Corrigendum: Mechanotransductive receptor Piezo1 as a promising target in the treatment of fibrosis diseases
- 1Department of Emergency Medicine, The Second Affiliated Hospital of Nanchang University, Nanchang, China
- 2The Second Affiliated Hospital of Nanchang University, The Second Clinical Medical College of Nanchang University, Nanchang, China
- 3Department of Ultrasound Medicine, The Second Affiliated Hospital of Nanchang University, Nanchang, China
- 4Department of Anesthesiology, The Second Affiliated Hospital of Nanchang University, Nanchang, China
Fibrosis could happen in every organ, leading to organic malfunction and even organ failure, which poses a serious threat to global health. Early treatment of fibrosis has been reported to be the turning point, therefore, exploring potential correlates in the pathogenesis of fibrosis and how to reverse fibrosis has become a pressing issue. As a mechanism-sensitive cationic calcium channel, Piezo1 turns on in response to changes in the lipid bilayer of the plasma membrane. Piezo1 exerts multiple biological roles, including inhibition of inflammation, cytoskeletal stabilization, epithelial-mesenchymal transition, stromal stiffness, and immune cell mechanotransduction, interestingly enough. These processes are closely associated with the development of fibrotic diseases. Recent studies have shown that deletion or knockdown of Piezo1 attenuates the onset of fibrosis. Therefore, in this paper we comprehensively describe the biology of this gene, focusing on its potential relevance in pulmonary fibrosis, renal fibrosis, pancreatic fibrosis, and cardiac fibrosis diseases, except for the role of drugs (agonists), increased intracellular calcium and mechanical stress using this gene in alleviating fibrosis.
1 Introduction
1.1 Fibrosis
The intricate balance between tissue repair and remodeling is disrupted in fibrosis, a pathological condition characterized by the aberrant accumulation of fibrous connective tissue within organs or tissues. This process, driven by a cascade of molecular events triggered by injury, inflammation, or underlying diseases, culminates in the excessive deposition of collagen and an altered extracellular matrix (ECM) composition (Wen D. et al., 2022). The lungs, liver, kidneys, and heart are among the organs susceptible to fibrotic transformations, with persistent injury perpetuating a cycle of escalating fibrogenesis, ultimately leading to compromised organ function and a continuum of deleterious consequences.
In the physiological processes of an organism, to maintain the normal functioning of tissues and organs, it is mandatory to ensure an appropriate reparative response, and fibrosis is considered to be a reparative response that restores the organ structure by replacing the destroyed tissues (Henderson et al., 2020; Wen J. H. et al., 2022). However, if this repair response is uncontrolled or over-activated, it can lead to pathological states such as organ fibrosis and abnormal function (Wen J. H. et al., 2022). Therefore, fibrosis is a pathological condition characterized by parenchymal cell necrosis as well as an unusual amount of hyperplasia and hyper-deposition of the extracellular matrix (Antar et al., 2023).
Fibrosis can develop in multiple organs and often occurs in the end stages of the disease. In the lung, fibrotic diseases include pneumoconiosis (Qi et al., 2021) and silicosis (Zhao Y. et al., 2022), whose etiology is known, and idiopathic pulmonary fibrosis (Cottin et al., 2019; Somogyi et al., 2019), whose etiology is not yet known. Pulmonary fibrosis is commonly the end stage of chronic lung diseases, such as silicosis (Handra et al., 2023) and idiopathic pulmonary fibrosis (Heukels et al., 2019) mentioned above. In chronic lung diseases, lung tissue will be progressively replaced by scar tissue, causing difficulty in breathing, and may eventually cause respiratory failure. Chronic liver diseases, such as chronic hepatitis B (Stalla et al., 2022), hepatitis C (Sebastiani et al., 2014), and alcoholic liver disease (Lackner and Tiniakos, 2019), are often accompanied by liver fibrosis at the end stage of the disease, eventually leading to severe damage to liver function and symptoms such as jaundice and hepatic ascites (Mansour and McPherson, 2018). Cardiac fibrosis is often the end stage of heart failure, prolonged myocardial damage can lead to fibrosis of myocardial tissue (González et al., 2018; Bacmeister et al., 2019). Similarly, chronic kidney disease is one of the common causes of renal fibrosis, prolonged damage to nephrons and glomeruli will gradually lead to fibrosis of the kidneys (Rayego-Mateos and Valdivielso, 2020; Panizo et al., 2021). Pancreatic fibrosis is a disease closely related to chronic pancreatitis. In patients with chronic pancreatitis, pancreatic tissue is gradually damaged, and pancreatic fibrosis is a manifestation of advanced pancreatitis (Shimizu, 2008; Swain et al., 2022).
In addition to the above-mentioned organs, fibrosis also often occurs in the skin (Andrews et al., 2016), bones and muscles (Mahdy, 2019), gastrointestinal tract (Wang J. et al., 2021), and other organs. In this review, we are focusing on Piezo1 and its potential contribution to the pathophysiology of pulmonary fibrosis, renal fibrosis, pancreatic fibrosis, and cardiac fibrosis diseases.
1.2 Introduction of Piezo1
Using stress-sensitive cells, Prof. Ardem Patapoutian uncovered a new sensor that is capable of responding to mechanical irritation in the skin and visceral organs (Dubin and Patapoutian, 2010; Kefauver et al., 2020). Thus, a new and completely unknown mechanosensitive ion channel, Piezo1, was discovered, followed by a second related gene, Piezo2 (Coste et al., 2010). Piezo proteins are a combination of Piezo1 and Piezo2. Piezo1 is a mechanosensitive cation channel protein situated on the membrane of cells and is a pivotal cytomechanical sensor that converts mechanical stimulation into galvanic signaling (Coste et al., 2010).
Piezo1 is a protein that can be engaged in the process of mechanosensation and mechanical force transformation. It forms ion channels on the cell surface and can perceive and react to mechanical stimulation around the cell (Huang et al., 2023). As mentioned above, Piezo1 channels perform an essential function in several physiological processes, including cell migration (Holt et al., 2021; Yu et al., 2021), vascular smooth muscle cell contraction (Chen et al., 2022a; Chen et al., 2022b; Porto Ribeiro et al., 2022), red blood cell morphology changes (Cahalan et al., 2015; Svetina et al., 2019), and sensory neuron perception of touch and pressure (Coste et al., 2010). In addition to the perception of mechanical stimuli, Piezo1 is engaged in the modulation of a wide range of cellular functions. For example, it regulates stem cell fate determination (Sugimoto et al., 2017; Qiu et al., 2023), cell proliferation and differentiation (He et al., 2018), skeletal muscle development and repair (Bernareggi et al., 2022), vascular endothelial cell permeability (Friedrich et al., 2019), and tumor cell invasion and metastasis (Jiang et al., 2022). It has also been found that Piezo1 mutations are also associated with several diseases, such as congenital erythrocytosis (Knight et al., 2019; Filser et al., 2021; Sochorcova et al., 2023) and familial pulmonary hypertension (Wang Z. et al., 2021; Liao et al., 2021; Porto Ribeiro et al., 2022). Currently, there are also a large number of studies that have identified a potential relationship between Piezo1 and fibrotic diseases (Zhang et al., 2021a; Braidotti et al., 2022a; He et al., 2022a; Swain et al., 2022).
In conclusion, Piezo1 is an important protein that has a critical role in mechanical force perception and regulation of cellular functions. Further studies are needed to gain insight into its specific role and regulatory mechanisms in physiological and pathological processes, which will not only facilitate our understanding of the mechanosensory mechanisms of Piezo1 but more importantly, can provide new methodologies to develop treatments for associated disorders.
1.3 Piezo1 and fibrosis
We all know that the main pathological changes in fibrosis are increased synthesis and insufficient degradation of extracellular matrix and that persistent fibrosis leads to structural destruction and functional decay of organs, but the mechanisms behind many fibrotic diseases are not yet understood by us. Several experiments now suggest that Piezo1 may have a potential relationship with fibrosis (Zhang et al., 2021a; He et al., 2021; Bartoli et al., 2022; Braidotti et al., 2022a; Zhao X. et al., 2022; Fang et al., 2022; Swain et al., 2022; Xing et al., 2023). In the pathological state, activation of Piezo1 channels by mechanical stimuli induces excessive ECM synthesis in the cells involved, leading to ECM deposition and promoting the progress of fibrosis. It was found that aberrant exposure of Piezo1 can be observed in fibrotic tissues and organs.
One investigator created a genetically engineered mouse model (He et al., 2022a) that specifically knocked out Piezo1 from bone marrow cells, intending to study the mechanism of the mechanosensitive protein Piezo1 in renal fibrosis, and finally found that mice with Piezo1 knockout alleviates renal fibrosis, suggesting that the development of targeting Piezo1 mechanical channels offers a possible approach to the management of renal fibrosis (He et al., 2022a; Zhao X. et al., 2022). In the pancreas, a hypertensive condition stimulates the opening of Piezo1 channels and the formation of fibrosis induced by stress (Swain et al., 2022). As for cardiomyocytes, experiments have identified a stress response after a myocardial injury that leads to the upregulation of Piezo1, which may be responsible for the positive feedback of fibrosis progression (Braidotti et al., 2022a). Experimental studies have demonstrated that Piezo1 has an active role in ARDS-associated pulmonary fibrosis exacerbated by mechanical stretch (MV) via mediation of calcium inward flow as well as ATP emission (Fang et al., 2022). Activation of Piezo1 channels can influence a range of signal pathways that play an important role in the progression of fibrotic disease (He et al., 2022a). For example, activation of Piezo1 can lead to calcium inward flow, which activates signal pathways such as TGF-β/Smad and p38-MAPK, which perform key functions in the onset and progression of fibrosis (Ding et al., 2021).
2 Structure and characteristics of Piezo1
Piezo1 and Piezo2 constitute the 2 major mechanically-activated (MA) channels identified in mammals. The Piezo1 protein was initially identified in mice (Coste et al., 2010). By comparison, the Piezo1 gene was found to be homologous in humans (Schrenk-Siemens et al., 2015), mice (Ikeda et al., 2014), chickens (Soattin et al., 2016), birds (Schneider et al., 2014), drosophila (He et al., 2018), African clawed frog meadowlark (Methfessel C Fau - Witzemann et al., 1986), and zebrafish (Faucherre et al., 2013). Piezo1 is broadly expressed in several human organs and tissues, encompassing vital organs such as the lungs (Xiong et al., 2022), the gastrointestinal system (Yang et al., 2022), and the skeleton (Qin et al., 2021; Xu et al., 2021), which strongly suggests that Piezo1 may have a critical function in the normal functioning of these organs, such as in respiration, digestion, and locomotion (Qin et al., 2021; Xu et al., 2021; Xiong et al., 2022; Yang et al., 2022). Structural similarities between mouse and human Piezo1 channels were observed by cryo-electron microscopy, providing a basis for further functional studies (Wang and Xiao, 2018; Xiao, 2020). Piezo1 and Piezo2 are respectively positioned on chromosome 16 and chromosome 18. In the human body, Piezo1 is comprised of 2,520 amino acids and Piezo2 is comprised of 2,752 amino acids (Gottlieb and Sachs, 2012).
The mechanosensitivity of Piezo1 channels is explained by a lever-like mechanism of mechanical action based on a unique three-leaf propeller-like homologous structure (Bae et al., 2015; Jiang et al., 2021a). The basic structure of the Piezo1 channel consists of multiple repeating structural domains, which include an N-terminal region, a membrane domain, and a C-terminal region (Kefauver et al., 2020; Fang et al., 2021; Tang et al., 2022). Based on the structure and function of the Piezo1 protein, some researchers have divided it into an ion-conducting pore portion, an anchor that acts as a conversion element: the CTD and bundles, and a mechanosensing portion consisting of the TM blades (Zhao et al., 2019). The channel can be in three active states: closed, open, and inactivated (Cox and Gottlieb, 2019). A mechanical stimulus acting on the cell membrane triggers the Piezo1 channel to shift from a closed state to an open state, allowing the flow of ions, such as calcium, potassium, and sodium ions (Gottlieb and Sachs, 2012).
The interaction of Piezo1 with the cytoskeleton in mechanosensing has been described in detail (Nourse and Pathak, 2017; Jiang et al., 2021b). The overexpression of Piezo1 channels in cells is characterized by rapid and complete inactivation, described as a pressure pulse in a split second (Coste et al., 2010; Wu et al., 2017), this character has also emerged as a signature of the Piezo1 channel. The structure of the Piezo1 channel facilitates our understanding of its mechanism in sensing mechanical stimuli and regulating the permeability of ion channels.
As an important force-sensitive channel, Piezo1 plays multiple physiological functions in cells. First, it plays a key role in maintaining the shape of red blood cells (Vaisey et al., 2022). By sensing extracellular mechanical forces, Piezo1 can regulate the morphology of the cell membrane and ensure the adaptability and functionality of red blood cells (Vaisey et al., 2022; Evtugina et al., 2023; Hatem et al., 2023). Secondly, Piezo1 is involved in the regulation of immune responses (Atcha et al., 2021a; Lai et al., 2022). The opening of its channels can trigger intracellular signal transmission, thereby affecting the activity of immune cells, which is crucial for maintaining the balance of the immune system (Solis et al., 2019a; Aykut et al., 2020; Atcha et al., 2021a; Geng et al., 2021; Leng et al., 2022). In addition, Piezo1 is also involved in the functional regulation of the cardiovascular system (Li et al., 2014; Douguet et al., 2019), and its channel activity is closely related to pathological conditions such as arrhythmia (Jiang F. et al., 2021; Rolland et al., 2023), suggesting that it plays an important role in cardiovascular biology.
One of the main functions of Piezo1 is to sense and respond to mechanical stimulation. The opening of its channel will lead to an increase in intracellular calcium ion concentration, thereby triggering multiple signaling pathways. This process not only affects the biological effects of cells, such as cell apoptosis, proliferation, and migration (Volkers et al., 2015; Liu S. et al., 2021; Dombroski et al., 2021; Shinge et al., 2022; Song et al., 2022). Piezo1 can also activate the protein kinase pathway and further regulate the activity of multiple cell signaling pathways (Blythe et al., 2019; Liu S. et al., 2021; Chen S. et al., 2022; Wang et al., 2022). In addition, Piezo1 can also regulate the activity of Na, and K-ATPase, further affecting intracellular ion balance and cell membrane stability (Shahidullah et al., 2022; Hirata et al., 2023). Recently, Shahidullah M and his colleagues studied the relationship between Piezo1 and Na, K-ATPase-mediated ion transport in mouse crystals. They found that after activation of Piezo1, Na, K-ATPase in cells will be affected (Shahidullah et al., 2022).
Therefore, Piezo1 has a variety of key physiological functions in cells. Its research will not only help to gain a deeper understanding of the basic mechanisms of cell biology but may also provide new therapeutic targets for the treatment of related diseases. Therefore, the function and regulatory mechanism of Piezo1 deserve further in-depth study.
