- 1Department of Biochemistry, Purdue University, West Lafayette, IN, United States
- 2Department of Chemistry and Biochemistry, Middlebury College, Middlebury, VT, United States
- 3Department of Chemistry and Biochemistry, University of Tampa, Tampa, FL, United States
Protein ubiquitylation is an essential post-translational modification that regulates nearly all aspects of eukaryotic cell biology. A diverse collection of ubiquitylation signals, including an extensive repertoire of polymeric ubiquitin chains, leads to a range of different functional outcomes for the target protein. Recent studies have shown that ubiquitin chains can be branched and that branched chains have a direct impact on the stability or the activity of the target proteins they are attached to. In this mini review, we discuss the mechanisms that control the assembly and disassembly of branched chains by the enzymes of the ubiquitylation and deubiquitylation machinery. Existing knowledge regarding the activities of chain branching ubiquitin ligases and the deubiquitylases responsible for cleaving branched chains is summarized. We also highlight new findings concerning the formation of branched chains in response to small molecules that induce the degradation of otherwise stable proteins and examine the selective debranching of heterotypic chains by the proteasome-bound deubiquitylase UCH37.
Introduction
Ubiquitin is a versatile post-translational signal that can impact the stability, activity, localization, and interaction properties of protein substrates in numerous ways. It is most commonly attached to lysine residues of proteins through an isopeptide bond, although a number of other conjugation sites and non-protein targets have been reported (Squair and Virdee, 2022; Dikic and Schulman, 2023). The versatile nature of ubiquitin as a modifier stems from its capacity to be incorporated into a number of distinct structures. Ubiquitin can be conjugated to substrates as a monomer on one or more sites, a modification referred to as monoubiquitylation or multi-monoubiquitylation, respectively. Alternatively, it can be polymerized to form a chain, in which the carboxy terminus of one ubiquitin (the donor) is linked to another ubiquitin (the acceptor), most commonly through an isopeptide bond at one of the eight amino groups on the surface of ubiquitin. At least 12 different sites of chain formation have been reported to date, including several serine and threonine residues (Swatek and Komander, 2016; Kelsall et al., 2019; Rodriguez Carvajal et al., 2021), resulting in a staggering number of potentially unique chain architectures that can be formed when variation in chain length and topology are taken into account.
Ubiquitin chains can be classified into two different general categories based on the types of ubiquitin linkages and the topology of the chain (Figure 1A). Homotypic chains are linked uniformly through the same acceptor site of ubiquitin (e.g., K48-linked chains), whereas heterotypic chains are linked through multiple sites and can be further classified as either mixed or branched. Mixed chains are composed of ubiquitin subunits that are modified on only a single acceptor site and are thus topologically equivalent to homotypic chains. By contrast, branched chains contain at least one ubiquitin subunit that is modified concurrently on more than one site, resulting in a branched or “forked” structure. While the structures and functions of many homotypic chains are well understood (Komander and Rape, 2012; Akutsu et al., 2016; Swatek and Komander, 2016), those of heterotypic chains are understood in much less detail. Nevertheless, considerable progress has been made in elucidating the architectures and physiological functions of branched chains over the past 5–6 years (French et al., 2021; Kolla, et al., 2022). They act as powerful degradation signals to ensure the timely removal of regulatory and misfolded proteins from cells, and they activate signaling pathways through degradation-independent mechanisms. In this mini review, we explore the molecular mechanisms that underlie the assembly and disassembly of branched chains, highlighting new findings regarding the chemically-induced formation of branched chains by small molecules and the cleavage of branched chains by UCH37. Although there have been many recent reports suggestive of branched chain architectures, we focus here on cases in which branched structures have been clearly demonstrated through the use of mass spectrometry and/or careful biochemical analysis.
