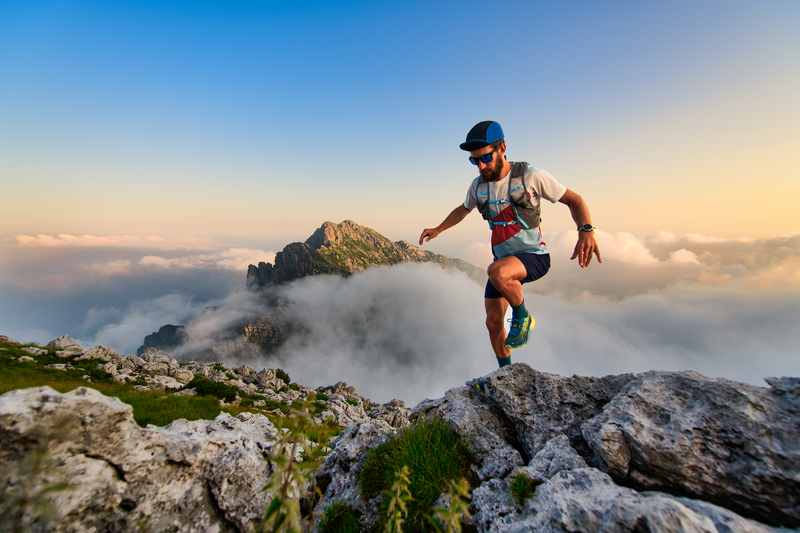
95% of researchers rate our articles as excellent or good
Learn more about the work of our research integrity team to safeguard the quality of each article we publish.
Find out more
REVIEW article
Front. Mol. Biosci. , 14 February 2023
Sec. Glycoscience
Volume 10 - 2023 | https://doi.org/10.3389/fmolb.2023.1146685
This article is part of the Research Topic Heparan Sulfate-Binding Proteins in Health and Disease View all 9 articles
Heparan sulfates (HSs) are the main components in the glycocalyx which covers endothelial cells and modulates vascular homeostasis through interactions with multiple Heparan sulfate binding proteins (HSBPs). During sepsis, heparanase increases and induces HS shedding. The process causes glycocalyx degradation, exacerbating inflammation and coagulation in sepsis. The circulating heparan sulfate fragments may serve as a host defense system by neutralizing dysregulated Heparan sulfate binding proteins or pro-inflammatory molecules in certain circumstances. Understanding heparan sulfates and heparan sulfate binding proteins in health and sepsis is critical to decipher the dysregulated host response in sepsis and advance drug development. In this review, we will overview the current understanding of HS in glycocalyx under septic condition and the dysfunctional heparan sulfate binding proteins as potential drug targets, particularly, high mobility group box 1 (HMGB1) and histones. Moreover, several drug candidates based on heparan sulfates or related to heparan sulfates, such as heparanase inhibitors or heparin-binding protein (HBP), will be discussed regarding their recent advances. By applying chemical or chemoenzymatic approaches, the structure-function relationship between heparan sulfates and heparan sulfate binding proteins is recently revealed with structurally defined heparan sulfates. Such homogenous heparan sulfates may further facilitate the investigation of the role of heparan sulfates in sepsis and the development of carbohydrate-based therapy.
Sepsis, a lethal syndrome caused by dysregulated host responses to infection, leads to millions of deaths annually (Singer et al., 2016). Several underlying mechanisms of sepsis have been identified, including overwhelming inflammation and immune suppression, resulting in cell damage and organ dysfunction. Heparan sulfates (HSs) are enriched in the protective cell surface glycocalyx layer and are shed in septic patients. Recent research identifies pro-inflammatory molecules that bind to HSs, including high mobility group box 1 (HMGB1) and extracellular histones. In addition, HSs are found to interact with bacterial-associated molecules, such as the lipopolysaccharide (LPS)/HMGB1 complex and the heparin-binding protein (HBP).
HSs are linear polysaccharides made in the Golgi compartment by various biosynthetic enzymes, consisting of repeating disaccharides of glucuronic acid (GlcA) and glucosamine (GlcN) or iduronic acid (IdoA). HSs can include two to over a hundred disaccharide units, depending on the polymerization process catalyzed by the exostosin glycosyltransferases 1/2 (EXT 1/2). The structures of HSs are diversified by sulfation patterns. Different sulfotransferases and epimerization of glucuronic acid to iduronic acid are involved in HS chain modification by N-deacetylase/N-sulfotransferase (NDST), C5 epimerase, and 2-/3-/6-O-sulfotransferases (2-/3-/6-OST) (Sarrazin et al., 2011). HSs are usually secreted by exocytosis and present on the cell surfaces alone or attached to proteoglycans (Figure 1).
FIGURE 1. HSs and HSBPs on glycocalyx in health and sepsis. (A) Heparan sulfates (HSs) present on the cell surfaces as proteoglycans, binding to perlecan, syndecans-1, and glypican. With other glycoproteins, including chondroitin sulfate (Tam et al., 2008) and hyaluronan (HA) bound to syndecans-1 and CD44, HS proteoglycans form the glycocalyx and regulate cell signaling by interacting with HS binding proteins (HSBPs) such as fibroblast growth factor receptor (FGFR), intracellular adhesion molecule-1 (iCAM-1). Binding to HSBPs requires specific HS structures; for example, FGFR binding requires 6-O-sulfated glucosamine and more than ten saccharide units. (B) During sepsis, HSs are released by upregulated heparanase. HSs mediate inflammation by binding to dysregulated HSBPs, such as extracellular histones, high mobility group box 1 (HMGB1), and interleukin 8 (IL-8). HSs also serve as co-receptors for immune cells by binding to p-selectin. Specific sulfation and length are also required for HS binding to heparanase and HMGB1.
Collectively with other glycoproteins, glycosaminoglycans (GAG), and plasma proteins, proteoglycans make up the glycocalyx that carpets the luminal side of endothelial cells lining every blood vessel in the vascular system (Zeng, 2017).
The gel-like layer of the glycocalyx is essential for normal physiology in several aspects: it regulates the permeability of the vasculature, serves as a mechanotransducer, and functions as an anti-inflammatory and anticoagulant coat to protect the underlying endothelium. The main components of the glycocalyx are membrane-bound proteoglycans, i.e., syndecans and glypicans, and soluble proteoglycan, i.e., perlecan. In the vasculature, HS proteoglycans represent over half the total amount of proteoglycans present in the glycocalyx (Ihrcke et al., 1993). Multiple functions of the glycocalyx are carried out by the interactions of HSs and hundreds of HS binding proteins (HSBPs), which cover a wide range of biological functions, such as mediating signaling pathways, maintaining glycocalyx integrity, and vascular homeostasis (Xu et al., 2018). For example, HSs regulate the activation of fibroblast growth factor 2 (FGF2) -FGF receptor 1 (FGFR1) signaling for the reconstitution of glycocalyx (Figure 1A) (Yang et al., 2017). The detailed functions of the glycocalyx can be found in other reviews (Ihrcke et al., 1993; Oshima et al., 2021).
It is well known that in pathologic conditions like sepsis, atherosclerosis, and kidney disease, the glycocalyx is damaged, resulting in vascular hyperpermeability and increased apoptosis (Figure 1B) (Garsen et al., 2016; Puerta-Guardo et al., 2016; Uchimido et al., 2019). Heparanase is an endo-β-glucuronidase that cleaves HSs. The expression and activity of heparanase are frequently associated with glycocalyx damage. Loss of glycocalyx HSs leads to the deterioration of glycocalyx function. The process is described as HSs shedding, which releases HSs in various sizes and sulfation patterns into circulation. HSs are responsible for multiple biological functions through interaction with various proteins in a homeostatic state; therefore HSs shedding interrupts crosstalk and communications between cells, contributing to abnormal inflammation, coagulation, and lipid metabolism systems under septic conditions (Hayashida et al., 2009; Arnold et al., 2020a). In an LPS-induced sepsis mouse model, pulmonary endothelial heparanase was activated in a TNF-α dependent manner (Schmidt et al., 2012). Consequently, the increase in heparanase activity leads to the development of acute lung injury during endotoxemia. In his study, the authors also demonstrate a high heparanase expression in biopsies of human lung tissue with diffuse alveolar damage, suggesting the potential contribution of heparanase to lung injury. Moreover, glycocalyx reconstitution is impaired in sepsis. A report showed that although the HS fragments are able to activate FGF2-FGFR1 signaling, HS biosynthesis by EXT1 and FGFR1 expression are downregulated in sepsis (Yang et al., 2017). Since the damaged glycocalyx cannot be repaired in sepsis, the loss of vascular homeostasis may contribute to long-term organ injury and dysfunction. This review focuses on the role of HSs and several dysregulated proteins in sepsis and therapeutic strategies reported preclinically and clinically.
HSs have been found to interact with various dysregulated proteins in preclinical and clinical trials, supporting its critical role in sepsis pathology. In this review, we will focus on two critical HSBPs, HMGB1 and extracellular histones. Other HSBPs that were studied in sepsis animal models or clinical trials are summarized in Table 1.
Although various HSBPs have been identified, many structure-function relationships between HSs and the proteins in sepsis remain unclear. As shown in Table 1, most studies were conducted with heparin, an HS mixture with anticoagulant activity. The structural complexity of heparin makes it challenging to elucidate the effect of size and sulfation patterns on specific protein binding. Furthermore, the dominating anticoagulant activity of heparin makes it impossible to take therapeutic advantage of its chemokine/cytokine binding, inhibition of adhesion molecules, and effects on lipid metabolism that heparin is also capable of. Therefore, it is suggested to use structurally defined HSs to investigate protein interactions and elucidate the impact of the interaction on sepsis (Axelsson et al., 2012). A research group used synthetic dodecasaccharides with or without 6-O-sulfation at the reducing end to reveal the importance of 6-O-sulfation on IL-8 inhibition by HSs (Jayson et al., 2015). Understanding HS structure preference to its binding proteins, as the study described above, is essential to optimize HS-based therapeutics in sepsis.
Damage associated molecular patterns (DAMPs) are nuclear, cellular, mitochondrial, or extracellular molecules passively released from damaged cells or actively released from immune cells in response to injury (Roh and Sohn, 2018; Gong et al., 2020). Several DAMPs, such as HMGB1, histones, extracellular cold-inducible RNA-binding protein (eCIRP), and heat shock proteins (HSPs), are recognized as critical inflammatory mediators that initiate and potentiate inflammation and can lead to organ dysfunction or death in sepsis (Denning et al., 2019; Gong et al., 2020). Similar to the electrostatic interactions between DNA and histones in the nucleus, these positively charged DAMPs are also reported to bind to HSs in sepsis.
Interestingly, the binding of DAMPs to HSs does not depend on electrostatic interactions alone. Recently, heparin was shown to trigger the formation of polymer complexes comprised of extracellular histones and cell-free DNA (cfDNA) in flowing blood. The polymer can be disrupted by DNase I and heparinase I/III, suggesting that heparin may form a tertiary complex with both negatively charged cfDNA and positively charged histone, although the mechanism is unknown (de Vries et al., 2019). In addition, the binding of heparin to HMGB1 is also shown to be independent of pH; other negatively charged molecules, such as sialic acid, do not bind to HMGB1 (Siddiqui et al., 2020). Their results suggest that factors other than electrostatic interaction contribute to HS/HMGB1 binding. A recent study from our group also indicated that the interaction between HMGB1 and extracellular histone 3 (H3) is HS size and sulfation pattern dependent in sepsis by applying a synthetic HS oligosaccharide library (Liao et al., 2023).
HMGB1 (Amphoterin, HMG-1) is a highly conserved chromatin-binding protein that regulates transcription factors and stabilizes nucleosomes in normal physiology (Wang et al., 2006; Andersson et al., 2018). HMGB1’s extracellular inflammatory activity in sepsis was first identified in 1999 by administering the bacterial endotoxin (lipopolysaccharide, LPS) in vitro and in vivo models (Wang et al., 1999). The elevation of plasma HMGB1 is delayed but prolonged in septic mice, which can be observed from 8 to 32 h after LPS (i.p.) injection and from 18 to 72 h after cecal ligation and puncture (CLP) induced sepsis (Wang et al., 1999; Yang et al., 2004). In septic patients, the serum HMGB1 was significantly higher than in healthy participants and can persist up to 10 days after sepsis diagnosis (Karlsson et al., 2008; Karakike et al., 2019). The HMGB1 kinetics suggest that it is a promising target for sepsis as it may offer a broad therapeutic window to treat established septic patients compared to other acute inflammatory cytokines or chemokines.
