- Instituto Superior de Investigaciones Biológicas (INSIBIO), CONICET-UNT and Instituto de Química Biológica “Dr. Bernabé Bloj”, Facultad de Bioquímica, Química y Farmacia, UNT, Tucumán, Argentina
The ability of siderophores to play roles beyond iron acquisition has been recently proven for many of them and evidence continues to grow. An earlier work showed that the siderophore enterobactin is able to increase copper toxicity by reducing Cu2+ to Cu+, a form of copper that is more toxic to cells. Copper toxicity is multifaceted. It involves the formation of reactive oxygen species (ROS), mismetallation of enzymes and possibly other mechanisms. Given that we previously reported on the capacity of enterobactin to alleviate oxidative stress caused by various stressors other than copper, we considered the possibility that the siderophore could play a dual role regarding copper toxicity. In this work, we show a bimodal effect of enterobactin on copper toxicity (protective and harmful) which depends on the siderophore concentration. We found that the absence of enterobactin rendered Escherichia coli cells more sensitive to copper, due to the reduced ability of those cells to cope with the metal-generated ROS. Consistently, addition of low concentrations of the siderophore had a protective effect by reducing ROS levels. We observed that in order to achieve this protection, enterobactin had to enter cells and be hydrolyzed in the cytoplasm. Further supporting the role of enterobactin in oxidative stress protection, we found that both oxygen and copper, induced the expression of the siderophore and also found that copper strongly counteracted the well-known downregulation effect of iron on enterobactin synthesis. Interestingly, when enterobactin was present in high concentrations, cells became particularly sensitive to copper most likely due to the Cu2+ to Cu+ reduction, which increased the metal toxicity leading to cell death.
Introduction
Metals such as iron, manganese, magnesium, copper, and others are co-factors for several enzymes involved in a wide range of biochemical processes. Therefore, they constitute essential micronutrients required by microbes (Arguello et al., 2013). However, when intracellular concentrations surpass the physiological needs, they become highly toxic (Chandrangsu et al., 2017). Consequently, microorganisms have evolved several homeostatic mechanisms to tightly regulate intracellular metal levels. These mechanisms involve a balanced control of uptake, efflux and metal sequestration (Arguello et al., 2013; Ladomersky and Petris, 2015; Chandrangsu et al., 2017). In E. coli, copper requirements are in the nanomolar range (Nies and Herzberg, 2013) and only few enzymes use copper [i.e., copper SOD, SodC; NADH dehydrogenase-2, NDH-2; cytochrome oxidase, CytBO(3); aromatic amine oxidase, MaoA; and 3-deoxy-D-arabino-heptulosonate-7-phosphate synthase, AroF] (Rensing and Grass, 2003). Protein chaperones are supposed to transport copper to the recipient enzymes thus preventing the adventitious mismetallation and its associated toxicity (Rensing and McDevitt, 2013). Excess of copper disturbs the cellular redox potential and destabilize iron-sulfur clusters of dehydratases and other vital enzymes. It also leads to the formation of incorrect disulfide bonds and catalyzes the generation of highly reactive hydroxyl free radicals that readily damage biomolecules, such as DNA, lipids and proteins (Dupont et al., 2011). E. coli, encounters copper mainly in the gut of warm-blooded animals where concentrations can be as high as 10 µM (Rensing and Grass, 2003). Pathogenic bacteria may also face high Cu+ in the phagolysosome in macrophages (Stafford et al., 2013). Copper homeostasis in E. coli involves the P-type ATPase CopA that carries out excess of Cu+ from the cytoplasm to the periplasm (Rensing et al., 2000). Next, the multi-component CusCFBA system transports the metal out of the cell (Franke et al., 2003). Since copper reducing conditions increase the metal toxicity, (Macomber et al., 2007; Porcheron et al., 2013; Tan et al., 2017), another mechanism for copper detoxification consists in the oxidation of Cu+ to Cu2+ by means of the multicopper oxidase CueO (Grass and Rensing, 2001). Unlike copper efflux, which has been thoroughly studied and shown to require energy input to overturn the chemical gradient, copper uptake is poorly understood and would occur by a so far unknown copper importer (Giachino and Waldron, 2020). Interestingly, it has been demonstrated that certain siderophores can influence copper uptake (Johnstone and Nolan, 2015). For example, yersiniabactin by complexing copper may favor a controlled entry of this metal into the cell when it is required. However, when copper is in excess, the siderophore sequesters the metal outside bacterial cells protecting them (Koh et al., 2017; Robinson et al., 2018). This strategy of minimizing metal toxicity while retaining the nutritional access has been termed “nutritional passivation” (Koh et al., 2017). Another siderophore with an apparent dual behavior regarding copper toxicity is enterobactin. Enterobactin is a tri-catecholate siderophore, naturally produced by all E. coli strains as well as many other enterobacteria. It is the molecule with the highest affinity for iron reported so far (Khan et al., 2018). Under certain conditions, enterobactin takes part in different processes such as Cu2+ reduction to Cu+, with the concomitant increase in copper toxicity (Li et al., 1994; Schweigert et al., 2001; Chaturvedi et al., 2012) and also in oxidative stress reduction caused by various stressors (Adler et al., 2012; Adler et al., 2014; Peralta et al., 2016). As copper toxicity is mediated in part by oxidative stress, we considered of value to reexamine the role of enterobactin regarding copper homeostasis. In this work, we show that E. coli cells lacking enterobactin had increased copper sensitivity as a consequence of the higher reactive oxygen species (ROS) levels. Supporting the role of enterobactin as a ROS scavenger, we observed higher transcriptional expression and production of the siderophore in aerobic conditions compared with anaerobic ones. We also found that copper promoted enterobactin transcriptional expression, even in the presence of an excess of iron. In addition, we provide evidence indicating that in order to reduce copper-induced oxidative stress, enterobactin had to be internalized into the cell cytoplasm and then hydrolyzed by the esterase Fes. As expected, we observed that addition of enterobactin in relatively low concentrations lowered ROS levels along with cells sensitivity. Interestingly, media supplementation with higher concentrations of enterobactin led to cell death, most likely because of copper reduction. Together, our observations indicate that enterobactin would have a protective effect against copper toxicity, however when the siderophore concentrations rise to certain levels it has the opposite effect.
Material and methods
Bacterial strains and growth conditions
The E. coli K-12 strains and plasmids used in this study are described in Table 1. Bacterial cultures were grown in aerobic conditions at 37°C in M9 minimal salt medium (Sigma-Aldrich) supplemented with 0.2% casamino acids, 0.2% glucose, 1 mM MgSO4, and 1 mg/ml vitamin B1. Solid media contained 1.5% agar. For growth curves, culture aliquots were taken at different times and OD at 600 nm was measured. Anaerobic environments were generated in a Gaspak jar system using AnaeroGen sachets (OXOID, Sigma-Aldrich). When required, antibiotics (Kanamycin 50 μg/ml, Ampicilin 50 μg/ml) were added to media.
General methods
Plasmid DNA was isolated using the Wizard Miniprep DNA purification system (Promega) according to the manufacturer’s instructions. Transformation of competent cells using the CaCl2 procedure was performed as described previously (Sambrook et al., 1989).