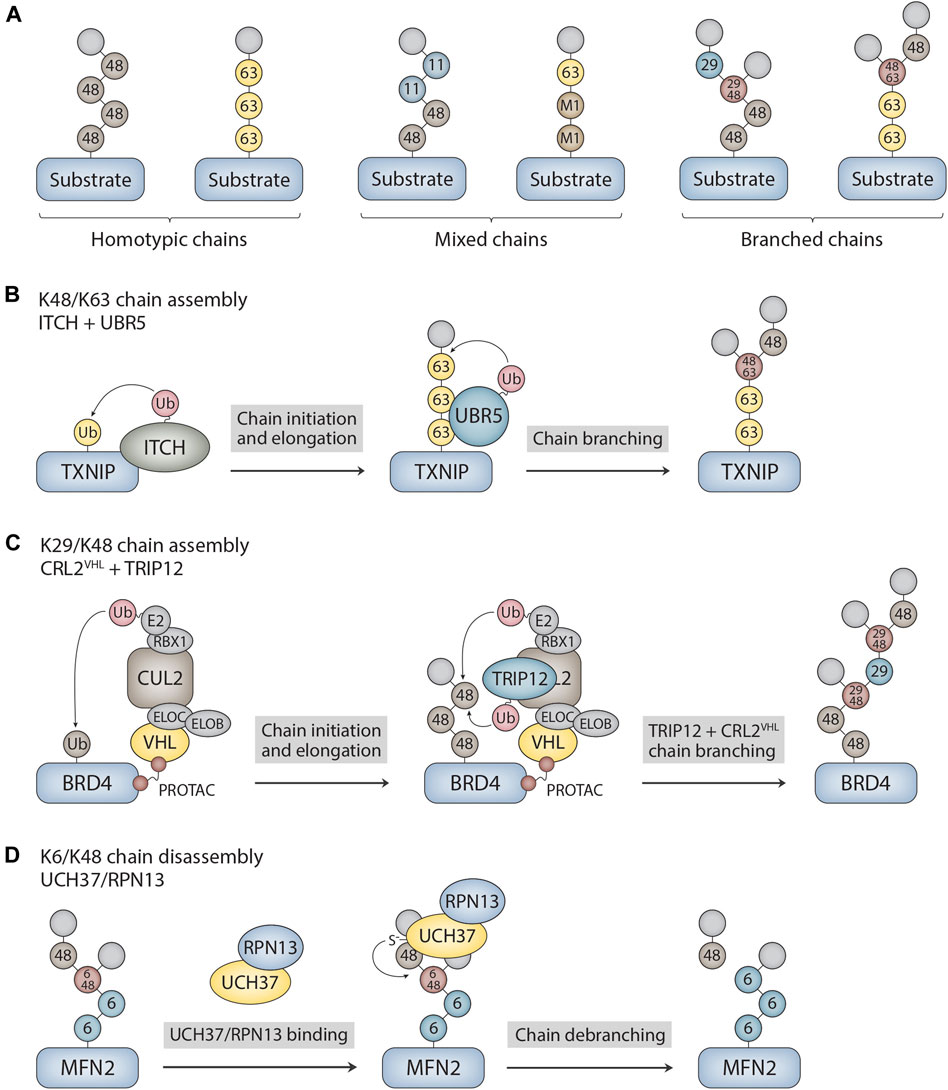
FIGURE 1. Ubiquitin chain architectures and mechanisms of branched chain assembly and disassembly. (A) Ubiquitin chains can be classified as either homotypic or heterotypic (mixed and branched) based on the types of ubiquitin linkages and how the subunits are connected to each other within the chain. Examples of homotypic K48- and K63-linked chains are shown. Other sites of chain formation include M1, K6, K11, T12, S20, T22, K27, K29, K33, and T55. Mixed K11/K48 and M1/K63 chains as well as branched K29/K48 and K48/K63 chains are shown as examples of heterotypic chains. The modified acceptor sites of the ubiquitin subunits are indicated in the figure. The terminal (unmodified) subunits are colored in light grey. (B) Mechanism of branched chain formation by the HECT ubiquitin ligases ITCH and UBR5. ITCH attaches homotypic K63-linked chains to TXNIP. UBR5 then binds to the K63 linkages through its UBA domain to nucleate the formation of K48 linkages, resulting in the assembly of branched K48/K63 chains. (C) PROTAC-dependent formation of branched chains by CRL2VHL and TRIP12. CRL2VHL assembles homotypic K48-linked chains on BRD4 and then recruits TRIP12, which attaches K29 linkages and further stimulates CRL2VHL activity. This cascade leads to the formation of complex branched K29/K48 chain architectures on BRD4. CLR2VHL subunits include VHL, elongin B (ELOB), elongin C (ELOC), CUL2, RBX1, and E2. (D) Mechanism of branched chain disassembly by the proteasome-bound UCH37/RPN13 complex. UCH37/RPN13 binds to both distal ubiquitin subunits that emanate from the K6/K48 branch point. Cleavage of the K48 linkage at the branch point is greatly stimulated by RPN13, resulting in trimming of the chain at the proximal K48 linkage and the release of homotypic K6- and K48-linked chains. The K6-linked chain attached to MFN2 is then presumably cleaved en bloc by RPN11, an essential proteasome-associated DUB component of the 19S regulatory particle, prior to MFN2 degradation. MFN2 was identified as a substrate of UCH37 in Du et al. (2022).
Assembly of branched chains
Ubiquitin signals are attached to substrates by the concerted actions of E1 ubiquitin-activating enzymes, E2 ubiquitin-conjugating enzymes, and E3 ubiquitin ligases. The human genome encodes ∼600 E3s (Li et al., 2008) with varying substrate specificities, enabling the modification of thousands of targets with ubiquitin monomers or chains of various configurations. Major classes of eukaryotic E3s include those containing a homologous to E6AP C-terminus (HECT), really interesting new gene (RING)/U-box, or RING-between-RING (RBR) domain (Zheng and Shabek, 2017; Lorenz, 2018; Walden and Rittinger, 2018). HECT and RBR E3s form a transient thioester intermediate with ubiquitin before transferring ubiquitin to the substrate and are generally thought to specify the type of ubiquitin chain linkage. By contrast, canonical RING/U-box E3s promote the direct transfer of ubiquitin from E2 to substrate and depend on the E2 to determine the type of chain linkage (Wright et al., 2016). In addition, several new classes of E3s with distinct mechanisms have recently been identified (Horn-Ghetko and Schulman, 2022), although the chain forming activities of these E3s have not been characterized in detail. While the mechanisms of homotypic chain formation are well established for many E2s and E3s (Deol et al., 2019), the events that underlie the formation of branched chains are not nearly as well understood.
