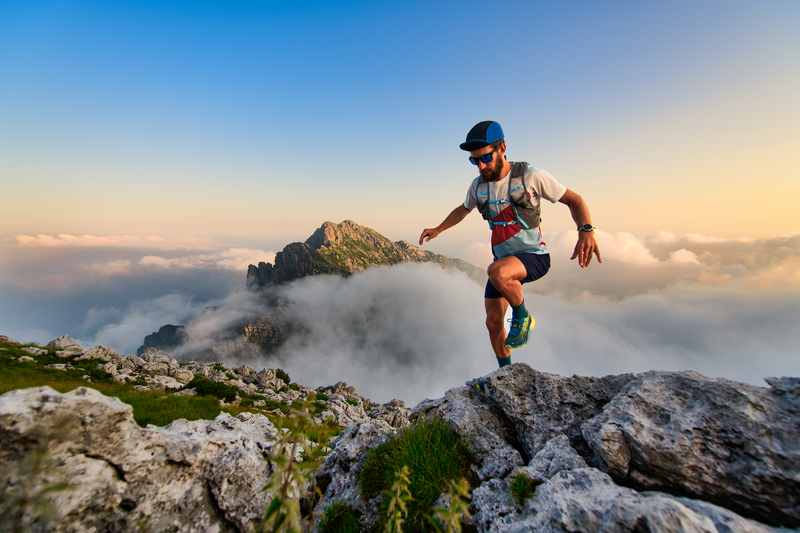
95% of researchers rate our articles as excellent or good
Learn more about the work of our research integrity team to safeguard the quality of each article we publish.
Find out more
MINI REVIEW article
Front. Mol. Biosci. , 29 June 2022
Sec. Lipids, Membranes and Membranous Organelles
Volume 9 - 2022 | https://doi.org/10.3389/fmolb.2022.923794
This article is part of the Research Topic In Celebration of Women in Science: Lipids, Membranes, and Membranous Organelles View all 11 articles
Methods of electron spin echo of pulse electron paramagnetic resonance (EPR) spectroscopy are increasingly employed to investigate biophysical properties of nitroxide-labeled biosystems at cryogenic temperatures. Two-pulse echo-detected ED-spectra have proven to be valuable tools to describe the librational dynamics in the low-temperature phases of both lipids and proteins in membranes. The motional parameter,
Steady-state, continuous wave electron paramagnetic resonance (cw-EPR) spectroscopy of nitroxide(NO)-labels (S = 1/2, I = 1) holds a prominent place in membrane biophysics (Berliner, 1976; Marsh, 1981; Berliner 1998; Hemminga and Berliner, 2007; Marsh, 2019). The success and relevance of spin-label EPR in biomembrane studies is due to the fact that its timescale is optimally sensitive to the nanoseconds and matches the timescale of various molecular motions occurring in membrane components. 9-GHz (X-band) spin-label cw-EPR has notably contributed to the study of the dynamics of proteins and lipids in membranes as well as in reconstituted lipid–protein complexes and in lipid model systems (Borbat et al., 2001; Marsh, 2008; Klare and Steinhoff, 2009; Guzzi and Bartucci, 2015; Sahu and Lorigan, 2021).