β-Galactosidase assays
The β-Galactosidase activities were determined following the method described by Zhou and Gottesman (1998), with a few modifications. Strain carrying the entE::lacZY transcriptional fusion was grown for 6 h in M9 medium at 37°C with aeration in presence or not (control) of 25 and 50 μM of CuSO4, FeCl3 or a combination of both metals, and assayed for β-Galactosidase activities. For this, 600 μl of these cultures were permeabilized with 0.1% SDS (24 μl) and chloroform (48 μl) for 20 min. Then, 100 μl of permeabilized cells were placed on 96-well microtiter plate and 100 μl of a 1.32 mg/ml solution of 2-nitrophenyl-β-D-galactopyranoside in buffer Z was added. Finally, the absorbance at 420 nm was measured for 20 min in a SpectraMax 250 spectrophotometer. Specific activity was calculated by dividing the slope of the line over time by the corresponding OD600nm and expressed as arbitrary units (AU).
Copper reducing power
In vitro copper reducing power of spent media obtained from different E. coli strains was measured using bathocuproine disulfonate (BCDS) (Sigma–Aldrich), following the method described by Volentini et al. (2011). This chelator forms a colored complex with cuprous ions, having a λmax at 480 nm (Poillon and Dawson, 1963). Cells were grown for 18 h at 37°C in M9 medium. Then, supernatants were filter sterilized and incubated with 100 μM CuSO4 and 200 μM BCDS. The absorbance at 480 nm was determined and Cu+ concentrations were calculated by comparison to a standard curve obtained in M9 medium supplemented with different Cu2+ concentrations and 25 mM ascorbic acid as a reducing agent.
Copper sensitivity assays
Minimal inhibitory concentrations (MIC) were determined in M9 medium supplemented with 0.2% casamino acids, 0.2% glucose, 1 mM MgSO4 and 1 μg/ml vitamin B1. 5 μl of double dilutions from a 30 mM CuSO4 solution were spotted on M9 agar plates, dried and a lawn of the corresponding strain was overlaid. The plates were incubated at 37°C for 24 h under aerobic conditions or for 48 h under anaerobic conditions. MIC values were determined as the maximum dilution of CuSO4 that showed a zone of clearing. Enterobactin (ENT), catalase (CAT) and FeCl3 were used at concentrations of 1 μM, 1 mg/ml and 100 μM, respectively.
Cu-inhibition assays were performed using overnight cultures of the wild-type or the indicated mutant strains diluted 1:200 into fresh M9 medium supplemented with 0.2% casamino acids, 0.2% glucose, 1 mM MgSO4, 1 mg/ml vitamin B1 and containing either 0, 25, 50, or 100 μM of CuSO4. Experiments were done in a final volume of 10 ml. OD 600nm was followed over a 24 h-period of time for growth curves. For the evaluation of the impact of incremental concentrations of enterobactin on copper toxicity, overnight cultures of the entE strain were diluted 1:200 into fresh M9 medium supplemented with 0.2% casamino acids, 0.2% glucose, 1 mM MgSO4 and 1 mg/ml vitamin B1, containing 100 μM of CuSO4 and 0, 10, 20, or 50 μM of pure enterobactin. Experiments were done in a final volume of 200 μl. Cultures were incubated at 37°C with shaking, and bacterial viability as CFU/ml in LBA was determined at different times (0, 3, 18, and 24 h). At 24 h, Cu+ concentration was determined in the supernatants using BCDS (Sigma–Aldrich), following the method described above.
Determination of catechol siderophore production
Catechol siderophore production was estimated according to the colorimetric method described by Arnow (1937). Briefly, cells were grown for 20 h at 37°C in M9 medium and supernatants filter sterilized. Then, 1 ml 0.5 N HCl, 1 ml nitrite-molybdate (1 g sodium nitrite and 1 g sodium molybdate dissolved in 10 ml water), 1 ml 1 N NaOH and 1 ml H2O were added to 1 ml of conditioned medium. Finally, the absorbance at 510 nm was determined and values were expressed as μM equivalents by comparison to a standard curve prepared using 2,3-dihydroxybenzoic acid (DHBA) (Sigma).
Measurement of reactive oxygen species levels
ROS levels were measured using the oxidation-sensitive fluorescent dye 2,7-dichlorodihydrofluorescein diacetate (DCFH-DA). DCFH-DA is a cell-permeable dye widely used for the detection of ROS. This probe is enzymatically hydrolyzed by the action of cellular esterases to DCFH and then oxidized by the action of intracellular ROS (species such as hydrogen peroxide, hydroxyl radical, peroxyl radicals and nitrogen radicals) into 2′,7′-dichlorofluorescein (DCF), a molecule highly fluorescent that can be easily detected at 530 nm when excited at 485 nm (Gomes et al., 2005). Cells grown in M9 medium with or without 25 μM CuSO4 and 1 μM of pure enterobactin, were washed twice and resuspended in 50 mM sodium phosphate buffer, pH 7 at a final OD600nm = 0.5. Then DCFH-DA was added at a final concentration of 10 μM and incubated for 30 min in darkness. After incubation, cells were washed, resuspended and sonicated in the same buffer. Fluorescence intensity was measured using a Perkin Elmer LS55 spectrofluorometer (excitation λ, 490 nm; emission λ, 519 nm) and results are expressed as relative fluorescence to that of the control.
Statistical analysis
Results are expressed as the mean ± standard deviation (SD). The statistical analysis of the data was performed using the InfoStat statistical software (InfoStat versión 2020, http://www.infostat.com.ar). Analysis of variance (ANOVA) using a general linear model was applied to determine the effects of the factors evaluated in each analysis and significant differences (p-value < 0.05) between mean values were determined by Tukey’s test.
Results
Absence of enterobactin increases copper toxicity
To test copper sensitivity in a condition with no enterobactin present, we made use of an E. coli strain harboring a mutation in the siderophore synthesis operon entCEBA (E. coli entE). Comparison of copper toxicity in E. coli entE and in its isogenic wild-type strain showed that wild-type growth in M9 medium was practically unaffected by supplementation with up to 100 μM CuSO4 (Figure 1A), while entE growth showed a dose-dependent inhibition response (Figure 1B). Interestingly, while iron addition restored entE growth to wild-type levels, it did not revert copper toxicity (Figure 1C). To rule out a pleiotropic effect caused by the mutation, we complemented entE strain with plasmid pNTR-entE and observed that the complemented strain showed an equivalent growth to that of the wild-type strain in all copper concentrations tested (25 and 50 µM is data not shown) (Figure 1A).
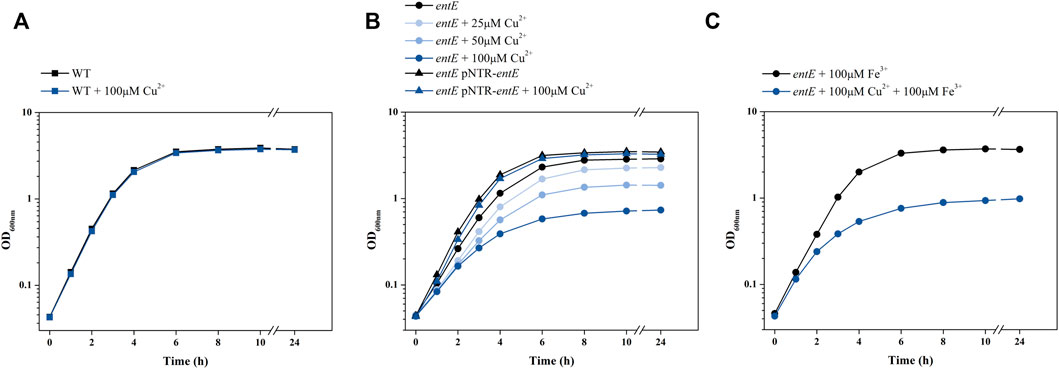
FIGURE 1. Effect of copper on E. coli growth. Growth curves obtained for E. coli wild-type (WT), entE mutant and entE mutant complemented with plasmid pNRT-entE. (A) Effect of copper addition on WT. (B) Effect of different copper concentrations on growth of entE and entE complemented with pNRT-entE. (C) Effect of simultaneous copper and iron supplementation on entE growth. Strains were cultured for 18 h in liquid aerated minimal M9 medium supplemented with 0, 25, 50, and 100 μM of CuSO4. Experiments were done at least in triplicates.
