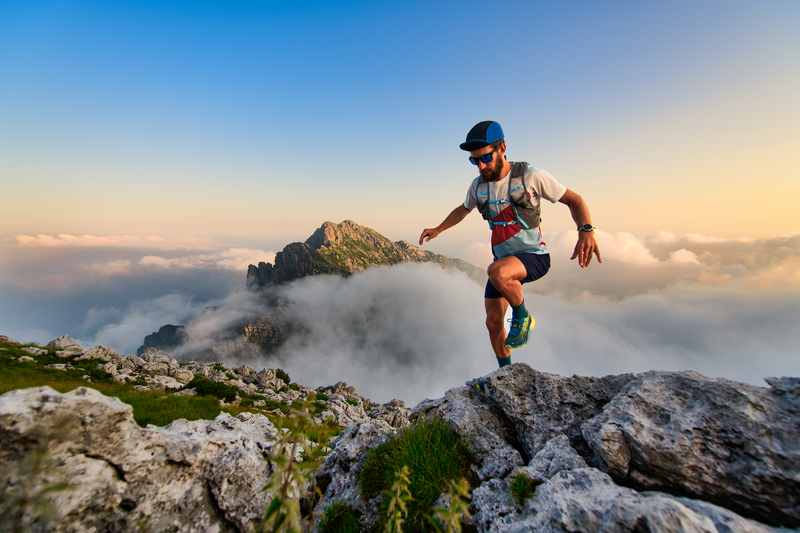
95% of researchers rate our articles as excellent or good
Learn more about the work of our research integrity team to safeguard the quality of each article we publish.
Find out more
REVIEW article
Front. Mol. Biosci. , 08 March 2023
Sec. Molecular Diagnostics and Therapeutics
Volume 10 - 2023 | https://doi.org/10.3389/fmolb.2023.1132353
This article is part of the Research Topic Biomechanics in Tissue Fibrosis: Molecular Mechanism & Potential Targets View all 6 articles
Skin fibrosis is a physiopathological process featuring the excessive deposition of extracellular matrix (ECM), which is the main architecture that provides structural support and constitutes the microenvironment for various cellular behaviors. Recently, increasing interest has been drawn to the relationship between the mechanical properties of the ECM and the initiation and modulation of skin fibrosis, with the engagement of a complex network of signaling pathways, the activation of mechanosensitive proteins, and changes in immunoregulation and metabolism. Simultaneous with the progression of skin fibrosis, the stiffness of ECM increases, which in turn perturbs mechanical and humoral homeostasis to drive cell fate toward an outcome that maintains and enhances the fibrosis process, thus forming a pro-fibrotic “positive feedback loop”. In this review, we highlighted the central role of the ECM and its dynamic changes at both the molecular and cellular levels in skin fibrosis. We paid special attention to signaling pathways regulated by mechanical cues in ECM remodeling. We also systematically summarized antifibrotic interventions targeting the ECM, hopefully enlightening new strategies for fibrotic diseases.
Dermal fibrosis is characterized by excessive deposition of ECM in the skin and belongs to a class of diseases caused by impaired tissue regeneration and faulty repair. It can occur in the normal repair of broken skin or as a significant hallmark in conditions such as systemic sclerosis (SSc) and keloids (Coentro et al., 2019). There are many diseases with this clinical manifestation of skin fibrosis. The etiology of these diseases varies and includes physical (radiation or mechanical stimulation), chemical, biological, and immune factors (Table 1; Figure 1). Numerous animal models have been developed and have been described in detail in Do NN’s review (Do and Eming, 2016).
FIGURE 1. Relationship between the etiology, regulation mechanisms and targeted therapy of skin fibrosis. With environmental or genetic pathogenic factors, fibroblasts are activated and secrete large amounts of extracellular matrix. This process is further enhanced by the matrix stiffness upregulation. The TGF-β signaling pathway acts as the center of this positve feedback. Targeted therapies to break this vicious circle is a promising pathway in skin fibrosis treatment.
Not only in the skin, but fibrosis can also occur in almost all organs, such as the lungs, liver, kidneys and heart. There is broad agreement between the pathological mechanisms of different fibrotic diseases, but there are still differences. Skin fibrosis shares many signaling pathways with other organ fibrosis in the molecular mechanism, such as transforming growth factor-β (TGF-β) signaling pathway and Hippo signaling pathway. However, the skin is on the surface of the human body and is susceptible to mechanical stresses from internal or external actions of the body. Therefore, the mechanical stress-related regulation of skin is a direction of interest. Compared to other tissues in the body, it is easier for people to artificially modify the mechanical microenvironment of the skin to inhibit skin fibrosis, which could provide a new insight for the development of therapies related to skin fibrosis.
Skin fibrosis is a public health issue for all humans, affecting more than 100 million people per year in developed countries, with a much higher prevalence in the worldwide population (Bayat et al., 2003). Skin fibrosis affects the normal physiological function of soft tissue and may also cause aesthetic problems and psychological distress (Bock et al., 2006). Despite the enormous market for anti-scar medications, estimated at over $12 billion annually in the United States (Sen et al., 2009), universally effective anti-scar treatment has been lacking thus far. How to reduce the occurrence of skin fibrosis, inhibit the progression of dermal and epidermal fibrotic diseases or even remove excess deposits of ECM in native tissues are significant concerns in the scientific and clinical fields. Elucidating the molecular mechanisms in the pathogenesis of skin fibrosis is crucial to developing therapeutic approaches.
The ECM is a collagen-based and well-organized dense meshwork of complex macromolecules containing proteins and polysaccharides secreted by resident cells such as fibroblasts. In addition to providing structural support for cells and tissues, the ECM has also been shown to significantly affect the proliferation, differentiation, and metabolism of parenchymal cells (Huang and Greenspan, 2012; Multhaupt et al., 2016; Tracy et al., 2016). There is clear evidence that ECM mechanical changes play vital roles in the occurrence and development of various kinds of skin fibrotic diseases, and increased ECM stiffness has been recognized as an important marker of fibrotic diseases (Hinz, 2009). Therefore, the mechanical properties of ECM have received increasing attention in the last decade. In the process of skin fibrosis, inflammatory cell infiltration, cytokine secretion, fibroblast proliferation and differentiation are the major biological events observed (Hsu et al., 2018). The combination of these events leads to ECM alterations in molecular composition and spatial structure, which is characterized as “ECM stiffness” from a macroscopic perspective. Altered ECM stiffness brings about changes in the extracellular mechanical microenvironment. The physical signals of matrix stiffening are sensed through receptors on the cell surface and further transduced intracellularly, triggering downstream signaling cascades and ultimately causing changes in transcription and posttranscription levels, leading to cellular metabolism and behavior alterations. Specifically, high ECM stiffness disrupts extracellular microenvironmental homeostasis, which is manifested by enhanced activation of mechanical signaling, increased levels of profibrotic cytokines, and consequently abnormally activated fibroblasts with promoted production of collagen fibers. Overall, this results in a positive feedback loop of “skin fibrosis–increased matrix stiffness–fibroblast activation–enhanced skin fibrosis” (Long et al., 2022). This loop accounts for the persistence and irreversibility of dermal fibrosis, making it a challenging and formidable task to inhibit the progression of skin fibrosis.
This review systematically summarizes the interrelationship between ECM stiffness and skin fibrosis, in order of histologic, cellular and molecular levels (Figure 1). We focused on the cellular and molecular mechanisms of the pathogenesis of skin fibrotic diseases mediated by ECM stiffness, especially mechanotransduction and regulation.
Mammalian skin mainly consists of three layers: the epidermis, the dermis, and the basement membrane (BM) that connects the epidermis and dermis (Fuchs and Raghavan, 2002; McGrath et al., 2004) (Figure 2A). The epidermis is composed of a keratinized stratified squamous epithelium that mainly contains keratinocytes in various developmental stages (McGrath et al., 2004; Rittié, 2016) (Figure 2A). Keratinocytes originate from the basal layer and become flattened dead corneocytes without a nucleus during maturation. During the maturation of keratinocytes, BM persistently produces new keratinocytes, pushing the old cells to the surface of the skin, which results in a stratum corneum with multiple layers of corneocytes on the surface of the skin. The main proteins expressed in different layers of the epidermis are also different (Bhattacharjee et al., 2019). Glycosaminoglycan (GAG) is the main component of epidermal ECM (Montagna et al., 1951), and includes hyaluronic acid (54%), heparan sulfate (33%) and chondroitin sulfate (13%) (Brown and Parkinson, 1983). In the stratum corneum, corneocytes act like bricks, while mixtures of extracellular lipids (ceramides, free fatty acids, and cholesterol) are similar to concrete, sticking the bricks together and conferring hydrophobic properties to the epidermis (Madison, 2003; McIntosh, 2003; Candi et al., 2005). The matrix components are highly organized, cross-linked, and closely integrated with keratinocytes. This matrix forms a barrier that resists mechanical stretching by external forces, reduces water loss from the body, and prevents invasion from pathogenic microorganisms (Cork, 1997; Holden et al., 2002; Baroni et al., 2012). Some studies found that initial stretching increased the proliferation of basal keratinocytes, leading to elongation of the basal layer and increased cellular density. The increased number of rete ridges suggests that they absorbed the impact of excessive proliferation, preserving the layered organization of the epidermis (Topczewska et al., 2019). In SSc, activated epidermal keratinocytes result in an increase in epidermal thickness (Nikitorowicz-Buniak et al., 2014; Russo et al., 2021). In atopic dermatitis, chronic skin injury causes hyperkeratosis of the epidermis in addition to dermal fibrosis (Lee et al., 2009). During the process of fibrosis, mechanical stimulation applied to the epidermis can be translocated to the dermis, activating fibroblasts by releasing cytokines such as connective tissue growth factor (CTGF or CNN2) and S100A9. This interaction demonstrates the integrity of skin structure and function (Nikitorowicz-Buniak et al., 2014).
FIGURE 2. Schematic of skin histology viewed in cross-section. Three layers of mammalian skin: epidermis, basement membrane and dermis. (A) The epidermis contains corneocytes on the surface and keratinocytes in various developmental stages. The ECM molecules include GAG, hyaluronic acid, heparan sulfate, chondroitin sulfate and various kinds of extracellular lipids. The dermis consists of blood vessels and nerves. Many kinds of cells, including fibroblasts and macrophages, participate in the process of fibrosis. (B) and (C) The basement membrane is composed of individual laminin and collagen type IV, which provide mechanical connections between the epidermis and dermis.
The epidermal BM is a reticular complex composed of individual laminin or collagen type IV (Uitto et al., 1989), which provides mechanical connections between the epidermis and dermis through two structurally independent networks consisting of laminin 332, collagen type IV and XVII and laminins with α5-chains (Behrens et al., 2012) (Figures 2B,C). The epidermal BM plays a crucial role in skin fibrosis. The information transduction of the epidermis and dermis in both directions relies on specific structures on the BM, such as adhesive patches and hemi-bridging granules. In pathological cases, the damaged epidermis can also release inflammatory factors and chemokines, which cause a local inflammatory response in the skin and dermal inflammatory cell infiltration in the area covered by the epidermis. Nikitorowicz-Buniak et al. (2014) found that the number and size of basal cells in BM significantly increased in SSc compared to healthy tissue.
The dermis is the inner layer of the skin, beneath the epidermis and BM. Compared with the epidermis, the dermis consists of blood vessels and nerves. The ECM is differentiated at different dermal levels and can be distinguished at the tissue level using histochemical staining, such as Herovici’s picropolychrome. Collagen, the most abundant protein in the dermal ECM, is secreted by fibroblasts and exists extracellularly as fibrin. The papillary dermis (PD) closest to the BM has a thin and loose arrangement of collagen fibers, which appear blue under Herovici’s picropolychrome staining; in the reticular dermis (RD), which is below the PD, collagen fibers are thicker and more densely arranged and stain purple (Watt and Fujiwara, 2011). The dermis is the main site of skin fibrosis. Using a combination of optical coherence tomography (OCT) and high-frequency ultrasound (HFUS) techniques, an increase in skin thickness during skin fibrosis was demonstrated (Ud-Din et al., 2019). Herovici staining demonstrated excessive collagen type III deposition in scar tissue and greater mechanical rigidity than normal tissue (Ud-Din et al., 2019). Elastin also undergoes significant changes during skin fibrosis, becoming broken in damaged skin, and is difficult to repair. Conversely, local tissue secretes more collagen, eventually leading to ECM stiffness (Wagenseil and Mecham, 2012). Furthermore, the molecular changes of the ECM during the fibrosis will be discussed later in Section 2.3. Interestingly, fibulin-5, an integrin-binding matricellular protein, can reduce tissue mechanical stiffness by promoting fiber assembly, as shown by the treatment of mice in a skin fibrosis model (Ikeda et al., 2009; Yanagisawa et al., 2009; Nakasaki et al., 2015). In normal tissue, the direction of collagen fiber is random (ultimately arranged in a mesh-like lattice under multiphoton microscopy) (Ueda et al., 2019).
The relationship between mechanical force and fibrosis was observed a long time ago. Although the detailed mechanism is unclear, antifibrotic therapy by modulating mechanical forces has been widely used in the clinic. In 1861, the anatomist Karl Langer summarized Dupuytren and Malgaigne’s earlier observations and pointed out that when puncturing cadaver skin with a conical spike, the puncture port was oval rather than circular (Carmichael, 2014). Further intensive puncturing of the skin surface and connecting the lines results in the formation of lines on the skin surface, now known as Langer’s lines (Abyaneh et al., 2014). This discovery helps reduce scar formation by making surgical incisions parallel to Langer’s lines (Paul, 2017). Langer’s lines also indicate the direction of maximum local skin tension, which is mainly affected by the arrangement direction of the main protein components of ECM, muscle contraction, and other factors (Silver et al., 2003; Bush et al., 2007; Casale et al., 2021). Ogawa et al. (2012) measured the distribution and skin stretching of 483 keloid patients and showed that mechanical force is an important factor driving keloid formation, even in genetically susceptible individuals. Hypertrophic scarring can be produced in experimental animal models by repeated mechanical force or sustained stretching at the incision edges (Shan et al., 2017). In addition, dilators are widely used in plastic and reconstructive surgery to obtain additional skin for grafting. This is because the continuous stretching force promotes the proliferation of the skin, including the ECM (Wang et al., 2015). In a large animal and phase I clinical study, Gurtner et al. (2011) showed that using a dynamic stress-shielding polymer device significantly reduced scar formation.
During skin fibrosis, abnormal changes occur in the number, variety, and metabolism of cellular populations, including resident cellular components and migratory cells in the skin (Smith and Chan, 2010; Deng et al., 2021). This process is often accompanied by changes in the cellular microenvironment, including mechanical properties and signaling molecular components of humoral components, and is observed macroscopically as immune inflammatory responses and immune cell infiltration (Pfisterer et al., 2021).
Fibroblasts and myofibroblasts are key parenchymal cell populations, and they play significant roles in maintaining the homeostasis of the skin’s mechanical microenvironment under physiological conditions. When subjected to abnormal mechanical stimulation, however, excessive activation, hyperproliferation, and differentiation of fibroblasts can lead to excessive ECM deposition, which contributes to the progression of fibrosis and further leads to the formation of keloids, scars and SSc (Macarak et al., 2021) (Figure 3). Myofibroblasts are typically activated fibroblasts that can be transformed by soluble growth factors such as TGF-β and mechanical signaling (Elson et al., 2019). They are the most critical cellular populations in tissue remodeling and wound healing. Their main feature is the expression of smooth muscle actin, which enables contractility. Considering the tight junctions of myofibroblasts with ECM components, such as collagen, myofibroblasts can alter the mechanical properties of the local ECM and promote wound contraction and scar contracture. There is an intermediate stage called the proto-myoblast stage during the activation of fibroblasts (Watsky et al., 2010). The rate of transformation to proto-myoblasts is related to mechanical changes, such as the stiffness of the tissue. The stiffness is mainly influenced by external stretching forces and the contractile forces exerted on the ECM by the myofibroblasts, which leads to a positive feedback mechanism maintaining steady states of proto-myoblasts and myoblasts (Figure 3). The mechanical changes can also activate the secretion of TGF-β, a crucial factor during fibrosis; the detailed mechanism will be discussed in Section 3.2.1 and Figure 5. Fibroblasts and myofibroblasts can participate in ECM remodeling by secreting matrix metalloproteinases (MMPs) and tissue inhibitors of metalloproteinases (TIMPs). MMPs are endopeptidases, which are capable of ECM degradation and bioactive molecules processing. MMPs and TIMPs are important factors in skin ECM remodeling, which will be discussed in detail in Section 2.3.1 (Leong et al., 2021). Recent studies have shown that M2 macrophages promote fibroblasts to differentiate into myofibroblasts through an acid-sensing ion channel 3—macrophage colony-stimulating factor–TGF-β1 positive feedback loop in keloid patients. This further proves that the pathogenesis of skin fibrosis is not a single factor but the result of the long-term joint action of mechanical homeostasis and the inflammatory response in tissues (Wu et al., 2022) (Figure 4).
FIGURE 3. The regulation in fibroblast activation. The fibroblasts derived from bone marrow can differentiate into an intermediate stage called protomyoblasts and then into myofibroblasts. Myoblasts can synthesize many kinds of molecules that contribute to mechanical changes in the ECM. In physiology, fibroblasts are vital to tissue homeostasis, while their excessive activation can lead to fibrosis in pathology. The rate of transformation to protomyoblasts and myofibroblasts is related to the mechanical changes in turn, which could lead to positive feedback. Mechanical changes can also activate the secretion of TGF-β, which is a crucial factor during fibrosis.
FIGURE 4. The positive feedback loop in skin fibrosis. ECM stiffness changes and inflammatory responses work together and lead to skin fibrosis. The mechanical property upregulation leads to the activation of TGF-β and other inflammatory factors. Inflammatory responses and inflammatory cell infiltration can promote the sedimentation of collagen and other ECM proteins. In general, a positive feedback loop in skin fibrosis is formed, and ECM stiffness plays a central role in this process.
In addition to fibroblasts, myofibroblasts can also be derived from other cell types. Single-cell sequencing identified a bone marrow-derived fibroblast subgroup (Deng et al., 2021). Bone marrow-derived progenitor cells enter the bloodstream and circulate to specific tissues, where they colonize and further differentiate into fibroblasts that promote local collagen deposition, especially during wound repair (Fathke et al., 2004; Ishii et al., 2005; Suga et al., 2014; Sinha et al., 2018). Many recent studies have indicated that myofibroblasts in skin fibrosis can also be adipocyte-derived (El Agha et al., 2017) (Figure 3).
Fibroblasts exhibit functional diversity due to different origins, anatomical locations, and tissue microenvironments (Griffin et al., 2020). In skin tissue, fibroblasts perform functional diversity in different anatomical localizations and microenvironments (Driskell and Watt, 2015). Fibroblasts in the papillary layer play an irreplaceable role in forming hair follicles and PD, while fibroblasts in the reticular layer play an important role in developing the reticular layer and part of the subcutaneous tissue in the skin. After skin damage, reticulofibroblasts first migrate to the damaged site, producing a collagen-rich dermis, but the hair follicles cannot regenerate. In contrast, papillary fibroblasts are involved in wound healing at a later stage. The study of fibroblast heterogeneity can provide a better understanding of the differences between skin scarring and fibrosis after wound healing.
Although ECM matrix thickening in skin fibrosis occurs mainly in the dermis, keratinocytes also play an important role in skin fibrosis. During wound healing and regeneration, keratinocytes migrate and proliferate at the wound edges at an early stage. Keratinocytes are observed to regulate fibroblast activation and ECM deposition by producing soluble inflammatory and growth factors in wound healing, hypertrophic scar formation, and other fibrotic processes (Shephard et al., 2004; Werner et al., 2007; Lee et al., 2019). In SSc, keratinocytes are found to promote fibroblast activation independent of TGF-β (McCoy et al., 2017). Keratinocytes can also modify the mechanical properties through the accumulation of collagen type I, increasing the expression of MMPs and decreasing the expression of tissue inhibitors of TIMPs (Russo et al., 2020).
Interestingly, keratinocyte proliferation, metabolism, and other cell behaviors are regulated by fibroblasts, forming a feedback loop. Fibroblasts inhibit keratinocyte apoptosis and promote keratinocyte survival and differentiation (El Ghalbzouri and Ponec, 2004; Peura et al., 2010; Fernandez et al., 2014). Fibroblasts also promote keratinocyte adhesion, possibly due to the secretion of soluble signaling molecules or transcription factors that freely diffuse to keratinocytes (Chowdhury et al., 2012; Quan et al., 2015; Yang et al., 2018).
EMT is the process by which epithelial cells lose polarity and acquire a mesenchymal phenotype. EMT can enhance cell migration, invasion capabilities, ECM matrix secretion, and antiapoptotic capabilities, making it an essential driver of tissue fibrosis (Kalluri and Neilson, 2003; Kalluri and Weinberg, 2009). EMT contributes to myofibroblast accumulation and increases myofibroblast contraction, migration, and ECM secretion ability. Integrin plays a significant role in fibrogenesis as a transmembrane receptor in EMT mediation and participates in the bidirectional signal transmission process between the external environment and cells (Yeh et al., 2012). Furthermore, evidence for microRNA-induced skin fibrosis via EMT is increasing. Some recent studies have shown that miRNA-21 and miRNA-200 increase the expression of the TGF-β type II receptor and contribute to EMT by interacting with the TGF-β pathway (Babalola et al., 2013). TGF-β can activate mesenchymal genes and inhibit epithelial gene expression through Smad or non-Smad signaling pathways, thus promoting the transdifferentiation of epithelial cells into mesenchymal cells (Derynck et al., 2014; Lamouille et al., 2014). Our research shows that additional stretching during skin regeneration can promote keratinocyte activation and EMT, suggesting that biomechanical force (BioF) can be a potential therapeutic target for skin fibrosis (Zhou et al., 2015).