Insights into the dynamics of spin-labeled membrane components emerged from the use of electron spin echo (ESE) methods of time-resolved, pulse-EPR spectroscopy (Freed, 2000; Bartucci et al., 2006; Dzuba, 2007). ESE methods are based on the use of resonant microwave power pulse sequences of defined short-time duration, typically 12–64 ns, separated by time intervals in which the microwaves are off, that produce an echo signal at a given delay time (Kevan and Bowman, 1990; Schweiger and Jeschke, 2001). The standard two-pulse sequence, π/2-τ-π-τ-primary echo (Figure 1A), allows experiments on the time domain of the interpulse time spacing τ, determined by the transverse phase memory time T2M of the spin-labels, and the two-pulse ESE technique is optimally sensitive to the spin-label dynamics in the nanoseconds timescale. The primary echo, recorded at 2τ from the first pulse, is the result of the refocusing of the spin magnetization after the action of the microwave pulses. The first π/2 pulse flips the magnetization by 90° into the X-Y plane perpendicular to the Z direction of the spectrometer magnetic field, B. The spins then dephase during τ, with the time constant T2M, until the inverting p pulse reverses the magnetization that will refocus after a time τ producing the echo signal. By integrating the echo while sweeping the static magnetic field, an echo-detected ED-EPR absorption spectrum is obtained, the lineshape of which reflects the angular orientation of the spins. For spin relaxation, the echo amplitude decays exponentially when the interpulse separation τ is incremented, and the corresponding collected ED-spectra show variations in the lineshapes (Figure 1A). Such ED-spectra directly reflect the amplitude and the rate of motion of spin-labeled biosystems and contain all the information on their dynamics. Low, cryogenic temperatures are required for ESE-based measurements because spin-labeled T2M-relaxation time is generally too fast to produce detectable echoes at an ambient temperature. Thus, ED-EPR spectra offer a convenient route to study the dynamics of spin-labeled biosystems at low temperatures, for samples cooled with liquid nitrogen down to 77 K or with helium below 77 K. Moreover, low-temperature studies are advantageous to reveal dynamical features that occur also at higher physiological temperatures where they cannot be resolved explicitly because they are hidden by large-amplitude motions.
FIGURE 1. (A) Two-pulse primary echo sequences and echo amplitudes decrease with increasing the interpulse delay time, τ; simulated examples of corresponding echo-detected ED-spectra of chain-labeled nitroxide in membranes. (B) Two-pulse (π/2-τ-π with microwave pulse widths of 32 and 64 ns) ED-EPR spectra of 5-PCSL in DPPC bilayers at T = 200 K recorded at incremented interpulse spacings τ (from top to bottom). Solid lines are the normalized experimental spectra, and dashed lines are simulations for isotropic librational motion. Underneath are reported the anisotropic part of the relaxation rate, W-spectra, obtained according to Eq. 1 from pairs of spectra with interpulse separations of τ1 = 168 and τ2 = 296 ns, τ1 = 168 ns and τ2 = 424 ns, or τ1 = 168 ns and τ2 = 552 ns. Schematic illustration of isotropic librational motion: the nitroxide molecule performs oscillations of small angular amplitude, α, about the three nitroxide axes. cw-EPR spectra of 5-PCSL in DPPC bilayers at 150, 220, and 260 K. ED-, W-, and cw-EPR spectra are taken from Aloi et al. (2017).
Here, we review results obtained on the low-temperature dynamics of spin-labeled lipid bilayers and natural Na,K-ATPase membranes from two-pulse ED-EPR spectra.
The pioneering work of Millhauser and Freed, (1984) showed the sensitivity of the two-pulse echo-induced EPR spectrum for each value of interpulse separation time τ to variation across the spectrum of the transverse relaxation time. With this ESE technique, the structure and dynamics of cholestane spin-label in oriented lipid multilayers were studied (Kar et al., 1985). Two-pulse ED-spectra have been used by Dzuba et al. (1992), Dzuba (1996), Dzuba (2000), and Kirilina et al. (2001) to investigate the motion of spin-probes in glassy media. The lineshapes, revealing anisotropic phase relaxation, showed a decrease of the amplitudes in the intermediate spectral regions at low and high field with increasing τ. The ED-spectra have been simulated by assuming the occurrence of librational motion, that is, an orientational molecular motion consisting of fast, low-amplitude oscillations near an equilibrium position.
An analogous dependence on τ has been observed later for the ED-spectra of chain-labeled lipids in model membranes (Bartucci et al., 2003; Erilov et al., 2004a; Erilov et al., 2004b). In these spectra, the regions at intermediate low and high fields, which correspond to the maximum variation of spin orientation with the static magnetic field, relax faster than the others, and the intensities decrease systematically with increasing the interpulse spacing, τ. Minor changes are instead observed in the outer peaks, which correspond to stationary turning points (Figure 1B).