Next, we explored the possibility that the observed phenotype in the mutant strain was a consequence of an altered metabolic flux caused by the blockade in enterobactin biosynthesis pathway. Catechols found in wild-type strain supernatants are primarily enterobactin and biosynthetic intermediates in fewer amounts (Winkelmann et al., 1994). While disruption of enterobactin biosynthesis pathway in entE strain causes the absence of enterobactin and would favor the accumulation of the catechol precursor 2,3-dihydroxybenzoic acid (DHBA) (Cox et al., 1970; Pollack et al., 1970; Crosa and Walsh, 2002; Raymond et al., 2003; Ma and Payne, 2012), entA and entB mutants, by blocking enterobactin synthesis at an earlier biosynthesis step, produce no catecholate intermediates (Supplementary Figure S1). Thus, we analyzed whether there was a correlation between copper sensitivity and copper reduction capacity of supernatants having different catechol levels. Compared with wild-type, entE supernatant had higher catechol content and higher copper reducing ability (Figures 2A,B). As expected, entA and entB supernatants had negligible catechol content and consequently little copper reduction power which was about 5–7 fold lower compared to that of the entE strain (Figures 2A,B). Next, we evaluated copper sensitivity for the mutants (entA, entB, and entE) determining the copper minimal inhibitory concentration (MIC) for each strain. Table 2 shows that entA and entB strains had the same sensitivity to copper than entE strain which was significantly higher than that of the wild-type strain. Results thus far indicate that the increased sensitivity of mutants impaired in enterobactin synthesis stemmed from the lack of the siderophore and not from a pleiotropic effect or a metabolic shift due to the mutations.
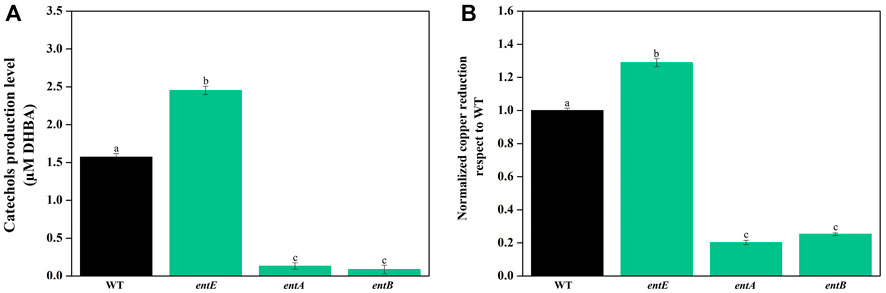
FIGURE 2. (A) Catechol content in supernatants of E. coli wild-type and mutants in the enterobactin system. Quantitation of catechol levels was performed using Arnow method with filter-sterilized supernatants obtained from wild-type (WT) and mutant strains impaired in enterobactin synthesis (entE, entA, and entB). Results are expressed as μM equivalents by comparison to a standard curve prepared using DHBA. Values are means ± SD for three independent experiments. (B) In vitro copper reducing power of supernatants of E. coli wild-type and mutants in the enterobactin system. Copper reducing activity was measured using bathocuproine disulfonate (BCDS). Values, normalized to Cu+ in WT supernatant, are the means ± SD of three independent experiments. Statistically significant differences between any two conditions compared are indicated with different letters (p < 0.05). Same letters indicate no statistically significant difference.
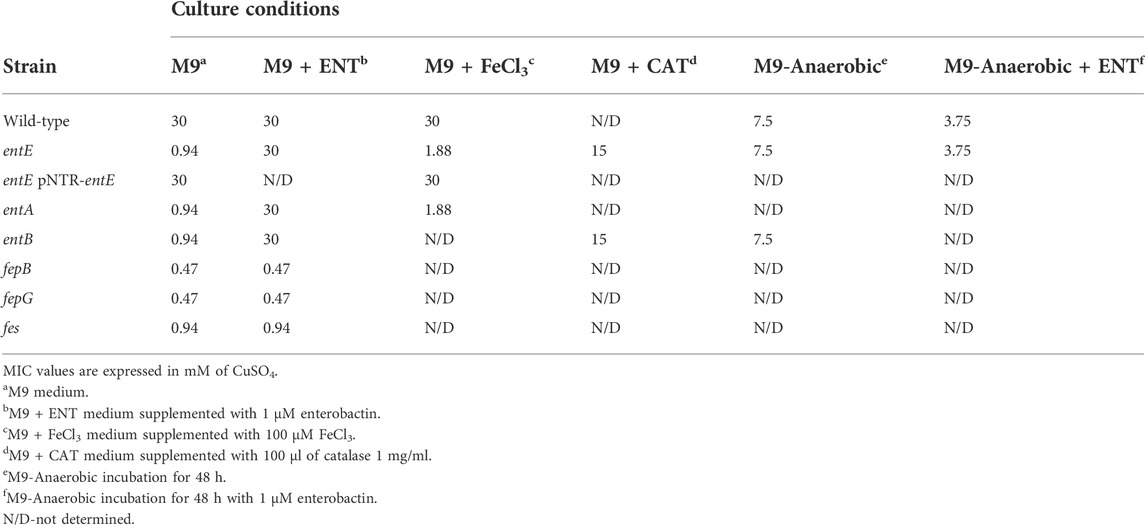
TABLE 2. MIC of CuSO4 against selected bacterial strains with different additives and under anaerobic culture conditions.
Enterobactin alleviates copper toxicity by reducing ROS
To test the role of enterobactin in copper toxicity, we supplemented media with pure siderophore in a concentration of 1 μM which is in the range of the physiological concentration found in our experimental settings (Figure 2A). Table 2 shows that 1 μM of enterobactin added to entE, entA, and entB cultures rendered these strains equally sensitive to copper as the wild-type strain. Based on previous findings associating enterobactin with oxidative stress protection (Peralta et al., 2016) and the observation that iron supplementation did not reduce copper toxicity considerably (Table 2; Figure 1C), we assumed that enterobactin would protect against copper toxicity by reducing oxidative stress rather than facilitating iron uptake. Hence, we measured ROS levels in wild-type and entE strains in presence of copper and enterobactin. Equally to what we previously reported (Adler et al., 2014), entE strain grown in M9 with no copper or other stressor added, shows increased ROS levels compared with the isogenic strain (Figure 3 left panel). While medium supplementation with sublethal copper concentrations (25 μM of CuSO4) further increased ROS levels in the mutant strain, ROS levels remained constant in the wild-type (Figure 3 central panel). Then, when medium was supplemented with 25 μM of CuSO4 and 1 μM enterobactin, ROS levels dropped only for the entE mutant, reaching levels equal to those of the wild-type (Figure 3 right panel). Next, we tested the impact of medium supplementation with catalase on copper toxicity and found that the enzyme evenly reduced the sensitivity of entE and entB to CuSO4 (Table 2). To further characterize the role of enterobactin, we studied the siderophore effect on copper toxicity under anaerobic conditions. We found that in these conditions the wild-type, entE and entB strains had the same sensitivity to copper and that the addition of 1 μM enterobactin to the medium did not protect entE mutant from copper toxicity (Table 2). Our observations imply enterobactin in reducing copper toxicity only under aerobic conditions, being this consistent with the proposed role as a ROS scavenger.
