- Department of Pharmaceutical Sciences, School of Pharmaceutical Sciences and Yunnan Key Laboratory of Pharmacology for Natural Products, Kunming Medical University, Kunming, China
Tumors are the leading cause of death all over the world, among which ovarian cancer ranks the third in gynecological malignancies. The current treatment for ovarian cancer is liable to develop chemotherapy resistance and high recurrence rate, in which a new strategy is demanded. Ferroptosis, a newly discovered manner of regulatory cell death, is shown to be induced by massive iron-dependent accumulation of lipid reactive oxygen species. With the in-depth study of ferroptosis, its associated mechanism with various tumors is gradually elucidated, including ovarian tumor, which probably promotes the application of ferroptosis in treating ovarian cancer. To this end, this review will focus on the history and current research progress of ferroptosis, especially its regulation mechanism, and its potential application as a novel treatment strategy for ovarian cancer.
1 Introduction
Malignant tumors, one of the major diseases that seriously endanger human health, are the leading cause of death and a major public health problem all over the world. The treatments for tumors commonly include surgical therapy, radiotherapy, and drug therapy (chemotherapy), which is referred to as systemic therapy.
Ovarian cancer ranks the third in incidence and the second in mortality rate among gynecological malignant tumors worldwide. Meanwhile, it is one of the three most diagnosed malignant tumors in the female reproductive system. As diagnosed, 90–95% of ovarian cancers are primary ovarian malignant tumors and 5–10% are primary metastatic ovarian malignancy detected at other proximal sites. Among primary ovarian malignant tumors, epithelial cutaneous ovarian cancer is most commonly observed, accounting for more than 90% (Brown et al., 2014), in which high-grade serous cancer (high-grade serous carcinoma, HGSC) accounts for 70% (Re id et al., 2017). Most ovarian cancer patients do not display specific symptoms at early onset, and 75% are identified as advanced stage at the time of diagnosis (Lheureux et al., 2019). In addition, most of them exhibit a poor prognosis because of drug resistance and adverse effects. Therefore, it remains a great challenge to realize early diagnosis and overcome drug resistance in the current investigation of ovarian cancer.
At present, comprehensive treatment in ovarian cancer is frequently adopted, that is, the initial surgical treatment, supplemented by chemotherapy with the diagnosis and treatment has entered the era of accurate and individualized comprehensive disease management. At present, surgery is not only the most effective treatment to remove tumors but also a necessary method to confirm the diagnosis and specific stages by pathological analysis. Ovarian cancer patients at an early stage are treated with comprehensive staging surgery in order to completely remove the tumor and identify the specific stages, and patients at the advanced stage are treated with debulking surgery to remove tumor maximally (China Anti-Cancer Association Gynecological Cancer Professional Committee, 2021). Owing to the spread of most ovarian cancer in the early stage, surgery can barely remove all malignant lesions. In addition, the tumor volume of some advanced patients has decreased significantly after drug treatment, which facilitates surgery. Therefore, systemic chemotherapy has become the most important auxiliary therapy for ovarian cancer. At present, the first-line chemotherapy regimen for ovarian cancer is the administration of platinum drugs combined with paclitaxel, and the targeted drugs bevacizumab and PARP inhibitor are usually used for the maintenance of treatment. In recent years, the neoadjuvant chemotherapy (NACT) (van Driel et al., 2018) such as immunotherapy which utilizes inhibitors of immune examination point has become research hotspot, and gradually participates in ovarian cancer treatment.
In recent years, a new type of regulatory cell death (RCD) has been reported, somewhere between cell necrosis and apoptosis (Luo et al., 2021), which was first named ferroptosis in 2012. The cellular accumulation of lipid peroxides depending on the existence of iron eventually leads to cell ferroptosis. Cells that die in this way displayed unique characteristics, including increased mitochondrial membrane density, decreased or disappeared mitochondrial cristae, and reduced mitochondrial volume. Furthermore, ferroptosis has been reported to be associated with tumors, neurological diseases, kidney injury, and other diseases (Li et al., 2020). However, current studies of the regulatory mechanism of ferroptosis, such as the Fenton reaction, reactive oxygen species (ROS) regulation mechanism, System-Xc−-GSH-GPX4 pathway, p53-related pathway, FSP1-COQ10-NAD (P) H pathway, Hippo pathway, and GCH1–BH4 pathway, are not enough. At present, research reports of ferroptosis in ovarian cancer are less than 100, including the cell iron level, transsulfuration pathway, and Hippo pathway with participating genes such as p53, SCD1, and FZD7. Some publications also explored the application of ferroptosis in treating patients with platinum and paclitaxel resistance. In the future, more targets and drugs related to ferroptosis are expected for the diagnosis, treatment, and prognosis of ovarian cancer. Therefore, we summarized the role of ferroptosis in tumors, especially ovarian cancer, to provide a new research direction and novel treatment strategy.
1.1 Research history of ferroptosis
In 2001, during the study of the role of glutamate oxidative toxicity on nerve cell death, researchers found that dead nerve cells presented features of cell necrosis and apoptosis and were prevented by macromolecular synthesis inhibitors. However, this type of death depends on the generation of oxidative stress, different from the classical mode of cell death, and is therefore named oxidative death (Tan et al., 2001). In 2003, Dolma et al. (2003) reported that when they were screening for genetically selective antitumor small molecule drugs, they identified a new compound (named erastin). They found cells treated with erastin did not induce DNA fragmentation, with no change in nuclear morphology observed, and this treatment was irreversible on cells. Therefore, researchers believe that erastin-induced a new nonapoptotic cell death. Then, in 2008, Yang and Stockwell (2008) used the oncogene RAS as a specific mutation in artificial lethal screening, and between the two new compounds identified (named RSL5 and RSL3), RSL3 can activate erastin-mediated cell death mechanisms and can be inhibited by iron complexes. In 2012, Dixon et al. (2012) defined ferroptosis for the first time when they found that the small molecule erastin in the cancer-causing RAS-selective lethal compound triggered a form of nonapoptotic cell death. This is iron-dependent, which is different from apoptosis, programmed necrosis, and other known forms of cell death, and is usually accompanied by a massive accumulation of ROS, oxidative stress). It was also found that erastin inhibited cellular uptake of cystine by targeting the cystine/glutamate reverse transporter, thereby reducing the synthesis of reduced glutathione (GSH) and depleting GSH, which can be inhibited by the lipophilic antioxidant small molecule iron statin (ferrostatin-1, Fer-1).
Studies have found that the deficiency of glutathione peroxidase 4 (GPX4) leads to the accumulation of lipid peroxidation and abnormal cell death, which was induced by oxidative stress and prevented by knocking down the AIF gene (Seiler et al., 2008). According to previous studies, GPX4 is widely expressed in mammals, belongs to one of the seven glutathione peroxidases in mammals, is the only known major antioxidant enzyme that directly reduces peroxidase in cell membranes and lipoproteins, and interacts with tocopherol (vitamin E) to inhibit lipid peroxidation (Yant et al., 2003). In 2014, GPX4 was selected by Yang et al. (2014) and was confirmed as a key regulator of ferroptosis activated by erastin and RSL3. Erastin deactivates GPX4 by exhausting GSH, leading to the production of cytoplasmic and lipid ROS, whereas RSL3 directly binds and inactivates GPX4, leading to ferroptosis, and can be inhibited by an RSL3 inhibitor, such as iron complexation (DFOM), a MEK inhibitor (U0126), and antioxidants. In 2015, Jiang et al. (2015) first reported a link between p53 and ferroptosis. One year later, Yang et al. (2016) reported that peroxidation of polyunsaturated fatty acids (PUFAs) occurred under the regulation of phosphorylase G2 (PHKG2) and lipoxygenase, respectively. In 2017, Doll et al. (2017) identified acyl-CoA synthetase long-chain family member 4 (ACSL4) as a key determinant of ferroptosis sensitivity, which can be used as a predictive marker of ferroptosis in different cellular environments and is an essential raw material for the production of PUFAs. In the following year, Ingold et al. (2018) found that selenium is present in GPX4 as the amino acid selenocysteine at position 21 and plays an indispensable role in GPX4 activity.
Then, in 2019, Bersuker et al. (2019) and Doll et al. (2019) investigated the mechanism of ferroptosis suppressor protein 1 [FSP1, previously known as the apoptosis-inducing factor mitochondrial 2 (AIFM2)] in ferroptosis, identifying FSP1 as a potent ferroptosis resistance factor. At the same time, another group (Wu et al., 2019; Yang et al., 2019) found that cell density was a nongenetic factor that could modulate the sensitivity of ferroptosis by regulating the Hippo pathway effector YAP/TAZ, and more specific regulatory mechanisms were subsequently found in kidney and ovarian cancer studies. In 2020, a new pathway of GCH1–BH4 was discovered (Kraft et al., 2020; Wei et al., 2020). Riegman et al. (2020) reported that cell swelling was observed with ferroptosis, suggesting that it is an osmotic process that can be prevented by the administration of osmoprotectants. Ferroptosis was also found to distribute between cells in a lipid peroxidation and iron-dependent manner, which was not prevented by treatment with osmoprotectants. It has also been shown that oleic acid in lymph protects melanoma cells from ferroptosis in an acyl-CoA synthetase long-chain family member 3 (ACSL3)-dependent manner and can increase their ability to form metastatic tumors (Ubellacker et al., 2020). The history of ferroptosis development is also demonstrated in Figure 1.
