- 1Department of Medical Genetics, National Institute for Genetic Engineering and Biotechnology, Tehran, Iran
- 2Research Institute for Children’s Health, Shahid Beheshti University of Medical Sciences, Tehran, Iran
- 3Pediatric Gastroenterology, Hepatology, and Nutrition Research Center, Research Institute for Children’s Health, Shahid Beheshti University of Medical Sciences, Tehran, Iran
- 4Pediatric Nephrology Research Center, Research Institute for Children’s Health, Shahid Beheshti University of Medical Science, Tehran, Iran
- 5Department of Microbiology, School of Medicine, Shahid Beheshti University of Medical Sciences, Tehran, Iran
- 6Pediatric Congenital Hematologic Disorders Research Center, Research Institute for Children’s Health, Shahid Beheshti University of Medical Sciences, Tehran, Iran
Background: Although the exact mechanisms of nonalcoholic fatty liver disease (NAFLD) are not fully understood, numerous pieces of evidence show that the variations in mitochondrial DNA (mtDNA) level and hepatic Fibroblast growth factor 21 (FGF21) expression may be related to NAFLD susceptibility.
Objectives: The main objective of this study was to determine relative levels of mtDNA copy number and hepatic FGF21 expression in a cohort of Iranian NAFLD patients and evaluate the possible relationship.
Methods: This study included 27 NAFLD patients (10 with nonalcoholic fatty liver (NAFL) and 17 with non-alcoholic steatohepatitis (NASH)) and ten healthy subjects. Total RNA and genomic DNA were extracted from liver tissue samples, and then mtDNA copy number and FGF21 expression levels were assessed by quantitative real-time PCR.
Results: The relative level of hepatic mtDNA copy number was 3.9-fold higher in patients than in controls (p < 0.0001). NAFLD patients showed a 2.9-fold increase in hepatic FGF21 expression compared to controls (p < 0.013). Results showed that hepatic FGF21 expression was positively correlated with BMI, serum ALT, and AST levels (p < 0.05). The level of mitochondrial copy number and hepatic FGF21 expression was not significantly associated with stages of change in hepatic steatosis. Finally, there was a significant correlation between FGF21 expression and mitochondrial copy number in NAFLD patients (p = 0.027).
Conclusion: Our findings suggest a considerable rise of hepatic FGF21 mRNA levels and mtDNA-CN and show a positive correlation between them in the liver tissue of NAFLD patients.
Introduction
Chronic liver diseases are rapidly growing as health priorities globally. Fatty liver disease can occur in the setting of both nonalcoholic fatty liver disease (NAFLD) and alcoholic liver disease (ALD) (Toshikuni et al., 2014). NAFLD is among the most prevalent chronic liver disorders worldwide, with a pooled global prevalence of 25.24% and over the past 2 decades there has been a steady increase in its incidence across many populations. (Mitra et al., 2020). NAFLD is characterized by steatosis affecting more than 5% of hepatocytes in individuals who do not consume excessive amounts of alcohol, do not have other liver diseases, and do not take steatogenic drugs. The histological spectrum of NAFLD comprises nonalcoholic fatty liver (NAFL), which involves steatosis without hepatocellular injury, and steatohepatitis (NASH), which involves inflammation and hepatocyte ballooning degeneration in addition to steatosis. Patients with NAFLD can progress to fibrosis, and ultimately, cirrhosis (Chalasani et al., 2012; Younossi et al., 2018). Patients with cirrhosis are at risk of potentially life-threatening liver-related complications such as portal hypertension, hepatic failure, and hepatocellular carcinoma (Ascha et al., 2010; Bhala et al., 2011; Nusrat et al., 2014). Knowledge about the mechanisms that are potentially involved in the pathogenesis of NAFLD is still incomplete. However, new evidence suggests that the pathogenesis of NAFLD involves complex mechanisms collectively referred to as the “multiple parallel hits hypothesis”. This theory proposes that multiple components act in parallel to contribute to the development of NAFLD, rather than in a linear series (Lonardo et al., 2017; Caturano et al., 2021). According to this theory, these factors are believed to play role in the development of NAFLD: insulin resistance (IR), genetic and epigenetic factors, mitochondrial dysfunction, endoplasmic reticulum stress, microbiota, chronic low-grade inflammation, and dysfunction of adipose tissue (Acierno et al., 2020).
The pathogenesis of NAFLD is impacted by changes in the mitochondria, such as mitochondrial DNA depletion, as well as modifications in the beta-oxidation and respiratory chain functions. (Pessayre and Fromenty, 2005). If mitochondrial and peroxisomal functions are unable to handle the increased lipid flow, it can cause respiratory oxidation to collapse, leading to disruption in lipid balance, production of harmful metabolites, and an excess of reactive oxygen species (ROS) (Begriche et al., 2006; Wang et al., 2020). These events contribute to oxidative stress, hepatic necro-inflammatory processes, and worsening of mitochondrial damage. In fact, it has been proven that mitochondrial dysfunction is directly associated with IR, obesity, and the release of pro-inflammatory cytokines levels like tumor necrosis factor-alpha (TNF-α) (Paradies et al., 2014). Furthermore, ROS and oxidized low-density lipoprotein (LDL) cholesterol particles can activate Kupffer and hepatic stellate cells, leading to the deposition of collagen and the progression of liver fibrosis (Cusi, 2009).
In addition, endoplasmic reticulum (ER) malfunction probably leads to accumulation of unfolded proteins inside the ER, increased protein synthesis, reduction of Adenosine triphosphate (ATP), and activation of the unfolded protein response (UPR). UPR is a compensatory response to decrease protein synthesis, increase protein trafficking capacity through the ER, and increase protein degradative pathways (Wang and Kaufman, 2014). UPR failure to solve the protein-folding defect, may induce hepatocytes apoptosis.
Based on the mentioned multi-hit hypothesis, and factors like ATP deficiency, increased lipid flow, and dysfunction of beta-oxidation which are directly linked to mitochondria, several studies have suggested that NAFLD might be a mitochondrial disease (Begriche et al., 2006; Dornas and Schuppan, 2020; Xu et al., 2021). This condition leads to mitochondrial damage and mitochondrial DNA copy number (mtDNA-CN) variations in hepatocytes (Pirola et al., 2015; Kamfar et al., 2016).