The proinflammatory activity of HMGB1 in sepsis can be attributed directly to its binding to receptors such as the Receptor for Advanced Glycation End-products (RAGE) and Toll-like Receptor 4 (TLR4) (Figure 2A). Also, HMGB1 can indirectly induce inflammation by interacting with other molecules, such as cytokines and bacterial products (Kang et al., 2014). For example, the HMGB1-RAGE axis is reported to activate macrophages to produce pro-IL-1β and pro-IL-18, trigger neutrophils-mediated injury, and increase the permeability of endothelial cells, mainly through the p38/ERK signaling pathway (Fiuza et al., 2003; He et al., 2012; Huang et al., 2012; Huebener et al., 2019). TLR4, on the other hand, binds to HMGB1 to induce the releases of TNF-α and IL-6 and promote the neutrophil extracellular trap (NET) formation (Yang et al., 2010; Tadie et al., 2013; Zheng et al., 2016). In addition, HMGB1 can bind to bacterial products, such as CpG-DNA or LPS, to facilitate their delivery into cells and further augment inflammation (Ivanov et al., 2007; Qin et al., 2009). Recently, a new mechanism of LPS delivery through coupling with HMGB1 was demonstrated by Deng and colleagues (Deng et al., 2018). Compared to LPS or HMGB1 alone, the LPS/HMGB1 complex induces dramatically higher TNF-α released from macrophages and increases LPS delivered into the cytosol. This resulted in increased caspase-11-dependent pyroptosis through binding to RAGE.
FIGURE 2. HMGB1 damage and proposed anti-HMGB1 mechanism of HSs in sepsis. (A) Infection sources, such as bacterial products, induce HMGB1 to be released from immune cells or damaged cells. HMGB1 binds to toll-like receptor 4 (TLR4) and receptor for advanced glycation end products (RAGE) on neutrophils and macrophages, inducing neutrophil extracellular trap (NET) formation and cytokines/chemokines release. HMGB1 also forms a complex with lipopolysaccharide (LPS), binding to RAGE on host cells and inducing cell pyroptosis. In addition, LPS forms a complex with apolipoprotein A-I (apoA-I), which can transport to the liver for clearance. (B) HSs reduce HMGB1-induced inflammation by inhibiting HMGB1/LPS complex binding to RAGE. Also, HSs facilitate apoA-I/LPS complex formation, replacing the LPS/HMGB1 complex. HSs are also proposed to reduce HMGB1/LPS complex formation and inhibit HMGB1/RAGE signaling in sepsis, yet the proposed mechanisms need further validation.
Extracellular HMGB1 has been found to bind to heparin/HS in previous studies, but the biological significance of the interaction between HMGB1 and HSs was not revealed until very recently. Heparin contributed significantly to the discovery of HMGB1, as HMGB1 was first isolated from the rat brain using heparin-Sepharose in 1987 (Rauvala and Pihlaskari, 1987). A few years later, it was discovered that HMGB1 was a ligand for syndecans, and binding was attributed to the HS chains, not chondroitin sulfate (Tam et al.) chains (Salmivirta et al., 1992). This result was confirmed in a later study that used mutant Chinese hamster ovary (CHO) cells, which lack HSs but have elevated CSs/dermatan sulfate (DSs). These mutant CHO cells could not bind HMGB1 compared to WT CHO cells (Xu et al., 2011). Moreover, treating human microvascular endothelial cells (HMVEC-c) with heparin lyases or protamine (a neutralizing heparin therapeutic) almost fully abolished HMGB1 stimulation, indicated by a significant reduction of phosphorylated Erk1/2 and p38. These results indicate that endothelial HSs are necessary for HMGB1 signaling. The binding site of HSs was further investigated by a biotinylation mapping strategy and mutagenesis studies, confirming that Lys-87, Lys-88, Lys-96, Lys-97, and Lys-150 contribute the most to HS binding to HMGB1. Interestingly, the authors later found that the mutant HMGB1 that lacks HS binding ability is still able to induce phosphorylation of Erk1/2 and p38 in vitro. RAGE, the receptor of HMGB1, was found to bind to HSs even in the absence of HMGB1. The RAGE/HS complex also can promote the oligomerization of RAGE, which is involved in MAPK/NF-κB activation (Zong et al., 2010; Xu et al., 2013). In addition to endothelial HSs, heparin has also been studied for HMGB1-RAGE binding. A study showed that heparin changes HMGB1 conformation by reducing β-sheet while inducing α-helix, with binding affinity KD 9.77 × 10−8 mol/L (Ling et al., 2011). Treating murine macrophage Raw264.7 with heparin significantly decreases HMGB1-induced inflammation, potentially by interrupting HMGB1-RAGE signaling. However, whether the event is also involved in endothelial HSs interaction with HMGB1-RAGE signaling remains to be determined, preferably with structured defined HSs instead of a heparin mixture.
To further elucidate the binding between HMGB1 and HSs, our group utilizes chemoenzymatic synthesis to generate an HS library with various length and sulfation patterns (Liu and Linhardt, 2014). Previously, Xu and colleagues showed that HMGB1 binding to heparin heavily depends on N-sulfated HSs (Xu et al., 2011). In the acute liver injury models, including acetaminophen-induced drug toxicity and liver ischemia-reperfusion induced liver injury, we demonstrated that binding to HMGB1 has a specific HS length and sulfation level requirement (Arnold et al., 2020b; Arnold et al., 2020c). In the NS2S repeating disaccharide HS group, at least 18 saccharides (octadecasaccharide, Figure 3) are required to bind to HMGB1, with KD at 168 nM (Arnold et al., 2020c). Reducing the HS length but maintaining the binding between HMGB1 and HSs is possible, but the sulfation degree needs to increase to NS2S6S (Arnold et al., 2020b). The binding between HMGB1 and HSs has shown therapeutic potential. Synthetic HSs reduced liver injury mediated by HMGB1 in both models of acute liver injury. The results set an example to further investigate HMGB1 and HSs relationship with structurally defined HSs and utilize this relationship for drug development.
FIGURE 3. The structure of octadecassacharide (18-mer). The octadeacassacharide (18-mer) is produced by chemoenzymatic synthesis (Liao et al., 2023).
Several studies have investigated HSs’ anti-HMGB1 activity in sepsis with various heparins and synthetic HSs (Figure 2B). Interestingly, most of the current evidence indicates that HSs’ beneficial binding effect on HMGB1 mainly targets the HMGB1/LPS—RAGE signaling pathway. For example, heparin treatment diminished the binding of HMGB1 to the cell surface under LPS stimulation in the murine macrophage Raw264.7 model (Li et al., 2015). Heparin also decreased the downstream p38/Erk1/2 pathway with a reduction of TNF-α induced by LPS/HMGB1 in vitro; however, it cannot affect inflammation induced by LPS alone. Also, Tang and colleagues indicated that heparin inhibits HMGB1/LPS binding. Inhibition leads to a reduction of LPS delivery to the cytosol, caspase-11 dependent pyroptosis, and sepsis-induced lethality (Tang et al., 2021). Moreover, the chemically modified non-anticoagulant heparin also could perform a similar effect as heparin, suggesting that the anti-inflammatory effect of heparin targeting HMGB1/LPS induced caspase-11 associated damage is independent of its anticoagulant activity.
To further elucidate the finding of HMGB1/LPS and HSs, our group used structurally defined HSs to determine their binding affinity between HMGB1 and investigated the reaction between HSs and HMGB1/LPS in sepsis. We found that HSs size dependently attenuated HMGB1/LPS induced inflammation (Liao et al., 2023). Interestingly, binding to HMGB1 by synthetic HS octadecassacharide (18-mer) is insufficient to dissociate the HMGB1/LPS complex. While the 18-mer binds to HMGB1, it cannot block HMGB1 binding to LPS in the endotoxemia model. Instead, HSs enlist the action of apolipoprotein A-I (ApoA-I), the main protein in high-density lipoprotein (HDL), to displace HMGB1 binding to LPS (Figure 2B). By increasing the non-toxic ApoA-I/LPS complex, the HS 18-mer reduces LPS/HMGB1-induced inflammation in sepsis. This protective mechanism of 18-mer was demonstrated in the LPS-induced endotoxemia and CLP mouse model. The mechanism involved in lipid modulation by HSs might contribute to the protective effect seen in septic mice co-administered atorvastatin and low molecular weight heparin (LMWH) (Jing et al., 2016). In this study, combined therapy of atorvastatin and LMWH significantly reduced lung injury, HMGB1, TNF-α, and IL-1β in CLP mice and improved survival rate. The potential therapeutic effect may be due to inducing HDL/apoA-I by atorvastatin and thus further augmenting heparin-facilitated apoA-I/LPS complex formation. Finally, in addition to findings of HSs on HMGB1/LPS complex, it is worth noting that the effect of HSs on HMGB1-RAGE induced damage cannot be excluded, as most of the synergetic effect of HMGB1/LPS complex was tested in a relatively low dose of HMGB1 and LPS.
Histones are highly conserved cationic nuclear proteins responsible for regulating the structure, stability, and availability of chromatin and DNA (Silk et al., 2017). Histones can be categorized into two subgroups; the core histones (H2A, H2B, H3, and H4) and the linker histones (H1 and H5) (Chen et al., 2014). The core histones have similar structures, binding to each other to form octameric cores and wrapping around DNA as nucleosomes, which are further connected by the linker histones as chromatins. The functional role of nuclear histones involves the regulation of epigenetic modifications and the replication of DNA.
During sepsis, histones are released as DAMPs from necrotic and apoptotic cells or activated immune cells, especially neutrophils, as part of neutrophil extracellular traps (NETs) (Chen et al., 2014). NETs are extracellular meshes composed of extracellular histones (H1, H2A, H2B, H3, and H4) and other proteins, including neutrophil elastase (NE), myeloperoxidase (MPO), and cathepsin G (Chapman et al., 2019). Specifically, citrullinated H3, which is generated by converting arginine to citrulline at residues 2, 8, and 17 by peptidyl-arginine deiminase 4 (PAD 4), has been used as a critical marker of NETs and proposed as a potential marker in sepsis (Li Y. et al., 2014; Thålin et al., 2020).
The detrimental effects of extracellular histones have been identified as crucial factors in sepsis, which can be attributed to direct interference of cell membrane integrity, inflammation, and coagulation (Figure 4A) (Xu et al., 2009; Li et al., 2021). First, extracellular histones exhibit direct cytotoxicity by binding to phospholipid-phosphodiester bonds of the endothelial and epithelial cell membrane, altering membrane permeability, and increasing calcium ion influx following cell death (Abrams et al., 2013; Xu et al., 2014). The disruption of calcium concentration by histones was also reported to cause cardiomyocyte damage in the CLP sepsis model (Kalbitz et al., 2015). Second, TLR2, TLR4, and TLR9 are implicated in histone-mediated damage and inflammation by activating NF-κB signaling pathways. Notably, activation of TLR2 and TLR4 by histones significantly increases cytokine TNF-α and IL-6; the mechanism was shown to contribute to septic kidney inflammation (Semeraro et al., 2011; Allam et al., 2012; Silk et al., 2017). Lastly, extracellular histones possess procoagulant activity, including promoting thrombin generation and activation, platelets activation, tissue factor upregulation, and fibrin clot formation (Ammollo et al., 2011; Simmons and Pittet, 2015; McDonald et al., 2017; Li et al., 2021). Of note, DAMP mechanisms of histones described above are intertwined; direct endothelial cell damage by histones also contributes to histone-mediated thrombosis, and impaired coagulation may further augment immune cell infiltration and proinflammatory chemokine/cytokines release.
