- 1Institute of Agricultural Sciences in the Tropics (Hans-Ruthenberg-Institute), University of Hohenheim, Stuttgart, Germany
- 2Tropical Forages Program, The Alliance of Bioversity International and the International Center for Tropical Agriculture (CIAT), Cali, Colombia
- 3Institute of Phytomedicine, University of Hohenheim, Stuttgart, Germany
- 4Institute of Chemistry, University of Hohenheim, Stuttgart, Germany
Introduction: Biological Nitrification Inhibition (BNI) is defined as the plant-mediated control of soil nitrification via the release of nitrification inhibitors. BNI of Brachiaria humidicola (syn. Urochloa humidicola) has been mainly attributed to root-exuded fusicoccane-type diterpenes, e.g., 3-epi-brachialactone. We hypothesized, however, that BNI of B. humidicola is caused by an assemblage of bioactive secondary metabolites.
Methods: B. humidicola root exudates were collected hydroponically, and metabolites were isolated by semi-preparative HPLC. Chemical structures were elucidated by HRMS as well as 1D and 2D NMR spectroscopy. Nitrification inhibiting potential of isolated metabolites was evaluated by a Nitrosomonas europaea based bioassay.
Results and discussion: Besides previously described brachialactone isomers and derivatives, five phenol and cinnamic acid derivatives were identified in the root exudates of B. humidicola: 2-hydroxy-3-(hydroxymethyl)benzaldehyde, vanillin, umbelliferone and both trans- and cis-2,6-dimethoxycinnamic acid. Notably, vanillin revealed a substantially higher nitrification inhibiting activity than 3-epi-brachialactone (ED50 ∼ 12.5 μg·ml−1, ED80 ∼ 20 μg·ml−1), identifying this phenolic aldehyde as novel nitrification inhibitor (NI). Furthermore, vanillin exudation rates were in the same range as 3-epi-brachialactone (1–4 μg·h−1·g−1 root DM), suggesting a substantial contribution to the overall inhibitory activity of B. humidicola root exudates. In relation to the verification of the encountered effects within soils and considering the exclusion of any detrimental impact on the soil microbiome, the biosynthetic pathway of vanillin via the precursor phenylalanine and the intermediates p-coumaric acid/ferulic acid (precursors of further phenolic NI) might constitute a promising BNI breeding target. This applies not only to Brachiaria spp., but also to crops in general, owing to the highly conserved nature of these metabolites.
1 Introduction
Perennial grasses have been described to control soil nitrification (Theron, 1951; Sylvester Bradley et al., 1988; Lata et al., 2004), an attribute contributing to the mitigation of nitrification related N losses, including NO3- leaching and N2O emissions (Subbarao et al., 2009; Byrnes et al., 2017). Biological Nitrification Inhibition (BNI), defined as the plant-exerted control of nitrifiers through the release of allelochemicals (Subbarao et al., 2006), is one mechanism explaining the reduction of soil nitrification rates (Coskun et al., 2017; Nardi et al., 2020). Several plants, including mostly graminaceous crops, have been screened for nitrification inhibiting secondary metabolites in plant tissue and especially root exudates (Coskun et al., 2017; Lu et al., 2021; Otaka et al., 2023). Notably, the tropical forage grass Brachiaria humidicola (syn. Urochloa humidicola) has been acknowledged to effectively control soil nitrification (Sylvester Bradley et al., 1988; Karwat et al., 2018; Nuñez et al., 2018). In B. humidicola, BNI has been attributed to different phenolic compounds, e.g., methyl coumarate and methyl ferulate, which are released during root turnover (Gopalakrishnan et al., 2007), as well as different fusicoccane-type diterpenes called brachialactones, which are actively exuded into the rhizosphere (Subbarao et al., 2009; Egenolf et al., 2020; Egenolf et al., 2021). It must be noted, however, that these nitrification inhibiting compounds have been determined solely under artificial conditions (hydroponics), while the evidence of their presence and activity in the rhizosphere or bulk soil of prevalent B. humidicola stands remains elusive. In fact, preliminary studies aiming at the in situ quantification of exudation and accumulation of the mentioned nitrification inhibitors (NI) in the rhizosphere of soil-grown B. humidicola plants via the sorption-filter technique described by Neumann et al. (2014) and different soil extraction approaches, were not successful (Egenolf, unpublished data). This may be attributed to well-known technical challenges during both in situ root exudate collection as well as soil extraction approaches (White, 1991; Neumann et al., 2009). On the other hand, it could be also ascribed to the overall low internal tissue concentrations of methyl coumarate, methyl ferulate (G. Subbarao, personal communication), and 3-epi-brachialactone [2–8 μg·g−1 root dry matter (DM) (Egenolf et al., 2021)], as well as low brachialactone and 3-epi-brachialactone exudation rates [0.4–4.0 μg·h−1·g−1 root DM (Subbarao et al., 2009; Egenolf et al., 2021)]. With this, the involvement of yet undiscovered active ingredients or alternative modes of action could be suggested. Consequently, complementary theories on soil nitrification control are broadly discussed, including microbial N immobilization resulting in the out-competition of ammonia oxidizers, as well as efficient uptake of NO3− by plants (Vázquez et al., 2020; Teutscherová et al., 2021; Egenolf et al., 2022). Here, however, it was our main ambition to verify the existence of so far undiscovered NI. More precisely, we hypothesize that root exudates of B. humidicola contain a broad range of secondary metabolites with nitrification inhibiting activity. Thereby, the objective was to collect root exudates in a hydroponic system (limiting confounding effects of an associated soil microbiome), identify major secondary metabolites present in the root exudates of B. humidicola and evaluate their nitrification inhibiting activity.