Circulating fibroblast growth factor 21 (FGF21), a member of the FGF family, is predominantly liver-derived and is involved in the hormonal regulation of glucose and lipid metabolism, energy homeostasis, insulin sensitivity, and other metabolic functions (Cuevas-Ramos et al., 2009). Numerous preclinical and clinical evidence suggested that aberrant FGF21 signaling may play a role in the pathogenesis and progression of NAFLD (Liu et al., 2015; Rusli et al., 2016; Tucker et al., 2019). Both FGF21 serum levels and FGF21 expression were discussed to be indicators of NAFLD (Falamarzi et al., 2022). It has been shown that FGF21 stimulates lipolysis by decreasing fat stores leading to reducing hepatic steatosis and lipotoxicity (Xu et al., 2009; Tanaka et al., 2015; Bao et al., 2018). Moreover, several studies have also reported that FGF21 reduces oxidative stress and endoplasmic reticulum stress (Ye et al., 2014; Boparai et al., 2015) and enhances mitochondrial function (Lee et al., 2016).
In this observational case-control study, considering the role of FGF21 and mitochondria in NAFLD pathogenesis, our first aim was to assess FGF21 expression and mtDNA-CN in Iranian NAFLD patients in different stages of the disease and compare those to healthy control group. Our second objective was to investigate the possible relation between the levels of FGF21 expression and mtDNA-CN in liver samples from Iranian NAFLD patients.
Methods
Study population
Over one and a half years, this study was carried out on NAFLD patients identified from a sub-specialist tertiary NAFLD clinic at the Khatam Ol-Anbia Hospital, Tehran, Iran. Participants were selected based on liver ultrasonography, clinical, and laboratory findings. Patients with other liver problems, cancer, family history of diabetes, history of alcohol drinking, viral hepatitis (B or C), steatogenic medications, and glucocorticoid therapy were carefully excluded. Control group consisted of healthy volunteers who did not have any history of liver and metabolic related disorders. A liver needle biopsy was used to obtain liver samples. two to three samples were obtained from each participant which were used for histological assessment and nucleic acid extraction. Relevant clinical and laboratory data were collected from the time of liver biopsy. Patients with NAFLD were classified into NAFL and NASH based on the histologic findings and were approved by at least two pathologists. The same method was used for control group classification and individuals with pathologic findings in their samples were excluded from the control group. The medical ethics committee approved the Protocol of Hamadan University of Medical Sciences (P/16/35/9/3481), and all the participants signed Written Informed Consent before participating in this research study. The study protocol conformed to the ethical guidelines of the World Medical Association Declaration of Helsinki.
Anthropometric assessment and results of biochemical analysis
Relevant clinical details such as age, gender, weight, and height were obtained from all patients at the time of liver biopsy. The body mass index (BMI) was calculated by the formula: weight (kg)/height2 (m2). Blood tests taken at the time of liver biopsy were used to determine related paraclinical parameters. An automated enzymatic procedure assayed with alanine transaminase (ALT), aspartate transaminase (AST), cholesterol, triglyceride, HDL cholesterol, and fasting blood glucose (FBS) levels. The Friedewald formula was used for calculation of LDL-cholesterol levels.
Histological assessment
Percutaneous liver biopsies were performed using a Menghini needle. Liver biopsies were all >15 mm in length and were read by two experienced hepatopathologists. And any disagreement was resolved with discussion between them. “NASH” was defined as steatosis with hepatocyte ballooning degeneration and inflammation with or without fibrosis (Yeh and Brunt, 2014). “NAFL” was defined as steatosis only, or steatosis with mild inflammation without hepatocyte ballooning degeneration.
DNA/RNA extraction
Genomic DNA and total RNA were extracted simultaneously from fresh liver samples using the AllPrep DNA/RNA Micro (Qiagen, Dubai, United Arab Emirates). According to the manufacturer’s instructions, hepatic tissue samples were first lysed and homogenized in a buffer for inhibition of DNases and RNases to obtain intact DNA and RNA. The lysate was passed through an AllPrep DNA spin column to selectively and efficiently isolate DNA. The column was then washed and DNA was eluted. Ethanol was added to the flow-through from the AllPrep DNA spin column to allow proper binding conditions for RNA, and the sample was then applied to RNeasy MinElute spin column, where total RNA binds into the membrane and contaminants were effectively washed away. Finally, RNA was then eluted in water.
Mitochondrial DNA copy number
Quantification of mtDNA-CN was assessed using quantitative real-time PCR (qPCR). According to the manufacturer’s Protocol, this assay was carried out using the SYBR master mix (Real qPCR 2x Mix, Amplicon, Wrocław, Poland). Amplification was done with two pair primers: ONP86/ONP89 and B-actin (Shakhssalim et al., 2013; Kamfar et al., 2016; Zabihi Diba et al., 2016). The first set of primers (86/89) was used to amplify a normal fragment in mtDNA, and the second set (B-actin) was used as an internal control for nucleic DNA. Primer-BLAST was used to check the primer specificity (Ye et al., 2012). The amplification was done for 40 cycles using the following conditions: 95°C for 15 min, then 95°C for 30 s, and 58°C for 1 min. All samples were run in triplicate. Relative levels of mtDNA-CN were measured by using the 2−ΔΔCT method (Jensen, 2012).
Hepatic mRNA expression of FGF21
RNA isolated from liver biopsy was reverse transcripted using the cDNA Synthesis Kit (Thermo Fisher Scientific, Waltham, MA, United States of America) and the obtained cDNA was used as template in the qPCR reaction. According to the manufacturer’s Protocol, the quantitative real-time PCR assay was carried out using specific primers in a 20 µL reaction volume containing SYBR Green master mix (Real QPCR 2x Mix, Amplicon, Wrocław, Poland). Each reaction was run in duplicate, and the accuracy of qPCR product size was confirmed by gel electrophoresis. Two primer pairs were designed to analyze FGF21 and B-actin as housekeeping genes (Table 1).
Statistical analysis
Analyses were performed using SPSS 18.0 software package (SPSS Inc., United States). Data were presented as means ± standard deviation (SD). The student's t-test was used for comparing normally distributed variables. Logistic regression was used to adjust for age, BMI, and lipid level confounders. Pearson correlation coefficient was carried out to describe the relationship of FGF21 expression and mtDNA-CN with variables related to NAFLD. In all statistical tests, p < 0.05 was regarded as statistically significant.