2 Regulatory mechanisms of ferroptosis
In this section, the key regulatory pathways and mechanisms of proteins involved in ferroptosis are elucidated (Figure 2), with an emphasis on the valuable targets for treatment.
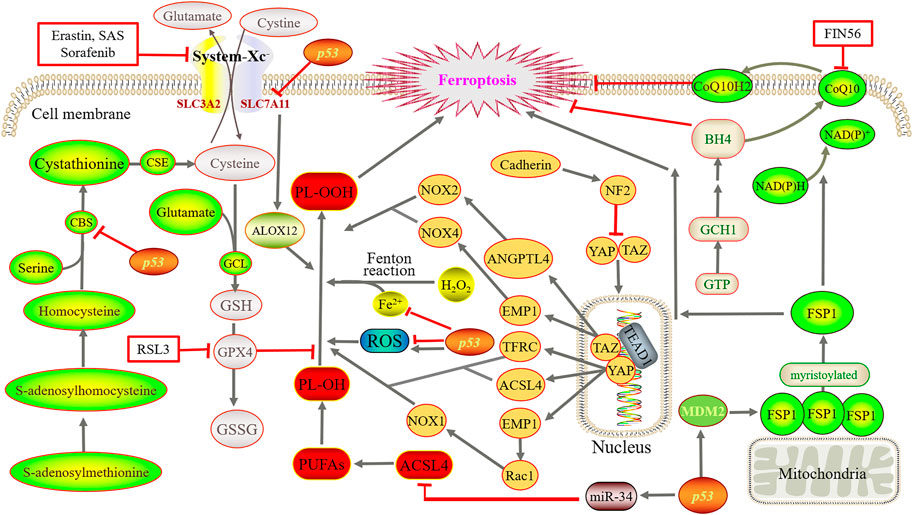
FIGURE 2. Main regulatory mechanisms of ferroptosis. Iron directs the Fenton reaction and induces large accumulation of ROS. Cystine is converted to cysteine for the synthesis of GSH. Then, GPX4 converts GSH to GSSG and reduces PL-OOH to PL-OH, thereby blocks the lipid peroxidation chain reaction. Using methionine as a sulfur donor, intermediate homocysteine, serine and cystathionine are converted into cysteine by the catalysis of CBS and CSE, simultaneously producing GSH. YAP and TAZ are abnormally activated, and bind to the TEAD after dephosphorylation. GCH1 overexpression promotes the generation of BH4. FSP1 oxidizes the terminal octadecylation modification of protein N to mediate lipid peroxidation. Simultaneously, FSP1 catalyzes the regeneration of ubiquinone and enters the circulation. By suppressing TFR1, ZIP14, ACSL4, SLC7A11 and CBS, p53 reduce or increase ROS as well as transactivate MDM2.
2.1 Iron reacts with Fenton
As the name suggested, the occurrence of ferroptosis depends on the existence of iron, which is essential for the normal maintenance of mammalian cells. Ingested iron absorbed by duodenal epithelial cells and red blood cell lysis produce Fe2+, and then, ceruloplasmin oxidizes Fe2+ to Fe3+. Fe3+ forms a binding substance with transferrin (TF), which is then transported through transferrin receptor 1 (TFR1) on the cell membrane surface and then endocytosed into cells. Later, Fe3+ is detached from the TF of the endosome and is finally reduced to Fe2+ by six-transmembrane epithelial antigen of prostate 3 (STEAP3) and then transported to the cytoplasm through the divalent metal ion transporter 1 (DMT1). In addition, Fe2+ constitutes labile iron pool (LIP) in cells as free iron ions, most of which are transferred to the mitochondria to participate in the formation of iron-dependent protein complexes, iron-sulfur clusters, heme, and other metabolic processes. However, heme oxygenase 1 (HO-1) catalyzes heme degradation to produce free iron, whose overexpression is able to accelerate erastin-induced ferroptosis. On the other hand, if Fe2+ is in excess in cells, it is stored as ferritin and released by the ferritin complex when needed or transported extracellularly by ferroportin 1 (FPN1) (Rockfield et al., 2017).
It is well known that iron deficiency often causes anemia, but excessive iron may imbalance iron homeostasis, thus driving the Fenton reaction to produce biotoxicity. Iron and hydrogen peroxide oxidize multiple substrates in biotoxic reactions called the Fenton reaction, which is able to produce hydroxyl radicals and higher oxidized states of iron (Winterbourn, 1995). The simplest representation of the Fenton reaction is as follows:
Iron directs the Fenton reaction to that of the hydrogen peroxide (H2O2) generation of a hydroxyl radical (HO.). When GPX4 is inhibited, HO causes PUFAs to produce peroxidized lipids, and then, oxygen further catalyzes lipid oxidation and disrupts the membrane (Green, 2019). Peroxidized lipids can also produce new free radicals under the catalysis of iron, undergo a chain reaction, and promote the further propagation of lipid peroxidation (Conrad and Pratt, 2019).
2.2 Reactive oxygen species
ROS include superoxide anions, hydrogen peroxide, singlet oxygen, and hydroxyl radicals, which drive lipid peroxidation and kill cells by destroying lipids, proteins, and DNA (Lin et al., 2018). Tumor cell sustained growth and proliferation require “hypermetabolism,” and cells undergo metabolic reprogramming and abnormalities in mitochondrial function, eventually producing ROS and conducting proliferation signals to promote tumor development (Wang et al., 2019), but too much ROS may cause cell death. In this process, the mitochondrial electron transport chain produces electron leakage to generate a superoxide anion, NADPH oxidase on the cell membrane produces a superoxide anion, a superoxide anion forms H2O2 under the action of superoxide dismutase, and H2O2 is catalyzed by iron to produce HO, which further induces lipid peroxidation (large accumulation of ROS accumulation) and eventually leads to ferroptosis.
2.3 System-Xc−-GSH-GPX4 pathway
System-Xc− is a heterodimeric amino acid reverse transporter that is widely distributed on the phospholipid bilayer. It is formed by solute carrier family members 11 (xCT) and solute carrier family 3 member 2 (SLC3A2, also called CD98hc) by disulfide bonds, also known as a cystine/glutamate reverse transporter. The former is a nutrient transporter protein that is frequently overexpressed in human malignancies (Koppula et al., 2018), and the latter uptakes extracellular cysteine while delivering equivalent amounts of glutamate to extracellular cells (Cao and Dixon, 2016). After cysteine enters the cell, cystine is converted to cysteine for the synthesis of GSH. Then, GPX4, which is active because of its selenocysteine content, converts GSH to oxidized GSH (GSSG) and reduces phospholipid hydrogen peroxide (PL-OOH) to phospholipid-alcohol (PL-OH), thereby blocking the lipid peroxidation chain reaction (Seibt et al., 2019). Studies have shown that erastin, sulfasalazine (SAS), and multiple kinase inhibitor sorafenib can block the function of xCT, deplete GSH, and thus induce ferroptosis (Gout et al., 2001; Dixon et al., 2012; Sun et al., 2016). However, the p53 gene can downregulate cystine uptake by inhibiting SLC7A11 transcription, which limits intracellular GSH production (Yant et al., 2003).
In addition, studies have shown that cysteine, a nonessential amino acid, can be synthesized through the transsulfuration pathway in some mammalian cells and then binds glutamate to generate GSH under the action of glutamate cysteine ligase. Using methionine as a sulfur donor, intermediate homocysteine, serine, and cystathionine are converted into cysteine by the catalysis of cystathionine β-synthase (CBS) and cystathionine gamma-lyase (CSE), simultaneously producing GSH and the gas signaling molecule hydrogen sulfide (McBean, 2012; Sbodio et al., 2019). In addition, homocysteine can be derived from dietary methionine, which is converted to S-adenosine methionine and is then catalyzed by methyltransferase to produce S-adenosine and further generate homocysteine. In particular, CBS (also known as L-serine hydrolase) catalyzes the condensation of serine and homocysteine to form cystathionine. CSE (also known as L-cystathionine cysteine-lyase) is the only enzyme in mammals that can directly produce cysteine using the cystathionine generated by CBS.
2.4 p53-related pathway
The expression of p53 in different species is highly conservative. Therefore, it is also called the guardian of genomes and cells, with a critical role. In 2015, it was reported by Jiang et al. (2015) for the first time that p53 was related to ferroptosis, indicating its dual effect on ferroptosis as involving iron metabolism, lipid metabolism, amino acid metabolism, and ROS regulation. At present, ferroptosis can be classified into two main categories: GPX4-centered and p53-centered. As p53 is a key regulator of cellular metabolism, it plays a vital role in ferroptosis regulation and is tightly related to ferroptosis initiation and progression (Liu and Gu, 2021; Liu and Gu, 2022). It is also related to the regulation mechanism in Section 2.1, Section 2.2, Section 2.3, and Section 2.5 of this review. It has been revealed that p53 suppresses TFR1 and Zrt- and Irt-like protein 14 (ZIP14) to reduce cell iron intake and p53 can also be reduced or increased in different conditions (Liu and Gu, 2022). In addition, p53 reduces ACSL4 by controlling miR-34 to suppress ferroptosis. On the one hand, p53 can inhibit SLC7A11 and CBS to reduce the synthesis of GSH, ultimately inducing ferroptosis. On the other hand, it can promote the release of ALOX12 by reducing SLC7A11, which, in turn, causes lipid peroxidation and finally ferroptosis. Furthermore, p53 can transactivate mouse double minute two homolog (MDM2), which activates FSP1 to promote ferroptosis.