2 Material and methods
2.1 Root exudate collection
Brachiaria humidicola cv. CIAT 679 plants (commercial name “Tully,” ranked as high BNI cultivar) were propagated and raised during 6 weeks in a growth chamber-based hydroponic system with a day length of 12 h (6:00–18:00 h), light intensity of 525 W·m−2, air humidity of 75% and day/night temperatures of 30/20°C. The nutrient solution contained (µM): NH4NO3 1200, KNO3 400, Ca(NO3)2 400, HNO3 600, K2HPO4 200, MgSO4 200, MgCl2 100, Na2SiO3 200, FeNa-EDTA 50, H3BO3 10.0, MnSO4 4.0, ZnSO4 4.0, CuSO4 1.0, Na2MoO4 1.0. The nutrient solution was exchanged every 2–3 days and pH of fresh nutrient solution was adjusted at 4.8. Root exudate collection was performed into fresh nutrient solution with a plant density of 4 plants L−1 of nutrient solution during 24 h. After root exudate collection, the nutrient solution was filtered to remove root debris and organic compounds were extracted by liquid phase extraction (by that eliminating salts contained in the nutrient solution as well as any root-derived impurities, e.g., proteins). For that, sodium chloride was added to the nutrient solution (polar phase) until saturation, facilitating the subsequent extraction of organic compounds with 300 ml of ethyl acetate (organic phase) per 1 L of nutrient solution in a separating funnel. The ethyl acetate phase was filtered through a layer of 2 cm of anhydrous Na2SO4 to remove any remaining water. The extraction of the nutrient solution was repeated. The two ethyl acetate extracts were pooled, concentrated in vacuo and stored at 4°C until further analysis. In total, root exudates of approximately 400 plants were pooled to obtain ∼3 mg of raw exudate, yielding between 100 and 200 μg pure compounds after semi-preparative fractionation (next section).
2.2 Root exudate fractionation
For HPLC-PDA analysis and semi-preparative HPLC fractionation, ethyl acetate extracts were dried under a N2 flow (30°C) and resuspended in H2O/acetonitrile (1/1, v/v) or H2O/isopropanol/acetonitrile (1/1/1, v/v/v), respectively. Root exudates were screened for major secondary metabolites based on PDA chromatograms obtained via HPLC-PDA analysis at a wavelength range of 200–600 nm (Accela HPLC/LTQ Velos MS, Thermo Scientific, Waltham, United States) using a Kinetex 2.6 μm XB-C18 100A reverse phase column (Phenomenex, Torrance, United States) with formate buffer (10 mM, pH 3.7) as polar and acetonitrile as nonpolar eluent (flow rate 0.5 ml·min−1).
Subsequently, selected major secondary compounds were isolated via semi-preparative HPLC (Knauer Smartline, Berlin, Germany), using an EC 250/10 Nucleodur PolarTec 5 µm reverse phase column (Macherey-Nagel, Düren, Germany) in a first, and a xSelect HSS Prep T3 5 µm 10 mm × 150 mm reverse phase column (Waters, Milford, United States) in a second step. Both fractionation steps were conducted with 0.01% trifluoroacetic acid as polar and acetonitrile as nonpolar eluent (flow rate 5 ml·min−1). The applied eluent gradients are provided in the Supplementary Tables S1–S3 (Supplementary Material).
2.3 HRMS
In order to accurately assess the molecular mass of purified secondary compounds high resolution mass-spectra were recorded on a QExactive Plus Electrospray Mass Spectrometer (Thermo Fisher Scientific Waltham, United States) coupled to an Agilent 1290 Ultra Performance Liquid Chromatography System. Measurement parameters were applied according to the standard protocols of our institution: ESI positive, HESI Source, Capillary Temp 360°C, Sheath gas 60, Aux gas 20, Probe Heater 380°C, Full scan: 100–800 m/z, resolution 35.000, MS2: resolution 17.500, NCE 15, 25, 35. The eluent gradient is provided in Supplementary Table S4 (Supplementary Material).
2.4 NMR spectroscopy
For the elucidation of molecular structures NMR-spectra were recorded on an Avance HD III 600 MHz spectrometer, equipped with a BBO Prodigy cryo-probe (Bruker, Billerica, United States). Metabolites were dissolved in methanol-d4 in standard 5 mm NMR tubes or 2 mm MATCH NMR tubes. The 1H and 13C chemical shifts were referenced to the residual solvent signal at δH/C 3.35 ppm/49.0 ppm. HSQC, HMBC, NOESY, COSY and selective 1D-TOCSY spectra were recorded using standard Bruker pulse sequences at 298 K. For processing and evaluation of NMR spectra, the software SpinWorks 4.2.8.0 (Copyright 2017, K. Marat, University of Manitoba, Canada) was used.
2.5 Assessment of nitrification inhibiting potential
Nitrification inhibiting activity was assessed by means of the Nitrosomonas europaea based bioluminescence assay developed by Subbarao et al. (2006) and adjusted by Nuñez et al. (2018). Briefly, the bioluminescent N. europaea IFO 14298 (ATCC 19178) pHLUX20 strain developed by Iizumi et al. (1998) was cultured in a kanamycin (50 μg·ml−1) supplemented phosphate buffered growth medium for 6 days at 50 rpm and 28°C. The growth medium was composed of (mM): KH2PO4 5.14, Na2HPO4 95.1, (NH4)2SO4 18.91, NaHCO3 5.95, CaCl2 0.034, MgSO4 0.041, Fe (III) EDTA 0.0027. Two hundred ml of liquid culture were centrifuged at 2,500 g for 20 min and the N. europaea pellet was resuspended in 50 ml of fresh culture medium. Compounds to be tested were dissolved in 1 µl DMSO, diluted to 100 µl with distilled H2O and then incubated with 125 μl N. europaea culture for 15 min (900 rpm, 15°C) prior to bioluminescence measurements. Bioluminescence was measured on 100 µl aliquots with two technical replicates on a Glomax 20/20 (Promega, Fitchburg, United States) integrating the flash luminescence reaction 2–10 s after automated injection of 25 µl of decanal (1%) in ethanol. Every measurement was repeated with three biological replicates and inhibition was calculated relative to the DMSO blank.