Results
Baseline characteristics of participants, including 27 patients with NAFLD (17 NASH, 10 NAFL) and ten healthy control subjects, are presented in Table2. The mean TG, ALT, AST, and BMI in NAFLD patients were significantly higher than in the control group (p < 0.05). No statistically significant difference was found in the other parameters between these two groups. According to the results, we observed a 3.9-fold increase in relative mtDNA-CN in the livers of NAFLD patients compared to healthy controls (p < 0.0001) (Figure1). A comparison of mtDNA-CN showed a 4.3 (p < 0.008) and 3.5-fold (p < 0.013) increase in patients with NAFL and NASH compared to control subjects, respectively. No substantial differences were observed in mtDNA-CN between the NAFL and NASH patients (p < 0.615) (Supplementary Table S1). No relation was observed after adjustment for age and BMI between mtDNA-CN and variables such as lipid levels and blood pressure (p > 0.05). In addition, no significant association was found between mtDNA-CN and age (p > 0.05) (Supplementary Table S2).
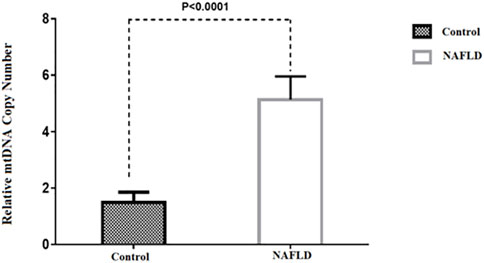
FIGURE 1. Comparison of the relative mtDNA copy number between NAFLD and control group. *p-value was computed t-test and data are shown as Mean ± SEM.
Our findings also indicated that FGF21 expression in hepatic tissue was 2.9-fold higher in patients with NAFLD than in control subjects (p = 0.013) (Figure 2). No significant difference was observed between patients with NAFL and NASH (p = 0.843) (Supplementary Table S1). Our results also showed a positive correlation between FGF21 expression and BMI (p = 0.035), AST (p = 0.02), and ALT (p < 0.01) levels (Supplementary Table S2). We found no sex difference in the expression of FGF21 between NAFL and NASH groups (p > 0.05). In addition, there was no significant relation between FGF21 expression and other anthropometric and biochemical measurements after adjusting for age and BMI in these groups.
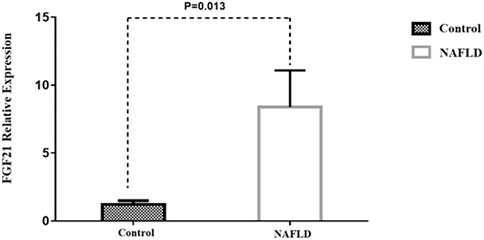
FIGURE 2. Comparison of the FGF21 relative expression between NAFLD and control group. *p-value was computed by t-test and data are shown as Mean ± SEM.
Finally, our findings in this study demonstrated a positive correlation between mtDNA-CN and FGF21expression levels in NAFLD patients (p = 0.027) (Figure 3). This comparison remained statistically significant after adjusting for age and BMI (p = 0.035).
Discussion
Recent studies have shown that NAFLD can be classified as a mitochondrial disease, in fact mitochondrial malfunction leads to abnormal hepatic fatty acid oxidation causing fat accumulation and hepatic steatosis. In addition to that, such mitochondria produce more ROS and less ATPs which cause more damages to mitochondria and hepatic cells making a destructive cycle (Koroglu et al., 2016; Dornas and Schuppan, 2020; Dabravolski et al., 2021). Thus, measuring relative mtDNA-CN as determining factor for mitochondrial damage and activity rate can be helpful for identifying the stage and prognosis of the disease (Cao et al., 2020; Filograna et al., 2021). On the other hand, in some stages of different mitochondrial disorders mtDNA-CN can be within the normal range and its changes should be interpreted along with clinical, histological, and other laboratory findings (Mavraki et al., 2023). However, despite its flows, mtDNA-CN is widely accepted among researchers as a useful method to assess mitochondrial function (Castellani et al., 2020; Zhang et al., 2022). Our results showed significant elevation in relative mtDNA-CN in NAFLD patients’ liver which were in accordance with our previous work (Kamfar et al., 2016). Also, Malik et al. demonstrated a rise in hepatic mtDNA content during the initial stages of hepatic steatosis in mice models (Malik et al., 2019). Chiappini et al. also found that the mtDNA to nuclear DNA (nDNA) ratio was higher in hepatic steatosis than in normal liver tissues (Chiappini et al., 2006). However, in contrast to these results Sookian et al. and Pirola et al. reported significant lower mtDNA/nDNA ratio in the liver of NAFLD patients compared to that of healthy control group (Sookoian et al., 2010; Pirola et al., 2015). Studies suggested that mtDNA-CN upregulation in humans with mitochondrial diseases, mostly concomitant with an overall rise in mitochondrial biogenesis, is regularly occurring and typically considered as a compensatory mechanism to support cellular bioenergetics (Hsin-Chen et al., 2000; Lee et al., 2018; Skuratovskaia et al., 2019; Filograna et al., 2021). We assumed that in NAFL patients this compensatory mechanism leads to mtDNA-CN upregulation and when this mechanism fails, due to disease progression and significant higher oxidative stress in NASH patients, the mtDNA-CN falls down. On the other hand, an increase in defective mitochondria can be considered detrimental rather than protective because of ROS accumulation as byproducts of the defective mitochondria (Sanyal et al., 2001; Shami et al., 2021). So, the precise involvement of mitochondrial biogenesis in these patients remains a topic of debate. In the present study we investigate NAFL and NASH patients separately in this regard. Although in our results relative mtDNA-CN was higher in NASH samples compared to NAFL ones, the difference was not significant. Thus, the interpretation of this data can be challenging, partly due to method-, specimen- and study design-related issues.
Some studies reported substantial depletion of mtDNA-CN in hepatic cells with aging in animal models (Barazzoni et al., 2000; Hartmann et al., 2011). Wachsmuth et al. suggested that mtDNA-CN decreased with age in human muscle tissue (Wachsmuth et al., 2016). However, Frahm et al. reported no age-related increase of mtDNA amount in brain, skeletal muscle and human heart (Frahm et al., 2005). Their result was in accordance with our present and previous studies (Kamfar et al., 2016) on human liver cells. This can have several reasons, for example, beside limited number of samples, our studies had a case-control design and we did not investigate liver mtDNA-CN in individuals through long duration of time. Also, evidence indicated that mtDNA-CN can vary between different cell types and answer differently to various physiologic and pathologic states including NAFLD (Tapia et al., 2018; Ma et al., 2020; Filograna et al., 2021) suggesting a dynamic nature for this parameter.
In the current study, we detected a significant increase in FGF21 expression in patients with higher BMI. This results were in concordance to many previous studies conducted on children (Reinehr et al., 2012) and adults (Dushay et al., 2010; Tyynismaa et al., 2011). According to our results, FGF21 expression rise was also significantly correlated to high AST and high ALT. Nakanishi et al. supported this result in their study which showed that FGF21 level was remarkably associated with AST and ALT elevation (Nakanishi et al., 2021). This emphasizes FGF21 role as an ameliorating agent which its production increases in liver injuries.