Given that members of all major classes of E3s have the ability to form branched polymers (Table 1), the mechanisms of chain branching are expected to be intricate and diverse. The general mechanisms of assembly can be grouped into four different categories. Members of the HECT and RBR classes of E3s, including NleL, UBE3C, Parkin, HECTD1, and WWP1, have the ability to form branched chains of various configurations in cooperation with a single E2 (Wang et al., 2006; Hospenthal et al., 2013; French et al., 2017; Swatek et al., 2019; Deol et al., 2020a; Chen et al., 2021; Harris et al., 2021). The anaphase-promoting complex (APC/C), a multisubunit RING E3, cooperates with two different E2s in a sequential fashion to produce branched K11/K48 polymers (Meyer and Rape, 2014; Yau et al., 2017). A similar mechanism has been described for the RING E3 cIAP1, which synthesizes branched chains containing K48/K63 and K11/K48 linkages in a manner that depends on the activities of UBE2D and UBE2N-UBE2V (Akizuki et al., 2023). Collaboration between pairs of E3s with distinct chain linkage preferences is another common mechanism of branched chain formation. For example, the HECT E3s ITCH and UBR5 collaborate to form branched K48/K63 chains on TXNIP (Figure 1B), whereas Ufd2, a U-box E3, cooperates with the HECT E3 Ufd4 to form branched K29/K48 chains on substrates of the ubiquitin fusion degradation pathway (Liu et al., 2017; Ohtake et al., 2018). Finally, it has been reported that yeast Ubc1 and its mammalian orthologue UBE2K promote the assembly of branched K48/K63 chains (Pluska et al., 2021), indicating that some E2s have an innate chain branching activity.
Regardless of the E2s and E3s involved, the initiation of chain branching requires the selection of the appropriate branch point linkage and location. Specificity for chain branching, as opposed to elongation, occurs through the recognition of an unbranched chain and the selection of an internal ubiquitin within the chain by the branching E2 or E3. For example, Ufd2 recognizes homotypic K29 chains formed by Ufd4 through its two N-terminal loops to nucleate the formation of branched K29/K48 chains (Liu et al., 2017). Likewise, HUWE1 recognizes homotypic K63 linkages assembled by TRAF6 through its UIM and UBA domains to produce branched K48/K63 chains (Ohtake et al., 2016). For E3s that have both a chain initiating and chain branching activity such as HECTD1 and WWP1, the mechanisms of branching are less clear. A noncovalent ubiquitin-binding site intrinsic to the E3 is likely responsible for orienting the internal acceptor ubiquitin within the chain to facilitate branching. It is unclear how such a site would be regulated to ensure that branching takes place at the appropriate time and location. Furthermore, in almost all cases where branching has been reported, it is unclear how the branch point ubiquitin is selected and how many branch points are present. New methods and approaches are needed to decipher the architectures of branched chains and to fully elucidate their mechanisms of synthesis.
Recent work has shown that branched chains are synthesized in response to small molecules that trigger the forced degradation of otherwise stable substrates. The VHL-based and BRD4-directed proteolysis-targeting chimera (PROTAC) MZ1 induces the formation of branched K29/K48 chains on BRD4. In this case, the mechanism of branched chain synthesis involves the PROTAC-dependent recruitment of CRL2VHL, a K48-specific cullin-RING E3, and TRIP12, a K29-specific HECT E3, to BRD4 (Kaiho-Soma et al., 2021) (Figure 1C). Similarly, the small molecule “degrader” LCL-161, which targets the inhibitor of apoptosis protein (IAP) family of E3s for self-ubiquitylation and degradation, triggers the formation of branched chains containing K11, K48, and K63 linkages. In this context, chain branching requires the activities of the UBE2D family of E2s and the K63-specific UBE2N-UBE2V E2 complex. An IAP-based PROTAC that targets the estrogen receptor (ER-α) also induces the formation of K48 and K63 chains in a UBE2N-dependent manner, suggesting a common mechanism of branched chain formation shared by IAP degraders and IAP-based PROTACs (Akizuki et al., 2023). Interestingly, in the case of branched K29/K48 chains assembled by CRL2VHL and TRIP12, TRIP12 appears to have a dual role in attaching K29 linkages to K48 chains synthesized by CRL2VHL and in further stimulating the K48 chain synthesis activity of CRL2VHL (Akizuki et al., 2023). This feedforward mechanism of branching results in the formation of complex chain architectures that are likely to have a direct impact on the degradation efficiency of BRD4 and other related PROTAC substrates (Figure 1C).
Given the therapeutic potential of PROTACs and other small molecule degraders, the mechanisms of chain branching on targeted substrates are of considerable interest. It is interesting to note that TRIP12 is not involved in the degradation of HIF-1α, an endogenous substrate of CRL2VHL, suggesting a unique mechanism of degradation by PROTACs that depends on the formation of branched K29/K48 chains (Akizuki et al., 2023). It has been proposed that the requirement for TRIP12 and branched K29/K48 chains can be explained by the fact that PROTAC-directed substrates are normally stable proteins that have not been evolutionarily optimized for degradation. Presumably, such suboptimal substrates require a different type of ubiquitin signal in order to be properly recognized and processed by the degradation machinery. Other classes of suboptimal or “hard-to-degrade” substrates that have been demonstrated or suggested to be degraded in a manner that depends on branched chains include aggregation-prone misfolded proteins, membrane-associated proteins, and proteins that are part of larger macromolecular complexes (Liu et al., 2017; Yau et al., 2017; Samant et al., 2018; Leto et al., 2019; Chen et al., 2021). The quality control pathways that stimulate chain branching E2s and E3s to recognize and modify these substrates in order to trigger their proteasomal degradation are not fully understood.