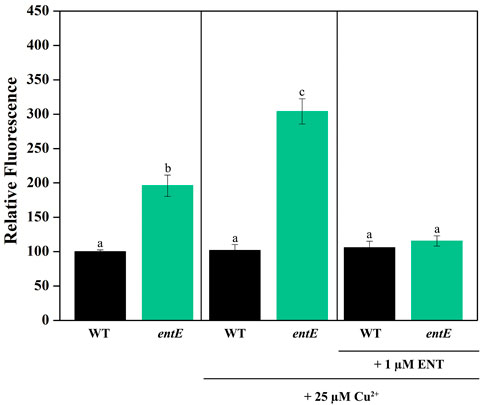
FIGURE 3. Measurement of ROS levels in E. coli wild-type and entE mutant. Impact of copper and enterobactin addition. Determination of ROS levels in wild-type (WT) and entE strains, grown in M9 with no copper added (left panel), with 25 µM of CuSO4 (central panel) and, with 25 µM CuSO4 plus 1 µM of enterobactin (right panel). Results are expressed as relative fluorescence to that of the control corresponding to WT strain grown in M9 medium. Values are means ± SD for three independent experiments. Statistically significant differences between any two conditions compared are indicated with different letters (p < 0.05). Same letters indicate no statistically significant difference.
Enterobactin reduce copper toxicity after its uptake and hydrolysis
Previously, we demonstrated that in order to reduce the oxidative stress caused by pyochelin, paraquat or H2O2, enterobactin has to reach the cell cytoplasm and be hydrolyzed by the esterase Fes. Then, the hydroxyl groups in the catechol moieties are freed from iron coordination and are able to directly stabilize radicals (Peralta et al., 2016). We hypothesized that enterobactin, once linearized in the cell cytoplasm would also protect E. coli against copper-generated ROS. To address this hypothesis, we determined copper MIC for E. coli mutant strains in enterobactin uptake (fepG and fepB) and enterobactin hydrolysis (fes) in media supplemented with pure enterobactin. Table 2 shows that mutant strains fepG, fepB and fes were also significantly more sensitive to copper damage when compared to the isogenic wild-type strain. Unlike with strains impaired in enterobactin synthesis (entA, entB, and entE), addition of 1 μM enterobactin to the medium did not revert copper sensitivity of fepG, fepB, and fes mutants (Table 2). Consistently, addition of copper to fepG and fes cultures increased ROS levels and supplementation with 1 μM enterobactin did not reduce this increment (Supplementary Figure S2). As the increased copper toxicity of fepG, fepB, and fes mutants was correlated with augmented ROS and since both variables were unresponsive to enterobactin addition, the hydrolyzed form of the siderophore results as the candidate molecule responsible for ROS reduction.
Oxidative stress-generating conditions induce enterobactin transcriptional expression
Regulation of enterobactin synthesis by oxidative stress would be consistent with its proposed antioxidant role (Adler et al., 2014; Peralta et al., 2016). In fact, in this work we show that the entE::lacZY chromosomal fusion activity (Figure 4A) and the catechol content in supernatants of the wild-type strain (Figure 4B), were significantly higher in aerobic culture conditions compared with anaerobic ones. Next, we wondered whether copper would also be able to induce enterobactin expression. For this, we used the same strain with the chromosomal entE::lacZY transcriptional fusion and grew it in M9 medium with the addition of CuSO4 (see materials and methods). Compared with the control with no copper added, medium supplementation with 25 μM or 50 μM of CuSO4 significantly increased entE transcriptional expression in a dose-dependent manner (Figure 5). As expected, addition of 25 μM or 50 μM of FeCl3 with no copper added, highly repressed the expression of enterobactin (Figure 5). However, when medium was supplemented with both metals (copper and iron), entE transcription was only repressed partially (Figure 5). By measuring the activity of the Fur regulated promoter ryhB in the entE strain, we proved that iron availability was not affected by the addition of copper to the medium (data not shown). Evidence showed that aerobic culture conditions and copper, triggered enterobactin transcription thus further supporting the involvement of the siderophore in oxidative stress protection.
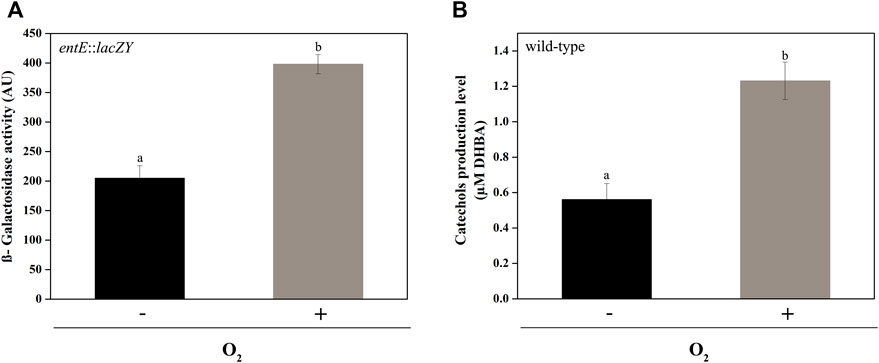
FIGURE 4. Comparison of entE expression and catechol production in aerobic and anaerobic culture conditions. (A) entE expression in aerobic and anaerobic culture conditions determined by means of β−Galactosidase assay using a strain with an entE::lacZY chromosomal fusion. (B) Determination of catechol production level in supernatants of E. coli wild-type in aerobic culture conditions compared with anaerobic ones. Quantitation was done using Arnow assay with filter-sterilized supernatants. Results are expressed as μM equivalents by comparison to a standard curve prepared using DHBA. Values are means ± SD for three independent experiments. Statistically significant differences between any two conditions compared are indicated with different letters (p < 0.05). Same letters indicate no statistically significant difference.
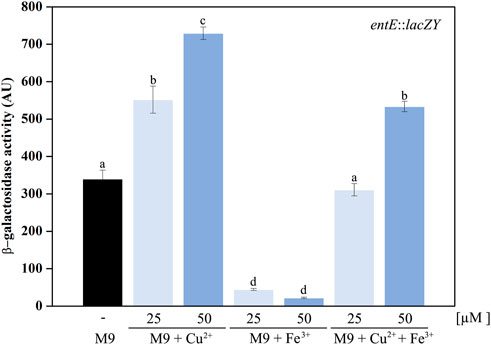
FIGURE 5. Induction of enterobactin transcriptional expression by copper. A strain with a chromosomal entE::lacZY transcriptional fusion was used as an indicator of enterobactin transcriptional expression. Data shows the control condition without CuSO4 or FeCl3 addition (black bar) and the effect of supplementing media with 25 and 50 µM CuSO4, 25 and 50 µM FeCl3 and simultaneous supplementation with both copper and iron. entE expression was determined by means of a β-Galactosidase assay described in the material and methods section. Values are means ± SD for three independent experiments. Statistically significant differences between any two conditions compared are indicated with different letters (p < 0.05). Same letters indicate no statistically significant difference.
