- 1Center for Gene Regulation in Health and Disease, Department of Biological, Geological, and Environmental Sciences, College of Arts and Sciences, Cleveland State University, Cleveland, OH, United States
- 2Case Comprehensive Cancer Center, Case Western Reserve University, Cleveland, OH, United States
- 3Department of Inflammation and Immunity, Lerner Research Institute, Cleveland Clinic, Cleveland, OH, United States
- 4Center for RNA Science and Therapeutics, Case Western Reserve University, Cleveland, OH, United States
Telomere maintenance is essential for genome integrity and chromosome stability in eukaryotic cells harboring linear chromosomes, as telomere forms a specialized structure to mask the natural chromosome ends from DNA damage repair machineries and to prevent nucleolytic degradation of the telomeric DNA. In Trypanosoma brucei and several other microbial pathogens, virulence genes involved in antigenic variation, a key pathogenesis mechanism essential for host immune evasion and long-term infections, are located at subtelomeres, and expression and switching of these major surface antigens are regulated by telomere proteins and the telomere structure. Therefore, understanding telomere maintenance mechanisms and how these pathogens achieve a balance between stability and plasticity at telomere/subtelomere will help develop better means to eradicate human diseases caused by these pathogens. Telomere replication faces several challenges, and the “end replication problem” is a key obstacle that can cause progressive telomere shortening in proliferating cells. To overcome this challenge, most eukaryotes use telomerase to extend the G-rich telomere strand. In addition, a number of telomere proteins use sophisticated mechanisms to coordinate the telomerase-mediated de novo telomere G-strand synthesis and the telomere C-strand fill-in, which has been extensively studied in mammalian cells. However, we recently discovered that trypanosomes lack many telomere proteins identified in its mammalian host that are critical for telomere end processing. Rather, T. brucei uses a unique DNA polymerase, PolIE that belongs to the DNA polymerase A family (E. coli DNA PolI family), to coordinate the telomere G- and C-strand syntheses. In this review, I will first briefly summarize current understanding of telomere end processing in mammals. Subsequently, I will describe PolIE-mediated coordination of telomere G- and C-strand synthesis in T. brucei and implication of this recent discovery.
Introduction
Eukaryotic genomes typically contain linear chromosomes, at the ends of which lie the telomere nucleoprotein complexes (Lu et al., 2013; Srinivas et al., 2020). In most eukaryotes, telomeres consist of simple repetitive sequences with the G-rich strand running 5′ to 3′ towards the chromosome end (Cross et al., 1989; Riethman et al., 1989; Podlevsky et al., 2008; Lyčka et al., 2023). Telomeres are essential for genome integrity and chromosome stability (O'Sullivan and Karlseder, 2010; Meena et al., 2015; Borges et al., 2022; Hackett et al., 2001; Murnane, 2010), mainly because telomeres form a specialized structure, masking the natural chromosome ends from DNA damage repair machineries and preventing the telomeric DNA from nucleolytic degradation and illegitimate DNA processes such as DNA recombination (de Lange, 2005; Muraki et al., 2012; Stewart et al., 2012; Ruis and Boulton, 2021). Proper chromosome end protection requires telomere proteins that directly or indirectly bind to the telomeric DNA (de Lange, 2005; Giraud-Panis et al., 2010b). Critically short telomeres cannot serve as an adequate docking site for telomere proteins, resulting in an “unprotected” telomere, which is recognized by the DNA damage response machinery to arrest cell growth. Therefore, most eukaryotic cells cannot continue to multiply (they enter replicative senescence) when telomeres are critically short (Shay and Wright, 2005; Aubert and Lansdorp, 2008; Vaiserman and Krasnienkov, 2020), and telomere maintenance mechanisms are essential for continued cell proliferation (Greider, 1998; Lulkiewicz et al., 2020; Rossiello et al., 2022). Conventional DNA polymerases always require a template and a primer and synthesize DNA in the 5′ to 3′ polarity, so they cannot fully replicate the ends of linear DNA molecules, leading to progressive telomere shortening with each round of DNA replication (Wellinger, 2014; Bonnell et al., 2021). Most eukaryotes use telomerase, a specialized reverse transcriptase to synthesize the telomere G-rich strand DNA de novo, thereby solving this “end replication” problem (Greider and Blackburn, 1987; Lingner et al., 1995; Shay and Wright, 2019). The telomerase core enzyme has a protein subunit, TERT, that has the reverse transcriptase catalytic activity, and an RNA subunit, TR, that provides the template for telomeric DNA synthesis (Autexier and Lue, 2006; Wyatt et al., 2010). Many telomere proteins control telomere length by regulating the access of telomerase to its telomere substrate (Smogorzewska and de Lange, 2004; Jafri et al., 2016; Aramburu et al., 2020).
In multicellular organisms such as humans, maintaining genome stability is critical for organism fitness as it helps suppress tumorigenesis (Guo et al., 2023). However, several eukaryotic pathogens that undergo antigenic variation would welcome a certain degree of telomere/subtelomere plasticity (Li, 2012). Trypanosoma brucei that causes human African trypanosomiasis, Plasmodium falciparum that causes malaria, and Pneumocystis jirovecii that causes pneumonia in immunodeficient patients are common in that they undergo antigenic variation to evade the host’s immune response (Li, 2012; Li, 2021), and genes encoding their major surface antigens involved in antigenic variation are located at subtelomeric regions (Stringer and Keely, 2001; Ralph et al., 2005; Schmid-Siegert et al., 2017; Cosentino et al., 2021). Similarly, in opportunistic pathogen Candida glabrata that causes mucosal and systemic infections in immunodeficient patients, the EPA gene family is also located at subtelomeric regions, where EPA encodes surface glycoproteins called epithelial adhesins that are important for host-pathogen interaction (De Las Penas et al., 2003).
Telomere dysfunction can induce genome instability (Hackett et al., 2001; Murnane, 2010). In addition, telomeres and subtelomeres behave like fragile sites and frequently experience increased levels of replication fork stalling and recombination (Sfeir et al., 2009; Glover et al., 2013; Zhang et al., 2013; Glousker and Lingner, 2021; Mirceta et al., 2022). Hence, locating the major surface antigen genes or contingency genes at subtelomeres presumably facilitate antigenic variation and/or adaptation of the microbial pathogen to its environment (Barry et al., 2003; López-Fuentes et al., 2018; Rahnama et al., 2021; Xu et al., 2021; Contreras et al., 2022). Specifically in T. brucei, variant surface glycoprotein (VSG) is the major surface antigen when the parasite proliferates in its mammalian host in extracellular spaces (Cross, 1975; Mugnier et al., 2016; Silva Pereira et al., 2022). T. brucei sequentially expresses distinct VSGs on its surface to evade the host’s immune response (Horn, 2014; McCulloch et al., 2017). While all >2,500 VSG genes and pseudogenes are located at subtelomeric regions (many in long VSG gene arrays) (Berriman et al., 2005; Cross et al., 2014; Müller et al., 2018), only those in VSG expression sites (ESs), polycistronic transcription units immediately upstream of the telomere repeats (de Lange and Borst, 1982; Berriman et al., 2002; Hertz-Fowler et al., 2008), are transcribed by RNA polymerase I in a strictly monoallelic manner (Cross, 1975; Gunzl et al., 2003; Gunzl et al., 2015). VSG switching can occur at the transcription level where the originally active VSG ES is silenced while a new one is derepressed (Myler P. et al., 1984; Myler P. J. et al., 1984). VSG switching can also occur through DNA recombination, where a new VSG sequence replaces the original active VSG sequence (Myler P. et al., 1984; Myler P. J. et al., 1984; Rudenko et al., 1996; McCulloch et al., 1997; Rudenko et al., 1998; Robinson et al., 1999). DNA double strand breaks (DSBs) at the active VSG vicinity has been shown to be a potent trigger for VSG switching (Alsford et al., 2009; Boothroyd et al., 2009; Glover et al., 2013; Li, 2021), as homology directed DNA recombination (HDR) repairs DSBs most accurately (Jasin and Rothstein, 2013; Haber, 2018; Wright et al., 2018), and HDR is very active in T. brucei (McCulloch and Barry, 1999; Robinson et al., 1999; Conway et al., 2002a; Barnes and McCulloch, 2007; Glover et al., 2008; Marin et al., 2018). Consistently, for known essential T. brucei telomere proteins, a transient depletion of the protein leads to an increased amount of DNA breaks at the telomere and subtelomere and more frequent VSG switching, indicating that these telomere proteins help maintain the telomere stability and suppress VSG switching (Jehi et al., 2014a; Jehi et al., 2014b; Jehi et al., 2016; Nanavaty et al., 2017; Afrin et al., 2020a; Afrin et al., 2020b; Saha et al., 2021; Rabbani et al., 2022; Gaurav et al., 2023). Furthermore, in telomerase null cells where the active ES-adjacent telomere is extremely short, a significant increase in the VSG switching rate is observed (Hovel-Miner et al., 2012). In P. falciparum, many var genes that encode the major surface antigen PfEMP1 involved in antigenic variation are also located at subtelomeres (Rubio et al., 1996; Hernandez-Rivas et al., 1997). In addition, DNA recombination frequently occurs at P. falciparum subtelomeres and contributes to divergence of var gene families (Calhoun et al., 2017), and this subtelomere plasticity is enhanced when a DSB is introduced (Calhoun et al., 2017; Zhang et al., 2019b). Nevertheless, although subtelomere plasticity facilitates antigenic variation, telomere maintenance in these eukaryotic parasites is also essential for genome integrity and parasite survival (Li et al., 2005; Yang et al., 2009; Glover et al., 2013; Jehi et al., 2014b; Jehi et al., 2016; Nanavaty et al., 2017; Afrin et al., 2020b; Saha et al., 2021; Rabbani et al., 2022; Gaurav et al., 2023). For example, inducing a DSB near or within the active VSG is detrimental to T. brucei, as only <10% of the population survives through VSG switching (Glover et al., 2013). Therefore, telomere/subtelomere plasticity is a double-edged sword, and telomere maintenance is essential for cell proliferation of eukaryotic parasites (Li, 2021). Interestingly, telomere maintenance mechanisms are not identical in the protozoan parasite T. brucei and its mammalian host.
A brief overview of telomere maintenance in mammalian cells
Telomere replication has multiple steps (Wu et al., 2012; Maestroni et al., 2017; Bonnell et al., 2021). The chromosome internal portion of the telomere can be replicated by conventional DNA polymerases during the S phase with the help from DNA helicases such as BLM, WRN, and RTEL1 (Opresko et al., 2002; Crabbe et al., 2004; Flanary, 2004; Lillard-Wetherell et al., 2004; Machwe et al., 2004; Uringa et al., 2011; Uringa et al., 2012; Vannier et al., 2013; Zimmermann et al., 2014) that disrupt the G-quadruplex structure formed by the telomere G-rich sequence (Gilson and Géli, 2007; Higa et al., 2017; Bryan, 2020). However, telomere ends are processed in a special way (Figure 1) (Lundblad, 2012; Bonetti et al., 2014). Mammalian telomere end processing has been extensively studied, although some regulatory mechanisms are not fully understood. After DNA replication by conventional DNA polymerases, the leading strand DNA replication product has a blunt end, while the lagging strand DNA replication results in a short 3′ overhang after the last primer is removed (Figure 1). Therefore, with each round of DNA replication by conventional DNA polymerase, telomere shortens progressively, hence the “End Replication Problem”. While telomerase can synthesize the G-rich telomere DNA de novo, it poses another problem. Telomerase uses single-stranded DNA with G-rich sequence as its substrate (Wallweber et al., 2003; Schmidt and Cech, 2015), so the blunt telomere end from the leading strand DNA replication cannot directly serve as a substrate for telomerase. In mammalian cells, these blunt ends are first converted by Apollo, a 5′ to 3′ exonuclease, to an end with a short 3′ overhang (van Overbeek and de Lange, 2006; Wu et al., 2010; Wu et al., 2012). Subsequently, Exo1, another 5′ to 3′ exonuclease, resects more 5′ telomere DNA, generating a longer 3′ overhang structure at both telomeres of the same chromosome (Wu et al., 2012). This allows telomerase to bind to the telomere end and extend the G-rich strand by de novo DNA synthesis. Subsequently, the C-rich telomere strand can be extended by DNA Polα/primase using the G-rich strand as template in a process called “C-strand fill-in” (Bonnell et al., 2021; Olson et al., 2022; He and Lim, 2023).