The ED-EPR spectra of lipid spin-labels in bilayers are successfully simulated according to the so-called “isotropic” model of librations (Erilov et al., 2004b). The model assumes that librations consist of independent and simultaneous rapid oscillations, each of small angular amplitude α and with correlation time τC, around each of the three perpendicular X-, Y-, and Z-axes of nitroxide (Figure 1B). For fast motion of small amplitude, that is, Δω2τ2c << 1, and for a polar orientation θ, φ of the magnetic field, B, relative to the nitroxide X-, Y-, and Z-axes, the amplitude of a two-pulse echo decay is approximatively described by
An alternative scheme of analyzing the dependence of the ED-EPR lineshapes on librational dynamics is given by the W-relaxation spectra. They are obtained from the experimental ED-spectra recorded at two different values, τ1 and τ2, of the interpulse delay by using the relation (Erilov et al., 2004b):
The W-spectra evaluated for different pairs of τ-values coincide within the noise level (Figure 1B), showing exponential anisotropic spin relaxation as a function of τ (especially on the low-field side), as expected for the isotropic model of librations. The relaxation rate W-curves are characterized by the maximum values, WL and WH, determined in the low- and high-field regions, respectively, of the ED-spectra. The difference in intensity at the two positions arises simply from the different inherent sensitivities of the two spectral regions to spin relaxation.
The relaxation rate WL or WH can also be used to characterize the librational dynamics in membranes. Indeed, they are related to the motional parameter
To fully describe the librational motion of spin-labels in membranes, it is desirable to know the mean-square angular amplitude,
An alternative approach to analyze ED-spectra is to evaluate the ratio of the echo amplitude at the two field positions with the largest and smallest anisotropies. For molecular librations, the resulting exponential decay rate Wanis is proportional to
In this section, we present results on the segmental librations of chain-labeled lipids in the low-temperature phases of model membranes. Bilayers composed of the most prevalent types of lipids present in the cell membrane of the three domains of life, that is, Eukarya, Bacteria, and Archaea, are considered (van Meer et al., 2008; Lombard et al., 2012). They include bilayers of diacylglycerophosphocholine and dialkylglycerophosphocholine lipids which consist of a phosphocholine (PC) polar head group and an apolar region formed by two fatty acid chains covalently bound to a glycerol moiety through ester or ether linkages, respectively (Figure 2A). For the ester-linked diacyl-PC bilayer forming lipids, we used dipalmitoylphosphatidylcholine (DPPC) and the unsaturated palmitoyloleoylphosphatidylcholine (POPC) and dioleoylphosphatidylcholine (DOPC) lipids. For ether-linked lipids, we used dihexadecyl phosphocholine (DHPC), which is analogous to DPPC.
FIGURE 2. (A) Chemical structure of the lipids DPPC, DHPC, POPC, and DOPC and of the chain-labeled phosphatidylcholine spin-label 5-PCSL and 16-PCSL. Characterization of the segmental librational motion in DPPC, DHPC, POPC, and DOPC membranes spin-labeled with 5- and 16-PCSL via the temperature dependence of the (i) amplitude-correlation time product,
From a biophysical standpoint, the single species lipid membranes show different properties and thermotropic phase behavior (Marsh, 2012). Notably, DPPC and DHPC form bilayers with gel to fluid main phase transition temperature Tm ca. 315 K but DHPC spontaneously forms lamellae gel phase with interdigitated chains, whereas DPPC forms noninterdigitated gel phase bilayers. POPC and DOPC, for the presence of cis-bonds in the lipid chain, form low-Tm bilayers, Tm being ca. 271 K for POPC and ca. 253 K for DOPC. For EPR measurements, the bilayers were spin-labeled with phosphatidylcholine lipids bearing the nitroxide group either at the 5th or at the 16th carbon atom positions of the sn-2 chain, namely, 5- and 16-PCSL, to probe, respectively, the first acyl chain segments and the terminal chain region of the hydrocarbon zone of the bilayers (Figure 2A). Lipids and spin-labeled lipids were purchased from Avanti Polar Lipids (Birmingham, AL).
Fast (τC from subnanoseconds to nanoseconds) librations of small amplitude (α < 20°) have been detected in DPPC, DHPC, POPC, and DOPC membranes in the low-temperature range of 77–270 K. However, the distinctive features of the lipid acyl chains and the different molecular chain packing between the membranes affect the characteristics of the librational motion.