2.6 Assessment of vanillin exudation in dependence of rhizosphere pH and nutritional N-form
Root exudation of vanillin by B. humidicola cv. CIAT 679 (commercial name “Tully”) was assessed as described by Egenolf et al. (2021). In brief, exudation patterns were investigated in a two-factorial hydroponic experiment. For factor “pH,” the pH of the trap solution was adjusted to target values of 4.2 and 6.8 by addition of HCl and Na2CO3, respectively. Factor “Trap solution” consisted of a NH4+ and NO3− treatment [see Experiment 1 in Egenolf et al. (2021)]. Root exudates were collected into fresh trap solution for 4 h and secondary metabolites were extracted via liquid-phase extraction as described above. Samples were then analyzed for vanillin via HPLC-PDA at 280 nm (Accela HPLC/PDA, Thermo Scientific, Waltham, United States) using a Kinetex 2.6 μm XB-C18 100A reverse phase column (Phenomenex, Torrance, United States) with formate buffer (10 mM, pH 3.7) as polar and acetonitrile as nonpolar eluent (flow rate 0.5 ml·min−1), and a commercial standard (Aldrich Chem. Co., Inc., Milwaukee, United States) in the range from 0.25–2.0 mg·L−1. The applied eluent gradients are provided in the Supplementary Table S5 (Supplementary Material).
2.7 Statistics
Statistical analysis was performed, and plots were created with R version 3.5.3 (R Core Team, 2018) using the packages “lsmeans,” “multcompView” and “ggplot2.” “lsmeans” package was used to perform an ANOVA and “multcompView” to evaluate statistical significance of the assessed treatments combinations on vanillin exudation rates using Tukey-Tests. Package “ggplot2” was used to create the figures.
3 Results
3.1 Isolation and identification of secondary metabolites
HPLC-PDA analysis of B. humidicola root exudates revealed eleven major peaks (Figure 1). At this stage, only few peaks showed distinct UV absorption spectra, indicating an overlay of several signals per peak. After fractionation of root exudates by semi-preparative HPLC and isolation of at least one compound per fraction, the major metabolites related to 7 out of 11 fractions were identified via HRMS and NMR spectroscopic techniques. Two fractions, namely, fractions 7 and 10, consisted of metabolites occurring in two different isomeric forms, totaling in 9 distinct secondary metabolites. The four cyclic diterpenes identified and classified as different brachialactone isomers and derivatives (fractions 4, 9 and 10) have been discussed earlier (Egenolf et al., 2020) and are not subject of this study. Remaining metabolites were classified as phenol and cinnamic acid derivatives. More precisely, the evaluation of HRMS, 1D and 2D NMR spectra identified fraction 1 (m/z 152) as 2-hydroxy-3-(hydroxymethyl)benzaldehyde (a), fraction 2 (m/z 152) as vanillin (b), fraction 3 (m/z 162) as umbelliferone (c) and fraction 7 (m/z 208) as a mixture of both trans- and cis-2,6-dimethoxycinnamic acid (d and e) (Figure 2). The HRMS recordings and the NMR-data confirming the chemical structures of these metabolites are provided in Supplementary Tables S6–S11 (Supplementary Material). The characterization of fractions 5, 6, 8 and 11 was not possible, due to impurity of the isolate, even after a second purification via semi-preparative HPLC.
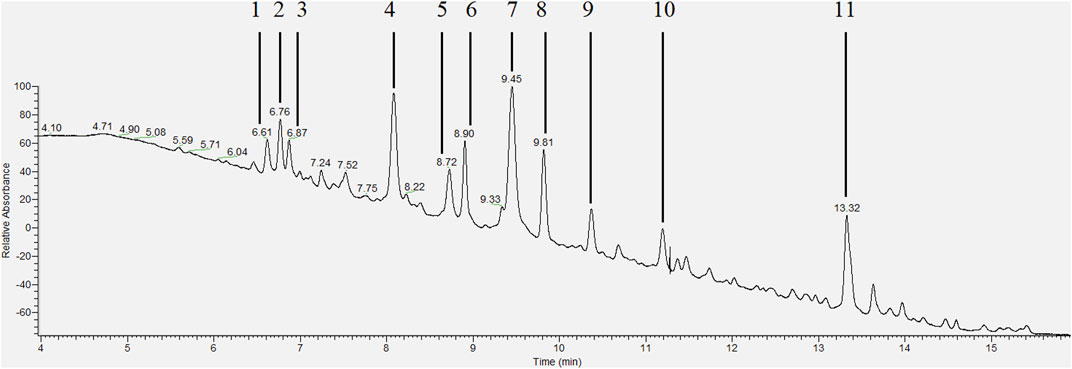
FIGURE 1. HPLC-PDA chromatogram (wavelength range 200–600 nm) of the extracted root exudates of Brachiaria humidicola with peaks corresponding to isolated fractions [adapted from Egenolf et al. (2020)].
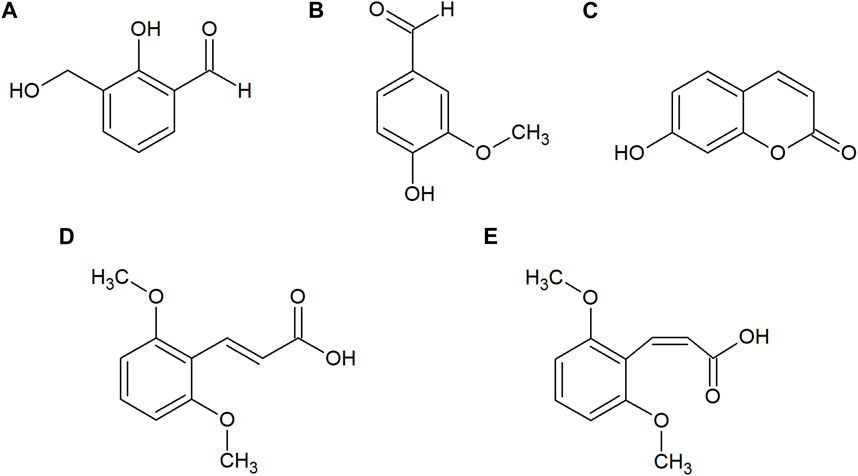
FIGURE 2. Chemical structures of 2-hydroxy-3-(hydroxymethyl)benzaldehyde (A), vanillin (B), umbelliferone (C), trans-2,6-dimethoxycinnamic acid (D) and cis-2,6-dimethoxycinnamic acid (E) isolated from the root exudates of B. humidicola.