Numerous evidence showed that circulatory FGF21 rise in NAFLD patients and discussed its role as a protective factor (Tucker et al., 2019; Tillman and Rolph, 2020). FGF21 elevation can be seen as another compensatory mechanism in these patients. However, we do not fully understand the molecular regulatory mechanisms behind its function yet (Watanabe et al., 2020; Tan et al., 2023). In our study we detected remarkable increase in its expression in liver tissue of NAFLD patients compared to of control group. This result was supported by other previous works (Kamfar et al., 2016; Liu et al., 2023). Li et al. suggested that FGF21 may mirror the severity and progression of NAFLD due to its association with obesity, triglyceride, and gama-glutamyltransferase (Li et al., 2010). They reported that hepatic FGF21 mRNA expression in NAFLD patients with grade 1 was 4-fold higher than that in grade 0 (p < 0.01), and grade 2–3 was 14.71-fold higher than that in grade 0 (p < 0.01). In another study conducted by Flisiak-Jackiewicz et al. serum FGF21 levels were higher significantly in obese children with NAFLD compared to obese children without the disease and had a positive correlation with steatosis grades in biopsies (Flisiak-Jackiewicz et al., 2019). However, we did not detect any significant difference in its expression between NAFL and NASH patients. This can be due to the failure of FGF21 related compensatory mechanisms in more advanced stages of the disease. In this regard Alisi et al. found that FGF21 levels increased progressively with the increase of hepatic steatosis, but when hepatic fat content reached the fourth quartile, FGF21 levels tended to decline (Alisi et al., 2013). The authors of that study suggested that decreased production of this molecule by hepatocytes due to their injury or death caused by lipotoxicity and hepatic inflammation may be the cause of its decline in adult patients with severe liver steatohepatitis. Their results were also in accordance with the study conducted by Dushay et al. who reported lower hepatic FGF21 expression in NASH compared to NAFLD and suggested it may reflect more advanced hepatic injury (Dushay et al., 2010).
Finally, we reported a positive correlation between mtDNA-CN and FGF21 expression levels in liver tissue samples of patients with NAFLD which as mentioned before might be a mitochondrial disease. Based on current results, these two factors may play critical roles at the early stages of disease in inhibiting NAFLD development to NASH. In a recent systematic review, Lin et al. reported that FGF21 is highly sensitive and specific for diagnosis of mitochondrial diseases (Lin et al., 2020). According to a study conducted by Ji et al. FGF21 expression in mitochondrial diseases increases as a compensatory mechanism in energy metabolism. Furthermore, they showed that FGF21 regulates energy homeostasis by increasing expression of mitochondrial genes and mtDNA-CN (Ji et al., 2015). A more accurate explanation of the relation between these two factors in NAFLD remains to be investigated. Despite the importance of this matter, there are currently no approved therapies for treating NAFLD or NASH globally (Francque and Vonghia, 2019). Exercise prescription is considered a central strategy in treatment (van der Windt et al., 2018). In confirmation of this proposed option, in one study, J Henkel et al. showed that exercise improved glucose tolerance in NAFLD by inducing FGF21 production by the liver (Henkel et al., 2019).
Our study holds some limitations. Firstly, limited number of participants is an important barrier for making a reliable conclusion that needed to be noticed. Secondly, because of the dangers of sample collection in this study we could not match our controls with patients perfectly. We tried to minimize the effect of this bias by adjusting for some confounding factors like age and BMI in our analysis. Thirdly, we did not collect data during the progression of the disease in individuals hence our results regarding changes in parameters during disease progression are subject to error. Finally, like any study using liver biopsy as a standard, miss-diagnosis of disease stage at biopsy can be caused by sampling error (Ratziu et al., 2005). The potential for sampling error in this study was minimized by collecting 2 to 3 biopsies >15 mm from each participant and consulting two hepatopathologists to examine each sample.
In the present study we did not collect data on FGF21 protein levels in liver tissues and blood samples of patients and just reported and analyzed FGF21 mRNA levels. It has been shown that the protein levels can be independent of associated mRNA levels in tissues and blood (Greenbaum et al., 2003; Silva and Vogel, 2016). This can be caused by several factors affecting FGF21 production like mRNA degradation, translation, and protein degradation (Battle et al., 2015; Bayoumi et al., 2021). Hence, making it challenging to find a direct cause-and-effect relationship between different gene expressions. We tried to tackle this issue by measuring mRNA levels which become affected prior to changes in protein levels and to help clarifying ambiguities. More studies with larger number of participants and measuring protein levels through disease progression are needed to track the changes more specifically.
As for studying mitochondrial changes we only collected data on mtDNA-CN in liver tissues of participants as previous literature suggested it to be a biomarker of mitochondrial function (Castellani et al., 2020; Zhang et al., 2022). However, further studies on mitochondrial changes with different methods like electron microscopy and evaluation of consequences of mtDNA-CN changes are needed.
Taken together, in this case-control study, we have shown a considerable rise in FGF21 expression and mtDNA-CN in NAFLD patients compared to healthy control group. While the data presented here suggest that mitochondrial dysfunction and FGF21 expression are involved in the disease mechanism, they are not conclusive in predicting prognosis or progression of the disease. Furthermore, our results suggested a positive correlation between hepatic FGF21 expression and mtDNA-CN in the liver tissue of NAFLD patients. Further research is needed to determine the exact relationship between mtDNA-CN and FGF21 with NAFLD susceptibility.
Data availability statement
The original contributions presented in the study are included in the article/Supplementary Materials, further inquiries can be directed to the corresponding author.
Ethics statement
The studies involving human participants were reviewed and approved by the Hamadan University of Medical Sciences (P/16/35/9/3481). The patients/participants provided their written informed consent to participate in this study.
Author contributions
All authors listed have made a substantial, direct, and intellectual contribution to the work and approved it for publication.
Funding
This study was supported by the Shahid Beheshti University of Medical Sciences, Tehran, Iran.
Acknowledgments
We are all very thankful to all generous donors for research purposes.
Conflict of interest
The authors declare that the research was conducted in the absence of any commercial or financial relationships that could be construed as a potential conflict of interest.
Publisher’s note
All claims expressed in this article are solely those of the authors and do not necessarily represent those of their affiliated organizations, or those of the publisher, the editors and the reviewers. Any product that may be evaluated in this article, or claim that may be made by its manufacturer, is not guaranteed or endorsed by the publisher.