FIGURE 4. Histone damage and proposed anti-histone mechanism of HSs in sepsis. (A) Histones are released from activated neutrophils, macrophages, and damaged host cells during sepsis. Specifically, citrullinated histone 3 (CitH3) can be used as a NET marker. Extracellular histones directly interrupt cell integrity by interacting with cell membranes and inducing calcium influx, causing cell death. Histones also bind to TLR2/4 on macrophages and platelets, inducing cytokine release or coagulation. (B) HSs reduce histone-induced inflammation and coagulation by directly neutralizing histones, inhibiting their interactions with cell membranes and TLR2/4 on macrophages and platelets.
As there are five subtypes of histones, it is important to note that the DAMP activity varies from H1 to H5. Specifically, H3 and H4 have been shown as the most proinflammatory and procoagulant extracellular histones (Xu et al., 2009; Ammollo et al., 2011). In human endothelial cells, H3 and H4 were indicated to be the most cytotoxic histones that significantly reduce cell viability. Also, H3 and H4 are the most potent histones to induce thrombin generation in platelet-poor plasma. Thus, developing anti-histone therapeutics may be more effective if focusing on targeting H3 and H4.
Since histones are basic proteins enriched with lysine and arginine at the N terminal tail, it is rational to use negatively charged heparin to neutralize circulating histones and attenuate the overwhelming inflammation response in sepsis (Li et al., 2021). The notion has been tested in numerous studies with unfractionated heparin (UFH) and low molecular weight heparin (LMWH); both heparins effectively neutralize extracellular histones and improve survival rates of mice that underwent direct histones or H3 (i.v.) injection, LPS, and CLP-induced sepsis (Iba et al., 2015; Hogwood et al., 2020). The protective effect of UFH/LMWH could be attributed to reducing the inflammatory response, such as NF-κB/MAPK dependent secretion of TNF-α and IL-6, and dampening endothelial and epithelial cell damage to protect against sepsis-induced kidney and lung injury (Figure 4B) (Wang et al., 2015; Wang et al., 2020). In addition, UFH was also found to reduce histone-mediated coagulopathy, demonstrated by reduced mRNA expression of tissue factor, fibrinogen, thrombomodulin, and thrombosis in mice lungs (Hogwood et al., 2020).
Interestingly, Wildhagen and colleagues demonstrated that the anti-histone-induced cytotoxicity of heparin is independent of its anticoagulant activity (Wildhagen et al., 2014). Using non-anticoagulant heparin derived from UFH, the authors showed that the protective effect against H3-induced cell death and binding affinity to H3 in vitro. The overall protective effect of the non-anticoagulant heparin was further confirmed in mouse survival studies with the LPS and CLP sepsis models. In addition, the sulfation pattern and length requirement of HSs for their anti-histone effect have been investigated. In a recent study, HS oligosaccharides in different sulfation patterns and sizes were prepared by depolymerization and gel filtration from UFH (Zhang et al., 2017). The authors demonstrated that HS oligosaccharides must be at least ten sugar residues long and have at least N-sulfo-glucosamine in order to bind to the mixture of calf thymus histones. The requirement of N-sulfation is confirmed by another group using the whole blood cell model, showing that N-desulfated heparin lost its ability to attenuate histone-induced elevation of IL-6, IL-8, and tissue factor (Hogwood et al., 2020). Interestingly, tetrasaccharide and decasaccharide provide similar lung protective effects against a sub-lethal dose of histone injection in mice, but neither of them improves the survival rate of mice administered a lethal dose of histone (Zhang et al., 2017). However, both tetrasaccharide and decasaccharide protect against low and high doses of histone-induced cytotoxicity in the human lung microvascular endothelial cell (HPMVEC-ST1.6R) model. In our recent study, we apply chemoenzymatic synthesized HS oligosaccharides to determine binding affinity by SPR, anti-histone effect by human endothelial cell EAHy.926, and direct lethal dose of histone injection and CLP models (Liao et al., 2023). Similar to previous findings, we confirmed that binding to histone H3 is HS size and N-sulfation dependent, with 18-mer performing the best to protect against histone-induced cell death, inflammation, and lethality in vitro and in vivo. The different findings regarding the protective effects of HS oligosaccharides may be due to the HS length and purity. Further investigation on the structure-activity relationship between HSs and histone is recommended to optimize HS-based therapy targeting histones in sepsis.
The characteristics of HSs have inspired therapeutic strategies in sepsis in three ways; first, developing HS-based treatments based on HSs’ interactions with numerous dysregulated proteins; second, preventing HS shedding with HSs derived or other small molecules; third, using HS fragments or HS related molecules as biomarkers in sepsis diagnosis. These three strategies have been tested in preclinical and clinical trials with promising results and are discussed below.
Recently, UFH and LMWH are also found to have potential protective effects in sepsis through interactions with critical proteins listed in Table 1, such as cytokines, chemokines, DAMPs, and adhesion molecules (Figures 4A, B). Interestingly, more studies attribute the protective effect to anti-inflammation rather than anticoagulation. Nevertheless, the anticoagulation effect of UFH is acknowledged in several studies, indicating that UFH reduces D-dimer, soluble fibrins, and thrombin-antithrombin (TAT) levels in plasma from LPS-challenged mice and healthy male volunteers (Pernerstorfer et al., 1999; Yang et al., 2019). Anti-inflammation effect of UFH/LMWH has been extensively investigated and revealed multiple pathways, such as neutralizing cytotoxic extracellular histones (Luan et al., 2014; Iba et al., 2015), inhibiting HMGB1/LPS mediated pyroptosis (Tang et al., 2021), interfering P-selectin/L-selectin associated neutrophil infiltration (Wang et al., 2002), and blocking inflammatory cascade by binding to IL-8, MCP-1, and HBP (Farrugia et al., 2018). However, the pro-infection effect of heparin was also reported in an in vitro study, showing that heparin facilitates LPS entry and activation of peripheral blood monocytes by binding to LPS-binding protein (LBP) and inducing IL-8 release (Heinzelmann and Bosshart, 2005). Such effect has also been observed with healthy volunteers given LPS and heparin, although in a small cohort (Heinzelmann and Bosshart, 2004). Nevertheless, UFH and LMWH are consistently shown to reduce organ injuries and improve survival in preclinical trials, suggesting an overall beneficial effect against sepsis. As inflammation and coagulation mechanisms are intertwined in sepsis, multi-functional heparin treatment such as UFH/LMWH is preferable as a drug candidate (Li and Ma, 2017; Hogwood et al., 2020). Further elucidation of the structure-function relationship of heparin in inflammation is required to advance HS-based therapy; investigations conducted with heparin derivatives and heparinoids are emerging.
The promising findings from preclinical studies with UFH/LMWH have inspired many clinical trials with septic patients, yet the results are conflicting (Table 2) (Li and Ma, 2017). Regarding large-scale randomized controlled trials, UFH/LMWH have been evaluated alone and in subgroup analysis combined with a once-approved sepsis treatment and two anticoagulants, including drotrecogin alfa (activated), tifacogin, and AT III in the 2010s (Warren et al., 2001; Abraham et al., 2003; Levi et al., 2007; Jaimes et al., 2009). Drotrecogin alfa (activated) is a recombinant activated protein C proposed to target extracellular histones in sepsis, and tifacogin is a recombinant tissue factor pathway inhibitor. The results show that UFH/LMWH is safe for septic patients except in combination with high-dose AT III therapy (Warren et al., 2001). The improvement of 28-day survival in severe sepsis was observed when giving UFH/LMWH in some trials, but the efficacy is not consistent. Further analysis by systemic reviews and meta-analysis with the above and other clinical trials have also been conducted, yet the conclusion is trending positive but is inconclusive (Wang C. et al., 2014; Zarychanski et al., 2015; Fan et al., 2016; Fu et al., 2022). Several clinical trials are still in progress, such as NCT04861922 (ClinicalTrial.org, retrieved on 12/1/2022).
The conflicting results between heparin clinical trials in sepsis may be due to several reasons. First, sepsis is a complex disease with changing definitions. Sepsis was first defined in 1991 as a systemic inflammatory response to infection, modified in 2001, and updated to life-threatening organ dysfunction caused by a dysregulated response to infection in 2016 (van der Poll et al., 2017). The updated definition of sepsis removes the category of “severe sepsis,” defined as “sepsis and acute organ dysfunction.” Population and treatment decisions may differ before and after 2016, making the results before 2016 less applicable to the current situation (van der Poll et al., 2017). Particularly, the HETRASE trial that evaluated heparin efficacy in sepsis included participants who are not defined as septic patients now. New clinical trials are required to evaluate heparin’s efficacy in sepsis to date. Second, sepsis is a heterogenous symptom. Some studies suggested that UFH/LMWH only benefit severe septic patients with disseminated intravascular coagulation (Reitsma et al., 2007), while other analysis only included Asians showed more benefit with heparin treatment (Fan et al., 2016; Yamakawa et al., 2016). Identification of interested subgroups for heparin is recommended. Third, as alluded to above, the beneficial effect of heparin other than anticoagulant activity may not be fully explored (Li and Ma, 2017). During the COVID-19 pandemic, a retrospective study reported that LMWH significantly increases D-dimer and reduces IL-6 in COVID-19 patients, although in a small cohort (Shi et al., 2020). It is plausible that LMWH can also reduce the overwhelming inflammatory response in septic patients. Such anti-inflammatory effects may require a higher dose of UFH/LMWH, yet the bleeding risk limits the therapeutic window. Lastly, UFH/LMWH are HS mixtures; sulfation degree and patterns vary between sources. While 6-O-sulfation is reported as a critical sulfation pattern responsible for anti-inflammatory effect, UFH/LMWH from porcine mucosa is shown to have much higher 6-O-sulfated glucosamines than those from bovine mucosa (80% vs. 55%) (Hayashida et al., 2009; Mauri et al., 2019). Variations of anti-inflammatory effects between UFH/LMWH from different sources may further complicate the result.
Preclinical studies have shown that the protective effect of UFH/LMWH is independent of anticoagulation. Non-anticoagulant heparins can be generated by various chemical modifications with UFH and have been tested in preclinical models (Figures 5C–F). The non-anticoagulant UFH is now commercially available as N-acetyl heparin (Figure 5C) (Sigma, Iduron, etc.) (Nagasawa et al., 1977). N-acetyl heparin has been referred to as non-anticoagulant heparins (NAH) in many studies, shown to protect against sepsis-induced lethality and organ damage by targeting HMGB1/LPS complex (Tang et al., 2021), heparanase (Lygizos et al., 2013), and histones (Hogwood et al., 2020). In addition, a 2-O, 3-O-desulfated heparin (ODSH) with minimal anticoagulant activity has been generated and shown to increase activated protein C and inhibit histones in septic mice, with the safety confirmed in dog clinical trials for sepsis peritonitis (Figure 5D) (Kowalska et al., 2014; Mauro et al., 2022). Moreover, Wildhagen and colleagues generated an antithrombin affinity-depleted heparin (AADH) by using an antithrombin column to remove the anticoagulant activity of UFH (Wildhagen et al., 2014). AADH was shown with a similar protective effect against histone H3-induced cytotoxicity in vitro as UFH, significantly reducing neutrophil infiltration and lung injury and improving the survival of septic mice. A first-in-human clinical trial is currently recruiting critically ill septic patients for AADH treatment (NCT05208112, ClinicalTrial.gov, retrieved on 1/1/23).
FIGURE 5. Structures of heparins. (A) Unfractionated heparin (UFH) and UFH derivatives, including low molecular weight heparin (LMWH), N-acetyl heparin, 2-O, 3-O-desulfated heparin (ODSH), and SST0001 are comprised of repeating disaccharide units. Glucosamine-glucuronic acid is more abundant that glucosamine-iduronic acid. Sulfation sites are varied as indicated. (B) The pentasaccharide binds to antithrombin III (AT III). The sequence is present in UFH and LMWH. (C,D,F) The structure of non-anticoagulant heparins. (C) The pentasaccharide in N-acetyl heparin. N-acetyl glucosamine eliminate anticoagulant activity. (D) The pentasaccharide in ODSH greatly reduces anticoagulant activity. (E) The structure of fondaparinux. (F) The pentasaccharide in SST0001 does not have anticoagulant activity.
