across species and lack of homology with other known proteins have prevented detailed identification of functional domains (Sanchez-Pulido and Ponting, 2011; Soares et al., 2011). In the present study, we examine the molecular basis of the recently reported ability of DISC1 to both detect and orchestrate the cellular response to oxidative stress induced by arsenic (Fuentes-Villalobos et al., 2019). The presence of a cysteine motif (C-X-C-X-C) in the C-terminal domain of DISC1 led us to investigate whether DISC1 could directly bind to arsenic. DISC1 C-terminal domain is especially relevant from a pathophysiological perspective because it encompasses polymorphisms recognized as risk factors for mental illness, such as the S704C and L807-frameshift mutant (Sachs et al., 2005; Hashimoto et al., 2006). The C-terminal domain is also deleted in the Scottish variant as a consequence of a balanced chromosomal translocation (Millar et al., 2000; Millar et al., 2001).
Here, we designed a C-terminal construct (residues 598-854, Figure 1C) encompassing the S (635-738) and C (691-836) structural regions identified by Korth and coworkers (Yerabham et al., 2017). Our C-terminal construct expresses recombinantly and predominantly forms tetramers in solution (Supplementary Figure S1). This matches well the observation by Korth and coworkers who reported that the S-region is predominantly tetrameric (Yerabham et al., 2017). Notably, the C-terminal contains a self-associating region rich in Ala, Gly, Leu, Pro, and Ser residues with high ß-strand propensity, reminiscent of amyloid proteins. It has recently been reported that this region may promote the aggregation and/or fibrillization of DISC1 in the right physiological environment (Cukkemane et al., 2021). Our purified samples presented no evidence of irreversible aggregation over time, but it should be noted that the samples were kept at relatively low concentration (2 μM) in conditions never exceeding room temperature. Nevertheless, the TEM micrographs clearly show that even after elution through SEC, a significant fraction of the samples form large amorphous aggregates, indicating the C-terminal domain has indeed a high-propensity to self-associate beyond tetrameric states (Figures 3A, B).
Our C-terminal construct was used in fluorescence-based assays to determine the affinity to trivalent arsenous acid, which is known to bind to the thiol groups of cysteines. We found that the WT variant (598-854) that contains a total 7 cysteines (i.e., three cysteines composing the C-X-C-X-C motif: C680, C682, and C684, and four isolated cysteines: C698, C762, C782, and C844), shows clear evidence of binding in the low micromolar range (Figure 2). We then compared the WT construct with a pseudo-WT variant named WT* where the four isolated cysteines (C698, C762, C782, and C844) were mutated to serine (Table 1). This WT* variant presented a similarly high affinity toward arsenous acid compared to the reference WT construct, although with a slightly lower maximum binding capacity (Supplementary Figure S3A, Table 1), indicating that arsenous acid is specifically binding to the three-cysteine motif (C680, C682, C684). Next, we used a series of single, double, and triple mutants to determine whether all three cysteines composing the motif were required to stably bind arsenous acid. Fluorescence assays recorded for the single mutants (C680S*, C682S*, and C684S*) reveal a large decrease in binding capacity compared to the WT or WT* variants (Supplementary Figure S3B, and Table 1), indicating all three cysteine of the motif participate to some degree in stabilizing interaction with arsenous acid. Notably, the consequence of the mutation was less important for C680 (Table 1), which suggests a lower contribution of the first cysteine of the motif toward binding. The structural model provided by TEM and Alphafold2 shows indeed that C680 is located at the C-terminal tip of an α-helix while C682 and C684 are positioned in a loop fully exposed to solvent and likely more readily available to bind arsenous acid (Figure 3D). Analysis of the binding assays recorded for the double and triple mutants shows binding capacities within the same range of those measured for the single mutants (Figure 2, Supplementary Figure S3C; Table 1), which confirms that all three cysteines of the motif participate to some degree in arsenous acid binding.