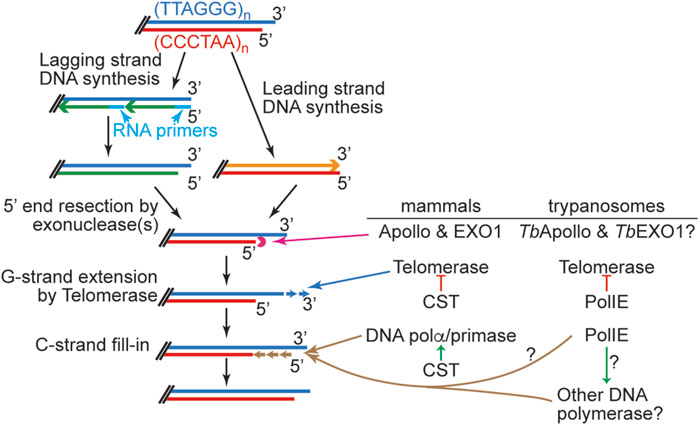
FIGURE 1. Left, A simplified summary of various steps in telomere end processing. Right, mammalian and trypanosome key telomere proteins involved in each step are listed. Enzymes directly involved in DNA degradation/elongation/replication are linked to the corresponding process with a long arrow. Red lines with a bar at the end stand for inhibitory effects. Short green arrows stand for stimulatory effects. Speculated functions are indicated with question marks.
Telomere length is heavily regulated by telomere proteins (Kim et al., 1999; Smogorzewska et al., 2000; Li and de Lange, 2003; Hockemeyer et al., 2005; Mason and Skordalakes, 2010; Takai et al., 2016; Aramburu et al., 2020; Bonetti et al., 2020). In mammalian cells, the Shelterin and CST complexes are key proteins associated with the telomere and play essential roles in telomere maintenance (Figure 2) (Liu et al., 2004a; de Lange, 2005; Giraud-Panis et al., 2010a; Lim and Cech, 2021). Shelterin has six protein components (de Lange, 2005): TRF1 (Chong et al., 1995) and TRF2 (Bilaud et al., 1997; Broccoli et al., 1997) bind to the duplex telomeric DNA (Bianchi et al., 1997; Bilaud et al., 1997; Broccoli et al., 1997), RAP1 (Li et al., 2000) interacts with TRF2, TPP1 (Liu et al., 2004b; Ye et al., 2004b; Houghtaling et al., 2004) and POT1 (Baumann and Cech, 2001; Baumann et al., 2002; Loayza and de Lange, 2003) bind the single-stranded telomere 3′ overhang as a heterodimer (Hwang et al., 2012; Xu et al., 2019), while TIN2 (Kim et al., 1999) interacts with TRF1, TRF2, and TPP1 (Ye et al., 2004a; O'Connor et al., 2006; Houghtaling et al., 2004) and serves as a bridge linking various Shelterin components together. The CST complex has three protein components: CTC1 (or CDC13 in budding yeast), STN1, and TEN1 (Rice and Skordalakes, 2016; Cai and de Lange, 2023). They bind single-stranded telomeric DNA as a trimer (Bhattacharjee et al., 2017; Cai et al., 2022), which structurally resembles the RPA complex (Miyake et al., 2009; Rice and Skordalakes, 2016; Barbour and Wuttke, 2023). Interestingly, TPP1, POT1, and CST components all have OB folds (Xin et al., 2008; Zhong et al., 2012; Bhattacharjee et al., 2016; Rice et al., 2017; Wang et al., 2023), which are commonly used to bind ssDNA or RNA (Theobald et al., 2003; Horvath, 2011; Nguyen et al., 2020).
Among OB fold-containing telomere proteins, TPP1 is a key telomerase recruiting factor (Rajavel et al., 2014; Sexton et al., 2014). The TEL patch domain of TPP1 directly interacts with TERT and helps recruit telomerase to the telomere end (Nandakumar et al., 2012; Grill et al., 2019; Sekne et al., 2022). TPP1 also helps stimulate telomerase processivity (Latrick and Cech, 2010; Nandakumar et al., 2012; Sandhu et al., 2021). On the other hand, POT1 seems to be able to both stimulate and inhibit telomerase action (Kelleher et al., 2005; Xu et al., 2019; Aramburu et al., 2020; Gu et al., 2021; Zade and Khattar, 2023). Binding of CST to the telomere 3′ overhang also inhibits the access of telomerase to its telomere substrate, hence suppressing telomere G-strand synthesis (Chen et al., 2012; Chen et al., 2013; Chen and Lingner, 2013; Feng et al., 2018; Zaug et al., 2021). On the other hand, CST promotes the telomere C-strand fill-in process by recruiting DNA Polα/primase to the telomere and specifying the origins for telomeric C-strand replication (Zaug et al., 2022).
Unique features of telomere maintenance in African trypanosomes
Protozoan parasites such as T. brucei and P. falciparum are eukaryotic pathogens and have linear genomes, but their telomere protein complexes are not completely conserved as those found in their mammalian host. In P. falciparum, SIR2 (Freitas-Junior et al., 2005; Merrick and Duraisingh, 2007; Mancio-Silva et al., 2008; Tonkin et al., 2009; Religa and Waters, 2012) and HP1 (Perez-Toledo et al., 2009; Hernandez-Rivas et al., 2010) homologs have been identified to associate with the telomere chromatin and help suppress subtelomeric gene expression. SIR2 homologs are histone deacetylases that help establish/maintain the heterochromatin structure (Xu et al., 2007; Jing and Lin, 2015), and HP1 homologs associate with heterochromatin and promote heterochromatin formation/maintenance/propagation (Zeng et al., 2010; Schoelz and Riddle, 2022). In addition, PfTRZ has been identified to bind the duplex telomeric DNA, subtelomeric var genes, and 5S rDNA loci (Bertschi et al., 2017). PfTRZ is also a remote functional homologue of TFIIIA and regulates 5S rRNA expression (Bertschi et al., 2017). PfAP2Tel is another protein identified to bind the telomeric DNA (Sierra-Miranda et al., 2017), and it contains an atypical AP2 domain that is frequently found in transcription factors of Apicomplexans (Painter et al., 2011). Finally, PfGBP2 has been identified to bind the telomere G-quadruplex structure and G-rich RNAs (Edwards-Smallbone et al., 2022). However, only PfTRZ has been shown to have an essential function in telomere maintenance (Bertschi et al., 2017). Functions of PfAP2Tel and PfGBP2 at the telomere are unclear. Nothing is known about telomere end processing in P. falciparum except that telomerase is a major factor for telomere maintenance (Figueiredo et al., 2005).
Much more has been learned about the T. brucei telomere protein complex and its function in telomere maintenance (Li, 2010; Li, 2015; Li, 2021; Li and Zhao, 2021). As shown in Figure 1, a number of proteins are involved in telomere end processing at various steps in mammalian cells. Similar processes are expected in T. brucei. Specifically, T. brucei has Apollo and EXO1 homologs, although their roles in telomere end processing have not been investigated. In addition, both T. brucei telomerase components, TbTERT and TbTR, have been identified, and it has been shown that telomerase-mediated telomere synthesis is the major mechanism of telomere maintenance (Dreesen et al., 2005; Gupta et al., 2013; Sandhu et al., 2013). Interestingly, T. brucei telomere length grows continuously during cell proliferation (Bernards et al., 1983), which is different from that in mammalian cells, where telomere lengths are maintained within a limited size range (Teixeira et al., 2004; Hug and Lingner, 2006). Therefore, T. brucei telomerase action is likely regulated by different mechanisms than that in mammals. Furthermore, the T. brucei Ku70/80 complex is also essential for proper telomere lengthening but not for telemeric silencing (Conway et al., 2002b; Janzen et al., 2004). Interestingly, although Ku homologs are typically involved in non-homologous end-joining pathway (NHEJ) (Frit et al., 2019), T. brucei only has HDR and microhomology-mediated end-joining (MMEJ) but not NHEJ (Burton et al., 2007; Glover et al., 2011). On the other hand, yeast and human Ku homologs have been shown to interact with the telomerase RNA directly and contribute to telomerase recruitment to the telomere (Stellwagen et al., 2003; Ting et al., 2005; Pfingsten et al., 2012; Hass and Zappulla, 2015). It is possible that T. brucei Ku has a similar function.
The T. brucei Shelterin-equivalent protein complex includes a TRF homolog (TbTRF) (Li et al., 2005), a RAP1 homolog (TbRAP1) (Yang et al., 2009), and TbTIF2 that is a functional homolog of mammalian TIN2 (Jehi et al., 2014b) (Figure 2). TbTRF directly interacts with TbRAP1 (Yang et al., 2009), TbTIF2 (Jehi et al., 2014b), and likely PolIE, too (Rabbani et al., 2022). Whether TbTRF recruits RecQ family DNA helicases (such as BLM and WRN) to the telomere to facilitate telomeric repeat replication as its mammalian homologs (Opresko et al., 2002; Crabbe et al., 2004; Lillard-Wetherell et al., 2004; Machwe et al., 2004; Zimmermann et al., 2014) is unclear. TbRECQ2, a RecQ helicase, has been shown to help repair DNA damage, and loss of TbRECQ2 leads to an increase in VSG switching rate (Devlin et al., 2016). Among T. brucei Shelterin components, TbRAP1 is a key regulator of VSG monoallelic expression, as depletion of TbRAP1 results in global VSG derepression for up to 1,000-fold, and TbRAP1’s dsDNA binding activity is essential for VSG silencing (Yang et al., 2009; Pandya et al., 2013; Afrin et al., 2020a; Afrin et al., 2020b). Since TbRAP1 helps compact telomere and subtelomere chromatin (Pandya et al., 2013), it is hypothesized that TbRAP1-mediated telomeric silencing is an epigenetic effect that relies on chromatin structure remodeling, although the underlying mechanism is not fully clear. Unexpectedly, TbRAP1 binds the active VSG RNA through its RRM domain (not present in vertebrate and yeast RAP1 homologs), which is essential for the full-level expression of the active VSG (Gaurav et al., 2023). TbTRF and TbRAP1 also help maintain the telomere/subtelomere stability by suppressing levels of telomere repeat-containing RNA (TERRA) (Rudenko and Van der Ploeg, 1989) and telomeric R-loop (Nanavaty et al., 2017; Saha et al., 2021). TERRA is expressed from the active VSG ES-adjacent telomere (Nanavaty et al., 2017; Saha et al., 2021), and it can form the three-stranded R-loop structure with the duplex telomeric DNA (Fernandes et al., 2021), while R-loops are known to have a tendency of inducing DNA breaks (Mackay et al., 2020; Brickner et al., 2022). Indeed, overexpressing RNaseH1 that degrades the RNA in the RNA:DNA hybrid in TbRAP1/TbTRF-depleted cells can reduce the level of telomeric R-loops, the amount of telomeric DNA break, and the VSG switching rate (Nanavaty et al., 2017; Saha et al., 2021). Therefore, it is clear that excessive amount of TERRA and telomeric R-loops reduces the telomere/subtelomere stability by causing more DNA damage and increases the VSG switching rate, at least transiently (Nanavaty et al., 2017; Saha et al., 2021). In contrast, it is unknown whether depletion of TERRA has any detrimental effect on parasite proliferation or telomere functions, as depletion of TERRA is difficult to achieve. Nevertheless, TbTRF and TbRAP1 probably do not use identical mechanisms to regulate TERRA and telomeric R-loop levels, as TbTRF can directly bind TERRA through its C-terminal Myb domain (that also binds the duplex telomeric DNA) while TbRAP1 does not bind TERRA (Li et al., 2005; Saha et al., 2021; Gaurav et al., 2023). TbTIF2 has also been shown to help maintain telomere/subtelomere stability by suppressing DNA breaks at the telomere vicinity (Jehi et al., 2014b), and TbTIF2 and TbTRF have both overlapping and distinct roles (Jehi et al., 2016), although TbTIF2’s effect on TERRA has not been examined in detail.