Synthetic heparin-like compounds are produced by chemical reactions and/or enzymatic synthesis. This allows researchers to have full control of HS length and sulfation pattern. Fondaparinux (Arixtra®) (Figure 5E), for example, is a chemically synthesized HS that only includes the essential pentasaccharide responsible for binding to ATIII (Samama and Gerotziafas, 2003). Compared to UFH/LMWH, fondaparinux is highly selective for anti-Xa activity vs. anti-IIa activity. Fondaparinux also has a longer half-life and higher bioavailability, although the synthesis is tedious, and an antidote is not available. In preclinical models, fondaparinux was shown to reduce E. coli-induced coagulopathy and inflammation in baboons (Keshari et al., 2020). In addition, fondaparinux mitigates white blood cell exhaustion and endothelial cell damage in LPS-challenged rats. However, it was less effective than LMWH (enoxaparin), suggesting that the size of fondaparinux is too short for the anti-inflammatory activity (Iba et al., 2012a). Non-anticoagulant fondaparinux was generated by oxidation of fondaparinux and shown to reduce inflammation in the kidney ischemia-reperfusion murine model but has not yet been tested in the sepsis model (Frank et al., 2006). Overall, fewer studies discuss the protective effect of fondaparinux in sepsis in preclinical and clinical settings. Based on current studies, fondaparinux’s anti-inflammatory activity seems less effective than UFH/LMWH. The difference is reasonable, as dysregulated protein targets such as HMGB1 require longer HSs and higher sulfation degree for binding and neutralization; fondaparinux is too short to bind to all inflammatory targets as UFH/LMWH (Liao et al., 2023).
Our group applies chemoenzymatic synthesis to produce structurally homogenous heparinoids. Using HS biosynthetic enzymes and sulfo donors in specific orders allows us to produce structurally defined HS oligosaccharides in various lengths and sulfation patterns. This method results in high purity and requires fewer synthetic steps than chemical synthesis (Liu and Linhardt, 2014). The chemoenzymatic synthesized HSs have high bioavailability and can also be selective to inflammation or coagulation targets with reversibility by protamine (Xu et al., 2017). More than a hundred synthetic HSs have been produced by chemoenzymatic synthesis, with or without anticoagulant activity, and used to elucidate the structure-function relationship between HSs and target proteins (Arnold et al., 2020a). In our previous studies, we used a synthetic HS library to investigate the binding requirement of HMGB1, showing that the anti-HMGB1 effect of HSs requires at least 18 saccharides or highly sulfated dodecassacharides (Arnold et al., 2020b; Arnold et al., 2020c). In a recent study, we demonstrate that a non-anticoagulant octadecasaccharide (18-mer) can be used as a multi-target therapy in LPS and CLP sepsis murine models by modulating pro-inflammatory extracellular H3, HMGB1, and anti-inflammatory ApoA-I (Figure 3) (Liao et al., 2023). Such structurally defined HS oligosaccharides may allow researchers to optimize carbohydrate-based therapy in sepsis by only choosing HS with the desired length and sulfation patterns. Proceeding to clinical trials for sepsis with synthetic heparin is recommended to further advance promising HS-based drug candidates.
Protecting the glycocalyx layer has been proposed as a potential anti-sepsis strategy (Iba and Levy, 2019; Uchimido et al., 2019). Heparins and their derivatives are shown as potential heparanase inhibitors (Vlodavsky et al., 2007; Ritchie et al., 2011). For example, SST0001 (Roneparstat), a non-anticoagulant heparin with N-acetylation and glycol split, has been tested in phase I clinical trial as heparanase inhibitors for multiple myeloma (Figure 5F) (Galli et al., 2018). Previously, we showed that heparanase could cleave the linkages between GlcA-GlcNS6S, GlcA-GlcNS3S, or GlcA2S-GlcNS, specifically reactive to GlcA-GlcNS6S in HSs (Peterson and Liu, 2010; Peterson and Liu, 2012). Thus, the HS sequences without the above disaccharides are probably resistant to heparanase and act as its competitive inhibitors.
Sulodexide, a highly purified portion from porcine intestinal mucosa, was reported as a heparanase inhibitor and is resistant to heparanase degradation. Sulodexide is comprised of HSs with iduronic acids and dermatan sulfate, which is not cleavable by heparanase. In a feces-induced peritonitis (FIP) model, sulodexide significantly improved survival in septic mice, facilitating glycocalyx restoration and reducing vascular permeability (Song et al., 2017). Our recent study using a largely heparanase-resistant synthetic octadecasaccharide also showed a renoprotective and anti-inflammatory effect (Liao et al., 2023). While the inhibition of heparanase by this octadecasaccharide is expected, further investigations are required to determine the interaction with heparanase in vivo.
Protein-based heparanase inhibitors have also been developed recently. A synthetic peptide 19-2.5, a peptide that was originally designed for anti-LPS treatment, was shown to reduce heparanase amount and activity in multiple organs, resulting in lessening circulating HS fragments in septic mice (Martin et al., 2015a). Heparanase-2 (HPSE2), an isoform of heparanase, is also identified as an endogenous heparanase inhibitor. In CLP-induced septic mice, HPSE2 was reported to reduce in plasma and kidney medullary capillaries. The reduction may indicate the loss of host regulation heparanase, as HPSE2 reduces HS shedding and LPS-induced TLR-4 signaling associated with heparanase activity (Kiyan et al., 2019). In addition, other drug modalities, such as neutralizing antibodies and siRNA, have also been proposed, further diversifying the choice of heparanase inhibitors (Vlodavsky et al., 2007). As each has different pharmacokinetics and metabolism pathways, multiple heparanase inhibitors available in clinics are ideal for clinicians to provide individualized treatment for septic patients. Although there are no approved heparanase inhibitors yet, the promising preclinical results from these drug candidates are expected to proceed further.
Significantly increased HS fragments in serum have been repeatedly observed in septic patients, which indicates glycocalyx degradation and thus is proposed as a potential diagnostic marker (Martin et al., 2015b; Hippensteel et al., 2019; Uchimido et al., 2019). The elevation of circulating HS fragments can be detected as soon as the diagnosis of sepsis (at day 0) and last to at least 7 days, although the temporal change along sepsis recovery or progression remains to be determined. Whether these detected HS fragments are beneficial or detrimental is controversial. HS shedding is likely a balancing act; an inductive host defense mechanism in certain circumstances but harmful if out of control. Specifically, overwhelming circulating HS fragments may stimulate inflammation when treating healthy cells in vitro. Yet, they can act as a neutralizer and exert a cell-protective effect in the presence of dysregulated HSBPs, such as histones or the LPS/HMGB1 complex. Appropriate and timely HS shedding in septic patients is potentially beneficial by neutralizing pro-inflammatory chemokines, cytokines, and DAMPs.
HBP draws clinical interest for its correlation to sepsis severity, with seven completed clinical trials regarding its role as a biomarker for predicting sepsis prognosis, facilitating early diagnosis, and validating severe sepsis since 2015 (ClinicalTrial.gov, retrieved on 1/1/23). HBP encompasses multiple biomarker-like characteristics, including rapid released by neutrophils in response to infection, high sensitive and specific to sepsis in the emergency departments, correlated to sepsis-induced lung and kidney dysfunction, and has been validated by various independent research groups in different countries (Fisher and Linder, 2017). Based on available results from clinical trials, HBP is significantly increased in septic patients, especially those with organ dysfunction (Kahn et al., 2019). However, HBP does not perform better than other proposed sepsis biomarkers, such as procalcitonin (PCT) and C-reactive protein (CRP) in the trial. In another study, HBP levels were dramatically elevated in septic patients but failed to be used as a single biomarker to predict 28-day sepsis survival (Katsaros et al., 2022). While HBP is promising as a diagnostic tool for early diagnosis of sepsis, further investigation is needed to elucidate its role and correlation to severe sepsis. Ideally, a biomarker corresponding to sepsis resolution and progression will greatly benefit disease management.
HSBPs, such as HMGB1 and histones, have been recognized as crucial modulators for inflammation and coagulation in sepsis. UFH/LMWH have been shown to be effective against sepsis by neutralizing dysregulated HSBPs, yet the mechanism is not fully understood. The efficacy has not been optimized due to its heterogeneous structure. Synthetic HSs with specific length and sulfation patterns are available recently, used as probes to elucidate mechanisms of HSBPs in sepsis and drug candidates to protect against sepsis-induced inflammation. Further investigations with homogenous HSs are expected to provide valuable insights into HS-based therapy. This may allow researchers to develop HS therapeutics with high specificity to various HSBPs and allow clinicians to optimize combined treatments for individuals based on the mechanisms of each drug. Ideally, developing HS-related biomarkers, such as HBP and circulating HS fragments, will significantly facilitate sepsis management by monitoring HS changes in sepsis, identifying the most benefitted subgroup for HS therapy, and evaluating the efficacy of HS-based therapies. Such combinations of HS-based therapies and diagnosis tools are promising to allow us to combat untreatable sepsis.
Y-EL, JL, and KA conceived the original conception and design of manuscript. Y-EL and KA wrote the manuscript. Y-EL generated the figures and tables, revised by JL and KA. All authors reviewed and approved the final manuscript.
This work was supported by R43GM144019. Y-EL is a recipient of predoctoral fellowship from the Government Scholarship to Study Abroad (GSSA) from Taiwan.
Figures were created with Biorender.com.
JL is a founder and chief scientific officer for Glycan Therapeutics. KA is a principal scientist at Glycan Therapeutics. The JL lab at UNC has received a gift from Glycan Therapeutics to support research in glycoscience. Y-EL declare no competing interests.
All claims expressed in this article are solely those of the authors and do not necessarily represent those of their affiliated organizations, or those of the publisher, the editors and the reviewers. Any product that may be evaluated in this article, or claim that may be made by its manufacturer, is not guaranteed or endorsed by the publisher.