Supplementary material
The Supplementary Material for this article can be found online at: https://www.frontiersin.org/articles/10.3389/fmolb.2023.1203019/full#supplementary-material
SUPPLEMENTARY TABLE S1 | Comparison of mtDNA-CN between NAFL and NASH patients and controls.
SUPPLEMENTARY TABLE S2 | Correlations of serum fgf21 expression with copy number anthropometric parameters and biochemical indexes.
References
Acierno, C., Caturano, A., Pafundi, P. C., Nevola, R., Adinolfi, L. E., and Sasso, F. C. (2020). Nonalcoholic fatty liver disease and type 2 diabetes: Pathophysiological mechanisms shared between the two faces of the same coin. Explor. Med. 1 (5). doi:10.37349/emed.2020.00019
Alisi, A., Ceccarelli, S., Panera, N., Prono, F., Petrini, S., De Stefanis, C., et al. (2013). Association between serum atypical fibroblast growth factors 21 and 19 and pediatric nonalcoholic fatty liver disease. PLoS One 8 (6), e67160. doi:10.1371/journal.pone.0067160
Ascha, M. S., Hanouneh, I. A., Lopez, R., Tamimi, T. A-R., Feldstein, A. F., and Zein, N. N. (2010). The incidence and risk factors of hepatocellular carcinoma in patients with nonalcoholic steatohepatitis. Hepatology 51 (6), 1972–1978. doi:10.1002/hep.23527
Bao, L., Yin, J., Gao, W., Wang, Q., Yao, W., and Gao, X. (2018). A long-acting FGF21 alleviates hepatic steatosis and inflammation in a mouse model of non-alcoholic steatohepatitis partly through an FGF21-adiponectin-IL17A pathway. Br. J. Pharmacol. 175 (16), 3379–3393. doi:10.1111/bph.14383
Barazzoni, R., Short, K. R., and Nair, K. S. (2000). Effects of aging on mitochondrial DNA copy number and cytochromec oxidase gene expression in rat skeletal muscle, liver, and heart. J. Biol. Chem. 275 (5), 3343–3347. doi:10.1074/jbc.275.5.3343
Battle, A., Khan, Z., Wang, S. H., Mitrano, A., Ford, M. J., Pritchard, J. K., et al. (2015). Genomic variation. Impact of regulatory variation from RNA to protein. Science 347 (6222), 664–667. doi:10.1126/science.1260793
Bayoumi, A., Elsayed, A., Han, S., Petta, S., Adams, L. A., Aller, R., et al. (2021). Mistranslation drives alterations in protein levels and the effects of a synonymous variant at the fibroblast growth factor 21 locus. Adv. Sci. 8 (11), 2004168. doi:10.1002/advs.202004168
Begriche, K., Igoudjil, A., Pessayre, D., and Fromenty, B. (2006). Mitochondrial dysfunction in NASH: Causes, consequences and possible means to prevent it. Mitochondrion 6 (1), 1–28. doi:10.1016/j.mito.2005.10.004
Bhala, N., Angulo, P., van der Poorten, D., Lee, E., Hui, J. M., Saracco, G., et al. (2011). The natural history of nonalcoholic fatty liver disease with advanced fibrosis or cirrhosis: An international collaborative study. Hepatology 54 (4), 1208–1216. doi:10.1002/hep.24491
Boparai, R. K., Arum, O., Miquet, J. G., Masternak, M. M., Bartke, A., and Khardori, R. K. (2015). Resistance to the beneficial metabolic effects and hepatic antioxidant defense actions of fibroblast growth factor 21 treatment in growth hormone-overexpressing transgenic mice. Int. J. Endocrinol. 2015, 282375. doi:10.1155/2015/282375
Cao, K., Wang, K., Yang, M., Liu, X., Lv, W., and Liu, J. (2020). Punicalagin improves hepatic lipid metabolism via modulation of oxidative stress and mitochondrial biogenesis in hyperlipidemic mice. Food Funct. 11 (11), 9624–9633. doi:10.1039/d0fo01545h
Castellani, C. A., Longchamps, R. J., Sun, J., Guallar, E., and Arking, D. E. (2020). Thinking outside the nucleus: Mitochondrial DNA copy number in health and disease. Mitochondrion 53, 214–223. doi:10.1016/j.mito.2020.06.004
Caturano, A., Acierno, C., Nevola, R., Pafundi, P. C., Galiero, R., Rinaldi, L., et al. (2021). Non-alcoholic fatty liver disease: From pathogenesis to clinical impact. Processes 9 (1), 135. doi:10.3390/pr9010135
Chalasani, N., Younossi, Z., Lavine, J. E., Diehl, A. M., Brunt, E. M., Cusi, K., et al. (2012). The diagnosis and management of non-alcoholic fatty liver disease: Practice guideline by the American association for the study of liver diseases, American college of gastroenterology, and the American gastroenterological association. Hepatology 55 (6), 2005–2023. doi:10.1002/hep.25762
Chiappini, F., Barrier, A., Saffroy, R., Domart, M-C., Dagues, N., Azoulay, D., et al. (2006). Exploration of global gene expression in human liver steatosis by high-density oligonucleotide microarray. Lab. Investig. 86 (2), 154–165. doi:10.1038/labinvest.3700374