Additional telomere proteins have also been identified in T. brucei that do not seem to have any homologs in mammals (Figure 2). TelAP1 is identified through its ability to bind a telomere sequence-containing DNA fragment and as a component in the TbTRF protein complex (Reis et al., 2018). It is not essential for T. brucei proliferation but plays a role in T. brucei differentiation from the infectious form proliferating in its mammalian host to the insect form proliferating in the midgut of its insect vector (Reis et al., 2018). So far, no telomere-specific OB fold-containing ssDNA binding factors have been identified in T. brucei (Rabbani et al., 2022). The T. brucei telomere chromatin has been purified and analyzed by Proteomics of Isolated Chromatin segments (PICh) (Dejardin and Kingston, 2009; Rabbani et al., 2022). In addition, the TbTRF-TbTIF2 protein complex has been purified and examined (Rabbani et al., 2022). Furthermore, proteins that directly bind the telomeric repeats were identified using a telomere sequence-containing DNA fragment (Reis et al., 2018). These studies helped identify T. brucei telomere proteins comprehensively, but no obvious homologs of TPP1, POT1, and CST have been identified, although further structural analysis may eventually identify homologs of these OB fold-containing telomere proteins. On the other hand, Leishmania RPA1 can bind the telomere ssDNA (Neto et al., 2007; Da Silveira et al., 2013; Pavani et al., 2014; Fernandes et al., 2020), and TbRPA1 is part of the telomere chromatin as shown by PICh (Rabbani et al., 2022), suggesting that TbRPA may fulfill at least part of CST functions, particularly since the CST trimeric complex structurally resembles the RPA complex (Miyake et al., 2009; Rice and Skordalakes, 2016; Barbour and Wuttke, 2023). However, more investigation is necessary to reveal TbRPA’s function in telomere end processing, if any.
Interestingly, two DNA polymerases have been identified to associate with the T. brucei telomere chromatin (Reis et al., 2018; Rabbani et al., 2022). One of these, PolIE, has been shown to coordinate telomere G- and C-strand syntheses, fulfilling similar functions as mammalian CST and DNA Polα/primase (Rabbani et al., 2022). PolIE is an A-type DNA polymerase that has been identified in the TbTRF protein complex and in the telomere chromatin (Rabbani et al., 2022). It also binds the telomere sequence-containing DNA fragment (Reis et al., 2018). Initially, PolIE was annotated to be a DNA polymerase theta homolog. However, careful sequence analysis indicates that PolIE actually belongs to the DNA polymerase I family (type A family) (Leal et al., 2020). PolIE is essential for T. brucei survival and participates in DNA damage repair (Leal et al., 2020; Rabbani et al., 2022). However, sequence alignment indicates that PolIE lacks the domains in human Polθ that are associated with lesion bypass activity (Leal et al., 2020), suggesting that PolIE lacks a translesion DNA synthesis activity (Leal et al., 2020), although this has not been experimentally confirmed.
PolIE can be depleted efficiently (>90%) by RNAi within 24 h, and PolIE-depleted cells quickly stop proliferating, indicating that PolIE is essential for T. brucei cell growth (Leal et al., 2020; Rabbani et al., 2022). However, PolIE-depleted cells do not arrest at specific stage of the cell cycle (Leal et al., 2020; Rabbani et al., 2022). Nevertheless, PolIE helps to repair DNA damage caused by UV irradiation, MMS, and cisplatin treatment, as PolIE-depleted cells survive more poorly than WT cells when treated with these DNA damaging agents (Leal et al., 2020; Rabbani et al., 2022). Depletion of PolIE also leads to a mild VSG derepression and an increased VSG switching rate, with increased amount of DNA damage at the telomere and subtelomere (Leal et al., 2020; Rabbani et al., 2022). Further analysis indicated that PolIE is essential for telomere end processing (Rabbani et al., 2022).
T. brucei telomeres have the expected terminal telomere 3′ overhang structure, but this overhang appears to be very short (∼12 nt in asynchronized cells) (Sandhu and Li, 2011), suggesting that G-rich and C-rich telomeric DNA syntheses are well coordinated with each other. Theoretically, the length of the telomere 3′ overhang changes at different steps of telomere end processing (Figure 1), and longer telomere 3′ overhang has been detected in S phase in yeast (Dionne and Wellinger, 1996; Fridholm et al., 2013). However, changes in telomere 3′ overhang length throughout the cell cycle have not been reported in T. brucei as synchronizing T. brucei cells under a physiological condition is challenging. The telomerase activity is also required for the telomere 3′ overhang structure (Sandhu and Li, 2017), indicating that telomerase is a major contributing factor for elongating the telomere 3′ overhang. The first sign suggesting that PolIE plays an important role in telomere end processing came from the observation that PolIE-depleted cells have a dramatically elongated telomere 3′ overhang, indicating an abnormal coordination between telomere G- and C-strand syntheses (Rabbani et al., 2022). It is known that telomeres in T. brucei can form a T-loop structure (Munoz-Jordan et al., 2001), although it is unknown whether T-loops can form at any stage of the cell cycle or only during a limited window in the cell cycle. Apparently, T-loop formation depends on the telomere 3′ overhang, and longer overhang is more prone to invade the duplex telomeric DNA. In addition, resolution of the T-loop can lead to formation of telomere circles (T-circles, Figure 3) (Pickett et al., 2009). Indeed, depletion of PolIE results in an increased amount of T-circles and telomere C-circles (Rabbani et al., 2022), where T circles are mostly double stranded with nicks on both strands, and C-circles are mostly single stranded (Figure 3) (Pickett et al., 2009; Henson et al., 2017). It is worth to mention that telomere C-circles are typically detected in ALT cells (Henson et al., 2009; Zhang et al., 2019a; Chen et al., 2021), which are telomerase negative human cancer cells that use alternative mechanisms to maintain their telomere length (Zhang and Zou, 2020). A high level of telomere C-circles typically suggests frequent telomere recombination, which has also been shown to be a key mechanism for telomere maintenance in ALT cells (Zhang and Zou, 2020). In PolIE-depleted cells, longer telomere 3′ overhang (Rabbani et al., 2022) presumably has a higher probability to invade the duplex telomere DNA region and leads to an increased T-loop formation, while resolution of the T-loop structure will result in circular DNA species. Depending on the exact invasion site and subsequent migration of the crossover region, this could lead to recombination events involving both telomeric and subtelomeric sequences. The increased amount of T-circles and C-circles in PolIE-depleted cells and the fact that PolIE depletion causes an increased VSG switching rate all suggest that PolIE normally helps suppress DNA recombination at the telomere and subtelomere by limiting the length of telomere 3′ overhang (Rabbani et al., 2022).
Using EdU-labeling to examine newly synthesized telomere G- and C-strand DNA levels, Rabbani et al. further discovered that the level of telomere G-strand synthesis is increased upon depletion of PolIE (Rabbani et al., 2022). Furthermore, this increase is telomerase-dependent (Rabbani et al., 2022). Therefore, PolIE normally suppresses telomerase action of elongating the telomere G-rich strand DNA. Interestingly, PolIE depletion-induced increase in the telomere 3′ overhang length and the telomere C-circle level is not telomerase-dependent, indicating that PolIE has additional functions other than suppressing telomerase (Rabbani et al., 2022). Telomere C-strand fill-in also affects the telomere 3′ overhang length, and depletion of PolIE causes a subtle decrease in nascent telomere C-strand DNA, suggesting that PolIE can also stimulate telomere C-strand fill-in (Rabbani et al., 2022).
T. brucei PolIE negatively affects telomere G-strand elongation and stimulates telomere C-strand fill-in (Rabbani et al., 2022), suggesting that it behaves similarly as mammalian CST. However, PolIE does not seem to be equivalent to CST. The main functions of CST in telomere end processing are two-fold. First, binding of CST on the telomere 3′ overhang physically sequesters the telomere terminal ssDNA and prevents telomerase from accessing the telomere substrate, hence inhibiting telomerase-mediated telomere G-strand synthesis (Zaug et al., 2021). PolIE also suppresses the telomerase action (Rabbani et al., 2022), but the underlying mechanisms are unclear. A recent proteomic study did not detect any interaction between TbTERT and PolIE (Davis et al., 2023), suggesting that PolIE may not directly affect the telomerase activity. On the other hand, PolIE, as a DNA polymerase, is expected to be able to bind the telomere DNA, as it is required for a proper telomere C-strand fill-in (Rabbani et al., 2022). However, whether PolIE also binds the telomere 3′ overhang and masks this substrate from telomerase binding is unknown.
Second, CST recruits DNA Polα/primase to the telomere and specify origins of DNA replication to complete the telomere C-strand fill-in (Zaug et al., 2022; He and Lim, 2023), but CST itself does not have DNA polymerase activity (Zaug et al., 2022). Sequence analysis showed that PolIE has the conserved DNA polymerase domain (Leal et al., 2020), suggesting that it does have a DNA polymerase activity, although this has not been experimentally verified. On the other hand, PolIE only has limited homology to the domains of human Polθ that are associated with the lesion bypass activity (Leal et al., 2020), suggesting that PolIE is not a translesion DNA polymerase, although further investigation is necessary to validate this. Therefore, PolIE may be directly involved in telomere C-strand synthesis, the same as human Polα/primase. However, whether the DNA polymerase activity of PolIE is required for telomere C-strand fill-in is unclear. It is also possible, though unlikely, that PolIE simply acts as CST and recruits another DNA polymerase to the telomere to actually fulfill the C-strand fill-in function.
Interestingly, T. brucei Polymerase-Primase Like 2 (PPL2) has also been identified from all three proteomic approaches described above aimed to identify telomere proteins (Reis et al., 2018; Rabbani et al., 2022), strongly suggesting that PPL2 is yet another telomere protein in T. brucei. PPL2 is essential and has translesion DNA polymerase activities that catalyzes error-prone bypass of 6-4 photoproduct, but it lacks a primase activity (Rudd et al., 2013). In addition, PPL2 is important for finishing DNA replication in the G2 phase (Rudd et al., 2013). These observations suggest that PPL2 may also be involved in telomere C-strand fill-in, which occurs after bulk DNA replication in S phase. Further investigation on PPL2’s function in telomere end processing will help us better understand mechanisms of telomere maintenance in this important eukaryotic pathogen.
In summary, telomere end processing (Figure 1) appears to be conserved from T. brucei to its mammalian host. However, T. brucei telomere protein complex has several distinct components than the human telomere complex. Even though TbTRF, TbRAP1, and TbTIF2 have telomere functions that are more comparable to their mammalian homologs, the sequence homology between these proteins and their mammalian counterparts is only limited within a few functional domains (Li et al., 2005; Yang et al., 2009; Jehi et al., 2014b). In addition, recently identified PolIE and PPL2 do not seem to have any strict homologs in the mammalian telomere complex. Nevertheless, T. brucei telomere proteins play essential roles in telomere maintenance, and further investigation will help us better understand how different eukaryotes use different players to achieve the same goals.
Discussion
Kinetoplastids are ancient organisms diverged from the mammalian branch in the evolutionary tree more than 500 million years ago. Recent studies on T. brucei telomere maintenance mechanisms suggest that TRF, RAP1, and TIN2 homologs have been evolved much earlier than TPP1/POT1 and CST homologs (Rabbani et al., 2022). Specifically, the DNA polymerase involved in telomere C-strand fill-in in T. brucei is a type A DNA polymerase that arose and diversified in the kinetoplastids lineage (Leal et al., 2020). Whether its ancestral is more closely related to PolI-like or Polθ/ν -like DNA polymerase is unclear. Nevertheless, a strictly conserved PolIE homolog appears to be absent in mammals.
T. brucei undergoes antigenic variation. Hence, vaccination is not effective, and chemotherapy is a key approach for treating African trypanosomiasis. Although subtelomere plasticity facilitates antigenic variation, telomere stability is critical for genome integrity and parasite proliferation. As T. brucei clearly uses distinct and essential telomere factors than its mammalian host for telomere end processing, the T. brucei-unique telomere proteins are promising targets for anti-trypanosome agents. Better understanding about the degree of effects of telomere plasticity and stability on VSG switching will further help us identify anti-parasite means that have clear detrimental effects on parasite growth without enhancing antigenic variation efficiency inadvertently. In addition, T. cruzi and Leishmania are kinetoplastid parasites that cause devastating human diseases affecting more than ten million people worldwide. They are closely related to T. brucei, and homologs of all essential telomere proteins are present in T. cruzi and Leishmania. It is expected that telomere maintenance mechanisms among these three trypanosomes are highly conserved, and knowledge gleaned from T. brucei on telomere protein functions and telomere end processing would also help eradication of T. cruzi and Leishmania.
