- 1Department of Nephrology, Sichuan Provincial People’s Hospital, University of Electronic Science and Technology of China, Chengdu, China
- 2Chinese Academy of Sciences Sichuan Translational Medicine Research Hospital, Chengdu, China
- 3Department of General Surgery and Pancreatic Injury and Repair Key Laboratory of Sichuan Province, The General Hospital of Western Theater Command (Chengdu Military General Hospital), Chengdu, Sichuan, China
- 4Department of Biochemistry and Molecular Biology, Mayo Clinic Arizona, Scottsdale, AZ, United States
Ferroptosis is a newly identified form of regulated cell death characterized by iron accumulation and lipid peroxidation. Ferroptosis plays an essential role in the pathology of numerous diseases and has emerged as a key area of focus in studies of chronic kidney disease (CKD). CKD is a major public health problem with high incidence and mortality that is characterized by a gradual loss of kidney function over time. The severity and complexity of CKD combined with the limited knowledge of its underlying molecular mechanism(s) have led to increased interest in this disease area. Here, we summarize recent advances in our understanding of the regulatory mechanism(s) of ferroptosis and highlight recent studies describing its role in the pathogenesis and progression of CKD. We further discuss the potential therapeutic benefits of targeting ferroptosis for the treatment of CKD and the major hurdles to overcome for the translation of in vitro studies into the clinic.
Introduction
Ferroptosis is an iron-dependent form of non-apoptotic cell death, first described in 2012 by Dixon and colleagues (Dixon et al., 2012). This form of regulated necrosis is characterized by lipid peroxidation at the plasma membrane and subcellular components, ultimately leading to cell rupture (Dixon et al., 2012; Yang and Sockwell, 2016; Latunde-Dada, 2017; Galluzzi et al., 2018; Gao et al., 2019; Hirayama et al., 2019; Li et al., 2020). Ferroptosis has distinct morphological and biochemical features that distinguishes it from other forms of cell death. Morphologically, ferroptosis is characterized by unique mitochondrial changes, including the rupture of mitochondria membranes, a reduction in mitochondrial crests and a decrease in mitochondria numbers (Otasevic et al., 2021). The contribution of mitochondria to ferroptosis has emerged as a promising target to prevent cell death through blocking ferroptosis. Biochemically, ferroptosis is characterized by an increased consumption of glutathione (GSH) and decreased activity of glutathione peroxidase 4 (GPX4) and system Xc− (a cysteine/glutamate antiporter system). Other biochemical features of ferroptosis include an increased production of reactive oxygen species (ROS), an accumulation of lipid peroxides and aberrant iron metabolism (Zheng and Conrad, 2020; Chen et al., 2021). In recent years, ferroptosis has emerged as a critical contributor and potential therapeutic target in the context of a range of pathologic states, including acute and chronic kidney disease, cardiovascular and neurodegenerative disease, stroke and chemotherapy-resistant cancers, amongst others (Xie et al., 2016; Stockwell et al., 2017; Conrad et al., 2021; Maremonti et al., 2022).
Chronic kidney disease (CKD) is a major global public health problem that afflicts 8%–16% of the population and is estimated to contribute to 5–10 million deaths annually (WHO, 2018; Xie et al., 2018; Chen et al., 2019). CKD is characterized by kidney damage (albuminuria) and a gradual decline in kidney function (estimated glomerular filtration rate (eGFR) < 60 ml/min/1.73 m2) (Levey et al., 2005). Cardiovascular disease is a significant adverse outcome in CKD patients that increases its mortality rates (Chandrajay, 2010). Despite improvements in the care of CKD patients and disease management, life expectancy remains low across all stages (Turin et al., 2012) and its global burden continues to rise (Xie et al., 2018). A deeper understanding of the molecular mechanism(s) governing CKD pathogenesis are required to delay its progression, improve diagnostics and to advance the discovery of novel therapeutics.
In recent years, ferroptosis has been widely studied in the context of kidney disease (Müller et al., 2017; Wenzel et al., 2017; Guan et al., 2021; Li et al., 2021) and has emerged as a major area of focus in CKD studies. Understanding the regulation of ferroptosis in CKD is a pre-requisite to reduce kidney cell death and its associated morbidity and mortality.
Herein, we review our latest understanding of ferroptosis in the kidneys and discuss its regulatory genes and links to CKD. We further describe challenges and future perspectives for the use of modulators of ferroptosis as much needed anti-CKD therapeutics.
General mechanisms of ferroptosis
Ferroptosis is regulated by a multitude of metabolic and signaling pathways, including system Xc− (Cysteine/Glutamate Antiporter), GPX4, iron homeostasis, ROS and lipid signaling. In this section, we summarize the role of these pathways during ferroptosis (Figure 1).
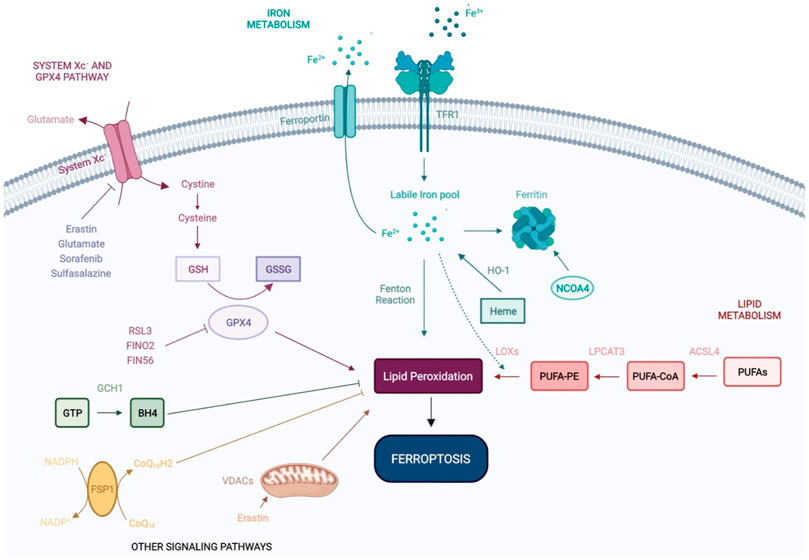
FIGURE 1. Molecular mechanisms of ferroptosis. Ferroptosis is an iron-dependent form of non-apoptotic cell death, regulated by a range of molecular mechanisms and metabolic pathways, including system Xc− and GPX4 signaling, and iron and lipid metabolism. Recent studies have shown that VDACs and other signaling pathways—FSP1-CoQ10-NADPH and GCH1-BH4 work cooperatively with the GPX4/glutathione system to regulate lipid peroxidation and ferroptosis. GSH: Glutathione; GSSG: Glutathione disulfide; GPX4: Glutathione Peroxidase 4; Fe2+: Ferrous iron; Fe3+: Ferric iron; TFR1: Transferrin Receptor 1; HO-1: Heme oxygenase-1; NCOA4: Nuclear receptor coactivator 4; LOXs: Lipoxygenases; LPCAT3: lysophosphatidylcholine acyltransferase 3; ACSL4: Acyl-CoA Synthetase Long Chain Family Member 4; PUFAs: Polyunsaturated fatty acids; PUFA-CoA: Polyunsaturated fatty acyl-coenzyme A; PUFA-PE: Polyunsaturated fatty acid-phosphatidylethanolamine; GTP: Guanosine triphosphate; BH4: tetrahydrobiopterin; GCH1: Guanosine triphosphate cyclohydrolase 1; FSP1: Ferroptosis suppressor protein 1; VDACs: Voltage-dependent anion channels; CoQ10: Coenzyme Q10.