Abraham, E., Reinhart, K., Opal, S., Demeyer, I., Doig, C., Rodriguez, A. L., et al. (2003). Efficacy and safety of tifacogin (recombinant tissue factor pathway inhibitor) in severe sepsis: A randomized controlled trial. J. Am. Med. Assoc. 290 (2), 238–247. doi:10.1001/jama.290.2.238
Abrams, S. T., Zhang, N., Manson, J., Liu, T., Dart, C., Baluwa, F., et al. (2013). Circulating histones are mediators of trauma-associated lung injury. Am. J. Respir. Crit. Care. Med. 187 (2), 160–169. doi:10.1164/rccm.201206-1037OC
Allam, R., Scherbaum, C. R., Darisipudi, M. N., Mulay, S. R., Hägele, H., Lichtnekert, J., et al. (2012). Histones from dying renal cells aggravate kidney injury via TLR2 and TLR4. J. Am. Soc. Nephrol. 23 (8), 1375–1388. doi:10.1681/ASN.2011111077
Ammollo, C. T., Semeraro, F., Xu, J., Esmon, N., and Esmon, C. (2011). Extracellular histones increase plasma thrombin generation by impairing thrombomodulin-dependent protein C activation. J. Thromb. Haemost. 9 (9), 1795–1803. doi:10.1111/j.1538-7836.2011.04422.x
Andersson, U., Yang, H., and Harris, H. (2018). Extracellular HMGB1 as a therapeutic target in inflammatory diseases. Expert Opin. Ther.Targets 22 (3), 263–277. doi:10.1080/14728222.2018.1439924
Arnold, K., Liao, Y.-E., and Liu, J. (2020a). Potential use of anti-inflammatory synthetic heparan sulfate to attenuate liver damage. Biomedicines 8 (11), 503. doi:10.3390/biomedicines8110503
Arnold, K., Xu, Y., Liao, Y.-E., Cooley, B. C., Pawlinski, R., and Liu, J. (2020b). Synthetic anticoagulant heparan sulfate attenuates liver ischemia reperfusion injury. Sci. Rep. 10 (1), 17187–17210. doi:10.1038/s41598-020-74275-7
Arnold, K., Xu, Y., Sparkenbaugh, E. M., Li, M., Han, X., Zhang, X., et al. (2020c). Design of anti-inflammatory heparan sulfate to protect against acetaminophen-induced acute liver failure. Sci. Transl. Med. 12 (535), eaav8075. doi:10.1126/scitranslmed.aav8075
Axelsson, J., Xu, D., Na Kang, B., Nussbacher, J. K., Handel, T. M., Ley, K., et al. (2012). Inactivation of heparan sulfate 2-O-sulfotransferase accentuates neutrophil infiltration during acute inflammation in mice. Blood 120 (8), 1742–1751. doi:10.1182/blood-2012-03-417139
Bentzer, P., Fisher, J., Kong, H. J., Mörgelin, M., Boyd, J. H., Walley, K. R., et al. (2016). Heparin-binding protein is important for vascular leak in sepsis. Intensive Care Med. Exp. 4 (1), 33–16. doi:10.1186/s40635-016-0104-3
Chapman, E. A., Lyon, M., Simpson, D., Mason, D., Beynon, R. J., Moots, R. J., et al. (2019). Caught in a trap? Proteomic analysis of neutrophil extracellular traps in rheumatoid arthritis and systemic lupus erythematosus. Front. Immunol. 423, 423. doi:10.3389/fimmu.2019.00423
Chen, R., Kang, R., Fan, X., and Tang, D. (2014). Release and activity of histone in diseases. Cell Death Dis. 5 (8), e1370. doi:10.1038/cddis.2014.337
Chen, V. C., Chao, L., Pimenta, D. C., Bledsoe, G., Juliano, L., and Chao, J. (2001). Identification of a major heparin-binding site in kallistatin. J. Biol. Chem. 276 (2), 1276–1284. doi:10.1074/jbc.M005791200
Chuang, K. I., Leung, B., Hsu, N., and Harris, H. W. (2011). Heparin protects against septic mortality via apoE-antagonism. Am. J. Surg. 202 (3), 325–335. doi:10.1016/j.amjsurg.2010.10.017
De Stoppelaar, S., Van't Veer, C., Roelofs, J., Claushuis, T., De Boer, O., Tanck, M., et al. (2015). Platelet and endothelial cell P-selectin are required for host defense against Klebsiella pneumoniae-induced pneumosepsis. J. Thromb. Haemost. 13 (6), 1128–1138. doi:10.1111/jth.12893
de Vries, J. C., Barendrecht, A. D., Clark, C. C., Urbanus, R. T., Boross, P., de Maat, S., et al. (2019). Heparin forms polymers with cell-free DnA Which elongate under Shear in flowing Blood. Sci. Rep. 9 (1), 18316–18411. doi:10.1038/s41598-019-54818-3
Deng, M., Tang, Y., Li, W., Wang, X., Zhang, R., Zhang, X., et al. (2018). The endotoxin delivery protein HMGB1 mediates caspase-11-dependent lethality in sepsis. Immunity 49 (4), 740–753. doi:10.1016/j.immuni.2018.08.016
Deng, Y., Foley, E. M., Gonzales, J. C., Gordts, P. L., Li, Y., and Esko, J. D. (2012). Shedding of syndecan-1 from human hepatocytes alters very low density lipoprotein clearance. Hepatology 55 (1), 277–286. doi:10.1002/hep.24626
Denning, N.-L., Aziz, M., Gurien, S. D., and Wang, P. (2019). DAMPs and NETs in sepsis. Front. Immunol. 10, 2536. doi:10.3389/fimmu.2019.02536
Fan, Y., Jiang, M., Gong, D., and Zou, C. (2016). Efficacy and safety of low-molecular-weight heparin in patients with sepsis: A meta-analysis of randomized controlled trials. Sci. Rep. 6 (1), 25984–25988. doi:10.1038/srep25984
Farrugia, B. L., Lord, M. S., Melrose, J., and Whitelock, J. M. (2018). The role of heparan sulfate in inflammation, and the development of biomimetics as anti-inflammatory strategies. J. Histochem. Cytochem. 66 (4), 321–336. doi:10.1369/0022155417740881
Felici, N., Liu, D., Maret, J., Restrepo, M., Borovskiy, Y., Hajj, J., et al. (2021). Long-term abnormalities of lipid profile after a single episode of sepsis. Front. Cardiovasc. Med. 8, 674248. doi:10.3389/fcvm.2021.674248
Fisher, J., and Linder, A. (2017). Heparin-binding protein: A key player in the pathophysiology of organ dysfunction in sepsis. J. Intern. Med. 281 (6), 562–574. doi:10.1111/joim.12604
Fisher, J., Russell, J. A., Bentzer, P., Parsons, D., Secchia, S., Mörgelin, M., et al. (2017). Heparin-binding protein (HBP): A causative marker and potential target for heparin treatment of human sepsis-induced acute kidney injury. Shock 48 (3), 313–320. doi:10.1097/SHK.0000000000000862
Fiuza, C., Bustin, M., Talwar, S., Tropea, M., Gerstenberger, E., Shelhamer, J. H., et al. (2003). Inflammation-promoting activity of HMGB1 on human microvascular endothelial cells. Blood 101 (7), 2652–2660. doi:10.1182/blood-2002-05-1300
Frank, R. D., Holscher, T., Schabbauer, G., Tencati, M., Pawlinski, R., Weitz, J. I., et al. (2006). A non-anticoagulant synthetic pentasaccharide reduces inflammation in a murine model of kidney ischemia-reperfusion injury. J. Thromb. Haemost. 96 (12), 802–806. doi:10.1160/th06-07-0418
Fu, S., Yu, S., Wang, L., Ma, X., and Li, X. (2022). Unfractionated heparin improves the clinical efficacy in adult sepsis patients: A systematic review and meta-analysis. BMC Anesthesiol. 22 (1), 28–12. doi:10.1186/s12871-021-01545-w
Galli, M., Chatterjee, M., Grasso, M., Specchia, G., Magen, H., Einsele, H., et al. (2018). Phase I study of the heparanase inhibitor roneparstat: An innovative approach for ultiple myeloma therapy. Haematologica 103 (10), e469–e472. doi:10.3324/haematol.2017.182865
Garsen, M., Rops, A. L., Li, J., Van Beneden, K., Van Den Branden, C., Berden, J. H., et al. (2016). Endothelial nitric oxide synthase prevents heparanase induction and the development of proteinuria. PLOS One 11 (8), e0160894. doi:10.1371/journal.pone.0160894
Gomes, R. N., Teixeira-Cunha, M. G., Figueiredo, R. T., Almeida, P. E., Alves, S. C., Bozza, P. T., et al. (2013). Bacterial clearance in septic mice is modulated by MCP-1/CCL2 and nitric oxide. Shock 39 (1), 63–69. doi:10.1097/SHK.0b013e31827802b5
Gong, T., Liu, L., Jiang, W., and Zhou, R. (2020). DAMP-sensing receptors in sterile inflammation and inflammatory diseases. Nat. Rev. Immunol. 20 (2), 95–112. doi:10.1038/s41577-019-0215-7
Goodall, K. J., Poon, I. K., Phipps, S., and Hulett, M. D. (2014). Soluble heparan sulfate fragments generated by heparanase trigger the release of pro-inflammatory cytokines through TLR-4. PLOS One 9 (10), e109596. doi:10.1371/journal.pone.0109596
Hayashida, K., Parks, W. C., and Park, P. W. (2009). Syndecan-1 shedding facilitates the resolution of neutrophilic inflammation by removing sequestered CXC chemokines. Blood 114 (14), 3033–3043. doi:10.1182/blood-2009-02-204966
He, Q., You, H., Li, X.-M., Liu, T.-H., Wang, P., and Wang, B.-E. (2012). HMGB1 promotes the synthesis of pro-IL-1β and pro-IL-18 by activation of p38 MAPK and NF-κB through receptors for advanced glycation end-products in macrophages. Asian pac. J. Cancer Prev. 13 (4), 1365–1370. doi:10.7314/apjcp.2012.13.4.1365
Heinzelmann, M., and Bosshart, H. (2004). Fondaparinux sodium lacks immunomodulatory effects of heparin. Am. J. Surg. 187 (1), 111–113. doi:10.1016/j.amjsurg.2003.03.005
Heinzelmann, M., and Bosshart, H. (2005). Heparin binds to lipopolysaccharide (LPS)-binding protein, facilitates the transfer of LPS to CD14, and enhances LPS-induced activation of peripheral blood monocytes. J. Immunol. 174 (4), 2280–2287. doi:10.4049/jimmunol.174.4.2280
Hippensteel, J. A., Anderson, B. J., Orfila, J. E., McMurtry, S. A., Dietz, R. M., Su, G., et al. (2019). Circulating heparan sulfate fragments mediate septic cognitive dysfunction. J. Clin. Investig. 129 (4), 1779–1784. doi:10.1172/JCI124485
Hogwood, J., Pitchford, S., Mulloy, B., Page, C., and Gray, E. (2020). Heparin and non-anticoagulant heparin attenuate histone-induced inflammatory responses in whole blood. PLOS One 15 (5), e0233644. doi:10.1371/journal.pone.0233644
Huang, W., Liu, Y., Li, L., Zhang, R., Liu, W., Wu, J., et al. (2012). HMGB1 increases permeability of the endothelial cell monolayer via RAGE and Src family tyrosine kinase pathways. Inflammation 35 (1), 350–362. doi:10.1007/s10753-011-9325-5
Huebener, P., Pradere, J.-P., Hernandez, C., Gwak, G.-Y., Caviglia, J. M., Mu, X., et al. (2019). The HMGB1/RAGE axis triggers neutrophil-mediated injury amplification following necrosis. J. Clin. Investig. 125 (2), 539–550. doi:10.1172/JCI76887
Hwaiz, R., Rahman, M., Syk, I., Zhang, E., and Thorlacius, H. (2015). Rac1-dependent secretion of platelet-derived CCL5 regulates neutrophil recruitment via activation of alveolar macrophages in septic lung injury. J. Leukoc. Biol. 97 (5), 975–984. doi:10.1189/jlb.4A1214-603R
Iba, T., Hashiguchi, N., Nagaoka, I., Tabe, Y., Kadota, K., and Sato, K. (2015). Heparins attenuated histone-mediated cytotoxicity in vitro and improved the survival in a rat model of histone-induced organ dysfunction. Intensive Care Med. Exp. 3 (1), 36–11. doi:10.1186/s40635-015-0072-z
Iba, T., and Levy, J. (2019). Derangement of the endothelial glycocalyx in sepsis. J. Thromb. Haemost. 17 (2), 283–294. doi:10.1111/jth.14371
Iba, T., Okamoto, K., Ohike, T., Tajirika, T., Aihara, K., Watanabe, S., et al. (2012a). Enoxaparin and fondaparinux attenuates endothelial damage in endotoxemic rats. J. Trauma Acute Care Surg. 72 (1), 177–182. doi:10.1097/TA.0b013e31821a83f0
Iba, T., Saito, D., Wada, H., and Asakura, H. (2012b). Efficacy and bleeding risk of antithrombin supplementation in septic disseminated intravascular coagulation: A prospective multicenter survey. Thromb. Res. 130 (3), e129–e133. doi:10.1016/j.thromres.2012.03.021
Ihrcke, N. S., Wrenshall, L. E., Lindman, B. J., and Platt, J. L. (1993). Role of heparan sulfate in immune system-blood vessel interactions. Immunol. Today 14 (10), 500–505. doi:10.1016/0167-5699(93)90265-M
Ishikawa, M., Yamashita, H., Oka, N., Ueda, T., Kohama, K., Nakao, A., et al. (2017). Antithrombin III improved neutrophil extracellular traps in lung after the onset of endotoxemia. J. Surg. Res. 208, 140–150. doi:10.1016/j.jss.2016.09.041
Ivanov, S., Dragoi, A.-M., Wang, X., Dallacosta, C., Louten, J., Musco, G., et al. (2007). A novel role for HMGB1 in TLR9-mediated inflammatory responses to CpG-DNA. Blood 110 (6), 1970–1981. doi:10.1182/blood-2006-09-044776
Jaimes, F., De La Rosa, G., Morales, C., Fortich, F., Arango, C., Aguirre, D., et al. (2009). Unfractioned heparin for treatment of sepsis: A randomized clinical trial (the HETRASE study). Crit. Care Med. 37 (4), 1185–1196. doi:10.1097/CCM.0b013e31819c06bc