Author contributions
BL: Conceptualization, Funding acquisition, Project administration, Resources, Supervision, Visualization, Writing–original draft, Writing–review and editing.
Funding
The author(s) declare financial support was received for the research, authorship, and/or publication of this article. This work is partly supported by NIH grants AI127562 (PI, Kim; Co-I, Li), AI066095 (PI, Li), GM147378 (PI, Li), and GRHD center at CSU.
Conflict of interest
The author declares that the research was conducted in the absence of any commercial or financial relationships that could be construed as a potential conflict of interest.
Publisher’s note
All claims expressed in this article are solely those of the authors and do not necessarily represent those of their affiliated organizations, or those of the publisher, the editors and the reviewers. Any product that may be evaluated in this article, or claim that may be made by its manufacturer, is not guaranteed or endorsed by the publisher.
References
Afrin, M., Gaurav, A. K., Yang, X., Pan, X., Zhao, Y., and Li, B. (2020a). TbRAP1 has an unusual duplex DNA binding activity required for its telomere localization and VSG silencing. Sci. Adv. 6, eabc4065. doi:10.1126/sciadv.abc4065
Afrin, M., Kishmiri, H., Sandhu, R., Rabbani, M. A. G., and Li, B. (2020b). Trypanosoma brucei RAP1 has essential functional domains that are required for different protein interactions. mSphere 5, 000277-20–e120. doi:10.1128/mSphere.00027-20
Alsford, S., Horn, D., and Glover, L. (2009). DNA breaks as triggers for antigenic variation in African trypanosomes. Genome Biol. 10, 223. doi:10.1186/gb-2009-10-6-223
Aramburu, T., Plucinsky, S., and Skordalakes, E. (2020). POT1-TPP1 telomere length regulation and disease. Comput. Struct. Biotechnol. J. 18, 1939–1946. doi:10.1016/j.csbj.2020.06.040
Aubert, G., and Lansdorp, P. M. (2008). Telomeres and aging. Physiol. Rev. 88, 557–579. doi:10.1152/physrev.00026.2007
Autexier, C., and Lue, N. F. (2006). The structure and function of telomerase reverse transcriptase. Annu. Rev. Biochem. 75, 493–517. doi:10.1146/annurev.biochem.75.103004.142412
Barbour, A. T., and Wuttke, D. S. (2023). RPA-like single-stranded DNA-binding protein complexes including CST serve as specialized processivity factors for polymerases. Curr. Opin. Struct. Biol. 81, 102611. doi:10.1016/j.sbi.2023.102611
Barnes, R. L., and McCulloch, R. (2007). Trypanosoma brucei homologous recombination is dependent on substrate length and homology, though displays a differential dependence on mismatch repair as substrate length decreases. Nucleic Acids Res. 35, 3478–3493. doi:10.1093/nar/gkm249
Barry, J. D., Ginger, M. L., Burton, P., and McCulloch, R. (2003). Why are parasite contingency genes often associated with telomeres? Int. J. Parasitol. 33, 29–45. doi:10.1016/s0020-7519(02)00247-3
Baumann, P., and Cech, T. R. (2001). Pot1, the putative telomere end-binding protein in fission yeast and humans. Science 292, 1171–1175. doi:10.1126/science.1060036
Baumann, P., Podell, E., and Cech, T. R. (2002). Human pot1 (protection of telomeres) protein: cytolocalization, gene structure, and alternative splicing. Mol. Cell Biol. 22, 8079–8087. doi:10.1128/mcb.22.22.8079-8087.2002
Bernards, A., Michels, P. A. M., Lincke, C. R., and Borst, P. (1983). Growth of chromosome ends in multiplying trypanosomes. Nature 303, 592–597. doi:10.1038/303592a0
Berriman, M., Ghedin, E., Hertz-Fowler, C., Blandin, G., Renauld, H., Bartholomeu, D. C., et al. (2005). The genome of the African trypanosome Trypanosoma brucei. Science , 309, 416–422. doi:10.1126/science.1112642
Berriman, M., Hall, N., Sheader, K., Bringaud, F., Tiwari, B., Isobe, T., et al. (2002). The architecture of variant surface glycoprotein gene expression sites in Trypanosoma brucei. Mol. Biochem. Parasitol. 122, 131–140. doi:10.1016/s0166-6851(02)00092-0
Bertschi, N. L., Toenhake, C. G., Zou, A., Niederwieser, I., Henderson, R., Moes, S., et al. (2017). Malaria parasites possess a telomere repeat-binding protein that shares ancestry with transcription factor IIIA. Nat. Microbiol. 2, 17033. doi:10.1038/nmicrobiol.2017.33
Bhattacharjee, A., Stewart, J., Chaiken, M., and Price, C. M. (2016). STN1 OB fold mutation alters DNA binding and affects selective aspects of CST function. PLoS Genet. 12, e1006342. doi:10.1371/journal.pgen.1006342
Bhattacharjee, A., Wang, Y., Diao, J., and Price, C. M. (2017). Dynamic DNA binding, junction recognition and G4 melting activity underlie the telomeric and genome-wide roles of human CST. Nucleic Acids Res. 45, 12311–12324. doi:10.1093/nar/gkx878
Bianchi, A., Smith, S., Chong, L., Elias, P., and de Lange, T. (1997). TRF1 is a dimer and bends telomeric DNA. EMBO J. 16, 1785–1794. doi:10.1093/emboj/16.7.1785
Bilaud, T., Brun, C., Ancelin, K., Koering, C. E., Laroche, T., and Gilson, E. (1997). Telomeric localization of TRF2, a novel human telobox protein. Nat. Genet. 17, 236–239. doi:10.1038/ng1097-236
Bonetti, D., Martina, M., Falcettoni, M., and Longhese, M. P. (2014). Telomere-end processing: mechanisms and regulation. Chromosoma 123, 57–66. doi:10.1007/s00412-013-0440-y
Bonetti, D., Rinaldi, C., Vertemara, J., Notaro, M., Pizzul, P., Tisi, R., et al. (2020). DNA binding modes influence Rap1 activity in the regulation of telomere length and MRX functions at DNA ends. Nucleic Acids Res. 48, 2424–2441. doi:10.1093/nar/gkz1203
Bonnell, E., Pasquier, E., and Wellinger, R. J. (2021). Telomere replication: solving multiple end replication problems. Front. Cell Dev. Biol. 9, 668171. doi:10.3389/fcell.2021.668171
Boothroyd, C. E., Dreesen, O., Leonova, T., Ly, K. I., Figueiredo, L. M., Cross, G. A. M., et al. (2009). A yeast-endonuclease-generated DNA break induces antigenic switching in Trypanosoma brucei. Nature 459, 278–281. doi:10.1038/nature07982
Borges, G., Criqui, M., and Harrington, L. (2022). Tieing together loose ends: telomere instability in cancer and aging. Mol. Oncol. 16, 3380–3396. doi:10.1002/1878-0261.13299
Brickner, J. R., Garzon, J. L., and Cimprich, K. A. (2022). Walking a tightrope: the complex balancing act of R-loops in genome stability. Mol. Cell 82, 2267–2297. doi:10.1016/j.molcel.2022.04.014
Broccoli, D., Smogorzewska, A., Chong, L., and de Lange, T. (1997). Human telomeres contain two distinct Myb-related proteins, TRF1 and TRF2. Nat. Genet. 17, 231–235. doi:10.1038/ng1097-231
Bryan, T. M. (2020). G-quadruplexes at telomeres: friend or foe. Molecules 25, 3686. doi:10.3390/molecules25163686
Burton, P., McBride, D. J., Wilkes, J. M., Barry, J. D., and McCulloch, R. (2007). Ku heterodimer-independent end joining in Trypanosoma brucei cell extracts relies upon sequence microhomology. Eukaryot. Cell 6, 1773–1781. doi:10.1128/EC.00212-07
Cai, S. W., and de Lange, T. (2023). CST-Polα/Primase: the second telomere maintenance machine. Genes Dev. 37, 555–569. doi:10.1101/gad.350479.123
Cai, S. W., Zinder, J. C., Svetlov, V., Bush, M. W., Nudler, E., Walz, T., et al. (2022). Cryo-EM structure of the human CST-Polα/primase complex in a recruitment state. Nat. Struct. Mol. Biol. 29, 813–819. doi:10.1038/s41594-022-00766-y
Calhoun, S. F., Reed, J., Alexander, N., Mason, C. E., Deitsch, K. W., and Kirkman, L. A. (2017). Chromosome end repair and genome stability in Plasmodium falciparum. mBio 8, e00547-17. doi:10.1128/mBio.00547-17
Chen, L. Y., and Lingner, J. (2013). CST for the grand finale of telomere replication. Nucleus 4, 277–282. doi:10.4161/nucl.25701
Chen, L. Y., Majerska, J., and Lingner, J. (2013). Molecular basis of telomere syndrome caused by CTC1 mutations. Genes Dev. 27, 2099–2108. doi:10.1101/gad.222893.113
Chen, L. Y., Redon, S., and Lingner, J. (2012). The human CST complex is a terminator of telomerase activity. Nature 488, 540–544. doi:10.1038/nature11269
Chen, Y. Y., Dagg, R., Zhang, Y., Lee, J. H. Y., Lu, R., Martin La Rotta, N., et al. (2021). The C-circle biomarker is secreted by alternative-lengthening-of-telomeres positive cancer cells inside exosomes and provides a blood-based diagnostic for ALT activity. Cancers (Basel) 13, 5369. doi:10.3390/cancers13215369
Chong, L., van Steensel, B., Broccoli, D., Erdjument-Bromage, H., Hanish, J., Tempst, P., et al. (1995). A human telomeric protein. Science 270, 1663–1667. doi:10.1126/science.270.5242.1663
Contreras, S. M., Zambrano Siri, R. T., Rivera, E. M., Cristaldi, C., Kamenetzky, L., Kim, K., et al. (2022). Architecture, chromatin and gene organization of Toxoplasma gondii subtelomeres. Epigenomes 6, 29. doi:10.3390/epigenomes6030029
Conway, C., McCulloch, R., Ginger, M. L., Robinson, N. P., Browitt, A., and Barry, J. D. (2002b). Ku is important for telomere maintenance, but not for differential expression of telomeric VSG genes, in African trypanosomes. J. Biol. Chem. 277, 21269–21277. doi:10.1074/jbc.M200550200
Conway, C., Proudfoot, C., Burton, P., Barry, J. D., and McCulloch, R. (2002a). Two pathways of homologous recombination in Trypanosoma brucei. Mol. Microbiol. 45, 1687–1700. doi:10.1046/j.1365-2958.2002.03122.x
Cosentino, R. O., Brink, B. G., and Siegel, T. N. (2021). Allele-specific assembly of a eukaryotic genome corrects apparent frameshifts and reveals a lack of nonsense-mediated mRNA decay. Nar. Genom Bioinform 3, lqab082. doi:10.1093/nargab/lqab082
Crabbe, L., Verdun, R. E., Haggblom, C. I., and Karlseder, J. (2004). Defective telomere lagging strand synthesis in cells lacking WRN helicase activity. Science 306, 1951–1953. doi:10.1126/science.1103619
Cross, G. A. M. (1975). Identification, purification and properties of clone-specific glycoprotein antigens constituting the surface coat of Trypanosoma brucei. Parasitology 71, 393–417. doi:10.1017/s003118200004717x
Cross, G. A. M., Kim, H. S., and Wickstead, B. (2014). Capturing the variant surface glycoprotein repertoire (the VSGnome) of Trypanosoma brucei Lister 427. Mol. Biochem. Parasitol. 195, 59–73. doi:10.1016/j.molbiopara.2014.06.004
Cross, S. H., Allshire, R. C., McKay, S. J., McGill, N. I., and Cooke, H. J. (1989). Cloning of human telomeres by complementation in yeast. Nature 338, 771–774. doi:10.1038/338771a0