3.2 Nitrification inhibitory potential of isolated fractions/metabolites
All isolated fractions or purified metabolites were assessed for their nitrification inhibiting activity in a N. europaea based liquid culture assay (Subbarao et al., 2006; Nuñez et al., 2018) at concentrations ranging from 0–40 μg·ml−1. Among all isolated fractions, vanillin (b, ED50 ∼ 12.5 μg·ml−1 ED80 ∼ 20 μg·ml−1) and the fractions 6 and 8 (ED50 ∼ 7.5–12.5 μg·ml−1 ED80 ∼ 15–25 μg·ml−1) showed the strongest inhibitory effects (Table 1). In contrast, only a slight inhibitory effect was detected for umbelliferone (c, ED50 ∼ 100 μg·ml−1), whereas 2-hydroxy-3-(hydroxymethyl)benzaldehyde (a) and both trans- and cis-2,6-dimethoxycinnamic acid (d and e) did not show any inhibitory, but a weak stimulatory effect on N. europaea (36%–46% at 20 μg·ml−1). The dose-response curves for vanillin (b), umbelliferone (c) and previously described 3-epi-brachialactone are displayed in Figure 3.
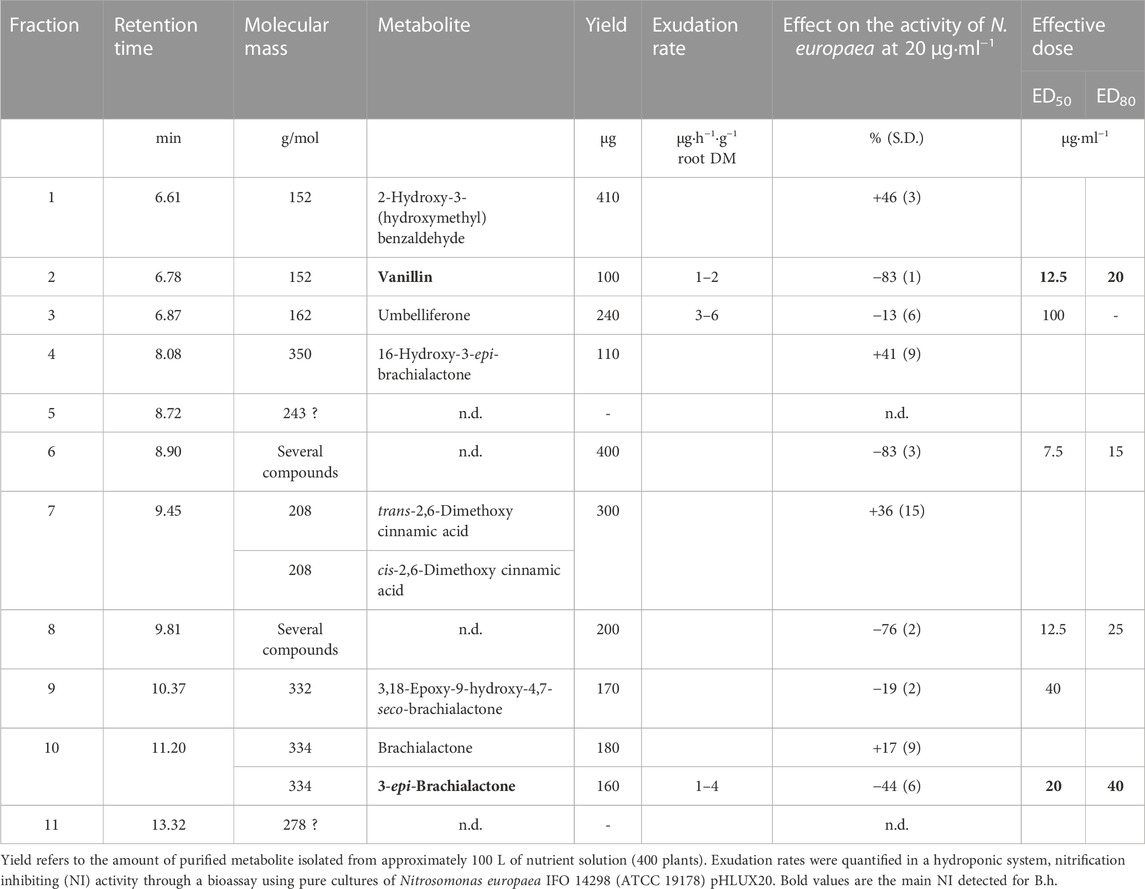
TABLE 1. Composition of Brachiaria humidicola root exudates and nitrification inhibitory activity of each fraction/metabolite.
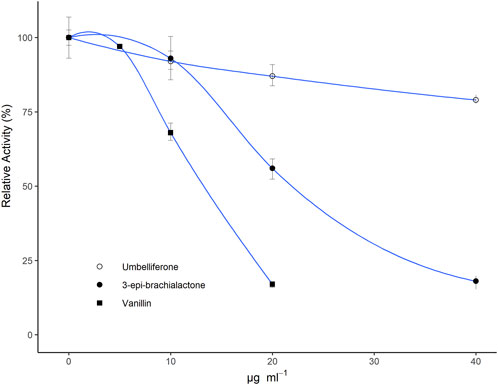
FIGURE 3. Relative metabolic activity (determined via bioluminescence measurements) of Nitrosomonas europaea IFO 14298 (ATCC 19178) pHLUX20 cultures in dependence of tested doses (10–40 μg·ml−1) of umbelliferone and vanillin in relation to previously assessed 3-epi-brachialactone (Egenolf et al., 2020). All metabolites were solubilized in DMSO and spiked to the assay medium. Results are displayed relative to a DMSO blank. Data represent the mean of three technical replications. Error bars represent standard errors (SE).