Disassembly of branched chains
The disassembly of branched chains and all other types of ubiquitin polymers requires the actions of deubiquitylases (DUBs), which are organized into seven different evolutionarily conserved families. The ∼100 DUBs encoded by the human genome are diverse in terms of their functions and biochemical activities. DUBs that target substrates modified with ubiquitin chains can cleave from the distal end of the chain (an exo-DUB activity), internally within the chain (an endo-DUB activity), or at the point of attachment between the substrate and the proximal ubiquitin to release an intact chain (an en bloc chain cleavage activity). Furthermore, while many DUBs show remarkable selectivity for the types of chain linkages they hydrolyze, others, especially those of the ubiquitin-specific protease (USP) family, are nonspecific and cleave chains of multiple linkage types and configurations (Mevissen and Komander, 2017; Clague et al., 2019). While several DUBs have been shown to cleave homotypic linkages within branched chains, as discussed in more detail below, UCH37/UCHL5 is currently the only known DUB to preferentially cleave at the branch point linkage between ubiquitin subunits within a chain.
UCH37 is a proteasome-associated DUB of the ubiquitin C-terminal hydrolase (UCH) family that was originally characterized as a K48-specific isopeptidase with exo-DUB activity that rescues substrates from degradation (Lam et al., 1997; de Poot et al., 2017). Recent studies, however, have shown that UCH37 has an unprecedented ability to selectively cleave at the branch point of a chain containing a K48 linkage (Deol et al., 2020b; Song et al., 2021). UCH37 efficiently debranches K6/K48, K11/K48, and K48/K63 chains (Deol et al., 2020b; Song et al., 2021), whereas it shows significantly weaker or no activity at all against homotypic and mixed chains containing K48 linkages (Yao et al., 2006; Bett et al., 2015; Lu et al., 2017; Deol et al., 2020b; Song et al., 2021). The debranching activity of UCH37 appears to promote rather than inhibit degradation of substrates by the proteasome (Deol et al., 2020b; Du et al., 2022), and it has been suggested that the function of debranching is, in part, to clear ubiquitin chains with complex higher order topologies from the proteasome, thus facilitating further rounds of substrate engagement and processing (Song et al., 2021). Notably, the debranching activity of UCH37 is greatly stimulated by its association with the proteasomal subunit RPN13 (Figure 1D), one of the three main ubiquitin receptors of the proteasome 19 S regulatory particle (Deol et al., 2020b).
How is the specificity of UCH37 for K48 branch points achieved? Although the precise mechanism is unknown, three key observations have shed light on the molecular basis of branch point cleavage. First, the UCH37/RPN13 complex binds to both distal ubiquitin subunits that emanate from the branch point of a K6/K48 chain to increase the affinity of the enzyme for the branch point (Song et al., 2021) (Figure 1D). The fact that the affinity of branched K11/K48 and K48/K63 chains for UCH37/RPN13 is similar to that of homotypic chains, however, indicates that binding specificity alone cannot fully explain the selectivity for branch point cleavage (Song et al., 2021). Second, RPN13 binds to UCH37 on a surface that includes the active-site crossover loop (Sahtoe et al., 2015; VanderLinden et al., 2015), a feature of all UCH DUBs that plays a crucial role in regulating access to the canonical active site of the enzyme. Through mechanisms that are not yet clear, the interaction between RPN13 and the UCH37 active-site crossover loop restricts the cleavage of homotypic chains and promotes the cleavage of branched chains (Song et al., 2021), thereby increasing the debranching specificity of the complex. Third, and perhaps most surprisingly, it has recently been demonstrated that UCH37 has a K48 chain binding site on the backside of the enzyme (at a site distant from the canonical UCH active site) that is required for chain debranching (Du et al., 2022). Molecular docking and chemical crosslinking experiments suggest that both branched and homotypic K48 chains sample the backside of the enzyme in multiple orientations, but only the branched chain configuration results in a catalytically competent arrangement in which the K48 linkage is accessible to the catalytic cysteine of UCH37 (Du et al., 2022).
Several other DUBs have been shown to cleave homotypic linkages within branched chains and thus have the ability to trim branched polymers in addition to cleaving homotypic chains. USP30, a DUB that preferentially disassembles homotypic K6 linkages (Cunningham et al., 2015; Gersch et al., 2017), also cleaves branched K6/K48 chains in vitro (Deol et al., 2020b). This activity is consistent with the role of USP30 in antagonizing ubiquitylation events mediated by Parkin (Bingol et al., 2014; Liang et al., 2015; Gersch et al., 2017; Ordureau et al., 2020), an E3 that assembles chains containing K6, K11, K48, and K63 linkages (Ordureau et al., 2014; Swatek et al., 2019). The ovarian tumor protease (OTU) family DUB Cezanne cleaves K11 linkages in the context of branched K11/K48 chains in vitro and has been shown to deubiquitylate APC/C substrates in vivo (Mevissen et al., 2016; Bonacci et al., 2018). TRABID/ZRANB1, another OTU family DUB, cleaves K29 linkages within branched K29/K48 chains attached to the E3 HECTD1, suggesting a DUB chain editing function (Harris et al., 2021). An editing function may allow DUBs that target substrates modified with branched chains to alter the landscape of the chains in a way that has a range of different functional outcomes for the target protein. It will be of interest, for example, to determine if chain debranching has the ability rescue certain substrates from degradation or to regulate protein activity through degradation-independent mechanisms.