3 A new hope for fibrosis diseases: Piezo1
3.1 Piezo1 and pulmonary fibrosis
Pulmonary fibrosis (PF) is a diffuse interstitial pulmonary disease featuring progressive inflammation and extracellular matrix deposition, resulting in irreversible damage caused by abnormal lung tissue repair (Thannickal et al., 2004; Henderson et al., 2020; Zhao Y. et al., 2022).
Several studies have demonstrated a strong relationship between epithelial-mesenchymal transition (EMT) with fibrosis (Qian et al., 2018; Rout-Pitt et al., 2018; Salton et al., 2019; Liu et al., 2022). Transforming growth factor beta (TGF-β) is thought to be closely associated with early embryonic development and organogenesis, and adult homeostasis (Xu et al., 2018), TGF-β overexpression can lead to excessive metabolic disorders and dysfunction, promoting EMT and ECM deposition (Su et al., 2020; Lee and Massagué, 2022), leading to fibrosis and cancer development (Hao et al., 2019; Andugulapati et al., 2020; Kim et al., 2020). Piezo1 is a mechanosensitive calcium channel, and immunohistochemical staining revealed widespread Piezo1 expression in mouse pulmonary tissues (Zhang Y. A. et al., 2021), epithelial cells, and endothelial cells (Zhong et al., 2018; Friedrich et al., 2019; Bhattacharya and Hough, 2019), and was suggested to play an important role in bleomycin-induced pulmonary fibrosis (Solis et al., 2019a; Solis et al., 2019b).
Jia-Qi Huang and his colleagues discovered through cell line studies and cell culture of rat lung cells that a positive response mechanism for the relationship of Piezo1 to TGF-β1 was found to exist in radiation-induced pulmonary fibrosis (Huang et al., 2021a) and has a critical role in the radiation-induced generation of EMT. It was found that upregulation of TGF-β1 was associated with the activation of Piezo1, some researchers have found through cell line studies (Lei et al., 2019; Huang Y. et al., 2021) and animal studies (Lei et al., 2019) that the Ca2+/HIF-1α signaling pathway can activate TGF-β1, and Piezo1 induced EMT by regulating TGF-β1 through the Ca2+/HIF-1α signaling pathway (Lei et al., 2019; Huang et al., 2021a; Huang Y. et al., 2021). TGF-β1 was able to inhibit C/EBPβ expression (Ramji and Foka, 2002), and C/EBPβ acts on the Piezo1 promoter to reduce the expression of Piezo1 (Huang et al., 2021a; Ghafouri-Fard et al., 2021). Research has also revealed that TGF-β acts through the smad3 signaling pathway to inhibit C/EBPβ on the expression of the Piezo1 promoter, resulting in upregulation of Piezo1 expression (Huang et al., 2021c).
Mechanical ventilation is essential in the treatment of some critical patients with respiratory illnesses, including acute respiratory distress syndrome (ARDS) (Walter et al., 2018; Pelosi et al., 2021; Shi et al., 2023). As mentioned previously, Piezo1 is strongly observed in both normal pulmonary epithelial cells and pulmonary endothelial cells (Bhattacharya and Hough, 2019; Shi et al., 2023). Classification of alveolar epithelial cells into type I (AT I) and type II(AT II). Caveolae are expressed in type I alveolar epithelium (Wicher et al., 2019; Jones and Minshall, 2020), and caveolae were found to be mechanosensory in the alveoli (Thompson et al., 2014; Wicher et al., 2019), stretch-induced Ca2+ signaling is dependent on Ca2+ entry through Piezo1 channels, allowing AT I cells to release ATP, resulting in the regulation of surfactant secretion in AT II cells (Diem et al., 2020; Lin et al., 2022).
Some researchers have found through animal trials (Zhang et al., 2021c) and cell line trials (Diem et al., 2020; He J. et al., 2022) that mechanical stretch can significantly induce Piezo1 activation in epithelial cells (Diem et al., 2020; Zhang et al., 2021c; He J. et al., 2022). Piezo1 can induce ATP release during the mechanical stretch, and the released ATP can, in turn, drive mechanical stretch to enhance EMT, thus exacerbating pulmonary fibrosis (Diem et al., 2020; Fang et al., 2022), and leading to more severe pulmonary fibrosis in ARDS during ventilation. Although Piezo1-mediated ATP release is essential in the exacerbation of pulmonary fibrosis by mechanical stretch (Miyamoto et al., 2014; Diem et al., 2020), the association of ATP with EMT and pulmonary fibrosis remains to be investigated.
When mechanical stretching was performed on pulmonary epithelial and endothelial cells, the extent of the injury was directly related to the duration of mechanical stretching, and the expression of Piezo1 was also proportional to it, indicating an association between Piezo1 and respiratory lung injury (Zhang Y. A. et al., 2021). After excessive mechanical stretching of the lung endothelium, Ca2+ inward flow activates Piezo1 channels and the adhesion junctions between endothelial cells are disrupted (Friedrich et al., 2019; Zhong et al., 2020; Jiang et al., 2021b). Using a mouse model induced by hyper-tidal volume mechanical ventilation (Zhang et al., 2021c), Yang Zhang and members of his experiments demonstrated that Piezo1 functions in the pathological processes in the epithelial cells of the lung in ventilator-induced pulmonary damage by activating the RhoA/ROCK1 pathway (Zhang Y. A. et al., 2021). In conclusion, Piezo1 performs a crucial function in lung injury due to mechanical stretch (MV).
When understanding the current relationship between Piezo1 and pulmonary fibrosis, we can find that in ATⅠ, ATⅠ-expressed caveolae can respond to mechanical signals through plasma membrane invagination, caveolae act as a mechanical sensor of Piezo1, Ca2+ inward flow activates pannexin-1 hemichannel to enter and localize to caveolae, acting on ATⅠ to release ATP (Diem et al., 2020); as shown in the Figure 1, among ATⅡ cells, after the mechanical signal activates Piezo1, calcium ion inward flow enters the cell, Piezo1 regulates TGF-β1 expression through Ca2+/HIF-1α signaling pathway, so that TGF-β1 expression is upregulated (Huang et al., 2021c; Zhang et al., 2022), and the upregulation of TGF-β1 can be activated through MAPK and smad-dependent signaling pathway (Guo et al., 2021) on the one hand EMT, which promotes lung fibrosis, and on the other hand, it may suppress the expression of C/EBPβ by the Smad3 pathway (Feinberg et al., 2004; Lourenço et al., 2020), thus inhibiting C/EBPβ from acting on the Piezo1 promoter and causing Piezo1 to be upregulated as well (Huang et al., 2021c).
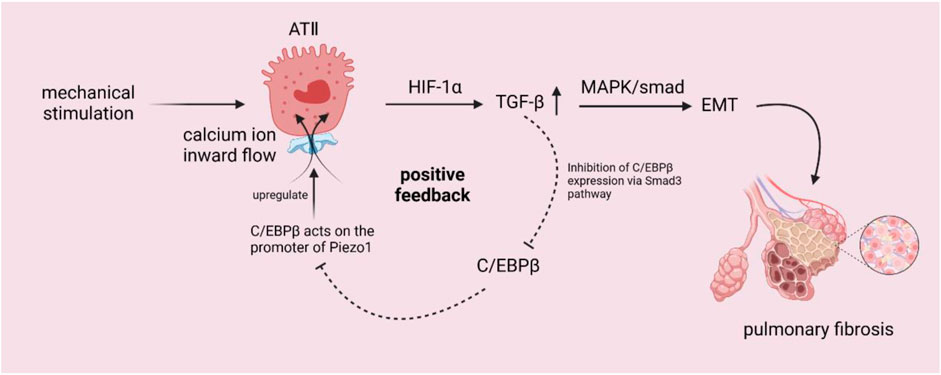
Figure 1. Schematic diagram of the mechanism by which mechanical stimulation of ATII cells activates Piezo1 channels to trigger related pathways. After activation of Piezo1 by mechanical signals, calcium ions inwardly flowed into the cells, and Piezo1 regulated TGF-β1 through the Ca2+/HIF-1α signaling pathway, leading to upregulation of TGF-β1. The upregulation of TGF-β1 could on the one hand activate the EMT through the MAPK and smad-dependent signaling pathways to promote lung fibrosis, on the other hand, and might inhibit C/EBPβ by the Smad3 pathway, which could inhibit the effect of C/EBPβ on the promoter of Piezo1, and result in the upregulation of Piezo1’s expression as well.
In addition, in animal trials on lung injury caused by mechanical ventilation in rats (Zhang et al., 2021c), mechanical ventilation can also stimulate Piezo1 channel activation, convert mechanical signals into biological signals, calcium ion inward flow, and elevated calcium in alveolar epithelial cells, leading to downregulation of non-apoptotic cytokine Bcl-2 expression (Liang et al., 2019) and alveolar cell necrosis. Piezo1 is also an upstream modulator of the RhoA/Rock1 pathway, activating this signaling pathway and inducing the onset of pulmonary fibrosis (Zhang et al., 2021c). In contrast, in respiratory lung injury secondary to ARDS, Piezo in the lung endothelium is activated by mechanical signaling and calcium ions flow inward, leading to disruption of the adhesion junctions (AJs) between endothelial cells and resulting in damage to the lung endothelial barrier (Liang et al., 2019; Jiang et al., 2021c).
Piezo1 is an ion channel protein widely expressed in various tissues and cell types, and its role in various disease processes has attracted much attention. Piezo1 is widely expressed in lung tissue, epithelial cells, and endothelial cells, and interacts with the TGF-β1 signaling pathway. In pulmonary fibrosis, the upregulation of Piezo1 is an important event, which may serve as a response to mechanical stimuli and play a key role in the occurrence and progression of fibrosis. Mechanical stretch activates Piezo1, leading to Ca2+ influx, activating the Ca2+/HIF-1α signaling pathway of TGF-β1, inducing epithelial-mesenchymal transition (EMT), and promoting pulmonary fibrosis. TGF-β1 also inhibits C/EBPβ through the smad3 signaling pathway, thereby upregulating the expression of Piezo1. Therefore, the increase in Piezo1 is accompanied by pulmonary fibrosis and further promotes the occurrence of fibrosis.
3.2 Piezo1 and renal fibrosis
Renal fibrosis is an irreversible pathology of long-term kidney disease and end-stage renal disease, manifested by improved production and insufficient breakdown of ECM within the renal tubules (Black et al., 2019; Bülow and Boor, 2019; Liang et al., 2022). Piezo1 which is a mechanosensitive cation channel (Coste et al., 2010) senses the stiffness from the external environment and converts mechanical signals into intracellular electrochemical signals (Lewis and Grandl, 2015; Kefauver et al., 2020; Xu et al., 2021). Piezo1 is expressed in endothelial and mural cells, proximal and distal curvilinear tubules of the renal vesicle (Peyronnet et al., 2013; Martins et al., 2016; Dalghi et al., 2019). Increased ECM synthesis and sclerosis of the cellular environment may exacerbate renal fibrosis (Chen et al., 2014; Imamura et al., 2018). One study using an animal model found that increased ECM synthesis and sclerosis can activate Piezo1 and exacerbate kidney fibrosis by the Piezo1-p38MAPK-YAP signaling pathway (Fu et al., 2021).
Macrophages have an essential function in renal fibrosis, and macrophages can transmit information to cells by sensing mechanical signals (Wei et al., 2022; Wu et al., 2022). It has been suggested that macrophages are multifunctional cells that possess pro- and anti-fibrotic effects (Wynn and Vannella, 2016; Tang et al., 2019). In the unilateral ureteral obstruction (UUO) model (Lee et al., 2023), Piezo1 deletion was observed followed by a crucial reduction in the CCL2-CCR2 signaling pathway and Notch pathway (He et al., 2022a), which inhibited the inflammation of macrophages and the progression of renal fibrosis. Macrophages are classified into M1 type (pro-fibrotic) and M2 type (anti-fibrotic) (Nishida et al., 2005). Piezo1 can activate the CCL2-CCR2 pathway via Notch, causing macrophage aggregation to trigger inflammation and thereby mediating ECM deposition and renal fibrosis (He et al., 2022a).
Recent research revealed that Piezo1 expressed markedly elevated in fibrotic kidneys, and treatment of the UUO model with GsMTx4, a blocker of Piezo1 (Velasco-Estevez et al., 2020), revealed a significant attenuation of renal fibrosis, indicating that Piezo1 has an essential function in renal fibrosis (Zhao X. et al., 2022). In addition, it has been found that mechanical stretch stimulation of Piezo1 induced fibrosis in human renal cortical proximal tubular epithelial cells (HK2 cells) (Zhang et al., 2021; Zhao X. et al., 2022) and primary cultured mouse proximal tubule cells (mptc) (Zhao X. et al., 2022), while inhibition of Piezo1 inhibited fibrosis through blocking the TGF-β1 signaling pathway, which suggests the role of Piezo1 in the fibrosis of renal tubular epithelial cells caused by mechanical stretch.
As is known, TGF-β1 is an important marker of EMT, but several studies have found no strong correlation between EMT and renal fibrosis in vivo (Galichon et al., 2013; Sheng and Zhuang, 2020). It has been suggested that TGF-β1 damages renal tubules through the smad signaling pathway, resulting in inadequate deposition and degradation of ECM, leading to renal fibrosis (Hu et al., 2018; Gifford et al., 2021).
As shown in Figure 2, the mechanical signal or activator of Piezo1, Yoda1, acted on HK2 cells and mptc, activated cellular piezo1 channels, TGF-β1 induced upregulation of fibronectin and α-SMA (Zhao X. et al., 2022), which increased ECM synthesis and also inhibited ECM degradation. The mechanical signal was delivered to ECM with calcium inward flow, activation of calpain2, which signals downstream of Piezo1, induces talin1 clearance and upper-regulation of integrin β1 protein (Bate et al., 2012; Zhao X. et al., 2022), and integrin and ECM bind more tightly and induce the development of renal fibrosis. When ECM stiffness increases, it may activate Yes-associated protein (YAP) (Dupont et al., 2011; Calvo et al., 2013), which acts as a transcription factor of the Hippo signaling pathway mechanically regulated by ECM stiffness. When Piezo1 is activated, a large amount of calcium ions inward flow may activate the P38-MAPK molecule, and P38-MAPK reactivates YAP, and YAP induces ECM deposition and promotes the process of renal fibrosis (Fu et al., 2021).