Cuevas-Ramos, D., Almeda-Valdes, P., Aguilar-Salinas, C. A., Cuevas-Ramos, G., Cuevas-Sosa, A. A., and Gomez-Perez, F. J. (2009). The role of fibroblast growth factor 21 (FGF21) on energy balance, glucose and lipid metabolism. Curr. diabetes Rev. 5 (4), 216–220. doi:10.2174/157339909789804396
Cusi, K. (2009). Nonalcoholic fatty liver disease in type 2 diabetes mellitus. Curr. Opin. Endocrinol. Diabetes Obes. 16 (2), 141–149. doi:10.1097/MED.0b013e3283293015
Dabravolski, S. A., Bezsonov, E. E., Baig, M. S., Popkova, T. V., Nedosugova, L. V., Starodubova, A. V., et al. (2021). Mitochondrial mutations and genetic factors determining NAFLD risk. Int. J. Mol. Sci. 22 (9), 4459. doi:10.3390/ijms22094459
Dornas, W., and Schuppan, D. (2020). Mitochondrial oxidative injury: A key player in nonalcoholic fatty liver disease. Am. J. Physiology-Gastrointestinal Liver Physiology 319 (3), G400–G11. doi:10.1152/ajpgi.00121.2020
Dushay, J., Chui, P. C., Gopalakrishnan, G. S., Varela-Rey, M., Crawley, M., Fisher, F. M., et al. (2010). Increased fibroblast growth factor 21 in obesity and nonalcoholic fatty liver disease. Gastroenterology 139 (2), 456–463. doi:10.1053/j.gastro.2010.04.054
Falamarzi, K., Malekpour, M., Tafti, M. F., Azarpira, N., Behboodi, M., and Zarei, M. (2022). The role of FGF21 and its analogs on liver associated diseases. Front. Med. (Lausanne) 9, 967375. doi:10.3389/fmed.2022.967375
Filograna, R., Mennuni, M., Alsina, D., and Larsson, N-G. (2021). Mitochondrial DNA copy number in human disease: The more the better? FEBS Lett. 595 (8), 976–1002. doi:10.1002/1873-3468.14021
Flisiak-Jackiewicz, M., Bobrus-Chociej, A., Wasilewska, N., Tarasow, E., Wojtkowska, M., and Lebensztejn, D. M. (2019). Can hepatokines be regarded as novel non-invasive serum biomarkers of intrahepatic lipid content in obese children? Adv. Med. Sci. 64 (2), 280–284. doi:10.1016/j.advms.2019.02.005
Frahm, T., Mohamed, S. A., Bruse, P., Gemünd, C., Oehmichen, M., and Meissner, C. (2005). Lack of age-related increase of mitochondrial DNA amount in brain, skeletal muscle and human heart. Mech. ageing Dev. 126 (11), 1192–1200. doi:10.1016/j.mad.2005.06.008
Francque, S., and Vonghia, L. (2019). Pharmacological treatment for non-alcoholic fatty liver disease. Adv. Ther. 36 (5), 1052–1074. doi:10.1007/s12325-019-00898-6
Greenbaum, D., Colangelo, C., Williams, K., and Gerstein, M. (2003). Comparing protein abundance and mRNA expression levels on a genomic scale. Genome Biol. 4 (9), 117. doi:10.1186/gb-2003-4-9-117
Hartmann, N., Reichwald, K., Wittig, I., Dröse, S., Schmeisser, S., Lück, C., et al. (2011). Mitochondrial DNA copy number and function decrease with age in the short-lived fish Nothobranchius furzeri. Aging Cell 10 (5), 824–831. doi:10.1111/j.1474-9726.2011.00723.x
Henkel, J., Buchheim-Dieckow, K., Castro, J. P., Laeger, T., Wardelmann, K., Kleinridders, A., et al. (2019). Reduced oxidative stress and enhanced FGF21 formation in livers of endurance-exercised rats with diet-induced NASH. Nutrients 11 (11), 2709. doi:10.3390/nu11112709
Hsin-Chen, L., Pen-Hui, Y., Ching-You, L., Chin-Wen, C., and Yau-Huei, W. (2000). Increase of mitochondria and mitochondrial DNA in response to oxidative stress in human cells. Biochem. J. 348 (2), 425–432. doi:10.1042/bj3480425
Jensen, E. C. (2012). Real-time reverse transcription polymerase chain reaction to measure mRNA: Use, limitations, and presentation of results. Anatomical Rec. 295 (1), 1–3. doi:10.1002/ar.21487
Ji, K., Zheng, J., Lv, J., Xu, J., Ji, X., Luo, Y. B., et al. (2015). Skeletal muscle increases FGF21 expression in mitochondrial disorders to compensate for energy metabolic insufficiency by activating the mTOR-YY1-PGC1α pathway. Free Radic. Biol. Med. 84, 161–170. doi:10.1016/j.freeradbiomed.2015.03.020
Kamfar, S., Alavian, S. M., Houshmand, M., Yadegarazari, R., Seifi Zarei, B., Khalaj, A., et al. (2016). Liver mitochondrial DNA copy number and deletion levels may contribute to nonalcoholic fatty liver disease susceptibility. Hepat. Mon. 16 (12), e40774. doi:10.5812/hepatmon.40774
Koroglu, E., Canbakan, B., Atay, K., Hatemi, I., Tuncer, M., Dobrucali, A., et al. (2016). Role of oxidative stress and insulin resistance in disease severity of non-alcoholic fatty liver disease. Turk J. Gastroenterol. 27 (4), 361–366. doi:10.5152/tjg.2016.16106
Lee, J. H., Kang, Y. E., Chang, J. Y., Park, K. C., Kim, H-W., Kim, J. T., et al. (2016). An engineered FGF21 variant, LY2405319, can prevent non-alcoholic steatohepatitis by enhancing hepatic mitochondrial function. Am. J. Transl. Res. 8 (11), 4750–4763.