Immune cells are important regulators of ECM reconstruction. Activated immune cells can produce multiple cytokines, including TGF-β and nuclear factor kappa-B (NF-κB), which directly promote the activation of fibroblasts (Mack, 2018). The immune cells involved in this process are highly diverse, including macrophages, neutrophils, T cells, B cells, and natural killer cells (Huang et al., 2020). Different immune cells promote skin fibrosis by diverse mechanisms, but the mammalian target of rapamycin (mTOR) signaling pathway plays a central role in inflammation-mediated skin fibrosis (Yoshizaki et al., 2010). In addition, changes in the local immune microenvironment brought about by inflammatory cell infiltration are often accompanied by increases in the levels of various cytokines, such as IL-6 (interleukin-6), IL-17, and IFNs (interferon) (Brown and O’Reilly, 2019), and are involved in the pathogenesis of fibrosis by activating JAK-STAT signaling pathway regulation (Huang et al., 2020).
Among the immune cells involved in fibrosis, macrophages have received the most extensive attention. Monocytes and macrophages are involved in the early response to inflammation (Guilliams et al., 2018). Macrophages can secrete tumor necrosis factor-α (TNF-α), TGF-β, interleukins (IL-1, IL-6, IL-8, IL-12), and various chemokines (Arango Duque and Descoteaux, 2014). Furthermore, these inflammatory factors can affect fibroblasts and promote ECM production (Yang and Plotnikov, 2021). Both M1 and M2 macrophages are involved in the development of fibrosis. M1 macrophages activate myofibroblasts by producing proinflammatory factors and chemokines (Braga et al., 2015). M2 macrophages mainly play an anti-inflammatory role but can produce TGF-β and promote ECM production (Mosser and Edwards, 2008; Sica and Mantovani, 2012). M2 macrophages promote wound healing and tissue regeneration after injury under physiological conditions. However, under pathological conditions, the stimuli that trigger the inflammatory response persist, and a large number of profibrotic factors, such as TGF-β and Galactin-3 secreted by M2 macrophages, play an important role in driving tissue fibrosis (Cao et al., 2014).
In addition, immune cells have been observed to directly interact with the ECM and actively participate in remodeling of the ECM. For example, macrophages release a variety of MMPs, such as MMP-10, MMP-12 and MMP-21, to promote ECM degradation (Skoog et al., 2006; Pellicoro et al., 2012; Feng et al., 2019). Th1 cells can secrete MMP-2 and MMP-9, enhancing the MMP secretion capacity of macrophages (Oviedo-Orta et al., 2008). Osteopontin (OPN), which contains an RGD motif and can bind to integrins, widely exists in the ECM (Oldberg et al., 1986). Macrophages, T cells, and NK cells can all express OPN (Murry et al., 1994; O’Regan et al., 1999) and further lead to skin fibrosis (Wu et al., 2012; Newe et al., 2021). In SSc, the overexpression of versican, which can bind to collagen type I and maintain the structural stability of ECM by CD14+ cells, is important for the formation of ECM stiffness (Masuda et al., 2013).
Mechanical forces and the immune system are dependent on each other. For example, the immune response is stimulated by increased ECM stiffness. Activated immune cells induce biomolecular secretion and lead to ECM stiffness increase directly or indirectly (Figure 4). Furthermore, mechanical stress can prolong the inflammatory response through a T-cell-dependent pathway, thereby promoting scarring (Wong et al., 2011). Prolonged changes in the immune microenvironment combined with changes in cellular mechanical stress lead to dysregulation of extracellular microenvironment homeostasis, ultimately leading to skin fibrotic lesions marked by excessive collagen deposition and ECM stiffness in the dermal ECM (Pakshir and Hinz, 2018; Pfisterer et al., 2021). In conclusion, during skin fibrosis, the positive feedback loop between ECM stiffness upregulation and immune activation is required for fibrogenesis and maintains the indispensable stimuli for fibrosis (Figure 4).
The dermal matrix is a “fishnet-like” structure composed of a complex series of proteins, mainly containing collagen types I and III (Smith et al., 1982). Dermal ECM-specific proteins, including collagen type IX, collagen type XII, fibril-associated collagens with interrupted triple helices (FACIT) and small leucine-rich proteoglycan (SLRP), remodel the mechanical properties of the ECM by regulating protein crosslinking, participate in communication between cells and the matrix, regulate cell behavior and even determine cell fates (Chermnykh et al., 2018; Dengjel et al., 2020; Potekaev et al., 2021).
Collagen is the most important component of skin ECM and the primary determinant of mechanical properties (Zhang et al., 1995; Ricard-Blum, 2011; Karayi et al., 2020). Collagen types I and III are the most abundant in the skin, and their gene transcription is significantly upregulated in fibrotic skin (Heino et al., 2009). Collagen type IV is the main component of BM (Ricard-Blum, 2011). Collagen types VI and VII help BM connect to the matrix of the papillary layer of the dermis by closely binding to collagen type I in the dermal matrix (Has et al., 2015). Collagen types IX and XII are fibrillar-associated collagens mediating collagen–collagen and collagen–other biomolecule connections. Collagen type XVII is a transmembrane collagen that regulates cell growth and metabolism (Has et al., 2015). Collagens can form a reticular structure that composes the main architecture of the ECM, mediates the communication between the ECM and cells, and participates in mechanosensitive signal transmission (Mak and Mei, 2017; Pozzi et al., 2017). FACIT, SLRP, and matricellular proteins can serve as molecular bridges that are important for the organization and stability of extracellular matrices. These molecules can effectively enhance the fibroblast response to TGF-β and regulate ECM stiffness by regulating fibril formation and collagen fiber cross-linking (Grässel and Bauer, 2013; Has et al., 2015; Chacón-Solano et al., 2022). In the development of skin fibrosis, ECM components change correspondingly. The expression levels of lumican and collagen type V were increased, while collagen fibril assembly was damaged (Zhou et al., 2021).
Lysyl oxidase (LOX), mainly produced by fibroblasts, can mediate the cross-linking of lysine and hydroxylysine of different collagen peptides to form a stable collagen network (Vallet and Ricard-Blum, 2019; Vorstandlechner et al., 2020). Collagen is cross-linked by the deamination of lysine residues with the catalysis of LOX. The increased cross-linked form of collagen has higher mechanical strength and stronger resistance to MMP degradation (Clarke et al., 2013). Collagen matrices with increased hydroxyallysine cross-link levels were less susceptible to MMP-1 degradation than the collagen matrices containing low hydroxyallysine levels (van der Slot-Verhoeven et al., 2005). Correspondingly, the use of LOXL2/LOXL3 inhibitor can reduce collagen oxidation and collagen crosslinking, which represents an innovative therapeutic approach for the treatment of fibrosis (Schilter et al., 2019). Huang et al. (2019) found that TGF-β can mediate ECM stiffness through LOXs using two in vitro models containing fibroblasts from SSc patients. LOXs can also directly induce the production of ECM at the transcriptional level by changing the metabolism of fibroblasts, thus directly participating in the process of fibrosis (Nguyen et al., 2021). The increase was mediated by LOX-induced c-Fos expression, the nuclear localization of c-Fos, and the overexpression of IL-6 in fibroblasts.
MMPs are calcium-dependent zinc-containing endopeptidases. Dermal fibroblasts and leukocytes are the main sources of MMPs, especially MMP-2, which is usually secreted in the form of inactive proMMP (Wang, 2018). Many MMPs, such as MMP-1,2,3,8,11,13, are able to hydrolyze ECM proteins and reduce the stiffness of the ECM. TIMPs are natural inhibitors of MMP. TIMP and MMP work together to form proMMP-2/TIMP-2/3/4 and MMP-9/TIMP-1 that maintain the balance of ECM synthesis and degradation under physiological conditions (Vafadari et al., 2016; Cheng et al., 2017). In SSc, it was observed that the decreases in MMP-1 and MMP-3 expression and the increase in TIMP-1 expression resulted in the inhibition of the structural protein hydrolysis of ECM (Leong et al., 2021). MMP-13 is produced by fibroblasts in adult gums and fetal skin wounds and promotes rapid collagen remodeling and scar-free healing (Ravanti et al., 2001; Toriseva et al., 2012).
Integrins are a family of transmembrane proteins (cell surface receptors) that mediate the interconnection between cells and the ECM. They mechanically anchor cells on the ECM and use their transmembrane structure to participate in the bidirectional signal transmission process between the external environment and cells (Henderson and Sheppard, 2013). Depending on their subtype, integrins have different functions, including collagen, laminin, leukocyte, and bilirubin receptors (Koivisto et al., 2018).
In addition to binding to the major structural proteins of the ECM, integrins can also bind to non-structural proteins such as a disintegrin and metalloproteinase (ADAM), thus directly contributing to the structural remodeling of the ECM (Watt and Fujiwara, 2011; Giebeler and Zigrino, 2016). Integrin can regulate ECM stiffness indirectly by activating latent TGF-β (Figure 5). The activated TGF-β induces the myofibroblastic differentiation. In addition, integrin contributes to autocrine TGF-β signaling (Asano et al., 2006a; Asano et al., 2006b). The integrin signaling pathway will be discussed in Section 4.2.
FIGURE 5. Integrin, Hippo and TGF-β signaling pathways mediate mechanical stress signaling to the cell. (A) TGF-β is secreted out of cells in an inactive form. Activated TGF-β can upregulate ECM stiffness through fibroblast activation. (B) Mechanical signals are involved in TGF-β activation.The interaction of activated TGF-β and TGFBR mediated the transmembrane transduction of ECM mechanical signals, which is transduced via two pathways, Smad and non-Smad, thereby regulating myofibroblast differentiation and the stiffness of the ECM. Integrins can signal through pathways such as FAK, ILK and Hippo, and there is crosstalk with the TGF-β pathway.
Many studies have shown that growth factors are widely involved in the initiation of fibroblasts and the maintenance of a steady myofibroblast population during various fibrotic diseases. TGF-β is a crucial regulatory signaling molecule in fibrosis. TGF-β1 expression significantly increases in keloids, SSc, and skin fibrosis caused by radiation factors (Verrecchia et al., 2006; Verrecchia and Mauviel, 2007). Abnormally increased secretion of TGF-β leads to excessive collagen deposition and ultimately scar formation. TGF-β was also found to downregulate the expression of MMP-1 and upregulate the expression of TIMP-3 to inhibit the degradation of ECM (Edwards et al., 1987; Ihn, 2002; Leivonen et al., 2013).
Platelet-derived growth factor (PDGF) and connective tissue growth factor (CTGF) have also been found to play important roles in the progression of fibrosis (Ihn, 2002). In gene-edited mice overexpressing PDGF-α, excessive proliferation of fibroblasts, ECM deposition, and a fibrotic phenotype were observed in multiple organs and tissues. PDGF can be a downstream regulator of TGF-β and work synergistically with it in the process of fibrosis by binding with PDGFR (Olson and Soriano, 2009). PDGFRs are receptor tyrosine kinases that dimerize after ligand binding, activating the intracellular tyrosine kinase domains. These activated domains autophosphorylate several tyrosine residues, creating docking sites for signaling proteins and adaptors that are responsible for the signal transduction process attracting fibroblasts and macrophages.
CTGF is induced by TGF-β and regulates fibroblast growth and ECM synthesis. CTGF is considered an important mediator in the pathogenesis of fibrosis and can strengthen the TGF-β/Smad3 signaling pathways (Verrecchia and Mauviel, 2007).
With the reconstruction of ECM in fibrogenesis, the cells embedded in the matrix are constantly exposed to abnormal mechanical stress (Jansen et al., 2018; Potekaev et al., 2021). The behavior of cells is tightly controlled by the mechanical environment, which eventually leads to the activation of fibroblasts, excessive ECM accumulation and ultimately fibrosis. The initiation of fibrosis will start a positive feedback loop (Figure 4), in which the constantly increasing tissue stiffness will lead to the persistence and self-reinforcement of the fibrosis process. This hypothesis explains the continuation of fibrotic diseases observed clinically and the difficulty in reversing the trend of fibrosis.
The positive feedback loop between fibroblasts, myofibroblasts and mechanical stress was described in detail earlier in Section 2.2 and Figure 3. In addition, mechanical signals can transmit into cell directly by membrane proteins such as integrin and piezo1. The matrix stiffness increase can also indirectly activate parenchymal cells in ECM by inducing TGF-β activation (Figure 5). During skin fibrosis, immune cells are activated and can further modulate fibroblasts or participate in the fibrotic process by directly engaging in ECM alterations. This process is also a positive feedback loop (Figure 4).
In the process of skin fibrosis, changes in ECM structure and composition regulate the activation of immune cells and induce immune cell infiltration (Lu et al., 2011; Hallmann et al., 2015; Simon and Bromberg, 2017). The proteolytic products of ECM proteins, including collagen, elastin, laminin, and hyaluronic acid, can act as inflammatory mediators (Adair-Kirk and Senior, 2008). Neutrophils bind to the 7S domain of collagen type IV through surface receptors. It is suggested that neutrophils may have chemotaxis to the collagen type IV hydrolyzed region in vivo (Senior et al., 1989). In addition, the mechanical force can act directly on immune cells and participate in the activation and aggregation of immune cells in fibrosis (Figure 4).
T and B cells perceive the mechanical microenvironment through mechanically sensitive T-cell receptor (TCR) and B-cell receptor (BCR) or antigen-presenting cell (APC) interactions (Huse, 2017; Saitakis et al., 2017). In 2D cell culture, the enhancement of culture surface rigidity can promote the activation, migration and proliferation of T cells (O'Connor et al., 2012). The sensing of mechanical signals may be related to CD3 (Judokusumo et al., 2012). CD3 (ε chain) can be attached to polymers under costimulation by CD28 (Riha and Rudd, 2010) and presented to primary T cells. Many proteins involved in this process, including interleukin-2 (IL-2) secretion and proliferation, interact directly or indirectly with the actin cytoskeleton for signaling (Bashour et al., 2014).
In addition, Majedi et al. found that in 3D cultured T cells, an ECM environment with a high elasticity modulus induced the expression of inflammatory factors such as IL-2, IFN-γ and TNF-α (Majedi et al., 2020). The enrichment of inflammatory factors can further induce tissue fibrosis. Yes-associated protein 1 (YAP), a well-established mechanosensitive protein, has also been found to play an important role in mechanical signal transduction between T cells and the ECM (Meng et al., 2020). Regulation of T-cell metabolism is mainly attributable to nuclear factor of activated T cells (NFAT)-driven transcription. YAP is expressed in activated T cells and negatively regulates T-cell proliferation and activation in response to the mechanical microenvironment by directly restricting NFAT1 translocation to the nucleus (Meng et al., 2020). However, YAP does not affect the early activation of T cells (Meng et al., 2020). We will discuss YAP-related signaling processes in detail in 4.2.3. Zeng et al. (2015) have shown that the rigidity of the substrate cultured in 2D can regulate the proliferation of B cells. The increase in matrix stiffness can promote or inhibit the proliferation of B cells, which is different in response to different proliferation stimuli. Macrophages have also been demonstrated to be regulated by mechanical signals. Under low substrate stiffness, the expression of CD86 on the cell surface of bone marrow-derived macrophages (BMMs) increased and secreted more proinflammatory factors, such as IL-1β and TNF-α. With increasing ECM stiffness, the expression levels of CD206, IL-4 and TGF-β in BMMs increased accordingly, while the synthesis of ROS decreased (Chen et al., 2020). In addition, the increase in matrix stiffness increases its migration capability (Hind et al., 2015), which is regulated by the PI3K-AKT1 and Rac signaling pathways. YAP-mediated mechanical transduction is also involved in macrophage-dependent inflammation (Meli et al., 2020).
Similarly, the mechanical properties of the matrix can affect neutrophil adhesion, diffusion and migration (Oakes et al., 2009). The difference in ECM stiffness leads to the migration of neutrophils to sites of injury. The magnitude of ECM stiffness determines the neutrophil migration rate and the final diffusion area (Oakes et al., 2009). Neutrophils migrate more slowly on harder substrates, but neutrophils eventually move farther, considering the longer migration duration (Oakes et al., 2009).
The increased matrix stiffness also enhanced the proinflammatory function of dendritic cells (DCs), and the glycolysis of DCs was enhanced to meet the energy requirements of DC activation and the raw material requirements for biosynthesis (Chakraborty et al., 2021). Zeng et al. (2015) also found that DCs receive extracellular mechanical signals through multiple signaling pathways. The Hippo signaling pathway is involved, as it has been shown that the Hippo signaling molecule MST1/2 regulates DC metabolism (Du et al., 2018). At the same time, DCs may mediate the transcription of target genes in response to substrate stiffness by upregulating the expression of the transcriptional coactivator with PDZ-binding motif (TAZ) gene and its Hippo signaling partner YAP and its translocation into the nucleus (Chakraborty et al., 2021).
Matrix stiffness can be used as an effective regulator for stem cells (Chermnykh et al., 2018). Studies by Engler et al. (2006) have shown that different levels of ECM stiffness can guide mesenchymal stem cells (MSCs) to differentiate into osteoblasts, myocytes, and nerve cells. This may be related to the anchoring density and anchoring force between stem cells and ECM (Park et al., 2011; Trappmann et al., 2012). The directional differentiation of tissue-resident MSCs is one of the important sources of myofibroblasts (Kramann et al., 2015). Continuous culture of MSC renewal on hydrogel with a rigid gradient of 1.0 ± 0.1 kPa/mm showed that MSCs migrated to a more rigid matrix (Tse and Engler, 2011). In addition, increased stiffness of the ECM induces EMT, indicating that epithelial cells can transdifferentiate into myofibroblasts by high mechanical stress (Leight et al., 2012).
We previously discussed the upregulation of ECM stiffness by TGF-β in detail (Section 2.2.1; Figure 3). Mechanical stress also has a regulatory effect on TGF-β, which forms a positive feedback loop. The mechanical properties of the ECM have an important impact on the activity and availability of TGF-β1. Mature TGF-β is a covalent homodimer. Its precursor protein is processed intracellularly after translation and cleaved to form latency-associated peptide (LAP) and mature TGF-β. LAP forms a complex with TGF-β in a non-covalently bound form and masks the active site of TGF-β. TGF-β is activated by dissociating LLC bound to LAP and/or ECM. This process can occur through various mechanisms, including integrin-LAP interaction-mediated TGF-β activation and mechanical tension-induced TGF-β activation (Mu et al., 2002; Frangogiannis, 2020) (Figure 5A). Pierre-JeanWipff et al. also found that myofibroblast contraction can directly activate TGF-β1 stored in the ECM (Wipff et al., 2007).
Integrin plays a central role in the activation of TGF-β during fibrosis (Henderson et al., 2013) (Figure 5B). Through the structural analysis of integrin and TFG-β, Ruoslahti and Pierschbacher et al. showed that TGF-β1 and TGFβ-3 bind to integrin based on their linear sequences of arginine, glycine, and aspartic acid (RGD sequence) (Ruoslahti and Pierschbacher, 1987) (Figure 5B). Munger’s research in the SSc mouse model showed that fibroblasts upregulated the expression of αvβ5 vitronectin receptor and led to the activation of latent TGF-β (Munger et al., 1999). Like many other ECM protein components, LAP contains RGD motifs that can be specifically bound by integrins. In addition, integrin αvβ8 activates by presenting the potential TGF-β complex to MMPs, resulting in the release of free TGF-β into the extracellular environment (Prieto et al., 1993). In mice, knockout of integrin subunits (β6, αv and β8) can activate TGF-β1 (Huang et al., 1996; Bader et al., 1998; Zhu et al., 2002), and mutations in the integrin binding sites in LAP produced the same effects as TGF-β1 knockout (Shull et al., 1992; Yang et al., 2007; Hinz, 2015).
Various signaling molecules, including integrins and TGF-β, can mediate mechanotransduction. Once the signal is transduced across the membrane into the cytoplasm and nucleus, it will cause a series of downstream changes (Figure 5). Figure 5B shows the complicated relationship among several most important signaling pathways.
The secretion and activation of TGF-β are regulated by immune cells and the extracellular mechanical microenvironment. Activated TGF-β binds to transforming growth factor-β receptor (TGFBR) on the surface of fibroblasts, upregulating α-SMA expression and promoting collagen secretion and cell proliferation. In addition, TGF-β acts as an inflammatory factor, inducing an inflammatory response (Li and Flavell, 2008) and indirectly promoting local tissue fibrosis. In skin fibrosis, the most classical signaling pathway is achieved by activating Smad transcription factors by TGFBR (Figure 6). TGF-β can also activate other proteins, such as Ras and Rho protein-mediated signaling pathways, called non-Smad signaling pathways (Figure 5).
FIGURE 6. Interaction of the TGF-β signaling pathway through Smad and non-Smad pathways in fibrosis. Mature TGF-β binds to TGFBR and acts on downstream molecules through the Smad and non-Smad pathways, thereby regulating ECM production. The downstream molecules of these pathways can interact with the molecules of the Smad pathway to inhibit or promote ECM production.
TGFBR has serine/threonine kinase activity and is activated upon binding to TGF-β, mediating different downstream signaling pathways through the phosphorylation of different substrates within its cells and ultimately resulting in phenotypic alterations, including fibroblast activation and EMT (Lamouille et al., 2014). The abundance of TGFBR in cell membrane species, including NIH-3T3 fibroblasts, can be regulated by endocytosis (Vander Ark et al., 2018). TGFBR is thought to be brought into the cell by endocytosis along with the cell membrane to form endocytic vesicles. Liposomes containing TGFBR can also bind to the cell membrane again and rapidly increase the cellular sensitivity to TGF-β (Huang and Chen, 2012).
TGFBR, a specific receptor of the TGF-β family, is divided into three types: TGFBR1, 2, and 3. TGFBR1 and TGFBR2 form stable complex receptor tetramers in the presence of TGF-β. First, TGF-β binds to TGFBR2 and activates its phosphokinase activity. Subsequently, TGFBR2 phosphorylates TGFBR1 (Budi et al., 2017; Yan et al., 2018) (Figure 6). TGFBR1 then phosphorylates R-Smads, after which the signal is transmitted intracellularly through the Smad signaling pathway and ultimately regulates the transcription of specific genes. TGFBR3 has no kinase activity but can present TGF-β to TGFBR2 and stabilize the complex between TGFBR1 and TGFBR2 (López-Casillas et al., 1993; Bilandzic and Stenvers, 2011). TGFBR3 is particularly important for TGF-β2 signaling (Kim et al., 2019; Kudipudi et al., 2019). However, the formation of the complex between TGFBR1 and TGFBR2 is inhibited when TGFBR3 alone binds to TGFBR1 or TGFBR2, thereby suppressing TGF-β-mediated Smad signaling (Tazat et al., 2015). In addition, the extracellular structural domain of TGFBR3 can be detached from the cell membrane, and further binding to TGF-β can block signal transduction into the cell (López-Casillas et al., 1994) (Figure 5B).