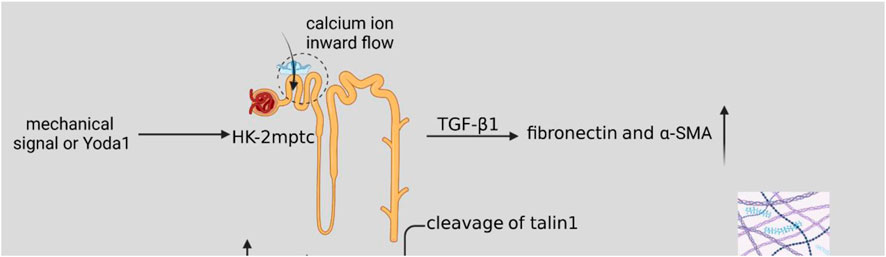
Figure 2. Schematic diagram of the Piezo1-related pathway mechanisms in renal fibrosis. Mechanical signaling or Yoda1 activates cellular Piezo1 channels after acting on HK2 cells and mptc. TGF-β1 induces upregulation of fibronectin and α-SMA, resulting in increased ECM synthesis. Activation of calpain2, which signals downstream of Piezo1, induces talin1 clearance and upper-regulation of integrin β1 protein, and increased ECM stiffness. A large number of calcium ions inward flow may activate P38-MAPK molecules, P38-MAPK then activates YAP, and YAP induces ECM deposition, which promotes the process of renal fibrosis.
Piezo1 is expressed in renal tubular and renal capsule endothelial cells and parietal cells. Upregulation of Piezo1 in renal fibrosis also occurs during fibrosis. Mechanical stretch or Piezo1 activators can lead to an increase in intracellular calcium ions, increased expression of TGF-β1, and promote ECM synthesis and renal fibrosis. It is worth mentioning that although TGF-β1 is an important marker of EMT, some studies have not found a strong correlation between EMT and renal fibrosis in vivo, indicating that Piezo1 may have a more complex role in renal fibrosis.
3.3 Piezo1 and pancreatic fibrosis
The pancreas is sensitive to mechanical injury (Romac et al., 2018), and pressure on the gland may lead to the development of pancreatitis (Wang et al., 2009; Romac et al., 2018; Swain et al., 2020; Swain et al., 2020) and fibrosis (Swain et al., 2022), so the pancreas can sense mechanical tension. When the pancreas is subjected to external mechanical injury, Piezo1 pathologically opens continuously, calcium ions flow in a large amount, intracellular calcium ion homeostasis is disrupted, and intracellular zymogen and lysosomal particles in the pancreatic follicle cells react abnormally, and lead to pancreatitis (Geokas et al., 1985; Tenner et al., 2013; Mayerle et al., 2019). Pancreatic fibrosis increases the risk of pancreatic cancer, and studies in recent years show that the progression from pancreatitis to pancreatic cancer may be interspersed with pancreatic fibrosis (Cannon et al., 2021; Huang et al., 2021). Excessive deposition of ECM produced primarily by activated pancreatic stellate cells (PSCs) triggers pancreatic fibrosis (Phillips et al., 2012; Thomas and Radhakrishnan, 2019; Huang et al., 2021; Hamada et al., 2022; Swain et al., 2022). PSCs can express Piezo1 (Kuntze et al., 2020; Swain et al., 2022), intracellular calcium ion concentration increases and TGF-β1 expression increases after a mechanical pull or the Piezo1 activator yoda1 acts on PSCs, and these phenomena disappear when the Piezo1 inhibitor GsMTx4 acts, so Piezo1 is critical in stress-induced pancreatic fibrosis (Swain et al., 2022).
However, one study found that Piezo1 is a rapidly inactivating pathway (Del Mármol et al., 2018; Shi et al., 2020) and that Piezo1 only causes a transient elevation of intracellular calcium ions (Swain et al., 2020), therefore, it is presumed that other mechanisms could lead to a sustained increase in intracellular calcium ions. TRPV4 was also found to be expressed in both mouse and human pancreatic follicles (Swain et al., 2020), and in the absence of TRPV4, Piezo1 triggers insufficient calcium inward flow signaling (Swain et al., 2020; Gorelick and Nathanson, 2020; Swain et al., 2022). It has been suggested that Piezo1 stimulates PLA2, which initiates the TRPV4 pathway (Swain et al., 2020), leading to a sustained increase in intracellular calcium ions, a sustained increase in intracellular calcium ion concentration will further activate intracellular protein kinases, leading to cellular self-digestion and damage to pancreatic cells (e.g., fibrosis). In addition to this, in human and mouse models, macrophages exacerbate fibrosis (Hu et al., 2020; LaRue et al., 2022) by producing TNF-α and TGF-β1 (Xue et al., 2015), while in macrophages, the mechanical pull is engaged in the inflammatory response and fibrosis by acting on Piezo1 (Solis et al., 2019a; Atcha et al., 2021b; Atcha et al., 2021a).
When patients suffer from chronic pancreatitis, it is usually associated with pancreatic fibrosis (Shimizu, 2008; Swain et al., 2022). First, after high-pressure acts on pancreatic alveolar cells, Piezo1 channels open and calcium ions flow inward into the cells, but some experiments have found that the opening of Piezo1 channels can only trigger transient calcium ion inward flow, which is not enough to cause pancreatitis, so only after prolonged high pressure acts on alveolar cells, Piezo1 channels open, inducing PLA2 channel activation, and then inducing TRPV4 channel opening, which eventually allows a continuous inward flow of calcium ions (Romac et al., 2018; Swain et al., 2020; Gorelick and Nathanson, 2020). The high intracellular concentration of calcium ions activates trypsin and disrupts zymogen granules, leading to damage of the alveolar cells and pancreatitis, complicated by pancreatic fibrosis (Figure 3) (Hu et al., 2016).
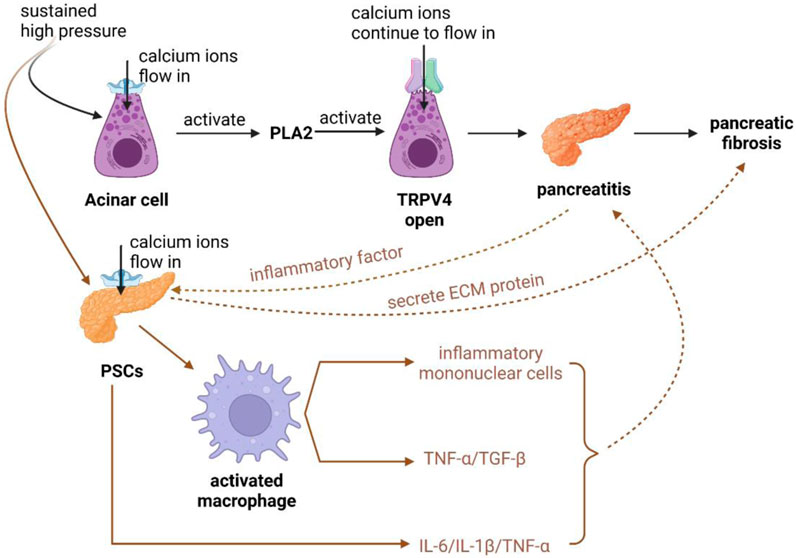
Figure 3. Schematic diagram of Piezo1 channels in pancreatic alveolar cells and PSCs cells associated with pancreatic fibrosis. (1) After prolonged high pressure is applied to the alveolar cells, the opening of Piezo1 channels activates PLA2 channels, which in turn induces the opening of TRPV4 channels, which ultimately allows for the sustained inward flow of calcium ions, causing pancreatitis with concomitant pancreatic fibrosis. (2) After the continuous action of high pressure on PSCs, PSCs were activated to secrete cellular inflammatory factors such as IL-6, IL-1β, TNF-α, etc., which could accelerate the damage of alveolar cells and lead to pancreatitis complicated by pancreatic fibrosis. Meanwhile, PSCs can activate macrophages to recruit inflammatory monocytes, and secrete TNF-α and TGF-β, which also accelerate the development of pancreatic fibrosis by promoting inflammation. (3) PSCs can secrete ECM proteins leading to pancreatic fibrosis, and after pancreatitis occurs, pancreatic alveolar cells can activate PSCs by secreting cellular inflammatory factors, accelerating the development of pancreatic fibrosis.
Pancreatic fibrosis is caused by ECM deposition proteins produced by PSCs, and at the same time, PSCs can secrete pro-inflammatory cytokines to aggravate pancreatitis complicated by fibrosis. PSCs express Piezo1, which is activated by the continuous action of high pressure on PSCs, secreting interleukin-6 (IL-6), interleukin-1β (IL-1β), tumor necrosis factor-α (TNF-α) and other cellular inflammatory factors, which can accelerate the damage of alveolar cells and lead to pancreatitis complicated by pancreatic fibrosis (Talukdar and Tandon, 2008; Piao et al., 2015; Hao et al., 2017). At the same time, PSCs can activate macrophages to recruit inflammatory monocytes. Meanwhile, PSCs can activate macrophages to recruit inflammatory monocytes (a regulator of fibrosis) and secrete tumor necrosis factor-α (TNF-α) and transforming growth factor-β (TGF-β), which likewise accelerate pancreatic fibrosis by promoting the onset of inflammation. After the onset of pancreatitis, pancreatic follicular cells can activate PSCs by secreting cytosolic inflammatory factors to accelerate the development of pancreatic fibrosis (Figure 3) (Kuntze et al., 2020; Swain et al., 2022).
In the pancreas, Piezo1 activation is triggered by external mechanical damage, leading to an abnormal increase in intracellular calcium ions and ultimately triggering pancreatic fibrosis. Thus, upregulation of Piezo1 precedes the onset of fibrosis. After pancreatic cells are mechanically damaged, the Piezo1 channel will continue to open, causing an increase in intracellular calcium ions, triggering the PLA2 pathway, and ultimately leading to the opening of the TRPV4 pathway, increasing intracellular calcium ion concentration, inducing cell self-digestion and pancreatic cell damage. Furthermore, macrophages exacerbate the development of fibrosis and pancreatitis through the production of inflammatory factors.
3.4 Piezo1 and cardiac fibrosis
When the heart is diseased, it is often accompanied by cardiac fibrosis (Frangogiannis, 2021; Bartoli et al., 2022), like heart failure (Liu M. et al., 2021; Oppedisano et al., 2021), myocardial infarction (Ma et al., 2021; Zaidi et al., 2021), and hypertension (Pinho, 2019; Siamwala et al., 2020). The key characteristic of cardiac fibrosis is ECM deposition. (Ma et al., 2018; Maruyama and Imanaka-Yoshida, 2022; Sarohi et al., 2022). Cardiac fibroblasts play a crucial part in the synthesis and metabolism of ECM. These fibroblasts secrete collagen proteins to form ECM. When pathological conditions persist, excessive ECM synthesis is induced by fibroblasts, leading to ECM deposition and subsequent cardiac fibrosis. This impairs cardiac compliance and diastolic function (Frangogiannis, 2021; Liu M. et al., 2021; Kurose, 2021; Shao et al., 2022). Additionally, under pathological conditions, fibroblasts can proliferate and differentiate into myofibroblasts (MFs), and prolonged injury can also contribute to the occurrence of cardiac fibrosis (Nagpal et al., 2016; Frangogiannis, 2019; Tarbit et al., 2019).
Studies indicated that Piezo1 is widely distributed in cardiac tissues and plays a crucial part in cardiac fibrosis. Piezo1 is expressed in cardiac fibroblasts (CF) (Stewart and Turner, 2021), and its dysregulation, either overexpression or silencing, can lead to calcium ion defects and ROS signaling dysregulation (Ma et al., 2013; Zhu et al., 2020; Jiang F. et al., 2021; Yan et al., 2022). Mechanical stimulation that activates Piezo1 channels can trigger calcium-mediated activation of calpains and calcineurin (Garcia-Dorado et al., 2012), leading to fibroblast-to-myofibroblast transition (Beech and Kalli, 2019; Xing et al., 2023).
Some studies have suggested a close relationship between Piezo1 and interleukin-6 (IL-6), which is a pro-fibrotic cytokine (Blythe et al., 2019). Thus, the activation of Piezo1 may induce fibroblast fibrosis through paracrine signaling involving IL-6 (Blythe et al., 2019; Emig et al., 2021; Malko et al., 2023). Experimental evidence has shown that Piezo1 activation can trigger calcium ion activation and promote fibroblast proliferation and differentiation into myofibroblasts, which are capable of secreting cytokines, including IL-6 (Bartoli et al., 2022; Braidotti et al., 2022a). Moreover, researchers have also found that Piezo1 activation results in increased intracellular calcium levels, subsequently activating downstream signaling pathways like p38- MAPK, resulting in elevated IL-6 levels (Figure 4) (Blythe et al., 2019; Bartoli et al., 2022; Braidotti et al., 2022a).
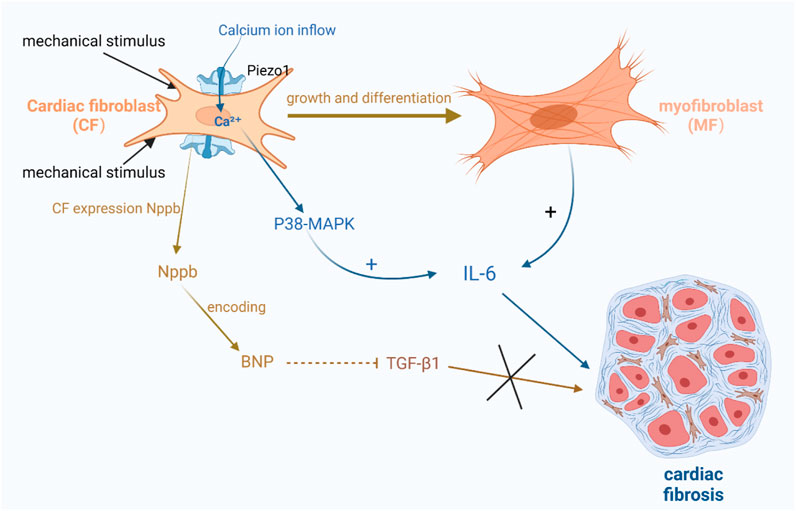
Figure 4. Schematic diagram of the mechanism by which Piezo1 channels in cardiac fibroblasts are associated with cardiac fibrosis. (1) Activation of Piezo1 triggers the activation of calcium ions and promotes fibroblasts into myofibroblasts, which are capable of secreting cytokines, including IL-6, etc. Increased calcium ions activate the downstream signaling pathway p38-MAPK, thereby increasing the level of IL-6. (2) After mechanical stimulation of Piezo1 channel opening, Nppb responded to mechanical stretching by expressing BNP, which inhibited TGF-β1 and also further inhibited the promotion of cardiac fibrosis by TGF-β1.
There is also evidence suggesting the involvement of brain natriuretic peptide (BNP) in cardiac fibrosis. BNP is abundantly present in cardiomyocytes and is known to inhibit collagen production and fibroblast proliferation (Hall, 2004; Goetze et al., 2020; Sun et al., 2023). Recent studies have identified BNP expression in fibroblasts. Fibroblasts express Nppb, which responds to mechanical stretch (Tsuruda et al., 2002; Ploeg et al., 2021). Nppb is the gene encoding BNP. Animal models have shown that the activation of Piezo1 using Yoda1, an agonist, increases Nppb and Tgf-β1. Conversely, silencing Piezo1 expression suppresses the expression of these two genes, indicating that Piezo1 mediates Nppb and Tgf-β1 in cardiac fibroblasts under mechanical stretch stimulation. Piezo1, as a mechanosensitive channel, has a crucial function in regulating the mechanical stress response in cardiac fibroblasts (Bartoli et al., 2022; Braidotti et al., 2022a). Upon mechanical stimulation and opening of the Piezo1 channel, Nppb reacts to it and expresses BNP, which inhibits TGF-β1 as well as further suppresses Acta2 induction by TGF-β1. Tgf-β1 is a gene involved in fibrosis and inflammation (Figure 4) (Paulus and Tschöpe, 2013; Tian et al., 2019).