Concluding remarks
Although considerable progress has been made in revealing the architectures and functions of branched ubiquitin chains in recent years, there are still many questions that remain unanswered. In most cases, the higher-order configurations of these chains, including the number of branch points and the length of the branches, are not well understood. The mechanisms that govern the selection of the branch point ubiquitin(s) and those that stimulate the branching of an otherwise unbranched polymer are also poorly understood. While the ubiquitin chain restriction (UbiCRest) method has proven to be somewhat useful in deciphering the order of ubiquitin linkages within a branched chain (Hospenthal et al., 2015; Harris et al., 2021; Akizuki et al., 2023), new techniques and approaches will ultimately be needed to reveal the precise architectures of branched chains and to fully elucidate their mechanisms of synthesis. It will be of great interest to determine if chain branching is a general property shared by many E2s and E3s or if branching is limited to a dedicated set of “E4”-like enzymes. Of note, HUWE1, UBR4, UBR5, and TRIP12 have been reported to have a branching activity in more than one context (Ohtake et al., 2016; Yau et al., 2017; Ohtake et al., 2018; Khan et al., 2021; Akizuki et al., 2023), perhaps indicating a division of labor between linkage-specific and architecture-specific E3s.
The discovery of UCH37 as the first DUB with remarkable specificity for cleaving at the branch point of a ubiquitin chain has paved the way for new and exciting areas of research. While other debranching enzymes have not been reported as of yet, it is noteworthy that branched chains have been detected in S. cerevisiae (Liu et al., 2017; Pluska et al., 2021), which lacks a UCH37 orthologue. Despite progress in understanding how branch point specificity is achieved by UCH37 at the molecular level, the precise role of RPN13 in contributing to this process remains elusive. Based on existing data, it seems likely that RPN13 stabilizes a conformation of UCH37 that is conducive to branched chain binding. It is also possible that RPN13 provides a binding site for the non-K48-linked ubiquitin that emanates from the branch point to stabilize a catalytic architecture favorable for debranching (Du et al., 2022). Structural work will almost certainly be needed in order to distinguish between these possibilities. Finally, it may be possible to harness the unique specificity of UCH37 for debranching in order to identify new targets of branched chains and to explore the biological functions of these enigmatic signals. Given the recent explosion in the number of reports of chain branching E2s and E3s, it seems probable that many such targets, both endogenous and therapeutic, will be discovered in the coming years.
Author contributions
JG, DX, and MF wrote and revised the manuscript. All authors contributed to the article and approved the submitted version.
Funding
This work was supported by funding from Middlebury College and the University of Tampa.
Acknowledgments
We thank Mike Emanuele (University of North Carolina at Chapel Hill) and Mike Carastro (University of Tampa) for comments on the manuscript. We apologize to those whose work could not be included in this article due to space limitations.
Conflict of interest
The authors declare that the research was conducted in the absence of any commercial or financial relationships that could be construed as a potential conflict of interest.
Publisher’s note
All claims expressed in this article are solely those of the authors and do not necessarily represent those of their affiliated organizations, or those of the publisher, the editors and the reviewers. Any product that may be evaluated in this article, or claim that may be made by its manufacturer, is not guaranteed or endorsed by the publisher.