Lee, K., Haddad, A., Osme, A., Kim, C., Borzou, A., Ilchenko, S., et al. (2018). Hepatic mitochondrial defects in a nonalcoholic fatty liver disease mouse model are associated with increased degradation of oxidative phosphorylation subunits*. Mol. Cell. Proteomics 17 (12), 2371–2386. doi:10.1074/mcp.RA118.000961
Li, H., Fang, Q., Gao, F., Fan, J., Zhou, J., Wang, X., et al. (2010). Fibroblast growth factor 21 levels are increased in nonalcoholic fatty liver disease patients and are correlated with hepatic triglyceride. J. Hepatol. 53 (5), 934–940. doi:10.1016/j.jhep.2010.05.018
Lin, Y., Ji, K., Ma, X., Liu, S., Li, W., Zhao, Y., et al. (2020). Accuracy of FGF-21 and GDF-15 for the diagnosis of mitochondrial disorders: A meta-analysis. Ann. Clin. Transl. Neurol. 7 (7), 1204–1213. doi:10.1002/acn3.51104
Liu, C., Schönke, M., Spoorenberg, B., Lambooij, J. M., van der Zande, H. J. P., Zhou, E., et al. (2023). FGF21 protects against hepatic lipotoxicity and macrophage activation to attenuate fibrogenesis in nonalcoholic steatohepatitis. eLife 12, e83075. doi:10.7554/eLife.83075
Liu, J., Xu, Y., Hu, Y., and Wang, G. (2015). The role of fibroblast growth factor 21 in the pathogenesis of non-alcoholic fatty liver disease and implications for therapy. Metabolism 64 (3), 380–390. doi:10.1016/j.metabol.2014.11.009
Lonardo, A., Nascimbeni, F., Maurantonio, M., Marrazzo, A., Rinaldi, L., and Adinolfi, L. E. (2017). Nonalcoholic fatty liver disease: Evolving paradigms. World J. Gastroenterology 23 (36), 6571–6592. doi:10.3748/wjg.v23.i36.6571
Ma, C., Liu, Y., He, S., Zeng, J., Li, P., Ma, C., et al. (2020). Association between leukocyte mitochondrial DNA copy number and non-alcoholic fatty liver disease in a Chinese population is mediated by 8-oxo-2'-deoxyguanosine. Front. Med. (Lausanne) 7, 536. doi:10.3389/fmed.2020.00536
Malik, A. N., Simões, I. C. M., Rosa, H. S., Khan, S., Karkucinska-Wieckowska, A., and Wieckowski, M. R. (2019). A diet induced maladaptive increase in hepatic mitochondrial DNA precedes OXPHOS defects and may contribute to non-alcoholic fatty liver disease. Cells 8 (10), 1222. doi:10.3390/cells8101222
Mavraki, E., Labrum, R., Sergeant, K., Alston, C. L., Woodward, C., Smith, C., et al. (2023). Genetic testing for mitochondrial disease: The United Kingdom best practice guidelines. Eur. J. Hum. Genet. 31 (2), 148–163. doi:10.1038/s41431-022-01249-w
Mitra, S., De, A., and Chowdhury, A. (2020). Epidemiology of non-alcoholic and alcoholic fatty liver diseases. Transl. Gastroenterol. Hepatol. 5, 16. doi:10.21037/tgh.2019.09.08
Nakanishi, K., Ishibashi, C., Ide, S., Yamamoto, R., Nishida, M., Nagatomo, I., et al. (2021). Serum FGF21 levels are altered by various factors including lifestyle behaviors in male subjects. Sci. Rep. 11 (1), 22632. doi:10.1038/s41598-021-02075-8
Nusrat, S., Khan, M. S., Fazili, J., and Madhoun, M. F. (2014). Cirrhosis and its complications: Evidence based treatment. World J. Gastroenterol. 20 (18), 5442–5460. doi:10.3748/wjg.v20.i18.5442
Paradies, G., Paradies, V., Ruggiero, F. M., and Petrosillo, G. (2014). Oxidative stress, cardiolipin and mitochondrial dysfunction in nonalcoholic fatty liver disease. World J. gastroenterology WJG 20 (39), 14205–14218. doi:10.3748/wjg.v20.i39.14205
Pessayre, D., and Fromenty, B. (2005). Nash: A mitochondrial disease. J. hepatology 42 (6), 928–940. doi:10.1016/j.jhep.2005.03.004
Pirola, C. J., Scian, R., Gianotti, T. F., Dopazo, H., Rohr, C., Martino, J. S., et al. (2015). Epigenetic modifications in the biology of nonalcoholic fatty liver disease: The role of DNA hydroxymethylation and TET proteins. Medicine 94 (36), e1480. doi:10.1097/MD.0000000000001480
Ratziu, V., Charlotte, F., Heurtier, A., Gombert, S., Giral, P., Bruckert, E., et al. (2005). Sampling variability of liver biopsy in nonalcoholic fatty liver disease. Gastroenterology 128 (7), 1898–1906. doi:10.1053/j.gastro.2005.03.084
Reinehr, T., Woelfle, J., Wunsch, R., and Roth, C. L. (2012). Fibroblast growth factor 21 (FGF-21) and its relation to obesity, metabolic syndrome, and nonalcoholic fatty liver in children: A longitudinal analysis. J. Clin. Endocrinol. Metabolism 97 (6), 2143–2150. doi:10.1210/jc.2012-1221
Rusli, F., Deelen, J., Andriyani, E., Boekschoten, M. V., Lute, C., van den Akker, E. B., et al. (2016). Fibroblast growth factor 21 reflects liver fat accumulation and dysregulation of signalling pathways in the liver of C57BL/6J mice. Sci. Rep. 6 (1), 30484. doi:10.1038/srep30484
Sanyal, A. J., Campbell-Sargent, C., Mirshahi, F., Rizzo, W. B., Contos, M. J., Sterling, R. K., et al. (2001). Nonalcoholic steatohepatitis: Association of insulin resistance and mitochondrial abnormalities. Gastroenterology 120 (5), 1183–1192. doi:10.1053/gast.2001.23256
Shakhssalim, N., Houshmand, M., Kamalidehghan, B., Faraji, A., Sarhangnejad, R., Dadgar, S., et al. (2013). The mitochondrial C16069T polymorphism, not mitochondrial D310 (D-loop) mononucleotide sequence variations, is associated with bladder cancer. Cancer Cell Int. 13 (1), 120. doi:10.1186/1475-2867-13-120
Shami, G. J., Cheng, D., Verhaegh, P., Koek, G., Wisse, E., and Braet, F. (2021). Three-dimensional ultrastructure of giant mitochondria in human non-alcoholic fatty liver disease. Sci. Rep. 11 (1), 3319. doi:10.1038/s41598-021-82884-z
Silva, G. M., and Vogel, C. (2016). Quantifying gene expression: The importance of being subtle. Mol. Syst. Biol. 12 (10), 885. doi:10.15252/msb.20167325
Skuratovskaia, D., Zatolokin, P., Vulf, M., Mazunin, I., and Litvinova, L. (2019). Interrelation of chemerin and TNF-α with mtDNA copy number in adipose tissues and blood cells in obese patients with and without type 2 diabetes. BMC Med. Genomics 12 (2), 40. doi:10.1186/s12920-019-0485-8
Sookoian, S., Rosselli, M. S., Gemma, C., Burgueño, A. L., Gianotti, T. F., Castaño, G. O., et al. (2010). Epigenetic regulation of insulin resistance in nonalcoholic fatty liver disease: Impact of liver methylation of the peroxisome proliferator-activated receptor γ coactivator 1α promoter. Hepatology 52 (6), 1992–2000. doi:10.1002/hep.23927