Enterobactin concentration dependency in copper toxicity
After establishing that physiological concentrations of enterobactin, at least those found in our experimental conditions (Figure 2), protected against oxidative stress (Table 2; Figure 3), we decided to perform a dose-response curve for enterobactin concentrations in presence of copper. For that, we measured CFU/mL at different time points of entE cultures supplemented with 100 μM CuSO4 and with increasing enterobactin concentrations (1, 10, 20, and 50 μM enterobactin). As controls, we used entE cultures with no enterobactin added in the presence and absence of copper.
As observed before (Figure 1), entE mutant with no enterobactin added showed higher copper sensitivity (log CFU/mL at 24 h of 11.89 vs. 8.69 for entE and entE + 100 μM CuSO4, respectively) (Figure 6A). When enterobactin was added in relatively low concentrations (1–10 μM), copper toxicity was clearly reduced at all time points measured. Medium enterobactin concentration (20 μM) still protected from copper toxicity at 18 and 24 h but to a lesser extent compared with 1 and 10 μM enterobactin. Finally, a high enterobactin concentration (50 μM) resulted radically harmful for E. coli cells (Figure 6A). As a control for this condition, we supplemented media with 50 µM enterobactin with no addition of copper and found an entE strain growth of 11.75 log CFU/ml at 24 h. Analysis of Cu+ levels in supernatants of 24 h old cultures, revealed a substantial increment of Cu+ in the condition having 50 μM of enterobactin compared with no enterobactin or 1, 10, or 20 μM enterobactin (Figure 6B). It is likely that low enterobactin concentrations protected cells from copper toxicity by reducing ROS and that higher concentrations of the siderophore were detrimental to cells because of the Cu2+ to Cu+ reduction which increases copper toxicity.
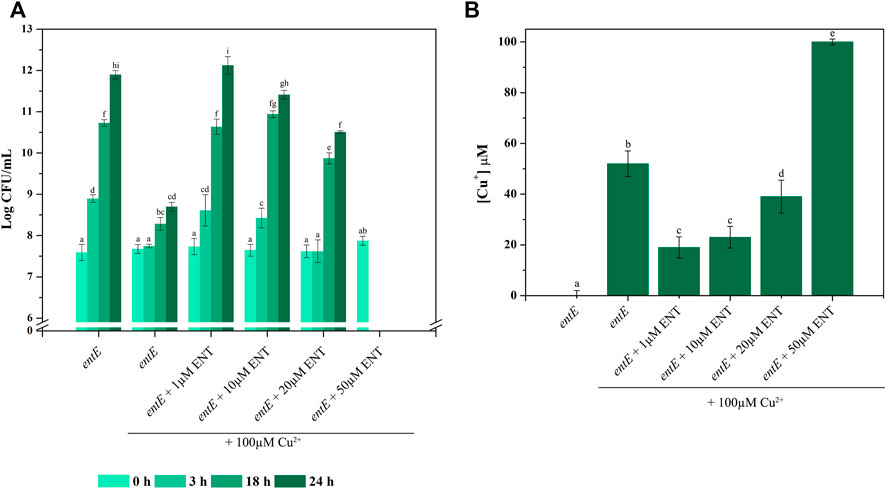
FIGURE 6. Concentration dependent effect of enterobactin on copper toxicity. (A) CFU/ml at different times (0, 3, 18, and 24 h) for E. coli entE strain grown in liquid aerated minimal M9 medium supplemented with 0, 1, 10, 20, and 50 µM of enterobactin (ENT) in presence of 100 µM of CuSO4. Data is plotted as the mean values ± SD of Log CFU/ml. Experiments were done in triplicates. (B) Cu+ concentration was determined by means of BCDS as described in material and methods. Data is plotted as the mean values ± SD of Cu+ concentrations determined at the end of the assay (24 h). Experiments were done in triplicates. Statistically significant differences between any two conditions compared are indicated with different letters (p < 0.05). Same letters indicate no statistically significant difference.
Discussion
We previously reported that the siderophore enterobactin protects E. coli against the oxidative stress generated by various stressors such as the Pseudomonad siderophore pyochelin, H2O2, and paraquat (Adler et al., 2012; Peralta et al., 2016). Given that copper involves the formation of ROS through Fenton-like reactions (Dupont et al., 2011), it is conceivable that enterobactin could play a role in copper tolerance. However, literature also implies enterobactin in reducing Cu2+ to Cu+ and therefore in increasing its toxicity (Chaturvedi et al., 2012). Thus, we considered necessary to evaluate E. coli copper sensitivity in conditions involving either no enterobactin, enterobactin in physiological levels and in increasing concentrations.
Our results clearly show that an E. coli strain unable to synthesize enterobactin (entE mutant) is substantially more susceptible to copper toxicity than the isogenic strain able to produce the siderophore (wild-type strain) (Figure 1). A pleiotropic effect in entE strain was ruled out by complementing the strain with plasmid pNTR-entE (Figure 1A; Table 2). The lack of correlation between the accumulation of catechols in mutants tested along with the copper reducing power and copper susceptibility, excluded the metabolic shift hypothesis (Figures 2A,B; Table 2). These observations further support that the deficiency of the siderophore in entE mutant is the underlying cause of increased copper toxicity. This susceptibility to copper was found to be dose dependent (Figure 1B) and not reverted by iron supplementation (Figure 1C; Table 2). Copper can be toxic to cells by generating oxidative stress and by displacing iron from Fe–S clusters. This causes and altered cellular redox potential and instability, inadequate conformation and malfunction of several vital iron-sulfur proteins (Macomber and Imlay, 2009). Hence, if increased copper toxicity in enterobactin deficient cells was due to iron displacement from Fe-S clusters, iron supplementation should have mitigated this effect. As addition of 100 µM FeCl3 to entE strain cultures did not revert the effect of 100 μM CuSO4 (Figure 1C; Table 2), we concluded that the lack of the siderophore did not lead to increased copper toxicity as a consequence of iron shortage.
Next, we evaluated the involvement of oxidative stress in the increased sensitivity to copper in cells lacking enterobactin. For that, we used the DCFH-DA probe which detects ROS, mainly hydrogen peroxide due to its higher stability (Kim and Xue, 2020). While the wild-type strain was able to maintain ROS levels constant upon copper supplementation, ROS levels increased in entE mutant, thus indicating that the strain was defective in dealing with the oxidative stress triggered by copper (Figure 3). Addition of 1 μM enterobactin decreased ROS in the entE strain to wild-type levels, corroborating the ability of the siderophore to reduce the oxidative stress (Figure 3). Moreover, addition of catalase, which alleviates oxidative stress derived from Fenton chemistry, significantly reduced the sensitivity of entE and entB strains (Table 2). Catalase decomposes hydrogen peroxide. Then, its ability to reduce cells sensitivity to copper indicates the relevance of this ROS species in the conditions tested. Furthermore, there was no difference in copper sensitivity for the wild-type and entE strains when they were cultured in anaerobic conditions either with no enterobactin added or with a 1 μM enterobactin supplementation (Table 2). These results further imply enterobactin in reducing oxidative stress caused by copper.