Piezo1 is widely distributed in cardiac tissue, and its dysregulation can lead to calcium ion defects and dysregulation of ROS signaling. Mechanical stimulation activates Piezo1 channels, triggering calcium-mediated activation of calpain and calcineurin, leading to the transformation of fibroblasts into myofibroblasts. Piezo1 is expressed in cardiac fibroblasts (CF), and activation of Piezo1 can trigger the transformation of fibroblasts into myofibroblasts, a key step in fibrosis. Therefore, the upregulation of Piezo1 plays a role in the fibrosis process. Furthermore, activation of Piezo1 may induce fibroblast fibrosis through paracrine signaling involving IL-6.
In summary, in most cases, Piezo1 activation is triggered by mechanical stimulation, both occur through calcium influx but induce fibrosis through different signaling pathways. In pulmonary, renal, and cardiac fibrosis, upregulation of Piezo1 may occur during the fibrotic process, whereas in pancreatic fibrosis, activation of Piezo1 is triggered by external mechanical injury and may occur before the fibrotic process. Notably, these processes may differ in different disease states and time points.
4 Piezo1 as a prospective treatment target for fibrotic diseases
Piezo1 attracts widespread attention as a potential target for fibrotic diseases. Fibrosis is a pathological condition involving excessive ECM deposition and abnormal remodeling of tissue structure. Several studies have attempted to inhibit the fibrotic process by inhibiting the activity of Piezo1 channels. The development of fibrosis is attenuated by interfering with Piezo1 channel function or blocking Piezo1 channel-related signal pathways, like the calcium pathway and intracellular signal pathways, through the use of specific Piezo1 channel antagonists or inhibitors. In addition to inhibiting Piezo1 channel activity, studies have also been conducted to enhance the function of Piezo1 channels through the use of agonists or promoters or to adjust the activity level of Piezo1 channels by the use of modulators, to achieve regulation of the fibrotic process. In addition to directly targeting Piezo1 channels, several studies are exploring other therapeutic strategies related to Piezo1. For example, researchers continue to identify downstream signaling pathways and molecular targets that can influence Piezo1 regulation and are banking on controlling downstream pathways and signals to achieve intervention in the fibrotic process.
4.1 Piezo1 as a prospective treatment target for pulmonary fibrosis disease
Recent studies have highlighted the great importance of Piezo1 channels in the EMT process, suggesting that they may serve as key components mediating TGF-β signaling and epithelial cell transformation. This not only contributes to a deeper understanding of EMT-related diseases such as pulmonary fibrosis but may also provide new targets for the development of therapeutic strategies (Huang et al., 2021a; Zhang Y. A. et al., 2021). Besides, Mechanical ventilation is extensively used in critically ill patients, but at the same time, it may trigger and exacerbate the progression of pulmonary fibrosis. It was discovered that Piezo1 channels are activated by mechanical stretch under conditions of mechanical ventilation, leading to a cascade of cellular signaling events. This process is mediated through the activation of the RhoA/ROCK1 signaling pathway, which in turn triggers an increase in intracellular calcium ion concentration and leads to Bcl-2 inhibition, which in turn induces apoptosis in type II lung cells (Liang et al., 2019; Zhang Y. A. et al., 2021; Jiang et al., 2021b). Mechanical stretch activation of Piezo1 induces type II lung cell apoptosis via Ca2+ inward flow (Zhang et al., 2021c). In ARDS, Piezo1 and Ca2+ inward flow are thought to have a potential role (Liang et al., 2019; Jiang et al., 2021c; Fang et al., 2022). Future in-depth studies are expected to reveal the fine mechanisms of these pathways and provide more insight into the development of therapeutic strategies.
In summary, the essential role of the Piezo1 pathway in lung diseases should not be overlooked, and further studies on its molecular mechanism will provide a basis for drug development and optimization of therapeutic approaches. This promising research direction is expected to bring new hope for the future development of lung disease treatment.
4.2 Piezo1 as a prospective treatment target for renal fibrosis disease
For the potential link between Piezo1 and renal fibrosis, several studies have provided evidence suggesting that Piezo1 can be activated by mechanical stretch, chemical stimuli, or increased synthesis of extracellular matrix (ECM). Additionally, inhibition of Piezo1 expression in animals has been demonstrated to alleviate fibrotic processes in the kidney, this provides preliminary evidence for the feasibility of Piezo1 as a prospective treatment for renal fibrosis (Zhao X. et al., 2022). These findings, along with the previously described signaling pathways associated with Piezo1 and renal fibrosis, strongly suggest that Piezo1 plays a significant part in renal fibrosis. Collagen deposition or cross-linking leads to increased ECM stiffness and accelerated ECM secretion, which in turn aggravates the renal fibrosis process, forming a vicious positive feedback loop. A potential therapeutic strategy has been proposed to target ECM stiffness-induced mechanotransduction signaling pathways. By interfering with the mechanotransduction signaling pathway, it is expected to inhibit the increase in ECM stiffness, thereby slowing down or reversing the process of renal fibrosis (Seghers et al., 2016; Fu et al., 2021; He et al., 2022a; Zhao X. et al., 2022). Although this therapeutic strategy still needs further research and validation, it provides a new direction and idea for the treatment of renal fibrosis.
4.3 Piezo1 as a prospective treatment target for pancreatic fibrosis disease
Elevated pancreatic duct pressure leads to fibrosis mediated by Piezo1-activated PSCs. In a mouse model, the action of Piezo1 activator Yoda1 on PSCs leads to increased fibrosis, while the action of Piezo1 inhibitor GsMTx4 attenuates the fibrotic response. It can be speculated that the blocker of Piezo1 is used to act on PSCs as a target to attenuate pancreatic fibrosis (Kuntze et al., 2020; Swain et al., 2022). In addition to this, it has been suggested that Piezo1 stimulates PLA2, which initiates the TRPV4 pathway, and we can also use the blocker of TRPV4 to attenuate the damage to pancreatic cells (Swain et al., 2020). Further studies will contribute to a better discovery of the mechanism of Piezo1 in pancreatic fibrosis and develop new therapeutic options (Zhan and Li, 2018; Swain et al., 2020; Gorelick and Nathanson, 2020; Swain et al., 2022).
4.4 Piezo1 as a prospective treatment target for cardiac fibrosis disease
Piezo1 takes a mechanosensing part in cardiac fibroblasts, and we suggest that Piezo1 may be a prospective target to attenuate fibrosis in abnormal pathological states of the heart and maybe a potential target to interfere with cardiac fibroblast function (Zhang et al., 2021a; Jiang F. et al., 2021; Braidotti et al., 2022a). Piezo1 has a crucial function in cardiac fibrosis and provides an idea for the attenuation, cessation, or prevention of cardiac fibrosis. On the one hand, we can start from the perspective that after the mechanical activation of Piezo1, the Nppb gene in fibroblasts expresses BNP to anti-fibroblasts, and through the anti-fibroblast effect of BNP, we can attenuate or even prevent the occurrence of fibroblasts ahead of time (Ploeg et al., 2021), and on the other hand, we can also start from the calcium inward flow triggered by Piezo1 and the P38-MAPK signaling pathway, which affects the release of cytokines related with fibroblasts formation, to modulate fibroblasts occurrence (Blythe et al., 2019; Emig et al., 2021). In addition, we have compiled a Table 1 detailing the pro fibrotic and antifibrotic effects of peizo1 in the context of renal, pancreatic, cardiac and pulmonary fibrosis.
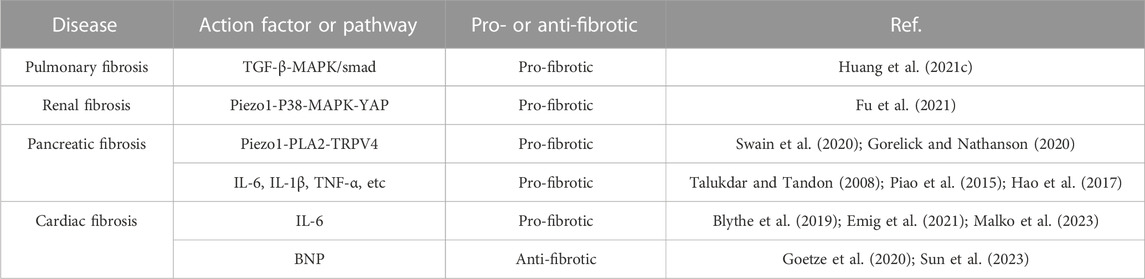
Table 1. Pro-or anti-fibrotic effects of Piezo1 in the context of pulmonary, renal, pancreatic, and cardiac fibrosis.
In conclusion, Piezo1 could be a potential target for pulmonary fibrosis, renal fibrosis, pancreatic fibrosis, and cardiac fibrosis. The difficulty associated with treating fibrotic diseases often lies in reversing them, and we are aware of the seriousness of persistent fibrosis in the heart, lungs, liver, and kidneys. Although Piezo1 provides us with a novel direction for treating fibrotic diseases, its current research and application are mostly limited to animal models. Considering the differences between humans and animals, it will take a long time to obtain effective results from Piezo1 for the treatment of fibrotic diseases, and we expect Piezo1 to bring hope to fibrotic patients sooner.
5 Summary and discussion
Fibrosis is a clinically advanced presentation of the majority of diseases and is a common phenomenon after organ damage with failure, severely affecting the wellbeing of patients. Therefore, using effective methods to inhibit or slow down the progression of disease fibrosis has attracted extensive attention from researchers. Due to the complex pathological mechanisms of fibrosis, it is crucial to further explore reliable therapeutic approaches. Piezo1, a key molecule in fibrosis, has been shown to exert an essential role in many types of fibrotic diseases. Hence, we expect that future studies should be devoted to further elucidating the specific mechanisms of Piezo1’s role in different fibrotic diseases, as well as its inter-regulatory relationship with other crucial signaling pathways. On this basis, the development of specific antagonists targeting Piezo1 will be a potential therapeutic strategy to provide new ideas for the clinical treatment of fibrotic diseases and open up new possibilities for the treatment of fibrotic diseases.
Author contributions
YX: Writing–original draft. YH: Writing–original draft. XC: Data curation, Writing–review and editing. BH: Writing–original draft. DJ: Data curation, Writing–review and editing. LW: Supervision, Writing–review and editing. SP: Supervision, Writing–review and editing. JH: Funding acquisition, Supervision, Writing–review and editing.
Funding
The author(s) declare financial support was received for the research, authorship, and/or publication of this article. This work was supported the grants from the doctoral start-up fund of the Second Affiliated Hospital of Nanchang University (B3150) and supported by Jiangxi Provincial Natural Science Foundation (20232BAB216042).
Acknowledgments
Thanks for the platform of the BioRender enables us to make high-quality figures (https://app.biorender.com/). And thanks for the support of the doctoral start-up fund of the Second Affiliated Hospital of Nanchang University (B3150) and supported by Jiangxi Provincial Natural Science Foundation (20232BAB216042).
Conflict of interest
The authors declare that the research was conducted in the absence of any commercial or financial relationships that could be construed as a potential conflict of interest.
Publisher’s note
All claims expressed in this article are solely those of the authors and do not necessarily represent those of their affiliated organizations, or those of the publisher, the editors and the reviewers. Any product that may be evaluated in this article, or claim that may be made by its manufacturer, is not guaranteed or endorsed by the publisher.
Abbreviations
MA, Mechanically-Activated; ECM, Extracellular matrix; TGF-β, Transforming Growth Factor-beta; MAPK, Mitogen-Activated Protein Kinase; TM, Transmembrane; CTD, C-terminal structural domain; CED, C-terminal extracellular structural domain; PF, Pulmonary fibrosis; EMT, Epithelial-mesenchymal transition; ARDS, Acute respiratory distress syndrome; MV, Mechanical stretch; ATI, Alveolar epithelial type I; ATII, Alveolar epithelial type II; AJs, Adhesion junctions; UUO, Unilateral ureteral obstruction; HK2 cells, Human renal cortical proximal tubular epithelial cells; mptc, Mouse proximal tubule cells; α-SMA, α-Smooth Muscle Actin; YAP, Yes-associated protein; PSCs, Pancreatic stellate cells; PLA2, Phospholipase A2; TRPV4, Transient receptor potential vanilloid 4; TNF-α, tumor necrosis factorα; IL-6, Interleukin-6; IL-1β, Interleukin-1β; MFs, Myofibroblasts; CF, Cardiac fibroblasts; BNP, Brain natriuretic peptide; ROS, Reactive Oxygen Species.