A temperature-dependent increase of the motional parameter
The linear and fully saturated acyl chains in DPPC and the interdigitated chains in DHPC impart a well compact and regular packing density to the lipid lamellae in the frozen state which restricts the librational dynamics, at least in the low-temperature regime. In contrast, the presence of double bonds in the hydrocarbon chain of the unsaturated lipids confers a loosened packing density to the bilayers which favors the segmental librations. In agreement with the results in Figure 2A, data on relaxation rates of stearic acid doxyl-labeled along the chain indicated more freedom of segmental chain librations in unsaturated POPC and DOPC bilayers compared to saturated DPPC bilayers (Surovtsev et al., 2012; Golysheva et al., 2018; Golysheva and Dzuba, 2020).
As seen for
In DHPC lamellae with interdigitated chains, the librations are restricted to small angular amplitude at both chain positions of labeling in the low-temperature regime. Only on entering the higher temperature regime, the angular amplitudes increase and are larger at the chain termini than at the beginning of the chain comparable to that in DPPC. Similar results have been obtained in lamellae with interdigitated chains formed by mixtures of DPPC and Lyso-palmitoilphosphatidylcholine or induced in DPPC by ethanol (Aloi and Bartucci, 2019). The behavior of the chain-labeled lipids in DHPC is consistent with the interdigitated phase in which the positional isomers at the chain termini are motionally restricted to an extent comparable to those in proximity of the polar/apolar interface (Boggs et al., 1989; Bartucci et al., 1993; Oranges et al., 2018). At highest temperatures, it is likely that 16-PCSL acquires significant freedom of motion relative to 5-PCSL since it is located in the interfacial region where the polar heads are spaced apart by interdigitation.
From Figure 2A, it can be seen that the rotational correlation time lies on the subnanosecond–nanosecond timescale, indicating that fast rapid segmental chain oscillations are detected in the considered model bilayers. On the whole, the differences in the librational dynamics in the various bilayers are attributable mostly to the variations in the angular amplitude rather than in the rotational correlation time. It is interesting to point out that the temperature dependence of
Membranous Na,K-ATPase is a complex transport system. The lipid bilayer sector is spanned by the sodium pump, a large integral protein (Figure 2B) that is responsible for maintenance of the electrochemical gradients of Na+ and K+ across the membrane in eukaryotes. Specific regions within the Na,K-ATPase membrane, including the protein, the cationic binding site, and the lipid bilayer environment, have been recently studied by cw- and pulse-EPR of spin-labels and spin-labeled lipids (Guzzi et al., 2009; Guzzi et al., 2015; Guo et al., 2018; Aloi et al., 2021).
The hydrophobic bilayer region of the sodium pump membrane has been investigated exploiting the affinity of ionized chain-labeled stearic acids (n-SASL) for the membrane (Bartucci et al., 2014; Guzzi et al., 2015). n-SASL was either purchased from Avanti Polar Lipids or synthesized as described elsewhere (Marsh and Watts, 1982). The studies in these samples include measurements of both the Na,K-ATPase membranes and the lipid model systems formed with the extracted membrane lipids and determination of the data at the lipid–protein interface as described in Bartucci et al. (2014) and Guzzi et al. (2015).
The temperature-dependent increase of the WL-relaxation parameter in Na,K-ATPase membranes is rather similar to that at the lipid–protein interface: the mobility is more evident for T > 180 K and independent on the label position (Figure 2B). It differs notably from that in bilayers of extracted lipids, where mobility is evident from a lower temperature (120 K) and more intense at the end of the chain (i.e., data for 14-SASL) than at the top (i.e., data for 5-SASL) (Guzzi et al., 2015). These features have been confirmed by the positional dependence of the transmembrane librational dynamics. Indeed, the profile of WL vs. label position is almost flat for lipid chains at the protein interface and in the Na,K-ATPase membrane where WL remains at a relatively low level, comparable to that at the top of the chain in the bilayer lipids. In the lipid bilayers, WL is larger toward the end of the chain, with a transition in the region of C10-C12.
Insights into the low-temperature dynamics of Na,K-ATPase have been gained from a comparison of the librational fluctuations of the extracted lipids and interfacial lipids with those of the protein alone studied with a maleimide spin-labels (5-MSL) covalently attached to cysteine–SH residues (Guzzi et al., 2009; Guzzi et al., 2015). The temperature dependence of the WL-rates for interfacial lipids resembles that of protein side-chains, but not that for the bilayer lipids (Figure 2B). Librational motions of lipids at the protein interface are coupled both to those of the protein and to those of the bilayer lipids: protein and membrane lipids communicate via the interfacial lipids. It is most likely that these librational oscillations could drive transitions between the different conformational substates in Na,K-ATPase, which are frozen at lower temperatures but contribute to the pathways between the principal enzymatic intermediates at higher temperatures.