Previously, we reported on the requirement of enterobactin uptake and hydrolysis for the reduction of oxidative stress triggered by stressors other than copper (Peralta et al., 2016). In this work, we show that strains impaired in enterobactin uptake and hydrolysis (fepG and fes), despite being able to produce the siderophore, had also increased copper sensitivity (Table 2) and higher ROS levels (Supplementary Figure S2) compared with the wild-type. Given that these mutants were unresponsive to enterobactin addition, it can be inferred that the hydrolyzed form of the siderophore is the molecule that scavenges ROS. This is in concordance with our previous results and with a recent report showing that enterobactin protects Salmonella cells from hydrogen peroxide after being linearized and exported to the extracellular compartment (Bogomolnaya et al., 2020). Casanova-Hampton et al. (2021) also linked the enterobactin synthesis and uptake system with copper tolerance. In that work, authors speculated that the increased copper toxicity found in the enterobactin system mutants could result from an impairment in iron homeostasis. However, in this work we provide evidence that iron shortage would not be accountable for the increased sensitivity and that the inability to cope with oxidative stress would be the underlying cause. Our results indicate that enterobactin would play a significant role in copper toxicity protection only under aerobic conditions where oxygen is available to form ROS. Hence, upregulation of enterobactin production in such conditions would be expected.
In fact, the analysis of entE::lacZY expression and catechol content in supernatants showed that these two variables were higher under aerobic conditions compared with anaerobic ones (Figures 4A,B). In addition, copper promoted the entE transcriptional expression in a dose dependent manner and counteracted the classical repression mediated by iron (Figure 5) (Hunt et al., 1994; Christoffersen et al., 2001). These results clearly show the relevance of the siderophore in conditions implying oxidative stress. Stressors such as paraquat and hydrogen peroxide were previously shown to indirectly induce enterobactin expression through ROS and probably involving the regulon soxRS (Peralta et al., 2016). Then, it is possible to assume that copper would act in a similar fashion (Kimura and Nishioka, 1997; Kershaw et al., 2005; Yamamoto and Ishihama, 2005). In agreement with this, the gene expression profile of E. coli MG1655 analyzed by oligonucleotide microarray showed that genes taking part in the biosynthesis and uptake of enterobactin are increased in E. coli cells when these are exposed to copper (Kershaw et al., 2005). Furthermore, Casanova-Hampton et al. (2021) recently showed that transcription of genes involved in the biosynthesis and uptake of enterobactin were significantly upregulated in E. coli during copper stress. Similarly, a microarray-based comparative analysis of the transcriptional response of Salmonella Typhimurium to copper, showed the transcriptional induction of enterobactin synthesis operon (entCEBA) upon exposure to copper (Pontel et al., 2014).
Finally, the impact of increasing enterobactin concentrations on copper toxicity was evaluated following CFU/ml over time. Figure 6A shows that medium supplementation with enterobactin in a range of 1–20 μM, protected cells against copper toxicity. This protection peaked at 1 μM and then slightly diminished as enterobactin concentration increased up to 20 μM. When 50 μM enterobactin was used, a dramatic change in CFU/ml was observed. The decrease in the protection against copper toxicity as enterobactin increased its concentration is inversely correlated with Cu+ found in supernatants (Figure 6B). Since medium supplementation with 50 μM enterobactin, with no copper added, did not negatively affect entE strain growth, we assume that the dramatic cell death observed at 50 μM enterobactin plus copper, resulted from the increased copper toxicity due to the higher presence of Cu+. Thus, it is likely that at low enterobactin concentrations, the ability of the siderophore to reduce the oxidative stress after its uptake and hydrolysis leads to less copper toxicity. However, as enterobactin concentration is augmented, the surplus of the siderophore is probably available for Cu2+ reduction to Cu+, hence favoring its toxicity. The balance between these two opposing effects would depend on the siderophore concentration, being the critical point between 20 and 50 μM. This level of enterobactin is substantially higher to that found in our experimental conditions and therefore it raises the question whether this situation would reflect an actual physiological condition. However, we cannot rule out a natural situation implying higher enterobactin levels leading to a detrimental effect if high copper concentrations are present. A simplified model of the proposed role of enterobactin in copper tolerance and toxicity in E. coli, is presented in Figure 7. To conclude, as enterobactin producing strains (intestinal commensals, intestinal pathogens, uropathogenic strains, free-living strains, etc.) have substantial genetic background differences, including the ability to produce other siderophores, and grow in dissimilar environments, we find the necessity of a broader study, covering such differences, in order to get a clearer picture of the biological relevance of enterobactin.
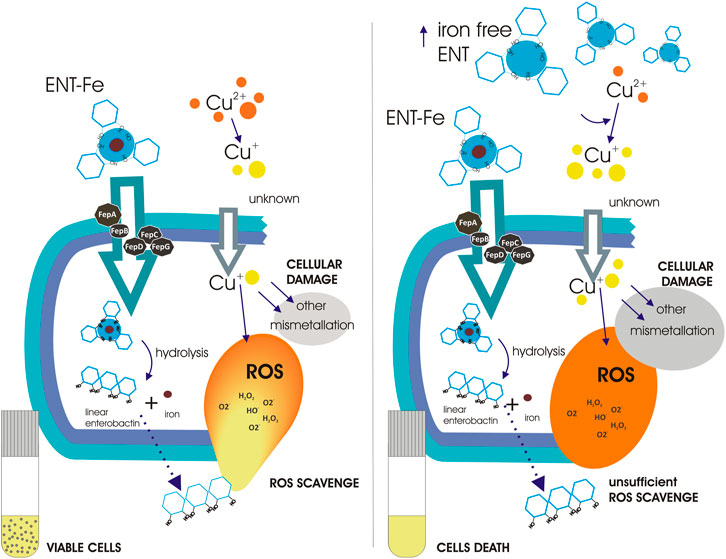
FIGURE 7. Schematic representation of the proposed role of enterobactin in copper tolerance and toxicity. Enterobactin enters cells as an iron-enterobactin complex. Uptake requires of the transport proteins FepA, FepB, FepC, FepD, and FepG. Once in the cytoplasm, the enterobactin-iron complex is hydrolyzed by Fes leaving enterobactin as a linear molecule, which is able to reduce ROS, and free iron for cellular metabolism. Linear enterobactin is possibly exported to the extracellular space of E. coli, where it would detoxify extracellular ROS, similarly as was recently reported for Salmonella (Bogomolnaya et al., 2020). Left panel shows that low enterobactin concentrations help cells to cope with copper-induced ROS. Even though, copper toxicity is multifaceted, enterobactin protection allows cells survival. Right panel when enterobactin is found in high concentrations, its ability to reduce Cu2+ to Cu+ enhances copper toxicity leading to cell death. The prevailing toxic mechanism of copper is unknown in this situation.
Data availability statement
The original contributions presented in the study are included in the article/Supplementary Material, further inquiries can be directed to the corresponding authors.
Author contributions
DP, JF, NB, and NC performed experiments. MP, PV, and CA conceived experiments. DP, JF, NB, NC, MP, PV, and CA analysed results. DP, JF, MP, NC, and CA wrote the manuscript. PV and CA provided funding.
Funding
This work was supported by grants PICT 2285 and 3552 from the Agencia Nacional de Promoción Científica y Tecnológica, PIP 908/15 from the Consejo Nacional de Investigaciones Científicas y Técnicas. DP was supported by a CONICET Postdoctoral fellowship. NC, MP, CA, and PV are Career Investigators from CONICET.