References
Akizuki, Y., Morita, M., Mori, Y., Kaiho-Soma, A., Dixit, S., Endo, A., et al. (2023). cIAP1-based degraders induce degradation via branched ubiquitin architectures. Nat. Chem. Biol. 19, 311–322. doi:10.1038/s41589-022-01178-1
Akutsu, M., Dikic, I., and Bremm, A. (2016). Ubiquitin chain diversity at a glance. J. Cell Sci. 129, 875–880. doi:10.1242/jcs.183954
Bett, J. S., Ritorto, M. S., Ewan, R., Jaffray, E. G., Virdee, S., Chin, J. W., et al. (2015). Ubiquitin C-terminal hydrolases cleave isopeptide- and peptide-linked ubiquitin from structured proteins but do not edit ubiquitin homopolymers. Biochem. J. 466, 489–498. doi:10.1042/BJ20141349
Bingol, B., Tea, J. S., Phu, L., Reichelt, M., Bakalarski, C. E., Song, Q., et al. (2014). The mitochondrial deubiquitinase USP30 opposes parkin-mediated mitophagy. Nature 510, 370–375. doi:10.1038/nature13418
Bonacci, T., Suzuki, A., Grant, G. D., Stanley, N., Cook, J. G., Brown, N. G., et al. (2018). Cezanne/OTUD7B is a cell cycle-regulated deubiquitinase that antagonizes the degradation of APC/C substrates. EMBO J. 37, e98701. doi:10.15252/embj.201798701
Chen, Y. H., Huang, T. Y., Lin, Y. T., Lin, S. Y., Li, W. H., Hsiao, H. J., et al. (2021). VPS34 K29/K48 branched ubiquitination governed by UBE3C and TRABID regulates autophagy, proteostasis and liver metabolism. Nat. Commun. 12, 1322. doi:10.1038/s41467-021-21715-1
Clague, M. J., Urbé, S., and Komander, D. (2019). Breaking the chains: Deubiquitylating enzyme specificity begets function. Nat. Rev. Mol. Cell Biol. 20, 338–352. doi:10.1038/s41580-019-0099-1
Cunningham, C. N., Baughman, J. M., Phu, L., Tea, J. S., Yu, C., Coons, M., et al. (2015). USP30 and Parkin homeostatically regulate atypical ubiquitin chains on mitochondria. Nat. Cell Biol. 17, 160–169. doi:10.1038/ncb3097
de Poot, S. A. H., Tian, G., and Finley, D. (2017). Meddling with fate: The proteasomal deubiquitinating enzymes. J. Mol. Biol. 429, 3525–3545. doi:10.1016/j.jmb.2017.09.015
Deol, K. K., Crowe, S. O., Du, J., Bisbee, H. A., Guenette, R. G., and Strieter, E. R. (2020b). Proteasome-bound UCH37/UCHL5 debranches ubiquitin chains to promote degradation. Mol. Cell 80, 796–809.e9. doi:10.1016/j.molcel.2020.10.017
Deol, K. K., Eyles, S. J., and Strieter, E. R. (2020a). Quantitative middle-down MS analysis of Parkin-mediated ubiquitin chain assembly. J. Am. Soc. Mass Spectrom. 31, 1132–1139. doi:10.1021/jasms.0c00058
Deol, K. K., Lorenz, S., and Strieter, E. R. (2019). Enzymatic logic of ubiquitin chain assembly. Front. Physiol. 10, 835. doi:10.3389/fphys.2019.00835
Dikic, I., and Schulman, B. A. (2023). An expanded lexicon for the ubiquitin code. Nat. Rev. Mol. Cell Biol. 24, 273–287. doi:10.1038/s41580-022-00543-1
Du, J., Babik, S., Li, Y., Deol, K. K., Eyles, S. J., Fejzo, J., et al. (2022). A cryptic K48 ubiquitin chain binding site on UCH37 is required for its role in proteasomal degradation. eLife 11, e76100. doi:10.7554/eLife.76100
French, M. E., Klosowiak, J. L., Aslanian, A., Reed, S. I., Yates, J. R., and Hunter, T. (2017). Mechanism of ubiquitin chain synthesis employed by a HECT domain ubiquitin ligase. J. Biol. Chem. 292, 10398–10413. doi:10.1074/jbc.M117.789479
French, M. E., Koehler, C. F., and Hunter, T. (2021). Emerging functions of branched ubiquitin chains. Cell Discov. 7, 6. doi:10.1038/s41421-020-00237-y
Gersch, M., Gladkova, C., Schubert, A. F., Michel, M. A., Maslen, S., and Komander, D. (2017). Mechanism and regulation of the Lys6-selective deubiquitinase USP30. Nat. Struct. Mol. Biol. 24, 920–930. doi:10.1038/nsmb.3475
Harris, L. D., Le Pen, J., Scholz, N., Mieszczanek, J., Vaughan, N., Davis, S., et al. (2021). The deubiquitinase TRABID stabilizes the K29/K48-specific E3 ubiquitin ligase HECTD1. J. Biol. Chem. 296, 100246. doi:10.1074/jbc.RA120.015162
Horn-Ghetko, D., and Schulman, B. A. (2022). New classes of E3 ligases illuminated by chemical probes. Curr. Opin. Struct. Biol. 73, 102341. doi:10.1016/j.sbi.2022.102341
Hospenthal, M. K., Freund, S. M., and Komander, D. (2013). Assembly, analysis, and architecture of atypical ubiquitin chains. Nat. Struct. Mol. Biol. 20, 555–565. doi:10.1038/nsmb.2547
Hospenthal, M. K., Mevissen, T. E. T., and Komander, D. (2015). Deubiquitinase-based analysis of ubiquitin chain architecture using Ubiquitin Chain Restriction (UbiCRest). Nat. Protoc. 10, 349–361. doi:10.1038/nprot.2015.018