Next, we collected a series of micrographs by TEM of DISC1 C-terminal domain, in the absence and in the presence of arsenous acid, to gain further insights into the molecular basis of DISC1 ability to bind arsenic (Figures 3A, B). 3D reconstruction performed on the smaller oligomeric species observed in the micrographs reveals spheroid complexes compatible with a tetrameric complex (Figure 3C). 3D reconstructed models obtained in the absence and in the presence of arsenous acid were found to be virtually identical indicating that binding to arsenous acid does not induce dissociation or major structural reorganization of the tetrameric complexes (Figure 3C). Finally, a series of structural predictions were run to complement the results obtained by TEM. Predictions by Alphafold2 for a tetrameric complex of the C-terminal domain of DISC1 matches very well the size and dimensions of the 3D reconstructed models (Figure 3C). The structural details of the prediction suggest a head-to-toe assembly of two dimeric units held together by a central helical bundle. The oligomeric interface consists of a series of helix-turn-helix motifs involving residues from the C-terminal region of the domain. This is in good agreement with recent report by Cukkemane et al. describing this region (residues 717-761) as a self-association core (Cukkemane et al., 2021). Prediction by Alphafold2 in terms of local secondary structure also matches well with data reported on truncated C-terminal fragments in complex with a camel nanobody and peptide fragments of ATF4 and NDE1 (Ye et al., 2017; Yerabham et al., 2018; Wang et al., 2019). Importantly, these predictions suggest that the cysteine motif is located in a loop fully exposed to solvent (Figure 3D), which provides a clear molecular framework explaining the ability of DISC1 to bind arsenous acid.
Altogether, these results provide insights into yet another functional facet of DISC1. As an arsenic binding protein, DISC1 could potentially act both as a cellular sensor and direct translational activator in response to the resulting oxidative stress. Our TEM data show no major conformational change of the C-terminal domain in the presence of arsenic but due to the rather low spatial resolution of this technique, one cannot exclude that arsenic may induce a subtle rearrangement of local structural motifs. Furthermore, in a cellular environment, arsenic may affect interactions between DISC1 and proteins partners such as girdin. Disruption of DISC1-girdin interaction as a result of arsenic binding would make DISC1 available to interact eiF3, which would eventually help to rescue the cell translational activity (Figures 1A, B).
Plasmid design and mutagenesis. DNA sequence encoding DISC1 WT construct (amino acids 598-854) and other variants (Table 1) were inserted into the pET28b (+) vector as His-tag fusion protein. All carry the additional mutations W602F and W752F, leaving a single tryptophan (W691) as a reporter for the fluorescence assays. These mutations were generated by QuickChange site directed mutagenesis and confirmed by DNA sequencing (Iowa State University DNA Sequencing Facility).
Protein Purification. All constructs were transformed into E. coli strain BL21 (DE3). Cells were grown in LB media at 37°C with kanamycin was added to a final concentration of (50 μg/mL). Growth was monitored by absorbance at wavelength 600 nm. At OD600 of 0.7, the temperature was reduced to 25°C and IPTG was added to a final concentration of 0.25 mM. Cells were collected after 16–20 h of induction and suspended in a lysis buffer. DNase (1 mg/mL), leupeptin (1 mg/mL), and PMSF (0.1 M) were all added to the solution. Cells were lysed by sonication and then centrifuged at 16,000 rpm for 1 h. Lysate was loaded into a Ni-NTA column equilibrated with W1 buffer (50 mM Tris-HCl, pH 8.0, 5 mM NaCl, 5 mM imidazole). Ni-NTA column was washed with 300 mL of W1 buffer and 150 mL of W2 buffer (50 mM Tris-HCl, pH 8.0, 5 mM NaCl, 30 mM imidazole) and 200 mL of salt buffer (50 mM Tris-HCl, pH 8.0, 400 mM NaCl, 30 mM imidazole). Samples were eluted with elution buffer (50 mM Tris-HCl, pH 8.0, 5 mM NaCl, 250 mM imidazole) then dialyzed overnight against 4 L of dialysis buffer (50 mM Tris-HCl, pH 8.0, 5 mM NaCl). The samples were then loaded into the Size Exclusion Chromatography (SEC) HiLoad® 26/600 Superdex® 200 pg pre-equilibrated with running buffer (50 mM of Tris-HCl, 10 mM of NaCl at pH 8.0) for further purification. Finally, purified proteins were examined by SDS-PAGE (15%, w/v) to ensure purity of at least 85%.
Tryptophan fluorescence assays. Fluorescence binding assays were performed using an excitation wavelength of 284 nm and emission wavelength of 348 nm. Ultraviolet (UV) light was applied as the light source with a PMT of 800. Arsenous acid stock solution was purchased from Thermo Fisher Scientific and diluted in fluorescence assay buffer (50 mM Tris-HCl, 10 mM NaCl at pH 8.0) and stored at room temperature. Protein samples were added to a final concentration of 2 μM in 2 mL quartz cuvettes with mini stir bar. A blank buffer reading was recorded as baseline and subtracted from the raw fluorescence signal. Each protein sample was titrated in triplicate to estimate average and standard deviations. Titration profiles were collectively fitted (i.e., including all variants presented in Table 1) to a two-site specific binding model in Graphpad Prism 8 to estimate the dissociation constants, Kd (high) and Kd (low), and maximum binding capacity.