Acknowledgments
We thank Dr. Fernando Soncini and Dr. Alejandro Vila for facilitating reagents and Dr. Mariana Grillo-Puertas for manuscript revision.
Conflict of interest
The authors declare that the research was conducted in the absence of any commercial or financial relationships that could be construed as a potential conflict of interest.
Publisher’s note
All claims expressed in this article are solely those of the authors and do not necessarily represent those of their affiliated organizations, or those of the publisher, the editors and the reviewers. Any product that may be evaluated in this article, or claim that may be made by its manufacturer, is not guaranteed or endorsed by the publisher.
Supplementary material
The Supplementary Material for this article can be found online at: https://www.frontiersin.org/articles/10.3389/fmolb.2022.961917/full#supplementary-material
References
Adler, C., Corbalan, N. S., Seyedsayamdost, M. R., Pomares, M. F., de Cristobal, R. E., Clardy, J., et al. (2012). Catecholate siderophores protect bacteria from pyochelin toxicity. PLoS One 7 (10), e46754. doi:10.1371/journal.pone.0046754
Adler, C., Corbalan, N. S., Peralta, D. R., Pomares, M. F., de Cristobal, R. E., and Vincent, P. A. (2014). The alternative role of enterobactin as an oxidative stress protector allows Escherichia coli colony development. PLoS One 9 (1), e84734. doi:10.1371/journal.pone.0084734
Arguello, J. M., Raimunda, D., and Padilla-Benavides, T. (2013). Mechanisms of copper homeostasis in bacteria. Front. Cell. Infect. Microbiol. 3, 73. doi:10.3389/fcimb.2013.00073
Arnow, L. E. (1937). Colorimetric determination of the components of 3, 4-dihydroxyphenylalanine-tyrosine mixtures. J. Biol. Chem. 118, 531–537. doi:10.1016/s0021-9258(18)74509-2
Bogomolnaya, L. M., Tilvawala, R., Elfenbein, J. R., Cirillo, J. D., and Andrews-Polymenis, H. L. (2020). Linearized siderophore products secreted via MacAB efflux pump protect Salmonella enterica serovar typhimurium from oxidative stress. mBio 11 (3), e00528. doi:10.1128/mBio.00528-20
Casanova-Hampton, K., Carey, A., Kassam, S., Garner, A., Donati, G. L., Thangamani, S., et al. (2021). A genome-wide screen reveals the involvement of enterobactin-mediated iron acquisition in Escherichia coli survival during copper stress. Metallomics. 13 (9), mfab052. doi:10.1093/mtomcs/mfab052
Chandrangsu, P., Rensing, C., and Helmann, J. D. (2017). Metal homeostasis and resistance in bacteria. Nat. Rev. Microbiol. 15 (6), 338–350. doi:10.1038/nrmicro.2017.15
Chaturvedi, K. S., Hung, C. S., Crowley, J. R., Stapleton, A. E., and Henderson, J. P. (2012). The siderophore yersiniabactin binds copper to protect pathogens during infection. Nat. Chem. Biol. 8 (8), 731–736. doi:10.1038/nchembio.1020
Christoffersen, C. A., Brickman, T. J., Hook-Barnard, I., and McIntosh, M. A. (2001). Regulatory architecture of the iron-regulated fepD-ybdA bidirectional promoter region in Escherichia coli. J. Bacteriol. 183 (6), 2059–2070. doi:10.1128/JB.183.6.2059-2070.2001
Cox, G. B., Gibson, F., Luke, R. K., Newton, N. A., O'Brien, I. G., and Rosenberg, H. (1970). Mutations affecting iron transport in Escherichia coli. J. Bacteriol. 104 (1), 219–226. doi:10.1128/jb.104.1.219-226.1970
Crosa, J. H., and Walsh, C. T. (2002). Genetics and assembly line enzymology of siderophore biosynthesis in bacteria. Microbiol. Mol. Biol. Rev. 66 (2), 223–249. doi:10.1128/MMBR.66.2.223-249.2002
Dupont, C. L., Grass, G., and Rensing, C. (2011). Copper toxicity and the origin of bacterial resistance: new insights and applications. Metallomics. 3 (11), 1109–1118. doi:10.1039/c1mt00107h
Franke, S., Grass, G., Rensing, C., and Nies, D. H. (2003). Molecular analysis of the copper-transporting efflux system CusCFBA of Escherichia coli. J. Bacteriol. 185 (13), 3804–3812. doi:10.1128/JB.185.13.3804-3812.2003
Giachino, A., and Waldron, K. J. (2020). Copper tolerance in bacteria requires the activation of multiple accessory pathways. Mol. Microbiol. 114 (3), 377–390. doi:10.1111/mmi.14522
Gomes, A., Fernandes, E., and Lima, J. L. (2005). Fluorescence probes used for detection of reactive oxygen species. J. Biochem. Biophys. Methods 65 (2-3), 45–80. doi:10.1016/j.jbbm.2005.10.003
Grass, G., and Rensing, C. (2001). CueO is a multi-copper oxidase that confers copper tolerance in Escherichia coli. Biochem. Biophys. Res. Commun. 286 (5), 902–908. doi:10.1006/bbrc.2001.5474
Hunt, M. D., Pettis, G. S., and McIntosh, M. A. (1994). Promoter and operator determinants for fur-mediated iron regulation in the bidirectional fepA-fes control region of the Escherichia coli enterobactin gene system. J. Bacteriol. 176 (13), 3944–3955. doi:10.1128/jb.176.13.3944-3955.1994
Johnstone, T. C., and Nolan, E. M. (2015). Beyond iron: non-classical biological functions of bacterial siderophores. Dalton Trans. 44 (14), 6320–6339. doi:10.1039/c4dt03559c
Kershaw, C. J., Brown, N. L., Constantinidou, C., Patel, M. D., and Hobman, J. L. (2005). The expression profile of Escherichia coli K-12 in response to minimal, optimal and excess copper concentrations. Microbiology 151 (4), 1187–1198. doi:10.1099/mic.0.27650-0
Khan, A., Singh, P., and Srivastava, A. (2018). Synthesis, nature and utility of universal iron chelator - siderophore: a review. Microbiol. Res. 212-213, 103–111. doi:10.1016/j.micres.2017.10.012
Kim, H., and Xue, X. (2020). Detection of total reactive oxygen species in adherent cells by 2', 7'-dichlorodihydrofluorescein diacetate staining. J. Vis. Exp. 160. doi:10.3791/60682
Kimura, T., and Nishioka, H. (1997). Intracellular generation of superoxide by copper sulphate in Escherichia coli. Mutat. Res. 389 (2-3), 237–242. doi:10.1016/s1383-5718(96)00153-2
Koh, E. I., Robinson, A. E., Bandara, N., Rogers, B. E., and Henderson, J. P. (2017). Copper import in Escherichia coli by the yersiniabactin metallophore system. Nat. Chem. Biol. 13 (9), 1016–1021. doi:10.1038/nchembio.2441
Ladomersky, E., and Petris, M. J. (2015). Copper tolerance and virulence in bacteria. Metallomics. 7 (6), 957–964. doi:10.1039/c4mt00327f
Li, Y., Trush, M. A., and Yager, J. D. (1994). DNA damage caused by reactive oxygen species originating from a copper-dependent oxidation of the 2-hydroxy catechol of estradiol. Carcinogenesis 15 (7), 1421–1427. doi:10.1093/carcin/15.7.1421