3.3 Vanillin exudation in dependence of rhizosphere pH and nutritional N-form
Both experimental factors had a significant effect on exudation rates of vanillin (N form: p = 0.0001; pH: p = 0.0336; N form*pH: p = 0.043). Especially the combination of NH4+ nutrition and low pH (4.2) prompted vanillin exudation (Figure 4), a finding in accordance with previous reports on NI exudation patterns in general (Subbarao et al., 2007b; Subbarao et al., 2009) and on 3-epi-brachialactone in specific (Egenolf et al., 2021).
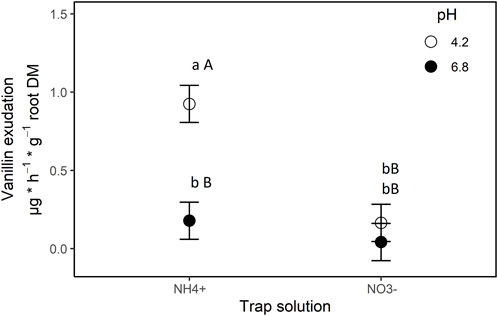
FIGURE 4. Exudation of vanillin by roots of B. humidicola into trap solutions differing in nitrogen source (x-axis) and pH (legend). Trap solutions were complete nutrient solutions with nitrogen being offered as ammonium (NH4+ treatment) or nitrate (NO3− treatment). Results represent least square means of four biological replications, error bars indicate standard error (SE) of the mean. Different uppercase letters indicates statistical difference for least-square means (α = 0.05) between N-treatments, lowercase letters indicate statistical differences between pH-treatments (α = 0.05).
4 Discussion
In this study, root exudates of B. humidicola were screened for novel nitrification inhibiting secondary metabolites. Besides the previously described NI brachialactone (Subbarao et al., 2009) and 3-epi-brachialactone (Egenolf et al., 2020), three additional fractions, namely, fraction 2 (vanillin), 6 and 8, possessed strong and substantially higher nitrification inhibiting activity (ED80 = 15–25 μg·ml−1) than both brachialactone isomers (Egenolf et al., 2020). With this, our hypothesis that the nitrification inhibiting activity of root exudates of B. humidicola is not only founded in the presence of brachialactones, but is also facilitated by additional NI (i.e., vanillin), could be verified.
Vanillin is the main flavoring agent of natural vanilla. In Vanilla spp., it occurs as vanillin-β-D-glucoside, with the aromatic aglycon vanillin accumulating to concentrations of 2%–2.5% after curing of pods. This phenolic aldehyde has also been described for a variety of crops, e.g., coffee, strawberries, tobacco, and grapes (Demian, 1993). The biosynthetic pathway of vanillin is linked to biosynthesis of lignin, one of the key structural polymers in plants. More precisely, two alternative biosynthetic pathways have been evidenced (Walton et al., 2003). The first pathway was proposed by Zenk (1965), suggesting β-oxidation of feruloyl-CoA to vanilloyl-CoA (analogous to fatty acid β-oxidation) and subsequent reduction to vanillin. The second pathway was proposed by Kanisawa et al. (1994), suggesting that vanillin-β-D-glucoside may arise directly from p-coumaric acid via p-hydroxybenzaldehyde (Podstolski et al., 2002; Walton et al., 2003). The link to lignin metabolism is obvious, as the common precursor to both vanillin biosynthetic pathways—p-coumaric acid—constitutes the central intermediate in the biosynthesis of the three monolignols p-coumaryl alcohol (principal monolignol in grasses), coniferyl alcohol (principal monolignol in gymnosperms) and sinapyl alcohol (Boerjan et al., 2003).
The biosynthetic pathway of vanillin via p-coumaric and/or ferulic acid deserves attention, as all three metabolites have been reported to possess an allelopathic potential (Singh et al., 2001; Reigosa and Malvido-Pazos, 2007), including herbicidal effects (Chuah et al., 2013). In leaf and root tissues of B. humidicola, various allelopathic metabolites have been identified. These comprise brachialactol, different flavones (especially quercetin glycosides) and saponins, but especially different phenolic acids, i.e., p-coumaric acid, p-hydroxy-benzoic acid and vanillic acid (Supplementary Table S12; Supplementary Material). These allelopathica have been proposed to be responsible for the suppression of companion plants (e.g., grass-legume mixtures) and responsible for the dominance of B. humidicola in many ecosystems (Souza Filho et al., 2005; Oliveira et al., 2017; Feitoza et al., 2018; Feitoza et al., 2020). However, with regard to nitrification inhibition, none of the discussed phenolic acids (p-coumaric acid, ferulic acid, p-hydroxy-benzoic acid, vanillic acid) showed inhibitory activity against N. europaea, which was tested in vitro with concentrations up to 100 mg·L−1 (data not shown). These results are in line with in vitro and soil incubation studies on the nitrification inhibitory potential of p-coumaric acid and ferulic acid by McCarty et al. (1991) and Wu et al. (1999), contradicting initial findings by Rice and Pancholy (1974). In contrast, different methyl-derivatives of these phenolic acids, namely, methyl coumarate and methyl ferulate (isolated from B. humidicola roots) and methyl 3-(4-hydroxyphenyl) propionate (a root exudate of Sorghum bicolor) have been verified as biological NI (Gopalakrishnan et al., 2007; Zakir et al., 2008). Considering that this pattern resembles the case of vanillic acid and vanillin (only the latter revealed a nitrification inhibiting activity, ED80 ∼ 20 μg·ml−1), it could be deduced that the biological activity of these phenolics is determined by the carboxylic acid/aldehyde functional group.