Jayaraman, S., Pérez, A., Miñambres, I., Sánchez-Quesada, J. L., and Gursky, O. (2022). Heparin binding triggers human VLDL remodeling by circulating lipoprotein lipase: Relevance to VLDL functionality in health and disease. Biochim. Biophys. Acta Mol. Cell Biol. Lipids 1867 (1), 159064. doi:10.1016/j.bbalip.2021.159064
Jayson, G. C., Hansen, S. U., Miller, G. J., Cole, C. L., Rushton, G., Avizienyte, E., et al. (2015). Synthetic heparan sulfate dodecasaccharides reveal single sulfation site interconverts CXCL8 and CXCL12 chemokine biology. Chem. Commun. 51 (72), 13846–13849. doi:10.1039/c5cc05222j
Jin, L., Batra, S., Douda, D. N., Palaniyar, N., and Jeyaseelan, S. (2014). CXCL1 contributes to host defense in polymicrobial sepsis via modulating T cell and neutrophil functions. J. Immunol. 193 (7), 3549–3558. doi:10.4049/jimmunol.1401138
Jing, F., Li, M., Ren, H., Zhang, J., Yao, Q., Chu, Y., et al. (2016). Effects of atorvastatin combined with low-molecular-weight heparin on plasma inflammatory cytokine level and pulmonary pathophysiology of rats with sepsis. Exp. Ther. Med. 12 (2), 1048–1054. doi:10.3892/etm.2016.3372
Joseph, P. R. B., Mosier, P. D., Desai, U. R., and Rajarathnam, K. (2015). Solution NMR characterization of chemokine CXCL8/IL-8 monomer and dimer binding to glycosaminoglycans: Structural plasticity mediates differential binding interactions. Biochem. J. 472 (1), 121–133. doi:10.1042/BJ20150059
Kahn, F., Tverring, J., Mellhammar, L., Wetterberg, N., Bläckberg, A., Studahl, E., et al. (2019). Heparin-binding protein as a prognostic biomarker of sepsis and disease severity at the emergency department. Shock 52 (6), e135–e145. doi:10.1097/SHK.0000000000001332
Kalbitz, M., Grailer, J. J., Fattahi, F., Jajou, L., Herron, T. J., Campbell, K. F., et al. (2015). Role of extracellular histones in the cardiomyopathy of sepsis. FASEB J. 29 (5), 2185–2193. doi:10.1096/fj.14-268730
Kang, R., Chen, R., Zhang, Q., Hou, W., Wu, S., Cao, L., et al. (2014). HMGB1 in health and disease. Mol. Asp. Med. 40, 1–116. doi:10.1016/j.mam.2014.05.001
Karakike, E., Adami, M.-E., Lada, M., Gkavogianni, T., Koutelidakis, I. M., Bauer, M., et al. (2019). Late peaks of HMGB1 and sepsis outcome: Evidence for synergy with chronic inflammatory disorders. Shock 52 (3), 334–339. doi:10.1097/SHK.0000000000001265
Karlsson, S., Pettilä, V., Tenhunen, J., Laru-Sompa, R., Hynninen, M., and Ruokonen, E. (2008). HMGB1 as a predictor of organ dysfunction and outcome in patients with severe sepsis. Intensive Care Med. 34 (6), 1046–1053. doi:10.1007/s00134-008-1032-9
Katsaros, K., Renieris, G., Safarika, A., Adami, E.-M., Gkavogianni, T., Giannikopoulos, G., et al. (2022). Heparin binding protein for the early diagnosis and prognosis of sepsis in the emergency department: The prompt multicenter study. Shock 57 (4), 518–525. doi:10.1097/SHK.0000000000001900
Kattan, O. M., Kasravi, F. B., Elford, E. L., Schell, M. T., and Harris, H. W. (2008). Apolipoprotein E-mediated immune regulation in sepsis. J. Immunol. 181 (2), 1399–1408. doi:10.4049/jimmunol.181.2.1399
Keshari, R. S., Silasi, R., Popescu, N. I., Georgescu, C., Chaaban, H., Lupu, C., et al. (2020). Fondaparinux pentasaccharide reduces sepsis coagulopathy and promotes survival in the baboon model of Escherichia coli sepsis. J. Thromb. Haemost. 18 (1), 180–190. doi:10.1111/jth.14642
Kiyan, Y., Tkachuk, S., Kurselis, K., Shushakova, N., Stahl, K., Dawodu, D., et al. (2019). Heparanase-2 protects from LPS-mediated endothelial injury by inhibiting TLR4 signalling. Sci. Rep. 9 (1), 13591. doi:10.1038/s41598-019-50068-5
Koopmann, W., Ediriwickrema, C., and Krangel, M. S. (1999). Structure and function of the glycosaminoglycan binding site of chemokine macrophage-inflammatory protein-1β. J. Immunol. 163 (4), 2120–2127. doi:10.4049/jimmunol.163.4.2120
Kowalska, M. A., Zhao, G., Zhai, L., David, G., Marcus, S., Krishnaswamy, S., et al. (2014). Modulation of protein C activation by histones, platelet factor 4, and heparinoids: New insights into activated protein C formation. Arterioscler. Thromb. Vasc. Biol. 34 (1), 120–126. doi:10.1161/ATVBAHA.113.302236
Lau, E. K., Paavola, C. D., Johnson, Z., Gaudry, J.-P., Geretti, E., Borlat, F., et al. (2004). Identification of the glycosaminoglycan binding site of the CC chemokine, MCP-1: Implications for structure and function in vivo. J. Biol. Chem. 279 (21), 22294–22305. doi:10.1074/jbc.M311224200
Levi, M., Levy, M., Williams, M. D., Douglas, I., Artigas, A., Antonelli, M., et al. (2007). Prophylactic heparin in patients with severe sepsis treated with drotrecogin alfa (activated). Am. J. Respir. Crit. Care. Med. 176 (5), 483–490. doi:10.1164/rccm.200612-1803OC
Li, L., Ling, Y., Huang, M., Yin, T., Gou, S.-M., Zhan, N.-Y., et al. (2015). Heparin inhibits the inflammatory response induced by LPS and HMGB1 by blocking the binding of HMGB1 to the surface of macrophages. Cytokine 72 (1), 36–42. doi:10.1016/j.cyto.2014.12.010
Li, P., Bledsoe, G., Yang, Z. R., Fan, H., Chao, L., and Chao, J. (2014a). Human kallistatin administration reduces organ injury and improves survival in a mouse model of polymicrobial sepsis. Immunol 142 (2), 216–226. doi:10.1111/imm.12242
Li, X., and Ma, X. (2017). The role of heparin in sepsis: Much more than just an anticoagulant. Br. J. Haematol. 179 (3), 389–398. doi:10.1111/bjh.14885
Li, Y., Liu, Z., Liu, B., Zhao, T., Chong, W., Wang, Y., et al. (2014b). Citrullinated histone H3: A novel target for the treatment of sepsis. Surgery 156 (2), 229–234. doi:10.1016/j.surg.2014.04.009
Li, Y., Wan, D., Luo, X., Song, T., Wang, Y., Yu, Q., et al. (2021). Circulating histones in sepsis: Potential outcome predictors and therapeutic targets. Front. Immunol. 12, 650184. doi:10.3389/fimmu.2021.650184
Liang, W. G., Triandafillou, C. G., Huang, T.-Y., Zulueta, M. M. L., Banerjee, S., Dinner, A. R., et al. (2016). Structural basis for oligomerization and glycosaminoglycan binding of CCL5 and CCL3. Proc. Natl. Acad. Sci. U.S.A. 113 (18), 5000–5005. doi:10.1073/pnas.1523981113
Liao, Y. E., Xu, Y., Arnold, K., Zhang, F., Li, J., Sellers, R., et al. (2023). Using heparan sulfate octadecasaccharide (18-mer) as a multi-target agent to protect against sepsis. Proc. Natl. Acad. Sci. U.S.A. 120 (4), e2209528120. doi:10.1073/pnas.2209528120
Libeu, C. P., Lund-Katz, S., Phillips, M. C., Wehrli, S., Hernáiz, M. J., Capila, I., et al. (2001). New insights into the heparan sulfate proteoglycan-binding activity of apolipoprotein E. J. Biol. Chem. 276 (42), 39138–39144. doi:10.1074/jbc.M104746200
Lin, W.-C., Chen, C.-W., Chao, L., Chao, J., and Lin, Y.-S. (2017). Plasma kallistatin in critically ill patients with severe sepsis and septic shock. PLOS One 12 (5), e0178387. doi:10.1371/journal.pone.0178387
Lin, W.-C., Chen, C.-W., Huang, Y.-W., Chao, L., Chao, J., Lin, Y.-S., et al. (2015). Kallistatin protects against sepsis-related acute lung injury via inhibiting inflammation and apoptosis. Sci. Rep. 5 (1), 12463. doi:10.1038/srep12463
Ling, Y., Yang, Z.-Y., Yin, T., Li, L., Yuan, W.-W., Wu, H.-S., et al. (2011). Heparin changes the conformation of high-mobility group protein 1 and decreases its affinity toward receptor for advanced glycation endproducts in vitro. Int. Immunopharmacol. 11 (2), 187–193. doi:10.1016/j.intimp.2010.11.014
Liu, J., and Linhardt, R. J. (2014). Chemoenzymatic synthesis of heparan sulfate and heparin. Nat. Prod. Rep. 31 (12), 1676–1685. doi:10.1039/c4np00076e
Luan, Z.-G., Naranpurev, M., and Ma, X.-C. (2014). Treatment of low molecular weight heparin inhibits systemic inflammation and prevents endotoxin-induced acute lung injury in rats. Inflammation 37 (3), 924–932. doi:10.1007/s10753-014-9812-6
Luo, J., Kato, M., Wang, H., Bernfield, M., and Bischoff, J. (2001). Heparan sulfate and chondroitin sulfate proteoglycans inhibit E-selectin binding to endothelial cells. J. Cell. Biochem. 80 (4), 522–531. doi:10.1002/1097-4644(20010315)80:4<522:aid-jcb1006>3.0.co;2-h
Lygizos, M. I., Yang, Y., Altmann, C. J., Okamura, K., Hernando, A. A., Perez, M. J., et al. (2013). Heparanase mediates renal dysfunction during early sepsis in mice. Physiol. Rep. 1 (6), e00153. doi:10.1002/phy2.153
Martin, L., De Santis, R., Koczera, P., Simons, N., Haase, H., Heinbockel, L., et al. (2015a). The synthetic antimicrobial peptide 19-2.5 interacts with heparanase and heparan sulfate in murine and human sepsis. PLOS One 10 (11), e0143583. doi:10.1371/journal.pone.0143583
Martin, L., Peters, C., Schmitz, S., Moellmann, J., Martincuks, A., Heussen, N., et al. (2015b). Soluble heparan sulfate in serum of septic shock patients induces mitochondrial dysfunction in murine cardiomyocytes. Shock 44 (6), 569–577. doi:10.1097/SHK.0000000000000462
Matan, M., King, D., Peled, E., Ackerman, S., Bar-Levi, Y., et al. (2018). -Heparanase level and procoagulant activity are reduced in severe sepsis. Eur. J. Haematol. 100 (2), 182–188. doi:10.1111/ejh.12997
Matsumoto, H., Ogura, H., Shimizu, K., Ikeda, M., Hirose, T., Matsuura, H., et al. (2018). The clinical importance of a cytokine network in the acute phase of sepsis. Sci. Rep. 8 (1), 13995. doi:10.1038/s41598-018-32275-8
Mauri, L., Marinozzi, M., Phatak, N., Karfunkle, M., Ange, St.K., Guerrini, M., et al. (2019). 1D and 2D-HSQC NMR: Two methods to distinguish and characterize heparin from different animal and tissue sources. Front. Med. (Lausanne) 6, 142. doi:10.3389/fmed.2019.00142
Mauro, K. D., Lambert, M. P., Kowalska, M. A., Thawley, V. J., Poncz, M., and Otto, C. M. (2022). Dose escalation trial of desulfated heparin (ODSH) in septic peritonitis. Front. Vet. Sci. 9, 862308. doi:10.3389/fvets.2022.862308
McDonald, B., Davis, R. P., Kim, S.-J., Tse, M., Esmon, C. T., Kolaczkowska, E., et al. (2017). Platelets and neutrophil extracellular traps collaborate to promote intravascular coagulation during sepsis in mice. Blood 129 (10), 1357–1367. doi:10.1182/blood-2016-09-741298
Nagasawa, K., Inoue, Y., and Kamata, T. (1977). Solvolytic desulfation of glycosaminoglycuronan sulfates with dimethyl sulfoxide containing water or methanol. Carbohydr. Res. 58 (1), 47–55. doi:10.1016/s0008-6215(00)83402-3
Nowak, J. E., Wheeler, D. S., Harmon, K. K., and Wong, H. R. (2010). Admission chemokine (CC motif) ligand 4 levels predict survival in pediatric septic shock. Pediatr. Crit. Care Med. 11 (2), 213–216. doi:10.1097/PCC.0b013e3181b8076c
Ode, Y., Aziz, M., and Wang, P. (2018). CIRP increases ICAM-1+ phenotype of neutrophils exhibiting elevated iNOS and NETs in sepsis. J. Leukoc. Biol. 103 (4), 693–707. doi:10.1002/JLB.3A0817-327RR
Olivares-Silva, F., Landaeta, R., Aránguiz, P., Bolivar, S., Humeres, C., Anfossi, R., et al. (2018). Heparan sulfate potentiates leukocyte adhesion on cardiac fibroblast by enhancing Vcam-1 and Icam-1 expression. Biochim. Biophys. Acta Mol. Basis Dis. 1864 (3), 831–842. doi:10.1016/j.bbadis.2017.12.002
Opal, S. M., Scannon, P. J., Vincent, J.-L., White, M., Carroll, S. F., Palardy, J. E., et al. (1999). Relationship between plasma levels of lipopolysaccharide (LPS) and LPS-binding protein in patients with severe sepsis and septic shock. J. Infect. Dis. 180 (5), 1584–1589. doi:10.1086/315093
Oshima, K., King, S. I., McMurtry, S. A., and Schmidt, E. P. (2021). “Endothelial heparan sulfate proteoglycans in sepsis: The role of the glycocalyx,” in Seminars in thrombosis and hemostasis (Thieme Medical Publishers, Inc.), 274–282.