System Xc− and glutathione peroxidase 4 pathways
System Xc− is a widely occurring cysteine (Cys)/glutamate (Glu) antiporter, first identified by Banai et al. (1986) and a known regulator of ferroptosis (Badgley et al., 1979) System Xc is composed of light and heavy chains that are encoded by SLC7A11 and SLC3A2, respectively (Lewerenz et al., 2013). System Xc− mediates the exchange of Glu and Cys across the plasma membrane, ultimately favoring reduced glutathione (GSH) synthesis (Koppula et al., 2018; Liu et al., 2021). Inhibition of system Xc− decreases GSH expression, leading to oxidative damage and ferroptosis. Erastin (the first identified inducer of ferroptosis), extracellular glutamate, sorafenib and sulfasalazine, can block system Xc− and trigger ferroptosis (Dixon et al., 2012; Gao et al., 2015; Zhao et al., 2020).
GPX4 is a peroxide-degrading enzyme that uses GSH as a substrate to produce glutathione disulfide (GSSG), ultimately preventing lipid peroxidation and maintaining redox homeostasis (Ingold et al., 2018). The genetic ablation of GPX4 (Seiler et al., 2008) or its pharmacological inhibition with RSL3 (Yang and Stockwell, 2008) leads to impaired antioxidant capacity and favors cell death by ferroptosis, independently of system Xc status (Yang et al., 2014). In addition to its ability to directly inactivate GPX4, erastin indirectly suppresses GPX4 through the upregulation of activating transcription factor 3 (ATF3) (Zhang et al., 2021). FINO2 and FIN56 have also been identified as direct- and indirect inhibitors of GPX4 activity, respectively (Gaschler et al., 2018).
Iron metabolism
Iron overload is one of the major hallmarks of ferroptosis. Iron is naturally present in the human body, however its active redox activity favors ROS production and lipid peroxidation, ultimately leading to ferroptosis (Hassannia et al., 2019; Battaglia et al., 2020; Li et al., 2020). Under physiological conditions, iron uptake and export are regulated by transferrin receptor and ferroportin respectively at the extracellular level, and by ferritin (iron storage) intracellularly. Ferritin is an intracellular protein that oxidizes ferrous iron (Fe2+) to ferric iron (Fe3+) avoiding the occurrence of the Fenton reaction and subsequent oxidative damage (Hassannia et al., 2019). Ferritin promotes iron storage, its degradation leading to the release of iron stores and subsequent ferroptosis (Rui et al., 2021). Controlling iron metabolism/homeostasis holds great potential for the control of ferroptosis. Accordingly, it has been shown that iron homeostasis is related to the nuclear receptor activator 4 (NCOA4), which can mediate ferritinophagy, a process in which ferritin is delivered to lysosomes and is selectively degraded via autophagy (Mancias et al., 2014; Gao et al., 2016; Hou et al., 2016; Torii et al., 2016; Buccarelli et al., 2018). Heme oxygenase-1 (HO-1), catalyzes the degradation of heme to produce Fe2+ and contributes to iron homeostasis (Chang et al., 2018; Tang et al., 2018). The activation of ferritinophagy and/or HO-1 overexpression increases free iron levels, leading to the accumulation of lipid peroxides and subsequent ferroptosis.
Lipid metabolism
Lipid metabolism is closely associated with ferroptosis and can be triggered by non-enzymatic (Fenton chemistry) and enzymatic mechanisms [lipoxygenases (LOXs)] (Doll and Conrad, 2017; Feng and Stockwell, 2018). Polyunstaturated fatty acids (PUFAs), such as arachidonic acid (AA) and adrenoyl acid are phospholipids that increase the susceptibility to lipid peroxidation during ferroptosis (Friedmann Angeli et al., 2014; Kagan et al., 2017; Wenzel et al., 2017), their abundance and localization impacting lipid peroxidation and the sensitivity of cells to ferroptosis (Doll and Conrad, 2017; Stockwell et al., 2017). More specifically, PUFA metabolism by Fe2+ and LOXs leads to the production of lipid peroxides that disturb membrane structure and function (Hassannia et al., 2019). In this regard, the fatty acid metabolism gene Acyl-CoA synthetase long-chain family member 4 (ASCL4) and lipid remodeling gene Lysophosphatidylcholine acyltransferase 3 (LPCAT3), regulate the insertion of PUFAs into cellular membranes and have been identified as pivotal biomarkers of ferroptosis (Dixon et al., 2015). ACSL4 silencing inhibits ferroptosis, whilst its overexpression modulates cellular lipid composition and sensitivity to ferroptosis (Yuan et al., 2016; Doll et al., 2017). Increasing the expression and/or catalytic activity of ASCL4, LPCAT3, and LOXs or the Fenton reaction increases the accumulation of lipid peroxides and ultimately, ferroptosis (Li and Li, 2020).
Other signaling pathways
Recent studies have revealed a range of signaling pathways that regulate ferroptosis in a multitude of cellular systems. The mevalonate pathway has been shown to inhibit ferroptosis in studies by Stockwell et al. (2017), and two independent studies identified FSP1 [ferroptosis suppressor protein 1, formerly named Gene apoptosis-inducing factor mitochondrial 2 (AIFM2)], as a novel ferroptosis resistance gene. FSP1 was shown to complement the loss (GPX4 KO) or inhibition (RLS3-treated) of the master regulator GPX4 (Bersuker et al., 2019; Doll et al., 2019). FSP1 NADH-dependent oxireductase is recruited to membranes of organelles where it converts oxidase CoQ10 (ubiquinone) into reduced CoQ10 (ubiquinol), preventing oxidative lipid production and its incorporation into membranes and lipoproteins. Collectively, this highlights new methods to suppress ferroptosis independently of GPX4.
In recent studies by Kraft et al. (2020), Guanosine triphosphate (GTP) cyclohydrolase 1 (GCH1), the rate-limiting enzyme in the synthesis of the antioxidant tetrahydrobiopterin (BH4), was identified as a highly potent suppressor of ferroptosis. More specifically, the overexpression of GCH1 favored BH4 synthesis, suppressing ferroptosis by abolishing lipid peroxidation. The GCH1-BH4 axis is a master regulator of ferroptosis resistance, controlling the endogenous production of BH4, the abundance of CoQ10, and the peroxidation of phospholipids independently of the GPX4/glutathione system.
Mitochondria drive the production of cellular ROS and play a central role in ferroptosis by promoting lipid peroxidation. Voltage-dependent anion channels (VDACs) transport iron and metabolites and are essential to ferroptosis (DeHart et al., 2018). Erastin inhibits VDACs, leading to mitochondrial dysfunction, ROS production and iron-mediated cell death (Yagoda et al., 2007).