References
Andrews, J. P., Marttala, J., Macarak, E., Rosenbloom, J., and Uitto, J. (2016). Keloids: the paradigm of skin fibrosis - pathomechanisms and treatment. Matrix Biol. 51, 37–46. doi:10.1016/j.matbio.2016.01.013
Andugulapati, S. B., Gourishetti, K., Tirunavalli, S. K., Shaikh, T. B., and Sistla, R. (2020). Biochanin-A ameliorates pulmonary fibrosis by suppressing the TGF-β mediated EMT, myofibroblasts differentiation and collagen deposition in in vitro and in vivo systems. Phytomedicine 78, 153298. doi:10.1016/j.phymed.2020.153298
Antar, S. A., Ashour, N. A., Marawan, M. E., and Al-Karmalawy, A. A. (2023). Fibrosis: types, effects, markers, mechanisms for disease progression, and its relation with oxidative stress, immunity, and inflammation. Int. J. Mol. Sci. 24 (4), 4004. doi:10.3390/ijms24044004
Atcha, H., Jairaman, A., Holt, J. R., Meli, V. S., Nagalla, R. R., Veerasubramanian, P. K., et al. (2021a). Mechanically activated ion channel Piezo1 modulates macrophage polarization and stiffness sensing. Nat. Commun. 12 (1), 3256. doi:10.1038/s41467-021-23482-5
Atcha, H., Meli, V. S., Davis, C. T., Brumm, K. T., Anis, S., Chin, J., et al. (2021b). Crosstalk between CD11b and Piezo1 mediates macrophage responses to mechanical cues. Front. Immunol. 12, 689397. doi:10.3389/fimmu.2021.689397
Aykut, B., Chen, R., Kim, J. I., Wu, D., Shadaloey, S. A. A., Abengozar, R., et al. (2020). Targeting Piezo1 unleashes innate immunity against cancer and infectious disease. Sci. Immunol. 5 (50), eabb5168. doi:10.1126/sciimmunol.abb5168
Bacmeister, L., Schwarzl, M., Warnke, S., Stoffers, B., Blankenberg, S., Westermann, D., et al. (2019). Inflammation and fibrosis in murine models of heart failure. Basic Res. Cardiol. 114 (3), 19. doi:10.1007/s00395-019-0722-5
Bae, C., Sachs, F., and Gottlieb, P. A. (2015). Protonation of the human PIEZO1 ion channel stabilizes inactivation. J. Biol. Chem. 290 (8), 5167–5173. doi:10.1074/jbc.M114.604033
Bartoli, F., Evans, E. L., Blythe, N. M., Stewart, L., Chuntharpursat-Bon, E., Debant, M., et al. (2022). Global PIEZO1 gain-of-function mutation causes cardiac hypertrophy and fibrosis in mice. Cells 11 (7), 1199. doi:10.3390/cells11071199
Bate, N., Gingras, A. R., Bachir, A., Horwitz, R., Ye, F., Patel, B., et al. (2012). Talin contains a C-terminal calpain2 cleavage site important in focal adhesion dynamics. PLoS One 7 (4), e34461. doi:10.1371/journal.pone.0034461
Beech, D. J., and Kalli, A. C. (2019). Force sensing by piezo channels in cardiovascular health and disease. Arterioscler. Thromb. Vasc. Biol. 39 (11), 2228–2239. doi:10.1161/ATVBAHA.119.313348
Bernareggi, A., Bosutti, A., Massaria, G., Giniatullin, R., Malm, T., Sciancalepore, M., et al. (2022). The state of the art of Piezo1 channels in skeletal muscle regeneration. Int. J. Mol. Sci. 23 (12), 6616. doi:10.3390/ijms23126616
Bhattacharya, J., and Hough, R. F. (2019). Piezo1 in the lung: at last. Am. J. Respir. Cell Mol. Biol. 60 (6), 609–610. doi:10.1165/rcmb.2018-0418ED
Black, L. M., Lever, J. M., and Agarwal, A. (2019). Renal inflammation and fibrosis: A double-edged sword. J. Histochem Cytochem 67 (9), 663–681. doi:10.1369/0022155419852932
Blythe, N. M., Muraki, K., Ludlow, M. J., Stylianidis, V., Gilbert, H. T. J., Evans, E. L., et al. (2019). Mechanically activated Piezo1 channels of cardiac fibroblasts stimulate p38 mitogen-activated protein kinase activity and interleukin-6 secretion. J. Biol. Chem. 294 (46), 17395–17408. doi:10.1074/jbc.RA119.009167
Braidotti, N., Chen, S. N., Long, C. S., Cojoc, D., and Sbaizero, O. (2022a). Piezo1 channel as a potential target for hindering cardiac fibrotic remodeling. Int. J. Mol. Sci. 23 (15), 8065. doi:10.3390/ijms23158065
Bülow, R. D., and Boor, P. (2019). Extracellular matrix in kidney fibrosis: more than just a scaffold. J. Histochem Cytochem 67 (9), 643–661. doi:10.1369/0022155419849388
Cahalan, S. M., Lukacs, V., Ranade, S. S., Chien, S., Bandell, M., and Patapoutian, A. (2015). Piezo1 links mechanical forces to red blood cell. Elife 4. doi:10.7554/eLife.07370
Calvo, F., Grande-Garcia, A., Hooper, S., Jenkins, R. P., Chaudhry, S. I., et al. (2013). Mechanotransduction and YAP-dependent matrix remodelling is required for the generation and maintenance of cancer-associated fibroblasts. Nat. Cell Biol. 15 (6), 637–646. doi:10.1038/ncb2756
Cannon, A., Thompson, C. M., Bhatia, R., Armstrong, K. A., Solheim, J. C., Kumar, S., et al. (2021). Molecular mechanisms of pancreatic myofibroblast activation in chronic pancreatitis and pancreatic ductal adenocarcinoma. J. Gastroenterol. 56 (8), 689–703. doi:10.1007/s00535-021-01800-4
Chen, W. C., Lin, H. H., and Tang, M. J. (2014). Regulation of proximal tubular cell differentiation and proliferation in primary culture by matrix stiffness and ECM components. Am. J. Physiol. Ren. Physiol. 307 (6), F695–F707. doi:10.1152/ajprenal.00684.2013
Chen, J., Miao, J., Zhou, D., Liao, J., Wang, Z., and Lin, Z. (2022a). Upregulation of mechanosensitive channel Piezo1 involved in high shear stress-induced pulmonary hypertension. Thromb. Res. 218, 52–63. doi:10.1016/j.thromres.2022.08.006
Chen, J., Rodriguez, M., Miao, J., Liao, J., Jain, P. P., Zhao, M., et al. (2022b). Mechanosensitive channel Piezo1 is required for pulmonary artery smooth muscle cell proliferation. Am. J. Physiol. Lung Cell Mol. Physiol. 322 (5), L737–l760. doi:10.1152/ajplung.00447.2021
Chen, S., Li, Z., Chen, D., Cui, H., Wang, J., Li, Z., et al. (2022c). Piezo1-mediated mechanotransduction promotes entheseal pathological new bone formation in ankylosing spondylitis. Ann. Rheum. Dis. 2022, 223428. doi:10.1136/ard-2022-223428
Coste, B., Mathur, J., Schmidt, M., Earley, T. J., Ranade, S., Petrus, M. J., et al. (2010). Piezo1 and Piezo2 are essential components of distinct mechanically activated cation channels. Science 330 (6000), 55–60. doi:10.1126/science.1193270
Cottin, V., Wollin, L., Fischer, A., Quaresma, M., Stowasser, S., and Harari, S. (2019). Fibrosing interstitial lung diseases: knowns and unknowns. Eur. Respir. Rev. 28 (151), 180100. doi:10.1183/16000617.0100-2018
Cox, C. D., and Gottlieb, P. A. (2019). Amphipathic molecules modulate PIEZO1 activity. Biochem. Soc. Trans. 47 (6), 1833–1842. doi:10.1042/BST20190372
Dalghi, M. G., Clayton, D. R., Ruiz, W. G., Al-Bataineh, M. M., Satlin, L. M., Kleyman, T. R., et al. (2019). Expression and distribution of PIEZO1 in the mouse urinary tract. Am. J. Physiol. Ren. Physiol. 317 (2), F303–F321. doi:10.1152/ajprenal.00214.2019
Del Mármol, J. I., Touhara, K. K., Croft, G., and MacKinnon, R. (2018). Piezo1 forms a slowly-inactivating mechanosensory channel in mouse embryonic stem cells. Elife 7. doi:10.7554/eLife.33149
Diem, K., Fauler, M., Fois, G., Hellmann, A., Winokurow, N., Schumacher, S., et al. (2020). Mechanical stretch activates piezo1 in caveolae of alveolar type I cells to trigger ATP release and paracrine stimulation of surfactant secretion from alveolar type II cells. FASEB J. 34 (9), 12785–12804. doi:10.1096/fj.202000613RRR
Ding, L., Li, Y., Yang, Y., Song, S., Qi, H., Wang, J., et al. (2021). Wenfei buqi tongluo formula against bleomycin-induced pulmonary fibrosis by inhibiting TGF-β/smad3 pathway. Front. Pharmacol. 12, 762998. doi:10.3389/fphar.2021.762998
Dombroski, J. A., Hope, J. M., Sarna, N. S., and King, M. R. (2021). Channeling the force: piezo1 mechanotransduction in cancer metastasis. Cells 10 (11), 2815. doi:10.3390/cells10112815
Douguet, D., Patel, A., Xu, A., Vanhoutte, P. M., and Honoré, E. (2019). Piezo ion channels in cardiovascular mechanobiology. Trends Pharmacol. Sci. 40 (12), 956–970. doi:10.1016/j.tips.2019.10.002
Dubin, A. E., and Patapoutian, A. (2010). Nociceptors: the sensors of the pain pathway. J. Clin. Invest. 120 (11), 3760–3772. doi:10.1172/JCI42843
Dupont, S., Morsut, L., Aragona, M., Enzo, E., Giulitti, S., Cordenonsi, M., et al. (2011). Role of YAP/TAZ in mechanotransduction. Nature 474 (7350), 179–183. doi:10.1038/nature10137
Emig, R., Knodt, W., Krussig, M. J., Zgierski-Johnston, C. M., Gorka, O., Groß, O., et al. (2021). Piezo1 channels contribute to the regulation of human atrial fibroblast mechanical properties and matrix stiffness sensing. Cells 10 (3), 663. doi:10.3390/cells10030663
Evtugina, N. G., Peshkova, A. D., Khabirova, A. I., Andrianova, I. A., Abdullayeva, S., Ayombil, F., et al. (2023). Activation of Piezo1 channels in compressed red blood cells augments platelet-driven contraction of blood clots. J. Thromb. Haemost. 21 (9), 2418–2429. doi:10.1016/j.jtha.2023.05.022
Fang, X. Z., Zhou, T., Xu, J. Q., Wang, Y. X., Sun, M. M., He, Y. J., et al. (2021). Structure, kinetic properties and biological function of mechanosensitive Piezo channels. Cell Biosci. 11 (1), 13. doi:10.1186/s13578-020-00522-z
Fang, X. Z., Li, M., Wang, Y. X., Zhang, P., Sun, M. M., Xu, J. X., et al. (2022). Mechanosensitive ion channel Piezo1 mediates mechanical ventilation-exacerbated ARDS-associated pulmonary fibrosis. J. Adv. Res. 2022. doi:10.1016/j.jare.2022.12.006
Faucherre, A., Nargeot, J., Mangoni, M. E., and Jopling, C. (2013). piezo2b regulates vertebrate light touch response. J. Neurosci. 33 (43), 17089–17094. doi:10.1523/JNEUROSCI.0522-13.2013
Feinberg, M. W., Watanabe, M., Lebedeva, M. A., Depina, A. S., Hanai, J. i., Mammoto, T., et al. (2004). Transforming growth factor-beta1 inhibition of vascular smooth muscle cell activation is mediated via Smad3. J. Biol. Chem. 279 (16), 16388–16393. doi:10.1074/jbc.M309664200
Filser, M., Giansily-Blaizot, M., Grenier, M., Monedero Alonso, D., Bouyer, G., Pérès, L., et al. (2021). Increased incidence of germline PIEZO1 mutations in individuals with idiopathic erythrocytosis. Blood 137 (13), 1828–1832. doi:10.1182/blood.2020008424
Frangogiannis, N. G. (2019). Cardiac fibrosis: cell biological mechanisms, molecular pathways and therapeutic opportunities. Mol. Asp. Med. 65, 70–99. doi:10.1016/j.mam.2018.07.001
Frangogiannis, N. (2021). Cardiac fibrosis. Cardiovasc Res. 117 (6), 1450–1488. doi:10.1093/cvr/cvaa324
Friedrich, E. E., Hong, Z., Xiong, S., Zhong, M., and Rehman, J. (2019). Endothelial cell Piezo1 mediates pressure-induced lung vascular hyperpermeability via disruption of adherens junctions. Proc. Natl. Acad. Sci. U. S. A. 116 (26), 12980–12985. doi:10.1073/pnas.1902165116
Fu, Y., Wan, P., Zhang, J., Li, X., Xing, J., Zou, Y., et al. (2021). Targeting mechanosensitive Piezo1 alleviated renal fibrosis through p38MAPK-YAP pathway. Front. Cell Dev. Biol. 9, 741060. doi:10.3389/fcell.2021.741060
Galichon, P., Finianos, S., and Hertig, A. (2013). EMT-MET in renal disease: should we curb our enthusiasm? Cancer Lett. 341 (1), 24–29. doi:10.1016/j.canlet.2013.04.018
Garcia-Dorado, D., Ruiz-Meana, M., Inserte, J., Rodriguez-Sinovas, A., and Piper, H. M. (2012). Calcium-mediated cell death during myocardial reperfusion. Cardiovasc Res. 94 (2), 168–180. doi:10.1093/cvr/cvs116
Geng, J., Shi, Y., Zhang, J., Yang, B., Wang, P., Yuan, W., et al. (2021). TLR4 signalling via Piezo1 engages and enhances the macrophage mediated host response during bacterial infection. Nat. Commun. 12 (1), 3519. doi:10.1038/s41467-021-23683-y
Geokas, M. C., Baltaxe, H. A., Banks, P. A., Silva, J., and Frey, C. F. (1985). Acute pancreatitis. Ann. Intern Med. 103 (1), 86–100. doi:10.7326/0003-4819-103-1-86
Ghafouri-Fard, S., Abak, A., Talebi, S. F., Shoorei, H., Branicki, W., Taheri, M., et al. (2021). Role of miRNA and lncRNAs in organ fibrosis and aging. Biomed. Pharmacother. 143, 112132. doi:10.1016/j.biopha.2021.112132
Gifford, C. C., Tang, J., Costello, A., Khakoo, N. S., Nguyen, T. Q., Goldschmeding, R., et al. (2021). Negative regulators of TGF-β1 signaling in renal fibrosis; pathological mechanisms and novel therapeutic opportunities. Clin. Sci. (Lond) 135 (2), 275–303. doi:10.1042/CS20201213