The Smad pathway regulates the production of the ECM matrix together with the non-Smad pathway. Collectively, there is crosstalk between the Smad and non-Smad pathways of TGF-β. For example, R-Smads are phosphorylated at multiple sites by other kinases (MAPKs, CDK2/4, and ROCK). The activity of R-Smads is finely regulated and accomplishes the integration of different signaling inputs (Wrighton et al., 2009; Liu and Feng, 2010). The proteins mentioned above involved in regulating R-Smads are regulated by the non-Smad pathway and act as substrates of the non-TGF-β signaling pathway. In addition, TGF-β-mediated downstream factors can act synergistically with signaling cascades such as Wnt and Notch (Derynck and Zhang, 2003). Thus, the decision to develop fibrotic disease results from a combination of different signals, not from a specific pathway alone, which is consistent with the fundamental laws of life.
In conjunction with the Smad and non-Smad pathways, TGF-β promotes the secretion of collagen type I, collagen type II, and fibronectin by fibroblasts in the human dermis (Varga et al., 1987). Posttranslational transport and modification of collagen are also extensively regulated by TGF-β, including proteins such as HSP47, PLOD2, and P4HA3 (Ishida and Nagata, 2011; Bourhis et al., 2012; Ishikawa and Bächinger, 2013). Extracellular meprin and LOX are involved in collagen maturation assembly. These two proteins are abundantly expressed at fibrotic sites and are induced by TGF-β (Broder et al., 2013; Biasin et al., 2014; Laczko and Csiszar, 2020). TGF-β also induces the expression of protease inhibitors, such as TIMP, and inhibits ECM hydrolysis (Edwards et al., 1987).
Smads are a family of structurally similar proteins that are the main carriers of intracellular signaling of the TGFBR (Itoh et al., 2000; Attisano and Tuen Lee-Hoeflich, 2001) (Figure 6). Smads can be classified into three subtypes based on their function, namely, receptor-regulated Smads (R-Smads), cochaperone Smads (Co-Smads), and inhibitory Smads (I-Smads) (Figure 5). Smads 1, 2, 3, and 5 and Smad8 are R-Smads located mainly in the cytoplasm and can be activated by phosphorylation of TGFBR. R-Smads can bind to specific DNA sequences or G/C-rich DNA regions (Shi and Massagué, 2003). Cooperating transcription factors can help stabilize Smad binding to DNA and enhance their specificity in recognizing DNA sequences. Smad4 is the only known Co-Smad expressed in humans and binds to activated R-Smads to cross the nuclear membrane. The remaining two Smads, Smad6 and Smad7, are mainly located in the nucleus and move to the plasma membrane in response to TGF-β stimulation. Activated Smad7 can bind to activated TGFBR to inhibit the phosphorylation of R-Smads (Shi and Massagué, 2003). I-Smads can also block TGF-β downstream signaling by preventing the nuclear translocation of R-Smads (Verrecchia et al., 2006; Verrecchia and Mauviel, 2007). In addition, Smad7 can act as an articulatory protein that promotes the binding of TGFBR1 to E3 ligases, thereby mediating the degradation of TGFBR1 via the ubiquitin pathway (Vander Ark et al., 2018).
Smad3 appears to be a key component of the signal transduction pathway involved in the fibrosis process. Smad3-deficient mice were found to be protected from radiation-induced skin fibrosis (Flanders, 2004). Using a combinatorial cDNA microarray promoter transactivation approach, Franck Verrecchia et al. identified Smad3/4 gene targets in cultured dermal fibroblasts: COL1A1, COL3A1, COL5A2, COL6A1, COL6A3, and TIMP-1 (Verrecchia et al., 2001). Thus, the TGF-β/Smad signaling pathway is essential for activating skin fibrillar collagen genes.
The Smad signaling pathway promotes the expression of proteins such as JunB (Jonk et al., 1998), PDGF (Taylor and Khachigian, 2000), and integrin, thus indirectly regulating the process of tissue fibrosis (Figure 5). In addition, the TGF-β/Smad signaling pathway inhibits the degradation of the ECM. The TGF-β/Smad signaling pathway also inhibits the transcription of MMP-1 (Yuan and Varga, 2001) and activates the expression of human plasminogen activator inhibitor-type 1 (PAI-1) (Dennler et al., 1998).
Ras is a small G protein that can hydrolyze GTP to GDP and then rebind to GTP with the assistance of guanine nucleotide exchange factor (GEF) and GTPase-activating protein (GAP). The different binding substrates result in two distinct states of Ras, which act as a switch in intracellular signal transmission. When Ras binds to GTP, the signaling pathway is “on”. When RAS hydrolyzes GTP to GDP, the signaling pathway is “off”. TGF-β1 increases mRNA levels and exogenous Ras promoter activity, thereby stimulating Ras (Brabletz and Brabletz, 2010; Su et al., 2020). Activated Ras activates Raf, which further activates mitogen-activated protein kinase kinase (MEK or MAPKK) and triggers a cascade reaction. Together, the proteins involved in this process form the Ras/Raf/MEK/extracellular signal-regulated kinase (ERK) signaling pathway (Figures 5, 6).
An essential feature of tissue fibrosis is the occurrence of EMT (Lamouille et al., 2014). Activation of the ERK signaling pathway is required for TGF-β-induced EMT (Xie et al., 2004). TGF-β can activate the ERK pathway through upstream factors (e.g., Ras/Raf) and through more downstream factors (e.g., MEK) (Xie et al., 2004). The ERK signaling pathway can lead to overexpression of FOXM1 at the protein and mRNA levels, which in turn leads to indirect upregulation of EMT-related transcription factors (e.g., ZEB1 and ZEB2) and induction of the EMT process by the downregulation of microRNA-200b (Brabletz and Brabletz, 2010).
Ras affects TGF-β Smad signaling mainly by regulating the activation level of Smad2/3 (Figure 6). Ras interacts with SPSB1, a negative regulator of TGFBR2 on the cell membrane, to promote the degradation of the SPSB1 protein. Decreased levels of SPSB1 stabilize TGFBR2, thereby enhancing Smad2/3 phosphorylation and signaling (Burch et al., 2010; Liu et al., 2018). Activation of ERK can increase or decrease Smad signaling (Adhikari et al., 2007; Lei et al., 2019). Ras can inhibit BMP-induced nuclear accumulation of Smad1 at four sites in the junctional structural domain (Kretzschmar et al., 1999). Through PI3K, Pak2 activation can lead to cell type-dependent activation of ERK (Hough et al., 2012) (Figure 5). This activated ERK phosphorylates Smad2 primarily within its junctional region, leading to Smad-mediated transcriptional activation. This phosphorylation process occurs mainly in the nucleus by Smad2.
TGF-β-activated kinase 1 (TAK1) is a member of the mitogen-activated protein kinase kinase kinase (MAPKKK) family (Xu and Lei, 2020). Activating TAK1 by TGF-β acts upstream of NF-κB and mitogen-activated protein kinases (MAPKs), thereby regulating ECM. Dynamic regulation of ubiquitination and deubiquitination plays an important role in TAK1-mediated activation of NF-κB (Lei et al., 2019). TRAF6 and TRAF4 are ubiquitin ligases (E3) (Figure 5). Upon induction of TGFBR, TRAF6/TRAF4 undergoes autoubiquitination and activates nuclear factor NF-κB via the cytokine interleukin 1 (Xie et al., 2004; Brabletz and Brabletz, 2010). After the recruitment of the bridging protein TAB1, TAK1 is autophosphorylated. It can affect the NF-κB pathway by regulating the IkappaB kinase (IKK)-subunit NFκB essential modulator and the NFκB-activating kinase IKKβ (Adhikari et al., 2007; Lei et al., 2019) (Figure 6).
The MAPK pathway is activated by the phosphorylation of TAK1 through a cascade reaction (Fang and Richardson, 2005) (Figure 6). MAPKs are a large family of serine-threonine kinases with three main subfamilies: ERKs, c-Jun N-terminal kinases (JNKs) or stress-activated protein kinases (SAPKs) and p38 MAPKs. JNK can also be regulated by the activation of TAK1 (Sorrentino et al., 2008) (Figure 6). Activation of both JNK and p38 is not Smad dependent (Yamashita et al., 2008). In fibroblasts, activation of JNK inhibits TNF-induced apoptosis (Ventura et al., 2004). Knockdown of focal adhesion kinase (FAK) in fibroblasts in mice and modeling of hypertrophic scar models revealed significant reductions in inflammatory responses and fibrosis in mice. In addition, JNK can induce fibrosis by stimulating the inflammatory response. This process involves the activation of AP-1 and NF-κB and the transcriptional upregulation of inflammation-related genes (Ip and Davis, 1998; Workman and Habelhah, 2013). Activation of p38 MAPK plays an important role in the differentiation of fibroblasts into myofibroblasts, and this process is regulated by mechanical signaling. p38 MAPK promotes the expression of collagen type I (Sato et al., 2002), and p38 inhibitors abrogated the upregulated expression of collagen type I in fibroblasts with SSc disease (Ihn et al., 2005; Matsushita et al., 2017).
Activation of p38 MAPK plays an important role in the differentiation of fibroblasts into myofibroblasts, and this process is regulated by mechanical signaling. Studies have shown that inhibition of p38 can downregulate the expression of myofibroblast-related genes (e.g., ED-A fibronectin) and genes encoding LOX, thereby inhibiting collagen maturation and reducing ECM. In contrast, culturing fibroblasts with p38 inhibitors inhibits their activation process (Dolivo et al., 2019). In addition, p38 transduces mechanical signaling via the transcription factor serum response factor (SRF) and phosphatase to differentiate fibroblasts (Wang et al., 2022). These effects can be replicated in several primary human dermal fibroblast cell lines. Stem cells of adipose tissue origin can inhibit hypertrophic scarring by downregulating p38 expression (Li et al., 2016a). Overall, JNKs and p38 MAPKs can exert antagonistic effects on cell proliferation and survival, depending on the type of cell, the strength of the signal, the duration of the signal, and the crosstalk between different signals (Wagner and Nebreda, 2009). JNK and p38 MAPK pathways regulate the activity and expression of key inflammatory mediators, which allows them to be potent promoters of fibrosis (Wagner and Nebreda, 2009).
A link between the p38/JNK and Smad pathways was established through the interaction between TAK1 and I-Smads (Figure 6). Smad6 inhibits TGF-β-induced activation of the TAK1-related signaling pathway, which occurs by blocking the ubiquitination of TRAF6 (Jung et al., 2013). Smad7 promotes TGF-β downstream of JNK and p38 MAPK activation (Mazars et al., 2001; Edlund et al., 2003). Specifically, Smad7 may direct TGF-β receptors to lipid rafts where TRAF6 is located, promoting TAK1 and downstream p38/JNK activation (Zhang, 2017). JNK and p38 signaling pathways have a facilitative effect on the Smad signaling pathway. In activated hepatic stellate cells, JNK in the TGF-β pathway can induce Smad2/3 phosphorylation (Yoshida et al., 2005). Similarly, p38 in rat myofibroblasts can phosphorylate Smad3 (Furukawa et al., 2003).
TGF-β has also been shown to activate AKT via PI3K and lead to EMT and cell migration. This process is not dependent on Smads (Bakin et al., 2000) (Figures 5, 6). In addition to TGFBR, AKT can also be activated by ubiquitinated TRAF6 (Yang et al., 2009). This suggests that TRAF6 can indirectly activate AKT through TGFBR activation.
The mTOR can be activated specifically by AKT. It can promote EMT and increase cell size and protein content, migration, and invasion (Lamouille and Derynck, 2007). In cancer cells, the TGF-β/PI3K/AKT pathway can activate mTOR complex 1 (mTORC1), mTOR complex 2 (mTORC2), and S6 kinase, thus promoting EMT (Lamouille et al., 2012). Considering that mTORC2 contributes to enhancing Akt activation, there is positive feedback in PI3K-AKT pathway (Lamouille et al., 2012) (Figure 6). The TGF-β/PI3K/AKT signaling pathway induces fibroblast proliferation and conversion to myofibroblasts (Wilkes et al., 2005). A complex regulatory relationship exists between AKT and Smads (Zhang, 2017).
TGF-β can activate Rho-like GTPases in a cell type-dependent manner, leading to key events in EMT, such as cell adhesion and cell migration (Mu et al., 2012). The Rho GTPase family, which includes RhoA, RhoB, Rac and Cdc42, is involved in many actin-related cellular processes to complete nuclear signaling (Phuyal and Farhan, 2019). TGF-β triggers the degradation of Rho through ligand-triggered TGFBR2 action, causing degradation of Rho, leading to direct phosphorylation of Par6 and promoting the recruitment of the ubiquitin ligase Smurf1, which targets RhoA for degradation (Zhang et al., 2004; Sutariya et al., 2016). The tumor microenvironment plays a crucial role in promoting EMT by controlling the subcellular localization and downstream signaling of Rac1/Cdc42 and Rac1b (Kalli et al., 2022). At the same time, TGF-β can activate RhoA independently of Smad2/3, leading to the activation of Rho-associated coiled-coil-containing protein kinase (ROCK) and EMT (Bhowmick et al., 2001) (Figure 6). ROCK phosphorylates myosin phosphatase, thereby inhibiting its phosphatase activity, leading to increased levels of phosphorylated myosin and induction of actomyosin-based contraction (Harvey et al., 2004).
In addition, there may have an interaction between Cdc42 and the TGF-β receptor complex (Barrios-Rodiles et al., 2005) (Figure 6). TGFBR3 is often considered to be a coreceptor that activates Cdc42 through interaction with the scaffolding protein β-arrestin2 and is presented to its signaling receptor (Mythreye and Blobe, 2009). Cdc42 can bind to p21-activated kinase 2 (PAK2) and induce PAK2 conformational changes, which lead to PAK2 activation. Furthermore, PAK2 blocks Smad2 and Smad3 activation in a kinase activity-dependent manner (Figure 6). Phosphorylation of Ser-417 on Smad2 by PAK2 inhibits the interaction of Smad2 with TGFBR1 (Yan et al., 2012).
Integrin β1 (ITGB1), also known as CD29, binds to integrins α1 and α2 to form integrin complexes, which are transmembrane collagen receptors. Within the cytoplasm, integrins bind to the actin cytoskeleton. Thus, integrins firmly attach the cytoskeleton to the ECM and promote cell adhesion.
Integrins can bind to many substrates in ECM. ITGB1 forms a heterodimer with integrin α3, which acts as a receptor for netrin-1 and reelin in the ECM. Kim and others showed that integrin α3β1 forms a complex at the apical junction with the TGFBR1 receptor and E-calmodulin. Upon TGF-β stimulation, integrin α3β1 triggers the formation of the pY654-β-catenin/pSmad2 complex and then upregulates EMT-related downstream gene expression (Kim et al., 2006; Kim et al., 2009a; Kim et al., 2009b). Numerous studies have shown that integrins αvβ3, αvβ5, αvβ6, and αvβ8 can bind to RGD sequences in LAP, thereby promoting the activation of latent TGF-β (Munger et al., 1999; Mu et al., 2002; Asano et al., 2006c; Henderson and Sheppard, 2013) (Figure 5B).
Integrins can directly participate in the downstream transduction of mechanical signals, ultimately regulating matrix remodeling, cell migration, and angiogenesis (Harburger and Calderwood, 2009). It is generally accepted that integrin α subunits are involved in extracellular to intracellular signaling, whereas β subunits are involved in intracellular to extracellular signaling (Hynes, 2002). Specifically, the process of β-subunit regulation is one in which intracellular signals modulate integrins to cause conformational changes in their extracellular structure, thereby increasing their affinity for extracellular ligands (Zhao et al., 2016). The cellular state receives regulation that can alter the affinity to the ECM and thus regulate the mechanical state (Figure 5B).
Talin, a cytoskeletal protein, is essential in integrin activation (Calderwood, 2004). Talin is concentrated in areas of cell-matrix intercontact, such as adhesive patches, and connects integrins to the actin cytoskeleton (Figure 5B). Each subunit consists of an amino-terminal head and a carboxy-terminal rod-like tail. The heads of these subunits can bind to integrins, while the tail contains an actin-binding site that binds directly to actin. Talin can be joined head to tail, creating a state of self-activated inhibition. Interestingly, integrin α and β subunits are close to each other in the quiescent state, forming a low ligand affinity conformation. Talin conformational changes are induced by PIP2 via charge action, leading to the direct binding of talin to the integrin β subunit (Wang, 2012). In the case of indirect binding, talin binds and activates PIPKIγ, which regulates the activity of vinculin and talin and ultimately leads to integrin activation, adhesive patch formation, enhanced cytoskeletal junctions, and mechanical signaling (Calderwood, 2004).
Recent studies have shown that the presence of kindlin promotes integrin activation by talin. Kindlin has a PTB structure similar to that of talin and can bind simultaneously with talin in different motifs of integrin β (Figure 5B). Inhibition of kindlin binding to integrin inhibits integrin activation, and conversely, coexpression of kindlin and talin promotes integrin activation. Specifically, all kindlin isoforms bind to the membrane distal NxxY motif in the cytoplasmic tail of the integrin β subunit via the F3 substructural domain at the PTB site, leading to conformational changes and activation of the integrin receptor (Chen et al., 2019).
Piezo1 also plays an important role in mechanical signaling. Our study showed that mechanical stretching (CMS) increased Piezo1 expression and activation in human dermal fibroblasts (HDFs) (He et al., 2021). In erythropoiesis, activation of Piezo1 leads to Ca2+ inward flow. Ca2+-dependent protein kinase C (PKC) is activated and subsequently activates the small GTPase Rap1. Rap1 is activated through the interaction of the Rap1-interacting adapter molecule (RIAM) and talin, ultimately leading to integrin activation (Aglialoro et al., 2020) (Figure 5B).
FAK, a tyrosine kinase with a binding domain with talin, can be recruited by paxillin to adhesion plaques and promote their homodimer formation (Mitra et al., 2005). The FAK Tyr397 site in the homodimer is autophosphorylated, which enhances binding to Src and a conformational change (Brami-Cherrier et al., 2014). In this FAK-Src complex, Src can transphosphorylate specific sites of FAK. Further phosphorylation of FAK exposes binding sites for Src homology 2 (SH2) domains and can further recruit Grb2 and PI3K (Mitra and Schlaepfer, 2006). Grb2 can further recruit SOS and activate the Ras/Raf/MEK/ERK signaling pathway (Figure 5B). PI3K binding to Src can activate the PI3K/AKT signaling pathway (Bolós et al., 2010) (Figure 5B). In addition, FAK regulates Rho GTPase activity, which leads to cytoskeletal remodeling and mediates directional cell movement (Tomar and Schlaepfer, 2009). FAK increases the expression of cellular myogenic markers and epithelial cell migration through the small G protein Cdc42 (Han et al., 2011; Wen et al., 2022). These signaling pathways are also present in TGF-β signaling (Figure 6). G proteins and small G proteins regulate FAK activity. Protein-activated receptor 1 (PAR1) and sphingosine-1-phosphate receptor 1 (S1P1) can upregulate FAK via downstream G protein activity (Thennes and Mehta, 2012).
FAK inhibitors reduce bleomycin-induced pulmonary fibrosis in mice (Zhao et al., 2016). Gurtner’s team showed that physical forces regulate fibrosis through the inflammatory FAK/ERK/MCP-1 pathway and that targeted inhibition of FAK inhibits mechanical force-induced hypertrophic scar formation (Wong et al., 2012).
Integrin-linked kinase (ILK) is another key node in integrin signaling (Legate et al., 2006). ILK, which is believed to be a pseudokinase, cannot phosphorylate any substrate directly (Boudeau et al., 2006; Byrne et al., 2017). ILK, PINCH, and parvin form a heterotrimeric complex, ILK-PINCH-PARVA (IPP). IPP is recruited to the adherent patch and acts as a hub for the integrin signaling network (Figure 5B). The complex connects the ECM to the actin cytoskeleton and protects ILK from proteasomal degradation (Legate et al., 2006). The ILK pathway is extensively involved in regulatory processes such as cell adhesion, apoptosis, proliferation, and migration (Martucci et al., 2021). In cancer, ILK promotes EMT and enhances the migration and invasion of cancer cells (McDonald and Dedhar, 2022). These cellular metabolism and behavior changes are facilitated by the activation of downstream signaling pathways such as AKT, Hippo, Wnt, and GSK-3β (Martucci et al., 2021). ILK can promote the transition from fibroblasts to myofibroblasts in the dermis with the transcription factor Snail1/Slug (Zoppi et al., 2018). The ILK-PI3K/AKT pathway regulates fibroblast migration (Li et al., 2016b).
The Hippo pathway is an evolutionarily highly conserved signaling pathway that plays key roles in organ development, epithelial homeostasis, tissue regeneration, wound healing, and immune regulation (Dey et al., 2020). Many of these actions are mediated by the transcriptional effectors YAP and TAZ. The NDR (nuclear Dbf2-related) kinase family members LATS1 (large tumour suppressor 1) and LATS2 (large tumour suppressor 2) are major regulators of YAP/TAZ. Many upstream proteins are involved in the regulation of LATS activity. These include the integrins mentioned earlier and TGFβ-related signaling proteins such as ILK, FAK-Src, and Rho (Piersma et al., 2015; Dey et al., 2020) (Figure 5B). In addition, YAP/TAZ can directly transduce mechanical signals of the ECM independently of the Hippo/LATS cascade (Figure 5B). This process requires the involvement of Rho GTPase and the tension of the actin cytoskeleton (Dupont et al., 2011). Notably, increased ECM stiffness can reduce DNA methylation in the promoter region of YAP, suggesting an epigenetic role of mechanical signaling in cellular alterations (Jang et al., 2021). YAP/TAZ can regulate the expression of profibrotic genes through TEAD transcription factors, specifically CTGF, PAI-1, and LOX (Dey et al., 2020). A clear and complete description of the Hippo/YAP signaling network was previously reviewed by Rognoni and Walko (Rognoni and Walko, 2019).