In this mini-review, we have illustrated the potential of ESE spectroscopy for the study of the nanosecond dynamics in bilayers and Na,K-ATPase membranes at cryogenic temperatures via two-pulse ED-spectra. Fast, low-amplitude librations that are readily detected and characterized at cryogenic temperatures must be present in the higher temperature phases of biomembranes, in addition to larger-scale rotational motions. The low, cryogenic temperatures contribute to highlight specific structural, dynamic, and kinetics features of biosystems, and spin-label pulse-EPR results deepen the biophysical characterization of membranes that are normally studied at higher temperatures. Therefore, ESE methods are increasingly used for studying complex macromolecular assemblies.
All authors listed have made a substantial, direct, and intellectual contribution to the work and approved it for publication.
The authors declare that the research was conducted in the absence of any commercial or financial relationships that could be construed as a potential conflict of interest.
All claims expressed in this article are solely those of the authors and do not necessarily represent those of their affiliated organizations, or those of the publisher, the editors, and the reviewers. Any product that may be evaluated in this article, or claim that may be made by its manufacturer, is not guaranteed or endorsed by the publisher.
Aloi, E., and Bartucci, R. (2022). Influence of Hydration on Segmental Chain Librations and Dynamical Transition in Lipid Bilayers. Biochimica Biophysica Acta (BBA) - Biomembr. 1864, 183805. doi:10.1016/j.bbamem.2021.183805
Aloi, E., and Bartucci, R. (2019). Interdigitated Lamellar Phases in the Frozen State: Spin-Label CW- and FT-EPR. Biophys. Chem. 253, 106229. doi:10.1016/j.bpc.2019.106229
Aloi, E., Guo, J. -H., Guzzi, R., Jiang, R. -W., Ladefoged, L. K., Marsh, D., et al. (2021). Geometry and Water Accessibility of the Inhibitor Binding Site of Na+-Pump: Pulse- and CW-EPR Study. Biophys. J. 120, 2679–2690.
Aloi, E., Guzzi, R., and Bartucci, R. (2019). Unsaturated Lipid Bilayers at Cryogenic Temperature: Librational Dynamics of Chain-Labeled Lipids from Pulsed and CW-EPR. Phys. Chem. Chem. Phys. 21, 18699–18705. doi:10.1039/c9cp03318a
Aloi, E., Oranges, M., Guzzi, R., and Bartucci, R. (2017). Low-Temperature Dynamics of Chain-Labeled Lipids in Ester- and Ether-Linked Phosphatidylcholine Membranes. J. Phys. Chem. B 121, 9239–9246. doi:10.1021/acs.jpcb.7b07386
Bartucci, R., Erilov, D. A., Guzzi, R., Sportelli, L., Dzuba, S. A., and Marsh, D. (2006). Time-resolved Electron Spin Resonance Studies of Spin-Labelled Lipids in Membranes. Chem. Phys. Lipids 141, 142–157. doi:10.1016/j.chemphyslip.2006.02.009
Bartucci, R., Guzzi, R., De Zotti, M., Toniolo, C., Sportelli, L., and Marsh, D. (2008). Backbone Dynamics of Alamethicin Bound to Lipid Membranes: Spin-Echo Electron Paramagnetic Resonance of TOAC-Spin Labels. Biophysical J. 94, 2698–2705. doi:10.1529/biophysj.107.115287
Bartucci, R., Guzzi, R., Esmann, M., and Marsh, D. (2014). Water Penetration Profile at the Protein-Lipid Interface in Na,K-ATPase Membranes. Biophysical J. 107, 1375–1382. doi:10.1016/j.bpj.2014.07.057