2.5 FSP1-CoQ10-NAD (P) H pathway
GPX4 has long been recognized as a core regulatory protein that suppresses the occurrence of ferroptosis. In 2019, Bersuker et al. (2019) and Doll et al. (2019) reported FSP1 as an important ferroptosis regulator protein and confirmed that the FSP1-CoQ10-NAD (P) H pathway was independent of System-Xc−-GSH-GPX4 pathway and cooperated with it to inhibit ferroptosis lead by lipid peroxidation. Using expression cloning methods, AIFM2 was identified by Doll’s group. This unknown inhibitory gene of ferroptosis was therefore renamed FSP1, which was originally described by Wu et al. (2002) as a proapoptotic gene. FSP1 is primarily attached to the outer mitochondrial membrane, and when octadecylation occurs, FSP1 moves to the plasma membrane.
FSP1 inhibition of ferroptosis is mediated by ubiquinone (CoQ10), which utilizes the terminal octadecylation modification of protein N to target cytoplasmic membrane, as an NADPH-dependent CoQ10 induces the redox function to cause oxidation or reduction. The oxidizing CoQ10 is a lipophilic radical trapper and functions as an antioxidant to inhibit lipid peroxidation to avoid further ferroptosis. The reduction reaction generates panthenol, which can capture free radicals and can further mediate lipid peroxidation to inhibit ferroptosis. At the same time, FSP1 catalyzes the regeneration of ubiquinone by NAD (P) H and takes the circulation. Studies also found (Shimada et al., 2016) that by binding and activating squalene synthase, small molecule compound FIN56 inhibits the methoxyeric pathway synthesis of CoQ10, which causes the consumption of CoQ10 and induction of ferroptosis.
2.6 GCH1–BH4 pathway
It was reported that recently, a new pathway (the GCH1–BH4 protection pathway) was identified, which was parallel with and independent from the two classical pathways, namely, System-Xc−-GSH-GPX4 and FSP1-CoQ10-NAD (P) H (Li L. et al., 2021). By increasing its expression, CoQ10 can be elevated to slow down the progression of ferroptosis (Kraft et al., 2020). GTP cyclohydrolase 1 (GCH1), originating from GTP, is able to remove lipid peroxidation, and its overexpression promotes the generation of tetrahydrobiopterin (BH4) and avoids RSL3-induced ferroptosis (Wei et al., 2020).
3 Application of ferroptosis in ovarian cancer
3.1 Elevated iron levels
RCD plays a key role in the normal growth and development as well as maintaining homeostasis of multicellular organisms. Ferroptosis is a unique type of RCD, and many studies have demonstrated the relationship of ferroptosis was closely with tumor treatment. In particular, elevated iron levels are associated with the occurrence of various malignant tumors such as stem cell cancer, lung cancer, ovarian cancer, and renal cell cancer (Toyokuni, 2009; Xia et al., 2019). Studies have shown a soluble molybdenum compound called sodium molybdate that induced the elevation of LIP in ovarian cancer cells (Mao et al., 2022). In the tumor tissue of HGSC patients, the iron efflux pump ferroportin (FPN) decreases whereas TFR1 and TF increase. As a result, the intracellular level of iron is increased (Basuli et al., 2017). A similar situation was observed in genetic models of ovarian cancer tumor-initiating cells, suggesting that tumor cell intracellular iron levels are also elevated in the early stages of ovarian cancer. In a recent study, GPX4 knockout reduced iron levels and decreased interleukin-6 and tumor necrosis factor expression in ovarian cancer cells (Li D. et al., 2021). It suggests that we probably could interfere with the iron metabolism of tumor cells in the early stage of ovarian cancer to kill tumor cells.
3.2 Genes
3.2.1 p53 gene
The p53 gene expresses the p53 protein, which acts as a DNA-binding transcription factor and selectively regulates the expression of certain p53 transcriptional target genes. Studies have shown (McBean, 2012) that the inhibition of SLC7A11 expression reduced cystine uptake and sensitized ovarian cancer tumor cells to ferroptosis. However, p533KR as an acetylation-deficient mutant of the p53 protein fails to induce cell cycle arrest, senescence, and apoptosis, which completely retains the ability to regulate SLC7A11 expression and induce ferroptosis in tumor cells under oxidative stress. Furthermore, PARP inhibitors can inhibit SLC7A11 expression in a p53-dependent manner (Hong et al., 2021). Quartuccio et al. (2015) studied a mouse model of tubal epithelial cells with p53 mutation, and the results showed that single p53 mutation only migrated normal cells and normal cells transformed into tumor cells when combining p53 mutation with the activation of K-ras. The expression of the p53 gene also promotes ferroptosis induced by superparamagnetic iron oxide (SPIO) with the incubation in human serum, which provides a theoretical basis for the development of iron nanomaterials as new tumor therapeutic drugs (Zhang Y. et al., 2021). According to the results, SPIO could also induce ferroptosis in human ovarian cancer stem cells by attenuated autophagy (Huang et al., 2020). Almost 96% of HGSC cases are detected with mutations in the p53 gene. Therefore, the interference with p53-mediated metabolic regulation has a significant impact on the treatment of ovarian cancer.
3.2.2 Stearyl-coenzyme A desaturase gene
Stearyl-coenzyme A desaturase (SCD) is also known as D9-fatty acyl-CoA desaturase, through which monounsaturated fatty acids are produced from saturated fatty acids. In humans, SCD includes two isoforms, namely, SCD5 and SCD1 (prevalent form). In ovarian cancer tumor cells, SCD1 is highly expressed, and studies have shown that the inhibition or deletion of SCD1 gene could induce apoptosis and ferroptosis. The coadministration of erastin with SCD1 inhibitor A939572 in mice with ovarian cancer tumor cells was much more effective than that of a single drug (Carbone and Melino, 2019; Tesfay et al., 2019). In addition, treatment with the SCD1 inhibitors MF-438, CAY10566, and A939572 increased the sensitivity of ovarian cancer cells to the ferroptosis inducers RSL3 and erastin (Wang et al., 2022a). Furthermore, SCD1 can induce ferroptosis in cancer cells mediated by the Menin-MLL inhibitor MI-463 (Kato et al., 2020).
3.2.3 Frizzleed-7
Overexpression of frizzleed-7 (FZD7) can activate the oncogenic factor P63, upregulate GPX4, and avoid ferroptosis in cells. The expression of FZD7 was directly associated with the expression of the GSH metabolism-related genes, namely, GSS, GSR, GPX2, and IDH (Wang et al., 2021a). Therefore, it is applicable to explore whether FZD7 could be used as a novel biomarker to evaluate the sensitivity of platinum-resistant ovarian cancer cells to ferroptosis.
In addition, the solute carrier family (SLC) is associated with inducing ovarian cancer tumor cells. The anesthetic lidocaine downregulates the expression of SLC7A11 in a dose-dependent manner by promoting the intracellular expression of microRNA-382-5p (miR-382-5p), and the inhibition of miR-382-5P blocked ferroptosis in ovarian cancer cells (Sun et al., 2021). The SNAI2 gene can directly bind to the SLC7A11 promoter to regulate SLC7A11 expression (Jin et al., 2022). The tumor suppressor miR-424-5p inhibits ferroptosis in ovarian cancer by targeting ACSL4 (Ma et al., 2021).
3.3 Transsulfuration pathway
Studies have confirmed that the high level of GSH (Nunes and Serpa, 2018) and the high expression of related proteases (Kigawa et al., 1998) are closely related to the chemotherapy resistance of ovarian cancer. Verschoor and Singh (2013) treated a model derived from the tetracycline-induced overexpression of the proto-oncogene ETS-1 in ovarian cancer cells in 2008 with xCT inhibitors and inhibitors of the transsulfuration pathway. It was found that the overexpression of ETS-1 was accompanied by an increase in intracellular GSH and a significant reduction of GSH levels in models treated with transsulfuration pathway inhibitors. It indicates that the transsulfuration pathway plays an important role in GSH synthesis in ovarian cancer cells. Then, Chakraborty et al. (2015) found that the expression of CBS was increased in epithelial ovarian cancer cells (EOC) and silencing the genes of CBS could inhibit the migration and invasion of EOC tumor cells. Liu et al. (2020) found that the antioxidant transcription factor nuclear factor erythroid 2-related factor 2 (NRF2) upregulates CBS expression in erastin-resistant cells but causes ferroptosis after RNAi knockdown. It shows that the cell activation of the reverse transsulfuration pathway by NRF2/CBS enhances erastin-induced ferroptosis resistance. Thus, we can further think about the potential of the transsulfuration pathway to treat ovarian cancer with ferroptosis inducers. In this pathway, cysteine deprivation induced ferroptosis in tumor cells of ovarian clear cell carcinoma (Novera et al., 2020).