Skin fibrosis and scar formation are common outcomes of surgery, pathological injury and some diseases. They are characterized by myofibroblast proliferation and ECM deposition that result in mechanical stiffness modulation. Basic and clinical research has shown that a variety of treatments are available for patients with skin fibrosis and scar formation, including surgical, drug and combination treatments. Although some existing drugs have been preliminary proved the efficiency in skin fibrosis, there is still a long way to develop a satisfied therapy (Kothari et al., 2019). Targeted therapies can significantly reduce side effects and improve the efficacy of treatment. With the in-depth study of the molecular mechanisms of skin fibrosis, molecular targeted therapy will become the key direction of skin fibrosis. The inhibition of TGF-β and integrin pathways are important in targeted therapies. Upregulation of MMP expression or activity is also a valuable direction. In addition, new therapeutic approaches, such as RNA and stem cell transplantation, have emerged.
As previously mentioned, TGF-β, a profibrotic factor controlled by mechanical stress and inflammatory factors, plays an important role in the physiopathological events of skin fibrosis and has become a central target for pharmacological intervention (Batlle and Massagué, 2019). TGF-β1 overexpression in keratin-forming cells induces skin inflammation, and significant epidermal hyperplasia occurs. The TGF-β1/Smad3 signaling pathway is activated during skin photoaging and induces the production of MMPs, leading to increased collagen type I and ECM deposition (Fisher et al., 2016). Recessive dystrophic epidermolysis bullosa (RDEB) is an inherited skin disease characterized by increased mechanical fragility of the skin. A comprehensive gene expression study by Knaup showed that untransformed RDEB keratinocytes also display elevated levels of TGF-β1, which affects the expression levels of collagen type VII (Knaup et al., 2011). Patients with SSc have extensive skin fibrosis and visceral organ involvement, for which the TGF-β signaling pathway is often thought to be the main mechanism (Verrecchia et al., 2006; Sargent et al., 2010). In SSc patients, TGF-β promotes myofibroblast differentiation by stimulating the expansion of KLRG1-ILC2s (natural ILC2s) and reducing IL10 production. Thus, TGF-β signaling pathway is an important therapeutic target in skin fibrosis.
Targeted ablation of TGFBR2 in mice induces the overproliferation of keratin-forming cells induced by external factors (Guasch et al., 2007). Han’s study demonstrated that the degree of skin inflammation was associated with TGF-β1 expression, and Enbrel and Rosiglitazone, an effective treatment for human psoriasis, can effectively alleviate skin inflammation (Han et al., 2010). The application of pirfenidone and IL10 combination therapy significantly reduced skin fibrosis in the SSc mouse model (Laurent et al., 2021). Rituximab (RTX) may improve skin and lung fibrosis in SSc patients via the TGF-β-Dkk-1 axis (Daoussis et al., 2016). Additionally, overexpression of TGF-β at different sites may have different effects. Acute induction of TGF-β1 overexpression in the suprabasal layer of the epidermis reduced epidermal overproliferation in the skin, whereas sustained induction of TGF-β1 overexpression in the basal layer did not cause significant changes in the epidermis (Wang et al., 1999). TGF-β can stimulate cancer-associated fibroblasts (CAFs) to play an important role in regulating the tumor ECM (Chen and Song, 2019). In addition, the treatment of fibrosis in other tissues may also inform the treatment of skin fibrosis. The small-molecule inhibitor pirfenidone has been approved for the treatment of pulmonary fibrotic disease (Behr et al., 2021). In lung fibroblasts, pirfenidone can significantly inhibit TGF-β1-stimulated fibroblast-mediated collagen gel contraction, migration, and collagen triple helix repeat containing protein 1(CTHRC1) release. CTHRC1 is able to activate fibroblasts and recruit M2 macrophages (Jin et al., 2019). Buserelin and oleuropein have been shown to reduce fibrosis in the lung and kidney in mouse models (Akhurst and Hata, 2012). However, their use in cutaneous fibrosis is still being explored.
Oxidative stress is influenced by TGF-β, which has shown a large role in the pathogenesis and treatment of dermal fibrosis. TGF-β activates several oxidative stress-related genes involved in the profibrotic pathway, acting through Smad and non-Smad pathways to enhance fibroblast recruitment and differentiation, leading to ECM deposition (Akhurst, 2012; Liu et al., 2012). A study of dermal fibroblasts from patients with scleroderma showed that following the application of antioxidants with oxidative stress inhibition, ERK1-2 and NF-kB activity reduced ECM deposition (Shroff et al., 2014). Similarly, simvastatin, arsenic trioxide and (PHTE) (Do and Eming, 2016)NQ have been shown in preliminary experiments to prevent skin fibrosis (Kavian et al., 2012; Bagnato et al., 2013). MMP exposure can also increase oxidative stress and stimulate fibroblast differentiation (Radisky et al., 2007). In summary, Oxidative stress is a potential target in skin fibrosis treatment.
Targeting the expression of genes downstream of the TGF-β1 signaling pathway, such as p53 and Smad3 and TGF-β1 oxidative stress-dependent genes, has also emerged as a therapeutic option for skin fibrosis (Samarakoon et al., 2013). Ebselenolide and enthesopine have been shown to reduce fibrosis in the lung and kidney in a mouse model (Shroff et al., 2014). In irradiated mice, exogenous interleukin 1β (IL1B) induces IL1B mRNA expression and rapidly increases MMPs but fails to reduce IL1-associated collagen accumulation (Liu et al., 2006).
MMP acts directly on the ECM and is regulated by mechanical stress and inflammatory factors involved in the proliferation of the ECM. Therapeutic studies targeting MMP have demonstrated its effectiveness to some extent. It has been shown that expression of MMP activity during wound healing can inhibit scar formation and may reduce fibrosis during healing. In most cases, MMP shows profibrotic activity, and MMP inhibitors may potentially treat fibrosis (Radisky et al., 2007). Core proteoglycans can treat pathological skin fibrosis by upregulating the expression of MMP-1 and MMP-3 mRNAs and decreasing the expression of collagen type I and type III mRNAs (Lee et al., 2015). After treatment with bone marrow mesenchymal stem cell (BM-MSC) transplantation, lesioned skin showed decreases in TGF-β1 and α-smooth muscle actin-positive cells, increased MMP expression, attenuated myofibroblast proliferation and ECM deposition, and collagen alignment similar to that of normal skin (Gourdie et al., 2006; Wu et al., 2014). MMP activation reduces excessive collagen deposition and subsequent scar formation to treat skin fibrotic disease (El Ayadi et al., 2020).
However, excessive activation of MMPs can lead to excessive hydrolysis of collagen, which can lead to safety risks such as bleeding, organ perforation or rupture. This requires special attention in the antifibrotic treatment of organs such as the lungs, liver, kidneys and intestines. In contrast, the skin, the largest organ in the body, has high regenerative and compensatory capacities, providing a wider margin of safety for the use of MMPs to degrade the overproduced ECM in fibrosis areas. Even when extreme cases are considered, the excessive degradation of the ECM in areas of skin fibrosis could not pose a risk to the patient’s life.
There is now a new understanding of the role of RNA in skin fibrosis. miRNAs are a group of small non-coding RNAs involved in skin fibrosis, including transforming growth factor-β signaling, ECM deposition, fibroblast proliferation and differentiation. There are both pro- and antifibrotic miRNAs. Antifibrotic miRNAs can be upregulated in treating skin fibrosis using mimetics and viral vectors. Conversely, profibrotic miRNAs can be downregulated using anti-miRNAs (Babalola et al., 2013). Since miR-486-3p mediates the overexpression of K17 protein under the regulation of the TGF-β/Smad pathway, thereby inducing glial cell proliferation, activating miR-486-3p may be a potential therapeutic approach for psoriasis (Jiang et al., 2017).
Although the molecular mechanisms of skin fibrosis have been extensively studied, the in vivo situation is complicated by the interplay of signaling networks, genetic-environmental interactions and variations in individual susceptibility. This makes it challenging to obtain similar results in clinical trials, and ultimately, only a small proportion can be successfully translated into therapeutic approaches. Interactions between different systemic signals have received increasing attention in the pathogenesis and progression of the disease. New research areas have been proposed to study mechano-immune and neuro-mechanical signaling networks. Exploring signaling cross-talk from the perspective of these areas may lead to discoveries in the pathogenesis of cutaneous fibrosis. To better mimic the in vivo microenvironment, three-dimensional cell cultures are now often used for in vitro studies of biomechanical mechanisms. However, the matrix materials currently available for cell culture may be cytotoxic and have limitations in terms of their mechanical properties. Material improvements for three-dimensional cell culture or the development of novel matrix materials may contribute to breakthroughs in biomechanical studies of fibrosis.
ECM stiffness, a central cue in the skin fibrosis process, may be an important factor to focus on in targeted therapies for the treatment of skin fibrosis. Compared to other organs, multiple delivery methods such as local injection and gel application can be easier to use in skin tissue and have a wider safety margin. Studies have demonstrated that reducing ECM stiffness by inhibiting the cross-linking of ECM structural proteins or causing appropriate hydrolysis of ECM can improve skin scarring. Targeted inhibition of the signaling pathways of TGF-β is also a valuable treatment therapy. Recently, a broader view of biological events, such as epigenetic and post-transcriptional regulation, may contribute to a deeper understanding of the mechanisms of skin fibrosis.
Figures were created with the authorization of QmlvcmVuZGVyLmNvbQ==.
All authors listed have made a substantial, direct, and intellectual contribution to the work and approved it for publication.
This study was supported by grants from National Natural Science Foundation of China (No. 82202449 to YG), National Natural Science Foundation of China (No. 81901963 to YZ), Shanghai “Rising Stars of Medical Talent” Youth Development Program (YZ), grants from the Shanghai Clinical Research Center of Plastic and Reconstructive Surgery supported by Science and Technology Commission of Shanghai Municipality (No. 22MC1940300 to QL), Shanghai Municipal Key Clinical Specialty (No. shslczdzk00901 to QL), Innovative research team of high-level local universities in Shanghai (No. SHSMU- ZDCX20210400 to QL), Young Physician Innovation Team Project of Shanghai Ninth People’s Hospital, Shanghai Jiao Tong university School of Medicine (No. QC202001 to YZ), The Fund for Excellent Young Scholars of Shanghai Ninth People’s Hospital, Shanghai JiaoTong University School of Medicine (No. JYYQ006 to YZ).
We are grateful to members of our laboratories for their input and discussions.
The authors declare that the research was conducted in the absence of any commercial or financial relationships that could be construed as a potential conflict of interest.
All claims expressed in this article are solely those of the authors and do not necessarily represent those of their affiliated organizations, or those of the publisher, the editors and the reviewers. Any product that may be evaluated in this article, or claim that may be made by its manufacturer, is not guaranteed or endorsed by the publisher.
Abyaneh, M-A. Y., Griffith, R., Falto-Aizpurua, L., and Nouri, K. (2014). Famous lines in history: Langer lines. JAMA Dermatol. 150 (10), 1087. doi:10.1001/jamadermatol.2014.659
Adair-Kirk, T. L., and Senior, R. M. (2008). Fragments of extracellular matrix as mediators of inflammation. Int. J. Biochem. Cell Biol. 40 (6-7), 1101–1110. doi:10.1016/j.biocel.2007.12.005
Adhikari, A., Xu, M., and Chen, Z. J. (2007). Ubiquitin-mediated activation of TAK1 and IKK. Oncogene 26 (22), 3214–3226. doi:10.1038/sj.onc.1210413
Aglialoro, F., Hofsink, N., Hofman, M., Brandhorst, N., and van den Akker, E. (2020). Inside out integrin activation mediated by PIEZO1 signaling in erythroblasts. Front. Physiology 11, 958. doi:10.3389/fphys.2020.00958
Akhurst, R. J., and Hata, A. (2012). Targeting the TGFβ signalling pathway in disease. Nat. Rev. Drug Discov. 11 (10), 790–811. doi:10.1038/nrd3810
Akhurst, R. J. (2012). The paradoxical TGF-β vasculopathies. Nat. Genet. 44 (8), 838–839. doi:10.1038/ng.2366
Arango Duque, G., and Descoteaux, A. (2014). Macrophage cytokines: Involvement in immunity and infectious diseases. Front. Immunol. 5, 491. doi:10.3389/fimmu.2014.00491
Arron, J. R. (2021). Biomarkers in systemic sclerosis: Mechanistic insights into pathogenesis and treatment. Curr. Opin. Rheumatol. 33 (6), 480–485. doi:10.1097/BOR.0000000000000827
Asano, Y., Ihn, H., Jinnin, M., Mimura, Y., and Tamaki, K. (2006). Involvement of alphavbeta5 integrin in the establishment of autocrine TGF-beta signaling in dermal fibroblasts derived from localized scleroderma. J. Invest Dermatol 126 (8), 1761–1769. doi:10.1038/sj.jid.5700331
Asano, Y., Ihn, H., Yamane, K., Jinnin, M., and Tamaki, K. (2006). Increased expression of integrin alphavbeta5 induces the myofibroblastic differentiation of dermal fibroblasts. Am. J. Pathol. 168 (2), 499–510. doi:10.2353/ajpath.2006.041306
Asano, Y., Ihn, H., Yamane, K., Jinnin, M., and Tamaki, K. (2006). Increased expression of integrin alphavbeta5 induces the myofibroblastic differentiation of dermal fibroblasts. Am. J. Pathology 168 (2), 499–510. doi:10.2353/ajpath.2006.041306
Babalola, O., Mamalis, A., Lev-Tov, H., and Jagdeo, J. (2013). The role of microRNAs in skin fibrosis. Arch. Dermatol Res. 305 (9), 763–776. doi:10.1007/s00403-013-1410-1
Bader, B. L., Rayburn, H., Crowley, D., and Hynes, R. O. (1998). Extensive vasculogenesis, angiogenesis, and organogenesis precede lethality in mice lacking all alpha v integrins. Cell. 95 (4), 507–519. doi:10.1016/s0092-8674(00)81618-9
Bagabir, R., Syed, F., Shenjere, P., Paus, R., and Bayat, A. (2016). Identification of a potential molecular diagnostic biomarker in keloid disease: Syndecan-1 (CD138) is overexpressed in keloid scar tissue. J. Investigative Dermatology 136.
Bagnato, G., Bitto, A., Irrera, N., Pizzino, G., Sangari, D., Cinquegrani, M., et al. (2013). Propylthiouracil prevents cutaneous and pulmonary fibrosis in the reactive oxygen species murine model of systemic sclerosis. Arthritis Res. Ther. 15 (5), R120. doi:10.1186/ar4300
Bakin, A. V., Tomlinson, A. K., Bhowmick, N. A., Moses, H. L., and Arteaga, C. L. (2000). Phosphatidylinositol 3-kinase function is required for transforming growth factor beta-mediated epithelial to mesenchymal transition and cell migration. J. Biol. Chem. 275 (47), 36803–36810. doi:10.1074/jbc.M005912200
Baroni, A., Buommino, E., De Gregorio, V., Ruocco, E., Ruocco, V., and Wolf, R. (2012). Structure and function of the epidermis related to barrier properties. Clin. Dermatology 30 (3), 257–262. doi:10.1016/j.clindermatol.2011.08.007
Barrios-Rodiles, M., Brown, K. R., Ozdamar, B., Bose, R., Liu, Z., Donovan, R. S., et al. (2005). High-throughput mapping of a dynamic signaling network in mammalian cells. Science 307 (5715), 1621–1625. doi:10.1126/science.1105776
Bashour, K. T., Gondarenko, A., Chen, H., Shen, K., Liu, X., Huse, M., et al. (2014). CD28 and CD3 have complementary roles in T-cell traction forces. Proc. Natl. Acad. Sci. U. S. A. 111 (6), 2241–2246. doi:10.1073/pnas.1315606111
Batlle, E., and Massagué, J. (2019). Transforming growth factor-β signaling in immunity and cancer. Immunity 50 (4), 924–940. doi:10.1016/j.immuni.2019.03.024
Bayat, A., McGrouther, D. A., and Ferguson, M. W. J. (2003). Skin scarringed. BMJ 326(7380), 88–92. doi:10.1136/bmj.326.7380.88
Behr, J., Prasse, A., Kreuter, M., Johow, J., Rabe, K. F., Bonella, F., et al. (2021). Pirfenidone in patients with progressive fibrotic interstitial lung diseases other than idiopathic pulmonary fibrosis (RELIEF): A double-blind, randomised, placebo-controlled, phase 2b trial. Lancet Respir. Med. 9 (5), 476–486. doi:10.1016/S2213-2600(20)30554-3
Behrens, D. T., Villone, D., Koch, M., Brunner, G., Sorokin, L., Robenek, H., et al. (2012). The epidermal basement membrane is a composite of separate laminin- or collagen IV-containing networks connected by aggregated perlecan, but not by nidogens*. J. Biol. Chem. 287 (22), 18700–18709. doi:10.1074/jbc.M111.336073
Bhattacharjee, O., Ayyangar, U., Kurbet, A. S., Ashok, D., and Raghavan, S. (2019). Unraveling the ECM-immune cell crosstalk in skin diseases. Front. Cell Dev. Biol. 7, 68. doi:10.3389/fcell.2019.00068
Bhowmick, N. A., Ghiassi, M., Bakin, A., Aakre, M., Lundquist, C. A., Engel, M. E., et al. (2001). Transforming growth factor-beta1 mediates epithelial to mesenchymal transdifferentiation through a RhoA-dependent mechanism. Mol. Biol. Cell. 12 (1), 27–36. doi:10.1091/mbc.12.1.27
Biasin, V., Marsh, L. M., Egemnazarov, B., Wilhelm, J., Ghanim, B., Klepetko, W., et al. (2014). Meprin β, a novel mediator of vascular remodelling underlying pulmonary hypertension. J. Pathol. 233 (1), 7–17. doi:10.1002/path.4303
Bilandzic, M., and Stenvers, K. L. (2011). Betaglycan: A multifunctional accessory. Mol. Cell Endocrinol. 339 (1-2), 180–189. doi:10.1016/j.mce.2011.04.014
Bock, O., Schmid-Ott, G., Malewski, P., and Mrowietz, U. (2006). Quality of life of patients with keloid and hypertrophic scarring. Arch. Dermatol Res. 297 (10), 433–438. doi:10.1007/s00403-006-0651-7
Bolognia, J. L., Jorizzo, J. L., and Rapini, R. P. (2007). Dermatology: 2-volume set. St Louis, MO: Mosby.
Bolós, V., Gasent, J. M., López-Tarruella, S., and Grande, E. (2010). The dual kinase complex FAK-Src as a promising therapeutic target in cancer. Onco Targets Ther. 3, 83–97. doi:10.2147/ott.s6909
Boudeau, J., Miranda-Saavedra, D., Barton, G. J., and Alessi, D. R. (2006). Emerging roles of pseudokinases. Trends Cell Biol. 16 (9), 443–452. doi:10.1016/j.tcb.2006.07.003
Bourhis, J-M., Mariano, N., Zhao, Y., Harlos, K., Exposito, J-Y., Jones, E. Y., et al. (2012). Structural basis of fibrillar collagen trimerization and related genetic disorders. Nat. Struct. Mol. Biol. 19 (10), 1031–1036. doi:10.1038/nsmb.2389
Brabletz, S., and Brabletz, T. (2010). The ZEB/miR-200 feedback loop–a motor of cellular plasticity in development and cancer? EMBO Rep. 11 (9), 670–677. doi:10.1038/embor.2010.117
Braga, T. T., Agudelo, J. S. H., and Camara, N. O. S. (2015). Macrophages during the fibrotic process: M2 as friend and foe. Front. Immunol. 6, 602. doi:10.3389/fimmu.2015.00602
Brami-Cherrier, K., Gervasi, N., Arsenieva, D., Walkiewicz, K., Boutterin, M-C., Ortega, A., et al. (2014). FAK dimerization controls its kinase-dependent functions at focal adhesions. EMBO J. 33 (4), 356–370. doi:10.1002/embj.201386399
Broder, C., Arnold, P., Vadon-Le Goff, S., Konerding, M. A., Bahr, K., Müller, S., et al. (2013). Metalloproteases meprin α and meprin β are C- and N-procollagen proteinases important for collagen assembly and tensile strength. Proc. Natl. Acad. Sci. U. S. A. 110 (35), 14219–14224. doi:10.1073/pnas.1305464110
Brown, K. W., and Parkinson, E. K. (1983). Glycoproteins and glycosaminoglycans of cultured normal human epidermal keratinocytes. J. Cell Sci. 61, 325–338. doi:10.1242/jcs.61.1.325
Brown, M., and O’Reilly, S. (2019). The immunopathogenesis of fibrosis in systemic sclerosis. Clin. Exp. Immunol. 195 (3), 310–321. doi:10.1111/cei.13238
Budi, E. H., Duan, D., and Derynck, D. (2017). Transforming growth factor-β receptors and Smads: Regulatory complexity and functional versatility. Trends Cell Biol. 27 (9), 658–672. doi:10.1016/j.tcb.2017.04.005
Burch, M. L., Yang, S. N., Ballinger, M. L., Getachew, R., Osman, N., and Little, P. J. (2010). TGF-beta stimulates biglycan synthesis via p38 and ERK phosphorylation of the linker region of Smad2. Cell Mol. Life Sci. 67 (12), 2077–2090. doi:10.1007/s00018-010-0315-9
Bush, J., Ferguson, M. W. J., Mason, T., and McGrouther, G. (2007). The dynamic rotation of Langer's lines on facial expression. Reconstr. Aesthetic Surg. 60 (4), 393–399. doi:10.1016/j.bjps.2006.06.008
Byrne, D. P., Foulkes, D. M., and Eyers, P. A. (2017). Pseudokinases: Update on their functions and evaluation as new drug targets. Future Med. Chem. 9 (2), 245–265. doi:10.4155/fmc-2016-0207
Chermnykh, E., Kalabusheva, E., and Vorotelyak, E. (2018). Extracellular matrix as a regulator of epidermal stem cell fate. Int. J. Mol. Sci. 19 (4), 1003. doi:10.3390/ijms19041003
Candi, E., Schmidt, R., and Melino, G. (2005). The cornified envelope: A model of cell death in the skin. Nat. Rev. Mol. Cell Biol. 6 (4), 328–340. doi:10.1038/nrm1619
Cao, Q., Wang, Y., and Harris, D. C. H. (2014). Macrophage heterogeneity, phenotypes, and roles in renal fibrosis. Kidney Int. Suppl. 4 (1), 16–19. doi:10.1038/kisup.2014.4
Carmichael, S. W. (2014). The tangled web of Langer's lines. Clin. Anat. 27 (2), 162–168. doi:10.1002/ca.22278
Casale, C., Imparato, G., Mazio, C., Netti, P. A., and Urciuolo, F. (2021). Geometrical confinement controls cell, ECM and vascular network alignment during the morphogenesis of 3D bioengineered human connective tissues. Acta Biomater. 131, 341–354. doi:10.1016/j.actbio.2021.06.022
Chacón-Solano, E., León, C., Carretero, M., García, M., Sánchez-Domínguez, R., Quero, F., et al. (2022). Mechanistic interrogation of mutation-independent disease modulators of RDEB identifies the small leucine-rich proteoglycan PRELP as a TGF-β antagonist and inhibitor of fibrosis. Matrix Biol. 111, 189–206. doi:10.1016/j.matbio.2022.06.007
Chakraborty, M., Chu, K., Shrestha, A., Revelo, X. S., Zhang, X., Gold, M. J., et al. (2021). Mechanical stiffness controls dendritic cell metabolism and function. Cell Rep. 34 (2), 108609. doi:10.1016/j.celrep.2020.108609
Chen, C., Manso, A. M., and Ross, R. S. (2019). Talin and kindlin as integrin-activating proteins: Focus on the heart. Pediatr. Cardiol. 40 (7), 1401–1409. doi:10.1007/s00246-019-02167-3
Chen, M., Zhang, Y., Zhou, P., Liu, X., Zhao, H., Zhou, X., et al. (2020). Substrate stiffness modulates bone marrow-derived macrophage polarization through NF-κB signaling pathway. Bioact. Mater 5 (4), 880–890. doi:10.1016/j.bioactmat.2020.05.004
Chen, X., and Song, E. (2019). Turning foes to friends: Targeting cancer-associated fibroblasts. Nat. Rev. Drug Discov. 18 (2), 99–115. doi:10.1038/s41573-018-0004-1
Cheng, Z., Limbu, M. H., Wang, Z., Liu, J., Liu, L., Zhang, X., et al. (2017). MMP-2 and 9 in chronic kidney disease. Int. J. Mol. Sci. 18, 776. doi:10.3390/ijms18040776
Chowdhury, S. R., Aminuddin, B. S., and Ruszymah, B. H. I. (2012). Effect of supplementation of dermal fibroblasts conditioned medium on expansion of keratinocytes through enhancing attachment. Indian J. Exp. Biol. 50 (5), 332–339.