Bartucci, R., Guzzi, R., Marsh, D., and Sportelli, L. (2003). Chain Dynamics in the Low-Temperature Phases of Lipid Membranes by Electron Spin-Echo Spectroscopy. J. Magnetic Reson. 162, 371–379. doi:10.1016/s1090-7807(03)00049-1
Bartucci, R., Pali, T., and Marsh, D. (1993). Lipid Chain Motion in an Interdigitated Gel Phase: Conventional and Saturation Transfer ESR of Spin-Labeled Lipids in Dipalmitoylphosphatidylcholine-Glycerol Dispersions. Biochemistry 32, 274–281. doi:10.1021/bi00052a035
Boggs, J. M., Rangaraj, G., and Watts, A. (1989). Behavior of Spin Labels in a Variety of Interdigitated Lipid Bilayers. Biochimica Biophysica Acta (BBA) - Biomembr. 981, 243–253. doi:10.1016/0005-2736(89)90034-5
Borbat, P. P., Costa-Filho, A. J., Earle, K. A., Moscicki, J. K., and Freed, J. H. (2001). Electron Spin Resonance in Studies of Membranes and Proteins. Science 291, 266–269. doi:10.1126/science.291.5502.266
De Simone, F., Guzzi, R., Sportelli, L., Marsh, D., and Bartucci, R. (2007). Marsh., D., Bartucci, RElectron Spin-Echo Studies of Spin-Labelled Lipid Membranes and Free Fatty Acids Interacting with Human Serum Albumin. Biochimica Biophysica Acta (BBA) - Biomembr. 1768, 1541–1549. doi:10.1016/j.bbamem.2007.02.019
Dzuba, S. A. (2000). Libration Motion of Guest Spin Probe Molecules in Organic Glasses: CW EPR and Electron Spin Echo Study. Spectrochimica Acta Part A Mol. Biomol. Spectrosc. 56, 227–234. doi:10.1016/s1386-1425(99)00234-6
Dzuba, S. A. (1996). Librational Motion of Guest Spin Probe Molecules in Glassy Media. Phys. Lett. A 213, 77–84. doi:10.1016/0375-9601(96)00081-3
Dzuba, S. A. (2007). Pulsed EPR in the Method of Spin Labels and Probes. Russ. Chem. Rev. 76, 699–713. doi:10.1070/rc2007v076n08abeh003722
Dzuba, S. A., Tsvetkov, Y. D., Yu, A. G., and Maryasov, A. G. (1992). Echo-induced EPR Spectra of Nitroxides in Organic Glasses: Model of Orientational Molecular Motions Near Equilibrium Position. Chem. Phys. Lett. 188, 217–222. doi:10.1016/0009-2614(92)90012-c
Erilov, D. A., Bartucci, R., Guzzi, R., Marsh, D., Dzuba, S. A., and Sportelli, L. (2004a). Echo-detected Electron Paramagnetic Resonance Spectra of Spin-Labeled Lipids in Membrane Model Systems. J. Phys. Chem. B 108, 4501–4507. doi:10.1021/jp037249y
Erilov, D. A., Bartucci, R., Guzzi, R., Marsh, D., Dzuba, S. A., and Sportelli, L. (2004b). Librational Motion of Spin-Labeled Lipids in High-Cholesterol Containing Membranes from Echo-Detected EPR Spectra. Biophysical J. 87, 3873–3881. doi:10.1529/biophysj.104.046631
Fenimore, P. W., Frauenfelder, H., McMahon, B. H., and Young, R. D. (2004). Bulk-solvent and Hydration-Shell Fluctuations, Similar to α- and β-fluctuations in Glasses, Control Protein Motions and Functions. Proc. Natl. Acad. Sci. U.S.A. 101, 14408–14413. doi:10.1073/pnas.0405573101
Freed, J. H. (2000). New Technologies in Electron Spin Resonance. Annu. Rev. Phys. Chem. 51, 655–689. doi:10.1146/annurev.physchem.51.1.655
Golysheva, E. A., De Zotti, M., Toniolo, C., Formaggio, F., and Dzuba, S. A. (2018). Low-temperature Dynamical Transition in Lipid Bilayers Detected by Spin-Label ESE Spectroscopy. Appl. Magn. Reson 49. doi: doi:10.1007/s00723-018-1066-2