Ferroptosis and chronic kidney disease
CKD is a major public health issue (WHO, 2018; Xie et al., 2018; Chen et al., 2019). The two main risk factors contributing to the increasing prevalence of CKD are diabetes and hypertension. Other causes include primary glomerulonephritis, inherited diseases such as polycystic kidney disease, kidney stones and repeated urinary infections (Couser et al., 2011). Despite recent interest into the molecular mechanisms regulating CKD pathogenesis and the contribution of environmental and genetic risk factors to disease susceptibility and heterogeneity (Cañadas-Garre et al., 2019), considerable knowledge gaps remain. As a consequence, therapeutic approaches for the management of CKD progression remain scarce.
The kidney is an iron metabolism-related organ. A loss of GPX4 activity (Friedmann Angeli et al., 2014), through genetic deletion leads to albuminuria, kidney tubular epithelial cell death and mortality within weeks (Friedmann Angeli et al., 2014). More specifically in the context of CKD, preclinical studies support the correlation between renal iron deposition, lipid deposition and ferroptosis in multiple forms of CKD, highlighting its clinical significance (Nankivell et al., 1992; Wang et al., 2001; Shah et al., 2007; Martines et al., 2013; Wenzel et al., 2017; Guan et al., 2021; Li et al., 2021). Improved understanding of the mechanisms regulating ferroptosis in the kidney and defining key genes regulating these processes will advance the discovery of new molecular targets for multiple forms of kidney disease.
Ferroptosis regulatory genes in chronic kidney disease
Ferroptosis regulates CKD and kidney function. The altered expression of genes that regulate ferroptosis may influence the incidence and predisposition to CKD. Table 1 summaries recent advances in this area.
To date, approximately 250 kidney function-associated loci have been identified, with around 50% showing relevance to kidney function in the context of CKD (Wuttke et al., 2019). Despite promise in this area, only a small number of these genes have been functionally characterized including UMOD (Trudu et al., 2013), DAB2 (Qiu et al., 2018), SHROOM3 (Miao et al., 2021), DACH1 (Doke et al., 2021) and MANBA (Gu et al., 2021). In recent genome-wide association studies for kidney disease, Dpep1 and Chmp1a, were identified as key regulators of ferroptosis (Guan et al., 2021). At the molecular level, Dpep1 and Chmp1 were shown to alter cellular iron trafficking, ultimately favoring the development of kidney disease. To our knowledge, this is the only study to-date that identifies two potential casual genes of CKD progression that are important regulators of ferroptosis. This highlights the potential therapeutic benefits of pharmacologically targeting ferroptosis through DPEP1 and/or CHMP1 in patients with kidney disease to prevent multiple forms of CKD.
Other ferroptosis regulatory genes that are not specifically identified as casual genes of CKD progression have been studied in the context of diabetic nephropathy (DN), renal fibrosis and autosomal dominant polycystic kidney disease (ADPKD). These are summarized in Table 1.
DN is the most common cause of mortality and morbidity in CKD patients (Iyengar et al., 2015). The main factors involved in DN pathogenesis include high glucose levels, oxidative stress and inflammatory responses (Perrone et al., 2021). Recent studies suggest that, in addition to other forms of programmed cell death, ferroptosis plays a crucial pathological role in the development of DN (Wang et al., 2020). In studies using in vitro (proximal kidney tubular cells), in vivo [streptozotocin (STZ)-induced DN mice model] and ex vivo (kidney biopsy samples) models, a significant reduction in the mRNA and protein expression of the ferroptosis-related molecules SLC7A11 and GPX4 were observed, leading to increased lipid peroxidation in DN compared to non-DN models (Kim et al., 2021). In addition, the changes associated with ferroptosis under diabetic conditions were ameliorated by specific ferroptosis inhibitors. Studies using STZ-induced DN and db/db mice further confirmed the involvement of ferroptosis in the progression of DN (Wang et al., 2020). More specifically, significant changes in the expression of the ferroptosis-associated markers ACSL4 and GPX4 were observed. This was accompanied by an increase in lipid peroxidation and iron content in DN mice. In vitro studies using known inducers of ferroptosis including erastin and RSL3, induced renal tubular cell death through increasing iron levels and ACSL4 expression, sensitizing cells to ferroptosis (Wang et al., 2020). Other studies showed that the increased expression of HO-1 favors the inhibition of oxidative stress and the restoration of redox balance, which may be beneficial for DN (Lee et al., 2009; Rodriguez et al., 2011). High-mobility box-1 (HMGB1) was shown to be activated in DN patients and mesangial cells in response to high glucose. In studies using high glucose-treated mesangial cells as in vitro model, increased translocation of HMGB1 to the nucleus was reported, which decreased Nrf2 expression and its subsequent downstream targets (Wu et al., 2021). Collectively, these findings suggest that HMGB1 acts as a positive regulator of ferroptosis via Nfr2 signaling. Targeting HMGB1 and ferroptosis therefore holds potential for the development of novel therapeutic strategies for DN.
Renal fibrosis is an important pathological process that contributes to the progression of CKD (Efstratiadis et al., 2009). The role of ferroptosis was demonstrated in studies using doxorubicin-induced renal fibrosis models (Fang et al., 2019). More specifically, increased expression of renal prostaglandin-endoperoxide synthase (Ptgs2) as a putative marker of ferroptosis was observed in the kidneys. Other ferroptosis-related molecules, including GPXs, iron and lipid peroxides have also been demonstrated (Sponsel et al., 1996; Himmelfarb, 2005; Zhang et al., 2022), corroborating the involvement of ferroptosis in the pathogenesis of renal fibrosis. Transforming growth factor β1 (TGF-β1) was shown to be a key mediator of renal fibrosis (Ikeda et al., 2014). More specifically, in vitro studies using renal tubular cells showed that TGF- β1 treatment increases the expression of SLC7A11 and GPX4, an effect that could be reversed by treatment with Ferrostain-1 (Fer-1), a well-characterized inhibitor of ferroptosis (Kim et al., 2021). The potential role and mechanisms underlying tubular cell ferroptosis during kidney fibrosis were demonstrated in kidney biopsies from patients with CKD and mouse models of fibrotic kidney disease unilateral ureter obstruction (UUO) or ischemia/reperfusion injury (IRI) nephropathy. Downregulation of the ferroptosis-associated marker GPX4 and upregulation of 4-hydroxynonenal (4-HNE) were observed (Li et al., 2017; Zhou et al., 2022). Moreover, inhibitors of ferroptosis were protective against kidney fibrosis in patients with CKD.