Da Silveira, R. C., Da Silva, M. S., Nunes, V. S., Perez, A. M., and Cano, M. I. (2013). The natural absence of RPA1N domain did not impair Leishmania amazonensis RPA-1 participation in DNA damage response and telomere protection. Parasitology 140, 547–559. doi:10.1017/S0031182012002028
Davis, J. A., Reyes, A. V., Nitika Saha, A., Wolfgeher, D. J., Xu, S. L., Truman, A. W., et al. (2023). Proteomic analysis defines the interactome of telomerase in the protozoan parasite, Trypanosoma brucei. Trypanos. Brucei. Front. Cell Dev. Biol. 11, 1110423. doi:10.3389/fcell.2023.1110423
Dejardin, J., and Kingston, R. E. (2009). Purification of proteins associated with specific genomic Loci. Cell 136, 175–186. doi:10.1016/j.cell.2008.11.045
de Lange, T. (2005). Shelterin: the protein complex that shapes and safeguards human telomeres. Genes Dev. 19, 2100–2110. doi:10.1101/gad.1346005
de Lange, T., and Borst, P. (1982). Genomic environment of the expression-linked extra copies of genes for surface antigens of Trypanosoma brucei resembles the end of a chromosome. Nature 299, 451–453. doi:10.1038/299451a0
De Las Penas, A., Pan, S. J., Castano, I., Alder, J., Cregg, R., and Cormack, B. P. (2003). Virulence-related surface glycoproteins in the yeast pathogen Candida glabrata are encoded in subtelomeric clusters and subject to RAP1- and SIR-dependent transcriptional silencing. Genes Dev. 17, 2245–2258. doi:10.1101/gad.1121003
Devlin, R., Marques, C. A., Paape, D., Prorocic, M., Zurita-Leal, A. C., Campbell, S. J., et al. (2016). Mapping replication dynamics in Trypanosoma brucei reveals a link with telomere transcription and antigenic variation. Elife 5, e12765. doi:10.7554/eLife.12765
Dionne, I., and Wellinger, R. J. (1996). Cell cycle-regulated generation of single-stranded G-rich DNA in the absence of telomerase. Proc. Natl. Acad. Sci. U. S. A. 93, 13902–13907. doi:10.1073/pnas.93.24.13902
Dreesen, O., Li, B., and Cross, G. A. M. (2005). Telomere structure and shortening in telomerase-deficient Trypanosoma brucei. Nuc Acids Res. 33, 4536–4543. doi:10.1093/nar/gki769
Edwards-Smallbone, J., Jensen, A. L., Roberts, L. E., Totañes, F. I. G., Hart, S. R., and Merrick, C. J. (2022). Plasmodium falciparum GBP2 is a telomere-associated protein that binds to G-quadruplex DNA and RNA. Front. Cell Infect. Microbiol. 12, 782537. doi:10.3389/fcimb.2022.782537
Feng, X., Hsu, S. J., Bhattacharjee, A., Wang, Y., Diao, J., and Price, C. M. (2018). CTC1-STN1 terminates telomerase while STN1-TEN1 enables C-strand synthesis during telomere replication in colon cancer cells. Nat. Commun. 9, 2827. doi:10.1038/s41467-018-05154-z
Fernandes, C. A. H., Morea, E. G. O., Dos Santos, G. A., da Silva, V. L., Vieira, M. R., Viviescas, M. A., et al. (2020). A multi-approach analysis highlights the relevance of RPA-1 as a telomere end-binding protein (TEBP) in Leishmania amazonensis. Biochim. Biophys. Acta Gen. Subj. 1864, 129607. doi:10.1016/j.bbagen.2020.129607
Fernandes, R. V., Feretzaki, M., and Lingner, J. (2021). The makings of TERRA R-loops at chromosome ends. Cell Cycle 20, 1745–1759. doi:10.1080/15384101.2021.1962638
Figueiredo, L. M., Rocha, E. P., Mancio-Silva, L., Prevost, C., Hernandez-Verdun, D., and Scherf, A. (2005). The unusually large Plasmodium telomerase reverse-transcriptase localizes in a discrete compartment associated with the nucleolus. Nucleic Acids Res. 33, 1111–1122. doi:10.1093/nar/gki260
Flanary, B. (2004). Regulation of murine telomere length via Rtel. Rejuvenation Res. 7, 168–170. doi:10.1089/rej.2004.7.168
Freitas-Junior, L. H., Hernandez-Rivas, R., Ralph, S. A., Montiel-Condado, D., Ruvalcaba-Salazar, O. K., Rojas-Meza, A. P., et al. (2005). Telomeric heterochromatin propagation and histone acetylation control mutually exclusive expression of antigenic variation genes in malaria parasites. Cell 121, 25–36. doi:10.1016/j.cell.2005.01.037
Fridholm, H., Astromskas, E., and Cohn, M. (2013). Telomerase-dependent generation of 70-nt-long telomeric single-stranded 3’ overhangs in yeast. Nucleic Acids Res. 41, 242–252. doi:10.1093/nar/gks1028
Frit, P., Ropars, V., Modesti, M., Charbonnier, J. B., and Calsou, P. (2019). Plugged into the Ku-DNA hub: the NHEJ network. Prog. Biophys. Mol. Biol. 147, 62–76. doi:10.1016/j.pbiomolbio.2019.03.001
Gaurav, A. K., Afrin, M., Yang, X., Saha, A., Sayeed, S. K. A., Pan, X., et al. (2023). The RRM-mediated RNA binding activity in T. brucei RAP1 is essential for VSG monoallelic expression. Nat. Commun. 14, 1576. doi:10.1038/s41467-023-37307-0
Gilson, E., and Géli, V. (2007). How telomeres are replicated. Nat. Rev. Mol. Cell Biol. 8, 825–838. doi:10.1038/nrm2259
Giraud-Panis, M. J., Pisano, S., Poulet, A., Le Du, M. H., and Gilson, E. (2010a). Structural identity of telomeric complexes. FEBS Lett. 584, 3785–3799. doi:10.1016/j.febslet.2010.08.004
Giraud-Panis, M. J., Teixeira, M. T., Géli, V., and Gilson, E. (2010b). CST meets shelterin to keep telomeres in check. Mol. Cell 39, 665–676. doi:10.1016/j.molcel.2010.08.024
Glousker, G., and Lingner, J. (2021). Challenging endings: how telomeres prevent fragility. Bioessays 43, e2100157. doi:10.1002/bies.202100157
Glover, L., Alsford, S., and Horn, D. (2013). DNA break site at fragile subtelomeres determines probability and mechanism of antigenic variation in African trypanosomes. PLoS Pathog. 9, e1003260. doi:10.1371/journal.ppat.1003260
Glover, L., Jun, J., and Horn, D. (2011). Microhomology-mediated deletion and gene conversion in African trypanosomes. Nucleic Acids Res. 39, 1372–1380. doi:10.1093/nar/gkq981
Glover, L., McCulloch, R., and Horn, D. (2008). Sequence homology and microhomology dominate chromosomal double-strand break repair in African trypanosomes. Nucleic Acids Res. 36, 2608–2618. doi:10.1093/nar/gkn104
Greider, C. W. (1998). Telomeres and senescence: the history, the experiment, the future. Curr. Biol. 8, R178–R181. doi:10.1016/s0960-9822(98)70105-8
Greider, C. W., and Blackburn, E. H. (1987). The telomere terminal transferase of Tetrahymena is a ribonucleoprotein enzyme with two kinds of primer specificity. Cell 51, 887–898. doi:10.1016/0092-8674(87)90576-9
Grill, S., Bisht, K., Tesmer, V. M., Shami, A. N., Hammoud, S. S., and Nandakumar, J. (2019). Two separation-of-function isoforms of human TPP1 dictate telomerase regulation in somatic and germ cells. Cell Rep. 27, 3511–3521. doi:10.1016/j.celrep.2019.05.073
Gu, P., Jia, S., Takasugi, T., Tesmer, V. M., Nandakumar, J., Chen, Y., et al. (2021). Distinct functions of POT1 proteins contribute to the regulation of telomerase recruitment to telomeres. Nat. Commun. 12, 5514. doi:10.1038/s41467-021-25799-7
Gunzl, A., Bruderer, T., Laufer, G., Schimanski, B., Tu, L. C., Chung, H. M., et al. (2003). RNA polymerase I transcribes procyclin genes and variant surface glycoprotein gene expression sites in Trypanosoma brucei. Eukaryot. Cell 2, 542–551. doi:10.1128/ec.2.3.542-551.2003
Gunzl, A., Kirkham, J. K., Nguyen, T. N., Badjatia, N., and Park, S. H. (2015). Mono-allelic VSG expression by RNA polymerase I in Trypanosoma brucei: expression site control from both ends? Gene 556, 68–73. doi:10.1016/j.gene.2014.09.047
Guo, S., Zhu, X., Huang, Z., Wei, C., Yu, J., Zhang, L., et al. (2023). Genomic instability drives tumorigenesis and metastasis and its implications for cancer therapy. Biomed. Pharmacother. 157, 114036. doi:10.1016/j.biopha.2022.114036
Gupta, S. K., Kolet, L., Doniger, T., Biswas, V. K., Unger, R., Tzfati, Y., et al. (2013). The Trypanosoma brucei telomerase RNA (TER) homologue binds core proteins of the C/D snoRNA family. FEBS Lett. 587, 1399–1404. doi:10.1016/j.febslet.2013.03.017
Haber, J. E. (2018). DNA repair: the search for homology. Bioessays 40, e1700229. doi:10.1002/bies.201700229
Hackett, J. A., Feldser, D. M., and Greider, C. W. (2001). Telomere dysfunction increases mutation rate and genomic instability. Cell 106, 275–286. doi:10.1016/s0092-8674(01)00457-3
Hass, E. P., and Zappulla, D. C. (2015). The Ku subunit of telomerase binds Sir4 to recruit telomerase to lengthen telomeres in S. cerevisiae. Elife 4, e07750. doi:10.7554/eLife.07750
He, Q., and Lim, C. J. (2023). Models for human telomere C-strand fill-in by CST-Polα-primase. Trends Biochem. Sci. S0968-0004 (23), 00174. doi:10.1016/j.tibs.2023.07.008
Henson, J. D., Cao, Y., Huschtscha, L. I., Chang, A. C., Au, A. Y., Pickett, H. A., et al. (2009). DNA C-circles are specific and quantifiable markers of alternative-lengthening-of-telomeres activity. Nat. Biotechnol. 27, 1181–1185. doi:10.1038/nbt.1587
Henson, J. D., Lau, L. M., Koch, S., Martin La Rotta, N., Dagg, R. A., and Reddel, R. R. (2017). The C-Circle Assay for alternative-lengthening-of-telomeres activity. Methods 114, 74–84. doi:10.1016/j.ymeth.2016.08.016
Hernandez-Rivas, R., Mattei, D., Sterkers, Y., Peterson, D. S., Wellems, T. E., and Scherf, A. (1997). Expressed var genes are found in Plasmodium falciparum subtelomeric regions. Mol. Cell Biol. 17, 604–611. doi:10.1128/mcb.17.2.604
Hernandez-Rivas, R., Perez-Toledo, K., Herrera Solorio, A. M., Delgadillo, D. M., and Vargas, M. (2010). Telomeric heterochromatin in Plasmodium falciparum. J. Biomed. Biotechnol. 2010, 290501. doi:10.1155/2010/290501
Hertz-Fowler, C., Figueiredo, L. M., Quail, M. A., Becker, M., Jackson, A., Bason, N., et al. (2008). Telomeric expression sites are highly conserved in Trypanosoma brucei. PLoS ONE 3, e3527. doi:10.1371/journal.pone.0003527
Higa, M., Fujita, M., and Yoshida, K. (2017). DNA replication origins and fork progression at mammalian telomeres. Genes (Basel) 8, E112. doi:10.3390/genes8040112
Hockemeyer, D., Sfeir, A. J., Shay, J. W., Wright, W. E., and de Lange, T. (2005). POT1 protects telomeres from a transient DNA damage response and determines how human chromosomes end. EMBO J. 24, 2667–2678. doi:10.1038/sj.emboj.7600733
Horn, D. (2014). Antigenic variation in African trypanosomes. Mol. Biochem. Parasitol. 195, 123–129. doi:10.1016/j.molbiopara.2014.05.001
Horvath, M. P. (2011). Structural anatomy of telomere OB proteins. Crit. Rev. Biochem. Mol. Biol. 46, 409–435. doi:10.3109/10409238.2011.609295