Ma, L., and Payne, S. M. (2012). AhpC is required for optimal production of enterobactin by Escherichia coli. J. Bacteriol. 194 (24), 6748–6757. doi:10.1128/JB.01574-12
Macomber, L., and Imlay, J. A. (2009). The iron-sulfur clusters of dehydratases are primary intracellular targets of copper toxicity. Proc. Natl. Acad. Sci. U. S. A. 106 (20), 8344–8349. doi:10.1073/pnas.0812808106
Macomber, L., Rensing, C., and Imlay, J. A. (2007). Intracellular copper does not catalyze the formation of oxidative DNA damage in Escherichia coli. J. Bacteriol. 189 (5), 1616–1626. doi:10.1128/JB.01357-06
Nies, D. H., and Herzberg, M. (2013). A fresh view of the cell biology of copper in enterobacteria. Mol. Microbiol. 87 (3), 447–454. doi:10.1111/mmi.12123
Peralta, D. R., Adler, C., Corbalan, N. S., Paz Garcia, E. C., Pomares, M. F., and Vincent, P. A. (2016). Enterobactin as part of the oxidative stress response repertoire. PLoS One 11 (6), e0157799. doi:10.1371/journal.pone.0157799
Poillon, W. N., and Dawson, C. R. (1963). On the nature of copper in ascorbate oxidase. I. The valence state of copper in the denatured and native enzyme. Biochim. Biophys. Acta 77, 27–36. doi:10.1016/0006-3002(63)90466-9
Pollack, J. R., Ames, B. N., and Neilands, J. B. (1970). Iron transport in Salmonella typhimurium: mutants blocked in the biosynthesis of enterobactin. J. Bacteriol. 104 (2), 635–639. doi:10.1128/jb.104.2.635-639.1970
Pontel, L. B., Scampoli, N. L., Porwollik, S., Checa, S. K., McClelland, M., and Soncini, F. C. (2014). Identification of a Salmonella ancillary copper detoxification mechanism by a comparative analysis of the genome-wide transcriptional response to copper and zinc excess. Microbiology 160 (8), 1659–1669. doi:10.1099/mic.0.080473-0
Porcheron, G., Garenaux, A., Proulx, J., Sabri, M., and Dozois, C. M. (2013). Iron, copper, zinc, and manganese transport and regulation in pathogenic enterobacteria: correlations between strains, site of infection and the relative importance of the different metal transport systems for virulence. Front. Cell. Infect. Microbiol. 3, 90. doi:10.3389/fcimb.2013.00090
Raymond, K. N., Dertz, E. A., and Kim, S. S. (2003). Enterobactin: an archetype for microbial iron transport. Proc. Natl. Acad. Sci. U. S. A. 100 (7), 3584–3588. doi:10.1073/pnas.0630018100
Rensing, C., and Grass, G. (2003). Escherichia coli mechanisms of copper homeostasis in a changing environment. FEMS Microbiol. Rev. 27 (2-3), 197–213. doi:10.1016/S0168-6445(03)00049-4
Rensing, C., and McDevitt, S. F. (2013). The copper metallome in prokaryotic cells. Met. Ions Life Sci. 12, 417–450. doi:10.1007/978-94-007-5561-1_12
Rensing, C., Fan, B., Sharma, R., Mitra, B., and Rosen, B. P. (2000). CopA: An Escherichia coli Cu(I)-translocating P-type ATPase. Proc. Natl. Acad. Sci. U. S. A. 97 (2), 652–656. doi:10.1073/pnas.97.2.652
Robinson, A. E., Lowe, J. E., Koh, E. I., and Henderson, J. P. (2018). Uropathogenic enterobacteria use the yersiniabactin metallophore system to acquire nickel. J. Biol. Chem. 293 (39), 14953–14961. doi:10.1074/jbc.RA118.004483
Saka, K., Tadenuma, M., Nakade, S., Tanaka, N., Sugawara, H., Nishikawa, K., et al. (2005). A complete set of Escherichia coli open reading frames in mobile plasmids facilitating genetic studies. DNA Res. 12 (1), 63–68. doi:10.1093/dnares/12.1.63
Sambrook, J., Fritsch, E. F., and Maniatis, T. (1989). Molecular cloning: a laboratory manual. Cold Spring Harbor, NY: Cold Spring Harbor Laboratory Press.
Schweigert, N., Zehnder, A. J., and Eggen, R. I. (2001). Chemical properties of catechols and their molecular modes of toxic action in cells, from microorganisms to mammals. Environ. Microbiol. 3 (2), 81–91. doi:10.1046/j.1462-2920.2001.00176.x
Stafford, S. L., Bokil, N. J., Achard, M. E., Kapetanovic, R., Schembri, M. A., McEwan, A. G., et al. (2013). Metal ions in macrophage antimicrobial pathways: emerging roles for zinc and copper. Biosci. Rep. 33 (4), e00049. doi:10.1042/BSR20130014
Tan, G., Yang, J., Li, T., Zhao, J., Sun, S., Li, X., et al. (2017). Anaerobic copper toxicity and iron-sulfur cluster biogenesis in Escherichia coli. Appl. Environ. Microbiol. 83 (16), e00867. doi:10.1128/AEM.00867-17
Volentini, S. I., Farias, R. N., Rodriguez-Montelongo, L., and Rapisarda, V. A. (2011). Cu(II)-reduction by Escherichia coli cells is dependent on respiratory chain components. Biometals. 24 (5), 827–835. doi:10.1007/s10534-011-9436-3
Winkelmann, G., Cansier, A., Beck, W., and Jung, G. (1994). HPLC separation of enterobactin and linear 2, 3-dihydroxybenzoylserine derivatives: a study on mutants of Escherichia coli defective in regulation (fur), esterase (fes) and transport (fepA). Biometals. 7 (2), 149–154. doi:10.1007/BF00140485
Yamamoto, K., and Ishihama, A. (2005). Transcriptional response of Escherichia coli to external copper. Mol. Microbiol. 56 (1), 215–227. doi:10.1111/j.1365-2958.2005.04532.x
Keywords: copper, tolerance, toxicity, enterobactin, ROS-reactive oxygen species
Citation: Peralta DR, Farizano JV, Bulacio Gil N, Corbalán NS, Pomares MF, Vincent PA and Adler C (2022) Less is more: Enterobactin concentration dependency in copper tolerance and toxicity. Front. Mol. Biosci. 9:961917. doi: 10.3389/fmolb.2022.961917
Received: 05 June 2022; Accepted: 26 July 2022;
Published: 16 August 2022.
Edited by:
Teresita Padilla-Benavides, Wesleyan University, United StatesReviewed by:
Nidia León-Sicairos, Autonomous University of Sinaloa, MexicoJorge Eugenio Vidal, University of Mississippi Medical Center, United States
Copyright © 2022 Peralta, Farizano, Bulacio Gil, Corbalán, Pomares, Vincent and Adler. This is an open-access article distributed under the terms of the Creative Commons Attribution License (CC BY). The use, distribution or reproduction in other forums is permitted, provided the original author(s) and the copyright owner(s) are credited and that the original publication in this journal is cited, in accordance with accepted academic practice. No use, distribution or reproduction is permitted which does not comply with these terms.
*Correspondence: Paula Andrea Vincent, dmluY2VudC5wYXVsYUBnbWFpbC5jb20=; Conrado Adler, YWRsZXJjb25yYWRvQGdtYWlsLmNvbQ==
†These authors share last authorship
‡These authors have contributed equally to this work