Ferroptosis has also been linked with ADPKD, a disease caused by mutations in polycystin-1 and -2 (PKD1 and PKD2) leading to the growth of cysts in the kidneys (Raaij et al., 2018; Zhang et al., 2021). Zhang et al. (2021) showed for the first time that ferroptosis regulates ADPKD progression, representing a promising therapeutic target (Zhang et al., 2021). Low levels of cell death were observed in Pdk1 mutant mice as a result of ferroptosis as opposed to apoptosis. Pdk1 mutant cells and kidney tissues from Pdk1 mouse models also showed decreased GSH expression and increased Transferrin receptor 1 (Tfr1), divalent metal transporter 1 (DMT1) and HO-1 expression. This resulted in high iron levels, low GSH and GPX4 activity, increased lipid peroxidation and ultimately ferroptosis. Erastin and Fer-1 prevented disease progression in Pkd1 mutant mice and 4-HNE expression, a lipid peroxidation product that increases in abundance during ferroptotic processes, increased in Pdk1 mutant renal epithelial cells, which regulated their proliferation via activation of Akt, S6, Stat3, and Rb. Collectively, these studies demonstrate the critical role of ferroptosis in the regulation of CKD progression, highlighting its promise as a novel therapeutic strategy.
Targeting ferroptosis in chronic kidney disease
A range of preclinical studies have shown the potential of inhibitors of ferroptosis to prevent kidney disease. These include Fer-1, Rosiglitazone, Deferasirox (DFX) and Deferoxamine mesylate (DFO), and are summarized in Table 2.
Under physiological conditions, iron homeostasis is maintained by hepcidin and iron regulatory proteins (Ueda et al., 1996). In CKD patients, iron homeostasis is disrupted as a result of altered iron uptake and/or insufficient iron export (Ueda et al., 1996). This leads to increased Fenton-mediated oxidative damage and renal injury (Naito et al., 2015). This suggests that iron accumulation in CKD patients occurs during ferroptosis. Therapeutic strategies to regulate the expression of iron metabolism-related proteins and alleviate ferroptosis through iron chelation (e.g., DFX or DFO) represent attractive approaches for the treatment of CKD. Indeed, studies (Table 2) using UUO or 5/6 nephrectomy-induced CKD rat/mouse models showed that DFX (Wang et al., 2022) and DFO (Li et al., 2017; Feng et al., 2021; Zhou et al., 2022) treatment reduced iron accumulation and renal injury through the regulation of the TGF-1β/Smad3 axis, inflammation and oxidative stress signaling pathways. As an alternative to iron chelators, the potential of Fer-1 to prevent ROS production and lipid peroxidation via hypoxia-inducible factor 1-alpha (HIF-1α)/HO-1 signaling was demonstrated in diabetic mouse models (Wiggin et al., 2008; Linkermann et al., 2014). In Pdk1 mutant mice, Fer-1 could inhibit ferroptotic cell death, Akt-mediated proliferation, S6, Stat3, and Rb signaling, delaying cyst growth in ADPKD mouse models (Zhang et al., 2021). Treatment of STZ-induced DN animal models with rosiglitazone (an ACSL4 inhibitor), led to a reduction in lipid peroxidation and iron content in the kidneys, improving kidney function (Bao et al., 2007; Wang et al., 2020; Li et al., 2022). In more recent studies, tectorigenin showed protective effects against kidney injury and fibrosis, two key factors during CKD pathogenesis (Bebber et al., 2020). In vitro, tectorigenin suppressed ferroptosis and TGF-β1-stimulated fibrosis in primary renal tubular epithelial cells (TECs). Consistent with these studies, UUO-mouse models administered tectorigenin showed attenuated tubular cell damage and fewer fibrotic lesions in the kidneys as a result of the inhibition of Smad3-mediated ferroptosis and fibrosis. More specifically, tectorigenin inhibited Smad3 phosphorylation and the expression of Nox4, a downstream modulator of ferroptosis. Moreover, isoflavone could indirectly restore the expression of GPX4, a negative regulator of ferroptosis. Collectively, these studies highlight the potential of tectorigenin as a therapeutic strategy for CKD. Future studies are now required to dissect the mechanisms of action and potential therapeutic applications of this compound.
From a therapeutic standpoint, pharmacological modulators of ferroptosis have been explored in vitro and in vivo CKD experiments (Table 2). Despite promising results, the efficacy of these modulators and their potential side effects requires assessment in future clinical studies.
Challenges and future perspectives
Numerous lines of evidence now support a role for ferroptosis in CKD, which is mediated by the iron-dependent accumulation of lipid peroxidation. Accordingly, we have discussed the major signaling pathways that regulate ferroptosis, its regulatory genes, the role of ferroptosis in CKD and potential therapeutic strategies to inhibit ferroptosis. Despite obvious progress, future studies must address the following concerns:
1) Ferroptosis is not an isolated event and is closely associated with other forms of cell death (apoptosis, necrosis and autophagy). The molecular mechanism(s) regulating this cross-talk require characterization to reveal potential antagonistic or synergistic roles in the context of kidney disease, and more specifically CKD.
2) Although a range of regulatory factors of ferroptosis have been described, they do not represent specific markers of ferroptosis. In this regard, reliable biomarkers, specifically in the context of kidney disease remains a challenge.
3) Accumulating evidence indicates that ferroptotic cell death can inhibit tumor growth and improve the efficacy of chemotherapeutic drugs. [99] However, in kidney disease, ferroptosis may negatively impact kidney health. Further mechanistic studies are therefore warranted.
4) Although the link between ferroptosis and acute kidney injury (AKI) has been widely explored and characterized, studies on ferroptosis and CKD remain limited. Indeed, the majority of studies to-date have used in vitro or in vivo models. However, as in vitro cell culture conditions and animal models of kidney diseases drastically differ, the interpretation and establishment of a link between ferroptosis and CKD can be challenging. Methods to translate scientific research into clinical applications should therefore be developed.
Author contributions
LW and Z-LL contributed to the conception and design. W-QZ and YW wrote the draft of this paper. H-JL prepared the figure and tables. All authors read and approved the final manuscript.
Funding
This work was supported by the National Natural Science Foundation of China (81770742), and the Sichuan Province Science and Technology Fundamental Project (2019YFS0538).
Conflict of interest
Author H-JL was employed by the Department of Biochemistry and Molecular Biology, Mayo Clinic Arizona.
The remaining authors declare that the research was conducted in the absence of any commercial or financial relationships that could be construed as a potential conflict of interest.
Publisher’s note
All claims expressed in this article are solely those of the authors and do not necessarily represent those of their affiliated organizations, or those of the publisher, the editors and the reviewers. Any product that may be evaluated in this article, or claim that may be made by its manufacturer, is not guaranteed or endorsed by the publisher.