At present, it remains impossible to estimate the individual contribution of each of the described metabolites to the overall allelopathic and nitrification inhibiting activity identified for B. humidicola. This is mainly related to the lack of data on internal concentrations and release rates through root turnover or active exudation in situ, as well as an insufficient understanding of their persistence and especially activity in soils. In this regard, data on root tissue concentrations have been provided for 3-epi-brachialactone [2–8 μg·g−1 root DM (Egenolf et al., 2021)], but not for vanillin (or possible vanillin glycosides), although the presence of all precursors strongly suggests internal vanillin pools (Oliveira et al., 2017). With regard to NI release, both 3-epi-brachialactone and vanillin exudation rates have been quantified in the same range of 1–4 μg·h−1·g−1 root DM (hydroponic studies) and to depend on external pH and cation feeding, suggesting active release via secondary transporters [see Figure 4 of this article and Egenolf et al. (2021)]. Whether the encountered exudation rates are sufficient to induce an accumulation of bioactive NI in soils, remains however to be addressed in subsequent studies, emphasizing that in situ exudation rates often lie several magnitudes higher than those observed in artificial systems (G. Neumann, personal communication). Furthermore, the allelopathic control of soil nitrification through the described putative NI, still has to be proven in situ, one possible approach constituting the simultaneous assessment and subsequent correlation of soil nitrification rates with the rhizosphere concentrations of the respective compounds.
In relation to the verification of the encountered effects within soils and considering the exclusion of any detrimental impact on the soil microbiome, the discussed phenolics might represent potential candidates responsible for BNI activity of B. humidicola. Especially the hypothesized metabolic pathways (1) phenylalanine → p-coumaric acid (→ methyl coumarate) → 4-hydroxybenzaldehyde → 3,4-dihydroxybenzaldehyde → vanillin as well as (2) phenylalanine → caffeic acid → ferulic acid (→ methyl ferulate) → vanilloylCoA → vanillin deserve further attention with respect to BNI breeding, including B. humidicola and other crops (Walton et al., 2003).
When breeding is concerned, potential co-benefits and trade-offs of the discussed phenolics with general allelopathic/antibiotic potentials must be considered. These comprise 1) potential non-target effects on the soil microbiome beyond ammonia oxidizers (e.g., poor N mineralization in extensive pasture systems), 2) allelopathic suppression of companion legumes within the pasture system, 3) feed quality in general (palatability), 4) harmful effects on animal health as some phenolic provoke secondary photosensitization of ruminants (Oliveira et al., 2017), and 5) effects on ruminal methane emissions reported for tannin-rich feeds (Verma et al., 2021).
Data availability statement
The original contributions presented in the study are included in the article/Supplementary Material, further inquiries can be directed to the corresponding author.
Author contributions
KE conducted the presented study, wrote the manuscript and modified it according to suggestions and corrections of the co-authors. JS and CB guided the isolation and purification of secondary metabolites. JS performed HPLC measurements. CB and JC recorded NMR spectra and elucidated the chemical structures. UB gave advise during the isolation of secondary metabolites and facilitated KE access to his lab facilities. JA was the leading scientist at CIAT, enabled KE access to his lab facilities and guided the performance of the bioassays. FR was leading senior scientist of the study. All authors contributed to the article and approved the submitted version.
Acknowledgments
The authors would like to thank Mr. Sébastian Mira and Mrs. Supriya Verma for their assistance during hydroponic cultivation of B. humidicola and root exudate collection. We are grateful to Mrs. Nadia Jimenez for conducting preliminary screenings for bioactive compounds. For their support during the bioassays, we are thankful to Mrs. Ashly Arevalo and Mr. Daniel Villegas. We thank Mr. Mario Wolf for his support during NMR analysis and Mrs. Iris Klaiber for her advice during HRMS analysis and interpretation. In addition, the authors acknowledge Dr. Guntur Subbarao for providing the N. europaea bioassay to CIAT and for the valuable discussions. Last but not least, we want to thank Prof. Georg Cadisch and Prof. Günter Neumann for their sound advice throughout the project.
Conflict of interest
The authors declare that the research was conducted in the absence of any commercial or financial relationships that could be construed as a potential conflict of interest.
Publisher’s note
All claims expressed in this article are solely those of the authors and do not necessarily represent those of their affiliated organizations, or those of the publisher, the editors and the reviewers. Any product that may be evaluated in this article, or claim that may be made by its manufacturer, is not guaranteed or endorsed by the publisher.