Transmission electron microscopy (TEM) measurements. Sample solution (4 μL) containing 10 μM protein was applied onto a glow-discharged carbon-coated copper grid (S160-4, Plano). After 2 min, the solution on the grid was blotted off by filter paper. The grid was then washed with 4 μL of 1% (w/v) uranyl acetate (UrAc) and blotted off immediately, and another 4 μL of 1% (w/v) UrAc was applied onto the grid for 1 min. TEM images were obtained using a TFS Talos 120 C (Thermo Scientific) with a voltage of 120 kV. Processing of the negatively stained images was performed using RELION 3.1 (Scheres, 2015). Contrast transfer function (CTF) was fitted using CTFFIND4 (Rohou and Grigorieff, 2015). For the tetrameric oligomers, 40 particles were selected from ten images (pixel size of 2.5 Å) and extracted with a box size of 80 pixels (200 Å). 2D classification was performed with a mask diameter of 180 Å.
Structural predictions. Secondary prediction was performed using RaptorX-SS8 (Wang et al., 2016), Jpred (Drozdetskiy et al., 2015), and s2D (Sormanni et al., 2015) using the sequence of the human DISC1 C-terminal domain (Uniprot Q9NRI5-1, residue 598-854) as template. Complete structural prediction of DISC1 598-854 was obtained for the DISC1 C-terminal domain using Alphafold2 (Jumper et al., 2021) provided by the High-Performance Computing facility at Iowa State University.
The raw data supporting the conclusion of this article will be made available by the authors, without undue reservation.
MW: Conceptualization, Data curation, Formal Analysis, Investigation, Methodology, Validation, Writing–original draft. TK: Data curation, Investigation, Validation, Writing–original draft. GW: Data curation, Investigation, Validation, Writing–original draft. JS: Data curation, Investigation, Validation, Writing–original draft. CS: Conceptualization, Methodology, Validation, Writing–review and editing. JR: Conceptualization, Formal Analysis, Funding acquisition, Methodology, Project administration, Resources, Supervision, Validation, Visualization, Writing–original draft, Writing–review and editing.
The author(s) declare financial support was received for the research, authorship, and/or publication of this article. This project is supported by funds from the Roy J. Carver Charitable Trust of Muscatine, Iowa, and from NIGMS R01 GM132561 (JR).
We acknowledge the Roy J. Carver High Resolution Microscopy Facility (Office of Biotechnology, Iowa State University, Ames, IA) for providing analytical instrumentation and we are thankful to Tracey P. Stewart for helping with TEM set up and data collection.
The authors declare that the research was conducted in the absence of any commercial or financial relationships that could be construed as a potential conflict of interest.
All claims expressed in this article are solely those of the authors and do not necessarily represent those of their affiliated organizations, or those of the publisher, the editors and the reviewers. Any product that may be evaluated in this article, or claim that may be made by its manufacturer, is not guaranteed or endorsed by the publisher.
The Supplementary Material for this article can be found online at: https://www.frontiersin.org/articles/10.3389/fmolb.2023.1308693/full#supplementary-material
Cukkemane, A., Becker, N., Zielinski, M., Frieg, B., Lakomek, N. A., Heise, H., et al. (2021). Conformational heterogeneity coupled with β-fibril formation of a scaffold protein involved in chronic mental illnesses. Transl. Psychiatry 11, 639. doi:10.1038/s41398-021-01765-1
Drozdetskiy, A., Cole, C., Procter, J., and Barton, G. J. (2015). JPred4: a protein secondary structure prediction server. Nucleic Acids Res. 43, W389–W394. doi:10.1093/nar/gkv332
Enomoto, A., Asai, N., Namba, T., Wang, Y., Kato, T., Tanaka, M., et al. (2009). Roles of disrupted-in-schizophrenia 1-interacting protein girdin in postnatal development of the dentate gyrus. Neuron 63, 774–787. doi:10.1016/j.neuron.2009.08.015