References
Badgley, M. A., Kremer, D. M., Carlo Maurer, H., DelGiorno, K. E., Lee, H. J., Purohit, V., et al. (1979). Cysteine depletion induces pancreatic tumor ferroptosis in mice. Science 2020 (6486), 85–89. doi:10.1126/science.aaw9872
Bannai, S. (1986). Exchange of cystine and glutamate across plasma membrane of human fibroblasts. J. Biol. Chem. 261 (5), 2256–2263. doi:10.1016/s0021-9258(17)35926-4
Bao, Y., Jia, R. H., Yuan, J., and Li, J. (2007). Rosiglitazone ameliorates diabetic nephropathy by inhibiting reactive oxygen species and its downstream-signaling pathways. Pharmacology 80 (1), 57–64. doi:10.1159/000103232
Battaglia, A. M., Chirillo, R., Aversa, I., Sacco, A., Costanzo, F., and Biamonte, F. (2020). Ferroptosis and cancer: Mitochondria meet the “iron maiden” cell death. Cells 9 (6), E1505. doi:10.3390/cells9061505
Bebber, C. M., Müller, F., Clemente, L. P., Weber, J., and von Karstedt, S. (2020). Ferroptosis in cancer cell biology. Cancers (Basel) 12 (1), E164. doi:10.3390/cancers12010164
Bersuker, K., Hendricks, J. M., Li, Z., Magtanong, L., Ford, B., Tang, P. H., et al. (2019). The CoQ oxidoreductase FSP1 acts parallel to GPX4 to inhibit ferroptosis. Nature 575 (7784), 688–692. doi:10.1038/s41586-019-1705-2
Buccarelli, M., Marconi, M., Pacioni, S., De Pasqualis, I., D’Alessandris, Q. G., Martini, M., et al. (2018). Inhibition of autophagy increases susceptibility of glioblastoma stem cells to temozolomide by igniting ferroptosis. Cell Death Dis. 9 (8), 841. doi:10.1038/s41419-018-0864-7
Cañadas-Garre, M., Anderson, K., Cappa, R., Skelly, R., Smyth, L. J., McKnight, A. J., et al. Genetic susceptibility to chronic kidney disease - some more pieces for the heritability puzzle. Front. Genetics2019 10 (MAY).
Chandrajay, D. (2010). Association of estimated glomerular filtration rate and albuminuria with all-cause and cardiovascular mortality in general population cohorts: A collaborative meta-analysis. Ann. Clin. Biochem. 47 (6), 578. doi:10.1258/acb.2010.201016
Chang, L. C., Chiang, S. K., Chen, S. E., Yu, Y. L., Chou, R. H., and Chang, W. C. (2018). Heme oxygenase-1 mediates BAY 11-7085 induced ferroptosis. Cancer Lett. 416, 124–137. doi:10.1016/j.canlet.2017.12.025
Chen, T. K., Knicely, D. H., and Grams, M. E. (2019). Chronic kidney disease diagnosis and management: A review. JAMA - J. Am. Med. Association2019 322 (13), 1294–1304. doi:10.1001/jama.2019.14745
Chen, X., Comish, P. B., Tang, D., and Kang, R. Characteristics and biomarkers of ferroptosis. Front. Cell Dev. Biol. 9. 637162. doi:10.3389/fcell.2021.637162
Conrad, M., Lorenz, S. M., and Proneth, B. (2021). Targeting ferroptosis: New hope for as-yet-incurable diseases. Trends Mol. Med. 27 (2), 113–122. doi:10.1016/j.molmed.2020.08.010
Couser, W. G., Remuzzi, G., Mendis, S., and Tonelli, M. (2011). The contribution of chronic kidney disease to the global burden of major noncommunicable diseases. Kidney Int. 80 (12), 1258–1270. doi:10.1038/ki.2011.368
DeHart, D. N., Fang, D., Heslop, K., Li, L., Lemasters, J. J., and Maldonado, E. N. (2018). Opening of voltage dependent anion channels promotes reactive oxygen species generation, mitochondrial dysfunction and cell death in cancer cells. Biochem. Pharmacol. 148, 155–162. doi:10.1016/j.bcp.2017.12.022
Dixon, S. J., Lemberg, K. M., Lamprecht, M. R., Skouta, R., Zaitsev, E. M., Gleason, C. E., et al. (2012). Ferroptosis: An iron-dependent form of nonapoptotic cell death. Cell 149 (5), 1060–1072. doi:10.1016/j.cell.2012.03.042
Dixon, S. J., Winter, G. E., Musavi, L. S., Lee, E. D., Snijder, B., Rebsamen, M., et al. (2015). Human haploid cell genetics reveals roles for lipid metabolism genes in nonapoptotic cell death. ACS Chem. Biol. 10 (7), 1604–1609. doi:10.1021/acschembio.5b00245
Doke, T., Huang, S., Qiu, C., Liu, H., Guan, Y., Hu, H., et al. (2021). Transcriptome-wide association analysis identifies DACH1 as a kidney disease risk gene that contributes to fibrosis. J. Clin. Invest. 131 (10), 141801. doi:10.1172/JCI141801
Doll, S., and Conrad, M. (2017). Iron and ferroptosis: A still ill-defined liaison. IUBMB Life 69 (6), 423–434. doi:10.1002/iub.1616
Doll, S., Freitas, F. P., Shah, R., Aldrovandi, M., da Silva, M. C., Ingold, I., et al. (2019). FSP1 is a glutathione-independent ferroptosis suppressor. Nature 575 (7784), 693–698. doi:10.1038/s41586-019-1707-0
Doll, S., Proneth, B., Tyurina, Y. Y., Panzilius, E., Kobayashi, S., Ingold, I., et al. (2017). ACSL4 dictates ferroptosis sensitivity by shaping cellular lipid composition. Nat. Chem. Biol. 13 (1), 91–98. doi:10.1038/nchembio.2239
Efstratiadis, G., Divani, M., Katsioulis, E., and Vergoulas, G. (2009). Renal fibrosis. Hippokratia 13 (4), 224–229.