3.4 Hippo pathway
The Hippo pathway, consisting of a range of protein kinases and transcription factors regulated by cell-to-cell contact and cell density, is highly conserved in the evolution of both lower and higher animals. It is a potent inhibitory mechanism in tumor progression involved (Sun and Chi, 2020; Wang et al., 2021b) in apoptosis as well as tumorigenesis, metastasis, and chemoresistance. Yes-associated protein 1 (YAP) and transcriptional coactivator with PDZ-binding motif (TAZ) are two major transcription coactivators of transcription enhancement-related domains (TEAD) in the Hippo pathway. They affect cell phenotypes that include promoting cell proliferation, self-renewal, and inhibiting apoptosis by regulating the expression of target genes. YAP/TAZ is considered a receptor of cell density. When cell density is low and cell-to-cell contact is limited, the Hippo pathway is turned off. YAP and TAZ are abnormally activated and translocated from the cytoplasm to the nucleus after dephosphorylation, where they bind to the transcription factor TEAD, trigger cell ferroptosis, and inhibit apoptosis. However, the opposite result occurs.
In epithelial malignant tumor cells, cadherin overexpression mediates high cell density-enhanced cell contacts that then activate the Hippo pathway through NF2 (also called merlin) tumor suppressor proteins, thereby inhibiting nuclear transposition and YAP activity, which ultimately inhibits sensitivity to ferroptosis. Among them, YAP promotes ferroptosis by upregulating ACSL4 and transferrin receptor (TFRC), which can be inhibited by the small molecule CA3 (Song et al., 2018). In head and neck cancer, overexpression of epithelial membrane protein 1 (EMP1) regulates the expression of Rac1 and NADPH oxidase 1 (NOX 1) through the Hippo-YAP pathway, further promoting RSL3-induced ferroptosis (Wang et al., 2022b). Endogenous glutamate was reported to inhibit the iron-death sensitivity (Zhang X. et al., 2021) of lung adenocarcinoma by inhibiting ADCY10-dependent YAP. However, in malignant kidney tumor cells, TAZ is highly expressed and regulates EMP1 expression, which then induces NADPH oxidase 4 (NOX4) to regulate ferroptosis.
It has also shown that the relatively high activity of YAP/TAZ promotes the invasion and metastasis of ovarian cancer cells and is often associated with poor prognosis (Yang and Chi, 2019). Cell density-dependent TAZ is the determinant factor of ferroptosis sensitivity in ovarian cancer. However, regulation is different from the pathway in kidney cancer. In ovarian cancer, TAZ regulates the level of its direct target gene angiopoietin-like 4 (ANGPTL4) to manage the activity of NADPH oxidase 2 (NOX2), finally leading to ferroptosis (Yang et al., 2020). YAP promotes ferroptosis in ovarian cancer malignant tumor cells by regulating its direct target gene, E3 ubiquitin ligase S phase protein-associated protein 2 (SKP2) (Yang W. H. et al., 2021). Furthermore, discoidin domain receptor tyrosine kinase 2 (DDR2) is upregulated in recurrent breast tumor cells, with sensitivity to ferroptosis enhanced by YAP/TAZ activation, which is unknown in ovarian cancer (Lin et al., 2021). In serous ovarian cancer, the TEAD family plays an important role, in which TEAD1 acts as a cotranscription factor in the Hippo pathway and the reduced TEAD2 expression may increase the accumulation of ROS, leading to ferroptosis (Ren et al., 2021). The currently studied proteins and genes of ferroptosis, especially in ovarian cancer, are summarized in Figure 3.
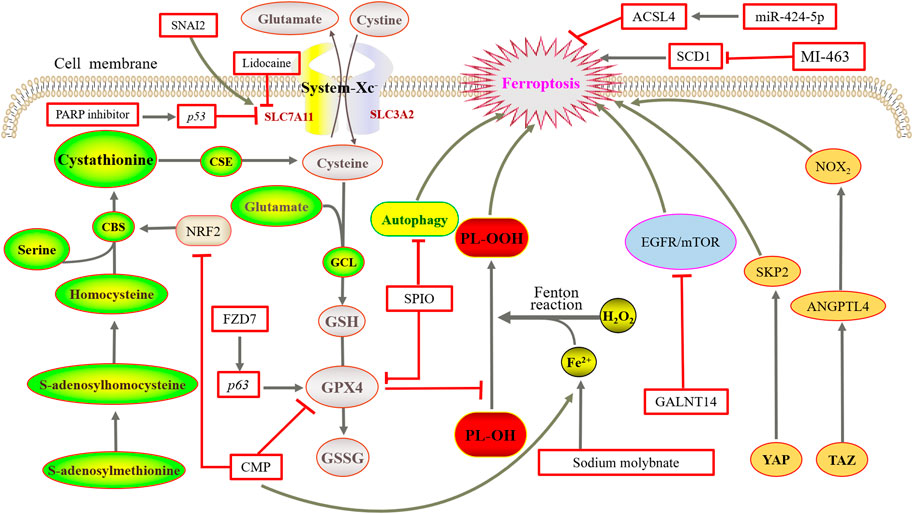
FIGURE 3. Mechanisms of ferroptosis in ovarian cancer. NRF2 upregulates CBS expression but causes ferroptosis after RNAi knockdown. Overexpression of FZD7 can activate the oncogenic factor P63, upregulate GPX4, and avoid undergoing ferroptosis. SPIO could also induce ferroptosis by attenuated autophagy. Sodium molybdate induced the elevation of LIP. The inhibition or deletion of SCD1 gene could induce ferroptosis. GALNT14 inhibits the EGFR/mTOR pathway, thereby downregulating ferroptosis. TAZ regulates the level of ANGPTL4 to manage the activity of NOX2, and finally leads to ferroptosis. YAP promotes ferroptosis by regulating SKP2.
4 Ferroptosis-related drugs
At present, sorafenib is a United States FDA-approved ovarian cancer treatment agent that can induce ferroptosis (Sun et al., 2017). Other studies are still undergoing to develop new and effective ferroptosis-related drugs for ovarian cancer treatment.
4.1 Chemoresistance
The coadministration of platinum drugs with paclitaxel is the first-line drug treatment regimen for ovarian cancer. However, most ovarian cancer patients frequently develop chemoresistance, especially the resistance by taking platinum drugs, leading to recurrence. Platinum drugs are nonspecific drugs for affecting cell cycle that bind to DNA after entering tumor cells to form Pt–DNA complexes, producing intracellular ROS, which leads to cell damage and death. A multicenter phase 2 randomized controlled trial evaluated the oral coadministration of sorafenib with topotecan as a maintenance treatment and found a statistically and clinically significant improvement in progression-free survival in patients with platinum-resistant ovarian cancer (Chekerov et al., 2018). Micelles made from arachidonic acid-conjugated amphipathic copolymers targeting GPX4 after wrapping of RSL3 enhance the ability of RSL3 to induce ferroptosis, suggesting that chemotherapy resistance could be overcome by reducing the dosage of platinum drugs used and coadministration with this copolymer (Konstorum et al., 2020).
The ferroptosis inducer erastin acts with cisplatin synergistically by ROS-mediated and activated apoptosis to inhibit the growth of ovarian cancer cells, and it could be used as a sensitizer to overcome cisplatin resistance (Cang et al., 2020; Cheng et al., 2021). A combination of cisplatin with mTOR inhibitors causes an additive effect of cell death, and GALNT14, a member of the family of acetyl-galactosyltransferases, regulates the stability of EGFR proteins to inhibit the EGFR/mTOR pathway, thereby downregulating self-induced ferroptosis in ovarian cancer cells (Li H.W. et al., 2022). This suggests that GALNT14 provides a strategy to overcome cisplatin resistance in ovarian cancer patients. The MAP30 protein isolated from bitter melon also exhibits an effect on tumor treatment and can be used as a supplement to enhance the chemotherapy effect. It is a natural AMPK activator that induces ferroptosis consistent with cisplatin, enhancing the antitumor response and antichemoresistance in ovarian cancer (Chan et al., 2020).
Furthermore, several studies have demonstrated that the acquired synthesis of cysteine and GSH affects carboplatin resistance in ovarian cancer. Lopes-Coelho et al. (2016) found that hepatocyte nuclear factor 1β (HNF1) promotes glutathione synthesis to avoid carboplatin resistance in ovarian clear cell carcinoma. By studying ovarian cancer cell lines, including ES2, OVCAR3, OVCAR8, A2780, and A2780cisR, Nunes et al. (2018) clarified that cysteine protects cells from hypoxia and carboplatin effect to develop ovarian cancer. Santos et al. (2019) hypothesized that chemotherapy resistance of carboplatin could be reversed by disrupting cysteine metabolism. They used selenium-containing Chrysin (SeChry) as a competitive inhibitor of xCT, testing the effect of SeChry on three different ovarian cancer cell lines (ES2, OVCAR3, and OVCAR8) and two nonmalignant cell lines (HaCaT and HK2). The results showed that SeChry depleted GSH and inhibited CBS, preventing the production of the antioxidant hydrogen sulfide. Then, a new nanomedicine is designed to improve the specificity of drugs to tumor cells, which has achieved good effects in the treatment of ovarian cancer.