Chung, L., and Utz, P. J. (2004). Antibodies in scleroderma: Direct pathogenicity and phenotypic associations. Curr. Rheumatol. Rep. 6 (2), 156–163. doi:10.1007/s11926-004-0061-9
Clarke, D. L., Carruthers, A. M., Mustelin, T., and Murray, L. A. (2013). Matrix regulation of idiopathic pulmonary fibrosis: The role of enzymes. Fibrogenesis Tissue Repair 6 (1), 20. doi:10.1186/1755-1536-6-20
Coentro, J. Q., Pugliese, E., Hanley, G., Raghunath, M., and Zeugolis, D. I. (2019). Current and upcoming therapies to modulate skin scarring and fibrosis. Adv. Drug Deliv. Rev. 146, 37–59. doi:10.1016/j.addr.2018.08.009
Cork, M. (1997). The importance of skin barrier function. J. Dermatological Treat. 8 (1), S7–S13. doi:10.3109/09546639709160948
Daoussis, D., Tsamandas, A., Antonopoulos, I., Filippopoulou, A., Papachristou, D. J., Papachristou, N. I., et al. (2016). B cell depletion therapy upregulates dkk-1 skin expression in patients with systemic sclerosis: Association with enhanced resolution of skin fibrosis. Arthritis Res. Ther. 18 (1), 118. doi:10.1186/s13075-016-1017-y
Deng, C-C., Hu, Y-F., Zhu, D-H., Cheng, Q., Gu, J-J., Feng, Q-L., et al. (2021). Single-cell RNA-seq reveals fibroblast heterogeneity and increased mesenchymal fibroblasts in human fibrotic skin diseases. Nat. Commun. 12 (1), 3709. doi:10.1038/s41467-021-24110-y
Dengjel, J., Bruckner-Tuderman, L., and Nyström, A. (2020). Skin proteomics - analysis of the extracellular matrix in health and disease. Expert Rev. Proteomics 17 (5), 377–391. doi:10.1080/14789450.2020.1773261
Dennler, S., Itoh, S., Vivien, D., ten Dijke, P., Huet, S., and Gauthier, J-M. (1998). Direct binding of Smad3 and Smad4 to critical TGFβ-inducible elements in the promoter of human plasminogen activator inhibitor-type 1 gene. EMBO J. 17 (11), 3091–3100. doi:10.1093/emboj/17.11.3091
Derynck, R., Muthusamy, B. P., and Saeteurn, K. Y. (2014). Signaling pathway cooperation in TGF-β-induced epithelial-mesenchymal transition. Curr. Opin. Cell Biol. 31, 56–66. doi:10.1016/j.ceb.2014.09.001
Derynck, R., and Zhang, Y. E. (2003). Smad-dependent and Smad-independent pathways in TGF-beta family signalling. Nature 425 (6958), 577–584. doi:10.1038/nature02006
Dey, A., Varelas, X., and Guan, K-L. (2020). Targeting the Hippo pathway in cancer, fibrosis, wound healing and regenerative medicine. Nat. Rev. Drug Discov. 19 (7), 480–494. doi:10.1038/s41573-020-0070-z
Do, N. N., and Eming, S. A. (2016). Skin fibrosis: Models and mechanisms. Curr. Res. Transl. Med. 64 (4), 185–193. doi:10.1016/j.retram.2016.06.003
Dolivo, D. M., Larson, S. A., and Dominko, T. (2019). Crosstalk between mitogen-activated protein kinase inhibitors and transforming growth factor-β signaling results in variable activation of human dermal fibroblasts. Int. J. Mol. Med. 43 (1), 325–335. doi:10.3892/ijmm.2018.3949
Driskell, R. R., and Watt, F. M. (2015). Understanding fibroblast heterogeneity in the skin. Trends Cell Biol. 25 (2), 92–99. doi:10.1016/j.tcb.2014.10.001
Du, X., Wen, J., Wang, Y., Karmaus, P. W. F., Khatamian, A., Tan, H., et al. (2018). Hippo/Mst signalling couples metabolic state and immune function of CD8α+ dendritic cells. Nature 558 (7708), 141–145. doi:10.1038/s41586-018-0177-0
Dupont, S., Morsut, L., Aragona, M., Enzo, E., Giulitti, S., Cordenonsi, M., et al. (2011). Role of YAP/TAZ in mechanotransduction. Nature 474 (7350), 179–183. doi:10.1038/nature10137
Edlund, S., Bu, S., Schuster, N., Aspenström, P., Heuchel, R., Heldin, N-E., et al. (2003). Transforming growth factor-beta1 (TGF-beta)-induced apoptosis of prostate cancer cells involves Smad7-dependent activation of p38 by TGF-beta-activated kinase 1 and mitogen-activated protein kinase kinase 3. Mol. Biol. Cell. 14 (2), 529–544. doi:10.1091/mbc.02-03-0037
Edwards, D. R., Murphy, G., Reynolds, J. J., Whitham, S. E., Docherty, A. J., Angel, P., et al. (1987). Transforming growth factor beta modulates the expression of collagenase and metalloproteinase inhibitor. EMBO J. 6 (7), 1899–1904. doi:10.1002/j.1460-2075.1987.tb02449.x
El Agha, E., Kramann, R., Schneider, R. K., Li, X., Seeger, W., Humphreys, B. D., et al. (2017). Mesenchymal stem cells in fibrotic disease. Cell Stem Cell. 21 (2), 166–177. doi:10.1016/j.stem.2017.07.011
El Ayadi, A., Jay, J. W., and Prasai, A. (2020). Current approaches targeting the wound healing phases to attenuate fibrosis and scarring. Int. J. Mol. Sci. 21 (3), 1105. doi:10.3390/ijms21031105
El Ghalbzouri, A., and Ponec, M. (2004). Diffusible factors released by fibroblasts support epidermal morphogenesis and deposition of basement membrane components. Wound Repair Regen. 12 (3), 359–367. doi:10.1111/j.1067-1927.2004.012306.x
Elder, G. H. (1998). Porphyria cutanea tarda. Semin. Liver Dis. 18 (1), 67–75. doi:10.1055/s-2007-1007142
Elson, E. L., Qian, H., Fee, J. A., and Wakatsuki, T. (2019). A model for positive feedback control of the transformation of fibroblasts to myofibroblasts. Prog. Biophysics Mol. Biol. 144, 30–40. doi:10.1016/j.pbiomolbio.2018.08.004
Engler, A. J., Sen, S., Sweeney, H. L., and Discher, D. E. (2006). Matrix elasticity directs stem cell lineage specification. Cell. 126 (4), 677–689. doi:10.1016/j.cell.2006.06.044
Fang, J. Y., and Richardson, B. C. (2005). The MAPK signalling pathways and colorectal cancer. Lancet Oncol. 6 (5), 322–327. doi:10.1016/S1470-2045(05)70168-6
Fathke, C., Wilson, L., Hutter, J., Kapoor, V., Smith, A., Hocking, A., et al. (2004). Contribution of bone marrow–derived cells to skin: Collagen deposition and wound repair. Stem Cells 22 (5), 812–822. doi:10.1634/stemcells.22-5-812
Feng, Y., Sun, Z-L., Liu, S-Y., Wu, J-J., Zhao, B-H., Lv, G-Z., et al. (2019). Direct and indirect roles of macrophages in hypertrophic scar formation. Front. Physiology 10, 1101. doi:10.3389/fphys.2019.01101
Fernandez, T. L., Van Lonkhuyzen, D. R., Dawson, R. A., Kimlin, M. G., and Upton, Z. (2014). In vitro investigations on the effect of dermal fibroblasts on keratinocyte responses to ultraviolet B radiation. Photochem Photobiol. 90 (6), 1332–1339. doi:10.1111/php.12317
Fett, N. (2013). Scleroderma: Nomenclature, etiology, pathogenesis, prognosis, and treatments: Facts and controversies. Clin. Dermatol 31 (4), 432–437. doi:10.1016/j.clindermatol.2013.01.010
Fisher, G. J., Shao, Y., He, T., Qin, Z., Perry, D., Voorhees, J. J., et al. (2016). Reduction of fibroblast size/mechanical force down-regulates TGF-β type II receptor: Implications for human skin aging. Aging Cell. 15 (1), 67–76. doi:10.1111/acel.12410
Flanders, K. C. (2004). Smad3 as a mediator of the fibrotic response. Int. J. Exp. Pathol. 85 (2), 47–64. doi:10.1111/j.0959-9673.2004.00377.x
Frangogiannis, N. (2020). Transforming growth factor-β in tissue fibrosis. J. Exp. Med. 217 (3), e20190103. doi:10.1084/jem.20190103
Fuchs, E., and Raghavan, S. (2002). Getting under the skin of epidermal morphogenesis. Nat. Rev. Genet. 3 (3), 199–209. doi:10.1038/nrg758
Furukawa, F., Matsuzaki, K., Mori, S., Tahashi, Y., Yoshida, K., Sugano, Y., et al. (2003). p38 MAPK mediates fibrogenic signal through Smad3 phosphorylation in rat myofibroblasts. Hepatol. Baltim. Md) 38 (4), 879–889. doi:10.1053/jhep.2003.50384
Giebeler, N., and Zigrino, P. (2016). A disintegrin and metalloprotease (ADAM): Historical overview of their functions. Toxins (Basel) 8 (4), 122. doi:10.3390/toxins8040122
Gourdie, R. G., Ghatnekar, G. S., O'Quinn, M., Rhett, M. J., Barker, R. J., Zhu, C., et al. (2006). The unstoppable connexin43 carboxyl-terminus: New roles in gap junction organization and wound healing. Ann. N. Y. Acad. Sci. 1080, 49–62. doi:10.1196/annals.1380.005
Grässel, S., and Bauer, R. J. (2013). Collagen XVI in health and disease. Matrix Biol. 32 (2), 64–73. doi:10.1016/j.matbio.2012.11.001
Griffin, M. F., Mascharak, S., Jardins, H. E., Mascharak, S., Borrelli, M. R., and Longaker, M. T. (2020). Understanding the impact of fibroblast heterogeneity on skin fibrosis. Dis. Models Mech. 13, dmm044164. doi:10.1242/dmm.044164
Guasch, G., Schober, M., Pasolli, H. A., Conn, E. B., Polak, L., and Fuchs, E. (2007). Loss of TGFbeta signaling destabilizes homeostasis and promotes squamous cell carcinomas in stratified epithelia. Cancer Cell. 12 (4), 313–327. doi:10.1016/j.ccr.2007.08.020
Guilliams, M., Mildner, A., and Yona, S. (2018). Developmental and functional heterogeneity of monocytes. Immunity 49.
Gurtner, G. C., Dauskardt, R. H., Wong, V. W., Bhatt, K. A., Wu, K., Vial, I. N., et al. (2011). Improving cutaneous scar formation by controlling the mechanical environment: Large animal and phase I studies. Ann. Surg. 254 (2), 217–225. doi:10.1097/SLA.0b013e318220b159
Hallmann, R., Zhang, X., Di Russo, J., Li, L., Song, J., Hannocks, M-J., et al. (2015). The regulation of immune cell trafficking by the extracellular matrix. Curr. Opin. Cell Biol. 36, 54–61. doi:10.1016/j.ceb.2015.06.006
Han, G., Williams, C. A., Salter, K., Garl, P. J., Li, A. G., and Wang, X-J. (2010). A role for TGFbeta signaling in the pathogenesis of psoriasis. J. Investigative Dermatology 130 (2), 371–377. doi:10.1038/jid.2009.252
Han, J-W., Lee, H-J., Bae, G-U., and Kang, J-S. (2011). Promyogenic function of integrin/FAK signaling is mediated by cdo, Cdc42 and MyoD. Cell. Signal. 23 (7), 1162–1169. doi:10.1016/j.cellsig.2011.03.001
Harburger, D. S., and Calderwood, D. A. (2009). Integrin signalling at a glance. J. Cell Sci. 122 (2), 159–163. doi:10.1242/jcs.018093
Harvey, S. A., Anderson, S. C., and SundarRaj, N. (2004). Downstream effects of ROCK signaling in cultured human corneal stromal cells: Microarray analysis of gene expression. Invest Ophthalmol. Vis. Sci. 45 (7), 2168–2176. doi:10.1167/iovs.03-1218
Has, C., and Nyström, A. (2015). “Section four - epidermal basement membrane in health and disease,” in Current topics in membranes. Editor J. H. Miner (Amsterdam: Basement Membranes), 762015, 117–170.
He, J., Fang, B., Shan, S., Xie, Y., Wang, C., Zhang, Y., et al. (2021). Mechanical stretch promotes hypertrophic scar formation through mechanically activated cation channel Piezo1. Cell Death Dis. 12 (3), 226. doi:10.1038/s41419-021-03481-6
Heino, J., Huhtala, M., Käpylä, J., and Johnson, M. S. (2009). Evolution of collagen-based adhesion systems. Int. J. Biochem. Cell Biol. 41 (2), 341–348. doi:10.1016/j.biocel.2008.08.021
Henderson, N. C., Arnold, T. D., Katamura, Y., Giacomini, M. M., Rodriguez, J. D., McCarty, J. H., et al. (2013). Targeting of αv integrin identifies a core molecular pathway that regulates fibrosis in several organs. Nat. Med. 19 (12), 1617–1624. doi:10.1038/nm.3282
Henderson, N. C., and Sheppard, D. (2013). Integrin-mediated regulation of TGFβ in fibrosis. Biochimica Biophysica Acta (BBA) - Mol. Basis Dis. 1832 (7), 891–896. doi:10.1016/j.bbadis.2012.10.005
Hind, L. E., Dembo, M., and Hammer, D. A. (2015). Macrophage motility is driven by frontal-towing with a force magnitude dependent on substrate stiffness. Integr. Biol. (Camb) 7 (4), 447–453. doi:10.1039/c4ib00260a
Hinz, B. (2015). The extracellular matrix and transforming growth factor-β1: Tale of a strained relationship. Matrix Biol. 47, 54–65. doi:10.1016/j.matbio.2015.05.006
Hinz, B. (2009). Tissue stiffness, latent TGF-beta1 activation, and mechanical signal transduction: Implications for the pathogenesis and treatment of fibrosis. Curr. Rheumatol. Rep. 11 (2), 120–126. doi:10.1007/s11926-009-0017-1
Holden, C., English, J., Hoare, C., Jordan, A., Kownacki, S., Turnbull, R., et al. (2002). Advised best practice for the use of emollients in eczema and other dry skin conditions. J. Dermatol. Treat. 13 (3), 103–106. doi:10.1080/09546630260199442
Hough, C., Radu, M., and Doré, J. J. (2012). Tgf-beta induced Erk phosphorylation of smad linker region regulates smad signaling. PLoS One 7 (8), e42513. doi:10.1371/journal.pone.0042513
Hsu, C-K., Lin, H-H., Harn, H. I. C., Hughes, M. W., Tang, M-J., and Yang, C-C. (2018). Mechanical forces in skin disorders. J. Dermatological Sci. 90 (3), 232–240. doi:10.1016/j.jdermsci.2018.03.004
Huang, A., and Glick, S. A. (2017). Genetic susceptibility to cutaneous radiation injury. Arch. Dermatol Res. 309 (1), 1–10. doi:10.1007/s00403-016-1702-3
Huang, E., Peng, N., Xiao, F., Hu, D., Wang, X., and Lu, L. (2020). The roles of immune cells in the pathogenesis of fibrosis. Int. J. Mol. Sci. 21 (15), 5203. doi:10.3390/ijms21155203
Huang, F., and Chen, Y-G. (2012). Regulation of TGF-β receptor activity. Cell & Biosci. 2 (1), 9. doi:10.1186/2045-3701-2-9
Huang, G., and Greenspan, D. S. (2012). ECM roles in the function of metabolic tissues. Trends Endocrinol. Metabolism 23 (1), 16–22. doi:10.1016/j.tem.2011.09.006
Huang, M., Liu, Z., Baugh, L., DeFuria, J., Maione, A., Smith, A., et al. (2019). Lysyl oxidase enzymes mediate TGF-β1-induced fibrotic phenotypes in human skin-like tissues. Lab. Invest 99 (4), 514–527. doi:10.1038/s41374-018-0159-8
Huang, X. Z., Wu, J. F., Cass, D., Erle, D. J., Corry, D., Young, S. G., et al. (1996). Inactivation of the integrin beta 6 subunit gene reveals a role of epithelial integrins in regulating inflammation in the lung and skin. J. Cell Biol. 133 (4), 921–928. doi:10.1083/jcb.133.4.921
Huse, M. (2017). Mechanical forces in the immune system. Nat. Rev. Immunol. 17 (11), 679–690. doi:10.1038/nri.2017.74
Hynes, R. O. (2002). Integrins: Bidirectional, allosteric signaling machines. Cell. 110 (6), 673–687. doi:10.1016/s0092-8674(02)00971-6
Ihn, H. (2002). Pathogenesis of fibrosis: Role of TGF-β and CTGF. Curr. Opin. Rheumatology 14 (6), 681–685. doi:10.1097/00002281-200211000-00009
Ihn, H., Yamane, K., and Tamaki, K. (2005). Increased phosphorylation and activation of mitogen-activated protein kinase p38 in scleroderma fibroblasts. J. Investigative Dermatology 125 (2), 247–255. doi:10.1111/j.0022-202X.2005.23766.x
Ikeda, M., Naitoh, M., Kubota, H., Ishiko, T., Yoshikawa, K., Yamawaki, S., et al. (2009). Elastic fiber assembly is disrupted by excessive accumulation of chondroitin sulfate in the human dermal fibrotic disease, keloid. Biochem. Biophysical Res. Commun. 390 (4), 1221–1228. doi:10.1016/j.bbrc.2009.10.125
Ip, Y. T., and Davis, R. J. (1998). Signal transduction by the c-jun N-terminal kinase (JNK) — From inflammation to development. Curr. Opin. Cell Biol. 10 (2), 205–219. doi:10.1016/s0955-0674(98)80143-9
Ishikawa, Y., and Bächinger, H. P. (2013). A molecular ensemble in the rER for procollagen maturation. Biochim. Biophys. Acta 1833 (11), 2479–2491. doi:10.1016/j.bbamcr.2013.04.008
Ishida, Y., and Nagata, K. (2011). Hsp47 as a collagen-specific molecular chaperone. Methods Enzymol. 499, 167–182. doi:10.1016/B978-0-12-386471-0.00009-2
Ishii, G., Sangai, T., Sugiyama, K., Ito, T., Hasebe, T., Endoh, Y., et al. (2005). In vivo characterization of bone marrow-derived fibroblasts recruited into fibrotic lesions. Stem Cells 23 (5), 699–706. doi:10.1634/stemcells.2004-0183
Itoh, S., Itoh, F., Goumans, M-J., and ten Dijke, P. (2000). Signaling of transforming growth factor-β family members through Smad proteins. Eur. J. Biochem. 267 (24), 6954–6967. doi:10.1046/j.1432-1327.2000.01828.x
Jang, M., An, J., Oh, S. W., Lim, J. Y., Kim, J., Choi, J. K., et al. (2021). Matrix stiffness epigenetically regulates the oncogenic activation of the Yes-associated protein in gastric cancer. Nat. Biomed. Eng. 5 (1), 114–123. doi:10.1038/s41551-020-00657-x
Jansen, K. A., Licup, A. J., Sharma, A., Rens, R., MacKintosh, F. C., and Koenderink, G. H. (2018). The role of network architecture in collagen mechanics. Biophysical J. 114 (11), 2665–2678. doi:10.1016/j.bpj.2018.04.043
Jiang, M., Sun, Z., Dang, E., Li, B., Fang, H., Li, J., et al. (2017). TGFβ/SMAD/microRNA-486-3p signaling Axis mediates keratin 17 expression and keratinocyte hyperproliferation in psoriasis. J. Investigative Dermatology 137 (10), 2177–2186. doi:10.1016/j.jid.2017.06.005
Jin, J., Togo, S., Kadoya, K., Tulafu, M., Namba, Y., Iwai, M., et al. (2019). Pirfenidone attenuates lung fibrotic fibroblast responses to transforming growth factor-β1. Respir. Res. 20 (1), 119. doi:10.1186/s12931-019-1093-z
Jonk, L. J. C., Itoh, S., Heldin, C-H., and ten Dijke, P. (1998). Identification and functional characterization of a Smad binding element (SBE) in the JunB promoter that acts as a transforming growth factor-beta, activin, and bone morphogenetic protein-inducible enhancer. J. Biol. Chem. 273 (33), 21145–21152. doi:10.1074/jbc.273.33.21145