Houghtaling, B. R., Cuttonaro, L., Chang, W., and Smith, S. (2004). A dynamic molecular link between the telomere length regulator TRF1 and the chromosome end protector TRF2. Curr. Biol. 14, 1621–1631. doi:10.1016/j.cub.2004.08.052
Hovel-Miner, G. A., Boothroyd, C. E., Mugnier, M., Dreesen, O., Cross, G. A. M., and Papavasiliou, F. N. (2012). Telomere length affects the frequency and mechanism of antigenic variation in Trypanosoma brucei. PLoS Pathog. 8, e1002900. doi:10.1371/journal.ppat.1002900
Hug, N., and Lingner, J. (2006). Telomere length homeostasis. Chromosoma 115, 413–425. doi:10.1007/s00412-006-0067-3
Hwang, H., Buncher, N., Opresko, P. L., and Myong, S. (2012). POT1-TPP1 regulates telomeric overhang structural dynamics. Structure 20, 1872–1880. doi:10.1016/j.str.2012.08.018
Jafri, M. A., Ansari, S. A., Alqahtani, M. H., and Shay, J. W. (2016). Roles of telomeres and telomerase in cancer, and advances in telomerase-targeted therapies. Genome Med. 8, 69. doi:10.1186/s13073-016-0324-x
Janzen, C. J., Lander, F., Dreesen, O., and Cross, G. A. M. (2004). Telomere length regulation and transcriptional silencing in KU80-deficient Trypanosoma brucei. Nucleic Acids Res. 32, 6575–6584. doi:10.1093/nar/gkh991
Jasin, M., and Rothstein, R. (2013). Repair of strand breaks by homologous recombination. Cold Spring Harb. Perspect. Biol. 5, a012740. doi:10.1101/cshperspect.a012740
Jehi, S. E., Li, X., Sandhu, R., Ye, F., Benmerzouga, I., Zhang, M., et al. (2014a). Suppression of subtelomeric VSG switching by Trypanosoma brucei TRF requires its TTAGGG repeat-binding activity. Nucleic Acids Res. 42, 12899–12911. doi:10.1093/nar/gku942
Jehi, S. E., Nanavaty, V., and Li, B. (2016). Trypanosoma brucei TIF2 and TRF suppress VSG switching using overlapping and independent mechanisms. PLoS One 11, e0156746. doi:10.1371/journal.pone.0156746
Jehi, S. E., Wu, F., and Li, B. (2014b). Trypanosoma brucei TIF2 suppresses VSG switching by maintaining subtelomere integrity. Cell Res. 24, 870–885. doi:10.1038/cr.2014.60
Jing, H., and Lin, H. (2015). Sirtuins in epigenetic regulation. Chem. Rev. 115, 2350–2375. doi:10.1021/cr500457h
Kelleher, C., Kurth, I., and Lingner, J. (2005). Human protection of telomeres 1 (POT1) is a negative regulator of telomerase activity in vitro. Mol. Cell Biol. 25, 808–818. doi:10.1128/MCB.25.2.808-818.2005
Kim, S. H., Kaminker, P., and Campisi, J. (1999). TIN2, a new regulator of telomere length in human cells. Nat. Genet. 23, 405–412. doi:10.1038/70508
Latrick, C. M., and Cech, T. R. (2010). POT1–TPP1 enhances telomerase processivity by slowing primer dissociation and aiding translocation. EMBO J. 29, 924–933. doi:10.1038/emboj.2009.409
Leal, A. Z., Schwebs, M., Briggs, E., Weisert, N., Reis, H., Lemgruber, L., et al. (2020). Genome maintenance functions of a putative Trypanosoma brucei translesion DNA polymerase include telomere association and a role in antigenic variation. Nucleic Acids Res. 48, 9660–9680. doi:10.1093/nar/gkaa686
Li, B. (2010). A newly discovered role of telomeres in an ancient organism. Nucleus 1, 260–263. doi:10.4161/nucl.1.3.11742
Li, B. (2012). Telomere components as potential therapeutic targets for treating microbial pathogen infections. Front. Oncol. 2, 156. doi:10.3389/fonc.2012.00156
Li, B. (2015). DNA double-strand breaks and telomeres play important roles in Trypanosoma brucei antigenic variation. Eukaryot. Cell 14, 196–205. doi:10.1128/EC.00207-14
Li, B. (2021). Keeping balance between genetic stability and plasticity at the telomere and subtelomere of Trypanosoma brucei. Front. Cell Dev. Biol. 9, 699639. doi:10.3389/fcell.2021.699639
Li, B., and de Lange, T. (2003). Rap1 affects the length and heterogeneity of human telomeres. Mol. Biol. Cell 14, 5060–5068. doi:10.1091/mbc.e03-06-0403
Li, B., Espinal, A., and Cross, G. A. M. (2005). Trypanosome telomeres are protected by a homologue of mammalian TRF2. Mol. Cell Biol. 25, 5011–5021. doi:10.1128/MCB.25.12.5011-5021.2005
Li, B., Oestreich, S., and de Lange, T. (2000). Identification of human Rap1: implications for telomere evolution. Cell 101, 471–483. doi:10.1016/s0092-8674(00)80858-2
Li, B., and Zhao, Y. (2021). Regulation of antigenic variation by Trypanosoma brucei telomere proteins depends on their unique DNA binding activities. Pathogens 10, 967–986. doi:10.3390/pathogens10080967
Lillard-Wetherell, K., Machwe, A., Langland, G. T., Combs, K. A., Behbehani, G. K., Schonberg, S. A., et al. (2004). Association and regulation of the BLM helicase by the telomere proteins TRF1 and TRF2. Hum. Mol. Genet. 13, 1919–1932. doi:10.1093/hmg/ddh193
Lim, C. J., and Cech, T. R. (2021). Shaping human telomeres: from shelterin and CST complexes to telomeric chromatin organization. Nat. Rev. Mol. Cell Biol. 22, 283–298. doi:10.1038/s41580-021-00328-y
Lingner, J., Cooper, J. P., and Cech, T. R. (1995). Telomerase and DNA end replication: no longer a lagging strand problem? Science 269, 1533–1534. doi:10.1126/science.7545310
Liu, D., O’Connor, M. S., Qin, J., and Songyang, Z. (2004a). Telosome, a mammalian telomere-associated complex formed by multiple telomeric proteins. J. Biol. Chem. 279, 51338–51342. doi:10.1074/jbc.M409293200
Liu, D., Safari, A., O’Connor, M. S., Chan, D. W., Laegeler, A., Qin, J., et al. (2004b). PTOP interacts with POT1 and regulates its localization to telomeres. Nat. Cell Biol. 6, 673–680. doi:10.1038/ncb1142
Loayza, D., and de Lange, T. (2003). POT1 as a terminal transducer of TRF1 telomere length control. Nature 424, 1013–1018. doi:10.1038/nature01688
López-Fuentes, E., Gutiérrez-Escobedo, G., Timmermans, B., Van Dijck, P., De Las Peñas, A., and Castaño, I. (2018). Candida glabrata’s genome plasticity confers a unique pattern of expressed cell wall proteins. J. Fungi (Basel) 4, 67. doi:10.3390/jof4020067
Lu, W., Zhang, Y., Liu, D., Songyang, Z., and Wan, M. (2013). Telomeres-structure, function, and regulation. Exp. Cell Res. 319, 133–141. doi:10.1016/j.yexcr.2012.09.005
Lulkiewicz, M., Bajsert, J., Kopczynski, P., Barczak, W., and Rubis, B. (2020). Telomere length: how the length makes a difference. Mol. Biol. Rep. 47, 7181–7188. doi:10.1007/s11033-020-05551-y
Lundblad, V. (2012). Telomere end processing: unexpected complexity at the end game. Genes Dev. 26, 1123–1127. doi:10.1101/gad.195339.112
Lyčka, M., Bubeník, M., Závodník, M., Peska, V., Fajkus, P., Demko, M., et al. (2023). TeloBase: a community-curated database of telomere sequences across the tree of life. Nucleic Acids Res., gkad672. doi:10.1093/nar/gkad672
Machwe, A., Xiao, L., and Orren, D. K. (2004). TRF2 recruits the Werner syndrome (WRN) exonuclease for processing of telomeric DNA. Oncogene 23, 149–156. doi:10.1038/sj.onc.1206906
Mackay, R. P., Xu, Q., and Weinberger, P. M. (2020). R-Loop physiology and pathology: a brief review. DNA Cell Biol. 39, 1914–1925. doi:10.1089/dna.2020.5906
Maestroni, L., Matmati, S., and Coulon, S. (2017). Solving the telomere replication problem. Genes (Basel) 8, 55. doi:10.3390/genes8020055
Mancio-Silva, L., Rojas-Meza, A. P., Vargas, M., Scherf, A., and Hernandez-Rivas, R. (2008). Differential association of Orc1 and Sir2 proteins to telomeric domains in Plasmodium falciparum. J. Cell Sci. 121, 2046–2053. doi:10.1242/jcs.026427
Marin, P. A., da Silva, M. S., Pavani, R. S., Machado, C. R., and Elias, M. C. (2018). Recruitment kinetics of the homologous recombination pathway in procyclic forms of Trypanosoma brucei after ionizing radiation treatment. Sci. Rep. 8, 5405. doi:10.1038/s41598-018-23731-6
Mason, M., and Skordalakes, E. (2010). Insights into Cdc13 dependent telomere length regulation. Aging (Albany NY) 2, 731–734. doi:10.18632/aging.100211
McCulloch, R., and Barry, J. D. (1999). A role for RAD51 and homologous recombination in Trypanosoma brucei antigenic variation. Genes Dev. 13, 2875–2888. doi:10.1101/gad.13.21.2875
McCulloch, R., Cobbold, C. A., Figueiredo, L., Jackson, A., Morrison, L. J., Mugnier, M. R., et al. (2017). Emerging challenges in understanding trypanosome antigenic variation. Emerg. Top. Life Sci. 1, 585–592. doi:10.1042/ETLS20170104
McCulloch, R., Rudenko, G., and Borst, P. (1997). Gene conversions mediating antigenic variation in Trypanosoma brucei can occur in variant surface glycoprotein expression sites lacking 70-base-pair repeat sequences. Mol. Cell. Biol. 17, 833–843. doi:10.1128/mcb.17.2.833
Meena, J., Rudolph, K. L., and Günes, C. (2015). Telomere dysfunction, chromosomal instability and cancer. Recent Results Cancer Res. 200, 61–79. doi:10.1007/978-3-319-20291-4_3
Merrick, C. J., and Duraisingh, M. T. (2007). Plasmodium falciparum Sir2: an unusual sirtuin with dual histone deacetylase and ADP-ribosyltransferase activity. Eukaryot. Cell 6, 2081–2091. doi:10.1128/EC.00114-07
Mirceta, M., Shum, N., Schmidt, M. H. M., and Pearson, C. E. (2022). Fragile sites, chromosomal lesions, tandem repeats, and disease. Front. Genet. 13, 985975. doi:10.3389/fgene.2022.985975
Miyake, Y., Nakamura, M., Nabetani, A., Shimamura, S., Tamura, M., Yonehara, S., et al. (2009). RPA-like mammalian Ctc1-Stn1-Ten1 complex binds to single-stranded DNA and protects telomeres independently of the Pot1 pathway. Mol. Cell 36, 193–206. doi:10.1016/j.molcel.2009.08.009
Mugnier, M. R., Stebbins, C. E., and Papavasiliou, F. N. (2016). Masters of disguise: antigenic variation and the VSG coat in Trypanosoma brucei. PLoS Pathog. 12, e1005784. doi:10.1371/journal.ppat.1005784
Müller, L. S. M., Cosentino, R. O., Förstner, K. U., Guizetti, J., Wedel, C., Kaplan, N., et al. (2018). Genome organization and DNA accessibility control antigenic variation in trypanosomes. Nature 563, 121–125. doi:10.1038/s41586-018-0619-8
Munoz-Jordan, J. L., Cross, G. A. M., de Lange, T., and Griffith, J. D. (2001). t-loops at trypanosome telomeres. EMBO J. 20, 579–588. doi:10.1093/emboj/20.3.579
Muraki, K., Nyhan, K., Han, L., and Murnane, J. P. (2012). Mechanisms of telomere loss and their consequences for chromosome instability. Front. Oncol. 2, 135. doi:10.3389/fonc.2012.00135