Supplementary material
The Supplementary Material for this article can be found online at: https://www.frontiersin.org/articles/10.3389/fmolb.2023.1192043/full#supplementary-material
References
Boerjan, W., Ralph, J., and Baucher, M. (2003). Lignin biosynthesis. Annu. Rev. Plant Biol. 54, 519–546. doi:10.1146/annurev.arplant.54.031902.134938
Byrnes, R. C., Nùñez, J., Arenas, L., Rao, I., Trujillo, C., Alvarez, C., et al. (2017). Biological nitrification inhibition by Brachiaria grasses mitigates soil nitrous oxide emissions from bovine urine patches. Soil Biol. Biochem. 107, 156–163. doi:10.1016/j.soilbio.2016.12.029
Chuah, T. S., Tan, P. K., and Ismail, B. S. (2013). Effects of adjuvants and soil microbes on the phytotoxic activity of coumarin in combination with p-vanillin on goosegrass (Eleusine indica L.) seedling emergence and growth. South Afr. J. Bot. 84, 128–133. doi:10.1016/j.sajb.2012.11.003
Coskun, D., Britto, D. T., Shi, W., and Kronzucker, H. J. (2017). Nitrogen transformations in modern agriculture and the role of biological nitrification inhibition. Nat. Plants 3, 17074. doi:10.1038/nplants.2017.74
Demian, B. A. (1993). Trace analysis of vanillin in tobacco. J. Liq. Chromatogr. 16, 3563–3574. doi:10.1080/10826079308019709
Egenolf, K., Conrad, J., Schöne, J., Braunberger, C., Beifuß, U., Walker, F., et al. (2020). Brachialactone isomers and derivatives of Brachiaria humidicola reveal contrasting nitrification inhibiting activity. Plant Physiol. Biochem. 154, 491–497. doi:10.1016/j.plaphy.2020.06.004
Egenolf, K., Schad, P., Arevalo, A., Villegas, D., Arango, J., Karwat, H., et al. (2022). Inter-microbial competition for N and plant NO3- uptake rather than BNI determine net nitrification under intensively managed Brachiaria humidicola. Biol. Fertil. Soils 58, 307–319. doi:10.1007/s00374-021-01606-9
Egenolf, K., Verma, S., Schöne, J., Klaiber, I., Arango, J., Cadisch, G., et al. (2021). Rhizosphere pH and cation-anion balance determine the exudation of nitrification inhibitor 3-epi-brachialactone suggesting release via secondary transport. Physiol. Plant 172, 116–123. doi:10.1111/ppl.13300
Feitoza, R., Lima, H., Oliveira, E., Oliveira, D. R., Moraes, L., Oliveira, A., et al. (2018). Structural and ultrastructural variations in roots of Calopogonium mucunoides Desv. treated with phenolic compounds from Urochloa humidicola (Rendle) Morrone & Zuloaga and phenolic commercial standards. South Afr. J. Bot. 116, 142–149. doi:10.1016/j.sajb.2018.03.005
Feitoza, R. B. B., Varela, R. M., Torres, A., Molinillo, J. M. G., Lima, H. R. P., Moraes, L. F. D., et al. (2020). Evaluation of the phytotoxicity of Urochloa humidicola roots by bioassays and microscopic analysis. Characterization of new compounds. J. Agric. Food Chem. 68, 4851–4864. doi:10.1021/acs.jafc.0c00307
Gopalakrishnan, S., Subbarao, G. V., Nakahara, K., Yoshihashi, T., Ito, O., Maeda, I., et al. (2007). Nitrification Inhibitors from the root tissues of Brachiaria humidicola, a tropical grass. J. Agric. Food Chem. 55, 1385–1388. doi:10.1021/jf062593o
Iizumi, T., Mizumoto, M., and Nakamura, K. (1998). A bioluminescence assay using Nitrosomonas europaea for rapid and sensitive detection of nitrification inhibitors. Applied and Environmental Microbiology 64 (10), 3656–3662. doi:10.1128/AEM.64.10.3656-3662.1998
Kanisawa, T., Tokoro, K., and Kawahara, S. (1994). Editors K. Kurihara, N. Suzuki, and H. Ogawa (Tokyo: Springer), 268.Olfaction Taste XI: Proceeding of the 11th International Symposium on Olfaction and Taste and of the 27th Japanese Symposium in Taste and Smell.
Karwat, H., Egenolf, K., Nuñez, J., Rao, I., Rasche, F., Arango, J., et al. (2018). Low 15N natural abundance in shoot tissue of Brachiaria humidicola is an indicator of reduced N losses due to biological nitrification inhibition (BNI). Front. Microbiol. 9, 2383. doi:10.3389/fmicb.2018.02383
Lata, J. C., Degrange, V., Raynaud, X., Maron, P. A., Lensi, R., and Abbadie, L. (2004). Grass populations control nitrification in savanna soils. Funct. Ecol. 18, 605–611. doi:10.1111/j.0269-8463.2004.00880.x
Lu, Y., Zhang, X., Ma, M., Zu, W., Kronzucker, H. J., and Shi, W. (2021). Syringic acid from rice as a biological nitrification and urease inhibitor and its synergism with 1,9-decanediol. Biol. Fertil. Soils 58, 277–289. doi:10.1007/s00374-021-01584-y
McCarty, G. W., Bremner, J. M., and Schmidt, E. L. (1991). Effects of phenolic acids on ammonia oxidation by terrestrial autotrophic nitrifying microorganisms. FEMS Microbiol. Lett. 85, 345–350. doi:10.1111/j.1574-6968.1991.tb04761.x
Nardi, P., Laanbroek, H. J., Nicol, G. W., Renella, G., Cardinale, M., Pietramellara, G., et al. (2020). Biological nitrification inhibition in the rhizosphere: determining interactions and impact on microbially mediated processes and potential applications. FEMS Microbiol. Rev. 44, 874–908. doi:10.1093/femsre/fuaa037
Neumann, G., Bott, S., Ohler, M. A., Mock, H.-P., Lippmann, R., Grosch, R., et al. (2014). Root exudation and root development of lettuce (Lactuca sativa L. cv. Tizian) as affected by different soils. Front. Microbiol. 5, 2. doi:10.3389/fmicb.2014.00002
Neumann, G., George, T. S., and Plassard, C. (2009). Strategies and methods for studying the rhizosphere - the plant science toolbox. Plant Soil 321, 431–456. doi:10.1007/s11104-009-9953-9
Nuñez, J., Arevalo, A., Karwat, H., Egenolf, K., Miles, J., Chirinda, N., et al. (2018). Biological nitrification inhibition activity in a soil-grown biparental population of the forage grass, Brachiaria humidicola. Plant Soil 426, 401–411. doi:10.1007/s11104-018-3626-5
Oliveira, D. R. D., Nepomuceno, D. D., Castro, R. N., Braz, R., and Carvalho, M. G. D. (2017). Special metabolites isolated from Urochloa humidicola (Poaceae). Acad Bras Cienc 89, 789–797. doi:10.1590/0001-3765201720160126
Otaka, J., Subbarao, G. V., MingLi, J., Ono, H., and Yoshihashi, T. (2023). Isolation and characterization of the hydrophilic BNI compound, 6-methoxy-2(3H)-benzoxazolone (MBOA), from maize roots. Plant Soil 489, 341–359. doi:10.1007/s11104-023-06021-7
Podstolski, A., Havkin-Frenkel, D., Malinowski, J., Blount, J. W., Kourteva, G., and Dixon, R. A. (2002). Unusual 4-hydroxybenzaldehyde synthase activity from tissue cultures of the vanilla orchid Vanilla planifolia. Phytochemistry 61, 611–620. doi:10.1016/S0031-9422(02)00285-6
R Core Team (2018). R: a language and environment for statistical computing. Available at: https://www.R-project.org.