Tan, H., Yue, T., Chen, Z., Wu, W., Xu, S., and Weng, J. (2023). Targeting FGF21 in cardiovascular and metabolic diseases: From mechanism to medicine. Int. J. Biol. Sci. 19 (1), 66–88. doi:10.7150/ijbs.73936
Tanaka, N., Takahashi, S., Zhang, Y., Krausz, K. W., Smith, P. B., Patterson, A. D., et al. (2015). Role of fibroblast growth factor 21 in the early stage of NASH induced by methionine-and choline-deficient diet. Biochimica Biophysica Acta (BBA)-Molecular Basis Dis. 1852 (7), 1242–1252. doi:10.1016/j.bbadis.2015.02.012
Tapia, M. A. P., Fragoso-Bargas, N., Rodríguez-Ríos, D., Lazo-de-la-Vega-Monroy, M. L., del Rocío Ibarra-Reynoso, L., Ruíz-Noa, Y., et al. (2018). Peripheral blood mitochondrial DNA copy number, a potential marker of non-alcoholic fatty liver disease? FASEB J. 32 (S1), lb112–lb. doi:10.1096/fasebj.2018.32.1_supplement.lb112
Tillman, E. J., and Rolph, T. (2020). FGF21: An emerging therapeutic target for non-alcoholic steatohepatitis and related metabolic diseases. Front. Endocrinol. 11, 601290. doi:10.3389/fendo.2020.601290
Toshikuni, N., Tsutsumi, M., and Arisawa, T. (2014). Clinical differences between alcoholic liver disease and nonalcoholic fatty liver disease. World J. Gastroenterol. 20 (26), 8393–8406. doi:10.3748/wjg.v20.i26.8393
Tucker, B., Li, H., Long, X., Rye, K. A., and Ong, K. L. (2019). Fibroblast growth factor 21 in non-alcoholic fatty liver disease. Metabolism 101, 153994. doi:10.1016/j.metabol.2019.153994
Tyynismaa, H., Raivio, T., Hakkarainen, A., Ortega-Alonso, A., Lundbom, N., Kaprio, J., et al. (2011). Liver fat but not other adiposity measures influence circulating FGF21 levels in healthy young adult twins. J. Clin. Endocrinol. Metab. 96 (2), E351–E355. doi:10.1210/jc.2010-1326
van der Windt, D. J., Sud, V., Zhang, H., Tsung, A., and Huang, H. (2018). The effects of physical exercise on fatty liver disease. Gene Expr. 18 (2), 89–101. doi:10.3727/105221617X15124844266408
Wachsmuth, M., Huebner, A., Li, M., Madea, B., and Stoneking, M. (2016). Age-related and heteroplasmy-related variation in human mtDNA copy number. PLoS Genet. 12 (3), 1005939. doi:10.1371/journal.pgen.1005939
Wang, J., He, W., Tsai, P-J., Chen, P-H., Ye, M., Guo, J., et al. (2020). Mutual interaction between endoplasmic reticulum and mitochondria in nonalcoholic fatty liver disease. Lipids Health Dis. 19, 72–19. doi:10.1186/s12944-020-01210-0
Wang, M., and Kaufman, R. J. (2014). The impact of the endoplasmic reticulum protein-folding environment on cancer development. Nat. Rev. Cancer 14 (9), 581–597. doi:10.1038/nrc3800
Watanabe, M., Risi, R., Camajani, E., Contini, S., Persichetti, A., Tuccinardi, D., et al. (2020). Baseline homa IR and circulating FGF21 levels predict NAFLD improvement in patients undergoing a low carbohydrate dietary intervention for weight loss: A prospective observational pilot study. Nutrients 12 (7), 2141. doi:10.3390/nu12072141
Xu, J., Lloyd, D. J., Hale, C., Stanislaus, S., Chen, M., Sivits, G., et al. (2009). Fibroblast growth factor 21 reverses hepatic steatosis, increases energy expenditure, and improves insulin sensitivity in diet-induced obese mice. Diabetes 58 (1), 250–259. doi:10.2337/db08-0392
Xu, J., Shen, J., Yuan, R., Jia, B., Zhang, Y., Wang, S., et al. (2021). Mitochondrial targeting therapeutics: Promising role of natural products in non-alcoholic fatty liver disease. Front. Pharmacol. 12, 796207. doi:10.3389/fphar.2021.796207
Ye, D., Wang, Y., Li, H., Jia, W., Man, K., Lo, C. M., et al. (2014). Fibroblast growth factor 21 protects against acetaminophen-induced hepatotoxicity by potentiating peroxisome proliferator-activated receptor coactivator protein-1α-mediated antioxidant capacity in mice. Hepatology 60 (3), 977–989. doi:10.1002/hep.27060
Ye, J., Coulouris, G., Zaretskaya, I., Cutcutache, I., Rozen, S., and Madden, T. L. (2012). Primer-BLAST: A tool to design target-specific primers for polymerase chain reaction. BMC Bioinforma. 13 (1), 134. doi:10.1186/1471-2105-13-134
Yeh, M. M., and Brunt, E. M. (2014). Pathological features of fatty liver disease. Gastroenterology 147 (4), 754–764. doi:10.1053/j.gastro.2014.07.056
Younossi, Z., Anstee, Q. M., Marietti, M., Hardy, T., Henry, L., Eslam, M., et al. (2018). Global burden of NAFLD and NASH: Trends, predictions, risk factors and prevention. Nat. Rev. Gastroenterology hepatology 15 (1), 11–20. doi:10.1038/nrgastro.2017.109
Zabihi Diba, L., Mohaddes Ardebili, S. M., Gharesouran, J., and Houshmand, M. (2016). Age-related decrease in mtDNA content as a consequence of mtDNA 4977 bp deletion. Mitochondrial DNA Part A 27 (4), 3008–3012. doi:10.3109/19401736.2015.1063046
Keywords: fibroblast growth factor 21, mitochondrial DNA, nonalcoholic fatty liver disease, FGF21, NAFLD
Citation: Houshmand M, Zeinali V, Hosseini A, Seifi A, Danaei B and Kamfar S (2023) Investigation of FGF21 mRNA levels and relative mitochondrial DNA copy number levels and their relation in nonalcoholic fatty liver disease: a case-control study. Front. Mol. Biosci. 10:1203019. doi: 10.3389/fmolb.2023.1203019
Received: 10 April 2023; Accepted: 22 May 2023;
Published: 06 June 2023.
Edited by:
Filippo M. Santorelli, Stella Maris Foundation (IRCCS), ItalyReviewed by:
Jogendra Singh Pawar, The Ohio State University, United StatesVaibhav Deshmukh, Washington University in St. Louis, United States
Rosalba Carrozzo, Bambino Gesù Children’s Hospital (IRCCS), Italy
Copyright © 2023 Houshmand, Zeinali, Hosseini, Seifi, Danaei and Kamfar. This is an open-access article distributed under the terms of the Creative Commons Attribution License (CC BY). The use, distribution or reproduction in other forums is permitted, provided the original author(s) and the copyright owner(s) are credited and that the original publication in this journal is cited, in accordance with accepted academic practice. No use, distribution or reproduction is permitted which does not comply with these terms.
*Correspondence: Sharareh Kamfar, a2FtZmFyc2hhcmFyZWhAZ21haWwuY29t