Goetze, J. P., Bruneau, B. G., Ramos, H. R., Ogawa, T., de Bold, M. K., and de Bold, A. J. (2020). Cardiac natriuretic peptides. Nat. Rev. Cardiol. 17 (11), 698–717. doi:10.1038/s41569-020-0381-0
González, A., Schelbert, E. B., Díez, J., and Butler, J. (2018). Myocardial interstitial fibrosis in heart failure: biological and translational perspectives. J. Am. Coll. Cardiol. 71 (15), 1696–1706. doi:10.1016/j.jacc.2018.02.021
Gorelick, F., and Nathanson, M. H. (2020). TRPV4 helps Piezo1 put the squeeze on pancreatic acinar cells. J. Clin. Invest. 130 (5), 2199–2201. doi:10.1172/JCI136525
Gottlieb, P. A., and Sachs, F. (2012). Piezo1: properties of a cation selective mechanical channel. Channels (Austin) 6 (4), 214–219. doi:10.4161/chan.21050
Guo, H., Jian, Z., Liu, H., Cui, H., Deng, H., Fang, J., et al. (2021). TGF-β1-induced EMT activation via both Smad-dependent and MAPK signaling pathways in Cu-induced pulmonary fibrosis. Toxicol. Appl. Pharmacol. 418, 115500. doi:10.1016/j.taap.2021.115500
Hall, C. (2004). Essential biochemistry and physiology of (NT-pro)BNP. Eur. J. Heart Fail 6 (3), 257–260. doi:10.1016/j.ejheart.2003.12.015
Hamada, S., Matsumoto, R., and Masamune, A. (2022). Pancreatic stellate cells and metabolic alteration: physiology and pathophysiology. Front. Physiol. 13, 865105. doi:10.3389/fphys.2022.865105
Handra, C. M., Gurzu, I. L., Chirila, M., and Ghita, I. (2023). Silicosis: new challenges from an old inflammatory and fibrotic disease. Front. Biosci. (Landmark Ed. 28 (5), 96. doi:10.31083/j.fbl2805096
Hao, W., Komar, H. M., Hart, P. A., Conwell, D. L., Lesinski, G. B., and Friedman, A. (2017). Mathematical model of chronic pancreatitis. Proc. Natl. Acad. Sci. U. S. A. 114 (19), 5011–5016. doi:10.1073/pnas.1620264114
Hao, Y., Baker, D., and Ten Dijke, P. (2019). TGF-β-Mediated epithelial-mesenchymal transition and cancer metastasis. Int. J. Mol. Sci. 20 (11), 2767. doi:10.3390/ijms20112767
Hatem, A., Poussereau, G., Gachenot, M., Pérès, L., Bouyer, G., and Egée, S. (2023). Dual action of Dooku1 on PIEZO1 channel in human red blood cells. Front. Physiol. 14, 1222983. doi:10.3389/fphys.2023.1222983
He, L., Si, G., Huang, J., Samuel, A. D. T., and Perrimon, N. (2018). Mechanical regulation of stem-cell differentiation by the stretch-activated Piezo channel. Nature 555 (7694), 103–106. doi:10.1038/nature25744
He, J., Fang, B., Shan, S., Xie, Y., Wang, C., Zhang, Y., et al. (2021). Mechanical stretch promotes hypertrophic scar formation through mechanically activated cation channel Piezo1. Cell Death Dis. 12 (3), 226. doi:10.1038/s41419-021-03481-6
He, Y., Deng, B., Liu, S., Luo, S., Ning, Y., Pan, X., et al. (2022a). Myeloid Piezo1 deletion protects renal fibrosis by restraining macrophage infiltration and activation. Hypertension 79 (5), 918–931. doi:10.1161/HYPERTENSIONAHA.121.18750
He, J., Shan, S., Li, Q., Fang, B., and Xie, Y. (2022b). Mechanical stretch triggers epithelial-mesenchymal transition in keratinocytes through Piezo1 channel. Front. Physiol. 13, 745572. doi:10.3389/fphys.2022.745572
Henderson, N. C., Rieder, F., and Wynn, T. A. (2020). Fibrosis: from mechanisms to medicines. Nature 587 (7835), 555–566. doi:10.1038/s41586-020-2938-9
Heukels, P., Moor, C. C., von der Thüsen, J. H., Wijsenbeek, M. S., and Kool, M. (2019). Inflammation and immunity in IPF pathogenesis and treatment. Respir. Med. 147, 79–91. doi:10.1016/j.rmed.2018.12.015
Hirata, Y., Cai, R., Volchuk, A., Steinberg, B. E., Saito, Y., Matsuzawa, A., et al. (2023). Lipid peroxidation increases membrane tension, Piezo1 gating, and cation permeability to execute ferroptosis. Curr. Biol. 33 (7), 1282–1294.e5. doi:10.1016/j.cub.2023.02.060
Holt, J. R., Zeng, W. Z., Evans, E. L., Woo, S. H., Ma, S., Abuwarda, H., et al. (2021). Spatiotemporal dynamics of PIEZO1 localization controls keratinocyte migration during wound healing. Elife 10, e65415. doi:10.7554/eLife.65415
Hu, Y., Sheng, Y., Yu, M., Li, K., Ren, G., Xu, X., et al. (2016). Antioxidant activity of Inonotus obliquus polysaccharide and its amelioration for chronic pancreatitis in mice. Int. J. Biol. Macromol. 87, 348–356. doi:10.1016/j.ijbiomac.2016.03.006
Hu, H. H., Chen, D. Q., Wang, Y. N., Feng, Y. L., Cao, G., Vaziri, N. D., et al. (2018). New insights into TGF-β/Smad signaling in tissue fibrosis. Chem. Biol. Interact. 292, 76–83. doi:10.1016/j.cbi.2018.07.008
Hu, F., Lou, N., Jiao, J., Guo, F., Xiang, H., and Shang, D. (2020). Macrophages in pancreatitis: mechanisms and therapeutic potential. Biomed. Pharmacother. 131, 110693. doi:10.1016/j.biopha.2020.110693
Huang, J. Q., Zhang, H., Guo, X. W., Lu, Y., Wang, S. N., Cheng, B., et al. (2021a). Mechanically activated calcium channel PIEZO1 modulates radiation-induced epithelial-mesenchymal transition by forming a positive feedback with TGF-β1. Front. Mol. Biosci. 8, 725275. doi:10.3389/fmolb.2021.725275
Huang, Y., Chen, Z., Lu, T., Bi, G., Li, M., Liang, J., et al. (2021b). HIF-1α switches the functionality of TGF-β signaling via changing the partners of smads to drive glucose metabolic reprogramming in non-small cell lung cancer. J. Exp. Clin. Cancer Res. 40 (1), 398. doi:10.1186/s13046-021-02188-y
Huang, C., Iovanna, J., and Santofimia-Castaño, P. (2021c). Targeting fibrosis: the bridge that connects pancreatitis and pancreatic cancer. Int. J. Mol. Sci. 22 (9), 4970. doi:10.3390/ijms22094970
Huang, Q., Zhu, W., Gao, X., Liu, X., Zhang, Z., and Xing, B. (2023). Nanoparticles-mediated ion channels manipulation: from their membrane interactions to bioapplications. Adv. Drug Deliv. Rev. 195, 114763. doi:10.1016/j.addr.2023.114763
Ikeda, R., Cha, M., Ling, J., Jia, Z., Coyle, D., and Gu, J. G. (2014). Merkel cells transduce and encode tactile stimuli to drive Aβ-afferent impulses. Cells 157, 664. doi:10.1016/j.cell.2014.02.026
Imamura, M., Moon, J. S., Chung, K. P., Nakahira, K., Muthukumar, T., Shingarev, R., et al. (2018). RIPK3 promotes kidney fibrosis via AKT-dependent ATP citrate lyase. JCI Insight 3 (3), e94979. doi:10.1172/jci.insight.94979
Jiang, Y., Yang, X., Jiang, J., and Xiao, B. (2021a). Structural designs and mechanogating mechanisms of the mechanosensitive piezo channels. Trends Biochem. Sci. 46 (6), 472–488. doi:10.1016/j.tibs.2021.01.008
Jiang, F., Yin, K., Wu, K., Zhang, M., Wang, S., Cheng, H., et al. (2021b). The mechanosensitive Piezo1 channel mediates heart mechano-chemo transduction. Nat. Commun. 12 (1), 869. doi:10.1038/s41467-021-21178-4
Jiang, L., Zhang, Y., Huang, T., Yan, K., Yang, W., et al. (2021c). Mechanosensitive Piezo1 channel activation promotes ventilator-induced lung injury via disruption of endothelial junctions in ARDS rats. Biochem. Biophys. Res. Commun. 556, 79–86. doi:10.1016/j.bbrc.2021.03.163
Jiang, Y., Zhang, H., Wang, J., Liu, Y., Luo, T., and Hua, H. (2022). Targeting extracellular matrix stiffness and mechanotransducers to improve cancer therapy. J. Hematol. Oncol. 15 (1), 34. doi:10.1186/s13045-022-01252-0
Jones, J. H., and Minshall, R. D. (2020). Lung endothelial transcytosis. Compr. Physiol. 10 (2), 491–508. doi:10.1002/cphy.c190012
Kefauver, J. M., Ward, A. B., and Patapoutian, A. (2020). Discoveries in structure and physiology of mechanically activated ion channels. Nature 587 (7835), 567–576. doi:10.1038/s41586-020-2933-1
Kim, B. N., Ahn, D. H., Kang, N., Yeo, C. D., Kim, Y. K., Lee, K. Y., et al. (2020). TGF-β induced EMT and stemness characteristics are associated with epigenetic regulation in lung cancer. Sci. Rep. 10 (1), 10597. doi:10.1038/s41598-020-67325-7
Knight, T., Zaidi, A. U., Wu, S., Gadgeel, M., Buck, S., and Ravindranath, Y. (2019). Mild erythrocytosis as a presenting manifestation of PIEZO1 associated erythrocyte volume disorders. Pediatr. Hematol. Oncol. 36 (5), 317–326. doi:10.1080/08880018.2019.1637984
Kuntze, A., Goetsch, O., Fels, B., Najder, K., Unger, A., Wilhelmi, M., et al. (2020). Protonation of Piezo1 impairs cell-matrix interactions of pancreatic stellate cells. Front. Physiol. 11, 89. doi:10.3389/fphys.2020.00089
Lackner, C., and Tiniakos, D. (2019). Fibrosis and alcohol-related liver disease. J. Hepatol. 70 (2), 294–304. doi:10.1016/j.jhep.2018.12.003
Lai, A., Cox, C. D., Chandra Sekar, N., Thurgood, P., Jaworowski, A., Peter, K., et al. (2022). Mechanosensing by Piezo1 and its implications for physiology and various pathologies. Biol. Rev. Camb Philos. Soc. 97 (2), 604–614. doi:10.1111/brv.12814
LaRue, M. M., Parker, S., Puccini, J., Cammer, M., Kimmelman, A. C., and Bar-Sagi, D. (2022). Metabolic reprogramming of tumor-associated macrophages by collagen turnover promotes fibrosis in pancreatic cancer. Proc. Natl. Acad. Sci. U. S. A. 119 (16), e2119168119. doi:10.1073/pnas.2119168119
Lee, J. H., and Massagué, J. (2022). TGF-β in developmental and fibrogenic EMTs. Semin. Cancer Biol. 86 (2), 136–145. doi:10.1016/j.semcancer.2022.09.004
Lee, H. J., Tomasini-Johansson, B. R., Gupta, N., and Kwon, G. S. (2023). Fibronectin-targeted FUD and PEGylated FUD peptides for fibrotic diseases. J. Control Release 360, 69–81. doi:10.1016/j.jconrel.2023.06.008
Lei, R., Li, J., Liu, F., Li, W., Zhang, S., Wang, Y., et al. (2019). HIF-1α promotes the keloid development through the activation of TGF-β/Smad and TLR4/MyD88/NF-κB pathways. Cell Cycle 18 (23), 3239–3250. doi:10.1080/15384101.2019.1670508
Leng, S., Zhang, X., Wang, S., Qin, J., Liu, Q., Liu, A., et al. (2022). Ion channel Piezo1 activation promotes aerobic glycolysis in macrophages. Front. Immunol. 13, 976482. doi:10.3389/fimmu.2022.976482
Lewis, A. H., and Grandl, J. (2015). Mechanical sensitivity of Piezo1 ion channels can be tuned by cellular membrane tension. Elife 4, e12088. doi:10.7554/eLife.12088
Li, J., Hou, B., Tumova, S., Muraki, K., Bruns, A., Ludlow, M. J., et al. (2014). Piezo1 integration of vascular architecture with physiological force. Nature 515 (7526), 279–282. doi:10.1038/nature13701
Liang, G. P., Xu, J., Cao, L. L., Zeng, Y. H., Chen, B. X., Yang, J., et al. (2019). Piezo1 induced apoptosis of type II pneumocytes during ARDS. Respir. Res. 20 (1), 118. doi:10.1186/s12931-019-1083-1
Liang, S., Wu, Y. S., Li, D. Y., Tang, J. X., and Liu, H. F. (2022). Autophagy and renal fibrosis. Aging Dis. 13 (3), 712–731. doi:10.14336/AD.2021.1027
Liao, J., Lu, W., Chen, Y., Duan, X., Zhang, C., Luo, X., et al. (2021). Upregulation of Piezo1 (piezo type mechanosensitive ion channel component 1) enhances the intracellular free calcium in pulmonary arterial smooth muscle cells from idiopathic pulmonary arterial hypertension patients. Hypertension 77 (6), 1974–1989. doi:10.1161/HYPERTENSIONAHA.120.16629
Lin, C., Zheng, X., Lin, S., Zhang, Y., Wu, J., and Li, Y. (2022). Mechanotransduction regulates the interplays between alveolar epithelial and vascular endothelial cells in lung. Front. Physiol. 13, 818394. doi:10.3389/fphys.2022.818394
Liu, S., Xu, X., Fang, Z., Ning, Y., Deng, B., Pan, X., et al. (2021a). Piezo1 impairs hepatocellular tumor growth via deregulation of the MAPK-mediated YAP signaling pathway. Cell Calcium 95, 102367. doi:10.1016/j.ceca.2021.102367
Liu, M., López de Juan Abad, B., and Cheng, K. (2021b). Cardiac fibrosis: myofibroblast-mediated pathological regulation and drug delivery strategies. Adv. Drug Deliv. Rev. 173, 504–519. doi:10.1016/j.addr.2021.03.021
Liu, W., Han, X., Li, Q., Sun, L., and Wang, J. (2022). Iguratimod ameliorates bleomycin-induced pulmonary fibrosis by inhibiting the EMT process and NLRP3 inflammasome activation. Biomed. Pharmacother. 153, 113460. doi:10.1016/j.biopha.2022.113460
Lourenço, A. R., Roukens, M. G., Seinstra, D., Frederiks, C. L., Pals, C. E., Vervoort, S. J., et al. (2020). C/EBPɑ is crucial determinant of epithelial maintenance by preventing epithelial-to-mesenchymal transition. Nat. Commun. 11 (1), 785. doi:10.1038/s41467-020-14556-x
Ma, Y. X., Li, W. H., and Xie, Q. (2013). Rosuvastatin inhibits TGF-beta1 expression and alleviates myocardial fibrosis in diabetic rats. Pharmazie 68 (5), 355–358.