Reigosa, M. J., and Malvido-Pazos, E. (2007). Phytotoxic effects of 21 plant secondary metabolites on Arabidopsis thaliana germination and root growth. J. Chem. Ecol. 33, 1456–1466. doi:10.1007/s10886-007-9318-x
Rice, E. L., and Pancholy, S. K. (1974). Inhibition of nitrification by climax ecosystems. III. Inhibitors other than tannins. Am. J. Bot. 61, 1095–1103. doi:10.1002/j.1537-2197.1974.tb12327.x
Singh, H. P., Batish, D. R., and Kohli, R. K. (2001). Allelopathy in agroecosystems. J. Crop Prod. 4, 1–41. doi:10.1300/J144v04n02_01
Souza Filho, A., Pereira, A., and Bayma, J. C. (2005). Aleloquímico produzido pela gramínea forrageira Brachiaria humidicola. Planta daninha 23, 25–32. doi:10.1590/S0100-83582005000100004
Subbarao, G. V., Ishikawa, T., Ito, O., Nakahara, K., Wang, H. Y., and Berry, W. L. (2006). A bioluminescence assay to detect nitrification inhibitors released from plant roots: a case study with Brachiaria humidicola. Plant Soil 288, 101–112. doi:10.1007/s11104-006-9094-3
Subbarao, G. V., Nakahara, K., Hurtado, M. P., Ono, H., Moreta, D. E., Salcedo, A. F., et al. (2009). Evidence for biological nitrification inhibition in Brachiaria pastures. Proc. Natl. Acad. Sci. U. S. A. 106, 17302–17307. doi:10.1073/pnas.0903694106
Subbarao, G. V., Wang, H. Y., Ito, O., Nakahara, K., and Berry, W. L. (2007b). NH4+ triggers the synthesis and release of biological nitrification inhibition compounds in Brachiaria humidicola roots. Plant Soil 290, 245–257. doi:10.1007/s11104-006-9156-6
Sylvester Bradley, R., Mosquera, D., and Méndez, J. E. (1988). Inhibition of nitrate accumulation in tropical grassland soils: effect of nitrogen fertilization and soil disturbance. J. Soil Sci. 39, 407–416. doi:10.1111/j.1365-2389.1988.tb01226.x
Teutscherová, N., Vázquez, E., Trubač, J., Villegas, D. M., Subbarao, G. V., Pulleman, M., et al. (2021). Gross N transformation rates in soil system with contrasting Urochloa genotypes do not confirm the relevance of BNI as previously assessed in vitro. Biol. Fertil. Soils 58, 321–331. doi:10.1007/s00374-021-01610-z
Theron, J. J. (1951). The influence of plants on the mineralization of nitrogen and the maintenance of organic matter in the soil. J. Agric. Sci. 41, 289–296. doi:10.1017/S0021859600049467
Vázquez, E., Teutscherova, N., Dannenmann, M., Töchterle, P., Butterbach-Bahl, K., Pulleman, M., et al. (2020). Gross nitrogen transformations in tropical pasture soils as affected by Urochloa genotypes differing in biological nitrification inhibition (BNI) capacity. Soil Biol. Biochem. 151, 108058. doi:10.1016/j.soilbio.2020.108058
Verma, S., Taube, F., and Malisch, C. S. (2021). Examining the variables leading to apparent incongruity between antimethanogenic potential of tannins and their observed effects in ruminants—a review. Sustainability 13, 2743. doi:10.3390/su13052743
Walton, N. J., Mayer, M. J., and Narbad, A. (2003). Vanillin. Phytochem. 63, 505–515. doi:10.1016/S0031-9422(03)00149-3
White, C. S. (1991). The role of monoterpenes in soil nitrogen cycling processes in ponderosa pine. Biogeochemistry 12, 43–68. doi:10.1007/BF00002625
Wu, E., Liu, X., and Zhu, X. (1999). The effect of groups in phenolic compounds on inhibition of nitrification in soil. Environ. Chem., 398–403.
Zakir, H. A. K. M., Subbarao, G. V., Pearse, S. J., Gopalakrishnan, S., Ito, O., Ishikawa, T., et al. (2008). Detection, isolation and characterization of a root-exuded compound, methyl 3-(4-hydroxyphenyl) propionate, responsible for biological nitrification inhibition by sorghum (Sorghum bicolor). New Phytol. 180, 442–451. doi:10.1111/j.1469-8137.2008.02576.x
Keywords: Urochloa humidicola (Poaceae), biological nitrification inhibition (BNI), forages, allelopathy, phenolics, Nitrosomonas europaea
Citation: Egenolf K, Schöne J, Conrad J, Braunberger C, Beifuß U, Arango J and Rasche F (2023) Root exudate fingerprint of Brachiaria humidicola reveals vanillin as a novel and effective nitrification inhibitor. Front. Mol. Biosci. 10:1192043. doi: 10.3389/fmolb.2023.1192043
Received: 22 March 2023; Accepted: 23 October 2023;
Published: 05 December 2023.
Edited by:
Wolfram Weckwerth, University of Vienna, AustriaReviewed by:
Michael Kertesz, The University of Sydney, AustraliaMalak M. Tfaily, University of Arizona, United States
Copyright © 2023 Egenolf, Schöne, Conrad, Braunberger, Beifuß, Arango and Rasche. This is an open-access article distributed under the terms of the Creative Commons Attribution License (CC BY). The use, distribution or reproduction in other forums is permitted, provided the original author(s) and the copyright owner(s) are credited and that the original publication in this journal is cited, in accordance with accepted academic practice. No use, distribution or reproduction is permitted which does not comply with these terms.
*Correspondence: Frank Rasche, ZnJhbmsucmFzY2hlQHVuaS1ob2hlbmhlaW0uZGU=
†Present address: Frank Rasche, International Institute of Tropical Agriculture (IITA), Nairobi, Kenya