Pernerstorfer, T., Hollenstein, U., Hansen, J.-B., Knechtelsdorfer, M., Stohlawetz, P., Graninger, W., et al. (1999). Heparin blunts endotoxin-induced coagulation activation. Circulation 100 (25), 2485–2490. doi:10.1161/01.cir.100.25.2485
Peterson, S. B., and Liu, J. (2010). Unraveling the specificity of heparanase utilizing synthetic substrates. J. Biol. Chem. 285 (19), 14504–14513. doi:10.1074/jbc.M110.104166
Peterson, S., and Liu, J. (2012). Deciphering mode of action of heparanase using structurally defined oligosaccharides. J. Biol. Chem. 287 (41), 34836–34843. doi:10.1074/jbc.M112.390161
Poluri, K. M., Joseph, P. R. B., Sawant, K. V., and Rajarathnam, K. (2013). Molecular basis of glycosaminoglycan heparin binding to the chemokine CXCL1 dimer. J. Biol. Chem. 288 (35), 25143–25153. doi:10.1074/jbc.M113.492579
Proudfoot, A. E., Handel, T. M., Johnson, Z., Lau, E. K., LiWang, P., Clark-Lewis, I., et al. (2003). Glycosaminoglycan binding and oligomerization are essential for the in vivo activity of certain chemokines. Proc. Natl. Acad. Sci. U.S.A. 100 (4), 1885–1890. doi:10.1073/pnas.0334864100
Puerta-Guardo, H., Glasner, D. R., and Harris, E. (2016). Dengue virus NS1 disrupts the endothelial glycocalyx, leading to hyperpermeability. PLOS Path 12 (7), e1005738. doi:10.1371/journal.ppat.1005738
Qin, Y.-H., Dai, S.-M., Tang, G.-S., Zhang, J., Ren, D., Wang, Z.-W., et al. (2009). HMGB1 enhances the proinflammatory activity of lipopolysaccharide by promoting the phosphorylation of MAPK p38 through receptor for advanced glycation end products. J. Immunol. 183 (10), 6244–6250. doi:10.4049/jimmunol.0900390
Rao, N. V., Argyle, B., Xu, X., Reynolds, P. R., Walenga, J. M., Prechel, M., et al. (2010). Low anticoagulant heparin targets multiple sites of inflammation, suppresses heparin-induced thrombocytopenia, and inhibits interaction of RAGE with its ligands. Am. J. Physiol. Cell. Physiol. 299 (1), C97–C110. doi:10.1152/ajpcell.00009.2010
Rauvala, H., and Pihlaskari, R. (1987). Isolation and some characteristics of an adhesive factor of brain that enhances neurite outgrowth in central neurons. J. Biol. Chem. 262 (34), 16625–16635. doi:10.1016/s0021-9258(18)49302-7
Reitsma, S., Slaaf, D. W., Vink, H., Van Zandvoort, M. A., and oude Egbrink, M. G. A. (2007). The endothelial glycocalyx: Composition, functions, and visualization. Pflugers Arch. 454 (3), 345–359. doi:10.1007/s00424-007-0212-8
Ritchie, J. P., Ramani, V. C., Ren, Y., Naggi, A., Torri, G., Casu, B., et al. (2011). SST0001, a chemically modified heparin, inhibits myeloma growth and angiogenesis via disruption of the heparanase/syndecan-1 axis. Clin. Cancer Res. 17 (6), 1382–1393. doi:10.1158/1078-0432.CCR-10-2476
Roh, J. S., and Sohn, D. H. (2018). Damage-associated molecular patterns in inflammatory diseases. Immune Netw. 18 (4), e27. doi:10.4110/in.2018.18.e27
Salmivirta, M., Rauvala, H., Elenius, K., and Jalkanen, M. (1992). Neurite growth-promoting protein (amphoterin, p30) binds syndecan. Exp. Cell Biol. 200 (2), 444–451. doi:10.1016/0014-4827(92)90194-d
Samama, M.-M., and Gerotziafas, G. T. (2003). Evaluation of the pharmacological properties and clinical results of the synthetic pentasaccharide (fondaparinux). Thromb. Res. 109 (1), 1–11. doi:10.1016/s0049-3848(03)00030-6
Sarrazin, S., Lamanna, W. C., and Esko, J. D. (2011). Heparan sulfate proteoglycans. Cold Spring Harb. Perspect. Biol. 3 (7), a004952. doi:10.1101/cshperspect.a004952
Schlömmer, C., Brandtner, A., and Bachler, M. (2021). Antithrombin and its role in host defense and inflammation. Int. J. Mol. Sci. 22 (8), 4283. doi:10.3390/ijms22084283
Schmidt, E. P., Yang, Y., Janssen, W. J., Gandjeva, A., Perez, M. J., Barthel, L., et al. (2012). The pulmonary endothelial glycocalyx regulates neutrophil adhesion and lung injury during experimental sepsis. Nat. Med. 18 (8), 1217–1223. doi:10.1038/nm.2843
Seidelin, J. B., Nielsen, O. H., and Strøm, J. (2002). Soluble L-selectin levels predict survival in sepsis. Intensive Care Med. 28 (11), 1613–1618. doi:10.1007/s00134-002-1501-5
Semeraro, F., Ammollo, C. T., Morrissey, J. H., Dale, G. L., Friese, P., Esmon, N. L., et al. (2011). Extracellular histones promote thrombin generation through platelet-dependent mechanisms: Involvement of platelet TLR2 and TLR4. Blood 118 (7), 1952–1961. doi:10.1182/blood-2011-03-343061
Semeraro, N., Ammollo, C. T., Semeraro, F., and Colucci, M. (2012). Sepsis, thrombosis and organ dysfunction. Thromb. Res. 129 (3), 290–295. doi:10.1016/j.thromres.2011.10.013
Seo, Y., Andaya, A., Bleiholder, C., and Leary, J. A. (2013). Differentiation of CC vs CXC chemokine dimers with GAG octasaccharide binding partners: An ion mobility mass spectrometry approach. J. Am. Chem. Soc. 135 (11), 4325–4332. doi:10.1021/ja310915m
Shi, C., Wang, C., Wang, H., Yang, C., Cai, F., Zeng, F., et al. (2020). The potential of low molecular weight heparin to mitigate cytokine storm in severe COVID-19 patients: A retrospective cohort study. Clin.Transl. Sci. 13 (6), 1087–1095. doi:10.1111/cts.12880
Siddiqui, S. S., Dhar, C., Sundaramurthy, V., Sasmal, A., Yu, H., Bandala-Sanchez, E., et al. (2020). Acidosis, zinc and HMGB1 in sepsis: A common connection involving sialoglycan recognition. bioRxiv 118 (10), e2018090118. doi:10.1073/pnas.2018090118
Silk, E., Zhao, H., Weng, H., and Ma, D. (2017). The role of extracellular histone in organ injury. Cell Death Dis. 8 (5), e2812. doi:10.1038/cddis.2017.52
Simmons, J., and Pittet, J.-F. (2015). The coagulopathy of acute sepsis. Curr. Opin. Anaesthesiol. 28 (2), 227–236. doi:10.1097/ACO.0000000000000163
Simpson, B. W., and Trent, M. S. (2019). Pushing the envelope: LPS modifications and their consequences. Nat. Rev. Microbiol. 17 (7), 403–416. doi:10.1038/s41579-019-0201-x
Singer, M., Deutschman, C. S., Seymour, C. W., Shankar-Hari, M., Annane, D., Bauer, M., et al. (2016). The third international consensus definitions for sepsis and septic shock (Sepsis-3). J. Am. Med. Assoc. 315 (8), 801–810. doi:10.1001/jama.2016.0287
Singh, A., Kett, W. C., Severin, I. C., Agyekum, I., Duan, J., Amster, I. J., et al. (2015). The interaction of heparin tetrasaccharides with chemokine CCL5 is modulated by sulfation pattern and pH. J. Biol. Chem. 290 (25), 15421–15436. doi:10.1074/jbc.M115.655845
Song, J. W., Zullo, J. A., Liveris, D., Dragovich, M., Zhang, X. F., and Goligorsky, M. S. (2017). Therapeutic restoration of endothelial glycocalyx in sepsis. J. Pharmacol. Exp. Ther. 361 (1), 115–121. doi:10.1124/jpet.116.239509
Stasi, A., Intini, A., Divella, C., Franzin, R., Montemurno, E., Grandaliano, G., et al. (2017). Emerging role of Lipopolysaccharide binding protein in sepsis-induced acute kidney injury. Nephrol. Dial. Transpl. 32 (1), 24–31. doi:10.1093/ndt/gfw250
Tadie, J.-M., Bae, H.-B., Jiang, S., Park, D. W., Bell, C. P., Yang, H., et al. (2013). HMGB1 promotes neutrophil extracellular trap formation through interactions with Toll-like receptor 4. Am. J. Physiol. Lung. Cell. Mol. Physiol. 304 (5), L342–L349. doi:10.1152/ajplung.00151.2012
Tam, S.-P., Kisilevsky, R., and Ancsin, J. B. (2008). Acute-phase-HDL remodeling by heparan sulfate generates a novel lipoprotein with exceptional cholesterol efflux activity from macrophages. PLOS One 3 (12), e3867. doi:10.1371/journal.pone.0003867
Tang, H., Ivanciu, L., Popescu, N., Peer, G., Hack, E., Lupu, C., et al. (2007). Sepsis-induced coagulation in the baboon lung is associated with decreased tissue factor pathway inhibitor. Am. J. Clin. Pathol. 171 (3), 1066–1077. doi:10.2353/ajpath.2007.070104
Tang, Y., Wang, X., Li, Z., He, Z., Yang, X., Cheng, X., et al. (2021). Heparin prevents caspase-11-dependent septic lethality independent of anticoagulant properties. Immunity 54 (3), 454–467.e6. doi:10.1016/j.immuni.2021.01.007
Thålin, C., Aguilera, K., Hall, N. W., Marunde, M. R., Burg, J. M., Rosell, A., et al. (2020). Quantification of citrullinated histones: Development of an improved assay to reliably quantify nucleosomal H3Cit in human plasma. J. Thromb. Haemost. 18 (10), 2732–2743. doi:10.1111/jth.15003
Uchimido, R., Schmidt, E. P., and Shapiro, N. I. (2019). The glycocalyx: A novel diagnostic and therapeutic target in sepsis. Crit. Care 23 (1), 16–12. doi:10.1186/s13054-018-2292-6
V Kumar, A., K Katakam, S., Urbanowitz, A.-K., and Gotte, M. (2015). Heparan sulphate as a regulator of leukocyte recruitment in inflammation. Curr. Protein Pept. Sci. 16 (1), 77–86. doi:10.2174/1573402111666150213165054
Valentin, S., Larnkjoer, A., Østergaard, P., Nielsen, J. I., Nordfang, O., and Larnkjer, A. (1994). Characterization of the binding between tissue factor pathway inhibitor and glycosaminoglycans. Thromb. Res. 75 (2), 173–183. doi:10.1016/0049-3848(94)90066-3
van der Poll, T., van de Veerdonk, F. L., Scicluna, B. P., and Netea, M. G. (2017). The immunopathology of sepsis and potential therapeutic targets. Nat. Rev. Immunol. 17 (7), 407–420. doi:10.1038/nri.2017.36