Ma, Z. G., Yuan, Y. P., Wu, H. M., Zhang, X., and Tang, Q. Z. (2018). Cardiac fibrosis: new insights into the pathogenesis. Int. J. Biol. Sci. 14 (12), 1645–1657. doi:10.7150/ijbs.28103
Ma, Y., Kuang, Y., Bo, W., Liang, Q., Zhu, W., Cai, M., et al. (2021). Exercise training alleviates cardiac fibrosis through increasing fibroblast growth factor 21 and regulating TGF-β1-smad2/3-MMP2/9 signaling in mice with myocardial infarction. Int. J. Mol. Sci. 22 (22), 12341. doi:10.3390/ijms222212341
Mahdy, M. A. A. (2019). Skeletal muscle fibrosis: an overview. Cell Tissue Res. 375 (3), 575–588. doi:10.1007/s00441-018-2955-2
Malko, P., Jia, X., Wood, I., and Jiang, L. H. (2023). Piezo1 channel-mediated Ca(2+) signaling inhibits lipopolysaccharide-induced activation of the NF-κB inflammatory signaling pathway and generation of TNF-α and IL-6 in microglial cells. Glia 71 (4), 848–865. doi:10.1002/glia.24311
Mansour, D., and McPherson, S. (2018). Management of decompensated cirrhosis. Clin. Med. (Lond) 18 (2), s60–s65. doi:10.7861/clinmedicine.18-2-s60
Martins, J. R., Penton, D., Peyronnet, R., Arhatte, M., Moro, C., Picard, N., et al. (2016). Piezo1-dependent regulation of urinary osmolarity. Pflugers Arch. 468 (7), 1197–1206. doi:10.1007/s00424-016-1811-z
Maruyama, K., and Imanaka-Yoshida, K. (2022). The pathogenesis of cardiac fibrosis: A review of recent progress. Int. J. Mol. Sci. 23 (5), 2617. doi:10.3390/ijms23052617
Mayerle, J., Sendler, M., Hegyi, E., Beyer, G., Lerch, M. M., and Sahin-Tóth, M. (2019). Genetics, cell biology, and pathophysiology of pancreatitis. Gastroenterology 156 (7), 1951–1968. doi:10.1053/j.gastro.2018.11.081
Methfessel C Fau - Witzemann, V., Takahashi, T., Mishina, M., Numa, S., and Sakmann, B. (1986). Patch clamp measurements on Xenopus laevis oocytes: currents through endogenous channels and implanted acetylcholine receptor and sodium channels. Pflugers Arch. 407 (6), 577–588. doi:10.1007/BF00582635
Miyamoto, T., Mochizuki, T., Nakagomi, H., Kira, S., Watanabe, M., Takayama, Y., et al. (2014). Functional role for Piezo1 in stretch-evoked Ca2⁺ influx and ATP release in urothelial cell cultures. J. Biol. Chem. 289 (23), 16565–16575. doi:10.1074/jbc.M113.528638
Nagpal, V., Rai, R., Place, A. T., Murphy, S. B., Verma, S. K., Ghosh, A. K., et al. (2016). MiR-125b is critical for fibroblast-to-myofibroblast transition and cardiac fibrosis. Circulation 133 (3), 291–301. doi:10.1161/CIRCULATIONAHA.115.018174
Nishida, M., Okumura, Y., Fujimoto, S. I., Shiraishi, I., Itoi, T., and Hamaoka, K. (2005). Adoptive transfer of macrophages ameliorates renal fibrosis in mice. Biochem. Biophys. Res. Commun. 332 (1), 11–16. doi:10.1016/j.bbrc.2005.04.083
Nourse, J. L., and Pathak, M. M. (2017). How cells channel their stress: interplay between Piezo1 and the cytoskeleton. Semin. Cell Dev. Biol. 71, 3–12. doi:10.1016/j.semcdb.2017.06.018
Oppedisano, F., Mollace, R., Tavernese, A., Gliozzi, M., Musolino, V., Macrì, R., et al. (2021). PUFA supplementation and heart failure: effects on fibrosis and cardiac remodeling. Nutrients 13 (9), 2965. doi:10.3390/nu13092965
Panizo, S., Martínez-Arias, L., Alonso-Montes, C., Cannata, P., Martín-Carro, B., Fernández-Martín, J. L., et al. (2021). Fibrosis in chronic kidney disease: pathogenesis and consequences. Int. J. Mol. Sci. 22 (1), 408. doi:10.3390/ijms22010408
Paulus, W. J., and Tschöpe, C. (2013). A novel paradigm for heart failure with preserved ejection fraction: comorbidities drive myocardial dysfunction and remodeling through coronary microvascular endothelial inflammation. J. Am. Coll. Cardiol. 62 (4), 263–271. doi:10.1016/j.jacc.2013.02.092
Pelosi, P., Ball, L., Barbas, C. S. V., Bellomo, R., Burns, K. E. A., Einav, S., et al. (2021). Personalized mechanical ventilation in acute respiratory distress syndrome. Crit. Care 25 (1), 250. doi:10.1186/s13054-021-03686-3
Peyronnet, R., Martins, J. R., Duprat, F., Demolombe, S., Arhatte, M., Jodar, M., et al. (2013). Piezo1-dependent stretch-activated channels are inhibited by Polycystin-2 in renal tubular epithelial cells. EMBO Rep. 14 (12), 1143–1148. doi:10.1038/embor.2013.170
Phillips, P. (2012). “Pancreatic stellate cells and fibrosis,” in Pancreatic cancer and tumor microenvironment. Editors P. J. Grippo, and H. G. Munshi (Trivandrum (India): Transworld Research Network).
Piao, R. L., Xiu, M., Brigstock, D. R., and Gao, R. P. (2015). An immortalized rat pancreatic stellate cell line RP-2 as a new cell model for evaluating pancreatic fibrosis, inflammation and immunity. Hepatobiliary Pancreat. Dis. Int. 14 (6), 651–659. doi:10.1016/s1499-3872(15)60415-5
Pinho, C. (2019). Cardiac fibrosis occurs before arterial hypertension becomes well defined? Arq. Bras. Cardiol. 112 (1), 65–66. doi:10.5935/abc.20180243
Ploeg, M. C., Munts, C., Prinzen, F. W., Turner, N. A., van Bilsen, M., and van Nieuwenhoven, F. A. (2021). Piezo1 mechanosensitive ion channel mediates stretch-induced Nppb expression in adult rat cardiac fibroblasts. Cells 10 (7), 1745. doi:10.3390/cells10071745
Porto Ribeiro, T., Barbeau, S., Baudrimont, I., Vacher, P., Freund-Michel, V., Cardouat, G., et al. (2022). Piezo1 channel activation reverses pulmonary artery vasoconstriction in an early rat model of pulmonary hypertension: the role of Ca(2+) influx and akt-eNOS pathway. Cells 11 (15), 2349. doi:10.3390/cells11152349
Qi, X. M., Luo, Y., Song, M. Y., Liu, Y., Shu, T., Liu, Y., et al. (2021). Pneumoconiosis: current status and future prospects. Chin. Med. J. Engl. 134 (8), 898–907. doi:10.1097/CM9.0000000000001461
Qian, W., Cai, X., Qian, Q., Zhang, W., and Wang, D. (2018). Astragaloside IV modulates TGF-β1-dependent epithelial-mesenchymal transition in bleomycin-induced pulmonary fibrosis. J. Cell Mol. Med. 22 (9), 4354–4365. doi:10.1111/jcmm.13725
Qin, L., Chen, S., Yang, D., Yi, W., Cao, H., et al. (2021). Roles of mechanosensitive channel Piezo1/2 proteins in skeleton and other tissues. Bone Res. 9 (1), 44. doi:10.1038/s41413-021-00168-8
Qiu, X., Deng, Z., Wang, M., Feng, Y., Bi, L., and Li, L. (2023). Piezo protein determines stem cell fate by transmitting mechanical signals. Hum. Cell 36 (2), 540–553. doi:10.1007/s13577-022-00853-8
Ramji, D. P., and Foka, P. (2002). CCAAT/enhancer-binding proteins: structure, function and regulation. Biochem. J. 365 (3), 561–575. doi:10.1042/BJ20020508
Rayego-Mateos, S., and Valdivielso, J. M. (2020). New therapeutic targets in chronic kidney disease progression and renal fibrosis. Expert Opin. Ther. Targets 24 (7), 655–670. doi:10.1080/14728222.2020.1762173
Rolland, L., Torrente, A. G., Bourinet, E., Maskini, D., Drouard, A., Chevalier, P., et al. (2023). Prolonged Piezo1 activation induces cardiac arrhythmia. Int. J. Mol. Sci. 24 (7), 6720. doi:10.3390/ijms24076720
Romac, J. M., Shahid, R. A., Swain, S. M., Vigna, S. R., and Liddle, R. A. (2018). Piezo1 is a mechanically activated ion channel and mediates pressure induced pancreatitis. Nat. Commun. 9 (1), 1715. doi:10.1038/s41467-018-04194-9
Rout-Pitt, N., Farrow, N., Parsons, D., and Donnelley, M. (2018). Epithelial mesenchymal transition (EMT): a universal process in lung diseases with implications for cystic fibrosis pathophysiology. Respir. Res. 19 (1), 136. doi:10.1186/s12931-018-0834-8
Salton, F., Volpe, M. C., and Confalonieri, M. (2019). Epithelial⁻Mesenchymal transition in the pathogenesis of idiopathic pulmonary fibrosis. Med. Kaunas. 55 (4), 83. doi:10.3390/medicina55040083
Sarohi, V., Chakraborty, S., and Basak, T. (2022). Exploring the cardiac ECM during fibrosis: A new era with next-gen proteomics. Front. Mol. Biosci. 9, 1030226. doi:10.3389/fmolb.2022.1030226
Schneider, E. R., Mastrotto, M., Laursen, W. J., Schulz, V. P., Goodman, J. B., Funk, O. H., et al. (2014). Neuronal mechanism for acute mechanosensitivity in tactile-foraging waterfowl. Proc. Natl. Acad. Sci. U. S. A. 111 (41), 14941–14946. doi:10.1073/pnas.1413656111
Schrenk-Siemens, K., Wende, H., Prato, V., Song, K., Rostock, C., Loewer, A., et al. (2015). PIEZO2 is required for mechanotransduction in human stem cell-derived touch receptors. Nat. Neurosci. 18 (1), 10–16. doi:10.1038/nn.3894
Sebastiani, G., Gkouvatsos, K., and Pantopoulos, K. (2014). Chronic hepatitis C and liver fibrosis. World J. Gastroenterol. 20 (32), 11033–11053. doi:10.3748/wjg.v20.i32.11033
Seghers, F., Yerna, X., Zanou, N., Devuyst, O., Vennekens, R., Nilius, B., et al. (2016). TRPV4 participates in pressure-induced inhibition of renin secretion by juxtaglomerular cells. J. Physiol. 594 (24), 7327–7340. doi:10.1113/JP273595
Shahidullah, M., Rosales, J. L., and Delamere, N. (2022). Activation of Piezo1 increases Na,K-ATPase-Mediated ion transport in mouse lens. Int. J. Mol. Sci. 23 (21), 12870. doi:10.3390/ijms232112870
Shao, J., Liu, J., and Zuo, S. (2022). Roles of epigenetics in cardiac fibroblast activation and fibrosis. Cells 11 (15), 2347. doi:10.3390/cells11152347
Sheng, L., and Zhuang, S. (2020). New insights into the role and mechanism of partial epithelial-mesenchymal transition in kidney fibrosis. Front. Physiol. 11, 569322. doi:10.3389/fphys.2020.569322
Shi, J., Hyman, A. J., De Vecchis, D., Chong, J., Lichtenstein, L., Futers, T. S., et al. (2020). Sphingomyelinase disables inactivation in endogenous PIEZO1 channels. Cell Rep. 33 (1), 108225. doi:10.1016/j.celrep.2020.108225
Shi, L., Dai, X., Yan, F., Lin, Y., Lin, L., Zhang, Y., et al. (2023). Novel lipidomes profile and clinical phenotype identified in pneumoconiosis patients. J. Health Popul. Nutr. 42 (1), 55. doi:10.1186/s41043-023-00400-7
Shimizu, K. (2008). Mechanisms of pancreatic fibrosis and applications to the treatment of chronic pancreatitis. J. Gastroenterol. 43 (11), 823–832. doi:10.1007/s00535-008-2249-7
Shinge, S. A. U., Zhang, D., Din, A. U., Yu, F., and Nie, Y. (2022). Emerging Piezo1 signaling in inflammation and atherosclerosis; a potential therapeutic target. Int. J. Biol. Sci. 18 (3), 923–941. doi:10.7150/ijbs.63819
Siamwala, J. H., Zhao, A., Barthel, H., Pagano, F. S., Gilbert, R. J., and Rounds, S. (2020). Adaptive and innate immune mechanisms in cardiac fibrosis complicating pulmonary arterial hypertension. Physiol. Rep. 8 (15), e14532. doi:10.14814/phy2.14532
Soattin, L., Fiore, M., Gavazzo, P., Viti, F., Facci, P., Raiteri, R., et al. (2016). The biophysics of piezo1 and piezo2 mechanosensitive channels. Biophys Chem. 208: 26–33. doi:10.1016/j.bpc.2015.06.013
Sochorcova, L., Hlusickova Kapralova, K., Fialova Kucerova, J., Pospisilova, D., Prochazkova, D., Jahoda, O., et al. (2023). Elevated erythroferrone distinguishes erythrocytosis with inherited defects in oxygen-sensing pathway from primary familial and congenital polycythaemia. Br. J. Haematol. 202 (3), 674–685. doi:10.1111/bjh.18891
Solis, A. G., Bielecki, P., Steach, H. R., Sharma, L., Harman, C. C. D., Yun, S., et al. (2019a). Mechanosensation of cyclical force by PIEZO1 is essential for innate immunity. Nature 573 (7772), 69–74. doi:10.1038/s41586-019-1485-8
Solis, A. G., Bielecki, P., Steach, H. R., Sharma, L., Harman, C. C. D., Yun, S., et al. (2019b). Author correction: mechanosensation of cyclical force by PIEZO1 is essential for innate immunity. Nature 575 (7784), E7. doi:10.1038/s41586-019-1755-5
Somogyi, V., Chaudhuri, N., Torrisi, S. E., Kahn, N., Müller, V., and Kreuter, M. (2019). The therapy of idiopathic pulmonary fibrosis: what is next? Eur. Respir. Rev. 28 (153), 190021. doi:10.1183/16000617.0021-2019
Song, S., Zhang, H., Wang, X., Chen, W., Cao, W., Zhang, Z., et al. (2022). The role of mechanosensitive Piezo1 channel in diseases. Prog. Biophys. Mol. Biol. 172, 39–49. doi:10.1016/j.pbiomolbio.2022.04.006
Stalla, F., Armandi, A., Marinoni, C., Fagoonee, S., Pellicano, R., and Caviglia, G. P. (2022). Chronic hepatitis B virus infection and fibrosis: novel non-invasive approaches for diagnosis and risk stratification. Minerva Gastroenterol. (Torino) 68 (3), 306–318. doi:10.23736/S2724-5985.21.02911-9
Stewart, L., and Turner, N. (2021). Channelling the force to reprogram the matrix: mechanosensitive ion channels in cardiac fibroblasts. Cells 10 (5), 990. doi:10.3390/cells10050990
Su, J., Morgani, S. M., David, C. J., Wang, Q., Er, E. E., Huang, Y. H., et al. (2020). TGF-β orchestrates fibrogenic and developmental EMTs via the RAS effector RREB1. Nature 577 (7791), 566–571. doi:10.1038/s41586-019-1897-5