Fang, X., Wang, H., Han, D., Xie, E., Yang, X., Wei, J., et al. (2019). Ferroptosis as a target for protection against cardiomyopathy. Proc. Natl. Acad. Sci. U. S. A. 116 (7), 2672–2680. doi:10.1073/pnas.1821022116
Feng, H., and Stockwell, B. R. (2018). Unsolved mysteries: How does lipid peroxidation cause ferroptosis? PLoS Biol. 16 (5), e2006203. doi:10.1371/journal.pbio.2006203
Feng, X., Wang, S., Sun, Z., Dong, H., Yu, H., Huang, M., et al. (2021). Ferroptosis enhanced diabetic renal tubular injury via HIF-1α/HO-1 pathway in db/db mice. Front. Endocrinol. 12, 626390. doi:10.3389/fendo.2021.626390
Friedmann Angeli, J. P., Schneider, M., Proneth, B., Tyurina, Y. Y., Tyurin, V. A., Hammond, V. J., et al. (2014). Inactivation of the ferroptosis regulator Gpx4 triggers acute renal failure in mice. Nat. Cell Biol. 16 (12), 1180–1191. doi:10.1038/ncb3064
Galluzzi, L., Vitale, I., Aaronson, S. A., Abrams, J. M., Adam, D., Agostinis, P., et al. Molecular mechanisms of cell death: Recommendations of the nomenclature committee on cell death 2018. Cell Death Differ. 25 (3), 486–541. doi:10.1038/s41418-017-0012-4
Gao, M., Monian, P., Pan, Q., Zhang, W., Xiang, J., and Jiang, X. (2016). Ferroptosis is an autophagic cell death process. Cell Res. 26 (9), 1021–1032. doi:10.1038/cr.2016.95
Gao, M., Monian, P., Quadri, N., Ramasamy, R., and Jiang, X. (2015). Glutaminolysis and transferrin regulate ferroptosis. Mol. Cell 59 (2), 298–308. doi:10.1016/j.molcel.2015.06.011
Gao, M., Yi, J., Zhu, J., Minikes, A. M., Monian, P., Thompson, C. B., et al. (2019). Role of mitochondria in ferroptosis. Mol. Cell 73 (2), 354–363.e3. e3. doi:10.1016/j.molcel.2018.10.042
Gaschler, M. M., Andia, A. A., Liu, H., Csuka, J. M., Hurlocker, B., Vaiana, C. A., et al. (2018). FINO2 initiates ferroptosis through GPX4 inactivation and iron oxidation. Nat. Chem. Biol. 14 (5), 507–515. doi:10.1038/s41589-018-0031-6
Gu, X., Yang, H., Sheng, X., Ko, Y. A., Qiu, C., Park, J., et al. (2021). Kidney disease genetic risk variants alter lysosomal beta-mannosidase (MANBA) expression and disease severity. Sci. Transl. Med. 13 (576), eaaz1458. doi:10.1126/scitranslmed.aaz1458
Guan, Y., Liang, X., Ma, Z., Hu, H., Liu, H., Miao, Z., et al. (2021). A single genetic locus controls both expression of DPEP1/CHMP1A and kidney disease development via ferroptosis. Nat. Commun. 12 (1), 5078–5117. Available from. doi:10.1038/s41467-021-25377-x
Hassannia, B., Vandenabeele, P., and Vanden Berghe, T. (2019). Targeting ferroptosis to iron out cancer. Cancer Cell 35 (6), 830–849. doi:10.1016/j.ccell.2019.04.002
Himmelfarb, J. (2005). Relevance of oxidative pathways in the pathophysiology of chronic kidney disease. Cardiol. Clin. 23 (3), 319–330. doi:10.1016/j.ccl.2005.03.005
Hirayama, T., Miki, A., and Nagasawa, H. (2019). Organelle-specific analysis of labile Fe(ii) during ferroptosis by using a cocktail of various colour organelle-targeted fluorescent probes. Metallomics. 11 (1), 111–117. doi:10.1039/c8mt00212f
Hou, W., Xie, Y., Song, X., Sun, X., Lotze, M. T., Zeh, H. J., et al. (2016). Autophagy promotes ferroptosis by degradation of ferritin. Autophagy 12 (8), 1425–1428. doi:10.1080/15548627.2016.1187366
Ikeda, Y., Ozono, I., Tajima, S., Imao, M., Horinouchi, Y., Izawa-Ishizawa, Y., et al. (2014). Iron chelation by deferoxamine prevents renal interstitial fibrosis in mice with unilateral ureteral obstruction. PLoS ONE 9 (2), e89355. doi:10.1371/journal.pone.0089355
Ingold, I., Berndt, C., Schmitt, S., Doll, S., Poschmann, G., Buday, K., et al. (2018). Selenium utilization by GPX4 is required to prevent hydroperoxide-induced ferroptosis. Cell 172 (3), 409–422.e21. e21. doi:10.1016/j.cell.2017.11.048
Iyengar, S. K., Sedor, J. R., Freedman, B. I., Kao, W. H. L., Kretzler, M., Keller, B. J., et al. (2015). Genome-wide association and trans-ethnic meta-analysis for advanced diabetic kidney disease: Family investigation of nephropathy and diabetes (FIND). PLoS Genet. 11 (8), e1005352. doi:10.1371/journal.pgen.1005352
Kagan, V. E., Mao, G., Qu, F., Angeli, J. P. F., Doll, S., Croix, C. S., et al. (2017). Oxidized arachidonic and adrenic PEs navigate cells to ferroptosis. Nat. Chem. Biol. 13 (1), 81–90. doi:10.1038/nchembio.2238
Kim, S., Kang, S. W., Joo, J., Han, S. H., Shin, H., Nam, B. Y., et al. (2021). Characterization of ferroptosis in kidney tubular cell death under diabetic conditions. Cell Death Dis. 12 (2), 160. doi:10.1038/s41419-021-03452-x
Koppula, P., Zhang, Y., Zhuang, L., and Gan, B. (2018). Amino acid transporter SLC7A11/xCT at the crossroads of regulating redox homeostasis and nutrient dependency of cancer. Cancer Commun. 38 (1), 12. doi:10.1186/s40880-018-0288-x
Kraft, V. A. N., Bezjian, C. T., Pfeiffer, S., Ringelstetter, L., Müller, C., Zandkarimi, F., et al. (2020). GTP cyclohydrolase 1/tetrahydrobiopterin counteract ferroptosis through lipid remodeling. ACS Cent. Sci. 6 (1), 41–53. doi:10.1021/acscentsci.9b01063
Latunde-Dada, G. O. Ferroptosis: Role of lipid peroxidation, iron and ferritinophagy. Biochimica Biophysica Acta - General Subjects2017 1861 (8), 1893–1900. doi:10.1016/j.bbagen.2017.05.019
Lee, S. C., Han, S. H., Li, J. J., Lee, S. H., Jung, D. S., Kwak, S. J., et al. (2009). Induction of heme oxygenase-1 protects against podocyte apoptosis under diabetic conditions. Kidney Int. 76 (8), 838–848. doi:10.1038/ki.2009.286
Levey, A. S., Eckardt, K. U., Tsukamoto, Y., Levin, A., Coresh, J., Rossert, J., et al. (2005). Definition and classification of chronic kidney disease: A position statement from kidney disease: Improving global outcomes (KDIGO). Kidney Int. 67 (6), 2089–2100. doi:10.1111/j.1523-1755.2005.00365.x
Lewerenz, J., Hewett, S. J., Huang, Y., Lambros, M., Gout, P. W., Kalivas, P. W., et al. (2013). The cystine/glutamate antiporter system xc- in health and disease: From molecular mechanisms to novel therapeutic opportunities. Antioxid. Redox Signal. 18 (5), 522–555. doi:10.1089/ars.2011.4391