Murnane, J. P. (2010). Telomere loss as a mechanism for chromosome instability in human cancer. Cancer Res. 70, 4255–4259. doi:10.1158/0008-5472.CAN-09-4357
Myler, P., Nelson, R. G., Agabian, N., and Stuart, K. (1984a). Two mechanisms of expression of a variant antigen gene of Trypanosoma brucei. Nature 309, 282–284. doi:10.1038/309282a0
Myler, P. J., Allison, J., Agabian, N., and Stuart, K. (1984b). Antigenic variation in African trypanosomes by gene replacement or activation of alternate telomeres. Cell 39, 203–211. doi:10.1016/0092-8674(84)90206-x
Nanavaty, V., Sandhu, R., Jehi, S. E., Pandya, U. M., and Li, B. (2017). Trypanosoma brucei RAP1 maintains telomere and subtelomere integrity by suppressing TERRA and telomeric RNA:DNA hybrids. Nucleic Acids Res. 45, 5785–5796. doi:10.1093/nar/gkx184
Nandakumar, J., Bell, C. F., Weidenfeld, I., Zaug, A. J., Leinwand, L. A., and Cech, T. R. (2012). The TEL patch of telomere protein TPP1 mediates telomerase recruitment and processivity. Nature 492, 285–289. doi:10.1038/nature11648
Neto, J. L., Lira, C. B., Giardini, M. A., Khater, L., Perez, A. M., Peroni, L. A., et al. (2007). Leishmania replication protein A-1 binds in vivo single-stranded telomeric DNA. Biochem. Biophys. Res. Commun. 358, 417–423. doi:10.1016/j.bbrc.2007.04.144
Nguyen, D. D., Kim, E. Y., Sang, P. B., and Chai, W. (2020). Roles of OB-fold proteins in replication stress. Front. Cell Dev. Biol. 8, 574466. doi:10.3389/fcell.2020.574466
O’Connor, M. S., Safari, A., Xin, H., Liu, D., and Songyang, Z. (2006). A critical role for TPP1 and TIN2 interaction in high-order telomeric complex assembly. Proc. Natl. Acad. Sci. U. S. A. 103, 11874–11879. doi:10.1073/pnas.0605303103
Olson, C. L., Barbour, A. T., and Wuttke, D. S. (2022). Filling in the blanks: how the C-strand catches up to the G-strand at replicating telomeres using CST. Nat. Struct. Mol. Biol. 29, 730–733. doi:10.1038/s41594-022-00818-3
Opresko, P. L., Von Kobbe, C., Laine, J. P., Harrigan, J., Hickson, I. D., and Bohr, V. A. (2002). Telomere binding protein TRF2 binds to and stimulates the Werner and Bloom syndrome helicases. J. Biol. Chem. 277, 41110–41119. doi:10.1074/jbc.M205396200
O’Sullivan, R. J., and Karlseder, J. (2010). Telomeres: protecting chromosomes against genome instability. Nat. Rev. Mol. Cell Biol. 11, 171–181. doi:10.1038/nrm2848
Painter, H. J., Campbell, T. L., and Llinás, M. (2011). The Apicomplexan AP2 family: integral factors regulating Plasmodium development. Mol. Biochem. Parasitol. 176, 1–7. doi:10.1016/j.molbiopara.2010.11.014
Pandya, U. M., Sandhu, R., and Li, B. (2013). Silencing subtelomeric VSGs by Trypanosoma brucei RAP1 at the insect stage involves chromatin structure changes. Nucleic Acids Res. 41, 7673–7682. doi:10.1093/nar/gkt562
Pavani, R. S., Fernandes, C., Perez, A. M., Vasconcelos, E. J., Siqueira-Neto, J. L., Fontes, M. R., et al. (2014). RPA-1 from Leishmania amazonensis (LaRPA-1) structurally differs from other eukaryote RPA-1 and interacts with telomeric DNA via its N-terminal OB-fold domain. FEBS Lett. 588, 4740–4748. doi:10.1016/j.febslet.2014.11.005
Perez-Toledo, K., Rojas-Meza, A. P., Mancio-Silva, L., Hernandez-Cuevas, N. A., Delgadillo, D. M., Vargas, M., et al. (2009). Plasmodium falciparum heterochromatin protein 1 binds to tri-methylated histone 3 lysine 9 and is linked to mutually exclusive expression of var genes. Nucleic Acids Res. 37, 2596–2606. doi:10.1093/nar/gkp115
Pfingsten, J. S., Goodrich, K. J., Taabazuing, C., Ouenzar, F., Chartrand, P., and Cech, T. R. (2012). Mutually exclusive binding of telomerase RNA and DNA by ku alters telomerase recruitment model. Cell 148, 922–932. doi:10.1016/j.cell.2012.01.033
Pickett, H. A., Cesare, A. J., Johnston, R. L., Neumann, A. A., and Reddel, R. R. (2009). Control of telomere length by a trimming mechanism that involves generation of t-circles. EMBO J. 28, 799–809. doi:10.1038/emboj.2009.42
Podlevsky, J. D., Bley, C. J., Omana, R. V., Qi, X., and Chen, J. J. (2008). The telomerase database. Nucleic Acids Res. 36, D339–D343. doi:10.1093/nar/gkm700
Rabbani, M. A. G., Tonini, M. L., Afrin, M., and Li, B. (2022). POLIE suppresses telomerase-mediated telomere G-strand extension and helps ensure proper telomere C-strand synthesis in trypanosomes. Nucleic Acids Res. 50, 2036–2050. doi:10.1093/nar/gkac023
Rahnama, M., Wang, B., Dostart, J., Novikova, O., Yackzan, D., Yackzan, A., et al. (2021). Telomere roles in fungal genome evolution and adaptation. Front. Genet. 12, 676751. doi:10.3389/fgene.2021.676751
Rajavel, M., Mullins, M. R., and Taylor, D. J. (2014). Multiple facets of TPP1 in telomere maintenance. Biochim. Biophys. Acta 1844, 1550–1559. doi:10.1016/j.bbapap.2014.04.014
Ralph, S. A., Scheidig-Benatar, C., and Scherf, A. (2005). Antigenic variation in Plasmodium falciparum is associated with movement of var loci between subnuclear locations. Proc. Natl. Acad. Sci. U. S. A. 102, 5414–5419. doi:10.1073/pnas.0408883102
Reis, H., Schwebs, M., Dietz, S., Janzen, C. J., and Butter, F. (2018). TelAP1 links telomere complexes with developmental expression site silencing in African trypanosomes. Nucleic Acids Res. 46, 2820–2833. doi:10.1093/nar/gky028
Religa, A. A., and Waters, A. P. (2012). Sirtuins of parasitic protozoa: in search of function(s). Mol. Biochem. Parasitol. 185, 71–88. doi:10.1016/j.molbiopara.2012.08.003
Rice, C., Shastrula, P. K., Kossenkov, A. V., Hills, R., Baird, D. M., Showe, L. C., et al. (2017). Structural and functional analysis of the human POT1-TPP1 telomeric complex. Nat. Commun. 8, 14928. doi:10.1038/ncomms14928
Rice, C., and Skordalakes, E. (2016). Structure and function of the telomeric CST complex. Comput. Struct. Biotechnol. J. 14, 161–167. doi:10.1016/j.csbj.2016.04.002
Riethman, H. C., Moyzis, R. K., Meyne, J., Burke, D. T., and Olson, M. V. (1989). Cloning human telomeric DNA fragments into Saccharomyces cerevisiae using a yeast-artificial-chromosome vector. Proc. Natl. Acad. Sci. U. S. A. 86, 6240–6244. doi:10.1073/pnas.86.16.6240
Robinson, N. P., Burman, N., Melville, S. E., and Barry, J. D. (1999). Predominance of duplicative VSG gene conversion in antigenic variation in African trypanosomes. Mol. Cell Biol. 19, 5839–5846. doi:10.1128/mcb.19.9.5839
Rossiello, F., Jurk, D., Passos, J. F., and d’Adda di Fagagna, F. (2022). Telomere dysfunction in ageing and age-related diseases. Nat. Cell Biol. 24, 135–147. doi:10.1038/s41556-022-00842-x
Rubio, J. P., Thompson, J. K., and Cowman, A. F. (1996). The var genes of Plasmodium falciparum are located in the subtelomeric region of most chromosomes. EMBO J. 15, 4069–4077. doi:10.1002/j.1460-2075.1996.tb00780.x
Rudd, S. G., Glover, L., Jozwiakowski, S. K., Horn, D., and Doherty, A. J. (2013). PPL2 translesion polymerase is essential for the completion of chromosomal DNA replication in the African trypanosome. Mol. Cell 52, 554–565. doi:10.1016/j.molcel.2013.10.034
Rudenko, G., Chaves, I., Dirks-Mulder, A., and Borst, P. (1998). Selection for activation of a new variant surface glycoprotein gene expression site in Trypanosoma brucei can result in deletion of the old one. Mol. Biochem. Parasitol. 95, 97–109. doi:10.1016/s0166-6851(98)00099-1
Rudenko, G., McCulloch, R., Dirksmulder, A., and Borst, P. (1996). Telomere exchange can be an important mechanism of variant surface glycoprotein gene switching in Trypanosoma brucei. Mol. Biochem. Parasitol. 80, 65–75. doi:10.1016/0166-6851(96)02669-2
Rudenko, G., and Van der Ploeg, L. H. (1989). Transcription of telomere repeats in protozoa. EMBO J. 8, 2633–2638. doi:10.1002/j.1460-2075.1989.tb08403.x
Ruis, P., and Boulton, S. J. (2021). The end protection problem-an unexpected twist in the tail. Genes Dev. 35, 1–21. doi:10.1101/gad.344044.120
Saha, A., Gaurav, A. K., Pandya, U. M., Afrin, M., Sandhu, R., Nanavaty, V., et al. (2021). TbTRF suppresses the TERRA level and regulates the cell cycle-dependent TERRA foci number with a TERRA binding activity in its C-terminal Myb domain. Nucleic Acids Res. 49, 5637–5653. doi:10.1093/nar/gkab401
Sandhu, R., and Li, B. (2011). Examination of the telomere G-overhang structure in Trypanosoma brucei. J. Vis. Exp. 47, 1959. doi:10.3791/1959
Sandhu, R., and Li, B. (2017). Telomerase activity is required for the telomere G-overhang structure in Trypanosoma brucei. Sci. Rep. 7, 15983. doi:10.1038/s41598-017-16182-y
Sandhu, R., Sanford, S., Basu, S., Park, M., Pandya, U. M., Li, B., et al. (2013). A trans-spliced telomerase RNA dictates telomere synthesis in Trypanosoma brucei. Cell Res. 23, 537–551. doi:10.1038/cr.2013.35
Sandhu, R., Sharma, M., Wei, D., and Xu, L. (2021). The structurally conserved TELR region on shelterin protein TPP1 is essential for telomerase processivity but not recruitment. Proc. Natl. Acad. Sci. U. S. A. 118, e2024889118. doi:10.1073/pnas.2024889118
Schmid-Siegert, E., Richard, S., Luraschi, A., Mühlethaler, K., Pagni, M., and Hauser, P. M. (2017). Mechanisms of surface antigenic variation in the human pathogenic fungus Pneumocystis jirovecii. MBio 8, e01470-17. doi:10.1128/mBio.01470-17
Schmidt, J. C., and Cech, T. R. (2015). Human telomerase: biogenesis, trafficking, recruitment, and activation. Genes Dev. 29, 1095–1105. doi:10.1101/gad.263863.115
Schoelz, J. M., and Riddle, N. C. (2022). Functions of HP1 proteins in transcriptional regulation. Epigenetics Chromatin 15, 14. doi:10.1186/s13072-022-00453-8
Sekne, Z., Ghanim, G. E., van Roon, A. M., and Nguyen, T. H. D. (2022). Structural basis of human telomerase recruitment by TPP1-POT1. Science 375, 1173–1176. doi:10.1126/science.abn6840
Sexton, A. N., Regalado, S. G., Lai, C. S., Cost, G. J., O’Neil, C. M., Urnov, F. D., et al. (2014). Genetic and molecular identification of three human TPP1 functions in telomerase action: recruitment, activation, and homeostasis set point regulation. Genes Dev. 28, 1885–1899. doi:10.1101/gad.246819.114
Sfeir, A., Kosiyatrakul, S. T., Hockemeyer, D., MacRae, S. L., Karlseder, J., Schildkraut, C. L., et al. (2009). Mammalian telomeres resemble fragile sites and require TRF1 for efficient replication. Cell 138, 90–103. doi:10.1016/j.cell.2009.06.021