Kaiho-Soma, A., Akizuki, Y., Igarashi, K., Endo, A., Shoda, T., Kawase, Y., et al. (2021). TRIP12 promotes small-molecule-induced degradation through K29/K48-branched ubiquitin chains. Mol. Cell 81, 1411–1424.e7. doi:10.1016/j.molcel.2021.01.023
Kelsall, I. R., Zhang, J., Knebel, A., Arthur, J. S. C., and Cohen, P. (2019). The E3 ligase HOIL-1 catalyses ester bond formation between ubiquitin and components of the Myddosome in mammalian cells. Proc. Natl. Acad. Sci. U. S. A. 116, 13293–13298. doi:10.1073/pnas.1905873116
Khan, O. M., Almagro, J., Nelson, J. K., Horswell, S., Encheva, V., Keyan, K. S., et al. (2021). Proteasomal degradation of the tumour suppressor FBW7 requires branched ubiquitylation by TRIP12. Nat. Commun. 12, 2043. doi:10.1038/s41467-021-22319-5
Kim, H. T., Kim, K. P., Lledias, F., Kisselev, A. F., Scaglione, K. M., Skowyra, D., et al. (2007). Certain pairs of ubiquitin-conjugating enzymes (E2s) and ubiquitin-protein ligases (E3s) synthesize nondegradable forked ubiquitin chains containing all possible isopeptide linkages. J. Biol. Chem. 282, 17375–17386. doi:10.1074/jbc.M609659200
Kolla, S., Ye, M., Mark, K. G., and Rapé, M. (2022). Assembly and function of branched ubiquitin chains. Trends biochem. Sci. 47, 759–771. doi:10.1016/j.tibs.2022.04.003
Komander, D., and Rape, M. (2012). The ubiquitin code. Annu. Rev. Biochem. 81, 203–229. doi:10.1146/annurev-biochem-060310-170328
Lam, Y. A., Xu, W., DeMartino, G. N., and Cohen, R. E. (1997). Editing of ubiquitin conjugates by an isopeptidase in the 26S proteasome. Nature 385, 737–740. doi:10.1038/385737a0
Leto, D. E., Morgens, D. W., Zhang, L., Walczak, C. P., Elias, J. E., Bassik, M. C., et al. (2019). Genome-wide CRISPR analysis identifies substrate-specific conjugation modules in ER-associated degradation. Mol. Cell 73, 377–389.e11. doi:10.1016/j.molcel.2018.11.015
Li, W., Bengtson, M. H., Ulbrich, A., Matsuda, A., Reddy, V. A., Orth, A., et al. (2008). Genome-wide and functional annotation of human E3 ubiquitin ligases identifies MULAN, a mitochondrial E3 that regulates the organelle’s dynamics and signaling. PloS One 3, e1487.
Liang, J. R., Martinez, A., Lane, J. D., Mayor, U., Clague, M. J., and Urbé, S. (2015). USP30 deubiquitylates mitochondrial Parkin substrates and restricts apoptotic cell death. EMBO Rep. 16, 618–627. doi:10.15252/embr.201439820
Liu, C., Liu, W., Ye, Y., and Li, W. (2017). Ufd2p synthesizes branched ubiquitin chains to promote the degradation of substrates modified with atypical chains. Nat. Commun. 8, 14274. doi:10.1038/ncomms14274
Lorenz, S. (2018). Structural mechanisms of HECT-type ubiquitin ligases. Biol. Chem. 399, 127–145. doi:10.1515/hsz-2017-0184
Lu, X., Nowicka, U., Sridharan, V., Liu, F., Randles, L., Hymel, D., et al. (2017). Structure of the Rpn13-Rpn2 complex provides insights for Rpn13 and Uch37 as anticancer targets. Nat. Commun. 8, 15540. doi:10.1038/ncomms15540
Mevissen, T. E. T., and Komander, D. (2017). Mechanisms of deubiquitinase specificity and regulation. Annu. Rev. Biochem. 86, 159–192. doi:10.1146/annurev-biochem-061516-044916
Mevissen, T. E. T., Kulathu, Y., Mulder, M. P. C., Geurink, P. P., Maslen, S. L., Gersch, M., et al. (2016). Molecular basis of Lys11-polyubiquitin specificity in the deubiquitinase Cezanne. Nature 538, 402–405. doi:10.1038/nature19836
Meyer, H. J., and Rape, M. (2014). Enhanced protein degradation by branched ubiquitin chains. Cell 157, 910–921. doi:10.1016/j.cell.2014.03.037
Oh, E., Mark, K. G., Mocciaro, A., Watson, E. R., Prabu, J. R., Cha, D. D., et al. (2020). Gene expression and cell identity controlled by anaphase-promoting complex. Nature 579, 136–140. doi:10.1038/s41586-020-2034-1
Ohtake, F., Saeki, Y., Ishido, S., Kanno, J., and Tanaka, K. (2016). The K48-K63 branched ubiquitin chain regulates NF-κB signaling. Mol. Cell 64, 251–266. doi:10.1016/j.molcel.2016.09.014
Ohtake, F., Tsuchiya, H., Saeki, Y., and Tanaka, K. (2018). K63 ubiquitylation triggers proteasomal degradation by seeding branched ubiquitin chains. Proc. Natl. Acad. Sci. U. S. A. 115, E1401–E1408. doi:10.1073/pnas.1716673115
Ordureau, A., Paulo, J. A., Zhang, J., An, H., Swatek, K. N., Cannon, J. R., et al. (2020). Global landscape and dynamics of Parkin and USP30-dependent ubiquitylomes in iNeurons during mitophagic signaling. Mol. Cell 77, 1124–1142.e10. doi:10.1016/j.molcel.2019.11.013