Golysheva, E. A., and Dzuba, S. A. (2020). Lipid Chain Mobility and Packing in DOPC Bilayers at Cryogenic Temperatures. Chem. Phys. Lipids 226, 104817. doi:10.1016/j.chemphyslip.2019.104817
Golysheva, E. A., Shevelev, G. Y., and Dzuba, S. A. (2017). Dynamical Transition in Molecular Glasses and Proteins Observed by Spin Relaxation of Nitroxide Spin Probes and Labels. J. Chem. Phys. 147, 064501. doi:10.1063/1.4997035
Guo, J. H., Jiang, R. W., Andersen, J. L., Esmann, M., and Fedosova, N. U. (2018). Spin‐labeled Derivatives of Cardiotonic Steroids as Tools for Characterization of the Extracellular Entrance to the Binding Site on Na + ,K + ‐ ATP Ase. FEBS J. 285, 2292–2305. doi:10.1111/febs.14480
Guzzi, R., and Bartucci, R. (2015). Electron Spin Resonance of Spin-Labeled Lipid Assemblies and Proteins. Archives Biochem. Biophysics 580, 102–111. doi:10.1016/j.abb.2015.06.015
Guzzi, R., Bartucci, R., Esmann, M., and Marsh, D. (2015). Lipid Librations at the Interface with the Na,K-ATPase. Biophysical J. 108, 2825–2832. doi:10.1016/j.bpj.2015.05.004
Guzzi, R., Bartucci, R., Sportelli, L., Esmann, M., and Marsh, D. (2009). Conformational Heterogeneity and Spin-Labeled −SH Groups: Pulsed EPR of Na,K-ATPase. Biochemistry 48, 8343–8354. doi:10.1021/bi900849z
Guzzi, R., Rizzuti, B., and Bartucci, R. (2012). Dynamics and Binding Affinity of Spin-Labeled Stearic Acids in β-Lactoglobulin: Evidences from EPR Spectroscopy and Molecular Dynamics Simulation. J. Phys. Chem. B 116, 11608–11615. doi:10.1021/jp3074392
Hemminga, M. A., and Berliner, L. J. (2007). ESR Spectroscopy in Membrane Biophysics. New York: Springer.
Isaev, N. P., and Dzuba, S. A. (2008). Fast Stochastic Librations and Slow Rotations of Spin Labeled Stearic Acids in a Model Phospholipid Bilayer at Cryogenic Temperatures. J. Phys. Chem. B 112, 13285–13291. doi:10.1021/jp805794c
Kar, L., Ney-Igner, E., and Freed, J. H. (1985). Electron Spin Resonance and Electron-Spin-Echo Study of Oriented Multilayers of L Alpha-Dipalmitoylphosphatidylcholine Water Systems. Biophysical J. 48, 569–595. doi:10.1016/s0006-3495(85)83814-5
Kevan, L., and Bowman, M. K. (1990). Modern Pulsed and Continuous-Wave Electron Spin Resonance. New York: Wiley.
Kirilina, E. P., Dzuba, S. A., Maryasov, A. G., and Tsvetkov, Y. D. (2001). Librational Dynamics of Nitroxide Molecules in a Molecular Glass Studied by Echo-Detected EPR. Appl. Magn. Reson. 21, 203–221. doi:10.1007/bf03162452
Klare, J. P., and Steinhoff, H.-J. (2009). Spin Labeling EPR. Photosynth. Res. 102, 377–390. doi:10.1007/s11120-009-9490-7
Laursen, M., Gregersen, J. L., Yatime, L., Nissen, P., and Fedosova, N. U. (2015). Structures and Characterization of Digoxin- and Bufalin-Bound Na + ,K + -ATPase Compared with the Ouabain-Bound Complex. Proc. Natl. Acad. Sci. U.S.A. 112, 1755–1760. doi:10.1073/pnas.1422997112
Lombard, J., López-García, P., and Moreira, D. (2012). The Early Evolution of Lipid Membranes and the Three Domains of Life. Nat. Rev. Microbiol. 10, 507–515. doi:10.1038/nrmicro2815
Marsh, D. (2019). Spin-Label Electron Paramagnetic Resonance Spectroscopy. Boca Raton, FL: CRC Press.