Shay, J. W., and Wright, W. E. (2005). Senescence and immortalization: role of telomeres and telomerase. Carcinogenesis 26, 867–874. doi:10.1093/carcin/bgh296
Shay, J. W., and Wright, W. E. (2019). Telomeres and telomerase: three decades of progress. Nat. Rev. Genet. 20, 299–309. doi:10.1038/s41576-019-0099-1
Sierra-Miranda, M., Vembar, S. S., Delgadillo, D. M., Ávila-López, P. A., Herrera-Solorio, A. M., Lozano Amado, D., et al. (2017). PfAP2Tel, harbouring a non-canonical DNA-binding AP2 domain, binds to Plasmodium falciparum telomeres. Cell Microbiol. 19, e12742. doi:10.1111/cmi.12742
Silva Pereira, S., Jackson, A. P., and Figueiredo, L. M. (2022). Evolution of the variant surface glycoprotein family in African trypanosomes. Trends Parasitol. 38, 23–36. doi:10.1016/j.pt.2021.07.012
Smogorzewska, A., and de Lange, T. (2004). Regulation of telomerase by telomeric proteins. Annu. Rev. Biochem. 73, 177–208. doi:10.1146/annurev.biochem.73.071403.160049
Smogorzewska, A., van Steensel, B., Bianchi, A., Oelmann, S., Schaefer, M. R., Schnapp, G., et al. (2000). Control of human telomere length by TRF1 and TRF2. Mol. Cell Biol. 20, 1659–1668. doi:10.1128/mcb.20.5.1659-1668.2000
Srinivas, N., Rachakonda, S., and Kumar, R. (2020). Telomeres and telomere length: a general overview. Cancers (Basel) 12, 558. doi:10.3390/cancers12030558
Stellwagen, A. E., Haimberger, Z. W., Veatch, J. R., and Gottschling, D. E. (2003). Ku interacts with telomerase RNA to promote telomere addition at native and broken chromosome ends. Genes Dev. 17, 2384–2395. doi:10.1101/gad.1125903
Stewart, J. A., Chaiken, M. F., Wang, F., and Price, C. M. (2012). Maintaining the end: roles of telomere proteins in end-protection, telomere replication and length regulation. Mutat. Res. 730, 12–19. doi:10.1016/j.mrfmmm.2011.08.011
Stringer, J. R., and Keely, S. P. (2001). Genetics of surface antigen expression in Pneumocystis carinii. Infect. Immun. 69, 627–639. doi:10.1128/IAI.69.2.627-639.2001
Takai, H., Jenkinson, E., Kabir, S., Babul-Hirji, R., Najm-Tehrani, N., Chitayat, D. A., et al. (2016). A POT1 mutation implicates defective telomere end fill-in and telomere truncations in Coats plus. Genes Dev. 30, 812–826. doi:10.1101/gad.276873.115
Teixeira, M. T., Arneric, M., Sperisen, P., and Lingner, J. (2004). Telomere length homeostasis is achieved via a switch between telomerase-extendible and -nonextendible states. Cell 117, 323–335. doi:10.1016/s0092-8674(04)00334-4
Theobald, D. L., Mitton-Fry, R. M., and Wuttke, D. S. (2003). Nucleic acid recognition by OB-fold proteins. Annu. Rev. Biophys. Biomol. Struct. 32, 115–133. doi:10.1146/annurev.biophys.32.110601.142506
Ting, N. S., Yu, Y., Pohorelic, B., Lees-Miller, S. P., and Beattie, T. L. (2005). Human Ku70/80 interacts directly with hTR, the RNA component of human telomerase. Nuc Acids Res. 33, 2090–2098. doi:10.1093/nar/gki342
Tonkin, C. J., Carret, C. K., Duraisingh, M. T., Voss, T. S., Ralph, S. A., Hommel, M., et al. (2009). Sir2 paralogues cooperate to regulate virulence genes and antigenic variation in Plasmodium falciparum. PLoS Biol. 7, e84. doi:10.1371/journal.pbio.1000084
Uringa, E. J., Lisaingo, K., Pickett, H. A., Brind’Amour, J., Rohde, J. H., Zelensky, A., et al. (2012). RTEL1 contributes to DNA replication and repair and telomere maintenance. Mol. Biol. Cell 23, 2782–2792. doi:10.1091/mbc.E12-03-0179
Uringa, E. J., Youds, J. L., Lisaingo, K., Lansdorp, P. M., and Boulton, S. J. (2011). RTEL1: an essential helicase for telomere maintenance and the regulation of homologous recombination. Nucleic Acids Res. 39, 1647–1655. doi:10.1093/nar/gkq1045
Vaiserman, A., and Krasnienkov, D. (2020). Telomere length as a marker of biological age: state-of-the-art, open issues, and future perspectives. Front. Genet. 11, 630186. doi:10.3389/fgene.2020.630186
Vannier, J. B., Sandhu, S., Petalcorin, M. I., Wu, X., Nabi, Z., Ding, H., et al. (2013). RTEL1 is a replisome-associated helicase that promotes telomere and genome-wide replication. Science 342, 239–242. doi:10.1126/science.1241779
van Overbeek, M., and de Lange, T. (2006). Apollo, an Artemis-related nuclease, interacts with TRF2 and protects human telomeres in S phase. Curr. Biol. 16, 1295–1302. doi:10.1016/j.cub.2006.05.022
Wallweber, G., Gryaznov, S., Pongracz, K., and Pruzan, R. (2003). Interaction of human telomerase with its primer substrate. Biochemistry 42, 589–600. doi:10.1021/bi026914a
Wang, H., Ma, T., Zhang, X., Chen, W., Lan, Y., Kuang, G., et al. (2023). CTC1 OB-B interaction with TPP1 terminates telomerase and prevents telomere overextension. Nucleic Acids Res. 51, 4914–4928. doi:10.1093/nar/gkad237
Wellinger, R. J. (2014). In the end, what’s the problem. Mol. Cell 53, 855–856. doi:10.1016/j.molcel.2014.03.008
Wright, W. D., Shah, S. S., and Heyer, W. D. (2018). Homologous recombination and the repair of DNA double-strand breaks. J. Biol. Chem. 293, 10524–10535. doi:10.1074/jbc.TM118.000372
Wu, P., Takai, H., and de Lange, T. (2012). Telomeric 3’ overhangs derive from resection by Exo1 and Apollo and fill-in by POT1b-associated CST. Cell 150, 39–52. doi:10.1016/j.cell.2012.05.026
Wu, P., van Overbeek, M., Rooney, S., and de Lange, T. (2010). Apollo contributes to G overhang maintenance and protects leading-end telomeres. Mol. Cell 39, 606–617. doi:10.1016/j.molcel.2010.06.031
Wyatt, H. D., West, S. C., and Beattie, T. L. (2010). InTERTpreting telomerase structure and function. Nucleic Acids Res. 38, 5609–5622. doi:10.1093/nar/gkq370
Xin, H., Liu, D., and Songyang, Z. (2008). The telosome/shelterin complex and its functions. Genome Biol. 9, 232. doi:10.1186/gb-2008-9-9-232
Xu, F., Zhang, Q., Zhang, K., Xie, W., and Grunstein, M. (2007). Sir2 deacetylates histone H3 lysine 56 to regulate telomeric heterochromatin structure in yeast. Mol. Cell 27, 890–900. doi:10.1016/j.molcel.2007.07.021
Xu, M., Kiselar, J., Whited, T. L., Hernandez-Sanchez, W., and Taylor, D. J. (2019). POT1-TPP1 differentially regulates telomerase via POT1 His266 and as a function of single-stranded telomere DNA length. Proc. Natl. Acad. Sci. U. S. A. 116, 23527–23533. doi:10.1073/pnas.1905381116
Xu, Z., Green, B., Benoit, N., Sobel, J. D., Schatz, M. C., Wheelan, S., et al. (2021). Cell wall protein variation, break-induced replication, and subtelomere dynamics in Candida glabrata. Mol. Microbiol. 116, 260–276. doi:10.1111/mmi.14707
Yang, X., Figueiredo, L. M., Espinal, A., Okubo, E., and Li, B. (2009). RAP1 is essential for silencing telomeric variant surface glycoprotein genes in Trypanosoma brucei. Cell 137, 99–109. doi:10.1016/j.cell.2009.01.037
Ye, J. Z., Donigian, J. R., van Overbeek, M., Loayza, D., Luo, Y., Krutchinsky, A. N., et al. (2004a). TIN2 binds TRF1 and TRF2 simultaneously and stabilizes the TRF2 complex on telomeres. J. Biol. Chem. 279, 47264–47271. doi:10.1074/jbc.M409047200
Ye, J. Z., Hockemeyer, D., Krutchinsky, A. N., Loayza, D., Hooper, S. M., Chait, B. T., et al. (2004b). POT1-interacting protein PIP1: a telomere length regulator that recruits POT1 to the TIN2/TRF1 complex. Genes Dev. 18, 1649–1654. doi:10.1101/gad.1215404
Zade, N. H., and Khattar, E. (2023). POT1 mutations cause differential effects on telomere length leading to opposing disease phenotypes. J. Cell Physiol. 238, 1237–1255. doi:10.1002/jcp.31034
Zaug, A. J., Goodrich, K. J., Song, J. J., Sullivan, A. E., and Cech, T. R. (2022). Reconstitution of a telomeric replicon organized by CST. Nature 608, 819–825. doi:10.1038/s41586-022-04930-8
Zaug, A. J., Lim, C. J., Olson, C. L., Carilli, M. T., Goodrich, K. J., Wuttke, D. S., et al. (2021). CST does not evict elongating telomerase but prevents initiation by ssDNA binding. Nucleic Acids Res. 49, 11653–11665. doi:10.1093/nar/gkab942
Zeng, W., Ball, A. R., and Yokomori, K. (2010). HP1: heterochromatin binding proteins working the genome. Epigenetics 5, 287–292. doi:10.4161/epi.5.4.11683
Zhang, J. M., and Zou, L. (2020). Alternative lengthening of telomeres: from molecular mechanisms to therapeutic outlooks. Cell Biosci. 10, 30. doi:10.1186/s13578-020-00391-6
Zhang, T., Zhang, Z., Shengzhao, G., Li, X., Liu, H., and Zhao, Y. (2019a). Strand break-induced replication fork collapse leads to C-circles, C-overhangs and telomeric recombination. PLoS Genet. 15, e1007925. doi:10.1371/journal.pgen.1007925
Zhang, X., Alexander, N., Leonardi, I., Mason, C., Kirkman, L. A., and Deitsch, K. W. (2019b). Rapid antigen diversification through mitotic recombination in the human malaria parasite Plasmodium falciparum. PLoS Biol. 17, e3000271. doi:10.1371/journal.pbio.3000271
Zhang, Y., Shin, S. J., Liu, D., Ivanova, E., Foerster, F., Ying, H., et al. (2013). ZNF365 promotes stability of fragile sites and telomeres. Cancer Discov. 3, 798–811. doi:10.1158/2159-8290.CD-12-0536
Zhong, F. L., Batista, L. F., Freund, A., Pech, M. F., Venteicher, A. S., and Artandi, S. E. (2012). TPP1 OB-fold domain controls telomere maintenance by recruiting telomerase to chromosome ends. Cell 150, 481–494. doi:10.1016/j.cell.2012.07.012
Keywords: telomere, telomere end processing, PolIE, telomerase, Trypanosoma brucei, antigenic variation, telomeric and subtelomeric DNA recombination
Citation: Li B (2023) Telomere maintenance in African trypanosomes. Front. Mol. Biosci. 10:1302557. doi: 10.3389/fmolb.2023.1302557
Received: 26 September 2023; Accepted: 15 November 2023;
Published: 24 November 2023.
Edited by:
Sunanda Bhattacharyya, University of Hyderabad, IndiaReviewed by:
Arthur J. Lustig, Tulane University, United StatesJean-Baptiste Boulé, INSERM U1154 Structure et Instabilité des Génomes, France
Copyright © 2023 Li. This is an open-access article distributed under the terms of the Creative Commons Attribution License (CC BY). The use, distribution or reproduction in other forums is permitted, provided the original author(s) and the copyright owner(s) are credited and that the original publication in this journal is cited, in accordance with accepted academic practice. No use, distribution or reproduction is permitted which does not comply with these terms.
*Correspondence: Bibo Li, b.li37@csuohio.edu