Judokusumo, E., Tabdanov, E., Kumari, S., Dustin, M. L., and Kam, L. C. (2012). Mechanosensing in T lymphocyte activation. Biophysical J. 102 (2), L5–L7. doi:10.1016/j.bpj.2011.12.011
Jung, S. M., Lee, J-H., Park, J., Oh, Y. S., Lee, S. K., Park, J. S., et al. (2013). Smad6 inhibits non-canonical TGF-β1 signalling by recruiting the deubiquitinase A20 to TRAF6. Nat. Commun. 4, 2562. doi:10.1038/ncomms3562
Kaewlai, R., and Abujudeh, H. (2012). Nephrogenic systemic fibrosis. Am. J. Roentgenol. 199 (1), W17–W23. doi:10.2214/AJR.11.8144
Kalli, M., Li, R., Mills, G. B., Stylianopoulos, T., and Zervantonakis, I. K. (2022). Mechanical stress signaling in pancreatic cancer cells triggers p38 MAPK- and JNK-dependent cytoskeleton remodeling and promotes cell migration via rac1/cdc42/myosin II. Mol. Cancer Res. 20 (3), 485–497. doi:10.1158/1541-7786.MCR-21-0266
Kalluri, R., and Neilson, E. G. (2003). Epithelial-mesenchymal transition and its implications for fibrosis. J. Clin. Investigation 112 (12), 1776–1784. doi:10.1172/JCI20530
Kalluri, R., and Weinberg, R. A. (2009). The basics of epithelial-mesenchymal transition. J. Clin. Investigation 119 (6), 1420–1428. doi:10.1172/JCI39104
Karayi, A. K., Basavaraj, V., Narahari, S. R., Aggithaya, M. G., Ryan, T. J., and Pilankatta, R. (2020). Human skin fibrosis: Up-regulation of collagen type III gene transcription in the fibrotic skin nodules of lower limb lymphoedema. Trop. Med. Int. Health 25 (3), 319–327. doi:10.1111/tmi.13359
Kavian, N., Marut, W., Servettaz, A., Nicco, C., Chéreau, C., Lemaréchal, H., et al. (2012). Reactive oxygen species-mediated killing of activated fibroblasts by arsenic trioxide ameliorates fibrosis in a murine model of systemic sclerosis. Arthritis Rheum. 64 (10), 3430–3440. doi:10.1002/art.34534
Kim, H., Lee, J., Kim, S., Seo, Y., Lee, J., Park, J., et al. (2007). Stromelysin-3 expression in the differential diagnosis of dermatofibroma and dermatofibrosarcoma protuberans: Comparison with factor XIIIa and CD34. Br. J. Dermatology 157 (2), 319–324. doi:10.1111/j.1365-2133.2007.08033.x
Kim, K. K., Kugler, M. C., Wolters, P. J., Robillard, L., Galvez, M. G., Brumwell, A. N., et al. (2006). Alveolar epithelial cell mesenchymal transition develops in vivo during pulmonary fibrosis and is regulated by the extracellular matrix. Proc. Natl. Acad. Sci. U. S. A. 103 (35), 13180–13185. doi:10.1073/pnas.0605669103
Kim, K. K., Wei, Y., Szekeres, C., Kugler, M. C., Wolters, P. J., Hill, M. L., et al. (2009). Epithelial cell alpha3beta1 integrin links beta-catenin and Smad signaling to promote myofibroblast formation and pulmonary fibrosis. J. Clin. Investigation 119 (1), 213–224. doi:10.1172/JCI36940
Kim, S. K., Henen, M. A., and Hinck, A. P. (2019). Structural biology of betaglycan and endoglin, membrane-bound co-receptors of the TGF-beta family. Exp. Biol. Med. (Maywood) 244 (17), 1547–1558. doi:10.1177/1535370219881160
Kim, Y., Kugler, M. C., Wei, Y., Kim, K. K., Li, X., Brumwell, A. N., et al. (2009). Integrin alpha3beta1-dependent beta-catenin phosphorylation links epithelial Smad signaling to cell contacts. J. Cell Biol. 184 (2), 309–322. doi:10.1083/jcb.200806067
Kirkpatrick, L. D., Shupp, J. W., Smith, R. D., Alkhalil, A., Moffatt, L. T., and Carney, B. C. (2021). Galectin-1 production is elevated in hypertrophic scar. Wound Repair Regen. 29 (1), 117–128. doi:10.1111/wrr.12869
Knaup, J., Gruber, C., Krammer, B., Ziegler, V., Bauer, J., and Verwanger, T. (2011). TGFβ-signaling in squamous cell carcinoma occurring in recessive dystrophic epidermolysis bullosa. Anal. Cell. Pathol. 34, 339–353. doi:10.3233/ACP-2011-0039
Koivisto, L., Bi, J., Häkkinen, L., and Larjava, H. (2018). Integrin αvβ6: Structure, function and role in health and disease. Int. J. Biochem. Cell Biol. 99, 186–196. doi:10.1016/j.biocel.2018.04.013
Kopecki, Z. (2020). Tumour serine proteases C1r and C1s as novel biomarkers and therapeutic targets in invasive sporadic and recessive dystrophic epidermolysis bullosa-associated cutaneous squamous cell carcinoma. Br. J. Dermatol 182 (3), 530–531. doi:10.1111/bjd.18419
Kothari, D., Patel, S., and Kim, S. K. (2019). Probiotic supplements might not be universally-effective and safe: A review. Biomed. Pharmacother. 111, 537–547. doi:10.1016/j.biopha.2018.12.104
Kramann, R., Schneider, R. K., DiRocco, D. P., Machado, F., Fleig, S., Bondzie, P. A., et al. (2015). Perivascular Gli1+ progenitors are key contributors to injury-induced organ fibrosis. Cell Stem Cell. 16 (1), 51–66. doi:10.1016/j.stem.2014.11.004
Kretzschmar, M., Doody, J., Timokhina, I., and Massagué, J. (1999). A mechanism of repression of TGFbeta/Smad signaling by oncogenic Ras. Genes Dev. 13 (7), 804–816. doi:10.1101/gad.13.7.804
Kudipudi, P. K., Galuska, S. P., Dietze, R., Scheiner-Bobis, G., Loveland, K. L., and Konrad, L. (2019). Betaglycan (TβRIII) is a key factor in TGF-β2 signaling in prepubertal rat sertoli cells. Int. J. Mol. Sci. 20 (24), 6214. doi:10.3390/ijms20246214
Kuwana, M., Gil-Vila, A., and Selva-O'Callaghan, A. (2021). Role of autoantibodies in the diagnosis and prognosis of interstitial lung disease in autoimmune rheumatic disorders. Ther. Adv. Musculoskelet. Dis. 13, 1759720x211032457. doi:10.1177/1759720X211032457
Laczko, R., and Csiszar, K. (2020). Lysyl oxidase (LOX): Functional contributions to signaling pathways. Biomolecules 10, 1093. doi:10.3390/biom10081093
Lamouille, S., Connolly, E., Smyth, J. W., Akhurst, R. J., and Derynck, R. (2012). TGF-β-induced activation of mTOR complex 2 drives epithelial–mesenchymal transition and cell invasion. J. Cell Sci. 125 (5), 1259–1273. doi:10.1242/jcs.095299
Lamouille, S., and Derynck, R. (2007). Cell size and invasion in TGF-beta-induced epithelial to mesenchymal transition is regulated by activation of the mTOR pathway. J. Cell Biol. 178 (3), 437–451. doi:10.1083/jcb.200611146
Lamouille, S., Xu, J., and Derynck, R. (2014). Molecular mechanisms of epithelial–mesenchymal transition. Nat. Rev. Mol. Cell Biol. 15 (3), 178–196. doi:10.1038/nrm3758
Laurent, P., Allard, B., Manicki, P., Jolivel, V., Levionnois, E., Jeljeli, M., et al. (2021). TGFβ promotes low IL10-producing ILC2 with profibrotic ability involved in skin fibrosis in systemic sclerosis. Ann. Rheumatic Dis. 80 (12), 1594–1603. doi:10.1136/annrheumdis-2020-219748
Lazzaroni, M. G., and Airò, P. (2018). Anti-RNA polymerase III antibodies in patients with suspected and definite systemic sclerosis: Why and how to screen. J. Scleroderma Relat. Disord. 3 (3), 214–220. doi:10.1177/2397198318786158
Lee, H. J., and Jang, Y. J. (2018). Recent understandings of biology, prophylaxis and treatment strategies for hypertrophic scars and keloids. Int. J. Mol. Sci. 19, 711. doi:10.3390/ijms19030711
Lee, J-H., Chen, S-Y., Yu, C-H., Chu, S-W., Wang, L-F., Sun, C-K., et al. (2009). Noninvasive in vitro and in vivo assessment of epidermal hyperkeratosis and dermal fibrosis in atopic dermatitis. JBO 14, 014008.
Lee, J. S., Kim, G. H., Lee, J. H., Ryu, J. Y., Oh, E. J., Kim, H. M., et al. (2022). MicroRNA-365a/b-3p as a potential biomarker for hypertrophic scars. Int. J. Mol. Sci. 23, 6117. doi:10.3390/ijms23116117
Lee, J. S., Kim, J. S., Lee, J. W., Choi, K. Y., Yang, J. D., Cho, B. C., et al. (2019). Effect of keratinocytes on myofibroblasts in hypertrophic scars. Aesth Plast. Surg. 43 (5), 1371–1380. doi:10.1007/s00266-019-01434-1
Lee, W. J., Ahn, H. M., Roh, H., Na, Y., Choi, I-K., Lee, J. H., et al. (2015). Decorin-expressing adenovirus decreases collagen synthesis and upregulates MMP expression in keloid fibroblasts and keloid spheroids. Exp. Dermatol. 24 (8), 591–597. doi:10.1111/exd.12719
Legate, K. R., Montañez, E., Kudlacek, O., and Fässler, R. I. L. K. (2006). ILK, PINCH and parvin: The tIPP of integrin signalling. Nat. Rev. Mol. Cell Biol. 7 (1), 20–31. doi:10.1038/nrm1789
Lei, C. Q., Wu, X., Zhong, X., Jiang, L., Zhong, B., and Shu, H. B. (2019). USP19 inhibits TNF-α- and IL-1β-triggered NF-κB activation by deubiquitinating TAK1. J. Immunol. 203 (1), 259–268. doi:10.4049/jimmunol.1900083
Leight, J. L., Wozniak, M. A., Chen, S., Lynch, M. L., and Chen, C. S. (2012). Matrix rigidity regulates a switch between TGF-β1-induced apoptosis and epithelial-mesenchymal transition. Mol. Biol. Cell. 23 (5), 781–791. doi:10.1091/mbc.E11-06-0537
Leivonen, S. K., Lazaridis, K., Decock, J., Chantry, A., Edwards, D. R., and Kähäri, V. M. (2013). TGF-β-elicited induction of tissue inhibitor of metalloproteinases (TIMP)-3 expression in fibroblasts involves complex interplay between Smad3, p38α, and ERK1/2. PLoS One 8 (2), e57474. doi:10.1371/journal.pone.0057474
Leong, E., Bezuhly, M., and Marshall, J. S. (2021). Distinct metalloproteinase expression and functions in systemic sclerosis and fibrosis: What we know and the potential for intervention. Front. Physiol. 12, 727451. doi:10.3389/fphys.2021.727451
Li, G., Li, Y-Y., and Sun, J-E. (2016). ILK-PI3K/AKT pathway participates in cutaneous wound contraction by regulating fibroblast migration and differentiation to myofibroblast. Lab. Invest 96 (7), 741–751. doi:10.1038/labinvest.2016.48
Li, M. O., and Flavell, R. A. (2008). TGF-Beta: A master of all T cell trades. TGF-β A Master All T Cell Trades. Cell. 134 (3), 392–404. doi:10.1016/j.cell.2008.07.025
Li, Y., Zhang, W., Gao, J., Liu, J., Wang, H., Li, J., et al. (2016). Adipose tissue-derived stem cells suppress hypertrophic scar fibrosis via the p38/MAPK signaling pathway. Stem Cell Res. Ther. 7 (1), 102. doi:10.1186/s13287-016-0356-6
Liu, J., Liao, S., Diop-Frimpong, B., Chen, W., Goel, S., Naxerova, K., et al. (2012). TGF-β blockade improves the distribution and efficacy of therapeutics in breast carcinoma by normalizing the tumor stroma. Proc. Natl. Acad. Sci. 109 (41), 16618–16623. doi:10.1073/pnas.1117610109
Liu, S., Iaria, J., Simpson, R. J., and Zhu, H. J. (2018). Ras enhances TGF-β signaling by decreasing cellular protein levels of its type II receptor negative regulator SPSB1. Cell Commun. Signal 16 (1), 10. doi:10.1186/s12964-018-0223-4
Liu, T., and Feng, X-H. (2010). Regulation of TGF-beta signalling by protein phosphatases. Biochem. J. 430 (2), 191–198. doi:10.1042/BJ20100427
Liu, W., Ding, I., Chen, K., Olschowka, J., Xu, J., Hu, D., et al. (2006). Interleukin 1beta (IL1B) signaling is a critical component of radiation-induced skin fibrosis. Radiat. Res. 165 (2), 181–191. doi:10.1667/rr3478.1
Llamas-Velasco, M., Mentzel, T., Ovejero-Merino, E., and Teresa Fernandez-Figueras, M. (2022). CD64 staining in dermatofibroma: A sensitive marker raising the question of the cell differentiation lineage of this neoplasm. J. Mol. Pathology 3(4), 190–195. doi:10.3390/jmp3040016
Long, Y., Niu, Y., Liang, K., and Du, Y. (2022). Mechanical communication in fibrosis progression. Trends Cell Biol. 32 (1), 70–90. doi:10.1016/j.tcb.2021.10.002
López-Casillas, F., Payne, H. M., Andres, J. L., and Massagué, J. (1994). Betaglycan can act as a dual modulator of TGF-beta access to signaling receptors: Mapping of ligand binding and GAG attachment sites. J. Cell Biol. 124 (4), 557–568. doi:10.1083/jcb.124.4.557
López-Casillas, F., Wrana, J. L., and Massagué, J. (1993). Betaglycan presents ligand to the TGF beta signaling receptor. Cell. 73 (7), 1435–1444. doi:10.1016/0092-8674(93)90368-z
Lu, P., Takai, K., Weaver, V. M., and Werb, Z. (2011). Extracellular matrix degradation and remodeling in development and disease. Cold Spring Harb. Perspect. Biol. 3, a005058. doi:10.1101/cshperspect.a005058
Macarak, E. J., Wermuth, P. J., Rosenbloom, J., and Uitto, J. (2021). Keloid disorder: Fibroblast differentiation and gene expression profile in fibrotic skin diseases. Exp. Dermatol 30 (1), 132–145. doi:10.1111/exd.14243
Mack, M. (2018). Inflammation and fibrosis. Matrix Biol. 68-69, 106–121. doi:10.1016/j.matbio.2017.11.010
Madison, K. C. (2003). Barrier function of the skin: “La raison d'Être” of the epidermis. J. Investigative Dermatology 121 (2), 231–241. doi:10.1046/j.1523-1747.2003.12359.x
Majedi, F. S., Hasani-Sadrabadi, M. M., Thauland, T. J., Li, S., Bouchard, L-S., and Butte, M. J. (2020). T-cell activation is modulated by the 3D mechanical microenvironment. Biomaterials 252, 120058. doi:10.1016/j.biomaterials.2020.120058
Mak, K. M., and Mei, R. (2017). Basement membrane type IV collagen and laminin: An overview of their biology and value as fibrosis biomarkers of liver disease. Anatomical Rec. 300 (8), 1371–1390. doi:10.1002/ar.23567
Martucci, N., Michalopoulos, G. K., and Mars, W. M. (2021). Integrin linked kinase (ILK) and its role in liver pathobiology. Gene Expr. 20 (3), 201–207. doi:10.3727/105221621X16113475275710
Masuda, A., Yasuoka, H., Satoh, T., Okazaki, Y., Yamaguchi, Y., and Kuwana, M. (2013). Versican is upregulated in circulating monocytes in patients with systemic sclerosis and amplifies a CCL2-mediated pathogenic loop. Arthritis Res. Ther. 15 (4), R74. doi:10.1186/ar4251
Matsushita, T., Date, M., Kano, M., Mizumaki, K., Tennichi, M., Kobayashi, T., et al. (2017). Blockade of p38 mitogen-activated protein kinase inhibits murine sclerodermatous chronic graft-versus-host disease. Am. J. Pathology 187 (4), 841–850. doi:10.1016/j.ajpath.2016.12.016
Mazars, A., Lallemand, F., Prunier, C., Marais, J., Ferrand, N., Pessah, M., et al. (2001). Evidence for a role of the JNK cascade in Smad7-mediated apoptosis. J. Biol. Chem. 276 (39), 36797–36803. doi:10.1074/jbc.M101672200
McCoy, S. S., Reed, T. J., Berthier, C. C., Tsou, P-S., Liu, J., Gudjonsson, J. E., et al. (2017). Scleroderma keratinocytes promote fibroblast activation independent of transforming growth factor beta. Rheumatology 56 (11), 1970–1981. doi:10.1093/rheumatology/kex280
McDonald, P. C., and Dedhar, S. (2022). New perspectives on the role of integrin-linked kinase (ILK) signaling in cancer metastasis. Cancers 14 (13), 3209. doi:10.3390/cancers14133209
McGrath, J. A., Eady, R. A. J., and Pope, F. M. (2004). Anatomy and organization of human skin. Rook’s Textb. dermatology 1, 32–380.
McIntosh, T. J. (2003). Organization of skin stratum corneum extracellular lamellae: Diffraction evidence for asymmetric distribution of cholesterol. Biophysical J. 85 (3), 1675–1681. doi:10.1016/S0006-3495(03)74597-4
Meli, V. S., Atcha, H., Veerasubramanian, P. K., Nagalla, R. R., Luu, T. U., Chen, E. Y., et al. (2020). YAP-mediated mechanotransduction tunes the macrophage inflammatory response. Sci. Adv 6, eabb8471. doi:10.1126/sciadv.abb8471
Meng, K. P., Majedi, F. S., Thauland, T. J., and Butte, M. J. (2020). Mechanosensing through YAP controls T cell activation and metabolism. J. Exp. Med. 217 (8), e20200053. doi:10.1084/jem.20200053
Mitra, S. K., Hanson, D. A., and Schlaepfer, D. D. (2005). Focal adhesion kinase: In command and control of cell motility. Nat. Rev. Mol. Cell Biol. 6 (1), 56–68. doi:10.1038/nrm1549
Mitra, S. K., and Schlaepfer, D. D. (2006). Integrin-regulated FAK–Src signaling in normal and cancer cells. Curr. Opin. Cell Biol. 18 (5), 516–523. doi:10.1016/j.ceb.2006.08.011
Montagna, W., Chase, H. B., and Melaragno, H. P. (1951). Histology and cytochemistry of human skin. I. Metachromasia in the mons pubis. J. Natl. Cancer Inst. 12 (3), 591–597.
Mosser, D. M., and Edwards, J. P. (2008). Exploring the full spectrum of macrophage activation. Nat. Rev. Immunol. 8 (12), 958–969. doi:10.1038/nri2448
Mu, D., Cambier, S., Fjellbirkeland, L., Baron, J. L., Munger, J. S., Kawakatsu, H., et al. (2002). The integrin alpha(v)beta8 mediates epithelial homeostasis through MT1-MMP-dependent activation of TGF-beta1. J. Cell Biol. 157 (3), 493–507. doi:10.1083/jcb.200109100
Mu, Y., Gudey, S. K., and Landström, M. (2012). Non-Smad signaling pathways. Cell Tissue Res. 347 (1), 11–20. doi:10.1007/s00441-011-1201-y
Multhaupt, H. A. B., Leitinger, B., Gullberg, D., and Couchman, J. R. (2016). Extracellular matrix component signaling in cancer. Adv. Drug Deliv. Rev. 97, 28–40. doi:10.1016/j.addr.2015.10.013
Munger, J. S., Huang, X., Kawakatsu, H., Griffiths, M. J. D., Dalton, S. L., Wu, J., et al. (1999). The integrin alpha v beta 6 binds and activates latent TGF beta 1: A mechanism for regulating pulmonary inflammation and fibrosis. Cell. 96 (3), 319–328. doi:10.1016/s0092-8674(00)80545-0
Murry, C. E., Giachelli, C. M., Schwartz, S. M., and Vracko, R. (1994). Macrophages express osteopontin during repair of myocardial necrosis. Am. J. Pathology 145 (6), 1450–1462.