Ordureau, A., Sarraf, S. A., Duda, D. M., Heo, J. M., Jedrychowski, M. P., Sviderskiy, V. O., et al. (2014). Quantitative proteomics reveal a feedforward mechanism for mitochondrial PARKIN translocation and ubiquitin chain synthesis. Mol. Cell 56, 360–375. doi:10.1016/j.molcel.2014.09.007
Pluska, L., Jarosch, E., Zauber, H., Kniss, A., Waltho, A., Bagola, K., et al. (2021). The UBA domain of conjugating enzyme Ubc1/Ube2K facilitates assembly of K48/K63-branched ubiquitin chains. EMBO J. 40, e106094. doi:10.15252/embj.2020106094
Rodriguez Carvajal, A., Grishkovskaya, I., Gomez Diaz, C., Vogel, A., Sonn-Segev, A., Kushwah, M. S., et al. (2021). The linear ubiquitin chain assembly complex (LUBAC) generates heterotypic ubiquitin chains. Elife 10, e60660. doi:10.7554/eLife.60660
Sahtoe, D. D., van Dijk, W. J., El Oualid, F., Ekkebus, R., Ovaa, H., and Sixma, T. K. (2015). Mechanism of UCH-L5 activation and inhibition by DEUBAD domains in RPN13 and INO80G. Mol. Cell 57, 887–900. doi:10.1016/j.molcel.2014.12.039
Samant, R. S., Livingston, C. M., Sontag, E. M., and Frydman, J. (2018). Distinct proteostasis circuits cooperate in nuclear and cytoplasmic protein quality control. Nature 563, 407–411. doi:10.1038/s41586-018-0678-x
Song, A., Hazlett, Z., Abeykoon, D., Dortch, J., Dillon, A., Curtiss, J., et al. (2021). Branched ubiquitin chain binding and deubiquitination by UCH37 facilitate proteasome clearance of stress-induced inclusions. eLife 10, e72798. doi:10.7554/eLife.72798
Squair, D. R., and Virdee, S. (2022). A new dawn beyond lysine ubiquitination. Nat. Chem. Biol. 18, 802–811. doi:10.1038/s41589-022-01088-2
Swatek, K. N., and Komander, D. (2016). Ubiquitin modifications. Cell Res. 26, 399–422. doi:10.1038/cr.2016.39
Swatek, K. N., Usher, J. L., Kueck, A. F., Gladkova, C., Mevissen, T. E. T., Pruneda, J. N., et al. (2019). Insights into ubiquitin chain architecture using Ub-clipping. Nature 572, 533–537. doi:10.1038/s41586-019-1482-y
Valkevich, E. M., Sanchez, N. A., Ge, Y., and Strieter, E. R. (2014). Middle-down mass spectrometry enables characterization of branched ubiquitin chains. Biochemistry 53, 4979–4989. doi:10.1021/bi5006305
VanderLinden, R. T., Hemmis, C. W., Schmitt, B., Ndoja, A., Whitby, F. G., Robinson, H., et al. (2015). Structural basis for the activation and inhibition of the UCH37 deubiquitylase. Mol. Cell 57, 901–911. doi:10.1016/j.molcel.2015.01.016
Walden, H., and Rittinger, K. (2018). RBR ligase-mediated ubiquitin transfer: A tale with many twists and turns. Nat. Struct. Mol. Biol. 25, 440–445. doi:10.1038/s41594-018-0063-3
Wang, M., Cheng, D., Peng, J., and Pickart, C. M. (2006). Molecular determinants of polyubiquitin linkage selection by a HECT ubiquitin ligase. EMBO J. 25, 1710–1719. doi:10.1038/sj.emboj.7601061
Wright, J. D., Mace, P. D., and Day, C. L. (2016). Noncovalent ubiquitin interactions regulate the catalytic activity of ubiquitin writers. Trends biochem. Sci. 41, 924–937. doi:10.1016/j.tibs.2016.08.003
Yao, T., Song, L., Xu, W., DeMartino, G. N., Florens, L., Swanson, S. K., et al. (2006). Proteasome recruitment and activation of the Uch37 deubiquitinating enzyme by Adrm1. Nat. Cell Biol. 8, 994–1002. doi:10.1038/ncb1460
Yau, R. G., Doerner, K., Castellanos, E. R., Haakonsen, D. L., Werner, A., Wang, N., et al. (2017). Assembly and function of heterotypic ubiquitin chains in cell-cycle and protein quality control. Cell 171, 918–933.e20. doi:10.1016/j.cell.2017.09.040
Keywords: ubiquitin, ubiquitylation (ubiquitination), branched ubiquitin chain, protein degradation, proteasome
Citation: Gregor JB, Xu D and French ME (2023) Assembly and disassembly of branched ubiquitin chains. Front. Mol. Biosci. 10:1197272. doi: 10.3389/fmolb.2023.1197272
Received: 30 March 2023; Accepted: 23 May 2023;
Published: 01 June 2023.
Edited by:
Qi Wu, Aarhus University, DenmarkReviewed by:
Catherine Lindon, University of Cambridge, United KingdomJerry Vriend, University of Manitoba, Canada
Copyright © 2023 Gregor, Xu and French. This is an open-access article distributed under the terms of the Creative Commons Attribution License (CC BY). The use, distribution or reproduction in other forums is permitted, provided the original author(s) and the copyright owner(s) are credited and that the original publication in this journal is cited, in accordance with accepted academic practice. No use, distribution or reproduction is permitted which does not comply with these terms.
*Correspondence: Michael E. French, bWZyZW5jaEB1dC5lZHU=
†These authors have contributed equally to this work and share first authorship