Li, D., and Li, Y. (2020). The interaction between ferroptosis and lipid metabolism in cancer. Signal Transduct. Target. Ther. 5 (1), 108. doi:10.1038/s41392-020-00216-5
Li, J., Cao, F., Yinliang, H., Huangjian, Z., Lintao, Z., Mao, N., et al. (2020). Ferroptosis: Past, present and future. Cell Death Dis. 11 (2), 88. doi:10.1038/s41419-020-2298-2
Li, J., Yang, J., Zhu, B., Fan, J., Hu, Q., and Wang, L. (2022). Tectorigenin protects against unilateral ureteral obstruction by inhibiting Smad3-mediated ferroptosis and fibrosis. Phytother. Res. 36 (1), 475–487. doi:10.1002/ptr.7353
Li, L. X., Fan, L. X., Zhou, J. X., Grantham, J. J., Calvet, J. P., Sage, J., et al. (2017). Lysine methyltransferase SMYD2 promotes cyst growth in autosomal dominant polycystic kidney disease. J. Clin. Invest. 127 (7), 2751–2764. doi:10.1172/JCI90921
Li, P., Jiang, M., Li, K., Li, H., Zhou, Y., Xiao, X., et al. (2021). Glutathione peroxidase 4–regulated neutrophil ferroptosis induces systemic autoimmunity. Nat. Immunol. 22 (9), 1107–1117. doi:10.1038/s41590-021-00993-3
Linkermann, A., Skouta, R., Himmerkus, N., Mulay, S. R., Dewitz, C., de Zen, F., et al. (2014). Synchronized renal tubular cell death involves ferroptosis. Proc. Natl. Acad. Sci. U. S. A. 111 (47), 16836–16841. doi:10.1073/pnas.1415518111
Liu, M. R., Zhu, W. T., and Pei, D. S. (2021). System xc -: A key regulatory target of ferroptosis in cancer. Invest. New Drugs 39 (4), 1123–1131. doi:10.1007/s10637-021-01070-0
Mancias, J. D., Wang, X., Gygi, S. P., Harper, J. W., and Kimmelman, A. C. (2014). Quantitative proteomics identifies NCOA4 as the cargo receptor mediating ferritinophagy. Nature 508 (7498), 105–109. doi:10.1038/nature13148
Maremonti, F., Meyer, C., and Linkermann, A. (2022). Mechanisms and models of kidney tubular necrosis and nephron loss. J. Am. Soc. Nephrol. 33 (3), 472–486. doi:10.1681/ASN.2021101293
Martines, A. M. F., Masereeuw, R., Tjalsma, H., Hoenderop, J. G., Wetzels, J. F. M., and Swinkels, D. W. (2013). Iron metabolism in the pathogenesis of iron-induced kidney injury. Nat. Rev. Nephrol. 9 (7), 385–398. doi:10.1038/nrneph.2013.98
Miao, Z., Balzer, M. S., Ma, Z., Liu, H., Wu, J., Shrestha, R., et al. (2021). Single cell regulatory landscape of the mouse kidney highlights cellular differentiation programs and disease targets. Nat. Commun. 12 (1), 2277. doi:10.1038/s41467-021-22266-1
Müller, T., Dewitz, C., Schmitz, J., Schröder, A. S., Bräsen, J. H., Stockwell, B. R., et al. (2017). Necroptosis and ferroptosis are alternative cell death pathways that operate in acute kidney failure. Cell. Mol. Life Sci. 74 (19), 3631–3645. doi:10.1007/s00018-017-2547-4
Naito, Y., Fujii, A., Sawada, H., Oboshi, M., Iwasaku, T., Okuhara, Y., et al. (2015). Association between renal iron accumulation and renal interstitial fibrosis in a rat model of chronic kidney disease. Hypertens. Res. 38 (7), 463–470. doi:10.1038/hr.2015.14
Nankivell, B. J., Boadle, R. A., and Harris, D. C. H. (1992). Iron accumulation in human chronic renal disease. Am. J. Kidney Dis. 20 (6), 580–584. doi:10.1016/s0272-6386(12)70222-6
Otasevic, V., Vucetic, M., Grigorov, I., Martinovic, V., and Stancic, A. (2021). Ferroptosis in different pathological contexts seen through the eyes of mitochondria, Oxid. Med. Cell Longev. 2021, 5537330. doi:10.1155/2021/5537330
Perrone, A., Giovino, A., Benny, J., and Martinelli, F. (2021). Advanced glycation end products. Oxidative Stress Diabet. Nephrop. 2021. doi:10.1155/2020/3818196
Qiu, C., Huang, S., Park, J., Park, Y. S., Ko, Y. A., Seasock, M. J., et al. (2018). Renal compartment–specific genetic variation analyses identify new pathways in chronic kidney disease. Nat. Med. 24 (11), 1721–1731. doi:10.1038/s41591-018-0194-4
Raaij, S. van, Swelm, R. van, Bouman, K., Cliteur, M., van den, H. M., Pertijs, J., et al. (2018). Tubular iron deposition and iron handling proteins in human healthy kidney and chronic kidney disease. Sci. Rep. 8 (1), 9353. doi:10.1038/s41598-018-27107-8
Rodriguez, F., Lopez, B., Perez, C., Fenoy, F. J., Hernandez, I., Stec, D. E., et al. (2011). Chronic tempol treatment attenuates the renal hemodynamic effects induced by a heme oxygenase inhibitor in streptozotocin diabetic rats. Am. J. Physiol. Regul. Integr. Comp. Physiol. 301, 1540–1548. doi:10.1152/ajpregu.00847.2010www.ajpregu.org
Rui, T., Wang, H., Li, Q., Cheng, Y., Gao, Y., Fang, X., et al. (2021). Deletion of ferritin H in neurons counteracts the protective effect of melatonin against traumatic brain injury-induced ferroptosis. J. Pineal Res. 70 (2), e12704. doi:10.1111/jpi.12704
Seiler, A., Schneider, M., Förster, H., Roth, S., Wirth, E. K., Culmsee, C., et al. (2008). Glutathione peroxidase 4 senses and translates oxidative stress into 12/15-lipoxygenase dependent- and AIF-mediated cell death. Cell Metab. 8 (3), 237–248. doi:10.1016/j.cmet.2008.07.005
Shah, S. v., Baliga, R., Rajapurkar, M., and Fonseca, V. A. (2007). Oxidants in chronic kidney disease. J. Am. Soc. Nephrol. 18 (1), 16–28. doi:10.1681/asn.2006050500
Sponsel, H. T., Alfrey, A. C., Hammond, W. S., Durr, J. A., Ray, C., and Anderson, R. J. (1996). Effect of iron on renal tubular epithelial cells. Kidney Int. 50 (2), 436–444. doi:10.1038/ki.1996.334
Stockwell, B. R., Friedmann Angeli, J. P., Bayir, H., Bush, A. I., Conrad, M., Dixon, S. J., et al. (2017). Ferroptosis: A regulated cell death nexus linking metabolism, redox biology, and disease. Cell 171 (2), 273–285. doi:10.1016/j.cell.2017.09.021
Tang, M., Chen, Z., Wu, D., and Linxi, C. (2018). Ferritinophagy/ferroptosis: Iron-related newcomers in human diseases. J. Cell. Physiol. 233 (12), 9179–9190. doi:10.1002/jcp.26954