Fuentes-Villalobos, F., Farkas, C., Riquelme-Barrios, S., Armijo, M. E., Soto-Rifo, R., Pincheira, R., et al. (2019). DISC1 promotes translation maintenance during sodium arsenite-induced oxidative stress. Biochim. Biophys. Acta Gene Regul. Mech. 1862, 657–669. doi:10.1016/j.bbagrm.2019.05.001
Hashimoto, R., Numakawa, T., Ohnishi, T., Kumamaru, E., Yagasaki, Y., Ishimoto, T., et al. (2006). Impact of the DISC1 Ser704Cys polymorphism on risk for major depression, brain morphology and ERK signaling. Hum. Mol. Genet. 15, 3024–3033. doi:10.1093/hmg/ddl244
Hikida, T., Gamo, N. J., and Sawa, A. (2012). DISC1 as a therapeutic target for mental illnesses. Expert Opin. Ther. Targets 16, 1151–1160. doi:10.1517/14728222.2012.719879
Hotta, Y., Ohnuma, T., Hanzawa, R., Shibata, N., Maeshima, H., Baba, H., et al. (2011). Association study between Disrupted-in-Schizophrenia-1 (DISC1) and Japanese patients with treatment-resistant schizophrenia (TRS). Prog. Neuropsychopharmacol. Biol. Psychiatry 35, 636–639. doi:10.1016/j.pnpbp.2011.01.011
Ishizuka, K., Kamiya, A., Oh, E. C., Kanki, H., Seshadri, S., Robinson, J. F., et al. (2011). DISC1-dependent switch from progenitor proliferation to migration in the developing cortex. Nature 473, 92–96. doi:10.1038/nature09859
Jumper, J., Evans, R., Pritzel, A., Green, T., Figurnov, M., Ronneberger, O., et al. (2021). Highly accurate protein structure prediction with Alphafold. Nature 596, 583–589. doi:10.1038/s41586-021-03819-2
Kim, J. Y., Duan, X., Liu, C. Y., Jang, M. H., Guo, J. U., Pow-anpongkul, N., et al. (2009). DISC1 regulates new neuron development in the adult brain via modulation of AKT-mTOR signaling through KIAA1212. Neuron 63, 761–773. doi:10.1016/j.neuron.2009.08.008
Mackie, S., Millar, J. K., and Porteous, D. J. (2007). Role of DISC1 in neural development and schizophrenia. Curr. Opin. Neurobiol. 17, 95–102. doi:10.1016/j.conb.2007.01.007
Mao, Y., Ge, X., Frank, C. L., Madison, J. M., Koehler, A. N., Doud, M. K., et al. (2009). Disrupted in schizophrenia 1 regulates neuronal progenitor proliferation via modulation of GSK3beta/beta-catenin signaling. Cell 136, 1017–1031. doi:10.1016/j.cell.2008.12.044
Millar, J. K., Christie, S., Anderson, S., Lawson, D., Hsiao-Wei Loh, D., Devon, R. S., et al. (2001). Genomic structure and localisation within a linkage hotspot of Disrupted in Schizophrenia 1, a gene disrupted by a translocation segregating with schizophrenia. Mol. Psychiatry 6, 173–178. doi:10.1038/sj.mp.4000784
Millar, J. K., Wilson-Annan, J. C., Anderson, S., Christie, S., Taylor, M. S., Semple, C. A., et al. (2000). Disruption of two novel genes by a translocation co-segregating with schizophrenia. Hum. Mol. Genet. 9, 1415–1423. doi:10.1093/hmg/9.9.1415
Ogawa, F., Kasai, M., and Akiyama, T. (2005). A functional link between Disrupted-In-Schizophrenia 1 and the eukaryotic translation initiation factor 3. Biochem. Biophys. Res. Commun. 338, 771–776. doi:10.1016/j.bbrc.2005.10.013
Roche, J., and Potoyan, D. A. (2019). Disorder mediated oligomerization of DISC1 proteins revealed by coarse-grained molecular dynamics simulations. J. Phys. Chem. B 123, 9567–9575. doi:10.1021/acs.jpcb.9b07467
Rohou, A., and Grigorieff, N. (2015). CTFFIND4: fast and accurate defocus estimation from electron micrographs. J. Struct. Biol. 192, 216–221. doi:10.1016/j.jsb.2015.08.008
Sachs, N. A., Sawa, A., Holmes, S. E., Ross, C. A., DeLisi, L. E., and Margolis, R. L. (2005). A frameshift mutation in Disrupted in Schizophrenia 1 in an American family with schizophrenia and schizoaffective disorder. Mol. Psychiatry 10, 758–764. doi:10.1038/sj.mp.4001667
Sanchez-Pulido, L., and Ponting, C. P. (2011). Structure and evolutionary history of DISC1. Hum. Mol. Genet. 20, R175–R181. doi:10.1093/hmg/ddr374
Scheres, S. H. W. (2015). Semi-automated selection of cryo-EM particles in RELION-1.3. J. Struc Biol. 189, 114–122. doi:10.1016/j.jsb.2014.11.010
Shen, S., Li, X. F., Cullen, W. R., Weinfeld, M., and Le, X. C. (2013). Arsenic binding to proteins. Chem. Rev. 113, 7769–7792. doi:10.1021/cr300015c