Mythreye, K., and Blobe, G. C. (2009). The type III TGF-beta receptor regulates epithelial and cancer cell migration through beta-arrestin2-mediated activation of Cdc42. Proc. Natl. Acad. Sci. U. S. A. 106 (20), 8221–8226. doi:10.1073/pnas.0812879106
Nakasaki, M., Hwang, Y., Xie, Y., Kataria, S., Gund, R., Hajam, E. Y., et al. (2015). The matrix protein Fibulin-5 is at the interface of tissue stiffness and inflammation in fibrosis. Nat. Commun. 6 (1), 8574. doi:10.1038/ncomms9574
Newe, M., Kant, T. A., Hoffmann, M., Rausch, J. S. E., Winter, L., Künzel, K., et al. (2021). Systemic mesalazine treatment prevents spontaneous skin fibrosis in PLK2-deficient mice. Naunyn Schmiedeb. Arch. Pharmacol. 394 (11), 2233–2244. doi:10.1007/s00210-021-02135-w
Nguyen, X-X., Nishimoto, T., Takihara, T., Mlakar, L., Bradshaw, A. D., and Feghali-Bostwick, C. (2021). Lysyl oxidase directly contributes to extracellular matrix production and fibrosis in systemic sclerosis. Am. J. Physiology-Lung Cell. Mol. Physiology 320 (1), L29–L40. doi:10.1152/ajplung.00173.2020
Nikitorowicz-Buniak, J., Shiwen, X., Denton, C. P., Abraham, D., and Stratton, R. (2014). Abnormally differentiating keratinocytes in the epidermis of systemic sclerosis patients show enhanced secretion of CCN2 and S100A9. J. Investigative Dermatology 134 (11), 2693–2702. doi:10.1038/jid.2014.253
O'Connor, R. S., Hao, X., Shen, K., Bashour, K., Akimova, T., Hancock, W. W., et al. (2012). Substrate rigidity regulates human T cell activation and proliferation. J. Immunol. 189 (3), 1330–1339. doi:10.4049/jimmunol.1102757
Oakes, P. W., Patel, D. C., Morin, N. A., Zitterbart, D. P., Fabry, B., Reichner, J. S., et al. (2009). Neutrophil morphology and migration are affected by substrate elasticity. Blood 114 (7), 1387–1395. doi:10.1182/blood-2008-11-191445
Ogawa, R., Okai, K., Tokumura, F., Mori, K., Ohmori, Y., Huang, C., et al. (2012). The relationship between skin stretching/contraction and pathologic scarring: The important role of mechanical forces in keloid generation. Wound Repair Regen. 20 (2), 149–157. doi:10.1111/j.1524-475X.2012.00766.x
Oldberg, A., Franzén, A., Heinegård, D., and FrAnzen, A. (1986). Cloning and sequence analysis of rat bone sialoprotein (osteopontin) cDNA reveals an Arg-Gly-Asp cell-binding sequence. Proc. Natl. Acad. Sci. 83 (23), 8819–8823. doi:10.1073/pnas.83.23.8819
Olson, L. E., and Soriano, P. (2009). Increased PDGFRalpha activation disrupts connective tissue development and drives systemic fibrosis. Dev. Cell. 16 (2), 303–313. doi:10.1016/j.devcel.2008.12.003
O’Regan, A. W., Chupp, G. L., Lowry, J. A., Goetschkes, M., Mulligan, N., and Berman, J. S. (1999). Osteopontin is associated with T cells in sarcoid granulomas and has T cell adhesive and cytokine-like properties in vitro. Vitro. J. Immunol. 162 (2), 1024–1031. doi:10.4049/jimmunol.162.2.1024
Ota, Y., and Kuwana, M. (2021). Updates on genetics in systemic sclerosis. Inflamm. Regen. 41 (1), 17. doi:10.1186/s41232-021-00167-6
Oviedo-Orta, E., Bermudez-Fajardo, A., Karanam, S., Benbow, U., and Newby, A. C. (2008). Comparison of MMP-2 and MMP-9 secretion from T helper 0, 1 and 2 lymphocytes alone and in coculture with macrophages. Immunology 124 (1), 42–50. doi:10.1111/j.1365-2567.2007.02728.x
Pakshir, P., and Hinz, B. (2018). The big five in fibrosis: Macrophages, myofibroblasts, matrix, mechanics, and miscommunication. Matrix Biol. 68-69, 81–93. doi:10.1016/j.matbio.2018.01.019
Park, J. S., Chu, J. S., Tsou, A. D., Diop, R., Tang, Z., Wang, A., et al. (2011). The effect of matrix stiffness on the differentiation of mesenchymal stem cells in response to TGF-β. Biomaterials 32 (16), 3921–3930. doi:10.1016/j.biomaterials.2011.02.019
Patel, V., and McGurk, M. (2017). Use of pentoxifylline and tocopherol in radiation-induced fibrosis and fibroatrophy. Br. J. Oral Maxillofac. Surg. 55 (3), 235–241. doi:10.1016/j.bjoms.2016.11.323
Paul, S. P. (2017). Biodynamic excisional skin tension (BEST) lines: Revisiting Langer’s lines, skin biomechanics, current concepts in cutaneous surgery, and the (lack of) science behind skin lines used for surgical excisions. J. Dermatological Res. 2 (1), 77–87. doi:10.17554/j.issn.2413-8223.2017.02.19
Pellicoro, A., Aucott, R. L., Ramachandran, P., Robson, A. J., Fallowfield, J. A., Snowdon, V. K., et al. (2012). Elastin accumulation is regulated at the level of degradation by macrophage metalloelastase (MMP-12) during experimental liver fibrosis. Hepatology 55 (6), 1965–1975. doi:10.1002/hep.25567
Peng, C., Jian, X., Xie, Y., Li, L., Ouyang, J., Tang, L., et al. (2022). Genomic alterations of dermatofibrosarcoma protuberans revealed by whole-genome sequencing. Br. J. Dermatology 186 (6), 997–1009. doi:10.1111/bjd.20976
Peterson, L. K., Jaskowski, T. D., Mayes, M. D., and Tebo, A. E. (2016). Detection of anti-U3-RNP/fibrillarin IgG antibodies by line immunoblot assay has comparable clinical significance to immunoprecipitation testing in systemic sclerosis. Immunol. Res. 64 (2), 483–488. doi:10.1007/s12026-015-8710-9
Petrof, G., Abdul-Wahab, A., Proudfoot, L., Pramanik, R., Mellerio, J. E., and McGrath, J. A. (2013). Serum levels of high mobility group box 1 correlate with disease severity in recessive dystrophic epidermolysis bullosa. Exp. Dermatol. 22 (6), 433–435. doi:10.1111/exd.12152
Peura, M., Siltanen, A., Saarinen, I., Soots, A., Bizik, J., Vuola, J., et al. (2010). Paracrine factors from fibroblast aggregates in a fibrin-matrix carrier enhance keratinocyte viability and migration. J. Biomed. Mater Res. A 95 (2), 658–664. doi:10.1002/jbm.a.32881
Pfendner, E. G., and Lucky, A. W. (1993). “Dystrophic epidermolysis bullosa,” in Seattle. GeneReviews is a registered trademark of the University of Washington. Editors M. P. Adam, D. B. Everman, G. M. Mirzaa, R. A. Pagon, and S. Wallace (Seattle: University of Washington).
Pfisterer, K., Shaw, L., Symmank, D., and Weninger, W. (2021). The extracellular matrix in skin inflammation and infection. Front. Cell Dev. Biol. 9, 682414. doi:10.3389/fcell.2021.682414
Phuyal, S., and Farhan, H. (2019). Multifaceted Rho GTPase signaling at the endomembranes. Front. Cell Dev. Biol. 7, 127. doi:10.3389/fcell.2019.00127
Piersma, B., Bank, R. A., and Boersema, M. (2015). Signaling in fibrosis: TGF-β, WNT, and YAP/TAZ converge. Front. Med. 2, 59. doi:10.3389/fmed.2015.00059
Potekaev, N. N., Borzykh, O. B., Medvedev, G. V., Pushkin, D. V., Petrova, M. M., Petrov, A. V., et al. (2021). The role of extracellular matrix in skin wound healing. J. Clin. Med. 10 (24), 5947. doi:10.3390/jcm10245947
Pozzi, A., Yurchenco, P. D., and Iozzo, R. V. (2017). The nature and biology of basement membranes. Matrix Biol. 57-58, 1–11. doi:10.1016/j.matbio.2016.12.009
Pratta, M., Paczesny, S., Socie, G., Barkey, N., Liu, H., Owens, S., et al. (2022). A biomarker signature to predict complete response to itacitinib and corticosteroids in acute graft-versus-host disease. Br. J. Haematol. 198 (4), 729–739. doi:10.1111/bjh.18300
Prieto, A. L., Edelman, G. M., and Crossin, K. L. (1993). Multiple integrins mediate cell attachment to cytotactin/tenascin. Proc. Natl. Acad. Sci. U. S. A. 90 (21), 10154–10158. doi:10.1073/pnas.90.21.10154
Quan, C., Cho, M. K., Shao, Y., Mianecki, L. E., Liao, E., Perry, D., et al. (2015). Dermal fibroblast expression of stromal cell-derived factor-1 (SDF-1) promotes epidermal keratinocyte proliferation in normal and diseased skin. Protein Cell. 6 (12), 890–903. doi:10.1007/s13238-015-0198-5
Radisky, D. C., Kenny, P. A., and Bissell, M. J. (2007). Fibrosis and cancer: Do myofibroblasts come also from epithelial cells via EMT? J. Cell Biochem. 101 (4), 830–839. doi:10.1002/jcb.21186
Ravanti, L., Toriseva, M., Penttinen, R., Crombleholme, T., Foschi, M., Han, J., et al. (2001). Expression of human collagenase-3 (MMP-13) by fetal skin fibroblasts is induced by transforming growth factor-β via p38 mitogen-activated protein kinase. FASEB J. 15 (6), 1098–1100. doi:10.1096/fsb2fj000588fje
Riha, P., and Rudd, C. E. (2010). CD28 co-signaling in the adaptive immune response. Self Nonself 1 (3), 231–240. doi:10.4161/self.1.3.12968
Rittié, L. (2016). Cellular mechanisms of skin repair in humans and other mammals. J. Cell Commun. Signal. 10 (2), 103–120. doi:10.1007/s12079-016-0330-1
Rognoni, E., and Walko, G. (2019). The roles of YAP/TAZ and the Hippo pathway in healthy and diseased skin. Cells 8, 411. doi:10.3390/cells8050411
Ruoslahti, E., and Pierschbacher, M. D. (1987). New perspectives in cell adhesion: RGD and integrins. Science 238 (4826), 491–497. doi:10.1126/science.2821619
Russo, B., Borowczyk, J., Boehncke, W-H., Truchetet, M-E., Modarressi, A., Brembilla, N. C., et al. (2021). Dysfunctional keratinocytes increase dermal inflammation in systemic sclerosis: Results from studies using tissue-engineered scleroderma epidermis. Arthritis & Rheumatology 73 (7), 1311–1317. doi:10.1002/art.41659
Russo, B., Brembilla, N. C., and Chizzolini, C. (2020). Interplay between keratinocytes and fibroblasts: A systematic review providing a new angle for understanding skin fibrotic disorders. Front. Immunol. 11, 648. doi:10.3389/fimmu.2020.00648
Saitakis, M., Dogniaux, S., Goudot, C., Bufi, N., Asnacios, S., Maurin, M., et al. (2017). Different TCR-induced T lymphocyte responses are potentiated by stiffness with variable sensitivity. eLife 6, e23190. doi:10.7554/eLife.23190
Samarakoon, R., Overstreet, J. M., and Higgins, P. J. (2013). TGF-Β signaling in tissue fibrosis: Redox controls, target genes and therapeutic opportunities. Cell Signal 25 (1), 264–268. doi:10.1016/j.cellsig.2012.10.003
Santos-Cortez, R. L. P., Hu, Y., Sun, F., Benahmed-Miniuk, F., Tao, J., Kanaujiya, J. K., et al. (2017). Identification of ASAH1 as a susceptibility gene for familial keloids. Eur. J. Hum. Genet. 25 (10), 1155–1161. doi:10.1038/ejhg.2017.121
Sargent, J. L., Milano, A., Bhattacharyya, S., Varga, J., Connolly, M. K., Chang, H. Y., et al. (2010). A TGFbeta-responsive gene signature is associated with a subset of diffuse scleroderma with increased disease severity. J. Investigative Dermatology 130 (3), 694–705. doi:10.1038/jid.2009.318
Sato, M., Shegogue, D., Gore, E. A., Smith, E. A., Trojanowska, M., and McDermott, P. J. (2002). Role of p38 MAPK in transforming growth factor β stimulation of collagen production by scleroderma and healthy dermal fibroblasts. J. Investigative Dermatology 118 (4), 704–711. doi:10.1046/j.1523-1747.2002.01719.x
Schilter, H., Findlay, A. D., Perryman, L., Yow, T. T., Moses, J., Zahoor, A., et al. (2019). The lysyl oxidase like 2/3 enzymatic inhibitor, PXS-5153A, reduces crosslinks and ameliorates fibrosis. J. Cell Mol. Med. 23 (3), 1759–1770. doi:10.1111/jcmm.14074
Senior, R. M., Hinek, A., Griffin, G. L., Pipoly, D. J., Crouch, E. C., and Mecham, R. P. (1989). Neutrophils show chemotaxis to type IV collagen and its 7S domain and contain a 67 kD type IV collagen binding protein with lectin properties. Am. J. Respir. Cell Mol. Biol. 1 (6), 479–487. doi:10.1165/ajrcmb/1.6.479
Sen, C. K., Gordillo, G. M., Roy, S., Kirsner, R., Lambert, L., Hunt, T. K., et al. (2009). Human skin wounds: A major and snowballing threat to public health and the economy. Wound Repair Regen. 17 (6), 763–771. doi:10.1111/j.1524-475X.2009.00543.x
Shan, S., Zhang, Y., Wu, M., Yi, B., Wang, J., and Li, Q. (2017). Naringenin attenuates fibroblast activation and inflammatory response in a mechanical stretch-induced hypertrophic scar mouse model. Mol. Med. Rep. 16 (4), 4643–4649. doi:10.3892/mmr.2017.7209
Shephard, P., Martin, G., Smola-Hess, S., Brunner, G., Krieg, T., and Smola, H. (2004). Myofibroblast differentiation is induced in keratinocyte-fibroblast Co-cultures and is antagonistically regulated by endogenous transforming growth factor-β and interleukin-1. Am. J. Pathology 164 (6), 2055–2066. doi:10.1016/s0002-9440(10)63764-9
Shi, Y., and Massagué, J. (2003). Mechanisms of TGF-β signaling from cell membrane to the nucleus. Cell. 113 (6), 685–700. doi:10.1016/s0092-8674(03)00432-x
Shroff, A., Mamalis, A., and Jagdeo, J. (2014). Oxidative stress and skin fibrosis. Curr. Pathobiol. Rep. 2 (4), 257–267. doi:10.1007/s40139-014-0062-y
Shull, M. M., Ormsby, I., Kier, A. B., Pawlowski, S., Diebold, R. J., Yin, M., et al. (1992). Targeted disruption of the mouse transforming growth factor-beta 1 gene results in multifocal inflammatory disease. Nature 359 (6397), 693–699. doi:10.1038/359693a0
Sica, A., and Mantovani, A. (2012). Macrophage plasticity and polarization: In vivo veritas. J. Clin. Investigation 122 (3), 787–795. doi:10.1172/JCI59643
Silver, F. H., Siperko, L. M., and Seehra, G. P. (2003). Mechanobiology of force transduction in dermal tissue. Skin Res. Technol. 9 (1), 3–23. doi:10.1034/j.1600-0846.2003.00358.x
Simon, T., and Bromberg, J. S. (2017). Regulation of the immune system by laminins. Trends Immunol. 38 (11), 858–871. doi:10.1016/j.it.2017.06.002
Sinha, M., Sen, C. K., Singh, K., Das, A., Ghatak, S., Rhea, B., et al. (2018). Direct conversion of injury-site myeloid cells to fibroblast-like cells of granulation tissue. Nat. Commun. 9 (1), 936. doi:10.1038/s41467-018-03208-w
Skoog, T., Ahokas, K., Orsmark, C., Jeskanen, L., Isaka, K., and Saarialho-Kere, U. (2006). MMP-21 is expressed by macrophages and fibroblasts in vivo and in culture. Exp. Dermatol. 15 (10), 775–783. doi:10.1111/j.1600-0625.2006.00460.x
Smith, G. P., and Chan, E. S. L. (2010). Molecular pathogenesis of skin fibrosis: Insight from animal models. Curr. Rheumatol. Rep. 12 (1), 26–33. doi:10.1007/s11926-009-0080-7
Smith, L. T., Holbrook, K. A., and Byers, P. H. (1982). Structure of the dermal matrix during development and in the adult. J. Investigative Dermatology 79 (1), 93–104. doi:10.1111/1523-1747.ep12545877
Sorrentino, A., Thakur, N., Grimsby, S., Marcusson, A., von Bulow, V., Schuster, N., et al. (2008). The type I TGF-beta receptor engages TRAF6 to activate TAK1 in a receptor kinase-independent manner. Nat. Cell Biol. 10 (10), 1199–1207. doi:10.1038/ncb1780
Srinagesh, H. K., Levine, J. E., and Ferrara, J. L. M. (2019). Biomarkers in acute graft-versus-host disease: New insights. Ther. Adv. Hematol. 10, 2040620719891358. doi:10.1177/2040620719891358
Stochmal, A., Czuwara, J., Trojanowska, M., and Rudnicka, L. (2020). Antinuclear antibodies in systemic sclerosis: An update. Clin. Rev. Allergy & Immunol. 58 (1), 40–51. doi:10.1007/s12016-018-8718-8
Strong Rodrigues, K., Oliveira-Ribeiro, C., de Abreu Fiuza Gomes, S., and Knobler, R.Diagnosis, and TreatmentAm (2018). Cutaneous graft-versus-host disease: Diagnosis and treatment. J. Clin. Dermatol 19 (1), 33–50. doi:10.1007/s40257-017-0306-9
Su, J., Morgani, S. M., David, C. J., Wang, Q., Er, E. E., Huang, Y. H., et al. (2020). TGF-β orchestrates fibrogenic and developmental EMTs via the RAS effector RREB1. Nature 577 (7791), 566–571. doi:10.1038/s41586-019-1897-5
Suga, H., Rennert, R. C., Rodrigues, M., Sorkin, M., Glotzbach, J. P., Januszyk, M., et al. (2014). Tracking the elusive fibrocyte: Identification and characterization of collagen-producing hematopoietic lineage cells during murine wound healing. Stem Cells 32 (5), 1347–1360. doi:10.1002/stem.1648
Sutariya, B., Jhonsa, D., and Saraf, M. N. (2016). TGF-Β: The connecting link between nephropathy and fibrosis. Immunopharmacol. Immunotoxicol. 38 (1), 39–49. doi:10.3109/08923973.2015.1127382
Swaminathan, S., Bose, C., Shah, S. V., Hall, K. A., and Hiatt, K. M. (2013). Gadolinium contrast agent-induced CD163+ ferroportin+ osteogenic cells in nephrogenic systemic fibrosis. Am. J. Pathol. 183 (3), 796–807. doi:10.1016/j.ajpath.2013.06.008
Tartaglia, G., Cao, Q., Padron, Z. M., and SouthImpaired Wound Healing, Fibrosis, A. P. (2021). Cancer: The paradigm of recessive dystrophic epidermolysis bullosa. Int. J. Mol. Sci 22.