Torii, S., Shintoku, R., Kubota, C., Yaegashi, M., Torii, R., Sasaki, M., et al. (2016). An essential role for functional lysosomes in ferroptosis of cancer cells. Biochem. J. 473 (6), 769–777. doi:10.1042/BJ20150658
Trudu, M., Janas, S., Lanzani, C., Debaix, H., Schaeffer, C., Ikehata, M., et al. (2013). Common noncoding UMOD gene variants induce salt-sensitive hypertension and kidney damage by increasing uromodulin expression. Nat. Med. 19 (12), 1655–1660. doi:10.1038/nm.3384
Turin, T. C., Tonelli, M., Manns, B. J., Ravani, P., Ahmed, S. B., and Hemmelgarn, B. R. (2012). Chronic kidney disease and life expectancy. Nephrol. Dial. Transpl. 27 (8), 3182–3186. doi:10.1093/ndt/gfs052
Ueda, N., Baliga, R., and Shah, S. V. (1996). Role of ‘catalytic’ iron in an animal model of minimal change nephrotic syndrome. Kidney Int. 49 (2), 370–373. doi:10.1038/ki.1996.54
Wang, H., Nishiya, K., Ito, H., Hosokawa, T., Hashimoto, K., and Moriki, T. (2001). Iron deposition in renal biopsy specimens from patients with kidney diseases. Am. J. Kidney Dis. 38 (5), 1038–1044. doi:10.1053/ajkd.2001.28593
Wang, J., Wang, Y., Liu, Y., Cai, X., Huang, X., Fu, W., et al. (2022). Ferroptosis, a new target for treatment of renal injury and fibrosis in a 5/6 nephrectomy-induced CKD rat model. Cell Death Discov. 8 (1), 127. doi:10.1038/s41420-022-00931-8
Wang, Y., Bi, R., Quan, F., Cao, Q., Lin, Y., Yue, C., et al. (2020). Ferroptosis involves in renal tubular cell death in diabetic nephropathy. Eur. J. Pharmacol. 888, 173574. doi:10.1016/j.ejphar.2020.173574
Wenzel, S. E., Tyurina, Y. Y., Zhao, J., stCroix, C. M., Dar, H. H., Mao, G., et al. (2017). PEBP1 wardens ferroptosis by enabling lipoxygenase generation of lipid death signals. Cell 171 (3), 628–641.e26. e26. doi:10.1016/j.cell.2017.09.044
Wiggin, T. D., Kretzler, M., Pennathur, S., Sullivan, K. A., Brosius, F. C., and Feldman, E. L. (2008). Rosiglitazone treatment reduces diabetic neuropathy in streptozotocin- treated DBA/2J mice. Endocrinology 149 (10), 4928–4937. doi:10.1210/en.2008-0869
Wu, Y., Zhao, Y., Yang, H. Z., Wang, Y. J., and Chen, Y. (2021). HMGB1 regulates ferroptosis through Nrf2 pathway in mesangial cells in response to high glucose. Biosci. Rep. 41 (2), BSR20202924. doi:10.1042/BSR20202924
Wuttke, M., Li, Y., Li, M., Sieber, K. B., Feitosa, M. F., Gorski, M., et al. (2019). A catalog of genetic loci associated with kidney function from analyses of a million individuals. Nat. Genet. 51 (6), 957–972. doi:10.1038/s41588-019-0407-x
Xie, Y., Bowe, B., Mokdad, A. H., Xian, H., Yan, Y., Li, T., et al. (2018). Analysis of the Global Burden of Disease study highlights the global, regional, and national trends of chronic kidney disease epidemiology from 1990 to 2016. Kidney Int. 94 (3), 567–581. doi:10.1016/j.kint.2018.04.011
Xie, Y., Hou, W., Song, X., Yu, Y., Huang, J., Sun, X., et al. (2016). Ferroptosis: Process and function. Cell Death Differ. 23 (3), 369–379. doi:10.1038/cdd.2015.158
Yagoda, N., von Rechenberg, M., Zaganjor, E., Bauer, A. J., Yang, W. S., Fridman, D. J., et al. (2007). RAS–RAF–MEK-dependent oxidative cell death involving voltage-dependent anion channels. Nature 447 (7146), 864–868. doi:10.1038/nature05859
Yang, W. S., and Sockwell, B. R. (2016). Ferroptosis: Death by lipid peroxidation. Trends Cell Biol. 26 (3), 165–176. doi:10.1016/j.tcb.2015.10.014
Yang, W. S., Sriramaratnam, R., Welsch, M. E., Shimada, K., Skouta, R., Viswanathan, V. S., et al. (2014). Regulation of ferroptotic cancer cell death by GPX4. Cell 156 (1–2), 317–331. doi:10.1016/j.cell.2013.12.010
Yang, W. S., and Stockwell, B. R. (2008). Synthetic lethal screening identifies compounds activating iron-dependent, nonapoptotic cell death in oncogenic-RAS-harboring cancer cells. Chem. Biol. 15 (3), 234–245. doi:10.1016/j.chembiol.2008.02.010
Yuan, H., Li, X., Zhang, X., Kang, R., and Tang, D. (2016). Identification of ACSL4 as a biomarker and contributor of ferroptosis. Biochem. Biophys. Res. Commun. 478 (3), 1338–1343. doi:10.1016/j.bbrc.2016.08.124
Zhang, X., Li, L. X., Ding, H., Torres, V. E., Yu, C., and Li, X. (2021). Ferroptosis promotes cyst growth in autosomal dominant polycystic kidney disease mouse models. J. Am. Soc. Nephrol. 32 (11), 2759–2776. doi:10.1681/ASN.2021040460
Zhang, Y., Mou, Y., Zhang, J., Suo, C., Zhou, H., Gu, M., et al. (2022). Therapeutic implications of ferroptosis in renal fibrosis. Front. Mol. Biosci. 9, 890766. doi:10.3389/fmolb.2022.890766https://www.frontiersin.org/articles/10.3389/fmolb.2022.890766/full
Zhao, Y., Li, Y., Zhang, R., Wang, F., Wang, T., and Jiao, Y. (2020). The role of Erastin in ferroptosis and its prospects in cancer therapy. Onco. Targets. Ther. 13, 5429–5441. doi:10.2147/OTT.S254995
Zheng, J., and Conrad, M. (2020). The metabolic underpinnings of ferroptosis. Cell Metab. 32 (6), 920–937. doi:10.1016/j.cmet.2020.10.011
Keywords: ferroptosis, chronic kidney disease (CKD), regulatory genes, review, therapeutic strategy
Citation: Zhuo W-Q, Wen Y, Luo H-J, Luo Z-L and Wang L (2022) Mechanisms of ferroptosis in chronic kidney disease. Front. Mol. Biosci. 9:975582. doi: 10.3389/fmolb.2022.975582
Received: 22 June 2022; Accepted: 28 July 2022;
Published: 24 August 2022.
Edited by:
Phillip Kantharidis, Monash University, AustraliaReviewed by:
Ke Yang, Shanghai Jiao Tong University, ChinaMohit Mathur, Visterra Inc., United States
Copyright © 2022 Zhuo, Wen, Luo, Luo and Wang. This is an open-access article distributed under the terms of the Creative Commons Attribution License (CC BY). The use, distribution or reproduction in other forums is permitted, provided the original author(s) and the copyright owner(s) are credited and that the original publication in this journal is cited, in accordance with accepted academic practice. No use, distribution or reproduction is permitted which does not comply with these terms.
*Correspondence: Zhu-Lin Luo, MzEyMDg1MDg4QHFxLmNvbQ==; Li Wang, U2N3YW5nbGk2MkAxNjMuY29t