It was also shown that the upregulation of the drug efflux transporter ABCB1 easily led to relapse of resistance to docetaxel therapy, but the ovarian cancer cell cycle remained in the G2/M phase after coadministration with erastin, and the relapse of resistance was reversed (Zhou et al., 2019). Promyelocytic leukemia protein (PML) can increase lipid peroxidation, regulate mitochondrial metabolism and iron metabolism in tumor cells, and then enhance the chemosensitivity of HGSC (Gentric et al., 2019).
4.2 Other
Artemisinin (ARS) is a classic antimalarial drug and can fight tumors (Efferth, 2017). The cellular response of ARS and its derivatives to tumor cells involves multiple cell death modes, including apoptosis, autophagy, ferroptosis, and necrosis. Artesunate (ART), derived from Artemisia annua leaves, has potent antiproliferative and cytotoxic effects on ovarian cancer cells (Greenshields et al., 2017). The strong anticancer effects of ART include that low concentrations of ART lead to ROS-independent cell cycle arrest in the G1 phase and inhibition of mTOR signaling; high concentrations of ART lead to ROS production, mediated by apoptosis and ferroptosis.
Furthermore, the plant extract uronic acid can promote ferroptosis through the activation of the JNK/p53 signaling pathway (Ruan et al., 2021). Carboxymethylated pachyman (CMP) inhibited HO-1 signaling, xCT, and GPX4, as well as upregulated Fe2+ expression by downregulating NRF2 expression, ultimately leading to the induction of ferroptosis in ovarian cancer cells. According to recent reports, sodium molybdate can modulate the production of nitric oxide to exhaust GSH, raise iron levels, and promote ferroptosis in ovarian cancer cells (Zheng et al., 2022). In the view of immunotherapy, it has found that checkpoint inhibitors induce CD8+ T cells to trigger ferroptosis in mice bearing ovarian tumor (Immunotherapy Activates, 2019). Furthermore, the combination of the Menin-MLL inhibitor MI-463 with the thioredoxin reductase inhibitor auranofin could synergistically increase the mortality of ovarian cancer tumor cells (Jing et al., 2022).
5 Discussion and perspective
Ferroptosis, discovered as a novel type of RCD, occurs in an iron-dependent manner after massive accumulation of ROS, presenting features different from other well known forms of cell death such as apoptosis and autophagy. The reported regulatory mechanisms of ferroptosis include the Fenton response, System-Xc−-GSH-GPX4 pathway, FSP1-CoQ10-NAD (P) H pathway, Hippo pathway, and GCH1–BH4 pathway. At present, most of these pathways have been studied extensively in malignant tumors, neurological diseases, and liver and kidney injury, except the latter two pathways, which are relatively rarely studied and showing no clear target or index for the treatment or prognosis in each disease. As a rising star, ferroptosis provides a new idea for the research of various diseases and plays a significantly important role in the remarkable success of tumor treatment. In recent years, a large number of studies have screened reliable mRNAs and genes to predict the prognosis of ovarian cancer patients by means of bioinformatics (Feng et al., 2022), comprehensive clinical analysis (Peng et al., 2021), and construction of ferroptosis-related gene models (Yang L. et al., 2021; Chen et al., 2021; Li X. X. et al., 2021; Zhang J. et al., 2021; Ye et al., 2021; You et al., 2021; Yu et al., 2021; Li Y. et al., 2022; Wang H. et al., 2022; Zou et al., 2022). It provides preciously applicable suggestions for exploring the therapeutic targets and prognostic indicators related to ferroptosis in ovarian cancer. At the same time, research of new compounds as inducers and inhibitors also provides a meaningful reference for the optimization of ovarian cancer treatment regimen. However, on the basis of the current research results, it is too soon to conclude the role of ferroptosis in the diagnosis, treatment, and prognosis of ovarian cancer for clinical purpose. In the future, inducers and inhibitors related to ferroptosis of ovarian cancer tumor cells, as well as the combined effects of new and classic drugs, the drug resistance of tumor cells, and the specific regulatory mechanisms, remain to be thoroughly studied.
Author contributions
ZT and YJ designed the review and revised the manuscript. ZT and HH dedicated in drafting the manuscript and performed the literature review. WS and YL participated in sorting and organizing the sentences and figures.
Funding
This review was supported by National Natural Science Foundation of China project (Grant No. 82160344) and Yunnan Fundamental Research Projects (Grant No. 202001AU070141 and Grant No.202101AY070001-068).
Conflict of interest
The authors declare that the research was conducted in the absence of any commercial or financial relationships that could be construed as a potential conflict of interest.
Publisher’s note
All claims expressed in this article are solely those of the authors and do not necessarily represent those of their affiliated organizations, or those of the publisher, the editors, and the reviewers. Any product that may be evaluated in this article, or claim that may be made by its manufacturer, is not guaranteed or endorsed by the publisher.
References
Basuli, D., Tesfay, L., Deng, Z., Paul, B., Yamamoto, Y., NinG, G., et al. (2017). Iron addiction: A novel therapeutic target in ovarian cancer. Oncogene 36 (29), 4089–4099. doi:10.1038/onc.2017.11
Bersuker, K., Hendricks, J. M., Li, Z., Magtanong, L., Ford, B., Tang, P. H., et al. (2019). The CoQ oxidoreductase FSP1 acts parallel to GPX4 to inhibit ferroptosis. Nature 575 (7784), 688–692. doi:10.1038/s41586-019-1705-2
Brown, J., Friedlander, M., Backes, F. J., Harter, P., O'Connor, D. M., de la Motte Rouge, T., et al. (2014). Gynecologic Cancer Intergroup (GCIG) consensus review for ovarian germ cell tumors. Int. J. Gynecol. Cancer 24 (9 Suppl. 3), S48–S54. doi:10.1097/IGC.0000000000000223
Cang, W., Wu, A., Di, W., and Qiu, L. (2020). Study of the iron death activator Erastin for enhancing cisplatin sensitivity in ovarian cancer cells by activating apoptosis [J]. Prog. Mod. Obstetrics Gynecol. 29 (10), 730–733. doi:10.13283/j.cnki.xdfckjz.2020.10.030
Cao, J. Y., and Dixon, S. J. (2016). Mechanisms of ferroptosis. Cell. Mol. Life Sci. 73 (11-12), 2195–2209. doi:10.1007/s00018-016-2194-1
Carbone, M., and Melino, G. (2019). Stearoyl CoA desaturase regulates ferroptosis in ovarian cancer offering new therapeutic perspectives. Cancer Res. 79 (20), 5149–5150. doi:10.1158/0008-5472.CAN-19-2453
Chakraborty, P. K., Xiong, X., Mustafi, S. B., Saha, S., Dhanasekaran, D., Mandal, N. A., et al. (2015). Role of cystathionine beta synthase in lipid metabolism in ovarian cancer. Oncotarget 6 (35), 37367–37384. doi:10.18632/oncotarget.5424
Chan, D. W., Yung, M. M., Chan, Y. S., Xuan, Y., Yang, H., Xu, D., et al. (2020). MAP30 protein from Momordica charantia is therapeutic and has synergic activity with cisplatin against ovarian cancer in vivo by altering metabolism and inducing ferroptosis. Pharmacol. Res. 161, 105157. doi:10.1016/j.phrs.2020.105157
Chekerov, R., Hilpert, F., Mahner, S., El-Balat, A., Harter, P., De Gregorio, N., et al. (2018). Sorafenib plus topotecan versus placebo plus topotecan for platinum-resistant ovarian cancer (TRIAS): A multicentre, randomised, double-blind, placebo-controlled, phase 2 trial. Lancet. Oncol. 19 (9), 1247–1258. doi:10.1016/S1470-2045(18)30372-3