Marsh, D. (2008). Electron Spin Resonance in Membrane Research: Protein-Lipid Interactions. Methods 46, 83–96. doi:10.1016/j.ymeth.2008.07.001
Marsh, D. (1981). “Electron Spin Resonance: Spin Labels,” in Biochemistry and Biophysics. Membrane SpectroscopyMolecular BiologyE. Grell (Berlin, Heidelberg, New York: Springer-Verlag), 31, 51–142. doi:10.1007/978-3-642-81537-9_2
Marsh, D., and Watts, A. (1982). Spin-labeling and Lipid-Protein Interactions in Membranes. In Lipid-Protein Interactions,. Editors P. C. Jost, and O. H. Griffith (New York: Wiley-Interscience), 2, 53–126.
Maslennikova, N. A., Golysheva, E. A., and Dzuba, S. A. (2021). Evidence for an Ordering Transition Near 120 K in an Intrinsically Disordered Protein, Casein. Molecules 26, 5971. doi:10.3390/molecules26195971
Millhauser, G. L., and Freed, J. H. (1984). Two‐dimensional Electron Spin Echo Spectroscopy and Slow Motions. J. Chem. Phys. 81, 37–48. doi:10.1063/1.447316
Oranges, M., Guzzi, R., Marsh, D., and Bartucci, R. (2018). Ether-linked Lipids: Spin-Label EPR and Spin Echoes. Chem. Phys. Lipids 212, 130–137. doi:10.1016/j.chemphyslip.2018.01.010
Sahu, I. D., and Lorigan, G. A. (2021). Probing Structural Dynamics of Membrane Proteins Using Electron Paramagnetic Resonance Spectroscopic Techniques. Biophysica 1, 106–125. doi:10.3390/biophysica1020009
Scarpelli, F., Bartucci, R., Sportelli, L., and Guzzi, R. (2011). Solvent Effect on Librational Dynamics of Spin-Labelled Haemoglobin by ED- and CW-EPR. Eur. Biophys. J. 40, 273–279. doi:10.1007/s00249-010-0644-5
Schweiger, A., and Jeschke, G. (2001). Principles of Pulse Electron Paramagnetic Resonance. Oxford: Oxford University Press.
Surovtsev, N. V., Ivanisenko, N. V., Kirillov, K. Y., and Dzuba, S. A. (2012). Low-temperature Dynamical and Structural Properties of Saturated and Monounsaturated Phospholipid Bilayers Revealed by Raman and Spin-Label EPR Spectroscopy. J. Phys. Chem. B 116, 8139–8144. doi:10.1021/jp3038895
van Meer, G., Voelker, D. R., and Feigenson, G. W. (2008). Membrane Lipids: where They Are and How They Behave. Nat. Rev. Mol. Cell. Biol. 9, 112–124. doi:10.1038/nrm2330
Keywords: model membranes, Na, K-ATPase, spin label, electron paramagnetic resonance, electron spin echo, echo-detected ED-spectra, librations
Citation: Bartucci R and Aloi E (2022) Librational Dynamics of Spin-Labeled Membranes at Cryogenic Temperatures From Echo-Detected ED-EPR Spectra. Front. Mol. Biosci. 9:923794. doi: 10.3389/fmolb.2022.923794
Received: 19 April 2022; Accepted: 19 May 2022;
Published: 29 June 2022.
Edited by:
Elena G. Govorunova, University of Texas Health Science Center at Houston, United StatesReviewed by:
Vasily Oganesyan, University of East Anglia, United KingdomCopyright © 2022 Bartucci and Aloi. This is an open-access article distributed under the terms of the Creative Commons Attribution License (CC BY). The use, distribution or reproduction in other forums is permitted, provided the original author(s) and the copyright owner(s) are credited and that the original publication in this journal is cited, in accordance with accepted academic practice. No use, distribution or reproduction is permitted which does not comply with these terms.
*Correspondence: Rosa Bartucci, cm9zYS5iYXJ0dWNjaUBmaXMudW5pY2FsLml0
†These authors have contributed equally to this work
Disclaimer: All claims expressed in this article are solely those of the authors and do not necessarily represent those of their affiliated organizations, or those of the publisher, the editors and the reviewers. Any product that may be evaluated in this article or claim that may be made by its manufacturer is not guaranteed or endorsed by the publisher.
Research integrity at Frontiers
Learn more about the work of our research integrity team to safeguard the quality of each article we publish.