Van Oosten, M., Rensen, P. C., Van Amersfoort, E. S., Van Eck, M., Van Dam, A.-M., Brevé, J. J., et al. (2001). Apolipoprotein E protects against bacterial lipopolysaccharide-induced lethality: A new therapeutic approach to treat gram-negative sepsis. J. Biol. Chem. 276 (12), 8820–8824. doi:10.1074/jbc.M009915200
Vlodavsky, I., Ilan, N., Naggi, A., and Casu, B. (2007). Heparanase: Structure, biological functions, and inhibition by heparin-derived mimetics of heparan sulfate. Curr. Pharm. Des. 13 (20), 2057–2073. doi:10.2174/138161207781039742
Vreugdenhil, A. C., Snoek, A. P., van‘t Veer, C., Greve, J.-W. M., and Buurman, W. A. (2001). LPS-binding protein circulates in association with apoB-containing lipoproteins and enhances endotoxin-LDL/VLDL interaction. J. Clin. Investig. 107 (2), 225–234. doi:10.1172/JCI10832
Wang, C., Chi, C., Guo, L., Wang, X., Guo, L., Sun, J., et al. (2014a). Heparin therapy reduces 28-day mortality in adult severe sepsis patients: A systematic review and meta-analysis. Crit. Care 18 (5), 563–569. doi:10.1186/s13054-014-0563-4
Wang, D., Sai, J., and Richmond, A. (2003). Cell surface heparan sulfate participates in CXCL1-induced signaling. Biochemistry 42 (4), 1071–1077. doi:10.1021/bi026425a
Wang, F., Zhang, N., Li, B., Liu, L., Ding, L., Wang, Y., et al. (2015). Heparin defends against the toxicity of circulating histones in sepsis. Front. Biosci. (Landmark Ed. 20 (8), 1259–1270. doi:10.2741/4370
Wang, H., Bloom, O., Zhang, M., Vishnubhakat, J. M., Ombrellino, M., Che, J., et al. (1999). HMG-1 as a late mediator of endotoxin lethality in mice. Science 285 (5425), 248–251. doi:10.1126/science.285.5425.248
Wang, H., Li, W., Goldstein, R., Tracey, K. J., and Sama, A. E. (2006). “"HMGB1 as a potential therapeutic target,” in Sepsis: New insights, new therapies: Novartis foundation symposium 280 (Wiley Online Library), 73–91.
Wang, L., Brown, J. R., Varki, A., and Esko, J. D. (2002). Heparin’s anti-inflammatory effects require glucosamine 6-O-sulfation and are mediated by blockade of L-and P-selectins. J. Clin. Investig. 110 (1), 127–136. doi:10.1172/JCI14996
Wang, L., Fuster, M., Sriramarao, P., and Esko, J. D. (2005). Endothelial heparan sulfate deficiency impairs L-selectin-and chemokine-mediated neutrophil trafficking during inflammatory responses. Nat. Immunol. 6 (9), 902–910. doi:10.1038/ni1233
Wang, L., Liu, Z., Dong, Z., Pan, J., and Ma, X. (2014b). Azurocidin-induced inhibition of oxygen metabolism in mitochondria is antagonized by heparin. Exp. Ther. Med. 8 (5), 1473–1478. doi:10.3892/etm.2014.1939
Wang, Z., Wang, L., Cao, C., Jin, H., Zhang, Y., Liu, Y., et al. (2020). Heparin attenuates histone-mediated cytotoxicity in septic acute kidney injury. Front. Med. (Lausanne) 7, 586652. doi:10.3389/fmed.2020.586652
Warren, B. L., Eid, A., Singer, P., Pillay, S. S., Carl, P., Novak, I., et al. (2001). Caring for the critically ill patient. High-Dose antithrombin III in severe sepsis: A randomized controlled trial. J. Am. Med. Assoc. 286 (15), 1869–1878. doi:10.1001/jama.286.15.1869
Wildhagen, K. C., García de Frutos, P., Reutelingsperger, C. P., Schrijver, R., Aresté, C., Ortega-Gómez, A., et al. (2014). Nonanticoagulant heparin prevents histone-mediated cytotoxicity in vitro and improves survival in sepsis. Blood 123 (7), 1098–1101. doi:10.1182/blood-2013-07-514984
Woodfin, A., Beyrau, M., Voisin, M.-B., Ma, B., Whiteford, J. R., Hordijk, P. L., et al. (2016). ICAM-1–expressing neutrophils exhibit enhanced effector functions in murine models of endotoxemia. Blood 127 (7), 898–907. doi:10.1182/blood-2015-08-664995
Xu, D., Arnold, K., and Liu, J. (2018). Using structurally defined oligosaccharides to understand the interactions between proteins and heparan sulfate. Curr. Opin. Struct. Biol. 50, 155–161. doi:10.1016/j.sbi.2018.04.003
Xu, D., Young, J. H., Krahn, J. M., Song, D., Corbett, K. D., Chazin, W. J., et al. (2013). Stable RAGE-heparan sulfate complexes are essential for signal transduction. ACS Chem. Biol. 8 (7), 1611–1620. doi:10.1021/cb4001553
Xu, D., Young, J., Song, D., and Esko, J. D. (2011). Heparan sulfate is essential for high mobility group protein 1 (HMGB1) signaling by the receptor for advanced glycation end products (RAGE). J. Biol. Chem. 286 (48), 41736–41744. doi:10.1074/jbc.M111.299685
Xu, J., Zhang, X., Pelayo, R., Monestier, M., Ammollo, C. T., Semeraro, F., et al. (2009). Extracellular histones are major mediators of death in sepsis. Nat. Med. 15 (11), 1318–1321. doi:10.1038/nm.2053
Xu, X., Takano, R., Nagai, Y., Yanagida, T., Kamei, K., Kato, H., et al. (2002). Effect of heparin chain length on the interaction with tissue factor pathway inhibitor (TFPI). Int. J. Biol. Macromol. 30 (3-4), 151–160. doi:10.1016/s0141-8130(02)00015-6
Xu, Y., Cai, C., Chandarajoti, K., Hsieh, P.-H., Li, L., Pham, T. Q., et al. (2014). Homogeneous low-molecular-weight heparins with reversible anticoagulant activity. Nat. Chem. Biol. 10 (4), 248–250. doi:10.1038/nchembio.1459
Xu, Y., Chandarajoti, K., Zhang, X., Pagadala, V., Dou, W., Hoppensteadt, D. M., et al. (2017). Synthetic oligosaccharides can replace animal-sourced low–molecular weight heparins. Sci. Transl. Med. 9 (406), eaan5954. doi:10.1126/scitranslmed.aan5954
Yamakawa, K., Umemura, Y., Hayakawa, M., Kudo, D., Sanui, M., Takahashi, H., et al. (2016). Benefit profile of anticoagulant therapy in sepsis: A nationwide multicentre registry in Japan. Crit. Care 20 (1), 229. doi:10.1186/s13054-016-1415-1
Yang, H., Hreggvidsdottir, H. S., Palmblad, K., Wang, H., Ochani, M., Li, J., et al. (2010). A critical cysteine is required for HMGB1 binding to Toll-like receptor 4 and activation of macrophage cytokine release. Proc. Natl. Acad. Sci. U.S.A. 107 (26), 11942–11947. doi:10.1073/pnas.1003893107
Yang, H., Ochani, M., Li, J., Qiang, X., Tanovic, M., Harris, H. E., et al. (2004). Reversing established sepsis with antagonists of endogenous high-mobility group box 1. Proc. Natl. Acad. Sci. U.S.A. 101 (1), 296–301. doi:10.1073/pnas.2434651100
Yang, X., Cheng, X., Tang, Y., Qiu, X., Wang, Y., Kang, H., et al. (2019). Bacterial endotoxin activates the coagulation cascade through gasdermin D-dependent phosphatidylserine exposure. Immunity 51 (6), 983–996. doi:10.1016/j.immuni.2019.11.005
Yang, Y., Haeger, S. M., Suflita, M. A., Zhang, F., Dailey, K. L., Colbert, J. F., et al. (2017). Fibroblast growth factor signaling mediates pulmonary endothelial glycocalyx reconstitution. Am. J. Respir. Cell Mol. Biol. 56 (6), 727–737. doi:10.1165/rcmb.2016-0338OC
Zarychanski, R., Abou-Setta, A. M., Kanji, S., Turgeon, A. F., Kumar, A., Houston, D. S., et al. (2015). Efficacy and safety of heparin in patients with sepsis: A systematic review and meta-analysis. Crit. Care 19 (1), P123–P201. doi:10.1186/cc14203
Zeng, Y. (2017). Endothelial glycocalyx as a critical signalling platform integrating the extracellular haemodynamic forces and chemical signalling. J. Cell Mol. Med. 21 (8), 1457–1462. doi:10.1111/jcmm.13081
Zhang, Y., Haeger, S. M., Yang, Y., Dailey, K. L., Ford, J. A., and Schmidt, E. P. (2017). Circulating heparan sulfate fragments attenuate histone-induced lung injury independently of histone binding. Shock 48 (6), 666–673. doi:10.1097/SHK.0000000000000907
Zheng, S., Pan, Y., Wang, C., Liu, Y., Shi, M., and Ding, G. (2016). HMGB1 turns renal tubular epithelial cells into inflammatory promoters by interacting with TLR4 during sepsis. J. Interferon Cytokine Res. 36 (1), 9–19. doi:10.1089/jir.2015.0067
Keywords: heparan sulfate, heparin, heparan sulfate binding proteins, HMGB1 (high mobility group box 1), histones
Citation: Liao Y-E, Liu J and Arnold K (2023) Heparan sulfates and heparan sulfate binding proteins in sepsis. Front. Mol. Biosci. 10:1146685. doi: 10.3389/fmolb.2023.1146685
Received: 17 January 2023; Accepted: 31 January 2023;
Published: 14 February 2023.
Edited by:
Kay Grobe, University of Münster, GermanyReviewed by:
Jing Zhao, China Agricultural University, ChinaCopyright © 2023 Liao, Liu and Arnold. This is an open-access article distributed under the terms of the Creative Commons Attribution License (CC BY). The use, distribution or reproduction in other forums is permitted, provided the original author(s) and the copyright owner(s) are credited and that the original publication in this journal is cited, in accordance with accepted academic practice. No use, distribution or reproduction is permitted which does not comply with these terms.
*Correspondence: Katelyn Arnold, YXJub2xkazJAZW1haWwudW5jLmVkdQ==
Disclaimer: All claims expressed in this article are solely those of the authors and do not necessarily represent those of their affiliated organizations, or those of the publisher, the editors and the reviewers. Any product that may be evaluated in this article or claim that may be made by its manufacturer is not guaranteed or endorsed by the publisher.
Research integrity at Frontiers
Learn more about the work of our research integrity team to safeguard the quality of each article we publish.