Chen, R., Cao, J., Jiang, W., Wang, S., and Cheng, J. (2021). Upregulated expression of CYBRD1 predicts poor prognosis of patients with ovarian cancer. J. Oncol. 2021, 7548406. doi:10.1155/2021/7548406
Cheng, Q., Bao, L., Li, M., Chang, K., and Yi, X. (2021). Erastin synergizes with cisplatin via ferroptosis to inhibit ovarian cancer growth in vitro and in vivo. J. Obstet. Gynaecol. Res. 47 (7), 2481–2491. doi:10.1111/jog.14779
China Anti -Cancer Association Gynecological Cancer Professional Committee (2021). Ovarian malignant tumor diagnosis and treatment guide. China Oncol. 31 (6), 490–500. doi:10.19401/j.cnki.1007-3639.2021.06.07
Conrad, M., and Pratt, D. A. (2019). The chemical basis of ferroptosis. Nat. Chem. Biol. 15 (12), 1137–1147. doi:10.1038/s41589-019-0408-1
Dixon, S. J., Lemberg, K. M., Lamprecht, M. R., Skouta, R., Zaitsev, E. M., Gleason, C. E., et al. (2012). Ferroptosis: An iron-dependent form of nonapoptotic cell death. Cell 149 (5), 1060–1072. doi:10.1016/j.cell.2012.03.042
Doll, S., Freitas, F. P., Shah, R., Aldrovandi, M., da Silva, M. C., Ingold, I., et al. (2019). FSP1 is a glutathione-independent ferroptosis suppressor. Nature 575 (7784), 693–698. doi:10.1038/s41586-019-1707-0
Doll, S., Proneth, B., Tyurina, Y. Y., Panzilius, E., Kobayashi, S., Ingold, I., et al. (2017). ACSL4 dictates ferroptosis sensitivity by shaping cellular lipid composition. Nat. Chem. Biol. 13 (1), 91–98. doi:10.1038/nchembio.2239
Dolma, S., Lessnick, S. L., Hahn, W. C., and Stockwell, B. R. (2003). Identification of genotype-selective antitumor agents using synthetic lethal chemical screening in engineered human tumor cells. Cancer Cell 3 (3), 285–296. doi:10.1016/s1535-6108(03)00050-3
Efferth, T. (2017). From ancient herb to modern drug: Artemisia annua and artemisinin for cancer therapy. Semin. Cancer Biol. 46, 65–83. doi:10.1016/j.semcancer.2017.02.009
Feng, S., Yin, H., Zhang, K., Shan, M., Ji, X., Luo, S., et al. (2022). Integrated clinical characteristics and omics analysis identifies a ferroptosis and iron-metabolism-related lncRNA signature for predicting prognosis and therapeutic responses in ovarian cancer. J. Ovarian Res. 15 (1), 10. doi:10.1186/s13048-022-00944-y
Gentric, G., Kieffer, Y., Mieulet, V., Goundiam, O., Bonneau, C., Nemati, F., et al. (2019). PML-regulated mitochondrial metabolism enhances chemosensitivity in human ovarian cancers. Cell Metab. 29 (1), 156–173. e10. doi:10.1016/j.cmet.2018.09.002
Gout, P. W., Buckley, A. R., Simms, C. R., and Bruchovsky, N. (2001). Sulfasalazine, a potent suppressor of lymphoma growth by inhibition of the x(c)- cystine transporter: A new action for an old drug. Leukemia 15 (10), 1633–1640. doi:10.1038/sj.leu.2402238
Green, D. R. (2019). The coming decade of cell death research: Five riddles. Cell 177 (5), 1094–1107. doi:10.1016/j.cell.2019.04.024
Greenshields, A. L., Shepherd, T. G., and Hoskin, D. W. (2017). Contribution of reactive oxygen species to ovarian cancer cell growth arrest and killing by the anti-malarial drug artesunate. Mol. Carcinog. 56 (1), 75–93. doi:10.1002/mc.22474
Hong, T., Lei, G., Chen, X., Li, H., Zhang, X., Wu, N., et al. (2021). PARP inhibition promotes ferroptosis via repressing SLC7A11 and synergizes with ferroptosis inducers in BRCA-proficient ovarian cancer. Redox Biol. 42, 101928. doi:10.1016/j.redox.2021.101928
Huang, Y., Lin, J., Xiong, Y., Chen, J., Du, X., Liu, Q., et al. (2020). Superparamagnetic iron oxide nanoparticles induce ferroptosis of human ovarian cancer stem cells by weakening cellular autophagy. J. Biomed. Nanotechnol. 16 (11), 1612–1622. doi:10.1166/jbn.2020.2991
Immunotherapy Activates (2019). Immunotherapy activates unexpected cell death mechanism. Cancer Discov. 9 (7), OF2. doi:10.1158/2159-8290.CD-NB2019-058
Ingold, I., Berndt, C., Schmitt, S., Doll, S., Poschmann, G., Buday, K., et al. (2018). Selenium utilization by GPX4 is required to prevent hydroperoxide-induced ferroptosis. Cell 172 (3), 409–422. e21. doi:10.1016/j.cell.2017.11.048
Jiang, L., Kon, N., Li, T., Wang, S. J., Su, T., Hibshoosh, H., et al. (2015). Ferroptosis as a p53-mediated activity during tumour suppression. Nature 520 (7545), 57–62. doi:10.1038/nature14344
Jin, Y., Chen, L., Li, L., Huang, G., Huang, H., Tang, C., et al. (2022). SNAI2 promotes the development of ovarian cancer through regulating ferroptosis. Bioengineered 13 (3), 6451–6463. doi:10.1080/21655979.2021.2024319
Jing, T., Guo, Y., and Wei, Y. (2022). Carboxymethylated pachyman induces ferroptosis in ovarian cancer by suppressing NRF1/HO-1 signaling. Oncol. Lett. 23 (5), 161. doi:10.3892/ol.2022.13281
Kato, I., Kasukabe, T., and Kumakura, S. (2020). Menin-MLL inhibitors induce ferroptosis and enhance the anti-proliferative activity of auranofin in several types of cancer cells. Int. J. Oncol. 57 (4), 1057–1071. doi:10.3892/ijo.2020.5116
Kigawa, J., Minagawa, Y., Cheng, X., and Terakawa, N. (1998). Gamma-glutamyl cysteine synthetase up-regulates glutathione and multidrug resistance-associated protein in patients with chemoresistant epithelial ovarian cancer. Clin. Cancer Res. 4 (7), 1737–1741.
Konstorum, A., Tesfay, L., Paul, B. T., Torti, F. M., Laubenbacher, R. C., Torti, S. V., et al. (2020). Systems biology of ferroptosis: A modeling approach. J. Theor. Biol. 493, 110222. doi:10.1016/j.jtbi.2020.110222
Koppula, P., Zhang, Y., Zhuang, L., and Gan, B. (2018). Amino acid transporter SLC7A11/xCT at the crossroads of regulating redox homeostasis and nutrient dependency of cancer. Cancer Commun. 38 (1), 12. doi:10.1186/s40880-018-0288-x
Kraft, V. A. N., Bezjian, C. T., Pfeiffer, S., Ringelstetter, L., Müller, C., Zandkarimi, F., et al. (2020). GTP cyclohydrolase 1/tetrahydrobiopterin counteract ferroptosis through lipid remodeling. ACS Cent. Sci. 6 (1), 41–53. doi:10.1021/acscentsci.9b01063
Lheureux, S., Gourley, C., Vergote, I., and Oza, A. M. (2019). Epithelial ovarian cancer. Lancet 393 (10177), 1240–1253. doi:10.1016/S0140-6736(18)32552-2
Li, D., Zhang, M., and Chao, H. (2021). Significance of glutathione peroxidase 4 and intracellular iron level in ovarian cancer cells-"utilization" of ferroptosis mechanism. Inflamm. Res. 70 (10-12), 1177–1189. doi:10.1007/s00011-021-01495-6
Li, H. W., Liu, M. B., Jiang, X., Song, T., Feng, S. X., Wu, J. Y., et al. (2022). GALNT14 regulates ferroptosis and apoptosis of ovarian cancer through the EGFR/mTOR pathway. Future Oncol. 18 (2), 149–161. doi:10.2217/fon-2021-0883
Li, J., Cao, F., Yin, H. L., Huang, Z. J., Lin, Z. T., Mao, N., et al. (2020). Ferroptosis: Past, present and future. Cell Death Dis. 11 (2), 88. doi:10.1038/s41419-020-2298-2
Li, L., Qiu, C., Hou, M., Wang, X., Huang, C., Zou, J., et al. (2021). Ferroptosis in ovarian cancer: A novel therapeutic strategy. Front. Oncol. 11, 665945. doi:10.3389/fonc.2021.665945
Li, X. X., Xiong, L., Wen, Y., and Zhang, Z. J. (2021). Comprehensive analysis of the tumor microenvironment and ferroptosis-related genes predict prognosis with ovarian cancer. Front. Genet. 12, 774400. doi:10.3389/fgene.2021.774400
Li, Y., Gong, X., Hu, T., and Chen, Y. (2022). Two novel prognostic models for ovarian cancer respectively based on ferroptosis and necroptosis. BMC Cancer 22 (1), 74. doi:10.1186/s12885-021-09166-9
Lin, C. C., Yang, W. H., Lin, Y. T., Tang, X., Chen, P. H., Ding, C. C., et al. (2021). DDR2 upregulation confers ferroptosis susceptibility of recurrent breast tumors through the Hippo pathway. Oncogene 40 (11), 2018–2034. doi:10.1038/s41388-021-01676-x