Sugimoto, A., Miyazaki, A., Kawarabayashi, K., Shono, M., Akazawa, Y., Hasegawa, T., et al. (2017). Piezo type mechanosensitive ion channel component 1 functions as a regulator of the cell fate determination of mesenchymal stem cells. Sci. Rep. 7 (1), 17696. doi:10.1038/s41598-017-18089-0
Sun, Y., Ma, M., Cao, D., Zheng, A., Zhang, Y., Su, Y., et al. (2023). Inhibition of fap promotes cardiac repair by stabilizing BNP. Circ. Res. 132 (5), 586–600. doi:10.1161/CIRCRESAHA.122.320781
Svetina, S., Švelc Kebe, T., and Božič, B. (2019). A model of piezo1-based regulation of red blood cell volume. Biophys. J. 116 (1), 151–164. doi:10.1016/j.bpj.2018.11.3130
Swain, S. M., Romac, J. M. J., Shahid, R. A., Pandol, S. J., Liedtke, W., Vigna, S. R., et al. (2020). TRPV4 channel opening mediates pressure-induced pancreatitis initiated by Piezo1 activation. J. Clin. Invest. 130 (5), 2527–2541. doi:10.1172/JCI134111
Swain, S. M., Romac, J. M. J., Vigna, S. R., and Liddle, R. A. (2022). Piezo1-mediated stellate cell activation causes pressure-induced pancreatic fibrosis in mice. JCI Insight 7 (8), e158288. doi:10.1172/jci.insight.158288
Talukdar, R., and Tandon, R. K. (2008). Pancreatic stellate cells: new target in the treatment of chronic pancreatitis. J. Gastroenterol. Hepatol. 23 (1), 34–41. doi:10.1111/j.1440-1746.2007.05206.x
Tang, P. M., Nikolic-Paterson, D. J., and Lan, H. Y. (2019). Macrophages: versatile players in renal inflammation and fibrosis. Nat. Rev. Nephrol. 15 (3), 144–158. doi:10.1038/s41581-019-0110-2
Tang, H., Zeng, R., Zhang, I., Ding, C., and Zhang, A. (2022). Piezo-type mechanosensitive ion channel component 1 (Piezo1): A promising therapeutic target and its modulators. J. Med. Chem. 65 (9), 6441–6453. doi:10.1021/acs.jmedchem.2c00085
Tarbit, E., Singh, I., Peart, J. N., and Rose'Meyer, R. B. (2019). Biomarkers for the identification of cardiac fibroblast and myofibroblast cells. Heart Fail Rev. 24 (1), 1–15. doi:10.1007/s10741-018-9720-1
Tenner, S., Baillie, J., DeWitt, J., and Vege, S. S.American College of Gastroenterology (2013). American College of gastroenterology guideline: management of acute pancreatitis. Am. J. Gastroenterol. 108 (9), 1400–1415. doi:10.1038/ajg.2013.218
Thannickal, V. J., Toews, G. B., White, E. S., Lynch, J. P., and Martinez, F. J. (2004). Mechanisms of pulmonary fibrosis. Annu. Rev. Med. 55, 395–417. doi:10.1146/annurev.med.55.091902.103810
Thomas, D., and Radhakrishnan, P. (2019). Tumor-stromal crosstalk in pancreatic cancer and tissue fibrosis. Mol. Cancer 18 (1), 14. doi:10.1186/s12943-018-0927-5
Thompson, M. A., Prakash Ys Fau - Pabelick, C. M., and Pabelick, C. M. (2014). The role of caveolae in the pathophysiology of lung diseases. Expert Rev. Respir. Med. 8, 111. doi:10.1586/17476348.2014.855610
Tian, M., Xiao, Y., Xue, J., Zhang, Y., Jia, Y., Luo, X., et al. (2019). The expression of BNP, ET-1, and TGF-β1 in myocardium of rats with ventricular arrhythmias. Int. J. Mol. Sci. 20 (23), 5845. doi:10.3390/ijms20235845
Tsuruda, T., Boerrigter, G., Huntley, B. K., Noser, J. A., Cataliotti, A., Costello-Boerrigter, L. C., et al. (2002). Brain natriuretic Peptide is produced in cardiac fibroblasts and induces matrix metalloproteinases. Circ. Res. 91 (12), 1127–1134. doi:10.1161/01.res.0000046234.73401.70
Vaisey, G., Banerjee, P., North, A. J., Haselwandter, C. A., and MacKinnon, R. (2022). Piezo1 as a force-through-membrane sensor in red blood cells. Elife 11, e82621. doi:10.7554/eLife.82621
Velasco-Estevez, M. A., Gadalla, K. K. E., Liñan-Barba, N., Cobb, S., Dev, K. K., and Sheridan, G. K. (2020). Inhibition of Piezo1 attenuates demyelination in the central nervous system. Glia 68 (2), 356–375. doi:10.1002/glia.23722
Volkers, L., Mechioukhi, Y., and Coste, B. (2015). Piezo channels: from structure to function. Pflugers Arch. 467 (1), 95–99. doi:10.1007/s00424-014-1578-z
Walter, J. M., Corbridge, T. C., and Singer, B. D. (2018). Invasive mechanical ventilation. South Med. J. 111 (12), 746–753. doi:10.14423/SMJ.0000000000000905
Wang, Y., and Xiao, B. (2018). The mechanosensitive Piezo1 channel: structural features and molecular bases underlying its ion permeation and mechanotransduction. J. Physiol. 596 (6), 969–978. doi:10.1113/JP274404
Wang, G. J., Gao, C. F., Wei, D., Wang, C., and Ding, S. Q. (2009). Acute pancreatitis: etiology and common pathogenesis. World J. Gastroenterol. 15 (12), 1427–1430. doi:10.3748/wjg.15.1427
Wang, J., Lin, S., Brown, J. M., van Wagoner, D., Fiocchi, C., and Rieder, F. (2021a). Novel mechanisms and clinical trial endpoints in intestinal fibrosis. Immunol. Rev. 302 (1), 211–227. doi:10.1111/imr.12974
Wang, Z., Chen, J., Babicheva, A., Jain, P. P., Rodriguez, M., Ayon, R. J., et al. (2021b). Endothelial upregulation of mechanosensitive channel Piezo1 in pulmonary hypertension. Am. J. Physiol. Cell Physiol. 321 (6), C1010–c1027. doi:10.1152/ajpcell.00147.2021
Wang, H., Yuan, Z., Wang, B., Li, B., Lv, H., He, J., et al. (2022). COMP (cartilage oligomeric matrix protein), a novel PIEZO1 regulator that controls blood pressure. Hypertension 79 (3), 549–561. doi:10.1161/HYPERTENSIONAHA.121.17972
Wei, J., Xu, Z., and Yan, X. (2022). The role of the macrophage-to-myofibroblast transition in renal fibrosis. Front. Immunol. 13, 934377. doi:10.3389/fimmu.2022.934377
Wen, D., Gao, Y., Ho, C., Yu, L., Zhang, Y., Lyu, G., et al. (2022a). Focusing on mechanoregulation Axis in fibrosis: sensing, transduction and effecting. Front. Mol. Biosci. 9, 804680. doi:10.3389/fmolb.2022.804680
Wen, J. H., Li, D. Y., Liang, S., Yang, C., Tang, J. X., and Liu, H. F. (2022b). Macrophage autophagy in macrophage polarization, chronic inflammation and organ fibrosis. Front. Immunol. 13, 946832. doi:10.3389/fimmu.2022.946832
Wicher, S. A., Prakash, Y. S., and Pabelick, C. M. (2019). Caveolae, caveolin-1 and lung diseases of aging. Expert Rev. Respir. Med. 13 (3), 291–300. doi:10.1080/17476348.2019.1575733
Wu, J., Young, M., Lewis, A. H., Martfeld, A. N., Kalmeta, B., and Grandl, J. (2017). Inactivation of mechanically activated Piezo1 ion channels is determined by the C-terminal extracellular domain and the inner pore helix. Cell Rep. 21 (9), 2357–2366. doi:10.1016/j.celrep.2017.10.120
Wu, Q., Sun, S., Wei, L., Liu, M., Liu, H., Liu, T., et al. (2022). Twist1 regulates macrophage plasticity to promote renal fibrosis through galectin-3. Cell Mol. Life Sci. 79 (3), 137. doi:10.1007/s00018-022-04137-0
Wynn, T. A., and Vannella, K. M. (2016). Macrophages in tissue repair, regeneration, and fibrosis. Immunity 44 (3), 450–462. doi:10.1016/j.immuni.2016.02.015
Xiao, B. (2020). Levering mechanically activated piezo channels for potential pharmacological intervention. Annu. Rev. Pharmacol. Toxicol. 60, 195–218. doi:10.1146/annurev-pharmtox-010919-023703
Xing, C., Bao, L., Li, W., and Fan, H. (2023). Progress on role of ion channels of cardiac fibroblasts in fibrosis. Front. Physiol. 14, 1138306. doi:10.3389/fphys.2023.1138306
Xiong, H., Yang, J., Guo, J., Ma, A., Wang, B., and Kang, Y. (2022). Mechanosensitive Piezo channels mediate the physiological and pathophysiological changes in the respiratory system. Respir. Res. 23 (1), 196. doi:10.1186/s12931-022-02122-6
Xu, X., Zheng, L., Yuan, Q., Zhen, G., Crane, J. L., Zhou, X., et al. (2018). Transforming growth factor-β in stem cells and tissue homeostasis. Bone Res. 6, 2. doi:10.1038/s41413-017-0005-4
Xu, X., Liu, S., Liu, H., Ru, K., Jia, Y., Wu, Z., et al. (2021). Piezo channels: awesome mechanosensitive structures in cellular mechanotransduction and their role in bone. Int. J. Mol. Sci. 22 (12), 6429. doi:10.3390/ijms22126429
Xue, J., Sharma, V., Hsieh, M. H., Chawla, A., Murali, R., Pandol, S. J., et al. (2015). Alternatively activated macrophages promote pancreatic fibrosis in chronic pancreatitis. Nat. Commun. 6, 7158. doi:10.1038/ncomms8158
Yan, J., Honglei, Y., Yun, W., Sheng, D., Yun, H., Anhua, Z., et al. (2022). Puerarin ameliorates myocardial remodeling of spontaneously hypertensive rats through inhibiting TRPC6-CaN-NFATc3 pathway. Eur. J. Pharmacol. 933, 175254. doi:10.1016/j.ejphar.2022.175254
Yang, H., Hou, C., Xiao, W., and Qiu, Y. (2022). The role of mechanosensitive ion channels in the gastrointestinal tract. Front. Physiol. 13, 904203. doi:10.3389/fphys.2022.904203
Yu, Y., Wu, X., Liu, S., Zhao, H., Li, B., Zhao, H., et al. (2021). Piezo1 regulates migration and invasion of breast cancer cells via modulating cell mechanobiological properties. Acta Biochim. Biophys. Sin. (Shanghai) 53 (1), 10–18. doi:10.1093/abbs/gmaa112
Zaidi, Y., Aguilar, E. G., Troncoso, M., Ilatovskaya, D. V., and DeLeon-Pennell, K. Y. (2021). Immune regulation of cardiac fibrosis post myocardial infarction. Cell Signal 77, 109837. doi:10.1016/j.cellsig.2020.109837
Zhan, L., and Li, J. (2018). The role of TRPV4 in fibrosis. Gene 642, 1–8. doi:10.1016/j.gene.2017.10.067
Zhang, Y., Su, S. A., Li, W., Ma, Y., Shen, J., Wang, Y., et al. (2021a). Piezo1-Mediated mechanotransduction promotes cardiac hypertrophy by impairing calcium homeostasis to activate calpain/calcineurin signaling. Hypertension 78 (3), 647–660. doi:10.1161/HYPERTENSIONAHA.121.17177
Zhang, Y. A., Jiang, L., Huang, T., Song, Y., Wang, L., et al. (2021b). Mechanosensitive cation channel Piezo1 contributes to ventilator-induced lung injury by activating RhoA/ROCK1 in rats. Respir. Res. 22 (1), 250. doi:10.1186/s12931-021-01844-3
Zhang, B., Chen, X., Ru, F., Gan, Y., Li, B., Xia, W., et al. (2021c). Liproxstatin-1 attenuates unilateral ureteral obstruction-induced renal fibrosis by inhibiting renal tubular epithelial cells ferroptosis. Cell Death Dis. 12 (9), 843. doi:10.1038/s41419-021-04137-1
Zhang, X., Hou, L., Li, F., Zhang, W., Wu, C., Xiang, L., et al. (2022). Piezo1-mediated mechanosensation in bone marrow macrophages promotes vascular niche regeneration after irradiation injury. Theranostics 12 (4), 1621–1638. doi:10.7150/thno.64963
Zhao, Q., Zhou, H., Li, X., and Xiao, B. (2019). The mechanosensitive Piezo1 channel: a three-bladed propeller-like structure and a lever-like mechanogating mechanism. Febs J. 286 (13), 2461–2470. doi:10.1111/febs.14711
Zhao, Y., Hao, C., Qu, Y., Guo, Y., Deng, X., et al. (2022). PD-1/PD-L1 inhibitor ameliorates silica-induced pulmonary fibrosis by maintaining systemic immune homeostasis. Biomed. Pharmacother. 148, 112768. doi:10.1016/j.biopha.2022.112768
Zhao, X., Kong, Y., Liang, B., Xu, J., Lin, Y., Zhou, N., et al. (2022). Mechanosensitive Piezo1 channels mediate renal fibrosis. JCI Insight. 7 (7), e152330. doi:10.1172/jci.insight.152330
Zhong, M., Komarova, Y., Rehman, J., and Malik, A. B. (2018). Mechanosensing Piezo channels in tissue homeostasis including their role in lungs. Pulm. Circ. 8 (2), 2045894018767393. doi:10.1177/2045894018767393
Zhong, M., Wu, W., Kang, H., Xiong, S., Gao, X., et al. (2020). Alveolar stretch activation of endothelial Piezo1 protects adherens junctions and lung vascular barrier. Am. J. Respir. Cell Mol. Biol. 62 (2), 168–177. doi:10.1165/rcmb.2019-0024OC
Keywords: Piezo1, Piezo2, therapeutic target, fibrosis, Ca2+
Citation: Xu Y, Huang Y, Cheng X, Hu B, Jiang D, Wu L, Peng S and Hu J (2023) Mechanotransductive receptor Piezo1 as a promising target in the treatment of fibrosis diseases. Front. Mol. Biosci. 10:1270979. doi: 10.3389/fmolb.2023.1270979
Received: 11 August 2023; Accepted: 26 September 2023;
Published: 12 October 2023.
Edited by:
Oscar Maiques, Queen Mary University of London, United KingdomReviewed by:
Bhola Shankar Pradhan, Łukasiewicz Research Network—PORT Polish Center for Technology Development, PolandHarikrishnan Venugopal, Albert Einstein College of Medicine, United States
Copyright © 2023 Xu, Huang, Cheng, Hu, Jiang, Wu, Peng and Hu. This is an open-access article distributed under the terms of the Creative Commons Attribution License (CC BY). The use, distribution or reproduction in other forums is permitted, provided the original author(s) and the copyright owner(s) are credited and that the original publication in this journal is cited, in accordance with accepted academic practice. No use, distribution or reproduction is permitted which does not comply with these terms.
*Correspondence: Jialing Hu, aHVqaWFsaW5nbmN1QDEyNi5jb20=; Shengliang Peng, NTI0NTgwNzI0QHFxLmNvbQ==; Lidong Wu, ZG9uZ2d1YXd1ODlAMTYzLmNvbQ==
†These authors have contributed equally to this work and share first authorship