Soares, D. C., Carlyle, B. C., Bradshaw, N. J., and Porteous, D. J. (2011). DISC1: structure, function, and therapeutic potential for major mental illness. ACS Chem. Neurosci. 2, 609–632. doi:10.1021/cn200062k
Sormanni, P., Camilloni, C., Fariselli, P., and Vendruscolo, M. (2015). The s2D method: simultaneous sequence-based prediction of the statistical populations of ordered and disordered regions in proteins. J. Mol. Biol. 427, 982–996. doi:10.1016/j.jmb.2014.12.007
Tomppo, L., Ekelund, J., Lichtermann, D., Veijola, J., Järvelin, M. R., and Hennah, W. (2012). DISC1 conditioned GWAS for psychosis proneness in a large Finnish birth cohort. PLoS One 7, 230643. doi:10.1371/journal.pone.0030643
Tomppo, L., Hennah, W., Miettunen, J., Järvelin, M. R., Veijola, J., Ripatti, S., et al. (2009). Association of variants in DISC1 with psychosis-related traits in a large population cohort. Arch. Gen. Psychiatry 66, 134–141. doi:10.1001/archgenpsychiatry.2008.524
Tropea, D., Hardingham, N., Millar, K., and Fox, K. (2018). Mechanisms underlying the role of DISC1 in synaptic plasticity. J. Physiol. 596, 2747–2771. doi:10.1113/JP274330
Wang, S., Li, W., Liu, S., and Xu, J. (2016). RaptorX-Property: a web server for protein structure property prediction. Nucleic Acids Res. 44, W430–W435. doi:10.1093/nar/gkw306
Wang, X., Ye, F., Wen, Z., Guo, Z., Yu, C., Huang, W. K., et al. (2019). Structural interaction between DISC1 and ATF4 underlying transcriptional and synaptic dysregulation in an iPSC model of mental disorders. Mol. Psychiatry 26, 1346–1360. doi:10.1038/s41380-019-0485-2
Ye, F., Kang, E., Yu, C., Qian, X., Jacob, F., Yu, C., et al. (2017). DISC1 regulates neurogenesis via modulating kinetochore attachment of Ndel1/Nde1 during mitosis. Neuron 96, 1041–1054. doi:10.1016/j.neuron.2017.10.010
Yerabham, A. S. K., Mas, P. J., Decker, C., Soares, D. C., Weiergräber, O. H., Nagel-Steger, L., et al. (2017). A structural organization for the Disrupted in Schizophrenia 1 protein, identified by high-throughput screening, reveals distinctly folded regions, which are bisected by mental illness-related mutations. J. Biol. Chem. 292, 6468–6477. doi:10.1074/jbc.M116.773903
Yerabham, A. S. K., Müller-Schiffmann, A., Ziehm, T., Stadler, A., Köber, S., Indurkhya, X., et al. (2018). Biophysical insights from a single chain camelid antibody directed against the Disrupted-in-Schizophrenia 1 protein. PLoS ONE 13, e0191162. doi:10.1371/journal.pone.0191162
Keywords: DISC1, arsenic binding protein, AKT/mTOR, disordered proteins, scaffolding proteins
Citation: Watanabe M, Khu TM, Warren G, Shin J, Stewart CE Jr and Roche J (2023) Evidence of DISC1 as an arsenic binding protein and implications regarding its role as a translational activator. Front. Mol. Biosci. 10:1308693. doi: 10.3389/fmolb.2023.1308693
Received: 06 October 2023; Accepted: 24 November 2023;
Published: 07 December 2023.
Edited by:
Christopher Jones, Western Sydney University, AustraliaReviewed by:
Patience Paul, Florida International University, United StatesCopyright © 2023 Watanabe, Khu, Warren, Shin, Stewart and Roche. This is an open-access article distributed under the terms of the Creative Commons Attribution License (CC BY). The use, distribution or reproduction in other forums is permitted, provided the original author(s) and the copyright owner(s) are credited and that the original publication in this journal is cited, in accordance with accepted academic practice. No use, distribution or reproduction is permitted which does not comply with these terms.
*Correspondence: Julien Roche, anJvY2hlQGlhc3RhdGUuZWR1
Disclaimer: All claims expressed in this article are solely those of the authors and do not necessarily represent those of their affiliated organizations, or those of the publisher, the editors and the reviewers. Any product that may be evaluated in this article or claim that may be made by its manufacturer is not guaranteed or endorsed by the publisher.
Research integrity at Frontiers
Learn more about the work of our research integrity team to safeguard the quality of each article we publish.