Lin, L. S., Song, J., Song, L., Ke, K., Liu, Y., Zhou, Z., et al. (2018). Simultaneous fenton-like ion delivery and glutathione depletion by MnO2 -based nanoagent to enhance chemodynamic therapy. Angew. Chem. Int. Ed. Engl. 57 (18), 4902–4906. doi:10.1002/anie.201712027
Liu, N., Lin, X., and Huang, C. (2020). Activation of the reverse transsulfuration pathway through NRF2/CBS confers erastin-induced ferroptosis resistance. Br. J. Cancer 122 (2), 279–292. doi:10.1038/s41416-019-0660-x
Liu, Y., and Gu, W. (2022). p53 in ferroptosis regulation: the new weapon for the old guardian. Cell Death Differ. 29 (5), 895–910. doi:10.1038/s41418-022-00943-y
Liu, Y., and Gu, W. (2021). The complexity of p53-mediated metabolic regulation in tumor suppression. Semin. Cancer Biol. S1044-579X(21)00060-2. doi:10.1016/j.semcancer.2021.03.010
Lopes-Coelho, F., Gouveia-Fernandes, S., Gonçalves, L. G., Nunes, C., Faustino, I., Silva, F., et al. (2016). HNF1β drives glutathione (GSH) synthesis underlying intrinsic carboplatin resistance of ovarian clear cell carcinoma (OCCC). Tumour Biol. 37 (4), 4813–4829. doi:10.1007/s13277-015-4290-5
Luo, M., Peng, H., and Guo, D. (2021). The mechanism of iron death and its application in tumor therapy [J]. Chem. Life 41 (01), 10–18. doi:10.13488/j.smhx.20200341
Ma, L. L., Liang, L., Zhou, D., and Wang, S. W. (2021). Tumor suppressor miR-424-5p abrogates ferroptosis in ovarian cancer through targeting ACSL4. Neoplasma 68 (1), 165–173. doi:10.4149/neo_2020_200707N705
Mao, G., Xin, D., Wang, Q., and Lai, D. (2022). Sodium molybdate inhibits the growth of ovarian cancer cells via inducing both ferroptosis and apoptosis. Free Radic. Biol. Med. 182, 79–92. doi:10.1016/j.freeradbiomed.2022.02.023
McBean, G. J. (2012). The transsulfuration pathway: A source of cysteine for glutathione in astrocytes. Amino Acids 42 (1), 199–205. doi:10.1007/s00726-011-0864-8
Novera, W., Lee, Z. W., Nin, D. S., Dai, M. Z. Y., Binte Idres, S., Wu, H., et al. (2020). Cysteine deprivation targets ovarian clear cell carcinoma via oxidative stress and iron-sulfur cluster biogenesis deficit. Antioxid. Redox Signal. 33 (17), 1191–1208. doi:10.1089/ars.2019.7850
Nunes, S. C., Ramos, C., Lopes-Coelho, F., Sequeira, C. O., Silva, F., Gouveia-Fernandes, S., et al. (2018). Cysteine allows ovarian cancer cells to adapt to hypoxia and to escape from carboplatin cytotoxicity. Sci. Rep. 8 (1), 9513. doi:10.1038/s41598-018-27753-y
Nunes, S. C., and Serpa, J. (2018). Glutathione in ovarian cancer: A double-edged sword. Int. J. Mol. Sci. 19 (7), 1882. doi:10.3390/ijms19071882
Peng, J., Hao, Y., Rao, B., and Zhang, Z. (2021). A ferroptosis-related lncRNA signature predicts prognosis in ovarian cancer patients. Transl. Cancer Res. 10 (11), 4802–4816. doi:10.21037/tcr-21-1152
Quartuccio, S. M., Karthikeyan, S., Eddie, S. L., Lantvit, D. D., O hAinmhire, E., Modi, D. A., et al. (2015). Mutant p53 expression in fallopian tube epithelium drives cell migration. Int. J. Cancer 137 (7), 1528–1538. doi:10.1002/ijc.29528
Reid, B. M., Permuth, J. B., and Sellers, T. A. (2017). Epidemiology of ovarian cancer: A review. Cancer Biol. Med. 14 (1), 9–32. doi:10.20892/j.issn.2095-3941.2016.0084
Ren, X., Wang, X., Peng, B., Liang, Q., Cai, Y., Gao, K., et al. (2021). Significance of TEAD family in diagnosis, prognosis and immune response for ovarian serous carcinoma. Int. J. Gen. Med. 27 (14), 7133–7143. doi:10.2147/IJGM.S336602
Riegman, M., Sagie, L., Galed, C., Levin, T., Steinberg, N., Dixon, S. J., et al. (2020). Ferroptosis occurs through an osmotic mechanism and propagates independently of cell rupture. Nat. Cell Biol. 22 (9), 1042–1048. doi:10.1038/s41556-020-0565-1
Rockfield, S., Raffel, J., Mehta, R., Rehman, N., and Nanjundan, M. (2017). Iron overload and altered iron metabolism in ovarian cancer. Biol. Chem. 398 (9), 995–1007. doi:10.1515/hsz-2016-0336
Ruan, F., Wang, Y., and Wang, J. (2021). To investigate the effect and mechanism of urin inducing iron death in OVCAR3 cells of ovarian cancer cell lines based on the JNK/p53 pathway [J]. Chin. J. Traditional Chin. Med. 39 (07), 62–64. + 267. doi:10.13193/j.issn.1673-7717.2021.07.016
Santos, I., Ramos, C., Mendes, C., Sequeira, C. O., Tome, C. S., Fernandes, D. G. H., et al. (2019). Targeting glutathione and cystathionine β-synthase in ovarian cancer treatment by selenium-chrysin polyurea dendrimer nanoformulation. Nutrients 11 (10), 2523. doi:10.3390/nu11102523
Sbodio, J. I., Snyder, S. H., and Paul, B. D. (2019). Regulators of the transsulfuration pathway. Br. J. Pharmacol. 176 (4), 583–593. doi:10.1111/bph.14446
Seibt, T. M., Proneth, B., and Conrad, M. (2019). Role of GPX4 in ferroptosis and its pharmacological implication. Free Radic. Biol. Med. 133, 144–152. doi:10.1016/j.freeradbiomed.2018.09.014
Seiler, A., Schneider, M., Förster, H., Roth, S., Wirth, E. K., Culmsee, C., et al. (2008). Glutathione peroxidase 4 senses and translates oxidative stress into 12/15-lipoxygenase dependent- and AIF-mediated cell death. Cell Metab. 8 (3), 237–248. doi:10.1016/j.cmet.2008.07.005
Shimada, K., Skouta, R., Kaplan, A., Yang, W. S., Hayano, M., Dixon, S. J., et al. (2016). Global survey of cell death mechanisms reveals metabolic regulation of ferroptosis. Nat. Chem. Biol. 12 (7), 497–503. doi:10.1038/nchembio.2079
Song, S., Xie, M., Scott, A. W., Jin, J., Ma, L., Dong, X., et al. (2018). A novel YAP1 inhibitor targets CSC-enriched radiation-resistant cells and exerts strong antitumor activity in esophageal adenocarcinoma. Mol. Cancer Ther. 17 (2), 443–454. doi:10.1158/1535-7163.MCT-17-0560
Sun, D., Li, Y. C., and Zhang, X. Y. (2021). Lidocaine promoted ferroptosis by targeting miR-382-5p/SLC7A11 Axis in ovarian and breast cancer. Front. Pharmacol. 12, 681223. doi:10.3389/fphar.2021.681223
Sun, J., Wei, Q., Zhou, Y., Wang, J., Liu, Q., Xu, H., et al. (2017). A systematic analysis of FDA-approved anticancer drugs. BMC Syst. Biol. 11 (Suppl. 5), 87. doi:10.1186/s12918-017-0464-7
Sun, T., and Chi, J. T. (2020). Regulation of ferroptosis in cancer cells by YAP/TAZ and Hippo pathways: The therapeutic implications. Genes Dis. 8 (3), 241–249. doi:10.1016/j.gendis.2020.05.004
Sun, X., Niu, X., Chen, R., He, W., Chen, D., Kang, R., et al. (2016). Metallothionein-1G facilitates sorafenib resistance through inhibition of ferroptosis. Hepatology 64 (2), 488–500. doi:10.1002/hep.28574
Tan, S., Schubert, D., and Maher, P. (2001). Oxytosis: A novel form of programmed cell death. Curr. Top. Med. Chem. 1 (6), 497–506. doi:10.2174/1568026013394741
Tesfay, L., Paul, B. T., Konstorum, A., Deng, Z., Cox, A. O., Lee, J., et al. (2019). Stearoyl-CoA desaturase 1 protects ovarian cancer cells from ferroptotic cell death. Cancer Res. 79 (20), 5355–5366. doi:10.1158/0008-5472.CAN-19-0369
Toyokuni, S. (2009). Role of iron in carcinogenesis: Cancer as a ferrotoxic disease. Cancer Sci. 100 (1), 9–16. doi:10.1111/j.1349-7006.2008.01001.x
Ubellacker, J. M., Tasdogan, A., Ramesh, V., Shen, B., Mitchell, E. C., Martin-Sandoval, M. S., et al. (2020). Lymph protects metastasizing melanoma cells from ferroptosis. Nature 585 (7823), 113–118. doi:10.1038/s41586-020-2623-z
van Driel, W. J., Koole, S. N., Sikorska, K., Schagen van Leeuwen, J. H., Schreuder, H. W. R., Hermans, R. H. M., et al. (2018). Hyperthermic intraperitoneal chemotherapy in ovarian cancer. N. Engl. J. Med. 378 (3), 1363–1364. doi:10.1056/NEJMc1802033
Verschoor, M. L., and Singh, G. (2013). Ets-1 regulates intracellular glutathione levels: Key target for resistant ovarian cancer. Mol. Cancer 12 (1), 138. doi:10.1186/1476-4598-12-138