Taylor, L. M., and Khachigian, L. M. (2000). Induction of platelet-derived growth factor B-chain expression by transforming growth factor-β involves transactivation by Smads *. J. Biol. Chem. 275 (22), 16709–16716. doi:10.1074/jbc.275.22.16709
Tazat, K., Hector-Greene, M., Blobe, G. C., and Henis, Y. I. (2015). TβRIII independently binds type I and type II TGF-β receptors to inhibit TGF-β signaling. Mol. Biol. Cell. 26 (19), 3535–3545. doi:10.1091/mbc.E15-04-0203
Thennes, T., and Mehta, D. (2012). Heterotrimeric G proteins, focal adhesion kinase, and endothelial barrier function. Microvasc. Res. 83 (1), 31–44. doi:10.1016/j.mvr.2011.05.004
Tomar, A., and Schlaepfer, D. D. (2009). Focal adhesion kinase: Switching between GAPs and GEFs in the regulation of cell motility. Curr. Opin. Cell Biol. 21 (5), 676–683. doi:10.1016/j.ceb.2009.05.006
Topczewska, J. M., Ledwon, J. K., Vaca, E. E., and Gosain, A. K. (2019). Mechanical stretching stimulates growth of the basal layer and rete ridges in the epidermis. J. Tissue Eng. Regen. Med. 13 (11), 2121–2125. doi:10.1002/term.2952
Toriseva, M., Laato, M., Carpén, O., Ruohonen, S. T., Savontaus, E., Inada, M., et al. (2012). MMP-13 regulates growth of wound granulation tissue and modulates gene expression signatures involved in inflammation, proteolysis, and cell viability. PLOS ONE 7 (8), e42596. doi:10.1371/journal.pone.0042596
Tracy, L. E., Minasian, R. A., and Caterson, E. J. (2016). Extracellular matrix and dermal fibroblast function in the healing wound. Adv. Wound Care (New Rochelle) 5 (3), 119–136. doi:10.1089/wound.2014.0561
Trappmann, B., Gautrot, J. E., Connelly, J. T., Strange, D. G. T., Li, Y., Oyen, M. L., et al. (2012). Extracellular-matrix tethering regulates stem-cell fate. Nat. Mater 11 (7), 642–649. doi:10.1038/nmat3339
Tsai, C-H., and Ogawa, R. (2019). Keloid research: Current status and future directions. Scars, Burns Heal. 5, 2059513119868659. doi:10.1177/2059513119868659
Tsai, C. W., Chao, C. C., Wu, V. C., Hsiao, C. H., and Chen, Y. M. (2007). Nephrogenic fibrosing dermopathy in a peritoneal dialysis patient. Kidney Int. 72 (10), 1294. doi:10.1038/sj.ki.5002460
Tse, J. R., and Engler, A. J. (2011). Stiffness gradients mimicking in vivo tissue variation regulate mesenchymal stem cell fate. PloS One 6 (1), e15978. doi:10.1371/journal.pone.0015978
Ud-Din, S., Foden, P., Stocking, K., Mazhari, M., Al-Habba, S., Baguneid, M., et al. (2019). Objective assessment of dermal fibrosis in cutaneous scarring, using optical coherence tomography, high-frequency ultrasound and immunohistomorphometry of human skin. Br. J. Dermatology 181 (4), 722–732. doi:10.1111/bjd.17739
Ueda, M., Saito, S., Murata, T., Hirano, T., Bise, R., Kabashima, K., et al. (2019). Combined multiphoton imaging and biaxial tissue extension for quantitative analysis of geometric fiber organization in human reticular dermis. Sci. Rep. 9 (1), 10644. doi:10.1038/s41598-019-47213-5
Uitto, J., Olsen, D. R., and Fazio, M. J. (1989). Extracellular matrix of the skin: 50 years of progress. J. Invest Dermatol 92, 61S–77S. doi:10.1111/1523-1747.ep13075039
Vafadari, B., Salamian, A., and Kaczmarek, L. (2016). MMP-9 in translation: From molecule to brain physiology, pathology, and therapy. Ther. J. Neurochem. 2, 91–114. doi:10.1111/jnc.13415
Vallet, S. D., and Ricard-Blum, S. (2019). Lysyl oxidases: From enzyme activity to extracellular matrix cross-links. Essays Biochem. 63 (3), 349–364. doi:10.1042/EBC20180050
van der Slot-Verhoeven, A. J., van Dura, E. A., Attema, J., Blauw, B., Degroot, J., Huizinga, T. W., et al. (2005). The type of collagen cross-link determines the reversibility of experimental skin fibrosis. Biochim. Biophys. Acta 1740 (1), 60–67. doi:10.1016/j.bbadis.2005.02.007
Vander Ark, A., Cao, J., and Li, X. (2018). TGF-β receptors: In and beyond TGF-β signaling. Cell. Signal. 52, 112–120. doi:10.1016/j.cellsig.2018.09.002
Varga, J., Rosenbloom, J., and Jimenez, S. A. (1987). Transforming growth factor β (TGFβ) causes a persistent increase in steady-state amounts of type I and type III collagen and fibronectin mRNAs in normal human dermal fibroblasts. Biochem. J. 247 (3), 597–604. doi:10.1042/bj2470597
Vasil, K. E., and Magro, C. M. (2007). Cutaneous vascular deposition of C5b-9 and its role as a diagnostic adjunct in the setting of diabetes mellitus and porphyria cutanea tarda. J. Am. Acad. Dermatology 56 (1), 96–104. doi:10.1016/j.jaad.2006.05.013
Ventura, J-J., Cogswell, P., Flavell, R. A., Baldwin, A. S., and Davis, R. J. (2004). JNK potentiates TNF-stimulated necrosis by increasing the production of cytotoxic reactive oxygen species. Genes & Dev. 18 (23), 2905–2915. doi:10.1101/gad.1223004
Verrecchia, F., Chu, M. L., and Mauviel, A. (2001). Identification of novel TGF-beta/Smad gene targets in dermal fibroblasts using a combined cDNA microarray/promoter transactivation approach. J. Biol. Chem. 276 (20), 17058–17062. doi:10.1074/jbc.M100754200
Verrecchia, F., Mauviel, A., and Farge, D. (2006). Transforming growth factor-β signaling through the Smad proteins: Role in systemic sclerosis. Autoimmun. Rev. 5 (8), 563–569. doi:10.1016/j.autrev.2006.06.001
Verrecchia, F., and Mauviel, A. (2007). Transforming growth factor-β and fibrosis. World J. Gastroenterol. 13 (22), 3056–3062. doi:10.3748/wjg.v13.i22.3056
Vorstandlechner, V., Laggner, M., Kalinina, P., Haslik, W., Radtke, C., Shaw, L., et al. (2020). Deciphering the functional heterogeneity of skin fibroblasts using single-cell RNA sequencing. FASEB J. 34 (3), 3677–3692. doi:10.1096/fj.201902001RR
Wagenseil, J. E., and Mecham, R. P. (2012). Elastin in large artery stiffness and hypertension. J. Cardiovasc Transl. Res. 5 (3), 264–273. doi:10.1007/s12265-012-9349-8
Wagner, E. F., and Nebreda, A. R. (2009). Signal integration by JNK and p38 MAPK pathways in cancer development. Nat. Rev. Cancer 9 (8), 537–549. doi:10.1038/nrc2694
Wang, J., Zhang, Y., Zhang, N., Wang, C., Herrler, T., and Li, Q. (2015). An updated review of mechanotransduction in skin disorders: Transcriptional regulators, ion channels, and microRNAs. Cell Mol. Life Sci. 72 (11), 2091–2106. doi:10.1007/s00018-015-1853-y
Wang, J-H. (2012). Pull and push: Talin activation for integrin signaling. Cell Res. 22 (11), 1512–1514. doi:10.1038/cr.2012.103
Wang, X. (2018). Matrix metalloproteinases, vascular remodeling, and vascular disease. Adv. Pharmacol. 81, 241–330. doi:10.1016/bs.apha.2017.08.002
Wang, X. J., Liefer, K. M., Tsai, S., O'Malley, B. W., and Roop, D. R. (1999). Development of gene-switch transgenic mice that inducibly express transforming growth factor beta1 in the epidermis. Proc. Natl. Acad. Sci. U. S. A. 96 (15), 8483–8488. doi:10.1073/pnas.96.15.8483
Wang, Y., Mack, J. A., Hascall, V. C., and Maytin, E. V. (2022). Transforming growth factor-β receptor–mediated, p38 mitogen-activated protein kinase–dependent signaling drives enhanced myofibroblast differentiation during skin wound healing in mice lacking hyaluronan synthases 1 and 3. Am. J. Pathology 2022.
Watsky, M. A., Weber, K. T., Sun, Y., and Postlethwaite, A. (2010). New insights into the mechanism of fibroblast to myofibroblast transformation and associated pathologies. Int. Rev. Cell Mol. Biol. 282, 165–192. doi:10.1016/S1937-6448(10)82004-0
Watt, F. M., and Fujiwara, H. (2011). Cell-extracellular matrix interactions in normal and diseased skin. Cold Spring Harb. Perspect. Biol. 3, a005124. doi:10.1101/cshperspect.a005124
Wen, C., Wang, C., Hu, C., Qi, T., Jing, R., Wang, Y., et al. (2022). REPS2 downregulation facilitates FGF-induced adhesion and migration in human lens epithelial cells through FAK/Cdc42 signaling and contributes to posterior capsule opacification. Cell. Signal. 97, 110378. doi:10.1016/j.cellsig.2022.110378
Werner, S., Krieg, T., and Smola, H. (2007). Keratinocyte–fibroblast interactions in wound healing. J. Investigative Dermatology 127 (5), 998–1008. doi:10.1038/sj.jid.5700786
Wilkes, M. C., Mitchell, H., Penheiter, S. G., Doré, J. J., Suzuki, K., Edens, M., et al. (2005). Transforming growth factor-beta activation of phosphatidylinositol 3-kinase is independent of Smad2 and Smad3 and regulates fibroblast responses via p21-activated kinase-2. Cancer Res. 65 (22), 10431–10440. doi:10.1158/0008-5472.CAN-05-1522
Wipff, P. J., Rifkin, D. B., Meister, J. J., and Hinz, B. (2007). Myofibroblast contraction activates latent TGF-beta1 from the extracellular matrix. J. Cell Biol. 179 (6), 1311–1323. doi:10.1083/jcb.200704042
Wong, V. W., Paterno, J., Sorkin, M., Glotzbach, J. P., Levi, K., Januszyk, M., et al. (2011). Mechanical force prolongs acute inflammation via T-cell-dependent pathways during scar formation. FASEB J. 25 (12), 4498–4510. doi:10.1096/fj.10-178087
Wong, V. W., Rustad, K. C., Akaishi, S., Sorkin, M., Glotzbach, J. P., Januszyk, M., et al. (2012). Focal adhesion kinase links mechanical force to skin fibrosis via inflammatory signaling. Nat. Med. 18 (1), 148–152. doi:10.1038/nm.2574
Woolen, S. A., Shankar, P. R., Gagnier, J. J., MacEachern, M. P., Singer, L., and Davenport, M. S. (2020). Risk of nephrogenic systemic fibrosis in patients with stage 4 or 5 chronic kidney disease receiving a group II gadolinium-based contrast agent: A systematic review and meta-analysis. JAMA Intern. Med. 180 (2), 223–230. doi:10.1001/jamainternmed.2019.5284
Workman, L. M., and Habelhah, H. (2013). TNFR1 signaling kinetics: Spatiotemporal control of three phases of IKK activation by posttranslational modification. Cell. Signal. 25 (8), 1654–1664. doi:10.1016/j.cellsig.2013.04.005
Wrighton, K. H., Lin, X., and Feng, X-H. (2009). Phospho-control of TGF-beta superfamily signaling. Cell Res. 19 (1), 8–20. doi:10.1038/cr.2008.327
Wu, J-J., Sun, Z-L., Liu, S-Y., Chen, Z-H., Yuan, Z-D., Zou, M-L., et al. (2022). The ASIC3-M-CSF-M2 macrophage-positive feedback loop modulates fibroblast-to-myofibroblast differentiation in skin fibrosis pathogenesis. Cell Death Dis. 13 (6), 1–14. doi:10.1038/s41419-022-04981-9
Wu, M., Schneider, D. J., Mayes, M. D., Assassi, S., Arnett, F. C., Tan, F. K., et al. (2012). Osteopontin in systemic sclerosis and its role in dermal fibrosis. J. Invest Dermatol 132 (6), 1605–1614. doi:10.1038/jid.2012.32
Wu, Q. (2019). Andrews' diseases of the skin: Clinical dermatology, 13th edition. J. Am. Acad. Dermatology 81 (6), e187. doi:10.1016/j.jaad.2019.08.041
Wu, Y., Huang, S., Enhe, J., Ma, K., Yang, S., Sun, T., et al. (2014). Bone marrow-derived mesenchymal stem cell attenuates skin fibrosis development in mice. Int. Wound J. 11 (6), 701–710. doi:10.1111/iwj.12034
Xie, J., Chen, L., Cao, Y., Wu, D., Xiong, W., Zhang, K., et al. (2021). Single-cell sequencing analysis and weighted Co-expression network analysis based on public databases identified that TNC is a novel biomarker for keloid. Front. Immunol. 12, 783907. doi:10.3389/fimmu.2021.783907
Xie, L., Law, B. K., Chytil, A. M., Brown, K. A., Aakre, M. E., and Moses, H. L. (2004). Activation of the Erk pathway is required for TGF-beta1-induced EMT in vitro. Neoplasia 6 (5), 603–610. doi:10.1593/neo.04241
Xu, Y. R., and Lei, C. Q. (2020). TAK1-TABs complex: A central signalosome in inflammatory responses. Front. Immunol. 11, 608976. doi:10.3389/fimmu.2020.608976
Yamashita, M., Fatyol, K., Jin, C., Wang, X., Liu, Z., and Zhang, Y. E. (2008). TRAF6 mediates Smad-independent activation of JNK and p38 by TGF-beta. Mol. Cell. 31 (6), 918–924. doi:10.1016/j.molcel.2008.09.002
Yan, X., Xiong, X., and Chen, Y. G. (2018). Feedback regulation of TGF-β signaling. Acta Biochim. Biophys. Sin. (Shanghai) 50 (1), 37–50. doi:10.1093/abbs/gmx129
Yan, X., Zhang, J., Sun, Q., Tuazon, P. T., Wu, X., Traugh, J. A., et al. (2012). p21-activated kinase 2 (PAK2) inhibits TGF-β signaling in madin-darby canine kidney (MDCK) epithelial cells by interfering with the receptor-smad interaction *. J. Biol. Chem. 287 (17), 13705–13712. doi:10.1074/jbc.M112.346221
Yanagisawa, H., Schluterman, M. K., and Brekken, R. A. (2009). Fibulin-5, an integrin-binding matricellular protein: Its function in development and disease. J. Cell Commun. Signal. 3 (3-4), 337–347. doi:10.1007/s12079-009-0065-3
Yang, L., Zhang, D., Wu, H., Xie, S., Zhang, M., Zhang, B., et al. (2018). Basic fibroblast growth factor influences epidermal homeostasis of living skin equivalents through affecting fibroblast phenotypes and functions. Skin. Pharmacol. Physiol. 31 (5), 229–237. doi:10.1159/000488992
Yang, S., and Plotnikov, S. V. (2021). Mechanosensitive regulation of fibrosis. Cells 10 (5), 994. doi:10.3390/cells10050994
Yang, W-L., Wang, J., Chan, C-H., Lee, S-W., Campos, A. D., Lamothe, B., et al. (2009). The E3 ligase TRAF6 regulates Akt ubiquitination and activation. Science 325 (5944), 1134–1138. doi:10.1126/science.1175065
Yang, X., Ren, H., Guo, X., Hu, C., and Fu, J. (2020). Radiation-induced skin injury: Pathogenesis, treatment, and management. Aging (Albany NY) 12 (22), 23379–23393. doi:10.18632/aging.103932
Yang, Z., Mu, Z., Dabovic, B., Jurukovski, V., Yu, D., Sung, J., et al. (2007). Absence of integrin-mediated TGFbeta1 activation in vivo recapitulates the phenotype of TGFbeta1-null mice. J. Cell Biol. 176 (6), 787–793. doi:10.1083/jcb.200611044
Yeh, Y-C., Lin, H-H., and Tang, M-J. (2012). A tale of two collagen receptors, integrin β1 and discoidin domain receptor 1, in epithelial cell differentiation. Am. J. Physiology-Cell Physiology 303 (12), C1207–C1217. doi:10.1152/ajpcell.00253.2012
Yin, X., Bu, W., Fang, F., Ren, K., and Zhou, B. (2022). Keloid biomarkers and their correlation with immune infiltration. Front. Genet. 13, 784073. doi:10.3389/fgene.2022.784073
Yoshida, K., Matsuzaki, K., Mori, S., Tahashi, Y., Yamagata, H., Furukawa, F., et al. (2005). Transforming growth factor-beta and platelet-derived growth factor signal via c-Jun N-terminal kinase-dependent Smad2/3 phosphorylation in rat hepatic stellate cells after acute liver injury. Am. J. Pathology 166 (4), 1029–1039. doi:10.1016/s0002-9440(10)62324-3
Yoshizaki, A., Yanaba, K., Yoshizaki, A., Iwata, Y., Komura, K., Ogawa, F., et al. (2010). Treatment with rapamycin prevents fibrosis in tight-skin and bleomycin-induced mouse models of systemic sclerosis. Arthritis & Rheumatism 62 (8), 2476–2487. doi:10.1002/art.27498
Yuan, W., and Varga, J. (2001). Transforming growth factor-beta repression of matrix metalloproteinase-1 in dermal fibroblasts involves Smad3. J. Biol. Chem. 276 (42), 38502–38510. doi:10.1074/jbc.M107081200
Zeng, Y., Yi, J., Wan, Z., Liu, K., Song, P., Chau, A., et al. (2015). Substrate stiffness regulates B-cell activation, proliferation, class switch, and T-cell-independent antibody responses in vivo. Eur. J. Immunol. 45 (6), 1621–1634. doi:10.1002/eji.201444777
Zhang, K., Garner, W., Cohen, L., Rodriguez, J., and Phan, S. (1995). Increased types I and III collagen and transforming growth factor-beta 1 mRNA and protein in hypertrophic burn scar. J. Invest Dermatol 104 (5), 750–754. doi:10.1111/1523-1747.ep12606979
Zhang, Y., Wang, H-R., and Wrana, J. L. (2004). Smurf1: A link between cell polarity and ubiquitination. Cell Cycle 3 (4), 391–392. doi:10.4161/cc.3.4.772
Zhang, Y. E. (2017). Non-Smad signaling pathways of the TGF-β family. Cold Spring Harb. Perspect. Biol. 9, a022129. doi:10.1101/cshperspect.a022129
Zhao, X-K., Cheng, Y., Liang Cheng, M., Yu, L., Mu, M., Li, H., et al. (2016). Focal adhesion kinase regulates fibroblast migration via integrin beta-1 and plays a central role in fibrosis. Sci. Rep. 6 (1), 19276. doi:10.1038/srep19276
Zhou, B., Tu, T., Gao, Z., Wu, X., Wang, W., and Liu, W. (2021). Impaired collagen fibril assembly in keloids with enhanced expression of lumican and collagen V. V. Arch. Biochem. Biophys. 697, 108676. doi:10.1016/j.abb.2020.108676
Zhou, J., Wang, J., Zhang, N., Zhang, Y., and Li, Q. (2015). Identification of biomechanical force as a novel inducer of epithelial-mesenchymal transition features in mechanical stretched skin. Am. J. Transl. Res. 7 (11), 2187–2198.
Zhu, J., Motejlek, K., Wang, D., Zang, K., Schmidt, A., and Reichardt, L. F. (2002). beta8 integrins are required for vascular morphogenesis in mouse embryos. Development 129 (12), 2891–2903. doi:10.1242/dev.129.12.2891
Zoppi, N., Chiarelli, N., Binetti, S., Ritelli, M., and Colombi, M. (2018). Dermal fibroblast-to-myofibroblast transition sustained by αvß3 integrin-ILK-Snail1/Slug signaling is a common feature for hypermobile Ehlers-Danlos syndrome and hypermobility spectrum disorders. Biochimica Biophysica Acta (BBA) - Mol. Basis Dis. 1864 (4), 1010–1023. doi:10.1016/j.bbadis.2018.01.005
ADAM a disintegrin and metalloproteinase
APC antigen-presenting cell
BCR B cell receptor
BioF biomechanical force
BM basement membrane
BMM bone marrow-derived macrophage
BM-MSC bone marrow mesenchymal stem cell
CAF cancer-associated fibroblasts
CMS mechanical stretch
Co-Smad co-chaperone Smad
CTGF/CNN2 connective tissue growth factor
DC dendritic cell
ECM extracellular matrix
EMT epithelial-mesenchymal transition
ERK extracellular signal-regulated kinase
FACIT fibril-associated collagens with interrupted triple helices
FAK focal adhesion kinase
GAG glycosaminoglycan
GAP GTPase-activating protein
GEF guanine nucleotide exchange factor
HDF human dermal fibroblast
HFUS high-frequency ultrasound
IFN interferon
IL interleukin
IL1B interleukin 1β
ILK integrin-linked kinase
IPP ILK-PINCH-PARVA
I-Smad inhibitory Smad
ITGB1 integrin β1
JNK c-Jun-N-terminal kinase
LAP latency-associated peptide
LOX lysyl oxidase
MAPK mitogen-activated protein kinase
MAPKK/MEK mitogen-activated protein kinase kinase
MAPKKK mitogen-activated protein kinase kinase
MMP matrix metalloproteinase
MSC mesenchymal stem cell
mTOR mammalian target of rapamycin
mTORC mTOR complex
NDR kinase nuclear Dbf2-related kinase
NFAT nuclear factor of activated T cells
LATS large tumour suppressor kinase
NF-κB Nuclear factor kappa-B
OCT optical coherence tomography
OPN osteopontin
PAR1 protein-activated receptor 1
PAK2 p21-activated kinase 2
PD papillary dermis
PDGF platelet-derived growth factor
PKC protein kinase C
RD reticular dermis
RDEB recessive dystrophic epidermolysis bullosa
RGD sequence Arg-Gly-Asp
RIAM Rap1-interacting adapter molecule
ROCK Rho-associated coiled-coil-containing protein kinase
R-Smad receptor-regulated Smad
RTX rituximab
RXFP1 relaxin family peptide receptor-1
S2P2 sphingosine-1-phosphate receptor 1
SH2 Src homology 2
SLRP small leucine-rich proteoglycan
SSc systemic sclerosis
TAK1 transforming growth factor-β-activated kinase 1
TAZ transcriptional coactivator with PDZ-binding motif
TCR T cell receptor
TGF-β transforming growth factor-β
TGFBR transforming growth factor-β receptor
TIMP tissue inhibitors of metalloproteinase
TNF-α tumor necrosis factor-α
YAP yes-associated protein 1
Keywords: skin fibrosis, extracellular matrix, stiffness, biomechanics, targeted therapy
Citation: Wang K, Wen D, Xu X, Zhao R, Jiang F, Yuan S, Zhang Y, Gao Y and Li Q (2023) Extracellular matrix stiffness—The central cue for skin fibrosis. Front. Mol. Biosci. 10:1132353. doi: 10.3389/fmolb.2023.1132353
Received: 27 December 2022; Accepted: 20 February 2023;
Published: 08 March 2023.
Edited by:
Georgina Gonzalez-Avila, National Institute of Respiratory Diseases-Mexico (INER), MexicoReviewed by:
Ramaswamy Krishnan, Beth Israel Deaconess Medical Center and Harvard Medical School, United StatesCopyright © 2023 Wang, Wen, Xu, Zhao, Jiang, Yuan, Zhang, Gao and Li. This is an open-access article distributed under the terms of the Creative Commons Attribution License (CC BY). The use, distribution or reproduction in other forums is permitted, provided the original author(s) and the copyright owner(s) are credited and that the original publication in this journal is cited, in accordance with accepted academic practice. No use, distribution or reproduction is permitted which does not comply with these terms.
*Correspondence: Yifan Zhang, emhhbmd5aWZhbjgyQDEyNi5jb20=; Ya Gao, Z2FveWFfc2p0dUAxMjYuY29t; Qingfeng Li, ZHIubGlxaW5nZmVuZ0BzaHNtdS5lZHUuY24=
†These authors have contributed equally to this work
Disclaimer: All claims expressed in this article are solely those of the authors and do not necessarily represent those of their affiliated organizations, or those of the publisher, the editors and the reviewers. Any product that may be evaluated in this article or claim that may be made by its manufacturer is not guaranteed or endorsed by the publisher.
Research integrity at Frontiers
Learn more about the work of our research integrity team to safeguard the quality of each article we publish.