Wang, H., Cheng, Q., Chang, K., Bao, L., and Yi, X. (2022). Integrated analysis of ferroptosis-related biomarker signatures to improve the diagnosis and prognosis prediction of ovarian cancer. Front. Cell Dev. Biol. 9, 807862. doi:10.3389/fcell.2021.807862
Wang, K., Jiang, J., Lei, Y., Zhou, S., Wei, Y., Huang, C., et al. (2019). Targeting metabolic-redox circuits for cancer therapy. Trends biochem. Sci. 44 (5), 401–414. doi:10.1016/j.tibs.2019.01.001
Wang, Y., Zhang, L., Yao, C., Ma, Y., and Liu, Y. (2022b). Epithelial membrane protein 1 promotes sensitivity to RSL3-induced ferroptosis and intensifies gefitinib resistance in head and neck cancer. Oxid. Med. Cell. Longev. 2022, 4750671. doi:10.1155/2022/4750671
Wang, Y., Zhao, G., Condello, S., Huang, H., Cardenas, H., Tanner, E. J., et al. (2021a). Frizzled-7 identifies platinum-tolerant ovarian cancer cells susceptible to ferroptosis. Cancer Res. 81 (2), 384–399. doi:10.1158/0008-5472.CAN-20-1488
Wang, Y., Zhao, Y., Ye, T., Yang, L., Shen, Y., Li, H., et al. (2021b). Ferroptosis signaling and regulators in atherosclerosis. Front. Cell Dev. Biol. 9, 809457. doi:10.3389/fcell.2021.809457
Wang, Y., Zhang, Y., Zhang, Y., Mou, J., and Wang, Y. (2022a). Progress in the treatment of stearyl-coenzymatic A desaturase 1 inhibitor in ovarian cancer [J]. Chin. J. Pract. Diagnostic Ther. 36 (05), 524–526. doi:10.13507/j.issn.1674-3474.2022.05.022
Wei, X., Yi, X., Zhu, X. H., and Jiang, D. S. (2020). Posttranslational modifications in ferroptosis. Oxid. Med. Cell. Longev. 2020, 8832043. doi:10.1155/2020/8832043
Winterbourn, C. C. (1995). Toxicity of iron and hydrogen peroxide: The Fenton reaction. Toxicol. Lett. 82-83, 969–974. doi:10.1016/0378-4274(95)03532-x
Wu, J., Minikes, A. M., Gao, M., Bian, H., Li, Y., Stockwell, B. R., et al. (2019). Intercellular interaction dictates cancer cell ferroptosis via NF2-YAP signalling. Nature 572 (7769), 402–406. doi:10.1038/s41586-019-1426-6
Wu, M., Xu, L. G., Li, X., Zhai, Z., and Shu, H. B. (2002). AMID, an apoptosis-inducing factor-homologous mitochondrion-associated protein, induces caspase-independent apoptosis. J. Biol. Chem. 277 (28), 25617–25623. doi:10.1074/jbc.M202285200
Xia, X., Fan, X., Zhao, M., and Zhu, P. (2019). The relationship between ferroptosis and tumors: A novel landscape for therapeutic approach. Curr. Gene Ther. 19 (2), 117–124. doi:10.2174/1566523219666190628152137
Yang, L., Tian, S., Chen, Y., Miao, C., Zhao, Y., Wang, R., et al. (2021). Ferroptosis-related gene model to predict overall survival of ovarian carcinoma. J. Oncol. 2021, 6687391. doi:10.1155/2021/6687391
Yang, W. H., and Chi, J. T. (2019). Hippo pathway effectors YAP/TAZ as novel determinants of ferroptosis. Mol. Cell. Oncol. 7 (1), 1699375. doi:10.1080/23723556.2019.1699375
Yang, W. H., Ding, C. C., Sun, T., Rupprecht, G., Lin, C. C., Hsu, D., et al. (2019). The Hippo pathway effector TAZ regulates ferroptosis in renal cell carcinoma. Cell Rep. 28 (10), 2501–2508. e4. doi:10.1016/j.celrep.2019.07.107
Yang, W. H., Huang, Z., Wu, J., Ding, C. C., Murphy, S. K., Chi, J. T., et al. (2020). A TAZ-ANGPTL4-NOX2 Axis regulates ferroptotic cell death and chemoresistance in epithelial ovarian cancer. Mol. Cancer Res. 18 (1), 79–90. doi:10.1158/1541-7786.MCR-19-0691
Yang, W. H., Lin, C. C., Wu, J., Chao, P. Y., Chen, K., Chen, P. H., et al. (2021). The Hippo pathway effector YAP promotes ferroptosis via the E3 ligase SKP2. Mol. Cancer Res. 19 (6), 1005–1014. doi:10.1158/1541-7786.MCR-20-0534
Yang, W. S., Kim, K. J., Gaschler, M. M., Patel, M., Shchepinov, M. S., Stockwell, B. R., et al. (2016). Peroxidation of polyunsaturated fatty acids by lipoxygenases drives ferroptosis. Proc. Natl. Acad. Sci. U. S. A. 113 (34), E4966–E4975. doi:10.1073/pnas.1603244113
Yang, W. S., SriRamaratnam, R., Welsch, M. E., Shimada, K., Skouta, R., Viswanathan, V. S., et al. (2014). Regulation of ferroptotic cancer cell death by GPX4. Cell 156 (1-2), 317–331. doi:10.1016/j.cell.2013.12.010
Yang, W. S., and Stockwell, B. R. (2008). Synthetic lethal screening identifies compounds activating iron-dependent, nonapoptotic cell death in oncogenic-RAS-harboring cancer cells. Chem. Biol. 15 (3), 234–245. doi:10.1016/j.chembiol.2008.02.010
Yant, L. J., Ran, Q., Rao, L., Van Remmen, H., Shibatani, T., Belter, J. G., et al. (2003). The selenoprotein GPX4 is essential for mouse development and protects from radiation and oxidative damage insults. Free Radic. Biol. Med. 34 (4), 496–502. doi:10.1016/s0891-5849(02)01360-6
Ye, Y., Dai, Q., Li, S., He, J., and Qi, H. (2021). A novel defined risk signature of the ferroptosis-related genes for predicting the prognosis of ovarian cancer. Front. Mol. Biosci. 8, 645845. doi:10.3389/fmolb.2021.645845
You, Y., Fan, Q., Huang, J., Wu, Y., Lin, H., Zhang, Q., et al. (2021). Ferroptosis-related gene signature promotes ovarian cancer by influencing immune infiltration and invasion. J. Oncol. 2021, 9915312. doi:10.1155/2021/9915312
Yu, Z., He, H., Chen, Y., Ji, Q., and Sun, M. (2021). A novel ferroptosis related gene signature is associated with prognosis in patients with ovarian serous cystadenocarcinoma. Sci. Rep. 11 (1), 11486. doi:10.1038/s41598-021-90126-5
Zhang, J., Xi, J., Huang, P., and Zeng, S. (2021). Comprehensive analysis identifies potential ferroptosis-associated mRNA therapeutic targets in ovarian cancer. Front. Med. 8, 644053. doi:10.3389/fmed.2021.644053
Zhang, X., Yu, K., Ma, L., Qian, Z., Tian, X., Miao, Y., et al. (2021). Endogenous glutamate determines ferroptosis sensitivity via ADCY10-dependent YAP suppression in lung adenocarcinoma. Theranostics 11 (12), 5650–5674. doi:10.7150/thno.55482
Zhang, Y., Xia, M., Zhou, Z., Hu, X., Wang, J., Zhang, M., et al. (2021). p53 promoted ferroptosis in ovarian cancer cells treated with human serum incubated-superparamagnetic iron oxides. Int. J. Nanomedicine 16, 283–296. doi:10.2147/IJN.S282489
Zheng, J., Guo, J., Wang, Y., Zheng, Y., Zhang, K., Tong, J., et al. (2022). Bioinformatic analyses of the ferroptosis-related lncRNAs signature for ovarian cancer. Front. Mol. Biosci. 8, 735871. doi:10.3389/fmolb.2021.735871
Zhou, H. H., Chen, X., Cai, L. Y., Nan, X. W., Chen, J. H., Chen, X. X., et al. (2019). Erastin reverses ABCB1-mediated docetaxel resistance in ovarian cancer. Front. Oncol. 9, 1398. doi:10.3389/fonc.2019.01398
Keywords: ovarian cancer, ferroptosis, ROS, System-Xc−, FSP1-CoQ10-NAD (P) H, GCH1–BH4, inducer, inhibitor
Citation: Tan Z, Huang H, Sun W, Li Y and Jia Y (2022) Current progress of ferroptosis study in ovarian cancer. Front. Mol. Biosci. 9:966007. doi: 10.3389/fmolb.2022.966007
Received: 10 June 2022; Accepted: 30 June 2022;
Published: 26 August 2022.
Edited by:
Yanqing Liu, Columbia University, United StatesReviewed by:
Qian Wang, College of Staten Island, United StatesLingli He, Harvard University, United States
Copyright © 2022 Tan, Huang, Sun, Li and Jia. This is an open-access article distributed under the terms of the Creative Commons Attribution License (CC BY). The use, distribution or reproduction in other forums is permitted, provided the original author(s) and the copyright owner(s) are credited and that the original publication in this journal is cited, in accordance with accepted academic practice. No use, distribution or reproduction is permitted which does not comply with these terms.
*Correspondence: Yinnong Jia, amlheWlubm9uZ0BrbW11LmVkdS5jbg==